DOI:
10.1039/D2EA00167E
(Tutorial Review)
Environ. Sci.: Atmos., 2023,
3, 444-473
A new advance in the pollution profile, transformation process, and contribution to aerosol formation and aging of atmospheric amines
Received
30th November 2022
, Accepted 31st January 2023
First published on 1st February 2023
Abstract
Amines, formed with the replacement of hydrogen atoms by NH3 molecules of volatile organic compounds, possess distinctive properties of alkalinity, volatility, water-solubility and oxidability and have attracted more and more attention due to their significant contributions to atmospheric pollution during the past few decades. To understand the environmental behaviors of amines in the atmospheric pollution process, this review mainly summarizes a new advance in sources and sinks of amines and their effects on atmospheric particulate matter (PM) formation and climate evolution within the last decade. First, the pollution profiles and source identification of amines are summarized. Some newfound sources of amines are correspondingly updated. Meanwhile, the current monitoring methods of amines are also evaluated. Second, the atmospheric oxidation reaction mechanisms of amines with various active radicals are discussed. Third, the combined data of field monitoring, laboratory experiments and theoretical calculations verified that amines contribute significantly to aerosol particles, including oxidation product condensation, direct dissolution, acid–base reactions and replacement reactions. Besides, we discussed how amines affect the climate directly or indirectly by generating brown carbon in PM, enhancing hygroscopicity and activating cloud condensation nuclei. All in all, the review provides new insights into the overall atmospheric circulation and final fate of amines as well as their effects on atmospheric aerosol particle formation and the climate.
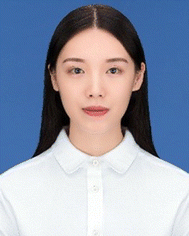 Xinlin Shen | Xinlin Shen received her B.S. degree in Environmental Engineering from the Guangdong University of Technology, China in 2020. She is currently pursuing a M.S. degree in Environmental Science and Engineering from 2020 to 2023 under the supervision of Prof. Taicheng An. Her research mainly focuses on atmospheric chemistry and reaction mechanisms of atmospheric amines. |
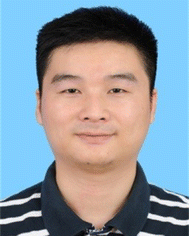 Jiangyao Chen | Prof. Jiangyao Chen received a PhD degree in environmental science from the Guangzhou Institute of Geochemistry, CAS, China. He is now a full professor at the Institute of Environmental Health and Pollution Control and School of Environmental Science and Engineering, Guangdong University of Technology. His research mainly focuses on the conversion mechanism, control technology and mechanism of VOCs. He has published 65 SCI papers in reputed journals, such as ES&T., Appl. Catal. B and Atmos. Chem. Phys., and 28 issued patents. He serves as the Youth Editorial board member of the Bulletin of Environmental Contamination and Toxicology and Chinese Chemical Letters. |
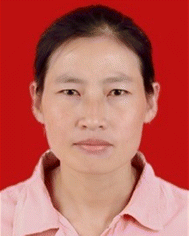 Guiying Li | Prof. Guiying Li received her PhD degree in environmental science from Griffith University, Australia. She is now a full professor at the Guangdong University of Technology. Her academic background covers both microbiology and environmental science. Her research interest mainly focuses on the development of photocatalysis and photoelectrocatalysis-based bactericidal methods and the application mechanism of photocatalytic bactericidal decomposition. She has published more than 300 SCI papers in reputed journals, such as Water Res., Appl. Catal. B, ES&T and J. Catal. She is the holder of 70 issued patents and the second award winner of the Natural Science Prize from the Ministry of Education (MOE), China. |
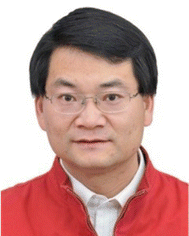 Taicheng An | Taicheng An is the founding director of the Institute of Environmental Health and Pollution Control and the Dean of the School of Environmental Sciences and Engineering, Guangdong University of Technology, China. He is the winner of the National Natural Science Funds for Distinguished Young Scholars and a Distinguished Professor of Chang Jiang Scholars. He is the recipient of the first award of Guangdong Province and second award of the Natural Science Prize from the MOE, China. Environmental geochemistry and health is one of his current research focuses. He has published more than 500 SCI papers in reputable journals, such as Nature Comm., PNAS, and JACS. He also served as an Associate Editor of Appl. Catal. B: Environ. and Crit. Rev. Env. Sci. Tec. |
Environmental significance
Atmospheric amines are one special group of alkaline volatile organic compounds, contributing significantly to aerosol particle formation through homogeneous and heterogeneous reactions. Amine associated aerosol particles show a vast potential impact on PM2.5, climate and human health. In this review, we summarized a new advance in atmospheric amines within the last decade, including their pollution profiles, source identification, monitoring methods, oxidation reaction mechanism, effects on the formation, growth and composition of aerosol particles, and ability to form brown carbon and enhance hygroscopicity, activating cloud condensation nuclei. The tutorial review provides new insights into the overall atmospheric circulation and final fate of amines as well as their effects on atmospheric PM formation and the climate.
|
1. Introduction
Exposure to air pollution has been considered the fifth largest human health risk factor globally, behind poor nutrition, dietary risk, high blood pressure and tobacco.1–3 New World Health Organization global air quality guidelines provide clear evidence of the damage that air pollution inflicts on human health, at even lower concentrations than previously understood.4 Volatile organic compounds (VOCs) are recognized as an important group of air pollutants. The tropospheric atmosphere of the earth contains ≥100
000 types of VOCs.5 The emissions from plant, marine and biomass combustion can globally produce a large number of natural VOCs. Anthropogenic activities such as industrial operations, vehicle emissions and cooking are also the main sources of VOCs in and around cities. These natural and anthropogenic VOCs may be oxidized by atmospheric oxidants (such as ˙OH, O3 and NO3˙),6–10 directly distribute to the particle phase,11 undergo heterogeneous reactions,12 or participate in new particle formation (NPF).13–17 The transformation of atmospheric VOCs plays an important role in the formation and growth of particulate matter (PM). PM can not only absorb and scatter solar and thermal radiation, but also act as cloud condensation nuclei (CCN) and ice nuclei,17 directly or indirectly affecting the global climate system. The transformation of VOCs into PM undergoes complex reaction pathways, leading to hundreds of organic compounds with different physicochemical properties in PM. Without a full understanding of the nature of VOCs, it is impossible to make out how they will affect the climate or human health. Therefore, more attention needs to be focused on the atmospheric chemistry mechanism of VOCs, especially certain specific VOCs such as amines. Their accurate description helps us to understand and quantify their impact on regional or global atmospheric quality.
Amines are a special group of VOCs, showing unique alkaline properties. They have always been detected from the ambient atmospheric environment18–20via offline21–24 and online25–27 methods. The sources of these ambient amines are also investigated. In addition to traditional sources, numerous new sources of amines have emerged. For example, with the development of post-combustion CO2 capture technology based on amine solution absorption to achieve carbon neutralization, the amines (e.g., mono, di-, tri-ethanolamine and piperazine (PZ)) released from the capture process become an important anthropogenic source of atmospheric amines.28–30 Meanwhile, alkyl amine surfactants can be used to capture ambient CO2 and be reversibly released under mild conditions.31 Amines have also been frequently reported in urban areas recently, which are closely related to human activities.18 An overview of the global distribution of amines is really needed to illustrate a better picture of their origins.
The atmospheric oxidation chemistry of amines is another very noteworthy research direction. Amines are easily oxidized by atmospheric reactive oxygen species (e.g., ˙OH, O3, NO3˙, ˙Cl) to aldehydes, amides, imines, nitrosamines and so on. Those oxidation processes have been well studied in the laboratory,1,32,33 and it was found that the oxidation chemistry of amines in the atmosphere mostly follows free radical chemistry. In recent years, RO2˙ formed by amines was found to be able to carry out subsequent reactions through the autoxidation process (i.e., hydrogen transfer)34–36 besides the bimolecular reaction. In addition, amines contribute significantly to atmospheric aerosol particles, especially secondary organic aerosols (SOAs). First, amines can promote the nucleation process of sulfuric acid (SA) or other organic acids.2,15,37 To date, many studies have shown that amines are more effective than NH3 in promoting NPF,38–41 although the concentration of atmospheric amines is estimated to be 2–3 orders of magnitude lower than that of NH3. Significantly increased studies have focused on the nucleation mechanism facilitated by amines. Positive conclusions have been obtained from measured data,14,15,42 laboratory experiments,38–41 and theoretical calculations.2,43 Therefore, a general overview of how amines participate in NPF in a complex atmospheric environment is needed. In addition to the contribution of amines to NPF, their role in promoting submicron aerosols as well as the browning process to produce brown carbon (BrC) has also been reported.44–46 BrC is a kind of nitrogenous organic matter that can show strong absorption of solar radiation in a particular wavelength range,47 and the appearance of BrC greatly changes atmospheric visibility and particle properties. However, it is not entirely clear how amines facilitate the production of SOAs and BrC. Therefore, it is important to summarize the updated knowledge of amines–BrC and explore the next steps. In the meantime, aerosol particle containing amines may enhance the hygroscopicity of particles and assisted in the activation of CCN.48,49 Their impact on the climate cannot be ignored and needs careful assessment.
There have also been a few reviews on atmospheric amines from around a decade ago.50–54 These reviews mainly focused on the traditional sources, atmospheric oxidation mechanisms and reaction kinetics of amines based on cognition at that time. As amines are recognized as an important atmosphere chemistry participator under the current circumstances, more and more researchers pay attention to such a group of VOCs, the amines. Tremendous new findings and deeper understandings of the sources and sinks of atmospheric amines during the last ten years have been summarized and reviewed in this paper. First, this review demonstrates the advance in monitoring methods, source apportionment and ambient pollution profiles of amines. Then, the oxidation reactions of amines with various atmospheric oxidants, including bimolecular reactions and autoxidation pathways are also summarized. Finally, the contributions of atmospheric amines to aerosol particles from different periods are highlighted. All in all, this review mainly aims to provide a detailed description of the atmospheric chemistry mechanism of amines and a series of evidence for their contributions to aerosol particles based on recent research progress of 10 years.
2. Pollution profiles and source apportionment of atmospheric amines
2.1 Monitoring methods of atmospheric amines
Cape et al.54 summarized two approaches to measure atmospheric organic nitrogen. One is determining the difference between total and inorganic nitrogen which is described as a “top-down” approach, and the other is the identification of the individual components and measuring them separately which was described as an alternative “bottom-up” approach. Their discussion of the latter is macroscopic which required the sum of each measured organic nitrogen compound, while our review focuses more on atmospheric amine measurements. As shown in Table 1, many advanced offline and online methods have been developed to accurately monitor trace amines in the atmosphere by improving sample collection,23 pretreatment,22,55 or detection methods.26,27,56–61 Offline monitoring methods can distinguish amines of different structures (i.e., isomers) and mainly consist of ion chromatography (IC)21–23,62,63 and gas chromatography-mass spectrometry (GC-MS).24,55 Chen et al. recently reported a GC-MS method that simultaneously detects several amines in both gas and particulate phases by optimizing the derivation conditions.64 However, offline monitoring methods often lack high time resolution due to preconcentration, derivatization and elution steps that may require hours. Besides, oxidation, reduction, complexation, precipitation, biochemistry and photochemistry processes may occur on the analytes from the period of sampling to determination. Hence, the final detection results show high inaccuracy. Although there has been a continuous improvement in sample collection and pre-treatment for offline monitoring methods, the undervaluation of amines is inevitable. Until now, many amines could not be detected because they were below the detection limits of these methods.
Table 1 Kinds, concentrations, and detection methods of atmospheric amines at different sites
Sampling sites |
|
Concentration |
Techniques |
Ref. |
Boreal forest in Finland (Hyytiälä SMEAR station) |
TMA (mean) |
59 pptv |
Hydronium-chemical ionization mass spectrometry (CIMS) |
25
|
Boreal forest in Finland (Hyytiälä SMEAR II station) |
DMA |
A few tens up to approximately 1500 ppqv |
Bisulphate-cluster based atmospheric pressure-CIMS |
66
|
Boreal forest in Finland (Hyytiälä SMEAR II station) |
EA + DMA |
Highest |
157 ± 20 pptv |
High-performance liquid chromatography-electrospray ionization-ion trap mass spectrometer (HPLC-ESIIT-MS) |
20
|
Mean |
42 ± 30 pptv |
PA + TMA |
Highest |
102 ± 61 pptv |
Mean |
21 ± 23 pptv |
DEA |
Highest |
15.5 ± 0.5 pptv |
Mean |
6.5 ± 5.6 pptv |
Urban air at a location in Atlanta |
MA |
<1 pptv |
AmPMS: chemical ionization + quadrupole mass spectrometer |
26
|
DMA |
<3 pptv |
TMA |
4–15 pptv (diurnal variation) |
C6-amine |
3–25 pptv (diurnal variation) |
The agricultural environment in Egbert, ON |
DMA + TMA + DEA |
Gas-phase |
1–10 pptv |
IC (the selectivity and detection limit of NR3/R3NH+ were improved using simple online sample preconcentration and conductivity inhibition prior to detection.) |
22
|
Cattle husbandry |
TMA |
4.21–7.64 ppb |
IC |
21
|
NH3 |
552–2601 ppb |
A cattle ranch in Chino, California, USA |
TMA |
3–6.8 ppb |
Extraction and analysis using weak cation exchange resin and IC |
23
|
NH3 |
19–1.5 parts per million (ppm) |
Wangdu, Hebei Province, China |
C2-amine |
14.6 ± 14.9 pptv |
Vocus PTR-ToF-MS |
27
|
Forests of the southeastern United States |
C3-amine |
1–10 pptv |
With ethanol as the ionization reagent-CIMS |
59
|
NH3 |
∼1–2 ppbv |
Polluted areas of the U.S. Midwest |
C1-amine |
1–4 pptv |
With ethanol as the ionization reagent-CIMS |
59
|
C3-amine |
5–10 pptv |
C4-amine |
10–50 pptv |
C5-amine |
10–100 pptv |
NH3 |
∼6 ppbv |
A suburb of Nanjing, China |
MA |
0.1–18.9 pptv (hourly average) |
Hydronium high resolution-ToF-CIMS (HR-ToF-CIMS) |
60
|
C2-amines |
0.1–29.9 pptv (hourly average) |
C3-amines |
0.1–9.3 pptv (hourly average) |
Shanghai |
C1-amine |
15.7 ± 5.9 pptv |
With protonated ethanol as the reagent ion-HR-ToF-CIMS |
61
|
C2-amine |
40.0 ± 14.3 pptv |
C3-amine |
1.1 ± 0.6 pptv |
C4-amine |
15.4 ± 7.9 pptv |
C5-amine |
3.4 ± 3.7 pptv |
C6-amine |
3.5 ± 2.2 pptv |
Autumn in Guangzhou |
The total concentration of nine amines in PM10 |
79.6–140.9 ng m−3 |
Gas chromatography-mass spectrometer (GC-MS) |
24
|
MA in PM10 |
29.2–70.1 ng m−3 (highest) |
Cape Verde Observatory |
MA |
Gas-phase |
0.8 ng m−3 |
IC |
62
|
Particle-phase |
0.2 ng m−3 |
DMA |
Gas-phase |
4.5 ng m−3 |
Particle-phase |
5.6 ng m−3 |
The atmosphere of the Yangtze River Delta, China |
MA (summer, particle-phase) |
15.0 ± 15.0 ng m−3 |
In situ gases and aerosol composition monitor |
69
|
DMA (summer, particle-phase) |
6.3 ± 6.9 ng m−3 |
DEA (summer, particle-phase) |
20.4 ± 30.1 ng m−3 |
TMA (summer, particle-phase) |
4.0 ± 5.9 ng m−3 |
Seoul, South Korea |
The total concentration of MA, EA, DEA, PA and butylamine (BA) in PM |
5.56 ± 2.76 ng m−3 |
Headspace solid-phase microextraction using gas chromatography plus tandem mass spectrometry |
55
|
Nanling Mountains in southern China |
The fall of 2016 ∑amines (MA, DMA, DEA and DBA) |
Gas-phase |
193.4 ± 111.7 ng m−3 |
Derivatization-GC-MS |
11
|
Particle-phase |
12.4 ± 10.8 ng m−3 |
The summer of 2017 ∑amines |
Gas-phase |
307.5 ± 196.7 ng m−3 |
Particle-phase |
20.7 ± 11.0 ng m−3 |
The vicinity of the CO2 Technology Center Mongstad |
DMA, TMA, MEA |
Several pptv |
|
PTR-QiToF-MS |
70
|
Shanghai (emitted from the ceiling duct) |
DMA |
Few human activities |
25.1 ± 12.8 μg m−3 |
IC |
67
|
More human activities |
370.4 μg m−3 |
TMA + DEA |
Few human activities |
1.7 ± 0.9 μg m−3 |
More human activities |
67.9 μg m−3 |
A rural site in central Germany |
C1, C2, C3, C4 and C6-amines |
1–5 pptv |
Chemical ionization–atmospheric pressure interface time-of-flight mass spectrometer (CI-APi-ToF) |
42
|
Helsinki, southern Finland (forest ecosystems) |
DMA + EA |
<9.5–54.9 pptv |
HPLC-ESIIT-MS |
58
|
TMA + PA |
3.9–26.9 pptv |
BA |
0.1–0.56 pptv |
DEA |
<0.08–1.3 pptv |
TEA |
<0.01–0.16 pptv |
Beijing |
TMA |
In dust PM |
(0.01 ± 0.001) − (0.05 ± 0.002) μg m−3 |
Solid phase extraction coupled with IC |
71
|
In haze PM |
(0.04 ± 0.001) − (0.08 ± 0.002) μg m−3 |
TMAO |
In dust PM |
(0.02 ± 0.001) − (0.09 ± 0.004) μg m−3 |
In haze PM |
(0.06 ± 0.002) − (0.14 ± 0.002) μg m−3 |
Kent, Ohio, USA |
DMA |
8 ± 3 pptv |
CIMS |
56
|
C3-amines |
16 ± 7 pptv |
NH3 |
517 ± 259 pptv |
The coastline of the East China Sea, South China Sea, and Yellow Sea |
NH3 |
Gas-phase |
1.2 ± 1.3 μg m−3 |
Ambient ion monitor-IC system (AIM-IC) |
72
|
Particle-phase |
(0.65 ± 0.71) - (5.4 ± 3.1) μg m−3 |
DMA |
Gas-phase |
11 ± 6.5 ng m−3 |
Particle-phase |
<4 to 100 ng m−3 |
TMA |
Gas-phase |
5.4 ± 2.4 ng m−3 |
Particle-phase |
<2 to 21 ng m−3 |
Wangdu, Hebei Province, China |
∑amines |
Burst periods |
625 ± 383 ng m−3 |
IC |
63
|
Nonburst periods |
164 ± 62.1 ng m−3 |
MA + DMA + TMA |
Average |
125 ± 21.0 ng m−3 |
DEA + TEA |
Average |
206 ± 292 ng m−3 |
Beijing |
NH3 |
2.8 ± 2.0 ppbv |
Water cluster-CIMS |
73
|
C1, C2, C3-amines |
5.2 ± 4.3 pptv |
China's marginal seas (over the Yellow and Bohai seas) |
NH3 |
Gas-phase |
0.53 ± 0.53 μg m−3 |
AIM-IC |
74
|
Particle-phase |
4.7 ± 7.2 μg m−3 |
DMA |
Gas-phase |
0.006 ± 0.006 μg m−3 |
Particle-phase |
0.065 ± 0.068 μg m−3 |
TMA |
Gas-phase |
0.031 ± 0.009 μg m−3 |
Particle-phase |
0.28 ± 0.18 μg m−3 |
To overcome the shortcomings of offline monitoring methods, a few online mass spectrometry technologies have been developed to measure atmospheric amines. These online mass spectrometry technologies are always composed of modules for sample lead-in, ion sources, analysis and detection. Chemical ionization (CI) is the most common soft ionization method which uses the molecular ion reaction to produce ions. The ionized products obtained from a CI source are mainly molecular ions with small fragments which make the analysis simple. The atmospheric samples are first introduced into the CI source and the ionization processes occur. Analyte molecules with higher proton affinities than reagent ions are protonated during ionization processes and then are detected as protonated ions.25,26,56 H2O is first used as a reagent ion in online mass spectrometry technology to detect atmospheric amines. Sellegri et al.25 and Hanson et al.26 separately used proton transfer reaction chemical ionization mass spectrometry (PTR-CIMS) and ambient pressure proton transfer mass spectrometry (AmPMS) to measure gas-phase amines. Both PTR-CIMS and AmPMS utilize protonated H2O ions (H3O+(H2O)n) as the ionization reagent, while a specific amine mass is identified by high proton affinity and the atmospheric hydrate distributions of the peak families formed by the tested amine and different numbers of H2O molecules (generally 1–4). In a real atmospheric environment, many organics show higher proton affinity to H2O than amines and detection interference occurs. Quite a few studies have been devoted to addressing this challenge. Recently, Pfeifer et al. described a new chemical ionization atmospheric pressure interface time-of-flight mass spectrometer (CI-APi-ToF) that used protonated H2O clusters for the selective ionization of dimethylamine (DMA).65 Besides, Yu et al. selectively monitored the amines online by CIMS with protonated ethanol and acetone ions.56 On the other hand, Wang et al. presented an application of the Vocus proton transfer reaction time of flight mass spectrometer (Vocus PTR-ToF-MS) to monitor gaseous DMA sensitively by adjusting the setting and using a permeation tube calibration method.27
To achieve online detection of low concentrations of amines, Sipilä et al. presented a CIMS method using bisulphate as the reagent ion and the method was capable of detecting DMA with concentrations even down to the level of parts per quadrillion by volume (ppqv).66 In addition to DMA, Simon et al. demonstrated a nitrate CIMS method using the NO3−·(HNO3)1–2·(DMA) cluster ion signal to simultaneously measure other substances relevant to NPF (such as SA and extremely low-volatility organic compounds).19 However, though these online mass spectrometry methods possess a low detection limit and high time resolution, they cannot accurately distinguish the isomers of amines (e.g., DMA and ethylamine (EA) both belong to C2-amines).
Both offline and online detection methods toward amines have their own advantages, disadvantages and scope of application. The former can provide high selectivity for the detection of atmospheric amines, but is limited by time resolution, while the latter has high time resolution and low detection limit, but can't distinguish isomers of amines in most cases. Two different groups of researchers (Kieloaho et al.20 and Sipilä et al.66) used offline and online methods to conduct the field measurement of ambient amines in a boreal forest at the SMEAR II station in Hyytiälä, southern Finland, respectively. In 2011, Kieloaho et al. reported the concentrations of five alkylamines (EA, DMA, propylamine (PA), trimethylamine (TMA) and diethylamine (DEA)) using a high-performance liquid chromatography-electrospray ionization ion trap mass spectrometer. The highest concentrations of EA + DMA (157 ± 20 parts per trillion by volume (pptv)), PA + TMA (102 ± 61 pptv) and DEA (15.5 ± 0.5 pptv) were obtained. The detection limit of this offline method was 0.2 pptv and the isomers of EA and DMA as well as PA and TMA could not be distinguished with this method. Two years later, Sipilä et al. developed an instrument (bisulphate-cluster-based atmospheric pressure chemical ionization mass spectrometer (APCI-MS)) to detect ambient amines online in the same place. They reported that the upper concentration of EA + DMA was merely ∼0.15 pptv. That is, although this method showed a much lower detection limit to EA + DMA (even down to 0.07 pptv) and higher time resolution than the above offline method, it could not distinguish the isomers of EA from DMA, yet. Thus, the future development of both offline and online methods must receive more attention to promote their ability of isomer discrimination.
2.2 Temporal and spatial distribution characteristics of atmospheric amines
Generally, the types and concentrations of atmospheric amines vary greatly along with seasons,11,67 day and night,25,27,59 or locations, and are related to source emission intensity, removal rates, atmospheric temperature and gas-particle transformation.
A number of studies have found that the total concentration of amines shows a typical temporal distribution characteristic with a general seasonal trend as follows: summer > autumn > spring > winter. The use of fertilizers and increasing temperatures are mainly responsible for the high amine concentration in the summer. In addition, seasonal variations have otherness amongst different amines on a more nuanced scale. Zhou et al. found that the seasonal variation of DMA was winter > autumn > summer > spring, while the level of TMA + DEA and triethylamine (TEA) was the highest in the winter and the lowest in the summer.67 Chen et al. also suggested that amine-containing particles are more abundant in the winter than in the summer.68 However, Kieloaho et al. reached different conclusions that DEA showed summer maxima, while EA + DMA (C2-amines) and TMA + PA (C3-amines) displayed autumn maxima in the forests of southern Finland.20 Furthermore, Liu et al. reported that there existed a seasonal variation in various amines led by different origins of air masses using the backward trajectory analyses at Nanling Mountains, southern China, which was strongly affected by long-distance transport from marine and continental air masses.11 Inversely, Choi et al. proposed a small seasonal difference in the total amine concentration in PM in Seoul.55
Atmospheric amines vary not only from season to season, but also from day to day. For example, Hanson et al. found that the highest concentration of TEA in the late afternoon was eight times the lowest concentration in the morning.26 This tremendous disparity may be related to emission sources (soil and vegetation) and temperature.42 Other meteorological conditions, such as humidity and wind direction, also affect the ambient concentration of amines. For instance, a peak concentration was observed for just TMA in the late afternoon in the forests of southern Finland,25 while C3-amines and C6-amines showed peak concentrations in the afternoon of the day in the urban air of Atlanta.26 Moreover, Wang et al. found that DMA displayed strong diurnal variation in Wangdu city, China.27 In contrast, Du et al. reported that the concentration of amines appeared to be independent of whether they were collected during the day or night.69
In addition to their temporal distribution characteristics, the spatial distribution characteristics of atmospheric amines also deserve our attention. It is generally believed that rural areas may be more important for amine emissions than urban areas,50 because amines are produced from agricultural activity and livestock operations.21,23,75,76 VandenBoer et al. reported 1–10 pptv of methyl and ethyl amines in rural Canada.22 Besides, Liu et al. found that MA and DMA were the dominant amines both in gas and PM2.5 in the Nanling Mountains, China.11 In recent years, more and more literature has reported the detection of amines in urban areas.26,56,61 In 2016, Huang et al. demonstrated and quantified the presence of atmospheric aliphatic amines in Shanghai city for the first time,77 and Yao et al. detected hundreds of C2-amines in Shanghai city in the same year.61 Subsequently, Feng et al. reported that methylamine (MA) and DMA were the most abundant amines in the urban area of Shanghai city.78 In another city, Dong et al. found that the total concentrations of carcinogenic heterocyclic aromatic amines were most abundant at commercial sites and the least at residential sites in Beijing City.79 In Seoul city of South Korea, Choi et al. measured five atmospheric amines and found that these amines could contribute to the formation of nitrosamines rather than nitramines.55 Amines have also been linked with fog events in urban cities. Liu et al. monitored TMA and TMA-N-oxide (TMAO) in dust as well as haze samples together of Beijing city.71 Zhang et al. observed the significantly enhanced effect of TMA in atmospheric submicron particles (especially particles larger than 0.5 mm) during fog events, while more ammonium appeared on clear days in the urban areas of Guangzhou city.57 However, Liu et al. found that MA, DMA and dibutylamine (DBA) were the dominant fractions in fine particles in the urban areas of Guangzhou city.24 Recently, Feng et al. observed four times higher amine concentrations in burst periods than in nonburst periods, and the ratio of ethyl-amines (EAs, sum of DEA and TEA) to methyl-amines (MAs, sum of MA, DMA and TMA) in burst periods was 5 times higher than that in nonburst periods in Wangdu County, Hebei Province.63
Besides rural and urban areas, the spatial distribution characteristics of atmospheric amines in coastal and forest areas are also worth mentioning. Gibb et al. showed that MAs accounted for up to one-fifth of the total measured gaseous alkaline matter and 12% of the total measured aerosol alkaline matter (submicron) in the northwest Arabian Sea.80 Amines are unstable nitrogenous species and their concentration in surface seawater may be mainly influenced by the structural activity and seasonal regulation of the microbial cycle.80,81 For example, rapid consumption of MAs by microorganisms may delay their release into the atmosphere.82 Pinxteren et al. presented measurements of MA, DMA and DEA in the sea surface microlayer, bulk seawater, gas, and the submicron particulate aerosol phase at the Cape Verde Atmospheric Observatory.62 They suggested that the amine abundance in the gas-phase reflected biological processes in seawater, while particulate amines did not show such a direct response. Amine emissions also vary in different coastal areas. The concentration of aerosol amines in Shanghai was lower than that of Huaniao Island,83 while it was approximately 4-fold higher than that on an isolated coast of Chongming Island.78
Similar to coastal areas, forest areas also show different pollution characteristics of atmospheric amines. Sintermann et al. presented that terrestrial vegetation during flowering could release amines and most likely prevailed during spring.84 Gentner et al. also found that the concentration of indole can reach 1–3 ppb in ambient air during a springtime flowering event.85 Besides, amines from non-flowering plants, fungi and decomposing organic matter also exhibited a wider amplitude during the year, with a probability to intensify towards the autumn. In addition, the change in vegetation composition may introduce, enrich or deplete amine-emitting species. Biomass burning (BB) is another important amine source in forest areas and showed temperature dependency, which showed a higher mass fraction in the evening with cooler temperatures and a lower mass fraction in the afternoon with warmer temperatures for aerosol-phase amines, but an opposite trend for gas-phase amines.48,59 Temperature dependency implies reversible processes of evaporation of amines from soil surfaces in daytime and deposition to soil surfaces at night time. It is also found that the concentrations of primary amines from micron and submicron particles are 2 orders of magnitude higher than those from the gas-phase. These results indicate that gas-particle conversion is one of the major processes that control ambient amines at forest sites. Furthermore, the nature of aged BB particles is different from that of newly formed BB particles. Place et al. also compared aged BB particle samples collected in St. John's, Canada and newly formed BB particle samples in the Lower Fraser Valley in British Columbia, and found them to have different amine emissions characteristics.86
In summary, atmospheric amines show obvious and different temporal and spatial distribution characteristics with increasing anthropogenic emissions. Seasonal differences are more likely related to environmental factors such as temperature and meteorology, while strong diurnal variations are more easily caused by human activities. Besides, a number of field observations confirm that human activities such as animal husbandry in rural areas and daily human life in densely populated urban areas lead to the emission of atmospheric amines. In addition to anthropogenic sources, natural sources such as the ocean and forest also show distinct spatial distribution characteristics. To further understand the temporal and spatial distribution of atmospheric amines in the atmospheric environment, source apportionment is necessary.
2.3 Source apportionment of atmospheric amines
Although various atmospheric amines have been detected by advanced offline and online methods, how to recognize their source apportionment has become a hot topic in the area of atmospheric chemistry. In general, atmospheric amines are from anthropogenic and natural sources.50,54 Ge et al.50 and Cape et al.54 have summarized many atmospheric amine sources discovered at that time, while our work is more likely to summarize newly discovered amine sources in the past decade.
In nature, the biodegradation of proteins, amino acids, or other nitrogen-containing compounds in oceans, forests, etc., is an important source of amines.48,68,74,81,87 Marine organisms are always considered both sources and sinks of amines.88 For example, TMA may be directly released from tissues or through decomposition during marine excretion or decay, and may also be consumed by some microorganisms as carbon and nitrogen sources and for energy metabolism.81,82,89 Another example is that TMAO is an osmotic regulatory compound for marine organisms,90 meanwhile, it is also an important precursor for MA.91 Besides, it has been found that the accumulation of amines in marine fine particles is positively correlated with algal blooms.87 Therefore, amine emissions over the ocean are thought to be primarily biologically driven. In addition to the ocean, the forest is also an important natural source of amines.20 Kieloaho et al. found that autumnal monoterpene emissions from the forest floor and litterfall maxima coincided with the elevated or peaked concentration of amines.20 The authors also proposed that similar to monoterpene concentrations in the forest air, amine concentrations seemed to be linked with vegetation, soil activity and litterfall, rather than with other trace gases in the atmosphere. BB is another important amine source from the forest. In a southeastern US forest, You et al. detected pptv-level amines which mostly came from BB.59 Besides, Köllner et al. found that TMA released from BB sources may transport over a long-range distance and further affect other areas' atmospheric environment.81 In general, amine emissions in coastal areas are related to biological drivers, while emissions in the forest are related to vegetation species, fluorescence and BB.
In recent decades, human activities have gradually increased as a more important amine source than natural activity. Du et al. confirmed that the main source of atmospheric amines in Chongming Island was anthropogenic sources such as industrial and biomass emissions, followed by marine sources including sea salt and marine biogenic sources.69 Similarly, You et al. reported higher concentrations of amines at polluted sites of coal-burning power plants and agricultural activities than at rural forested sites.59 Hence, agricultural and industrial activities significantly contribute to atmospheric amines. As early as the 1990s, Schade et al. proposed that animal husbandry was one of the main sources of MAs.75 Studies showed that TMA accounted for 3/4 of MA emissions from animal husbandry and the concentrations of TMA in fishing industrial areas were far in excess of industrial limits.76 Afterward, it was believed that amines were from animals' exhalation on cattle farms,21 and Sun et al. found that TMA was an important substrate in the gastrointestinal tract of ruminants.89 In addition to agricultural activities, amines are also emitted from industrial activities. Zheng et al. detected small molecule amines in Nanjing city, China, and deduced that these amines were mainly emitted from nearby steel mills and petrochemical refinery facilities.60 Moreover, Michalski et al. found that amines can be discharged from the food industry such as factories of wine, beer, cheeses, meat, fermented products, fish and other seafood.92 Besides, due to the intensified greenhouse effect, amines have been used as a solvent to capture CO2, increasing the industrial emission of amines,28,93–95 especially monoethanolamine (MEA)30 and PZ.96 Recent studies even pointed out that the use of herbicides with amines in their formulations could lead to non-negligible atmospheric amine emissions.97 Besides the sources mentioned above, human activities of daily living are also non-neglected anthropogenic sources of amines. For example, transportation56,78 and cooking56,79 are both recognized as release sources of amines. Motor vehicle emission is one of the important mobile sources.63,73,98 Feng et al. observed that the widely used ethanol gasoline vehicles in Hebei Province, China can primarily emit EAs.63 Yang et al. further compared the amine emission strengths of different power sources, and found that the total emission strength of diesel trucks was the greatest followed by those of gasoline cars and natural gas cars.98 Besides, a high level of alkylamines was found in the exhaust ducts of buildings in Shanghai, China.18,67 Furthermore, anthropogenic and natural sources always emit amines together with other inorganic pollutants, causing complex pollution in the atmospheric environment.60 Therefore, future work should be conducted not only by continuing the source apportionment of amines, but also by assessing the impact of synergistic emissions of amines and other gaseous pollutants.
3. Oxidation kinetics and mechanisms of atmospheric amines
3.1 Reaction kinetics
Free radical reactions are triggered when gaseous amines are attacked by atmospheric oxidants (e.g., ˙OH, NO3˙, O3, ˙Cl and other radicals), and amines are initially converted to low volatile organic products which may condense into SOAs. The most studied kinetics of amines are their reactions with ˙OH or O3, while the data on the reaction rates of amines with NO3˙ and ˙Cl are still scarce. In Table 2, we summarize the reaction rate coefficients of common atmospheric amines with oxidants and the corresponding measurement methods which mainly include the absolute rate method99,100 and relative rate method.101,102 The reaction rate constants of alkylamines with ˙OH (10−11–10−10 cm3 molecule−1 s−1) are found to be much higher than that with O3 (10−21–10−17 cm3 molecule−1 s−1).52 In daytime, the reaction of amines with ˙OH is the most important sink of amines due to very fast reaction rates. O3 may play a non-negligible role in the degradation of amines at night or in areas with serious O3 pollution, while NO3˙ is also an important oxidant of the night time oxidation of amines.103 ˙Cl is normally generated through the multiphase reaction of sea salt. But in recent years, the precursors ClNO2 and Cl2 have also been detected in inland areas. However, the relative reaction data are still insufficient, although it is generally believed that the reaction rate of ˙Cl with amines is fast enough to be comparable with that of ˙OH. Recently, Onel et al. reported that the absolute rate coefficient of ˙OH + MEA was obtained as (7.61 ± 0.76) × 10−11 cm3 molecule−1 s−1 at room temperature, and decreased by about 40% at 510 K.99,104 Soon afterwards, Onel et al. presented the first determination of the rate coefficient of ˙OH + PZ, which had a negative temperature dependence and was parametrized as (2.37 ± 0.03) × 10−10 (T/298)(−1.76±0.08) cm3 molecule−1 s−1 × 105 MEA and PZ are both widely used solvents in post-combustion carbon dioxide capture technology, while the reaction rate coefficient of ˙OH + PZ is more than 3 times that of ˙OH + MEA. Such studies on the temperature dependence of the reaction of atmospheric oxidants with amines can provide more information on the reaction mechanism of amines, especially for combustion related amine emissions.
Table 2 Literature on the reaction rate coefficient of amines with different oxidants
Amine types |
Oxidizing agent |
The reaction rate coefficient (cm3 molecule−1 s−1) |
Method |
Ref. |
MEA |
˙OH |
(7.61 ± 0.76) × 10−11(indoor temperature) |
Laser-induced fluorescence monitoring ˙OH attenuation (absolute rate method) |
99
|
(7.73 ± 0.24) × 10−11(T/295) −(0.79±0.11) (296–510K) |
(7.02 ± 0.46) × 10−11(295 ± 3K) |
PTR-MS relative rate method |
101
|
O3 |
(1.09 ± 0.05) × 10−18 |
PTR-MS absolute rate method |
101
|
PZ |
˙OH |
(2.38 ± 0.28) × 10−10 (N2) (298K) |
Laser-induced fluorescence monitoring ˙OH attenuation (absolute rate method |
105
|
(2.37 ± 0.03) × 10−10(T/298) −(1.76±0.08) (N2) (298–500K) |
˙Cl |
4.7 × 10−10 (298K) |
A combination of quantum chemistry calculations and kinetics modeling |
123
|
MA |
˙OH |
(1.97 ± 0.11) × 10−11(N2) (298K) |
Laser-induced fluorescence |
104
|
(1.88 ± 0.11) × 10−11(O2 + N2) (298K) |
(1.83 ± 0.14) × 10−11(OD, O2 + N2) (298K) |
(1.889 ± 0.053) × 10−11(T/298) −(0.56±0.10) (298–600K) |
˙Cl |
(2.90 ± 0.44) × 10−10 |
Laser flash photolysis resonance fluorescence technique |
124
|
DMA |
˙OH |
(6.27 ± 0.63) × 10−11(N2) (298K) |
Laser-induced fluorescence |
104
|
(5.80 ± 0.70) × 10−11(O2 + N2) (298K) |
(6.75 ± 0.74) × 10−11(OD, O2 + N2) (298K) |
(6.39 ± 0.35) × 10−11(T/298) −(0.75±0.18) (298–600K) |
˙Cl |
(3.89 ± 0.58) × 10−10 |
Laser flash photolysis resonance fluorescence technique |
124
|
EA |
˙OH |
(2.50 ± 0.13) × 10−11(N2) (298K) |
Laser-induced fluorescence |
104
|
(2.75 ± 0.18) × 10−11(OD, O2 + N2) (298K) |
(2.54 ± 0.08) × 10−11(T/298) −(0.68±0.10) (298–600K) |
TMA |
˙OH |
(5.78 ± 0.48) × 10−11(N2) (298K) |
Laser-induced fluorescence |
104
|
˙Cl |
(3.68 ± 0.55) × 10−10 |
Laser flash photolysis resonance fluorescence technique |
124
|
O3 |
(5.73 ± 0.15) × 10−11(T/298) − (0.71 ± 0.10) (298–600K) |
PTR-MS-absolute rate method (second-order rate constant equation) |
100
|
Ethenamine |
˙Cl |
2.7 × 10−10 |
A quantum chemical method and kinetics modeling |
125
|
N-Methylethanolamine (MMEA) |
˙OH |
(8.51 ± 0.65) × 10−11(T/298) − (0.79 ± 0.22) (∼300–500K) |
Laser-induced fluorescence |
119
|
N, N-Dimethylethanolamine (DMEA) |
˙OH |
(6.85 ± 0.25) × 10−11(T/298) − (0.44 ± 0.12) (∼300–500K) |
Laser-induced fluorescence |
119
|
1, 2-Dimethylpropylamine |
˙OH |
(5.08 ± 1.02) × 10−11 |
Relative dynamics |
102
|
Tert-amyl amine |
˙OH |
(0.86 ± 0.17) × 10−11 |
Relative dynamics |
102
|
DEA |
˙OH |
(7.36 ± 1.47) × 10−11 |
Relative dynamics |
102
|
O3 |
(8.20 ± 1.01) × 10−17 |
Pseudo first-order rate constant |
115
|
N, N-Dimethylethylamine |
˙OH |
(7.37 ± 1.47) × 10−11 |
Relative dynamics |
102
|
N, N-Dimethylpropylamine |
˙OH |
(10.97 ± 1.78) × 10−11 |
Relative dynamics |
102
|
N, N-Dimethylisopropylamine |
˙OH |
(9.79 ± 1.75) × 10−11 |
Relative dynamics |
102
|
N, N-Diethylmethylamine |
˙OH |
(8.92 ± 1.54) × 10−11 |
Relative dynamics |
102
|
Aniline |
˙Cl |
1.7 × 10−10 |
A quantum chemical method and kinetics modeling |
125
|
Indole |
˙OH |
7.9 × 10−11 |
A combination of quantum chemistry calculations and kinetics modeling |
122
|
˙Cl |
2.9 × 10−10 |
A combination of quantum chemistry calculations and kinetics modeling |
TEA |
˙OH |
(10.86 ± 1.88) × 10−11 |
Relative dynamics |
102
|
It is difficult to generalize the reaction kinetics of heterogeneous reactions of atmospheric amines compared with those of homogeneous reactions. The kinetics of heterogeneous reactions cover a wide range, from promoting the formation of clusters of NPF precursors106,107 to contributing to the formation of BrC.108,109 However, there is no denying that the addition of a small amount of atmospheric amines can increase the rates of these reactions by orders of magnitude. The complexity of heterogeneous reactions and the particularity of each case make it difficult to simply classify their kinetics. In contrast, it is more meaningful to find out the contribution of heterogeneous mechanisms of amines to SOAs at different stages first, and then discuss the kinetics with sufficient data.
3.2 Oxidation mechanisms
3.2.1 The initial step of oxidation reaction mechanisms.
Since 1978, many atmospheric scientists have simulated the oxidation behaviours of typical atmospheric amines and their potential effects in the atmosphere using smog chamber-mass spectrometry systems, including photodegradation of amines triggered by ˙OH,32,33,101,110–113 reactions induced by NO3˙,32,33,103,110,114 or dark reactions induced by O3.1,32,33,101,110,115
In general, the atmospheric reaction of amines with ˙OH follows free radical chemistry. The reactions are initiated by attacking amines to form C-center radicals (derived from C–H abstraction) or N-center radicals (derived from N–H abstraction). For tertiary amines, the initiation reaction can only abstract C–H to form C-center radicals, while primary and secondary amines can also form N-center radicals because they have N–H bonds (Fig. 1).116 MEA contains a hydroxyl group, which also follows radical chemistry and produces imine NH
CHCH2OH, isocyanate HNCO and other products through cleavage or condensation reactions.101,117,118 The branching ratios rαC–H and rN–H for ˙OH reacting with amines vary according to the types and numbers of substituents.104,119 For example, Onel et al. suggested that the branching ratio of an N-center radical was about 0.09, which was much smaller than that of C-center radical formation in the reaction of ˙OH + PZ.105 Ren et al. further confirmed that abstraction of ˙OH + PZ predominantly occurs from C–H sites by theoretical kinetics investigation.120 It is difficult to detect short-life intermediates such as transition states intermediates, distinguish the interactions between molecules (e.g., molecular clusters), and reveal the microscopic mechanism of atmospheric transformation of amines. Hence, we need theoretical studies to investigate the initial steps of atmospheric oxidation of amines. Borduas et al. studied the gas-phase reaction of the simplest reduction of organic nitrogen such as MA using ab initio calculations, and found that ˙OH extraction of N–H was competitive, but the potential energy of C–H extraction was the lowest and most likely to occur.121 However, Xue et al. found that ˙OH addition was the dominant pathway for the reaction of ˙OH with indole which is a polyfunctional heterocyclic secondary amine.122
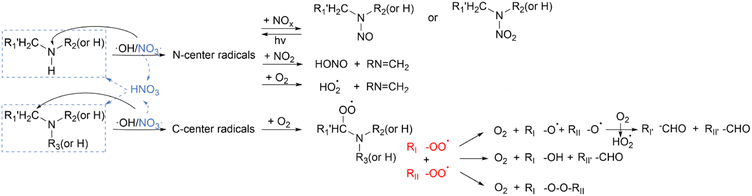 |
| Fig. 1 Reaction pathways of atmosphere amines attacked by ˙OH/NO3˙. | |
Amines are also attacked by O3. Gai et al. believed that amines might react with O3 through the electrophilic reaction mechanism.115 Tuazon et al. considered that amine ozonation could be attributed to a mechanism for forming energy-rich amine oxides in the initial step, and subsequently proceeded with an intramolecular hydrogen transfer to produce a hydroxyl group which broke to produce C-center radicals or N-center radicals.100 The authors also found that CH3 group breaking was an important route because it was not easy for amine oxides to transfer hydrogen atoms within themselves, producing CH3NO2 ultimately. Some people have different opinions (Fig. 2). Furuhama et al. found that the rapid rate constant of the reaction between TMA and O3 could be explained by the circular transition state generated in the initial stage of the reaction, which supported that the H-abstraction with diradical O3 from amines was the initial step, rather than the formation of energy-rich amine oxides.126 Recently, Tong et al. reported a series of products containing nitrogen and oxygen from the decomposition processes of DEA and TEA ozonation.1 According to their experimental data and thermodynamic calculations, DEA was first formed in the decomposition of TEA by O3, followed by the formation of N-ethylamine, which partly confirmed why products of tertiary amine included secondary amine products (Fig. 3).
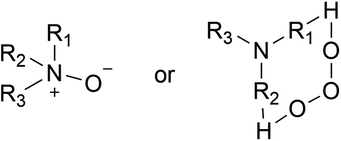 |
| Fig. 2 Possible initial steps in the reaction of amines with O3. | |
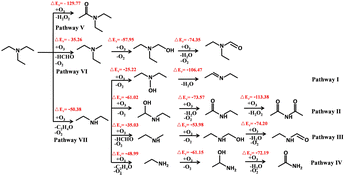 |
| Fig. 3 Proposed ozonolysis pathways and mechanism of DEA and TEA by Tong et al. in ref. 1. | |
The NO3˙ reaction with amines mainly represents the night time chemistry of atmospheric amines.112 The initial step and products of the reactions are similar to those of ˙OH and amine reactions.103 Notably, NO3˙ snatching hydrogen from amines produces nitric acid (HNO3), which will promote acid–base reactions and produce aminium nitrate.112
The reaction of ˙Cl with amines has gradually attracted the attention of researchers in recent years. There are some experimental and computational studies exploring its initial steps.122–125,127–129 In 2003, Rudić et al. first revealed that hydrogen-abstraction occurred with roughly equal probability from both the carbon and nitrogen sites of MA in the reaction of ˙Cl with MA through isotope experiments.127 Szűcs et al. also found that the hydrogen-abstraction of carbon and nitrogen sites of MA was exothermic low-barrier, but the substitution reactions were endothermic high-barrier using an accurate composite ab initio approach.129 Nevertheless, Nicovich et al. predicted theoretically that the reactions of ˙Cl with MA and DMA proceeded predominantly by hydrogen-abstraction from nitrogen sites, which was unlike the situation of ˙OH reactions.124 Furthermore, Xie et al. and Ma et al. suggested that the reaction of ˙Cl with MEA or PZ exclusively produced N-center radicals.123,128 Meanwhile, Ma et al. first proposed that the charge transfer mechanism was the main reason for the favorable formation of N-center radicals in the reactions of ˙Cl with amines.123 For unsaturated amines, they may also undergo addition reactions via a ˙Cl addition.122,125 For example, Xie et al. considered that both ethenamine+˙Cl and aniline+˙Cl reactions mainly produced delocalized radicals with the radical center on the C-site and N-site via a ˙Cl addition and the hydrogen-abstraction from the nitrogen site, respectively.125 Besides, the reaction of ethenamine+˙Cl first showed that the products of hydrogen-abstraction from the N-site could be favorably formed via addition intermediates in addition to the direct H-abstraction pathway. Recently, Xue et al. also found that both ˙Cl addition and hydrogen-abstraction from N-sites were feasible for the reaction of indole with ˙Cl, while ˙OH addition was the dominant pathway in the case of the ˙OH reaction.122 Obviously, the reaction of ˙Cl with amines is quite different from that of ˙OH. Hence, to assess the fate of atmospheric amines more accurately, it is necessary to consider both the atmospheric concentrations of ˙Cl and ˙OH and their initial reaction steps with atmospheric amines.
The atmospheric reactions of amines mentioned above involve common atmospheric oxidants. But in the actual atmosphere, diverse intermediate free radicals formed by other VOCs also exist. Under certain pollution conditions, amines may also react with stabilized Criegee intermediates (sCIs) which are formed by the reaction of unsaturated carbon–carbon double bonds of olefins with O3.39,130–133 Earlier researchers have found that ozonation of olefins enhances the formation of both NPF and SOAs in the presence of amines. Berndt et al. observed the SA-independent process of particle formation in the case of α-pinene and limonene ozonolysis in the presence of trace amines.39 Duporte et al. also observed an increase in NPF and SOA formation when α-pinene was oxidized by O3 in the presence of DMA.130 But they didn't reveal the reaction mechanisms of sCIs and amines. Recently, theoretical calculations have been used to discover the sCI-amine reaction mechanism. Kumar et al. elucidated that DMA acted as an agent to initiate oligomerization of Criegee intermediates by reacting with an initial one to form a peroxide functionality, which further reacted with Criegee intermediates and formed high molecular weight oligomers.132 This may account for an appreciable fraction of aerosol particles in California's central valley, New York City, and Paris areas. Compared to that for NH3, the rate constants for Criegee-amine would increase with the methyl substitution of NH3 derivatives increasing.132–134 But Chhantyal-Pun et al. suggested that Criegee-amine reactions were not fast enough to compete with ˙OH-initiated removal of NH3 and CH3NH2 from the Earth's troposphere unless the rates were significantly enhanced by complexation with water molecules.135 Ma et al. also investigated the synergistic effect of NH2 and OH groups on the Criegee reaction in the presence of MEA, and found the reaction of sCIs with NH2 groups of MEA molecules was prior to that with OH groups.131 Besides, they demonstrated that sCIs reacted with NH2 groups of MEA molecules directly mediated by water molecules, resulting in additional products at the gas–liquid interface. The reaction of sCIs with MEA might assist in sCI removal in a dry environment, due to its higher rate than Criegee hydration. At present, there are not many studies on sCI-amine chemistries, especially there is a lack of experimental data, which requires further research on the reaction under different conditions. More studies of amines and other important atmospheric intermediate free radicals are needed in the future to supplement what is currently missing.
3.2.2 Bimolecular reactions and autooxidation pathways.
The N-center radicals and C-center radicals generated in the initial step of amine oxidation may be followed by bimolecular reactions or autooxidation reactions. But these two radicals will undergo different subsequent reactions. For N-center radicals, they appear to only undergo bimolecular reactions with NOx to form carcinogens, nitramines or nitrosamines (Fig. 1). However, the role of O2 in the above reaction under low NOx concentrations is controversial. Lindley et al. considered that (CH3)2N˙ would produce nitramine and nitrosamine with NOx rapidly even at a low concentration of NOx and high concentration of O2, and the reaction rate constant with (CH3)2N˙ of the former was 107 times higher than that of the latter.136 But, Alam et al. found that although the rate constant of CH3NH˙ + O2 was much lower than that of CH3NH˙ + NOx under tropospheric conditions, the reaction path of CH3NH˙ + O2 was the dominant process in the atmospheric oxidation process with NOx concentration lower than 100 parts per billion (ppb).137 Normally, nitrosamines will be rapidly photolyzed; hence many previous experimental studies did not focus on the fate of nitrosamines. But in recent years, researchers have found that the stability of nitrosamines produced by different precursors is not completely consistent. Many smog chambers and other laboratory studies have proved that secondary and tertiary amines react with NO2 and NO to produce nitramines or nitrosamines, while primary amines only convert to nitramines.138 From the condensed phase chemistry, the secondary amines easily form stable nitrosamines, while the nitrosamines in primary fatty amines are very unstable.138 The standard aqueous nitrification of primary amines results in deamination; therefore it is generally assumed that primary fatty amines do not form nitrosamines in the gas-phase.138 In fact, primary amines can also form nitrosamines in the atmosphere, but they are rapidly isomerized.113,139–141 Tang et al. found that the nitrosamines formed by MA (CH3NHNO) and EA (CH3CH2NHNO) might undergo isomerization (CH2NHNOH and CH3CHNHNOH, respectively) and subsequent reactions with O2 to form the corresponding imines (CH2
NH and CH3CH
NH, respectively).139,140 However, da Silva considers that CH3N
NOH isomers are the major reaction products of nitrosamines formed by MA, from which water is further eliminated to form diazomethane (CH2NN).141 Similarly, Tan et al. studied the ˙OH reaction with tert-butylamine in the presence of NOx, and found that the formed nitrosamines isomerized into corresponding (CH3)3CN
NOH.113 All in all, the nitrosamines formed by primary amine oxidation are not rapidly photolyzed, but transformed into other structures. Besides, PZ is a six-membered ring that has two amine groups, whose potential to form nitrosamines is also discussed in different studies. Onel et al. considered that the branching ratio of an N-center radical is merely about 0.09 in the reaction of ˙OH + PZ, which represents an upper limit for carcinogenic compound production.105 However, Ma et al. found that the nitrosamine yield of ˙Cl + PZ is higher than that of MEA when [NO] is <5 ppb by quantum chemical methods and kinetics modelling.123 They considered that the N-center radicals formed by PZ are more favorable to react with NO than the N-center radicals formed by MEA, indicating that the yield of N-center radicals cannot directly be used as a metric for the yield of the corresponding carcinogenic nitrosamine. Their group further found that the delocalized character of N-center radicals formed by indole is the reason why they wouldn't further form nitrosamine.122 It is evident that the potential, efficiency and stability of N-center radical forming nitrosamines are highly dependent on the structure and the functional groups of parent amines.
As shown in Fig. 1, the C-center radicals will further react with O2 to form a peroxy radical (RO2˙). Rissanen et al. found that the reaction rates of the CH2NH2 radical and CH3CHNH2 radical with O2 were at about 10−11 cm3 molecule−1 s−1.142 RO2˙ formed by tertiary amines will lead to ˙OH recycling, while this is not the case for primary and secondary amines.119,138 RO2˙ further undergoes bimolecular reactions with NO, ˙OH, or other RO2˙ to form RO˙.33,116 As shown in Fig. 1, two RO2˙ reactions follow three possible mechanisms and form products including amides, alcohol-amines, peroxides, RO˙ and so on.33 O2 may take the hydrogen from RO˙ to form HO2˙ and amide. In addition to this, RO˙ may also break the N–C or ˙OC–C bond to form an aldehyde and N-center radical or amide and alkyl radical.116 Price et al. observed that oligomers were generated only in the oxidation reaction of TMA, indicating that the functional groups and molecular structure of aldehydes (aldehyde-amide formed by TMA) played an important role in the formation of oligomers, which were produced by peroxy radical chemistry, while ketone functional groups (ketone-amide formed by amines whose branched chain has more than two carbons) showed an inhibitory effect.33 The authors subsequently studied the temperature effect and confirmed the formation mechanism of TMA oligomers in aerosols.112 In addition, they found that RO2˙ + HO2˙ reactions also occurred at low temperature besides the RO2˙ + RO2˙ reaction which produced oligomers. This indicates that multiple bimolecular reactions may exist simultaneously in the atmospheric environment.
In recent years, it has been found that RO2˙ formed by VOCs also undergoes autoxidation reactions (hydrogen transfer)143 besides bimolecular reactions and the branching ratios of these reactions can reach very high values. But the current research on autoxidation is limited to theoretical calculations, and measured and experimental data are lacking. This is because real-time monitoring of RO2˙ is very difficult, due to its very short lifetime. However, the contribution of such reactions cannot be ruled out, because in certain environments the products produced by them generally have a high O/C ratio and are sufficiently low in volatility to form SOAs with incalculable effects. During the last few years, there were some theoretical calculations made on the oxidation of amines.34–36 Current studies have shown that the autoxidation of amines is achieved through the intramolecular 1,5-hydrogen transfer of N-containing RO2˙ (Fig. 4). Moller et al. studied the oxidation rate of amines with ˙OH to reveal the branching ratio of this path.34 They found that the main oxidation reaction product of TMA was double hydrogen peroxide amide (produced by TMA undergoing a thrice intramolecular 1, 5-H shift). In addition to hydroperoxamide products, autoxidation pathways also lead to the atmospheric circulation of ˙OH as shown in Fig. 4. This is consistent with our previous discussion that only tertiary amines can undergo ˙OH recycling. Ma et al. demonstrated that N could promote the occurrence of the H-transfer reaction, which was the initial step of the autoxidation mechanism.35 What is noteworthy is that the aforementioned autoxidation pathway of amines is closely related to the structure of amines. The yield of hydroperoxamide may be reduced for secondary amines, such as DMA and DEA, due to competition from HO2˙ loss reactions.34 Besides, the pathway of dissociation and fragmentation will compete with the autoxidation pathway in the TEA reaction system which is also a tertiary amine as well as TMA.35 This may result from the long branch chain of TEA. Moreover, high concentrations of NO also inhibits the autooxidation of N-containing RO2˙.34 Although the autooxidation pathway of amines is restrictive and has potential competition, it has certain significance in explaining the fate of amines in the atmosphere. For example, Berndt et al. extended the study of Moller et al.34 on TMA autooxidation by comparing it with the results of four terpenes and three aromatics which were considered important reaction systems in the atmosphere, and revealed that the ˙OH + TMA reaction was a very efficient atmospheric autooxidation system.36 Hence, more attention needs to be given to the autoxidation pathways of amines in the future.
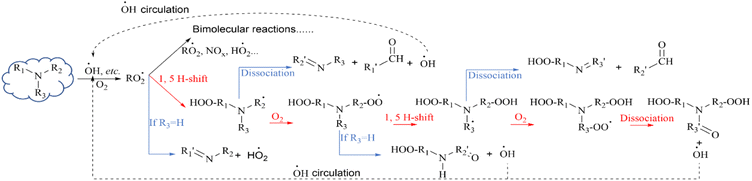 |
| Fig. 4 Autoxidation processes of atmosphere amines. | |
The secondary oxidation of amines and products should also be considered. Malloy et al. proposed that primary fatty amines and their carbonyl-containing oxidation products (amides) could generate imines through a mechanism like the Schiff-base reaction.103 Specifically, the parent amine N attacks the carbonyl carbon of the product amide and finally produces imines (Fig. 5). Besides, when Tuazon et al. studied the oxidation experiment of DMA and O3, they found that the consumption of amine/O3 > 1 in an experiment with excess amine, indicating that DMA has other loss pathways.100 They found DMA and formaldehyde in a consumption ratio of 2
:
1 through the reaction of amine-aldehyde condensation. Zhu et al. also found that a quick Schiff-base reaction would happen between primary amines and formaldehyde in emission monitoring instruments on the suction line, while secondary amines and tertiary amines didn't react with formaldehyde.144 Beyond this, Louie et al. found that single molecule water was sufficient to catalyse MA/DMA/NH3 and formaldehyde to generate methanolamine using theoretical calculations, and the DMA + CH2O + H2O reaction pathway was considered a particularly important factor causing atmospheric DMA loss under low sunlight conditions with low ˙OH concentrations.145
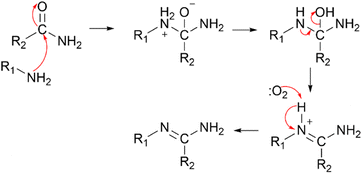 |
| Fig. 5 Primary amines form imines through the Schiff base reaction mechanism. | |
In conclusion, the homogeneous oxidation reaction mechanisms of amines with various atmospheric oxidants have been widely investigated. The discovery of the new mechanism heavily relied on the development of advanced detection means or theoretical calculation methods. Moreover, the incomplete matching theory and measured results also imply that there is still a lot of research space for the reaction mechanism of atmospheric amines. In addition, current studies have not paid enough attention to the interaction mechanism between parent amines and their products, which may have different contributions to the formation and growth of SOAs. More attention should be paid to this direction in the future.
4. Contribution of atmospheric amines to aerosols
In general, the contribution of atmospheric amines to aerosols includes the process from formation to aging. In other words, amines not only participate in NPF, but also contribute to subsequent submicron aerosols including condensation of the oxidation products of amines, direct dissolution, acid–base reactions and replacement reactions. In addition, amines are important precursors of BrC (a special kind of SOA). Furthermore, aminium salts in aerosols can enhance the hygroscopic capacity and increase CCN activity. Considering that the homogeneous and heterogeneous contributions of atmospheric amines to aerosols are complicated and multifaceted, we attempt to review them categorically.
4.1 Atmospheric amines associated with NPF
NPF involves a two-step process, nucleation-the formation of a critical nucleus, followed by growth to a larger size which should at least be detected.146 The formation of a critical nucleus is entropy-limited, and the Gibbs free energy barrier needs to be overcome before the spontaneous transition to a new stage. At the critical nucleus, the change of Gibbs free energy reaches the maximum, which reflects the nucleation and growth process of aerosols on the side. Besides, nanoparticles have a strong Kelvin effect, i.e., a highly increased equilibrium vapor pressure above the surface, which is another major constraint in NPF. Particle formation can be initialized by forming clusters of acid–base molecules with strong non-covalent bonds. NPF usually requires higher concentrations of SA.14,147–151 However, under practical conditions, the combination of SA and H2O cannot form strongly bound clusters. Additional stable vapor molecules are essential for NPF, especially for highly alkaline substances such as NH3 and amines.14,39,53,84,151–154 Quantum chemical studies have also predicted that clusters containing only SA and H2O can't grow and must be stabilized by NH3, amines, or organic vapors.106,151,155–157 Otherwise, the high NPF rates measured in the boundary layer cannot be explained. Quantum chemical calculations have shown that the limiting steps for acid–base NPF depend largely on the formation of the smallest (acid)1–2(base)1–2 clusters, as they provide the basis for further cluster growth.158,159
In field measurements, amines have been observed to participate in SA-induced NPF. In urban and remote forest NPF events, aminium ions are commonly detected on fine particles ranging from a few nm to a dozen nm, accounting for up to half of the positive ions detected.107,160 For example, the days of NPF measured in Finland peaked with the peak of the total alkylamine concentration in the autumn.20 Besides, the NPF rates were related to nucleation of SA-DMA-H2O, including SA dimers and SA-DMA clusters in Shanghai.147 Meanwhile, the field measurements on dairy farms discovered that high nucleation rates were associated with significant SA dimer concentrations, whereas they were inversely correlated with NH3 and amine concentrations in the gas-phase which might be effectively consumed in nucleation clusters.42 In addition to alkylamines, alkanol amines may also be important for NPF. MEA can hydrate more easily than alkylamines. It has been reported that MEA-dominated prenucleation clusters near MEA emission sources were at least 3 orders of magnitude higher than TMA and DMA concentrations, indicating that alkanol amines may play an important role in atmospheric nucleation in high humidity environments.161
Although the concentration of atmospheric amines may be 2–3 orders of magnitude lower than that of NH3, the difference in free energy between amine-containing and NH3-containing clusters complexing with SA/HSO4− is sufficient to overcome this mass balance effect, indicating that amines may be more important than NH3 in neutral/ion-induced SA/HSO4−-water nucleation.106 Within the peak concentration range of atmospheric NH3, NH3 has a measurable promotion effect on nucleation rates, which can increase the number of particles by 1–2 orders of magnitude under relatively dry conditions. However, tert-butylamine, whose concentration is one order of magnitude lower than that of NH3, can increase the number of particles by 2 orders of magnitude under the same conditions.38 Similarly, even a few pptv of DMA can increase the NPF rates by several orders of magnitude compared to NH3.107 Though DMA is one of the strongest enhancing agents for SA-base NPF confirmed by ambient observations and experiments, Ma et al. found that the enhancing potential of PZ was greater than that of DMA and MEA.162 Considering the differences in efficiency of the various pathways for the removal of different amines in the atmosphere (e.g., oxidative decomposition, formation of carcinogenic nitramines or nitrosamines and participation in NPF), we should take each practical situation into account to assess the environmental impact and risk of amines. The importance of amines and NH3 in NPF depends on the stability of amines and NH3 to SA clusters and the abundance of amines and NH3 in the atmosphere. For example, Li et al. found that a binary SA-DMA nucleation system dominated near industrial sources with high DMA concentrations, while high NH3 concentrations could significantly affect cluster formation in the near agricultural field.15 Besides, NPF events observed in a highly polluted suburb of the North China Plain of Beijing were found to have a high NPF rate, in which 68% is associated with SA-DMA-H2O, while the remaining 32% of NPF events are thought to be attributed to SA-NH3.14 In addition to the monitoring data, laboratory studies have shown that amines can enhance SA-H2O nucleation in the atmosphere more efficiently than NH3.38–41 Ortega et al. found that clusters formed by DMA and SA were more stable than those by NH3.155 The higher alkalinity,41,163 the combining capacity with a neutral or charged cluster,40,106,159 the assisted ability of SA nucleation under water-free conditions or in the presence of several H2O molecules157,161 and an abundance of functional groups39,164 are the reasons why most amines are more effective than NH3 in SA nucleation. Furthermore, we are curious about the effect of SA concentration. Zhang et al. computed that it was easier for DMA to approach the air-nanoparticle interface than NH3 to form DMA-SA clusters, and the efficiency in promoting aerosol growth was independent of the concentration of gaseous SA.43 They further discovered that MAs were involved in two types of heterogeneous reactions in aqueous particles with different SA concentrations.2 Amines were neutralized by H3O+ to form aminium salts within a few picoseconds at high SA concentrations, while they mainly proceeded by hydrolysis reactions to produce ionic pairs of aminium and OH− at low SA concentrations. In summary, amines can interact with SA at an air-particle interface to promote the formation of aerosol particles (Fig. 6). Amines also appear to facilitate SA-nucleation more readily than less volatile oxidation products. For example, Berndt et al. studied the effects of amines or organic oxidation products on the nucleation system of SA-H2O, and found that NPF caused by SA-amine interaction was much more effective than that caused by SA-organic oxidation products.39
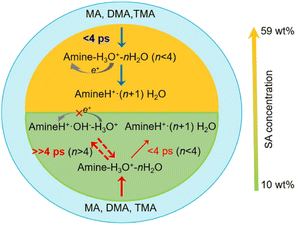 |
| Fig. 6 Methylamines participate in two types of reactions at low and high SA concentrations.2 | |
Although amines have a strong effect on NPF, the actual atmospheric reactions tend to be more complex. Therefore, the synergistic effect of mixed amines with other atmospheric compounds should be considered to understand and predict their importance in different atmospheric environments. For example, since the concentration of NH3 is usually higher than that of amines in the atmospheric environment, NH3 replacement by amines is one of the important reaction pathways in the formation of SA clusters.159 Elm et al. proposed that the presence of two kinds of bases increased the likelihood of forming larger clusters due to an increase in the concentration of available vapors.152 Glasoe et al. reported that NPF was significantly affected by the synergistic interaction between NH3 and amines.163 However, their stability does not explain this phenomenon. For instance, Temelso et al. found that a mixed base system was not more stable than a system containing only alkylamines.165 Recently, Myllys et al. found evidence of the synergy with NH3 to form more clusters than intermolecular interaction of the amines, and NH3 could be a bridging agent in SA-amine clusters by studying the Gibbs free energy of SA-amine-NH3 clusters.166 They also proved that NH3 was usually easier than DMA to protonate and ammonium ions were located in the center of the cluster structure, thus forming strong intermolecular interactions with surrounding molecules and ions. Besides, Li et al. calculated that SA-DMA clusters tended to form core–shell structures, in which DMA was located in the outer layer due to steric hindrances of the two alkyl groups, while NH3 could pass through the gap between clusters relatively freely and optimize interaction sites with SA.15 Furthermore, Shen et al. found that the formation of initial clusters was dominated by the alkalinity of amines, but with the increase of clusters, steric hindrance played an increasingly significant role.37 The results suggested that NPF potential couldn't just be determined by the strength of the base. Substituting a portion of a relatively strong base in the atmosphere with a weaker base might enhance NPF due to base synergy rather than reduce it. Therefore, different amines have different primary and secondary roles at different stages of nucleation, and several amines and NH3 with different alkalinities and structures will synergistically enhance acid-driven NPF.
In addition to promoting the nucleation of SA, amines have also been reported to enhance the nucleation of other acids in the atmosphere.37,167–171 For example, Ning et al. found that DMA could structurally stabilize iodic acid (IA, a critical driver for marine NPF) via hydrogen and halogen bonds, and enhance the formation rate of IA clusters by five orders of magnitude.171 Besides, there were several studies suggesting the potentially important roles of NH3 and amines in the nucleation system containing nitric acid recently.172,173 The potentiality of enhancing the nucleation of amines into atmospheric acids is related to the hydrogen bond capacity (number of hydrogen bonds) of amines and acids and the alkalinity and steric hindrance of amines. There are different primary and secondary relationships and mechanisms under different conditions and systems.
4.2 Condensation of the oxidation products of amines to SOAs
The low volatility products generated by the oxidation of amines will condense onto submicron aerosols. The specific mechanisms have been discussed in detail in Section 3. It was found that the oxidation of amines yielded amides and imides,103,114 which would condense onto SOAs and then might be photolyzed or oxidized to HCN, etc.174 Recently, Tong et al. reported the importance of aldehydes in promoting SOA formation and growth from the reaction of amine products.1 Besides, Silva et al. considered that NO3˙ reacting with TMA could explain why the midnight peak of fine particles associated with amines.114 Moreover, tertiary amines were easier to form a mass of non-saline SOAs than other amines.110 The acid–base reaction was the main way of reaction of NO3˙ with BA and DEA, while TMA tended to form non-salt SOAs under dry conditions.32 Furthermore, Price et al. found that only TMA would generate oligomers through peroxy free radicals.33 This might be part of the explanation for why TMA formed more non-salt SOAs than other amines. Besides, amines also affect submicron aerosols in other different ways. First, the reactions of amines with dicarbonyl aldehydes contribute to the formation of BrC (a part of SOAs) and such reactions will be discussed in detail in the next section. Second, amines have an effect on other atmospheric reactions and climates. For example, Duporte et al. confirmed that the presence of DMA enhanced the nucleation of α-pinene ozonation and the formation of SOAs.130 The photooxidation process of amines induced by ˙OH also changes the surface structure of existing visible particles in the atmosphere, such as soot particles.175
4.3 Other reactions and secondary aerosols
On account of possessing the inherent characteristics of high alkalinity and polarity, the fate of atmospheric amines goes far beyond being oxidized or facilitating NPF. Yu et al. suggested that aerosol uptake with an uptake coefficient of 0.03 reduced the lifetime of MAs by ∼30% over oceans and much more over the major continents, resulting in the shortened lifetime of amines compared to merely oxidation reactions.176 In some circumstances, due to lower concentrations of atmospheric oxidants, relatively weak photochemistry and more complex sources and meteorology, other factors besides oxidizing capacity play more important roles in affecting the uptake of amines by aerosol particles.87 Many atmospheric observations have detected the presence of amines in aerosols. Youn et al. found that DMA is the most abundant amine in aerosols and cloud water in semi-arid (Tucson, Arizona) and marine (Nucleation on the central coast of California) areas,177 while Lidbury et al. found that the DMA-derived SOA is among the most abundant SOAs detected in marine fine particles over the North and tropical Atlantic Oceans.91 Besides, Huang et al. reported that DMA and DEA are the dominant alkylamines in atmospheric aerosol particles collected in Tung Chung, Hong Kong.178 In addition, Liu et al. observed MAs and MA-N-oxides in aerosol samples from Beijing,71 while Feng et al. detected eight amines in urban samples of Shanghai.78 Recently, Du et al. conducted a field observation and found four amines in the aerosols of the Yangtze River Delta, China.69 Notably, the gas-particle transformation always results in amines distributing in the particle phase. The concentrations of MA, EA, and aniline in PM all over the world are estimated at about several to dozens of ng m−3. Field observation68,179,180 and laboratory simulation110,115 indicate that secondary amines are more easily detected in atmospheric PM samples. This is either because they tend to accumulate in the sample size range, or they are chemically stabler than the salts formed by primary and tertiary amines, or favored thermodynamic equilibrium of secondary aminium salts in less acidic aqueous droplets.153,179,181,182 Besides, dialkylamines are less prone to oxidation than trialkylamines.115,181 In conclusion, competitive mechanisms of gas-particle distribution and oxidative degradation may favour the selective accumulation of dialkylaminium salts in environmental aerosol samples relative to homologous species. Some studies also found that TMA is one of the important precursors of DMA in particles, which may also partly account for the phenomenon mentioned above.74,180
Atmospheric amines can be incorporated into aerosol particles through direct dissolution, the homogeneous or heterogeneous reaction with acidic substances or the displacement reaction with NH3. The gas-particle transformation of amines is complicated because a solid phase and at least three types of liquid phases should be considered for unprotonated amines (free-base is represented as Am) and/or aminium salts (protonated Am is represented as AmH) in atmospheric particles.180,183 Three types of liquid phases are considered individually or simultaneously possible for absorptive uptake of atmospheric amines, including a mostly water phase, a mostly (by mass) organic phase that has at least some polarity (e.g., predominantly a secondary organic aerosol, which may contain significant water on a mole fraction basis), and a mostly organic phase that is less polar (e.g., predominantly a primary organic aerosol, containing little water).180,183 The simplest is that gas-phase amines may be physically adsorbed onto PM.64,87 However, other processes may be more important than physical adsorption. For example, Chen et al. found that cyclohexylamine is more likely to enter the condensed phase by direct dissolution, while DBA is more likely to enter the particle phase by reacting with acid gas.64 Hence, they suggested that the absorption process in the aqueous phase plays a more important role in the gas-particle transformation of amines than physical adsorption.
4.3.1 Direct dissolution.
Direct dissolution is a way for gas-phase amines to participate in the growth of secondary aerosols. Liu et al. found that about 7.4% of gaseous amines are distributed into the particle phase, and direct dissolution is the key step of gas-particle distribution in high relative humidity (RH).11 High RH would enhance the gas-particle transformation of amines, which promotes gas-phase amine dissolution in the aqueous phase of PM.11,68,87 Zhou et al. observed the enhancement of gas-phase TMA to particles by cloud and fog processing in both field and laboratory simulations.87 Furthermore, aerosol aminiums have a peak at 0.56–1.0 μm (referred to as droplet mode) and a peak at 0.18–0.32 μm (referred to as condensation mode). Yu et al. also considered that DMA and TMA in PM exhibit an accumulation mode with a median mass aerodynamic diameter of 0.7 ± 0.1 μm, which is referred to as droplet mode associated with cloud processing.184 Nielsen et al. suggested that just protonation would greatly change the degradation pattern of dissolved amines compared to pure gas-phase systems.185 In addition to being dissolved in the aqueous phase, many studies suggested that amines exist in an unprotonated state in the liquid organic phase.68,72,180,186 Xie et al. considered that particulate DMA and TMA in the <0.2 μm size range overwhelmingly existed in the liquid organic phase as unprotonated TMA and DMA, but those in the >0.2 μm size range mainly exist in the aqueous (or solid) phase.180 Chen et al. also found that the mixing state of amine-containing particles is favorable for the uptake of amines via different pathways including absorptive uptake on organic aerosols.68 Besides, Gao et al. inferred that the observed TMA in PM in marine atmospheres is probably overwhelmed by primary sea spray organic aerosols and existed mainly in either the organic phase or mixed phase.186 However, more thermodynamic parameters in the liquid organic phase as well as the information of major organic compounds at the molecular level are required for examining the analysis comprehensively.
4.3.2 Acid–base reaction.
The acid–base reaction of amines with acidic substances will promote the absorption of amines in PM. Ge et al. believed that gaseous amines might condense onto particles after reacting with acidic substances under low RH conditions.50,51 Smog chamber simulation experiments have shown that the reaction of amines with nitric acid could produce granular aminium nitrate and finally achieved the distribution balance between the gas-phase and particle-phase.110,114 Zhang et al. found that nitrates and sulphates have a stronger correlation with TMA during fogging, while NH3 dominates on sunny days in Guangzhou.57 Pratt et al. proposed that amines are closely related to nitrates and sulphates in summer and less so in autumn in the United States.187 Franziska et al. also demonstrated that TMA might take part in neutralizing acidic aerosols along with ammonia, which would be enhanced in the vicinity of clouds and fog through dissolution of TMA in droplets and subsequent acid–base reactions.81 Chen et al. also found that the reaction between Am and aqueous-phase acid in the water phase likely promotes water absorption.64 Youn et al. observed that the DMA
:
NH4+ molar ratios in aerosols have peaks between 0.18 and 1.0 μm, suggesting that DMA competes better with NH3 in those sizes in terms of reactive uptake by particles.177 The competitive uptake of DMA against NH3 on acidic aerosols generally follows thermodynamic equilibria and appears to be sensitive to DMA
:
NH3 molar ratios.72 Chen et al. found that the molar ratio of DMAH+ to (DMA + DMAH+) is about 0.31 with a corresponding DMA
:
NH3 ratio of about 1.8 × 10−3, about 0.80 with a corresponding DMA
:
NH3 ratio of about 1.3 × 10−2, and about 0.56 in the remaining cases.72 Atmospheric amines will be more effective in neutralizing acidic substances in a smaller size range. For example, Yu et al. first found that there is a peak of DMA+ and TMA+ in the particle size range of 0.01–0.1 μm, implying that the two ions likely play an important role in neutralizing acidic species in smaller particles.184 Besides, Xie et al. proposed a weaker neutralization potential of DMA and TMA for acidic species against NH4+ in larger size particles at 0.8 ± 0.2 μm.180 Normally, amines also have a preference for different acidic substances. For instance, Youn et al. observed that the DMA concentration in PM is proportional to the sulfate concentration, while not significantly correlating with the nitrate concentration.177 However, Liu et al. proposed that amines (mainly DMA) are mostly likely in the form of aminium nitrate while NH3 is found mostly likely in the form of ammonia sulfate.11 Chen et al. suggested that the higher measured partition fraction of DBA than the predicted value is likely due to the reaction with gaseous HCl.64 Besides inorganic acids, Fairhurst et al. studied the reactions of amines with a series of solid diacids.188,189 They found that butylaminium salts formed from evaporation of aqueous solutions of n-BA with C3, C5 and C7 diacids are viscous liquids, suggesting that ionic liquids form on the surface during the reactions of gas-phase amines with odd carbon diacids.189 It is possible that ionic liquids may be formed via reactions of amines with the acidic components of semi-solid SOAs, which should be noticed. However, Erupe et al. found that aminium salts are formed from acid–base interactions and amine-N-oxides are formed by direct oxidation by O3 which only accounted for minor amounts of particulate amines (1.5%).190 This suggests that a large proportion of particulate amines have other sources.
4.3.3 Replacement reaction.
Alkylamines can react with ammonium sulphates (AS) as an ammonia replacement reaction, while ammonium bisulphates can also undergo acid–base reactions with amines.40,191,192 However, as the particle size grows, the corresponding surface to volume ratio decreases and the time required to achieve complete conversion of ammonium salts to aminium salts becomes excessively long. Complete exchange of NH3 in ammonium bisulfate and ammonium nitrate clusters (sub-3 nm) by amines is expected to occur within several seconds to minutes in the ambient atmosphere,193 while exchange of NH3 of ammonium nitrate in 20–500 nm particles is measurable within a few hours.194 Besides the effect of particle size, the aerosol phase state also affects the occurrence of the displacement reaction.192,195 The exchange rates of aqueous particles are generally higher than those of their corresponding solid counterparts. For example, Chan et al. found that the heterogeneous uptake of about 40 ppm TEA by multiple aqueous ammonium salts leads to an increase in the particle mass of over 90%. at 50 or 75% RH, while crystalline solid particles of (NH4)2SO4 and (NH4)2C2O4 experienced small mass increases of <5%, both of which are attributed to the hindered mass transfer of TEA in crystalline solids.192 However, TEA reacted with an amorphous solid NH4NO3 particle at <3% RH as effectively as if it were in an aqueous NH4NO3 droplet (50% RH). The exchange of NH3/amine vapors in the particle phase is reversible when the salt particles are in an aqueous state at elevated RH. Overall, the phase states of ammonium and aminium salt particles crucially determine the replacement reaction rates and final product properties and identities.195
All in all, atmospheric amines contribute to formed aerosols via abundant ways. They may directly participate in the formation of aerosols through dissolving in PM, undergoing acid–base reactions with acidic substances in PM, or displacing NH3 in PM. In addition, atmospheric amines also contribute to SOAs indirectly. A portion of the oxidation products of amines with low volatility will condense onto the PM. Amines may merely make aerosols grow and become larger, or may even change the internal structure of aerosols. The latter could change the light-absorbing capacity, viscosity and hygroscopicity of PM, and then affect the climate.
4.4 Contribution of amines to BrC formation
BrC is a part of atmospheric particles that absorbs light like black carbon (BC). Different from BC, BrC is a kind of organic matter that can show strong absorption of solar radiation in the blue visible and near-ultraviolet regions (between 200 and 550 nm), and its absorption in the ultraviolet band increases with the shortening of wavelength.47 Many reactions of amines with dicarbonyl aldehydes were reported in atmospheric multiphase or aqueous phase systems, finding that the presence of amines accelerated the browning process in the aqueous-phase or aerosol-phase (Fig. 7). The formation rates of BrC from heterogeneous reactions of amines with dicarbonyl aldehydes are several orders of magnitude faster than those in bulk solution.108,109 The reactions of amines with dicarbonyl aldehydes are even more effective than the replacement reaction of amines and ammonium salts. For example, De Haan et al. suggested that gas-phase MA was more effective when reacting with aqueous-phase methylglyoxal than ammonium salts.109 Ammonium salts are important N-containing substances and have a potential contribution to the formation of BrC. For example, De Haan et al. observed the formation of BrC when methylglyoxal was exposed to aerosols containing amines or AS before and after cloud processing.108 But amines may be more effective than ammonium salts in promoting BrC formation. For example, Powelson et al. suggested that amines were more effective than AS to form BrC via reactions with small carbonyl compounds on a per mole basis generally.46 Besides, increased BrC formation on both ammonium sulphate and methylammonium sulphate seed particles was observed in the presence of methylglyoxal and MA, regardless of wet or dry particles.108
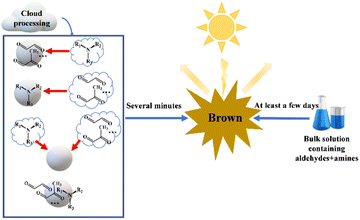 |
| Fig. 7 The patterns of the formation of brown carbon between amines and aldehydes. | |
Many studies have shown how amines and dicarbonyl aldehydes form BrC. De Haan et al. found that glyoxal would irreversibly trap volatile amines in the aerosol-phase and then converted them into oligomers.44 Furthermore, they explored the effect of MA on aerosols containing methylglyoxal, and the results showed that reactive absorption of MA into clouds and aqueous aerosols might affect the optical and physical properties of SOAs by converting existing oligomers into surface-active, light-absorbing nitrogen-containing oligomers and hygroscopic cationic imidazoles, even if the size of the SOA remains constant.196 Besides, the structure of aldehydes will affect the efficiency of BrC formation. Marrero-Ortiz et al. found that the single scattering albedo for methylglyoxal-amine mixtures was smaller than that of glyoxal-amine mixtures and increased with the methyl substitution of amines. Their conclusion suggested that BrC formed from the particle-phase reaction of methylglyoxal with MA was the most likely to contribute to atmospheric warming because its nucleophilic addition reactions were most efficient (facilitating nucleophilic attack through electrostatic attraction between carbonyl and amine groups, forming N-heterocyclic rings).197 In addition, BrC formation may be promoted in the presence of other carbonyl compounds. For example, Rodriguez et al. found a significantly increased yield of imidazole products (precursor of BrC) in the amine + methylglyoxal reaction in the presence of formaldehyde and acetaldehyde in cloud droplets.198 However, the BrC formation of aldehyde-amine-ammonium sulfate browning reactions is found to be at least 10 times lower than that of wood burning because its mass absorption coefficient is lower than that of wood burning and only responsible for <10% of light absorption.46
In summary, amines can promote BrC formation both in the gas-phase and aerosol-phase, especially during cloud processing. The heterogeneous reactions of amines and dicarbonyl aldehydes yield BrC much more quickly than their bulk solution. There is still much research space in this area because there are various circumstances which can form BrC in a complex atmospheric environment.
4.5 Influence of amines on the hygroscopicity/CCN activity of aerosols
The interaction with water vapors often plays an important role in the growth of atmospheric nanoparticles. Hence, hygroscopicity is an important parameter for ultrafine particles. Recently, Corral et al. observed DMA in cloud water over the northwest Atlantic, implying that DMA might enhance the hygroscopicity of particles and assisted the activation of CCN.48 Alkylaminium sulfates (AASs) are highly hygroscopic and absorb water even at low RH.53,199,200 Many studies characterized the hygroscopic growth factor (HGF) of alkylamine salts by using a humidifier tandem differential mobility analyzer (HTDMA).49,160,182,201 The HGF is defined as the ratio Dp,RH/Dp,0, where Dp,RH and Dp,0 represent the mobility diameters at higher RH values and of dry particles, respectively. The hygroscopic growth of aminium sulfate particles is distinct from that of AS whose deliquescence only occurs at RH greater than 79%.53,182 Qiu et al. found that aerosols of all AASs including monomethylaminium sulfate (MMAS), dimethyaminium sulfate (DMAS), trimethylaminium sulfate (TMAS), diethylaminium sulfate (DEAS) and triethylaminium sulfate (TEAS) exhibit a monotonic increase in size when exposed to increasing RH (in the range of 10-90%) without a clear deliquescence point or the deliquescence point is much lower than that of AS, suggesting that AASs can readily absorb water even at low RH to lead to aerosol volume and mass increases.182 Furthermore, even a small mass fraction of AASs such as MMAS and DMAS can considerably lower the deliquescence point and enhance water adsorption on AS particles, which may as a result of the replacement reaction of alkylamines with AS aerosols lead to a transition from the crystalline to an amorphorous phase and an improved water uptake, considerably enhancing their direct and indirect climate forcing.182 Therewith, Clegg et al. converted the HGF determined by Qiu et al.182 to a moles of water per mole of solute basis and obtained the relationships between the concentration and equilibrium RH (water activity) for solutions of the five AASs.202 Chu et al. also defined water-to-solute molar ratios (WSRs) and the hygroscopicity was expressed as the WSR as a function of RH;199 however, the WSRs of MMAS, DMAS and TMAS particles they obtained through the Raman spectra are significantly higher than those of Clegg et al..202 Chu et al. suggested that the parameter Dp,0 defined by Clegg et al. at RH ∼12% would underestimate the WSRs. Besides, Chu et al. found that AASs are more hygroscopic than their corresponding ammonium counterparts within <3–90% RH, and the deliquescence points of MMAS and monoethylaminium sulfate (MEAS) were 37–43% and 12–17%, respectively, while DMAS and TMAS exhibit a continuous hygroscopic trend without either efflorescence or deliquescence. During the drying process (RH 90–<3%), the amines of MMAS, MEAS and monoethanolamine sulfate at an aminium/sulfate (A/S) molar ratio of 2.0 would not evaporate and the particles are stable, whereas the amines of DMAS and TMAS would evaporate at similar molar ratios, resulting in 1.9–1.5 and 1.7–1.0, respectively.199,200 However, Tian et al. found that monoethanolamine sulfate is less hygroscopic than MEAS, AS, and ammonium bisulfate.200 They also calculated the uptake coefficients of MEA, which are estimated to be (3.23 ± 0.64)×10−3 and (9.89 ± 2.62)×10−4 at 40% and 70% RH, respectively, and the negative correlation of the uptake coefficients of MEA and RH is likely due to the increased mass accommodation and Henry's law solubility at lower RH.200 Besides, Sauerwein et al. suggested that the number of methyl or ethyl groups in the aminium ion might have a stronger lowering effect on water activity than the alkyl chain length in concentrated solutions.203 Besides the effect of the structure of amines on the hygroscopic capacity of aminium salts, the potential impact of acids cannot be ignored. Lavi et al.’s measurements of the volatility of alkylaminium carboxylate salts show high thermal stability from organic acid–amine reactions, with measured vapor pressures of alkylaminium monocarboxylate salts of ∼10−9 Pa and alkylaminium dicarboxylate salts of ∼10−6 Pa.201 They measured the hygroscopicity of nucleation mode alkylaminium carboxylate (10–15 nm) and accumulation mode (100 nm) particles, and found that these salts have no deliquescence point. Besides, the measured HGFs of 10–15 nm particles at 90% RH are 1.49 ± 0.07 and 1.61 ± 0.21 for acetate and propionate salts, respectively, which are comparable to the HGF of 10 nm AS particles (1.49), while the HGFs of 100 nm of these salts are higher (1.83 ± 0.09 and 1.77 ± 0.13, respectively) than that of AS (1.58).201 Furthermore, Gomez-Hernandez et al. found that the deliquescence point is apparent for several alkylaminium dicarboxylates but not for alkylaminium monocarboxylates which were dependent on their molecular functionality.49 The HGF at 90% RH is in the range of 1.3 to 1.8 for alkylaminium monocarboxylates and 1.1 to 2.2 for alkylaminium dicarboxylates, depending on the molecular functionality (i.e., the carboxylic or OH functional group in organic acids and methyl substitution in alkylamines).49 Besides, the measured hygroscopicity of alkylaminium carboxylates increases with a decreasing acid to base ratio. Recently, Liu et al. incorporated surface tension into the conventional Köhler model, and found that the critical supersaturation follows a consistent order of AS > MMAS > DMAS > TMAS over 10 nm and MMAS > DMAS > AS > TMAS for ultra-small particles with ∼3 nm.204 According to their results, the value of AS is higher at large size and contributes more to CCN activity, but MMAS and DMAS would show higher hygroscopic capacity in primary nanoparticles. Clearly, the potential hydrate formation, unknown phase-state, and particle-shape effect represent fundamental limitations in measurements of the hygroscopic growth and CCN activity of alkylaminium aerosols.
Conventionally, Köhler theory is employed to predict the critical supersaturation of aerosols, a threshold above which particle growth by water vapor uptake and formation of a stable cloud droplet become thermodynamically favorable.205 Organic salt formation from the reactive uptake of amines increases the effective van't Hoff factor of the solute, thereby decreasing the water vapor saturation required for a particle to develop into a CCN.160,206 Gomez-Hernandez et al. found that the activation diameters of alkylaminium carboxylates range from 74 to 219 nm at a supersaturation value of 0.19%, suggesting that alkylaminium carboxylates can readily form cloud droplets under atmospherically relevant conditions.49
All in all, hygroscopicity affects the optical (i.e., scattering and absorption) properties of aerosols, which impact not only visibility and air quality but also direct radiative forcing, and the CCN activation efficiency of aerosols is related to cloud microphysics and precipitation efficiency as well as to the indirect radiative forcing in climate projections, which is highly uncertain. Alkylaminium salts may play an important role in both hygroscopicity and CCN activation, and aerosol particle containing alkylaminium salts have significant impacts on the climate by direct interactions with solar radiation and indirect contributions to cloud formation.
5. Future perspectives
The review mainly summarizes the environmental concentrations, source analysis and pollution profiles of atmospheric amines. But due to the unique characteristics of amines of low concentrations, strong reactivity, regional distribution, viscosity and alkalinity, some new advanced monitoring methods and instruments have yet to be demonstrated. According to amine emission at a specific location, we suggest that multiple detection methods can be used simultaneously for long-term, continuous monitoring. In addition, various atmospheric amines may have different sinks in each specific atmospheric environment, and then produce different atmospheric effects due to the formation of various transformation products from the homogeneous and heterogeneous reactions of amines with various reactive species in the atmosphere. Therefore, it is necessary to comprehensively evaluate the temporal and spatial distribution characteristics of atmospheric amines, which requires more measured data. In particular, the indoor source of amines generated by human daily life is worthy of attention which has been overlooked. If amines are taken into account, indoor pollution may need to be reconsidered. For example, squalene is a kind of ozone-active substance in human skin lipids and has been a hot research area for indoor pollution in recent years.6,207–209 When the effects of indoor amines are considered in these studies, there may be surprising results. Besides, most studies on the migration and transformation of atmospheric amines only consider the concentration of oxidants, types of temperature, and air humidity at present. But the migration and transformation in actual meteorological environments have not been thought about too much yet, which also should be taken into account in the subsequent work.
Then, the various atmospheric oxidation reactions of amines in the atmosphere are introduced. We mainly summarize the kinetics and mechanisms of the homogeneous reactions of amines with different oxidants in the atmosphere. We should take temperature into account when studying dynamics because partial amines are discharged from industrial processes or biomass combustion. Besides, the oxidation of amines by some other intermediate free radicals shouldn't be ignored, and sCIs are the classic example.131–133 However, the reactions of amines with sCIs or other intermediate radicals and the autoxidation mechanisms of amines still need more monitoring data and simulation experiments to be confirmed. In addition to radicals, more attention also should be paid to the secondary oxidation mechanism of parent amines and their degradation products in the future which is often ignored and underestimated. Specifically, some small molecule aldehydes are not only the important products of amine oxidation, but also the main products of olefin and benzene oxidation in the atmosphere, which have the potential for aldehyde–amine reactions. After the initial step, radicals formed by amine oxidation are consumed by bimolecular reactions or autooxidation pathways. The structure of amines affects their atmospheric transformation pathways as well as the branching ratios of each pathway. Therefore, we expect more studies comparing the structural effects of amines in the future.
The contributions of amines to aerosol particles were also summarized from various homogeneous and heterogeneous reactions, and the focus domains include NPF, the growth of submicron aerosols including oxidation product condensation, direct dissolution, acid–base reactions and replacement reactions, the formation of BrC, and the ability to enhance hygroscopicity and activate CCN. First, SA is found to be a very important precursor to NPF, and the existence of atmospheric amines was also found to promote this process. Future studies should focus on the synergistic effects of multiple amines or NH3 on NPF and the promoting effects of amines on the nucleation of other organic acids. In addition to facilitating NPF, amines also have a non-negligible contribution to submicron aerosols. Amines can enter aerosol particles directly or indirectly through direct dissolution, acid–base reactions, or replacement reactions. In addition, the low volatile oxidation products from amine oxidation also facilitate the growth of SOAs or change the structure of SOAs. Besides, the contribution of amines to SOAs in the presence of inorganic gases should also be properly considered. Although it has been known that amines have a non-negligible contribution to the generation and growth of SOAs, how specific amines or their products contribute to the processes has not been studied in detail. For another, BrC is a kind of special SOA which is light-absorbing. Amines may contribute to the browning process of glyoxal or methylglyoxal in heterogeneous or aqueous phases, especially in cloud processing. The rates are much faster than in their bulk solution. RH and the pH value influence these processes significantly and future studies should pay more attention to the influence of these factors on the formation of BrC. Besides, future work also should comprehensively estimate BrC caused by amines–aldehydes or biomass combustion and the light absorption of different BrC, so as to identify the contribution of amines to this aspect. The effects of amines in aerosol particles on the climate do more than promote the formation of BrC. Alkylaminium salts may play an important role in both hygroscopicity and CCN activation, and aerosol particle containing alkylaminium salts have significant impacts on the climate by direct interactions with solar radiation and indirect contributions to cloud formation. The composition of PM in the atmosphere is complex, and it is necessary to study the CCN activation capacity of alkylaminium salts in more complex or real situations in the future.
All in all, given their environmental behaviour in the atmosphere, amines may also have negative effects on human health during their life cycle.76,210 To fully understand the effects of amines and their degradation intermediates on human health, future studies should consider different exposure pathways, such as skin, ingestion, and inhalation. On the other hand, the acute and chronic effects of amines on the human body also need to be considered in the near future.
Conflicts of interest
There are no conflicts to declare.
Acknowledgements
This work was financially supported by the National Natural Science Foundation of China (42020104001 and 42177354), Local Innovative and Research Teams Project of the Guangdong Pearl River Talents Program (2017BT01Z032), Key-Area Research and Development Program of Guangdong Province (2019B110206002), and Guangdong Basic and Applied Basic Research Foundation (2019B151502064).
References
- D. Tong, J. Chen, D. Qin, Y. Ji, G. Li and T. An, Mechanism of atmospheric organic amines reacted with ozone and implications for the formation of secondary organic aerosols, Sci. Total Environ., 2020, 737, 139830 CrossRef CAS.
- W. Zhang, J. Zhong, Q. Shi, L. Gao, Y. Ji, G. Li, T. An and J. S. Francisco, Mechanism for Rapid Conversion of Amines to Ammonium Salts at the Air–Particle Interface, J. Am. Chem. Soc., 2021, 143, 1171–1178 CrossRef CAS.
- B. C. McDonald, J. A. de Gouw, J. B. Gilman, S. H. Jathar, A. Akherati, C. D. Cappa, J. L. Jimenez, J. Lee-Taylor, P. L. Hayes, S. A. McKeen, Y. Y. Cui, S.-W. Kim, D. R. Gentner, G. Isaacman-VanWertz, A. H. Goldstein, R. A. Harley, G. J. Frost, J. M. Roberts, T. B. Ryerson and M. Trainer, Volatile chemical products emerging as largest petrochemical source of urban organic emissions, Science, 2018, 359, 760–764 CrossRef CAS PubMed.
-
WHO global air quality guidelines, Particulate Matter (PM2.5 and PM10), Ozone, Nitrogen Dioxide, Sulfur Dioxide and Carbon Monoxide, World Health Organization, Geneva, 2021, Licence: CC BY-NC-SA 3.0 IGO, https://www.who.int/publications/i/item/9789240034228) Search PubMed.
- A. H. Goldstein and I. E. Galbally, Known and unexplored organic constituents in the earth's atmosphere, Environ. Sci. Technol., 2007, 41, 1514–1521 CrossRef CAS.
- J. Y. Xiong, Z. C. He, X. C. Tang, P. K. Misztal and A. H. Goldstein, Modeling the Time-Dependent Concentrations of Primary and Secondary Reaction Products of Ozone with Squalene in a University Classroom, Environ. Sci. Technol., 2019, 53, 8262–8270 CrossRef CAS.
- G. Y. Wang, S. M. Jia, X. L. Niu, H. Q. Tian, Y. R. Liu, Z. Xie, C. Liu, Y. C. Dong, Y. Su, J. L. Yu, G. F. Shi, X. F. Chen, L. Li and P. Zhang, Potential factors and mechanism of particulate matters explosive increase induced by free radicals oxidation, J. Environ. Sci., 2019, 81, 205–213 CrossRef CAS.
- B. Shi, W. G. Wang, L. Zhou, J. L. Li, J. Wang, Y. Chen, W. Y. Zhang and M. F. Ge, Kinetics and mechanisms of the gas-phase reactions of OH radicals with three C-15 alkanes, Atmos. Environ., 2019, 207, 75–81 CrossRef CAS.
- C. M. Kenseth, Y. L. Huang, R. Zhao, N. F. Dalleska, C. Hethcox, B. M. Stoltz and J. H. Seinfeld, Synergistic O-3 + OH oxidation pathway to extremely low-volatility dimers revealed in beta-pinene secondary organic aerosol, Proc. Natl. Acad. Sci. U. S. A., 2018, 115, 8301–8306 CrossRef CAS PubMed.
- C. Li, Q. He, A. P. S. Hettiyadura, U. Kafer, G. Shmul, D. Meidan, R. Zimmermann, S. S. Brown, C. George, A. Laskin and Y. Rudich, Formation of Secondary Brown Carbon in Biomass Burning Aerosol Proxies through NO3 Radical Reactions, Environ. Sci. Technol., 2020, 54, 1395–1405 CrossRef CAS.
- F. X. Liu, X. H. Bi, G. H. Zhang, X. F. Lian, Y. Z. Fu, Y. X. Yang, Q. H. Lin, F. Jiang, X. M. Wang, P. A. Peng and G. Y. Sheng, Gas-to-particle partitioning of atmospheric amines observed at a mountain site in southern China, Atmos. Environ., 2018, 195, 1–11 CrossRef CAS.
- H. Su, Y. Cheng and U. Poschl, New Multiphase Chemical Processes Influencing Atmospheric Aerosols, Air Quality, and Climate in the Anthropocene, Acc. Chem. Res., 2020, 53, 2034–2043 CrossRef CAS PubMed.
- R. Yin, C. Yan, R. Cai, X. Li, J. Shen, Y. Lu, S. Schobesberger, Y. Fu, C. Deng, L. Wang, Y. Liu, J. Zheng, H. Xie, F. Bianchi, D. R. Worsnop, M. Kulmala and J. Jiang, Acid–Base Clusters during Atmospheric New Particle Formation in Urban Beijing, Environ. Sci. Technol., 2021, 55, 10994–11005 CrossRef CAS PubMed.
- S. Yang, Z. Liu, P. S. Clusius, Y. Liu, J. Zou, Y. Yang, S. Zhao, G. Zhang, Z. Xu, Z. Ma, Y. Yang, J. Sun, Y. Pan, D. Ji, B. Hu, C. Yan, M. Boy, M. Kulmala and Y. Wang, Chemistry of new particle formation and growth events during wintertime in suburban area of Beijing: Insights from highly polluted atmosphere, Atmos. Res., 2021, 255, 105553 CrossRef CAS.
- H. Li, A. Ning, J. Zhong, H. Zhang, L. Liu, Y. Zhang, X. Zhang, X. C. Zeng and H. He, Influence of atmospheric conditions on sulfuric acid-dimethylamine-ammonia-based new particle formation, Chemosphere, 2020, 245, 125554 CrossRef CAS.
- L. Dada, K. Lehtipalo, J. Kontkanen, T. Nieminen, R. Baalbaki, L. Ahonen, J. Duplissy, C. Yan, B. W. Chu, T. Petaja, K. Lehtinen, V. M. Kerminen, M. Kulmala and J. Kangasluoma, Formation and growth of sub-3-nm aerosol particles in experimental chambers, Nat. Protoc., 2020, 15, 1013–1040 CrossRef CAS.
- S. H. Lee, H. Gordon, H. Yu, K. Lehtipalo, R. Haley, Y. X. Li and R. Y. Zhang, New Particle Formation in the Atmosphere: From Molecular Clusters to Global Climate, J. Geophys. Res. Atmos., 2019, 124, 7098–7146 CrossRef CAS.
- Y. Chang, Y. Gao, Y. Lu, L. Qiao, Y. Kuang, K. Cheng, Y. Wu, S. Lou, S. Jing, H. Wang and C. Huang, Discovery of a Potent Source of Gaseous Amines in Urban China, Environ. Sci. Technol. Lett., 2021, 8, 725–731 CrossRef CAS.
- M. Simon, M. Heinritzi, S. Herzog, M. Leiminger, F. Bianchi, A. Praplan, J. Dommen, J. Curtius and A. Kürten, Detection of dimethylamine in the low pptv range using nitrate chemical ionization atmospheric pressure interface time-of-flight (CI-APi-TOF) mass spectrometry, Atmos. Meas. Tech., 2016, 9, 2135–2145 CrossRef CAS.
- A. J. Kieloaho, H. Hellen, H. Hakola, H. E. Manninen, T. Nieminen, M. Kulmala and M. Pihlatie, Gas-phase alkylamines in a boreal Scots pine forest air, Atmos. Environ., 2013, 80, 369–377 CrossRef CAS.
- U. Kuhn, J. Sintermann, C. Spirig, M. Jocher, C. Ammann and A. Neftel, Basic biogenic aerosol precursors: Agricultural source attribution of volatile amines revised, Geophys. Res. Lett., 2011, 38, L16811 CrossRef.
- T. C. VandenBoer, M. Z. Markovic, A. Petroff, M. F. Czar, N. Borduas and J. G. Murphy, Ion chromatographic separation and quantitation of alkyl methylamines and ethylamines in atmospheric gas and particulate matter using preconcentration and suppressed conductivity detection, J. Chromatogr. A, 2012, 1252, 74–83 CrossRef CAS PubMed.
- M. L. Dawson, V. Perraud, A. Gomez, K. D. Arquero, M. J. Ezell and B. J. Finlayson-Pitts, Measurement of gas-phase ammonia and amines in air by collection onto an ion exchange resin and analysis by ion chromatography, Atmos. Meas. Tech., 2014, 7, 2733–2744 CrossRef.
- F. X. Liu, X. H. Bi, G. H. Zhang, L. Peng, X. F. Lian, H. Y. Lu, Y. Z. Fu, X. M. Wang, P. A. Peng and G. Y. Sheng, Concentration, size distribution and dry deposition of amines in atmospheric particles of urban Guangzhou, China, Atmos. Environ., 2017, 171, 279–288 CrossRef CAS.
- K. Sellegri, B. Umann, M. Hanke and F. Arnold, Deployment of a ground-based CIMS apparatus for the detection of organic gases in the boreal forest during the QUEST campaign, Atmos. Chem. Phys., 2005, 5, 357–372 CrossRef CAS.
- D. R. Hanson, P. H. McMurry, J. Jiang, D. Tanner and L. G. Huey, Ambient Pressure Proton Transfer Mass Spectrometry: Detection of Amines and Ammonia, Environ. Sci. Technol., 2011, 45, 8881–8888 CrossRef CAS PubMed.
- Y. Wang, G. Yang, Y. Lu, Y. Liu, J. Chen and L. Wang, Detection of gaseous dimethylamine using vocus proton-transfer-reaction time-of-flight mass spectrometry, Atmos. Environ., 2020, 243, 117875 CrossRef CAS.
- S. R. Shewchuk, A. Mukherjee and A. K. Dalai, Selective carbon-based adsorbents for carbon dioxide capture from mixed gas streams and catalytic hydrogenation of CO2 into renewable energy source: A review, Chem. Eng. Sci., 2021, 243, 116735 CrossRef CAS.
- X. Chen, G. Huang, C. An, Y. Yao and S. Zhao, Emerging N-nitrosamines and N-nitramines from amine-based post-combustion CO2 capture – A review, Chem. Eng. J., 2018, 335, 921–935 CrossRef CAS.
- X. Ge, S. L. Shaw and Q. Zhang, Toward Understanding Amines and Their Degradation Products from Postcombustion CO2 Capture Processes with Aerosol Mass Spectrometry, Environ. Sci. Technol., 2014, 48, 5066–5075 CrossRef CAS PubMed.
- N. Belman, J. N. Israelachvili, Y. L. Li, C. R. Safinya, J. Bernstein and Y. Golan, The Temperature-Dependent Structure of Alkylamines and Their Corresponding Alkylammonium-Alkylcarbamates, J. Am. Chem. Soc., 2009, 131, 9107–9113 CrossRef CAS.
- X. C. Tang, D. Price, E. Praske, S. A. Lee, M. A. Shattuck, K. Purvis-Roberts, P. J. Silva, A. Asa-Awuku and D. R. Cocker, NO3 radical, OH radical and O-3-initiated secondary aerosol formation from aliphatic amines, Atmos. Environ., 2013, 72, 105–112 CrossRef CAS.
- D. J. Price, C. H. Clark, X. Tang, D. R. Cocker, K. L. Purvis-Roberts and P. J. Silva, Proposed chemical mechanisms leading to secondary organic aerosol in the reactions of aliphatic amines with hydroxyl and nitrate radicals, Atmos. Environ., 2014, 96, 135–144 CrossRef CAS.
- K. H. Moller, T. Berndt and H. G. Kjaergaard, Atmospheric Autoxidation of Amines, Environ. Sci. Technol., 2020, 54, 11087–11099 CrossRef.
- F. Ma, H.-B. Xie, M. Li, S. Wang, R. Zhang and J. Chen, Autoxidation Mechanism for Atmospheric Oxidation of Tertiary Amines: Implications for Secondary Organic Aerosol Formation, Chemosphere, 2020, 273, 129207 CrossRef PubMed.
- T. Berndt, K. H. Moller, H. Herrmann and H. G. Kjaergaard, Trimethylamine Outruns Terpenes and Aromatics in Atmospheric Autoxidation, J. Phys. Chem. A, 2021, 125, 4454–4466 CrossRef CAS PubMed.
- J. Shen, J. Elm, H.-B. Xie, J. Chen, J. Niu and H. Vehkamaki, Structural Effects of Amines in Enhancing Methanesulfonic Acid-Driven New Particle Formation, Environ. Sci. Technol., 2020, 54, 13498–13508 CrossRef CAS PubMed.
- T. Berndt, F. Stratmann, M. Sipila, J. Vanhanen, T. Petaja, J. Mikkila, A. Gruner, G. Spindler, R. L. Mauldin, J. Curtius, M. Kulmala and J. Heintzenberg, Laboratory study on new particle formation from the reaction OH + SO2: influence of experimental conditions, H2O vapour, NH3 and the amine tert-butylamine on the overall process, Atmos. Chem. Phys., 2010, 10, 7101–7116 CrossRef CAS.
- T. Berndt, M. Sipila, F. Stratmann, T. Petaja, J. Vanhanen, J. Mikkila, J. Patokoski, R. Taipale, R. L. Mauldin and M. Kulmala, Enhancement of atmospheric H2SO4/H2O nucleation: organic oxidation products versus amines, Atmos. Chem. Phys., 2014, 14, 751–764 CrossRef.
- J. Zhao, J. N. Smith, F. L. Eisele, M. Chen, C. Kuang and P. H. McMurry, Observation of neutral sulfuric acid-amine containing clusters in laboratory and ambient measurements, Atmos. Chem. Phys., 2011, 11, 10823–10836 CrossRef CAS.
- H. Yu, R. McGraw and S.-H. Lee, Effects of amines on formation of sub-3 nm particles and their subsequent growth, Geophys. Res. Lett., 2012, 39, L02807 CrossRef.
- A. Kurten, A. Bergen, M. Heinritzi, M. Leiminger, V. Lorenz, F. Piel, M. Simon, R. Sitals, A. C. Wagner and J. Curtius, Observation of new particle formation and measurement of sulfuric acid, ammonia, amines and highly oxidized organic molecules at a rural site in central Germany, Atmos. Chem. Phys., 2016, 16, 12793–12813 CrossRef.
- W. N. Zhang, Y. M. Ji, G. Y. Li, Q. J. Shi and T. C. An, The heterogeneous reaction of dimethylamine/ammonia with sulfuric acid to promote the growth of atmospheric nanoparticles, Environ. Sci.: Nano, 2019, 6, 2767–2776 RSC.
- D. O. De Haan, M. A. Tolbert and J. L. Jimenez, Atmospheric condensed-phase reactions of glyoxal with methylamine, Geophys. Res. Lett., 2009, 36, L11819 CrossRef.
- D. O. De Haan, L. N. Hawkins, J. A. Kononenko, J. J. Turley, A. L. Corrigan, M. A. Tolbert and J. L. Jimenez, Formation of Nitrogen-Containing Oligomers by Methylglyoxal and Amines in Simulated Evaporating Cloud Droplets, Environ. Sci. Technol., 2011, 45, 984–991 CrossRef CAS PubMed.
- M. H. Powelson, B. M. Espelien, L. N. Hawkins, M. M. Galloway and D. O. De Haan, Brown Carbon Formation by Aqueous-Phase Carbonyl Compound Reactions with Amines and Ammonium Sulfate, Environ. Sci. Technol., 2014, 48, 985–993 CrossRef CAS PubMed.
- T. W. Kirchstetter, T. Novakov and P. V. Hobbs, Evidence that the spectral dependence of light absorption by aerosols is affected by organic carbon, J. Geophys. Res., 2004, 109, D21208 CrossRef.
- A. F. Corral, Y. Choi, B. L. Collister, E. Crosbie, H. Dadashazar, J. P. DiGangi, G. S. Diskin, M. Fenn, S. Kirschler, R. H. Moore, J. B. Nowak, M. A. Shook, C. T. Stahl, T. Shingler, K. L. Thornhill, C. Voigt, L. D. Ziemba and A. Sorooshian, Dimethylamine in cloud water: a case study over the northwest Atlantic Ocean, Environ. Sci.: Atmos., 2022, 2, 1534–1550 CAS.
- M. Gomez-Hernandez, M. McKeown, J. Secrest, W. Marrero-Ortiz, A. Lavi, Y. Rudich, D. R. Collins and R. Zhang, Hygroscopic Characteristics of Alkylaminium Carboxylate Aerosols, Environ. Sci. Technol., 2016, 50, 2292–2300 CrossRef CAS PubMed.
- X. L. Ge, A. S. Wexler and S. L. Clegg, Atmospheric amines - Part I. A review, Atmos. Environ., 2011, 45, 524–546 CrossRef CAS.
- X. L. Ge, A. S. Wexler and S. L. Clegg, Atmospheric amines - Part II. Thermodynamic properties and gas/particle partitioning, Atmos. Environ., 2011, 45, 561–577 CrossRef CAS.
- D. Lee and A. S. Wexler, Atmospheric amines - Part III: Photochemistry and toxicity, Atmos. Environ., 2013, 71, 95–103 CrossRef CAS.
- C. Qiu and R. Y. Zhang, Multiphase chemistry of atmospheric amines, Phys. Chem. Chem. Phys., 2013, 15, 5738–5752 RSC.
- J. N. Cape, S. E. Cornell, T. D. Jickells and E. Nemitz, Organic nitrogen in the atmosphere — Where does it come from? A review of sources and methods, Atmos. Res., 2011, 102, 30–48 CrossRef CAS.
- N. R. Choi, J. Y. Lee, Y. G. Ahn and Y. P. Kim, Determination of atmospheric amines at Seoul, South Korea via gas chromatography/tandem mass spectrometry, Chemosphere, 2020, 258, 127367 CrossRef CAS PubMed.
- H. Yu and S. H. Lee, Chemical ionisation mass spectrometry for the measurement of atmospheric amines, Environ. Chem., 2012, 9, 190–201 CrossRef CAS.
- G. Zhang, X. Bi, L. Y. Chan, L. Li, X. Wang, J. Feng, G. Sheng, J. Fu, M. Li and Z. Zhou, Enhanced trimethylamine-containing particles during fog events detected by single particle aerosol mass spectrometry in urban Guangzhou, China, Atmos. Environ., 2012, 55, 121–126 CrossRef CAS.
- H. Hellen, A. J. Kieloaho and H. Hakola, Gas-phase alkyl amines in urban air; comparison with a boreal forest site and importance for local atmospheric chemistry, Atmos. Environ., 2014, 94, 192–197 CrossRef CAS.
- Y. You, V. P. Kanawade, J. A. de Gouw, A. B. Guenther, S. Madronich, M. R. Sierra-Hernandez, M. Lawler, J. N. Smith, S. Takahama, G. Ruggeri, A. Koss, K. Olson, K. Baumann, R. J. Weber, A. Nenes, H. Guo, E. S. Edgerton, L. Porcelli, W. H. Brune, A. H. Goldstein and S. H. Lee, Atmospheric amines and ammonia measured with a chemical ionization mass spectrometer (CIMS), Atmos. Chem. Phys., 2014, 14, 12181–12194 CrossRef.
- J. Zheng, Y. Ma, M. D. Chen, Q. Zhang, L. Wang, A. F. Khalizov, L. Yao, Z. Wang, X. Wang and L. X. Chen, Measurement of atmospheric amines and ammonia using the high resolution time-of-flight chemical ionization mass spectrometry, Atmos. Environ., 2015, 102, 249–259 CrossRef CAS.
- L. Yao, M. Y. Wang, X. K. Wang, Y. J. Liu, H. F. Chen, J. Zheng, W. Nie, A. J. Ding, F. H. Geng, D. F. Wang, J. M. Chen, D. R. Worsnop and L. Wang, Detection of atmospheric gaseous amines and amides by a high-resolution time-of-flight chemical ionization mass spectrometer with protonated ethanol reagent ions, Atmos. Chem. Phys., 2016, 16, 14527–14543 CrossRef CAS.
- M. van Pinxteren, K. W. Fomba, D. van Pinxteren, N. Triesch, E. H. Hoffmann, C. H. L. Cree, M. F. Fitzsimons, W. von Tumpling and H. Herrmann, Aliphatic amines at the Cape Verde Atmospheric Observatory: Abundance, origins and sea-air fluxes, Atmos. Environ., 2019, 203, 183–195 CrossRef CAS.
- X. Feng, C. Wang, Y. Feng, J. Cai, Y. Zhang, X. Qi, Q. Li, J. Li and Y. Chen, Outbreaks of Ethyl-Amines during Haze Episodes in North China Plain: A Potential Source of Amines from Ethanol Gasoline Vehicle Emission, Environ. Sci. Technol. Lett., 2022, 9, 306–311 CrossRef CAS.
- Y. Chen, Q. Lin, G. Li and T. An, A new method of simultaneous determination of atmospheric amines in gaseous and particulate phases by gas chromatography-mass spectrometry, J. Environ. Sci., 2021, 273, 027 Search PubMed.
- J. Pfeifer, M. Simon, M. Heinritzi, F. Piel, L. Weitz, D. Wang, M. Granzin, T. Müller, S. Bräkling, J. Kirkby, J. Curtius and A. Kürten, Measurement of ammonia, amines and iodine compounds using protonated water cluster chemical ionization mass spectrometry, Atmos. Meas. Tech., 2020, 13, 2501–2522 CrossRef CAS.
- M. Sipilä, N. Sarnela, T. Jokinen, H. Junninen, J. Hakala, M. P. Rissanen, A. Praplan, M. Simon, A. Kürten, F. Bianchi, J. Dommen, J. Curtius, T. Petäjä and D. R. Worsnop, Bisulfate - cluster based atmospheric pressure chemical ionization mass spectrometer for high-sensitivity (<100 ppqV) detection of atmospheric dimethyl amine: proof-of-concept and first ambient data from boreal forest, Atmos. Meas. Tech., 2015, 8, 4001–4011 CrossRef.
- S. Zhou, J. Lin, X. Qin, Y. Chen and C. Deng, Determination of atmospheric alkylamines by ion chromatography using 18-crown-6 as mobile phase additive, J. Chromatogr. A, 2018, 1563, 154–161 CrossRef CAS PubMed.
- Y. Chen, M. Tian, R. J. Huang, G. Shi, H. Wang, C. Peng, J. Cao, Q. Wang, S. Zhang, D. Guo, L. Zhang and F. Yang, Characterization of urban amine-containing particles in southwestern China: seasonal variation, source, and processing, Atmos. Chem. Phys., 2019, 19, 3245–3255 CrossRef CAS.
- W. Du, X. P. Wang, F. Q. Yang, K. X. Bai, C. Wu, S. J. Liu, F. L. Wang, S. J. Lv, Y. B. Chen, J. Z. Wang, W. L. Liu, L. J. Wang, X. Y. Chen and G. H. Wang, Particulate Amines in the Background Atmosphere of the Yangtze River Delta, China: Concentration, Size Distribution, and Sources, Adv. Atmos. Sci., 2021, 38, 1128–1140 CrossRef CAS.
- T. Mikoviny, C. J. Nielsen, W. Tan, A. Wisthaler, L. Zhu, A. K. Morken and T. N. Nilsen, Ambient Measurements of Amines by PTR-QiTOF: Instrument Performance Assessment and Results from Field Measurements in the Vicinity of TCM, Mongstad, Energy Procedia, 2017, 114, 1017–1021 CrossRef CAS.
- Q. Y. Liu and T. T. Zhang, Determination of Methylamines and Methylamine-N-oxides in Particulate Matter Using Solid Phase Extraction Coupled with Ion Chromatography, Chinese J. Anal. Chem., 2018, 46, 524–529 CrossRef CAS.
- D. Chen, X. Yao, C. K. Chan, X. Tian, Y. Chu, S. L. Clegg, Y. Shen, Y. Gao and H. Gao, Competitive Uptake of Dimethylamine and Trimethylamine against Ammonia on Acidic Particles in Marine Atmospheres, Environ. Sci. Technol., 2022, 56, 5430–5439 CrossRef CAS PubMed.
- S. Zhu, C. Yan, J. Zheng, C. Chen, H. Ning, D. Yang, M. Wang, Y. Ma, J. Zhan, C. Hua, R. Yin, Y. Li, Y. Liu, J. Jiang, L. Yao, L. Wang, M. Kulmala and D. R. Worsnop, Observation and Source Apportionment of Atmospheric Alkaline Gases in Urban Beijing, Environ. Sci. Technol., 2022, 56, 17545–17555 CrossRef CAS PubMed.
- D. Chen, Y. Shen, J. Wang, Y. Gao, H. Gao and X. Yao, Mapping gaseous dimethylamine, trimethylamine, ammonia, and their particulate counterparts in marine atmospheres of China's marginal seas – Part 1: Differentiating marine emission from continental transport, Atmos. Chem. Phys., 2021, 21, 16413–16425 CrossRef CAS.
- G. W. Schade and P. J. Crutzen, Emission of aliphatic amines from animal husbandry and their reactions: Potential source of N2O and HCN, J. Atmos. Chem., 1995, 22, 319–346 CrossRef CAS.
- S. G. Seo, Z. K. Ma, J. M. Jeon, S. C. Jung and W. B. Lee, Measurements of key offensive odorants in a fishery industrial complex in Korea, Atmos. Environ., 2011, 45, 2929–2936 CrossRef CAS.
- X. Huang, C. Deng, G. Zhuang, J. Lin and M. Xiao, Quantitative analysis of aliphatic amines in urban aerosols based on online derivatization and high performance liquid chromatography, Environ. Sci.: Processes Impacts, 2016, 18, 796–801 RSC.
- H. Feng, X. Ye, Y. Liu, Z. Wang, T. Gao, A. Cheng, X. Wang and J. Chen, Simultaneous determination of nine atmospheric amines and six inorganic ions by non-suppressed ion chromatography using acetonitrile and 18-crown-6 as eluent additive, J. Chromatogr. A, 2020, 1624, 461234 CrossRef CAS PubMed.
- X. L. Dong, D. M. Liu and S. P. Gao, Seasonal variations of atmospheric heterocyclic aromatic amines in Beijing, China, Atmos. Res., 2013, 120, 287–297 CrossRef.
- S. W. Gibb, R. F. C. Mantoura and P. S. Liss, Ocean-atmosphere exchange and atmospheric speciation of ammonia and methylamines in the region of the NW Arabian Sea, Global Biogeochem. Cycles, 1999, 13, 161–177 CrossRef CAS.
- F. Köllner, J. Schneider, M. D. Willis, T. Klimach, F. Helleis, H. Bozem, D. Kunkel, P. Hoor, J. Burkart, W. R. Leaitch, A. A. Aliabadi, J. P. D. Abbatt, A. B. Herber and S. Borrmann, Particulate trimethylamine in the summertime Canadian high Arctic lower troposphere, Atmos. Chem. Phys., 2017, 17, 13747–13766 CrossRef.
- I. Lidbury, J. C. Murrell and Y. Chen, Trimethylamine and trimethylamine N-oxide are supplementary energy sources for a marine heterotrophic bacterium: implications for marine carbon and nitrogen cycling, ISME J., 2015, 9, 760–769 CrossRef CAS PubMed.
- X. F. Huang, S. J. Kao, J. Lin, X. F. Qin and C. R. Deng, Development and validation of a HPLC/FLD method combined with online derivatization for the simple and simultaneous determination of trace amino acids and alkyl amines in continental and marine aerosols, PLoS One, 2018, 13, 13 Search PubMed.
- J. Sintermann and A. Neftel, Ideas and perspectives: on the emission of amines from terrestrial vegetation in the context of new atmospheric particle formation, Biogeosciences, 2015, 12, 3225–3240 CrossRef.
- D. R. Gentner, E. Ormeño, S. Fares, T. B. Ford, R. Weber, J. H. Park, J. Brioude, W. M. Angevine, J. F. Karlik and A. H. Goldstein, Emissions of terpenoids, benzenoids, and other biogenic gas-phase organic compounds from agricultural crops and their potential implications for air quality, Atmos. Chem. Phys., 2014, 14, 5393–5413 CrossRef.
- B. K. Place, A. T. Quilty, R. A. Di Lorenzo, S. E. Ziegler and T. C. VandenBoer, Quantitation of 11 alkylamines in atmospheric samples: separating structural isomers by ion chromatography, Atmos. Meas. Tech., 2017, 10, 1061–1078 CrossRef CAS.
- S. Zhou, H. Li, T. Yang, Y. Chen, C. Deng, Y. Gao, C. Chen and J. Xu, Characteristics and sources of aerosol aminiums over the eastern coast of China: insights from the integrated observations in a coastal city, adjacent island and surrounding marginal seas, Atmos. Chem. Phys., 2019, 19, 10447–10467 CrossRef CAS.
- C. Müller, Y. Iinuma, J. Karstensen, D. van Pinxteren, S. Lehmann, T. Gnauk and H. Herrmann, Seasonal variation of aliphatic amines in marine sub-micrometer particles at the Cape Verde islands, Atmos. Chem. Phys., 2009, 9, 9587–9597 CrossRef.
- J. Sun, M. A. Mausz, Y. Chen and S. J. Giovannoni, Microbial trimethylamine metabolism in marine environments, Environ. Microbiol., 2019, 21, 513–520 CrossRef PubMed.
- S. W. Gibb and A. D. Hatton, The occurrence and distribution of trimethylamine-N-oxide in Antarctic coastal waters, Mar. Chem., 2004, 91, 65–75 CrossRef CAS.
- I. Lidbury, M. A. Mausz, D. J. Scanlan and Y. Chen, Identification of dimethylamine monooxygenase in marine bacteria reveals a metabolic bottleneck in the methylated amine degradation pathway, ISME J., 2017, 11, 1592–1601 CrossRef CAS PubMed.
- R. Michalski, P. Pecyna-Utylska and J. Kernert, Determination of ammonium and biogenic amines by ion chromatography. A review, J. Chromatogr. A, 2021, 1651, 462319 CrossRef CAS PubMed.
- S. Manzoor, M. Karl, A. Simperler and A. Korre, Model study on the influence of plant design, photochemistry and meteorology on atmospheric concentrations of nitrosamines and nitramines in vicinity of an amine-based CO2 capture facility, Int. J. Greenh. Gas Control, 2017, 65, 203–217 CrossRef CAS.
- M. Karl, T. Svendby, S. E. Walker, A. S. Velkena, N. Castell and S. Solberg, Modelling atmospheric oxidation of 2-aminoethanol (MEA) emitted from post-combustion capture using WRF-Chem, Sci. Total Environ., 2015, 527–528, 185–202 CrossRef CAS PubMed.
- M. Karl, N. Castell, D. Simpson, S. Solberg, J. Starrfelt, T. Svendby, S. E. Walker and R. F. Wright, Uncertainties in assessing the environmental impact of amine emissions from a CO2 capture plant, Atmos. Chem. Phys., 2014, 14, 8533–8557 CrossRef.
- G. Rochelle, E. Chen, S. Freeman, D. Van Wagener, Q. Xu and A. Voice, Aqueous piperazine as the new standard for CO2 capture technology, Chem. Eng. J., 2011, 171, 725–733 CrossRef CAS.
- S. M. Sharkey, A. M. Hartig, A. J. Dang, A. Chatterjee, B. J. Williams and K. M. Parker, Amine Volatilization from Herbicide Salts: Implications for Herbicide Formulations and Atmospheric Chemistry, Environ. Sci. Technol., 2022, 56, 13644–13653 CrossRef CAS PubMed.
- D. Yang, S. Zhu, Y. Ma, L. Zhou, F. Zheng, L. Wang, J. Jiang and J. Zheng, Emissions of Ammonia and Other Nitrogen-Containing Volatile Organic Compounds from Motor Vehicles under Low-Speed Driving Conditions, Environ. Sci. Technol., 2022, 56, 5440–5447 CrossRef CAS PubMed.
- L. Onel, M. A. Blitz and P. W. Seakins, Direct Determination of the Rate Coefficient for the Reaction of OH Radicals with Monoethanol Amine (MEA) from 296 to 510 K, J. Phys. Chem. Lett., 2012, 3, 853–856 CrossRef CAS PubMed.
- E. C. Tuazon, R. Atkinson, S. M. Aschmann and J. Arey, Kinetics and products of the gas-phase reactions of O 3 with amines and related compounds, Res. Chem. Intermed., 1994, 20, 303–320 CrossRef CAS.
- N. Borduas, J. P. D. Abbatt and J. G. Murphy, Gas Phase Oxidation of Monoethanolamine (MEA) with OH Radical and Ozone: Kinetics, Products, and Particles, Environ. Sci. Technol., 2013, 47, 6377–6383 CrossRef CAS PubMed.
- I. Barnes, P. Wiesen and M. Gallus, Rate Coefficients for the Reactions of OH Radicals with a Series of Alkyl-Substituted Amines, J. Phys. Chem. A, 2016, 120, 8823–8829 CrossRef CAS PubMed.
- Q. G. J. Malloy, L. Qi, B. Warren, D. R. Cocker, M. E. Erupe and P. J. Silva, Secondary organic aerosol formation from primary aliphatic amines with NO3 radical, Atmos. Chem. Phys., 2009, 9, 2051–2060 CrossRef CAS.
- L. Onel, L. Thonger, M. A. Blitz, P. W. Seakins, A. J. C. Bunkan, M. Solimannejad and C. J. Nielsen, Gas-Phase Reactions of OH with Methyl Amines in the Presence or Absence of Molecular Oxygen. An Experimental and Theoretical Study, J. Phys. Chem. A, 2013, 117, 10736–10745 CrossRef CAS PubMed.
- L. Onel, M. Dryden, M. A. Blitz and P. W. Seakins, Atmospheric Oxidation of Piperazine by OH has a Low Potential To Form Carcinogenic Compounds, Environ. Sci. Technol. Lett., 2014, 1, 367–371 CrossRef CAS.
- T. Kurtén, V. Loukonen, H. Vehkamäki and M. Kulmala, Amines are likely to enhance neutral and ion-induced sulfuric acid-water nucleation in the atmosphere more effectively than ammonia, Atmos. Chem. Phys., 2008, 8, 4095–4103 CrossRef.
- J. Almeida, S. Schobesberger, A. Kurten, I. K. Ortega, O. Kupiainen-Maatta, A. P. Praplan, A. Adamov, A. Amorim, F. Bianchi, M. Breitenlechner, A. David, J. Dommen, N. M. Donahue, A. Downard, E. Dunne, J. Duplissy, S. Ehrhart, R. C. Flagan, A. Franchin, R. Guida, J. Hakala, A. Hansel, M. Heinritzi, H. Henschel, T. Jokinen, H. Junninen, M. Kajos, J. Kangasluoma, H. Keskinen, A. Kupc, T. Kurten, A. N. Kvashin, A. Laaksonen, K. Lehtipalo, M. Leiminger, J. Leppa, V. Loukonen, V. Makhmutov, S. Mathot, M. J. McGrath, T. Nieminen, T. Olenius, A. Onnela, T. Petaja, F. Riccobono, I. Riipinen, M. Rissanen, L. Rondo, T. Ruuskanen, F. D. Santos, N. Sarnela, S. Schallhart, R. Schnitzhofer, J. H. Seinfeld, M. Simon, M. Sipila, Y. Stozhkov, F. Stratmann, A. Tome, J. Trostl, G. Tsagkogeorgas, P. Vaattovaara, Y. Viisanen, A. Virtanen, A. Vrtala, P. E. Wagner, E. Weingartner, H. Wex, C. Williamson, D. Wimmer, P. L. Ye, T. Yli-Juuti, K. S. Carslaw, M. Kulmala, J. Curtius, U. Baltensperger, D. R. Worsnop, H. Vehkamaki and J. Kirkby, Molecular understanding of sulphuric acid-amine particle nucleation in the atmosphere, Nature, 2013, 502, 359–363 CrossRef CAS PubMed.
- D. O. De Haan, L. N. Hawkins, H. G. Welsh, R. Pednekar, J. R. Casar, E. A. Pennington, A. de Loera, N. G. Jimenez, M. A. Symons, M. Zauscher, A. Pajunoja, L. Caponi, M. Cazaunau, P. Formenti, A. Gratien, E. Pangui and J.-F. Doussin, Brown Carbon Production in Ammonium- or Amine-Containing Aerosol Particles by Reactive Uptake of Methylglyoxal and Photolytic Cloud Cycling, Environ. Sci. Technol., 2017, 51, 7458–7466 CrossRef CAS PubMed.
- D. O. De Haan, E. Tapavicza, M. Riva, T. Q. Cui, J. D. Surratt, A. C. Smith, M. C. Jordan, S. Nilakantan, M. Almodovar, T. N. Stewart, A. de Loera, A. C. De Haan, M. Cazaunau, A. Gratien, E. Pangui and J. F. Doussin, Nitrogen-Containing, Light-Absorbing Oligomers Produced in Aerosol Particles Exposed to Methylglyoxal, Photolysis, and Cloud Cycling, Environ. Sci. Technol., 2018, 52, 4061–4071 CrossRef CAS PubMed.
- S. M. Murphy, A. Sorooshian, J. H. Kroll, N. L. Ng, P. Chhabra, C. Tong, J. D. Surratt, E. Knipping, R. C. Flagan and J. H. Seinfeld, Secondary aerosol formation from atmospheric reactions of aliphatic amines, Atmos. Chem. Phys., 2007, 7, 2313–2337 CrossRef CAS.
- X. Tang, D. Price, E. Praske, D. N. Vu, K. Purvis-Roberts, P. J. Silva, D. R. Cocker and A. Asa-Awuku, Cloud condensation nuclei (CCN) activity of aliphatic amine secondary aerosol, Atmos. Chem. Phys., 2014, 14, 5959–5967 CrossRef.
- D. J. Price, M. Kacarab, D. R. Cocker, K. L. Purvis-Roberts and P. J. Silva, Effects of temperature on the formation of secondary organic aerosol from amine precursors, Aerosol Sci. Technol., 2016, 50, 1216–1226 CrossRef CAS.
- W. Tan, L. Zhu, T. Mikoviny, C. J. Nielsen, A. Wisthaler, P. Eichler, M. Müller, B. D'Anna, N. J. Farren, J. F. Hamilton, J. B. C. Pettersson, M. Hallquist, S. Antonsen and Y. Stenstrøm, Theoretical and Experimental Study on the Reaction of tert-Butylamine with OH Radicals in the Atmosphere, J. Phys. Chem. A, 2018, 122, 4470–4480 CrossRef CAS PubMed.
- P. J. Silva, M. E. Erupe, D. Price, J. Elias, Q. G. J. Malloy, Q. Li, B. Warren and D. R. Cocker, Trimethylamine as precursor to secondary organic aerosol formation via nitrate radical reaction in the atmosphere, Environ. Sci. Technol., 2008, 42, 4689–4696 CrossRef CAS PubMed.
- Y. B. Gai, M. F. Ge and W. G. Wang, Rate Constants for the Gas Phase Reactions of Ozone with Diethylamine and Triethylamine, Acta Phys. Chim. Sin., 2010, 26, 1768–1772 CAS.
- J. N. Pitts, D. Grosjean, K. Van Cauwenberghe, J. P. Schmid and D. R. Fitz, Photo-oxidation of aliphatic-amines under simulated atmospheric conditions – formation of nitrosamines, nitramines, amides, and photo-chemical oxidant, Environ. Sci. Technol., 1978, 12, 946–953 CrossRef CAS.
- M. Karl, C. Dye, N. Schmidbauer, A. Wisthaler, T. Mikoviny, B. D'Anna, M. Muller, E. Borras, E. Clemente, A. Munoz, R. Porras, M. Rodenas, M. Vazquez and T. Brauers, Study of OH-initiated degradation of 2-aminoethanol, Atmos. Chem. Phys., 2012, 12, 1881–1901 CrossRef CAS.
- G. da Silva, Atmospheric chemistry of 2-aminoethanol (MEA): reaction of the NH2˙CHCH2OH radical with O2, J. Phys. Chem. A, 2012, 116, 10980–10986 CrossRef CAS PubMed.
- L. Onel, M. A. Blitz, J. Breen, A. R. Rickardcd and P. W. Seakins, Branching ratios for the reactions of OH with ethanol amines used in carbon capture and the potential impact on carcinogen formation in the emission plume from a carbon capture plant, Phys. Chem. Chem. Phys., 2015, 17, 25342–25353 RSC.
- Z. Ren and G. da Silva, Atmospheric Oxidation of Piperazine Initiated by OH: A Theoretical Kinetics Investigation, ACS Earth Space Chem., 2019, 3, 2510–2516 CrossRef CAS.
- N. Borduas, J. P. D. Abbatt, J. G. Murphy, S. So and G. da Silva, Gas-Phase Mechanisms of the Reactions of Reduced Organic Nitrogen Compounds with OH Radicals, Environ. Sci. Technol., 2016, 50, 11723–11734 CrossRef CAS PubMed.
- J. Xue, F. Ma, J. Elm, J. Chen and H. B. Xie, Atmospheric oxidation mechanism and kinetics of indole initiated by ˙OH and ˙Cl: a computational study, Atmos. Chem. Phys., 2022, 22, 11543–11555 CrossRef CAS.
- F. Ma, Z. Ding, J. Elm, H.-B. Xie, Q. Yu, C. Liu, C. Li, Z. Fu, L. Zhang and J. Chen, Atmospheric Oxidation of Piperazine Initiated by ·Cl: Unexpected High Nitrosamine Yield, Environ. Sci. Technol., 2018, 52, 9801–9809 CrossRef CAS PubMed.
- J. M. Nicovich, S. Mazumder, P. L. Laine, P. H. Wine, Y. Tang, A. J. C. Bunkan and C. J. Nielsen, An experimental and theoretical study of the gas phase kinetics of atomic chlorine reactions with CH3NH2, (CH3)2NH, and (CH3)3N, Phys. Chem. Chem. Phys., 2015, 17, 911–917 RSC.
- H. B. Xie, F. Ma, Q. Yu, N. He and J. Chen, Computational Study of the Reactions of Chlorine Radicals with Atmospheric Organic Compounds Featuring NHx–π-Bond (x = 1, 2) Structures, J. Phys. Chem. A, 2017, 121, 1657–1665 CrossRef CAS PubMed.
- A. Furuhama, T. Imamura, S. Maeda and T. Taketsugu, Theoretical study of initial reactions of amine (CH3)(n)NH(3-n) (n=1, 2, 3) with ozone, Chem. Phys. Lett., 2018, 692, 111–116 CrossRef CAS.
- S. Rudić, C. Murray, J. N. Harvey and A. J. Orr-Ewing, The product branching and dynamics of the reaction of chlorine atoms with methylamine, Phys. Chem. Chem. Phys., 2003, 5, 1205–1212 RSC.
- H. B. Xie, F. Ma, Y. Wang, N. He, Q. Yu and J. Chen, Quantum Chemical Study on ·Cl-Initiated Atmospheric Degradation of Monoethanolamine, Environ. Sci. Technol., 2015, 49, 13246–13255 CrossRef CAS PubMed.
- T. Szűcs and G. Czakó, Benchmark ab initio stationary-point characterization of the complex potential energy surface of the multi-channel Cl + CH3NH2 reaction, Phys. Chem. Chem. Phys., 2021, 23, 10347–10356 RSC.
- G. Duporte, M. Riva, J. Parshintsev, E. Heikkinen, L. M. F. Barreira, N. Myllys, L. Heikkinen, K. Hartonen, M. Kulmala, M. Ehn and M. L. Riekkola, Chemical Characterization of Gas- and Particle-Phase Products from the Ozonolysis of alpha-Pinene in the Presence of Dimethylamine, Environ. Sci. Technol., 2017, 51, 5602–5610 CrossRef CAS PubMed.
- X. Ma, X. Zhao, Y. Wei, W. Wang, F. Xu, Q. Zhang and W. Wang, Effect of multifunctional compound monoethanolamine on Criegee intermediates reactions and its atmospheric implications, Sci. Total Environ., 2020, 715, 136812 CrossRef CAS PubMed.
- M. Kumar and J. S. Francisco, Elucidating the molecular mechanisms of Criegee-amine chemistry in the gas phase and aqueous surface environments, Chem. Sci., 2019, 10, 743–751 RSC.
- H. F. Mull, G. J. R. Aroeira, J. M. Turney and H. F. Schaefer, The atmospheric importance of methylamine additions to Criegee intermediates, Phys. Chem. Chem. Phys., 2020, 22, 22555–22566 RSC.
- R. Chhantyal-Pun, R. J. Shannon, D. P. Tew, R. L. Caravan, M. Duchi, C. Wong, A. Ingham, C. Feldman, M. R. McGillen, M. A. H. Khan, I. O. Antonov, B. Rotavera, K. Ramasesha, D. L. Osborn, C. A. Taatjes, C. J. Percival, D. E. Shallcross and A. J. Orr-Ewing, Experimental and computational studies of Criegee intermediate reactions with NH3 and CH3NH2, Phys. Chem. Chem. Phys., 2019, 21, 14042–14052 RSC.
- W. Chao, C. Yin, K. Takahashi and J. J.-M. Lin, Effects of water vapor on the reaction of CH2OO with NH3, Phys. Chem. Chem. Phys., 2019, 21, 22589–22597 RSC.
- C. R. C. Lindley, J. G. Calvert and J. H. Shaw, Rate studies of the reactions of the (CH3)2N radical with O2, NO, and NO2, Chem. Phys. Lett., 1979, 67, 57–62 CrossRef CAS.
- M. A. Alam, Z. Ren and G. da Silva, Nitramine and nitrosamine formation is a minor pathway in the atmospheric oxidation of methylamine: a theoretical kinetic study of the CH3NH + O2 reaction, Int. J. Chem. Kinet., 2019, 51, 723–728 CrossRef CAS.
- L. Onel, M. Blitz, M. Dryden, L. Thonger and P. Seakins, Branching Ratios in Reactions of OH Radicals with Methylamine, Dimethylamine, and Ethylamine, Environ. Sci. Technol., 2014, 48, 9935–9942 CrossRef CAS PubMed.
- Y. Tang, M. Hanrath and C. J. Nielsen, Do primary nitrosamines form and exist in the gas phase? A computational study of CH3NHNO and (CH3)2NNO, Phys. Chem. Chem. Phys., 2012, 14, 16365–16370 RSC.
- Y. Tang and C. J. Nielsen, Theoretical Study on the Formation and Photolysis of Nitrosamines (CH3CH2NHNO and (CH3CH2)2NNO) under Atmospheric Conditions, J. Phys. Chem. A, 2013, 117, 126–132 CrossRef CAS PubMed.
- G. da Silva, Formation of Nitrosamines and Alkyldiazohydroxides in the Gas Phase: The CH3NH + NO Reaction Revisited, Environ. Sci. Technol., 2013, 47, 7766–7772 CrossRef CAS PubMed.
- M. P. Rissanen, A. J. Eskola, T. L. Nguyen, J. R. Barker, J. Liu, J. Liu, E. Halme and R. S. Timonen, CH2NH2 + O2 and CH3CHNH2 + O2 reaction kinetics: photoionization mass spectrometry experiments and master equation calculations, J. Phys. Chem. A, 2014, 118, 2176–2186 CrossRef CAS PubMed.
- S. N. Wang, M. Riva, C. Yan, M. Ehn and L. M. Wang, Primary Formation of Highly Oxidized Multifunctional Products in the OH-Initiated Oxidation of Isoprene: A Combined Theoretical and Experimental
Study, Environ. Sci. Technol., 2018, 52, 12255–12264 CrossRef CAS PubMed.
- L. Zhu, T. Mikoviny, A. Wisthaler and C. J. Nielsen, A Sampling Line Artifact in Stack Emission Measurement of Alkanolamine-enabled Carbon Capture Facility: Surface Reaction of Amines with Formaldehyde, Energy Procedia, 2017, 114, 1022–1025 CrossRef CAS.
- M. K. Louie, J. S. Francisco, M. Verdicchio, S. J. Klippenstein and A. Sinha, Dimethylamine Addition to Formaldehyde Catalyzed by a Single Water Molecule: A Facile Route for Atmospheric Carbinolamine Formation and Potential Promoter of Aerosol Growth, J. Phys. Chem. A, 2016, 120, 1358–1368 CrossRef CAS PubMed.
- M. Kulmala, How Particles Nucleate and Grow, Science, 2003, 302, 1000–1001 CrossRef CAS PubMed.
- L. Yao, O. Garmash, F. Bianchi, J. Zheng, C. Yan, J. Kontkanen, H. Junninen, S. B. Mazon, M. Ehn, P. Paasonen, M. Sipila, M. Y. Wang, X. K. Wang, S. Xiao, H. F. Chen, Y. Q. Lu, B. W. Zhang, D. F. Wang, Q. Y. Fu, F. H. Geng, L. Li, H. L. Wang, L. P. Qiao, X. Yang, J. M. Chen, V. M. Kerminen, T. Petaja, D. R. Worsnop, M. Kulmala and L. Wang, Atmospheric new particle formation from sulfuric acid and amines in a Chinese megacity, Science, 2018, 361, 278–281 CrossRef CAS PubMed.
- K. Lehtipalo, C. Yan, L. Dada, F. Bianchi, M. Xiao, R. Wagner, D. Stolzenburg, L. R. Ahonen, A. Amorim, A. Baccarini, P. S. Bauer, B. Baumgartner, A. Bergen, A. K. Bernhammer, M. Breitenlechner, S. Brilke, A. Buchholz, S. B. Mazon, D. X. Chen, X. M. Chen, A. Dias, J. Dommen, D. C. Draper, J. Duplissy, M. Ehn, H. Finkenzeller, L. Fischer, C. Frege, C. Fuchs, O. Garmash, H. Gordon, J. Hakala, X. C. He, L. Heikkinen, M. Heinritzi, J. C. Helm, V. Hofbauer, C. R. Hoyle, T. Jokinen, J. Kangasluoma, V. M. Kerminen, C. Kim, J. Kirkby, J. Kontkanen, A. Kurten, M. J. Lawler, H. J. Mai, S. Mathot, R. L. Mauldin, U. Molteni, L. Nichman, W. Nie, T. Nieminen, A. Ojdanic, A. Onnela, M. Passananti, T. Petaja, F. Piel, V. Pospisilova, L. L. J. Quelever, M. P. Rissanen, C. Rose, N. Sarnela, S. Schallhart, S. Schuchmann, K. Sengupta, M. Simon, M. Sipila, C. Tauber, A. Tome, J. Trostl, O. Vaisanen, A. L. Vogel, R. Volkamer, A. C. Wagner, M. Y. Wang, L. Weitz, D. Wimmer, P. L. Ye, A. Ylisirnio, Q. Z. Zha, K. S. Carslaw, J. Curtius, N. M. Donahue, R. C. Flagan, A. Hansel, I. Riipinen, A. Virtanen, P. M. Winkler, U. Baltensperger, M. Kulmala and D. R. Worsnop, Multicomponent new particle formation from sulfuric acid, ammonia, and biogenic vapors, Sci. Adv., 2018, 4, eaau5363 CrossRef CAS PubMed.
- P. H. McMurry, M. Fink, H. Sakurai, M. R. Stolzenburg, R. L. Mauldin, J. Smith, F. Eisele, K. Moore, S. Sjostedt, D. Tanner, L. G. Huey, J. B. Nowak, E. Edgerton and D. Voisin, A criterion for new particle formation in the sulfur-rich Atlanta atmosphere, J. Geophys. Res. Atmos., 2005, 110, D22S02 Search PubMed.
- M. Sipilä, T. Berndt, T. Petäjä, D. Brus, J. Vanhanen, F. Stratmann, J. Patokoski, R. L. Mauldin, A.-P. Hyvärinen, H. Lihavainen and M. Kulmala, The Role of Sulfuric Acid in Atmospheric Nucleation, Science, 2010, 327, 1243–1246 CrossRef PubMed.
- M. Kulmala, J. Kontkanen, H. Junninen, K. Lehtipalo, H. E. Manninen, T. Nieminen, T. Petäjä, M. Sipilä, S. Schobesberger, P. Rantala, A. Franchin, T. Jokinen, E. Järvinen, M. Äijälä, J. Kangasluoma, J. Hakala, P. P. Aalto, P. Paasonen, J. Mikkilä, J. Vanhanen, J. Aalto, H. Hakola, U. Makkonen, T. Ruuskanen, R. L. Mauldin, J. Duplissy, H. Vehkamäki, J. Bäck, A. Kortelainen, I. Riipinen, T. Kurtén, M. V. Johnston, J. N. Smith, M. Ehn, T. F. Mentel, K. E. J. Lehtinen, A. Laaksonen, V. M. Kerminen and D. R. Worsnop, Direct Observations of Atmospheric Aerosol Nucleation, Science, 2013, 339, 943–946 CrossRef CAS PubMed.
- J. Elm, Clusteromics I: Principles, Protocols, and Applications to Sulfuric Acid-Base Cluster Formation, ACS Omega, 2021, 6, 7804–7814 CrossRef CAS PubMed.
- L. Wang, V. Lal, A. F. Khalizov and R. Y. Zhang, Heterogeneous Chemistry of Alkylamines with Sulfuric Acid: Implications for Atmospheric Formation of Alkylaminium Sulfates, Environ. Sci. Technol., 2010, 44, 2461–2465 CrossRef CAS PubMed.
- J. Julin, B. N. Murphy, D. Patoulias, C. Fountoukis, T. Olenius, S. N. Pandis and I. Riipinen, Impacts of Future European Emission Reductions on Aerosol Particle Number Concentrations Accounting for Effects of Ammonia, Amines, and Organic Species, Environ. Sci. Technol., 2018, 52, 692–700 CrossRef CAS PubMed.
- I. K. Ortega, O. Kupiainen, T. Kurtén, T. Olenius, O. Wilkman, M. J. McGrath, V. Loukonen and H. Vehkamäki, From quantum chemical formation free energies to evaporation rates, Atmos. Chem. Phys., 2012, 12, 225–235 CrossRef CAS.
- P. Paasonen, T. Olenius, O. Kupiainen, T. Kurten, T. Petaja, W. Birmili, A. Hamed, M. Hu, L. G. Huey, C. Plass-Duelmer, J. N. Smith, A. Wiedensohler, V. Loukonen, M. J. McGrath, I. K. Ortega, A. Laaksonen, H. Vehkamaki, V. M. Kerminen and M. Kulmala, On the formation of sulphuric acid - amine clusters in varying atmospheric conditions and its influence on atmospheric new particle formation, Atmos. Chem. Phys., 2012, 12, 9113–9133 CrossRef CAS.
- V. Loukonen, T. Kurtén, I. K. Ortega, H. Vehkamäki, A. A. H. Pádua, K. Sellegri and M. Kulmala, Enhancing effect of dimethylamine in sulfuric acid nucleation in the presence of water – a computational study, Atmos. Chem. Phys., 2010, 10, 4961–4974 CrossRef CAS.
- J. Elm, Elucidating the Limiting Steps in Sulfuric Acid–Base New Particle Formation, J. Phys. Chem. A, 2017, 121, 8288–8295 CrossRef CAS PubMed.
- T. Olenius, O. Kupiainen-Määttä, I. K. Ortega, T. Kurtén and H. Vehkamäki, Free energy barrier in the growth of sulfuric acid–ammonia and sulfuric acid–dimethylamine clusters, J. Chem. Phys., 2013, 139, 084312 CrossRef CAS PubMed.
- J. N. Smith, K. C. Barsanti, H. R. Friedli, M. Ehn, M. Kulmala, D. R. Collins, J. H. Scheckman, B. J. Williams and P. H. McMurry, Observations of aminium salts in atmospheric nanoparticles and possible climatic implications, Proc. Natl. Acad. Sci. U.S.A., 2010, 107, 6634–6639 CrossRef CAS PubMed.
- P. Ge, G. Luo, W. Huang, H. Xie, J. Chen and Y. Luo, Theoretical study of the hydration effects on alkylamine and alkanolamine clusters and the atmospheric implication, Chemosphere, 2020, 243, 125323 CrossRef CAS PubMed.
- F. Ma, H.-B. Xie, J. Elm, J. Shen, J. Chen and H. Vehkamäki, Piperazine Enhancing Sulfuric Acid-Based New Particle Formation: Implications for the Atmospheric Fate of Piperazine, Environ. Sci. Technol., 2019, 53, 8785–8795 CrossRef CAS PubMed.
- W. A. Glasoe, K. Volz, B. Panta, N. Freshour, R. Bachman, D. R. Hanson, P. H. McMurry and C. Jen, Sulfuric acid nucleation: An experimental study of the effect of seven bases, J. Geophys. Res. Atmos., 2015, 120, 1933–1950 CrossRef CAS.
- H. B. Xie, J. Elm, R. Halonen, N. Myllys, T. Kurten, M. Kulmala and H. Vehkamaki, Atmospheric Fate of Monoethanolamine: Enhancing New Particle Formation of Sulfuric Acid as an Important Removal Process, Environ. Sci. Technol., 2017, 51, 8422–8431 CrossRef CAS PubMed.
- B. Temelso, E. F. Morrison, D. L. Speer, B. C. Cao, N. Appiah-Padi, G. Kim and G. C. Shields, Effect of Mixing Ammonia and Alkylamines on Sulfate Aerosol Formation, J. Phys. Chem. A, 2018, 122, 1612–1622 CrossRef CAS PubMed.
- N. Myllys, S. Chee, T. Olenius, M. Lawler and J. Smith, Molecular-Level Understanding of Synergistic Effects in Sulfuric Acid–Amine–Ammonia Mixed Clusters, J. Phys. Chem. A, 2019, 123, 2420–2425 CrossRef CAS PubMed.
- C. X. Xu, S. Jiang, Y. R. Liu, Y. J. Feng, Z. H. Wang, T. Huang, Y. Zhao, J. Li and W. Huang, Formation of atmospheric molecular clusters of methanesulfonic acid–Diethylamine complex and its atmospheric significance, Atmos. Environ., 2020, 226, 117404 CrossRef CAS.
- M. L. Dawson, M. E. Varner, V. Perraud, M. J. Ezell, R. B. Gerber and B. J. Finlayson-Pitts, Simplified mechanism for new particle formation from methanesulfonic acid, amines, and water via experiments and ab initio calculations, Proc. Natl. Acad. Sci. U. S. A., 2012, 109, 18719–18724 CrossRef CAS PubMed.
- A. Pysanenko, K. Fárníková, J. Lengyel, E. Pluhařová and M. Fárník, Molecular-level insight into uptake of dimethylamine on hydrated nitric acid clusters, Environ. Sci.: Atmos., 2022, 2, 1292–1302 CAS.
- C. J. Bready, V. R. Fowler, L. A. Juechter, L. A. Kurfman, G. E. Mazaleski and G. C. Shields, The driving effects of common atmospheric molecules for formation of prenucleation clusters: the case of sulfuric acid, formic acid, nitric acid, ammonia, and dimethyl amine, Environ. Sci.: Atmos., 2022, 2, 1469–1486 CAS.
- A. Ning, L. Liu, S. Zhang, F. Yu, L. Du, M. Ge and X. Zhang, The critical role of dimethylamine in the rapid formation of iodic acid particles in marine areas, NPJ Clim. Atmos. Sci., 2022, 5, 92 CrossRef CAS.
- L. Liu, F. Yu, L. Du and X. Zhang, Rapid sulfuric acid–dimethylamine nucleation enhanced by nitric acid in polluted regions, Proc. Natl. Acad. Sci. U.S.A., 2021, 118, e2108384118 CrossRef CAS PubMed.
- K. Chen, K. Zhang and C. Qiu, Potential enhancement in atmospheric new particle formation by amine-assisted nitric acid condensation at room temperature, Atmos. Environ., 2022, 287, 119252 CrossRef CAS.
- N. Borduas, G. da Silva, J. G. Murphy and J. P. D. Abbatt, Experimental and Theoretical Understanding of the Gas Phase Oxidation of Atmospheric Amides with OH Radicals: Kinetics, Products, and Mechanisms, J. Phys. Chem. A, 2015, 119, 4298–4308 CrossRef CAS PubMed.
- C. Chen, O. Y. Enekwizu, X. Ma, Y. L. Jiang, A. F. Khalizov, J. Zheng and Y. Ma, Effect of organic coatings derived from the OH-initiated oxidation of amines on soot morphology and cloud activation, Atmos. Res., 2020, 239, 104905 CrossRef CAS.
- F. Yu and G. Luo, Modeling of gaseous methylamines in the global atmosphere: impacts of oxidation and aerosol uptake, Atmos. Chem. Phys., 2014, 14, 12455–12464 CrossRef.
- J. S. Youn, E. Crosbie, L. C. Maudlin, Z. Wang and A. Sorooshian, Dimethylamine as a major alkyl amine species in particles and cloud water: Observations in semi-arid and coastal regions, Atmos. Environ., 2015, 122, 250–258 CrossRef CAS PubMed.
- R. Huang, W. B. Li, Y. Wang, Q. Wang, W. T. Jia, K. Ho, J. Cao, G. Wang, X. D. Chen, I. Ei-Haddad, Z. Zhuang, X. Wang, A. S. H. Prévôt, C. O'Dowd and T. Hoffmann, Determination of alkylamines in atmospheric aerosol particles. a comparison of gas chromatography-mass spectrometry and ion chromatography approaches, Atmos. Meas. Tech., 2014, 7, 2027–2035 CrossRef.
- M. C. Facchini, S. Decesari, M. Rinaldi, C. Carbone, E. Finessi, M. Mircea, S. Fuzzi, F. Moretti, E. Tagliavini, D. Ceburnis and C. D. O'Dowd, Important Source of Marine Secondary Organic Aerosol from Biogenic Amines, Environ. Sci. Technol., 2008, 42, 9116–9121 CrossRef CAS PubMed.
- H. Xie, L. Feng, Q. Hu, Y. Zhu, H. Gao, Y. Gao and X. Yao, Concentration and size distribution of water-extracted dimethylaminium and trimethylaminium in atmospheric particles during nine campaigns - Implications for sources, phase states and formation pathways, Sci. Total Environ., 2018, 631–632, 130–141 CrossRef CAS PubMed.
- S. Angelino, D. T. Suess and K. A. Prather, Formation of aerosol particles from reactions of secondary and tertiary alkylamines: Characterization by aerosol time-of-flight mass spectrometry, Environ. Sci. Technol., 2001, 35, 3130–3138 CrossRef CAS PubMed.
- C. Qiu and R. Zhang, Physiochemical Properties of Alkylaminium Sulfates: Hygroscopicity, Thermostability, and Density, Environ. Sci. Technol., 2012, 46, 4474–4480 CrossRef CAS PubMed.
- J. F. Pankow, Phase considerations in the gas/particle partitioning of organic amines in the atmosphere, Atmos. Environ., 2015, 122, 448–453 CrossRef CAS.
- P. Yu, Q. Hu, K. Li, Y. Zhu, X. Liu, H. Gao and X. Yao, Characteristics of dimethylaminium and trimethylaminium in atmospheric particles ranging from supermicron to nanometer sizes over eutrophic marginal seas of China and oligotrophic open oceans, Sci. Total Environ., 2016, 572, 813–824 CrossRef CAS PubMed.
- C. J. Nielsen, H. Herrmann and C. Weller, Atmospheric chemistry and environmental impact of the use of amines in carbon capture and storage (CCS), Chem. Soc. Rev., 2012, 41, 6684–6704 RSC.
- Y. Gao, D. Chen, Y. Shen, Y. Gao, H. Gao and X. Yao, Mapping gaseous dimethylamine, trimethylamine, ammonia, and their particulate counterparts in marine atmospheres of China's marginal seas – Part 2: Spatiotemporal heterogeneity, causes, and hypothesis, Atmos. Chem. Phys., 2022, 22, 1515–1528 CrossRef CAS.
- K. A. Pratt, L. E. Hatch and K. A. Prather, Seasonal Volatility Dependence of Ambient Particle Phase Amines, Environ. Sci. Technol., 2009, 43, 5276–5281 CrossRef CAS PubMed.
- M. C. Fairhurst, M. J. Ezell and B. J. Finlayson-Pitts, Knudsen
cell studies of the uptake of gaseous ammonia and amines onto C3–C7 solid dicarboxylic acids, Phys. Chem. Chem. Phys., 2017, 19, 26296–26309 RSC.
- M. C. Fairhurst, M. J. Ezell, C. Kidd, P. S. J. Lakey, M. Shiraiwa and B. J. Finlayson-Pitts, Kinetics, mechanisms and ionic liquids in the uptake of n-butylamine onto low molecular weight dicarboxylic acids, Phys. Chem. Chem. Phys., 2017, 19, 4827–4839 RSC.
- M. E. Erupe, A. Liberman-Martin, P. J. Silva, Q. G. J. Malloy, N. Yonis, D. R. Cocker and K. L. Purvis-Roberts, Determination of methylamines and trimethylamine-N-oxide in particulate matter by non-suppressed ion chromatography, J. Chromatogr. A, 2010, 1217, 2070–2073 CrossRef CAS PubMed.
- C. Qiu, L. Wang, V. Lal, A. F. Khalizov and R. Y. Zhang, Heterogeneous Reactions of Alkylamines with Ammonium Sulfate and Ammonium Bisulfate, Environ. Sci. Technol., 2011, 45, 4748–4755 CrossRef CAS PubMed.
- L. P. Chan and C. K. Chan, Displacement of Ammonium from Aerosol Particles by Uptake of Triethylamine, Aerosol Sci. Technol., 2012, 46, 236–247 CrossRef CAS.
- B. R. Bzdek, D. P. Ridge and M. V. Johnston, Amine exchange into ammonium bisulfate and ammonium nitrate nuclei, Atmos. Chem. Phys., 2010, 10, 3495–3503 CrossRef CAS.
- J. A. Lloyd, K. J. Heaton and M. V. Johnston, Reactive Uptake of Trimethylamine into Ammonium Nitrate Particles, J. Phys. Chem. A, 2009, 113, 4840–4843 CrossRef CAS PubMed.
- L. P. Chan and C. K. Chan, Role of the Aerosol Phase State in Ammonia/Amines Exchange Reactions, Environ. Sci. Technol., 2013, 47, 5755–5762 CrossRef CAS PubMed.
- D. O. De Haan, A. Pajunoja, L. N. Hawkins, H. G. Welsh, N. G. Jimenez, A. De Loera, M. Zauscher, A. D. Andretta, B. W. Joyce, A. C. De Haan, M. Riva, T. Q. Cui, J. D. Surratt, M. Cazaunau, P. Formenti, A. Gratien, E. Pangui and J. F. Doussin, Methylamine's Effects on Methylglyoxal-Containing Aerosol: Chemical, Physical, and Optical Changes, ACS Earth Space Chem., 2019, 3, 1706–1716 CrossRef CAS.
- W. Marrero-Ortiz, M. Hu, Z. F. Du, Y. M. Ji, Y. J. Wang, S. Guo, Y. Lin, M. Gomez-Hermandez, J. F. Peng, Y. X. Li, J. Secrest, M. L. Zamora, Y. Wang, T. C. An and R. Y. Zhang, Formation and Optical Properties of Brown Carbon from Small alpha-Dicarbonyls and Amines, Environ. Sci. Technol., 2019, 53, 117–126 CrossRef CAS PubMed.
- A. A. Rodriguez, A. de Loera, M. H. Powelson, M. M. Galloway and D. O. Haan, Formaldehyde and Acetaldehyde Increase Aqueous-Phase Production of Imidazoles in Methylglyoxal/Amine Mixtures: Quantifying a Secondary Organic Aerosol Formation Mechanism, Environ. Sci. Technol. Lett., 2017, 4, 234–239 CrossRef CAS.
- Y. Chu, M. Sauerwein and C. K. Chan, Hygroscopic and phase transition properties of alkyl aminium sulfates at low relative humidities, Phys. Chem. Chem. Phys., 2015, 17, 19789–19796 RSC.
- X. Tian, Y. Chu and C. K. Chan, Reactive Uptake of Monoethanolamine by Sulfuric Acid Particles and Hygroscopicity of Monoethanolaminium Salts, Environ. Sci. Technol. Lett., 2022, 9, 16–21 CrossRef CAS.
- A. Lavi, E. Segre, M. Gomez-Hernandez, R. Zhang and Y. Rudich, Volatility of Atmospherically Relevant Alkylaminium Carboxylate Salts, J. Phys. Chem. A, 2015, 119, 4336–4346 CrossRef CAS PubMed.
- S. L. Clegg, C. Qiu and R. Zhang, The deliquescence behaviour, solubilities, and densities of aqueous solutions of five methyl- and ethyl-aminium sulphate salts, Atmos. Environ., 2013, 73, 145–158 CrossRef CAS.
- M. Sauerwein, S. L. Clegg and C. K. Chan, Water Activities and Osmotic Coefficients of Aqueous Solutions of Five Alkylaminium Sulfates and Their Mixtures with H2SO4 at 25oC, Aerosol Sci. Technol., 2015, 49, 566–579 CrossRef CAS.
- L. Liu and H. Li, Hygroscopicity of ultrafine particles containing ammonium/alkylaminium sulfates: A Köhler model investigation with correction of surface tension, Atmos. Environ., 2023, 294, 119500 CrossRef CAS.
- Y. Ma, S. D. Brooks, G. Vidaurre, A. F. Khalizov, L. Wang and R. Zhang, Rapid modification of cloud-nucleating ability of aerosols by biogenic emissions, Geophys. Res. Lett., 2013, 40, 6293–6297 CrossRef CAS.
- A. Lavi, N. Bluvshtein, E. Segre, L. Segev, M. Flores and Y. Rudich, Thermochemical, Cloud Condensation Nucleation Ability, and Optical Properties of Alkyl Aminium Sulfate Aerosols, J. Phys. Chem. C, 2013, 117, 22412–22421 CrossRef CAS.
- M. Zhang, J. Xiong, Y. Liu, P. K. Misztal and A. H. Goldstein, Physical–Chemical Coupling Model for Characterizing the Reaction of Ozone with Squalene in Realistic Indoor Environments, Environ. Sci. Technol., 2021, 55, 1690–1698 CrossRef CAS PubMed.
- G. C. Morrison, A. Eftekhari, F. Majluf and J. E. Krechmer, Yields and Variability of Ozone Reaction Products from Human Skin, Environ. Sci. Technol., 2021, 55, 179–187 CrossRef CAS PubMed.
- C. Arata, N. Heine, N. Wang, P. K. Misztal, P. Wargocki, G. Bekö, J. Williams, W. W. Nazaroff, K. R. Wilson and A. H. Goldstein, Heterogeneous Ozonolysis of Squalene: Gas-Phase Products Depend on Water Vapor Concentration, Environ. Sci. Technol., 2019, 53, 14441–14448 CrossRef CAS PubMed.
- Z. Qiu, G. Li and T. An, In vitro toxic synergistic effects of exogenous pollutants-trimethylamine and its metabolites on human respiratory tract cells, Sci. Total Environ., 2021, 783, 146915 CrossRef CAS PubMed.
|
This journal is © The Royal Society of Chemistry 2023 |
Click here to see how this site uses Cookies. View our privacy policy here.