DOI:
10.1039/D2VA00147K
(Critical Review)
Environ. Sci.: Adv., 2022,
1, 705-724
Current progress in the environmental analysis of poly- and perfluoroalkyl substances (PFAS)†
Received
24th June 2022
, Accepted 4th September 2022
First published on 21st September 2022
Abstract
Per- and polyfluoroalkyl substances (PFASs) are a class of persistent organic pollutants (POPs). They are widely used in industrial and consumer applications and are known for their persistence, long-distance migration and toxicity. Various PFASs have been manufactured and distributed over the years at a global scale. Decades of relevant research on these emerging contaminants has revealed that PFASs are bio-accumulative and possibly carcinogenic to animals as well as humans. Following regulations and public concern about their impact on ecosystems and uncertain environmental fate, some legacy PFASs have been banned in some regions e.g. Europe, and their industrial production has switched to alternative fluoroalkyl substances. Recently, novel PFAS classes have been identified in numerous environmental matrices. The high variety of legacy and emerging PFASs across the ecosystems is alarming and calls for an efficient monitoring strategy for the quantitative determination of known substances as well as the elucidation and discovery of new compounds. This is crucial for PFAS management and risk assessment in the environment and merits the attention of regulators. This review reports and discusses the most recent analytical method development for PFASs in air, water, abiotic solid matrices and biological matrices. Various instrumental analysis techniques and screening approaches are presented, explored, and compared on the basis of their efficiency and applicability, together with sampling, pre-treatment and extraction methods. Additionally, promising non-target and non-specific approaches are addressed as the key-element in future PFAS analysis.
Environmental significance
Per- and polyfluoroalkyl substances (PFASs) are persistent and bioaccumulative chemicals that cause adverse reproductive and developmental effects in mammals and vertebrates. This group of substances has attracted the attention of regulators and policy makers all around the world. In this review, we have assembled the most recent, state-of-the-art analytical methods for the identification of these chemicals in all major environmental matrices. We report and critically describe the strengths, limitations and future potential of sampling and extraction techniques, instrumental methodologies and screening approaches in the field of PFAS analysis, as part of their environmental monitoring.
|
1. Introduction
Per- and poly-fluoroalkyl substances (PFASs) are a group of highly fluorinated aliphatic chemicals that have been broadly used in several industrial and household applications since the 1940s due to their high stability, and water/lipid resistance.1 PFASs are identified as emerging contaminants2 and are persistent, bio-accumulative and possibly carcinogenic to animals as well as humans.3 The increased half-lives of many PFAS in both wildlife and humans render them highly hazardous to the environment.4 In search of additional data, the monitoring of PFASs is essential. This will ultimately promote environmental regulation and help assess the environmental fate of many PFASs. However, because of their unique physicochemical properties PFASs tend to accumulate at trace levels in various environmental matrices, which hinders the analytical procedure for their identification and quantification. Several analytical regimes have been developed for the determination of PFAS in a variety of matrices, including sediments, ground- and freshwater,5 fish and other aquatic organisms,6 birds7 and mammals.8
Solid phase extraction (SPE) and liquid–liquid extraction (LLE) are techniques that have been applied in the extraction, purification and pre-concentration of PFASs in environmental samples in recent years.9 The phase-out of legacy compounds and their replacement with structurally similar PFASs has been the most common industry policy in the last few decades.10 This fact poses a great environmental danger, since most emerging PFASs also show high toxicity, yet are to this day not routinely monitored or part of any regulatory guideline.11 Additionally, many PFASs undergo transformation in wastewater treatment plants as well as metabolic alteration in humans and livestock (Table 1). To date there are nearly 5000 PFASs that are broadly used in industrial and commercial applications.12 Currently, targeted methodologies only cover a small fraction of the existing PFASs, while the vast majority of PFAS precursors remains unknown.13 Establishing analytical approaches that employ untargeted screening to elucidate and, ultimately, identify unknown PFASs are a necessary step toward the understanding of the full scale environmental risk and hazardous potential of these contaminants. To date, liquid chromatography (LC) coupled with mass spectrometric (MS) or tandem mass spectrometric (MS/MS) detection has been the gold standard for targeted PFAS determination.14 Benefiting from the latest improvements in the field of high resolution mass spectrometry (HRMS), novel PFASs have been continuously identified in a variety of environmental matrices.15 Recently, time-of-flight mass spectrometry (TOF-MS), Fourier transform ion cyclotron resonance mass spectrometry (FTICR-MS) and orbitrap instrumentations have been utilized for structure proposal of unknown PFAS molecules from more than twenty established classes16 and contribute to the developing field of emerging PFAS discovery via suspect and non-target screening.17
Table 1 List of poly- and perfluoroalkyl substances (PFAS) and their structure
Group |
Compound name |
Acronym |
Structure |
Perfluoroalkyl carboxylic acids (PFCAs) |
Trifluoroacetic acid (n = 1) |
TFA |
|
Perfluoropropanoic acid (n = 2) |
PFPrA |
Perfluorobutanoic acid (n = 3) |
PFBA |
Perfluoropentanoic acid (n = 4) |
PFPeA |
Perfluorohexanoic acid (n = 5) |
PFHxA |
Perfluoroheptanoic acid (n = 6) |
PFHpA |
Perfluorooctanoic acid (n = 7) |
PFOA |
Perfluorononanoic acid (n = 8) |
PFNA |
Perfluorodecanoic acid (n = 9) |
PFDA |
Perfluoroundecanoic acid (n = 10) |
PFUnDA |
Perfluorododecanoic acid (n = 11) |
PFDoDA |
Perfluorotridecanoic acid (n = 12) |
PFTrDA |
Perfluorotetradecanoic acid (n = 13) |
PFTeDA |
Perfluorohexadecanoic acid (n = 15) |
PFHxDA |
Perfluorooctadecanoic acid (n = 17) |
PFODA |
Perfluoroalkyl sulphonic acids (PFSAs) |
Perfluorobutane sulphonic acid (n = 4) |
PFBS |
|
Perfluoropentane sulphonic acid (n = 5) |
PFPeS |
Perfluorohexane sulphonic acid (n = 6) |
PFHxS |
Perfluoroheptane sulphonic acid (n = 7) |
PFHpS |
Perfluorooctane sulphonic acid (n = 8) |
PFOS |
Perfluorononane sulphonic acid (n = 9) |
PFNS |
Perfluorodecane sulphonic acid (n = 10) |
PFDS |
Perfluorododecane sulphonic acid (n = 12) |
PFDoDS |
Perfluoroalkane sulphonamides (FASAs) |
Perfluorooctane sulphonamide (n = 8, R1 = H, R2 = H) |
FOSA |
|
N-Methyl fluorobutane sulphonamide (n = 4, R1 = H, R2 = H) |
MeFBSA |
N-Methyl fluorooctane sulphonamide (n = 8, R1 = CH3, R2 = H) |
MeFOSA |
N-Ethyl fluorooctane sulphonamide (n = 8, R1 = C2H5, R2 = H) |
EtFOSA |
N-Alkyl perfluoroalkane sulphonamido acetic acids (FASAAs) |
Perfluorooctane sulphonamidoacetic acid (R1 = H) |
FOSAA |
|
N-Methyl fluorooctane sulphonamido acetic acid (R1 = CH3) |
MeFOSAA |
N-Ethyl fluorooctane sulphonamido acetic acid (R1 = C2H5) |
EtFOSAA |
N-Alkyl perfluoroalkane sulphonamido ethanols (FASEs) |
2-(N-Methyl fluorooctane sulphonamido)-ethanol (R1 = CH3) |
MeFOSE |
|
2-(N-Ethyl fluorooctane sulphonamido)-ethanol (R1 = C2H5) |
EtFOSE |
Perfluoroalkyl phosphonic acids (PFPAs) |
Perfluorohexane phosphonic acid (n = 6) |
PFHxPA |
|
Perfluorooctane phosphonic acid (n = 8) |
PFOPA |
Perfluorodecane phosphonic acid (n = 10) |
PFDPA |
Perfluoroalkyl phosphinic acids (PFPiAs) |
6 : 6 Perfluoroalkyl phosphinic acid (m = 6, n = 6) |
6 : 6 PFPiA |
|
6 : 8 Perfluoroalkyl phosphinic acid (m = 6, n = 8) |
6 : 8 PFPiA |
8 : 8 Perfluoroalkyl phosphinic acid (m = 8, n = 8) |
8 : 8 PFPiA |
Perfluoroalkyl iodides (PFAIs) |
Perfluorohexyl iodide (n = 6) |
PFHxI |
|
Perfluorooctyl iodide (n = 8) |
PFOI |
Perfluorodecyl iodide (n = 10) |
PFDI |
Perfluoroether carboxylic acids (PFECAs) |
Hexafluoropropylene oxide dimer acid |
HFPO-DA (trade name: GenX) |
|
Hexafluoropropylene oxide trimer acid |
HFPO-TA |
|
4,8-Dioxa-3H-perfluorononanoic acid |
ADONA |
|
Perfluoroether sulphonic acids (PFESAs) |
6 : 2 Chlorinated polyfluorinated ether sulphonic acid (n = 6) |
6 : 2 Cl-PFESA (trade name: F-53B) |
|
8 : 2 Chlorinated polyfluorinated ether sulphonic acid (n = 8) |
8 : 2 Cl-PFESA |
10 : 2 Chlorinated polyfluorinated ether sulphonic acid (n = 10) |
10 : 2 Cl-PFESA |
Perfluorooctane sulphonamido ethanol-based phosphate esters (SAmPAPs) |
Phosphate diester of N-ethylperfluorooctane sulphonamido ethanol (R1 = R, R2 = R, R3 = H) |
SAmPAP diester |
|
Phosphate triester of N-ethylperfluorooctane sulphonamido ethanol (R1 = R, R2 = R, R3 = R) |
SAmPAP triester |
Cyclic perfluoroalkyl sulphonic acids (cyclic PFSAs) |
Perfluoromethylcyclohexane sulphonic acids (R1 = CH3) |
PFMeCHS |
|
Perfluoroethylcyclohexane sulphonic acids (R1 = C2H5) |
PFECHS |
Fluorotelomer sulphonic acids (FTSAs) |
n : 2 Fluorotelomer sulphonic acids (n = 4, 6, 8, 10) |
n : 2 FTSA |
|
Fluorotelomer carboxylic acids (FTCAs) |
n : 2 Fluorotelomer carboxylic acids (n = 6, 8, 10) |
n : 2 FTCA |
|
n : 3 Fluorotelomer carboxylic acids (n = 5, 7) |
n : 3 FTCA |
|
Fluorotelomer unsaturated carboxylic acids (FTUCAs) |
n : 2 Fluorotelomer unsaturated carboxylic acids (n = 6, 8, 10) |
n : 2 FTUCA |
|
Fluorotelomer olefins (FTOs) |
n : 2 Fluorotelomer olefins (n = 6, 8, 10) |
n : 2 FTO |
|
Fluorotelomer alcohols (FTOHs) |
n : 2 Fluorotelomer alcohols (n = 4, 6, 8, 10, 12) |
n : 2 FTOH |
|
Fluorotelomer iodides (FTIs) |
n : 2 Fluorotelomer iodides (n = 4, 6, 8) |
n : 2 FTI |
|
Fluorotelomer acrylates (FTACs) |
n : 2 Fluorotelomer acrylates (n = 4, 6, 8, 10, 12) |
n : 2 FTAC |
|
Fluorotelomer methacrylates (FTMACs) |
n : 2 Fluorotelomer methacrylates (n = 6, 8) |
n : 2 FTMAC |
|
Polyfluoroalkyl phosphate monoesters (monoPAPs) |
n : 2 Polyfluoroalkyl phosphate monoesters (n = 4, 6, 8, 10) |
n : 2 monoPAP |
|
Polyfluoroalkyl phosphate diesters (diPAPs) |
n : 2 Polyfluoroalkyl phosphate diesters (m = n = 4, 6, 8, 10) |
n : 2 diPAP |
|
4 : 2/n : 2 Polyfluoroalkyl phosphate diesters (m = 4, n = 4, 6) |
4 : 2/n : 2 diPAP |
6 : 2/n : 2 Polyfluoroalkyl phosphate diesters (m = 6, n = 6, 8, 10, 12, 14) |
6 : 2/n : 2 diPAP |
8 : 2/n : 2 Polyfluoroalkyl phosphate diesters (m = 8, n = 8, 10, 12) |
8 : 2/n : 2 diPAP |
10 : 2/10 : 2 Polyfluoroalkyl phosphate diesters (m = 10, n = 10) |
10 : 2/10 : 2 diPAP |
The objective of the present review was to investigate the newest monitoring trends and the most recent analytical methods for the identification and determination of PFASs in diverse environmental matrices, including untargeted and non-specific approaches. The advantages and shortcomings of the existing analytical techniques and their performance with several sample types are discussed in detail. We also present the most commonly used instrumental analysis approaches, extraction methods and matrix types, and literature reported limits of detection and quantification, as well as sampling techniques (Fig. 1).
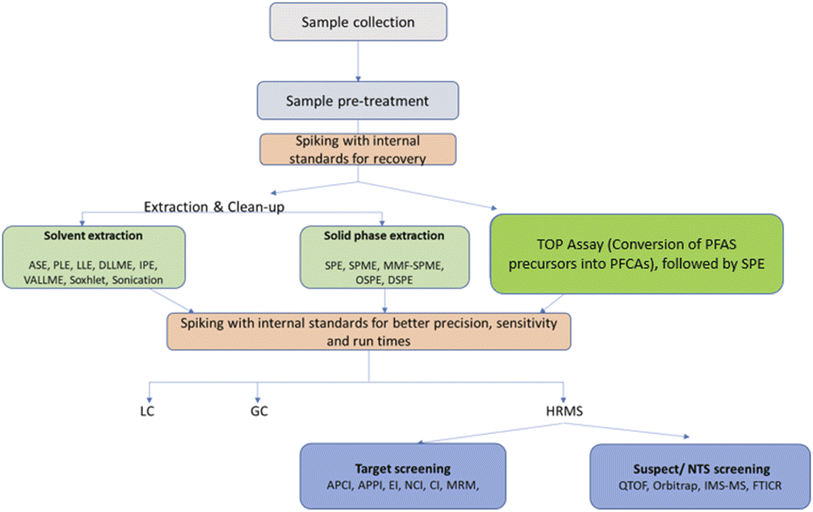 |
| Fig. 1 Graphic illustration of PFAS analysis regimes. | |
2. Air samples
Ionic and zwitterionic perfluoroalkyl acids (PFAAs) as well as neutral fluorotelomer alcohols (FTOHs) are the two most abundant classes in air particles. Both of these PFAS families are considered to be highly volatile and therefore find their way to airborne matter. Perfluoroalkane sulphonamido ethanols (FASEs) and perfluoroalkane sulphonamides (FASAs) have also been identified in air samples. PFASs in collected air samples are measured by gas chromatography-mass spectrometry (GC-MS) or liquid chromatography-mass spectrometry (LC-MS/MS).
2.1. Collection and storage
Both active and passive sampling methods are utilized in the collection of PFASs in air samples.18 Sorbent-impregnated polyurethane foam (SIP) disks and simple polyurethane foam (PUF) disks are the most common tools in airborne PFAS sampling, thanks to their easy handling and consistent efficiency.19 Simple passive air samplers have been allegedly used at site locations where active sampling was not possible.20 For instance, in the last decade, high volume PUF samplers equipped with quartz-fibre filters (QFFs)/glass-fibre filters (GFFs) and Amberlite® XAD®-2 resin were designed for the acquisition and storage of big particle masses containing volatile FTOHs.21 Conventional low volume active air samplers are equipped with solid phase extraction (SPE) cartridges to enable the collection of PFAS classes with diverse physicochemical properties and volatilities. All purpose, strongly hydrophilic, reversed-phase, water-wettable polymers, such as OASIS HLB (Waters Inc., Milford, MA, USA), or hydroxylated polystyrene-divinylbenzene copolymers, such as ISOLUTE ENV+ (Biotage AB, Uppsala, Sweden) have been traditionally used for neutral PFAS sampling.22 Typically, the sampling volume for air particles is in the range of 2 to 200 m3 for indoor spaces and 300 to 2500 m3 for outdoor areas.18 To detect airborne PFASs in the ppb range, field blanks are also collected during the sampling campaigns. Eliminating background contamination during all stages prior to analysis, including washing, storage and transport and pre-treatment of samplers, is crucial too. Last but not least, cryogenic samplers23 as well as the application of sorbents that are specific to PFASs24 have been utilized as sampling tools in recent years. Quality control (QC) is an integral element of PFAS analysis in air samples. All samplers are individually placed in polypropylene (PP) containers or wrapped with aluminum foil and stored at −20 °C up to the sample extraction stage in the laboratory.
2.2. Extraction, clean-up and concentration
In recent years, mainly SPE cartridges were used for indoor air monitoring, due to their easy and standardizable handling as well as low solvent volumes, especially for the monitoring of polyfluoroalkyl phosphate diesters (diPAPs).9 In most of the methods, SPE cartridges are precleaned with methanol or ethyl acetate, then dried with nitrogen before use.9 For XAD and PUF methods, preliminary Soxhlet extraction is performed with organic solvents such as methanol and dichloromethane.25 1
:
1 mixtures of acetone and petroleum ether have also been employed for the extraction of analytes from SIP disks.26 These disks are generally saturated with XAD-like resins, and then stored in solvent-rinsed glass jars until used.20 On the other hand, GFFs and QFFs are individually wrapped with aluminum foil, and then baked for 18–24 h at 450 °C for the removal of contaminants.27 Overall, XAD-like resins have been employed more broadly than GFFs and QFFs and are considered to be the norm when it comes to disk saturation materials.
2.3. Instrumental analysis and measurement results
Neutral PFASs are usually determined by GC-MS coupled with electron ionisation (EI) or, in fewer cases, with chemical ionisation (CI) in selected ion monitoring (SIM) mode.28 The golden standard for ionic PFAS detection involves analysis by HPLC-MS/MS with electrospray ionisation (ESI)9 and is therefore the go-to instrumental technique for PFAS screening in air samples. In both cases negative ionization (ESI−) is preferred. For GC, a WAX column can be employed to improve analyte separation.22 Ionic PFASs are adequately separated by a C18 column with an aqueous and methanol/acetonitrile mobile phase buffered with 5 mM ammonium acetate. As Gremmel et al. stated, in negative ESI, FTOHs, FASEs and FOSEs form acetate adducts ([M + Ac]−), which are only stable at lower temperatures.29 Therefore, the addition of ammonium acetate to the mobile phase is a common practice, as part of the latest analytical regimes. Particularly for short-chain (C2–C4) perfluorinated carboxylic acids (PFCAs), Tian et al. suggested the use of an ion exchange column (Shodex RSpak JJ-50 2D; Showa Denko America, Inc., New York, NY, USA) compatible with HPLC.30 According to their results, the ion-exchange resin beads improved the recovery of these substances. Historically, PFASs have been significantly less monitored in air samples than they have been monitored in aqueous and biological samples. Mainly, there are records about airborne PFASs from Central and Northern Europe, Northern America and Antarctica. Overall, perfluoroalkyl sulfonic acid (PFSA) concentrations in outdoor air samples have shown increasing trends in the last decade, unlike FTOHs, FASAs, FASEs and PFCAs, whose levels have been stable for the same period.31 Due to their volatile properties, FOTHs comprise the class that has been more thoroughly investigated. Rauert et al. reported that FTOHs were detected at fairly high concentrations during the 2009–2015 Global Atmospheric Passive Sampling (GAPS) survey, ranging from <0.4 to 21 pg m−3 on the glacial terain, and 40 to 238 pg m−3 in industrial and urban areas.31 In the framework of another study conducted at the northernmost continuously inhabited place in the world, Alert (Qikiqtaaluk Region, Nunavut, Canada) between 2006 and 2014, FTOHs, FASAs and FASEs were detected at concentrations <0.17–30 pg m−3, <0.014–0.82 pg m−3 and <0.10–4.8 pg m−3, respectively.32 As for indoor spaces, PFCAs and PFSAs have been detected primarily in households, while FASA/FASE levels were higher in more visited places, such as hotels. In their relevant study from 2018 Yao et al. reported that the yields of these substances were in range of nondetectable (ND) up to almost 2500 pg m−3.33 FTOH numbers, on the other hand, were alarmingly higher, ranging from 246 to 62
100 pg m−3, as presented in the same case survey.
2.4. Discussion
In general, passive samplers coupled to SIP disks are primarily used for the monitoring of outdoor air masses, while SPE cartridges are also occasionally employed for dust monitoring in closed spaces.34 Sampling and extraction methodologies for air samples are improved for zwitterionic, cationic, and anionic compounds, yet there is space for improvement regarding emerging PFASs. Nevertheless, the main challenge when monitoring PFASs in air matrices lies in the fact that there is no standardised methodology. Assessing the technical difficulties regarding the collection of indoor as well as outdoor air particles will greatly help towards the development of a universally applicable and standardized sampling protocol. Last but not least, multiresidue analytical methods for anionic and neutral PFASs in particles remain limited. To characterise the environmental fate and long range transport of PFASs, new analytical methods are needed for the less studied air matrices.
3. Aqueous matrices
Almost every established PFAS class has been historically detected in several parts of the aquatic continuum, including drinking water, surface water, groundwater, wastewater, snowmelt, landfill leachate, and brackish as well as sea water. The United States Geological Survey (USGS, https://www.usgs.gov/) published in 2006 a detailed description of the different aqueous samples sources, types as well as all available sampling techniques for various aqueous samples up to that point35. Special focus has been put on the analysis of PFOS and PFOA as well as their alternatives in aqueous samples. Suwannakot et al. reported the development of metal–organic framework (MOF)-based probes for the direct and rapid detection and quantification of PFOA by mass spectrometry.36 Four water-resistant MOFs—ZIF-8, UiO-66, MIL88-A, and Tb2(BDC)3—were coated on poly(dopamine) precoated stainless steel needles and used to rapidly preconcentrate PFOA from water for direct analysis by nanoelectrospray ionization mass spectrometry. MOF-functionalized probes can be potentially used for the rapid (<5 min) and sensitive quantification of PFOA-like molecules such as GenX and ADONA at low ng L−1 levels in environmental water samples (i.e., tap water, rainwater, and seawater) with no sample preparation.
3.1. Collection and storage
This report comprises the basis of today's syllabus for sampling, transporting and storing different water samples. Sampling of drinking water, surface water, and wastewater samples is fairly easy and is performed utilizing pre-cleaned buckets or stainless steel grab samplers. As sampling technology advanced through the last few years various autosamplers are available in the market, for instance Liquiport 2010 CSP44 by Endress and Hauser AG, Reinach, Switzerland.37 Lake and open ocean water samples are collected by more sophisticated circuits, such as a conductivity-temperature-depth meter (CTD) systems equipped with narrow, cylindrical, long plastic tubes (commercially available as “Niskin-X bottles”) capped at both ends.2 The collecting depth for samples is specifically delineated in the sampling protocol of each study. As for groundwater sample collection, digging trenches through the soil is the most popular technique. Usually, two samples are collected from the upper aquifer, while at least three samples are collected from the lower aquifer. Samples are collected utilizing simple vacuum tubes.38 Typically, the sampling volume ranges between 0.1 and 2 litres. Afterwards, samples are transferred to a pre-washed high-density polyethylene (HDPE),39 or polypropylene (PP) container.40 They remain stored at either 4 °C (simple refrigeration) or −20 °C (deep freeze) until further analysis.41 Field blanks are HPLC grade water or distilled water, unless otherwise noted in the analytical protocol.39 All-inclusive, the authors would like to strongly suggest the use of stainless steel tools as well as verified PFAS-free plastic parts for sampling, and transportation as well as storage of aqueous samples, since this is a very important component of the quality assurance and quality control (QA/QC) process.
3.2. Extraction, clean-up and concentration
SPE cartridges have been predominantly used for water sample enrichment and clean-up, as well as improved sensitivity of the analytical procedure,9 and are up to this day the norm for analyte extraction from PFAS-burdened aqueous samples. HLB series or Strata-X cartridges (Phenomenex, Torrance, CA, USA) are widely employed for targeted multi-class PFAS analysis.42 Methanol is frequently used as the elution solvent. Oasis WAX (Waters, Inc.) and Strata X-AW (Phenomenex) are also used for high throughput PFAS analysis of water samples.14 For heavily contaminated samples, such as wastewater matrices, an additional clean-up step with ENVI-Carb can be applied after SPE clean-up.39 Some additional techniques that have been applied more sporadically, yet with satisfying recoveries for the extraction of analytes from aqueous matrices are multiple monolithic fibre solid-phase microextraction (MMF-SPME), liquid–liquid extraction (LLE) and dispersive liquid–liquid microextraction (DLLME). MMF-SPME utilizing a monolith-based adsorbent that generates anion-exchange as well as fluorophilic interactions with PFCAs was evaluated for the precise detection of long-chain PFCAs in environmental water and milk samples in the pg L−1 range.43 LLE and its main ecological application, DLLME, are often applied for the clean-up of PFAS burdened aqueous samples. Recent DLLME approaches42 utilise less extraction solvent, yet achieve sufficient recoveries (80.6–121% for house water, river water and urine samples) and relatively low detection limits (0.6–8.7 ng L−1 for water and urine samples). Vortex-assisted liquid–liquid microextraction (VALLME), another recent LLE extension, employs a vortex mixer instead of dispersive solvent, a simpler alternative to the two different solvent systems employed in traditional DLLME methods. This technique was applied for legacy perfluoroalkyl sulphonic acids (PFSAs) and perfluoroalkane sulfonamides (FASAs) analyses in seawater by LC-LTQ-Orbitrap HRMS,44 as well as PFOS determination in tap, river and well water samples.45 A direct injection (DI) approach followed by liquid chromatography tandem mass spectrometry (DI-LC-MS/MS) for targeted PFAS analysis of various water samples including drinking water, ground water, river water, lake water and wastewater reported with satisfactory sensitivity has been recently reported.37 Jia et al. introduced multiple interactions into the conventional SPME process via a dual-functional modification of MIL-101(Cr),46 namely amination and subsequent fluorination, which was then used as an adsorbent for the efficient enrichment of PFAS prior to analysis by LC-MS/MS. Ultrasensitive quantitative analysis was achieved for nine selected PFASs with high linearities above 0.9941 in the ranges of 0.5–1500 ng L−1, low limits of detection of 0.004–0.12 ng L−1, and satisfactory repeatability and reproducibility with a relative standard deviation (RSD) <11.6%, as well as excellent performance in complicated water samples (recovery ratio of 76.2–108.6%). Another selective extraction method with low cost, simplicity, and significant solvent savings was developed by Shi et al.47 for the detection of long-chain PFASs, based on their specific protein affinities. Bovine serum albumin (BSA) was selected to extract PFASs from aqueous samples, which were then desorbed using MeOH and ultimately analyzed by LC-MS/MS. Protein affinity properties were confirmed to be the major extraction mechanism, which significantly increased the selectivity for PFASs. The recoveries of this method for fifteen long-chain legacy PFASs and three chlorinated polyfluoroether sulfonic acids in tap water and river water samples with three spiked levels were 73.3–122.3 and 83.8–119.4%, respectively. The method limits of quantification in the two real matrix samples were 4.7–133.3 ng L−1. Last but not least, Cao et al. presented in 2019 a simple yet efficient method, inspired by the sea-spray aerosol enrichment in nature, for preconcentrating PFASs in tap water.48 In this method, in situ H2 microbubbles were generated by electrochemical water reduction using a porous Ni foam electrode. These H2 bubbles were able to pick up PFASs as they rose through the water column that contained low concentration PFASs, simulating gas bubbles in the ocean that serve to scavenge the surface active material, carrying it to the air-ocean interface. When these H2 bubbles reached the water surface, they burst and produced aerosol droplets that were enriched in PFASs. In this manner, the authors achieved a ∼1000-fold preconcentration for ten legacy PFASs in the concentration range from 1 pM to 1 nM (or ∼0.5 ng L−1 to 500 ng L−1) in only 10 minutes, employing a simple mechanism that simulates natural processes.
3.3. Instrumental analysis and measurement results
The gold standard for instrumental analysis of PFASs in aquatic matrices, especially for target screening, remains HPLC-MS/MS.14,40 Nevertheless, in some cases HRMS such as orbitrap or time-of-flight (TOF)-MS are employed for quantitative and qualitative analyses.49 MS is generally operated in ESI-negative mode due to the fact that most legacy and emerging PFASs are anionic. Although neutral PFASs such as FASAs, FASEs and FTOHs can still be ionized using ESI, atmospheric pressure chemical ionisation (APCI) and atmospheric pressure photoionisation (APPI) have also been tested.16 LC-MS/MS is the benchmark analytical technique for PFAS identification in aquatic matrices due to its improved accuracy and precision, great selectivity, and generic applicability, despite its relatively high operational cost and limited sample throughput in comparison to LC-HRMS.
Mean concentrations of seven long-chain PFCAs (C6–C12) and two PFSAs (C4 and C6) in Australian wastewater treatment plant (WWTP) influents and effluents were found to be in the range of 0.3 to 25 ng L−1.39 The effluent concentration levels were particularly alarming. Elevated levels of iconic PFASs, namely PFOS and PFOA have been repeatedly reported in studies about PFAS monitoring in Asian bodies. An important study involving water samples collected in 2016 from 65 rivers and 34 coastal drain outlets around the Bohai Sea, China50 indicated high PFOS and PFOA levels as well as increasing trends in the concentrations of these two chemicals. Various tap water samples were analysed for the determination of 14 legacy target compounds, including four PFSAs (C4C10) and 10 PFCAs (C5–C14), in South Korea in 2017.51 With reported concentrations up to 189.6 ng L−1, this particular case study raised serious concerns about the presence of PFASs in households, hospitals and other human foundations. The investigation of global concentrations, patterns and trends of novel PFASs in aqueous samples, including cyclic PFSAs, perfluoroalkyl phosphonic acids (PFPAs), perfluoroether sulphonic acids (PFESAs) and perfluoroether carboxylic acids (PFECAs) has been a very important topic in relative literature. Well-known as well as novel PFASs, including 6
:
2 chlorinated polyfluorinated ether sulphonic acid (6
:
2 Cl-PFESA, commercially known as F-53B) and 6
:
2 FTSA, detected in ground water samples collected from 13 non-industrialised cities in Jiangsu Province, China, were 2.7–556 ng L−1.52 Hexafluoropropylene oxide dimer acid (HFPO-DA, commercially known as GenX), hexafluoropropylene oxide trimer acid (HFPO-TA) and 6
:
2 Cl-PFESA, have been widely detected in developed as well as developing countries, such as China, the United States, the United Kingdom, Sweden, Germany, the Netherlands and South Korea. This validates last decade's hypothesis that the PFOS and PFOA alternatives' levels will eventually be comparable to the original PFAS concentrations in Asia, Europe, and America. The surprisingly high frequency of appearance (FoA) of F-53B (>95%) in several Chinese water bodies has raised the concerns of regulators over the past decade.17 The screening of short-chain PFCAs is still a developing field of research. In one of the few available publications on that subject, it was reported that (C2–C8) PFCAs were detected in the range of 0.056 mg L−1 (PFPrA) to 2.2 mg L−1 (TFA) from aquifer water collected at polluted sites in the state of Baden-Wuerttemberg, Germany.53 PFOA and TFA were the most abundant analytes. Nevertheless, it has to be noted that some researchers constrict the term “Short-chain PFCAs” to PFCAs whose molecule's carbon chain strictly consists of 2 to 5 carbon atoms.
3.4. Discussion
The most notable development for PFAS analysis in aqueous matrices in recent years can be observed in the field of extraction and clean-up techniques. More specifically, the specialization of LLE towards the development of DLLME and VALLE as well as micro-SPE significantly decreased the required sample volume and the amount of extraction solvent needed,49 enabling greener applications of established techniques. Multiresidue and simultaneous analytical methods have been developed and validated for the analysis of a wide range of emerging PFASs, such as 6
:
2 Cl-PFESA, HFPO-DA and HFPO-TA, together with legacy PFASs. Various technical difficulties remain though, namely method optimization for all target analytes. This is a multifaceted process and hence still very challenging. Additionally, short-chain PFASs have been proven to be significantly more affected by matrix effects that cause ionization and signal suppression, resulting in higher LODs.53 Since short-chain PFAS (C6 or shorter) have been progressively manufactured and used at the global scale as alternatives for long-chain homologues, yet are more volatile and diffusive, more research in order to determine their environmental fate and toxicology is a necessity. Valid evidence shows that short-chain PFASs are more likely to accumulate in wastewater treatment facilities.54 Therefore, developing robust and multiresidue analytical methods is essential for grasping the chemical properties of both original and novel PFASs in aqueous matrices.
4. Abiotic solid matrices
The extended use of aqueous film-forming foam (AFFF) on firefighting training grounds has been the primary source of PFAS contamination of land areas.55 PFAS research in abiotic solid matrices, which include dust particles, soil, and sediments, mainly focuses on the quantitative identification of compounds in various soil matrices55 while constantly aiming towards the optimization of extraction and clean-up methods for emerging PFAS.56 On the other hand PFAS monitoring in dust is a novel field of research, therefore methods for dust analysis call for further exploration. Nevertheless, a key study from Finland showed that polyfluoroalkyl phosphate esters (PAPs) and FTOHs were the predominant PFASs in children's bedroom floor dust.57 In the same study PFOS dust levels were found to be higher in rooms with plastic floors compared to wooden floor spaces, strengthening the hypothesis that PFASs tend to sorb primarily into plastics. Finally, earth core and borehole samples have been investigated to estimate time series and temporal trends of legacy and emerging PFAS.58
4.1. Collection and storage
Dust samples are generally collected utilizing simple household tools, such as a vacuum cleaner bag or a precleaned bristle brush.57 As soil cores are regarded, the prescribed sampling depth plays a determining role, taking into account the sorbing potential and the overall volatility of various PFAS homologues, making them settle at different depth layers into the soil. Surface soil sampling is carried out by using a stainless-steel trowel or a shovel precleared with methanol.59 This applies to the top 15 cm of the soil. For a given area, each soil sample generally consists of a series of sub-samples. This is carried out for representative sampling purposes. Typically, up to five sub-samples are collected from the center of the given site up to 100 m2 and 1 soil core is extracted from each of the four corners of the area.60 Common tools for surface sediment sampling include a Van Veen grab sampler and Ponar grab sampler, while MC-400 Multi-corer samplers are used for the collection of more complex core samples that consist of particles with various diameters.61 Core samples are routinely dissected into parts with 0.5–2 cm of thickness each, using a stainless-steel knife before further treatment.62 In general, after sampling, solid samples are transported to the laboratory wrapped in aluminum foil. Pre-treatment includes drying and calculation of the water content, sieving, homogenization, and storing in PP containers refrigerated at 4 °C or frozen at −20 °C until analysis.63 Solid commercial products such as textile, fabrics and food packaging parts are bought from local retailers. Non-specific, generic and unbiased selection of these consumable samples is a necessity for the reproduction of real case scenarios.64
4.2. Extraction and clean-up
With the exception of a promising extraction method developed by Wang et al. in 2018,65 no worth-mentioning advancements in pre-treatment approaches can be seen in the extraction field for abiotic matrices. According to the aforementioned study, solvent extraction proved to achieve better analyte recoveries for the target compounds than SPE. Nevertheless, SPE has several advantages over other extraction techniques (i.e. LLE) such as low solvent consumption, enormous saving of time, increased extraction efficiency, decreased evaporation volumes, higher selectivity, cleaner extracts, greater reproducibility, avoidance of emulsion formation, and easier automation. Therefore, SPE schemes are still incorporated in protocols for PFAS extraction and enrichment from abiotic solid matrices as an additional step.39 Particularly for dust samples, methods tend to be more sophisticated and involve sequences of extraction steps as well as fractionating with several solvents,9 due to the fact that these matrices are routinely analysed for both volatile and non-volatile PFAS; Generally, pre-treatment methods consist of Soxhlet extraction, PLE, and supported liquid extraction (SLE), followed by additional clean-up procedures for heavily contaminated matrices, i.e. sludge samples, using graphite carbon materials such as ENVI-Carb, SLE or ion pair extraction (IPE).19 The most commonly used combination is SLE followed by ENVI-Carb or an SPE cartridge (e.g., OASIS WAX, OASIS HLB or C18) under neutral or basic conditions. For sludge matrices, an additional clean-up step with ENVI-Carb can be applied after SPE clean-up.39 Arguably, the main focus of generic pre-treatment processes for sediments and soils should be newly discovered and suspect cationic and zwitterionic PFASs that are characterized by increased hydrophobicity.56
4.3. Instrumental analysis and measurement results
For ionic PFASs, instrumentation used as the gold standard for target screening is HPLC-ESI(−)-MS/MS, as in the case of aqueous matrices analysis. LC conditions are generally similar to those applied for air and aqueous matrices. A few studies essentially employed Orbitrap-MS63 or TOF-MS.66 Nevertheless, it was recently reported that an alkyl-perfluorinated C8 column (Epic FO LB, ES Industries, Inc., West Berlin, NJ, USA) achieved better chromatographic separation of PFAS isomers than a RP C18 column.67 This has to be further tested in upcoming studies. GC-MS is the predominant method for volatile PFAS analysis in solids and is, therefore, advised to be employed as the method of choice for PFAS analysis in solid matrices. Although LC-MS offers several advantages over GC-MS such as quicker and less extensive extraction procedures and the ability to identify and measure a broader range of compounds, GC-MS offers high reproducibility of generated mass spectra using electron ionization (EI), compared to liquid chromatography. The electron impact ionization process, used in GC-MS, is a hard ionization that results in the production of very reproducible mass spectra from one instrument to another.
Studies from the last 15 years have been steadily reporting PFOS and PFOA terrestrial sediment concentrations in the range of ND to 623 ng g−1 dry weight (dw) and ND to 16 ng g−1 dw, respectively.68 This demonstrates the vast difference in sediment contamination by PFOS and that it is less of an environmental threat than PFOA, on an average. Marine sediment core samples collected in developing Asian counties, such as China and Korea, displayed similar trends, in terms of PFOS/PFOA ratios and abundance.58 It should be noted, that surface sediment layers were characterized by significantly higher ΣPFAS values compared to borehole samples, which was also the case for core sediment samples from the Great Lakes region of North America.69 diPAPs and PFPiAs were detected in the upper most sediment layer from the same region during monitoring campaigns between 2006 and 2009. In the framework of per- and polyfluorinated chemical long-term monitoring, emerging PFASs, such as 6
:
2 Cl-PFESA and its analogues, and 6
:
2 fluorotelomer sulphamide alkylbetaines (FTABs), have also been detected in abiotic solid matrices and other parts of the aquatic continuum rarely traced perfluorooctane sulphonamido ethanol-based phosphate (SAm-PAP) diester and triester were also detected in freshwater sediments from Lake Tai, Yangtze Delta area, China.15 As the occurrence of PFASs in dust samples is regarded, both the levels and profile of ΣPFAS depend on the location as well as anthropogenic activity at the various collection sites. Samples collected from hotels, hospitals and public buildings are predominantly characterized by higher levels of FTOHs, while samples from households contain much fewer FTOHs. In the latter samples short-chain PFCAs are the most abundant PFAS class.33 Last but not least, PAPs and, especially, diPAPs are regularly detected in dust samples in notable concentrations, according to the literature.57
4.4. Discussion
Time series analyses as well as numerical simulations point out towards the fact that sediments, soils and sludges are arguably the most important environmental sink for PFASs, followed by surface water bodies and open seas.70 A fair amount of emerging PFAS homologues have been detected in recent studies.56 These substances tend to exhibit strong adsorption to solid matrices.71 Dust is one of the most important means of human exposure to PFAS, since it can easily coalesce with indoor particles, calling for a robust and versatile method for PFAS determination in trace concentrations. Overall, the urge for a continuous development of techniques for the extraction of legacy as well as newly discovered PFAS classes, for both volatile as well as non-volatile compounds, is a priority.
5. Biological samples
The main focal point of PFAS screening in biological samples has been the development of efficient extraction and clean-up techniques, since biological samples consist of complex matrices42. IPE and alkaline digestion followed by LLE have been the main pre-treatment methods.72 Eluates are commonly subjected to an additional clean-up stage with an SPE cartridge containing HLB, WAX or ENVI-Carb. In recent years, accelerated solvent extraction (ASE) has been applied for the extraction of analytes from complex biological matrices due to its green profile and technical advantages, compared to traditional extraction methods13 Multiple studies have investigated the occurrence of established as well as new PFASs and their isomers from most of the known classes. Special focus has been given to homologues from PFECAs and PFESAs,73 perfluoroethylcyclohexane sulphonate (PFECHS)74, PFPAs and perfluorophosphinates (PFPiAs),75 polyfluoroalkyl phosphate monoesters (monoPAPs) and diPAP76 families. Additionally, rare cationic and zwitterionic compounds were recently identified in various fish tissues.77 In the prism of green chemistry, several eco-friendly techniques, including focused ultrasound solid–liquid extraction (FUSLE) and turbulent flow chromatography (TFC) are assessed.42 Overall, plasma, serum and breast milk are the most thoroughly studied biological matrices so far,78 but many recent studies are focusing on urine, hair and nail for human biomonitoring79 as well as wildlife to help improve chemical management in the environment.7
5.1. Collection and storage
Biological samples cover a variety of complex matrices such as tissues of mammals, birds, fish, seafood, invertebrates, vegetables, and eggs, as well as biological fluids such as urine, blood, plasma etc.80 Birds, fish and invertebrate samples are commonly captured using nest traps,81 gill nets and bottom trawls.50 They can also be purchased from local markets. After sampling, organs and tissues, including the brain, liver, heart, gonads, adipose and muscle tissue, among others, are carefully transported to the analytical laboratory. Afterwards, they get homogenized with precleaned stainless-steel tools. Bird egg samples are processed by separating the yolk from the albumen and homogenizing the two parts.82 Human milk, urine and blood samples are collected and stored in polypropylene bags and tubes.80 After sampling, most biological samples are stored in polypropylene containers and remain frozen at −20 °C or hyper freezed at −80 °C until analysis.
5.2. Extraction, clean-up and concentration
The most widely used methods for analyte extraction from biota and human matrices are SPE, SLE, LLE, and IPE; alkaline digestion and acetonitrile protein precipitation have been also employed for the clean-up of biological matrices.79 A recent study by Androulakakis et al. involved generic pre-treatment methods and the utilization of accelerated solvent extraction (ASE) for the extraction of PFASs from various top predator livers as well as fish muscle tissues that were collected in Northern Europe.13 The advantages of ASE in comparison to conventional extraction techniques lie in the faster extraction of analytes, the more efficient contact between analytes and solvents, and the smaller consumption of solvents. All these methods result in cleaner extracts using a greener approach. Finally, the most modern trend to report in the field of human biomonitoring, is the reduction and/or simplification of pre-treatment steps and the usage of online SPE systems, yet this is still a fairly new field. Moreover, Hou et al.83 developed a novel method based on the integration of SPME and nanoelectrospray ionization mass spectrometry (nanoESI-MS) for the rapid and ultrasensitive analysis of 14 legacy PFCAs and PFSAs in blood and other biological samples. More specifically, a novel SPME probe with an F-functionalized covalent organic framework (COF) coating was designed for highly selective enrichment of trace PFASs from complex samples. After extraction, the loaded COFs-SPME probe was directly applied to nanoESI-MS analysis under ambient and open-air conditions. For the 14 investigated PFASs, the limits of detection and quantification varied from 0.02 to 0.8 ng L−1 and 0.06 to 3 ng L−1, respectively, for blood matrices. These novel extraction techniques should find more applications in the analysis of environmental and biological samples, since they make up for the gaps of conventional methods while aiding to separate, identify, and quantify PFAS in a more ecological and effective manner.
5.3. Instrumental analysis and measurement results
The mainstream analytical technique for PFAS analysis of biological samples is LC-MS/MS. LC-MS/MS should be the guideline for instrumental analysis of biota for the same reasons that are mentioned in the previous sections of this review, namely its excellent accuracy and precision, optimum selectivity, and generic applicability. To a smaller extent HPLC instrumentations coupled to orbitrap or time-of-flight (TOF)-MS are used for biota analysis. Last decade's most prominent advantage in the field of PFAS analysis in wildlife and human matrices is the application of online SPE or dual column systems coupled with HPLC-MS/MS.84 Temporal trends and time series analyses of human serum and blood samples for the biomonitoring of PFOS and PFOA in the Northern hemisphere clearly depict reduced levels and a low frequency of appearance of these chemicals, although their occurrence in the environment remains steadily high.85 The overall increasing trend of PFAS levels in human and wildlife samples from China, especially close to sites where PFASs are used or produced, constitutes one of the biggest environmental challenges at the global scale and a major concern for regulators.50 Next to the established and in most countries banned legacy PFAS, novel PFAS have been detected in diverse biological samples. The rising levels of these compounds next to their increasing detection rate is the aftereffect of the phasing out of original PFASs and the industrial shift towards alternatives.76 Recently, 6
:
2 Cl-PFESA was detected for the first time in the liver of a Eurasian otter from the East Anglia region, UK, at a concentration of 3.3 ng g−1 ww, in the framework of a study of assessing PFAS occurrence patterns in top predators and their prey from Northern Europe13 Both 6
:
2 and 8
:
2 Cl-PFESA as well as PFOS had bioaccumulated and been biomagnified in the marine ecosystem of the Bohai Sea on the east coast of Mainland China, as demonstrated in the findings of a recent research by Chen et al. 78 6
:
2 Cl-PFESA and, to a lesser extent, HFPO-TA were detected in various tissues from black spotted frogs near a PFAS mass production and manufacturing unit in China,73 validating once again the response of PFAS concentrations in the environment to the production rate of fluorochemicals in the area. PFPAs and PFPiAs are two groups of emerging PFASs that have raised the concerns of scientists, regulators and policy makers over the recent years, due to their increasing FoA and proven toxicity, bioaccumulation potential, and mobility. 141 samples of dolphins and fish as well as hunting birds collected between 2004 and 2011 in aquatic and terrestrial ecosystems of North America were analysed for PFPAs and PFPiAs.75 PFPiAs were detected in all animals at fair levels, while most PFPAs were found below the LOD. In the field of human biomonitoring, the vast majority of PFAS related studies that were published since 2000 cover the determination of legacy and emerging PFASs in the blood and serum of adults and infants. Fewer studies focus on organs, breast milk, urine, hair and nail samples.80 Nevertheless, it has been scientifically established that nail matrix acts as the best indicator for human exposure to PFAS, with PFOS tested as a case study compound.86 In another recent study, 6
:
2 Cl-PFESA was detected in most of the analyzed urine, hair and nail samples that were derived from two populations with different exposure rates and conditions.87
5.4. Discussion
Method development is a priority for the assessment of the exposure of wildlife and humans to legacy and emerging PFASs through robust and multiresidue analysis of these chemicals. Particularly in Asian countries where most fluoropolymer production takes place, and thousands of precursors, metabolites and biotransformation products of legacy PFASs are in the environment, it is crucial to measure organisms' exposure to PFAS alternatives such as PFESAs, PFECAs and PFPiAs through biomonitoring and chemicals management. The recent discovery of hundreds of novel PFASs belonging to more than 10 different classes in biota, including mammals, birds, fish and mussels from around the globe, by non-target mass spectrometry strategies is alarming for regulators and warrants for the urge of revolutionary techniques in the field of untargeted PFAS analysis in biological samples. A recent study involving the NTS screening of pooled fish liver samples from China77 pointed out the fact that analytical advancements in the field of PFAS analysis cannot cope with the rapid industrial production of PFAS alternatives. The development of ethical and non-invasive sample collection techniques, more efficient clean-up methods, and improved ionisation techniques for MS analysis are essentially needed. Although, numerous publications on targeted analysis of wildlife and humans for legacy and novel PFAS determination by LC-MS/MS have been reported since 2015,88 the wide variety of physicochemical properties of PFAS hindered the development of a sufficient pretreatment method that eliminates or at least significantly reduces matrix effect complex tissues and other biological in samples. Isotope dilution76 and matrix-matched calibration curves have been important tools towards better quantitation and overall analytical performance.84 In order to lessen matrix effects, the volume of the initial sample and the amount of the injected extract in the instrument sequence need to be reduced. This is not always feasible, though. Yet, the biggest challenge in targeted PFAS analysis remains the fact that less than 80 reference standards are currently available, while matrices that are representative of all possible biological samples are also not available. The ratio of commercially available reference standards to known PFASs does not exceed 0.015%, up to this day.89 Last but not least, the loss of sensitivity caused by ionisation suppression due to ESI approaches adds to non-detection, which is often unrealistic. Pre-clean-up lyophilization, sample freezing after SPE extraction,84 graphite carbon (e.g., ENVI-Carb) clean-up76 and/or addition of 1-methyl piperazine to the LC-MS/MS mobile phase84 could possibly reduce the lipid content of the final extract, but none of the above can act as a full remedy for complex and fatty matrices. As mentioned above, the high production rhythm of the unmonitored alternatives, whose physicochemical properties, toxicology, long-range transport effect and bioaccumulation potential are unknown, calls for sophisticated, robust and efficient risk assessment of suspect and newly discovered compounds and their environmental threat.
6. Untargeted analyses
6.1. Suspect screening
Highly sensitive and specific analytical methods have contributed greatly to targeted PFAS determination and quantification in environmental samples. However, the ramping number of legacy and emerging PFAS in society, currently, warrants for HRMS techniques that aim for the discovery of unknown or suspect compounds in the environment, without the necessity of reference standards. The timely discovery of novel, yet possibly hazardous PFAS contributes to early warning strategies via their risk assessment and the monitoring of their early life-cycle, prohibiting—if necessary—the global distribution of these compounds. Some of the most acknowledged and reliable databases for PFAS suspect screening include SFISHFLUORO on the NORMAN Suspect List Exchange,76 as well as EPAPFASINV, PFASKEMI and PFASOECD on the U.S. Environmental Protection Agency (EPA) CompTox Chemistry Dashboard.22 Though it has to be noted that at least 20% of the ∼5000 PFAS listed in these databases has no molecular formula and/or structural information. Suspect screening can be performed against databases containing either exact mass and isotope patterns,90 generated molecular formulae, MS/MS data retrieved from the literature and open source databases (e.g. Massbank,37,79 and MZCloud91), or in silico MS/MS predictions (e.g. MetFrag37 and CFM-ID37). Typically, it is applicable during the step of full-scan acquisition, where m/z ratios are acquired over defined mass ranges with a specified scanning frequency and/or resolving power (the ability of the instrument to separate two adjacent peaks, RP).
Suspect screening can be further utilized for the proposal of per- and polyfluorinated molecular formulae, taking advantage of HRMS instruments' mass accuracy (≤5 ppm, or even <1 ppm, depending on its calibration efficiency).14 Lastly, suspect screening can be performed in the phase of MS/MS structural characterization by isolating a specific parent ion, fragmenting it by collision-induced dissociation (CID) or electron-capture dissociation,92 and collecting the product ions in full-scan mode for complete MS/MS spectra attainment. The rapid scan of numerous samples and the possibility of retrospective screening of stored samples,93 are a key advancement of this untargeted HRMS strategy over conventional MS/MS techniques.
6.2. Non-target screening
For over a decade, nontarget HRMS methodologies act as powerful tools for the discovery of numerous PFASs that are endlessly manufactured and discharged into the ecosystems at the global scale.17 Just recently, more than 950 unknown PFASs, disregarding possible branched isomers, were detected in various environmental media.17 The proposed structures of the aforementioned analytes contribute greatly to next-level PFAS research and the risk assessment of these substances and their side-products.42 The main focus of formula elucidation and structure proposal of unknown PFASs via NTS methodologies has found applications in AFFF and surfactant samples. Several novel PFASs in screened AFFF fractions were identified using fast atom bombardment (FAB)-MS and quadrupole TOF-MS.49 Additional and so-far-unknown PFAS from various classes including anions, zwitterions, cations and neutral species were also identified in AFFF samples and fluorocarbon surfactants.94 More case studies involve the implementation of NTS pathways on freshwater, drinking water and wastewater matrices.66 Additionally, airborne particulate matter,95 human blood samples,96 and various fish tissues77 were screened for the detection of unmonitored emerging PFASs. Four new PFAS classes consisting of more than 165 homologues, in total, were also reported in pooled fish samples collected downstream from a fluorochemical industry, in 2018.77
In a revolutionary study from 2021, Heuckeroth et al.97 demonstrated the usefulness of a hard ionization source (ICP-MS/MS) as a fluorine-specific detector in combination with ESI-MS for the identification of fluorine containing compounds. Simultaneous hyphenation of HPLC-ICP-MS/MS with HR-ESI-MS was applied to evaluate biodegradation products of organofluorine compounds by sewage sludge. The data were analyzed in a NT approach using MZmine. Due to the fluorine-specific detection by ICP-MS/MS, more than 5000 peaks (features) of the ESI-MS were reduced to 15 features. Of these, one was identified as a PFAS degradation compound of 8
:
2 FTOH without using targeted analysis. Another unconventional NTS study alternated between scan events with high and low collision energies (CE) for the screening of parent ions as probable PFA precursors by TOF-MS.94 This study led to the discovery of 5 anionic, 30 cationic, 15 zwitterionic and 40 neutral PFAS, raising the number of newly established fluorosubstances in the last five years by another 90. This type of scanning needs to be further tested together with in-source fragmentation flagging scans for anionic PFAS.77 Both of these scanning strategies could be powerful tools in the developing field of PFAS NTS screening. Multidimensional analysis techniques such as GC × GC or LC × LC coupled to TOF-MS that have been developed for non-target analysis of organic contaminants in dust samples,98 could be potentially applicable in the development of PFAS NTS strategies for environmental matrices. So far, the main pre-treatment methodologies for target analysis have been applied in NTS strategies too. The most widely used clean-up and extraction approaches that have been adopted as part of NTS pathways are SPE,77 and SLE96 (also coupled to activated carbon filtration66), as well as filtration/dilution combinations.94 Nevertheless, the main fault of these methods lies in the fact that they have only been assessed for anionic compounds, that have comprised the majority of target analytes for decades. Thus, the loss of cationic, zwitterionic and neutral PFASs with different physicochemical properties during NTS is inevitable.66,95 The above was demonstrated in the framework of a study analysing firefighting foams in soils. Analyte recoveries of cationic and zwitterionic PFASs were found to be out of the 70–170% range when using standardized pre-treatment techniques optimised for anionic PFASs.56 Therefore, the urge to carefully select should be one of the main focal points in the improvement of future NTS strategies within PFAS research. The employment of generic, non-intrusive pre-treatment methods, together with extraction procedures that are able to capture diverse PFASs, while simultaneously reducing matrix effects and signal interferences are a priority in future PFAS research.95 Last but not least, FluoroMatch Flow has been introduced as the first software to automate a comprehensive PFAS non-targeted analysis workflow. The latest format FluoroMatxh 2.0 covers all steps of data processing for PFAS discovery in liquid chromatography—high-resolution tandem mass spectrometry samples. These steps include feature detection, feature blank filtering, exact mass matching to catalogued PFASs, mass defect filtering, homologous series detection, retention time pattern analysis, class-based MS/MS screening, fragment screening, and predicted MS/MS from SMILES structures. In addition, a comprehensive confidence level criterion is implemented to help users understand annotation certainty and integrate various layers of evidence to reduce overreporting. In an application of this revolutionary tool to aqueous film forming foam analysis, Koelmel et al. discovered over one thousand likely PFASs including previously unreported species. Furthermore, the authors were able to filter out 96% of features which were likely not PFASs, yet contained some fluorinated carbon atoms in their molecule.99
Overall, in-depth non-target workflows have good chances of discovering potential PFAS transformation products, intermediates, and manufacturing impurities, as well as PFASs not listed in available suspect databases, yet several elucidation schemes have led to identifications that remain tentative. It is a necessity that, in frequent cases, NTS must be followed by authentic chemical standard synthesis for utter confirmation, and further targeted and quantitative monitoring by highly sensitive MS/MS instrumentations, as well as toxicology studies, towards a holistic risk assessment of newly discovered substances. NTS is closely connected with chemical prioritization schemes. Robust, effective yet cost-benefit chemical prioritization schemes are indefeasible aspects of modern chemicals monitoring. When finally deciding what PFASs ought to be prioritized, scientists, regulators and policy makers greatly affect the big-scale synthesis of fluorochemicals and their environmental footprint. Therefore, the current monitoring degree, toxicological testing, detection frequency and possible increasing trends in environmental samples for the watched substances should be taken into consideration.100
6.3. TOPA and TOF for PFAS and precursor analysis
A vast and constantly increasing number of PFAS homologues, including legacy compounds and their precursors currently exist, often in just trace concentrations, in environmental matrices. Most common PFAS precursor compounds include fluorotelomer alcohols, amides and fluorotelomer sulfonates. The majority of these substances can be degraded primarily to PFCAs and secondarily to PFSAs.101 Telomer precursors are a very wide family of PFAS compounds, most of them sharing a polyfluorinated n:2 structure (n = no of perfluorinated carbon (C) bound to two C without fluorine (F)). These substances have been used in numerous industrial and commercial products. Among precursors being analyzed on a routine basis 4
:
2, 6
:
2 and 8
:
2 FTS can be mentioned. Moreover various FTOH and PAP are also sometimes studied, though other analytical methods are commonly needed. Precursors in this regard may include more advanced structures with ester, ether, urethane, ethoxylate and phosphate linkages among others.102 Precursor biotic or abiotic transformation to PFAS end products has important implications for PFAS remediation efforts. By solely focusing on target PFAS removal, without consideration of the total precursor pool, an unanticipated increase in the concentrations of target PFAS may occur over time, resulting in potential future liability.103
Among the “known” precursors, those forming PFOS (so called PreFOS) as the major end-product have attracted much attention, and in many cases these have been produced in large quantities. Moreover, as for the PreFOS precursor, compounds with the ability to form other perflourinated sulphonates e.g. PFHxS do also exist. Besides the “known” ones there are also numerous “unknowns”, not included in conventional analyses or potentially not identified.
Although no regulatory criteria have been established for PFAS precursors, concerns regarding their migration and transformation into PFAAs are growing. These substances can include compounds that originated in the original PFAS formulations or are polyfluorinated intermediate transformation products. The number of precursors quantifiable with commercially available analytical methods (using LC-MS/MS) is very limited. The USEPA Method 537.1 (ref. 104) for PFAS analysis in drinking water only includes two precursors: N-methylperfluoro-1-octanesulfonamidoacetic acid and N-ethylperfluoro-1-octanesulfonamidoacetic acid. The lack of quantification is due in large part to the absence of available analytical standards for these precursor compounds. This limitation for quantifying PFAA precursors using current standard methods restricts our understanding of the occurrence and extent of PFAA precursors and their potential to be transformed into PFAAs. The fact that the majority of these precursors are unknown, due to commercial unavailability of their standards for quantification creates the biggest analytical challenge in the field of PFAS analysis. For that, and in order to mitigate leaks in the environmental fate of precursors, as a result of their possible biodegradation as intermediates, new methods have been developed to detect fluoro-substances.
However, transformation studies published to date are available for only of a small portion of these precursors, and therefore, much uncertainty exists regarding the extent to which precursor transformation occurs on a global scale, which environmental compartments represent the majority of transformation, relevant environmental conditions that affect transformation processes, and transformation rates and pathways.
The most well-known methods include total oxidisable precursor (TOP) assays,105 fluorine-19 nuclear magnetic resonance (19F NMR) spectroscopy,106 inductively coupled plasma-mass spectrometry (ICP-MS/MS),107 and X-ray photoelectron spectroscopy (XPS).108 Methods for total fluorine (TF) analysis have also been developed for the discovery of PFAS precursors in complex environmental matrices.
These methods include total organic fluorine (TOF) analysis, particle-induced gamma ray emission (PIGE) spectroscopy and combustion ion chromatography (CIC). After PFASs are extracted from the matrices of interest by SPE, TOF yield can be measured by PIGE.109 PIGE is a very fast technique and effective for quantitative analysis, but the requirement of gamma radiation for nuclear activation makes it costly and rarerly applied. Another reason for its low applicability lies in the fact that it only measures fluorine yield on the surface. The latter is also linked to likely overestimations of the values due to surface coating. CIC involves the adsorption of both PFAS and their precursors on an activated carbon matrix (ACM). The ACM gets then combusted and the released fluorine from every fluorinated substance is measured. Through that procedure, the control of the mass balance is possible, yet no individual components can be traced.110 Extractable organic fluorine (EOF) assay using CIC has been used for the analysis of water matrices, sediments and various biological samples since it was first reported by Miyake et al. in 2007.111 Adsorbable organic fluorine (AOF) assay utilises activated carbon adsorbent and also employs CIC but to the authors‘ knowledge there has not been a direct comparison between EOF and AOF approaches so far.
The TOP assay, originally developed by Houtz and Sedlak in 2012,105 is perhaps the most promising and therefore most widely used non-specific method to report. It achieves the best detection selectivity from the available non-specific methods, but only for precursors that can be oxidised to certain perfluoroalkyl carboxylic and sulfonic acids (PFAAs). This is performed by comparing a given matrix prior and after oxidation by hydroxyl radicals. This method can be facilitated by utilizing a simple LC-MS/MS system, yet is highly precise and can effectively target possible precursors of specific PFAAs. However, the oxidation of precursor compounds (known and unknown) may result in intermediate fluorinated compounds or other PFAS that are not measured as part of the analysis, contextually. Thus measuring increases or decreases in only the PFASs included in a given analytical protocol may solely represent a portion of the potentially transformable precursors in the sample.
Recently, TOP assay has been applied to various water samples to evaluate the tradeoff between selectivity and inclusivity in PFAS analyses.112 Additionally, it was implemented in the analysis of effluent wastewater samples for the assessment of the environmental fate of per- and polyfluoroalkyl ether acids.113 Ultrashort-chain perfluoroalkyl carboxylic acids were included in the TOP assay protocol in 2019,53 while zwitterionic, cationic, and anionic PFAS were integrated into the TOP assay groundwater protocol the same year.114 TOF, on the other hand, was applied to detect organic and inorganic fluoride from seawater and blood samples.111 Extraction was performed by SPE and IPE with methyl tert-butyl ether (MTBE) and hexane as solvents. CIC was then applied for the determination of PFAS and precursors in both sample types.
Although not yet commercially available, other lines of evidence, such as LC-QToF, have been established and used by academia to semi-quantitatively identify precursors.112 Using TOPA in conjunction with non-target LC-QToF can greatly improve the understanding of PFAS precursors. Quantitative analysis of individual precursors will become more consistent and potentially commercially available in the near future as more standards for precursors become available.
Disclaimer
The content of this article reflects only the authors’ views and the Research Executive Agency is not responsible for any use that may be made of the information it contains.
Conflicts of interest
The authors declare no conflict of interest.
References
- X. Song, R. Vestergren, Y. Shi, J. Huang and Y. Cai, Emissions, Transport, and Fate of Emerging Per- and Polyfluoroalkyl Substances from One of the Major Fluoropolymer Manufacturing Facilities in China, Environ. Sci. Technol., 2018, 52, 9694–9703 CrossRef CAS PubMed
.
- N. Yamashita, K. Kannan, S. Taniyasu, Y. Horii, T. Okazawa, G. Petrick and T. Gamo, Analysis of Perfluorinated Acids at Parts-Per-Quadrillion Levels in Seawater Using Liquid Chromatography-Tandem Mass Spectrometry, Environ. Sci. Technol., 2004, 38, 5522–5528 CrossRef CAS PubMed
.
- L. Ahrens, Polyfluoroalkyl compounds in the aquatic environment: a review of their occurrence and fate, J. Environ. Monit., 2011, 13, 20–31 Search PubMed
.
- Y. Zhang, S. Beesoon, L. Zhu and J. W. Martin, Biomonitoring of perfluoroalkyl acids in human urine and estimates of biological half-life, Environ. Sci. Technol., 2013, 47, 10619–10627 Search PubMed
.
- H. Joerss, C. Apel and R. Ebinghaus, Emerging per- and polyfluoroalkyl substances (PFASs) in surface water and sediment of the North and Baltic Seas, Sci. Total Environ., 2019, 686, 360–369 CrossRef CAS PubMed
.
- M. Babut, P. Labadie, C. Simonnet-Laprade, G. Munoz, M. C. Roger, B. J. D. Ferrari, H. Budzinski and E. Sivade, Per- and poly-fluoroalkyl compounds in freshwater fish from the Rhone River: Influence of fish size, diet, prey contamination and biotransformation, Sci. Total Environ., 2017, 605–606, 38–47 CrossRef CAS PubMed
.
- M. C. Russell, S. R. Newton, K. M. McClure, R. S. Levine, L. P. Phelps, A. B. Lindstrom and M. J. Strynar, Per- and polyfluoroalkyl substances in two different populations of northern cardinals, Chemosphere, 2019, 222, 295–304 CrossRef CAS PubMed
.
- G. Boisvert, C. Sonne, F. F. Riget, R. Dietz and R. J. Letcher, Bioaccumulation and biomagnification of perfluoroalkyl acids and precursors in East Greenland polar bears and their ringed seal prey, Environ. Pollut., 2019, 252, 1335–1343 Search PubMed
.
- T. Groffen, R. Lasters, F. Lemiere, T. Willems, M. Eens, L. Bervoets and E. Prinsen, Development and validation of an extraction method for the analysis of perfluoroalkyl substances (PFASs) in environmental and biotic matrices, J. Chromatogr. B: Anal. Technol. Biomed. Life Sci., 2019, 1116, 30–37 Search PubMed
.
- Z. Wang, J. C. DeWitt, C. P. Higgins and I. T. Cousins, A Never-Ending Story of Per- and Polyfluoroalkyl Substances (PFASs)?, Environ. Sci. Technol., 2017, 51, 2508–2518 Search PubMed
.
- X. Cao, C. Wang, Y. Lu, M. Zhang, K. Khan, S. Song, P. Wang and C. Wang, Occurrence, sources and health risk of polyfluoroalkyl substances (PFASs) in soil, water and sediment from a drinking water source area, Ecotoxicol. Environ. Saf., 2019, 174, 208–217 Search PubMed
.
- R. C. Buck, J. Franklin, U. Berger, J. M. Conder, I. T. Cousins, P. de Voogt, A. A. Jensen, K. Kannan, S. A. Mabury and S. P. van Leeuwen, Perfluoroalkyl and polyfluoroalkyl substances in the environment: terminology, classification, and origins, Integr. Environ. Assess. Manage., 2011, 7, 513–541 CrossRef CAS PubMed
.
- A. Androulakakis, N. Alygizakis, G. Gkotsis, M. C. Nika, V. Nikolopoulou, E. Bizani, E. Chadwick, A. Cincinelli, D. Classen, S. Danielsson, R. Dekker, G. Duke, N. Glowacka, H. A. H. Jansman, O. Krone, T. Martellini, P. Movalli, S. Persson, A. Roos, E. O'Rourke, U. Siebert, G. Treu, N. W. van den Brink, L. A. Walker, R. Deaville, J. Slobodnik and N. S. Thomaidis, Determination of 56 per- and polyfluoroalkyl
substances in top predators and their prey from Northern Europe by LC-MS/MS, Chemosphere, 2021, 287, 131775 Search PubMed
.
- S. Dalahmeh, S. Tirgani, A. J. Komakech, C. B. Niwagaba and L. Ahrens, Per- and polyfluoroalkyl substances (PFASs) in water, soil and plants in wetlands and agricultural areas in Kampala, Uganda, Sci. Total Environ., 2018, 631–632, 660–667 CrossRef CAS
.
- F. Xiao, Emerging poly- and perfluoroalkyl substances in the aquatic environment: A review of current literature, Water Res., 2017, 124, 482–495 CrossRef CAS PubMed
.
- J. F. Ayala-Cabrera, F. Javier Santos and E. Moyano, Negative-ion atmospheric pressure ionisation of semi-volatile fluorinated compounds for ultra-high-performance liquid chromatography tandem mass spectrometry analysis, Anal. Bioanal. Chem., 2018, 410, 4913–4924 CrossRef CAS PubMed
.
- Y. Pan, H. Zhang, Q. Cui, N. Sheng, L. W. Y. Yeung, Y. Sun, Y. Guo and J. Dai, Worldwide Distribution of Novel Perfluoroether Carboxylic and Sulfonic Acids in Surface Water, Environ. Sci. Technol., 2018, 52, 7621–7629 Search PubMed
.
- Z. Xie, Z. Wang, W. Mi, A. Moller, H. Wolschke and R. Ebinghaus, Neutral poly-/perfluoroalkyl substances in air and snow from the Arctic, Sci. Rep., 2015, 5, 8912 CrossRef CAS PubMed
.
- A. Jahnke and U. Berger, Trace analysis of per- and polyfluorinated alkyl substances in various matrices—How do current methods perform?, J. Chromatogr. A, 2009, 1216, 410–421 Search PubMed
.
- M. Shoeib, T. Harner, S. Lee, D. Lane and J. Zhu, Sorbent-Impregnated Polyurethane Foam Disk for Passive Air Sampling of Volatile Fluorinated Chemicals, Anal. Chem., 2008, 80, 675–682 CrossRef CAS PubMed
.
- J. L. Barber, U. Berger, C. Chaemfa, S. Huber, A. Jahnke, C. Temme and K. C. Jones, Analysis of per- and polyfluorinated alkyl substances in air samples from Northwest Europe, J. Environ. Monit., 2007, 9, 530–541 RSC
.
- J. A. Padilla-Sanchez, E. Papadopoulou, S. Poothong and L. S. Haug, Investigation of the Best Approach for Assessing Human Exposure to Poly- and Perfluoroalkyl Substances through Indoor Air, Environ. Sci. Technol., 2017, 51, 12836–12843 Search PubMed
.
- N. Yu, H. Wen, X. Wang, E. Yamazaki, S. Taniyasu, N. Yamashita, H. Yu and S. Wei, Nontarget Discovery of Per- and Polyfluoroalkyl Substances in Atmospheric Particulate Matter and Gaseous Phase Using Cryogenic Air Sampler, Environ. Sci. Technol., 2020, 54, 3103–3113 CrossRef CAS PubMed
.
- R. Wu, H. Lin, E. Yamazaki, S. Taniyasu, M. Sörengård, L. Ahrens, P. K. S. Lam, H. Eun and N. Yamashita, Simultaneous analysis of neutral and ionizable per- and polyfluoroalkyl substances in air, Chemosphere, 2021, 280, 130607 CrossRef CAS PubMed
.
- Z. Lu, R. Lu, H. Zheng, J. Yan, L. Song, J. Wang, H. Yang and M. Cai, Risk exposure assessment of per- and polyfluoroalkyl substances (PFASs) in drinking water and atmosphere in central eastern China, Environ. Sci. Pollut. Res. Int., 2018, 25, 9311–9320 CrossRef CAS
.
- J. Martin, D. Muir, C. Moody, D. Ellis, W. Kwan, K. Solomon and S. Mabury, Collection of Airborne Fluorinated Organics and Analysis by Gas Chromatography/Chemical Ionization Mass Spectrometry, Anal. Chem., 2002, 74, 584–590 CrossRef CAS
.
- M. Koblizkova, S. Genualdi, S. C. Lee and T. Harner, Application of sorbent impregnated polyurethane foam (SIP) disk passive air samplers for investigating organochlorine pesticides and polybrominated diphenyl ethers at the global scale, Environ. Sci. Technol., 2012, 46, 391–396 Search PubMed
.
- M. Shoeib, T. Harner and P. Vlahos, Perfluorinated Chemicals in the Arctic Atmosphere, Environ. Sci. Technol., 2006, 40, 7577–7583 Search PubMed
.
- C. Gremmel, T. Fromel and T.
P. Knepper, HPLC-MS/MS methods for the determination of 52 perfluoroalkyl and polyfluoroalkyl substances in aqueous samples, Anal. Bioanal. Chem., 2017, 409, 1643–1655 CrossRef CAS PubMed
.
- Y. Tian, Y. Yao, S. Chang, Z. Zhao, Y. Zhao, X. Yuan, F. Wu and H. Sun, Occurrence and Phase Distribution of Neutral and Ionizable Per- and Polyfluoroalkyl Substances (PFASs) in the Atmosphere and Plant Leaves around Landfills: A Case Study in Tianjin, China, Environ. Sci. Technol., 2018, 52, 1301–1310 CrossRef CAS
.
- C. Rauert, M. Shoieb, J. K. Schuster, A. Eng and T. Harner, Atmospheric concentrations and trends of poly- and perfluoroalkyl substances (PFAS) and volatile methyl siloxanes (VMS) over 7 years of sampling in the Global Atmospheric Passive Sampling (GAPS) network, Environ. Pollut., 2018, 238, 94–102 CrossRef CAS PubMed
.
- F. Wong, M. Shoeib, A. Katsoyiannis, S. Eckhardt, A. Stohl, P. Bohlin-Nizzetto, H. Li, P. Fellin, Y. Su and H. Hung, Assessing temporal trends and source regions of per- and polyfluoroalkyl substances (PFASs) in air under the Arctic Monitoring and Assessment Programme (AMAP), Atmos. Environ., 2018, 172, 65–73 CrossRef CAS
.
- Y. Yao, Y. Zhao, H. Sun, S. Chang, L. Zhu, A. C. Alder and K. Kannan, Per- and Polyfluoroalkyl Substances (PFASs) in Indoor Air and Dust from Homes and Various Microenvironments in China: Implications for Human Exposure, Environ. Sci. Technol., 2018, 52, 3156–3166 CrossRef CAS PubMed
.
- T. Shoeib, Y. Hassan, C. Rauert and T. Harner, Poly- and perfluoroalkyl substances (PFASs) in indoor dust and food packaging materials in Egypt: Trends in developed and developing countries, Chemosphere, 2016, 144, 1573–1581 Search PubMed
.
-
USGS, Chapter A4. Collection of water samples, Techniques of Water-Resources Investigations, 2006, Version 2.0 Search PubMed
.
- P. Suwannakot, F. Lisi, E. Ahmed, K. Liang, R. Babarao, J. J. Gooding and W. A. Donald, Metal-Organic Framework-Enhanced Solid-Phase Microextraction Mass Spectrometry for the Direct and Rapid Detection of Perfluorooctanoic Acid in Environmental Water Samples, Anal. Chem., 2020, 92, 6900–6908 Search PubMed
.
- L. Ciofi, L. Renai, D. Rossini, C. Ancillotti, A. Falai, D. Fibbi, M. C. Bruzzoniti, J. J. Santana-Rodriguez, S. Orlandini and M. Del Bubba, Applicability of the direct injection liquid chromatographic tandem mass spectrometric analytical approach to the sub-ngL(-1) determination of perfluoro-alkyl acids in waste, surface, ground and drinking water samples, Talanta, 2018, 176, 412–421 CrossRef CAS
.
- T. Meyer, A. O. De Silva, C. Spencer and F. Wania, Fate of perfluorinated carboxylates and sulfonates during snowmelt within an urban watershed, Environ. Sci. Technol., 2011, 45, 8113–8119 CrossRef CAS PubMed
.
- C. Gallen, G. Eaglesham, D. Drage, T. H. Nguyen and J. F. Mueller, A mass estimate of perfluoroalkyl substance (PFAS) release from Australian wastewater treatment plants, Chemosphere, 2018, 208, 975–983 CrossRef CAS PubMed
.
- J. Janda, K. Nodler, H. J. Brauch, C. Zwiener and F. T. Lange, Robust trace analysis of polar (C2–C8) perfluorinated carboxylic acids by liquid chromatography-tandem mass spectrometry: method development and application to surface water, groundwater and drinking water, Environ. Sci. Pollut. Res. Int., 2019, 26, 7326–7336 CrossRef CAS PubMed
.
- Z. H. Deng, C. G. Cheng, X. L. Wang, S. H. Shi, M. L. Wang and R. S. Zhao, Preconcentration and Determination of Perfluoroalkyl Substances (PFASs) in Water Samples by Bamboo Charcoal-Based Solid-Phase Extraction Prior to Liquid Chromatography-Tandem Mass Spectrometry, Molecules, 2018, 23, 902–913 CrossRef
.
- M. Lorenzo, J. Campo and Y. Picó, Analytical challenges to determine emerging persistent organic pollutants in aquatic ecosystems, TrAC, Trends Anal. Chem., 2018, 103, 137–155 Search PubMed
.
- Y. Huang, H. Li, M. Bai and X. Huang, Efficient extraction of perfluorocarboxylic acids in complex samples with a monolithic adsorbent combining fluorophilic and anion-exchange interactions, Anal. Chim. Acta, 2018, 1011, 50–58 Search PubMed
.
- E. Concha-Grana, G. Fernandez-Martinez, P. Lopez-Mahia, D. Prada-Rodriguez and S. Muniategui-Lorenzo, Fast and sensitive determination of per- and polyfluoroalkyl substances in seawater, J. Chromatogr. A, 2018, 1555, 62–73 CrossRef CAS PubMed
.
- A. Papadopoulou, I. P. Roman, A. Canals, K. Tyrovola and E. Psillakis, Fast screening of perfluorooctane sulfonate in water using vortex-assisted liquid-liquid microextraction coupled to liquid chromatography-mass spectrometry, Anal. Chim. Acta, 2011, 691, 56–61 Search PubMed
.
- Y. Jia, J. Qian and B. Pan, Dual-Functionalized MIL-101(Cr) for the Selective Enrichment and Ultrasensitive Analysis of Trace Per- and Poly-fluoroalkyl Substances, Anal. Chem., 2021, 93, 11116–11122 CrossRef CAS PubMed
.
- Y. Shi, J. Wang and Y. Cai, A Highly Selective Extraction Approach for Per- and Polyfluoroalkyl Substances Based on Protein Affinity, Anal. Chem., 2020, 92, 8675–8679 CrossRef CAS PubMed
.
- Y. Cao, C. Lee, E. T. J. Davis, W. Si, F. Wang, S. Trimpin and L. Luo, 1000-Fold Preconcentration of Per- and Polyfluorinated Alkyl Substances within 10 Minutes via Electrochemical Aerosol Formation, Anal. Chem., 2019, 91, 14352–14358 CrossRef CAS PubMed
.
- R. Troger, P. Klockner, L. Ahrens and K. Wiberg, Micropollutants in drinking water from source to tap – Method development and application of a multiresidue screening method, Sci. Total Environ., 2018, 627, 1404–1432 CrossRef PubMed
.
- H. Chen, J. Han, J. Cheng, R. Sun, X. Wang, G. Han, W. Yang and X. He, Distribution, bioaccumulation and trophic transfer of chlorinated polyfluoroalkyl ether sulfonic acids in the marine food web of Bohai, China, Environ. Pollut., 2018, 241, 504–510 CrossRef CAS PubMed
.
- H. Park, G. Choo, H. Kim and J. E. Oh, Evaluation of the current contamination status of PFASs and OPFRs in South Korean tap water associated with its origin, Sci. Total Environ., 2018, 634, 1505–1512 CrossRef CAS PubMed
.
- C. Wei, Q. Wang, X. Song, X. Chen, R. Fan, D. Ding and Y. Liu, Distribution, source identification and health risk assessment of PFASs and two PFOS alternatives in groundwater from non-industrial areas, Ecotoxicol. Environ. Saf., 2018, 152, 141–150 CrossRef CAS PubMed
.
- J. Janda, K. Nodler, M. Scheurer, O. Happel, G. Nurenberg, C. Zwiener and F. T. Lange, Closing the gap – inclusion of ultrashort-chain perfluoroalkyl carboxylic acids in the total oxidizable precursor (TOP) assay protocol, Environ. Sci.: Processes Impacts, 2019, 21, 1926–1935 RSC
.
- O. Arvaniti, A. Asimakopoulos, M. Dasenaki, E. Ventouri, A. Stasinakis and N. S. Thomaidis, Simultaneous determination of eighteen perfluorinated compounds in dissolved and particulate phases of wastewater, and in sewage sludge by liquid chromatography-tandem mass spectrometry, Anal. Methods, 2014, 6, 1341–1349 Search PubMed
.
- J. S. Skaar, E. M. Raeder, J. L. Lyche, L. Ahrens and R. Kallenborn, Elucidation of contamination sources for poly- and perfluoroalkyl substances (PFASs) on Svalbard (Norwegian Arctic), Environ. Sci. Pollut. Res. Int., 2019, 26, 7356–7363 Search PubMed
.
- G. Munoz, P. Ray, S. Mejia-Avendano, S. Vo Duy, D. Tien Do, J. Liu and S. Sauve, Optimization of extraction methods for comprehensive profiling of perfluoroalkyl and polyfluoroalkyl substances in firefighting foam impacted soils, Anal. Chim. Acta, 2018, 1034, 74–84 Search PubMed
.
- K. Winkens, G. Giovanoulis, J. Koponen, R. Vestergren, U. Berger, A. M. Karvonen, J. Pekkanen, H. Kiviranta and I. T. Cousins, Perfluoroalkyl acids and their precursors in floor dust of children's bedrooms - Implications for indoor exposure, Environ. Int., 2018, 119, 493–502 Search PubMed
.
- A. Shen, S. Lee, K. Ra, D. Suk and H. B. Moon, Historical trends of perfluoroalkyl substances (PFASs) in dated sediments from semi-enclosed bays of Korea, Mar. Pollut. Bull., 2018, 128, 287–294 CrossRef CAS PubMed
.
- L. Tang, X. Liu, G. Yang, J. Xia, N. Zhang, D. Wang, H. Deng, M. Mao, X. Li and B. Ni, Spatial distribution, sources and risk assessment of perfluoroalkyl substances in surface soils of a representative densely urbanized and industrialized city of China, Catena, 2021, 198, 105059 CrossRef CAS
.
- A. Ahmadireskety, B. F. Da Silva, T. G. Townsend, R. A. Yost, H. M. Solo-Gabriele and J. A. Bowden, Evaluation of extraction workflows for quantitative analysis of per- and polyfluoroalkyl substances: A case study using soil adjacent to a landfill, Sci. Total Environ., 2021, 760, 143944 Search PubMed
.
- G. Codling, S. Hosseini, M. B. Corcoran, S. Bonina, T. Lin, A. Li, N. C. Sturchio, K. J. Rockne, K. Ji, H. Peng and J. P. Giesy, Current and historical concentrations of poly and perfluorinated compounds in sediments of the northern Great Lakes - Superior, Huron, and Michigan, Environ. Pollut., 2018, 236, 373–381 Search PubMed
.
- E. Pignotti and E. Dinelli, Distribution and partition of endocrine disrupting compounds in water and sediment: Case study of the Romagna area (North Italy), J. Geochem. Explor., 2018, 195, 66–77 CrossRef CAS
.
- T. Ruan, Y. Lin, T. Wang, R. Liu and G. Jiang, Identification of Novel Polyfluorinated Ether Sulfonates as PFOS Alternatives in Municipal Sewage Sludge in China, Environ. Sci. Technol., 2015, 49, 6519–6527 CrossRef CAS PubMed
.
- M. Kotthoff, J. Muller, H. Jurling, M. Schlummer and D. Fiedler, Perfluoroalkyl and polyfluoroalkyl substances in consumer products, Environ. Sci. Pollut. Res. Int., 2015, 22, 14546–14559 CrossRef CAS PubMed
.
- Q. W. Wang, G. P. Yang, Z. M. Zhang and J. Zhang, Optimization of sample preparation and chromatography for the determination of perfluoroalkyl acids in sediments from the Yangtze Estuary and East China Sea, Chemosphere, 2018, 205, 524–530 CrossRef CAS PubMed
.
- S. Newton, R. McMahen, J. A. Stoeckel, M. Chislock, A. Lindstrom and M. Strynar, Novel Polyfluorinated Compounds Identified Using High Resolution Mass Spectrometry Downstream of Manufacturing Facilities near Decatur, Alabama, Environ. Sci. Technol., 2017, 51, 1544–1552 CrossRef CAS PubMed
.
- H. Zhang, B. Wen, W. Wen, Y. Ma, X. Hu, Y. Wu, L. Luo and S. Zhang, Determination of perfluoroalkyl acid isomers in biosolids, biosolids-amended soils and plants using ultra-high performance liquid chromatography tandem mass spectrometry, J. Chromatogr. B: Anal. Technol. Biomed. Life Sci., 2018, 1072, 25–33 Search PubMed
.
- H. Lee and S. A. Mabury, Sorption of Perfluoroalkyl Phosphonates and Perfluoroalkyl Phosphinates in Soils, Environ. Sci. Technol., 2017, 51, 3197–3205 CrossRef CAS PubMed
.
- R. Guo, D. Megson, A. L. Myers, P. A. Helm, C. Marvin, P. Crozier, S. Mabury, S. P. Bhavsar, G. Tomy, M. Simcik, B. McCarry and E. J. Reiner, Application of a comprehensive extraction technique for the determination of poly- and perfluoroalkyl substances (PFASs) in Great Lakes Region sediments, Chemosphere, 2016, 164, 535–546 CrossRef CAS PubMed
.
- C. Su, Y. Lu, T. Wang, X. Lu, S. Song, L. Li, K. Khan, C. Wang and R. Liang, Dynamic multimedia fate simulation of Perfluorooctane Sulfonate (PFOS) from 1981 to 2050 in the urbanizing Bohai Rim of China, Environ. Pollut., 2018, 235, 235–244 CrossRef CAS PubMed
.
- Y. Zhi and J. Liu, Sorption and desorption of anionic, cationic and zwitterionic polyfluoroalkyl substances by soil organic matter and pyrogenic carbonaceous materials, Chem. Eng. J., 2018, 346, 682–691 Search PubMed
.
- K. J. Hansen, L. A. Clemen, M. E. Ellefson and H. O. Johnson, Compound-Specific, Quantitative Characterization
of Organic Fluorochemicals in Biological Matrices, Environ. Sci. Technol., 2001, 35, 766–770 Search PubMed
.
- Q. Cui, Y. Pan, H. Zhang, N. Sheng, J. Wang, Y. Guo and J. Dai, Occurrence and Tissue Distribution of Novel Perfluoroether Carboxylic and Sulfonic Acids and Legacy Per/Polyfluoroalkyl Substances in Black-Spotted Frog (Pelophylax nigromaculatus), Environ. Sci. Technol., 2018, 52, 982–990 Search PubMed
.
- A. O. De Silva, C. Spencer, B. F. Scott, S. Backus and D. C. Muir, Detection of a cyclic perfluorinated acid, perfluoroethylcyclohexane sulfonate, in the Great Lakes of North America, Environ. Sci. Technol., 2011, 45, 8060–8066 CrossRef CAS PubMed
.
- A. O. De Silva, C. Spencer, K. C. Ho, M. Al Tarhuni, C. Go, M. Houde, S. R. de Solla, R. A. Lavoie, L. E. King, D. C. Muir, P. A. Fair, R. S. Wells and G. D. Bossart, Perfluoroalkylphosphinic Acids in Northern Pike (Esox lucius), Double-Crested Cormorants (Phalacrocorax auritus), and Bottlenose Dolphins (Tursiops truncatus) in Relation to Other Perfluoroalkyl Acids, Environ. Sci. Technol., 2016, 50, 10903–10913 CrossRef CAS PubMed
.
- I. Zabaleta, E. Bizkarguenaga, A. Prieto, M. Ortiz-Zarragoitia, L. A. Fernandez and O. Zuloaga, Simultaneous determination of perfluorinated compounds and their potential precursors in mussel tissue and fish muscle tissue and liver samples by liquid chromatography-electrospray-tandem mass spectrometry, J. Chromatogr. A, 2015, 1387, 13–23 CrossRef CAS PubMed
.
- Y. Liu, M. Qian, X. Ma, L. Zhu and J. W. Martin, Nontarget Mass Spectrometry Reveals New Perfluoroalkyl Substances in Fish from the Yangtze River and Tangxun Lake, China, Environ. Sci. Technol., 2018, 52, 5830–5840 CrossRef CAS PubMed
.
- S. P. van Leeuwen and J. de Boer, Extraction and clean-up strategies for the analysis of poly- and perfluoroalkyl substances in environmental and human matrices, J. Chromatogr. A, 2007, 1153, 172–185 CrossRef CAS PubMed
.
- J. Li, F. Guo, Y. Wang, J. Zhang, Y. Zhong, Y. Zhao and Y. Wu, Can nail, hair and urine be used for biomonitoring of human exposure to perfluorooctane sulfonate and perfluorooctanoic acid?, Environ. Int., 2013, 53, 47–52 CrossRef CAS PubMed
.
- C. Lau, K. Anitole, C. Hodes, D. Lai, A. Pfahles-Hutchens and J. Seed, Perfluoroalkyl acids: a review of monitoring and toxicological findings, Toxicol. Sci., 2007, 99, 366–394 Search PubMed
.
- M. Sebastiano, W. Jouanneau, P. Blevin, F. Angelier, C. Parenteau, J. Gernigon, J. C. Lemesle, F. Robin, P. Pardon, H. Budzinski, P. Labadie and O. Chastel, High levels of fluoroalkyl substances and potential disruption of thyroid hormones in three gull species from South Western France, Sci. Total Environ., 2021, 765, 144611 Search PubMed
.
- J. D. Crosse, R. F. Shore, K. C. Jones and M. G. Pereira, Long term trends in PBDE concentrations in gannet (Morus bassanus) eggs from two UK colonies, Environ. Pollut., 2012, 161, 93–100 CrossRef CAS PubMed
.
- Y. J. Hou, J. Deng, K. He, C. Chen and Y. Yang, Covalent Organic Frameworks-Based Solid-Phase Microextraction Probe for Rapid and Ultrasensitive Analysis of Trace Per- and Polyfluoroalkyl Substances Using Mass Spectrometry, Anal. Chem., 2020, 92, 10213–10217 CrossRef CAS PubMed
.
- K. Gao, J. Fu, Q. Xue, Y. Li, Y. Liang, Y. Pan, A. Zhang and G. Jiang, An integrated method for simultaneously determining 10 classes of per- and polyfluoroalkyl substances in one drop of human serum, Anal. Chim. Acta, 2018, 999, 76–86 Search PubMed
.
- M. Land, C. A. de Wit, A. Bignert, I. T. Cousins, D. Herzke, J. H. Johansson and J. W. Martin, What is the effect of phasing out long-chain per- and polyfluoroalkyl substances on the concentrations of perfluoroalkyl acids and their precursors in the environment? A systematic review, Environ. Evid., 2018, 7, 4–36 CrossRef
.
- Y. Wang, Y. Zhong, J. Li, J. Zhang, B. Lyu, Y. Zhao and Y. Wu, Occurrence of perfluoroalkyl substances in matched human serum, urine, hair and nail, J. Environ. Sci. (China), 2018, 67, 191–197 CrossRef CAS PubMed
.
- Y. Wang, Y. Shi, R. Vestergren, Z. Zhou, Y. Liang and Y. Cai, Using hair, nail and urine samples for human exposure assessment of legacy and emerging per- and polyfluoroalkyl substances, Sci. Total Environ., 2018, 636, 383–391 CrossRef CAS PubMed
.
- Y. Liu, T. Ruan, Y. Lin, A. Liu, M. Yu, R. Liu, M. Meng, Y. Wang, J. Liu and G. Jiang, Chlorinated Polyfluoroalkyl Ether Sulfonic Acids in Marine Organisms from Bohai Sea, China: Occurrence, Temporal Variations, and Trophic Transfer Behavior, Environ. Sci. Technol., 2017, 51, 4407–4414 Search PubMed
.
-
OECD, Toward a New Comprehensive Global Database of Per- and Polyfluoroalkyl Substances (PFASs): Summary Report on Updating the OECD 2007 List of Per- and Polyfluoroalkyl Substances (PFASs), 2018 Search PubMed
.
- H. Barrett, X. Du, M. Houde, S. Lair, J. Verreault and H. Peng, Suspect and Nontarget Screening Revealed Class-Specific Temporal Trends (2000–2017) of Poly- and Perfluoroalkyl Substances in St. Lawrence Beluga Whales, Environ. Sci. Technol., 2021, 55, 1659–1671 Search PubMed
.
- K. Kuchibhotla and B. Bathellier, Neural encoding of sensory and behavioral complexity in the auditory cortex, Curr. Opin. Neurobiol., 2018, 52, 65–71 CrossRef CAS PubMed
.
- S. Mejia-Avendano, G. Munoz, S. Vo Duy, M. Desrosiers, T. P. Benoi, S. Sauve and J. Liu, Novel Fluoroalkylated Surfactants in Soils Following Firefighting Foam Deployment During the Lac-Megantic Railway Accident, Environ. Sci. Technol., 2017, 51, 8313–8323 CrossRef CAS PubMed
.
- N. A. Alygizakis, P. Oswald, N. S. Thomaidis, E. L. Schymanski, R. Aalizadeh, T. Schulze, M. Oswaldova and J. Slobodnik, NORMAN digital sample freezing platform: A European virtual platform to exchange liquid chromatography high resolution-mass spectrometry data and screen suspects in “digitally frozen” environmental samples, TrAC, Trends Anal. Chem., 2019, 115, 129–137 CrossRef CAS
.
- F. Xiao, S. A. Golovko and M. Y. Golovko, Identification of novel non-ionic, cationic, zwitterionic, and anionic polyfluoroalkyl substances using UPLC-TOF-MS(E) high-resolution parent ion search, Anal. Chim. Acta, 2017, 988, 41–49 Search PubMed
.
- N. Yu, H. Guo, J. Yang, L. Jin, X. Wang, W. Shi, X. Zhang, H. Yu and S. Wei, Non-Target and Suspect Screening of Per- and Polyfluoroalkyl Substances in Airborne Particulate Matter in China, Environ. Sci. Technol., 2018, 52, 8205–8214 Search PubMed
.
- A. Rotander, A. Karrman, L. M. Toms, M. Kay, J. F. Mueller and M. J. Gomez Ramos, Novel fluorinated surfactants tentatively identified in firefighters using liquid chromatography quadrupole time-of-flight tandem mass spectrometry and a case-control approach, Environ. Sci. Technol., 2015, 49, 2434–2442 Search PubMed
.
- S. Heuckeroth, T. N. Nxumalo, A. Raab and J. Feldmann, Fluorine-Specific Detection Using ICP-MS Helps to Identify PFAS Degradation Products in Nontargeted Analysis, Anal. Chem., 2021, 93, 6335–6341 CrossRef CAS PubMed
.
- X. Ouyang, J. M. Weiss, J. de Boer, M. H. Lamoree and P. E. G. Leonards, Non-target analysis of household dust and laundry dryer lint using comprehensive two-dimensional liquid chromatography coupled with time-of-flight mass spectrometry, Chemosphere, 2017, 166, 431–437 CrossRef CAS PubMed
.
- J. P. Koelmel, P. Stelben, C. A. McDonough, D. A. Dukes, J. J. Aristizabal-Henao, S. L. Nason, Y. Li, S. Sternberg, E. Lin, M. Beckmann, A. J. Williams, J. Draper, J. P. Finch, J. K. Munk, C. Deigl, E. E. Rennie, J. A. Bowden and K. J. Godri Pollitt, FluoroMatch 2.0-making automated and comprehensive non-targeted PFAS annotation a reality, Anal. Bioanal. Chem., 2022, 414, 1201–1215 Search PubMed
.
- Y. Gao, X. Li, X. Li, Q. Zhang and H. Li, Simultaneous determination of 21 trace perfluoroalkyl substances in fish by isotope dilution ultrahigh performance liquid chromatography tandem mass spectrometry, J. Chromatogr. B: Anal. Technol. Biomed. Life Sci., 2018, 1084, 45–52 Search PubMed
.
-
OECD, Lists of PFOS, PFAS, PFOA, PFCA, Related Compounds and Chemicals that May Degrade to PFCA, in Environment, Health and Safety Publications Series on Risk Management, 2007, vol. 21, p. 157 Search PubMed
.
- J. Liu and S. Mejia Avendano, Microbial degradation of polyfluoroalkyl chemicals in the environment: a review, Environ. Int., 2013, 61, 98–114 CrossRef CAS PubMed
.
- Y. Wang, W. Chang, L. Wang, Y. Zhang, Y. Zhang, M. Wang, Y. Wang and P. Li, A review of sources, multimedia distribution and health risks of novel fluorinated alternatives, Ecotoxicol. Environ. Saf., 2019, 182, 109402 Search PubMed
.
-
EPA, Method 537.1: A Solid Phase Extraction (SPE) Liquid Chromatography/tandem Mass Spectrometry (LC/MS/MS) Method for the Determination of Selected Per- and Polyfluorinated Alkyl Substances (PFAS) in Drinking Water, in EPA Journal for the Analysis of Per- and Polyfluorinated Alkyl Substances (PFAS), 2018, vol. 32 Search PubMed
.
- E. F. Houtz and D. L. Sedlak, Oxidative conversion as a means of detecting precursors to perfluoroalkyl acids in urban runoff, Environ. Sci. Technol., 2012, 46, 9342–9349 Search PubMed
.
- A. O. Okaru, T. S. Brunner, S. M. Ackermann, T. Kuballa, S. G. Walch, M. Kohl-Himmelseher and D. W. Lachenmeier, Application of (19)F NMR Spectroscopy for Content Determination of Fluorinated Pharmaceuticals, J. Anal. Methods Chem., 2017, 2017, 9206297 Search PubMed
.
- W. Guo, L. Jin, S. Hu and Q. Guo, Method Development for the Determination of Total Fluorine in Foods by Tandem Inductively Coupled Plasma Mass Spectrometry with a Mass-Shift Strategy, J. Agric. Food Chem., 2017, 65, 3406–3412 CrossRef CAS PubMed
.
- M. Boča, P. Barborík, M. Mičušík and M. Omastová, X-ray photoelectron spectroscopy as detection tool for coordinated or uncoordinated fluorine atoms demonstrated on fluoride systems NaF, K2TaF7, K3TaF8, K2ZrF6, Na7Zr6F31 and K3ZrF7, Solid State Sci., 2012, 14, 828–832 CrossRef
.
- A. Wagner, B. Raue, H. J. Brauch, E. Worch and F. T. Lange, Determination of adsorbable organic fluorine from aqueous environmental samples by adsorption to polystyrene-divinylbenzene based activated carbon and combustion ion chromatography, J. Chromatogr. A, 2013, 1295, 82–89 CrossRef CAS PubMed
.
- M. Ateia, A. Maroli, N. Tharayil and T. Karanfil, The overlooked short- and ultrashort-chain poly- and perfluorinated substances: A review, Chemosphere, 2019, 220, 866–882 CrossRef CAS PubMed
.
- Y. Miyake, N. Yamashita, P. Rostkowski, M. K. So, S. Taniyasu, P. K. Lam and K. Kannan, Determination of trace levels of total fluorine in water using combustion ion chromatography for fluorine: a mass balance approach to determine individual perfluorinated chemicals in water, J. Chromatogr. A, 2007, 1143, 98–104 CrossRef CAS PubMed
.
- C. A. McDonough, J. L. Guelfo and C. P. Higgins, Measuring Total PFASs in Water: The Tradeoff between Selectivity and Inclusivity, Curr. Opin. Environ. Sci. Health, 2019, 7, 13–18 Search PubMed
.
- C. Zhang, Z. R. Hopkins, J. McCord, M. J. Strynar and D. R. U. Knappe, Fate of Per- and Polyfluoroalkyl Ether Acids in the Total Oxidizable Precursor Assay and Implications for the Analysis of Impacted Water, Environ. Sci. Technol. Lett., 2019, 6, 662–668 CrossRef CAS PubMed
.
- D. Martin, G. Munoz, S. Mejia-Avendano, S. V. Duy, Y. Yao, K. Volchek, C. E. Brown, J. Liu and S. Sauve, Zwitterionic, cationic, and anionic perfluoroalkyl and polyfluoroalkyl substances integrated into total oxidizable precursor assay of contaminated groundwater, Talanta, 2019, 195, 533–542 CrossRef CAS PubMed
.
|
This journal is © The Royal Society of Chemistry 2022 |
Click here to see how this site uses Cookies. View our privacy policy here.