DOI:
10.1039/D1QI00149C
(Research Article)
Inorg. Chem. Front., 2021,
8, 2549-2560
Diversifying the luminescence of phenanthro-diimine ligands in zinc complexes†
Received
2nd February 2021
, Accepted 12th March 2021
First published on 7th April 2021
Abstract
Strongly blue fluorescent 1-phenyl-2-(pyridin-2-yl)-1H-phenanthro[9,10-d]imidazole (L1) is a facile block for the construction of multichromophore organic molecules, and simultaneously serves as a chelating diimine ligand. The coordination of L1 to zinc halides enhances the intraligand charge transfer and decreases the emission energy. For the iodide derivative, intra- and intermolecular heavy atom effects lead to a dual singlet–triplet emission with a temperature-dependent ratio of fluorescence and phosphorescence bands in the crystalline state. Decoration of the anthracene core with pyridyl-phenanthroimidazole units (L2 and L3) changes the localization of the lowest energy electronic transitions to the former polyaromatic motif. The solid-state photophysical characteristics of L2 and L3-based compounds strongly depend on the intermolecular interactions between the constituting π-systems (phenanthrene and anthracene), which are perturbed by the ZnX2 coordinated fragments. Modulation of π-stacking contacts in these molecular materials containing L2 and L3 chromophores forms a basis for dynamic optical properties, responsive to mechanical, thermal, or chemical stimuli.
Introduction
Organic platforms, which offer a range of molecular materials with versatile solid-state photophysical behavior without tedious synthetic modifications, are particularly attractive systems for the development of tunable and stimuli-responsive luminophores.1–8 An efficient and straightforward approach to influence the electronic structures of π-conjugated chromophores and to modulate intermolecular interactions is the employment of coordination bonding. In this respect, readily available Zn(II) salts are considered as attractive and inexpensive modifiers of organic emitters decorated with nitrogen and oxygen donor functions. Due to the stable d10 closed shell configuration, the zinc(II) metal center does not participate in the metal to ligand charge transfer upon electronic excitation. Therefore, the complexation of an organic motif with the Zn(II) ion typically enhances intra-ligand fluorescence as a result of increased molecular rigidity and suppression of photoinduced electron transfer reactions. The binding of this metal can also perturb the intramolecular charge transfer (ICT) that distinctly changes the emission energy of the complex compared to that of the free ligand.9–12 Besides, the coordination-driven assembly of two or more chromophore ligands can lead to secondary interactions between the corresponding π-systems and produce aggregates with dynamic optical properties and interligand charge-transfer processes.13–15 In solution, such easily detectable responses allow for efficient luminescence sensing of the Zn(II) ion by a variety of organic probes.16–22
In the solid state, in addition to intramolecular effects, the non-covalent intermolecular interactions, e.g. hydrogen/halogen bonding and π-stacking, govern the organization of the constituting conjugated moieties, and often play a decisive role in modulating the physical characteristics of the bulk materials.23 It is not surprising that the combination of a metal ion or of a simple MXn fragment with an extended π-molecule has a strong influence on the supramolecular arrangement of organic building blocks, and introduces supplementary intermolecular connectivity with the participation of the metal cation and X anions.24 For instance, the complexation of an amine-functionalized pyridinyl-pyrazole ligand with ZnCl2 shows an efficient alteration of the hydrogen bonding network and of the phase behavior caused by the metal halide unit.25 Tuning the π–π stacking contacts between the chromophores of a chiral pyrene-containing histidine by means of Zn(II) coordination has been used to switch the circularly polarized luminescence of the nanoassemblies.26 On the other hand, packing-assisted heteroleptic π-interactions between electron-poor and -rich ligands can yield solid donor–acceptor complexes with improved charge transfer properties.27 Furthermore, dense π–π stacking between aromatic linkers in Zn(II)-assembled metal–organic frameworks and coordination polymers has been identified as an important factor for enhancing long room temperature phosphorescence (RTP).28–30 In general, triplet emission rarely occurs in zinc complexes under ambient conditions because of the inefficient spin–orbit coupling (SOC) and small heavy atom effect (HAE) caused by this metal ion;31,32 the majority of the reported examples of phosphorescent materials correspond to the polymer-like species.28–30,33–38 To solve this problem, a recently proposed approach to increase the SOC, promote intersystem crossing (ISC) and populate the triplet excited state, relying on an external HAE induced by Zn-bound heavier halides or halide counterions has been applied.32,39,40 This facile strategy, which does not require modification of the organic ligand by forming covalent C–Br/I bonds, allowed RTP with quantum yields of up to 9%.39 It is noteworthy that the effect of intermolecular interactions on the photoluminescence properties of organic molecular materials is frequently manifested by remarkable mechano-, vapo- and thermo-responsive behaviors, which can be triggered or tuned as well through the coordination to various metal ions including zinc(II).41–44
In the current work, we have selected a family of easy to prepare phenanthrene-based pyridyl-imidazole ligands and their anthracene derivatives (Fig. 1), which are known to be fluorescent in solution.45 Binding these chromophores to some ZnX2 salts (X = Cl, I, OAc) has a substantial impact on the solid state photophysical behavior. A range of intermolecular contacts were found to be subtly dependent on the anions X and allowed realizing such phenomena as temperature-variable dual emission and mechanochromic luminescence, which could not be attained for the parent organic compounds.
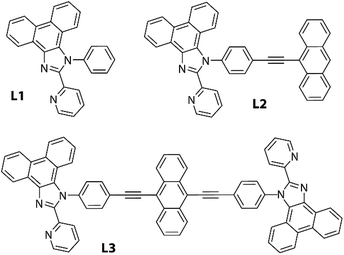 |
| Fig. 1 Ligands L1–L3 used in this study. | |
Results and discussion
Preparation of zinc complexes
Ligands L1 and L2, containing a pyridyl-imidazole coordinating function fused with a polyaromatic phenanthrene motif, were readily obtained as described earlier.45,46L3, which comprises diethynylanthracene decorated with two diimine motifs, was synthesized in a good yield analogously to L2 (Scheme S1, see the experimental details in the ESI†).
The coordination of congener bidentate pyridyl-imidazole ligands to zinc(II) ions has been described previously for a number of polymeric and molecular complexes.47–54 Treatment of ligands L1–L3 with ZnX2 salts in dichloromethane/methanol or diethyl ether mixtures at room temperature afforded the corresponding complexes Zn(L1)X2 (1X, X = Cl 1Cl, I 1I, OAc 1OAc), Zn(L2)X2 (2X, X = Cl 2Cl, I 2I, OAc 2OAc) and Zn2(L3)(OAc)4 (3OAc), which were isolated as stable crystalline solids (75–97%), Scheme 1. The halide congeners of 3OAc are very poorly soluble that prevented their purification and characterization. The 1H NMR spectra of the halide complexes measured in chloroform show well-resolved patterns, which indicate that these species retain the proposed structures in solution (Fig. S1–S3†).
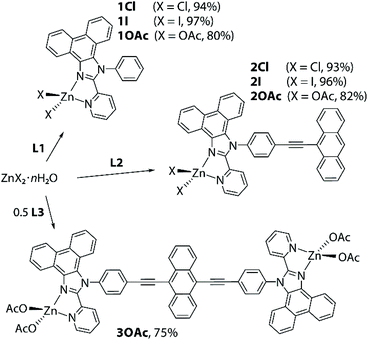 |
| Scheme 1 Synthesis of the ZnX2 complexes with L1–L3 ligands (reaction conditions: methanol or diethyl ether/dichloromethane, 2 h, 298 K). | |
The acetate compounds, particularly 2OAc and 3OAc, show concentration dependent behavior in solution, which is tentatively attributed to the reversible dissociation of Zn(OAc)2 motifs. Similar coordination–decoordination has been observed for a terpyridine-derived complex.9 Thus, in the case of 2OAc, lowering the concentration ultimately allows the identification of the signals of the free ligand (Fig. S2†) that confirms the hypothesis. The presence of two metal centers in 3OAc essentially leads to somewhat different dynamics and explains the lack of ligand L3 in a solution of the complex. It should be mentioned that multiple recrystallizations of 3OAc result in the appearance of substantial amounts of uncoordinated L3, which is in line with the limited stability of zinc acetate compounds 1–3OAc in solution.
Photophysical studies in solution
The optical properties of L1 and L2 were described in an earlier publication.45 The lowest energy absorption and emission bands of L1 are assigned to a mixture of πphen → π*phen/π*py transitions. The coordination of zinc halide units to L1 causes a bathochromic shift of bands in both 1Cl and 1I (Table 1 and Fig. 2), which is in accordance with the reported effect of zinc complexation.55–58 The metal perturbation evidently leads to an appreciable πphen → π*py intraligand charge transfer (ILCT) character and stabilization of the π*py orbitals (LUMO) as confirmed by TD-DFT analysis (see the predicted low energy excitations and frontier molecular orbitals of 1X in Table S1† and Fig. 3, S4†). These ILCT transitions, which predominantly occur between the HOMO and the LUMO, account for the disappearance of the vibronic structure of the main emission band of these complexes (λem = 430–434 nm). The calculated wavelengths of 1Cl and 1I correlate well with the predicted values (Table S2†). Both 1Cl and 1I show a weak emission band at 371 nm that presumably corresponds to the free ligand due to some degree of dissociation, although the presence of L1 was not detected in the NMR spectra of these complexes. The lifetime measured at the major band and quantum yield of 1Cl (Φem = 0.45, τobs = 2.3 ns) are close to those of the free ligand, and expectedly confirm the fluorescence origin of the emission. Such behavior in solution is typical of zinc(II) diimine complexes, which exhibit intraligand fluorescence, somewhat bathochromically shifted, often with higher intensity compared to that of the parent ligands.58–61 Halide ligands at the zinc metal show little influence on the emission wavelength of 1Cl and 1I, but drastically affect the intensity of emission that has also been noticed for the related ZnX2 (X = Cl, I) compounds.55 Replacing chloride with iodide decreases the quantum yield from 0.45 (1Cl) to 0.02 (1I). Under oxygen-free conditions, a rather weak lower energy band (λem = 518, 555 nm) can be detected for 1I (Fig. 2, the decay profile is shown in Fig. S5†). A long lifetime of 2.7 μs suggests that this signal arises from the triplet manifold with substantial ligand-centered nature (i.e.3ππ*), as pointed by discernible vibronic progression (ca. 1290 cm−1).
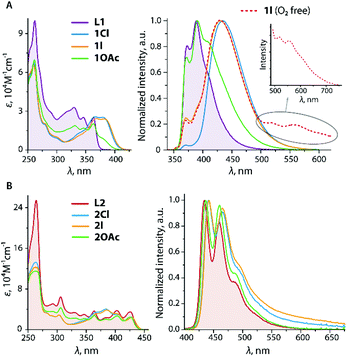 |
| Fig. 2 Absorption (left) and normalized emission (right) spectra of L1 (filled graphs), 1X (A) and L2, 2X (B) recorded in 1,2-dichloroethane (298 K). | |
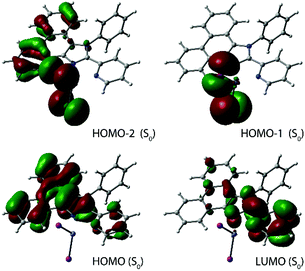 |
| Fig. 3 Frontier molecular orbitals for the ground states of complex 1I (in chloroform, the singly occupied orbitals for S1 and T1 states are shown in Fig. S4†). | |
Table 1 Photophysical properties of ligands L1–L3 and their zinc complexes in solution (1,2-dichloroethane, 298 K)
|
λ
abs, nm (ε, 104 M−1 cm−1) |
λ
em,a nm |
Φ
|
τ, ns |
k
r,b s−1 |
k
nr,c s−1 |
λ
exc 365 nm for 1Cl, L3 and 3OAc and λexc 330 nm for other compounds.
k
r values were estimated by Φ/τobs.
k
nr values were estimated by (1 − Φ)/τobs.
Emission data in dichloromethane from ref. 45.
Data obtained under deaerated conditions.
In aerated solution.
sh stands for shoulder.
|
L1
45 |
261 (10.0), 316 (3.0), 330 (3.3), 346 (2.5), 362 (2.5) |
370, 388 |
0.37 |
1.9 |
1.9 × 108 |
3.3 × 108 |
1Cl
|
261 (6.8), 367 (2.6), 379 (2.6) |
371, 434 |
0.45 |
2.3 |
2.0 × 108 |
2.4 × 108 |
1I
|
260 (7.6), 369 (2.8), 382 (2.8) |
371, 430, (518, 555)e |
0.02f |
<1@433 nm (2700@560 nm)e |
|
|
1OAc
|
262 (6.8), 322 (2.2), 347 (2.1), 363 (2.4) |
372, 390, 407 |
0.57 |
2.2 |
2.6 × 108 |
2.0 × 108 |
L2
45 |
264 (25.4), 292 (5.2), 306 (6.4), 330 (3.4), 347 (3.0), 363 (3.7), 402 (3.8), 425 (3.6) |
433, 459, 486 |
0.65 |
3.3 |
2.0 × 108 |
1.1 × 108 |
2Cl
|
262 (13.3), 303 (2.9), 384 (4.1), 429 (2.5) |
442, 465 |
0.40 |
6.4 |
6.3 × 107 |
9.4 × 107 |
2I
|
263 (12.3), 303 (2.9), 385 (3.9), 429 (2.5) |
442, 465 |
0.20 |
5.4 |
3.7 × 107 |
1.5 × 108 |
2OAc
|
263 (11.6), 306 (4.3), 364 (2.8), 402 (2.7), 426 (2.5) |
436, 460, 487 |
0.58 |
3.6 |
1.6 × 108 |
1.2 × 108 |
L3
|
262 (14.1), 274 (13.4), 319 (8.2), 346 (3.4), 363 (3.5), 447 (4.6), 473 (5.0) |
486, 515, 550shg |
0.60 |
2.6 |
2.3 × 108 |
1.5 × 108 |
3OAc
|
262 (17.3), 274 (15.7), 319 (8.0), 345 (3.5), 363 (3.8), 447 (4.8), 473 (5.2) |
485, 516, 550shg |
0.65 |
2.6 |
2.5 × 108 |
1.3 × 108 |
The decrease of the fluorescence intensity for 1I and the appearance of the triplet emission indicate that a rather fast ISC (S1 → Tn) takes place resulting in the population of the triplet excited state. This process is likely facilitated by the internal heavy atom effect caused by two iodine ligands. According to the population analysis, the HOMO and the LUMO of 1I (Fig. 3), which constitute 98% of the S0 → S1 transition (f = 0.54, Table S1†), are distributed over the pyridyl-imidazole and phenanthrene motifs (21% and 76% for the HOMO, 83% and 14% for the LUMO) with a minor contribution of the iodides (3% for the HOMO). Also, the ZnI2 unit plays a negligible role in the formation of the lowest and the highest singly occupied molecular orbitals (LSOMO and HSOMO) predicted for the S1 and T1 states (Fig. S4†). The iodides, however, have a major contribution to the HOMO−1/−2 (Fig. 3) and might account for some admixture of the halide → π* charge transfer (XLCT) in the excitation and increase the SOC. It should be mentioned that only a few zinc complexes have demonstrated phosphorescence in solution under ambient conditions.31,62
The absorption and emission profiles of 1OAc (Fig. 2) are generally closer to those of the free ligand L1, and show only some contribution of low energy charge transfer transitions that reflects a weaker binding of the Zn(OAc)2 motif and its relatively easy dissociation in solution.
In the case of L245 and L3, the electronic transitions between the HOMO and LUMO, which are localized within the polyaromatic anthracene chromophore and the phenylene-acetylene spacers (Fig. 4), are responsible for the excitation and emission.
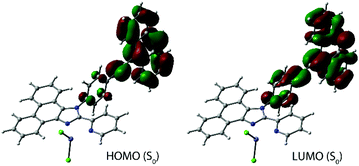 |
| Fig. 4 Frontier molecular orbitals of complex 2Cl (in chloroform). | |
Symmetrical substitution of anthracene in L3 results in some extension of the conjugated π-system, and therefore decreases the optical band gap ΔEcalc (HOMO–LUMO) to 2.9 eV compared to that of L2 (3.3 eV). Overall, the fluorescence spectra of these ligands (Fig. 2 and 5) resemble those of the corresponding phenylethynyl anthracences.63 Due to the lack of electronic communication of the emissive motif with the metal-coordinating parts of the ligand, the emission bands of the ligands L2 and L3 and respective zinc complexes 2X, 3OAc (Fig. 2 and 5) are very similar and reveal the clearly resolved vibrational structure arising from their ππ*an character (Table S1;†Fig. 4, S6 and S7†). The effect of metal binding to L2 and L3 on the photophysics is mainly seen in a decrease in the quantum yield of halides, ΦemL2 > 2Cl > 2I, which probably suggests some excitation energy transfer to the predominantly dark triplet state of the phenanthroimidazole fragment.
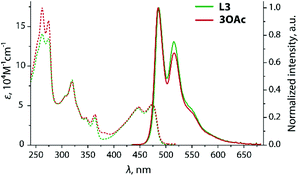 |
| Fig. 5 Absorption (dotted lines) and normalized emission (solid lines) spectra of L3 and 3OAc recorded in 1,2-dichloroethane (298 K). | |
Structural and photophysical studies in the solid state
The pertinent luminescence data are listed in Table 2. The emission properties of the titled complexes in the solid state substantially depend on the molecular packing, and therefore their discussion is carried out together with systematic crystallographic analysis. The crystal data are summarized in Tables S3 and S4,† powder diffraction patterns are presented in Fig. S8.† In all complexes, zinc atoms are found in a typical pseudo tetrahedral coordination environment, comprising two nitrogen donors forming a five membered chelate ring, and two halide or acetate ligands. The pyridyl ring and the phenanthroimidazole system are expectedly virtually in the same plane, together with the metal center. The bond lengths and angles around the zinc ion (Tables S5 and S6†) are not exceptional and lie in the range determined for similar compounds.9,25,55,58 In view of the photophysical behavior of bulk crystalline samples, we mainly focus on the mutual arrangement of the chromophores and their intermolecular interactions.
Table 2 Photophysical properties of ligands L1–L3 and their zinc complexes in the solid state (298 K)
|
λ
em nm |
Φ
|
τ, nsa |
k
r,b s−1 |
k
nr,c s−1 |
Average emission lifetime for the two exponential decay τav = (A1τ12 + A2τ22)/(A1τ1 + A2τ2), Ai are pre-exponential factors.
k
r values were estimated by Φ/τobs.
k
nr values were estimated by (1 − Φ)/τobs.
|
L1
|
413 |
0.12 |
3.0 |
4.0 × 107 |
2.9 × 108 |
1Cl_a
|
460 |
0.28 |
2.7 |
1.0 × 108 |
2.7 × 108 |
1Cl_b
|
464(sh), 473 |
0.19 |
1.7 |
1.1 × 108 |
4.8 × 108 |
1I_a
|
469 |
0.01 |
0.5 |
2.0 × 107 |
2.0 × 109 |
1I_b
|
470, 554, 596 |
0.03 |
1.0, 105 |
|
|
1OAc
|
478 |
0.14 |
2.0c |
7.0 × 107 |
4.3 × 108 |
L2
|
497 |
0.03 |
2.9 |
1.0 × 107 |
3.3 × 108 |
2Cl
|
471 |
0.03 |
1.6 |
1.9 × 107 |
6.1 × 108 |
2I
|
506(sh), 598 |
0.06 |
1.3, 3.6 |
|
|
2OAc
|
497 |
0.02 |
1.6 |
1.3 × 107 |
6.1 × 108 |
L3_y (yellow) |
543 |
0.04 |
2.6 |
1.5 × 107 |
3.7 × 108 |
L3_r (red) |
597 |
0.03 |
2.3 |
1.3 × 107 |
4.2 × 108 |
3OAc_y
|
562 |
0.03 |
3.5 |
8.6 × 106 |
2.8 × 108 |
3OAc_r
|
611 |
0.06 |
3.4 |
1.8 × 107 |
2.8 × 108 |
L1 and 1X series.
The fluorescence of crystalline L1 (λem = 413 nm, Φem = 0.12, Fig. 6) is less intense and is slightly red shifted with respect to the characteristics of 1,2-dichloroethane solution (λem max = 388 nm, Φem = 0.37). In the absence of stacking interactions in the structure of L1,45 the radiative relaxation is evidently of an intramolecular ππ* nature as in the fluid medium.
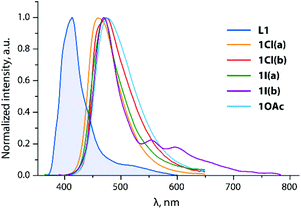 |
| Fig. 6 Normalized solid state emission spectra of L1 (filled graph) and 1X (298 K, λexc = 350 nm). | |
Crystallization of compounds 1X resulted in different modifications depending on the solvents used. The solvatomorphs of the chloride, 1Cl_a (dichloromethane solvate) and 1Cl_b (chloroform solvate), exhibit noticeable π-stacking interactions between the phenanthrene systems (Fig. S9†).
The acetate 1OAc (Fig. S10†) and the dichloromethane solvate of the iodide complex 1I_a (Fig. 7) show π–π contacts, which involve both polyaromatic and the pyridine motifs of adjacent molecules. However, the emission maxima of these crystalline species demonstrate a rather minor variation (λem = 460–478 nm, Fig. 6) indicating that stacking in this case has a moderate influence on the properties of the excited state. Analogously to the solution behavior, the luminescence of 1X in the solid form is manifested by broad structureless bands, which are observed at lower energies than those of the parent L1. The excitation spectra of L1 and 1X (Fig. S11†) follow the same trend that complies with the change in the nature of the S1 state of complexes and the substantial ILCT character of the emission of 1X. The chloride and acetate compounds 1X are somewhat more efficient fluorophores (Φem = 0.14–0.28) than the free ligand primarily due to the increased radiative decay rate of ca. 1.0 × 108 s−1 (Table 2). As in solution, the iodide 1I in the solid state (in both forms 1I_a and 1I_b) exhibits the lowest quantum yield due to the ISC (S1 → Tn) transition to the dark triplet state, similar to zinc halide compounds with pyridylimidazo-pyridine ligands.55 While 1I_a does not show room temperature phosphorescence, the solvent-free modification to 1I_b is dually emissive (Fig. 6). It demonstrates a dominating high energy fluorescence band (λem = 470 nm), which is accompanied by a low energy structured signal (λem = 554, 596; total Φem = 0.03) with a long observed lifetime of ca. 100 μs. In contrast to 1I_a, no appreciable π-interactions are seen in 1I_b. Instead, 1I_b forms short intermolecular contacts I⋯π (ca. 3.5 Å, Fig. 7) between the iodide ligand and the imidazole ring (cf. 4.121 Å for analogous separation in 1I_a). This unique arrangement presumably accounts for the enhancement of the external HAE in 1I_b that causes larger SOC and results in detectable intraligand triplet emission under ambient conditions. The importance of the HAE and of close halide⋯chromophore contacts in promoting SOC has been recently shown for zinc halide coordination polymers prepared via a 1,3,5-tris(1-imidazolyl)benzene luminescent linker.38
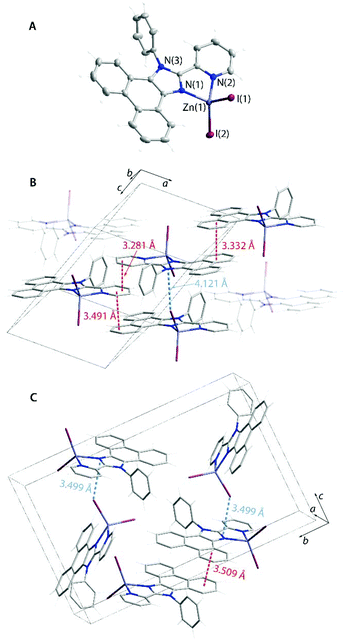 |
| Fig. 7 Molecular view of 1I_a (A), and packing fragments of 1I_a (B) and of 1I_b (C). Crystallization dichloromethane molecules in 1I_a are omitted for clarity. The intermolecular π–π distances are measured between the planes of the phenanthrene motifs. The intermolecular π–I distance is measured between I and the imidazole plane. Thermal ellipsoids are shown at the 50% probability level. | |
Decreasing the temperature has a drastic influence on the ratio of fluorescence and phosphorescence intensities of form 1I_b (Fig. 8A and Table S7†). Upon cooling the crystals of 1I_b, the low energy band shows a drastic growth that produces white-orange luminescence, whereas the observed lifetime of the red shifted band increases from ca. 100 μs at 298 K to 2.2 ms at 77 K. Taking into account that SOC induced by the HAE is proportional in the first approximation to Z4/r3 (Z is the atomic number and r is the distance between the chromophore and the heavy atom),64 and then the rate of ISC, kisc ∝ |ΨT1|HSO|ΨS1|2/(ΔES1–T1)2, is related to the distance as 1/r6. This implies that the probability of populating the T1 state is highly sensitive to intermolecular separation for 1I_b assuming the external HAE.
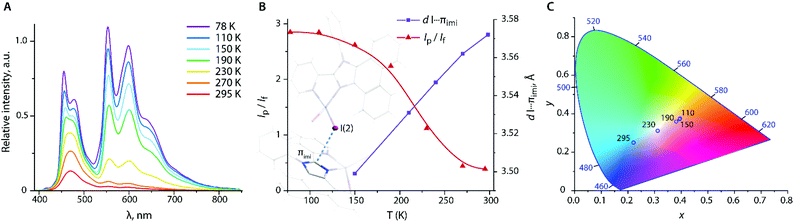 |
| Fig. 8 (A) Variable temperature solid state emission spectra of 1I_b; (B) temperature dependences of the ratio of intensities of the phosphorescence and fluorescence bands (Ip/If), and of the intermolecular distance between the iodine atom and the plane of imidazole (d I⋯πimi); and (C) CIE 1931 coordinates of the emission of 1I_b at different temperatures. | |
To assess the correlation of the emission with the structural parameters and temperature, we performed variable-temperature XRD studies. Indeed, the intermolecular distance I⋯πimi is nearly linearly dependent on the temperature and decreases from 3.572 Å at 295 K to 3.499 Å at 150 K (Fig. 8B, Table S7†) that could be one of the decisive factors that determines the increase of phosphorescence vs. fluorescence. In addition to the triplet luminescence, which is uncommon for zinc complexes,31,32,65,661I_b represents an interesting example of a reversibly responsive material, the emission color of which (Fig. 8C) is regulated by the temperature-dependent ratio of the singlet and triplet radiative decays.
Form 1I_a also exhibits luminescence thermochromism (Fig. S12†), although in a less pronounced manner compared to 1I_b. In the absence of short I⋯π contacts, a notable increase of the phosphorescence band of 1I_a starts at around 150 K that can be attributed to the rigidification of the structure and moderate intra- and intermolecular heavy atom effects.
L2 and 2X series.
The emission of the solid ligand L2 (λem = 497 nm, Fig. 9A) is unstructured and is found at a longer wavelength in comparison with that in solution (λem = 433 nm, Fig. 2). Substantial quenching of luminescence in the solid state (ca. 20-fold decrease vs. solution, Table 1) and the decrease in energy likely arise from certain π-interactions between the chromophore anthracene fragments, however, the assignment to either perturbed monomer or excimer emission is ambiguous on the basis of the available data.67,68 The quantum yield, observed lifetimes, and the excitation and emission profiles of L2 and 2OAc are very similar (Fig. 9 and S13†), whereas the fluorescence maxima nearly coincide that suggests resembling resemblance in the packing and the nature of the excited states. The hypsochromic shift of the fluorescence band of the crystalline chloride complex 2Cl (λem = 471 nm, Fig. 9A) confirms the monomer anthracene emission. Indeed, the structure of 2Cl (Fig. S14†) confirms that the anthracene π-system is not involved in appreciable stacking interactions, which are found between the phenanthrene fragments only. Mechanical grinding of the pristine sample 2Cl produces an amorphous material exhibiting green-yellow luminescence (λem = 532 nm, Fig. 9B and Table S8†). Together with the visibly changed excitation spectrum (Fig. S13†), it points to the alteration of molecular packing, which conceivably results in the emergence of π-interactions between the anthracene chromophores. On the other hand, grinding of 2OAc has a little influence on the fluorescence spectrum (Fig. S15†).
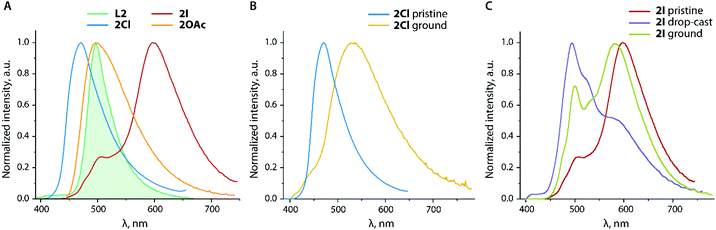 |
| Fig. 9 Normalized solid state emission spectra of (A) L2 (filled graph) and 2X (298 K, λexc = 400 nm for 2I, λexc = 350 nm for other samples); (B) pristine and ground 2Cl (298 K); and (C) pristine, ground and dropcast 2I (298 K). | |
Unlike 2Cl and 2OAc, the crystalline iodide congener 2I is a dual emitter with the dominating band with the maximum at 598 nm (Fig. 9A) and the observed lifetime of 3.6 ns. Such a significant red shift is not typical of anthracene excimer fluorescence.67,69,70 The crystal structure of 2I shows short π–π contacts between the emissive anthracene and phenanthrene fragments (Fig. 10) that makes the formation of an exciplex or intermolecular charge transfer (anthracene → phenanthroimidazole) possible. The excitation spectrum of 2I monitored at 620 nm clearly differs from those of other 2X species and shows an intense red shifted band (Fig. S13†) that assumes ground state aggregation. The minor high energy band in the emission of 2I (λem = 506 nm) plausibly arises from the locally excited state, i.e. corresponding to the monomer anthracene luminescence. This signal might originate for instance from the surface/peripheral molecules, which experience less intermolecular interactions.71,72 The impact of mechanical stimulus on the emission pattern of 2I is rather moderate and results in the growth of some blue shifted bands (Fig. 9C), evidently due to the high stability of the crystalline packing mode. However, the fluorescence of a film-like sample prepared by fast evaporation of a dichloromethane solution of 2I is essentially switched mainly to the monomer origin with the maximum at 494 nm and a distinguishable vibrational structure (ca. 1160 cm−1) due to the lack of intermolecular interactions. The excitation spectrum of the dropcast sample 2I is similar to those of 2OAc and ground 2Cl, and therefore supports the disruption of charge-transfer anthracene–phenanthrene stacking in the amorphous state.
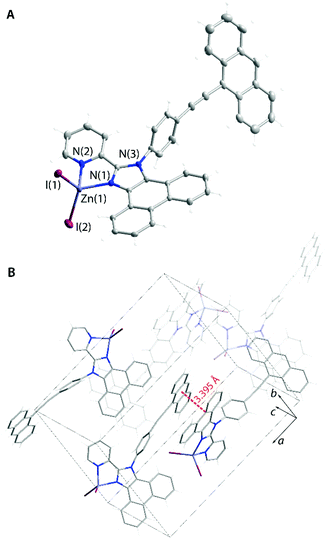 |
| Fig. 10 Molecular view (A) and unit cell packing of 2I (B). Crystallization of chloroform molecules are omitted for clarity. The intermolecular π–π distance is measured between the plane of the anthracene and the C(8) atom of phenanthrene. Thermal ellipsoids are shown at the 50% probability level. | |
L3 and complex 3OAc.
Depending on the conditions, ligand L3 and the zinc acetate derivative 3OAc can be isolated by at least two modifications (see the ESI), which show distinctly different luminescence characteristics (Fig. 11). The minor yellow crystalline forms of L3_ycr and 3OAc_ycr were structurally characterized (Fig. S16† and Fig. 12).
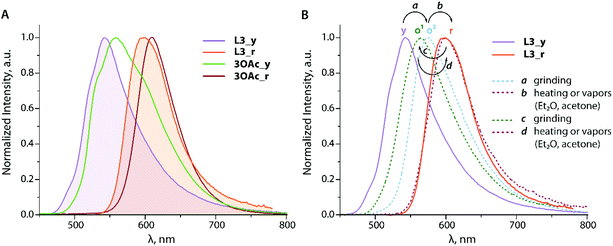 |
| Fig. 11 Normalized solid state emission spectra of L3 (filled graphs) and complex 3OAc at 298 K (A) and variation of the emission spectra of L3 under different stimuli (B). | |
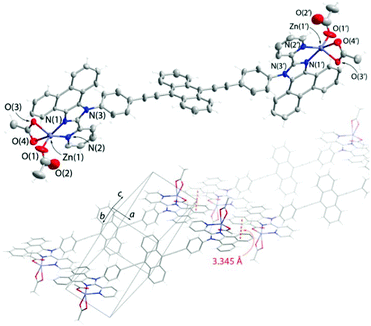 |
| Fig. 12 Molecular view and packing fragment of 3OAc_y. Crystallization solvent was disordered and omitted from the refinement model. The intermolecular π–π distances were measured between the planes of the phenanthrene motifs. Symmetry transformations used to generate equivalent atoms (′): −x, 1 − y, −z. Thermal ellipsoids are shown at the 50% probability level. | |
Although these single crystals do not represent the bulk yellow modifications L3_y/3OAc_y, the photophysics of which was analyzed, similar colours of the samples suggest that they have similar arrangements in the solid. According to the XRD data, the diethynyl anthracene fragments of the chromophore do not show appreciable stacking interactions, and therefore the observed yellow fluorescence (λem = 543/562 nm, Φem = 0.04/0.03 for L3_y/3OAc_y) presumably corresponds to the monomer emission, broadened and red shifted with respect to the solution spectra (Fig. 5).
Although we could not obtain crystals of L3_r/3OAc_r suitable for structural analysis, the colours of these solids and of their luminescence (λem = 597/611 nm, Φem = 0.03/0.06 for L3_r/3OAc_r) propound aggregation with the involvement of the emissive anthracene centers, reminiscent of the crystalline 9,10-bis(phenylethynyl)anthracene (BPEA).73 The emissions of both forms of the complex are visibly red shifted compared to those of the corresponding L3_y/r forms of the ligand (Fig. 11A). The lowest energy maxima of the excitation spectra for the yellow and orange-red modifications vary by more than 60 nm (Fig. S17†), and thus corroborate that different molecular packings generate the excited states operating in these materials.
Ligand L3 demonstrates distinct stimuli-responsive behaviour. Mechanical grinding of yellow L3_y produces a metastable orange material (o1, λem = 575 nm). Brief heating (up to ca. 300 °C) or exposure to vapors of diethyl ether further transforms this solid into the red form, the emission of which (λem = 598 nm) is nearly identical to that of the independently prepared L3_r (Fig. 11B). Grinding the red form leads to another metastable orange modification (o2, λem = 564 nm), the process is also reversible thermally by means of vapors. The transitions between different forms apparently result from the perturbation of intermolecular interactions, which involved the anthracene chromophore.
Albeit phenanthroimidazole fragments in L3 and 3OAc are not expected to contribute to the frontier orbitals according to the theoretical studies (Fig. S7†), apparently, they influence the intermolecular organization and mechano-/vapo-responsive behaviour. The coordinating ability of the pendant groups can be used to tune these features as the binding of zinc acetate e.g. bathochromically shifts the emission of both phases from 543/597 nm (L3) to 562/611 nm (3OAc, Table 2). The photophysical properties of the yellow and orange-red forms of L3/3OAc remind those of a film (yellow) and crystals (orange-red) of BPEA.72,74 On the other hand, the fluorescence mechanochromism of some functionalized BPEA-s is clearly less expressed.75,76
Conclusions
In this study, we have analysed the photophysical behaviour of readily available fluorophores L1–L3, which comprise a pyridyl-imidazole coordinating function fused with a polyaromatic phenanthrene motif, and their zinc(II) complexes 1X–3X derived from simple ZnX2 salts (X = Cl, I, OAc). In solution, the optical properties of organic emitters and the corresponding metal-containing species are governed by the intraligand charge transfer and ππ* electronic transitions of the singlet parentage, localized either on the phenanthrene-diimine system (L1 and 1X) or within the alkynyl-anthracene chromophore fragment (L2 and L3-based compounds). However, the ZnX2 units cause little perturbance to the intraligand fluorescence of L2 and L3 in fluid medium, and coordination of zinc halide units to L1 red shifts the lowest energy absorption and emission bands in 1Cl and 1I due to the enhanced πphen → π*py CT. In the case of 1I, the intramolecular heavy atom effect associated with iodide ligands facilitates ISC resulting in a significant decrease in the fluoresce intensity and in the phosphorescence emission, seldom observed for zinc coordination complexes in solution.
The investigation of luminescence properties in the solid state was supported by extensive structural characterization of the title compounds. Systematic crystallographic studies highlight a strong impact of ZnX2 groups on non-covalent intermolecular interactions, and as a consequence, on the optical features of these materials. In particular, the iodide complex 1I demonstrates packing-dependent dual (singlet–triplet) emission, which has been correlated with short intermolecular I⋯π contacts. In turn, due to the temperature variability of this distance and the alteration of the phosphorescence vs. fluorescence ratio, the solvent-free crystals of 1I exhibit a distinct emission color change from blue to yellow-orange in a temperature range of 150–300 K.
Switching the emissive center to the anthracene moiety in L2 and L3-based species provides more possibilities for responsive properties based on the ground state π–π interactions (anthracene–phenanthrene and anthracene–anthracene). It is noteworthy that L3 and 3OAc exist in several forms exhibiting different fluorescence performances (λem alters from 543 to 597 nm for L3), which can be interconverted by means of various stimuli (mechanical and thermal treatment and exposure to a solvent). Overall, accessible preparation of organic chromophores with coordinating ability and their combination with optically innocent metal ions such as zinc offer a wide range of possibilities for the development of easy to synthesize molecular materials with diverse photophysical functionalities.
Author contributions
D. T. and K. S. K. contributed equally and performed the synthesis, and structural and NMR spectroscopic characterization. T. E. and P. H. were responsible for computational analysis. N.K. carried out PXRD measurements. K. S. K., J. R. S. and A. S. M. performed the photophysical studies. S. P. T., E. V. G. and I. O. K. planned the research.
Conflicts of interest
There are no conflicts to declare.
Acknowledgements
Financial support from the Academy of Finland (decision 317903, I.O.K.; Flagship Programme, Photonics Research and Innovation PREIN, decision 320166) and the Russian Science Foundation (grant 19-73-20055, J.R.S., photophysical studies) is gratefully acknowledged. The work was carried out using equipment of St Petersburg State University Research Park (Centres of Optical and Laser Materials Research, Chemical Analysis and Materials Research, X-ray Diffraction) and the Analytical Centre for Nano- and Biotechnologies (Peter the Great St. Petersburg Polytechnic University, with financial support from the Ministry of Education and Science of Russian Federation).
Notes and references
- M. Shimizu and T. Hiyama, Organic Fluorophores Exhibiting Highly Efficient Photoluminescence in the Solid State, Chem. – Asian J., 2010, 5, 1516–1531 CrossRef CAS.
- Z. Chi, X. Zhang, B. Xu, X. Zhou, C. Ma, Y. Zhang, S. Liu and J. Xu, Recent advances in organic mechanofluorochromic materials, Chem. Soc. Rev., 2012, 41, 3878–3896 RSC.
- Z. Ma, Z. Wang, M. Teng, Z. Xu and X. Jia, Mechanically Induced Multicolor Change of Luminescent Materials, ChemPhysChem, 2015, 16, 1811–1828 CrossRef CAS.
- J. Mei, N. L. C. Leung, R. T. K. Kwok, J. W. Y. Lam and B. Z. Tang, Aggregation-Induced Emission: Together We Shine, United We Soar!, Chem. Rev., 2015, 115, 11718–11940 CrossRef CAS PubMed.
- X. Huang, L. Qian, Y. Zhou, M. Liu, Y. Cheng and H. Wu, Effective structural modification of traditional fluorophores to obtain organic mechanofluorochromic molecules, J. Mater. Chem. C, 2018, 6, 5075–5096 RSC.
- S. Xu, Y. Duan and B. Liu, Precise Molecular Design for High-Performance Luminogens with Aggregation-Induced Emission, Adv. Mater., 2020, 32, 1903530 CrossRef CAS PubMed.
- Y. Xie and Z. Li, The development of mechanoluminescence from organic compounds: breakthrough and deep insight, Mater. Chem. Front., 2020, 4, 317–331 RSC.
- M. K. Bera, P. Pal and S. Malik, Solid-state emissive organic chromophores: design, strategy and building blocks, J. Mater. Chem. C, 2020, 8, 788–802 RSC.
- T. Tsukamoto, R. Aoki, R. Sakamoto, R. Toyoda, M. Shimada, Y. Hattori, M. Asaoka, Y. Kitagawa, E. Nishibori, M. Nakano and H. Nishihara, A simple zinc(II) complex that features multi-functional luminochromism induced by reversible ligand dissociation, Chem. Commun., 2017, 53, 3657–3660 RSC.
- R. Geng, X. Hou, Y. Sun, C. Yan, Y. Wu, H.-L. Zhang and X. Shao, Driving π-plane to π-bowl through lateral coordination at room temperature, Mater. Chem. Front., 2018, 2, 1456–1461 RSC.
- Y. Watanabe, W. Sungnoi, A. O. Sartorio, M. Zeller and A. Wei, A zinc-responsive fluorophore based on 5′-(p-hydroxyphenyl)-pyridylthiazole, Mater. Chem. Front., 2020, 4, 899–904 RSC.
- A. Zavaleta, A. O. Lykhin, J. H. S. K. Monteiro, S. Uchida, T. W. Bell, A. de Bettencourt-Dias, S. A. Varganov and J. Gallucci, Full Visible Spectrum and White Light Emission with a Single, Input-Tunable Organic Fluorophore, J. Am. Chem. Soc., 2020, 142, 20306–20312 CrossRef CAS PubMed.
- T. Ogawa, J. Yuasa and T. Kawai, Highly Selective Ratiometric Emission Color Change by Zinc-Assisted Self-Assembly Processes, Angew. Chem., Int. Ed., 2010, 49, 5110–5114 CrossRef CAS PubMed.
- Y. Imai and J. Yuasa, Off–off–on chiroptical property switching of a pyrene luminophore by stepwise helicate formation, Chem. Commun., 2019, 55, 4095–4098 RSC.
- S. Iseki, K. Nonomura, S. Kishida, D. Ogata and J. Yuasa, Zinc-Ion-Stabilized Charge-Transfer Interactions Drive Self-Complementary or Complementary Molecular Recognition, J. Am. Chem. Soc., 2020, 142, 15842–15851 CrossRef CAS PubMed.
- Z. Xu, J. Yoon and D. R. Spring, Fluorescent chemosensors for Zn2+, Chem. Soc. Rev., 2010, 39, 1996–2006 RSC.
- J. Wang, W. Lin and W. Li, Single Fluorescent Probe Displays a Distinct Response to Zn2+ and Cd2+, Chem. – Eur. J., 2012, 18, 13629–13632 CrossRef CAS PubMed.
- Y. Dong, R. Fan, W. Chen, P. Wang and Y. Yang, A simple quinolone Schiff-base containing CHEF based fluorescence ‘turn-on’ chemosensor for distinguishing Zn2+ and Hg2+ with high sensitivity, selectivity and reversibility, Dalton Trans., 2017, 46, 6769–6775 RSC.
- S. Ullmann, R. Schnorr, M. Handke, C. Laube, B. Abel, J. Matysik, M. Findeisen, R. Rüger, T. Heine and B. Kersting, Zn2+-Ion Sensing by Fluorescent Schiff Base Calix[4]arene Macrocycles, Chem. – Eur. J., 2017, 23, 3824–3827 CrossRef CAS PubMed.
- W. L. Turnbull and L. G. Luyt, Amino-Substituted 2,2′-Bipyridine Ligands as Fluorescent Indicators for ZnII and Applications for Fluorescence Imaging of Prostate Cells, Chem. – Eur. J., 2018, 24, 14539–14546 CrossRef CAS PubMed.
- S. N. Ansari, A. K. Saini, P. Kumari and S. M. Mobin, An imidazole derivative-based chemodosimeter for Zn2+ and Cu2+ ions through “ON–OFF–ON” switching with intracellular Zn2+ detection, Inorg. Chem. Front., 2019, 6, 736–745 RSC.
- G. Ambrosi, M. Paz Clares, I. Pont, M. Formica, V. Fusi, A. Ricci, P. Paoli, P. Rossi, E. García-España and M. Inclán, Zn2+ and Cu2+ complexes of a fluorescent scorpiand-type oxadiazole azamacrocyclic ligand: crystal structures, solution studies and optical properties, Dalton Trans., 2020, 49, 1897–1906 RSC.
- S. Varughese, Non-covalent routes to tune the optical properties of molecular materials, J. Mater. Chem. C, 2014, 2, 3499–3516 RSC.
- E. R. T. Tiekink, Supramolecular assembly based on “emerging” intermolecular interactions of particular interest to coordination chemists, Coord. Chem. Rev., 2017, 345, 209–228 CrossRef CAS.
- L. K. Hiscock, D. Joekar, B. Balonova, M. Tomas Piqueras, Z. W. Schroeder, V. Jarvis, K. E. Maly, B. A. Blight and L. N. Dawe, Structures, Phase Behavior, and Fluorescent Properties of 3-Phenyl-1-(pyridin-2-yl)-1H-pyrazol-5-amine and Its ZnCl2 Complex, Inorg. Chem., 2019, 58, 16317–16321 CrossRef CAS PubMed.
- D. Niu, Y. Jiang, L. Ji, G. Ouyang and M. Liu, Self-Assembly through Coordination and π-Stacking: Controlled Switching of Circularly Polarized Luminescence, Angew. Chem., Int. Ed., 2019, 58, 5946–5950 CrossRef CAS PubMed.
- K. Prasad, D. Samanta, R. Haldar and T. K. Maji, Excitation Energy Transfer Supported Amplified Charge-Transfer Emission in an Anthracenedicarboxylate- and Bipyridophenazine-Based Coordination Complex, Inorg. Chem., 2018, 57, 2953–2956 CrossRef CAS PubMed.
- D. Li, X. Yang and D. Yan, Cluster-Based Metal–Organic Frameworks: Modulated Singlet–Triplet Excited States and Temperature-Responsive Phosphorescent Switch, ACS Appl. Mater. Interfaces, 2018, 10, 34377–34384 CrossRef CAS PubMed.
- X.-G. Yang, Z.-M. Zhai, X.-M. Lu, J.-H. Qin, F.-F. Li and L.-F. Ma, Hexanuclear Zn(II)-Induced Dense π-Stacking in a Metal–Organic Framework Featuring Long-Lasting Room Temperature Phosphorescence, Inorg. Chem., 2020, 59, 10395–10399 CrossRef CAS PubMed.
- X.-G. Yang, X.-M. Lu, Z.-M. Zhai, J.-H. Qin, X.-H. Chang, M.-L. Han, F.-F. Li and L.-F. Ma, π-Type halogen bonding enhanced the long-lasting room temperature phosphorescence of Zn(ii) coordination polymers for photoelectron response applications, Inorg. Chem. Front., 2020, 7, 2224–2230 RSC.
- K. D. Oyler, F. J. Coughlin and S. Bernhard, Controlling the Helicity of 2,2′-Bipyridyl Ruthenium(II) and Zinc(II) Hemicage Complexes, J. Am. Chem. Soc., 2007, 129, 210–217 CrossRef CAS PubMed.
- F. Kobayashi, R. Ohtani, S. Teraoka, M. Yoshida, M. Kato, Y. Zhang, L. F. Lindoy, S. Hayami and M. Nakamura, Phosphorescence at Low Temperature by External Heavy-Atom Effect in Zinc(II) Clusters, Chem. – Eur. J., 2019, 25, 5875–5879 CrossRef CAS PubMed.
- X. Yang and D. Yan, Long-afterglow metal–organic frameworks: reversible guest-induced phosphorescence tunability, Chem. Sci., 2016, 7, 4519–4526 RSC.
- X. Yang and D. Yan, Direct white-light-emitting and near-infrared phosphorescence of zeolitic imidazolate framework-8, Chem. Commun., 2017, 53, 1801–1804 RSC.
- X.-G. Yang, Z.-M. Zhai, X.-M. Lu, Y. Zhao, X.-H. Chang and L.-F. Ma, Room temperature phosphorescence of Mn(ii) and Zn(ii) coordination polymers for photoelectron response applications, Dalton Trans., 2019, 48, 10785–10789 RSC.
- Y. Zhao, X.-G. Yang, X.-M. Lu, C.-D. Yang, N.-N. Fan, Z.-T. Yang, L.-Y. Wang and L.-F. Ma, {Zn6} Cluster Based Metal–Organic Framework with Enhanced Room-Temperature Phosphorescence and Optoelectronic Performances, Inorg. Chem., 2019, 58, 6215–6221 CrossRef CAS PubMed.
- X.-G. Yang, Z.-M. Zhai, X.-Y. Liu, J.-Y. Li, F.-F. Li and L.-F. Ma, Sulfur heteroatom-based MOFs with long-lasting room-temperature phosphorescence and high photoelectric response, Dalton Trans., 2020, 49, 598–602 RSC.
- Z.-X. Liu, J.-Q. Wang, Y. Mu, Q. Wei, J.-H. Li and G.-M. Wang, Room-Temperature Phosphorescence with Variable Lifetime of Halogen-Comprising Coordination Polymers, Inorg. Chem., 2020, 59, 17870–17874 CrossRef CAS PubMed.
- J.-Q. Wang, Y. Mu, S.-D. Han, J. Pan, J.-H. Li and G.-M. Wang, Room-Temperature Phosphorescence with Excitation-Energy Dependence and External Heavy-Atom Effect in Hybrid Zincophosphites, Inorg. Chem., 2019, 58, 9476–9481 CrossRef CAS PubMed.
- Y. Mu, J.-Q. Wang, S.-D. Han, J. Pan, J.-H. Li and G.-M. Wang, Enhanced Room-Temperature Phosphorescence of an Organic Ligand in 3D Hybrid Materials Assisted by Adjacent Halogen Atom, Inorg. Chem., 2020, 59, 972–975 CrossRef CAS PubMed.
- R. Gao, Y. Zhao, X. Yang and D. Yan, Stimuli-responsive fluorescence based on the solid-state bis[2-(2-benzothiazoly)phenolato]zinc(II) complex and its fiber thin film, RSC Adv., 2015, 5, 56470–56477 RSC.
- T. Tsukamoto, R. Aoki, R. Sakamoto, R. Toyoda, M. Shimada, Y. Hattori, Y. Kitagawa, E. Nishibori, M. Nakano and H. Nishihara, Mechano-, thermo-, solvato-, and vapochromism in bis(acetato-κ1O)[4′-(4-(diphenylamino)phenyl)](2,2′:6′,2′′-terpyridine-κ3N,N′,N′′)zinc(II) and its polymer, Chem. Commun., 2017, 53, 9805–9808 RSC.
- Y. Ma, Y. Dong, S. Liu, P. She, J. Lu, S. Liu, W. Huang and Q. Zhao, Chameleon-Like Thermochromic Luminescent Materials with Controllable Response Behaviors for Multilevel Security Printing, Adv. Opt. Mater., 2020, 8, 1901687 CrossRef CAS.
- L. Tom and M. R. P. Kurup, A reversible thermo-responsive 2D Zn(ii) coordination polymer as a potential self-referenced luminescent thermometer, J. Mater. Chem. C, 2020, 8, 2525–2532 RSC.
- K. S. Kisel, T. Eskelinen, W. Zafar, A. I. Solomatina, P. Hirva, E. V. Grachova, S. P. Tunik and I. O. Koshevoy, Chromophore-functionalized phenanthro-diimine ligands and their Re(I) complexes, Inorg. Chem., 2018, 57, 6349–6361 CrossRef CAS PubMed.
- K. Qiu, M. Ouyang, Y. Liu, H. Huang, C. Liu, Y. Chen, L. Ji and H. Chao, Two-photon photodynamic ablation of tumor cells by mitochondria-targeted iridium(III) complexes in aggregate states, J. Mater. Chem. B, 2017, 5, 5488–5498 RSC.
- Z.-W. Wang, C.-C. Ji, J. Li, Z.-J. Guo, Y.-Z. Li and H.-G. Zheng, Synthesis, X-ray Structures, and Fluorescent Properties of Coordination Networks Constructed from 2-(2-Pyridinyl-benzimidazolyl) Acetic Anion, Cryst. Growth Des., 2009, 9, 475–482 CrossRef CAS.
- M. G. B. Drew, D. A. Tocher, K. Chowdhury, S. Chowdhury and D. Datta, Zinc(II) mediated synthesis of an N-substituted 2-(2-pyridyl)imidazole from a 1,2-diketone and 2-(aminomethyl)pyridine and its ligational behaviour, New J. Chem., 2004, 28, 323–325 RSC.
- H.-Y. Liu, H. Wu, J.-F. Ma, Y.-Y. Liu, B. Liu and J. Yang, SynthesesStructures, and Photoluminescence of Zinc(II) Coordination Polymers Based on Carboxylates and Flexible Bis-[(pyridyl)-benzimidazole] Ligands, Cryst. Growth Des., 2010, 10, 4795–4805 CrossRef CAS.
- C.-J. Wang, K.-F. Yue, W.-H. Zhang, J.-C. Jin, X.-Y. Huang and Y.-Y. Wang, Three new d10 coordination polymers based on 2-(2-pyridyl)benzimidazole ligand: Synthesis, structures and properties, Inorg. Chem. Commun., 2010, 13, 1332–1336 CrossRef CAS.
- A. S. Burlov, A. S. Antsyshkina, G. G. Sadkov, V. V. Chesnokov, Y. V. Koshchienko, D. A. Garnovskii, I. S. Vasil'chenko, A. I. Uraev, G. S. Borodkin, V. S. Sergienko and A. D. Garnovskii, Coordination compounds of ambidentate 1-(H)alkyl-2-(2-pyridyl)benzimidazoles. Synthesis and crystal structure, Russ. J. Coord. Chem., 2010, 36, 906–912 CrossRef CAS.
- N. Kundu, S. M. T. Abtab, S. Kundu, A. Endo, S. J. Teat and M. Chaudhury, Triple-Stranded Helicates of Zinc(II) and Cadmium(II) Involving a New Redox-Active Multiring Nitrogenous Heterocyclic Ligand: Synthesis, Structure, and Electrochemical and Photophysical Properties, Inorg. Chem., 2012, 51, 2652–2661 CrossRef CAS PubMed.
- S. Chen, R.-Q. Fan, X.-M. Wang and Y.-L. Yang, Novel bright blue emissions IIB group complexes constructed with various polyhedron-induced 2-[2′-(6-methoxy-pyridyl)]-benzimidazole derivatives, CrystEngComm, 2014, 16, 6114–6125 RSC.
- F. Li, M.-L. Sun, X. Zhang and Y.-G. Yao, Diverse Structures and Luminescence Properties of Nine Novel Zn/Cd(II)-Organic Architectures Assembled by Two Different Rigid Ligands, Cryst. Growth Des., 2019, 19, 4404–4416 CrossRef CAS.
- G. A. Ardizzoia, S. Brenna, S. Durini, B. Therrien and M. Veronelli, Synthesis, Structure, and Photophysical Properties of Blue-Emitting Zinc(II) Complexes with 3-Aryl-Substituted 1-Pyridylimidazo[1,5-a]pyridine Ligands, Eur. J. Inorg. Chem., 2014, 2014, 4310–4319 CrossRef CAS.
- X.-M. Wang, S. Chen, R.-Q. Fan, F.-Q. Zhang and Y.-L. Yang, Facile luminescent tuning of ZnII/HgII complexes based on flexible, semi-rigid and rigid polydentate Schiff bases from blue to green to red: structural, photophysics, electrochemistry and theoretical calculations studies, Dalton Trans., 2015, 44, 8107–8125 RSC.
- X.-m. Liu, X.-y. Mu, H. Xia, L. Ye, W. Gao, H.-y. Wang and Y. Mu, Synthesis, Structures, and Luminescent Properties of d10 Group 12 Metal Complexes with Substituted 2,2′-Bipyridine Ligands, Eur. J. Inorg. Chem., 2006, 2006, 4317–4323 CrossRef.
- G. Volpi, E. Priola, C. Garino, A. Daolio, R. Rabezzana, P. Benzi, A. Giordana, E. Diana and R. Gobetto, Blue fluorescent zinc(II) complexes based on tunable imidazo[1,5-a]pyridines, Inorg. Chim. Acta, 2020, 509, 119662 CrossRef CAS.
- V. N. Kozhevnikov, O. V. Shabunina, D. S. Kopchuk, M. M. Ustinova, B. König and D. N. Kozhevnikov, Facile synthesis of 6-aryl-3-pyridyl-1,2,4-triazines as a key step toward highly fluorescent 5-substituted bipyridines and their Zn(II) and Ru(II) complexes, Tetrahedron, 2008, 64, 8963–8973 CrossRef CAS.
- G. Li, D. Zhang, G. Liu and S. Pu, A highly selective fluorescent probe for Cd2+ and Zn2+ based on a new diarylethene with quinoline–benzimidazole conjugated system, Tetrahedron Lett., 2016, 57, 5205–5210 CrossRef CAS.
- J. Yuasa, A. Mitsui and T. Kawai, π–π* Emission from a tetrazine derivative complexed with zinc ion in aqueous solution: a unique water-soluble fluorophore, Chem. Commun., 2011, 47, 5807–5809 RSC.
- A. Barbieri, G. Accorsi and N. Armaroli, Luminescent complexes beyond the platinum group: the d10 avenue, Chem. Commun., 2008, 2185–2193 RSC.
- F. Zhong and J. Zhao, Phenyleneanthracene derivatives as triplet energy acceptor/emitter in red light excitable triplet-triplet-annihilation upconversion, Dyes Pigm., 2017, 136, 909–918 CrossRef CAS.
-
S. P. McGlynn, T. Azumi and M. Kinoshita, Molecular Spectroscopy of the Triplet State, Prentice-Hall, Englewood Cliffs, NJ, 1969 Search PubMed.
- Q.-D. Liu, R. Wang and S. Wang, Blue phosphorescent Zn(ii) and orange phosphorescent Pt(ii) complexes of 4,4′-diphenyl-6,6′-dimethyl-2,2′-bipyrimidine, Dalton Trans., 2004, 2073–2079 RSC.
- T. E. Kokina, M. I. Rakhmanova, N. A. Shekhovtsov, L. A. Glinskaya, V. Y. Komarov, A. M. Agafontsev, A. Y. Baranov, P. E. Plyusnin, L. A. Sheludyakova, A. V. Tkachev and M. B. Bushuev, Luminescent Zn(ii) and Cd(ii) complexes with chiral 2,2′-bipyridine ligands bearing natural monoterpene groups: synthesis, speciation in solution and photophysics, Dalton Trans., 2020, 49, 7552–7563 RSC.
- H. Ihmels, D. Leusser, M. Pfeiffer and D. Stalke, Solid-State Photolysis of Anthracene-Linked Ammonium Salts: The Search for Topochemical Anthracene Photodimerizations, Tetrahedron, 2000, 56, 6867–6875 CrossRef CAS.
- Y. Mizobe, M. Miyata, I. Hisaki, Y. Hasegawa and N. Tohnai, Anomalous Anthracene Arrangement and Rare Excimer Emission in the Solid State: Transcription and Translation of Molecular Information, Org. Lett., 2006, 8, 4295–4298 CrossRef CAS PubMed.
- G. E. Berkovic and Z. Ludmer, Anomalous excimer fluorescence from anthracene derivatives crystallizing in chiral crystal structures, J. Chem. Soc., Chem. Commun., 1981, 768–769 RSC.
- J. C. Amicangelo and W. R. Leenstra, Excimer Formation in the Interlayer Region of Arene-Derivatized Zirconium Phosphonates, J. Am. Chem. Soc., 2003, 125, 14698–14699 CrossRef CAS PubMed.
- T. Seko, K. Ogura, Y. Kawakami, H. Sugino, H. Toyotama and J. Tanaka, Excimer emission of anthracene, perylene, coronene and pyrene microcrystals dispersed in water, Chem. Phys. Lett., 1998, 291, 438–444 CrossRef CAS.
- Y. Yagita and K. Matsui, Size-dependent optical properties of 9,10-bis(phenylethynyl)anthracene crystals, J. Lumin., 2015, 161, 437–441 CrossRef CAS.
- A. Li, J. Wang, S. Xu, Z. Huo, Y. Geng, W. Xu and H. Cui, Distinct stimuli-responsive behavior for two polymorphs of 9,10-bis(phenylethynyl)anthracene under pressure based on intermolecular interactions, Dyes Pigm., 2019, 170, 107603 CrossRef CAS.
- B. Manna, A. Nandi and R. Ghosh, Ultrafast Singlet Exciton Fission Dynamics in 9,10-Bis(phenylethynyl)anthracene Nanoaggregates and Thin Films, J. Phys. Chem. C, 2018, 122, 21047–21055 CrossRef CAS.
- Y. Matsunaga and J.-S. Yang, Multicolor Fluorescence Writing Based on Host–Guest Interactions and Force-Induced Fluorescence-Color Memory, Angew. Chem., Int. Ed., 2015, 54, 7985–7989 CrossRef CAS PubMed.
- Y. Sagara, Y. C. Simon, N. Tamaoki and C. Weder, A mechano- and thermoresponsive luminescent cyclophane, Chem. Commun., 2016, 52, 5694–5697 RSC.
Footnotes |
† Electronic supplementary information (ESI) available: Synthesis, computational and photophysical details. CCDC 2060299–2060311. For ESI and crystallographic data in CIF or other electronic format see DOI: 10.1039/d1qi00149c |
‡ Equal contribution. |
|
This journal is © the Partner Organisations 2021 |
Click here to see how this site uses Cookies. View our privacy policy here.