DOI:
10.1039/D1NA00233C
(Review Article)
Nanoscale Adv., 2021,
3, 4019-4028
Silicon nanoparticles (SiNPs) in sustainable agriculture: major emphasis on the practicality, efficacy and concerns
Received
28th March 2021
, Accepted 29th May 2021
First published on 31st May 2021
Abstract
Silicon (Si), a beneficial element for plants, is known for its prophylactic effect under stress conditions. Many studies have documented the role of biogenic silica (bulk-Si) in alleviating biotic and abiotic stresses in plants. The scarce amount of the plant-available form of Si (monosilicic acid) in most of the cultivated soil and the limited efficacy of silicate fertilizers (bulk-Si) are the major concerns for the exploration of Si-derived benefits. In this regard, recent advances in nanotechnology have opened up new avenues for crop improvement, where plants can derive benefits associated with Si nanoparticles (SiNPs). Most of the studies have shown the positive effect of SiNPs on the growth and development of plants specifically under stress conditions. In contrast, a few studies have also reported their toxic effects on some plant species. Hence, there is a pertinent need for elaborative research to explore the utility of SiNPs in agriculture. The present review summarizes SiNP synthesis, application, uptake, and role in stimulating plant growth and development. The advantages of SiNPs over conventional bulk-Si fertilizers in agriculture, their efficacy in different plant species, and safety concerns have also been discussed. The gaps in our understanding of various aspects of SiNPs in relation to plants have also been highlighted, which will guide future research in this area. The increased attention towards SiNP-related research will help to realize the true potential of SiNPs in agriculture.
Introduction
Silicon (Si) is the second most abundant element in the earth's crust, and its importance in agriculture has increased multifold.1 Regardless of its abundance in the earth's crust, most of the Si exists in the unavailable form as silicon dioxide. Plants uptake Si in the form of silicic acid or mono silicic acid [Si(OH)4 or H4SiO4], and this is the only available form for plant uptake.2 Silicic acid concentration varies from 0.1 to 0.6 mM in soil solution at <pH = 9.3 The Si level in the leaves of plants ranges from 0.1 to 10% on a dry weight basis.2 The variation in Si content was found lower within plant species compared to the variation observed among different plant species.4 The ability of roots to uptake Si is the major cause leading to differences in Si accumulation among different plant species.5 Uptake of Si by roots occurs via the apoplastic and symplastic routes. A group of aquaporin transporters, viz., NIPs (Nod26-like intrinsic proteins), are involved in the symplastic transport, and these transporters have been characterized in many dicots and monocots.2 The uptake, transport, accumulation, and mechanism of Si action have been reviewed recently in detail by Imtiaz et al.6
To date, extensive research studies are available demonstrating the positive effects of Si on plant growth, particularly under biotic or abiotic stresses.1 For instance, Si supplementation has been observed to increase abiotic stress tolerance in crops such as rice,7 wheat,8 tomato,9 and sorghum.10 Similarly, Si is known to protect against many plant diseases. Schurt et al.11 demonstrated that Si application increased sheath blight resistance in rice. Several such pieces of evidence have led to innovative uses of Si as a fertilizer for improving plant growth under stress conditions.12 By considering the important role of Si in plant growth under stress conditions, Si-research has gained huge interest among the research community.12 In this regard, considerable efforts are made across the globe to elucidate its role and mechanisms underlying stress tolerance in plants.2
Based on the type of silicate present, Si-fertilizers have been categorized as calcium silicate fertilizer, sodium silicate fertilizer, potassium silicate, steel-slag-based silicate, and mineral-based fertilizer.13–16 Among these, sodium silicates and potassium silicates are most widely used in agriculture. Menzies et al.17 demonstrated that the use of Si-fertilizers (potassium silicate) significantly decreases the infection of powdery mildew disease in three crop species viz. muskmelon, cucumber, and zucchini squash. However, the amount of Si released from these bulk fertilizers as well as the amount of Si available for plant uptake is questionable. For example, in the case of wollastonites (consist of 23% Si), the release of Si is highly dependent on soil properties such as pH, organic content, and the presence of metal oxides in soil. Further, the Si released from Si-fertilizers might undergo polymerization or form complexes with metal oxides, resulting in a lower amount of plant-available Si.18 Hogan et al.19 reported that the amount of Si released from sodium metasilicate fertilizer, Pro-Tekt (a commercially available fertilizer), and ash-based fertilizer was significantly lower than that from natural sources of Si such as rice husk and rice husk amendments. However, there is no doubt that the use of these silicate fertilizers has played an important role in enhancing plant growth and development under various stresses.12 But, farmers have been using these commercially available silicate fertilizers for a long time, and yet a clear picture of plant-available Si is still lacking. Not only these fertilizers are costly compared with rice husk amendments, but also the percentage of Si released from them is also unclear. Hence, the need has been felt by researchers to find some alternative approaches that will increase the efficiency of Si availability, uptake, transport, and accumulation in plant tissues besides being inexpensive.20
In this context, the use of nanotechnology in Si research has emerged as an important alternative approach to increase the efficiency of the use of Si fertilizers in crop improvement. Nanoparticles are relatively more soluble and reactive because of their small size (1–100 nm) and large surface area.20 Si-nanoparticles (SiNPs) can be synthesized through different approaches; Jal et al.21 prepared SiNPs from commercially available silica gel by using a precipitation method. Research is now inclined towards using plant materials to synthesize SiNPs, which include several plant species such as rice, sugarcane, and bamboo;22–25 for example, rice husk is the major source for the green or plant-based synthesis of SiNPs.21–25 SiNPs resemble the silica present in plant phytoliths. SiNPs supplied to the plants enter plant roots by symplastic and apoplastic pathways and are further transported to the other parts of plants.25 Despite being the emerging field in Si research, there are not enough studies available comparing SiNP and silicate fertilizer (bulk-Si) uptake and transport in plants. There is debate regarding the effects of SiNPs and bulk-Si fertilizers on plants. For example, one study claimed that the effects of SiNPs and silicate fertilizers on plants yielded almost similar results; however, silicon nanoparticles had more influence on silicon accumulation, lignification of the cell wall, and formation of stress enzymes.26 But, more studies are required to conclude which Si amendment is better for agriculture practices.
The use of SiNPs in agriculture has been suggested to possibly eliminate the requirement of conventional bulk fertilizers.27 Besides, due to their smaller size and higher absorption capacity, they can easily penetrate the cell wall barrier and enter the plant system, or get easily absorbed and transported by transporters.28 The present review focuses on the current status of the use of SiNPs in agriculture. Besides, we also discussed the potential, efficiency, and biosafety concerns related to the use of SiNPs for plant growth and development under stress conditions.
Major approaches for silicon nanoparticle (SiNP) synthesis
Recently, researchers' interest in nanobiotechnology has paved the way towards discoveries in the field of biology.29 SiNPs being small, with an average diameter of approximately 7 nm and wide absorption surface area (∼400 m2), can easily pass through the bio-membranes compared to crystalline bulk-Si.29 SiNPs are being commercially synthesized from metals and metal oxides using different techniques such as sputtering, lithography, laser ablation, mechanical milling, sonication, milling, UV irradiation, sol–gel technique, reverse micro-emulsion, Stober's method, atomic condensation, spray pyrolysis, aerosol process, bio-reduction, and flame synthesis.30,31 These techniques are categorized into two major approaches, viz. top-down approach (physical method) and bottom-up approach (chemical method).32,33 In the top-down approach, the dimension of the original size of Si is reduced with the help of physical methods such as special size reduction techniques. The bottom-up method (chemical approach) uses a common route to synthesize SiNPs from an atomic or molecular scale. Even though the top-up approach is simpler, it is not widely used because of the imperfection of the surface structure, whereas the bottom-down approach is widely used to synthesize SiNPs.32 Apart from physical and chemical methods, biological methods are also used to synthesize SiNPs, which come under the bottom-up approach34 (Fig. 1). Biological methods such as plant-mediated or green synthesis and microbial synthesis are cost-efficient and eco-friendly.35
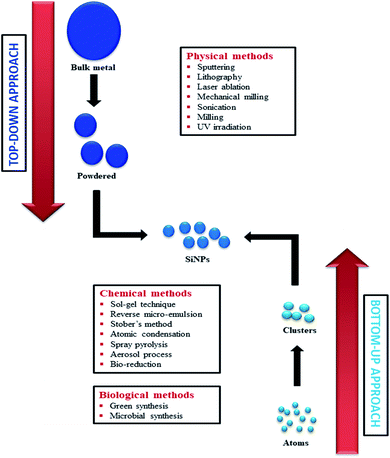 |
| Fig. 1 Simplified flowcharts of major approaches for silicon nanoparticle (SiNP) synthesis. | |
Most commonly used techniques for the synthesis of SiNPs include green synthesis, microbial synthesis, Stober's method, reverse micro-emulsion, sol–gel synthesis, and flame synthesis.36,37 Green synthesis (plant-mediated synthesis) is one of the simplest and cheapest methods for the synthesis of SiNPs from plant tissues such as leaf, root, fruit, husk, and bark.37,38 For example, Adinarayana et al.38 explored this method to synthesize SiNPs from Equisetum arvense through a microwave-assisted facile green route. Furthermore, microbial synthesis involves the synthesis of SiNPs from microbes such as bacteria and viruses. For instance, the bacterium Actinobacter39 and tobacco mosaic virus (TMV)40 produce Si or SiO2 on the nano-scale and have been used for the synthesis of SiNPs.
Stöber et al.41 introduced a chemical method to produce spherical SiNPs with sizes less than 50 nm to 2000 nm, and this method involved the hydrolysis of alkyl silicates followed by condensation of Si(OH)2 in alcoholic solutions. This method is commonly used to synthesize non-porous SiNPs and mesoporous SiNPs (MSNs). In the microemulsion method, oil-in-water micelles or water-in-oil reverse micelles are formed which are stabilized by surfactant molecules that act as nanoreactors for SiNP synthesis.42 Inside the nanoreactors, SiNPs are formed from Si precursors by hydrolysis and condensation. This method is usually employed to synthesize core–shell SiNPs, non-porous SiNPs, MSNs, and hollow mesoporous silica nanoparticles (HMSNs).42 Another standard method for the production of SiNPs is flame synthesis,43 which involves the decomposition of Si precursors in high-temperature flame. This method faces troubles in controlling the particle size, phase composition, and morphology of SiNPs. In contrast, this is a widely used method to produce commercially available SiNPs. In contrast to flame synthesis, the sol–gel technique can control particle size, morphology, and size distribution by monitoring reaction parameters.36 In this method, pure silica particles are synthesized through hydrolysis and condensation of Si(OR)4 or inorganic salts in the presence of an acid or base.36 Hence, these advances in the methods and techniques for SiNP synthesis have led to the production of SiNPs on a commercial scale, as well as effective utilization of SiNPs in the fields of agriculture, biology, and medicine.
Green synthesis of SiNPs: practicality
Previously, we have mentioned that different methods are used for the synthesis of SiNPs, viz. synthetic and biological. Although the SiNPs synthesized using a synthetic approach are superior to those synthesized by the biological approach, synthetic approaches are neither environmentally friendly nor cost-efficient. In this regard, green synthesis of SiNPs is considered eco-friendly as well as cost-effective.26 In the case of green synthesis of SiNPs, plant extracts and biological waste material are used as precursors.23,27 Also, different types of microorganisms such as fungi,44 bacteria,45 and diatoms46 can be used for the synthesis of SiNPs. Agro-wastes such as sugarcane bagasse,23 ash of agro-waste,47 and the waste from rice such as straw and husk are widely used in various studies for the green synthesis of SiNPs.48
The use of different plant-based materials and agro-wastes for the synthesis of SiNPs has been considered as the best option as far as cost-effectiveness and environmental safety are considered. In this context, many studies have documented the green synthesis of SiNPs from fly ash, plant extracts, and agro-wastes including rice husk, sugarcane bagasse, and corn cob husk (see Table 1). In most of the studies, SiNPs are synthesized in two steps that involve the extraction of sodium silicates by using NaOH treatment followed by a sol–gel method which involves the formation of silica gel by neutralizing inorganic salts such as sodium silicates in the presence of HCl.36 Adinarayana et al.38 synthesized SiNPs from Equisetum arvense by pyrolyzing the extract at high temperature in a microwave oven and obtained SiNPs with an average diameter of 2–3 nm along with fluorescence activity. Similarly, Bose et al.37 synthesized SiNPs from rice husk. In this case, powdered rice husk mixed with NaOH is heated in a microwave oven (2.45 GHz, 600 W) for an hour to synthesize SiNPs, which were used as sustainable fluorophores for white light emission. In a study conducted by Adebisi et al.49 a modified sol–gel method was used for the preparation of SiNPs from maize stalks. This method involves acid treatment, calcination at 700 °C, modified sol–gel, and post-filtration treatments. Besides improving plant health, SiNPs have also been used for the detection of Fe(III) ions,38 as an antimicrobial agent,36 as feedstock for silicon production,45 and as nano-composites that can be used in dental filling and drug delivery.36
Table 1 Details of major studies demonstrating green synthesis of silicon nanoparticles (SiNPs)a
S. no. |
Substrate |
Nanoparticles' shape/arrangement |
Size |
Characterization |
Reference |
X-ray fluorescence (XRF); X-ray diffraction (XRD); field emission scanning electron microscopy (FESEM); transmission electron microscopy (TEM); Fourier-transform infrared spectroscopy (FTIR); thermogravimetric analysis (TGA); atomic force microscopy (AFM); Dynamic Light Scattering (DLS); energy dispersive X-ray spectroscopy (EDAX); small-angle X-ray scattering (SAXS).
|
1 |
Coal fly ash |
Amorphous mesoporous SiNPs |
190–250 nm and pore size 3.5 to 4.5 nm |
XRF, XRD, FESEM, TEM, porosity analyzer, FT-IR, TGA |
116
|
2 |
Fly ash |
Amorphous, aggregated spherical SiNPs |
20–70 nm |
FT-IR, Raman spectroscopy, XRD, FESEM-EDS, TEM, AFM |
47
|
3 |
Sugarcane bagasse |
Amorphous, spherical SiNPs |
Around 30 nm |
FT-IR, XRD, SEM and Micromeritics analyzer |
23
|
4 |
Cynodon dactylon
|
Amorphous, spherical SiNPs |
7 to 80 nm |
UV-vis spectroscopy, FT-IR, DLS, XRD, SEM, TEM, EDAX |
35
|
5 |
Bamboo |
Crystalline, porous SiNPs |
Around 200 nm |
SEM, TEM, XRD |
22
|
6 |
Rice husk |
Biogenic porous SiNPs |
25 to 30 nm |
XRD, SEM, TEM, SAXS |
48
|
7 |
Sugarbeet bagasse |
Spheroid shaped SiNPs |
38 to 190 nm |
SEM-EDS, TEM, DLS, FT-IR, XPS, Raman spectroscopy |
117
|
8 |
Sugarcane waste ash |
Amorphous, mesoporous SiNPs |
<1 to 100 μm |
XRD, XRF, SEM, TGA, FT-IR, particle size analyzer |
118
|
9 |
Maize stalk |
Amorphous |
30 nm |
XRD, SEM, EDS, TEM, TGA, FTIR, Raman spectroscopy |
49
|
10 |
Corn cob husk |
Spherical shape |
40 to 70 nm |
SEM, STEM, EDX, FT-IR |
119
|
Important characteristics of SiNPs
Silicon nanoparticles (Si-NPs) consist of 46.83% Si and 53.33% oxygen.50 Moreover, SiNPs are inert to pH variations, less toxic, optically transparent and some of them are degradable.51 These particles possess the largest range of accessible sizes and can be synthesized from different processes.52 However, the size of SiNPs can be modified by varying reaction parameters such as mixing speed, ammonia or sodium hydroxide concentration, and the rate of tetra-ethyl-orthosilicate addition.42 SiNPs have a large surface area and intrinsic surface reactivity which make them highly feasible for modification with chemical introduction.53 The most important characteristics of SiNPs include ease of synthesis, small size, large surface area, large pore volume, tunable surface modification, and high stability, which make them some of the best candidates for drug delivery. Furthermore, the fabrication of SiNPs is simple, scalable, and cost-effective. Besides, they show excellent biocompatibility, less toxicity, and are “Generally Recognized As Safe” (GRAS). Additionally, the size of nanoparticles plays a vital role in toxicity, so the small size of SiNPs makes them comparatively non-toxic in nature.54
Based on the structural morphology, SiNPs can be divided into four types, viz. non-porous, mesoporous (MSNs), hollow mesoporous (HMSNs), and core–shell SiNPs. Non-porous SiNPs are a solid colloidal material that can be synthesized by base-catalyzed hydrolysis of a Si source and can be used for the delivery of cargos through conjugation or encapsulation. In contrast to this, MSNs have a pore size of around 2 to 20 nm and are widely used for drug delivery.55,56 Hollow mesoporous SiNPs (HMSNs) are spherical-shaped and have a large hole in the center, and they can be used for loading antigens to activate the immune system.57 Core–shell SiNPs are spherical silica particles having solid cores and mesoporous shells.58 The shape and size of SiNPs play an important role in their use and activity. The most common shapes of SiNPs are nanospheres and nanorods. Nanorods can be further divided into short rod nanoparticles and long rod nanoparticles. The porous structure of SiNPs may also be different in different SiNPs. According to Selvarajan et al.42 three types of porous structures may be present in SiNPs, viz. hexagonal pores, radial wrinkled pores, and worm-like pores.
SiNPs: mode of application and worries
In plants, SiNPs are applied by three different modes, namely foliar, soil, and seed priming. In the case of foliar application, SiNPs (in the liquid form) are sprayed on the shoot tissues (leaf surfaces), which are then subsequently absorbed via leaf epidermal cells or stomata59 (Fig. 2). Following the uptake of SiNPs, a binary film is formed at the epidermal cell wall which imparts a structural color to the plants.60 SiNPs can also be applied directly to the soil/nutrient solution, and the effectiveness of this method depends on soil characteristics such as pH, texture, salt content, and the duration of agrochemical release by the NPs (Fig. 2). However, the foliar application of SiNPs has been observed to be less effective compared to the soil-application of SiNPs.61 This might be due to the reduced uptake of Si-NPs by leaf tissues compared to root tissues.61 Roots release many exudates which form a complex with the NPs which subsequently bind to membrane transporters or carrier proteins and pass through ion channels, aquaporins, or are endocytosed.62
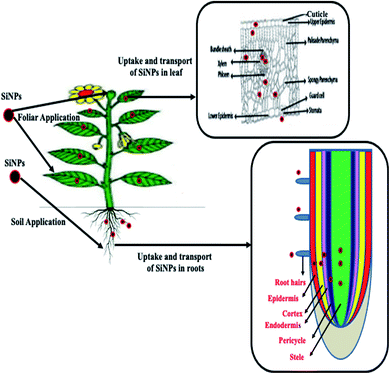 |
| Fig. 2 Diagram showing the mechanism of uptake and transport of SiNPs in leaf and root tissues. In the leaf tissue, SiNPs penetrate through the cuticle and then enter the leaf mesophyll via passing through the epidermis layer, and from the leaf mesophyll tissue they enter the vascular tissues. In the root, SiNPs are taken up by root hairs, subsequently, they pass through the epidermis and cortex by following the apoplastic and symplastic pathways, and finally reach the xylem and phloem, and then they are further transported to the upper parts of the plant system. | |
Besides these two methods, SiNPs can also be applied by seed priming. Recent studies have demonstrated that seed priming with SiNPs has significantly improved the growth and productivity in different plant species such as wheat28 and tomato.63 However, many authors suggested that NP application by seed priming is of greater importance compared to other techniques such as soil and foliar application.64–67 Seed priming provides controlled hydration until the germination process starts, and stimulates a particular physiological state of plants before the sowing of seeds.68 Despite the availability of these three different modes of SiNP application to plants, very little information exists regarding the effectiveness of these three approaches. There is limited or almost no comparative study demonstrating the efficiency of these methods of SiNP application in plant growth. Hence, efforts have to be made to elucidate the relative efficiency of these three methods in the plant growth of different plant species to harness the true potential of Si-fertilizers in crop improvement.
Until now, most of the studies have documented either the beneficial effects or ineffectiveness of SiNPs on plant growth62 (Table 2). However, there are only a few studies that have reported the toxic effects of SiNPs on plants.69,70 These studies suggested that the toxic effects of SiNPs are mostly due to pH changes in the growth media resulting from the addition of SiNPs. For example, Slomberg and Schoenfisch70 showed that by excluding the changes in pH by SiNP addition, the toxic effect of SiNPs on Arabidopsis growth was completely alleviated. Similarly, Rui et al.69 also suggested that the SiNP-induced change of pH in growth media is responsible for their toxic effect on cotton. Based on the above studies, it can be concluded that by adjusting the pH of the growth media, Si-NPs can be considered either beneficial or ineffective for different plant species. However, concrete evidence is still required to fully understand the beneficial, non-beneficial, or toxic effects of SiNPs on different plants.
Table 2 Details of studies showing beneficial effects of silicon nanoparticles (SiNPs) on plant growth and stress tolerance in Si accumulators, non-accumulators and intermediatesa
S. no. |
Plant species |
Size of SiO2-NPs used |
Positive effects on plant growth/stress tolerance |
Accumulator/non-accumulator/intermediate |
Reference |
Silicon-dioxide nanoparticles (SiO2-NPs).
|
1 |
Banana |
20–35 nm |
Drought and salt tolerance |
Accumulator |
120
|
2 |
Barley |
20–30 nm |
Drought tolerance |
Intermediate |
121
|
3 |
Basil |
20–30 nm |
Salt tolerance |
Intermediate |
122
|
4 |
Coriander |
20–35 nm |
Heavy metal tolerance |
Intermediate |
123
|
5 |
Cucumber |
10 nm |
Drought and salt tolerance |
Intermediate |
124
|
6 |
Cumin |
10–20 nm |
Drought tolerance |
Intermediate |
125
|
7 |
Faba bean |
40 nm |
Salt tolerance |
Non-accumulator |
126
|
8 |
Faba bean |
10 nm |
Enhanced seed germination and growth |
Non-accumulator |
127
|
9 |
Faba bean |
10 nm |
Salt tolerance |
Non-accumulator |
128
|
10 |
Lentil |
20–30 nm |
Salt tolerance |
|
129
|
11 |
Maize |
∼170 |
Arsenic and oxidative stress tolerance |
Accumulator |
83
|
12 |
Maize |
5–15 nm |
Aluminum tolerance |
Accumulator |
130
|
13 |
Mango |
5–15 nm |
Salt tolerance |
Accumulator |
101
|
14 |
Oat |
20–30 nm |
Multiple trait improvement |
Intermediate |
65
|
15 |
Pea |
∼170 |
Chromium tolerance |
Intermediate |
79
|
16 |
Potato |
50 nm |
Salt tolerance |
Non-accumulator |
131
|
17 |
Pumpkin |
12 nm |
Salt tolerance |
Intermediate |
132
|
18 |
Rice |
230.4 nm |
Fluoride tolerance |
Accumulator |
133
|
20 |
Rice |
20–30 nm |
Heavy metal tolerance |
Accumulator |
134
|
21 |
Rice |
30–40 nm |
Cadmium tolerance |
Accumulator |
135
|
22 |
Rice |
30–40 nm |
Arsenic tolerance |
Accumulator |
77
|
23 |
Rice |
30–60 nm |
Salt tolerance |
Accumulator |
76
|
24 |
Strawberry |
10–20 nm |
Salt tolerance |
Non-accumulator |
136
|
25 |
Sugarbeet |
20–30 nm |
Drought tolerance |
|
137
|
26 |
Sunflower |
20–30 nm |
Seed germination |
Non-accumulator |
96
|
27 |
Tomato |
20 nm |
Salt tolerance |
Non-accumulator |
95
|
28 |
Tomato |
5–15 nm |
Salt tolerance |
Non-accumulator |
138
|
29 |
Tomato |
5–15 nm |
Weed protection |
Non-accumulator |
92
|
30 |
Tomato |
5–15 nm |
Arsenic tolerance |
Non-accumulator |
91
|
31 |
Tomato |
5–15 nm |
Root-knot nematode resistance |
Non-accumulator |
139
|
32 |
Tomato |
5–15 nm |
Salt tolerance |
Non-accumulator |
140
|
33 |
Tomato |
5–15 nm |
Salt tolerance |
Non-accumulator |
138
|
34 |
Wheat |
<50 nm |
UV-B stress tolerance |
Intermediate |
104
|
35 |
Wheat |
<50 nm |
Cadmium tolerance |
Intermediate |
28
|
36 |
Wheat |
<50 nm |
Cadmium tolerance |
Intermediate |
141
|
37 |
Wheat |
<50 nm |
Cadmium tolerance |
Intermediate |
142
|
38 |
Wheat |
<50 nm |
Heat tolerance |
Intermediate |
143
|
39 |
Wheat |
<50 nm |
Plant growth and physiological traits |
Intermediate |
144
|
Bulk-Si vs. SiNPs: what are their similarities or differences in plants
The unique properties of nanomaterials have attracted considerable attention in the fields of agriculture and environmental safety. The differences in physical and chemical properties of SiNPs relative to their bulk counterparts are due to their smaller size, higher surface area/weight ratio, and structure.71,72 SiNPs possessing a smaller diameter and highly reactive surface-to-volume ratio can easily pass through leaf stomata and pores of cell walls.73 Besides, they are considered more effective than other Si forms because of their higher density in reactive areas.74 Hence, it is a prerequisite to elucidate how differently SiNPs interact with the environment. Recently, Nazaralian et al.75 compared the effects of SiNPs and bulk-Si and demonstrated that SiNPs were more effective for Si uptake, translocation and accumulation, lignification of cell walls, and stress enzyme production and gene expression in fenugreek. In addition, some recent studies have also documented that SiNPs showed better results in plant growth and mitigation of abiotic stresses than the bulk-Si.76–79 These authors suggested that differences in outcomes might exist due to the extraordinarily unique properties of SiNPs compared to bulk-Si.
Si-fertilizers in bulk form have reduced solubility and low bioavailability, and hence are not directly utilized by plants.80 In contrast, SiNPs possess higher bioavailability than traditional Si-fertilizers, and these NPs can penetrate leaves and cell wall pores very easily and are immediately absorbed by plants. Suriyaprabha et al.61 observed that the rapid uptake of SiNPs relative to bulk-Si provided higher seed coat resistance in maize plants. The rice plant, being a Si-accumulator, deposits higher Si in cell walls, and does not allow the heavy metal to enter cells.12,81 This has been demonstrated as an important mechanism utilized by the rice plant for providing tolerance against heavy metal stress.82 Recently, the effects of SiNPs and bulk-Si application on arsenate and oxidative stress were compared in maize crops where SiNPs were shown to have higher potential for reducing the arsenate stress.83 Similar findings were also reported in rice by Liu et al. 2015.84 They suggested that this is because of the higher bioavailability of Si from SiNPs than that from bulk-Si which leads to alleviation of metal stress.
From the above information, it is clear that there are differences in the uptake, translocation, and accumulation of SiNPs and bulk-Si through different routes of absorption and transport. Although a few studies have provided evidence for the involvement of both the symplastic and apoplastic pathways in the transport of SiNPs, little is known regarding the differences or similarities in the uptake and transport pathways between SiNPs and bulk-Si in different plant species. Hence, research efforts are needed to provide clear information regarding the routes/pathways followed by SiNPs and bulk-Si in their absorption and transport in plants.
Efficacy of SiNPs in diverse crops: accumulators vs. non-accumulators
Based on Si accumulation in their shoots, plant species have been classified as Si-accumulators, non-accumulators, and intermediates.85 In general, accumulators such as rice and sugarcane can accumulate 10 to 15% Si (by weight), whereas non-accumulators viz. tomato and sunflower possess >0.5% Si.86 To this end, intermediates such as cucumber can accumulate Si between 0.5 and 1.5%. However, this difference in Si accumulation among these different categories of plant species results from distinct mechanisms involved in the uptake and transport of Si.2 In general, Si absorption in accumulators, intermediates, and non-accumulators occurs through active, passive, and rejective mechanisms, respectively.87 For example, most monocots/accumulators (such as rice, wheat, ryegrass, barley, maize, and banana) use an active process for the absorption of Si. In contrast, cucumber (intermediate) follows the passive mechanism for Si absorption.87 However, some non-accumulators such as tomato and faba bean use the rejective approach, which excludes Si from their roots.88 Besides, accumulators, such as rice, possess efficient Si-transporters and specialized silica cells for the increased uptake and accumulation of Si. The non-accumulators usually do not possess silica-cells, and many dicot families have been documented to have lost the Si-transporter AQPs.89 Although, several studies have shown that the application of Si in the bulk form provides a beneficial effect not only for high Si-accumulating plants such as rice and sugarcane, but also for poor accumulators such as tomato, cucumber, strawberry, and orange.2,12 However, the beneficial effects derived from conventional Si fertilizers on plant growth and stress tolerance were comparatively higher in Si-accumulators than in low/non-accumulators.2,8 This has been suggested to be due to a higher ability of Si-accumulators to accumulate more Si in their tissues relative to non-accumulators.90
In contrast, recent studies have also shown the benefits of SiNPs in the enhancement of growth and stress tolerance in non-accumulators as well as intermediate plants (Table 2). For example, the application of SiNPs has been reported to significantly increase tolerance in different plants against various biotic and abiotic stresses, for instance, providing resistance against arsenic stress,91 parasitic weed,92 root-knot nematode,93 fungal disease,94 and salt stress.95 These studies have demonstrated that SiNP-mediated enhancement of plant stress tolerance results mainly from their positive effects on photosynthesis, antioxidant defense systems, ROS scavenging, and oxidative damage.87,89,91 The positive effects of Si have been recently explained to result from its beneficial influence on nutrient homeostasis and carbon metabolism.96,97 Similarly, pre-sowing treatment of SiNPs significantly increased the germination of sunflower seeds.98 To this end, SiNPs have been demonstrated to positively affect the growth and stress tolerance of Si-accumulators such as rice99,100 (Table 2) and sugarcane.101 The higher efficiency of SiNPs than bulk-Si in non-accumulators has been suggested to result from different pathway mechanisms adopted in the uptake and transport of SiNPs. SiNPs have a lower size and surface-to-volume ratio and hence do not need transporters for their absorption; they can easily pass through the pores of the cell wall and leaf stomata102,103 (Fig. 2). There might be similar pathways involved in the uptake and transport of Si-NPs in accumulators and non-accumulators, which in turn will lead to higher accumulation of Si equally in both these categories of plant species, ultimately resulting in equal Si-derived benefits. Besides, many authors have observed higher efficiency of SiNPs in accumulators compared to bulk-Si.81,83,104 Hence, different studies in diverse plant species, viz. accumulators, non-accumulators, and intermediates, have demonstrated that SiNPs have great potential for positively influencing plant growth, especially under various biotic and abiotic stress conditions (Table 2).
Safety concerns associated with nanoparticle (NP) application
Toxicity of nanoparticles (NPs) to living organisms (plants and animals) is the major issue concerning their practical use in different fields such as agriculture, textile production, medicine, and sciences. NPs are unique in terms of their particle size, shape, dispersity, and charge, and these factors determine their toxicity. Nano-range particles (<100 nm) have been observed to be more toxic than larger-sized particles with the same chemical composition.105 Adhesion of NPs to plant roots was found to interfere with water and mineral transport resulting in phytotoxicity.106 Since NPs are produced from bulk materials, their entry into terrestrial and aquatic ecosystems has been anticipated.107 NPs could enter the food chain through various routes including nutrients, pesticides, or environmental pollutants.108 These NPs can enter living organisms via dermal, respiratory, and digestive tract routes depending on their physico-chemical properties, and can access vital organs.109 Many researchers believe that the observed toxicity of NPs to plants is based on plant–NP physical interactions. Alteration of the root surface and clogging of root openings by NPs are some of the hypotheses believed to result in reduced hydraulic conductivity and nutrient uptake.110,111
To date, only limited studies have been carried out to understand the toxicity effects of metal oxide NPs such as Fe3O4, CeO2, SiO2, TiO2, and ZnO on a few crop plants.108 It has been revealed that all plants treated with NPs do not exhibit toxicity effects, and these studies have also documented positive or no consequences in plants treated with NPs.112–114 However, SiNP application to plants is considered safe, since silica is widely distributed in plant tissues such as leaf epidermis and root endodermis. But there are some reports which have documented the negative impacts of SiNPs on plants. For example, Si-NPs of size 50 nm and 200 nm caused decreased growth in Arabidopsis.70 Similarly, plant height and biomass were reduced in Bt-cotton by the application of SiNPs.69 The root meristem cells of Allium cepa at a SiNP concentration of 100 μg mL−1 showed significant DNA damage.115 Therefore, SiNPs of a particular size and standardized concentration may prove beneficial to plants, while improper size or too high concentrations may result in severe toxic effects. Besides, the effect of Si-NPs varies among plant species. Hence, it is of great interest to elucidate the detailed role and mechanism involved in SiNP-mediated regulation of plant growth in different species. Moreover, all the factors (size, concentration, surface area, physicochemical properties, plant species, plant age, and NP stability) need to be critically considered to get the maximum benefits from SiNPs in agriculture. SiNPs may also help in restricting the use of agrochemicals, pesticides, and chemical fertilizers responsible for large-scale pollution.
Conclusion
Nanotechnology is an emerging area of interdisciplinary research in several fields such as agriculture, medicine, and pharmaceuticals. Recently, SiNPs applied to crop plants as nano-fertilizers have been observed to substantially improve plant growth under multiple stress conditions. Among the different methods used for SiNP synthesis, biological methods, viz. green and microbial synthesis, have emerged as cost-effective and environmentally safe approaches. Studies have demonstrated that SiNPs are more efficient than bulk-Si as a fertilizer for plants. It has been confirmed that SiNPs showed similar performance for the enhancement of plant growth under stress conditions in both accumulators and low/non-accumulators, which was in contrast to the case of bulk-Si. However, the mechanism and other aspects related to the positive effect of SiNPs on plant growth need to be understood. Undoubtedly, increased attention and efforts to understand SiNP-mediated enhanced plant growth under stress conditions will yield favorable and sustainable results for future agriculture and global food security.
Author contributions
All authors have equally contributed to the development of this manuscript.
Funding
This research received no external funding.
Conflicts of interest
There are no conflicts to declare.
Acknowledgements
Javaid Akhter Bhat is thankful to the Nanjing Agricultural University for providing support to his research projects. Rupesh Deshmukh is thankful to the Department of Biotechnology, Government of India, for the financial support in the form of a Ramalingaswami Fellowship.
References
- R. K. Deshmukh, J. F. Ma and R. R. Bélanger, Front. Plant Sci., 2017, 8, 1858 CrossRef PubMed.
- S. M. Zargar, R. Mahajan, J. A. Bhat, M. Nazir and R. Deshmukh R, 3 Biotech, 2019, 9, 73 CrossRef PubMed.
-
C. T. G. Knight and S. D. Kinrade, in Silicon in agriculture, studies in plant science, ed. Datnoff L. E., Snyder G. H. and Korndörfer G. H., Elsevier, Amsterdam, 2001, 8, pp. 57–84 Search PubMed.
-
M. Broadley, P. Brown, I. Cakmak, J. F. Ma, Z. Rengel and F. Zhao, in Marschner's mineral nutrition of higher plants, ed. Marschner P., Elsevier, Amsterdam, 2011, pp. 257–261 Search PubMed.
- J. F. Ma and N. Yamaji, Silicon uptake and accumulation in higher plants, Trends Plant Sci., 2006, 11, 392–397 CrossRef CAS PubMed.
- M. Imtiaz, M. S. Rizwan, M. A. Mushtaq, M. Ashraf, S. M. Shahzad, B. Yousaf, D. A. Saeed, M. Rizwan, M. A. Nawaz, S. Mehmood and S. Tu, J. Environ. Manage., 2016, 183, 521–529 CrossRef CAS PubMed.
- M. Mahdieh, N. Habibollahi, M. Amirjani, M. Abnosi and M. Ghorbanpour, J. Soil Sci. Plant Nutr., 2015, 15, 1050–1060 CAS.
- A. L. Tuna, C. Kaya, D. Higgs, B. Murillo-Amador, S. Aydemir and A. R. Girgin, Environ. Exp. Bot., 2008, 62, 10–16 CrossRef CAS.
- Z. M. Almutairi ZM, Plant Omics, 2016, 9, 106 Search PubMed.
- L. Yin, S. Wang, K. Tanaka, S. Fujihara, A. Itai, X. Den and S. Zhang, Plant, Cell Environ., 2016, 39, 245–258 CrossRef CAS PubMed.
- D. A. Schurt, M. F. Cruz, K. J. Nascimento, M. C. Filippi and F. A. Rodrigues, Trop. Plant Path., 2014, 39, 457–463 CrossRef.
- J. A. Bhat, S. M. Shivaraj, P. Singh, D. B. Navadagi, D. K. Tripathi, P. K. Dash, A. U. Solanke, H. Sonah and R. Deshmukh, Plants, 2019, 8, 71 CrossRef CAS PubMed.
- T. Kanto, A. Miyoshi, T. Ogawa, K. Maekawa and M. Aino, J. Gen. Plant Pathol., 2006, 72, 137–142 CrossRef CAS.
- D. Ning, Y. Liang, Z. Liu, J. Xiao and A. Duan, PLoS One, 2016, 11, e0168163 CrossRef PubMed.
- R. T. Rashad and R. A. Hussien, Commun. Soil Sci. Plant Anal., 2020, 51, 1078–1088 CrossRef CAS.
- M. Zhao, Y. Liu, H. Li, Y. Cai, M. K. Wang, Y. Chen, T. Xie and G. Wang, Environ. Sci. Pollut. Res., 2017, 24, 21700–21709 CrossRef CAS PubMed.
- J. Menzies, P. Bowen, D. Ehret and A. D. Glass, J. Am. Soc. Hortic. Sci., 1992, 117, 902–905 CAS.
- T. Babu, B. Tubana, L. Datnoff, J. Yzenas and K. Maiti, Commun. Soil Sci. Plant Anal., 2016, 47, 1559–1577 CrossRef CAS.
- B. Hogan, F. Mcdermott and O. Schmidt, J. Plant Nutr. Soil Sci., 2018, 181, 231–239 CrossRef CAS.
- F. Asgari, A. Majd, P. Jonoubi and F. Najafi, Plant Physiol. Biochem., 2018, 127, 152–160 CrossRef CAS.
- P. Jal, M. Sudarshan, A. Saha, S. Patel and B. Mishra, Colloids Surf., A, 2004, 240, 173–178 CrossRef CAS.
- V. Kumar, P. Tiwari, L. Krishnia, R. Kumari, A. Singh, A. Ghosh and P. K. Tyagi, Adv. Mater. Lett., 2016, 7, 271–276 CrossRef CAS.
- N. K. Mohd, N. N. A. N. Wee and A. A. Azmi, AIP Conf. Proc., 2017, 1885, 020123 CrossRef.
- S. Snehal and P. Lohani, J. Pharmacogn. Phytochem., 2018, 7, 3383–3393 CAS.
- C. N. H. Thuc and H. H. Thuc, Nanoscale Res. Lett., 2013, 8, 1–10 CrossRef PubMed.
- S. Nazaralian, A. Majd, S. Irian, F. Najafi, F. Ghahremaninejad, T. Landberg and M. Greger, Plant Physiol. Biochem., 2017, 115, 25–33 CrossRef CAS PubMed.
- R. Raliya, V. Saharan, C. Dimkpa and P. Biswas, J. Agric. Food Chem., 2017, 66, 6487–6503 CrossRef PubMed.
- A. Hussain, M. Rizwan, Q. Ali and S. Ali, Environ. Sci. Pollut. Res., 2019, 26, 7579–7588 CrossRef CAS PubMed.
- Y. Shang, M. K. Hasan, G. J. Ahammed, M. Li, H. Yin and J. Zhou, Molecules, 2019, 24, 2558 CrossRef CAS PubMed.
- A. Liberman, N. Mendez, W. C. Trogler and A. C. Kummel, Surf. Sci. Rep., 2014, 69, 132–158 CrossRef CAS PubMed.
- S. P. C. C. Gonçalves, M. Strauss and D. S. T. Martinez, Environ. Sci. Technol., 2018, 52, 13845–13853 CrossRef PubMed.
-
K. J. Klabunde and R. M. Richards, Nanoscale materials in chemistry: John Wiley & Sons. 2009 Search PubMed.
- E. Reverchon and R. Adami, J. Supercrit. Fluids, 2006, 37, 1–22 CrossRef CAS.
- D. Sharma, S. Kanchi and K. Bisetty, Arabian J. Chem., 2019, 12, 3576–3600 CrossRef CAS.
- R. H. Babu, P. Yugandhar and N. Savithramma, Bull. Mater. Sci., 2018, 41, 1–8 CrossRef CAS.
- I. A. Rahman and V. Padavettan, J. Nanomater., 2012, 132424 Search PubMed.
- S. Bose, M. A. Ganayee, B. Mondal, A. Baidya, S. Chennu, J. S. Mohanty and T. Pradeep, ACS Sustainable Chem. Eng., 2018, 6, 6203–6210 CrossRef CAS.
- T. Adinarayana, A. Mishra, I. Singhal and D. R. K. Reddy, Nanoscale Adv., 2020, 2, 4125–4132 RSC.
- S. Singh, U. M. Bhatta, P. Satyam, A. Dhawan, M. Sastry and B. Prasad, J. Mater. Chem., 2008, 18, 2601–2606 RSC.
- W. Shenton, T. Douglas, M. Young, G. Stubbs and S. Mann, Adv. Mater., 1999, 11, 253–256 CrossRef CAS.
- W. Stöber, A. Fink and E. Bohn, J. Colloid Interface Sci., 1968, 26, 62–69 CrossRef.
- V. Selvarajan, S. Obuobi and P. L. R. Ee, Front. Chem., 2020, 8, 602 CrossRef CAS PubMed.
- S. Shekar, M. Sander, R. C. Riehl, A. J. Smith, A. Braumann A and M. Kraft, Chem. Eng. Sci., 2012, 70, 54–66 CrossRef CAS.
- E. Vetchinkina, E. Loshchinina, M. Kupryashina, A. Burov, T. Pylaev and V. Nikitina, PeerJ, 2018, 6, e5237 CrossRef PubMed.
- E. Vetchinkina, E. Loshchinina, M. Kupryashina, A. Burov and V. Nikitina, Ind. Eng. Chem. Res., 2019, 58, 17207–17218 CrossRef CAS.
- N. Asmathunisha and K. Kathiresan, Colloids Surf., B, 2013, 103, 283–287 CrossRef CAS PubMed.
- V. K. Yadav and M. Fulekar, Mater. Today: Proc., 2019, 18, 4351–4359 Search PubMed.
- W. Wang, J. C. Martin, X. Fan, A. Han, Z. Luo and L. Sun, ACS Appl. Mater. Interfaces, 2012, 4, 977–981 CrossRef CAS PubMed.
- J. A. Adebisi, J. O. Agunsoye, S. A. Bello, M. Haris, M. M. Ramakokovhu, M. O. Daramola and S. B. Hassan, Part. Sci. Technol., 2020, 38, 667–675 CrossRef CAS.
-
F. Buyuknalcaci, Y. Polat, T. Negawo, E. Döner, M. Alam, T. Hamouda and A. Kilic, Polymer-based Nanocomposites for Energy and Environmental Applications, Elsevier, 2018, pp. 635–661 Search PubMed.
-
Y. Lian, W. Zhang, L. Ding, X. Zhang, Y. Zhang and X. D. Wang, Novel Nanomaterials for Biomedical, Environmental and Energy Applications, Elsevier, 2019, pp. 241–273 Search PubMed.
-
M. Schroffenegger and E. Reimhult, Comprehensive Nanoscience and Nanotechnology, Academic Press, Oxford, 2nd edn, 2019, pp. 145–170 Search PubMed.
-
C. Hussain and S. Mitra, Comprehensive Sampling and Sample Preparation, Elsevier Inc., 2012, pp. 389–418 Search PubMed.
-
O. Esim, S. Kurbanoglu, A. Savaser, S. A. Ozkan and Y. Ozkan, New Developments in Nanosensors for Pharmaceutical Analysis, Elsevier, 2019, pp. 273–301 Search PubMed.
- S. E. Lehman, I. A. Mudunkotuwa, V. H. Grassian and S. C. Larsen, Langmuir, 2016, 32, 731–742 CrossRef CAS PubMed.
- L. Tang and J. Cheng, Nano today, 2013, 8, 290–312 CrossRef CAS PubMed.
- J. Y. Lee, M. K. Kim, T. L. Nguyen and J. Kim, ACS Appl. Mater. Interfaces, 2020, 12, 34658–34666 CrossRef CAS PubMed.
- A. Baliś and S. Zapotoczny, Nanomaterials, 2018, 8, 230 CrossRef PubMed.
- H. M. Laane, Plants, 2018, 7, 45 CrossRef CAS PubMed.
- G. Strout, S. D. Russell, D. P. Pulsifer, S. Erten, A. Lakhtakia and D. W. Lee, Ann. Bot., 2013, 112, 1141–1148 CrossRef CAS PubMed.
- R. Suriyaprabha, G. Karunakaran, R. Yuvakkumar, V. Rajendran and N. Kannan, Metal-Org. Nano-Metal Chem., 2014, 44, 1128–1131 CrossRef CAS.
- A. Rastogi, D. K. Tripathi, S. Yadav, D. K. Chauhan, M. Živčák, M. Ghorbanpour, N. I. El-Sheery and M. Brestic, 3 Biotech, 2019, 9, 1–11 CrossRef PubMed.
- M. M. Lu, D. M. De Silva, E. Peralta, A. Fajardo and M. Peralta, Philippine e-J. Appl. Res. Devel., 2015, 5, 11–22 Search PubMed.
- H. Abou-Zeid and G. Ismail, Egypt. J. Bot., 2018, 58, 73–85 Search PubMed.
- F. Asgari, A. Majd, P. Jonoubi and F. Najafi, Plant Physiol. Biochem., 2018, 127, 152–160 CrossRef CAS PubMed.
- M. Rizwan, S. Ali, M. Z. Rehman, S. Malik, M. Adrees, M. F. Qayyum, S. A. Alamri, M. N. Alyemeni and P. Ahmad, Acta Physiol. Plant., 2019, 41, 1–12 CrossRef CAS.
- N. Sundaria, M. Singh, P. Upreti, R. P. Chauhan, J. Jaiswal and A. Kumar, J. Plant Growth Regul., 2019, 38, 122–131 CrossRef CAS.
- K. Jisha, K. Vijayakumari and J. T. Puthur, Acta Physiol. Plant., 2013, 35, 1381–1396 CrossRef.
- Y. Rui, X. Gui, X. Li, S. Liu and Y. Han, J. Nanobiotechnol., 2014, 12, 50 CrossRef PubMed.
- D. L. Slomberg and M. H. Schoenfisch, Environ. Sci. Technol., 2012, 46, 10247–10254 CAS.
- N. O'Farrell, A. Houlton and B. R. Horrocks, Int. J. Nanomed., 2006, 1, 451 CrossRef PubMed.
- E. Roduner, Chem. Soc. Rev., 2006, 35, 583–592 RSC.
- R. Nair, S. H. Varghese, B. G. Nair, T. Maekawa, Y. Yoshida and D. S. Kumar, Plant Sci., 2010, 179, 154–163 CrossRef CAS.
-
R. S. El-Serafy and A. El-Sheshtawy, Improving seed germination of althaea rosea L. Under salt stress by seed soaking with silicon and nano silicon, 2017 Search PubMed.
- S. Nazaralian, A. Majd, S. Irian, F. Najafi, F. Ghahremaninejad, T. Landberg and M. Greger, Plant Physiol. Biochem., 2017, 115, 25–33 CrossRef CAS PubMed.
- M. E. Abdel-Haliem, H. S. Hegazy, N. S. Hassan and D. M. Naguib, Ecol. Eng., 2017, 99, 282–289 CrossRef.
- J. Cui, Y. Li, Q. Jin and F. Li, Environ. Sci.: Nano, 2020, 7, 162–171 RSC.
- D. K. Tripathi, S. Singh, V. P. Singh, S. M. Prasad, N. K. Dubey and D. K. Chauhan, Plant Physiol. Biochem., 2017, 110, 70–81 CrossRef CAS PubMed.
- D. K. Tripathi, V. P. Singh, S. M. Prasad, D. K. Chauhan, N. K. Dubey and A. K. Rai, Ecotoxicol. Environ. Saf., 2015, 113, 133–144 CrossRef CAS PubMed.
- J. A. Raven, New Phytol., 2003, 158, 419–421 CrossRef.
- S. Kumar, Y. Milstein, Y. Brami, M. Elbaum and R. Elbaum, New Phytol., 2017, 213, 791–798 CrossRef CAS PubMed.
- C. Zhang, L. Wang, W. Zhang and F. Zhang, Plant Soil, 2013, 372, 137–149 CrossRef CAS.
- D. K. Tripathi, S. Singh, V. P. Singh, S. M. Prasad, D. K. Chauhan and N. K. Dubey, Front. Environ. Sci., 2016, 4, 46 Search PubMed.
- J. Liu, H. Cai, C. Mei and M. Wang, Front. Environ. Sci. Eng., 2015, 9, 905–911 CrossRef CAS.
- E. Takahashi, J. Ma and Y. Miyake, Comments Agric. Food Chem., 1990, 2, 99–102 CAS.
- K. E. Richmond and M. Sussman, Curr. Opin. Plant Biol., 2003, 6, 268–272 CrossRef CAS PubMed.
- E. Takahashi, J. Ma and Y. Miyake, Comments Agric. Food Chem., 1990, 2, 99–102 CAS.
- M. Nikolic, N. Nikolic, Y. Liang, E. A. Kirkby and V. Römheld, Plant Physiol., 2007, 143, 495–503 CrossRef CAS PubMed.
- R. K. Deshmukh, J. Vivancos, G. Ramakrishnan, V. Guérin, G. Carpentier, H. Sonah, C. Labbé, P. Isenring, F. J. Belzile and R. R. Bélanger, Plant J., 2015, 83, 489–500 CrossRef CAS.
- M. A. Ahanger, J. A. Bhat, M. H. Siddiqui, J. Rinklebe and P. Ahmad, J. Exp. Bot., 2020, 71, 6758–6774 CrossRef CAS.
- M. González-Moscoso, N. V. Martínez-Villegas, G. Cadenas-Pliego, A. Benavides-Mendoza, M. C. Rivera-Cruz, S. González-Morales and A. Juárez-Maldonado, Foods, 2019, 8, 612 CrossRef PubMed.
- M. M. Madany, A. M. Saleh, T. H. Habeeb, W. N. Hozzein and H. AbdElgawad, Environ. Sci.: Nano, 2020, 7, 1415–1430 RSC.
- Z. V. Udalova, G. Folmanis, M. Fedotov, L. Pelgunova, E. Y. Krysanov, F. Khasanov and S. Zinovieva, Dokl. Biochem. Biophys., 2020, 495, 329–333 CrossRef CAS PubMed.
- A. Derbalah, M. Shenashen, A. H. A. Mohamed and S. El Safty, Egyptian J. Basic Appl. Sci., 2018, 5, 145–150 CrossRef.
- Z. M. Almutairi, Plant Omics, 2016, 9, 106 CAS.
- S. Hussain, L. Shuxian, M. Mumtaz, I. Shafiq, N. Iqbal, M. Brestic, M. Shoaib, Q. Sisi, W. Li, X. Mei, C. Bing, M. Zivcak, A. Rastogi, M. Skalicky, V. Hejnak, L. Weiguo and Y. Wenyu, J. Hazard. Mater., 2021, 401, 123256 CrossRef CAS PubMed.
- Y. Zhang, S. Yu, H.-j. Gong, H.-l. Zhao, H.-l. Li and Y.-h. Hu,
et al., Beneficial effects of silicon on photosynthesis of tomato seedlings under water stress, J. Integr. Agric., 2018, 17, 2151–2159 CrossRef CAS.
- M. Janmohammadi and N. Sabaghnia, Botanica, 2015, 21, 13–21 Search PubMed.
- A. Banerjee, A. Singh, M. Sudarshan and A. Roychoudhury, Chemosphere, 2021, 262, 127826 CrossRef CAS.
- M. Rizwan, S. Ali, M. Z. Rehman, S. Malik, M. Adrees, M. F. Qayyum, S. A. Alamri, M. N. Alyemeni and P. Ahmad, Acta Physiol. Plant., 2019, 41, 1–12 CrossRef CAS.
- N. I. Elsheery, M. N. Helaly, H. M. El-Hoseiny and S. M. Alam-Eldein, Agronomy, 2020, 10, 558 CrossRef CAS.
- R. Nair, S. H. Varghese, B. G. Nair, T. Maekawa, Y. Yoshida and D. S. Kumar, Plant Sci., 2010, 179, 154–163 CrossRef CAS.
- J. Wang and N. Naser, Electroanalysis, 1994, 6, 571–575 CrossRef CAS.
- D. K. Tripathi, S. Singh, V. P. Singh, S. M. Prasad, N. K. Dubey and D. K. Chauhan, Plant Physiol. Biochem., 2017, 110, 70–81 CrossRef CAS PubMed.
- B. Fadeel and A. E. Garcia-Bennett, Adv. Drug Delivery Rev., 2010, 62, 362–374 CrossRef CAS PubMed.
- J. E. Cañas, M. Long, S. Nations, R. Vadan, L. Dai, M. Luo, R. Ambikapathi, E. H. Lee and D. Olszyk, Environ. Toxicol. Chem., 2008, 27, 1922–1931 CrossRef PubMed.
- R. Owen and R. D. Handy, Environ. Sci. Technol., 2007, 41, 5582–5588 CrossRef.
- C. M. Rico, S. Majumdar, M. Duarte-Gardea, J. R. Peralta-Videa and J. L. Gardea-Torresdey, J. Agric. Food Chem., 2011, 59, 3485–3498 CrossRef CAS PubMed.
- D. B. Warheit, B. R. Laurence, K. L. Reed, D. H. Roach, G. A. Reynolds and T. R. Webb, Toxicol. Sci., 2004, 77, 117–125 CrossRef CAS PubMed.
- S. Asli and P. M. Neumann, Plant, Cell Environ., 2009, 32, 577–584 CrossRef CAS PubMed.
- J. E. Cañas, M. Long, S. Nations, R. Vadan, L. Dai, M. Luo, R. Ambikapathi, E. H. Lee and D. Olszyk, Environ. Toxicol. Chem., 2008, 27, 1922–1931 CrossRef PubMed.
- L. Changmei, Z. Chaoying, W. Junqiang, W. Guorong and T. Mingxuan, Soybean Sci., 2002, 21, 168–171 CrossRef.
- R. C. Monica and R. Cremonini, Caryologia, 2009, 62, 161–165 CrossRef.
- R. Nair, S. H. Varghese, B. G. Nair, T. Maekawa, Y. Yoshida and D. S. Kumar, Plant Sci., 2010, 179, 154–163 CrossRef CAS.
- R. Liman, Y. Acikbas, I. H. Ciğerci, M. M. Ali and M. D. Kars, Bull. Environ. Contam. Toxicol., 2020, 104, 215–221 CrossRef CAS.
- M. E. Aphane, F. J. Doucet, R. A. Kruger, L. Petrik and E. M. van der Merwe, Waste Biomass Valorization, 2019, 1–15 Search PubMed.
- N. O. San, C. Kurşungöz, Y. Tümtaş, O. Yaşa, B. Ortaç and T. Tekinay, Particuology, 2014, 17, 29–35 CrossRef CAS.
- R. H. Alves, T. V. dS. Reis, S. Rovani and D. A. Fungaro, J. Chem., 2017, 6129035 Search PubMed.
- A. Piela, E. Żymańczyk-Duda, M. Brzezińska-Rodak, M. Duda, J. Grzesiak, A. Saeid, M. Mironiuk and M. Klimek-Ochab, Bioorg. Chem., 2020, 99, 103773 CrossRef CAS.
- L. M. Mahmoud, M. Dutt, A. M. Shalan, M. E. El-Kady, M. S. El-Boray, Y. M. Shabana and J. W. Grosser, S. Afr. J. Bot., 2020, 132, 155–163 CrossRef CAS.
- M. Ghorbanpour, H. Mohammadi and K. Kariman, Environ. Sci.: Nano, 2020, 7, 443–461 RSC.
- M. Kalteh, Z. T. Alipour, S. Ashraf, M. Marashi Aliabadi and A. Falah Nosratabadi, J. Chem. Health Risks, 2018, 4, 49–55 Search PubMed.
- H. Fatemi, B. E. Pour and M. Rizwan, Environ. Sci. Pollut. Res., 2021, 28, 1417–1425 CrossRef CAS PubMed.
- A. Alsaeedi, H. El-Ramady, T. Alshaal, M. El-Garawany, N. Elhawat and A. Al-Otaibi, Plant Physiol. Biochem., 2019, 139, 1–10 CrossRef CAS PubMed.
- M. Salajegheh, M. Yavarzadeh, A. Payandeh and M. M. Akbarian, Banat’s J. Biotechnol., 2020, 10, 19–25 Search PubMed.
- A. M. A. Qados and A. E. Moftah, J. Exp. Agric. Int., 2015, 509–524 Search PubMed.
- G. Roohizadeh, A. Majd and S. Arbabian, Trop. Plant Res., 2015, 2, 85–89 Search PubMed.
- F. El-Emary and M. Amer, J. Plant Prod., 2018, 9, 955–964 CrossRef.
- N. Sabaghnia and M. Janmohammadi, Annales UMCS, Biologia, 2015, 69, 39–55 Search PubMed.
- A. de Sousa, A. M. Saleh, T. H. Habeeb, Y. M. Hassan, R. Zrieq, M. A. M. Wadaan, W. N. Hozzein, S. Selim, M. Matos and H. AbdElgawad, Sci. Total Environ., 2019, 693, 133636 CrossRef CAS.
- M. Gowayed, H. S. Al-Zahrani and E. M. Metwali, Int. J. Agric. Biol., 2017, 19, 183–194 CrossRef.
- M. H. Siddiqui, M. H. Al-Whaibi, M. Faisal and A. A. Al Sahli, Environ. Toxicol. Chem., 2014, 33, 2429–2437 CrossRef CAS PubMed.
- A. Banerjee, A. Singh, M. Sudarshan and A. Roychoudhury, Chemosphere, 2021, 262, 127826 CrossRef CAS PubMed.
- B. Hussain, Q. Lin, Y. Hamid, M. Sanaullah, L. Di, M. B. Khan, Z. He and X. Yang, Sci. Total Environ., 2020, 712, 136497 CrossRef CAS PubMed.
- J. Cui, T. Liu, F. Li, J. Yi, C. Liu and H. Yu, Environ. Pollut., 2017, 228, 363–369 CrossRef CAS PubMed.
- S. Avestan, M. Ghasemnezhad, M. Esfahani and C. S. Byrt, Agronomy, 2019, 9, 246 CrossRef CAS.
- S. Namjoyan, A. Sorooshzadeh, A. Rajabi and M. Aghaalikhani, Acta Physiol. Plant., 2020, 42, 1–16 CrossRef.
- M. Haghighi, Z. Afifipour and M. Mozafarian, J. Microbiol., Biotechnol. Environ. Sci., 2012, 6, 87–90 Search PubMed.
- A. S. Ardakani, Nematology, 2013, 15, 671–677 CAS.
- F. M. Isfahani, A. Tahmourespour, M. Hoodaji, M. Ataabadi and A. Mohammadi, Pol. J. Environ. Stud., 2019, 28, 153–163 CrossRef.
- S. Ali, M. Rizwan, A. Hussain, M. Z. Rehman, B. Ali, B. Yousaf, L. Wijaya L, M. N. Alyemeni and P. Ahmad, Plant Physiol. Biochem., 2019, 140, 1–8 CrossRef CAS PubMed.
- Z. S. Khan, M. Rizwan, M. Hafeez, S. Ali, M. Adrees, M. F. Qayyum, S. Khalid, M. Z. Rehman and M. A. Sarwar, Environ. Sci. Pollut. Res., 2020, 27, 4958–4968 CrossRef CAS PubMed.
- A. Younis, H. Khattab and M. Emam, Biol. Plant., 2020, 64, 343–352 CrossRef CAS.
- J. Karimi and S. Mohsenzadeh, Russ. J. Plant Physiol., 2016, 63, 119–123 CrossRef CAS.
|
This journal is © The Royal Society of Chemistry 2021 |
Click here to see how this site uses Cookies. View our privacy policy here.