DOI:
10.1039/C9QI01504C
(Research Article)
Inorg. Chem. Front., 2020,
7, 4580-4592
Luminescent Ir(III)–Ln(III) coordination polymers showing slow magnetization relaxation†
Received
20th November 2019
, Accepted 13th January 2020
First published on 13th January 2020
Abstract
A series of iridium(III)–lanthanide(III) bimetallic coordination polymers were successfully obtained by hydrothermal reactions based on the Ir unit [Ir(ppy)2(Hdcbpy)], namely, [Ln{Ir(ppy)2(dcbpy)}2(NO3)(H2O)n]·solvent (Ir2Ln, n = 5, Ln = Gd and Dy; and n = 4, Ln = Er and Yb) and [Ln2(OH){Ir(ppy)2(dcbpy)}4(NO3)(H2O)4]·solvent (Ir4Ln2, Ln = Gd, Dy, Er, and Yb). Compounds Ir2Ln show chain structures in which the lanthanide ions are bridged by the Ir(ppy)2(dcbpy)− ligands through the carboxylate groups. Compounds Ir4Ln2 have double-chain structures, in which the dimers of Yb2(OH) are connected by the Ir(ppy)2(dcbpy)− ligands. Magnetic studies reveal that Ir2Dy and Ir4Dy2 exhibit slow magnetic relaxation behaviour in zero dc field, which is characteristic of single molecule magnets, but Ir2Er, Ir4Yb2, Ir4Er2 and Ir4Yb2 require a field to induce such characteristics. Moreover, since the iridium(III) moiety can serve as an “antenna” to effectively sensitize the f–f transitions of the lanthanide ions, near-infrared luminescence has been observed for the YbIII and ErIII complexes. As far as we know, this is the first observation of SMM behaviour and NIR luminescence in iridium(III)–lanthanide(III) bimetallic coordination polymers.
Introduction
The exponential growth of studies related to multifunctional coordination polymers integrating several physical and chemical properties into one single crystalline structure has become a hot topic of research over the last two decades.1 Magneto-luminescent molecular materials belong to one family of those multifunctional materials which are gaining increasing attention due to their potential applications in several areas such as quantum computing, medical imaging and sensors.2,3 One of the most effective strategies to combine magnetism with luminescence is the synthesis of lanthanide complexes which exhibit not only large unquenched orbital angular momentum and strong spin–orbit coupling that are ideal for the construction of single-molecule magnets (SMMs),4,5 but also excellent luminescence properties including narrow line-like emission, high quantum yield and long lifetime.2,6 Since the direct excitation of a lanthanide ion requires a Laporte forbidden f–f transition, indirect excitation via light-harvesting antennas or sensitizing chromophores is generally employed.7 In comparison with the traditional organic chromophores being used as sensitizing groups,8 using transition metal complexes allows more design flexibility, offering longer emission lifetime and extends the optical excitation window to lower energy region.9 Their promising characteristics have therefore attracted substantial attention in recent years. Lanthanide ions that are near-infrared (NIR) emitters (NdIII, YbIII, ErIII, and PrIII) have been involved in most of the work in this area and are useful in optical communication, medical imaging and biological labelling.9,10
Among the transition metals, the IrIII complexes with energy levels modifiable through the cyclometalated ligands stand out as sensitizers for NIR emission from lanthanide ions.11 The advantage is that the excitation is done in the visible region of the spectrum instead of the destructive UV light normally needed for organics. In 2008, De Cola and co-workers first reported a tetrametallic iridium–ytterbium complex showing NIR emission from Yb sensitized by the Ir complex.12 Since then, several IrIII–LnIII bimetallic complexes showing NIR emission have been reported.13 Furthermore, only three IrIII–LnIII (Ln = Yb, Er, and Nd) coordination polymers exhibiting NIR luminescence were reported by Luo et al. in 2014, which successfully extended the dimension of luminescent IrIII–LnIII system.14
On the contrary, luminescent IrIII–LnIII single molecule magnets (SMMs) have been scarcely described. The only examples, as far as we are aware, are Ir6Ln (Ln = Dy, Er, and Yb) clusters which have been reported by us and exhibit simultaneously slow magnetization relaxation from LnIII and luminescence dominated by IrIII.15 However, no efficient energy transfer between Ir and Ln was observed.
Owing to our long-term interest in magneto-optical bifunctional materials,15,16 herein we report two new series of IrIII–LnIII coordination polymers based on the metalloligand Ir(ppy)2(Hdcbpy) (Scheme 1), namely, [Ln{Ir(ppy)2(dcbpy)}2(NO3)(H2O)n]·solvent (Ir2Ln, n = 5, Ln = Gd and Dy; and n = 4, Ln = Er and Yb) and [Ln2(OH){Ir(ppy)2(dcbpy)}4(NO3)(H2O)4]·solvent (Ir4Ln2, Ln = Gd, Dy, Er, and Yb). Ir2Ln show chain structures, in which the LnIII ions are cross-linked by the Ir(ppy)2(dcbpy)− ligands via the two carboxylate groups. For Ir4Ln2, dimers of Ln2(OH) are observed, which are further connected by the carboxylate groups of the Ir(ppy)2(dcbpy)− ligands, forming a ladder-like chain structure. Interestingly, both single molecule magnetism and Ir → Ln energy transfer accompanied by near-infrared emission were demonstrated to co-exist in Ir2Ln and Ir4Ln2 (Ln = Er and Yb).
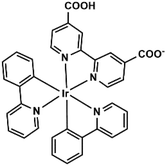 |
| Scheme 1 The molecular structure of the metalloligand Ir(ppy)2(Hdcbpy). | |
Results and discussion
Crystal structures
Compounds [Ln{Ir(ppy)2(dcbpy)}2(NO3)(H2O)n]·solvent (Ir2Ln, n = 5, Ln = Gd and Dy; and n = 4, Ln = Er and Yb) and [Ln2(OH) {Ir(ppy)2(dcbpy)}4(NO3)(H2O)4]·solvent (Ir4Ln2, Ln = Gd, Dy, Er, and Yb) were synthesized by solvothermal reactions of Ir(ppy)2(Hdcbpy) and Ln(NO3)3·6H2O in H2O/MeOH using different concentrations of NaOH and temperatures. Powder XRD measurements revealed that Ir2Ln (Ln = Gd, Dy, Er, and Yb) and Ir4Ln2 (Ln = Gd, Dy, Er, and Yb) are isostructural compounds. However, single crystals of good quality were obtained only for compounds Ir2Dy, Ir2Er, Ir2Yb and Ir4Yb2. The crystallographic data for these four compounds are given in Table 1.
Table 1 Crystallographic data for compounds Ir2Dy, Ir2Er, Ir2Yb and Ir4Yb2
|
Ir2Dy
|
Ir2Er
|
Ir2Yb
|
Ir4Yb2
|
R
1 = ∑||Fo| − |Fc||/∑|Fo|.
wR2 = [∑w(Fo2 − Fc2)2/∑w(Fo2)2]1/2.
|
Formula |
C68H54N8O13DyIr2 |
C140H122N18O35Er2Ir4 |
C140H122N18O35Yb2Ir4 |
C280H236N34O61Yb4Ir8 |
M
|
1738.09 |
3719.87 |
3731.43 |
7282.77 |
Crystal system |
Triclinic |
Triclinic |
Triclinic |
Monoclinic |
Space group |
P![[1 with combining macron]](https://www.rsc.org/images/entities/char_0031_0304.gif) |
P![[1 with combining macron]](https://www.rsc.org/images/entities/char_0031_0304.gif) |
P![[1 with combining macron]](https://www.rsc.org/images/entities/char_0031_0304.gif) |
P21/c |
a (Å) |
8.5559(13) |
8.6129(14) |
8.5775(16) |
36.569(3) |
b (Å) |
18.370(3) |
18.343(3) |
18.360(3) |
8.6347(5) |
c (Å) |
23.830(4) |
23.903(4) |
23.823(4) |
43.991(3) |
α (o) |
70.638(3) |
70.841(4) |
70.887(5) |
90 |
β (o) |
80.107(3) |
80.828(4) |
81.179(6) |
112.317(2) |
γ (o) |
77.866(3) |
77.440(3) |
77.676(5) |
90 |
V (Å3) |
3433.3(9) |
3465.9(10) |
3449.0(11) |
12 850.4(14) |
Z
|
2 |
1 |
1 |
2 |
ρ
calcd (g cm−3) |
1.681 |
1.782 |
1.797 |
1.882 |
μ (mm−1) |
5.009 |
5.106 |
5.270 |
5.653 |
F (000) |
1684.0 |
1812.0 |
1816.0 |
7076.0 |
R
int
|
0.0495 |
0.0482 |
0.0432 |
0.0617 |
T
max, Tmin |
0.634, 0.350 |
0.629, 0.350 |
0.621, 0.350 |
0.728, 0.360 |
R
1 a, wR2 b [I > 2σ(I)] |
0.1105, 0.2755 |
0.0795, 0.1847 |
0.0485, 0.1178 |
0.0844, 0.1952 |
R
1 a, wR2 b (all data) |
0.1273, 0.2852 |
0.1093, 0.2019 |
0.0621, 0.1244 |
0.1012, 0.2036 |
GoF on F2 |
1.110 |
1.031 |
0.998 |
1.022 |
(Δρ)max, (Δρ)min (e Å−3) |
5.05, −8.18 |
4.86, −6.94 |
2.22, −1.97 |
5.32, −3.22 |
CCDC |
1956646
|
1956647
|
1956648
|
1956649
|
Ir2Ln are isomorphous compounds that crystallize in the triclinic P
space group, though the numbers of the coordination water molecules are slightly different (n = 5 for Ln = Gd and Dy; and n = 4 for Ln = Er and Yb). Therefore, Ir2Yb is selected as a representative for the description of the structures. The asymmetric unit of Ir2Yb consists of two Ir(ppy)2(dcbpy)− ligands, one YbIII, one NO3−, four coordinated water molecules, two MeOH and 0.5 lattice water molecule. As shown in Fig. 1a and b, the Yb atom is seven-coordinated by three carboxylate oxygen atoms from three ligands (O1, O3A, and O5) and four coordination water molecules. One of the coordination water molecules is disordered over two sites (O4W and O4W′) (Fig. 1b). Continuous Shape Measure (CShM) analysis17 suggests that the geometries of the two possible [YbO7] polyhedra are slightly deviated from an ideal capped trigonal prism (CShM = 1.17) and pentagonal bipyramid (CShM = 1.25), respectively. The Yb–O bond lengths are in the range of 2.191(7)–2.452(18) Å, and the O–Yb–O angles are in the range of 67.4(5)–149.8(3)°.
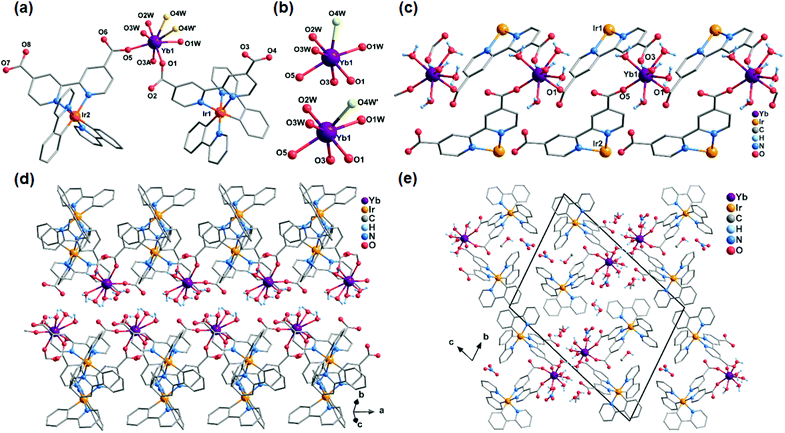 |
| Fig. 1 (a) The building unit of the structure of Ir2Yb. (b) Coordination environments of the YbIII ion. (c) The single chain structure of the compound Ir2Yb. The ppy ligands are omitted for clarity. (d) The double-chain structure of Ir2Yb. (e) Packing diagram of the structure of Ir2Yb viewed along the a-axis. All H atoms except those attached to the oxygen atoms are omitted for clarity. | |
Two Ir(ppy)2(dcbpy)− ligands are crystallographically distinguished. One ligand (Ir1) adopts a bi-dentate coordination mode by using two of its four carboxylate oxygen atoms (O1 and O3), and connects the neighbouring Yb centres into an infinite chain running along the a-axis. The other ligand (Ir2) adopts a mono-dentate coordination mode and binds the Yb atom through its carboxylate oxygen atom O5 (Fig. 1c). The Yb⋯Yb distance within the chain is 8.58 Å. The chain is polar with the Ir(ppy)2(dcbpy)− ligands sitting on one side and the coordination water molecules on the other side. Two equivalent chains are connected through extensive hydrogen bonding interactions between the coordination water molecules, forming a supramolecular double chain which is non-polar (Fig. 1d). The shortest interchain Yb⋯Yb separation is 6.20 Å. The double chains are stacked along the a-axis with the space between the double-chains filled with lattice water and methanol molecules and nitrate anions (Fig. 1e). Extensive H-bonds are observed among the aromatic groups, the non-coordinated carboxylate oxygen atoms, the coordination and lattice water molecules and the nitrate anions. A three-dimensional supramolecular network is thus constructed.
Compounds Ir2Dy and Ir2Er show similar 3D supramolecular structures made up of 1D chain (Fig. S3 and S4†). However, a significant difference is found in the coordination environment of DyIII in Ir2Dy. Unlike the seven-coordinated lanthanide ion in Ir2Yb and Ir2Er (Fig. S4†), the Dy atom is eight-coordinated by three carboxylate oxygen atoms from three ligands (O1, O3A, and O5) [Dy–O: 2.270(16)–2.376(16) Å] and five coordinated water molecules (O1W, O2W, O3W, O4W, and O5W) [Dy–O: 2.332(19)–2.48(3) Å] (Fig. 2). This can be related to the lanthanide contract effect, the DyIII ion with larger ionic radius shows higher coordination number. The geometry of DyIII is best described as a slightly distorted triangular dodecahedron (D2d) with a CShM value of 0.94.
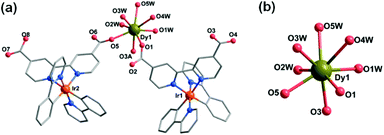 |
| Fig. 2 (a) The building unit of the structure of Ir2Dy. (b) The coordination environment of the DyIII ion. | |
The compound Ir4Yb2 crystallizes in the monoclinic space group P21/c. Its asymmetric unit contains four Ir(ppy)2(dcbpy)− ligands, two YbIII, one OH−, one NO3−, four coordinated water molecules, four free MeOH molecules and two and a half lattice water molecules. As shown in Fig. 3, both the crystallographically distinct Yb atoms have seven coordinated environments, viz. four carboxylate oxygen atoms, one bridging coordinated OH− and two oxygen atoms from water (Fig. 3b). Both YbIII ions adopt pentagonal bipyramidal geometry, as suggested by the CShM values S(D5h) = 1.80 (Yb1) and S(D5h) = 0.96 (Yb2). The neighbouring [YbO7] polyhedra are linked via the OH− group, forming a dimer of {Yb2(OH)(COO)8(H2O)4} (Yb–O–Yb = 159.6(1)° and Yb⋯Yb = 4.3962(11) Å). All of the Ir(ppy)2(dcbpy)− ligands adopt a bi-dentate coordination mode, unlike those in Ir2Ln, and connect the {Yb2(OH)(COO)8(H2O)4} dimers into a one-dimensional double-chain structure (Fig. 3c). The intrachain Yb⋯Yb distance between the dimers is 8.63 Å. The double chains are stacked in the lattice. The lattice solvent molecules and the nitrate anions occupy the inter-chain spaces, and are involved in the extensive H-bond network.
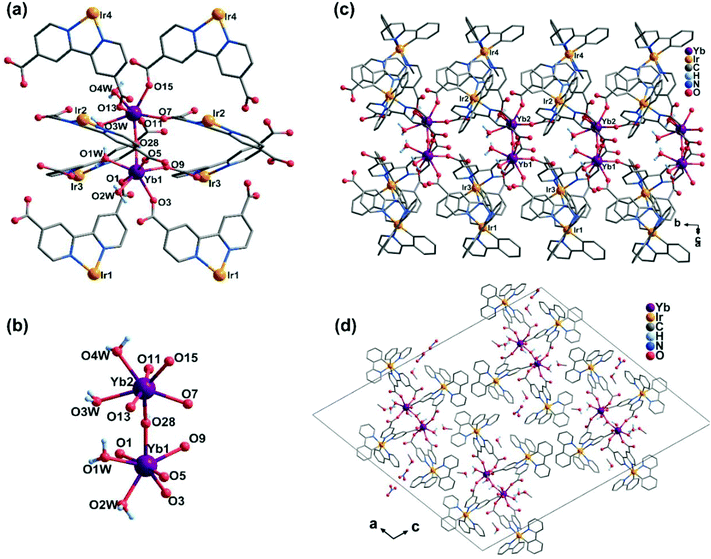 |
| Fig. 3 (a) The building unit of the structure of Ir4Yb2. The ppy ligands are omitted for clarity. (b) Coordination environments of the YbIII ions. (c) The double-chain structure of the compound Ir4Yb2. (d) Packing diagram of the structure of Ir4Yb2 viewed along the b-axis. All H atoms except those attached to the oxygen atoms are omitted for clarity. | |
Although single crystals of good quality were not obtained for compounds Ir2Gd, Ir4Gd2, Ir4Dy2 and Ir4Er2, the unit cell parameters were confirmed by Pawley fitting of their powder diffraction patterns using TOPAS 5.0 program (Table S8, Fig. S5–S10†).
Obviously, the structure of Ir4Yb2 is significantly different from that of Ir2Yb. A careful analysis reveals, however, that the double chain in Ir4Yb2 can be viewed as two single chains in Ir2Yb connected by the hydroxyl groups. The involvement of an OH− group in Ir4Yb2 can be explained by the more basic reaction condition used for the synthesis of Ir4Yb2.
Notably, IrIII–LnIII coordination polymers containing the same ligand Ir(ppy)2(Hdcbpy) are still rare. The only examples, as far as we are aware, are Ln[Ir(ppy)2(dcbpy)]2(OH)·H2O (Ln = Gd, Yb, Er, and Nd)14 and Y[Ir(ppy)2(dcbpy)]2(OH).18 These compounds possess identical chain structures that are remarkably different from those of Ir2Yb and Ir4Yb2, in which the dimers of edge-sharing {LnO7} or {YO7} pentagonal bipyramid via two OH− groups are further connected by the carboxylate groups in the metalloligand. Therefore compounds Ir2Ln and Ir4Ln2 provide new structural types of the IrIII–LnIII coordination polymers based on Ir(ppy)2(Hdcbpy).
Magnetic properties
Variable temperature dc magnetic susceptibilities of Ir2Ln and Ir4Ln2 (Ln = Gd, Dy, Er, and Yb) were measured in the temperature range of 2–300 K in an applied field of 1 kOe. Plots of χMT versus T (χM is the molar magnetic susceptibility per LnIII ion) for these compounds are shown in Fig. 4. At 300 K, the χMT values are 7.73 and 7.84 cm3 K mol−1 for Ir2Gd and Ir4Gd2, respectively, which are close to the expected value of 7.88 cm3 K mol−1 for an isolated GdIII ion (S = 7/2, L = 0, 8S7/2, J = 7/2, g = 2). Upon cooling, the χMT value of Ir2Gd decreases continuously in the range of 300–50 K and then decreases smoothly to the minimum value of 6.88 cm3 K mol−1 at 2 K. In contrast, for compound Ir4Gd2, the χMT value decreases to 20 K followed by a rapid drop to the minimum value of 5.91 cm3 K mol−1 at 2 K. The low temperature feature could be attributed to the occurrence of the GdIII⋯GdIII antiferromagnetic exchange interactions, and is given by the negative values of the Weiss constants (θ) as −0.04 K for Ir2Gd and −0.54 K for Ir4Gd2 (Fig. S11†). The stronger antiferromagnetic interactions in Ir4Gd2 may be due to short distances of the GdIII ions in the dimer connected via the OH− group. The field dependence of the magnetization at 2 K shows a rapid increase at low field and then slowly reaches the corresponding maximum values of 6.67Nβ per Gd for Ir2Gd and 6.80Nβ per Gd for Ir4Gd2 at 70 kOe, which are close to the theoretical saturation value of 7Nβ for an isolated GdIII ion.
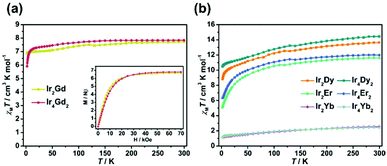 |
| Fig. 4 (a) Temperature dependence of χMT per Gd on cooling in a field of 1 kOe for Ir2Gd and Ir4Gd2. Inset: M vs. H plots of the two compounds at 2 K. (b) Temperature dependence of χMT per Ln on cooling in a field of 1 kOe for Ir2Ln and Ir4Ln2 (Ln = Dy, Er, and Yb). | |
The dc magnetic susceptibilities of compounds Ir2Ln and Ir4Ln2 (Ln = Dy, Er, and Yb) were measured under the same conditions. As shown in Fig. 4b, all the compounds show a similar behaviour. The room temperature χMT values per Ln are 13.65 (Ir2Dy), 14.44 (Ir4Dy2), 11.60 (Ir2Er), 11.98 (Ir4Er2), 2.53 (Ir2Yb) and 2.45 (Ir4Yb2) cm3 K mol−1, which are consistent with the theoretical values of 14.17 (DyIII, 6H15/2), 11.48 (ErIII, 4I15/2) and 2.57 (YbIII, 2F7/2) cm3 K mol−1. The decrease in the χMT value on lowering the temperature is attributed to the thermal depopulation of the excited MJ sublevels and/or the weak antiferromagnetic exchange interactions between the metal centres.19 The magnetization values of compounds Ir2Ln and Ir4Ln2 at 2 K and 70 kOe are significantly smaller than the expected saturation values for the corresponding lanthanide ions, revealing the existence of a significant magnetic anisotropy20 which is further confirmed by the non-superimposed M vs. H/T plots at different temperatures (Fig. S12 and S13†).
In order to assess the effect of coordination configuration on the dynamic magnetic properties, the alternative-current (ac) magnetic susceptibilities of Ir2Ln and Ir4Ln2 (Ln = Dy, Er, and Yb) were measured using a Quantum Design PPMS in the frequency range of 10–10
000 Hz. Under the zero dc field, only Ir2Dy and Ir4Dy2 showed clear frequency dependence of in-phase (χ′) and out-of-phase (χ′′) susceptibilities below ca. 11 K, indicating a zero-field SMM with slow magnetization relaxation (Fig. 5a and c). However, the peak value of χ′′ did not change significantly with increasing temperature, indicating that the magnetic relaxation mainly originated from the quantum tunnelling process.21 The Cole–Cole plots can be well fit by the generalized Debye model to extract the relaxation time (τ) (Tables S9 and S14†).22 The distribution parameters (α) are in the range of 0.34–0.37 for Ir2Dy and in the range of 0.25–0.45 for Ir4Dy2, suggesting multiple relaxation processes with broad distribution of the relaxation times. Moreover, the ln
τ vs. T−1 curve can be fit by considering the Orbach and quantum tunnelling process using eqn (1),23 and determining the parameters Ueff = 5.2 K, τ0 = 1.6 × 10−5 s, and τQTM = 3.8 × 10−5 s for Ir2Dy (Fig. 5b), and Ueff = 23.5 K, τ0 = 1.17 × 10−6 s, and τQTM = 7.34 × 10−4 s for Ir4Dy2 (Fig. 5d), respectively.
| τ−1 = τQTM−1 + τ0−1 exp(−Ueff/kT) | (1) |
| τ−1 = τQTM−1 + AT + τ0−1 exp(−Ueff/kT) | (2) |
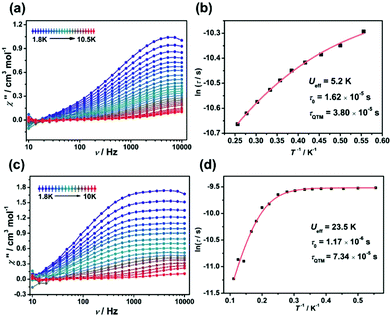 |
| Fig. 5 Out-of-phase (χ′′) ac susceptibilities of Ir2Dy (a) and Ir4Dy2 (c) under zero dc field. Plots of ln(τ) vs. 1/T for Ir2Dy (b) and Ir4Dy2 (d). | |
In order to suppress the QTM and get further insight into the magnetic relaxation dynamics of complexes Ir2Dy and Ir4Dy2, we obtained the ac magnetic susceptibilities under an applied dc bias field of 2 kOe. Variable-frequency ac magnetic susceptibilities of Ir2Dy are shown in Fig. S15 and S16.† With increasing temperature, the position of χ′′ peak did not change below 5.4 K, indicating a complicated relaxation process. The Cole–Cole plots were generated using the frequency dependent ac susceptibilities in the temperature region of 5.4–7.5 K, and can be well fit by employing the sum of two modified Debye functions. For the fast relaxation (FR) process, the low value of the relaxation width (α1 ≈ 0, Table S10†) suggests a narrow distribution of relaxation times, and the temperature independence of ln
τ also indicates that only the quantum tunnelling mechanism is involved.24 However, the slow relaxation (SR) processes show a linear temperature dependence of ln
τ corresponding to an Orbach relaxation mechanism,25 leading to the parameters Ueff = 38.1 K and τ0 = 2.93 × 10−6 s according to the Arrhenius law (Fig. S16d†).
For compounds Ir4Dy2, the frequency-dependent χ′′ exhibits more clear peaks than in zero-dc field and the positions of maxima change from high to low frequency as the temperature is decreased. Although there are two crystallographic sites differing in environments in Ir4Dy2, no dual magnetization relaxation processes were distinguished. It is possible to fit the Cole–Cole plots with only one relaxation process (Fig. S18 and 19, Table S12†).26 Furthermore, the temperature dependent relaxation time profiles can be fit as a sum of the contributions of the Orbach, quantum tunnelling, and direct mechanisms (eqn (2)).27 The best fit led to the following parameters: Ueff = 21.4 K, τ0 = 2.89 × 10−6 s, τQTM = 7.98 × 10−4 s and A = 193.9 K−1 s−1. Obviously, the parameters of Orbach and quantum tunnelling processes do not change significantly under an applied field, but the direct process induced by the applied field results in the decrease of the magnetic relaxation rate.
The magnetization dynamics of compounds Ir2Er and Ir4Er2 were also explored. Unfortunately, under zero external field, neither peaks nor frequency dependence of χ′ and χ′′ was found at 2 K, indicating the presence of significant QTM effect. When an external dc field was applied, both χ′ and χ′′ were found and they showed frequency-dependence, which are typical of a field-induced SMM behaviour (Fig. S20–S23, Tables S13 and S14†). As shown in Fig. S21 and S23,† the maximum of χ′′ gradually shifted to high frequencies with increasing temperature, while no maximum was observed above 2.2 K. The relaxation time τ can be extracted by fitting the Cole–Cole plots using the generalized Debye model assuming a single relaxation process. The ln
τ values increase linearly with temperature in the range of 1.8–2.6 K, and an Arrhenius law fitting results in the energy barriers of 13.6 K (τ0 = 2.72 × 10−8 s) for Ir2Er and 11.8 K (τ0 = 6.68 × 10−8 s) for Ir4Er2.
The temperature-dependent magnetic dynamics of Ir2Yb and Ir4Yb2 were also investigated under an external dc field (Fig. 6, S24–S27, Tables S15 and S16†). For Ir2Yb, the frequency scan of χ′′ exhibited single peaks below 6 K and the maxima gradually shifted to high frequencies upon heating. By assuming a single relaxation process, the relaxation time τ can again be extracted by fitting the Cole–Cole plots using the generalized Debye model. The ln
τ vs. T−1 curve can be reasonably fit by using eqn (2), leading to the parameters Ueff = 24.4 K, τ0 = 2.88 × 10−7 s, and A = 687.9 K−1 s−1. However, two distinct peaks at low temperatures were observed in the temperature-dependent magnetic dynamics of Ir4Yb2, indicating dual relaxation processes by accounting for the different combinations of contributions from the direct and Orbach relaxation processes. The ln
τ vs. T−1 profile can be fit in the whole temperature range,28 and the resulting parameters are Ueff = 22.2 K, τ0 = 2.03 × 10−7 s and A = 8678 K−1 s−1 for the FR process, while Ueff = 28.3 K, τ0 = 2.5 × 10−8 s and A = 682 K−1 s−1 for the SR process. The observation of dual magnetization relaxation processes in Ir4Yb2 could result from the presence of two crystallographic sites with two different environments in the structure. A similar phenomenon was also observed in a few other Yb dimer complexes.29
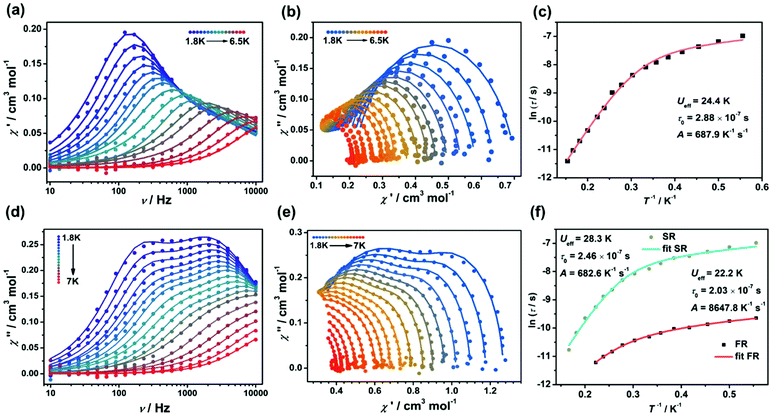 |
| Fig. 6 Frequency dependence of out-of-phase (χ′′) ac susceptibilities at different temperatures for Ir2Yb (a) and Ir4Yb2 (d) under an external dc field. The Cole–Cole plots of the ac susceptibilities for Ir2Yb (b) and Ir4Yb2 (e). Plots of ln(τ) vs. 1/T for Ir2Yb (c) and Ir4Yb2 (f). | |
To the benefit of the D5h symmetry, especially the Kramers DyIII and ErIII ions, the observation of high energy barrier to moment reversal in Ln–SMMs was reported by several groups.30–32 Therefore, a small anisotropy barrier in Ir–Ln bimetallic complexes is not expected. This may be explained by the orientation of the easy-axis anisotropy deviating significantly from an idealized C5 axis, which has been observed in other lanthanide complexes.33
Optical properties
The UV-vis spectra of the free metalloligand and Ir–Ln coordination polymers in the solid state were transformed from the diffuse reflectance spectra using the Kubelka–Munk equation: F(R) = (1 − R)2/2R. As shown in Fig. S28,† the absorptions in the high energy region (250–330 nm) are associated with the π–π* transition of the aromatic ligands (Hdcbpy and ppy) and the intense absorption bands at 330–450 nm can be explained by the mixed spin-allowed 1LLCT (ligand-to-ligand charge-transfer) and 1MLCT (metal-to-ligand charge-transfer). The lower energy absorptions (ca. 450–550 nm) originate from the spin forbidden 3MLCT and are common in cyclometalated iridium derivatives.34 The absorption profiles of all Ir–Ln complexes show a similar electronic transition to the IrIII unit. However, the most significant difference is that weak absorption peaks located at 755 nm (13
245 cm−1) and 654 nm (15
290 cm−1) were observed for Ir2Dy and Ir2Er, respectively, corresponding to the f–f transitions of the DyIII ions (5H15/2 to 6F3/2) and ErIII ions (4I15/2 to 4F9/2).35 Although the structures are different, a similar phenomenon was also observed for Ir4Dy2 and Ir4Er2.
Upon excitation in the visible region at 375 nm, Ir(ppy)2(Hdcbpy) exhibits a strong emission band at 605 nm, corresponding to the 3MLCT transition as a consequence of the heavy-atom effect. As is to be expected, the solid-state luminescence spectra of compounds Ir2Ln and Ir4Ln2 (Ln = Gd, Dy, Er, and Yb) are similar to that of the free metalloligand, indicating that the phosphorescence of polymers arises from the Ir(III) unit (Fig. 7 and Table 2). However, the emission maxima of Ir–Ln compounds were blue-shifted with respect to the parent metalloligand, which may be attributed to the deprotonation of the carboxylate groups upon coordination, leading to a decrease in the intermolecular H-bond interactions in the polymers.36
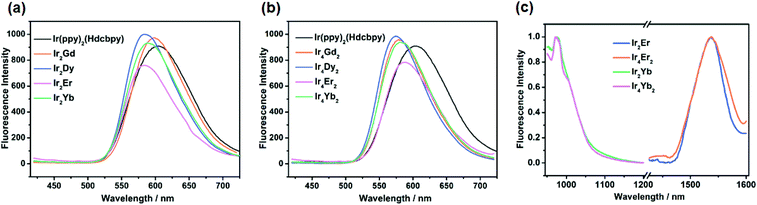 |
| Fig. 7 (a) The emission spectra of Ir(ppy)2(Hdcbpy) and Ir2Ln in the solid-state (λex = 375 nm). (b) The emission spectra of Ir(ppy)2(Hdcbpy) and Ir4Ln2 (Ln = Gd, Dy, Er, Yb) in the solid-state (λex = 375 nm). (c) The solid-state NIR emission spectra of the Ir–Yb (left) and Ir–Er (right) complexes (λex = 500 nm). | |
Table 2 Magnetic and photophysical parameters of Ir2Ln and Ir4Ln2 (Ln = Gd, Dy, Er, and Yb)
|
dc field |
Relaxation processes |
Fitting parameters |
λ
em/nm |
τ/μs |
QY/% |
Ir(ppy)2(Hdcbpy) |
— |
— |
— |
605 |
6.60 |
4.22 |
Ir2Gd
|
— |
— |
— |
598 |
8.69 |
5.83 |
Ir4Gd2
|
— |
— |
— |
578 |
7.74 |
8.08 |
Ir2Dy
|
0 kOe |
Orbach, QTM |
U
eff = 5.2 K, τ0 = 1.62 × 10−5 s, τQTM = 3.80 × 10−5 s |
583 |
4.79 |
6.00 |
2.0 kOe |
QTM (FR) |
τ
QTM = 2.25 × 10−5 |
|
|
|
|
Orbach(SR) |
U
eff = 38.1 K, τ0 = 2.93 × 10−6 s |
|
|
|
Ir4Dy2
|
0 kOe |
Orbach, QTM |
U
eff = 23.5 K, τ0 = 1.17 × 10−6 s |
575 |
0.66 |
11.94 |
|
|
τ
QTM = 7.34 × 10−4 s |
|
|
|
2.0 kOe |
Orbach, QTM; |
U
eff = 21.4 K, τ0 = 2.89 × 10−6 s |
|
|
|
|
direct |
τ
QTM = 7.98 × 10−4 s, A = 193.9 K−1 s−1 |
|
|
|
Ir2Er
|
1.0 kOe |
Orbach |
U
eff = 13.6 K, τ0 = 2.72 × 10−8 s |
584 |
6.37 |
0.68 |
|
|
|
1538 |
1.40 |
— |
Ir4Er2
|
1.5 kOe |
Orbach |
U
eff = 11.8 K, τ0 = 6.68 × 10−8 s |
588 |
5.47 |
1.08 |
|
|
|
|
1538 |
1.37 |
— |
Ir2Yb
|
1.0 kOe |
Orbach, direct |
U
eff = 24.4 k, τ0 = 2.88 × 10−7 s, A = 687.9 K−1 s−1 |
590 |
2.23 |
5.60 |
|
|
|
975 |
0.92 |
— |
Ir4Yb2
|
1.5 kOe |
Orbach, direct (FR) |
U
eff = 22.2 K, τ0 = 2.03 × 10−7 s |
582 |
1.24 |
7.91 |
|
|
A = 8647.8 K−1 s−1 |
975 |
0.86 |
— |
|
Orbach, direct (SR) |
U
eff = 28.3 K, τ0 = 2.46 × 10−8 s, A = 682.6 K−1 s−1 |
|
|
|
Further research on the photoluminescence efficiencies and time-resolved luminescence decay of the IrIII unit and Ir–Ln compounds was carried out, and the data are summarized in Table 2. Owing to the mismatch in the energy levels, the GdIII ions cannot accept energy from the triplet state of the Ir(III) unit,37 so the photoluminescence efficiencies of Ir2Gd and Ir4Gd2 can be used as the reference for the other Ir–Ln (Ln = Dy, Yb, and Er) complexes. The quantum yield increases slightly from 4.22% to 5.83% (Ir2Gd) and 8.08% (Ir4Gd2), which is attributed to the rigidity of the coordination framework that reduces self-quenching and the possibility of nonradiative transitions.38 In addition, the long lifetime compared to the parent linker (6.60 μs) also implies that no d–f energy transfer occurs in Ir2Gd and Ir4Gd2 (Fig. S29†), while for the other luminescent Ir–Ln (Ln = Dy, Er, and Yb) coordination polymers, the lifetimes were shortened to varying degrees, which indicates that the energy effectively transfers from the light-harvesting Ir unit to the lanthanide centres. Moreover, according to the emission spectrum, the triplet energy level of Ir(ppy)2(Hdcbpy) is 16
600 cm−1, which is suitable for sensitizing the NIR lanthanide ions YbIII and ErIII, but is too low to sensitize the low-lying excited state (4F9/2, 21
000 cm−1) of DyIII ions.35,39
In order to detect whether a lanthanide ion can be sensitized by the metalloligand Ir(ppy)2(Hdcbpy), upon irradiation at 500 nm, the near-infrared emission spectra of Ir–Ln (Ln = Er and Yb) compounds were examined in the solid-state. For the YbIII complexes, the emission bands occurring at 978 nm can be assigned to the 2F5/2 → 2F7/2 transition, while in Ir4Yb2, the emission peak shows a shoulder at 1005 nm, indicating that the local symmetry environment around the metal centre is affected by crystal-field splitting,40 which is in agreement with the two independent YbIII ions in the single crystal structure. The emission spectra of the ErIII complexes show an emission peak at around 1530 nm, which is attributed to the 4I13/2 → 4I15/2 transition.41 Moreover, the luminescence decay is well fit to a bi-exponential curve, affording lifetimes of 0.92 μs for Ir2Yb, 0.86 μs for Ir4Yb2, 1.40 μs for Ir2Er and 1.37 μs for Ir4Er2 (Fig. S30 and S31†). Noting that the quantum yields of Ir2Er (ϕ = 0.68%) and Ir4Er2 (ϕ = 1.08%) in the solid state are markedly lower than those of the other Ir–Ln (Ln = Gd, Dy, and Yb) complexes, the energy transfer between the metalloligand and erbium could be more efficient. This can be explained by the effective energy overlap between the 3MLCT state of Ir(ppy)2(Hdcbpy) and the f–f excited states of erbium.
Conclusion
In this work, we report a series of iridium(III)–lanthanide(III) bimetallic coordination polymers with single-chain (for Ir2Ln) and double-chain (for Ir4Ln2) structures. Magnetic studies demonstrate that complexes Ir2Dy and Ir4Dy2 exhibit SMM behaviour in zero dc field, while complexes Ir2Er, Ir2Yb, Ir4Er2 and Ir4Yb2 show field-induced slow magnetic relaxation. The complex Ir4Yb2 is of particular interest because of the field-induced dual relaxation processes, which may originate from the two different coordinated geometries of lanthanide ions. Moreover, in the complexes Ir2Er, Ir2Yb, Ir4Er2 and Ir4Yb2, the Ir unit can act as a good light-harvesting antenna to effectively sensitize Ln-based near-infrared (NIR) luminescence by energy transfer from the Ir unit to the Ln3+ ions. As far as we know, this is the first observation of SMM behaviour and NIR luminescence coexisting in iridium(III)–lanthanide(III) bimetallic CPs.
Experimental section
Materials and physical measurements
All the starting materials were of reagent grade and were obtained from the commercial sources and used without further purification. Powder X-ray diffraction (PXRD) data were obtained using a Bruker D8 ADVANCE X-ray powder diffractometer (Cu-Kα) at room temperature. Infrared spectra were recorded on a Bruker Tensor 27 spectrometer using KBr pellets in the range of 400–4000 cm−1. Thermogravimetric analysis (TGA) was performed under a nitrogen atmosphere in the temperature range of 25–600 °C at a heating rate of 5 °C min−1 using a METTLER TOLEDO TGA/DSC 1 instrument. Elemental analyses for C, H and N were performed with a PerkinElmer 240C elemental analyzer. The UV-vis spectra were recorded on a PerkinElmer Lambda 950 UV/Vis/NIR spectrometer using powder samples. The steady fluorescence spectra in the visible region were obtained using a PerkinElmer LS55 fluorescence spectrometer and a Xenon lamp as the excitation source. The emission lifetime and the absolute luminescent quantum yields in the solid state at room temperature were determined using a HORIBA FM-4P-TCSPC spectrometer. The steady state near infrared (NIR) emission spectra were recorded on an Edinburgh FLS980 fluorescence spectrometer equipped with a Hamamatsu R5509-72 photomultiplier. The magnetic susceptibility data were obtained using Quantum Design SQUID MPMS-XL7, VSM and PPMS magnetometer for freshly prepared samples. The diamagnetic contributions of the samples were estimated from Pascal's constants.
Synthesis of [Ln{Ir(ppy)2(dcbpy)}2(NO3)(H2O)n]·solvent (Ir2Ln, n = 5, Ln = Gd and Dy; n = 4, Ln = Er and Yb)
Compounds Ir2Ln (Ln = Gd, Dy, Er, and Yb) were synthesized under similar optimized experimental conditions. A typical procedure for the preparation of [Yb{Ir(ppy)2(dcbpy)}2(NO3)(H2O)4]·2CH3OH·0.5H2O (Ir2Yb) is described below. Ir(ppy)2(Hdcbpy) (7.4 mg, 0.01 mmol) was suspended in 2 mL of distilled water and dissolved in 5 μL of aqueous NaOH (1 M). Then Yb(NO3)3·6H2O (9.34 mg, 0.02 mmol) in 7 mL of MeOH was added. After keeping the vial at 60 °C for 2 days, orange rod crystals were obtained. The crystalline product of Ir2Yb was separated by filtration and washed with distilled water and a minimum amount of methanol. Yield: 29%. Elemental analyses (%) calcd for C70H61YbIr2N9O17.5, C, 45.06; H, 3.29; N, 6.76; found: C, 45.09; H, 3.12; N, 6.74. IR (KBr, cm−1): 3384 (m), 3105 (m), 3062 (m), 3043 (m), 1606 (s), 1585 (s), 1546 (m), 1477 (s), 1423 (s), 1380 (s), 1317 (w), 1288 (m), 1269 (m), 1234 (m), 1031 (w), 783 (m), 759 (s), 738 (w), 730 (w), 696 (w).
For [Gd{Ir(ppy)2(dcbpy)}2(NO3)(H2O)5]·2CH3OH·0.5H2O (Ir2Gd): yield: 18%. Elemental analyses (%) calcd for C70H61GdIr2N9O17.5, C, 45.45; H, 3.32; N, 6.81; found: C, 44.89; H, 3.27; N, 6.81. IR (KBr, cm−1): 3355 (m), 3107 (w), 3059 (m), 2925 (m), 1631 (s), 1585 (s), 1544 (s), 1476 (s), 1421 (s), 1367 (s), 1270 (m), 1233 (m), 1160 (w), 864 (m), 781 (m), 758 (m), 694 (m), 670(w).
For [Dy{Ir(ppy)2(dcbpy)}2(NO3)(H2O)5]·2CH3OH·0.5H2O (Ir2Dy): yield: 41%. Elemental analyses (%) calcd for C70H61DyIr2N9O17.5, C, 45.32; H, 3.31; N, 6.79; found: C, 43.93; H, 3.26; N, 6.63. IR (KBr, cm−1): 3423 (s), 3107 (w), 3049 (w), 1610 (s), 1546 (m), 1477 (s), 1427 (s), 1379 (s), 1313 (m), 1274 (m), 1234 (m), 1161 (w), 1062 (w), 1031 (w), 761 (m), 734 (w), 698 (w), 628 (w), 603 (w), 567 (w).
For [Er{Ir(ppy)2(dcbpy)}2(NO3)(H2O)4]·2CH3OH·0.5H2O (Ir2Er): yield: 37%. Elemental analyses (%) calcd for C70H61ErIr2N9O17.5, C, 45.20; H, 3.30; N, 6.77; found: C, 44.76; H, 3.24; N, 6.83. IR (KBr, cm−1): 3375 (m), 3103 (m), 3043 (m), 1606 (s), 1585 (s), 1546 (m), 1514 (w), 1477 (s), 1421 (s), 1377 (s), 1286 (m), 1269 (m), 1232 (m), 1161 (w), 1031 (w), 781 (m), 759 (s), 738 (w), 729 (w), 696 (w).
Thermal analyses reveal that the weight losses at 90 °C are 4.2% (for Ir2Dy), 4.4% (for Ir2Er) and 3.9% (for Ir2Yb) (Fig. S32†), which is in agreement with the release of lattice solvents (calcd 3.9% for 2CH3OH + 0.5H2O). The weight loss for Ir2Gd (5.6%) is higher than those for others, suggesting that one coordination water molecule could also be released below this temperature (calcd 4.9%). Between 90 and 300 °C slow weight losses occur corresponding to the partial removal of the coordination water molecule. Above 300 °C, the organic ligands start to decompose and the framework structures collapse.
Synthesis of [Ln2{Ir(ppy)2(dcbpy)}4(NO3)2(H2O)4]·solvent (Ir4Ln2; Ln = Gd, Dy, Er, and Yb)
Compounds Ir4Ln2 (Ln = Gd, Dy, Er, and Yb) were synthesized under similar optimized experimental conditions. A typical preparation procedure for [Yb2(OH){Ir(ppy)2(dcbpy)}4(NO3)(H2O)4]·2CH3OH·2.5H2O (Ir4Yb2) is as follows. Ir(ppy)2(Hdcbpy) (7.4 mg, 0.01 mmol) was suspended in 2 mL of distilled water and dissolved in 10 μL of aqueous NaOH (1 M). Then Yb(NO3)3·6H2O (9.34 mg, 0.02 mmol) in 7 mL of MeOH was added. An immediate precipitate of a green powder was obtained. After keeping the vial at 80 °C for 2 days, orange rod crystals were obtained. The crystalline product of Ir4Yb2 was separated by filtration and washed with distilled water and a minimum amount of methanol. Yield: 23%. Elemental analyses (%) calcd for C138H110N17Yb2Ir4O28.5, C, 46.33; H, 3.10; N, 6.66; found: C, 45.82; H, 3.16; N, 6.83. IR (KBr, cm−1): 3400 (s), 3103 (m), 3043 (m), 1608 (s), 1546 (m), 1477 (s), 1427 (s), 1379 (s), 1272 (w), 1232 (m), 1161 (w), 1031 (w), 866 (w), 784 (m), 761 (m), 734 (w), 698 (w), 630 (w).
For [Gd2(OH){Ir(ppy)2(dcbpy)}4(NO3)(H2O)4]·2CH3OH·2.5H2O (Ir4Gd2): yield: 15%. Elemental analyses (%) calcd for C138H110N17Gd2Ir4O28.5, C, 46.74; H, 3.13; N, 6.71; found: C, 45.97; H, 3.48; N, 6.99. IR (KBr, cm−1): 3357 (s), 3105 (m), 3042 (m), 1604 (s), 1583 (s), 1562 (m), 1544 (s), 1476 (s), 1420 (s), 1365 (s), 1317 (m), 1269 (w), 1235 (m), 782 (m), 729 (w), 694 (w), 629 (w).
For [Dy2(OH){Ir(ppy)2(dcbpy)}4(NO3)(H2O)4]·2CH3OH·2.5 H2O (Ir4Dy2): yield: 26%. Elemental analyses (%) calcd for C138H110N17Dy2Ir4O28.5, C, 46.61; H, 3.12; N, 6.70; found: C, 45.74; H, 3.21; N, 6.68. IR (KBr, cm−1): 3429 (m), 3107 (m), 3053 (m), 1610 (s), 1548 (m), 1477 (s), 1425 (s), 1379 (s), 1271 (w), 1234 (w), 1162 (w), 1033 (w), 761 (s), 734 (w), 698 (w), 669 (w), 628 (w), 559 (w), 426 (w).
For [Er2(OH){Ir(ppy)2(dcbpy)}4(NO3)(H2O)4]·3CH3OH·2.5H2O (Ir4Er2): yield: 28%. Elemental analyses (%) calcd For C139H114N17Er2Ir4O29.5, C, 46.40; H, 3.19; N, 6.62; found: C, 45.50; H, 3.57; N, 6.48. IR (KBr, cm−1): 3402 (m), 3107 (m), 3045 (m), 1608 (s), 1546 (m), 1477 (s), 1427 (s), 1380 (s), 1271 (w), 1234 (w), 1161 (w), 1031 (w), 784 (m), 761 (m), 734 (w), 698 (w), 630 (w).
Thermal analyses reveal that the weight losses at 100 °C are 2.8% for Ir4Gd2, Ir4Dy2 and Ir4Yb2 (Fig. S33†), which is in agreement with the release of two CH3OH and 2.5H2O molecules (calcd 3.0–3.1%). The weight loss for Ir4Er2 is 4.1%, corresponding to the release of three CH3OH and 2.5 H2O molecules (calcd 3.9%). The number of solvent molecules for Ir4Yb2 is less than that obtained from the single crystal structural determination, suggesting that the lattice solvents are partially released at room temperature. The weight losses between 100 and 300 °C are attributed to the partial release of the coordination water molecules, and above 300 °C the structure begins to collapse.
X-ray crystallographic analyses
Suitable single crystals were selected for indexing and obtaining intensity data using a Bruker SMART APEX D8 diffractometer equipped with a graphite-monochromated Mo Kα radiation source (λ = 0.71073 Å) at 150 K.42 Adsorption corrections were carefully performed for all data. The structures were solved by direct methods and refined on F2 by full-matrix least squares using SHELXTL.43 All non-hydrogen atoms were located in the Fourier maps and were refined with anisotropic thermal parameters. All H atoms were either placed in the calculated positions or found in the F-maps and refined isotropically.
In the case of Ir2Dy, the residual electron densities in the solvent-accessible void due to heavily disordered solvent molecules and nitrate anions were determined using the PLATON/SQUEEZE program.44 In cases of Ir2Er, Ir2Yb and Ir4Yb2, the lattice solvent molecules and nitrate anions were found in the Fourier map. The hydrogen atoms of disordered water molecules were not placed. Quite large (2.2–5.3 e A3) residual electron density peaks were located near the Ln or Ir atoms in the corresponding structures, and could not be reasonably modelled with the disordered atoms. The crystallographic data and refinement details are listed in Table 1. The selected bond lengths and angles for Ir2Dy, Ir2Er, Ir2Yb and Ir4Yb2 are given in Tables S1–S7.†
Conflicts of interest
There are no conflicts to declare.
Acknowledgements
Financial support from the National Key R&D Program of China (2017YFA0303203, 2018YFA0306004) and the National Natural Science Foundation of China (21731003) is acknowledged. MK is funded by the CNRS (France).
Notes and references
-
(a) Y. Cui, B. Li, H. He, W. Zhou, B. Chen and G. Qian, Metal-Organic Frameworks as Platforms for Functional Materials, Acc. Chem. Res., 2016, 49, 483–493 CrossRef CAS;
(b) S. Roy, A. Chakraborty and T. K. Maji, Lanthanide-organic frameworks for gas storage and as magneto-luminescent materials, Coord. Chem. Rev., 2014, 273–274, 139–164 CrossRef CAS;
(c) Y.-S. Meng and T. Liu, Manipulating Spin Transition To Achieve Switchable Multifunctions, Acc. Chem. Res., 2019, 52, 1369–1379 CAS.
-
(a) J. Long, Y. Guari, R. A. S. Ferreira, L. D. Carlos and J. Larionova, Recent advances in luminescent lanthanide based Single-Molecule Magnets, Coord. Chem. Rev., 2018, 363, 57–70 CrossRef CAS;
(b) J.-H. Jia, Q.-W. Li, Y.-C. Chen, J.-L. Liu and M.-L. Tong, Luminescent single-molecule magnets based on lanthanides: Design strategies, recent advances and magneto-luminescent studies, Coord. Chem. Rev., 2019, 378, 365–381 CrossRef CAS;
(c) F. Pointillart, B. le Guennic, O. Cador, O. Maury and L. Ouahab, Lanthanide Ion and Tetrathiafulvalene-Based Ligand as a “Magic” Couple toward Luminescence, Single Molecule Magnets, and Magnetostructural Correlations, Acc. Chem. Res., 2015, 48, 2834–2842 CrossRef CAS PubMed.
-
(a) M. M. Paquette, D. Plaul, A. Kurimoto, B. O. Patrick and N. L. Frank, Opto-Spintronics: Photoisomerization-Induced Spin State Switching at 300 K in Photochrome Cobalt-Dioxolene Thin Films, J. Am. Chem. Soc., 2018, 140, 14990–15000 CrossRef CAS PubMed;
(b) J.-L. Wang, Q. Liu, Y.-S. Meng, X. Liu, H. Zheng, Q. Shi, C.-Y. Duan and T. Liu, Fluorescence modulation via photoinduced spin crossover switched energy transfer from fluorophores to FeII ions, Chem. Sci., 2018, 9, 2892–2897 RSC;
(c) M. Estrader, J. S. Uber, L. A. Barrios, J. Garcia, P. Lloyd-Williams, O. Roubeau, S. J. Teat and G. Aromí, A Magneto-optical Molecular Device: Interplay of Spin Crossover, Luminescence, Photomagnetism, and Photochromism, Angew. Chem., Int. Ed., 2017, 56, 15622–15627 CrossRef CAS PubMed;
(d) T. Delgado, M. Meneses-Sánchez, L. Piñeiro-López, C. Bartual-Murgui, M. C. Muñoz and J. A. Real, Thermo- and photo-modulation of exciplex fluorescence in a 3D spin crossover Hofmann-type coordination polymer, Chem. Sci., 2018, 9, 8446–8452 RSC;
(e) X.-D. Huang, M. Kurmoo, S.-S. Bao, K. Fan, Y. Xu, Z.-B. Hu and L.-M. Zheng, Coupling photo-, mechano- and thermochromism and single-ion-magnetism of two mononuclear dysprosium-anthracene-phosphonate complexes, Chem. Commun., 2018, 54, 3278–3281 RSC;
(f) J. Yuan, S.-Q. Wu, M.-J. Liu, O. Sato and H.-Z. Kou, Rhodamine 6G-Labeled Pyridyl Aroylhydrazone Fe(II) Complex Exhibiting Synergetic Spin Crossover and Fluorescence, J. Am. Chem. Soc., 2018, 140, 9426–9433 CrossRef CAS PubMed.
-
(a) B. M. Day, F.-S. Guo and R. A. Layfield, Cyclopentadienyl Ligands in Lanthanide Single-Molecule Magnets: One Ring To Rule Them All?, Acc. Chem. Res., 2018, 51, 1880–1889 CrossRef CAS PubMed;
(b) M. Ren and L.-M. Zheng, Lanthanide-based Single Molecule Magnets, Acta Chim. Sin., 2015, 73, 1091–1113 CrossRef CAS;
(c) Z.-H. Zhu, M. Guo, X.-L. Li and J.-K. Tang, Molecular magnetism of lanthanide: Advances and perspectives, Coord. Chem. Rev., 2019, 378, 350–364 CrossRef CAS.
-
(a) Y.-S. Meng, S.-D. Jiang, B.-W. Wang and S. Gao, Understanding the Magnetic Anisotropy toward Single-Ion Magnets, Acc. Chem. Res., 2016, 49, 2381–2389 CrossRef CAS PubMed;
(b) D. N. Woodruff, R. E. P. Winpenny and R. A. Layfield, Lanthanide Single-Molecule Magnets, Chem. Rev., 2013, 113, 5110–5148 CrossRef CAS PubMed;
(c) K. Liu, X.-J. Zhang, X.-X. Meng, W. Shi, P. Cheng and A. K. Powell, Constraining the coordination geometries of lanthanide centers and magnetic building blocks in frameworks: a new strategy for molecular nanomagnets, Chem. Soc. Rev., 2016, 45, 2423–2439 RSC;
(d) H.-L. Wang, B.-W. Wang, Y.-Z. Bian, S. Gao and J.-Z. Jiang, Single-molecule magnetism of tetrapyrrole lanthanide compounds with sandwich multiple-decker structures, Coord. Chem. Rev., 2016, 306, 195–216 CrossRef CAS;
(e) F.-S. Guo, B. M. Day, Y.-C. Chen, M.-L. Tong, A. Mansikkamäki and R. A. Layfield, Magnetic hysteresis up to 80 kelvin in a dysprosium metallocene single-molecule magnet, Science, 2018, 362, 1400–1403 CrossRef CAS PubMed.
- J. G. Bünzli, On the design of highly luminescent lanthanide complexes, Coord. Chem. Rev., 2015, 293–294, 19–47 CrossRef.
-
(a) M. Pan, W.-M. Liao, S.-Y. Yin, S.-S. Sun and C.-Y. Su, Single-Phase White-Light-Emitting and Photoluminescent Color-Tuning Coordination Assemblies, Chem. Rev., 2018, 118, 8889–8935 CrossRef CAS PubMed;
(b) Y. Hasegawa and Y. Kitagawa, Thermo-sensitive luminescence of lanthanide complexes, clusters, coordination polymers and metal-organic frameworks with organic photosensitizers, J. Mater. Chem. C, 2019, 7, 7494–7511 RSC.
-
(a) A. T. Bui, A. Roux, A. Grichine, A. Duperray, C. Andraud and O. Maury, Twisted Charge-Transfer Antennae for Ultra-Bright Terbium(III) and Dysprosium(III) Bioprobes, Chem. – Eur. J., 2018, 24, 3408–3412 CrossRef CAS PubMed;
(b) D. Kovacs, D. Phipps, A. Orthaber and K. E. Borbas, Highly luminescent lanthanide complexes sensitised by tertiary amide-linked carbostyril antennae, Dalton Trans., 2018, 47, 10702–10714 RSC;
(c) M. Ren, Z.-L. Xu, S.-S. Bao, T.-T. Wang, Z.-H. Zheng, R. A. Ferreira, L.-M. Zheng and L. D. Carlos, Lanthanide salen-type complexes exhibiting single ion magnet and photoluminescent properties, Dalton Trans., 2016, 45, 2974–2982 RSC;
(d) Y. Ning, X.-S. Ke, J.-Y. Hu, Y.-W. Liu, F. Ma, H.-L. Sun and J.-L. Zhang, Bioinspired Orientation of β-Substituents on Porphyrin Antenna Ligands Switches Ytterbium(III) NIR Emission with Thermosensitivity, Inorg. Chem., 2017, 56, 1897–1905 CrossRef CAS PubMed;
(e) B. S. K. Chong and E. G. Moore, Quantitative Sensitization Efficiencies in NIR-Emissive Homoleptic Ln(III) Complexes Using 2-(5-Methylpyridin-2-yl)-8-hydroxyquinoline, Inorg. Chem., 2018, 57, 14062–14072 CrossRef CAS PubMed;
(f) Q. Zou, X.-D. Huang, J. C. Liu, S.-S. Bao and L.-M. Zheng, Lanthanide anthracene complexes: slow magnetic relaxation and luminescence in DyIII, ErIII and YbIII based materials, Dalton Trans., 2019, 48, 2735–2740 RSC.
- F.-F. Chen, Z.-Q. Chen, Z.-Q. Bian and C.-H. Huang, Sensitized luminescence from lanthanides in d–f bimetallic complexes, Coord. Chem. Rev., 2010, 254, 991–1010 CrossRef CAS.
-
(a) C. Giansante, P. Ceroni, V. Balzani and F. Vögtle, Self-Assembly of a Light-Harvesting Antenna Formed by a Dendrimer, a RuII Complex, and a NdIII Ion, Angew. Chem., Int. Ed., 2008, 47, 5422–5425 CrossRef CAS PubMed;
(b) H.-B. Xu, X.-L. Chen, J.-G. Deng, Z.-H. Deng, S.-L. Huang, M. Kurmoo and M.-H. Zeng, Sensitized near infrared emission through supramolecular d → f energy transfer within an ionic Ru(II)-Er(III) pair, Dalton Trans., 2018, 47, 2073–2078 RSC.
-
(a) Y. You and W. Nam, Photofunctional triplet excited states of cyclometalated Ir(III) complexes: beyond electroluminescence, Chem. Soc. Rev., 2012, 41, 7061–7084 RSC;
(b) D. R. Martir and E. Zysman-Colman, Supramolecular iridium(III) assemblies, Coord. Chem. Rev., 2018, 364, 86–117 CrossRef.
- M. Mehlstäubl, G. S. Kottas, S. Colella and L. De Cola, Sensitized near-infrared emission from ytterbium(III) via direct energy transfer from iridium(III) in a heterometallic neutral complex, Dalton Trans., 2008, 2385–2388 RSC.
-
(a) F. Chen, Z. Bian, B. Lou, E. Ma, Z. Liu, D. Nie, Z. Chen, J. Bian, Z. Chen and C. Huang, Sensitised near-infrared emission from lanthanides using an iridium complex as a ligand in heteronuclear Ir2Ln arrays, Dalton Trans., 2008, 5577–5583 RSC;
(b) F. Chen, Z. Bian, Z. Liu, D. Nie, Z. Chen and C. Huang, Highly Efficient Sensitized Red Emission from Europium(III) in Ir-Eu Bimetallic Complexes by 3MLCT Energy Transfer, Inorg. Chem., 2008, 47, 2507–2513 CrossRef CAS PubMed;
(c) L.-Y. Zhang, Y.-J. Hou, M. Pan, L. Chen, Y.-X. Zhu, S.-Y. Yin, G. Shao and C.-Y. Su, Near-infrared (NIR) emitting Nd/Yb(III) complexes sensitized by MLCT states of Ru(II)/Ir(III) metalloligands in the visible light region, Dalton Trans., 2015, 44, 15212–15219 RSC;
(d) F.-F. Chen, H.-B. Wei, Z.-Q. Bian, Z.-W. Liu, E. Ma, Z.-N. Chen and C.-H. Huang, Sensitized Near-Infrared Emission from Ir-III-Ln(III) (Ln = Nd, Yb, Er) Bimetallic Complexes with a ((NO)-O-boolean AND)((NO)-O-boolean AND) Bridging Ligand, Organometallics, 2014, 33, 3275–3282 CrossRef CAS;
(e) A. Jana, S. J. A. Pope and M. D. Ward, D → f energy transfer in heteronuclear Ir(III)/Ln(III) near-infrared luminescent complexes, Polyhedron, 2017, 127, 390–395 CrossRef CAS.
- L. Li, S. Zhang, L. Xu, Z.-N. Chen and J. Luo, Highly sensitized near-infrared luminescence in Ir-Ln heteronuclear coordination polymers via light-harvesting antenna of Ir(III) unit, J. Mater. Chem. C, 2014, 2, 1698–1703 RSC.
-
(a) D. Zeng, M. Ren, S.-S. Bao, L. Li and L.-M. Zheng, A luminescent heptanuclear DyIr6 complex showing field-induced slow magnetization relaxation, Chem. Commun., 2014, 50, 8356–8359 RSC;
(b) D. Zeng, K. Fan, L.-P. Wang, S.-S. Bao, M. Ren and L.-M. Zheng, Octahedral erbium and ytterbium ion encapsulated in phosphorescent iridium complexes showing field-induced magnetization relaxation, J. Magn. Magn. Mater., 2019, 484, 139–145 CrossRef CAS.
-
(a) X.-D. Huang, Y. Xu, K. Fan, S.-S. Bao, M. Kurmoo and L.-M. Zheng, Reversible SC-SC Transformation involving [4 + 4] Cycloaddition of Anthracene: A Single-Ion to Single-Molecule Magnet and Yellow-Green to Blue-White Emission, Angew. Chem., Int. Ed., 2018, 57, 8577–8581 CrossRef CAS PubMed;
(b) X.-D. Huang, J.-G. Jia, M. Kurmoo, S.-S. Bao and L.-M. Zheng, Interplay of anthracene luminescence and dysprosium magnetism by steric control of photodimerization, Dalton Trans., 2019, 48, 13769–13779 RSC.
-
(a) M. Pinsky and D. Avnir, Continuous Symmetry Measures. 5. The Classical Polyhedra, Inorg. Chem., 1998, 37, 5575–5582 CrossRef CAS PubMed;
(b) D. Casanova, P. Alemany, J. M. Bofill and S. Alvarez, Shape and Symmetry of Heptacoordinate Transition-Metal Complexes: Structural Trends, Chem. – Eur. J., 2003, 9, 1281–1295 CrossRef CAS PubMed.
- L. Li, S. Zhang, L. Xu, J. Wang, L. Shi, Z. Chen, M. Hong and J. Luo, Effective visible-light driven CO2 photoreduction via a promising bifunctional iridium coordination polymer, Chem. Sci., 2014, 5, 3808–3813 RSC.
-
(a) L. Norel, L. E. Darago, B. L. Guennic, K. Chakarawet, M. I. Gonzalez, J. H. Olshansky, S. Rigaut and J. R. Long, A Terminal Fluoride Ligand Generates Axial Magnetic Anisotropy in Dysprosium Complexes, Angew. Chem., Int. Ed., 2018, 57, 1933–1938 CrossRef CAS PubMed;
(b) E. Moreno-Pineda, G. Taran, W. Wernsdorfer and M. Ruben, Quantum tunnelling of the magnetisation in single-molecule magnet isotopologue dimers, Chem. Sci., 2019, 10, 5138–5145 RSC.
- X.-J. Zhang, S. Liu, V. Vieru, N. Xu, C. Gao, B.-W. Wang, W. Shi, L. F. Chibotaru, S. Gao, P. Cheng and A. K. Powell, Coupling Influences SMM Properties for Pure 4 f Systems, Chem. – Eur. J., 2018, 24, 6079–6086 CrossRef CAS PubMed.
- K. L. M. Harriman, J. J. Le Roy, L. Ungur, R. J. Holmberg, I. Korobkova and M. Murugesu, Cycloheptatrienyl trianion: an elusive bridge in the search of exchange coupled dinuclear organolanthanide single-molecule magnets, Chem. Sci., 2017, 8, 231–240 RSC.
-
(a) K. S. Cole and R. H. Cole, Dispersion and absorption in dielectrics: Alternating current characteristics, J. Chem. Phys., 1941, 9, 341–351 CrossRef CAS;
(b) Y.-N. Guo, G.-F. Xu, Y. Guo and J.-K. Tang, Relaxation dynamics of dysprosium(III) single molecule magnets, Dalton Trans., 2011, 40, 9953–9963 RSC;
(c)
N. F. Chilton, CC-Fit, The University of Manchester, Manchester, UK, 2014, http://www.nfchilton.com/cc-fit.html Search PubMed.
-
(a) S.-M. Chen, J. Xiong, Y.-Q. Zhang, Q. Yuan, B.-W. Wang and S. Gao, A soft phosphorus atom to “harden” an erbium(III) single-ion magnet, Chem. Sci., 2018, 9, 7540–7545 RSC;
(b) K. Katoh, S. Yamashita, N. Yasuda, Y. Kitagawa, B. K. Breedlove, Y. Nakazawa and M. Yamashita, Control of the Spin Dynamics of Single-Molecule Magnets by using a Quasi One-Dimensional Arrangement, Angew. Chem., Int. Ed., 2018, 57, 9262–9267 CrossRef CAS PubMed.
-
(a) J.-J. Lu, Y.-Q. Zhang, X.-L. Li, M. Guo, J.-F. Wu, L. Zhao and J.-K. Tang, Influence of Magnetic Interactions and Single-Ion Anisotropy on Magnetic Relaxation within a Family of Tetranuclear Dysprosium Complexes, Inorg. Chem., 2019, 58, 5715–5724 CrossRef CAS PubMed;
(b) R. Boča, C. Rajnák, J. Titiš and D. Valigura, Field Supported Slow Magnetic Relaxation in a Mononuclear Cu(II) Complex, Inorg. Chem., 2017, 56, 1478–1482 CrossRef PubMed.
-
(a) C. Schlesier, L. Spree, A. Kostanyan, R. Westerström, A. Brandenburg, A. U. B. Wolter, S.-F. Yang, T. Greber and A. A. Popov, Strong carbon cage influence on the single molecule magnetism in Dy-Sc nitride clusterfullerenes, Chem. Commun., 2018, 54, 9730–9733 RSC;
(b) H.-L. Gao, S.-X. Huang, X.-P. Zhou, Z. Liu and J.-Z. Cui, Magnetic properties and structure of tetranuclear lanthanide complexes based on 8-hydroxylquinoline Schiff base derivative and β-diketone coligand, Dalton Trans., 2018, 47, 3503–3511 RSC.
-
(a) J. Vallejo, J. Cano, I. Castro, M. Julve, F. Lloret, O. Fabelo, L. Cañadillas-Delgado and E. Pardo, Slow magnetic relaxation in carbonato-bridged dinuclear lanthanide(III) complexes with 2,3-quinoxalinediolate ligands, Chem. Commun., 2012, 48, 7726–7728 RSC;
(b) K. L. M. Harriman and M. Murugesu, An Organolanthanide Building Block Approach to Single-Molecule Magnets, Acc. Chem. Res., 2016, 49, 1158–1167 CrossRef CAS PubMed.
- K. Kumar, O. Stefanczyk, S. Chorazy, K. Nakabayashi, B. Sieklucka and S.-i. Ohkoshi, Effect of Noble Metals on Luminescence and Single-Molecule Magnet Behavior in the Cyanido-Bridged Ln-Ag and Ln-Au (Ln = Dy, Yb, Er) Complexes, Inorg. Chem., 2019, 58, 5677–5687 CrossRef CAS PubMed.
-
(a) Y.-Q. Zhai, Y.-F. Deng and Y.-Z. Zheng, Pseudotetrahedral cobalt(II) complexes with PNP-ligands showing uniaxial magnetic anisotropy, Dalton Trans., 2018, 47, 8874–8878 RSC;
(b) J. Li, S. Gomez-Cocá, B. S. Dolinar, L. Yang, F. Yu, M. Kong, Y.-Q. Zhang, Y. Song and K. R. Dunbar, Hexagonal Bipyramidal Dy(III) Complexes as a Structural Archetype for Single-Molecule Magnets, Inorg. Chem., 2019, 58, 2610–2617 CrossRef CAS PubMed.
-
(a) L. Mandal, S. Biswas, G. Cosquer, Y.-B. Shen and M. Yamashita, Anion-driven structures and SMM behavior of dinuclear terbium and ytterbium complexes, Dalton Trans., 2018, 47, 17493–17499 RSC;
(b) H.-Q. Tian, B.-L. Wang, J. Lu, H.-T. Liu, J.-H. Su, D.-C. Li and J.-M. Dou, Consecutive one-/two-step relaxation transformations of single-molecule magnets via coupling dinuclear dysprosium compounds with chloride bridges, Chem. Commun., 2018, 54, 12105–12108 RSC.
-
(a) S. G. McAdams, A. Ariciu, A. K. Kostopoulos, J. P. S. Walsh and F. Tuna, Molecular single-ion magnets based on lanthanides and actinides: Design considerations and new advances in the context of quantum technologies, Coord. Chem. Rev., 2017, 346, 216–239 CrossRef CAS;
(b) J.-L. Liu, Y.-C. Chen and M.-L. Tong, Symmetry strategies for high performance lanthanide-based single-molecule magnets, Chem. Soc. Rev., 2018, 47, 2431–2453 RSC.
-
(a) J.-L. Liu, Y.-C. Chen, Y.-Z. Zheng, W.-Q. Lin, L. Ungur, W. Wernsdorfer, L. F. Chibotaru and M.-L. Tong, Switching the anisotropy barrier of a single-ion magnet by symmetry change from quasi-D5h to quasi-Oh, Chem. Sci., 2013, 4, 3310–3316 RSC;
(b) J. Liu, Y.-C. Chen, J.-L. Liu, V. Vieru, L. Ungur, J. Jia, L. F. Chibotaru, Y.-H. Lan, W. Wernsdorfer, S. Gao, X.-M. Chen and M.-L. Tong, A Stable Pentagonal Bipyramidal Dy(III) Single-Ion Magnet with a Record Magnetization Reversal Barrier over 1000 K, J. Am. Chem. Soc., 2016, 138, 5441–5450 CrossRef CAS PubMed;
(c) Y.-C. Chen, J.-L. Liu, W. Wernsdorfer, D. Liu, L. F. Chibotaru, X.-M. Chen and M.-L. Tong, Hyperfine-Interaction-Driven Suppression of Quantum Tunneling at Zero Field in a Holmium(III) Single-Ion Magnet, Angew. Chem., Int. Ed., 2017, 56, 4996–5000 CrossRef CAS PubMed.
-
(a) Y.-S. Ding, N. F. Chilton, R. E. P. Winpenny and Y.-Z. Zheng, On Approaching the Limit of Molecular Magnetic Anisotropy: A Near-Perfect Pentagonal Bipyramidal Dysprosium(III) Single-Molecule Magnet, Angew. Chem., Int. Ed., 2016, 55, 16071–16074 CrossRef CAS PubMed;
(b) L. Ungur and L. F. Chibotaru, Strategies toward High-Temperature Lanthanide-Based Single-Molecule Magnets, Inorg. Chem., 2016, 55, 10043–10056 CrossRef CAS PubMed;
(c) S. K. Gupta, T. Rajeshkumar, G. Rajaraman and R. Murugavel, An air-stable Dy(III) single-ion magnet with high anisotropy barrier and blocking temperature, Chem. Sci., 2016, 7, 5181–5191 RSC.
-
(a) J. D. Rinehart and J. R. Long, Exploiting single-ion anisotropy in the design of f-element single-molecule magnets, Chem. Sci., 2011, 2, 2078–2085 RSC;
(b) M. Ren, S.-S. Bao, B.-W. Wang, R. A. S. Ferreira, L.-M. Zheng and L. D. Carlos, Lanthanide phosphonates with pseudo-D5h local symmetry exhibiting magnetic and luminescence bifunctional properties, Inorg. Chem. Front., 2015, 2, 558–566 RSC;
(c) M. Ren, S.-S. Bao, R. A. S. Ferreira, L.-M. Zheng and L. D. Carlos, A layered erbium phosphonate in pseudo-D5h symmetry exhibiting field-tunable magnetic relaxation and optical correlation, Chem. Commun., 2014, 50, 7621–7624 RSC;
(d) X.-C. Huang, M. Zhang, D. Wu, D. Shao, X.-H. Zhao, W. Huang and X.-Y. Wang, Single molecule magnet behavior observed in a 1-D dysprosium chain with quasi-D5h symmetry, Dalton Trans., 2015, 44, 20834–20838 RSC;
(e) J. Long, A. N. Selikhov, E. Mamontova, K. A. Lyssenko, Y. Guari, J. Larionova and A. A. Trifonov, Single-molecule magnet behaviour in a Dy(III) pentagonal bipyramidal complex with a quasi-linear Cl-Dy-Cl sequence, Dalton Trans., 2019, 48, 35–39 RSC;
(f) M. Li, H. Wu, Q. Yang, H. Ke, B. Yin, Q. Shi, W. Wang, Q. Wei, G. Xie and S. Chen, Experimental and Theoretical Interpretation on the Magnetic Behavior in a Series of Pentagonal-Bipyramidal DyIII Single-Ion Magnets, Chem. – Eur. J., 2017, 23, 17775–17787 CrossRef CAS PubMed.
- S. Lamansky, P. Djurovich, D. Murphy, F. Abdel-Razzaq, R. Kwong, I. Tsyba, M. Bortz, B. Mui, R. Bau and M. E. Thompson, Synthesis and Characterization of Phosphorescent Cyclometalated Iridium Complexes, Inorg. Chem., 2001, 40, 1704–1711 CrossRef CAS PubMed.
- W. T. Carnall, P. R. Fields and K. Rajnak, Electronic Energy Levels in the Trivalent Lanthanide Aquo Ions. I. Pr3+, Nd3+, Pm3+, Sm3+, Dy3+, Ho3+, Er3+, and Tm3+, J. Chem. Phys., 1968, 49, 4424–4442 CrossRef CAS.
-
(a) J. B. Waern, C. Desmarets, L. Chamoreau, H. Amouri, A. Barbieri, C. Sabatini, B. Ventura and F. Barigelletti, Luminescent Cyclometalated RhIII, IrIII, and (DIP)2RuII Complexes with Carboxylated Bipyridyl Ligands: Synthesis, X-ray Molecular Structure, and Photophysical Properties, Inorg. Chem., 2008, 47, 3340–3348 CrossRef CAS PubMed;
(b) W. Jiang, Y. Gao, Y. Sun, F. Ding, Y. Xu, Z. Bian, F. Li, J. Bian and C. Huang, Zwitterionic Iridium Complexes: Synthesis, Luminescent Properties, and Their Application in Cell Imaging, Inorg. Chem., 2010, 49, 3252–3260 CrossRef CAS PubMed;
(c) M. Ho, Y. Chen, T. Chen, P. Chang, Y. Yu, K. Cheng, C. Shih, G. Lee and H. Sheu, Synthesis, structure and oxygen-sensing properties of Iridium(III)-containing coordination polymers with different cations, Dalton Trans., 2012, 41, 2592–2600 RSC;
(d) L. Li, S. Zhang, L. Xu, L. Han, Z. Chen and J. Luo, An Intensely Luminescent Metal-Organic Framework Based on a Highly Light-Harvesting Dyclo-Metalated Iridium(III) Unit Showing Effective Detection of Explosives, Inorg. Chem., 2013, 52, 12323–12325 CrossRef CAS PubMed.
-
(a) W. T. Carnall, P. R. Fields and K. Rajnak, Spectral Intensities of the Trivalent Lanthanides and Actinides in Solution. II. Pm3+, Sm3+, Eu3+, Gd3+, Tb3+, Dy3+, and Ho3+, J. Chem. Phys., 1968, 49, 4412–4423 CrossRef CAS;
(b) D. Sykes and M. D. Ward, Visible-light sensitisation of Tb(III) luminescence using a blue-emitting Ir(III) complex as energy-donor, Chem. Commun., 2011, 47, 2279–2281 RSC;
(c) A. Jana, B. J. Crowston, J. R. Shewring, L. K. McKenzie, H. E. Bryant, S. W. Botchway, A. D. Ward, A. J. Amoroso, E. Baggaley and M. D. Ward, Heteronuclear Ir(III)–Ln(III) Luminescent Complexes: Small-Molecule Probes for Dual Modal Imaging and Oxygen Sensing, Inorg. Chem., 2016, 55, 5623–5633 CrossRef CAS PubMed.
-
(a) Y. Wang, T. Yang, X. Liu, G. Li, W. Che, D. Zhu and Z. Su, New cationic Ir(III) complexes without “any soft substituents”: aggregation-induced emission and piezochromic luminescence, J. Mater. Chem. C, 2018, 6, 12217–12223 RSC;
(b) D. G. Congrave, Y.-T. Hsu, A. S. Batsanov, A. Beeby and M. R. Bryce, Sky-blue emitting bridged diiridium complexes: beneficial effects of intramolecular π–π stacking, Dalton Trans., 2018, 47, 2086–2098 RSC;
(c) L. He, D. Ma, L. Duan, Y. Wei, J. Qiao, D. Zhang, G. Dong, L. Wang and Y. Qiu, Control of Intramolecular π–π Stacking Interaction in Cationic Iridium Complexes via Fluorination of Pendant Phenyl Rings, Inorg. Chem., 2012, 51, 4502–4510 CrossRef CAS PubMed.
-
(a) E. G. Moore, A. P. S. Samuel and K. N. Raymond, From Antenna to Assay: Lessons Learned in Lanthanide Luminescence, Acc. Chem. Res., 2009, 42, 542–552 CrossRef CAS PubMed;
(b) S. V. Eliseeva and J. G. Buenzli, Lanthanide luminescence for functional materials and bio-sciences, Chem. Soc. Rev., 2010, 39, 189–227 RSC.
-
(a) D. Li, F.-F. Chen, Z.-Q. Bian, Z.-W. Liu, Y.-L. Zhao and C.-H. Huang, Sensitized near-infrared emission of YbIII from an IrIII–YbIII bimetallic complex, Polyhedron, 2009, 28, 897–902 CrossRef CAS;
(b) J. E. Jones, R. L. Jenkins, R. S. Hicks, A. J. Hallett and S. J. A. Pope, Water-soluble, luminescent iridium(III)-ytterbium(III) complexes using dipyrido[3,2-a: 2′,3′-c]phenazine derivatives as bridging units, Dalton Trans., 2012, 41, 10372–10381 RSC.
-
(a) J. G. Bünzli and S. V. Eliseeva, Lanthanide NIR luminescence for telecommunications, bioanalyses and solar energy conversion, J. Rare Earths, 2010, 28, 824–842 CrossRef;
(b) Y. Xu, S.-S. Bao, X.-D. Huang and L.-M. Zheng, Homochiral Erbium Coordination Polymers: Salt-Assisted Conversion from Triple to Quadruple Helices, Cryst. Growth Des., 2018, 18, 4045–4053 CrossRef CAS.
-
SAINT, Program for Data Extraction and Reduction, Siemens Analytical X-ray Instruments, Madison, WI, 1994–1996 Search PubMed.
-
SHELXTL (version 5.0), Reference Manual, Siemens Industrial Automation, Analytical Instruments, Madison, WI, 1995 Search PubMed.
- A. L. Spek, Single-crystal structure validation with the program PLATON, J. Appl. Crystallogr., 2003, 36, 7–13 CrossRef CAS.
Footnote |
† Electronic supplementary information (ESI) available: Synthetic details, structural data, IR, PXRD, TG. CCDC 1956646–1956649. For ESI and crystallographic data in CIF or other electronic format see DOI: 10.1039/c9qi01504c |
|
This journal is © the Partner Organisations 2020 |
Click here to see how this site uses Cookies. View our privacy policy here.