Introductory Lecture: Mechanochemistry, a versatile synthesis strategy for new materials
Received
28th August 2014
, Accepted 2nd September 2014
First published on 2nd September 2014
Abstract
Mechanochemistry deals with reactions induced by the input of mechanical energy – for example by impacts within a vibratory ball mill. The technique has a long history with significant contributions from Ostwald, Carey Lea and, notably, Faraday. Mechanochemistry has subsequently seen application in a variety of areas of materials science including mechanical alloying in metallurgy, the synthesis of complex organic molecules and, more recently, the discovery and development of new solid forms of active pharmaceutical ingredients. This paper overviews the broad areas of application of mechanochemistry, some key features which make it a particularly attractive approach to materials synthesis and some mechanistic aspects highlighted within the literature. A significant part, however, will focus on recent applications in the area of pharmaceuticals and its important role in exploring the rich variety of solid forms available for small, drug-like, molecules.
1. Introduction
Mechanochemistry is a long-established method for the synthesis of solids and molecules. Its chemical importance was recorded by Faraday, who in 1820 demonstrated the reduction of AgCl to Ag by grinding in a mortar and pestle a mixture of AgCl and Zn.1 Significant progress followed, especially through the work of Carey Lea (1823–1897),2 and the position of mechanochemistry within the framework of chemistry as a whole was established by Ostwald who noted the equivalency of electrochemistry, thermochemistry, photochemistry and mechanochemistry.3–5
Long held as an efficient process for the synthesis of metallic alloys, a recent extensive review by James et al.6 has highlighted several of the emergent areas now being actively pursued with regard to the application of mechanical energy in driving various transformations – indeed a working definition from that review is “mechanochemistry refers to reactions, normally of solids, induced by the impact of mechanical energy such as grinding in ball mills”. A formal definition by IUPAC states “a mechano-chemical reaction is a chemical reaction that is induced by mechanical energy”.7 Although a narrow definition of mechanochemistry may lead to a suggestion that the making and breaking of covalent bonds would be a prerequisite for a process to fall under the definition of “mechanochemistry”,8 following the appreciation of the importance of “non-covalent bonds” in supramolecular chemistry it seems reasonable to include changes in crystal packing to be a mechanochemical event. Links to mechanochemical activation by ultrasound have also been proposed.9,10
This paper will briefly summarise recent developments in mechanochemistry, particularly in its application in pharmaceutical materials science and its role in the development of new solid drug dosage forms, to complement earlier literature which has covered extensively metals and alloys and inorganic (ionic) systems. As we will describe later, the amount of energy which can be imparted to a system under mechanical activation can be significant – certainly sufficient to break chemical bonds6 – but can also be reduced when working with systems such as soft molecular crystals in order to minimise chemical activation while still enabling processes such as the conversion of a crystalline solid to an amorphous product,11 or the readjustment of molecules within a lattice. Such readjustments may lead to the introduction of lattice imperfections,12 to polymorphic transformations13 or to the intimate mixing of two separate crystalline molecular solids to create a crystalline multicomponent product indistinct from that which would be obtained by a conventional solution crystallisation.14 Particularly advantageous in mechanochemical transformations is the avoidance of the need for large volumes of solvent (as required, for example, in the recrystallization of poorly soluble molecules), and hence a strong “Green Chemistry” aspect to mechanochemistry is recognised.15,16 In terms of commercial interest, the review by James et al. describes a search of patent applications for the terms “mechanochemical” and “mechanochemistry” which revealed a significant increase in usage in the patent literature from 1980 onwards.6
2. Areas of application and development
The areas in which mechanochemistry has been applied with success are varied and include: catalysts,17 nanoparticles,18–20 organic synthesis,21–27 MOFs,28 oxides,29 biomaterials,30,31 supramolecular chemistry,32–34 process engineering,35 dyes and pigments,36 fluorophores,37 exfoliation of graphene nanosheets38 and modification of fullerenes (see Fig. 1).39 Numerous further examples are given in the review by James et al.6 and several other reviews on the application of mechanochemistry are available.28,40–47 In particular, the contributions of V. V. Boldyrev and his colleagues to both experimental and theoretical aspects of mechanochemistry have been important to the subject.3,9,48,49
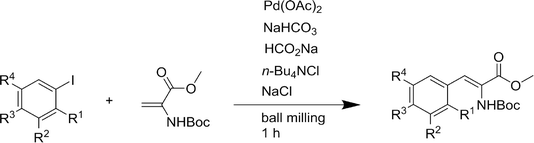 |
| Fig. 1 General scheme for the Heck reaction under ball milling conditions. Reproduced from ref. 6. | |
From a synthetic chemistry viewpoint an important observation is that substances can react by a significantly different pathway in the solid state than they would in solution, leading to new products or to much higher selectivities.50,51 This ability to generate a much broader product-spectrum, as we will demonstrate later, is also particularly important in areas other than chemical transformations e.g. in crystal polymorph conversions – and it is to be noted that the addition of very small amounts of liquid may have a significant impact on the outcome of a solid–solid reaction, even though quantities are much less than a conventional solvent-based reaction might require – see later.
A key area of active research remains the understanding of the processes by which mechanically induced reactions occur.52 Given the variety of materials of interest, ranging from metals and ceramics to pharmaceuticals, the specific details of how a process occurs will likely be varied and complex.3,53,54 Also to be distinguished is the time-scale over which an event is studied. During the few milliseconds following the impact of two solids at high velocity, significant local consequences will ensue. Hot spots, magma–plasma regions, rapid induction of defects (point, linear and planar) and then propagation of these defects through the crystal are some of the concepts which have evolved. The distribution of imparted energy will also be different in those solids with high rigidity and few pathways for energy dissipation (so that the effects at the specific point of impact will be great) than it is in those systems where structural movement (e.g. slippage of crystal planes) may allow the energy (and its consequences) to be distributed over a much larger distance.3 During this early stage, the making and breaking of chemical bonds would seem possible. Following the initial short-term consequences, there will also be the longer term events associated with the final energy dissipation and relaxation of the system. The slow crystallisation of an intermediate amorphous phase may be such an example (see Fig. 2 and 3).11,52,55,56 It is possible, then, to envisage that an individual particle, for example within a milled pharmaceutical material, may be completely amorphous initially, but over time may change to consist of domains of crystalline solid embedded within an amorphous shell (or more likely vice versa where, for example, humidity from the surrounding atmosphere results in each amorphous particle crystallising from the outside). This model might suggest that a description of a product as 25% amorphous does not mean that 25% of all the particles are amorphous and 75% are not – but that each individual particle within the product consists of domains of crystalline and amorphous in a 3
:
1 ratio.
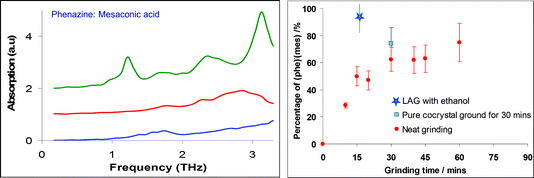 |
| Fig. 2 (a) Terahertz spectra obtained from (top to bottom): a 1 : 1 mesaconic acid–phenazine cocrystal; mesaconic acid; phenazine. (b) Analysis (ex situ) of the extent of formation of the cocrystal from a mixture of phenazine and mesaconic acid, as a function of neat grinding time, as measured using the characteristic cocrystal band at 1.1 THz (red circles). The blue star, by comparison, shows close to 100% conversion in 15 minutes as a result of the presence of liquid in a LAG experiment. The blue square represents the percentage of a cocrystal sample that remained crystalline during dry grinding.56 | |
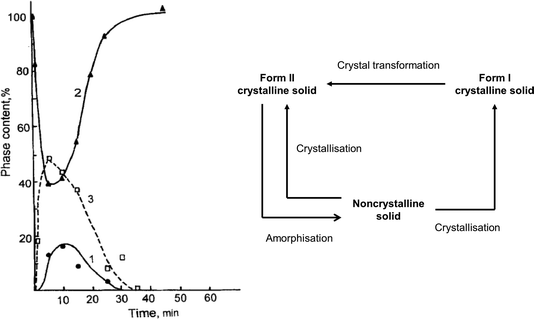 |
| Fig. 3 (a) Schematic of the different pathways occurring during the mechanochemical treatment of sulphathiaziole. Mechanical treatment resulted in the partial amorphisation of the drug and the interconversion of Forms I and II. (b) Time profile for the phase interconversion for sulphathiazole: 1 – indicating generation of Form I; 2 – initial loss and subsequent regeneration of Form III; 3 – the existence of a transient amorphous phase. Figure adapted from ref. 55. | |
A key factor for potential commercial exploitation in many instances would seem to be difficulties associated with scale-up. The progression from gram-scale, lab-based synthesis to kilogram-scale or higher outputs is an issue that has been addressed in many industrial applications3 and more recently in the area of pharmaceuticals, where twin extrusion approaches are employed (Fig. 4).57,58 Furthermore, it is noteworthy that frequently the generation of a new material (e.g. in the case of molecular crystals) at the gram level can allow, through subsequent seeding of a supersaturated solution, production via a conventional solvent-based crystallisation route.59
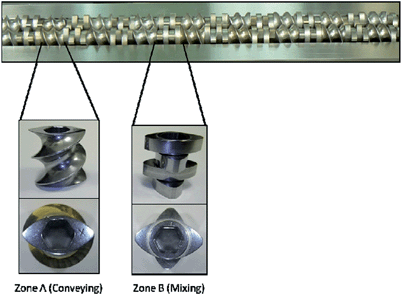 |
| Fig. 4 Typical twin extrusion configuration used for the formation of cocrystals. The conveying and mixing zones are shown. Typical output rate is approximately 0.5 g min−1. PXRD suggests comparable properties for the product to that obtained by conventional solution growth. The possibility of simultaneously blending the cocrystal product within a polymer matrix is an important additional advantage of the method. Reproduced from ref. 58. | |
It is also necessary to recognise the need for methods of structurally characterising the products of mechanochemical processes. When applied to chemical conversions which may occur, molecular identity can be established by conventional analytical methods (e.g. solution NMR and mass spectrometry). For solid–solid transformations, where the identity of crystalline intermediates or final states is required, major improvements in crystal structure determination from powder X-ray diffraction data (frequently coupled with solid-state NMR) have been pivotal.60–64 In addition, the structural analysis of individual crystallites that result from mechanochemical processes is possible through utilisation of the electron diffraction facility of the transmission electron microscope.65–67
3. Pharmaceutical materials
Within various areas of process engineering (including food science, chemicals and pharmaceuticals) particle size reduction is an important step.35,68 Approaches to size reduction include compression (e.g. rollers) or impact (e.g. impact mills and ball mills). All methods involve transfer of varying amounts of energy to the particle(s), and as a result other consequences can accompany the size reduction including the introduction of defects (such as dislocations, point defects and stacking faults)12 and in particular amorphisation69 or polymorphic transformations.70
The work of Descamps and others with regard to pharmaceutical materials has clearly demonstrated the critical role of the glass transition temperature, Tg, in determining whether or not a persistent amorphous phase will be generated.11,71–73 The combination of increased milling intensity and the absence of any plasticisers, which might lower Tg (either as liquid or vapour, e.g. water, from the surrounding atmosphere), will encourage the formation of an amorphous product (Fig. 5). The distinction between amorphous and crystalline products is particularly important for pharmaceutical applications given the inherent increased transient solubility (and hence improved bioavailability) associated with amorphous compared to crystalline solid forms.69,74–76
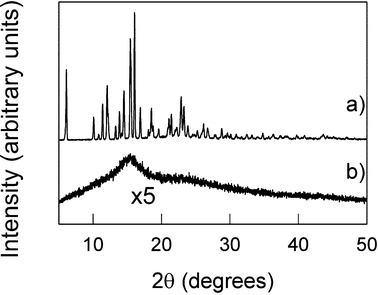 |
| Fig. 5 Demonstration of the amorphisation of trehelose as a result of milling at room temperature within a high energy planetary micro-mill. PXRD patterns (a) before and (b) after milling. Adapted from ref. 11. | |
Frequently, the amorphisation of a material can be a desirable outcome, especially as a means of increasing the transient solubility of the material or of eliminating compression issues with the crystalline equivalent.68 This attribute is frequently used in the pharmaceutical industry, and the complex nature of the resulting solids has been extensively studied (especially with regard to the instability of the amorphous form with respect to the crystalline state).74
Polymorphic transformations from the stable to a metastable form (again with increased apparent solubility) can also be a useful consequence of the application of mechanical energy (Fig. 6).13,77–79 Jet-milling, a particle-size reduction method frequently used within the pharmaceutical industry where particle–particle collisions occur within a carrier gas (typically air), has, for example, been found to induce polymorph interconversions along with the size reductions.
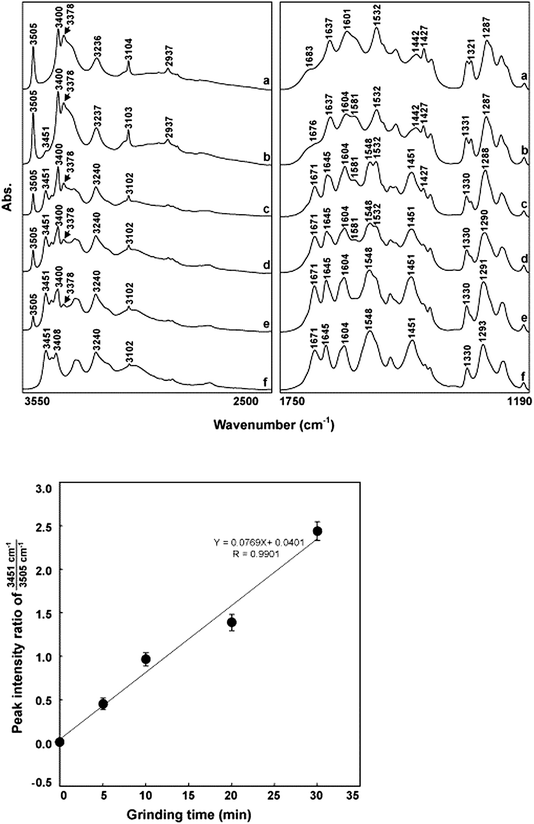 |
| Fig. 6 (a) Grinding-time dependence of the FTIR spectra for famotidine showing from top to bottom the emergence of the absorption at 3451 cm−1 which is used to monitor the formation of Form A of the material. (b) Plot of peak intensity ratio (I3451/I3505) for the spectra above indicating a possible zero order rate law for the conversion. Reproduced from ref. 78 with permission from Elsevier. | |
With grinding experiments, an approach for directing the polymorphic outcome through addition of a small amount of liquid was described by Trask et al. for the cases of anthranilic acid and succinic acid (see Fig. 7).80 For anthranilic acid, interconversion between the three known polymorphic forms occurred depending upon the choice of the added liquid.81 In the case of succinic acid, while the β-polymorph appeared stable to neat grinding, addition of a few drops of a low polarity liquid (e.g. hexane) caused conversion to the α-polymorph. More polar liquids (e.g. water, acetonitrile or methanol) appeared not to induce the transformation. The actual mechanism of the solid–solid transformation is an area of ongoing interest, especially where the change appears to be crystal–crystal in nature.82
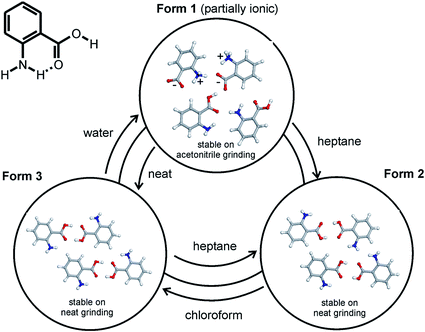 |
| Fig. 7 Schematic of the observed transformations between the three polymorphic forms of anthranilic acid with the pathways depending on the conditions under which mechanochemical treatment was performed. | |
An additional reason for interest in mechanochemical processes comes from the fact that the majority of drugs (80–90%) are formulated as tablets, which are seen as a reliable and general way of delivering medicines, and that the tableting process itself requires the application of significant applied pressures (see Fig. 8).55,83–90 Importantly, tablets contain other components (excipients), which are added to enhance performance e.g. lubricants, dissolving agents and so on. When pressure is applied during compression, there is a risk that surface–surface reactions between the components and drug, such as that between dicalcium phosphate dihydrate and aspirin, will lead to enhanced instability.91
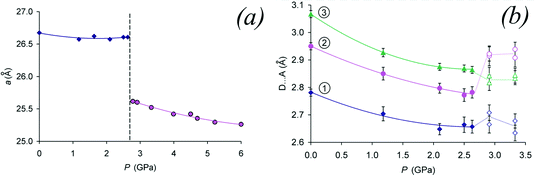 |
| Fig. 8 (a) Hydrostatic pressure induced transition for chlorpropamide in a saturated ethanol solution. The phase change results in a first order change at approximately 3.5 GPa. The change is illustrated for the a-axis. (b) Changes in non-hydrogen atom positions as the transition pressure is reached. Adapted from ref. 46. | |
More recent interest has focused on the formation of cocrystals (see Fig. 9) by milling or grinding.92–94 One of the earliest examples of a cocrystal obtained by grinding is that of quinhydrone reported in the work of Wohler published in 1844.95 The advantage for this system compared to solution crystallization was shown by Patil et al.,96 who demonstrated that simple grinding of mixtures was significantly superior to attempted solution growth, where self oxidation–reduction lowered the efficiency of complex formation. Subsequently, numerous examples of host–complex formation by solid–solid mixing were reported by Toda and co-workers.50 Work by Etter and colleagues also demonstrated how solid–solid grinding could be used to produce hydrogen bonded cocrystals of adenine and thymine derivatives, where a marked selectivity amongst various base pairs was observed.14 Hollingsworth et al.51 used grinding as a means of creating cocrystals of dinitriles and urea, as part of a wide study of inclusion crystals, in cases where solution growth was not possible. Pedireddi et al. demonstrated that in some cases a third component is necessary in order for a cocrystallisation reaction to take place97 and Kuroda and her colleagues studied the influence of the addition of a third component on the optical properties of the resulting cocrystals.98
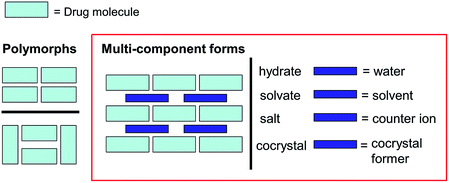 |
| Fig. 9 Schematic of the characteristics of polymorphs and multicomponent systems. Cocrystals represent a sub-set of the multicomponent group, frequently that characterised by the requirement that the components be neutral and solid at room temperature. | |
Frequently, the solid state properties of a pure drug can present processing or application problems e.g. particle flow, thermal stability, stability to moisture or ability to be compacted,68 and, increasingly, poor solubility is an issue.99,100 The challenge with a badly behaving drug, therefore, is to be able to control the properties of the molecule in the solid state without recourse to chemical modification by covalent chemistry. The use of multicomponent systems, especially pharmaceutical cocrystals, and more recently ionic cocrystals,101,102 has been demonstrated to be an effective strategy in such instances.103–112 In terms of property improvement with pharmaceutical cocrystals, various examples are known including: enhancing stability against hydration of an anhydrate form (see Fig. 10),113–116 developing forms with improved compression properties (for tablet formation) (see Fig. 11 and 12),117,118 significantly improving the bioavailability of poorly soluble drugs and controlled release strategies e.g. for insulin.119 Trask has reported on the potential intellectual property implications of pharmaceutical cocrystals,120 and recent interest has also focused on regulatory guidelines.121 In non-pharmaceutical applications, benefits of cocrystallisation have been shown for fluorescent materials37,122 and explosives.123,124
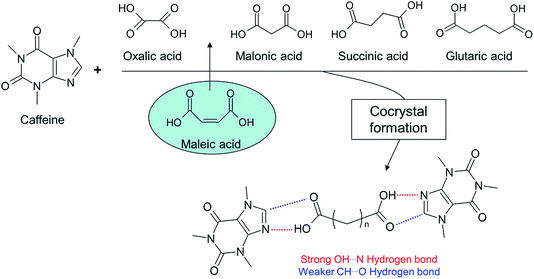 |
| Fig. 10 Supramolecular synthon approach used in the design of cocrystals of caffeine with various dicarboxylic acids. This approach lead to a variety of solid forms of caffeine which could be tested for stability under conditions of high humidity. Mechanochemistry was the technique used to both screen for cocrystal formation and also prepare powders for humidity assessment. | |
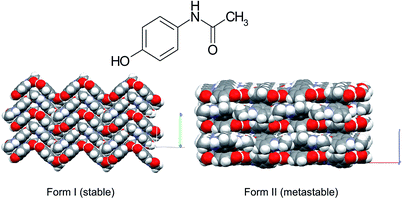 |
| Fig. 11 Packing diagrams for Form I and Form II paracetamol. Whilst Form I is the stable phase it does not compress well during tablet formation. The metastable Form II has a layered structure more appropriate for compression, but readily transforms to Form I. | |
 |
| Fig. 12 Micrographs of tablets made from (a) paracetamol Form I and (b to d) cocrystals of paracetamol with theophylline, naphthalene and oxalic acid respectively. Tablet (a) is poorly formed as a result of the inappropriate packing of the molecules in the lattice, whereas the cocrystal structures are amenable to deformation during tableting.118 | |
An early indication of the potential to form pharmaceutical cocrystals by grinding was provided by Caira et al. in 1995 who used grinding to prepare a series of crystalline products containing sulfonamide (similar to those obtained from solution) in a facile manner.125 They also reported detailed solid state kinetic data based on powder X-ray measurements and were able to show a distinct selectivity between reactants during grinding.
An ongoing challenge, however, is selecting possible coformers which might cocrystallise with the API rather than remain as a separate and distinct crystalline phase. Whilst various strategies have been developed to predict the likelihood of cocrystal formation,126–129 experimental screening for viable coformers remains vital (Fig. 13).130–132 While solution growth remains the ideal approach – especially with regard to scale-up in later stages of development – significant differences in solubility between the drug and coformer often presents difficulties.115,116,133–135 Mechanochemistry has emerged as a powerful tool in the experimental screening area, and has the advantage of minimising the need for large volumes of solvent (Fig. 14). It has also emerged as a powerful way of searching for different polymorphic forms of cocrystals135,136 as well as for various stoichiometric compositions e.g. 1
:
1, 1
:
2 and 2
:
1.59 Furthermore, mechanochemistry has been used to identify chiral agents suitable for converting a racemate into two distinct diastereomeric cocrystal phases137 as well as for coformer exchange.138
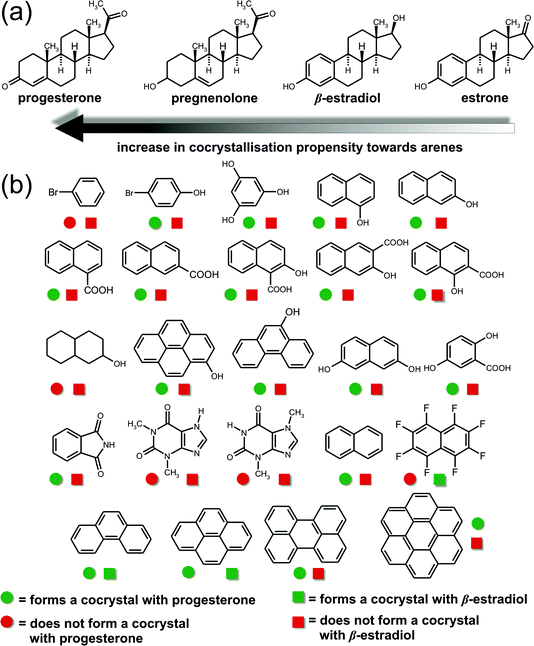 |
| Fig. 13 The use of mechanochemistry to explore the various possible combinations of coformers for a family of steroid drugs. The various outcomes are delineated by the accompanying legend. See ref. 161 for details. | |
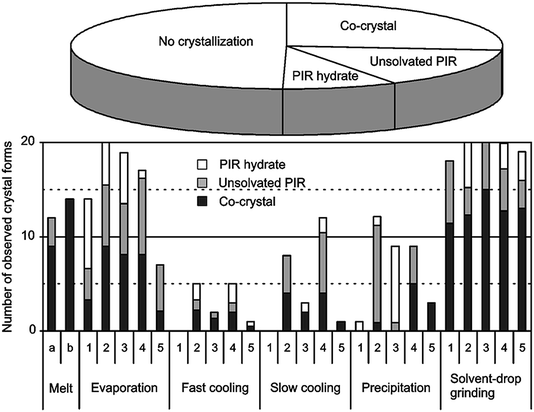 |
| Fig. 14 Overview of the results obtained by various cocrystallisation attempts for piroxicam. Solvent-drop grinding (LAG) is shown in this case to be the most efficient and straightforward screening method. The numbers 1–5 indicate the various solvents used in the experiments. Reproduced with permission from ref. 142. | |
An extension to simple (i.e. neat or dry) grinding of mixtures was reported by Shan et al. who added a few drops of liquid to the reaction mixture (liquid assisted grinding, LAG) (see Fig. 15).139 Initial attention focused on LAG as a means of accelerating cocrystallisation kinetics, although it was subsequently shown to provide a means of increasing product diversity. For example, the outcome of mechanochemical formation of cocrystals has been compared with that from using other approaches to cocrystallsation. Trask et al. reported three possible outcomes in the case of cocrystals with caffeine: (i) solution growth and milling produced identical products; (ii) a different stoichiometric product could be obtained by milling compared to solution; and (iii) different polymorphic forms were possible.59 Numerous recent publications have demonstrated that LAG is likely to be the most efficient way of screening for cocrystal formation.140–144 The key advantage of LAG over solution based approaches was interpreted by Davey,145,146 Childs147 and others148 on the basis of solution phase chemistry (Fig. 16). Other developments, such as ion- and liquid-assisted grinding (ILAG)149 and vapour digestion of solid mixtures,150,151 have also been reported.
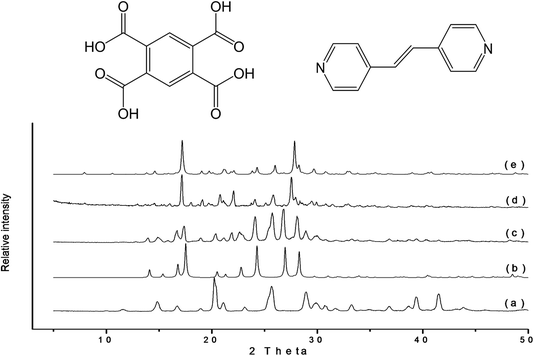 |
| Fig. 15 Demonstration of the enhanced kinetics of cocrystal formation upon addition of a few drops of methanol to the grinding jar. PXRD patterns for (a) tetracarboxylic acid, (b) bipyridyl, (c) outcome of neat grinding for 60 minutes (as the sum of the reactants), (d) the product obtained when a few drops of methanol are added and (e) the simulated pattern for the cocrystal. | |
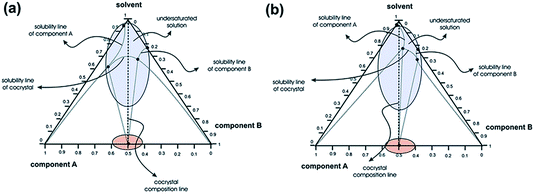 |
| Fig. 16 Typical three-component phase diagrams contrasting (a) congruent and (b) incongruent solubility profiles indicating the difficulties which will arise during conventional evaporative solution growth crystallisation. The fact that LAG experiments, with minimal amounts of solvent present, places the system close to the cocrystal composition illustrates the ability of LAG to form cocrystals when solids of very different solubility are investigated. Reproduced from ref. 34. | |
Further insight has been given by the recent work of Bučar et al. for the case of caffeine and benzoic acid.152 No cocrystals for this pair of molecules were known despite a strong expectation based on studies of analogous systems153 and despite extensive experimental efforts to generate such a species over many years. A systematic study using a large range of cocrystallisation methods including neat grinding, liquid assisted grinding, melt crystallization and various solution methods was employed (Fig. 17). All failed to produce the elusive cocrystal even though theoretical work had clearly confirmed a cocrystal was thermodynamically feasible. During grinding experiments, however, the addition of a few seed crystals of an isostructural fluorobenzoic acid cocrystal readily generated the elusive cocrystal, with the inference being that, as in solution crystallisation, a high barrier to nucleation of a particular form can frequently exist.
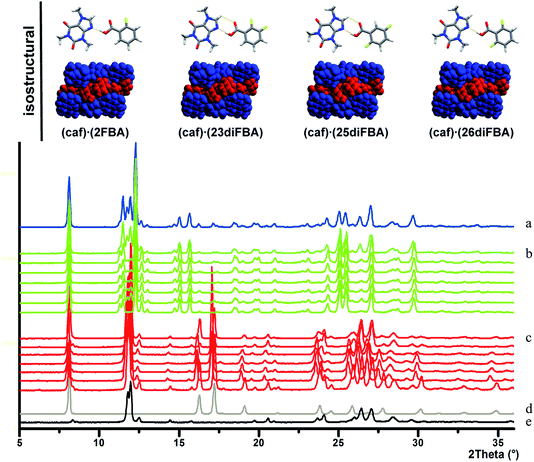 |
| Fig. 17 Top. Isostructural cocrystals of caffeine with various fluorobenzoic acids which have similar packing to that predicted for the likely structure of the caffeine–benzoic acid cocrystal. Bottom. PXRD traces of (a) product without deliberate addition of seeds, but prepared in an environment where the caffeine–benzoic acid cocrystal had previously been obtained, (b) products of various seeding reactions, (c) products of various unseeded reactions, (d) benzoic acid and (e) caffeine. The main points to note include the similarity of the patterns in (a) and (b) and that those in (c) are a simple combination of the starting materials in (d) and (e). See ref. 152 for more details. | |
Accompanying the recent developments in mechanochemistry has been the development of techniques to follow the course of solid–solid reactions using both ex situ and in situ approaches.56,122,154–158Ex situ analysis has frequently suggested that there may be distinct intermediates generated (Fig. 18).154,159 The application of synchrotron X-ray diffraction for in situ studies has indeed confirmed the presence of crystalline intermediates and a possible role of amorphous phases.157 To better understand the processes occurring during milling, several recent efforts have concerned developing methods to follow in situ the course of the reaction. Noteworthy has been the use of synchrotron X-ray diffraction. In a study of the milling of a mixture of carbamazepine and succinic acid, Halasz et al.157 showed that, in the absence of any added liquid, the outcome was the gradual disappearance of reflections of the two components and apparent amorphisation. A similar experiment, but now with the addition of 50 μL of acetonitrile showed that within 10 seconds of milling, reflections associated with a triclinic (cbz)(sac) cocrystal emerged. The reaction was complete within 4 minutes. Such rapidity was to be compared with other solid–solid grinding systems (e.g. the reaction of ZnO with imidazoles) where reaction timescales were much longer. Similar in situ studies were conducted for suberic acid and nicotinamide demonstrating that formation of a 2
:
1 cocrystal during grinding proceeds via a 1
:
1 cocrystal intermediate (see Fig. 19). Friščić and his colleagues have also used in situ methods, based on the changes in fluorescence122 accompanying cocrystallisation, to monitor directly events within the grinding jar.
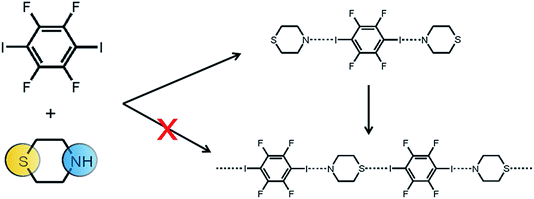 |
| Fig. 18 The discrete trimer units and extended polymer chains (based on halogen bond interactions) believed to be formed during the LAG reaction. When the strength of the halogen bond is weak the product is limited to the trimer unit. | |
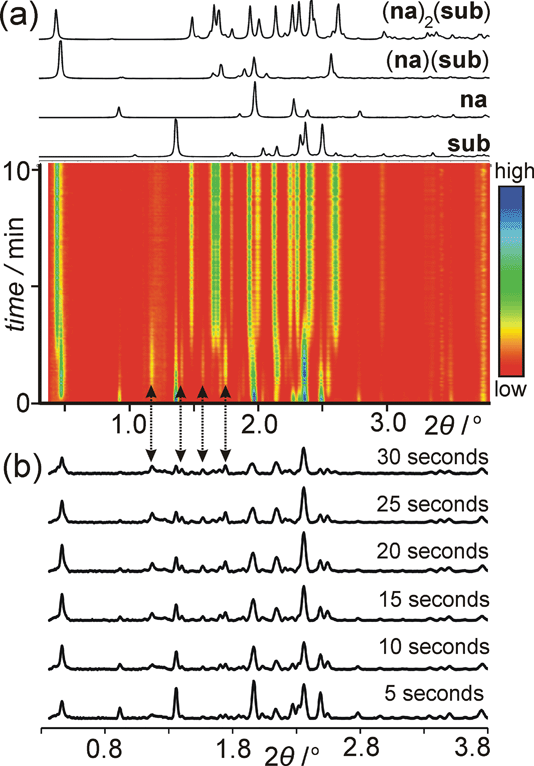 |
| Fig. 19
In situ synchrotron data for the milling of suberic acid and nicotinamide in a 2 : 1 ratio. (a) The PXRD patterns of the phases expected to be formed during milling along with the data obtained as a function of time. The inset shows the particular region at 0.4 to 0.5 degrees two-theta showing the initial formation of the 1 : 1 cocrystal followed by the 2 : 1 product. (b) The specific reflections indicated by arrows indicate the intermediate phase which is not detected by conventional ex situ studies. See ref. 157 for further details. | |
4. Concluding remarks
In the case of pharmaceutical solids, recent work using atomic force microscopy (AFM), terahertz spectroscopy and transmission electron microscopy (TEM) suggests that these techniques will be important for characterising mechanically treated samples and understanding changes which occur during such processing. For example, there are particle–particle effects – especially important during compression in a tablet containing various components. In the case of aspirin (acetylsalicylic acid) and dicalcium phosphate, when the excipient was attached to the tip of the AFM cantilever and different pressures and contact times under various humidity conditions there was evidence for enhanced reactivity induced by surface contact with excipient.91 Terahertz spectroscopy has emerged as a sensitive method of monitoring changes in the solid form during mechanical activation,56,156 whereas TEM has been shown to be a powerful technique for characterising the size and shape of particulates generated mechanochemically, as well as determining their crystal form (see Fig. 20). TEM can also be used to identify defects in pharmaceutical crystals (see Fig. 21), and will enable a greater understanding of how defects introduced into crystals during mechanical processing influence material properties – for example a link between defects in theophylline crystals and crystal fracture has been established.160
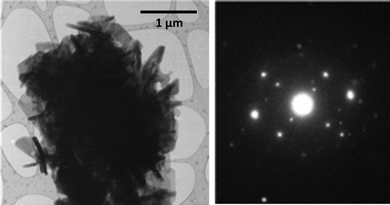 |
| Fig. 20 (a) Transmission electron micrograph and (b) electron diffraction pattern obtained from a sample of a theophylline:L-malic acid cocrystal prepared by LAG. The method is able to monitor particle size as well as polymorphic form.160 | |
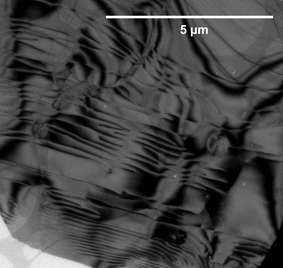 |
| Fig. 21 Extensive network of dislocations in a sample of theophylline as revealed by transmission electron microscopy. In general, such dislocations can result from the mechanical treatment of crystals. | |
Mechanochemistry, as mentioned at the outset, provides many opportunities for developing environmentally-friendly processes (especially the avoidance of large volumes of solvent). It also provides routes to materials not readily obtained by other methods. It has a long history and no doubt will continue to demonstrate useful applications whilst at the same time generate considerable activity in rationalising the mechanistic aspects behind the many types of transformations observed.
References
- L. Takacs, The mechanochemical reduction of AgCl with metals revisiting an experiment of M. Faraday, J. Therm. Anal. Calorim., 2007, 90, 81–84 CrossRef CAS.
- L. M. Takacs, Carey Lea, the father of mechanochemistry, Bull. Hist. Chem., 2003, 28, 26–34 CAS.
- V. V. Boldyrev, Mechanochemistry and mechanical activation of solids, Russ. Chem. Rev., 2006, 75, 177–189 CrossRef CAS PubMed.
-
P. Baláž, Mechanochemistry in Nanoscience and Minerals Engineering, Springer-Verlag, Berlin, Germany, 2008 Search PubMed.
- L. Takacs, The historical development of mechanochemistry, Chem. Soc. Rev., 2013, 42, 7649–7659 RSC.
- S. L. James, C. J. Adams, C. Bolm, D. Braga, P. Collier, T. Friščić, F. Grepioni, K. D. M. Harris, G. Hyett and W. Jones,
et al., Mechanochemistry: opportunities for new and cleaner synthesis, Chem. Soc. Rev., 2012, 41, 413–447 RSC.
-
A. D. McNaught and A. Wilkinson, IUPAC Compendium of Chemical Terminology, Blackwell Scientific Publications, Oxford, 1997 Search PubMed.
- G. Kaupp, Mechanochemistry: the varied applications of mechanical bond-breaking, CrystEngComm, 2009, 11, 388–403 RSC.
- V. V. Boldyrev, Mechanochemistry and sonochemistry, Ultrason. Sonochem., 1995, 2, S143–S145 CrossRef CAS.
- G. Cravotto, E. C. Gaudino and P. Cintas, On the mechanochemical activation by ultrasound, Chem. Soc. Rev., 2013, 42, 7521–7534 RSC.
- M. Descamps, J. F. Willart, E. Dudognon and V. Caron, Transformation of pharmaceutical compounds upon milling and comilling: The role of Tg, J. Pharm. Sci., 2007, 96, 1398–1407 CrossRef CAS PubMed.
- R. Hüttenrauch, S. Fricke and P. Zielke, Mechanical activation of pharmaceutical systems, Pharm. Res., 1985, 02, 302–306 CrossRef.
- H. Li, J. G. Stowell, X. He, K. R. Morris and S. R. Byrn, Investigations on solid–solid phase transformation of 5-methyl-2-[(4-methyl-2-nitrophenyl)amino]-3-thiophenecarbonitrile, J. Pharm. Sci., 2007, 96, 1079–1089 CrossRef CAS PubMed.
- M. C. Etter, S. M. Reutzel and C. G. Choo, Self-organization of adenine and thymine in the solid state, J. Am. Chem. Soc., 1993, 115, 4411–4412 CrossRef CAS.
- G. W. V. Cave, C. L. Raston and J. L. Scott, Recent advances in solventless organic reactions: towards benign synthesis with remarkable versatility, Chem. Commun., 2001, 2159–2169 RSC.
- A. S. Cannon and J. C. Warner, Noncovalent derivatization: green chemistry applications of crystal engineering, Cryst. Growth Des., 2002, 2, 255–257 CAS.
- K. Ralphs, C. Hardacre and S. L. James, Application of heterogeneous catalysts prepared by mechanochemical synthesis, Chem. Soc. Rev., 2013, 42, 7701–7718 RSC.
- F. K. Urakaev and V. V. Boldyrev, Theoretical estimation of conditions for the preparation of nanoscale systems in mechanochemical reactors, Russ. J. Phys. Chem., 2005, 79, 563–572 CAS.
- V. K. Smolyakov, O. V. Lapshin and V. V. Boldyrev, Mechanochemical synthesis of nanosize products in heterogeneous systems: macroscopic kinetics, Int. J. Self-Propag. High-Temp. Synth., 2008, 17, 20–29 CrossRef CAS.
- P. Baláž, M. Achimovičová, M. Baláž, P. Billik, Z. Cherkezova-Zheleva, J. M. Criado, F. Delogu, E. Dutková, E. Gaffet and F. J. Gotor,
et al., Hallmarks of mechanochemistry: from nanoparticles to technology, Chem. Soc. Rev., 2013, 42, 7571–7637 RSC.
- M. C. Etter, G. M. Frankenbach and J. Bernstein, Solid-state nucleophilic aromatic substitution reaction of a carboxylic acid cocrystal, Tetrahedron Lett., 1989, 30, 3617–3620 CrossRef CAS.
- M. A. Mikhailenko, T. P. Shakhtshneider and V. V. Boldyrev, On the mechanism of mechanochemical synthesis of phthalylsulphathiazole, J. Mater. Sci., 2004, 39, 5435–5439 CrossRef CAS.
- R. Trotzki, M. M. Hoffmann and B. Ondruschka, Studies on the solvent-free and waste-free Knoevenagel condensation, Green Chem., 2008, 10, 767–772 RSC.
- A. Bruckmann, A. Krebs and C. Bolm, Organocatalytic reactions: effects of ball milling, microwave and ultrasound irradiation, Green Chem., 2008, 10, 1131–1141 RSC.
- V. Štrukil, M. D. Igrc, L. Fábián, M. Eckert-Maksic, S. L. Childs, D. G. Reid, M. J. Duer, I. Halasz, C. Mottillo and T. Friščić, A model for a solvent-free synthetic organic research laboratory: click-mechanosynthesis and structural characterization of thioureas without bulk solvents, Green Chem., 2012, 14, 2462–2473 RSC.
- J. Stojaković, B. S. Farris and L. R. MacGillivray, Vortex grinding for mechanochemistry: application for automated supramolecular catalysis and preparation of a metal–organic framework, Chem. Commun., 2012, 48, 7958–7960 RSC.
- G.-W. Wang, Mechanochemical organic synthesis, Chem. Soc. Rev., 2013, 42, 7668–7700 RSC.
- T. Friščić, Supramolecular concepts and new techniques in mechanochemistry: cocrystals, cages, rotaxanes, open metal–organic frameworks, Chem. Soc. Rev., 2012, 41, 3493–3510 RSC.
- V. Šepelák, A. Düvel, M. Wilkening, K.-D. Becker and P. Heitjans, Mechanochemical reactions and syntheses of oxides, Chem. Soc. Rev., 2013, 42, 7507–7520 RSC.
- S. M. Hick, C. Griebel, D. T. Restrepo, J. H. Truitt, E. J. Buker, C. Bylda and R. G. Blair, Mechanocatalysis for biomass-derived chemicals and fuels, Green Chem., 2010, 12, 468–474 RSC.
- T. Kleine, J. Buendia and C. Bolm, Mechanochemical degradation of lignin and wood by solvent-free grinding in a reactive medium, Green Chem., 2013, 15, 160–166 RSC.
-
D. Braga, D. D'Addario, L. Maini, M. Polito, S. Giaffreda, K. Rubini, F. Grepioni. Applications of Crystal Engineering Strategies in Solvent-free Reactions: Toward a Supramolecular Green Chemistry, in Frontiers in Crystal Engineering, ed. E. R. T. Tiekink and J. J. Vittal, John Wiley & Sons, Ltd, Chichester, UK, 2006 Search PubMed.
- A. M. Belenguer, T. Friščić, G. M. Day and J. K. M. Sanders, Solid-state dynamic combinatorial chemistry: reversibility and thermodynamic product selection in covalent mechanosynthesis, Chem. Sci., 2011, 2, 696–700 RSC.
- A. Delori, T. Friščić and W. Jones, The role of mechanochemistry and supramolecular design in the development of pharmaceutical materials, CrystEngComm, 2012, 14, 2350–2362 RSC.
- C. F. Burmeister and A. Kwade, Process engineering with planetary ball mills, Chem. Soc. Rev., 2013, 42, 7660–7667 RSC.
- D.-K. Bučar, S. Filip, M. Arhangelskis, G. O. Lloyd and W. Jones, Advantages of mechanochemical cocrystallisation in the solid-state chemistry of pigments: colour-tuned fluorescein cocrystals, CrystEngComm, 2013, 15, 6289–6291 RSC.
- D. Yan, A. Delori, G. O. Lloyd, T. Friščić, G. M. Day, W. Jones, J. Lu, M. Wei, D. G. Evans and X. Duan, A cocrystal strategy to tune the luminescent properties of stilbene-type organic solid-state materials, Angew. Chem., Int. Ed., 2011, 50, 12483–12486 CrossRef CAS PubMed.
- N. G. Shang, P. Papakonstantinou, S. Sharma, G. Lubarsky, M. Li, D. W. McNeill, A. J. Quinn, W. Zhou and R. Blackley, Controllable selective exfoliation of high-quality graphene nanosheets and nanodots by ionic liquid assisted grinding, Chem. Commun., 2012, 48, 1877–1879 RSC.
- S.-E. Zhu, F. Li and G.-W. Wang, Mechanochemistry of fullerenes and related materials, Chem. Soc. Rev., 2013, 42, 7535–7570 RSC.
- N. Blagden, D. J. Berry, A. Parkin, H. Javed, A. Ibrahim, P. T. Gavan, L. L. De Matos and C. C. Seaton, Current directions in co-crystal growth, New J. Chem., 2008, 32, 1659–1672 RSC.
- P. Baláž and E. Dutková, Fine milling in applied mechanochemistry, Miner. Eng., 2009, 22, 681–694 CrossRef PubMed.
- X. Guo, D. Xiang, G. Duan and P. Mou, A review of mechanochemistry applications in waste management, Waste Manage., 2010, 30, 4–10 CrossRef PubMed.
- A. L. Black, J. M. Lenhardt and S. L. Craig, From molecular mechanochemistry to stress-responsive materials, J. Mater. Chem., 2011, 21, 1655–1663 RSC.
-
E. V. Boldyreva, Mechanochemistry of organic solids: where are we now?, VI International Conference on Mechanochemistry and Mechanical Alloying (INCOME 2008), NMLJamshedpur, India, 2011, pp. 17–30 Search PubMed.
- A. Nasser and U. Mingelgrin, Mechanochemistry: A review of surface reactions and environmental applications, Appl. Clay Sci., 2012, 67–68, 141–150 CrossRef CAS PubMed.
- E. Boldyreva, Mechanochemistry of inorganic and organic systems: what is similar, what is different?, Chem. Soc. Rev., 2013, 42, 7719–7738 RSC.
- G. Majano, L. Borchardt, S. Mitchell, V. Valtchev and J. Perez-Ramirez, Rediscovering zeolite mechanochemistry – A pathway beyond current synthesis and modification boundaries, Microporous Mesoporous Mater., 2014, 194, 106–114 CrossRef CAS PubMed.
- V. V. Boldyrev and K. Tkáčová, Mechanochemistry of solids: past, present, and prospects, J. Mater. Synth. Process., 2000, 8, 121–132 CrossRef CAS.
- V. V. Boldyrev, Mechanochemical modification and synthesis of drugs, J. Mater. Sci., 2004, 39, 5117–5120 CrossRef CAS.
- F. Toda, K. Tanaka and A. Sekikawa, Host–guest complex formation by a solid–solid reaction, J. Chem. Soc., Chem. Commun., 1987, 279–280 RSC.
- M. D. Hollingsworth, B. D. Santarsiero, H. Oumar-Mahamat and C. J. Nichols, New series of 1
:
1 layered complexes of α,ω-dinitriles and urea, Chem. Mater., 1991, 3, 23–25 CrossRef CAS.
- T. Friščić and W. Jones, Recent advances in understanding the mechanism of cocrystal formation via grinding, Cryst. Growth Des., 2009, 9, 1621–1637 Search PubMed.
- V. V. Boldyrev, Hydrothermal reactions under mechanochemical action, Powder Technol., 2002, 122, 247–254 CrossRef CAS.
- A. A. L. Michalchuk, I. A. Tumanov and E. V. Boldyreva, Complexities of mechanochemistry: elucidation of processes occurring in mechanical activators via implementation of a simple organic system, CrystEngComm, 2013, 15, 6403–6412 RSC.
- T. P. Shakhtshneider, Phase transformations and stabilization of metastable states of molecular crystals under mechanical activation, Solid State Ionics, 1997, 101–103, 851–856 CrossRef CAS.
- K. L. Nguyen, T. Friščić, G. M. Day, L. F. Gladden and W. Jones, Terahertz time-domain spectroscopy and the quantitative monitoring of mechanochemical cocrystal formation, Nat. Mater., 2007, 6, 206–209 CrossRef CAS PubMed.
- C. Medina, D. Daurio, K. Nagapudi and F. Alvarez-Nunez, Manufacture of pharmaceutical co-crystals using twin screw extrusion: a solvent-less and scalable process, J. Pharm. Sci., 2010, 99, 1693–1696 CAS.
- D. Daurio, K. Nagapudi, L. Li, P. Quan and F. Alvarez-Nunez, Application of twin screw extrusion to the manufacture of cocrystals: scale-up of AMG 517–sorbic acid cocrystal production, Faraday Discuss., 2014 10.1039/C3FD00153A.
- A. V. Trask, J. van de Streek, W. D. S. Motherwell and W. Jones, Achieving polymorphic and S. diversity in cocrystal formation: Importance of solid-state grinding, powder X-ray structure determination, and seeding, Cryst. Growth Des., 2005, 5, 2233–2241 CAS.
- E. Y. Cheung, S. J. Kitchin, K. D. M. Harris, Y. Imai, N. Tajima and R. Kuroda, Direct Structure determination of a multicomponent molecular crystal prepared by a solid-state grinding procedure, J. Am. Chem. Soc., 2003, 125, 14658–14659 CrossRef CAS PubMed.
- S. Karki, L. Fábián, T. Friščić and W. Jones, Powder X-ray diffraction as an emerging method to structurally characterize organic solids, Org. Lett., 2007, 9, 3133–3136 CrossRef CAS PubMed.
- E. Salager, G. M. Day, R. S. Stein, C. J. Pickard, B. Elena and L. Emsley, Powder crystallography by combined crystal structure prediction and high-resolution 1H solid-state NMR spectroscopy, J. Am. Chem. Soc., 2010, 132, 2564–2566 CrossRef CAS PubMed.
- K. D. M. Harris, Powder diffraction crystallography of molecular solids, Top. Curr. Chem., 2012, 315, 133–178 CrossRef CAS.
- M. Arhangelskis, G. O. Lloyd and W. Jones, Mechanochemical synthesis of pyrazine:dicarboxylic acid cocrystals and a study of dissociation by quantitative phase analysis, CrystEngComm, 2012, 14, 5203–5208 RSC.
- U. Kolb, T. E. Gorelik, E. Mugnaioli and A. Stewart, Structural characterization of organics using manual and automated electron diffraction, Polym. Rev., 2010, 50, 385–409 CrossRef CAS.
- M. D. Eddleston, K. E. Hejczyk, E. G. Bithell, G. M. Day and W. Jones, Polymorph identification and crystal structure determination by a combined crystal structure prediction and transmission electron microscopy approach, Chem.–Eur. J., 2013, 19, 7874–7882 CrossRef CAS PubMed.
- M. D. Eddleston, K. E. Hejczyk, E. G. Bithell, G. M. Day and W. Jones, Determination of the crystal structure of a new polymorph of theophylline, Chem.–Eur. J., 2013, 19, 7883–7888 CrossRef CAS PubMed.
-
Y. Qiu, Y. Chen and G. G. Z. Zhang, Developing solid oral dosage forms: Pharmaceutical theory and practise, Academic Press, USA, 2009, p. 943 Search PubMed.
- N. S. Trasi and S. R. Byrn, Mechanically induced amorphization of drugs: A study of the thermal behavior of cryomilled compounds, AAPS PharmSciTech, 2012, 13, 772–784 CrossRef CAS PubMed.
- G. Bruni, V. Berbenni, F. Sartor, C. Milanese, A. Girella, D. Franchi and A. Marini, Quantification methods of amorphous/crystalline fractions in high-energy ball milled pharmaceutical products, J. Therm. Anal. Calorim., 2012, 108, 235–241 CrossRef CAS.
- S. Desprez and M. Descamps, Transformations of glassy indomethacin induced by ball-milling, J. Non-Cryst. Solids, 2006, 352, 4480–4485 CrossRef CAS PubMed.
- J. F. Willart, N. Descamps, V. Caron, F. Capet, F. Danede and M. Descamps, Formation of lactose–mannitol molecular alloys by solid state vitrification, Solid State Commun., 2006, 138, 194–199 CrossRef CAS PubMed.
- V. Caron, J. F. Willart, F. Danede and M. Descamps, The implication of the glass transition in the formation of trehalose/mannitol molecular alloys by ball milling, Solid State Commun., 2007, 144, 288–292 CrossRef CAS PubMed.
- B. C. Hancock and M. Parks, What is the true solubility advantage for amorphous pharmaceuticals?, Pharm. Res., 2000, 17, 397–404 CrossRef CAS.
- S. B. Murdande, M. J. Pikal, R. M. Shanker and R. H. Bogner, Solubility advantage of amorphous pharmaceuticals: I. A thermodynamic analysis, J. Pharm. Sci., 2010, 99, 1254–1264 CrossRef CAS PubMed.
- A. Newman, G. Knipp and G. Zografi, Assessing the performance of amorphous solid dispersions, J. Pharm. Sci., 2012, 101, 1355–1377 CrossRef CAS PubMed.
- M. Pudipeddi and A. T. M. Serajuddin, Trends in solubility of polymorphs, J. Pharm. Sci., 2005, 94, 929–939 CrossRef CAS PubMed.
- S.-Y. Lin, W.-T. Cheng and S.-L. Wang, Thermodynamic and kinetic characterization of polymorphic transformation of famotidine during grinding, Int. J. Pharm., 2006, 318, 86–91 CrossRef CAS PubMed.
- P. L. D. Wildfong, K. R. Morris, C. A. Anderson and S. M. Short, Demonstration of a shear-based solid-state phase transformation in a small molecular organic system: chlorpropamide, J. Pharm. Sci., 2007, 96, 1100–1113 CrossRef CAS PubMed.
- A. V. Trask, N. Shan, W. D. S. Motherwell, W. Jones, S. Feng, R. B. H. Tan and K. J. Carpenter, Selective polymorph transformation via solvent-drop grinding, Chem. Commun., 2005, 880–882 RSC.
-
N. Madusanka, Solid state transformations in single-component and multi-component pharmaceutical crystals, M.Phil thesis, University of Cambridge, UK, 2012.
- I. Halasz, Single-crystal-to-single-crystal reactivity: Gray, rather than black or white, Cryst. Growth Des., 2010, 10, 2817–2823 CAS.
- H. G. Brittain, Effects of mechanical processing on phase composition, J. Pharm. Sci., 2002, 91, 1573–1580 CrossRef CAS PubMed.
- E. V. Boldyreva, V. Dmitriev and B. C. Hancock, Effect of pressure up to 5.5 GPa on dry powder samples of chlorpropamide form-A, Int. J. Pharm., 2006, 327, 51–57 CrossRef CAS PubMed.
- I. D. H. Oswald, I. Chataigner, S. Elphick, F. P. A. Fabbiani, A. R. Lennie, J. Maddaluno, W. G. Marshall, T. J. Prior, C. R. Pulham and R. I. Smith, Putting pressure on elusive polymorphs and solvates, CrystEngComm, 2009, 11, 359–366 RSC.
- M. D. Moore, A. M. Steinbach, I. S. Buckner and P. L. D. Wildfong, A structural investigation into the compaction behavior of pharmaceutical composites using powder X-ray diffraction and total scattering analysis, Pharm. Res., 2009, 26, 2429–2437 CrossRef CAS PubMed.
- I. S. Buckner, R. A. Friedman and D. E. Wurster, Using compression calorimetry to characterize powder compaction behavior of pharmaceutical materials, J. Pharm. Sci., 2010, 99, 861–870 CAS.
- V. Mazel, C. Delplace, V. Busignies, V. Faivre, P. Tchoreloff and N. Yagoubi, Polymorphic transformation of anhydrous caffeine under compression and grinding: a re-evaluation, Drug Dev. Ind. Pharm., 2011, 37, 832–840 CrossRef CAS PubMed.
- R. Roopwani and I. S. Buckner, Understanding deformation mechanisms during powder compaction using principal component analysis of compression data, Int. J. Pharm., 2011, 418, 227–234 CrossRef CAS PubMed.
- N. P. A. Christensen, C. Cornett and J. Rantanen, Role of excipients on solid-state properties of piroxicam during processing, J. Pharm. Sci., 2012, 101, 1202–1211 CrossRef CAS PubMed.
- A. M. Cassidy, C. E. Gardner, T. Auffret, B. Aldous and W. Jones, Decoupling the effects of surface chemistry and humidity on solid-state hydrolysis of aspirin in the presence of dicalcium phosphate dihydrate, J. Pharm. Sci., 2012, 101, 1496–1507 CrossRef CAS PubMed.
- D. Braga and F. Grepioni, Solventless reactions: Reactions between or within molecular crystals, Angew. Chem., Int. Ed., 2004, 43, 4002–4011 CrossRef CAS PubMed.
- A. V. Trask and W. Jones, Crystal engineering of organic cocrystals by the solid-state grinding approach, Top. Curr. Chem., 2005, 254, 41–70 CAS.
- D. Braga, L. Maini and F. Grepioni, Mechanochemical preparation of co-crystals, Chem. Soc. Rev., 2013, 42, 7638–7648 RSC.
- F. Wöhler, Untersuchungen über das Chinon, Justus Liebigs Ann. Chem., 1844, 51, 145–163 CrossRef.
- A. O. Patil, D. Y. Curtin and I. C. Paul, Solid-state formation of quinhydrones from their components. Use of solid–solid reactions to prepare compounds not accessible from solution, J. Am. Chem. Soc., 1984, 106, 348–353 CrossRef CAS.
- V. R. Pedireddi, W. Jones, A. P. Chorlton and R. Docherty, Creation of crystalline supramolecular arrays: a comparison of co-crystal formation from solution and by solid-state grinding, Chem. Commun., 1996, 987–988 RSC.
- R. Kuroda, Y. Imai and N. Tajima, Generation of a co-crystal phase
with novel coloristic properties via solid state grinding procedures, Chem. Commun., 2002, 2848–2849 RSC.
- N. Blagden, M. de Matas, P. T. Gavan and P. York, Crystal engineering of active pharmaceutical ingredients to improve solubility and dissolution rates, Adv. Drug Delivery Rev., 2007, 59, 617–630 CrossRef CAS PubMed.
- S. J. Bethune, N. Schultheiss and J.-O. Henck, Improving the poor aqueous solubility of nutraceutical compound pterostilbene through cocrystal formation, Cryst. Growth Des., 2011, 11, 2817–2823 CAS.
- D. Braga, F. Grepioni, L. Maini, S. Prosperi, R. Gobetto and M. R. Chierotti, From unexpected reactions to a new family of ionic co-crystals: the case of barbituric acid with alkali bromides and cesium iodide, Chem. Commun., 2010, 46, 7715–7717 RSC.
- D. Braga, F. Grepioni, G. I. Lampronti, L. Maini and A. Turrina, Ionic co-crystals of organic molecules with metal halides: A new prospect in the solid formulation of active pharmaceutical ingredients, Cryst. Growth Des., 2011, 11, 5621–5627 CAS.
- O. Almarsson and M. J. Zaworotko, Crystal engineering of the composition of pharmaceutical phases. Do pharmaceutical co-crystals represent a new path to improved medicines?, Chem. Commun., 2004, 1889–1896 RSC.
- G. P. Stahly, Diversity in single- and multiple-component crystals. The search for and prevalence of polymorphs and cocrystals, Cryst. Growth Des., 2007, 7, 1007–1026 CAS.
- N. Shan and M. J. Zaworotko, The role of cocrystals in pharmaceutical science, Drug Discovery Today, 2008, 13, 440–446 CrossRef CAS PubMed.
- N. A. Meanwell, The emerging utility of co-crystals in drug discovery and development, Annu. Rep. Med. Chem., 2008, 43, 373–404 CAS.
- G. P. Stahly, A Survey of Cocrystals Reported Prior to 2000, Cryst. Growth Des., 2009, 9, 4212–4229 CAS.
- T. Friščić and W. Jones, Benefits of cocrystallization in pharmaceutical materials science: an update, J. Pharm. Pharmacol., 2010, 62, 1547–1559 CrossRef PubMed.
- N. Qiao, M. Li, W. Schlindwein, N. Malek, A. Davies and G. Trappitt, Pharmaceutical cocrystals: An overview, Int. J. Pharm., 2011, 419, 1–11 CrossRef CAS PubMed.
- H. G. Brittain, Pharmaceutical cocrystals: The coming wave of new drug substances, J. Pharm. Sci., 2013, 102, 311–317 CrossRef CAS PubMed.
- R. Thakuria, A. Delori, W. Jones, M. P. Lipert, L. Roy and N. Rodríguez-Hornedo, Pharmaceutical cocrystals and poorly soluble drugs, Int. J. Pharm., 2013, 453, 101–125 CrossRef CAS PubMed.
- J. W. Steed, The role of co-crystals in pharmaceutical design, Trends Pharmacol. Sci., 2013, 34, 185–193 CrossRef CAS PubMed.
- A. V. Trask, W. D. S. Motherwell and W. Jones, Pharmaceutical cocrystallization: Engineering a remedy for caffeine hydration, Cryst. Growth Des., 2005, 5, 1013–1021 CAS.
- A. V. Trask, W. D. S. Motherwell and W. Jones, Physical stability enhancement of theophylline via cocrystallization, Int. J. Pharm., 2006, 320, 114–123 CrossRef CAS PubMed.
- M. D. Eddleston, R. Thakuria, B. J. Aldous and W. Jones, An investigation of the causes of cocrystal dissociation at high humidity, J. Pharm. Sci., 2014, 103, 2859–2864 CrossRef CAS PubMed.
- M. D. Eddleston, N. Madusanka and W. Jones, Cocrystal dissociation in the presence of water: A general approach for identifying stable cocrystal forms, J. Pharm. Sci., 2014, 103, 2865–2870 CrossRef CAS PubMed.
- C. C. Sun and H. Hou, Improving mechanical properties of caffeine and methyl gallate crystals by cocrystallization, Cryst. Growth Des., 2008, 8, 1575–1579 CAS.
- S. Karki, T. Friščić, L. Fábián, P. R. Laity, G. M. Day and W. Jones, Improving mechanical properties of crystalline solids by cocrystal formation: new compressible forms of paracetamol, Adv. Mater., 2009, 21, 3905–3909 CrossRef CAS.
- M. L. Brader, M. Sukumar, A. H. Pekar, D. S. McClellan, R. E. Chance, D. B. Flora, A. L. Cox, L. Irwin and S. R. Myers, Hybrid insulin cocrystals for controlled release delivery, Nat. Biotechnol., 2002, 20, 800–804 CrossRef CAS PubMed.
- A. V. Trask, An overview of pharmaceutical cocrystals as intellectual property, Mol. Pharmaceutics, 2007, 4, 301–309 CrossRef CAS PubMed.
- S. Aitipamula, R. Banerjee, A. K. Bansal, K. Biradha, M. L. Cheney, A. R. Choudhury, G. R. Desiraju, A. G. Dikundwar, R. Dubey and N. Duggirala,
et al., Polymorphs, salts, and cocrystals: What's in a name?, Cryst. Growth Des., 2012, 12, 2147–2152 CAS.
- M. Frenette, G. Cosa and T. Friščić, Characterisation of organic solid forms and real-time in situ monitoring of their transformations using solid-state fluorescence, CrystEngComm, 2013, 15, 5100–5106 RSC.
- K. B. Landenberger and A. J. Matzger, Cocrystal engineering of a prototype energetic material: supramolecular chemistry of 2,4,6-trinitrotoluene, Cryst. Growth Des., 2010, 10, 5341–5347 CAS.
- J. P. Shen, X. H. Duan, Q. P. Luo, Y. Zhou, Q. Bao, Y. J. Ma and C. H. Pei, Preparation and characterization of a novel cocrystal explosive, Cryst. Growth Des., 2011, 11, 1759–1765 CAS.
- M. R. Caira, L. R. Nassimbeni and A. F. Wildervanck, Selective formation of hydrogen bonded cocrystals between a sulfonamide and aromatic carboxylic acids in the solid state, J. Chem. Soc., Perkin Trans. 2, 1995, 2213–2216 RSC.
- L. Fábián, Cambridge Structural Database Analysis of Molecular Complementarity in Cocrystals, Cryst. Growth Des., 2009, 9, 1436–1443 Search PubMed.
- D. Musumeci, C. A. Hunter, R. Prohens, S. Scuderi and J. F. McCabe, Virtual cocrystal screening, Chem. Sci., 2011, 2, 883–890 RSC.
- G. Springuel, B. Norberg, K. Robeyns, J. Wouters and T. Leyssens, Advances in pharmaceutical co-crystal screening: effective co-crystal screening through structural resemblance, Cryst. Growth Des., 2012, 12, 475–484 CAS.
- P. A. Wood, N. Feeder, M. Furlow, P. T. A. Galek, C. R. Groom and E. Pidcock, Knowledge-based approaches to co-crystal design, CrystEngComm, 2014, 16, 5839–5848 RSC.
- V. André, D. Braga, F. Grepioni and M. T. Duarte, Crystal forms of the antibiotic 4-aminosalicylic acid: solvates and molecular salts with dioxane, morpholine, and piperazine, Cryst. Growth Des., 2009, 9, 5108–5116 Search PubMed.
- S. R. Bysouth, J. A. Bis and D. Igo, Cocrystallization via planetary milling: Enhancing throughput of solid-state screening methods, Int. J. Pharm., 2011, 411, 169–171 CrossRef CAS PubMed.
- H. Abourahma, J. M. Urban, N. Morozowich and B. Chan, Examining the robustness of a theophylline cocrystal during grinding with additives, CrystEngComm, 2012, 14, 6163–6169 RSC.
- E. Gagnière, D. Mangin, F. Puel, A. Rivoire, O. Monnier, E. Garcia and J. P. Klein, Formation of co-crystals: kinetic and thermodynamic aspects, J. Cryst. Growth, 2009, 311, 2689–2695 CrossRef PubMed.
- E. Gagnière, D. Mangin, S. Veesler and F. Puel, Co-crystallization in solution and scale-up issues, RSC Drug Discovery Ser., 2012, 16, 188–211 Search PubMed.
- M. D. Eddleston, S. Sivachelvam and W. Jones, Screening for polymorphs of cocrystals: a case study, CrystEngComm, 2013, 15, 175–181 RSC.
- N. Madusanka, M. D. Eddleston, M. Arhangelskis and W. Jones, Polymorphs, hydrates and solvates of a co-crystal of caffeine with anthranilic acid, Acta Crystallogr., Sect. B: Struct. Sci., Cryst.
Eng. Mater., 2014, 70, 72–80 CAS.
- M. D. Eddleston, M. Arhangelskis, T. Friščić and W. Jones, Solid state grinding as a tool to aid enantiomeric resolution by cocrystallisation, Chem. Commun., 2012, 48, 11340–11342 RSC.
- D. Braga, F. Grepioni and G. I. Lampronti, Supramolecular metathesis: co-former exchange in co-crystals of pyrazine with (R,R)-, (S,S)-, (R,S)- and (S,S/R,R)-tartaric acid, CrystEngComm, 2011, 13, 3122–3124 RSC.
- N. Shan, F. Toda and W. Jones, Mechanochemistry and co-crystal formation: effect of solvent on reaction kinetics, Chem. Commun., 2002, 2372–2373 RSC.
- S. A. Myz, T. P. Shakhtshneider, K. Fucke, A. P. Fedotov, E. V. Boldyreva, V. V. Boldyrev and N. I. Kuleshova, Synthesis of co-crystals of meloxicam with carboxylic acids by grinding, Mendeleev Commun., 2009, 19, 272–274 CrossRef CAS PubMed.
- C. B. Aakeröy, A. B. Grommet and J. Desper, Co-crystal screening of diclofenac, Pharmaceutics, 2011, 3, 601–614 CrossRef PubMed.
- K. Fucke, S. A. Myz, T. P. Shakhtshneider, E. V. Boldyreva and U. J. Griesser, How good are the crystallisation methods for co-crystals? A comparative study of piroxicam, New J. Chem., 2012, 36, 1969–1977 RSC.
- K. Yamamoto, S. Tsutsumi and Y. Ikeda, Establishment of cocrystal cocktail grinding method for rational screening of pharmaceutical cocrystals, Int. J. Pharm., 2012, 437, 162–171 CrossRef CAS PubMed.
- H.-L. Lin, T.-K. Wu and S.-Y. Lin, Screening and characterization of cocrystal formation of metaxalone with short-chain dicarboxylic acids induced by solvent-assisted grinding approach, Thermochim. Acta, 2014, 575, 313–321 CrossRef CAS PubMed.
- R. A. Chiarella, R. J. Davey and M. L. Peterson, Making co-crystals – The utility of ternary phase diagrams, Cryst. Growth Des., 2007, 7, 1223–1226 CAS.
- K. Chadwick, R. Davey, G. Sadiq, W. Cross and R. Pritchard, The utility of a ternary phase diagram in the discovery of new co-crystal forms, CrystEngComm, 2009, 11, 412–414 RSC.
- S. L. Childs, N. Rodríguez-Hornedo, L. S. Reddy, A. Jayasankar, C. Maheshwari, L. McCausland, R. Shipplett and B. C. Stahly, Screening strategies based on solubility and solution composition generate pharmaceutically acceptable cocrystals of carbamazepine, CrystEngComm, 2008, 10, 856–864 RSC.
- T. Friščić, S. L. Childs, S. A. A. Rizvi and W. Jones, The role of solvent in mechanochemical and sonochemical cocrystal formation: a solubility-based approach for predicting cocrystallisation outcome, CrystEngComm, 2009, 11, 418–426 RSC.
- T. Friščić, D. G. Reid, I. Halasz, R. S. Stein, R. E. Dinnebier and M. J. Duer, Ion- and liquid-assisted grinding: improved mechanochemical synthesis of metal–organic frameworks reveals salt inclusion and anion templating, Angew. Chem., Int. Ed., 2010, 49, 712–715 CrossRef PubMed.
- D. Braga and F. Grepioni, Making crystals from crystals: a green route to crystal engineering and polymorphism, Chem. Commun., 2005, 3635–3645 RSC.
- D. Braga, S. L. Giaffreda, F. Grepioni, M. R. Chierotti, R. Gobetto, G. Palladino and M. Polito, Solvent effect in a “solvent free” reaction, CrystEngComm, 2007, 9, 879–881 RSC.
- D.-K. Bučar, G. M. Day, I. Halasz, G. G. Z. Zhang, J. R. G. Sander, D. G. Reid, L. R. MacGillivray, M. J. Duer and W. Jones, The curious case of (caffeine)·(benzoic acid): how heteronuclear seeding allowed the formation of an elusive cocrystal, Chem. Sci., 2013, 4, 4417–4425 RSC.
- S. Heiden, L. Troebs, K.-J. Wenzel and F. Emmerling, Mechanochemical synthesis and structural characterisation of a theophylline-benzoic acid cocrystal (1
:
1), CrystEngComm, 2012, 14, 5128–5129 RSC.
- D. Cinčić, T. Friščić and W. Jones, A stepwise mechanism for the mechanochemical synthesis of halogen-bonded cocrystal architectures, J. Am. Chem. Soc., 2008, 130, 7524–7525 CrossRef PubMed.
- I. A. Tumanov, A. F. Achkasov, E. V. Boldyreva and V. V. Boldyrev, Following the products of mechanochemical synthesis step by step, CrystEngComm, 2011, 13, 2213–2216 RSC.
- Y. Du, Y. Xia, H. Zhang and Z. Hong, Using terahertz time-domain spectroscopical technique to monitor cocrystal formation between piracetam and 2,5-dihydroxybenzoic acid, Spectrochim. Acta, Part A, 2013, 111, 192–195 CrossRef CAS PubMed.
- I. Halasz, A. Puškarić, S. A. J. Kimber, P. J. Beldon, A. M. Belenguer, F. Adams, V. Honkimäki, R. E. Dinnebier, B. Patel and W. Jones,
et al., Real-Time In Situ Powder X-ray diffraction monitoring of mechanochemical synthesis of pharmaceutical cocrystals, Angew. Chem., Int. Ed., 2013, 52, 11538–11541 CrossRef CAS PubMed.
- T. Friščić, I. Halasz, P. J. Beldon, A. M. Belenguer, F. Adams, S. A. J. Kimber, V. Honkimäki and R. E. Dinnebier, Real-time and in situ monitoring of mechanochemical milling reactions, Nat. Chem., 2012, 5, 66–73 CrossRef PubMed.
- S. Karki, T. Friščić and W. Jones, Control and interconversion of cocrystal stoichiometry in grinding: Stepwise mechanism for the formation of a hydrogen-bonded cocrystal, CrystEngComm, 2009, 11, 470–481 RSC.
- M. D. Eddleston, E. G. Bithell and W. Jones, Transmission electron microscopy of pharmaceutical materials, J. Pharm. Sci., 2010, 99, 4072–4083 CAS.
- T. Friščić, R. W. Lancaster, L. Fábián and P. G. Karamertzanis, Tunable recognition of the steroid α-face by adjacent π-electron density, Proc. Natl. Acad. Sci. U. S. A., 2010, 107, 13216–13221 CrossRef PubMed.
|
This journal is © The Royal Society of Chemistry 2014 |
Click here to see how this site uses Cookies. View our privacy policy here.