DOI:
10.1039/C4CC90211D
(Editorial)
Chem. Commun., 2014,
50, 12417-12419
Structure of catalyst particles from in-situ electron microscopy: a web themed issue
Received
2nd June 2014
, Accepted 2nd June 2014
Heterogeneous catalysis is a chemical process performed at a solid–liquid or solid–gas interface.1,2 Atoms of a catalyst surface directly participate into the chemical process but do not become a part of any products. The participation of these catalyst surface atoms offers a new pathway with activation barrier lower than that without using a catalyst. Thus, a thermodynamically favorable chemical process is typically performed at a mild condition such as a low temperature or/and a low pressure of reactants through a pathway with low activation barrier if a catalyst is used. As activation barrier is an intrinsic parameter of a catalyst, design of a catalyst with a lower activation barrier is one of the main tasks in the field of catalysis.
Although a catalytic process is performed at the surface of a catalyst particle buried in a gaseous or liquid reactants and a solid catalyst, there is no catalyst only consisting of a layer of the catalyst atoms which directly participate into the chemical process. Here we call the layer of catalyst atoms directly participating into the chemical process the active catalyst atoms. Active catalyst atoms of a heterogeneous catalyst are supported on a nonmetallic or metallic support. In many cases, the support is an oxide particle. Such oxide particles can be inert or active. If it is inert, its function is to increase the dispersion of the catalyst such as a precious metal. In some cases, the support is a reducible oxide, which is typically a part of catalyst. Both the supported particle and the reducible oxide support directly participate into the catalytic reaction.
Pretreatment or/and activation are necessary steps to prepare active sites for a catalyst. For example, reduction of an oxidized surface of a metal nanoparticle in H2 pretreatment is a necessary process in the generation of active sites for catalysis. On the other hand, oxidation of a surface could be a step to form an active oxide for catalysis or to remove weakly bound species which are introduced in disposition–precipitate (dp) or impregnation.2 As these oxidation or reduction processes are typically performed at a temperature higher than 300 °C, a reaction between the catalyst precursor and support, a phase transformation, and change of chemical composition at 300 °C or higher are a typical consequence of this oxidation or reduction process. Tracking the chemical and structural evolution of support is significant for understanding (1) the role of support and (2) how an active phase at macroscopic level and active sites in microscopic scale are formed in the process of pretreatment or activation. Clearly, information on structural evolution and phase formation of (1) metal or oxide nanoparticles, (2) interface between metal or oxide nanoparticles and their support, and (3) support, is critical for understanding formation of catalysts and interpreting the catalytic mechanism. Unfortunately, scanning tunneling microscopy (STM) cannot provide any information on the structure of the three locations since it is a surface-sensitive technique. Alternatively, transmission electron microscopy (TEM) is the appropriate technique for identifying structures of subsurface and bulk of catalyst particles and their support at atomic scale. It offers chemical, structural, and compositional information which is critical for understanding the preparation of catalysts and formation of catalytic sites.
Evolution of catalyst structure and necessity of identifying catalyst structure in situ
As described above, a process of formation of active catalytic sites typically involves evolution of structure, composition, and oxidation state of different sections of a catalyst particle. The structural and chemical information of a catalyst before a pretreatment (or a catalytic reaction) may not represent the authentic information of the catalyst during the pretreatment since a specific temperature or pressure is necessary to retain a phase in some cases. From this point of view, structural characterization of materials in the process of pretreatment or activation is critical. In addition, deactivation of catalysts is a general issue of industrial catalysts. One classical example is the accumulation of atomic carbon on Ni catalyst during CH4 reforming and the formation of coke on an anode in high-temperature fuel cells.3 Mechanistic understanding of the deactivation process requires tracking of the evolution of the structure during deactivation instead of only the structure after deactivation.
Another motivation for the in situ studies is the understanding of materials growth. For example, catalytic study of catalyst particles with specific shape or composition is one main approach to deconvolute the interplaying structural and chemical parameters determining catalytic performances (activity and selectivity). Through this deconvolution, how a specific parameter such as size or shape of metal or oxide nanoparticles could directly impact catalytic activity and selectivity can be elucidated. The success of this approach relies on the precise control of these structural and chemical parameters of catalyst particles through fundamental understanding of the growth process. From this point of view, tracking of the growth process is critical for this approach applied in fundamental studies of heterogeneous catalysis.
In situ study is also the key for catching the structural and chemical information of the active phase of a catalyst for understanding the catalytic mechanism. As a catalytic phase could only be retained at a specific temperature or/and even a certain pressure of reactant,4 the structural and chemical information can be characterized only when the reaction conditions (a specific temperature or/and pressure) are preserved. Thus, characterization of active phase during catalysis is the key for understanding catalytic performance since a point-to-point information of a structure and its corresponding function can allow us to clearly build a correlation between catalyst structure and its catalytic performance. For example, a phase transition from Co3O4 to CoO is undergone in the annealing to a high temperature.5 The generation of a new phase during catalysis results in an increase of catalytic selectivity for production of N2 significantly in the catalytic reaction of reducing nitric oxide with CO. Another example is the generation of iron carbide (FexCy) from catalyst precursor iron oxide in a mixture of CO and H2.6–9 The active phase of Fe-based catalyst of Fischer–Tropsch synthesis, iron carbide is formed through phase transformation of iron oxide in CO and H2 at high temperature.
In situ studies of catalyst particles with environmental electron microscopy offering structural, compositional, and chemical information
A prototype study of materials in certain environments using electron microscopy can be tracked back to the very early efforts in imaging a biological sample in its hydrated state in the 1930s.10 To achieve authentic information of materials under their working conditions in gas environments, special instrumentation has been employed toward examining them under semi-working or real working conditions so that point-to-point information can be built for appropriately understanding the catalytic mechanism. Significant progress has been made in instrumentation of environmental transmission electron microscopy (E-TEM) in recent decades.11 Three different approaches have realized the in situ visualization of particles in a gas environment. They are gas injection,12–14 the use of a window,15–23 and having local high pressure through differential pumping.24–30 The main instrumentation is the creation of a local gaseous environment for materials at room temperature or higher.
E-TEM in both broad beam and focused beam mode has been used in elucidating the structures of sub-surface and bulk of catalyst particles. Its atom-resolved imaging, spectroscopy and diffraction have been powerfully integrated to provide structural and chemical information of different sections of a catalyst particle (Fig. 1). In the field of heterogeneous catalysis, E-TEM has been used to track phase formation of the active phase, sintering of nanoparticles, deactivation and poisoning effect of catalysts, oxidation or reduction in pretreatment, restructuring of bimetallic nanoparticles, observation of catalyst particles during catalysis, and many other topics. Other than in situ studies of heterogeneous catalysts, it has been used in in situ observation of growth of nanocrystals in vapor or liquid phases, in situ measurements of mechanical properties such as in situ straining experiments, cathodoluminescence, current-induced transport, etc.31
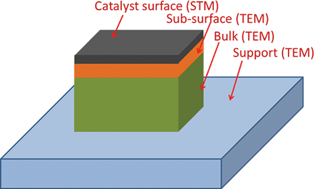 |
| Fig. 1 Schematic showing different sections of a catalyst particle. | |
In this themed issue of Chem Comm, publications related to in situ studies using E-TEM are collected. Due to the limits of the timeline, unfortunately this theme issue only collected work from some of the groups interested in situ studies. The excellent work of these groups who didn't contribute to this themed issue can be found in literatures or their group pages.
Acknowledgements
The encouragement from Editor, Dr Robert D. Eagling for editing this themed issue is highly appreciated. We appreciate Alan Holder's help in handling the submission and review process of manuscripts submitted to this themed issue.
This work was supported by the Chemical Sciences, Geosciences and Biosciences Division, Office of Basic Energy Sciences, Office of Science, U.S. Department of Energy under Grant No. DE-FG02-12ER16353.
References
-
G. A. Somorjai and Y. Li, Introduction to Surface Chemistry and Catalysis, Wiley-VCH, 2nd edn, 2010 Search PubMed.
-
G. K. Ertl, H. Knözinger, F. Schuth and J. Weitkamp, Handbook of Heterogeneous Catalysis, Wiley-VCH, 2008 Search PubMed.
- W. Wang, C. Su, Y. Z. Wu, R. Ran and Z. P. Shao, Progress in Solid Oxide Fuel Cells with Nickel-Based Anodes Operating on Methane and Related Fuels, Chem. Rev., 2013, 113, 8104 CrossRef CAS PubMed.
- F. Tao, S. Dag, L.-W, Wang, Z. Liu, D. R. Butcher, H. Bluhm, M. Salmeron and G. A. Somorjai, Break-Up of Stepped Platinum Catalyst Surfaces by High CO Coverage, Science, 2010, 327, 850 CrossRef CAS PubMed.
- S. R. Zhang, J. Shan, Y. Zhu, L. Nguyen, W. Huang, H. Yoshida, S. Takeda and F. Tao, Restructuring Transition Metal Oxide Nanorods for 100% Selectivity in Reduction of Nitric Oxide with Carbon Monoxide, Nano Lett., 2013, 13, 3310 CrossRef CAS PubMed.
- E. de Smit and B. M. Weckhuysen, The renaissance of iron-based Fischer–Tropsch synthesis: on the multifaceted catalyst deactivation behaviour, Chem. Soc. Rev., 2008, 37, 2758 RSC.
- G. B. Yu, B. Sun, Y. Pei, S. Xie, S. Yan, M. Qiao, K. Fan, X. Zhang and B. Zong, FexOy@C Spheres as an Excellent Catalyst for Fischer–Tropsch Synthesis, J. Am. Chem. Soc., 2010, 132, 935 CrossRef CAS PubMed.
- B. Sun, M. H. Qiao, K. N. A. Fan, J. Ulrich and F. Tao, Fischer–Tropsch Synthesis over Molecular Sieve Supported Catalysts, ChemCatChem, 2011, 3, 542 CrossRef CAS PubMed.
- A. Y. Khodakov, W. Chu and P. Fongarland, Advances in the development of novel cobalt Fischer–Tropsch catalysts for synthesis of long-chain hydrocarbons and clean fuels, Chem. Rev., 2007, 107, 1692 CrossRef CAS PubMed.
- L. Marton, Bull. Acad. R. Belg. Cl. Sci., 1935, 21, 553 Search PubMed.
-
P. Butler and K. Hale, Practical Methods in Electron Microscopy, North Holland, 1981, vol. 9, pp. 239–308 Search PubMed.
- K. T. Kohlmann, M. Thiemann and W. H. Brünger, E-beam induced X-ray mask repair with optimized gas nozzle geometry, Microelectron. Eng., 1991, 13, 279 CrossRef CAS.
- S. Matsui and T. Ichihashi,
In situ observation on electron-beam-induced chemical vapor deposition by transmission electron microscopy, Appl. Phys. Lett., 1988, 53, 842 CrossRef CAS PubMed.
- K. Kishita, H. Sakai, H. Tanaka, H. Saka, K. Kuroda, M. Sakamoto, A. Watabe and T. Kamino, Development of an analytical environmental TEM system and its application, J. Electron Microsc. Tech., 2009, 58, 331 CrossRef CAS PubMed.
- S. Giorgio, S. Sao Joao, S. Nitsche, D. Chaudanson, G. Sitja and C. R. Henry, Environmental electron microscopy (ETEM) for catalysts with a closed E-cell with carbon windows, Ultramicroscopy, 2006, 106, 503 CrossRef CAS PubMed.
- G. M. Parkinson, High resolution in situ controlled atmosphere transmission electron microscopy (CATEM) of heterogeneous catalysts, Catal. Lett., 1989, 2, 303 CrossRef CAS.
- H. L. L. Xin, K. Y. Niu, D. H. Alsem and H. M. Zheng, In Situ TEM Study of Catalytic Nanoparticle Reactions in Atmospheric Pressure Gas Environment, Microsc. Microanal., 2013, 19, 1558 CrossRef CAS PubMed.
- S. Mehraeen,
et al., A (S)TEM Gas Cell Holder with Localized Laser Heating for In Situ Experiments, Microsc. Microanal., 2013, 19, 470 CrossRef CAS PubMed.
- S. B. Vendelbo, P. J. Kooyman, J. F. Creemer, B. Morana, L. Mele, P. Dona, B. J. Nelissen and S. Helveg, Method for local temperature measurement in a nanoreactor for in situ high-resolution electron microscopy, Ultramicroscopy, 2013, 133, 72 CrossRef CAS PubMed.
- H. M. Zheng, R. K. Smith, Y.-w. Jun, C. Kisielowski, U. Dahmen and A. P. Alivisatos, Observation of Single Colloidal Platinum Nanocrystal Growth Trajectories, Science, 2009, 324, 1309 CrossRef CAS PubMed.
- H. G. Liao, L. K. Cui, S. Whitelam and H. M. Zheng, Real-Time Imaging of Pt3Fe Nanorod Growth in Solution, Science, 2012, 336, 1011 CrossRef CAS PubMed.
- J. M. Yuk, J. Park, P. Ercius, K. Kim, D. J. Hellebusch, M. F. Crommie, J. Yong Lee, A. Zettl and A. P. Alivisatos, High-Resolution EM of Colloidal Nanocrystal Growth Using Graphene Liquid Cells, Science, 2012, 336, 61 CrossRef CAS PubMed.
- T. Yaguchi, M. Suzuki, A. Watabe, Y. Nagakubo, K. Ueda and T. Kamino, Development of a high temperature-atmospheric pressure environmental cell for high-resolution TEM, J. Electron Microsc. Tech., 2011, 60, 217 CrossRef CAS PubMed.
-
R. Sharma and P. A. Crozier, in Handbook of Microscopy for Nanotechnology, ed. N. Yao and Z. L. Wang, Kluwer Academic Publishers, New
York, 2005, pp. 531–563 Search PubMed.
-
P. R. Swann and N. J. Tighe, paper presented at the Proc. 5th Eur. Reg. Cong Electron Microscopy, 1972.
-
R. C. Doole, G. M. Parkinson and J. M. Stead, paper presented at the Inst. Phys. Conf. Ser. 119, 1991.
- R. Sharma and K. Weiss, Microsc. Res. Tech., 1998, 42, 270 CrossRef CAS.
- T. C. Lee, D. K. Dewald, J. A. Eades, I. M. Robertson and H. K. Birnbaum, An environmental cell transmission electron microscope, Rev. Sci. Instrum., 1991, 62, 1438 CrossRef CAS PubMed.
- E. D. Boyes and P. L. Gai, Environmental high resolution electron microscopy and applications to chemical science, Ultramicroscopy, 1997, 67, 219 CrossRef CAS.
-
N. Yao, et al., Proc. 49th Annual Meeting of Microscopy Society of America, San Francisco Press, 1991, pp. 1028–1029 Search PubMed.
-
G. H. Dehm, J. M. Howe and J. Zweck, In-situ Electron Microscopy: Applications in Physics, Chemistry, and Materials Science, Wiley-VCH, 2012 Search PubMed.
|
This journal is © The Royal Society of Chemistry 2014 |
Click here to see how this site uses Cookies. View our privacy policy here.