DOI:
10.1039/D3BM01772A
(Minireview)
Biomater. Sci., 2024,
12, 621-633
Natural products applied in acute kidney injury treatment: polymer matters
Received
31st October 2023
, Accepted 6th December 2023
First published on 6th December 2023
Abstract
Acute kidney injury (AKI) is a global health threat due to its high morbidity and mortality. There is still a lack of effective therapeutic methods to deal with AKI clinically. Natural products with outstanding accessibility and bioactivity are potential candidates for AKI treatment. Natural product-based prodrugs or nano-structures with improved properties are frequently fabricated for maximizing bioavailability and decreasing side effects, in which natural polymers are selected as carriers, or natural drugs are loaded as cargos on designed polymers. In this review, the etiologies of AKI are briefly presented, and emerging natural products delivered rationally for AKI therapy, as either carriers or cargos, are both introduced. Moreover, the challenges of the future development of nature-based nanodrugs or prodrugs for AKI have also been discussed.
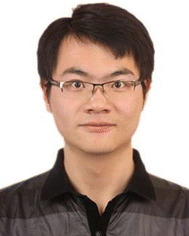 Bo Yu | Bo Yu received his bachelor's degree from Zhejiang University in 2020. After that, he joined Prof. Jian Ji's group in the Department of Polymer Science and Engineering, Zhejiang University as a PhD candidate. His research focuses on the design of bio-responsive materials for AKI therapy. |
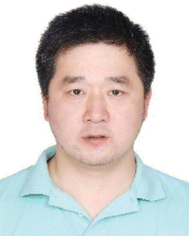 Qiao Jin | Prof. Dr Qiao Jin is currently the associate director of the Institute of Biomedical Macromolecules in Zhejiang University. He received his Ph.D in 2010 at Zhejiang University and worked as a postdoc follow at the University of Marburg in Germany from 2011 to 2012. He joined Zhejiang University in 2012 and was appointed as a professor in 2021. His main research interest is focused on stimuli-responsive polymers and smart drug delivery systems for cancer therapy and antibacterial applications. |
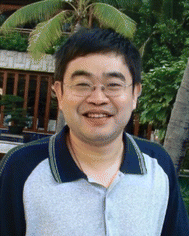 Jian Ji | Prof. Jian Ji is the director of the Institute of Biomedical Macromolecules in Zhejiang University and a Qiushi Chair Professor in the Department of Polymer Science and Engineering, Zhejiang University. He received his PhD degree in polymer science from Zhejiang University in 1997 and became a full professor in 2004. In 2010, he received the National Science Fund for Distinguished Young Scholars from the National Science Foundation of China. In 2015, he received the CheungKong Scholars award from the Ministry of Education. He is a fellow of the Royal Society of Chemistry and became an associate editor of the Journal of Materials Chemistry B in 2018. His research focuses on the development of advanced biomimetic biomaterials via self-assembly, surface modification of cardiovascular biomaterials, design of a signaling surface to trigger cell behavior, development of polymer-based gene delivery systems via self-assembly and novel biomimetic polymeric nano-carriers for drug delivery. |
Introduction
Acute kidney injury (AKI) is a syndrome with a rapid loss of renal function which is prevalent around the world.1 The multiple etiologies make it common in most wards in hospitals, especially in intensive care units (ICUs).2–5 However, existing clinical methods to deal with AKI including fluid replacement and electrolyte correction are limited and even rare to combat it in the initial period.6 The retarded intervention of AKI leaves patients with a high risk of death. Even if the sufferers could get through the most dangerous period, chronic kidney diseases are also hard to avoid with the progress of AKI.7,8 Considering its morbidity and severity, multiple efforts have been devoted to exploiting novel approaches for the early diagnosis and effective treatment of AKI.9,10
Although great efforts have been made towards treating AKI, the efficient delivery of therapeutic agents to the kidneys to achieve renal accumulation is still attracting increasing research interest.11 Although a great amount of admininistrated agents tend to arrive at kidneys since they are major organs for excretion, the renal accumulation and retention of agents are still complicated.12 In particular, for nano-medicines with countless functions and advantages, due to their relatively large size, the nanoparticles prefer to accumulate in the liver, leading to very low kidney accumulation.13 At the same time, the glomerular basement membrane (GBM) acts as an additional barrier for the kidneys specifically.14 So far, several strategies have been put forward for renal drug delivery, which could be described as passive and active targeting methods.13 Passive targeting strategies include size, water solubility and surface charge, and active targeting strategies focus on specific receptors overexpressed on injured renal tissues. In general, materials with high hydrophilicity and a smaller size are more liable to be excreted renally; the ideal size cut-off for glomerular filtration is around 5 nm in healthy kidneys, equating to about 30–50 kDa in molecular weight.15–17 Additionally, it is also possible for nanoparticles with larger sizes to reach renal tissues, especially injured lesions via endocytosis or a damaged GBM, reported in recent works.13,18,19 Considering the negatively charged GBM, materials with a positive surface have more opportunities to cross the GBM to realize renal accumulation.20,21 Meanwhile, AKI is a typical inflammatory process accompanied with injuries; several inflammation- or damage-related receptors are overexpressed on the membranes of affected cells, providing another way to actively bind to those cells and effectively accumulate in injured lesions.20
Among the approaches reported, natural products as well as their derivatives constitute an important branch in both diagnosis and therapy of AKI.22–25 Natural products, usually extracted from plants which are traditionally medically used, are abundant in nature and easy to access. The effectiveness and biosafety are also verified in long-term use.26–28 Considering the unparalleled advantages of natural products mentioned above, an increasing number of modern research studies find specific potentials of natural products for AKI treatment, and have previously been well reviewed.22,23 However, the fact is still neglected that the application of natural products is often related to polymers. Several natural polymers, with inherent affinity for renal tissues, were applied for the precise renal accumulation. On the other hand, many other kinds of natural drugs like polyphenols, with renal-protective capacities, are hard to be administered directly because of their poor solubility, stability or delivery efficiency. Specifically, the hydrophobic products need to be dispersed in water for intravenous administration, and many unprotected products may be adhered to proteins and deactivated in a comprehensive environment in the human system. The biodistribution of natural products is also required to be altered to make full use of them and to avoid potential risks if the agents are metabolized in an undesired way. To resolve the problems above, natural drugs are usually administered with polymers to form designed prodrugs or nanocarriers with improved performance.29–31
Focusing on the extraordinary affinity between natural products and polymers, we reviewed recent reports on AKI treatment that applied natural polymers directly as carriers, or that delivered natural products via polymer-based structures and tried to draw out a reference for future design and application of natural products in AKI-related realms, considering the essential roles of natural products in AKI and the importance of rational design of delivery systems of them.
Brief etiologies of AKI
The kidneys are the major places for metabolism to maintain the homeostasis of body fluids, electrolytes, osmolality and pH in human bodies.32 In each small independent functional unit, named nephron in the kidneys, the glomerular membrane filters the fluid and small molecules from the blood to form crude urine, which is concentrated by tubules called reabsorption and gathered in collecting tubes before going to the bladder. The intense interchange of small molecules and ions in renal tubules requires lots of energy, which is supported by the high density of mitochondria and high intensity of metabolism in tubular cells.33 That is to say, insufficient nutriment supply will easily lead to lethal damage, called acute tubular necrosis (ATN). Therefore, a temporary or long-lasting blockage of blood supply is a major pathogenesis to AKI, and the injury will be worsened when the blood reperfuses suddenly, which is called ischemia-reperfusion (IR) induced AKI.34 For example, the disorder of or operation on major organs like the heart, lungs or liver may lead to this injury. At the same time, since the kidneys should deal with most body fluids, the systematic disturbance will exert a tremendous pressure on renal cells; in this way, the high levels of inflammatory factors in the circulation system caused by sepsis or rhabdomyolysis are common inducers of kidney disorder.35,36 Finally, renal cells, including tubular and glomerular cells, are susceptible to nephrotoxins like some kinds of antibiotics (gemcitabine, polymyxin, etc.), chemotherapeutic agents (cisplatin) and contrast agents.37–40 Both glomerular and tubular cells may be affected by the side effect of those nephrotoxins in hospitals or from drug abuse, and this is the reason why the administered dose of several medicines is limited even though a better therapeutic outcome could be expected at a higher dose level.
Among all kinds of AKI, oxidative stress and inflammation are typical pathological features, and thus major therapeutic targets (Fig. 1).41 In some AKI subtypes like those induced by IR, ischemia usually disrupts the normal aerobic metabolism, and leads to leakage of reactive oxygen species (ROS) like superoxide anions and hydrogen peroxide, which could destroy DNA, oxidize lipids and proteins, generate toxic reactive nitrogen species (RNS) and trigger severe inflammatory response.42 The ion channels are also interfered with during this process, and an uncontrollable influx of metal ions like Ca2+ after blood reperfusion will create further disturbance for more production of ROS.43 The overproduced ROS usually lead to abnormal cell necrosis, which may also be caused by some nephrotoxins. During necrosis, the plasma membrane collapses rapidly and toxic pro-inflammatory mediators are released at the same time to recruit immune cells for the clearance of debris. The infiltrated immune cells will secrete more ROS when activated, and the self-amplified inflammation is initiated.44 Therefore, anti-oxidative and anti-inflammatory therapies have been mainstream in AKI strategies recently. Lots of anti-oxidative and anti-inflammatory medicines were reported to be effective for AKI remission, like N-acetylcysteine (NAC), glucocorticoids and others, but few were applied in the clinic.6
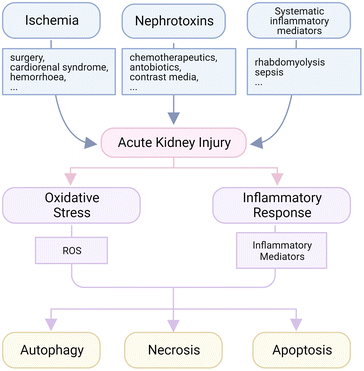 |
| Fig. 1 Typical pathologies of AKI treatment. | |
The necrosis of injured tubular cells has been recognized as the major cause of the loss of renal function, but emerging evidence has implied that the etiology is probably not alone, and might be accompanied by apoptosis and possibly autophagic cell death.45 Apoptosis is reported to be detected in the kidneys after ischemia, toxin exposure, inflammation and sepsis, and uncontrolled apoptosis is believed to be a contributor to renal failure.45–47 In mitochondria where the apoptosis-related signals are integrated, the permeabilization of membranes is regulated by Bcl-2 family proteins, among which Bax and Bak are primary members to increase membrane permeabilization in renal cells. Bcl-2 and Bcl-XL, another two members in the Bcl-2 family, counteract Bax and Bak to maintain the balance. The ratio of Bax to Bcl-2 is usually upregulated to a high pro-apoptotic state during AKI, and leads to the activation of caspase-9 initiating unbalanced apoptosis of renal tubular cells. Receptors of the tumor necrosis factor (TNF) superfamily, including Fas, TNF receptor 1 and Fn14, are also expressed on renal tubular cells. Fas ligands and TNF-like weak inducer of apoptosis (TWEAK) can activate downstream caspases and derive apoptosis induction.
Autophagy is regarded as a cytoprotective process to degrade the damaged organelles and molecules under stress, which is proved to be vitally involved in AKI.48–51 Several Atg protein complexes are involved in the formation of autophagosomes (Fig. 2), for example the ULK1/2 complex regulated by nutrient- and energy-sensitive kinases mammalian target of rapamycin (mTOR) and adenosine monophosphate kinase (AMPK), and Beclin-1/class III PI3K complex, ubiquitin-like conjugation systems (ATG16L1 complex and lipidation of microtubule-associated protein-1 LC3). Although the roles of autophagy are still not clear in AKI, ROS, endoplasmic reticulum (ER) stress and energy depletion in AKI are all triggers of autophagy, and efforts were made toward the management of autophagy for effective renal repairment.
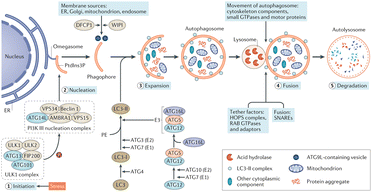 |
| Fig. 2 Autophagy pathways, process and molecular machinery. Reproduced from ref. 51 with permission from Springer Nature, copyright 2020. | |
The classical inflammation-related NFκB pathway plays an important role in the pathogenesis of AKI.52 Animal models revealed that the inhibition of NFκB could suppress immune response, decrease M1 macrophage infiltration and alleviate kidney injury.53–55 Consequently, mediators involved in this pathway, like p50, p52, and p65, have attracted wide attention for anti-inflammatory therapy of AKI.
Thanks to the recent biological breakthrough to gain a full understanding of AKI, many more researchers have concentrated on clarifying the mechanisms underneath the AKI therapy, and by applying biomolecules like siRNAs for the inhibition of specific pathways, more novel biological signals were revealed.56–62 Advanced strategies with more precise targets have been developed for effective disease management, which might be the future direction for the related designs. In the meantime, the effective delivery of those molecules has become a new demand and challenge.
Natural polymers for renal-targeted delivery
Kidney targeting, as well as injured-kidney targeting, is a bottleneck for the efficient delivery of nephroprotective agents. Based on the normal or pathological structure of the kidneys, several natural polymers with certain specific affinities or physical features are applied for higher targeting outcomes, among which polysaccharides are the most prevalent candidates. Several polysaccharides, including fucoidan, hyaluronic acid, chitosan and cyclodextrin, were reported for higher renal accumulation or clearance, based on the specific chemical recognition or physical properties (Table 1).
Table 1 Natural polymers reported for renal targeting
Natural polymers |
Renal targeting mechanism |
Loaded agent |
AKI model |
Ref. |
Fucoidan |
Target P-selectin |
Curcumin |
LPS |
63
|
Ferulic acid |
Cisplatin |
64
|
Proanthocyanidin |
Cisplatin |
65
|
Hyaluronic acid |
Target CD44 |
Curcumin |
IR |
66
|
Bilirubin |
IR |
67
|
Bilirubin, BAPTA-AM |
IR |
68
|
IL-10 |
IR |
69
|
Hyaluronic acid mixed with chitosan |
Target CD44 |
SS31 |
LPS |
70
|
Chitosan modified with α-cyclam-p-toluic acid |
Target CXCR4, positive charge |
siRNA against p53 |
IR |
71
|
Chitosan |
Positive charge |
Lutein, celastrol |
Cisplatin |
72
|
Curcumin |
LPS |
73
|
SS31 peptide, L-serine |
IR |
74
|
MSCs |
IR |
75
|
Cyclodextrin |
Small size, high hydrophilicity |
Multiple fluorescent molecules |
Cisplatin, gentamicin, diatrizoate |
76–78
|
Fucoidan
Fucoidan, a marine polysaccharide mainly composed of L-fucose and sulfate groups, has a specifically high affinity for P-selectin overexpressed on activated endothelial cells during multiple inflammations including AKI.79,80 Interestingly, fucoidan also shows a wide range of biological activities including anti-inflammatory, antioxidant and anti-tumor effects. For this reason, fucoidan was recently applied as a bioactive macromolecular carrier for treatment of AKI. Shu et al. designed curcumin-loaded micelles via octenyl succinic anhydride-grafted fucoidan, which were proved to have sustained drug release behavior and excellent physicochemical stability with a diameter of around 100 nm.63 The cellular internalization and kidney-targeting in LPS-induced AKI models both showed that the antioxidant and anti-inflammatory abilities were elevated due to the precise targeting. Gao et al. fabricated a ferulic acid (FA)-loaded fucoidan nanoparticle with a size of 158.6 ± 4.5 nm for the treatment of cisplatin-induced AKI.64 After being loaded on fucoidan, FA shows limited cytotoxicity and enhanced therapeutic effects. Similarly, fucoidan-proanthocyanidin nanoparticles were also reported by the same group, with a similar renal accumulation and therapeutic outcome in a cisplatin-induced AKI model, expanding the application of fucoidans as carriers for complex molecules with condensed rings.65
Hyaluronic acid
Hyaluronic acid (HA), a biocompatible and biodegradable natural polysaccharide, contains an inherent ability to specifically target CD44 receptors, which are found to be overexpressed in injured tubuloepithelial cells during AKI. Based on the abundance of carboxylic and hydroxyl groups, HA is available for further modification and applied as a natural drug carrier for AKI targeting. Curcumin was handily conjugated to HA by Hu et al. via esterification reaction.66 The elevated uptake by inflamed renal tubular epithelial cells and specific accumulation in injured renal tissues were verified both in vitro (HK-2 cells) and in vivo (mice IR induced AKI model). Since the loaded curcumin is released via physiological degradation of the ester bond, a prolonged drug release in circulation is also expected for this complex. In another work reported by Huang et al., HA was coated on the surface of ε-polylysine–bilirubin conjugated nanoparticles for IRI-injured kidney targeting (Fig. 3).67 The constructed nanoparticles showed favorable stability and high biocompatibility, together with specific kidney targeting and protective ability. The direct conjugation of anti-oxidative bilirubin on HA is also feasible via the linker adipic dihydride. HA became amphiphilic after the modification of hydrophobic bilirubin to form nanoparticles, which is responsive to ROS because of the conversion from bilirubin to biliverdin with the improvement on solubility.68 1,2-Bis(2-aminophenoxy)ethane-N,N,N′,N′-tetraacetic acid acetoxymethyl ester (BAPTA-AM), a calcium ion chelator for blockage of calcium channels intracellularly, was successfully encapsulated in the aforementioned carrier via a flash nanocomplexation micromixing method. HA was also mixed with chitosan together for the delivery of SS31.70 The complex based on electrostatic balance could become disintegrated once endocytosed in lysosomes, and released SS31 could further retain the mitochondria function to relieve injury. Besides those HA-based particles, injectable hydrogels were also fabricated from HA modified with host–guest interacted molecules.69 Anti-inflammatory interleukin-10 (IL-10) was then loaded into the hydrogel, which was injected under the kidney capsule. The gel was totally degraded 15 days after injection, resulting in a high efficiency of delivery and sustained release of cytokines, proved effective at suppressing IR induced AKI and systematic inflammation.
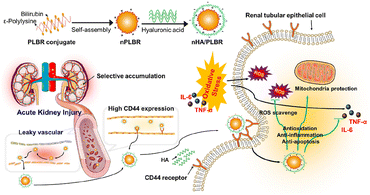 |
| Fig. 3 Illustrative scheme of ε-polylysine-bilirubin coated with HA with both passive and active renal targeting abilities for the AKI remission via various ways. HA is applied for renal-targeting based on its affinity with CD44. Reproduced from ref. 67 with permission from Elsevier, copyright 2021. | |
Chitosan
Specific affinities with AKI-related biomarkers were also found in modified chitosan-based materials. Tang et al. modified chitosan with α-cyclam-p-toluic acid to construct a novel siRNA carrier (C–CS).71 Interestingly, this combination was found to be bound with C–X–C chemokine receptor type 4 (CXCR4). The nanoparticles were then fabricated via the self-assembly between synthesized polymer C–CS and siRNA against p53. The enhanced accumulation and retention of nanoparticles in renal tubules, as well as satisfactory anti-inflammatory and renal protective effects were found in the IR-induced model. Some reports pointed out that positively charged agents are more feasible to cross the negative GBM.74
In most cases, chitosan is used for enhanced renal accumulation because of its positive charge. In a recent work reported by Pang et al., lutein and celastrol were loaded into chitosan via pH-sensitive citraconic anhydride linkers.72 The renal protective ability of fabricated nanomicelles was verified in cisplatin-induced AKI models, via NFκB p65 and p38 mitogen-activated protein kinase (MAPK) inflammatory signaling pathways. Similarly, Wang et al. conjugated the natural antioxidant curcumin to chitosan oligosaccharide (LMWC) for the treatment of lipopolysaccharide (LPS)-induced AKI.73 Due to the hydrophilia of LMWC and its ability to bind to the megalin receptor which mediated specifically cellular internalization of LMWC, the accumulation of curcumin was enhanced in tubular epithelium cells. Biomolecules are also suitable for the chitosan-based delivering system. Moreover, a mitochondria-targeted anti-oxidative peptide, SS31, was conjugated to L-serine-modified chitosan via the ROS-responsive thioketal linker (Fig. 4).74 The modification of L-serine gave the complex an efficient renal targeting ability via its special affinity for Kidney Injury Molecule (KIM)-1 overexpressed on the membrane of injured tubular epithelium cells. The subsequent precise targeting and therapy of mitochondria by SS31 could relieve renal injury and inhibit apoptosis of tubular cells in the IR induced model. Modified chitosan is also a great scaffold for cell delivery. Injectable thermosensitive hydrogel could be fabricated by chitosan chloride to deliver adipose-derived mesenchymal stem cells (ADMSCs) into IR injured kidneys.75 The hydrogel could improve the retention and survival of ADMSCs, reduce the apoptosis of tubular cells, and promote the proliferation and recovery of injured tissues.
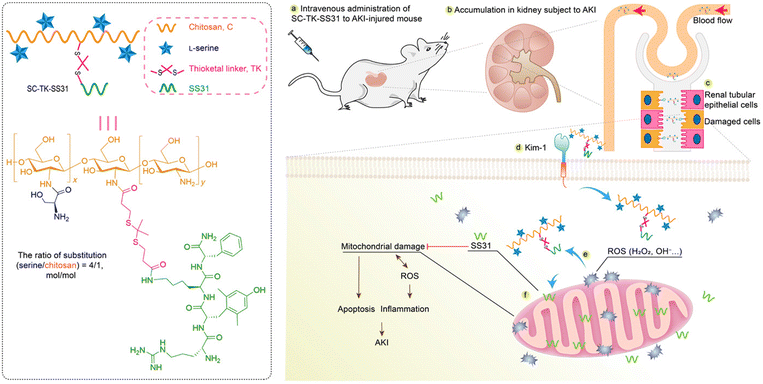 |
| Fig. 4 Illustrative scheme of SS31 and L-serine loaded chitosan for ROS-responsive protection of mitochondria during AKI. L-Serine is conjugated for renal targeting based on its affinity with overexpressed KIM-1 receptors on injured cells. Mitochondrial protective SS31 is conjugated to chitosan via ROS-responsive thioketal linkers. Positively charged chitosan is beneficial for renal accumulation and capable of peptide loading. Reproduced from ref. 74 (Open Access). | |
Cyclodextrin
Higher hydrophilicity usually leads to more kidney excretion. Cyclodextrins are widely used to improve the water solubility due to their hydrophilic shells and hydrophobic cavities, in which other molecules could be inserted with appropriate sizes. Among the family of cyclodextrins, β-cyclodextrin is most commercially accessible, and the further modification of hydroxypropyl (HPβCD) results in higher water solubility because of the destruction of hydrogen bonds. Therefore, HPβCD is an ideal platform with high renal clearance.81 A series of probes for AKI diagnosis were then designed based on click-reaction with propynyl-HPβCD (Fig. 5).76–78 The synthesized renal clearable probes could be responsive to a wide range of biomarkers including neutrophil gelatinase associated lipocalin (NGAL), N-acetyl-β-D-glucosaminidase (NAG), superoxide anions, caspase-3 and γ-glutamyl transferase, and could regain the ability for near-infrared fluorescence (NIRF), chemiluminescence, or photoacoustic detection ex or in vivo, assisting an early diagnosis of AKI. Such excellent works pointed out a convenient way to quickly improve the water solubility of small molecules and enhance the renal clearance as a result.
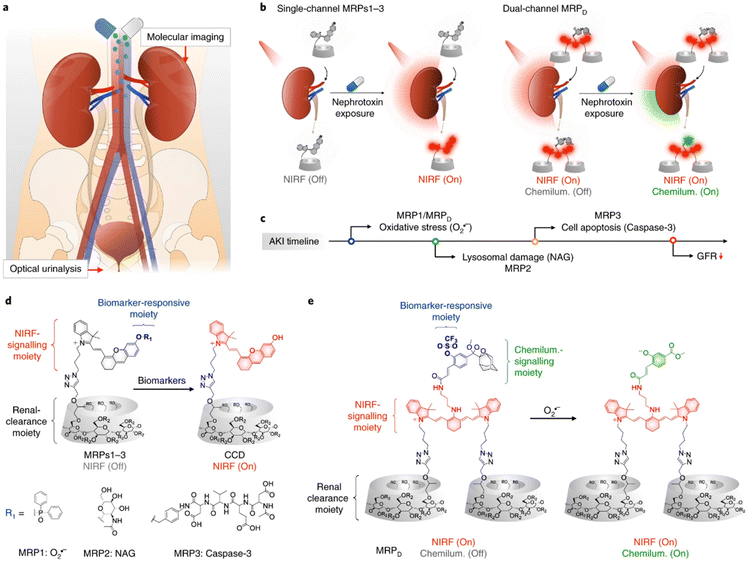 |
| Fig. 5 The synthesis of HPβCD-based probes with high renal clearance efficiency for early diagnosis of AKI. Reproduced from ref. 77 with permission from Springer Nature, copyright 2019. (a) Schematic graph of diagnosis ex/in vivo; (b) illustrative scheme of different structures responsive to multiple bio-signals; (c) the earliest effective detection timepoint of different probes; (d) detailed molecular design of probes responsive to single biomarkers; (e) detailed molecular design of probes responsive to dual biomarkers. | |
Polymer-delivered natural products for AKI therapy
Despite natural polymers mentioned above for targeted kidney delivery based on hydrophilicity, surface charge or specific affinity, more natural products are directly applied for AKI mitigation based on their multiple anti-oxidative, anti-inflammatory and anti-apoptotic potential. Several renal protective natural products are macromolecules (like alginate and fucoidan), which could be applied as carriers to load other agents for comprehensive combinative therapy. However, there are still a great number of natural molecules with remarkable bioactivities that are difficult to be delivered to lesions, because of their poor solubility or stability. Different kinds of polymer-based carriers were fabricated for the delivery of those molecules. Besides the improved dispersity, versatile platforms with various properties could also be constructed after further modification of the polymers, which emerged as another superiority to load natural products on tailored polymers (Table 2).
Table 2 Polymer-delivered natural products reported for AKI therapy
|
Natural product |
Carrier |
AKI model |
Mechanism reported |
Ref. |
Flavonoids |
Baicalein |
Silk fibroin peptide |
Cisplatin |
Increase GSH and SOD, inhibit DNA damage, Inhibit cGAS-STING pathway |
82
|
Quercetin |
— |
LPS |
Increase Sirt1 inhibit NFκB pathway |
83
|
PEG conjugated PEI |
IR |
Anti-oxidation |
84
|
Myricetin |
HS-15 |
Cisplatin |
Increase GSH, SOD and MDA activity, inhibit cGAS-STING pathway |
85
|
Curcuminoids |
Curcumin |
PEG-b-PAGA, PDMAEMA-r-PAAPBA |
Cisplatin |
Anti-oxidation, activate autophagy, decrease ER stress, inhibit apoptosis |
86
|
SA modified dextran |
LPS |
Anti-oxidation, inhibit apoptosis, decrease Bax, increase Bcl-2 |
87
|
PVP |
Cisplatin |
High renal accumulation |
15
|
Coordinate with ferric ions |
Glycerol |
Anti-oxidation |
88
|
Bisdemethoxycurcumin |
Star-shaped polyglutamate |
Folic acid |
Inhibit apoptosis and necroptosis, inhibit ferroptosis |
89
|
Other polyphenols |
Tannic acid |
Coordinate with phosphotungstic acid |
Cisplatin, Glycerol |
Anti-oxidation, increase SOD, inhibit apoptosis |
90
|
Gallic acid |
Coordinate with ferric ions, Stabilized by PVP |
Cisplatin, Glycerol |
Anti-oxidation, increase SOD |
91
|
Ethyl caffeate |
L-Serine modified polyglutamic acid |
IR |
Anti-oxidation |
92
|
Rosmarinic acid |
L-Serine modified G4-PAMAM |
IR |
Anti-oxidation, inhibit apoptosis |
93
|
Urolithin A |
Gambogic acid modified PLGA |
Cisplatin |
Decrease Nrf2 and P53 |
94
|
Other natural products |
Triptolide |
PLGA-b-mPEG |
IR |
Decrease C3 complement, decrease p-ERK |
95
|
Flavonoids
Flavonoids are a large subgroup of natural polyphenols, which are composed of two benzene rings linked by a heterocyclic ring containing oxygen. Although the anti-inflammatory and anti-cancer advantages of diet-derived flavonoids have drawn increasing attention, the bioavailability of these is in general described to be low.96 Recently, multiple carriers have been designed for the efficient delivery of flavonoids for AKI therapy.97 Liu et al. used silk fibroin peptide nanofibers for the delivery of baicalein (Fig. 6).82 The aqueous dispersity and storage stability were both improved compared to baicalein alone. Then, the fabricated nanofibers were also verified to protect against cisplatin-induced injuries both in vitro and in vivo. For other models like sepsis-induced AKI, quercetin, as another kind of flavonoid, was reported to be effective. As reported by Lu et al., the pretreatment of quercetin nanoparticles could obviously restrain LPS-induced cell apoptosis and inflammation, which may be ascribed to elevated expression of Sirt1 and quenched NF/κB activation.83 Huang et al. synthesized polyethylene glycol (PEG) conjugated polyethyleneimine (PEI) for the delivery of quercetin.84 The size of fabricated nanoparticles was around 20.92 nm measured by DLS. They further investigated the protective potential of those particles in IR-induced mice; the suppressed levels of blood urea nitrogen (BUN), serum creatine (sCr) and cystatin C demonstrated the eloquent therapeutic effect of quercetin-loaded nanoparticles. Similarly, myricetin, another natural flavonoid, was loaded on the nano-micelle formed from the nonionic surfactant Solutol HS-15.85 The myricetin-loaded nano-micelles could suppress the inflammatory response, alter the activities of antioxidant enzymes and restore the renal function in the cisplatin-induced AKI model, which was ascribed to the inhibition of the cGAS-STING pathway according to the authors.
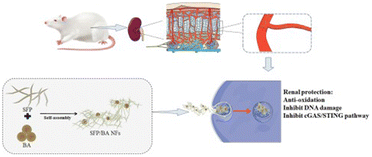 |
| Fig. 6 Illustrative scheme of baicalein-loaded silk fibroin peptide nanofibers. Reproduced from ref. 82 with permission from Elsevier, copyright 2022. | |
Curcuminoids
Curcumin (CUR) as well as its derivatives are widely used in foods, cosmetics, and traditional medicine, with reported antioxidant, anti-inflammatory, anticancer and antimicrobial activities.98,99 However, the poor solubility and low stability both limit their therapeutic application.100 Besides several works listed above,66,73 emerging works have delivered CUR using different kinds of polymers to extend its bioactivity to the AKI realm. Lan et al. fabricated a CUR-loaded hierarchical nanodrug delivery system (Fig. 7) via the assembly of two individual copolymers, one was the block-polymer of PEG and polyacrylic esters with grafted alginic acid (AGA), named PEG-b-PAGA, and the other was the random copolymer of polyacrylic esters with phenylboronic acid and 2-(dimethylamino)ethyl groups (PDMAEMA-r-PAAPBA).86 The fabricated nanoparticles were shelled by negatively charged PEG-b-PAGA, and the boronate ester formed between phenylboronic acid and AGA could respond to both ROS and acidic microenvironment and present a positively charged sub-shell of PDMAEMA. The switch of surface charge could prolong the circulation period and enrich the accumulation in cisplatin injured renal tissues. The protective effect was ascribed mainly to the antioxidative and subsequent anti-inflammatory potential of CUR and boronate esters. Hu et al. conjugated CUR to sialic acid (SA)-modified dextran via a matrix metalloproteinase-2 (MMP-2)-responsive PVGLIG peptide.87 The solubility of CUR was significantly increased after conjugation, and the uptake by inflamed vascular endothelial cells was improved due to the specific interaction between SA and overexpressed E-selectin on those cells. The high density of MMP-2 in inflamed tissues would further break the designed peptide linker to release CUR. In vivo AKI models induced by LPS revealed that the micelle could adjust the oxidative stress, suppress the expression of the apoptosis-promoting Bax protein, and upregulate the anti-apoptosis Bcl-2 protein. By some reasonable designs, the bioavailability of curcumin in kidney tissues could be precisely adjusted. Wei et al. have loaded curcumin on a series of polyvinylpyrrolidone (PVP) polymers with different sizes, and found that particles with a smaller size between 5 and 8 nm had higher renal accumulation compared with larger ones (20–50 nm), providing a key point for the rational design of carriers for AKI therapy.15 It is also worth mentioning that curcumin was reported to be feasibly coordinated with low-toxic ferric ions to form polymer nanodots with ultra-small size (less than 10 nm).88 These fabricated nanodots retained relatively high anti-oxidative ability, and could restore the function of kidneys insulted by glycerol in mice models.
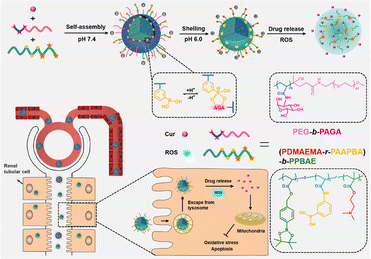 |
| Fig. 7 Illustrative scheme of the chemical structure, fabrication and application of the hierarchical nanodrug delivery system to load curcumin (Cur). A carrier responsive to both oxidative and acidic environments is fabricated based on boronate esters. Reproduced from ref. 86 with permission from ACS Publications, copyright 2022. | |
Another member of the curcuminoid family, bisdemethoxycurcumin (BDMC), was also exploited for AKI treatment. Crosslinked star-shaped polyglutamate was applied for the delivery of BDMC.89 The fabricated conjugate (St–PGA–CL–BDMC) had a hydrodynamic diameter of around 40.2 ± 2.4 nm, resulting in a high renal accumulation percentage. The apoptosis and necroptosis induced by Tweal/TNFα/IFNγ were found to be suppressed by St–PGA–CL–BDMC. Furthermore, the expression of ferroptosis markers was found to be decreased after the treatment of St–PGA–CL–BDMC in a folic acid-induced AKI mouse model, showing another possible mechanism for renal protection of curcuminoids.
Other polyphenols
Polyphenols are a large family including a wide range of natural products; in addition to those listed above, other polyphenols, like the tannins and derivatives of hydroxybenzoic acid, hydroxycinnamic acid, phenolic acid and chlorogenic acids, were also combined with polymers or applied for polymer formation for the AKI therapy. Tannic acid, a unique natural sourced macromolecule with multiple phenyl groups, showed strong coordination ability with lots of metal ions and strong anti-oxidative and antibacterial potential. An ultra-small nanodot (TWND) was developed based on phosphotungstic acid and tannic acid, with an average diameter of about 8.2 nm (Fig. 8).90 TWNDs could enter the mitochondria of the renal tubules without additional targeting decorations, and a high ratio of W(V) to W(VI) endowed TWNDs with high efficiency for ROS elimination. At the same time, tungsten provided a potential application on X-ray computed tomography (CT) imaging fields based on its higher sensitivity. The therapeutic effect of TWNDs was also verified in both cisplatin- and glycerol-induced AKI models. The outstanding binding and therapeutic potential is also inherited by gallic acid. Another self-assembled ultra-small nanodot was reported, denoted as FGP, consisting of iron ions, gallic acid and polyvinylpyrrolidone.91 The size as small as around 3.5 nm guarantees a high renal clearance efficiency of FGPs, and the verified broad-spectrum ROS and RNS scavenging ability resulted in a positive therapeutic outcome of cisplatin- and glycerol-induced AKI, which was superior to the small molecule drug (amifostine).
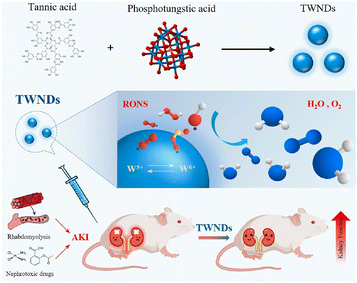 |
| Fig. 8 Illustrative scheme of an ultra-small nanodot based on phosphotungstic acid and tannic acid, and its mechanism of renal protection. Reproduced from ref. 90 (open access). | |
Caffeic acid and its esters are compounds found in several natural medicinal plants and proved to suppress the activation of the NFκB pathway with downstream inflammatory mediators.101,102 In our research group, we integrated injured kidney-targeted L-serine with antioxidative ethyl caffeate (EC) by the host–guest interaction of polyglutamic acid decorated with adamantane and β-cyclodextrin separately.92 EC was conjugated via ROS-responsive phenylboronate esters to release 4-hydroxybenzyl alcohol as a byproduct after stimulation, which was a fair antioxidant itself. The multi-step ROS-scavenging strategy showed excellent effectiveness in preserving the integrity and functions of kidneys injured by IR in mice models. Rosmarinic acid (RA), an ester of caffeic acid and 3,4-dihydroxy-phenylacetic acid, has been reported with many bioactive properties as well and was applied in other disease models.103,104 A fourth-generation poly-amidoamine-based amphiphilic polymer (G4-PAMAM) was an ideal drug carrier for hydrophobic molecules like RA with a controllable size and feasible post-modification potential.93 After the decoration of L-serine, RA-loaded G4-PAMAM could target AKI kidneys and improve the renal function, repair damaged renal tissue, and decrease oxidative stress, inflammatory response and apoptosis of tubular cells in the IR-induced AKI model.
Urolithin A (UA) is a gut metabolite of tannin ellagic acid, with a proved positive influence on AKI mice. When UA was orally delivered by gambogic acid-modified polylactic-co-glycolic acid (PLGA) nanoparticles which target transferrin receptor (TfR1) expressed in the villous epithelium, duodenal crypts and Peyer's patches of the small intestine, the internalization was significantly elevated and cisplatin-induced AKI was also relieved.94 This interesting work focused on the AKI management via orally delivery methods, providing a novel approach for convenient treatment.
Other natural products
Triptolide (TP) is a promising anti-inflammatory natural agent with the reported ability to down-regulate the expression of C3 complement and phosphor-extracellular signal-regulated kinase (p-ERK).105,106 To deal with the low solubility of TP, amphiphilic poly(lactide-co-glycolide)-block-poly(ethylene glycol) methyl ether (PLGA-b-PEG) was applied to formulate the nanoparticle for the delivery of TP.95 Lower hepatotoxicity, reproductive toxicity and immunotoxicity were found after loading, providing a new therapeutic strategy for renal diseases.
Challenges and prospects
AKI is a common clinical disease drawing extensive attention for its severity. The management of AKI is intractable considering to both target kidneys efficiently and maintain the homeostasis during and after injury. Natural products with multiple bioactivities are ideal candidates to meet the requirements above with comparatively lower biotoxicity. Although emerging excellent works have been reported to design complexes containing natural products for AKI therapy, almost all of them were just proofs of concept and hardly for clinical use. Several challenges may include those listed below (Fig. 9):
1. The kinds of natural products applied against AKI are limited. As listed in this review, most of the renal targeting natural polymer carriers are polysaccharides, while most of the renal protective natural agents are polyphenols. Although they are two major species in the family of natural products, there are lots of other natural materials to be exploited.
2. Multiple methods of administration are required. It is an obscure way for orally administered nanoparticles or other prodrugs to go from the gut to kidneys, and that is why almost all the complexes reported were administered intravenously, but surprisingly, there have still been several attempts focusing on orally-delivered strategies for AKI therapy, which doubtlessly leads to high adherence of patients and more convenience for treatment.94,107
3. The tools for efficient renal accumulation and retention are still insufficient. Passive and active strategies were applied for kidney targeting. For passive targeting, it is widely accepted that ultra-small size (lower molecular weight) is an important factor for renal excretion, which seems to be contradictory with polymers usually with higher molecular weight and hydrodynamic diameters. Additionally, the surface charge also determines the distribution of administrated materials. Positively charged particles were reported to be easily transported by the glomerular basement membrane.74 However, natural products with positive charges for carriers are rare besides chitosan, and the biosafety and biostability of such positively charged materials are still challenges. Active targeting methods were then exploited, like the post-modification of other ligands which could bind with receptors overexpressed on injured renal cells. Nevertheless, the decoration may require redundant organic synthesis, and the nature of carriers themselves may be influenced at the same time, let alone the scarcity of ideal ligands and the erratic specificity. Based on the significant progress of the development of functional biomaterials, a rational design of the carrier is critical to optimize the therapeutic outcome, and switchable size as well as surface potential is worth trying for novel systems.108–110
4. Precise subcellular targeting therapies are less common in AKI therapy. It is revealed that the oxidative stress in mitochondria and ER stress responses act prominently during the injury process.111 The precise delivery to those specific organelles brings higher requirement to the elegant design of carriers. Recent works reported the subcellular organelle-targeting potential of natural products, which might be inspirational in relevant designs in AKI therapy.112
5. The pivotal point of AKI management is mostly confined to ROS scavenging. ROS and accompanying RNS are key mediators during the inflammation and injury process. The regulation of such reactive species is a hot topic in lots of fields, including AKI. As demonstrated above, the anti-oxidative potential of natural products endows them with the possibility for AKI alleviation, but also leads to a large number of homogeneous research studies. More and more works are reporting out more detailed biological pathways and mediators interfering with natural products, but they are still insufficient. Much more bioactive functions of natural products need to be exploited for more delicate designs which could make full use of those products. Meanwhile, the physicochemical properties are also valuable for novel designs, for example, the coordination ability of natural polyphenols or alginates with metal ions, like platin, may be applied for the treatment of AKI induced by cisplatin.113,114
6. Large-animal models are rare in related studies. Many research studies were limited to rodent models; however, pre-clinical large animal models like porcine or nonhuman primates could provide more instructive results for translational research, considering the difference in innate immunity, adaptive immunity and gut microbiota among different species.115
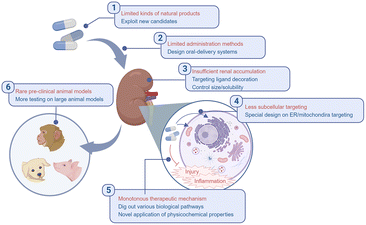 |
| Fig. 9 The challenges and potential solutions of polymer-related natural products against AKI. | |
Conclusions
The fight against AKI will become more and more urgent in the near future because of its multifactorial pathogenesis which is related to all other systems in the human body, although there is still a lack of effective methods to mitigate its process. Natural products, most of which have been traditionally used for a long period, are potent candidates for AKI related therapies because they are easy to access and have low toxicities. Various natural products were delivered and fabricated as prodrugs or nano-structures for AKI therapy, in which they were applied for both renal targeting and injury remission, and from which the traces of polymers are easy to be found. Generally, for those natural polymers used as targeting carriers, polysaccharides are the dominating polymers selected, usually with high affinity for specific receptors and high hydrophilicity. Different kinds of cargos including hydrophobic agents, RNAs and peptides were all successfully loaded on the natural polymers, depicting a broad applicability of the platform. For those natural agents used for treatment, polyphenols are usually used; based on the antioxidant and anti-inflammatory potential, they are loaded as cargos via chemical covalent bonds, hydrophobic interactions or host–guest interactions. Specially, a complex could be directly formed when the natural products have specific coordination with metal ions like polyphenols. Apart from those with renal targeting abilities themselves, several targeting-ligands were decorated on the fabricated polymers to enhance the renal accumulation. Even so, the simple way for renal targeting is to design and fabricate particles with smaller sizes like nanodots, and more effective methods are still in great demand. All in all, natural products have been traditionally used for a long period of time, and their rational application against AKI is a novel, challenging but meaningful attempt, providing a brand-new approach to deal with kidney injury as well as similar diseases.
Conflicts of interest
There are no conflicts to declare.
Acknowledgements
This work was financially supported by the Fundamental Research Funds for the Central Universities (26-2023-00074) and the National Natural Science Foundation of China (52022090). Fig. 1, Fig. 9 and the TOC figure were created with biorender.com.
References
- E. A. J. Hoste, J. A. Kellum, N. M. Selby, A. Zarbock, P. M. Palevsky, S. M. Bagshaw, S. L. Goldstein, J. Cerdá and L. S. Chawla, Nat. Rev. Nephrol., 2018, 14, 607–625 CrossRef CAS PubMed
.
- M. A. Perazella, Kidney Int., 2012, 81, 1172–1178 CrossRef CAS PubMed
.
- L. Awdishu, Curr. Opin. Crit. Care, 2017, 23, 484–490 CrossRef PubMed
.
- H. Seethapathy, S. Zhao, D. F. Chute, L. Zubiri, Y. Oppong, I. Strohbehn, F. B. Cortazar, D. E. Leaf, M. J. Mooradian, A. C. Villani, R. J. Sullivan, K. Reynolds and M. E. Sise, Clin. J. Am. Soc. Nephrol., 2019, 14, 1692–1700 CrossRef CAS PubMed
.
- M. Joannidis, W. Druml, L. G. Forni, A. B. J. Groeneveld, P. Honore, H. M. Oudemans-van Straaten, C. Ronco, M. R. C. Schetz and A. J. Woittiez, Intensive Care Med., 2010, 36, 392–411 CrossRef PubMed
.
- M. Joannidis, Acta Clin. Belg., 2007, 62, 353–356 CrossRef PubMed
.
- A. Abebe, K. Kumela, M. Belay, B. Kebede and Y. Wobie, Sci. Rep., 2021, 11, 15672 CrossRef CAS PubMed
.
- M. Ostermann and M. H. Rosner, Nat. Rev. Nephrol., 2023, 19, 77–78 CrossRef PubMed
.
-
S. G. Demirjian and E. P. Paganini, Controversies in Acute Kidney Injury, 2011, vol. 174, pp. 242–251 Search PubMed
.
- Y. Zhao, M. Pu, Y. Wang, L. Yu, X. Song and Z. He, J. Controlled Release, 2021, 336, 233–251 CrossRef CAS PubMed
.
- C.-P. Liu, Y. Hu, J.-C. Lin, H.-L. Fu, L. Y. Lim and Z.-X. Yuan, Med. Res. Rev., 2019, 39, 561–578 CrossRef PubMed
.
- Z.-X. Yuan, Z. Shang, J. Gu and L. He, Future Med. Chem., 2019, 11, 2237–2240 CrossRef CAS PubMed
.
- H. N. Geo, D. D. Murugan, Z. Chik, A. Norazit, Y. Y. Foo, B. F. Leo, Y. Y. Teo, S. Kadir, Y. Chan, H. J. Chai, M. Medel, N. A. Abdullah, E. J. Johns, M. J. Vicent, L. Y. Chung and L. V. Kiew, J. Controlled Release, 2022, 343, 237–254 CrossRef CAS PubMed
.
- T. Benzing and D. Salant, N. Engl. J. Med., 2021, 384, 1437–1446 CrossRef CAS PubMed
.
- H. Wei, D. Jiang, B. Yu, D. Ni, M. Li, Y. Long, P. A. Ellison, C. M. Siamof, L. Cheng, T. E. Barnhart, H.-J. Im, F. Yu, X. Lan, X. Zhu, Q. He and W. Cai, Bioact. Mater., 2023, 19, 282–291 CAS
.
- A. Ruggiero, C. H. Villa, E. Bander, D. A. Rey, M. Bergkvist, C. A. Batt, K. Manova-Todorova, W. M. Deen, D. A. Scheinberg and M. R. McDevitt, Proc. Natl. Acad. Sci. U. S. A., 2010, 107, 12369–12374 CrossRef CAS PubMed
.
- H. Soo Choi, W. Liu, P. Misra, E. Tanaka, J. P. Zimmer, B. Itty Ipe, M. G. Bawendi and J. V. Frangioni, Nat. Biotechnol., 2007, 25, 1165–1170 CrossRef PubMed
.
- F. Oroojalian, A. H. Rezayan, F. Mehrnejad, A. H. Nia, W. T. Shier, K. Abnous and M. Ramezani, Mater. Sci. Eng., C, 2017, 79, 770–782 CrossRef CAS PubMed
.
- R. M. Williams, J. Shah, B. D. Ng, D. R. Minton, L. J. Gudas, C. Y. Park and D. A. Heller, Nano Lett., 2015, 15, 2358–2364 CrossRef CAS PubMed
.
- M. E. M. Dolman, S. Harmsen, G. Storm, W. E. Hennink and R. J. Kok, Adv. Drug Delivery Rev., 2010, 62, 1344–1357 CrossRef CAS PubMed
.
- P. Zhou, X. Sun and Z. Zhang, Acta Pharm. Sin. B, 2014, 4, 37–42 CrossRef PubMed
.
- C.-Y. Fang, D.-Y. Lou, L.-Q. Zhou, J.-C. Wang, B. Yang, Q.-J. He, J.-J. Wang and Q.-J. Weng, Acta Pharmacol. Sin., 2021, 42, 1951–1969 CrossRef CAS PubMed
.
- H. G. Kang, H. K. Lee, K. B. Cho and S. I. Park, Medicina, 2021, 57, 1266 CrossRef PubMed
.
- Y. Cai, C. M. Huang, M. Y. Zhou, S. Q. Xu, Y. W. Xie, S. H. Gao, Y. T. Y. Yang, Z. R. Deng, L. B. Zhang, J. C. Shu, T. D. Yan and C. C. Wan, Phytomedicine, 2022, 104, 154306 CrossRef CAS PubMed
.
- Y. Q. Yang, Y. Y. Nan, Q. H. Chen, Z. X. Xiao, Y. T. Zhang, H. A. Zhang, Q. Huang and K. L. Ai, J. Mater. Chem. B, 2023, 11, 8081–8095 RSC
.
- M. S. Butler, Nat. Prod. Rep., 2005, 22, 162–195 RSC
.
- Z. Guo, Acta Pharm. Sin. B, 2017, 7, 119–136 CrossRef PubMed
.
- E. Patridge, P. Gareiss, M. S. Kinch and D. Hoyer, Drug Discovery Today, 2016, 21, 204–207 CrossRef CAS PubMed
.
- B. Li, H. Shao, L. Gao, H. Li, H. Sheng and L. Zhu, Drug Delivery, 2022, 29, 2130–2161 CrossRef CAS PubMed
.
- J. K. Patra, G. Das, L. F. Fraceto, E. V. R. Campos, M. D. P. Rodriguez-Torres, L. S. Acosta-Torres, L. A. Diaz-Torres, R. Grillo, M. K. Swamy, S. Sharma, S. Habtemariam and H.-S. Shin, J. Nanobiotechnol., 2018, 16, 71 CrossRef PubMed
.
- W. Jia, L. Zhou, L. Li, P. Zhou and Z. Shen, Pharmaceuticals, 2023, 16, 101 CrossRef CAS PubMed
.
- J. A. Kellum, P. Romagnani, G. Ashuntantang, C. Ronco, A. Zarbock and H.-J. Anders, Nat. Rev. Dis. Primers, 2021, 7, 52 CrossRef PubMed
.
- D. León-Aparicio, C. Salvador, O. E. Aparicio-Trejo, A. Briones-Herrera, J. Pedraza-Chaverri, L. Vaca, A. Sampieri, T. Padilla-Flores, Z. López-González, J. C. León-Contreras, R. Hernández-Pando and L. I. Escobar, Int. J. Mol. Sci., 2019, 20, 4995 CrossRef PubMed
.
- F. T. Billings, C. Yu, J. G. Byrne, M. R. Petracek and M. Pretorius, Cardiorenal Med., 2014, 4, 12–21 CrossRef CAS PubMed
.
- J. Sun, J. X. Zhang, J. K. Tian, G. M. Virzi, K. Digvijay, L. Cueto, Y. J. Yin, M. H. Rosner and C. Ronco, J. Am. Soc. Nephrol., 2019, 30, 1151–1161 CrossRef CAS PubMed
.
- S. Ma, R. G. Evans, N. Iguchi, M. Tare, H. C. Parkington, R. Bellomo, C. N. May and Y. R. Lankadeva, Microcirculation, 2019, 26, e12483 CrossRef PubMed
.
- H. Izzedine and M. A. Perazella, Kidney Int. Rep., 2017, 2, 504–514 CrossRef PubMed
.
- Y. Wang, K. X. Liu, X. S. Xie and B. Song, Clin. Imaging, 2021, 69, 354–362 CrossRef PubMed
.
- M. A. Perazella and M. H. Rosner, Clin. J. Am. Soc. Nephrol., 2022, 17, 1220–1233 CrossRef CAS PubMed
.
- H. Z. Wu and J. G. Huang, Curr. Drug Metab., 2018, 19, 559–567 CrossRef CAS PubMed
.
- K. Hosohata, Int. J. Mol. Sci., 2016, 17, 1826 CrossRef PubMed
.
- L. Su, J. Zhang, H. Gomez, J. A. Kellum and Z. Peng, Autophagy, 2023, 19, 401–414 CrossRef CAS PubMed
.
- A. Görlach, K. Bertram, S. Hudecova and O. Krizanova, Redox Biol., 2015, 6, 260–271 CrossRef PubMed
.
- Z. A. Radi, Toxicol. Pathol., 2018, 46, 930–943 CrossRef CAS PubMed
.
- A. Havasi and S. C. Borkan, Kidney Int., 2011, 80, 29–40 CrossRef CAS PubMed
.
- M. El Mouedden, G. Laurent, M. P. Mingeot-Leclercq, H. S. Taper, J. Cumps and P. M. Tulkens, Antimicrob. Agents Chemother., 2000, 44, 665–675 CrossRef CAS PubMed
.
- E. J. Nouwen, M. Q. Zhu and M. E. Debroe, Kidney Int., 1994, 46, 1462–1462 Search PubMed
.
- J. Cui, X. Y. Bai and X. M. Chen, Adv. Exp. Med. Biol., 2020, 1207, 469–480 CrossRef CAS PubMed
.
- G. P. Kaushal and S. V. Shah, Kidney Int., 2016, 89, 779–791 CrossRef CAS PubMed
.
- M. Jiang, Q. Wei, G. Dong, M. Komatsu, Y. Su and Z. Dong, Kidney Int., 2012, 82, 1271–1283 CrossRef CAS PubMed
.
- C. Tang, M. J. Livingston, Z. Liu and Z. Dong, Nat. Rev. Nephrol., 2020, 16, 489–508 CrossRef CAS PubMed
.
- N. Song, F. Thaiss and L. Guo, Front. Immunol., 2019, 10, 815 CrossRef CAS PubMed
.
- D. Kumar, S. K. Singla, V. Puri and S. Puri, PLoS One, 2015, 10, e115947 CrossRef PubMed
.
- F. L. Johnson, N. S. A. Patel, G. S. D. Purvis, F. Chiazza, J. Chen, R. Sordi, G. Hache, V. V. Merezhko, M. Collino, M. M. Yaqoob and C. Thiemermann, J. Am. Heart Assoc., 2017, 6, e005092 CrossRef PubMed
.
- L. L. Lv, P. M.-K. Tang, C. J. Li, Y. K. You, J. Li, X.-R. Huang, J. Ni, M. Feng, B. C. Liu and H.-Y. Lan, Kidney Int., 2017, 91, 587–602 CrossRef CAS PubMed
.
- X. Y. Xiong, B. Tang, T. T. Ji, X. Y. Li and S. J. Bai, Am. J. Transl. Res., 2021, 13, 4296–4308 CAS
.
- W. Qin, W. Xie, X. Yang, N. Xia and K. L. Yang, Med. Sci. Monit., 2016, 22, 818–823 CrossRef CAS PubMed
.
- H. J. Kim, S. H. Kim, M. Kim, H. Baik, S. J. Park, M. S. Kang, D. H. Kim, B. W. Kim, S. D. Markowitz and K. B. Bae, Am. J. Physiol.: Renal Physiol., 2020, 319, F1054–F1066 CrossRef CAS PubMed
.
- B. F. Palmer and D. J. Clegg, Clin. J. Am. Soc. Nephrol., 2022, 18, 279–289 CrossRef PubMed
.
- C. Z. Liu, X. X. Wang, X. N. Wang, Y. F. Zhang, W. J. Min, P. Yu, J. H. Miao, W. W. Shen, S. Q. Chen, S. Zhou, X. L. Li, P. Meng, Q. Y. Wu, F. F. Hou, Y. H. Liu, P. Yang, C. Wang, X. Lin, L. Tang, X. F. Zhou and L. L. Zhou, Theranostics, 2022, 12, 7158–7179 CrossRef CAS PubMed
.
- L. W. Maines, C. L. Green, S. N. Keller, L. R. Fitzpatrick and C. D. Smith, Int. J. Nephrol. Renovasc. Dis., 2022, 15, 323–334 CrossRef CAS PubMed
.
- Q. Z. Chen, J. F. Ma, X. N. Yang, Q. Y. Li, Z. F. Lin and F. H. Gong, Front. Pharmacol., 2020, 11, 241 CrossRef CAS PubMed
.
- G. F. Shu, C. Y. Lu, Z. X. Wang, Y. Y. Du, X. L. Xu, M. Xu, Z. W. Zhao, M. J. Chen, Y. Y. Dai, Q. Y. Weng, S. J. Fang, K. Fan, D. Liu, Y. Z. Du and J. S. Ji, Nanomedicine, 2021, 32, 102342 CrossRef CAS PubMed
.
- X. T. Gao, J. Wang, Y. Q. Wang, S. Liu, K. H. Dong, J. Wu, X. C. Wu, D. Y. Shi, F. Y. Wang and C. L. Guo, Int. J. Biol. Macromol., 2022, 223, 1083–1093 CrossRef CAS PubMed
.
- X. T. Gao, Y. L. Yin, S. Liu, K. H. Dong, J. Wang and C. L. Guo, Int. J. Biol. Macromol., 2023, 245, 125541 CrossRef CAS PubMed
.
- J. B. Hu, S. J. Li, X. Q. Kang, J. Qi, J. H. Wu, X. J. Wang, X. L. Xu, X. Y. Ying, S. P. Jiang, J. You and Y. Z. Du, Carbohydr. Polym., 2018, 193, 268–280 CrossRef CAS PubMed
.
- Z. W. Huang, Y. N. Shi, Y. Y. Zhai, C. C. Du, J. Zhai, R. J. Yu, L. F. Kou, J. Xiao, Y. Z. Zhao and Q. Yao, J. Controlled Release, 2021, 334, 275–289 CrossRef CAS PubMed
.
- Y. Wang, M. Pu, J. Yan, J. Zhang, H. Wei, L. Yu, X. Yan and Z. He, ACS Nano, 2023, 17, 472–491 CrossRef CAS PubMed
.
- D. E. Soranno, C. B. Rodell, C. Altmann, J. Duplantis, A. Andres-Hernando, J. A. Burdick and S. Faubel, Am. J. Physiol.: Renal Physiol., 2016, 311, F362–F372 CrossRef CAS PubMed
.
- D. Liu, F. Jin, G. Shu, X. Xu, J. Qi, X. Kang, H. Yu, K. Lu, S. Jiang, F. Han, J. You, Y. Du and J. Ji, Biomaterials, 2019, 211, 57–67 CrossRef CAS PubMed
.
- W. M. Tang, S. Panja, C. M. Jogdeo, S. Y. Tang, L. Ding, A. Yu, K. W. Foster, D. Dsouza, Y. S. Chhonker, H. Jensen-Smith, H. S. Jang, E. I. Boesen, D. J. Murry, B. Padanilam and D. Oupicky, Biomaterials, 2022, 285, 121562 CrossRef CAS PubMed
.
- M. X. Pang, S. C. Duan, M. M. Zhao, Q. Q. Jiao, Y. M. Bai, L. L. Yu, B. Du and G. Y. Cheng, Toxicol. Appl. Pharmacol., 2022, 450, 116155 CrossRef CAS PubMed
.
- D. W. Wang, S. J. Li, X. Y. Tan, J. H. Wang, Y. Hu, Z. Tan, J. Liang, J. B. Hu, Y. G. Li and Y. F. Zhao, Carbohydr. Polym., 2021, 256, 117556 CrossRef CAS PubMed
.
- D. Liu, G. Shu, F. Jin, J. Qi, X. Xu, Y. Du, H. Yu, J. Wang, M. Sun, Y. You, M. Zhu, M. Chen, L. Zhu, Q. Shen, X. Ying, X. Lou, S. Jiang and Y. Du, Sci. Adv., 2020, 6, eabb7422 CrossRef CAS PubMed
.
- J. Gao, R. Liu, J. Wu, Z. Liu, J. Li, J. Zhou, T. Hao, Y. Wang, Z. Du, C. Duan and C. Wang, Biomaterials, 2012, 33, 3673–3681 CrossRef CAS PubMed
.
- P. H. Cheng, W. Chen, S. H. Li, S. S. He, Q. Q. Miao and K. Y. Pu, Adv. Mater., 2020, 32, 1908530 CrossRef CAS PubMed
.
- J. Huang, J. Li, Y. Lyu, Q. Miao and K. Pu, Nat. Mater., 2019, 18, 1133–1143 CrossRef CAS PubMed
.
- J. S. Huang, J. G. Huang, P. H. Cheng, Y. Y. Jiang and K. Y. Pu, Adv. Funct. Mater., 2020, 30, 2003628 CrossRef CAS
.
- B. Li, M. Juenet, R. Aid-Launais, M. Maire, V. Ollivier, D. Letourneur and C. Chauvierre, Adv. Healthcare Mater., 2017, 6, 1601200 CrossRef PubMed
.
- K. Singbartl, S. A. Green and K. Ley, FASEB J., 2000, 14, 48–54 CrossRef CAS PubMed
.
- T. Loftsson, P. Jarho, M. Másson and T. Järvinen, Expert Opin. Drug Delivery, 2005, 2, 335–351 CrossRef CAS PubMed
.
- S. Liu, X. T. Gao, Y. Q. Wang, J. Wang, X. J. Qi, K. H. Dong, D. Y. Shi, X. C. Wu and C. L. Guo, Int. J. Pharm., 2022, 626, 122161 CrossRef CAS PubMed
.
- S. Lu, S. Zhou, J. W. Chen, J. Zheng, J. Ren, P. Y. Qi, Z. Q. Zhu and Z. Z. Li, J. Biomed. Nanotechnol., 2021, 17, 230–241 CrossRef CAS PubMed
.
- K. T. Huang, C. T. Wu, Y. Chang, F. M. Ho, C. K. Chiang and S. W. Liu, Biochem. Biophys. Res. Commun., 2022, 608, 122–127 CrossRef CAS PubMed
.
- X. J. Qi, J. Wang, F. S. Fei, X. T. Gao, X. C. Wu, D. Y. Shi and C. L. Guo, Mol. Pharm., 2023, 20, 136–146 CrossRef CAS PubMed
.
- T. Y. Lan, H. L. Guo, X. Lu, K. D. Geng, L. Wu, Y. J. Luo, J. F. Zhu, X. C. Shen, Q. Q. Guo and S. Z. Wu, Biomacromolecules, 2022, 23, 5253–5266 CrossRef CAS PubMed
.
- J. B. Hu, D. Liu, J. Qi, K. J. Lu, F. Y. Jin, X. Y. Ying, J. You and Y. Z. Du, Biomater. Sci., 2018, 6, 3397–3409 RSC
.
- R. Zhang, L. Cheng, Z. L. Dong, L. Q. Hou, S. H. Zhang, Z. Q. Meng, O. Betzer, Y. H. Wang, R. Popovtzer and Z. Liu, Mater. Horiz., 2021, 8, 1314–1322 RSC
.
- G. Cordoba-David, A. Duro-Castano, R. C. Castelo-Branco, C. Gonzalez-Guerrero, P. Cannata, A. B. Sanz, M. J. Vicent, A. Ortiz and A. M. Ramos, Sci. Rep., 2020, 10, 2056 CrossRef CAS PubMed
.
- Q. Huang, Y. Yang, T. Zhao, Q. Chen, M. Liu, S. Ji, Y. Zhu, Y. Yang, J. Zhang, H. Zhao, Y. Nan and K. Ai, Bioact. Mater., 2023, 21, 381–393 CAS
.
- D. Y. Zhang, H. K. Liu, T. He, M. R. Younis, T. H. Tu, C. Yang, J. Zhang, J. Lin, J. L. Qu and P. Huang, Small, 2021, 17, 2005113 CrossRef CAS PubMed
.
- F. Jia, B. Yu, J. Li, F. Cai, G. Fu, Q. Jin and J. Ji, Adv. Healthcare Mater., 2023, 12, 2301615 CrossRef CAS PubMed
.
- J. J. Li, Q. J. Duan, X. N. Wei, J. P. Wu and Q. Q. Yang, Small, 2022, 18, 2204388 CrossRef CAS PubMed
.
- D. X. Zou, R. Ganugula, M. Arora, M. B. Nabity, D. Sheikh-Hamad and M. N. V. R. Kumar, Am. J. Physiol.: Renal Physiol., 2019, 317, F1255–F1264 CrossRef CAS PubMed
.
- X. Deng, T. Zeng, J. Li, C. Huang, M. Yu, X. Wang, L. Tan, M. Zhang, A. Li and J. Hu, Biomater. Sci., 2019, 7, 5312–5323 RSC
.
- S. J. Maleki, J. F. Crespo and B. Cabanillas, Food Chem., 2019, 299, 125124 CrossRef CAS PubMed
.
- P. Peng, J. R. Zou, B. Zhong, G. X. Zhang, X. F. Zou and T. P. Xie, Pharmacology, 2023, 108, 27–36 CrossRef CAS PubMed
.
- S. Llano, S. Gómez, J. Londoño and A. Restrepo, Phys. Chem. Chem. Phys., 2019, 21, 3752–3760 RSC
.
- Y. Cai, C. Huang, M. Zhou, S. Xu, Y. Xie, S. Gao, Y. Yang, Z. Deng, L. Zhang, J. Shu, T. Yan and C. C. Wan, Phytomedicine, 2022, 104, 154306 CrossRef CAS PubMed
.
- S. Sabet, A. Rashidinejad, L. D. Melton and D. J. McGillivray, Trends Food Sci. Technol., 2021, 110, 253–266 CrossRef CAS
.
- Y. M. Chiang, C. P. Lo, Y. P. Chen, S. Y. Wang, N. S. Yang, Y. H. Kuo and L. F. Shyur, Br. J. Pharmacol., 2005, 146, 352–363 CrossRef CAS PubMed
.
- Y. Li, S. Sheng, B. Yu, F. Jia, K. Wang, H. Han, Q. Jin, Y. Wang and J. Ji, Chin. J. Polym. Sci., 2022, 40, 1101–1109 CrossRef CAS
.
- C. H. Chung, W. Jung, H. Keum, T. W. Kim and S. Jon, ACS Nano, 2020, 14, 6887–6896 CrossRef CAS PubMed
.
- M. Ghasemzadeh Rahbardar and H. Hosseinzadeh, Naunyn-Schmiedeberg's Arch. Pharmacol., 2020, 393, 1779–1795 CrossRef CAS PubMed
.
- Y. Hong, W. Zhou, K. Li and S. H. Sacks, Kidney Int., 2002, 62, 1291–1300 CrossRef CAS PubMed
.
- H.-J. Kim, K. Ravichandran, A. Ozkok, Q. Wang, Z. He, A. Jani, D. Ljubanovic, I. S. Douglas and C. L. Edelstein, J. Pharmacol. Exp. Ther., 2014, 349, 518–525 CrossRef PubMed
.
- B. Surnar, A. S. Shah, S. Guin, N. Kolishetti, A. Fornoni and S. Dhar, Biomacromolecules, 2021, 22, 4244–4250 CrossRef CAS PubMed
.
- Q. Wen, Q. Cai, P. Fu, D. Chang, X. Xu, T.-J. Wen, G.-P. Wu, W. Zhu, L.-S. Wan, C. Zhang, X.-H. Zhang, Q. Jin, Z.-L. Wu, C. Gao, H. Zhang, N. Huang, C.-Z. Li and H. Li, Chin. Chem. Lett., 2023, 34, 107592 CrossRef CAS
.
- X. Deng, K. Chen, K. Pang, X. Liu, M. Gao, J. Ren, G. Yang, G. Wu, C. Zhang, X. Ni, P. Zhang, J. Ji, J. Liu, Z. Mao, Z. Wu, Z. Xu, H. Zhang and H. Li, Chin. Chem. Lett., 2023, 108861, DOI:10.1016/j.cclet.2023.108861
.
- D. Liu, E. J. Cornel and J. Du, Adv. Funct. Mater., 2020, 31, 2007330 CrossRef
.
- A. W. Porter, J. L. Brodsky and T. M. Buck, Am. J. Physiol.: Cell Physiol., 2022, 323, C1697–C1703 CrossRef CAS PubMed
.
- J. Zhang, B. Gao, B. Ye, Z. Sun, Z. Qian, L. Yu, Y. Bi, L. Ma, Y. Ding, Y. Du, W. Wang and Z. Mao, Adv. Mater., 2023, 35, 2208571 CrossRef CAS PubMed
.
- J. Yang, T. Su, H. Zou, G. Yang, J. Ding and X. Chen, Angew. Chem., Int. Ed., 2022, 61, e202211136 CrossRef CAS PubMed
.
- T. Imai, K. Fujii, S. Shiraishi and M. Otagiri, J. Pharm. Sci., 1997, 86, 244–247 CrossRef CAS PubMed
.
- B. Packialakshmi, I. J. Stewart, D. M. Burmeister, K. K. Chung and X. M. Zhou, Ren. Fail., 2020, 42, 1042–1058 CrossRef PubMed
.
|
This journal is © The Royal Society of Chemistry 2024 |