DOI:
10.1039/D3TC03058J
(Perspective)
J. Mater. Chem. C, 2023,
11, 14527-14539
On the fundamentals of organic mixed ionic/electronic conductors
Received
25th August 2023
, Accepted 12th October 2023
First published on 12th October 2023
Abstract
The first Telluride Science meeting (formerly TSRC) on organic mixed ionic and electronic conductors (OMIECs), Oct 3–7, 2022, brought together researchers across the field to understand the fundamental processes and identify out-standing questions related to this exciting class of materials. OMIECs are organic materials that promote the transport of mobile electronic charge carriers while simultaneously supporting ionic transport and ionic–electronic coupling. These properties open up broad areas of applications from energy to bioelectronics. Devices include batteries, supercapacitors, actuators, electrochromic displays, and organic electrochemical transistors (OECTs). They possess the key strengths of traditional organic electronic materials, such as synthetic tunability and low-temperature processing. Despite the recent advances in devices and applications achieved with such materials, many challenges and gaps in understanding remain. These topics hold the key to designing next-generation materials and devices that continue to push the limits of performance and stability and facilitate novel functionality. This perspective aims to summarize the current understanding, conversations, and debates that made this TSRC particularly engaging, enabling new directions and searching for missing pieces of the OMIEC puzzle.
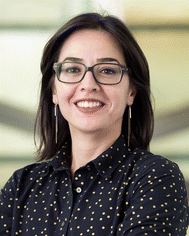
Sahika Inal
| Sahika Inal is an Associate Professor of Bioengineering with co-affiliations in Electrical Engineering and Materials Science and Engineering programs at King Abdullah University of Science and Technology (KAUST). She has a BSc degree in Textile Engineering from Istanbul Technical University (Turkey), an MSc in Polymer Science, and a PhD in Experimental Physics from the University of Potsdam, Germany. She completed her postdoctoral training at the Center of Microelectronics of Provence of the Ecole Nationale Supérieure des Mines de Saint-Étienne (France). Launched in 2016, Inal lab at KAUST exploits the functionalities of organic electronic materials, investigates ionic/electronic charge transport, and designs electronic devices that record/stimulate biological signals. Sahika is a Fellow of the Royal Society of Chemistry and has received recognitions, including the ACS PMSE Young Investigator Award 2022, Beilby Medal and Prize 2022, and the Journal of Materials Chemistry Lectureship (2022). She is the author of 110+ publications, and her work has been cited more than 10 000 times. |
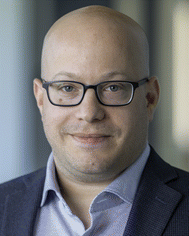
Jonathan Rivnay
| Jonathan Rivnay is a Professor of Biomedical Engineering and Materials Science & Engineering at Northwestern University. Jonathan earned his BSc in 2006 from Cornell University. He then moved to Stanford University, where he earned a MSc and PhD in Materials Science and Engineering, studying the structure and electronic transport properties of organic electronic materials. In 2012, he joined the Department of Bioelectronics at the Ecole des Mines de Saint-Etienne in France as a Marie Curie postdoctoral fellow, working on conducting polymer-based devices for bioelectronics. Jonathan spent 2015–2016 as a member of the research staff in the Printed Electronics group at the Palo Alto Research Center (PARC, a Xerox Co.) before joining the faculty at Northwestern in 2017. He is a recipient of a Faculty Early Career Development (CAREER) award from the National Science Foundation (2018), a research fellowship from the Alfred P. Sloan Foundation (2019), and was named a Materials Research Society Outstanding Early Career Investigator (2020). |
The materials – search for model systems
As in other fields within materials science, it is important to identify model systems that will enable accessible and repeatable fundamental studies. At their most basic level, OMIECs support electronic transport through an electronic transport pathway (i.e., along and between conjugated polymer backbones or between nearby redox centers), and ionic transport occurs through free volume or an ion transport pathway. The ions are needed to ensure charge neutrality when the conjugated or redox system is doped (chemically or electrochemically).
The opportunity and the challenge with organic systems is that such an arrangement can be achieved through many routes: blends, composites, single component systems with varied side chains, block co-polymers, etc. A single model system has eluded the field as a whole, and different questions demand different model systems. Poly(3-hexylthiophene) (P3HT) is the most studied system in this area. Many fundamental studies have focused on electrochemistry in organic electrolytes, such as TBAPF6 in acetonitrile. Controlled electrochemical doping led to highly conducting films.1 P3HT, in combination with salts (e.g., LiClO4) and poly(ethyleneoxide) (PEO), finds an audience in energy applications or other areas where dry mixed conduction is sought.2
Poly(ethylendioxythiophene):poly(styrenesulfonate) (PEDOT:PSS) is the most studied OMIEC, a combination of a doped conducting polymer and a polyelectrolyte, and can be regarded as a polyelectrolyte complex. PEDOT:PSS with aqueous electrolytes, including NaCl or KCl, has been well studied owing to their commercial availability and ubiquity in the community of researchers interested in aqueous operation or the role of hydration, e.g., for OECTs or actuators. More recently, conjugtaed backbones with ionic side groups (so-called conjugated polyelectrolytes) and oligoether side chains fill yet another need and allow operation in aqueous electrolytes. Additional materials strategies from side-chain free polymers and radical polymers are also gaining popularity.
Even so, single component systems are still largely represented by a few chemical structures. While polythiophene backbones are the p-type work-horse of the community, naphthalene diimide (NDI) acceptor units combined with thiophene donors can be regarded as the n-type model system. The electrochemistry of this polymer (e.g., NDI-T) is quite complex but points to a backbone with localized charges on the NDI units.3 Another n-type mixed conductor, the ladder-type poly(benzimidazobenzophenanthroline) (BBL), stands as one of the few side-chain free mixed conductors and has shown high performance in OECTs. Pure p-type redox polymers bear, for example, triarylamine or carbazole redox units.4 On the small molecules front, the films of oligoether tethered fullerenes,5 thiophene-flanked diketopyrrolopyrrole units,6 polycyclic systems consisting of naphthalene bis-isatin dimer core with rhodanine terminals,7 or thiophene-EDOT based cores8 are among the structures that can be electrochemically doped and de-doped in aqueous media, while they are yet to be commonly used by different research teams. All these systems can be modified with additives, dopants, and through processing and post-processing.
No one system can answer all the key questions, and no one system is completely ideal (i.e., using PEDOT:PSS in commercial formulations means some additives may not be known to the researcher, and small batch novel synthesized polymers can have variable levels of impurities, defects, and distributions of molecular weights). Nevertheless, some parallels and generalities can be gleaned, putting these diverse materials on a broader spectrum of understanding that benefits the community at large.
The operating environment dictates properties
The composition, structure, and properties of OMIECs are complex and interrelated. Compositionally, all species play a critical role. Structurally, OMIECs often form semi- or para-crystalline domains in an amorphous matrix. Multicomponent OMIECs can undergo phase separation, such as the segregation into conjugated polymer-rich and polyelectrolyte-rich domains in PEDOT:PSS.9,10 Ions and additives affect the degree of crystallinity, coherence length within the crystalline domains, phase separation, and phase purity, amongst other structural parameters.11 Harsh processing techniques can enhance crystallinity and increase the ratio of conjugated polymer to polyelectrolyte in multicomponent OMIECs.12
OMIECs have applications in a variety of operating environments. The permeable nature of OMIECs makes them sensitive to operating environments that often include contact with, or immersion in, electrolytes when operated in electrochemical devices. Here again, all species play a critical role, and the role of ion and solvent transport between the OMIEC and its environment must be considered. Contacting electrolytes (e.g., solid polymer, aqueous, organic, or ionic liquid) interact with OMIECs in different ways and to different degrees, depending on characteristics of the solvent (polarity, miscibility) and the ion (size, solvation, polarizability).
The passive swelling (just due to contact with the electrolyte, no electrochemical potential applied) and active swelling (driven by electrochemical potential) of OMIECs must be considered to understand the morphology of the film during operation. During passive swelling, ions and solvent penetrate and swell the OMIEC. The choice of water versus acetonitrile electrolytes leads to different degrees of swelling, e.g., determined by in situ spectroscopic ellipsometry coupled with cyclic voltammetry.13,14 The degree of swelling strongly depends on the miscibility of the electrolyte and the OMIEC, which is often determined by the OMIEC side chain chemistry (oligoether, alkyl, tethered ion) and architecture (length, branching).15–18 The miscibility of solvent and ions in the OMIEC can vary, resulting in differential solvent and ion uptake.19 Further, solvated ions may shed or retain solvation shells when injected into OMIEC. The degree of swelling may differ between amorphous and crystalline domains20 or between conjugated polymer-rich and polyelectrolyte-rich phases.21 Ion and solvent uptake into the crystalline domains expands and modifies the crystal structure, in some cases enhancing or disrupting crystallinity dependent on both the OMIEC, electrolyte, and electronic charge density.20,22–27
If the as-prepared OMIEC contains mobile ions that differ from those in the contacting electrolyte, ion exchange will occur, such as ion-proton exchange in PEDOT:PSS.21 In some systems, larger concentrations of fixed ionic (polyelectrolyte) charge in the OMIEC introduces Donnan exclusion, limiting ion uptake to oppositely charged counterions.21 In such cases, di- and poly-valent counterions can chelate with the fixed charge groups, becoming trapped and inducing ionic crosslinks.28 But in most high-performing OMIECs, Donnan exclusion is suppressed or absent, and cation and anion transport, along with solvent transport, contribute to OMIEC swelling.21,29
A change in the electrochemical potential of the OMIEC can actively modulate how much it swells. The electrochemical oxidation or reduction of the OMIEC modulates the electronic carrier and the counterbalancing ion (dopant) density. This accumulated charge, in turn, can modulate optoelectronic properties – which is core to electroactive functionality of OMIECs in a variety of applications, e.g. electrochemical displays but also electrochemically switchable nanoantennas as metasurfaces.30 Dopant modulation can occur by both ion uptake and expulsion.14,29 This modulation of electrostatics also changes the miscibility of the OMIEC and electrolyte surroundings, bringing in or expelling solvent and charge-balanced populations of anions and cations, beyond just the dopant transport.21
The degree of active swelling can vary greatly depending on the electrolyte choice and the OMIEC chemical structure. Microscopically, electrochemical cycling can induce crystallite expansion and contraction.20,24,25 Extreme active swelling can lead to dissolution/delamination,31 but can also be leveraged in reversible swelling/gel formation.32 The effects of active swelling on composition, structure, and properties are often not fully reversible.33
Additionally, under operating conditions, impurities can play a large role. Dissolved oxygen can limit the electrochemical stability window and accelerate OMIEC degradation.34,35 Further, OMIECs with shallow HOMO levels can be spontaneously doped (oxidized).36 Residual catalysts within the OMIEC can determine its electronic conductivity.37 The contacting electrodes also affect the OMIEC, with electrode material (along with oxygen and potential) dictating the potential stability window and degradation kinetics of the OMIEC.34
All this demands the use of multimodal in situ characterization in various operating conditions to capture and interrogate the complexities. Structure, composition, and properties must be mapped across relevant environments and potentials. This requires simultaneous (or parallel) probing of electronic (stored charge, electrical conductivity), compositional (individual anions, cations, and solvent), structural (micro-, mesoscale, macroscopic), spectral, and mechanical properties of OMIECs in application-relevant electrolyte environments under controlled potentials. Some popular in situ characterization tools are electrochemical UV-VIS-IR absorption spectroscopy, quartz crystal microbalance with dissipation monitoring (QCM-D), X-ray characterization, X-ray photoelectron spectroscopy (XPS), spectroscopic ellipsometry, and scan-probe microscopies.38
Where are the ions in the structure?
A large effort has been made to understand where ions reside within the volume of OMIECs during electrochemical doping/dedoping. However, getting a holistic picture of ions has proven challenging due to the heterogeneous microstructure of OMIECs and the experimental challenges of working with (especially aqueous) electrolytes in situ. To understand ion arrangement in OMIECs, the driving force for ion intercalation has to be considered, favourable microstructures have to be found that feature efficient ionic–electronic coupling, and finally, it has to be understood how ions are distributed at device length scales where electric fields can drive their motion.
The ability for ions to penetrate OMIECs is highly dependent on the mixing enthalpy.39 It has been shown that polar sidechains are one powerful way to facilitate bulk ion penetration when using atomic ions (e.g., Na+, Cl−, etc.).40 Volumetric doping may also be achieved by modifying the charge distribution on ionic species.41 There is also evidence to suggest that charge neutral ion pairs can diffuse into OMIECs42 and ions can remain in OMIEC films following doping/dedoping cycles21,29 indicating mixing between ions and OMIECs is thermodynamically favorable.
We also note that the number of charge-compensated ion-electron/hole pairs scales with the applied potential.43,44 This indicates that the doping level is thermodynamically tied to the electric potential, where the energy for changing the doping level may be tied to the entropy for different carrier densities45 voltage-dependent enthalpy of mixing between ions and OMIECs,39,46 and/or the chemical/quantum capacitance of the conjugated polymer.47,48
Clarifying where ions reside within the microstructure of OMIECs is essential to understand the coupling between ionic and electronic charges. From an energetic standpoint, we would expect electrons/holes to first populate the lower energy sites of the microstructure within the ordered regions.49 This is also supported by electrochemical cyclic voltammetry of different amorphous and semicrystalline P3HT films (regioregular vs. regio-random, as-cast versus well-crystallized).1,50 However, during chemical doping, reactants must diffuse through the amorphous region of the film, leading to doping/dedoping of the amorphous region first.51,52 Similarly, ion conduction in OMIECs requires free volume for ions, which are often hydrated, to move. Thus, there is likely to be a balance between doping of the lowest energy sites first vs. doping the sites accessible to ions.
There are many reports stating that the degree of swelling during doping/dedoping is largest in the amorphous parts of the microstructure.53,54 However, at higher doping densities, ions can disrupt the crystals, leading to reversible26,51 and irreversible51,55,56 phase changes. In some cases, ion pathways form within the microstructure via self-assembly, minimizing changes to the microstructure during doping/dedoping.22
One must also consider the rearrangement of ions across device length scales. While typical models for OECT operation use C* as the governing quantity to solve for carrier densities,57 this model ignores the ion drift under the electric field. Ions are charged and will also move under the same applied potential driving hole current across the OECT channel. Recently, lateral ion drift along the channel has been taken into account to accurately capture experimentally measured potential drops along the channel.58,59 Similarly, ionic and electronic drift and diffusion have been modeled in PEDOT:PSS by treating the OMIEC as a composite of a pure electrolyte phase and pure electronic phase with a local electric field at their interface.60 While this model works well for PEDOT:PSS, it might be challenging to extend to homopolymer OMIECs which may not have a distinct electrolyte phase.
The role of swelling and ion motion
Another common thread through many of our discussions was the role of swelling in OMIECs. There is broad agreement that swelling is the factor that distinguishes OMIEC applications from dry organic semiconductor applications. Even then, there is no broad consensus on how swelling should be defined. The literature distinguishes “passive” (swelling at the open circuit potential) from “active” (applied bias-induced swelling) swelling. But many newer, low threshold voltage materials are partially doped in ambient so that demarcation becomes ambiguous. Unfortunately, quantifying swelling at the level needed to understand what is occurring on the microscale is quite difficult. Bulk swelling is typically measured via electrochemical quartz crystal microbalance with dissipation monitoring (EQCM-D). EQCM-D provides great insight into total mass, but mass does not distinguish exactly which species are moving. It could be a cation, anion, or solvent moving or some complex combination of the three that give rise to the total mass change.29 For this reason, there is a need for more tools that identify which species are moving under what conditions. Ion motion can be studied indirectly through moving front experiments, though this may not be appropriate for systems in which doping occurs, also, via ion expulsion. X-ray fluorescence experiments have also provided valuable insights,21 yet there remains a need for operando studies or adoption of ambient XPS techniques to shed light on ionic composition during operation.
Even once the identity of mobile species is resolved, real questions remain about where the swelling occurs. There is a common observation that bulk (QCM-D) swelling is larger than the observed swelling of the semicrystalline lattice.25 This leads to speculation that most swelling occurs in the amorphous regions, which could disrupt the percolative electronic network.20 New emerging techniques could start proving or disproving this hypothesis.61 However, that level of swelling, which can range from 20 to hundreds of percent, is hard to understand from a mechanical standpoint. When a film is swelling more than 100%, a persistent question is what holds the film (often decorated with highly hydrophilic side chains) together? We discussed ionic vs. covalent cross-linking, hydrogen bonding, or even electrostatic effects of the polaron, among other options, and agree that this issue requires further investigation.
Controlling mixed conduction
Molecular design trends
The transition from organic field effect transistors (OFETs) to OECTs saw the utilization of many similar materials, whereby the need to facilitate ion transport in water was often met by replacing alkyl side chains with oligoether ones or with the addition of ionic units.62–65 However, emerging studies are showing that mixed conduction can be achieved without side chains altogether.66,67 So, the logical question arises: are side chains (ion-free or bearing) necessary?
It has been shown that side-chain engineering modulates the mixed conduction capability of polymer films, typically portrayed as a matter of balancing ionic and electronic conduction. However, recent work showed that BBL, not only exhibits facile mixed conduction capability, but outperforms the widely studied n-type NDI-T2 based polymers, bearing hydrophilic side chains.68 Tang et al. showed that BBL is not unique in its ability to exhibit mixed conduction in the absence of solvating side chains. The side-chain free poly(benzodifurandione) synthesized via oxidative polymerization in the presence of quinone oxidants appears to be a candidate for bridging the performance gap between n-type and p-type mixed conductors.69
What remains evident is that predicting mixed conductor performance based on chemical structure alone is challenging. To understand and utilize the underlying process, it is vital to venture out of what is conventionally known (and assumed) and explore every aspect of polymer chemistry that facilitates and governs mixed conduction.
The role of molecular weight and dispersity
Another outstanding challenge in OMIECs is controlling their molecular weight and dispersity (Đ and shape of the molecular distribution), and understanding how these critical molecular parameters influence performance in devices. In semiconducting polymers for OFETs, it is well established, particularly for P3HT, that the molecular weight and dispersity strongly influence the charge mobility. High molecular weight polymers generally have higher mobility due to an increased fraction of tie chains.70 It was shown that even a small fraction of low molecular weight polymers in polydisperse samples resulted in a drop in mobility.71 On the other hand, for mixed transport, the relationship between molecular weight and dispersity, and performance is yet to be established and is likely to be polymer-specific. For example, Wu et al. found that higher molecular weight BBL led to increased performance in OECTs.72 As described above, the OMIEC system includes the salt – thus, the identity of the electrolyte also affects trends related to molecular weight.20 In multi-component systems, such as PEDOT:PSS, the molecular weight of PSS did not affect the OECT performance, but having a higher Đ led to a 4-fold increase in [μC*].73 For establishing clear structure–property relationships for new OMIECs, it will, therefore, be crucial to compare polymers with similar molecular weights and dispersity. This task, however, is likely to be complicated by synthetic challenges, including differences in solubility between the polymers, difficulty in reproducibly synthesizing π-conjugated polymers with controlled molecular weight and dispersity, a similar defect distribution, and variable degrees of purity (e.g., residual catalyst content37).
Post-processing and additives
A different way to modulate mixed conduction that does not require synthetic means is through post-processing. These approaches include molecular doping, solvent blending, and temperature annealing. These are well established for conventional organic semiconductors and their electronic devices, such as OFETs, organic photovoltaics and thermoelectric generators but have largely been unexplored for OMIECs and OECTs, in particular.
Molecular doping strategies have been applied to OMIECs, both for p- and n-type materials, which have been successful in modulating current densities, shifting threshold voltages, minimizing side reactions, and improving long-term stability in OECTs.74–76 Other small molecule additives have been employed to improve the output characteristics of OECTs.77 Moreover, the de-doping of PEDOT:PSS has been achieved by promoting interactions between amine groups and PSS-, allowing the generate a polymer for use in enhancement mode OECTs.78 While progress has been done in this regard, little is understood about dopant-water interactions (or more generally dopant-solvent interactions), how the dopant interacts with the material itself, and how this extra molecule interacts with the ions in the electrolyte.
On the other hand, solvent blending has been more widely utilized, with co-solvents added to PEDOT:PSS dispersions to improve the conductivity through a change in morphology and.11 The co-solvent approach has also been successful in improving performance of OECTs bearing hole- or electron-conducting OMIECs as the active material. For example, acetone was used as a “bad solvent” to induce aggregation in an n-type NDI-T2 copolymer.79 Chlorobenzene was added to a p-type T2-T copolymer to improve the morphology by extending the drying time of the film after spin coating.75 Both examples use chloroform as base solvent, which solubilizes the backbone of the polymers better than the additive solvent. Choosing to solubilize either the side chains or the backbone has very different effects on device performance. Solubilizing side chains with polar solvents increases backbone aggregation, which improves the OECT mobility.80 This effect is observed not only in polymers but also in small molecule semiconductors.81
Another approach to improve device performance is via an increase of charge density, which can be achieved by chemical dopants. NDI-T2 based polymers were admixed with a Lewis acid, ammonium salt tetra-n-butylammonium fluoride, and the additive acted as a simultaneous molecular dopant and morphology-additive, with the combined effects significantly enhancing OECT performance.74 Organic salts added to the polymer casting solution, with no energetically plausible mechanism of charge transfer to the OMIEC, improved the backbone planarity and charge delocalization by coupling to the backbone.82 The salt-added films show a distinctly porous morphology where the interconnectivity is affected by the salt type and responsible for faster OECT operation.
Another additive type that modulates mixed conduction is ion transporting materials such as cellulose nanofibrils. The ion conductors improved the ion mobility of, e.g., PEDOT:PSS83 and a polythiophene with oligoether side chains.84 Besides conductivity enhancing ability, the nanocellulose can act as a reinforcing agent, leading to mechanically adaptive materials that show a reversible decrease in stiffness upon swelling.
The effect of temperature is known to improve crystallinity in organic semiconductors, which in turn can improve field effect mobilities. For OMIECs, for which electrolyte-carrying ions are needed for conductivity modulation, thermal annealing has so far been considered detrimental, as shown by Flagg, et al. in a p-type polymer. By improving crystallinity, the resistance of the active layer to ion penetration is increased. This leads to a lower OECT performance by decreasing mobility.63 However, a recent report showed that operation with a large anion (PF6 or TFSI) is possible after annealing the film up to 300 °C.85 The positive or negative effects of annealing differ from one backbone to another, and seem to be directly tied to their glass transition and melting temperature, rather than to the nature of the side chains themselves.
Many devices comprise OMIEC thin films (on a substrate) with a thickness of typically less than 100 nanometers, which ensures that ions can rapidly reach the complete volume of the material, essential for, e.g., achieving a high switching speed. For some devices such as fiber based OECTs, the material (e.g. highly aligned PEDOT:PSS microfibers86) may act as both the OMIEC as well as the de facto substrate and must hence feature adequate mechanical robustness. The OMIEC material then tends to have dimensions of at least several micrometers, which considerably reduces the speed of ion ingression, but could be mitigated through the use of porous materials.87
Emergent phenomena owing to high charge densities
OMIECs display a finite potential window of high electrical conductivity, with the conductance decreasing dramatically beyond a critical gate voltage-controlled charge carrier density. While this phenomenon is reversible and not due to chemical or electrochemical degradation of the OMIEC's π-system, the precise cause is not fully understood yet.
Measurements of in situ conductance coupled with cyclic voltammetry show bell-shaped conductance profiles for redox polymer systems.4 For P3HT in TBAPF6/acetonitrile, for example, a plateau-like conductance is found over a large electrochemical potential range of 0.4 up to 0.9 V vs. Fc/Fc+ where polaron and bipolaron species are formed.1 A possible explanation for the behavior may be associated with band filling,88–90 while others have proposed that energetic/structural disorder at high carrier densities leads to decreased charge carrier mobility.1,91–93 For a further discussion on plateau-like conductivity and the decrease at very high potentials, we refer to electrochemical literature.94
In BBL, the maximum electrical conductivity coincides with a change in the polarity of the Seebeck coefficient as the carrier density approaches 1 electron/repeating unit.95 This is explained by the formation of multiply charged species and the opening of a hard Coulomb gap around the Fermi energy caused by double occupation and Coulomb interactions occurring at high doping levels. It was also found that the soft Coulomb gap previously proposed to explain the drop in electrical conductivity is of minor importance,96 particularly for a rigid polymer like BBL.
Recent work has also shown that the decrease in conductance at high charge carrier density is associated with electron–cation interactions occurring at the electrolyte and organic semiconductor interface that alter the potential energy landscape in which electronic charges move.97 In fullerene (C60) single crystals gated through ionic liquids, the degree of conductance suppression is considerably affected by the size of the ions. Small ions support activated transport and a complete conductance suppression at around 1 electron per C60, while larger ions decrease the extent of suppression and allow exploring transport up to 1.7 s per C60.
Although the decrease in electrical conductivity may pose challenges for conventional logic gates and sensors, it also leads to a unique transistor current response with a Gaussian shape. This response can simulate the activation and deactivation of sodium channels present in biological neurons, creating conductance-based organic electrochemical neurons that accurately replicate crucial biological neural features.98 Therefore, these neurons can serve as event-based neuromorphic sensing and processing components, displaying exceptional biorealistic capabilities.
Modeling and simulation
Both quantum chemistry and molecular dynamics (MD) simulations have been used to interrogate the transport mechanisms and structure–function relationships of OMIECs. Recent work61 shows how grazing-incidence resonant X-ray diffraction (GIRXRD) complemented by periodic DFT calculations allows the determination of the mean position of the ion relative to the polymer backbone in the crystalline regions, which somewhat surprisingly is found near the lamellae midpoint (and not close to the polymer backbone). Multiscale methods combining MD and Kinetic Monte Carlo (KMC) have been instrumental as a tool to describe charge transport and percolation in conjugated materials and will continue to be key in OMIEC research. A multiscale transport model based on information from the structural and electrical characterization of PEDOT:PSS blends was also recently reported to explain the exponential-like conductivity scaling at different PEDOT:PSS ratios.99 Charge transport from one PEDOT-rich grain to the next happens thanks to isolated/dispersed PEDOT chains in a PSS-rich matrix, with PSS-conjugated groups enhancing tunneling efficiency.
A coarse-grained multiscale model was used to systematically investigate the effects of side chain polarity in a series of random OMIEC copolymers.19 The highest charge mobility (and doping) is associated with the highest fraction of polar side chains. The results where a similar fraction of polar and apolar side-chains were mixed point to a significant drop in charge mobility due to a shorter polaron delocalization length arising from the increased torsional disorder along the polymer backbone.
Multiscale models also shed light on time-dependent ion-charge interactions in mixed-conducting materials. Recent work100 has incorporated DFT calculations and MD simulations to capture the rapid charge fluctuation on the polymer backbone due to the presence of solvent and ions. While this approach does not consider charge transfer across polymer chains, it captures both ion-induced instantaneous polarization and slow (∼1 ns) charge reorganization due to conformational changes in polymer chains. Interactions across polymer chains dominate charge dynamics, which are expected to play a major role in bulk polymer aggregates or crystallites. While promising, this study highlights how representing the interaction between ionic and electronic charge carriers remains the most challenging task for any computational model of OMIECs. Although some methodological barriers still exist for reliably reproducing amorphous and semi-crystalline morphologies and ion-polaron coupling, ample opportunities exist for using already mature methodologies to study other structure-function and morphology-function relationships in OMIECs. Such efforts are made urgent by the extreme complexity and potential variety of OMIEC materials (ionic species, polymer backbone and connectivity, side chain polarity, length, and frequency). Despite the number of unknowns in the field, we believe a systematic OMIEC optimization effort guided by computational screening will soon be within reach as the community gradually uncovers relationships and correlations.
Spanning different systems
Despite some generalities across systems and common tools to study them, different systems and applications are governed by very different phenomena. For example, electronic transport is mechanistically different in non-conjugated versus conjugated redox polymers. Specifically for non-conjugated redox polymers, the electronic charge is localized on pendant groups where electrons can hop from site to site.101 In contrast, conjugated polymers distribute charge through the polymer's pi-conjugated backbone.
For electron conduction in non-conjugated redox polymers and small radical molecules, it is important to consider the proximity of the redox sites. As the site-to-site distance decreases, the conductivity increases. For example, a 1000-fold difference in the solid-state conductivity occurs when changing the hopping distance from 5.7 to 11.6 Å in certain crystals of small radical molecules.102 For non-conjugated redox polymers, the flexibility of the polymer chain must be considered in addition to redox site proximity.103 As the chain's flexibility increases, the diffusivity of the polymer increases, and there are more opportunities for redox sites to come within closer proximity through thermal motion. For example, the highly flexible and low-Tg radical-containing polymer poly(4-glycidyloxy-2,2,6,6-tetramethylpiperidine-1-oxyl) exhibited the highest solid-state conductivity within its class of 28 S m−1. In the case of carbazole redox polymers (polyvinylcarbazole), conductivities as high as 2 × 10−2 S cm−1 could be obtained upon chemical doping.4 In this case, the simultaneous crosslinking and doping of the starting materials into bicarbazole redox dimers could be proven by a combination of UV-vis-NIR spectroscopy and in situ and ex situ conductivity measurements.
In contrast to dry conditions, non-conjugated redox polymers exhibit mixed ion–electron–solvent transport in electrochemical cells. The charge transport is affected by polymer–solvent and polymer–ion interactions, swelling, and the ion's solvation shell.104–106 For example, EQCM-D experiments revealed that both anions and cations could participate in the polymer's redox behavior, which should be considered in future designs.104 Further, electrolyte choice can yield 1000× changes in the charge storage of non-conjugated redox polymers,105 indicating a rich future design space for electrolytes.
The combination of ac-impedance spectroscopy and dc measurements of mixed conductors (PEDOT:PSS and conjugated polyelectrolytes) can help to decouple electronic and ionic charge transport as a function of relative humidity and corresponding water uptake.15,107 The influence of relative humidity was particularly prominent for the ion conductivity, which reached 10−2 S cm−1 in the wet state. The electronic conductivity is less sensitive to humidity but requires the existence of electronic percolation pathways throughout the films, which is directly interrelated with the morphology upon exposure to humidity. It is interesting to note that the humidity. Response of the polyelectrolyte phases could be used to tune mechanical properties and make actuators.108
When translating OMIECs into dry systems, the advantages conferred by solvent-assisted ion transport are compromised, and instead, the segmental mobility of the ion-conducting chain becomes a determining factor in the intrinsic limitations of ion transport. In the case of side-chain-based OIMECs, it becomes essential to carefully consider the structure of the side chains, ensuring optimal ion solvation and promoting fast dynamics to facilitate ion motion. Recent joint computational and experimental studies have provided valuable insights into the intricacies of Li-salt-doped OMIECs based on oligoethylene glycol-substituted thiophene derivatives.109–112 These reports on dry OMIECs highlight the challenge of effectively separating the dynamics of the side chains responsible for ion transport from the more rigid conjugated core imparting semicrystallinity for efficient electronic transport.
The rate at which an OMIEC transports ions is affected by whether or not the ions are contained within a solvent shell, as is typically the case for PEDOT:PSS and oligoether side chain containing polymers in aqueous electrolytes.56,63,113,114 In other cases, for example, for P3HT with polymer electrolytes, the ion moves without a solvent shell, where solubility interactions dominate.115 It is typically, but not always, observed that a solvent shell improves ion transport. Nevertheless, a single perspective unifying the two extremes and providing a predictive structure–property relationship remains challenging.
Conclusions and perspectives
While our focus has been on conjugated polymers and organic small molecules, it is clear that the library of OMIECs continues to expand by the day. Small molecules, for example, have found an important place in precision molecular design and the extreme case of poly-crystalline morphologies. MOFs, COFs, and porous conjugated polymers, while their integration into devices presents barriers currently, afford three-dimensional frameworks where porosity can be controlled at the molecular scale, and chemical functionality can be finely dialed in. As for electrolytes, ionic liquids, as first investigated in electrolyte-gated transistors, are now numerous, and biocompatible versions with various ionic units may introduce new characteristics to the same OMIEC devices. Broadening the library of mixed conductors beyond polymers – perhaps even introducing hybrid systems – will thus open new applications for OMIEC devices.
The quest for model systems is useful but may be futile given the breadth of conditions and target applications under the OMIEC tent. Nevertheless, they prove useful for fundamental studies. The OMIEC must be considered a complete system, including the extrinsic or intrinsic ions, additives, and solvents, as appropriate. In addition, given the volatility of the solvents or the extrinsic nature of some electrolytes, multi-modal and operando characterization is needed to make direct device-material connections. Many differences in molecular design and film microstructures are not considered or well controlled, adding further challenges. Moving forward, questions remain about the exact location, interactions, and compositions (ionic) within OMIEC materials and how to model them properly. Can such understanding unlock designs for ideal materials? More stable formulations? Or allow for less common and highly non-linear responses toward novel applications? It's likely, but the community needs to tackle these questions collaboratively to get there.
Conflicts of interest
There are no conflicts to declare.
Acknowledgements
The authors and attendees thank the Telluride Science organization for their hospitality and support. Further sponsorship was provided by the Journal of Materials Chemistry C. The full list of TS workshop attendees was: the authors of this perspective (listed in alphabetical order), in addition to: C. D. Frisbie, C. Luscombe, C. K. Ober, A. Salleo, I. Zozoulenko, who engaged in fruitful discussion.
References
- D. Neusser, C. Malacrida, M. Kern, Y. M. Gross, J. Van Slageren and S. Ludwigs, High Conductivities of Disordered P3HT Films by an Electrochemical Doping Strategy, Chem. Mater., 2020, 32(14), 6003–6013, DOI:10.1021/acs.chemmater.0c01293.
- A. E. Javier, S. N. Patel, D. T. Hallinan, V. Srinivasan and N. P. Balsara, Simultaneous Electronic and Ionic Conduction in a Block Copolymer: Application in Lithium Battery Electrodes, Angew. Chem., Int. Ed., 2011, 50(42), 9848–9851, DOI:10.1002/anie.201102953.
- Y. M. Gross, D. Trefz, C. Dingler, D. Bauer, V. Vijayakumar, V. Untilova, L. Biniek, M. Brinkmann and S. Ludwigs, From Isotropic to Anisotropic Conductivities in P(NDI2OD-T2) by (Electro-)Chemical Doping Strategies, Chem. Mater., 2019, 31(9), 3542–3555, DOI:10.1021/acs.chemmater.9b00977.
- C. Malacrida, Y. Lu, K. Dirnberger, S. Gámez-Valenzuela, M. C. Ruiz Delgado and S. Ludwigs, Towards Highly Conducting Bicarbazole Redox Polymer Films with Plateau-like Conductivities, J. Mater. Chem. C, 2020, 8(43), 15393–15405, 10.1039/D0TC03090B.
- C. G. Bischak, L. Q. Flagg, K. Yan, C.-Z. Li and D. S. Ginger, Fullerene Active Layers for N-Type Organic Electrochemical Transistors, ACS Appl. Mater. Interfaces, 2019, 11(31), 28138–28144, DOI:10.1021/acsami.9b11370.
- K. Liu, P. Li, Y. Lei, Z. Zhang, X. Pan, S. K. So and T. Lei, J-Type Self-Assembled Supramolecular Polymers for High-Performance and Fast-Response n-Type Organic Electrochemical Transistors, Adv. Funct. Mater., 2023, 33(22), 2300049, DOI:10.1002/adfm.202300049.
- J. Duan, G. Zhu, L. Lan, J. Chen, X. Zhu, C. Chen, Y. Yu, H. Liao, Z. Li, I. McCulloch and W. Yue, Electron-Deficient Polycyclic Molecules via Ring Fusion for n-Type Organic Electrochemical Transistors, Angew. Chem., Int. Ed., 2023, 62(1), e202213737, DOI:10.1002/anie.202213737.
- Z. S. Parr, R. B. Rashid, B. D. Paulsen, B. Poggi, E. Tan, M. Freeley, M. Palma, I. Abrahams, J. Rivnay and C. B. Nielsen, Semiconducting Small Molecules as Active Materials for P-Type Accumulation Mode Organic Electrochemical Transistors, Adv. Electron. Mater., 2020, 6(6), 2000215, DOI:10.1002/aelm.202000215.
- M. Modarresi, J. F. Franco-Gonzalez and I. Zozoulenko, Computational Microscopy Study of the Granular Structure and pH Dependence of PEDOT:PSS, Phys. Chem. Chem. Phys., 2019, 21(12), 6699–6711, 10.1039/C8CP07141A.
- A. M. Nardes, M. Kemerink, R. A. J. Janssen, J. A. M. Bastiaansen, N. M. M. Kiggen, B. M. W. Langeveld, A. J. J. M. van
Breemen and M. M. de
Kok, Microscopic Understanding of the Anisotropic Conductivity of PEDOT:PSS Thin Films, Adv. Mater., 2007, 19(9), 1196–1200, DOI:10.1002/adma.200602575.
- J. Rivnay, S. Inal, B. A. Collins, M. Sessolo, E. Stavrinidou, X. Strakosas, C. Tassone, D. M. Delongchamp and G. G. Malliaras, Structural Control of Mixed Ionic and Electronic Transport in Conducting Polymers, Nat. Commun., 2016, 7(1), 11287, DOI:10.1038/ncomms11287.
- N. Kim, S. Kee, S. H. Lee, B. H. Lee, Y. H. Kahng, Y.-R. Jo, B.-J. Kim and K. Lee, Highly Conductive PEDOT:PSS Nanofibrils Induced by Solution-Processed Crystallization, Adv. Mater., 2014, 26(14), 2268–2272, DOI:10.1002/adma.201304611.
- C. Dingler, R. Walter, B. Gompf and S. Ludwigs, In Situ Monitoring of Optical Constants, Conductivity, and Swelling of PEDOT:PSS from Doped to the Fully Neutral State, Macromolecules, 2022, 55(5), 1600–1608, DOI:10.1021/acs.macromol.1c02515.
- L. G. Kaake, B. Gompf and S. Ludwigs, Electrochemical and Solvent-Driven Swelling in a Conducting Polymer Film, Chem. Mater., 2023, 35(11), 4532–4540, DOI:10.1021/acs.chemmater.3c00849.
- M. Wieland, C. Dingler, R. Merkle, J. Maier and S. Ludwigs, Humidity-Controlled Water Uptake and Conductivities in Ion and Electron Mixed Conducting Polythiophene Films, ACS Appl. Mater. Interfaces, 2020, 12(5), 6742–6751, DOI:10.1021/acsami.9b21181.
- M. Moser, J. Gladisch, S. Ghosh, T. C. Hidalgo, J. F. Ponder, R. Sheelamanthula, Q. Thiburce, N. Gasparini, A. Wadsworth, A. Salleo, S. Inal, M. Berggren, I. Zozoulenko, E. Stavrinidou and I. McCulloch, Controlling Electrochemically Induced Volume Changes in Conjugated Polymers by Chemical Design: From Theory to Devices, Adv. Funct. Mater., 2021, 2100723, DOI:10.1002/adfm.202100723.
- A. Savva, R. Hallani, C. Cendra, J. Surgailis, T. C. Hidalgo, S. Wustoni, R. Sheelamanthula, X. Chen, M. Kirkus, A. Giovannitti, A. Salleo, I. McCulloch and S. Inal, Balancing Ionic and Electronic Conduction for High-Performance Organic Electrochemical Transistors, Adv. Funct. Mater., 2020, 30(11), 1907657, DOI:10.1002/adfm.201907657.
- S. Moro, N. Siemons, O. Drury, D. A. Warr, T. A. Moriarty, L. M. A. Perdigão, D. Pearce, M. Moser, R. K. Hallani, J. Parker, I. McCulloch, J. M. Frost, J. Nelson and G. Costantini, The Effect of Glycol Side Chains on the Assembly and Microstructure of Conjugated Polymers, ACS Nano, 2022, 16(12), 21303–21314, DOI:10.1021/acsnano.2c09464.
- A. Khot and B. M. Savoie, How SIDE-CHAIN Hydrophilicity Modulates Morphology and Charge Transport in Mixed Conducting Polymers, J. Polym. Sci., 2022, 60(3), 610–620, DOI:10.1002/pol.20210773.
- J. Tropp, D. Meli, R. Wu, B. Xu, S. B. Hunt, J. D. Azoulay, B. D. Paulsen and J. Rivnay, Revealing the Impact of Molecular Weight on Mixed Conduction in Glycolated Polythiophenes through Electrolyte Choice, ACS Mater. Lett., 2023, 5(5), 1367–1375, DOI:10.1021/acsmaterialslett.2c01114.
- R. Wu, B. D. Paulsen, Q. Ma, I. McCulloch and J. Rivnay, Quantitative Composition and Mesoscale Ion Distribution in P-Type Organic Mixed Ionic–Electronic Conductors, ACS Appl. Mater. Interfaces, 2023, 15(25), 30553–30566, DOI:10.1021/acsami.3c04449.
- T. J. Quill, G. LeCroy, D. M. Halat, R. Sheelamanthula, A. Marks, L. S. Grundy, I. McCulloch, J. A. Reimer, N. P. Balsara, A. Giovannitti, A. Salleo and C. J. Takacs, An Ordered, Self-Assembled Nanocomposite with Efficient Electronic and Ionic Transport, Nat. Mater., 2023, 22(3), 362–368, DOI:10.1038/s41563-023-01476-6.
- B. D. Paulsen, R. Wu, C. J. Takacs, H. Steinrück, J. Strzalka, Q. Zhang, M. F. Toney and J. Rivnay, Time-Resolved Structural Kinetics of an Organic Mixed Ionic–Electronic Conductor, Adv. Mater., 2020, 32(40), 2003404, DOI:10.1002/adma.202003404.
- B. D. Paulsen, A. Giovannitti, R. Wu, J. Strzalka, Q. Zhang, J. Rivnay and C. J. Takacs, Electrochemistry of Thin Films with In Situ/Operando Grazing Incidence X-Ray Scattering: Bypassing Electrolyte Scattering for High Fidelity Time Resolved Studies, Small, 2021, 17(42), 2103213, DOI:10.1002/smll.202103213.
- L. Q. Flagg, L. E. Asselta, N. D’Antona, T. Nicolini, N. Stingelin, J. W. Onorato, C. K. Luscombe, R. Li and L. J. Richter, In Situ Studies of the Swelling by an Electrolyte in Electrochemical Doping of Ethylene Glycol-Substituted Polythiophene, ACS Appl. Mater. Interfaces, 2022, 14(25), 29052–29060, DOI:10.1021/acsami.2c06169.
- C. G. Bischak, L. Q. Flagg, K. Yan, T. Rehman, D. W. Davies, R. J. Quezada, J. W. Onorato, C. K. Luscombe, Y. Diao, C.-Z. Li and D. S. Ginger, A Reversible Structural Phase Transition by Electrochemically-Driven Ion Injection into a Conjugated Polymer, J. Am. Chem. Soc., 2020, 142(16), 7434–7442, DOI:10.1021/jacs.9b12769.
- N. Siemons, D. Pearce, C. Cendra, H. Yu, S. M. Tuladhar, R. K. Hallani, R. Sheelamanthula, G. S. LeCroy, L. Siemons, A. J. P. White, I. McCulloch, A. Salleo, J. M. Frost, A. Giovannitti and J. Nelson, Impact of Side-Chain Hydrophilicity on Packing, Swelling, and Ion Interactions in Oxy-Bithiophene Semiconductors, Adv. Mater., 2022, 34(39), 2204258, DOI:10.1002/adma.202204258.
- S. Ghosh, J. Rasmusson and O. Inganäs, Supramolecular Self-Assembly for Enhanced Conductivity in Conjugated Polymer Blends: Ionic Crosslinking in Blends of Poly(3,4-Ethylenedioxythiophene)-Poly(Styrenesulfonate) and Poly(Vinylpyrrolidone), Adv. Mater., 1998, 10(14), 1097–1099, DOI:10.1002/(SICI)1521-4095(199810)10:14
1097::AID-ADMA1097
3.0.CO;2-M.
- L. Q. Flagg, C. G. Bischak, R. J. Quezada, J. W. Onorato, C. K. Luscombe and D. S. Ginger, P-Type Electrochemical Doping Can Occur by Cation Expulsion in a High-Performing Polymer for Organic Electrochemical Transistors, ACS Mater. Lett., 2020, 2(3), 254–260, DOI:10.1021/acsmaterialslett.9b00501.
- J. Karst, M. Floess, M. Ubl, C. Dingler, C. Malacrida, T. Steinle, S. Ludwigs, M. Hentschel and H. Giessen, Electrically Switchable Metallic Polymer Nanoantennas, Science, 2021, 374(6567), 612–616, DOI:10.1126/science.abj3433.
- A. A. Szumska, I. P. Maria, L. Q. Flagg, A. Savva, J. Surgailis, B. D. Paulsen, D. Moia, X. Chen, S. Griggs, J. T. Mefford, R. B. Rashid, A. Marks, S. Inal, D. S. Ginger, A. Giovannitti and J. Nelson, Reversible Electrochemical Charging of N-Type Conjugated Polymer Electrodes in Aqueous Electrolytes, J. Am. Chem. Soc., 2021, 143(36), 14795–14805, DOI:10.1021/jacs.1c06713.
- J. Gladisch, E. Stavrinidou, S. Ghosh, A. Giovannitti, M. Moser, I. Zozoulenko, I. McCulloch and M. Berggren, Reversible Electronic Solid–Gel Switching of a Conjugated Polymer, Adv. Sci., 2020, 7(2), 1901144, DOI:10.1002/advs.201901144.
- J. Gladisch, V. K. Oikonomou, M. Moser, S. Griggs, I. McCulloch, M. Berggren and E. Stavrinidou, An Electroactive Filter with Tunable Porosity Based on Glycolated Polythiophene, Small Sci., 2022, 2(4), 2100113, DOI:10.1002/smsc.202100113.
- E. A. Schafer, R. Wu, D. Meli, J. Tropp, M. Moser, I. McCulloch, B. D. Paulsen and J. Rivnay, Sources and Mechanism of Degradation in P-Type Thiophene-Based Organic Electrochemical Transistors, ACS Appl. Electron. Mater., 2022, 4(4), 1391–1404, DOI:10.1021/acsaelm.1c01171.
- S. Zhang, P. Ding, T. Ruoko, R. Wu, M. Stoeckel, M. Massetti, T. Liu, M. Vagin, D. Meli, R. Kroon, J. Rivnay and S. Fabiano, Toward Stable p-Type Thiophene-Based Organic Electrochemical Transistors, Adv. Funct. Mater., 2023, 2302249, DOI:10.1002/adfm.202302249.
- A. Giovannitti, R. B. Rashid, Q. Thiburce, B. D. Paulsen, C. Cendra, K. Thorley, D. Moia, J. T. Mefford, D. Hanifi, D. Weiyuan, M. Moser, A. Salleo, J. Nelson, I. McCulloch and J. Rivnay, Energetic Control of Redox-Active Polymers toward Safe Organic Bioelectronic Materials, Adv. Mater., 2020, 32(16), 1908047, DOI:10.1002/adma.201908047.
- S. Griggs, A. Marks, D. Meli, G. Rebetez, O. Bardagot, B. D. Paulsen, H. Chen, K. Weaver, M. I. Nugraha, E. A. Schafer, J. Tropp, C. M. Aitchison, T. D. Anthopoulos, N. Banerji, J. Rivnay and I. McCulloch, The Effect of Residual Palladium on the Performance of Organic Electrochemical Transistors, Nat. Commun., 2022, 13(1), 7964, DOI:10.1038/s41467-022-35573-y.
- R. Wu, M. Matta, B. D. Paulsen and J. Rivnay, Operando Characterization of Organic Mixed Ionic/Electronic Conducting Materials, Chem. Rev., 2022, 122(4), 4493–4551, DOI:10.1021/acs.chemrev.1c00597.
- L. G. Kaake, B. D. Paulsen, C. D. Frisbie and X.-Y. Zhu, Mixing at the Charged Interface of a Polymer Semiconductor and a Polyelectrolyte Dielectric, J. Phys. Chem. Lett., 2010, 1(5), 862–867, DOI:10.1021/jz900471g.
- A. Giovannitti, D.-T. Sbircea, S. Inal, C. B. Nielsen, E. Bandiello, D. A. Hanifi, M. Sessolo, G. G. Malliaras, I. McCulloch and J. Rivnay, Controlling the Mode of Operation of Organic Transistors through Side-Chain Engineering, Proc. Natl. Acad. Sci. U. S. A., 2016, 113(43), 12017–12022, DOI:10.1073/pnas.1608780113.
- L. Q. Flagg, R. Giridharagopal, J. Guo and D. S. Ginger, Anion-Dependent Doping and Charge Transport in Organic Electrochemical Transistors, Chem. Mater., 2018, 30(15), 5380–5389, DOI:10.1021/acs.chemmater.8b02220.
- T. J. Quill, G. LeCroy, A. Melianas, D. Rawlings, Q. Thiburce, R. Sheelamanthula, C. Cheng, Y. Tuchman, S. T. Keene, I. McCulloch, R. A. Segalman, M. L. Chabinyc and A. Salleo, Ion Pair Uptake in Ion Gel Devices Based on Organic Mixed Ionic–Electronic Conductors, Adv. Funct. Mater., 2021, 2104301, DOI:10.1002/adfm.202104301.
- C. M. Proctor, J. Rivnay and G. G. Malliaras, Understanding Volumetric Capacitance in Conducting Polymers, J. Polym. Sci., Part B: Polym. Phys., 2016, 54(15), 1433–1436, DOI:10.1002/polb.24038.
- A. V. Volkov, K. Wijeratne, E. Mitraka, U. Ail, D. Zhao, K. Tybrandt, J. W. Andreasen, M. Berggren, X. Crispin and I. V. Zozoulenko, Understanding the Capacitance of PEDOT:PSS, Adv. Funct. Mater., 2017, 27(28), 1700329, DOI:10.1002/adfm.201700329.
- M. Cucchi, A. Weissbach, L. M. Bongartz, R. Kantelberg, H. Tseng, H. Kleemann and K. Leo, Thermodynamics of Organic Electrochemical Transistors, Nat. Commun., 2022, 13(1), 4514, DOI:10.1038/s41467-022-32182-7.
- P. Shiri, D. Neusser, C. Malacrida, S. Ludwigs and L. G. Kaake, Mixed Ion-Carrier Diffusion in Poly(3-Hexyl Thiophene)/Perchlorate Electrochemical Systems, J. Phys. Chem. C, 2021, 125(1), 536–545, DOI:10.1021/acs.jpcc.0c09527.
- S. Yu and E. L. Ratcliff, Tuning Organic Electrochemical Transistor (OECT) Transconductance toward Zero Gate Voltage in the faradaic Mode, ACS Appl. Mater. Interfaces, 2021, 13(42), 50176–50186, DOI:10.1021/acsami.1c13009.
- I. Sahalianov, S. K. Singh, K. Tybrandt, M. Berggren and I. Zozoulenko, The Intrinsic Volumetric Capacitance of Conducting Polymers: Pseudo-Capacitors or Double-Layer Supercapacitors?, RSC Adv., 2019, 9(72), 42498–42508, 10.1039/C9RA10250G.
- L. G. Kaake, P. F. Barbara and X.-Y. Zhu, Intrinsic Charge Trapping in Organic and Polymeric Semiconductors: A Physical Chemistry Perspective, J. Phys. Chem. Lett., 2010, 1(3), 628–635, DOI:10.1021/jz9002857.
- K. Bruchlos, D. Trefz, A. Hamidi-Sakr, M. Brinkmann, J. Heinze, A. Ruff and S. Ludwigs, Poly(3-Hexylthiophene) Revisited – Influence of Film Deposition on the Electrochemical Behaviour and Energy Levels, Electrochim. Acta, 2018, 269, 299–311, DOI:10.1016/j.electacta.2018.02.126.
- C. Chen, I. E. Jacobs, K. Kang, Y. Lin, C. Jellett, B. Kang, S. B. Lee, Y. Huang, M. BaloochQarai, R. Ghosh, M. Statz, W. Wood, X. Ren, D. Tjhe, Y. Sun, X. She, Y. Hu, L. Jiang, F. C. Spano, I. McCulloch and H. Sirringhaus, Observation of Weak Counterion Size Dependence of Thermoelectric Transport in Ion Exchange Doped Conducting Polymers Across a Wide Range of Conductivities, Adv. Energy Mater., 2023, 13(9), 2202797, DOI:10.1002/aenm.202202797.
- S. Inal, G. G. Malliaras and J. Rivnay, Optical Study of Electrochromic Moving Fronts for the Investigation of Ion Transport in Conducting Polymers, J. Mater. Chem. C, 2016, 4(18), 3942–3947, 10.1039/C5TC04354A.
- C. Cendra, A. Giovannitti, A. Savva, V. Venkatraman, I. McCulloch, A. Salleo, S. Inal and J. Rivnay, Role of the Anion on the Transport and Structure of Organic Mixed Conductors, Adv. Funct. Mater., 2019, 29(5), 1807034, DOI:10.1002/adfm.201807034.
- R. Giridharagopal, L. Q. Flagg, J. S. Harrison, M. E. Ziffer, J. Onorato, C. K. Luscombe and D. S. Ginger, Electrochemical Strain Microscopy Probes Morphology-Induced Variations in Ion Uptake and Performance in Organic Electrochemical Transistors, Nat. Mater., 2017, 16(7), 737–742, DOI:10.1038/nmat4918.
- J. O. Guardado and A. Salleo, Structural Effects of Gating Poly(3-Hexylthiophene) through an Ionic Liquid, Adv. Funct. Mater., 2017, 27(32), 1701791, DOI:10.1002/adfm.201701791.
- A. Savva, C. Cendra, A. Giugni, B. Torre, J. Surgailis, D. Ohayon, A. Giovannitti, I. McCulloch, E. Di Fabrizio, A. Salleo, J. Rivnay and S. Inal, Influence of Water on the Performance of Organic Electrochemical Transistors, Chem. Mater., 2019, 31(3), 927–937, DOI:10.1021/acs.chemmater.8b04335.
- D. A. Bernards and G. G. Malliaras, Steady-State and Transient Behavior of Organic Electrochemical Transistors, Adv. Funct. Mater., 2007, 17(17), 3538–3544, DOI:10.1002/adfm.200601239.
- V. Kaphle, P. R. Paudel, D. Dahal, R. K. Radha Krishnan and B. Lüssem, Finding the Equilibrium of Organic Electrochemical Transistors, Nat. Commun., 2020, 11(1), 2515, DOI:10.1038/s41467-020-16252-2.
- P. R. Paudel, V. Kaphle, D. Dahal, R. K. Radha Krishnan and B. Lüssem, Tuning the Transconductance of Organic Electrochemical Transistors, Adv. Funct. Mater., 2021, 31(3), 2004939, DOI:10.1002/adfm.202004939.
- K. Tybrandt, I. V. Zozoulenko and M. Berggren, Chemical Potential–Electric Double Layer Coupling in Conjugated Polymer–Polyelectrolyte Blends, Sci. Adv., 2017, 3(12), eaao3659, DOI:10.1126/sciadv.aao3659.
- L. Q. Flagg, J. W. Onorato, C. K. Luscombe, V. Bhat, C. Risko, B. Levy-Wendt, M. F. Toney, C. R. McNeill, G. Freychet, M. Zhernenkov, R. Li and L. J. Richter, Resonant X-Ray Diffraction Reveals the Location of Counterions in Doped Organic Mixed Ionic Conductors, Chem. Mater., 2023, 35(10), 3960–3967, DOI:10.1021/acs.chemmater.3c00180.
- A. Giovannitti, I. P. Maria, D. Hanifi, M. J. Donahue, D. Bryant, K. J. Barth, B. E. Makdah, A. Savva, D. Moia, M. Zetek, P. R. F. Barnes, O. G. Reid, S. Inal, G. Rumbles, G. G. Malliaras, J. Nelson, J. Rivnay and I. McCulloch, The Role of the Side Chain on the Performance of N-Type Conjugated Polymers in Aqueous Electrolytes, Chem. Mater., 2018, 30(9), 2945–2953, DOI:10.1021/acs.chemmater.8b00321.
- L. Q. Flagg, C. G. Bischak, J. W. Onorato, R. B. Rashid, C. K. Luscombe and D. S. Ginger, Polymer Crystallinity Controls Water Uptake in Glycol Side-Chain Polymer Organic Electrochemical Transistors, J. Am. Chem. Soc., 2019, 141(10), 4345–4354, DOI:10.1021/jacs.8b12640.
- A. Giovannitti, K. J. Thorley, C. B. Nielsen, J. Li, M. J. Donahue, G. G. Malliaras, J. Rivnay and I. McCulloch, Redox-Stability of Alkoxy-BDT Copolymers and Their Use for Organic Bioelectronic Devices, Adv. Funct. Mater., 2018, 28(17), 1706325, DOI:10.1002/adfm.201706325.
- N. A. Kukhta, A. Marks and C. K. Luscombe, Molecular Design Strategies toward Improvement of Charge Injection and Ionic Conduction in Organic Mixed Ionic–Electronic Conductors for Organic Electrochemical Transistors, Chem. Rev., 2022, 122(4), 4325–4355, DOI:10.1021/acs.chemrev.1c00266.
- H. Sun, M. Vagin, S. Wang, X. Crispin, R. Forchheimer, M. Berggren and S. Fabiano, Complementary Logic Circuits Based on High-Performance n-Type Organic Electrochemical Transistors, Adv. Mater., 2018, 30(9), 1704916, DOI:10.1002/adma.201704916.
- X. Wu, T. L. D. Tam, S. Chen, T. Salim, X. Zhao, Z. Zhou, M. Lin, J. Xu, Y. Loo and W. L. Leong, All-Polymer Bulk-Heterojunction Organic Electrochemical Transistors with Balanced Ionic and Electronic Transport, Adv. Mater., 2022, 34(42), 2206118, DOI:10.1002/adma.202206118.
- J. Surgailis, A. Savva, V. Druet, B. D. Paulsen, R. Wu, A. Hamidi-Sakr, D. Ohayon, G. Nikiforidis, X. Chen, I. McCulloch, J. Rivnay and S. Inal, Mixed Conduction in an N-Type Organic Semiconductor in the Absence of Hydrophilic Side-Chains, Adv. Funct. Mater., 2021, 31(21), 2010165, DOI:10.1002/adfm.202010165.
- H. Tang, Y. Liang, C. Liu, Z. Hu, Y. Deng, H. Guo, Z. Yu, A. Song, H. Zhao, D. Zhao, Y. Zhang, X. Guo, J. Pei, Y. Ma, Y. Cao and F. Huang, A Solution-Processed n-Type Conducting Polymer with Ultrahigh Conductivity, Nature, 2022, 611(7935), 271–277, DOI:10.1038/s41586-022-05295-8.
- K. Gu, C. R. Snyder, J. Onorato, C. K. Luscombe, A. W. Bosse and Y.-L. Loo, Assessing the Huang–Brown Description of Tie Chains for Charge Transport in Conjugated
Polymers, ACS Macro Lett., 2018, 7(11), 1333–1338, DOI:10.1021/acsmacrolett.8b00626.
- S. Himmelberger, K. Vandewal, Z. Fei, M. Heeney and A. Salleo, Role of Molecular Weight Distribution on Charge Transport in Semiconducting Polymers, Macromolecules, 2014, 47(20), 7151–7157, DOI:10.1021/ma501508j.
- H. Wu, C. Yang, Q. Li, N. B. Kolhe, X. Strakosas, M. Stoeckel, Z. Wu, W. Jin, M. Savvakis, R. Kroon, D. Tu, H. Y. Woo, M. Berggren, S. A. Jenekhe and S. Fabiano, Influence of Molecular Weight on the Organic Electrochemical Transistor Performance of Ladder-Type Conjugated Polymers, Adv. Mater., 2022, 34(4), 2106235, DOI:10.1002/adma.202106235.
- C.-Y. Lo, Y. Wu, E. Awuyah, D. Meli, D. M. Nguyen, R. Wu, B. Xu, J. Strzalka, J. Rivnay, D. C. Martin and L. V. Kayser, Influence of the Molecular Weight and Size Distribution of PSS on Mixed Ionic–Electronic Transport in PEDOT:PSS, Polym. Chem., 2022, 13(19), 2764–2775, 10.1039/D2PY00271J.
- A. F. Paterson, A. Savva, S. Wustoni, L. Tsetseris, B. D. Paulsen, H. Faber, A. H. Emwas, X. Chen, G. Nikiforidis, T. C. Hidalgo, M. Moser, I. P. Maria, J. Rivnay, I. McCulloch, T. D. Anthopoulos and S. Inal, Water Stable Molecular N-Doping Produces Organic Electrochemical Transistors with High Transconductance and Record Stability, Nat. Commun., 2020, 11(1), 3004, DOI:10.1038/s41467-020-16648-0.
- T. C. Hidalgo Castillo, M. Moser, C. Cendra, P. D. Nayak, A. Salleo, I. McCulloch and S. Inal, Simultaneous Performance and Stability Improvement of a P-Type Organic Electrochemical Transistor through Additives, Chem. Mater., 2022, 34(15), 6723–6733, DOI:10.1021/acs.chemmater.2c00632.
- I. C. Kwak, Y. Lee, M. J. Kim, Y. J. Choi, D. G. Roe, M. S. Kang, H. Y. Woo and J. H. Cho, Solid-State Homojunction Electrochemical Transistors and Logic Gates on Plastic, Adv. Funct. Mater., 2023, 33(13), 2211740, DOI:10.1002/adfm.202211740.
- J. Nightingale, C. Pitsalidis, A.-M. Pappa, E. Tan, K. Stewart, R. M. Owens and J.-S. Kim, Small Molecule Additive for Low-Power Accumulation Mode Organic Electrochemical Transistors, J. Mater. Chem. C, 2020, 8(26), 8846–8855, 10.1039/D0TC02149K.
- S. T. Keene, T. P. A. van der Pol, D. Zakhidov, C. H. L. Weijtens, R. A. J. Janssen, A. Salleo and Y. van de Burgt, Enhancement-Mode PEDOT:PSS Organic Electrochemical Transistors Using Molecular De-Doping, Adv. Mater., 2020, 32(19), 2000270, DOI:10.1002/adma.202000270.
- A. Savva, D. Ohayon, J. Surgailis, A. F. Paterson, T. C. Hidalgo, X. Chen, I. P. Maria, B. D. Paulsen, A. J. Petty, J. Rivnay, I. McCulloch and S. Inal, Solvent Engineering for High-Performance n-Type Organic Electrochemical Transistors, Adv. Electron. Mater., 2019, 5(8), 1900249, DOI:10.1002/aelm.201900249.
- D. Jeong, I. Jo, S. Lee, J. H. Kim, Y. Kim, D. Kim, J. R. Reynolds, M. Yoon and B. J. Kim, High-Performance n -Type Organic Electrochemical Transistors Enabled by Aqueous Solution Processing of Amphiphilicity-Driven Polymer Assembly, Adv. Funct. Mater., 2022, 2111950, DOI:10.1002/adfm.202111950.
- G. Zhu, J. Chen, J. Duan, H. Liao, X. Zhu, Z. Li, I. McCulloch and W. Yue, Fluorinated Alcohol-Processed N-Type Organic Electrochemical Transistor with High Performance and Enhanced Stability, ACS Appl. Mater. Interfaces, 2022, 14(38), 43586–43596, DOI:10.1021/acsami.2c13310.
- D. Ohayon, L. Q. Flagg, A. Giugni, S. Wustoni, R. Li, T. C. Hidalgo Castillo, A.-H. Emwas, R. Sheelamanthula, I. McCulloch, L. J. Richter and S. Inal, Salts as Additives: A Route to Improve Performance and Stability of n-Type Organic Electrochemical Transistors, ACS Mater. Au, 2023, 3(3), 242–254, DOI:10.1021/acsmaterialsau.2c00072.
- A. Malti, J. Edberg, H. Granberg, Z. U. Khan, J. W. Andreasen, X. Liu, D. Zhao, H. Zhang, Y. Yao, J. W. Brill, I. Engquist, M. Fahlman, L. Wågberg, X. Crispin and M. Berggren, An Organic Mixed Ion–Electron Conductor for Power Electronics, Adv. Sci., 2016, 3(2), 1500305, DOI:10.1002/advs.201500305.
- M. Mone, Y. Kim, S. Darabi, S. Zokaei, L. Karlsson, M. Craighero, S. Fabiano, R. Kroon and C. Müller, Mechanically Adaptive Mixed Ionic–Electronic Conductors Based on a Polar Polythiophene Reinforced with Cellulose Nanofibrils, ACS Appl. Mater. Interfaces, 2023, 15(23), 28300–28309, DOI:10.1021/acsami.3c03962.
- S. Samal, H. Roh, C. E. Cunin, G. G. Yang and A. Gumyusenge, Molecularly Hybridized Conduction in DPP-Based Donor–Acceptor Copolymers toward High-Performance Iono-Electronics, Small, 2023, 19(18), 2207554, DOI:10.1002/smll.202207554.
- Y. Kim, H. Noh, B. D. Paulsen, J. Kim, I. Jo, H. Ahn, J. Rivnay and M. Yoon, Strain-Engineering Induced Anisotropic Crystallite Orientation and Maximized Carrier Mobility for High-Performance Microfiber-Based Organic Bioelectronic Devices, Adv. Mater., 2021, 33(10), 2007550, DOI:10.1002/adma.202007550.
- A. I. Hofmann, I. Östergren, Y. Kim, S. Fauth, M. Craighero, M.-H. Yoon, A. Lund and C. Müller, All-Polymer Conducting Fibers and 3D Prints via Melt Processing and Templated Polymerization, ACS Appl. Mater. Interfaces, 2020, 12(7), 8713–8721, DOI:10.1021/acsami.9b20615.
- D. Ofer, R. M. Crooks and M. S. Wrighton, Potential Dependence of the Conductivity of Highly Oxidized Polythiophenes, Polypyrroles, and Polyaniline: Finite Windows of High Conductivity, J. Am. Chem. Soc., 1990, 112(22), 7869–7879, DOI:10.1021/ja00178a004.
- M. J. Panzer and C. D. Frisbie, Polymer Electrolyte Gate Dielectric Reveals Finite Windows of High Conductivity in Organic Thin Film Transistors at High Charge Carrier Densities, J. Am. Chem. Soc., 2005, 127(19), 6960–6961, DOI:10.1021/ja051579.
- H. Zeng, M. Mohammed, V. Untilova, O. Boyron, N. Berton, P. Limelette, B. Schmaltz and M. Brinkmann, Fabrication of Oriented n-Type Thermoelectric Polymers by Polarity Switching in a DPP-Based Donor–Acceptor Copolymer Doped with FeCl3, Adv. Electron. Mater., 2021, 7(5), 2000880, DOI:10.1002/aelm.202000880.
- B. D. Paulsen and C. D. Frisbie, Dependence of Conductivity on Charge Density and Electrochemical Potential in Polymer Semiconductors Gated with Ionic Liquids, J. Phys. Chem. C, 2012, 116(4), 3132–3141, DOI:10.1021/jp2093934.
- S. Wang, M. Ha, M. Manno, C. Daniel Frisbie and C. Leighton, Hopping Transport and the Hall Effect near the Insulator–Metal Transition in Electrochemically Gated Poly(3-Hexylthiophene) Transistors, Nat. Commun., 2012, 3(1), 1210, DOI:10.1038/ncomms2213.
- I. N. Hulea, H. B. Brom, A. J. Houtepen, D. Vanmaekelbergh, J. J. Kelly and E. A. Meulenkamp, Wide Energy-Window View on the Density of States and Hole Mobility in Poly(p-Phenylene Vinylene), Phys. Rev. Lett., 2004, 93(16), 166601, DOI:10.1103/PhysRevLett.93.166601.
- J. Heinze, B. A. Frontana-Uribe and S. Ludwigs, Electrochemistry of Conducting Polymers—Persistent Models and New Concepts, Chem. Rev., 2010, 110(8), 4724–4771, DOI:10.1021/cr900226k.
- K. Xu, T. Ruoko, M. Shokrani, D. Scheunemann, H. Abdalla, H. Sun, C. Yang, Y. Puttisong, N. B. Kolhe, J. S. M. Figueroa, J. O. Pedersen, T. Ederth, W. M. Chen, M. Berggren, S. A. Jenekhe, D. Fazzi, M. Kemerink and S. Fabiano, On the Origin of Seebeck Coefficient Inversion in Highly Doped Conducting Polymers, Adv. Funct. Mater., 2022, 32(20), 2112276, DOI:10.1002/adfm.202112276.
- J. Liu, G. Ye, B. V. D. Zee, J. Dong, X. Qiu, Y. Liu, G. Portale, R. C. Chiechi and L. J. A. Koster, N-Type Organic Thermoelectrics of Donor-Acceptor Copolymers: Improved Power Factor by Molecular Tailoring of the Density of States, Adv. Mater., 2018, 30(44), 1804290, DOI:10.1002/adma.201804290.
- T. He and C. D. Frisbie, Sub-Band Filling, Mott-like Transitions, and Ion Size Effects in C60 Single Crystal Electric Double Layer Transistors, ACS Nano, 2022, 16(3), 4823–4830, DOI:10.1021/acsnano.2c00222.
- P. C. Harikesh, C.-Y. Yang, H.-Y. Wu, S. Zhang, M. J. Donahue, A. S. Caravaca, J.-D. Huang, P. S. Olofsson, M. Berggren, D. Tu and S. Fabiano, Ion-Tunable Antiambipolarity in Mixed Ion–Electron Conducting Polymers Enables Biorealistic Organic Electrochemical Neurons, Nat. Mater., 2023, 22(2), 242–248, DOI:10.1038/s41563-022-01450-8.
- S. T. Keene, W. Michaels, A. Melianas, T. J. Quill, E. J. Fuller, A. Giovannitti, I. McCulloch, A. A. Talin, C. J. Tassone, J. Qin, A. Troisi and A. Salleo, Efficient Electronic Tunneling Governs Transport in Conducting Polymer-Insulator Blends, J. Am. Chem. Soc., 2022, 144(23), 10368–10376, DOI:10.1021/jacs.2c02139.
- A. Landi, M. Reisjalali, J. D. Elliott, M. Matta, P. Carbone and A. Troisi, Simulation of Polymeric Mixed Ionic and Electronic Conductors with a Combined Classical and Quantum Mechanical Model, J. Mater. Chem. C, 2023, 11(24), 8062–8073, 10.1039/D2TC05103F.
- T. Ma, A. D. Easley, R. M. Thakur, K. T. Mohanty, C. Wang and J. L. Lutkenhaus, Nonconjugated Redox-Active Polymers: Electron Transfer Mechanisms, Energy Storage, and Chemical Versatility, Annu. Rev. Chem. Biomol. Eng., 2023, 14(1), 187–216, DOI:10.1146/annurev-chembioeng-092220-111121.
- Z. Liang, S.-N. Hsu, Y. Tan, H. Tahir, H. J. Kim, K. Liu, J. F. Stoehr, M. Zeller, L. Dou, B. M. Savoie and B. W. Boudouris, Significant Charge Transport Effects Due to Subtle Molecular Changes in Nitroxide Radical Single Crystals, Cell Rep. Phys. Sci., 2023, 4(5), 101409, DOI:10.1016/j.xcrp.2023.101409.
- K. Sato, R. Ichinoi, R. Mizukami, T. Serikawa, Y. Sasaki, J. Lutkenhaus, H. Nishide and K. Oyaizu, Diffusion-Cooperative Model for Charge Transport by Redox-Active Nonconjugated Polymers, J. Am. Chem. Soc., 2018, 140(3), 1049–1056, DOI:10.1021/jacs.7b11272.
- S. Wang, F. Li, A. D. Easley and J. L. Lutkenhaus, Real-Time Insight into the Doping Mechanism of Redox-Active Organic Radical Polymers, Nat. Mater., 2019, 18(1), 69–75, DOI:10.1038/s41563-018-0215-1.
- T. Ma, C.-H. Li, R. M. Thakur, D. P. Tabor and J. L. Lutkenhaus, The Role of the Electrolyte in Non-Conjugated Radical Polymers for Metal-Free Aqueous Energy Storage Electrodes, Nat. Mater., 2023, 22(4), 495–502, DOI:10.1038/s41563-023-01518-z.
- T. Ma, A. D. Easley, S. Wang, P. Flouda and J. L. Lutkenhaus, Mixed Electron-Ion-Water Transfer in Macromolecular Radicals for Metal-Free Aqueous Batteries, Cell Rep. Phys. Sci., 2021, 2(5), 100414, DOI:10.1016/j.xcrp.2021.100414.
- R. Merkle, P. Gutbrod, P. Reinold, M. Katzmaier, R. Tkachov, J. Maier and S. Ludwigs, Mixed Conductivity of Polythiophene-Based Ionic Polymers under Controlled Conditions, Polymer, 2017, 132, 216–226, DOI:10.1016/j.polymer.2017.10.064.
- C. Dingler, H. Müller, M. Wieland, D. Fauser, H. Steeb and S. Ludwigs, From Understanding Mechanical Behavior to Curvature Prediction of Humidity-Triggered Bilayer Actuators, Adv. Mater., 2021, 33(9), 2007982, DOI:10.1002/adma.202007982.
- B. X. Dong, C. Nowak, J. W. Onorato, J. Strzalka, F. A. Escobedo, C. K. Luscombe, P. F. Nealey and S. N. Patel, Influence of Side-Chain Chemistry on Structure and Ionic Conduction Characteristics of Polythiophene Derivatives: A Computational and Experimental Study, Chem. Mater., 2019, 31(4), 1418–1429, DOI:10.1021/acs.chemmater.8b05257.
- J. W. Onorato, Z. Wang, Y. Sun, C. Nowak, L. Q. Flagg, R. Li, B. X. Dong, L. J. Richter, F. A. Escobedo, P. F. Nealey, S. N. Patel and C. K. Luscombe, Side Chain Engineering Control of Mixed Conduction in Oligoethylene Glycol-Substituted Polythiophenes, J. Mater. Chem. A, 2021, 9(37), 21410–21423, 10.1039/D1TA05379E.
- B. X. Dong, Z. Liu, M. Misra, J. Strzalka, J. Niklas, O. G. Poluektov, F. A. Escobedo, C. K. Ober, P. F. Nealey and S. N. Patel, Structure Control of a π-Conjugated Oligothiophene-Based Liquid Crystal for Enhanced Mixed Ion/Electron Transport Characteristics, ACS Nano, 2019, 13(7), 7665–7675, DOI:10.1021/acsnano.9b01055.
- Z. Wang, C. Wang, Y. Sun, K. Wang, J. W. Strzalka, S. N. Patel, P. F. Nealey, C. K. Ober and F. A. Escobedo, Ion Transport in 2D Nanostructured π-Conjugated Thieno[3,2-b]Thiophene-Based Liquid Crystal, ACS Nano, 2022, 16(12), 20714–20729, DOI:10.1021/acsnano.2c07789.
- L.-S. Hornberger, D. Neusser, C. Malacrida, L. G. Kaake and S. Ludwigs, How Charge Trapping Affects the Conductivity of Electrochemically Doped Poly(3-Hexylthiophene) Films, Appl. Phys. Lett., 2021, 119(16), 163301, DOI:10.1063/5.0056484.
- R. M. Thakur, A. D. Easley, S. Wang, Y. Zhang, C. K. Ober and J. L. Lutkenhaus, Real Time Quantification of Mixed Ion and Electron Transfer Associated with the Doping of Poly(3-Hexylthiophene), J. Mater. Chem. C, 2022, 10(18), 7251–7262, 10.1039/D2TC00001F.
- P. Shiri, E. J. S. Dacanay, B. Hagen and L. G. Kaake, Vogel–Tammann–Fulcher Model for Charging Dynamics in an Organic Electrochemical Transistor, J. Mater. Chem. C, 2019, 7(41), 12935–12941, 10.1039/C9TC02563D..
|
This journal is © The Royal Society of Chemistry 2023 |
Click here to see how this site uses Cookies. View our privacy policy here.