DOI:
10.1039/D4TB02372B
(Paper)
J. Mater. Chem. B, 2025,
13, 1326-1337
In situ dressing based on a D–π–A structured aggregation-induced emission photosensitizer for healing infected wounds†
Received
22nd October 2024
, Accepted 2nd December 2024
First published on 4th December 2024
Abstract
Photodynamic antimicrobial therapy (aPDT) can effectively kill bacteria without promoting drug resistance. However, the phototoxicity of photosensitizers in aPDT against normal cells hinders their practical applications. In this work, we report the utilization of an aggregation-induced emission (AIE)-active photosensitizer, DTTPB, to develop antibacterial dressing for effective eradication of both Gram-positive and Gram-negative bacteria. The D–π–A structure of DTTPB facilitates efficient ROS generation in the aggregate state, addressing the limitations of a traditional photosensitizer. Notably, DTTPB demonstrates good biocompatibility towards normal cells, which minimizes its phototoxicity to normal tissues. To demonstrate its practical implications, DTTPB is combined with Carbomer 940 to create an injectable hydrogel dressing (DTTPB@gel). DTTPB@gel not only adheres to wounds but also maintains the antimicrobial properties of DTTPB, which together contributes to its enhanced wound-healing performance. Biocompatibility and toxicity assessments confirm the safety of this novel material, highlighting its potential as a practical and effective treatment for bacterial infections in wounds. The results underscore the importance of innovative antimicrobial strategies in fighting against antibiotic resistance, paving the way for safer and more effective therapeutic options.
Introduction
Bacteria are omnipresent in nature, including in water, food, air, and soil and on various surfaces, and are found in patients.1 Even the human body harbours a diverse array of bacteria.2 Typically, the resident microbiota and the immune system function synergistically to safeguard the human body from pathogenic bacteria.3 However, an overgrowth of pathogenic bacteria, or their presence in individuals with wounds, immune deficiencies, or dysbiosis, can result in a range of diseases, including skin inflammation,4 diarrhea,5 sepsis,6 severe pneumonia, meningitis, shock, and even death.7
Antibiotics are widely used for both prevention and treatment of bacterial infections.8 Their inhibitions on bacterial growth involve multiple pathways, including disrupting bacterial membranes, targeting peptidoglycan synthesis, interfering with metabolic processes, and affecting nucleic acid or protein synthesis, ultimately leading to pathogen death.9 Since the discovery of penicillin in 1928, significant progress has been made in controlling bacterial infections.10 However, the misuse of antibiotics has led many invasive pathogenic microorganisms to develop mechanisms to evade both antibiotics and the host's innate immune system, resulting in multi-drug resistant (MDR) strains,11 such as fidaxomicin-resistant Enterococcus faecalis (K-1476)12 and methicillin-resistant Staphylococcus aureus (MRSA).13 Therefore, the development of diagnostic and therapeutic agents that do not induce drug resistance is crucial for managing clinical infections.
Photodynamic antimicrobial therapy (aPDT) presents distinct advantages over conventional antibiotics.14 This technique employs reactive oxygen species (ROS) sensitized by photosensitizers in the presence of light to kill pathogenic bacteria.15 The ROS-mediated inactivation mechanism of aPDT enables effective bacterial eradication while circumventing the development of drug resistance.16 Therefore, aPDT represents a highly effective strategy for managing bacterial infections.
Traditional photosensitizers, such as phenothiazine salts, porphyrins, phthalocyanines, diketopyrroles, and anthocyanins, are widely used in photodynamic anticancer and antibacterial research.17 However, these photosensitizers often possess large π-conjugated coplanar structures, leading to strong π–π interactions in the aggregate state or at high concentrations. This can cause the aggregation-caused quenching (ACQ) effect, which attenuates excited-state energy through non-radiative pathways.18 As a result, the fluorescence intensity and ROS sensitization efficiency are significantly reduced, diminishing their bactericidal efficacy.
In 2001, Prof. Ben Zhong Tang reported and termed a novel luminescent phenomenon of aggregation-induced emission (AIE).19,20 Unlike ACQ materials, AIE luminophores are non-luminescent in solutions, but display significant fluorescence in the aggregate state. Comprehensive investigations uncovered restricted intramolecular motion (RIM)21 as the main cause for the AIE effect. The discovery and exploration of AIE systems have greatly accelerated the advancement of precision medicine and light-based healthcare.22 Recently, some AIE materials have been reported to exhibit photosensitizing attributes, and are widely employed in PDT applications. The AIE characteristic allows for highly efficient production of ROS in the aggregate state, and endows AIE photosensitizers with enhanced stability under irradiation, which are advantageous for prolonged PDT treatment. These unique photophysical properties make AIE materials highly promising for biomedical applications,23,24 offering advantages such as improved fluorescence stability and increased therapeutic efficacy,25 and attempts have been made to explore their potentials in aPDT.
Hydrogels are commonly used as wound dressings due to their advantage of keeping wounds moist,26 which facilitates oxygen penetration and thus promotes wound healing.27 However, ordinary hydrogels do not possess antimicrobial properties, and when pathogenic microorganisms colonize the wound, the wound-healing effect of these hydrogels is greatly reduced.28 Thus, photosensitizers with excellent aPDT performance in the gel matrix are highly desirable.
Recently, antibacterial dressings based on AIE photosensitizers are garnering increasing attention. These dressings make use of the unique optical properties of AIE-active materials to achieve highly sensitive detection of bacteria29 and efficient antibacterial effect.30 These research studies focus on elevating the antibacterial performance, but the potent phototoxicity to normal tissues has seldom been investigated. Building on the foundations laid by previous studies, the present research places particular emphasis on improving the safety aspect of antimicrobial materials.31
Previously, we reported a novel AIE photosensitizer, DTTPB, and employed it for antimicrobial studies. In these investigations, DTTPB not only exhibited high antibacterial activity but also achieved a significant enhancement in biosafety. The low toxicity towards human cells ensured its heightened safety during application, and encouraged us to explore its potential as antibacterial dressing.
In this study, we report the utilization of DTTPB with a D–π–A structure as antibacterial dressing, and describe its ability to kill both Gram-positive and Gram-negative bacteria. To explore the practical application, DTTPB was fabricated into an injectable hydrogel (DTTPB@gel) wound dressing through mixing with Carbomer 940. Owing to the AIE attribute, DTTPB retained the ability of highly efficient sensitizing ROS in the hydrogel matrix, and the hydrogel property ensured the adhesion of DTTPB@gel to the wound. Together, these attributes added to the convenience of applying the photosensitizer to inhibit and destroy bacteria and thus promoted wound healing.
Results and discussion
Photophysical and photosensitizing properties of DTTPB
DTTPB was synthesized following previously reported procedures.32 In DTTPB, the triphenylamine segment and dithienyl units functioned as electron donors, the carbon–carbon double bonds acted as π-bridges, and the pyridine served as the electron acceptor, thus establishing a D–π–A structural motif (Fig. 1a). This arrangement was beneficial for reducing the energy gap between the singlet and triplet excited states, which facilitated intersystem crossing and enhanced the ROS generation efficiency. The particle size distribution of the material was characterized by dynamic light scattering (Fig. 1b). The results indicated the narrow size distribution of DTTPB in PBS with most of the particles centered at around 424 nm. This uniform particle size distribution suggested the good stability and homogeneity of these particles, which was crucial for practical applications. DTTPB exhibited a broadband absorption in the 300–750 nm range that peaked at 498 nm (Fig. 1c) and a red emission peak at 724 nm, which further extended to near-infrared regions (Fig. 1d). The strong and broad absorption band covering the visible light regions was advantageous for white-light PDT applications.
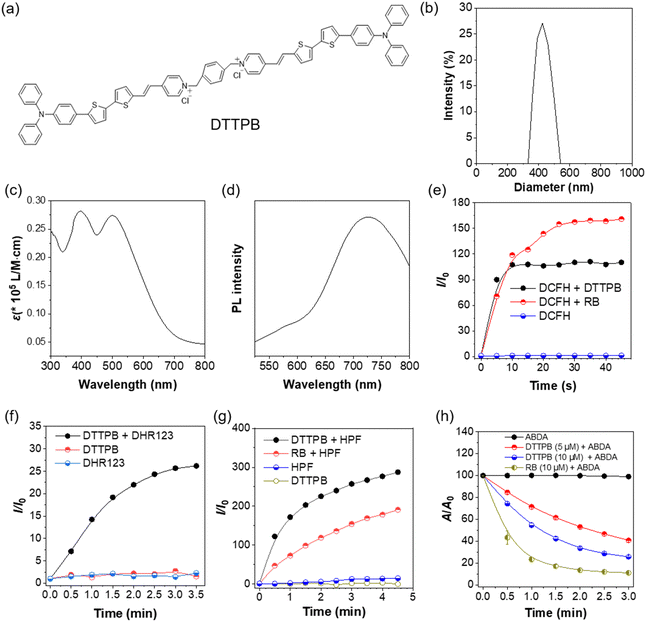 |
| Fig. 1 (a) Molecular structure of DTTPB. (b) Dynamic light scattering of DTTPB (10 μm) in PBS (pH = 7.4). (c) and (d) Absorption and fluorescence spectra of the DTTPB (10 μm, λex = 505 nm). (e) Detection of ROS generated by light irradiation of DTTPB using DCFH. I: fluorescence intensity at 525 nm; I0: fluorescence intensity at 525 nm prior to light irradiation. (f) Detection of O2− generated by light irradiation of DTTPB using DHR123. I: fluorescence intensity at 526 nm; I0: fluorescence intensity at 526 nm prior to light irradiation. (g) Detection of OH generated by light irradiation of DTTPB using HPF. I: fluorescence intensity at 515 nm; I0: fluorescence intensity at 515 nm prior to light irradiation. (h) Detection of 1O2 generated by light irradiation of DTTPB using ABDA. A: absorbance at 378 nm; A0: absorbance at 378 nm prior to light irradiation. | |
To evaluate the ROS-sensitizing ability of DTTPB for PDT applications, a series of ROS detection assays were conducted. A general ROS indicator, 2,7-dichlorodihydrofluorescein (DCFH), was used to assess the overall ROS production of DTTPB under white-light OLED irradiation (36 mW cm−2). For comparison, a commercially available photosensitizer, Rose Bengal (RB), was also tested under the same conditions. As shown in Fig. 1e and Fig. S1a (ESI†), the fluorescence intensity of DCFH alone remained constant, indicating no ROS generation. However, in the presence of DTTPB, the fluorescence at 521 nm increased by 106-fold within 10 s of irradiation (Fig. S1b, ESI†), clearly demonstrating the high ROS sensitizing efficiency of DTTPB. Under the same experimental conditions, RB could also sensitize ROS production efficiently.
To gain deeper insight into the ROS species, dihydrorhodamine 123 (DHR123), N,N-dimethylformamide (HPF) and 9,10-anthracenediyl-bis(methylene)dipropanoic acid (ABDA) were employed to detect the presence of superoxide radical (O2˙−), hydroxyl radical (˙OH), and singlet oxygen (1O2), respectively (Fig. 1f–h). Compared with the control groups, the fluorescence at 526 nm in the “DTTPB + DHR123” group significantly increased in a time-dependent manner (Fig. 1f and Fig. S2a, ESI†), which confirmed the efficient generation of O2˙− and indicated sustained O2˙− generation. In contrast, without DTTPB, the fluorescence intensity remained largely unchanged (Fig. S2b, ESI†), confirming that the observed O2˙− production was sensitized by DTTPB. Additionally, the minimal fluorescence changes in the control experiments without irradiation (Fig. S2c, ESI†) further verified that light exposure was necessary for the activation of DTTPB to generate ROS.
When using HPF to detect ˙OH, the “DTTPB + HPF” group showed a remarkable increase in fluorescence as the irradiation time was prolonged (Fig. 1g and Fig. S3a, ESI†). This highlighted the ability of DTTPB to generate ˙OH, a crucial factor for oxidative stress and antibacterial activity. For comparison, the ˙OH sensitization of RB was tested under the same conditions, from which a similar trend was observed in the “RB + HPF” group (Fig. 1g and Fig. S3b, ESI†), but the degree of fluorescence increase was lower. This confirmed DTTPB as an effective photosensitizer for ˙OH production and its photosensitization efficiency for ˙OH was higher than that of RB. The control group with only DTTPB (Fig. S3c, ESI†) or HPF (Fig. S3d, ESI†) displayed minimal fluorescence enhancement, further validating the DTTPB-sensitized ROS generation in the “DTTPB + HPF” group. By employing ABDA as a sensor, the 1O2 generation capacity of DTTPB was also evaluated (Fig. 1h). Upon treatment with DTTPB (5 μM and 10 μM) and light irradiation, the absorbance of ABDA decayed gradually with increasing irradiation time. The performance of DTTPB was comparable to that of RB, confirming its potential as an efficient 1O2 generator for PDT applications. The results indicated that DTTPB could effectively sensitize the production of type I ROS of O2˙− and ˙OH upon white-light irradiation. Notably, DTTPB could sensitize ˙OH more effectively than RB. Moreover, DTTPB could sensitize the production of type II ROS of 1O2, and its sensitizing efficacy was comparable to that of RB. The above results demonstrated that DTTPB could sensitize both type I and type II ROS, which make it an excellent candidate for aPDT applications.
Imaging of bacteria
Before conducting antibacterial treatments, we first investigated the imaging capability of DTTPB on different bacteria. DTTPB was co-cultured with various bacteria to assess its imaging ability. Escherichia coli (E. coli) was used as a model for Gram-negative bacteria, while Staphylococcus epidermidis (S. epidermidis) was used as a model for Gram-positive bacteria. As shown in Fig. 2a, DTTPB demonstrated good staining ability for both E. coli and S. epidermidis. After incubating DTTPB with E. coli and S. epidermidis in the dark for 30 min, clear red fluorescence was observed for both types of bacteria. This indicated that DTTPB could effectively stain both Gram-positive and Gram-negative bacteria, and might be used for the imaging of most pathogenic bacteria.
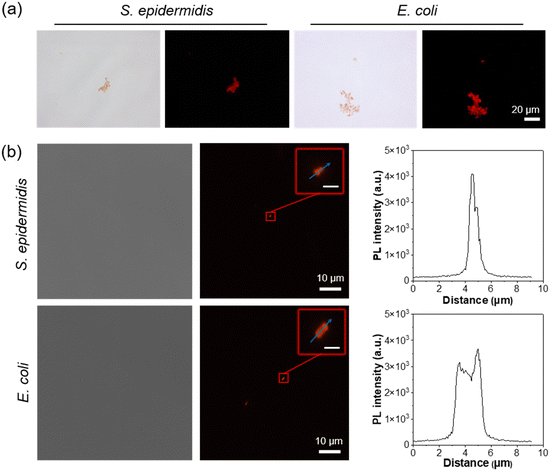 |
| Fig. 2 (a) Bright-field and fluorescence images of S. epidermidis and E. coli incubated with 5 μM of DTTPB for 10 min. Scale bars: 20 μm. (b) Confocal images and bright-field images of S. epidermidis and E. coli incubated with 5 μM of DTTPB for 10 min. Scale bars for the insets of Fig. 2: 1 μm. | |
To further explore DTTPB's staining capability, we stained different bacteria with DTTPB in PBS buffer and imaged these bacteria using a confocal laser scanning microscope. As illustrated in Fig. 2b, both S. epidermidis and E. coli were clearly stained. The fluorescence intensity of DTTPB in the S. epidermidis cell membrane was notably higher than that of E. coli. This can be ascribed to the thicker peptidoglycan cell wall of Gram-positive bacteria compared to that of Gram-negative bacteria, which exists as a sandwiched structure together with an inner phospholipid bilayer and an asymmetric lipopolysaccharide layer in the outer membrane. The outer membrane of Gram-negative bacteria may act as a barrier, affecting compound binding. DTTPB molecules targeted the cell membrane and the cell wall, thus resulting in the higher fluorescence in Gram-positive bacteria.
To validate our hypothesis, we incubated 5 μM and 10 μM of DTTPB with HeLa cells for one hour (Fig. S4, ESI†) and examined the fluorescence under a confocal microscope. The results showed that DTTPB primarily bound to the cell membrane, which preliminarily confirmed the membrane-targeting of DTTPB. Additionally, we measured the Zeta potentials of E. coli and S. epidermidis after incubation with DTTPB for 30 min. As shown in Fig. S5 (ESI†), compared to the control group (bacteria in PBS solution), DTTPB shifted the Zeta potentials of both S. epidermidis and E. coli towards more positive values. Specifically, the Zeta potential of S. epidermidis was shifted from −29.6 mV to −19.5 mV after light exposure, while that of E. coli was shifted from −35.2 mV to −30.9 mV. This shift was attributed to the electrostatic interactions between the positively charged amphiphilic AIE molecules and the negatively charged bacterial surfaces, as well as the significant differences in the cell wall structures between the Gram-positive and Gram-negative bacteria.
Photodynamic antibacterial studies
Given the excellent photosensitizing performance of DTTPB and its ability to image both Gram-positive and Gram-negative bacteria, we further explored its aPDT applications. By using S. epidermidis and E. coli as model strains, we evaluated its antibacterial efficacy. The aPDT killing efficiency of DTTPB against the target bacteria were quantitatively analysed using the plate count method. As shown in Fig. 3a–c, bacteria grew and formed colonies in the dark. However, with increasing concentrations of DTTPB, the bacterial growth was gradually inhibited. Notably, when combined with white-light irradiation, the killing effect of DTTPB on S. epidermidis was significantly enhanced, with a low inhibitory concentration of 1 μM. These results indicated that DTTPB was an effective bacterial inhibitor against both Gram-positive and Gram-negative bacteria, and that PDT further enhanced its antibacterial efficacy.
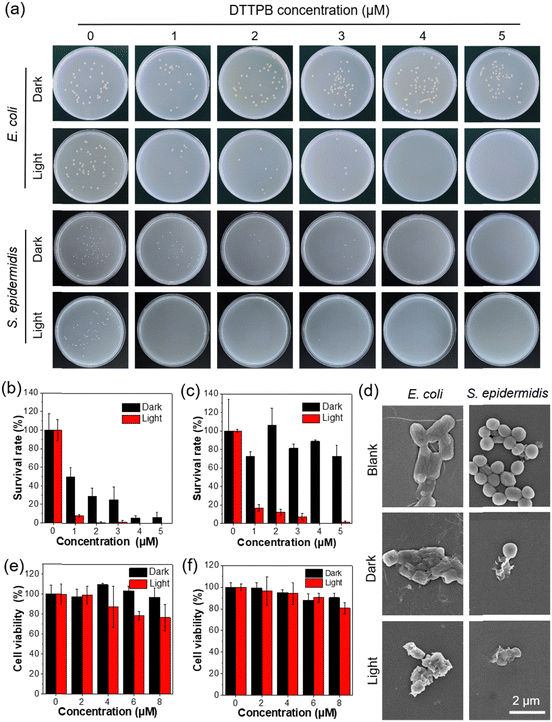 |
| Fig. 3 (a)–(c) Determination of the antibacterial effect of DTTPB by the plate count method. (a) Representative plates of the plate count assay. DTTPB of different concentrations (0, 1, 2, 3, 4, and 5 μM) were used to stain bacterial cultures for 30 min before spreading on the plates. (b) and (c) Statistical analysis of the colony numbers of (b) S. epidermidis and (c) E. coli in the plate count assay. (d) SEM images of S. epidermidis and E. coli after DTTPB treatment with (light) or without (dark) light irradiation. (e and f) Determination of the cytotoxicity of DTTPB on (e) NIH/3T3 and (f) HUVEC cells by the MTT assay. | |
To directly observe the damage inflicted by DTTPB on bacteria, we conducted scanning electron microscopy (SEM) analysis on DTTPB-treated bacteria cells. As illustrated in Fig. 3d, DTTPB induced significant membrane deformation and envelope fusion in both S. epidermidis and E. coli. The application of white-light irradiation notably enhanced the disruption of bacterial envelopes. These results indicated that DTTPB acted as an effective bacterial inhibitor with bactericidal properties against both Gram-positive and Gram-negative bacteria. Furthermore, PDT could substantially augment its bactericidal efficacy without inducing bacterial resistance.
To evaluate the cytotoxicity of DTTPB, we conducted MTT assays on NIH/3T3 fibroblasts and human umbilical vein endothelial cells (HUVEC), both with and without light irradiation (Fig. 3e and 3f). Cells were treated with various concentrations (0, 2, 4, and 8 μM) of DTTPB for one hour, followed by exposure to white-light irradiation (36 mW cm−2) for 10 min. Control cells were kept in the dark for the same duration. DTTPB exhibited minimal impact on the viability of NIH/3T3 and HUVEC cells in the dark. Even upon light irradiation, the HUVEC viability continued to exceed 78% at 8 μM, which was much higher than that required for effective aPDT (4 μM). These results demonstrated the favourable biocompatibility of DTTPB on normal cells, which minimized its influence on normal cells during aPDT applications. The excellent aPDT effect and good normal cell compatibility of DTTPB makes it an excellent candidate for wound-healing applications.
Wound-healing study of DTTPB
To evaluate the photodynamic antibacterial and wound healing capabilities of DTTPB, we established a mixed-infection wound model by incorporating E. coli and S. epidermidis. Surgical incisions of 6 mm diameter were created on mouse dorsal skin. On day 0, an equal volume of E. coli and S. epidermidis mixture was inoculated into the wounds. Afterwards, these mice were subjected to different treatments. Through regular observation of wounds in mice receiving different treatments (Fig. 4a), no significant difference among the groups was found on the 3rd day. However, by the 5th day, compared to the control group (no drug treatment post-infection), the wound area in the DTTPB group was significantly smaller than the NC group (p < 0.01). This outcome could be attributed to the bactericidal ability of DTTPB. On the 7th day, hematoxylin and eosin (H&E) staining was conducted on these wounds to depict the histological analysis of the wounds (Fig. 4b). The control and “Dark” groups exhibited signs of tissue damage or inflammation, indicative of ongoing infection. In contrast, the “Light” group displayed a thick epithelial layer, relatively complete tissue and obvious granulation tissue, with high cell density, indicating a more active healing reaction. At high magnification, fibroblasts and collagen fibers increased significantly, indicating that DTTPB mediated the aPDT-promoted wound healing.
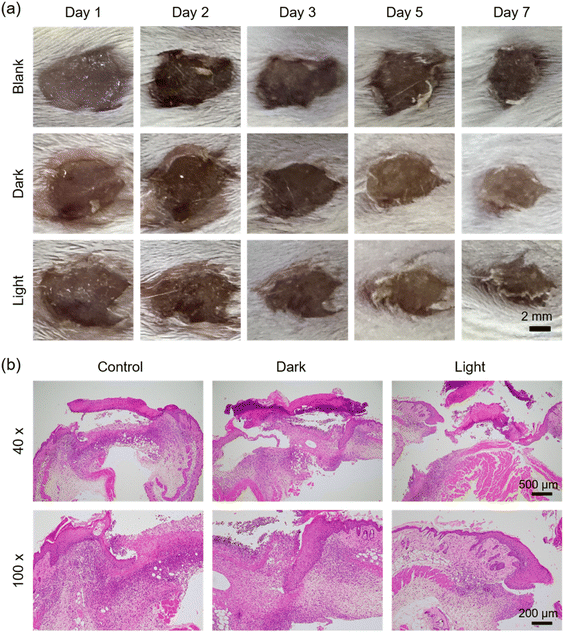 |
| Fig. 4 Wound healing assay in mice treated with DTTPB-mediated PDT. (a) Evaluation of the in vivo antimicrobial effect of DTTPB-mediated PDT against E. coli- and S. epidermidis-infected mouse wounds. (b) H&E staining of E. coli- and S. epidermidis-infected wounds in the presence of PBS, DTTPB without light irradiation (Dark), or DTTPB with white-light irradiation (Light). | |
The comparative analysis of these histological sections highlighted the potential of DTTPB-mediated PDT in promoting wound repair and antimicrobial action in vivo. Fig. S6a (ESI†) shows the corresponding change in the wound area calculated from wound images over the course of 7 days, indicating a significant reduction in wound size in the “Light” group compared to the “Dark” and “Blank” groups. Fig. S6b (ESI†) demonstrates the body weight of BALB/c mice across different treatment groups, with no significant weight changes (p > 0.05), indicating no systemic toxicity. To further investigate the potential damage caused by DTTPB, organs from both the control and PDT-treated mice were collected and subjected to H&E staining. As depicted in Fig. S7 (ESI†), the DTTPB treatment, with or without light irradiation, did not inflict any additional damage to the mouse heart, liver, lungs, kidneys, or spleen. These results demonstrated the exceptional biocompatibility and great feasibility of employing DTTPB in PDT for promoting the healing of infected wounds.
Performance and antibacterial capacity of the DTTPB@gel
Hydrogels are commonly employed as wound dressings due to their excellent adhesion and moisturizing properties, which are desirable for wound healing.33 Additionally, the gelation process of hydrogels can promote the AIE effect,34,35 and are thus expected to efficiently elevate the ROS sensitization. Inspired by the superior performance of hydrogels, we combined DTTPB with Carbomer 940 to develop a hydrogel, DTTPB@gel, for aPDT applications. Various amounts of Carbomer 940 were added to 10 mL of a DTTPB (10 μM) solution to prepare gel solutions with Carbomer 940 concentrations of 0.5%, 1%, 2%, and 4%, respectively. These mixtures were stirred for 6 hours at room temperature. Subsequently, 1 mL of triethanolamine was added, and the mixture was sonicated for 10 min. After cooling, the hydrogels were stored at 4 °C. Additionally, gel dressings with different pH values were prepared by adjusting the amount of triethanolamine, following similar steps.
Shear-thinning is an important characteristic for gels, which allows the antibacterial dressing to exhibit enhanced spreadability, contact properties, and comfort during practical application. Thus, the shear-thinning property of DTTPB@gel was assessed under different pH values (Fig. 5a) and Carbomer 940 concentrations (Fig. 5b). The results demonstrated that the gel exhibited a clear shear-thinning behaviour at pH values ranging from 3.5 to 9.4 (Fig. 5a), where the viscosity was decreased significantly as the shearing rate was increased. Notably, at higher pH values, the initial viscosity of the gel was greater, indicating a pH-dependent rheological response. This suggested that pH variations directly impacted the viscosity of the gel, with higher pH levels leading to a more viscous gel and more pronounced shear-thinning characteristics. The observed shear-thinning behaviour enhanced the spreadability, making it suitable for practical uses such as wound dressing or drug delivery. Similarly, as the concentration of Carbomer 940 was increased from 0.5% to 4%, the initial viscosity of the gel also rose (Fig. 5b), further confirming the concentration's role in enhancing the gel's viscosity. All samples exhibited shear-thinning behaviour, and gels with higher Carbomer 940 concentrations demonstrated more substantial initial viscosities and more prominent shear-thinning effects. This indicated that both pH and Carbomer 940 concentration were critical factors in tuning the rheological properties of the gel, making it adaptable for different biomedical applications. Under an applied shear force, the flowability of the dressing increased, thereby improving its application efficacy, enhancing drug release efficiency, and promoting wound healing and protection. These advantages rendered the DTTPB@gel antibacterial dressings with great potential for wound care and treatment.
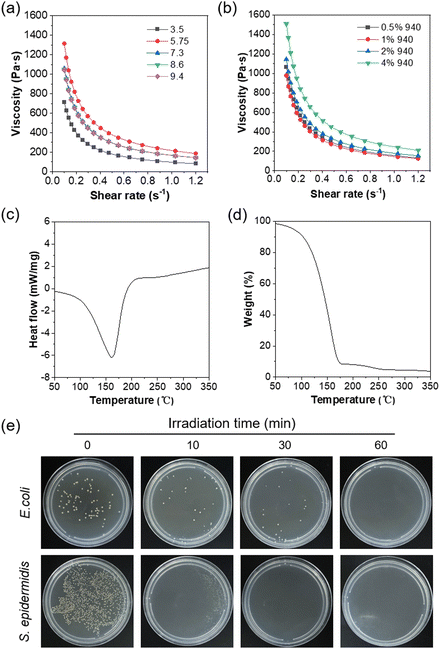 |
| Fig. 5 (a) Plot of the viscosity versus shear rate for the gel at different pH values. (b) Plot of the viscosity versus shear rate for the gel with varying carbomer content. (c) DSC and (d) TGA curves of DTTPB@gel. (e) Determination of the antibacterial effect of DTTPB@gel by the plate count method. | |
To further investigate the thermal stability of the hydrogels, differential scanning calorimetry (DSC) and thermogravimetric analysis (TGA) were performed (Fig. 5c and d). In the DSC cure of DTTPB@gel, a prominent endothermic peak was observed in the range of 150–200 °C (Fig. 5c), suggesting a phase transition or thermal degradation within this temperature range. In the TGA curve, the hydrogel exhibited a gradual decrease in mass with rising temperature, followed by a sharp drop beyond 250 °C (Fig. 5d), signifying the rapid thermal decomposition. By approximately 350 °C, the sample was nearly completely decomposed. The results suggested that the thermal stability of DTTPB@gel maintained at temperatures of up to 250 °C, beyond which significant degradation occurred. Together, the endothermic peak and thermal decomposition profile suggested that the hydrogel could withstand moderate heating without significant loss of material, but underwent rapid decomposition at higher temperatures, which was crucial for determining its potential applications in thermally stable environments.
In the next place, the antibacterial property of DTTPB@gel was evaluated through photodynamic antibacterial experiments to examine the aPDT efficacy of DTTPB in a gel matrix. The viability of bacteria with different treatments was examined by the plate count method. The results demonstrated that DTTPB@gel exhibited excellent antibacterial efficacy. As shown in Fig. 5e, prolonged light exposure significantly inhibited bacterial growth. These results indicated that DTTPB@gel effectively maintained its photodynamic antibacterial performance and demonstrated substantial efficacy in practical applications.
Wound-healing study of DTTPB@gel
To evaluate the photodynamic antibacterial and wound healing capabilities of DTTPB@gel in an in vivo infected-wound model, a mixed bacterial infection involving E. coli and S. epidermidis was established. Over the course of the experiment, the progression of wound healing in different treatment groups was closely monitored, and the results are displayed in Fig. 6a. On day 3, the wound images indicated no substantial differences in healing across the groups, with wounds remaining similar in size and appearance. On day 5, a notable divergence appeared: the wounds receiving DTTPB@gel and light irradiation (DTTPB@gel + L) demonstrated significantly enhanced wound healing compared to both the untreated control group and the dark control group. The accelerated wound closure, reduced signs of inflammation, and a visible reduction in infection were demonstrative of the high efficacy of DTTPB@gel in promoting wound healing, which should be attributed to the synergistic effects of DTTPB-sensitized ROS generation under light activation and the hydrogel's ability to provide a favourable environment for tissue repair. By day 7, the DTTPB@gel + L group achieved the most substantial healing improvements, with nearly complete wound closure and minimal signs of residual infection (p < 0.05), surpassing the performance of both the untreated control and the DTTPB + L group (DTTPB without gel). This outcome highlighted the importance of the hydrogel component, which not only enhanced the photosensitizer's retention at the wound site but also facilitated moisture retention, thus promoting a faster and more efficient wound healing process. Additionally, the hydrogel acted as a protective barrier, preventing secondary infections and further aiding in the healing process. As shown in Fig. S8 (ESI†), H&E staining of the E. coli and S. epidermidis infected wounds in the presence of PBS (Control), DTTPB@gel without light irradiation (Dark), or DTTPB@gel with white-light irradiation (Light) further supports the findings. The tissue samples from the Light group exhibited significantly reduced inflammation and accelerated tissue regeneration compared to the Control and Dark groups, as indicated by the more organized and healthier tissue structure observed in the magnified 100× images.
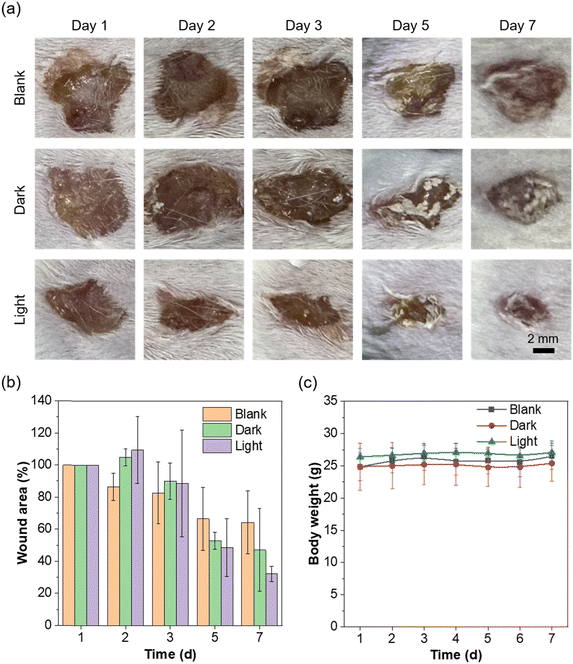 |
| Fig. 6 The wound healing assay of mice treated with DTTPB@gel-mediated PDT. (a) Evaluation of the in vivo antimicrobial effect of DTTPB@gel-mediated PDT against E. coli- and S. epidermidis-infected mouse wounds. (b) Corresponding change in wound area calculated from wound images. The error bars (n = 3) represent the mean ± SD. (c) Body weight of BALB/c mice with various treatments. The error bars (n = 3) represent the mean ± SD. | |
Moreover, the overall health and safety of the mice were assessed through regular monitoring of their body weights (Fig. 6c) and histological analysis of their major organs (Fig. S9, ESI†). These evaluations revealed that no significant difference in body weight was observed (p > 0.05), indicating that the PDT treatment was well-tolerated and did not induce any systemic toxicity. Histological assessments confirmed the absence of notable morphological abnormalities or damage in vital organs such as the liver, kidneys, and lungs, underscoring the biocompatibility and safety of DTTPB@gel-mediated PDT. These findings collectively suggested that DTTPB@gel, when activated by light, held significant promise as an effective, biocompatible, and safe treatment for bacterial infections in wounds. The combination of the photosensitizer's ROS generation under light exposure and the hydrogel's wound-healing properties presents a powerful therapeutic strategy.
Conclusions
In summary, we explored the potential of employing an AIE-active photosensitizer, DTTPB, for illuminating and ablating both Gram-positive and Gram-negative bacteria through aPDT. Leveraging the AIE properties of DTTPB and the distinct membrane structures of Gram-positive and Gram-negative bacteria, confocal laser-scanning microscopy revealed that DTTPB rapidly stained both bacterial types. DTTPB was capable of eradicating Gram-positive bacteria at low concentrations and Gram-negative bacteria at higher concentrations. Furthermore, the integration of DTTPB with Carbomer 940 yielded an antibacterial hydrogel dressing (DTTPB@gel). In vivo experimental results demonstrated that DTTPB@gel not only possessed exceptional aPDT potency but also promoted wound healing in mice infected with bacteria, which should be attributed to the hydrogel's adhesive and hydrating properties. This study demonstrated the potential of DTTPB@gel-mediated PDT as a novel and robust alternative to conventional antimicrobial approaches, particularly in addressing the growing global challenge of antibiotic resistance. The study is expected to pave the way for further clinical research and potential applications in wound care and infection management.
Experimental
Materials
All chemical reagents were obtained from Macklin and used without further purification. Thiazolyl Blue (MTT) was purchased from Coolaber Technology Co. (China). DMEM medium was bought from Procell Life Science & Technology Co. Phosphate buffered saline (PBS), penicillin and streptomycin were purchased from Beyotime Biotech Inc. Fetal bovine serum (FBS) and Trypsin-EDTA (0.25%) were purchased from Gibco. 2′,7′-Dichlorodihydrofluorescein diacetate (DCFH-DA) was purchased from Aladdin. DHE, DHR123 and HPF were purchased from Maokang Bio (China). Milli-Q water was supplied by Milli-Q Plus System (Millipore Corporation, United States).
Total ROS generating ability test.
The transformation of DCFH-DA to DCFH was first conducted. The activated DCFH-DA solution (DCFH, 40 μM) was added into the sample solution (PBS) containing DTTPB (5 μM). Upon irradiation with a white-light source (400–800 nm, 36 mW cm−2) for different time intervals, the fluorescence of DCF at 521 nm was measured in a fluorescence spectrophotometer at the excitation of 480 nm. DCFH alone was tested under the same experimental procedures as the control.
1O2 generating ability test.
1O2 production was investigated using 9,10-anthracenediyl-bis(methylene)dipropanoic acid (ABDA) as an indicator. Typically, a mixture of ABDA (100 μM) and photosensitizers (10 μM) in PBS with 1% DMSO was exposed to white-light irradiation (36 mW cm−2). The decomposition of ABDA was monitored by recording the decrease in absorbance at 378 nm every 30 seconds.
˙OH detection.
The generation of ˙OH was evaluated using HPF as an indicator. HPF stock solution (5 mM) was diluted to 5 μM in PBS containing AIEgens (10 μM) and RB (10 μM). After irradiation with white light (36 mW cm−2), HPF fluorescence was monitored in the range of 510–610 nm with an excitation wavelength of 490 nm at different time intervals. The fluorescence intensity at 515 nm was recorded to indicate the rate of ˙OH generation.
O2˙− measurement.
O2˙− generation was measured using DHR123 as an indicator. DHR123 stock solution (5 mM) was diluted to 5 μM in PBS containing the AIEgens sample solution (2 μM). After irradiation with white light (36 mW cm−2), the fluorescence of DHR123 was monitored in the range of 500–620 nm with an excitation wavelength of 495 nm at different time intervals. The fluorescence intensity at 526 nm was recorded to indicate the rate of O2˙− generation.
Bacterial culture.
Gram-positive S. epidermidis (ATCC 12228) and Gram-negative E. coli (ATCC 25922) were employed for the experiments.
Bacterial culture conditions.
Bacterial stock solutions were stored at −80 °C. For use, they were inoculated into LB liquid medium and incubated overnight at 37 °C with shaking at 200 rpm. The following day, the cultures were subcultured into fresh LB medium at a 1% inoculum ratio, and further incubated under the same conditions until required for experiments.
Bacterial imaging.
Bacteria cultured to an OD600 of 0.01 were centrifuged (10
000 rpm, 1 min), washed twice with PBS, and then resuspended in PBS. DTTPB (final concentration 10 μM) was added, and the bacteria were incubated in the dark at 37 °C with shaking at 200 rpm for 30 min. The bacteria were then resuspended in PBS (1 mL), and a 5 μL aliquot of the suspension was transferred to a glass slide. Imaging E. coli was performed using fluorescence microscopy.
Cell imaging.
Cells were seeded into culture dishes at a density of 5 × 105 cells per dish. The cells were cultured in DMEM supplemented with 10% fetal bovine serum and 1% penicillin–streptomycin. The culture dishes were incubated overnight at 37 °C in a humidified atmosphere containing 5% CO2 to allow cell attachment and growth to appropriate confluency. For staining, 5 μM and 10 μM DTTPB solutions were freshly prepared. Cells were then incubated with 5 μM or 10 μM DTTPB solution at 37 °C for 1 hour. After incubation, the cells were washed three times with pre-cooled phosphate-buffered saline (PBS). The stained cells were imaged using a confocal laser scanning microscope. The excitation wavelength was set to 505 nm, and fluorescence was captured using a 620–720 nm emission filter to detect the fluorescence signal of DTTPB.
SEM imaging.
The bacterial cultures were grown to an OD600 of 0.1 for S. epidermidis and 0.25 for E. coli. Afterward, the cultures were centrifuged, and the supernatant was discarded. This step was repeated three times. Subsequently, 5 μM of DTTPB was added to the bacterial suspensions, which were incubated in dark at 37 °C with shaking at 200 rpm for 30 min. Following incubation, the bacterial cultures were exposed to white light (36 mW cm−2) for 10 min. After illumination, the samples were rested for 60 min, centrifuged, washed, and resuspended in 1 mL of 4% paraformaldehyde solution for fixation. The fixed bacteria were stored overnight at 4 °C. The next day, the bacteria were subjected to a second round of centrifugation, resuspension, and washing, which was repeated three times to remove any excess reagents. To dehydrate the bacteria, a series of ethanol solutions of increasing concentrations (20% to 100%, in 20% increments) were used, with each step lasting 10 min. After dehydration, the bacteria were resuspended in anhydrous ethanol and mixed thoroughly. Finally, a small volume of the bacterial suspension was deposited on a conductive adhesive surface, preparing the sample for SEM imaging.
Zeta potential measurement.
The bacterial suspension was diluted to an OD600 of 0.1, and 100 μL of the suspension was added to 900 μL of PBS containing DTTPB, resulting in a final DTTPB concentration of 5 μM. The mixture was incubated at 37 °C with shaking for 30 min, followed by exposure to white light (36 mW cm−2) for 10 min. The bacterial suspension was then centrifuged to remove the supernatant, washed three times with PBS, and resuspended in 1 mL of ultrapure water. The zeta potential of the bacterial suspension was measured using a Zetasizer Nano ZS analyzer. Control groups consisted of bacterial suspensions either untreated with DTTPB or not exposed to light, tested under the same conditions. Each sample was tested independently in triplicate.
MTT assay.
Cells were harvested and diluted to a concentration of 6 × 104 cells per mL. 100 μL of cell suspension was inoculated into a 96-well cell culture plate and incubated at 37 °C with 5% CO2. On the following day, the culture medium was replaced with 100 μL of DMEM containing varying concentrations of DTTPB (0, 2, 4, 6, 8 μM). After incubating the cells at 37 °C with 5% CO2 for 1 hour, the photosensitizer-containing medium was removed and the cells were washed three times with PBS, followed by replenishment with fresh DMEM medium. Afterwards, the cells were either stored under dark conditions or irradiated with white light (400–800 nm, 36 mW cm−2) for 10 min, followed by culturing in the incubator for 24 h. On the next day, the medium was removed from the 96-well plate and 10 μL of MTT solution were added to each well. After incubation for 4 hours, the medium was discarded and 100 μL of DMSO was added to dissolve the formazan crystals. The absorbance at 490 nm was measured, and the cell viability was assessed by comparing the absorbance to the blank control group. Each DTTPB concentration was tested in four replicates, with untreated cells serving as the control.
In vivo antibacterial and wound healing assessment.
Animals received care, following the guidance suggestions for the Care and Use of Laboratory Animals. The procedures were approved by the Animal Care and Use Committee (Ethics Committee of the Academy of Medical Sciences of Zhengzhou University) with a protocol code of ZZU-LAC20220401 [04]. Eight to ten-week-old BALB/c mice were used to establish wound infections with a mixture of E. coli and S. epidermidis (108 CFU mL−1). All in vivo experiments were conducted under sterile conditions in a laminar flow hood, with prior disinfection of personnel and equipment. The mice were divided into three groups: Control, DTTPB, and DTTPB + Light (n = 3 per group). For the experiment, a 6-mm diameter wound was created on the dorsal side of each mouse, and 50 μL of bacterial suspension was applied to the wound. In the Control group, wounds were left untreated. In the DTTPB group, wounds were treated with 50 μL of DTTPB (5 μM) or DTTPB@gel. In the DTTPB + Light group, the wounds were treated with DTTPB@gel and exposed to white light at an irradiance of 36 mW cm−2 for 10 min. Treatment was administered for 3 days. On the seventh day post-treatment, all wounds were photographed, and the mice were euthanized. Wound tissues and mouse organs were collected for bacterial enumeration and H&E staining.
Statistical analysis.
The data analysis was carried out using IBM SPSS Statistics 26. For statistical analysis, one-way ANOVA was performed, followed by SNK test for post hoc comparisons. The significance levels were defined as follows: Ns (P > 0.05), indicating no significant difference; p ≤ 0.05, indicating a significant difference; p ≤ 0.01, indicating a highly significant difference; and p ≤ 0.001, indicating an extremely significant difference. The results were presented as means ± standard deviation (SD).
Author contributions
Yu Ma: conceptualization, methodology, data curation, formal analysis, writing – original draft. Jiawei You: investigation, visualization, writing – review & editing. Jianquan Hou: writing – review & editing. Yupeng Shi: writing – review & editing. Engui Zhao: project administration, supervision, funding acquisition, writing – review & editing. Led the project, secured funding, and supervised the entire study.
Data availability
The data supporting the findings of this article are available in the ESI† or from the corresponding author E. Z upon reasonable request.
Conflicts of interest
There are no conflicts to declare.
Acknowledgements
The authors acknowledge the financial support by the National Natural Science Foundation of China (22005050) and Guangdong University Featured Innovation Program Project (2024KTSCX109).
Notes and references
- F. Jia, J. Wang, L. Zhang, J. Zhou, Y. He, Y. Lu, K. Liu, W. Yan and K. Wang, J. Pept. Sci., 2021, 27, e3294 CrossRef CAS PubMed.
- M. Loftus, S. A.-D. Hassouneh and S. Yooseph, Sci. Rep., 2021, 11, 2828 CrossRef CAS PubMed.
- Y. Belkaid and T. Hand, Cell, 2014, 157, 121–141 CrossRef CAS PubMed.
- V. D. Khadka, L. Markey, M. Boucher and T. D. Lieberman, J. Invest. Dermatol., 2024, 144, 2541–2552.e10 CrossRef CAS PubMed.
- J. B. Kaper, J. P. Nataro and H. L. T. Mobley, Nat. Rev. Microbiol., 2004, 2, 123–140 CrossRef CAS PubMed.
- L. Li, L. Chen, Y. Lu, B. Li, R. Hu, L. Huang, T. Zhang, X. Wei, Z. Yang and C. Mao, Aggregate, 2023, 4, e200 CrossRef CAS.
- K. E. Rudd, S. C. Johnson, K. M. Agesa, K. A. Shackelford, D. Tsoi, D. R. Kievlan, D. V. Colombara, K. S. Ikuta, N. Kissoon and S. Finfer,
et al.
, Lancet, 2020, 395, 200–211 CrossRef PubMed.
- B. M. Aiesh, M. A. Nazzal, A. I. Abdelhaq, S. A. Abutaha, S. H. Zyoud and A. Sabateen, Sci. Rep., 2023, 13, 5040 CrossRef CAS PubMed.
- H. von Döhren, Protein Sci., 2004, 13, 3059–3060 CrossRef.
- A. Fleming, Bull. W. H. O., 2001, 79, 780–790 CAS.
- C. T. Walsh and T. A. Wencewicz, J. Antibiot., 2014, 67, 7–22 CrossRef CAS PubMed.
- L. Guan, M. Beig, L. Wang, T. Navidifar, S. Moradi, F. Motallebi Tabaei, Z. Teymouri, M. Abedi Moghadam and M. Sedighi, Ann. Clin. Microbiol. Antimicrob., 2024, 23, 80 CrossRef CAS PubMed.
- N. A. Turner, B. K. Sharma-Kuinkel, S. A. Maskarinec, E. M. Eichenberger, P. P. Shah, M. Carugati, T. L. Holland and V. G. Fowler, Nat. Rev. Microbiol., 2019, 17, 203–218 CrossRef CAS PubMed.
- M. R. Hamblin and T. Hasan, Photochem. Photobiol. Sci., 2004, 3, 436–450 CrossRef CAS PubMed.
- J. Li, Z. Zhuang, X. Lou, Z. Zhao and B. Z. Tang, Chem. Biomed. Imaging, 2023, 1, 785–795 CrossRef CAS PubMed.
- F. Wang, P.-Y. Ho, C. Kam, Q. Yang, J. Liu, W. Wang, E. Zhao and S. Chen, Aggregate, 2023, 4, e312 CrossRef CAS.
- J. P. Celli, B. Q. Spring, I. Rizvi, C. L. Evans, K. S. Samkoe, S. Verma, B. W. Pogue and T. Hasan, Chem. Rev., 2010, 110, 2795–2838 CrossRef CAS PubMed.
- Y. Liu, L. Mao, S. Yang, M. Liu, H. Huang, Y. Wen, F. Deng, Y. Li, X. Zhang and Y. Wei, Dyes Pigm., 2018, 158, 79–87 CrossRef CAS.
- J. Luo, Z. Xie, J. W. Y. Lam, L. Cheng, H. Chen, C. Qiu, H. S. Kwok, X. Zhan, Y. Liu, D. Zhu and B. Z. Tang, Chem. Commun., 2001, 1740–1741 RSC.
- E. Zhao and S. Chen, Mater. Chem. Front., 2021, 5, 3322–3343 RSC.
- J. Mei, N. L. C. Leung, R. T. K. Kwok, J. W. Y. Lam and B. Z. Tang, Chem. Rev., 2015, 115, 11718–11940 CrossRef CAS PubMed.
- H. Wang, Q. Li, P. Alam, H. Bai, V. Bhalla, M. R. Bryce, M. Cao, C. Chen, S. Chen and X. Chen,
et al.
, ACS Nano, 2023, 17, 14347–14405 CrossRef CAS PubMed.
- P.-Y. Ho, T. Y. Chou, C. Kam, W. Huang, Z. He, A. H. W. Ngan and S. Chen, Sci. Adv., 2023, 9, eabn5390 CrossRef PubMed.
- M.-Y. Wu, A. Y. H. Wong, J.-K. Leung, C. Kam, K. L.-K. Wu, Y.-S. Chan, K. Liu, N. Y. Ip and S. Chen, Proc. Natl. Acad. Sci. U. S. A., 2021, 118, e2106143118 CrossRef CAS PubMed.
- D. Ding, K. Li, B. Liu and B. Z. Tang, Acc. Chem. Res., 2013, 46, 2441–2453 CrossRef CAS PubMed.
- X. Chen, M. Zhao, Q. Xie, S. Zhou, X. Zhong, J. Zheng, R. Yang, X. Du, J. Xia and Y. Liao, Aggregate, 2024, 5, e406 CrossRef CAS.
- M. Kuddushi, A. A. Shah, C. Ayranci and X. Zhang, J. Mater. Chem. B, 2023, 11, 6201–6224 RSC.
- L. Wang, L. Duan, G. Liu, J. Sun, M.-A. Shahbazi, S. C. Kundu, R. L. Reis, B. Xiao and X. Yang, Adv. Sci., 2023, 10, 2207352 CrossRef CAS PubMed.
- Z. Fan, Y. Liu, Y. Ye and Y. Liao, Aggregate, 2023, 4, e620 Search PubMed.
- S. Deng, Z. Peng, F. Zhou, G. Zhong, Z. Cai, X. Tang, C. Xu, L. Zheng, B. Z. Tang and J. Zhang, Aggregate, 2023, 4, e575 CrossRef.
- F. Wang, Y. Shi, P. Ho, E. Zhao, C. Kam, Q. Zhang, X. Zhao, Y. Pan and S. Chen, Bioeng. Transl. Med., 2023, 8, e10539 CrossRef CAS PubMed.
- M.-Y. Wu, M. Gu, J.-K. Leung, X. Li, Y. Yuan, C. Shen, L. Wang, E. Zhao and S. Chen, Small, 2021, 17, 2101770 CrossRef CAS PubMed.
- V. Gounden and M. Singh, Gels, 2024, 10, 43 CrossRef CAS PubMed.
- W. Lu, S. Wei, H. Shi, X. Le, G. Yin and T. Chen, Aggregate, 2021, 2, e37 CrossRef CAS.
- J. Liu, J. Feng, Y. Yu, L. Xu, Q. Liu, H. Zhang, J. Shen and W. Qi, J. Phys. Chem. C, 2020, 124, 23844–23851 CrossRef CAS.
|
This journal is © The Royal Society of Chemistry 2025 |
Click here to see how this site uses Cookies. View our privacy policy here.