DOI:
10.1039/D2BM01473D
(Minireview)
Biomater. Sci., 2023,
11, 2974-2987
Development of substrates for the culture of human pluripotent stem cells
Received
11th September 2022
, Accepted 22nd February 2023
First published on 15th March 2023
Abstract
Although human pluripotent stem cell (hPSC) lines were initially established in culture using feeder cells, the development of culture media and substrates is essential for safe, stable, high-quality, and efficient production of large numbers of cells. Many researchers are now culturing hPSCs in chemically defined media and on culture substrates without feeder cells. In this review, we first discuss the problems with Matrigel, which has long been used as a culture substrate. Then, we summarize the development of extracellular matrix proteins for hPSCs, which are now the mainstream alternative, and synthetic substrates that are expected to be the future mainstream alternative. We also highlight three-dimensional culture for suitable mass production of hPSCs.
1. Introduction
Human pluripotent stem cells (hPSCs), including human embryonic stem cells (hESCs) and human induced pluripotent stem cells (hiPSCs), have great potential for a wide range of applications in regenerative medicine and as tools for human disease modeling and drug screening.
In 1981, two groups first established embryonic stem cells derived from the inner cell mass of mouse blastocysts independently.1,2 In terms of humans, Thomson et al. succeeded in 1998,3 but it required nearly 20 years. A reason for this is the difference in the culture medium and substrate requirements between mouse embryonic stem cells and hESCs.
Mouse embryonic stem cells are easily maintained in an undifferentiated state and proliferate under feeder-free conditions by adding leukemia inhibitory factor (LIF) to the culture medium.4 Because of the strong differentiation inhibitory effect of LIF, there is little requirement for culture substrates, and a gelatin coating is usually sufficient. However, it is difficult to inhibit spontaneous differentiation of hESCs in culture. Therefore, it is necessary to remove differentiated cells manually. Additionally, dissociated cells have weak adhesion to the substrate. Therefore, culture methods, including the culture medium and substrate, have been developed for undifferentiated expansion of hESCs. With the development of hESC culture technology, the establishment of mouse induced pluripotent stem cell (iPSC) lines was first reported in 2006,5 and the establishment of human iPSC lines was reported in the following year.6,7
In terms of hPSC culture media, high-quality products (capable of maintaining homogenous and undifferentiated hPSCs) are currently being developed and marketed, the price of which is expected to decrease while maintaining current performance. However, in terms of culture substrates, there has been a shift from feeder cells and Matrigel to human recombinant proteins, but synthetic substrates are not widely used. Synthetic substrates are expected to be a promising field because of their cost, homogeneity, and stable supply.
The following discussion focuses on the development of culture substrates. The development of stable chemically defined media will lead to proper evaluation of culture substrates and encourage the development of culture substrates. Therefore, we summarize the history of chemically defined medium development before the development of culture substrates. Finally, we briefly mention three-dimensional (3D) culture that is expected to be the future for mass culture of hPSCs. To understand the importance and progress of this field, we recommend reading several referenced reviews.8–12
2. Development of chemically defined medium for hPSCs
Culture medium containing fetal bovine serum (FBS) was used with mouse embryonic fibroblasts (MEFs) as feeders in the early studies after establishing hESC lines. In some cases, embryonic fibroblast-derived cell lines such as STO and SNL were used as an alternative to MEFs. Because of batch variations in FBS, it was necessary to periodically validate a suitable batch for stable culture. Additionally, such culture systems contain non-human (xeno) cells and proteins, which are unsuitable for clinical applications in regenerative medicine, requiring the development of both culture media and substrates.
In 2001, the first hESC culture method without feeder cells was reported.13 This study used Matrigel as a culture substrate instead of feeder cells in combination with conditioned medium from MEFs (MEF-CM). In 2004, Amit et al. reported a new culture method using serum-free medium with KnockOut Serum Replacement (KSR) (Thermo Fisher Scientific) instead of FBS on MEFs.14 KSR contains animal components, but has less batch variation than FBS. Additionally, the study described the development of a feeder-free culture system using fibronectin as a culture substrate instead of MEFs and the addition of basic fibroblast growth factor (bFGF), transforming growth factor β (TGFβ), and LIF to medium containing KSR. At approximately the same time, the effects of cytokines on hESC self-renewal began to be elucidated.15–18 The cytokine with the most common effect was bFGF with nodal/activin/TGFβ being the second most common.
Based on these findings, non-commercial and commercially available chemically defined media for hPSCs have been reported.19–27 However, some of these media are reportedly difficult to reproduce. To address this issue, the world's leading research teams in hESC research, the International Stem Cell Initiative Consortium, evaluated eight representative chemically defined culture media. They found that only two media, mTeSR1 (STEMCELL Technologies) and StemPro (Thermo Fisher Scientific), could maintain many cell lines in an undifferentiated state for ten passages.28 As a result, reliable synthetic culture media were identified, allowing the efficient development of culture substrates.
In 2012, Chen et al. re-evaluated media components and reported E8 medium, which does not contain a serum albumin component.29 Indeed, E8 medium consists of only eight components: Dulbecco's modified Eagle's medium/F12, L-ascorbic acid, selenium, transferrin, NaHCO3, insulin, bFGF, and TGFβ. This medium was later marketed as Essential 8 (Thermo Fisher Scientific) and TeSR-E8 (STEMCELL Technologies), which have been adopted by many researchers.
Many chemically defined media are currently available, providing options to best suit specific research needs. Although CTS-Grade Xeno-Free KSR is currently available, most researchers culture cells in chemically defined media under feeder-free conditions. Table 1 lists the media we routinely use in our laboratory with stable results. At present, we are using improved products Essential 8 Flex and CTS Essential 8 (Thermo Fisher Scientific).
Table 1 Commercially available chemically defined media for hPSC culture
Media |
Vendor catalog |
No. |
mTeSR1 (cGMP) |
STEMCELL Technologies |
85850 |
mTeSR Plus (cGMP) |
STEMCELL Technologies |
100-0276 |
TeSR-E8 |
STEMCELL Technologies |
05990 |
TeSR-AOF |
STEMCELL Technologies |
100-0401 |
StemFlex medium |
Thero Fisher Scientific |
A3349401 |
Essential 8 flex medium |
Thero Fisher Scientific |
A2858501 |
CTS essential 8 medium |
Thero Fisher Scientific |
A2656101 |
StemFit AK02N |
Ajinomoto |
AK02N |
StemFit AK03N |
Ajinomoto |
AK03N |
Watanabe et al. found that the ROCK inhibitor Y-27632 suppresses apoptosis of dissociated hESCs and permits cell survival.30 This effect allows seeded cells to adhere to the culture substrate during passaging and promotes the formation of hESC clusters in suspension culture. The effects of Y-27632 are transient and often insufficient at low cell-seeding densities. Furthermore, long-term exposure to Y-27632 reportedly alters the metabolism of hPSCs,31 suggesting that Y-27632 should be handled with care to proceed with precision bioprocesses using hPSCs.
Because chemically defined culture media are now the mainstream for scientists, we excluded reports using MEF-CM and other similar media despite their recent publications, because MEF-CM contains adhesion factors and ECM portions that are necessary for cell adhesion.
3. Matrigel use and problems
As described above, the first feeder-free culture system for undifferentiated hESCs was reported in 2001, which used Matrigel (Corning) with MEF-CM.13 Matrigel and similar commercial products named Geltrex (Thermo Fisher Scientific) and Cultrex BME (R&D Systems) are extracts of the Engelbreth-Holm-Swarm sarcoma cell line. We collectively refer to them as Matrigel hereafter.
Matrigel is thawed at 4 °C and undergoes gelation at 22–37 °C for 30 min. The resulting gel does not readily re-dissolve on cooling. Due to its inherent bioactivity, Matrigel has been used for various applications for different cell types.32–34 As a thin gel coating, Matrigel has been used to culture and expand various cells, including hPSCs. Thicker Matrigel coatings allow 3D organizational structures, including organoids.
Matrigel has long been the gold standard culture substrate for hPSCs because it is less expensive than laminin, vitronectin, and other culture substrates. Moreover, many researchers have confirmed its reproducibility. Because Matrigel has been widely used for cells other than hPSCs, it is expected that the conversion of hPSCs from undifferentiated growth to induced differentiation occurs rapidly.
However, Matrigel is subject to batch variations that can cause problems for data reproducibility. Although hESC-qualified Matrigel is now available, its performance still needs to be confirmed before use in culture protocols.
A significant reason is that Matrigel is a crude extract consisting of laminin (∼60%), collagen IV (∼30%), entactin (∼8%), and heparin sulfate proteoglycan perlecan (∼2%–3%).35 Further detailed results from LC-MS analysis showed that Matrigel contains more than 14
000 unique peptides and 1850 unique proteins.36 Many of these have been identified as constitutive proteins, but include growth factors.36–38 As a result, Matrigel acts as a reservoir of these growth factors, affecting cultured cells and their microenvironment. Additionally, Matrigel has potential risks of pathogenic contamination, such as lactate dehydrogenase-elevating virus,39 and non-human immunogenic epitopes such as N-glycolylneuraminic acid.40 Therefore, the development of media and culture substrates adapted to clinical applications is needed.
4. Extracellular matrix (ECM)-purified proteins
4.1 Integrins
Integrins are major transmembrane receptors for cell adhesion to ECM proteins and play important roles in specific cell–cell adhesions.41 Integrins consist of αβ heterodimers. In mammals, 24 distinct integrins comprise 18 α-chains and eight β-chains. A suitable ECM can be estimated by the specific expression pattern of integrins in cells (Fig. 1a).
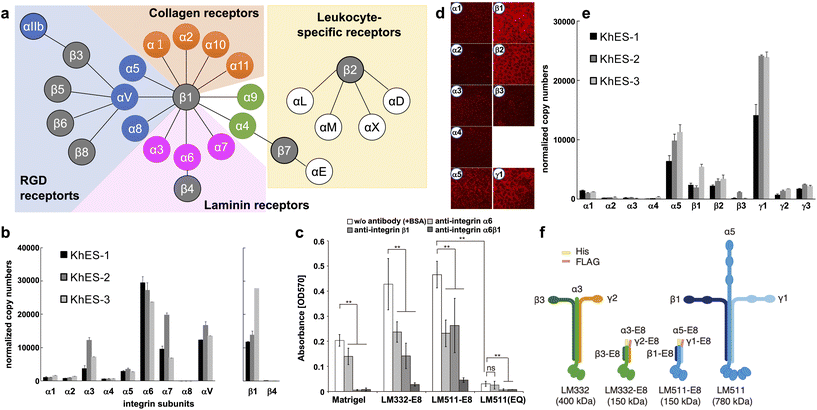 |
| Fig. 1 Characterization of integrins and laminins expressed in hPSCs. (a) Integrin receptor family. Integrins are αβ heterodimers. In mammals, 24 distinct integrins exist by the combination of 18 α subunits and eight β subunits. Receptors for RGD (blue), laminin (magenta), collagen (orange), and leukocyte-specific (yellow) are shown. (b) Determination of integrin isoforms expressed on hESCs. qPCR analysis used total RNA from three hESC lines cultured on MEF feeders. Copy numbers were normalized to qPCR of plasmids with cDNA of each integrin subunit as the template. hESC highly express α6β1 integrins. (c) Inhibition of integrin binding on LM-E8s. Completely dissociated H9 hESCs were incubated with an integrin-specific blocking antibody for 30 min in mTeSR1 medium. Cell attachment to both LM-E8s was significantly reduced by the addition of antibodies against α6 and/or β1 integrins. LM511(EQ), an inactive form of LM511-E8, dramatically abrogated cell adhesion. Error bars indicate the s.d. of five individual assays. **P < 0.05; ns, non-significant; two-way ANOVA or Tukey's test. (d and e) Laminin isoforms produced by hESCs on MEF feeders. hESCs predominantly produce laminin-511/-521 (α5, β1, β2, and γ1). (d) Immunostaining of human laminin subunits. Images were obtained at the same exposure time. (e) qPCR analysis of laminin subunits expressed on hESCs. Total RNA of three hESC lines was used for the analysis. Copy numbers were normalized using plasmids with cDNA of each laminin subunit as the template. (f) Schematic representations of two LM-E8s and the original intact laminin isoforms. A 6×His-tag and FLAG-tag were attached to the N-termini of α and γ chains, respectively, of LM-E8s to facilitate purification. The molecular weights of individual proteins are shown in parentheses. [(a) Adapted with permission.41 Copyright 2002, Cell Press. (b, d and e) Adapted with permission.42 Copyright 2008, Elsevier. (c and f) Reproduced with permission.43 Copyright 2012, SpringerNature.] | |
Many integrins are commonly expressed in hPSCs. H1 hESCs express high levels of α6 and β1, moderate levels of α2, and low levels of α1, α3, and β4. Nearly all cells (97%–99%) are positive for both α6 and β1 that may form a dimer specific for laminin.13 We also obtained similar results from three hESC lines (KhES-1, KhES-2, and KhES-3).42 As shown in Fig. 1b, we classified the α-chain into several categories: the laminin-binding type (α3, α6, and α7), collagen-binding type (α1 and α2), and RGD-binding type (α4, α5, α8, and αV). The α6 chain is expressed most abundantly in all three hESC lines. α3 and α7 chains are also expressed in the three hESC lines and at relatively high levels in KhES-2 cells. In terms of the two β chains of the laminin-binding type, the β1 chain, but not the β4 chain, is expressed. Similar results have been obtained from other hESC and hiPSCs.43 The importance of α6β1 integrin in hPSC adhesion was confirmed by the inhibitory effects of α6 and β1-neutralizing antibodies (Fig. 1c)43 and shRNAs.44 Based on the integrin expression pattern and Fig. 1a, laminin is the most likely candidate cell culture substrate, followed by RGD-binding types such as vitronectin. In addition, integrin α6β1 has the strongest affinity for laminin-511/521 and specific affinities for laminin-332 and -111.45 Finally, we showed that hPSCs adhere to all these laminin isoforms, which sustains their survival.42
4.2 Laminins
Laminins are the main component of the ECM in basement membranes, which are heterotrimeric glycoproteins composed of three covalently linked chains termed α, β, and γ.46 To date, 15 laminins have been identified, of which two isoforms, laminin-511/521, are popularly used for hPSC culture.42,43,47,48 Laminin-511/521 have a more robust adhesion activity for hPSCs than Matrigel and vitronectin, and confer a higher cell survival rate during passaging.
We examined the expression of laminin subunits in hESCs cultured on MEF feeders by immunostaining.42 hESCs were stained with antibodies against individual laminin subunits. We only found immunofluorescence signals for α5, β1, β2, and γ1 chains around cell peripheries (Fig. 1d). These results were confirmed by qPCR (Fig. 1e). Among the five α-subunit chains, α5 was abundantly expressed in all three hESC lines. Among the three β-subunits, β1 and β2 were highly expressed in all hESC lines examined at similar levels. Among the three γ-subunits, γ1 was highly expressed in all three hESC lines. These results indicate that hESCs predominantly produce laminin-511/-521 that has high affinity for integrin α6β1. This suggests that hPSCs themselves may actively produce laminins to adhere to the substrate.
Because of its superior performance, laminin-521 is currently more commonly used for culture of hPSCs. In 2014, Rodin et al. reported interesting differences between hPSCs cultured on laminin-521 and -511.48 Although they found little difference in cell–cell adhesion between the two laminins, cell viability 24 hours after seeding was higher for laminin-521. They speculated that the higher cell motility of laminin-521 resulted in higher cell viability. After seeding, adherent cells more rapidly formed colonies on laminin-521 than other culture substrates, resulting in higher cell viability and better subsequent proliferation.
Recombinant E8 fragments of laminin isoforms (LM-E8s) (Fig. 1f) are the minimum fragments that confer integrin-binding activity at the same level as intact laminin.43,49 Intact laminin is a multifunctional molecule that binds to basement membrane molecules such as nidogen and heparan sulfate proteoglycans. Conversely, LM-E8s contain only the integrin-binding site. Therefore, LM-E8s are recombinant proteins specialized for cell adhesion. Interestingly, LM-E8s have a more robust cell adhesion activity than Matrigel, vitronectin, and even intact laminin, allowing efficient expansion culture of cells at the single cell level without using Y-27632 and with a >200-fold increase in the number of hPSCs compared with that obtained by conventional culture over 1 month.43 These hPSCs maintained high-level expression of pluripotency markers, had a normal karyotype, and could differentiate into all three germ layers in culture and in vivo. Furthermore, we found that the solid adhesive activity of LM-E8s accelerated cell adhesion by simple addition to the culture medium while passaging without precoating culture vessels, which is usually required.50 LM-E8s for hPSC culture are available as iMatrix-511 (Matrixome/Nippi, Japan).
Furthermore, several groups recently reported the successful establishment of hESC lines for clinical use using laminin without support cells.51–54 These cell lines are manufactured in current good manufacturing practice-grade facilities and expected to be used in future clinical trials.
4.3 Vitronectin
Vitronectin is 75 kDa glycoprotein in serum and the ECM.55 The N-terminal of vitronectin contains an RGD sequence that interacts with integrins αVβ5 and α5β1 involved in cell attachment, spreading, and migration. Braam et al. showed that recombinant vitronectin supports hESC self-renewal via αVβ5 integrin in chemically defined medium.56 Furthermore, truncated vitronectin (VTN-N), which omits the N-terminal somatomedin B domain, has been used with Essential 8 serum-free defined medium (Thermo Fisher Scientific or Stem Cell Technologies).29 VTN-N markedly enhances the proliferation and adherence of hPSCs compared with wildtype vitronectin, even in Essential 8 medium.
Vitronectin is not significantly larger than laminin-511/521 that is approximately 750 kDa. Recombinant laminin requires production in human cell lines, whereas recombinant vitronectin can be produced in other expression systems such as E. coli. Therefore, purified recombinant vitronectin can be produced at a relatively low cost. Whether vitronectin has a comparable adhesion ability for hPSCs with Matrigel depends on the cell line.43 However, adding Y-27632 to the culture medium during passaging for one to several days improves hPSC adhesion to vitronectin.
4.4 E-cadherin
E-cadherin is a transmembrane glycoprotein involved in Ca2+-dependent cell–cell adhesion.57 In hPSCs, E-cadherin has a role in cell–cell adhesion, survival, self-renewal, and pluripotency.58,59 E-cadherin-Fc-chimeric protein as a culture substrate supports hESC self-renewal in mTeSR1 medium.60 However, this culture method has an acute problem in terms of detachment conditions during cell passaging. Without Ca2+, enzymes such as trypsin reduce cell surface expression of E-cadherin in hESCs and decrease cell viability. Thus, during passaging, cells should be treated with a mild, enzyme-free detachment solution for an optimal time. Additionally, E-cadherin-Fc-chimera protein with laminin-521 allows clonal derivation, clonal survival, and long-term self-renewal of hPSCs under completely chemically defined conditions without a ROCK inhibitor.48
5 Synthetic substrates
Synthetic substrates are superior to natural substrates in terms of product homogeneity and stable supply. Additionally, most natural substrates are recombinant proteins that tend to have high manufacturing costs. Therefore, developing synthetic substrates suitable for hPSC culture is essential for the practical application of regenerative medicine. Synthetic substrates are divided into two main types by their characteristics: (1) synthetic substrates loaded with oligopeptides with cell adhesion properties, and (2) synthetic substrates with cell-adhesive properties. Some studies have used recombinant proteins instead of synthetic peptides, but we have omitted them in this review because of their production cost. Synthemax, the most commonly used synthetic substrate for hPSC culture, is described first.
5.1 Synthemax
A synthetic peptide-acrylate surface can be constructed by conjugating peptides derived from the biologically active region of extracellular matrix proteins, which supports the self-renewal and pluripotency of hESCs in xeno-free chemically defined medium X-VIVO 10 (Lonza).61 This study tested acrylate surfaces with bound peptides from laminin, bone sialoprotein (BSP), BSP-linker, vitronectin, and two types of fibronectin. A synthetic peptide-acrylate surface with vitronectin or BSP-derived peptides had the best functionality. Corning incorporated marketed the culture vessel as Synthemax Surface with RGD peptide containing a sequence from vitronectin. Additionally, Synthemax Surface supports undifferentiated growth of hiPSCs in mTeSR1 medium.62 We demonstrated that Synthemax Surface supports efficient expansion of hPSCs by both colony and single cell passaging in the undifferentiated state. In this study, we succeeded in culturing the cells using chemically defined and xeno-free culture media mTeSR1, TeSR2, NutriStem XF (Stemgent/ReproCELL and Biological Industries), and PSGro (StemRD).63 Additionally, Synthemax Surface has successfully induced differentiation of hPSCs into various cell types such as cardiomyocytes,61 definitive endodermal cells,62 retinal cells,64 oligodendrocyte progenitor cells,65 and insulin-producing cells.66 However, spontaneous differentiation of hPSCs is reportedly more likely to emerge with Synthemax than other culture substrates;67,68 accordingly, sufficient consideration should be given to its versatility in terms of hPSC lines, culture media, and other culture conditions when using Synthemax.
Currently, Synthemax Surface culture vessels are no longer manufactured and Synthemax II SC Substrate (Corning), a self-coating product, is available as an alternative. We have compared the two products and confirmed that they have comparable performance.
5.2. Synthetic substrates loaded with oligopeptides with cell-adhesive properties
We have summarized synthetic substrate-coated surfaces for hPSC culture in Table 2.
Table 2 Lists of synthetic substrate-coating surfaces used for hPSC culture
Synthetic coatings |
Protein derivation |
Peptide sequences |
Medium |
Ref. |
Acrylate polymer |
VN |
Ac-KGG![[P with combining low line]](https://www.rsc.org/images/entities/char_0050_0332.gif) ![[Q with combining low line]](https://www.rsc.org/images/entities/char_0051_0332.gif) ![[V with combining low line]](https://www.rsc.org/images/entities/char_0056_0332.gif) ![[T with combining low line]](https://www.rsc.org/images/entities/char_0054_0332.gif) ![[R with combining low line]](https://www.rsc.org/images/entities/char_0052_0332.gif) ![[G with combining low line]](https://www.rsc.org/images/entities/char_0047_0332.gif) ![[D with combining low line]](https://www.rsc.org/images/entities/char_0044_0332.gif) ![[V with combining low line]](https://www.rsc.org/images/entities/char_0056_0332.gif) ![[F with combining low line]](https://www.rsc.org/images/entities/char_0046_0332.gif) ![[T with combining low line]](https://www.rsc.org/images/entities/char_0054_0332.gif) ![[M with combining low line]](https://www.rsc.org/images/entities/char_004d_0332.gif) ![[P with combining low line]](https://www.rsc.org/images/entities/char_0050_0332.gif) |
X-VIVO10 + GF medium, mTeSR1, TeSR2, NutriStem, PSGro |
61–63
|
Acrylate polymer |
BSP |
Ac-KGG![[N with combining low line]](https://www.rsc.org/images/entities/char_004e_0332.gif) ![[G with combining low line]](https://www.rsc.org/images/entities/char_0047_0332.gif) ![[E with combining low line]](https://www.rsc.org/images/entities/char_0045_0332.gif) ![[P with combining low line]](https://www.rsc.org/images/entities/char_0050_0332.gif) ![[R with combining low line]](https://www.rsc.org/images/entities/char_0052_0332.gif) ![[G with combining low line]](https://www.rsc.org/images/entities/char_0047_0332.gif) ![[D with combining low line]](https://www.rsc.org/images/entities/char_0044_0332.gif) ![[T with combining low line]](https://www.rsc.org/images/entities/char_0054_0332.gif) ![[Y with combining low line]](https://www.rsc.org/images/entities/char_0059_0332.gif) ![[R with combining low line]](https://www.rsc.org/images/entities/char_0052_0332.gif) ![[A with combining low line]](https://www.rsc.org/images/entities/char_0041_0332.gif) ![[Y with combining low line]](https://www.rsc.org/images/entities/char_0059_0332.gif) |
X-VIVO10 + GF medium |
61
|
Streptavidin/biotin system alkanethiol |
Heparin-binding peptides (VN) |
GKKQRFRHRNRKG |
mTeSR1 + Y-27632 |
69
|
Polyacrylamide hydrogels |
VN |
GKKQRFRHRNRKG |
mTeSR1 + Y-27632 |
83
|
Poly(OEGMA-co-HEMA) |
VN |
Ac-KGG![[P with combining low line]](https://www.rsc.org/images/entities/char_0050_0332.gif) ![[Q with combining low line]](https://www.rsc.org/images/entities/char_0051_0332.gif) ![[V with combining low line]](https://www.rsc.org/images/entities/char_0056_0332.gif) ![[T with combining low line]](https://www.rsc.org/images/entities/char_0054_0332.gif) ![[R with combining low line]](https://www.rsc.org/images/entities/char_0052_0332.gif) ![[G with combining low line]](https://www.rsc.org/images/entities/char_0047_0332.gif) ![[D with combining low line]](https://www.rsc.org/images/entities/char_0044_0332.gif) ![[V with combining low line]](https://www.rsc.org/images/entities/char_0056_0332.gif) ![[F with combining low line]](https://www.rsc.org/images/entities/char_0046_0332.gif) ![[T with combining low line]](https://www.rsc.org/images/entities/char_0054_0332.gif) ![[M with combining low line]](https://www.rsc.org/images/entities/char_004d_0332.gif) ![[P with combining low line]](https://www.rsc.org/images/entities/char_0050_0332.gif) |
mTeSR1 |
70
|
Polyvinyl alcohol-coitaconic acid hydrogels (PVA-IA) |
VN |
KGGP![[Q with combining low line]](https://www.rsc.org/images/entities/char_0051_0332.gif) ![[V with combining low line]](https://www.rsc.org/images/entities/char_0056_0332.gif) ![[T with combining low line]](https://www.rsc.org/images/entities/char_0054_0332.gif) ![[R with combining low line]](https://www.rsc.org/images/entities/char_0052_0332.gif) ![[G with combining low line]](https://www.rsc.org/images/entities/char_0047_0332.gif) ![[D with combining low line]](https://www.rsc.org/images/entities/char_0044_0332.gif) ![[V with combining low line]](https://www.rsc.org/images/entities/char_0056_0332.gif) ![[F with combining low line]](https://www.rsc.org/images/entities/char_0046_0332.gif) ![[T with combining low line]](https://www.rsc.org/images/entities/char_0054_0332.gif) ![[M with combining low line]](https://www.rsc.org/images/entities/char_004d_0332.gif) ![[P with combining low line]](https://www.rsc.org/images/entities/char_0050_0332.gif) |
mTeSR1, Essential 8 |
67 and 68 |
Polydopamine (pDA) |
VN |
CGG![[K with combining low line]](https://www.rsc.org/images/entities/char_004b_0332.gif) ![[K with combining low line]](https://www.rsc.org/images/entities/char_004b_0332.gif) ![[Q with combining low line]](https://www.rsc.org/images/entities/char_0051_0332.gif) ![[R with combining low line]](https://www.rsc.org/images/entities/char_0052_0332.gif) ![[F with combining low line]](https://www.rsc.org/images/entities/char_0046_0332.gif) ![[R with combining low line]](https://www.rsc.org/images/entities/char_0052_0332.gif) ![[H with combining low line]](https://www.rsc.org/images/entities/char_0048_0332.gif) ![[R with combining low line]](https://www.rsc.org/images/entities/char_0052_0332.gif) ![[N with combining low line]](https://www.rsc.org/images/entities/char_004e_0332.gif) ![[R with combining low line]](https://www.rsc.org/images/entities/char_0052_0332.gif) ![[K with combining low line]](https://www.rsc.org/images/entities/char_004b_0332.gif) ![[G with combining low line]](https://www.rsc.org/images/entities/char_0047_0332.gif) |
mTeSR1, StemPro, Essential 8 |
71
|
Polydopamine-carboxymethyl chitosan (CMC) |
VN |
Ac-KGG![[P with combining low line]](https://www.rsc.org/images/entities/char_0050_0332.gif) ![[Q with combining low line]](https://www.rsc.org/images/entities/char_0051_0332.gif) ![[V with combining low line]](https://www.rsc.org/images/entities/char_0056_0332.gif) ![[T with combining low line]](https://www.rsc.org/images/entities/char_0054_0332.gif) ![[R with combining low line]](https://www.rsc.org/images/entities/char_0052_0332.gif) ![[G with combining low line]](https://www.rsc.org/images/entities/char_0047_0332.gif) ![[D with combining low line]](https://www.rsc.org/images/entities/char_0044_0332.gif) ![[V with combining low line]](https://www.rsc.org/images/entities/char_0056_0332.gif) ![[F with combining low line]](https://www.rsc.org/images/entities/char_0046_0332.gif) ![[T with combining low line]](https://www.rsc.org/images/entities/char_0054_0332.gif) ![[M with combining low line]](https://www.rsc.org/images/entities/char_004d_0332.gif) ![[P with combining low line]](https://www.rsc.org/images/entities/char_0050_0332.gif) |
mTeSR1 |
72
|
Polydopamine-carboxymethyl chitosan |
VN, BSP |
Ac-KGG![[P with combining low line]](https://www.rsc.org/images/entities/char_0050_0332.gif) ![[Q with combining low line]](https://www.rsc.org/images/entities/char_0051_0332.gif) ![[V with combining low line]](https://www.rsc.org/images/entities/char_0056_0332.gif) ![[T with combining low line]](https://www.rsc.org/images/entities/char_0054_0332.gif) ![[R with combining low line]](https://www.rsc.org/images/entities/char_0052_0332.gif) ![[G with combining low line]](https://www.rsc.org/images/entities/char_0047_0332.gif) ![[D with combining low line]](https://www.rsc.org/images/entities/char_0044_0332.gif) ![[T with combining low line]](https://www.rsc.org/images/entities/char_0054_0332.gif) ![[Y with combining low line]](https://www.rsc.org/images/entities/char_0059_0332.gif) ![[R with combining low line]](https://www.rsc.org/images/entities/char_0052_0332.gif) ![[A with combining low line]](https://www.rsc.org/images/entities/char_0041_0332.gif) ![[Y with combining low line]](https://www.rsc.org/images/entities/char_0059_0332.gif) |
mTeSR1 |
73
|
Poly(acrylamide-co-propargyl acrylamide) (PAPA) |
|
cRGDfK |
Essential 8 |
74
|
Poly(vinyl alcohol-co-itaconic acid)-polyethylene glycol (PVI-PEG) |
VN |
GCGGKGG![[P with combining low line]](https://www.rsc.org/images/entities/char_0050_0332.gif) ![[Q with combining low line]](https://www.rsc.org/images/entities/char_0051_0332.gif) ![[V with combining low line]](https://www.rsc.org/images/entities/char_0056_0332.gif) ![[T with combining low line]](https://www.rsc.org/images/entities/char_0054_0332.gif) ![[R with combining low line]](https://www.rsc.org/images/entities/char_0052_0332.gif) ![[G with combining low line]](https://www.rsc.org/images/entities/char_0047_0332.gif) ![[D with combining low line]](https://www.rsc.org/images/entities/char_0044_0332.gif) ![[V with combining low line]](https://www.rsc.org/images/entities/char_0056_0332.gif) ![[F with combining low line]](https://www.rsc.org/images/entities/char_0046_0332.gif) ![[T with combining low line]](https://www.rsc.org/images/entities/char_0054_0332.gif) ![[M with combining low line]](https://www.rsc.org/images/entities/char_004d_0332.gif) ![[P with combining low line]](https://www.rsc.org/images/entities/char_0050_0332.gif) |
Essential 8 |
75
|
“Polyvinyl alcohol-coitaconic acid hydrogels (PVA-IA)” |
LN (b4) |
PMQKMRGDVFSP |
Essential 8 |
76
|
Klim et al. screened >500 unique surfaces based on 18 bioactive peptides. The heparin-binding peptide from vitronectin, GKKQRFRHRNRKG, which interacts with glycosaminoglycans, supported the self-renewal of several hPSC lines for 3 months.69 This study used mTeSR1 medium supplemented with 10 μM ROCK inhibitor Y-27632. In 2013, Deng et al. tethered a vitronectin-derived peptide (Ac-KGGPQVTRGDVFTMP) onto a poly(OEGMA-co-HEMA) film on Au-coated glass and cultured hPSCs for ten passages in mTeSR1 medium.70 In 2015, Higuchi et al. grafted a vitronectin oligopeptide (KGGPQVTRGDVFTMP) onto a polyvinyl alcohol-co-itaconic acid hydrogel.67 They reported maintaining hPSC cultures on the hydrogel in mTeSR1 and Essential 8 media. Park et al. studied the effect of immobilizing two vitronectin peptides on polydopamine, VN1 (CGGPQVTRGDVFTMP)61 and VN2 (CGGKKQRFRHRNRKG).69 Dimer formation via disulfide bonds of the VN2 peptide enables long-term culture of hPSCs in the undifferentiated state in mTeSR1, StemPro, and Essential 8 media, thus, VN2 facilitates better hPSC attachment than VN1.71 Interestingly, VN2 becomes near 100% dimerized with polydopamine, whereas VN1 remains a monomer. Chen et al. reported a similar effect using a dual-chain version of another vitronectin oligopeptide.68 In 2016, Zhou et al. reported that a polydopamine-based surface with an immobilized vitronectin peptide (Ac-KGGPQVTRGDVFTMP) through EDC/NHS coupling technology supports hPSC self-renewal in mTeSR1 medium.72 They further investigated the ability of peptide sequences to sustain hPSC survival by modifying the surrounding region of the RGD sequence. They found that the Ac-KGGPQVTRGDTYRAY sequence better supports hPSC self-renewal than the original vitronectin peptide.73 In 2018, Lambshead et al. prepared a poly(acrylamide-co-propargyl acrylamide) coating on culture plates through high-intensity UV light irradiation and then conjugated cRGDfK peptide, which supported long-term self-renewal of hPSCs in Essential 8 medium.74 Interestingly, Klim et al. had previously reported that RGDfK is unsuitable for hPSC culture.69 The major difference between the two studies is the method of synthetic coating. Vitronectin-based peptide sequences are most used in this field, but may need to be re-examined in combination with synthetic coating and peptide sequences. More recently, poly(vinyl alcohol-co-itaconic acid) hydrogels were used as a base cell culture surface onto which vitronectin-derived peptides were grafted with PEG joint nanosegments. This device reduced the amount of vitronectin-derived peptides required to maintain undifferentiated hPSCs from 200 μg ml−1 to 50 μg ml−1.75 Thus, most synthetic substrates use vitronectin-derived peptides. In addition, Sung et al. used a peptide sequence (PMQKMRGDVFSP) derived from laminin-b4 to successfully culture hPSCs in Essential 8 medium for more than ten passages.76
5.3 Synthetic substrates with cell-adhesive properties
The cost of peptide synthesis, the stability of the biomolecule-attached substrate, and the difficulty in scaling up during production are all considered in practical applications of hPSCs. Therefore, the establishment of a pure synthetic surface on which the culture substrate itself yields a cell-adhesive activity for chemically defined and xeno-free culture of hPSCs is also expected. However, there have been only a few recent publications on this approach.
In 2010, Villa-Diaz et al. first reported that this type of synthetic polymer coating, which maintained long-term growth of hESCs in various culture media, was poly[2-(methacryloyloxy)ethyl dimethyl-(3-sulfopropyl)ammonium hydroxide].77 They also found variability in the response of cell lines to MEF-CM, human cell-CM, and StemPro. In StemPro medium, long-term culture of undifferentiated cells was only successful for H9 hESCs. Thus, further improvements in media or synthetic substrates are needed for versatility. Mei et al. prepared a poly(2-hydroxyethyl methacrylate) coating to 22 kinds of acrylate monomers and performed high-throughput screening. These surfaces with different crosslinking densities showed diverse hydrophobicity and hydrophilicity. After incubation with human serum, the “Hit 9” polymer allowed hPSC culture in an undifferentiated state in mTeSR1 medium.78 Brafman et al. used a high-throughput screening approach to identify polymers that support hPSC self-renewal.79 They first selected approximately 90 polymers varying in chemical composition and molecular weight. Then, they found that poly(methyl vinyl ether-alt-maleic anhydride) was most capable of supporting long-term maintenance of hPSCs in StemPro medium. In 2011, Irwin et al. prepared a synthetic polymer hydrogel of aminopropyl methacrylamide (APMAAm), which supported undifferentiated growth of hESCs in mTeSR1 medium.80 Interestingly, they found that bovine serum albumin in mTeSR1 medium played a crucial role in hESC attachment to the APMAAm hydrogel interface. In 2013, Chang et al. reported the potential of synthetic hydrogels containing heparin-mimicking and sodium poly(4-vinylbenzenesulfonate) moieties to support hPSC self-renewal in StemPro medium.81 A synthetic matrix was developed by copolymerizing polyacrylamide and sodium poly(4-vinylbenzenesulfonate) at a molar ratio of 6
:
2.
At present, few suitable synthetic substrates for hPSCs have been reported. However, future approaches based on molecular mechanisms of hPSC adhesion to the culture substrate may necessitate studies of synthetic materials.
5.4 Stem cell niche between synthetic substrates and hPSCs
It is crucial to consider the microenvironment called the niche surrounding hPSCs and culture substrates rather than just the simple binding of hPSCs to substrates.
Matrigel has been widely used for feeder-free culture of hPSCs, and its main component is laminin, an isoform of which is laminin-111. However, we previously showed that the recombinant laminin-111 isoform has much weaker adhesion to hESCs than Matrigel or laminin-511/332.42 The reason for this has not been clearly explained. However, it has been suggested that Matrigel contains many network-forming proteins that allow stem cells to adhere to their substrates and efficiently adsorb secreted molecules from hPSCs and medium components, thereby altering the microenvironment to be more suitable for stem cell self-renewal.82
Several studies have reported that optimal stiffness maintains an undifferentiated state of hPSCs. In 2012, Musah et al. synthesized a polyacrylamide hydrogel with various elastic moduli (0.7, 3, and 10 kPa) and grafted the glycosaminoglycan-binding peptide GKKQRFRHRNRKG.69 They found that a rigid substrate of 10 kPa supported long-term self-renewal of hPSC lines in mTeSR1 medium.83 In 2015, Higuchi et al. prepared polyvinyl alcohol-co-itaconic acid hydrogels of varying elasticities, which were grafted with a vitronectin-derived oligopeptide (KGGPQVTRGDVFTMP).67 A hydrogel with optimal elasticity of 25 kPa and grafted with high concentrations (500–1500 μg ml−1) of the vitronectin-derived peptide maintained hPSC cultures at similar efficiency to Matrigel under xeno-free conditions. Conversely, hPSCs cultured on the stiffest substrate (30.4 kPa) tended to differentiate after 5 days of culture. In 2020, Paiva et al. fabricated polyacrylamide soft substrates with moduli of 3, 12, and 25 kPa. VTN-N protein was covalently crosslinked to culture hPSCs in mTeSR1 medium.84 They found that hPSCs lost pluripotency below 25 kPa, whereas the cells maintained self-renewal with proliferation above 25 kPa.
It is well known that surface wettability affects the composition, orientation, and conformation of the protein layer on the substrate surface and has important implications in stem cell behavior. Although it is generally believed that hydrophilic surfaces are conducive for cell adhesion, little research has focused on wettability. One report examined biomaterials and hydrophilicity in culture dishes to cultivate hPSCs.78
6. Three-dimensional culture of hPSCs
A large number of cells are required to advance clinical applications in regenerative medicine.85 For example, cell therapies for myocardial infarction and type 1 diabetes require more than 1 × 109 cardiomyocytes or β cells, respectively. Additionally, producing many cells in a single manufacturing process is advantageous in terms of cost and quality control. In 2017, Tohyama et al. reported the efficient differentiation of more than 1.5 × 109 cardiomyocytes from hPSCs using a large-scale 2D culture.86 However, such hPSC manufacturing methods are labor-intensive, significantly limiting process scalability and potentially causing unexpected batch-to-batch variations. Therefore, development of automated 3D culture using bioreactors is essential to bring cell viability, homogeneity, and differentiation efficiency in line with industrialization.
6.1 Modalities of hPSC 3D culture
There are three primary 3D culture methods:85 hPSC aggregate culture, microcarrier-based culture, and microencapsulation-based culture. The advantages and disadvantages of each 3D cell culture modality for hPSC expansion are summarized in Table 3. Importantly, the use of bioreactors makes the scale-up of any 3D culture method much easier than 2D culture methods.
Table 3 Advantages and disadvantages of different culture systems for hPSC expansion
Culture methods |
Advantages |
Disadvantages |
2D culture |
Easy visualization/monitoring |
Labor-intensive |
Suitable for small- to mid-scale |
High variability |
Increased cell growth efficiency |
Limited scalability |
|
Difficult automation |
Aggregates |
Scalable |
Limited control of aggregate size |
Easy handling |
Cell damage due to physical forces |
High reproducibility |
Agglomeration of aggregates |
High differentiation efficiency |
Low viability of reaggregated cells |
Less expensive |
|
Microcarriers |
Scalable |
High material cost (microcarrier) |
Easy visualization/monitoring |
Cell-bead separation step required |
Unlimited mass and gas diffusion |
Cell damage due to physical forces |
High reproducibility |
Controlling microcarrier (agglomeration/clumping) |
Microcapsulation |
Scalable |
High material cost (encapsulation) |
Protection from physical forces |
Limited diffusion in aggregate core |
High reproducibility |
Difficult to monitor cultures |
|
Limited mass and gas diffusion |
|
Cell harvesting (decapsulation) |
Microcarrier and microencapsulation culture methods, which differ from cell-aggregate methods, require materials other than hPSCs. Therefore, the cost of materials is higher and a separation step from the material may be required to recover hPSCs. For example, microcarrier-based methods require microcarrier beads and materials to coat the beads, such as laminin-521,87 vitronectin,88,89 or synthetic peptides (Ac-KGGPQVTRGDVFTMP) derived from vitronectin.90
Several solutions have recently been proposed for the separation of hPSCs from these materials, including dissolvable microcarriers91 and temperature-sensitive hydrogels.92 In 2021, Fattahi et al. reported an alternative approach using a microencapsulation-based method. Briefly, hPSCs were expanded in microcapsules and induced to differentiate directly into pancreatic β cells.93 An isolation step is unnecessary because these microcapsules can be directly utilized for therapy and drug evaluation.
Here, we focus on the simplest 3D culture method to produce hPSCs as aggregates without specialized devices such as coated substrates or microcarriers. In such aggregate culture, hPSCs are cultured and grown in an undifferentiated state under optimized culture conditions.94–101
6.2 Aggregate culture
Significant challenges have been found for 3D aggregate culture that are not present in 2D cultures, such as reduced cell viability due to agitation-induced shear stress, surface foaming,95 and aggregation and sedimentation of cell clusters.98,101 In 3D cultures, large aggregates are easily formed by fusion of cell aggregates. The size of aggregates becomes uncontrollable, resulting in their failure to disperse uniformly in the medium and spontaneous differentiation within the aggregates. Additionally, nutrients and oxygen are not adequately supplied in large aggregates, and necrosis is often seen in the core of aggregates.102,103 A proposed solution is to reduce the shear stress associated with bubbling at the air-medium interface by adding an anti-foaming agent such as Pluronic.95 Krawetz et al. used another approach with frequent passaging as single cells to reduce aggregation growth with a ROCK inhibitor in the culture medium.96
6.3 Aggregate culture using gellan gum
We have developed a new culture system in which cells are not damaged by agitation by adding a polymer, low-acyl gellan gum (GG), to the culture medium to suppress sedimentation of aggregates and by culturing the cells in a gas-permeable membrane.104Fig. 2a and b show that GG consists of a linear anionic tetrasaccharide repeating unit, forming double helical structures that assemble into firm and brittle aqueous gels in the presence of cationic ions.105 Most polymers such as methylcellulose have elevated viscosity and difficulty in handling at high concentrations. Nevertheless, they cannot completely inhibit sedimentation (Fig. 2c, lower panel). In contrast, adding GG does not increase apparent viscosity, but completely inhibits bead sedimentation at low concentrations (i.e., approximately 0.02%; Fig. 2c, upper panel). In such a medium, polystyrene beads remain in suspension without the need for agitation (Fig. 2d). To demonstrate the capability of scaling up our novel 3D sphere culture system, we tested 200 ml culture bags made of a gas-permeable membrane. KhES-1 hESC spheres were subcultured at 1.32 × 107 cells per bag, which increased by more than 10-fold on day 5, yielding 1.43 × 108 cells per bag from three independent experiments (Fig. 2e and f).
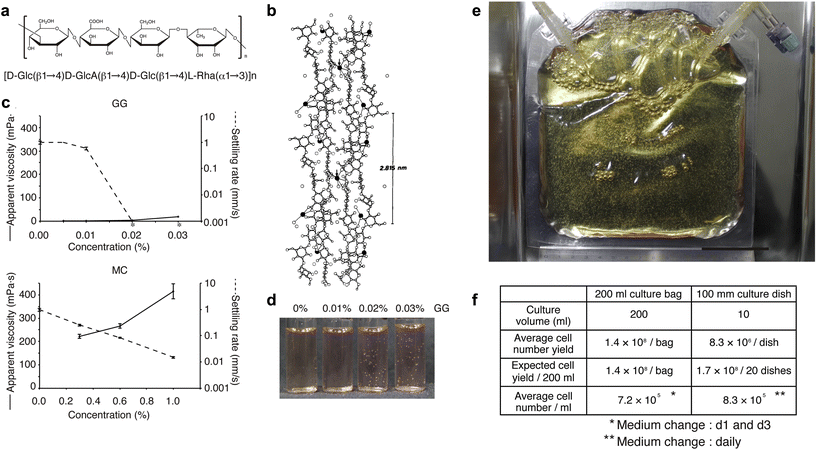 |
| Fig. 2 Characterizations of low-acyl gellan gum (GG) polymer and validation of 3D hPSC sphere culture using GG. (a) Chemical structure of the repeat unit of low-acyl gellan gum (GG). (b) Stereo view of GG. Two adjacent upward and downward-pointing GG double helices are crosslinked at the arrows by calcium ions (filled circles). (c) Apparent viscosities and settling rates of GG and methylcellulose (MC). The average and SD from three experiments are shown (n = 3). Asterisks indicate no settling. For both GG and MC, the settling ability of beads decreases with increasing concentration (until the beads float). However, the viscosity of the solution increases with increasing concentration for MC, while the viscosity remains almost the same for GG. (d) Settled or suspended polystyrene beads in the culture medium under various concentrations of GG. At GG above 0.02%, polystyrene beads do not require dynamic agitation and remain suspended. (e and f) Validation of 3D hPSC sphere culture using GG. (e) Trial for large-scale sphere culture using 200 ml gas-permeable membrane bags. The scale bar represents 5 cm. (f) Comparison of cell yield calculated from the average cell number obtained in 3D sphere or adherent culture using the KhES-1 cell line. [(b) Reproduced with permission.105 Copyright 1990, Elsevier; others reproduced with permission.104 Copyright 2014, Cell Press.] | |
Furthermore, most researchers have focused on the initial process of 3D culture, i.e., the transition from 2D to 3D culture, and have not significantly examined passaging methods in 3D culture. Treatment with enzymes, such as accutase and TrypLE Select, dissociate hPSC colonies into single cells or small aggregates for subculture. They induce considerable hPSC loss because of the sensitivity of these cells to physical stresses and single cell dissociation.
We have proposed a solution to the difficulty of passaging in 3D culture.104 We developed a mechanical subculture method in which the cell aggregates are filtered through a 50 μm nylon mesh. These treatments are simple and cost effective with improved hPSC survival. After passaging, these meshes produce spheres with a diameter of approximately 80 μm, which expand into larger spheres of 220–250 μm in 4–5 days, depending on the cell line. In 2017, Abecasis et al. applied these treatments to hPSCs, resulting in a better cell growth rate with a 70 μm nylon mesh than a 40 μm mesh.106 The mesh size may need to be changed depending on the 3D culture system. This protocol for aggregate dissociation and passaging is notably less laborious and time consuming than enzymatic or chemical dissociation protocols by avoiding additional incubation times and washing steps that ultimately compromise cell recovery yields.
6.4 Other materials improving aggregate culture
Dextran sulfate (DS), a type of mucopolysaccharide, effectively controls the aggregation of hPSCs in 3D culture to enable the formation of colonies with a relatively uniform size.107,108 DS suppresses expression of ICAM1 and activates the Wnt signaling pathway, resulting in downregulated expression of E-cadherin to prevent excessive colony aggregation.109 Furthermore, a combination of DS and polyvinyl alcohol reportedly promotes hPSC proliferation in 3D culture while inhibiting colony aggregation.110
Chen et al. reported a simple and non-destructive method for 3D culture of hESCs using the vitronectin-modified thermoresponsive polymer poly(N-isopropylacrylamide).111 At 37 °C, thermoresponsive polymer worms are promoted to bridge hESCs, aiding in the natural ability of hESCs to form aggregates. However, at 25 °C, the polymer worms facilitate aggregate breakdown. The thermoresponsive nature of the worms enables cyclical dissociation and propagation of hPSCs. In addition, alternative and cheaper culture substrates, such as recombinant fibronectin fragments112 and hyaluronic acid, are being considered because of the high cost of reported substrates.113,114
7. Conclusions and perspectives
The development of ECM proteins including their fragments is relatively mature. Laminin is the most versatile. In particular, we recommend LM-E8s that provide better adhesion than other culture substrates, and they can be used without precoating culture vessels. Laminin-521 often performs optimally to provide a cell adhesion substrate for hPSCs. Vitronectin is less versatile than laminin with varying adhesiveness among cell lines, but in some cases, it provides more stable results than other ECM proteins.
Synthemax is the only synthetic substrate commercially marketed and evaluated by users. However, Synthemax is still expensive and tends to induce spontaneous differentiation more often than coated ECM proteins,55,67 suggesting the need for further development. Some synthetic substrates do not facilitate adequate adhesion of hPSCs, and other improvements are needed. For example, it is possible to improve the cell adhesion to substrates by increasing the adhesion area of cells and substrates, such as by making the surface of the culture vessel convex or concave. Additionally, cell dissociation solutions that are milder for hPSCs than conventional cell dissociation solutions have been developed, such as L7 hPSC Passing Solution (Lonza) and ReLeSR (STEMCELL Technologies), which may promote cell adhesion to the culture substrate.
Conversely, if cell-substrate adhesion is too strong, thermoresponsive, chemically defined hydrogels115 can be used. Such hydrogels permit gentle, reagent-free cell passaging under transient modulation of the ambient temperature from 37 °C to 15 °C for 30 min.
Appropriate culture substrates for undifferentiated proliferation and induced differentiation of hPSCs might differ. Nevertheless, culture substrates that can be used for both proliferation and differentiation116,117 may produce the necessary differentiated cells most efficiently. In many cases, the goal is not to grow hPSCs per se but to efficiently produce differentiated cells that meet the objective. Therefore, a culture substrate that allows this process to be performed simultaneously on a single culture substrate is an approach that is only possible with synthetic substrates.
Although many issues remain to be solved for 3D culture of hPSCs compared with 2D culture, it is suitable for mass culture and expected to produce many differentiated cells and tissues. These differentiation products may be useful for clinical applications in regenerative medicine and are expected to come in various forms for transplantation, including cell suspensions, cell-seeded scaffolds, cell sheets, or mixtures in combination with devices.12
Differentiated tissues derived from hPSCs would be useful for pharmacological and toxicological evaluations. In recent years, there has been a worldwide movement to minimize animal testing in drug discovery and evaluation. For example, in the United States, the Environmental Protection Agency Administrator announced a policy in 2019 to eliminate animal testing of mammals as much as possible by 2035.118 hPSC-derived organoids have a structure and function similar to human biological tissue and are expected to replace animal testing in drug evaluation.119 In addition, models using patients’ hiPSCs will enable the discovery and evaluation of new drugs, which was not possible in the past.
In conclusion, although the development of culture substrates has helped realize the potential of hPSCs, further innovation is expected for the industrialization of hPSCs.
Conflicts of interest
The authors declare no conflict of interest in this manuscript.
Acknowledgements
The authors thank the past and present members of our laboratories and facility. We also thank Mitchell Arico from Edanz for editing a draft of this manuscript. E. K. was partially supported by a Grant-in-Aid for Scientific Research (C) from the Ministry of Education, Culture, Sports, Science and Technology of Japan (project number: JP19K12755).
References
- M. J. Evans and M. H. Kaufman, Establishment in culture of pluripotential cells from mouse embryos, Nature, 1981, 292, 154–156 CrossRef CAS PubMed
.
- G. R. Martin, Isolation of a pluripotent cell line from early mouse embryos cultured in medium conditioned by teratocarcinoma stem cells, Proc. Natl. Acad. Sci. U. S. A., 1981, 78, 7634–7638 CrossRef CAS PubMed
.
- J. A. Thomson, J. Itskovitz-Eldor, S. S. Shapiro, M. A. Waknitz, J. J. Swiergiel, V. S. Marshall and J. M. Jones, Embryonic stem cell lines derived from human blastocysts, Science, 1988, 282, 1145–1147 CrossRef PubMed
.
- A. G. Smith, J. K. Heath, D. D. Donaldson, G. G. Wong, J. Moreau, M. Stahl and D. Rogers, Inhibition of pluripotential embryonic stem cell differentiation by purified polypeptides, Nature, 1988, 336, 688–690 CrossRef CAS PubMed
.
- K. Takahashi and S. Yamanaka, Induction of pluripotent stem cells from mouse embryonic and adult fibroblast cultures by defined factors, Cell, 2006, 126, 663–676 CrossRef CAS PubMed
.
- K. Takahashi, K. Tanabe, M. Ohnuki, M. Narita, T. Ichisaka, K. Tomoda and S. Yamanaka, Induction of pluripotent stem cells from adult human fibroblasts by defined factors, Cell, 2007, 131, 861–872 CrossRef CAS PubMed
.
- J. Yu, M. A. Vodyanik, K. Smuga-Otto, J. Antosiewicz-Bourget, J. L. Frane, S. Tian, J. Nie, G. A. Jonsdottir, V. Ruotti, R. Stewart, I. I. Slukvin and J. A. Thomson, Induced pluripotent stem cell lines derived from human somatic cells, Science, 2007, 318, 1917–1920 CrossRef CAS PubMed
.
- A. Higuchi, Q. D. Ling, Y. A. Ko, Y. Chang and A. Umezawa, Biomaterials for the feeder-free culture of human embryonic stem cells and induced pluripotent stem cells, Chem. Rev., 2011, 111, 3021–3035 CrossRef CAS PubMed
.
- A. Higuchi, Q. D. Ling, S. Kumar, M. Munusamy, A. A. Alarfajj, A. Umezawa and G. J. Wu, Design of polymeric materials for culturing human pluripotent stem cells: Progress toward feeder-free and xeno-free culturing, Prog. Polym. Sci., 2014, 39, 1348–1374 CrossRef CAS
.
- S. Schmidt, A. Lilienkampf and M. Bradley, New substrates for stem cell control, Philos. Trans. R. Soc., B, 2018, 373, 20170223 CrossRef PubMed
.
- A. Polanco, B. Kuang and S. Yoon, Bioprocess Technologies that Preserve the Quality of iPSCs, Trends Biotechnol., 2020, 38, 1128–1140 CrossRef CAS PubMed
.
- S. E. Tannenbaum and B. E. Reubinoff, Advances in hPSC expansion towards therapeutic entities: A review, Cell Proliferation, 2022, 55, e13247 CrossRef PubMed
.
- C. Xu, M. S. Inokuma, J. Denham, K. Golds, P. Kundu, J. D. Gold and M. K. Carpenter, Feeder-free growth of undifferentiated human embryonic stem cells, Nat. Biotechnol., 2001, 19, 971–974 CrossRef CAS PubMed
.
- M. Amit, C. Shariki, V. Margulets and J. Itskovitz-Eldor, Feeder layer- and serum-free culture of human embryonic stem cells, Biol. Reprod., 2004, 70, 837–845 CrossRef CAS PubMed
.
- D. James, A. J. Levine, D. Besser and A. Hemmati-Brivanlou, TGFbeta/activin/nodal signaling is necessary for the maintenance of pluripotency in human embryonic stem cells, Development, 2005, 132, 1273–1282 CrossRef CAS PubMed
.
- I. Klimanskaya, Y. Chung, L. Meisner, J. Johnson, M. D. West and R. Lanza, Human embryonic stem cells derived without feeder cells, Lancet, 2005, 365, 1636–1641 CrossRef CAS PubMed
.
- G. Wang, H. Zhang, Y. Zhao, J. Li, J. Cai, P. Wang, S. Meng, J. Feng, C. Miao, M. Ding, D. Li and H. Deng, Noggin and bFGF cooperate to maintain the pluripotency of human embryonic stem cells in the absence of feeder layers, Biochem. Biophys. Res. Commun., 2005, 330, 934–942 CrossRef CAS PubMed
.
- R. H. Xu, R. M. Peck, D. S. Li, X. Feng, T. Ludwig and J. A. Thomson, Basic FGF and suppression of BMP signaling sustain undifferentiated proliferation of human ES cells, Nat. Methods, 2005, 2, 185–190 CrossRef CAS PubMed
.
- M. K. Furue, J. Na, J. P. Jackson, T. Okamoto, M. Jones, D. Baker, R. Hata, H. D. Moore, J. D. Sato and P. W. Andrews, Heparin promotes the growth of human embryonic stem cells in a defined serum-free medium, Proc. Natl. Acad. Sci. U. S. A., 2008, 105, 13409–13414 CrossRef CAS PubMed
.
- Y. Li, S. Powell, E. Brunette, J. Lebkowski and R. Mandalam, Expansion of human embryonic stem cells in defined serum-free medium devoid of animal-derived products, Biotechnol. Bioeng., 2005, 91, 688–698 CrossRef CAS PubMed
.
- Y. Liu, Z. Song, Y. Zhao, H. Qin, J. Cai, H. Zhang, T. Yu, S. Jiang, G. Wang, M. Ding and H. Deng, A novel chemical-defined medium with bFGF and N2B27 supplements supports undifferentiated growth in human embryonic stem cells, Biochem. Biophys. Res. Commun., 2006, 346, 131–139 CrossRef CAS PubMed
.
- J. Lu, R. Hou, C. J. Booth, S. H. Yang and M. Snyder, Defined culture conditions of human embryonic stem cells, Proc. Natl. Acad. Sci. U. S. A., 2006, 103, 5688–5693 CrossRef CAS PubMed
.
- L. Vallier, M. Alexander and R. A. Pedersen, Activin/Nodal and FGF pathways cooperate to maintain pluripotency of human embryonic stem cells, J. Cell Sci., 2005, 118, 4495–4509 CrossRef CAS PubMed
.
- S. Yao, S. Chen, J. Clark, E. Hao, G. M. Beattie, A. Hayek and S. Ding, Long-term self-renewal and directed differentiation of human embryonic stem cells in chemically defined conditions, Proc. Natl. Acad. Sci. U. S. A., 2006, 103, 6907–6912 CrossRef CAS PubMed
.
- L. Wang, T. C. Schuiz, E. S. Sherrer, D. S. Dauphin, S. Shin, A. M. Nelson, C. B. Ware, M. Zhan, C. Z. Song, X. Chen, S. N. Brimble, A. McLean, M. J. Galeano, E. W. Uhl, K. A. D'Amour, J. D. Chesnut, M. S. Rao, C. A. Blau and A. J. Robins, Self-renewal of human embryonic stem cells requires insulin-like growth factor-1 receptor and ERBB2 receptor signaling, Blood, 2007, 110, 4111–4119 CrossRef CAS PubMed
.
- T. E. Ludwig, V. Bergendahl, M. E. Levenstein, J. Yu, M. D. Probasco and J. A. Thomson, Feeder-independent culture of human embryonic stem cells, Nat. Methods, 2006, 3, 637–646 CrossRef CAS PubMed
.
- T. E. Ludwig, M. E. Levenstein, J. M. Jones, W. T. Berggren, E. R. Mitchen, J. L. Frane, L. J. Crandall, C. A. Daigh, K. R. Conard, M. S. Piekarczyk, R. A. Llanas and J. A. Thomson, Derivation of human embryonic stem cells in defined conditions, Nat. Biotechnol., 2006, 24, 185–187 CrossRef CAS PubMed
.
- International Stem Cell Initiative Consortium; V. Akopian, P. W. Andrews, S. Beil, N. Benvenisty, J. Brehm, M. Christie, A. Ford, V. Fox, P. J. Gokhale, L. Healy, F. Holm, O. Hovatta, B. B. Knowles, T. E. Ludwig, R. D. McKay, T. Miyazaki, N. Nakatsuji, S. K. Oh, M. F. Pera, J. Rossant, G. N. Stacey and H. Suemori, Comparison of defined culture systems for feeder cell free propagation of human embryonic stem cells, In Vitro Cell. Dev. Biol.: Anim., 2010, 46, 247–258 CrossRef PubMed
.
- G. Chen, D. R. Gulbranson, Z. Hou, J. M. Bolin, V. Ruotti, M. D. Probasco, K. Smuga-Otto, S. E. Howden, N. R. Diol, N. E. Propson, R. Wagner, G. O. Lee, J. Antosiewicz-Bourget, J. M. Teng and J. A. Thomson, Chemically defined conditions for human iPSC derivation and culture, Nat. Methods, 2011, 8, 424–429 CrossRef CAS PubMed
.
- K. Watanabe, M. Ueno, D. Kamiya, A. Nishiyama, M. Matsumura, T. Wataya, J. B. Takahashi, S. Nishikawa, K. Muguruma and Y. Sasai, A ROCK inhibitor permits survival of dissociated human embryonic stem cells, Nat. Biotechnol., 2007, 25, 681–686 CrossRef CAS PubMed
.
- S. I. Vernardis, K. Terzoudis, N. Panoskaltsis and A. Mantalaris, Human embryonic and induced pluripotent stem cells maintain phenotype but alter their metabolism after exposure to ROCK inhibitor, Sci. Rep., 2017, 7, 42138 CrossRef CAS PubMed
.
- G. Benton, I. Arnaoutova, J. George, H. K. Kleinman and J. Koblinski, Matrigel: from discovery and ECM mimicry to assays and models for cancer research, Adv. Drug Delivery Rev., 2014, 79–80, 3–18 CrossRef CAS PubMed
.
- S. Kaur, I. Kaur, P. Rawal, D. M. Tripathi and A. Vasudevan, Non-matrigel scaffolds for organoid cultures, Cancer Lett., 2021, 504, 58–66 CrossRef CAS PubMed
.
- M. T. Kozlowski, C. J. Crook and H. T. Ku, Towards organoid culture without Matrigel, Commun. Biol., 2021, 4, 1387 CrossRef PubMed
.
- H. K. Kleinman, M. L. McGarvey, J. R. Hassell, V. L. Star, F. B. Cannon, G. W. Laurie and G. R. Martin, Basement membrane complexes with biological activity, Biochemistry, 1986, 25, 312–318 CrossRef CAS PubMed
. Corning® Matrigel® Matrix Frequently Asked Questions.
- C. S. Hughes, L. M. Postovit and G. A. Lajoie, Matrigel: a complex protein mixture required for optimal growth of cell culture, Proteomics, 2010, 10, 1886–1890 CrossRef CAS PubMed
.
- N. C. Talbot and T. J. Caperna, Proteome array identification of bioactive soluble proteins/peptides in Matrigel: relevance to stem cell responses, Cytotechnology, 2015, 67, 873–883 CrossRef CAS PubMed
.
- S. Vukicevic, H. K. Kleinman, F. P. Luyten, A. B. Roberts, N. S. Roche and A. H. Reddi, Identification of multiple active growth factors in basement membrane Matrigel suggests caution in interpretation of cellular activity related to extracellular matrix components, Exp. Cell Res., 1992, 202, 1–8 CrossRef CAS PubMed
.
- J. A. Carlson Scholz, R. Garg, S. R. Compton, H. G. Allore, C. J. Zeiss and E. M. Uchio, Poliomyelitis in MuLV-infected ICR-SCID mice after injection of basement membrane matrix contaminated with lactate dehydrogenase-elevating virus, Comp. Med., 2011, 61, 404–411 Search PubMed
.
- M. J. Martin, A. Muotri, F. Gage and A. Varki, Human embryonic stem cells express an immunogenic nonhuman sialic acid, Nat. Med., 2005, 11, 228–232 CrossRef CAS PubMed
.
- R. O. Hynes, Integrins: bidirectional, allosteric signaling machines, Cell, 2002, 110, 673–687 CrossRef CAS PubMed
.
- T. Miyazaki, S. Futaki, K. Hasegawa, M. Kawasaki, N. Sanzen, M. Hayashi, E. Kawase, K. Sekiguchi, N. Nakatsuji and H. Suemori, Recombinant human laminin isoforms can support the undifferentiated growth of human embryonic stem cells, Biochem. Biophys. Res. Commun., 2008, 375, 27–32 CrossRef CAS PubMed
.
- T. Miyazaki, S. Futaki, H. Suemori, Y. Taniguchi, M. Yamada, M. Kawasaki, M. Hayashi, H. Kumagai, N. Nakatsuji, K. Sekiguchi and E. Kawase, Laminin E8 fragments support efficient adhesion and expansion of dissociated human pluripotent stem cells, Nat. Commun., 2012, 3, 1236 CrossRef PubMed
.
- L. G. Villa-Diaz, J. K. Kim, A. Laperle, S. P. Palecek and P. H. Krebsbach, Inhibition of Focal Adhesion Kinase Signaling by Integrin α6β1 Supports Human Pluripotent Stem Cell Self-Renewal, Stem Cells, 2016, 34, 1753–1764 CrossRef CAS PubMed
.
- R. Nishiuchi, J. Takagi, M. Hayashi, H. Ido, Y. Yagi, N. Sanzen, T. Tsuji, M. Yamada and K. Sekiguchi, Ligand-binding specificities of laminin-binding integrins: a comprehensive survey of laminin-integrin interactions using recombinant α3β1, α6β1, α7β1 and α6β4 integrins, Matrix Biol., 2006, 25, 189–197 CrossRef CAS PubMed
.
- J. H. Miner and P. D. Yurchenco, Laminin functions in tissue morphogenesis, Annu. Rev. Cell Dev. Biol., 2004, 20, 255–284 CrossRef CAS PubMed
.
- S. Rodin, A. Domogatskaya, S. Ström, E. M. Hansson, K. R. Chien, J. Inzunza, O. Hovatta and K. Tryggvason, Long-term self-renewal of human pluripotent stem cells on human recombinant laminin-511, Nat. Biotechnol., 2010, 28, 611–615 CrossRef CAS PubMed
.
- S. Rodin, L. Antonsson, C. Niaudet, O. E. Simonson, E. Salmela, E. M. Hansson, A. Domogatskaya, Z. Xiao, P. Damdimopoulou, M. Sheikhi, J. Inzunza, A. S. Nilsson, D. Baker, R. Kuiper, Y. Sun, E. Blennow, M. Nordenskjöld, K. H. Grinnemo, J. Kere, C. Betsholtz, O. Hovatta and K. Tryggvason, Clonal culturing of human embryonic stem cells on laminin-521/E-cadherin matrix in defined and xeno-free environment, Nat. Commun., 2014, 5, 3195 CrossRef PubMed
.
- H. Ido, A. Nakamura, R. Kobayashi, S. Ito, S. Li, S. Futaki and K. Sekiguchi, The requirement of the glutamic acid residue at the third position from the carboxyl termini of the laminin gamma chains in integrin binding by laminins, J. Biol. Chem., 2007, 282, 11144–11154 CrossRef CAS PubMed
.
- T. Miyazaki, T. Isobe, N. Nakatsuji and H. Suemori, Efficient Adhesion Culture of Human Pluripotent Stem Cells Using Laminin Fragments in an Uncoated Manner, Sci. Rep., 2017, 7, 41165 CrossRef CAS PubMed
.
- H. Main, M. Hedenskog, G. Acharya, O. Hovatta and F. Lanner, Karolinska Institutet Human Embryonic Stem Cell Bank, Stem Cell Res., 2020, 45, 101810 CrossRef CAS PubMed
.
- E. Kawase, K. Takada, R. Nakatani, S. Yamazaki and H. Suemori, Generation of clinical-grade human embryonic stem cell line KthES11 according to Japanese regulations, Stem Cell Res., 2021, 54, 102383 CrossRef CAS PubMed
.
- T. Souralova, D. Rehakova, M. Jeseta, L. Tesarova, J. Beranek, P. Ventruba, A. Hampl and I. Koutna, The Manufacture of Xeno- and Feeder-Free Clinical-Grade Human Embryonic Stem Cell Lines: First Step for Cell Therapy, Int. J. Mol. Sci., 2022, 23, 12500 CrossRef CAS PubMed
.
- K. Takada, R. Nakatani, E. Moribe, S. Yamazaki-Fujigaki, M. Fujii, M. Furuta, H. Suemori and E. Kawase, Efficient derivation and banking of clinical-grade human embryonic stem cell lines in accordance with Japanese regulations, Regen. Ther., 2022, 21, 553–559 CrossRef CAS PubMed
.
- I. Schvartz, D. Seger and S. Shaltiel, Vitronectin, Int. J. Biochem. Cell Biol., 1999, 31, 539–544 CrossRef CAS PubMed
.
- S. R. Braam, L. Zeinstra, S. Litjens, D. Ward-van Oostwaard, S. van den Brink, L. van Laake, F. Lebrin, P. Kats, R. Hochstenbach, R. Passier, A. Sonnenberg and C. L. Mummery, Recombinant vitronectin is a functionally defined substrate that supports human embryonic stem cell self-renewal via alphavbeta5 integrin, Stem Cells, 2008, 26, 2257–2265 CrossRef CAS PubMed
.
- M. Takeichi, Morphogenetic roles of classic cadherins, Curr. Opin. Cell Biol., 1995, 7, 619–627 CrossRef CAS PubMed
.
- A. M. Eastham, H. Spencer, F. Soncin, S. Ritson, C. L. Merry, P. L. Stern and C. M. Ward, Epithelial-mesenchymal transition events during human embryonic stem cell differentiation, Cancer Res., 2007, 67, 11254–11262 CrossRef CAS PubMed
.
- D. Li, J. Zhou, L. Wang, M. E. Shin, P. Su, X. Lei, H. Kuang, W. Guo, H. Yang, L. Cheng, T. S. Tanaka, D. E. Leckband, A. B. Reynolds, E. Duan and F. Wang, Integrated biochemical and mechanical signals regulate multifaceted human embryonic stem cell functions, J. Cell Biol., 2010, 191, 631–644 CrossRef CAS PubMed
.
- M. Nagaoka, K. Si-Tayeb, T. Akaike and S. A. Duncan, Culture of human pluripotent stem cells using completely defined conditions on a recombinant E-cadherin substratum, BMC Dev. Biol., 2010, 10, 60 CrossRef PubMed
.
- Z. Melkoumian, J. L. Weber, D. M. Weber, A. G. Fadeev, Y. Zhou, P. Dolley-Sonneville, J. Yang, L. Qiu, C. A. Priest, C. Shogbon, A. W. Martin, J. Nelson, P. West, J. P. Beltzer, S. Pal and R. Brandenberger, Synthetic peptide-acrylate surfaces for long-term self-renewal and cardiomyocyte differentiation of human embryonic stem cells, Nat. Biotechnol., 2010, 28, 606–610 CrossRef CAS PubMed
.
- S. Jin, H. Yao, J. L. Weber, Z. K. Melkoumian and K. Ye, A synthetic, xeno-free peptide surface for expansion and directed differentiation of human induced pluripotent stem cells, PLoS One, 2012, 7, e50880 CrossRef CAS PubMed
.
- E. Kawase, Efficient Expansion of Dissociated Human Pluripotent Stem Cells Using a Synthetic Substrate, Methods Mol. Biol., 2016, 1307, 61–69 CrossRef PubMed
.
- B. A. Tucker, K. R. Anfinson, R. F. Mullins, E. M. Stone and M. J. Young, Use of a synthetic xeno-free culture substrate for induced pluripotent stem cell induction and retinal differentiation, Stem Cells Transl. Med., 2013, 2, 16–24 CrossRef CAS PubMed
.
- Y. Li, A. Gautam, J. Yang, L. Qiu, Z. Melkoumian, J. Weber, L. Telukuntla, R. Srivastava, E. M. Whiteley and R. Brandenberger, Differentiation of oligodendrocyte progenitor cells from human embryonic stem cells on vitronectin-derived synthetic peptide acrylate surface, Stem Cells Dev., 2013, 22, 1497–1505 CrossRef CAS PubMed
.
- P. Y. Lin, S. H. Hung, Y. C. Yang, L. C. Liao, Y. C. Hsieh, H. J. Yen, H. E. Lu, M. S. Lee, I. M. Chu and S. M. Hwang, A synthetic peptide-acrylate surface for production of insulin-producing cells from human embryonic stem cells, Stem Cells Dev., 2014, 23, 372–379 CrossRef CAS PubMed
.
- A. Higuchi, S. H. Kao, Q. D. Ling, Y. M. Chen, H. F. Li, A. A. Alarfaj, M. A. Munusamy, K. Murugan, S. C. Chang, H. C. Lee, S. T. Hsu, S. S. Kumar and A. Umezawa, Long-term xeno-free culture of human pluripotent stem cells on hydrogels with optimal elasticity, Sci. Rep., 2015, 5, 18136 CrossRef CAS PubMed
.
- Y. M. Chen, L. H. Chen, M. P. Li, H. F. Li, A. Higuchi, S. S. Kumar, Q. D. Ling, A. A. Alarfaj, M. A. Munusamy, Y. Chang, G. Benelli, K. Murugan and A. Umezawa, Xeno-free culture of human pluripotent stem cells on oligopeptide-grafted hydrogels with various molecular designs, Sci. Rep., 2017, 7, 45146 CrossRef CAS PubMed
.
- J. R. Klim, L. Li, P. J. Wrighton, M. S. Piekarczyk and L. L. Kiessling, A defined glycosaminoglycan-binding substratum for human pluripotent stem cells, Nat. Methods, 2010, 7, 989–994 CrossRef CAS PubMed
.
- Y. Deng, X. Zhang, X. Zhao, Q. Li, Z. Ye, Z. Li, Y. Liu, Y. Zhou, H. Ma, G. Pan, D. Pei, J. Fang and S. Wei, Long-term self-renewal of human pluripotent stem cells on peptide-decorated poly(OEGMA-co-HEMA) brushes under fully defined conditions, Acta Biomater., 2013, 9, 8840–8850 CrossRef CAS PubMed
.
- H. J. Park, K. Yang, M. J. Kim, J. Jang, M. Lee, D. W. Kim, H. Lee and S. W. Cho, Bio-inspired oligovitronectin-grafted surface for enhanced self-renewal and long-term maintenance of human pluripotent stem cells under feeder-free conditions, Biomaterials, 2015, 50, 127–139 CrossRef CAS PubMed
.
- P. Zhou, F. Wu, T. Zhou, X. Cai, S. Zhang, X. Zhang, Q. Li, Y. Li, Y. Zheng, M. Wang, F. Lan, G. Pan, D. Pei and S. Wei, Simple and versatile synthetic polydopamine-based surface supports reprogramming of human somatic cells and long-term self-renewal of human pluripotent stem cells under defined conditions, Biomaterials, 2016, 87, 1–17 CrossRef CAS PubMed
.
- P. Zhou, B. Yin, R. Zhang, Z. Xu, Y. Liu, Y. Yan, X. Zhang, S. Zhang, Y. Li, H. Liu, Y. A. Yuan and S. Wei, Molecular basis for RGD-containing peptides supporting adhesion and self-renewal of human pluripotent stem cells on synthetic surface, Colloids Surf., B, 2018, 171, 451–460 CrossRef CAS PubMed
.
- J. W. Lambshead, L. Meagher, J. Goodwin, T. Labonne, E. Ng, A. Elefanty, E. Stanley, C. M. O'Brien and A. L. Laslett, Long-Term Maintenance of Human Pluripotent Stem Cells on cRGDfK-Presenting Synthetic Surfaces, Sci. Rep., 2018, 8, 701 CrossRef PubMed
.
- A. A. Alarfaj, A. H. Hirad, M. A. Munusamy, S. S. Kumar and A. Higuchi, Human embryonic stem cells cultured on hydrogels grafted with extracellular matrix protein-derived peptides with polyethylene glycol joint nanosegments, IET Nanobiotechnol., 2022, 16, 295–304 CrossRef PubMed
.
- T. C. Sung, M. W. Lu, Z. Tian, H. H. Lee, J. Pan, Q. D. Ling and A. Higuchi, Poly(vinyl alcohol- co-itaconic acid) hydrogels grafted with several designed peptides for human pluripotent stem cell culture and differentiation into cardiomyocytes, J. Mater. Chem. B, 2021, 9, 7662–7673 RSC
.
- L. G. Villa-Diaz, H. Nandivada, J. Ding, N. C. Nogueira-de-Souza, P. H. Krebsbach, K. S. O'Shea, J. Lahann and G. D. Smith, Synthetic polymer coatings for long-term growth of human embryonic stem cells, Nat. Biotechnol., 2010, 28, 581–583 CrossRef CAS PubMed
.
- Y. Mei, K. Saha, S. R. Bogatyrev, J. Yang, A. L. Hook, Z. I. Kalcioglu, S. W. Cho, M. Mitalipova, N. Pyzocha, F. Rojas, K. J. Van Vliet, M. C. Davies, M. R. Alexander, R. Langer, R. Jaenisch and D. G. Anderson, Combinatorial development of biomaterials for clonal growth of human pluripotent stem cells, Nat. Mater., 2010, 9, 768–778 CrossRef CAS PubMed
.
- D. A. Brafman, C. W. Chang, A. Fernandez, K. Willert, S. Varghese and S. Chien, Long-term human pluripotent stem cell self-renewal on synthetic polymer surfaces, Biomaterials, 2010, 31, 9135–9144 CrossRef CAS PubMed
.
- E. F. Irwin, R. Gupta, D. C. Dashti and K. E. Healy, Engineered polymer-media interfaces for the long-term self-renewal of human embryonic stem cells, Biomaterials, 2011, 32, 6912–6919 CrossRef CAS PubMed
.
- C. W. Chang, Y. Hwang, D. Brafman, T. Hagan, C. Phung and S. Varghese, Engineering cell-material interfaces for long-term expansion of human pluripotent stem cells, Biomaterials, 2013, 34, 912–921 CrossRef CAS PubMed
.
- N. T. Kohen, L. E. Little and K. E. Healy, Characterization of Matrigel interfaces during defined human embryonic stem cell culture, Biointerphases, 2009, 4, 69–79 CrossRef PubMed
.
- S. Musah, S. A. Morin, P. J. Wrighton, D. B. Zwick, S. Jin and L. L. Kiessling, Glycosaminoglycan-binding hydrogels enable mechanical control of human pluripotent stem cell self-renewal, ACS Nano, 2012, 6, 10168–10177 CrossRef CAS PubMed
.
- S. Paiva, P. Joanne, C. Migdal, E. L. Soler, Y. Hovhannisyan, A. Nicolas and O. Agbulut, Polyacrylamide Hydrogels with Rigidity-Independent Surface Chemistry Show Limited Long-Term Maintenance of Pluripotency of Human Induced Pluripotent Stem Cells on Soft Substrates, ACS Biomater. Sci. Eng., 2020, 6, 340–351 CrossRef CAS PubMed
.
- M. Serra, C. Brito, C. Correia and P. M. Alves, Process engineering of human pluripotent stem cells for clinical application, Trends Biotechnol., 2012, 30, 350–359 CrossRef CAS PubMed
.
- S. Tohyama, J. Fujita, C. Fujita, M. Yamaguchi, S. Kanaami, R. Ohno, K. Sakamoto, M. Kodama, J. Kurokawa, H. Kanazawa, T. Seki, Y. Kishino, M. Okada, K. Nakajima, S. Tanosaki, S. Someya, A. Hirano, S. Kawaguchi, E. Kobayashi and K. Fukuda, Efficient Large-Scale 2D Culture System for Human Induced Pluripotent Stem Cells and Differentiated Cardiomyocytes, Stem Cell Rep., 2017, 9, 1406–1414 CrossRef CAS PubMed
.
- A. T. Lam, J. Li, A. K. Chen, W. R. Birch, S. Reuveny and S. K. Oh, Improved Human Pluripotent Stem Cell Attachment and Spreading on Xeno-Free Laminin-521-Coated Microcarriers Results in Efficient Growth in Agitated Cultures, BioRes. Open Access, 2015, 4, 242–257 CrossRef CAS PubMed
.
- S. M. Badenes, T. G. Fernandes, C. S. Cordeiro, S. Boucher, D. Kuninger, M. C. Vemuri, M. M. Diogo and J. M. Cabral, Defined Essential 8™ Medium and Vitronectin Efficiently Support Scalable Xeno-Free Expansion of Human Induced Pluripotent Stem Cells in Stirred Microcarrier Culture Systems, PLoS One, 2016, 11, e0151264 CrossRef PubMed
.
- Y. Fan, F. Zhang and E. S. Tzanakakis, Engineering Xeno-Free Microcarriers with Recombinant Vitronectin, Albumin and UV Irradiation for Human Pluripotent Stem Cell Bioprocessing, ACS Biomater. Sci. Eng., 2017, 3, 1510–1518 CrossRef CAS PubMed
.
- Y. Fan, M. Hsiung, C. Cheng and E. S. Tzanakakis, Facile engineering of xeno-free microcarriers for the scalable cultivation of human pluripotent stem cells in stirred suspension, Tissue Eng., Part A, 2014, 20, 588–599 CrossRef CAS PubMed
.
- A. L. Rodrigues, C. A. V. Rodrigues, A. R. Gomes, S. F. Vieira, S. M. Badenes, M. M. Diogo and J. M. S. Cabral, Dissolvable Microcarriers Allow Scalable Expansion And Harvesting Of Human Induced Pluripotent Stem Cells Under Xeno-Free Conditions, Biotechnol. J., 2019, 14, e1800461 CrossRef PubMed
.
- S. Derakhti, S. H. Safiabadi-Tali, G. Amoabediny and M. Sheikhpour, Attachment and detachment strategies in microcarrier-based cell culture technology: A comprehensive review, Mater. Sci. Eng., C, 2019, 103, 109782 CrossRef CAS PubMed
.
- P. Fattahi, A. Rahimian, M. Q. Slama, K. Gwon, A. M. Gonzalez-Suarez, J. Wolf, H. Baskaran, C. D. Duffy, G. Stybayeva, Q. P. Peterson and A. Revzin, Core-shell hydrogel microcapsules enable formation of human pluripotent stem cell spheroids and their cultivation in a stirred bioreactor, Sci. Rep., 2021, 11, 7177 CrossRef CAS PubMed
.
- V. C. Chen, S. M. Couture, J. Ye, Z. Lin, G. Hua, H. I. Huang, J. Wu, D. Hsu, M. K. Carpenter and L. A. Couture, Scalable GMP compliant suspension culture system for human ES cells, Stem Cell Res., 2012, 8, 388–402 CrossRef CAS PubMed
.
- D. E. Kehoe, D. Jing, L. T. Lock and E. S. Tzanakakis, Scalable stirred-suspension bioreactor culture of human pluripotent stem cells, Tissue Eng., Part A, 2010, 16, 405–421 CrossRef CAS PubMed
.
- R. Krawetz, J. T. Taiani, S. Liu, G. Meng, X. Li, M. S. Kallos and D. E. Rancourt, Large-scale expansion of pluripotent human embryonic stem cells in stirred-suspension bioreactors, Tissue Eng., Part C, 2010, 16, 573–582 CrossRef CAS PubMed
.
- R. Olmer, A. Haase, S. Merkert, W. Cui, J. Palecek, C. Ran, A. Kirschning, T. Scheper, S. Glage, K. Miller, E. C. Curnow, E. S. Hayes and U. Martin, Long term expansion of undifferentiated human iPS and ES cells in suspension culture using a defined medium, Stem Cell Res., 2010, 5, 51–64 CrossRef CAS PubMed
.
- R. Olmer, A. Lange, S. Selzer, C. Kasper, A. Haverich, U. Martin and R. Zweigerdt, Suspension culture of human pluripotent stem cells in controlled, stirred bioreactors, Tissue Eng., Part C, 2012, 18, 772–784 CrossRef CAS PubMed
.
- H. Singh, P. Mok, T. Balakrishnan, S. N. Rahmat and R. Zweigerdt, Up-scaling single cell-inoculated suspension culture of human embryonic stem cells, Stem Cell Res., 2010, 4, 165–179 CrossRef CAS PubMed
.
- D. Steiner, H. Khaner, M. Cohen, S. Even-Ram, Y. Gil, P. Itsykson, T. Turetsky, M. Idelson, E. Aizenman, R. Ram, Y. Berman-Zaken and B. Reubinoff, Derivation, propagation and controlled differentiation of human embryonic stem cells in suspension, Nat. Biotechnol., 2010, 28, 361–364 CrossRef CAS PubMed
.
- R. Zweigerdt, R. Olmer, H. Singh, A. Haverich and U. Martin, Scalable expansion of human pluripotent stem cells in suspension culture, Nat. Protoc., 2011, 6, 689–700 CrossRef CAS PubMed
.
- M. Amit, J. Chebath, V. Margulets, I. Laevsky, Y. Miropolsky, K. Shariki, M. Peri, I. Blais, G. Slutsky, M. Revel and J. Itskovitz-Eldor, Suspension culture of undifferentiated human embryonic and induced pluripotent stem cells, Stem Cell Rev. Rep., 2010, 6, 248–259 CrossRef PubMed
.
- J. Wu, M. R. Rostami, D. P. Cadavid Olaya and E. S. Tzanakakis, Oxygen transport and stem cell aggregation in stirred-suspension bioreactor cultures, PLoS One, 2014, 9, e102486 CrossRef PubMed
.
- T. G. Otsuji, J. Bin, A. Yoshimura, M. Tomura, D. Tateyama, I. Minami, Y. Yoshikawa, K. Aiba, J. E. Heuser, T. Nishino, K. Hasegawa and N. Nakatsuji, A 3D sphere culture system containing functional polymers for large-scale human pluripotent stem cell production, Stem Cell Rep., 2014, 2, 734–745 CrossRef CAS PubMed
.
- R. Chandrasekaran and V. G. Thailambal, The influence of calcium ions, acetate and l -glycerate groups on the gellan double-helix, Carbohydr. Polym., 1990, 12, 431–442 CrossRef CAS
.
- B. Abecasis, T. Aguiar, É. Arnault, R. Costa, P. Gomes-Alves, A. Aspegren, M. Serra and P. M. Alves, Expansion of 3D human induced pluripotent stem cell aggregates in bioreactors: Bioprocess intensification and scaling-up approaches, J. Biotechnol., 2017, 246, 81–93 CrossRef CAS PubMed
.
- Y. Y. Lipsitz, P. D. Tonge and P. W. Zandstra, Chemically controlled aggregation of pluripotent stem cells, Biotechnol. Bioeng., 2018, 115, 2061–2066 CrossRef CAS PubMed
.
- D. E. S. Nogueira, C. A. V. Rodrigues, M. S. Carvalho, C. C. Miranda, Y. Hashimura, S. Jung, B. Lee and J. M. S. Cabral, Strategies for the expansion of human induced pluripotent stem cells as aggregates in single-use Vertical-Wheel™ bioreactors, J. Biol. Eng., 2019, 13, 74 CrossRef PubMed
.
- H. Wu, X. Tang, Y. Wang, N. Wang, Q. Chen, J. Xie, S. Liu, Z. Zhong, Y. Qiu, P. Situ, M. A. Zern, J. Wang, H. Chen and Y. Duan, Dextran sulfate prevents excess aggregation of human pluripotent stem cells in 3D culture by inhibiting ICAM1 expression coupled with down-regulating E-cadherin through activating the Wnt signaling pathway, Stem Cell Res. Ther., 2022, 13, 218 CrossRef CAS PubMed
.
- X. Tang, H. Wu, J. Xie, N. Wang, Q. Chen, Z. Zhong, Y. Qiu, J. Wang, X. Li, P. Situ, L. Lai, M. A. Zern, H. Chen and Y. Duan, The combination of dextran sulphate and polyvinyl alcohol prevents excess aggregation and promotes proliferation of pluripotent stem cells in suspension culture, Cell Proliferation, 2021, 54, e13112 CAS
.
- X. Chen, A. B. Prowse, Z. Jia, H. Tellier, T. P. Munro, P. P. Gray and M. J. Monteiro, Thermoresponsive worms for expansion and release of human embryonic stem cells, Biomacromolecules, 2014, 15, 844–855 CrossRef CAS PubMed
.
- X. Chen, L. Harkness, Z. Jia, A. Prowse, M. J. Monteiro and P. P. Gray, Methods for Expansion of Three-Dimensional Cultures of Human Embryonic Stem Cells Using a Thermoresponsive Polymer, Tissue Eng., Part C, 2018, 24, 146–157 CrossRef CAS PubMed
.
- B. L. Ekerdt, C. M. Fuentes, Y. Lei, M. M. Adil, A. Ramasubramanian, R. A. Segalman and D. V. Schaffer, Thermoreversible Hyaluronic Acid-PNIPAAm Hydrogel Systems for 3D Stem Cell Culture, Adv. Healthcare Mater., 2018, 7, e1800225 CrossRef PubMed
.
- H. J. Johnson, S. Chakraborty, R. J. Muckom, N. P. Balsara and D. V. Schaffer, A scalable and tunable thermoreversible polymer for 3D human pluripotent stem cell biomanufacturing, iScience, 2022, 25, 104971 CrossRef CAS PubMed
.
- R. Zhang, H. K. Mjoseng, M. A. Hoeve, N. G. Bauer, S. Pells, R. Besseling, S. Velugotla, G. Tourniaire, R. E. Kishen, Y. Tsenkina, C. Armit, C. R. Duffy, M. Helfen, F. Edenhofer, P. A. de Sousa and M. Bradley, A thermoresponsive and chemically defined hydrogel for long-term culture of human embryonic stem cells, Nat. Commun., 2013, 4, 1335 CrossRef PubMed
.
- Y. Deng, S. Wei, L. Yang, W. Yang, M. S. Dargusch and Z.-G. Chen, A Novel Hydrogel Surface Grafted With Dual Functional Peptides for Sustaining Long-Term Self-Renewal of Human Induced Pluripotent Stem Cells and Manipulating Their Osteoblastic Maturation, Adv. Funct. Mater., 2018, 28, 1705546 CrossRef
.
- F. Etezadi, M. N. T. Le, H. Shahsavarani, A. Alipour, N. Moazzezy, S. Samani, A. Amanzadeh, S. Pahlavan, S. Bonakdar, M. A. Shokrgozar and K. Hasegawa, Optimization of a PDMS-Based Cell Culture Substrate for High-Density Human-Induced Pluripotent Stem Cell Adhesion and Long-Term Differentiation into Cardiomyocytes under a Xeno-Free Condition, ACS Biomater. Sci. Eng., 2022, 8, 2040–2052 CrossRef CAS PubMed
.
- D. Grimm, EPA plan to end animal testing splits scientists, Science, 2019, 365, 1231 CrossRef CAS PubMed
.
- X. Y. Tang, S. Wu, D. Wang, C. Chu, Y. Hong, M. Tao, H. Hu, M. Xu, X. Guo and Y. Liu, Human organoids in basic research and clinical applications, Signal Transduction Targeted Ther., 2022, 7, 168 CrossRef PubMed
.
|
This journal is © The Royal Society of Chemistry 2023 |
Click here to see how this site uses Cookies. View our privacy policy here.