DOI:
10.1039/C5RA01029B
(Paper)
RSC Adv., 2015,
5, 37682-37690
Development of ionic liquid based electromembrane extraction and its application to the enrichment of acidic compounds in pig kidney tissues
Received
18th January 2015
, Accepted 20th April 2015
First published on 21st April 2015
Abstract
The ionic liquid-based electromembrane extraction (IL-EME) is presented for the determination of acidic compounds in complex biological samples for the first time. In this work, the IL was held in the hollow fiber pores serving as the supported liquid membrane (SLM). An aqueous acceptor solution was placed in the lumen of hollow fiber. The acidic analytes migrated in the electric field, and were eventually enriched in the acceptor phase. In this extraction mode, electrokinetic migration served as the primary driving force, which significantly increased the extraction efficiency, resulting in a considerable increase in the extraction rate. Four chlorophenoxy acid herbicides, namely, 2,4-dichlorophenoxy acetic acid, 2-(2,4-dichlorophenox)propionic acid, 4-chloromethylphenoxyacetic acid, and 2,4,5-trichlorophenoxyacetic acid were used as the model analytes to test the applicability of IL-EME for acidic compounds. And the pig kidney was chosen as the sample matrix. Prior to the microextraction, some pretreatments including homogenating, shaking together with NaOH and centrifugating were needed for the kidney tissues. Important parameters affecting the extraction efficiency, such as the type of SLM, voltage, sample solution and acceptor phase pH, extraction time, temperature, stirring rate and salt addition were also investigated and optimized. Under the optimized conditions, the extraction procedure was completed within 15 min with 78–90 folds enrichment factors and the spike recoveries were in the range of 87.5–97.8%. Moreover, high linearities over 10.0 μg L−1 to 640 μg L−1 were obtained. The correlation coefficients exceeded than 0.9900.
1 Introduction
Sample pretreatment, which involved preconcentration of target analytes from various matrices, is a basic and crucial step in the entire analytical procedure. In recent years, a number of alternative, miniaturized extraction techniques have been successfully developed. Liquid-phase microextraction (LPME), specifically termed in 1997, was developed stemming from a concept to perform liquid–liquid microextraction and injection in only one commonly used microsyringe.1 This technique has been paid great attention since it came out. At present, several operating modes have been developed for LPME, including single-drop microextraction (SDME),1 hollow fiber liquid-phase microextraction (HF-LPME)2 and dispersive liquid–liquid microextraction (DLLME).3 In HF-LPME, a porous hollow fiber is used to protect microdroplets of extract solvent, which effectively overcomes the drop instability with merits including relatively lower precision and low sensitivity of SDME. It exhibits excellent clean-up ability compared with SDME and DLLME, and therefore can be applied to more complex matrix samples. In HF-LPME, the organic solvent is immobilized in the wall pores of the hollow fiber serving as an interface between the sample solution and the acceptor phase. Therefore, the selection of an appropriate solvent is vital to achieving efficient extraction. Toluene, undecane, 1-octanol and dihexyl ether are the most commonly used solvents in HF-LPME.4–6 However, because of their low viscosity and high volatility, there is a potential solvent loss when the extraction is performed at a high stirring rate, relatively high extraction temperature, and a prolongation of extraction time. The extraction abilities of these solvents for polar compounds are also limited. Recently, room temperature ionic liquids (ILs) have been proposed as an alternative to traditional organic solvents. ILs are widely used in extraction because of their distinct physical and chemical properties including low volatility and toxicity, high viscosity, adjustable polarity and high extractability for organic and inorganic compounds. However, the application of ILs in HF-LPME remains limited compared with that in SDME and DLLME, because it requires a long extraction time (30 min to 8 h).7–9 This prolonged extraction may be due to the high viscosity of ILs, which causes resistance during mass transfer. Therefore, a satisfactory solution for this problem must be found. In 2006, Pedersen-Bjergaard and Rasmussen proposed a new concept called electro membrane isolation (EMI).10 In this method, an electric field is introduced into the extraction setup. The charged analytes then electrokinetically migrate across a SLM immobilized in the hollow fiber pores. Electrokinetic migration, instead of passive diffusion, serves as the main driving force. The reported results suggest that electrokinetic migration is a significantly efficient transport mechanism, providing high recovery in a very short time.11,12 By adapting this novel concept, we attempted to apply electric field to IL based HF-LPME to address the mass transfer limitation, and then the ionic liquid based EME (IL-EME) was developed. This method has been successfully applied to the enrichment of basic compounds in human urine.13 And compared with traditional organic solvent (2-ethylnitrobenzene) based EME, IL-EME provided a higher enrichment factor, and similar repeatability and accuracy, which could be contributed to the better extraction ability of ILs for ionic analytes.13
In this research, the applicability of IL-EME method for acidic compounds in more complex sample matrix was tested using chlorophenoxyacid herbicides (CPAHs) as model drugs. CPAHs, physiological toxic compounds, are used to control the growth control of weeds in crops. These compounds are some of the most widely used herbicides in agriculture and gardening accounting for approximately 5.1% of the total herbicides consumption14 because of their low price and potent weed eradication.15 Most of CPAHs have pKa values around 3.0. Therefore these compounds are generally soluble in environmental water and can be retained in soil resulting in persistent environment pollution. Given their potential teratogenic and carcinogenic effects, a valid determination method must be developed to detect these compounds at trace. At present, several countries and unions have established standards of maximum residue level (MRL) for CPAHs in crops and environmental samples. However, reports on CPAHs residue in animal tissue remain scarce. According to metabolism researches, CPAHs are excreted through the renal route causing highest tissue residues in the kidneys in animal body.16,17 Therefore, the pig kidney was used as sample matrix providing a potential way of monitoring the human exposure to CPAHs from our daily diet.
In this study, an IL-EME method was applied for the enrichment of acidic compounds for the first time. The residue of four CPAHs, i.e., 2,4-dichlorophenoxyacetic acid (2,4-D), 2-(2,4-dichlorophenox)propionic acid (2,4-DP), 4-chloromethylphenoxy acetic acid (MCPA) and 2,4,5-trichlorophenoxyacetic acid (2,4,5-T), in pig kidney tissues were determined with IL-EME method, which confirmed the applicability of IL-EME method.
2 Experimental
2.1 Chemical and reagents
Reference standards, 2,4-D, 2,4-DP and MCPA was purchased from the Tianjing Heowns Biochemical Technology Co. (Tianjing, China), while 2,4,5-T from J&K Chemical Ltd. (Beijing, China) and their structures, pKa, log
P (ref. 18 and 19) were shown in Table 1. Chromatographic-grade methanol and acetonitrile were purchased from Merck Co. (Darmstadt, Germany). 1-Butyl-3-methylimidazolium-hexafluorophosphate ([C4MIm][PF6]), 1-hexyl-3-methylimidazolium-hexafluorophosphate ([C6MIm][PF6]), and 1-octyl-3-methylimidazolium-hexafluorophosphate ([C8MIm][PF6]) were obtained from Lanzhou Institute of Chemical Physics of CAS (Lanzhou, China). Other chemicals are of analytical grade and obtained from Tianjin Chemical Reagent Co. (Tianjin, China). Ultrapure water was used for the preparation of mobile phase and sample solution.
Table 1 Chemical structures, pKa, log
P and distribution ratio of CAPHs
Compound name |
Abbreviation |
Chemical structure |
pKa (ref. 18 and 19) |
log P (ref. 18 and 19) |
Distribution ratio |
Octanol |
C4a |
C6b |
C8c |
C4 is short for [C4MIm][PF6]. C6 is short for [C6MIm][PF6]. C8 is short for [C8MIm][PF6]. |
2,4-Dichlorophenoxy acetic acid |
2,4-D |
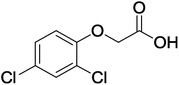 |
3.0 |
2.4 |
0.19 |
0.078 |
0.26 |
0.16 |
2-(2,4-Dichlorophenox) propionic acid |
2,4-DP |
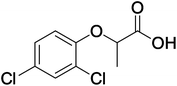 |
3.0 |
3.4 |
0.11 |
0.04 |
0.25 |
0.28 |
4-Chloro-methylphenoxy acetic acid |
MCPA |
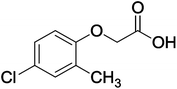 |
3.1 |
2.8 |
0.25 |
0.14 |
0.33 |
0.20 |
2,4,5-Trichlorophenoxy acetic acid |
2,4,5-T |
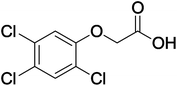 |
2.9 |
3.3 |
0.23 |
0.14 |
0.30 |
0.26 |
2.2 Chromatographic condition
Chromatographic analysis was performed on Agilent 1200 HPLC system comprising a G1312A binary pump, a G1315D diode-array detector and a G1328B manual injector. Chromatographic separation of the analytes was performed on a Kromasil C18 column (5 μm, 4.6 mm × 250 mm i.d.) (Dalian Institute of Chemical Physics, Chinese Academy of Sciences, Dalian, China). Agilent ChemStation was employed to acquire and process chromatographic data. The mobile phase consisted of 5 mmol L−1 ammonium acetate buffer solution containing 0.1% formic acid (pH = 3.1) and acetonitrile containing 10% methanol in a ratio of 48
:
52 (v/v). The flow rate was 1.0 mL min−1. The column temperature was maintained at 25 °C and the detection wavelength was 230 nm.
2.3 Preparation of standard solution and biological matrix
The stock solutions of the reference standards at the concentration of 1.0 mg mL−1 were separately prepared in methanol and stored at 4 °C before use. The working solutions were freshly prepared by diluting the stock solutions with ultrapure water.
The pig kidney samples were obtained from six different local retailers in Lanzhou city, Gansu province, China. The sample solution of kidney tissues was prepared as follows: a pig kidney was cut into small fragments, and then 5 g of the fragments were accurately weighed and grinded into semi-liquid consistency with a blender. Thereafter, 10 mL of 10 mmol L−1 NaOH solution was added to the homogenate followed by a vigorous hand shaking for 2 min and centrifugation at 4000 rpm for 20 min. The supernatant was withdrawn by a syringe, while 5 mL of 10 mmol L−1 NaOH solution was added into the residue, and then the above procedure was repeated for two times. The three supernatants were combined and the pH was adjusted to 11, and then the whole solution was diluted to a total volume of 25 mL.
2.4 IL-EME setup and procedure
The experimental setup of IL-EME adopted from the Pedersen-Bjergaard10 and Yamini20 was illustrated in Fig. 1. The DC power was supplied by a DC–AC converter with programmable voltage in the range of 1.5–12 V. Platinum wires (Tianjin Aidahengsheng Technology Co., Ltd, Tianjing, China) with a diameter of 0.2 mm and with a diameter of 0.5 mm, both of which were connected to the DC–AC converter, were used as positive electrode and negative electrode, respectively. The PP Q2/1 polypropylene hollow fibers (Membrana, Wuppertal, Germany) with an internal diameter of 600 μm, a wall thickness of 200 μm and a pore size of 0.2 μm were used to immobilize the artificial liquid membrane and hold the acceptor solution.
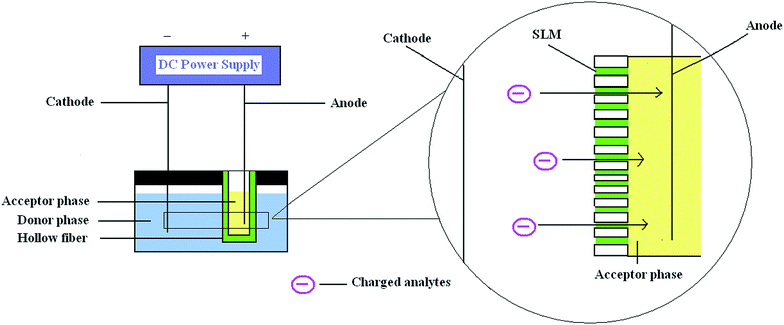 |
| Fig. 1 Set-up of IL-EME. | |
The extraction procedure was performed as follows: 3 mL of sample solution was placed in a 4.0 mL-glass vial. The vial was placed on a magnetic stirrer. A 3.5 cm segment of hollow fiber was dipped in the ionic liquid for 5 min to form an artificial liquid membrane with the aid of sonication. Excessive solvent on the surface of the hollow fiber was gently wiped using filter paper. The upper end of the hollow fiber was connected to a 1.0 cm length glass capillary. During the extraction procedure, a small part of ILs may migrate in the electrical field. Considering the loss of extraction solvent, 1 μL of the IL was used for SLM replenishment. About 15 μL of the acceptor solution was injected into the lumen of the hollow fiber using a microsyringe. The lower end of the hollow fiber was then heat-sealed. Afterwards, the fiber was placed in the sample solution. Finally, the positive electrode was placed in the lumen of the hollow fiber (acceptor phase) through the guiding tube, and the negative electrode was placed in the sample solution. IL-EME was performed for 15 min at a voltage of 3 V and at a stirring rate of 1000 rpm. After extraction, the acceptor solution was withdrawn with a microsyringe and quickly transferred to a 0.5 mL Eppendorf tube. Exactly, 10 μL of a 2.0 mg L−1 salicylic acid solution was then used as an internal standard (IS) and added to the acceptor phase to eliminate the air-dry and injection errors. The mixture solution was air-dried. The residue was then dissolved in 20 μL methanol. Exactly 15 μL of the dissolving solution was then injected for HPLC analysis. Given its low cost, the used fiber was discarded after extraction to eliminate possible carry-over effect.
To investigate the performance and reliability of the method, some parameters including enrichment factor, linear range, repeatability and accuracy of method were determined. All the experiments were carried out in the sample solution of kidney tissues.
3 Results and discussion
3.1 The application of ILs in IL-EME
According to the reported studies on electromembrane extraction,21,22 the composition of SLM corresponds to the following factors: immiscibility with water; relatively low vapor pressure to minimize the loss of SLM during extraction, and certain chemical properties that enables the transport of charged analytes through the SLM. Ionic liquids completely satisfy the above points. Compared with that in traditional IL-HF-LPME, an electric field was introduced into the IL-HF-LPME. In this case, the driving force for extraction is the electrokinetic migration instead of the passive diffusion. Therefore, the extraction efficiency should be dramatically improved and the extraction time is expected to be shortened.
3.2 Optimization of IL-EME procedure
According to the theoretical model reported in a previous research,23 the flux of the target compounds across the SLM depends on the diffusion coefficient of the analytes in the SLM. This coefficient is determined by the SLM type, membrane thickness, applied voltage across the SLM, ion balance and temperature. Therefore, several factors such as SLM type, voltage, pH values of the sample solution and the acceptor phase, and salt concentration, were optimized in the present research. A total of 3 mL of the working solution containing 200 μg L−1 of the analytes was used for all optimization experiments. Three parallel experiments were performed for each influencing factors. The peak area ratios of analytes to IS were used to evaluate the extraction efficiency.
3.2.1 Selection of SLM. The flux of analyte across the SLM is directly proportional to the diffusion coefficient of the ionic analyte in SLM. For a certain group of analytes, the diffusion coefficients depend on the type of SLM. Therefore, the type of SLM is vital to the extraction efficiency. In the current research, two aspects should be considered. First, SLM should be sufficiently stable and durable for extraction procedure. The low volatility is one of their most significant characteristics, which could be stable for a relatively long extraction time. Therefore, the loss of SLM during the extraction caused by evaporation could be minimized. According to the previous research,24 the conductivity of alkylimidazolium hexafluorophosphate-based ILs decreases with the increase in the length of 3-alkyl side chain. Therefore, [C8MIm][PF6] can provide the most stable SLM under the electric field. Second, the extraction efficiency of ionic analytes increased with the increase of the diffusion coefficient, which is proportional to the distribution ratio, thus, theoretically, high distribution can bring high extraction efficiency. But not to be ignored, the high distribution ratio also causes target analytes to be trapped in the SLM. Therefore, the highest distribution ratio may not get the highest extraction efficiency of EME. According to our experiment, the highest distribution ratio is obtained from [C6MIm][PF6] (Table 1). However, as shown in Fig. 2, the use of [C8MIm][PF6] as SLM yields the highest extraction efficiencies. The use of the traditional organic solvent 1-octanol as the SLM in EME procedure was also investigated under the same conditions. Extraction was not achieved when a 3 V electric field was applied. However, when the voltage was increased to 9 V, and an extraction efficiency similar to that of [C8MIm][PF6] was obtained.
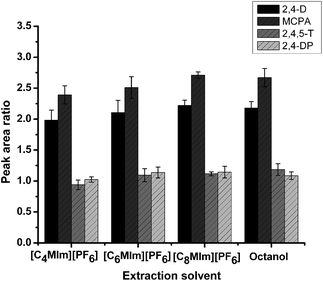 |
| Fig. 2 Effect of extraction solvent on the peak area ratio of CAPHs to salicylic acid. Extraction conditions: voltage 3 V; pH of sample solution 11; pH of acceptor phase 12; temperature 45 °C; extraction time 15 min; stirring rate 1000 rpm; salt addition 15%. | |
3.2.2 Effect of voltage. In EME, electrokinetic migration of charged analytes is believed to be the main transport mechanism. On the basis of the theoretical mode, the flux of analytes increased with the increase in applied potential. Thus, the effect of voltage ranging from 1.5 to 9 V was optimized. The results (Fig. 3) show that a lower voltage can provide higher extraction efficiency. This phenomenon can be explained as follows: the entire IL-EME setup was an electric circuit, in which the SLM served as an electric resistance, and the charged analytes migrated following the electric current. This current must be kept at a small value to inhibit electrolysis. Given the relatively low resistance of the ILs, which serves as the SLM, the applied potential should be higher at low voltage. So, 1.5 V voltage can provide efficient electric field intensity for migration of ionized analytes. As also can be seen from the Fig. 3, the efficiency is almost constant in the range of 1.5 to 6 V taking the RSD into consideration. But when the voltage increases further, the SLM becomes unstable and the current increases thus causing the electrolysis, and the extraction efficiency is reduced.
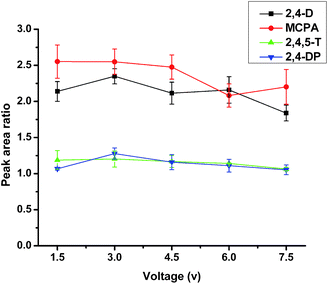 |
| Fig. 3 Effect of voltage on the peak area ratio of CAPHs to salicylic acid. The SLM was composed of [C8MIm][PF6], and other extraction conditions were the same as Fig. 2. | |
As shown in Fig. 3, the repeatability of a set of independent IL-EME experiments is relatively low. This phenomenon can be explained by using the perspective stated in one research:25 in this extraction mode, the total charge of extracted analytes is equal to the electric charge. According to Faraday's law, the electric charges for different current in the same time will be different. At constant voltage, the electric current in the system varies with the resistance of the extraction system, which is given by the SLM in non-uniform extraction units. Therefore, a careful operation would be required to reduce the variation.
3.2.3 Effect of pHs of sample and acceptor solution. In IL-EME, the analytes were extracted in the ionized form, which is determined by the pH values of sample solution and the acceptor phase. Therefore, the pH values of sample solution (from 7.0–13.0) were optimized while those of the acceptor solution were kept at 12.0. The results are shown in Fig. 4. The highest extraction efficiency was obtained at pH 11.0. The pH value of the sample solution was then maintained at 11.0, whereas the pH values of acceptor phase (ranging from 9.0–13.0) were optimized. As shown in Fig. 5, the best extraction efficiency was achieved at pH 12.0. The results can be explained as follows: first, a strong alkaline environment kept the analytes in ionized form. Therefore, when pH decreased, the analytes were not completely ionized causing back-extraction of analytes in the molecular form. Second, according to the theoretical mode, χ, which is defined as the ratio of total ionic concentration in sample solution to that in the acceptor solution (ion balance), is inversely proportional to the flux of analytes. Therefore, better extraction was achieved when the ionic concentration of acceptor phase was higher than that of the sample solution. In this case, the pH of acceptor solution should be higher than that of the sample solution.
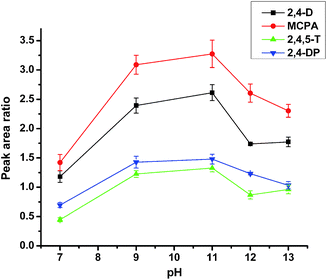 |
| Fig. 4 Effect of pH of sample solution on the peak area ratio of CAPHs to salicylic acid. The SLM was composed of [C8MIm][PF6], and other extraction conditions were the same as Fig. 2. | |
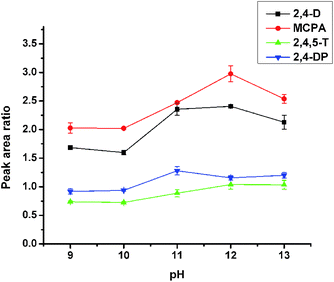 |
| Fig. 5 Effect of pH of acceptor solution on the peak area ratio of CAPHs to salicylic acid. The SLM was composed of [C8MIm][PF6], and other extraction conditions were the same as Fig. 2. | |
3.2.4 Effect of extraction time. The short extraction time is one of the major advantages of EME. In this section, the effect of extraction time (from 5 to 45 min) is investigated. Fig. 6a shows the change trend of the extraction efficiency with the extraction time. Initially, the extraction efficiency dramatically increased when the extraction time was increased from 5 to 15 min then reached its maximum at 15 min.
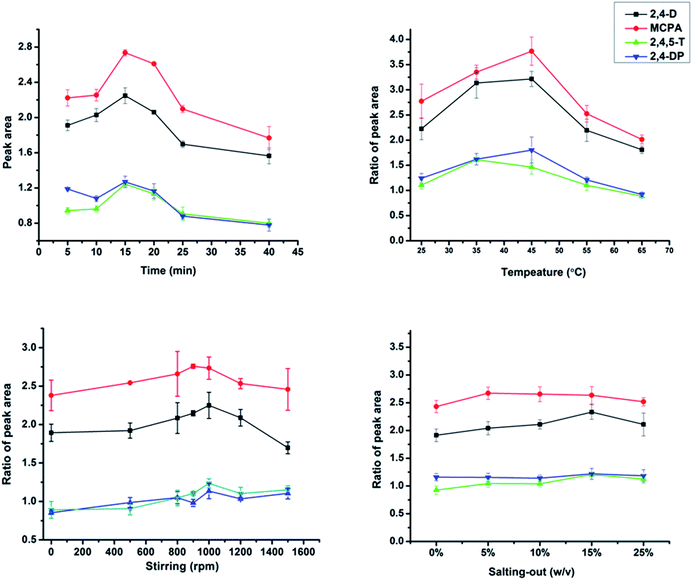 |
| Fig. 6 Effect of (a) extraction time, (b) temperature, (c) stirring rate and (d) salt addition on the peak area ratio of CAPHs to salicylic acid. The SLM was composed of [C8MIm][PF6], and other extraction conditions were the same as Fig. 2. | |
Then, the efficiency decreased with the continued increase in time. This phenomenon may be due to the back-diffusion of analytes to the SLM. Along with the increase in extraction time, the concentration of target analytes increased causing oversaturation in acceptor phase.26 So the analytes may diffuse back to the donor phase (sample solution) in the force of concentration gradient. Finally, 15 min was chosen as the extraction time.
3.2.5 Effect of temperature. The effect of temperature (25–65 °C) was evaluated to obtain the best extraction efficiency. The results (Fig. 6b) show that the best extraction efficiency was achieved at 45 °C. Temperature increased the solubility of the charged CPAHs in SLM and decreased the viscosity of SLM, which improves the rate of mass transfer. When the temperature is too high, the resistance of the whole setup would decrease causing an increase in current. The high current will cause electrolysis and instability of SLM.Therefore, the temperature must be kept at appropriate level. Therefore, 45 °C was chosen as the extraction temperature for the subsequent experiments.
3.2.6 Effect of stirring rate. In this research, the volume of the sample solution was set at 3.0 mL. This volume is a relative large one for an electrical field-enhanced extraction. Therefore, appropriate stirring should be performed to increase the convection between the two electrodes. In this section, the effect of the stirring rate (from 0 to 1500 rpm) was evaluated. The results in Fig. 6c, show that changes in the stirring rate caused slight changes in the extraction efficiency. Based on these results, 1000 rpm yielded the best extraction efficiency and was therefore chosen as the optimal stirring rate.
3.2.7 Effect of salt addition. In conventional liquid–liquid extraction, salt addition decreases the solubility of analytes in aqueous sample, thus increasing their partition in the organic phase. However, in IL-EME, salt addition may have a different effect. The effect of NaCl addition (0–30% (w/v)) to the sample solution was evaluated. As shown in Fig. 6d, the extraction efficiency of the analytes changed slightly with the increase of the NaCl concentration. Relatively high extraction efficiency was obtained at 15%. This result suggests that the extraction efficiency is not affected by a high ionic strength environment. Therefore, the proposed method was suitable for the subsequent experiments including method validation and application in real sample. These experiments were performed in kidney tissue.
3.3 Validation of the method
Enrichment factor (EF) (Table 2) is calculated according to the following equation: EF = Cd/Co, where Cd is the concentration of analyte dissolved in methanol for HPLC analysis, and Co is the concentration of analyte originally presented in the sample solution.
Table 2 Enrichment factors, linearities, LODs, LOQs and spiked recoveries of CAPHs in kidney tissue
Compound |
EF |
Correlation equation (linear range) |
r |
LOD (μg L−1) |
LOQ (μg L−1) |
Recovery (n = 3) |
Added (μg g−1) |
Mean recovery (%) |
RSD (%) |
2,4-D |
90 |
Y = 2.8411X − 0.4615 (10.0–640 μg L−1) |
0.9979 |
2.0 |
7.0 |
0.2 |
97.5 |
7.8 |
1.0 |
96.0 |
5.6 |
1.6 |
94.1 |
8.9 |
2,4-DP |
78 |
Y = 1.5501X − 0.1981 (10.0–640 μg L−1) |
0.9977 |
3.5 |
11.0 |
0.2 |
95.0 |
7.7 |
1.0 |
97.5 |
6.9 |
1.6 |
97.8 |
8.1 |
MCPA |
87 |
Y = 3.6095X − 0.6276 (10.0–640 μg L−1) |
0.9975 |
2.0 |
7.0 |
0.2 |
87.5 |
3.2 |
1.0 |
94.5 |
7.5 |
1.6 |
95.3 |
2.7 |
2,4,5-T |
85 |
Y = 1.3783X − 0.2423 (10.0–640 μg L−1) |
0.9972 |
3.5 |
11.0 |
0.2 |
97.5 |
4.6 |
1.0 |
96.5 |
8.3 |
1.6 |
94.7 |
5.8 |
The calibration standard working solutions were prepared by spiking five levels of the analytes and 120 μg L−1 salicylic acid to the sample solution of kidney tissues. The samples were extracted with the optimized IL-EME procedure, and then analyzed by HPLC. The calibration curves were constructed by plotting the peak area ratio of analytes to IS against their concentration ratio at five different concentrations in three parallel experiments. The correlation equations, correlation coefficients, linear ranges, limits of detection (LODs) and limits of quantitation (LOQs) are calculated and summarized in Table 2.
The precision of the instrument was evaluated by performing intra-day (n = 5) and inter-day assays (n = 3) replicate injection of 2,4-D standard solution. Intra-day assay precision was measured for continuous injections during the same day whereas inter-day assay precision was measured on 3 consecutive days. The RSD value of peak area was 0.44% for intra-day precision, and 2.48% for inter-day precision.
Five parallel determination of a kidney sample spiked 1.0 μg g−1 of the four target analytes under optimal conditions gave relative standard deviation of 4.56, 7.82, 6.73 and 5.31% for 2,4-D, 2,4-DP, MCPA and 2,4,5-T, respectively.
The accuracy of the method was confirmed by spike recovery test. Three levels (0.2, 1.0, 1.6 μg g−1) of the analytes were separately spiked to the pig kidney tissues containing 1.0 μg g−1 of the four analytes at first. Then the three sets of spiked samples were extracted with the same method as that optimized above and analyzed by HPLC. The recoveries of the analytes are illustrated in Table 2.
3.4 Method comparison
The proposed method was compared with other microextraction methods for the determination of CPAHs including DLLME,27 auto solid phase extraction,28 dynamic HF-LPME,29 suspended LPME30 and reverse-micelle DLLME.31 As Table 3 shows, the developed method has the merits of low cost and almost no consumption of organic solvent. And the method provides satisfying extraction efficiency in a more complex sample matrix comparing with the other reported methods. Especially pointed out, although the extraction time is much longer than that of DLLME which is thought to be the fast extraction mode in LPME, it is several times shorter than that of conventional HF-LPME.
Table 3 Comparison of EF-IL-HF-LPME with other microextraction methods for the determination of CPAHs
Method |
Analyte |
Sample matrix |
LOD (μg L−1) |
Linear range (μg L−1) |
Time (min) |
Volume and sort of extraction solvent |
Reference |
DLLME-LC |
2,4-D |
Tap water/river water |
0.13 |
0.16–400 |
1.5 |
25 μL 1,1,2,2-tetracloroethane |
27 |
2,4,5-T |
0.11 |
Auto SPE-LC-MS |
2,4-D |
River water |
0.005 |
0.05–50 |
30 |
750 μL methanol |
28 |
2,4,5-T |
0.002 |
MCPA |
0.01 |
D-HF-LPME-HPLC |
2,4-D |
Tap water/river water/well water |
0.50 |
1.00–100 |
30 |
900 μL 1-octanol |
29 |
MCPA |
0.50 |
SDME-LC |
2,4-D |
Tap water/well water |
0.30 |
5.00–500 |
45 |
25 μL 1-octanol |
30 |
MCPA |
0.40 |
2,4-DP |
0.30 |
Reverse-micelle DLLME-HPLC |
2,4-D |
Tap water/river water/sea water |
0.80 |
1.50–200 |
— |
20 mg DeA |
31 |
MCPA |
0.50 |
1.00–200 |
EF-IL-HF-LPME |
2,4-D |
Kidney tissues |
2.00 |
10.0–640 |
15 |
15 μL NaOH solution |
This method |
2,4-DP |
3.50 |
2,4,5-T |
2.00 |
MCPA |
3.50 |
3.5 Application in real sample
The sample solution of the kidney tissue was directly introduced into HPLC, where no IL-EME procedure was applied. From the obtained chromatogram (Fig. 7A), a number of large, overlapping peaks from matrix background were observed. But these peaks were eliminated (Fig. 7B) after IL-EME. The disappearance of these peaks can be attributed to the high clean-up ability and selective extraction performance of IL-EME. Six sets of pig kidney purchased from different retailers were processed and subsequently extracted by following the above optimized procedure in triplicate. The four CPAHs were not found in each set of samples. Fig. 7C shows the typical chromatogram of the spiked sample solution at a concentration of 0.2 μg g−1 after extraction.
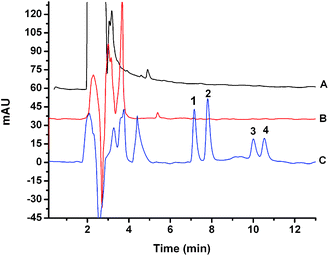 |
| Fig. 7 Chromatograms of (A) direct injection of kidney tissue extracts solution, (B) blank kidney tissue after IL-EME procedure, and (C) spiked samples containing 0.2 μg g−1 target analytes extraction time. | |
4. Conclusion
For the first time, an IL based EME method was applied to the determination of acidic compounds in a relatively complex biological sample. In this mode, electromigration appeared to be the main driving force for extraction instead of passive diffusion, which completely eliminates the difficulty in mass transfer. With this method, the charged CPAHs were enriched in a small volume of the acceptor phase. Moreover, the adverse effect of sample matrix was minimized. Good extraction efficiency and high recovery were obtained in a relatively short time. Sensitivity and linearity were also satisfactory. According to the research, the IL-EME is a reliable method for the enrichment of acidic compounds. As can be concluded, the proposed method is fast, simple and reliable for the detection of trace CPAHs in complex body tissues.
Acknowledgements
The work was supported by the National Nature Science Foundation of China (nos 21105106 and 21075127) and Natural Science Foundation of Gansu Province (no. 1107RJZA146).
Notes and references
- Y. He and H. K. Lee, Anal. Chem., 1997, 69, 4634 CrossRef CAS.
- S. Pedersen-Bjergaard and K. E. Rasmussen, Anal. Chem., 1999, 71, 2650 CrossRef CAS.
- M. Rezaee, Y. Assadi, M. R. M. Hosseinia, E. Aghaee, F. Ahmadi and S. Berijani, J. Chromatogr. A, 2006, 1116, 1 CrossRef CAS PubMed.
- Q. Xiao and B. Hu, J. Chromatogr. B: Anal. Technol. Biomed. Life Sci., 2010, 878, 1599 CrossRef CAS PubMed.
- M. Ramos Payán, M. Á. Bello López, R. Fernández-Torres, M. Callejón Mochón and J. L. Gómez Ariza, Talanta, 2010, 82, 854 CrossRef PubMed.
- Y. Yang, J. Chen and Y.-P. Shi, J. Chromatogr. B: Anal. Technol. Biomed. Life Sci., 2010, 878, 2811 CrossRef CAS PubMed.
- X. Ma, M. Huang, Z. Li and J. Wu, J. Hazard. Mater., 2011, 194, 24 CrossRef CAS PubMed.
- Y. Tao, J.-F. Liu, X.-L. Hu, H.-C. Li, T. Wang and G.-B. Jiang, J. Chromatogr. A, 2009, 1216, 6259 CrossRef CAS PubMed.
- J. F. Peng, J. F. Liu, X. L. Hu and G. B. Jiang, J. Chromatogr. A, 2007, 1139, 165 CrossRef CAS PubMed.
- S. Pedersen-Bjergaard and K. E. Rasmussen, J. Chromatogr. A, 2006, 1109, 183 CrossRef CAS PubMed.
- M. Balchen, L. Reubsaet and S. Pedersen-Bjergaard, J. Chromatogr. A, 2008, 1194, 143 CrossRef CAS PubMed.
- L. E. E. Eibak, A. Gjelstad, K. E. Rasmussen and S. Pedersen-Bjergaard, J. Pharm. Biomed. Anal., 2012, 57, 33 CrossRef PubMed.
- J.-N. Sun, J. Chen and Y.-P. Shi, J. Chromatogr. A, 2014, 1352, 1 CrossRef CAS PubMed.
- I. Rodríguez Pereiro, R. González Irimia, E. Rubí Cano and R. Cela Torrijos, Anal. Chim. Acta, 2004, 524, 249 CrossRef PubMed.
- M. I. Catalina, J. Dallüge, R. J. J. Vreuls and U. A. T. Brinkman, J. Chromatogr. A, 2000, 877, 153 CrossRef CAS.
- K. Grossmann, Trends Plant Sci., 2000, 5, 506 CrossRef CAS.
- H. Aydın, N. Özdemir and N. Uzunören, Forensic Sci. Int., 2005, 153, 53 CrossRef PubMed.
- A. Moral, C. Caballo, M. D. Sicilia and S. Rubio, Anal. Chim. Acta, 2012, 709, 59 CrossRef CAS PubMed.
- M. E. León-González, N. Rosales-Conrado, L. V. Pérez-Arribas and L. M. Polo-Díez, J. Chromatogr. A, 2010, 1217, 7507 CrossRef PubMed.
- S. Seidi, Y. Yamini and M. Rezazadeh, J. Pharm. Biomed. Anal., 2011, 56, 859 CrossRef CAS PubMed.
- A. Gjelstad, T. M. Andersen, K. E. Rasmussen and S. Pedersen-Bjergaard, J. Chromatogr. A, 2007, 1157, 38 CrossRef CAS PubMed.
- M. Rezazadeh, Y. Yamini and S. Seidi, J. Sep. Sci., 2012, 35, 571 CrossRef CAS PubMed.
- A. Gjelstad, K. E. Rasmussen and S. Pedersen-Bjergaard, J. Chromatogr. A, 2007, 1174, 104 CrossRef CAS PubMed.
- W. C. Su, C. H. Chou, D. S. H. Wong and M. H. Li, Fluid Phase Equilib., 2007, 252, 74 CrossRef CAS PubMed.
- A. Šlampová, P. Kubáň and P. Boček, J. Chromatogr. A, 2012, 1234, 32 CrossRef PubMed.
- K. Alhooshani, C. Basheer, J. Kaur, A. Gjelstad, K. E. Rasmussen, S. Pedersen-Bjergaard and H. K. Lee, Talanta, 2011, 86, 109 CrossRef CAS PubMed.
- W.-C. Tsai and S.-D. Huang, J. Chromatogr. A, 2009, 1216, 7846 CrossRef CAS PubMed.
- M. Takino, S. Daishima and T. Nakahara, Analyst, 2001, 126, 602 RSC.
- A. Esrafili, Y. Yamini, M. Ghambarian, M. Moradi and S. Seidi, J. Sep. Sci., 2011, 34, 957 CrossRef CAS PubMed.
- J. Hassan, M. Shamsipur, A. Es'haghi and S. Fazili, Chromatographia, 2011, 73, 999 CAS.
- M. Tayyebi, Y. Yamini and M. Moradi, J. Sep. Sci., 2012, 35, 2491 CrossRef CAS PubMed.
|
This journal is © The Royal Society of Chemistry 2015 |
Click here to see how this site uses Cookies. View our privacy policy here.