DOI:
10.1039/D4IM00014E
(Review Article)
Ind. Chem. Mater., 2024,
2, 514-532
Aqueous Zn–CO2 batteries: a route towards sustainable energy storage
Received
31st January 2024
, Accepted 11th April 2024
First published on 16th April 2024
Abstract
In recent years, the concept of rechargeable aqueous Zn–CO2 batteries has attracted extensive attention owing to their dual functionality of power supply and simultaneous conversion of CO2 into value-added chemicals or fuels. The state-of-the-art research has been mainly focused on the exploration of working mechanisms and catalytic cathodes but hardly applies an integrative view. Although numerous studies have proven the feasibility of rechargeable aqueous Zn–CO2 batteries, challenges remain including the low CO2 conversion efficiency, poor battery capacity, and low energy efficiency. This review systematically summarizes the working principles and devices, and the catalytic cathodes used for Zn–CO2 batteries. The challenges and prospects in this field are also elaborated, providing insightful guidance for the future development of rechargeable aqueous Zn–CO2 batteries with high performance.
Keywords: Zn–CO2 battery; CO2 reduction reaction; Working mechanism; Electrocatalysts.
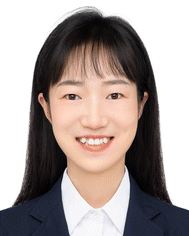 Yanxiu Liu | Yanxiu Liu received her BE degree from Nanjing Tech University in 2020 and ME degree from Yancheng Institute of Technology in 2023. She is currently a PhD candidate under the supervision of Prof. Yu Zhang in the School of Mechanical and Power Engineering at East China University of Science and Technology. Her research mainly focuses on the electrocatalyst synthesis and mechanism studies for metal–CO2 batteries. |
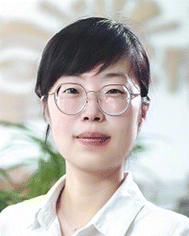 Yu Zhang | Yu Zhang is currently a professor from East China University of Science and Technology (ECUST) in Shanghai, China. She obtained her BS degree from Nanjing University in 2012 and PhD degree from Nanyang Technological University, Singapore in 2017. She then worked as a postdoctoral fellow at the Hong Kong University of Science and Technology and Georgia Institute of Technology. In 2020, she joined the School of Mechanical and Power Engineering in ECUST. Her research expertise lies in the development of electrode materials and devices for rechargeable batteries and electrocatalysis. |
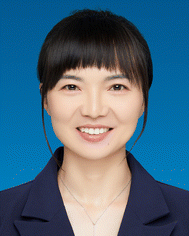 Zhihong Tian | Zhihong Tian is a Yellow River Scholar and professor at Henan University. She obtained her first PhD degree in engineering at Zhengzhou University in 2018, and a second PhD degree in natural science at Max Planck Institute of Colloids and Interfaces/Potsdam University in 2019 in Germany. After finishing her PhD studies, she worked as a postdoc in Professor Markus Antonietti's group at Max Planck Institute of Colloids and Interfaces in Germany. In January 2021, she joined the Engineering Research Center for Nanomaterials at Henan University. Her research interests are the structural design and performance optimization of porous carbon materials. |
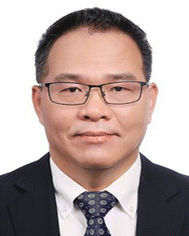 Tianxi Liu | Dr Tianxi Liu is currently a full professor at the School of Chemical and Material Engineering, Jiangnan University. He received his PhD (1998) from Changchun Institute of Applied Chemistry, Chinese Academy of Sciences, China. His research is mainly engaged in polymer nanocomposites, new energy materials and devices, and nanofibers and their composites. |
1 Introduction
Energy management has become an increasing concern due to the limited reserves of fossil fuels, which can potentially be compensated by the steep rise of sustainable electricity generation, mediating its fluctuating and volatile nature assumed.1 Furthermore, CO2 released during the combustion of fossil fuels has been regarded as the main “greenhouse gas” causing increasingly serious climate-related issues.2,3 However, it is also a source of carbon (as demonstrated by natural photosynthesis) and balancing generation and fixation is an obvious mandate.
This opens the opportunity to tackle those challenges with new global chemical engineering tools or industries. An electrochemical CO2 reduction reaction (CO2RR) has been recently demonstrated to be an effective strategy to use excess sustainable electricity for upgrading CO2 into value-added chemicals (such as HCOOH or C2H4).4–10 Notably, compared with metal-ion or metal–air batteries,11–16 metal–CO2 batteries do not only reduce CO2 into products of high value but also provide energy during the discharging process, which is regarded as an extra advantage compared to the individual electrochemical CO2 conversion.17,18 Therefore, metal–CO2 batteries on a larger scale can become a powerful tool for both energy and raw material management.19,20
In pioneering work, Takechi et al. for the first time reported in 2011 that the capacity of a Li–O2 battery was greatly improved after the introduction of CO2 to the gas phase.21 Subsequently, the batteries using CO2 as the working gas received widespread attention.18,19,22 As for the working mechanism, the Li metal anode gets oxidized and releases electrons to form Li+ and CO2 gets reduced to Li2CO3 and C-species during the discharging process; Li2CO3 and C-species are decomposed or oxidized and release CO2 during the charging process.23,24 However, the practical implementation of Li–CO2 batteries suffers from the complex reaction mechanism, deposition of solid products, and the high cost and safety concerns of organic electrolytes, driving the research community to search for alternative metal–CO2 batteries.25,26 Compared with non-aqueous Na/Li–CO2 batteries, Zn–CO2 batteries possess favorable merits including good safety and low cost due to the use of aqueous electrolytes.27,28 Besides, the cathodic reaction does not produce solid products during the discharge process, which is beneficial for improving the cycle life.
Recently, Zn metal has been described as one of the potential candidates for use as the anode in batteries due to its low price, high theoretical capacity (825 mA h g−1), and the ability to use sustainable aqueous electrolytes.29,30 The function of aqueous Zn–CO2 batteries involves various simultaneous electrochemical reactions including CO2RR during the discharging process and oxygen evolution reactions (OER) or the oxidation of liquid products of CO2RR during the charging process. The advantage of the Zn–CO2 battery is that it could combine CO2 reduction and energy storage. However, cathodes are additionally required to inhibit the competitive hydrogen evolution reaction (HER) against the CO2RR by electrocatalyst design.31,32 Since Xie et al. developed a reversible Zn–CO2 battery using Pd nanosheets as catalysts for the cathode,33 different types of catalysts have been investigated for rechargeable aqueous Zn–CO2 batteries. For a pre-review, Table 1 summarizes the catalytic cathodes and the performance of recently reported first trials to reach the diverse working units of a Zn–CO2 battery. However, the development of a whole Zn–CO2 battery is still rather the exception, and anodic sides as well as integration into an operative true battery system must be further investigated. Herein, mainly device engineering towards thin-film or flow batteries is discussed, as most work is still done with laboratory-level H-cell set-ups.
Table 1 Summary on the components and performance of Zn–CO2 battery
Catalytic cathodes |
Discharge |
FE (%) |
Power density |
Stability (h) |
Catalyst loading |
Energy efficiency (%) |
Ref. |
Product |
(mW cm−2) |
(mg cm−2) |
Metal-free catalysts |
CB-NGC-2 |
CO (g) |
80.4 |
0.51 |
— |
1 |
— |
34
|
C-BN@600 |
CH3OH (l) |
50.1 |
5.42 |
300 |
20 |
57.5 |
35
|
P@NCA |
CO (g) |
92 |
0.8 |
20 |
— |
— |
36
|
Non-noble metal catalysts |
Fe@NPC |
CO (g) |
70.1 |
3 |
40 |
1 |
— |
37
|
ZnTe/ZnO@C |
HCOOH (l) |
68 |
0.93 |
36 |
0.2 |
— |
38
|
Ni4N/Ni3ZnC0.7 |
CO (g) |
68.9 |
0.85 |
15 |
1 |
— |
39
|
Cu-HDA |
C2 products |
— |
6.48 |
60 |
1.5 |
— |
40
|
La0.5Sr0.5Fe0.6MnO3 |
CO (g) |
82 |
1.27 |
80 |
2 |
— |
41
|
SnO2/MXene |
HCOOH (l) |
85 |
4.28 |
60 |
1 |
41.18 |
42
|
BiC/HCS |
HCOOH (l) |
92 ± 2 |
7.2 ± 0.5 |
65 |
1 |
68.9 |
43
|
PNCB |
HCOOH (l) |
80 |
1.43 |
20 |
0.96 |
— |
44
|
Sn/NCNFs |
CO (g) |
97.6 |
1.38 |
35 |
1 |
— |
45
|
Noble metal-based catalysts |
Ag As/CC |
CO (g) |
95.3 |
2.16 |
50 |
0.5 |
— |
46
|
Coralloid Au |
CO (g) |
63 |
0.7 |
68 |
— |
— |
47
|
BiPdC |
HCOOH (l) |
52.64 |
0.42 |
45 |
1 |
— |
48
|
Single-atom catalysts |
Fe-SA/BNC |
CO (g) |
98.9 |
1.18 |
27 |
1 |
66.26 |
49
|
Fe–N4O–C/Gr |
CO (g) |
90.1 |
0.96 |
74 |
0.28 |
— |
50
|
CoPc@DNHCS-8 |
CO (g) |
94 |
1.02 |
40 |
1 |
— |
51
|
Pd1-O-CB |
CO (g) |
— |
1.72 |
100 |
0.83 |
— |
52
|
FeNC NSs-1000 |
CO (g) |
90 |
1.05 |
30 |
1 |
— |
53
|
This review will cover the reaction mechanisms, battery configurations, and catalytic cathodes of the reported aqueous Zn–CO2 battery, as shown in Fig. 1. The challenges and perspectives are also provided to enable the reader for an own judgement about the future development of high-performance Zn–CO2 batteries.
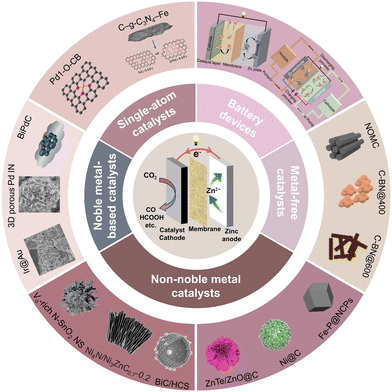 |
| Fig. 1 A “city-map” of topics and catalysts to develop a future aqueous Zn–CO2 battery. Reprinted with permission. Reproduced from ref. 29 with permission from WILEY-VCH, copyright 2019. Reproduced from ref. 33 with permission from WILEY-VCH, copyright 2018. Reproduced from ref. 35 with permission from Elsevier, copyright 2023. Reproduced from ref. 38 with permission from American Chemical Society, copyright 2022. Reproduced from ref. 39 with permission from Elsevier, copyright 2022. Reproduced from ref. 43 with permission from Elsevier, copyright 2022. Reproduced from ref. 48 with permission from American Chemical Society, copyright 2022. Reproduced from ref. 52 with permission from Elsevier, copyright 2023. Reproduced from ref. 69 with permission from WILEY-VCH, copyright 2021. Reproduced from ref. 84 with permission from WILEY-VCH, copyright 2023. Reproduced from ref. 88 with permission from WILEY-VCH, copyright 2022. Reproduced from ref. 104 with permission from WILEY-VCH, copyright 2020. Reproduced from ref. 138 with permission from WILEY-VCH, copyright 2020. | |
2 Working principles and devices
Despite the lower energy density than that of the Li–CO2 battery, the Zn–CO2 battery has some relevant advantages. It mainly uses aqueous electrolytes without generating solid discharge products, featuring a safer, more environmentally friendly, and greener operation.54,55 During the discharging process, the Zn foil is oxidized into Zn2+ at the anode and forms zincate (Zn(OH)42−) in the alkaline electrolyte, together with the simultaneous occurrence of CO2RR at the cathode. During the charging process, Zn2+ ions are redeposited at the anode, and the OER by water splitting occurs in the current systems at the cathode. The theoretical voltage of the Zn–CO2 battery is actually lower than that of the Zn–O2 battery by replacing the oxygen reduction reaction (ORR) with the CO2RR,56 but practically, it might avoid the unchangeable high overpotentials (= energy losses) in the OER while loading the battery. It also simplifies the activation of the otherwise inert ZnO by its electrochemical dissolution with, e.g., formic acid, the potential product of CO2-reduction.
We underline here that a more feasible practical Zn–CO2 battery is presumably better understood as an open asymmetric flow battery: in the deloading phase, CO2 is filled in for conversion into its reduction products to flow out, while in the loading phase, water has to be added, and oxygen flows out.
In the laboratory evaluation in an H-cell or a thin film battery set-up, we can also focus on the oxidation of formic acid, ethanol, or other liquid reduction products instead of the OER, as they are kept in the cell. Considering that a bipolar membrane (BPM) is generally used as the separator, the products on both sides cannot cross and are not affected by each other (except pH changes). Therefore, analysis of different catalytic cathodes and their selectivity towards different reaction products is the first step to fix the working mode of a Zn–CO2 battery during the charging and discharging process. For instance, when CO2 is reduced to HCOOH during the discharging process, the reactions occur as follows (eqn (1)–(8)):33
Cathodic reaction during the discharging process (catholyte: 1 M NaCl, 0.1 M HCOONa, sat. CO2):
| CO2 + 2H+ + 2e− → HCOOH | (1) |
Anodic reaction during the discharging process (anolyte: alkaline electrolyte):
| Zn2+ + 4OH− → Zn(OH)42− | (3) |
| Zn(OH)42− → ZnO + 2OH− + H2O | (4) |
Overall reaction of the discharging process:
| Zn + CO2 + 2H+ + 2OH− → ZnO + HCOOH + H2O | (5) |
Cathodic reaction during the charging process:
| HCOOH → CO2 + 2H+ + 2e− | (6) |
Anodic reaction during the charging process:
| ZnO + H2O + 2e− → Zn + 2OH− | (7) |
Overall reaction of the charging process:
| ZnO + HCOOH + H2O → Zn + CO2 + 2H+ + 2OH− | (8) |
According to these reactions, we see that replacing the oxygen in a metal air battery with the save CO
2 takes some of the theoretical voltage, but simplifies the activation of the otherwise inert ZnO by its electrochemical dissolution in aqueous formic acid. With liquid CO
2 products and avoiding OER contributions, the cell could be run as a classical film battery.
For the reduction of CO2 into CO as an example of gaseous products during the CO2RR, the reaction mechanism is shown in eqn (9)–(18):29
Cathodic reaction during the discharging process (catholyte: KHCO3 sat. CO2):
| CO2 + 2H+ + 2e− → CO + H2O | (9) |
Anodic reaction during the discharging process (anolyte: alkaline electrolyte):
| Zn2+ + 4OH− → Zn(OH)42− | (11) |
| Zn(OH)42− → ZnO + 2OH− + H2O | (12) |
Overall reaction of the discharging process:
| Zn + CO2 + 2H+ + 2OH− → Zn(OH)42− + CO + 2H2O | (13) |
Cathodic reaction during the charging process:
| H2O → 1/2O2 + 2H+ + 2e− | (14) |
Anodic reaction during the charging process:
| Zn(OH)42− → Zn2+ + 4OH− | (15) |
| ZnO + H2O + 2OH− → Zn(OH)42− | (17) |
Overall reaction of the charging process:
| Zn(OH)42− + H2O → Zn + 1/2O2 + 2H+ + 4OH− | (18) |
As mentioned above, the OER with sluggish reaction kinetics during the charging process tends to reduce the energy efficiency of the battery. In addition, the high charging voltage can reduce the stability of catalysts. Meanwhile, ZnO can be produced and precipitated in the anodic side during the cycles, which retard the Zn plating/stripping processes and contaminate the electrolyte, resulting in poor cycling performance. Recently, Liu
et al. have replaced the OER with the oxidation of reducing molecules, which can reduce the charging voltage from 2.45 V to 1.35 V at 4.29 mA cm
−2. During the charging process, the reducing molecules can be oxidized to N
2, acetone, or sulfate. This coupling can also effectively improve the stability of catalysts, benefiting the long cycling performance of the battery.
57
Here, we see that the cell runs in an open fashion: the battery cycle produces CO and oxygen from CO2 and water, and one part of the invested energy is stored as electricity and the other part is stored as chemical energy: the cell is bifunctional. Ideally, it is then in the hand of the scientists to run and switch between a “closed” and “open” operation modes, depending on the actual wish to create chemicals or store electricity.
Recent studies have focussed on the design of electrochemical cells and materials due to their key role in improving the performance, including the current density, faradaic efficiency (FE), and stability of the CO2RR to a maximal possible extent.58 Working in an H-cell (Fig. 2a) is the current lab practice for evaluating the catalysts in the Zn–CO2 battery. The cell is generally divided into an anode chamber and a cathode chamber, which are connected to each other by a connecting channel and separated by ion exchange membranes. At present, the BPM is widely used to maintain the pH due to the different electrolytes used in each channel. The working electrode and reference electrode are placed in the cathode chamber, and the counter electrode is placed in the anode chamber. Such a structure ensures to some extent that the products on both sides are not cross-contaminated during the reaction process, and different electrolytes can be used on both sides. The separation of the different reactions improves the stability of the electrocatalysts. As the mass transfer efficiency in the H-cell is relatively low due to the larger geometric distances involved, the current density achieved in the H-cell is generally far below the requirement of a practical device.
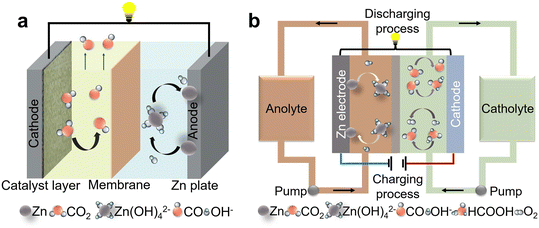 |
| Fig. 2 Schematic diagram of the H-cell (a) and flow cell (b). | |
For a real Zn–CO2 battery targeted for practical applications, an open and asymmetric flow system has to be designed, where CO2 is continuously filled in and the reductive products flow out; meanwhile, water has to be added due to the consumption for O2 generation (Fig. 2b). Compared to the widely used H-cell, the flow cell has much smaller distances between the anode and the cathode, which is beneficial for improving transport, minimizing polarization, and thereby improving power density and energy efficiency. Another issue to address in flow batteries is that conditions such as pH and substrate concentrations change along the channel length. For example, the pH around the cathode could be >7 even when the electrolyte is an acid-based solution, originating from the fast reaction kinetics.59,60 In such cases, the competing HER can be inhibited, while formic acid can be produced instead of formate, without the necessity of further acidification and distillation. In addition, the battery can run in a nearly constant mode (but at a lower voltage) with the usage of a flowing fluid reservoir. Therefore, further improvements of Zn–CO2 batteries in terms of energy efficiency and stability are expected by employing flow cells or other specifically designed electrolytic cells.41,61
3 Catalytic cathodes
As discussed, the electrocatalysts on the cathodes play a very important role in the reaction kinetics and reaction products of the CO2RR. In the Zn–CO2 battery, catalytic cathodes with diverse chemical and electronic structures were applied, which greatly affect the working mechanism, power density, and cycling stability of the battery. We can classify the reported approaches into four types of catalysts: metal-free catalysts, non-noble metal catalysts, noble metal-based catalysts, and single-atom catalysts.
3.1 Metal-free catalysts
Carbon-based materials have been the main metal-free catalysts investigated for the Zn–CO2 battery due to their good electrical conductivity, large specific surface area, high chemical inertness, and the simplicity to adjust their structure.62,63 In addition, they are easily available in various forms such as graphenes, carbon nanotubes, or heteroatom-containing carbonized derivatives from organic precursors.64–66 Normally, pristine carbon materials are electrochemically inert to the CO2RR because all the unipolar and only weakly polarizable carbon structures have no clear path to strongly adsorb and activate the CO2 molecules. Therefore, the electronic structure of C materials has been generally optimized by introducing heteroatoms (e.g., N, B, and P) into the carbon skeleton and constructing carbon defects, which all provide the active sites. It is well analyzed and understood that the doping of heteroatoms increases adsorption enthalpies, reduces the reaction energy barrier of the CO2RR, and enhances, for instance, the adsorption of the critical intermediates (e.g., one electron–proton *COOH) near the active sites.67,68
For example, Gao et al. used the mesoporous silica species SBA-15 as a template to synthesize a nitrogen-doped ordered mesoporous carbon (NOMC), which exhibited abundant parallel channels.69 The resulting high specific surface area (794.6 m2 g−1) and abundant nitrogen active sites of the NOMC were shown to be beneficial for the transport of reactants during both the CO2RR and OER processes. Specifically, the current density of the NOMC (−15.9 mA cm−2) was higher than the control samples including ordered regular mesoporous carbon (OMC) (−2.7 mA cm−2) and nitrogen-doped carbon (NC) (−2.1 mA cm−2) for the CO2RR at −0.8 V vs. RHE. Further theoretical calculations suggested that N-doping could significantly reduce the energy barrier required for *COOH formation, which was considered responsible for the enhanced CO2-to-CO activity of the NOMC. In addition, the FE of the NOMC for the CO2RR was close to 100% (Fig. 3a) with stable running for 80 h at −0.47 V. Meanwhile, the overpotential of the OER at 10 mA cm−2 was as low as 586 mV (Fig. 3b), attributed to both the N-doping and exposed surface of the catalyst. The Zn–CO2 battery could achieve a peak power density of 0.71 mW cm−2 and an energy efficiency of 52.8%. Besides, it maintained 76% of FECO at a discharge current density of 1 mA cm−2 and stable cycling for 300 times. Of course, the FE is relatively low for a real-life battery, but the concept was pioneering.
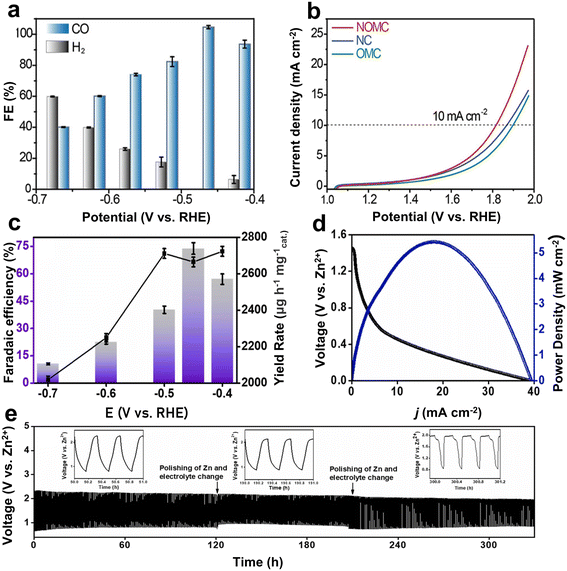 |
| Fig. 3 (a) FECO in the potentiostatic electrolysis. (b) OER performance evaluated by the linear sweep voltammetry curves of NOMC, OMC, and NC in a 0.8 M KHCO3 electrolyte with a scan rate of 10 mV s−1. Reproduced from ref. 69 with permission from Wiley-VCH, copyright 2021. (c) Bar graph representing the FE and yield rate of CH3OH as a product calculated from UV at different potentials. (d) Discharge curve and corresponding power density of the cell at a scan rate of 5 mV s−1. (e) Galvanostatic charge–discharge cycles at 1 mA cm−2. Reproduced from ref. 35 with permission from Elsevier, copyright 2023. | |
In addition to the widely studied N, co-doping with other non-metal elements (e.g., B, P, and S) can differently modify the electronic structure by inducing the polarization of carbon near heteroatoms, thereby enhancing the CO2RR activity.35,70,71 For instance, Kaur et al. designed and synthesized a B/N-doped carbon with tubular morphology (C-BN@600) as the catalytic cathode for an aqueous Zn–CO2 battery.35 The higher surface area of the nanotubular morphology provided more electrochemically active sites, and the presence of heteroatoms B and N improved the mass and charge transport at the electrode-electrolyte interface and thus faster kinetics. As the main adsorption sites, B with insufficient electrons could adsorb the O of CO2, while N with a lone pair of electrons binds to C of CO2. Compared with N in composite materials, B showed a lower adsorption free energy for the intermediates *COOH and *CO. The better CO2RR electrocatalytic ability of C-BN@600 was reflected in a more positive onset potential and larger current density. Meanwhile, the synergistic effect of B/N promoted the effective adsorption of intermediates and accelerated the electron transfer to liberate CH3OH with an FE of 74% (Fig. 3c). The assembled Zn–CO2 battery exhibited a power density of 5.42 mW cm−2 and enabled stable cycling for more than 300 h (Fig. 3d and e).
3.2 Non-noble metal catalysts
Although the metal-free catalysts are widely studied with the merits of low cost, chemical inertness, and good conductivity, their catalytic performance is fundamentally limited due to the active sites only based on covalent chemistry. Non-noble metals (e.g., Fe, Co, and Ni) are good choices, as they are easily available, with abundant active sites, and have been demonstrated to show good catalytic activity and high selectivity.72–75 In particular, supporting non-noble metal species with carbon-based materials can maintain good dispersion of metals and reduces the agglomeration of high surface area species.76,77 Adjusting the carbon skeleton can also change the electron density in the metal center and improve the electrochemical performance of the composite material.78,79
Metal–organic frameworks (MOFs) have attracted attention as precursors of non-noble metal-based catalysts due to their ordered structural morphology and unique coordinative sites.80 After carbonization, heteroatom-doped metal/carbon composites can be obtained with high porosity, high specific surface area, and tunable pore size.81–83 Teng et al. prepared a ZnTe-MOF-derived ZnTe/ZnO@C (Fig. 4a) as the electrocatalyst, which showed a selectivity of 83% towards formate at −1.1 V (vs. RHE) (Fig. 4b).38 The high performance was attributed by the authors to the special flower-like structure of ZnTe/ZnO@C, which could provide abundant active sites. Further theoretical calculations revealed that the synergistic effect between ZnTe sites and ZnO was also conducive to the performance improvement. In particular, ZnTe(111) was favorable to the first step of proton-coupled electron transfer and easily adsorbed the *OCHO intermediate (−1.55 eV), and the subsequent proton-coupled electron transfer to form *HCOOH occurred on ZnO(100). Meanwhile, ZnTe/ZnO@C also had good catalytic performance for OER in the neutral carbonate solution (10 mA cm−2 at 1.9 V) (Fig. 4c). After being assembled into a Zn–CO2 battery, the battery exhibited stability for 36 h and a peak power density of 0.93 mW cm−2 (Fig. 4d and e). Actually, a typical, obvious hysteresis between loading and de-loading curves can be observed (Fig. 4e), indicating that a potential battery use is inhibited by high polarization and suboptimal kinetics. Hence, the peak power density is much lower than that of practical batteries, including lithium-ion batteries. The presented concept of a Zn–CO2 battery is interesting from an academic view, but needs engineering developments towards a real battery application.
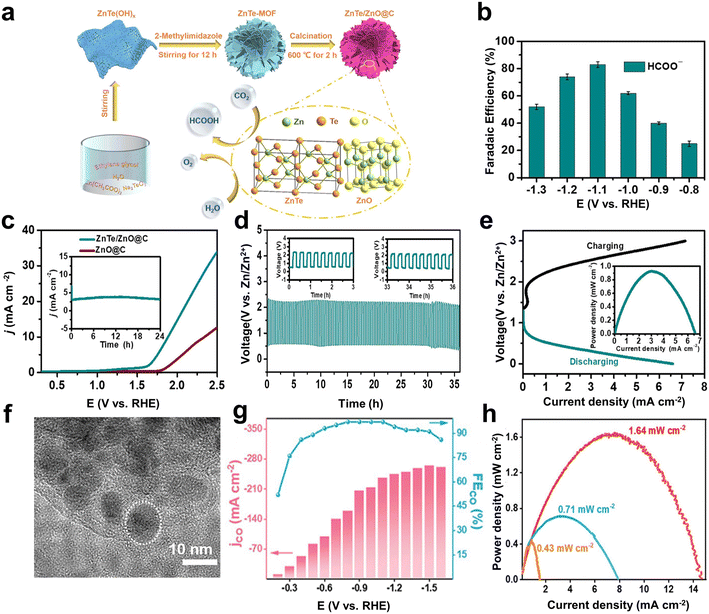 |
| Fig. 4 (a) Schematic illustration for the preparation of ZnTe/ZnO@C as a bifunctional electrocatalyst for the selective CO2 reduction coupled with the OER. (b) Variations in FEHCOO− with potentials. (c) LSV curves for the OER for ZnTe/ZnO@C and ZnO@C electrodes in 0.5 M KHCO3. The inset shows the results of OER stability measurements on ZnTe/ZnO@C for a period of 24 h at 1.7 V. (d) Galvanostatic discharge–charge curves at 1 mA cm−2 for 108 cycles over 36 h. (e) Charge–discharge polarization curves for a Zn–CO2 battery. The curve in the inset shows the power density at different current densities. Reproduced from ref. 38 with permission from American Chemical Society, copyright 2022. (f) High-resolution TEM (HRTEM) image of Ni@N–C. (g) FECO% and JCO with different applied potentials of Ni@N–C in the flow cell. (h) Power density curves of Zn–CO2 battery. Reproduced from ref. 84 with permission from Wiley-VCH, copyright 2023. | |
In addition, Wang et al. used SBA-15 as a template to prepare nickel-based metal–organic frameworks (Ni-MOFs), giving finally Ni nanoparticles encapsulated in N-doped carbon shells (Ni@N–C) via pyrolysis and etching (Fig. 4f).84 The doping of N could adjust the local electronic structure in the carbon shell, enabling the stronger CO2 adsorption, faster electron transfer rate, and more exposed active sites in synergy with metallic Ni. As revealed by the theoretical calculations, the Fcc-Ni@N–C had weak van der Waals interaction between N–C and metallic Ni and tended to evolve into more stable Top-Ni@N–C after the adsorption of CO2. The other model of Hcp-Ni@N–C had a large thermodynamic barrier in the step of CO2 adsorption, because the CO2 bonding to one N atom caused the breaking of one N–Ni bond. Therefore, the most thermodynamically stable configuration was N–C located on the top of Ni slabs (Top-Ni@N–C), which had the lowest thermodynamic barrier to the formation of *COOH and the desorption of CO. As a result, Ni@N–C exhibited a large current density of 244 mA cm−2 and a high FEco of 97% at −1.1 V (vs. RHE) in the flow cell (Fig. 4g). As the catalytic cathode for the Zn–CO2 battery, a maximum power density of 1.64 mW cm−2 and a cycling performance of 45 h were achieved (Fig. 4h).
Compared to N coordination, the P atom has a multi-electron p orbital and relatively weak electronegativity, providing additional properties in tuning the electronic structure of substrates.85–87 Liu et al. introduced Fe–P nanocrystals into N-doped carbon polyhedrons (Fe–P@NCPs) (Fig. 5a).88 Compared with N-doped carbon polyhedrons (NCPs), the Fe–N doped carbon polyhedrons (Fe-NCPs) and Fe–P@NCPs showed better CO2RR performance. These results indicated that Fe–N and Fe–P active sites could play important roles toward the CO2RR. As mentioned above, the P atom had relatively weak electronegativity, allowing electrons to transfer less against a diodic surface potential. As a result, the Fe–P unit, as the activation center of CO2, could easily transfer more electrons for CO2 reduction. As a result, Fe–P@NCPs showed a higher CO selectivity (FECO = 95%) (Fig. 5b). In addition, Fe–P@NCPs showed an overpotential of 840 mV at 10 mA cm−2 for the OER in aqueous KHCO3 (0.1 M) as the electrolyte (Fig. 5c). The Zn–CO2 battery (referred to H-cell) with the Fe–P@NCP cathode showed a peak power density of 0.85 mW cm−2 but maintained outstanding stability over 7 days without degradation (Fig. 5d and e). It has to be mentioned that the alkaline electrolyte in the H-cell will dominantly react and buffer with CO2. Therefore, the flow cell coupled with a gas diffusion electrode, which can employ the alkaline electrolyte, is a desirable configuration for the Zn–CO2 battery.
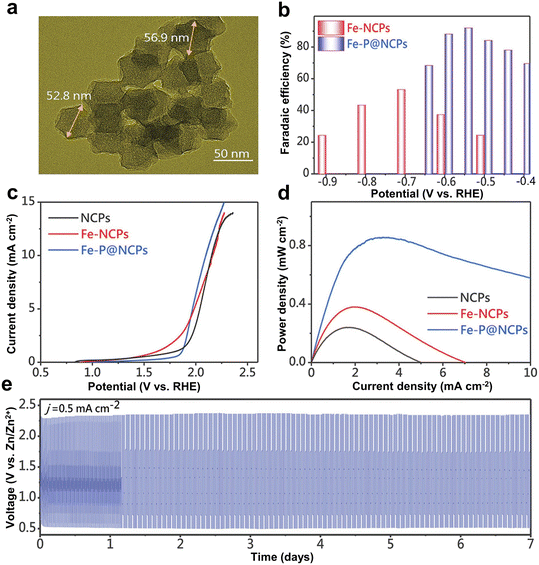 |
| Fig. 5 (a) TEM image of the synthesized Fe–P@NCPs. (b) FECO values at different applied potentials for Fe-NCPs and Fe–P@NCPs. (c) OER evaluation by the LSV curves in a 0.1 M KHCO3 solution. (d) Power density curves of the aqueous Zn–CO2 cell based on different samples. (e) Galvanostatic discharge–charge cycling curves at 0.5 mA cm−2 for Fe–P@NCPs. Reproduced from ref. 88 with permission from Wiley-VCH, copyright 2022. | |
It has also been reported that introducing solar energy into an electrochemical device (e.g., Li–CO2, Li–O2, Zn–air) can improve the energy efficiency and reaction kinetics of the CO2RR and ORR.89–92 Liu et al. developed an ultra-thin Cu2O/CuCoCr-LDH (layered double hydroxide) photocathode.93 Benefiting from the ultra-thin p–n heterojunction nanosheets on the photocathode, it could not only effectively separate photogenerated electrons and holes and promote electron transfer, but also provide abundant CO2 adsorption and reaction sites. As a result, the Cu2O/CuCoCr-LDH showed a good selectivity (FE = 90.14%) and a yield of 1167.6 μmol g−1 h−1 towards CO. As the cathode of a model Zn–CO2 battery under light, the battery had a discharge voltage of 1.22 V (higher than the discharge voltage of a light-free Zn–CO2 battery of 0.59 V), and exhibited good cycling stability within 54 h.
Bi-based catalysts have demonstrated good adsorption properties for *OCHO intermediates, and thus, can selectively catalyze the reduction of CO2 to formate.94–96 The *OCHO intermediate can be stabilized by regulating the electron accumulation of Bi nanoparticles by supports containing pyrrolic-N. Besides, benefitting from the combined effect of special structural design and good conductivity, the catalyst exhibits faster reaction kinetics and high formate formation rate.44 For example, Yang et al. demonstrated that Bi clusters dispersed on the hollow carbon spheres (BiC/HCS) (Fig. 6a) could stabilize the *OCHO intermediate, which can facilitate the CO2RR to form formate and inhibit the competitive HER as well.43 The maximum FEformate at −0.6 V (vs. RHE) was 97 ± 2% (Fig. 6b). The Zn–CO2 battery based on the BiC/HCS cathode showed a peak power of 7.2 ± 0.5 mW cm−2 and a cycling performance of 200 times. In addition, the introduction of other metals (such as Cu, Ti, and In) into Bi-based materials enhanced the adsorption of *OCHO intermediates and improved the FE of formic acid.97–99 For instance, a Bi/In bimetal catalyst turned out to be favorable for producing the key intermediate *OCHO from CO2 to formate, and synergistically reduced the free energy from *OCHO to *HCOOH, thereby promoting the production of HCOOH.99
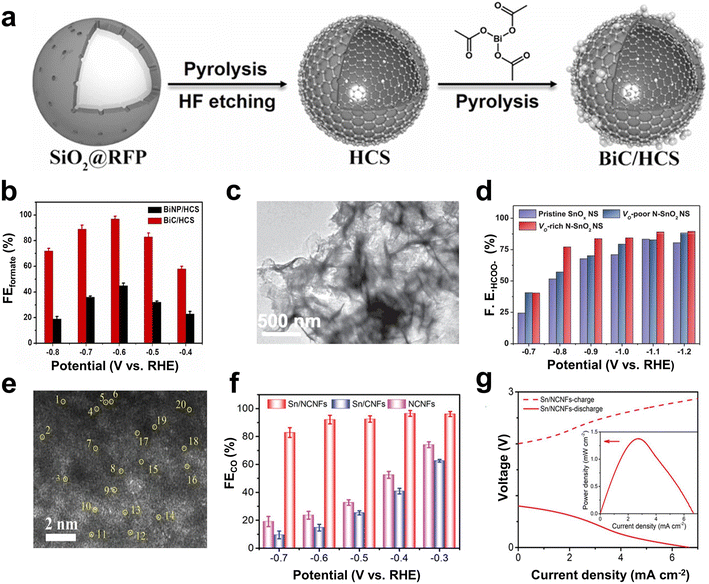 |
| Fig. 6 (a) Schematic illustration of the synthesis procedure. (b) FEformate values at different applied potentials. Reproduced from ref. 43 with permission from Elsevier, copyright 2022. (c) TEM images of the VO-rich N-SnO2 NS. (d) FEHCOO− of the pristine SnOx NS, the VO-poor N–SnO2 NS and VO-rich N–SnO2 NS at different potentials. Reproduced from ref. 104 with permission from Wiley-VCH, copyright 2020. (e) HAADF-STEM image of Sn/NCNFs with atomic-level Sn atoms highlighted by yellow circles. (f) FECO of Sn/NCNFs, Sn/CNFs, and NCNFs at different potentials in a flow cell. (g) Polarization curves and power density (inset) of Sn/NCNFs. Reproduced from ref. 45 with permission from Wiley-VCH, copyright 2022. | |
In addition, In- and Sn-based metal catalysts have been proven to have good selectivity towards formate.100–102 Teng et al. designed an In/ZnO@C hollow nanocube electrocatalyst.103 Through in situ doping, In could be evenly distributed on the cube, and the hollow cube structure provided more exposed active sites. Therefore, In/ZnO@C exhibited a good catalytic activity and formic acid selectivity. At −1.2 V (vs. RHE), the partial current density for formate production reached 23.5 mA cm−2 with an FE of 90%. As an electrocatalytic cathode for the aqueous rechargeable Zn–CO2 battery, the battery reached a power density of 1.32 mW cm−2 and was cycled for 51 h at a current density of 1 mA cm−2. Li et al. prepared N-doped SnO2 nanosheets with oxygen vacancies (VO-rich N–SnO2 NS) (Fig. 6c).104 The electronic junction and geometric structure of SnO2 were adjusted via N doping and VO, which was expected to be beneficial for the protonation pathway of *OCHO while inhibiting the adsorption of *H on the SnO2 surface. At a potential of −0.9 V, VO-rich N–SnO2 NS exhibited good CO2RR performance, and the FE of HCOO− was about 83% (Fig. 6d). However, by regulating the electronic structure of the active site, the binding energy of the active center Sn to *OCHO and *COOH intermediates could be changed, which in turn changed the CO2RR product.105 Hu et al. demonstrated that Sn–N as the active site in porous N-doped carbon nanofibers (Sn/NCNFs) (Fig. 6e) accelerated the dissociation and proton transfer of H2O, thereby promoting the formation of *COOH intermediates.45 Meanwhile, the pyrrolic N adjacent to the Sn–N active site helped to reduce the formation energy barrier of the *COOH intermediate, thereby improving the kinetics of the CO2RR. As a result, Sn/NCNFs exhibited a high CO2RR activity, and the FE of CO was as high as 96.5% (Fig. 6f). The peak power density of the Zn–CO2 battery with Sn/NCNFs as the cathode reached 1.38 mW cm−2 (Fig. 6g).
3.3 Noble metal-based catalysts
Despite scarcity and high cost, noble metal catalysts are still widely used in the field of electrochemistry due to their excellent catalytic activity, high stability and high selectivity.106–108 In this field, it is a challenge to use less noble metals for the same reactivity. For that, it has been demonstrated that the catalytic performance of noble metal catalysts can be optimized through crystal facet control and nanostructure engineering.109–113 In addition, strategies such as alloying other metals or loading on conductive substrates are used to leverage the catalytic performance of precious metals while reducing costs.114,115 Currently, noble metal catalysts including Pd, Au, and Ag are still typical choices for the aqueous Zn–CO2 battery.46,48,116
Pd catalysts can not only reduce CO2 to HCOOH, but also oxidize HCOOH to CO2.117,118 Therefore, Pd-based catalysts are suitable as bifunctional catalytic cathodes for the Zn–CO2 battery. In 2018, Xie et al. proposed and prepared a reversible Zn–CO2 battery for the first time (Fig. 7a).33 The battery used Pd nanosheets as the bifunctional catalytic cathode (Fig. 7b) and realized reversible conversion between CO2 and HCOOH during the discharging and the charging process. In order to obtain good catalytic performance for the CO2RR and the oxidation of formic acid (FAO), respectively, the morphology and structure of Pd-based catalysts were carefully modified. The experimental results showed that Pd nanosheets rich in edges and pores promoted the CO2RR towards HCOOH with an FE of 90% (partial current density was 15 mA cm−2) (Fig. 7c). Moreover, FAO could produce a stable current of 4.3 mA cm−2 at a voltage of 0.3 V, and gas chromatography proved that the product was CO2. A Zn–CO2 battery based on Pd nanosheets as the cathode showed an energy efficiency of 81.2% and a cycling durability of more than 100 cycles.
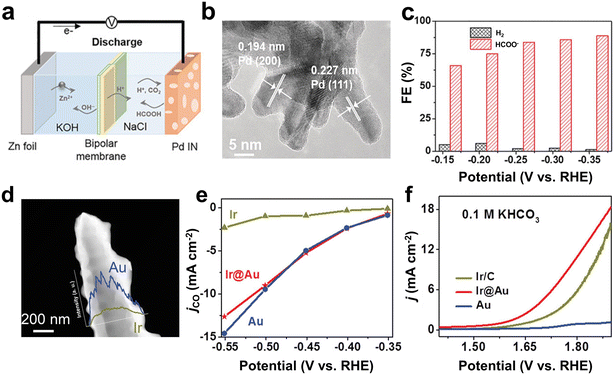 |
| Fig. 7 (a) Schematic of the reversible aqueous Zn–CO2 battery. (b) HRTEM image of the as-prepared Pd nanosheet. (c) FE of different products at various potentials. Reproduced from ref. 33 with permission from Wiley-VCH, copyright 2018. (d) HAADF image and corresponding linear elemental mapping of gold and iridium. (e) Partial current density of CO (jCO) in potentiostatic electrolysis. (f) OER performance evaluated by the LSV curves. Reproduced from ref. 29 with permission from WILEY-VCH, copyright 2019. | |
To explore bi-functional noble metal-based catalytic cathodes for Zn–CO2 batteries, Wang et al. prepared Ir@Au bimetallic nanomaterials by combining chemical deposition and electrochemical deposition (Fig. 7d).29 In particular, it was found that pristine Ir showed a higher partial current density of HER than that of Ir@Au and Au, while the partial current density to CO was significantly lower than that of the other two samples (Fig. 7e). In comparison, the partial current density of CO from pure Au was slightly higher than that of Ir@Au. Therefore, the results showed that the introduction of Au could inhibit the occurrence of HER in Ir@Au and generate a higher selectivity of Au for CO. In addition, Ir@Au performed comparably to Ir/C catalysts as a commercial OER catalyst. Accordingly, the Ir@Au bimetallic nanomaterial exhibited a pleasing selectivity for CO (−0.5 V, FE ≈ 85%) and good OER performance with a current density of 10 mA cm−2 at 1.79 V (Fig. 7f). As a catalytic cathode of Zn–CO2, the battery had an energy efficiency of 68% and a cycling stability of 30 h. It is however clear that Ir is a very rare element and thereby not favorable for large-scale practical applications.
3.4 Single-atom catalysts
Single-atom catalysts (SACs) have received great attention due to their atom efficiency, as well as their unique catalytic properties. Compared to the nanoparticle-based catalysts, the single-atom metal active sites distributed on a conductive substrate can achieve maximum atomic utilization.119–121 Importantly, the catalytic activity and selectivity in a specific metal atom can be fine-tuned in a wider range through its coordination environment.122–125 Usually, metals are introduced into heteroatom-doped carbon materials by methods such as pyrolysis and electrochemical deposition to adjust the electronic structure of the active center, thereby modifying the CO2RR and OER performance of the catalyst.126–128
For example, Li et al. constructed N- and O-coordinated single-atom Pd catalysts (Pd1-N-CB and Pd1-O-CB, respectively).52 The results showed that the asymmetric coordination and strong regulation of O to the Pd center enabled Pd1-O-CB to show excellent CO2RR selectivity for CO at lower potentials (99.6% under −0.6 V vs. RHE). The theoretical calculations further proved that the O-coordinated Pd1-O-CB provided more electrons than the N-coordinated Pd1-N-CB to the *COOH intermediate, and thus achieved the lowest free energy of the potential-determining step (PDS) (ΔG = 1.02 eV). In addition, the desorption of *CO on Pd1-O-CB was easier than that of Pd nanoparticles, preventing CO product inhibition of Pd active sites. As the cathode of a Zn–CO2 battery, the Pd1-O-CB cathode showed a power density of 1.72 mW cm−2 and an endurance of at least 100 h for CO production.
Currently, the metal/N-doped carbon (M–N–C) is a widely studied class of electrocatalysts. The doping with N can reduce the formation energy of CO2RR intermediates such as *COOH and *OCHO on the M–N active site, thus showing higher selectivity.129–131 Compared with noble metals, single-atom catalysts of transition metals such as Fe, Co, and Ni have more economic benefits.132–135 For example, Gong et al. anchored cobalt phthalocyanine on N-doped hollow carbon spheres with carbon defects (CoPc@DNHCS-8) (Fig. 8a).51 The N-doping strategy provided the electron-withdrawing effect of the carbon matrix, resulting in the formation of Co(II) sites. Under cathodic bias, Co(II) could obtain electrons and be reduced to Co(I), which was generally considered to be the catalytic active site for CO2 reduction. The CO2 could gain electrons and be activated at the Co(I) site to form the intermediate *COOH (Fig. 8b). As a result, CoPc@DNHCS-8 showed a high FECO of 95.68%. The Zn–CO2 battery based on CoPc@DNHCS-8 exhibited a maximum power density of 1.02 mW cm−2 and a cycling stability of 40 h (Fig. 8c).
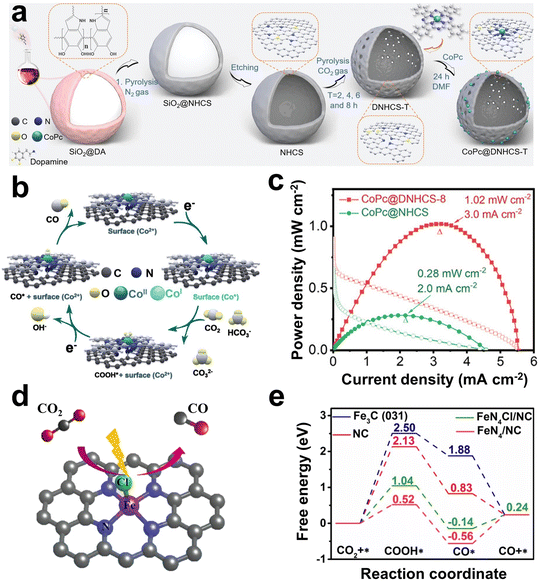 |
| Fig. 8 (a) Scheme of the formation of CoPc@DNHCS-T series (T = 2–8). (b) Mechanism model diagram of CO2-to-CO reaction pathway. (c) Power density curves of the Zn–CO2 battery based on CoPc@DNHCS-8 and CoPc@NHCS as the cathode. Reproduced from ref. 51 with permission from Wiley-VCH, copyright 2022. (d) Schematic structural model of FeN4Cl/NC. (e) Free-energy profiles of reaction intermediates in the CO2RR on different catalysts. Reproduced from ref. 134 with permission from Elsevier, copyright 2021. | |
Single-atom Fe-based catalysts are another promising choice, due to the abundance of iron and its low onset potential in the CO2RR.136,137 However, some studies have proven that in the coordination form of Fe–N4, the active sites are inhibited due to the strong adsorption of *CO intermediates.138–140 Ni et al. found that the true active sites for CO2 reduction were associated with intrinsic defects rather than the Fe–N4 centers. Further calculations showed that these defects, coupled with Fe–N4, demonstrated a diminished energy barrier for CO2 reduction and concurrently mitigated hydrogen evolution activity.138 In addition to the N atoms, other types of heteroatoms such as Cl, B, P, and O are often used to coordinate with single metal atoms.49,50,134 For example, Li et al. adjusted the electronic structure of Fe in Fe–N4 by introducing an axial chlorine (Cl) ligand (Fig. 8d), thereby promoting the desorption of *CO and inhibiting the adsorption of *H.134 Further DFT calculations showed that the formation energy barrier of *COOH on FeN4Cl in N-doped carbon (FeN4Cl/NC) (1.04 eV) was lower than that on NC (2.13 eV) and Fe3C (2.50 eV), but higher than that on Fe–N4/NC (0.52 eV) (Fig. 8e). Besides, desorption of *CO to CO had a lower energy barrier on FeN4Cl/NC (0.38 eV) than that on Fe–N4/NC (0.80 eV). In addition, the adsorption energy of *H on FeN4Cl/NC was higher than that of Fe–N4/NC and Fe3C, indicating that the introduction of Cl atoms inhibited HER. Therefore, FeN4Cl/NC exhibited excellent CO2RR performance, showing a high FECO of 90.5% at a promising current density of 10.8 mA cm−2 with an overpotential of 490 mV. As the cathode of an aqueous Zn–CO2 battery, the battery showed a power density of 0.545 mW cm−2 and a cycling stability of 15 h.
4 Summary and outlook
Overall, we reviewed a very interesting collection of current studies which indicate the high potential of aqueous Zn–CO2 batteries as a green and sustainable energy storage and conversion technology. The use of compressed CO2 saves more, showing better solubility in aqueous solvents, than the current Zn–O2 battery (which is very difficult to cycle effectively), and it conceptually avoids the high overpotentials and, thereby, energy losses of ORR and OER. In addition, CO2 reduction products such as formic acid promote the redissolution of ZnO, thereby improving the cyclability. The battery however can also be run in an asymmetric open flow mode, thus combining energy storage with the electrochemical production of CO2-based chemicals in discharging while oxidizing water to O2 in the charging process. This disruptive concept that combines CO2 conversion and energy supply comes with the disadvantage of losing energy in the OER again while being flexible to switch between electricity and chemical generation, depending on the actual demand.
As the Zn–metal anode is well understood and the state of the art, research efforts mainly focus on the development of efficient electrocatalytic (flow) cathodes. In view of the different redox reactions of the Zn–CO2 battery during the charging and discharging processes, the electrocatalytic cathode needs to be able to simultaneously promote the CO2RR to defined products while suppressing the HER as the competing, here parasitic reaction. For this purpose, traditional noble metal-based catalysts (Pd, Ag, etc.), but more scalable, also metal-free carbon-based material catalysts, non-noble metal catalysts, and supported single-atom catalysts were developed as potential cathode materials for a potential Zn–CO2 battery.
However, the research on aqueous Zn–CO2 batteries is still in its infancy, and a number of questions should be addressed. First, the catalytic cathodes determine whether the CO2RR product during the discharging process is a gas-phase or liquid-phase product. C
O is, in the consumer space, a critical product which comes with safety measures, but higher-value reduction products such as methanol also come with a higher energy storage density (6 electrons instead of only 2). A liquid-phase product could be oxidized to regenerate CO2, enabling a closed battery system. In an open, switchable system, the reaction that occurs at the cathode during the charging process is the OER, and at the anode, it is the redeposition of Zn2+. The catalytic performance of the electrocatalyst for the OER will critically affect the energy efficiency of the overall system.
Second, most of the current research studies are conducted with H-cells, and the voltage hysteresis between loadings is correspondingly high, mostly due to the polarization issues and long charge transport distances involved. Going to a real, flat and thin battery geometry comes with extra problems, which however should be tackled before calling such systems “batteries”. One of the problems is the limited solubility of CO2 in aqueous electrolytes, which is not an issue in high-solvent volume H-cells, but requests either a three-phase boundary gas electrode or a flow cell geometry for the practical solutions. Improving the solubility of CO2 in electrolytes and/or pressurized cells (“soda cells”) could also be a focus for future experiments. As it is still unclear whether dissolved CO2 or HCO3− in the electrolyte operates as the carbon source in the discharging process, further research might consider investigating the underlying mechanims by providing the specific carbon sources. This would extend the engineering operation range of such devices. Besides, further research could focus on the improvement of reaction kinetics on the anode side, guiding, for instance, the uniform deposition of Zn2+ on the anode surface. This problem is general for all metal anodes in batteries.
For further study, some additional strategies to improve the overall performance of Zn–CO2 batteries are proposed: (1) developing high-performance catalytic cathodes with low overpotentials for both CO2RR and OER; (2) improving the stability of catalytic cathodes, which are capable of standing the repeated reduction and oxidation processes as the bifunctional catalysts; (3) improving the stability of Zn electrodes, which also plays a key role in enhancing the cycling performance of Zn–CO2 batteries; (4) replacing the BPM with other ion exchange membranes using electrolytes with close pH values on both sides, since the BPM requires an additional voltage of ∼0.83 V for water dissociation.
In summary, the aqueous Zn–CO2 battery shows great potential in energy storage and CO2 utilization, but current systems are still far from true application, and in-depth research efforts beyond “only” cathodic electrocatalysis are still limited. We however strongly believe that these issues can be successfully addressed in a more holistic fashion, and that Zn–CO2 batteries have a serious potential as a disruptive device technology on a large scale to switch on demand between CO2 utilization and sustainable energy storage.
Conflicts of interest
The authors declare no conflict of interest.
Acknowledgements
This work was supported by the National Natural Science Foundation of China (No. 22109044, 52373205, 52003251, 52102166), Natural Science Foundation of Shanghai, China (No. 22ZR1418500), start-up funds from the East China University of Science and Technology, and Henan Center for Outstanding Overseas Scientists (GZS2022014). The computing work in this paper is supported by the Public Service Platform of High Performance Computing by the Network and Computing Center of HUST.
References
- D. Schimel, B. B. Stephens and J. B. Fisher, Effect of increasing CO2 on the terrestrial carbon cycle, Proc. Natl. Acad. Sci. U. S. A., 2015, 112, 436–441 CrossRef CAS PubMed.
- S. C. Doney, V. J. Fabry, R. A. Feely and J. A. Kleypas, Ocean acidification: the other CO2 problem, Annu. Rev. Mar. Sci., 2009, 1, 169–192 CrossRef PubMed.
- S. L. Wells and J. DeSimone, CO2 technology platform: an important tool for environmental problem solving, Angew. Chem., Int. Ed., 2001, 40, 518–527 CrossRef CAS PubMed.
- M. Liu, Y. Pang, B. Zhang, P. D. Luna, O. Voznyy, J. Xu, X. Zheng, C. T. Dinh, F. Fan, C. Cao, F. P. G. de Arquer, T. S. Safaei, A. Mepham, A. Klinkova, E. Kumacheva, T. Filleter, D. Sinton, S. O. Kelley and E. H. Sargent, Enhanced electrocatalytic CO2 reduction via field-induced reagent concentration, Nature, 2016, 537, 382–386 CrossRef CAS PubMed.
- Z. Lyu, S. Zhu, M. Xie, Y. Zhang, Z. Chen, R. Chen, M. Tian, M. Chi, M. Shao and Y. Xia, Controlling the surface oxidation of Cu nanowires improves their catalytic selectivity and stability toward C2+ products in CO2 reduction, Angew. Chem., Int. Ed., 2021, 60, 1909–1915 CrossRef CAS PubMed.
- S. Yang, H. An, S. Arnouts, H. Wang, X. Yu, J. de Ruiter, S. Bals, T. Altantzis, B. M. Weckhuysen and W. van der Stam, Halide-guided active site exposure in bismuth electrocatalysts for selective CO2 conversion into formic acid, Nat. Catal., 2023, 6, 796–806 CrossRef CAS.
- Y. Li, E. P. Delmo, G. Hou, X. Cui, M. Zhao, Z. Tian, Y. Zhang and M. Shao, Enhancing local CO2 adsorption by L-histidine incorporation for selective formate production over the wide potential window, Angew. Chem., Int. Ed., 2023, 62, e202313522 CrossRef CAS PubMed.
- Z. Wang, Y. Li, X. Zhao, S. Chen, Q. Nian, X. Luo, J. Fan, D. Ruan, B.-Q. Xiong and X. Ren, Localized alkaline environment via in situ electrostatic confinement for enhanced CO2-to-ethylene conversion in neutral medium, J. Am. Chem. Soc., 2023, 145, 6339–6348 CrossRef CAS PubMed.
- J. Mao, Y. Wang, B. Zhang, Y. Lou, C. Pan, Y. Zhu and Y. Zhang, Advances in electrocarboxylation reactions with CO2, Green Carbon, 2024 DOI:10.1016/j.greenca.2024.02.001.
- C. Deng, C. Qi, X. Wu, G. Jing and H. Zhao, Unveiling the relationship between structural evaluation and catalytic performance of InOOH during electroreduction of CO2 to formate, Green Carbon, 2024 DOI:10.1016/j.greenca.2024.02.003.
- H. Ang, W. Zhang, H. T. Tan, H. Chen and Q. Yan, Copper oxide supported on platinum nanosheets array: high performance carbon-free cathode for lithium-oxygen cells, J. Power Sources, 2015, 294, 377–385 CrossRef CAS.
- W. Zhang, J. Zhu, H. Ang, H. Wang, H. T. Tan, D. Yang, C. Xu, N. Xiao, B. Li, W. Liu, X. Wang, H. H. Hng and Q. Yan, Fe-based metallopolymer nanowall-based composites for Li–O2 battery cathode, ACS Appl. Mater. Interfaces, 2014, 6, 7164–7170 CrossRef CAS PubMed.
- C. C. Li, W. Zhang, H. Ang, H. Yu, B. Y. Xia, X. Wang, Y. H. Yang, Y. Zhao, H. H. Hng and Q. Yan, Compressed hydrogen gas-induced synthesis of Au–Pt core–shell nanoparticle chains towards high-performance catalysts for Li–O2 batteries, J. Mater. Chem. A, 2014, 2, 10676–10681 RSC.
- W. Zhang, Y. Huang, Y. Liu, L. Wang, S. Chou and H. Liu, Strategies toward stable nonaqueous alkali metal–O2 batteries, Adv. Energy Mater., 2019, 9, 1900464 CrossRef.
- J. Li, Z. Liu, S. Han, P. Zhou, B. Lu, J. Zhou, Z. Zeng, Z. Chen and J. Zhou, Hetero nucleus growth stabilizing zinc anode for high-biosecurity zinc-ion batteries, Nano-Micro Lett., 2023, 15, 237 CrossRef CAS PubMed.
- X. Xie, J. Li, Z. Xing, B. Lu, S. Liang and J. Zhou, Biocompatible zinc battery with programmable electro-cross-linked electrolyte, Natl. Sci. Rev., 2023, 10, nwac281 CrossRef CAS PubMed.
- S. Xu, S. K. Das and L. A. Archer, The Li–CO2 battery: a novel method for CO2 capture and utilization, RSC Adv., 2013, 3, 6656–6660 RSC.
- W. I. Al Sadat and L. A. Archer, The O2-assisted Al/CO2 electrochemical cell: a system for CO2 capture/conversion and electric power generation, Sci. Adv., 2016, 2, e1600968 CrossRef PubMed.
- Z. Xie, X. Zhang, Z. Zhang and Z. Zhou, Metal–CO2 batteries on the road: CO2 from contamination gas to energy source, Adv. Mater., 2017, 29, 1605891 CrossRef PubMed.
- C. J. Fetrow, C. Carugati, X.-D. Zhou and S. Wei, Electrochemistry of metal-CO2 batteries: opportunities and challenges, Energy Storage Mater., 2022, 45, 911–933 CrossRef.
- K. Takechi, T. Shiga and T. Asaoka, A Li–O2/CO2 battery, Chem. Commun., 2011, 47, 3463–3465 RSC.
- S. K. Das, S. Xu and L. A. Archer, Carbon dioxide assist for non-aqueous sodium–oxygen batteries, Electrochem. Commun., 2013, 27, 59–62 CrossRef CAS.
- Y. Liu, R. Wang, Y. Lyu, H. Li and L. Chen, Rechargeable Li/CO2–O2 (2: 1) battery and Li/CO2 battery, Energy Environ. Sci., 2014, 7, 677–681 RSC.
- Z. Zhang, X.-G. Wang, X. Zhang, Z. Xie, Y.-N. Chen, L. Ma, Z. Peng and Z. Zhou, Verifying the rechargeability of Li-CO2 batteries on working cathodes of Ni nanoparticles highly dispersed on N-doped graphene, Adv. Sci., 2018, 5, 1700567 CrossRef PubMed.
- J. Xie, Q. Liu, Y. Huang, M. Wu and Y. Wang, A porous Zn cathode for Li–CO2 batteries generating fuel-gas CO, J. Mater. Chem. A, 2018, 6, 13952–13958 RSC.
- Y. Xing, Y. Yang, D. Li, M. Luo, N. Chen, Y. Ye, J. Qian, L. Li, D. Yang, F. Wu, R. Chen and S. Guo, Crumpled Ir nanosheets fully covered on porous carbon nanofibers for long-life rechargeable Lithium–CO2 batteries, Adv. Mater., 2018, 30, 1803124 CrossRef PubMed.
- Y. Jiao, J. Qin, H. M. K. Sari, D. Li, X. Li and X. Sun, Recent progress and prospects of Li-CO2 batteries: mechanisms, catalysts and electrolytes, Energy Storage Mater., 2021, 34, 148–170 CrossRef.
- C. Xu, Y. Dong, Y. Shen, H. Zhao, L. Li, G. Shao and Y. Lei, Fundamental understanding of nonaqueous and hybrid Na–CO2 batteries: challenges and perspectives, Small, 2023, 19, 2206445 CrossRef CAS PubMed.
- X. Wang, J. Xie, M. A. Ghausi, J. Lv, Y. Huang, M. Wu, Y. Wang and J. Yao, Rechargeable Zn–CO2 electrochemical cells mimicking two-step photosynthesis, Adv. Mater., 2019, 31, 1807807 CrossRef PubMed.
- R. Yang, J. Xie, Q. Liu, Y. Huang, J. Lv, M. A. Ghausi, X. Wang, Z. Peng, M. Wu and Y. Wang, A trifunctional Ni–N/P–O–codoped graphene electrocatalyst enables dual-model rechargeable Zn–CO2/Zn–O2 batteries, J. Mater. Chem. A, 2019, 7, 2575–2580 RSC.
- N. Sreekanth, M. A. Nazrulla, T. V. Vineesh, K. Sailaja and K. L. Phani, Metal-free boron-doped graphene for selective electroreduction of carbon dioxide to formic acid/formate, Chem. Commun., 2015, 51, 16061–16064 RSC.
- Y. Jia, L. Zhang, A. Du, G. Gao, J. Chen, X. Yan, C. L. Brown and X. Yao, Defect graphene as a trifunctional catalyst for electrochemical reactions, Adv. Mater., 2016, 28, 9532–9538 CrossRef CAS PubMed.
- J. Xie, X. Wang, J. Lv, Y. Huang, M. Wu, Y. Wang and J. Yao, Reversible aqueous Zinc–CO2 batteries based on CO2–HCOOH interconversion, Angew. Chem., Int. Ed., 2018, 57, 16996–17001 CrossRef CAS PubMed.
- X. Hao, X. An, A. M. Patil, P. Wang, X. Ma, X. Du, X. Hao, A. Abudula and G. Guan, Biomass-derived N-doped carbon for efficient electrocatalytic CO2 reduction to CO and Zn–CO2 batteries, ACS Appl. Mater. Interfaces, 2021, 13, 3738–3747 CrossRef CAS PubMed.
- S. Kaur, M. Kumar, D. Gupta, P. P. Mohanty, T. Das, S. Chakraborty, R. Ahuja and T. C. Nagaiah, Efficient CO2 utilization and sustainable energy conversion via aqueous Zn-CO2 batteries, Nano Energy, 2023, 109, 108242 CrossRef CAS.
- W. Zheng, D. Wang, Y. Zhang, S. Zheng, B. Yang, Z. Li, R. D. Rodriguez, T. Zhang, L. Lei, S. Yao and Y. Hou, Promoting industrial-level CO2 electroreduction kinetics via accelerating proton feeding on a metal-free aerogel electrocatalyst, Nano Energy, 2023, 105, 107980 CrossRef CAS.
- W. Yang, Z. Xue, J. Yang, J. Xian, Q. Liu, Y. Fan, K. Zheng, P. Liao, H. Su, Q. Liu, G. Li and C.-Y. Su, Fe nanoparticles embedded in N-doped porous carbon for enhanced electrocatalytic CO2 reduction and Zn-CO2 battery, Chin. J. Catal., 2023, 48, 185–194 CrossRef CAS.
- X. Teng, J. Lu, Y. Niu, S. Gong, M. Xu, T. J. Meyer and Z. Chen, Selective CO2 reduction to formate on a Zn-based electrocatalyst promoted by tellurium, Chem. Mater., 2022, 34, 6036–6047 CrossRef CAS.
- J. Wang, Z. Li, Z. Zhu, J. Jiang, Y. Li, J. Chen, X. Niu, J. S. Chen and R. Wu, Tailoring the interactions of heterostructured Ni4N/Ni3ZnC0.7 for efficient CO2 electroreduction, J. Energy Chem., 2022, 75, 1–7 CrossRef CAS.
- D. Xiang, K. Li, K. Miao, R. Long, Y. Xiong and X. Kang, Amine-functionalized Copper catalysts: hydrogen bonding mediated electrochemical CO2 reduction to C2 products and superior rechargeable Zn-CO2 battery performance, Acta Phys.-Chim. Sin., 2024, 40(8), 2308027 Search PubMed.
- H. Liao, H. Xie, S. Zhai, L. Fu, Y. Zhang, S. Hao, B. Chen, C. He and Z. Shao, A partially Fe-substituted
perovskite electrode for enhancing Zn-CO2 batteries, Chem. Eng. J., 2023, 474, 145594 CrossRef CAS.
- L. Han, X. Peng, H.-T. Wang, P. Ou, Y. Mi, C.-W. Pao, J. Zhou, J. Wang, X. Liu, W.-F. Pong, J. Song, Z. Lin, J. Luo and H. L. Xin, Chemically coupling SnO2 quantum dots and MXene for efficient CO2 electroreduction to formate and Zn–CO2 battery, Proc. Natl. Acad. Sci. U. S. A., 2022, 119, e2207326119 CrossRef CAS PubMed.
- M. Yang, S. Liu, J. Sun, M. Jin, R. Fu, S. Zhang, H. Li, Z. Sun, J. Luo and X. Liu, Highly dispersed Bi clusters for efficient rechargeable Zn−CO2 batteries, Appl. Catal., B, 2022, 307, 121145 CrossRef CAS.
- Y. Wang, L. Xu, L. Zhan, P. Yang, S. Tang, M. Liu, X. Zhao, Y. Xiong, Z. Chen and Y. Lei, Electron accumulation enables Bi efficient CO2 reduction for formate production to boost clean Zn-CO2 batteries, Nano Energy, 2022, 92, 106780 CrossRef CAS.
- X. Hu, Y. Liu, W. Cui, X. Yang, J. Li, S. Zheng, B. Yang, Z. Li, X. Sang, Y. Li, L. Lei and Y. Hou, Boosting industrial-level CO2 electroreduction of N-doped carbon nanofibers with confined Tin-Nitrogen active sites via accelerating proton transport kinetics, Adv. Funct. Mater., 2023, 33, 2208781 CrossRef CAS.
- W. Wang, S. Gong, R. Lu, H. Wang, J. Liu, X. Zhu, B. Liu and X. Lv, In situ growth of Ag aerogels mediating effective electrocatalytic CO2 reduction and Zn-CO2 batteries, Chem. Eng. Sci., 2023, 280, 119042 CrossRef CAS.
- S. S. Gao, M. M. Jin, J. Q. Sun, X. J. Liu, S. S. Zhang, H. Y. Li, J. Luo and X. P. Sun, Coralloid Au enables high-performance Zn-CO2 battery and self-driven CO production, J. Mater. Chem. A, 2021, 9, 21024–21031 RSC.
- S. Gao, S. Chen, Q. Liu, S. Zhang, G. Qi, J. Luo and X. Liu, Bifunctional BiPd alloy particles anchored on carbon matrix for reversible Zn–CO2 battery, ACS Appl. Nano Mater., 2022, 5, 12387–12394 CrossRef CAS.
- S. Liu, M. Jin, J. Sun, Y. Qin, S. Gao, Y. Chen, S. Zhang, J. Luo and X. Liu, Coordination environment engineering to boost electrocatalytic CO2 reduction performance by introducing boron into single-Fe-atomic catalyst, Chem. Eng. J., 2022, 437, 135294 CrossRef CAS.
- S. Chen, J. Chen, Y. Li, S. Tan, X. Liao, T. Zhao, K. Zhang, E. Hu, F. Cheng and H. Wang, Fe–N4O–C nanoplates covalently bonding on graphene for efficient CO2 electroreduction and Zn–CO2 batteries, Adv. Funct. Mater., 2023, 33, 2300801 CrossRef CAS.
- S. Gong, W. Wang, C. Zhang, M. Zhu, R. Lu, J. Ye, H. Yang, C. Wu, J. Liu, D. Rao, S. Shao and X. Lv, Tuning the metal electronic structure of anchored cobalt phthalocyanine via dual-regulator for efficient CO2 electroreduction and Zn–CO2 batteries, Adv. Funct. Mater., 2022, 32, 2110649 CrossRef CAS.
- J. Li, L.-W. Chen, Y.-C. Hao, M. Yuan, J. Lv, A. Dong, S. Li, H. Gu, A.-X. Yin, W. Chen, P. Li and B. Wang, Asymmetric coordinated single-atom Pd sites for high performance CO2 electroreduction and Zn–CO2 battery, Chem. Eng. J., 2023, 461, 141865 CrossRef CAS.
- D. Lin, T. Wang, Z. Zhao, Y. Liu, H. Song, X. Yang, Z. Li, S. Yao, X. Hu, L. Lei, B. Yang and Y. Hou, Molten-salt-assisted synthesis of single-atom iron confined N-doped carbon nanosheets for highly efficient industrial-level CO2 electroreduction and Zn-CO2 batteries, Nano Energy, 2023, 113, 108568 CrossRef CAS.
- M. K. Aslam, H. Wang, S. Chen, Q. Li and J. Duang, Progress and perspectives of metal (Li, Na, Al, Zn and K)–CO2 batteries, Mater. Today Energy, 2023, 31, 101196 CrossRef CAS.
- J. Xie, Z. Zhou and Y. Wang, Metal–CO2 batteries at the crossroad to practical energy storage and CO2 recycle, Adv. Funct. Mater., 2020, 30, 1908285 CrossRef CAS.
- S. Ibraheem, S. Chen, J. Li, W. Li, X. Gao, Q. Wang and Z. Wei, Three-dimensional Fe,N-decorated carbon-supported NiFeP nanoparticles as an efficient bifunctional catalyst for rechargeable Zinc–O2 batteries, ACS Appl. Mater. Interfaces, 2019, 11, 699–705 CrossRef CAS PubMed.
- Y. Liu, Y. An, J. Zhu, L. Zhu, X. Li, P. Gao, G. He and Q. Pang, Integrated energy storage and CO2 conversion using an aqueous battery with tamed asymmetric reactions, Nat. Commun., 2024, 15, 977 CrossRef CAS PubMed.
- S. Liang, N. Altaf, L. Huang, Y. Gao and Q. Wang, Electrolytic cell design for electrochemical CO2 reduction, J. CO2 Util., 2020, 35, 90–105 CrossRef CAS.
- Y. Xie, P. Ou, X. Wang, Z. Xu, Y. Li, Z. Wang, J. Huang, J. Wicks, C. McCallum, N. Wang, Y. Wang, T. Chen, B. Lo, D. Sinton, J. Yu, Y. Wang and E. Sargent, High carbon utilization in CO2 reduction to multi-carbon products in acidic media, Nat. Catal., 2022, 5, 564–570 CrossRef CAS.
- X. Zou and J. Gu, Strategies for efficient CO2 electroreduction in acidic conditions, Chin. J. Catal., 2023, 52, 14–31 CrossRef CAS.
- K. Wang, Y. Wu, X. Cao, L. Gu and J. Hu, A Zn–CO2 flow battery generating electricity and methane, Adv. Funct. Mater., 2020, 30, 1908965 CrossRef CAS.
- D. S. Su, J. Zhang, B. Frank, A. Thomas, X. Wang, J. Paraknowitsch and R. Schlögl, Metal-free heterogeneous catalysis for sustainable chemistry, ChemSusChem, 2010, 3, 169–180 CrossRef CAS PubMed.
- J. Wu, W. Pisula and K. Müllen, Graphenes as potential material for electronics, Chem. Rev., 2007, 107, 718–747 CrossRef CAS PubMed.
- X.-K. Kong, Z.-Y. Sun, M. Chen, C.-L. Chen and Q.-W. Chen, Metal-free catalytic reduction of 4-nitrophenol to 4-aminophenol by N-doped graphene, Energy Environ. Sci., 2013, 6, 3260–3266 RSC.
- K. Gong, F. Du, Z. Xia, M. Durstock and L. Dai, Nitrogen-doped carbon nanotube arrays with high electrocatalytic activity for oxygen reduction, Science, 2009, 323, 760–764 CrossRef CAS PubMed.
- E. Gottlieb, K. Matyjaszewski and T. Kowalewski, Polymer-based synthetic routes to carbon-based metal-free catalysts, Adv. Mater., 2019, 31, 1804626 CrossRef PubMed.
- H. Wang, Y. Chen, X. Hou, C. Ma and T. Tan, Nitrogen-doped graphenes as efficient electrocatalysts for the selective reduction of carbon dioxide to formate in aqueous solution, Green Chem., 2016, 18, 3250–3256 RSC.
- X. Duan, J. Xu, Z. Wei, J. Ma, S. Guo, S. Wang, H. Liu and S. Dou, Metal-free carbon materials for CO2 electrochemical reduction, Adv. Mater., 2017, 29, 1701784 CrossRef PubMed.
- S. Gao, Y. Liu, Z. Xie, Y. Qiu, L. Zhuo, Y. Qin, J. Ren, S. Zhang, G. Hu, J. Luo and X. Liu, Metal-free bifunctional ordered mesoporous carbon for reversible Zn-CO2 batteries, Small Methods, 2021, 5, 2001039 CrossRef CAS PubMed.
- C. Chen, X. Sun, X. Yan, Y. Wu, H. Liu, Q. Zhu, B. B. A. Bediako and B. Han, Boosting CO2 electroreduction on N, P-Co-doped carbon aerogels, Angew. Chem., 2020, 132, 11216–11222 CrossRef.
- H. Yang, Y. Wu, Q. Lin, L. Fan, X. Chai, Q. Zhang, J. Liu, C. He and Z. Lin, Composition tailoring via N and S Co-doping and structure tuning by constructing hierarchical pores: metal-free catalysts for high-performance electrochemical reduction of CO2, Angew. Chem., 2018, 130, 15702 CrossRef.
- Y. Zhang, X. Wang, S. Zheng, B. Yang, Z. Li, J. Lu, Q. Zhang, N. M. Adli, L. Lei, G. Wu and Y. Hou, Hierarchical cross-linked carbon aerogels with transition metal-nitrogen sites for highly efficient industrial-level CO2 electroreduction, Adv. Funct. Mater., 2021, 31, 2104377 CrossRef CAS.
- D. R. Chen, M. Chitranshi, V. Shanov and M. Schulz, Electrochemically activated CNT sheet as a cathode for Zn-CO2 batteries, Int. J. Mol. Sci., 2022, 23, 12602 CrossRef CAS PubMed.
- F. Pan, W. Deng, C. Justiniano and Y. Li, Identification of champion transition metals centers in metal and nitrogen-codoped carbon catalysts for CO2 reduction, Appl. Catal., B, 2018, 226, 463–472 CrossRef CAS.
- T. N. Huan, N. Ranjbar, G. Rousse, M. Sougrati, A. Zitolo, V. Mougel, F. Jaouen and M. Fontecave, Electrochemical reduction of CO2 catalyzed by Fe-N-C materials: a structure–selectivity study, ACS Catal., 2017, 7, 1520–1525 CrossRef CAS.
- Y. Qu, Z. Li, W. Chen, Y. Lin, T. Yuan, Z. Yang, C. Zhao, J. Wang, C. Zhao, X. Wang, F. Zhou, Z. Zhuang, Y. Wu and Y. Li, Direct transformation of bulk copper into copper single sites via emitting and trapping of atoms, Nat. Catal., 2018, 1, 781–786 CrossRef CAS.
- Y. Mun, K. Kim, S. Kim, S. Lee, S. Lee, W. Choi, S. Kim, J. Han and J. Lee, A novel strategy to develop non-noble metal catalyst for CO2 electroreduction: hybridization of metal-organic polymer, Appl. Catal., B, 2018, 236, 154–161 CrossRef CAS.
- S. Liang, L. Huang, Y. Gao, Q. Wang and B. Liu, Electrochemical reduction of CO2 to CO over transition metal/N-doped carbon catalysts: the active sites and reaction mechanism, Adv. Sci., 2021, 8, 2102886 CrossRef CAS PubMed.
- H.-J. Zhu, M. Lu, Y.-R. Wang, S.-J. Yao, M. Zhang, Y.-H. Kan, J. Liu, Y. Chen, S.-L. Li and Y.-Q. Lan, Efficient electron transmission in covalent organic framework nanosheets for highly active electrocatalytic carbon dioxide reduction, Nat. Commun., 2020, 11, 497 CrossRef CAS PubMed.
- A. E. Baumann, D. A. Burns, B. Liu and V. S. Thoi, Metal-organic framework functionalization and design strategies for advanced electrochemical energy storage devices, Commun. Chem., 2019, 2, 86 CrossRef.
- Q. Ren, H. Wang, X.-F. Lu, Y.-X. Tong and G.-R. Li, Recent progress on MOF-derived heteroatom-doped carbon-based electrocatalysts for oxygen reduction reaction, Adv. Sci., 2018, 5, 1700515 CrossRef PubMed.
- Y. V. Kaneti, J. Tang, R. R. Salunkhe, X. Jiang, A. Yu, K. C. W. Wu and Y. Yamauchi, Nanoarchitectured design of porous materials and nanocomposites from metal-organic frameworks, Adv. Mater., 2017, 29, 1604898 CrossRef PubMed.
- M. Liu, X. Lu, C. Guo, Z. Wang, Y. Li, Y. Lin, Y. Zhou, S. Wang and J. Zhang, Architecting a mesoporous N-doped graphitic carbon framework encapsulating CoTe2 as an efficient oxygen evolution electrocatalyst, ACS Appl. Mater. Interfaces, 2017, 9, 36146–36153 CrossRef CAS PubMed.
- F. Wang, G. Wang, P. Deng, Y. Chen, J. Li, D. Wu, Z. Wang, C. Wang, Y. Hua and X. Tian, Ultrathin nitrogen-doped carbon encapsulated Ni nanoparticles for highly efficient electrochemical CO2 reduction and aqueous Zn-CO2 batteries, Small, 2023, 19, 2301128 CrossRef CAS PubMed.
- M. Sun, H. Liu, J. Qu and J. Li, Earth-rich transition metal phosphide for energy conversion and storage, Adv. Energy Mater., 2016, 6, 1600087 CrossRef.
- M. Peng, S. Ci, P. Shao, P. Cai and Z. Wen, Cu3P/C nanocomposites for efficient electrocatalytic CO2 reduction and Zn-CO2 battery, J. Nanosci. Nanotechnol., 2019, 19, 3232–3236 CrossRef CAS PubMed.
- K. P. Singh, E. J. Bae and J.-S. Yu, Fe–P: a new class of electroactive catalyst for oxygen reduction reaction, J. Am. Chem. Soc., 2015, 137, 3165–3168 CrossRef CAS PubMed.
- S. Liu, L. Wang, H. Yang, S. Gao, Y. Liu, S. Zhang, Y. Chen, X. Liu and J. Luo, Nitrogen-doped carbon polyhedrons confined Fe–P nanocrystals as high-efficiency bifunctional catalysts for aqueous Zn-CO2 batteries, Small, 2022, 18, 2104965 CrossRef CAS PubMed.
- D.-H. Guan, X.-X. Wang, M.-L. Li, F. Li, L.-J. Zheng, X.-L. Huang and J.-J. Xu, Light/electricity energy conversion and storage for a hierarchical porous In2S3@CNT/SS cathode towards a flexible Li-CO2 battery, Angew. Chem., Int. Ed., 2020, 59, 19518–19524 CrossRef CAS PubMed.
- Z. Li, M.-L. Li, X.-X. Wang, D.-H. Guan, W.-Q. Liu and J.-J. Xu, In situ fabricated photo-electro-catalytic hybrid cathode for light-assisted lithium–CO2 batteries, J. Mater. Chem. A, 2020, 8, 14799–14806 RSC.
- Z. Zhu, X. Shi, G. Fan, F. Li and J. Chen, Photo-energy conversion and storage in an aprotic Li-O2 battery, Angew. Chem., 2019, 131, 19197–19202 CrossRef.
- K. Wang, Z. Mo, S. Tang, M. Li, H. Yang, B. Long, Y. Wang, S. Song and Y. Tong, Photo-enhanced Zn–air batteries with simultaneous highly efficient in situ H2O2 generation for wastewater treatment, J. Mater. Chem. A, 2019, 7, 14129–14135 RSC.
- X. Liu, S. Tao, J. Zhang, Y. Zhu, R. Ma and J. Lu, Ultrathin p–n type Cu2O/CuCoCr-layered double hydroxide heterojunction nanosheets for photo-assisted aqueous Zn–CO2 batteries, J. Mater. Chem. A, 2021, 9, 26061–26068 RSC.
- J. Fan, X. Zhao, X. Mao, J. Xu, N. Han, H. Yang, B. Pan, Y. Li, L. Wang and Y. Li, Large-area vertically aligned bismuthene nanosheet arrays from galvanic replacement reaction for efficient electrochemical CO2 conversion, Adv. Mater., 2021, 33, 2100910 CrossRef CAS PubMed.
- Y. Wang, Z. Huang, Y. Lei, J. Wu, Y. Bai, X. Zhao, M. Liu, L. Zhan, S. Tang, X. Zhang, F. Luo and X. Xiong, Bismuth with abundant defects for electrocatalytic CO2 reduction and Zn–CO2 batteries, Chem. Commun., 2022, 58, 3621–3624 RSC.
- H. Wang, M. K. Aslam, Z. Nie, K. Yang, X. Li, S. Chen, Q. Li, D. Chao and J. Duan, Dual-anion regulation for reversible and energetic aqueous Zn–CO2 batteries, Small Methods, 2023, 2300867 CrossRef PubMed.
- W. Wu, J. Zhu, Y. Tong, S. Xiang and P. Chen, Electronic structural engineering of bimetallic Bi-Cu alloying nanosheet for highly-efficient CO2 electroreduction and Zn-CO2 batteries, Nano Res., 2024, 17, 3684–3692 CrossRef CAS.
- A. Xu, X. Chen, D. Wei, B. Chu, M. Yu, X. Yin and J. Xu, Regulating the electronic structure of bismuth nanosheets by titanium doping to boost CO2 electroreduction and Zn–CO2 batteries, Small, 2023, 19, 2302253 CrossRef CAS PubMed.
- Q. Wang, X. Yang, H. Zang, C. Liu, J. Wang, N. Yu, L. Kuai, Q. Qin and B. Geng, InBi bimetallic sites for efficient electrochemical reduction of CO2 to HCOOH, Small, 2023, 19, 2303172 CrossRef CAS PubMed.
- Y. Huang, X. Mao, G. Yuan, D. Zhang, B. Pan, J. Deng, Y. Shi, N. Han, C. Li, L. Zhang, L. Wang, L. He, Y. Li and Y. Li, Size-dependent selectivity of electrochemical CO2 reduction on converted In2O3 nanocrystals, Angew. Chem., 2021, 133, 15978–15982 CrossRef.
- X. Zheng, P. De Luna, F. P. García de Arquer, B. Zhang, N. Becknell, M. B. Ross, Y. Li, M. N. Banis, Y. Li, M. Liu, O. Voznyy, C. T. Dinh, T. Zhuang, P. Stadler, Y. Cui, X. Du, P. Yang and E. H. Sargent, Sulfur-modulated tin sites enable highly selective electrochemical reduction of CO2 to formate, Joule, 2017, 1, 794–805 CrossRef CAS.
- S. Liu, J. Xiao, X. F. Lu, J. Wang, X. Wang and X. W. Lou, Efficient electrochemical reduction of CO2 to HCOOH over Sub-2 nm SnO2 quantum wires with exposed grain boundaries, Angew. Chem., Int. Ed., 2019, 58, 8499–8503 CrossRef CAS PubMed.
- X. Teng, Y. Niu, S. Gong, M. Xu, X. Liu, L. Ji and Z. Chen, In/ZnO@C hollow nanocubes for efficient electrochemical reduction of CO2 to formate and rechargeable Zn–CO2 batteries, Mater. Chem. Front., 2021, 5, 6618–6627 RSC.
- Z. Li, A. Cao, Q. Zheng, Y. Fu, T. Wang, K. T. Arul, J.-L. Chen, B. Yang, N. M. Adli, L. Lei, C.-L. Dong, J. Xiao, G. Wu and Y. Hou, Elucidation of the synergistic effect of dopants and vacancies on promoted selectivity for CO2 electroreduction to formate, Adv. Mater., 2021, 33, 2005113 CrossRef CAS PubMed.
- W. Ni, Y. Gao, Y. Lin, C. Ma, X. Guo, S. Wang and S. Zhang, Nonnitrogen coordination environment steering electrochemical
CO2-to-CO conversion over single-atom tin catalysts in a wide potential window, ACS Catal., 2021, 11, 5212–5221 CrossRef CAS.
- Y. Shi, Z. Lyu, M. Zhao, R. Chen, Q. N. Nguyen and Y. Xia, Noble-metal nanocrystals with controlled shapes for catalytic and electrocatalytic applications, Chem. Rev., 2021, 121, 649–735 CrossRef CAS PubMed.
- Y. Chen, C. W. Li and M. W. Kanan, Aqueous CO2 reduction at very low overpotential on oxide-derived Au nanoparticles, J. Am. Chem. Soc., 2012, 134, 19969–19972 CrossRef CAS PubMed.
- D. Mateo, I. Esteve-Adell, J. Albero, J. F. S. Royo, A. Primo and H. Garcia, 111 oriented gold nanoplatelets on multilayer graphene as visible light photocatalyst for overall water splitting, Nat. Commun., 2016, 7, 11819 CrossRef PubMed.
- N. Hoshi, T. Mizumura and Y. Hori, Significant difference of the reduction rates of carbon dioxide between Pt(111) and Pt(110) single crystal electrodes, Electrochim. Acta, 1995, 40, 883–887 CrossRef CAS.
- S. Mezzavilla, S. Horch, I. E. L. Stephens, B. Seger and I. Chorkendorff, Structure sensitivity in the electrocatalytic reduction of CO2 with gold catalysts, Angew. Chem., 2019, 131, 3814–3818 CrossRef.
- S. Dai, T.-H. Huang, W.-I. Liu, C.-W. Hsu, S.-W. Lee, T.-Y. Chen, Y.-C. Wang, J.-H. Wang and K.-W. Wang, Enhanced CO2 electrochemical reduction performance over Cu@AuCu catalysts at high noble metal utilization efficiency, Nano Lett., 2021, 21, 9293–9300 CrossRef CAS PubMed.
- A. R. Woldu, From low to high-index facets of noble metal nanocrystals: a way forward to enhance the performance of electrochemical CO2 reduction, Nanoscale, 2020, 12, 8626–8635 RSC.
- J. Wang, S. Kattel, C. J. Hawxhurst, J. H. Lee, B. M. Tackett, K. Chang, N. Rui, C.-J. Liu and J. G. Chen, Enhancing activity and reducing cost for electrochemical reduction of CO2 by supporting palladium on metal carbides, Angew. Chem., Int. Ed., 2019, 58, 6271–6275 CrossRef CAS PubMed.
- X. Huang, X. Zhou, S. Wu, Y. Wei, X. Qi, J. Zhang, F. Boey and H. Zhang, Reduced graphene oxide-templated photochemical synthesis and in situ assembly of Au nanodots to orderly patterned Au nanodot chains, Small, 2010, 6, 513–516 CrossRef CAS PubMed.
- S. Gao, S. Hao, Z. Huang, Y. Yuan, S. Han, L. Lei, X. Zhang, R. Shahbazian-Yassar and J. Lu, Synthesis of high-entropy alloy nanoparticles on supports by the fast moving bed pyrolysis, Nat. Commun., 2020, 11, 2016 CrossRef CAS PubMed.
- S. Gao, M. Jin, J. Sun, X. Liu, S. Zhang, H. Li, J. Luo and X. Sun, Coralloid Au enables high-performance Zn–CO2 battery and self-driven CO production, J. Mater. Chem. A, 2021, 9, 21024–21031 RSC.
- V. Celorrio, P. M. Quaino, E. Santos, J. Flórez-Montaño, J. J. L. Humphrey, O. Guillén-Villafuerte, D. Plana, M. J. Lázaro, E. Pastor and D. J. Fermín, Strain effects on the oxidation of CO and HCOOH on Au–Pd core–shell nanoparticles, ACS Catal., 2017, 7, 1673–1680 CrossRef CAS.
- D. Gao, H. Zhou, F. Cai, D. Wang, Y. Hu, B. Jiang, W.-B. Cai, X. Chen, R. Si, F. Yang, S. Miao, J. Wang, G. Wang and X. Bao, Switchable CO2 electroreduction via engineering active phases of Pd nanoparticles, Nano Res., 2017, 10, 2181–2191 CrossRef CAS.
- S. Yang, J. Kim, Y. J. Tak, A. Soon and H. Lee, Single-atom catalyst of platinum supported on titanium nitride for selective electrochemical reactions, Angew. Chem., Int. Ed., 2016, 55, 2058–2062 CrossRef CAS PubMed.
- Y. H. Li, S. N. Zhao and S. Q. Zang, Programmable kernel structures of atomically precise metal nanoclusters for tailoring catalytic properties, Exploration, 2023, 3, 20220005 CrossRef PubMed.
- T. Tang, Z. Wang and J. Guan, Achievements and challenges of copper-based single-atom catalysts for the reduction of carbon dioxide to C2+ products, Exploration, 2023, 3, 20230011 CrossRef PubMed.
- J. Wu, L. Xiong, B. Zhao, M. Liu and L. Huang, Densely populated single atom catalysts, Small Methods, 2020, 4, 1900540 CrossRef CAS.
- L. Zhao, Y. Zhang, L.-B. Huang, X.-Z. Liu, Q.-H. Zhang, C. He, Z.-Y. Wu, L.-J. Zhang, J. Wu, W. Yang, L. Gu, J.-S. Hu and L.-J. Wan, Cascade anchoring strategy for general mass production of high-loading single-atomic metal-nitrogen catalysts, Nat. Commun., 2019, 10, 1278 CrossRef PubMed.
- G. Zhang, Y. Jia, C. Zhang, X. Xiong, K. Sun, R. Chen, W. Chen, Y. Kuang, L. Zheng, H. Tang, W. Liu, J. Liu, X. Sun, W.-F. Lin and H. Dai, A general route via formamide condensation to prepare atomically dispersed metal–nitrogen–carbon electrocatalysts for energy technologies, Energy Environ. Sci., 2019, 12, 1317–1325 RSC.
- J. Jones, H. Xiong, A. T. DeLaRiva, E. J. Peterson, H. Pham, S. R. Challa, G. Qi, S. Oh, M. H. Wiebenga, X. I. Pereira Hernández, Y. Wang and A. K. Datye, Thermally stable single-atom platinum-on-ceria catalysts via atom trapping, Science, 2016, 353, 150–154 CrossRef CAS PubMed.
- Y. Pan, S. Liu, K. Sun, X. Chen, B. Wang, K. Wu, X. Cao, W.-C. Cheong, R. Shen, A. Han, Z. Chen, L. Zheng, J. Luo, Y. Lin, Y. Liu, D. Wang, Q. Peng, Q. Zhang, C. Chen and Y. Li, A bimetallic Zn/Fe polyphthalocyanine-derived single-atom Fe-N4 catalytic site: a superior trifunctional catalyst for overall water splitting and Zn–Air batteries, Angew. Chem., Int. Ed., 2018, 57, 8614–8618 CrossRef CAS PubMed.
- J. Chen, H. Li, C. Fan, Q. Meng, Y. Tang, X. Qiu, G. Fu and T. Ma, Dual single-atomic Ni-N4 and Fe-N4 sites constructing janus hollow graphene for selective oxygen electrocatalysis, Adv. Mater., 2020, 32, 2003134 CrossRef CAS PubMed.
- Z. Zeng, A. G. Mohamed, A. X. Zhang and Y. Wang, Wide potential CO2-to-CO electroreduction relies on pyridinic-N/Ni–Nx sites and its Zn–CO2 battery application, Energy Technol., 2021, 9, 2100205 CrossRef CAS.
- C. Zhao, X. Dai, T. Yao, W. Chen, X. Wang, J. Wang, J. Yang, S. Wei, Y. Wu and Y. Li, Ionic exchange of metal–organic frameworks to access single nickel sites for efficient electroreduction of CO2, J. Am. Chem. Soc., 2017, 139, 8078–8081 CrossRef CAS PubMed.
- X. Li, W. Bi, M. Chen, Y. Sun, H. Ju, W. Yan, J. Zhu, X. Wu, W. Chu, C. Wu and Y. Xie, Exclusive Ni–N4 sites realize near-unity CO selectivity for electrochemical CO2 reduction, J. Am. Chem. Soc., 2017, 139, 14889–14892 CrossRef CAS PubMed.
- Y. Pan, R. Lin, Y. Chen, S. Liu, W. Zhu, X. Cao, W. Chen, K. Wu, W.-C. Cheong, Y. Wang, L. Zheng, J. Luo, Y. Lin, Y. Liu, C. Liu, J. Li, Q. Lu, X. Chen, D. Wang, Q. Peng, C. Chen and Y. Li, Design of single-atom Co–N5 catalytic site: a robust electrocatalyst for CO2 reduction with nearly 100% CO selectivity and remarkable stability, J. Am. Chem. Soc., 2018, 140, 4218–4221 CrossRef CAS PubMed.
- Y. Zhang, L. Jiao, W. Yang, C. Xie and H.-L. Jiang, Rational fabrication of low-coordinate single-atom Ni electrocatalysts by MOFs for highly selective CO2 reduction, Angew. Chem., Int. Ed., 2021, 60, 7607–7611 CrossRef CAS PubMed.
- J. Chen, Z. Li, X. Wang, X. Sang, S. Zheng, S. Liu, B. Yang, Q. Zhang, L. Lei, L. Dai and Y. Hou, Promoting CO2 electroreduction kinetics on atomically dispersed monovalent ZnI sites by rationally engineering proton-feeding centers, Angew. Chem., Int. Ed., 2022, 61, e202111683 CrossRef CAS PubMed.
- Z. Li, R. Wu, S. Xiao, Y. Yang, L. Lai, J. S. Chen and Y. Chen, Axial chlorine coordinated iron-nitrogen-carbon single-atom catalysts for efficient electrochemical CO2 reduction, Chem. Eng. J., 2022, 430, 132882 CrossRef CAS.
- Y. Zhao, Z. Pei, X. F. Lu, D. Luan, X. Wang and X. W. Lou, Rationally designed nitrogen-doped carbon macroporous fibers with loading of single cobalt sites for efficient aqueous Zn-CO2 batteries, Chem Catal., 2022, 2, 1480–1493 CrossRef CAS.
- J. Gu, C.-S. Hsu, L. Bai, H. M. Chen and X. Hu, Atomically dispersed Fe3+ sites catalyze efficient CO2 electroreduction to CO, Science, 2019, 364, 1091–1094 CrossRef CAS PubMed.
- C. Zhang, S. Yang, J. Wu, M. Liu, S. Yazdi, M. Ren, J. Sha, J. Zhong, K. Nie, A. S. Jalilov, Z. Li, H. Li, B. I. Yakobson, Q. Wu, E. Ringe, H. Xu, P. M. Ajayan and J. M. Tour, Electrochemical CO2 reduction with atomic Iron-dispersed on nitrogen-doped graphene, Adv. Energy Mater., 2018, 8, 1703487 CrossRef.
- W. Ni, Z. Liu, Y. Zhang, C. Ma, H. Deng, S. Zhang and S. Wang, Electroreduction of carbon dioxide driven by the intrinsic defects in the carbon plane of a single Fe–N4 site, Adv. Mater., 2021, 33, 2003238 CrossRef CAS PubMed.
- C. Guo, T. Zhang, X. Liang, X. Deng, W. Guo, Z. Wang, X. Lu and C.-M. L. Wu, Single transition metal atoms on nitrogen-doped carbon for CO2 electrocatalytic reduction: CO production or further CO reduction?, Appl. Surf. Sci., 2020, 533, 147466 CrossRef CAS.
- W. Ju, A. Bagger, X. Wang, Y. Tsai, F. Luo, T. Möller, H. Wang, J. Rossmeisl, A. S. Varela and P. Strasser, Unraveling mechanistic reaction pathways of the electrochemical CO2 reduction on Fe–N–C single-site catalysts, ACS Energy Lett., 2019, 4, 1663–1671 CrossRef CAS.
|
This journal is © Institute of Process Engineering of CAS 2024 |
Click here to see how this site uses Cookies. View our privacy policy here.