DOI:
10.1039/D4QI00326H
(Review Article)
Inorg. Chem. Front., 2024,
11, 4499-4527
225Ac/213Bi radionuclide generators for the separation of 213Bi towards clinical demands
Received
2nd February 2024
, Accepted 5th May 2024
First published on 10th June 2024
Abstract
Bismuth-213 (213Bi) is a promising radionuclide for clinical application in targeted alpha therapy of cancers. 225Ac/213Bi radionuclide generators are of particular interest for the production/separation of 213Bi. This review aims to give an overview of the current status of 225Ac production, 225Ac/213Bi radionuclide generator systems, the types of adsorbents, separation conditions, separation mechanisms, and critical challenges. The review also provides guidance for investigating emerging new sorbents that can be developed to overcome the limitations of currently used sorbents.
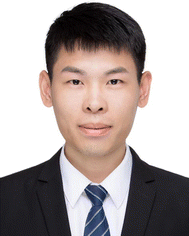 Hongshan Zhu | Hongshan Zhu (born in 1993) obtained his PhD in Chemistry at KU Leuven in 2023 after which he moved to the Dalian Institute of Chemical Physics, Chinese Academy of Sciences to pursue a postdoctoral position under the guidance of Prof. Zhimou Guo and Prof. Xinmiao Liang. His research mainly focuses on the separation of medical alpha-emitting radionuclides from irradiated radionuclides and exploring the radiation stability of materials. He published 13 papers in international journals (h-index = 10) and is co-inventor of 1 patent application. |
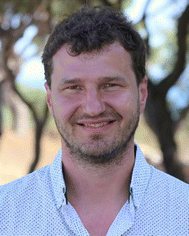 Stephan Heinitz | Stephan Heinitz (born in 1982) obtained his PhD in 2013 from the University of Berne, Switzerland, in collaboration with PSI. The work was devoted towards purification of irradiated liquid metal targets and behaviour of polonium in lead–bismuth eutectic. He continued as PSI PostDoc in radioactive target development where he was involved in lanthanide purification for different research institutes such as CERN and INFN. In 2018, he joined SCK CEN as project leader for the production of the medical isotope 225Ac. He published 120 papers in international journals (h-index = 17) and is co-inventor of 2 patents (applications). |
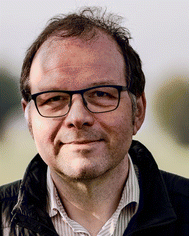 Koen Binnemans | Prof. Koen Binnemans (born in 1970) is an inorganic chemist who is combining fundamental and applied research in the fields of metallurgical chemistry and hydrometallurgy. He is head of the SOLVOMET group at the Department of Chemistry of KU Leuven (Belgium). He has more than 30 years of experience working with rare earths and is recognised as a world-leading specialist in this field. He has published 600 papers in international journals. His work has been cited 35 500 times (h-index = 89) according to Web of Science. He has been awarded two ERC Advanced Grants (SOLCRIMET and CIRMET). |
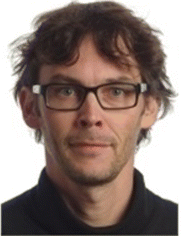 Steven Mullens | Steven Mullens (born in 1972) is a senior researcher at the Flemish Institute for Technological Research. His research focusses on shaping and surface modification of ceramic, metallic and hybrid materials by 3D-printing, coatings and granulation. These structures are utilized in various applications, including biomaterials, advanced sorbent materials, and as structured catalysts. He has been involved for more than 20 years in several academic and industrial projects. He has published 120 papers in international journals (h-index = 34) and is co-inventor of 14 patents (applications). |
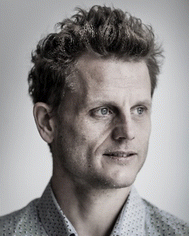 Thomas Cardinaels | Thomas Cardinaels (born in 1979) obtained his PhD in Chemistry in 2006 at KU Leuven where he continued research in the field of metal-containing liquid crystals as PostDoc. In 2009 he moved to SCK CEN expanding expertise in nuclear fuel materials. Since 2015 he is head of the Radiochemistry group at SCK CEN which he combines with a position as Associate Professor in Chemistry at KU Leuven. His research aims at developing radiochemical process technology for sustainable radioactive waste solutions, and to produce medical radioisotopes and radiopharmaceuticals. He published 100 papers (h-index = 24) and is co-inventor of 6 patents (applications). |
1. Introduction
The use of radionuclides is becoming increasingly important for medical diagnosis, and therapeutic applications.1,2 The diagnostic imaging can enable precise visualization of physiological processes.1,2 Radiopharmaceutical therapy (RPT), in particular, is a safe and effective method to treat various cancers via delivering radioactive atoms to tumor-associated targets (Fig. 1).2 The radiation emitted by the radionuclides kills cancer cells locally, which ensures targeted therapeutic outcomes while minimizing radiation exposure to non-targeted tissues.3 RPT offers several advantages over conventional therapeutic techniques, including targeted therapy, metastatic disease treatment, high efficacy, and minimal toxicity.4 These benefits make it an attractive alternative or complementary option to traditional cancer treatments like chemotherapy, radiotherapy, and surgery.2 The therapeutic efficacies of radionuclides vary based on their fundamental nuclear properties, including their decay pathway, energy emissions, effective range, half-life, and chemical behavior.5 The penetration range and the linear energy transfer (LET) are critical factors, as the potent pathway of malignant cell destruction is related to the direct interaction between ionization events and the DNA string in the nucleus.6 The emitted particles should possess a suitable penetration range (usually several cell diameters).1,2,6 A more extensive penetration range can result in the death of the surrounding healthy cells and induce unnecessary dose. Alternatively, a low penetration range is insufficient for damaging malignant cells.1,2,6 The LET indicates the energy deposited per unit length of the track. The LET radiations are usually divided into two classes.1,2,6 High LET radiations, like neutrons and alpha particles, have stronger biological effects due to their higher ionization events within tissues, while low LET radiations (e.g., X-rays and gamma rays) produce fewer biological effects. However, the biological effects are also impacted by other factors, as described in previous reviews.7–9
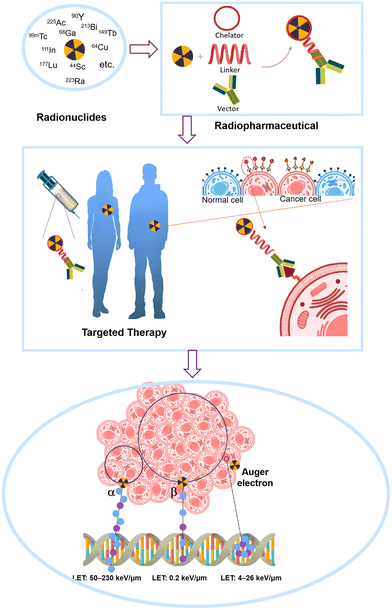 |
| Fig. 1 Simplified illustration of how radiopharmaceutical targeting works and damage from radioactive emissions: α-emitters, β-emitters, and Auger-electron emitters. | |
Radionuclides used in radiopharmaceutical therapy are typically classified as alpha, beta, and Auger-electron emitters (Fig. 1).10,11 Alpha particles, positively charged helium nuclei, are highly effective ionization agents with a short penetration range of 40–100 μm, corresponding to roughly 2–6 cell diameters. They possess a high LET of 50–230 keV μm−1, which enables maximum malignant cell destruction with reduced cytotoxicity to surrounding normal cells.12,13 Moreover, the distance between two strands in a DNA structure coincides with the distance between two consecutive alpha ionizations, indicating that a single alpha particle can kill one cell.14 In contrast, beta particles (electrons) with a long penetration range of 0.3–12 mm (several hundred cells in diameter) may damage healthy tissues surrounding the malignant cells. Additionally, beta particles also have a low LET of only 0.2 keV μm−1.11 Hundreds of beta particles are possibly needed to break a double-stranded DNA structure.13 Although Auger electrons possess a higher LET of 4–26 keV μm−1, their limited penetration range of 2–500 nm restricts their efficacy to a single cell, as they must cross the malignant cell membrane to reach the nucleus.15 Auger elections can damage the cancer cells via cross-dose effect, bystander effect, generation of hydroxyl free radicals, cell membrane damage, and direct or indirect DNA damage.16 Consequently, more recent research has focused on radiopharmaceuticals with alpha particles because of their more favorable radiobiological properties.13
The alpha-emitting radiopharmaceuticals for targeted alpha therapy (TAT) (Fig. 1) are primarily made of radioactive isotopes, chelating agents, linkers, and biological-targeting vectors.17 Chelator is used to attach the isotope to the vector, while linkers can connect the radionuclide-chelator to the vector. Disease-targeting vectors can act as carriers of radionuclides to the targeted cancer cells.18 The chemical purity of 213Bi should be high, as impurities can affect chelation and reduce stability and effectiveness. The half-life of radionuclides should be suitable for the therapeutic window (referring to the drug dose needed), which should match the biological kinetics of the targeted malignant cells without producing toxic effects in any patients.13 Typically, the half-life of radionuclides needs to be carefully balanced to avoid overexposure on one hand, and on the other hand allow adequate time for radionuclide and radiopharmaceutical production and delivery to the patients while ensuring cancer cells receive a sufficient dose.13 Other preferred characteristics are related to the decay process, in which radionuclides must undergo alpha decay, preferably with a high alpha decay ratio, and produce no long-lived intermediates, ultimately decaying into a stable, non-hazardous element. Additionally, they should have high specific activity (the activity per unit mass of a radionuclide) and abundance (significant quantities of the radioactive substance present within a sample).19 However, the potential detachment of daughter radionuclides due to recoil effects resulting from multiple alpha decays should be mentioned to reduce unnecessary dose for patients.
While there are over 100 radionuclides capable of emitting alpha particles, only a handful of alpha-emitter radionuclides are suitable for therapeutic applications.13,20–23 These radionuclides have suitable characteristics, including the correct nuclear properties, chemical properties (e.g., stable complexes with biomolecules), or accessibility (e.g., regularly available and affordable). 223Ra was the first therapeutic alpha-emitting radionuclide approved by the Food and Drug Administration (FDA) for clinical prostate cancer treatment in 2013.24 Among all radionuclides, 225Ac and its daughter 213Bi have been attractive candidates for TAT because of their high specific activity, effective half-life, high alpha decay ratio (the ratio of alpha particles emitted by a radioactive substance relative to other types of radiation), absence of long-lived intermediates, and stable complexes with the availability of chelators.25 Geerlings M. W. et al. suggested the use of 225Ac and 213Bi for therapy in 1993.26 Clinical applications of 225Ac have been reported for treating targeted leukemia, glioma, neuroendocrine tumors, and prostate cancer, whereas 213Bi has shown potential in clinical trials of leukemia, lymphoma, malignant melanoma, glioma, neuroendocrine tumors, fungal infections and infectious diseases.25,27 However, the limited availability and accessibility of 225Ac for clinical use pose a significant challenge, as its production and purification can be both challenging and expensive.28–30 Being less cytotoxic than 225Ac, the chemical properties and the presence of an alpha-emitting daughter (213Po) make 213Bi much easier to incorporate into future clinical trials.19 The supply of 225Ac also impacts the application of 213Bi for TAT since 213Bi is obtained by the 225Ac decay (Fig. 2). While 213Bi is a valuable radionuclide for TAT, currently its application is very limited mainly due to the availability of its parent 225Ac. Furthermore, the application of 213Bi is the cost as it requires about 1000 times more activity per patient dose compared to 225Ac.28,31,32 As a result, research efforts have been focused on developing more efficient and cost-effective routes to produce larger quantities of 225Ac.28–30,33 Additionally, the separation of 213Bi from 225Ac is also crucial. The chemical and radiochemical purity is indispensable for precise labeling at high specific activity, ensuring optimal diagnostic accuracy and therapeutic efficacy while minimizing radiation exposure in nuclear medicine applications.
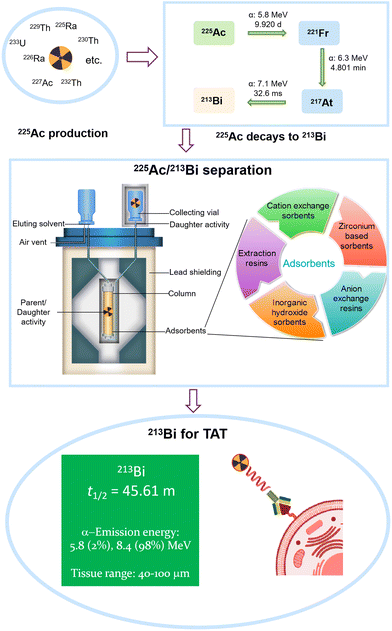 |
| Fig. 2 Schematic diagram of the process from 225Ac production to 213Bi for targeted alpha therapy. | |
213Bi is generally separated by the 225Ac/213Bi radionuclide generators. These generators are widely used to produce and separate daughter radionuclides with short half-lives from their long-lived parent radionuclides.34 A schematic diagram of the representative radionuclide generator is shown in Fig. 2, composed of shielding containers, adsorbent material, radionuclide(s), and eluent.34 Their use can ensure the availability of short-lived daughter radionuclides for medical applications in hospitals that are located far away from nuclide production facilities (e.g., nuclear reactors, accelerators, and actinide stocks).34,35 Moreover, radionuclide generators are capable of providing short-lived daughter radionuclides with high specific activity and in a carrier-free form at a relatively low cost.35,36 The choice of separation systems for separating 213Bi depends on the adsorption and desorption performance of the adsorbents used.37,38 This review sheds light on the various separation techniques used in previous studies, offering insights into selecting appropriate separation systems for specific isotopes of interest.
The adsorbent is the crucial component that plays a key role in facilitating the separation of radionuclides, and a variety of adsorbent materials have been utilized in the 225Ac/213Bi radionuclide generators.37–49 While these materials can be suitable for certain conditions, most of them still have drawbacks such as limited shelf-life (e.g., less than one day), radiolytic damage (e.g., scission of functional groups), nanoparticle aggregation, insufficient active sites, low adsorption capacity, long diffusion time, poor structural stability, and difficulties in their regeneration.37–49 Therefore, there is still an urgent need to investigate new emerging adsorbents that can meet the stringent requirements posed in clinical applications. The advantages and disadvantages of the reported adsorbents are introduced in this review.
This review aims to consolidate current knowledge and provide a comprehensive overview of 225Ac/213Bi radionuclide generators, their working principles, and the adsorbents involved (Fig. 2). The present paper provided a brief overview of the current status of 225Ac production, highlighting its current limitations and prospects for the future. The physical and chemical properties of 225Ac and 213Bi are introduced to facilitate a better understanding of the separation mechanisms employed in 225Ac/213Bi radionuclide generators. A detailed discussion of previously used generator systems and adsorbents provides the readers with a historical context and helps them understand the evolution of the separation techniques used for 213Bi from 225Ac, as well as identify the advantages and limitations of such adsorbents. By covering these aspects, this review serves as a valuable resource for researchers, clinicians, and other stakeholders involved in 225Ac/213Bi radionuclide generators and radiopharmaceutical development.
2. Actinium-225 production
Currently, there is limited availability of carrier-free 225Ac for preclinical and clinical trials.50 Approximately 4 TBq (216 Ci) of 225Ac per year is required to meet the demand for cancer treatment, and the activity for 213Bi should be increased accordingly.51 Various approaches have been considered or examined to produce 225Ac, which can be broadly divided into two categories: direct and in-direct production routes.50 In the direct route, 225Ac is the primary product of a nuclear reaction. In contrast, the indirect route involves the production of either 229Th or 225Ra, which subsequently decay to create 225Ac.50Fig. 3 illustrates the numerous relevant nuclear reactions involved in 225Ac production.33,52–62
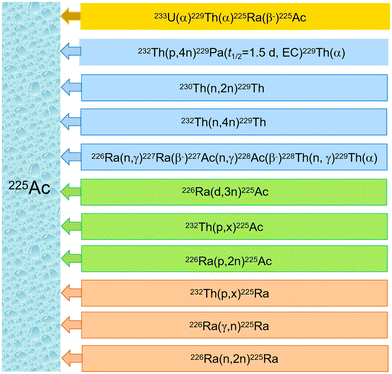 |
| Fig. 3 Nuclear reactions for 225Ac production. | |
Currently, the primary global supply of 225Ac is facilitated by the decay of 229Th sources with a long half-life of 7920 (34) years, primarily from the decay of fissile 233U with a half-life of 159
200 years.41,50,63–66233U is produced by the neutron irradiation of 232Th for its applications in advanced fuel cycles for Th-breeders.52Fig. 4 depicts the decay chain starting from 233U.41,50,64–66 However, only a limited number of 229Th and 233U stocks are available because of non-proliferation restrictions on the management of fissile material.67 It is estimated that no more than 63 GBq (1.7 Ci) per year of 225Ac can be produced worldwide by the decay of 229Th.52,68 Several institutions can generate limited quantities of 225Ac by this method; for instance, 26.6 GBq (720 mCi) per year produced by Oak Ridge National Lab (ORNL) in Oak Ridge, USA, ∼26.4 (715 mCi) per year produced by Institute of Physics and Power Engineering (IPPE) in Obninsk, Russia, and 13.1 GBq (350 mCi) per year produced by the Institute for Transuranium Elements (ITU) in Karlsruhe, Germany.30,31,62,67,69,70 In addition, the Belgian Nuclear Research Centre (SCK CEN) in Mol, Belgium, has a limited quantity of high-purity 229Th became available from legacy thorium sources originating from reactor irradiated 226Ra. 225Ac from this material has been used to evaluate the alternative adsorbents in 225Ac/213Bi generators and preclinical studies.31,47,71,72 There is also an additional small source of 229Th that is currently becoming available at Canadian Nuclear Laboratories.73 The separation process of 225Ac from 225Ra and 229Th was illustrated in the previous review52 and will thus not be covered within this work.
Several alternative strategies to produce 229Th have been considered or investigated over time. 229Th can be produced from the proton irradiations of 232Th with the reaction of 232Th(p,4n)229Pa (t1/2 = 1.5 d, EC) 229Th.33,53 However, this process produces a limited supply of 229Th because of the low cross-sections (e.g., 162 ± 14 mb at a proton energy of 29.7 ± 0.5 MeV), and the concerns regarding co-production of 230Th.29,53,74 Furthermore, the handling and separation process is challenging due to a broad range of interfering isotopes such as 228Pa and 230Pa. The irradiation of 230Th and 232Th using fast neutrons was also considered for 230Th(n,2n)229Th or 232Th(n,4n)229Th, but these methods only provide isotopically diluted 229Th and further investigations must be conducted to verify their feasibility.29 Yet another approach is the thermal neutron irradiation of 226Ra, described as follows: 226Ra(n,γ)227Ra(β−)227Ac(n,γ)228Ac(β−)228Th(n, γ)229Th, which involves three neutron captures and two beta(−) decays via a series of alternative pathways.54 However, the production of 229Th via this approach can only be efficiently conducted in high flux reactors and requires significant infrastructure due to the complicated process and the handling of large activities of intermediate products, such as 227Ac and 228Th. Likewise, 229Th can also be produced by the irradiation of 227Ac, 228Ra and 228Th, based on the irradiation route of 226Ra.54
The production of 225Ac by the proton-induced irradiation of 232Th via232Th (p,x)225Ac or 232Th(p,x)225Ra(β−)225Ac in a proton accelerator at energies between 66 and 500 MeV is widely considered a promising method.52,55–57 The preparation of 232Th targets does not pose difficulties, and this method is currently efficiently applied for the production of a higher amount of 225Ac (e.g., 11.2 TBq of 225Ac per month in TRIUMF in principle).52 However, it should be noted that the high energy proton spallation produces a broad variety of other radionuclides that require complex chemical separation procedures. Additionally, co-production of 227Ac with a half-life of 21.772 years is inevitable and hampers the radionuclidic purity of 225Ac (225/227Ac atom ratio 1
:
1 at end of irradiation).58,59 To circumvent this, separating 225Ra from 232Th can be used as the mother isotope for 225Ac, which can significantly enhance the radionuclidic purity of 225Ac.58 However, the activity of 225Ac obtained from 225Ra this way is an order of magnitude lower than that directly produced from 232Th irradiation.55,59 The presence of small amounts of 227Ac and its descendants (e.g.227Th) within 225Ac appears to be acceptable for maintaining the purity of 213Bi from 225Ac/213Bi radionuclide generators.31,48,58,75 Hence, the production of 225Ac in this manner might be beneficial for the application of 213Bi.58
The irradiation of 226Ra (t1/2 = 1600 years) with protons, deuterons, fast neutrons and gamma rays can be applied or suggested to create 225Ac through different nuclear reactions, which has also garnered much attention from scientists.60 However, the 226Ra targets also face a series of challenges with respect to the limited availability of the starting material, high infrastructure costs and considerable safety hazards (e.g., 222Rn gas and emission of high energy gamma rays).76,77 Proton-irradiation of 226Ra, resulting in the 226Ra(p,2n)225Ac reaction, is the most promising direct method for the large-scale production of 225Ac, which can generate 225Ac with significantly less interference from 227Ac.62 The co-production of 226Ac (29.37 h) and 224Ac (2.78 h) probably exerts less effect than the 227Ac contaminant because of their relatively short half-lives, but increases the cooling time after irradiation to achieve the required 225Ac isotopic purity.52 Additionally, the 226Ra(p,2n)225Ac reaction has a high cross-section (710 mb [maximum] at 16.8 MeV beam energy) by comparison to 232Th(p,x)225Ac production.52,62,70 Irradiating 226Ra by deuterons, resulting in the reaction 226Ra(d,3n)225Ac, has been suggested as a promising approach.60,61 This reaction can produce relatively high production yields compared to 226Ra(p,2n)225Ac, as predicted by model calculations.60 However, this method is hampered by the availability of suitable accelerators that offer deuteron beams of sufficiently high energy.61 Similarly, the fast neutron reaction pathway via226Ra(n,2n)225Ra has a promisingly high cross-section (860 mb at 16.6 MeV), but the challenge remains in the availability of a suitable facility providing the required fast neutron fluxes.78
The use of photonuclear reactions to induce 226Ra(γ,n)225Ra(β−)225Ac reaction is another potential route for 225Ac production.77,79 This reaction involves irradiating 226Ra with bremsstrahlung photons to generate 225Ra. Based on the properties of the target configuration to thermalize emitted neutrons, the obtained 225Ac will be free from other actinium isotopes after a series of consecutive milking operations of the irradiated target. However, the production yield is relatively low compared to other methods, and the efficiency of this production method will depend on factors such as target mass, the energy and intensity of the electron/bremsstrahlung used etc.
Other potential methods have also been discussed in previous reviews.52 Moreover, studies have demonstrated the separation of 225Ac from large target masses.52,80 In summary, the production and separation of 225Ac with large quantities and high purity are crucial for developing the 225Ac- and 213Bi-radiopharmaceuticals.
3. Physical and chemical properties of 225Ac and 213Bi
Understanding the physical and chemical characteristics of 225Ac3+ and 213Bi3+ ions is crucial for identifying the separation mechanisms of the adsorbents and optimizing the separation procedures. These insights can be exploited to develop improved separation methods and alternative adsorbents for separating 225Ac and 213Bi.
3.1. Decay characteristics of 225Ac
225Ac is a highly cytotoxic radiometal with a half-life of 9.920 days and is considered a pure alpha emitter.64,81 It undergoes decay via a cascade involving six short-lived radionuclide daughters (221Fr, 217At, 213Bi, 213Po, 209Pb, and 209Bi), ultimately resulting in the long-lived 209Bi (t1/2 = 1.9 × 1019 years) (Fig. 4).25,64 The 225Ac decay process predominantly involves the emission of four alpha and two beta particles. Most intermediates in the decay path have high energies, demonstrated by the significant radiations of 5.8–8.4 MeV for alpha emitters and 0.6–2.0 MeV for beta emitters.64 The cumulative energy of four alpha particles can reach about 28 MeV, which can be harnessed for cancer treatment.82 However, the leaching of its daughters from chelating agents is a concern regarding the potential toxicity to healthy cells. The high recoil energy of an alpha particle, ranging from 100–200 keV, is challenging for the radiation stability of chelating agents, as it exceeds the binding energy of the coordination complex.64 In addition to alpha particles emission, two useful gamma emissions are produced by the disintegration of 221Fr (218 keV, 11.6% emission probability) and 213Bi (440 keV, 26.1% emission probability), which can be applied for vivo imaging.25
3.2. Actinium/lanthanum chemistry
Non-radioactive lanthanum is usually used as a surrogate of actinium, owing to its several similar chemical behaviors in aqueous.81,83 Here, the properties of actinium and lanthanum together were displayed. The stable valence for actinium ions is +III (Ac3+), and Ac3+ (an electronic configuration of [Rn]5f0) is considered the largest trivalent cation among the elements in the periodic table.83 The stable lanthanum valence is also +III (La3+ with an electronic configuration of [Xe]4f0). The first hydrolysis constant of the Ac3+ is in the pH range of 8.6–10.4, and the La3+ is at pH = ∼8.5.81,84–86Fig. 5a shows the distribution species of La as a function of pH.87 Typically, the separation of 225Ac and 213Bi occurs under acidic conditions (pH < 5), where hydrolysis of 225Ac minimally impacts on the separation process. According to the hard and soft acids and bases (HSAB) theory, the Ac3+ and La3+ both belong to hard Lewis acids, and the absolute chemical hardness for Ac3+ and La3+ is 14.4 and 15.4 eV, respectively.88–91 Hence, such metal ions are possibly preferred to “hard” nonpolarizable, electronegative Lewis bases.83 One of the main differences between Ac3+ and La3+ is the ionic radius, with a 6-coordinate ionic radius of 0.112 nm for Ac3+ and 0.103 nm for La3+.83 Due to its larger size, Ac3+ possesses a lower charge density.83 Ferrier et al. reported that the Ac3+ could directly coordinate with 10.9 ± 0.5 water molecules, and Ac–O(H2O) distance is about 2.63 Å.92,93 The detailed information on coordination properties for actinium can be found in a review paper.81 Allen et al. proposed that the hydration number of La3+ is 9.2 at low chloride concentrations, whereas the distance of the La–O(H2O) bond is 2.54 Å.94
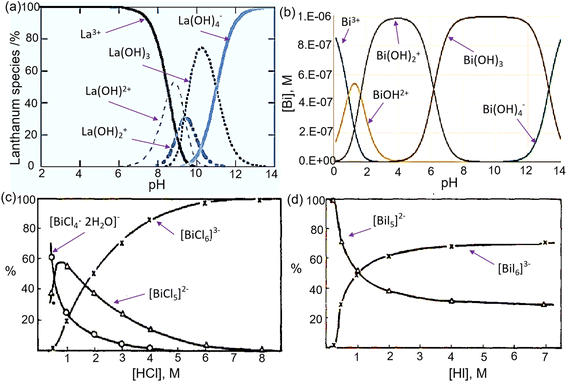 |
| Fig. 5 Distribution species of La3+ as a function of pH (a) (reproduced from ref. 95 with permission from the Royal Society of Chemistry, copyright 2006); distribution species of Bi3+ (10−6 mol L−1) as a function of pH (b) (reproduced (adapted) from ref. 96. with permission from the American Chemical Society, Copyright 2022 ); and distribution species of Bi3+ as a function of HCl (c) and HI concentrations (d) (reproduced (adapted) from ref. 97 with permission from Elsevier, copyright 1979). | |
3.3. Decay characteristics of 213Bi
The radiometal 213Bi, with a half-life of 45.61 min, is a mixed alpha/beta emitter.64 It decays via a branched pathway involving alpha and beta disintegration to the near-stable 209Bi.25,64 In particular, 213Bi (97.8%) decays primarily via the beta emission to a pure alpha-emitter 213Po with an ultra-short half-life of 3.708 μs (Fig. 4). 213Po emits alpha particles with a high energy of 8.4 MeV and a path length of 85 μm in human tissue, which is mainly responsible for the therapy's efficacy.25 Moreover, the 213Bi decay is accompanied by the emission of 440 keV gamma rays (26%), which is possibly beneficial for quantitative tumor uptake and dose calculations for clinical medical treatment.25 Approximately 2.2% of 213Bi decays via alpha emission to 209Tl, a beta-emitting isotope. The decay of 209Tl follows with the emission of high-energy gamma rays (∼1567 keV). Indeed, it has a low probability, which might exhibit a limited effect on patients. When working with a high amount of 213Bi, shielding is a possible consideration to protect personnel and the environment from these potentially harmful gamma rays.
3.4. Bismuth chemistry
Bismuth, with an electronic configuration of [Xe]4f145d106s26p3, is readily able to accept electron pairs due to the poor shielding of the f-orbital electrons and has a high affinity for extended coordination due to its unoccupied orbits.98,99 The trivalent cation (Bi3+), with an ionic radius of 0.096–0.117 nm (CN = 5–8), is the most stable oxidation due to the inner pair effect. Even the pentavalent cation is recognized as an oxidative reagent.1,100 Bi3+ is usually classified as a borderline acid, and it has a high affinity for oxygen and nitrogen donors and forms strong complexes with sulfur and halogens.100,101 Chelating agents, including oxygen, nitrogen or thiolate donors, have the ability to form very stable complexes with Bi3+, with coordination numbers ranging from three to nine.100 The first pKa of Bi3+ is approximately 1.1, indicating that the Bi3+ undergoes hydrolysis in acidic solutions, which is an important consideration when separating 213Bi from 225Ac.102 The speciation diagram of Bi3+ as a function of pH is shown in Fig. 5b. The eluents with halide ions are usually utilized to elute 213Bi due to the formation of negatively charged Bi-halide complexes (Fig. 5c and d) from cation exchange platforms, and the strength of Bi-halide complexes increases in the expected order of F− < Cl− < Br− < I−.101 In a 0.1 M NaI/0.1 M HCl solution, often used as an elution solution, the dominant species include BiI4− and BiI52−.69
4. Principle of 225Ac/213Bi radionuclide generators
4.1 Ingrowth of 225Ac and 213Bi
Generally, 229Th and 225Ra serve as the grandparent and parent for the indirect production of 225Ac, respectively. The optimal elution frequency and schedule may vary depending on the decay properties of the radionuclides and the specific clinical needs of patients. The ingrowth of 225Ra (from 0) and 225Ac (from 0) from 229Th was calculated, and the results are shown in Fig. 6a.34,103 Clearly, 225Ac and 225Ra can reach secular equilibrium with 229Th after four months, while more than 80% of 225Ac are available after two months. Fig. 6b shows the decay of 225Ra to 225Ac, with a transient equilibrium time of 17 days. The optimal elution time for 225Ac is about every 10 days. Fig. 6c displays the ingrowth of 213Bi from the decay of 225Ac. The secular equilibrium time is about 5 h, whereas more than 80% and 90% of 213Bi can be obtained in about 2 and 3 h, respectively.42 Typically, the generators are eluted every 2–3 h.37,104
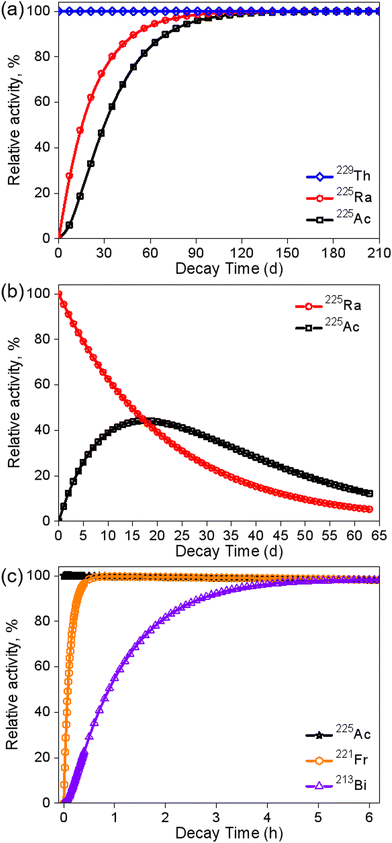 |
| Fig. 6 Theoretical 229Th-decay and 225Ra/225Ac-ingrowth (a); theoretical 225Ra-decay and 225Ac-ingrowth (b); theoretical 225Ac-decay and 221Fr/213Bi-ingrowth (c). | |
4.2 Adsorption mechanisms
Understanding the separation mechanisms of adsorbents is crucial for selecting suitable adsorbents for radionuclide generators that produce high-purity radioisotopes with optimal efficiency and selectivity. These separation mechanisms can be divided into two categories: adsorption and desorption.34 Unfortunately, there is limited research on the adsorption and desorption mechanisms of adsorbents towards 225Ac, possibly due to the absence of stable, non-radioactive isotopes of this element. In this review, the general sorption mechanisms have been illustrated by using previous studies on the separation of similar metal ions and radionuclides. The primarily sorption mechanisms are as follows: electrostatic interactions, ion exchange, and surface complexation.105,106 Physical adsorption mechanisms may also occur, such as pore filling, hydrolysis, and precipitation; however, current literature indicates that these mechanisms have a limited impact on Ac3+/Bi3+ adsorption.105,106 Additionally, the primary objective of this section is to shed light on the role of surface functional groups. As such, a detailed discussion on these physical adsorption mechanisms is not included in this section.
Electrostatic attractive and repulsive interactions, mainly occurs between the protonated/deprotonated function groups and metal ion species (Fig. 7a).105 Positively charged metal ions (e.g., Ac3+, La3+, and Bi3+) are attracted by negatively charged functional groups (e.g., –SO3−, –PO3−, and –COO−) and are repelled by positively charged functional groups (e.g., R-N(CH3)3+, R-N(CH2CH3)3+, and R-NH(CH2CH3)2+).38,39,47,107–114 This type of interaction is highly governed by the solution pH. Typically, the surface charge of the adsorbents becomes negative when the solution pH exceeds the point of zero charges (pHpzc), which is beneficial for the adsorption of cations. If the pH of the solution is lower than the pHpzc, the surface charge becomes positive.105 Apart from the impact on the charge of the functional groups, the solution pH also influences the metal ion speciation.47,114,115 This is because the concentration of H+ ions can affect the oxidation states, solubility, ionization, and forms of metal ions. Apart from the pH, other solution parameters, including the ionic strength, and the presence of co-existing ions that can complexate, play a major role in the type of interaction.116
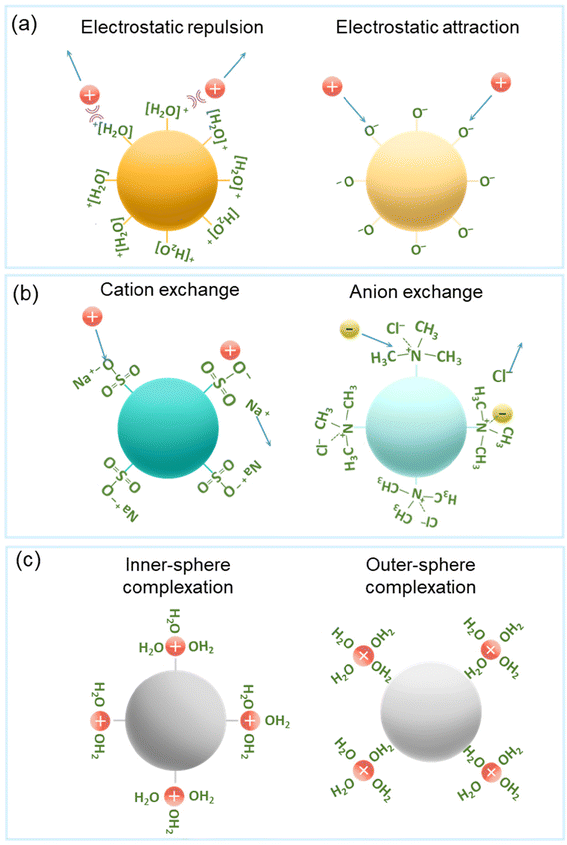 |
| Fig. 7 Schematic diagrams of the adsorption mechanisms: electrostatic interaction (a), ion exchange (b), and surface complexation (c). | |
Ion exchange is a process in which cations (or anions) in a solution replace dissimilar and displaceable ions of the same charge contained in a solid materials (Fig. 7b). During cation exchange, cations from the liquid can replace positively charged metal ions attached to the adsorbents.105 Conversely, during anion exchange, anions from the liquid can replace negatively charged metal ions attached to the adsorbents. For a number of commercially available ion exchange resins, a selectivity series is available to indicate the affinity to converse from one ion form to the other. In cation exchange resins, sulfonic acid groups (strong acidity), phosphonic (medium acidity), and carboxylic (weak acidity) acid groups are the main functional groups.39,47,107–110,112–114,117 Meanwhile, anion exchange resins utilize quaternary ammonium and amine groups to facilitate ion exchange.38,111 For example, Ac3+ ions are adsorbed onto the cation exchange resin by cation exchange, while the Bi-halide complexes are adsorbed onto the anion exchange resins via the anion exchange.26,38,111,118
Surface complexation is a crucial adsorption mechanism involving inner- and outer-sphere complexation (Fig. 7c).105,116,119 Inner-sphere complexation occurs when metal ions bind to specific active adsorption sites on a surface, owing to a strong bond between the adsorbing species and active adsorption sites.105 In contrast, outer-sphere complexation occurs when adsorbing metal ions interact with active adsorption sites via water molecules. This interaction is highly sensitive to the ion strength, which can alter the inner and outer-sphere complexations.
The adsorption of metal ions which preferably interact by inner-sphere complexation, is less impacted by changes in the ionic strength.116 This is in contrast to metals that predominantly interact by outer-sphere complexation, for which the adsorption decreases with increasing ionic strength, due to the increased competition with other ions that are present in solution.116
The contribution of the three main types of interaction in the total adsorption process will be dependent on numerous factors, including the pH, the ionic strength, the type of ions, and the introduction of competing or complexing ions and chelators.39–41,69,107,120–125 The desorption mechanism is illustrated when introducing the previously used adsorbents for 225Ac and 213Bi separation.
4.3 Types of 225Ac/213Bi radionuclide generators
Understanding the separation process of 225Ac/213Bi radionuclide generators is crucial for selecting or designing the appropriate adsorbents to use in these generators. This knowledge enables researchers to optimize the efficiency and effectiveness of the separation process, thereby ensuring high purity (e.g., the 225Ac impurity less than 10−4%) for the desired radionuclides and prolonging the lifespan of the generators. Fig. 8a presents several general requirements for radionuclide generators that can be used as a guide to evaluate the advantages and disadvantages of current 225Ac/213Bi radionuclide generators.34 Various types of radionuclide generators have been examined for their efficacy in 225Ac and 213Bi separation.28,48,100,120 The generator columns for the 225Ac and 213Bi separation can be typically classified into two types based on the method of operation and properties of the adsorbents: regular (direct) and inverse columns.34,39,44,48 Considering the number of columns involved, the generator systems can be primarily classified into single-column and double-column systems. The second column is typically used to concentrate and further purify the 213Bi eluate from the first column in order to fulfill radiopharmaceutical requirements.49,107 In this review, the generator types (Fig. 8b) are divided into the following categories: (1) single-column direct 225Ac/213Bi generators; (2) two-column direct 225Ac/213Bi generators; (3) single-column inverse 225Ac/213Bi generators; (4) multicolumn selectivity inversion generators (MSIGs); and (5) millifluidics-controlled electrodeposition-based 225Ac/213Bi generators.37,39,41–47,49,107,108,126–130
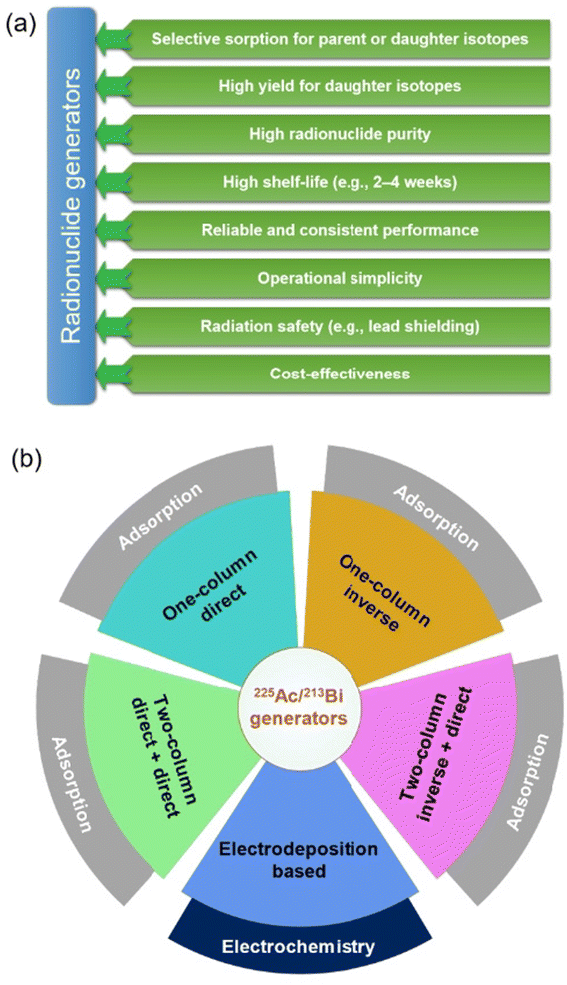 |
| Fig. 8 Requirements of radionuclide generators (a) and the types of 225Ac/213Bi radionuclide generators (b). | |
4.3.1 Single-column direct 225Ac/213Bi generators.
Direct radionuclide generators, which are the most popular generators for the separation of medical radionuclides, can simplify the separation process and shorten the separation time.34,43 The adsorbents in a direct column should have a strong adsorption affinity for the mother isotope, while the daughter isotope can be selectively eluted at regular intervals using appropriate eluents, which must be devoid of the mother isotope. The separation process of the direct generator systems typically consists of the following several steps (Fig. 9a): (1) column preconditioning, (2) column rinsing, (3) 225Ac/213Bi stock solution loading, (4) elution of 213Bi, and (5) column regeneration.26
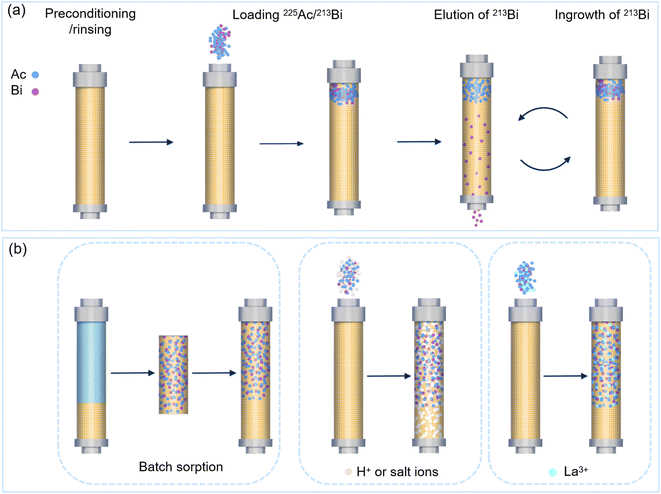 |
| Fig. 9 Separation process of the direct generator system (a) and the loading process to distribute the 225Ac in a large mass of sorbents (b) (La3+ acted as a competition ion for Ac3+ sorption). | |
Column preconditioning is an essential but easily overlooked step because it removals any impurities that can interfere with the separation process. During the preconditions, the adsorbent materials are activated in order to have an optimal performance in both ad- and desorption. This step is typically performed using solutions such as HCl (e.g. 1.5 M HCl for AG MP-50) and HNO3 (e.g. 8 M HNO3 for AG 50WX4).41,45,120,131 Afterward, any excess acid attached to the column can be removed by rinsing the column to ensure that the adsorbent is within the appropriate pH range for optimal separation and to remove any impurities introduced during the preconditioning process.45
There are various methods for loading 225Ac onto an adsorption column. The most straightforward method is to pass the 225Ac stock solution through the column, allowing the 225Ac to be adsorbed on the adsorbent (Fig. 9a). In case the adsorbent has a very high affinity, such as cation exchange resins with sulfonic acid groups, 225Ac adsorption will predominantly occur at the top layer of the column. This localized adsorption might lead to severe radiolytic damage of the adsorbents.48 For example, the AG MP-50 column can be irreversibly damaged within a few hours when more than 4 GBq of 225Ac is loaded on the top layer of the sorbents, which was mainly due to the scission of sulfonic acid groups, discoloration, and the damage of polymer structures.48,71 In an alternative loading method, about two-thirds of the adsorbent is removed from the column, mixed with the 225Ac solution, and shaken for a period to ensure a more homogeneously spreading of 225Ac on the adsorbent (Fig. 9b).39 Thereafter, the column is filled with the adsorbent material onto which 225Ac solution is more uniformly loaded. This method aims to lengthen the lifetime of the adsorbents by increasing the contact area between the radionuclides and sorbents. However, it can also lengthen the handling time of the radionuclides and probably increases the risk of personnel and the environment being exposed to radiation. Hence, a novel strategy is to distribute the 225Ac throughout a large mass of adsorbents by reducing the sorption capacity for 225Ac. This can be achieved by increasing the acid or salt concentrations of the 225Ac stock solution (Fig. 9b).69 Similarly, another method for dispersing 225Ac across a large mass of adsorbents is to use a high-acid solution to elute the column. For example, a 2 M HCl solution can be used to distribute 225Ac throughout half of the AG MP-50 column.107 Additionally, introducing 225Ac surrogates, such as La3+, is expected to reduce the sorption capacity of sorbents for 225Ac because of the increased adsorption competition between La3+ and Ac3+ (Fig. 9b).
The fourth step in the process is the selective desorption of 213Bi. Therefore, a stripping solution is used to selectively desorb 213Bi without desorbing the 225Ac. As explained above, elution with halide ions is typically used to milk 213Bi owing to the formation of 213Bi-halide complexes.43,120,121 Additionally, various chelating agents, such as diethylenetriamine pentaacetate (DTPA) and 1,4,7,10-tetraazacyclododecane-1,4,7,10-tetraacetic acid (DOTA), can be used to elute 213Bi.107
However, the use of a single column in the separation process poses high requirements on the adsorbents with regard to their high affinity for 225Ac and a low sorption capacity for 213Bi complexes to ensure the production of 213Bi with a high yield and purity. As most of the adsorbents are prone to radiolytic damage, a safe and sustainable operation to obtain high-activity 213Bi for medical applications is compromised.48,109,132–134
4.3.2 Two-column direct 225Ac/213Bi generators.
A two-column direct 225Ac/213Bi generator system is used to increase the purity and concentration of 213Bi eluates.39,45,107,129 The separation process of the first column is similar to that of a single-column direct 225Ac/213Bi generator. The 213Bi eluate from the first column is passed into the second column (Fig. 10a), also called the guard column or concentrated column, where the adsorbent has a higher affinity for 225Ac than for 213Bi-halide complexes. In such separation systems, the 213Bi-halide complexes with low volume and suitable conditions for 225Ac sorption onto the second column can be directly passed through the second column. However, when the volume of 213Bi eluates from the first column is high (e.g., >5 mL), both 213Bi and 225Ac need to be adapted for adsorption on the second column, and subsequently 213Bi can be selectively eluted, which can further increase the 213Bi concentration and purification.
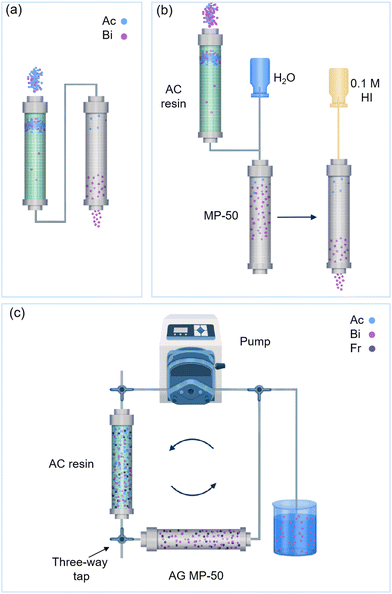 |
| Fig. 10 Separation process of two-column direct 225Ac/213Bi generator, highlighting the functions of second column in further purification (a), both purification and concentration (b), and purification (automatic) (c). | |
Wu et al. designed a two-column direct 225Ac/213Bi generator (Fig. 10b) by employing the actinide resin (AC resin, silica gel impregnated with di-2-ethylhexyl-methane-bis-phosphonic acid) in the first column and MP-50 as the adsorbents in the second columns, respectively.39 The first column was filled with AC resin and equilibrated with 0.5 M HNO3. Two-thirds of the AC resin sorbed the 225Ac in the 0.5 M HNO3 solution for 30 min under gentle agitation at room temperature before packing it to the generator column. Thereafter, the AC resin was equilibrated with 1.0 M HCl. Moreover, 1.0 M HCl could eluate 213Bi from the AC resin. The breakthrough of the 225Ac for the first column was less than 0.05%. Subsequently, the 213Bi eluate from the first column was diluted using water, and the 213Bi was adsorbed onto the second column containing MP 50. Finally, the 213Bi that adsorbed on MP-50 was eluted using 0.1 M HI. No 225Ac was detected in the 213Bi eluate after the MP-50 column.39
Recently, a new type of two-column direct 225Ac/213Bi generator was developed at the Institute for Nuclear Research of the Russian Academy of Sciences (INR RAS) (Fig. 10c).107,129 The parent 225Ac is adsorbed onto the first column containing AC resin, while the 221Fr can be selectively and continuously washed with HNO3 and NH4Cl, which can be called a 225Ac/221Fr generator. Next, 221Fr is adsorbed onto the second column using the AG MP-50 resin and decays to 213Bi. After a certain period, 213Bi can be milked from the second column by using 0.1 M HCl/0.1 M KI, DOTA or DTPA.
Two-column generator aims to ensure the high purity and concentration of 213Bi. The requirements for the sorbents used in the first column are less strict in terms of 225Ac breakthrough. In other words, the 213Bi purity from the first column may not satisfy the requirements in medical applications, but adsorbents must operate for a long time when loading high-activity 225Ac. The AG MP-50 and AC resins are well-known materials used in the second column because of their high affinity for 225Ac, which can improve the purity of 213Bi. It should be noted that the two-column direct 225Ac/213Bi generator is slightly more complex than the single-column direct 225Ac/213Bi generator. However, this generator remains useful in cases where an ideal sorbent for a single-column direct 225Ac/213Bi generator is unavailable.
4.3.3 Single-column inverse 225Ac/213Bi generators.
Another approach to achieve selectivity is the inverse generator, where the sorbent must have a high affinity towards Bi3+/Bi-complexes over 225Ac.38,40,44 Inverse generator systems typically comprise the following steps (Fig. 11a): (1) preconditioning the column, (2) rinsing the column, (3) loading the 225Ac/213Bi stock solution, (4) stocking 225Ac in a vial, (5) eluting 213Bi, and (6) regenerating the column. During the 225Ac/213Bi loading step, 213Bi can be selectively adsorbed onto the column when the 225Ac/213Bi stock solution passes through the column, but no 225Ac is adsorbed onto the sorbent (ideal condition). Subsequently, the 213Bi can be eluted with various elutes, such as HNO3, NaOAc, HCl, halide ions, and DTPA.38,40 Typically, this generator produces a higher 213Bi yield in a smaller eluate volume than direct generators. However, compared to direct generators, 213Bi eluates from inverse generators usually have a higher 225Ac impurity because of the small amount of 225Ac adsorbed onto the column.38,40,44,48 It is challenging to find a suitable sorbent for the inverse generators to produce 213Bi with a high purity that is suitable for radiopharmaceuticals. However, the sorbents used in inverse generators receive a relatively lower radiation dose than those used in direct generators. This is because stocking 225Ac in a vial shortens the contact time between the sorbents and the radionuclides. One example of an inverse generator is the Bi-generator from the Pacific Northwest National Laboratory (PNNL), which uses organic anion-absorbing resins incorporated into a cartridge.38 This generator also possibly provides additional 213Bi purification to avoid the influence of 225Ra, 221Fr, 229Th, and 227Ac. One drawback of the inverse generators is the more complex separation process and the longer time for handling and manipulating radionuclides. This can be overcome to some extent by implementing automation in the separation equipment.38 Vasiliev et al. reported an automatic separation scheme (Fig. 11b) that can minimize the irradiation exposure for the personnel handling radionuclides.44 Analogous to the some direct generators, a second column (guard column) is required for the further purification of the 213Bi eluate to satisfy the radiochemical requirements.49
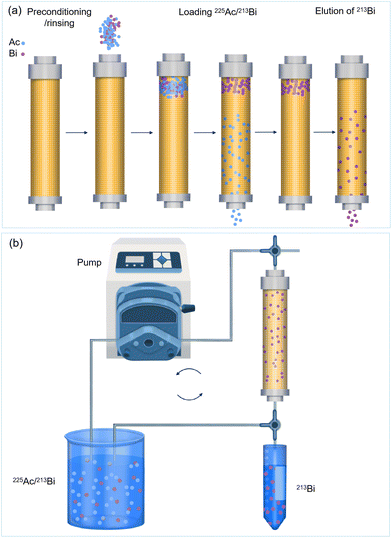 |
| Fig. 11 Simple separation process of the inverse 225Ac/213Bi generator (a) and an automatic separation scheme (b). | |
4.3.4 Multicolumn selectivity inversion generators.
The multicolumn selectivity inversion generators (MSIGs) system typically comprises a primary separation column and a guard column (Fig. 12a).49,75,135–137 The primary separation column also refers to an inverse column with a strong affinity for 213Bi or 213Bi-halide complexes and a weak/no adsorption affinity for 225Ac. The guard column is usually used to improve the purity of 213Bi originating from the primary separation column (inverse generator) while maintaining a minimal influence on the 213Bi yield. Here, 213Bi from the inverse generator can pass through the guard column, which adsorbs the possible 225Ac impurity. Apart from the production of high-purity 213Bi, this separation technique minimizes the irradiation of radionuclides onto the sorbents. Additionally, it is possible to selectively adsorb 213Bi onto a small column, resulting in a high 213Bi concentration. Generally, the AG MP-50 and its analogs with a low radiation stability can be utilized as the guard column owing to the decreased irradiation time.49 The UTEVA-AG MP-50 generator is a representative MSIG used for the 225Ac/213Bi separation.49 The UTEVA resin used in the primary separation column has a high affinity for 213Bi–chloride complexes while exhibiting a low affinity for 225Ac. Subsequently, the 213Bi eluate is passed through the AG MP-50 column to improve its purity. At INR RAS, an automatic MSIG was developed by employing inorganic materials in the primary column, as shown in Fig. 12b.75 The commercial MSIG system has been approved by the US Food and Drug Administration for use in the 99mTc generator in NorthStar.138
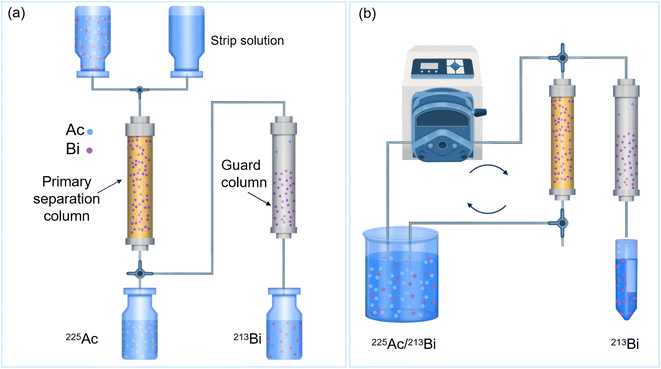 |
| Fig. 12 Simple separation process of MSIG (a) and an automatic separation scheme (b). | |
4.3.5 Millifluidics-controlled electrodeposition-based 225Ac/213Bi generators.
The different generators described above are all based on adsorption. Alternatively, electrochemistry is also a useful separation technology. This new type of radionuclide generator can selectively separate the target isotope onto an electrode owing to the electroplating mechanism.34,46 Following that, recovery of the target isotope from this electrode can be achieved by applying an external potential. The radionuclide deposited on the metal substrate can show high radiolytic stability. Fig. 13 shows a schematic diagram of the electrochemical radionuclide generator.139 Recently, researchers at the Los Alamos National Laboratory (Los Alamos, United States) developed a millifluidics-controlled electrodeposition-based 225Ac/213Bi generator using nickel and titanium electrodes.46 After 15 min reaction duration, 70%–98% of 213Bi was deposited on the nickel plates. By applying an outside potential (2.0 V for 10 min at pH 4.0–5.5), 60%–94% of 213Bi (Bi/Bi3+, +0.317 vs. SHE) could be recovered.140 This new generator exhibits high durability and reliability, which are necessary for separating high-activity 225Ac and 213Bi radionuclides. However, such generators may have limitations. The separation process requires electrochemical and radiochemical skills. Additionally, strict adherence to operating procedures (e.g., concentration of reactants or electrolytes, voltage and current, temperature, impurities, electrode surface, pH levels, and mass transfer) is essential because of the sensitivity of the electrochemical process.34 Further optimization of the process and the related set up will be required to assess the potential of this approach as radiopharmaceutical generators.
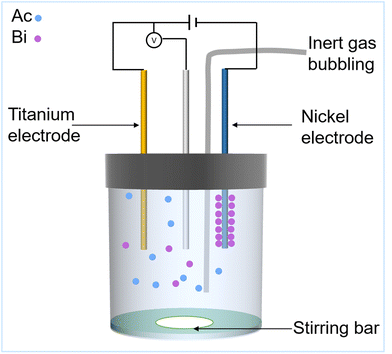 |
| Fig. 13 Schematic diagram of the electrochemical radionuclide generator setup. | |
4.4.
213Bi elution
The conditions for the 213Bi elution are determined by the nature of the adsorbent materials, but also by the 213Bi labeling conditions. Table 1 provides an overview of conditions for 213Bi eluates and their corresponding labeling conditions from previous research.39–41,69,107,120–125 While the elution occurs at acid pH (pH < 2), labeling is performed at higher pH values (pH 4.0–8.4). To bridge this pH gap, a buffer solution (often citrate or acetate) is added, providing the additional benefit of preventing 213Bi hydrolysis due to weak complexation.1,37 In case I− ions to eluate 213Bi, demetallized L-ascorbic acid is added to decolorize the eluate.41 The labeling process generally lasts about 1–10 min. Considering the short half-life of 213Bi, achieving a high 213Bi yield in the eluate is crucial and should be a requirement for 225Ac/213Bi radionuclide generators. Adjusting the pH values enables elution with a high-acidic concentration, such as 2 M HCl, to be suitable for the labeling conditions. The influence of salt concentrations on 213Bi labeling may be minimal. Chelators are also used as eluents to elute 213Bi from the radionuclide generators. This is believed to reduce the loss of 213Bi during the labeling process.107 However, it should be carefully examined whether other chelators can form selective complexes with 213Bi rather than 225Ac. The 213Bi eluates shown in Table 1 can be adjusted to a standard labeling condition from the previous research. Therefore, the choice of such or similar elution is expected to have a minimal effect on the labeling process.39–41,69,107,120–125
Table 1 A representative summary of the 213Bi eluates and their corresponding labeling conditions
213Bi elution |
Labelling conditions |
Ref. |
Chelators/molecules |
Standard labelling conditions |
0.1 M HCl/0.1 M NaI |
CHX-A-DTPA-HuM195 |
∼0.23 M NH4OAc, pH 4–5, RT, 10 min |
41
|
CHX-A′′-DTPA-chelated anti-EGFR-mAb |
0.4 M NH4OAc, pH 5.5, RT, 7 min |
69 and 122 |
DOTATATE |
0.15 M Tris buffer, 2.6 mM ascorbic acid, pH 8.4, 95 °C, 5 min |
123
|
0.1 M HI |
Anti-Tac-CHX-A-DTPA |
NH4OAc, pH 5.0–5.5, RT, 6 min |
39
|
CHX-A′′-DTPA conjugated scFv or diabody |
NH4OAc, pH 5.0, RT, 10 min |
124
|
0.5 M HCl |
BSA-DOTA |
Saturated Na2CO3 solution, pH 5.5–7, RT, 10 min |
121
|
1 HCl |
Picolinate containing acyclic ligand |
Phosphate-buffered saline (PBS, c(PO43−) = 0.01 M) and morpholino ethane sulfonic acid, pH 5.5–6.5, RT, 1–2 min |
120
|
Picolinate containing macrocyclic ligand |
2 M HCl |
2D11-DTPA |
0.1 M citrate buffer, pH 5.5, RT, 15 min |
125
|
MOPC-DTPA |
0.05 M NH4OAc (pH 4.4) |
Cyclohexyl-DTPA-derivatives |
RT, 10 min |
40
|
DTPA, acetate buffer, pH 2–5.3, RT |
— |
— |
107
|
5. Sorbents
Various adsorbents have been explored for use in the 225Ac/213Bi radionuclide generators. These adsorbents have been categorized into six types in this review. Before explaining in more detail these classes, a short section will be devoted to explain in general the different types of adsorption mechanisms.
5.1 Cation exchange sorbents with sulfonic acid groups
Cation exchange resins with sulfonic acid groups (Fig. 14a) are the commonly used adsorbents in direct 225Ac/213Bi generators, due to the strong affinity of the sulfonic acid groups towards 225Ac and a weak affinity for 213Bi complexes, such as Bi-halide and Bi-DTPA complexes.39,107,110,126 The adsorption mechanisms can be attributed to a combination of electrostatic attractions between the negatively charged sulfonic acid groups and the positively charged of 225Ac species, and via the ion exchange between 225Ac and hydrogen or salt ions (e.g., Na+) attached to the sulfonic acid groups. These resins typically comprise sulfonic acid groups bonded to styrene-divinylbenzene copolymer backbone, such as, AG 50X4 (4% cross-linkages),131 AG 50X8 (8% cross-linkages),127 Dowex 50W-X8 (8% cross-linkages),26 and AG MP-50 (macroporous).41 The crosslinking degree of the resin determines the rigidity and pore size of the resin bead. A resin with a lower cross-linkage (2%–8%) has a more open structure permeable to higher molecular weight substances than a highly cross-linked, and more rigid resin (8%–16%). It also has a lower physical resistance to shrinking and swelling, so that it absorbs more water and swells to a larger wet diameter than a highly cross-linked resin of equivalent dry diameter. Other important characteristics of the resin are the porosity and bead size, which will have an impact on the kinetics of the sorption process. In dry conditions, the resin matrix is compact. When contacted with water, the channels and the pores of the resin will be filled with water, and the beads will swell. Macroporous resins, with additional pore sizes (mesoporous) in the range of 100 nm, offer transport channels that will facilitate the transport and fasten the gel diffusion. Another consideration to take into account when selecting a resin material, if its resistance towards irradiation. High crosslinking degrees typically have a better stability towards radiation.36,48,134
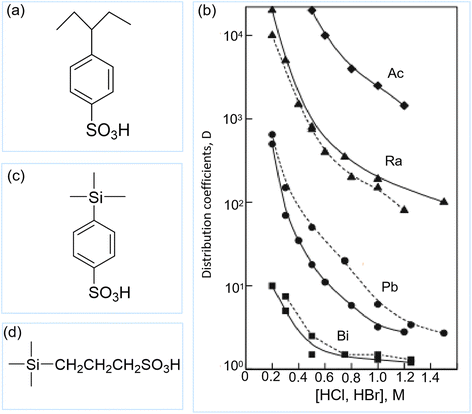 |
| Fig. 14 Schematic structure of cation exchange resin (a); distributing coefficients of Dowex 50-X8 as a function of HCl (dashed lines) and HBr (solid lines) concentrations (b) (reproduced (adapted) from ref. 126 with permission from Springer Nature, copyright 2010); and schematic structures of Isolute SCX (c) and SCX-2 (d). | |
Dowex 50W-X8, a spherical-shaped strong cation exchange resin used in direct generators, has been reported by Samad et al. and Geerlings et al.26,118 In these studies, 225Ac (in a 1 M HNO3 or 2 M HCl solution) was adsorbed onto the Dowex 50W-X8 column, while 213Bi was eluted as BiCi4− or Bi2Cl82− by using a 0.5–2 M HCl solution as the eluent.26,118 However, they did not provide any information regarding the yield and purity of 213Bi. Guseva and Dogadkin used the same column materials and showed a large difference in adsorption capacity between Ac3+ and Bi3+ (in 0.25 M HCl), due to the formation of Bi-halide complexes (Fig. 14b).126 HBr and HCl (1–2 mL of 0.25–0.3 M) were used as eluates, resulting in a 213Bi yield of over 90%.126 The 213Bi yield could be further maximized to 95% by using 1 mL of 10−3–10−2 DTPA at pH 1.8–2.2.126 Moore et al. reported that the 213Bi yield achieved using AG-50X8 column could reach 85% ± 6% by using 0.1 M KI/HCl as the eluent, with a 225Ac breakthrough of 0.01% ± 0.02%.127 A resin with lower crosslinking degree (AG 50X4) was investigated by Boll et al.131225Ac adsorption was performed in 1 M HNO3 stock solution, while desorption was performed in a 0.15 M HI, leading to 213Bi yield between 91%–98% with an 225Ac to 213Bi ratio of 10−5–10−4 and a 225Ra to 213Bi ratio of 10−3.36,100,131
However, cross-linkages typically determine the radiation stability of cation exchange resins. The limited resistance of such resins to radiolysis hindered their applicability due to their relatively lower cross-linking degree. Macroporous AG MP-50 is currently the most widely used resin, owing to its enhanced thermal stability and high radiation stability compared to similar resins.48 In medical applications, an 225Ac/213Bi radionuclide generator should load high activity of 225Ac (4 GBq). However, under this condition, columns that contain 200 mg of AG MP-50 cannot operate for more than one day.48 Although the radiolytic stability of AG MP-50 is higher than that of its counterparts with a lower degree of cross-linking, it cannot separate 225Ac/213Bi contents sufficiently in terms of radiolytic stability for medical applications. This is because a high amount (e.g., 4 GBq) of acidic 225Ac solution is efficiently sorbed to a tiny layer at the top of the AG MP-50 resin.48 Although the AG MP-50 resin provided a high yield (e.g., 76%) and purity (e.g., 225Ac impurity less than 10−4%) of 213Bi, it failed to function after a few days when ∼0.74 GBq (20 mCi) 225Ac was sorbed on the resin.39,48,100 Various approaches have been employed to reduce the impact of radiation on adsorbents.41,141 Based on the unit of radiation dose (J kg−1), increasing the mass of adsorbents for 225Ac can decrease the absorbed dose.41 McDevitt et al. introduced a technique to evenly distribute the 225Ac over large parts of the AG MP-50.41 An 225Ac solution is first contacted with the AG MP-50 slurry for 30 min under gentle agitation to ensure uniform adsorption. Subsequently, the AG MP-50 slurry with 225Ac is poured inside a column comprising a small amount of washed AG MP-50 which did not come into contact with 225Ac and which can act as a catch plug to reduce the 225Ac breakthrough during milking.41 This technique reduces the radiation dose applied to AG MP-50 resin. The 225Ac/213Bi radionuclide generator, which has been developed by the Institute for Transuranium Elements (ITU), Karlsruhe, Germany, also employs this method and is capable of reliable operation when loaded with activities up to 4 GBq of 225Ac.25,28 The 213Bi yield can reach 76% ± 3% in a 0.6 mL of 0.1 M NaI/0.1 M HCl solution with an 225Ac breakthrough activity of less than 2 × 10−5%.37 Additionally, in a 4 M HNO3 solution, the 225Ac content can be distributed across approximately 60% of the resin. This distribution is ascribed to the competitive adsorption of the H+ ions to the resin (due to the low pH), leading to a decrease of the 225Ac adsorption capacity.69 However, highly acidic solutions should be avoided as all of the 225Ac from the cation exchange resins with sulfonic acid groups might be eluted; for example, 6 M HNO3 and 8 M HNO3 should be used for Dowex 50-X8 and AG MP-50, respectively.141,142 An alternative strategy to obtain a more even distribution is the increase of the ionic strength by adding salts, as demonstrated by the decreased adsorption capacity of AG MP-50 towards La3+ (as a surrogate of Ac3+).47
Cation exchange resins with sulfonic acid groups have also been employed in guard columns for further purification of the 213Bi eluate, which can significantly reduce the radiolytic damage to such adsorbents owing to a limited contact time. As explained above, a system combining an AC resin column and an AG MP-50 column has been reported, aiming to further purify and concentrate 213Bi.39 Additionally, AG MP-50 resin can also be used in a 221Fr/213Bi generator. The 221Fr content is eluted from the first column, such as the T-5 (100) column (explained below) and AC column.45,107
Apart from a polymeric backbone, also inorganic materials have been used as a matrix to graft sulfonic acids, mainly aiming to improve the irradiation stability. Moore et al. reported the use of Isolute SCX (benzenesulfonic acid bonded silica) and SCX-2 (propylsulfonic acid bonded silica) in direct 225Ac/213Bi radionuclide generators (Fig. 14c and d).127 The Isolute SCX and SCX-2 resins are initially equilibrated with 1 M acetic acid. The 225Ac content in a 0.1 M HNO3 solution is adsorbed onto the Isolute SCX or SCX-2 resins, and then the resin with 225Ac is packed to the generator column containing a 0.15 mL catch plug. The 213Bi content is milked using 0.1 M KI/HCl for a 1-bed volume. The 213Bi yield reached 67% ± 9% and 72% ± 1% when using the SCX-2 column and SCX column, respectively.127 Compared to the reference AG-50 X8 column with a yield of 85% ± 6%, the 213Bi recovery from Isolute SCX and SCX-2 is relatively lower. However, the 225Ac breakthrough from SCX-2 (0.002% ± 0.002%) and SCX (0.002% ± 0.002%) is relatively lower than AG-50 X8 (0.01% ± 0.02%). Additionally, these columns filled with Isolute SCX and SCX-2 might surfer less damage. However, compared to the AG MP-50 resin in the ITU system, there is at least a hundred fold increase in the 225Ac breakthrough of Isolute SCX and SCX-2.37 Furthermore, the 213Bi eluate from the Isolute SCX and SCX-2 must be purified using a guard column for medical applications, which can be achieved using a two-column direct 225Ac/213Bi generator.
Despite the promising sorption performance of such inorganic materials with grafted sulfonic acids, further research is required to have a good view on their potential in 225Ac/213Bi radionuclide generators. Although inorganic materials themselves are highly stable against radiolytic damage, the use of organic linkers (e.g., benzenesulfonic acid and propylsulfonic acids) might lead to issues by irradiation. Also the leaching of the metal oxides at (very) low pH (e.g., pH < 1) is a reason for caution.143 One option to overcome the drawbacks mentioned, is to graft sulfonic acid groups directly onto an inorganic material surface without an organic linker. However, few synthesis strategies have been described so far, without a full investigation of their separation performance. Their separation performance should be investigated in detail as the chemical properties of such functional groups might be altered, thereby resulting in poor separation performance. Testa and Parola conducted a minireview on sulfonic acid-functionalized inorganic materials and provided detailed information regarding the grafting of sulfonic acid groups onto silica, titania, zirconia, and alumina.144
5.2 Extraction chromatographic resins
Extraction chromatographic resins comprise extractants that are dispersed in the pores of inert solid supports (e.g., porous polymer and silica). The extractant is mainly responsible for selectively binding the target analyte(s) during chromatographic separation.145,146 Such resins have been widely utilized for separating radioactive isotopes, such as actinides and lanthanides, owing to their high selectivity, excellent capacity, and easy handling.107,120,145–147 Several commercial examples of extraction chromatographic resins include actinide, TRU, DGA, LN and UTEVA resins.145
Extracting agents with diphosphonic acid groups typically exhibit remarkable complexing properties towards actinide ions.147 Actinide resin (AC resin), also referred to as DIPEX™ resin in literature, is an extraction chromatographic extraction resin based on P,P′-di(2-ethylhexyl)methanediphosphonic acid (H2DEH[MDP]) (Fig. 15a) and an inert support.39,107,120,148 Compared to the polymeric support, the extract impregnated into silica exhibited substantially low metal sorption kinetics and was more susceptible to radiolytic degradation in an aqueous solution.149 Therefore, polymeric supports are preferred over silica for H2DEH[MDP]. AC resin has been used in different separation systems.39 Owing to its high affinity towards 225Ac (as shown in Fig. 15b), the AC resin can be used in a single-column direct 225Ac/213Bi generator under a low 225Ac activity.120 In a small column (bead volume (BV) = 0.35), the 213Bi yield can reach 85%, and the 225Ac breakthrough is less than 0.0001% for the first 1.5 mL of eluate when using the 1 M HCl as the eluent. In a large column, at least 6 mL of 1 M HCl is needed to elute a high proportion of 213Bi, which results in a low concentration of 213Bi eluate.120 This is why the second column with MP-50 has been typically used to concentrate 213Bi.39 Chen et al. reported that 0.32 mL of 2 M HCl can milk 90% of 213Bi in a 0.35 mL column with DIPEX resins, whereas 1.2 mL of 1 M HCl can only milk 80% of 213Bi.130 However, the radiopurity of 213Bi was not reported quantitatively. Although the 225Ac breakthrough increased with a rise in HCl concentration, this can result in a higher 213Bi concentration. Meanwhile, they also used an AC resin in a two-column direct 225Ac/213Bi generator or 225Ac/221Fr generator, as explained above.39,129 However, if 200 mCi 225Ac or more is used to fill the first two-thirds of a 1 mL AC resin column, the column cannot be operated for more than two days.48 To reduce the radiolytic damage to the AC resins, a new separation technique was developed using an AC resin and AG MP-50 resin by distributing 225Ac content over a large mass of the resins, as explained in the generator section.39 However, another disadvantage is the relatively slow sorption kinetics; thus, batch sorption of high-activity of 225Ac onto AC resins is necessitated before column chromatography to reduce the column volume.120 Additionally, AC resins can also be used in guard columns to purify the 213Bi eluate from the first inverse generator (such as Termoxide adsorbents, UTEVA, and surface-modified carbon columns). Horwitz et al. reported that DIPEX resin extractant loss remains below 4% for every 100 units of free column volume (FCV), in which about 60% of them occur during the initial rinsing when the FCY is 25 unites.148 Although the extractant loss is expected to have little effect on the separation performance of resins if the working part does not exceed 20% of the total resin capacity, the interference of such extractants in the 213Bi labeling process should be noted. The low radiation stability and leaching of extractants might hinder the applicability of these resins for the separation of high-activity 225Ac and 213Bi.48,149
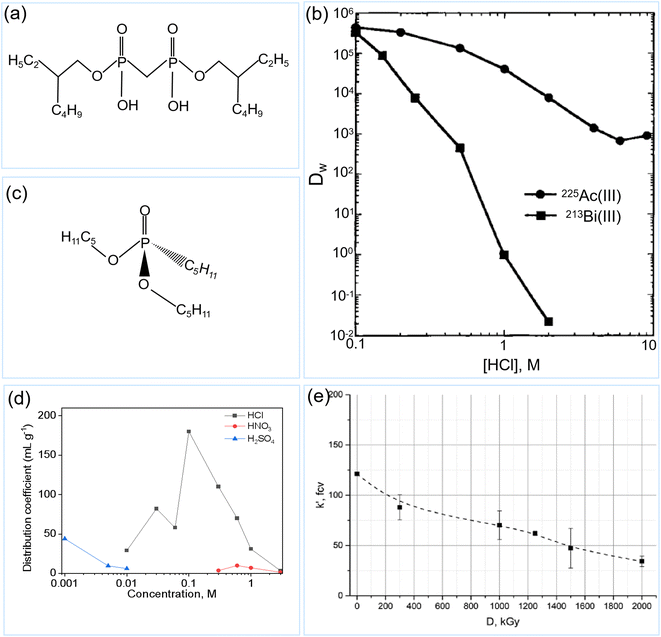 |
| Fig. 15 Schematic structure of H2DEH[MDP] (a); distributing coefficients of AC resin for 225Ac3+ and 213Bi3+ (b) (reproduced (adapted) from ref. 39 with permission from De Gruyter, copyright 1997); schematic structure of DAAP (c); adsorption capacity of 207Bi onto UTEVA as a function of acid concentrations (data from the Tables 5–7 of ref. 150) (d) and adsorption capacity of 213Bi onto UTEVA resin as a function of absorbed dose (e) (reproduced (adapted) from ref. 48 with permission from Taylor & Francis, copyright 2021). | |
The uranium and TEtraValent actinides (UTEVA) resin is formed by impregnating diamyl amylphosphonate (DAAP) (Fig. 15c) into a polymeric acrylic resin.145 The Bi3+ adsorption on UTEVA resin is the function of nature and concentration of the acid in solution, as shown in Fig. 15d.150 It can be seen that the Bi3+ in HCl media has a stronger adsorption affinity onto UTEVA resin than in HNO3 and H2SO4 media. This increased adsorption is due to the formation of Bi-chloride complexes. Maximum Kd values for 213Bi are obtained when a 0.1 M HCl feed comprising 225Ac/213Bi is passed through the UTEVA column, while no 225Ac sorption is observed under these conditions.49 Then, the 213Bi is eluted using a NaCl/NaOAc buffer (pH = 4) with a 225Ac impurity of less than 0.01%. Owing to the limited purification of the 213Bi eluate from the UTEVA column, a guard column with AG MP-50 has been used for further purification of 213Bi, with a 225Ac impurity of less than <10−7%.49 However, UTEVA is highly sensitive to radiolytic damage. With an increase in the absorbed dose, the sorption performance for 213Bi decreased quickly, as shown in Fig. 15e.48 Even though such materials used in the inverse generators are expected to absorb relatively low radiation doses, the choice of high radiation stability materials is crucial in preventing the contamination that could arise from the degradation of the decomposed parts.
5.3 Zirconium-based materials with phosphate/phosphonate groups
Zirconium-based materials with phosphate (–PO4H) and/or phosphonate (–PO3H) groups exhibit high applicability to the direct 225Ac/213Bi generators.108,109,112,113 Their general formula is as follows: Zr(phosphonate)x(HPO4)2−x·nH2O, where x ranges between of 0–2; n is the number of water molecules; and the example of a phosphonate is the N-(phosphonomethyl)iminodiacetic acid.112 Inorganic zirconium phosphate powders are typically dispersed into polymer matrices (e.g., polyacrylonitrile (PAN)) to increase mechanical and chemical stabilities and avoid particle agglomeration. Zr(HPO4)2-PAN has been reported in a patent for use in 225Ac/213Bi generators.109,112 The 225Ac and 213Bi can bind to the phosphate/phosphonate present on the adsorbent surface via electrostatic and coordination interactions, thereby leading to the formation of a stable complex. After adsorption, 213Bi can be eluted by using the DTPA and NaI solutions.109,112 The 213Bi yield exceeds 80%, and the 225Ac impurity remains below 0.01% when using the 0.01 M DTPA/0.005 M HNO3 eluent. A further column filled with the same adsorbents can be used for further purification, which leads to an 225Ac impurity of less than 0.0001%.109 These promising preliminary results have sparked interest in zirconium-based adsorbent materials and their potential applications in 225Ac/213Bi radionuclides.108 However, there is a lack of publications providing comprehensive information on the separation performance of these materials towards Ac3+ and Bi3+. This knowledge gap poses a challenge for researchers and industry professionals to fully understand and optimize the use of such adsorbents in separation processes. Furthermore, investigating suitable eluents and their effect on 213Bi yield is crucial.
5.4 Anion exchange resins
Anion exchange resins (e.g., Anex, Dowex-1, and 3M anion exchange discs) have also been used to separate 225Ac and 213Bi in an inverse generator (also known as PNNL Bi-generator) via selective adsorption of 213Bi instead of 225Ac by using a suitable concentration of halide ions and hydrogen ions (e.g., 0.25–1.0 M HCl).38,111 Such anion exchange resins typically have quaternary amine (e.g., –CH2N(CH3)3+ and –CH2CH2N(CH2CH3)3+) and amine (e.g., –NH3+ and –CH2CH2NH(CH2CH3)2+) functional groups. The formation of negatively charged Bi chloro-complexed (e.g., in 0.5 M HCl) enables the adsorption to the resin by electrostatic attraction, while other interfering cations such as rare earth, radium, francium, and actinium ions cannot be extracted.40,111 The 213Bi is typically eluted using a striping solution (e.g., sodium acetate and DTPA) with high pH values (e.g., pH = 4–4.5), and the 213Bi yield can reach 85%–93%.38,40,111 The 213Bi eluate can be directly used for radiolabeling.40 A PNNL automated generator with anion exchange resins has also been designed and evaluated.38 For each operation, 2%–3% of 225Ac is adsorbed onto the interstitial space. The activity ratio of 225Ac to 213Bi is about 0.07% in the 213Bi eluate. This generator cannot detect other cation impurities except for Na+ sourced from sodium acetate.38 Regarding high 225Ac impurity, 213Bi eluate from the anion exchange resin must be further purified to meet the requirements of radiopharmaceutical applications.
5.5 Inorganic hydroxide sorbents
Inorganic hydroxide materials have attracted considerable attention for use in the separation of 225Ac and 213Bi, owing to their high radiation stability. Betenekov et al. and Vasiliev et al. investigated the performance of mixed oxides of titania/zirconia and zirconia/yttria annealed Termoxid-5 (T-5) and Termoxid-39 (T-39), respectively.44,45,128 Here, T-5 has been used in a direct 225Ac/221Fr generators. The operational pH range for T-5 column is 5.5–7.3, which ensures the high affinity of adsorbents for 225Ac (Fig. 16a), while 1 M NH4Cl was used to elute about 50% of 221Fr (Fig. 16b), implying that approximately 50% of 221Fr decays into 213Bi within the column, where it remains.45 The 221Fr was then be adsorbed onto the second column (221Fr/213Bi column) with ferrocyanide-based sorbents. Such as T-35 (hydrated zirconium oxide modified with nickel–potassium ferrocyanide).45 The accumulated 213Bi in the second column, which results from the decay of 221Fr, was eluted using 1 M HCl. However, the 225Ac impurity in the 213Bi eluate is relatively high (∼0.02%–0.11% of its initial activity).45 Therefore, the authors recommend using a small guard column with AC resin for further purification of the 213Bi eluate.45
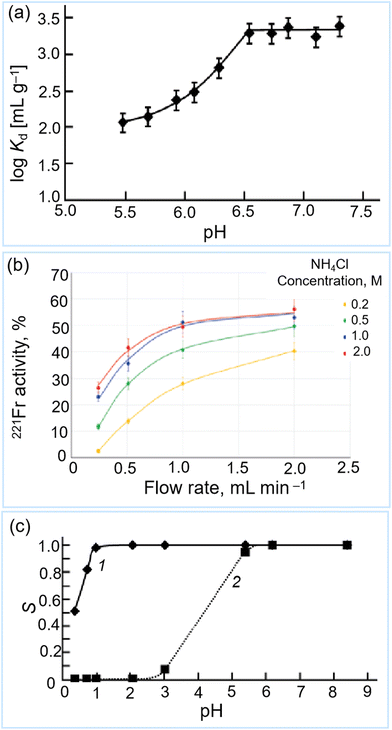 |
| Fig. 16 Separation performance of T-5(450) for 225Ac in aqueous with ∼1 M NH3/NH4Cl buffer (a) (reproduced (adapted) from ref. 128 with permission from Springer Nature, copyright 2019); (b) the 221Fr activity desorbed from the T-5(100) column as a function of flow rate of NH4Cl (reproduced (adapted) from ref. 45 with permission from Springer Nature, copyright 2020); (c) 207Bi sorption onto T-39(950) sorbent from (1) 0.1 M NaNO3 and (2) 0.1 M NaCl +0.1 M KI (reproduced (adapted) from ref. 128 with permission from Springer Nature, copyright 2019). | |
T-39 cannot be employed as a sorbent in a direct 225Ac/213Bi generator due to the lack of suitable eluents. However, Betenekov et al. believed that the use of T-39 should exhibit better performance in an inverse 225Ac/213Bi generator owing to its selective adsorption performance for 213Bi at low pH values (pH < ∼2).128 Further research has been conducted by the same group to enhance the understanding and performance of the materials and processes involved. Vasiliev et al. have tested the separation performance of T-39 for 225Ac and 213Bi by conducting batch and dynamic experiments.44 The 213Bi, in a 0.1 M HNO3 solution, can be adsorbed on the T-39 (Fig. 16c), and then desorbed by 1 M HCl. Subsequently, they developed a new generator with a circulation mode system to regenerate the column for subsequent use. A high 213Bi yield of 45%–98% can be obtained by increasing the adsorbent mass in the column. Additionally, 90% of 213Bi can be eluted using 0.3 mL eluate in a 200 mg column. The 225Ac impurity can be reduced to 0.01% by increasing the salt concentrations. Due to the low purity of 213Bi in the eluate, a guard column was used to further purify the 213Bi eluate from T-39 column.75 These results demonstrated that T-39 has the potential for producing clinical 225Ac quantities (up to 6 GBq) in an inverse 225Ac/213Bi generator, due to its high radiolytic stability and good sorption/desorption performance.44 Although leaching does not have a profound impact on the 213Bi yield, high leaching of Y and Zr under the acidic solutions (e.g., 1 M HCl, 1 M HNO3, and 0.1 M HNO3) is of most concern, as it can contaminate the 213Bi eluate.44
5.6 Surface-modified carbon materials
Carbon materials are becoming increasingly popular for their application in the separation of high-activity radionuclides, owing to their specific surface area, chemical stability, resistance to harsh conditions, thermal stability, tailorable surface chemistry, mechanical strength, and environmental friendliness.151 However, carbon materials typically exhibit low sorption capacities because they comprise insufficient functional groups, which are decomposed under high pyrolysis temperatures. To address this limitation, the use of grafting and the addition of impregnated functional groups on the surface of carbon materials have been proposed as effective strategies.151 Although direct grafting of appropriate functional groups on the carbon structures is an ideal strategy, interference from other functional groups must be considered.117,152 Impregnating extractants into the porous carbon structures can produce one suitable active group; however, the leaching of the extractants from the carbon structures should be taken into account.153
Zhu et al. investigated surface-modified activated carbon materials for use in the separation of La3+ (which is certified as a surrogate for Ac3+) and Bi3+.47 These materials with oxygen-containing groups can selectively adsorb Bi3+ rather than La3+/Ac3+ by adjusting the adsorption conditions, such as pH (Fig. 17a) and salt concentration (Fig. 17b).47 A preliminary column test shows that a 3 M HCl solution could be used to elute 213Bi from the surface-modified carbon materials.47 By adjusting the carbon structures, a 1 M HCl solution was used for 213Bi eluting from the inverse generator using surface-modified carbon materials.114 The 213Bi yield can exceed 90% with a 225Ac impurity of less than 0.04% in small volume (0.6–1 mL). Furthermore, AG MP-50 resin can be used in a guard column to purify 213Bi eluate from this inverse generator.114 Although it has been established that surface-modified carbon materials can be utilized in inverse 225Ac/213Bi radionuclide generators, they exist as irregular powders, which may limit their applicability in columns. Further efforts are still needed to optimize the morphology of carbon structures for separating 225Ac and 213Bi. Surface-modified carbon materials exhibit a high sorption affinity for La3+/Ac3+ and Bi3+ ions at relatively high pH values, which allows them to satisfy the adsorption requirements in direct generators. However, choosing suitable eluents for the selective desorption of 213Bi is a problem that has not been solved.47
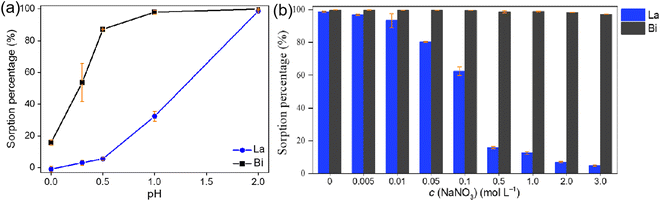 |
| Fig. 17 Sorption percentage of surface-modified activated carbon for La3+ and Bi3+ as a function of pH (a) or NaNO3 concentration (b).47 | |
Carbon structures can be used as supports for extractants to improve the irradiation stability compared to that achieved using polymeric supports. It should be noted that oxygen-containing groups are expected to be grafted onto the surface of carbon materials due to the high irradiation that results in the formation of free radicals under acidic conditions. The interference of such functional groups in the separation process should be examined. However, for the inverse generator, this issue seems to have a low impact on the 213Bi separation. Extractions such as H2DEH[MDP] and DAAP are expected to be impregnated into carbon structures for evaluating the separation performance of 225Ac/213Bi radionuclide generators.49,147,148,153
6. Discussion and outlook
Using 213Bi for targeted alpha therapy is a promising approach for cancer treatment because of its appropriate physical and chemical properties. However, several existing limitations, such as the limited supply of its parent isotope 225Ac and the development of appropriate adsorbents for 225Ac/213Bi radionuclide generators, must be overcome to widely use 213Bi at the clinical level. The primary source of 225Ac is the decay of 229Th, which is isolated from the limited 233U stockpile. However, the limited availability of 229Th and 233U hinders the application of 225Ac and 213Bi. As briefly discussed in this review, various other nuclear reactions have been explored and assessed to produce substantial amounts of 225Ac in reactors, accelerators, and cyclotrons. Such methods offer the possibility of supplying high amounts of 225Ac and 213Bi radionuclides in the near future.
This review highlights the need for continuous research and development in the separation of 213Bi from 225Ac by using the radionuclide generators. Different radionuclide generator systems, based on adsorption or electrochemistry, have been examined for the 213Bi separation. Single-column direct 225Ac/213Bi generators should be the primary research objective because they are more convenient for hospital operators even though they have a strict set of requirements for the adsorbent materials. Table 2 illustrates representative sorbents that have been used or investigated in the 225Ac/213Bi radionuclide generators.48 Different loading methods have been developed to distribute 225Ac across a large mass of adsorbents in order to minimize radiation damage to the adsorbents. Although such techniques can increase the radiation stability of the adsorbents to some extent, they might impact the 213Bi yield, concentration, and purity. A MSIG generator with automated equipment is also a promising technology for separating high-activity 213Bi from 225Ac, given the limited radiation stability of the current adsorbents used in direct generators. Adsorbents in inverse generators receive a reduced irradiation dose by allowing the ingrowth of 213Bi to occur in the stored solution stored in a vial instead of on the adsorbents. Additionally, a guard column must be used to further purify the 213Bi eluate from residual 225Ac impurity. This process ensures that the obtained 213Bi eluate meets the required chemical purity for the labeling procedure. Since radionuclide generators should produce a high 213Bi eluate concentration, the 213Bi eluate from the first column can occasionally be concentrated by the guard column. Typically, the adsorbents used in the direct generators are also employed in the guard column. The operation process of such generators should be further studied, and the equipment should be designed according to the number of manual interventions required by the operator, making it user-friendly. This review introduces almost all of the separation systems, which can be chosen based on the separation properties of the adsorbents.
Table 2 The characteristics of the representative adsorbents in 225Ac/213Bi radionuclide generators48
Adsorbents |
Generator type |
Eluents |
213Bi yield (%) |
Volume (mL) |
225Ac break-through (%) |
Chemical/radiolytic stability |
Ref. |
AG MP-50 |
Direct |
0.1 M NaI/0.1 M HCl |
76 ± 3 |
0.6 |
<2 × 10−5 |
High/low |
37
|
Ac resin |
Direct |
1 M HCl |
85 |
1.5 |
<10−4 |
Low/low |
120
|
Isolute SCX-2 |
Direct |
0.1 M KI/HCl |
67 ± 9 |
— |
<0.004 |
Low/medium |
127
|
Isolute SCX |
Direct |
0.1 M KI/HCl |
72 ± 1 |
— |
<0.004 |
Low/medium |
127
|
Ac resin + AG MP-50 |
Direct |
1 M HCl/0.1 M HI |
>85 |
— |
— |
Low/low |
39
|
UTEVA resin + AG 50 W-X8 |
Inverse |
0.75 M NaCl/0.5 M sodium acetate, pH = 4.0 |
87 ± 3 |
2 |
<10−7 |
Low/low |
49
|
Termoxide-39 |
Inverse |
1 M HCl |
80 |
0.5 |
<0.01 |
Low/high |
44 and 48 |
Sulfonated carbon |
Inverse |
1 M HCl |
94 ± 3 |
0.6–1 |
<0.04 |
High/high |
114
|
An-exchanger |
Inverse |
Sodium acetate, pH 4–5.5 |
85–93 |
1 |
0.07 |
High/low |
38
|
Adsorbents are a crucial component of radionuclide generators. Therefore, in order to select the appropriate adsorbents, it is essential to thoroughly understand their separation properties for 225Ac and 213Bi. This can be achieved through batch adsorption experiments and column tests. Appropriate separation systems for 225Ac and 213Bi can be readily determined by considering the separation properties of adsorbents. If the adsorbents exhibit a stronger affinity for 225Ac than for 213Bi complexes, they can be employed in the direct column. The need for a guard column depends on the breakthrough of 225Ac. Conversely, if 213Bi is referentially adsorbed over 225Ac, the adsorbent materials can be used in the inverse generator. A guard column is commonly used to enhance the purification of the 213Bi eluate obtained from the inverse generator. However, there is insufficient data on the adsorption and desorption of the previously used adsorbents.
Previous studies have primarily focused on examining the sorption performance of Ac3+/Bi3+ in relation to various acid concentrations. However, other important parameters for the adsorption performance, such as such as salt concentration, adsorption time, and halide ions, have been less studied. The impact of such factors on the adsorption process should be considered in order to provide comprehensive information on the separation mechanism. Typically, sulfonic and phosphonic acid groups exhibit a strong affinity for 225Ac in highly acidic solutions, whereas the phosphate and carboxylic groups exhibit weak affinity, typically at pH levels below 2. Therefore, materials containing sulfonic and phosphonic acid groups are primarily used in direct and guard columns because they have a stronger adsorption affinity for 225Ac than for Bi complexes. However, the interference of other functional groups should also be examined to ensure that they do not significantly affect the separation process. Phosphate groups may be a viable option for use in the direct generator because they are present in the structures of inorganic materials, zirconium hydrogen phosphate. However, halide ions do not appear to be ideal for 213Bi elution due to the limited 213Bi yield based on the batch experiments. Further studies are required to investigate the use of halide ions as eluents for eluting 213Bi from materials that contain phosphate groups. Chelators, such as DTPA, are usually used to elute 213Bi from the zirconium hydrogen phosphate. The possibility of other chelators preferentially forming complexes with 213Bi over 225Ac should also be considered and examined. Owing to the limited eluent options, it has been challenging to use materials that contain carboxylic groups in direct generators. Therefore, suitable alternative eluents for 213Bi elution should be investigated to achieve 225Ac/213Bi separation in direct generators.
Anion functional groups can be used for the selective adsorption of 213Bi over 225Ac, making them suitable for inverse generators. Moreover, the materials containing cation exchange groups can also be used in inverse 225Ac/213Bi generators by adjusting the sorption conditions, such as pH and salt concentrations. However, it should be noted that the same functional groups usually exhibited slightly different properties in different structures. Thus, evaluating new alternative adsorbents at the laboratory level is necessary. Future studies should also thoroughly investigate the separation mechanisms of potential adsorbents.
Radiation stability is an important factor to consider when selecting adsorbents and separation systems. Typically, the radiolytic degradation of adsorbents has the following negative impacts: (1) it contaminates the 213Bi elute owing to the decay of the adsorbent structures and functional groups; (2) it increases the 225Ac breakthrough; (3) it decreases the 213Bi yield owing to the alpha-recoil damage to the adsorbent structure; (4) it decreases the flow rates in the column owing to the formation of small fragments.154 Such erratic performance can limit the applications of such materials. Adsorbents that cannot withstand prolonged radiation exposure are unsuitable for use in direct generators over extended periods (e.g., 2–4 weeks) because of the radiolytic damage caused by 225Ac (e.g., 4 GBq) and its descendants on the adsorbents. However, such materials may be used in guard columns owing to the reduced irradiation time. Although the adsorbents used in inverse generators have relatively lower radiolytic stability requirements than those used in direct generators, it is still crucial to consider this aspect. Adsorbent material must remain radiation-resistant and stable throughout the process to prevent degradation or breakdown. Furthermore, the adsorbents may receive increased radiation doses in automatic systems due to prolonged cycling times. Therefore, it is imperative to aim for adsorbents with high radiation stability.
Future research should prioritize exploring and designing new sorbents with enhanced properties, in terms of sorption capacity, temperature, separation selectivity, radiation stability, and regeneration to address the limitations of previously used adsorbents. In addition, the interference radionuclides (e.g.227Ac) from the production of 225Ac should be investigated during the separation process to obtain the high-purity 213Bi. Also, the 225Ac/213Bi generator is a more complex system than it sounds as some descendants (e.g.221Fr) need to be taken into account when designing the separation system. Thus, investigating the separation performance of adsorbents for such descendants should be undertaken.
To identify the most suitable materials, it is essential to thoroughly understand the fundamental mechanisms that govern the separation process comprehensively. Collaboration between radiochemists, materials scientists, and medical professionals is critical for developing 225Ac/213Bi radionuclide generators and ensuring their safe and effective implementation in clinical practice. Ongoing research and collaborative efforts will lead to significant progress in this field, ultimately benefiting patients who require novel and effective therapeutic options.
Conflicts of interest
There are no conflicts to declare.
Acknowledgements
The SCK CEN Academy and VITO are acknowledged for funding. Furthermore, the authors would like to acknowledge the comments and modifications of Prof. Dr Thierry Verbiest, Prof. Dr Tatjana Vogt, Prof. Dr Maria Seo, Prof. Dr Vera Meynen, and Dr Sofía Riaño.
References
- T. I. Kostelnik and C. Orvig, Radioactive Main Group and Rare Earth Metals for Imaging and Therapy, Chem. Rev., 2019, 119(2), 902–956 CrossRef CAS PubMed.
- G. Sgouros, L. Bodei, M. R. McDevitt and J. R. Nedrow, Radiopharmaceutical therapy in cancer: clinical advances and challenges, Nat. Rev. Drug Discovery, 2020, 19, 589–608 CrossRef CAS PubMed.
- S. St James, B. Bednarz, S. Benedict, J. C. Buchsbaum, Y. Dewaraja, E. Frey, R. Hobbs, J. Grudzinski, E. Roncali, G. Sgouros, J. Capala and Y. Xiao, Current Status of Radiopharmaceutical Therapy, Int. J. Radiat. Oncol., Biol., Phys., 2021, 109(4), 891–901 CrossRef PubMed.
- K. Chakraborty, J. Mondal, J. M. An, J. Park and Y. K. Lee, Advances in Radionuclides and Radiolabelled Peptides for Cancer Therapeutics, Pharmaceutics, 2023, 15(3), 971 CrossRef CAS PubMed.
- N. H. Álvarez, D. Bauer, J. Hernández-Gil and J. S. Lewis, Recent Advances in Radiometals for Combined Imaging and Therapy in Cancer, ChemMedChem, 2021, 16(19), 2909–2941 CrossRef PubMed.
- A. I. Kassis, Therapeutic radionuclides: biophysical and radiobiologic principles, Semin. Nucl. Med., 2008, 38(5), 358–366 CrossRef PubMed.
- Q. T. Luu and P. DuChateau, The relative biologic effectiveness versus linear energy transfer curve as an output-input relation for linear cellular systems, Math. Biosci. Eng., 2009, 6(3), 591–602 Search PubMed.
- J. S. Wang, H. J. Wang and H. L. Qian, Biological effects of radiation on cancer cells, Mil. Med. Res., 2018, 5, 20 Search PubMed.
- G. Sgouros, Y. K. Dewaraja, F. Escorcia, S. A. Graves, T. A. Hope, A. Iravani, N. Pandit-Taskar, B. Saboury, S. S. James and P. B. Zanzonico, Tumor Response to Radiopharmaceutical Therapies: The Knowns and the Unknowns, J. Nucl. Med., 2021, 62(Suppl 3), 12S–22S CAS.
- J. Pellico, P. J. Gawne and R. T. M. de Rosales, Radiolabelling of nanomaterials for medical imaging and therapy, Chem. Soc. Rev., 2021, 50, 3355–3423 RSC.
- M. Czerwińska, A. Bilewicz, M. Kruszewski, A. Wegierek-Ciuk and A. Lankoff, Targeted Radionuclide Therapy of Prostate Cancer-From Basic Research to Clinical Perspectives, Molecules, 2020, 25(7), 1743 CrossRef PubMed.
- Y. Dekempeneer, M. Keyaerts, A. Krasniqi, J. Puttemans, S. Muyldermans, T. Lahoutte, M. D'Huyvetter and N. Devoogdt, Targeted alpha therapy using short-lived alpha-particles and the promise of nanobodies as targeting vehicle, Expert Opin. Biol. Ther., 2016, 16(8), 1035–1047 CrossRef CAS PubMed.
- M. G. Ferrier and V. Radchenko, An Appendix of Radionuclides Used in Targeted Alpha Therapy, J. Med. Imaging Radiat. Sci., 2019, 50(4 Suppl 1), S58–S65 CrossRef PubMed.
- J. S. Jaggi, E. Henke, S. V. Seshan, B. J. Kappel, D. Chattopadhyay, C. May, M. R. McDevitt, D. Nolan, V. Mittal, R. Benezra and D. A. Scheinberg, Selective alpha-particle mediated depletion of tumor vasculature with vascular normalization, PLoS One, 2007, 2(3), e267 CrossRef PubMed.
- S. Poty, L. C. Francesconi, M. R. McDevitt, M. J. Morris and J. S. Lewis, α-Emitters for Radiotherapy: From Basic Radiochemistry to Clinical Studies-Part 1, J. Nucl. Med., 2018, 59(6), 878–884 CrossRef CAS PubMed.
- A. Ku, V. J. Facca, Z. Cai and R. M. Reilly, Auger electrons for cancer therapy – a review, EJNMMI Radiopharm. Chem., 2019, 4, 27 CrossRef PubMed.
- D. J. Fiszbein, V. Brown, N. A. Thiele, J. J. Woods, L. Wharton, S. N. MacMillan, V. Radchenko, C. F. Ramogida and J. J. Wilson, Tuning the Kinetic Inertness of Bi3+ Complexes: The Impact of Donor Atoms on Diaza-18-Crown-6 Ligands as Chelators for 213Bi Targeted Alpha Therapy, Inorg. Chem., 2021, 60(12), 9199–9211 CrossRef CAS PubMed.
- S. V. Gudkov, N. Y. Shilyagina, V. A. Vodeneev and A. V. Zvyagin, Targeted Radionuclide Therapy of Human Tumors, Int. J. Mol. Sci., 2016, 17(1), 33 CrossRef PubMed.
- E. R. Birnbaum, R. W. Atcher, K. D. John, O. Rixe and J. P. Norenberg, Alpha-Emitters and Targeted Alpha Therapy in Oncology: from Basic Science to Clinical Investigations, Targeted Oncol., 2018, 13, 189–203 CrossRef PubMed.
- Targeted Alpha Therapy Working Group, C. Parker, V. Lewington, N. Shore, C. Kratochwil, M. Levy, O. Linden, W. Noordzij, J. Park and F. Saad, Targeted Alpha Therapy, an Emerging Class of Cancer Agents: A Review, JAMA Oncol., 2018, 4, 1765–1772 CrossRef PubMed.
- L. Wang, L. Song and X. Dai, Advances in Targeted Alpha Therapy: Preparation and Separation of Alpha Radionuclides, J. Nucl. Radiochem., 2022, 44(3), 326–338, DOI:10.7538/hhx.2022.YX.2020095.
- H. Cui and L. Shen, Alpha-Emitting Metallic Radiopharmaceuticals: Current Status and Future Prospects, J. Nucl. Radiochem., 2020, 42(6), 524–538, DOI:10.7538/hhx.2020.YX.2020076.
- S. Yamamoto, K. Kato, N. Fujita, M. Yamashita, T. Nishimoto, H. Kameyama and S. Abe, Detection of alpha radionuclides in air from patients during Ra-223 alpha radionuclide therapy, Sci. Rep., 2018, 8(1), 10976 CrossRef PubMed.
- M. Pacilio, G. Ventroni, G. De Vincentis, B. Cassano, R. Pellegrini, E. Di Castro, V. Frantellizzi, G. A. Follacchio, T. Garkavaya, L. Lorenzon, P. Ialongo, R. Pani and L. Mango, Dosimetry of bone metastases in targeted radionuclide therapy with alpha-emitting 223Ra-dichloride, Eur. J. Nucl. Med. Mol. Imaging, 2016, 43, 21–33 CrossRef CAS PubMed.
- A. Morgenstern, C. Apostolidis, C. Kratochwil, M. Sathekge, L. Krolicki and F. Bruchertseifer, An Overview of Targeted Alpha Therapy with 225Actinium and 213Bismuth, Curr. Radiopharm., 2018, 11(3), 200–208 CrossRef CAS PubMed.
- M. W. Geerlings, F. M. Kaspersen, C. Apostolidis and R. V. Der Hout, The feasibility of 225Ac as a source of α-particles in radioimmunotherapy, Nucl. Med. Commun., 1993, 14(2), 121–125 CrossRef CAS PubMed.
- E. Dadachova, Future Vistas in Alpha Therapy of Infectious Diseases, J. Med. Imaging Radiat. Sci., 2019, 50, S49–S52 CrossRef PubMed.
- A. Morgenstern, C. Apostolidis and F. Bruchertseifer, Supply and Clinical Application of Actinium-225 and Bismuth-213, Semin. Nucl. Med., 2020, 50(2), 119–123 CrossRef PubMed.
- J. W. Engle, The Production of Ac-225, Curr. Radiopharm., 2018, 11(3), 173–179 CrossRef CAS PubMed.
- R. A. Boll, D. Malkemus and S. Mirzadeh, Production of actinium-225 for alpha particle mediated radioimmunotherapy, Appl. Radiat. Isot., 2005, 62(5), 667–679 CrossRef CAS PubMed.
- S. Ahenkorah, I. Cassells, C. M. Deroose, T. Cardinaels, A. R. Burgoyne, G. Bormans, M. Ooms and F. Cleeren, Bismuth-213 for Targeted Radionuclide Therapy: From Atom to Bedside, Pharmaceutics, 2021, 13(5), 599 CrossRef CAS PubMed.
- D. A. Mulford, D. A. Scheinberg and J. G. Jurcic, The Promise of Targeted α-Particle Therapy, J. Nucl. Med., 2005, 46, 199S–204S Search PubMed.
- N. Burahmah, J. R. Griswold, L. H. Heilbronn and S. Mirzadeh, Transport model predictions of 225Ac production cross sections via energetic p, d and α irradiation of 232Th targets, Appl. Radiat. Isot., 2021, 172, 109676 CrossRef CAS PubMed.
- A. Dash and R. Chakravarty, Pivotal role of separation chemistry in the development of radionuclide generators to meet clinical demands, RSC Adv., 2014, 4(81), 42779–42803 RSC.
- A. Dash, F. F. Knapp Jr and M. R. A. Pillai, Industrial radionuclide generators: a potential step towards accelerating radiotracer investigations in industry, RSC Adv., 2013, 3, 14890–14909 RSC.
- S. Mirzadeh, Generator-produced alpha-emitters, Appl. Radiat. Isot., 1998, 49(4), 345–349 CrossRef CAS.
- A. Morgenstern, F. Bruchertseifer and C. Apostolidis, Bismuth-213 and actinium-225 – generator performance and evolving therapeutic applications of two generator-derived alpha-emitting radioisotopes, Curr. Radiopharm., 2012, 5(3), 221–227 CrossRef CAS PubMed.
- L. A. Bray, J. M. Tingey, J. R. DesChane, O. B. Egorov, T. S. Tenforde, D. S. Wilbur, D. K. Hamlin and P. M. Pathare, Development of a Unique Bismuth (Bi-213) Automated Generator for Use in Cancer Therapy, Ind. Eng. Chem. Res., 2000, 39(9), 3189–3194 CrossRef CAS.
- C. Wu, M. W. Brechbiel and O. A. Gansow, An Improved Generator for the Production of 213Bi from 225Ac, Radiochim. Acta, 1997, 79(2), 141–144 CrossRef CAS.
- P. M. Pathare, D. K. Hamlin, D. S. Wilbur, M. W. Brechbiel and L. A. Bray, Synthesis and radiolabeling of a biotin-CHX-B chelate for Bi-213, J. Labelled Compd. Radiopharm., 1998, 41(7), 595–603 CrossRef CAS.
- M. R. McDevitt, R. D. Finn, G. Sgouros, D. Ma and D. A. Scheinberg, An 225Ac/213Bi generator system for therapeutic clinical applications: construction and operation, Appl. Radiat. Isot., 1999, 50(5), 895–904 CrossRef CAS PubMed.
- D. Ma, M. R. McDevitt, R. D. Finn and D. A. Scheinberg, Breakthrough of 225Ac and its radionuclide daughters from an 225Ac/213Bi generator: development of new methods, quantitative characterization, and implications for clinical use, Appl. Radiat. Isot., 2001, 55(5), 667–678 CrossRef CAS PubMed.
- L. I. Guseva and N. N. Dogadkin, Development of a tandem generator system 229Th/225Ac/213Bi for repeated production of short-lived α-emitting radionuclides, Radiochemistry, 2009, 51, 169–174 CrossRef CAS.
- A. N. Vasiliev, S. V. Ermolaev, E. V. Lapshina, B. L. Zhuikov and N. D. Betenekov,
225Ac/213Bi generator based on inorganic sorbents, Radiochim. Acta, 2019, 107(12), 1203–1211 CrossRef CAS.
- N. D. Betenekov, S. V. Ermolaev and A. K. Skasyrskaya, Development of a 225Ac/213Bi Generator with Two Inorganic Sorbents. Processes Involving Radionuclides of the 225Ac Subfamily, Radiochemistry, 2020, 62(4), 524–531 CrossRef.
-
M. E. Fassbender, 225Ac/213Bi Generator Based on Millifluidics Controlled Electrodeposition. LA-UR-21-29374 2021, project #20200165ER, DOI:10.2172/1822690.
- H. Zhu, S. Heinitz, S. Eyley, W. Thielemans, K. Binnemans, S. Mullens and T. Cardinaels, Sorption and desorption performance of La3+/Bi3+ by surface-modified activated carbon for potential application in medical 225Ac/213Bi generators, Chem. Eng. J., 2023, 464, 142456 CrossRef CAS.
- A. N. Vasiliev, V. A. Zobnin, Y. S. Pavlov and V. M. Chudakov, Radiation Stability of Sorbents in Medical 225Ac/213Bi Generators, Solvent Extr. Ion Exch., 2021, 39(4), 353–372 CrossRef CAS.
- D. R. McAlister and E. P. Horwitz, Automated two column generator systems for medical radionuclides, Appl. Radiat. Isot., 2009, 67(11), 1985–1991 CrossRef CAS PubMed.
- R. Eychenne, M. Chérel, F. Haddad, F. Guérard and J.-F. Gestin, Overview of the Most Promising Radionuclides for Targeted Alpha Therapy: The “Hopeful Eight”, Pharmaceutics, 2021, 13(6), 906 CrossRef CAS PubMed.
- D. Iwahashi, Y. Sasaki, T. Shinohara and N. Takaki, Semi-Permanent Mass Production of Ac-225 for Cancer Therapy by the (3n,x) Reaction in Pressurized Water Reactor, Processes, 2023, 12, 83 CrossRef.
- A. K. H. Robertson, C. F. Ramogida, P. Schaffer and V. Radchenko, Development of 225Ac Radiopharmaceuticals: TRIUMF Perspectives and Experiences, Curr. Radiopharm., 2018, 11, 156–172 CrossRef PubMed.
- C. U. Jost, J. R. Griswold, S. H. Bruffey, S. Mirzadeh, D. W. Stracener and C. L. Williams, Measurement of cross sections for the 232Th(P,4n)229Pa reaction at low proton energies, AIP Conf. Proc., 2013, 1525, 520–524 CrossRef CAS.
- S. Hogle, R. A. Boll, K. Murphy, D. Denton, A. Owens, T. J. Haverlock, M. Garland and S. Mirzadeh, Reactor production of Thorium-229, Appl. Radiat. Isot., 2016, 114, 19–27 CrossRef CAS PubMed.
- J. W. Weidner, S. G. Mashnik, K. D. John, B. Ballard, E. R. Birnbaum, L. J. Bitteker, A. Couture, M. E. Fassbender, G. S. Goff, R. Gritzo, F. M. Hemez, W. Runde, J. L. Ullmann, L. E. Wolfsberg and F. M. Nortier, Appl. Radiat. Isot., 2012, 70, 2590–2595 CrossRef CAS PubMed.
- A. K. H. Robertson, K. Ladouceur, M. Nozar, L. Moskven, C. F. Ramogida, J. D’Auria, V. Sossi and P. Schaffer, Design and simulation of a thorium target for 225Ac production, AIP Conf. Proc., 2017, 1845, 020019 CrossRef.
- S. V. Ermolaev, B. L. Zhuikov, V. M. Kokhanyuk, V. L. Matushko, S. N. Kalmykov, R. A. Aliev, I. G. Tananaev and B. F. Myasoedov, Production of actinium, thorium and radium isotopes from natural thorium irradiated with protons up to 141 MeV, Radiochim. Acta, 2012, 100, 223–229 CrossRef CAS.
- A. K. H. Robertson, B. L. McNeil, H. Yang, D. Gendron, R. Perron, V. Radchenko, S. Zeisler, P. Causey and P. Schaffer,
232Th-Spallation-Produced 225Ac with Reduced 227Ac Content, Inorg. Chem., 2020, 59, 12156–12165 CrossRef CAS PubMed.
- J. W. Weidner, S. G. Mashnik, K. D. John, F. Hemez, B. Ballard, H. Bach, E. R. Birnbaum, L. J. Bitteker, A. Couture, D. Dry, M. E. Fassbender, M. S. Gulley, K. R. Jackman, J. L. Ullmann, L. E. Wolfsberg and F. M. Nortier, Proton-induced cross sections relevant to production of 225Ac and 223Ra in natural thorium targets below 200 MeV, Appl. Radiat. Isot., 2012, 70, 2602–2607 CrossRef CAS PubMed.
- A. Morgenstern, K. Abbas, F. Bruchertseifer and C. Apostolidis, Production of Alpha Emitters for Targeted Alpha Therapy, Curr. Radiopharm., 2008, 1, 135–143 CrossRef CAS.
- M. Shi, V. Jakobsson, L. Greifenstein, P.-L. Khong, X. Chen, R. P. Baum and J. Zhang, Alpha-peptide receptor radionuclide therapy using actinium-225 labeled somatostatin receptor agonists and antagonists, Front. Med., 2022, 9, 1034315 CrossRef PubMed.
- C. Apostolidis, R. Molinet, J. McGinley, K. Abbas, J. Möllenbeck and A. Morgenstern, Cyclotron production of Ac-225 for targeted alpha therapy, Appl. Radiat. Isot., 2005, 62, 383–387 CrossRef CAS PubMed.
- Z. Varga, A. Nicholl and K. Mayer, Determination of the 229Th half-life, Phys. Rev. C: Nucl. Phys., 2014, 89, 064310 CrossRef.
- Y.-S. Kim and M. W. Brechbiel, An overview of targeted alpha therapy, Tumor Biol., 2012, 33, 573–590 CrossRef CAS PubMed.
- M. F. McLaughlin, J. Woodward, R. A. Boll, A. J. Rondinone, S. Mirzadeh and J. D. Robertson, Gold-coated lanthanide phosphate nanoparticles for an 225Ac in vivo alpha generator, Radiochim. Acta, 2013, 101, 595–600 CrossRef CAS.
- A. K. H. Robertson, C. F. Ramogida, C. Rodríguez-Rodríguez, S. Blinder, P. Kunz, V. Sossi and P. Schaffer, Multi-isotope SPECT imaging of the 225Ac decay chain: feasibility studies, Phys. Med. Biol., 2017, 62, 4406 CrossRef CAS PubMed.
- R. A. Aliev, S. V. Ermolaev, A. N. Vasiliev, V. S. Ostapenko, E. V. Lapshina, B. L. Zhuikov, N. V. Zakharov, V. V. Pozdeev, V. M. Kokhanyuk, B. F. Myasoedov and S. N. Kalmykov, Solvent Extr. Ion Exch., 2014, 32, 468–477 CrossRef CAS.
- D. Iwahashi, K. Kawamoto, Y. Sasaki and N. Takaki, Neutronic Study on Ac-225 Production for Cancer Therapy by (n,2n) Reaction of Ra-226 or Th-230 Using Fast Reactor Joyo, Processes, 2022, 10(7), 1239 CrossRef.
- C. Apostolidis, R. Molinet, G. Rasmussen and A. Morgenstern, Production of Ac-225 from Th-229 for Targeted α Therapy, Anal. Chem., 2005, 77(19), 6288–6291 CrossRef CAS PubMed.
- J. R. Griswold, D. G. Medvedev, J. W. Engle, R. Copping, J. M. Fitzsimmons, V. Radchenko, J. C. Cooley, M. E. Fassbender, D. L. Denton, K. E. Murphy, A. C. Owens, E. R. Birnbaum, K. D. John, F. M. Nortier, D. W. Stracener, L. H. Heilbronn, L. F. Mausner and S. Mirzadeh, Large scale accelerator production of 225Ac: Effective cross sections for 78–192 MeV protons incident on 232Th targets, Appl. Radiat. Isot., 2016, 118, 366–374 CrossRef CAS PubMed.
- H. Zhu, S. Heinitz, S. Eyley, W. Thielemans, E. Derveaux, P. Adriaensens, K. Binnemans, S. Mullens and T. Cardinaels, Gamma radiation effects on AG MP-50 cation exchange resin and sulfonated activated carbon for bismuth-213 separation, RSC Adv., 2023, 13, 30990–31001 RSC.
- S. Boden, K. Vints, S. Cagno, D. Maertens, K. Van Hecke and T. Cardinaels, Thorium-229 quantified in historical Thorium-228 capsules, Appl. Radiat. Isot., 2017, 120, 40–44 CrossRef CAS PubMed.
- R. Perron, D. Gendron and P. W. Causey, Construction of a thorium/actinium generator at the Canadian Nuclear Laboratories, Appl. Radiat. Isot., 2020, 164, 109262 CrossRef CAS PubMed.
- J. R. Griswold, C. U. Jost, D. W. Stracener, S. H. Bruffey, D. Denton, M. Garland, L. Heilbronn and S. Mirzadeh, Production of 229Th for medical applications: Excitation functions of low-energy protons on 232Th targets, Phys. Rev. C, 2018, 98(4), 044607 CrossRef CAS.
- B. L. Zhuikov and S. V. Ermolaev, Radioisotope research and development at the Linear Accelerator of the Institute for Nuclear Research of RAS, Phys.-Usp., 2021, 64(12), 1311–1322 CrossRef CAS.
- T. Higashi, K. Nagatsu, A. B. Tsuji and M.-R. Zhang, Research and Development for Cyclotron Production of 225Ac from 226Ra—The Challenges in a Country Lacking Natural Resources for Medical Applications, Processes, 2022, 10(6), 1215 CrossRef CAS.
- S. Heinitz, J. Mermans, D. Maertens, H. Skliarova, A. Aerts, T. Cardinaels, C. Gueibe, J. Rutten, N. Ireland, D. Kuznicki and S. Kuznicki, Adsorption of radon on silver exchanged zeolites at ambient temperatures, Sci. Rep., 2023, 13, 6811 CrossRef CAS PubMed.
-
J. Morrell, Next-generation isotope production via deuteron breakup, Ph.D. Thesis, Department of Nuclear Engineering, Univerrsity of California, Berkeley, 2021 Search PubMed.
- O. D. Maslov, A. V. Sabel'nikov and S. N. Dmitriev, Preparation of 225Ac by 226Ra(γ, n) photonuclear reaction on an electron accelerator, MT-25 microtron, Radiochemistry, 2006, 48, 195–197 CrossRef CAS.
- J. T. Harvey, NorthStar Perspectives for Actinium-225 Production at Commercial Scale, Curr. Radiopharm., 2018, 11(3), 180–191 CrossRef CAS PubMed.
- G. J. P. Deblonde, M. Zavarin and A. B. Kersting, The coordination properties and ionic radius of actinium: A 120-year-old enigma, Coord. Chem. Rev., 2021, 446, 214130 CrossRef CAS.
- J. Kunikowska and L. Królicki, Targeted alpha-Emitter Therapy of Neuroendocrine Tumors, Semin. Nucl. Med., 2020, 50(2), 171–176 CrossRef PubMed.
- N. A. Thiele and J. J. Wilson, Actinium-225 for Targeted α Therapy: Coordination Chemistry and Current Chelation Approaches, Cancer Biother. Radiopharm., 2018, 33(8), 336–348 CrossRef PubMed.
- D. M. Ziv and I. A. Shestakova, Investigation of the solubility of certain actinium compounds. II. Determination of the solubility and evaluation of the relative basicity of actinium hydroxide, Sov. Radiochem. (Engl. Transl.), 1965, 7, 176 Search PubMed.
-
C. F. Baes and R. E. Mesmer, The hydrolysis of cations, Wiley, New York, 1976. https://archive.org/details/hydrolysisofcati0000baes Search PubMed.
- E. V. Kulikov, A. F. Novgorodov and D. Schumann, Hydrolysis of 225Actinium trace quantities, J. Radioanal. Nucl. Chem., 1992, 164, 103–108 CrossRef CAS.
- B. V. Egorova, M. S. Oshchepkov, Y. V. Fedorov, O. A. Fedorova, G. S. Budylin, E. A. Shirshin and S. N. Kalmykov, Complexation of Bi3+, Ac3+, Y3+, Lu3+, La3+ and Eu3+ with benzo-diaza-crown ether with carboxylic pendant arms, Radiochim. Acta, 2016, 104(8), 555–565 CrossRef CAS.
- R. G. Pearson, Hard and Soft Acids and Bases, J. Am. Chem. Soc., 1963, 85(22), 3533–3539 CrossRef CAS.
- R. G. Parr and R. G. Pearson, Absolute hardness: companion parameter to absolute electronegativity, J. Am. Chem. Soc., 1983, 105(26), 7512–7516 CrossRef CAS.
- R. G. Pearson, Absolute electronegativity and hardness: application to inorganic chemistry, Inorg. Chem., 1988, 27(4), 734–740 CrossRef CAS.
- B. Zielińska and A. Bilewicz, The hydrolysis of actinium, J. Radioanal. Nucl. Chem., 2004, 261, 195–198 CrossRef.
- M. G. Ferrier, E. R. Batista, J. M. Berg, E. R. Birnbaum, J. N. Cross, J. W. Engle, H. S. La Pierre, S. A. Kozimor, J. S. L. Pacheco, B. W. Stein, S. C. E. Stieber and J. J. Wilson, Spectroscopic and computational investigation of actinium coordination chemistry, Nat. Commun., 2016, 7, 12312 CrossRef CAS PubMed.
- M. G. Ferrier, B. W. Stein, E. R. Batista, J. M. Berg, E. R. Birnbaum, J. W. Engle, K. D. John, S. A. Kozimor, J. S. L. Pacheco and L. N. Redman, Synthesis and Characterization of the Actinium Aquo Ion, ACS Cent. Sci., 2017, 3(3), 176–185 CrossRef CAS PubMed.
- P. G. Allen, J. J. Bucher, D. K. Shuh, N. M. Edelstein and I. Craig, Coordination Chemistry of Trivalent Lanthanide and Actinide Ions in Dilute and Concentrated Chloride Solutions, Inorg. Chem., 2000, 39(3), 595–601 CrossRef CAS PubMed.
- F. Bouyer, N. Sanson, M. Destarac and C. Gérardin, Hydrophilic block copolymer-directed growth of lanthanum hydroxide nanoparticles, New J. Chem., 2006, 30(3), 399–408 RSC.
- T. G. Levitskaia, N. P. Qafoku, M. E. Bowden, R. M. Asmussen, E. C. Buck, V. L. Freedman and C. I. Pearce, A Review of Bismuth(III)-Based Materials for Remediation of Contaminated Sites, ACS Earth Space Chem., 2022, 6(4), 883–908 CrossRef CAS.
- B. Y. Spivakov, E. S. Stoyanov, L. A. Gribov and Y. A. Zolotov, Raman laser spectroscopic studies of bismuth(III) halide complexes in aqueous solutions, J. Inorg. Nucl. Chem., 1979, 41(4), 453–455 CrossRef CAS.
- B. D. Jadhav and S. K. Pardeshi, In situ quenching of monoaryl bismuth(III) dihalides with nitrogen donor ligands: Isolation of adducts and their spectral characterization, J. Mol. Struct., 2018, 1173, 876–884 CrossRef CAS.
- G. G. Briand and N. Burford, Bismuth Compounds and Preparations with Biological or Medicinal Relevance, Chem. Rev., 1999, 99(9), 2601–2658 CrossRef CAS PubMed.
- S. Hassfjell and M. W. Brechbiel, The Development of the α-Particle Emitting Radionuclides 212Bi and 213Bi, and Their Decay Chain Related Radionuclides, for Therapeutic Applications, Chem. Rev., 2001, 101(7), 2019–2036 CrossRef CAS PubMed.
- A. H. Bond and R. D. Rogers, Bismuth coordination chemistry: a brief retrospective spanning crystallography to clinical potential, J. Coord. Chem., 2021, 74(1–3), 129–151 CrossRef CAS.
- D. B. Kleja, J. P. Gustafsson, V. Kessler and I. Persson, Bismuth(III) Forms Exceptionally Strong Complexes with Natural Organic Matter, Environ. Sci. Technol., 2022, 56(5), 3076–3084 CrossRef CAS PubMed.
- H. Bateman, The solution of a system of differential equations occurring in the theory of radioactive transformations, Proc. Cambridge Philos. Soc., 1910, 15, 423–427 CAS.
- C. Kratochwil, F. L. Giesel, F. Bruchertseifer, W. Mier, C. Apostolidis, R. Boll, K. Murphy, U. Haberkorn and A. Morgenstern,
213Bi-DOTATOC receptor-targeted alpha-radionuclide therapy induces remission in neuroendocrine tumours refractory to beta radiation: a first-in-human experience, Eur. J. Nucl. Med. Mol. Imaging, 2014, 41, 2106–2119 CrossRef CAS PubMed.
- M. A. H. Badsha, M. Khan, B. Wu, A. Kumar and I. M. C. Lo, Role of surface functional groups of hydrogels in metal adsorption: From performance to mechanism, J. Hazard. Mater., 2021, 408, 124463 CrossRef CAS PubMed.
- S. Liu, J. Huang, W. Zhang, L. Shi, K. Yi, H. Yu, C. Zhang, S. Li and J. Li, Microplastics as a vehicle of heavy metals in aquatic environments: A review of adsorption factors, mechanisms, and biological effects, J. Environ. Manage., 2022, 302, 113995 CrossRef CAS PubMed.
- S. Ermolaev, A. Skasyrskaya and A. Vasiliev, A Radionuclide Generator of High-Purity Bi-213 for Instant Labeling, Pharmaceutics, 2021, 13(6), 914 CrossRef CAS PubMed.
- L. Ondrák, K. O. Fialová, M. Sakmár, M. Vlk, K. Štamberg, B. Drtinová, M. Šlouf, F. Bruchertseifer, A. Morgenstern and J. Kozempel, Preparation and characterization of α-zirconium phosphate as a perspective material for separation of 225Ac and 213Bi, J. Radioanal. Nucl. Chem., 2023, 332, 1475–1481 CrossRef.
-
L. Ondrák, K. O. Fialová, M. Vlk, M. Sakmár and J. Kozempel, Sorbent, Zařízení a sada pro separac 213Bi ze smeši 225Ac a produktů jeho radioaktivní přeměny, CZ35955, 2022, https://patents.google.com/patent/CZ35955U1.
- T. K. Nikula, M. R. McDevitt, R. D. Finn, C. Wu, R. W. Kozak, K. Garmestani, M. W. Brechbiel, M. J. Curcio, C. G. Pippin, L. Tiffany-Jones, M. W. Geerlings, C. Apostolidis, R. Molinet, M. W. Geerlings, O. A. Gansow and D. A. Scheinberg, Alpha-Emitting Bismuth Cyclohexylbenzyl DTPA Constructs of Recombinant Humanized Anti-CD33 Antibodies: Pharmacokinetics, Bioactivity, Toxicity and Chemistry, J. Nucl. Med., 1999, 40(1), 166–176 CAS.
-
L. A. Bray and J. R. DesChane, Bismuth generator method, US5749042, 1998, https://patents.google.com/patent/US5749042A.
-
P. Sylvester, Ion exchange materials for use in a Bi-213 generator, WO2004001767, 2003, https://patents.google.com/patent/WO2004001767.
-
L. Ondrák, K. Fialová, M. Vlk, M. Sakmár and J. Kozempel, Sorbent a sada pro separaci 225Ac a 213Bi, CZ35214, 2021, https://patents.google.com/patent/CZ35214U1.
- H. Zhu, S. Heinitz, S. Eyley, W. Thielemans, K. Binnemans, S. Mullens and T. Cardinaels, Selective separation of Bi3+ from La3+/Ac3+ by sorption on sulfonated carbon materials for use in an inverse 225Ac/213Bi radionuclide generator: Batch and column tests, Chem. Eng. J., 2023, 468, 143416 CrossRef CAS.
- B. A. Marinho, R. O. Cristóvão, R. Djellabi, J. M. Loureiro, R. A. R. Boaventura and V. J. P. Vilar, Photocatalytic reduction of Cr(VI) over TiO2-coated cellulose acetate monolithic structures using solar light, Appl. Catal., B, 2017, 203, 18–30 CrossRef CAS.
- Y. Zhang, C. Zhu, F. Liu, Y. Yuan, H. Wu and A. Li, Effects of ionic strength on removal of toxic pollutants from aqueous media with multifarious adsorbents: A review, Sci. Total Environ., 2019, 646, 265–279 CrossRef CAS PubMed.
- V. Beaugeard, J. Muller, A. Graillot, X. Ding, J.-J. Robin and S. Monge, Acidic polymeric sorbents for the removal of metallic pollution in water: A review, React. Funct. Polym., 2020, 152, 104599 CrossRef CAS.
- O. E. Samad, J. Dalmasso, G. Barci-Funel and G. Ardisson, Fast Radiochemical Separation and γ Spectroscopy of Short-lived Thallium Isotopes, Radiochim. Acta, 1993, 62(1–2), 65–70 CrossRef.
- X. Yang, Y. Wan, Y. Zheng, F. He, Z. Yu, J. Huang, H. Wang, Y. S. Ok, Y. Jiang and B. Gao, Surface functional groups of carbon-based adsorbents and their roles in the removal of heavy metals from aqueous solutions: A critical review, Chem. Eng. J., 2019, 366, 608–621 CrossRef CAS PubMed.
- I. L. Sinenko, T. P. Kalmykova, D. V. Likhosherstova, B. V. Egorova, A. D. Zubenko, A. N. Vasiliev, S. V. Ermolaev, E. V. Lapshina, V. S. Ostapenko, O. A. Fedorova and S. N. Kalmykov,
213Bi production and complexation with new picolinate containing ligands, J. Radioanal. Nucl. Chem., 2019, 321(2), 531–540 CrossRef CAS.
- P. P. Boldyrev, S. M. Deev, V. A. Golovachenko, V. A. Zagryadskii, A. S. Zakharov, V. I. Nikolaev, R. F. Nurtdinov, M. A. Proshin, D. Y. Chuvilin and Y. A. Yashin, Determination of the labeling yield and stability of the complexes Bi-BSA-DOTA and Bi-BSA-DTPA, Radiochemistry, 2014, 56, 194–199 CrossRef CAS.
- B. Pfost, C. Seidl, M. Autenrieth, D. Saur, F. Bruchertseifer, A. Morgenstern, M. Schwaiger and R. Senekowitsch-Schmidtke, Intravesical alpha-radioimmunotherapy with 213Bi-anti-EGFR-mAb defeats human bladder carcinoma in xenografted nude mice, J. Nucl. Med., 2009, 50(10), 1700–1708 CrossRef CAS PubMed.
- H. S. Chan, M. W. Konijnenberg, T. Daniels, M. Nysus, M. Makvandi, E. de Blois, W. A. Breeman, R. W. Atcher, M. de Jong and J. P. Norenberg, Improved safety and efficacy of 213Bi-DOTATATE-targeted alpha therapy of somatostatin receptor-expressing neuroendocrine tumors in mice pre-treated with L-lysine, EJNMMI Res., 2016, 6, 83 CrossRef PubMed.
- G. P. Adams, C. C. Shaller, L. L. Chappell, C. Wu, E. M. Horak, H. H. Simmons, S. Litwin, J. D. Marks, L. M. Weiner and M. W. Brechbiel, Delivery of the α-emitting radioisotope bismuth-213 to solid tumors via single-chain Fv and diabody molecules, Nucl. Med. Biol., 2000, 27(4), 339–346 CrossRef CAS PubMed.
- F. M. Kaspersen, E. Bos, A. V. Doornmalen, M. W. Geerlings, C. Apostolidis and R. Molinet, Cytotoxicity of 213Bi- and 225Ac-immunoconjugates, Nucl. Med. Commun., 1995, 16(6), 468–476 CrossRef CAS PubMed.
- L. I. Guseva and N. N. Dogadkin, A generator system for production of medical alpha-radionuclides Ac-225 and Bi-213, J. Radioanal. Nucl. Chem., 2010, 285(3), 667–673 CrossRef CAS.
- M. A. Moore, R. M. Counce, J. S. Watson and H. Hall, The Performance of two silica based ion exchange resins in the separation of 213Bi from its parent solution of 225Ac, Appl. Radiat. Isot., 2018, 141, 68–72 CrossRef CAS PubMed.
- N. D. Betenekov, E. I. Denisov, A. N. Vasiliev, S. V. Ermolaev and B. L. Zhuikov, Prospects for the Development of an 225Ac/213Bi Generator Using Inorganic Hydroxide Sorbents, Radiochemistry, 2019, 61, 211–219 CrossRef CAS.
- S. V. Ermolaev and A. K. Skasyrskaya, Motion of Genetically Related 221Fr and 213Bi Radionuclides in a Chromatographic Medium, Radiochemistry, 2019, 61, 44–54 CrossRef CAS.
- J. Chen, Y. Liu, M. Xu and Z. Liu, Preparation and use of the 225Ac- 213Bi generator, Chin. J. Nucl. Med. Mol. Imaging, 2022, 42(6), 330–334 Search PubMed.
- R. A. Boll, S. Mirzadeh and S. J. Kennel, Optimizations of Radiolabeling of Immunoproteins with 213Bi, Radiochim. Acta, 1997, 79(2), 145–150 CrossRef CAS.
-
A. R. Kazanjian and D. R. Horrell, Radiation effects on ion-exchange resins. Part III. Alpha irradiation of Dowex 50 W; US AEC, Rocky Flats Division, Golden, Colorado, RFP-2298, 1975 Search PubMed.
- K. K. S. Pillay, A review of the radiation stability of ion exchange materials, J. Radioanal. Nucl. Chem., 1986, 102, 247–268 CrossRef CAS.
- A. M. Dessouki, A. H. Zahran, A. M. Rabie and S. I. Amer, Some investigations on the radiation stability of a strongly acidic cation exchange resin, Radiat. Phys. Chem., 1989, 33(6), 545–549 CrossRef CAS.
-
E. P. Horwitz and A. H. Bond, Multicolumn selectivity inversion generator for production of ultrapure radionuclides, WO2003086569, 2003, https://patents.google.com/patent/WO2003086569A1/en.
- E. P. Horwitz, D. R. McAlister, A. H. Bond and R. E. Barrans Jr, Novel Extraction of Chromatographic Resins Based on Tetraalkyldiglycolamides: Characterization and Potential Applications, Solvent Extr. Ion Exch., 2005, 23(3), 319–344 CrossRef CAS.
- E. Horwitz and A. Bond, Purification of radionuclides for nuclear medicine: the multicolumn selectivity inversion generator concept, Czech. J. Phys., 2003, 53(Suppl 1), A713–A716 CrossRef CAS.
- T. J. Ruth, The Shortage of Technetium-99m and Possible Solutions, Annu. Rev. Nucl. Part. Sci., 2020, 70(1), 77–94 CrossRef CAS.
- A. Dash and R. Chakravarty, Electrochemical Separation: Promises, Opportunities, and Challenges To Develop Next-Generation Radionuclide Generators To Meet Clinical Demands, Ind. Eng. Chem. Res., 2014, 53(10), 3766–3777 CrossRef CAS.
- G. T. Cheek and D. Peña, Electrochemical Investigations of L-Cysteine Interactions with Bismuth Ions, J. Electrochem. Soc., 2020, 167, 155522 CrossRef CAS.
-
C. Apostolidis, B. Brandalise, R. Carlos-Marquez, W. Janssens, R. Molinet and T. Nikula, Method of loading a radioelement generator with mother radionuclide, US20050008553, 2005, https://patents.google.com/patent/US20050008553A1.
- J. Fitzsimmons, B. Foley, B. Torre, M. Wilken, C. S. Cutler, L. Mausner and D. Medvedev, Optimization of Cation Exchange for the Separation of Actinium-225 from Radioactive Thorium, Radium-223 and Other Metals, Molecules, 2019, 24(10), 1921 CrossRef CAS PubMed.
- W. Yantasee, G. E. Fryxell, K. Pattamakomsan, T. Sangvanich, R. J. Wiacek, B. Busche, R. S. Addleman, C. Timchalk, W. Ngamcherdtrakul and N. Siriwon, Selective capture of radionuclides (U, Pu, Th, Am and Co) using functional nanoporous sorbents, J. Hazard. Mater., 2019, 366, 677–683 CrossRef CAS PubMed.
- M. L. Testa and V. La Parola, Sulfonic Acid-Functionalized Inorganic Materials as Efficient Catalysts in Various Applications: A Minireview, Catalysts, 2021, 11(10), 1143 CrossRef CAS.
- E. R. Bertelsen, J. A. Jackson and J. C. Shafer, A Survey of Extraction Chromatographic f-Element Separations Developed by E. P. Horwitz, Solvent Extr. Ion Exch., 2020, 38(3), 251–289 CrossRef CAS.
- L. E. Jassin, Radiochemical separation advancements using extraction chromatography: A review of recent Eichrom Users’ Group Workshop presentations with a focus on matrix interferences, J. Radioanal. Nucl. Chem., 2005, 263, 93–96 CrossRef CAS.
- M. L. Dietz and E. P. Horwitz, Applications of Extraction Chromatography in the Development of Radionuclide Generator Systems for Nuclear Medicine, Ind. Eng. Chem. Res., 2000, 39(9), 3181–3188 CrossRef CAS.
- E. P. Horwitz, R. Chiarizia and M. L. Dietz, DIPEX: A new extraction chromatographic material for the separation and preconcentration of actinides from aqueous solution, React. Funct. Polym., 1997, 33(1), 25–36 CrossRef CAS.
- R. Chiarizia and E. P. Horwitz, Radiolytic stability of some recently developed ion exchange and extraction chromatographic resins containing diphosphonic acid groups, Solvent Extr. Ion Exch., 2000, 18(1), 109–132 CrossRef CAS.
- G. M. Marinov, A. P. Marinova, D. V. Medvedev, J. A. Dadakhanov, M. M. Milanova, S. Happel, V. I. Radchenko and D. V. Filosofov, Determination of distribution coefficients (Kd) of various radionuclides on UTEVA resin, Radiochim. Acta, 2016, 104(10), 735–742 CrossRef CAS.
- C. H. Kwak, C. Lim, S. Kim and Y.-S. Lee, Surface modification of carbon materials and its application as adsorbents, J. Ind. Eng. Chem., 2022, 116, 21–31 CrossRef CAS.
- J. M. Fonseca, L. Spessato, A. L. Cazetta, C. da Silva and V. d. C. Almeida, Sulfonated carbon: synthesis, properties and production of biodiesel, Chem. Eng. Process., 2022, 170, 108668 CrossRef CAS.
- M. G. Sanku, K. Forsberg and M. Svärd, Impregnation of preparative high-performance solid phase extraction chromatography columns by organophosphorus acid compounds, J. Chromatogr. A, 2022, 1676, 463278 CrossRef CAS PubMed.
-
A. H. Bond, J. J. Hines, J. E. Young and E. P. Horwitz, Automated radionuclide separation system and method, US6787042, 2002, https://patents.google.com/patent/US6787042B2.
|
This journal is © the Partner Organisations 2024 |
Click here to see how this site uses Cookies. View our privacy policy here.