Nanoscience and nanotechnology for water remediation: an earnest hope toward sustainability
Received
4th February 2024
, Accepted 21st March 2024
First published on 22nd March 2024
Abstract
Water pollution and the global freshwater crisis are the most alarming concerns of the 21st century, as they threaten the sustainability and ecological balance of the environment. The growth of global population, climate change, and expansion of industrial processes are the main causes of these issues. Therefore, effective remediation of polluted water by means of detoxification and purification is of paramount importance. To this end, nanoscience and nanotechnology have emerged as viable options that hold tremendous potential toward the advancement of wastewater treatment methods to enhance treatment efficiency along with augmenting water supply via utilization of unconventional water sources. Materials at the nano level have shown great promise toward water treatment applications owing to their unique physicochemical properties. In this focus article, we highlight the role of new fundamental properties at the nano scale and material properties that are drastically increased due to the nano dimension (e.g. volume–surface ratio) and highlight their impact and potential toward water treatment. We identify and discuss how nano-properties could improve the three main domains of water remediation: the identification of pollutants, their adsorption and catalytic degradation. After discussing all the beneficial aspects we further discuss the key challenges associated with nanomaterials for water treatment. Looking at the current state-of-the-art, the potential as well as the challenges of nanomaterials, we believe that in the future we will see a significant impact of these materials on many water remediation strategies.
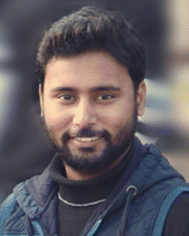
Subhajit Dutta
| Dr Subhajit Dutta obtained his BSc (chemistry) degree from the University of Calcutta in 2014 and MSc (chemistry) degree from the University of Calcutta in 2016. Thereafter, he joined the Indian Institute of Science Education and Research (IISER), Pune as a PhD student under the supervision of Prof. Sujit K. Ghosh and completed his PhD in 2022. Subsequently, Subhajit joined BCMaterials (Basque center for materials, applications and nanostructures; Spain) to pursue his post-doctoral research work under Prof. Stefan Wuttke. His current research interests focus on the development of next-generation functional porous materials that foster environmental sustainability. |
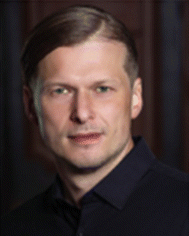
Stefan Wuttke
| Prof. Stefan Wuttke created the research group “WuttkeGroup for Science,” initially hosted at the Institute of Physical Chemistry at the University of Munich (LMU, Germany). Currently, he is an Ikerbasque Professor at the Basque Center for Materials, Applications, and Nanostructures (BCMaterials, Spain). His research interests focus on the design, synthesis, and functionalization of MOFs and their nanometric counterparts to target diverse applications. At the same time, he aims to establish a basic understanding of the chemical and physical elementary processes involved in the synthesis, functionalization, and application of these hybrid materials. |
1. Introduction
Water stands as the fundamental resource, crucial for the existence and development of all living organisms.1,2 Despite covering approximately 71% of the Earth's surface, only a mere 0.5% constitutes freshwater suitable for drinking. The contemporary era, marked by technological advancements and overpopulation, has led to unprecedented environmental challenges, including global warming, water pollution, and ocean acidification. These issues stem from the adverse impacts of rapid growth in industrial and agricultural sectors worldwide.3–6 As a consequence, freshwater resources are depleting due to the extensive release and infiltration of wastewaters into natural water reserves.7,8 The United Nations estimates that the global population will surpass 10 billion by 2050, and that 2 billion people lack or have limited access to safe drinking water already in 2024.9 Moreover, hazardous contaminants in water significantly disrupt the balance of aquatic ecosystems. Therefore, proper disposal, separation, and detoxification of wastewater are imperative for preserving ecological symbiosis.10–14
The primary sources of water pollutants include industrial waste, oil spills, nuclear waste leakage, mining activities, and agricultural products, which introduce toxic chemicals into natural water bodies.15–20 These pollutants can be broadly categorized into inorganic and organic types based on their chemical compositions. Inorganic pollutants, comprising toxic heavy metals and radioactive elements, pose greater threats due to their charged nature, resulting in high solubility and mobility in water.21–23 Additionally, these contaminants can accumulate in the food chain and in the human body where a narrow range lies between deficiency and toxicity, posing a grave threat due to their toxicity and lethality even at lower concentrations.24–26 On the other hand, organic pollutants, such as hydrocarbons, black waters, insecticides and herbicides, agricultural waste products, dyes, oils and pharmaceuticals persistently enter the environment in large volumes.27–29 Despite being, for the most part, less persistent than inorganic pollutants, their continuous generation makes them pseudo-persistent in environmental ecosystems.
To reduce the health and environmental risks, various water treatment techniques, including filtration, chemical precipitation, and coagulation, have been developed.30–33 These techniques employ a plethora of different materials characterized by different properties, such as oxidizing agents, coagulants, flocculants, ion exchange resins, and pH-adjusting substances. However, these methods face limitations, such as expensive facilities, high maintenance and complex instrumentation, or require materials that are too costly or whose production is environmentally unsustainable, making them often less sustainable, in particular in developing countries.34–36 Novel and simpler methods with improved performance from both energetic and economic perspectives are highly needed. To this end, recent advancements in chemistry and materials science have the potential to advance water treatment technology.
In particular, emergence of nanoscience and nanotechnology over the past few decades has played a key role in shaping current environmental remediation science and technology.37–42 To begin with, it is important to understand the scope and differences between nanoscience and nanotechnology. Typically, nanoscience represents a broad multidisciplinary area of scientific research, dealing with particles and structures in the dimensions of 1–100 nm.43 Nanoscience deals with precise control over nanoscale assembly of atoms and molecules into larger structures, which determines the extent of different physiochemical properties such as the thermal, optical, electrical and mechanical properties of nanomaterials. The field of nanoscience allows mutual cooperation of physicists, chemists, biologists, and material scientists to understand and overcome critical scientific challenges.44–46 On the other hand, nanotechnology is generally defined as the application of nanoscience and nanomaterials. Hence, it works in continuation with nanoscience toward engineering and manufacturing of nanomaterials and nanomaterial-based devices for practical applications.47
The transformation from bulk materials to nano-scale materials inevitably induces unique material characteristics such as size- and surface-dependent physiochemical behaviours and size-dependent quantum effects.48,49 Consequently, nanomaterials are found to have unique electronic, optical and mechanical properties. Overall, their properties can be divided into two large groups: (i) enhanced properties and (ii) emergent novel properties.
(i) Enhancement of bulk properties
Typically, the bulk counterparts have these properties; however, the reduction in size to the nano-level induces an exponential enhancement of properties such as surface area, the number of catalytic centres, and the number of binding sites and as a result reactivity and superparamagnetic properties.
(ii) Emergence of novel properties
Typically, bulk materials do not have these properties and they appear at the nano-scale. For instance, quantum effects in nanoparticles result in size-dependent positioning of energy levels of QDs, resulting in a shift of absorbed light wavelength, plasmon resonance of Au and Ag nanoparticles and their size-dependent shift.
As a result, nanoscience attracts huge research interest for many applications (Fig. 1), but surprisingly water treatment applications remain underexplored so far (Fig. 1). To date, the enhancement in nano-properties has been mostly exploited for water treatment, presenting vast unexplored opportunities and a lot of space for further improvements both in nanoscience and nanotechnology for water treatment.
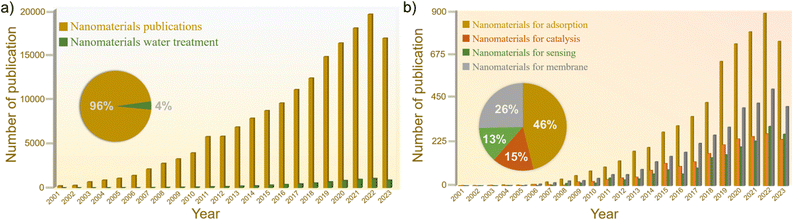 |
| Fig. 1 Chronological evolution of the nanomaterials field and/or nanomaterials for water treatment: (a) comparison between overall publications in nanomaterials and nanomaterials for water treatment; (b) numerical comparison between publication trends for various water treatment processes using nanomaterials. | |
It is worth mentioning that the advantages of the properties vary significantly among different nanomaterials, highlighting the impact of material nature on the focus of investigation. For instance, the surface area increases significantly for almost all materials when switching from the bulk to the nano scale. In contrast, properties like the melting point or fluorescence exhibit diverse changes. Organic nanoparticles show a substantial change in the melting point compared to bulk, while metal nanoparticles show no change. Quantum dots display tunable size-dependent fluorescence, whereas organic molecules show no such dependence. Therefore, adequate information and control over such material–property relationships are crucial for the development of efficient nanomaterials for water treatment.
Therefore, in this focus article, we wish to discuss the state-of-the-art as well as provide a critical assessment of the benefits and challenges of using nanomaterials and nanotechnology for water remediation.
2. The promise of “nano” in water treatment
The unique chemical, physical and optical properties of nanomaterials and their judicious utilization via nanotechnology have opened up new horizons in water treatment research and technologies (Fig. 2).50–58 For instance, the enhanced light absorbing ability of functional nanomaterials made them an excellent platform for photothermal water treatment in solar desalination, nano-photonics-based membrane distillation, disinfection applications and so on.59–64 Moreover, traditional water treatment methods involve utilization of toxic chemicals as disinfectants that pose critical threats from generation of carcinogenic by-products and iron- and aluminium-based coagulants in large volume which are typically dumped into landfills after use and rarely regenerated.65–68 To this end, employing nanomaterials in water treatment not only reduces the potential toxicity footprints but also enhances the overall working capacity and sustainability via multicycle regeneration with unaltered performance.
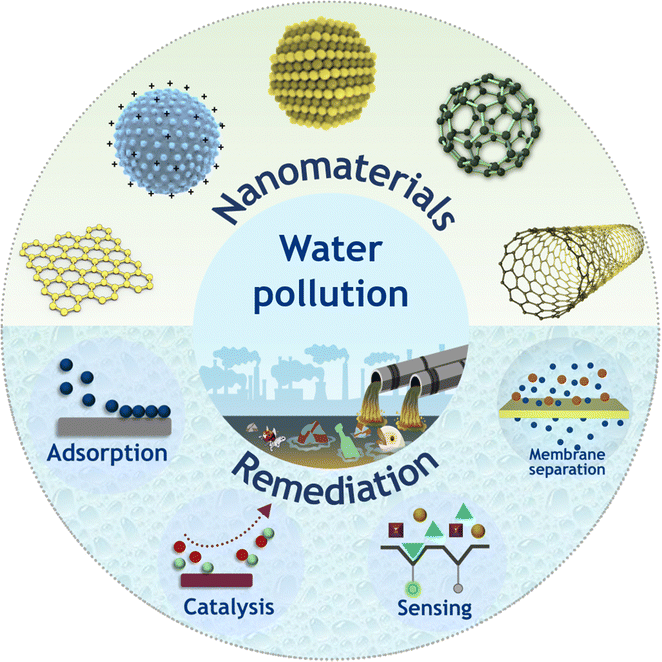 |
| Fig. 2 Schematic illustration of various nanomaterials and their potential applicability in different water remediation processes. | |
Individual or combined merits of enhanced and emergent nano-properties provide extraordinary water remediation abilities in terms of adsorptive, catalytic and sensory properties.57–61 Hence, it is important to discuss the origin of such properties and why “nano” materials help to address the existing challenges.
Adsorptive properties
Nanomaterials have emerged as an important platform for adsorbing water pollutants owing to their unique properties.
High surface area and tunable surface properties.
The high surface area that originates from the high surface to volume ratio together with tunable surface properties provide a large number of active sites for the adsorption of water pollutants and enhanced sorbate–sorbent interactions.69–71 Hence, a small amount of nanomaterials can exhibit very high adsorption kinetics and removal capacity.
Particle size.
The smaller size and closer intraparticle distance allow the nanoparticles to reach and adequately interact with micro/nano-scaled water pollutants at the molecular level. With a greater extent of interaction, the nanomaterials’ ability toward adsorbing a wide range of contaminants from water enhances.
Porosity.
The higher porosity of nanomaterials compared to their bulks is found to enhance the interaction at both internal and external surfaces, leading to improved adsorption capacity and diffusion rates. In this regard, porous nanomaterials such as activated carbon nanofibers, zeolites, metal–organic frameworks (MOFs), etc. offer tunable porous structures and porous surfaces that allow control over sorption kinetics and capacity.72–77
Moreover, the properties of nano-sorbents can be judicially tuned for achieving target-specific pollutant adsorption via establishing precise structure–property relationships. For instance, significant pitfalls of conventional water treatment methods have been associated with several toxic ionic contaminants (perchlorate, nitrate, etc.), heavy metal-based oxo-anions (e.g. chromate, arsenate, and selenite) and emerging pollutants including per- and polyfluoroalkyl substances (PFAS), etc. owing to their poor selectivity and lack of active functional sites.78–80 To this end, nanomaterials are found to offer superior selectivity toward targeted pollutants and enhanced catalytic activity toward cleaving the C–F bond and sorptive efficiency for the removal of PFAS. Furthermore, nano-adsorbents can be easily integrated with other macroscopic structures or used in existing water treatment by loading into porous granules or via coating filter media. The cumulative or individual merits of these adsorptive properties make nanomaterials superior in real-time water treatment applications.
Catalytic properties
Nanomaterials with a high surface-to-volume ratio can induce a high degree of catalytic activity owing to the presence of a greater number of catalytically active sites and their facile accessibility compared to their bulk counterparts.81 In addition, the higher reactivity of nano-catalysts resulted in improved degradation or transformation of environmental pollutants with better kinetics and selectivity than that of their bulk materials.82–84
Typically, catalytic degradation processes involve the following stages: adsorption of the pollutant molecules, absorption of the irradiated light, and eventually charge transport and separation and degradation of pollutants.85 From a fundamental perspective, the reason behind the superior photocatalytic efficiency of nano-catalyst materials can be attributed to two main nano-properties.
Quantum size effect.
Upon decreasing the particle size to below the nanoscale range, one of the immediate effects observed on the particles is quantum confinement.86 Depending on the electronic properties and the size of the materials, the CB and the VB convert into different energy levels, inducing positive electric potential in the VB and negative electric potential in the CB. Consequently, the oxidation and reduction potentials of the holes and the electrons get increased, resulting in improved oxidation reactivity of nano-photocatalysts (e.g., ZnO and TiO2).87
Higher surface area.
An increased surface area improves the efficiency of the first step of photocatalytic degradation – adsorption of pollutants, and the last steps – charge separation and catalytic degradation. The reactivity is typically governed by the time required for the holes and electrons to reach the catalyst surface.88 In the nanoscale range, the particle diameter becomes considerably small, which renders in a much rapid charge transport from the interior to the surface of the nanoparticles, reducing the possibilities of electron–hole recombination and commencing the redox reaction. For example, the photocatalytic efficiencies of ZnO and TiO2 nanoparticles are found to be much higher with the decrease of the particle size.89,90
It is worth noting that the catalytic activities of nano-materials can be significantly influenced by several factors including (i) size, (ii) shape, (iii) the preparation medium, (iv) the reaction conditions, and (v) the distribution of the nanoparticles. Precise monitoring of all these factors is crucial in order to achieve ultimate control over the catalytic properties.86
Sensory properties
Precise monitoring of water pollutants is regarded as one of the toughest scientific and technological tasks because of the extremely low concentrations of micropollutants along with the higher complexity of natural waters and wastewater systems. To this end, nanomaterials have shown tremendous potential toward the selective sensing of various water pollutants with higher efficiency and detection limits. From the fundamental point of view, the higher surface area, surface activity and the electronic properties of nanomaterials play a crucial role in their effectiveness for sensing applications.
Surface effects.
The higher surface area of nanomaterials provides huge numbers of active/functional sites available for interactions with the targeted analytes, resulting in improved signal-to-noise ratios. Such improved signal-to-noise ratios are critical for sensing pollutants with very low/diluted concentrations and decreasing the limit of detection. Moreover, the increased surface area allows greater adsorption of pollutants, consequently enhancing the sensitivity of the sensor.
Electronic effects.
Size-dependent quantum effects allow tuning the electronic structure by adjusting the particle size, leading to unique optical properties. Such strategy is often employed for fluorescence-based sensory probes where the presence of targeted pollutants can be monitored via the change in fluorescence intensity or wavelength. In addition, semiconducting nanomaterials are widely used for electrochemical sensing in which the electrical conductivity and the energy-bandgap can be precisely controlled by tuning the size, composition and functionalization of the nanomaterials, achieving a higher selectivity of the sensory probe.
Overall, water remediation is a very diverse topic as different aquatic systems present different levels and types of pollutants which necessitates the development of target specific nanomaterials and technologies with high precision. From the design and selection perspective, the judicious choice of respective building units for both nanomaterials (at the molecular level) and nanotechnologies (at the macroscopic level) holds huge importance toward the development of high-performance water treatment methods or devices.91–93 It is important to point out that two nanomaterials with similar elemental composition can exhibit completely different water treatment efficiency and effectiveness upon alteration of their physiochemical parameters such as shape, size, surface functionality etc.94,95 In addition, the nano-effects of nanocrystalline materials can also be fine-tuned by tuning the molecular building units as well as the synthetic parameters during synthesis or post-synthesis, resulting in alteration of adsorptive, magnetic, and catalytic activities.96–98 Controlled manipulation of surface functionalization of nanoparticles has shown tremendous potential not only to improve stability and dispersibility of nanoparticles but also for effective transformation of nanomaterials into macroscopic structures in the form of hybrid composites, membranes, etc. for real-time applicability.99–103 Moreover, surface functionalization can induce higher binding affinity and selectivity toward target contaminants, resulting in improved catalytic and oxidative activity, sorption capacity, paramagnetism and antimicrobial activity. On the other hand, the core–shell structure of nanomaterials affords additional advantages toward multifunctionality by controlling the stability, reactivity, and tunability at the nanoscale.104 Hence, an in-depth understanding and appropriate selection of various shapes, sizes and surface functionalities along with the core–shell nanostructures can result in highly efficient nanomaterials and nanotechnologies.
3. Chemistry of nanoparticles in an aqueous environment
The systematic correlation of the physicochemical properties of nanoparticles with their performance in water is the key toward achieving improved water treatments. Upon entering into water, the immediate interactions occur at the solid–liquid interface between the nanoparticle surface and the water medium, which consequently control the nano-abilities of the material in the immersed state.105 The solid–liquid interfacial interactions between the nanoparticles and the water medium are governed by the surface hydrophobicity and hydrophilicity along with the zeta potential of the nanoparticles (Fig. 3). The water treatment performance of nanomaterials significantly depends also on their affinity toward water.106,107 Again, the nanoparticle surface plays a crucial role as the amount of polar or Lewis acidic/basic functional groups present on the surface determines the extent of hydrogen bonding interactions with water molecules and thus controls the overall affinity toward water. From the nano-perspective, the nano-effect allows a higher degree of facial hydrophilic/hydrophobic interactions, which help the nanoparticles to be dispersed easily in water medium, resulting in a greater extent of contact with the contaminants and higher efficiency compared to their bulk components.
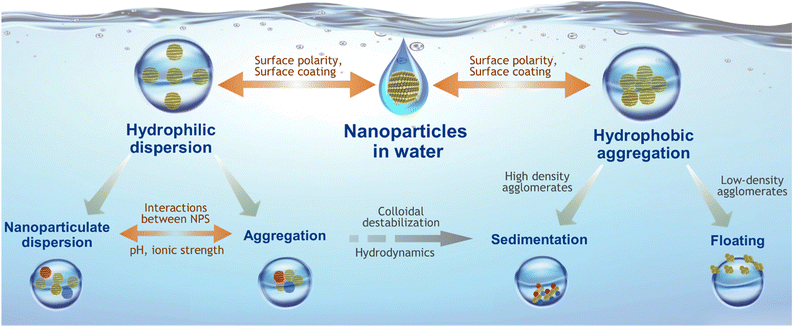 |
| Fig. 3 Properties of nanoparticles in water. | |
Moreover, structural and physical properties such as lattice defects, surface curvature, roughness, etc. also play crucial roles in interfacial interactions and solubility of nanoparticles. The overall behaviour of nanoparticles in an aqueous medium is typically governed by their chemical stability and dispersion stability of the materials.108,109 In particular, the dispersion stability of nanoparticles determines their exposure duration in water, whereas the chemical stability controls their overall physiochemical properties under water.110 Dispersion stability of nanoparticles is one of the key factor for determining the exposure and fate of these materials in an aquatic environment. It is well established that the size-variation at the nano-scale will allow relatively bigger agglomerates of nanoparticles to act differently from the well dispersed ones.111 The dispersion stability of nanoparticles can be influenced by several parameters, including their surface properties, solution chemistry (e.g., pH of the medium, ionic composition, ionic strength), nanoparticle concentration etc.110 From the molecular point of view, the dispersion stability depends on the interparticle collision frequency and collision efficiency between two nanoparticles. In aquatic environments, although the interparticle collision frequency can originate from both Brownian motion and the local mechanical stirring, Brownian motion prevails toward controlling the aggregation and dispersion extents of the nanoparticles.112–114 Moreover, the sticking efficiency also depends on the type of interfacial interactions (attractive or repulsive) existing between colliding nanoparticles. For instance, a stronger repulsive interaction results in reduced sticking efficiency and higher particle dispersion. On the other hand, a stronger attractive interaction induces higher sticking efficiency and higher aggregation of the nanoparticles.
At the nanoscale, the type and extent of attractive and repulsive interactions are governed by the existing electrostatic interactions and van der Waals interactions between nanoparticles.115 The electrostatic interactions occur between two electronically charged/polarized surfaces via electronic cloud diffusion. Hence, the ionic strength and pH of the medium, surface charge, and interparticle distances are the important factors, controlling the degree of electrostatic interactions in the water medium.116 Typically, electrostatic interactions in a homogeneous dispersion of the same nanomaterial are repulsive in nature owing to the similar electric surface potential of the nanoparticles. On the other hand, the van der Waals interactions between nanoparticles are constant and attractive in nature. Hence, the resultant interactions originating from the repulsive electrostatic interactions and attractive van der Waals interactions determine the extent of colloidal dispersion and dispersion stability at the nano-level, thus significantly affecting the remediation potential of the nanomaterials.
4. Water remediation using nanoscience
Nanoscience is actively being explored toward improvement of the existing water treatment processes and even to develop new processes. However, for the real-world water treatment scenario, it is important to precisely define the target pollutants, their behaviour and safe-limits in order to develop sustainable and affordable nano-remediation technologies. Target-specific designing of nanomaterials by fine-tuning their size, electronic properties and functionalities has shown tremendous promise in terms of selectivity and sensitivity toward several environmental contaminants even at very low concentration levels. Thus, defining the permitted level of the concentration is important for an appropriate selection of nanomaterials toward developing the efficient water treatment process.
In the following section, we will highlight the key role of nanoscience in water pollutant remediation.
4.1 Adsorption and separation
Among the commonly utilized nano-adsorbents, carbon nanotubes (CNTs) are accounted as a promising alternative of activated carbon owing to their ability to effectively remove both toxic organic and inorganic water pollutants.117–121 Owing to the advantage of controlled pore size distribution and a high surface-active site to volume ratio, CNTs have already shown improvement in pollutants removal efficiency compared to traditional powdered or granular activated carbons (Fig. 4).122 In particular, CNTs are found to have active binding sites for the adsorption of bulky organic pollutants, which promote selective interactions between CNTs and the incoming pollutants via intermolecular electrostatic interactions, π–π bonding, hydrogen bonding, etc. Extensive studies have revealed that the removal of pollutants depends on both the type of sorbate molecules and the surface functional groups.123 Moreover, their characteristic π-rich surface can act as both an electron donor and acceptor toward toxic polar aromatic pollutants such as phenols and nitroaromatics (Fig. 4).124 While hydrophobic graphitic surfaces are found to exhibit higher removal efficiency toward organic pollutants, the adsorption of inorganic pollutants such as toxic metal ions is primarily dominated by the surface functionalities via electrostatic interactions, which can be reversed controllably by tuning the pH of the medium, enabling reusability of the nanomaterials (Fig. 4).125,126 Since CNTs are associated with significantly higher cost compared to the activated carbon materials, current research has been dedicated toward the development of energy efficient and economically sustainable materials for pollutant degradation.
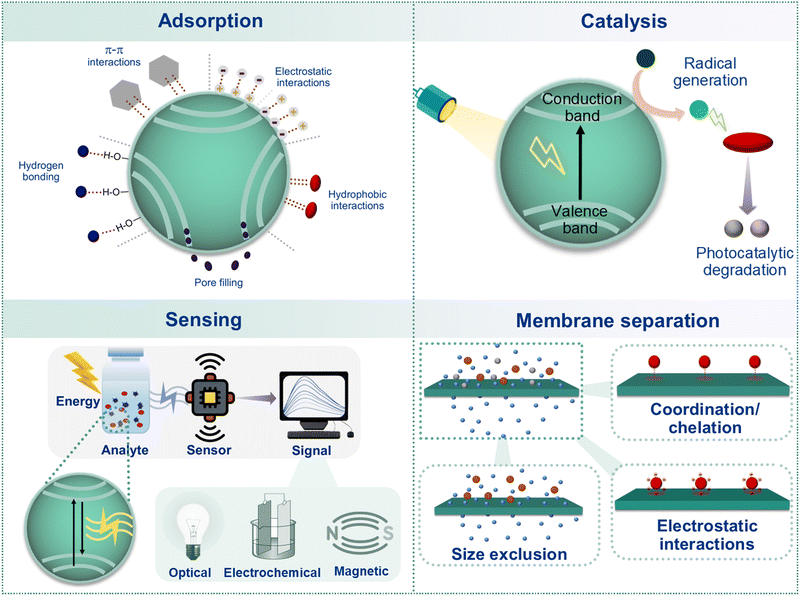 |
| Fig. 4 Schematic illustration of nano-scale mechanistic insights of different water remediation processes. | |
To this end, another allotropy of carbon, namely graphene, has emerged as a cost-effective alternative which can be synthesized by the exfoliation of graphite.122 In the last few decades, there has been significant utilization of graphene and graphene-based materials, such as graphene oxide (GO) for water pollutant remediation applications. This is attributed to their unique structural features, enabling the efficient remediation of a broad range of water pollutants.127–130 Compared to CNTs, graphene and its derivatives offer several advantages as sorbents for water treatment. Firstly, the single-layered graphene intrinsically possesses two accessible basal planes for effective interactions with the incoming pollutants, whereas in the case of CNTs, the inner walls are not available for the incoming pollutants. Secondly, GO-based materials involve simpler synthetic methods which avoid the utilization of metallic catalysts and energy intensive purification steps, resulting in more sustainable environmental footprints.122 Moreover, these materials are found to exhibit significant improvements in sorption capacity compared to their bulk carbon materials and pristine graphene analogues. In this regard, water treatment using GO-based materials has shown tremendous potential owing to their intrinsic functionalized surface area (rich with polar oxygen-groups) which not only imparts high reactivity toward polar pollutants but also avoids the additional treatments by toxic acids to introduce hydrophilic character.130 A variety of studies have demonstrated the utilization of the combined merits of polar functionalities and high surface area of GO toward an effective removal of toxic inorganic species such as metal ions (Pb(II), Cd(II), Zn(II), Hg(II), etc.) from water via electrostatic interactions and coordinative binding.131–134 Both GO and graphene nanosheets (GNs) can be hybridized with other functional materials such as metal oxides to produce hybrid composite materials which showed high target specificity toward contaminants.122
Apart from the carbon-based nano-materials, nanosized metal oxides such as nano-TiO2, nanomagnetites Fe3O4 have emerged as affordable sorbents for efficient remediation of toxic heavy metals and radionuclides.135–137 Similar to the organic counterparts, these nanomaterials also offer a high surface area and tunable surface structures such as edges, corners, vacancies, crystallographic facets, etc. which can be strategically controlled in order to maximize the adsorption performance. In addition, the development of nanomagnetites that possess superparamagnetic properties, enables easy segregation of the sorbent materials after water treatment via a weak magnetic field.138,139 This unique feature allows the development of next-generation nanoparticles with core–shell structures where the shell allows the introduction of desired functionalities, and the magnetic core helps in rapid particle separation.139 However, the significant surface energy of the metal oxides at the nanometer level inevitably leads to particle agglomeration because of their strong van der Waals interactions, resulting in reduced performance in terms of both sorption capacity and selectivity.140 An effective way to overcome these technical bottlenecks is to hybridize the metal oxide nanoparticles with different porous supports to form hierarchical macroscopic objects. The commonly utilized supports include synthetic polymer hosts, cross-linked ion-exchange resins, porous manganese oxide complexes, Al2O3 membranes, etc.122 However, the fabrication of these hybrid sorbent materials is still at the nascent phase and several technical limitations need to be addressed including a slow fabrication process and performance optimization via interplay between the nanoparticles and the porous support of the composite materials.
4.2 Photocatalytic degradation
Nanomaterials with high surface-to-volume ratio can induce in high degree of catalytic activity owing to the presence of greater catalytically active sites and their facile accessibility compared to their bulk counterparts.81,141 Hence, much scientific attention has been paid toward the development of photocatalytically active nanomaterials which can operate under visible or UV light in water. There are two prime mechanistic theories for photocatalysis: (1) single-step oxidative degradation of pollutant molecules via initiation of free radicals, induced by the generation of electron (e−)–hole (h+) pairs at the nano-catalyst surface; and (2) adsorption followed by photocatalytic degradation where the pollutant molecule initially gets adsorbed on the photocatalyst surface and subsequently photo-degraded via the generated radicals or the superficial electron–hole pairs (Fig. 4).142,143 Moreover, the photodegradation kinetics and the overall performance can be governed by the nature of the catalyst surface, size of the nanoparticles, accessibility of the surface-active sites and adsorption ability toward the organic molecules.144
To this end, semiconductor materials have exhibited tremendous promise as photocatalysts for the treatment of various organic pollutants.145 The electronic structure of the semiconductor materials and the difference between their energy levels, namely the valence band (VB) and the conduction band (CB), known as the bandgap, determine the photocatalytic activity of the materials. Upon irradiation by photons having energy ≥ bandgap, electrons from the VB get excited and jump to the CB, creating a positively charged hole in the VB. In semiconductor materials, both the electrons and the holes act as reducing and oxidizing species, respectively, toward the incoming pollutant molecules. It is important to note that the crystallinity, photon absorption efficiency, and the rate of electron–hole pair separation of a photocatalyst also play a crucial role in determining the overall efficacy of the photocatalyst. In this regard, TiO2 has gained much scientific attention over other semiconducting materials owing to its promising chemical properties, higher physiochemical stability and low cost. Furthermore, hetero-atom doping (e.g. carbon, nitrogen, and sulphur) in TiO2 has shown promise toward photocatalytic degradation. Multi-doped TiO2 composite materials are found to exhibit great efficiency toward the photocatalytic reduction of toxic heavy-metal ions, such as chromium(VI) in water.146 Moreover, metal sulfides (e.g. WS2, MoS2, CoS2, ZnS, etc.) and their heterojunction composites (e.g. ZnIn2S4/TiO2) have shown tremendous potential toward the photocatalytic degradation of water pollutants.147 In addition, in pursuit of eco-friendlier and sustainable options, metal-free 2D materials including various g-C3N4 nanosheets, black phosphorus (BP), and their heterojunction composite materials such as oxygen-enriched porous g-C3N4 nanosheets assembled with black phosphorus, have been investigated toward water remediation owing to their broad visible light absorption range, chemical stability, high surface area and non-toxic nature.148,149
Other catalytic methods including electrocatalysis, photoelectrocatalysis and piezocatalysis have also been widely studied. To this end, metal-oxide electrode materials such as cobalt oxide (Co3O4) and bimetallic oxide electrodes (e.g. Co3O4–La2O3) have been proven to act as exceptional catalysts for conducting heterogeneous catalysis processes owing to their unique structural and electronic properties.150
Moreover, photoelectrocatalytic processes have emerged as a viable advanced oxidation method for mineralization and decomposition of various aquatic pollutants. Several materials including TiO2, zinc oxide, bismuth vanadium oxide (BiVO4) and their composite materials have exhibited significant photoelectrocatalytic activity toward the degradation of organic pollutants.151 Importantly, precise control over the semiconducting properties and electronic band structure of the catalyst is of paramount importance in determining the oxidation (photoanode) or reduction (photocathode) efficiency. In the quest of achieving improved efficiency and eco-sustainability, piezocatalytic processes have been explored in recent times. In particular, Bi-based materials have attracted huge attention owing to their both photocatalytic and piezocatalytic activities that elegantly blend the advantages of both processes, resulting in improved degradation efficiency. Among different materials, Bi-based materials have attracted much attention owing to their layered structures comprising (Bi2O2)2+ layers and interlayer ions that offer lesser eco-toxicity footprints. Moreover, Bi-catalysts can show piezoelectric activity even under mechanical vibrations because of the lone-pair electrons of Bi3+, providing a sustainable alternative as lead-free piezoelectric materials.
From the material designing perspective, several controlling factors including bandgap, carrier transport, chemical stability and surface area are highly important. Although significant efforts are being devoted toward the development of photocatalysts with small bandgaps, a rapid recombination of the charge carriers (hole–electron) can happen because of the small bandgap, thus affecting the photocatalytic activity. Hence, precise control to achieve a fine balance between the bandgap and charge recombination is of paramount importance in order to develop an efficient photocatalyst.152
4.3 Sensing
From the mechanistic point of view, sensors can operate via three main signal transduction mechanisms, namely optical, magnetic and electrochemical sensing (Fig. 4). Depending on the structural, electronic and physiochemical properties of the nanomaterials along with the type of analytes and the interfacial interactions between the sensor and the analyte, different signal transduction methods can be employed.
Nanomaterials such as CNTs, dye-doped nanoparticles, quantum dots (QDs), carbon nanostructures (carbon nanotubes, graphene, etc.), magnetic nanoparticles, metal nanoparticles, etc. are widely utilized as nano-sensor materials owing to their unique optical, electrochemical and magnetic properties.148 For example, QDs offer a wide absorption range with a narrow and stable fluorescence emission range which can be tuned via tuning the chemical compositions and particle size and shape, resulting in targeted multiplex detection with a single excitation source.153–155 In addition, the strategic inclusion of organic dyes into porous silica or polymeric nanoparticles is found to induce high luminesce properties owing to the confined effect on the dye molecules.156 On the other hand, noble metal nanoparticles such as nano-Au and nano-Ag have been extensively exploited as sensory probes owing to their wide range of shapes, facile surface functionalization and high extinction coefficients (ε > 3 × 1011 M−1 cm−1).157 The colloidal solutions of nano-Au and nano-Ag particles exhibit size-dependent chromism behaviour. For instance, the spherical nano-Au particles with diameter in the range of ∼5–50 nm exhibit an intense red colour in the solution phase, however, it transforms into purple by increasing the particle size up to ∼100 nm. In the same line, a wide range of nanostructured metal oxide materials including iron oxides, zirconium oxides, titanium oxides, cerium oxides, tin oxides, zinc oxides, etc. have been explored toward effective recognition of aquatic pollutants.
In addition, magnetic metal oxides such as maghemite (γ-Fe3O4) and magnetite (Fe3O4) also have attracted significant attention toward water remediation processes owing to their ease in structural functionalization and less energy-economic footprints.158 Apart from the QDs and metal oxides, functional organic materials such as CNTs and graphene are employed as sensory probes owing to their high electrical and thermal conductivity and excellent mechanical strength. In particular, these materials have shown excellent improvement in sensitivity and electrochemical detection limits for glassy carbon electrodes toward several analytes.159,160
5. Water remediation by membrane separation
Membrane-based nanotechnology is one of the most crucial methods for the global water treatment and purification.161 Although membrane-based separation does not directly deal with nano-properties at the atomic or molecular level, it involves the utilization of nanoscience, nanomaterials and nanocomposites toward the development of membrane-based nanotechnology water treatment and separation processes. Nanomaterials present several advantages in membrane-based nanotechnology.
Enhanced pore structure
Incorporation of nanomaterials into water-treatment membranes allows fine tuning of the membrane-pore structures at the nanoscale which can subsequently result in improved permeability and selectivity of the membrane, enabling effective pollutant separation from water.
Improved flux rates
Judicious choice of the nanomaterials, polymer matrices and the support layers can lead to a better water flux rate through the membrane, enabling higher interactions between the contaminants and the active materials. Such strategy is highly critical for methods such as nanofiltration and ultrafiltration where very high-water flow is desired.
Selective filtration
The liberty in flexible designing of both nanomaterials and the membranes allows for control over the functionalization and porosity of the membrane which enables selective removal/separation of the targeted contaminants from water (Fig. 4).
The separation performance of water-treatment membranes is significantly governed by the physiochemical properties of the membrane material, thus overcoming the trade-off between the solute rejection and solvent permeation.162 Amongst different membrane nanotechnologies, thin film nanocomposite (TFNC) membranes, aligned CNT membranes and biomimetic membranes have shown tremendous promise in overcoming the above-mentioned trade-off. In the case of both biomimetic (also known as aquaporin) and CNT-based membranes, the intrinsic nanochannels assist in preferential water diffusion with very high permeation rates, yielding a high volume of purified water.163–165 Such selectivity and high flux are attributed to the nanosized channels with a unique hourglass shape, size and surface composition of the nanotubes. In fact, it is observed that even with very lower loading of aligned CNTs and aquaporins, the resultant water flux along the membrane is much higher than that of the commercially available reverse osmosis (RO) membranes.166,167 Such high flux is found to significantly reduce the energy-economic footprints in seawater desalination and wastewater treatment by RO. In addition to high flux water channels, aquaporins can provide advantages of selective ion channels which can selectively pull out ions from the water.
Lately, there has been an introduction of various nanoparticles into polymeric thin films to create TFNC membranes, aiming to harness the additional nano-scale properties of nanomaterials. The fabrication of TFNC membranes with nanoparticles during interfacial polymerization or via surface attachment has been found to improve the separation performance, antimicrobial activity and antifouling properties of the membranes.168,169 Moreover, the separation performance of TFNC membranes can also be tuned by tuning the size and composition of the nanoparticles, enabling target-specific membrane designing for the efficient treatment of a wide-range of aquatic pollutants. TFNC membranes fabricated from zeolite nanoparticles have shown promise in water treatment due to the molecular sieving properties of zeolites.170 The zeolite nanoparticles are found to enhance the permeability of the membrane owing to their nano-scale, hydrophilic pores that induce preferential water flux while rejecting hydrated ions. Moreover, significant efforts have been devoted toward the development of reactive nano-composite membranes which are mainly formulated with nano-TiO2 particles, combined with either polymeric membranes or ceramic membranes.171 Such a reactive nano-composite membrane is found to facilitate simultaneous dual-treatment, i.e. separation and catalytic degradation of aquatic contaminants along with improved membrane fouling properties.
Another important aspect of membrane nanotechnology is controlling the fouling properties of the membranes during water treatment processes. To address this issue, various nanomaterials including TiO2-nanoparticles, nano-alumina, and CNTs have been utilized as additional supports in polymeric membranes via surface self-assembly.172,173 However, installation of these nanomaterials via covalent bonding has been regarded as the most effective method in order to maximize the nanomaterial utilization and generation of active sites. The incorporation of the nanomaterials inevitably increases the fouling resistance of the nanocomposite membranes, owing to the antimicrobial activity and surface hydrophilicity of the nanomaterials.
The integration of nanotechnology with water treatment membranes provides wide opportunities in achieving enhanced membrane performance in terms of both improved fouling resistance and selective removal. Strategic utilization of functional membranes via nanotechnology has shown great potential in enhancing the water permeability, catalytic activity, flux recovery and robustness (chemical, physical, thermal and mechanical) of the water treatment methods compared to those of the current state-of-the-art methods. For example, ceramic membranes with reactive surfaces exhibited good potential toward the removal of selective pollutants in nanofiltration and reverse-osmosis methods with high durability. Moreover, the introduction of photocatalytically active and antimicrobial nanoparticles in such membranes are found to show significant self-cleaning and catalytic water treatment performance. The mixed-matrix-membranes offer enhanced mechanical stability, fouling resistance, and filtration performance as an individual matrix or as a support-matrix for TFNC membranes. Integration of each of these membranes with nanotechnology-enabled water treatments shows promise to provide great platforms toward practically viable alternatives.13,160
6. Challenges
Importantly, despite the huge potential of nanomaterials in water treatment, the attractive nano-properties come with intrinsic challenges that have to be addressed.174–181
Environmental toxicity
The entry of nanomaterials into aquatic and terrestrial environments inevitably induce additional toxicity that threatens environmental symbiosis. The type and extent of ecotoxicity of nanomaterials vastly depend on the type of nanomaterials, their chemical composition, functionalization and the fate of the nanomaterials in water. Because of their smaller size and high surface reactivity, nanomaterials often undergo significant bioaccumulations by the aquatic organisms, which consequently leads to biomagnification in the food chain, potentially impacting higher trophic levels. Moreover, nanoparticles can undergo disintegration under water which may cause release of toxic particles, ions and other by-products that could potentially induce different toxicological effects than the original nanoparticles. For example, silver or lead nanoparticles may release toxic ions upon disintegration, which can also be extremely dangerous to aquatic organisms, disrupting the balance of microbial systems and affecting the overall health of ecosystems.
Agglomeration
Because of their higher surface reactivity, nanoparticles are often found to agglomerate to form larger particles or clusters under water, significantly limiting their potential toward water treatment processes. Upon formation of the larger agglomerate particles, the effective surface area and surface reactivity of the nanomaterials decrease, thus decreasing the water treatment performance. Moreover, agglomerated nanoparticles exhibit lower mobility under water and can quickly settle down which reduces their ability to reach and interact with targated pollutants in water.
Recyclability
While nanomaterials can offer exciting properties, several of them can contribute to hindering their effective recovery and reuse from water. As mentioned before, agglomerated nanoparticles can form larger particles that settle quickly, making it challenging to separate them from the water for recycling. Moreover, several nanomaterials tend to lose their surface properties over successive usage cycles, resulting in a significant decrease in water treatment performance and their overall recyclability. Finally, nanomaterials can undergo structural degradation over multicycle usage under harsh water conditions which not only reduces their recyclability performance but also induces acute toxicity to the water body. Hence, the development of environmentally friendly and energy-efficient methods for recovering and reusing nanoparticles in water treatment that can be implemented at the real-world scale is still a significant challenge.
Property controllability
Presice control of the nano-properties is the key for the successful use of nanomaterials in water treatment. However, achieving higher controllability in the nanoscale range poses several challenges. For example, the standout feature of nanomaterials lies on their high surface area and surface activities, which contribute to efficient sorptive interaction or catalytic activity toward water pollutants. However, these same properties can make the nanomaterials undergo interparticulate binding with other particles/bio-organisms and/or agglomeration within the same nanoparticles. Controllability over surface functional properties of nanomaterials under water is extremely difficult. Hence, the target-specificity of the nanomaterials can be significantly affected as the reactive surface-functionalities can interact/bind with other molecules and get saturated even before interacting with the water pollutants. Moreover, precise control over structural defects and defect densities is crucial, yet highly challenging in order to control the nano-properties. Addressing these challenges demands multidisciplinary approach that allows precise understanding of the environmental demands and development of next generation nanomaterials.
Complex characterization
Nanomaterials’ characterization in the water medium presents additional challenges due to the complex interactions of the nanomaterials and the advanced characterization techniques required for it. The properties and behaviour of nanomaterials can be significantly influenced by water chemistry, temperature, and the presence of other dissolved or suspended substances. Moreover, the agglomerating ability of nanomaterials can significantly affect the dispersion stability of the nanoparticles in water, thus leading to higher complexity in characterization. The compositional complexity of the real-world water matrix creates additional challenges for performing analytical techniques under water. On the other hand, the standardization of nano-properties in the water matrix is often challenging and requires highly qualified experts. Accounting on these challenges, the characterization techniques under water are often found to be relatively expensive and need trained experts.
Cost
From the real-world applicability scenario, the economic feasibility and commercialization of a ‘nano’ product are key concerns. The synthetic methods of nanomaterials can be complex and require expensive equipment and expertise which can lead to high production costs, especially for large-scale manufacturing. Moreover, the utilization of costly raw materials and complexity associated with the surface functionalization or modification result in increased costs in terms of materials’ synthesis and processing. Hence, continued scientific and technological efforts must be devoted toward overcoming these cost barriers in order to push real-world utilization of nanomaterials.
7. Conclusion
Sustainable access to clean and drinking water is one of the most crucial challenges of the 21st century which necessitates the development of next-generation water treatment approaches and technological reforms. Continued development of nanoscience and nanotechnology has shown promise to provide an ideal platform toward achieving novel and efficient water treatment methods. Thanks to the scientific and technological efforts devoted over the last few decades, significant progress has been made toward the fundamental understanding and development of nanoscience and nanotechnology. Despite their several advantages and unique properties, nanomaterials still suffer from several key challenges that need to be overcome in order to establish their long-term sustainability and industrialization. Significant attention must be paid toward the development of green synthesis of nanomaterials with minimal toxicity footprints toward the environment. Moreover, the current research direction also focuses on designing and developing multifunctional nanomaterials that can overcome multiple water treatment challenges simultaneously. From the practical applicability point of view, it is important to exploring ways toward the integration of nanomaterials with the existing water treatment technologies in order to enhance the overall treatment efficiency. Significant efforts have been made to overcome the issues associated with large-scale production of nanomaterials, which in combination with improved cost and reproducibility can show the way toward their sustainable production and profitable commercialization. Moreover, current research and methodologies possess serious pitfalls in recycling the nanoparticles and nano-composite materials owing to operational difficulties. Apart from magnetic separation-based techniques, most of the nano-material-integrated methods lack fast, affordable and energy-efficient NP removing and recycling techniques. Future research should focus on the development of energy-efficient recycling processes for nanomaterials in water. Moreover, systematic evaluation of the nanomaterials' fate in the environment should be paid much attention in future research in order to achieve environmental sustainability. From the practical implementation point of view, integration of nanotechnology-enabled water treatment methods with other existing techniques, like biological and chemical purification can pave the way toward achieving more efficient water purification methods. Considering that nano-engineered water-treatment methods possess difficulty in mass-scale adaptation, significant research and development efforts must be devoted toward the hybridization of traditional methods (e.g. desalination) and the emerging nano-engineered water-treatment methods.
Conflicts of interest
There are no conflicts to declare.
Acknowledgements
This work was supported by the Spanish State Research Agency (AEI) and the European Regional Development Fund (ERDF) through the project PID2020–15935RB-C42. The European Union's Horizon 2020 research and Innovation programmes is also acknowledged for the funding of the LC-SC3-RES-25-2020 4AirCRAFT (ref. 101022633) project. In addition, the 4AirCRAFT project was also supported by the Japan Science and Technology Agency (JST) and Mission Innovation Challenge was supported by the Sao Paulo Research Foundation (FAPESP). J. A. and S. D. thank the Ministerio de Ciencia e Innovación and the European Union-Next GenerationEU for Juan de la Cierva Formación (FJC2021-048154-I) and (JDC2022-049611-I) research contract, respectively. Furthermore, A. S. and S. W. acknowledge the Human Frontier Science Program (HFSP) for their support (RGP0047/2022).
Notes and references
- M. A. Shannon, P. W. Bohn, M. Elimelech, J. G. Georgiadis, B. J. Mariñas and A. M. Mayes, Nature, 2008, 452, 301–310 CrossRef CAS PubMed.
- C. J. Vörösmarty, P. Green, J. Salisbury and R. B. Lammers, Science, 2000, 289, 284–288 CrossRef PubMed.
- S. Fajal, S. Dutta and S. K. Ghosh, Mater. Horiz., 2023, 10, 4083–4138 RSC.
- P. C. D. Milly, J. Betancourt, M. Falkenmark, R. M. Hirsch, Z. W. Kundzewicz, D. P. Lettenmaier and R. J. Stouffer, Science, 2008, 319, 573–574 CrossRef CAS PubMed.
- S. Rojas and P. Horcajada, Chem. Rev., 2020, 120, 8378–8415 CrossRef CAS PubMed.
- Resolution A/RES/64/292; United Nations General Assembly, July 2010.
- P. A. Kobielska, A. J. Howarth, O. K. Farha and S. Nayak, Coord. Chem. Rev., 2018, 358, 92–107 CrossRef CAS.
- S. Dutta, S. Let, S. Sharma, D. Mahato and S. K. Ghosh, Chem. Rec., 2021, 21, 1666–1680 CrossRef CAS PubMed.
- World Population Prospects: The 2015 Revision, Methodology of the United Nations Population Estimates and Projections; Working Paper No. ESA/P/WP.242; Department of Economic and Social Affairs, Population Division, United Nations, 2015.
- C. A. Martínez-Huitle and S. Ferro, Chem. Soc. Rev., 2006, 35, 1324–1340 RSC.
- Q. Gao, J. Xu and X.-H. Bu, Coord. Chem. Rev., 2019, 378, 17–31 CrossRef CAS.
- Q. Zhang, Y. Cui and G. Qian, Coord. Chem. Rev., 2019, 378, 310–332 CrossRef CAS.
- S. Dutta, R. F. de Luis, J. Goscianska, A. Demessence, R. Ettlinger and S. Wuttke, Adv. Funct. Mater., 2023, 2304790 CrossRef.
- A. Fuchs, F. Knechtel, H. Wang, Z. Ji, S. Wuttke, O. M. Yaghi and E. Ploetz, J. Am. Chem. Soc., 2023, 145(26), 14324–14334 CrossRef CAS PubMed.
- F. Ahmadijokani, A. Ghaffarkhah, H. Molavi, S. Dutta, Y. Lu, S. Wuttke, M. Kamkar, O. J. Rojas and M. Arjmand, Adv. Funct. Mater., 2023, 2305527 CrossRef.
- A. Valverde, D. Payno, L. Lezama, J. M. Laza, S. Wuttke and R. F. de Luis, Adv. Sustainable Syst., 2022, 6, 2200024 CrossRef CAS.
- S. Mukherjee, D. Sensharma, O. T. Qazvini, S. Dutta, L. K. Macreadie, S. K. Ghosh and R. Babarao, Coord. Chem. Rev., 2021, 437, 213852 CrossRef CAS.
- I. Ali, Chem. Rev., 2012, 112(10), 5073–5091 CrossRef CAS PubMed.
- F. Carolin C, T. Kamalesh, P. S. Kumar and G. Rangasamy, Ind. Eng. Chem. Res., 2023, 62(22), 8575–8601 CrossRef.
- S. Dutta, P. Samanta, B. Joarder, S. Let, D. Mahato, R. Babarao and S. K. Ghosh, ACS Appl. Mater. Interfaces, 2020, 12(37), 41810–41818 CrossRef CAS PubMed.
- R. Iranpour, M. Stenstrom, G. Tchobanoglous, D. Miller, J. Wright and M. Vossoughi, Science, 1999, 285, 706–711 CrossRef CAS PubMed.
- M. W. Skougstad and M. J. Fishman, Toxicol. Environ. Chem. Rev., 1978, 2, 219–236 CrossRef CAS.
- P. Samanta, S. Let, W. Mandal, S. Dutta and S. K. Ghosh, Inorg. Chem. Front., 2020, 7, 1801–1821 RSC.
- P. B. Tchounwou, C. G. Yedjou, A. K. Patlolla and D. J. Sutton, EXS, 2012, 133–164 Search PubMed.
- S. N. Groudev, S. G. Bratcova and K. Komnitsas, Miner. Eng., 1999, 12, 261–270 CrossRef CAS.
- H. Galal-Gorchev, Food Addit. Contam., 1993, 10, 115–128 CrossRef CAS PubMed.
-
Organic Pollutants in Water, ed. I. H. Suffet and M. Malaiyandi, American Chemical Society, Washington, DC, 1986, vol. 214 Search PubMed.
- M. Mon, R. Bruno, J. Ferrando-Soria, D. Armentano and E. Pardo, J. Mater. Chem. A, 2018, 6, 4912–4947 RSC.
- M. Patel, R. Kumar, K. Kishor, T. Mlsna, C. U. Pittman Jr. and D. Mohan, Chem. Rev., 2019, 119(6), 3510–3673 CrossRef CAS PubMed.
- B. Bolto and J. Gregory, Water Res., 2007, 41, 2301–2324 CrossRef CAS PubMed.
- N. Savage and M. S. Diallo, J. Nanopart. Res., 2005, 7, 331–342 CrossRef CAS.
- J.-Q. Jiang, N. Graham, C. André, G. H. Kelsall and N. Brandon, Water Res., 2002, 36, 4064–4078 CrossRef CAS PubMed.
- L. Giusti, Waste Manage., 2009, 29, 2227–2239 CrossRef CAS PubMed.
- G. Montes-Hernandez, N. Concha-Lozano, F. Renard and E. Quirico, J. Hazard. Mater., 2009, 166, 788–795 CrossRef CAS PubMed.
- R. T. Yang, A. J. Hernandez-Maldonado and F. H. Yang, Science, 2003, 301, 79–81 CrossRef CAS PubMed.
- Y. S. Tao, H. Kanoh, L. Abrams and K. Kaneko, Chem. Rev., 2006, 106, 896–910 CrossRef CAS PubMed.
- D. Rudolph, M. Ischyropoulou, J. Pfeifer, J. Napp, U. Schepers, F. Alves and C. Feldmann, Nanoscale Adv., 2024, 6, 973–984 RSC.
- M. M. Falinski, R. S. Turley, J. Kidd, A. W. Lounsbury, M. Lanzarini-Lopes, A. Backhaus, H. E. Rudel, M. K. M. Lane, C. L. Fausey, A. C. Barrios, J. E. LoyoRosales, F. Perreault, W. S. Walker, L. B. Stadler, M. Elimelech, J. L. GardeaTorresdey, P. Westerhoff and J. B. Zimmerman, Environ. Sci.: Nano, 2020, 7, 3255–3278 RSC.
- J. Li, X. Cai, P. Jiang, H. Wang, S. Zhang, T. Sun, C. Chen and K. Fan, Adv. Mater., 2024, 36, 2307337 CrossRef CAS PubMed.
- F. Ahmadijokani, H. Molavi, A. Bahi, S. Wuttke, M. Kamkar, O. J. Rojas, F. Ko and M. Arjmand, Chem. Eng. J., 2023, 457, 141176 CrossRef CAS.
- M. Panahi-Sarmad, S. Samsami, A. Ghaffarkhah, S. A. Hashemi, S. Ghasemi, M. Amini, S. Wuttke, O. Rojas, K. C. Tam, F. Jiang, M. Arjmand, F. Ahmadijokani and M. Kamkar, Adv. Funct. Mater., 2023, 2304473 CrossRef.
- J. Andreo, R. Ettlinger, O. Zaremba, Q. Pena, U. Lächelt, R. F. de Luis, R. Freund, S. Canossa, E. Ploetz, W. Zhu, C. S. Diercks, H. Gröger and S. Wuttke, J. Am. Chem. Soc., 2022, 144(17), 7531–7550 CrossRef CAS PubMed.
- H. Goesmann and C. Feldmann, Angew. Chem., Int. Ed., 2010, 49, 1362–1395 CrossRef CAS PubMed.
- M. Ischyropoulou, K. Sabljo, L. Schneider, C. M. Niemeyer, J. Napp, C. Feldmann and F. Alves, Adv. Mater., 2023, 35, 2305151 CrossRef CAS PubMed.
- M. M. Modena, B. Rühle, T. P. Burg and S. Wuttke, Adv. Mater., 2019, 31, 1901556 CrossRef PubMed.
- R. Freund, U. Lächelt, T. Gruber, B. Rühle and S. Wuttke, ACS Nano, 2018, 12(3), 2094–2105 CrossRef CAS PubMed.
- J. Deng, X. Lu, L. Liu, L. Zhang and O. G. Schmidt, Adv. Energy Mater., 2016, 6, 1600797 CrossRef.
-
R. Jose Varghese, E. H. M. Sakho, S. Parani, S. Thomas, O. S. Oluwafemi and J. Wu, Nanomaterials for Solar Cell Applications, Elsevier, 2019, pp. 75–95 Search PubMed.
- E. Roduner, Chem. Soc. Rev., 2006, 35, 583 RSC.
- F. Perreault, A. F. de Faria and M. Elimelech, Chem. Soc. Rev., 2015, 44, 5861–5896 RSC.
- J. Li, T. Zhao, T. Chen, Y. Liu, C. N. Ong and J. Xie, Nanoscale, 2015, 7, 7502–7519 RSC.
- Q. Peña, A. Wang, O. Zaremba, Y. Shi, H. W. Scheeren, J. M. Metselaar, F. Kiessling, R. M. Pallares, S. Wuttke and T. Lammers, Chem. Soc. Rev., 2022, 51, 2544–2582 RSC.
- Y. Guo, K. Xu, C. Wu, J. Zhao and Y. Xie, Chem. Soc. Rev., 2015, 44, 637–646 RSC.
- F. D. Duman and R. S. Forgan, J. Mater. Chem. B, 2021, 9, 3423–3449 RSC.
- L. Yan, F. Zhao, S. Li, Z. Hu and Y. Zhao, Nanoscale, 2011, 3, 362–382 RSC.
- V. A. Oyanedel-Craver and J. A. Smith, Environ. Sci. Technol., 2008, 42, 927–933 CrossRef CAS PubMed.
- P. Kumari, M. Alam and W. A. Siddiqi, Sustainable Mater. Technol., 2019, 22, e00128 CrossRef CAS.
- L. N. Pincus, F. Melnikov, J. S. Yamani and J. B. Zimmerman, J. Hazard. Mater., 2018, 358, 145–154 CrossRef CAS PubMed.
- Z. Lei, B. Hu, P. Zhu, X. Wang and B. Xu, Nano Energy, 2024, 122, 109307 CrossRef CAS.
- L. N. Pincus, A. W. Lounsbury and J. B. Zimmerman, Acc. Chem. Res., 2019, 52, 1206–1214 CrossRef CAS PubMed.
- A. K. Rana, Y. Kumar Mishra, V. K. Gupta and V. K. Thakur, Sci. Total Environ., 2021, 797, 149129 CrossRef CAS PubMed.
- S. Guo, K. Heck, S. Kasiraju, H. Qian, Z. Zhao, L. C. Grabow, J. T. Miller and M. S. Wong, ACS Catal., 2017, 8, 503–515 CrossRef.
- M. Sharma, M. Poddar, Y. Gupta, S. Nigam, D. K. Avasthi, R. Adelung, R. Abolhassani, J. Fiutowski, M. Joshi and Y. K. Mishra, Mater. Today Chem., 2020, 17, 100336 CrossRef CAS; T. Arunkumar, Y. Ao, Z. Luo, L. Zhang, J. Li, D. Denkenberger and J. Wang, Renewable Sustainable Energy Rev., 2019, 115, 109409 CrossRef.
- K. Simeonidis, S. Mourdikoudis, E. Kaprara, M. Mitrakas and L. Polavarapu, Environ. Sci.: Water Res. Technol., 2016, 2, 43–70 RSC.
- N. Chaukura, S. S. Marais, W. Moyo, N. Mbali, L. C. Thakalekoala, T. Ingwani, B. B. Mamba, P. Jarvis and T. T. I. Nkambule, J. Environ. Chem. Eng., 2020, 8, 103659 CrossRef CAS.
- L. Al-Issai, W. Elshorbagy, M. A. Maraqa, M. Hamouda and A. M. Soliman, Water, 2019, 11, 559 CrossRef CAS.
- M. Zhang, X. Wang, T. T. Du, H. H. Wang, H. Z. Hao, Y. Y. Wang, Y. Li and T. W. Hao, Water Res., 2019, 162, 1–10 CrossRef CAS PubMed.
- M. Auffan, J. Rose, O. Proux, D. Borschneck, A. Masion, P. Chaurand, J. L. Hazemann, C. Chaneac, J. P. Jolivet, M. R. Wiesner, A. V. Geen and J. Y. Bottero, Langmuir, 2008, 24, 3215–3222 CrossRef CAS PubMed.
- S. Pacheco, M. Medina, F. Valencia and J. Tapia, J. Environ. Eng., 2006, 132, 342–349 CrossRef CAS.
- K. Yang and B. Xing, Environ. Pollut., 2007, 145, 529–537 CrossRef CAS PubMed.
- Y. K. Seo, J. W. Yoon, J. S. Lee, Y. K. Hwang, C. H. Jun, J. S. Chang, S. Wuttke, P. Bazin, A. Vimont, M. Daturi, S. Bourrelly, P. L. Llewellyn, P. Horcajada, C. Serre and G. Ferey, Adv. Mater., 2012, 24, 806–810 CrossRef CAS PubMed.
- F. Ahmadijokani, H. Molavi, A. Bahi, R. Fernández, P. Alaee, S. Wu, S. Wuttke, F. Ko and M. Arjmand, Adv. Funct. Mater., 2022, 32, 2207723 CrossRef CAS.
- S. Mukherjee, S. Dutta, Y. D. More, S. Fajal and S. K. Ghosh, Dalton Trans., 2021, 50, 17832–17850 RSC.
- S. Dutta, W. Mandal, A. V. Desai, S. Fajal, G. K. Dam, S. Mukherjee and S. K. Ghosh, Mol. Syst. Des. Eng., 2023, 8, 1483–1491 RSC.
- R. Freund, O. Zaremba, G. Arnauts, R. Ameloot, G. Skorupskii, M. Dincă, A. Bavykina, J. Gascon, A. Ejsmont, J. Goscianska, M. Kalmutzki, U. Lächelt, E. Ploetz, C. S. Diercks and S. Wuttke, Angew. Chem., Int. Ed., 2021, 60, 23975–24001 CrossRef CAS PubMed.
- S. Canossa and S. Wuttke, Adv. Funct. Mater., 2020, 30, 2003875 CrossRef CAS.
- X. Yu, J. Andreo, M. Walden, J. F. D. Campo, L. Basabe-Desmonts, F. Benito-Lopez, T. P. Burg and S. Wuttke, Small, 2024, 8, 2300603 CrossRef CAS PubMed.
- S. Sarkar, J. E. Greenleaf, A. Gupta, D. Uy and A. K. SenGupta, Annu. Rev. Chem. Biomol. Eng., 2012, 3, 497–517 CrossRef CAS PubMed.
- N. B. Saleh, A. Khalid, Y. Tian, C. Ayres, I. V. Sabaraya, J. Pietari, D. Hanigan, I. Chowdhury and O. G. Apul, Environ. Sci.: Water Res. Technol., 2019, 5, 198–208 RSC.
- M. R. Hoffmann, S. T. Martin, W. Choi and D. W. Bahnemann, Chem. Rev., 1995, 95, 69–96 CrossRef CAS.
- A. E. Danks, S. R. Hall and Z. Schnepp, Mater. Horiz., 2016, 3, 91–112 RSC.
- P. D. Dongare, A. Alabastri, S. Pedersen, K. R. Zodrow, N. J. Hogan, O. Neumann, J. Wu, T. Wang, A. Deshmukh, M. Elimelech, Q. Li, P. Nordlander and N. J. Halas, Proc. Natl. Acad. Sci. U. S. A., 2017, 114, 6936 CrossRef CAS PubMed.
- J. K. Stolarczyk, S. Bhattacharyya, L. Polavarapu and J. Feldmann, ACS Catal., 2018, 8(4), 3602–3635 CrossRef CAS.
- S. C. Perry, D. Pangotra, L. Vieira, L.-I. Csepei, V. Sieber, L. Wang, C. P. de León and F. C. Walsh, Nat. Rev. Chem., 2019, 37(3), 442–458 CrossRef.
- P. Singh, B. Mohan, V. Madaan, R. Ranga, P. Kumari, S. Kumar, V. Bhankar, P. Kumar and K. Kumar, Environ. Sci. Pollut. Res., 2022, 29, 69294–69326 CrossRef CAS PubMed.
- J. C. Colmenares, R. Luque, J. M. Campelo, F. Colmenares, Z. Karpiński and A. A. Romero, Materials, 2009, 2(4), 2228–2258 CrossRef CAS.
- J. Wang, H. Yang, L. Jiang, S. Liu, Z. Hao, J. Cheng and G. Ouyang, Catal. Sci. Technol., 2018, 8, 5024–5033 RSC.
- D. S. Kim and S.-Y. Kwak, Appl. Catal., A, 2007, 323, 110–118 CrossRef CAS.
- H. Lin, C. P. Huang, W. Li, C. Ni, S. I. Shah and Y.-H. Tseng, Appl. Catal., B, 2006, 68, 1–11 CrossRef CAS.
-
M. F. Ashby, Materials selection in mechanical design, Pergamon Press, 1992 Search PubMed.
-
M. F. Ashby, Materials and the Environment: Eco-informed Material Choice, Elsevier Science, 2009 Search PubMed.
- M. F. Ashby, Y. J. M. Bréchet, D. Cebon and L. Salvo, Mater. Des., 2004, 25, 51–67 CrossRef.
- L. M. Gilbertson, J. B. Zimmerman, D. L. Plata, J. E. Hutchison and P. T. Anastas, Chem. Soc. Rev., 2015, 44, 5758–5777 RSC.
- M. M. Falinski, D. L. Plata, S. S. Chopra, T. L. Theis, L. M. Gilbertson and J. B. Zimmerman, Nat. Nanotechnol., 2018, 13, 708–714 CrossRef CAS PubMed.
- T. Luttrell, S. Halpegamage, J. Tao, A. Kramer, E. Sutter and M. Batzill, Sci. Rep., 2014, 4, 4043 CrossRef PubMed.
- N. S. Allen, N. Mahdjoub, V. Vishnyakov, P. J. Kelly and R. J. Kriek, Polym. Degrad. Stab., 2018, 150, 31–36 CrossRef CAS.
- J. Dhal, B. Mishra and G. Hota, Int. J. Environ. Sci. Technol., 2015, 12, 1845–1856 CrossRef CAS.
- S. Mallakpour and M. Madani, Prog. Org. Coat., 2015, 86, 194–207 CrossRef CAS.
- U. N. Maiti, W. J. Lee, J. M. Lee, Y. Oh, J. Y. Kim, J. E. Kim, J. Shim, T. H. Han and S. O. Kim, Adv. Mater., 2014, 26, 40–67 CrossRef CAS PubMed.
- T. Tosco, M. Petrangeli Papini, C. Cruz Viggi and R. Sethi, J. Cleaner Prod., 2014, 77, 10–21 CrossRef CAS.
- J.-M. Jian, C. Zhang, F. Wang, X. Lu, F. Wang and E. Y. Zeng, Environ. Pollut., 2019, 251, 425–433 CrossRef CAS PubMed.
- S. Zhang, T. Shao, S. S. K. Bekaroglu and T. Karanfil, Environ. Sci. Technol., 2009, 43, 5719–5725 CrossRef CAS PubMed.
- A. Vanderkooy, Y. Chen, F. Gonzaga and M. A. Brook, ACS Appl. Mater. Interfaces, 2011, 3, 3942–3947 CrossRef CAS PubMed.
- A. Nagar and T. Pradeep, ACS Nano, 2020, 14(6), 6420–6435 CrossRef CAS PubMed.
- W. Wu, R. F. Giese and C. J. Van Oss, Colloids Surf., B, 1999, 14, 47–55 CrossRef CAS.
- C. J. Van Oss, J. Mol. Recognit., 2003, 16, 177–190 CrossRef CAS PubMed.
- Y. Xu, Y. Qin, S. Palchoudhury and Y. Bao, Langmuir, 2011, 27(14), 8990–8997 CrossRef CAS PubMed.
- Y. Zhang, Y. Chen, P. Westerhoff, K. Hristovski and J. C. Crittenden, Water Res., 2008, 42, 2204–2212 CrossRef CAS PubMed.
- R. P. Singh, K. Sharma and K. Mausam, Mater. Today Proc., 2020, 26, 2021–2025 CrossRef CAS.
- K. L. Chen, S. E. Mylon and M. Elimelech, Environ. Sci. Technol., 2006, 40(5), 1516–1523 CrossRef CAS PubMed.
- M. Elimelech and C. R. O'Melia, Langmuir, 1990, 6(6), 1153–1163 CrossRef CAS.
- R. Hogg, T. W. Healy and D. W. Fuerstenau, Trans. Faraday Soc., 1966, 62, 1638–1651 RSC.
- M. V. Smoluchowski, Versuch einer mathematischen theorie der koagulation, Z. Phys. Chem., 1917, 92, 129–155 Search PubMed.
- N. Barroso, S. Dutta, J. Andreo, G. Beobide, O. Castillo, A. Luque, S. Pérez-Yáñez and S. Wuttke, J. Mater. Chem. A, 2023, 11, 21300–21311 RSC.
- J. Brant, H. Lecoanet and M. R. Wiesner, J. Nanopart. Res., 2005, 7, 545–553 CrossRef CAS.
- A. Stafiej and K. Pyrzynska, Microchem. J., 2008, 89, 29–33 CrossRef CAS.
- Y.-H. Li, S. Wang, J. Wei, X. Zhang, C. Xu, Z. Luan, D. Wu and B. Wei, Chem. Phys. Lett., 2002, 357, 263–266 CrossRef CAS.
- C. Lu and C. Liu, J. Chem. Technol. Biotechnol., 2006, 81, 1932–1940 CrossRef CAS.
- Y.-H. Li, J. Ding, Z. Luan, Z. Di, Y. Zhu, C. Xu, D. Wu and B. Wei, Carbon, 2003, 41, 2787–2792 CrossRef CAS.
- K. Anitha, S. Namsani and J. K. Singh, J. Phys. Chem. A, 2015, 119, 8349–8358 CrossRef CAS PubMed.
- C. Santhosh, V. Velmurugan, G. Jacob, S. K. Jeong, A. N. Grace and A. Bhatnagar, Chem. Eng. J., 2016, 306, 1116–1137 CrossRef CAS.
- K. Yang and B. S. Xing, Chem. Rev., 2010, 110, 5989–6008 CrossRef CAS PubMed.
- B. Pan and B. S. Xing, Environ. Sci. Technol., 2008, 42, 9005–9013 CrossRef CAS PubMed.
- G. P. Rao, C. Lu and F. Su, Sep. Purif. Technol., 2007, 58, 224–231 CrossRef CAS.
- W. Gao, M. Majumder, L. B. Alemany, T. N. Narayanan, M. A. Ibarra, B. K. Pradhan and P. M. Ajayan, ACS Appl. Mater. Interfaces, 2011, 3, 1821–1826 CrossRef CAS PubMed.
- R. Sitko, B. Zawisza and E. Malicka, TrAC, Trends Anal. Chem., 2013, 51, 33–43 CrossRef CAS.
- A. Stafiej and K. Pyrzynska, Sep. Purif. Technol., 2007, 58, 49–52 CrossRef CAS.
- J. Zhao, Z. Wang, J. C. White and B. Xing, Environ. Sci. Technol., 2014, 48, 9995–10009 CrossRef CAS PubMed.
- G. Zhao, J. Li, X. Ren, C. Chen and X. Wang, Environ. Sci. Technol., 2011, 45, 10454–10462 CrossRef CAS PubMed.
- Y.-C. Lee and J.-W. Yang, J. Ind. Eng. Chem., 2012, 18, 1178–1185 CrossRef CAS.
- J. Li, S. Zhang, C. Chen, G. Zhao, X. Yang, J. Li and X. Wang, ACS Appl. Mater. Interfaces, 2012, 4, 4991–5000 CrossRef CAS PubMed.
- Y. Wang, S. Liang, B. Chen, F. Guo, S. Yu and Y. Tang, PLoS One, 2013, 8, e65634 CrossRef CAS PubMed.
- X.-J. Hu, Y.-G. Liu, G.-M. Zeng, S.-H. You, H. Wang, X. Hu, Y.-M. Guo, X.-F. Tan and F.-Y. Guo, J. Colloid Interface Sci., 2014, 435, 138–144 CrossRef CAS PubMed.
- J. E. Van Benschoten, B. E. Reed, M. R. Matsumoto and P. J. McGarvey, Water Environ. Res., 1994, 66, 168–174 CrossRef CAS.
- J. A. Coston, C. C. Fuller and J. A. Davis, Geochim. Cosmochim. Acta, 1995, 59, 3535–3547 CrossRef CAS.
- A. Agrawal and K. K. Sahu, J. Hazard. Mater., 2006, 137, 915–924 CrossRef CAS PubMed.
- A. R. Mahdavian and M. A.-S. Mirrahimi, Chem. Eng. J., 2010, 159, 264–271 CrossRef CAS.
- X. Zhao, L. Lv, B. Pan, W. Zhang, S. Zhang and Q. Zhang, Chem. Eng. J., 2011, 170, 381–394 CrossRef CAS.
- L. Cumbal and A. K. SenGupta, Environ. Sci. Technol., 2005, 39, 6508–6515 CrossRef CAS PubMed.
- S. Banerjee, S. C. Pillai, P. Falaras, K. E. O’Shea, J. A. Byrne and D. D. Dionysiou, J. Phys. Chem. Lett., 2014, 5(15), 2543–2554 CrossRef CAS PubMed.
- C. Kormann, D. W. Bahnemann and M. R. Hoffmann, Environ. Sci. Technol., 1991, 25, 494–500 CrossRef CAS.
- D. F. Ollis, C.-Y. Hsiao, L. Budiman and C.-L. Lee, J. Catal., 1984, 88, 89–96 CrossRef CAS.
- Y. Yu, C. Y. Jimmy, C.-Y. Chan, Y.-K. Che, J.-C. Zhao, L. Ding, W.-K. Ge and P.-K. Wong, Appl. Catal., B, 2005, 61, 1–11 CrossRef CAS.
- N. Zhang, Y. Zhang, X. Pan, M.-Q. Yang and Y.-J. Xu, J. Phys. Chem. C, 2012, 116, 18023–18031 CrossRef CAS.
- X. F. Lei, X. X. Xue, H. Yang, C. Chen, X. Li, M. C. Niu, X. Y. Gao and Y. T. Yang, Appl. Surf. Sci., 2015, 332, 172–180 CrossRef CAS.
- I. Som, M. Roy and R. Saha, ChemCatChem, 2020, 12, 3409–3433 CrossRef CAS.
- J. Hu, T. Yang, J. Chen, X. Yang, J. Qu and Y. Cai, Chem. Eng. J., 2022, 430, 133039 CrossRef CAS.
- J. Hu, C. Chen, H. Yang, F. Yang, J. Qu, X. Yang, W. Sun, L. Dai and C. M. Li, Appl. Catal., B, 2022, 317, 121723 CrossRef CAS.
- J. Hu, C. Chen, T. Hu, J. Li, H. Lu, Y. Zheng, X. Yang, C. Guo and C. M. Li, J. Mater. Chem. A, 2020, 8, 19484–19492 RSC.
- W. Zhao, G. Wang, P. Li, Y. Shu, H. Wang, Y. Zhou, Z. Meng and W. Zhu, ACS ES&T Water, 2024 DOI:10.1021/acsestwater.3c00575.
- Y. Cheng, Y. Zhang, Z. Wang, R. Guo, J. You and H. Zhang, Nanoscale, 2023, 15, 18571–18580 RSC.
- X. Qu, J. Brame, Q. Li and P. J. J. Alvarez, Acc. Chem. Res., 2013, 46(3), 834–843 CrossRef CAS PubMed.
- X. Wu, H. Liu, J. Liu, K. N. Haley, J. A. Treadway, J. P. Larson, N. Ge, F. Peale and M. P. Bruchez, Nat. Biotechnol., 2003, 21, 41–46 CrossRef CAS PubMed.
- T.-W. Sung and Y.-L. Lo, Sens. Actuators, B, 2012, 165, 119–125 CrossRef CAS.
- P. J. Vikesland and K. R. Wigginton, Environ. Sci. Technol., 2010, 44(10), 3656–3669 CrossRef CAS PubMed.
- S. Link and M. A. El-Sayed, J. Phys. Chem. B, 1999, 103(40), 8410–8426 CrossRef CAS.
- M. Hnaiein, W. M. Hassen, A. Abdelghani, C. Fournier-Wirth, J. Coste, F. Bessueille, D. Leonard and N. Jaffrezic-Renault, Electrochem. Commun., 2008, 10, 1152–1154 CrossRef CAS.
- H. Fan, Y. Li, D. Wu, H. Ma, K. Mao, D. Fan, B. Du, H. Li and Q. Wei, Anal. Chim. Acta, 2012, 711, 24–28 CrossRef CAS PubMed.
- J. Dong, X. Fan, F. Qiao, S. Ai and H. Xin, Anal. Chim. Acta, 2013, 761, 78–83 CrossRef CAS PubMed.
- Y. Yang, P. Dementyev, N. Biere, D. Emmrich, P. Stohmann, R. Korzetz, X. Zhang, A. Beyer, S. Koch, D. Anselmetti and A. Gölzhäuser, ACS Nano, 2018, 12(5), 4695–4701 CrossRef CAS PubMed.
- R. Zhang, J. Tian, S. Gao and B. V. D. Bruggen, J. Mater. Chem. A, 2020, 8, 8831–8847 RSC.
- J. K. Holt, H. G. Park, Y. M. Wang, M. Stadermann, A. B. Artyukhin, C. P. Grigoropoulos, A. Noy and O. Bakajin, Science, 2006, 312, 1034–1037 CrossRef CAS PubMed.
- M. S. Mauter and M. Elimelech, Environ. Sci. Technol., 2008, 42, 5843–5859 CrossRef CAS PubMed.
- B. L. de Groot and H. Grubmuller, Science, 2001, 294, 2353–2357 CrossRef CAS PubMed.
- M. M. Pendergast and E. M. V. Hoek, Energy Environ. Sci., 2011, 4, 1946–1971 RSC.
- M. Shahbabaei and T. Tang, Phys. Chem. Chem. Phys., 2022, 24, 29298–29327 RSC.
- H. M. Hegab, A. ElMekawy, T. G. Barclay, A. Michelmore, L. Zou, D. Losic, C. P. Saint and M. Ginic-Markovic, Sci. Rep., 2017, 7, 7490 CrossRef PubMed.
- B. H. Jeong, E. M. V. Hoek, Y. S. Yan, A. Subramani, X. F. Huang, G. Hurwitz, A. K. Ghosh and A. Jawor, J. Membr. Sci., 2007, 294, 1–7 CrossRef CAS.
- M. M. Modena, P. Hirschle, S. Wuttke and T. P. Burg, Small, 2018, 14, 1800826 CrossRef PubMed.
- G. Liu, J. Gao, H. Ai and X. Chen, Small, 2012, 9, 1533–1545 CrossRef PubMed.
- M. Salta, J. A. Wharton, P. Stoodley, S. P. Dennington, L. R. Goodes, S. Werwinski, U. Mart, R. J. K. Wood and K. R. Stokes, Philos. Trans. R. Soc., A, 2010, 368, 4729–4754 CrossRef CAS PubMed.
- E. Kabir, V. Kumar, K.-H. Kim, A. C. K. Yip and J. R. Sohn, J. Environ. Manage., 2018, 225, 261–271 CrossRef CAS PubMed.
- I. Lynch, C. Weiss and E. Valsami-Jones, Nano Today, 2014, 9, 266 CrossRef CAS.
- T. Xia, M. Kovochich, M. Liong, L. Madler, B. Gilbert, H. Shi, J. I. Yeh, J. I. Zink and A. E. Nel, ACS Nano, 2008, 2, 2121 CrossRef CAS PubMed.
- P. Cronholm, H. L. Karlsson, J. Hedberg, T. Lowe, K. Elihn, I. O. Wallinder and L. Moller, Toxicol. Lett., 2012, 211, S201 CrossRef.
- H. Zhang, Z. Ji, T. Xia, H. Meng, C. Low-Kam, R. Liu, S. Pokhrel, S. Lin, X. Wang, Y. P. Liao, M. Wang, L. Li, R. Rallo, R. Damoiseaux, D. Telesca, L. Madler, Y. Cohen, J. I. Zink and A. E. Nel, ACS Nano, 2012, 6, 4349 CrossRef CAS PubMed.
- J. Goscianska, R. Freund and S. Wuttke, Adv. Funct. Mater., 2022, 32(14), 2107826 CrossRef CAS.
- H. Hyung, J. D. Fortner, J. B. Hughes and J. H. Kim, Environ. Sci. Technol., 2007, 41, 179 CrossRef CAS PubMed.
- S. Lin, J. Reppert, Q. Hu, J. S. Hudson, M. L. Reid, T. A. Ratnikova, A. M. Rao, H. Luo and P. C. Ke, Small, 2009, 5, 1128 CrossRef CAS PubMed.
- S. Lin, T. Yu, Z. Yu, X. Hu and D. Yin, Adv. Mater., 2018, 30, 1705691 CrossRef PubMed.
- T. Xia, Y. Zhao, T. Sager, S. George, S. Pokhrel, N. Li, D. Schoenfeld, H. Meng, S. Lin, X. Wang, M. Wang, Z. Ji, J. Zink, L. Madler, V. Castranova, S. Lin and A. E. Nel, ACS Nano, 2011, 5(2), 1223–1235 CrossRef CAS PubMed.
|
This journal is © The Royal Society of Chemistry 2024 |
Click here to see how this site uses Cookies. View our privacy policy here.