DOI:
10.1039/D1GC03127A
(Tutorial Review)
Green Chem., 2022,
24, 1049-1066
Recent progress on immobilization technology in enzymatic conversion of marine by-products to concentrated omega-3 fatty acids
Received
27th August 2021
, Accepted 17th December 2021
First published on 17th December 2021
Abstract
Marine by-products (heads, frames, trimmings, viscera, skin and scales) have been extensively investigated as sources of marine omega-3 fatty acids (mainly eicosapentaenoic acid and docosahexaenoic acid). Traditionally, extraction of fish oil and enrichment of omega-3 fatty acids are performed at high temperatures, which require high energy input and may lead to product decomposition. Enzymatic extraction and concentration have been studied as green alternative techniques, and immobilized enzymes are of particular interest for use due to their advantages including high stability, reusability and feasible separation from products. In this tutorial review, recent research progress on immobilization of lipases used for enrichment of omega-3 fatty acids in fish oil is presented and discussed. The frequently used immobilization methods and the commonly studied properties of immobilized lipases are summarized. In comparison to free lipases, immobilized lipases generally have improved stability towards heat, pH and organic solvents due to increased rigidity in their conformation. The activity and selectivity of immobilized lipases are significantly affected by the immobilization method, the support material and the reaction medium. The omega-3 fatty acids in fish oil are mainly concentrated in the form of free fatty acids, ethyl esters or acylglycerols through hydrolysis or alcoholysis of triacylglycerols catalyzed by the immobilized lipases.
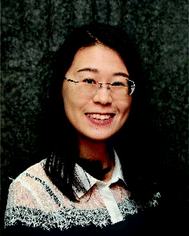 Yi Liu | Dr Yi Liu holds a BSc degree in chemistry education from Jiangsu University, China, and a PhD degree in green chemistry from Memorial University of Newfoundland, Canada. Her PhD research was focused on the synthesis of a novel compound from the monomer of chitin derived from shells of crustaceans, and related studies of its properties and applications. Since 2018, Dr Liu joined the Centre for Aquaculture and Seafood Development, Fisheries and Marine Institute of Memorial University of Newfoundland as a Postdoctoral Fellow. Her research interests include marine bioprocessing and valorization of marine by-products into high-value products. |
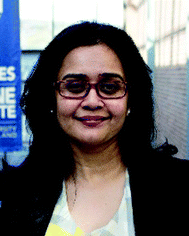 Deepika Dave | Dr Deepika Dave is a Research Scientist in the Centre for Aquaculture and Seafood Development at the Fisheries and Marine Institute of Memorial University of Newfoundland. Dr Dave has over 22 years of increasingly responsible experience in engineering, science, management, research, training and facilitation. Dr Dave specializes in the areas of marine bioprocessing and bioconversion, blue biotechnology and waste utilization, environmental engineering and energy production and management. She is a principal investigator for the delivery of the product and process design, industrial and development services at the Marine Institute's Marine Bioprocessing Facility. |
Introduction
Due to the rise of public awareness of healthy diets in recent decades, there is an exponentially increasing consumption of nutritional supplements, among which omega-3 fatty acids (omega-3 FAs) are one of the most in-demand products. Omega-3 (n-3) FAs are a group of polyunsaturated fatty acids (PUFAs) of which the last double bond ends at the third carbon counted from the methyl group end (as indicated by * in Fig. 1). Omega-3 FAs have been reported to have a variety of health benefits, including reduction of inflammation, relief of depression, prevention of cardiovascular diseases and dementia (e.g. Alzheimer's disease), and lowering the risk of diabetes and several types of cancer. They are also important for the health of pregnant women and the neural and visual development of infants.1–3
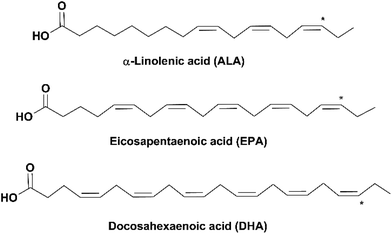 |
| Fig. 1 Structures of ALA, EPA and DHA (* indicates the location of the last double bond of omega-3 FAs). | |
Three omega-3 FAs are most closely related to human health: α-linolenic acid (18:3, n-3, ALA), eicosapentaenoic acid (20:5, n-3, EPA) and docosahexaenoic acid (22:6, n-3, DHA) (Fig. 1). ALA is an essential FA, which is vital for the human body but can only be ingested from diets such as plant seeds and vegetable oils. EPA and DHA can be synthesized from ALA in the human body through metabolism; however, the conversion rate is very low.4 Therefore, it is recommended to obtain EPA and DHA from external sources. Fish oil is the main source of EPA and DHA. Depending on the fish species, the amount of EPA and DHA varies in the range of 5–26% of the total lipid content in the fish.5 The adequate dietary intake of EPA and DHA recommended by the World Health Organization is 250–500 mg day−1 for healthy adults and 300 mg day−1 (at least 200 mg DHA) for pregnant or lactating women.6 According to the Dietary Guidelines for Americans, healthy adults should guarantee an average intake of 250 mg day−1 EPA and DHA by eating at least 8 oz of seafood per week, and pregnant or lactating women should consume 8–12 oz.7 However, this is challenging for most people worldwide due to a variety of reasons, such as dietary habits, geographical unavailability of seafood and economic limitation. In addition, fulfillment of the recommended amount of EPA and DHA from fish might lead to the high intake of cholesterol, saturated FAs and some contaminants (e.g. methylmercury, polychlorinated biphenyls and dioxins).8–10 Therefore, nutritional supplements of refined and concentrated omega-3 FAs derived from fish oil have been developed in the market.
Currently, most of the marine omega-3 FA supplements are produced from oily fish, such as anchovy, sardine and mackerel. However, there is an emerging risk of overfishing of these species in recent years, which leads to the consideration of marine by-products as an alternative source of omega-3 FAs. Globally, more than 70% of the fish harvested are processed for filleting, heading or gutting.11 The by-products generated (heads, frames, trimmings, viscera, skin and scales) account for more than 50% of the fish body.12 The by-products of some fish species have been reported abundant in EPA and DHA, such as salmon, trout and tuna,13–16 thus being appropriate candidates for extraction of fish oil and production of omega-3 FA products.
Extraction of fish oil and concentration of omega-3 FAs
The conventional procedure of industrial production of marine omega-3 FA supplements is shown in Fig. 2. Traditionally, fish oil is extracted by heating raw fish materials to 90–100 °C for 10–20 min and pressing the mixture.17 The use of high temperatures leads to high energy cost and promotes side reactions of the lipids. Therefore, alternative green extraction methods have been investigated, such as enzymatic hydrolysis. During enzymatic extraction, fish materials are treated with a protease, which breaks the peptide bonds of proteins and thereby releases the oil embedded in the protein network. In comparison to heat treatment, the temperature required for enzymatic hydrolysis is relatively low (40–65 °C, depending on the optimal operation range of the enzyme), so the energy consumption is reduced and the nutritional components in the oil can be preserved.
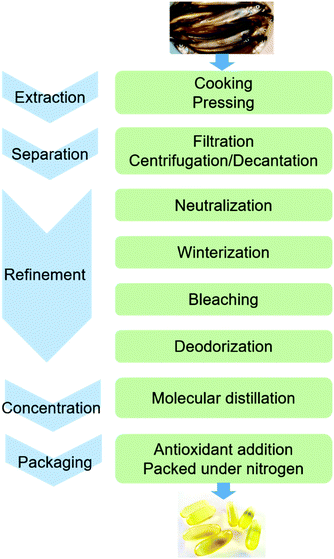 |
| Fig. 2 The conventional procedure of industrial production of marine omega-3 FA supplements. | |
Besides EPA and DHA, fish oil contains several other omega-3 FAs, including ALA, stearidonic acid (18:4, n-3), eicosatetraenoic acid (20:4, n-3) and docosapentaenoic acid (22:5, n-3). However, enrichment of these omega-3 FAs from fish oil has been rarely studied. Therefore, in the present review the “omega-3 FAs” derived from fish oil only include EPA and DHA. The omega-3 FAs exist in the form of triacylglycerols (TAGs) in the natural fish oil, which usually contains approximately 18% EPA and 12% DHA after basic refinement and therefore is called “1812 TAG fish oil”.18 As the nutraceutical and pharmaceutical industries pursue more concentrated products (50–90% EPA and DHA), an enrichment procedure is performed. Part or all of the FAs are removed from the glycerol backbone in the TAG molecule, and EPA and DHA are separated from other FAs and collected in the form of free fatty acids (FFAs), FA esters or acylglycerols. If an EPA and DHA concentration of above 90% is required, more delicate technologies are applied for the separation, such as high-performance liquid chromatography.19
A large number of techniques have been developed for the concentration of EPA and DHA, such as molecular distillation, urea complexation, supercritical fluid extraction and enzymatic enrichment. Currently, the most commonly used method in industry is molecular distillation. The TAGs in fish oil are reacted with an alcohol (usually ethanol) at 80–90 °C to generate ethyl esters (EEs) of different FAs, which are subsequently distilled at 140–160 °C under vacuum and separated based on their different boiling points. Since the boiling point of a compound is affected by its chain length and molecular weight, it is difficult to separate EPA- and DHA-derived EEs from other fractions with similar molecular structures. Consequently, the total concentration of EPA and DHA in the products usually doesn't exceed 70% after molecular distillation.20 Moreover, this process is energy-intensive due to the high temperatures used, and omega-3 FAs are vulnerable to side reactions, such as oxidation, polymerization and degradation. In recent decades, enzymatic processes using lipases have been intensively studied as an alternative method for the enrichment of omega-3 FAs.21 Lipases are a type of enzyme that can catalyze a variety of reactions of lipids, including hydrolysis, transesterification/alcoholysis, interesterification, esterification and acidolysis. The reaction conditions (temperature and pH) required in lipase-catalyzed reactions are usually very mild. The regio- and FA selectivity varies among different lipases, which can be used to generate products with specific structures. For example, in the study by He et al. on enzymatic ethanolysis of sardine oil to produce monoacylglycerols (MAGs), the amount of 2-MAGs formed was 5.6-fold higher than 1-MAGs due to the use of 1,3-specific lipase Candida antarctica lipase B (CALB). Since the majority of DHA (64.4%) in sardine oil was located at sn-2 position, the concentration of DHA was effectively increased from 9.04% in the original oil to 57.13% in the 2-MAGs.22
Use of immobilized enzymes
Since the 1970s, enzyme immobilization has been studied as a way to improve the performance of enzymes.23 In comparison to free enzymes, separation of immobilized enzymes from the reaction medium is easier, thus facilitating its recovery and reuse, and preventing product contamination by the enzyme. After immobilization, the interaction between the enzyme and the support reinforces the rigidity of the lipase's conformation, so the lipase has more resistance towards heat or denaturants and therefore becomes more stable. Moreover, immobilization has been reported to improve the catalytic performance of enzymes, such as activity, selectivity and specificity.24 Due to these benefits, a variety of commercial immobilized enzymes have been developed and widely applied in multiple industries, including food, cosmetics, nutraceuticals, pharmaceuticals and biodiesel. Meanwhile, research on enzyme immobilization is being constantly performed in the academic area, with the aim of finding more effective enzymes, support materials or immobilization methods to further improve the efficiency of immobilized enzymes and reduce the manufacturing cost.
In this tutorial review, we will provide a summary of the scientific efforts to date on immobilization of enzymes and their application in production of concentrated omega-3 FAs from marine by-products. Since there haven't been any published reports on the use of immobilized proteases for extraction of fish oil, this review is focused on enrichment of omega-3 FAs in fish oil catalyzed by immobilized lipases. The main purpose of this tutorial review is to serve as a starting point for researchers who are interested in utilizing immobilization technology in enzymatic transformation of marine biomass for the development of blue economy.
Enzyme immobilization
Immobilization methods
In general, there are four types of methods frequently used for enzyme immobilization: adsorption, covalent attachment, cross-linking and entrapment (Fig. 3). The description of each method, their advantages and disadvantages are summarized in Table 1.
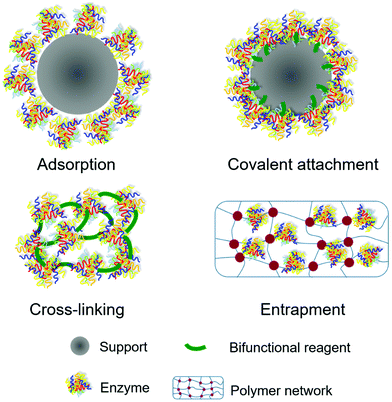 |
| Fig. 3 The frequently used enzyme immobilization methods. | |
Table 1 A summary of the frequently used immobilization methods, their advantages and disadvantages
Immobilization method |
Description |
Advantages |
Disadvantages |
Adsorption |
van der Waals forces; hydrophobic interactions; hydrogen bonds; ionic binding |
• Simple operation |
• Enzyme leaching |
• Low operating cost |
• Diluted activity |
• Retention of activity |
|
• Reversible process |
|
Covalent attachment |
Connection of the enzyme and support via covalent bonding using a bifunctional reagent |
• Relatively simple operation |
• Diluted activity |
• Relatively little enzyme leaching |
• Irreversible process |
|
• Loss of activity |
Cross-linking |
Assembly of enzyme molecules via covalent bonding with a crosslinker |
• Absence of a support |
• Irreversible process |
• Concentrated enzyme activity |
• Loss of activity |
• Relatively little enzyme leaching |
|
Entrapment |
Inclusion of enzyme molecules in a polymer network |
• Optimum microenvironment |
• Diluted activity |
• Relatively little enzyme leaching |
• Irreversible process |
|
• Mass transfer limitation |
Adsorption.
Enzymes can be adsorbed on the support through two ways: physical and ionic adsorption. Physical adsorption of enzymes onto the support is achieved through weak intermolecular forces, such as van der Waals forces, hydrophobic interactions and hydrogen bonds. Ionic adsorption (ionic binding), which is generally stronger than physical adsorption, is achieved through weak ionic interactions between the enzyme and the surface of the support. The adsorption method is relatively simple to perform and low in operating cost. Since physical and ionic interactions are weak, the adsorption process is reversible. Therefore, if the enzyme is permanently deactivated, the support can be recovered for reuse after desorption of the enzyme. During an adsorption process, there is little change in enzyme conformation, so the enzyme activity can be maintained without significant loss.25 However, physical or ionic adsorption might be not strong enough to tightly fix the enzyme on the support, so the enzyme is liable to leak from the surface of the support as affected by the reaction environment (e.g. pH, temperature, reactant and product concentrations, and ionic strength).26
Covalent attachment.
In the covalent attachment method, a bifunctional reagent (e.g. glutaraldehyde) is often used to act as a bridge to link the enzyme and the support. The surface of the support is “activated” through covalent bonding with one reactive end of the bifunctional reagent, and the enzyme forms covalent bonds with the other reactive end of the reagent. Covalent bonding is much stronger than physical and ionic adsorption, and can effectively prevent enzyme leaching. However, the bonding process may result in changes in enzyme conformation and the consequent loss of activity.
Cross-linking.
Generally, in a cross-linking process, the free enzyme is dissolved in a buffer solution, followed by crystallization at its optimal pH or precipitation by adding salts or water-miscible organic solvents. Subsequently, a bifunctional reagent (“crosslinker”) is added, and the mixture is gently stirred for a certain time and left still to allow for the formation of cross-linking enzyme crystals or aggregates. Similar to covalent attachment, the cross-linking method involves covalent bonding between the enzyme and a bifunctional reagent. However, there is no use of any support, and the crosslinker connects two enzyme molecules via its two reactive ends. The absence of a support reduces the manufacturing cost and prevents dilution of the enzyme activity, which occurs in the other three methods due to the large proportion of the non-catalytic support in the immobilized enzyme system (above 95% of the total mass).27
Entrapment.
The entrapment of an enzyme is performed by adding the enzyme to the reaction medium when carrying out the polymerization of the support matrix. The entrapped enzyme is physically restrained inside the polymer network instead of on the surface of the support, thus being protected from leaching. A microenvironment (e.g. pH, ionic strength, and reactant and product concentrations) that is optimum for the enzyme can be created inside the network by modifying the polymer materials.28 However, the reactant will have limited access to the active sites of the enzyme due to the surrounding of the matrix. If the pore size of the matrix is too large, enzyme leakage may still happen. Therefore, covalent bonding between the enzyme and matrix might be added to further stabilize the enzyme and prevent leakage.29
Terminology and analysis
Evaluation of an immobilization process.
As summarized by Sheldon and van Pelt,29 the terms most commonly used to evaluate an enzyme immobilization process are immobilization yield, efficiency and recovery, which should be calculated as follows:
Immobilization yield (%) = \cr \tab\quad (Immobilized activity/Starting activity) × 100 |
Immobilization efficiency (%) = \cr \tab\quad (Observed activity/Immobilized activity) × 100 |
Activity recovery (%) = \cr \tab\quad (Observed activity/Starting activity) × 100 |
It needs to be emphasized that the “immobilized activity” is different from the “observed activity”. The “observed activity” of the immobilized enzyme is measured using an assay (see subsection Activity). The “immobilized activity” should be determined by subtracting the residual activity in the solutions after immobilization and wash from the starting activity of the free enzyme. These definitions sometimes lead to confusion and inaccuracy of calculations. Besides calculation based on the activity of the enzymes, in some studies the immobilization yield was calculated as the ratio of the amount of the proteins immobilized on the support to the amount of the proteins added initially.30,31 The protein content is usually measured by the Bradford or Lowry method using bovine serum albumin as the standard.32,33
Activity.
The hydrolytic activity is often measured as a necessary parameter to characterize a lipase. The assay most frequently used is to spectrophotometrically measure the absorbance at 348 nm, which is attributed to p-nitrophenol released from the hydrolysis of p-nitrophenyl butyrate catalyzed by the lipase. One international unit (U) of the lipase activity is defined as the amount of the lipase required to produce 1 μmol of p-nitrophenol per minute under the assay conditions. Alternatively, p-nitrophenyl acetate or p-nitrophenyl palmitate can also be used as the substrate in this assay. There have been other assays reported which used soy oil, olive oil, palm olefin or tributyrin as the substrate for the hydrolysis.34–39 The obtained FFAs were titrated with a sodium or potassium hydroxide solution, and the activity was defined as the amount of the lipase required to produce 1 μmol of FFAs per minute under the assay conditions.
When an enzyme is used to catalyze the targeted reaction, its activity in this process can be expressed as the amount of the desired products generated per minute per gram or milligram of the enzyme. For example, when EPA and DHA are concentrated as FFAs during the hydrolysis of fish oil, the activity of a lipase can be defined as the amount of EPA and DHA released per minute per milligram of the lipase. However, it should be noted that for reactions in which EPA and DHA are not converted and remain in the acylglycerols, this activity is usually not discussed.
Stability.
Improvement in stability is a prominent advantage brought by immobilization of enzymes. Therefore, the thermal and pH stability of immobilized enzymes are often analyzed and compared with free enzymes. The thermal stability is determined by incubating the enzyme diluted/suspended in a buffer solution (usually at pH 7) at a fixed temperature for different amounts of time, or at different temperatures for a fixed amount of time, or a combination of both (i.e. incubation of the enzyme solution at different temperatures, and samples are taken at different time intervals). The pH stability is usually studied by incubating the enzyme diluted/suspended in buffer solutions with different pH values at a fixed temperature (e.g. 25 °C) for a certain amount of time. The residual/relative activity is usually calculated in the stability analysis, which is the ratio of the activity measured at the given time during the incubation to the activity of the untreated enzyme at time zero.
In some research on enzymatic hydrolysis and alcoholysis of fish oil, a biphasic system composed of a buffer solution and organic solvents and co-solvents was used.40–43 Therefore, the stability of the lipase in organic solvents was investigated. The lipase was diluted or suspended in a buffer solution (usually at pH 7) containing a certain amount of the organic solvent and incubated for a certain amount of time. The incubation temperature should be the same as that used in the targeted reaction. For example, if the hydrolysis of fish oil is catalyzed by the lipase at 25 °C, then this temperature should be used in the organic solvent stability analysis.
Selectivity.
The selectivity (e.g. regioselectivity and chemoselectivity) of lipases can have significant influence on the structures and properties of the concentrated omega-3 FA products. For example, 2-MAGs abundant in omega-3 FAs have been reported with numerous nutritional benefits and to be readily absorbed by human body.44,45 In the study by Haq et al., ethanolysis of TAGs in the oil derived from Atlantic salmon frames was performed using 1,3-specific lipases to remove the FAs at the sn-1,3 positions of the glycerol backbone.46 The highest yield of 41.81% 2-MAG (containing 21.34% omega-3 FAs) was obtained using Lipozyme TLIM.
During an enzymatic process where omega-3 FAs in fish oil are converted to FFAs or FA esters, the selectivity of the lipase is often expressed as the ratio of the amount of the desired products to other undesired products (e.g. saturated and monounsaturated FAs). For example, in the study by Fernández-Lorente et al. on hydrolysis of sardine oil, the selectivity of the immobilized lipases towards EPA and DHA over oleic acid and palmitic acid ((EPA + DHA)/(OA + PA)) was investigated by calculating the ratio of the amount of EPA and DHA to that of OA and PA released.47 Some lipases can discriminate between EPA and DHA, and therefore are used to enrich only EPA or DHA in fish oil. The selectivity of these lipases towards EPA or DHA over the other is often studied, which is expressed as the ratio of the amount of the desired omega-3 FA to the other (i.e. EPA/DHA or DHA/EPA). It should be noted that the selectivity of a lipase will change along with the reaction time due to the change in the amount of products released. In most studies, the selectivity was usually measured at the first stage of the reaction when 10% or 15% of the substrate was converted.
Reusability.
Reusability is an important advantage of immobilized enzymes and a critical factor to evaluate their potential for commercialization. An immobilized enzyme that can be commercialized must be capable of being used multiple times without significant loss in its activity. In some studies, the reusability of an immobilized enzyme was investigated by repeatedly using the same portion of the enzyme in the activity assay. However, since the actual catalytic performance of an enzyme is dependent on the nature of the substrate and the reaction environment (substrate concentration, pH, temperature, etc.), which can be quite different from that in the assay, the result obtained might not accurately indicate the reusability of the enzyme in the targeted process. It is more reasonable to examine the reusability by collecting the immobilized enzyme at the end of the targeted reaction and re-introducing it into a fresh reaction medium for another run. The reusability can be determined through either the relative activity of the immobilized enzyme or the progress of the reaction, such as the degree of hydrolysis or the content of desired products.
Structural characterization.
In recent years, some materials have been investigated as new types of supports for immobilization of enzymes, such as chitosan and magnetic nanoparticles. In order to better understand the structures of immobilized enzymes on these supports, some instrumental analyses have been performed. The Fourier-transform infrared spectrum of an immobilized enzyme is often analyzed and compared with that of the original support material. The successful immobilization can be confirmed by detecting the appearance or intensity increase of characteristic absorption bands of amide, amino or carboxyl groups derived from the enzyme. If the enzyme is immobilized through covalent attachment, the formation of new covalent bonds may lead to the appearance of a new absorption band in the infrared spectrum. For example, when glutaraldehyde is used to link the enzyme and support, an absorption peak at 1640–1690 cm−1 can be observed, which is attributed to C
N stretching vibration due to the formation of a Schiff base (Fig. 4).
 |
| Fig. 4 Immobilization of an enzyme through covalent attachment using glutaraldehyde. | |
The morphology of an immobilized enzyme can be studied through scanning electron microscopy or transmission electron microscopy. When nanoparticles are used as the support, X-ray diffraction analysis is often performed to confirm the crystal structures of the support. Specifically, for immobilized enzymes on magnetic nanoparticles, their supermagnetism property can be studied through vibrating sample magnetometry.
Concentration of omega-3 FAs in fish oil
Enzymatic hydrolysis
Release of EPA and DHA as FFAs.
Theoretically, one TAG molecule in fish oil can be hydrolyzed to one glycerol and three FFA molecules (Fig. 5). Some FA esters on the glycerol backbone have long chains or double bonds which lead to steric hindrance for lipases, therefore are hydrolyzed at a slower speed in comparison to others. In addition, some lipases exhibit regio- and FA selectivity during hydrolysis. Consequently, the corresponding FFAs are possibly generated at different time intervals during the reaction, and thereby concentrated in the collected fractions.
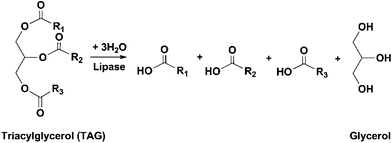 |
| Fig. 5 Enzymatic hydrolysis of TAGs. | |
Use of lipases immobilized on hydrophobic porous supports.
Fernández-Lorente et al. studied hydrolysis of sardine oil using three immobilized lipases (CALB, Thermomyces lanuginose lipase (TLL) and Rhizomucor miehei lipase (RML)).47 Since pure sardine oil is viscous and tends to remain on the surface of reaction devices and immobilized lipases, a biphasic aqueous–organic system was developed, in which the fish oil was dissolved in the organic phase for easier manipulation. However, the interfacial interaction between the organic solvent and the lipase in the aqueous phase may lead to inactivation of the lipase due to the disruption of its secondary structure.48 In addition, the organic solvent may interfere with the water-enzyme interaction that is essential for the lipase to function.49 Immobilization of the lipase inside a porous support can effectively diminish these negative effects brought by the organic solvent. A lipase dissolved in an aqueous medium is equilibrated between two forms (Fig. 6): a closed form (the active site is covered by a polypeptide chain) and an open form (the active site is exposed). The interaction between a lipase and a hydrophobic support tends to shift the equilibrium towards the open form, and the activity of the lipase is consequently increased, which is a phenomenon called “overactivation” or “hyperactivation”.50 In the present study, the three lipases were immobilized on a hydrophobic porous support (octyl-Sepharose) through physical adsorption. The activity of immobilized CALB, TLL and RML was significantly increased to 120, 1000 and 2000% of the free lipases respectively, due to the hyperactivation effect and inhibition of interfacial inactivation by the hydrophobic porous support. In the hydrolysis of sardine oil, the selectivity of the three immobilized lipases towards EPA and DHA over oleic acid (OA) and palmitic acid (PA) (two abundant FAs in sardine oil) was studied. Immobilized CALB showed the highest selectivity with an (EPA + DHA)/(OA + PA) ratio of 4.2, while for immobilized TLL and RML this ratio was approximately 1. However, immobilized TLL and RML were more selective towards EPA over DHA, with an EPA/DHA ratio of approximately 4.5. In comparison, the EPA/DHA ratio was 1.5 using immobilized CALB, indicating its similar preference towards the two omega-3 FAs.
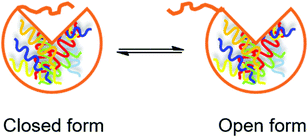 |
| Fig. 6 An equilibrium between closed and open forms of a lipase in an aqueous medium. | |
The same group carried out another study that compared immobilization of seven lipases on octyl-Sepharose through physical adsorption with cyanogen bromide (CNBr)-activated Sepharose through covalent attachment.51 Due to the hyperactivation effect by the hydrophobic support, most lipases immobilized on octyl-Sepharose had higher activity in the hydrolysis of sardine oil in comparison to those immobilized on CNBr-activated Sepharose. During the first stage (15–120 min) of hydrolysis, EPA was released at a faster speed than DHA for all immobilized lipases tested. The use of Rhizopus oryzae (ROL) and Yarrowia lipolytica (YLL) lipases immobilized on octyl-Sepharose resulted in the highest EPA/DHA ratio of 9.8 and 10.5, respectively. In order to further improve the activity and EPA/DHA selectivity, the researchers modified the protein surface of the immobilized lipases.52 After CALB, TLL and RML were immobilized on octyl-Sepharose or CNBr-activated Sepharose, they were modified using three methods (Fig. 7), including (1) amination, in which the carboxyl groups of the lipases were activated by a carbodiimide and reacted with ethylenediamine to generate more amino groups on the surface; (2) succinylation, in which the lysine residues of the lipases were reacted with succinic anhydride to increase the number of carboxyl groups on the surface; and (3) coating of the lipase surface using polyethylenimine (PEI) to modify the microenvironment around the active site. The effect of these modifications was largely dependent on the lipase species and the immobilization method. The activity of immobilized CALB on CNBr-activated Sepharose was increased the most by 110% after amination. The EPA/DHA ratio achieved after the hydrolysis of sardine oil using immobilized RML on CNBr-activated Sepharose was significantly improved to 34 after succinylation, in comparison to 7.5 without any modification.
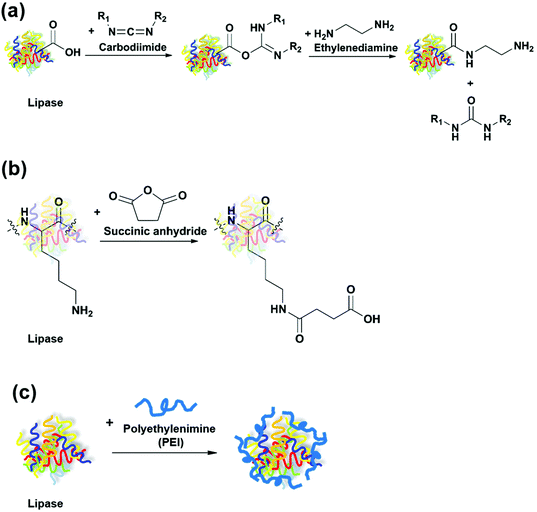 |
| Fig. 7 Modification of lipases through (a) amination; (b) succinylation; and (c) polyethylenimine (PEI) coating. | |
As claimed by Fernández-Lorente et al., addition of organic co-solvents to a biphasic reaction system could lead to conformational changes of immobilized lipases, and thereby alter their activity and selectivity. However, desorption of lipases from the supports was also promoted by the co-solvents.53 Cross-linking of immobilized lipases was investigated as an approach to reduce desorption, based on the assumption that the cross-linked lipase would desorb only after all the lipase molecules were simultaneously leached from the support.53 CALB, RML, TLL and Bacillus thermocatenolatus lipase (BTL) were immobilized on octyl-Sepharose, followed by amination and cross-linking via dextran aldehyde. The cross-linked lipases were treated with 50% propanol or detergents to test their ability against desorption, and 85–95% of the lipase activity was retained. All immobilized lipases showed adequate stability when incubated in 50% 2-propanol at pH 7 and 25 °C, with a half-life of approximately 60 h. Cross-linked RML was selected to catalyze the hydrolysis of sardine oil, and the EPA/DHA ratio was significantly improved from 3.45 without adding any co-solvent to 22.4 by adding 50% 2-propanol.
Use of lipases immobilized on ionic supports.
The efficiency of immobilization through ionic adsorption is dependent on the charges of the support and the amino acid residues on the surface of the lipase. In the study by Pereira et al., HPL was adsorbed on a variety of anion exchangers with 100% immobilization yield, but could not be immobilized on any cationic exchanger, indicating that the surface of this lipase was abundant in negatively charged amino acid residues.54 Due to the relatively strong ionic binding, most of the immobilized HPL on ionic supports was only partially desorbed when exposed to salt solutions. In comparison, all HPL physically adsorbed on hydrophobic supports was fully desorbed by a detergent within 45 min. In the hydrolysis of sardine oil, HPL immobilized via ionic adsorption showed 2-fold higher selectivity towards EPA over DHA in comparison to its counterpart immobilized via physical adsorption.
Júnior et al. investigated the influence of solid-phase amination on immobilization of Geotrichum candidum lipase (GCL) on two cation exchangers (carboxymethyl agarose and sulfopropyl agarose).55 The solid-phase amination was performed by adsorbing GCL on a solid support (octyl-Sepharose) first to prevent its precipitation and protect the active site, followed by amination and desorption of the aminated GCL (GCL-A) using a detergent. Amination was observed to improve the thermal stability of the lipase at 30–50 °C and alter its activity profile in pH range of 3–10. GCL-A was fully immobilized on both ionic supports with above 80% of activity retained. In comparison, GCL without amination could not be immobilized on the ionic supports, possibly due to the lack of positively charged groups on its surface. The thermal stability at 30–50 °C and stability in organic solvents (methanol, propanol and cyclohexane) were improved for GCL-A immobilized on both ionic supports. In comparison to GCL-A immobilized on carboxymethyl agarose, GCL-A immobilized on sulfopropyl agarose was more stable in organic solvents, possibly because the ionic binding between sulfopropyl and amino groups resulted in more rigidity of the lipase conformation, which inhibited the interfacial inactivation by the organic solvents. Both immobilized GCL-A were approximately 2.3-fold more active and 3.5-fold more selective towards EPA over DHA compared to GCL-A in the hydrolysis of sardine oil. This indicates that the binding of GCL on the ionic supports resulted in certain distortion of its active site which promoted its catalytic performance in the hydrolysis of fish oil.
Use of lipases immobilized through one-point and multipoint covalent attachment.
Immobilization of lipases on CNBr-activated supports has been reported to be relatively weak since the covalent attachment only occurs on the terminal amino groups of the lipase.51 Therefore, this type of immobilization was claimed as “one-point covalent attachment”. In comparison, glyoxyl-functionalized supports have been widely used for lipase immobilization through “multipoint covalent attachment”, as the aldehyde groups in glyoxyl-functionalized supports can form covalent bonds with multiple types of amino groups of the lipase. Pereira et al. compared the effect of one-point and multipoint covalent attachment on the stability of immobilized Hypocrea pseudokoningii lipase (HPL).41 The free HPL was aminated first to increase the number of amino groups on its surface, followed by immobilization on CNBr-activated agarose or glyoxyl-agarose. In comparison to HPL immobilized via one-point covalent attachment, HPL immobilized via multipoint attachment was significantly more stable at a relatively high temperature (50 and 60 °C) and in organic solvents (methanol, ethanol and propanol). The stronger multipoint covalent bonding resulted in more rigidity of the conformation of immobilized HPL, and thereby improved its stability. When used to catalyze the hydrolysis of sardine oil, immobilized HPL on glyoxyl-agarose was 1.8-fold more active and 2.3-fold more selective towards EPA over DHA in comparison to immobilized HPL on CNBr-activated agarose, possibly because the conformational change of the lipase caused by the stronger multipoint covalent attachment was more favorable to the hydrolysis process.
Use of immobilized lipases hyperactivated by detergents.
The use of some detergents at low concentrations, such as Triton X-100 and sucrose laurate, has been reported to result in hyperactivation (see the definition in section Use of lipases immobilized on hydrophobic porous supports) of immobilized lipases due to the interaction between the detergent's hydrophobic moiety and the lipase.56 However, the presence of detergents in the reaction system may inhibit the contact of oil droplets with the active site of the lipase. Filice et al. investigated different methods for immobilization of RML in the presence of a detergent, intending to stabilize the open form of RML so as to retain the hyperactivated RML after removal of the detergent.57 Among the three detergents tested, sucrose laurate showed the most prominent hyperactivation effect for RML by increasing its activity by 1000% and therefore was selected for the subsequent study. The free RML was immobilized on octyl-Sepharose and then desorbed using 0.5% sucrose laurate, resulting in a 20-fold increase in its activity. The obtained RML solution containing the detergent was mixed with different supports for immobilization. Three immobilization methods were studied, including (1) ionic adsorption of RML on anion exchangers; (2) one-point covalent attachment of RML on CNBr-activated Sepharose; and (3) multipoint covalent attachment of RML on glyoxyl-Sepharose. After immobilization, the detergent was removed by filtration and wash. Among all immobilized RML obtained, immobilized RML on Q Sepharose (RML-Sepharose Q) via multipoint anion exchange retained most of the hyperactivation effect (95.7%), while RML immobilized through the other two methods lost more than 90% of the hyperactivation effect. RML-Sepharose Q retained 100 and 80% of its activity after incubation at 25 °C for 3 weeks and 37 °C for 24 h respectively, indicating its superior stability. The use of RML-Sepharose Q in the hydrolysis of sardine oil resulted in a low omega-3 FAs/(OA + PA) ratio of 1.06, but a high EPA/DHA ratio of 4.2.
Use of lipases immobilized on chitosan.
Chitosan is a natural biopolymer with many attractive properties, such as non-toxicity, biocompatibility, biodegradability and antibacterial activity.58 Furthermore, chitosan is abundant in amino and hydroxyl groups, so it can be readily modified with different functional groups. Due to these advantages, chitosan and its derivatives have been widely investigated as supports for enzyme immobilization with applications in food, pharmaceutical and engineering industries.59 Urrutia et al. reported the immobilization of CALB and RML on hydrophobic chitosan materials through physical adsorption.30 Chitosan was cross-linked with epichlorohydrin and modified with butyl-, octyl-, or dodecyl groups to increase its hydrophobicity. The increase in alkyl chain length led to increased hydrophobicity of the chitosan support and improved activity of the immobilized lipase. The immobilization yield for CALB was similar using all three chitosan supports, but decreased for RML with the increase of the alkyl chain length of the support. The alkyl group likely hindered the access for lipase molecules to the chitosan support, and this effect was more evident for longer alkyl chains and larger lipase molecules (i.e. RML in this study). During the hydrolysis of menhaden (a species of forage fish) oil, the use of CALB immobilized on dodecyl- and octyl-chitosan resulted in the release of a similar amount of EPA + DHA (approximately 90–100 mM), which was higher in comparison to CALB immobilized on butyl-chitosan (approximately 40 mM). However, the amount of EPA + DHA released using immobilized RML was increased with the decrease of the alkyl chain length, following the order of dodecyl-, octyl- and butyl-chitosan (approximately 50, 400 and 580 mM, respectively). Both immobilized CALB and RML were more selective towards DHA and produced PUFAs containing approximately 90% and 70–88% DHA, respectively. The amount of DHA released by using immobilized RML was smaller in comparison to immobilized CALB, possibly because RML is sn-1,3 specific while DHA in menhaden oil mainly exists at the sn-2 position. The reusability was studied for CALB and RML immobilized on dodecyl- and butyl-chitosan, respectively. During five cycles, the total mass of EPA + DHA released per gram of biocatalyst had no significant change using immobilized CALB but decreased drastically after the second run using immobilized RML, possibly due to the lower stability of immobilized RML at the reaction temperature (35 °C).
Use of oriented-immobilized lipases.
In most studies on immobilization of lipases, the lipase is randomly positioned on the support. This may bring about issues such as block of the active site and low reproducibility of the immobilization protocol. Mohammadi et al. investigated oriented immobilization of RML on two epoxy-functionalized silica nanoparticles (Santa Barbara amorphous (SBA-15) and Mobil crystalline material (MCM-41)).31 Among the six histidine residues in the primary structure of RML, only number 42 (H42) has an affinity for metal ions due to its imidazole group and thereby can be adsorbed through the chelate effect. For oriented immobilization, the epoxy groups on the silica materials were partially modified with iminodiacetic acid and copper(II) sulfate to introduce copper ions (Cu2+) to the supports (Fig. 8). During the first stage of immobilization, RML was oriented and adsorbed on the support through chelation of H42 to Cu2+ ions. Afterwards, further immobilization of RML was carried out through covalent bonding between the carboxyl groups around H42 and the epoxy groups on the support. The oriented immobilized RML showed slightly lower stability towards heat and organic solvents in comparison to their counterparts randomly immobilized. This is likely because the number of epoxy groups available for covalent attachment was decreased in oriented immobilization, thus resulting in the less rigid conformation of the immobilized lipase. Nevertheless, in the hydrolysis of menhaden oil at 25 °C, the selectivity of the oriented immobilized RML on SBA-15 towards EPA over DHA was significantly improved, allowing for the release of EPA and DHA mixture with almost 95% of EPA purity during the first stage of the process at pH 5. The same group performed another study on oriented immobilization of RML on epoxy- and Cu2+-functionalized silica gel.42 The activity and EPA/DHA selectivity of the oriented immobilized RML was the highest when the hydrolysis of menhaden oil was conducted at 25 °C and pH 7, allowing for the release of EPA and DHA mixture with almost 87% of EPA purity. The immobilized RML was recovered by filtration and washed with cyclohexane, and could be reused up to 5 cycles with 80% of the activity retained.
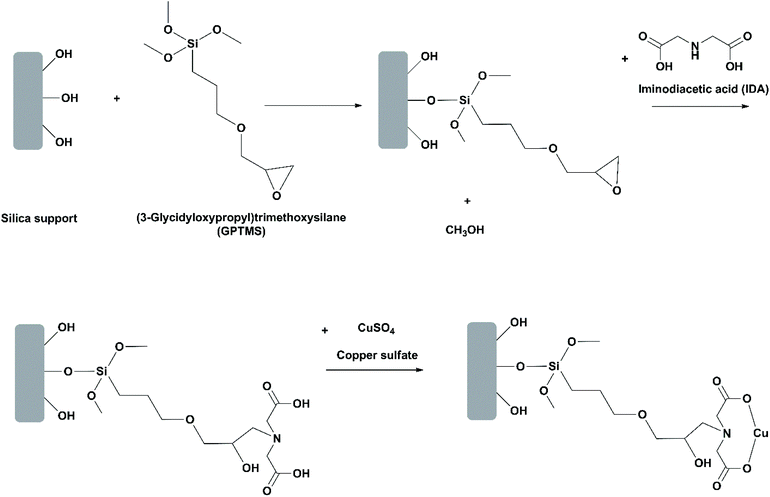 |
| Fig. 8 Functionalization of silica materials with epoxy groups and copper(II) ions. | |
Ashjari et al. studied oriented immobilization of ROL on epoxy- and Cu2+-functionalized silica supports (silica gel, SBA-15 and MCM-41) through chelation of histidine residue number 134 (H134) on the surface of ROL to Cu2+ ions.60 In order to further stabilize the lipase on the support, ROL was aminated to increase the number of amino groups on the surface which can form more covalent bonds with the epoxy groups on the support. After oriented immobilization, the aminated ROL was more stable at 45–55 °C and in organic solvents (1-propanol, 2-propanol and dioxane) compared to the non-aminated ROL. The use of aminated ROL immobilized on SBA-15 led to the highest EPA/DHA ratio of 16.1 in the hydrolysis of menhaden oil at 4 °C and pH 5. All the immobilized derivatives of aminated ROL showed adequate reusability with above 90% of the activity retained after five cycles, in comparison to 65–80% for the immobilized derivatives of non-aminated ROL.
Yousefi et al. performed oriented immobilization of aminated and non-aminated RML on epoxy- and nickel(II) ions (Ni2+)-functionalized agarose, and compared them with their counterparts randomly immobilized through one-point covalent attachment.61 Both aminated and non-aminated RML after oriented immobilization showed significantly improved EPA/DHA selectivity in the hydrolysis of fish oil. The highest EPA/DHA ratio of 32.9 was obtained by using the aminated and oriented RML derivative at pH 5 and 4 °C, allowing for the release of EPA and DHA mixture with almost 97% of EPA purity during the first stage of the reaction. The aminated and non-aminated RML after oriented immobilization could be reused five runs with 9 and 30% loss of activity, respectively. In comparison, the use of immobilized RML on CNBr-activated Sepharose resulted in an EPA/DHA ratio of 10.6 in the hydrolysis, and only 50% of its activity was retained after reused five cycles.
As indicated by these studies, oriented immobilization might be able to improve the EPA/DHA selectivity of a lipase in the hydrolysis of fish oil in comparison to random immobilization. Amination potentially further stabilizes the lipase on the support through reinforced covalent bonding, thereby increasing the reusability of the immobilized lipase.
Retention of EPA and DHA in acylglycerols.
Generally, omega-3 FAs in commercial supplements are provided in the form of fatty acid ethyl esters (FAEEs). Therefore, if EPA and DHA are produced as FFAs in the hydrolysis of fish oil, a subsequent step of esterification is usually required. (Alternatively, alcoholysis of fish oil can be performed to directly obtain the esters of omega-3 FAs. See section Enzymatic transesterification/alcoholysis.) However, it has been reported that EPA and DHA present in the form of TAGs have higher bioavailability.62–64 In addition, FAEEs will be hydrolyzed in the stomach and result in unpleasant fishy burps. The alcohol generated may cause health issues or religious conflicts.4 Consequently, re-esterification of free EPA and DHA or their esters with glycerol has been applied as an approach to produce omega-3 concentrates in the form of TAGs. Research has been performed on selective hydrolysis of fish oil using lipases, with the aim to remove other FAs and retain mainly EPA or DHA on the glycerol backbone. The acylglycerols (Fig. 9) will be collected and purified by winterization (an oil refinement method to reduce turbidity in oils, based on the differences in melting points of oils, fats and waxes), solvent extraction, urea fractionation, distillation or chromatography separation. The EPA and DHA fractions will then be reacted with the FFAs or esters of omega-3 FAs or other FAs of interest to prepare the products with desired structures.
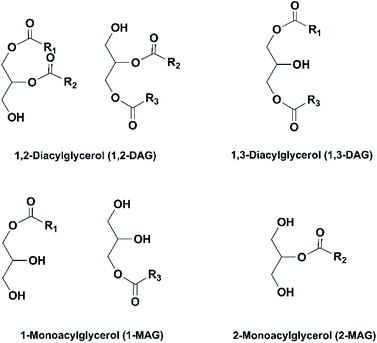 |
| Fig. 9 Acylglycerols possibly produced in enzymatic hydrolysis and alcoholysis of TAGs. | |
Use of lipases immobilized through physical adsorption.
Rice et al. reported the immobilization of Candida cylindracea lipase (CCL) on microporous polypropylene fibers through physical adsorption.65 The immobilized lipase selectively catalyzed the hydrolysis of medium-chain saturated and monounsaturated FAs (C14, C16, C16:1, C18:1) in menhaden oil at 40 °C and pH 7, and retained over 90% of the initial EPA and DHA in the acylglycerols. The selectivity of CCL towards short- and medium-chain FAs was likely due to the steric hindrance around the active site of the lipase, which inhibited its access to the ester bonds of long-chain FAs in the oil. Akanbi et al. claimed that Candida Antarctica lipase A (CALA) was FA-selective in the hydrolysis of anchovy oil, with sequential selectivity towards saturated, monounsaturated and polyunsaturated FAs in the order of increased number of double bonds.66 After immobilized on octadecyl resin via physical adsorption, immobilized CAL-A retained its FA selectivity and showed increased activity due to the hyperactivation effect (see the definition in section Use of lipases immobilized on hydrophobic porous supports). In the hydrolysis of tuna oil using immobilized CALA, EPA and DHA were concentrated from approximately 8 and 25% originally in the oil to 15 and 35% in the acylglycerols, respectively. The immobilized CALA could be reused five cycles without significant loss in the activity.
Use of lipases immobilized through covalent attachment.
Cui et al. immobilized bovine pancreatic lipase on N-succinyl-chitosan beads through covalent attachment using 1-ethyl-3-(3-dimethylaminopropyl)carbodiimide (EDC)/N-hydroxysuccinimide (NHS) as the activating agents (Fig. 10).38 The immobilization process was optimized with the maximum activity recovery of 74.55% achieved. In comparison to the free lipase, the immobilized lipase showed high activity over a wider range of temperatures and pH. The immobilized lipase was used to selectively catalyze the hydrolysis of saturated and monounsaturated FAs in fish oil, and EPA and DHA were concentrated from 4.3 and 11.9% originally in the oil to 6.5 and 20.6% in the acylglycerols, respectively.
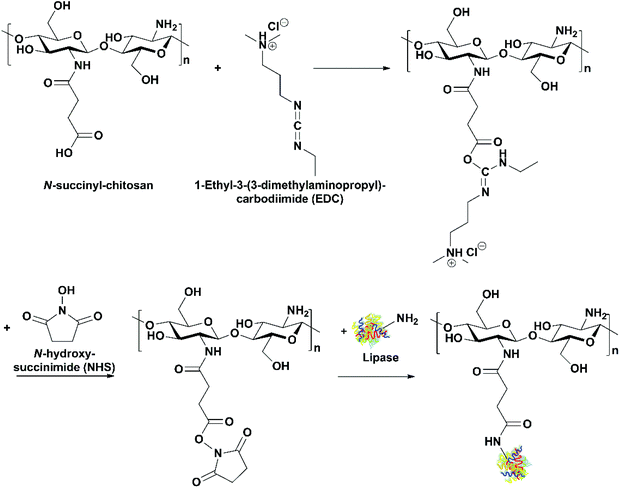 |
| Fig. 10 Immobilization of a lipase on N-succinyl-chitosan through EDC/NHS as the activating agents. | |
Use of lipases immobilized through cross-linking.
Yan et al. investigated the efficacy of cross-linked Geotrichum sp. lipase in enrichment of omega-3 FAs in fish oil.67 The immobilization process was performed in the presence of PEI, with the aim to increase the number of amino groups available for cross-linking and stabilize the lipase through the ionic exchange between the cationic groups in PEI and the anionic groups on the surface of the lipase. The lipase was dissolved in a phosphate buffer and mixed with PEI, followed by the addition of acetone for lipase aggregation and glutaraldehyde as the cross-linker. No leakage was observed from the cross-linked lipase (PEI-CLEAs) during filtration and wash. The stability of PEI-CLEAs was higher at 45–55 °C and in organic solvents (acetone, tert-butyl alcohol and octane) in comparison to the cross-linked lipase without PEI (CLEAs) and the free lipase. In the hydrolysis of fish oil, the use of PEI-CLEAs increased EPA and DHA content from 6.94 and 0.97% in the original oil to 12.69 and 3.88% in the acylglycerols, respectively. After reused five cycles, PEI-CLEAs still afforded 72% of relative degree of hydrolysis, while only 46% was obtained for CLEAs after three cycles. The vigorous stirring during the reaction generated gas bubbles, which possibly led to interfacial inactivation of the lipase. The ionic interaction between the lipase and PEI was likely to increase the rigidity of the immobilized lipase and prevent this interfacial inactivation. However, the mechanical force during centrifugation and wash might have broken the structure of the cross-linked lipase and thereby reduced its catalytic performance.
Use of lipases immobilized through entrapment.
Okada et al. entrapped CRL in chitosan-alginate-calcium chloride (CaCl2) beads through an ionotropic gelatin method.68 The lipase was dissolved in a solution of sodium alginate and mixed with a chitosan solution containing CaCl2. During the reaction, alginate was cross-linked with chitosan via ionic binding, and CRL was immobilized inside the polymer network. In comparison to free CRL, immobilized CRL showed improved stability at 20–60 °C and pH 2–10. Two-round hydrolysis of sardine oil was performed using free and immobilized CRL. After the first round of hydrolysis, the FFAs released were neutralized and removed by centrifugation. The immobilized CRL was collected through filtration and reused in the second hydrolysis of the concentrated oil. Since the free lipase could not be recovered, fresh free CRL was added to the reaction system for the next round. The free and immobilized CRL similarly concentrated EPA from 25.2% in the original oil to 40.2 and 39.6%, and DHA from 7.18% to 15.5 and 15.3% in the acylglycerols, respectively.
Use of lipases immobilized on nanomaterials.
During recent decades, nanomaterials have been extensively studied as support materials for enzyme immobilization due to their high surface area to volume ratio, which leads to higher enzyme loading and reduces mass transfer limitations in comparison to traditional bulk materials.69 Matuoog et al. reported the immobilization of Thermomyces lanuginosus lipase (TLL) on multi-walled carbon nanotubes through physical adsorption.37 The effects of TLL loading, pH, temperature and time during immobilization were investigated, and the maximum immobilization efficiency of 97.78% was obtained after optimization of the process. The use of immobilized TLL effectively enriched DHA from approximately 7% originally in the fish oil to 31% in the acylglycerols, in comparison to 19.7% by using free TLL. After reused six cycles, immobilized TLL still afforded above 80% of relative degree of hydrolysis, indicating its adequate reusability.
When magnetic nanoparticles are used as the support, they can be fast separated from the reaction mixture by magnetic attraction, which facilitates the recovery of immobilized enzymes. Iron(II,III) oxide (Fe3O4) is the most commonly used type of magnetic nanoparticles for enzyme immobilization due to its relatively easy synthesis and remarkable superparamagnetic properties. Matuoog et al. immobilized TLL on Fe3O4 nanoparticles that were coated with (3-aminopropyl)triethoxysilane (APTES) and activated by glutaldehyde.70 An immobilization efficiency of 98.7% and an activity recovery of 109.5% were achieved from the optimized immobilization process. In the hydrolysis of fish oil using immobilized TLL, the content of DHA was increased from 7% originally in the oil to 41% in the acylglycerols. The relative degree of hydrolysis was slightly decreased to 82% after the immobilized lipase was reused six cycles.
Liu et al. carried out oriented immobilization of Yarrowia lipolytica lipase LIP2 on APTES-coated Fe3O4 nanoparticles in a reverse micelle system.71 A reverse micelle system is composed of water (in a relatively small amount), an organic solvent (in a relatively large amount) and a surfactant.72 After vigorous mixing, the surfactant molecules interact with water and the organic solvent molecules via their polar and non-polar ends, respectively. Consequently, numerous aqueous-phase droplets are dispersed in the organic phase. If the aqueous phase contains lipase molecules, the active site of the lipase will be oriented towards the hydrophobic phase of the organic solvent. In the present study, due to the interfacial activation by the organic phase, the activity recovery of the immobilization performed in the reverse micelle system was as high as 382%, in comparison to 29% of that in an aqueous system. A variety of surfactants and co-surfactants were studied about their influence on immobilization. The highest activity recovery of 540% was achieved by using the nonionic surfactant, Span 20, at a water/surfactant ratio of 1. The activity of the oriented immobilized lipase was further increased with the addition of hexanol, propanol or octanol as the co-surfactant, and the maximum activity was obtained by using hexanol at a co-surfactant/surfactant ratio of 2. In the hydrolysis of fish oil, the degree of hydrolysis reached 40% by using the oriented immobilized lipase, and DHA was concentrated from approximately 19% in the original oil to 36.8% in the acylglycerols. In comparison, the use of the free lipase resulted in 27.3% of hydrolysis and 31.6% of DHA in the acylglycerols. The oriented immobilized lipase could be reused twenty cycles and still afforded 80% of relative degree of hydrolysis.
Use of bioimprinted lipases.
Molecular bioimprinting is an effective method to improve the catalytic activity of enzymes in non-aqueous media (Fig. 11). The imprint molecules interact with the enzyme, promote its conformational changes and occupy its active site in a way similar to the enzyme–substrate interaction. The enzyme-imprint material complex is lyophilized, immobilized or precipitated in a non-aqueous medium. The complex is collected and the imprint material is removed by an organic solvent. The enzyme retains the open form due to the rigidity, so the active site remains exposed for the substrate to access.73,74 In the study by Yan et al., fish oil or oleic acid was added as the imprint material during immobilization of GCL on macroporous NKA resin (crosslinked polystyrene adsorption resin).75 The purified lipase was dissolved in a microemulsion composed of the imprint material and a phosphate buffer, followed by the addition of the support and incubation at 4 °C for 4 h. The immobilized lipase was collected through filtration and washed using tert-butyl alcohol to remove the imprint material. IMLAF (immobilized GCL that was bioimprinted using fish oil) and IMLAOA (immobilized GCL that was bioimprinted using oleic acid) showed similar stability at 45–55 °C and in organic solvents (acetone, diethyl ether, tert-butyl alcohol, hexane, heptane and octane), which were improved in comparison to free GCL. In the hydrolysis of fish oil, the use of IMLAF led to the highest degree of hydrolysis and initial reaction rate, as well as the shortest time required to reach the highest degree of hydrolysis, followed by IMLAOA, immobilized GCL without bioimprinting, and free GCL. Therefore, the hydrolytic efficiency of immobilized GCL was effectively improved by bioimprinting. In comparison to oleic acid which is only one among the various FAs that fish oil contains, fish oil is multi-component-based and resulted in more effective activation of the lipase for the hydrolysis of fish oil. The effect of a two-phase medium on immobilization of GCL using fish oil as the imprint material was investigated by replacing the phosphate buffer with a mixture of phosphate buffer and octane. The resulting immobilized GCL (IMLAOF) showed a higher degree of hydrolysis and initial reaction rate in the hydrolysis of fish oil in comparison to IMLAF, indicating its further improved hydrolytic efficiency. The content of EPA and DHA was increased by IMLAOF from 6.94 and 0.97% in the original fish oil to 12.65 and 3.85% in the acylglycerols, respectively. Both IMLAF and IMLAOF still afforded 80% of relative degree of hydrolysis after reused five times.
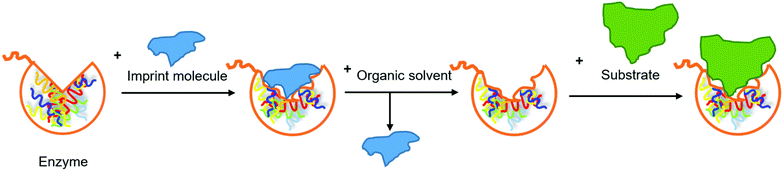 |
| Fig. 11 Molecular bioimprinting of an enzyme. | |
Generally, bioimprinted enzymes are used in a non-aqueous or micro-aqueous environment (an environment that is mostly composed of an organic solvent and contains a very small amount of water), as in aqueous solutions the bioimprinted molecules will have conformational changes due to the interaction with water molecules and therefore lose their structural memory.76 In order to stabilize the bioimprinted lipase and extend its use to aqueous media, Sampath et al. investigated cross-linking of bioimprinted CRL via glutaraldehyde.35 CRL was bioimprinted using oleic acid, followed by the addition of bovine serum albumin as the co-feeder and PEI as the co-aggregator. The obtained aggregates of bioimprinted CRL (CRL-CLEA) was cross-linked by adding glutaraldehyde and collected through centrifugation. The maximum activity recovery of approximately 55% and aggregation yield of 99% were achieved at a CRL: oleic acid ratio of 1
:
5, CRL: bovine serum albumin ratio of 1
:
1, CRL: PEI ratio of 1
:
1, with the addition of 340 μL of glutaraldehyde and after 45 min of cross-linking. In the hydrolysis of sardine oil, 35.97% of hydrolysis was achieved by using CRL-CLEA, and EPA was effectively enriched from 11.81% in the original oil to 45.75% in the acylglycerols, in comparison to 3.45% of hydrolysis and 18.16% of EPA in the acylglycerols by using free CRL. The LC-MS analysis indicated that EPA was mainly present in the form of MAGs after the reaction using CRL-CLEA. The content of DHA was decreased from the original 6.1% to 5.04% in the acylglycerols using CRL-CLEA, indicating that the DHA glycerol ester was slightly hydrolyzed. During the reuse of CRL-CLEA, the EPA or DHA content in the acylglycerols was slightly decreased by approximately 10% till the 5th cycle, but then drastically reduced by approximately 50 and 30% in the 6th and 7th cycle, respectively. The continuous stirring and repeated wash resulted in compaction of the lipase molecules, so the access of sardine oil to the active site of CRL was inhibited and the lipase activity was reduced.
Enzymatic transesterification/alcoholysis
Enzymatic transesterification/alcoholysis has been widely investigated as a method to prepare FA esters from TAGs (Fig. 12). For omega-3 FA concentrates that are intended for human consumption, ethanol is the most commonly used alcohol due to its relatively low toxicity. Omega-3 FAs can either be converted to ethanol esters (EEs) or maintained on the glycerol backbone as acylglycerols (Fig. 9). It should be noted that during enzymatic alcoholysis, irreversible deactivation of lipases by alcohol has been frequently observed, which can be restricted by stepwise addition of the alcohol.77,78
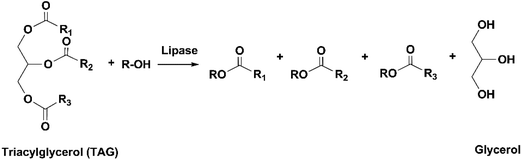 |
| Fig. 12 Enzymatic transesterification/alcoholysis of TAGs. | |
Release of EPA and DHA as EEs.
Moreno-Pérez et al. reported the immobilization of CALB, TLL and RML on an anion exchanger (Duolite A568) via ionic adsorption and two hydrophobic supports (Lewatit VPOC1600 and Sepabeads C18) via physical adsorption.43 The immobilized lipases were used to catalyze the ethanolysis of sardine oil in two organic solvents (cyclohexane or tert-amyl alcohol). The lipases immobilized on the hydrophobic supports were stabilized and showed higher activity and selectivity in comparison to their counterparts immobilized on the anion exchanger. Immobilized TLL on Sepabeads C18 exhibited the highest initial activity and EPA/DHA selectivity, with an EE-EPA/EE-DHA ratio of 29 achieved. Modification of the immobilized lipases was performed through amination, succinylation or coating with polymers to further improve their catalytic performance. PEI coating significantly improved the stability of the Sepabeads C18 derivatives of TLL and RML in cyclohexane and tert-amyl alcohol, respectively, with no loss in their transesterification activity after incubation at 45 °C for 24 h. After the ethanolysis of sardine oil catalyzed by TLL immobilized on Sepabeads C18 with PEI coating, the product mixture was abundant in EE-EPA (80%) with less than 5% of EE-DHA. The same group further studied this process in a solvent-free system.79 The use of immobilized Lecitase Ultra on Sepabeads C18 resulted in the highest EE-EPA/EE-DHA ratio of 43, indicating that almost pure EE-EPA was produced during the first stage of the reaction. TLL and Lecitase Ultra immobilized on Sepabeads C18 showed adequate reusability in the solvent-free system, with 80 and 100% of the activity retained after four and five cycles, respectively.
Cipolatti et al. prepared polyurethane nanoparticles modified with polyethylene glycol (PU-PEG), and used this material as the support for the immobilization of TLL.80 PEG modification was claimed to increase the mechanic resistance of the PU support. The immobilization yield was as high as 98–100% using all PU supports modified with PEG with different molecular weight (PEG 400, 4000, or 6000). However, the recovered activity of immobilized TLL on PU-PEG-400 was much lower (37.6%) in comparison to others (100%), possibly due to the agglomeration of the relatively small PEG 400 molecules. All the immobilized TLL showed improved stability towards heat and pH. In the ethanolysis of sardine oil at 28 °C, the EPA/DHA selectivity was increased for all immobilized TLL compared to free TLL, especially immobilized TLL on PU-PEG 6000, which afforded the highest EE-EPA/EE-DHA ratio of 31.8. In the further study by the same group, PEI or trehalose coating was carried out to further stabilize the immobilized lipase.81 Coating with 20% PEI promoted the hyperactivation effect (see the definition in section Use of lipases immobilized on hydrophobic porous supports), and the resulting immobilized TLL showed the highest activity and thermal stability in comparison to its counterparts coated with 10% PEI or trehalose. During the ethanolysis of sardine oil, the amount of EE-EPA or EE-DHA produced using immobilized TLL coated with PEI was at least four times higher than that using non-coated immobilized TLL. The highest EPA/DHA selectivity was obtained using the immobilized TLL coated with 20% PEI, with an EE-EPA/EE-DHA ratio of 45.8.
Retention of EPA and DHA in acylglycerols.
Pawongrat et al. prepared MAGs enriched with omega-3 FAs through glycerolysis of tuna oil using the immobilized lipase AK from Pseudomonas fluorescence.34 During the synthesis of acylglycerols, acyl-migration often occurs, in which the acyl groups uncontrollably move from one position to another on the glycerol backbone. If the glycerolysis reaction is catalyzed by an immobilized lipase, acyl-migration can be restrained by using certain types of supports in the immobilization.82 In the present study, Accurel EP-100 was selected as the support as it doesn't have superficial charges and thereby won't catalyze acyl-migration.83 A MAG yield of 24.5% was achieved from the optimized glycerolysis process catalyzed by the immobilized lipase AK. The omega-3 FA content was increased from 31.87% originally in the tuna oil (4.2% of EPA and 27.9% of DHA in 99.3% of TAG) to 55.9% (7.7% of EPA and 48.2% of DHA) in the MAG products. However, the side reaction of oil hydrolysis was significantly promoted due to the addition of water in the reaction medium, resulting in the formation of 41.6% of FFAs. The concentration of omega-3 FAs in the FFAs was 36.4% (7.9% of EPA and 28.5% of DHA). Therefore, in 100 g product mixture, the amount of omega-3 FFAs was 15.14 g (36.4% × 41.6% × 100 g), which was even higher than that of omega-3 MAGs (13.70 g, 55.9% × 24.5% × 100 g). In another study performed by the same group, water was not added in the glycerolysis reaction, and the immobilized lipase AK was treated with three drying methods to remove the residual water.84 However, it was observed that the activity of the immobilized lipase in glycerolysis of tuna oil was reduced along with the decrease of the water content. Therefore, it was concluded that a certain amount of water was essential for this lipase to catalyze the glycerolysis reaction; however, the existence of water might promote the hydrolysis of TAGs to FFAs at the same time.
Structured TAGs (STAGs) that contain EPA and DHA at the sn-2 position and medium-chain FAs at the sn-1 and 3 positions have been reported to be more readily absorbed by the human body in comparison to non-structured TAGs with similar FA compositions.85 Muñío et al. prepared 2-MAGs abundant in DHA as the precursor of STAGs through the ethanolysis of fish oil catalyzed by 1,3-specific lipases (lipase D from Rhizopus oryzae and lipase Rd from Rhizopus delemar) immobilized on Accurel EP-100.86 In the ethanolysis of cod liver oil, only 2-MAGs were detected with no formation of FFAs or 1(3)-MAGs, which facilitated the separation of the 2-MAG products from the EEs. The PUFA content in the 2-MAGs was 39.5 and 36% using immobilized lipase D and Rd, respectively, which was similar to that of the sn-2 position in the original cod liver oil (39.1%). Therefore, almost all PUFAs at the sn-2 position of TAGs in the oil were preserved in the 2-MAG products, due to that the lipases are 1,3-specific and acyl-migration was effectively restricted by the support and the solvent (acetone).87 Since DHA in cod liver oil is mainly distributed at the sn-2 position, it was effectively enriched from 11.1% in the original oil to 29.1% in the 2-MAGs. In comparison, the concentration of EPA in the 2-MAGs (10.4%) was similar to that originally in the oil (9.5%), possibly because EPA in cod liver oil is mainly located at the sn-1(3) position. The yield of 2-MAGs obtained by using immobilized lipase D and Rd was even higher than that by using the commercial immobilized lipase Novozym 435. However, when the reaction was scaled up, Novozym 435 was selected to use due to its higher stability during the reuse.88 Therefore, good stability and reusability are essential for an immobilized enzyme to be developed for large-scale applications and commercialization.
Enzymatic esterification
Besides hydrolysis and alcoholysis of esters, lipases also catalyze the esterification of FFAs (Fig. 13). Some lipases have the selectivity towards saturated and monounsaturated FAs and discriminate against PUFAs in the esterification process, and therefore can be used for the enrichment of omega-3 FAs in the form of FFAs.89 Jonzo et al. immobilized two lipases (Lip A and B, purified from commercial CRL) on Duolite A 568 through ionic adsorption with an immobilization yield of above 99%.36 The two immobilized lipases were used to catalyze the esterification of cholesterol with FFAs that were obtained from the hydrolysis of TAGs extracted from sardine mill effluent. During the reaction, palmitic acid (a saturated FA) was observed to be esterified first, followed by palmitoleic acid and oleic acid (monounsaturated FAs). The majority of DHA remained unreacted and was enriched by Lip A and B from 7.4% originally in the oil to 32% and 25.3% in the FFAs collected after the reaction, respectively.
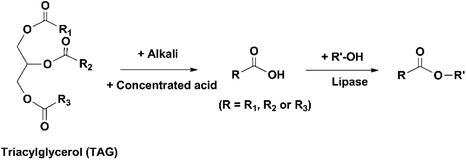 |
| Fig. 13 Enzymatic esterification of FFAs derived from TAGs. | |
Mbatia et al. concentrated omega-3 FAs in Nile perch viscera oil using two methods, esterification of FFAs and hydrolysis of FAEEs, catalyzed by two immobilized lipases (TLL and Pseudomonas cepacia lipase (PCL)) on Accurel MP100.90 The FFAs were prepared by saponification of the oil and acidification of the produced FA salts. A part of the FFAs was treated with ethanol to form FAEEs. The FFAs were esterified and the FAEEs were hydrolyzed using the immobilized lipases, with the aim to leave EPA and DHA unreacted. In both reactions, immobilized TLL showed stronger discrimination against DHA, while PCL was more effective in retaining EPA. The use of immobilized TLL resulted in the enrichment of DHA from 9 mol% in the original oil to 27 mol% in the FAEEs collected after the hydrolysis and 39% in the FFAs collected after the esterification. The use of immobilized PCL concentrated EPA from 3 mol% originally in the oil to 11 mol% after the hydrolysis and 16 mol% after the esterification. Although the omega-3 FAs were more concentrated in the FFAs collected after the esterification, their recovery was higher after the hydrolysis. After the esterification and hydrolysis, 68 and 84% of DHA were recovered by using immobilized TLL, and 70 and 92% of EPA were recovered by immobilized PCL, respectively.
It should be noted that compared to direct hydrolysis or alcoholysis of TAGs in fish oil, enrichment of omega-3 FAs through esterification requires extra procedures to prepare the FFAs derived from the TAGs, which involves the use of hazardous chemicals (concentrated acids, alkalis and organic solvents) and consumption of energy for heating. Therefore, this method has relatively little potential to be developed for industrial production and is thereby less investigated.
Conclusions
During recent decades, marine by-products have been investigated as sources of EPA and DHA due to the enormously growing demand for omega-3 FA supplements and development of the blue economy. To produce marine omega-3 FA products, fish oil is extracted from the fish materials and refined, followed by the enrichment of EPA and DHA. Immobilization has been intensively investigated as a green technology to improve the catalytic performance and reusability of lipases in enzymatic concentration of marine omega-3 FAs. The immobilized lipases were mainly applied to catalyze the hydrolysis or alcoholysis of TAGs in fish oil to obtain concentrated EPA and/or DHA in the form of FFAs, EEs or acylglycerols. As indicated in this review, immobilization results in conformational distortion of a lipase and changes the accessibility of the substrate to the active site, which can either positively or negatively influence the activity and selectivity of the lipase. The properties and catalytic performance of an immobilized lipase are closely affected by multiple factors, such as the immobilization conditions, the conformation of the lipase, the nature of the support and the composition of the reaction medium. Therefore, it is necessary to investigate different immobilization processes when aiming to optimize the functionality of an immobilized lipase.
With the evolution of chemistry towards being cleaner and more sustainable, the enzymatic enrichment of omega-3 FAs using immobilized lipases are essential to meet the green chemistry principles of design for energy efficiency and catalysis, and more research should be performed to improve the processes. For example, reusability is an important feature of immobilized enzymes and a critical factor to evaluate their commercialization potential. The immobilized enzymes should have appropriate reusability to be sustainable for use, and leverage the cost and workload of the immobilization process. However, this property has not been sufficiently investigated in the research area we discussed. Future work should be focused on the design of highly active and stable immobilized enzymes which can be used in large-scale and consecutive production of concentrated omega-3 FAs from marine by-products. The immobilization methods need to be economically and technically feasible and scalable for industrial production. A techno-economic assessment should be performed to analyze the viability of the immobilized enzymes for commercial use.
Abbreviations
1-MAG | 1-Monoacylglycerol |
2-MAG | 2-Monoacylglycerol |
1,2-DAG | 1,2-diacylglycerol |
1,3-DAG | 1,3-diacylglycerol |
EDC | 1-Ethyl-3-(3-dimethylaminopropyl)carbodiimide |
APTES | (3-aminopropyl)triethoxysilane |
ALA | α-Linolenic acid |
BTL |
Bacillus thermocatenolatus lipase |
CCL |
Candida cylindracea lipase |
CALA |
Candida antarctica lipase A |
CALB |
Candida antarctica lipase B |
DHA | Docosahexaenoic acid |
EPA | Eicosapentaenoic acid |
EE | Ethyl ester |
FA | Fatty acid |
FAEE | Fatty acid ethyl ester |
FFA | Free fatty acid |
GCL |
Geotrichum candidum lipase |
HPL |
Hypocrea pseudokoningii lipase |
MAG | Monoacylglycerol |
NHS |
N-Hydroxysuccinimide |
OA | Oleic acid |
PA | Palmitic acid |
PEG | Polyethylene glycol |
PEI | Polyethylenimine |
PU | Polyurethane |
PUFA | Polyunsaturated fatty acid |
PCL |
Pseudomonas cepacia lipase |
RML |
Rhizomucor miehei lipase |
ROL |
Rhizopus oryzae lipase |
STAGs | Structured triacylglycerols |
TLL |
Thermomyces lanuginose lipase |
YLL |
Yarrowia lipolytica lipase |
Conflicts of interest
There are no conflicts to declare.
Acknowledgements
This research was supported by the Ocean Frontier Institute, through an award from the Canada First Research Excellence Fund. The authors also acknowledge the Harris Centre, Memorial University of Newfoundland, for their financial support through the MMSB Waste Management Applied Research Fund.
References
- P. C. Calder, JPEN, J. Parenter. Enteral Nutr., 2015, 39, 18S–32S CrossRef PubMed.
- P. C. Calder, Proc. Nutr. Soc., 2018, 77, 52–72 CrossRef CAS PubMed.
- F. Shahidi and P. Ambigaipalan, Annu. Rev. Food Sci. Technol., 2018, 9, 345–381 CrossRef CAS PubMed.
-
T. Balle and D. Cowan, in Omega-6/3 Fatty Acids: Functions, Sustainability Strategies and Perspectives, eds. F. De Meester, R. R. Watson and S. Zibadi, Humana Press, 1st edn, 2013, pp. 341–352 Search PubMed.
- V. G. Alfio, C. Manzo and R. Micillo, Molecules, 2021, 26, 1002 CrossRef CAS PubMed.
-
Food and Agriculture Organization of the United Nations, Fats and fatty acids in human nutrition-Report of an expert consultation, Geneva, Switzerland, 2010 Search PubMed.
-
U.S. Department of Agriculture and U.S. Department of Health and Human Services, Dietary guidelines for Americans, 2020–2025, 2020 Search PubMed.
- J. L. Domingo, Crit. Rev. Food Sci. Nutr., 2016, 56, 979–988 CrossRef CAS PubMed.
-
Linus Pauling Institute, Essential Fatty Acids, https://lpi.oregonstate.edu/mic/other-nutrients/essential-fatty-acids, (accessed 25 July 2021).
- F. Shahidi and U. N. Wanasundara, Trends Food Sci. Technol., 1998, 9, 230–240 CrossRef CAS.
- N. Rubio-Rodríguez, M. Sara, S. Beltrán, I. Jaime, M. T. Sanz and J. Rovira, J. Food Eng., 2012, 109, 238–248 CrossRef.
-
T. Rustad, in Maximising the Value of Marine By-Products, ed. F. Shahidi, CRC Press, Boca Ratón, US, 2007, pp. 3–21 Search PubMed.
- Y. Liu, V. V. Ramakrishnan and D. Dave, Biocatal. Agric. Biotechnol., 2020, 30, 101866 CrossRef.
- S. Ferdosh, Z. I. Sarker, N. Norulaini, A. Oliveira, K. Yunus, A. J. Chowdury, J. Akanda and M. Omar, J. Food Process. Preserv., 2015, 39, 432–441 CrossRef CAS.
- L. Fiori, M. Solana, P. Tosi, M. Manfrini, C. Strim and G. Guella, Food Chem., 2012, 134, 1088–1095 CrossRef CAS PubMed.
- D. Dave and W. Routray, J. Cleaner Prod., 2018, 180, 617–641 CrossRef.
- R. Ciriminna, F. Meneguzzo, R. Delisi and M. Pagliaro, Sustainable Chem. Pharm., 2017, 5, 54–59 CrossRef CAS.
- J. A. Kralovec, S. Zhang, W. Zhang and C. J. Barrow, Food Chem., 2012, 131, 639–644 CrossRef CAS.
-
S. P. J. Namal Senanayake, in Separation, Extraction and Concentration Processes in the Food, Beverage and Nutraceutical Industries, ed. S. S. H. Rizvi, Woodhead Publishing, 2013, pp. 483–505 Search PubMed.
- Puro Omega, Concentrating omega-3 oils–Supercritical fluid technology versus molecular distillation, https://www.puroomega.com/wpcontent/uploads/2016/06/Lembke2012-NutraCos-Supercritical-versus-Molecular-Distillation-March-2012.pdf, (accessed 27 July 2021).
- N. Castejón and F. J. Señoráns, New Biotechnol., 2020, 57, 45–54 CrossRef PubMed.
- Y. He, J. Li, S. Kodali, T. Balle, B. Chen and Z. Guo, Bioresour. Technol., 2017, 224, 445–456 CrossRef CAS PubMed.
- A. M. Klibanov, Anal. Biochem., 1979, 93, 1–25 CrossRef CAS PubMed.
- R. C. Rodrigues, J. J. Virgen-Ortíz, J. C. S. dos Santos, Á. Berenguer-Murcia, A. R. Alcantara, O. Barbosa, C. Ortiz and R. Fernandez-Lafuente, Biotechnol. Adv., 2019, 37, 746–770 CrossRef CAS PubMed.
- T. Jesionowski, J. Zdarta and B. Krajewska, Adsorption, 2014, 20, 801–821 CrossRef CAS.
- A. R. Ismail and K. H. Baek, Int. J. Biol. Macromol., 2020, 163, 1624–1639 CrossRef CAS PubMed.
- R. A. Sheldon, Appl. Microbiol. Biotechnol., 2011, 92, 467–477 CrossRef CAS PubMed.
- H. H. Nguyen and M. Kim, Appl. Sci. Converg. Technol., 2017, 26, 157–163 CrossRef.
- R. A. Sheldon and S. van Pelt, Chem. Soc. Rev., 2013, 42, 6223–6235 RSC.
- P. Urrutia, R. Arrieta, L. Alvarez, C. Cardenas, M. Mesa and L. Wilson, Int. J. Biol. Macromol., 2018, 108, 674–686 CrossRef CAS PubMed.
- M. Mohammadi, Z. Habibi, S. Dezvarei, M. Yousefi, S. Samadi and M. Ashjari, Process Biochem., 2014, 49, 1314–1323 CrossRef CAS.
- M. M. Bradford, Anal. Biochem., 1976, 72, 248–254 CrossRef CAS PubMed.
- O. H. Lowry, N. J. Rosebrough, A. L. Farr and R. J. Randall, J. Biol. Chem., 1951, 193, 265–275 CrossRef CAS.
- R. Pawongrat, X. Xu and A. H-Kittikun, Food Chem., 2007, 104, 251–258 CrossRef CAS.
- C. Sampath, P. D. Belur and R. Iyyasami, Enzyme Microb. Technol., 2018, 110, 20–29 CrossRef CAS PubMed.
- M. D. Jonzo, A. Hiol, I. Zagol, D. Druet and L. C. Comeau, Enzyme Microb. Technol., 2000, 27, 443–450 CrossRef CAS PubMed.
- N. Matuoog, K. Li and Y. Yan, Mater. Res. Express, 2017, 4, 125402 CrossRef.
- S. Cui, Q. Zhou, X. Wang, S. Yang, K. Chen, Z. Dai, Y. Huang and T. Zhou, J. Food Biochem., 2017, 41, e12395 CrossRef.
- L. T. M. Hien, Ho Chi Minh City Open Uni. J. Sci., 2018, 8, 35–42 Search PubMed.
- W. G. Morais Júnior, G. Fernández-Lorente, J. M. Guisán, E. J. Ribeiro, M. M. De Resende and B. Costa Pessela, Biocatal. Biotransform., 2017, 35, 63–73 CrossRef.
- M. G. Pereira, F. D. A. Facchini, A. M. Polizeli, A. C. Vici, J. A. Jorge, B. C. Pessela, G. Férnandez-Lorente, J. M. Guisán and M. L. T. M. Polizeli, J. Mol. Catal. B: Enzym., 2015, 121, 82–89 CrossRef CAS.
- M. Mohammadi, Z. Habibi, S. Dezvarei, M. Yousefi and M. Ashjari, Food Bioprod. Process., 2015, 94, 414–421 CrossRef CAS.
- S. Moreno-Pérez, J. M. Guisan and G. Fernandez-Lorente, J. Am. Oil Chem. Soc., 2014, 91, 63–69 CrossRef.
- Y. Zhang, X. Wang, D. Xie, S. Zou, Q. Jin and X. Wang, Food Chem., 2018, 250, 60–66 CrossRef CAS PubMed.
- M. C. Michalski, C. Genot, C. Gayet, C. Lopez, F. Fine, F. Joffre, J. L. Vendeuvre, J. Bouvier, J. M. Chardigny and K. Raynal-Ljutovac, Prog. Lipid Res., 2013, 52, 354–373 CrossRef CAS PubMed.
- M. Haq, P. Pendleton and B. S. Chun, Waste Biomass Valorization, 2020, 11, 153–163 CrossRef CAS.
- G. Fernández-Lorente, C. Pizarro, D. López-Vela, L. Betancor, A. V. Carrascosa, B. Pessela and J. M. Guisan, J. Am. Oil Chem. Soc., 2011, 88, 819–826 CrossRef.
-
M. Bechtold and S. Panke, in Comprehensive Chirality, eds. E. M. Carreira and H. Yamamoto, Elsevier Science, 1st edn, 2012, pp. 71–100 Search PubMed.
- A. M. Klibanov, Nature, 2001, 409, 241–246 CrossRef CAS PubMed.
- A. Bastida, P. Sabuquillo, P. Armisen, R. Fernández-Lafuente, J. Huguet and J. M. Guisán, Biotechnol. Bioeng., 1998, 58, 486–493 CrossRef CAS PubMed.
- G. Fernández-Lorente, L. Betancor, A. V. Carrascosa and J. M. Guisán, J. Am. Oil Chem. Soc., 2011, 88, 1173–1178 CrossRef.
- G. Fernández-Lorente, L. Betancor, A. V. Carrascosa, J. M. Palomo and J. M. Guisan, J. Am. Oil Chem. Soc., 2012, 89, 97–102 CrossRef.
- G. Fernandez-Lorente, M. Filice, D. Lopez-Vela, C. Pizarro, L. Wilson, L. Betancor, Y. Avila and J. M. Guisan, J. Am. Oil Chem. Soc., 2011, 88, 801–807 CrossRef CAS.
- M. G. Pereira, F. D. A. Facchini, L. E. C. Filó, A. M. Polizeli, A. C. Vici, J. A. Jorge, G. Fernandez-Lorente, B. C. Pessela, J. M. Guisan and M. L. T. M. Polizeli, Process Biochem., 2015, 50, 561–570 CrossRef CAS.
- W. G. de Morais Júnior, C. R. F. Terrasan, G. Fernández-Lorente, J. M. Guisán, E. J. Ribeiro, M. M. de Resende and B. C. Pessela, Eur. Food Res. Technol., 2017, 243, 1375–1384 CrossRef.
- G. Fernandez-Lorente, J. Palomo, Z. Cabrera, R. Fernandez-Lafuente and J. Guisán, Biotechnol. Bioeng., 2007, 97, 242–250 CrossRef CAS PubMed.
- M. Filice, M. Marciello, L. Betancor, A. V. Carrascosa, J. M. Guisan and G. Fernandez-Lorente, Biotechnol. Prog., 2011, 27, 961–968 CrossRef CAS PubMed.
- L. A. Frank, G. R. Onzi, A. S. Morawski, A. R. Pohlmann, S. S. Guterres and R. V. Contri, React. Funct. Polym., 2020, 147, 104459 CrossRef CAS.
- M. L. Verma, S. Kumar, A. Das, J. S. Randhawa and M. Chamundeeswari, Environ. Chem. Lett., 2020, 18, 315–323 CrossRef CAS.
- M. Ashjari, M. Mohammadi and R. Badri, J. Mol. Catal. B Enzym., 2015, 122, 147–155 CrossRef CAS.
- M. Yousefi, M. Marciello, J. M. Guisan, G. Fernandez-Lorente, M. Mohammadi and M. Filice, Molecules, 2020, 25, 545 CrossRef CAS PubMed.
- J. Dyerberg, P. Madsen, J. M. Møller, I. Aardestrup and E. B. Schmidt, Prostaglandins, Leukotrienes Essent. Fatty Acids, 2010, 83, 137–141 CrossRef CAS PubMed.
- J. Neubronner, J. P. Schuchardt, G. Kressel, M. Merkel, C. Von Schacky and A. Hahn, Eur. J. Clin. Nutr., 2011, 65, 247–254 CrossRef CAS PubMed.
- S. Ghasemifard, G. M. Turchini and A. J. Sinclair, Prog. Lipid Res., 2014, 56, 92–108 CrossRef CAS PubMed.
- K. E. Rice, J. Watkins and C. G. Hill, Biotechnol. Bioeng., 1999, 63, 33–45 CrossRef CAS PubMed.
- T. O. Akanbi and C. J. Barrow, Food Chem., 2017, 229, 509–516 CrossRef CAS PubMed.
- J. Yan, X. Gui, G. Wang and Y. Yan, Appl. Biochem. Biotechnol., 2012, 166, 925–932 CrossRef CAS PubMed.
- T. Okada and M. T. Morrissey, J. Food Sci., 2008, 73, 146–150 CrossRef PubMed.
- M. N. Gupta, M. Kaloti, M. Kapoor, K. Solanki, M. N. Gupta, M. Kaloti, M. Kapoor and K. Solanki, Artif. Cells, Blood Substitutes, Biotechnol., 2011, 39, 98–109 CrossRef CAS PubMed.
- N. Matuoog, K. Li and Y. Yan, J. Food Biochem., 2018, 42, e12549 CrossRef.
- T. Liu, Y. Zhao, X. Wang, X. Li and Y. Yan, Bioresour. Technol., 2013, 132, 99–102 CrossRef CAS PubMed.
-
A. Sadana, in Bioseparations of Proteins: Unfolding/Folding and Validations, ed. S. Ahuja, Academic Press, 1st edn, 1998, vol. 1, pp. 287–312 Search PubMed.
-
A. Vaidya and L. Fischer, in Immobilization of Enzymes and Cells, ed. J. M. Guisán, Humana Press, 2nd edn, 2006, pp. 175–183 Search PubMed.
- L. M. de S. Brandão, M. S. Barbosa, R. L. Souza, M. M. Pereira, Á. S. Lima and C. M. F. Soares, Biotechnol Prog., 2021, 37, e3064 CrossRef PubMed.
- J. Yan, S. Liu, J. Hu, X. Gui, G. Wang and Y. Yan, Bioresour. Technol., 2011, 102, 7154–7158 CrossRef CAS PubMed.
- A. V. Gutierrez, M. Hedström and B. Mattiasson, Biotechnol. Rep., 2016, 11, 12–17 CrossRef PubMed.
- L. Deng, X. Xu, G. G. Haraldsson, T. Tan and F. Wang, J. Am. Oil Chem. Soc., 2005, 82, 341–347 CrossRef CAS.
- Y. Watanabe, Y. Shimada, A. Sugihara and Y. Tominaga, J. Biosci. Bioeng., 1999, 88, 622–626 CrossRef CAS PubMed.
- S. Moreno-Perez, D. F. M. Turati, J. P. Borges, P. Luna, F. J. Señorans, J. M. Guisan and G. Fernandez-Lorente, J. Agric. Food Chem., 2017, 65, 117–122 CrossRef CAS PubMed.
- E. P. Cipolatti, S. Moreno-Pérez, L. T. A. Souza, A. Valério, J. M. Guisán, P. H. H. de Araújo, C. Sayer, J. L. Ninow, D. de Oliveira and B. C. Pessela, J. Mol. Catal. B: Enzym., 2015, 122, 163–169 CrossRef CAS.
- E. P. Cipolatti, A. Valério, J. L. Ninow, D. de Oliveira and B. C. Pessela, Biochem. Eng. J., 2016, 112, 54–60 CrossRef CAS.
- A. M. Fureby, C. Virto, P. Adlercreutz and B. Mattiasson, Biocatal. Biotransform., 1996, 14, 89–111 CrossRef CAS.
- E. Hita, A. Robles, B. Camacho, A. Ramírez, L. Esteban, M. J. Jiménez, M. M. Muñío, P. A. González and E. Molina, Process Biochem., 2007, 42, 415–422 CrossRef CAS.
- R. Pawongrat, X. Xu and A. H-Kittikun, J. Sci. Food Agric., 2008, 88, 256–262 CrossRef CAS.
- M. S. Christensen, C. E. Høy, C. C. Becker and T. G. Redgrave, Am. J. Clin. Nutr., 1995, 61, 56–61 CrossRef CAS PubMed.
- M. del M. Muñío, L. Esteban, A. Robles, E. Hita, M. J. Jiménez, P. A. González, B. Camacho and E. Molina, Process Biochem., 2008, 43, 1033–1039 CrossRef.
- U. Schmid, U. J. Bornscheuer, M. M. Soumanou, G.
P. McNeill and R. D. Schmid, J. Am. Oil Chem. Soc., 1998, 75, 1527–1531 CrossRef CAS.
- L. Esteban, M. del M. Muñío, A. Robles, E. Hita, M. J. Jiménez, P. A. González, B. Camacho and E. Molina, Biochem. Eng. J., 2009, 44, 271–279 CrossRef CAS.
- L. Martín Valverde, P. A. González Moreno, A. Rodríguez Quevedo, E. Hita Peña, M. J. Jiménez Callejón, L. Esteban Cerdán, E. Molina Grima and A. Robles Medina, J. Am. Oil Chem. Soc., 2012, 89, 1633–1645 CrossRef.
- B. Mbatia, B. Mattiasson, F. Mulaa and P. Adlercreutz, Eur. J. Lipid Sci. Technol., 2011, 113, 717–723 CrossRef CAS.
|
This journal is © The Royal Society of Chemistry 2022 |
Click here to see how this site uses Cookies. View our privacy policy here.