DOI:
10.1039/D1EN00872B
(Critical Review)
Environ. Sci.: Nano, 2022,
9, 40-60
Dimension dependency of tungsten oxide for efficient gas sensing
Received
20th September 2021
, Accepted 17th November 2021
First published on 30th November 2021
Abstract
Air pollution is a serious concern worldwide as it affects living beings and the environment due to the release of hazardous gases and particulate matter. The major cause of pollution is toxic gases which are released by industries, automobiles, waste and stubble burning, etc. In order to detect and monitor toxic gases like CO, NOx, H2S, SO2, NH3etc., metal oxide-based gas sensors are developed owing to their exotic properties such as high sensitivity, rapid response, and recyclability as well as long stability. Among these metal oxides, tungsten oxide (WO3) is a transition metal oxide having enormous potential in gas-sensing applications. It is perceived that the morphology of tungsten oxide plays a significant role in the effective detection of these hazardous gases. The low dimension (0D, 1D, 2D) and hierarchical structures of WO3 exhibit enhanced sensitivity and are utilized for efficient sensing of various gases. In this review, we have comprehensively discussed the effect of the morphology of tungsten oxide nanostructures and their application in detection of gases such as H2, NH3, H2S, CO, O3, VOCs etc. Further, the sensing mechanism along with the impact of surface functionalization and doping has been discussed. The challenges in the field and future prospects have also been discussed.
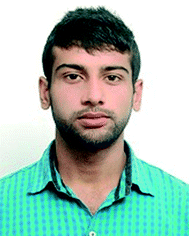 Mr. Aditya Yadav | Mr. Aditya Yadav is a research scholar pursuing his Ph.D. at the Academy of Scientific and Innovative Research (AcSIR). He obtained his master's degree in Physics from Maharishi Dayanand University, Rohtak, India. His current research activity is focused on the fabrication of metal oxide thin films and nanostructure-based efficient gas sensors for detection of pollutant gases. |
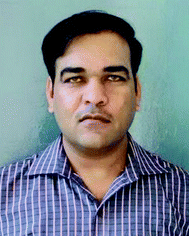 Preetam Singh | Dr. Preetam Singh is Principal Scientist at the CSIR-National Physical Laboratory, New Delhi, India. He received his Ph.D. degree in Condensed Matter Physics (Experimental) from Indian Institute of Technology Roorkee (IIT Roorkee), India, in 2008. He has worked as a Postdoctoral Fellow at Inha University, Incheon, Republic of Korea, during 2008–2009. He has expertise in the growth of high-quality thin films of metals, metal oxide semiconductors by PVD and CVD techniques and their characterization. His current research activity is focused on the fabrication of metal oxide thin films and nanostructures for the application of solid-state gas sensors. |
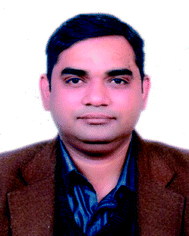 Govind Gupta | Prof. Dr. Govind Gupta is Senior Principal Scientist and Professor, Academy of Scientific and innovative Research (AcSIR), Head, Sensor Devices and Metrology Group, CSIR-National Physical Laboratory (National Measurement Institute of India), New Delhi, India, and Professor, Academy of Scientific and Innovative Research (AcSIR). He received his Ph.D. in Solid State Physics from G. B. Pant Univ. Ag. and Tech., Pantnagar, India, in 2000. His core area of expertise is growth of III-nitrides, metal oxide and 2D materials, and fabrication of smart optical and gas sensors. He has published >250 research articles in SCI journals. He is a Senior Member of the IEEE (USA) and Associate Academician of Asia Pacific Academy of Materials (APAM). He has received numerous awards and fellowships including the MRSI medal, Young Scientist Medal, National Academy of Sciences, India, BOYSCAST fellowship, etc. |
Environmental significance
Air pollution is a serious concern worldwide as it affects living beings and the environment due to the release of hazardous gases and particulate matter. The major cause of pollution is toxic gases which are released by industries, automobiles, waste and stubble burning, etc. In order to detect and monitor toxic gases like CO, NOx, H2S, SO2, NH3etc., metal oxide-based gas sensors are developed owing to their exotic properties such as high sensitivity, rapid response, and recyclability as well as long stability. We have focused on the dimensionality of WO3, which is one of the best suited for the detection of gases such as H2, NH3, H2S, CO, O3, VOCs etc. The low dimension (0D, 1D, 2D) and hierarchical structures of WO3 exhibit enhanced sensitivity and are utilized for efficient sensing of various gases. The impact of structure dimensionality and surface morphologies on the gas-sensing behaviour is discussed. Further, the influence of surface functionalization and doping has also been discussed. The challenges in the field and future perspectives have also been discussed which can be useful for development of faster and efficient gas sensors.
|
1. Introduction
Several industrial and domestic activities like automobile discharge, burning fossil fuels etc. emit gases which are harmful, affect the environment and cause air pollution.1 The majority of the gases emitted as industrial waste are poisonous like CO, NOx, SO2, NH3, H2S, etc. and to determine the concentration of these harmful/poisonous gases, special devices called gas sensors have been explored.2 Among the various types of gas sensors, metal oxide (SnO2, ZnO, WO3, MoO3etc.)-based gas sensors have been utilized to accurately detect these hazardous gases (CO, NOx, etc.) in parts per million (ppm) or in sub-parts per billion (sub ppb) range. These metal oxide gas sensors are portable, highly sensitive, fast responsive, stable, inexpensive and offer accurate and reliable data.3,4 Their smaller size and high sensitivity in identifying extremely low concentrations of a variety of gaseous chemical species are the significant factors which make them promising candidates in the field of gas sensors.5,6 Among various available metal oxide semiconducting materials (i.e., ZnO, SnO2, CuO, TiO2, NiO etc.), tungsten oxide (WO3) emerges as one of the best sensing materials due to its chemical and thermal stability at room temperature. WO3 has a wide bandgap which varies between 2.6 and 3.2 eV.7 It is an intrinsically n-type bulk semiconductor having a cubic perovskite-like structure with a tungsten atom at the centre of each octahedron and oxygen atoms at the corners. Tungsten oxide is also available in various structures which are temperature dependent; for example, it is in the tetragonal phase for temperatures >740 °C, orthorhombic in the temperature range 330–740 °C, monoclinic in the temperature range 17–330 °C and triclinic in the temperature range −50 to 17 °C.7 In addition, it can easily be synthesized into nanoparticles and nanofilms by simple and inexpensive methods like solvothermal, hydrothermal etc.8 Diverse sizes and shapes have been attained from 0D to 3D which have shown incredible enhancement in the sensing response towards various gases. It was observed that the sensing response of the fabricated device is strongly dependent on the morphology of the grown structure. In this review, we focus on the size- and shape (morphology)-dependent sensing response of tungsten oxide nanostructures towards efficient detection of various gases. In addition, the growth process of tungsten oxide structures using different techniques has also been summarized. The impact of morphology on the parameters like selectivity, sensitivity, response time and stability of the fabricated device has also been discussed which highlights the influence of dimensionality and surface morphology on the functioning of these gas sensors. Further, to enhance the performance of the gas-sensing devices, strategies such as heterojunctions, doping and surface functionalization using noble metals are discussed. The challenges and future prospects related to tungsten oxide-based gas sensors have also been discussed.
2. Morphology-dependent growth of WO3
It has been described that the dimensionality and surface morphology of the sensing film plays an important role in enhancement of the sensing performance of the device. As of now, diverse shape- and size-based tungsten oxide has been grown using different techniques such as thermal evaporation,9,10 magnetron sputtering,11 chemical vapour deposition,12,13 hydrothermal,14,15 template-assisted growth,16,17etc. Each of the above-mentioned techniques has a set of parameters that control the morphology of the synthesized WO3. Further, nanoscale structures are highly desired due to the high surface-to-volume ratio which plays a significant role in sensing. On the basis of dimension in the nanoscale, structural dimensions are sorted into various kinds: zero-dimensional (0D) structures (i.e., nanoparticles,18 quantum dots,19 nanoclusters20), one-dimensional (1D) structures (i.e., nanowires,13 nanofibers,21 nanorods,22 nanotubes10,23), two-dimensional (2D) structures (i.e., nanosheets,24 nanobelts,25 superlattices26) and three dimensional (3D) and hierarchical structures (i.e., nanoflowers27etc.). Composite structures which are created by one or more than one low-dimensional structures are known as 3D structures. It is perceived that tungsten oxide-based gas sensing is significantly dependent upon the method of preparation and the dimensionality of the grown material. Hence, it is essentially required to discuss the dimensionality-dependent sensing of WO3-based structures for various gases. In this review, we have discussed the dimension-dependent tungsten oxide structures (0D, 1D, 2D, and 3D) and their response towards various gases.
2.1. Zero-dimensional (0D) structure
Zero-dimensional structures are spherical or near-spherical structures and also the simplest. Gas adsorption and diffusion are not favoured by the low specific surface area and porous structure of 0D tungsten oxide. Thereby, such 0D structures like quantum dots and tiny nanoparticles lead to unsatisfactory performance in gas sensing but are popular in the application in electronic and optical devices and catalysis.28,29 The detection of the targeted gas may be increased due to the agglomeration and coarsening of such nanoparticles while fabricating the gas sensor. Recently, Yu et al. prepared colloidal quantum dots of WO3 using a solvothermal method with a precursor of WCl6 mixed with ethanol, oleyl amine and oleic acid,30 which were utilized as a sensing layer to detect H2S gas in chemiresistive gas sensors. By adjusting the reaction temperature, the grain size distribution of WO3−x quantum dots was pre-controlled from 1.3 nm to 4.5 nm. The fabricated WO3−x quantum dot-based sensors showed high stability, high sensitivity and the lowest detection limit at room temperature towards formaldehyde due to rich oxygen vacancy defects and their very small size.31 For the appropriate use of rich oxygen vacancies of nanoparticles or quantum dots of WO3, other sensing materials such as graphene or reduced graphene are used to disperse these quantum dots.32 Recently, Li et al.15 utilized a hydrothermal method to synthesize highly dispersible nanoparticles of WO3 with varying grain sizes between 10 and 50 nm. Similarly, Santos et al.33 synthesized WO3 nanoparticles with a size varying from 46 nm to 234 nm using a hydrothermal technique for biosensing applications. Zhang et al.34 synthesized microspheres of WO3 by using a hydrothermal method and found that uniform microspheres have better dispersion (Fig. 1).
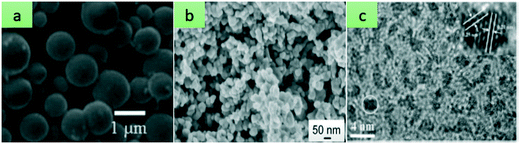 |
| Fig. 1 Tungsten oxide with different 0D nanostructures: (a) microspheres,34 (b) nanoparticles,15 and (c) quantum dots.29 The figure is adapted with permission from ref. 15, 29 and 34. Copyright (2021) Elsevier. | |
2.2. One-dimensional (1D) structures
One-dimensional structures are those which have two dimensions in the nanoscale, with the other one being of non-nanoscale size, such as nanowires, nanotubes etc. These one dimensional structures are also under goes effective transformation of chemical species on the surface which makes them suitable for gas sensing applications.35 1D structures also have a unique feature, i.e., specific electron flow in a particular dimension, as their length is larger than their diameter. For most of the gas sensor devices, the preferred size of the structure is in the millimetre (mm) range; therefore, a single 1D structure does not meet the required size and joining multiple 1D structures will hinder the electron flow which could be solved by using an ultralong 1D structure. Recently, Saidi et al. have grown WO3 nanowires13 by an aerosol-assisted chemical vapour deposition technique and showed that they could be the best to detect volatile organic compounds (VOCs). Another simple and versatile technique considered for the development of several fibres and nanotubes over a wide variety of applications is electrospinning.36 Leng et al. synthesized WO3 nanofibers by an electrospinning technique for ammonia sensors operable at 500 °C.37 Long et al. have synthesized substrate-free WO3 nanorod arrays for NH3 (ref. 38) sensing using a hydrothermal process which showed a high response of 8.3 for NH3 (50 ppm) at 200 °C. Also, Punetha et al. synthesized WO3 nanorods14 using a hydrothermal process which at 250 °C showed a response of 4.64 for a concentration of 5 ppm NH3 gas. San et al.10 fabricated WO3 nanotube arrays using a thermal evaporation method onto a Si substrate which showed a maximum response of 7.3 at 150 °C towards 10 ppm H2S gas (Fig. 2).
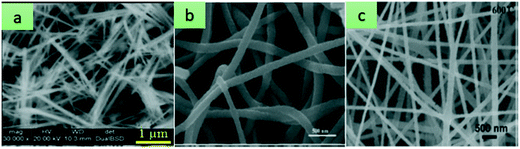 |
| Fig. 2 Tungsten oxide with different 1D nanostructures: (a) nanowires,13 (b and c) nanofibers.37,39 The figure is adapted with permission from ref. 13, 37 and 39. Copyright (2021) Elsevier. | |
2.3. Two-dimensional (2D) structures
Two-dimensional structures are those which have only one dimension in the nanoscale such as thin films, nanosheets etc. Such structures offer a high surface-to-volume ratio, modulated surface activities, rich oxygen vacancies and surface polarisation which are useful for giving a high sensor response.40 Due to strong interconnection and abundant active sites, they have more channels for electron transfer which can enhance the reaction ratio between the sensing material and the target gas.41 In addition, porous sub-microstructures play a major role in enhancing the gas-sensing performance of the thin films. Recently, thickness-dependent NO2 sensing of tungsten oxide film prepared by reactive ion magnetron sputtering has been reported by Prajapati et al.11 The fabricated sensors display high sensitivity and selectivity towards NO2 gas, and a detection limit of 16 ppb is achieved. In addition, Preiß et al.42 has prepared porous WO3 thin films by picosecond laser deposition which exhibits an increase in the porosity on increasing the oxygen pressure that leads to enhanced sensitivity. WO3 nanoflake (vertically oriented) array films were also synthesized by an electrochemical technique which has been utilized for room-temperature H2S sensing.43 Various other techniques such as solvothermal44 and hydrothermal45 have also been employed for synthesizing WO3 structures. The hydrothermal technique is used to develop various kinds of structures. WO3 nanoplates with thickness varying from 90 to 150 nm grown by a hydrothermal technique have been deposited directly onto a soda lime glass substrate for NO2 gas detection.45 Similarly, Zhang et al.46 synthesized WO3 nanoplates with a length of 100–170 nm and thickness of 30–50 nm with thickness varying from tens to hundreds of nanometers.47–49 Also, under the assistance of the surface templates polyethylene oxide–polypropylene oxide–polyethylene oxide, 2D tungsten oxide nanosheets having a thickness of 10 nm and rich in defects were synthesized for the detection of NO2 gas.50 Further, 2D WO3 nanosheets were prepared by utilizing a facile wet-chemical synthesis process, i.e. sonication of tungsten particles in an acidic environment followed by thermal treatment.51 In addition, Rahmani et al. prepared WO3 nanosheets by anodization of tungsten film for H2 sensing in the range of 0.06% to 1%.52 Recently, Yuan53 used a porous silicon (PS) surface as a template to grow 2D WO3 hollow nanoscale structures by using a spin coating technique; the fabricated device displays high sensitivity and high response characteristics towards NO2 gas. Huang et al.54 synthesized Ru-loaded nanosheets of tungsten oxide using a facile impregnation technique for hydrogen gas-sensing application (Fig. 3).
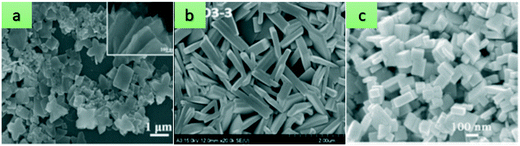 |
| Fig. 3 Tungsten oxide with different 2D nanostructures: (a) nanosheets,50 (b and c) nanoplates.45,46 The figure is adapted with permission from ref. 45, 46 and 50. Copyright (2021) Elsevier. | |
2.4. Three-dimensional (3D) and hierarchical structures
Three-dimensional WO3 is an assembly of nanoplates, nanoparticles, nanosheets, and nanorods to form structures like microflowers, microspheres, urchin-like structures and other irregular structures. Such structures help in achieving better gas-sensing performance due to their high porosity, larger specific surface area and unique morphology.55 3D structures mainly consist of polyhedra and hierarchical structures which do not exhibit anisotropic growth and usually have complicated structures with diverse properties. The synthesis of WO3 polyhedra mainly includes octahedral structures which are typically enclosed by surfaces with relatively high energy. Hence, such types of surfaces tend to be unstable during the reaction and thus are converted into low-energy surfaces which are more stable.56 Mesoporous tungsten oxide (M-WO3) can be functionalized by noble metal (Au, Ag, Pt etc.) particles or heterojunction formation, i.e., coupling with other sensing materials, thereby enhancing the performance. Ma et al. have used homogeneously distributed Pt nanoparticles (4 nm) onto a WO3 matrix for the detection of CO.57 Wu et al.58 synthesized palladium-doped M-WO3 for H2 gas sensors using a hard template method which showed a sensor response of 11.78 for 5000 ppm H2 gas at room temperature. Hydrothermal, microwave-assisted hydrothermal and sonochemical methods are convenient techniques to prepare 3D microspheres of WO3,59,60 urchin-like structures,61,62 microflowers63,64 and other WO3 structures.65,66 Recently, Li et al. used a 1-pot hydrothermal method to synthesize polyethyleneimine-mediated WO3 nanoparticles,67 which showed a sensing response of 251.7 for 5 ppm NO2 at 100 °C. Also, Wei et al.68 developed cauliflower-like WO3 nanostructures using a hydrothermal synthesis process and the developed sensor exhibits good selectivity towards CO gas at 270 °C. Behera et al.69 synthesized WO3 nanorods by a thermal oxidation technique for NO2 gas sensing with a lower detection limit of 2 ppm. Recently, Ding et al.70 prepared pure WO3 urchin-like hollow spheres using a hydrothermal method with diameter ranging between 1.5 and 2 μm.
Hierarchical structures are those which are formed by assembling 0D, 1D and 2D structures. Song et al., using a solvothermal method,71 synthesized WO3 nanotubes. Adhikari et al.72 developed cauliflower-like hierarchical tungsten oxide structures using a microwave hydrothermal method. Tong et al.73 prepared nanoporous WO3 nanorod bundles. Wang et al.,74 using a microwave-assisted solvothermal method, synthesized WO3 with a flower-like architecture which was developed by inter-crossing nanorods, where the flower-like structure has a high surface area and more interspacing which is significantly useful for gas-sensing applications. Also, Wang et al.,75 by calcining the acid-treated hydrothermal precursor, fabricated flower-like WO3 which displayed a high response and good selectivity towards NO2 gas. You et al.76 used an acid-treated CaWO4 hollow microsphere precursor to synthesize hierarchical WO3 hollow microspheres with a porous structure of size 1 nm to 170 nm, which were found to be highly sensitive for NO2 gas. Utilizing the hydrothermal method, Shen et al.77 developed WO3 flocky microspheres having a particle size of 3–4 μm. A facile template-free method was used by Zhang et al.78 to synthesize WO3 core–shell microspheres where the core was made up of a group of nanoparticles enclosed by a shell layer which are self-assembled by nearly 15 mm thick ultrafine nanoplates (Fig. 4).
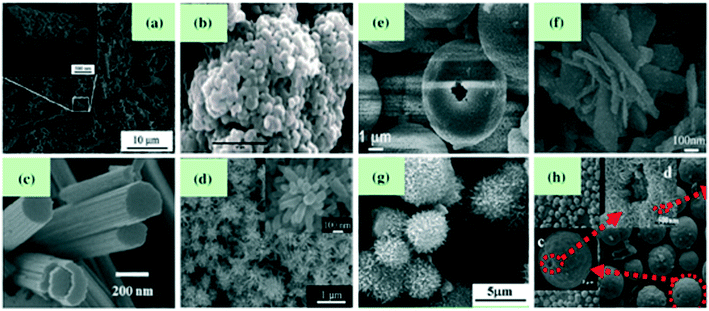 |
| Fig. 4 Tungsten oxide with various hierarchical structures: (a and b) Nanotubes71 and cauliflower-like structure72 (group of 0D nanostructures), (c and d) nanorod bundles73 and flower-like architectures74 (group of 1D nanostructures), (e and f) hollow microspheres76 and flower-like structures75 (group of 2D nanostructures), (g) flocky microspheres (group of 0D and 1D nanostructures),77 (h) core–shell microspheres (group of 0D and 2D nanostructures).78 The figure is adapted with permission from ref. 71–78. Copyright (2021) Elsevier. | |
3. Gas-sensing mechanism of WO3
The concept of the gas-sensing mechanism of tungsten oxide can be explained by the variation in resistance of the sensing film on contact with the target gas. Initially, in the oxygen environment, atmospheric oxygen will be adsorbed on the surface of tungsten oxide as a material by the process of physical adsorption normally at temperatures above 200 °C. The oxygen will capture the electrons from the surface of tungsten oxide, becoming an oxygen ion (like O2−, O−, O2−)79 and thereby creating an electron depletion region in WO3 grains. Hence, the potential barrier for electron transport across WO3 grains increases, which will determine the initial resistance of the tungsten oxide thin film, as shown in Fig. 5(a). Then, the target gas adsorbed on the WO3 surface will interact with the oxygen ions and change the resistance of the metal oxide.
In an oxidizing gas80 environment, for example, NO gas will react with oxygen ions to emit N2 and the gas gives oxygen atoms to the material, decreasing the oxygen vacancies in the material. Therefore, the potential barrier will increase and thereby increase the electrical resistance of the n-type semiconductor as a function of time.
| NO(gas) + e− → NO−(ads) | (1) |
| 2NO−(ads) → N2(gas) + 2O−(ads) | (2) |
In a reducing gas environment, for example, CO (ref.
81) gas will react with oxygen ions to emit CO
2 and the captured electrons will be released back to the tungsten oxide semiconductor, as shown in
Fig. 5(b). Therefore, the potential barrier will decrease, thereby decreasing the electrical resistance of the tungsten oxide semiconductor as a function of time.
| CO(g) + O−(ad) → CO2(g) + e−, | (3) |
| CO + 2O− → CO32− → CO2 + 1/2 O2 + 2e−. | (4) |
The performance of the tungsten oxide-based gas sensor is classified by various performance parameters such as sensitivity, selectivity, response and recovery time, and stability.
Sensitivity is the comparison of resistance of the sensing element in the presence of gas to that in air. Mathematically, sensitivity is Ra/Rg and Rg/Ra for reducing and oxidizing gases, respectively. The resistance of the gas sensor in the reference gas is represented by Ra and the resistance of the gas sensor in the target gas is represented by Rg.82 Percentage sensitivity83 is given by
| S (%) = [(Ra − Rg)/Ra] × 100. | (5) |
The property by which the gas sensor senses only the specific or targeted gas among other gases is known as selectivity.
84 Stability
85 accounts for the overall repeatable capacity of the sensor device. With successive measurements, if the device is able to recover back to its base value and reaches the same peaks in the same time frame, then the device is said to be fit for further use. Response time
86 is the time (total) taken by the gas sensor to reach the 90% value of its change in resistance after introducing the target gases. Recovery time
86 is the total time taken by the gas sensor to recover back to 90% of the initial resistance value upon removal of the target gases. Temperature and humidity are also important factors influencing the performance of metal oxide gas sensors.
87 The conduction mechanism of WO
3 humidity sensor depends on H
+ or H
3O
+ resulting from the dissociation of adsorbed water on the metal oxide surface, and these H
+ or H
3O
+ ions hops between adjacent hydroxyl groups. In this process water adsorbing on the metal oxide surface will not donate electrons to the sensing layers which will reduce the sensitivity and enhance the recovery time of WO
3 sensors. Further, the adsorption of water will lead to less chemisorption of oxygen species on the surface due to the decrease of surface area which is responsible for the sensor response. Moreover, at high temperature, the sensing response initially increases and will reach a maximum value and then decreases rapidly with increasing temperature. In addition, at high temperatures, small grains tend to agglomerate into large entities, decreasing both the surface area and catalytic properties of the material.
88 Thus, it is requisite to keep a balance between decreasing grain sizes and stability.
3.1. Gas sensing with tungsten oxide structures
Tungsten oxide is an important material to detect hazardous gases. These gases are mainly of two types: oxidizing and reducing gases. Gases that have the capability of accepting electrons from the surface of metal oxide are called oxidizing gases (O2, NO2, CO2etc.). Oxygen adsorbs swiftly on the surface of the metal oxide. Gases that have the capability to donate electrons and which can desorb the chemisorbed oxygen ions and physisorbed hydroxyl ions from the metal oxide surface are called reducing gases (CO, H2, NH3, H2S etc.). WO3 can sense various kinds of gases: (1) flammable gases that have a strong possibility of exploding on mixing with oxygen, e.g., H2; (2) toxic gases, which are the by-product of industries, can cause a prompt response in human beings, e.g., CO, NH3, H2S; (3) inorganic gases, the environment has a constant concentration of gases and variation in their concentration will cause a series of changes, e.g., O3; and (4) organic gases that can be subdivided into the above three types and are known as volatile organic compounds (VOCs).
3.1.1. Hydrogen (H2).
Tungsten oxide is considered as the most promising material to detect hydrogen gas.89 H2 is an odourless, tasteless and non-toxic gas in nature. However, the high concentration of H2 gas in the atmosphere can cause a lack of oxygen in the environment, which may affect human health with symptoms including headache, vomiting, unconsciousness etc. It is hazardous to health if the concentration surmounts the threshold limit value (TLV) of 500–700 ppm (causes death after 30–60 min) and causes nearly instant death in concentrations of 1000–2000 ppm. Therefore, monitoring of the concentration of H2 gas is necessary, which can be done by a hydrogen gas sensor. Rahmani et al.52 synthesized WO3 by anodization of tungsten film which showed an optimum response of about 80% for 1% H2 exposure at the temperature of 250 °C; the response time and recovery time were 120 s and 235 s respectively. Wu et al.58 perceived that bare WO3 powder is not appropriate to detect even 5000 ppm H2 gas at room temperature (RT) due to inconsistent sensing. They utilized a hard template method and used Pd-loaded mesoporous WO3 (Pd/M-WO3) as the sensing material, which exhibited the highest hydrogen sensing ability in comparison to WO3 powder and synthetic M-WO3. Different types of structures were utilized to fabricate sensors and the performance was compared to analyse the hydrogen sensitivity, as shown in Fig. 6(a). It was discussed that the doping in mesoporous WO3 with Pd effectively improves the specific surface area, which leads to absorption of large amounts of oxygen on the surface as well as inside the material, improving the sensing efficiency (from 1.01 to 11.78) with an extremely short recovery time (10 s) and making the fabricated sensor an ideal device highly selective for H2, as shown in Fig. 6(b).
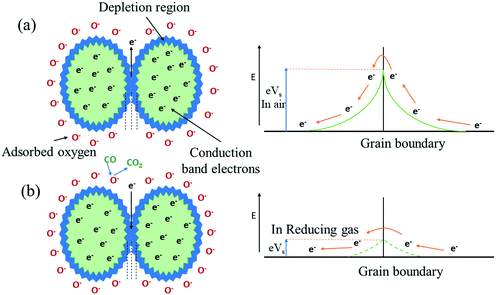 |
| Fig. 5 Mechanism of semiconductor gas sensors for an n-type material in (a) an oxygen environment and (b) a reducing gas (CO) environment. | |
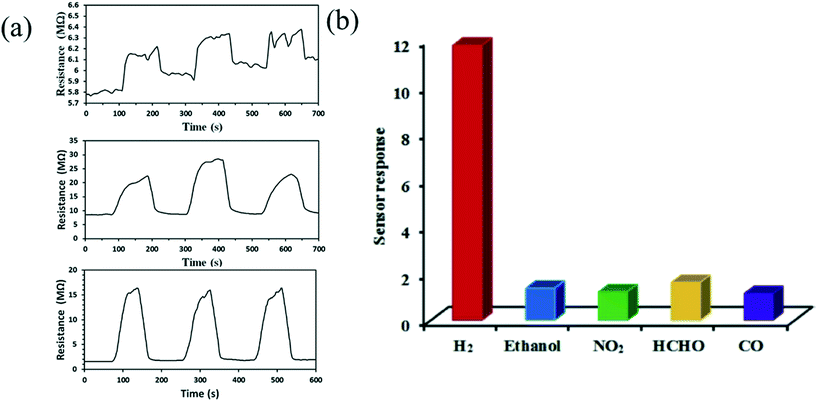 |
| Fig. 6 (a) Variation in resistance of WO3, MWO3, and Pd/MWO3 to hydrogen (5000 ppm) at RT. (b) Response of WO3 towards various gases at RT.58 The figure is adapted with permission from ref. 58. Copyright (2021) Elsevier. | |
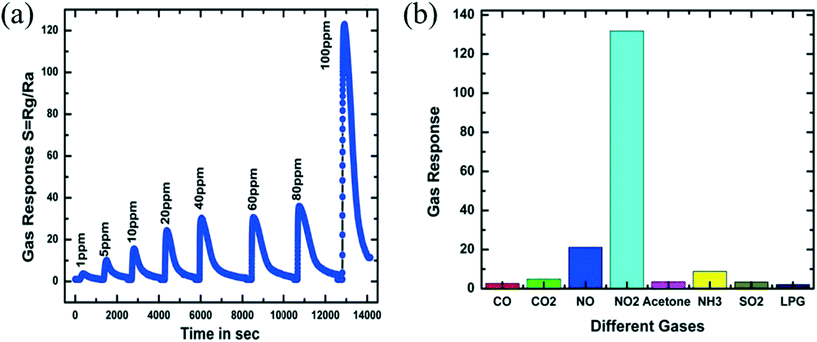 |
| Fig. 7 (a) Sensor response curves of a WO3 film sensor towards various concentrations of NO2 at 100 °C, (b) responses of WO3 film for different gases (for 100 ppm) at 100 °C.45 The figure is adapted with permission from ref. 45. Copyright (2021) Elsevier. | |
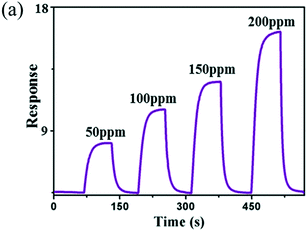 |
| Fig. 8 (a) Sensor response curve of WO3 arrays towards NH3 for different concentrations at a temperature of 200 °C.38 The figure is adapted with permission from ref. 38. Copyright (2021) Elsevier. | |
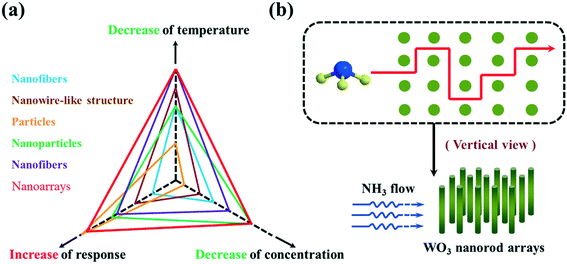 |
| Fig. 9 (a) Comparison of various tungsten oxide-based NH3 sensors. (b) The ammonia-sensing mechanism of WO3 nanorods.38 The figure is adapted with permission from ref. 38. Copyright (2021) Elsevier. | |
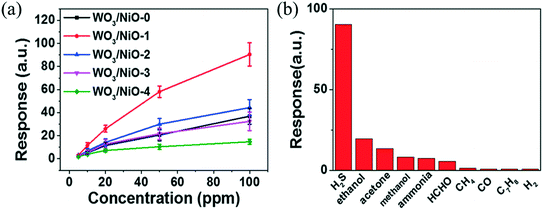 |
| Fig. 10 (a) Response curves for mesoporous WO3/NiO−x (x = 0, 1.0, 2.0, 3.0, and 4.0) samples for H2S at 250 °C. (b) Responses of the mesoporous WO3/NiO−x-based sensor for different gases (50 ppm) at 250 °C.110 The figure is adapted with permission from ref. 114. Copyright (2020) American Chemical Society. | |
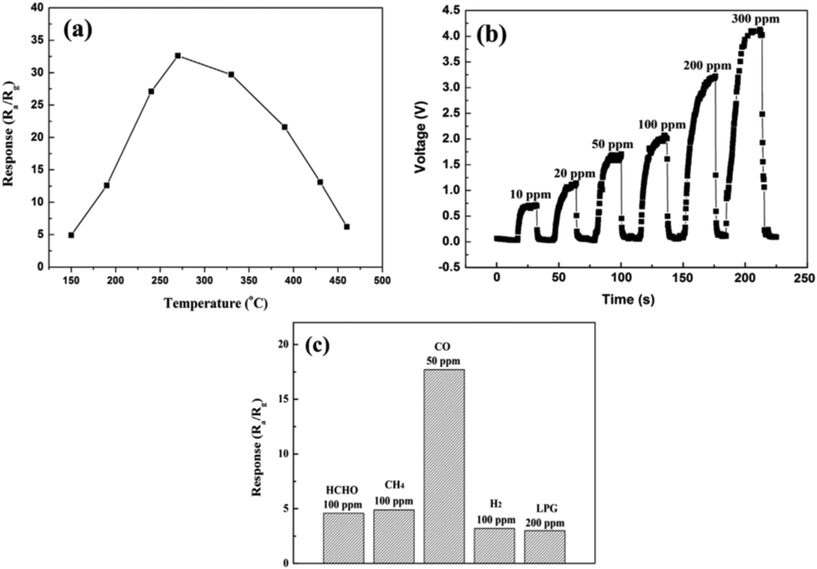 |
| Fig. 11 (a) Response curve for CO gas at different temperatures, (b) sample response towards different concentrations of CO, (c) selectivity for CO at 270 °C.113 The figure is adapted with permission from ref. 117. Copyright (2021) Elsevier. | |
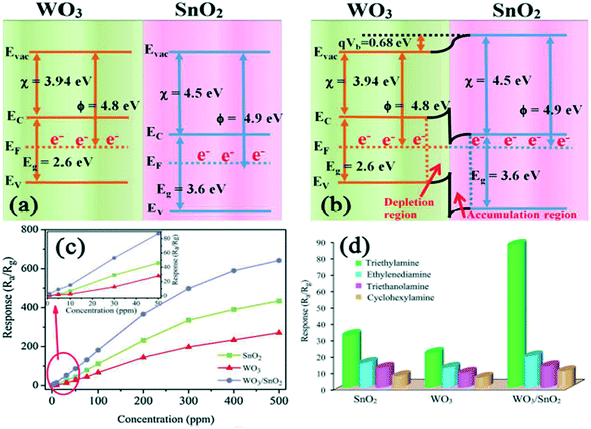 |
| Fig. 12 Band bending of WO3 and SnO2 in air (a) and TEA gas (b). (c) Response of the sensors based on single SnO2, WO3 and WO3–SnO2 composites to TEA gas at 220 °C (the inset shows the calibration curve from 1 to 50 ppm) and selectivity towards 50 ppm interference gases (d).164 The figure is adapted with permission from ref. 164. Copyright (2021) Elsevier. | |
Recently, Chang et al.90 used WO3-based structure decorated with Pt nanoparticles and the sensor is fabricated via rapid thermal evaporation process. The performance of the device was tested for H2 gas and it showed a very high response of 1.41 × 106 with the lowest detection limit of 1 ppm H2 at 200 °C and a short response/recovery time of 201/26 s. Kadir et al.91 reported a nanoporous WO3 Schottky diode-based gas sensor which was fabricated by depositing a platinum catalytic contact and applying H2 gas (anodic oxidation). The I–V characteristics and dynamic responses of the sensor shows a larger voltage shift in the presence of H2 gas at 200 °C. The observed voltage shift is attributed to the reduction of the Schottky barrier height which can be easily estimated by the thermionic emission equation. However, Schottky diode-based gas sensors are more sensitive towards hydrogen gas. Yaacob et al.92 have also reported the gasochromic response of WO3 films coated with catalytic Pt towards H2 gas. The Pt/WO3 thin films exhibited gasochromic characteristics in the visible–NIR (400–900 nm) spectral range where the total absorbance was increased by approximately 15% when exposed to 0.06% H2 gas, which is further enhanced to 60% in the presence of 1% H2 gas in air. However, the observed response was reduced by ∼ 40% when the same concentration of H2 gas in air with humidity of 6% was exposed. The sensor exhibits low recovery time at room temperature, but at 100 °C the fabricated device displays a highly sensitive, stable and repeatable response towards low concentrations of H2 gas. A detailed overview of the gas-sensing performance for H2 gas using WO3 structures is summarized in Table 1.
Table 1 Gas-sensing performance of H2 using various WO3 structures
Sensing materials |
Synthesis method |
Target gas |
Concentration/sensor response |
Operating temp. |
Response/recovery time |
Ref. |
Pd-loaded mesoporous WO3 |
Hard template |
H2 |
5000 ppm/11.78 |
RT |
80 s/10 s |
58
|
Nanoparticle WO3 |
Thermal evaporation |
H2 |
1 ppm/1.41 × 106 |
200 °C |
201 s/26 s |
90
|
PdO-decorated WO3 nanospindle |
Hydrothermal |
H2 |
50 ppm/76 |
150 °C |
1 s/214 s |
119
|
3.1.2. Nitrogen oxides (NOx).
Nitrogen oxides exist in various forms such as N2O, NO, NO2, N2O3, N2O4 and N2O5. Except for nitrogen dioxide, all these gases are unstable and convert into NO2 easily. Nitrogen dioxide is a reddish-brown coloured toxic gas and also a pronounced air pollutant. It also affects humans and animals. Detection of NO2 is important for environmental protection and human health. It is problematic for humans when its concentration is over the TLV of 3 ppm.93 Unloaded WO3 is described as the most capable metal oxide for NO2 gas sensing from most reported data in the literature.94–96 To achieve this, the gas-sensing measurements were performed in a relatively high-temperature environment.97 Akiyama et al.98 detected 80 ppm NO2 at 300 °C and found that WO3 is highly sensitive for NO2 gas. Behera et al.69 fabricated WO3 nanorods using thermal oxidation which showed a response of 2.02 for 10 ppm NO2 at 225 °C and a lower detection level of 2 ppm NO2 when operated at an optimal working temperature of 250 °C. By using a hydrothermal method, Li et al.67 prepared WO3 nanoparticles which showed a sensing response of 251.7 at 100 °C and the response/recovery time of ∼11/124 s for a concentration of 5 ppm NO2. Also, Shendage et al. utilized a similar method to grow WO3 nanoplates (with average thickness ∼110 nm and length ∼750 nm) and directly deposited on a soda lime substrate, which exhibited a gas response of ∼10 for 5 ppm of NO2 gas at 100 °C.45 The sensing response of WO3 nanoplates for NO2 is shown in Fig. 7(a) along with a comparison of the sensing response with other gases at 100 °C in Fig. 7(b).
Recently, tungsten oxide nanosheets have also been utilized to detect an ultra-low concentration of NO2 (40 ppb) at 150 °C with an extraordinary response of 30.51 Yuan et al.53 used a spin coating technique to synthesize hollow nanoscale structures of WO3 on the surface of porous silicon (PS), which displayed a distinguished response and high sensitivity up to the ppb level of NO2. Prajapati et al. have shown precise measurement up to 39 ppb at 150 °C by utilizing an 85 nm thick WO3 thin film, while the fabricated device can detect up to 16 ppb.11 In recent studies, researchers have used 1D and 2D WO3 structures for detecting various concentrations of NO2 gas with different sensor response100 at operating temperature, as summarised in Table 2.
Table 2 Gas-sensing performance of NO2 using various WO3 structures
Sensing materials |
Synthesis method |
Target gas |
Concentration/sensor response |
Operating temp. (°C) |
Response/recovery time |
Ref. |
WO3 nanoplates |
Hydrothermal |
NO2 |
5 ppm/10 |
100 |
— |
45
|
WO3 nanoparticles |
Hydrothermal |
NO2 |
5 ppm/251.7 |
100 |
11 s/124 s |
67
|
WO3 nanorods |
Thermal oxidation |
NO2 |
10 ppm/2.02 |
250 |
— |
69
|
WO3 nanosheet |
Hydrothermal |
NO2 |
50 ppb/6 |
140 |
140 s/75 s |
50
|
Unloaded WO3 |
Atmospheric plasma spraying |
NO2 |
450 ppb/77 |
130 |
— |
94
|
Au@WO3 CSNSs |
Hydrothermal and calcination processes |
NO2 |
1 ppm/20 |
100 |
4 s/218 s |
99
|
Polyaniline−WO3 thin-film |
Hydrothermal |
NO2 |
2 ppm/1.18 |
50 |
— |
101
|
3.1.3. Ammonia (NH3).
NH3 is the most alkaline hazardous gas in the environment; it is colourless and lighter than air as well. Besides the vast usability of NH3 in areas such as fertilizers, chemical industries, medical diagnosis, food processing and environmental protection, it is also hazardous for humans as it causes lung disease, poisoning, and a burning sensation when its concentration exceeds the TLV of 25 ppm.102 In an earlier study towards NH3, Au-doped WO3 was used by Maekawa et al.103 in 1992. A better response of 18.2 for 10 ppm of NH3 vapour at 200 °C (ref. 104) was shown by using WO3 films prepared by thermal deposition than the sensors synthesized by other methods like hard template and electrospinning methods.105,106 A linear relationship for NH3 gas detection was realized using WO3 nanowires, i.e., the sensitivity increased linearly with increasing temperature.53 The sensitivity for 1500 ppm NH3 at 200–300 °C (the optimum working temperature for NH3 detection) was found to be 9.67 with a lowest response and recovery time of 7 s and 8 s, respectively. Long et al.38 proposed a substrate-free fabrication of WO3 nanoarrays using a hydrothermal method and showed a high response of 8.3 for 50 ppm NH3 at 200 °C. The response of WO3 towards different concentrations of NH3 is illustrated in Fig. 8. Further, the comparison of various WO3 nanostructures and mechanism of NH3 sensing using tungsten oxide nanoarrays are shown in Fig. 9, where the orderly arrangement of nanorods was exploited. The flow of NH3 gas through nanorod arrays in a zigzag way caused frequent contact with WO3 which led to an enhanced response.
Wang et al.107 used a flame melting biconical taper method to prepare WO3 nanorods which exhibited very good sensitivity and selectivity towards NH3 gas. The spectrum shift of tungsten oxide nanorods is found to be 16.23 nm when the concentration of NH3 gas varies from low (0) to very high (11
640 ppm) concentrations. Recently, Fan et al.108 have grown WO3 nanoplates using an ultrasonic spray method and showed a high response of 34 at room temperature when exposed to 100 ppm NH3; the fabricated device was found to be sensitive even to 5 ppm NH3 (Table 3).
Table 3 Gas-sensing performance of NH3 using various WO3 structures
Sensing materials |
Synthesis methods |
Target gas |
Concentration/sensor response |
Operating temp. (°C) |
Response/recovery time |
Ref. |
WO3 nanoarrays |
Hydrothermal |
NH3 |
50 ppm/8.3 |
200 |
— |
38
|
WO3 film |
Thermal deposition |
NH3 |
10 ppm/18.2 |
200 |
— |
104
|
WO3 nanoplates |
Ultrasonic spray |
NH3 |
100 ppm/34 |
RT |
— |
108
|
rGO/WO3 nanowire composites |
Hydrothermal |
NH3 |
500 ppm/35 |
300 |
37 s/711 s |
109
|
Pd loaded WO3 film |
Photochemical |
NH3 |
50 ppm |
300 |
10 s/20 s |
110
|
3.1.4. Hydrogen sulphide (H2S).
H2S is a colourless, toxic, corrosive, flammable and extremely hazardous gas. It is released by the biological (natural) decomposition of organic waste and occurs naturally in crude petroleum, hot springs and natural gas. It is used in large quantities to extract heavy water. H2S can cause paralysis in humans if exposed to 100 ppm and can cause death at concentrations higher than 250 ppm.111 In an earlier study, the sensitivity of WO3 towards H2S was reported by Barrett et al.112 in 1990. Tungsten oxide nanocrystalline films were grown with a Pt sensitizer which enhances the active sites, thereby enhancing the response. It was observed that the nanocrystalline WO3 thick films displayed an excellent response towards H2S gas at room temperature.113 Yu et al.30 used quantum dots of WO3 as a sensing layer in a chemiresistive H2S gas sensor, which displayed a response of ∼57 towards 50 ppm H2S at 80 °C with the response/recovery time of 47/126 s. Recently, Xiao et al.114 used p–n heterojunction-based WO3/NiO sensors, which exhibited a response of 50 for a concentration of 50 ppm H2S at 250 °C with response and recovery times of 4 s and 66 s, respectively. Nickel concentration-dependent response is shown in Fig. 10(a) and the sensing response of H2S (50 ppm) and other gases is shown in Fig. 10(b).
San et al.10 synthesized tungsten oxide thin films onto silicon substrates by using a sputtering technique and these thin films were covered with WO3 nanotubes grown by a thermal evaporation method which exhibited a maximum response of 7.3 at 150 °C towards 10 ppm H2S gas. Also, Pt–Na2W4O13-loaded WO3 nanotubes were prepared by using the electrospinning method which unveiled the sensing response of >203 for 1 ppm H2S with response and recovery time of <10 s and <30 s, respectively, under 95% humidity.115 Poongodi et al.43 used vertically oriented WO3 nanoflakes with an electrodeposition technique to detect H2S (0.1–10 ppm), which revealed that the response of the device is directly correlated with temperature, i.e., 2.8% to 9.8% (at 100 °C), 12.8% to 68.9% (at 200 °C) and 26.2% to 85% (at 300 °C) (Table 4).
Table 4 Gas-sensing performance of H2S using various WO3 structures
Sensing materials |
Synthesis method |
Target gas |
Concentration/sensor response |
Operating temp. (°C) |
Response/recovery time |
Ref. |
WO3 quantum dots |
Colloidal synthesis |
H2S |
50 ppm/57 |
80 |
47 s/126 s |
30
|
WO3 nanotubes |
Thermal evaporation |
H2S |
10 ppm/7.3 |
150 |
— |
10
|
WO3 nanoflakes |
Electrodeposition |
H2S |
0.1–10 ppm/2.8% up to 85% |
100–300 |
— |
43
|
Pt–Na2 W4O13 loaded WO3 nanotubes |
Electrospinning |
H2S |
1 ppm/203.5 |
450 |
<10s/<30s |
115
|
WO3/NiO |
Evaporation-induced multicomponent co-assembly |
H2S |
50 ppm/— |
250 |
4 s/66 s |
114
|
PdO loaded microspheres WO3 nanoplates |
Solvothermal |
H2S |
25 ppm/1029 |
190 |
1 s/− |
44
|
3.1.5. Carbon monoxide (CO).
CO is a colourless and odourless toxic gas which is produced due to incomplete combustion of fuels (automobile exhaust emission). This gas is toxic, as large amounts of this gas such as 600 ppm can cause coma in human beings by blocking the oxygen transport of blood in haemoglobin and higher levels of CO exposure can cause death. The threshold limit value for CO is at a concentration of 10 ppm for 8 hours of exposure.116 However, a concentration below the immediately dangerous to life and health (IDLH) level of CO can cause negative health effects on humans. The sensitivity of WO3 towards CO appears to be relatively low. Wei et al.117 used cauliflower-like WO3 (CFL-WO3) structures obtained using a hydrothermal process and reported the response of 16.6 at 270 °C for 50 ppm CO gas. The gas-sensing properties of CFL-WO3 sensor were depending on the operating temperature and it was observed that 270 °C is the optimal temperature for detection of 200 ppm CO gas, as shown in Fig. 11(a). Furthermore, the dynamic responses of CFL-WO3 towards distinct concentrations of CO gas were examined, which is shown in Fig. 11(b). Subsequently, the gas sensor response towards the different concentrations of gases at 270 °C was examined and it was found that the sensor displayed high selectivity towards CO gas in the presence of other gases like HCHO, CH4, H2, and LPG (Fig. 11(c)).
Ma et al.57 synthesized mesoporous WO3 nanocomposites which revealed a good response to low concentrations of CO at low working temperature with a very short response/recovery time (16 s/1 s), good sensitivity and high selectivity. Using density functional theory calculation, Tian et al.118 indicated that tungsten oxide has high sensitivity towards CO gas. The theoretical analysis of gas interaction with the sensing film can abridge the research in achieving new results with completely different perspectives; however, these calculations are beyond the scope of the present review (Table 5).
Table 5 Gas-sensing performance of CO using various WO3 structures
Sensing materials |
Synthesis method |
Target gas |
Concentration/sensor response |
Operating temp. |
Response/recovery time |
Ref. |
Cauliflower-like WO3 structure |
Hydrothermal |
CO |
50 ppm/16.6 |
270 °C |
9–15 s/5–9 s |
117
|
Mesoporous WO3 nanocomposites |
Chemical route |
CO |
100 ppm/10.1 ± 1.0 |
125 °C |
16 s/1 s |
57
|
3.1.6. Ozone (O3).
The most significant component of the ozone layer consists of O3. This component protects the earth from harmful and excess ultraviolet rays that can harm human beings and other species. The fluctuation in the concentration of this gas can cause a number of changes. O3 is highly toxic towards organisms, which is little known. A very small amount of O3 like 10 ppm can cause a decline in oxygen level in the human blood which can stimulate an increase in respiratory rate. Cantalini et al.120 fabricated WO3 thin films using various methods and the grown films were capable of measuring O3 in a range of 10 ppb to 160 ppb. In the recent past, the sensitivity/response towards O3 is no longer the focus of researchers as the high sensitivity/response to O3 has been investigated in earlier studies.
3.1.7. Volatile organic components.
Volatile organic gases which come from combustion and human breathing are also polluting the enviornment. These organic gases can present a flammability, toxicity and asphyxiation risk. They can cause headache and fatigue while high concentrations of gases like CH4 can even cause death due to suffocation. To detect these harmful organic gases, WO3-based nanostructures are in the focus of research nowadays. The availability of a large number of gases makes it unrealistic to discuss all of them; here we have compiled some of the recent studies. A direct comparison of the performance of WO3 structures toward various VOCs is not possible; therefore Table 6 indicates WO3 structures with target gases without mentioning the specific parameters.
Table 6 Various studies on the sensitivity of WO3 structures towards volatile organic gases
Sensing materials |
Target gases |
Ref. |
Films of WO3 |
Liquid petroleum gas (LPG) |
121
|
Flower-like WO3 |
Toluene, ethanol, n-butanol acetone, methanol |
122
|
Porous WO3 |
Acetone, ethanol, formaldehyde, methanol |
123
|
Nanoplates of WO3 |
Cyclohexene |
124
|
WO3 spheres |
Ethyl acetate, ethanol methanol, isopropanol, toluene |
125
|
WO3 octahedral |
Benzene |
126
|
4. Effort to enhance the gas-sensing properties of WO3
We have discussed that the gas-sensing properties of WO3-based gas sensors can effectively be enhanced on the basis of dimensionalities. For further improving the gas-sensing behaviour, WO3 nanomaterials were optimized which improves the performance of the fabricated sensing devices. The resistive-type gas sensor response is determined by the change of resistivity which is derived after the reaction on the surface of the sensing material between the adsorbed oxygen species and the target gas molecules. Therefore, it can be concluded that the sensing property of WO3 is enhanced by the transfer of induced electrons. For further improving the gas-sensing properties, basic strategies such as crystal facet engineering, noble metal functionalization and elemental doping might be adopted. Also, the electron transfer rate could be regulated by the formation of a heterojunction though coupling with the sensing material, thereby enhancing the sensing performance.
More recently, to achieve higher selectivity, rapid response and high reliability of WO3 nanomaterials, special attention has been paid towards the crystal facet engineering127,128 of metal oxide semiconductor (MOS) nanocrystals due to their surface atomic arrangement, active sites and dangling bonds. Two different hierarchical structures of pure WO3 were synthesized by Wei et al.129via a hydrothermal process: daisy-like hexagonal WO3 (Dh-WO3) and rose-like monoclinic WO3. The Dh-WO3 sensor exhibits outstanding acetone sensing at 260 °C from the dominant (002) facets and the intracrystalline tunnel structure of the hexagonal phase. Both WO3 nanocrystals have the same matrix material but differ in crystalline phase.
4.1. Impact of doping
Doping in WO3 has been a crucial technique to increase the gas-sensing performance. The creation of defects in the lattice, change in the bandgap and increase in the oxygen vacancies are done by doping with many non-metallic elements (Si,130 C,131 N (ref. 132)) and metallic elements (Fe,133 Cr,134,135 Co,136 Sb,137 Sn,138 Gd,139 Cu,140 and alkali metal,141 Pd (ref. 142)) into WO3, resulting in enhanced sensing properties. Due to the similarity in the radius of Fe (0.0645 nm) and W (0.0620 nm) ions, Fe is easily doped in WO3, giving a highly stable structure without having any distortion.143 Fe-doped mesoporous WO3 hollow nanospheres were observed to be selective towards NO2 gas133 and also a slight distortion was induced that created active sites as a result of a number of defects which acted as active sites. Detection of even 10 ppb of NO2 at low operating temperature (100–140 °C) could be achieved with a good signal-to-noise ratio by these active sites.
4.2. Impact of surface functionalization
Surface functionalization is an important process for enhancing the performance of WO3-based gas devices. Functionalization can be attained by using noble metals such as Pt, Ag, Pd, Ru, and Au, resulting in improved sensitivity, lowering the operating temperature and tuning selectivity. The role of ‘noble metal nanoparticles’ in enhancing the gas-sensing performance can be explained by catalytic oxidation and electronic sensitization.144,145 In catalytic oxidation, the noble metal catalyst dissociates molecular oxygen in order to enrich the quantities of adsorbed oxygen molecules; the active oxygen species are thus deposited onto the WO3 surface. Hence, the depletion layer is increased with more band electrons. As a result, the variation in resistance is apparently better than that of the pure counterparts. The gas-sensing performance can also be improved due to the interaction between the noble metals and WO3 (called electronic sensitization) as it leads to shifting of the Fermi level of WO3. Au/WO3 core–shell nanospheres were prepared by using Au nanoparticles (20–25 nm diameter) and shells of WO3 with a thickness of 30–50 nm (ref. 146) which evidenced four times higher response than pure WO3 nanospheres, i.e., 136 towards 5 ppm NO2 at an operating temperature of 100 °C with excellent NO2 selectivity and long-term stability. Zhang et al. have fabricated an on-chip bilayer Au nanoparticle-decorated WO3 nanoporous thin film by using polystyrene which is stacked layer by layer with periodic Au sputtering deposition.147 A fast response and recovery time of 9 and 16 s, respectively, was observed at 150 °C that showed a sensitivity of 96.0 for 1 ppm NO2. Also, Yang et al.148 have prepared Au-functionalized WO3 nanofibers by an electrospinning method. The film showed excellent sensitivity towards n-butanol with a sensor response of ∼152.7 for 100 ppm at 250 °C and response and recovery time varying between 5–43 s and 10–122 s, respectively. Zhang et al.149 synthesized bare and PtAg-loaded WO3 nanorods for CO detection. PtAg nanoparticles having a diameter of 20–30 nm were loaded on the surface of WO3 nanorods, which resulted in improved performance for CO sensing. The sensor response was found to be 2.79 toward 100 ppm CO with response and recovery time of 75 s and 24 s, respectively. Moreover, first-principles analysis based on density functional theory (DFT) showed that the best adsorption sites of CO on the surface of Pt (111) and Ag (111) are the top sites and the adsorption energy of Pt (111) is better than that of Ag (111) on each site. Gas sensitivity improved due to the special sensitization mechanism of Pt and Ag and their strong interaction. Furthermore, a Pt-sensitized mesoporous WO3 crystalline framework was synthesized by Ma et al.150 by co-assembly of hydrophobic platinum precursor, amphiphilic poly-b-polystyrene and hydrophilic tungsten precursor. This device showed excellent sensitivity for CO gas for the concentration ranging from 100 to 500 ppm at a temperature of 125 °C and humidity level of 60% RH. By loading 1% Pt nanoparticles, Wang et al. have displayed the high sensing properties of mesoporous WO3 for high concentrations of NH3 (50–1500 ppm).151 It was observed that due to the catalytic behaviour of Pt nanoparticles, Pt-loaded WO3 identified NH3 at a significantly lower operating temperature (75 °C) than that of a pure WO3 sensor (200 °C).
4.3. Impact of heterojunctions
The gas-sensing performance can be significantly upgraded by coupling various composites with WO3, i.e., creating a heterojunction interface. This interface is created between the two different kinds of materials where the Fermi levels could equilibrate to the same energy.152,153 Hence, the sensing performance is enhanced because of synergistic effects and also due to the interfaces derived from the diverse components. Furthermore, an extended region of charge depletion and charge transfer will ensue. WO3-based heterojunctions have been reported by the coupling of WO3 with various materials like metal oxides,154–158 polymers,67,159 carbon nanomaterials,160,161 and also one other important approach to enhance the sensing performance of WO3 is to establish ternary heterojunctions with liquid metals162etc. Taking SnO2 as an example from n-type metal oxides, the heterojunction of WO3 and SnO2 was formed where the electron transport mechanism of the heterojunction was found to be different from that of individual WO3 or SnO2 (where the bandgap and work function of WO3 is 2.6 eV and 4.41 eV, respectively, while for SnO2, it is 3.6 eV and 4.18 eV, respectively). The energy band structure of the heterojunction is configured in Fig. 12(a and b), which demonstrates that the position of the Fermi level in WO3 is relatively higher than in SnO2. Therefore, electrons will be transferred from WO3 to SnO2, forming a potential barrier across the sensor surface which hinders the movement of electrons. Thus, more active electrons are available for adsorbed oxygen molecules, increasing the conductivity of the sensor and improving the sensor response and selectivity in return towards TEA (triethylamine) as compared to the single WO3 and SnO2 (Fig. 12(c and d)). To increase the sensing properties, a similar mechanism is followed by the other WO3 heterojunctions as well.
Recently, a simple ultrasonic method has been employed for the preparation of rGO/WO3 nanocomposites at room temperature which showed a detection limit of 1.14 ppm NH3, excellent selectivity, very high response of 680 and good response/recovery time (18/24 s).161 Surface engineering techniques can also further increase the selectivity of WO3 gas sensors. The microwave-assisted gas–liquid interfacial solvothermal method was adopted by Gui et al.163 to synthesize flower-like WO3 with active (−112) facets, which showed high sensitivity towards TEA gas at room temperature with a response as high as 180.7 times for 100 ppm TEA along with response and recovery time of 264 and 72 s, respectively. Recently, Han et al. synthesized a WS2–WO3 heterojunction via a sonochemical oxidation process at room temperature, which showed a detection limit of 50 ppb for NO2 gas and unveiled an enhanced response of 16.7 for 5 ppm NO2 gas with a quick response time of 34 s; the fabricated device showed good recoverability compared to bare WS2 sensor.165Table 7 summarizes recent results of WO3-based heterostructures and nanomaterials towards various gases and their sensing performance.
Table 7 Gas-sensing performance of WO3 heterostructures and nanomaterials
Material |
Synthesis technique |
Targeted gas and concentration |
Operating temp. (°C) |
Response |
Response and recovery time |
LOD |
Ref. |
Gas response S = Ra/Rb.
Gas response S = (Ra − Rb)/Rg.
|
WO3–NiO hollow nanoflower |
Hydrothermal |
Xylene/50 ppb–100 ppm |
300 |
354.7a |
51 s/57 s |
0.05 ppm |
154
|
WO3–SnO2 nanospheres |
Hydrothermal |
Acetone 1000 ppm |
240 |
16.9a |
51 s/11 s |
— |
155
|
WO3–ZnO ordered mesoporous |
Hydrothermal |
NO2 200 ppm |
200 |
186a |
— |
250 ppb |
156
|
WO3–Fe2O3 nanoplates |
Solution-based method |
Acetone 100 ppm |
260 |
105.8a |
— |
1 ppm |
157
|
WO3–CuO composite |
Hydrothermal |
H2S/5 ppm |
100 |
223a |
70 s/450 s |
— |
158
|
GO(rGO)/WO3 nanofiber |
Electrospinning |
Acetone/100 ppm |
375 |
35.9a |
4 s/10 s |
— |
160
|
GO(rGO)/WO3 porous composite |
Ultrasonication |
NH3/100 ppm |
RT |
14.53a |
18 s/24 s |
1.14 ppm |
161
|
PPy/WO3 nanoparticles |
Chemical oxidation polymerization |
TEA/100 ppm |
RT |
680b |
49 s/− |
5.4 ppm |
159
|
PEI/WO3 nanoparticles |
Hydrothermal |
NO2 5 ppm |
100 |
251.7a |
>10 s/>120 s |
50 ppb |
67
|
WS2/WO3 composites |
Drop casting |
NH3 |
150 |
— |
— |
1 ppm |
166
|
Rh-decorated WO3 nanorods |
Glancing angle deposition |
Acetone/0.2–1000 ppm |
300 |
75.9 |
12 s/8 s |
131 ppt |
167
|
Au-loaded mesoporous WO3 |
Nanocasting |
n-Butanol 100 ppm |
300 |
5.67a |
10 s/35 s |
— |
168
|
Au-impregnated WO3 nanorods |
Modified precipitation/impregnation |
NO2 0.125–5 ppm |
250 |
836.6 |
64.2 s/− |
— |
169
|
Ag/WO3 nanosheets |
Precipitation |
HCHO 100 ppm |
300 |
20.8a |
5 s/5 s |
10 ppm |
170
|
Pt/WO3 mesoporous |
Nanocasting method |
NH3 200 ppm |
125 |
13.61a |
43 s/272 s |
— |
171
|
Pd-loaded mesoporous WO3 |
Hard-template method |
H2 5000 ppm |
RT |
11.78a |
80 s/10 s |
1000 ppm |
58
|
5. Challenges and future perspectives
In the present review, we have summarized the impact of structure dimensionality and surface morphology on the gas-sensing behaviour of WO3-based sensors. Substantial efforts have been carried out to address the challenges associated with the structure and performance of WO3-based devices; however, there is still scope for further improvement, especially in the area of sensitivity and response time. We have systematically discussed the role of WO3-based sensors in the detection of various gases from industrial/natural waste such as NOx, NH3, H2S, CO and other volatile organic compounds. The advancement in sensor technology is useful in detecting different pollutant gases which will be beneficial to restrict the level of various toxic gases in the environment. To detect environmental pollutant gases, the role of surface morphology and structural changes has been discussed in the case of gas sensing using WO3 as a base material. Various nanostructures or morphologies including 0D, 1D, 2D, and 3D such as nanoparticles, nanosheets, nanofibers, microspheres, microflowers, etc. (having high surface area which provides more active sites for the reaction between absorbed oxygen and target gas molecules55) have been evaluated for detection of different gases. It was observed that the surface morphology and structure depend on the growth/synthesis techniques and deposition conditions. In addition, the annealing temperature also plays a significant role in controlling the morphology and the surface roughness which can alter the performance of a fabricated sensor. In addition, the gas-sensing mechanism for various nanostructure-based sensors developed by using WO3 is discussed. However, the stability, selectivity, sensitivity and response/recovery time of the gas-sensing device still require further attention. Moreover, the role of surface functionalization, heterostructure, and doping has been discussed which can be utilized to enhance the sensitivity, stability, selectivity, and detectivity of the device and may be exploited for the commercialization of the technology. The other significant issue related to WO3-based devices is their high operating temperature; efforts are essentially required to develop systems/devices which produce better sensor response at or slightly above room temperature. A lower operating temperature will result in less power consumption and will lead to the development of energy-efficient devices.
More recently, 2D materials172 including graphene, transition metal dichalcogenides, etc. are playing a significant role in the field of gas sensing and are potential competitors to these metal oxide sensors. These 2D material are efficiently detecting various gases at low or even room temperature; however the recovery time is a serious issue in these devices. Hence, efforts are essentially required to develop different kinds of structures specifically designed to enhance the sensing properties of WO3 with a large surface area (vertically aligned, flower-like, and hierarchical dendrites etc.) with lower operating temperatures.
It is established that most target gases (except a few) are detected at a higher operating temperature which requires circuital complications due to the use of a heater. However, it could be disastrous if there is an existence of flammable gases such as H2 along with the target gas. WO3-based gas sensors have proven to be very efficient at higher temperature; however there is a requirement to substantially reduce the operating temperature without compromising the sensing performance.
In addition, there is huge scope of these metal oxide-based sensors in the area of healthcare monitoring where the growth of metal oxides on flexible/wearable substrates needs to be exploited.173 Further, for industrial and space applications, the necessity is to develop sensors that are capable of operating under harsh environmental conditions. In addition, surface modification (loading of nanoparticles and doping with different elements), binary and ternary heterostructures for efficient gas detection with state-of-the-art performance parameters at lower operating temperature should be utilized.
Additionally, the synthesizing techniques of these sensor films play an important role in their performance; however, this dependency is not universal. Several methods are technically mature, yet they use harmful agents with energy consumption which is harmful for the environment and goes against “green science”. Hence, it is expected that researchers will focus on the designability during synthesis and consider all the effects including the economic and environmental effects. Enormous efforts have been invested to understand the surface morphology/dimensionality of WO3 materials and their correlation with the detection of target gas; however, there is still a possibility for improvement. Hence, there is no doubt that the enhancement in the sensing performance will continue to play a vital role in controlling global worsening conditions in the future and would prove to be a great help to provide a clean environment.
Conflicts of interest
The authors declare no financial interest.
Acknowledgements
We acknowledge the Director, CSIR-NPL, New Delhi, for his encouragement and support. AY would like to acknowledge UGC for financial assistance via a Junior Research Fellowship.
References
-
https://medium.com/@anasbaig/everyday-activities-that-cause-air-pollution5ed490625635
.
- Z. J. Li, H. Li, Z. L. Wu, M. K. Wang, J. T. Luo, H. Torun, P. A. Hu, C. Yang, M. Grundmann, X. T. Liu and Y. Q. Fu, Advances in designs and mechanisms of semiconducting metal oxide nanostructures for high-precision gas sensors operated at room temperature, Mater. Horiz., 2019, 6, 470–506 RSC.
- I. D. Kim, A. Rothschild and H. L. Tuller, Advances and new directions in gas-sensing devices, Acta Mater., 2013, 61, 974–1000 CrossRef CAS.
- Y. F. Sun, S. B. Liu, F. L. Meng, J. Y. Liu, Z. Jin, L. T. Kong and J. H. Liu, Metal Oxide Nanostructures and Their Gas Sensing Properties: A Review, Sensors, 2012, 12, 2610–2631 CrossRef CAS PubMed.
- H. Wang, W. P. Lusting and J. Li, Sensing and capture of toxic and hazardous gases and vapors by metal-organic frameworks, Chem. Soc. Rev., 2018, 47, 4729–4756 RSC.
- Z. H. Xiao, L. B. Kong, S. C. Ruan, X. L. Li, S. J. Yu, X. Y. Li, Y. Jiang, Z. J. Yao, S. Ye, C. H. Wang, T. S. Zhang, K. Zhou and S. A. Li, Recent development in nanocarbon materials for gas sensor applications, Sens. Actuators, B, 2018, 274, 235–267 CrossRef CAS.
- M. C. Rao, Structure and properties of WO3 thin films for Electrochromic device application, J. Non-oxide Glasses, 2013, 5, 1–8 Search PubMed.
- H. Long, W. Zeng and H. Zhang, The Solvothermal Synthesis of the Cobweb-like WO3 and its Enhanced Gas-sensing Property, Mater. Lett., 2017, 188, 334–337 CrossRef CAS.
- M. M. El-Nahass, M. M. Saadeldin, H. A. M. Ali and M. Zaghllol, Electrochromic properties of amorphous and crystalline WO3 thin films prepared by thermal evaporation technique, Mater. Sci. Semicond. Process., 2015, 29, 201–205 CrossRef CAS.
- X. San, Y. Lu, G. Wang, D. Meng, X. Gong and Q. Jin, In situ growth of WO3 nanotube arrays and their H2S gas sensing properties for reduced operating temperature, Mater. Lett., 2020, 271, 127716 CrossRef CAS.
- C. S. Prajapati and N. Bhat, ppb level detection of NO2 using a WO3 thin film-based sensor: material optimization, device fabrication and packaging, RSC Adv., 2018, 8, 6590–6599 RSC.
- J. J. Zhang, W. X. Zhang, Z. H. Yang, Z. B. Yu, X. B. Zhang, T. C. Chang and A. Javey, Vertically aligned tungsten oxide nanorod film with enhanced performance in photoluminescence humidity sensing, Sens. Actuators, B, 2014, 202, 708–713 CrossRef CAS.
- T. Saidi, D. Palmowski, S. Babicz-Kiewlicz, T. G. Welearegay, N. El Bari, R. Ionescu, J. Smulko and B. Bouchikhi, Exhaled breath gas sensing using pristine and functionalized WO3 nanowire sensors enhanced by UV-light irradiation, Sens. Actuators, B, 2018, 273, 1719–1729 CrossRef CAS.
- D. Punetha and S. K. Pandey, Optimization in NH3 gas response of WO3 nanorods based sensor array, IEEE Sens. J., 2019, 1–4 Search PubMed.
- T. T. Li, Y. B. Shen, S. K. Zhao, X. X. Zhong, W. Zhang and C. Han, Sub-ppm level NO2 sensing properties of polyethyleneimine-mediated WO3 nanoparticles synthesized by a one-pot hydrothermal method, J. Alloys Compd., 2019, 783, 103–112 CrossRef CAS.
- J. Zhang, J. P. Tu, G. F. Cai, G. H. Du, X. L. Wang and P. C. Liu, Enhanced electrochromic performance of highly ordered, macroporous WO3 arrays electrodeposited using polystyrene colloidal crystals as template, Electrochim. Acta, 2013, 99, 1–8 CrossRef CAS.
- E. Lue'vano-Hipo'lito, A. Martı'nez-de la Cruz, Q. L. Yu and H. J. H. Brouwers, Precipitation synthesis of WO3 for NOx removal using PEG as template, Ceram. Int., 2014, 40, 12123–12128 CrossRef.
- M. Heydari, S. M. Ghoreishi and A. Khoobi, Chemometrics-assisted determination of Sudan dyes using zinc oxide nanoparticle-based electrochemical sensor, Food Chem., 2019, 283, 68–72 CrossRef CAS PubMed.
- H. Kaur, Aptamer Conjugated Quantum Dots for Imaging Cellular Uptake in Cancer Cells, J. Nanosci. Nanotechnol., 2019, 19, 3798–3803 CrossRef CAS PubMed.
- Q. Yuan, Y. Yao, X. Zhang, J. Yuan, B. Sun and X. Gao, The Gold Nanocluster Protects Neurons Directly or via Inhibiting Cytotoxic Secretions of Microglia Cell, J. Nanosci. Nanotechnol., 2019, 19, 1986–1995 CrossRef CAS PubMed.
- O. Balaban, I. Grygorchak, N. Mitina, A. Zaichenko, B. Lukiyanets, V. Glasunova, A. Borysiuk, M. Larkin, O. Hevus and N. Pokladok,
et al. Fabrication of 1D-Nanofiber/Fe2O3 Composites with Tailored Magnetic Properties, J. Nanosci. Nanotechnol., 2019, 19, 3871–3878 CrossRef CAS PubMed.
- K. Kumar, A. Priya, A. Arun, S. Hait and A. Chowdhury, Antibacterial and natural room-light driven photocatalytic activities of CuO nanorods, Mater. Chem. Phys., 2019, 226, 106–112 CrossRef CAS.
- S. F. Liu, S. Lin and T. M. Swager, An Organocobalt–Carbon Nanotube Chemiresistive Carbon Monoxide Detector, ACS Sens., 2016, 1, 354–357 CrossRef CAS PubMed.
- L. Wu and D. Yang, Dielectric Properties and Thermal Conductivity of Poly(vinylidene fluoride)-Based Composites with Graphite Nanosheet and Nickel Particle, J. Nanosci. Nanotechnol., 2019, 19, 3591–3596 CrossRef CAS PubMed.
- Y. Qiao, C. Shi, X. Wang, P. Wang, Y. Zhang, D. Wang, R. Qiao, X. Wang and J. Zhong, Electrospun Nanobelt-Shaped Polymer Membranes for Fast and High-Sensitivity Detection of Metal Ions, ACS Appl. Mater. Interfaces, 2019, 11, 5401–5413 CrossRef CAS PubMed.
- D. J. Sprouster, C. Sun, Y. Zhang, S. N. Chodankar, J. Gan and L. E. Ecker, Irradiation-Dependent Helium Gas Bubble Superlattice in Tungsten, Sci. Rep., 2019, 9, 2277 CrossRef CAS PubMed.
- D. Meng, D. Liu, G. Wang, Y. Shen, X. San, M. Li and F. Meng, Low-temperature formaldehyde gas sensors based on NiO-SnO2 heterojunction microflowers assembled by thin porous nanosheets, Sens. Actuators, B, 2018, 273, 418–428 CrossRef CAS.
- P. Y. Dong, G. H. Hou, X. G. Xi, R. Shao and F. Dong, WO3-based photocatalysts: morphology control, activity enhancement and multifunctional applications, Environ. Sci.: Nano, 2017, 4, 539–557 RSC.
- Y. Yao, Q. Zhao, W. Wei, Z. Chen, Y. Zhu, P. Zhang, Z. Zhang and Y. Gao, WO3 Quantum-dots Electrochromism, Nano Energy, 2020, 68, 104350 CrossRef CAS.
- H. X. Yu, Z. L. Song, Q. Liu, X. Ji, J. Q. Liu, S. M. Xu, H. Kan, B. H. Zhang, J. Y. Liu, J. J. Jiang, L. Miao and H. Liu, Colloidal synthesis of tungsten oxide quantum dots for sensitive and selective H2S gas detection, Sens. Actuators, B, 2017, 248, 1029–1036 CrossRef CAS.
- Y. H. Li, Q. Q. Zhang, X. S. Li, H. Bai, W. T. Li, T. T. Zeng and G. C. Xi, Ligand-free and size-controlled synthesis of oxygen vacancy-rich WO3-x quantum dots for efficient room-temperature formaldehyde gas sensing, RSC Adv., 2016, 6, 95747 RSC.
- P. V. Adhyapak, A. D. Band, P. More and N. R. Munirathnam, Nanostructured WO3/grapheme composites for sensing NOx at room temperature, RSC Adv., 2018, 89, 34035–34040 RSC.
- L. Santos, C. Silveira, E. Elangovan, J. P. Neto, D. Nunes, L. Pereira, R. Martins, J. Viegas, J. J. G. Moura, S. Todorovic, M. G. Almeida and E. Fortunato, Synthesis of WO3 nanoparticles for biosensing applications, Sens. Actuators, B, 2016, 223, 186–194 CrossRef CAS.
- Y. D. Zhang, W. W. He, H. X. Zhao and P. J. Li, Template-free to fabricate highly sensitive and selective acetone gas sensor based on WO3 microspheres, Vacuum, 2013, 95, 30–34 CrossRef CAS.
- S. An, S. Park, H. Ko and C. Lee, Enhanced NO2 gas sensing properties of WO3 nanorods encapsulated with ZnO, Appl. Phys. A: Mater. Sci. Process., 2012, 108, 53–58 CrossRef CAS.
- J. J. Xue, J. W. Xie, W. Y. Liu and Y. N. Xia, Electrospun nanofibers: new concepts, materials, and applications, Acc. Chem. Res., 2017, 50, 1976–1987 CrossRef CAS PubMed.
- J. Leng, X. Xu, N. Lv, H. Fan and T. Zhang, Synthesis and gas-sensing characteristics of WO3 nanofibers via electrospinning, J. Colloid Interface Sci., 2011, 356, 54–57 CrossRef CAS PubMed.
- H. W. Long, Y. Q. Li and W. Zeng, Substrate-free synthesis of WO3 nanorod arrays and their superb NH3-sensing performance, Mater. Lett., 2017, 209, 342–344 CrossRef CAS.
- J. Zhang, D. Leng, L. Zhang, G. Li, F. Ma, J. Gao, H. Lu and B. Zhu, Porosity and oxygen vacancy engineering of mesoporous WO3 nanofibers for fast and sensitive low-temperature NO2 sensing, J. Alloys Compd., 2021, 853, 157339 CrossRef CAS.
- R. Vargas-Bernal, Electrical Properties of Two-Dimensional Materials Used in Gas Sensors, Sensors, 2019, 19, 1295 CrossRef CAS PubMed.
- P. G. Choi, N. Izu, N. Shirahata and Y. Masuda, Fabrication and H2-Sensing Properties of SnO2 Nanosheet Gas Sensors, ACS Omega, 2018, 3, 14592–14596 CrossRef CAS PubMed.
- E. M. Preiß, A. Krauß, V. Kekkonen, N. Barsan and H. Seide, Characterization of WO3 thin films prepared by picosecond laser deposition for gas sensing, Sens. Actuators, B, 2017, 248, 153–159 CrossRef.
- S. Poongodi, P. S. Kumar, D. Mangalaraj, N. Ponpandian, P. Meena, Y. Masuda and C. Lee, Electrodeposition of WO3 nanostructured thin films for relectrochromic and H2S gas sensor applications, J. Alloys Compd., 2017, 719, 71–81 CrossRef CAS.
- C. Wang, Y. Zhang, X. Sun, Y. Sun, F. Liu, X. Yan, C. Wang, P. Sun and G. Lu, Preparation of Pd/PdO loaded WO3 microspheres for H2S detection, Sens. Actuators, B, 2020, 321, 128629 CrossRef CAS.
- S. S. Shendage, V. L. Patil, S. A. Vanalakar, S. P. Patil, N. S. Harale, J. L. Bhosale, J. H. Kim and P. S. Patil, Sensitive and selective NO2 gas sensor based on WO3 nanoplates, Sens. Actuators, B, 2017, 240, 426–433 CrossRef CAS.
- H. L. Zhang, J. Q. Yang, D. Li, W. Guo, Q. Qin, L. J. Zhu and W. J. Zheng, Template-free facile preparation of monoclinic WO3 nanoplates and their high photocatalytic activities, Appl. Surf. Sci., 2014, 305, 274–280 CrossRef CAS.
- J. Y. Zheng, G. Song, J. Hong, T. K. Van, A. U. Pawar, D. Y. Kim, C. W. Kim, Z. Haider and Y. S. Kang, Facile Fabrication of WO3 Nanoplates Thin Films with Dominant Crystal Facet of (002) for Water Splitting, Cryst. Growth Des., 2014, 14, 6057–6066 CrossRef CAS.
- C. Q. Yang, Q. Zhu, S. P. Zhang, Z. J. Zou, K. Tian and C. S. Xie, A comparative study of microstructures on the photoelectric properties of tungsten trioxide films with plate-like arrays, Appl. Surf. Sci., 2014, 297, 116–124 CrossRef CAS.
- C. K. Wang, C. K. Lin, C. L. Wu, S. C. Wang and J. L. Huang, Synthesis and characterization of electrochromic plate-like tungsten oxide films by acidic treatment of electrochemical anodized tungsten, Electrochim. Acta, 2013, 112, 24–31 CrossRef CAS.
- Z. B. Wang, D. Wang and J. Sun, Controlled synthesis of defect-rich ultrathin two-dimensional WO3 nanosheets for NO2 gas detection, Sens. Actuators, B, 2017, 245, 828–834 CrossRef CAS.
- H. Khan, A. Zavabeti, Y. C. Wang, C. J. Harrison, B. J. Carey, M. Mohiuddin, A. F. Chrimes, I. A. D. Castro, B. Y. Zhang, Y. M. Sabri, S. K. Bhargava, J. Z. Qu, T. Daeneke, S. P. Russo, Y. X. Li and K. Kalantar-zadeh, Quasi physisorptive two-dimensional tungsten oxide nanosheets with extraordinary sensitivity and selectivity to NO2, Nanoscale, 2017, 9, 19162–19175 RSC.
- M. B. Rahmani, M. H. Yaacob and Y. M. Sabri, Hydrogen sensors based on 2D WO3 nanosheets prepared by anodization, Sens. Actuators, B, 2017, 251, 57–64 CrossRef CAS.
-
L. Yuan, Research on porous silicon-based nano-tungsten oxide multilevel structure material gas sensor (Master's thesis), Tianjin University, Tianjin, China, 2017 Search PubMed.
- D. Huang, W. Yuan, S. Fan, C. Tian, Z. Hua, X. Tian, Y. Wu and E.-P. Li, Hydrogen sensing mechanism of Ru-loaded WO3 nanosheets, Sens. Actuators, B, 2019, 304, 127339 CrossRef.
- H. Zhang, M. Yao, L. Bai, W. Xiang, H. Jin, J. Lia and F. Yuan, Synthesis of uniform octahedral tungsten trioxide by RF induction thermal plasma and its application in gas sensing, CrystEngComm, 2013, 15, 1432–1438 RSC.
- H. W. Long, W. Zeng, Y. Q. Li, W. G. Chen, B. Miao and C. X. Wang, Nanosci. Nanotechnol. Lett., 2014, 6, 1–6 CrossRef.
- J. H. Ma, Y. Ren, X. R. Zhou, L. L. Liu, Y. H. Zhu, X. W. Cheng, P. C. Xu, X. X. Li, Y. H. Deng and D. Y. Zhao, Pt nanoparticles sensitized ordered mesoporous WO3 semiconductor: gas sensing performance and mechanism study, Adv. Funct. Mater., 2018, 28, 1705268 CrossRef.
- C. H. Wu, Z. Zhu, S. Y. Huang and R. J. Wu, Preparation of palladium-doped mesoporous WO3 for hydrogen gas sensors, J. Alloys Compd., 2019, 776, 965–973 CrossRef CAS.
- C. Y. Wang, X. Zhang, Q. Rong, N. N. Hou and H. Q. Yu, Ammonia sensing by closely packed WO3 microspheres with oxygen vacancies, Chemosphere, 2018, 204, 202–209 CrossRef CAS PubMed.
- J. W. Li, X. Liu, J. S. Cui and J. B. Sun, Hydrothermal synthesis of self-assembled hierarchical tungsten oxides hollow spheres and their gas sensing properties, ACS Appl. Mater. Interfaces, 2015, 7, 10108–10114 CrossRef CAS PubMed.
- Y. Yao, M. L. Yin, J. Q. Yan and S. Z. Liu, P-type sub-tungsten-oxide based urchin-like nanostructure for superior room temperature alcohol sensor, Appl. Surf. Sci., 2018, 441, 277–284 CrossRef CAS.
- Y. X. Zhang, W. Zeng and Y. Q. Li, NO2 and H2 sensing properties for urchin-like hexagonal WO3 based on experimental and first-principle investigations, Ceram. Int., 2019, 45, 6043–6050 CrossRef CAS.
- C. Wang, M. D. Ding, X. Y. Kou, L. L. Guo, C. H. Feng, X. Li, H. Zhang, P. Sun, Y. F. Sun and G. Y. Lu, Detection of nitrogen dioxide down to ppb levels using flower-like tungsten oxide nanostructures under different annealing temperatures, J. Colloid Interface Sci., 2016, 483, 314–320 CrossRef CAS PubMed.
- Z. X. Cai, H. Y. Li, J. C. Ding and X. Guo, Hierarchical flowerlike WO3 nanostructures assembled by porous nanoflakes for enhanced NO gas sensing, Sens. Actuators, B, 2017, 246, 225–234 CrossRef CAS.
- J. J. Qi, S. Gao, K. Chen, J. Yang, H. W. Zhao, L. Guo and S. H. Yang, vertically aligned, double-sided, and self-supported 3D WO3 nanocolumn bundles for low-temperature gas sensing, J. Mater. Chem. A, 2015, 3, 18019–18026 RSC.
- Z. W. Jin, P. F. Hu, W. Xu, J. Zhou, W. W. Guo, Y. Chen and C. J. Qiu, Hydrothermal synthesis and gas sensing properties of hybrid WO3 nano-materials using octadecylamine, J. Alloys Compd., 2019, 785, 1047–1055 CrossRef CAS.
- T. T. Li, Y. B. Shen, S. K. Zhao, X. X. Zhong, W. Zhang, C. Han, D. Z. Wei, D. Meng and Y. X. Ao, Sub-ppm level NO2 sensing properties of polyethyleneimine-mediated WO3 nanoparticles synthesized by a one-pot hydrothermal method, J. Alloys Compd., 2019, 783, 103–112 CrossRef CAS.
- S. H. Wei, L. X. Han, M. Y. Wang, H. H. Zhang, W. M. Du and M. H. Zhou, Hollow cauliflower-like WO3 nanostructures: Hydrothermal synthesis and their CO sensing properties, Mater. Lett., 2017, 186, 259–262 CrossRef CAS.
- B. Behera and S. Chandra, Synthesis of WO3 nanorods by thermal oxidation technique for NO2 gas sensing application, Mater. Sci. Semicond. Process., 2018, 86, 79–84 CrossRef CAS.
- Q. Ding, Y. Wang, P. Guo, J. Li, C. Chen, T. Wang, K. Sun and D. He, Cr-Doped Urchin-Like WO3 Hollow Spheres: The Cooperative Modulation of Crystal Growth and Energy-Band Structure for High-Sensitive Acetone Detection, Sensors, 2020, 20, 3473 CrossRef CAS PubMed.
- C. W. Song, C. Li, Y. Y. Yin, J. K. Xiao, X. N. Zhang, M. Y. Song and W. Dong, Preparation and gas sensing properties of partially broken WO3 nanotubes, Vacuum, 2015, 114, 13–16 CrossRef CAS.
- R. Adhikari, G. Gyawali, T. H. Kim, T. Sekino and S. W. Lee, EDTA mediated microwave hydrothermal synthesis of WO3 hierarchical structure and its photoactivity under simulated solar light, J. Environ. Chem. Eng., 2014, 2, 1365–1370 CrossRef CAS.
- P. V. Tong, N. D. Hoa, V. V. Quang, N. V. Duy and N. V. Hieu, Diameter controlled synthesis of tungsten oxide nanorod bundles for highly sensitive NO2 gas sensors, Sens. Actuators, B, 2013, 183, 372–380 CrossRef CAS.
- Z. Y. Wang, P. Sun, T. L. Yang, Y. Gao, X. W. Li, G. Y. Lu and Y. Du, Flower-like WO3 architectures synthesized via a microwave-assisted method and their gas sensing properties, Sens. Actuators, B, 2013, 186, 734–740 CrossRef CAS.
- C. Wang, R. Z. Sun, X. Li, Y. F. Sun, P. Sun, F. M. Liu and G. Y. Lu, Hierarchical flower-like WO3 nanostructures and their gas sensing properties, Sens. Actuators, B, 2014, 204, 224–230 CrossRef CAS.
- L. You, X. He, D. Wang, P. Sun, Y. F. Sun, X. S. Liang, Y. Du and G. Y. Lu, Ultrasensitive and low operating temperature NO2 gas sensor using nanosheets assembled hierarchical WO3 hollow microspheres, Sens. Actuators, B, 2012, 173, 426–432 CrossRef CAS.
- Y. Shen, D. F. Ding and Y. Z. Deng, Fabrication, and characterization of WO3 flocky microspheres induced by ethanol, Powder Technol., 2011, 211, 114–119 CrossRef CAS.
- L. Zhang, X. C. Tang, Z. G. Lu, Z. M. Wang, L. X. Li and Y. H. Xiao, Facile synthesis, and photocatalytic activity of hierarchical
WO3 core–shell microspheres, Appl. Surf. Sci., 2011, 258, 1719–1724 CrossRef CAS.
- T. V. Belysheva, L. P. Bogovtseva, E. A. Kazachkov and N. V. Serebryakova, Gas-sensing properties of doped In2O3 films as sensors for NO2 in air, J. Anal. Chem., 2003, 58, 583–587 CrossRef CAS.
- N. Yamazoe, New approaches for improving semiconductor gas sensors, Sens. Actuators, B, 1991, 5, 7–19 CrossRef CAS.
- G. Jimenez-Cadena, J. Riu and F. X. Rius, Gas sensors based on nanostructured materials, Analyst, 2007, 132, 1083–1099 RSC.
- K. Wetchakun, T. Samerjai, N. Tamaekong, C. Liewhiran, C. Siriwong, V. Kruefu, A. Tuantranont, S. Phanichphant and A. Wisitsoraat, Semiconducting metal oxides as sensors for environmentally hazardous gases, Sens. Actuators, B, 2011, 160, 580–591 CrossRef CAS.
- S. J. Patil, A. V. Patil, V. G. Dighavkar, K. S. Thakare, R. Y. Borase, S. J. Nandre, N. G. Deshpande and R. R. Ahire, Semiconductor metal oxide compounds-based gas sensors: a literature review, Front. Mater. Sci., 2015, 9, 14–37 CrossRef.
- G. Korotcenkov and B. K. Cho, Engineering approaches for the improvement of conductometric gas sensor parameters: Part 1. Improvement of sensor sensitivity and selectivity (short survey), Sens. Actuators, B, 2013, 188, 709–728 CrossRef CAS.
- Y. Liu,
et al., Solid-state gas sensors for high temperature applications – a review, J. Mater. Chem. A, 2014, 26, 9919–9943 RSC.
- X. Liu, S. Cheng, H. Liu, S. Hu, D. Zhang and H. Ning, A survey on gas sensing technology, Sensor, 2012, 12, 9635–9665 CrossRef PubMed.
- Y. V. Kaneti, J. Yue, X. Jiang and A. Yu, Controllable Synthesis of ZnO Nanoflakes with Exposed (10
0) for Enhanced Gas Sensing Performance, J. Phys. Chem. C, 2013, 25, 13153–13162 CrossRef.
- V. Oison, L. Saadi, C. Lambert-Mauriat and R. Hayn, Mechanism of CO and O3 sensing on WO3 surfaces: First principal study, Sens. Actuators, B, 2011, 166, 505–510 CrossRef.
- P. J. Shaver, Activated tungsten oxide gas detectors, Appl. Phys. Lett., 1967, 11, 255–257 CrossRef CAS.
- C. H. Chang, T. C. Chou, W. C. Chen, J. S. Niu, K. W. Lin, S. Y. Cheng, J. H. Tsai and W. C. Liu, Study of a WO3 thin film-based hydrogen gas sensor decorated with platinum nanoparticles, Sens. Actuators, B, 2020, 317, 128145 CrossRef CAS.
- R. A. Kadir, W. Zhang, Y. Wang, J. Z. Ou, W. Wlodarski, A. P. O'Mullane, G. Bryant, M. Taylor and K. Kalantar-zadeh, anodized nanoporous WO3 Schottky contact structures for hydrogen and ethanol sensing, J. Mater. Chem. A, 2015, 3, 7994–8001 RSC.
- M. H. Yaacob,
et al., Absorption spectral response of nanotextured WO3 thin flms with Pt catalyst towards H2, Sens. Actuators, B, 2009, 137, 115–120 CrossRef.
- Air Quality Ontario, Ministry of Environment, Ontario, Canada. www.airqualityontario.com/science/pollutants/nitrogen.cfm, 2010 (accessed 10.12.10).
- C. Zhang, M. Debliquy, A. Boudiba, H. Liao and C. Coddet, sensing properties of atmospheric plasma sprayed WO3 coating for sub-ppm NO2 detection, Sens. Actuators, B, 2010, 144, 280 CrossRef CAS.
- D. Meng, T. Yamazaki, Y. Shen, Z. Liu and T. Kikuta, Preparation of WO3 nanoparticles and application to NO2 sensor, Appl. Surf. Sci., 2009, 256, 1050–1053 CrossRef CAS.
- E. K. Heidari, C. Zamani, E. Marzbanrad, B. Raissi and S. Nazarpour, WO3-based NO2 sensors fabricated through low frequency AC electrophoretic deposition, Sens. Actuators, B, 2010, 146, 165–170 CrossRef CAS.
- T. Akamatsu, T. Itoh, N. Izu and W. Shin, NO and NO2 Sensing Properties of WO3 and Co3O4 Based Gas Sensors, Sensors, 2013, 13, 12467–12481 CrossRef PubMed.
- M. Akiyama, J. Tamaki, N. Miura and N. Yamazoe, Tungsten Oxide-Based Semiconductor Sensor Highly Sensitive to NO and NO2, Chem. Lett., 1991, 9, 1611–1614 CrossRef.
- S. Zhao, Y. Shen, P. Zhou, X. Zhong, C. Han, Q. Zhao and D. Wei, Design of Au@WO3 core−shell structured nanospheres for ppb-level NO2 sensing, Sens. Actuators, B, 2019, 282, 917–926 CrossRef CAS.
- Y. Zhang, W. Zeng and Y. Li, NO2 and H2 sensing properties for urchin-like hexagonal WO3 based on experimental and first-principal investigations, Ceram. Int., 2019, 45, 6043–6050 CrossRef CAS.
- W. He, Y. Zhao and Y. Xiong, Bilayer Polyaniline−WO3 Thin-Film Sensors Sensitive to NO2, ACS Omega, 2020, 5, 9744–9751 CrossRef CAS PubMed.
- Ammonia. www.en.wikipedia.org/wiki/Ammonia, 2010 (accessed 10.12.10).
- T. Maekawa, J. Tamaki, N. Miura and N. Yamazoe, Gold-loaded tungsten oxide sensor for detection of ammonia in air, Chem. Lett., 1992, 4, 639–642 CrossRef.
- A. Ponzoni, E. Comini, M. Ferroni and G. Sberveglieri, Nanostructured WO3 deposited by modified thermal evaporation for gas-sensing applications, Thin Solid Films, 2005, 81, 490 Search PubMed.
- E. K. Heidari, C. Zamani, E. Marzbanrad, B. Raissi and S. Nazarpour, WO3-based NO2 sensors fabricated through low frequency AC electrophoretic deposition, Sens. Actuators, B, 2010, 146, 165 CrossRef CAS.
- J. Leng, X. Xu, N. Lv, H. Fan and T. Zhang, Synthesis and gas-sensing characteristics of WO3 nanofibers via electrospinning, J. Colloid Interface Sci., 2011, 356, 54–57 CrossRef CAS PubMed.
- Q. Wang, H. Fu, J. Ding, C. Yang and S. Wang, Sensitivity enhanced microfiber interferometer ammonia gas sensor by using WO3 nanorods coatings, Opt. Laser Technol., 2020, 125, 106036 CrossRef CAS.
- G. Fan, D. Chen, T. Li, S. Yi, H. Ji, Y. Wang, Z. Zhang, G. Shao, B. Fan, H. Wang, H. Xu, H. Lu, Y. Zhou, R. Zhang and J. Sun, Enhanced room-temperature ammonia-sensing properties of polyaniline-modified WO3 nanoplates derived via ultrasonic spray process, Sens. Actuators, B, 2020, 312, 127892 CrossRef CAS.
- C. M. Hung, D. Q. Dat, N. V. Duy, V. V. Quang, N. V. Toan, N. V. Hieu and N. D. Hoa, Facile synthesis of ultrafine rGO/WO3 nanowire nanocomposites for highly sensitive toxic NH3 gas sensors, Mater. Res. Bull., 2020, 125, 110810 CrossRef CAS.
- C. Castillo, G. Cabello, B. Chornik, Y. Huentupil and G. E. Buono-Core, Characterization of photochemically grown Pd loaded WO3 thin films and its evaluation as ammonia gas sensor, J. Alloys Compd., 2020, 825, 154166 CrossRef CAS.
- M. Kaur, N. Jain, K. Sharma, S. Bhattacharya, M. Roy, A. K. Tyagi, S. K. Gupta and J. V. Yakhmi, Room-temperature H2S gas sensing at ppb level by single crystal In2O3 whiskers, Sens. Actuators, B, 2008, 133, 456–461 CrossRef CAS.
- E. P. S. Barrett, G. C. Georgiades and P. A. Sermon, The mechanism of operation of WO3-based H2S sensors, Sens. Actuators, B, 1990, 1, 1–6 CrossRef.
- J. L. Solis, S. Saukko, L. B. Kish, C. G. Granqvist and V. Lantto, Nanocrystalline tungsten oxide thick-films with high sensitivity to H2S at room temperature, Sens. Actuators, B, 2001, 77, 316–321 CrossRef CAS.
- X. Xiao, X. Zhou, J. Ma, Y. Zhu, X. Cheng, W. Luo and Y. Deng, Rational Synthesis and Gas Sensing Performance of Ordered Mesoporous Semiconducting WO3/NiO Composites, ACS Appl. Mater. Interfaces, 2019, 11, 26268–26276 CrossRef CAS PubMed.
- D. H. Kim, J. S. Jiang, W. T. Koo, S. J. Choi, H. J. Cho, M. H. Kim, S. J. Kim and I. D. Kim, Bioinspired cocatalysts decorated WO3 nanotube toward unparalleled hydrogen sulfide chemresistor, ACS Sens., 2018, 3, 1164–1173 CrossRef CAS PubMed.
- AQS, Department of the Environment, Transport and the Regions, The Scottish Executive, The National Assembly for Wales and The Department of the Environment Northern Ireland, The Air Quality Strategy for England Scotland, Wales and Northern Ireland, 2000 (accessed 10.12.10).
- S. H. Wei, L. X. Han, M. Y. Wang, H. H. Zhang, W. M. Du and M. H. Zhou, Hollow cauliflower-like WO3 nano structures: Hydrothermal synthesis and their CO sensing properties, Mater. Lett., 2017, 186, 259–262 CrossRef CAS.
- F. H. Tian, L. H. Zhao, X. Y. Xue, Y. Y. Shen, X. F. Jia, S. G. Chen and Z. H. Wang, DFT study of CO sensing mechanism on hexagonal WO3 (0 0 1) surface: The role of oxygen vacancy, Appl. Surf. Sci., 2014, 311, 362–368 CrossRef CAS.
- L. Cai,
et al., Highly sensitive H2 sensor based on PdO-decorated WO3 nanospindle p-n heterostructure, Int. J. Hydrogen Energy, 2020, 45(55), 31327–31340 CrossRef CAS.
- C. Cantalini, W. Wlodarski, Y. Li, M. Passacantando, S. Santucci, E. Comini, G. Faglia and G. Sberveglieri, Investigation on the O3 sensitivity properties of WO3 thin films prepared by sol–gel, thermal evaporation and r.f. sputtering techniques, Sens. Actuators, B, 2000, 64, 182–188 CrossRef CAS.
- R. S. Khadayate, J. V. Sali and S. B. Rane, Preparation and Characterization of WO3-Based Liquid Petroleum Gas, J. Manuf. Mater. Process., 2017, 22, 277–280 CrossRef.
- J. R. Huang, X. J. Xu, C. P. Gu, M. Yang, M. Yang and J. H. Liu, Large-scale synthesis of hydrated tungsten oxide 3D architectures by a simple chemical solution route and their gas-sensing properties, J. Mater. Chem., 2011, 21, 13283–13289 RSC.
- Z. Xie, Y. G. Zhu and J. Xu, Porous WO3 with enhanced photocatalytic and selective gas sensing properties, CrystEngComm, 2011, 13, 6393–6398 RSC.
- X. Q. Gao, X. T. Su, C. Yang, F. Xiao, J. D. Wang, X. D. Cao, S. J. Wang and L. Zhang, Hydrothermal synthesis of WO3 nanoplates as highly sensitive cyclohexene sensor and high-efficiency MB photocatalyst, Sens. Actuators, B, 2013, 181, 537–543 CrossRef CAS.
- Z. H. Li, J. C. Li, L. L. Song, H. Q. Gong and Q. Niu, Ionic liquid-assisted synthesis of WO3 particles with enhanced gas sensing properties, J. Mater. Chem. A, 2013, 1, 15377–15382 RSC.
- H. B. Zhang, M. S. Yao and L. Y. Bai, Synthesis of uniform octahedral tungsten trioxide by RF induction thermal plasma and its application in gas sensing, CrystEngComm, 2013, 15, 1432–1438 RSC.
- M. Yin, L. Yu and S. Liu, Synthesis of thickness-controlled cuboid WO3 nanosheets and their exposed facets-dependent acetone sensing properties, J. Alloys Compd., 2017, 696, 490–497 CrossRef CAS.
- Q.-Q. Jia, H.-M. Ji, D.-H. Wang, X. Bai, X.-H. Sun and Z.-G. Jin, Exposed facets induced enhanced acetone selective sensing property of nanostructured tungsten oxide, J. Mater. Chem. A, 2014, 2, 13602–13611 RSC.
- S. Wei, S. Li, R. Wei, S. Liu and W. Du, Different morphologies of WO3 and their exposed facets-dependent acetone sensing properties, Sens. Actuators, B, 2021, 329, 129188 CrossRef CAS.
- M. Righettoni, A. Tricoli and S. E. Pratsinis, Thermally stable, silica-doped WO3 for sensing of acetone in the human breath, Chem. Mater., 2010, 22, 3152–3157 CrossRef CAS.
- J. Y. Shen, L. Zhang, J. Ren, J. C. Wang, H. C. Yao and Z. J. Li, Highly enhanced acetone sensing performance of porous C-doped WO3 hollow spheres by carbon spheres as templates, Sens. Actuators, B, 2017, 239, 597–607 CrossRef CAS.
- A. Kumar and S. Keshri, Effect of N5+ ion implantation on optical and gas sensing properties of WO3 films, Surf. Interface Anal., 2015, 47, 1020–1028 CrossRef CAS.
- Z. Y. Zhang, M. Haq, Z. Wen, Z. Z. Ye and L. P. Zhu, Ultrasensitive ppb-level NO2 gas sensor based on WO3 hollow nanosphers doped with Fe, Appl. Surf. Sci., 2018, 434, 891–897 CrossRef CAS.
- F. Li, S. P. Ruan, N. Zhang, Y. Y. Yin, S. J. Guo, Y. Chen, H. F. Zhang and C. N. Li, Synthesis and characterization of Cr-doped WO3 nanofibers for conductometric sensors with high xylene sensitivity, Sens. Actuators, B, 2018, 265, 355–364 CrossRef CAS.
- Z. Y. Zhu, L. J. Zheng, J. Chen, M. H. Liang, Y. T. Tian and D. C. Yang, Cr doped WO3 nanofibers enriched with surface oxygen vacancies for highly sensitive detection of the 3-hydroxy-2-butanone biomarker, J. Mater. Chem. A, 2018, 6, 21419–21427 RSC.
- Z. W. Liu, B. Liu, W. Y. Xie, H. Li, R. Zhou, Q. H. Li and T. H. Wang, enhanced selective acetone sensing characteristics based on Co-doped WO3 hierarchical flower-like nanostructures assembled with nanoplates, Sens. Actuators, B, 2016, 235, 614–621 CrossRef CAS.
- J. J. Qi, K. Chen, Y. Xing, H. Fan, H. W. Zhao, J. Yang, L. D. Li, B. Y. Yan, J. Zhou, L. Guo and S. H. Yang, Application of 3D hierarchical monoclinic-type structural Sb-doped WO3 towards NO2 gas detection at low temperature, Nanoscale, 2018, 10, 7440–7450 RSC.
- C. Wang, L. L. Guo, N. Xie, X. Y. Kou, Y. F. Sun, X. H. Chuai, S. M. Zhang, H. W. Song, Y. Wang and G. Y. Lu, Enhanced nitrogen oxide sensing performance based on tin-doped tungsten oxide nanoplates by a hydrothermal method, J. Colloid Interface Sci., 2018, 512, 740–749 CrossRef CAS PubMed.
- J. Kaur, K. Anand, A. Kaur and R. C. Singh, Sensitive and selective acetone sensor based on Gd doped WO3-reduced grapheme oxide nanocomposite, Sens. Actuators, 2018, 258, 1022–1035 CrossRef CAS.
- X. Bai, H. M. Ji, P. Gao, Y. Zhang and X. H. Sun, Morphology, phase structure and acetone sensitive properties of copper-doped tungsten oxide sensors, Sens. Actuators, B, 2014, 193, 100–106 CrossRef CAS.
- Z. H. Wang, X. X. Fan, D. M. Han and F. B. Gu, Structural and electronic engineering of 3D WO3 by alkali metal doping for improved NO2 sensing performance, Nanoscale, 2016, 8, 10622–10631 RSC.
- L. Ping, Z. Zhang, Z. Zhuang, J. Guo, Z. Fang, S. L. Fereja and W. Chen, Pd-Doping-Induced Oxygen Vacancies in One-Dimensional Tungsten Oxide Nanowires for Enhanced Acetone Gas Sensing, Anal. Chem., 2021, 93, 7465–7472 CrossRef PubMed.
- J. Y. Shen, M. D. Wang, Y. F. Wang, J. Y. Hu, Y. Y. Zhu, Y. X. Zhang, Z. J. Li and H. C. Yao, Iron and carbon co doped WO3 with hierarchical walnut-like microstructure for highly sensitive and selective acetone sensor, Sens. Actuators, B, 2018, 256, 27–37 CrossRef CAS.
- P. Rai, S. M. Majhi, Y. T. Yu and J. H. Lee, Noble metal@metal oxide semiconductor core@shell nano-archiectrures as a new platform for gas sensor applications, RSC Adv., 2015, 5, 76229–76248 RSC.
- D. Huang, W. Yuan, S. Fan, C. Tian, Z. Hua, X. Tian, Y. Wu and E.-P. Li, Hydrogen Sensing Mechanism of Ru-loaded WO3 Nanosheets, Sens. Actuators, B, 2019, 304, 127339 CrossRef.
- S. K. Zhao, Y. B. Shen, P. F. Zhou, X. X. Zhong, C. Han, Q. Zhao and D. Z. Wei, Design of Au@WO3 core-shell structured nanospheres for ppb-level NO2 sensing, Sens. Actuators, B, 2019, 282, 917–926 CrossRef CAS.
- H. W. Zhang, Y. Y. Wang, X. G. Zhu, Y. Li and W. P. Cai, Bilayer Au nanoparticle-decorated WO3 porous thin films: on-chip fabrication and enhanced NO2 gas sensing performances with high selectivity, Sens. Actuators, B, 2019, 280, 192–200 CrossRef CAS.
- X. Yang, V. Salles, Y. V. Kaneti, M. Liu, M. Maillard, C. Journet, X. Jiang and A. Brioude, Fabrication of Highly Sensitive Gas Sensor Based on Au Functionalized WO3 Composite Nanofibers by Electrospinning, Sens. Actuators, B, 2015, 220, 1112–1119 CrossRef CAS.
- Y. Zhang, Y. Wang, L. Zhu, R. Zhang and J. Cao, Enhanced CO sensing performance of WO3 nanorods with PtAg nanoparticles modification: A combined experimental and first-principle study, Vacuum, 2021, 193, 110526 CrossRef CAS.
- J. H. Ma, Y. Ren, X. R. Zhou, L. L. Liu, Y. H. Zhu, X. W. Cheng, P. C. Xu, X. X. Li, Y. H. Deng and D. Y. Zhao, Pt nanoparticles sensitized ordered mesoporous WO3 semiconductors: gas sensing performance and mechanism study, Adv. Funct. Mater., 2018, 28, 1705268 CrossRef.
- Y. Wang, J. Liu, X. Cui, Y. Gao, J. Ma, Y. Sun, P. Sun, F. Liu, X. Liang, T. Zhang and G. Lu, NH3 gas sensing performance enhanced by Pt-loaded on mesoporous WO3, Sens. Actuators, B, 2017, 238, 473–481 CrossRef CAS.
- D. R. Miller, S. A. Akbar and P. A. Morris, Nanoscale metal oxide-based heterojunctions for gas sensing: A review, Sens. Actuators, B, 2014, 204, 250–272 CrossRef CAS.
- T. M. Liu, W. Zeng and Z. C. Wang, Quasi-one-dimensional metal-oxide-based heterostructural gas-sensing materials: A review, Sens. Actuators, B, 2015, 221, 1570–1585 CrossRef.
- H. Y. Gao, Q. Yu, K. Chen, P. Sun, F. M. Liu, X. Yan, F. M. Liu and G. Y. Lu, Ultrasensitive gas sensor based on hollow tungsten trioxide-nickel oxide (WO3-NiO) nanoflowers for fast and selective xylene detection, J. Colloid Interface Sci., 2019, 535, 458–468 CrossRef CAS PubMed.
- Y. Y. Zhu, H. J. Wang, J. K. Liu, M. L. Yin, L. M. Yu, J. Zhou, Y. Liu and F. Qiao, High-performance gas sensor based on the WO3-SnO2 nanosphere composites, J. Alloys Compd., 2019, 782, 789–795 CrossRef CAS.
- J. H. Sun, L. X. Sun, N. Han, J. L. Pan, W. Q. Liu, S. L. Bai, Y. J. Feng, R. X. Luo, D. Q. Li and A. F. Chen, Ordered mesoporous WO3/ZnO nanocomposites with isotype heterojunctions for sensitive detection of NO2, Sens. Actuators, B, 2019, 285, 68–75 CrossRef CAS.
- D. Y. Xue, F. Y. Zong, J. M. Zhang, X. P. Lin and Q. H. Li, Synthesis of Fe2O3/WO3 nanocomposites with enhanced sensing performance to acetone, Chem. Phys. Lett., 2019, 716, 61–68 CrossRef CAS.
- L. Yin, G. P. Qu, P. Guo, R. Zhang, J. Sun and D. L. Chen, Construction and enhanced low-temperature H2S-sensing performance of novel hierarchical CuO@WO3nanocomposites, J. Alloys Compd., 2019, 785, 367–373 CrossRef CAS.
- J. H. Sun, X. Shu, Y. L. Tian, Z. F. Tong, S. L. Bai, R. X. Luo, D. Q. Li and A. F. Chen, Preparation of polypyrrole@WO3 hybrids with p-n heterojunction and sensing performance to trimethylamine at room temperature, Sens. Actuators, B, 2017, 238, 510–517 CrossRef CAS.
- J. N. Zhang, H. B. Lu, C. Yan, Z. B. Yang, G. Q. Hu, J. Z. Gao, F. Yin and C. L. Wang, Fabrication of conductive graphene oxide-WO3 composite nanofibers by electrospinning and their enhanced acetone gas sensing properties, Sens. Actuators, B, 2018, 264, 128–138 CrossRef CAS.
- G. Jeevitha, R. Abhinayaa, D. Mangalaraj, N. Ponpandian, P. Meena, V. Mounasamy and S. Madanagurusamy, Porous reduced graphene oxide (rGO/WO3 nanocomposites for the enhanced detection of NH3 at room temperature), Nanoscale Adv., 2019, 1, 1799–1811 RSC.
- J. Han, J. Yang, J. Tang, M. B. Ghasemian, L. J. Hubble, N. Syed, T. Daeneke and K. Kalantar-Zadeh, Liquid metals for tuning gas sensitive layers, J. Mater. Chem. C, 2019, 7, 6375–6382 RSC.
- Y. H. Gui, K. Tian, J. X. Liu, L. Yang, H. Z. Zhang and Y. Wang, Superior trimethylamine detection at room temperature by {−112} faceted WO3 gas sensor, J. Hazard. Mater., 2019, 380, 120876 CrossRef CAS PubMed.
- V. K. Tomer, S. Devi, R. Malik, S. P. Nehra and S. Duhan, Highly sensitive and selective Volatile organic amine (VOA) sensors using mesoporous WO3-SnO2 nanohybrids, Sens. Actuators, B, 2016, 229, 321–330 CrossRef CAS.
- Y. Han, Y. Liu, C. Su, X. Chen, B. Li, W. Jiang, M. Zeng, N. Hu, Y. Su, Z. Zhou and Z. G. Zhu, Hierarchical WS2–WO3 Nanohybrids with P–N Heterojunctions for NO2 Detection, ACS Appl. Nano Mater., 2021, 4, 1626–1634 CrossRef CAS.
- F. Perrozzi, S. M. Emamjomeh, V. Paolucci, G. Taglieri, L. Ottaviano and C. Cantalini, Thermal staility of WS2 flakes and gas sensing properties of WS2/WO3 composite to H2, NH3 and NO2, Sens. Actuators, B, 2017, 243, 812–822 CrossRef CAS.
- Y. G. Song, J. Y. Park, J. M. Suh, Y.-S. Shim, S. Y. Yi, H. W. Jang, S. Kim, J. M. Yuk, B.-K. Ju and C.-Y. Kang, Heterojunction Based on Rh-Decorated WO3 Nanorods for Morphological Change and Gas Sensor Application Using the Transition Effect, Chem. Mater., 2019, 31, 207–215 CrossRef CAS.
- Y. L. Wang, B. Zhang, J. Liu, Q. Y. Yang, X. B. Cui, Y. Gao, X. H. Chuai, F. M. Liu, P. Sun, X. S. Liang, Y. F. Sun and G. Y. Lu, Au-loaded mesoporous WO3: preparation and n-butanol sensing performances, Sens. Actuators, B, 2016, 236, 67–76 CrossRef CAS.
- S. Kabcum, N. Kotchasak, D. Channei, A. Tuantranont, A. Wisitsoraat, S. Phanichphant and C. Liewhiran, Highly sensitive and selective NO2 sensor based on Au-impregnated WO3 nanorods, Sens. Actuators, B, 2017, 252, 523–536 CrossRef CAS.
- H. M. Yu, J. Z. Li, Z. Y. Li, Y. W. Tian and Z. D. Yang, Enhanced formaldehyde sensing performance based on Ag@WO3 2D nanocomposites, Powder Technol., 2019, 343, 1–10 CrossRef CAS.
- Y. L. Wang, J. Liu, X. B. Cui, Y. Gao, J. Ma, Y. F. Sun, P. Sun, F. M. Liu, X. S. Liang, T. Zhang and G. Y. Lu, NH3 gas sensing performance enhanced by Pt-loaded on mesoporous WO3, Sens. Actuators, B, 2017, 238, 473–481 CrossRef CAS.
- S. J. Choi and I. D. Kim, Recent Developments in 2D Nanomaterials for Chemiresistive-Type Gas Sensors, Electron. Mater. Lett., 2018, 14, 221–260 CrossRef CAS.
- X. Zheng and H. Cheng, Flexible and stretchable metal oxide gas sensors for healthcare, Sci. China: Technol. Sci., 2019, 62, 209–223 CrossRef CAS.
|
This journal is © The Royal Society of Chemistry 2022 |
Click here to see how this site uses Cookies. View our privacy policy here.