DOI:
10.1039/D1TA02493K
(Review Article)
J. Mater. Chem. A, 2021,
9, 18887-18905
Recent advances in wearable self-powered energy systems based on flexible energy storage devices integrated with flexible solar cells
Received
25th March 2021
, Accepted 7th June 2021
First published on 7th June 2021
Abstract
Wearable electronics are considered to be an important technology in next-generation smart electronics. Meanwhile, the ever-increasing energy consumption and the growing environmental awareness have highlighted the requirements of green and renewable energy. Integrating flexible photovoltaic cells (PVCs) with flexible energy storage devices (ESDs) to construct self-sustaining energy systems not only provides a promising strategy to address the energy and environmental issues, but also enables the entire system to be operated continuously without external charging, which is considered to be a promising direction for future wearable electronics. Herein, we summarize the recent progress in wearable self-sustaining energy systems based on flexible ESDs integrated with flexible PVCs. First, the recent developments in flexible PVCs and their characteristics are summarized. Following that, we discuss the advances in flexible ESDs used in wearable electronics. Then, we give a short discussion on the integration of flexible ESDs with flexible PVCs. Thereafter, various functional applications of these self-sustaining energy systems in wearable electronics are introduced. Finally, the main challenges and future prospects of self-powered energy systems for wearable electronics are discussed.
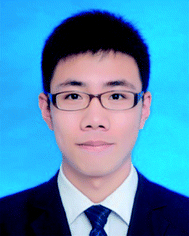 Jiangqi Zhao | Dr Jiangqi Zhao is currently an Associate Professor at the College of Materials Science and Engineering at Sichuan University. He obtained his BE and PhD from Sichuan University, in 2014 and 2020, respectively. He was a visiting scholar in the Department of Electrical Engineering and Computer Sciences at the University of California, Berkeley, during 2017–2019. He worked as a Research Assistant at the City University of Hong Kong in 2020–2021. His research interests include biomass materials, flexible energy storage devices, sweat sensors, wearable electronics, etc. |
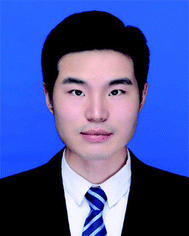 Jiajia Zha | Jiajia Zha received his BS from Southeast University in 2017. After receiving his MS from Fudan University in 2020, he moved to the Department of Electrical Engineering at the City University of Hong Kong in 2020 to pursue his PhD under the supervision of Asst. Prof. Chaoliang Tan. His research interests mainly include nanoelectronics/optoelectronics based on 2D materials. |
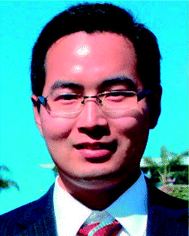 Zhiyuan Zeng | Dr Zhiyuan Zeng obtained his BE and ME from Central South University, Zhejiang University, in 2006 and 2008, respectively. He completed his PhD under Prof. Hua Zhang at Nanyang Technological University, Singapore (2013). He joined Dr Haimei Zheng's group as a Post-doctoral Fellow at the Lawrence Berkeley National Laboratory (2013–2017). After working for Applied Materials Inc. in Silicon Valley (2017–2019), he joined the City University of Hong Kong as an Assistant Professor in 2019. His current research interest focuses on battery intercalation strategies involving the preparation of single-layer transition metal dichalcogenides and their applications in energy storage, catalysis, etc. |
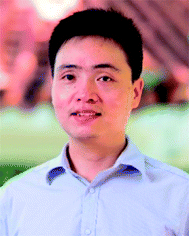 Chaoliang Tan | Dr Chaoliang Tan is currently an Assistant Professor in the Department of Electrical Engineering at the City University of Hong Kong. He received his BE and ME from the Hunan University of Science and Technology and South China Normal University in 2009 and 2012, respectively. He received his PhD from Nanyang Technological University in 2016. After working as a Research Fellow in the same group for about one year, he then worked as a Postdoctoral Researcher at the University of California, Berkeley, for two years. His research focuses on 2D materials for electronics/optoelectronics and engineering of layered materials for biomedicine, energy storage, etc. |
1. Introduction
Wearable electronics have received increasing attention and experienced rapid growth in recent years.1,2 These devices have been widely used in multifunctional entertainment, intelligent monitoring, personal healthcare and exercise management, and gradually change people's lifestyles.3–5 At the same time, the huge consumption of energy caused by the rapid development of electronic devices along with the growing environmental awareness has highlighted the requirements for new technology to collect and store green and renewable energy.6 Solar energy is the most abundant, sustainable and cleanest energy in the world.7 Along with the continuous improvement of conversion technology from light to electricity, solar energy accounts for an increasing proportion of the energy system, and holds promise as an ideal alternative to conventional fossil fuel energy in the future.8,9 However, because of the non-continuous and unstable nature of sunlight, it is not stable for solar cells to directly supply power to wearable electronics.10 Combining photovoltaic cells (PVCs) with energy storage devices (ESDs) to realize a self-powered system provides a feasible solution to address the imposed restrictions of sunlight availability and acts as a reliable source of energy.11 In this regard, self-sustaining energy technology is considered to be a highly promising direction for future wearable electronics since it can enable electronics to operate continuously without external power supply.12 At the same time, building integrated self-powered systems enables the development of wearable electronic devices toward intelligence and miniaturization.12
Up to now, different types of self-powered energy systems with a great variety of flexible PVCs and flexible ESDs have been developed as reliable energy sources used in wearable electronics.13–16 Although these wearable electronics with multiple functions have been demonstrated to be used in various applications, these demonstrations are still far from actual commercial products, which is caused by the complex fabrication process, high cost, single function, immature design, etc. In addition, in some previous review papers, the authors usually only focus on a certain part (energy harvest, energy storage, wearable sensors, etc.), and the discussion on their applications in the area of wearable devices is limited.17–20 Overall, the development of the field of self-powered wearable energy systems is still in its infancy. It is quite urgent to explore more advanced functional materials and efficient integration strategies to promote the development of self-powered energy systems to realize large-scale production and market applications. The conventional concept of a “self-powered system” which has been proposed before only accounts for a small part of the full context, and especially ignores the energy conversion efficiency, reliability, durability and challenges of the entire systems.17–20 The lack of a comprehensive review article, which summarizes the progress of self-powered systems and the problems faced, drives us to summarize the recent progress from the perspective of entire self-powered energy systems.
Herein, we provide a comprehensive summary of the recent progress and future prospects of wearable self-powered energy systems by categorizing different composed modules. In this regard, firstly, flexible PVCs, including silicon solar cells (SSCs), organic solar cells (OSCs), dye-sensitized solar cells (DSSCs) and perovskite solar cells (PSCs), are introduced based on different light conversion mechanisms. Then, according to different energy storage mechanisms, recently developed flexible ESDs, such as supercapacitors (SCs), lithium-ion batteries (LIBs) and zinc-ion batteries (ZIBs), and their application in wearable electronics are summarized. Thereafter, we give a short discussion on the integration of flexible ESDs with flexible PVCs. Following this section, various functional applications of self-powered energy systems in wearable electronics, such as pulse monitoring, sweat monitoring and gas detection, are presented. Finally, to facilitate further research and technology development, some significant challenges and further research directions of self-powered wearable systems are discussed. A brief summary is schematically shown in Fig. 1, which illustrates the main studies of wearable self-powered energy systems.
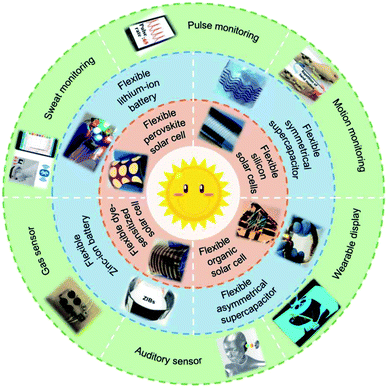 |
| Fig. 1 Schematic illustration of the important applications of flexible ESDs integrated with PVCs. | |
2. Flexible photovoltaic cells in self-powered wearable electronics
Photovoltaic cells have become ideal alternatives to common energy sources because of their advantages of light weight, compact structure and high conversion efficiency. With the advent of new functional materials and progress of fabrication technologies, as well as the growing demand in the wearable market, different types of flexible photovoltaic cells, including SSCs, OSCs, DSSCs and PSCs, have been continuously enriched. The performance of various kinds of flexible PVCs is summarized in Table 1.
Table 1 Summary of the types, materials and capacities and flexibility of flexible ESDs
Types |
Materials |
Specific power |
PCE |
Ref. |
SSC |
Nanostructured silicon |
— |
12.4% |
26
|
SSC |
P3HT/PCBM |
— |
3.27% |
29
|
SSC |
Amorphous silicon |
— |
1.4% |
30
|
OSC |
PEDOT:PSS |
10 W g−1 |
4.2% |
38
|
OSC |
PBDTTT-OFT/PC71BM |
11.46 W g−1 |
10.5% |
39
|
DSSC |
N719 TNARs |
— |
4.54% |
45
|
DSSC |
TiO2 |
— |
3.27% |
46
|
DSSC |
TNAR/Ti N-719 |
— |
3.82% |
47
|
PSC |
Perovskite |
23 W g−1 |
12% |
56
|
PSC |
Perovskite |
— |
14.78% |
57
|
PSC |
Perovskite |
— |
12.2% |
59
|
2.1. Flexible silicon solar cells (SSCs)
Benefiting from the high natural abundance, excellent reliability and good power conversion efficiency (PCE), SSCs, the earliest developed solar cells, have long dominated the photovoltaic market.21 According to the working mechanisms, silicon-based solar cells can be divided into monocrystalline, polycrystalline and amorphous SSCs.22 In contrast to early rigid SSCs, flexible ones have been processed in recent years through depositing silicon on flexible substrates or embedding microscale SSCs into flexible substrates.23,24 In 2011, Yoon et al. reported flexible concentrator photovoltaics fabricated by embedding microscale SSCs into luminescent waveguides.25 As shown in Fig. 2a, the incident light could be reflected in the flexible matrix and redirected onto the microscale SSCs to increase the power of the obtained SSCs by more than 300%. This unusual design endows the resulting SSCs with ultrathin, mechanically bendable formats. Later on, Lee et al. prepared printable nanostructured SSCs from wafer-based materials directly (Fig. 2b).26 This strategy can reduce the cost of materials and facilitate programmable, large-scale distribution. Ultrathin micro-SSCs integrated with flexible substrates (poly-ethylene terephthalate) exhibited a PCE of 12.4%. Compared with crystalline silicon, amorphous silicon costs less and is easier to be processed into thin films. In 1991, Kishi et al. demonstrated a flexible solar cell for the first time by coating amorphous silicon on a transparent plastic substrate with an output power of 275 mW g−1 at that time.27 From then on, amorphous silicon has been extensively studied and used in mass-producing flexible solar cells (Fig. 2c).28 In addition to the above flat flexible solar cells, flexible fiber-shaped SSCs, with the features of light weight, foldability and knittability, are also an important research direction. In 2009, Lee et al. fabricated a solar power wire by coating P3HT/PCBM on stainless wires (Fig. 2d), and the PCE was up to 3.27%.29 Furthermore, Plentz et al. reported a flexible energy harvesting textile fabricated by coating amorphous thin-film SSCs on glass fiber fabrics (Fig. 2e).30 The textile solar cell exhibits a PCE of up to 1.4% and a pseudo-efficiency of up to 2.1%. With the continuous development of flexible SSCs, their application studies in flexible wearable electronics are also increasing. So far, a variety of self-powered wearable devices based on flexible SSCs have been developed.17,31,32 As illustrated in Fig. 2f, Ostfeld et al. designed a flexible power source by integrating commercial SSCs with lithium-ion batteries.33 Under an irradiance of 100 mW cm−2, the self-charging system can be charged to 4.1 V at a current of around 40 mA h, and demonstrates good stability during the cycles of charge and discharge. Moreover, the integrated device can be flexed in the hand and attached onto a jacket sleeve (Fig. 2g), to collect energy from the surrounding environment and power pulse oximeter components.
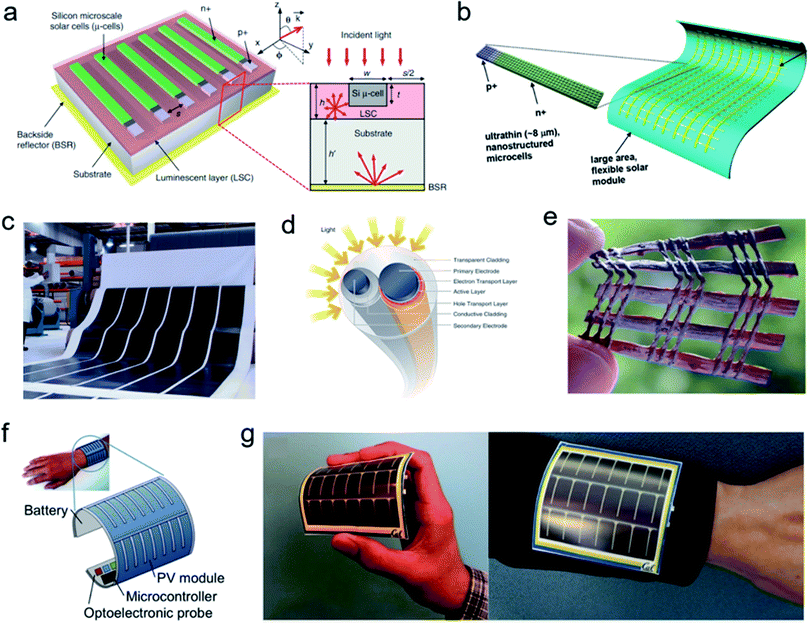 |
| Fig. 2 (a) Schematic illustration of a flexible SSC consisting of a solar microcell array.25 Copyright 2011, Springer Nature. (b) Schematic illustration of nanostructured silicon solar microcells.26 Copyright 2014, American Chemical Society. (c) Photograph of mass-production of flexible SSCs.28 Copyright 2008, Wiley-VCH. (d) Schematic of a fiber-shaped SSC.29 Copyright 2009, American Association for the Advancement of Science. (e) Photograph of a textile glass fiber fabric.30 Copyright 2016, Elsevier. (f) Illustration of a self-powered activity-tracking wristband. Photographs of a self-charging system being flexed in the hand (left) and on a jacket sleeve (right).33 Copyright 2016, Springer Nature. | |
2.2. Flexible organic solar cells (OSCs)
In the past few decades, flexible OSCs have received increasing attention because of their light weight, good flexibility and low cost.34,35 Compared with SSCs, the good flexibility of organic materials makes OSCs more suitable for application in flexible electronics.36,37 In general, flexible OSCs consist of a flexible substrate, transport electrode, active layer and metal electrode, as shown in Fig. 3a.38 Kaltenbrunner et al. fabricated ultrathin flexible OSCs (less than 2 μm thick) by coating a highly conductive PEDOT:PSS film on a PET substrate. As shown in Fig. 3b, the prepared OSCs have an extremely high specific power of 10 W g−1 and a PCE of 4.2%. More importantly, the OSCs can withstand elastic compression deformations of different amplitudes and withstand more than 300% tensile strain (Fig. 3b). Furthermore, Park et al. designed double-grating-OSCs on an ultra-thin and flexible substrate (Fig. 3c).39 The obtained OSCs not only exhibit shiny outer surfaces and ultra-flexibility (Fig. 3d), but also possess a high power of 11.46 W g−1 and an outstanding PCE of 10.5%. In addition, the authors further integrated the OSCs with organic electrochemical transistors to fabricate self-powered ultra-flexible electronic devices. The fabricated self-powered ultra-flexible electronic devices can measure biometric signals with very high signal-to-noise ratios when located on skin or other tissues (Fig. 3e).
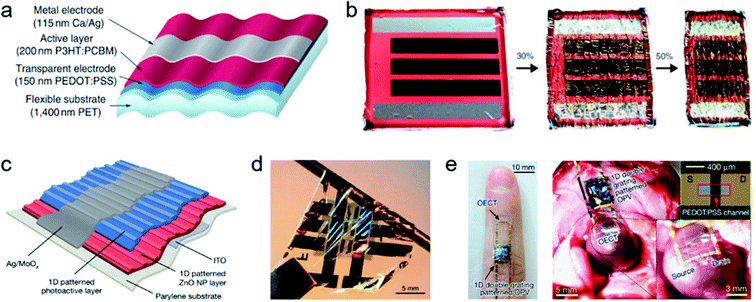 |
| Fig. 3 (a) Schematic illustration of a flexible OSC. (b) Bending flexibility demonstrated by wrapping a flexible OSC.38 Copyright 2012, Springer Nature. (c) Structure of a flexible OSC device. (d) Photograph of the flexible OSC wrapped over a spatula rod and pulled with tweezers. (e) Photograph of a self-powered electronic attached to a finger and attached to the heart of a rat (left), and enlarged images of the channel area (right, top) and of the source-drain electrode (right, bottom).39 Copyright 2018, Springer Nature. | |
2.3. Flexible dye-sensitized solar cells (DSSCs)
Because of the low cost, abundant raw materials, and high photoelectrical conversion efficiency, DSSCs have caught more and more peoples' attention.40 Compared with DSSCs based on rigid and brittle conductive glass, flexible DSSCs possess unique advantages of light weight, low production cost, and compatible with roll-to-roll manufacturing technology.41 Consequently, considerable research work has been done on the development of flexible DSSCs on transparent conductive oxide-coated polymer substrates.42–44 In the work done by Xu et al., a flexible DSSC was prepared based on a CuS transparent conductive film (Fig. 4a),45 in which the CuS film shows good electrocatalytic performance, resulting in a PCE of 4.54% for the prepared DSSC. This flexible DSSC exhibited superior mechanical robustness and kept a stable current density in the bending cycling measurement. In another attempt, Wu et al. reported a large-scale flexible DSSC module, fabricating by deposition TiO2 on a plastic substrate.46 The flexible DSSC exhibited a large effective working area of 100 mm × 100 mm (Fig. 4b) with a PCE of 3.27%. In the work reported by Zhou et al., a highly flexible, conductive and catalytic Pt network has been used as a transparent counter electrode for flexible DSSCs.47 The Pt networks can be transferred onto flexible substrates and possess excellent mechanical flexibility in bending and twisting tests. Therefore, the obtained wearable DSSCs with a PCE of 3.82% could be adhered onto human fingers with good working stability (Fig. 4c). Furthermore, Wen et al. demonstrated a fiber-shaped DSSC through coating dye-adsorbed TiO2 nanotube arrays on a Ti wire (Fig. 4d).48 Then they demonstrated the application of the fiber-shaped DSSC in wearable electronics by integrating with triboelectric nanogenerators to form an all-fiber-shaped energy harvesting module. The hybridized energy harvesting module was able to be conveniently woven into textiles to get wearable smart clothes (Fig. 4e).
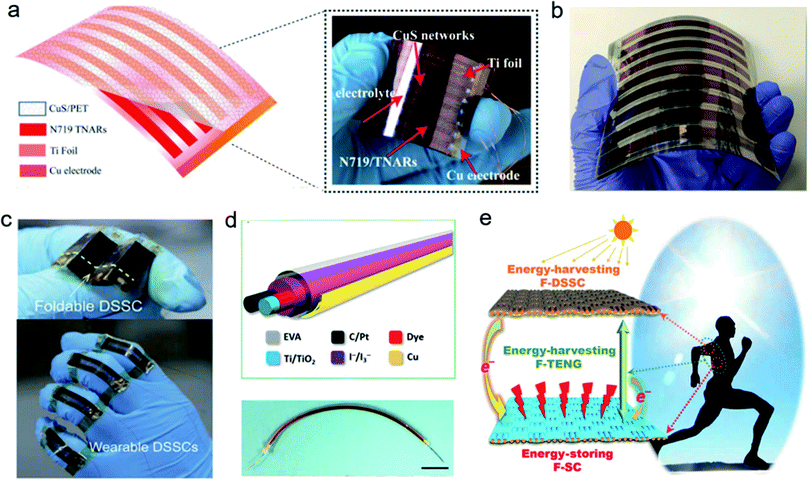 |
| Fig. 4 (a) Schematic illustration (left) and corresponding photograph (right) of a flexible DSSC.45 Copyright 2018, Elsevier. (b) Photograph of a flexible DSSC module with the dimensions of 100 mm × 100 mm.46 Copyright 2016, Elsevier. (c) Photograph of wearable DSSCs affixed to human fingers.47 Copyright 2015, Royal Society of Chemistry. (d) Schematic diagram (up) and photograph (down) of a single fiber-shaped DSSC. (e) A view of the application of a fiber-based DSSC in a self-powered system.48 Copyright 2016, American Association for the Advancement of Science. | |
2.4. Flexible perovskite solar cells (PSCs)
Thanks to their flexibility, light weight and excellent photovoltaic efficiencies, PSCs are regarded as a promising alternative to conventional photovoltaics.49,50 In addition, the photoactive layer in PSCs can be processed at rather low temperature, making PSCs ideal platforms for fabricating flexible devices.51,52 Planar PSC perovskite batteries generally have a sandwich structure that contains a photoactive layer between the two charge transport layers, as shown in Fig. 5a.53 In addition, there are some reports about double-twisted PSCs based on conductive yarn (e.g., carbon nanotube fibers).54,55Fig. 5b shows a schematic of this type of PSC structure.55 Kaltenbrunner et al. prepared a flexible PSC by introducing a chromium oxide–chromium interlayer and a transparent polymer electrode.56 The resulting PSCs achieve a PCE of 12% and a specific power as high as 23 W g−1. In the work reported by Park et al., solution-processed Li-doped SnO2 has been developed at low temperature, which served as an effective electron transport layer in flexible PSCs.57 The prepared PSCs exhibit a high PCE of 14.78% and good flexibility. Furthermore, the PSC could be fixed on the wrist and power a small fan successfully (Fig. 5d). Flexible PSCs with light weight and desirable efficiency are desirable for wearable power sources, giving them huge application potential in self-powered wearable electronics.58 For instance, Kim et al. prepared a flexible PSC by depositing a TiOx layer on a cheap polyethylene naphthalate (PEN) substrate.59 Benefiting from the reasonable structural design and fast electron transport, the prepared PSC achieves a high PCE of 12.2%. As shown in Fig. 5e, the PSC shows excellent flexibility and exhibits outstanding stability during different bending tests. Based on the above advantages, the authors envisioned using this flexible PSC to construct a self-powered smart watch (Fig. 5f).
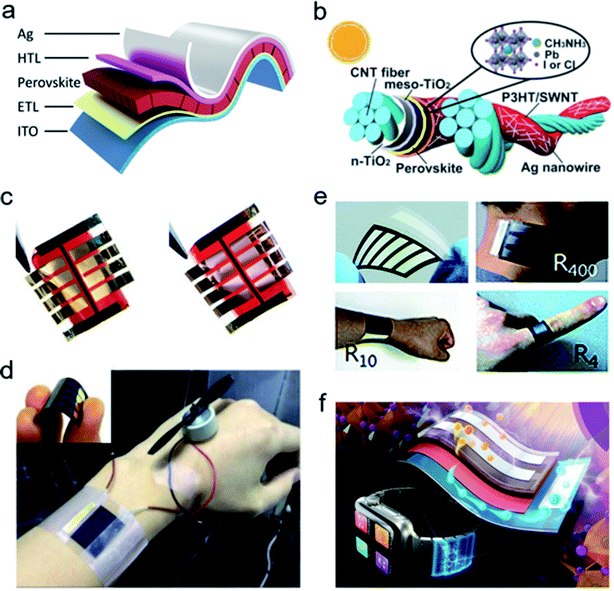 |
| Fig. 5 (a) Schematic illustration of a planar PSC.53 Copyright 2019, Wiley-VCH. (b) Schematic of a double-twisted fibrous PSC.55 Copyright 2015, Wiley-VCH. (c) Freestanding 3 μm-thick solar cells with gold top metal.56 Copyright 2015, Springer Nature. (d) Photographs of a flexible PSC fixed on the wrist and powering a small fan.57 Copyright 2016, Elsevier. (e) The photographs of a flexible PSC attached on the human neck, wrist, and finger, respectively. (f) A view of the self-powered smart watch based on flexible PSCs.59 Copyright 2015, Royal Society of Chemistry. | |
3. Flexible energy storage devices (ESDs) in self-powered wearable electronics
Limited by the non-continuous and unstable nature of sunlight, the output of PVCs is unstable and unlikely to power electronics directly. Thus, ESDs have also been introduced into self-powered energy systems. Combining PVCs with ESDs to construct a self-sustaining energy system not only breaks through the restrictions of the sunlight intermittency, but also enables the electronics without external charging to operate continuously. At the same time, the growing market of wearable and portable electronics stimulates huge demand for ESDs that are endowed with both high electrochemical performance and good flexibility. In the past decade, flexible ESDs including different kinds of batteries and SCs have already made great progress with the help of the development of new energy materials and device structures. The performance of various kinds of ESDs is summarized in Table 2.
Table 2 Summary of the types, materials, capacities and flexibility of flexible ESDs
Types |
Materials |
Capacities |
Energy density |
Ref. |
SSC |
Polyelectrolyte-wrapped graphene/CNT |
269 mF cm−2 |
5.91 μW h cm−2 |
67
|
SSC |
PEDOT on stainless steel |
158.2 mF cm−2 |
10.3 μW h cm−2 |
68
|
SSC |
MnO2 nanoflakes on Ni nanocoral |
52.9 mF cm−2 |
11.1 mW h cm−3 |
69
|
ASC |
Peptide–Co9S8 nanobricks |
1.3 F cm−2 |
79 W h kg−1 |
74
|
ASC |
Zinc–nickel–cobalt ternary oxides |
564 mF cm−2 |
53.33 μW h cm−2 |
75
|
ASC |
NiCo LDH@GSs@CF-NF |
1729.413 mF cm−2 |
109.6μW h cm−2 |
78
|
LIB |
CNT/LMO |
2.2 mA h m−1 |
|
87
|
LIB |
CNT–Si |
0.22 mA h cm−1 |
4.5 mW h cm−2 |
88
|
LIB |
LiFePO4 |
140.4 mA h g−1 |
|
90
|
ZIB |
α-MnO2 |
306 mA h g−1 |
6.18 mW h cm−2 |
97
|
ZIB |
CNT@MnO2 |
302.1 mA h g−1 |
53.8 mW h cm−3 |
98
|
ZIB |
COG@MnO2 |
369.73 mA h g−1 |
20.5 mW h cm−3 |
100
|
3.1. Flexible supercapacitors (SCs)
Owing to their high power density, high charge and discharge rate and long cycle life, SCs have attracted considerable attention recently.60 In particular, flexible SCs with a small volume, light weight, good safety and good mechanical durability are increasingly used in various wearable electronics.61,62 According to whether the materials of the two electrodes are the same or not, SCs can be divided into symmetrical SCs and asymmetric SCs.
3.1.1. Flexible symmetrical supercapacitors (SSCs).
For SSCs, the electrodes on both sides are made of the same material. Generally, the electrodes of SSCs are made of carbon-based materials, including activated carbon particles, carbon nanotubes (CNTs), carbon nanofibers (CNFs) and graphene.63,64 As shown in Fig. 6a, the energy storage mechanism of SSCs is generally based on electric double layer capacitance (EDLC), and the electrochemical performances are mainly dependent on the electrical conductivity, pore structures and the specific surface area of the electrode materials.20 By using flexible electrodes and quasi-solid electrolytes, flexible quasi-solid-state SCs can be prepared and used in a self-powered system (Fig. 6b).65 In addition, two yarn/fiber electrodes can be assembled into yarn/fiber shaped SCs, which can be further weaved into textile SCs (Fig. 6c).66 For instance, Kou et al. prepared core-sheath fibre electrodes consisting of polyelectrolyte-wrapped graphene/CNT by a coaxial wet-spinning assembly approach.67 Then, these core-sheath fibres are used directly as electrodes to fabricated two-ply yarn SCs with an ultra-high capacitance of 269 mF cm−2 and an energy density of 5.91 mW h cm−2. On this basis, a cloth SC is further interwoven from two individual core-sheath fibres (Fig. 6d). Furthermore, Du et al. prepared yarn-based SCs using composite electrodes, which are fabricated by depositing poly(3,4-ethyl-enedioxythiophene) (PEDOT) on stainless steel yarns.68 The all-solid-state yarn-based SCs achieve an excellent areal specific capacitance of 158.2 mF cm−2 as well as good stability. Moreover, the authors incorporated the yarn-based SCs with linear sensors into an all-in-one wearable textile system (Fig. 6e). The self-powered and self-sensing wearable textile system displayed high flexibility and outstanding sensitive to detect human motion (Fig. 6f). In recent years, printing technology, with the features of enabling scalable and feasible fabrication, has aroused more and more research interest in the fabrication of SCs. For example, Lin et al. prepared a flexible all-solid-state planar symmetrical SC through an inkjet-printing method.69 Benefiting from the unique nanocoral structure, the as-fabricated flexible planar SSCs exhibit an areal capacitance of up to 52.9 mF cm−2 and excellent versatility. Meanwhile, symmetrical SCs can be designed into different artistic symbols and further integrated with commercial polycrystalline silicon solar cells to form a self-powered T-shirt. As shown in Fig. 6g and h, the SCs, in the patterns of HKUST (the logo of the Hong Kong University of Science), are able to power 41 LED arrays arranged in the shape of three letters of “UST”.
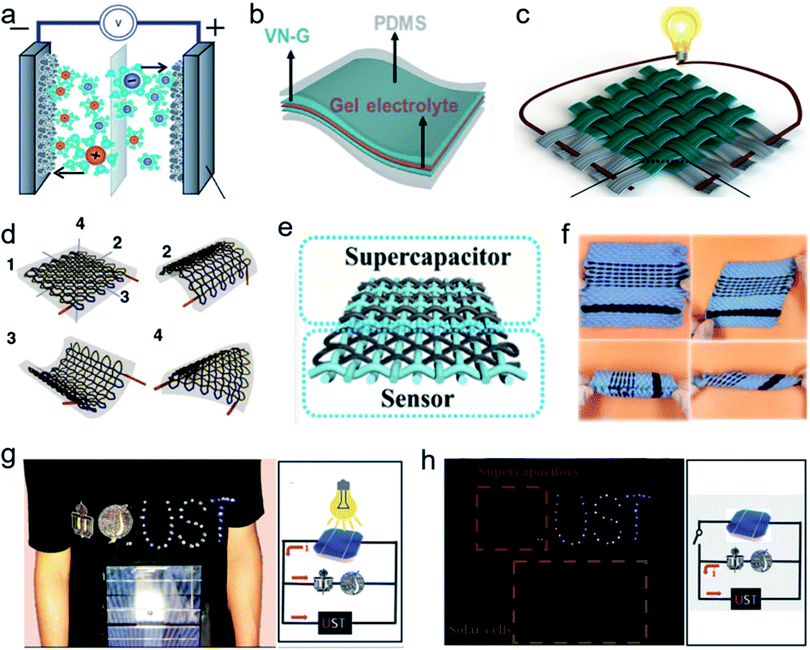 |
| Fig. 6 (a) Schematics of an all-carbon EDLC.20 Copyright 2018, Wiley-VCH. (b) Schematic illustration of a flexible SSC device.65 Copyright 2019, Tsinghua University Press. (c) Schematic illustration of a woven SSC using activated carbon fiber threads.66 Copyright 2018, Wiley-VCH. (d) Schematic illustration of a cloth woven from fiber shaped SCs with bending different angles.67 Copyright 2014, Springer Nature. (e) Schematic illustration and (f) images of a yarn-based wearable system consisting of linear sensors and yarn-based SCs.68 Copyright 2020, American Chemical Society. Photographs of a wearable self-powered system on a T-shirt that could be lit by: (g) solar cell panels under illumination or (h) SCs under dark conditions.69 Copyright 2017, Wiley-VCH. | |
3.1.2. Flexible asymmetric supercapacitors (ASCs).
For ASCs, the materials of the two electrodes are different. One of the electrodes is usually a carbon-based material, while the other is usually an electrochemically active material, such as conducting polymers and metal oxides/nitrides/sulfides.70 Different from EDLCs, these electrochemically active materials can offer pseudocapacitance through reversible electrochemical reactions, which endows the asymmetric SCs with a higher capacitance and energy density.71–73 As illustrated in Fig. 7a, Xiong et al. fabricated a flexible ASC based on active carbon and core–shell structured peptide–Co9S8 nanobricks.74 The obtained ASC shows a capacitance of 1.3 F cm−2 and good cycling stability. In addition, the ASC can be combined with a TENG to form a flexible self-powered TENG/SC system. Furthermore, Guo et al. prepared a fiber-shaped ASC based on yarn electrodes (Fig. 7b).75 The obtained ASC exhibits high specific capacitance (564 mF cm−2), outstanding energy density (53.33 μW h cm−2) and excellent mechanical flexibility. Thanks to their higher energy density and lightness, flexible asymmetric SCs have wide application prospects in the field of self-powered wearable electronics. For instance, Tian et al. reported a magnesium ion quasi-solid-state ASC through printable technology. The printed ASC shows an excellent energy density of 13.1 mW h cm−3.76 On this basis, the ASCs are combined with amorphous Si solar cells to form a flexible self-charging integrated unit (Fig. 7c), which exhibits good mechanical robustness, excellent photo-charging cycling stability, and high overall energy conversion efficiency (Fig. 7d). Yun et al. reported an all-transparent stretchable ASC, consisting of Au/Ag embedded polydimethylsiloxane (PDMS) and WO3 nanotube/PEDOT:PSS electrodes as well as a polyacrylamide (PAAm)-based hydrogel electrolyte.77 The prepared ASC shows a maximum specific capacitance of 471.0 F g−1. As exhibited in Fig. 7e, the fabricated ASC is further integrated into a wearable patch device to serve as an electrochromic coloration device plus an electrochemical ESD. Furthermore, Gao et al. fabricated a fiber-shaped ASC from 3D copper foam, graphene sheets and layered double hydroxides.78 The graphene sheets act as bridges to increase the surface area and decrease the interface resistance, resulting in a high capacity (1729.413 mF cm−2) and a rate capability (87.1% retention from 1 to 20 mA cm−2). More importantly, the fiber-shaped ASCs with good flexibility can be knitted into clothes or implanted in wearable electronic devices, as schematically illustrated in Fig. 7f.
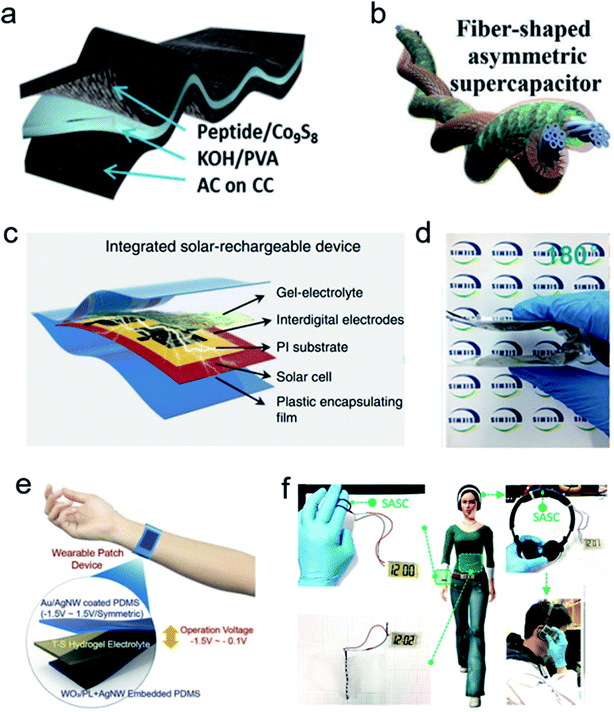 |
| Fig. 7 (a) Schematic illustration of a flexible solid-state ASC.74 Copyright 2019, Elsevier. (b) The schematic diagram of a fiber-shaped ASC device.75 Copyright 2018, Elsevier. (c) Schematic illustration of an integrated solar-charging self-powered unit, and (d) digital photo showing its bending condition.76 Copyright 2019, Springer Nature. (e) Schematic illustration of a wearable patch device based on transparent ASCs.77 Copyright 2019, American Chemical Society. (f) A view of application of fiber-shaped ASCs in various electronic devices, including a watch, headphone and lab cloth.78 Copyright 2018, American Chemical Society. | |
3.2. Flexible batteries
Batteries can convert chemical energy into electrical energy. Typically, a battery consists of a cathode, an anode, a separator and an electrolyte. Compared with SCs, batteries (such as LIBs and ZIBs) generally have higher capacity and energy density, which makes them more widely used in wearable and portable electronics.
3.2.1. Flexible lithium-ion batteries (LIBs).
Ascribed to their high energy density and working voltage and long cycle life, LIBs play a dominant role in the market of electric vehicles, portable electronics and large-scale energy-storage systems.79,80 However, conventional LIBs are typically rigid and heavy, and have inherent safety hazards, which considerably restrict their extensive application in the area of wearable electronics.81 In recent years, more and more flexible LIBs have been developed based on the usage of flexible electrodes as well as the improvement of electrolytes.82–84 In the work reported by Gaikwad et al., flexible electrodes were prepared by using a CNT coating porous membrane, as shown in Fig. 8a.85 The prepared electrode possesses increasing tensile strengths of ≈5.5–7.0 MPa and good stability during bending tests. The final batteries exhibited enhanced mechanical performance and stability. In addition, several attempts have been made to fabricate fiber-shaped LIBs, which are more soft, flexible and durable. According to the electrode position, fiber-shaped batteries can be mainly categorized into core–shell (Fig. 8b) and intertwined structures (Fig. 8c).86,87 Furthermore, fiber-shaped LIBs can be knitted into textiles (Fig. 8d), which is highly desirable for making wearable electronics.88 Tajima et al. developed a flexible LIB and integrated it with a flexible organic light-emitting diode (LED) panel or a flexible printed circuit to construct a wrist-wearable display (Fig. 8e).89 The flexible LIB can retain a stable electrochemical performance during a bending test, which helps to fabricate a wearable display. For self-sustaining systems composed of batteries and solar cells, the overall photo-electric conversion and storage efficiency are very important. Xu et al. demonstrated a self-charging device by using PSC-based direct photo-charging LIBs, as schematically shown in Fig. 8f.90 The developed PSC-LIB exhibits a high overall photo-electric conversion and a storage efficiency of 7.80% as well as excellent cycling stability, holding promise for various potential wearable applications.
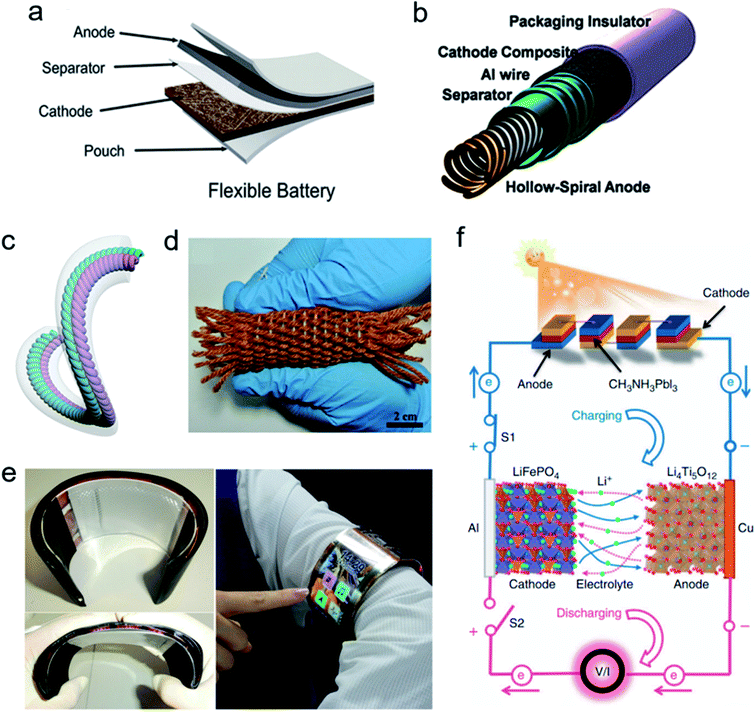 |
| Fig. 8 (a) Schematic of a flexible LIB.85 Copyright 2014, Wiley-VCH. (b) Schematic illustration of a cable LIB with a hollow anode system.86 Copyright 2012, Wiley-VCH. (c) Schematic illustration of a fiber-shaped ZIB with an intertwined structure.87 Copyright 2014, Wiley-VCH. (d) Photograph of fiber-shaped LIBs being woven into a textile.88 Copyright 2014, American Chemical Society. (e) Photographs of a self-powered wearable display integrated with flexible LIBs and a flexible display.89 Copyright 2014, Society for Information Display. (f) Schematic diagram of a PSC-LIB self-sustaining system.90 Copyright 2015, Macmillan Publishers Limited. | |
3.2.2. Flexible zinc-ion batteries (ZIBs).
The inadequate energy density of SCs has hindered their applications in miniaturized electronics.91 LIBs have a relatively higher energy density and dominate the commercial battery market, especially for portable electronic devices and electric vehicles. However, the inherent security issue of LIBs limits their application in wearable electronics.92 Recently, rechargeable ZIBs, using non-toxic aqueous electrolytes, have attracted significant attention due to their high safety, light weight, cost-effectiveness and eco-friendliness.93,94 Particularly, quasi-solid ZIBs coupled with hydrogel electrolytes show high capacity and good flexibility, making them a promising alternative to LIBs.95,96 Therefore, rechargeable ZIBs have developed rapidly in recent years, and begun to shine light on the wearable field. In 2018, Li et al. reported a solid-state ZIB using a polymer electrolyte, which is extremely safe (Fig. 9a).97 The obtained ZIB not only delivers a high specific capacity (306 mA h g−1), areal energy density (6.18 mW h cm−2) and power density (148.2 mW cm−2), but also displays an extremely safe performance after different kinds of damage, including cutting, bending, hammering, puncturing, sewing and washing in water. In addition, flexible ZIBs could power various wearable electronics, including smart watches (Fig. 9b) and smart insoles (Fig. 9c). In addition, Li et al. fabricated a high-performance yarn ZIB using fiber electrodes and a polymer electrolyte (Fig. 9d).98 The PAM electrolyte endows the ZIB with a high specific capacity (302.1 mA h g−1) and a high volumetric energy density (53.8 mW h cm−3) as well as the advantages of being waterproof and stretchable. The obtained yarn ZIB can be further woven into a battery textile and power a LED belt and flexible electroluminescent panel (Fig. 9e). On this basis, Mo et al. developed an anti-freezing hydrogel electrolyte, which allows ZIBs to work under low temperature (−20 °C).99 Thus, anti-freezing ZIBs have high potential to power portable and wearable electronics in extremely cold environments (Fig. 9f). The current electrode materials are mostly made of synthetic materials, which are usually expensive and not environmentally friendly. In response to this problem, Zhao et al. reported a quasi-solid-state ZIB battery derived from biomass garbage floating on the ocean.100 The electrodes possess a special honeycomb-like structure with multi-level open channels, which contributes to electrolyte diffusion and ion transportation. As a result, the obtained ZIBs exhibit a high capacity of 306.7 mA h g−1, good rate capability, and excellent cycling stability. Furthermore, as shown in Fig. 9g, the obtained ZIBs were further combined with a flexible solar cell to form a self-sustaining power bar, which can harvest and store clean and sustainable energy from the surrounding environment and power wearable electronics, including a watch or a badge. This work provides a new strategy for the preparation of biomass-based ZIBs.
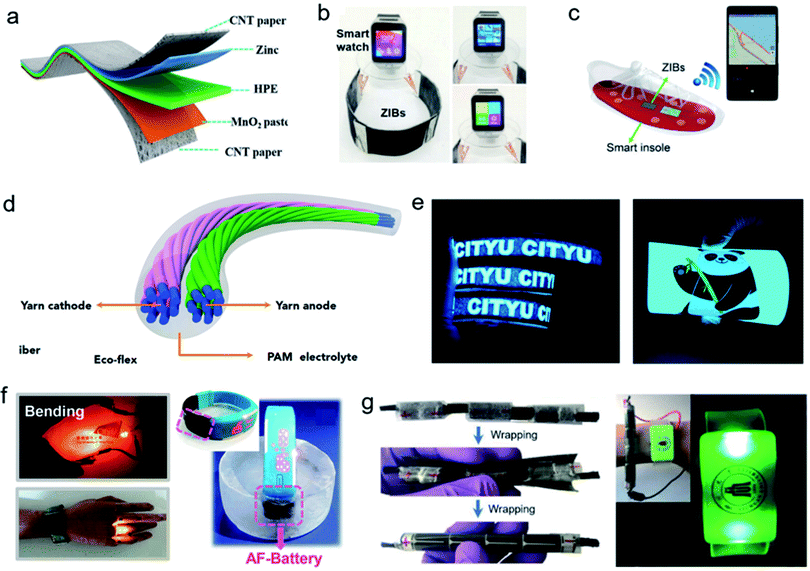 |
| Fig. 9 (a) Schematic illustration of a flexible planer ZIB. (b) Photographs of three flexible ZIBs connected in series and powering a commercial smart watch. (c) Schematic diagram of the application of flexible ZIBs in a self-powered smart insole.97 Copyright 2018, Royal Society of Chemistry. (d) Schematic diagram of a yarn ZIB. (e) Photographs of various electroluminescent panels powered by yarn batteries.98 Copyright 2018, American Chemical Society. (f) Photographs of a yellow LED powered by flexible ZIBs in contact with a demonstrator's wrist (left), and a watch powered by flexible ZIBs while being sealed in solid ice (right).99 Copyright 2019, Royal Society of Chemistry. (g) Photographs of a self-sustaining power bar consisting of a flexible SSC and three flexible ZIBs (left), and a watch powered by the self-sustaining power bar (right).100 Copyright 2020, Royal Society of Chemistry. | |
4. Integration of self-powered energy systems
With respect to self-powered energy systems, the integration process of PVCs and ESDs is quite vital. It not only affects the overall energy collection/storage efficiency of the fabricated self-powered energy systems, but also decides the appearance, flexibility, and durability of the final products. Based on the current fabrication methods, the integration of a self-powered system can be classified into two categories; they are a combination of two independent PVCs and ESDs or the integral production of PVCs and ESDs, respectively.
4.1. Combination of two independent PVCs and ESDs
In most self-powered energy systems, researchers only focus on PVCs or ESDs separately instead of studying the two parts as a whole item. For instance, Zhao et al. fabricated a high-performance ZIB based on defective MnO2−x nanosheet-grown carbon cloth (MnO2−x@CC), which not only showed excellent flexibility with an ultrahigh energy density of 5.11 mW h cm−2, but also presented a high safety in a wide temperature range under various severe conditions.101 Subsequently, they attached flexible PSCs to the surface of the obtained flexible ZIBs to construct a safe, self-powered wristband which could power a commercial smart bracelet (Fig. 10a). Moreover, cylindrical ZIBs could be prepared directly from ocean floating biomass garbage.100 Furthermore, three obtained ZIBs were wrapped with a commercial flexible solar cell to form a self-sustaining power bar (Fig. 9g). In this kind of integration method, researchers usually choose suitable flexible PVCs to match the prepared ESDs. In this way, the resulting self-powered energy systems show better flexibility, which is suitable for fabricating wearable electronics. As shown in Fig. 10b, the self-powered wristband with desirable flexibility can be conveniently worn on the subjects' wrist during sports including outdoor running and indoor biking. However, as mentioned before, PVCs and ESDs are prepared independently, and are not specifically designed for each other. Therefore, the energy loss between these two parts will lead to a low energy transfer efficiency from PVCs to ESDs.90,102
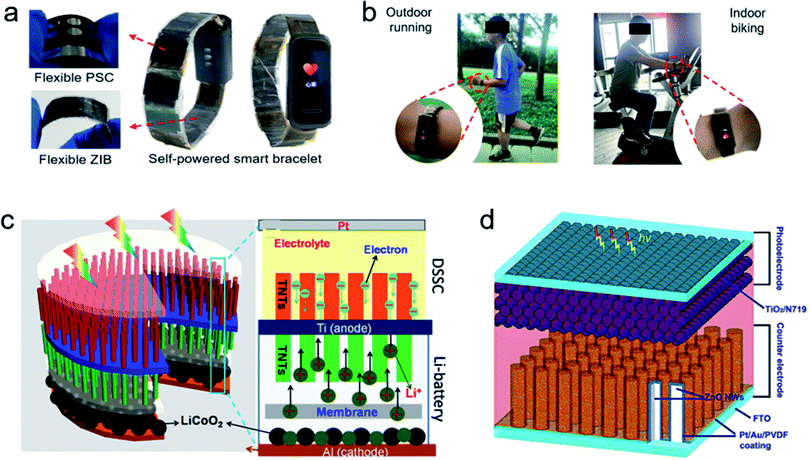 |
| Fig. 10 (a) Digital photographs demonstrating the integration process of a self-powered smart bracelet from flexible ZIBs with flexible PSCs and a commercial bracelet. (b) Digital photographs showing a subject wearing the self-powered smart bracelet on the wrist during outdoor running and indoor biking.101 Copyright 2021, American Chemical Society. (c) Detailed structure and working principle of the integrated self-powered energy system.103 Copyright 2012, American Chemical Society. (d) Scheme of a TiO2 dye-sensitized solar cell with energy storage function by modifying the CE with PVDF/ZNWA nanocomposites.104 Copyright 2013, Wiley-VCH. | |
4.2. Integral production of PVCs and ESDs
Another integration strategy is to fabricate PVCs and ESDs at the same time as a whole device, and they can even share electrodes. In 2012, Guo et al. put forward a novel approach to fabricate an integrated power pack by hybridizing energy harvest and storage processes.103 As shown in Fig. 10c, this power pack incorporates a series-wound DSSC and a LIB on the same Ti foil that has double-sided TiO2 nanotube arrays. Attributed to this unique structural design, the integrated power pack was able to be charged to about 3 V in around 8 min, and the discharge capacity was about 38.89 μA h under a discharge density of 100 μA. Furthermore, in the work reported by Zhang et al., an integrated self-powered system with dual functions of photocurrent output and energy storage has been developed.104 As shown in Fig. 10d, the counter electrodes of DSSCs are modified with PVDF/ZnO nanowire array nanocomposites for the purpose of obtaining higher energy storage ability. The Pt/Au catalytic layer for a DSSC and the charge storage interface share the same PVDF surface coated with ZnO nanowires. In this integration strategy, since PVCs and ESDs are prepared as a whole device, the energy loss between the two parts is relatively small, which helps to improve the total energy conversion and storage efficiency of the whole system. However, at the time of writing, most of this class of all-in-one self-powered systems are rigid, which hinders their applications in the field of wearable electronics.103,104
5. Applications of self-powered energy systems in wearable electronics
Based on the various flexible PVCs and ESDs discussed above, numerous self-powered wearable electronics with different functions have been reported and are summarized in Table 3. According to different usage scenarios, the main application fields of self-powered wearable electronics include motion monitoring, pulse monitoring, sweat monitoring, gas monitoring and so on.
Table 3 Summary of the types and performance of sensors involved in different self-powered systems
Functions |
Sensors |
Materials |
Sensitivities |
Ref. |
Motion monitoring |
Strain sensor |
rGO@PU |
25–30 kPa−1 |
109
|
Pulse monitoring |
Strain sensor |
|
|
119
|
Sweat monitoring |
Electrochemical sensor |
Glucose oxidase |
3.29 nA μM−1 |
102
|
Gas detection |
Gas sensor |
SnO2 |
13.2–1.54 wt% |
134
|
5.1. Motion monitoring
The real time monitoring of the motion information (including gait, motion state, position, etc.) is important for sports training and assessing the physical condition of the elderly or disabled.105 With the rapid development of technology and the increase of people's demands, more and more smart wearable electronics, such as smart watches, smart bracelets, electronic skin and so on, have been developed and equipped with motion monitoring functions.106–108 However, most of the built-in batteries for these wearable electronics are rigid and considerably bulky and require external charging or frequent replacement. Recently, Li et al. presented a self-powered wearable sensing system by integrating a flexible PSC, lithium-ion capacitor (LIC) and strain sensors (Fig. 11a).109 The combined flexible PSC-LIC module achieves an overall energy conversion efficiency of 8.41% and a high output voltage of 3 V. In addition, the strain sensors in this study are prepared by coating of a thin reduced graphene oxide film onto the backbone of a PU sponge, with the advantages of cost-effectiveness, low-voltage operation, wearability, and ultrasensitivity. Thus, the self-powered sensing system can be placed on the wrist (Fig. 11b) and work for 5 h continuously and monitor physiological signals, such as finger motion (Fig. 11c).
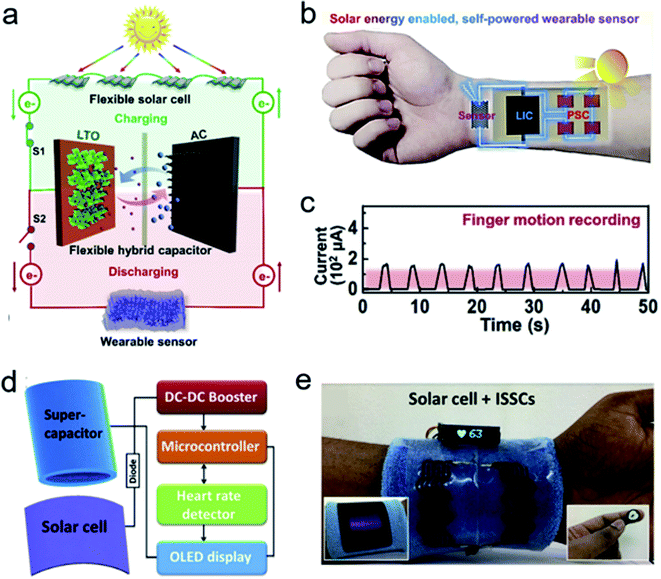 |
| Fig. 11 (a) Schematic illustration of a self-powered sensing system consisting of PSCs, LICs and a wearable sensor. (b) Schematic diagram of the self-powered sensing system in contact with a wrist for motion monitoring. (c) The measurement results of finger motion.109 Copyright 2019, Elsevier Ltd. (d) System-level block diagram of a self-powered sensor system consisting of a flexible PVC, a SC, a microcontroller, a pulse rate sensor and an OLED display. (e) Photographs of the self-powered sensor system for real time pulse monitoring.119 Copyright 2019, Elsevier Ltd. | |
5.2. Pulse monitoring
The heart rate is an unequivocal sign of a person's heart health.110 Heart rate monitoring can provide the personal health status, early disease warning, and therapeutic treatments to patients.111 Traditional heart rate monitoring needs electrocardiograph (ECG)/pulse monitoring devices and professional technical operations, resulting in inconvenience in daily life and restricting medical applications.112 Recently, many research teams have developed flexible pressure sensors to monitor the pulse.113–115 Furthermore, self-powered sensors can be obtained by integrating pressure sensors with energy harvesting and storage models, which is of great significance in promoting mobile health and telemedicine.116–118 For example, Rajendran et al. presented a self-powered monitoring system by integrating a flexible PVC, a printed SC, a microcontroller, a pulse rate sensor and an OLED display (Fig. 11d).119 With the help of a stretchable sweat band, the self-powered monitoring system can be worn comfortably on the wrist (Fig. 11e). In addition, the sensor is fixed on a finger for pulse rate monitoring and the results can be displayed real time. The test results illustrate that as the exercise intensity increases, the heart rate increases accordingly.
5.3. Sweat monitoring
Sweat is a type of body fluid secreted by the sweat glands of the human body.120 It contains rich substances, such as electrolyte ions, metabolic small molecules, nutrient elements and medicine molecules.121,122 Benefiting from easy access and rich composition, sweat detection holds great opportunities for monitoring of an individual's health conditions at the molecular level.123–126 Zhao et al. developed a fully integrated and self-powered smart watch, which contains flexible PSCs and rechargeable ZIBs in the forms of a “watch strap”, electrochemical glucose sensors, customized circuits, and display units integrated into a “dial” platform, as shown in Fig. 12a.102 Thanks to the superior flexibility of the power devices and sensors, the integrated smart watch can be comfortably worn on the wrist (Fig. 12b). More importantly, the fully integrated smart watch can realize energy harvesting/storage, biosensing, and signal processing, integrated in a single platform. Under outdoor sunlight, the self-powered smart watch can be charged up to 6.0 V within 1 h, and achieves a cruising duration of up to 8 h. In addition, it takes around 2 h to charge the battery to 4.2 V under room light, and the stored energy can support the watch for around 1 h. As shown in Fig. 12c, volunteers can wear the self-powered smart watch during indoor cycling and outdoor running and get the real-time data of glucose levels in sweat. The as-developed fully integrated and self-powered smart watch provides a promising protocol for health monitoring and fitness management, and offers a new opportunity for the construction of wearable self-powered energy systems.
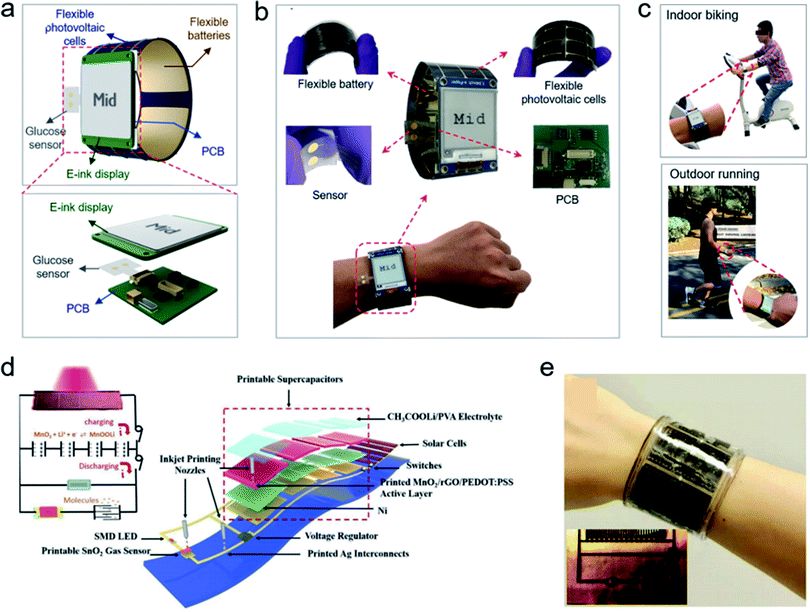 |
| Fig. 12 (a) Schematic illustrations and (b) images of a self-powered smart watch consisting of flexible SSCs, ZIBs, a glucose sensor, a printed circuit board and an electronic ink display. (c) Photographs of a subject wearing the self-powered smart watch during indoor cycling (upper panel) and outdoor running status (lower panel).102 Copyright 2019, American Chemical Society (further permissions related to the material excerpted should be directed to the ACS). (d) Schematic illustrations of a self-powered gas sensor system fabricated by a printable approach. (e) Photos of the self-powered gas sensor system in contact with a wristband.134 Copyright 2018, Wiley-VCH. | |
5.4. Gas detection
Some toxic gases exist in the environment where we live, such as formaldehyde, phenol and carbon monoxide.127 The detection of these toxic gases in a simple and quick method is very important to protect our health and safety. In addition, some gases are important markers of the body.128,129 By detecting these gases, we can learn about the health status of our body. Many research teams have tried to develop self-powered gas sensor systems, which allowed people to detect some special gases quickly and conveniently.130–133 Lin et al. reported a self-powered smart sensor system integrated with printable SCs, printed interconnects, printed gas sensors and embedded SSCs, for ethanol and acetone gas detection.134 The structure of this fully integrated self-powered platform is illustrated in Fig. 11d. More interestingly, the whole system is designed into a planar architecture and further packaged into a flexible and wearable wristband fashion, as shown in Fig. 11e. The prepared SnO2 gas sensors deliver a sensitivity of 13.2–1.54 vt% of ethanol and 3.1–6.07 vt% of acetone. When the system is exposed to ethanol or acetone, the resistance of the SnO2 sensor will decrease, leading to the increase of voltage applied on the LED. Thus, the LED can be turned on as a warning signal.
6. Conclusions and prospects
Wearable electronics with good flexibility, safety and multiple functions have witnessed impressive growth and can find wide applications in motion monitoring, pulse monitoring, sweat monitoring, gas monitoring and so on. At the same time, the fast development of wearable devices has in turn promoted the development of flexible ESDs. In addition, the increasing consumption of energy and the growing environmental awareness have highlighted the demand for green energy harvesting from the environment, which spurred the development of self-powered energy systems that combine energy harvesting and storage modules. In this review, we have summarized the most recent progress in the fabrication of wearable self-powered energy systems based on flexible ESDs integrated with flexible PVCs. In this regard, firstly, recently developed flexible solar cells, which were categorized into four main different groups, including SSCs, OSCs, DSSCs and PSCs, were discussed. Afterward, recent advances in flexible ESDs, such as SCs, LIBs, ZIBs, and so on, were reported. In the following parts, various applications of current self-powered energy systems in wearable electronics have also been summarized.
The rapid advances and achievements in wearable self-powered energy systems have demonstrated their promising potential toward practical applications in future wearable electronics. However, several significant challenges still exist and further efforts are needed to achieve the commercial development of self-powered energy systems for wearable electronics. First, due to the limitation of energy density, ESDs, including various SCs and batteries, often occupy most of the space and weight of current wearable and portable electronics, which go against the miniaturization of wearables.135 Second, most of the materials reported so far for energy harvest and storage devices are synthetic materials, such as CNTs, graphene, metal oxide nanoparticles, MXenes, metal–organic frameworks (MOFs), etc. These materials face many problems, such as a complex synthesis process, low yield, and environmental incompatibility. Third, although there are many long-term cycling test standards and good results for individual parts, such as solar cells, batteries, and sensors of self-powered energy systems, there are rare reports on the long-term durability test for entire self-powered energy systems. Fourth, safety is a very critical issue for wearable electronics, especially under some extreme conditions. Most ESDs in current wearable electronics still pose serious security risks of toxicity and flammability to consumers. Fifth, the development in the field of self-powered wearable systems is still at the early stage of lab research. There are relatively few practical self-powered wearable electronics developed at present, and their costs are relatively high, which is a very big obstacle to their promotion in the consumer market.
In response to some of the challenges listed above, a lot of work can be done in the near future. Firstly, more efforts need to be continuously devoted to the miniaturization of wearable electronics. To achieve this goal, it is a must to improve the conversion efficiency of PVCs, the energy density of ESDs, and the overall energy storage efficiency to reduce the size of these components. Secondly, with the increasing awareness of environmental protection, future wearable electronic products must meet the environmentally friendly requirements. On the one hand, wearable electronics should give priority to using renewable green materials. Besides, new technologies need to be developed to process and recycle waste electronic devices to reduce environmental pollution. Thirdly, in order to be quickly recognized by consumers, the evaluation standards for self-powered wearable electronics, such as the overall energy conversion efficiency, comfort, durability, and safety, should be set up as soon as possible. It is a collaborative process which needs to be completed by the relevant enterprises and scientific research units. After that, the replaceable components of wearable electronics produced by different companies should be unified. In addition, for those wearable devices in direct contact with the human body, the safety issue of electronics is the priority among priorities in future research. First, nontoxic and non-flammable aqueous electrolytes or solid electrolytes should be developed and used in building ESDs to ensure the safety of wearable electronics. Moreover, the biocompatibility analysis of flexible substrates and active materials, including their long-term toxicity analysis, must be carried out simultaneously. Furthermore, the durability of self-powered wearable electronics should be concerned, which includes but not limited to the long-term working stability under different bending, rubbing and even washing conditions. It is highly recommended that the stability performance and fatigue life under dynamically mechanical deformations such as bending, folding, twisting, stretching, and compressing should be systematically tested. Finally, it is necessary to improve the preparation method of the materials as well as the assembly technologies and efficiency of integrated systems, to realize the large-scale production of wearable electronics. Along with the continuously increasing research and progress on energy harvest and storage devices as well as integration technology, we believe that self-powered wearable electronics will emerge in our daily lives and change our lifestyle in the near future.
Conflicts of interest
There are no conflicts to declare.
Acknowledgements
C. T. acknowledges the financial support from the Start-Up Grant (Project No. 9610495) from the City University of Hong Kong and National Natural Science Foundation of China (Project No. 22005259). Z. Z. acknowledges the Start-Up Grant support from the City University of Hong Kong (Project No. 9610435) and ECS scheme (CityU 9048163) from the Research Grant Council of Hong Kong.
References
- J. S. Heo, J. Eom, Y. H. Kim and S. K. Park, Small, 2018, 14, 1703034 CrossRef PubMed
.
- M. G. Honarvar and M. Latifi, J. Text. Inst., 2016, 108, 631–652 CrossRef
.
- S. Khan, S. Ali and A. Bermak, Sensors, 2019, 19, 1230 CrossRef CAS PubMed
.
- Q. Shi, B. Dong, T. He, Z. Sun, J. Zhu, Z. Zhang and C. Lee, InfoMat, 2020, 2, 1131–1162 CrossRef
.
- X. Chen, L. Yin, J. Lv, A. J. Gross, M. Le, N. G. Gutierrez, Y. Li, I. Jeerapan, F. Giroud, A. Berezovska, R. K. O'Reilly, S. Xu, S. Cosnier and J. Wang, Adv. Funct. Mater., 2019, 29, 1905785 CrossRef CAS
.
- A. Paco and T. Lavrador, J. Environ. Manage., 2017, 197, 384–392 CrossRef PubMed
.
- M. A. Green and A. Ho-Baillie, ACS Energy Lett., 2017, 2, 822–830 CrossRef CAS
.
- R. J. Detz, J. N. H. Reek and B. C. C. van der Zwaan, Energy Environ. Sci., 2018, 11, 1653–1669 RSC
.
- H. Weldekidan, V. Strezov and G. Town, Renewable Sustainable Energy Rev., 2018, 88, 184–192 CrossRef CAS
.
- Z. Gao, C. Bumgardner, N. Song, Y. Zhang, J. Li and X. Li, Nat. Commun., 2016, 7, 11586 CrossRef CAS PubMed
.
- P. Dong, M.-T. F. Rodrigues, J. Zhang, R. S. Borges, K. Kalaga, A. L. M. Reddy, G. G. Silva, P. M. Ajayan and J. Lou, Nano Energy, 2017, 42, 181–186 CrossRef CAS
.
- R. Liu, J. Wang, T. Sun, M. Wang, C. Wu, H. Zou, T. Song, X. Zhang, S. T. Lee, Z. L. Wang and B. Sun, Nano Lett., 2017, 17, 4240–4247 CrossRef CAS PubMed
.
- K. Zhang and Y. Yang, Adv. Funct. Mater., 2017, 27, 1703331 CrossRef
.
- Y. Cho, S. Pak, Y. G. Lee, J. S. Hwang, P. Giraud, G. H. An and S. Cha, Adv. Funct. Mater., 2020, 30, 1908479 CrossRef CAS
.
- J. Huang, S. Peng, J. Gu, G. Chen, J. Gao, J. Zhang, L. Hou, X. Yang, X. Jiang and L. Guan, Mater. Horiz., 2020, 7, 2085–2096 RSC
.
- J. Sun, Y. Li, J. Sun, Z. Zhu, Y. Zhai and S. Dong, Chem. Commun., 2019, 55, 12060–12063 RSC
.
- S. A. Hashemi, S. Ramakrishna and A. G. Aberle, Energy Environ. Sci., 2020, 13, 685–743 RSC
.
- Q. Xue, J. Sun, Y. Huang, M. Zhu, Z. Pei, H. Li, Y. Wang, N. Li, H. Zhang and C. Zhi, Small, 2017, 13, 1701827 CrossRef PubMed
.
- W. Liu, M. S. Song, B. Kong and Y. Cui, Adv. Mater., 2017, 29, 1603436 CrossRef PubMed
.
- Z. Liu, F. Mo, H. Li, M. Zhu, Z. Wang, G. Liang and C. Zhi, Small Methods, 2018, 2, 1800124 CrossRef
.
- J. Bullock, M. Hettick, J. Geissbühler, A. J. Ong, T. Allen, C. M. Sutter-Fella, T. Chen, H. Ota, E. W. Schaler, S. De Wolf, C. Ballif, A. Cuevas and A. Javey, Nat. Energy, 2016, 1, 1–7 Search PubMed
.
- P. K. Enaganti, P. K. Dwivedi, A. K. Srivastava and S. Goel, Prog. Photovoltaics, 2020, 28, 725–735 Search PubMed
.
- K. Ruan, K. Ding, Y. Wang, S. Diao, Z. Shao, X. Zhang and J. Jie, J. Mater. Chem. A, 2015, 3, 14370–14377 RSC
.
- C. Zhang, Y. Song, M. Wang, M. Yin, X. Zhu, L. Tian, H. Wang, X. Chen, Z. Fan, L. Lu and D. Li, Adv. Funct. Mater., 2017, 27, 1604720 CrossRef
.
- J. Yoon, L. Li, A. V. Semichaevsky, J. H. Ryu, H. T. Johnson, R. G. Nuzzo and J. A. Rogers, Nat. Commun., 2011, 2, 343 CrossRef PubMed
.
- S.-M. Lee, R. Biswas, W. Li, D. Kang, L. Chan and J. Yoon, ACS Nano, 2014, 8, 10507–10516 CrossRef CAS PubMed
.
- H. h. Y. Kishi, K. Murata, H. TanaKa, S. Kouzuma, M. Morizane, Y. Fukuda, H. Nishiwaki, K. Nakano, A. Takeoka, M. Ohnishi and Y. Kuwano, Sol. Energy Mater., 1991, 23, 312–318 CrossRef
.
- M. Pagliaro, R. Ciriminna and G. Palmisano, ChemSusChem, 2008, 1, 880–891 CrossRef CAS PubMed
.
- M. R. Lee, R. D. Eckert, K. Forberich, G. Dennler, C. J. Brabec and R. A. Gaudiana, Science, 2009, 324, 232–235 CrossRef CAS PubMed
.
- J. Plentz, G. Andrä, T. Pliewischkies, U. Brückner, B. Eisenhawer and F. Falk, Mater. Sci. Eng., B, 2016, 204, 34–37 CrossRef CAS
.
- C. Bao, W. Zhu, J. Yang, F. Li, S. Gu, Y. Wang, T. Yu, J. Zhu, Y. Zhou and Z. Zou, ACS Appl. Mater. Interfaces, 2016, 8, 23868–23875 CrossRef CAS PubMed
.
- P. Du, X. Hu, C. Yi, H. C. Liu, P. Liu, H.-L. Zhang and X. Gong, Adv. Funct. Mater., 2015, 25, 2420–2427 CrossRef CAS
.
- A. E. Ostfeld, A. M. Gaikwad, Y. Khan and A. C. Arias, Sci. Rep., 2016, 6, 26122 CrossRef CAS PubMed
.
- J. H. Seo, I. Hwang, H. D. Um, S. Lee, K. Lee, J. Park, H. Shin, T. H. Kwon, S. J. Kang and K. Seo, Adv. Mater., 2017, 29, 1701479 CrossRef PubMed
.
- W. Song, X. Fan, B. Xu, F. Yan, H. Cui, Q. Wei, R. Peng, L. Hong, J. Huang and Z. Ge, Adv. Mater., 2018, 30, 1800075 CrossRef PubMed
.
- X. Meng, L. Zhang, Y. Xie, X. Hu, Z. Xing, Z. Huang, C. Liu, L. Tan, W. Zhou, Y. Sun, W. Ma and Y. Chen, Adv. Mater., 2019, 31, 1903649 CrossRef CAS PubMed
.
- Y. Li, G. Xu, C. Cui and Y. Li, Adv. Energy Mater., 2018, 8, 1701791 CrossRef
.
- M. Kaltenbrunner, M. S. White, E. D. Glowacki, T. Sekitani, T. Someya, N. S. Sariciftci and S. Bauer, Nat. Commun., 2012, 3, 770 CrossRef PubMed
.
- S. Park, S. W. Heo, W. Lee, D. Inoue, Z. Jiang, K. Yu, H. Jinno, D. Hashizume, M. Sekino, T. Yokota, K. Fukuda, K. Tajima and T. Someya, Nature, 2018, 561, 516–521 CrossRef CAS PubMed
.
- K. Sharma, V. Sharma and S. S. Sharma, Nanoscale Res. Lett., 2018, 13, 381 CrossRef PubMed
.
- G. Li, L. Sheng, T. Li, J. Hu, P. Li and K. Wang, J. Mater. Sci. Eng., 2019, 177, 80–98 CAS
.
- M. N. Mustafa and Y. Sulaiman, J. Mater. Sci. Eng., 2020, 212, 332–338 CAS
.
- S. Yang, S. Sha, H. Lu, J. Wu, J. Ma, D. Wang and Z. Sheng, Colloids Surf., A, 2020, 594, 124665 CrossRef CAS
.
- B. D. Choudhury, C. Lin, S. M. A. Z. Shawon, J. Soliz-Martinez, J. Gutierrez, M. N. Huda, F. Cesano, K. Lozano, J. Z. Zhang and M. J. Uddin, ACS Appl. Energy Mater., 2021, 4, 870–878 CrossRef CAS
.
- Z. Xu, T. Li, Q. Liu, F. Zhang, X. Hong, S. Xie, C. Lin, X. Liu and W. Guo, Sol. Energy Mater. Sol. Cells, 2018, 179, 297–304 CrossRef CAS
.
- C. Wu, B. Chen, X. Zheng and S. Priya, Sol. Energy Mater. Sol. Cells, 2016, 157, 438–446 CrossRef CAS
.
- R. Zhou, W. Guo, R. Yu and C. Pan, J. Mater. Chem. A, 2015, 3, 23028–23034 RSC
.
- Z. Wen, M.-H. Yeh, H. Guo, J. Wang, Y. Zi, W. Xu, J. Deng, L. Zhu, X. Wang, C. Hu, L. Zhu, X. Sun and Z. L. Wang, Sci. Adv., 2016, 2, 1600097 CrossRef PubMed
.
- Y. Rong, Y. Hu, A. Mei, H. Tan, M. I. Saidaminov, S. I. Seok, M. D. McGehee, E. H. Sargent and H. Han, Science, 2018, 361, 6408 CrossRef PubMed
.
- J.-P. Correa-Baena, M. Saliba, T. Buonassisi, M. Grätzel, A. Abate, W. Tress and A. Hagfeldt, Science, 2017, 358, 739–744 CrossRef CAS PubMed
.
- H. S. Jung, G. S. Han, N.-G. Park and M. J. Ko, Joule, 2019, 3, 1850–1880 CrossRef CAS
.
- F. Di Giacomo, A. Fakharuddin, R. Jose and T. M. Brown, Energy Environ. Sci., 2016, 9, 3007–3035 RSC
.
- K. Huang, Y. Peng, Y. Gao, J. Shi, H. Li, X. Mo, H. Huang, Y. Gao, L. Ding and J. Yang, Adv. Energy Mater., 2019, 9, 1901419 CrossRef CAS
.
- Q. Li, A. Balilonda, A. Ali, R. Jose, F. Zabihi, S. Yang, S. Ramakrishna and M. Zhu, Sol. RRL, 2020, 4, 2000269 CrossRef CAS
.
- R. Li, X. Xiang, X. Tong, J. Zou and Q. Li, Adv. Mater., 2015, 27, 3831–3835 CrossRef CAS PubMed
.
- M. Kaltenbrunner, G. Adam, E. D. Glowacki, M. Drack, R. Schwodiauer, L. Leonat, D. H. Apaydin, H. Groiss, M. C. Scharber, M. S. White, N. S. Sariciftci and S. Bauer, Nat. Mater., 2015, 14, 1032–1039 CrossRef CAS PubMed
.
- M. Park, J.-Y. Kim, H. J. Son, C.-H. Lee, S. S. Jang and M. J. Ko, Nano Energy, 2016, 26, 208–215 CrossRef CAS
.
- X. Meng, Z. Cai, Y. Zhang, X. Hu, Z. Xing, Z. Huang, Z. Huang, Y. Cui, T. Hu, M. Su, X. Liao, L. Zhang, F. Wang, Y. Song and Y. Chen, Nat. Commun., 2020, 11, 3016 CrossRef CAS PubMed
.
- B. J. Kim, D. H. Kim, Y.-Y. Lee, H.-W. Shin, G. S. Han, J. S. Hong, K. Mahmood, T. K. Ahn, Y.-C. Joo, K. S. Hong, N.-G. Park, S. Lee and H. S. Jung, Energy Environ. Sci., 2015, 8, 916–921 RSC
.
- K. Yang, X. Shuai, H. Yang, J. Yan and K. Cen, Acta Phys.-Chim. Sin., 2019, 35, 755–765 Search PubMed
.
- Z. Niu, L. Liu, L. Zhang, W. Zhou, X. Chen and S. Xie, Adv. Energy Mater., 2015, 5, 1500677 CrossRef
.
- H. Yang, Z. BO, X. Shuai, J. Yan and K. Cen, Acta Phys.-Chim. Sin., 2019, 35, 200–207 Search PubMed
.
- K. Wang, M. Xu, Y. Gu, Z. Gu, J. Liu and Q. H. Fan, Nano Energy, 2017, 31, 486–494 CrossRef CAS
.
- Y.-J. Gu, W. Wen and J.-M. Wu, J. Mater. Chem. A, 2018, 6, 21078–21086 RSC
.
- L. Yu, Y. Yi, T. Yao, Y. Song, Y. Chen, Q. Li, Z. Xia, N. Wei, Z. Tian, B. Nie, L. Zhang, Z. Liu and J. Sun, Nano Res., 2018, 12, 331–338 CrossRef
.
- C. Shen, Y. Xie, B. Zhu, M. Sanghadasa, Y. Tang and L. Lin, Sci. Rep., 2017, 7, 14324 CrossRef
.
- L. Kou, T. Huang, B. Zheng, Y. Han, X. Zhao, K. Gopalsamy, H. Sun and C. Gao, Nat. Commun., 2014, 5, 3754 CrossRef CAS PubMed
.
- X. Du, M. Tian, G. Sun, Z. Li, X. Qi, H. Zhao, S. Zhu and L. Qu, ACS Appl. Mater. Interfaces, 2020, 12, 55876–55883 CrossRef CAS PubMed
.
- Y. Lin, Y. Gao and Z. Fan, Adv. Mater., 2017, 29, 1701736 CrossRef PubMed
.
- Y. Shao, M. F. El-Kady, J. Sun, Y. Li, Q. Zhang, M. Zhu, H. Wang, B. Dunn and R. B. Kaner, Chem. Rev., 2018, 118, 9233–9280 CrossRef CAS PubMed
.
- Q. Jiang, N. Kurra, M. Alhabeb, Y. Gogotsi and H. N. Alshareef, Adv. Energy Mater., 2018, 8, 1703043 CrossRef
.
- M. Boota and Y. Gogotsi, Adv. Energy Mater., 2019, 9, 1802917 CrossRef
.
- Z. Lai, A. Chaturvedi, Z. Shi, J. Zhao, T. H. Tran, B. Chen, Y. Huang, X. Cao, Q. He, Z. Zeng, C. Tan and H. Zhang, Small, 2021, 17, 2006866 CrossRef CAS PubMed
.
- W. Xiong, K. Hu, Z. Li, Y. Jiang, Z. Li, Z. Li and X. Wang, Nano Energy, 2019, 66, 104149 CrossRef CAS
.
- J. Guo, Q. Zhang, J. Sun, C. Li, J. Zhao, Z. Zhou, B. He, X. Wang, P. Man, Q. Li, J. Zhang, L. Xie, M. Li and Y. Yao, J. Power Sources, 2018, 382, 122–127 CrossRef CAS
.
- Z. Tian, X. Tong, G. Sheng, Y. Shao, L. Yu, V. Tung, J. Sun, R. B. Kaner and Z. Liu, Nat. Commun., 2019, 10, 4913 CrossRef PubMed
.
- T. G. Yun, M. Park, D. H. Kim, D. Kim, J. Y. Cheong, J. G. Bae, S. M. Han and I. D. Kim, ACS Nano, 2019, 13, 3141–3150 CrossRef CAS PubMed
.
- L. Gao, J. Song, J. U. Surjadi, K. Cao, Y. Han, D. Sun, X. Tao and Y. Lu, ACS Appl. Mater. Interfaces, 2018, 10, 28597–28607 CrossRef CAS PubMed
.
- Y. Zhang, Y. Zhao, J. Ren, W. Weng and H. Peng, Adv. Mater., 2016, 28, 4524–4531 CrossRef CAS PubMed
.
- Z. Zhou, L. Ma and C. Tan, Chem. Res. Chin. Univ., 2021, 42, 662–670 Search PubMed
.
- D. Chen, Z. Lou, K. Jiang and G. Shen, Adv. Funct. Mater., 2018, 28, 1805596 CrossRef
.
- G. Qian, X. Liao, Y. Zhu, F. Pan, X. Chen and Y. Yang, ACS Energy Lett., 2019, 4, 690–701 CrossRef CAS
.
- H. Cha, J. Kim, Y. Lee, J. Cho and M. Park, Small, 2018, 14, 1702989 CrossRef PubMed
.
- M.-S. Balogun, H. Yang, Y. Luo, W. Qiu, Y. Huang, Z.-Q. Liu and Y. Tong, Energy Environ. Sci., 2018, 11, 1859–1869 RSC
.
- A. M. Gaikwad, B. V. Khau, G. Davies, B. Hertzberg, D. A. Steingart and A. C. Arias, Adv. Energy Mater., 2015, 5, 1401389 CrossRef
.
- Y. H. Kwon, S. W. Woo, H. R. Jung, H. K. Yu, K. Kim, B. H. Oh, S. Ahn, S. Y. Lee, S. W. Song, J. Cho, H. C. Shin and J. Y. Kim, Adv. Mater., 2012, 24, 5192–5197 CrossRef CAS PubMed
.
- Y. Zhang, W. Bai, X. Cheng, J. Ren, W. Weng, P. Chen, X. Fang, Z. Zhang and H. Peng, Angew. Chem., Int. Ed., 2014, 53, 14564–14568 CrossRef CAS PubMed
.
- W. Weng, Q. Sun, Y. Zhang, H. Lin, J. Ren, X. Lu, M. Wang and H. Peng, Nano Lett., 2014, 14, 3432–3438 CrossRef CAS PubMed
.
- R. Tajima, T. Miwa, T. Oguni, A. Hitotsuyanagi, H. Miyake, H. Katagiri, Y. Goto, Y. Saito, J. Goto, M. Kaneyasu, M. Hiroki, M. Takahashi and S. Yamazaki, J. Soc. Inf. Disp., 2014, 22, 237–244 CrossRef CAS
.
- J. Xu, Y. Chen and L. Dai, Nat. Commun., 2015, 6, 8103 CrossRef CAS PubMed
.
- P. Zhang, Y. Li, G. Wang, F. Wang, S. Yang, F. Zhu, X. Zhuang, O. G. Schmidt and X. Feng, Adv. Mater., 2019, 31, 1806005 CrossRef PubMed
.
- W. Qiu, Y. Li, A. You, Z. Zhang, G. Li, X. Lu and Y. Tong, J. Mater. Chem. A, 2017, 5, 14838–14846 RSC
.
- X. Xu, Y. Chen, D. Zheng, P. Ruan, Y. Cai, X. Dai, X. Niu, C. Pei, W. Shi, W. Liu, F. Wu, Z. Pan, H. Li and X. Cao, Small, 2021, 17, 2101901 Search PubMed
.
- H. Pan, Y. Shao, P. Yan, Y. Cheng, K. S. Han, Z. Nie, C. Wang, J. Yang, X. Li, P. Bhattacharya, K. T. Mueller and J. Liu, Nat. Energy, 2016, 1, 16039 CrossRef CAS
.
- Y. Zeng, X. Zhang, Y. Meng, M. Yu, J. Yi, Y. Wu, X. Lu and Y. Tong, Adv. Mater., 2017, 29, 1700274 CrossRef PubMed
.
- Y. Huang, J. Liu, J. Wang, M. Hu, F. Mo, G. Liang and C. Zhi, Angew. Chem., Int. Ed., 2018, 57, 9810–9813 CrossRef CAS PubMed
.
- H. Li, C. Han, Y. Huang, Y. Huang, M. Zhu, Z. Pei, Q. Xue, Z. Wang, Z. Liu, Z. Tang, Y. Wang, F. Kang, B. Li and C. Zhi, Energy Environ. Sci., 2018, 11, 941–951 RSC
.
- H. Li, Z. Liu, G. Liang, Y. Huang, Y. Huang, M. Zhu, Z. Pei, Q. Xue, Z. Tang, Y. Wang, B. Li and C. Zhi, ACS Nano, 2018, 12, 3140–3148 CrossRef CAS PubMed
.
- F. Mo, G. Liang, Q. Meng, Z. Liu, H. Li, J. Fan and C. Zhi, Energy Environ. Sci., 2019, 12, 706–715 RSC
.
- J. Zhao, W. Wu, X. Jia, T. Xia, Q. Li, J. Zhang, Q. Wang, W. Zhang and C. Lu, J. Mater. Chem. A, 2020, 8, 18198–18206 RSC
.
- J. Zhao, Z. Xu, Z. Zhou, S. Xi, Y. Xia, Q. Zhang, L. Huang, L. Mei, Y. Jiang, J. Gao, Z. Zeng and C. Tan, ACS Nano, 2021, 15, 10597–10608 CrossRef PubMed
.
- J. Zhao, Y. Lin, J. Wu, H. Y. Y. Nyein, M. Bariya, L. C. Tai, M. Chao, W. Ji, G. Zhang, Z. Fan and A. Javey, ACS Sens., 2019, 4, 1925–1933 CrossRef CAS PubMed
.
- W. Guo, X. Xue, S. Wang, C. Lin and Z. L. Wang, Nano Lett., 2012, 12, 2520–2523 CrossRef CAS PubMed
.
- X. Zhang, X. Huang, C. Li and H. Jiang, Adv. Mater., 2013, 25, 4093–4096 CrossRef CAS PubMed
.
- B. Shi, Microprocessors and Microsystems, 2021, 81, 103791 CrossRef
.
- J. Kim, A. S. Campbell, B. E. de Avila and J. Wang, Nat. Biotechnol., 2019, 37, 389–406 CrossRef CAS PubMed
.
- J. C. Yang, J. Mun, S. Y. Kwon, S. Park, Z. Bao and S. Park, Adv. Mater., 2019, 31, 1904765 CrossRef CAS PubMed
.
- K. Xu, Y. Lu and K. Takei, Adv. Mater. Technol., 2019, 4, 1800628 CrossRef
.
- C. Li, S. Cong, Z. Tian, Y. Song, L. Yu, C. Lu, Y. Shao, J. Li, G. Zou, M. H. Rümmeli, S. Dou, J. Sun and Z. Liu, Nano Energy, 2019, 60, 247–256 CrossRef CAS
.
- J. F. Thayer, F. Ahs, M. Fredrikson, J. J. Sollers III and T. D. Wager, Neurosci. Biobehav. Rev., 2012, 36, 747–756 CrossRef PubMed
.
- N. Xiao, W. Yu and X. Han, Measurement, 2020, 164, 108102 CrossRef
.
- F. El-Amrawy and M. I. Nounou, J. Healthc. Inform. Res., 2015, 21, 315–320 CrossRef PubMed
.
- J. H. Park, D. G. Jang, J. W. Park and S. K. Youm, Sensors, 2015, 15, 23402–23417 CrossRef PubMed
.
- R. Martinek, J. Nedoma, M. Fajkus, R. Kahankova, J. Konecny, P. Janku, S. Kepak, P. Bilik and H. Nazeran, Sensors, 2017, 17, 890 CrossRef PubMed
.
- K.-Y. Shin, J. S. Lee and J. Jang, Nano Energy, 2016, 22, 95–104 CrossRef CAS
.
- H. Ouyang, J. Tian, G. Sun, Y. Zou, Z. Liu, H. Li, L. Zhao, B. Shi, Y. Fan, Y. Fan, Z. L. Wang and Z. Li, Adv. Mater., 2017, 29, 1703456 CrossRef PubMed
.
- Z. Lin, J. Chen, X. Li, Z. Zhou, K. Meng, W. Wei, J. Yang and Z. L. Wang, ACS Nano, 2017, 11, 8830–8837 CrossRef CAS PubMed
.
- D. Y. Park, D. J. Joe, D. H. Kim, H. Park, J. H. Han, C. K. Jeong, H. Park, J. G. Park, B. Joung and K. J. Lee, Adv. Mater., 2017, 29, 1702308 CrossRef PubMed
.
- V. Rajendran, A. M. V. Mohan, M. Jayaraman and T. Nakagawa, Nano Energy, 2019, 65, 104055 CrossRef CAS
.
- M. Bariya, Z. Shahpar, H. Park, J. Sun, Y. Jung, W. Gao, H. Y. Y. Nyein, T. S. Liaw, L. C. Tai, Q. P. Ngo, M. Chao, Y. Zhao, M. Hettick, G. Cho and A. Javey, ACS Nano, 2018, 12, 6978–6987 CrossRef CAS PubMed
.
- M. Bariya, H. Y. Y. Nyein and A. Javey, Nat. Electron., 2018, 1, 160–171 CrossRef
.
- J. Heikenfeld, Electroanalysis, 2016, 28, 1242–1249 CrossRef CAS
.
- H. Y. Nyein, M. Bariya, L. Kivimäki, S. Uusitalo, T. S. Liaw, E. Jansson, C. H. Ahn, J. A. Hangasky, J. Zhao, Y. Lin, T. Happonen, M. Chao, C. Liedert, Y. Zhao, L.-C. Tai, J. Hiltunen and A. Javey, Sci. Adv., 2019, 5, eaaw9906 CrossRef CAS PubMed
.
- W. Gao, S. Emaminejad, H. Y. Y. Nyein, S. Challa, K. Chen, A. Peck, H. M. Fahad, H. Ota, H. Shiraki, D. Kiriya, D. H. Lien, G. A. Brooks, R. W. Davis and A. Javey, Nature, 2016, 529, 509–514 CrossRef CAS PubMed
.
- S. Emaminejad, W. Gao, E. Wu, Z. A. Davies, H. Y. Y. Nyein, S. Challa, S. P. Ryan, H. M. Fahad, K. Chen, Z. Shahpar, S. Talebi, C. Milla, A. Javey and R. W. Davis, Proc. Natl. Acad. Sci. U. S. A., 2017, 114, 4625–4630 CrossRef CAS PubMed
.
- L. C. Tai, W. Gao, M. Chao, M. Bariya, Q. P. Ngo, Z. Shahpar, H. Y. Y. Nyein, H. Park, J. Sun, Y. Jung, E. Wu, H. M. Fahad, D. H. Lien, H. Ota, G. Cho and A. Javey, Adv. Mater., 2018, 30, 1707442 CrossRef PubMed
.
- X. Liu, N. Li, M. Li, H. Chen, N. Zhang, Y. Wang and K. Zheng, Coord. Chem. Rev., 2020, 404, 213109 CrossRef CAS
.
- A. D. Wilson, Metabolites, 2015, 5, 140–163 CrossRef CAS PubMed
.
- T. Stacewicz, Z. Bielecki, J. Wojtas, P. Magryta, J. Mikolajczyk and D. Szabra, Opto-Electron. Rev., 2016, 24, 82–94 CAS
.
- M. W. Hoffmann, L. Mayrhofer, O. Casals, L. Caccamo, F. Hernandez-Ramirez, G. Lilienkamp, W. Daum, M. Moseler, A. Waag, H. Shen and J. D. Prades, Adv. Mater., 2014, 26, 8017–8022 CrossRef CAS PubMed
.
- Y. Kim, S. Lee, J. G. Song, K. Y. Ko, W. J. Woo, S. W. Lee, M. Park, H. Lee, Z. Lee, H. Choi, W. H. Kim, J. Park and H. Kim, Adv. Funct. Mater., 2020, 30, 2003360 CrossRef CAS
.
- K. Zhao, G. Gu, Y. Zhang, B. Zhang, F. Yang, L. Zhao, M. Zheng, G. Cheng and Z. Du, Nano Energy, 2018, 53, 898–905 CrossRef CAS
.
- S. Cui, Y. Zheng, T. Zhang, D. Wang, F. Zhou and W. Liu, Nano Energy, 2018, 49, 31–39 CrossRef CAS
.
- Y. Lin, J. Chen, M. M. Tavakoli, Y. Gao, Y. Zhu, D. Zhang, M. Kam, Z. He and Z. Fan, Adv. Mater., 2019, 31, 1804285 CrossRef PubMed
.
- F. Zabihi, M. Tebyetekerwa, Z. Xu, A. Ali, A. K. Kumi, H. Zhang, R. Jose, S. Ramakrishna and S. Yang, J. Mater. Chem. A, 2019, 7, 26661–26692 RSC
.
|
This journal is © The Royal Society of Chemistry 2021 |
Click here to see how this site uses Cookies. View our privacy policy here.