DOI:
10.1039/D1RA07958A
(Paper)
RSC Adv., 2021,
11, 38115-38119
Influence of water-soluble pillararene hosts on Kemp elimination†
Received
29th October 2021
, Accepted 22nd November 2021
First published on 26th November 2021
Abstract
Since pillar[5]arene was first discovered in 2008, it has developed into a multifunctional supramolecular host. Its application covers many fields from drug delivery and chemical sensing to the construction of molecular machines, and so on. Supramolecular catalysis based on pillar[n]arenes is one of the hot research topics that has emerged in recent years. In this paper, we have synthesized two water-soluble pillar[5]arenes with peripheral rims bearing opposite charges and investigated their influence on Kemp elimination reaction of 1,2-phenylisoxazole derivatives. It is found that both hosts have a moderate rate acceleration effect on the reaction, and the positively charged host H1 has a greater impact on the reaction rate than the negatively charged host H2.
1 Introduction
Enzymes are powerful and efficient catalysts that can promote the rapid progress of chemical reactions in organisms and maintain the normal operation of the body with high substrate specificity and selectivity.1 These properties of enzymes have prompted chemists to search for artificial supramolecular hosts that can mimic these functions. Since the concept of supramolecular chemistry was proposed in the 1980s,2 myriads of artificial supramolecular hosts have been synthesized, such as crown ethers,3 cyclodextrins,4 calixarenes,5 cucurbiturils,6 pillararenes,7 deep cavity cavitands,8 and self-assembled metal–organic cages.9,10 These hosts can accommodate guests that are complementary to their shape and sizes through various noncovalent interactions. Encapsulated guests often behave distinctly compared to the free state.11 Therefore, their reactivity would be greatly influenced and modulated by supramolecular hosts.
So far, many unique reactions catalyzed or mediated by supramolecular hosts have been reported,12–15 and the utilization of well-defined nanospace to generate new chemical reactions or obtain novel products has been achieved. There are several strategies that have been frequently employed in modulating chemical reactivities, e.g. stabilization of transition state, destabilization of ground state, substrate preorganization, local concentration enrichment, desolvation, extra catalytic site incorporation.16,17 Chemists have been versed in performing chemical reactions using these approaches.18 Nevertheless, it remains challenging to approach the efficiency and complicacy of natural enzymes.19
Pillar[n]arenes, which were first introduced in 2008 by Ogoshi group,7 have been developed into a versatile supramolecular host. Pillar[n]arenes can recognize guest molecules selectively in organic solvents, due to the possession of an electron-rich cavity and the combination of various noncovalent interactions such as dipole–dipole, C–H⋯π, π–π interactions.20–22 Owing to the easy synthesis and excellent binding performance of pillar[n]arenes, researchers have discovered their applications ranging from drug delivery,23 chemical sensing24 to molecular machines.25 Catalysis by pillar[n]arenes is also one of the hot research topics, which emerged in the last few years.26,27 One of advantages of pillar[n]arenes is their tubular structures which allow for reactant ingress and product egress.28 However, the key is to control the guest exchange dynamic that favours the product formation. So far, there are very limited reports investigating the cavity of pillar[n]arene in influencing chemical reactions in aqueous media. Moreover, not only catalysis was achieved,29 but also in some cases, inhibitions were observed for the reaction occurred inside the pillararene cavity.30–32
Herein, we report the effects of two oppositely charged water-soluble pillar[5]arene hosts33,34 on Kemp elimination reaction of 1,2-phenylisoxazole derivatives (Scheme 1). Previously, Michael Ward35 and co-workers investigated the effect of water-soluble metal–organic cages [Co8L12]16+ on Kemp elimination reaction, and their results showed that the existence of cage has a huge rate acceleration (kcat/kuncat = 2 × 105) on the reaction. We assume the hydrophobic cavity of pillar[5]arene will have similar effect on this reaction. It was found that our two hosts bearing opposite charges on their rims have influenced the reaction rate to different extent. The charged host can generate an electrostatic potential field (EPF) under the coulombic interaction to control the chemical reaction, which has been considered and applied by external electric field or high concentrated ionic aggregates.36,37 The same is true for our two hosts, which will also generate EPF and affect the reactions of the encapsulated guests.
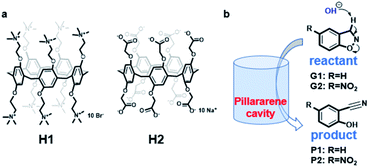 |
| Scheme 1 (a) Structure of the pillar[5]arene hosts; (b) illustration of the Kemp elimination reaction within the pillar[5]arene cavity. | |
2 Experimental
2.1 Materials
All solvents and reagents used in this study are chemically pure. 1,4-Diethoxy benzene was purchased from Tokyo Chemical Industry Co. Ltd., polyformaldehyde and ammonium hydroxide solution were purchased from Shanghai Titan Scientific Co. Ltd (China), ethyl bromoacetate, triphenylphosphine, carbon tetrabromide and s-trioxane were purchased from the Saen Chemical Technology (Shanghai) Co. Ltd, boron tribromide and boron trifluoride diethyl etherate were purchased from Shanghai Macklin Biochemical Co. Ltd. Guests G1 and G2 were purchased from Aladdin Chemical Reagent Co. Ltd (Shanghai, China) and Bide Pharmatech Ltd (Shanghai). All solvents were purchased from Shanghai Titan Scientific Co. Ltd (China) and used directly without further purification. Hosts H1 and H2 were synthesized according to the reported procedures.33,34
2.2 Binding affinity measurements
Binding constants were measured by 1H NMR titrations with a Bruker Avance 400 MHz spectrometer and ITC experiments by a GE MicroCal iTC200. In the 1H NMR experiments, guest concentration was maintained to be 5 × 10−4 M, and then different equivalents of host were added successively. With the addition of host, the signal peaks of guest shifted upfield. The addition was stopped when the peaks of guest no longer shifted. Fitting of the data was performed using Origin 2021 according to the following equation: |
Δδ = (Δδ∞/[G]0) (0.5[H]0 + 0.5([G]0 + 1/Ka) − (0.5([H]02 + (2[H]0(1/Ka − [G]0)) + (1/Ka + [G]0)2)0.5))
| (1) |
where Δδ is the chemical shift changes of H1 in G1, Δδ∞ is the chemical shift changes when G1 is completely complexed, [G]0 is the fixed initial concentration of G1, and [H]0 is the varying concentrations of the host. In the ITC experiments, the guest concentration (5 × 10−4 M) was kept constant, and then a total volume of 25 μL hosts (5 × 10−3 M) were injected in 25 aliquots.
2.3 Kinetic measurements
UV-Vis spectroscopy by a Shimadzu UV 1780 UV-Vis Spectrophotometer was employed for the kinetic measurements of Kemp elimination reaction. During the tests, host concentrations were maintained to be 1 × 10−4 M in a 3 mL cuvette, and then 10 μL of guest (3 × 10−2 M) was added to keep the molar ratio of host-guest at 1
:
1. Subsequently, 10 equiv. NaOH solution was added to the above mixture to initiate the reaction. The absorption peaks of products P1 and P2 appear at 325 nm and 378 nm, respectively, and the tests were stopped when the absorption of the product was no longer enhanced. In the control experiments of free guests, the guest concentration was also kept to be 1 × 10−4 M. Fitting of the data was performed using Origin 2021 mono-exponential growth function, |
y = A1 × exp(−x/t1) + y0
| (2) |
where y is the absorption or yield of product and x is corresponding time. The rate constant k is the inverse of the life time t1.
3 Results and discussion
Binding experiments between the host H1 and guest G1 were followed using 1H NMR spectroscopy in D2O solution. First, we kept the total concentration of G1 and H1 at 6 mM, and measured the job's plot by changing their molar ratio. With the increase of H1 content, upfield shifts of the proton peaks of G1 appeared, which signified that there is host-guest interaction between them and indicated the formation of a 1
:
1 inclusion complex (Fig. S1 and S2†). Next, the binding constant between H1 and G1 was obtained through 1H NMR titrations. The peaks of G1 shifted upfield in the titration experiment between H1 and G1. Fitting the data by eqn (1) gave the binding constant as 51 M−1 (Fig. S3 and S4†). Similarly, the binding constant between H2 and G1 was calculated to be 24 M−1 (Fig. S5 and S6†). Meanwhile, ITC was used to test the binding between the hosts and G1, and the values of binding constant are 698 M−1 (H1–G1) and 641 M−1 (H2–G1), respectively (Fig. S27 and S28†). There is an order of magnitude difference in binding constants measured by 1H NMR and ITC, which is attributed to the fact that heat of dilution and assembly process may also occur during the ITC experiments. Overall, the binding of the Kemp elimination substrate G1 with the water-soluble pillar[5]arene is not strong, which is mainly caused by the lack of charges on the substrate; the neutral guest can tumble freely within the tubular cavity of pillar[5]arene. In terms of G2, similar binding mode was observed upon mixing it with H1 and H2 in 1
:
1 ratio (Fig. S7 and S8†).
Although weak binding between the above hosts and guests were observed, we assume the charge difference on the rims will still influence the rate of Kemp elimination. Ultraviolet-Visible (UV-Vis) spectroscopy is a common method for quantitatively studying compounds in solution, and we used it to study the Kemp elimination reaction of 1,2-phenylisoxazole derivatives. In the spectra of free G1, the absorption peak of G1 is around 280 nm. When the reaction started, a new absorption peak corresponding to P1 appeared at 325 nm. As time goes by, the absorption peak of G1 gradually decreased and the P1's gradually increased (Fig. S12†). After that, the positively charged host H1 and the negatively charged host H2 were added separately, and their corresponding UV-Vis spectra were obtained (Fig. S13 and S14†). Preliminary results showed that not only the presence or the absence but also the kind of the hosts affected the speed of the reaction. After accurate data fitting by eqn (2), three kinetic curves and corresponding rate constants were obtained (Fig. S18–S20†), which showed that the hosts could accelerate the reaction rate of G1 by 5.8 (kH1–G1/kfree G1) and 2.9 (kH2–G1/kfree G1) times, respectively (Fig. 1). The results of G2 were similar to that of G1. In the spectra of G2, the absorption peak of P2 is at 378 nm. Due to the poor solubility of G2, the reaction curve of free G2 cannot be well fitted to obtain specific rate constant (Fig. S21†). But it is certain that in the presence of the hosts, the rate acceleration caused by H1 is 4 times (kH1–G2/kH2–G2) faster than that of H2 (Fig. S22 and S23†). The above results indicate that the presence of the host can indeed speed up the reaction rate, and the positively charged host H1 is more efficient than the negatively charged H2.
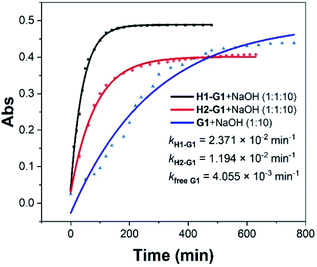 |
| Fig. 1 Kinetic curves for the UV-Vis experiments of G1 with 10 equiv. of NaOH in the presence and absence of the water-soluble pillar[5]arene hosts H1 and H2. | |
In addition to UV-Vis, 1H NMR was also used to track the above reactions. 10 equiv. NaOH solution was added in the UV-Vis experiments, but since the concentrations of the host and guest were increased to 1 × 10−3 M in 1H NMR experiments, the corresponding concentration of NaOH increased to 1 × 10−2 M. This concentration in 1H NMR was so high that the reaction proceeded too fast to be recorded by 1H NMR. Therefore, the concentration of NaOH was reduced to 2 equivalents. In the process of G1, the proton signals of G1 gradually disappeared and the peaks of P1 gradually appeared (Fig. S9–S11†). The specified two peaks (the peaks of G1 (7.82 ppm) and P1 (6.56 ppm)) were integrated in the 1H NMR spectra to obtain the corresponding reaction yields. Further data processing gave a yield–time curve and further fitted three kinetic curves (Fig. S24–S26†). It was found that the presence of hosts accelerated the reaction rate by 4.4 (kH1–G1/kfree G1) and 3.2 (kH2–G1/kfree G1) times (Fig. 2). These results were consistent with that obtained by UV-Vis. Due to the poor solubility of G2 in water, no reliable data was obtained in the 1H NMR experiments.
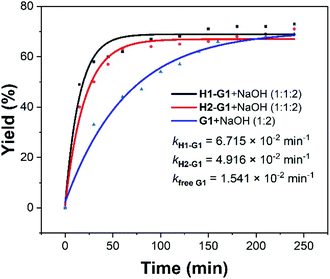 |
| Fig. 2 Kinetic curves for the 1H NMR experiments of G1 with 2 equiv. of NaOH in the presence and absence of the water-soluble pillar[5]arene hosts H1 and H2. | |
The mechanism on how the pillar[5]arene hosts influence the rate of Kemp elimination reaction is proposed. Due to the poor water-solubility of substrates G1 and G2, the reaction rate of the guest in aqueous solution is relatively slow. However, they can be enclosed in the cavity of water-soluble pillararene through hydrophobic interaction in the presence of supramolecular host, which increases the solubility of the reactants, hence the reaction rate will be increased. In addition, the reaction in this paper is a type of E2 elimination reaction, which involves a negatively charged transition state (TS). The EPF generated by the positive H1 can stabilize the negatively charged TS and reduce activation energy of the reaction. Moreover, the high positive charge in H1 results in accumulation of hydroxide ions around the host surface, affording a very high local concentration of anionic OH− close to the bound guest in the cavity. Although the EPF of H2 cannot stabilize the TS, it can remove negatively charged products through electrostatic repulsion, thus playing a catalytic role (Fig. 3).
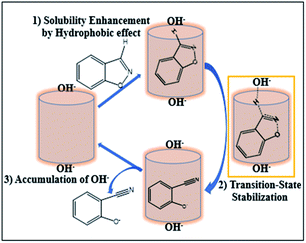 |
| Fig. 3 Proposed possible mechanism of the Kemp elimination reaction in the presence of water-soluble pillararene hosts. | |
4 Conclusions
In summary, Kemp elimination reaction of 1,2-phenylisoxazole derivatives in aqueous phase was investigated using two water-soluble pillar[5]arenes with different rim charges. Our results by UV-Vis and 1H NMR showed that the positively charged host H1 brought a roughly 6-fold rate acceleration to the reaction, and the negatively charged host H2 would also accelerate the reaction but not as fast as H1. Although pillararene hosts didn't give a significant rate acceleration on Kemp elimination in this case, our studies in this article provides an example for the use of pillararene cavity to modulate chemical reactions, and expands the functions and applications of pillararenes. Our laboratory is also actively seeking other reactions that can be catalyzed by pillararenes with greater extent.
Author contributions
Q. Liu performed the experiments and wrote the original draft. X. Tian, Y. Shen and X. Huang completed partial synthesis of the hosts. K. Wang and X. Hu designed and supervised the project. All authors reviewed and revised the manuscript.
Conflicts of interest
There are no conflicts to declare.
Acknowledgements
This work was supported by the Natural Science Foundation of Jiangsu Province (No. BK20200432, BK20211179), and the Fundamental Research Funds for the Central Universities (No. NE2019002, NS2021040).
Notes and references
- T. Wang, X. Fan, C. Hou and J. Liu, Curr. Opin. Struct. Biol., 2018, 51, 19–27 CrossRef CAS PubMed.
- J.-M. Lehn, Angew. Chem., Int. Ed., 1988, 27, 89–112 CrossRef.
- G. W. Gokel, W. M. Leevy and M. E. Weber, Chem. Rev., 2004, 104, 2723–2750 CrossRef CAS PubMed.
- Y.-M. Zhang, Y.-H. Liu and Y. Liu, Adv. Mater., 2020, 32, 1806158 CrossRef CAS PubMed.
- Y. Razuvayeva, R. Kashapov and L. Zakharova, Supramol. Chem., 2020, 32, 178–206 CrossRef CAS.
- R.-C. Mutihac, A. A. Bunaciu, H.-J. Buschmann and L. Mutihac, J. Incl. Phenom. Macro., 2020, 98, 137–148 CrossRef CAS.
- T. Ogoshi, S. Kanai, S. Fujinami, T.-A. Yamagishi and Y. Nakamoto, J. Am. Chem. Soc., 2008, 130, 5022–5023 CrossRef CAS PubMed.
- S. Mosca, Y. Yu and J. Rebek, Nat. Protoc., 2016, 11, 1371–1387 CrossRef CAS PubMed.
- Q.-F. Sun, J. Iwasa, D. Ogawa, Y. Ishido, S. Sato, T. Ozeki, Y. Sei, K. Yamaguchi and M. Fujita, Science, 2010, 328, 1144–1147 CrossRef CAS PubMed.
- C. T. McTernan, T. K. Ronson and J. R. Nitschke, J. Am. Chem. Soc., 2019, 141, 6837–6842 CrossRef CAS PubMed.
- A. Galan and P. Ballester, Chem. Soc. Rev., 2016, 45, 1720–1737 RSC.
- Y. Fang, J. A. Powell, E. Li, Q. Wang, Z. Perry, A. Kirchon, X. Yang, Z. Xiao, C. Zhu, L. Zhang, F. Huang and H.-C. Zhou, Chem. Soc. Rev., 2019, 48, 4707–4730 RSC.
- G. Olivo, G. Capocasa, D. Del Giudice, O. Lanzalunga and S. Di Stefano, Chem. Soc. Rev., 2021, 50, 7681–7724 RSC.
- C. Gaeta, P. La Manna, M. De Rosa, A. Soriente, C. Talotta and P. Neri, ChemCatChem, 2021, 13, 1638–1658 CrossRef CAS.
- M. Petroselli, Y.-Q. Chen, J. J. Rebek and Y. Yu, Green Synth. Catal., 2021, 2, 123–130 CrossRef.
- K. Wang, J. H. Jordan, X.-Y. Hu and L. Wang, Angew. Chem., Int. Ed., 2020, 59, 13712–13721 CrossRef CAS PubMed.
- W. Liu and J. F. Stoddart, Chem, 2021, 7, 919–947 CAS.
- B. Mitschke, M. Turberg and B. List, Chem, 2020, 6, 2515–2532 CAS.
- Z. Dong, Q. Luo and J. Liu, Chem. Soc. Rev., 2012, 41, 7890–7908 RSC.
- C. Han, G. Yu, B. Zheng and F. Huang, Org. Lett., 2012, 14, 1712–1715 CrossRef CAS PubMed.
- N. L. Strutt, H. Zhang, M. A. Giesener, J. Lei and J. F. Stoddart, Chem. Commun., 2012, 48, 1647–1649 RSC.
- X. Shu, S. Chen, J. Li, Z. Chen, L. Weng, X. Jia and C. Li, Chem. Commun., 2012, 48, 2967–2969 RSC.
- G. Yu, W. Yu, Z. Mao, C. Gao and F. Huang, Small, 2015, 11, 919–925 CrossRef CAS PubMed.
- P.-Y. Li, Y. Chen, C. H. Chen and Y. Liu, Soft Matter, 2019, 15, 8197–8200 RSC.
- C. Biagini, S. D. P. Fielden, D. A. Leigh, F. Schaufelberger, S. Di Stefano and D. Thomas, Angew. Chem., Int. Ed., 2019, 58, 9876–9880 CrossRef CAS PubMed.
- K. Wang, X. Tian, J. H. Jordan, K. Velmurugan, L. Wang and X.-Y. Hu, Chin. Chem. Lett., 2021 DOI:10.1016/j.cclet.2021.06.026.
- K. Wang, J. H. Jordan, K. Velmurugan, X. Tian, M. Zuo, X.-Y. Hu and L. Wang, Angew. Chem., Int. Ed., 2021, 60, 9205–9214 CrossRef CAS PubMed.
- D. Zhang, J. Cheng, L. Wei, W. Song, L. Wang, H. Tang and D. Cao, J. Phys. Chem. Lett., 2020, 11, 2021–2026 CrossRef CAS PubMed.
- D. G. Liz, A. M. Manfredi, M. Medeiros, R. Montecinos, B. Gomez-Gonzalez, L. Garcia-Rio and F. Nome, Chem. Commun., 2016, 52, 3167–3170 RSC.
- J.-C. Gui, Z.-Q. Yan, Y. Peng, J.-G. Yi, D.-Y. Zhou, D. Su, Z.-H. Zhong, G.-W. Gao, W.-H. Wu and C. Yang, Chin. Chem. Lett., 2016, 27, 1017–1021 CrossRef CAS.
- G. Yu, J. Zhou, J. Shen, G. Tang and F. Huang, Chem. Sci., 2016, 7, 4073–4078 RSC.
- D. Xia, P. Wang and B. Shi, Org. Biomol. Chem., 2017, 15, 7618–7622 RSC.
- T. Ogoshi, M. Hashizume, T.-A. Yamagishi and Y. Nakamoto, Chem. Commun., 2010, 46, 3708–3710 RSC.
- Y. Ma, X. Ji, F. Xiang, X. Chi, C. Han, J. He, Z. Abliz, W. Chen and F. Huang, Chem. Commun., 2011, 47, 12340–12342 RSC.
- W. Cullen, M. C. Misuraca, C. A. Hunter, N. H. Williams and M. D. Ward, Nat. Chem., 2016, 8, 231–236 CrossRef CAS PubMed.
- A. C. Aragones, N. L. Haworth, N. Darwish, S. Ciampi, N. J. Bloomfield, G. G. Wallace, I. Diez-Perez and M. L. Coote, Nature, 2016, 531, 88–91 CrossRef CAS PubMed.
- Y. Pocker and R. F. Buchholz, J. Am. Chem. Soc., 1970, 92, 4033–4038 CrossRef CAS.
Footnote |
† Electronic supplementary information (ESI) available. See DOI: 10.1039/d1ra07958a |
|
This journal is © The Royal Society of Chemistry 2021 |
Click here to see how this site uses Cookies. View our privacy policy here.