DOI:
10.1039/D1NR00244A
(Paper)
Nanoscale, 2021,
13, 4660-4669
A novel electrochemical lung cancer biomarker cytokeratin 19 fragment antigen 21-1 immunosensor based on Si3N4/MoS2 incorporated MWCNTs and core–shell type magnetic nanoparticles†
Received
13th January 2021
, Accepted 2nd February 2021
First published on 2nd February 2021
Abstract
Lung cancer is one of deadliest and most life threatening cancer types. Cytokeratin 19 fragment antigen 21-1 (CYFRA 21-1) is a significant biomarker for the diagnosis of non-small cell lung cancer (NSCLC). Due to these reasons, a novel electrochemical immunosensor based on a silicon nitride (Si3N4)–molybdenum disulfide (MoS2) composite on multi-walled carbon nanotubes (Si3N4/MoS2–MWCNTs) as an electrochemical sensor platform and core–shell type magnetic mesoporous silica nanoparticles@gold nanoparticles (MMSNs@AuNPs) as a signal amplifier was presented for CYFRA21-1 detection in this study. Capture antibody (Ab1) immobilization on a Si3N4/MoS2–MWCNT modified glassy carbon electrode (Si3N4/MoS2–MWCNTs/GCE) was firstly successfully performed by stable electrostatic/ionic interactions between the –NH2 groups of the capture antibody and the polar groups of Si3N4/MoS2. Then, specific antibody–antigen interactions between the electrochemical sensor platform and the signal amplifier formed a novel voltammetric CYFRA21-1 immunosensor. The prepared composite materials and electrochemical sensor surfaces were characterized by transmission electron microscopy (TEM), scanning electron microscopy (SEM), X-ray diffraction (XRD), X-ray photoelectron spectroscopy (XPS), Fourier transform infrared spectroscopy (FTIR), cyclic voltammetry (CV) and electrochemical impedance spectroscopy (EIS). A linearity range of 0.01–1.0 pg mL−1 and a low detection limit (LOD) of 2.00 fg mL−1 were also obtained for analytical applications. Thus, the proposed immunosensor based on Si3N4/MoS2–MWCNTs and MMSNs@AuNPs has great potential for medical diagnosis of lung cancer.
1. Introduction
Lung cancer is the most common malignant tumor in the world. It is difficult to diagnose in the early period because it usually gives nonspecific findings. Because of this, patients with lung cancer have a high mortality rate.1 According to the World Health Organization, this rate can be about 90–95%.2 Lung cancer, which is spreading rapidly in the world, is defined as one of the most common types of cancer after prostate cancer in men and breast cancer in women. Lung cancer, whose most significant cause is shown to be smoking, can manifest itself especially with shortness of breath, wheezing, sudden weight loss or various pains. Thus, a rapid and sensitive diagnosis is significant for patients with lung cancer. The cancer severity directly corresponds to tumor marker levels in the plasma of cancer patients, suggesting that the recognition of these markers has attracted intense interest.3,4 Cytokeratins are intermediary filaments that form the skeleton of epithelial cells and are divided into different subtypes according to their molecular weights. In general, it can be said that low molecular weight cytokeratins belong to the simple and glandular epithelium, while high molecular weight cytokeratins belong to the multilayer and epidermal type epithelium.5 Cytokeratin-19 (CK-19) is the lowest molecular weight cytokeratin with a weight of 40 kDa and is found in simple epithelial cells. CYFRA21-1 is a soluble fragment of CK19 and it is released in cells during apoptosis’ intermediate stage.6 In addition, CYFRA21-1 serves as a biomarker in several malignancies.7 Furthermore, a high expression rate in the plasma of many patients with non-small cell lung cancer shows CYFRA21-1's presence.7 In addition, CYFRA21-1's pre-treatment is importantly related to the stage of disease and its high concentration is a negative prognostic indicator of patients with NSCLC.8 Thus, the sensitive and selective detection of CYFRA21-1 has started to attract great attention.
Up to now, traditional methods such as fluorometry,7,9 surface plasmon resonance analysis,10 and the immunoradiometric assay method11 have been used for CYFRA21-1 detection. In addition, immunosensors based on voltammetry have been reported for the detection of lung cancer biomarkers.12,13 These immunosensors have some positive properties such as selectivity, sensitivity and lower costs in comparison with traditional methods.14,15 In particular, voltammetry has gained attention as an important analytical method used for precision and sensitive detection in recent years.16–19
Immunosensors can be designed in two ways such as (i) sandwich-type with a label and (ii) label-free. In particular, sandwich-type immunosensors are frequently used as a result of high sensitivity and stable sensor signals.20,21 In these sandwich-type immunosensors, after the conjugation of primary antibodies to a sensor platform modified with a nanomaterial/nanocomposite and secondary antibodies likewise to a nanomaterial/nanocomposite surface, the target antigens are sandwiched between primary and secondary antibodies.22,23 In the literature, significant immunosensors for many different purposes have been reported. For example, a photothermal–thermoelectric coupled immunoassay of α-fetoprotein was performed using Seebeck effect transduction. The developed photothermal–thermoelectric coupled immunosensor demonstrated a LOD of 0.39 ng mL−1.24 Secondly, MXene quantum dot-encapsulated liposomes for sandwich-type photothermal immunoassay of prostate-specific antigen (PSA) were prepared using a near-infrared camera. An MXene quantum dot-based photothermal immunosensor showed a linearity range from 1.0 ng mL−1 to 50 ng mL−1 with a LOD of 0.4 ng mL−1 for PSA.25 In another study, a near-IR light-activated non-enzymatic signal-off photoelectrochemical immunosensor for α-fetoprotein analysis was constructed using branched polyethylenimine modified upconversion nanoparticle@CdTe quantum dots. This photoelectrochemical immunosensor showed a linearity range from 10.0 pg mL−1 to 50.0 ng mL−1 with a LOD of 1.2 pg mL−1.26 Finally, a paper electrode-based flexible pressure sensor modified with carbon nanotubes was developed for immunoassay of carcinoembryonic antigen with digital multimeter readout. This pressure sensor-based technique showed a linearity range of 0.5–60.0 ng mL−1 with a LOD of 167.0 pg mL−1.27 In addition, some CYFRA 21-1 detections were performed using immunosensor technology. For instance, a photoelectrochemical immunosensor based on In2O3/In2S3/CdIn2S4 was developed for CYFRA 21-1 detection.28 A linearity range of 0.50 pg mL−1–50.0 ng mL−1 and a LOD of 0.16 pg mL−1 were also obtained. In another study, a label-free electrochemical immunosensor for CYFRA 21-1 recognition based on graphene and AuNPs was prepared and applied to clinical serum samples.29 A linearity range of 0.25–800 ng mL−1 and a low LOD of 100.0 pg mL−1 were obtained. The graphene–AuNP composite demonstrated high biological activity and specific surface area in CYFRA 21-1 analysis. Fluorometry based immunosensing for CYFRA 21-1 determination was also presented and a LOD of 0.008 ng mL−1 was calculated. A florimetric immunosensor was designed by a hydrothermal method for the preparation of carbon quantum dots using Citrus lemon pericarp.9
In this study, a novel sandwich-type voltammetric immunosensor based on Si3N4/MoS2–MWCNTs and core–shell type magnetic mesoporous silica nanoparticles@gold nanoparticles for CYFRA 21-1 analysis was developed. Considering the literature in terms of CYFRA 21-1 analysis, it is seen that conventional methods such as fluorometry and surface plasmon resonance analysis have been developed. Nonetheless, these methods have some problems such as high cost equipment, slowness and the need for experienced staff. Thanks to the immunosensor we developed in this study, highly sensitive analysis was performed in a shorter time. The developed immunosensor in this study was prepared using Si3N4/MoS2–MWCNTs and MMSNs@AuNPs. Because the preparation procedures of these composites were low-cost and caused the least waste generation, it is possible to state that an environmentally friendly immunosensor is developed. In addition, the developed immunosensor showed a LOD of 2.00 fg mL−1 and perfect selective responses in the presence of other substances in comparison with conventional methods. Hence, the prepared immunosensor can provide a chance in terms of early detection of lung cancer via this biosensor.
2. Experimental
2.1. Materials
Cytokeratin fragment antigen 21-1 (CYFRA21-1), CYFRA21-1 primary antibody (anti-CYFRA21-1-Ab1), CYFRA21-1 secondary antibody (anti-CYFRA21-1-Ab2), carcinoembryonic antigen (CEA), ascorbic acid (AA), dopamine hydrochloride (DA), uric acid (UA), bovine serum albumin (BSA), sodium sulfide (Na2S), ammonium heptamolybdate tetrahydrate (AHMT, (NH4)6Mo7O24), citric acid (CA, C6H8O7), silicon nitride (Si3N4), MWCNTs (25–30 nm in diameter and 1.0–5.0 μm in length), dimethylformamide (DMF), cetyltrimethylammonium bromide (CTAB), 3,3′,5,5′-tetramethylbenzidine (TMB), tetraethyl orthosilicate (TEOS), and (3-aminopropyl)triethoxysilane (APTES) were purchased from Sigma-Aldrich. Phosphate buffer solution (PBS, 0.1 M, pH 7.0) as a supporting electrolyte and dilution buffer was also utilized.
2.2. Instrumentation
SEM and XRD images were obtained using a ZEISS EVO 50 SEM analytical microscope and Rigaku X-ray diffractometer, respectively. A JEOL 2100 TEM was used for TEM measurement. XPS analysis was carried out using a PHI 5000 Versa Probe. Gamry Reference 600 work-station (Gamry, USA) was utilized for differential pulse voltammetry (DPV), CV and EIS measurements. FTIR measurements were performed using a Bruker Tensor 27 FT-IR with a DTGS detector.
2.3. Preparation of MoS2, Si3N4/MoS2 and Si3N4/MoS2–MWCNT composites
The hydrothermal method was performed for MoS2 synthesis. Na2S as a sulfur source was utilized in the MoS2 synthesis process. After the preparation of a mixture of AHMT (1.50 g) and CA (0.50 g) in 25.0 mL distilled water, the dispersion was stirred constantly for 20 min. After that, Na2S (0.50 g) was added to the above dispersion and the dispersion was mixed with a magnetic stirrer until the green color turned red. In a subsequent experiment, the prepared solution was transferred into a Teflon autoclave at 200 °C for 120 min. After 120 min, the black dispersion was centrifuged at 5000 rpm and washed with distilled water and MoS2 was stored at 25 °C.30
MoS2 (250.0 mg) and Si3N4 powder (250.0 mg) were transferred to a milling jar. Then, the prepared material was milled for 15 h with a rotating speed of 200 rpm and collected. After that, the material was tagged as Si3N4/MoS2. After the preparation of a mixture of MWCNTs (50.0 mg) and Si3N4/MoS2 (10.0 mg) in DMF (100.0 mL), the mixture was sonicated for 90 min and the Si3N4/MoS2–MWCNT composite was filtered.
2.4. Si3N4/MoS2–MWCNT composite as an electrochemical sensor platform with anti-CYFRA21-1-Ab1 and antigen CYFRA21-1 immobilization
A triple electrode system including a glassy carbon electrode (GCE) as an indicator electrode, Ag/AgCl/KCl(sat) as a reference electrode and platinum wire as an auxiliary electrode was utilized for all electrochemical measurements. The cleaning protocol of glassy carbon electrodes was firstly performed according to our previous paper.31 A Si3N4/MoS2–MWCNT composite modified GCE (Si3N4/MoS2–MWCNTs/GCE) was developed by the dropping of composite dispersion (25.0 μL) on the clean GCE surfaces. Then, the solvent on the Si3N4/MoS2–MWCNT/GCE was removed with an IR lamp.
The anti-CYFRA21-1-Ab1 immobilization on the Si3N4/MoS2–MWCNT/GCE was successfully performed by stable electrostatic/ionic interactions between the –NH2 groups of anti-CYFRA21-1-Ab1 (30.0 μL, 50.0 μg mL−1) and the polar groups of Si3N4/MoS2 at 37.0 °C for 30 min, generating anti-CYFRA21-1-Ab1/Si3N4/MoS2–MWCNTs/GCE. Then, BSA (2.0% w/v) was utilized to prevent the non-specific interactions and the anti-CYFRA21-1-Ab1/Si3N4/MoS2–MWCNT/GCE was immersed in BSA dispersion at 37.0 °C for 30 min. After that, CYFRA21-1 antigens with different concentrations were incubated on the anti-CYFRA21-1-Ab1/Si3N4/MoS2–MWCNT/GCE at 37.0 °C for 30 min, tagged as CYFRA21-1/anti-CYFRA21-1-Ab1/Si3N4/MoS2–MWCNTs/GCE. Lastly, the developed immunosensor platform based on Si3N4/MoS2–MWCNTs was washed with 0.1 M PBS (pH 7.0) to remove unbound CYFRA21-1 proteins.
2.5. Preparation of MMSNs, MMSNs@AuNPs and conjugation of MMSNs@AuNPs with secondary antibody
After the preparation of 3.0 mol L−1 FeCl3 solution in 3.0 mol L−1 HCl, Na2SO3 solution (2.0 mol L−1, 3.0 mL) was slowly added to FeCl3 solution under stirring. After the color transformation from red to yellow, NH4OH (100.0 mL, 1.0 mol L−1) was slowly added to the above solution under stirring. After that, oleic acid (200.0 μL) was added and the resulting dispersion was stirred with a magnetic stirrer. Finally, the black precipitate (Fe3O4NPs) was dried at 25 °C.
Magnetic mesoporous silica nanoparticles (MMSNs) were prepared by a surfactant-templated seed-mediated growth method.32 After the addition of Fe3O4 NPs in chloroform (1.0 mL, 10.0 mg mL−1) to CTAB (0.10 mol L−1) solution, the ultrasonication treatment was performed to remove chloroform for 45 min. The prepared solution was added to the mixture including 25.0 mL distilled water, ethylene glycol (15.0 mL) and NH4OH (1.0 mL). After that, incubation was carried out at 60 °C for 20 min. Decane (1.0 mL) and TMB (1.0 mL) were added to the incubated solution and stirred for 90 min for homogenization. Lastly, TEOS (500.0 μL) was slowly added and the resulting reaction was followed for 120 min at 100 °C. After centrifugation at 5000 rpm for 40 min, the prepared MMSNs were transferred into NH4NO3 solution (50.0 mg in 50.0 mL ethanol) and stirred for 1 h at 70 °C. This treatment was repeated four times and the product was filtered and dried at 50 °C.
Secondly, amino modified MMSNs (MMSNs–NH2) were prepared.33 After the preparation of MMSN dispersion (60.0 mg in 30.0 mL ethanol), APTES (200.0 μL) was added to the dispersion and stirred at 80 °C for 18 h. Then, MMSN–NH2 was collected with a magnet and washed four times with ethanol. After the prepared MMSN–NH2 (60.0 mg) was dispersed in distilled water (40.0 mL) by ultrasonication for 40 min; HAuCl4 solution (40.0 mmol L−1) was added to the above dispersion under stirring. Then, NaBH4 (20.0 mL, 0.1 mol L−1) was added and stirred at 100 rpm for 60 min. MMSNs@AuNPs were washed with distilled water four times. After the preparation of anti-CYFRA21-1-Ab2 (30.0 μL, 50.0 μg mL−1), secondary anti-CYFRA21-1-Ab2 was conjugated to MMSNs@AuNPs via amino-gold affinity between anti-CYFRA21-1-Ab2 and MMSNs@AuNP dispersions at 37.0 °C for 30 min.34 After centrifugation at 5000 rpm, MMSNs@AuNPs conjugated to anti-CYFRA21-1-Ab2 (MMSNs@AuNPs/anti-CYFRA21-1-Ab2) was stored in pH 7.0, 0.1 M PBS.
2.6. Electrochemical measurements
Antibody–antigen interactions between MMSNs@AuNPs/anti-CYFRA21-1-Ab2 and CYFRA21-1/anti-CYFRA21-1-Ab1/Si3N4/MoS2-MWCNTs/GCE provided an electrochemical CYFRA21-1 immunosensor. The above prepared MMSNs@AuNPs/anti-CYFRA21-1-Ab2 solution (30.0 μL, 15.0 mg mL−1) was incubated on the CYFRA21-1/anti-CYFRA21-1-Ab1/Si3N4/MoS2–MWCNT/GCE for the immune reaction of 30 min. Then, the prepared electrochemical immunosensor for CYFRA21-1 antigen's recognition was stored in pH 7.0, 0.1 M PBS (3.0 mL). In order to perform voltammetric measurements, 0.1 M PBS including 1.0 mM H2O2 was prepared owing to H2O2's usage in medical diagnosis.35 Before the voltammetric measurements, argon gas (99.999%) was passed to remove dissolved oxygen for 15 min. The voltammograms were recorded without pressure fluctuations at 25 °C in an enclosed cabinet to prevent from external environmental influences. The preparation procedures are shown in Scheme 1, including Si3N4/MoS2–MWCNTs, MMSNs@AuNPs, the immobilization of the capture antibody, the secondary antibody, and the antigen and the final electrochemical immunosensor development. According to Scheme 1, we can explain the most important purposes of the prepared sensor platform (Si3N4/MoS2–MWCNTs) as follows: (i) providing anti-CYFRA21-1-Ab1 immobilization via electrostatic/ionic interactions between the –NH2 groups of anti-CYFRA21-1-Ab1 and the polar groups of Si3N4/MoS2, (ii) providing the increase of surface conductivity via MWCNTs. After the conjugation of anti-CYFRA21-1-Ab2 to the signal amplifier via amino-gold affinity, voltammetric measurements were carried out in 0.1 M PBS including 1.0 mM H2O2 in the potential range from +0.1 V to +0.5 V. The related electrochemical reaction mechanism for H2O2 in the potential range is also provided in Scheme 1 as H2O2↔O2+2H++2e−.
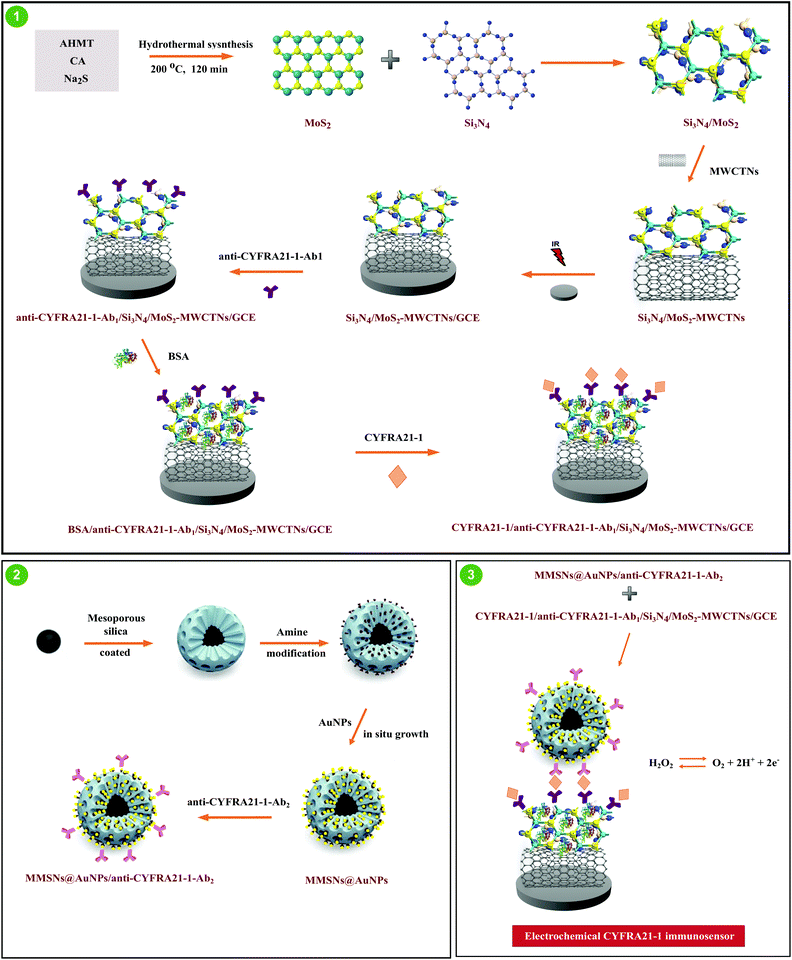 |
| Scheme 1 Preparation procedure of the voltammetric CYFRA21-1 immunosensor. | |
2.7. Sample preparation
CYFRA21-1 free plasma samples were supplied from the Blood Bank in Turkey. The sample preparation protocol is explained in detail in the ESI.†36
3. Results and discussion
3.1. Characterization of Si3N4, MoS2, Si3N4/MoS2 and the Si3N4/MoS2–MWCNT composite as a sensor platform
Firstly, FTIR measurements were carried out for spectroscopic characterization (Fig. 1A). The obvious two absorption bands attributed to 920 cm−1 and 1040 cm−1 indicated Si–N group's stretching vibration.37 For MoS2, the absorption band at 485 cm−1 corresponded to Mo–S's stretching vibration.38 Lastly, specific bands such as Si–N and Mo–O confirmed the successful synthesis of the Si3N4/MoS2 composite. Then, XRD measurements were performed in the range from 2θ = 10° to 2θ = 80° (Fig. 1B). According to the XRD pattern of Si3N4, the diffraction peaks at 2θ = 12.90°, 20.49°, 22.98°, 27.03°, 35.51°, 38.95°, 42.95° and 58.13° were attributed to the (100), (101), (110), (021), (120), (121), (031) and (222) planes, respectively, indicating a hexagonal crystal structure. For MoS2, the diffraction peaks at 2θ = 13.85°, 33.21°, 40.08° and 59.03° were assigned to the (002), (100), (103) and (110) planes, respectively, indicating a hexagonal crystal structure.39,40 When we investigate the XRD pattern of the Si3N4/MoS2 composite, the obvious peaks corresponding to Si3N4 and MoS2 were observed for the composite structure. Nonetheless, the peak intensities of the Si3N4/MoS2 composite were lower than that of Si3N4, suggesting the structural changes and the decrease in particle size resulting from the milling process. After that, Raman spectroscopy (Fig. 1C), another spectroscopic technique, was used with a 785 nm wavelength laser. In the Raman spectrum of Si3N4, the peaks at 255 cm−1, 520 cm−1 and 880 cm−1 were assigned to silicon nitride's A, Eg, A1g vibrational modes. For MoS2, the peaks at 370 cm−1 and 410 cm−1 suggested MoS2's E12g and A1g active vibration bands.30,41 In the Raman spectrum of the Si3N4/MoS2 composite, specific absorption bands belonging to Si3N4 and MoS2 were obtained, and the peak intensities at 255 cm−1 and 410 cm−1 were lower in comparison with Si3N4 and MoS2. This situation is owing to some surface defects and Si3N4/MoS2 composite formation.
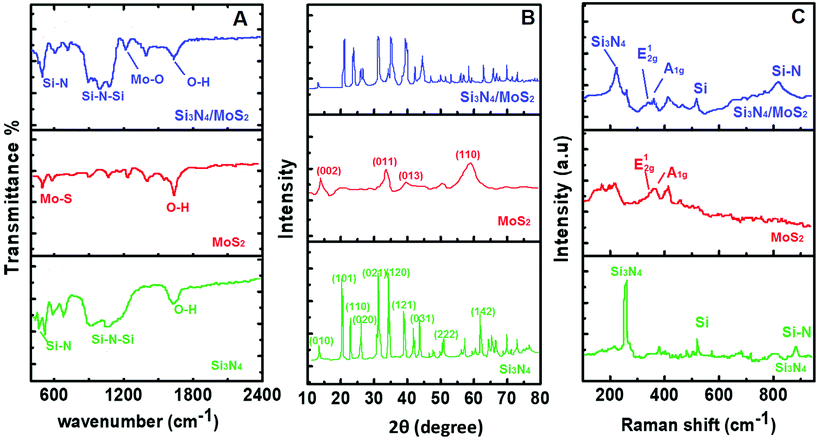 |
| Fig. 1 (A) FTIR spectra, (B) XRD patterns, and (C) Raman spectra of Si3N4, MoS2 and the Si3N4/MoS2 composite. | |
The morphological properties of Si3N4, MoS2 and the Si3N4/MoS2 composite were investigated by SEM (Fig. S1†). The average particle sizes of Si3N4, MoS2 and the Si3N4/MoS2 composite with a polyhedral shape were obtained as about 172 nm, 709 nm and 20 nm, respectively. In addition, the SEM image (Fig. S2A†) of Si3N4/MoS2–MWCNTs showed that Si3N4/MoS2 particles were homogeneously distributed on MWCNTs. Finally, the EDX spectra (Fig. S2B†) of Si3N4/MoS2–MWCNTs were recorded, suggesting the uniform distribution of Si, N, Mo, and S on MWCNTs. Then, the TEM images of the Si3N4/MoS2 composite were obtained (Fig. 2). According to Fig. 2A, the incorporation of Si3N4 particles into MoS2 was successfully realized and the heterojunction interaction occurred between Si3N4 and MoS2 (Fig. 2B). Si3N4 particles incorporating MoS2 were identified from their laminar morphology (Fig. 2C). According to Fig. 2C, lattice spaces such as 0.70, 0.43, and 0.36 nm were attributed to the (010), (011), and (020) planes belonging to Si3N4 and 0.63 nm corresponded to the (002) plane belonging to MoS2.42
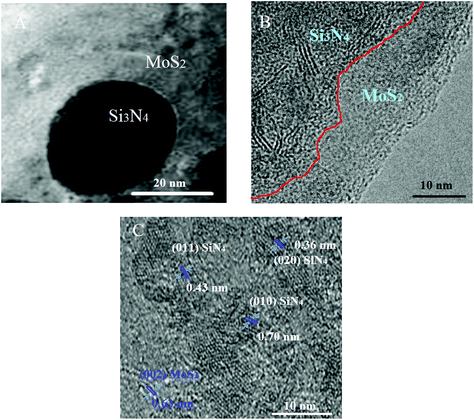 |
| Fig. 2 TEM images (A–C) of the Si3N4/MoS2 composite. | |
XPS characterization (Fig. S3†) was performed to show the chemical functionality of the Si3N4/MoS2 composite. According to the Mo 3d high-resolution spectra, the peaks at 231.7 eV (Mo 3d3/2) and 228.3 eV (Mo 3d5/2) were attributed to Mo4+'s orbital features. In addition, the peak at 226.3 eV was related to the S 2s interactions in MoS2.43 S 2p spectra demonstrated four peaks at 160.36, 162.01, 163.04 and 163.98 eV. The peaks at 162.01 and 163.04 were in harmony with S 2p3/2 and S 2p1/2, confirming S2− of MoS2.44 The peak at 163.98 eV was related to the edge sulfur of MoS2 and a novel peak at 160.36 eV indicated the silicon–sulfur (Si–S) interactions. In the Si 2p spectrum, the peak at 101.6 eV was attributed to the Si4+ presence in Si3N4.44 Finally, when we want to investigate the N 1s spectrum, the peaks at 398.12 and 395.22 eV corresponded to nitrogen binding to silicon (N–Si) on Si3N4 and the Mo–N interactions, respectively.45 Thus, the XPS results verified the presence of the Si3N4/MoS2 composite in harmony with Fig. 2. BET measurements of Si3N4, Si3N4/MoS2 and Si3N4/MoS2–MWCNTs were performed to show the determination of surface areas (Fig. S4†). Si3N4, Si3N4/MoS2 and the Si3N4/MoS2–MWCNT composite showed a type IV adsorption–desorption isotherm. The surface areas of Si3N4, Si3N4/MoS2 and Si3N4/MoS2–MWCNTs were calculated as 10.148 m2 g−1, 16.742 m2 g−1 and 34.894 m2 g−1, respectively. Hence, it is concluded that Si3N4/MoS2–MWCNTs with the highest surface area can be used as a sensor platform for effective and greater immobilization of capture antibodies.
3.2. Characterization of Fe3O4 NPs, MMSNs and MMSNs@AuNPs
Firstly, TEM characterization was performed for Fe3O4 NPs, MMSNs and MMSNs@AuNPs (Fig. 3). Fig. 3A indicates the presence of spherical Fe3O4 NPs with the average particle sizes of 25–35 nm. According to Fig. 3B and C, a clear crinkle structure with average particle sizes of 45–50 nm was observed for MMSNs. After AuNP incorporation into MMSNs, the spherical shape of MMSNs appeared to be preserved (Fig. 3D). Fig. 3E confirms the successful incorporation of AuNPs such as the shell formation on MMSNs. Thus, SEM and TEM images confirmed the successful confination of the dispersed AuNPs with a high density by in situ HAuCl4 growth on MMSNs. In addition, not only the porous and magnetic mesoporous silicon structure increased the diffusion rate of AuNPs, but also active sites helped in easy immobilization of secondary antibodies. Finally, the EDX image (Fig. 3F) showed the presence of elements such as Fe, O, Si and Au.
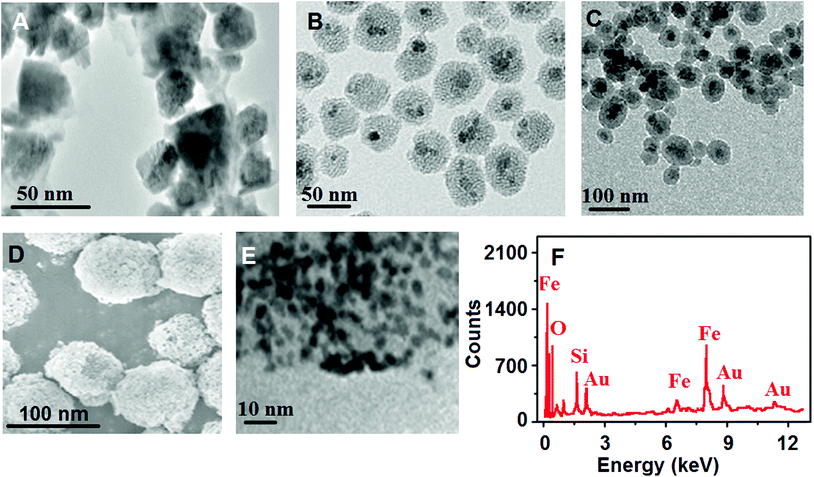 |
| Fig. 3 TEM images of (A) Fe3O4 NPs and (B and C) MMSNs, (D) SEM image of MMSNs@AuNPs, (E) HRTEM image of MMSNs@AuNPs, and (F) EDX spectra of MMSNs@AuNPs. | |
Secondly, FTIR characterization was performed for confirmation of the MMSNs and MMSN@AuNP formation (Fig. S5A†). The specific absorption bands in the range from 582 cm−1 to 556 cm−1 indicated Fe3O4 NPs’ coating with mesoporous silicon in the FTIR spectrum of MMSNs. In addition, the absorption bands at 1076 cm−1, 457 cm−1 and 928 cm−1 corresponded to the stretching Si–O–Si, bending Si–O–Si and bending Si–OH, respectively. The peak at 1557 cm−1 in the FTIR spectrum of MMSN–NH2 corresponded to N–H, indicating the successful modification of MMSNs with amino groups. Thus, we can say that the core–shell type magnetic mesoporous silica nanoparticles@gold nanoparticles were successfully prepared. According to the XRD patterns (Fig. S5B†), the diffraction peaks at 2θ = 29.82°, 36.84°, 42.86°, 57.33° and 62.87° for Fe3O4 NPs were assigned to the (220), (222), (400), (511) and (440) planes, respectively. In addition, for MMSNs@AuNPs, the peaks at 2θ = 38.34°, 44.93°, 64.11°, 78.18° and 82.39° were related to the (111), (200), (220), (311) and (222) planes of AuNPs, showing AuNPs’ presence on MMSNs. In addition, the XRD pattern of MMSNs@AuNPs demonstrated that the peak intensities in the XRD pattern of Fe3O4 NPs decreased with the formation of MMSNs. Thus, these results showed that MMSNs@AuNPs were successfully prepared. Finally, XPS measurements were performed for confirmation of MMSNs@AuNPs (Fig. S5C†). The Fe 2p region was highlighted by Fe 2p1/2 and Fe 2p3/2, appearing at 721.8 and 719.2 eV, respectively. Hence, the Fe3O4 NP presence was verified in the Fe 2p region. For the Au 4f narrow region, the peaks at 82.4 and 87.1 eV confirmed AuNPs’ formation on MMSNs. Lastly, a large peak at 103.1 eV for Si 2p showed silicon's presence on MMSNs@AuNPs.
3.3. Electrochemical characterization of the proposed voltammetric immunosensor
Electrochemical characterization of the modified sensor platform (CYFRA21-1/anti-CYFRA21-1-Ab1/Si3N4/MoS2–MWCNTs/GCE) was progressively investigated in 1.0 mM [Fe(CN)6]3− containing 0.1 M KCl by CV and EIS. A bare GCE demonstrated small anodic and cathodic peak signals in 1.0 mM [Fe(CN)6]3− (curve a of Fig. S6A†). The more anodic and cathodic peak signals were observed in the MWCNTs/GCE due to the enhanced electrical conductivity on the electrode surface46 (curve b of Fig. S6A†). Because of the hetero-structured Si3N4/MoS2 providing more active centers and easy electron transfer on MWCNTs (curve c of Fig. S6A†), an increased electrochemical activity was obtained by Si3N4/MoS2–MWCNTs/GCE.47 After anti-CYFRA21-1-Ab1 immobilization on the Si3N4/MoS2–MWCNTs/GCE by electrostatic/ionic interactions, the anodic and cathodic peak intensities decreased in comparison with curve c, suggesting the successful anti-CYFRA21-1-Ab1 immobilization (curve d of Fig. S6A†). After the sequential immobilization treatments of BSA and CYFRA21-1 antigens, the anodic and cathodic peak signals further decreased (curve e and f of Fig. S6A†). Hence, the obstructive effect on electron transfer started to become more noticeable. Hence, the successful attachments of primary, secondary and antigen proteins to the electrode surface were observed from the CV results.
Then, EIS measurements were performed in 1.0 mM [Fe(CN)6]3− containing 0.1 M KCl to confirm the CV results (Fig. S6B†). The EIS graph of the bare GCE was shown in curve a of Fig. S6B.† When the resistance values were investigated on MWCNT/GCE (curve b of Fig. S6B†) and Si3N4/MoS2–MWCNT/GCE (curve c of Fig. S6B†), the values gradually reduced, suggesting that the electron transfer occurred more easily in harmony with the CV results. After anti-CYFRA21-1-Ab1 immobilization on Si3N4/MoS2–MWCNTs/GCE, the resistance values on the electrode surface appeared to be higher (curve d of Fig. S6B†). According to curves e and f of Fig. S6B,† the blocking effect on electron transfer was in harmony with Fig. S6A.† Hence, the successful immunosensor construction was presented for CYFRA21-1 recognition.
In addition, several immunosensors including AuNPs/anti-CYFRA21-1-Ab2 and MMSNs@AuNPs/anti-CYFRA21-1-Ab2 were developed to observe the effect of signal amplification (Fig. S6C†). First of all, the sensor platforms (Si3N4/MoS2–MWCNTs/GCE) including anti-CYFRA21-1-Ab1 and antigen CYFRA21-1 were subjected to an immune reaction for 30 min with MMSNs@AuNPs/anti-CYFRA21-1-Ab2, AuNPs/anti-CYFRA21-1-Ab2 and anti-CYFRA21-1-Ab2, respectively. Then, all immunosensors were tested in 1.0 mM H2O2 in pH 7.0, 0.1 M PBS and in the absence of H2O2. H2O2 as a redox probe was selected due to its use in biomolecules’ medical diagnosis.35 H2O2 oxidation into O2 at about +0.30 V was followed using the above voltammetric immunosensors. The signal amplifier containing only anti-CYFRA21-1-Ab2 showed a small signal (about 2.0 μA) for 1.0 mM H2O2 (curve b of Fig. S6C†). When we used AuNPs/anti-CYFRA21-1-Ab2 (curve c of Fig. S6C†) and MMSNs@AuNPs/anti-CYFRA21-1-Ab2 (curve d of Fig. S6C†) for immunosensor development, peak signals corresponding to 8.0 μA and 13.0 μA were obtained, respectively. Due to the high specific surface area and good electrical conductivity of AuNPs,48,49 an enhanced electrochemical activity was observed in comparison with curve b of Fig. S6C.† Furthermore, owing to the synergistic effect providing a larger surface area between AuNPs and MMSNs,50,51 the anti-CYFRA21-1-Ab2 conjugation process was realized more efficiently on MMSNs@AuNPs. Thus, we selected MMSNs@AuNPs/anti-CYFRA21-1-Ab2 signal amplification for subsequent immunosensor application.
3.4. Optimization for voltammetric measurements
The effects of pH, immune reaction time, H2O2 and MMSNs@AuNPs/anti-CYFRA21-1-Ab2 solution concentrations were evaluated in detail in Fig. S7.†
3.5. Linearity range
Fig. 4 demonstrates differential pulse voltammograms with concentration increase and the obtained linearity was found to be y (μA) = 87.824x (pg mL−1) + 2.3747 (inset of Fig. 4). The quantification limit (LOQ) and LOD were calculated as 0.01 pg mL−1 and 2.00 fg mL−1, respectively using eqn (1) and (2) below:where S is the standard deviation of intercept and m is the slope of the calibration equation. Table 1 shows the comparative work between the voltammetric CYFRA21-1 immunosensor and the other analytical methods. The prepared immunosensor suggested a low LOD, confirming that this biosensor quantifies the CYFRA21-1 concentration with high selectivity. This performance resulted from the high surface area, good electrical conductivity and synergistic effect between AuNPs and MMSNs, providing many binding sites for the antigen and antibody. In addition, the preparation procedures of Si3N4/MoS2–MWCNTs and MMSNs@AuNPs were low-cost and the least waste generation occurred, presenting an environmentally friendly immunosensor.
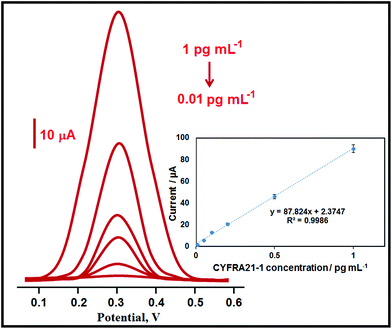 |
| Fig. 4 Concentration effect (from 0.01 to 1.0 pg ml−1 CYFRA21-1) on immunosensor signals, inset: the calibration curve for the voltammetric CYFRA21-1 immunosensor. | |
Table 1 Comparison of the voltammetric CYFRA21-1 immunosensor with other techniques
Material/method |
Linearity ranges |
LOD |
Ref. |
Graphene@gold nanoparticles |
0.25–800.0 ng mL−1 |
100.0 pg mL−1 |
29
|
APTES/ZrO2–RGO/ITO |
2.00–22.0 ng mL−1 |
0.12 ng mL−1 |
52
|
QPs-LFTS |
1.30–480.0 ng mL−1 |
0.16 ng mL−1 |
53
|
CdSe/ZnS quantum dots |
0.50–128.0 ng mL−1 |
0.20 ng mL−1 |
54
|
Poly(thionine)–Au composite |
0.50 ng mL−1–10.0 fg mL−1 |
4.60 fg mL−1 |
55
|
Polydopamine/tungsten oxide nanocomposite |
0.50 pg mL−1–10.0 ng mL−1 |
2.50 fg mL−1 |
56
|
AuNPs/amperometry |
50.0 fg mL−1–100.0 ng mL−1 |
38.0 fg mL−1 |
57
|
MMSNs@AuNPs
|
0.01–1.0 pg mL
−1
|
2.0 fg mL
−1
|
This study
|
3.6. Recovery
Firstly, recovery experiments were performed to show the voltammetric CYFRA21-1 immunosensor's high selectivity. The recovery was calculated using eqn (3) below: | Recovery = found CYFRA21-1, pg mL−1/real CYFRA21-1, pg mL−1 | (3) |
The close values to 100.00% (Table S1†) confirmed the voltammetric CYFRA21-1 immunosensor's high selectivity. Furthermore, the standard addition method was applied to prepare plasma samples (sample preparation in the ESI†) to verify the immunosensor's high selectivity. The obtained calibration equation by the standard addition method was y (μA) = 87.843x (pg mL−1) + 10.1791. The close slope values between direct calibration and standard addition methods showed that this biosensor can quantify the CYFRA21-1 concentration with high selectivity without the interference effect in plasma medium.
3.7. Selectivity, stability, reproducibility and reusability
For selectivity test, 6 different protein solution mixtures were prepared: (i) 0.100 pg mL−1 CYFRA21-1, (ii) 0.100 pg mL−1 CYFRA21-1 + 10.00 pg mL−1 CEA, (iii) 0.100 pg mL−1 CYFRA21-1 + 10.00 pg mL−1 AA, (iv) 0.100 pg mL−1 CYFRA21-1 + 10.00 pg mL−1 DA, (v) 0.100 pg mL−1 CYFRA21-1 + 10.00 pg mL−1 UA, (vi) 0.100 pg mL−1 CYFRA21-1 + 10.00 pg mL−1 BSA. After that, 6 different voltammetric CYFRA21-1 immunosensors were separately developed using these 6 antigen protein solutions according to section 2.5 and section 2.6. The prepared 6 immunosensors were applied to 1.0 mM H2O2 solution including in pH 7.0, 0.1 M PBS. According to Fig. S8A,† 0.34% of relative standard deviation (RSD) showed a high selectivity of the voltammetric CYFRA21-1 immunosensor.
The stability of one voltammetric CYFRA21-1 immunosensor was investigated in a 4 °C storage environment for seven weeks. The observed signals during seven weeks were about 98.61% of the first current signal (Fig. S8B†). Thus, a voltammetric CYFRA21-1 immunosensor with high stability was presented for clinical applications.
For the reproducibility test, 10 voltammetric CYFRA21-1 immunosensors having 0.100 pg mL−1 CYFRA21-1 were independently prepared according to sections 2.5, 2.4 and 2.6 and used towards 1.0 mM H2O2 solution. 0.27% of RSD confirmed the high reliability of the immunosensor production procedure.
Finally, the reusability of the voltammetric CYFRA21-1 immunosensor was investigated. The current signals were constantly recorded during 25 times usage of only one prepared CYFRA21-1 immunosensor and the RSD value of the recorded current signals was obtained as 0.15%. Hence, a voltammetric CYFRA21-1 immunosensor with a high degree of reusability was prepared in the present study.
3.8. Precision and accuracy
Three concentrations (0.100, 0.200 and 0.500 pg mL−1 CYFRA21-1) in the linearity range were determined on the same day (intra-day precision) and six consecutive days (inter-day precision) (Table S2†). The calculated RSD values were 0.049–0.366 for intra-day and 0.098–0.485 for inter-day precision. Therefore, a low RSD demonstrated high precision of the voltammetric CYFRA21-1 immunosensor. Accuracy was investigated as % relative error between the found and added concentrations for CYFRA21-1 (Bias %). Hence, low Bias % (Table S2†) confirmed that the accuracy of the voltammetric CYFRA21-1 immunosensor was high.
4. Conclusions
In this study, a selective, sensitive, stable and reproducible new-type voltammetric immunosensor based on a silicon nitride (Si3N4)–molybdenum disulfide (MoS2) composite on multi-walled carbon nanotubes (Si3N4/MoS2–MWCNTs) as an electrochemical sensor platform and core–shell type magnetic mesoporous silica nanoparticles@gold nanoparticles (MMSNs@AuNPs) as a signal amplifier were prepared and applied to cytokeratin 19 fragment antigen 21-1 detection in plasma samples. The prepared CYFRA21-1 biosensor had a more satisfactory sensitivity and showed a lower detection limit of 2.0 fg mL−1 in comparison with other detection methods. Thus, the development of an environmentally friendly immunosensor offers an alternative biosensor for clinical diagnosis.
Conflicts of interest
There are no conflicts of interest to declare.
Acknowledgements
Mehmet Lütfi YOLA would like to thank the Turkish Academy of Sciences (TUBA- GEBIP) for their invaluable support.
References
- M. Chen, C. Hou, D. Huo, M. Yang and H. Fa, Anal. Methods, 2015, 7, 9466–9473 RSC.
- L. Wang, N. Liu and Z. Ma, J. Mater. Chem. B, 2015, 3, 2867–2872 RSC.
- F. Yang, J. Han, Y. Zhuo, Z. Yang, Y. Chai and R. Yuan, Biosens. Bioelectron., 2014, 55, 360–365 CrossRef CAS.
- S. Holdenrieder, B. Wehnl, K. Hettwer, K. Simon, S. Uhlig and F. Dayyani, Br. J. Cancer, 2017, 116, 1037–1045 CrossRef CAS.
- Z. W. Baloch, S. Abraham, S. Roberts and V. A. LiVolsi, Hum. Pathol., 1999, 30, 1166–1171 CrossRef CAS.
- H.-H. Wu, P.-H. Wang, J.-Y. Yeh, Y.-J. Chen, M.-S. Yen, R.-L. Huang, Y.-J. Tsai and C.-C. Yuan, Taiwan. J. Obstet. Gynecol., 2014, 53, 30–34 CrossRef.
- S. Boeck, C. Wittwer, V. Heinemann, M. Haas, C. Kern, P. Stieber, D. Nagel and S. Holdenrieder, Br. J. Cancer, 2013, 108, 1684–1694 CrossRef CAS.
- Y. Xu, L. Xu, M. Qiu, J. Wang, Q. Zhou, L. Xu, J. Wang and R. Yin, Sci. Rep., 2015, 5, 1–10 Search PubMed.
- N. A. Alarfaj, M. F. El-Tohamy and H. F. Oraby, Nanoscale Res. Lett., 2020, 15, 12 CrossRef CAS.
- N. F. Chiu and H. T. Yang, Front. Bioeng. Biotechnol., 2020, 8, 234 CrossRef.
- B. Nakata, T. Takashima, Y. Ogawa, T. Ishikawa and K. Hirakawa, Br. J. Cancer, 2004, 91, 873–878 CrossRef CAS.
- H. Yang, J. Bao, D. Huo, Y. Zeng, X. Wang, M. Samalo, J. Zhao, S. Zhang, C. Shen and C. Hou, Talanta, 2021, 224, 121816 CrossRef CAS.
- A. Khanmohammadi, A. Aghaie, E. Vahedi, A. Qazvini, M. Ghanei, A. Afkhami, A. Hajian and H. Bagheri, Talanta, 2020, 206, 120251 CrossRef CAS.
- H.-H. Jeong, N. Erdene, J.-H. Park, D.-H. Jeong, H.-Y. Lee and S.-K. Lee, Biosens. Bioelectron., 2013, 39, 346–351 CrossRef CAS.
- X. Luo and J. J. Davis, Chem. Soc. Rev., 2013, 42, 5944–5962 RSC.
- H. Karimi-Maleh, F. Karimi, S. Malekmohammadi, N. Zakariae, R. Esmaeili, S. Rostamnia, M. L. Yola, N. Atar, S. Movaghgharnezhad and S. Rajendran, J. Mol. Liq., 2020, 310, 113185 CrossRef CAS.
- H. Karimi-Maleh and O. A. Arotiba, J. Colloid Interface Sci., 2020, 560, 208–212 CrossRef CAS.
- H. Karimi-Maleh, F. Karimi, M. Alizadeh and A. L. Sanati, Chem. Rec., 2020, 20, 682–692 CrossRef CAS.
- H. Karimi-Maleh, M. Sheikhshoaie, I. Sheikhshoaie, M. Ranjbar, J. Alizadeh, N. W. Maxakato and A. Abbaspourrad, New J. Chem., 2019, 43, 2362–2367 RSC.
- H. Medetalibeyoglu, M. Beytur, O. Akyıldırım, N. Atar and M. L. Yola, Sens. Actuators, B, 2020, 319, 128195 CrossRef CAS.
- X. Pei, B. Zhang, J. Tang, B. Liu, W. Lai and D. Tang, Anal. Chim. Acta, 2013, 758, 1–18 CrossRef CAS.
- M. L. Yola and N. Atar, Nanoscale, 2020, 12, 19824–19832 RSC.
- S. Lv, K. Zhang, L. Zhu and D. Tang, Anal. Chem., 2019, 92, 1470–1476 CrossRef.
- L. Huang, J. Chen, Z. Yu and D. Tang, Anal. Chem., 2020, 92, 2809–2814 CrossRef CAS.
- G. Cai, Z. Yu, P. Tong and D. Tang, Nanoscale, 2019, 11, 15659–15667 RSC.
- Z. Luo, Q. Qi, L. Zhang, R. Zeng, L. Su and D. Tang, Anal. Chem., 2019, 91, 4149–4156 CrossRef CAS.
- Z. Yu, Y. Tang, G. Cai, R. Ren and D. Tang, Anal. Chem., 2018, 91, 1222–1226 CrossRef.
- T. Wu, J. Feng, S. Zhang, L. Liu, X. Ren, D. Fan, X. Kuang, X. Sun, Q. Wei and H. Ju, Biosens. Bioelectron., 2020, 169, 112580 CrossRef CAS.
- Y. Zeng, J. Bao, Y. Zhao, D. Huo, M. Chen, M. Yang, H. Fa and C. Hou, Talanta, 2018, 178, 122–128 CrossRef CAS.
- G. Nagaraju, C. N. Tharamani, G. T. Chandrappa and J. Livage, Nanoscale Res. Lett., 2007, 2, 461 CrossRef CAS.
- M. L. Yola and N. Atar, Mater. Sci. Eng., C, 2019, 96, 669–676 CrossRef CAS.
- S. Angelos, N. M. Khashab, Y.-W. Yang, A. Trabolsi, H. A. Khatib, J. F. Stoddart and J. I. Zink, J. Am. Chem. Soc., 2009, 131, 12912–12914 CrossRef CAS.
- W. Luo, C. Zhu, S. Su, D. Li, Y. He, Q. Huang and C. Fan, ACS Nano, 2010, 4, 7451–7458 CrossRef CAS.
- B. Đ. Glišić, U. Rychlewska and M. I. Djuran, Dalton Trans., 2012, 41, 6887–6901 RSC.
- V. Stanković, S. Đurđić, M. Ognjanović, J. Mutić, K. Kalcher and D. M. Stanković, J. Electroanal. Chem., 2020, 876, 114487 CrossRef.
- M. L. Yola and N. Atar, Biosens. Bioelectron., 2019, 126, 418–424 CrossRef CAS.
- K. Sardar, R. Bounds, M. Carravetta, G. Cutts, J. S. J. Hargreaves, A. L. Hector, J. A. Hriljac, W. Levason and F. Wilson, Dalton Trans., 2016, 45, 5765–5774 RSC.
- A. S. Krishna Kumar, S.-J. Jiang and J. K. Warchoł, ACS Omega, 2017, 2, 6187–6200 CrossRef CAS.
- D. Duphil, S. Bastide and C. Lévy-Clément, J. Mater. Chem., 2002, 12, 2430–2432 RSC.
- H. Yu, Y. Liu and S. L. Brock, Inorg. Chem., 2008, 47, 1428–1434 CrossRef CAS.
- H. Zhao, Y. Dong, P. Jiang, H. Miao, G. Wang and J. Zhang, J. Mater. Chem. A, 2015, 3, 7375–7381 RSC.
- K. D. Rasamani, F. Alimohammadi and Y. Sun, Mater. Today, 2017, 20, 83–91 CrossRef CAS.
- D. Ganta, S. Sinha and R. T. Haasch, Surf. Sci. Spectra, 2014, 21, 19–27 CrossRef CAS.
- A. Rauf, M. S. A. Sher Shah, G. H. Choi, U. B. Humayoun, D. H. Yoon, J. W. Bae, J. Park, W.-J. Kim and P. J. Yoo, ACS Sustainable Chem. Eng., 2015, 3, 2847–2855 CrossRef CAS.
- X. D. Huang, X. F. Gan, F. Zhang, Q. A. Huang and J. Z. Yang, Electrochim. Acta, 2018, 268, 241–247 CrossRef CAS.
- M. L. Yola and N. Atar, Electrochim. Acta, 2014, 119, 24–31 CrossRef CAS.
- G. Angamuthu, D. Bosubabu, K. Ramesha and V. Rengarajan, Appl. Mater. Today, 2021, 22, 100916 CrossRef.
- Y. Zhao, D. Huo, J. Bao, M. Yang, M. Chen, J. Hou, H. Fa and C. Hou, Sens. Actuators, B, 2017, 244, 1037–1044 CrossRef CAS.
- Y. Ge, J. Wu, H. Ju and S. Wu, Talanta, 2014, 120, 218–223 CrossRef CAS.
- S. Wang, M. Zhang and W. Zhang, ACS Catal., 2011, 1, 207–211 CrossRef CAS.
- Y. Lin, Z. Li, Z. Chen, J. Ren and X. Qu, Biomaterials, 2013, 34, 2600–2610 CrossRef CAS.
- S. Kumar, J. G. Sharma, S. Maji and B. D. Malhotra, Biosens. Bioelectron., 2016, 78, 497–504 CrossRef CAS.
- Z. Chen, R. Liang, X. Guo, J. Liang, Q. Deng, M. Li, T. An, T. Liu and Y. Wu, Biosens. Bioelectron., 2017, 91, 60–65 CrossRef CAS.
- L. Ding, X. Chen, L. He, F. Yu, S. Yu, J. Wang, Y. Tian, Y. Wang, Y. Wu, L.-E. Liu and L. Qu, Microchim. Acta, 2020, 187, 171 CrossRef CAS.
- H. Wang, X. Gao and Z. Ma, Sci. Rep., 2017, 7, 1023 CrossRef.
- R. Atchudan, N. Muthuchamy, T. Edison, S. Perumal, R. Vinodh, K. H. Park and Y. R. Lee, Biosens. Bioelectron., 2019, 126, 160–169 CrossRef CAS.
- H. Wang and Z. Ma, Microchim. Acta, 2017, 184, 1045–1050 CrossRef CAS.
Footnote |
† Electronic supplementary information (ESI) available. See DOI: 10.1039/d1nr00244a |
|
This journal is © The Royal Society of Chemistry 2021 |
Click here to see how this site uses Cookies. View our privacy policy here.