DOI:
10.1039/D0MH01317J
(Review Article)
Mater. Horiz., 2021,
8, 145-167
Polyphenol scaffolds in tissue engineering
Received
14th August 2020
, Accepted 17th September 2020
First published on 17th September 2020
Abstract
Polyphenols are a class of ubiquitous compounds distributed in nature, with fascinating inherent biocompatible, bioadhesive, antioxidant, and antibacterial properties. The unique polyphenolic structures based on catechol or pyrogallol moieties allow for strong non-covalent interactions (e.g., multiple hydrogen bonding, electrostatic, and cation–π interactions) as well as covalent interactions (e.g., Michael addition/Schiff-base reaction, radical coupling reaction, and dynamic coordination interactions with boronate or metal ions). This review article provides an overview of the polyphenol-based scaffolds including the hydrogels, films, and nanofibers that have emerged from chemical and functional signatures during the past years. A full description of the structure–function relationships in terms of their utilization in wound healing, bone regeneration, and electroactive tissue engineering is also carefully discussed, which may pave the path towards the rational design and facile preparation of next-generation polyphenol scaffolds for tissue engineering applications.
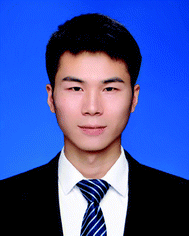
Xueqian Zhang
| Xueqian Zhang received his master's degree from the State Key Laboratory of Polymer Materials Engineering of Sichuan University in 2019. He currently works at the College of Polymer Science and Engineering of Sichuan University as a research assistant. His current research interests focus on the fabrication of ultra-small functional polyphenol nanoparticles for biomedical applications. |
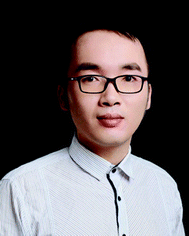
Zhipeng Gu
| Zhipeng Gu received his PhD in Materials Science in 2014 from Sichuan University. He was a postdoctoral researcher at the Department of Neurosurgery, West China Hospital, Sichuan University. From 2016 to 2019, he worked at the School of Biomedical Engineering at Sun Yat-sen University. He currently works at the College of Polymer Science and Engineering of Sichuan University. His main research aim is to develop various functional polymers for biomedical applications. |
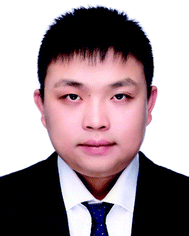
Yiwen Li
| Yiwen Li received his BS in Chemistry from the University of Science and Technology of China (2008) and PhD in Polymer Science from the University of Akron (2013). After two years’ postdoctoral training at University of California, San Diego, Yiwen began his independent career at Sichuan University in 2016, where he is currently a professor at the College of Polymer Science and Engineering, and the State Key Laboratory of Polymer Materials Engineering. His current research interests focus on bioinspired polymers including synthetic melanin and polyphenolic materials. He has published 100+ peer-reviewed articles and served as the associate editor for Materials Express (ASP) and as an editorial board member for Giant (Elsevier) and Molecules (MDPI). |
1. Introduction
Millions of patients suffer from tissue loss or organ failure due to accidents or diseases every year. Tissue or organ transplantation is a generally accepted therapy to treat these patients.1 However, this approach is severely limited by a critical donor shortage.1,2 In 1993, Langer et al. reported an alternative strategy for the repair and regeneration of lost tissues of patients, which was regarded as the original concept of tissue engineering.3 Inspired by this, different kinds of approaches have been proposed and employed to develop functional biological substitutes according to the combined principles of engineering and biology. So far, a variety of tissues have been rationally engineered for further clinical treatments including fabricated artery, bladder, skin, blood vessels, cartilage, bone, and ligament.1,4–6
The construction of new tissues using tissue engineering strategies mainly involve three basic components: proteins (growth factors and extracellular matrix (ECM) proteins), cells, and scaffolds.7 Among them, bioactive scaffolds, which are a critical element in tissue engineering, play similar roles as ECM in natural tissues, bring cells together and control the tissue structure, regulate the function of the cells, and support the cell attachment, proliferation, and differentiation.1 The architectural design of scaffolds usually focuses on a highly porous and three-dimensional (3D) hierarchical structure, which allows cells to accumulate and grow inside, organizes the cells into a 3D functional tissue, and enables the efficient mass transfer of nutrients, oxygen transfer, and waste exchange.7 Many different materials from natural biomass to synthetic biopolymers have been investigated and utilized for scaffold fabrication in tissue engineering. These materials generally possess some unique properties, including high elasticity and flexibility, good biocompatibility and biodegradability.1,8,9 Natural biopolymers are usually generated during the growth cycles of all organisms and are generally available in large quantities. Most natural biopolymers have been known in the biomedical field before the advent of tissue engineering as a research topic.10,168 The group includes proteins such as collagen and fibrin, polysaccharides such as chitosan (CS) and hyaluronic acid (HA), and some derivatives of ECM.11 The inherent bioactivities of these natural biopolymers can promote cell adhesion, proliferation, and tissue recovery. Scaffolds could also be constructed from synthetic biopolymers. A commonly used synthetic biopolymer is poly(lactic acid) (PLA), an aliphatic polyester, which could degrade within the human body to form lactic acid, a naturally occurring chemical that could be easily removed from the body.1 Similar materials include polyglycolic acid (PGA), polycaprolactone (PCL), poly(lactic-co-glycolic acid) (PLGA), and polyurethane (PU).12–17 These synthetic biopolymers have a long history of use in biomedical applications and are considered safe in many situations.1,18 Nevertheless, there are still some limitations as these polymeric biomaterials generally present poor hydrophilicity, bioinert properties, and the lack of biological signals exhibited in the ECM, which significantly limit their performances in tissue engineering applications. Thus, it is of great necessity to develop highly bioactive scaffolds to replace, remodel, or support the damaged tissues, which can be rationally achieved by the incorporation of bioactive components into the scaffold systems. There are several established synthetic strategies applied to improve the bioactivity of scaffold materials, including the chemical grafting of bioactive components onto polymeric networks, the physical blending of bioactive components with the polymeric matrix, as well as directly functionalizing the scaffold surfaces with bioactive components.19,20
Polyphenols are a class of chemical compounds consisting of two or more phenol structural units and are widely distributed in plants and marine organisms.21,22,167,169 To date, more than 8000 polyphenolic compounds have been characterized and identified, and most of them contain multiple phenolic moieties such as catechol, resorcinol, pyrogallol, or hydroxyquinol connected by the ester or more stable C–C bonds (Fig. 1). In particular, catechol and resorcinol units usually possess two, and pyrogallol and hydroxyquinol units have three phenolic hydroxy groups, respectively. The mixing of these units together may generate functional polyphenols with complex molecular structures. Therefore, polyphenols can be simply classified into three main groups: dihydroxyphenols, trihydroxyphenols, as well as the dihydroxyphenol and trihydroxyphenol mixed system. Owing to the presence of multiple phenolic moieties, these compounds can interact with a variety of biomolecules through covalent interactions (e.g., Michael addition, Schiff-base reaction, radical coupling reaction, and coordination interactions) and non-covalent interactions (e.g., hydrogen bonding, electrostatic interactions, and π–π interactions). They also exhibit a wide range of unique intrinsic properties including antioxidant, antimicrobial, and bioadhesive properties.20,23–25 Therefore, polyphenols have been regarded as promising candidates for the design and fabrication of bioactive scaffolds. By incorporating polyphenols into the aforementioned natural or synthetic polymeric materials, a more biocompatible and bioactive scaffold could be produced. Thus, polyphenols have been continuously explored in many ways for tissue engineering applications. Considering that catechol and pyrogallol moieties are the primary structural units of polyphenols, this review paper will mainly focus on the catechol-containing (e.g., dopamine (DA) and norepinephrine (NE)) biomaterials and pyrogallol-containing (e.g., pyrogallol (PG), gallic acid (GA), and tannic acid (TA)) biomaterials, which have been well documented in recent years. These polyphenol-based biomaterials can be easily fabricated into various dimensional scaffold structures, such as one-dimensional (1D) nanofibers, two-dimensional (2D) surface coating films, and 3D microspheres/hydrogels.
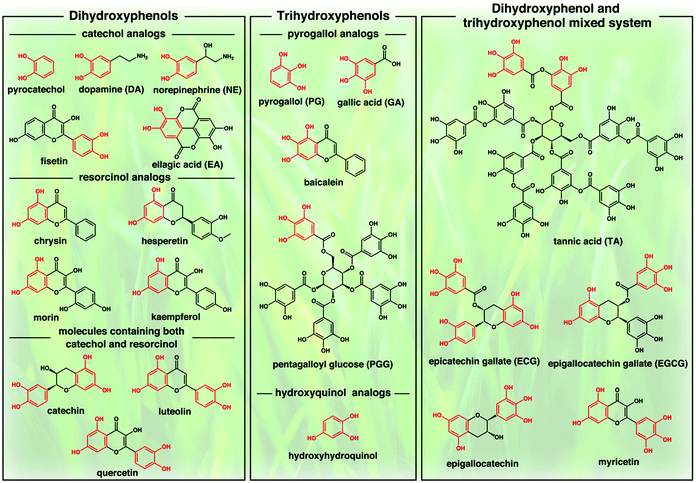 |
| Fig. 1 Chemical structures of typical polyphenols. | |
The purpose of this review is to give a brief overview of the structural and functional features, fabrication strategies, and various tissue engineering applications of polyphenol-based scaffolds (Fig. 2). In the first section, we carefully discussed the use of polyphenols as the structural and functional synthons for the construction of bioactive scaffold composite materials. In the second section, we focused on the detailed fabrication strategies and structural engineering approaches towards different kinds of polyphenol-based scaffolds. In the last section, the various uses of polyphenol-based scaffolds in tissue engineering will be carefully discussed. We believe that this review paper could promote the further development of polyphenol-based scaffolds from the fundamental design to clinical translation, which would attract significant research interests from biological, chemistry, materials, and medicine fields.
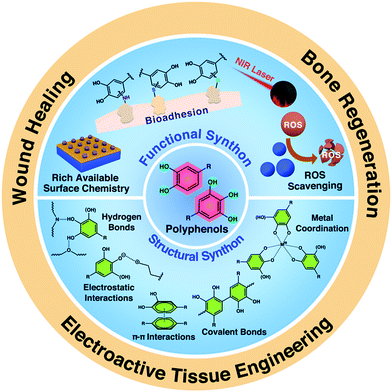 |
| Fig. 2 Overview of the structural and functional features, and tissue engineering applications of polyphenol-based scaffolds. | |
2. Polyphenols in bioactive scaffolds
In recent years, polyphenol-based scaffolds have attracted increasing attention in tissue engineering due to their unique structural and functional features. In particular, the presence of multiple phenolic moieties within the scaffolds could form a series of covalent and non-covalent interactions with various biomacromolecules and bioactive components to enhance the biological activities of materials.26–28 For example, polyphenolic structures can promote the self-polymerization in the presence of oxidizing reagents or undergo the oxidative transition of catechol/pyrogallol to quinone under alkaline conditions. Diverse nucleophiles, including amine, thiol, or imidazole groups, can be covalently conjugated onto polyphenols via Michael addition or Schiff-base chemistry.29,30 As a result, the incorporation of polyphenols can endow the scaffolds with a variety of excellent properties, including adhesiveness, antioxidation, radical scavenging, and antibacterial properties. In this section, we will discuss the use of kinds of polyphenol synthons to design bioactive scaffolds for tissue engineering applications, which are also summarized in Table 1 in detail.
Table 1 Typical polyphenol-based scaffolds in tissue engineering
Polyphenols |
Scaffolds |
Structure |
Involved Property |
Classification |
Applications |
Ref. |
PDA: polydopamine, BMP-2: bone morphogenetic protein-2, SCPP: strontium-doped calcium polyphosphate, SF: silk fibroin, CA-PCL-DA-g-AH: poly(citric acid-co-polycaprolactone-co-dopamine)-g-aniline hexamer, GO: graphene oxide, PNIPAAm: poly(N-isopropylacrylamide), SN: silver nitrate, PNE: poly(norepinephrine), PGG: pentagalloyl glucose. |
DA |
HA–catechol conjugates |
Polyphenol-grafted polymers |
Adhesiveness, hydrophilicity |
Films |
Neuronal tissue engineering |
31
|
HA–catechol hydrogels |
Polyphenol-grafted polymers |
Cohesiveness |
Hydrogels |
Neuronal tissue engineering |
31
|
Gelatin methacrylate–DA hydrogels |
Polyphenol-grafted polymers |
Adhesiveness |
Hydrogels |
Neuronal tissue engineering |
46
|
PDA microcapsules |
Coating of polyphenol onto the scaffolds |
Adhesiveness, hydrophilicity |
Microcapsules |
Bone regeneration |
35
|
PDA/BMP-2/PLGA scaffolds |
Coating of polyphenol onto the scaffolds |
Adhesiveness, hydrophilicity |
Films |
Bone regeneration |
29
|
SCPP/PDA/SF scaffolds |
Coating of polyphenol onto the scaffolds |
Adhesiveness |
Films |
Bone regeneration |
47
|
CA-PCL-DA-g-AH elastomer films |
Polyphenol-grafted polymers |
Adhesiveness, hydrophilicity |
Films |
Cardiovascular tissue, neuronal tissue engineering |
19
|
CS/PDA/GO composite hydrogels |
Polyphenol-grafted polymers |
Adhesiveness, reducing properties |
Hydrogels |
Cardiovascular tissue, neuronal tissue engineering |
34
|
TA |
TA/PNIPAAm multilayer films |
Coating of polyphenol onto the scaffolds |
Adhesiveness |
Films |
Bone regeneration |
44
|
Polypyrrole/TA/Fe3+ hydrogels |
Polyphenol/polymer complexes |
Adhesiveness, antioxidant |
Hydrogels |
Spinal cord injury repair |
48
|
Gelatin–TA–SN hydrogels |
Polyphenol-grafted polymers |
Adhesiveness, antibacterial, hydrophilicity |
Hydrogels |
Wound healing |
42
|
CS/SF/TA/Fe3+ cryogels |
Polyphenol/polymer complexes |
Hemostasis, photothermal performance, antibacterial |
Hydrogels |
Wound healing |
49
|
TA-DNA hydrogels |
Polyphenol/polymer complexes |
Adhesiveness, hemostasis |
Hydrogels |
Wound healing |
50
|
NE |
Collagen–CaCO3–PNE composite scaffolds |
Polyphenol/polymer complexes |
Adhesiveness, hydrophilicity, fluorescence |
Nanofibers |
Bone regeneration |
51
|
Catechin |
Catechin-PCL nanofibrous scaffolds |
Coating of polyphenol onto the scaffolds |
Adhesiveness, antioxidation |
Nanofibers |
Bone regeneration |
52
|
PGG |
PGG-treated collagen (or elastin) scaffolds |
Coating of polyphenol onto the scaffolds |
Antioxidant, anti-inflammatory |
Films |
Cardiovascular tissue engineering |
53
|
2.1 Structural contribution
Covalent interactions.
(a) Michael addition/Schiff-base reaction and radical coupling reaction.
For catechol-containing compounds, the catechol groups can be oxidized to o-catecholquinone in the presence of oxidizing reagent (e.g., NaIO4, H2O2, enzyme, and O2) (Fig. 3a).31,32 The oxidized catechol moieties are highly reactive and can bond to the tissue/cell by chemically reacting with nucleophilic functional groups such as amino groups, thiol groups, or imidazole groups of proteins and peptides to form stable interfacial covalent bonds with the tissue/cell surfaces via Michael addition and/or Schiff-base reactions (Fig. 3c-i).33,34 On the other hand, the oxidized catechol moieties can also undergo a coupling reaction for achieving the covalent linkage to form catechol-catechol adducts, which are subsequently polymerized into oligomers and polymers (Fig. 3c-ii).29,31 Such a unique chemical cross-linking reaction has been widely employed to generate 3D polymeric networking scaffolds through the oxidative cross-linking reactions of catechol(s)-tethered biomacromolecules (e.g., poly-(ethylene glycol) (PEG), HA, and CS).29,31,35
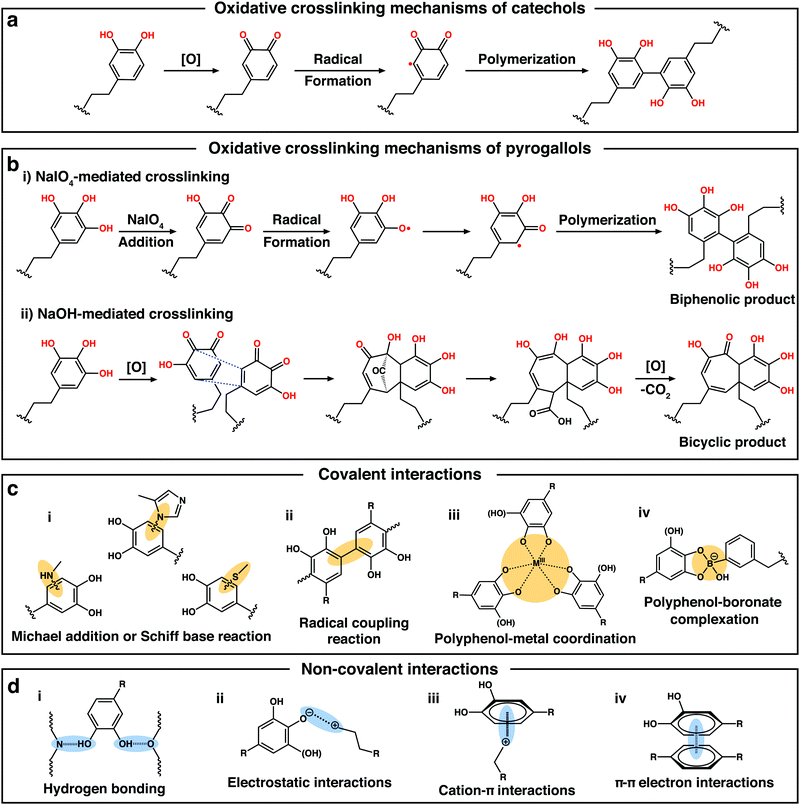 |
| Fig. 3 (a) Proposed oxidative crosslinking mechanisms of catechol. (b) Proposed oxidative crosslinking mechanisms of the two oxidative processes of pyrogallols triggered by NaIO4 or NaOH. Reproduced with permission.36 Copyright 2018, Wiley-VCH. (c and d) Diverse covalent and non-covalent interactions in polyphenol-containing compounds. | |
Similar to catechol, in the presence of a strong oxidizing reagent such as NaIO4, pyrogallol can also be oxidized into o-galloquinone by oxygen transfer and subsequent free radical formation, further triggering the dimerization reaction to form biphenolic compounds (Fig. 3b-i).36 However, under weak oxidative conditions, the covalent bonds of pyrogallols preferably undergo tautomerization of the reactive o-galloquinone into carbonyl-bridged intermediates and the subsequent generation of purpurogallin (benzotropolone) (Fig. 3b-ii). Purpurogallin can be further oxidized into purpurogallin-quinone and quinhydrone structures. Note that these types of quinone-intermediates generated by the oxidation processes can also form covalent reactions with amine or thiol groups of biomolecules via the Schiff-base reaction.36 In 2007, inspired by the strong underwater adhesion of Mytilus edulis foot protein 5 (Mefp-5) in mussels, which is rich in 3,4-dihydroxy-1-phenylalanine and lysine amino acids, Messersmith and coworkers reported that PDA, the first synthetic surface-independent coating material, could be facilely produced via the oxidative self-polymerization of DA under alkaline conditions in the presence of air.33 After the oxidative polymerization, the polycatechol structure of PDA could also undergo reactions with various functional groups, including thiol and amine to form covalently-conjugated functional layers via Schiff-base or Michael addition reactions.29,35,37 Thus, PDA usually exhibits a strong binding affinity to various bioactive molecules containing thiol and/or amine groups, such as thiolated peptides,38 polysaccharides,39 cell adhesive proteins,18 anticancer drugs, antibodies,37 or growth factor proteins (e.g., basic fibroblast growth factor and vascular endothelial growth factor).40 This feature makes PDA widely applicable in many scaffolds for improving their physical and biological properties for tissue engineering applications. For example, Xu et al. fabricated poly-(lactic-co-glycolic acid)/β-tricalcium phosphate (PLGA/β-TCP) composite scaffolds with the surface coating of PDA.41 Mouse pre-osteogenic cell (MC3T3-E1 cell line) culture results indicated that the PDA/PLGA/β-TCP scaffolds could significantly promote cell adhesion and proliferation, and improve the osteogenesis in comparison with the non-coated scaffolds. Besides PDA, TA, a kind of plant-derived polyphenol compound with multiple pyrogallol moieties, has also been widely applied to improve the bioactivity of scaffolds. Similar to PDA, TA can also chemically bond with nucleophiles including amines, thiols, and imidazole in proteins or peptides to form strong covalent interactions.42–44 The pyrogallol moiety of TA not only participated in the cross-linking reactions of polymer hydrogels with improved mechanical properties45 but can also chemically bond with tissues/proteins to engineer the scaffolds for tissue engineering.
(b) Polyphenol–metal coordination.
Apart from several covalent interactions mentioned above, polyphenols can also form dynamic coordination interactions with metals or metal ions. When the phenolic group is deprotonated, an oxygen center is generated with a high charge density, making polyphenols as good ligands for metal cation complexation.54 Thus, the pyrogallol/catechol groups in polyphenols can chelate a variety of metal cations (e.g., Mg2+, Ca2+, Al3+, and Fe3+) or metal oxides (e.g., Al2O3, Fe3O4, TiO2, SiO2, and ZnO) to form different polyphenol-metal coordination complexes (Fig. 3c-iii).26,55–60 Based on these metal-phenol coordination networks, researchers have developed a novel strategy for the surface engineering of the scaffolds, which is an assembly of metal-phenolic networks (MPNs).61 Ejima et al. reported the one-step coating of diverse interfaces using the coordination MPN of TA and Fe3+.62 The deposition process was independent of the substrate, which could perform in a range of organic/inorganic templates from 2D substrates to biological particles. Interestingly, such a unique TA–Fe3+ coordination MPN exhibited pH-dependent disassembly behavior due to the reversible transitions between mono-, bis-, and tris-complex states. At pH 7.4, the coordination networks between TA and Fe3+ were stable as the tris-complex form, while in strong acidic environments (pH ≈ 2.0), most of the hydroxyl groups were protonated, which led to the rapid disassembly of the MPNs. The simple preparation and biologically tunable physicochemical properties of the MPN coatings provide a useful platform for the surface engineering of the biomaterial scaffolds.
In addition, when polyphenols are used for the surface functionalization of inorganic/metallic scaffolds, the presence of metal cations/metal in the surfaces also allows the formation of metal–phenol coordination between the scaffolds and phenol groups, resulting in robust bonding with the inorganic/metallic surfaces, thus endowing the scaffolds with enhanced biological properties. For this reason, many researchers have used polyphenols for inorganic/metallic scaffolds’ surface modification. For example, in our previous study, we fabricated strontium-doped calcium polyphosphate/PDA/silk fibroin (SCPP/PDA/SF) scaffolds by using PDA as the interface bridge between SCPP and SF.47 Molecular dynamics simulation indicated that aside from hydrogen bonding and electrostatic interactions between PDA and SCPP, the catechol group formed the coordination complexes with Ca2+ ions, which also contributes to the strong adhesion. In another work, Huang and co-workers found that the surfaces of 316L stainless steel stents coated with PDA showed significantly enhanced adhesion and function of human umbilical vein endothelial cells (HUVECs),63 suggesting the potential of PDA as coatings for functionalizing the surface of metallic scaffolds for applications in tissue engineering.
(c) Polyphenol–boronate complexation.
Polyphenol–boronate complexation is another dynamic covalent interaction (Fig. 3c-iv), which has been continuously explored in many ways in recent years.64–66 Boronic acids can bind with 1,2- and/or 1,3-diols to form cyclic ester species (i.e., boronate esters) by boronate-diol complexation.67 Owing to the favorable syn–peri-planar arrangement of the aromatic hydroxyl groups combined with the electron-donating properties, phenolic moieties show great potential of reacting with boronic acid to form boronate ester complexes.54,68 Similar to polyphenol–metal complexes, the polyphenol–boronate complexes also possess an outstanding pH-responsive feature. These complexes are stable at alkaline pH but dissociate at acidic pH and/or in the presence of exogenous competing cis-diols (e.g., mannitol).68–70 Taking advantage of the dynamic nature of polyphenol-boronate complexation chemistry, Caruso and coworkers designed a pH and cis-diol dual-responsive boronate-phenolic network (BPN) capsule system via the complexation of phenylboronic acid and TA to form robust films on particulate templates.68 BPN capsules were stable in biological environments, even in the presence of competing carbohydrates, which endowed the BPN capsules with the potential to be exploited in biological applications. In another interesting study, Lee's group developed a smart adhesive hydrogel that transitioned reversibly between its adhesive and non-adhesive states in response to pH changes.71 This adhesive contained network-bound DA and phenylboronic acid that could robustly stick to borosilicate glass with a strong adhesion energy of 2000 mJ m−2 at pH 3. When the pH was increased to 9, the adhesion energy was decreased by more than one order of magnitude to 180 mJ m−2 owing to the formation of intermolecular catechol–boronate complexes, which reduced the interfacial adhesion sites. Based on the above, polyphenol–boronate complexation also plays pivotal roles in the preparation of functional materials and surfaces, exhibiting great potential in tissue engineering.72,73
Non-covalent interactions.
(a) Hydrogen bonding.
Hydrogen bonding, a typical intermolecular interaction, usually has a bonding energy in the range of 0.2–40 kcal mol−1.74 When the pH value is lower than the pKa of polyphenols, most of the phenolic hydroxyl groups in polyphenols (e.g., PDA, TA, and epigallocatechin gallate (EGCG)) are protonated and can act as excellent hydrogen-bond donors, which are capable of rapidly forming extensive intermolecular hydrogen bonds with many biomacromolecules (e.g., CS, cellulose nanofibril, and collagen) (Fig. 3d-i).75–77 The reversible hydrogen bonds between hydroxyl groups can usually provide physically crosslinked networks within phenol-containing materials, endowing them with self-healing ability and improved mechanical properties.26,55,78 More importantly, after being modified with phenolic groups on the surface, the scaffolds can be bonded with a wide range of bioactive molecules (e.g., cell adhesive proteins or peptides, and growth factors secreted by the cells) through hydrogen bonding interactions, which directly initiates cell attachment and spreading.18 Besides, the hydrogen bonding between phenolic groups and water molecules can also improve the surface hydrophilicity of the scaffolds, which offers another important support for facilitating cell attachment and proliferation.31
(b) Electrostatic interactions.
The phenolic groups in polyphenols can be partially ionized when the pH is higher than the pKa, leading to abundant negatively charged groups.35,79 In this way, polyphenols are usually expected to electrostatically assemble with cationic polymers (e.g., CS, poly(dimethyldiallylamide) (PDDA), and poly(N-isopropylacrylamide) (PNIPAAm)) to engineer the scaffold surface via the layer-by-layer (LbL) self-assembly method.35,44,80 The procedure is based on the alternative assembly of oppositely charged molecules on the substrates via electrostatic interactions on the surfaces (Fig. 3d-ii). Notably, compared with the conventional surface modification method, the entire process of the LbL self-assembly method could be performed in under mild conditions without involving any toxic reagents, which is beneficial for improving the biocompatibility of the scaffolds. Valmikinathan et al. prepared temperature-responsive films using TA and thermo-responsive PNIPAAm via the LbL self-assembly technique.44 Multilayered films were successfully fabricated by the alternative deposition of PNIPAAm and TA on the tissue culture plates. The cell culture results demonstrated that these multilayer structures could promote cell attachment with uniform distribution on the tissue culture plate surface.
(c) Cation–π and π–π interactions.
Due to the presence of electron-rich π orbitals in the phenol groups, polyphenols can also form cation–π interactions with a variety of cations (e.g., Na+, K+, NH4+, and species containing positive charges), which can also act as significant contributing factors to their adhesion (Fig. 3d-iii).67 Lee and co-workers reported a simple, multifunctional scaffold surface modification via the catechin coating for bone regeneration.52 The chemical mechanism of the surface coating proved to be the physical dimeric self-assembly of catechin molecules mediated by cation–π interactions and some other interactions between the physically-stacked dimers and the substrates (e.g., π–π interaction and hydrogen bonding). Apart from cation–π interactions, the π–π electron interactions between the benzene ring of polyphenols and another benzyl moiety also exist widely in phenol-containing materials (Fig. 3d-iv).34,81 Taken together, the cation–π and π–π interaction-based non-covalent polyphenol chemistry can provide an attractive platform to engineer functional biomaterial scaffolds for tissue engineering.
2.2 Functional contribution
Bioadhesion.
As mentioned before, polyphenols have a strong binding affinity to a variety of surfaces due to their strong covalent and non-covalent interactions with almost all types of materials.82–87 In particular, the high binding affinity of oxidized catechol/pyrogallol groups to various nucleophiles in proteins is very useful, which can endow polyphenol-based scaffolds with strong cell/tissue adhesiveness. Cho et al. developed a bioinspired hydrogel scaffold by the in situ crosslinking of PG-modified HA in NaOH solution.36 Several types of quinone-intermediates generated during the crosslinking process can cause the Schiff-base reaction with amine or thiol groups in proteins, resulting in robust tissue attachment and efficient cell transplantation (Fig. 4a). The resulting HA-PG hydrogel was able to tightly adhere onto the surface of various organs including the heart, kidney, and liver. Besides, it was also found that the surface coating by catechol/pyrogallol-functionalized polymers could not only enhance the cell attachment but also promote cell proliferation and differentiation, which performed similar functions as that of cell adhesion motifs in ECM.88 Interestingly, on comparing the adhesion performance between catechol- and pyrogallol-functionalized polymers, the latter ones usually exhibited a seven-fold enhancement in underwater adhesion conditions.89 The higher binding strength of pyrogallol-functionalized polymers was ascribed to the stronger tridentate-related interfacial interactions between the polymer and the substrate, and greater cohesive interactions inside the polymers.
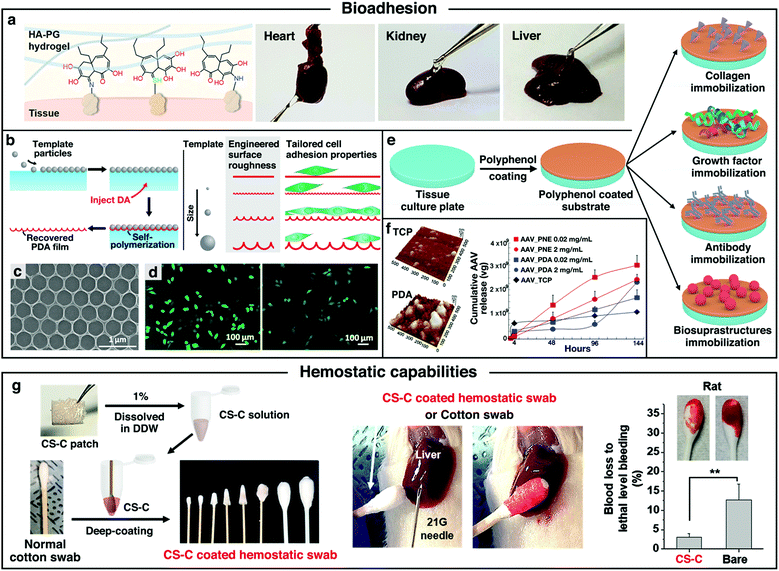 |
| Fig. 4 (a) Schematic illustration of tissue adhesion behaviors of NaOH-induced HA–PG hydrogel. Reproduced with permission.36 Copyright 2018, Wiley-VCH. (b) Schematic illustration of the preparation method towards patterned PDA films. (c) SEM images of a patterned PDA film. (d) Fluorescence microscopy images of HeLa-GFP with Hoechst-stained nuclei cultured on: 0.5 μm patterned PDA films (left) and nonpatterned PDA films (right). Reproduced with permission.90 Copyright 2017, American Chemical Society. (e) Schematic representation of the polyphenol coating and subsequent secondary treatment towards the functional scaffolds. (f) AFM images of PDA coated surface and polystyrene tissue culture plate (TCP) surface with AAV immobilization, and the cumulative quantities of AAV released from each substrate at different time point. Reproduced with permission.94 Copyright 2014, American Chemical Society. (g) Schematic illustration of the preparation and hemostatic effects testing of adhesive CS-C/cotton composite hemostatic swabs. Reproduced with permission.95 Copyright 2018, American Chemical Society. | |
In addition, it has been reported that cell adhesion onto biomaterial scaffolds can be modulated by tuning the topography of the substrate. To further improve the cell adhesion, Chen et al. fabricated PDA films with controllable surface patterns via the polymerization of DA at the air–water interface of a floating bed of spherical particles (Fig. 4b).90 Subsequent dissolution of the spherical templates could generate free-standing PDA films with tunable indentations. The surface morphology of the patterned PDA film showed a stable hexagonally close-packed pattern structure (Fig. 4c). Cell-adhesion studies showed that the cell adhesion of HeLa cells was significantly enhanced (∼43%) on the 0.5 μm-patterned PDA films compared to that on the non-patterned PDA films (Fig. 4d). The increase in the contact area with the cells of the patterned films might be the explanation for the increase in cell adhesion.
Moreover, after the surface coating of polyphenols onto the scaffolds, the resulting biomaterial surface showed high reactivity to nucleophiles (i.e., amine, thiol, and imidazole groups) via Schiff-base or Michael addition reactions, which provided a versatile platform for post-modification with various functional molecules.91–93 Through the proper selection of post-modification reactants, polyphenol coatings can be used to create a variety of ad-layers, such as bioinert and bioactive surfaces via the grafting of macromolecules.33 For tissue engineering applications, secondary reactions can be applied to immobilize a variety of biomolecules onto the surface to develop a bioactive scaffold with new functions (Fig. 4e). To date, there are four main categories of immobilized biomolecules: (1) collagen; (2) growth factors (neurotrophic and angiogenic factors); (3) antibody or drugs; (4) biosuprastructures (such as viruses). Among them, the immobilization of collagen or growth factors on the scaffolds is usually aimed at mimicking the natural ECM, which was regarded as an efficient route to improve the biocompatibility of the scaffolds.29,38,43 Collagen is a class of well-known proteins that occur ubiquitously in multicellular animals. Owing to the typical triple helix structure, type I collagens can interact with the cell surface receptors (e.g., integrins, mannose receptors, and osteoblast receptors), thus regulating many cellular processes including adhesion, proliferation, and migration.93 For growth factor immobilization, owing to the presence of the catechol or pyrogallol functional group with high binding affinity to proteins, polyphenol-modified scaffolds could significantly reduce the initial burst of loaded growth factors and improve the release profiles for sustained delivery. Compared with traditional methods used for scaffold surface modifications (e.g., chemical conjugation and physical adsorption), the strong adhesion and facile engineering process of polyphenol-assisted surface modification might be an easy and efficient method to deposit growth factors or proteins onto the scaffolds. Based on the discussions above, the polyphenol-mediated surface functionalization method towards tissue engineering bioactive scaffolds with enhanced biocompatibility is of great research interest.
In addition to the immobilization of collagen and growth factors, polyphenol is also a good candidate for intermediating antibodies or drugs onto the surfaces of the scaffolds. For instance, Liu et al. developed a specific immunological tissue engineering scaffold by grafting the agonistic rat anti-human CD40 antibody (CD40mAb) onto the surfaces of PLLA fibers through the PDA motif-mediated crosslinking reaction (PLLA–PDA–CD40mAb).37 The cancer therapeutic effect of the PLLA–PDA–CD40mAb scaffolds on tumor cell apoptosis was examined in vivo. It was found that the tumor volumes of the mice treated with the PLLA–PDA–CD40mAb scaffold were the smallest among all the groups, indicating that the PLLA–PDA–CD40mAb membranes could effectively inhibit the tumor growth. Another advancement in utilizing polyphenol chemistry to immobilize biomolecules onto the scaffold surface is to control the sustained release of biosuprastuctures (such as viruses) to provide efficient ex vivo gene delivery. For example, Kim et al. designed a substrate-mediated viral delivery system by combining adeno-associated viral (AAV) vectors and adhesive catecholamine (i.e., PDA or PNE) surfaces.94 Anchoring AAV vectors on sticky catecholamine substrates could result in more viral immobilization than that on the unmodified tissue culture polystyrene (TCP) surface. Importantly, compared to the unmodified TCP, the adhesive catecholamine interfaces exhibited sustained release of the viral vectors without initial burst at the early stage (Fig. 4f). Thus, the delivery platform could efficiently tune the viral release at fixed rates and induce the gene expression in a controlled manner, which indicated the potential of the bioinspired adhesive catecholamine interfaces as a promising platform tool to deliver genes to the target cells.
Besides the cell/tissue adhesion and post-modification features, polyphenols also exhibited hemostatic ability. It was reported that the adhesive catechol-functionalized CS could form self-sealing membranes by rapid complexation with the serum proteins in the blood. The binding kinetics towards serum proteins was extremely fast, suggesting that CS–catechol has a good hemostatic ability.95 After that, the hemostatic capabilities of catechol-functionalized polymers have been continuously explored in many ways. As an interesting example, a hemostatic swab based on catechol-modified CS (CS-C) was developed by Lee and coworkers.95 To make the swab, commercial cotton-ball swabs were immersed in the CS-C solution and further lyophilized (Fig. 4g). A rat liver hemorrhaging model showed that the CS-C cotton swabs exhibited excellent hemostatic effect compared to the one using unmodified commercial swabs. In another work, a haemostatic hypodermic needle has also been presented by dropping the CS-C solution onto a needle surface and drying it by needle rotation under ambient conditions.96 Intravenous or intramuscular injection was then performed to study the capabilities of these haemostatic needles in vivo. Compared to the bare needles, the needles bearing the CS-C coatings did not cause any bleeding and the punctured site was immediately self-sealed upon the removal of the haemostatic needle, resulting in the complete prevention of blood loss. This might be accounted for by the formation of serum proteins and CS-C complexations, which acted as a physical barrier upon bleeding. The exceptional hemostatic capabilities of polyphenols can be applied to develop hemostatic materials for wound healing, which is also an important application in tissue engineering.
Antioxidant and anti-inflammatory.
Many works have demonstrated that polyphenols can be applied as efficient antioxidants to scavenge free radicals both in vitro and in vivo.97–101 There are two main reaction mechanisms for the radical scavenging activity of polyphenols: hydrogen atom donation and electron transfer-proton transfer (Fig. 5a).23,99 For the former issue, owing to their special chemical structure (hydroxyl group(s) on a benzene ring(s)), polyphenols can scavenge free radicals via hydrogen atom transfer from the active hydroxyl groups of the polyphenol to the free radical (Fig. 5a-i).23,102 As a result, a phenolic radical is generated and a stable quinone structure is formed. Note that the resulting phenolic radicals are resonance stabilized, which do not easily initiate new reactions but could further react with other free radicals, thus acting as radical scavengers.24 Electron transfer-proton transfer is another reaction pathway towards the radical scavenging activity of polyphenols (Fig. 5a-ii). They can scavenge free radicals by donating the transfer electrons to the free radicals, thus stabilizing the reactive species.
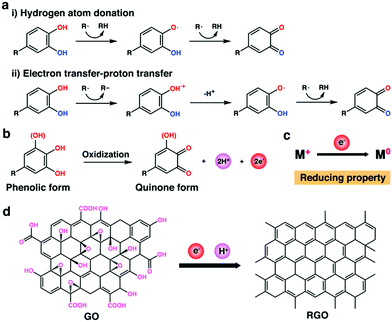 |
| Fig. 5 (a) Mechanisms of the free radical scavenging activity of polyphenols: (i) hydrogen atom donation; (ii) electron transfer-proton transfer. R˙: free radical. (b) Schematic representation of polyphenol getting oxidized to quinone with the subsequent release of electrons and freely reactive hydrogen atom. (c) Mechanism for the reducing property of polyphenols. M: metal. (d) Reduction mechanism of graphene oxide (GO) to reduced graphene oxide (RGO) by polyphenols.106 | |
The body usually produces reactive oxygen species (ROS) that play vital roles in a variety of biological and biochemical processes. However, in some special biochemical processes, such as inflammatory response, the activated phagocytic cells (e.g., macrophages and granulocytes) could generate high levels of ROS and the overproduction of these free radicals will lead to oxidative stress, which might cause damage to lipids, proteins, and DNA, finally leading to impaired cell function and adverse inflammatory changes.24,99,102,103 Fortunately, the introduction of polyphenols can endow the scaffolds with cytoprotective and anti-inflammatory properties.104 Wang et al. fabricated a multifunctional surface for biodegradable MgZnYNd alloy substrates with TA coating.102 They demonstrated that the TA-coated surface can capture the ROS, thus endowing excellent antioxidant properties of the alloy scaffolds. They further analyzed the viabilities of HUVECs treated with and without TA coating upon H2O2 damage. The results showed that when H2O2 was applied to damage the cells, more dead cells could be observed on the surface of the unmodified alloy substrates compared with that on the one with TA coating, indicating that TA coating could introduce strong antioxidant activity and inhibit the H2O2-induced cell apoptosis.102 Recently, our group developed ultra-small and precisely structured polyphenol-functionalized polyhedral oligomeric silsesquioxanes (POSS) nanoparticles (GA-POSS) by accurately functionalizing the POSS surface with GA units via thiol-Michael “click” reactions.105 The 2,2-diphenyl-1-picrylhydrazyl (DPPH) free radical scavenging study demonstrated that the resulting GA-POSS nanoparticles exhibited strong free radical scavenging capacity and fast radical scavenging rates, which was due to the high density of the polyphenol units on the nanoparticle surface and the ultrasmall size (∼5 nm) of the particles. The antioxidant performances of the GA-POSS nanoparticles for protecting the cells from oxidative stress were further evaluated. The results indicated that the level of ROS of the cells could be significantly inhibited by treating these with GA-POSS nanoparticles, suggesting that the polyphenol nanoparticles possess great potentials in inhibiting oxidative stress-induced pathologies.
PGG is another kind of well-characterized polyphenol with antioxidant, antidiabetic, and anti-inflammatory activities.21,53 Chow et al. designed a facile strategy for protecting the scaffolds from diabetes-related complications through the pre-implantation treatment of collagen and elastin scaffolds with PGG.53 By virtue of the unique features, PGG was able to chemically stabilize the scaffolds, slow down their enzymatic degradation, and reduce the scaffold-bound advanced glycation end products (AGEs) levels, thereby supporting the future use of diabetes-resistant scaffolds for cardiovascular tissue engineering in patients with diabetes. Importantly, after implantation in diabetic rats, less cell infiltration in the PGG-treated scaffolds was observed, suggesting that PGG could suppress the acceleration of AGE accumulation and inflammation. These results indicated that PGG can be applied as an anti-inflammatory agent in biomaterial scaffolds.53
In addition, it has been reported that polyphenols can also act as an effective reducing agent due to the presence of catechol/pyrogallol groups within their structures. Under basic conditions, these polyphenolic groups could take part in the redox reactions by forming quinones that simultaneously donate electrons and liberate freely reactive hydrogen atom (Fig. 5b).23 The donated electrons can make polyphenols an effective reducing agent by reducing some oxidized molecules (e.g., graphene oxide (GO) and oxidized metal ions in metal salts) to the reduced states (Fig. 5c and d).106–108 This mechanism is somewhat similar to that of polyphenols as antioxidants. Cataldo et al. have demonstrated that TA could be used as an effective reducing agent to further generate colloidal silver nanoparticles in silver salt solutions.107 They found that the resulting colloidal silver was more stable than those prepared by the reduction of silver salt with NaBH4 since TA could also serve as the protective groups. Xu et al. designed an effective approach for the reduction of GO by employing DA as a reducing agent (Fig. 5d).106 Compared with conventional reducing agents, including hydrazine, dimethylhydrazine, and hydroquinone, the DA-based reducing agent was low-cost and environment-friendly. In addition, the PDA adlayer can also be applied to graft thiol- or amino-functionalized molecules via Michael addition/Schiff-base reaction, providing a versatile means to further stabilize and decorate the resulting reduced GO surface for further functionalization. Benefiting from the reducibility of polyphenols, many multifunctional composite scaffolds could be constructed.34,42,108,109 For example, GO could be incorporated into the polyphenol-containing scaffolds and be reduced to reduced graphene oxide (RGO) by polyphenols to endow the scaffolds with electrical conductivity for electroactive tissue engineering.34 As another example, silver ions could be doped into polyphenol-containing scaffolds and be reduced to silver nanoparticles, thus endowing the scaffolds with improved antibacterial properties.42
Photothermal performance.
The high photothermal conversion capability of some polyphenols is another attractive feature. As a typical example, PDA, a melanin-like material, has attracted extensive attention due to its high photothermal conversion efficiency.110,111 Naturally-occurring eumelanin is well-known to have broadband monotonic absorption ranging from the ultraviolet to the visible region. The absorbed photon energy will be converted non-radiatively into heat within a short time.112,113 Such a broad absorption and high photothermal conversion capability protects living organisms from ultraviolet injury.110,114,115 Due to a similar structure as that of eumelanin, PDA also exhibits a similar absorption property and photothermal conversion capability. In particular, high absorption in the near-infrared (NIR) region (700–1300 nm) of the light spectrum is quite critical for biotherapy owing to the deep tissue penetration of NIR light. Indeed, due to the strong NIR absorption as well as excellent biocompatibility, PDA has gained great attention as a photothermal therapeutic agent for photothermal therapeutic (PTT).116 In particular, the promising in vivo PTT and synergetic therapy performances of PDA-based synthetic melanin nanoparticles (SMNPs) have been well documented and summarized elsewhere.110 Despite the intense interest in these non-toxic PDA-based SMNPs, there is still a great challenge that needs to be overcome. The relatively low NIR light absorption and photothermal effects of PDA-based SMNPs has limited their further application, which might be because of their structural heterogeneity and complexity. Very recently, our group proposed several methods to further improve the total photothermal effects of PDA-based SMNPs.116–119 For example, we designed a new family of SMNPs with superior photothermal effects via the one-pot copolymerization of arginine and DA under mild conditions (Fig. 6a).116 Compared with conventional PDA-based synthetic melanin agents, the resulting arginine-doped SMNPs demonstrated enhanced total photothermal efficiencies (i.e., ∼60% increase). The mechanisms of photothermal effect enhancement in these arginine-doped SMNPs could be summarized in two aspects: on the one hand, the construction of donor–acceptor microstructures within the SMNPs by arginine–DA conjugates could decrease their energy band gap and promote the electron transfer process, resulting in enhanced NIR light absorption of the SMNPs for more income energy. On the other hand, the introduction of the arginine unit within the PDA structures could destroy the efficient interunit π-conjugation within the SMNPs, which were able to increase the free radical concentration within the SMNPs and then inhibited the non-thermal radiative transition process. In addition, we found that the NIR light absorbance and photothermal effect of SMNPs could also be rationally improved by doping with various typical metal ions (e.g., Fe3+, Cu2+, Zn2+, and Ga3+), which might be attributed to the increased absorption cross-section areas via the coordination between metal ions and catechol groups.119 Moreover, we also found that both light absorption ability and photothermal effects were gradually enhanced by increasing the doping content of the metal ions, which might be due to the generation of a relatively narrower energy bandgap.
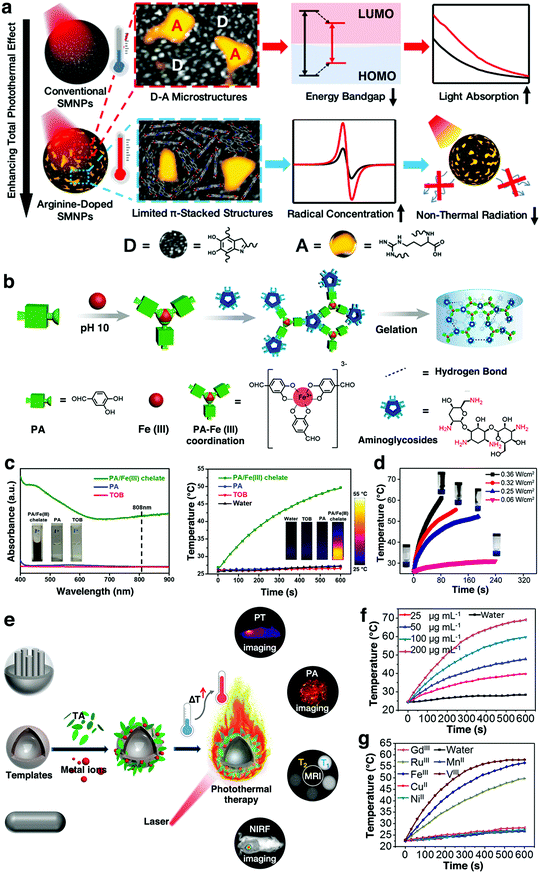 |
| Fig. 6 (a) Schematic illustration of the design of arginine-doped SMNPs with enhanced photothermal effect. LUMO: lowest unoccupied molecular orbital; HOMO: highest occupied molecular orbital. Reproduced with permission.116 Copyright 2019, American Chemical Society. (b) Schematic illustration of the preparation of the PA/Fe3+/aminoglycoside smart hydrogels. (c) UV-vis-NIR spectra and photothermal effect testing of the PA/Fe3+ chelate, PA, and tobramycin (TOB) (2.5 mg mL−1) from the gel. (d) Temperature curve of the smart hydrogel irradiated at various NIR laser power densities. Reproduced with permission.122 Copyright 2019, American Chemical Society. (e) Schematic illustration of the cooperation of adhesive MITAs with diverse templates for advanced applications. (f) Photothermal effect of Fe3+–TA solutions at different concentrations under laser irradiation. (g) Photothermal effect of different MITAs upon laser irradiation. Reproduced with permission.120 Copyright 2018, American Chemical Society. | |
Apart from PDA, some other polyphenols have also been reported for coordination with various metal ions to form photothermal materials. It has been found that many metal-phenolic networks (such as Fe3+–TA,120 Fe3+–ellagic acid (Fe3+–EA),121 Fe3+–protocatechualdehyde (Fe3+–PA),122 and Fe3+–EGCG networks123) exhibit strong near-infrared (NIR) light absorption and high photothermal capability. The photothermal mechanism of the networks was due to the strong coordination phenol hydroxyl-metal charge transfer, which exhibited strong absorption and high heat capacity in the NIR region.121,123 Very recently, our group reported a simple assembly of smart hydrogels by the direct gelation of PA, Fe3+, and aminoglycosides via two types of dynamic chemical bonds (i.e., Fe3+–(catecholate)3 coordination bond and imine bond) (Fig. 6b).122 The PA/Fe3+ chelate displayed favorable photothermal convention features, as evident by the UV-vis-NIR absorption and photothermal tests (Fig. 6c). As a result, the smart hydrogels also exhibited promising NIR light absorption and photothermal effects (Fig. 6d). In another work, Liu et al. developed a family of photothermal materials based on various metal ion/TA assemblies (MITAs) (Fig. 6e). MITAs from Fe3+, V3+, and Ru3+ could all afford excellent photothermal efficiency (η ≈ 40%) (Fig. 6f and g).120 Compared with the currently existing photothermal agents, MITAs were highlighted by the merits including green synthesis and facile incorporation of diagnostic metal ions, particularly, the excellent adhesion. Importantly, the special adhesion enables MITAs that were suited as a photothermal platform for versatile cooperation with other therapy approaches and diagnostic techniques so as achieve various advanced applications, including photothermal imaging (PTI), photoacoustic imaging (PAI), T1- and T2-weighted magnetic resonance imaging (MRI), and near-infrared fluorescence (NIRF) imaging together with photothermal therapy.
Other functions.
(a) Hydrophilicity.
The hydrophilicity has also been regarded as an important role in the interaction between biomaterials and tissues.20,124 For tissue engineering applications, good hydrophilicity of the scaffold is usually required due to a large number of cells that need to adhere and grow on the scaffold surfaces. Many researchers have demonstrated that surfaces coated with polyphenolic compounds were able to enhance the total hydrophilic property of the scaffolds. This rule was workable even on some bioinert low-surface-energy substrates, such as poly(ethylene) (PE), poly(tetrafluoroethylene) (PTFE), silicone rubber, and poly(dimethyl-siloxane) (PDMS), resulting in cell-adhesive surfaces from cell-resistant surfaces.124 In addition, with the introduction of hydrophilic polyphenolic groups, the hydration properties of the scaffolds have also been improved, which can promote complete adhesion.19
(b) Self-healing.
One of the key functions of the scaffolds is to encapsulate cells for the in situ repairing of the damaged tissues.125,126 However, sometimes scaffolds might be subject to unexpected conditions during the service periods when suffering unrecoverable deformation or mechanical loading.34 To solve this problem, a series of self-healing scaffolds have been developed in the recent years.125–127 Catechol/pyrogallol-containing molecules possess various reversible interactions including hydrogen bonding, electrostatic interaction, phenol-metal ion coordination, and π–π stacking interactions between the chains, which can be used to design self-healing scaffolds.34,70,128 Wang et al. reported a regenerative self-healing and self-sealing film by using a range of phenolic compounds (e.g., pyrocatechol and pyrogallol) and an amine-rich polymer such as polyethyleneimine (PEI).129 Pyrocatechol/pyrogallol was spontaneously oxidized and reacted with PEI to form a film in the liquid/air interface due to the presence of atmospheric oxygen. Upon the damage of the film, the underlying solution was re-exposed to oxygen in the air, further generating a new film that attached to the surroundings with the driving force of non-covalent bonds (e.g., hydrogen bonds and π–π interactions). This could lead to the self-healing regeneration at the air–water interface of the film.129
(c) Fluorescence.
Recent studies have shown that the co-polymerization of DA and PEI under alkaline oxidative conditions resulted in the PEI–PDA copolymer with enhanced fluorescence emission properties.130,131 PEI can react with DA via either Michael addition reaction or Schiff-base reaction to reduce the π–π stacking interaction between the catechol groups of PDA, finally resulting in fluorescence emission.130 Dhand et al. fabricated nanofibrous collagen scaffolds by the electrospinning of collagen-containing catecholamines (DA and NE) and Ca2+.51 The photoluminescence properties of catecholamine-crosslinked scaffolds were investigated and the results indicated that the mats displayed intense blue (405 nm) and green (488 nm) emission. Thus, the polyphenol-containing compounds with excellent photoluminescent properties can be used as non-invasive real-time probes to investigate scaffold degradation patterns and tissue integration without sacrificing animals.51
(d) Antibacterial.
Several kinds of plant polyphenols have been widely regarded as antimicrobial agents to provide resistance against microorganisms.21,108 Previously, studies that have been carried out by Kyaw and co-workers revealed that TA has potential antibacterial activity against one of the most antibiotic drug-resistant “superbugs”, the methicillin-resistant Staphylococcus aureus (MRSA) strain.132 One possible antibacterial mechanism was proposed that TA could interact with the bacterial cell wall, which played an important role in the osmotic protection of the cell, leading to the decrease in the tolerance of the cell to both high ionic strength and low osmotic pressure.23,42 Zhao et al. reported that epigallocatechin gallate (EGCE) was able to directly bind to the peptidoglycan of S. aureus, thus affecting cell integrity.133 The bacterial cell wall peptidoglycan layer was essential for bacteria viability, which could offer important sites for interactions with antibiotics.23 For these reasons, polyphenols have also gained great attention as antibacterial agents for tissue engineering applications. For example, Guo et al. designed a bioadhesive (Gel–TA–SN) derived from the reaction of TA and gelatin under oxidizing conditions and then crosslinked by silver nitrate.42 TA could be conjugated to the gelatin side chain via a one-step Michael addition reaction under oxidizing conditions. The antimicrobial capabilities of the Gel–TA–SN hydrogels have been evaluated. After 24 h incubation with S. aureus and E. coli, the bacterial inhibition ratios of all the Gel–TA–SN groups were all close to 100%, while the control group was close to 0, indicating the strong antibacterial performances of the Gel–TA–SN hydrogels. Compared with conventional antibacterial approaches by doping antimicrobial agents into a biomaterial to combat infections, the Gel–TA–SN systems showed long-term sustained action from TA incorporated into the gelatin network.42
3. Fabrication strategies
3.1 Structure of polyphenol-based scaffolds
Based on many excellent properties and unique structures, polyphenols have been regarded as important synthons for the construction of various functional scaffolds. In general, polyphenol-containing scaffolds can be fabricated by coating, grafting, and blending polyphenols with biopolymers (Fig. 7). Coating polyphenols onto the scaffold surface could not only enhance the scaffold surface bioactivity for cell adhesion but can also immobilize bioactive materials for some special applications. Grafting or blending catechol/pyrogallol moieties into biopolymers can also introduce numerous bioactive properties into the scaffolds. The following sections focus on these three approaches towards the fabrication of polyphenol-based scaffolds.
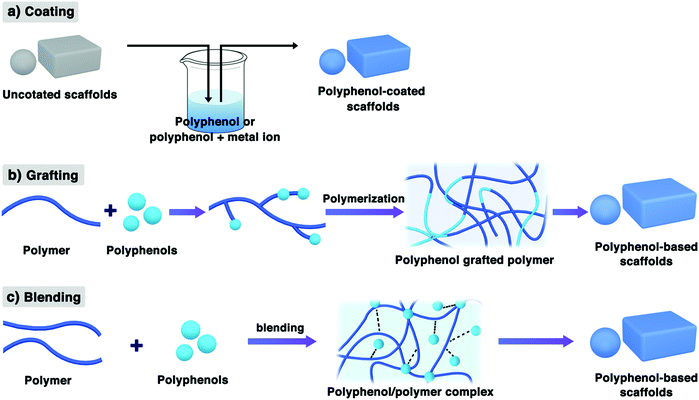 |
| Fig. 7 Schematic representation of the three fabrication methods towards polyphenol-based scaffolds. | |
Coating of polyphenol onto polymer scaffolds.
Surface chemistry is essential for improving the functionality of biomaterial scaffolds, which plays an important role in tissue engineering applications. Several typical methods have been applied for the surface modification of scaffolds to improve the cell affinity such as covalent chemical conjugation and physical adsorption.29 Although widely implemented in research, many established methods still have limitations for widespread practical use, where the chemical conjugation methods usually require multistep and complicated procedures such as surface activation or other means of activation through pretreatment with oxygen plasma, irradiation, or chemicals.134,135 Physical adsorption methods are sometimes inefficient and unstable. Therefore, the development of simple, reliable, and versatile strategies for scaffold surface modification is required to overcome the aforementioned shortcomings.
Based on the excellent properties discussed in the above sections, the coating of polyphenolic compounds onto the scaffold surface may provide an easy, environment-friendly, solvent-free, and highly efficient approach for surface functionalization.33,136,137 The simple immersion of substrates or scaffolds in a dilute aqueous solution of catechol- or pyrogallol-containing molecules buffered to an alkaline pH condition and the phenolic compounds can be oxidized to quinone intermediates and then self-polymerized to the oligomer, finally resulting in the spontaneous deposition of a thin film coating onto the scaffold substrates (Fig. 7a).33 This approach was regarded as a universal route towards the deposition of multifunctional coatings onto the surfaces.138–140 The resulting coatings could retain their excellent intrinsic biochemical properties, such as superior bioadhesion, antibacterial, and antioxidant properties. Also, polyphenols (e.g., TA and EGCG) can coordinate with various metal cations, such as Fe3+ or Cu2+, which also could result in metal-polyphenolic networks.27,141 Tsai et al. showed that PDA coating surfaces enhanced the cell affinity towards several types of biodegradable polymer surfaces, such as PLLA, PCL, PLGA, and PU.18 Interestingly, the authors have also studied the impact of PDA deposition on chondrocyte adhesion for various DA monomer incubation times. The results showed that PDA deposition for even 5 min was sufficient for full cellular spreading, which suggested that the DA coating time should be as short as possible to minimize the thickness of the PDA layer. A lengthy PDA coating is not necessary and may be undesirable for tissue engineering applications.18
In addition to DA, pyrogallol-containing compounds have also been observed to undergo oxidative self-polymerization under weakly alkaline conditions and to form multifunctional coatings on various substrates. Sileika et al. performed a series of tests on the coating potentials of pure pyrogallol analogs such as PG, EGCG, epicatechin gallate (ECG), as well as the crude extracts of natural polyphenols such as red wine (RWE), cacao bean (CBE), and green tea (GTE) (Fig. 8a).142 The immersion of polycarbonate substrates into buffered saline of each compound could generate a uniform colorless polyphenol coating on the surface. The resulting coatings commonly exhibited antibacterial and antioxidant properties. Interestingly, the thickness of the coated layer increased with the incubation time and the coating behaviors of TA and PG were quite different (Fig. 8b). The maximum thickness of approximately 20 nm occurred after 8 h of incubation in PG and 65 nm in TA (2 mg mL−1 in buffered saline).
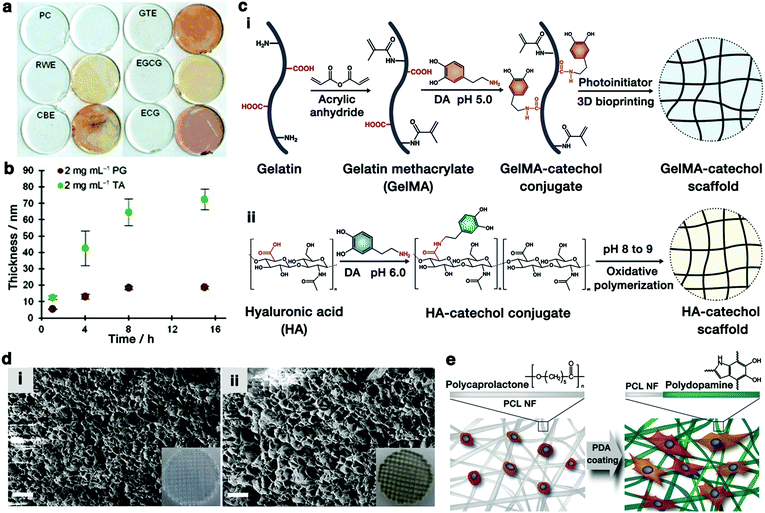 |
| Fig. 8 (a) Coatings of various natural polyphenols deposited onto clear PC disks. In each pair of disks, the right image is a visualization of the thin-layer coatings after treating with silver nitrate. (b) Thickness of the coating layer as a function of time: PG on gold and TA on TiO2. Reproduced with permission.142 Copyright 2013, Wiley-VCH. (c) Schematic diagram of the preparation of (i) 3D-bioprinted GelMA-DA (GelMA-catechol) scaffold46 and (ii) HA-catechol scaffold.31 (d) SEM images (cross-sectional view) of (i) GelMA and (ii) GelMA–DA porous scaffold (scale bar = 10 μm). The inset images are photographs of the corresponding scaffolds. Reproduced with permission.46 Copyright 2018, American Chemical Society. (e) Schematic representation of cell adhesion on PDA-coated PCL nanofibers (PCL NFs). Reproduced with permission.159 Copyright 2010, Elsevier. | |
Polyphenol-grafted polymers.
Polyphenol-grafted polymers were also widely used to fabricate desirable scaffolds. Many researchers have reported that phenolic molecules with a pendant amine group (e.g., DA and NE) can be introduced onto the polymeric backbone via covalent bonding reactions (Fig. 7b).143–146 The obtained polyphenol-conjugated biopolymers can be further employed to fabricate functional scaffolds by coating onto the scaffold surfaces or crosslinking into hydrogels.30,31,147,148 For instance, Zhou et al. fabricated gelatin methacrylate-DA (GelMA-DA) neural scaffolds by 3D-bioprinting using a custom-designed stereolithography-based printer.46 To prepare the biocompatible printable ink (GelMA-DA), DA was coupled with the carboxyl group of GelMA via a covalent bonding reaction. Subsequently, this ink was 3D-printed through photo-cross-linking under UV light to obtain the GelMA-DA scaffolds (Fig. 8c-i). Interestingly, as shown in Fig. 8d, the apparent pore size and the porous framework in the GelMA-DA hydrogel were looser than those in the GelMA hydrogel. GelMA-DA was synthesized from GelMA in an acidic environment (pH 5.0), leading to a relatively lower cross-linking density. Compared to the GelMA hydrogel, the GelMA-DA hydrogel possessed larger pore sizes and greater swelling ability, which could benefit nutrient storage and enhance cell proliferation or differentiation.46 In another work, Hong et al. fabricated the HA-catechol hydrogels via catechol chemistry. Under acidic conditions (pH ≈ 6), DA was coupled with the carboxyl group of HA to prepare the HA-catechol conjugate.31 Subsequently, the HA-catechol conjugate directly formed a hydrogel via the chemical crosslinking between the conjugated catechol moieties in an alkaline buffer (pH 8 to 9) (Fig. 8c-ii). This catechol crosslinking strategy has also been widely applied to prepare bioactive scaffolds by direct coupling of either amine or carboxyl-functionalized phenol-containing molecules onto the biomacromolecules (e.g., alginate, CS, gelatin, and HA) at the first step, followed by the oxidative cross-linking of the catechol/pyrogallol groups under alkaline conditions. Importantly, this method provided a convenient and environment-friendly route towards polyphenol-conjugated scaffolds.55,147,148
Polyphenol/polymer complexes.
The physical blending of polymeric biomaterials with polyphenols represented another effective way to improve the materials’ bioactivity for tissue engineering applications (Fig. 7c). For instance, Dhand et al. designed bone-like composite structures by the electrospinning of collagen-doping catecholamines (i.e., DA and NE) and CaCl2, followed by the exposure to ammonium carbonate.51 The presence of hydrogen bonding interactions between catecholamines and collagen could promote inter-fiber adhesion and form welded junctions between the fibers. Besides, during electrospinning, divalent cations (Ca2+) could trigger the partial oxidative polymerization of catecholamines to polycatecholamines with inherently adhesive properties, thus leading to a dense collagen network with soldered junctions. Subsequent mineralization by exposing the mats to (NH4)2CO3 further induced the oxidative polymerization of catecholamines and the in situ precipitation of CaCO3. As a result, this in situ crosslinked collagen–CaCO3–catecholamine composite scaffold could display enhanced bone cell attachment, proliferation, differentiation, and expression of osteogenic proteins compared to the pristine collagen.51
3.2 Classification of polyphenol-based scaffolds
Biopolymeric scaffolds are fabricated into macrostructures with different shapes and functions to mimic the nature of the ECM of the tissues. To achieve desirable properties, polymeric scaffolds are usually fabricated into different kinds of forms with distinct morphologies, including hydrogels, films, and nanofibers. These polyphenol-based scaffolds will be discussed in this section.
Hydrogels.
Hydrogels are 3D cross-linked networks of polymer chains that can absorb a large amount of water without dissolving. Due to their structural similarity to natural soft tissues and ECM, hydrogels have been widely used in numerous applications such as tissue engineering and drug delivery.10,149–151 More importantly, hydrogels can be injected into the body, which enable the direct delivery of cells or other bioactive components in a minimally invasive manner. Hydrogels can usually be divided into natural hydrogels (e.g., HA, collagen, CS, fibrin, and gelatin) and synthetic hydrogels (such as PEG, polyethylene oxide (PEO), and poly(vinyl alcohol) (PVA)).1,10 Although various types of hydrogels have been successfully used for tissue engineering,1,3 their physical and biological properties can still be significantly improved by the involvement of polyphenolic molecules.
So far, many efforts have been devoted to the fabrication of polyphenol-based composite hydrogels via catechol- or pyrogallol-based chemistry. For example, a variety of catechol-conjugated hydrogels including heparin–catechol, HA–catechol, and gelatin–catechol have been reported.31,152 The phenolic moieties not only improve the bioactivity of the biopolymers but also serve to crosslink with biomolecules to develop a 3D hydrogel. For instance, Lee et al. prepared divalent ion-free alginate hydrogels by conjugating DA to the alginate backbones (alginate-catechol) in the pH range of 4.5–5.5 and then the tethered catechol underwent oxidative cross-linking under basic conditions in the presence of a chemical oxidizing agent.30 The conjugated catechol groups could be oxidized to quinone, triggered by alkaline pH, and then the catechol–catechol dimer is formed, resulting in the crosslinked alginate-catechol hydrogel. The oxidizing agents, such as sodium periodate (NaIO4), used in this study, could accelerate the conversion of catechol to quinone, and facilitate polymerization and hydrogel formation. The resulting alginate-catechol hydrogels exhibited tunable biophysical and mechanical properties and improved the biocompatibility due to the absence of cations within the gel matrices.
Films.
Polymer films, a kind of 2D materials, could efficiently enable the attachment of the cells for cell growth and proliferation. Several strategies have been applied to fabricate bioactive polymer films, such as chemical-vapor-deposited coating,153 LbL assembly,35,44,75 and in situ polymerization on the surface.33 Recently, polyphenol-based functional films have attracted increasing interests in tissue engineering due to their excellent adhesive and biocompatible properties. Note that the polyphenol-based films could show several advantages towards tissue engineering applications: (1) the films are intrinsically adhesive, which are able to enhance cell attachment and spreading; (2) the surface of the films remains reactive to amine and thiol groups via Schiff-base reaction or Michael addition, which can be used to further create a variety of functional layers via secondary reactions. A typical example is that the PDA layers have been widely used to immobilize proteins or peptides, and growth factors to mimic ECM; (3) the films are usually hydrophilic, which is also an important factor for facilitating the attachment and proliferation of cells. All these advantages demonstrated the potential of the polyphenol films in tissue engineering.
Nanofibers.
Nanofibers are a kind of 1D materials with the diameter in few nanometers. They usually possess large surface-area-to-volume ratio, high porosity, and good interconnectivity, which are also similar to the features of natural ECM, and therefore, can be regarded as a class of ideal scaffolds for cell attachment and efficient mass transfer of nutrients and gases.4 There are several established strategies to fabricate nanofibers, such as electrospinning, template synthesis, self-assembly, and thermal-induced phase separation.2 Among them, electrospinning is the most commonly used method to prepare functional nanofibers owing to its simplicity, versatility, and the ability to generate ultrathin fibers with controllable compositions and diameters.51,154–157
In general, polyphenols can be introduced into the polymer nanofibers during the electrospinning process to generate polyphenol-containing nanofibrous scaffolds with improved properties. On the one hand, the strong covalent and non-covalent interactions of the catechol/pyrogallol groups with polymers can provide physically and chemically crosslinked networks, endowing the nanofibrous scaffolds with strong mechanical behaviors. On the other hand, the polyphenols can enhance the adhesive properties of the electrospun nanofibers and facilitate the infiltration of the culture media into the pores of the nanofibers in vitro, which could greatly promote cell attachment, penetration, and proliferation. In addition, polyphenol-containing nanofibrous scaffolds can also be directly fabricated by immersing the nanofibers into a polyphenol solution.158,159 Since the nanofibers are randomly oriented and present porous networking structures, the polyphenols can cover the surface of the 3D nanofiber mesh as a shell and enhance cell infiltration, adhesion, and proliferation (Fig. 8e).159
4. Applications
It has been demonstrated that the polyphenol-containing scaffolds can enhance cell adhesion, proliferation, and differentiation because of their excellent adhesive and biocompatible properties. These promising features allow these functional scaffolds to be applied in different kinds of tissue engineering applications, including wound healing, bone regeneration, and electroactive (e.g., cardiac myoblasts, skeletal myoblasts, and nerve cells) tissue engineering.
4.1 Wound healing
Skin is an important natural barrier organ for protecting the body against pathogens and excessive water loss and could lose its protective functions upon damages.160 A slight wound naturally heals through the wound healing process without any specific treatments. However, more severely damaged skin is quite difficult to heal naturally. If there is a bacterial infection present, severe wound inflammation and even death might occur.49 Therefore, facile and efficient strategies for promoting wound healing and curing skin damage and trauma are often highly desirable. In particular, polyphenol-functionalized materials are attracting distinguished attention in this area due to their intrinsic properties, such as tissue adhesion, hemostasis, radical scavenging, and antibacterial properties.
In general, the whole wound healing process is usually divided into four distinguished phases, including hemostasis, inflammation, proliferation, and remodeling.49,161 The polyphenol-functionalized wound dressing biomaterials could have great impacts on all the phases, particularly in the hemostasis phase. On the one hand, the incorporated phenolic compounds can act as a tissue sealant due to the tissue adhesion property to enhance the hemostasis effect;96,162 on the other hand, the catechol/pyrogallol groups can also form complexes with the serum proteins in the blood, which act as a physical barrier to effectively stop bleeding.95 As an example, a polyphenol-based hemostatic hydrogel has been developed by incorporating TA into DNA (Fig. 9a).50 The hydrogel was called TNA hydrogel (TA + DNA), where TA was used as a “molecular glue” to interact with the phosphate backbone of DNA. Owing to the rich polyphenol groups in TA and TNA with strong tissue adhesive property, it exhibited enhanced hemostatic capability due to the sealing effect of TA. The mouse liver bleeding model showed superior hemostatic capability (time to hemostasis: 53 s) compared to other groups (97 s for DNA) (Fig. 9b).50
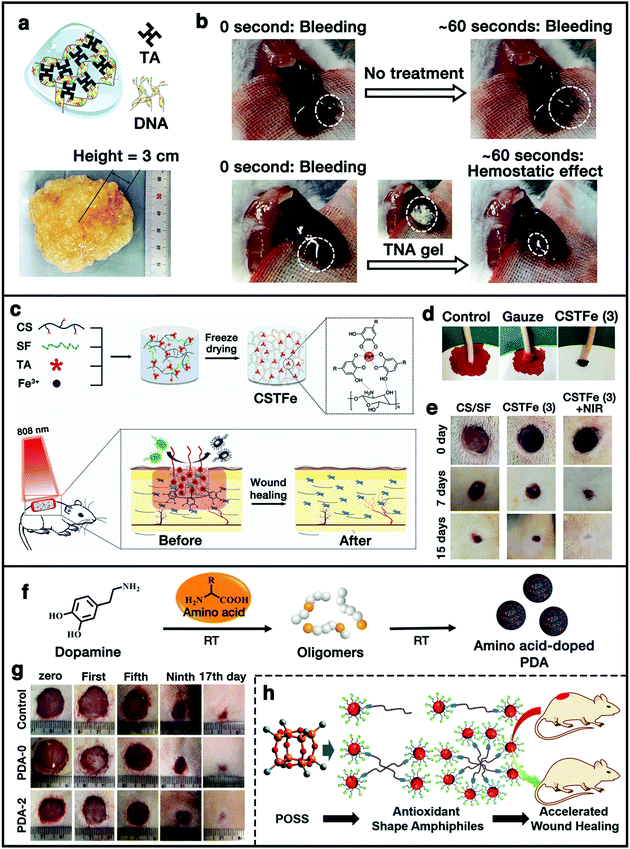 |
| Fig. 9 (a and b) Structure and hemostatic capability of the TA/DNA hydrogel. Reproduced with permission.50 Copyright 2015, Wiley-VCH. (c) Schematic illustration of the preparation of the cryogel and its application as a wound dressing material. (d) Hemostatic capacity evaluation of the cryogel. (e) Photographs of the wounds accompanied by an infection with S. aureus following cryogel treatment with and without NIR irradiation. Reproduced with permission.49 Copyright 2019, Wiley-VCH. (f) Synthesis of amino acid-doped PDA nanoparticles. (g) Optical images of wound recovery at first, fifth, ninth, and 17th day. Reproduced with permission.163 Copyright 2020, Chinese Chemical Society. (h) Schematic illustration of polyphenol-functionalized POSS-based antioxidant shape amphiphiles for accelerated wound healing. Reproduced with permission.98 Copyright 2020, The Royal Society of Chemistry. | |
Besides the hemostasis effect, phenolic compounds can act as efficient antibacterial agents to inhibit the growth of bacteria. Moreover, phenolic compounds also possess high efficiency of light-heat conversion, and thus, can be used as photothermal agents for antibacterial therapy by converting light energy to heat energy under the irradiation of an NIR laser. In addition, the bioactive polyphenol is also beneficial for cell proliferation. Yu et al. developed a multifunctional wound dressing cryogel by mixing CS, SF, TA, and ferric ion (Fe3+) via the freeze-drying method (Fig. 9c).49 Apart from the excellent blood absorption and blood clotting property (Fig. 9d), the cryogel also exhibited excellent antibacterial activity towards both Gram-negative and positive bacteria, which was attributed to the high photothermal behavior of the TA/Fe3+ complex. In addition, the animal study further demonstrated that the bioactive cryogel could promote cell proliferation, and enhance wound repair and skin regeneration (Fig. 9e).49 More recently, we reported a facile fabrication approach towards synthetic melanin-like PDA nanoparticles with tunable structure and antioxidant properties via the copolymerization of DA and basic amino acid (Fig. 9f).163 The doped amino acid could destroy the compact π-stacked microstructures within PDA, resulting in enhanced free-radical scavenging and antioxidant properties. A cutaneous wound model demonstrated that the arginine-doped PDA nanoparticles have the potential to protect the cells from ROS-induced damage and accelerate the wound healing process (Fig. 9g). In another work, we synthesized a family of polyphenol-functionalized POSS-based shape amphiphiles with various macromolecular architectures for efficient and accelerated wound healing (Fig. 9h).98 Multiple GA units were incorporated onto the POSS head of the shape amphiphiles via thiol–ene “click” chemistry to directly introduce the antioxidant feature. Also, several kinds of azido-functionalized PEG (PEG-(N3)n) chains were used to couple with alkyne-functionalized POSS nanoparticles to afford shape amphiphilic products, which also greatly promoted the biocompatibility and solubility of the whole systems in water. Animal studies were performed to further confirm the efficiency of the antioxidant shape amphiphiles in the acceleration of the wound healing process. It was observed that the wound area treated with the 500 μg mL−1 antioxidant sample showed almost full closure at 15 d, while ∼10% of the wound area in the control group did not heal, which indicated that the polyphenol-functionalized POSS-based antioxidant shape amphiphile materials could promote wound healing with a faster speed. All the results above suggested the promising utilization of polyphenol-containing functional scaffolds in wound dressing.
4.2 Bone regeneration
Bone fracture is one of the most critical public health issues, generally caused by traumatic bone injury, tumor resection, and osteitis. Owing to the drawbacks associated with autografts and allografts, bone tissue engineering as a promising therapeutic technique has been widely investigated and employed for repairing bone defects and reconstructing bone tissues.2,7,19 For bone tissue-engineering scaffolds, osteogenic properties are needed for promoting stem cell-mediated osteogenesis as well as proper physical and mechanical microenvironments. To this end, the scaffolds are usually modified with osteoinductive growth factors or other biomolecules.
Polyphenols can also be applied as a versatile affinity tag in the scaffolds for immobilizing proteins or growth factors on the biomaterials to mimic ECM, which offers new opportunities in bone tissue engineering. For instance, Ko et al. reported PDA-mediated immobilization of bone morphogenetic protein-2 (BMP-2)-derived peptides onto the PLGA scaffolds via catechol chemistry for enhancing the osteogenic differentiation of human adipose-derived stem cells (hADSCs) (Fig. 10a).29 They evaluated the osteogenic differentiation of hADSCs on the PDA–BMP-2–PLGA substrates in vitro. The results revealed that the expression of osteogenic markers (OPN and COL I) was increased in the hADSCs cultured on the PDA–BMP-2–PLGA substrates, indicating that the immobilization of BMP-2 peptides via PDA coating could enhance osteogenic differentiation and calcium mineralization of the hADSCs. Interestingly, the expression of osteogenic markers was significantly higher in the PDA–PLGA group than in the bare PLGA group, indicating that PDA can enhance the cellular activity, which was consistent with the discussions in the previous sections. Furthermore, the in vivo osteogenic differentiation of hADSCs has also been examined by the transplantation of hADSCs using PDA–BMP-2–PLGA scaffolds in the mouse model of the critically-sized calvarial bone defect. The PDA–BMP-2–PLGA scaffold could enhance mineralized tissue formation in the defects compared with the bare PLGA scaffolds, suggesting that the PDA–BMP-2–PLGA scaffolds could significantly promote bone formation in vivo.29
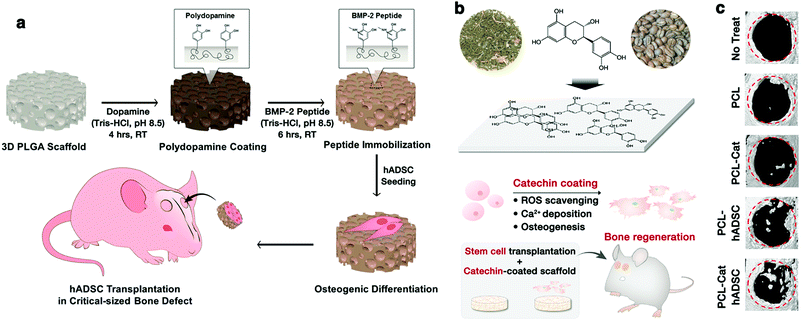 |
| Fig. 10 (a) Schematic illustration of PDA-assisted immobilization of osteoinductive BMP-2 peptides on PLGA scaffolds for enhancing osteogenic differentiation and bone formation of the hADSCs. Reproduced with permission.29 Copyright 2013, American Chemical Society. (b) Schematic illustration of the catechin hydrate surface coating and its multifunctional application in tissue engineering. (c) In vivo bone regeneration by hADSCs transplantation with catechin-coated PCL scaffolds in critically-sized calvarial bone defect mouse model. Cat: catechin. Reproduced with permission.52 Copyright 2017, American Chemical Society. | |
In another example, Lee et al. developed a functionalized tissue-engineering scaffold by surface modification via catechin coating for bone regeneration by the stem cells (Fig. 10b).52 The intrinsic biochemical functions of catechin endowed the scaffolds with antioxidant and calcium-binding abilities, resulting in improved adhesion, proliferation, mineralization, and osteogenic differentiation of the hADSCs. A mouse model with a critically-sized calvarial bone defect demonstrated that catechin-coated polymer nanofiber scaffolds significantly promoted in vivo bone formation by hADSCs transplantation (Fig. 10c). In addition, biomaterial scaffolds for bone regeneration usually require high biomechanical properties when used in higher-load bearing bone repair. It has been reported that polyphenols can also be employed as adhesion molecules to modify the scaffold surface so as to immobilize biological macromolecules for improving the biomechanical properties.47
4.3 Electroactive tissue engineering
It has been reported that the electroactivity of materials is a vital property that can enhance the proliferation and differentiation of electrical-signal-sensitive cells, such as cardiac myoblasts, skeletal myoblasts, and nerve cells. Therefore, mimicking the highly conductive properties of tissue is also critical in electroactive tissue engineering scaffold designs.81,164,165 To date, a variety of conducting hydrogels have been developed by combining conducting polymers with non-conducting hydrogels for electroactive tissue engineering applications. Due to the excellent adhesiveness and bioactivity, phenolic moieties can also act as a crosslinker, and thus can combine the intrinsic biochemical properties and high electroactivity of the conducting biopolymers into scaffolds.88,166 For instance, Jing et al. fabricated CS/GO composite hydrogels by the incorporation of PDA as cross-linking and reducing agents (Fig. 11a).34 The various covalent and non-covalent interactions endowed the composite hydrogels with strong adhesiveness. During the oxidative polymerization of DA, GO was partially reduced to conductive graphene by PDA, endowing the composite hydrogels with good electrical conductivity. The electrical conductivity of the hydrogels achieved a value of 1.22 mS cm−1, which matched with that of the native myocardium. Furthermore, this hydrogel could enhance the viability and proliferation of human embryonic stem cell-derived fibroblasts and cardiomyocytes, suggesting that the conductive hydrogels have great potential in electroactive tissue engineering applications.34
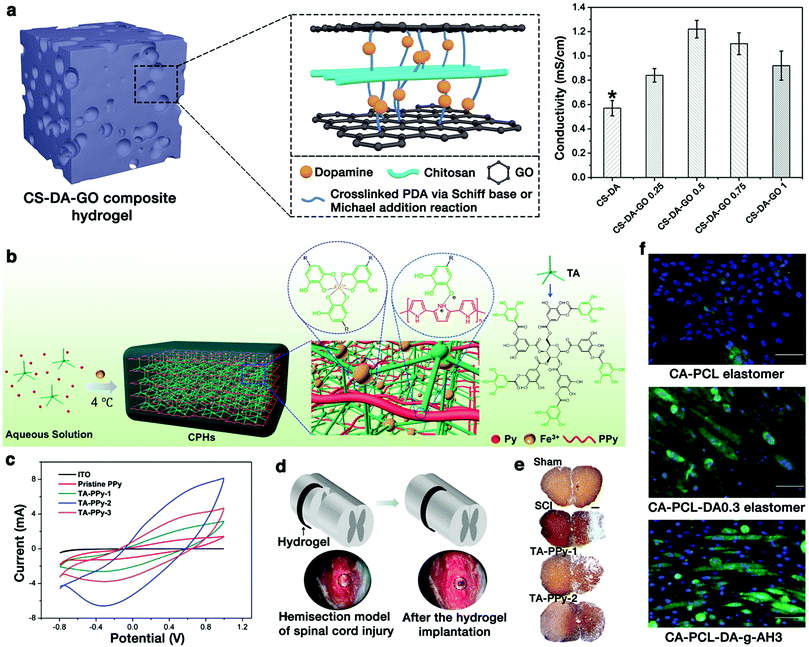 |
| Fig. 11 (a) Schematic illustration and conductivity results of the CS–DA–GO composite hydrogels. Reproduced with permission.34 Copyright 2017, Elsevier. (b) Schematic illustration of the formation of a CPH. (c) Cyclic voltammograms of the ITO bare electrode, pristine PPy, and different hydrogels. (d) A semitubular CPH was implanted as a bridge to cover the spinal cord hemisection gap. (e) Images of transverse spinal cord sections stained with Tuj1. Reproduced with permission.48 Copyright 2018, American Chemical Society. (f) Immunofluorescence staining of C2C12 cells cultured on different elastomers for 5 days. Reproduced with permission.19 Copyright 2017, American Chemical Society. | |
More recently, Zhou et al. developed a porous, highly conductive, and biocompatible conducting polymer hydrogel (CPH) using TA, Fe3+, and the conductive polypyrrole (PPy) chain for spinal cord injury (SCI) repair (Fig. 11b).48 The intermolecular electrostatic interactions between the protonated nitrogen groups of PPy and the phenol hydroxy groups of TA could lead to a rapid gelation process. An excess amount of Fe3+ ions promoted the polymerization of pyrrole and formed ionically cross-linked networks via coordinate interactions with TA. CPH exhibited an excellent electronic conductivity (0.05–0.18 S cm−1) (Fig. 11c), which was in the range of the conductivities for the spinal cord tissue (10−2 to 10−1 S cm−1). The therapeutic efficacy of CPHs in vivo was examined by implanting a “C”-shape, semi-tubular CPH patch as a highly conductive bridge across a spinal cord hemisection gap in a severe mouse SCI model (Fig. 11d). Compared to the control group, the higher electroactivity gel (TA-PPy-2) group promoted more nerve tissue preservation and significantly reduced the cavity size (Fig. 11e), suggesting that these hydrogels could promote the SCI repair in vivo.
In another study, the DA-incorporated composite scaffold based on a hexamethylene diisocyanate (HDI)-crosslinked poly(citric acid-co-PCL-co-DA) (CA–PCL–DA) prepolymer and an aniline hexamer (AH) was developed by Ma and coworkers.19 For the scaffold system, PDA was chemically grafted into the PU elastomers’ networks to enhance the materials’ bioactivity, while aniline oligomers were introduced to improve the materials’ electroactivity. The authors chose C2C12 cells to evaluate the effects of these elastomers on the myogenic differentiation capacity of myoblasts. As shown in Fig. 11f, the myotube number was much higher on CA–PCL–DA and CA–PCL–DA-g-AH3 than on the CA–PCL film, indicating the vital role that DA and HA play in promoting myogenic differentiation. Catechol groups could enhance the C2C12 cells’ adhesion. The AH segment could endow the film with electrical conductivity, which can manipulate the cell behavior, such as protein distribution, gene expression, and action potential. This dual bioactive PU elastomer, simultaneously possessing bioactive catechol groups and electroactivity, synergistically promoted the myogenic differentiation of C2C12 cells, revealing that this polyphenol-incorporated biomaterial demonstrated a great potential for skeletal muscle tissue engineering.19
5. Summary and perspectives
In summary, polyphenol-based biomaterial scaffolds have been widely investigated because of their multiple reactive-groups and excellent functional properties including adhesiveness, antioxidant properties, antimicrobial capabilities, and biocompatible properties. With specific design and fabrication strategies, they could be used as promising tools for tissue engineering applications. Although many achievements have been well documented, there are still many challenges that should be taken into account in the future so as to advance this important field of research. Firstly, due to the high chemical reactivity of phenolic moieties, polyphenols are easily oxidized in air. Thus, exploring the long-term stability of polyphenol-based scaffolds and/or their storage might be a critical issue. Secondly, the choice of polyphenols for designing scaffolds is still blind in most cases due to the imprecise regulation and unclear theoretical basis of the structure–function performances. For this reason, detailed investigations on the structure–function relationship between catechol- and pyrogallol-containing molecules should be seriously considered prior to the construction of novel functional polyphenol-based scaffolds. Thirdly, the fabrication of most polyphenol-based scaffolds usually requires sophisticated chemical synthesis operations such as protection/deprotection reactions. More efforts on the development of simple and low-cost fabrication strategies (such as one-pot approach) of polyphenol-based scaffolds are still highly desirable. Finally, owing to the widely distribution in living species such as plants or animals, apart from the above widely studied functions, polyphenols (e.g., DA, epinephrine, and grape polyphenols) also possess many other excellent properties that play vital roles in a variety of biological and biochemical processes. Thus, the exploration of more potential properties of polyphenols and the design of biomimetic polyphenol-based scaffolds is also one of the future trends. For the ultimate goal of clinical translation of tissue engineering, further innovations are essential regarding the development of more kinds of polyphenol-based tissue engineering scaffolds with promising bioactive properties.
Conflicts of interest
The authors declare no conflict of interest.
Acknowledgements
The authors are indebted to many scientists whose research results are cited here. This work was supported by the National Natural Science Foundation of China (21774079 and 21975167), the National Key R&D Program of China (2019YFA0904500), the Program of the Science & Technology Department of Guangzhou, China (201803020039), and the Fundamental Research Funds for Central Universities.
References
- K. Y. Lee and D. J. Mooney, Chem. Rev., 2001, 101, 1869–1879 CrossRef CAS
.
- J. M. Holzwarth and P. X. Ma, Biomaterials, 2011, 32, 9622–9629 CrossRef CAS
.
- R. Langer and J. Vacanti, Science, 1993, 260, 920–926 CrossRef CAS
.
- D. Sundaramurthi, U. M. Krishnan and S. Sethuraman, Polym. Rev., 2014, 54, 348–376 CrossRef CAS
.
- H. Yu, J. Peng, Y. Xu, J. Chang and H. Li, ACS Appl. Mater. Interfaces, 2016, 8, 703–715 CrossRef CAS
.
- O. Lam Van Ba, S. Aharony, O. Loutochin and J. Corcos, Adv. Drug Delivery Rev., 2015, 82, 31–37 CrossRef
.
- J. Malda, J. Visser, F. P. Melchels, T. Jüngst, W. E. Hennink, W. J. A. Dhert, J. Groll and D. W. Hutmacher, Adv. Mater., 2013, 25, 5011–5028 CrossRef CAS
.
- P. Kord Forooshani and B. P. Lee, J. Polym. Sci., Part A: Polym. Chem., 2017, 55, 9–33 CrossRef CAS
.
- Z. Li, J. Hu, L. Yang, X. Zhang, X. Liu, Z. Wang and Y. Li, Nanoscale, 2020, 12, 11395–11415 RSC
.
- Y. Zou, L. Zhang, L. Yang, F. Zhu, M. Ding, F. Lin, Z. Wang and Y. Li, J. Controlled Release, 2018, 273, 160–179 CrossRef CAS
.
- P. B. Malafaya, G. A. Silva and R. L. Reis, Adv. Drug Delivery Rev., 2007, 59, 207–233 CrossRef CAS
.
- M. Li, W. Liu, J. Sun, Y. Xianyu, J. Wang, W. Zhang, W. Zheng, D. Huang, S. Di, Y.-Z. Long and X. Jiang, ACS Appl. Mater. Interfaces, 2013, 5, 5921–5926 CrossRef CAS
.
- L. Tytgat, L. Van Damme, J. Van Hoorick, H. Declercq, H. Thienpont, H. Ottevaere, P. Blondeel, P. Dubruel and S. Van Vlierberghe, Acta Biomater., 2019, 94, 340–350 CrossRef CAS
.
- H. S. Yoo, E. A. Lee, J. J. Yoon and T. G. Park, Biomaterials, 2005, 26, 1925–1933 CrossRef CAS
.
- S. Lee, J. H. Ryu, M. J. Do, E. Namkoong, H. Lee and K. Park, ACS Appl. Mater. Interfaces, 2020, 12, 4285–4294 CrossRef CAS
.
- X. Jing, H.-Y. Mi, X.-C. Wang, X.-F. Peng and L.-S. Turng, ACS Appl. Mater. Interfaces, 2015, 7, 6955–6965 CrossRef CAS
.
- T. Miao, J. Wang, Y. Zeng, G. Liu and X. Chen, Adv. Sci., 2018, 5, 1700513 CrossRef
.
- W. B. Tsai, W. T. Chen, H. W. Chien, W. H. Kuo and M. J. Wang, Acta Biomater., 2011, 7, 4187–4194 CrossRef CAS
.
- X. Zhao, R. Dong, B. Guo and P. X. Ma, ACS Appl. Mater. Interfaces, 2017, 9, 29595–29611 CrossRef CAS
.
- F. Reitzer, M. Allais, V. Ball and F. Meyer, Adv. Colloid Interface Sci., 2018, 257, 31–41 CrossRef CAS
.
- A. Shavandi, A. E.-D. A. Bekhit, P. Saeedi, Z. Izadifar, A. A. Bekhit and A. Khademhosseini, Biomaterials, 2018, 167, 91–106 CrossRef CAS
.
- H. Wang, C. Wang, Y. Zou, J. Hu, Y. Li and Y. Cheng, Giant, 2020, 3, 100022 CrossRef
.
- C. Papuc, G. V. Goran, C. N. Predescu, V. Nicorescu and G. Stefan, Compr. Rev. Food Sci. Food Saf., 2017, 16, 1243–1268 CrossRef CAS
.
- S. Oliver, O. Vittorio, G. Cirillo and C. Boyer, Polym. Chem., 2016, 7, 1529–1544 RSC
.
- Q. Dai, H. Geng, Q. Yu, J. Hao and J. Cui, Theranostics, 2019, 9, 3170–3190 CrossRef CAS
.
- A. H. Hofman, I. A. van Hees, J. Yang and M. Kamperman, Adv. Mater., 2018, 30, 1704640 CrossRef
.
- M. A. Rahim, S. L. Kristufek, S. Pan, J. J. Richardson and F. Caruso, Angew. Chem., Int. Ed., 2019, 58, 1904–1927 CrossRef CAS
.
- Z. Li, T. Wang, F. Zhu, Z. Wang and Y. Li, Chin. Chem. Lett., 2020, 31, 783–786 CrossRef CAS
.
- E. Ko, K. Yang, J. Shin and S.-W. Cho, Biomacromolecules, 2013, 14, 3202–3213 CrossRef CAS
.
- C. Lee, J. Shin, J. S. Lee, E. Byun, J. H. Ryu, S. H. Um, D. I. Kim, H. Lee and S. W. Cho, Biomacromolecules, 2013, 14, 2004–2013 CrossRef CAS
.
- S. Hong, K. Yang, B. Kang, C. Lee, I. T. Song, E. Byun, K. I. Park, S.-W. Cho and H. Lee, Adv. Funct. Mater., 2013, 23, 1774–1780 CrossRef CAS
.
- H. A. Lee, E. Park and H. Lee, Adv. Mater., 2020, 1907505 CrossRef CAS
.
- H. Lee, S. M. Dellatore, W. M. Miller and P. B. Messersmith, Science, 2007, 318, 426–430 CrossRef CAS
.
- X. Jing, H. Y. Mi, B. N. Napiwocki, X. F. Peng and L. S. Turng, Carbon, 2017, 125, 557–570 CrossRef CAS
.
- Z. Wang, C. Li, J. Xu, K. Wang, X. Lu, H. Zhang, S. Qu, G. Zhen and F. Ren, Chem. Mater., 2015, 27, 848–856 CrossRef CAS
.
- J. H. Cho, J. S. Lee, J. Shin, E. J. Jeon, S. An, Y. S. Choi and S. Cho, Adv. Funct. Mater., 2018, 28, 1705244 CrossRef
.
- X. Liu, H. Zhang, R. Cheng, Y. Gu, Y. Yin, Z. Sun, G. Pan, Z. Deng, H. Yang, L. Deng, W. Cui, H. A. Santos and Q. Shi, Mater. Horiz., 2018, 5, 1082–1091 RSC
.
- K. Yang, J. S. Lee, J. Kim, Y. Bin Lee, H. Shin, S. H. Um, J. B. Kim, K. I. Park, H. Lee and S. W. Cho, Biomaterials, 2012, 33, 6952–6964 CrossRef CAS
.
- S. Hong, J. H. Sunwoo, J. S. Kim, H. Tchah and C. Hwang, Biomater. Sci., 2019, 7, 139–148 RSC
.
- Z. Wang, Y. Zou, Y. Li and Y. Cheng, Small, 2020, 16, 1907042 CrossRef CAS
.
- Z. Xu, N. Wang, P. Liu, Y. Sun, Y. Wang, F. Fei, S. Zhang, J. Zheng and B. Han, Molecules, 2019, 24, 4397 CrossRef CAS
.
- J. Guo, W. Sun, J. P. Kim, X. Lu, Q. Li, M. Lin, O. Mrowczynski, E. B. Rizk, J. Cheng, G. Qian and J. Yang, Acta Biomater., 2018, 72, 35–44 CrossRef CAS
.
- D. Choi, M. Komeda, J. Heo, J. Hong, M. Matsusaki and M. Akashi, ACS Biomater. Sci. Eng., 2018, 4, 1833–1842 CAS
.
- C. M. Valmikinathan, W. Chang, J. Xu and X. Yu, Biofabrication, 2012, 4, 035006 CrossRef
.
- W. Kim and G. H. Kim, Chem. Eng. J., 2018, 334, 2308–2318 CrossRef CAS
.
- X. Zhou, H. Cui, M. Nowicki, S. Miao, S.-J. Lee, F. Masood, B. T. Harris and L. G. Zhang, ACS Appl. Mater. Interfaces, 2018, 10, 8993–9001 CrossRef CAS
.
- X. Wang, Z. Gu, B. Jiang, L. Li and X. Yu, Biomater. Sci., 2016, 4, 678–688 RSC
.
- L. Zhou, L. Fan, X. Yi, Z. Zhou, C. Liu, R. Fu, C. Dai, Z. Wang, X. Chen, P. Yu, D. Chen, G. Tan, Q. Wang and C. Ning, ACS Nano, 2018, 12, 10957–10967 CrossRef CAS
.
- Y. Yu, P. Li, C. Zhu, N. Ning, S. Zhang and G. J. Vancso, Adv. Funct. Mater., 2019, 29, 1904402 CrossRef
.
- M. Shin, J. H. Ryu, J. P. Park, K. Kim, J. W. Yang and H. Lee, Adv. Funct. Mater., 2015, 25, 1270–1278 CrossRef CAS
.
- C. Dhand, S. T. Ong, N. Dwivedi, S. M. Diaz, J. R. Venugopal, B. Navaneethan, M. H. U. T. Fazil, S. Liu, V. Seitz, E. Wintermantel, R. W. Beuerman, S. Ramakrishna, N. K. Verma and R. Lakshminarayanan, Biomaterials, 2016, 104, 323–338 CrossRef CAS
.
- J. S. Lee, J. S. Lee, M. S. Lee, S. An, K. Yang, K. Lee, H. S. Yang, H. Lee and S.-W. Cho, Chem. Mater., 2017, 29, 4375–4384 CrossRef CAS
.
- J. P. Chow, D. T. Simionescu, H. Warner, B. Wang, S. S. Patnaik, J. Liao and A. Simionescu, Biomaterials, 2013, 34, 685–695 CrossRef CAS
.
- L. Q. Xu, K.-G. Neoh and E.-T. Kang, Prog. Polym. Sci., 2018, 87, 165–196 CrossRef CAS
.
- W. Zhang, R. Wang, Z. Sun, X. Zhu, Q. Zhao, T. Zhang, A. Cholewinski, F. K. Yang, B. Zhao, R. Pinnaratip, P. K. Forooshani and B. P. Lee, Chem. Soc. Rev., 2020, 49, 433–464 RSC
.
- H. H. Kristoffersen, J. E. Shea and H. Metiu, J. Phys. Chem. Lett., 2015, 6, 2277–2281 CrossRef CAS
.
- S. Yamamoto, S. Uchiyama, T. Miyashita and M. Mitsuishi, Nanoscale, 2016, 8, 5912–5919 RSC
.
- Z. Jia, Y. Zeng, P. Tang, D. Gan, W. Xing, Y. Hou, K. Wang, C. Xie and X. Lu, Chem. Mater., 2019, 31, 5625–5632 CrossRef CAS
.
- J. Guo, B. L. Tardy, A. J. Christofferson, Y. Dai, J. J. Richardson, W. Zhu, M. Hu, Y. Ju, J. Cui, R. R. Dagastine, I. Yarovsky and F. Caruso, Nat. Nanotechnol., 2016, 11, 1105–1111 CrossRef CAS
.
- F. Cavalieri, E. Colombo, E. Nicolai, N. Rosato and M. Ashokkumar, Mater. Horiz., 2016, 3, 563–567 RSC
.
- K. Li, G. Xiao, J. J. Richardson, B. L. Tardy, H. Ejima, W. Huang, J. Guo, X. Liao and B. Shi, Adv. Sci., 2019, 6, 1801688 CrossRef
.
- H. Ejima, J. J. Richardson, K. Liang, J. P. Best, M. P. van Koeverden, G. K. Such, J. Cui and F. Caruso, Science, 2013, 341, 154–157 CrossRef CAS
.
- Z. Yang, Q. Tu, Y. Zhu, R. Luo, X. Li, Y. Xie, M. F. Maitz, J. Wang and N. Huang, Adv. Healthcare Mater., 2012, 1, 548–559 CrossRef CAS
.
- C. Wang, H. Sang, Y. Wang, F. Zhu, X. Hu, X. Wang, X. Wang, Y. Li and Y. Cheng, Nano Lett., 2018, 18, 7045–7051 CrossRef CAS
.
- X. Cheng, M. Li, H. Wang and Y. Cheng, Chin. Chem. Lett., 2020, 31, 869–874 CrossRef CAS
.
- S. Liu, J. Pan, J. Liu, Y. Ma, F. Qiu, L. Mei, X. Zeng and G. Pan, Small, 2018, 14, 1703968 CrossRef
.
- C. Zhang, B. Wu, Y. Zhou, F. Zhou, W. Liu and Z. Wang, Chem. Soc. Rev., 2020, 49, 3605–3637 RSC
.
- J. Guo, H. Sun, K. Alt, B. L. Tardy, J. J. Richardson, T. Suma, H. Ejima, J. Cui, C. E. Hagemeyer and F. Caruso, Adv. Healthcare Mater., 2015, 4, 1796–1801 CrossRef CAS
.
- A. Gennari, C. Gujral, E. Hohn, E. Lallana, F. Cellesi and N. Tirelli, Bioconjugate Chem., 2017, 28, 1391–1402 CrossRef CAS
.
- L. He, D. E. Fullenkamp, J. G. Rivera and P. B. Messersmith, Chem. Commun., 2011, 47, 7497–7499 RSC
.
- A. R. Narkar, B. Barker, M. Clisch, J. Jiang and B. P. Lee, Chem. Mater., 2016, 28, 5432–5439 CrossRef CAS
.
- W. J. Yang, P. Zhou, L. Liang, Y. Cao, J. Qiao, X. Li, Z. Teng and L. Wang, ACS Appl. Mater. Interfaces, 2018, 10, 18560–18573 CrossRef CAS
.
- W. L. A. Brooks and B. S. Sumerlin, Chem. Rev., 2016, 116, 1375–1397 CrossRef CAS
.
- T. Steiner, Angew. Chem., Int. Ed., 2002, 41, 48–76 CrossRef CAS
.
- A. Sundaramurthy, M. Vergaelen, S. Maji, R. Auzély-Velty, Z. Zhang, B. G. De Geest and R. Hoogenboom, Adv. Healthcare Mater., 2014, 3, 2040–2047 CrossRef CAS
.
- M. Shin, J. H. Galarraga, M. Y. Kwon, H. Lee and J. A. Burdick, Acta Biomater., 2019, 95, 165–175 CrossRef CAS
.
- Y. Shen, F. Zhu, W. Shen, Q. Fan, Y. Li and Y. Cheng, Chem. J. Chinese U, 2020, 41, 633–638 Search PubMed
.
- K. Li, L. N. Skolrood, T. Aytug, H. Tekinalp and S. Ozcan, ACS Sustainable Chem. Eng., 2019, 7, 14341–14346 CrossRef CAS
.
- L. Liu, H. Shi, H. Yu, R. Zhou, J. Yin and S. Luan, Biomater. Sci., 2019, 7, 5035–5043 RSC
.
- X. Shi, P. Yang, X. Peng, C. Huang, Q. Qian, B. Wang, J. He, X. Liu, Y. Li and T. Kuang, Polymer, 2019, 170, 65–75 CrossRef CAS
.
- H. Yan, L. Li, Z. Wang, Y. Wang, M. Guo, X. Shi, J.-M. Yeh and P. Zhang, ACS Biomater. Sci. Eng., 2020, 6, 634–646 CrossRef CAS
.
- N. Pandey, A. Hakamivala, C. Xu, P. Hariharan, B. Radionov, Z. Huang, J. Liao, L. Tang, P. Zimmern, K. T. Nguyen and Y. Hong, Adv. Healthcare Mater., 2018, 7, 1701069 CrossRef
.
- D. X. Oh, S. Kim, D. Lee and D. S. Hwang, Acta Biomater., 2015, 20, 104–112 CrossRef CAS
.
- Q. Ye, F. Zhou and W. Liu, Chem. Soc. Rev., 2011, 40, 4244–4258 RSC
.
- A. Cholewinski, F. (Kuo) Yang and B. Zhao, Mater. Horiz., 2019, 6, 285–293 RSC
.
- L. Liu, X. Tian, Y. Ma, Y. Duan, X. Zhao and G. Pan, Angew. Chem., 2018, 130, 8004–8008 CrossRef
.
- J. Cui, Y. Wang, A. Postma, J. Hao, L. Hosta-Rigau and F. Caruso, Adv. Funct. Mater., 2010, 20, 1625–1631 CrossRef CAS
.
- L. Han, X. Lu, M. Wang, D. Gan, W. Deng, K. Wang, L. Fang, K. Liu, C. W. Chan, Y. Tang, L.-T. Weng and H. Yuan, Small, 2017, 13, 1601916 CrossRef
.
- K. Zhan, C. Kim, K. Sung, H. Ejima and N. Yoshie, Biomacromolecules, 2017, 18, 2959–2966 CrossRef CAS
.
- X. Chen, C. Cortez-Jugo, G. H. Choi, M. Björnmalm, Y. Dai, P. J. Yoo and F. Caruso, Bioconjugate Chem., 2017, 28, 75–80 CrossRef CAS
.
- W. Cheng, X. Zeng, H. Chen, Z. Li, W. Zeng, L. Mei and Y. Zhao, ACS Nano, 2019, 13, 8537–8565 CrossRef CAS
.
- H.-C. Yang, R. Z. Waldman, M.-B. Wu, J. Hou, L. Chen, S. B. Darling and Z.-K. Xu, Adv. Funct. Mater., 2018, 28, 1705327 CrossRef
.
- Y. Hu, W. Dan, S. Xiong, Y. Kang, A. Dhinakar, J. Wu and Z. Gu, Acta Biomater., 2017, 47, 135–148 CrossRef CAS
.
- E. Kim, S. Lee, S. Hong, G. Jin, M. Kim, K. I. Park, H. Lee and J.-H. Jang, ACS Appl. Mater. Interfaces, 2014, 6, 8288–8294 CrossRef CAS
.
- M. Shin, J. H. Ryu, K. Kim, M. J. Kim, S. Jo, M. S. Lee, D. Y. Lee and H. Lee, ACS Biomater. Sci. Eng., 2018, 4, 2314–2318 CrossRef CAS
.
- M. Shin, S.-G. Park, B.-C. Oh, K. Kim, S. Jo, M. S. Lee, S. S. Oh, S.-H. Hong, E.-C. Shin, K.-S. Kim, S.-W. Kang and H. Lee, Nat. Mater., 2017, 16, 147–152 CrossRef CAS
.
- S. Xiang, P. Yang, H. Guo, S. Zhang, X. Zhang, F. Zhu and Y. Li, Macromol. Rapid Commun., 2017, 38, 1700446 CrossRef
.
- Z. Li, J. Zhang, Y. Fu, L. Yang, F. Zhu, X. Liu, Z. Gu and Y. Li, J. Mater. Chem. B, 2020, 8, 7018–7023 RSC
.
- J. Zhang, Y. Fu, P. Yang, X. Liu, Y. Li and Z. Gu, Adv. Mater. Interfaces, 2020, 2000632 CrossRef CAS
.
- H. Liu, Y. Yang, Y. Liu, J. Pan, J. Wang, F. Man, W. Zhang and G. Liu, Adv. Sci., 2020, 7, 1903129 CrossRef CAS
.
- J. Hu, L. Yang, P. Yang, S. Jiang, X. Liu and Y. Li, Biomater. Sci., 2020, 8, 4940–4950 RSC
.
- P. Wang, J. Liu, X. Luo, P. Xiong, S. Gao, J. Yan, Y. Li, Y. Cheng and T. Xi, J. Mater. Chem. B, 2019, 7, 7314–7325 RSC
.
- T. Zhou, L. Yan, C. Xie, P. Li, L. Jiang, J. Fang, C. Zhao, F. Ren, K. Wang, Y. Wang, H. Zhang, T. Guo and X. Lu, Small, 2019, 15, 1805440 CrossRef
.
- X. Li, P. Gao, J. Tan, K. Xiong, M. F. Maitz, C. Pan, H. Wu, Y. Chen, Z. Yang and N. Huang, ACS Appl. Mater. Interfaces, 2018, 10, 40844–40853 CrossRef CAS
.
- Z. Li, H. Li, J. Zhang, X. Liu, Z. Gu and Y. Li, Chinese J. Polym. Sci., 2020, 38, 1149–1156 CrossRef CAS
.
- L. Q. Xu, W. J. Yang, K. G. Neoh, E. T. Kang and G. D. Fu, Macromolecules, 2010, 43, 8336–8339 CrossRef CAS
.
- F. Cataldo, O. Ursini and G. Angelini, Eur. Chem. Bull., 2013, 2, 700–705 CAS
.
- J. Luo, J. Lai, N. Zhang, Y. Liu, R. Liu and X. Liu, ACS Sustainable Chem. Eng., 2016, 4, 1404–1413 CrossRef CAS
.
- S. Wang, C. Duan, W. Yang, X. Gao, J. Shi, J. Kang, Y. Deng, X.-L. Shi and Z.-G. Chen, Nanoscale, 2020, 12, 11936–11946 RSC
.
- Y. Liu, K. Ai and L. Lu, Chem. Rev., 2014, 114, 5057–5115 CrossRef CAS
.
- L. Huang, M. Liu, H. Huang, Y. Wen, X. Zhang and Y. Wei, Biomacromolecules, 2018, 19, 1858–1868 CrossRef CAS
.
- Y. Liu, K. Ai, J. Liu, M. Deng, Y. He and L. Lu, Adv. Mater., 2013, 25, 1353–1359 CrossRef CAS
.
- Z. Yuan, C. Lin, Y. He, B. Tao, M. Chen, J. Zhang, P. Liu and K. Cai, ACS Nano, 2020, 14, 3546–3562 CrossRef CAS
.
- H. Fan, X. Yu, Y. Liu, Z. Shi, H. Liu, Z. Nie, D. Wu and Z. Jin, Soft Matter, 2015, 11, 4621–4629 RSC
.
- C. Wang, D. Wang, T. Dai, P. Xu, P. Wu, Y. Zou, P. Yang, J. Hu, Y. Li and Y. Cheng, Adv. Funct. Mater., 2018, 28, 1802127 CrossRef
.
- P. Yang, S. Zhang, N. Zhang, Y. Wang, J. Zhong, X. Sun, Y. Qi, X. Chen, Z. Li and Y. Li, ACS Appl. Mater. Interfaces, 2019, 11, 42671–42679 CrossRef CAS
.
- X. Wang, L. Yang, P. Yang, W. Guo, Q.-P. Zhang, X. Liu and Y. Li, Sci. China: Chem., 2020, 63, 1295–1305 CrossRef CAS
.
- Y. Zou, X. Chen, P. Yang, G. Liang, Y. Yang, Z. Gu and Y. Li, Sci. Adv., 2020, 6, eabb4696 CrossRef CAS
.
- Y. Zou, T. Wu, N. Li, X. Guo and Y. Li, Polymer, 2020, 186, 122042 CrossRef CAS
.
- T. Liu, M. Zhang, W. Liu, X. Zeng, X. Song, X. Yang, X. Zhang and J. Feng, ACS Nano, 2018, 12, 3917–3927 CrossRef CAS
.
- G. Zhao, H. Wu, R. Feng, D. Wang, P. Xu, P. Jiang, K. Yang, H. Wang, Z. Guo and Q. Chen, ACS Appl. Mater. Interfaces, 2018, 10, 3295–3304 CrossRef CAS
.
- M. Li, H. Wang, J. Hu, J. Hu, S. Zhang, Z. Yang, Y. Li and Y. Cheng, Chem. Mater., 2019, 31, 7678–7685 CrossRef CAS
.
- X. Chen, Z. Yi, G. Chen, X. Ma, W. Su, X. Cui and X. Li, J. Mater. Chem. B, 2019, 7, 4066–4078 RSC
.
- S. H. Ku, J. Ryu, S. K. Hong, H. Lee and C. B. Park, Biomaterials, 2010, 31, 2535–2541 CrossRef CAS
.
- M. Liu, X. Zeng, C. Ma, H. Yi, Z. Ali, X. Mou, S. Li, Y. Deng and N. He, Bone Res., 2017, 5, 17014 CrossRef CAS
.
- T. Wu, C. Cui, Y. Huang, Y. Liu, C. Fan, X. Han, Y. Yang, Z. Xu, B. Liu, G. Fan and W. Liu, ACS Appl. Mater. Interfaces, 2020, 12, 2039–2048 CrossRef CAS
.
- R. Dong, X. Zhao, B. Guo and P. X. Ma, ACS Appl. Mater. Interfaces, 2016, 8, 17138–17150 CrossRef CAS
.
- M. Shan, C. Gong, B. Li and G. Wu, Polym. Chem., 2017, 8, 2997–3005 RSC
.
- Y. Wang, J. P. Park, S. H. Hong and H. Lee, Adv. Mater., 2016, 28, 9961–9968 CrossRef CAS
.
- P. Yang, S. Zhang, X. Chen, X. Liu, Z. Wang and Y. Li, Mater. Horiz., 2020, 7, 746–761 RSC
.
- C. Zhao, F. Zuo, Z. Liao, Z. Qin, S. Du and Z. Zhao, Macromol. Rapid Commun., 2015, 36, 909–915 CrossRef CAS
.
- B. M. Kyaw, S. Arora and C. S. Lim, Braz. J. Microbiol., 2012, 43, 938–945 CrossRef CAS
.
- W. H. Zhao, Z. Q. Hu, Y. Hara and T. Shimamura, Antimicrob. Agents Chemother., 2002, 46, 2266–2268 CrossRef CAS
.
- G. Marletta, G. Ciapetti, C. Satriano, S. Pagani and N. Baldini, Biomaterials, 2005, 26, 4793–4804 CrossRef CAS
.
- N. D. Leipzig, R. G. Wylie, H. Kim and M. S. Shoichet, Biomaterials, 2011, 32, 57–64 CrossRef CAS
.
- S. H. Hong, S. Hong, M.-H. Ryou, J. W. Choi, S. M. Kang and H. Lee, Adv. Mater. Interfaces, 2016, 3, 1500857 CrossRef
.
- S. Hong, J. Yeom, I. T. Song, S. M. Kang, H. Lee and H. Lee, Adv. Mater. Interfaces, 2014, 1, 1400113 CrossRef
.
- H. A. Lee, Y. Ma, F. Zhou, S. Hong and H. Lee, Acc. Chem. Res., 2019, 52, 704–713 CrossRef CAS
.
- Q. Wei and R. Haag, Mater. Horiz., 2015, 2, 567–577 RSC
.
- H. K. Park, D. Lee, H. Lee and S. Hong, Mater. Horiz., 2020, 7, 1387–1396 RSC
.
- M. Shin, E. Park and H. Lee, Adv. Funct. Mater., 2019, 29, 1903022 CrossRef CAS
.
- T. S. Sileika, D. G. Barrett, R. Zhang, K. H. A. Lau and P. B. Messersmith, Angew. Chem., Int. Ed., 2013, 52, 10766–10770 CrossRef CAS
.
- R. Wang, J. Li, W. Chen, T. Xu, S. Yun, Z. Xu, Z. Xu, T. Sato, B. Chi and H. Xu, Adv. Funct. Mater., 2017, 27, 1604894 CrossRef
.
- W. Shen, R. Wang, Q. Fan, X. Gao, H. Wang, Y. Shen, Y. Li and Y. Cheng, CCS Chem., 2020, 2, 146–157 CrossRef CAS
.
- E. Faure, C. Falentin-Daudré, C. Jérôme, J. Lyskawa, D. Fournier, P. Woisel and C. Detrembleur, Prog. Polym. Sci., 2013, 38, 236–270 CrossRef CAS
.
- A. M. Elseman, W. Sharmoukh, S. Sajid, P. Cui, J. Ji, S. Dou, D. Wei, H. Huang, W. Xi, L. Chu, Y. Li, B. Jiang and M. Li, Adv. Sci., 2018, 5, 1800568 CrossRef
.
- H. J. Park, Y. Jin, J. Shin, K. Yang, C. Lee, H. S. Yang and S. W. Cho, Biomacromolecules, 2016, 17, 1939–1948 CrossRef CAS
.
- J. H. Ryu, Y. Lee, W. H. Kong, T. G. Kim, T. G. Park and H. Lee, Biomacromolecules, 2011, 12, 2653–2659 CrossRef CAS
.
- J. Gao, Y. Yuan, Q. Yu, B. Yan, Y. Qian, J. Wen, C. Ma, S. Jiang, X. Wang and N. Wang, Chem. Commun., 2020, 56, 3935–3938 RSC
.
- K. Molnar, A. Jedlovszky-Hajdu, M. Zrinyi, S. Jiang and S. Agarwal, Macromol. Rapid Commun., 2017, 38, 1700147 CrossRef
.
- S. Jiang, N. Helfricht, G. Papastavrou, A. Greiner and S. Agarwal, Macromol. Rapid Commun., 2018, 39, 1700838 CrossRef
.
- F. Lee, J. E. Chung, K. Xu and M. Kurisawa, ACS Macro Lett., 2015, 4, 957–960 CrossRef CAS
.
- P. Moni, H. S. Suh, M. Dolejsi, D. H. Kim, A. C. Mohr, P. F. Nealey and K. K. Gleason, Langmuir, 2018, 34, 4494–4502 CrossRef CAS
.
- S. A. Sell, M. J. McClure, K. Garg, P. S. Wolfe and G. L. Bowlin, Adv. Drug Delivery Rev., 2009, 61, 1007–1019 CrossRef CAS
.
- S. Jian, J. Zhu, S. Jiang, S. Chen, H. Fang, Y. Song, G. Duan, Y. Zhang and H. Hou, RSC Adv., 2018, 8, 4794–4802 RSC
.
- S. Agarwal, S. Jiang and Y. Chen, Macromol. Mater. Eng., 2019, 304, 1800548 CrossRef
.
- L. Liu, H. Bakhshi, S. Jiang, H. Schmalz and S. Agarwal, Macromol. Rapid Commun., 2018, 39, 1800082 CrossRef
.
- S. H. Ku and C. B. Park, Adv. Healthcare Mater., 2013, 2, 1445–1450 CrossRef CAS
.
- S. H. Ku and C. B. Park, Biomaterials, 2010, 31, 9431–9437 CrossRef CAS
.
- Z. Fan, B. Liu, J. Wang, S. Zhang, Q. Lin, P. Gong, L. Ma and S. Yang, Adv. Funct. Mater., 2014, 24, 3933–3943 CrossRef CAS
.
- D. Stern and H. Cui, Adv. Healthcare Mater., 2019, 8, 1900104 CrossRef
.
- J. Shin, S. Choi, J. H. Kim, J. H. Cho, Y. Jin, S. Kim, S. Min, S. K. Kim, D. Choi and S. Cho, Adv. Funct. Mater., 2019, 29, 1903863 CrossRef CAS
.
- P. Yang, Z. Gu, F. Zhu and Y. Li, CCS Chem., 2020, 2, 128–138 CrossRef CAS
.
- B. Guo and P. X. Ma, Biomacromolecules, 2018, 19, 1764–1782 CrossRef CAS
.
- A. Burnstine-Townley, Y. Eshel and N. Amdursky, Adv. Funct. Mater., 2020, 30, 1901369 CrossRef CAS
.
- M. Shin, K. H. Song, J. C. Burrell, D. K. Cullen and J. A. Burdick, Adv. Sci., 2019, 6, 1901229 CrossRef CAS
.
- C. M. Ewulonu, X. Liu, M. Wu and Y. Huang, J. Bioresour. Bioprod., 2019, 4, 3–10 CAS
.
- N. Zhang, Z. Li, Y. Xiao, Z. Pan, P. Jia, G. Feng, C. Bao, Y. Zhou and L. Hua, J. Bioresour. Bioprod., 2020, 5, 67–77 CrossRef
.
- H. Li, Y. Liang, P. Li and C. He, J. Bioresour. Bioprod., 2020, 5, 170–188 Search PubMed
.
|
This journal is © The Royal Society of Chemistry 2021 |
Click here to see how this site uses Cookies. View our privacy policy here.