DOI:
10.1039/C9NA00800D
(Minireview)
Nanoscale Adv., 2020,
2, 1410-1416
Thermo-responsive plasmonic systems: old materials with new applications
Received
26th December 2019
, Accepted 11th March 2020
First published on 18th March 2020
Abstract
Thermo-responsive plasmonic systems of gold and poly(N-isopropylacrylamide) have been actively studied for several decades but this system keeps reinventing itself, with new concepts and applications which seed new fields. In this minireview, we show the latest few years development and applications of this intriguing system. We start from the basic working principles of this puzzling system which shows different plasmon shifts for even slightly different chemistries. We then present its applications to colloidal actuation, plasmon/meta-film tuning, and bioimaging and sensing. Finally we briefly summarize and propose several promising applications of the ongoing effort in this field.
1. Introduction
Active plasmonics has attracted great interest in the plasmonics community and numerous efforts have been devoted to this with many emerging concepts and applications.1,2 Different from passive plasmonic nanostructures, which are solely made of metals with fixed configurations, active plasmonics combines metallic nanostructures with functional materials, which brings tuneability to the plasmonic system. With such active tuneability, active modulation of the light flow at the nanoscale is possible, which triggers a series of applications in information technologies,3 energy harvesting,4 (bio)chemical sensing5,6 and security.7,8 The functional materials used commonly include responsive polymers,9 DNA origami,10 liquid crystals11 and optoelectronic materials.12 This active plasmonics concept also links with nonlinear optics,13 ultrafast optics14 and quantum plasmonics15 to form many extended directions.
Responsive (macro)molecules are typical materials that have been vigorously utilized in active plasmonics as they provide optical means for the (multiplexed) sensing of external stimuli, such as pH,16,17 temperature,18,19 light,20,21 electric22,23 and magnetic fields.24 One of the most studied stimulus materials are synthetic polymers showing a lower critical solution temperature (LCST) such as poly(N-isopropylacrylamide) (PNIPAM), which promotes reversible (de)solvation when the temperature is (above) below the LCST. This has been studied since the late 1990s, and is still actively investigated (Fig. 1) for different applications,25 such as sensing,26 catalysis,27,28 drug delivery,29 tissue engineering,30 artificial muscles,31etc.
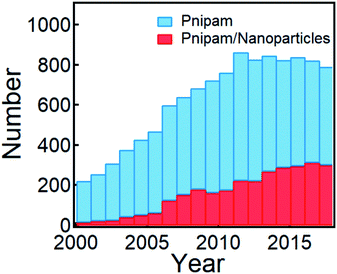 |
| Fig. 1 Publications that involve PNIPAM and PNIPAM/nanoparticles within the last two decades (from 2000 to 2018, search updated on December 12, 2019 from Web of Science). | |
Early research on PNIPAM mainly focused on block co-polymers containing PNIPAM segments or polymer core–shell hybrids.32–34 Later, this extended to inorganic cores such as silica,35 iron oxides36 and noble metals such as platinum for catalysis applications.28 With the development of nanophotonics in the 1990s, plasmonic nanoparticles (NPs) were also hybridized with PNIPAM.37 In this early research, the PNIPAM brushes/chains were either chemically grafted-from or grafted-to the metallic nanoparticle surfaces.38–40 On changing the temperature across the LCST of PNIPAM, the refractive index and volume of PNIPAM hydrogel changes, which modifies the optical plasmon resonances. Using this principle, many new designs and materials for thermo-responsive plasmonic systems bloomed.41–43 Although now well-known, this field is still developing and many new concepts and intriguing applications are still emerging,44–50 which deserve revisiting.
In this minireview, we introduce recent understanding and developments of such thermo-responsive plasmonic systems. Although silver (Ag) NPs may show better plasmonic performances,51–53 we mainly focus on the systems made of gold (Au) NPs and PNIPAM as the latter is more stable and reliable. Nevertheless, the working principle of thermal responsiveness is the same for both plasmonic NPs. We firstly analyse the physical chemistry aspects of this hybrid plasmonic system (Section 2), then recent applications for nanoactuation, plasmon tuning, metamaterials, and chemical sensing will be highlighted (Section 3). Lastly, we will conclude this minireview with a brief summary and perspectives of this growing field (Section 4).
2. Fundamental physical chemistry of Au NP/PNIPAM hybrid system
The temperature responsiveness of the Au/PNIPAM hybrid varies greatly in different systems. In most cases, only a very small plasmon shift54–57 or change in attenuation of transmission58,59 is observed when cycling the temperature while in other cases, the plasmon shift is very large.45,60,61 This inconsistency has recently been revealed as due to extra free PNIPAM in solution which is the main driving force promoting particle aggregation.62,63 Besides this, PNIPAM grafting density differences on Au surfaces also play some role, which has been thoroughly reviewed in previous literature.64 Beyond this, the electrostatic repulsion (Ucs) between nanoparticles seems to be crucial as it limits the closest separation between the Au NPs.65 Particles are stabilized with high surface charges even though the PNIPAM chains coil around them.60 The hydration of the PNIPAM chains coated on the particles' surfaces provides another shielding even if the zeta potential (Ψ) is low. However, when the PNIPAM chains are triggered through the phase transition upon heating, the nanoparticle surface becomes hydrophobic, which leads to aggregation. This seemly simple aggregation is actually a variable and complicated process. In one sense this system is simply switching between dispersed Au NPs and aggregated Au NP clusters. However, if the pH is lower than 3, this Au/PNIPAM hybrid system shows two transitions in each heating and cooling cycle (Fig. 2a), which corresponds to the aggregation of Au NPs and co-aggregation of Au clusters and PNIPAM beads. Intriguingly, a redshift of plasmons is observed during the cooling cycle (stage iii), which is due to the transformation from solid clusters (Fig. 2b) to hollow vesicles (Fig. 2c).60 Clearly, the assembly pathways of AuNPs/PNIPAM hybrids can be altered by changing the aggregation kinetics of PNIPAM chains, which is related to the amount of PNIPAM, pH, and salt in this system.
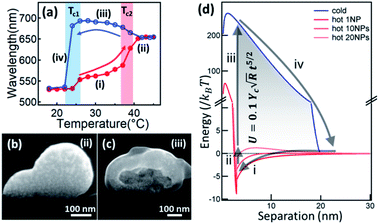 |
| Fig. 2 Physical understanding of the temperature-sensitive reversible assembly of Au@PNIPAM. (a) Plasmon resonance change with temperature (red on increasing T, blue on decreasing T). (b and c) SEM cross-sectional images of Au/PNIPAM clusters of different configurations at different stages (modified from ref. 60. Copyright permission from RSC). (d) Energy diagram of the Au/PNIPAM hybrid system with changing separation between the Au NPs. Red lines represents the aggregation of Au NPs while blue line represents the disaggregation. Process (i) happens when T > Tc, and process (ii–iv) happen instantaneously when T decreases below Tc. The energy stored in this cycle is expressed as the formula shown in the shaded region, where γc is Young's modulus of PNIAPM, R is radius of Au NPs, and t is thickness of PNIPAM coating (modified from ref. 45. Copyright permission from PNAS). | |
The existence of a steric potential (Ue) from the polymer chains makes the aggregation events reversible. This takes effect when the particle separation becomes smaller than the PNIPAM coating thickness, with steric hindrance working against the van de Waals potential (UvdW) to reach a new equilibrium.66 During cycles of this colloidal transition, significant amounts (∼1000 kBT per NP) of elastic energy in the polymer chains are stored and released, in a reversible energy cycle (Fig. 2d). The expansion force is thus large (∼nN) with fast dynamics of the polymer phase transition (∼μs).45,67 With such large forces and fast response, many novel applications emerge, such as plasmonic nanoactuators,44–46,68,69 plasmon tuning,70,71 fast plasmonic color switching films and metafilms,49,72,73 and plasmonic sensing.16,47,48,74 These will be discussed in the next Section.
3. Applications
3.1 Colloidal actuation
Collective movement or self-assembly of colloidal particles has long been an interesting topic for the colloidal chemistry community but not until recently has attention been shifted to nano-/micro-swimmers and nanoactuators for the applications of drug delivery, nanosurgery and nanomachines.75 As colloidal swimmers are largely covered in previous reviews,76 here we mainly focus on static colloidal actuators that can change their shape or volume with external stimuli.77 Typical colloidal actuators mostly are composite materials that involve soft hydrogels and hard inorganics. Typically PNIPAM is applied for reversible (dis)assembly of Au NPs. In such a way, actuation can be realized in different dimensions, depending on their assembly configurations (Table 1). As most of the hydrogels have to work in aqueous environment, these thermosensitive nanoactuators are mainly applied as valves for microfluidics78,79 and actuators for DNA origami.46
Table 1 Au/PNIPAM colloidal actuation system of different types
Early attempts by using photothermal effects to control microfluidic valves were based on composites of PNIPAM and photo-absorbing materials such as graphene79 and Au NPs,69 but the photothermal response is slow due to their large sizes. One way to improve its response speed is to use electrical thermal actuation.80 Here first patterned microelectrodes are made with PNIPAM brushes in the microfluidic channels. The thermal effect is induced by applying voltage to the electrodes, which then causes the shrinkage of PNIPAM (Fig. 3a). This works well for valve switching (Fig. 3b) and its speed can potentially be improved to ∼ms (Fig. 3c).81 But it requires the construction of wiring in the aqueous environment to form circuits (Fig. 3a), which increases the complexity of microfluidic chips. Another way to improve the photothermal response is to optically address the microfluidic valve with assistance of nanoplasmonics which provides unique advantages of simplicity, low cost, high integration and high speed. These are commonly made of Au/PNIPAM hybrid NPs which form clusters upon heating and expand upon cooling. This is a fast (∼μs) switching mechanism that can be remotely triggered by light, making it a light-induced actuating nanotransducer (ANT).45
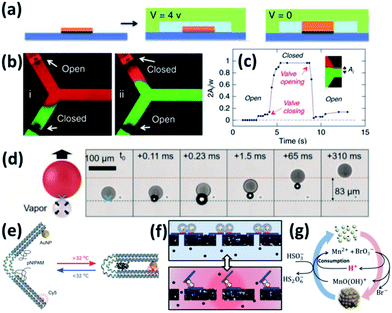 |
| Fig. 3 Colloidal actuation systems based on Au/PNIPAM. (a) Scheme of electric thermal valve action. (b) Fluorescence imaging of the microfluidics with electric thermo-controlled opening and closing of the valve. (c) Time response of the microvalve with electric thermal heating (modified from ref. 81. Copyright permission from Springer Nature). (d) Scheme of gas expansion mechanism for locomotion, with time-stamped microscope video frames taken with a high-speed camera (modified from ref. 82. Copyright permission from Wiley-VCH). (e) PNIPAM assisted actuation of DNA flexor (modified from ref. 46. Copyright permission from Wiley-VCH). (f) Scheme of Au/PNIPAM assisted actuation of filter valve (modified from ref. 45. Copyright permission from PNAS). (g) Chemomechanic oscillation with pH oscillation chemistry (modified from ref. 83. Copyright permission from ACS). | |
These ANT NPs can be dispersed in microdroplets, which drive their directional movement due to the formation of Janus-type droplets.82 Such Janus droplets can accumulate heat on one side and generate microbubbles which propel their directional movement (Fig. 3d).
Another application of plasmonic nanoactuators is that they can help with DNA origami actuation, which is probed by changes of photoluminescence (Fig. 3e).46 The key is to engineer the DNA origami to accommodate dye molecules and thiol groups at the opposite ends and click chemistry for PNIPAM at the joint. This optical control of DNA origami flexors can potentially serve as optical gating for nanofiltering (Fig. 3f).45
Actuating Au NP/PNIPAM systems autonomously with constant energy input provides possibility for understanding and mimicking biological systems. With the coupling of pH oscillation reactions, Au@PNIPAM NPs show continuous cycling of aggregation and disaggregation (Fig. 3g) with energy efficiency up to 34%. Rather different from ANTs, the energy input here that continuously powers such actuation is supported by the chemical potentials, making it a chemomechanical energy transducer (CoMET).83
3.2 Tuneable plasmonics and metafilms
As the plasmonic property of Au NPs is highly dependent on their separation and surrounding media,9 the colloidal actuation of plasmonic nanoparticles simultaneously induces changes of their optical properties. With colloidal actuation of PNIPAM, this has been largely applied for tuning of surface plasmons and metamaterials.9 The basic principle for the thermo-responsive tuning is the expansion and contraction of PNIPAM, which changes the separation between each Au NPs so the plasmon resonances changes accordingly. Another tuning strategy is keep the PNIPAM stable at fixed temperature but grow the Au cores within the PNIPAM so that their lattice constant is modified, which tunes the plasmon resonances (Fig. 4a).70,84 A further set of plasmonic nanoresonators such as Au NP on Au films,85 can also be tuned with PNIPAM actuation. The separation between Au NP and Au film changes in the out-of-plane direction with the expansion and contraction of the PNIPAM spacer, which reversibly tunes plasmon resonances (Fig. 4b).72 This plasmon tuning based on single Au NPs on mirror (NPoM) can be controlled with light due to the photothermal effect, which shows fast response (∼ms) due to rapid heating and cooling in a nanoscale volume.44
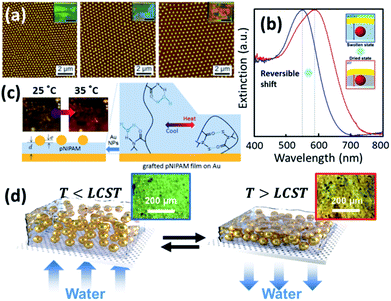 |
| Fig. 4 Thermosensitive plasmonics and metafilms based on Au/PNIPAM systems. (a) 2D arrays of Au/PNIPAM metafilms with tunable separation (modified from ref. 70. Copyright permission from RSC). (b) Reversible tuning of plasmon coupling with Au films overcoated on AuNP/PNIPAM hybrid films (modified from ref. 72. Copyright permission from ACS). (c) Change of NP optical interference with PNIPAM film thickness modulation (modified from ref. 49. Copyright permission from Wiley-VCH). (d) Switching of the reflection of hybrid Au/PNIPAM metafilms with temperature (modified from ref. 86. Copyright permission from Wiley-VCH). | |
PNIPAM can also be applied for the tuning of plasmonic metafilms. A similar structure to Au NPoMs but with much thicker PNIPAM spacing layer (grown via atomic transfer radical polymerization) shows interference between Au NP scattering and Au film reflection. Such interference leads to different colours of the metafilms at different temperatures as the optical path difference changes with PNIPAM thickness (Fig. 4c).49 Plasmonic metafilms made of PNIPAM and Au NP composites also show dramatic color changes across the LCST of PNIPAM (Fig. 4d). These plasmonic metal films again can be tuned with an unfocussed halogen lamp as it produces strong photothermal heating within the films, thus contracting the composite metafilms. As a result, the filling ratio of the metafilms increases which results in a redshift of plasmons.86
3.3 Bioimaging and sensing
As the change of plasmons also indicates the change of local environment, it can serve as a plasmonic ruler for sensing applications.87,88 The most direct sensing parameter is the temperature due to the thermosensitive polymer (PNIPAM). However, this is only applicable for qualitative sensing as it show a nonlinear plasmon response vs. temperature with strong hysteresis.60 This can be improved for quantitative temperature sensing if Au nanorods or bipyramids are used.89 Besides temperature sensing, other aspects that can potentially be exploited are the sensing of solvents, pH and ion concentration since these parameters influence the LCST of PNIPAM, which then affect plasmon shifts.
Another commonly applied sensing strategy with plasmons is to utilize their field enhancement effects to enable surface enhanced spectroscopies, such as Raman (SERS).90 The added value of using PNIPAM is that the temperature responsive shrinking and expansion of PNIPAM gels also provides a molecular trapping and release mechanism for SERS switching, which shows improved sensitivity (Fig. 5a).26,47 Such sensing can be applied in biological contexts enabling tags for cell imaging and cancer detection (Fig. 5b).74
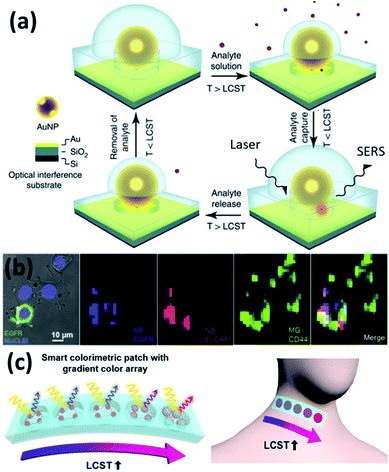 |
| Fig. 5 Plasmonic sensors based on Au/PNIPAM hybrids. (a) Au/PNIPAM nanostructure assisted single molecule detection (modified from ref. 26. Copyright permission from Springer Nature). (b) Au/PNIPAM hydrogel based SERS sensing and imaging of three tumor-associated biomarkers: epidermal growth factor receptor (EGFR), epithelial cell adhesion molecule (EpCAM), and homing cell adhesion molecule (CD44) (modified from ref. 74. Copyright permission from Wiley-VCH). (c) Au/PNIPAM hybrid gel films function as body temperature sensor (modified from ref. 48. Copyright permission from Springer Nature). | |
Compared to SERS sensing, plasmonic colorimetric sensing provides an alternative route towards the sensing of chemicals that does not require high accuracy.91 It is much simpler and straightforward for on-site testing, which greatly improves the efficiency and convenience without any sophisticated instrumentation. Ko and colleagues developed a wearable colorimetric sensor for body temperature based on Au/PNIPAM hybrid gel films (Fig. 5c). They show temperature visualization over a wide temperature range of 25–40 °C with a response time of ∼1 s.48
4. Summary and outlook
We have reviewed developments of thermo-responsive plasmonic systems in recent years. Clearly this system is still developing with ever more interdisciplinary focus. Future efforts will likely focus more on the application side of this system. Actuators based on PNIPAM systems will be one of the main areas to optimise. These Au/PNIPAM hybrid systems are biocompatible and water soluble, and work over the body temperature range, making them ideal candidates for many bio-related applications. Current systems are either too slow or the forces too weak due to small Young's modulus of PNIPAM. One way to improve this is to incorporate activated nanogels into the system,92 while another way is to adopt metallic nanoparticles to boost the force output.45 The challenge is how to apply these in specific nanomechanical and biomechanical systems, which needs further nano-engineering. Colorimetric sensing based on plasmons is another intriguing area due to its sensitivity and simplicity. However, the colour saturation of plasmons is poor due to the high losses of metals, which potentially can be made up by combining particles with gain materials. Sensing should not be restricted to simple temperature ranges as it can potentially also involve many other physicochemical parameters such as solvent, ion strength, pH and refractive index. As for plasmon tuning, this Au/PNIPAM system seems to be not immediately attractive as they are less compatible with electronics due to their working environment (with water). Thus applications in microfluidics, biomedicine and drug delivery are a sensible direction since both Au and PNIPAM are biocompatible and functional in the aqueous phase. This smart plasmonic system will continue to grow with many new applications such as microfluidics, SERS tags, nanomedicine and nanomachines.93
Conflicts of interest
There are no conflicts to declare.
Acknowledgements
This research is supported by the National Natural Science Foundation of China (NSFC, 21703160, 11974265), Wuhan Science and Technology Project (2019010701011420) and Fundamental Research Funds for the Central Universities (2042018kf0254, 2042018kf0038), as well as UK EPSRC grants EP/L027151/1, EP/N016920/1, EP/P029426/1, and EP/R020965/1.
Notes and references
-
A. V. Krasavin, K. F. MacDonald and N. I. Zheludev, in Nanophotonics with surface plasmons, ed. V. M. Shalaev and S. Kawata, Elsevier, Amsterdam, 2007, vol. 109 Search PubMed
.
-
A. V. Zayats and S. A. Maier, Active plasmonics and tuneable plasmonic metamaterials, ed. A. V. Zayats and S. A. Maier, John Wiley & Sons, New Jersey, 2013, p. 313 Search PubMed
.
- Z. Fei, A. S. Rodin, G. O. Andreev, W. Bao, A. S. McLeod, M. Wagner, L. M. Zhang, Z. Zhao, M. Thiemens, G. Dominguez, M. M. Fogler, A. H. C. Neto, C. N. Lau, F. Keilmann and D. N. Basov, Nature, 2012, 487, 82 CrossRef CAS PubMed
.
- K. Ueno, T. Oshikiri, Q. Sun, X. Shi and H. Misawa, Chem. Rev., 2018, 118, 2955 CrossRef CAS PubMed
.
- J. R. Mejía-Salazar and O. N. Oliveira, Chem. Rev., 2018, 118, 10617 CrossRef PubMed
.
- J. N. Anker, W. P. Hall, O. Lyandres, N. C. Shah, J. Zhao and R. P. Van Duyne, Nat. Mater., 2008, 7, 442 CrossRef CAS PubMed
.
- A. Kristensen, J. K. W. Yang, S. I. Bozhevolnyi, S. Link, P. Nordlander, N. J. Halas and N. A. Mortensen, Nat. Rev. Mater., 2016, 2, 16088 CrossRef
.
- Y. Zheng, C. Jiang, S. H. Ng, Y. Lu, F. Han, U. Bach and J. J. Gooding, Adv. Mater., 2016, 28, 2330 CrossRef CAS PubMed
.
- N. Jiang, X. Zhuo and J. Wang, Chem. Rev., 2018, 118, 3054 CrossRef CAS PubMed
.
- N. Liu and T. Liedl, Chem. Rev., 2018, 118, 3032 CrossRef CAS PubMed
.
- G. Y. Si, Y. Zhao, E. Sok, P. Leong and Y. Jun Liu, Materials, 2014, 7, 1296 CrossRef CAS PubMed
.
- B. Lee, W. Liu, C. H. Naylor, J. Park, S. C. Malek, J. S. Berger, A. T. C. Johnson and R. Agarwal, Nano Lett., 2017, 17, 4541 CrossRef CAS PubMed
.
- M. Mesch, B. Metzger, M. Hentschel and H. Giessen, Nano Lett., 2016, 16, 3155 CrossRef CAS PubMed
.
- K. F. MacDonald, Z. L. Sámson, M. I. Stockman and N. I. Zheludev, Nat. Photonics, 2008, 3, 55 CrossRef
.
- D. C. Marinica, M. Zapata, P. Nordlander, A. K. Kazansky, P. M. Echenique, J. Aizpurua and A. G. Borisov, Sci. Adv., 2015, 1, e1501095 CrossRef PubMed
.
- S. Wu, M. Zhu, Q. Lian, D. Lu, B. Spencer, D. J. Adlam, J. A. Hoyland, K. Volk, M. Karg and B. R. Saunders, Nanoscale, 2018, 10, 18565 RSC
.
- B. Liu, X. Lu, Z. Qiao, L. Song, Q. Cheng, J. Zhang, A. Zhang, Y. Huang and T. Chen, Langmuir, 2018, 34, 13047 CrossRef CAS PubMed
.
- J.-E. Lee, K. Chung, J. Lee, K. Shin and D. H. Kim, Adv. Funct. Mater., 2015, 25, 6716 CrossRef CAS
.
- Y. Liu, X. Han, L. He and Y. Yin, Angew. Chem., Int. Ed., 2012, 51, 6373 CrossRef CAS PubMed
.
- W. M. Wilson, J. W. Stewart and M. H. Mikkelsen, Nano Lett., 2018, 18, 853 CrossRef CAS PubMed
.
- R. Klajn, K. J. M. Bishop and B. A. Grzybowski, Proc. Natl. Acad. Sci. U. S. A., 2007, 104, 10305 CrossRef CAS PubMed
.
- Q. Liu, Y. Yuan and I. I. Smalyukh, Nano Lett., 2014, 14, 4071 CrossRef CAS PubMed
.
- P. A. Kossyrev, A. Yin, S. G. Cloutier, D. A. Cardimona, D. Huang, P. M. Alsing and J. M. Xu, Nano Lett., 2005, 5, 1978 CrossRef CAS PubMed
.
- M. Wang, C. Gao, L. He, Q. Lu, J. Zhang, C. Tang, S. Zorba and Y. Yin, J. Am. Chem. Soc., 2013, 135, 15302 CrossRef CAS PubMed
.
- M. Karg, A. Pich, T. Hellweg, T. Hoare, L. A. Lyon, J. J. Crassous, D. Suzuki, R. A. Gumerov, S. Schneider, I. I. Potemkin and W. Richtering, Langmuir, 2019, 35, 6231 CrossRef CAS PubMed
.
- Y. Zheng, A. H. Soeriyadi, L. Rosa, S. H. Ng, U. Bach and J. Justin Gooding, Nat. Commun., 2015, 6, 8797 CrossRef CAS PubMed
.
- M. Zeltner, A. Schätz, M. L. Hefti and W. J. Stark, J. Mater. Chem., 2011, 21, 2991 RSC
.
- C.-W. Chen and M. Akashi, J. Polym. Sci., Part A: Polym. Chem., 1997, 35, 1329 CrossRef CAS
.
-
D. Subhash, H. Mody, R. Banerjee, D. Bahadur and R. Srivastava, Poly(n-isopropylacrylamide) based polymer nanogels for drug delivery applications, presented at 2011 11th IEEE International Conference on Nanotechnology, Portland, OR, USA, 15–18 Aug 2011 Search PubMed
.
- A. Galperin, T. J. Long and B. D. Ratner, Biomacromolecules, 2010, 11, 2583 CrossRef CAS PubMed
.
- T. H. Kim, J. G. Choi, J. Y. Byun, Y. Jang, S. M. Kim, G. M. Spinks and S. J. Kim, Sci. Rep., 2019, 9, 7905 CrossRef PubMed
.
- M. Ballauff and Y. Lu, Polymer, 2007, 48, 1815 CrossRef CAS
.
- S. Zhou and B. Chu, J. Phys. Chem. B, 1998, 102, 1364 CrossRef CAS
.
- C. Wu and X. Qiu, Phys. Rev. Lett., 1998, 80, 620 CrossRef CAS
.
- J. Liu, R. Pelton and A. N. Hrymak, J. Colloid Interface Sci., 2000, 227, 408 CrossRef CAS PubMed
.
- N. Shamim, L. Hong, K. Hidajat and M. S. Uddin, J. Colloid Interface Sci., 2006, 304, 1 CrossRef CAS PubMed
.
- D. J. Kim, S. M. Kang, B. Kong, W.-J. Kim, H.-j. Paik, H. Choi and I. S. Choi, Macromol. Chem. Phys., 2005, 206, 1941 CrossRef CAS
.
- Y. Zuo, J. Zhao, Y. Gao and Y. Zhang, J. Mater. Sci., 2017, 52, 9584 CrossRef CAS
.
- M. Karg, I. Pastoriza-Santos, J. Pérez-Juste, T. Hellweg and L. Liz-Marzán, Small, 2007, 3, 1222 CrossRef CAS PubMed
.
- H. Lange, B. H. Juárez, A. Carl, M. Richter, N. G. Bastús, H. Weller, C. Thomsen, R. von Klitzing and A. Knorr, Langmuir, 2012, 28, 8862 CrossRef CAS PubMed
.
- M. Karg, Y. Lu, E. Carbó-Argibay, I. Pastoriza-Santos, J. Pérez-Juste, L. M. Liz-Marzán and T. Hellweg, Langmuir, 2009, 25, 3163 CrossRef CAS PubMed
.
- M. Karg, T. Hellweg and P. Mulvaney, Adv. Funct. Mater., 2011, 21, 4668 CrossRef CAS
.
- K. Volk, J. Fitzgerald, M. Retsch and M. Karg, Adv. Mater., 2015, 27, 7332 CrossRef CAS PubMed
.
- S. Cormier, T. Ding, V. Turek and J. J. Baumberg, Adv. Opt. Mater., 2018, 6, 1701281 CrossRef
.
- T. Ding, V. K. Valev, A. R. Salmon, C. J. Forman, S. K. Smoukov, O. A. Scherman, D. Frenkel and J. J. Baumberg, Proc. Natl. Acad. Sci. U. S. A., 2016, 113, 5503 CrossRef CAS PubMed
.
- V. A. Turek, R. Chikkaraddy, S. Cormier, B. Stockham, T. Ding, U. F. Keyser and J. J. Baumberg, Adv. Funct. Mater., 2018, 28, 1706410 CrossRef
.
- R. A. Álvarez-Puebla, R. Contreras-Cáceres, I. Pastoriza-Santos, J. Pérez-Juste and L. M. Liz-Marzán, Angew. Chem., Int. Ed., 2009, 48, 138 CrossRef PubMed
.
- A. Choe, J. Yeom, R. Shanker, M. P. Kim, S. Kang and H. Ko, NPG Asia Mater., 2018, 10, 912 CrossRef CAS
.
- T. Ding, C. Rüttiger, X. Zheng, F. Benz, H. Ohadi, G. A. E. Vandenbosch, V. V. Moshchalkov, M. Gallei and J. J. Baumberg, Adv. Opt. Mater., 2016, 4, 877 CrossRef CAS
.
- Y. Wang and T. Ding, Nanoscale, 2019, 11, 10589 RSC
.
- P.-G. Yin, Y. Chen, L. Jiang, T.-T. You, X.-Y. Lu, L. Guo and S. Yang, Macromol. Rapid Commun., 2011, 32, 1000 CrossRef CAS PubMed
.
- Y. Yan, L. Liu, Z. Cai, J. Xu, Z. Xu, D. Zhang and X. Hu, Sci. Rep., 2016, 6, 31328 CrossRef CAS PubMed
.
- W. Lewandowski, M. Fruhnert, J. Mieczkowski, C. Rockstuhl and E. Górecka, Nat. Commun., 2015, 6, 6590 CrossRef PubMed
.
- S. Rittikulsittichai, A. G. Kolhatkar, S. Sarangi, M. A. Vorontsova, P. G. Vekilov, A. Brazdeikis and T. Randall Lee, Nanoscale, 2016, 8, 11851 RSC
.
- M. Karg, I. Pastoriza-Santos, L. M. Liz-Marzán and T. Hellweg, ChemPhysChem, 2006, 7, 2298 CrossRef CAS PubMed
.
- H.-J. Li, P.-Y. Li, L.-Y. Li and O. He, Molecules, 2018, 23, 921 CrossRef PubMed
.
- R. Contreras-Cáceres, J. Pacifico, I. Pastoriza-Santos, J. Pérez-Juste, A. Fernández-Barbero and L. M. Liz-Marzán, Adv. Funct. Mater., 2009, 19, 3070 CrossRef
.
- M.-Q. Zhu, L.-Q. Wang, G. J. Exarhos and A. D. Q. Li, J. Am. Chem. Soc., 2004, 126, 2656 CrossRef CAS PubMed
.
- S. T. Jones, Z. Walsh-Korb, S. J. Barrow, S. L. Henderson, J. del Barrio and O. A. Scherman, ACS Nano, 2016, 10, 3158 CrossRef CAS PubMed
.
- F. Deng, Y. Wang, X. Lu and T. Ding, Phys. Chem. Chem. Phys., 2019, 21, 15742 RSC
.
- D. Fava, M. A. Winnik and E. Kumacheva, Chem. Commun., 2009, 18, 2571 RSC
.
- F. Han, A. H. Soeriyadi, S. R. C. Vivekchand and J. J. Gooding, ACS Macro Lett., 2016, 5, 626 CrossRef CAS
.
- Y. Chen, Z. Wang, Y. W. Harn, S. Pan, Z. Li, S. Lin, J. Peng, G. Zhang and Z. Lin, Angew. Chem., Int. Ed., 2019, 58, 11910 CrossRef CAS PubMed
.
- M. I. Gibson and R. K. O'Reilly, Chem. Soc. Rev., 2013, 42, 7204 RSC
.
- V. A. Turek, S. Cormier, B. Sierra-Martin, U. F. Keyser, T. Ding and J. J. Baumberg, Adv. Opt. Mater., 2018, 6, 1701270 CrossRef
.
- K. J. Bishop, C. E. Wilmer, S. Soh and B. A. Grzybowski, Small, 2009, 5, 1600 CrossRef CAS PubMed
.
- S. Murphy, S. Jaber, C. Ritchie, M. Karg and P. Mulvaney, Langmuir, 2016, 32, 12497 CrossRef CAS PubMed
.
- S. Bang, J. Kim, G. Yoon, T. Tanaka and J. Rho, Micromachines, 2018, 9, 560 CrossRef PubMed
.
- S. R. Sershen, G. A. Mensing, M. Ng, N. J. Halas, D. J. Beebe and J. L. West, Adv. Mater., 2005, 17, 1366 CrossRef CAS
.
- T. Honold, K. Volk, A. Rauh, J. Fitzgerald and M. Karg, J. Mater. Chem. C, 2015, 3, 11449 RSC
.
- T. Ding, A. W. Rudrum, L. O. Herrmann, V. Turek and J. J. Baumberg, Nanoscale, 2016, 8, 15864 RSC
.
- Y. Brasse, M. Mueller, M. Karg, C. Kuttner, T. A. F. Koenig and A. Fery, ACS Appl. Mater. Interfaces, 2017, 10, 3133 CrossRef PubMed
.
- M. Wei and M. J. Serpe, Part. Part. Syst. Charact., 2019, 36, 1800326 CrossRef
.
- G. Bodelon, V. Montes García, C. Fernández-López, I. Pastoriza-Santos, J. Pérez-Juste and L. Liz-Marzán, Small, 2015, 11, 4149 CrossRef CAS PubMed
.
- L. Xu, F. Mou, H. Gong, M. Luo and J. Guan, Chem. Soc. Rev., 2017, 46, 6905 RSC
.
- G. A. Ozin, I. Manners, S. Fournier-Bidoz and A. Arsenault, Adv. Mater., 2005, 17, 3011 CrossRef CAS
.
- A. A. Shah, B. Schultz, W. Zhang, S. C. Glotzer and M. J. Solomon, Nat. Mater., 2014, 14, 117 CrossRef PubMed
.
- A. D. Jadhav, B. Yan, R.-C. Luo, L. Wei, X. Zhen, C.-H. Chen and P. Shi, Biomicrofluidics, 2015, 9, 034114 CrossRef PubMed
.
- Y. Cheng, K. Ren, C. Huang and J. Wei, Sens. Actuators, B, 2019, 298, 126908 CrossRef
.
- C. Yu, S. Mutlu, P. Selvaganapathy, C. H. Mastrangelo, F. Svec and J. M. J. Fréchet, Anal. Chem., 2003, 75, 1958 CrossRef CAS PubMed
.
- L. D'Eramo, B. Chollet, M. Leman, E. Martwong, M. Li, H. Geisler, J. Dupire, M. Kerdraon, C. Vergne, F. Monti, Y. Tran and P. Tabeling, Microsyst. Nanoeng., 2018, 4, 17069 CrossRef
.
- A. R. Salmon, S. Cormier, W. Wang, C. Abell and J. J. Baumberg, Adv. Opt. Mater., 2019, 7, 1900951 CrossRef CAS
.
- F. Deng, J. Feng and T. Ding, ACS Appl. Mater. Interfaces, 2019, 11, 42580 CrossRef CAS PubMed
.
- R. Contreras-Caceres, A. Sánchez Iglesias, M. Karg, I. Pastoriza-Santos, J. Pérez-Juste, J. Pacifico, T. Hellweg, A. Fernández-Barbero and L. Liz-Marzán, Adv. Mater., 2008, 20, 1666 CrossRef CAS
.
- S.-Y. Ding, J. Yi, J.-F. Li, B. Ren, D.-Y. Wu, R. Panneerselvam and Z.-Q. Tian, Nat. Rev. Mater., 2016, 1, 16021 CrossRef CAS
.
- S. Cormier, T. Ding, V. Turek and J. J. Baumberg, Adv. Opt. Mater., 2018, 6, 1800208 CrossRef
.
- K. M. Mayer and J. H. Hafner, Chem. Rev., 2011, 111, 3828 CrossRef CAS PubMed
.
- M. E. Stewart, C. R. Anderton, L. B. Thompson, J. Maria, S. K. Gray, J. A. Rogers and R. G. Nuzzo, Chem. Rev., 2008, 108, 494 CrossRef CAS PubMed
.
- Y. Liu, X. Dai, S. Mallawaarachchi, H. Hapuarachchi, Q. Shi, D. Dong, S. H. Thang, M. Premaratne and W. Cheng, J. Mater. Chem. C, 2017, 5, 10926 RSC
.
- L. Tong, H. Wei, S. Zhang and H. Xu, Sensors, 2014, 14, 7959 CrossRef CAS PubMed
.
-
C. Kuttner, in Plasmonics, ed. T. Gric, Intechopen, 2018, p. 1 Search PubMed
.
- L.-W. Xia, R. Xie, X.-J. Ju, W. Wang, Q. Chen and L.-Y. Chu, Nat. Commun., 2013, 4, 2226 CrossRef PubMed
.
- L. Reinišová, S. Hermanová and M. Pumera, Nanoscale, 2019, 11, 6519 RSC
.
|
This journal is © The Royal Society of Chemistry 2020 |
Click here to see how this site uses Cookies. View our privacy policy here.