DOI:
10.1039/C9CC01047E
(Highlight)
Chem. Commun., 2019,
55, 5408-5419
Recent advances of 1,2,3,5-tetrakis(carbazol-9-yl)-4,6-dicyanobenzene (4CzIPN) in photocatalytic transformations
Received
4th February 2019
, Accepted 8th April 2019
First published on 25th April 2019
Abstract
1,2,3,5-Tetrakis(carbazol-9-yl)-4,6-dicyanobenzene (4CzIPN) is a typical donor–acceptor fluorophore, with carbazolyl as an electron donor and dicyanobenzene as an electron acceptor. It has emerged as a powerful organophotocatalyst since 2016. Excellent redox window, good chemical stability and broad applicability make 4CzIPN an attractive metal-free photocatalyst. In this review, the recent advances of the application of 4CzIPN as a photoredox catalyst in the past three years (2016–2018) for various organic reactions are summarized.
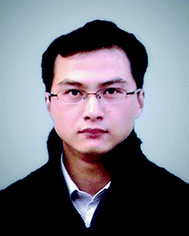
Wei-Min He
| Wei-Min He was born in Anqing, China, on November 22, 1982, and received his PhD degree from the Hunan University in 2012. He was a research scholar at the University of California, Santa Barbara before joining the Hunan University, as an assistant professor in 2013. He moved to Hunan University of Science and Engineering in 2016 and is currently an associate professor. His research focuses on green organic synthesis. |
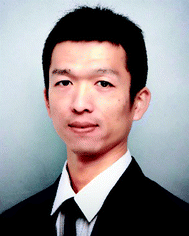
Bing Yu
| Bing Yu obtained his PhD degree from the Nankai University in 2014. He then moved to the Beijing Institute of Technology (2014–2015), the Seoul National University (2015–2016) and the Tokyo Institute of Technology (2016–2017) as a postdoctoral researcher. He joined Zhengzhou University in 2017. His research interest is developing novel approaches for green and efficient organic synthesis. |
1. Introduction
From the standpoint of green and sustainable chemistry, the development of clean, economical and efficient chemical processes has attracted extensive interest from both academia and industry.1 Over the past few decades, radical chemistry has emerged as a powerful tool for the rapid construction of complicated organic molecules due to the formation of versatile open shell reactive species.2 Accordingly, a plethora of methods could be applied to trigger radical reactions, such as thermolysis, radiation, photolysis, electrolysis and redox systems.3 Among these strategies, photocatalysis has been regarded as one of the most clean and promising methods, which has been extensively applied in radical chemistry.4 In particular, the past few years have witnessed the significant renaissance of photocatalysis in the field of organic chemistry since the pioneer works reported by MacMillan5 and Yoon6 in 2008. Despite the classic photoredox catalysts like ruthenium and iridium polypyridyl complexes displaying tremendous potential in organic synthetic chemistry,7 inexpensive and transition-metal-free organic photocatalysts also revealed extensive utilities. Notably, recent reviews on organic photoredox catalysis have summarized the synthetic applications of Eosin Y, 9-mesityl-10-methylacridinium ions, and other organic dyes.8 Recently, 1,2,3,5-tetrakis(carbazol-9-yl)-4,6-dicyanobenzene (4CzIPN) was found to be a versatile photocatalyst for various reactions (Scheme 1). As this is a fast-growing field, a summary of 4CzIPN for organic transformation is highly desired. In this article, the recent trend of using 4CzIPN as a visible-light photocatalyst in organic synthesis is reviewed.
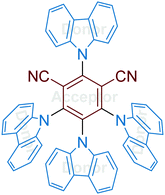 |
| Scheme 1 Structure of 1,2,3,5-tetrakis(carbazol-9-yl)-4,6-dicyanobenzene (4CzIPN). | |
2. Properties of 4CzIPN
The family of carbazoyl dicyanobenzenes (CDCBs), with carbazolyl (Cz) as an electron donor and dicyanobenzene as an electron acceptor, were first reported as highly efficient light harvesters for organic light-emitting diodes by Adachi and co-workers in 2012.9 Among these, 4CzIPN is one of the most promising compounds for photocatalysis. As shown in Fig. 1, the carbazolyl groups are remarkably distorted from the dicyanobenzene ring with a large dihedral angle of about 60° due to the steric hindrance. Density functional theory (DFT) calculations reveal that the highest occupied molecular orbitals (HOMOs) and the lowest unoccupied molecular orbitals (LUMOs) of 4CzIPN are localized on the donor moiety Cz and acceptor moiety dicyanobenzene, respectively (Fig. 1A and B). The separated HOMOs and LUMOs give rise to a small energy gap (ΔEST) between S1 and T1 states. Consequently, the non-overlapping frontier molecular orbitals (FMOs) make 4CzIPN an efficient thermally activated delayed fluorescence (TADF) material. More importantly, the spatially separated HOMOs and LUMOs allow an independent fine tuning of both orbitals through the modification of both the electron donor and electron acceptor moieties of the cyanobenzene scaffold, by which their photophysical and electrochemical properties can be tuned for specific catalytic purposes.10
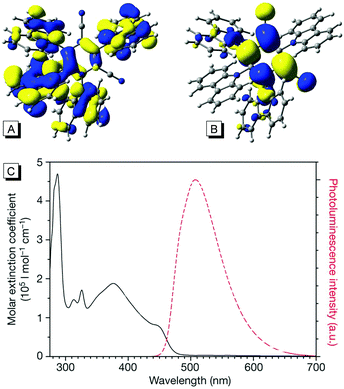 |
| Fig. 1 (A) HOMOs of 4CzIPN; (B) LUMOs of 4CzIPN; (C) UV-visible and photoluminescence spectra of 4CzIPN. Reprinted in part with permission from ref. 9a. Copyright 2012 Springer Nature Publishers Limited. | |
In addition, the ultraviolet-visible absorption spectrum and the photoluminescence spectrum of 4CzIPN in toluene are presented in Fig. 1C, suggesting a maximum emission at 507 nm. Importantly, a high photoluminescence quantum yield (up to 94.6%) and a long life-time in the excited state (5.1 μs) were observed. The photoredox potentials of 4CzIPN were also reported9a,11 (see Table 1).
Table 1 Comparison of the redox potentials and excited state lifetime of common photocatalystsa
Name |
E
1/2(P+/P*) (V) |
E
1/2(P*/P−) (V) |
E
1/2(P+/P) (V) |
E
1/2(P/P−) (V) |
τ (μs) |
Ref. |
All potentials are given in volts versus the saturated calomel electrode (SCE). Measurements were performed in acetonitrile at room temperature. EY = Eosin Y. RB = Rose Bengal. Ir-1 = fac-Ir(ppy)3. Ir-2 = [Ir[dF(CF3)ppy]2(dtbbpy)](PF6). Ru-1 = [Ru(bpy)3](PF6)2. τ = excited state lifetime.
|
4CzIPN |
−1.04 |
+1.35 |
+1.52 |
−1.21 |
5.1 |
9a
|
EY |
−1.11 |
+0.83 |
+0.78 |
−1.06 |
|
8c
|
RB |
−0.99 |
+0.66 |
+0.78 |
−1.11 |
|
12
|
Ir-1 |
−1.73 |
+0.31 |
+0.77 |
−2.19 |
1.9 |
13
|
Ir-2 |
−0.89 |
+1.21 |
+1.69 |
−1.37 |
2.3 |
14
|
Ru-1 |
−0.81 |
+0.77 |
+1.29 |
−1.33 |
1.1 |
15
|
Photocatalysts such as organic dyes (e.g., Eosin Y, Rose Bengal) and polypyridyl complexes (e.g., fac-Ir(ppy)3, [Ir[dF(CF3)ppy]2(dtbbpy)](PF6), and [Ru(bpy)3](PF6)2) have found tremendous applications for photocatalytic transformations in recent decades. These compounds are excellent photocatalysts for various organic transformations. They are poor single-electron oxidants/reductants in the ground states; however, the excited states under visible-light irradiation are potent single electron transfer reagents. Generally, wide redox potential windows, long-lived excited states, and high fluorescence quantum yields of the photocatalysts might be beneficial to their catalytic activities.
As summarized in Table 1, the redox potentials and excited state lifetime of the above-mentioned photocatalysts were compared with those of 4CzIPN. Despite being an inexpensive organic molecule, 4CzIPN shares several common photophysical features with Ru- and Ir-polypyridyl catalysts such as long excited state lifetime, wide redox window, and high fluorescence quantum yield. For example, 4CzIPN bears both oxidation and reduction potentials similar to that of the Ir-catalyst [Ir[dF(CF3)ppy]2(dtbbpy)](PF6), rendering it a highly desirable photocatalyst. Moreover, the redox properties of 4CzIPN analogues could also be easily tuned by deliberate molecular design and modifications of the electron donor and acceptor moieties according to the established structure–property relationships.10b Overall, the above-mentioned unique properties make 4CzIPN not only a metal-free alternative to transition metal photocatalysts but also an irreplaceable powerful photocatalyst in modern synthesis.
3. 4CzIPN/metal (Ni or Pd) dual-photoredox systems
3.1 4CzIPN in radical generation and metal catalyst regeneration
The application of 4CzIPN as a photocatalyst for organic reactions just started in 2016 by Zhang and co-workers.11 For the energetically demanding photoredox/Ni dual catalytic decarboxylative arylation, the suggested reaction mechanism (Scheme 2) revealed that a photocatalyst with high oxidative potential (>+1.1 V) was necessary for the generation of reactive radical ˙R2via a single electron transfer (SET) from the precursors like carboxylic acids 2, alkyltrifluoroborates 4, silicates 8, and glyoxylic acid monohydrate 14, etc.16 On the other hand, reduced photocatalyst [4CzIPN]˙− underwent a SET to regenerate the Ni(0) catalyst species. Accordingly, reactions following this general mechanism will be summarized in this section.
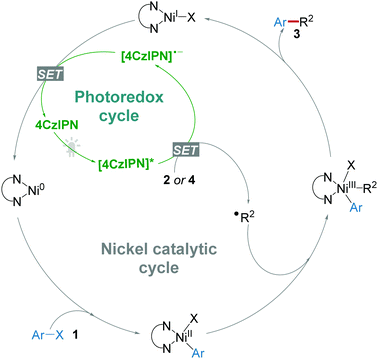 |
| Scheme 2 The proposed mechanism of photoredox/Ni dual catalytic decarboxylative arylation. | |
Given that the reductive potential of photoexcited 4CzIPN ([4CzIPN]*) is +1.35 V (vs. SCE), while the oxidative potential of amino acid N-tert-butoxycarbonyl-proline (N-Boc-pro) is +0.95 V (vs. SCE), it should be feasible for [4CzIPN]* to obtain an electron from N-Boc-pro. After the evaluation of rationally designed CDCB-based donor–acceptor (D–A) fluorophores, 4CzIPN was demonstrated to be a stable and active photoredox catalyst for the photoredox/Ni dual catalytic decarboxylative arylation of aryl halides 1 and carboxylic compounds 2 (Scheme 3a) and cross-coupling of alkyltrifluoroborates 4 with aryl halides (Scheme 3b) under light irradiation from a compact fluorescence lamp (CFL).
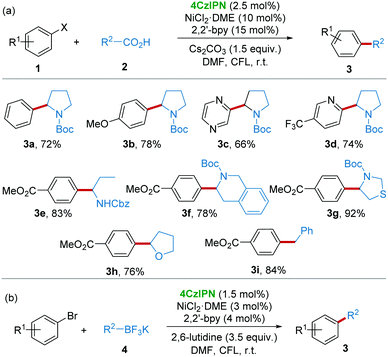 |
| Scheme 3 4CzIPN/Ni catalyzed arylation of aryl halides with carboxylic compounds and alkyltrifluoroborates. | |
Subsequently in 2016, the group of Ollivier and Fensterbank17 found that the photooxidation of benzyl bis(catecholato)silicates 5 (E1/2(Ox/red) = +0.3 to ∼+0.9 V vs. SCE) with 4CzIPN (E1/2([4CzIPN]*/[4CzIPN]˙−) = +1.35 V vs. SCE) was possible to generate benzyl radicals, which could be trapped by TEMPO 6 (2,2,6,6-tetramethyl-1-piperidinyloxy) affording 7 under the irradiation of blue LED light (Scheme 4a). Therefore, a 4CzIPN/Ni dual-catalytic system was applied for the reaction of various alkyl bis(catecholato)silicates 8 as radical precursors with radical acceptors 9 such as allylsulfones, alkenyl halides, β-bromostyrene, etc. (Scheme 4b).
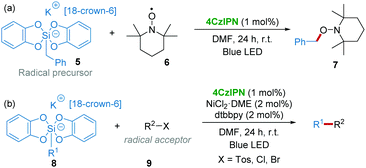 |
| Scheme 4 4CzIPN/Ni catalyzed arylation of aryl halides with silicates. | |
Having known that aryl–NiII complexes, formed from aromatic halides and nickel catalysts (Scheme 2), are good radical acceptors for the in situ generated radicals from carboxylic acids, alkyltrifluoroborates, and silicates, some other approaches were also developed by using the 4CzIPN/Ni system for the construction of C–C bonds. For instance, Molander's group reported that the 4CzIPN/Ni dual-catalytic system could be utilized in the synthesis of natural and unnatural flavanones 12 from trifluoroboratochromanones 11 with aryl and heteroaryl bromides 10 under mild conditions (weak base, ambient temperature, and visible-light) (Scheme 5).18
 |
| Scheme 5 4CzIPN/Ni catalyzed arylation of aryl bromides with trifluoroborates. | |
Moreover, glyoxylic acid monohydrate 14 was successfully applied as a formyl radical precursor via photoinduced decarboxylation, which then coupled with aryl iodides/bromides 13 to synthesize various arene and heteroarene carbaldehydes 15 under the dual catalysis of 4CzIPN/Pd (Scheme 6).19 A plausible reaction mechanism was proposed, which is similar to the mechanism of the 4CzIPN/Ni system shown in Scheme 2. This procedure provides a practical method for the construction of aryl aldehydes under mild conditions.
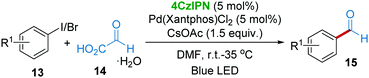 |
| Scheme 6 4CzIPN/Pd catalyzed formylation of aryl halides. | |
In 2017, Mariano and Wang et al.20 demonstrated that readily available 2,2-diethoxyacetic acid 16 has a low oxidative potential (+0.95 V vs. SCE, Cs salt of 16 in CH3CN), which should be a promising radical precursor in the presence of photoexcited 4CzIPN ([4CzIPN]*). Furthermore, studies established a formylation of organic (pseudo)halides with 16 as a radical precursor to generate ˙CH2(OEt)2 radical 18 by the catalysis of the 4CzIPN/Ni dual-catalytic system under the irradiation of blue LED light (Scheme 7a). As shown in the proposed mechanism (Scheme 7b), the reaction of radical 18 and the Ni(0) complex produced diacetal-nickel(I) intermediate 19, followed by an oxidative addition of 1 affording Ni(III) complexes 20. After the reductive elimination, aryl diacetal 21 was obtained, which could then be converted into aryl aldehyde 17 by acidic workup. This work represents a cost-effective formylation strategy for the synthesis of aromatic aldehydes starting from substantial aryl chlorides and avoiding the use of toxic CO and tin compounds under extremely mild conditions.
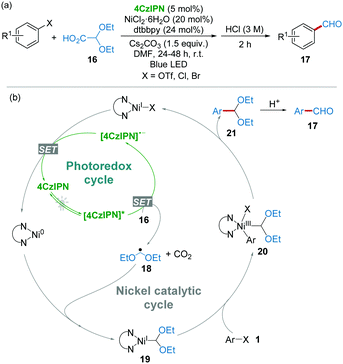 |
| Scheme 7 4CzIPN/Ni catalyzed formylation of aryl halides and triflates. | |
In medicinal chemistry, the introduction of C-glycoside moieties into drug molecules has become an effective strategy for the development of novel pharmaceutical compounds, which may display unique activities as antitumor and antibiotic agents.21 In particular, C-acyl-glycoside is one of the most attractive compounds towards complex bioactive molecules.22 However, the direct synthesis of C-acyl-glycoside using easily available carboxylic acid as an acyl source is very challenging.23 Recently, Molander and co-workers24 used high-throughput experimentation techniques demonstrating that dimethyl dicarbonate (DMDC) is a good activator for various carboxylic acids for the formylation reaction, in which glycosyl substituted 1,4-dihydropyridines 23 were employed as radical precursors25 under blue LED light with 4CzIPN/Ni dual catalysis (Scheme 8a). Notably, a free hydroxy group and steroidal moieties (24a and 24b) can be well tolerated in this elegant procedure. Subsequently, a similar strategy was applied to the arylation of highly functionalized saccharides 26 from aryl bromide 25 and glycosyl substituted 1,4-dihydropyridines 23 with 4CzIPN/Ni dual catalysis under visible-light irradiation (Scheme 8b).26
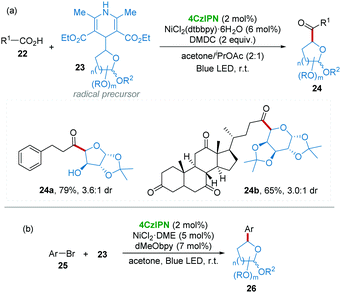 |
| Scheme 8 4CzIPN/Ni catalyzed synthesis of acylated/arylated glycosides. | |
The construction of the C–S bond has been an important issue in heteroatom chemistry, material science and medicinal chemistry.27 Alkynyl sulphides could be obtained by using umpolung-type strategies including sulfur umpolung and alkyne umpolung.28 However, methods for the direct synthesis of alkynyl sulphides are rare, because the direct reaction of thiols and terminal alkynes generally gives the thiol–yne click reaction products.29 In 2017, Collins and co-workers realized the oxidative coupling of bromoalkynes 27 and thiols 28 to synthesize thioalkynes 29 using continuous flow techniques involving 4CzIPN/Ni dual photocatalysis.30 It is believed that the thiyl radical is generated from thiols 28 under the catalysis of 4CzIPN under LED light. The procedure was highly efficient (30 min, 50–96% yields) and showed high reproducibility (gram scale). In particular, 19-membered macrocyclic alkynyl sulphide 29a could be synthesized, albeit in 28% yield under flow conditions, via an intramolecular coupling by using this dual photocatalytic system (Scheme 9).
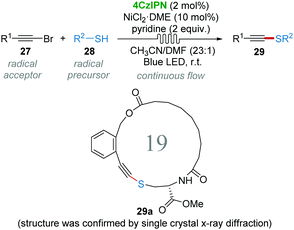 |
| Scheme 9 4CzIPN/Ni catalyzed synthesis of thioalkynes. | |
In 2018, direct C–H oxidation of 2-arylpyridines and 2-arylbenzothiazoles 30 towards phenols 31 with the metallaphotoredox system under visible-light with O2 as a green oxidant was reported by Singh's group (Scheme 10).31 In this protocol, BrCCl3 and toluene (or cyclohexane) were found to be significant for this transformation. It was proposed that ˙CCl3 radical 35 was first produced from BrCCl3 catalyzed by 4CzIPN, which then induced benzyl radical 36 (or cyclohexyl radical 37) from toluene (or cyclohexane). Subsequently, radical 36 (or 37) was trapped by O2 to produce intermediate peroxo radical 38 (or 39), which then released hydroxyl radical 40 (HO˙).
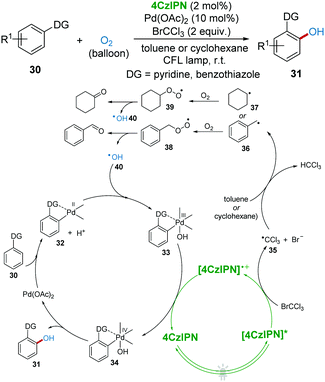 |
| Scheme 10 4CzIPN/Pd catalyzed hydroxylation. | |
3.2 4CzIPN in photocatalytic metal catalyst regeneration
The transformation of carbon dioxide (CO2) into valuable products under mild conditions has drawn huge attention in the past few decades.32 Selective hydrocarboxylation of styrenes with CO2 is one of the most challenging reactions.33 Recently, König and co-workers reported a ligand-controlled selective Markovnikov and anti-Markovnikov hydrocarboxylation of styrenes with CO2 under mild conditions. As shown in Scheme 11a, the hydrocarboxylation products could be tuned by using different ligands.34 For example, when 1,4-bis-(diphenylphosphino)butane (dppb, L1) as a ligand gave anti-Markovnikov product 42 (linear/branched ≥ 97
:
3), neocuproine (L2) as a ligand afforded Markovnikov product 43 (branched/linear = 100
:
0) by 4CzIPN/Ni dual catalysis.
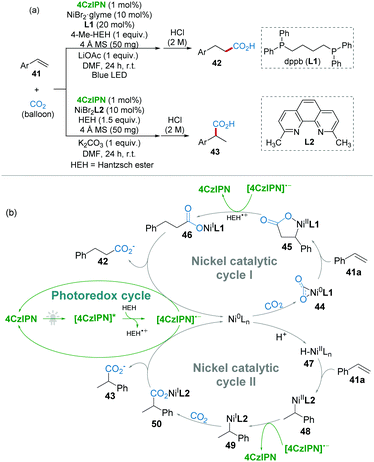 |
| Scheme 11 4CzIPN/Ni catalyzed hydrocarboxylation of styrene with CO2. | |
In the mechanistic studies, it was found that radical inhibitors could not inhibit the reaction, suggesting that radical intermediates are not essential. On this basis, a possible mechanism with two pathways was proposed as shown in Scheme 11b. For the anti-Markovnikov reaction, the Ni(0) species might first coordinate with CO2 generating Aresta's complex 44,35 which was followed by alkene insertion affording 5-membered nickelalactone 45. After a SET step, nickel carboxylate 46 was produced, and then anti-Markovnikov product 42 was released via a subsequent reduction (nickel catalytic cycle I). On the other hand, H2 was detected in the reaction indicating that a nickel hydride intermediate (H–NiII) was plausible. For the Markovnikov reaction, the reaction pathway (nickel catalytic cycle II) involving nickel hydride intermediate 47 was also reasonable.
4. Metal-free catalytic system
Compared with the classic photocatalysts with strong excited state reduction potential (i.e. Ru/Ir complexes), 4CzIPN has prominent features such as low cost and easy preparation.9a,11 Therefore, 4CzIPN was applied as an excellent organophotocatalyst for various transformations under the irradiation of visible-light. With the catalysis of 4CzIPN, radical precursors could be converted into reactive radicals, which could be trapped by organic substrates, such as unsaturated C
C or C
N bonds, rendering metal-free photocatalytic approaches. Therefore, reactions employing 4CzIPN as the sole metal-free photocatalyst are summarized in this section.
4.1 Reactions involving C
C bonds
After demonstrating that 2,2-diethoxyacetic acid 16 as a radical precursor could generate ˙CH2(OEt)2 radical 18 by 4CzIPN catalysis under the irradiation of blue LED light (Scheme 7a),20 the research group of Mariano and Wang36 subsequently developed a regioselective hydroformylation reaction of aryl olefins with diethoxyacetic acid 16 as the formylation reagent using a continuous flow system (Scheme 12). In their proposed mechanism, relatively stable benzylic radical 52 was produced via the anti-Markovnikov addition of radical 18 to the C
C bond, which then underwent a SET process affording anion 53. Anion 53 was easily converted into the desired aldehyde 51 by protonation and acid catalyzed hydrolysis.
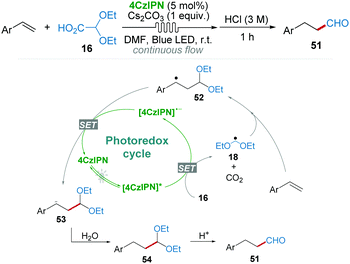 |
| Scheme 12 4CzIPN catalyzed hydroformylation reaction of aryl olefins. | |
Almost at the same time, Molander's group disclosed a mild and efficient method for the construction of gem-difluoroalkenes 56 from trifluoromethyl-substituted alkenes 55 which underwent radical defluorinative alkylation with the photocatalysis of 4CzIPN (Scheme 13).37 Various radical precursors including alkyl bis(catecholato)silicates, organotrifluoroborate, and α-silylamine were investigated in this procedure. Key intermediate 58 was formed via the radical addition and the SET process, followed by an E1cB-type fluoride elimination delivering gem-difluoroalkenes 56.
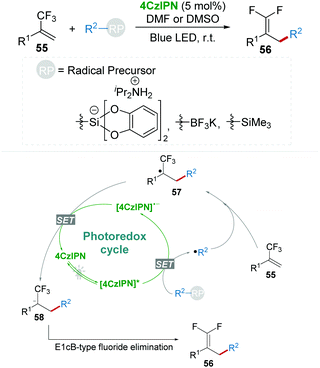 |
| Scheme 13 4CzIPN catalyzed defluorinative alkylation. | |
Considering the importance of cyclopropyl in pharmaceutical active molecules, direct cyclopropanation from easily available compounds is highly valuable. Consequently, Molander's group38 developed a radical cyclopropanation reaction of alkenes 59via a single-electron photocatalysis approach with 4CzIPN catalysis under visible-light (Scheme 14). In this work, triethylammonium bis(catecholato)iodomethylsilicate 60 was applied as a cyclopropanating reagent, which generated a halomethyl radical in the reaction. The mechanistic studies revealed that the addition of this halomethyl radical to 59 was followed by a SET process using 4CzIPN, which converted the resulting radical adduct 62 to an anion 63 that undergoes anionic 3-exo-tet cyclization producing the product 61.
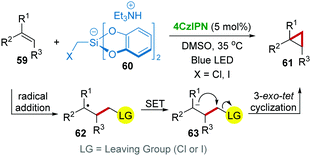 |
| Scheme 14 4CzIPN catalyzed cyclopropanation of alkenes. | |
Subsequently, the 4CzIPN photocatalytic system was further applied to the cyclopropanation reaction of homoallylic tosylate 64 and radical precursors 65 under blue light (Scheme 15).39 This method is a more general cyclopropanation strategy because various radicals are applicable for the radical addition with the C
C bond of 64.
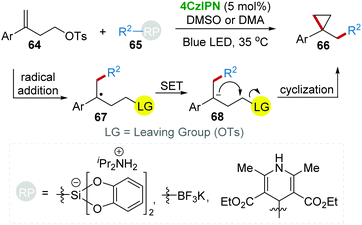 |
| Scheme 15 4CzIPN catalyzed radical/polar annulation reactions of homoallylic tosylate. | |
Due to the similar bond dissociation energies (BDEs) of Si–H and C–H bonds, the selective activation of Si–H bonds is challenging.40 Recently, Wu and co-workers41 demonstrated that the combination of photocatalyst 4CzIPN and hydrogen atom transfer (HAT) catalysts 72 and 73 was an efficient catalytic system for the hydrosilylation of alkenes through Si–H activation (Scheme 16). As Si is more electropositive than C, Si–H bonds are generally more hydridic than C–H bonds. Therefore, it is feasible to generate silyl radicals via selective Si–H activation by using a highly electrophilic HAT catalyst.42 In this work, the hydrosilylation of both electron-deficient 69 and electron-rich alkenes 70 toward the corresponding products 74 and 75 was achieved by using different HAT catalysts 72 or 73 incorporated with 4CzIPN under mild blue light irradiation.
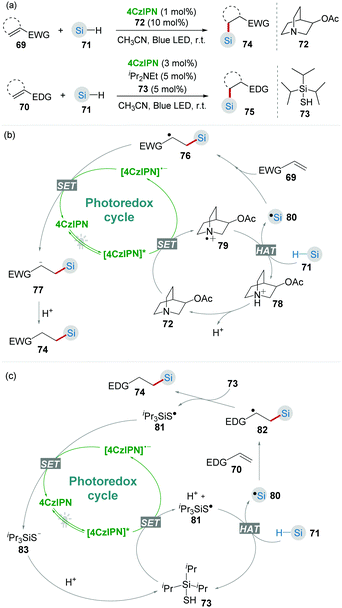 |
| Scheme 16 4CzIPN/HAT catalyzed hydrosilylation of alkenes. | |
CO2 is an abundant, renewable C1 feedstock, and an important electrophile for organic synthesis. Transformations of CO2 under mild conditions have drawn considerable attention in the past few decades.43 In the proposed reaction pathway of combined photoredox system (4CzIPN/HAT) catalyzed hydrosilylation of olefins (Scheme 16b), silyl radical addition adduct 76 underwent a single-electron reduction giving the corresponding nucleophilic anion 77. Inspired by this key step, Wu's group44 realized the silacarboxylation of alkenes 84 with silane and CO2 under similar photocatalytic conditions (Scheme 17). Significantly, the unprefunctionalized C(sp3)–H alkanes also proceeded smoothly to react with alkene and CO2 under identical conditions delivering the important γ-amino acid motifs as products.
 |
| Scheme 17 4CzIPN/HAT catalyzed silacarboxylation of alkenes with silane and CO2. | |
Cascade radical reactions have emerged as a powerful method for the rapid construction of complex scaffolds in the past few decades.45 In particular, photocatalysis methods are powerful and sustainable synthetic protocols to initiate the radical cascade reactions.46 Very recently, the research group of Cai reported a series of visible-light promoted radical cascade reactions using 4CzIPN as a metal-free photocatalyst (Scheme 18). For example, the trifluoromethylation/aryl migration reaction of α,α-diaryl allylic alcohols 86 and CF3SO2Na (Langlois reagents) 87 with 4CzIPN photocatalysis produced β-trifluoromethyl-α-aryl ketones 88 as products (Scheme 18a).47 This method avoids the use of transition metal catalysts, expensive trifluoromethyl sources and strong oxidants.48 Similarly, the same catalytic system could also be applied to the reaction of N-arylacrylamides 89 and Langlois reagent 87, affording CF3-containing oxindoles (Scheme 18b).49 In addition, using dimethyl sulfoxide 91 as a radical precursor, α-aryl-γ-methylsulfinyl ketones 92 were constructed from α,α-diaryl allylic alcohols 86 by 4CzIPN photocatalysis. In this work, phenyliodonium bis(trifluoroacetate) (PhI(OCOCF3)2, PIFA) and 1,3,5-trimethoxybenzene were added to form the hypervalent iodine(III) compound intermediate as an oxidant. The kinetic isotope effect demonstrated that C–H bond cleavage might be the rate-determining step (Scheme 18c).50
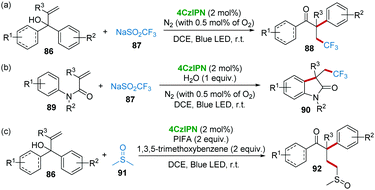 |
| Scheme 18 4CzIPN catalyzed radical cascade reactions. | |
Very recently, Studer's group reported the 1,2-amidoalkynylation of unactivated alkenes 93 with 4CzIPN photocatalysis.51 Troc-protected α-aminoxy acids 94 (Troc = 2,2,2-trichloroethoxycarbonyl) were used as precursors for the formation of amidyl radicals 99. The nucleophilic addition of N-radicals 99 with alkenes 93 afforded the adduct radicals 100, which were then trapped by hypervalent iodine(III) compounds to eventually provide amidoalkynylation products 96 (Scheme 19). Notably, various mono-, di- and trisubstituted unactivated alkenes are employed as substrates for the construction of diverse β-alkynylated Troc-amides under mild conditions.
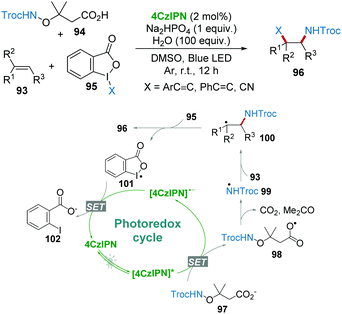 |
| Scheme 19 4CzIPN catalyzed 1,2-amidoalkynylation of unactivated alkenes. | |
4.2 Reactions involving C
N bonds
Besides the above-mentioned unsaturated C
C bonds, the C
N bonds were also reported as radical acceptors for novel transformations under 4CzIPN photocatalysis. On the other hand, it is also possible to generate α-amino radical anions from C
N bonds via a SET process, rendering a possible radical–radical coupling reaction. For instance, Molander's group reported a C–C bond formation reaction from imines 103 and ammonium alkyl bis(catecholato)silicates 104 using 4CzIPN as the sole photocatalyst in DMSO without any additives (Scheme 20).52
 |
| Scheme 20 4CzIPN catalyzed alkylation of imines. | |
In this procedure, the single-electron oxidation of silicates 104 produced C-centered radicals 106. Subsequently, two reaction pathways should be possible (Scheme 21): (a) The addition of radical 106 to imine 103, followed by a single-electron reduction of the resulting N-centered radical 107; or (b) a “persistent radical”, i.e., α-amino radical anion 108 was produced by the single-electron reduction of 103, followed by a radical–radical coupling with radical 106. In the two possible pathways, although the reduction of imine 103 to the corresponding α-amino radical anion 108 was unfavourable, pathway b could not be excluded in this process.
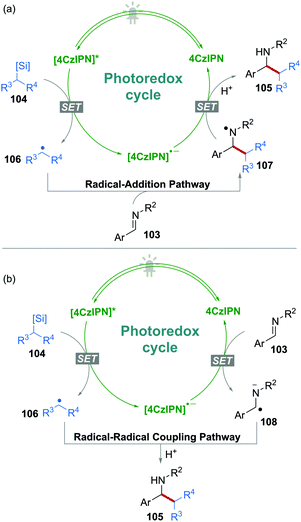 |
| Scheme 21 Two possible reaction pathways. | |
In 2018, the group of Weng and Lu53 disclosed 4CzIPN-catalyzed decarboxylative benzylation of imines 109 under the irradiation of visible-light (Scheme 22). A variety of primary, secondary, and tertiary arylacetic acids 110 were successfully applied as precursors of benzyl radicals, enabling the facile benzylation of a variety of imines affording diverse β-arylethylamines 111. In the mechanistic studies, some imine reduction and imine dimer by-products were observed, thus ruling out the radical-addition pathway.
 |
| Scheme 22 4CzIPN catalyzed decarboxylative benzylation of imines. | |
In 2018, Yu's group developed the 4CzIPN-catalyzed hydrocarboxylation of imines (or enamides) 112 with a CO2 atmosphere to synthesize α,α-disubstituted α-amino acids 113 under irradiation of visible-light (Scheme 23).54 The traditional umpolung hydrocarboxylation of imines with CO2 is very challenging;55 however, it is hypothesized that α-amino carbanions 114 could be generated by the visible-light-driven reduction of imines 112, followed by the nucleophilic addition of CO2 to give carboxylates 115.
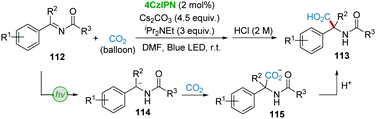 |
| Scheme 23 4CzIPN catalyzed hydrocarboxylation of imines. | |
The research group of Zhou and Wang56 used the one-pot approach to realize the synthesis of α,α-difluoroketone hydrazones 119 from aldehydes 116, hydrazines 117, and bromodifluorinated reagents 118via visible-light photoredox catalysis (Scheme 24). The condensation of aldehydes 116 and hydrazines 117 produced the corresponding hydrazones 120, followed by a single-electron oxidation to generate radical 121 or 122. On the other hand, the single-electron reduction of BrCF2R4118 under photoredox conditions afforded radical 123. The radical addition of 122 and 123 furnished intermediate 124, which then converted into the desired product 119 by deprotonation.
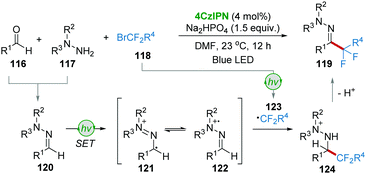 |
| Scheme 24 4CzIPN catalyzed one-pot synthesis of α,α-difluoroketone hydrazones. | |
Sherwood et al. reported an improved, one-pot Minisci reaction using carboxylic acids and N-containing heterocycles including quinoline, pyridine, quinaldine, isoquinoline, quinazolinone, purine, phthalazine, quinoxaline, 7-azaindoles, benzimidazole, benzothiazole, caffeine, nebularine, etc. with the 4CzIPN photocatalysis system (Scheme 25).57 Carboxylic acids 126 first undergo N-(acyloxy)phthalimides (NAPs) 128 formation in the presence of N-hydroxyphthalimide (NHPI) and N,N′-diisopropylcarbodiimide (DIC) and DMAP. Subsequently, the NAPs generate the alkyl radical releasing CO2 and phthalimide under photocatalytic conditions, followed by radical addition with heterocycles 125 giving the C–C bond formation product 127.
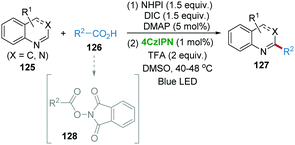 |
| Scheme 25 4CzIPN catalyzed one-pot Minisci reaction. | |
In Molander's recent report, 4CzIPN was also found to be an efficient photocatalyst for the direct C–H functionalization of heteroarene 129 with tert-butyltrifluoroborate 130 affording product 131 in the presence of K2S2O8 and trifluoracetic acid (TFA) (Scheme 26).58 However, when primary alkyltrifluoroborate was employed as a substrate, the yield was very poor, probably due to the extremely high oxidation potential of primary alkyltrifluoroborates.
 |
| Scheme 26 4CzIPN catalyzed direct C–H functionalization. | |
4.3 Miscellaneous reactions
In 2018, the group of Cramail and Landais described a 4CzIPN photocatalyzed oxidative decarboxylation of oxamic acids 132 with alcohol 133 or amine 136 to synthesize urethanes 135 and ureas 137 (Scheme 27).59 In this strategy, a hypervalent iodine reagent (BI-OAc, 134) was employed as an oxidant. Adduct 138, resulting from 132 and 134, underwent a free-radical decarboxylation under visible-light, generating carbamoyl radical 140, followed by oxidation which furnished the key isocyanate intermediate 141. Finally, the addition of alcohol 133 or amine 136 to 141 delivered the corresponding products urethanes 135 and ureas 137.
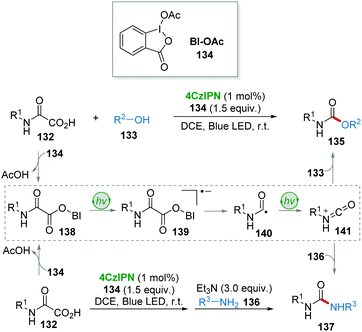 |
| Scheme 27 4CzIPN catalyzed oxidative decarboxylation coupling. | |
Due to the importance of deuterium- and tritium-labelled pharmaceutical compounds for drug discovery, in 2017 MacMillan and co-workers60 reported an efficient dual photo-/HAT-catalysis system for the installation of deuterium (D) and tritium (T) at the α-amino C(sp3)–H of 18 drug molecules in a single step using isotopically labelled water (D2O or T2O) as the source of the hydrogen isotope (Scheme 28). For example, commercially available antidepressant drug clomipramine 142 was deuterated to give the corresponding product 143 in 76% yield using 4CzIPN as a photocatalyst and thiol 73 as a HAT catalyst.
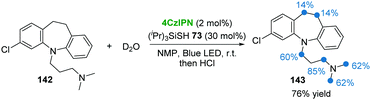 |
| Scheme 28 Dual photo-/HAT-catalysis system for C(sp3)–H deuteration (gram scale). | |
Recently, the research group of Xie and Zhu disclosed an elegant synergistic photo-/HAT-catalysis system to realize the transition-metal-free, site-specific trifluoromethylthiolation of tertiary ethers 144 with 4CzIPN as a photocatalyst and BINOL-based phosphorothiol 146 as a HAT catalyst (Scheme 29).61 Based on the calculation of singly occupied molecular orbital (SOMO) energies of several kinds of thiyl radicals, compound 146 with significantly high electrophilic reactivity was proved to be a potential HAT catalyst. Under photocatalysis, 146 was converted into a thiyl radical to abstract the most hydridic C–H bond of the ether providing radical 148. Radical 148 underwent a homolytic cleavage of the C–O bond forming tertiary alkyl radical 149, which then reacted with 145 resulting in product 147via the radical–radical pathway or the radical-addition pathway.
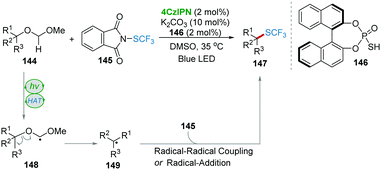 |
| Scheme 29 Trifluoromethylthiolation of tertiary ethers. | |
Leonori and co-workers62 described a 4CzIPN-involved photocatalysis approach for the remote C(sp3)–H functionalization of amides and amines 150 with polarized SOMOphiles (X–Y) 151 (Scheme 30). Substrate 150 suffered from a SET oxidation and fragmentation giving amidyl radical 153, followed by enthalpy-favorable 1,5-HAT resulting in the key radical 154. Then, the SH2 reaction between 154 and SOMOphiles 151 furnished the targeted products 152. This procedure provides an efficient method for the incorporation of F, Cl, SPh, CN, and alkyne functionalities into amides and amines via a cascade process of 1,5-HAT and SH2-functionalization.
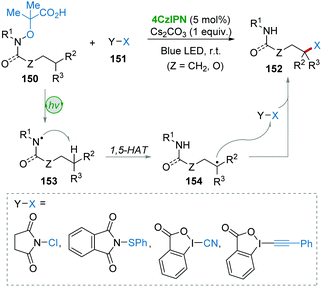 |
| Scheme 30 4CzIPN-catalyzed remote C(sp3)–H functionalization. | |
5. Conclusions
Visible-light promoted organic synthesis with metal complexes (Ru and Ir, etc.) as photocatalysts has gained huge attention in the past few decades. To a great extent, 1,2,3,5-tetrakis(carbazol-9-yl)-4,6-dicyanobenzene (4CzIPN) is an excellent and inexpensive metal-free alternative for those noble photocatalysts. In this review, the recent advances of the application of 4CzIPN as a photoredox catalyst for the generation of various radicals in organic reactions were summarized. For instance, benzyl radicals, formyl radicals, ˙CH2(OEt)2 radical, ˙CCl3 radical, silyl radicals, amidyl radicals, alkyl radicals, carbamoyl radicals, thiyl radicals, etc. from many different precursors could be achieved via 4CzIPN photocatalysis. However, 4CzIPN photocatalysis offers far more than a metal-free alternative to the classic noble complexes. As a typical donor–acceptor molecule, 4CzIPN has shown an excellent redox window, good chemical stability and broad applicability. It is convenient for researchers to modify the charge transfer characteristics of 4CzIPN analogues with specific redox potentials by changing the donors and acceptors.10 Overall, 4CzIPN has been used as a photocatalyst for organic transformation just since 2016. The application of simple and economical 4CzIPN as a metal-free photocatalyst will open new avenues for the exploration of novel visible-light-promoted transformations.
Conflicts of interest
There are no conflicts to declare.
Acknowledgements
We gratefully acknowledge the National Natural Science Foundation of China (No. 21501010) and the Hunan Provincial Natural Science Foundation of China (No. 2019JJ20008) for the financial support.
Notes and references
-
(a) X. Feng, T. Yang, X. He, B. Yu and C.-W. Hu, Appl. Organomet. Chem., 2018, 32, e4314 CrossRef;
(b) G.-P. Yang, D. Dilixiati, T. Yang, D. Liu, B. Yu and C.-W. Hu, Appl. Organomet. Chem., 2018, 32, e4450 CrossRef;
(c) G.-P. Yang, X. He, B. Yu and C.-W. Hu, Appl. Organomet. Chem., 2018, 32, e4532 CrossRef;
(d) G.-P. Yang, S.-X. Shang, B. Yu and C.-W. Hu, Inorg. Chem. Front., 2018, 5, 2472 RSC;
(e) C. Jing, X. Chen, K. Sun, Y. Yang, T. Chen, Y. Liu, L. Qu, Y. Zhao and B. Yu, Org. Lett., 2019, 21, 486 CrossRef CAS PubMed;
(f) G.-P. Yang, X. Wu, B. Yu and C.-W. Hu, ACS Sustainable Chem. Eng., 2019, 7, 3727 CrossRef CAS;
(g) L.-Y. Xie, Y. Duan, L.-H. Lu, Y.-J. Li, S. Peng, C. Wu, K.-J. Liu, Z. Wang and W.-M. He, ACS Sustainable Chem. Eng., 2017, 5, 10407 CrossRef CAS;
(h) L.-Y. Xie, Y.-J. Li, J. Qu, Y. Duan, J. Hu, K.-J. Liu, Z. Cao and W.-M. He, Green Chem., 2017, 19, 5642 RSC;
(i) L.-Y. Xie, S. Peng, J.-X. Tan, R.-X. Sun, X. Yu, N.-N. Dai, Z.-L. Tang, X. Xu and W.-M. He, ACS Sustainable Chem. Eng., 2018, 6, 16976 CrossRef CAS;
(j) L.-Y. Xie, S. Peng, F. Liu, Y.-F. Liu, M. Sun, Z. Tang, S. Jiang, Z. Cao and W.-M. He, ACS Sustainable Chem. Eng., 2019, 7, 7193 CrossRef CAS.
-
(a) M. Yan, J. C. Lo, J. T. Edwards and P. S. Baran, J. Am. Chem. Soc., 2016, 138, 12692 CrossRef CAS PubMed;
(b) M.-H. Huang, W.-J. Hao, G. Li, S.-J. Tu and B. Jiang, Chem. Commun., 2018, 54, 10791 RSC;
(c) L. J. Sebren, J. J. Devery and C. R. J. Stephenson, ACS Catal., 2014, 4, 703 CrossRef CAS PubMed;
(d) E. Godineau and Y. Landais, Chem. – Eur. J., 2009, 15, 3044 CrossRef CAS PubMed;
(e) S. Barata-Vallejo, M. V. Cooke and A. Postigo, ACS Catal., 2018, 8, 7287 CrossRef CAS;
(f) G. Qiu, L. Lai, J. Cheng and J. Wu, Chem. Commun., 2018, 54, 10405 RSC;
(g) K. Hofman, N.-W. Liu and G. Manolikakes, Chem. – Eur. J., 2018, 24, 11852 CrossRef CAS PubMed;
(h) Y. Zhao and W. Xia, Chem. Soc. Rev., 2018, 47, 2591 RSC;
(i) K. Duan, X. Yan, Y. Liu and Z. Li, Adv. Synth. Catal., 2018, 360, 2781 CrossRef CAS;
(j) M. D. Kärkäs, ACS Catal., 2017, 7, 4999 CrossRef;
(k) J.-J. Guo, A. Hu and Z. Zuo, Tetrahedron Lett., 2018, 59, 2103 CrossRef CAS;
(l) G. Fang, X. Cong, G. Zanoni, Q. Liu and X. Bi, Adv. Synth. Catal., 2017, 359, 1422 CrossRef CAS;
(m) A. N. Hancock and C. H. Schiesser, Chem. Commun., 2013, 49, 9892 RSC;
(n) B. Zhang and A. Studer, Chem. Soc. Rev., 2015, 44, 3505 RSC;
(o) J.-P. Goddard, C. Ollivier and L. Fensterbank, Acc. Chem. Res., 2016, 49, 1924 CrossRef CAS PubMed;
(p) X. Chen, X. Li, X.-L. Chen, L.-B. Qu, J.-Y. Chen, K. Sun, Z.-D. Liu, W.-Z. Bi, Y.-Y. Xia, H.-T. Wu and Y.-F. Zhao, Chem. Commun., 2015, 51, 3846 RSC;
(q) S. A. Morris, J. Wang and N. Zheng, Acc. Chem. Res., 2016, 49, 1957 CrossRef CAS PubMed;
(r) X.-L. Chen, X. Li, L.-B. Qu, Y.-C. Tang, W.-P. Mai, D.-H. Wei, W.-Z. Bi, L.-K. Duan, K. Sun, J.-Y. Chen, D.-D. Ke and Y.-F. Zhao, J. Org. Chem., 2014, 79, 8407 CrossRef CAS PubMed;
(s) K. Nakajima, Y. Miyake and Y. Nishibayashi, Acc. Chem. Res., 2016, 49, 1946 CrossRef CAS PubMed;
(t) W.-M. Zhao, X.-L. Chen, J.-W. Yuan, L.-B. Qu, L.-K. Duan and Y.-F. Zhao, Chem. Commun., 2014, 50, 2018 RSC;
(u) I. Ghosh, L. Marzo, A. Das, R. Shaikh and B. König, Acc. Chem. Res., 2016, 49, 1566 CrossRef CAS PubMed;
(v) Y. Liu, X.-L. Chen, F.-L. Zeng, K. Sun, C. Qu, L.-L. Fan, Z.-L. An, R. Li, C.-F. Jing, S.-K. Wei, L.-B. Qu, B. Yu, Y.-Q. Sun and Y.-F. Zhao, J. Org. Chem., 2018, 83, 11727 CrossRef CAS PubMed.
-
(a) H. Hu, X. Chen, K. Sun, J. Wang, Y. Liu, H. Liu, B. Yu, Y. Sun, L. Qu and Y. Zhao, Org. Chem. Front., 2018, 5, 2925 RSC;
(b) H. Hu, X. Chen, K. Sun, J. Wang, Y. Liu, H. Liu, L. Fan, B. Yu, Y. Sun, L. Qu and Y. Zhao, Org. Lett., 2018, 20, 6157 CrossRef CAS PubMed;
(c) M. H. Muhammad, X.-L. Chen, B. Yu, L.-B. Qu and Y.-F. Zhao, Pure Appl. Chem., 2019, 91, 33 CAS;
(d) K. Sun, X.-L. Chen, S.-J. Li, D.-H. Wei, X.-C. Liu, Y.-L. Zhang, Y. Liu, L.-L. Fan, L.-B. Qu, B. Yu, K. Li, Y.-Q. Sun and Y.-F. Zhao, J. Org. Chem., 2018, 83, 14419 CrossRef CAS PubMed;
(e) L.-H. Lu, S.-J. Zhou, W.-B. He, W. Xia, P. Chen, X. Yu, X. Xu and W.-M. He, Org. Biomol. Chem., 2018, 16, 9064 RSC;
(f) R. Li, X. Chen, S. Wei, K. Sun, L. Fan, Y. Liu, L. Qu, Y. Zhao and B. Yu, Adv. Synth. Catal., 2018, 360, 4807 CrossRef.
-
(a) J.-R. Chen, X.-Q. Hu, L.-Q. Lu and W.-J. Xiao, Chem.
Soc. Rev., 2016, 45, 2044 RSC;
(b) J.-R. Chen, X.-Q. Hu, L.-Q. Lu and W.-J. Xiao, Acc. Chem. Res., 2016, 49, 1911 CrossRef CAS PubMed;
(c) X.-Q. Hu, J.-R. Chen and W.-J. Xiao, Angew. Chem., Int. Ed., 2017, 56, 1960 CrossRef CAS PubMed;
(d) J. Xuan, Z.-G. Zhang and W.-J. Xiao, Angew. Chem., Int. Ed., 2015, 54, 15632 CrossRef CAS PubMed.
- D. A. Nicewicz and D. W. C. MacMillan, Science, 2008, 322, 77 CrossRef CAS PubMed.
- M. A. Ischay, M. E. Anzovino, J. Du and T. P. Yoon, J. Am. Chem. Soc., 2008, 130, 12886 CrossRef CAS PubMed.
-
(a) C. K. Prier, D. A. Rankic and D. W. C. MacMillan, Chem. Rev., 2013, 113, 5322 CrossRef CAS PubMed;
(b) M. H. Shaw, J. Twilton and D. W. C. MacMillan, J. Org. Chem., 2016, 81, 6898 CrossRef CAS PubMed.
-
(a) W. Xu, X. Dai, H. Xu and J. Weng, Chin. J. Org. Chem., 2018, 38, 2807 CrossRef;
(b) Z. Heng and L. Aiwen, Asian J. Org. Chem., 2018, 7, 1164 CrossRef;
(c) D. P. Hari and B. König, Chem. Commun., 2014, 50, 6688 RSC;
(d) N. A. Romero and D. A. Nicewicz, Chem. Rev., 2016, 116, 10075 CrossRef CAS PubMed;
(e) V. Vij, V. Bhalla and M. Kumar, Chem. Rev., 2016, 116, 9565 CrossRef CAS PubMed;
(f) S. P. Pitre, C. D. McTiernan and J. C. Scaiano, Acc. Chem. Res., 2016, 49, 1320 CrossRef CAS PubMed;
(g) D. A. Nicewicz and T. M. Nguyen, ACS Catal., 2014, 4, 355 CrossRef CAS;
(h) D. Ravelli, M. Fagnoni and A. Albini, Chem. Soc. Rev., 2013, 42, 97 RSC;
(i) S. P. Pitre, C. D. McTiernan and J. C. Scaiano, ACS Omega, 2016, 1, 66 CrossRef CAS;
(j) S. Fukuzumi and K. Ohkubo, Org. Biomol. Chem., 2014, 12, 6059 RSC;
(k) J.-B. Peng, X. Qi and X.-F. Wu, ChemSusChem, 2016, 9, 2279 CrossRef CAS PubMed.
-
(a) H. Uoyama, K. Goushi, K. Shizu, H. Nomura and C. Adachi, Nature, 2012, 492, 234 CrossRef CAS PubMed;
(b) M. Yokoyama, K. Inada, Y. Tsuchiya, H. Nakanotani and C. Adachi, Chem. Commun., 2018, 54, 8261 RSC.
-
(a) J. Lu, B. Pattengale, Q. Liu, S. Yang, W. Shi, S. Li, J. Huang and J. Zhang, J. Am. Chem. Soc., 2018, 140, 13719 CrossRef CAS;
(b) E. Speckmeier, T. G. Fischer and K. Zeitler, J. Am. Chem. Soc., 2018, 140, 15353 CrossRef CAS PubMed.
- J. Luo and J. Zhang, ACS Catal., 2016, 6, 873 CrossRef CAS.
- C. R. Lambert and I. E. Kochevar, Photochem. Photobiol., 1997, 66, 15 CrossRef CAS.
- L. Flamigni, A. Barbieri, C. Sabatini, B. Ventura and F. Barigelletti, Top. Curr. Chem., 2007, 281, 143 CrossRef CAS.
- M. S. Lowry, J. I. Goldsmith, J. D. Slinker, R. Rohl, R. A. Pascal, G. G. Malliaras and S. Bernhard, Chem. Mater., 2005, 17, 5712 CrossRef CAS.
- A. Joshi-Pangu, F. Levesque, H. G. Roth, S. F. Oliver, L. C. Campeau, D. Nicewicz and D. A. DiRocco, J. Org. Chem., 2016, 81, 7244 CrossRef CAS PubMed.
- I. Karakaya, D. N. Primer and G. A. Molander, Org. Lett., 2015, 17, 3294 CrossRef CAS PubMed.
- C. Leveque, L. Chenneberg, V. Corce, C. Ollivier and L. Fensterbank, Chem. Commun., 2016, 52, 9877 RSC.
- J. K. Matsui and G. A. Molander, Org. Lett., 2017, 19, 436 CrossRef CAS PubMed.
- B. Zhao, R. Shang, W.-M. Cheng and Y. Fu, Org. Chem. Front., 2018, 5, 1782 RSC.
- H. Huang, X. Li, C. Yu, Y. Zhang, P. S. Mariano and W. Wang, Angew. Chem., Int. Ed., 2017, 56, 1500 CrossRef CAS PubMed.
- Y. Yang and B. Yu, Chem. Rev., 2017, 117, 12281 CrossRef CAS PubMed.
- T. Kawamata, M. Nagatomo and M. Inoue, J. Am. Chem. Soc., 2017, 139, 1814 CrossRef CAS PubMed.
- C. Zhao, X. Jia, X. Wang and H. Gong, J. Am. Chem. Soc., 2014, 136, 17645 CrossRef CAS PubMed.
- S. O. Badir, A. Dumoulin, J. K. Matsui and G. A. Molander, Angew. Chem., Int. Ed., 2018, 57, 6610 CrossRef CAS PubMed.
- L. Buzzetti, A. Prieto, S. R. Roy and P. Melchiorre, Angew. Chem., Int. Ed., 2017, 56, 15039 CrossRef CAS PubMed.
- A. Dumoulin, J. K. Matsui, A. Gutierrez-Bonet and G. A. Molander, Angew. Chem., Int. Ed., 2018, 57, 6614 CrossRef CAS PubMed.
-
(a) J. Zhu, W. C. Yang, X. D. Wang and L. Wu, Adv. Synth. Catal., 2018, 360, 386 CrossRef CAS;
(b) O. M. Mulina, A. I. Ilovaisky and A. O. Terent'ev, Eur. J. Org. Chem., 2018, 4648 CrossRef CAS;
(c) P. Devendar and G.-F. Yang, Top. Curr. Chem., 2017, 375, 82 CrossRef PubMed;
(d) L.-Q. Lu, T.-R. Li, Q. Wang and W.-J. Xiao, Chem. Soc. Rev., 2017, 46, 4135 RSC;
(e) Z. Qiao and X. Jiang, Org. Biomol. Chem., 2017, 15, 1942 RSC;
(f) C. Wu, L. Hong, H. Shu, Q.-H. Zhou, Y. Wang, M. Sun, S. Jiang, Z. Cao and W.-M. He, ACS Sustainable Chem. Eng., 2019 DOI:10.1021/acssuschemeng.9b00708;
(g) A. M. Faisca Phillips and A. J. L. Pombeiro, ChemCatChem, 2018, 10, 3354 CrossRef CAS.
-
(a) J. T. Reeves, K. Camara, Z. S. Han, Y. Xu, H. Lee, C. A. Busacca and C. H. Senanayake, Org. Lett., 2014, 16, 1196 CrossRef CAS PubMed;
(b) B. Mohan, S. Hwang, H. Woo and K. H. Park, Synthesis, 2015, 3741 CAS.
- C. E. Hoyle and C. N. Bowman, Angew. Chem., Int. Ed., 2010, 49, 1540 CrossRef CAS PubMed.
- J. Santandrea, C. Minozzi, C. Cruche and S. K. Collins, Angew. Chem., Int. Ed., 2017, 56, 12255 CrossRef CAS PubMed.
- S. S. Shah, A. Paul, M. Bera, Y. Venkatesh and N. D. P. Singh, Org. Lett., 2018, 20, 5533 CrossRef CAS PubMed.
-
(a) A. Tortajada, F. Juliá-Hernández, M. Börjesson, T. Moragas and R. Martin, Angew. Chem., Int. Ed., 2018, 57, 15948 CrossRef CAS PubMed;
(b) B. Yu, B. Zou and C.-W. Hu, J. CO2 Util., 2018, 26, 314 CrossRef CAS;
(c) S.-S. Wang and G.-Y. Yang, Chem. Rev., 2015, 115, 4893 CrossRef CAS PubMed;
(d) W.-Z. Zhang and X.-B. Lu, Chin. J. Catal., 2012, 33, 745 CrossRef CAS;
(e) T. Sakakura, J.-C. Choi and H. Yasuda, Chem. Rev., 2007, 107, 2365 CrossRef CAS PubMed;
(f) B. Yu, D. Kim, S. Kim and S. H. Hong, ChemSusChem, 2017, 10, 1080 CrossRef CAS PubMed;
(g) B. Yu and L.-N. He, ChemSusChem, 2015, 8, 52 CrossRef CAS PubMed.
-
(a) J. Choi, P. Martín-Gago and G. C. Fu, J. Am. Chem. Soc., 2014, 136, 12161 CrossRef CAS PubMed;
(b) H. Seo, A. Liu and T. F. Jamison, J. Am. Chem. Soc., 2017, 139, 13969 CrossRef CAS PubMed.
- Q.-Y. Meng, S. Wang, G. S. Huff and B. König, J. Am. Chem. Soc., 2018, 140, 3198 CrossRef CAS PubMed.
- B. Yu, Z.-F. Diao, C.-X. Guo and L.-N. He, J. CO2 Util., 2013, 1, 60 CrossRef CAS.
- H. Huang, C. Yu, Y. Zhang, Y. Zhang, P. S. Mariano and W. Wang, J. Am. Chem. Soc., 2017, 139, 9799 CrossRef CAS PubMed.
- S. B. Lang, R. J. Wiles, C. B. Kelly and G. A. Molander, Angew. Chem., Int. Ed., 2017, 56, 15073 CrossRef CAS PubMed.
- J. P. Phelan, S. B. Lang, J. S. Compton, C. B. Kelly, R. Dykstra, O. Gutierrez and G. A. Molander, J. Am. Chem. Soc., 2018, 140, 8037 CrossRef CAS PubMed.
- J. A. Milligan, J. P. Phelan, V. C. Polites, C. B. Kelly and G. A. Molander, Org. Lett., 2018, 20, 6840 CrossRef CAS PubMed.
- H. Qrareya, D. Dondi, D. Ravelli and M. Fagnoni, ChemCatChem, 2015, 7, 3350 CrossRef CAS.
- R. Zhou, Y. Y. Goh, H. W. Liu, H. R. Tao, L. H. Li and J. Wu, Angew. Chem., Int. Ed., 2017, 56, 16621 CrossRef CAS PubMed.
-
(a) M. H. Shaw, V. W. Shurtleff, J. A. Terrett, J. D. Cuthbertson and D. W. C. MacMillan, Science, 2016, 352, 1304 CrossRef CAS PubMed;
(b) C. Le, Y. Liang, R. W. Evans, X. Li and D. W. C. MacMillan, Nature, 2017, 547, 79 CrossRef CAS PubMed.
-
(a) N. Zhang, B. Zou, G.-P. Yang, B. Yu and C.-W. Hu, J. CO2 Util., 2017, 22, 9 CrossRef CAS;
(b) B. Yu, B.-B. Cheng, W.-Q. Liu, W. Li, S.-S. Wang, J. Cao and C.-W. Hu, Adv. Synth. Catal., 2016, 358, 90 CrossRef CAS;
(c) S. Wang and C. Xi, Chem. Soc. Rev., 2019, 48, 382 RSC;
(d) S. Castro, J. Albo and A. Irabien, ACS Sustainable Chem. Eng., 2018, 6, 15877 CrossRef CAS.
- J. Hou, A. Ee, H. Cao, H.-W. Ong, J.-H. Xu and J. Wu, Angew. Chem., Int. Ed., 2018, 57, 17220 CrossRef CAS PubMed.
-
(a) F. Liu, J.-Y. Wang, P. Zhou, G. Li, W.-J. Hao, S.-J. Tu and B. Jiang, Angew. Chem., Int. Ed., 2017, 56, 15570 CrossRef CAS PubMed;
(b) M.-H. Huang, W.-J. Hao and B. Jiang, Chem. – Asian J., 2018, 13, 2958 CrossRef CAS PubMed.
-
(a) D. De Keukeleire and S. L. He, Chem. Rev., 1993, 93, 359 CrossRef CAS;
(b) E. H. Oh, H. J. Kim and S. B. Han, Synthesis, 2018, 3346 CAS;
(c) D. Ravelli, S. Protti and M. Fagnoni, Chem. Rev., 2016, 116, 9850 CrossRef CAS PubMed.
- S. Cai, Y. Tian, J. Zhang, Z. Liu, M. Lu, W. Weng and M. Huang, Adv. Synth. Catal., 2018, 360, 4084 CrossRef CAS.
-
(a) X. Liu, F. Xiong, X. Huang, L. Xu, P. Li and X. Wu, Angew. Chem., Int. Ed., 2013, 52, 6962 CrossRef CAS PubMed;
(b) H.-L. Huang, H. Yan, G.-L. Gao, C. Yang and W. Xia, Asian J. Org. Chem., 2015, 4, 674 CrossRef CAS;
(c) P. Xu, K. Hu, Z. Gu, Y. Cheng and C. Zhu, Chem. Commun., 2015, 51, 7222 RSC;
(d) H. Egami, R. Shimizu, Y. Usui and M. Sodeoka, Chem. Commun., 2013, 49, 7346 RSC.
- M. Lu, Z. Liu, J. Zhang, Y. Tian, H. Qin, M. Huang, S. Hu and S. Cai, Org. Biomol. Chem., 2018, 16, 6564 RSC.
- M. Lu, H. Qin, Z. Lin, M. Huang, W. Weng and S. Cai, Org. Lett., 2018, 20, 7611 CrossRef CAS PubMed.
- H. Jiang and A. Studer, Chem. – Eur. J., 2019, 25, 516 CrossRef CAS PubMed.
- N. R. Patel, C. B. Kelly, A. P. Siegenfeld and G. A. Molander, ACS Catal., 2017, 7, 1766 CrossRef CAS PubMed.
- J. Guo, Q.-L. Wu, Y. Xie, J. Weng and G. Lu, J. Org. Chem., 2018, 83, 12559 CrossRef CAS PubMed.
- T. Ju, Q. Fu, J.-H. Ye, Z. Zhang, L.-L. Liao, S.-S. Yan, X.-Y. Tian, S.-P. Luo, J. Li and D.-G. Yu, Angew. Chem., Int. Ed., 2018, 57, 13897 CrossRef CAS PubMed.
-
(a) T. Mita, J. Chen, M. Sugawara and Y. Sato, Angew. Chem., Int. Ed., 2011, 50, 1393 CrossRef CAS PubMed;
(b) A. A. Sathe, D. R. Hartline and A. T. Radosevich, Chem. Commun., 2013, 49, 5040 RSC;
(c) T. Mita, M. Sugawara, K. Saito and Y. Sato, Org. Lett., 2014, 16, 3028 CrossRef CAS PubMed;
(d) C.-X. Guo, W.-Z. Zhang, H. Zhou, N. Zhang and X.-B. Lu, Chem. – Eur. J., 2016, 22, 17156 CrossRef CAS PubMed.
- J.-X. Li, L. Li, M.-D. Zhou and H. Wang, Org. Chem. Front., 2018, 5, 1003 RSC.
- T. C. Sherwood, N. Li, A. N. Yazdani and T. G. M. Dhar, J. Org. Chem., 2018, 83, 3000 CrossRef CAS PubMed.
- J. K. Matsui, D. N. Primer and G. A. Molander, Chem. Sci., 2017, 8, 3512 RSC.
- G. G. Pawar, F. Robert, E. Grau, H. Cramail and Y. Landais, Chem. Commun., 2018, 54, 9337 RSC.
- Y. Y. Loh, K. Nagao, A. J. Hoover, D. Hesk, N. R. Rivera, S. L. Colletti, I. W. Davies and D. W. C. MacMillan, Science, 2017, 358, 1182 CrossRef CAS PubMed.
- W. Xu, J. Ma, X.-A. Yuan, J. Dai, J. Xie and C. Zhu, Angew. Chem., Int. Ed., 2018, 57, 10357 CrossRef CAS PubMed.
- S. P. Morcillo, E. M. Dauncey, J. H. Kim, J. J. Douglas, N. S. Sheikh and D. Leonori, Angew. Chem., Int. Ed., 2018, 57, 12945 CrossRef CAS PubMed.
|
This journal is © The Royal Society of Chemistry 2019 |
Click here to see how this site uses Cookies. View our privacy policy here.