DOI:
10.1039/D2TA00690A
(Perspective)
J. Mater. Chem. A, 2022,
10, 17988-17999
On the challenges of materials and electrochemical characterization of concentrated electrolytes for redox flow batteries
Received
25th January 2022
, Accepted 23rd July 2022
First published on 25th July 2022
Abstract
High concentration electrolytes are of growing interest in energy storage technologies, such as redox flow batteries, where multimolar active species concentrations are believed to be necessary for economic viability. However, conventional approaches for materials and electrochemical characterization of multicomponent solutions often assume dilute conditions and, consequently, are challenged by the molar-scale concentrations of redoxmers (e.g., organics, coordination complexes) and supporting salt. Further, emergent behaviors (e.g., solution structuring, molecular aggregation, phase changes) can be present at elevated concentrations, which confound traditional interpretations. Accordingly, different methods and techniques are required to determine electrochemical and transport descriptors and to link these macroscopic properties to microscopic phenomena. In this perspective, we describe the need for and difficulties inherent to experimental measurements of concentrated electrolytes; we highlight recent progress in terms of scientific insight and method development; and we suggest new directions for the research community with a particular focus on nonaqueous redox flow batteries.
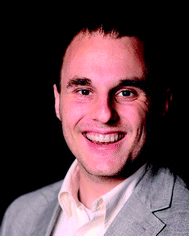 Alexis M. Fenton Jr | Alexis Maguin Fenton Jr is a recent graduate of the Chemical Engineering (ChemE) Department at the Massachusetts Institute of Technology, where he worked with Prof. Fikile Brushett on developing automated voltammetric methods to diagnose redox electrolyte behavior in batteries. He recently defended his PhD dissertation on the same subject, and during his candidacy, he was a MathWorks Engineering Fellow while serving as the ChemE Teaching Development Fellow. Prior to his graduate studies, Alexis received his B. S. in Chemical Engineering at Rice University. |
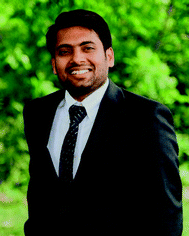 Rahul Kant Jha | Rahul Kant Jha is a PhD student in the Department of Chemistry at University of Michigan. Prior to this, he received his B. S. degree in Chemistry from Birla Institute of Technology and his M. S. degree in Chemistry from IISER Bhopal, India. Before moving to Michigan in August 2021, he worked as a PhD student at University of Kentucky for two years under the supervision of Prof. Susan Odom on developing organic redoxmers for nonaqueous redox flow battery applications. His research interests include energy storage systems and approaches to polymer recycling/upcycling. |
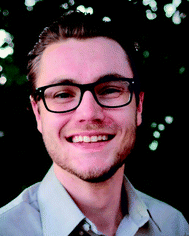 Bertrand J. Neyhouse | Bertrand Neyhouse is a National Science Foundation Graduate Research Fellow pursuing his PhD in Chemical Engineering at the Massachusetts Institute of Technology. His research in the Brushett Group centers on the design of redox flow batteries, applying chemical / electrochemical engineering principles to better understand design tradeoffs for constituent materials. Prior to graduate school, Bertrand received his B. S. in Chemical Engineering from Ohio University. |
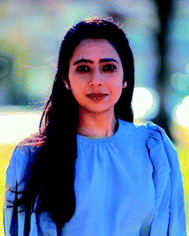 Aman Preet Kaur | Aman Preet Kaur is currently a Research Assistant Professor in the Department of Chemistry at University of Kentucky (UK). She works on studying structure/property relationships of redox-active molecules for energy storage applications, such as lithium-ion batteries and redox flow batteries. She received her PhD in chemistry under the supervision of Prof. Mark S. Meier from UK. She later continued as post-doctoral researcher with Prof. Susan A. Odom. Her research interests are in design, synthesis, and characterization of conjugated organic materials for applications that access multiple states of oxidation. |
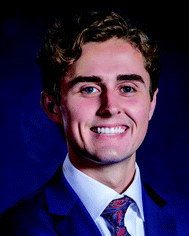 Daniel A. Dailey | Daniel Allen Dailey is an Honors student at the University of Kentucky (UK) pursuing a B. S. in Chemical Engineering and Mathematics minor alongside undergraduate certificates in Environmental Engineering, Lean Systems, and Power and Energy. He worked on the design, synthesis, and characterization of redox-active organic species under the supervision of Prof. Susan A. Odom from 2019–2022. He also worked on a pilot–plant scale rare-earth extraction project under the supervision of Prof. Rick Q. Honaker during 2020, to which he returned in May 2022. He will lead UK's efforts in using synthetic biology for rare-earth extraction in Fall 2022. |
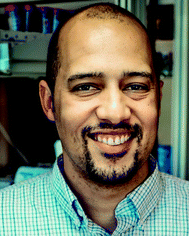 Fikile R. Brushett | Fikile Brushett is an Associate Professor of Chemical Engineering at the Massachusetts Institute of Technology (MIT). Prior to joining MIT, he received his PhD from the University of Illinois at Urbana-Champaign and was a Director's Postdoctoral Fellow at Argonne National Laboratory. He leads a research group advancing the science and engineering of electrochemical technologies for a sustainable energy economy. Their current efforts focus on redox flow batteries for grid storage and electrochemical processes for carbon management and chemical manufacturing. Brushett also serves as the Research Integration co-Lead for the Joint Center for Energy Storage Research, a DOE-funded energy innovation hub. |
1. Introduction
Growing global concern regarding environmental damage caused by the combustion of fossil fuels has prompted a shift toward a more sustainable energy infrastructure.1 Indeed, the rapid advancement and decreasing cost of renewable energy technologies (e.g., solar photovoltaic, wind) has led to significant increases in their deployment within the energy sector.2 However, the intermittent nature of these resources necessitates the implementation of cost-effective energy management solutions, including large-scale storage systems that are capable of facilitating the reliable delivery of renewable electricity and fortifying the existing grid infrastructure.
While a range of electrochemical technologies are under consideration for stationary energy storage applications, redox flow batteries (RFBs) are particularly attractive, as their system architecture enables independent scaling of power and energy components, long service lifetimes, simplified manufacturing, and improved safety.3,4 A key limitation of current state-of-the-art aqueous RFB chemistries is their upfront cost, which is driven by low energy densities as compared to enclosed rechargeable battery technologies (e.g., lithium-ion batteries) and by the use of relatively expensive charge-storage materials (e.g., vanadium).4 Transitioning from aqueous electrolytes to nonaqueous electrolytes enables access to a wider window of electrochemical stability and a diverse array of supporting salts and solvents.5 Further, replacing transition metal salts with redoxmers—loosely defined as metal-centered coordination complexes, redox-active organic monomers, oligomers, polymers, and colloids6—enables tuning of performance-relevant properties, including solubility, stability, and redox potential, through molecular functionalization. Moreover, if appropriately selected, these compounds have the potential to be produced at-scale for comparatively low cost, making them attractive for use in nonaqueous RFBs.7,8
Despite these promises, nonaqueous RFBs are a nascent storage concept, and there is considerable uncertainty about the materials and performance requirements for technological feasibility. Techno-economic modeling efforts have suggested that high redoxmer concentrations (ca. 3–5 M) are needed to offset the expense of nonaqueous electrolytes, though improvements to other areas of the battery system (e.g., higher cell voltages, lower resistance reactors, reduced balance-of-plant costs) may enable lower active species concentrations.6,9 Further, while increased redoxmer concentrations augment the battery energy density, they can also significantly impact electrochemical and physiochemical properties of the electrolytes, which, in turn, adversely impact battery operation and efficiency.10 Understanding the behavior of concentrated solutions also requires more complex mathematical treatments, as dilute solution theories do not capture solute–solute interactions.11 The critical concentrations at which electrochemical and transport properties change is a function of—among other factors—electrolyte chemistry (e.g., solvent and solute identities), operating conditions (e.g., temperature), and electrochemical state (e.g., electrolyte state-of-charge (SOC) and state-of-health (SOH)).10,12,13
Accurate quantitative analyses of concentrated solutions are necessary yet challenging to perform, as electrolyte behavior often changes in unique and non-intuitive ways with increasing redoxmer concentration (e.g., formation of aggregates12). Further, activity coefficients—often assumed to be unity for simplicity—may assume a range of values at higher solute concentrations, necessitating additional analyses to accurately model concentrated electrolyte behavior. As such, there is a need to develop systematic approaches to understand, characterize, and ultimately engineer high concentration electrolytes suitable for high-performance RFBs. This perspective highlights ongoing work that assesses RFB electrolytes with high redoxmer concentrations to contextualize opportunities for improved methods and workflows, as illustrated in Fig. 1.
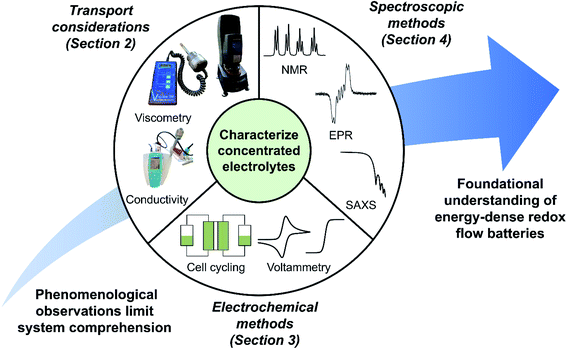 |
| Fig. 1 Pictorial overview of the topics addressed within this perspective. A concerted set of techniques can be used to evaluate transport and electrochemical phenomena—along with solution structure—in concentrated electrolytes, enabling the foundational understandings needed for judicious engineering of energy-dense RFBs. The electron paramagnetic resonance (EPR) spectrum is adapted with permission from N. H. Attanayake, J. A. Kowalski, K. V. Greco, M. D. Cassleman, J. D. Milshtein, S. J. Chapman, S. R. Parkin, F. R. Brushett, S. A. Odom, Tailoring Two-Electron-Donating Phenothiazines To Enable High-Concentration Redox Electrolytes for Use in Nonaqueous Redox Flow Batteries, Chem. Mater., 31(12), 4353–4363. Copyright 2019 American Chemical Society.14 The small-angle X-ray scattering (SAXS) spectrum, in turn, is adapted with permission from J. Maes, N. Castro, K. De Nolf, W. Walravens, B. Abécassis, Z. Hens, Size and Concentration Determination of Colloidal Nanocrystals by Small-Angle X-ray Scattering, Chem. Mater., 30(12), 3952–3962. Copyright 2018 American Chemical Society.15 | |
More specifically, we aim to describe the foundational approaches needed to robustly evaluate concentrated electrolytes by: (1) discussing the impact of high redoxmer concentrations on electrolyte rheology and species transport, alongside methods to measure these properties in the context of RFBs; (2) describing electrochemical methods for studying RFB redoxmers and considering their efficacy toward evaluating concentrated solutions; and (3) highlighting the strengths and drawbacks of existing and proposed spectroscopic analyses of concentrated electrolytes. This work is not an exhaustive treatment of the subject matter; rather, it seeks to underscore key features and analyses of concentrated redoxmer electrolyte performance while providing references to more detailed studies. While we focus on nonaqueous RFBs—as increasing redoxmer concentrations are critical to the success of this storage concept—many approaches discussed are also applicable to aqueous RFBs; where appropriate, we also highlight advances within this subfield.
2. Rheological and transport phenomena considerations for concentrated electrolytes
2.1. Current methods
Concentrated electrolytes present complex design tradeoffs which must be considered in electrolyte formulation, device operation, and reactor modeling. Understanding their associated rheological and transport phenomena can provide preliminary insight into possible flow cell behavior, and techniques that probe these processes should be integrated into the suite of RFB electrolyte diagnostics. To this end, we discuss implications of density, conductivity, redox species mobility, and rheology alongside current and future characterization methods.
Electrolyte density (mass per volume) plays a key role in computing mass and momentum transport through the electrochemical cell, electrolyte reservoirs, pumps, and manifolding.16 The density also relates the molarity of a compound to its molality, informing colligative properties as well as the gravimetric and volumetric charge capacities for a given electrolyte. Despite the simplicity of the concept, solution density is important to quantify in concentrated electrolyte formulations, as it is connected to key properties as well as other processes (e.g., solvent self-diffusion).10
Ionic conductivity considers the combined mobilities of all dissolved charged species and impacts the rates of charge transport through the electrolyte phase. At low solute concentrations (i.e., dilute solutions), the Einstein relation equates ionic mobility with diffusivity, which in turn relates to viscosity.17 At higher solute concentrations, where the dilute solution assumption is invalid, the tradeoff between conductivity and viscosity persists, but the governing equations may vary based on solute–solute interactions and relative ion mobilities (i.e., transference). Quantifying relationships between ion mobility and concentration with commercial conductivity probes and electroanalytical measurements in bespoke cells (e.g., electrochemical impedance spectroscopy18) provides valuable insights into ion transport rates in concentrated electrolytes.
Similarly, solute concentration also affects redoxmer mobility, as dissolved species diffuse more slowly through increasingly viscous solutions; consequently, this elevates concentration overpotentials (i.e., resistance to reactant transport) in redox flow cells.19 Redoxmer transport properties are primarily quantified through fundamental electroanalytical20 and spectroscopic methods,21 while in flow cells, advective mass transport rates can be quantified via limiting currents obtained through polarization experiments (vide infra).22 Advection serves as a tunable handle to enhance redoxmer mass transport rates in flow cells, enabling higher limiting currents and accessible capacities. Losses in species mobility at increasing concentrations may necessitate higher flow rates to maintain specific performance parameters; however, increases in viscosity result in higher pressure drops (increased hydraulic resistance) throughout the fluidic loops, exacerbating tradeoffs between the pumping power required to generate sufficient electrolyte velocities and the associated improvements in cell performance.
Species interactions at increased concentrations can also lead to higher order—nanoscale and mesoscale—structural changes in the electrolyte, which impact rheological responses including increases in viscosity.13 Quantification of rheological properties extends from measuring Newtonian viscosity to more completely characterizing the fluid response to variable shear rates. In general, RFB electrolytes containing small molecule redox species are largely Newtonian and weakly shear-dependent, often making simpler tools sufficient for most rheological characterizations. For example, probe viscometers have been used to quantify the viscosity in relatively concentrated electrolytes (ca. 1 M active species) to understand tradeoffs in transport properties (i.e., viscosity, conductivity, diffusivity).18,19 Flow viscometers, which offer control over fluid dynamic conditions, have also been applied to provide insight into the steady shear response in similar electrolytes, confirming the Newtonian behavior in such systems.13 Conversely, macromolecular redox species (i.e., polymers, colloids) typically exhibit higher viscosities and non-Newtonian characteristics in concentrated electrolytes, such as shear-thinning or shear-thickening behavior.23–25 Such electrolytes may necessitate higher pumping power to achieve acceptable mass transport rates, potentially limiting overall performance. Further, non-Newtonian electrolytes can induce clogging/gelling in flow channels as a result of aggregation and rapid velocity changes, which impose larger local pressure drops and may lead to system failure (e.g., leaks, over-pressurization).26 In these cases, rheometers may be needed to reliably characterize fluid properties, as these instruments enable high precision measurements in multiple geometries over a wide range of shear rates and deformation profiles.27,28 These conditions can also be selected to mimic fluid dynamic environments within the flow cell.
The transport properties discussed throughout this section also tend to vary with the electrolyte SOC, changing more dramatically at elevated redoxmer concentrations.29–31 Thus, quantifying electrolyte properties at different SOCs and supporting salt concentrations presents an additional handle to tune the electrolyte structure and evince essential chemical and electrochemical features; for example, these quantitative relationships have previously been used to monitor operando SOCs using in-line density and viscosity measurements in vanadium RFBs.32,33
2.2. Future approaches
Methods for investigating rheological and transport phenomena are relatively mature, as they are integral to a range of disciplines (e.g., fluid mechanics, soft matter physics, electroanalytical chemistry); as such, the tools discussed here are likely to be sufficient for the characterization of modern electrolyte formulations. Future approaches must continue leveraging existing workflows to evaluate transport properties in tandem and across varying conditions (e.g., species concentrations, SOC) to elicit design tradeoffs and better understand structure–property relationships. Additionally, the electrochemical and spectroscopic methods discussed in subsequent sections may further advance these measurements by informing connections between the chemical environment, transport properties, and electrochemical performance. Complementary to experimental tools, simulation-based approaches (e.g., molecular dynamics (MD)) have shown promise for interrogating structure–property relationships and predicting transport property profiles in complex electrochemical environments.34–36 We anticipate these methods will provide deeper theoretical insights for emerging electrolytes.
3. Electrochemical methods to study concentrated electrolytes
3.1. Current methods
Electrochemical characterization plays an essential role in evaluating RFB performance, with approaches ranging from first-principles to device-level analyses.14,37,38 Fundamental studies typically utilize electroanalytical techniques (e.g., cyclic voltammetry,14,37 rotating disk voltammetry38) to evaluate electrochemical and transport properties of redoxmers at dilute active species concentrations (ca. 1–10 mM38–43). Different technique sets, in turn, are required to evaluate the behavior of redoxmers at higher concentrations due to the presence of larger ohmic losses, greater electric field effects, chemistry-dependent phenomena (e.g., aggregation), and possibly convection.12,44 Diagnostic flow cell configurations can provide valuable information about high concentration electrolyte behavior.37,45–48 In particular, the zero-gap cell architecture used in a number of studies results in a lower ohmic resistance through reduced electrode-to-electrode distances and higher surface area electrodes;37,46 this, in turn, enables sufficiently high currents able to charge and discharge concentrated redoxmer systems. Symmetric cell cycling—where only one redox couple is added to both half-cells, each with its own distinct reservoir—can simulate the performance of a single redox couple (e.g., polarization behavior, long-term stability),37 while single electrolyte cells, which utilize a single reservoir for both half-cells, can study the polarization behavior of individual electrolytes at a fixed SOC.46
Combining these platforms with other techniques (e.g., electrochemical impedance spectroscopy) as well as variable operating conditions (e.g., different fluid flow rates) can elicit multifaceted responses, which, in turn, can provide insight into kinetic, ohmic, and mass transfer resistances that collectively result in overpotential losses (i.e., lower device efficiency), as well as inform the modes/rates of capacity fade.37,46 Beyond symmetric and single electrolyte cells, full-cell cycling—where different redox couples are used in each half-cell—introduces additional complications (e.g., crossover, electrolyte compatibility) but is a necessary step in the development and demonstration of viable RFB systems.45 While these complicating features mean that full cell cycling is less suited for understanding individual redox couple behavior, such experiments enable quantification of important performance metrics such as cycling efficiency and power density.48
In contrast to cell cycling, fundamental electrochemical techniques are less equipped to interrogate concentrated electrolytes, in part because of potential distortions that arise at larger currents (e.g., distorted peak-to-peak separation in CVs).20,49 These effects can be mitigated using ohmic compensation,19,50,51 though in cases where compensation is conducted post-experiment, some aspects cannot be fully corrected, such as distorted scan rates and turnaround potentials (aptly illustrated in Fig. 7.1 of ref. 52). Further, more complex levels of theory—potentially accounting for solute–solute interactions, migration, non-unity activity coefficients, and homogeneous electron transfer (e.g., the Dahms–Ruff equation)—are necessary to describe concentrated electrolyte behavior.11,20,44 Aggregation of redoxmers (e.g., dimerization, oligomerization, contact networks) has also been reported with increasing concentration—sometimes at concentrations as low as tens of mM—where the manner and extent of these effects can be chemistry-specific, further complicating analytical work-ups.12,36 Potentially for these reasons, most current approaches forego electroanalytical techniques like voltammetry. Rather, studies primarily rely upon spectroscopic, rheological, and conductivity measurements to characterize the dynamics of these concentrated electrolytes,10,53 in turn revealing the need to advance electrochemical methods.
3.2. Future approaches
Employing voltammetric techniques whose signal is not appreciably distorted under relevant electrolyte conditions is one strategy to overcome the aforementioned limitations. In this vein, microelectrode voltammetry (working electrode radius ca. 5–50 μm) holds particular promise for electroanalytical interrogation, as the smaller current magnitude (ca. nA) negates ohmic distortions. Researchers have already capitalized on these advantages to probe electrolytes which are challenging to study using macroelectrodes (working electrode radius ca. 1 mm), such as high resistance electrolytes.49 The method is also largely non-invasive due to the small electrode size49 and the near-zero conversion of bulk species.54 Although not a unique advantage to concentrated electrolytes, microelectrode voltammetry also exhibits distinct oxidative and reductive current plateaus, making it a potentially powerful tool to quantify SOC and SOH.54,55 A visual example of the ability for microelectrode voltammetry to probe solutions at higher concentrations, along with its ability to estimate solution SOC and SOH, is depicted in Fig. 2.
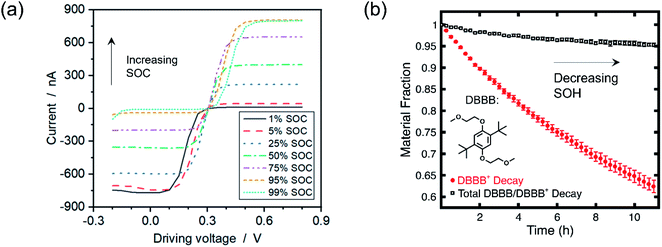 |
| Fig. 2 (a) Microelectrode voltammogram of 500 mM ferri-/ferrocyanide at various SOCs in an aqueous solution of 1 M KCl. Adapted with permission from ref. 55. Copyright 2019 American Chemical Society. (b) Solution SOH as predicted from microelectrode voltammograms of 5 mM of electrochemically oxidized 2,5-di-tert-butyl-1,4-bis(2-methoxyethoxy)benzene (DBBB) in a solution of 1 M lithium bis(trifluoromethane)sulfonamide in propylene carbonate. Republished with permission of IOP Publishing, Ltd, from A Method for Evaluating Soluble Redox Couple Stability Using Microelectrode Voltammetry, Kowalski et al., 167, 16, 2020; permission conveyed through Copyright Clearance Center, Inc.54 | |
Fig. 2a illustrates the ability for microelectrodes to accurately estimate the SOC of solutions containing 500 mM redoxmers, though the authors were able to probe concentrations up to 1.3 M.55 While these experiments were conducted in an aqueous solution—where dilute solution theory arguably breaks down at higher solute concentrations given the neat concentration of water (ca. 56 M, compared to, for example, propylene carbonate (ca. 12 M) and acetonitrile (ca. 19 M))—they nonetheless demonstrate the potential for microelectrodes to assess more concentrated analyte solutions. Fig. 2b, in turn, depicts a framework for simultaneously estimating the SOC (not directly shown) and SOH of a solution containing relatively dilute redox couples (≤25 mM) undergoing decay, further demonstrating the promise microelectrodes hold in probing RFB electrolytes.54
Despite these potential advantages, microelectrodes have not been widely employed to study RFB-relevant concentrated electrolytes. This lack of implementation may arise in part from the limited use of the technique by the greater RFB field (as compared to macroelectrode voltammetry), the need for specialized equipment (e.g., sensitive potentiostats, expensive or home-made electrodes) that may not be universally accessible, and the greater sensitivity to external interferences (e.g., mechanical vibrations). Further, existing microelectrode theory has been well-developed for dilute solutions,56 but the theory behind microelectrode models becomes complicated in the concentrated solution regime; it requires many parameters to be accurately estimated (e.g., solute–solute interaction coefficients),11 it may necessitate the formulation of additional modes of mass transport (e.g., migration, faradaic convection),57 and it may require knowledge of chemical-specific phenomena such as aggregation and nano-heterogeneity.12,58
Custom-made cell configurations (e.g., microfluidic electrochemical devices59) may also find utility in evaluating concentrated electrolytes by further quantifying transport descriptors. For example, a Hittorf cell can be constructed to estimate the cation transference number for a concentrated binary electrolyte.60,61 Similar configurations and associated theoretical treatment may ultimately be extended—and potentially combined with microelectrode voltammetry studies—to evaluate the behavior of ternary or quaternary electrolytes, though a large number of unknown parameters will be introduced when considering additional species, likely resulting in more complex mathematical treatment.11,61 Solution structure (e.g., contact–ion pairs) must also be considered and evaluated when probing high concentration electrolytes.58 In sum, once the aforementioned advances are achieved, microelectrode voltammetry and other bespoke methods may be able to complement rheological analysis, cycling methods, and spectroscopy to more deeply probe the behavior of concentrated electrolytes in nonaqueous RFBs.
4. Spectroscopic methods to investigate concentrated electrolytes
4.1. Current methods
Spectroscopy has been widely used in the study of electrolytes, as this family of analytical techniques is more sensitive to composition than conventional electrochemical methods. Traditionally, multiple techniques like ultraviolet-visible spectrophotometry (UV-vis), nuclear magnetic resonance (NMR), and electron paramagnetic resonance (EPR) are used for examining electrolyte structure–property relationships either ex situ, operando, or post mortem.37,62–64 A combination of these spectroscopic techniques can provide a more holistic picture of the chemical and electrochemical environment.14,47 For example, researchers strategically combined operando NMR, EPR, and electrochemical studies to probe the performance of aqueous electrolytes containing 2,6-dihydroxyanthraquinone (DHAQ) (Fig. 3a and b).63,65,66 Although these studies were conducted with aqueous electrolytes, we anticipate that similar approaches can be employed in nonaqueous electrolytes, as ex situ NMR and EPR studies have been successfully conducted for nonaqueous RFB systems.14,67 Indeed, the more complex molecular structures of organic solvents and supporting salts may result in more discernible features—particularly for NMR—that could aid investigations. However, these additional signals may also lead to convolutions that challenge analyses. In addition, some of these powerful analytical methods (e.g., NMR, EPR) may be more challenging to engage operando because of the need for longer access times, which may not be feasible in centralized user facilities, along with the need for specialized device-spectrometer configurations.
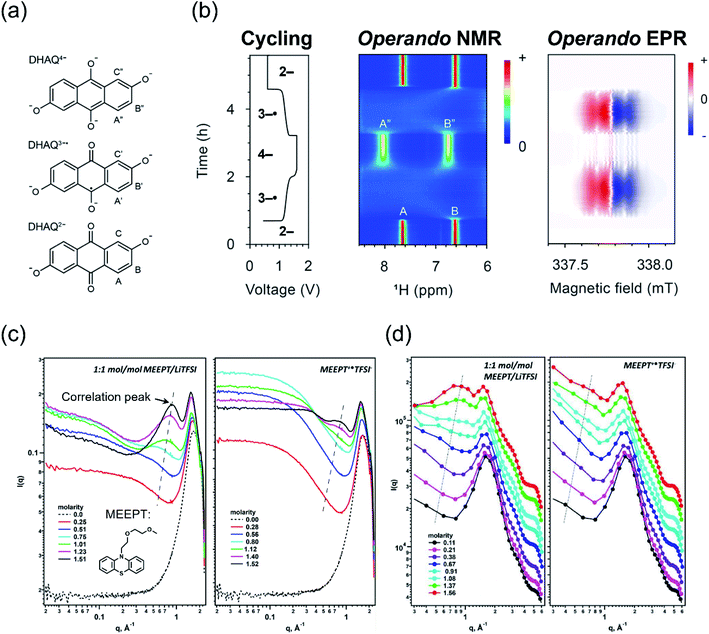 |
| Fig. 3
Operando
1H NMR and EPR spectra acquired during electrochemical cycling containing (a) structures of the DHAQ2−, DHAQ3−˙, and DHAQ4− anions—upper-case letters are proton labels—along with (b) one complete cycle with a potential hold of 1.6 V during charging and 0.6 V during discharging, along with the associated 1H NMR and EPR spectra vs. cycling time. The bolded numbers on the voltage profile indicate the predominant oxidation state of DHAQ, and the color bars indicate the intensity of the resonance in arbitrary units. Adapted with permission from ref. 63viahttps://pubs.acs.org/doi/10.1021/jacs.0c10650; further inquiries related to the material excerpted should be directed to the American Chemical Society. Copyright 2021 American Chemical Society. Also included are (c) SAXS spectra of neutral and charged MEEPT as a function of molarity and (d) MD simulations of the SAXS spectra. In both (c) and (d), the correlation peaks are accentuated via trend lines. Reprinted from Journal of Molecular Liquids, Volume 334, Shkrob et al., Crowded electrolytes containing redoxmers in different states of charge: Solution structure, properties, and fundamental limits on energy density, 116533, Copyright 2021, with permission from Elsevier.10 | |
Despite extensive implementation on dilute solutions, some techniques may lack the ability to probe concentrated electrolytes in a simple or accessible fashion. UV-vis serves as an exemplar technique; for an anthraquinone derivative in an acidic aqueous electrolyte, concentrations of up to 200 mM can be probed with a path length of 100 μm.68 This threshold concentration will likely change depending on species identity and solution composition, but in this instance, spectrometer oversaturation ultimately sets the limit on allowable redoxmer concentration. That said, other spectroscopic techniques are better-suited to evaluate concentrated electrolytes. NMR is one such method, as the signal-to-noise ratio is proportional to the amount of species present, though the linewidth may broaden for solutions with greater viscosities (via slower diffusion).69 Indeed, pulsed-field gradient (PFG) NMR has been utilized to quantify solvent self-diffusion, but generally care should be taken when using PFG NMR to estimate chemical and electrochemical properties (e.g., transference numbers).10,70 EPR is similarly well-suited and provides a strong complement to NMR; radical species, which are common to organic redoxmers, obfuscate NMR spectra but are readily observed in EPR spectra. Despite the utility of these techniques, not all NMR and EPR analyses probe RFB electrolytes in their native environment. For example, many NMR studies use deuteration, potentially necessitating dilution that can disrupt the aggregation of active material and supporting salt at higher concentrations.12,36
Recently, scattering experiments—such as small-angle neutron scattering or small-angle X-ray scattering (SAXS)—have been paired with other techniques (either experimental or simulation-based) to probe the microscopic solution structure of concentrated electrolytes to quantify aggregation.36,58,63,65,71,72 For example, Shkrob et al. reported the application of SAXS to analyze the unique modes of interactions that occur in neutral and charged nonaqueous electrolytes at higher redoxmer concentrations (Fig. 3c). Using N-[2-(2-methoxyethoxy)ethyl]phenothiazine (MEEPT) as a model compound in solutions of lithium bis(trifluoromethane)sulfonamide supporting salt and acetonitrile, the authors found that a gel-like regime forms between solute concentrations of ca. 1.5–2 M, decreasing the fluidity (i.e., ease of flow), diffusivity, and ionic conductivity.10 Classical MD simulations, along with other experimental techniques, can also be conducted to gain further insight into the electrolyte solution structure to validate SAXS studies (Fig. 3d). As an example, the combination of MD and EPR enabled researchers to deduce that contact networks lead to the increased stability of an oxidized dialkoxybenzene radical cation via entrapment.36
In sum, the variety of techniques highlighted here demonstrate the ability to probe the microscopic behavior of concentrated solutions using a suite of spectroscopic tools. They also illustrate how redoxmers may behave in concentrated solutions, although the effects of these dynamic networks on bulk rheological and mass transport properties (e.g., viscosity, effective diffusion coefficient) are not yet fully elucidated. While some techniques, like NMR and EPR, have been deployed for operando monitoring,63–66 others (e.g., SAXS) have only been conducted off-line and ex situ for nonaqueous RFBs at the time of writing,36,71 limiting the diagnostic information that can be generated in real-time. As such, greater insights into the microscopic environment of these electrolytes may be possible with further advances in operando workflows and configurations.73
4.2. Future approaches
Although significant advances have been made towards understanding the behavior of concentrated electrolytes, opportunities exist for characterizing electrolytes in operating systems. For example, present studies have evaluated homogeneous solution behavior at high redoxmer concentrations, but to fully characterize RFB performance, heterogeneous interactions between high concentration electrolytes and the flow field or electrode (along with other RFB components) must also be evaluated. Synchrotron X-ray radiography may potentially elucidate these dynamics in nonaqueous RFBs, similar to how researchers have studied electrolyte flow through carbon felt electrodes for vanadium RFBs.74,75 For example, the configuration described in Eifert et al. may be integrated with other spectroscopic techniques to evaluate other pertinent phenomena (e.g., aggregation).74
Overall, the variety of spectroscopic studies conducted thus far demonstrates the possibility of examining the experimental behavior of concentrated electrolytes—in some cases operando.36,71 Integration of real-time SAXS studies for nonaqueous RFBs may enable a deeper understanding of the solution structure within an operating cell, which can be used to connect macroscopic behavior with specific microscopic phenomena at various SOCs and SOHs.73 The insights developed from such studies could then be applied to optimize the performance of high-concentration electrolytes through molecular engineering and cell design.
5. Conclusions
This perspective highlights the challenges associated with employing high redoxmer concentrations for RFBs and discusses current and emerging methodologies for investigating concentrated electrolytes. Though increased redoxmer concentrations are concomitant with higher energy densities, other important electrolyte and transport characteristics (e.g., viscosity, conductivity, diffusivity) also evolve in nuanced ways that are sometimes detrimental to battery performance. The rheological, chemical, and electrochemical properties of concentrated electrolytes are interrelated and thus, when evaluated together, provide necessary insight for understanding cell-level behavior. Previous studies have partially elucidated the influence of redoxmer concentration on electrolyte behavior, but knowledge gaps remain in understanding the relationship between microscopic properties and macroscopic behavior in these concentrated solutions across the wide range of operating conditions encountered in practical technology embodiments. These gaps may be systematically narrowed by implementing suitable electroanalytical methods (e.g., microelectrode voltammetry, diagnostic flow cell cycling) and by advancing additional operando measurements, such as real-time scattering studies. Overall, greater mechanistic knowledge of concentrated redoxmer-based electrolytes will enable effective workflows; this, in turn, can be used to identify electrolytes that improve RFB performance and further the development of cost-effective stationary energy storage systems.
Conflicts of interest
The Brushett and Odom groups have several patents and patent applications related to nonaqueous redox flow batteries under consideration with the US Patent and Trademark Office.
Author contribution
A. M. F., Jr performed the conceptualization, writing − original draft, writing − review and editing, and visualization. R. K. J. performed the conceptualization, writing − original draft, writing − review and editing, and visualization. B. J. N. performed the conceptualization, writing − original draft, writing − review and editing, and visualization. A. P. K. performed the conceptualization, writing − original draft, writing − review and editing, and visualization. D. A. D. performed the conceptualization, writing − original draft, writing − review and editing, and visualization. S. A. O. performed the conceptualization, funding acquisition, project administration, resources, supervision, writing − original draft, writing − review and editing. F. R. B. performed the conceptualization, funding acquisition, project administration, resources, supervision, writing − original draft, writing − review and editing.
Acknowledgements
This work is dedicated to the memory of Professor Susan A. Odom, a fierce, creative, and passionate scientist who helped pioneer the implementation of redoxmers in energy storage systems. This work is funded by the National Science Foundation (NSF) under Award Number 1805566 and is also partially supported as a part of the Joint Center for Energy Storage Research, an Energy Innovation Hub funded by the U.S. Department of Energy, Office of Science, Basic Energy Sciences. A. M. F. Jr gratefully acknowledges funding from the Massachusetts Institute of Technology School of Engineering MathWorks Engineering Fellowship. R. K. J. gratefully acknowledges support from the NSF Established Program to Stimulate Competitive Research. B. J. N. gratefully acknowledges the NSF Graduate Research Fellowship Program under grant number 2141064. Any opinion, findings, and conclusions or recommendations expressed in this material are those of the authors and do not necessarily reflect the views of the NSF. We thank Nicholas Matteucci, Alexander Quinn, and Christopher Mallia—all of the Brushett Group—for their constructive feedback of this manuscript, and we thank Kyle Lennon for taking the photograph of the rheometer in Fig. 1.
References
-
Global Warming of 1.5 °C. An IPCC Special Report on the Impacts of Global Warming of 1.5 °C above Pre-Industrial Levels and Related Global Greenhouse Gas Emission Pathways, in the context of Strengthening the Global Response to the Threat of Climate Change, Sustainable Development, and Efforts to Eradicate Poverty, ed. V. Masson-Delmotte, P. Zhai, H.-O. Pörtner, D. Roberts, J. Skea, P. R. Shukla, A. Pirani, W. Moufouma-Okia, C. Péan, R. Pidcock, S. Connors, J. B. R. Matthews, Y. Chen, X. Zhou, M. I. Gomis, E. Lonnoy, T. Maycock, M. Tignor and T. Waterfield, 2019 Search PubMed.
-
M. Bolinger and J. Seel, Utility-Scale Solar: Emperical Trends in Project Technology, Cost, Performance, and PPA Pricing in the United States - 2018 Edition, 2018 Search PubMed.
- A. Z. Weber, M. M. Mench, J. P. Meyers, P. N. Ross, J. T. Gostick and Q. Liu, Redox Flow Batteries: A Review, J. Appl. Electrochem., 2011, 41, 1137–1164, DOI:10.1007/s10800-011-0348-2.
- B. R. Chalamala, T. Soundappan, G. R. Fisher, M. R. Anstey, V. V. Viswanathan and M. L. Perry, Redox Flow Batteries: An Engineering Perspective, Proc. IEEE, 2014, 102(6), 976–999, DOI:10.1109/JPROC.2014.2320317.
- S.-H. Shin, S.-H. Yun and S.-H. Moon, A Review of Current Developments in Non-Aqueous Redox Flow Batteries: Characterization of Their Membranes for Design Perspective, RSC Adv., 2013, 3, 9095–9116, 10.1039/C3RA00115F.
- R. M. Darling, K. G. Gallagher, J. A. Kowalski, S. Ha and F. R. Brushett, Pathways to Low-Cost Electrochemical Energy Storage: A Comparison of Aqueous and Nonaqueous Flow Batteries, Energy Environ. Sci., 2014, 7, 3459–3477, 10.1039/c4ee02158d.
- V. Dieterich, J. D. Milshtein, J. L. Barton, T. J. Carney, R. M. Darling and F. R. Brushett, Estimating the Cost of Organic Battery Active Materials: A Case Study on Anthraquinone Disulfonic Acid, Transl. Mater. Res., 2018, 5(3), 034001, DOI:10.1088/2053-1613/aacb0e.
- T. D. Gregory, M. L. Perry and P. Albertus, Cost and Price Projections of Synthetic Active Materials for Redox Flow Batteries, J. Power Sources, 2021, 499, 229965, DOI:10.1016/j.jpowsour.2021.229965.
- R. Dmello, J. D. Milshtein, F. R. Brushett and K. C. Smith, Cost-Driven Materials Selection Criteria for Redox Flow Battery Electrolytes, J. Power Sources, 2016, 330, 261–272, DOI:10.1016/j.jpowsour.2016.08.129.
- I. A. Shkrob, L. A. Robertson, Z. Yu, R. S. Assary, L. Cheng, L. Zhang, E. Sarnello, X. Liu, T. Li, A. P. Kaur, T. M. Suduwella, S. A. Odom, Y. Wang, R. H. Ewoldt, H. M. Farag and Y. Z, Crowded Electrolytes Containing Redoxmers in Different States of Charge: Solution Structure, Properties, and Fundamental Limits on Energy Density, J. Mol. Liq., 2021, 334, 116533, DOI:10.1016/j.molliq.2021.116533.
-
J. Newman and K. E. Thomas-Alyea, Electrochemical Systems, 3rd ed., John Wiley & Sons. Inc., Hoboken, 2004 Search PubMed.
- T. J. Carney, S. J. Collins, J. S. Moore and F. R. Brushett, Concentration-Dependent Dimerization of Anthraquinone Disulfonic Acid and Its Impact on Charge Storage, Chem. Mater., 2017, 29, 4801–4810, DOI:10.1021/acs.chemmater.7b00616.
- Y. Wang, A. P. Kaur, N. H. Attanayake, Z. Yu, T. M. Suduwella, L. Cheng, S. A. Odom and R. H. Ewoldt, Viscous Flow Properties and Hydrodynamic Diameter of Phenothiazine-Based Redox-Active Molecules in Different Supporting Salt Environments, Phys. Fluids, 2020, 32(8), 083108, DOI:10.1063/5.0010168.
- N. H. Attanayake, J. A. Kowalski, K. V. Greco, M. D. Casselman, J. D. Milshtein, S. J. Chapman, F. R. Brushett and S. A. Odom, Tailoring Two-Electron-Donating Phenothiazines To Enable High-Concentration Redox Electrolytes for Use in Nonaqueous Redox Flow Batteries, Chem. Mater., 2019, 31(12), 4353–4363, DOI:10.1021/acs.chemmater.8b04770.
- J. Maes, N. Castro, K. De Nolf, W. Walravens, B. Abécassis and Z. Hens, Size and Concentration Determination of Colloidal Nanocrystals by Small-Angle X-Ray Scattering, Chem. Mater., 2018, 30(12), 3952–3962, DOI:10.1021/acs.chemmater.8b00903.
- B. Kumar Chakrabarti, E. Kalamaras, A. Kumar Singh, A. Bertei, J. Rubio-Garcia, V. Yufit, M. K. Tenny, B. Wu, F. Tariq, S. Y. Hajimolana, P. N. Brandon, C. T. J. Low, L. E. P. Roberts, Y.-M. Chiang and F. R. Brushett, Modelling of Redox Flow Battery Electrode Processes at a Range of Length Scales: A Review, Sustainable Energy Fuels, 2020, 4(11), 5433–5468, 10.1039/D0SE00667J.
-
W. M. Deen, Analysis of Transport Phenomena, Oxford University Press, 2nd edn, 2011 Search PubMed.
- J. D. Milshtein, J. L. Barton, T. J. Carney, J. A. Kowalski, R. M. Darling and F. R. Brushett, Towards Low Resistance Nonaqueous Redox Flow Batteries, J. Electrochem. Soc., 2017, 164(12), A2487–A2499, DOI:10.1149/2.0741712jes.
- J. L. Barton, J. D. Milshtein, J. J. Hinricher and F. R. Brushett, Quantifying the Impact of Viscosity on Mass-Transfer Coefficients in Redox Flow Batteries, J. Power Sources, 2018, 399, 133–143, DOI:10.1016/j.jpowsour.2018.07.046.
-
A. J. Bard and L. R. Faulkner, Electrochemical Methods: Fundamentals and Applications, 2nd ed., John Wiley & Sons. Inc., New York, 2001 Search PubMed.
- H. Sun, W. Chen and A. E. Kaifer, A Simple Method Based on NMR Spectroscopy and Ultramicroelectrode Voltammetry for the Determination of the Number of Electrons in Faradaic Processes, Organometallics, 2006, 25(7), 1828–1830, DOI:10.1021/om050917c.
- J. D. Milshtein, K. M. Tenny, J. L. Barton, J. Drake, R. M. Darling and F. R. Brushett, Quantifying Mass Transfer Rates in Redox Flow Batteries, J. Electrochem. Soc., 2017, 164(11), E3265–E3275, DOI:10.1149/2.0201711jes.
- N. Gavvalapalli, J. Hui, K. J. Cheng, T. Lichtenstein, M. Shen, J. S. Moore and J. Rodríguez-López, Impact of Redox-Active Polymer Molecular Weight on the Electrochemical Properties and Transport Across Porous Separators in Nonaqueous Solvents, J. Am. Chem. Soc., 2014, 136(46), 16309–16316, DOI:10.1021/ja508482e.
- M. Burgess, E. Chénard, K. Hernández-Burgos, G. Nagarjuna, R. S. Assary, J. Hui, J. S. Moore and J. Rodríguez-López, Impact of Backbone Tether Length and Structure on the Electrochemical Performance of Viologen Redox Active Polymers, Chem. Mater., 2016, 28(20), 7362–7374, DOI:10.1021/acs.chemmater.6b02825.
- E. C. Montoto, G. Nagarjuna, J. Hui, M. Burgess, N. M. Sekerak, K. Hernández-Burgos, T.-S. Wei, M. Kneer, J. Grolman, K. J. Cheng, J. A. Lewis, J. S. Moore and J. Rodríguez-López, Redox Active Colloids as Discrete Energy Storage Carriers, J. Am. Chem. Soc., 2016, 138(40), 13230–13237, DOI:10.1021/jacs.6b06365.
- V. A. Iyer, J. K. Schuh, E. C. Montoto, V. Pavan Nemani, S. Qian, G. Nagarjuna, J. Rodríguez-López, R. H. Ewoldt and K. C. Smith, Assessing the Impact of Electrolyte Conductivity and Viscosity on the Reactor Cost and Pressure Drop of Redox-Active Polymer Flow Batteries, J. Power Sources, 2017, 361, 334–344, DOI:10.1016/j.jpowsour.2017.06.052.
- D. T. N. Chen, Q. Wen, P. A. Janmey, J. C. Crocker and A. G. Yodh, Rheology of Soft Materials, Annu. Rev. Condens. Matter Phys., 2010, 1, 301–322, DOI:10.1146/annurev-conmatphys-070909-104120.
-
C. W. Macosko, Rheology: Principles, Measurements, and Applications, Wiley-VCH, Inc., New York, 1996 Search PubMed.
- Q. Xu, T. S. Zhao and C. Zhang, Effects of SOC-Dependent Electrolyte Viscosity on Performance of Vanadium Redox Flow Batteries, Appl. Energy, 2014, 130, 139–147, DOI:10.1016/j.apenergy.2014.05.034.
- T. Struckmann, P. Kuhn and S. Ressel, A Combined in situ Monitoring Approach for Half Cell State of Charge and State of Health of Vanadium Redox Flow Batteries, Electrochim. Acta, 2020, 362, 137174, DOI:10.1016/j.electacta.2020.137174.
- S. Ressel, F. Bill, L. Holtz, N. Janshen, A. Chica, T. Flower, C. Weidlich and T. Struckmann, State of Charge Monitoring of Vanadium Redox Flow Batteries Using Half Cell Potentials and Electrolyte Density, J. Power Sources, 2018, 378, 776–783, DOI:10.1016/j.jpowsour.2018.01.006.
- P. C. Ghimire, A. Bhattarai, T. M. Lim, N. Wai, M. Skyllas-Kazacos and Q. Yan,
In situ Tools Used in Vanadium Redox Flow Battery Research—Review, Batteries, 2021, 7(3), 53, DOI:10.3390/batteries7030053.
- O. Nolte, I. A. Volodin, C. Stolze, M. D. Hager and U. S. Schubert, Trust Is Good, Control Is Better: A Review on Monitoring and Characterization Techniques for Flow Battery Electrolytes, Mater. Horiz., 2021, 8(7), 1866–1925, 10.1039/D0MH01632B.
- D. J. Siegel, L. Nazar, Y.-M. Chiang, C. Fang and N. P. Balsara, Establishing a Unified Framework for Ion Solvation and Transport in Liquid and Solid Electrolytes, Trends Chem., 2021, 3(10), 807–818, DOI:10.1016/j.trechm.2021.06.004.
- Z. Li, L. A. Robertson, I. A. Shkrob, K. C. Smith, L. Cheng, L. Zhang, J. S. Moore and Y. Z, Realistic Ion Dynamics through Charge Renormalization in Nonaqueous Electrolytes, J. Phys. Chem. B, 2020, 124(15), 3214–3220, DOI:10.1021/acs.jpcb.0c01197.
- I. A. Shkrob, T. Li, E. Sarnello, L. A. Robertson, Y. Zhao, H. Farag, Z. Yu, J. Zhang, S. R. Bheemireddy, Y. Z, R. S. Assary, R. H. Ewoldt, L. Cheng and L. Zhang, Self-Assembled Solute Networks in Crowded Electrolyte Solutions and Nanoconfinement of Charged Redoxmer Molecules, J. Phys. Chem. B, 2020, 124, 10226–10236, DOI:10.1021/acs.jpcb.0c07760.
- M.-A. Goulet and M. J. Aziz, Flow Battery Molecular Reactant Stability Determined by Symmetric Cell Cycling Methods, J. Electrochem. Soc., 2018, 165(7), A1466–A1477, DOI:10.1149/2.0891807jes.
- C. G. Armstrong, R. W. Hogue and K. E. Toghill, Application of the Dianion Croconate Violet for Symmetric Organic Non-Aqueous Redox Flow Battery Electrolytes, J. Power Sources, 2019, 440, 227037, DOI:10.1016/j.jpowsour.2019.227037.
- D. G. Kwabi, K. Lin, Y. Ji, E. F. Kerr, M.-A. Goulet, D. De Porcellinis, D. P. Tabor, D. A. Pollack, A. Aspuru-Guzik, R. G. Gordon and M. J. Aziz, Alkaline Quinone Flow Battery with Long Lifetime at pH 12, Joule, 2018, 2(9), 1894–1906, DOI:10.1016/j.joule.2018.07.005.
- N. H. Attanayake, T. M. Suduwella, Y. Yan, A. P. Kaur, Z. Liang, M. S. Sanford and S. A. Odom, Comparative Study of Organic Radical Cation Stability and Coulombic Efficiency for Nonaqueous Redox Flow Battery Applications, J. Phys. Chem. C, 2021, 125(26), 14170–14179, DOI:10.1021/acs.jpcc.1c00686.
- N. H. Attanayake, Z. Liang, Y. Wang, A. P. Kaur, S. R. Parkin, J. K. Mobley, R. H. Ewoldt, J. Landon and S. A. Odom, Dual Function Organic Active Materials for Nonaqueous Redox Flow Batteries, Mater. Adv., 2021, 2(4), 1390–1401, 10.1039/D0MA00881H.
- C. S. Sevov, R. E. M. Brooner, E. Chénard, R. S. Assary, J. S. Moore, J. Rodríguez-López and M. S. Sanford, Evolutionary Design of Low Molecular Weight Organic Anolyte Materials for Applications in Nonaqueous Redox Flow Batteries, J. Am. Chem. Soc., 2015, 137(45), 14465–14472, DOI:10.1021/jacs.5b09572.
- A. A. Shinkle, A. E. S. Sleightholme, L. T. Thompson and C. W. Monroe, Electrode Kinetics in Non-Aqueous Vanadium Acetylacetonate Redox Flow Batteries, J. Appl. Electrochem., 2011, 41(10), 1191–1199, DOI:10.1007/s10800-011-0314-z.
- E. J. F. Dickinson, J. G. Limon-Petersen, N. V. Rees and R. G. Compton, How Much Supporting Electrolyte Is Required to Make a Cyclic Voltammetry Experiment Quantitatively “Diffusional”? A Theoretical and Experimental Investigation, J. Phys. Chem. C, 2009, 113, 11157–11171, DOI:10.1021/jp901628h.
- D. G. Kwabi, Y. Ji and M. J. Aziz, Electrolyte Lifetime in Aqueous Organic Redox Flow Batteries: A Critical Review, Chem. Rev., 2020, 120(14), 6467–6489, DOI:10.1021/acs.chemrev.9b00599.
- J. D. Milshtein, J. L. Barton, R. M. Darling and F. R. Brushett, 4-Acetamido-2,2,6,6-Tetramethylpiperidine-1-Oxyl as a Model Organic Redox Active Compound for Nonaqueous Flow Batteries, J. Power Sources, 2016, 327, 151–159, DOI:10.1016/j.jpowsour.2016.06.125.
- J. D. Milshtein, A. P. Kaur, M. D. Casselman, J. A. Kowalski, S. Modekrutti, P. L. Zhang, N. H. Attanayake, C. F. Elliott, S. R. Parkin, C. Risko, F. R. Brushett and S. A. Odom, High Current Density, Long Duration Cycling of Soluble Organic Active Species for Non-Aqueous Redox Flow Batteries, Energy Environ. Sci., 2016, 9(11), 3531–3543, 10.1039/c6ee02027e.
-
B. J. Neyhouse and F. R. Brushett, From the Synthesis Vial to the Full Cell: Electrochemical Methods for Characterizing Active Materials for Redox Flow Batteries, Encyclopedia of Energy Storage, 2022, 2, pp. 453–465, DOI:10.1016/B978-0-12-819723-3.00058-5.
- A. M. Bond, Past, Present and Future Contributions of Microelectrodes to Analytical Studies Employing Voltammetric Detection: A Review, Analyst, 1994, 119(11), 1–21, 10.1039/AN994190001R.
- M. B. Rooney, D. C. Coomber and A. M. Bond, Achievement of Near-Reversible Behavior for the [Fe(CN)6]3-/4- Redox Couple Using Cyclic Voltammetry at Glassy Carbon, Gold, and Platinum Macrodisk Electrodes in the Absence of Added Supporting Electrolyte, Anal. Chem., 2000, 72(15), 3486–3491, DOI:10.1021/ac991464m.
-
J. D. Milshtein, Electrochemical Engineering of Low-Cost and High-Power Redox Flow Batteries, Massachusetts Institute of Technology, Cambridge, MA, 2017 Search PubMed.
-
R. G. Compton; E. Laborda and K. R. Ward, Understanding Voltammetry: Simulation of Electrode Processes, Imperial College Press, London, 2014 Search PubMed.
- J. Zhang, R. E. Corman, J. K. Schuh, R. H. Ewoldt, I. A. Shkrob and L. Zhang, Solution Properties and Practical Limits of Concentrated Electrolytes for Nonaqueous Redox Flow Batteries, J. Phys. Chem. C, 2018, 122(15), 8159–8172, DOI:10.1021/acs.jpcc.8b02009.
- J. A. Kowalski, A. M. Fenton Jr, B. J. Neyhouse and F. R. Brushett, A Method for Evaluating Soluble Redox Couple Stability Using Microelectrode Voltammetry, J. Electrochem. Soc., 2020, 167(16), 160513, DOI:10.1149/1945-7111/abb7e9.
- C. Stolze, J. P. Meurer, M. D. Hager and U. S. Schubert, An Amperometric, Temperature-Independent, and Calibration-Free Method for the Real-Time State-of-Charge Monitoring of Redox Flow Battery Electrolytes, Chem. Mater., 2019, 31(15), 5363–5369, DOI:10.1021/acs.chemmater.9b02376.
-
R. G. Compton and C. E. Banks, Understanding Voltammetry, 2nd ed., Imperial College Press, London, 2011 Search PubMed.
- J. Liu and C. W. Monroe, Solute-Volume Effects in Electrolyte Transport, Electrochim. Acta, 2014, 133, 447–460, DOI:10.1016/j.electacta.2014.05.009.
- O. Borodin, L. Suo, M. Gobet, X. Ren, F. Wang, A. Faraone, J. Peng, M. Olguin, M. Schroeder, M. S. Ding, E. Gobrogge, A. von Wald Cresce, S. Munoz, J. A. Dura, S. Greenbaum, C. Wang and K. Xu, Liquid Structure with Nano-Heterogeneity Promotes Cationic Transport in Concentrated Electrolytes, ACS Nano, 2017, 11(10), 10462–10471, DOI:10.1021/acsnano.7b05664.
- O. A. Ibrahim, M. Navarro-Segarra, P. Sadeghi, N. Sabaté, J. P. Esquivel and E. Kjeang, Microfluidics for Electrochemical Energy Conversion, Chem. Rev., 2022, 122(7), 7236–7266, DOI:10.1021/acs.chemrev.1c00499.
- T. Hou and C. W. Monroe, Composition-Dependent Thermodynamic and Mass-Transport Characterization of Lithium Hexafluorophosphate in Propylene Carbonate, Electrochim. Acta, 2020, 332, 135085, DOI:10.1016/j.electacta.2019.135085.
- A. A. Wang, T. Hou, M. Karanjavala and C. W. Monroe, Shifting-Reference Concentration Cells to Refine Composition-Dependent Transport Characterization of Binary Lithium-Ion Electrolytes, Electrochim. Acta, 2020, 358, 136688, DOI:10.1016/j.electacta.2020.136688.
- M.-A. Goulet, L. Tong, D. A. Pollack, D. P. Tabor, S. A. Odom, A. Aspuru-Guzik, E. E. Kwan, R. G. Gordon and M. J. Aziz, Extending the Lifetime of Organic Flow Batteries via Redox State Management, J. Am. Chem. Soc., 2019, 141(20), 8014–8019, DOI:10.1021/jacs.8b13295.
- E. W. Zhao, E. Jónsson, R. B. Jethwa, D. Hey, D. Lyu, A. Brookfield, P. A. A. Klusener, D. Collison and C. P. Grey, Coupled in situ NMR and EPR Studies Reveal the Electron Transfer Rate and Electrolyte Decomposition in Redox Flow Batteries, J. Am. Chem. Soc., 2021, 143(4), 1885–1895, DOI:10.1021/jacs.0c10650.
- E. Wang, E. W. Zhao and C. P. Grey, New Magnetic Resonance and Computational Methods to Study Crossover Reactions in Li-Air and Redox Flow Batteries Using TEMPO, J. Phys. Chem. C, 2021, 125(50), 27520–27533, DOI:10.1021/acs.jpcc.1c07886.
- E. W. Zhao, T. Liu, E. Jónsson, J. Lee, I. Temprano, R. B. Jethwa, A. Wang, H. Smith, J. Carretero-González, Q. Song and C. P. Grey, In situ NMR Metrology Reveals Reaction Mechanisms in Redox Flow Batteries, Nature, 2020, 579, 224–228, DOI:10.1038/s41586-020-2081-7.
- Y. Jing, E. W. Zhao, M.-A. Goulet, M. Bahari, E. M. Fell, S. Jin, A. Davoodi, E. Jónsson, M. Wu, C. P. Grey, R. G. Gordon and M. J. Aziz, In situ Electrochemical Recomposition of Decomposed Redox-Active Species in Aqueous Organic Flow Batteries, Nat. Chem., 2022 DOI:10.1038/s41557-022-00967-4.
- J. A. Kowalski, M. D. Casselman, A. P. Kaur, J. D. Milshtein, C. F. Elliott, S. Modekrutti, N. H. Attanayake, N. Zhang, S. R. Parkin, C. Risko, F. R. Brushett and S. A. Odom, A Stable Two-Electron-Donating Phenothiazine for Application in Nonaqueous Redox Flow Batteries, J. Mater. Chem. A, 2017, 5, 24371–24379, 10.1039/C7TA05883G.
- L. Tong, Q. Chen, A. A. Wong, R. Gómez-Bombarelli, A. Aspuru-Guzik, R. G. Gordon and M. J. Aziz, UV-vis Spectrophotometry of Quinone Flow Battery Electrolyte for in situ Monitoring and Improved Electrochemical Modeling of Potential and Quinhydrone Formation, Phys. Chem. Chem. Phys., 2017, 19(47), 31684–31691, 10.1039/C7CP05881K.
-
T. D. W. Claridge, High-Resolution NMR Techniques, 3rd ed., Elsevier, Amsterdam, 2016 Search PubMed.
- A. K. Sethurajan, S. A. Krachkovskiy, I. C. Halalay, G. R. Goward and B. Protas, Accurate Characterization of Ion Transport Properties in Binary Symmetric Electrolytes Using in situ NMR Imaging and Inverse Modeling, J. Phys. Chem. B, 2015, 119(37), 12238–12248, DOI:10.1021/acs.jpcb.5b04300.
- L. A. Robertson, Z. Li, Y. Cao, I. A. Shkrob, M. Tyagi, K. C. Smith, L. Zhang, J. S. Moore and Y. Z, Observation of Microheterogenaiety in Highly Concentrated Nonaqueous Electrolyte Solutions, J. Am. Chem. Soc., 2019, 141, 8041–8046, DOI:10.1021/jacs.9b02323.
- Z. Yu, N. P. Balsara, O. Borodin, A. A. Gewirth, N. T. Hahn, E. J. Maginn, K. A. Persson, V. Srinivasan, M. F. Toney, K. Xu, K. R. Zavadil, L. A. Curtiss and L. Cheng, Beyond Local Solvation Structure: Nanometric Aggregates in Battery Electrolytes and Their Effect on Electrolyte Properties, ACS Energy Lett., 2022, 7(1), 461–470, DOI:10.1021/acsenergylett.1c02391.
- K. Qian, R. E. Winans and T. Li, Insights into the Nanostructure, Solvation, and Dynamics of Liquid Electrolytes through Small-Angle X-Ray Scattering, Adv. Energy Mater., 2021, 11(4), 2002821, DOI:10.1002/aenm.202002821.
- L. Eifert, N. Bevilacqua, K. Köble, K. Fahy, L. Xiao, M. Li, K. Duan, A. Bazylak, P.-C. Sui and R. Zeis, Synchrotron X-Ray Radiography and Tomography of Vanadium Redox Flow Batteries—Cell Design, Electrolyte Flow Geometry, and Gas Bubble Formation, ChemSusChem, 2020, 13, 3154–3165, DOI:10.1002/cssc.202000541.
- K. Köble, L. Eifert, N. Bevilacqua, K. F. Fahy, A. Bazylak and R. Zeis, Synchrotron X-Ray Radiography of Vanadium Redox Flow Batteries – Time and Spatial Resolved Electrolyte Flow in Porous Carbon Electrodes, J. Power Sources, 2021, 492, 229660, DOI:10.1016/j.jpowsour.2021.229660.
|
This journal is © The Royal Society of Chemistry 2022 |