DOI:
10.1039/B314469K
(Tutorial Review)
Lab Chip, 2004,
4, 98-103
Microfabrication and microfluidics for tissue engineering: state of the art and future opportunities
Received
10th November 2003
, Accepted 16th February 2004
First published on 10th March 2004
Abstract
An introductory overview of the use of microfluidic devices for tissue engineering is presented. After a brief description of the background of tissue engineering, different application areas of microfluidic devices are examined. Among these are methods for patterning cells, topographical control over cells and tissues, and bioreactors. Examples where microfluidic devices have been employed are presented such as basal lamina, vascular tissue, liver, bone, cartilage and neurons. It is concluded that until today, microfluidic devices have not been used extensively in tissue engineering. Major contributions are expected in two areas. The first is growth of complex tissue, where microfluidic structures ensure a steady blood supply, thereby circumventing the well-known problem of providing larger tissue structures with a continuous flow of oxygen and nutrition, and withdrawal of waste products. The second, and probably more important function of microfluidics, combined with micro/nanotechnology, lies in the development of in vitro physiological systems for studying fundamental biological phenomena.
Helene Andersson | Helene Andersson was born in Sweden, in 1974. She received her PhD in microsystems technology from the Royal Institute of Technology in Sweden 2001. In 2002 she joined Silex Microsystems as business manager for life sciences. She is also associate professor at the Royal Institute of Technology in the microsystems technology group and research associate at the MESA+ Institute in Holland. Her current research interests are microfluidic devices for cell and tissue analysis. |
Albert van den Berg | Albert van den Berg received his Masters degree in applied physics from the University of Twente, The Netherlands in 1983. In 1988 he finished his thesis at the same university on the topic of chemically modified ISFETs. From 1988 to 1990 he was at the Swiss Center for Microelectronics and Microtechnology (CSEM) in Neuchâtel, Switzerland, as project manager in the chemical sensor department. From 1990–1993 he did research on miniaturized chemical sensors and sensor systems at the IMT, University of Neuchâtel, Switzerland. From 1993 until 1999 he was research coordinator Micro Total Analysis Systems (μTAS) at MESA, University of Twente, a topic that was recently extended to Miniaturized Chemical Systems (MiCS). In 1998 he was appointed as full professor on Miniaturized Systems for (Bio)Chemical Analysis at the faculty of Electrical Engineering. Albert van den Berg is member of the μTAS, Transducers and Nanotech steering committees, editor of the section μTAS of Sensors and Actuators B and member of the advisory board of Lab on a Chip. He was chairman of the μTAS conference in 1994 and 2000, chairman of the 2003 GRC on microfluidics and chairman of the international Nanotech 2003 conference. In 2002 he received the Simon Stevin Master award from the Dutch Technical Science foundation. His current research interests focus on theory, technologies, new devices and applications of micro- and nanofluidics for miniaturized (bio)chemical synthesis and analysis systems, with a special interest in single-cell based systems. |
1 Introduction to tissue engineering
One of the first fruits of small scale engineering in biology has been DNA chips, which capture an unprecedented amount of information about all the genes being expressed in the cell.1,2 The next has been to use microtechniques to create microfluidic chips to bring just about any biological assay that works on a molecular level into a chip.3–5 This so called Lab-on-a-Chip approach has greatly speeded up cumbersome reactions and allowed scientists to control the accuracy of the tests more precisely. Eventually the chips could include several layers of parallel reactions, allowing, for instance, many different assays on a small sample of biological material.
The field of tissue engineering (TE) is also developing much more sophisticated abilities based on micro- and nanofabrication techniques. Cells need to be placed on a scaffold that gives them structures before they can be coaxed into forming tissues. With the ability to devise more precise scaffolds, tissue engineers can create tissues that are more complex and have e.g. a built-in blood supply. In the past, the tissue engineering field has managed to create viable skin, bone, cartilage, bladders, and blood vessels.6 Muscle tissue is currently under development, including heart muscle and more complex structures based on bone and cartilage.6
The ultimate goal is building large pieces of tissue and whole organs, with the aim of solving the organ shortage problem which keeps many people waiting for donor organs that are in very short supply. However, creating organs is no easy task, and requires a detailed understanding of the underlying biology, as well as the ability to manipulate many factors in the cells. Maybe the greatest challenge in building a whole organ is the problem of ordering all the cells in a thick (3D) living structure. It is difficult to provide larger structures with a steady flow of oxygen and nutrition. One approach to overcome this barrier is using microfabrication to create an entire blood supply for the tissue as it is being built. Thus, as microfluidics is becoming more important in the field of tissue engineering the motivation of writing this review is to provide an evaluation of what has been achieved and realized so far.
The term tissue engineering was initially defined in 1988 by the attendees of the first NSF sponsored meeting in 1988 and later in 1993 summarized by Langer and Vacanti7 as ‘an interdisciplinary field that applies the principles of engineering and life sciences toward the development of biological substitutes for the repair or regeneration of tissue or organ functions’. Tissue engineering has now emerged as a potential alternative to tissue or organ transplantation. With this technology, tissue loss or organ failure can be treated either by implantation of an engineered biological substitute or alternatively with ex vivo perfusion systems. In Fig. 1 a schematic representation of the tissue engineering approach is shown.6 The tissue engineered products may be fully functional at the time of treatment (e.g. liver assist devices, encapsulated islets), or have the potential to integrate and form the expected functional tissue upon implantation (e.g. chondrocytes embedded in a matrix carrier). Cells are a key element for tissue regeneration and repair due to their proliferation and differentiation, cell–cell signaling, biomolecule production and formation of extracellular matrix. For more details on tissue engineering, several reviews have recently been published covering this topic.8
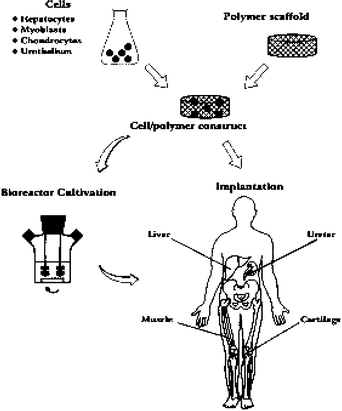 |
| Fig. 1 Schematic representation of the tissue engineering approach. Specific cell populations are harvested from the appropriate tissue and seeded on a biodegradable polymer scaffold. The cell–polymer constructs may undergo a period of dynamic tissue culture in a bioreactor prior to implantation. Organized structural and functional tissues may be produced in this way.7 | |
Scaffolds are porous, degradable structures fabricated from either natural materials (collage, fibrin) or synthetic polymers (polyglycolide, polylactide).9 They can be sponge-like sheets, gels, or highly complex structures with intricate pores and channels fabricated using micro or nanotechnologies. Virtually all scaffolds in tissue engineering are intended to degrade slowly after implantation in the patient and be replaced by new tissue.
A crucial mainstay of tissue engineering is the biomaterial from which scaffolds are fashioned. Many biomaterials direct the growth of cells in culture. The ideal biomaterial for a scaffold would selectively interact with the specific adhesion and growth factor receptors expressed by target cells in the surrounding tissues required for repair of damaged tissue. The scaffold could guide migration of these target cells into the injury site and stimulate their growth and differentiation, finally degrading in response to matrix remodeling enzymes released by the cells as tissue repair progresses.10 Significant opportunities and challenges exist in the creation and characterization of biomaterials. Current methods of synthesis and characterization of these materials are covered in ref. 11.
2 Methods for cell patterning and cultivation
Microfabrication techniques are used to generate patterns of cells on surfaces. This cellular patterning is a necessary component for tissue engineering and fundamental cell biology studies. Photolithographic techniques are highly developed and have been widely used for patterning of cells. However, this technique has some disadvantages for certain applications, especially biocompatibility. Recently, a set of alternative techniques such as microcontact printing, microfluidic patterning using microchannels and laminar flow patterning has been developed for biological applications.
2.1.1 Photolithography.
Photolithography has been widely used for patterning cells on hard materials.12–14 Materials of interest, such as cell-adhesion proteins, are patterned using lift-off techniques. Examples of cell-adhesion materials include polylysine, fibronectin, and collagen. Complex matrices such as Matrigel (a solubilized basement membrane preparation extracted from EHS mouse sarcoma (Beckton–Dickinson)) can also be used to achieve cell adhesion. Finally, the surface is incubated with cell solution and the desired cell pattern can be obtained.
2.1.3 Microfluidic patterning using microchannels.
Microchannels can be formed by contacting the PDMS structure with a substrate, and these channels deliver the fluid to restricted areas on the substrate. The microchannels can selectively deliver the materials for cell adhesion or cell suspension to desired areas of a substrate.18,19 When two or more streams of laminar flow are joined into a single stream the combined streams flow parallel to each other without any turbulent mixing. The only mixing takes place by diffusion across the interface. This ability to generate and sustain parallel streams of different solutions in microchannels provides a unique opportunity to pattern cells and their environments.20,21 This method can also be used to study the subcellular processes by positioning the interface between two adjacent streams over a single cell;22 see Fig. 2. For example, two different dyes or drugs can be applied to different regions of the same cell.
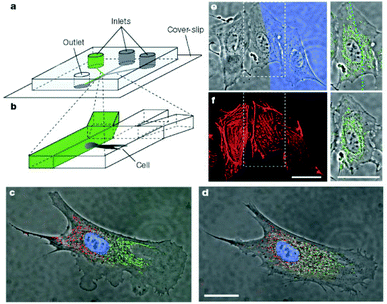 |
| Fig. 2 Differential manipulation of regions of a single bovine capillary endothelial cell using laminar flows. (a,b) Experimental set-up. (c) Fluorescence images of a single cell after treatment of its right pole with Mitotracker Green FM and its left pole with Mitotracker red CM-H2Xros. The entire cell is treated with the DNA-binding dye Hoechst 33342. (d) Image of the same cell but taken 2.5 h later, showing intermixing of the red and green subpopulations of mitochondria. (e) Treatment of a portion of a single cell with latrunculin A. The blue region (Dextran-70.000 Cascade Blue stain) reveals the flow of medium containing latrunculin A. Right, enlarged view of the middle cell and its mitochondria (green). (f) Phalloidin-Alexa 594-labelled image of the same cell immediately after 10 min of treatment with latrunculin A. The cell in the middle, which was only partly in the stream containing latrunculin, shows disruption of actin microfilaments that is limited to this region (scale bars 25 µm).22 | |
2.2 Topographical control over cells
The core of the tissue-engineered replacement is the biomaterial construct or scaffold, in which a given cell population is seeded. One of the challenges in tissue engineering is to find a more suitable method for the fabrication of scaffolds of defined architecture to guide cell growth and development. A tissue engineered construct that has a well controlled microstructure will better maintain cell morphology, differentiation and functionality over long periods of time.23 A key feature of these constructs will be the replication of in vivo geometry and dimensional size scale that will aid in the maintenance of an in vivo like cell phenotype. Using microtechnologies it is possible create implants with precise microarchitectures for immunoisolation, cellular ingrowth and drug delivery.24 There have been many studies showing topographical control over cells and tissues which are reviewed by Singhvi.23 Deutsch et al. have shown that three dimensional surface topography of culture substrates significantly affects in vitro cardiac myocyte orientation, see Fig. 3.25 By creating culture surfaces with topographic features corresponding to cellular dimensions, the cells seemed to exhibit a more in vivo like cellular morphology.25 It has also been shown that microtexture has significant effects on cell adhesion.26 Van Kooten et al. have shown that DNA profiles were influenced by microtexture.27 The arrangement of cells in controlled two or three dimensional arrangements has been shown to have beneficial effects on cell differentiation, maintenance, and functional longevity.28,29 It has also been shown that topography can influence and direct cell migration.30,31
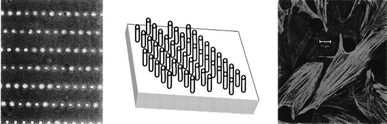 |
| Fig. 3 Micropegs can be used to create microarchitectures which direct cell attachment and morphology.24 | |
2.3 Bioreactors
Culturing cells up to higher cell density is one of the most important tasks to achieve physiologically meaningful functions for tissue engineering. In order to reach this goal, continuous nutrition and oxygen supply and waste removal through the culture medium must be ensured. The first generation of bioreactors for culturing cells were designed simply to pump nutrient liquid through the assembling tissue. The next wave of bioreactors designed for growing blood vessels and cartilage subjected the nascent tissue to compression, shear stresses, and even pulsative flow of the culture medium. Such stresses profoundly improve the mechanical properties of engineered vessels, cartilage, and cardiac muscle.32 In conventional cell culture systems it can be quite difficult to enable sufficient circulation.33,34 However, microfluidic structures can easily offer microscale controllable fluid circuits. Several two dimensional microfluidic devices for cell culturing have been presented.35 However, only a few multilayer structures have been presented.36,37 A PDMS based multi-layer microfluidic device enabling perfusion culture of mammalian cells has been presented in ref. 36. The multi-layer device with an oxygen chamber showed good performance in culturing cells at high densities. Vozzi et al. have constructed 3D scaffolds using biodegradable poly(DL-lactide-co-glycolide)
(PGLA).37 Once the membranes were fabricated 3D structures were assembled by stacking layers together. No biological experiments have been shown on these constructs yet.
3 Applications of cell patterning and cultivation
3.1 Basal lamina
The basal lamina performs several important functions in a variety of tissues. In the glomerulus of the kidney, the basal lamina separates endothelial cells from podocytes and acts as a selectively permeable barrier to plasma molecules. In the skin, the basal lamina functions as a barrier that separates cells from underlying connective tissue (dermis). Although sponges, foams, and matrices with defined porosities are useful for the fabrication of relatively large and highly porous three dimensional analogs of the extracellular matrix, their use is limited for the creation of a basal lamina. A microfabricated approach has been used to produce analogs of the basal lamina with complex topographic features.38 A test pattern of ridges and channels (40–310 µm) similar to the invaginations found in a native basal lamina was laser machined into the surface of polyimide master chip. Negative replicates of the chip were produced in PDMS and these replicates were used as templates for the production of thin (21 µm) membranes of collagen or gelatin. These novel microfabricated analogs of the basal lamina will probably help to elucidate the influence of topography on epithelial cell proliferation and differentiation.
3.2 Vascular tissue
One of the principal constraints on the size of tissues engineered in vitro that do not have their own blood supply is the short distance over which oxygen can diffuse before being consumed. Once implanted in the patient, cells in the engineered tissue will consume the available oxygen within a few hours, but it will take several days for the growth of new blood vessels that will deliver oxygen and nutrients to the implants. Microfluidic networks have been designed, built and tested, and have produced highly uniform flow patterns which mimic large-scale physiological properties such as total flow rate, as well as small-scale phenomena such as fluid velocity in the capillaries.39 A fluid dynamic model which approximates the behavior of blood flow in the target organ was used as the basis of the lithographic masks used to pattern the blood vessel network. Polymer films were cast in master molds and were integrated to form thick 3D scaffolds for cell seeding and expansion. Non-degradable biocompatible templates or biodegradable templates based on copolymers of polylactic and polyglycolic acid or other restorable polymer systems were used. Cell seeding results demonstrate successful attachment and proliferation of endothelial cells in microfluidics channels. The ultimate goal is to scale up the technology to a full, 3D system with the blood volume and cell density required for a complete organ.39
3.3 Liver
Hepatocytes, the cells that carry out most of the metabolic and biosynthetic processes in liver, rapidly lose liver-specific functions when maintained under standard in vitro cell culture conditions.40 A variety of culture methods have been developed to foster retention of hepatocytic function including bioreactors.41–43 Still, not all of the important functions of liver can yet be replicated at desired levels, promoting continued development of new culture methods. Bathia et al. utilized conventional co-cultures of primary rat hepatocytes and murine 3T3-J2 fibroblasts to investigate the role of increasing fibroblast density on hepatic function.42 Microfabrication techniques were used to localize both cell populations in patterned configurations on rigid substrates.42 Powers et al. have presented a bioreactor that enables both morphogenesis of 3D tissue structures under continuous perfusion and repeated in situ observation by light microscopy.43 Three dimensional scaffolds were created by deep reactive ion etching of silicon wafers to create an array of channels (through-holes) with cell-adhesive walls; see Fig. 4. Scaffolds were combined with a cell-retaining filter and support in a reactor housing designed to deliver a continuous perfusate across the top of the array and through the 3D tissue mass in each channel. It was demonstrated that this system enables the formation of hepatocellular aggregates reminiscent of structures seen in hepatic acini. The aggregates maintained their structure and viability at least 2 weeks in bioreactor culture, providing a promising platform for the studies of in vivo physiology and pathology in an in vitro environment.43
![(a) Schematic of scaffold and reactor housing.41
(b) The silicon microfluidic chip in a polycarbonate housing [www.easternplastics.com].](/image/article/2004/LC/b314469k/b314469k-f4.gif) |
| Fig. 4 (a) Schematic of scaffold and reactor housing.41
(b) The silicon microfluidic chip in a polycarbonate housing [www.easternplastics.com]. | |
3.4 Bone
Bone-tissue engineering aims to heal bone defects with autologous cells and tissue without the donor site morbidity and expense associated with harvesting autologous bone. One such strategy involves seeding autologous osteogenic cells in vitro throughout a scaffold to create a scaffold–cell hybrid. The main challenge in preparing a useful tissue engineering construct is to obtain a ubiquitous distribution of cells, and hence new tissue, throughout the entire 3-dimensional scaffold volume. Recently a new processing technique for creating biodegradable polymer scaffolds with geometry similar to that of trabecular bone was presented.44 This marks the first report of successful three-dimensional bone-tissue engineering repair using autologous marrow cells without the use of supplementary growth factors. As yet, there have been no reports on the use of microfluidic structures in bone-tissue engineering.
3.5 Cartilage
Within the field of tissue engineering, considerable effort has been directed towards the development of substrates which will support articular cartilage regeneration. Maintenance of the chondrogenic phenotype is fundamental to cell-based engineering in neocartilage tissue. Differentiation is routinely maintained in chondrocyte populations through the restriction of cell spreading both in monolayers and bioreactors45,46 as well as in suspension cultures.47 Micropatterning techniques have also used to fabricate scaffolds specially designed to support chondrogenesis. A micropatterned porous polysaccharide scaffold has been shown to promote high-density chondrocyte culture, attachment and biosynthesis.48 Cells applied to these scaffolds were observed to maintain the chondrocyte phenotype as evidenced by their morphology and ability to produce type II collagen.48
3.6 Neurons
The control of neuronal cell position and outgrowth is of fundamental interest in the development of applications ranging form cellular biosensors to tissue engineering. For extra cellular signal recording from nerve cells in vitro, the use of microelectrode arrays is common. These devices employ glass or silicon substrates, onto which electrode arrays made of gold, platinum or indium tin oxide are fabricated. With such systems, simultaneous recordings from multiple sites of electrogenic cultures have been reported.49–52 Sufficient electrical coupling between the cell and the electrode for signal recording is achieved only when a neuron is located directly on the top of the electrode. In order to manipulate the growth of neuronal cells, photolithographic techniques can be applied to provide guiding structures (topographical or chemical) on the device substrate.53–55 A microfluidic multicompartment neuronal culturing device that can be used for a number of neuroscience research applications has recently been reported upon;53 see Fig. 5. The ability to direct the sites of neuronal attachment and the orientation of neurite outgrowth by micropatterning techniques, combined with fluidically isolated compartments within the culture area, offers significant advantages over standard open culture methods and other conventional methods for manipulating distinct neuronal microenvironments.53
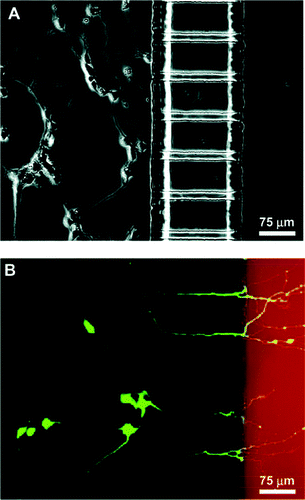 |
| Fig. 5 Demonstration of neuronal culture inside the microfabricated device and effectiveness of neuritic insult containment. (a) Phase micrograph of neurons extending processes to the neuritic chamber after 4 days in culture. (b) Epifluorescence micrography of the same region with cells stained with calcein AM (green) and isolation of Texas red dextran (red) in the neuritic chamber. Only live cells can take up calcein and become fluorescently labeled. The micrograph clearly demonstrates selective labeling of neurons that have processes extending into the neuritic side.53 | |
4 Conclusions and outlook
Building artificial organs is a challenge; it requires detailed understanding of fundamental biology and the ability to precisely manipulate cells. The real limit today for building a whole organ is the problem of ordering cells in a thick living structure. The creation of tissues containing hierarchical cell–cell interactions under appropriate conditions will take in vitro systems closer to living systems. The increasingly intimate combination of engineering and biology offers the prospect of sophisticated physiological in vitro models of many different human tissues. So, what can microtechnology and microfluidics do for the field of tissue engineering? The fundamental problem is that these technologies clearly have great potential in tissue engineering, but have not yet shown their ultimate utility in this field. Some key issues to their utility in tissue engineering is (1) whether they will allow one to accomplish something that cannot be accomplished without these techniques (this has yet to be demonstrated experimentally), (2) how to utilize these technologies with the materials required for tissue engineering, and (3) how to fabricate real 3D (e.g. millimetre in thickness) structures with these technologies, as this will likely be required for tissue engineering utility. Point (1) is particularly relevant at this time since significant advances have been made in creating complex 3D tissue structures comprising multiple cell types56,57 without using these techniques. In conclusion the authors believe that microfabrication and microfluidics will be extremely useful in tissue engineering, but the definitive proof of this assumption is still lacking.
Acknowledgements
Financial support from STW (project TMM 6016, “NanoSCAN”) is gratefully acknowledged.
References
- D. Blohm and A. Guiseppi-Elie, New developments in microarray technology, Curr. Opin. Biotechnol., 2001, 12, 41–47 CrossRef CAS.
- W. Freeman, D. Robertson and K. Vrana, Fundamentals of DNA hybridization arrays for gene expression analysis, BioTechniques, 2000, 29, 1042–1055 Search PubMed.
- G. Sanders and A. Manz, Chip-based microsystems for genomic and proteomic analysis, Trends Anal. Chem., 2000, 19, 364–378 CrossRef CAS.
- N. Jeon, H. Baskaran, S. Dertinger, G. Whitesides, L. Van de Water and M. Toner, Neutrophil chemotaxis in linear and complex gradients of interleukin-8 formed in a microfabricated device, Nat. Biotechnol., 2002, 20(8), 826–830 CAS.
- A. Fu, C. Spence, A. Scherer, F. Arnold and S. Quake, A microfabricated fluorescence-activated cell sorter, Nat. Biotechnol., 1999, 17(11), 1109–1111 CrossRef CAS.
- J. Marler, J. Upton, R. Langer and J. Vacanti, Transplantation of cells in matrices for tissue generation, Adv. Drug Delivery Rev., 1998, 33, 165–182 CrossRef CAS.
- R. Langer and J. Vacanti, Tissue engineering, Science, 1993, 260, 920–926 CAS.
- E. Ochoa and J. Vancanti, An overview of the pathology and approaches to tissue engineering, Ann. N. Y. Acad. Sci., 2002, 979, 10–26.
- L. Griffith, Polymeric biomaterials, Acta Mater., 2000, 48, 263–277 CrossRef CAS.
- J. Hubell, Bioactive biomaterials, Curr. Opin. Biotechnol., 1999, 10, 123–129 CrossRef.
- N. Peppas and R. Langer, New challenges in biomaterials, Science, 1994, 263, 1715–1720 CAS.
- S. Britland, P. Clark and G. Moores, Micropatterned substratum adhesiveness: a model for morphogenic cues controlling cell behavior, Exp. Cell Res., 1992, 198, 124–129 CrossRef CAS.
- S. Bhatia, M. Yarmusch and M. Toner, Controlling cell interactions by micropatterning in co-cultures: hepatocytes and 3T3 fibroblasts, J. Biomed. Mater. Res., 1997, 34, 189–199 CrossRef CAS.
- K. Healy, C. Thomas, A. Rezania, J. Kim, P. McKeown, B. Lom and P. Hockberger, Kinetics of bone cell organization and mineralization on materials with patterned surface chemistry, Biomaterials, 1996, 17, 195–208 CrossRef CAS.
- A. Kumar and G. Whitesides, Features of gold having micrometer to centimeter dimensions can be formed through a combination of stamping with an elastomeric stamp and alkanethiol ink followed by chemical etching, Appl. Phys. Lett., 1993, 273, 347–349.
- G. Lopez, M. Albers, S. Schreiber, R. Carroll, E. Peralta and G. Whitesides, Convenient methods for patterning the adhesion of mammalian cells to surfaces using self-assembled monlayers of alkanethiolates on gold, J. Am. Chem. Soc., 1993, 115, 5877–5878 CrossRef CAS.
- M. Mrksich, L. Dike, J. Tien, D. Ingber and G. Whitesides, Using microcontact printing to pattern the attachment of mammalian cells to self-assembled monolayers of alkanethiolates on transparent films of gold and silver, Exp. Cell Res., 1997, 235, 305–313 CrossRef CAS.
- A. Folch, A. Ayon, O. Hurtado, M. Schmidt and M. Toner, Molding of deep poly(dimethylsiloxane) microstructures for microfluidics and biological applications, J. Biomech. Eng., 1999, 121, 28–34 CAS.
- A. Folch and M. Toner, Cellular micropatterns on biocompatible material, Biotechnol. Prog., 1998, 14, 388–392 CrossRef CAS.
- S. Takayama, J. McDonald, E. Ostuni, M. Liang, P. Kenis, R. Ismagilov and G. Whitesides, Pattterning cells and their environments using multiple laminar flows in capillary networks, Proc. Natl. Acad. Sci. USA, 1999, 96, 5545–5548 CrossRef CAS.
- S. Takayama, E. Ostuni, X. Qian, J. McDonald, X. Jiang, P. LeDuc, M. Wu, D. Ingber and G. Whitesides, Topographical micropatterning of poly(dimethylsiloxane) using laminar flows of liquids in capillaries, Adv. Mater., 2001, 13, 570–574 CrossRef CAS.
- S. Takayama, E. Ostuni, LeDuc, K. Naruse, D. Ingber and G. Whitesides, Subcellular positioning of small molecules, Nature, 2001, 411, 1016 CrossRef CAS.
- R. Singhvi, G. Stephanopoulos and D. Wang, Review: Effects of substratum morphology on cell physiology, Biotechnol. Bioeng, 1994, 43, 764–771.
- T. Desai, Micro- and nanoscale structures for tissue engineering constructs, Med. Eng. Phys., 2000, 22, 595–606 CrossRef CAS.
- J. Deutsch, D. Motlagh, B. Russell and T. Desai, Fabrication of microtextured membranes for cardiac myocyte attachment and orientation, J. Biomed. Mater. Res. (Appl. Biomater.), 2000, 53–57 Search PubMed.
- E. Den Braber, J. de Ruijter, L. Ginsel, A. von Recom and J. Jansen, Orientation of ECM protein deposition, fibroblast cytoskeleton, and attachment complex components on silicone microgrooved surfaces, J. Biomed. Mater. Res., 1998, 40(2), 291–300 CrossRef CAS.
- T. Van Kooten, J. Whitesides and A. von Recum, Influence of silicone surface texture on human skin fibroblast proliferation as determined by cell cycle analysis, J. Biomed. Mater. Res., 1998, 43, 1–14 CrossRef CAS.
- R. Singhvi, A. Kumar, G. Lopez, G. Stephanopoulos, D. Wang, G. Whitesides and D. Ingber, Engineering cell shape and function, Science, 1994, 264, 696–698 CAS.
- R. Kapur, J. Calvert and A. Rudolph, Electrical, chemical, and topological addressing of mammalian cells with microfabricated systems, J. Biomech. Eng., 1999, 121, 65–72 CAS.
- R. Flemming, C. Murphy, G. Abrams, S. Goodman and P. Nealy, Effects of synthetic micro- and nano-structured surfaces on cell behavior, Biomaterials, 1999, 20(6), 573–588 CrossRef CAS.
- M. Dalby, M. Riehle, S. Yarwood, C. Wilkinson and A. Curtis, Nucleus alignment and cell signaling in fibroblasts: response to a microgrooved topography, Exp. Cell Res., 2003, 284, 274–282 CrossRef CAS.
- A. Grodzinsky, M. Levenston, E. Jin and H. Frank, Cartilage tissue remodeling in response to mechanical forces, Annu. Rev. Biomed. Eng., 2000, 2, 691 Search PubMed.
- E. Leclerc, Y. Sakai and T. Fujii, Three dimensional PDMA microstructure for 3T3-L1 fibroblast and HEP G2 cells perfusion culture, MicroTAS, 2002, 826–827 Search PubMed.
- N. Szita, A. Zanxotto, P. Boccazzi, A. Sinskey, M. Schmidt and K. Jensen, Monitoring cell growth, oxygen, and pH in microfermentors, MicroTAS, 2002, 7–9 Search PubMed.
- H. Andersson and A. van den Berg, Microfluidic devices for cellomics: a review, Sens. Actuators B, 2003, 92/3, 315–325 CrossRef.
- E. Leclerc, Y. Sakai and T. Fujii, A multi-layer PDMS microfluidic device for tissue engineering applications, Transducers, 2003, 415–418 Search PubMed.
- G. Vozzi, C. Flaim, A. Ahluwalia and S. Bhatia, Fabrication of PLGA scaffolds using soft lithography and microsyringe deposition, Biomaterials, 2003, 24, 2533–2540 CrossRef CAS.
- G. Pins, M. Toner and J. Morgan, Microfabrication of an analog of the basal lamina: biocompatible membranes with complex topographies, FASEB, 2000, 14, 593–602 Search PubMed.
- J. Borenstein, H. Terai, K. King, E. Weinberg, M. Kaazempur-Mofrad and J. Vacanti, Microfabrication technology for vascular tissue engineering, Biomed. Microdev., 2002, 4(3), 167–175 Search PubMed.
- E. LeCluyse, P. Bullock and A. Parkinson, Strategies for restoration and maintenance of normal hepatic structure and function in long-term cultures of rat hepatocytes, Adv. Drug Delivery Rev., 1996, 22, 133–186 CrossRef CAS.
- A. Tilles, F. Berthiaume, M. Yarmush, R. Tompkins and M. Toner, Bioengineering of liver assist devices, J. Hepatobiliary Pancreat. Surg., 2002, 9, 686–696 Search PubMed.
- S. Bathia, U. Balis, M. Yarmush and M. Toner, Microfabrication of hepatocyte/fibroblast co-cultures: role of homotypic cell interactions, Biotechnol. Prog., 1998, 14, 378–387 CrossRef.
- M. Powers, K. Domansky, M. Kaazempur-Mofrad, A. Kalezi, A. Capitano, A. Upadhyaya, P. Kurzawski, K. Wack, D. Stolz, R. Kamm and L. Griffith, A microfabricated array bioreactor for perfused 3D liver culture, Biotech. Bioeng., 2002, 78(3), 257–269 CrossRef CAS.
- C. Holy, J. Fialkov, J. Davies and M. Shoichet, Use of a biomimetic strategy to engineer bone, J. Biomed. Mater. Res., 2003, 65 A(4), 447–453 CrossRef CAS.
- E. Petersen, K. Fishbein, McFarland and R. Spencer, 31P NMR spectroscopy of developing cartilage produced from chick chondrocytes in a hollow-fiber reactor, Magn. Reson. Med., 2000, 44, 367–372 CrossRef CAS.
- K. Potter, J. Butler, W. Horton and R. Spencer, Response of engineered cartilage tissue to biochemical agents as studied by proton magnetic resonance microscopy, Arthritis Rheum., 2000, 43, 1580–1590 CrossRef CAS.
- L. Freed, I. Martin and G. Vunjak-Novakovic, Frontiers in tissue engineering. In vitro modulation of chondrogenesis, Clin. Orthop., 1999, 367, 46–58 Search PubMed.
- E. Petersen, R. Spencer and E. McFarland, Microengineering neocartilage scaffolds, Biotech. Bioeng, 2002, 78(7), 802–805 CrossRef.
- M. Bove, S. Martinoia, G. Verreschi, M. Giugliano and M. Grattarola, Analysis of the signals generated by networks of neurons coupled to planar arrays of microtransducers in simulated experiments, Biosens. Bioelectron., 1998, 13, 601–612 CrossRef CAS.
- U. Egert, B. Schlosshauser, S. Fennrich, W. Nisch, M. Fejtl, T. Knott, T. Muller and H. Hämmerle, A novel organotypic long-term culture of the rat hippocampus on substrate-integrated multielectrode arrays, Brain Res., 1998, 2, 229–242 CrossRef CAS.
- P. Thiebaud, C. Beuret, M. Koudelka-Hep, M. Bove, S. Martinoia, M. Grattarola, H. Jahnsen, R. Rebaudo, M. Balestrino, J. Zimmer and Y. Dupont, An array of Pt-tip microelectrodes for extracellular monitoring of activity of brain slices, Biosens. Bioelectron., 1999, 14, 61–65 CrossRef CAS.
- C. Sprösser, M. Denyer, S. Britland, W. Knoll and A. Offenhäusser, Electrical recordings from rat cardiac muscle cells using field-effect transistors, Phys. Rev., 1999, E60, 2171–2176 Search PubMed.
- A. Taylor, S. Rhee, C. Tu, D. Cribbs, C. Cotman and N. Jeon, Microfluidic multicomponent device for neuroscience research, Langmuir, 2003, 19, 1551–1556 CrossRef CAS.
- D. Kleinfeld, K. Kahler and P. Hockberger, Controlled outgrowth of dissociated neurons on patterned substrates, J. Neurosci., 1988, 11, 4098–4120 Search PubMed.
- A. Oliva, C. James, C. Kingman, H. Craighead and G. Banker, Patterning axonal guidance molecules using a novel strategy for microcontact printing, Neurochem. Res., 2003, 11, 1639–1648.
- J. Lai, C. Yoon, J. Yoo, T. Wulf and A. Atala, Phenotypic and functional characterization of in vivo tissue engineered smooth muscle from normal and pathological bladders, J. Urol., 2002, 168, 1853–1857 Search PubMed.
- T. Grikscheit, E. Ochoa, A. Ramsanahie, E. Alsberg, D. Mooney, E. Whang and J. Vacanti, Tissue engineered large intestine resembles native colon with appropriate in vitro physiology and architecture, Ann. Surg., 2003, 238, 35–41 CrossRef.
|
This journal is © The Royal Society of Chemistry 2004 |