DOI:
10.1039/D4QI01945H
(Review Article)
Inorg. Chem. Front., 2024,
11, 7238-7255
Targeting protein aggregation: the promising application of polyoxometalates in neurodegenerative diseases
Received
1st August 2024
, Accepted 5th September 2024
First published on 7th September 2024
Abstract
With the global aging trend, the incidence of neurodegenerative diseases (NDDs), including Alzheimer's disease (AD), Parkinson's disease (PD) and amyotrophic lateral sclerosis (ALS), has greatly increased. NDDs are sporadic and rare inherited diseases of the nervous system characterized by loss of neurons and slow progressive degeneration in different regions of the nervous system. A common feature of many NDDs is the formation of persistent and irreversible protein aggregates during their pathogenesis, such as amyloid β (Aβ) and tau aggregates in AD, α-synuclein aggregates in PD and TDP-43 aggregates in ALS. Hence, therapeutic approaches aiming to inhibit the formation or promote the clearance of protein aggregates have emerged in recent years, some of which have advanced to clinical trials. Polyoxometalates (POMs), formed by the condensation reaction of early transition-metal oxo anions, are prime candidates for constructing functional nanoclusters covering a wide range of fields including catalysis, energy storage and biomedicine. A series of modified POMs obtained by high-throughput screening or rational design are able to modulate protein aggregation via stereoselectivity. Here we summarize and discuss recent progress in the therapeutic applications of POMs to target protein aggregation in NDDs.
1. Introduction
Polyoxometalates (POMs) are a class of metal–oxo clusters formed by discrete early transition metals, M (such as V, Mo, W, Nb, Sb, etc.), and oxygen in their highest oxidation states.1 Since 1934, scholars have classified POMs mainly as six basic structures, Keggin (XM12O40n−), Dawson (X2M18O62n−), Anderson (XM6O24n−), Waugh (XM9O32n−), Silverton (XM12O4210−) and Lindqvist (M6O192−) (X = Si, PV, B, Ge, AsV, Al, etc.; M = V, Mo, W, Nb, Sb, etc.) (Fig. 1a–c).2 Among these structural formulas, “X” refers to heteroatoms, also known as central atoms. POMs contain the following characteristics. Firstly, POMs are relatively stable, with strong electron acceptance and transfer abilities, and can form derivatives of different structures by coupling with metal cations or organic groups.3 Secondly, POMs have excellent redox properties. Thirdly, POMs are easily soluble in water and have relatively high thermal stability. Therefore, the unique properties of POMs enable them to exhibit diversity in terms of size, structure, and physicochemical capabilities. POMs and their functionalized materials have broad application prospects in cutting-edge technology fields such as biomedicine, photomagnetic systems, environmental protection, catalysts and energy storage.4–7
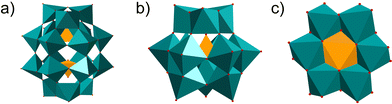 |
| Fig. 1 Overview of POMs with the most common archetypes. (a) Wells–Dawson structure; (b) Keggin structure; (c) Anderson structure. | |
In the past few decades, POMs have been found to possess anti-tumor, antiviral and antibacterial activities and exhibit therapeutic potential in the treatment of cancer and infectious diseases as a new generation of inorganic metal drugs.8–10 In 1974, Jasmin et al. first found that (NH4)17Na[NaSb9W21O86] had an inhibitory effect on tumors caused by viruses.11 Since then, the development of the bioactivity of POMs has attracted considerable attention, and many POM derivatives have been synthesized. Firstly, POMs can be used as a photosensitizer to promote apoptosis of tumor cells by photothermal therapy (PTT), which can induce local irreversible damage to the tumor cell membrane within a short period of time.12,13 In 2024, Tang et al. designed and synthesized POM-nanozyme-integrated nanomotors (POMotors) based on P2W18Fe4 and polydopamine (PDA).14 The tumor inhibition rate of POMotors reached 93.86% after 10 min of near infrared (NIR) irradiation at 808 nm, which significantly improved the therapeutic effect of PTT on tumor growth. Secondly, POMs can also be used in chemodynamic therapy (CDT) to inhibit tumor growth by depleting glutathione and converting endogenous H2O2 into OH, which is harmful to tumor cells.15,16 In 2024, Ge et al. synthesized Mn-POM-based single-atom catalysts (Mn-POM-SACs), anchoring the SACs firmly on the POM supports through oxygen atom coordination.17 Mn-POM-SACs exhibited outstanding catalytic activities (e.g. peroxidase-like, oxidase-like, catalase-like, and glutathione peroxidase-like activities), which produced cytotoxic ROS and depleted glutathione for cancer therapy. In addition, the redox nature of POMs reduces inflammation by eliminating inflammation-related reactive oxygen species (ROS).18 Thirdly, POMs have demonstrated potent antiviral effects against various viruses, including human immunodeficiency virus, herpes simplex virus, dengue virus, and influenza virus.19 As early as 2020, Qi et al. discovered that Keggin-type Cs2K4Na[SiW9Nb3O40] (POM-12) could inhibit the infection of Flaviviridae viruses due to destruction of the viral envelope, which disrupted the integrity of the viruses and promoted the RNA genome of viral particles being easily degraded by RNase.9 Finally, recent studies have also demonstrated that Mo-POMs exhibit highly effective anti-biofilm and bactericidal properties.20 In 2023, Qu et al. synthesized Mo-POM nanoclusters doped with Cu (Cu-POM NCs) as an efficient bioorthogonal catalyst.21 Through the biofilm-responsive self-assembly behavior, Cu-POM NCs facilitated the efficient synthesis of antibacterial molecules and PTT selectively triggered by H2S in pathogens, which is beneficial for the inhibition of bacterial tolerance and elimination of biofilms. However, the applications of POMs and their derivatives are not limited to cancer and infectious diseases. Recent studies have uncovered the novel bioactivities of POMs and extended their applications to the treatment of neurodegenerative diseases (NDDs).22
A typical example of NDDs linked with the therapeutic potential of POMs is Alzheimer's disease (AD), which is marked by the aggregation of β-amyloid (Aβ). The interaction of POMs with Aβ is closely related to the structure, volume and electrostatic attraction of POMs.23 Interestingly, due to the tunability of the POM structure, different types of metals as well as organic or inorganic groups can be easily introduced into POMs. Therefore, a variety of functional POM compounds have been synthesized to reduce their toxicity and improve their biological activity. For an up to date and excellent review on the therapeutic application of POMs for combating Aβ aggregation in AD, as well as correlations between anti-amyloid activities and structural features of POMs, refer to Ma et al.24
In addition to AD, many other NDDs are also associated with the formation of persistent and irreversible protein aggregates. Accordingly, POMs have common application potential in various NDDs by targeting and modulating different protein aggregates. In this review, we summarized various types of POM-based nanomaterials with well-defined structures as therapeutic candidates for NDDs, including AD, prion disease, Parkinson's disease (PD) and amyotrophic lateral sclerosis (ALS). We hope that this review will be beneficial for understanding the relationship between POM structures and their anti-NDD properties, and provide implications for the design and precise synthesis of POM-based drug candidates for NDD treatment.
2. NDDs and pathogenic protein aggregates
With the global aging trend, the prevalence of NDDs is increasing.25 NDDs are caused by the loss of neurons and the myelin sheath and worsen over time, leading to structural damage and dysfunction of the nervous system. Studies have found that NDDs are related to oxidative stress, mitochondrial dysfunction, neuroinflammation, apoptosis and other pathophysiological changes.26 Recently, studies have shown that misfolding, aggregation and accumulation of proteins in neurons are at the center of the pathogenesis of NDDs (Fig. 2), such as AD, PD, ALS, and etc.27,28
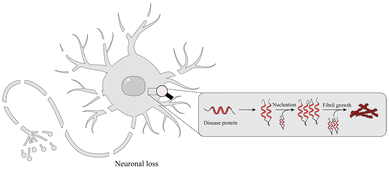 |
| Fig. 2 NDDs and pathogenic protein aggregates. NDD-associated proteins form fibrils that aggregate in neurons through a range of secondary mechanisms, including oxidative stress and neuroinflammation, eventually leading to neuronal death. | |
2.1. AD
2.1.1. Aβ amyloid aggregation associated with AD.
AD is an age-related neurodegenerative disease with cognitive decline and impaired functioning that affects personal every day and social activities.29 It is estimated that AD accounts for 60 to 80% of all dementia cases. Most patients exhibit late-onset AD, and only 5% of patients inherit the condition and exhibit early onset AD between the ages of 30 and 60.30 The main pathological features of AD are characterized by Aβ-containing extracellular plaques and tau-containing intracellular neurofibrillary tangles.31,32 Aβ is a series of small peptide isoforms ranging from 38 to 43 amino acids, which are cleaved from amyloid precursor protein (APP) by β-secretase and γ-secretase (Fig. 3).33,34 Among all Aβ isoforms, Aβ40 and Aβ42 are the most important ones that form Aβ aggregates, with Aβ42 bearing two extra residues at the C-terminus compared to Aβ40. Aβ usually exists as a stable monomer in the normal physiological state, but forms multimers and ultimately amyloid fibrils consisting of β-sheet structures in the pathological state.35,36 According to the amyloid hypothesis, mutations in genes involved in Aβ biosynthesis, aggregation and degradation could lead to an imbalance between Aβ production and clearance, which further results in abnormal accumulation of Aβ aggregates in the brain.37 The N- and C-termini of Aβ, His13–Lys16 (HHQK), hinge or turning region and hydrophobic region (KLVFF) play key roles in Aβ oligomeric and fiber propagation.38 Therefore, diverse strategies aimed at mitigating Aβ-induced neurotoxicity have been proposed for the treatment of AD.23
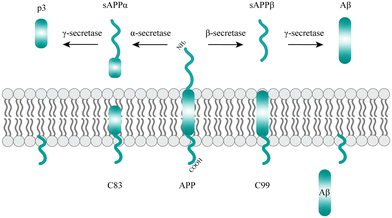 |
| Fig. 3 Aβ polypeptide is produced from APP though stepwise cleavage by secretases. | |
2.1.2. S100A9 amyloid aggregation associated with AD.
Neuroinflammation, which is defined as activation of the innate immune system typically within the brain or spinal cord, has been demonstrated as a major driver of multiple NDDs, including AD and ALS.39,40 The pro-inflammatory S100A9 protein contributes significantly to the amyloid-neuroinflammatory cascade in NDDs.41 S100A9 is a member of the calcium-binding protein and S100 protein family. Stimulation of inflammatory signaling pathways by S100A9 has been found in many diseases related to inflammatory processes.42 Overexpression of S100A9 is closely associated with neuronal death and the formation of Aβ amyloid.43 Currently, studies have found that S100A9 itself is highly neurotoxic, causing rapid neuronal damage and death. S100A9 amyloid aggregates are more stable and more resistant to proteases, which are unfavorable for clearance. As a result, S100A9 interacts with Aβ to promote the formation of fibrillar amyloid β structures, completing the vicious cycle of the amyloid-neuroinflammatory cascade.44
2.2 Prion disease
Prion disease is a rare neurodegenerative disease in humans and animals caused by the misfolding and aggregation of prion protein (PrP).45 PrP has two major isoforms: the normal cellular prion protein (PrPc) and the pathogenic scrapie prion protein (PrPSc).46 PrPc is a soluble glycoprotein that is very sensitive to protease digestion. PrPc contains an N-terminal flexible tail and a globular C-terminal structural domain that includes a low content of β-sheet structures (3%) and three α-helices.47 In contrast, PrPSc is the main component of prions characterized by resistance to protease digestion, insolubility and high levels of β-sheet structure.48 During the pathogenesis of prion disease, PrPc is conformationally transformed into PrPSc, which in turn acts as an infectious template that recruits PrPc for conformational conversion.49 Therefore, the inhibition or clearance of pathogenic PrPSc aggregates is of particular significance to the treatment of prion disease.
2.3 PD
The typical motor features of PD include bradykinesia, rigidity, resting tremor and postural instability due to impaired activity in the basal ganglia caused by the loss of dopaminergic neurons in the substantia nigra (SN).50 However, patients often exhibit a variety of non-motor symptoms, including constipation, hypotension, rapid eye movements, sleep disturbances and cognitive impairment.51 The neuronal protein α-synuclein encoded by the SNCA gene plays an important role in PD.52 Of the major PD-causative gene mutations, six have been identified in the SNCA gene, namely, A53T, A30P, E46K, H50Q, G51D and A53E.53 α-Synuclein interacts with a variety of proteins to perform many functions, such as regulation of phospholipase D and tyrosine hydroxylase. However, when α-synuclein is mutated, it is prone to misfold and form neurotoxic protein aggregates in the brain.54
2.4 ALS
ALS is a type of NDD with progressive muscle weakness resulting from the loss of upper and lower motor neurons.55 ALS patients eventually die due to respiratory failure. Approximately 10% of ALS cases are inherited in families, and 90% of ALS cases are sporadic with uncertain etiology. Known disease-causative mutations have been identified in 15% of ALS cases.56 Many of these mutations occur in genes encoding RNA binding proteins, such as TDP-43 and FUS. Mutated TDP-43 and FUS proteins found in ALS patients are prone to form protein aggregates via a process known as liquid–liquid phase separation (LLPS).57 It has been shown that TDP-43 and FUS are key components of insoluble aggregates in patient brains. TDP-43 and FUS are mainly localized in the nucleus to exert their functions such as regulation of splicing and stabilization of RNA. However, mutated TDP-43 and FUS proteins are retained in the cytoplasm and further result in the formation of neurotoxic protein aggregates as the concentration of cytoplasmic proteins increases.58–60 Both the loss of their original functions in the nucleus and the gain of neurotoxic functions in the cytoplasm contribute to neuronal death in ALS.61
Recent studies have shown that POMs are potential candidates for the treatment of AD due to their low toxicity and therapeutic activity.62 A series of POMs and transition metal functionalized POM derivatives have been reported as inhibitors of Aβ protein aggregation. Next, we focus on the current progress of POMs with therapeutic potential in AD and prion disease (Table 1), as well as the future prospects of POMs in PD and ALS.
Table 1 Summary of POMs that target NDDs
Category |
Neurodegenerative disease |
Protein aggregates |
POMs |
Molecular mechanism |
Ref. |
1 |
AD |
Aβ |
K8[P2CoW17O61] |
K8[P2CoW17O61] can act as a photocatalyst for Aβ monomers and oligomers to inhibit Aβ-induced toxicity |
64, 75 and 82 |
2 |
AD |
Aβ |
α-Na9H[SiW9O34], K8[P2CoW17O61], K7[PTi2W10O40], K8[β-SiW11O39] |
Interactions between the large surface of POMs and Aβ oligomers may inhibit the formation of Aβ fibrils by blocking direct contact between Aβ monomers |
64
|
3 |
AD |
Aβ |
(NH4)42{Mo132O372(OAc)30} |
Due to their polyanionic nature and rigid cagelike structures, Zn2+ and Cu2+ are able to strongly interact with (NH4)42{Mo132O372(OAc)30}, which inhibits metal-ion-induced aggregation of Aβ |
74
|
4 |
AD |
Aβ |
K8[P2NiW17O61], K8[P2CuW17O61] |
Electrostatic and histidine-chelating effects promote strong binding between POMds and Aβ monomers, significantly inhibiting Aβ aggregation |
82
|
5 |
AD |
Aβ |
[MnMo9]-D-Phe, [MnMo9]-L-Phe |
Firstly, chiral POMs can bind to the cationic cluster from His13 to Lys16 (HHQK). Secondly, Leu17 and Val18 on Aβ can interact with hydrocarbon chains. Thirdly, two adjacent phenylalanine residues (F19 and F20) form a strong hydrophobic region and specifically interact with hydrophobic amino acids. Finally, chiral POMs bind to the Aβ13–23 segment and exhibit strong enantioselectivity in inhibiting Aβ aggregation |
76
|
6 |
AD |
Aβ |
[(CH3)2NH2]15{α-P2W15Zr3(L-tartH)[α-P2W16]} |
Chiral POMs selectively inhibit Aβ aggregation, and in terms of inhibitory activity, D-POMs are significantly higher than L-POMs |
84
|
7 |
AD |
Aβ |
[CoL(H2O)]2[CoL]2[HAsVMoV6MoVI6O40] |
CAM can disassemble β-sheet-rich fibrils and metal-induced or self-assembled Aβ aggregates, and further inhibit ROS. Thus, it could protect neurons from synaptic toxicity caused by metal-induced Aβ aggregates |
66
|
8 |
AD |
Aβ |
(Me4N)3{PW11(SiC3H6NH2)2PtCl2} |
Inhibition of Aβ aggregation by PtII–PW11 is attributed to multiple interactions, including coordination interactions between Pt2+ and amino acids, electrostatic interactions, hydrogen bonding and van der Waals forces |
38
|
9 |
AD |
Aβ |
POM@peptide |
Due to the relatively large size of POM@peptide, its binding site would cover the N-terminal hydrophobic segment of Aβ monomers. Therefore, POM@peptide can inhibit the formation of Aβ aggregates |
85
|
10 |
AD |
Aβ |
AuNPs@POMD-8pep |
AuNPs@POMD-8pep has protease-like activity to deplete Aβ aggregation and superoxide dismutase (SOD)-like activity to scavenge Aβ-induced ROS |
86
|
11 |
AD |
Aβ |
CeONP@POMs |
CeONP@POMs have proteolytic enzyme activity. When binding to Aβ fibrils, the stability of Aβ fibrils is reduced and they are decomposed into oligomers or monomers. Then, Aβ monomers can be hydrolyzed by CeONP@POMs |
87
|
12 |
AD |
Aβ |
rPOMDs@MSNs@copolymers |
Due to their strong NIR absorption, rPOMDs@MSNs@copolymers are capable of generating local hyperthermia under NIR laser irradiation. Furthermore, rPOMDs@MSNs@copolymers are capable of scavenging Aβ-induced ROS |
88
|
13 |
AD |
Aβ |
(H2dap)6[Cd4Cl2(B-α-AsW9O34)2]·8H2O |
(H2dap)6[Cd4Cl2(B-α-AsW9O34)2]·8H2O can efficiently modulate the β-sheet-rich fibrils of Aβ and inhibit the production of ROS from Aβ aggregates |
89
|
14 |
AD |
Aβ |
K8{[Co(H2O)4][HP2Mo5O23]2}·8H2O |
As a conformational modulator, K8{[Co(H2O)4][HP2Mo5O23]2}·8H2O can prevent Aβ aggregation and suppress the generation of ROS |
90
|
15 |
AD |
Aβ |
AuNPs@POM@PEG |
The synergetic effect between AuNPs and POMs effectively inhibits Aβ aggregation |
17
|
16 |
AD |
Aβ |
Peptide@Mo-POMs |
Peptide@Mo-POMs can suppress Zn2+-induced Aβ aggregation and disaggregate Aβ fibrils |
91
|
17 |
AD |
S100A9 |
[N(CH3)4]6[Nb10O28], [N(CH3)4]7[TiNb9O28] |
Both POMs interact with positively charged Lys-rich regions on the surface of S100A9 |
41
|
18 |
Prion disease |
PrPSc |
H4[PW12O40] |
Separation of PrPSc from PrPC is achieved by precipitation with H4[PW12O40] |
92
|
19 |
Prion disease |
PrPSc |
Na4[SiW12O40], K5[BW12O40], Na6[H2W12O40] |
Size-specific electrostatic interactions between Keggin POMs and multiple PrPSc oligomers lead to PrPSc deposition |
93
|
20 |
Prion disease |
PrPSc |
(NH4)6[H2W12O40], K5[BW12O40] |
BTA and HTA can significantly reduce the number of amyloid fibrils; this is associated with an increased negative charge of POMs |
94
|
21 |
Prion disease |
PrPSc |
Na7[PW11O39]·12H2O |
Na7[PW11O39]·12H2O can selectively bind to PrPScvia electrostatic interactions |
95
|
22 |
Prion disease |
PrPSc |
Ag3PW12O40 |
As a Keggin-type POM, Ag3PW12O40 can specifically interact with PrPSc to inhibit its formation |
96
|
23 |
Prion disease |
PrPSc |
[PW11VO40]4−, [PW10V2O40]5−, [PMo11VO40]4−, [PMo10V2O40]5− |
[PW10V2O40]5− shows the strongest oxidation effect on the Met residue of PrPSc |
97
|
3. Application of POMs in AD via targeting Aβ
As a class of metal–oxo clusters (MOCs), POMs have shown good application prospects in biomedicine since 1998.63 The molecular properties of POMs affecting recognition and reactivity with their biomolecular targets are variable, including polarity, redox potential, surface charge distribution, shape and acidity.64 Recent studies have shown that POMs can restrain Aβ aggregation to reduce Aβ-induced cytotoxicity through non-covalent interactions such as hydrogen bonding, electrostatic attraction, π–π stacking and hydrophobic interactions.65 At the same time, POMs can cross the blood–brain barrier (BBB) and be metabolized within a period of time; this provides a novel strategy for intervening in the pathology of AD.66 In recent years, countless potential drugs have demonstrated low efficacy in clinical trials due to their inability to penetrate the BBB, which becomes a significant challenge in drug development for neurological disorders.67 The BBB is a dynamic, semipermeable, and highly selective system located in the brain microvasculature of most vertebrates. Its primary function is to separate the blood from the extracellular fluid of the brain, which plays a crucial role in regulating the transport of substances essential for proper brain function.68 The transmission pathways of the BBB mainly involve diffusional transmission, the transporter protein pathway, receptor-mediated transcytosis, adsorptive transcytosis and the cell-mediated pathway. POMs mainly cross the BBB through adsorptive transcytosis, which relies on electrostatic interactions with the surface of the cell membrane.69 Additionally, the easily adjustable and structurally variable nature of POMs enables diverse modifications, such as chirality, nanocarriers, and liposomes, which can further enhance the penetration ability of POMs.70,71 For example, in 2023, Perich et al. combined AuNPs, PEG and POMs to synthesize AuNPs@POM@PEG for the treatment of AD.72 PEG can improve biocompatibility by reducing BBB damage and protein absorption. In addition, the drug nanocarrier AuNPs with a smaller size and targeting molecule modification can more easily pass through the BBB, further promoting the absorption of POMs.73 Therefore, more and more attention has been paid to the applications of POMs and their derivatives in the treatment of AD.
3.1. Pure POMs
Pure POMs exhibit rich chemical compositions and nanostructures.74 Studies have shown that polyoxytungstates (POTs), polyoxymolybdates (POMos), polyoxyvanadates (POVs) and polyoxoniobates (PONbs) are able to inhibit protein aggregation.64,74–79 In 2011, Qu's group demonstrated for the first time that the Wells–Dawson structure of POMs could effectively inhibit Aβ aggregation.64 This finding also proved that phosphotungstate with the Wells–Dawson structure had the highest inhibitory effect and a POM with the Keggin structure had a moderate to high inhibitory effect on Aβ aggregation, while a smaller POM with an Anderson structure showed no effect, suggesting that the POM–Aβ interaction mainly relied on size-dependent electrostatic interactions.24 In 2014, Chen et al. synthesized three representative POM nanoclusters based on Mo132 for countering Aβ40 aggregation (Fig. 4).74 Recent studies found that POMs carrying a large amount of negative charges preferentially bound to the positively charged Lys and Arg residues on the Aβ protein surface.65 For example, the Aβ12–28 fragment is positively charged with a cationic His13–Lys16 (HHQK) cluster. In addition, POMs can bind to Aβ monomers and inhibit the formation of their aggregates by electrostatic attraction.38 In 2022, Gao et al. modified a Wells–Dawson structured POM with thiazolidinethione (POMD-TZ) to improve the binding specificity to the Lys side chain of Aβ.77 POMD-TZ could selectively covalently bind to the Lys16 site on Aβ and reduce amyloid aggregation via site-directed chemical modification (Fig. 5). In conclusion, the size, surface charge and ligands of POM complexes may play important roles in Aβ recognition and inhibition of amyloid aggregation.74 It also raises the question of the necessity for POM modification for better recognition and inhibition of Aβ aggregation.
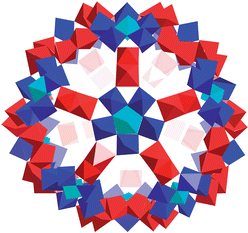 |
| Fig. 4 The basic spherical shape of anionic clusters. | |
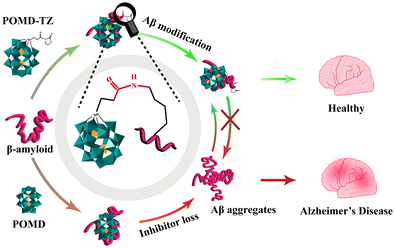 |
| Fig. 5 POMD-TZ can specifically modify Aβ at the Lys16 site and inhibit Aβ aggregation. | |
3.2. Modified POMs
3.2.1. Transition metal hybrid POMs.
As drug candidates, pure POMs are limited by cytotoxicity and instability, which may restrict their applications in vivo.80 Therefore, researchers started to search for more suitable inhibitors or functionalized POMs, and further explored their biological mechanisms and theories.81 In 2014, Qu's group designed and synthesized a series of transition metal functionalized POMs with an improved inhibitory effect on Aβ aggregation compared to Wells–Dawson type POMs (Fig. 6a).82 They found that the order of inhibitory effects followed the trend of POMds (Dawson type POM derivatives)-Dawson-Ni > POMds-Dawson-Co > POM-Dawson. The inhibition of nickel metallized POM derivatives achieved sixfold improvement. Indeed, the surface negative charge densities of POMs are controlled by multiple factors, including a redox active metal, transition metal substitution, charge density and geometry, which are closely related to the binding between POMs and Aβ.83 The POMds-binding site in Aβ was the cationic HHQK cluster, rather than His or another single amino acid. POM-Dawson contained six negative charges, while POMds had eight negative charges so that the electrostatic attraction to the cationic HHQK clusters was increased (Fig. 7).82 In addition, POMds-Dawson-Ni and POMds-Dawson-Co showed better inhibition of Aβ-mediated peroxidase-like activity, suggesting that the modified POMs could be used as bifunctional drugs against AD. Meanwhile, the POM-binding site in Aβ12–28 was a positively charged cavity-like domain mainly surrounded by the amino acids His13, Gln15 and Lys16.76 Through histidine chelation between transition metals and cationic HHQK clusters, POMds strongly bound to Aβ monomers, which prevented direct contact between Aβ monomers to restrain their nucleation and fiber growth (Fig. 8).82 Through electrostatic attraction and histidine chelation, POMs and their derivatives can more effectively target Aβ protein to prevent aggregation.
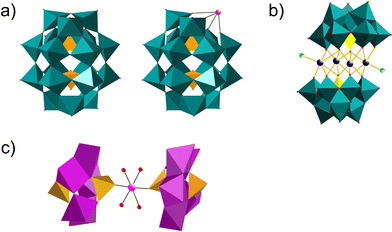 |
| Fig. 6 Structures of transition metal hybrid POMs. (a) Wells–Dawson structure and Wells–Dawson POMds with defined histidine-chelating metals (such as Ni, Co, Cu, Fe and Mn). (b) Polyhedral/ball-and-stick representation of (H2dap)6[Cd4Cl2(B-α-AsW9O34)2]·8H2O. (c) A combined polyhedral/ball-and-stick representation of K8{[Co(H2O)4][HP2Mo5O23]2}·8H2O. (PO4 polyhedra: light orange; WO6 polyhedra: teal; MoO6 octahedra: violet; AsO4: yellow; the histidine-chelating metal is shown as a purple ball. The Cd, Cl, O, C, N and H atoms are shown as dark blue, light green, red, grey, dark blue and white balls, respectively.) | |
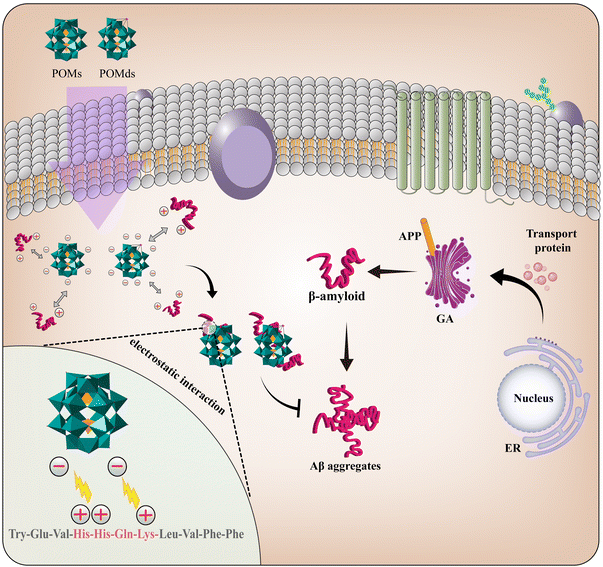 |
| Fig. 7 POMds can inhibit Aβ aggregation by binding to the cationic HHQK clusters on Aβ monomers through electrostatic interactions. Compared to POMs-Dawson, which contains six negative charges, POMds have eight negative charges to increase the electrostatic attraction to the HHQK site. | |
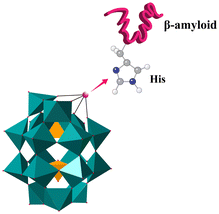 |
| Fig. 8 POMds strongly bind to Aβ monomers by histidine chelation between transition metals and cationic HHQK clusters. | |
Photocatalysis is a low cost, high efficiency and energy saving technology. As a novel type of photocatalyst, POMs have broad application prospects related to photocatalysis, including the treatment of AD.98 In 2013, Li et al. discovered that K8[P2CoW17O61] had unparalleled photosensitive properties.75 When generating ROS through UV excitation, POMs could serve as photocatalysts to weaken hydrogen bonds between amino acid residues. Thus, the secondary structure of the Aβ peptide was disrupted, and the α-helix configuration was lost. Therefore, POMs could degrade Aβ monomers and oligomers and block Aβ-induced cytotoxicity by photocatalysis.99
Misfolded protein accumulation is a critical step involved in the onset and progression of AD.100 The basic structure of the toxic substance is β-sheet Aβ aggregates formed by a process of misfolded aggregation. Furthermore, ROS generated by toxic aggregates is a crucial neurodegenerative factor. In 2022, Hua et al. successfully designed and synthesized a nano-POM, (H2dap)6[Cd4Cl2(B-α-AsW9O34)2]·8H2O (dap = 1,2-diaminopropane), based on a tetra-Cd cluster sandwiched by a trivacant Keggin type tungstoarsenate (Fig. 6b).89 It could both modulate the β-sheet-rich fibrils of Aβ peptides efficiently and inhibit the production of ROS from Aβ aggregates to relieve the neurotoxicity of Aβ aggregates.
In recent work, a POM based on a {Co(H2O)4}2+ complex and two Strandberg-type fragments, [P2Mo5O23]6−, sandwich-type K8{[Co(H2O)4][HP2Mo5O23]2}·8H2O (abbreviated as CoPM), was synthesized and structurally characterized (Fig. 6c).90 Because POM fragments and Co complexes interact synergistically, CoPM could modulate the conformation. As a conformational modulator, CoPM could prevent Aβ from aggregating and prevent Cu2+–Aβ species from producing ROS.
3.2.2. Ligand-modified POMs.
3.2.2.1. Chiral ligands.
New possibilities for POMs in the field of biomedicine are constantly being developing as the properties of POMs are different from other inorganic compounds in various aspects, e.g. chirality. Chirality is a basic feature of nature. The basic substances that constitute living organisms are chiral, such as amino acids, sugars, proteins, DNA, etc.101 Molecules containing asymmetric carbon substitutions are called chiral molecules.102 Chiral drugs are also a kind of common chiral molecule. Chiral POMs are a class of chiral cluster molecules with precise asymmetric structures. By combining their advantages with chirality, POMs have been widely used in stereoselective catalysis, chiral recognition, sensing, biological simulations, nonlinear materials and biomedicine.103,104 Aβ consists of an α-helix/β-sheet structure, which is sensitive to the chiral environment. Therefore, chiral recognition plays a crucial role in the recognition and inhibition of Aβ aggregation.105 In 2019, Gao et al. synthesized a series of POMs functionalized with chiral amino acids, including negatively charged amino acids (D-glutamic acid and L-glutamic acid), positively charged amino acids (D-histidine and L-histidine), and hydrophobic amino acids (D-leucine, L-leucine, D-phenylalanine, and L-phenylalanine) (Fig. 9a).76 Subsequently, these chiral POMs were screened based on binding affinity and enantioselectivity to Aβ. They found that POMs modified with D-phenylalanine (POM-D-Phe) interacted with two hydrophobic amino acids (Phe4 and Phe19) in Aβ1–40 to form a strongly hydrophobic region, leading to inhibition of Aβ aggregation by a specific hydrophobic amino acid interaction. Compared with POMs-L-Phe, POMs-D-Phe formed two additional hydrogen bonds with Aβ located at Ser8 and His13.
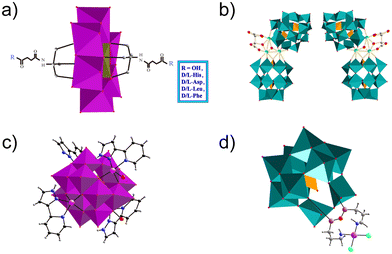 |
| Fig. 9 Structures of ligand modified POMs. (a) Rationally designed D/L-amino acid-functionalized POM derivatives. (b) The polyhedral/ball-and-stick representation of [(CH3)2NH2]15{[α-P2W15O55(H2O)]Zr3(μ3-O)(H2O)(L/D-tartH)[α-P2W16O59]}. (c) The polyhedral/ball-and-stick representation of [CoL(H2O)]2[CoL]2[HAsVMoV6MoVI6O40] monomer. (d) The polyhedral/ball-and-stick representation of (Me4N)3[PW11O40(SiC3H6NH2)2PtCl2] (MoO6 octahedra: violet; MnO6 polyhedra: dark yellow; PO4 polyhedra: light orange; WO6 polyhedra: teal. The Zr, Co, Si, Pt, Cl, O, C and N atoms are shown as green, pink, plum, purple, light green, red, grey and dark blue, respectively). | |
Given the critical influence of chirality on Aβ aggregation, incorporating enantioselective recognition into POMs is a reasonable modification during the design of Aβ inhibitors. Recently, Hu et al. prepared a couple of inherently enantiomerically pure POMs, [(CH3)2NH2]15{[α-P2W15O55(H2O)]Zr3(μ3-O)(H2O)(L-tartH)[α-P2W16O59]} (L-POM) and [(CH3)2NH2]15{[α-P2W15O55(H2O)]Zr3(μ3-O)(H2O)(D-tartH)[α-P2W16O59]} (D-POM), as novel chiral Aβ inhibitors (Fig. 9b).84 Owing to their chiral conformation and similar size to typical α-helix peptides, the chiral POM enantiomers exhibited a different binding affinity with Aβ40, leading to a noticeable enantioselective inhibitory effect on Aβ40 aggregation. In contrast to L-POM, D-POM displayed a higher Aβ40-binding affinity and greater brain biodistribution. D-POM had the ability to significantly reduce Aβ40 accumulation, scavenge ROS and rescue memory deficits in a mouse model of AD. Therefore, chiral POMs have improved enantioselectivity and specificity for their chiral biological targets and are promising modified POMs for the recognition of Aβ protein for AD diagnosis and treatment.
3.2.2.2. Other ligands.
In 2018, Ma et al. designed a modified POM, [CoL(H2O)]2[CoL]2[HAsVMoV6MoVI6O40] [CAM, L = 2-(1H-pyrazole-3-yl)pyridine], to decompose Aβ aggregates, with L as the Aβ targeting group and POM as the conformational modifier (Fig. 9c).66 X-ray crystallography showed that CAM consisted of an ε-Keggin unit and four coordination units. CAM could disaggregate the β-sheet-rich fibrils and metal-induced or self-aggregated Aβ aggregates, and inhibit the production of ROS. Therefore, it protected the neurons from synaptic toxicity induced by Zn2+– or Cu2+–Aβ aggregates or Aβ self-aggregation. Molecular simulations indicated that the appropriate sizes of CAM and β-sheet cavities were beneficial for the interaction between CAM and Aβ aggregates. Additionally, the hydrogen bonding preference of amino acid residues in the cavity provided a prerequisite for the interaction. Thus, CAM represents a new type of modified POM with a unique mechanism for targeting and inhibiting Aβ aggregates. The concept of this study may be applicable to other NDDs caused by protein misfolding and aggregation.
In 2019, Liu's group designed and prepared an organic platinum substituted POM, (Me4N)3[PW11O40(SiC3H6NH2)2PtCl2] (abbreviated as PtIIPW11) for inhibiting the aggregation of Aβ42 (Fig. 9d).38 PtIIPW11 at a dose of 8 μM exhibited a significant neuroprotective effect on neurotoxicity induced by Aβ42 aggregation, leading to an increase in cell viability from 49% to 67%. More importantly, PtIIPW11 significantly reduced Aβ42 deposition and rescued memory loss in a mouse model of AD without obvious cytotoxicity, demonstrating its potential as a drug for AD. The ability of PtIIPW11 to inhibit the aggregation of Aβ42 was attributed to synergistic interactions, including electrostatic attraction, hydrogen bonding, and van der Waals forces between Pt2+ in PtIIPW11 and the amino group in Aβ42. Therefore, achiral ligand POMs are also an important class of modified POMs that could effectively inhibit Aβ aggregation.
3.2.3. POM-based nanocomposites.
With the development of nanotechnology and materials science, the study of POMs is no longer limited to purely inorganic POMs, but also includes POM-based nanohybrid compounds, which can reduce cytotoxicity and improve the structural stability of POMs by functional combination or encapsulation of organic structures.106 Metals such as platinum (Pt), cobalt (Co), tungsten (W), gold (Au), cerium (Ce) and vanadium (V) in complexes, compounds, and biological nanoparticles have been widely utilized to modify POMs.2 In 2015, Gao et al. were inspired by the catalytic mechanism of serine protease to design and construct a bifunctional nanoenzyme, AuNPs@POMD-pep (AuNPs: gold nanoparticles, POMD: POM with Wells–Dawson structure, pep: peptide of N-acetyl-Cys-LPFFD).107 The strong binding between AuNPs@POMD-pep and Aβ monomers, caused by electrostatic, hydrogen bonding, and hydrophobic interactions, significantly reduced the concentration of free monomers, driving the equilibrium away from aggregation. The interactions between POMD and Aβ are primarily driven by electrostatic and hydrogen bonding, while the peptide and Aβ mainly engage in hydrophobic interactions. After that, Gao et al. developed AuNPs@POMD-8pep with improved targeting ability via 8pep (octa-peptides of N-acetyl-Cys-LPFFD) on the basis of AuNPs@POMD-pep (Fig. 10a).86In vitro experiments showed that AuNPs@POMD-8pep had serine protease and superoxide dismutase (SOD) activity, which could hydrolyze Aβ monomers and eliminate excess ROS generated within cells (Fig. 11). In 2016, Guan et al. synthesized three different types of POMs/cerium dioxide (CeONP@POMD, CeONP@POMK and CeONP@POMA) as mimetics of metalloproteinase MMP (Fig. 10b).87 The experimental results confirmed that CeONP@POMD had the highest efficiency for hydrolyzing Aβ and reducing the overproduction of ROS that could trigger cell death.108 Therefore, Ce/POM hybrid nanoparticles inhibited Aβ-induced cytotoxicity by both degrading Aβ aggregates and eliminating excess ROS.
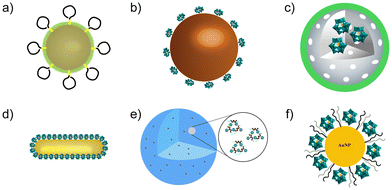 |
| Fig. 10 Structures of POM-based nanocomposites. (a) A bifunctional nanoenzyme, AuNPs@POMD-8pep. (b) CeONP@POMD. (c) rPOMs@MSNs@copolymers. (d) AuP nanorods. (e) Peptide@Mo-POMs. (f) AuNPs@POM@PEG. | |
 |
| Fig. 11 Aβ aggregation destroys the activity of NMDA receptors, leading to the generation of extracellular ROS and excess calcium influx into neurons, eventually causing mitochondrial dysfunction. ROS plays a key role in Aβ-mediated neuronal apoptosis. Dysfunctional mitochondria will further release ROS, which stimulates the production of pro-apoptotic proteins and causes apoptosis of nerve cells. AuNPs@POMD-8pep has serine protease and SOD activity, which can hydrolyze Aβ monomers and eliminate excess ROS generated within cells to treat AD. | |
POMs can also be used in PTT, which has received widespread attention in AD treatment due to its non-invasive nature and minimal damage to surrounding normal tissues. In 2018, Ma et al. designed and synthesized a NIR-responsive POM-based nanoplate with redox activation (rPOMs@MSNs@copolymers), which had a high photothermal effect and antioxidant activity (Fig. 10c).88 Due to the strong NIR absorption of POMs (rPOM), rPOMs@MSNs@copolymers could convert light to local heat to decompose Aβ fibers under NIR laser irradiation. Furthermore, Aβ-induced ROS was eliminated by the antioxidant activity of rPOMs. Similarly, in 2017, Li et al. proposed a novel strategy through the self-assembly of peptide (Ac-QKLVFF-NH2) coupled Au nanorods (AuP), which inhibited Aβ aggregates by PTT (Fig. 10d).109 The AuP can effectively dissociate the amyloid protein deposits under NIR laser irradiation in mouse cerebrospinal fluid, which protects cells from Aβ-induced toxicity. These studies may provide new insights for developing POM-based AD therapy in combination with PTT.
In 2019, peptide modified Mo POM nanoparticles (Mo-POMs) were synthesized by the self-assembly of Aβ targeting peptide (Ac-QKLVFF-NH2) and Mo-POMs (Fig. 10e).91 Importantly, by modification with the Aβ targeting peptide, the Mo-POM nanoparticles exhibited enhanced BBB penetration and a high binding affinity with Aβ species. Directed interactions between the peptide@Mo-POM nanoparticles and Aβ resulted in the suppression of Aβ aggregation and disaggregation of Aβ fibrils by stabilizing the native structure of Aβ monomers. Furthermore, in vitro experiments revealed that peptide@Mo-POMs could target and be well absorbed by PC12 cells to inhibit intracellular Aβ aggregation and reduce the Aβ aggregate-induced cytotoxicity.
Recently, a tri-component nanohybrid system, AuNPs@POM@PEG, was produced based on gold nanoparticles (AuNPs) covered with POMs (Fig. 10f).17 The presence of PEG increased the stability of the nanosystem, which could be preserved for 14 weeks, demonstrating good long-term stability in physiological media. AuNPs@POM@PEG could effectively inhibit Aβ aggregation via a synergetic effect between AuNPs and POM components that allowed inhibition levels of up to 75%. AuNPs@POM@PEG could cross the BBB at similar levels to other nanovehicles reported for drug delivery into the brain. These results suggest that AuNPs@POM@PEG or other POM-functionalized AuNPs could serve as promising candidates for the treatment of AD, which could be further extended to other NDDs.
In summary, protein aggregation plays a crucial role in the pathogenesis of AD. Among the six classic POMs, the Wells–Dawson structured POM shows the best inhibitory effect on Aβ protein aggregation. Organic or inorganic hybridization of POMs, such as transition metal and rare Earth metal substitution, and organic ligand modification, can effectively improve the inhibitory effect on protein aggregation. In addition, the combination of POMs and nanomaterials can improve the inhibitory effect on protein aggregation. The complex system may prompt the design of new multifunctional materials (tracking, drug loading, targeted drug release, etc.) for AD treatment. We speculate that the size, surface charge and ligands of POMs may play important roles in the recognition and inhibition of Aβ aggregation. Further work is needed to elucidate the exact inhibitory mechanisms through which these POMs act. In addition, the challenge lies in reducing potential cytotoxicity and improving stability before POMs can be applied in clinical treatment.
4. Application of POMs in AD via targeting S100A9
The pro-inflammatory S100A9 protein is recognized as an important contributor to neuroinflammation associated with the pathogenesis of AD. Studies have shown that the expression of S100A9 is upregulated in neuritic plaques and glial cells. Upregulated S100A9 can activate the p38 mitogen-activated protein kinase cascade, NF-kB or calcium-dependent signal transduction and stimulate the production of proinflammatory cytokines, leading to inflammation.110 Unlike other pro-inflammatory factors, S100A9 has the unique ability to spontaneously self-assemble amyloids under physiological conditions. During inflammation, S100A9 levels continue to rise, further leading to amyloid formation and aggregates.111 Therefore, S100A9 contributes to the development of AD via driving a self-reinforcing cycle that sustains inflammation and amyloid aggregation. In 2021, Chaudhary et al. demonstrated that two selected nanosized niobium POMs (Nb10 and TiNb9) served as effective inhibitors of S100A9 amyloid protein assembly (Fig. 12).41 Molecular dynamics simulations revealed that both Nb10 and TiNb9 bound to the S100A9 homo-dimer by forming ionic interactions with the positively charged Lys residue-rich patches on the protein surface. The region encompassing Lys50 to Lys54, characterized by high amyloid propensity, was the key sequence involved in S100A9 amyloid self-assembly. As the concentration of POMs increased, the long fibrillar structure of S100A9 completely disappeared, while short elongated stretches were formed.41 Thus, an alternative strategy for POM-based AD therapy is to inhibit the aggregation of S100A9 and ameliorate the self-reinforcing cycle of inflammation and amyloid aggregation.
 |
| Fig. 12 Structures of (a) decaniobate Nb10 = [Nb10O28]6− and (b) monotitanoniobate TiNb9 = [TiNb9O28]7−. NbO6 polyhedra: blue, TiO6 polyhedra: light green, and oxygen atoms: red balls. | |
5. Application of POMs in prion disease
As mentioned before, conformational conversion of soluble PrPc into insoluble PrPSc is a central aspect of prion disease. Therapeutic strategies to treat prion disease have been focused on the recognition of PrPSc aggregates for inhibition or clearance. Initially, Lee et al. developed a conformation-dependent immunoassay (CDI) for the detection of the infectious isoform of PrPSc.112 Na4[SiW12O40], K5[BW12O40] and Na6[H2W12O40] contained anions with the same Keggin structure as that of [PW12O40]3−, while central PIV atom substitution resulted in an increasing negative charge along the [SiW12O40]4−, [BW12O40]5− and [H2W12O40]6− series. They were able to selectively precipitate PrPSc from tissue homogenates by surface charge, facilitating the detection of prion infection. In addition, Keggin POMs, which carry a negative charge and exhibit conformational selectivity, could generate electrostatic interactions between multiple PrPSc oligomers. Their binding sites were located in the clefts of one or more positively charged residues on PrPSc. At the same time, the size, surface property and charge density of the POMs have an impact on the binding affinity with PrPSc.113 For example, the large sized POM inhibits the precipitation of PrPSc. POMs can be used not only for the detection of prions, but also for the inhibition of prion aggregation. The phosphotungstate anion (PTA), one of the Keggin type POMs, is widely used to detect and inhibit PrPSc aggregation. PTA can selectively bind to PrPScvia electrostatic interactions.95 Other studies have shown that PrPSc from the brain of patients binds to apolipoprotein B (apoB) in very low-density lipoprotein (VLDL) and low-density lipoprotein (LDL), which enhances the participation of lipoproteins in PrPSc aggregation.114 However, the affinity between PTA and PrPSc is stronger than the affinity between PrPSc and apoB. Thus, PTA can separate PrPSc from lipoproteins by strongly binding to PrPSc, preventing lipoproteins from participating and PrPSc from aggregating.
Due to the excellent redox properties, POMs also have the ability to oxidize PrPSc, which has a potential inhibitory effect on the aggregation of amyloid peptides. Recently, Li et al. developed vanadium-substituted Keggin POMs.97 The oxidation of PrPSc by POMs depended not only on the electron acceptance capacity of POMs, but also on their stability. Vanadium substitution affected the band structure of POMs and conferred improved stability on them. At the same time, the substitution of W/Mo by vanadium disrupted the symmetrical electron distribution of POMs, resulting in an enhanced local electron distribution on the bridging O atom, which strengthened the hydrogen bond to the histidine site of PrPSc (Fig. 13). Therefore, vanadium-substituted POMs showed a good inhibitory effect on PrPSc aggregation and resulting neurotoxicity by oxidation.
 |
| Fig. 13 Interaction modes between V-substituted W/Mo-POMs and PrP. | |
6. Application of POMs in PD
As a chronic NDD, PD is difficult to diagnose due to the site of pathology and clinical phenotype.115 Therefore, the development of real-time quantitative methods for PD biomarkers is essential for clinical diagnosis.116 In 2023, Shetty et al. designed and constructed a polyoxometalate–γ-cyclodextrin metal–organic framework (POM-γCD MOF), which could be used as an excellent electrocatalyst to develop highly selective sensors for detecting the in situ release of dopamine that served as a major biomarker of PD.117 POM-γCD MOF generated multiple modes of signals such as electrochemical and colorimetric signals, which could be converted into digital signals by a smartphone read-out platform.
In addition to AD and prion disease, the application of POMs in the treatment of other NDDs has also begun. Recently, Kalita et al. found that hen egg white lysozyme (Lyz) could be converted into amyloid fibrils; this was considered to be an excellent model protein for the study of NDD-associated protein aggregation.118 Utilizing this model, they showed that Keggin POMs such as phosphomolybdic acid and silicomolybdic acid, inhibited the aggregation of Lyz via hydrogen bonding and electrostatic interactions. It also indicates the possibility of suppressing NDD-associated protein aggregates by Keggin POMs. In addition, MOCs are widely recognized as novel nanoagents, which have broad application prospects in nanomedicine due to their ultrafine size, low toxicity and high renal clearance.119 In our recent study, we rationally introduced chirality into titanium oxide nanoclusters by chiral ligand exchange to synthesize R-Ti10Cd6 and S-Ti10Cd6 (R = R-hydrobenzoin, S = S-hydrobenzoin).120 Furthermore, we found that R-Ti10Cd6 but not S-Ti10Cd6 could effectively recover the loss of dopaminergic neurons and defects in dopamine-dependent locomotion behaviors in a C. elegans model of PD. As an important class of MOCs, POMs may have similar bioactivity that might be employed to explore their therapeutic application in PD.121 Indeed, we have screened a series of POMs in a C. elegans model of PD and identified two types of POMs that were able to inhibit α-synuclein aggregation and recover the defects in locomotion behaviors (Chen et al., unpublished data). Therefore, the application of POMs and their derivatives could be extended to the treatment of PD in the future.
7. Application of POMs in ALS
LLPS of biomolecules has been used to explain the formation of membraneless condensates such as stress granules and protein aggregates, and serves as a mechanism underlying the pathogenesis of NDDs that are closely associated with the transformation of soluble proteins into insoluble aggregates and amyloid fibrils.122,123 As mentioned before, mutated FUS and TDP-43 mislocalize in the cytoplasm and form protein aggregates via LLPS, leading to the development of ALS.124 Most recently, Zhang et al. demonstrated that graphene quantum dots (GQDs) could not only inhibit FUS LLPS but also disassemble FUS droplets in vitro through electrostatic and hydrophobic interactions.125 Similarly, halogen-doped GQDs inhibited abnormal LLPS of TDP-43 and reduced TDP-43 aggregates.126 As a type of MOC with a negative charge and large surface, POMs exist in the form of completely hydrophilic surfaces, with a uniform shape and intact molecular structures in dilute solutions.127 POMs can broadly attract positive charges, which confer on them the ability to selectively attract high-valence or monovalent ions with smaller hydrated sizes. POMs actually possess the ability to modulate LLPS of solutions through electrostatic, hydrophobic and counterion-mediated attractions.128 Therefore, POMs may also have potential in ALS treatment via suppressing the abnormal LLPS of FUS and TDP-43 proteins.
8. Conclusion and outlook
NDDs are characterized by the progressive loss of neurons associated with protein deposits and show changes to the physicochemical properties of the brain and peripheral organs. The most common protein aggregates involved in the pathogenesis of NDDs are Aβ and S100A9 in AD, prion protein in prion disease, α-synuclein in PD, and TDP-43 and FUS in ALS.129,130 Among these, the deposition and accumulation of Aβ lead to the formation of amyloid plaques, which are one of the basic features of AD patients, leading to neuronal toxicity in the brain.131 In the normal brain, there is a balance between Aβ production and clearance. However, with aging, mutations in genes associated with Aβ aggregation lead to a series of problems such as a decrease in the number of degradative enzymes, a decrease in the number of receptors that mediate the clearance or reduction of Aβ and others that cause protein misfolding and aggregation with uncontrolled chronic neuroinflammation.132 However, the increase in Aβ aggregation leads to synapsis loss and dysfunction, mitochondrial damage, oxidative stress, and the dysregulation of homeostasis.133 Therefore, inhibiting the formation of protein aggregates or removing the formed aggregates represents a promising therapeutic strategy for NDDs.
An increasing number of studies have demonstrated that POMs possess the ability to selectively recognize and inhibit protein aggregation, which means POMs have potential for application in NDD treatment. The mechanisms underlying the therapeutic effect of POMs can be summarized as follows. Firstly, as an inorganic nanomaterial with a large negative charge, POMs can cross the BBB to target Aβ. Through electrostatic interactions and histidine chelation, POMs and POMds strongly bind to Aβ monomers carrying positively charged cationic HHQK clusters and inhibit the formation of Aβ aggregates. Similarly, POMs can bind to the region with concentrated positive charge on the surface of S100A9 protein and inhibit the formation of S100A9 aggregates. Secondly, protein aggregates are usually chiral molecules, such as Aβ. Modified POMs with a corresponding chiral conformation can potently improve the interaction between POMs and protein aggregates by enantioselectivity, leading to the enhanced recognition of protein aggregates. Thirdly, POMs can be used as a photocatalyst to generate free radicals, which destroy the secondary structure of Aβ aggregates and depolymerize them. Fourthly, POMs act as NIR photothermal agents to convert light into local heat, leading to the decomposition of Aβ aggregates. Finally, through the antioxidant properties of POMs, excess ROS produced by Aβ aggregates can be eliminated to avoid initiating cell death. Overall, POMs have a significant effect on inhibiting or clearing protein aggregates due to their diverse functions.
However, challenges also remain with the rational and optimal design and application of POMs in NDDs. Firstly, a spatial structure relationship between protein monomers (e.g. Aβ40 and Aβ42 monomer) or normal isoforms (e.g. PrPc) and POMs versus the relationship between protein aggregates and POMs should be further investigated to ensure POMs selectively and preferentially target protein aggregates rather than monomer or normal isoforms so that their normal physiological function will not be disturbed. Secondly, the introduction of other ligands or properties (e.g. chirality, targeting peptide, antioxidant and hydrolyzing activity) to rationally modify POMs is necessary to improve the enantioselectivity or inhibitory effect towards protein aggregates. Additional instant structure and interaction analysis of POMs with pathogenic proteins will be helpful for understanding their biological mechanisms and theories, which will greatly promote the design of functional POM materials for targeting protein aggregation. Thirdly, the therapeutic effect of POMs is important, but the potential toxicity of POMs should also be carefully evaluated. Both of them could include studies at multiple levels, including cell and animal models that are closer to clinical trials. Finally, protein aggregates are a common hallmark and pathogenic factor of NDDs. While current studies of POMs are limited to protein aggregates associated with AD and prion disease only, future studies could modify the specificity of POMs and extend it to target α-synuclein, TDP-43 and FUS aggregates for therapeutic applications in PD and ALS. Addressing these issues will advance the applications of POMs to target NDDs in the future.
Data availability
No primary research results, software or code have been included and no new data were generated or analysed as part of this review.
Conflicts of interest
There are no conflicts to declare.
Acknowledgements
We are grateful for the support of this work by the National Natural Science Foundation of China (NSFC 31970755 and 21801226), the Natural Science Foundation of Zhejiang Province (LY21C120001 and LY20B010002) and the Zhejiang Normal University Fund.
References
- J. C. Raabe, F. Jameel, M. Stein, J. Albert and M. J. Poller, Heteroelements in polyoxometalates: a study on the influence of different group 15 elements on polyoxometalate formation, Dalton Trans., 2024, 53, 454–466 RSC.
- F. Carvalho and M. Aureliano, Polyoxometalates Impact as Anticancer Agents, Int. J. Mol. Sci., 2023, 24, 5043 CrossRef CAS.
- X. Wang, S. Wei, C. Zhao, X. Li, J. Jin, X. Shi, Z. Su, J. Li and J. Wang, Promising application of polyoxometalates in the treatment of cancer, infectious diseases and Alzheimer's disease, J. Biol. Inorg. Chem., 2022, 27, 405–419 CrossRef CAS PubMed.
- L. Guo, L. He, Q. Zhuang, B. Li, C. Wang, Y. Lv, J. Chu and Y. F. Song, Recent Advances in Confining Polyoxometalates and the Applications, Small, 2023, 19, e2207315 CrossRef.
- R. Yousefi, S. Asgari, A. Banitalebi Dehkordi, G. Mohammadi Ziarani, A. Badiei, F. Mohajer, R. S. Varma and S. Iravani, MOF-based composites as photoluminescence sensing platforms for pesticides: Applications and mechanisms, Environ. Res., 2023, 226, 115664 CrossRef CAS.
- J. Pisk and D. Agustin, Molybdenum, Vanadium, and Tungsten-Based Catalysts for Sustainable (ep)Oxidation, Molecules, 2022, 27, 6011 CrossRef CAS.
- W. Tang, T. Qiu, Z. Hu, Y. Li, R. Yao, Y. Wang, X. Lang, H. Tan, Y. Li and Q. Jiang, Monodisperse Manganese-Vanadium-Oxo Clusters with Extraordinary Lithium Storage, Adv. Sci., 2024, e2402616, DOI:10.1002/advs.202402616.
- A. Bijelic, M. Aureliano and A. Rompel, Polyoxometalates as Potential Next-Generation Metallodrugs in the Combat Against Cancer, Angew. Chem., Int. Ed., 2019, 58, 2980–2999 CrossRef CAS.
- Y. Qi, L. Han, Y. Qi, X. Jin, B. Zhang, J. Niu, J. Zhong and Y. Xu, Anti-flavivirus activity of polyoxometalate, Antiviral Res., 2020, 179, 104813 CrossRef CAS.
- A. Bijelic, M. Aureliano and A. Rompel, The antibacterial activity of polyoxometalates: structures, antibiotic effects and future perspectives, Chem. Commun., 2018, 54, 1153–1169 RSC.
- C. Jasmin, J. C. Chermann, G. Herve, A. Teze, P. Souchay, C. Boy-Loustau, N. Raybaud, F. Sinoussi and M. Raynaud, In vivo inhibition of murine leukemia and sarcoma viruses by the heteropolyanion 5-tungsto-2-antimoniate, J. Natl. Cancer Inst., 1974, 53, 469–474 CrossRef CAS.
- X. Kong, Q. Chen, G. Wan, Y. Yang, H. Yu, B. Li and L. Wu, Hyaluronic Acid-Enwrapped Polyoxometalate Complex for Synergistic Near Infrared-II Photothermal/Chemo-Therapy and Chemodynamic Therapy, Biomacromolecules, 2022, 23, 3752–3765 CrossRef CAS PubMed.
- W. Wang, Y. Song, J. Chen, Y. Yang, J. Wang, Y. Song, J. Ni, M. Tang, J. Zhao, Y. Sun, T. Sun and J. Peng, Polyoxometalate-covalent organic framework hybrid materials for pH-responsive photothermal tumor therapy, J. Mater. Chem. B, 2022, 10, 1128–1135 RSC.
- M. Tang, J. Ni, Z. Yue, T. Sun, C. Chen, X. Ma and L. Wang, Polyoxometalate-Nanozyme-Integrated Nanomotors (POMotors) for Self-Propulsion-Promoted Synergistic Photothermal-Catalytic Tumor Therapy, Angew. Chem., Int. Ed., 2024, 63, e202315031 CrossRef CAS.
- Y. Song, Y. Sun, M. Tang, Z. Yue, J. Ni, J. Zhao, W. Wang, T. Sun, L. Shi and L. Wang, Polyoxometalate Modified by Zeolite Imidazole Framework for the pH-Responsive Electrodynamic/Chemodynamic Therapy, ACS Appl. Mater. Interfaces, 2022, 14, 4914–4920 CrossRef CAS.
- F. Lu, M. Wang, N. Li and B. Tang, Polyoxometalate-Based Nanomaterials Toward Efficient Cancer Diagnosis and Therapy, Chemistry, 2021, 27, 6422–6434 CrossRef CAS PubMed.
- X. Ge, Y. Yin, X. Wang, Y. Gao, X. Guan, J. Sun, J. Ouyang and N. Na, Multienzyme-Like Polyoxometalate-Based Single-Atom Enzymes for Cancer-Specific Therapy Through Acid-Triggered Nontoxicity-to-Toxicity Transition, Small, 2024, 20, e2401073 CrossRef.
- D. Wang, Y. Wang, X. Zhang, Q. Lv, G. Ma, Y. Gao, S. Liu, C. Wang, C. Li, X. Sun and J. Wan, A Polyoxometalate-Encapsulated Metal-Organic Framework Nanoplatform for Synergistic Photothermal-Chemotherapy and Anti-Inflammation of Ovarian Cancer, Molecules, 2022, 27, 8350 CrossRef CAS.
- Z. Li, Y. Duan, Y. Yu, Y. Su, M. Zhang, Y. Gao, L. Jiang, H. Zhang, X. Lian, X. Zhu, J. Ke, Q. Peng and X. Chen, Sodium Polyoxotungstate Inhibits the Replication of Influenza Virus by Blocking the Nuclear Import of vRNP, Microorganisms, 2024, 12, 1017 CrossRef CAS.
- Y. Wang, X. Kong, F. Li, B. Li, L. Wu, K. Chen and Y. Wu, Mo(154) Synergistically Enhanced Antibiofilm and Antibacterial Effects of Spermine via Coassembly, ACS Appl. Bio Mater., 2022, 5, 5281–5288 CrossRef CAS PubMed.
- H. Zhao, C. Zhao, Z. Liu, J. Yi, X. Liu, J. Ren and X. Qu, A Polyoxometalate-Based Pathologically Activated Assay for Efficient Bioorthogonal Catalytic Selective Therapy, Angew. Chem., Int. Ed., 2023, 62, e202303989 CrossRef CAS PubMed.
- H. Moorthy, L. P. Datta, S. Samanta and T. Govindaraju, Multifunctional Architectures of Cyclic Dipeptide Copolymers and Composites, and Modulation of Multifaceted Amyloid-beta Toxicity, ACS Appl. Mater. Interfaces, 2022, 14, 56535–56547 CrossRef CAS.
- Z. Du, M. Li, J. Ren and X. Qu, Current Strategies for Modulating Abeta Aggregation with Multifunctional Agents, Acc. Chem. Res., 2021, 54, 2172–2184 CrossRef CAS.
- M. Ma, Z. Liu, H. Zhao, H. Zhang, J. Ren and X. Qu, Polyoxometalates: metallodrug agents for combating amyloid aggregation, Natl. Sci. Rev., 2024, 11, nwae226 CrossRef.
- K. Singh, P. Sethi, S. Datta, J. S. Chaudhary, S. Kumar, D. Jain, J. K. Gupta, S. Kumar, A. Guru and S. P. Panda, Advances in gene therapy approaches targeting neuro-inflammation in neurodegenerative diseases, Ageing Res. Rev., 2024, 98, 102321 CrossRef CAS.
- O. Binvignat and J. Olloquequi, Excitotoxicity as a Target Against Neurodegenerative Processes, Curr. Pharm. Des., 2020, 26, 1251–1262 CrossRef CAS PubMed.
- H. Adam, S. C. B. Gopinath, M. K. M. Arshad, T. Adam, S. Subramaniam and U. Hashim, An Update on Parkinson's Disease and its Neurodegenerative Counterparts, Curr. Med. Chem., 2024, 31, 2770–2787 CrossRef.
- M. Perez-Berlanga, V. I. Wiersma, A. Zbinden, L. De Vos, U. Wagner, C. Foglieni, I. Mallona, K. M. Betz, A. Clery, J. Weber, Z. Guo, R. Rigort, P. de Rossi, R. Manglunia, E. Tantardini, S. Sahadevan, O. Stach, M. Hruska-Plochan, F. H. Allain, P. Paganetti and M. Polymenidou, Loss of TDP-43 oligomerization or RNA binding elicits distinct aggregation patterns, EMBO J., 2023, 42, e111719 CrossRef CAS.
- Z. Cao, F. Kong, J. Ding, C. Chen, F. He and W. Deng, Promoting Alzheimer's disease research and therapy with stem cell technology, Stem Cell Res. Ther., 2024, 15, 136 CrossRef.
- A. A. Rostagno, Pathogenesis of Alzheimer's Disease, Int. J. Mol. Sci., 2022, 24, 107 CrossRef PubMed.
- Y. Chen, Y. He, J. Han, W. Wei and F. Chen, Blood-brain barrier dysfunction and Alzheimer's disease: associations, pathogenic mechanisms, and therapeutic potential, Front. Aging Neurosci., 2023, 15, 1258640 CrossRef CAS PubMed.
- D. S. Knopman, H. Amieva, R. C. Petersen, G. Chetelat, D. M. Holtzman, B. T. Hyman, R. A. Nixon and D. T. Jones, Alzheimer disease, Nat. Rev. Dis. Primers, 2021, 7, 33 CrossRef PubMed.
- W. He, X. Shi and Z. Dong, The roles of RACK1 in the pathogenesis of Alzheimer's disease, J. Biomed. Res., 2024, 38, 137–148 CrossRef PubMed.
- K. S. Orobets and A. L. Karamyshev, Amyloid Precursor Protein and Alzheimer’s Disease, Int. J. Mol. Sci., 2023, 24, 14794 CrossRef CAS PubMed.
- D. Hefter, S. Ludewig, A. Draguhn and M. Korte, Amyloid, APP, and Electrical Activity of the Brain, Neuroscientist, 2020, 26, 231–251 CrossRef PubMed.
- X. Kong, G. Wan, B. Li and L. Wu, Recent advances of polyoxometalates in multi-functional imaging and photothermal therapy, J. Mater. Chem. B, 2020, 8, 8189–8206 RSC.
- E. Fedele, Anti-Amyloid Therapies for Alzheimer's Disease and the Amyloid Cascade Hypothesis, Int. J. Mol. Sci., 2023, 24, 14499 CrossRef CAS.
- J. Zhao, K. Li, K. Wan, T. Sun, N. Zheng, F. Zhu, J. Ma, J. Jiao, T. Li, J. Ni, X. Shi, H. Wang, Q. Peng, J. Ai, W. Xu and S. Liu, Organoplatinum-Substituted Polyoxometalate Inhibits beta-amyloid Aggregation for Alzheimer's Therapy, Angew. Chem., Int. Ed., 2019, 58, 18032–18039 CrossRef CAS.
- S. Thakur, R. Dhapola, P. Sarma, B. Medhi and D. H. Reddy, Neuroinflammation in Alzheimer's Disease: Current Progress in Molecular Signaling and Therapeutics, Inflammation, 2023, 46, 1–17 CrossRef CAS PubMed.
- A. D. Calma, N. Pavey, P. Menon and O. S. Vucic, Neuroinflammation in amyotrophic lateral sclerosis: pathogenic insights and therapeutic implications, Curr. Opin. Neurol., 2024, 37, 585–592 CrossRef CAS PubMed.
- H. Chaudhary, I. A. Iashchishyn, N. V. Romanova, M. A. Rambaran, G. Musteikyte, V. Smirnovas, M. Holmboe, C. A. Ohlin, Z. M. Svedruzic and L. A. Morozova-Roche, Polyoxometalates as Effective Nano-inhibitors of Amyloid Aggregation of Pro-inflammatory S100A9 Protein Involved in Neurodegenerative Diseases, ACS Appl. Mater. Interfaces, 2021, 13, 26721–26734 CrossRef CAS.
- M. Pruenster, R. Immler, J. Roth, T. Kuchler, T. Bromberger, M. Napoli, K. Nussbaumer, I. Rohwedder, L. M. Wackerbarth, C. Piantoni, K. Hennis, D. Fink, S. Kallabis, T. Schroll, S. Masgrau-Alsina, A. Budke, W. Liu, D. Vestweber, C. Wahl-Schott, J. Roth, F. Meissner, M. Moser, T. Vogl, V. Hornung, P. Broz and M. Sperandio, E-selectin-mediated rapid NLRP3 inflammasome activation regulates S100A8/S100A9 release from neutrophils
via transient gasdermin D pore formation, Nat. Immunol., 2023, 24, 2021–2031 CrossRef CAS PubMed.
- Y. Tian, R. Cao, B. Che, D. Sun, Y. Tang, L. Jiang, Q. Bai, Y. Liu, L. A. Morozova-Roche and C. Zhang, Proinflammatory S100A9 Regulates Differentiation and Aggregation of Neural Stem Cells, ACS Chem. Neurosci., 2020, 11, 3549–3556 CrossRef CAS PubMed.
- M. Leri, D. Sun, Z. M. Svedruzic, D. Sulskis, V. Smirnovas, M. Stefani, L. Morozova-Roche and M. Bucciantini, Pro-inflammatory protein S100A9 targeted by a natural molecule to prevent neurodegeneration onset, Int. J. Biol. Macromol., 2024, 276, 133838 CrossRef CAS PubMed.
- D. L. Ritchie and M. A. Barria, Prion Diseases: A Unique Transmissible Agent or a Model for Neurodegenerative Diseases?, Biomolecules, 2021, 11, 107 CrossRef.
- S. Thellung, A. Corsaro, I. Dellacasagrande, M. Nizzari, M. Zambito and T. Florio, Proteostasis unbalance in prion diseases: Mechanisms of neurodegeneration and therapeutic targets, Front. Neurosci., 2022, 16, 966019 CrossRef PubMed.
- A. C. Gill and A. R. Castle, The cellular and pathologic prion protein, Handb. Clin. Neurol., 2018, 153, 21–44 Search PubMed.
- C. J. Atkinson, K. Zhang, A. L. Munn, A. Wiegmans and M. Q. Wei, Prion protein scrapie and the normal cellular prion protein, Prion, 2016, 10, 63–82 CrossRef CAS PubMed.
- C. Zhu and A. Aguzzi, Prion protein and prion disease at a glance, J. Cell Sci., 2021, 134, jcs245605 CrossRef CAS.
- J. C. Bozelli Jr., E. Kamski-Hennekam, G. Melacini and R. M. Epand, alpha-Synuclein and neuronal membranes: Conformational flexibilities in health and disease, Chem. Phys. Lipids, 2021, 235, 105034 CrossRef PubMed.
- S. Salim, F. Ahmad, A. Banu and F. Mohammad, Gut microbiome and Parkinson's disease: Perspective on pathogenesis and treatment, J. Adv. Res., 2023, 50, 83–105 CrossRef CAS PubMed.
- H. R. Morris, M. G. Spillantini, C. M. Sue and C. H. Williams-Gray, The pathogenesis of Parkinson's disease, Lancet, 2024, 403, 293–304 CrossRef CAS.
- H. Y. Li, D. S. Liu, Y. B. Zhang, H. Rong and X. J. Zhang, The interaction between alpha-synuclein and mitochondrial dysfunction in Parkinson's disease, Biophys. Chem., 2023, 303, 107122 CrossRef CAS.
- P. Calabresi, G. Di Lazzaro, G. Marino, F. Campanelli and V. Ghiglieri, Advances in understanding the function of alpha-synuclein: implications for Parkinson's disease, Brain, 2023, 146, 3587–3597 CrossRef PubMed.
- L. T. Ghaffari, D. Trotti, A. R. Haeusler and B. K. Jensen, Breakdown of the central synapses in C9orf72-linked ALS/FTD, Front. Mol. Neurosci., 2022, 15, 1005112 CrossRef CAS PubMed.
- T. Meyer, Amyotrophic lateral sclerosis (ALS) - diagnosis, course of disease and treatment options, Dtsch. Med. Wochenschr., 2021, 146, 1613–1618 CrossRef CAS PubMed.
- P. Chakraborty and M. Zweckstetter, Role of aberrant phase separation in pathological protein aggregation, Curr. Opin. Struct. Biol., 2023, 82, 102678 CrossRef CAS PubMed.
- J. R. Peinado, K. Chaplot, T. S. Jarvela, E. M. Barbieri, J. Shorter and I. Lindberg, Sequestration of TDP-43(216–414) Aggregates by Cytoplasmic Expression of the proSAAS Chaperone, ACS Chem. Neurosci., 2022, 13, 1651–1665 CrossRef CAS PubMed.
- D. M. Ho, M. Shaban, F. Mahmood, P. Ganguly, L. Todeschini, D. Van Vactor and S. Artavanis-Tsakonas, cAMP/PKA signaling regulates TDP-43 aggregation and mislocalization, Proc. Natl. Acad. Sci. U. S. A., 2024, 121, e2400732121 CrossRef CAS PubMed.
- H. Motaln, U. Cercek, A. Yamoah, P. Tripathi, E. Aronica, A. Goswami and B. Rogelj, Abl kinase-mediated FUS Tyr526 phosphorylation alters nucleocytoplasmic FUS localization in FTLD-FUS, Brain, 2023, 146, 4088–4104 CrossRef PubMed.
- L. Chen, FUS mutation is probably the most common pathogenic gene for JALS, especially sporadic JALS, Rev. Neurol., 2021, 177, 333–340 CrossRef CAS PubMed.
- S. F. Razavi, F. F. Bamoharram, T. Hashemi, K. Shahrokhabadi and A. Davoodnia, Nanolipid-loaded Preyssler polyoxometalate: Synthesis, characterization and invitro inhibitory effects on HepG2 tumor cells, Toxicol. In Vitro, 2020, 68, 104917 CrossRef CAS PubMed.
- J. T. Rhule, C. L. Hill, D. A. Judd and R. F. Schinazi, Polyoxometalates in Medicine, Chem. Rev., 1998, 98, 327–358 CrossRef CAS.
- J. Geng, M. Li, J. Ren, E. Wang and X. Qu, Polyoxometalates as inhibitors of the aggregation of amyloid beta peptides associated with Alzheimer's disease, Angew. Chem., Int. Ed., 2011, 50, 4184–4188 CrossRef CAS.
- A. Gil and J. J. Carbo, Computational Modelling of the Interactions Between Polyoxometalates and Biological Systems, Front. Chem., 2022, 10, 876630 CrossRef CAS PubMed.
- X. Ma, J. Hua, K. Wang, H. Zhang, C. Zhang, Y. He, Z. Guo and X. Wang, Modulating Conformation of Abeta-Peptide: An Effective Way to Prevent Protein-Misfolding Disease, Inorg. Chem., 2018, 57, 13533–13543 CrossRef CAS PubMed.
- S. M. Lombardo, M. Schneider, A. E. Tureli and N. Gunday Tureli, Key for crossing the BBB with nanoparticles: the rational design, Beilstein J. Nanotechnol., 2020, 11, 866–883 CrossRef CAS PubMed.
- F. Sanchez-Cano, L. C. Hernandez-Kelly and A. Ortega, The Blood-Brain Barrier: Much More Than a Selective Access to the Brain, Neurotoxic. Res., 2021, 39, 2154–2174 CrossRef CAS PubMed.
- A. Alahmari, Blood-Brain Barrier Overview: Structural and Functional Correlation, Neural Plast., 2021, 2021, 6564585 Search PubMed.
- Z. Cheng, M. Li, R. Dey and Y. Chen, Nanomaterials for cancer therapy: current progress and perspectives, J. Hematol. Oncol., 2021, 14, 85 CrossRef PubMed.
- N. Song, M. Lu, J. Liu, M. Lin, P. Shangguan, J. Wang, B. Shi and J. Zhao, A Giant Heterometallic Polyoxometalate Nanocluster for Enhanced Brain-Targeted Glioma Therapy, Angew. Chem., Int. Ed., 2024, 63, e202319700 CrossRef CAS.
- M. Perxes Perich, S. Palma-Florez, C. Sole, S. Goberna-Ferron, J. Samitier, P. Gomez-Romero, M. Mir and A. Lagunas, Polyoxometalate-Decorated Gold Nanoparticles Inhibit beta-Amyloid Aggregation and Cross the Blood-Brain Barrier in a microphysiological Model, Nanomaterials, 2023, 13, 2697 CrossRef CAS PubMed.
- Y. Fan, C. Xu, N. Deng, Z. Gao, Z. Jiang, X. Li, Y. Zhou, H. Pei, L. Li and B. Tang, Understanding drug nanocarrier and blood-brain barrier interaction based on a microfluidic microphysiological model, Lab Chip, 2023, 23, 1935–1944 RSC.
- Q. Chen, L. Yang, C. Zheng, W. Zheng, J. Zhang, Y. Zhou and J. Liu, Mo polyoxometalate nanoclusters capable of inhibiting the aggregation of Abeta-peptide associated with Alzheimer's disease, Nanoscale, 2014, 6, 6886–6897 RSC.
- M. Li, C. Xu, J. Ren, E. Wang and X. Qu, Photodegradation of beta-sheet amyloid fibrils associated with Alzheimer's disease by using polyoxometalates as photocatalysts, Chem. Commun., 2013, 49, 11394–11396 RSC.
- N. Gao, Z. Du, Y. Guan, K. Dong, J. Ren and X. Qu, Chirality-Selected Chemical Modulation of Amyloid Aggregation, J. Am. Chem. Soc., 2019, 141, 6915–6921 CrossRef CAS.
- N. Gao, Z. Liu, H. Zhang, C. Liu, D. Yu, J. Ren and X. Qu, Site-Directed Chemical Modification of Amyloid by Polyoxometalates for Inhibition of Protein Misfolding and Aggregation, Angew. Chem., Int. Ed., 2022, 61, e202115336 CrossRef CAS PubMed.
- M. Aureliano, N. I. Gumerova, G. Sciortino, E. Garribba, A. Rompel and D. C. Crans, Polyoxovanadates with emerging biomedical activities, Coord. Chem. Rev., 2021, 447, 214143 CrossRef CAS.
- M. Aureliano, N. I. Gumerova, G. Sciortino, E. Garribba, C. C. McLauchlan, A. Rompel and D. C. Crans, Polyoxidovanadates’ interactions with proteins: An overview, Coord. Chem. Rev., 2022, 454, 214334 CrossRef.
- A. V. Konkova, I. V. Savina, D. V. Evtushok, T. N. Pozmogova, M. V. Solomatina, A. R. Nokhova, A. Y. Alekseev, N. V. Kuratieva, I. V. Eltsov, V. V. Yanshole, A. M. Shestopalov, A. A. Ivanov and M. A. Shestopalov, Water-Soluble Polyoxometal Clusters of Molybdenum(V) with Pyrazole and Triazole: Synthesis and Study of Cytotoxicity and Antiviral Activity, Molecules, 2023, 28, 8079 CrossRef CAS PubMed.
- P. Gao, Y. Wu and L. Wu, Co-assembly of polyoxometalates and peptides towards biological applications, Soft Matter, 2016, 12, 8464–8479 RSC.
- N. Gao, H. Sun, K. Dong, J. Ren, T. Duan, C. Xu and X. Qu, Transition-metal-substituted polyoxometalate derivatives as functional anti-amyloid agents for Alzheimer's disease, Nat. Commun., 2014, 5, 3422 CrossRef PubMed.
- K. P. Sullivan, Q. Yin, D. L. Collins-Wildman, M. Tao, Y. V. Geletii, D. G. Musaev, T. Lian and C. L. Hill, Multi-Tasking POM Systems, Front. Chem., 2018, 6, 365 CrossRef PubMed.
- S. Hu, X. Ning, J. Lv, Y. Wei, H. Zhang and M. Li, Enantioselective modulation of amyloid burden and memory deficits by chiral polyoxometalates for Alzheimer's disease treatment, Inorg. Chem. Front., 2023, 10, 5347–5356 RSC.
- M. Li, C. Xu, L. Wu, J. Ren, E. Wang and X. Qu, Self-assembled peptide-polyoxometalate hybrid nanospheres: two in one enhances targeted inhibition of amyloid beta-peptide aggregation associated with Alzheimer's disease, Small, 2013, 9, 3455–3461 CrossRef CAS PubMed.
- N. Gao, K. Dong, A. Zhao, H. Sun, Y. Wang, J. Ren and X. Qu, Polyoxometalate-based nanozyme: Design of a multifunctional enzyme for multi-faceted treatment of Alzheimer's disease, Nano Res., 2016, 9, 1079–1090 CrossRef CAS.
- Y. Guan, M. Li, K. Dong, N. Gao, J. Ren, Y. Zheng and X. Qu, Ceria/POMs hybrid nanoparticles as a mimicking metallopeptidase for treatment of neurotoxicity of amyloid-beta peptide, Biomaterials, 2016, 98, 92–102 CrossRef CAS PubMed.
- M. Ma, N. Gao, Y. Sun, X. Du, J. Ren and X. Qu, Redox-Activated Near-Infrared-Responsive Polyoxometalates Used for Photothermal Treatment of Alzheimer's Disease, Adv. Healthcare Mater., 2018, 7, e1800320 CrossRef PubMed.
- J. Hua, X. Wei, X. Ma, J. Jiao, B. Chai, C. Wu, C. Zhang and Y. Niu, A {Cd4Cl2O14} cluster functionalized sandwich-type tungstoarsenate as a conformation modulator for misfolding Aβ peptides, CrystEngComm, 2022, 24, 1171–1176 RSC.
- M. Wang, J. Hua, P. Zheng, Y. Tian, S. Kang, J. Chen, Y. Duan and X. Ma, A Nanoscale Cobalt Functionalized Strandberg-Type Phosphomolybdate with β-Sheet Conformation Modulation Ability in Anti-Amyloid Protein Misfolding, Inorganics, 2023, 11, 4317 Search PubMed.
- Y. Liu, J. Sun, Y. Gong, H. Zhou, X. Chen, X. Zhu, Y. Zhao, Y. Wen, X. Qin and J. Liu, Peptide-Modified Mo Polyoxometalate Nanoparticles Suppress Zn2+-Induced Aβ Aggregation, ChemNanoMat, 2019, 5, 897–910 CrossRef CAS.
- J. G. Safar, H. Wille, M. D. Geschwind, C. Deering, D. Latawiec, A. Serban, D. J. King, G. Legname, K. H. Weisgraber, R. W. Mahley, B. L. Miller, S. J. DeArmond and S. B. Prusiner, Human prions and plasma lipoproteins, Proc. Natl. Acad. Sci. U. S. A., 2006, 103, 11312–11317 CrossRef CAS PubMed.
- I. S. Lee, J. R. Long, S. B. Prusiner and J. G. Safar, Selective Precipitation of Prions by Polyoxometalate Complexes, J. Am. Chem. Soc., 2005, 127, 13802–13803 CrossRef CAS PubMed.
- H. Wille, M. Shanmugam, M. Murugesu, J. Ollesch, G. Stubbs, J. R. Long, J. G. Safar and S. B. Prusiner, Surface charge of polyoxometalates modulates polymerization of the scrapie prion protein, Proc. Natl. Acad. Sci. U. S. A., 2009, 106, 3740–3745 CrossRef CAS PubMed.
- D. J. Levine, J. Stohr, L. E. Falese, J. Ollesch, H. Wille, S. B. Prusiner and J. R. Long, Mechanism of scrapie prion precipitation with phosphotungstate anions, ACS Chem. Biol., 2015, 10, 1269–1277 CrossRef CAS PubMed.
- W. Liang, C. Huang and L. Ma, Controllable synthesis of polyoxometalates nanocubes and their specific interactions with prion proteins, Sci. China, Ser. B: Chem., 2009, 52, 2156–2160 CrossRef CAS.
- B. Li, C. Xu, Y. Lv, G. Liu, X. Sun, Z. Sun, X. Xu, W. Chen, L. He and Y. F. Song, Vanadium-Substituted Polyoxometalates Regulate Prion Protein Fragment 106–126 Misfolding by an Oxidation Strategy, ACS Appl. Mater. Interfaces, 2023, 15, 34497–34504 CrossRef CAS PubMed.
- X. Chen, H. Wu, X. Shi and L. Wu, Polyoxometalate-based frameworks for photocatalysis and photothermal catalysis, Nanoscale, 2023, 15, 9242–9255 RSC.
- X. Zhao, S. Zhang, J. Yan, L. Li, G. Wu, W. Shi, G. Yang, N. Guan and P. Cheng, Polyoxometalate-Based Metal-Organic Frameworks as Visible-Light-Induced Photocatalysts, Inorg. Chem., 2018, 57, 5030–5037 CrossRef CAS PubMed.
- I. G. Ju, S. Lee, J. G. Choi, N. Kim, E. Huh, J. K. Lee and M. S. Oh, Aerial part of Houttuynia cordata reverses memory impairment by regulating amyloid beta accumulation and neuroinflammation in Alzheimer's disease model, Phytother. Res., 2023, 37, 2854–2863 CrossRef CAS PubMed.
- W. Jung, J. Kwon, W. Cho and J. Yeom, Chiral Biomaterials for Nanomedicines: From Molecules to Supraparticles, Pharmaceutics, 2022, 14, 1951 CrossRef CAS PubMed.
- H. Alkadi and R. Jbeily, Role of Chirality in Drugs: An Overview, Infect. Disord.: Drug Targets, 2018, 18, 88–95 CAS.
- G. Nystrom, M. Arcari and R. Mezzenga, Confinement-induced liquid crystalline transitions in amyloid fibril cholesteric tactoids, Nat. Nanotechnol., 2018, 13, 330–336 CrossRef CAS PubMed.
- Z. Zhu, M. Wei, B. Li and L. Wu, Constructing chiral polyoxometalate assemblies via supramolecular approaches, Dalton Trans., 2021, 50, 5080–5098 RSC.
- A. R. Foley and J. A. Raskatov, Understanding and controlling amyloid aggregation with chirality, Curr. Opin. Chem. Biol., 2021, 64, 1–9 CrossRef CAS PubMed.
- D. Chang, Y. Li, Y. Chen, X. Wang, D. Zang and T. Liu, Polyoxometalate-based nanocomposites for antitumor and antibacterial applications, Nanoscale Adv., 2022, 4, 3689–3706 RSC.
- N. Gao, H. Sun, K. Dong, J. Ren and X. Qu, Gold-nanoparticle-based multifunctional amyloid-beta inhibitor against Alzheimer's disease, Chemistry, 2015, 21, 829–835 CrossRef CAS PubMed.
- E. C. Cheung and K. H. Vousden, The role of ROS in tumour development and progression, Nat. Rev. Cancer, 2022, 22, 280–297 CrossRef CAS PubMed.
- M. Li, Y. Guan, A. Zhao, J. Ren and X. Qu, Using Multifunctional Peptide Conjugated Au Nanorods for Monitoring beta-amyloid Aggregation and Chemo-Photothermal Treatment of Alzheimer's Disease, Theranostics, 2017, 7, 2996–3006 CrossRef CAS PubMed.
- C. Wang, A. G. Klechikov, A. L. Gharibyan, S. K. Warmlander, J. Jarvet, L. Zhao, X. Jia, V. K. Narayana, S. K. Shankar, A. Olofsson, T. Brannstrom, Y. Mu, A. Graslund and L. A. Morozova-Roche, The role of pro-inflammatory S100A9 in Alzheimer's disease amyloid-neuroinflammatory cascade, Acta Neuropathol., 2014, 127, 507–522 CrossRef CAS PubMed.
- M. Leri, H. Chaudhary, I. A. Iashchishyn, J. Pansieri, Z. M. Svedruzic, S. Gomez Alcalde, G. Musteikyte, V. Smirnovas, M. Stefani, M. Bucciantini and L. A. Morozova-Roche, Natural Compound from Olive Oil Inhibits S100A9 Amyloid Formation and Cytotoxicity: Implications for Preventing Alzheimer's Disease, ACS Chem. Neurosci., 2021, 12, 1905–1918 CrossRef CAS PubMed.
- I. S. Lee, J. R. Long, S. B. Prusiner and J. G. Safar, Selective precipitation of prions by polyoxometalate complexes, J. Am. Chem. Soc., 2005, 127, 13802–13803 CrossRef CAS PubMed.
- H. Wille, M. Shanmugam, M. Murugesu, J. Ollesch, G. Stubbs, J. R. Long, J. G. Safar and S. B. Prusiner, Surface charge of polyoxometalates modulates polymerization of the scrapie prion protein, Proc. Natl. Acad. Sci. U. S. A., 2009, 106, 3740–3745 CrossRef CAS PubMed.
- J. G. Safar, H. Wille, M. D. Geschwind, C. Deering, D. Latawiec, A. Serban, D. J. King, G. Legname, K. H. Weisgraber, R. W. Mahley, B. L. Miller, S. J. Dearmond and S. B. Prusiner, Human prions and plasma lipoproteins, Proc. Natl. Acad. Sci. U. S. A., 2006, 103, 11312–11317 CrossRef CAS PubMed.
- E. Mikula, J. Katrlik and L. R. Rodrigues, Electrochemical Aptasensors for Parkinson's Disease Biomarkers Detection, Curr. Med. Chem., 2022, 29, 5795–5814 CrossRef CAS PubMed.
- D. G. Coughlin and D. J. Irwin, Fluid and Biopsy Based Biomarkers in Parkinson's Disease, Neurotherapeutics, 2023, 20, 932–954 CrossRef CAS PubMed.
- S. S. Shetty, B. Moosa, L. Zhang, B. Alshankiti, W. Baslyman, V. Mani, N. M. Khashab and K. N. Salama, Polyoxometalate-cyclodextrin supramolecular entities for real-time in situ monitoring of dopamine released from neuroblastoma cells, Biosens. Bioelectron., 2023, 229, 115240 CrossRef CAS PubMed.
- K. Kalita, S. J. Phukan, S. Garai and K. Sankaranarayanan, Polyoxometalates Mediated Amyloid Fibrillation Dynamics and Restoration of Enzyme Activity of Hen Egg White Lysozyme Treated under Cold Atmospheric Pressure Plasma, ACS Omega, 2024, 9, 3423–3429 CAS.
- Y. Shi, Z. Wu, M. Qi, C. Liu, W. Dong, W. Sun, X. Wang, F. Jiang, Y. Zhong, D. Nan, Y. Zhang, C. Li, L. Wang and X. Bai, Multiscale Bioresponses of Metal Nanoclusters, Adv. Mater., 2024, 36, e2310529 CrossRef PubMed.
- L.-C. Meng, J.-Y. Chen, Z.-M. Feng, Z.-G. Jiang, Z. Jin and C.-H. Zhan, Archimedean heterologous helixes in Ti10Cd6-oxo nanoclusters: double-helical self-assembly and therapeutic application in Parkinson's disease, Inorg. Chem. Front., 2024, 11, 3527–3537 RSC.
- M. J. W. Budych, K. Staszak, A. Bajek, F. Pniewski, R. Jastrząb, M. Staszak, B. Tylkowski and K. Wieszczycka, The future of polyoxymetalates for biological and chemical apllications, Coord. Chem. Rev., 2023, 493, 215306 CrossRef CAS.
- P. H. Peng, K. W. Hsu and K. J. Wu, Liquid-liquid phase separation (LLPS) in cellular physiology and tumor biology, Am. J. Cancer Res., 2021, 11, 3766–3776 CAS.
- H. Zhang, X. Ji, P. Li, C. Liu, J. Lou, Z. Wang, W. Wen, Y. Xiao, M. Zhang and X. Zhu, Liquid-liquid phase separation in biology: mechanisms, physiological functions and human diseases, Sci. China: Life Sci., 2020, 63, 953–985 CrossRef PubMed.
- B. Portz, B. L. Lee and J. Shorter, FUS and TDP-43 Phases in Health and Disease, Trends Biochem. Sci., 2021, 46, 550–563 CrossRef CAS PubMed.
- H. Zhang, J. Gu, Y. Zhang, H. Guo, S. Zhang, J. Song, C. Liu, L. Wang, D. Li and B. Dai, Graphene Quantum Dots Modulate Stress Granule Assembly and Prevent Abnormal Phase Transition of Fused in Sarcoma Protein, ACS Nano, 2023, 17, 10129–10141 CrossRef CAS PubMed.
- H. Zhang, H. Guo, D. Li, Y. Zhang, S. Zhang, W. Kang, C. Liu, W. Le, L. Wang, D. Li and B. Dai, Halogen doped graphene quantum dots modulate TDP-43 phase separation and aggregation in the nucleus, Nat. Commun., 2024, 15, 2980 CrossRef CAS PubMed.
- Z. D. Wang, S. Liang, Y. Yang, Z. N. Liu, X. Z. Duan, X. Li, T. Liu and H. Y. Zang, Complex phase transitions and phase engineering in the aqueous solution of an isopolyoxometalate cluster, Nat. Commun., 2023, 14, 2767 CrossRef CAS PubMed.
- Z. D. Wang, K. Bo, C. L. Zhong, Y. H. Xin, G. L. Lu, H. Sun, S. Liang, Z. N. Liu and H. Y. Zang, Multifunctional Polyoxometalates-Based Ionohydrogels toward Flexible Electronics, Adv. Mater., 2024, 36, e2400099 CrossRef PubMed.
- K. Jagaran and M. Singh, Nanomedicine for Neurodegenerative Disorders: Focus on Alzheimer's and Parkinson's Diseases, Int. J. Mol. Sci., 2021, 22, 9082 CrossRef CAS PubMed.
- P. Hivare, K. Mujmer, G. Swarup, S. Gupta and D. Bhatia, Endocytic pathways of pathogenic protein aggregates in neurodegenerative diseases, Traffic, 2023, 24, 434–452 CrossRef CAS PubMed.
- H. Yu and J. Wu, Amyloid-beta: A double agent in Alzheimer's disease?, Biomed. Pharmacother., 2021, 139, 111575 CrossRef CAS PubMed.
- D. Bhardwaj, C. Mitra, C. A. Narasimhulu, A. Riad, M. Doomra and S. Parthasarathy, Alzheimer's Disease-Current Status and Future Directions, J. Med. Food, 2017, 20, 1141–1151 CrossRef CAS PubMed.
- S. I. Liyanage and D. F. Weaver, Misfolded proteins as a therapeutic target in Alzheimer's disease, Adv. Protein Chem. Struct. Biol., 2019, 118, 371–411 Search PubMed.
Footnote |
† These authors contributed equally to this work. |
|
This journal is © the Partner Organisations 2024 |
Click here to see how this site uses Cookies. View our privacy policy here.