DOI:
10.1039/D4QI01670J
(Research Article)
Inorg. Chem. Front., 2024,
11, 7426-7436
Engineered iron-doped MOF nanosheets: acid-induced lattice strain for enhanced rate performance in asymmetric supercapacitors†
Received
2nd July 2024
, Accepted 18th September 2024
First published on 20th September 2024
Abstract
The development of electrode materials is the key to realizing efficient energy storage. In order to solve the problems of low conductivity and poor cycling stability of existing metal–organic frameworks (MOFs), element doping and chemical etching strategies are effective strategies. In this work, we propose a strategy to modify the surface of MOFs via Fe doping and HCl etching strategies. The Jahn–Teller effect was induced and the electronic configuration of Co was optimized by doping Fe3+ ions with [Fe(CN)6]3−. In addition, HCl etching induces lattice strain, enhances the interaction between Fe and Co, and provides a fast charge transfer rate. This synergistic effect enhances the conductivity of the Co MOF, introduces more electrochemically active sites, and further accelerates the electrochemical reaction kinetics. In particular, the specific capacity of e-Fe-MOF CNs-30 at 1 A g−1 is as high as 1431 C g−1, and the capacity retention rate is 84.2%. Additionally, an e-Fe-MOF CNs-30//AC asymmetric supercapacitor was assembled, which has a high energy density of 83.75 W h kg−1 and a superior cycling stability of about 91.66% after 5000 cycles. The structural design of these MOFs significantly improves the low energy density and cycle life of MOF-based supercapacitors and provides insights into the electronic structure regulation and lattice strain engineering of low conductivity MOF electrodes.
Introduction
With the advancement of science and technology, the energy crisis has become a global challenge, and the extensive combustion of fossil fuels has significantly impacted the environment. Consequently, the development of renewable and clean energy technologies has become crucial.1,2 Nonetheless, the utilization of these energy sources is constrained by factors such as climate and geographical location.3 To overcome these limitations, the research and development of energy storage technologies, particularly supercapacitors (SCs), are of paramount importance. SCs combine the high energy storage capacity of batteries with the rapid charge–discharge capabilities of capacitors and have been applied across various domains,4,5 such as portable electronic devices, hybrid vehicles, smart grids, and other fields.6,7 Nonetheless, the relatively low energy density of SCs poses a constraint on their more extensive use. To advance the development of SCs, the key lies in designing efficient electrode materials to enhance their energy density.
Metal–organic frameworks (MOFs) are a type of crystal composed of metal ions and organic ligands connected through coordination bonds, characterized by high specific surface area, high porosity, and controllable topological morphology.8,9 These characteristics enable their widespread use in electrochemical energy storage.10–12 The limited conductivity and stability of most MOFs impede their use in SCs. Currently, there are many strategies to improve or compensate for these inherent defects of MOF materials. For example, Bai et al.13 prepared a high-capacity Co3O4/g-C3N4 p–n junction with enhanced conductivity and charge transfer by simulating solar irradiation. The Co3O4 phase undergoes lattice distortion after illumination, and the high single-ion magnetic anisotropy and low self-selection configuration of Co3+ ions enhance the covalent component for the Co–O σ bond. The covalent configuration of the bond further increases the number of reactive centers, which is beneficial for greatly improving the conductivity and charge transfer efficiency of the material. Meanwhile, Yao et al.14 obtained a NiMn-MOF@CoOOH composite electrode. The introduction of the NiMn-MOF resulted in multiple lattice strains on the surface of CoOOH, accelerating ion conduction and introducing additional electrochemically active sites. Thus, NiMn-MOF@CoOOH reaches an excellent specific capacity of 1771 F g−1 at 1 A g−1. It is worth noting that both lattice strain and doping engineering are effective methods for designing high-performance electrode materials, but they always exist in a single form. For instance, Zhou et al.15 constructed a porous and defect-rich FexBi2−xS3 solid solution structure using Fe-doped Bi2O2CO3 porous nanosheets, and the resulting composite phase exhibited significantly higher energy storage performance than its single phase. This can be attributed to Fe solutes being randomly dispersed in the Bi2S3 framework, which can effectively regulate the electronic structure of Bi elements and introduce rich defects due to the presence of Fe(II). These results provide a scientific basis for designing ideal performance MOF materials using synergistic effects.
In this work, we propose an innovative strategy to address the challenges of MOF materials in terms of conductivity and cycling stability: (1) the synergistic effect of Fe doping and HCl etching is utilized to “double enhance” the MOF conductivity. On the one hand, the [Fe(CN)6]3− coordinate introduced by Fe doping triggers the Jahn–Teller (J–T) effect, which induces the formation of empty orbitals by Co3+ ions, generating the free motion of additional electrons to enhance the MOF electrical conductivity. On the other hand, the negative effect of the J–T effect on the MOF nanosheet structure is mitigated and synergized by using the lattice strain generated by HCl etching. The lattice strain not only stabilizes the MOF nanosheet structure, but also changes the electron density around Co to enhance the MOF conductivity together with the J–T effect. (2) The lattice strain is utilized to modulate the MOF nanostructure, thus extending the cycle life of the MOF. The lattice strain generates compressive stresses that cause the MOF nanosheets to curl. This structure can significantly increase the specific surface area of the MOF, expose more active sites to promote electrochemical reactions, and alleviate the surface volume expansion caused by long-term cycling, thus prolonging the cycle life of MOF materials. The e-Fe-MOF CNs-30 prepared based on this strategy exhibited a specific capacity of up to 1431 C g−1 at 1 A g−1. The constructed asymmetric supercapacitor (ASC) achieved a maximum energy density of 83.75 W h kg−1 at a power density of 1600 W kg−1 and maintained a capacitance retention of 91.66% after 5000 cycles, which is a performance superior to those of most of the currently reported electrode materials. This work not only provides a new idea for the design of high energy density supercapacitors based on MOF-based materials, but also provides potential and application of lattice strain engineering in materials science.
Results and discussion
Morphology and structure
The MOF-derived e-Fe-MOF CNs materials were synthesized by the “coprecipitation–ion–exchange–HCl etching” method, as shown in Fig. 1. In comparison with the traditional synthesis methods, such as hydrothermal/solvent heating, microwave-assisted, ultrasound-assisted, mechanochemical, and electrochemical methods,16 this room-temperature synthesis method can drastically reduce the operation difficulty and minimize the energy loss. Firstly, Co MOF nanosheets were synthesized by coordinating Co2+ ions with 2-methylimidazole (2-MIM) ligands by the coprecipitation method at room temperature. Then, [Fe(CN)6]3− ions undergo an anion exchange reaction in the Co MOF, introducing an Fe-doped Co MOF. The electronic structure of Co was regulated by doping transition metal Fe ions with a radius similar to that of the Co ions. The e-Fe-MOF CNs were obtained by immersing in HCl solution at room temperature and adjusting the etching time at different intervals. The lattice strain engineering in etching was mainly reflected in two aspects: (1) the strong interaction between Fe and Co is generated after etching, and the rapid charge transfer further optimizes the electronic structure of Co; (2) the etching suppresses the negative impact of the J–T effect in doping and maintains the stability of the two-dimensional (2D) structure of the MOF, and the three-dimensional (3D) structure transformation is realized. During this process, the apparent properties of the NF substrate are changed. As shown in Fig. S1,† the initial Co MOF grown on NF appears bright purple. After Fe doping, the color of NF changes to dark green. The retention of this dark green color is dependent on the HCl etching time, with a longer etching time resulting in a lighter shade of dark green.
 |
| Fig. 1 Schematic diagram of the preparation of e-Fe-MOF CNs. | |
The scanning electron microscopy (SEM) images in Fig. 2a–c and S2† show that samples with different etching times preserve the triangular nanosheet morphology of their precursors well. In particular, the triangular nanosheets of e-Fe-MOF CNs had undergone varying degrees of curling. The magnified images of e-Fe-MOF CNs-15, e-Fe-MOF CNs-30 and e-Fe-MOF CNs-60 confirmed this point well (insets of Fig. 2a–c). This indicates that HCl etching causes lattice strain, resulting in compressive stress acting from the edge to the center of the triangular nanosheets, resulting in this unique curly structure.17 Compared to 2D MOFs, this 3D structure significantly increases the specific surface area and exposes a greater number of active sites on the surface for redox reactions, further promoting the occurrence of electrochemical reactions.18
 |
| Fig. 2 Structural characterization of e-Fe-MOF CNs-15, e-Fe-MOF CNs-30, and e-Fe-MOF CNs-60. (a–c) SEM images (a1, b1, c1: IFFT image, a2, b2, c2: FFT image, and a3, b3, c3: intensity profiles of the (211) crystal plane). (d) XRD pattern. (e) Elemental mapping images. (f) EDS spectrum of e-Fe-MOF CNs-30. | |
The structure of the etched samples was studied in more detail through a series of characterization processes. Transmission electron microscopy (TEM) images reveal that e-Fe-MOF CNs exhibit curling in random areas of the triangular nanosheets (Fig. S3a, b and d†). Furthermore, it is observed that as the etching time increases, the curling becomes more pronounced, which is in agreement with the SEM results. Moreover, the lattice fringes with crystal plane spacing of 0.473 nm, 0.482 nm, and 0.492 nm can be observed from high-resolution transmission electron microscopy (HRTEM) images (Fig. S3c†), which can be attributed to the (211) crystal plane of the Co MOF. It is worth noting that the standard spacing of the (211) crystal plane is 0.496 nm, and the sample plane spacing observed after 15, 30 and 60 min of HCl etching is smaller than the standard spacing. This implies the occurrence of lattice contraction, confirming the generation of lattice strain. Then, the selected area electron diffraction (SAED) pattern further reveals a low crystallinity of e-Fe-MOF CNs-30 (inset of Fig. S3c†). The X-ray diffraction (XRD) pattern shows the crystal structure of the sample before and after etching (Fig. 2d). After successfully creating the Co MOF precursor (Fig. S4†), the etched samples displayed diffraction peaks that matched the (100) and (111) planes of ZIF-67 (JCPDs PDF#43-0144) at 7.25° and 12.58° incidence angles.19 The absence of iron-related diffraction peaks in the Fe-doped Co MOF sample could be attributed to its minimal iron content.20 In addition, the diffraction peak intensities in some planes of the samples at different etching times showed a nonlinear relationship with the etching time. The prolongation of etching time may increase the etching depth of the sample surface, but it may also be accompanied by irregular changes in the grain structure and arrangement in the sample, which affect the crystallinity of certain grain surfaces in the sample, and make the corresponding changes in the diffraction peak intensities show an irregular trend.21,22 Notably, the characteristic reflections of e-Fe-MOF CNs-15, e-Fe-MOF CNs-30 and e-Fe-MOF CNs-60 at 17.86° on the (211) crystal plane increased to 18.84°, 18.42° and 18.22°, respectively. The lattice contraction rates were 4.8%, 2.7%, and 0.7%, which further prove the generation of lattice strain.23 Additionally, the elements Co, Fe, C, N, and O are evenly dispersed throughout the entire area of the curled nanosheet (Fig. 2e and S5†). Among them, the Fe content in e-Fe-MOF CNs-30 is 4.89%, which proves the successful doping of the Fe element (Fig. 2f).
To illustrate the composition and internal functional group changes of the etching samples, we obtained the Fourier-transform IR (FTIR) spectra, as shown in Fig. 3a and S6a.† The Raman spectra in Fig. 3b and S6b† reveal two significant peaks at 2105 cm−1 and 2150 cm−1, corresponding to the CN vibration in Fe3+/2+-CN-Co3+/2+ of the etching samples. These peaks, which are characteristic of the CN groups in K3[Fe(CN)6] at 2085 cm−1, shifted to 2100 cm−1 and 2139 cm−1 due to the influence of compressive stress.24–26 Besides, a splitting peak appears at 2083 cm−1, which is responsible for the introduction of hydrogen bonds by HCl etching, caused by hydrogen bond vibration. The UV-Vis spectra in Fig. S6c† for the Co MOF, Fe-doped Co MOF, and e-Fe-MOF CNs-30 indicate that the characteristic absorption peaks for the Co MOF are at 541 and 589 nm. The introduction of Fe and HCl etching do not alter these precursor peaks. Additionally, the specific surface area is crucial for the electrochemical performance of materials. After doping and etching, the thicknesses of multiple nanosheets in the samples were estimated, and their average thicknesses were reduced from ∼200 nm to ∼2 nm, which resulted in a size effect and improved the surface area to volume ratio of the MOF. Consequently, the specific surface area for e-Fe-MOF CNs-30 rose to 678 m2 g−1, a significant jump from the initial 71 m2 g−1 of the Co MOF. In addition, the pore structure of the MOF was significantly changed. Abundant mesopores and macropores mainly existed in the pristine Co MOF, and the pore distribution was mainly concentrated in 13.04 nm mesopores and 115.36 nm macropores. However, the modified e-Fe-MOF CNs-30 exhibits a more complex porous structure, which contains three types of pores, namely, micropores, mesopores, and macropores, with 1.4 nm micropores and 3.8 nm mesopores showing the main distribution (Fig. 3c and S7†). In this porous structure, micropores can provide sufficient active sites for ion storage, mesopores act as channels for ion migration and transport, and macropores provide the necessary space for ion buffering. This variety of porous structure gives e-Fe-MOF CNs-30 an excellent specific capacity.
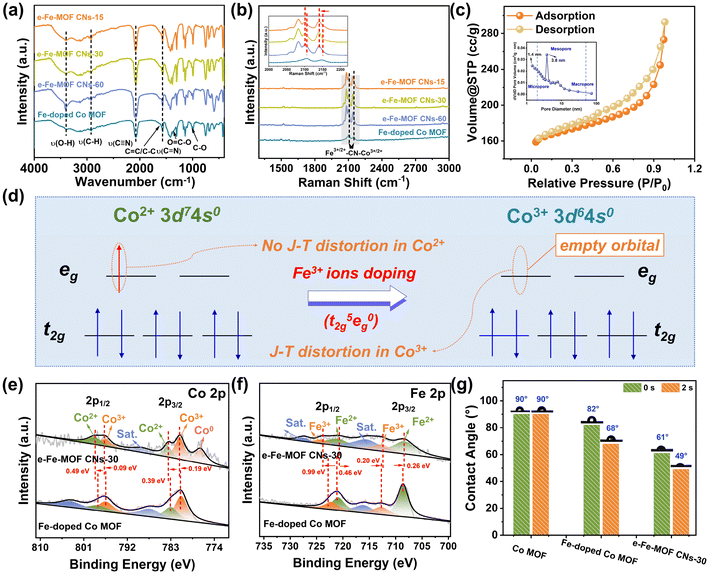 |
| Fig. 3 Characterization data of the Co MOF, Fe-doped Co MOF, e-Fe-MOF CNs-15, e-Fe-MOF CNs-30, and e-Fe-MOF CNs-60. (a) FTIR spectra. (b) Raman spectra. (c) N2 adsorption–desorption isotherm curves of e-Fe-MOF CNs-30. (d) Possible crystal field study before and after Fe3+ doping in Co MOF. XPS spectra of (e) Co 2p and (f) Fe 2p. (g) Static contact angle. | |
To further investigate the chemical valence state changes of the materials after Fe doping and HCl etching, X-ray photoelectron spectroscopy (XPS) measurements were examined. As shown in Fig. S8a,† the binding energy peak of Fe doping can be clearly observed. In addition, the full survey scan confirmed that Co, Fe, C, N and O still exist in e-Fe-MOF CNs-30 after further etching (Fig. S8b†). As seen in Fig. S9,† the Co MOF doped with Fe3+ ions in K3[Fe(CN)6] does not cause any changes in the positions of Co2+ and Co3+ ions binding energies, but the peak binding energy of Co3+ ions increased significantly after doping. This indicates that more Co2+ ions are converted into Co3+ ions. At this point, the 3d orbital electrons of Co2+ and Co3+ are 3d7 and 3d6, respectively, with corresponding electron configurations of t2g6eg1 and t2g6eg0. This can be attributed to the 3d orbital electron number of the central ion [Fe(CN)6]3− in the complex of 3d5 with an electron configuration of t2g5eg0.27 The electron distribution in the ion is asymmetric, causing J–T distortion. Due to the J–T effect, the eg orbital of Co3+ generates an empty orbital, which promotes the formation of free electrons in the original eg orbital of Co3+, thereby enhancing the conductivity (Fig. 3d).28 This may also be the reason for the slight change in the microstructure of the Co MOF after doping (Fig. S10†).29
Meanwhile, the characteristic peaks at 783.4 eV and 798.6 eV corresponding to Co(II) 2p3/2 and Co(II) 2p1/2 and 781 eV and 796.4 eV corresponding to Co(III) 2p3/2 and Co(III) 2p1/2 can be observed in the Co 2p spectrum of e-Fe-MOF CNs-30. The characteristic peaks located at 788.9 eV and 776.8 eV were satellite peaks of Co 2p and Co(0). It may be due to the reducibility of HCl, which resulted in some Co2+/Co3+ ions being reduced to Co0 ions. Moreover, the etched material exhibits a shift towards higher binding energy, indicating a decrease in electron density near Co2+/Co3+ ions (Fig. 3e). As shown in Fig. 3f, the characteristic peaks at 708.3 eV and 720.9 eV in the Fe 2p spectrum correspond to the satellite peaks of Fe(III) 2p3/2 and Fe(II) 2p1/2 at 712.5 eV and 723.9 eV, respectively. Among them, most of the characteristic peaks in the Fe 2p spectrum of e-Fe-MOF CNs-30 shift towards lower binding energies. The phenomenon indicates an increase in electron density near Fe2+/Fe3+ ions. The above results confirm that lattice strain leads to electron transfer from Co to Fe, and the strong interaction between Fe and Co enhances electron mobility and further enhances conductivity.30,31 The lattice strain seems to effectively suppress the negative effects of the J–T effect. Therefore, after Fe doping caused a structural change in the Co MOF, HCl etching also maintained the nanosheet structure of the Co MOF. The fitted peaks of O 1s at 531.04, 531.74, and 532.74 eV can be attributed to the characteristic peaks of M–O, O–H, and absorbed O. Moreover, the peaks observed at 528.8 eV in the Fe-doped Co MOF are likely due to the presence of lattice O (OL) (Fig. S11†).32 With the creation of an acidic environment after etching, the OL disappears in e-Fe-MOF CNs-30.33 The corresponding C 1s, N 1s and O 1s of the Co MOF, Fe-doped Co MOF, and e-Fe-MOF CNs-30 are shown in Fig. S12 and S13.†
In order to analyze the hydrophilicity of the as-prepared materials, static contact angle tests were conducted (Fig. 3g). The initial contact angles of the Co MOF, Fe-doped Co MOF and e-Fe-MOF CNs-30 were 90°, 82°, and 61°, respectively. After 2 s, the contact angles of the Fe-doped Co MOF and e-Fe-MOF CNs-30 decreased to 68° and 49°, respectively, while the contact angle of the Co MOF remained unchanged. All these results imply that e-Fe-MOF CNs-30 has the best hydrophilicity. Due to the polarity of HCl molecules, rapid ion diffusion within the electrode is guaranteed, making it easier to come in contact with the active sites.34
The electrochemical performances of the prepared electrodes were tested in a three-electrode system to explore their electrochemical behavior. As shown in Fig. 4a, all of these CV curves exhibit two pairs of redox peaks, indicating that the electrode undergoes two reversible redox reactions during energy storage. Among them, the electrode etched for 15 min is conspicuous by the presence of oxidation peaks due to its similar current density of oxidation peaks in both reactions. The redox peaks at lower and higher potentials (O1/R1 and O2/R2) correspond to the redox reactions of Fe2+/Fe3+ ions and Co2+/Co3+ ions, respectively. However, with the increase of etching time, the electrode morphology changed slightly, resulting in small shifts in their oxidation and reduction potentials, respectively.35,36 In addition, the enhancement of the redox peak current densities for Fe and Co can be attributed to the increased exposure of a specific crystalline plane in the electrodes and the expansion of the electrochemically active surface area.37,38 Meanwhile, the e-Fe-MOF CNs-30 electrode has a larger CV integrated area compared to e-Fe-MOF CNs-15 and e-Fe-MOF CNs-60 at 2 mV s−1, which means that the active substance in the e-Fe-MOF CNs-30 electrode is maximally oxidized or reduced, and the amount of charge generated during the reaction is maximized to obtain the largest specific capacity. Then, we also conducted the CV tests of the Co MOF and Fe-doped Co MOF, and the results were as expected; the CV curve area of the e-Fe-MOF CNs-30 electrode is significantly larger than those of the other two electrodes (Fig. S14†). As the scan rate increases, there is no significant distortion in the shape of the CV curves of all the electrodes, indicating a faster transport rate of ions and protons (Fig. S15 and S16†). Furthermore, we evaluated the specific capacity values of all electrodes through GCD tests. In Fig. 4b, a clear discharge plateau is observed in the GCD curves of all electrodes, indicating their battery-type behaviors.39 The e-Fe-MOF CNs-30 electrode has the longest discharge time at 1 A g−1 compared to the other electrodes, which is consistent with the results of the CV curve analysis. Based on the discharge time (Fig. 4c), the specific capacities of the e-Fe-MOF CNs-15, e-Fe-MOF CNs-30 and e-Fe-MOF CNs-60 electrodes were 940 C g−1, 1431 C g−1, and 1048 C g−1, respectively.
 |
| Fig. 4 Comparison of the electrochemical performances of e-Fe-MOF CNs-15, e-Fe-MOF CNs-30, and e-Fe-MOF CNs-60. (a) CV curves at 2 mV s−1. (b) GCD curves at 1 A g−1. (c) Specific capacity at 1 A g−1. (d) Nyquist plots (inset: magnified high-frequency region and circuit diagram). The e-Fe-MOF CNs-30 electrode: (e) cycling stability after 5000 charge–discharge cycles, (f) linear relationships between log(i) and log(v), (g) capacitive contribution at 10 mV s−1, and (h) contribution ratios at various scan rates. | |
The charge transfer ability of electrodes was further analyzed by electrochemical impedance spectroscopy (EIS), as shown in Fig. 4d. e-Fe-MOF CNs-30 has the smallest charge transfer resistance (Rct) value of 5.743 Ω. This suggests that properly curled MOF nanosheets provide the maximum number of ion/electron transport channels. The detailed values are shown in Table S1.† Subsequently, the cycling stability of three electrodes with different etching times was tested. The capacitance retention of the e-Fe-MOF CNs-30 electrode was 84.2% at a current density of 50 A g−1, and also achieved a high coulombic efficiency of 97.95% (Fig. 4e). The coulombic efficiency curves showed small fluctuations due to the trace amount of HCl that may still remain inside the e-Fe-MOF CNs-30 electrode. During a long charge/discharge cycle test in an alkaline KOH electrolyte, KOH and HCl will undergo a neutralization reaction with the equation KOH + HCl → KCl + H2O. This reaction will continuously deplete the active ions in the electrolyte. During discharge, if there are insufficient active ions in the electrolyte, the electrode may not be able to fully release the stored charge, resulting in fluctuations in coulombic efficiency.40,41 Meanwhile, the capacitance retention rates of the other two electrodes of e-Fe-MOF CNs-15 and e-Fe-MOF CNs-60 were 77.77% and 84.2%, respectively, at the same current density (Fig. S17†). The specific capacity of e-Fe-MOF CNs-30 exceeds those of most MOF-based electrode materials reported in alkaline electrolytes (Table S2†). Furthermore, we investigated the morphology of the e-Fe-MOF CNs-30 electrode after the cycling stability test. As shown in Fig. S18,† the curled nanosheet structure was well maintained after 5000 charge–discharge cycles. This configuration offers ample room to accommodate volume fluctuations during charging and discharging, thereby preserving the stability of the electrode structure.
The energy storage mechanism of electrodes is crucial, so the CV curves of all samples are analyzed using the relationship between the current (i) and scan rate (v), and the equations are as follows:42
where
i and
v are the cathodic/anodic peak current (A) and scan rate (mV s
−1), respectively. In the case of small changes in scan rates,
a and
b can be considered as constants. The range of
b values is usually between 0.5 and 1. When the
b value is close to 0.5, the charge storage behavior is controlled by the diffusion-controlled process. If the
b value is close to 1, capacitive controlled behavior dominates.
43 For e-Fe-MOF CNs-30, the
b values at the anodic and cathodic peaks are 0.5 and 0.41, respectively (
Fig. 4f), indicating a diffusion-controlled charge storage mechanism in electrodes. Subsequently, the capacitive contribution ratio is calculated using the following equation:
44where
i is the current under a constant potential (V) and
k1 and
k2 are adjustable parameters. The purple area indicates a diffusion-controlled proportion of 94% at 10 mV s
−1 (
Fig. 4g). With the increase of the scan rate, the proportion of capacitance control (depicted in pink) steadily rises, culminating at 22% at a scan rate of 20 mV s
−1. This suggests that the energy storage mechanism in e-Fe-MOF CNs-30 is predominantly governed by the diffusion-controlled process, as illustrated in
Fig. 4h. The capacitive contributions for the other electrodes are detailed in Fig. S19,
† demonstrating similar dynamic behavior.
Electrochemical performances of the assembled ASC
The feasibility of e-Fe-MOF CNs-30 electrodes for practical applications was further evaluated by assembling an ASC. The ASC was assembled using e-Fe-MOF CNs-30, activated carbon (AC), and cellulose as the positive electrode, negative electrode, and separator, respectively (Fig. 5a). Firstly, we tested the electrochemical properties of the AC negative electrode in a 1 M KOH electrolyte (Fig. S20†). The CV curves of the AC electrode display a shape close to a rectangle within the −1–0 V potential window, signifying double layer characteristics. According to the discharge time in the GCD curves, the specific capacitance of the AC electrode is calculated to be 204 F g−1 at 1 A g−1. Secondly, we calculated the mass of the AC negative electrode according to the eqn (S2)† and tested the electrochemical properties of the assembled ASC. The potential windows of e-Fe-MOF CNs-30 and AC electrodes are 0–0.5 V and −1–0 V at 10 mV s−1, respectively (Fig. 5b). Then, the CV curves at various voltages were tested at a scan rate of 20 mV s−1 (Fig. 5c). As the voltage window increases from 0–1.6 V to 0–1.8 V, an obvious polarization phenomenon occurs. Therefore, the working voltage window of the ASC is set to 0–1.6 V. Moreover, there is no significant distortion in the curve at different scan rates (Fig. 5d), which indicates that the ASC has good rate capability. According to the GCD curves at different current densities (Fig. 5e), the maximum specific capacitance of the ASC can reach 235.5 F g−1 at 2 A g−1 and maintain 126.25 F g−1 at 20 A g−1 (Fig. 5f).
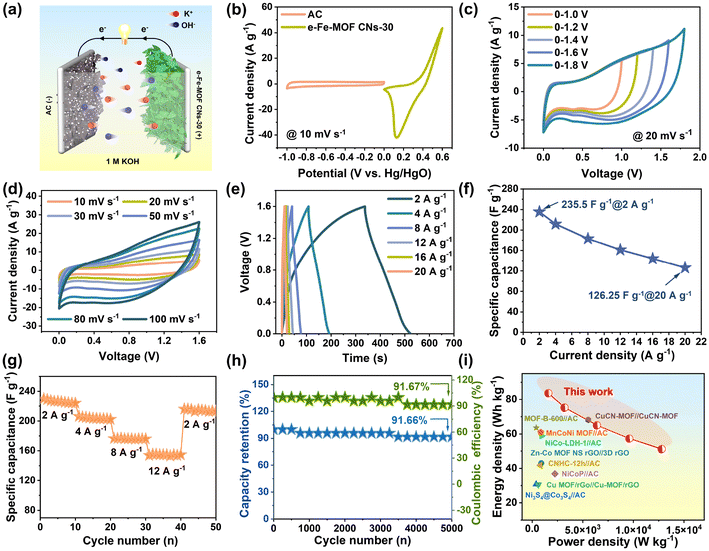 |
| Fig. 5 Electrochemical performance of e-Fe-MOF CNs-30//AC ASC. (a) The composition of the ASC device. (b) CV curves were collected for e-Fe-MOF CNs-30 and AC at 10 mV s−1. (c) CV curves at different voltages at 20 mV s−1. (d) CV curves at various scan rates. (e) GCD curves at various current densities. (f) The specific capacitance at different current densities. (g) Rate capability. (h) Cycling stability after 5000 charge–discharge cycles. (i) Ragone plots. | |
Furthermore, the rate performance was a significant parameter for the ASC. We also tested the ASC under a series of current densities in 50 cycles (Fig. 5g). After the current density increases from 2 to 12 A g−1 and then returns to 2 A g−1, the specific capacitance has no significant decrease and exhibits excellent electrochemical reversibility. After 5000 cycles, the capacitance retention is 91.66% of the initial specific capacitance. Besides, the coulombic efficiency remains at 91.67% after the cycling process (Fig. 5h). Due to the repeated shuttle of K+ or OH− in the electrolyte between the positive and negative electrodes during a long-term charge–discharge cycle at high current density, the electrode volume will inevitably expand, which will greatly shorten the cycle life of the device. Thus, the 3D curled NS structure of e-Fe-MOF CNs-30 can effectively alleviate volume expansion and greatly extend the cycling life of the device. Finally, the energy and power density of the e-Fe-MOF CNs-30//AC ASC were determined at different densities. The ASC device achieves a peak energy density of 83.75 W h kg−1 at a power density of 1600 W kg−1. Furthermore, it sustains an energy density of 44.88 W h kg−1 at an elevated power density of 16 kW kg−1, outperforming many previously reported devices (Fig. 5i and Table S3†).45–56
Considering the practical application of the SCs as an energy storage power source, we connected the device in series and parallel to observe its power supply to microelectronic devices. Fig. 6a and b show the CV and GCD curves of the series and parallel devices. The working voltage window of a series device is twice that of a single device, while the discharge time of a parallel device is twice that of a single device. The two devices were connected in series to drive a pink light emitting diode (LED) successfully. Meanwhile, the electronic watch with a working voltage of 1.5 V can also work normally (Fig. 6c and d). This further substantiates the viability of e-Fe-MOF CNs-30 as a positive electrode material for SCs in practical applications.
 |
| Fig. 6 The practical applications of the ASC device. (a and b) CV and GCD curves of a single device and two devices connected in series and parallel at 20 mV s−1 and at 4 A g−1. (c) Optical image of two devices connected in series driving an LED. (d) Optical images of a single device driving an electronic watch in 10 min. | |
Conclusions
In conclusion, we have successfully improved the inherent defects of Co MOFs by Fe doping and HCl etching strategies. On the one hand, HCl etching realizes the transformation of the MOF from a 2D to 3D structure by regulating and triggering the lattice strain of the MOF nanostructure, which provides a large specific surface area and increases the number of surface accessible active sites. The curled nanosheet structure mitigated the volume expansion of the MOF during the cycling process and prolonged the cycle life. In particular, the MOF structure changes upon Fe doping and is restored upon HCl etching. The lattice strain plays a dual role in regulating and stabilizing the structure. On the other hand, Fe doping in the MOF optimizes the electronic structure of Co, generating the J–T effect. This effect induces empty orbitals after the conversion of Co2+ ions to Co3+ ions, and the electrons in the original orbitals become free electrons, ultimately improving the electrical conductivity. The lattice strain induced by HCl etching facilitates charge transfer between Fe and Co, further promoting the enhancement of electrical conductivity. In both aspects, Fe doping and HCl etching show a synergistic effect. Therefore, the specific capacity of e-Fe-MOF CNs-30 reaches up to 1431 C g−1 at 1 A g−1. It also has excellent cycling stability, maintaining 84.2% of its original capacitance after 5000 cycles. In addition, the assembled e-Fe-MOF CNs-30//AC ASC can reach a maximum energy density and power density of 83.75 W h kg−1 and 16 kW kg−1, respectively. This work provides not only a valuable scientific basis for modified MOF materials, but also a precious reference value for their application in future energy storage and conversion technologies.
Data availability
The data supporting this article have been included as part of the ESI.†
Conflicts of interest
There are no conflicts to declare.
Acknowledgements
This work is supported by the National Natural Science Foundation of China (NSFC, No. 52402100), the China Postdoctoral Science Foundation (No. 2023M740552), and the Heilongjiang Postdoctoral Foundation (No. LBH-Z23067). Y. Z. acknowledges the technical support from the Analysis and Testing Center of NEFU.
References
- B. Chettiannan, E. Dhandapani, G. Arumugam, R. Rajendran and M. Selvaraj, Metal-organic frameworks: a comprehensive review on common approaches to enhance the energy storage capacity in supercapacitor, Coord. Chem. Rev., 2024, 518, 216048 CrossRef CAS.
- C. Han, Y. Zhao, Y. Yuan, Z. Guo, G. Chen, J. Yang, Q. Bao, L. Guo and C. Chen, Transition metal-based layered double hydroxides and their derivatives for efficient oxygen evolution reaction, Int. J. Hydrogen Energy, 2024, 63, 918–936 CrossRef CAS.
- W. Elvis and P. Meukam, Impact of the climate change on the site suitability for solar farms: Case study of Cameroon, Renewable Energy, 2024, 225, 120310 CrossRef.
- Y. Yuan, C. Han, L. Guo, X. Wu and Y. Zhao, Exploring the mechanism of magnetic field in supercapacitors: material classification, material nanostructures, and electrochemical properties, J. Mater. Chem. A, 2024, 12, 6165–6189 RSC.
- W. Zhao, Y. Zeng, Y. Zhao and X. Wu, Recent advances in metal-organic framework-based electrode materials for supercapacitors: A review, J. Energy Storage, 2023, 62, 106934 CrossRef.
- M. Xu, X. Zhu, Y. Lai, A. Xia, Y. Huang, X. Zhu and Q. Liao, Production of hierarchical porous bio-carbon based on deep eutectic solvent fractionated lignin nanoparticles for high-performance supercapacitor, Appl. Energy, 2024, 353, 122095 CrossRef CAS.
- Y. Zhao, X. He, R. Chen, Q. Liu, J. Liu, J. Yu, J. Li, H. Zhang, H. Dong, M. Zhang and J. Wang, A flexible all-solid-state asymmetric supercapacitors based on hierarchical carbon cloth@CoMoO4@NiCo layered double hydroxide core-shell heterostructures, Chem. Eng. J., 2018, 352, 29–38 CrossRef CAS.
- Y. Xie, X. Wu, Y. Shi, Y. Peng, H. Zhou, X. Wu, J. Ma, J. Jin, Y. Pi and H. Pang, Recent Progress in 2D Metal–Organic Framework–Related Materials, Small, 2024, 20, 2305548 CrossRef CAS PubMed.
- Y. Yuan, C. Han, Y. Fu, Z. Ye, Q. Shen, W. Feng and Y. Zhao, Structural energy storage composites based on etching engineering Fe-doped Co MOF electrode toward high energy density supercapacitors, J. Power Sources, 2024, 609, 234688 CrossRef CAS.
- M. Saleem, F. Ahmad, M. Fatima, A. Shahzad, M. S. Javed, S. Atiq, M. A. Khan, M. Danish, O. Munir and S. M. B. Arif, Exploring new frontiers in supercapacitor electrodes through MOF advancements, J. Energy Storage, 2024, 76, 109822 CrossRef.
- R. Balamurugan and A. C. Bose, Surface-Sulfurized Zn-MOF Grown on Ni-Foam with Various Sulfurizing Agents for Aqueous Hybrid Supercapacitor Device Fabrication, ACS Appl. Energy Mater., 2024, 7, 974–985 CrossRef CAS.
- R. Razaq, M. M. U. Din, D. R. Småbråten, V. Eyupoglu, S. Janakiram, T. O. Sunde, N. Allahgoli, D. Rettenwander and L. Deng, Synergistic Effect of Bimetallic MOF Modified Separator for Long Cycle Life Lithium–Sulfur Batteries, Adv. Energy Mater., 2024, 14, 2302897 CrossRef CAS.
- L. Bai, H. Huang, S. Zhang, L. Hao, Z. Zhang, H. Li, L. Sun, L. Guo, H. Huang and Y. Zhang, Photocatalysis–Assisted Co3O4/g–C3N4 p-n Junction All–Solid–State Supercapacitors: A Bridge between Energy Storage and Photocatalysis, Adv. Sci., 2020, 7, 2001939 CrossRef CAS PubMed.
- S. Yao, Y. Jiao, C. Lv, Y. Kong, S. Ramakrishna and G. Chen, Lattice-strain engineering of CoOOH induced by NiMn-MOF for high-efficiency supercapacitor
and water oxidation electrocatalysis, J. Colloid Interface Sci., 2022, 623, 1111–1121 CrossRef CAS.
- F. Zhou, X. Wang, R. Jing, X. Li, Q. Zhang, Z. Li, Y. Du, Z. Xiao and L. Wang, Hierarchical FexBi2-xS3 solid solutions for boosted supercapacitor performance, Nano Res., 2023, 17, 3997–4005 CrossRef.
- Q. Zhang, S. Yan, X. Yan and Y. Lv, Recent advances in metal-organic frameworks: Synthesis, application and toxicity, Sci. Total Environ., 2023, 902, 165944 Search PubMed.
- Z. Li, Q. Wang, X. Bai, M. Wang, Z. Yang, Y. Du, G. E. Sterbinsky, D. Wu, Z. Yang, H. Tian, F. Pan, M. Gu, Y. Liu, Z. Feng and Y. Yang, Doping-modulated strain control of bifunctional electrocatalysis for rechargeable zinc-air batteries, Energy Environ. Sci., 2021, 14, 5035–5043 RSC.
- Z. Gong, X. Wang, W. Pi, N. Yao, Z. Fang, H. Bao and Q. Wu, Lattice contraction-driven design of highly efficient and stable O-NiFe layered double hydroxide electrocatalysts for water oxidation, Mater. Today Phys., 2024, 43, 101399 CrossRef CAS.
- N. Li, G. Qu, X. Zhang, S. Zhao, C. Wang, G. Zhao, P. Hou and X. Xu, Amorphous Ni-Co-S nanocages assembled with nanosheet arrays as cathode for high-performance zinc ion battery, Chin. Chem. Lett., 2022, 33, 3272–3276 CrossRef CAS.
- Z. Li, Z. Jiang, W. Zhu, C. He, P. Wang, X. Wang, T. Li and L. Tian, Facile preparation of CoSe2 nano-vesicle derived from ZIF-67 and their application for efficient water oxidation, Appl. Surf. Sci., 2020, 504, 144368 CrossRef CAS.
- R. Xin, M. Kim, P. Cheng, A. Ashok, S. Chowdhury, T. Park, A. Alowasheeir, M. S. Hossain, J. Tang, J. W. Yi, Y. Yamauchi, Y. V. Kaneti and J. Na, Enlarging the porosity of metal–organic framework-derived carbons for supercapacitor applications by a template-free ethylene glycol etching method, J. Mater. Chem. A, 2023, 11, 12759–12769 RSC.
- G. Zhang, T. Xiong, M. Yan, L. He, X. Liao, C. He, C. Yin, H. Zhang and L. Mai, α-MoO3- by plasma etching with improved capacity and stabilized structure for lithium storage, Nano Energy, 2018, 49, 555–563 CrossRef CAS.
- W. Cheng, X. Zhao, H. Su, F. Tang, W. Che, H. Zhang and Q. Liu, Lattice-strained metal-organic-framework arrays for bifunctional oxygen electrocatalysis, Nat. Energy, 2019, 4, 115–122 CrossRef CAS.
- H. Ding, S. Zhao, X. Wang, C. Qian, T. Zou, X. Li, H. Li, F. Jiang, Y. Liu, H. Cao, Z. Fang and Y. Zhu, Simple fabrication of (CoFe)Se2@NC electrode materials derived from MOF materials and the electrochemical properties for supercapacitor, Colloids Surf., A, 2023, 668, 131462 CrossRef CAS.
- X. Lu, H. Xu, T. Yang, X. Chen, Z. Cheng, Q. Hou, X. Lin, S. Liu, S. Wei and Z. Wang, Co3+-rich CoFe-PBA encapsulated in ultrathin MoS2 sheath as integrated core-shell architectures for highly efficient OER, J. Alloys Compd., 2023, 942, 169004 CrossRef CAS.
- H. Yang, J. Liu, Z. Chen, R. Wang, B. Fei, H. Liu, Y. Guo and R. Wu, Unconventional bi-vacancies activating inert Prussian blue analogues nanocubes for efficient hydrogen evolution, Chem. Eng. J., 2021, 420, 127671 CrossRef CAS.
- J. Luo, X. Wang, J. Zhang and Y. Zhou, Fe-doped Co3O4 anchored on hollow carbon nanocages for efficient electrocatalytic oxygen evolution, J. Fuel Chem. Technol., 2023, 51, 571–579 CrossRef CAS.
- H. N. Dhandapani, R. Madhu, A. De, M. A. Salem, B. Ramesh Babu and S. Kundu, Tuning the Surface Electronic Structure of Amorphous NiWO4 by Doping Fe as an Electrocatalyst for OER, Inorg. Chem., 2023, 62, 11817–11828 CrossRef PubMed.
- Z. Hou, J. Wang, N. Dai, S. Yao, S. Wang, Y. Ji, X. Gao, H. Zhang, Z. Tang and Y. Sun, Eliminating the Mn 3d Orbital Degeneracy to Suppress the Jahn-Teller Distortion for Stable MnO2 Cathode, Adv. Energy Mater., 2024, 14, 2302477 CrossRef CAS.
- J. Jiang, F. Sun, S. Zhou, W. Hu, H. Zhang, J. Dong, Z. Jiang, J. Zhao, J. Li and W. Yan, Atomic-level insight into super-efficient electrocatalytic oxygen evolution on iron and vanadium co-doped nickel (oxy) hydroxide, Nat. Commun., 2018, 9, 2885 CrossRef PubMed.
- Y. Yan, J. Lin, K. Huang, X. Zheng, L. Qiao, S. Liu, J. Cao, S. C. Jun, Y. Yamauchi and J. Qi, Tensile strain-mediated spinel ferrites enable superior oxygen evolution activity, J. Am. Chem. Soc., 2023, 145, 24218–24229 CrossRef CAS PubMed.
- P. Prasad, S. Kiran, M. Meenu and A. S. Prasad, Sucrose mediated synthesis and XPS analysis of nanocrystalline LaCrO3 perovskite oxide, Mater. Today: Proc., 2023, 80, 1209–1213 CAS.
- J. Xu, X. Zhang, S. Sun, R. Fu, F. Cheng, P. Wei, J. Luo, Q. Li, C. Fang and H. Lin, The ordered lattice host framework induced guest Li+ disordering in high performance cobalt-free Ni-rich cathode materials, J. Colloid Interface Sci., 2024, 669, 877–885 CrossRef CAS PubMed.
- D. Pawar, D. Malavekar, A. Lokhande and C. Lokhande, Facile synthesis of layered reduced graphene oxide/polyaniline (rGO/PANI) composite electrode for flexible asymmetric solid-state supercapacitor, J. Energy Storage, 2024, 79, 110154 CrossRef.
- L. Sun, L. Chai, L. Jing, Y. Chen, K. Zhuo and J. Wang, 2,6-Diaminoanthraquinone modified MXene (Ti3C2Tx)/graphene as the negative electrode materials for ionic liquid-based asymmetric supercapacitors, Green Energy Environ., 2024, 8, 004 Search PubMed.
- B. Tao, L. C. Yule, E. Daviddi, C. L. Bentley and P. R. Unwin, Correlative Electrochemical Microscopy of Li-Ion (de)intercalation at a Series of Individual LiMn2O4 Particles, Angew. Chem., Int. Ed., 2019, 58, 4606–4611 CrossRef CAS PubMed.
- Y. Wang, T. Zhou, K. Jiang, P. Da, Z. Peng, J. Tang, B. Kong, W. B. Cai, Z. Yang and G. Zheng, Reduced Mesoporous Co3O4 Nanowires as Efficient Water Oxidation Electrocatalysts and Supercapacitor Electrodes, Adv. Energy Mater., 2014, 4, 201400696 Search PubMed.
- C. Zhu, Y. Song, X. Dong, G. Li, A. Chen, W. Chen, G. Wu, S. Li, W. Wei and Y. Sun, Ampere-level CO2 reduction to multicarbon products over a copper gas penetration electrode, Energy Environ. Sci., 2022, 15, 5391–5404 RSC.
- M. Mottakin, V. Selvanathan, M. S. Su'ait, S. A. Razali, M. A. Islam, M. A. Ibrahim, G. Muhammad and M. Akhtaruzzaman, Enhancing pseudocapacitive energy storage system performance with electrodeposited CuSx and CoSx biphasic transitional metal sulfide (TMS) based nanostructured electrode on nickel foam, J. Phys. Chem. Solids, 2024, 186, 111795 CrossRef CAS.
- Y. Yang, C. Wu, X. X. He, J. Zhao, Z. Yang, L. Li, X. Wu, L. Li and S. L. Chou, Boosting the Development of Hard Carbon for Sodium-Ion Batteries: Strategies to Optimize the Initial Coulombic Efficiency, Adv. Funct. Mater., 2023, 34, 2302277 CrossRef.
- B. Wang, Z. Zhang, F. Yuan, D. Zhang, Q. Wang, W. Li, Z. Li, Y. A. Wu and W. Wang, An insight into the initial Coulombic efficiency of carbon-based anode materials for potassium-ion batteries, Chem. Eng. J., 2022, 428, 131093 CrossRef CAS.
- J. Choi, J. Lim, D. Kim, S. Park, B. Yan, D. Ko, Y. Cho, L. Y. S. Lee and Y. Piao, CoFe Prussian blue analogues on 3D porous N-doped carbon nanosheets boost the intercalation kinetics for a high-performance quasi-solid-state hybrid capacitor, J. Mater. Chem. A, 2022, 10, 14501–14512 RSC.
- R. Balamurugan and A. C. Bose, Surface-sulfurized Zn-MOF Grown on Ni-foam with Various Sulfurizing Agents for Aqueous Hybrid Super capacitor Device Fabrication, ACS Appl. Energy Mater., 2024, 7, 974–985 CrossRef CAS.
- Y. Liu, Q. Liu, X. Liu, C. Zhao, R. Su, X. Luo, Z. Liu and A. Ying, Russian-Doll-Like Porous Carbon as Anode Materials for High-Performance Potassium-Ion Hybrid Capacitors, Small, 2022, 19, 2206895 CrossRef PubMed.
- D. Huang, L. Chen, L. Yue, F. Yang, H. Guo and W. Yang, Nitrogen-doped carbon-enriched MOF and derived hierarchical carbons as electrode for excellent asymmetric aqueous supercapacitor, J. Alloys Compd., 2021, 867, 158764 CrossRef CAS.
- S. Kavian, S. Hajati and M. Moradi, High-rate supercapacitor based on NiCo-MOF-derived porous NiCoP for efficient energy storage, J. Mater. Sci.: Mater. Electron., 2021, 32, 13117–13128 CrossRef CAS.
- S. Krishnan, A. K. Gupta, M. K. Singh, N. Guha and D. K. Rai, Nitrogen-rich Cu-MOF decorated on reduced graphene oxide nanosheets for hybrid supercapacitor applications with enhanced cycling stability, Chem. Eng. J., 2022, 435, 135042 CrossRef CAS.
- S. Rajasekaran, S. D. K R, B. S. Reghunath, B. Saravanakumar, J. J. William and D. Pinheiro, Sm-MOF/rGO/PANI composite as an electrode material for supercapacitor applications, Electrochim. Acta, 2023, 467, 143031 CrossRef CAS.
- R. Sahoo, S. Ghosh, S. Chand, S. C. Pal, T. Kuila and M. C. Das, Highly scalable and pH stable 2D Ni-MOF-based composites for high performance supercapacitor, Composites, Part B, 2022, 245, 110174 CrossRef CAS.
- X. Liang, Y. Chen, Z. Jiao, M. Demir, M. Du and J. Han, MXene-transition metal sulfide composite electrodes for supercapacitors: Synthesis and electrochemical characterization, J. Energy Storage, 2024, 88, 111634 CrossRef.
- J. Wang, Y. Ma, X. Kang, H. Yang, B. Liu, S. Li, X. Zhang and F. Ran, A novel moss-like 3D Ni-MOF for high performance supercapacitor electrode material, J. Solid State Chem., 2022, 309, 122994 CrossRef CAS.
- W. Zhang, X. Guo, Y. Wang, Y. Zheng, J. Zhao, H. Xie, Z. Zhang and Y. Zhao, Self-Assembly of Ni-Doped Co-MOF Spherical Shell Electrode for a High-Performance Supercapacitor, Energy Fuels, 2022, 36, 1716–1725 CrossRef CAS.
- G. Liu, L. Liu, G. Li, S. Wu, J. He, Y. Zhou, M. Demir and P. Ma, Temperature–Dependent Electrochemical
Performance of Ta–Substituted SrCoO3 Perovskite for Supercapacitors, Chem. – Eur. J., 2024, 30, e202303267 CrossRef CAS PubMed.
- Y. Zhao, X. He, R. Chen, Q. Liu, J. Liu, D. Song, H. Zhang, H. Dong, R. Li, M. Zhang and J. Wang, Hierarchical NiCo2S4@CoMoO4 core-shell heterostructures nanowire arrays as advanced electrodes for flexible all-solid-state asymmetric supercapacitors, Appl. Surf. Sci., 2018, 453, 73–82 CrossRef CAS.
- S. Kumar, S. Sekar, A. K. Kaliamurthy and S. Lee, Bifunctional rGO-NiCo2S4 MOF hybrid with high electrochemical and catalytic activity for supercapacitor and nitroarene reduction, J. Mater. Res. Technol., 2021, 12, 2489–2501 CrossRef CAS.
- J. He, Y. Zhou, S. Wu, L. Jin, J. Cao, M. Demir and P. Ma, Cr-Substituted SrCoO3-δ Perovskite with Abundant Oxygen Vacancies for High-Energy and Durable Low-Temperature Antifreezing Flexible Supercapacitor, Inorg. Chem., 2024, 63, 13755–13765 CrossRef CAS PubMed.
|
This journal is © the Partner Organisations 2024 |
Click here to see how this site uses Cookies. View our privacy policy here.