DOI:
10.1039/D4NA00172A
(Review Article)
Nanoscale Adv., 2024,
6, 2993-3008
Applications of self-assembled peptide hydrogels in anti-tumor therapy
Received
29th February 2024
, Accepted 29th April 2024
First published on 30th April 2024
Abstract
Peptides are a class of active substances composed of a variety of amino acids with special physiological functions. The rational design of peptide sequences at the molecular level enables their folding into diverse secondary structures. This property has garnered significant attention in the biomedical sphere owing to their favorable biocompatibility, adaptable mechanical traits, and exceptional loading capabilities. Concurrently with advancements in modern medicine, the diagnosis and treatment of tumors have increasingly embraced targeted and personalized approaches. This review explores recent applications of self-assembled peptides derived from natural amino acids in chemical therapy, immunotherapy, and other adjunctive treatments. We highlighted the utilization of peptide hydrogels as delivery systems for chemotherapeutic drugs and other bioactive molecules and then discussed the challenges and prospects for their future application.
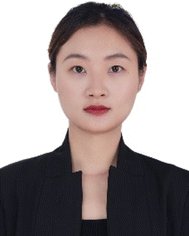 Yue Hua | Yue Hua is currently a PhD candidate at the School of Medicine at Southeast University under the supervision of Prof. Yang Shen. Her current research interest focuses on the design, synthesis and mechanism of peptides related to gynecological tumors. |
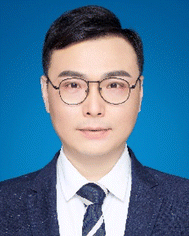 Yang Shen | Yang Shen is the director of the Department of Obstetrics and Gynecology, Southeast University. He received his PhD degree in 2008 from the Nanjing University of Chinese Medicine and was a visiting scholar at Stanford University (2014–2015). His research interest focuses on gynecological tumor-targeted drug delivery systems, the innovation of endoscopy technology, and artificial intelligence in the diagnosis of gynecological tumors. |
1. Introduction
Cancer continues to be a major public health issue worldwide. Approximately 19.29 million new cases of cancer were estimated by 2020.1 While traditional chemoradiotherapy can suppress tumor growth under specific conditions, its efficacy significantly varies among individuals.2 Over the past few decades, numerous strategies have been explored to improve the effectiveness of antitumor treatments; however, limited success has been observed in both preclinical and clinical investigations.3 Due to their lack of specificity, only a small amount of drug molecules can circulate to the tumor site, while the majority are absorbed by normal tissues, leading to significant toxic side effects.4 Conversely, tumor cells may develop resistance to these medications following repeated administration, resulting in tumor recurrence and progression.5 Therefore, it is imperative to explore innovative and effective antitumor therapeutics.
Hydrogels, three-dimensional network polymers capable of swelling in water but insoluble therein, are classified as natural hydrogels (composed of hyaluronic acid, collagen, sodium alginate, peptides, etc.) or synthetic hydrogels (comprising polyacrylamide, polyethylene glycol, etc.) depending on the source of raw materials.6 Peptide hydrogels, self-assembled through dehydration and condensation of natural amino acids, exhibit excellent biocompatibility and metabolic properties.7 Their outstanding water retention properties and grid-like structure enable these materials to mimic cell growth conditions while facilitating the delivery of various bioactive substances and medications.8 Moreover, self-assembled peptides exhibit lower immunogenicity compared to antibodies and can more readily penetrate tissues owing to their reduced molecular weight, rendering them promising candidates for cancer therapy.9 Various non-covalent interactions, including hydrogen bonds, hydrophilicity, hydrophobicity, π–π stacking, electrostatic interactions, and metal coordination bonds, facilitate the formation of diverse secondary structures in peptides, such as nanoparticles, fibers, and micelles.10 With advancement in peptide synthesis technology, the sequence, structure and properties of peptide hydrogels can be precisely regulated to achieve specific functions and applications,11 making it possible to actively target various diseases actively. The mechanical properties of peptides can adapt to the acidic microenvironment and abundant bioactive substances in the tumor microenvironment, facilitating their intelligent assembly or degradation.12 This review outlines recent advances and innovative strategies involving self-assembled peptide hydrogels in cancer chemotherapy, radiotherapy, and immunotherapy, with the aim of bolstering the development of more efficacious anti-tumor treatment modalities (Scheme 1).
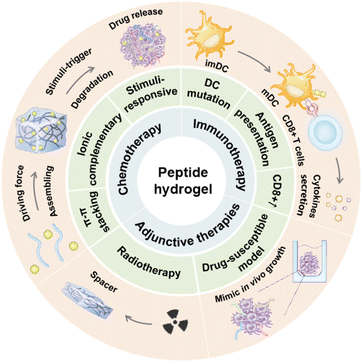 |
| Scheme 1 Applications of self-assembled peptide hydrogels in anti-tumor therapies. | |
2. Delivery of chemical agents
Surgery remains the primary therapeutic approach for most malignant tumors. However, postoperative chemotherapy is commonly adjunctive to eradicate residual tumor cells.13 Paclitaxel (PTX), doxorubicin (DOX), cisplatin (CDDP), and other agents serve as first-line treatments, inducing disease remission in certain patients. However, their systemic administration is associated with evident neurologic, circulatory, and digestive toxicities.14,15 In contrast, traditional chemical drugs, such as the active ingredients of traditional Chinese medicine, have fewer adverse effects, but their poor water solubility and low bioavailability limit their clinical application.16,17
Peptide hydrogels offer several advantages as drug delivery platforms:8,18–21 (i) high water content: peptide hydrogels, due to their abundant water molecules, can accommodate both water-soluble and insoluble medications; (ii) high-drug loading capacity: the 3D network structure of peptide hydrogels enables the accommodation of a large quantity of drugs, enhancing drug loading efficiency; (iii) increased drug stability: preventing environmental degradation of drugs can improve their stability and bioavailability; and (iv) targeted delivery: by adding specific ligands or molecular markers to the peptide, it is possible to achieve targeted delivery to specific cells or tissues, minimizing adverse effects on normal tissues and enhancing the accuracy of treatment. (v) Excellent biocompatibility: peptide hydrogels generally exhibit high biocompatibility, evoking minimal immune responses or rejection. This section reviews the latest applications of peptide hydrogels utilizing diverse self-assembly techniques in cancer drug therapy. Table 1 summarizes the representative self-assembled units, response mechanisms and applications in drug therapy for carcinomas.
Table 1 Self-assembled strategies of peptide hydrogels and their applications in drug delivery
Self-assembled unit |
Precursor |
Driving force |
Mechanism |
Drugs |
References |
FF |
Fmoc–F–FF–DOPA |
π–π stacking |
Thermolysin or WQ9-2 triggers the bond formation between Fmoc–F and FF–DOPA |
CXCL10 and TRAIL |
23 and 24 |
|
Fmoc–FF/(FY)3 |
π–π stacking and electrostatic interactions |
DMSO triggers the self-assembly of Fmoc–FF/(FY)3 and then the solvent was removed after the gelation |
DOX |
25
|
|
FEFFFK |
π–π stacking and electrostatic interactions |
Heating–cooling process |
DOX |
26
|
|
HCPT-FFFK-cyclen |
π–π stacking and electrostatic interactions |
Heating–cooling process |
HCPT and CRB |
27 and 28 |
CRB-FFFK-cyclen |
|
HCPT-FFERGD |
π–π stacking and electrostatic interactions |
HCPT-peptide interacts with cisplatin to form nanofibers or nanoparticles |
HCPT and Cisplatin |
38
|
|
CRB-FFE-YSV |
π–π stacking and electrostatic interactions |
Heating–cooling process |
CRB and YSV |
43
|
Nap-GFF |
Nap-GFFY |
π–π stacking |
Heating–cooling process |
T317 |
29
|
|
Nap-GFFYTKPR |
π–π stacking |
Heating–cooling process |
Tuftsin and OVA |
30
|
|
Nap-GFFKEH |
π–π stacking |
The zinc ion triggers the gelation of the peptide and the ester bond between lysine and BLT could be cleaved by esterase to release BLT |
BLT |
31
|
ID-GFFKEH |
|
Nap-GFFYGRGD |
π–π stacking |
Heating–cooling process |
DOX |
36 and 37 |
Nap-GFFYGRGDH |
RADA |
RADA16 |
Electrostatic interactions |
The hydrophobic alanine residues rapidly gather close to each other to reduce the energy of the system, while the aspartate and arginine residues, which are able to ionize, are arranged in the outer layer of the assembly by electrostatic attractions |
EM, PTX, DOX, and CUR |
44–46
|
|
RADA32-GG-KLAKLAKKLAKLAK |
Electrostatic interactions |
The α-helical structures of KLA disturb the formation of beta-sheets, but extended sequence RADA32 promotes the formation of self-assemblies |
KLA |
47
|
|
Melittin-(RADA)6 |
Electrostatic interactions |
The backbone peptide is degraded in response to proteinase K and drugs will be released from hydrogels gradually |
KN93 and DOX |
48 and 49 |
Melittin-(RADA)8 |
DRF3 |
RLDIKVEFRLDIKVEF |
Electrostatic interactions |
The repeated RLDIKVEF sequence enhances the stability and self-assembly ability of peptides |
OVA |
50
|
FKFEY |
FKFEYYSV |
π–π stacking and electrostatic interactions |
Heating–cooling process |
HCPT |
51
|
Taxol-YSV |
Taxol-EYSV |
Electrostatic interactions |
The peptide solution was transformed into a transparent hydrogel using a bottom-up method |
Taxol |
52
|
2.1 Self-assembled peptides based on π–π-stacking
Molecules containing aromatic ring structures tend to pile up on each other through π–π-stacking, forming organized fibers or nanostructures that facilitate the formation of self-assembled hydrogels.22
The Phe–Phe (F–F) hydrogelator is one of the most researched dipeptides due to its excellent self-assembly ability. Zhang et al. developed an oligopeptide hydrogelator, Fmoc–F–FF–DOPA, capable of bonding Fmoc–F and FF–DOPA upon thermolysin activation. CXCL10, a chemokine that could recruit activated T cells, was encapsulated in the hydrogels, exhibiting sustained release (89.23 ± 4.37%) over 12 days.23 Jiang et al. chose another protease WQ9-2 to trigger the hydrogelation of Fmoc–F–FF–DOPA, resulting in transparent hydrogels 10 minutes after protease addition.24 However, although chemotherapeutic drugs such as DOX could be wrapped in pure Fmoc–FF hydrogels, it appeared to be homogeneous only at a high concentration (0.018 mol L−1). When Fmoc–FF was coupled with hexapeptide (FY)3, the mixed hydrogels exhibited a complicated web of entangled fibers. The maximum amount of DOX that could be added in Fmoc–FF/(FY)3 gels was 2.32 mg mL−1. Due to the positive charge of the drug and the negative charge of the peptide at the C-terminus, the encapsulation ratio of DOX could reach 100%.25 Qi et al. synthesized the hexapeptide FEFFFK, forming interleaved nanofibers in a β-sheet structure to encapsulate DOX, resulting in a transparent hydrogel upon macroscopic observation.26 As positively charged cyclen might aid small-molecule drugs in entering the nucleus and consuming intracellular ATP, Guo et al. developed an HCPT-FFFK-cyclen hydrogel to enhance the nuclear delivery and accumulation of HCPT. They demonstrated that HCPT in the hydrogel group could persist for more than 12 h.27 Additionally, they combined chlorambucil (CRB) with FFF, which was proven to be useful for creating self-assembling peptides via π–π interactions. Compared with free CRB, CRB-FFFK-cyclen exhibited a much lower IC50 against A549 cells (23.8 μM vs. 279.8 μM),28 indicating that drug-FFFK-cyclen hydrogels had excellent tumor-targeting capability and biostability.
By adding 2-naphthylacetic acid to the N-terminus of FF dipeptides, the synthesized NapFF showed a stronger self-assembly capability than FF itself. Feng et al. synthesized Nap-GFFY hydrogels to encapsulate the liver X receptor (LXR) ligand (T317), which increased IFN-γ production by activating LXR. T317 was uniformly dispersed into the hydrogel and Nap-GFFY-T317 hydrogel was kept intact at a low concentration of proteinase K. However, at a high concentration (1 mg mL−1), Nap-GFFY hydrogel was entirely broken down, suggesting that the hydrogel was susceptible to cellular proteases.29 Similarly, Li et al. utilized Nap-GFFY to improve the stability of tuftsin, a natural tetrapeptide known for its immunotherapy efficacy, by self-assembly into hydrogels.30 Additionally, the N-terminal Nap could be replaced by other groups. He et al. substituted Nap with indomethacin (ID) as ID could not only regulate the hydrophilicity of the peptide sequence but also possessed anti-inflammatory properties. Compared with Nap-GFFKEH, ID-GFFKEH had a lower gelation concentration (0.6 vs. 1.0% wt%). Later, ID-GFFKEH was conjugated with bicalutamide (BLT) through a cleavable–ester bond, which could be cleaved by a carboxylic ester hydrolase to release BLT. The cumulative release rate of BLT was pH- and esterase-dependent, reaching 63.4% at 72 h with 50 U mL−1 ester.31 Song et al. modified the C-terminus of NapFF with 2-aminoethyl sulfate to create a sulfated sequence S1. This sequence self-assembled into nanofibers on the surfaces of tumor cells to sequester vascular endothelial growth factor (VEGF), thereby inhibiting the growth, invasion and angiogenesis of cancer cells.32
RGD, a short peptide made up of the amino acids arginine (R), glycine (G), and aspartic acid (D), is widely present in the extracellular matrix proteins. It can prevent tumor cells from adhering to the extracellular matrix by competitively binding to integrin receptors or directly inducing tumor cell death, resulting in the suppression of tumor cell proliferation, invasion, and metastasis.33–35 Xue et al. connected NapGFFY and RGD through glycine to synthesize nanofibers with good self-assembly capability and water solubility. When DOX was added into the NapGFFYGRGD solution, it transformed into nanospheres on the surface of peptide nanofibers.36 Similarly, NapGFFYGRGDH (1-RGDH) synthesized by Mei et al. exhibited an outstanding DOX encapsulation efficiency of up to 92%, with a drug loading capacity of 12%.37 Yang et al. reported an excellent nuclear targeting system based on the self-assembled motif FFERGD for dual drug delivery. In comparison to free cisplatin, the cytotoxicity of complexes was more than 60 times higher, benefiting from the nanostructures formed by the mixture of HCPT-FFERGD and cisplatin which promoted the endocytosis of cells.38 To enhance tissue penetration and tumor cell targeting, CRGDK/RGPD/EC, a novel derivative of RGD, was developed and termed internalizing RGD (iRGD). The peptide iRGD first binds to the integrin receptor, then undergoes proteolytic cleavage and changes into CRGDK/R with the ability to bind to neuropilin-1 (NRP-1) overexpressed on the tumor surface, and finally internalizes into the cells.39,40 Wang et al. conjugated DOX with functional iRDG, resulting in a 40.2% increase in lifespan and a 54% increase in cell uptake compared to the RGD delivery system.41 In addition, Hu et al. coupled PTX with iRGD and achieved greater tumor penetration and anticancer effects.42
2.2 Self-assembled peptides based on ionic complementary strategies
Ionic complementary self-assembled peptides (ISAPs) are a special type of peptide containing both positively and negatively charged amino acid residues in their sequences. Stable interactions such as electrostatic interactions, hydrogen bonds, or van der Waals forces between peptide molecules may aid in their self-assembly to form nanofibers or granular structures, which is greatly important for drug delivery.53–55
RADA16-I, a typical ISAPS, is made up of positively charged Arg (R), negatively charged Ala (A), and the non-polar amino acid Asp (D). Alternating R and A in RADA16-I creates its hydrophilicity, ensuring system stability in aqueous solution, while the hydrophobic zone formed by folded D residues is ideal for encapsulating hydrophobic medications.56 Despite the potential of natural anthraquinone emodin (EM) in anti-oxidation, anti-tumor, and immune system regulation, its poor solubility limited its application in tumor therapy. Wei et al. demonstrated that RADA16-I-loaded EM could form a homogeneous hydrogel both in vitro and in vivo. Compared with free EM, the RADA16-I-EM hydrogel had higher cell uptake rates but lower side effects.44 Based on its excellent self-assembly capability and injectable properties, RADA16-I was used for PTX encapsulation. 48 h after the addition of PTX, the RADA16-I solution turned into a colloidal suspension. Moreover, 40–45% of the integrated PTX was released from 1% RADA16-I hydrogel within 24 h. Comparatively, the half-release period of 0.5% hydrogel was only 8–10 h, suggesting that a higher peptide concentration could delay the release rate of the loaded drug, thereby prolonging the half-release duration of PTX.45 Karavasili et al. applied RADA16 hydrogel for the treatment of highly malignant glioblastoma multiforme (GBM). DOX and curcumin (CUR) were encased in the hydrogel at the same time. Within 4 h, there was a blast release of DOX (73%) and CUR (49%). As mentioned before, the hydrophobic amino acid residues of RADA16-I could assemble into nanofibers and CUR might interact with these fibers, resulting in a slow-release trend over time, while the relatively hydrophilic DOX was released faster from the RADA16-I hydrogel.46
Additionally, RADA-16 could be directly functionalized by extending its C-terminus to functional peptide motifs. Liu et al. utilized two RADA16 sequences to couple a pro-apoptotic peptide KLAKLAKKLAKLAK (KLA) with Gly–Gly, as the α-helical structure of KLA could disrupt the folding of a single RADA16 molecule. The synthetic RADA-KLA hydrogel could not only generate nanofibers, but also trigger cell death, block adhesion and facilitate migration of hepatoma cells.47 Dai et al. chose to couple RADA motifs with melittin, a 26-amino-acid peptide that is primarily found in bee venom, which could kill tumor cells with slight hemolytic toxicity. Melittin-(RADA)4, Melittin-(RADA)6 and Melittin-(RADA)8 had all been synthesized, but only Melittin-(RADA)6 could gelate quickly and exert anti-tumor effects efficiently. Moreover, KN93, a particular inhibitor of CAMKII, was encased in Melittin-(RADA)6 hydrogels with about 100% loading efficiency.48 Similarly, Jin et al. added DOX to the Melittin-(RADA)8 hydrogel matrix to improve the immunosuppressive tumor microenvironment. The presence of protease K caused a nearly 10% increase in the rate of DOX release at 48 h compared with its absence (88.8% vs. 77.7%), which became larger for the backbone peptide from Melittin-(RADA)8 hydrogels (43.7% vs. 100%).49
In addition to RADA16, other combinations of hydrophilic and hydrophobic amino acids could also form self-assembling hydrogels. Luo et al. designed an octapeptide RLDIKVEF, in which four hydrophobic amino acids (Lys, Ile, Val, and Phe) were uniformly inserted between four hydrophilic amino acids (Arg, Asp, Lys, and Glu). In their study, RLDIKVEF was repeated (DRF3) to improve the stability of peptide structures and could assemble into nanofibers instantly in neutral solution. Ovalbumin (OVA) antigen was encapsulated into the hydrogel and then incubated in PBS at 37 °C. When the concentration of DRF3 reached 10 mg mL−1, the release rate of OVA was 97% and remained stable during the later period.50 Liu et al. added an amphiphilic peptide FKFEY to the N-terminus of YSV, which exerted an anti-tumor effect by inhibiting histone deacetylase. Since YSV normally displayed biological functions at high concentrations, the synthesized FKFEYYSV (1-YSV) presented a 2-fold increase in anti-tumor activity over single YSV. Moreover, hydrophobic chemotherapeutic drug 10-hydroxycamptothecin (HCPT) could preserve structural integrity in 1-YSV hydrogel for 5 days. The cumulative release of HCPT from 1-YSV/HCPT hydrogel and aqueous solution was studied and results showed that HCPT released explosively (54.71%) from the aqueous solution but only 6.52% from hydrogels at 1 h, and it took 12 h for nearly half of the HCPT to be released from 1-YSV/HCPT hydrogel. Meanwhile, approximately 60% 1-YSV was released from the hydrogel by 96 h, indicating that it was the degradation of hydrogels that resulted in the continuous release of HCPT.51 Ren et al. designed an amphiphilic molecule by coupling YSV with a hydrophobic anticancer drug Taxol, and Glu (E) was added between these two moieties to enhance the solubility of the whole sequence. When the gel-forming concentration was 0.4 wt%, the Taxol-EYSV hydrogels were slowly eroded and Taxol was released linearly at a rate of 35 μg every day. Furthermore, the hydrogel showed noticeably greater anti-tumor efficacy than the free drug at higher doses although there was a little difference at low concentrations, which could be explained by the enhanced solubility of taxol with hydrogels.52 Similarly, Yang et al. coupled CRB and YSV with FFE and the synthesized CRB-FFE-YSV hydrogel not only improved the stability of YSV but also effectively synergized the antitumor effects of CRB and YSV, prompting the development of novel anti-tumor combination strategies.43 Although CTCE-9980 demonstrated strong anti-metastatic effects in preclinical studies, its ability to inhibit tumor growth was limited.57,58 To address this, Chen et al. designed a peptide-drug conjugate (CTCE-DTX) that not only self-assembled into nanoparticles in aqueous environments but also targeted tumor cells with high expression of CXCR4. This design aimed to enhance the efficacy of docetaxel (DTX) while inhibiting bone metastasis in the case of triple-negative breast cancer.59
2.3 Stimuli-responsive peptide hydrogels
Due to the interaction of noncovalent forces, the reticular structure of peptide hydrogels is fragile and reversible. These hydrogels can undergo morphological transformations in response to stimuli such as enzymes, pH and ionic concentration.60,61 Common hydrogels mainly release drugs through their own dispersion or material degradation, which is unpredictable and uncontrollable.62 However, stimuli-responsive hydrogels are able to present significant changes in gel-solution transitions, permeability, and mechanical strength in response to the tumor microenvironment.63 This section will introduce recent advances in stimuli-responsive hydrogels for targeted drug delivery in different environments.
2.3.1 Enzyme-responsive hydrogels.
The substrates of enzymes used for living activities are mostly made up of amino acids. Enzyme-responsive hydrogels refer to specific chemical reactions of substrate molecules such as peptides and derivatives under the catalysis of enzymes. These interactions promote their spatial organization, leading to the formation of structures such as nanofibers, nanoparticles, and micelles, ultimately resulting in a macroscopic phase transition.64,65
Phosphatase enzymes, such as alkaline phosphate (ALP), enhance substrate hydrophobicity by cleaving phosphate groups. Through non-covalent interactions, hydrophobic substrates can self-assemble into 3D network architectures in aqueous phases to create hydrogels.66 Alkaline phosphate (ALP) is a crucial member of the phosphatase family, which can catalyze the hydrolysis of phosphate groups in various molecules.67 Studies have shown that alkaline phosphatase is overexpressed in various malignancies, such as cervical cancer, ovarian cancer, and liver cancer.68 Phosphorylated tyrosine (Yp), a specific substrate of ALP, can be dephosphorylated and then assembles into hydrogels by π–π stacking interaction.69 Yang et al. designed a series of functional peptide assemblies composed of an NBD fluorophore, a self-assembling sequence (GFF), and a specific EGFR-binding sequence (YHWYGYTPQNVI), among which NBD-GFFpYHWYGYTPQNVI (pY1) presented the optimal dephosphorylation reaction in response to ALP, forming highly tangled nanofibers within 5 min and reaching a balance in assemblies at 12 h. Moreover, when co-cultured with OVA, pY1 could effectively block the endocytosis of OVAs (Fig. 1).70 Xu et al. combined Fmoc-pYGGDADA (1a) with vancomycin, and 1a assembled into a hydrogel when treated with ALP. In in vitro experiments, the half-inhibitory concentration of vancomycin, 1a and 1b alone against HeLa cells was more than 500 μmol L−1, and the half-inhibitory concentration was less than 300 μmol L−1 when the same molar ratio of 1a and vancomycin was added, indicating that the co-assembly of vancomycin and 1a improved the anti-tumor activity.71 Cheng et al. reported a dual-enzyme responsive hydrogel by covalently combining NapFFYp and AZD8055, a novel ATP-competitive mTOR inhibitor. After 12 h of incubation with ALP, the nanoparticles of Nap-AZD-Yp transformed into nanofibers, which became denser after addition of carboxylesterases (CEs) and then AZD was released from the assembly.72 Gao et al. designed a trident compound with two medications (CPT and hydroxychloroquine, HCQ) attached to the side chain of Lys in the self-assembling sequence NapFFKKYp. Similarly, after dephosphorylation of ALP and hydrolysis of CES, released HCQ impaired cancer cell self-protection by suppressing autophagy, rendering cancer cells more susceptible to the chemotherapeutic effect of CPT.73
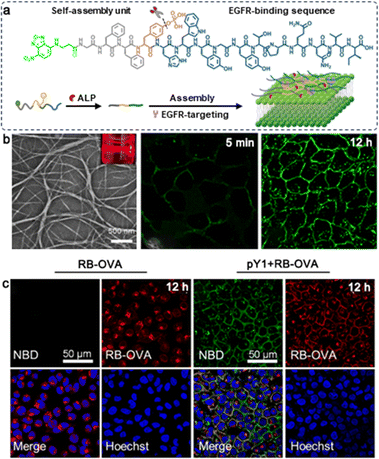 |
| Fig. 1 (a) Schematic illustration of ALP-triggered assembly of pY1 and its targeting of EGFR on the surface of the cell membrane. (b) TEM images of pY1 incubated with ALP (10 U mL−1) for 3 hours, and CLSM images of HeLa cells after incubation with pY1. (c) The cellular distribution of RB-OVA with (right two columns) or without pY1(left two columns). Modified with permission from ref. 70. Copyright 2023. Journal of the American Chemical Society. | |
In contrast, the hydrophilicity of the substrate is enhanced by kinase activity. The structure of the hydrogel is broken, after which the hydrogel is transformed into a solution. Protein kinase A (PKA) is a ubiquitous enzyme found in all cells, playing a vital role in carbohydrate and lipid metabolism. Webber et al. designed an amphiphile peptide (KRRASVAGK[C12]-NH2) using the RRXSO motif found in PKA substrates, where X could be any residue and O must be the hydrophobic sequence. Under the circumstances of PKA, the serine in the sequence was phosphorylated and the formed nanofibers disappeared.74 As salt-inducible kinase 2 (SIK2) is overexpressed in ovarian cancer, a SIK2-responsive hydrogel with the sequence (NapFFEELYRTQSSSNL, abbreviated as Nap-S) containing the specific substrate LYRTQSSSNL for SIK2 was developed. Similarly, the nanofibers of Nap-S turned into particles when treated with SIK2, leading to macroscopic gel-to-sol transitions. Hydrogels released about 42.5% of the SIK2 inhibitor (HG) when incubated with SKOv3-SIK2 cells for 64 h, but this value dropped below 20% when incubated with SKOv3-EV, indicating that Nap-S hydrogels could selectively disassemble to release HG in the presence of SIK2.75 He et al. reported an enterokinase (ENTK)-responsive system of magnetic nanoparticles coated with Mito-Flag, a mitochondrial-targeting peptide. After the hydrolysis of ENTK, Mito-Flag transformed into networks with MNPs encapsulated in them. The enrichment of MNP in mitochondria can enhance the production of reactive oxygen species, consequently leading to mitochondrial dysfunction.76 Zhang et al. designed a peptide probe that responds to matrix metalloproteinase (MMP-2). This probe can form nanofibers and anchor to cell membranes upon exposure to MMP-2, effectively inhibiting tumor cell growth and metastasis.77
2.3.2 pH-responsive hydrogels.
In normal cells, glucose is oxidatively phosphorylated to produce energy. However, the rapid growth of tumor cells allows them to metabolize glucose through the glycolytic pathway, and the lactate generated makes the surrounding environment acidic. Moreover, the tumor vasculature cannot effectively provide enough oxygen and nutrients to the tumor tissue, further exacerbating the acidity of the tumor microenvironment (TME).78–80 Mei et al. combined the self-assembling peptide Nap-GFFY with RGD and appended histidine (H) to the C-terminus of the whole sequence, as the imidazole residue of H could become protonated below pH 6.6, thereby breaking the gelation balance and hastening the erosion process of hydrogels under mildly acidic conditions. At a pH of 7.4, Nap-GFFYGRGDH co-assembled with DOX could form dense nanofibers. The density of nanofibers gradually diminished with decreased pH values. When pH was 5.5, no nanofibers were observed, demonstrating the pH-responsive behavior of the mixed hydrogels.37 Furthermore, Nap was replaced by hydrophobic indomethacin which could be turned into hydrogels under neutral conditions. However, the DOX loading capacity of IDM-GFFYGRGDH was much higher than that of Nap-GFFYGRGDH.81
FEFEFKFK is considered to be a pH-sensitive octapeptide, which can assemble into nanofibers and degrade in an acidic environment. Raza et al. replaced one lysine (K) with R and the synthesized FEFEFRFK (FER-8) hydrogel could be loaded with up to 9 mg mL−1 PTX. A burst release of PTX was observed at the beginning and then remained stable over the next 6 days.82 Zhang et al. designed an in situ self-assembled hydrogel (MTX-KKFKFEFEF(DA)) in which the poorly soluble agent MTX and pH-responsive DA were connected to peptides by amide bonds. MTX-KKFKFEFEF(DA) was observed to form transparent hydrogels in the acidic TME. What's more, DA moieties were detachable at the tumor site, allowing tumor cells to more effectively absorb the positively charged MTX-KKFKFEFEF(DA) hydrogel.83
For persistent and effective administration of DOX, Baek et al. reported a short nucleo-peptide AdeFFF by adding hydrophilic adenine to the self-assembling element FFF. In an alkaline solution, AdeFFF powder crystallized, while under neutral conditions, it self-assembled to produce hydrogels which could be broken down by protease K. Although DOX in the solution group presented an explosive release on day 1, as time went on, the hydrogel gradually degraded. DOX in the AdeFFF hydrogel group was continuously released, and the cell viability dropped to 20% on day 5, but with 63% of DOX in the hydrogel, suggesting that AdeFFF hydrogel had a long-lasting inhibitory effect on tumor cell activity.84 Zhu et al. designed a dual responsive peptide CKIKIKIK-IDPPT-KIOIKIKC-NH2 (IC1-R) composed of self-assembling sequences with cysteine as the two ends and -IDPPT- as the center. Compared with its low concentration, a high concentration of IC1-R formed a more compact fiber-like structure, but disappeared when the environment became acidic. In a neutral solution with glutathione, the cumulative release of PTX from IC1-R hydrogel was less than 10% on day 7, while at pH 5.8, this value increased to 89.63% at a peptide concentration of 0.5 mg mL−1 (Fig. 2).85
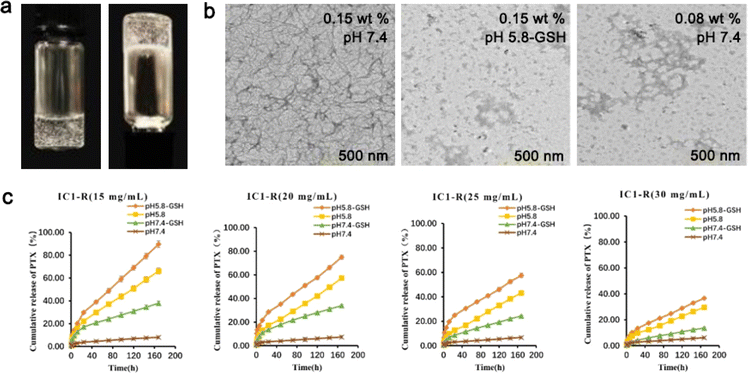 |
| Fig. 2 (a) Optical images of IC1-R under neutral conditions. (b) TEM images of different concentrations of IC1-R in different buffer solutions. (c) Cumulative release of PTX from different concentrations of IC1-R hydrogel with or without glutathione at different pH values. Modified with permission from ref. 85. Copyright 2020. Biomaterials Science. | |
2.3.3 GSH-responsive hydrogels.
Previous studies have shown that the amount of glutathione (GSH) in tumor cells is much higher than that in normal cells and the disulfide bond can be cleaved by GSH.86 Wu et al. reported a GSH-responsive hydrogel HCPT-SA-FFEssEE with hydrophobic drugs conjugated to redox-responsive properties, which could not only improve the solubility of HCPT but also enhance tumor targeting.87 Similarly, an amphiphilic peptide naphthyl-P(Cys–SS–CH2CH2COOH) was designed to encapsulate CDDP. Through measurement of the release profile of CDDP, it was found that the cumulative release of CDDP was 21%, 28% and 40% at pH 7.5, 6.5 and 5.5, respectively. When GSH was added, these values increased to 64%, 67% and 72%, indicating that the synthesized hydrogel was pH/redox dual-responsive.88 Wang et al. designed an in situ self-assembly system (LND-GFFYK(TPP)-ss-PEG) that targets mitochondria to induce mitochondrial dysfunction and autophagy by releasing mitochondrial-targeting peptides and loaded-drugs upon cleavage by GSH.89
2.3.4 Metal cation-responsive hydrogels.
In prostate cancer tissues, the concentration of zinc ions is approximately 10 times higher than that in normal tissues.90 Therefore, it is rational and necessary to design a kind of zinc ion-responsive peptide hydrogel. A forky peptide D3F3 was developed to interact with DOX, which was then triggered by zinc ions to form nanofibers and co-assemble into hydrogels. Although the IC50 value of free DOX in DU-145 cells was 12.46 μg mL−1 and the pure D3F3 peptide had little cytotoxicity, the mixed DOX- D3F3 hydrogel exhibited a much lower IC50 value with zinc ions, indicating that it was the cationic ion-responsive hydrogel enhancing the anti-tumor efficacy of DOX.91 Considering that histidine (H) could chelate zinc ions to form coordination bonds, the self-assembled peptide was capped with ID, and a cleavable ester bond was utilized to connect K with BLT, a classical non-steroidal anti-androgen medication (Fig. 3). In prostate cancer tissue with high zinc ion concentrations, ID-GFFKEH-BLT (ID-1-BLT) could form dense nanofibers. In the acidic TME local decomposition of ID-1-BLT hydrogels led to the internalization of nanofibers, which were subsequently cleaved by a carboxylic ester hydrolase (CES) in the cytoplasm, thus releasing BLT for anti-tumor activity. Moreover, in situ delivery of ID-1-BLT ensured sustained levels of pharmaceuticals in the prostate tissue over 96 h, whereas injections of aqueous solution led to the quick removal of BLT within 4 h.31
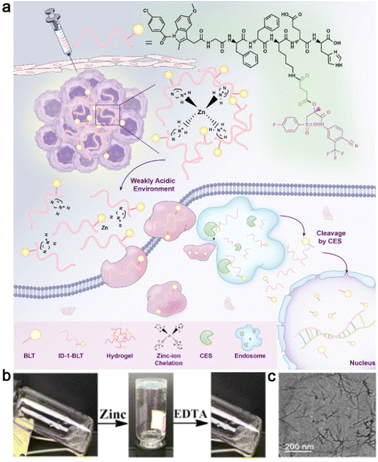 |
| Fig. 3 (a) Schematic representation of zinc-ion-triggered transition of ID-1-BLT for the treatment of prostate cancer. (b) Morphological transition of ID-1-BLT in the presence of zinc ions or EDTA. (c) A TEM image of ID-1-BLT at a concentration of 1.2 wt%. Modified with permission from ref. 31. Copyright 2019. Nanoscale. | |
3. Enhancement of tumor immunotherapy
Tumor immunotherapy is a therapeutic method that breaks immunological tolerance by activating immune cells and enhancing the anti-tumor immune response.92 Compared with traditional chemoradiotherapy, tumor immunotherapy has the advantages of strong specificity and few side effects.93 At present, a variety of immune checkpoint inhibitors such as CTLA-4 monoclonal antibody ipilimumab, PD-1 monoclonal antibody nivolumab and pembrolizumab, and PD-L1 monoclonal antibodies atezolizumab and avelumab have been used in clinical practice.94–97 However, while amplifying the immune effect, immune checkpoint inhibitors may disturb the body's normal immune tolerance, which manifests as excessive activation and expansion of T cells, causing immunopathological damage to normal cells.98 Adoptive cellular immunotherapy is a type of passive tumor immunotherapy in which immune cells from the patient's tumor are transformed, activated, and amplified in vitro before being reinfused into the patient's body to eliminate the tumor.99 Tumor-infiltrating lymphocytes (TILs), chimeric antigen receptor T cells (CAR-T) and TCR-modified T cells (TCR-T) are the most promising methods in clinical application.100–103 However, the limited number of immune cells and the difficulties in extraction and expansion limit the scale and efficacy of treatment.104 Peptide hydrogels' regulatory and diverse biological properties enable them to transport immunomodulatory factors and cells to targeted areas and recruit endogenous immune cells with fewer side effects.105
Tuftsin, a short peptide secreted by the liver, can promote the phagocytosis and chemotaxis of immune cells and boost immunological regulation. To increase Tuftsin's resistance to enzymatic digestion, Li et al. attached it to the self-assembling peptide Nap-GDFDFDYTKPR and then incubated it with bone marrow dendritic cells (BMDCs) and Raw264.7 cells at a concentration of 100 μM. When compared to the control group, the hydrogel of Nap-GDFDFDYTKPR (Comp. 3) significantly increased the expression of CD40 and cytokines, indicating that it could successfully stimulate the maturation of dendritic cells (DCs) and expression of particular types of cytokines. In addition, the ratio of CD8+ T cells in the Comp. 3 group was 4.16 times greater than that in the control group, demonstrating Comp. 3's capacity to elicit a potent CD8+ T immunological response (Fig. 4).30
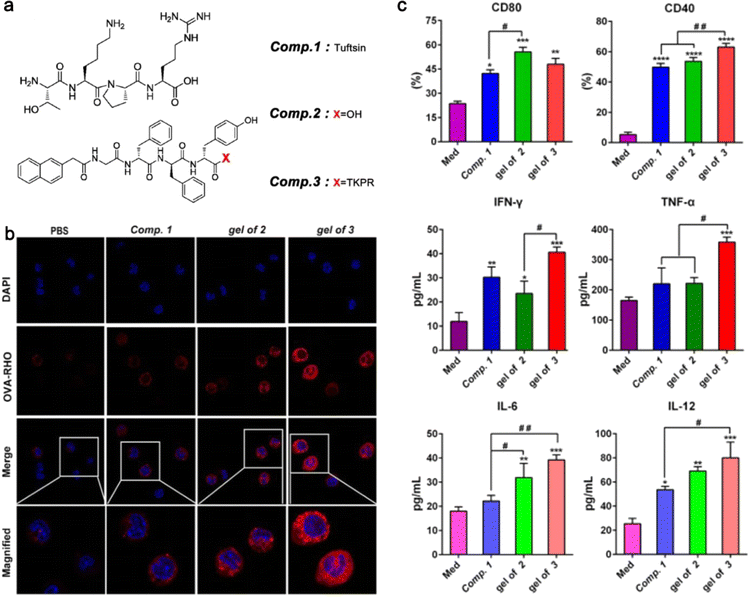 |
| Fig. 4 (a) The structure of the assembling peptide NapGDFDFDYTKPR. (b) Confocal laser scanning microscopy images of primary peritoneal macrophages incubated with Rho-OVA under the stimulation of Comp. 1, the gel of 2, or the gel of 3. (c) The levels of cell markers and cytokines expressed by BMDCs. Modified with permission from ref. 30. Copyright 2020. Journal of Nanobiotechnology. | |
CAR-T is a brand-new, precisely targeted treatment for cancer, which is used for genetically altering T cells to prevent tumor cells from evading the immune system. However, with the present culture methods, it could take 2–4 weeks to develop CAR-T cells.106 Jie et al. generated artificial immune cell matrix scaffolds based on the self-assembled peptide KFKFEFEFGGKLDV to preserve and augment the CAR-T-cell phenotype. In comparison to the conventional methodology, the processing time of CAR-T was shortened to 3 days using the all-in-one nanoscale matrix scaffolds. Additionally, when compared to conventional CAR-T therapy, local delivery of CAR-T cells from the scaffolds greatly improved long-term retention, inhibited tumor development, and boosted the infiltration of effector T cells.107
Due to the high specificity and affinity of antibodies to their targets, antibody-based drugs have shown great promise for cancer diagnostics and treatment.108 However, conventional antibody-drug conjugates (ADCs) and antibody-modified nanomaterials frequently suffer from low drug loading and loss of antibody function due to the covalent modification of antibodies.109 Liang et al. utilized NBD-βA-GDFDFPDY to co-assemble with a HER2-specific affibody and the drug loading in the supramolecular nanofibers was more than 30 wt%.110
As the immunosuppressive TME creates tremendous obstacles for effective anti-tumor therapy, it may be promising to remodel the TME by immune-supportive methods.111 Jin et al. designed a melittin-RADA32 hybrid peptide hydrogel, which could not only load DOX efficiently but also promote the maturation of DCs, thus augmenting the expression of the surface markers CD80 and CD86 in a concentration-dependent manner, as well as the cytokines associated with DC maturation such as IL2, IL-6, IL12, and TNFα.49 Tumor-associated macrophages (TAMs), as important innate immune cells, not only reverse the immunosuppressive tumor microenvironment but also promote the adaptive immune response.112 The CD47 receptor is highly expressed on tumor cells, providing a “don't eat me” anti-phagocytic signal.113 Lv et al. reported a matrix metalloproteinase-2 (MMP-2)-responsive CD47 blockage peptide, which self-assembled into nanofibers in situ after being cut by MMP-2. The nanofibers, which inhibit the typical CD47 “don't eat me” signal, promoted the phagocytosis of tumor cells by macrophages, thereby inducing effective antigen presentation and initiating T cell-mediated adaptive immune response to inhibit tumor growth.114 Xiao et al. reported an amphiphilic peptide molecule (RCPAs) that reprograms macrophages by inducing the repolarization of M2 macrophages to the Mi phenotype. This occurs through the inhibition of endoplasmic reticulum stress and oxidative stress. The repolarization enhances anti-tumor activity and immunomodulatory functions, leading to the remodelling of the immune microenvironment.115
Peptides that target two related or complementary targets are known as bifunctional peptides. In addition to preserving the functional activity of single target peptides, conjugated peptides also extend the peptides' stability and half-life.116 Wang et al. linked RGD and a CD3-targeting peptide with GNNQQNY, which could assemble into nanofibers at high concentrations (>400 μM). In contrast to CD3 antibody and inactivated T cell groups, which demonstrated statistically insignificant cytotoxic effects, the TRA peptide (AKMGEGGWGANDY-GNNQQNY-RGD) enhanced T cell-mediated tumor death in B16F10 melanoma cells (up to 43%).117
Vaccination has shown great promise in cancer immunotherapy, but inducing a robust and broad therapeutic CD8+ T-cell immunity against tumors remains challenging due to the inherent heterogeneity of tumor antigen expression.118,119 Jia et al. used arginine and fluorine phenylalanine as building blocks to synthesize a series of supramolecular self-assembling peptides. Among these peptides, 4RDP not only enhanced antigen uptake and maturation of DCs, but also facilitated lysosomal escape and antigen cross-presentation in DCs.120 Shi et al. modified the structure of the MAGE-A1 antigen peptide (SQYGEPRKL) by covalently linking it with TLR2 agonist N–Ac PamCS through different linkers. The conjugates could self-assemble into nanoparticles based on their hydrophilicity and hydrophobicity properties, acting as dual adjuvants and facilitating passive antigen targeting to DC cells.121
4. Peptide hydrogels as other adjunctive therapies
4.1 In radiotherapy
Radiotherapy is one of the primary treatment options for tumors. Although irradiation of the target area can have a positive anti-tumor impact, it is easy to induce radiation damage to the surrounding healthy tissues.122 In recent years, some studies have sought to use injectable hydrogels to achieve spacing, which can not only boost the dose to the target area but also lower the risk of radiation injury to surrounding tissues during radiotherapy.123 However, the hydrogels that can be used as spacer materials are mainly composed of a polyethylene glycol (PEG) matrix and hyaluronic acid (HA) matrix, and it has also been reported that collagen can also be used as a spacer material.124–126 Since this article mainly describes the application of peptide-based hydrogels in anti-tumor therapies, the relevant content will not be repeated.
4.2 In three-dimensional culture
The primary method for verifying the in vitro efficacy of anti-cancer medications is cell culture, while cells grown on 2D platforms cannot mimic the structural connections of tissues. Additionally, cell viability, proliferation, differentiation, and sensitivity to pharmacological stimulation can also be impacted. As a cross-linked network with high water content, supramolecular peptide hydrogel systems can not only carry a variety of therapeutic drugs but also be used to build the microenvironment required for cell growth, providing a 3D support to some extent.127 In this section, we will briefly introduce the latest research on peptide hydrogels in cell three-dimensional culture, providing a theoretical basis for the screening of tumor-sensitive drugs.
Song et al. prepared a classical RADA16-I peptide with isosmotic sucrose solution to build a solid 3D cell culture. In RADA16-I hydrogel integrin β1 was highly expressed, which was associated with the formation of tight intracellular junctions, indicating that the hydrogel offered a favorable microenvironment for the growth of ovarian cancer cells. Notably, the cells in the RADA16 group stretched out irregularly into the hydrogel, suggesting that this peptide hydrogel provided a suitable environment for tumor cells to grow. Besides, CDDP and PTX both showed considerably reduced inhibitory rates in RADA16-I hydrogel cultures compared with 2D cell cultures with the same cell counts, which was beneficial for screening chemotherapeutic drugs sensitive to tumor models.128 However, the low pH properties of RADA16-I have the potential to damage the encapsulated cells and host tissues. Therefore, Huang et al. chose another self-assembling peptide FLIVIGSIIGPGGDGPGGD (h9e) to mix with a complete culture medium, constructing a hydrogel system for 3D cultivation of breast cancer cells. Interestingly, the gel-solution transformation of h9e could be achieved by repeated pipetting. Although the cell proliferation rate was significantly slowed down in this hydrogel system, the cell viability was much higher and no obvious apoptosis was observed.129 Nagai et al. developed a self-assembling peptide [CH3CONH]-RLDLRLALRLDLR-[CONH2] (SPG-178) which could maintain stability in neutral solution and be sterilized using an autoclave. C2C12 cells in SPG-178 hydrogels expanded about 12-fold within 8 days, suggesting that the hydrogel culture system provided a well-suited interface for cell adhesion and survival.130 To transport C3H10t1/2 mesenchymal stem cells, Haines-Butterick et al. used the 20-residue peptide VKVKVKVKVKVDPLPTKVEVKVKV (MAX8). When cells were added to the MAX1 solution, the salt in the DMEM electrostatically filtered the charges on the lysine side chains, causing the peptide to fold and subsequently self-assemble. The gel state was disrupted during syringe injection, but as soon as the shear action disappeared, the gel quickly reset and returned to its original mechanical stiffness, making it the perfect vehicle for delivering cells to specific biological areas during tissue regeneration.131
5. Conclusion and outlook
Compared with other natural polymers, peptides exhibit favorable biosafety, easy degradation and metabolism in vivo, while producing less toxicity and side effects. Different self-assembly strategies enable peptides to form stable nanostructures, which can not only improve the solubility and encapsulation efficiency of medications but also increase the accumulation of drugs in cells following endocytosis. In response to the acidic and hypoxic microenvironment, high concentrations of ROS, glutathione, and other tumor cell-specific secretases, stimulus-responsive peptide hydrogels can depolymerize precisely at the tumor site, releasing drugs and minimizing systemic toxic side effects caused by small molecule chemotherapeutics. However, challenges persist in this field. Even though peptides can be cleaved by different enzymes, the release sequence from hydrogels is typically erratic and unmanageable. Moreover, degradation and loss of structural integrity over time are still major challenges for their applications. In some research, artificial amino acids are used to retain the secondary structure of peptides.132–134 Additionally, even though various responsive peptide hydrogels have demonstrated controllable drug release in both in vitro and in vivo, improving the accuracy of drug release volume and rate is still a key problem to be solved. What's more, due to their non-immunogenic nature, most peptide hydrogels have been primarily investigated as vaccine adjuvants in immunotherapy. Besides, due to the complexity of the components and the uncontrollable regulation of the immune system, the clinical translation of self-assembling peptide vaccines is also facing challenges. At the cellular level, it remains to be elucidated how peptide assemblies interact with organelles and regulate cellular signaling pathways.
Currently, commercialized hydrogel preparations are primarily utilized for mucosal medication delivery (oral and ocular) or wound healing, and the research on tumor treatment remains largely experimental. Difficulties in clinical translation mainly focus on the short circulating half-lives of peptides, poor chemical and physical stability in serum, and limited volume for local administration. Although there are some difficulties in the clinical application of self-assembled peptide hydrogels, with the progress of material design and synthesis technology, these limitations are expected to be solved and become an auxiliary means of anti-tumor therapy. In summary, self-assembling peptide hydrogels are a new type of smart material with great development prospects and application value.
Author contributions
Yue Hua: conceptualization, writing – original draft, writing – review & editing. Yang Shen: supervision, funding acquisition, writing – review & editing.
Conflicts of interest
There are no conflicts to declare.
Acknowledgements
This work was supported by the National Natural Science Foundation of China (Grants 82072078) and Postgraduate Research & Practice Innovation Program of Jiangsu Province (KYCX23_0325).
References
- R. L. Siegel, K. D. Miller, N. S. Wagle and A. Jemal, Cancer statistics, 2023, Ca-Cancer J. Clin., 2023, 73(1), 17–48 CrossRef PubMed.
- C. M. Tilsed, S. A. Fisher, A. K. Nowak, R. A. Lake and W. J. Lesterhuis, Cancer chemotherapy: insights into cellular and tumor microenvironmental mechanisms of action, Front. Oncol., 2022, 12, 960317 CrossRef CAS PubMed.
- Z. Li, W. Xu, J. Yang, J. Wang, J. Wang, G. Zhu, D. Li, J. Ding and T. Sun, A Tumor Microenvironments-Adapted Polypeptide Hydrogel/Nanogel Composite Boosts Antitumor Molecularly Targeted Inhibition and Immunoactivation, Adv. Mater., 2022, 34(21), 2200449 CrossRef CAS PubMed.
- G. Wei, Y. Wang, G. Yang, Y. Wang and R. Ju, Recent progress in nanomedicine for enhanced cancer chemotherapy, Theranostics, 2021, 11(13), 6370–6392 CrossRef CAS PubMed.
- Q. Yang, J. Xu, J. Gu, H. Shi, J. Zhang, J. Zhang, Z. S. Chen, X. Fang, T. Zhu and X. Zhang, Extracellular Vesicles in Cancer Drug Resistance: Roles, Mechanisms, and Implications, Adv. Sci., 2022, 9(34), 2201609 CrossRef CAS PubMed.
- T. C. Ho, C. C. Chang, H. P. Chan, T. W. Chung, C. W. Shu, K. P. Chuang, T. H. Duh, M. H. Yang and Y. C. Tyan, Hydrogels: Properties and Applications in Biomedicine, Molecules, 2022, 27(9), 2902 CrossRef CAS PubMed.
- M. Sedighi, N. Shrestha, Z. Mahmoudi, Z. Khademi, A. Ghasempour, H. Dehghan, S. F. Talebi, M. Toolabi, V. Preat, B. Chen, X. Guo and M. A. Shahbazi, Multifunctional Self-Assembled Peptide Hydrogels for Biomedical Applications, Polymers, 2023, 15(5), 1160 CrossRef CAS PubMed.
- K. Z. Lee, J. Jeon, B. Jiang, S. V. Subramani, J. Li and F. Zhang, Protein-Based Hydrogels and Their Biomedical Applications, Molecules, 2023, 28(13), 4988 CrossRef CAS PubMed.
- T. Abdullah, K. Bhatt, L. J. Eggermont, N. O’Hare, A. Memic and S. A. Bencherif, Supramolecular Self-Assembled Peptide-Based Vaccines: Current State and Future Perspectives, Front. Chem., 2020, 8, 598160 CrossRef PubMed.
- Y. Gao, L. Wang, X. Zhang, Z. Zhou, X. Shen, H. Hu, R. Sun and J. Tang, Advances in Self-Assembled Peptides as Drug Carriers, Pharmaceutics, 2023, 15(2), 482 CrossRef CAS PubMed.
- S. Yu, Y. Huang, B. Shen, W. Zhang, Y. Xie, Q. Gao, D. Zhao, Z. Wu and Y. Liu, Peptide hydrogels: Synthesis, properties, and applications in food science, Compr. Rev. Food Sci. Food Saf., 2023, 22(4), 3053–3083 CrossRef CAS PubMed.
- M. Delfi, R. Sartorius, M. Ashrafizadeh, E. Sharifi, Y. Zhang, P. De Berardinis, A. Zarrabi, R. S. Varma, F. R. Tay, B. R. Smith and P. Makvandi, Self-assembled peptide and protein nanostructures for anti-cancer therapy: Targeted delivery, stimuli-responsive devices and immunotherapy, Nano Today, 2021, 38, 101119 CrossRef CAS PubMed.
- K. M. Heinhuis, W. Ros, M. Kok, N. Steeghs, J. H. Beijnen and J. H. M. Schellens, Enhancing antitumor response by combining immune checkpoint inhibitors with chemotherapy in solid tumors, Ann. Oncol., 2019, 30(2), 219–235 CrossRef CAS PubMed.
- V. Schirrmacher, From chemotherapy to biological therapy: A review of novel concepts to reduce the side effects
of systemic cancer treatment (Review), Int. J. Oncol., 2019, 54(2), 407–419 CrossRef CAS PubMed.
- Y. Ferro, S. Maurotti, M. G. Tarsitano, O. Lodari, R. Pujia, E. Mazza, L. Lascala, R. Russo, A. Pujia and T. Montalcini, Therapeutic Fasting in Reducing Chemotherapy Side Effects in Cancer Patients: A Systematic Review and Meta-Analysis, Nutrients, 2023, 15(12), 2666 CrossRef CAS PubMed.
- X. Zhang, H. Qiu, C. Li, P. Cai and F. Qi, The positive role of traditional Chinese medicine as an adjunctive therapy for cancer, BioSci. Trends, 2021, 15(5), 283–298 CrossRef CAS PubMed.
- Y. Xiang, Z. Guo, P. Zhu, J. Chen and Y. Huang, Traditional Chinese medicine as a cancer treatment: Modern perspectives of ancient but advanced science, Cancer Med., 2019, 8(5), 1958–1975 CrossRef PubMed.
- Y. Wang, H.-Q. Lv, X. Chao, W.-X. Xu, Y. Liu, G.-X. Ling and P. Zhang, Multimodal therapy strategies based on hydrogels for the repair of spinal cord injury, Mil. Med. Res., 2022, 9(1), 16 Search PubMed.
- X. Q. Dou and C. L. Feng, Amino Acids and Peptide-Based Supramolecular Hydrogels for Three-Dimensional Cell Culture, Adv. Mater., 2017, 29(16), 1604062 CrossRef PubMed.
- P. Worthington, S. Langhans and D. Pochan, beta-hairpin peptide hydrogels for package delivery, Adv. Drug Delivery Rev., 2017, 110–111, 127–136 CrossRef CAS PubMed.
- J. Chen and X. Zou, Self-assemble peptide biomaterials and their biomedical applications, Bioact. Mater., 2019, 4, 120–131 Search PubMed.
- L. Zhu, Q. Lu, T. Bian, P. Yang, Y. Yang and L. Zhang, Fabrication and Characterization of pi-pi Stacking Peptide-Contained Double Network Hydrogels, ACS Biomater. Sci. Eng., 2023, 9(8), 4761–4769 CrossRef CAS PubMed.
- J. Zhang, C. Chen, A. Li, W. Jing, P. Sun, X. Huang, Y. Liu, S. Zhang, W. Du, R. Zhang, Y. Liu, A. Gong, J. Wu and X. Jiang, Immunostimulant hydrogel for the inhibition of malignant glioma relapse post-resection, Nat. Nanotechnol., 2021, 16(5), 538–548 CrossRef CAS PubMed.
- T. Jiang, S. Shen, T. Wang, M. Li, B. He and R. Mo, A Substrate-Selective Enzyme-Catalysis Assembly Strategy for Oligopeptide Hydrogel-Assisted Combinatorial Protein Delivery, Nano Lett., 2017, 17(12), 7447–7454 CrossRef CAS PubMed.
- E. Gallo, C. Diaferia, E. Rosa, G. Smaldone, G. Morelli and A. Accardo, Peptide-Based Hydrogels and Nanogels for Delivery of Doxorubicin, Int. J. Nanomed., 2021, 16, 1617–1630 CrossRef PubMed.
- Y. Qi, H. Min, A. Mujeeb, Y. Zhang, X. Han, X. Zhao, G. J. Anderson, Y. Zhao and G. Nie, Injectable Hexapeptide Hydrogel for Localized Chemotherapy Prevents Breast Cancer Recurrence, ACS Appl. Mater. Interfaces, 2018, 10(8), 6972–6981 CrossRef CAS PubMed.
- Q. Guo, Y. Liu, Z. Wang, J. Zhang, G. Mu, W. Wang and J. Liu, Supramolecular nanofibers increase the efficacy of 10-hydroxycamptothecin by enhancing nuclear accumulation and depleting cellular ATP, Acta Biomater., 2021, 122, 343–353 CrossRef CAS PubMed.
- Q. Guo, Y. Liu, G. Mu, L. Yang, W. Wang, J. Liu and J. Liu, A peptide–drug hydrogel to enhance the anti-cancer activity of chlorambucil, Biomater. Sci., 2020, 8(20), 5638–5646 RSC.
- K. Feng, C. Ma, Y. Liu, X. Yang, Z. Yang, Y. Chen, T. Xu, C. Yang, S. Zhang, Q. Li, Z. Wei, D. Zhao, P. Zeng, J. Han, J. Gao, Y. Chen and Y. Duan, Encapsulation of LXR ligand by D-Nap-GFFY hydrogel enhances anti-tumorigenic actions of LXR and removes LXR-induced lipogenesis, Theranostics, 2021, 11(6), 2634–2654 CrossRef CAS PubMed.
- X. Li, Y. Wang, S. Wang, C. Liang, G. Pu, Y. Chen, L. Wang, H. Xu, Y. Shi and Z. Yang, A strong CD8+ T cell-stimulating supramolecular hydrogel, Nanoscale, 2020, 12(3), 2111–2117 RSC.
- S. He, L. Mei, C. Wu, M. Tao, Z. Zhai, K. Xu and W. Zhong, In situ hydrogelation of bicalutamide-peptide conjugates at prostate tissue for smart drug release based on pH and enzymatic activity, Nanoscale, 2019, 11(11), 5030–5037 RSC.
- J. Song, L. Shao, H. Yu, C. Meng and G. Li, Self-Assembly of Sulfate-Containing Peptides Sequesters VEGF for Inhibiting Cancer Cell Invasion, Biomacromolecules, 2024 DOI:10.1021/acs.biomac.4c00168.
- R. J. Slack, S. J. F. Macdonald, J. A. Roper, R. G. Jenkins and R. J. D. Hatley, Emerging therapeutic opportunities for integrin inhibitors, Nat. Rev. Drug Discovery, 2022, 21(1), 60–78 CrossRef CAS PubMed.
- T. Kuwada, M. Shiokawa, Y. Kodama, S. Ota, N. Kakiuchi, Y. Nannya, H. Yamazaki, H. Yoshida, T. Nakamura, S. Matsumoto, Y. Muramoto, S. Yamamoto, Y. Honzawa, K. Kuriyama, K. Okamoto, T. Hirano, H. Okada, S. Marui, Y. Sogabe, T. Morita, T. Matsumori, A. Mima, Y. Nishikawa, T. Ueda, K. Matsumura, N. Uza, T. Chiba and H. Seno, Identification of an Anti-Integrin alphavbeta6 Autoantibody in Patients With Ulcerative Colitis, Gastroenterology, 2021, 160(7), 2383–2394 CrossRef CAS PubMed.
- M. Li, Y. Wang, M. Li, X. Wu, S. Setrerrahmane and H. Xu, Integrins as attractive targets for cancer therapeutics, Acta Pharm. Sin. B, 2021, 11(9), 2726–2737 CrossRef CAS PubMed.
- Q. Xue, H. Ren, C. Xu, G. Wang, C. Ren, J. Hao and D. Ding, Nanospheres of doxorubicin as cross-linkers for a supramolecular hydrogelation, Sci. Rep., 2015, 5(1), 8764 CrossRef CAS PubMed.
- L. Mei, K. Xu, Z. Zhai, S. He, T. Zhu and W. Zhong, Doxorubicin-reinforced supramolecular hydrogels of RGD-derived peptide conjugates for pH-responsive drug delivery, Org. Biomol. Chem., 2019, 17(15), 3853–3860 RSC.
- Y. Cai, H. Shen, J. Zhan, M. Lin, L. Dai, C. Ren, Y. Shi, J. Liu, J. Gao and Z. Yang, Supramolecular “Trojan Horse” for Nuclear Delivery of Dual Anticancer Drugs, J. Am. Chem. Soc., 2017, 139(8), 2876–2879 CrossRef CAS PubMed.
- Z. Davoodi and F. Shafiee, Internalizing RGD, a great motif for targeted peptide and protein delivery: a review article, Drug Delivery Transl. Res., 2022, 12(10), 2261–2274 CrossRef CAS PubMed.
- I. Winer, S. Wang, Y.-E. K. Lee, W. Fan, Y. Gong, D. Burgos-Ojeda, G. Spahlinger, R. Kopelman and R. J. Buckanovich, F3-Targeted Cisplatin-Hydrogel Nanoparticles as an Effective Therapeutic That Targets Both Murine and Human Ovarian Tumor Endothelial
Cells In vivo, Cancer Res., 2010, 70(21), 8674–8683 CrossRef CAS PubMed.
- K. Wang, X. Zhang, Y. Liu, C. Liu, B. Jiang and Y. Jiang, Tumor penetrability and anti-angiogenesis using iRGD-mediated delivery of doxorubicin-polymer conjugates, Biomaterials, 2014, 35(30), 8735–8747 CrossRef CAS PubMed.
- H. Hu, B. Wang, C. Lai, X. Xu, Z. Zhen, H. Zhou and D. Xu, iRGD-paclitaxel conjugate nanoparticles for targeted paclitaxel delivery, Drug Dev. Res., 2019, 80(8), 1080–1088 CrossRef CAS PubMed.
- L. Yang, C. Zhang, C. Ren, J. Liu, Y. Zhang, J. Wang, F. Huang, L. Zhang and J. Liu, Supramolecular Hydrogel Based on Chlorambucil and Peptide Drug for Cancer Combination Therapy, ACS Appl. Mater. Interfaces, 2018, 11(1), 331–339 CrossRef PubMed.
- W. Wei, J. Tang, H. Li, Y. Huang, C. Yin, D. Li and F. Tang, Antitumor Effects of Self-Assembling Peptide-Emodin in situ Hydrogels in vitro and in vivo, Int. J. Nanomed., 2021, 16, 47–60 CrossRef PubMed.
- J. Liu, Controlled release of paclitaxel from a self-assembling peptide hydrogel formed in situ and antitumor study in vitro, Int. J. Nanomed., 2011, 2143–2153 CrossRef CAS PubMed.
- C. Karavasili, E. Panteris, I. S. Vizirianakis, S. Koutsopoulos and D. G. Fatouros, Chemotherapeutic Delivery from a Self-Assembling Peptide Nanofiber Hydrogel for the Management of Glioblastoma, Pharm. Res., 2018, 35(8), 166 CrossRef PubMed.
- T. Liu, P. Li, H. Jin, Q. Ding, Z. Zou and G. Peng, Influence of designer self-assembling nanofiber scaffolds containing anti-cancer peptide motif on hepatoma carcinoma cells, J. Biomed. Mater. Res., Part A, 2017, 105(8), 2329–2334 CrossRef CAS PubMed.
- X. Dai, J. Meng, S. Deng, L. Zhang, C. Wan, L. Lu, J. Huang, Y. Hu, Z. Zhang, Y. Li, J. F. Lovell, G. Wu, K. Yang and H. Jin, Targeting CAMKII to reprogram tumor-associated macrophages and inhibit tumor cells for cancer immunotherapy with an injectable hybrid peptide hydrogel, Theranostics, 2020, 10(7), 3049–3063 CrossRef CAS PubMed.
- H. Jin, C. Wan, Z. Zou, G. Zhao, L. Zhang, Y. Geng, T. Chen, A. Huang, F. Jiang, J.-P. Feng, J. F. Lovell, J. Chen, G. Wu and K. Yang, Tumor Ablation and Therapeutic Immunity Induction by an Injectable Peptide Hydrogel, ACS Nano, 2018, 12(4), 3295–3310 CrossRef CAS PubMed.
- R. Luo, Y. Wan, X. Luo, G. Liu, Z. Li, J. Chen, D. Su, N. Lu and Z. Luo, A Rapid Self-Assembly Peptide Hydrogel for Recruitment and Activation of Immune Cells, Molecules, 2022, 27(2), 419 CrossRef CAS PubMed.
- J. Liu, C. Wu, G. Dai, F. Feng, Y. Chi, K. Xu and W. Zhong, Molecular self-assembly of a tyroservatide-derived octapeptide and hydroxycamptothecin for enhanced therapeutic efficacy, Nanoscale, 2021, 13(9), 5094–5102 RSC.
- C. Ren, Y. Gao, Y. Guan, Z. Wang, L. Yang, J. Gao, H. Fan and J. Liu, Carrier-Free Supramolecular Hydrogel Composed of Dual Drugs for Conquering Drug Resistance, ACS Appl. Mater. Interfaces, 2019, 11(37), 33706–33715 CrossRef CAS PubMed.
- J. K. Wychowaniec, R. Patel, J. Leach, R. Mathomes, V. Chhabria, Y. Patil-Sen, A. Hidalgo-Bastida, R. T. Forbes, J. M. Hayes and M. A. Elsawy, Aromatic Stacking Facilitated Self-Assembly of Ultrashort Ionic Complementary Peptide Sequence: beta-Sheet Nanofibers with Remarkable Gelation and Interfacial Properties, Biomacromolecules, 2020, 21(7), 2670–2680 CrossRef CAS PubMed.
- W. Guo, Y. Ma, L. Hu, Y. Feng, Y. Liu, X. Yi, W. Zhang and F. Tang, Modification Strategies for Ionic Complementary Self-Assembling Peptides: Taking RADA16-I as an Example, Polymers, 2022, 14(23), 5221 CrossRef CAS PubMed.
- J. Lee, M. Ju, O. H. Cho, Y. Kim and K. T. Nam, Tyrosine-Rich Peptides as a Platform for Assembly and Material Synthesis, Adv. Sci., 2019, 6(4), 1801255 CrossRef PubMed.
- F. Gelain, Z. Luo and S. Zhang, Self-Assembling Peptide EAK16 and RADA16 Nanofiber Scaffold Hydrogel, Chem. Rev., 2020, 120(24), 13434–13460 CrossRef CAS PubMed.
- L. Wang, J. Zhou, J. Wang, X. Wang, H. Dong, L. Zhao, J. Wu and J. Peng, Hepatic Stellate Cell-Targeting Micelle Nanomedicine for Early Diagnosis and Treatment of Liver Fibrosis, Adv. Healthcare Mater., 2024, e2303710 CrossRef PubMed.
- A. Akonnor, M. Makise and A. Kuniyasu, CXCR4-Targeted Necrosis-Inducing Peptidomimetic for Treating Breast Cancer, ACS Omega, 2023, 8(27), 24467–24476 CrossRef CAS PubMed.
- C. Li, J. Lang, Y. Wang, Z. Cheng, M. Zu, F. Li, J. Sun, Y. Deng, T. Ji, G. Nie and Y. Zhao, Self-assembly of CXCR4 antagonist peptide-docetaxel conjugates for breast tumor multi-organ metastasis inhibition, Acta Pharm. Sin. B, 2023, 13(9), 3849–3861 CrossRef PubMed.
- D. Gupta, V. Gupta, D. Nath, C. Miglani, D. Mandal and A. Pal, Stimuli-Responsive Self-Assembly Disassembly in Peptide Amphiphiles to Endow Block-co-Fibers and Tunable Piezoelectric Response, ACS Appl. Mater. Interfaces, 2022, 15(21), 25110–25121 CrossRef PubMed.
- M. Vazquez-Gonzalez and I. Willner, Stimuli-Responsive Biomolecule-Based Hydrogels and Their Applications, Angew Chem. Int. Ed. Engl., 2020, 59(36), 15342–15377 CrossRef CAS PubMed.
- M. Amiri, P. Khazaeli, A. Salehabadi and M. Salavati-Niasari, Hydrogel beads-based nanocomposites in novel drug delivery platforms: Recent trends and developments, Adv. Colloid Interface Sci., 2021, 288, 102316 CrossRef CAS PubMed.
- A. C. Marques, P. J. Costa, S. Velho and M. H. Amaral, Stimuli-responsive hydrogels for intratumoral drug delivery, Drug Discovery Today, 2021, 26(10), 2397–2405 CrossRef CAS PubMed.
- F. K. Shieh, S. C. Wang, C. I. Yen, C. C. Wu, S. Dutta, L. Y. Chou, J. V. Morabito, P. Hu, M. H. Hsu, K. C. Wu and C. K. Tsung, Imparting functionality to biocatalysts via embedding enzymes into nanoporous materials by a de novo approach: size-selective sheltering of catalase in metal-organic framework microcrystals, J. Am. Chem. Soc., 2015, 137(13), 4276–4279 CrossRef CAS PubMed.
- N. E. Davis, S. Ding, R. E. Forster, D. M. Pinkas and A. E. Barron, Modular enzymatically crosslinked protein polymer hydrogels for in situ gelation, Biomaterials, 2010, 31(28), 7288–7297 CrossRef CAS PubMed.
- H. Hu, Y. W. Tong and Y. He, Current insight into enhanced strategies and interaction mechanisms of hydrogel materials for phosphate removal and recovery from wastewater, Sci. Total Environ., 2023, 892, 164514 CrossRef CAS PubMed.
- K. Tyagi, R. Kumari and V. Venkatesh, Alkaline phosphatase (ALP) activatable small molecule-based prodrugs for cancer theranostics, Org. Biomol. Chem., 2023, 21(21), 4455–4464 RSC.
- X. Du, J. Zhou, J. Shi and B. Xu, Supramolecular Hydrogelators and Hydrogels: From Soft Matter to Molecular Biomaterials, Chem. Rev., 2015, 115(24), 13165–13307 CrossRef CAS PubMed.
- A. Pietraszek, G. Ledwojcik, J. Lewandowska-Lancucka, W. Horak, R. Lach, A. Latkiewicz and A. Karewicz, Bioactive hydrogel scaffolds reinforced with alkaline-phosphatase containing halloysite nanotubes for bone repair applications, Int. J. Biol. Macromol., 2020, 163, 1187–1195 CrossRef CAS PubMed.
- Y. Ding, D. Zheng, L. Xie, X. Zhang, Z. Zhang, L. Wang, Z. W. Hu and Z. Yang, Enzyme-Instructed Peptide Assembly Favored by Preorganization for Cancer Cell Membrane Engineering, J. Am. Chem. Soc., 2023, 145(8), 4366–4371 CrossRef CAS PubMed.
- X. D. Junfeng Shi, D. Yuan, R. Haburcak, D. Wu, N. Zhou and B. Xu, Enzyme transformation to modulate the ligand-receptor interactions between small molecules, Chem. Commun., 2015, 51(23), 4899–4901 RSC.
- X. Cheng, T. Xia, W. Zhan, H. D. Xu, J. Jiang, X. Liu, X. Sun, F. G. Wu and G. Liang, Enzymatic Nanosphere-to-Nanofiber Transition and Autophagy Inducer Release Promote Tumor Chemotherapy, Adv. Healthcare Mater., 2022, 11(23), e2201916 CrossRef PubMed.
- G. Gao, Y.-W. Jiang, W. Zhan, X. Liu, R. Tang, X. Sun, Y. Deng, L. Xu and G. Liang, Trident Molecule with Nanobrush–Nanoparticle–Nanofiber Transition Property Spatially Suppresses Tumor Metastasis, J. Am. Chem. Soc., 2022, 144(26), 11897–11910 CrossRef CAS PubMed.
- M. J. Webber, C. J. Newcomb, R. Bitton and S. I. Stupp, Switching of Self-Assembly in a Peptide Nanostructure with a Specific Enzyme, Soft Matter, 2011, 7(20), 9665–9672 RSC.
- Y. Hua, H. Yin, X. Liu, J. Xie, W. Zhan, G. Liang and Y. Shen, Salt-Inducible Kinase 2-Triggered Release of Its Inhibitor from Hydrogel to Suppress Ovarian Cancer Metastasis, Adv. Sci. (Weinheim, Ger.), 2022, 9(22), e2202260 Search PubMed.
- H. He, J. Guo and B. Xu, Enzymatic Delivery of Magnetic Nanoparticles into Mitochondria of Live Cells, ChemNanoMat, 2021, 7(10), 1104–1107 CrossRef CAS PubMed.
- W. Zhang, J. J. Hu, R. Liu, J. Dai, L. Yuan, Y. Liu, B. Chen, M. Gong, F. Xia and X. Lou, A Peptide-Conjugated Probe with Cleavage-Induced Morphological Change for Treatment on Tumor Cell Membrane, Adv. Sci., 2023, 10(11), e2207228 CrossRef PubMed.
- K. E. de Visser and J. A. Joyce, The evolving tumor microenvironment: From cancer initiation to metastatic outgrowth, Cancer Cell, 2023, 41(3), 374–403 CrossRef CAS PubMed.
- Y. Xiao and D. Yu, Tumor microenvironment as a therapeutic target in cancer, Pharmacol. Ther., 2021, 221, 107753 CrossRef CAS PubMed.
- D. C. Hinshaw and L. A. Shevde, The Tumor Microenvironment Innately Modulates Cancer Progression, Cancer Res., 2019, 79(18), 4557–4566 CrossRef CAS PubMed.
- L. Mei, S. He, Z. Liu, K. Xu and W. Zhong, Co-assembled supramolecular hydrogels of doxorubicin and indomethacin-derived peptide conjugates for synergistic inhibition of cancer cell growth, Chem. Commun., 2019, 55(30), 4411–4414 RSC.
- F. Raza, Y. Zhu, L. Chen, X. You, J. Zhang, A. Khan, M. W. Khan, M. Hasnat, H. Zafar, J. Wu and L. Ge, Paclitaxel-loaded pH responsive hydrogel based on self-assembled peptides for tumor targeting, Biomater. Sci., 2019, 7(5), 2023–2036 RSC.
- J. Zhang, W. Lin, L. Yang, A. Zhang, Y. Zhang, J. Liu and J. Liu, Injectable and pH-responsive
self-assembled peptide hydrogel for promoted tumor cell uptake and enhanced cancer chemotherapy, Biomater. Sci., 2022, 10(3), 854–862 RSC.
- K. Baek, A. D. Noblett, P. Ren and L. J. Suggs, Self-assembled nucleo-tripeptide hydrogels provide local and sustained doxorubicin release, Biomater. Sci., 2020, 8(11), 3130–3137 RSC.
- Y. Zhu, L. Wang, Y. Li, Z. Huang, S. Luo, Y. He, H. Han, F. Raza, J. Wu and L. Ge, Injectable pH and redox dual responsive hydrogels based on self-assembled peptides for anti-tumor drug delivery, Biomater. Sci., 2020, 8(19), 5415–5426 RSC.
- P. Zhang, J. Wu, F. Xiao, D. Zhao and Y. Luan, Disulfide bond based polymeric drug carriers for cancer chemotherapy and relevant redox environments in mammals, Med. Res. Rev., 2018, 38(5), 1485–1510 CrossRef CAS PubMed.
- C. Wu, R. Li, Y. Yin, J. Wang, L. Zhang and W. Zhong, Redox-responsive supramolecular hydrogel based on 10-hydroxy camptothecin-peptide covalent conjugates with high loading capacity for drug delivery, Mater. Sci. Eng., C, 2017, 76, 196–202 CrossRef CAS PubMed.
- Q. Xu, X. Li, J. Yang, Y. Zhang, X. Deng, G. Li and Q. Yuan, Naphthyl-Poly(S-((2-carboxyethyl)thio)-l-cysteine) Peptide Amphiphiles with Different Degrees of Polymerization: Synthesis, Self-Assembly, pH/Reduction-Triggered Drug Release, and Cytotoxicity, Mol. Pharm., 2023, 20(2), 1256–1268 CrossRef CAS PubMed.
- Z. Wang, Q. Wang, H. Cao, Z. Wang, D. Wang, J. Liu, T. Gao, C. Ren and J. Liu, Mitochondrial Localized In Situ Self-Assembly Reprogramming Tumor Immune and Metabolic Microenvironment for Enhanced Cancer Therapy, Adv. Mater., 2024, 36(15), e2311043 CrossRef PubMed.
- M. Ussia, M. Urso, M. Kratochvilova, J. Navratil, J. Balvan, C. C. Mayorga-Martinez, J. Vyskocil, M. Masarik and M. Pumera, Magnetically Driven Self-Degrading Zinc-Containing Cystine Microrobots for Treatment of Prostate Cancer, Small, 2023, 19(17), e2208259 CrossRef PubMed.
- M. Tao, J. Liu, S. He, K. Xu and W. Zhong, In situ hydrogelation of forky peptides in prostate tissue for drug delivery, Soft Matter, 2019, 15(20), 4200–4207 RSC.
- M. Corrado and E. L. Pearce, Targeting memory T cell metabolism to improve immunity, J. Clin. Invest., 2022, 132(1), 148546 CrossRef PubMed.
- R. Rui, L. Zhou and S. He, Cancer immunotherapies: advances and bottlenecks, Front. Immunol., 2023, 14, 1212476 CrossRef CAS PubMed.
- S. J. Wang, S. K. Dougan and M. Dougan, Immune mechanisms of toxicity from checkpoint inhibitors, Trends Cancer, 2023, 9(7), 543–553 CrossRef CAS PubMed.
- A. Cortellini, M. Tucci, V. Adamo, L. S. Stucci, A. Russo, E. T. Tanda, F. Spagnolo, F. Rastelli, R. Bisonni, D. Santini, M. Russano, C. Anesi, R. Giusti, M. Filetti, P. Marchetti, A. Botticelli, A. Gelibter, M. A. Occhipinti, R. Marconcini, M. G. Vitale, L. Nicolardi, R. Chiari, C. Bareggi, O. Nigro, A. Tuzi, M. De Tursi, N. Petragnani, L. Pala, S. Bracarda, S. Macrini, A. Inno, F. Zoratto, E. Veltri, B. Di Cocco, D. Mallardo, M. G. Vitale, D. J. Pinato, G. Porzio, C. Ficorella and P. A. Ascierto, Integrated analysis of concomitant medications and oncological outcomes from PD-1/PD-L1 checkpoint inhibitors in clinical practice, J. Immunother. Cancer, 2020, 8(2), 001361 Search PubMed.
- B. Rowshanravan, N. Halliday and D. M. Sansom, CTLA-4: a moving target in immunotherapy, Blood, 2018, 131(1), 58–67 CrossRef CAS PubMed.
- Y. Liu and P. Zheng, Preserving the CTLA-4 Checkpoint for Safer and More Effective Cancer Immunotherapy, Trends Pharmacol. Sci., 2020, 41(1), 4–12 CrossRef CAS PubMed.
- D. B. Johnson, C. A. Nebhan, J. J. Moslehi and J. M. Balko, Immune-checkpoint inhibitors: long-term implications of toxicity, Nat. Rev. Clin. Oncol., 2022, 19(4), 254–267 CrossRef PubMed.
- E. Leon, R. Ranganathan and B. Savoldo, Adoptive T cell therapy: Boosting the immune system to fight cancer, Semin. Immunol., 2020, 49, 101437 CrossRef CAS PubMed.
- Z. Zhao, Y. Chen, N. M. Francisco, Y. Zhang and M. Wu, The application of CAR-T cell therapy in hematological malignancies: advantages and challenges, Acta Pharm. Sin. B, 2018, 8(4), 539–551 CrossRef PubMed.
- F. J. Lowery, S. Krishna, R. Yossef, N. B. Parikh, P. D. Chatani, N. Zacharakis, M. R. Parkhurst, N. Levin, S. Sindiri, A. Sachs, K. J. Hitscherich, Z. Yu, N. R. Vale, Y. C. Lu, Z. Zheng, L. Jia, J. J. Gartner, V. K. Hill, A. R. Copeland, S. K. Nah, R. V. Masi, B. Gasmi, S. Kivitz, B. C. Paria, M. Florentin, S. P. Kim, K. I. Hanada, Y. F. Li, L. T. Ngo, S. Ray, M. L. Shindorf, S. T. Levi, R. Shepherd, C. Toy, A. Y. Parikh, T. D. Prickett, M. C. Kelly, R. Beyer, S. L. Goff, J. C. Yang, P. F. Robbins and S. A. Rosenberg, Molecular signatures of antitumor neoantigen-reactive T cells from metastatic human cancers, Science, 2022, 375(6583), 877–884 CrossRef CAS PubMed.
- M. H. Gee, A. Han, S. M. Lofgren, J. F. Beausang, J. L. Mendoza, M. E. Birnbaum, M. T. Bethune, S. Fischer, X. Yang, R. Gomez-Eerland, D. B. Bingham, L. V. Sibener, R. A. Fernandes, A. Velasco, D. Baltimore, T. N. Schumacher, P. Khatri, S. R. Quake, M. M. Davis and K. C. Garcia, Antigen Identification for Orphan T Cell Receptors Expressed on Tumor-Infiltrating Lymphocytes, Cell, 2018, 172(3), 549–563 CrossRef CAS PubMed.
- D. Gumber and L. D. Wang, Improving CAR-T immunotherapy: Overcoming the challenges of T cell exhaustion, EBioMedicine, 2022, 77, 103941 CrossRef CAS PubMed.
- M. Martinez and E. K. Moon, CAR T Cells for Solid Tumors: New Strategies for Finding, Infiltrating, and Surviving in the Tumor Microenvironment, Front. Immunol., 2019, 10, 128 CrossRef CAS PubMed.
- Z. Zhang, C. He and X. Chen, Designing Hydrogels for Immunomodulation in Cancer Therapy and Regenerative Medicine, Adv. Mater., 2023, e2308894 Search PubMed.
- R. C. Sterner and R. M. Sterner, CAR-T cell therapy: current limitations and potential strategies, Blood Cancer J., 2021, 11(4), 69 CrossRef PubMed.
- J. Jie, D. Mao, J. Cao, P. Feng and P. Yang, Customized Multifunctional Peptide Hydrogel Scaffolds for CAR-T-Cell Rapid Proliferation and Solid Tumor Immunotherapy, ACS Appl. Mater. Interfaces, 2022, 14(33), 37514–37527 CrossRef CAS PubMed.
- H. Lou and X. Cao, Antibody variable region engineering for improving cancer immunotherapy, Cancer Commun., 2022, 42(9), 804–827 CrossRef PubMed.
- Z. Chen, R. K. Kankala, Z. Yang, W. Li, S. Xie, H. Li, A. Z. Chen and L. Zou, Antibody-based drug delivery systems for cancer therapy: Mechanisms, challenges, and prospects, Theranostics, 2022, 12(8), 3719–3746 CrossRef CAS PubMed.
- C. Liang, L. Zhang, W. Zhao, L. Xu, Y. Chen, J. Long, F. Wang, L. Wang and Z. Yang, Supramolecular Nanofibers of Drug-Peptide Amphiphile and Affibody Suppress HER2+ Tumor Growth, Adv. Healthcare Mater., 2018, 7(22), e1800899 CrossRef PubMed.
- G. M. Allen, N. W. Frankel, N. R. Reddy, H. K. Bhargava, M. A. Yoshida, S. R. Stark, M. Purl, J. Lee, J. L. Yee, W. Yu, A. W. Li, K. C. Garcia, H. El-Samad, K. T. Roybal, M. H. Spitzer and W. A. Lim, Synthetic cytokine circuits that drive T cells into immune-excluded tumors, Science, 2022, 378(6625), eaba1624 CrossRef CAS PubMed.
- X. Shen, S. Zhou, Y. Yang, T. Hong, Z. Xiang, J. Zhao, C. Zhu, L. Zeng and L. Zhang, TAM-targeted reeducation for enhanced cancer immunotherapy: Mechanism and recent progress, Front. Oncol., 2022, 12, 1034842 CrossRef CAS PubMed.
- M. E. W. Logtenberg, F. A. Scheeren and T. N. Schumacher, The CD47-SIRPalpha Immune Checkpoint, Immunity, 2020, 52(5), 742–752 CrossRef CAS PubMed.
- M. Y. Lv, W. Y. Xiao, Y. P. Zhang, L. L. Jin, Z. H. Li, Z. Lei, D. B. Cheng and S. D. Jin, In situ self-assembled peptide enables effective cancer immunotherapy by blockage of CD47, Colloids Surf., B, 2022, 217, 112655 CrossRef CAS PubMed.
- Q. Xiao, J. Huang, X. Wang, Z. Chen, W. Zhang, F. Liu, J. Li, Z. Yang, J. Zhan and Y. Cai, Supramolecular Peptide Amphiphile Nanospheres Reprogram Tumor-associated Macrophage to Reshape the Immune Microenvironment for Enhanced Breast Cancer Immunotherapy, Small, 2023, e2307390 CrossRef PubMed.
- A. V. Joglekar, M. T. Leonard, J. D. Jeppson, M. Swift, G. Li, S. Wong, S. Peng, J. M. Zaretsky, J. R. Heath, A. Ribas, M. T. Bethune and D. Baltimore, T cell antigen discovery via signaling and antigen-presenting bifunctional receptors, Nat. Methods, 2019, 16(2), 191–198 CrossRef CAS PubMed.
- M. D. Wang, G. T. Lv, H. W. An, N. Y. Zhang and H. Wang,
In Situ Self-Assembly of Bispecific Peptide for Cancer Immunotherapy, Angew Chem. Int. Ed. Engl., 2022, 61(10), e202113649 CrossRef CAS PubMed.
- H. Song, Q. Su, Y. Nie, C. Zhang, P. Huang, S. Shi, Q. Liu and W. Wang, Supramolecular assembly of a trivalent peptide hydrogel vaccine for cancer immunotherapy, Acta Biomater., 2023, 158, 535–546 CrossRef CAS PubMed.
- N. Falcone, M. Ermis, D. G. Tamay, M. Mecwan, M. Monirizad, T. G. Mathes, V. Jucaud, A. Choroomi, N. R. de Barros, Y. Zhu, N. E. Vrana, H. B. Kraatz, H. J. Kim and A. Khademhosseini, Peptide Hydrogels as Immunomaterials and Their Use in Cancer Immunotherapy Delivery, Adv. Healthcare Mater., 2023, 12(27), e2301096 CrossRef PubMed.
- S. Jia, S. Ji, J. Zhao, Y. Lv, J. Wang, D. Sun and D. Ding, A Fluorinated Supramolecular Self-Assembled Peptide as Nanovaccine Adjuvant for Enhanced Cancer Vaccine Therapy, Small Methods, 2023, 7(5), e2201409 CrossRef PubMed.
- W. Shi, Z. Tong, S. Chen, Q. Qiu, J. Zhou and H. Qian, Development of novel self-assembled vaccines based on tumour-specific antigenic peptide and TLR2 agonist for effective breast cancer immunotherapy via activating CD8(+) T cells and enhancing their function, Immunology, 2023, 169(4), 454–466 CrossRef CAS PubMed.
- D. E. Citrin, Recent Developments in Radiotherapy, N. Engl. J. Med., 2017, 377(11), 1065–1075 CrossRef CAS PubMed.
- L. E. Miller, J. A. Efstathiou, S. K. Bhattacharyya, H. A. Payne, E. Woodward and M. Pinkawa, Association of the Placement of a Perirectal Hydrogel Spacer With the Clinical Outcomes of Men Receiving Radiotherapy for Prostate Cancer: A Systematic Review and Meta-analysis, JAMA Netw. Open, 2020, 3(6), e208221 CrossRef PubMed.
- S. Vaggers, B. P. Rai, E. C. P. Chedgy, A. de la Taille and B. K. Somani, Polyethylene glycol-based hydrogel rectal spacers for prostate brachytherapy: a systematic review with a focus on technique, World J. Urol., 2021, 39(6), 1769–1780 CrossRef CAS PubMed.
- J. Zhang, Y. Zhu, Y. Zhang, W. Lin, J. Ke, J. Liu, L. Zhang and J. Liu, A balanced charged hydrogel with anti-biofouling and antioxidant properties for treatment of irradiation-induced skin injury, Mater. Sci. Eng., C, 2021, 131, 112538 CrossRef CAS PubMed.
- S. R. Hadigal and A. K. Gupta, Application of Hydrogel Spacer SpaceOAR Vue for Prostate Radiotherapy, Tomography, 2022, 8(6), 2648–2661 CrossRef PubMed.
- A. J. Dominijanni, M. Devarasetty, S. D. Forsythe, K. I. Votanopoulos and S. Soker, Cell Viability Assays in Three-Dimensional Hydrogels: A Comparative Study of Accuracy, Tissue Eng., Part C, 2021, 27(7), 401–410 CrossRef CAS PubMed.
- H. Song, G. H. Cai, J. Liang, D. S. Ao, H. Wang and Z. H. Yang, Three-dimensional culture and clinical drug responses of a highly metastatic human ovarian cancer HO-8910PM cells in nanofibrous microenvironments of three hydrogel biomaterials, J. Nanobiotechnol., 2020, 18(1), 90 CrossRef CAS PubMed.
- H. Huang, Y. Ding, X. S. Sun and T. A. Nguyen, Peptide hydrogelation and cell encapsulation for 3D culture of MCF-7 breast cancer cells, PLoS One, 2013, 8(3), e59482 CrossRef CAS PubMed.
- Y. Nagai, H. Yokoi, K. Kaihara and K. Naruse, The mechanical stimulation of cells in 3D culture within a self-assembling peptide hydrogel, Biomaterials, 2012, 33(4), 1044–1051 CrossRef CAS PubMed.
- K. R. Lisa Haines-Butterick, M. Branco, D. Salick, R. Rughani, M. Pilarz, S. Matthew, D. J. P. Lamm and P. Joel, Schneider, Controlling hydrogelation kinetics by peptide design for three-dimensional encapsulation and injectable delivery of cells, Proc. Natl. Acad. Sci. U. S. A., 2007, 104(19), 7791–7796 CrossRef PubMed.
- Y. Shi, P. Sang, J. Lu, P. Higbee, L. Chen, L. Yang, T. Odom, G. Daughdrill, J. Chen and J. Cai, Rational Design of Right-Handed Heterogeneous Peptidomimetics as Inhibitors of Protein-Protein Interactions, J. Med. Chem., 2020, 63(21), 13187–13196 CrossRef CAS PubMed.
- H. Tadano, T. Tsukahara, E. Mizushima, A. Akamatsu, K. Watanabe, I. Nojima, T. Kubo, T. Kanaseki, Y. Hirohashi, N. Sato and T. Torigoe, Development of an artificial antibody specific for HLA/peptide complex derived from cancer stem-like cell/cancer-initiating cell antigen DNAJB8, Br. J. Cancer, 2020, 123(9), 1387–1394 CrossRef CAS PubMed.
- C. Jiang, J. Li, W. Zhang, Z. Zhuang, G. Liu, W. Hong, B. Li, X. Zhang and C. C. Chao, Potential association factors for developing effective peptide-based cancer vaccines, Front. Immunol., 2022, 13, 931612 CrossRef CAS PubMed.
|
This journal is © The Royal Society of Chemistry 2024 |