DOI:
10.1039/D4NA00145A
(Review Article)
Nanoscale Adv., 2024,
6, 3714-3732
Revolutionizing cancer therapy using tetrahedral DNA nanostructures as intelligent drug delivery systems
Received
17th February 2024
, Accepted 24th May 2024
First published on 28th May 2024
Abstract
DNA nanostructures have surfaced as intriguing entities with vast potential in biomedicine, notably in the drug delivery area. Tetrahedral DNA nanostructures (TDNs) have received worldwide attention from among an array of different DNA nanostructures due to their extraordinary stability, great biocompatibility, and ease of functionalization. TDNs could be readily synthesized, making them attractive carriers for chemotherapeutic medicines, nucleic acid therapeutics, and imaging probes. Their varied uses encompass medication delivery, molecular diagnostics, biological imaging, and theranostics. This review extensively highlights the mechanisms of functional modification of TDNs and their applications in cancer therapy. Additionally, it discusses critical concerns and unanswered problems that require attention to increase the future application of TDNs in developing cancer treatment.
1. Introduction
Cancer is a leading cause of death worldwide, with 18 million new cases and 10 million deaths in 2018 alone.1 A reliable and efficient drug delivery system is crucially required to prevent cancer formation, multidrug resistance, metastasis, and recurrence. Several studies have been carried out to create and advance theranostic nano-platforms with diverse uses. An ideal nano-drug delivery system (NDDS) possesses the following integrated features: (1) safeguarding drugs from enzymatic degradation within the body; (2) traversing various physiological barriers; (3) achieving precise and controllable drug release; (4) mitigating the adverse effects of the administered drug; and (5) ensuring safety and compatibility with the human body. Organic nanocarriers such as liposomes,2 polymeric micelles,3 dendrimers,4 metallic nanomaterials,5 and inorganic nanoparticles like carbon nanotubes6 and mesoporous silica7 are some of the several nanoscale drug delivery systems (NDDSs) which are available in the market.8 Despite the clinical use of some NDDSs, the treatment of cancer remains challenging due to their heterogeneity, inadequate biocompatibility, and ineffective drug administration. Therefore, it is essential to develop novel NDDSs that demonstrate exceptional clinical efficacy to enhance the therapeutic index of drug delivery systems.
Deoxyribonucleic acid (DNA) has attracted much interest as a potential solution to the problems stated above because of its programmability, small size, excellent biocompatibility, and predictable secondary structure.9 Additionally, DNA nanotechnology, a field that makes use of DNA's biomolecular self-assembly properties, has several uses across many fields, including synthetic biology, chemical analysis, and medication delivery.10 Functional nanostructures with great spatial programmability, such as a DNA nanodevice that is compatible with the immune system or a DNA-based smart drug delivery vehicle, could be readily built by forming specified base pairs in DNA.11 A DNA nano-platform that incorporates nanowires, nanotubes, nanosheets, polymers, quantum dots, gold nanoparticles (AuNPs), and iron oxides can detect cancer early and provide treatment promptly.12
A “tetrahedral DNA nanostructure” (TDN) is a 3D nanostructure that possesses a pyramidal shape and is produced by the complementary interaction of four individual DNA strands.13 The TDN has been suggested to be a potential vehicle for pharmaceuticals because of its exceptional stability, biocompatibility, abundant sites for functional modifications, compatibility with many medications, and outstanding rates of cellular absorption.14 In the past few decades, various DNA nanostructures have been reported and widely applied in chemical biology, analytical chemistry, medicine, and materials science. The TDN is a widely used structure due to its geometry.15 The shape of the TDN allows minimum interaction with the plasma membrane while entering from the corner angle, thus minimizing the repelling charge forces.16 The small size of the TDN makes it a suitable candidate to cross the blood–brain barrier, making it a suitable candidate for targeting neurodegenerative disorders as well.17 One limitation with small size is that the loading capacity of the TDN is lower compared to that of other larger DNA nanostructures. However, the functionalization of the TDN can improve targeted delivery of the drug and hence increase the delivery efficiency. This review specifically examines the functional alterations of targeted drug nanocarriers, the design of intelligent nanomedicine drug delivery systems (NDDSs), and the potential of TDN-based drug delivery systems for treating tumors.
2. Development and assessment of tetrahedral DNA nanostructures
Following the initial discovery by Turberfield and others, the single-step synthesis of the TDN has gained significant popularity as a DNA nanostructure, finding various applications.13 Typically, a TDN consists of only four oligonucleotides that spontaneously arrange themselves into a well-defined tetrahedral structure by forming complementary base pairs.18 TDN synthesis, equivalent to other DNA nanostructures, requires a thermal annealing process where all four DNA oligonucleotides are combined in an appropriate solution. Various analytical methods may be used to confirm and describe the successful creation of TDNs. Currently, gel electrophoresis under non-denaturing conditions is the most basic method used to verify the binding of specified cDNA by observing a change in bands.15 Dynamic light scattering (DLS) is a commonly used method for measuring the size and zeta potential of TDNs,19 as well as assessing the uniformity of nanostructures in a solution. This approach accurately measures the hydrodynamic dimensions at the nanoscale level.20 The atomic force microscopy (AFM) and transmission electron microscopy (TEM) techniques provide direct observation of the structure and conformation of TDNs.21 In general, these methodologies provide valuable insights and verification of the TDN structure, enabling a greater understanding of their general characteristics.
3. Functional modifications of TDNs
TDNs are mostly used as duplexes and double bundles, with a specific emphasis on investigating duplex TDNs. The functional modifications of TDNs include several components, including fluorescent dyes,21 bioligand molecules,22 functional proteins,23 small molecule anticancer drugs,21 and even nucleic acid molecules,24 among other examples (Table 1). There are four fundamental methods based on the site for altering the TDNs by manipulating the functional groups or molecules: vertex modification, mosaic modification, capsule modification, and cantilever modification (Fig. 1).
Table 1 TDN modifications and their applications for drug delivery
Classification |
Example |
Modification |
Application |
DNA tetrahedron function |
Ref. |
Small molecule |
Doxorubicin (DOX) |
Mosaic |
The effective chemotherapy medication doxorubicin (DOX) inhibits DNA replication and induces cell death; it is extensively used to treat a variety of malignancies, including leukemia, and breast and lung cancers |
Facilitates targeted delivery, reduces systemic toxicity |
25
|
Paclitaxel (PTX) |
Mosaic |
The chemotherapeutic medication paclitaxel (PTX) inhibits cancer cell development and affects microtubule activity; it is used to treat a variety of malignancies, including breast, ovarian, and lung cancers |
Enhances drug efficacy, enables controlled release |
26
|
Platinum drugs |
Mosaic |
In cancer treatment, platinum medicines, including cisplatin, are often used to treat a variety of malignancies by decreasing tumor development by DNA crosslink formation, cell division disruption, and apoptosis induction |
Facilitates targeted delivery, enhances the therapeutic effect |
27
|
5-Fluorouracil |
Vertex |
Inhibiting DNA synthesis and cell proliferation, 5-fluorouracil is a chemotherapeutic medicine that is used to treat a variety of malignancies, such as colorectal, breast, and skin cancers |
Facilitates targeted delivery, reduces the dosage needed |
28
|
Methylene blue |
Mosaic |
Methylene blue is a medicinal remedy for methemoglobinemia, a disorder in which the blood's oxygen-carrying ability is diminished because of abnormally high amounts of methemoglobin, and a biological stain for demonstrating cellular structures in laboratories |
Enhances cellular uptake, improves drug efficacy |
29
|
Triphenylphosphine |
Vertex |
In organic synthesis, triphenylphosphine is a versatile reagent that is commonly employed to facilitate a variety of reactions, including Staudinger reduction and Wittig olefination |
Facilitates targeted delivery, enhances the therapeutic effect |
30
|
Protein or peptide sequence |
Cetuximab |
Vertex |
Inhibiting the action of the epidermal growth factor receptor (EGFR) inhibits cancer cell proliferation; this monoclonal antibody, cetuximab, is used in the treatment of certain kinds of colorectal and head and neck malignancies |
Facilitates targeted delivery, enhances the therapeutic effect |
31
|
Cytochrome c |
Capsule |
The mitochondrial electron transport chain relies on cytochrome c, a protein essential to cellular respiration |
Facilitates cellular uptake, improves drug efficacy |
32
|
Streptavidin |
Capsule |
Protein purification, immunoassays, and molecular biology detection techniques are only a few examples of the various biotechnological uses for streptavidin because of its high biotin affinity |
Enhances cellular uptake, reduces the dosage needed |
33
|
Vertex |
D-(KLAKLAK)2 |
Vertex |
A synthetic peptide termed D-(KLAKLAK)2 can cause apoptosis in cancer cells while preserving normal cells, which makes it a promising candidate for targeted cancer treatment |
Facilitates cellular uptake, enhances the therapeutic effect |
34
|
Angiopep-2 |
Cantilever |
By effectively penetrating the blood–brain barrier and improving the transport of medicinal substances, the peptide angiopep-2 indicates an encouraging potential as a medication delivery vehicle |
Facilitates cellular uptake, enhances drug delivery |
19
|
KillerRed |
Cantilever |
The genetically encoded photosensitizer KillerRed has potential uses in cancer treatment and precise control in biological research since it may cause specific cell damage or death when exposed to light, which makes it an attractive tool in optogenetics |
Enhances drug efficacy, enables precise control |
35
|
Tumor-penetrating peptide |
Vertex |
Tumor-penetrating peptides improve targeted therapy for cancer treatment by enhancing medication delivery by effective traversal of the firm extracellular matrix of tumors |
Facilitates cellular uptake, improves drug delivery |
36
|
Nucleic acid sequence |
AS1411 |
Vertex |
A DNA aptamer called AS1411 can limit tumor development by binding specifically to nucleolin, a protein that is overexpressed in cancer cells. This makes it an intriguing option for use in cancer treatment |
Facilitates targeted delivery, enhances the therapeutic effect |
37
|
sgc8c |
Vertex |
A potential route for precision cancer therapy is the use of SGC8C in tumor research, which entails specific therapeutic interventions to disrupt certain signaling pathways |
Facilitates targeted delivery, enhances the therapeutic effect |
38
|
ZY11-targeting aptamer |
Vertex |
Precision medicine could advance the treatment of cancer if therapeutic chemicals are delivered to tumor cells via the ZY11-targeting aptamer |
Facilitates targeted delivery, enhances the therapeutic effect |
39
|
17E DNAzyme |
Vertex |
By targeting and cleaving certain tumor-associated RNA molecules, the 17E DNAzyme provides an effective way of preventing tumor development and progression and could be used in cancer treatment |
Facilitates targeted delivery, enhances the therapeutic effect |
39
|
siRNA |
Cantilever |
A potential therapeutic strategy for cancer therapy, small interfering RNA (siRNA), has many potential uses in tumors, such as targeted gene silencing, which inhibits tumor development by reducing certain oncogenes or by encouraging apoptosis |
Facilitates targeted delivery, enhances the therapeutic effect |
25
|
Vertex |
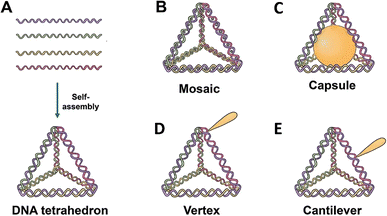 |
| Fig. 1 Structural representation of Tetrahedral DNA Nanostructures (TDNs) and their potential for drug loading. (A) Formation of a TDN through self-assembly. Each single strand comprises a complementary sequence to another single strand, resulting in the formation of a tetrahedral structure. (B) Modification in the mosaic region of the tetrahedron for chemotherapy drugs (e.g., doxorubicin, paclitaxel, actinomycin D). (C) Modification in the capsule region for nanoparticles (e.g., AuNPs), cytochrome c, or peptides (e.g., melittin). (D) Modification in the vertex region for molecules such as CpG, aptamers, monoclonal antibodies (mAbs), 5-fluorouracil, or peptides, and (E) modification in the cantilever region for ligands like folate, siRNA, KillerRed, or camptothecin. | |
3.1 Vertex modification
Functional groups at the vertex position of a TDN can be modified in various ways including sulfhydryl groups,40 amino groups,41 bioactive molecules,34 or specific sequences41 for molecular recognition. One such example is the addition of azide groups34 for subsequent click reactions. One of the four ssDNA strands is modified at the 5′ or 3′ end, and further, the self-assembly of the TDN is carried out for vertex modification. Zhan et al.42 linked a DNA aptamer-modified TDN-based delivery system containing the antimetabolite 5-fluorouracil (5-FU) (AS1411-T-5-FU) to enhance the treatment efficacy and targeting of breast cancer. The anticancer drug AS1411 can decrease Bcl-2 expression, bind selectively to nucleolin, and inhibit NF-κB signalling.43,44 Studies on cell uptake showed that compared to T-5-FU, AS1411-T-5-FU is more effective in targeting breast cancer cells. Additionally, the effects of AS1411-T-5-FU and 5-FU on cell growth inhibition and associated protein expression were evaluated. An analysis of the mitochondrial apoptotic pathway revealed that AS1411-T-5-FU might hasten apoptosis by upregulating Bax and caspase-3 expression while downregulating Bcl-2 expression. DNA materials based on aptamers improve internalization efficiency, bind specifically to cancer cells, and exhibit great recognition selectivity. To accomplish mitochondrial targeting, Yan et al.34 varied the amounts of D-(KLAKLAK)2 (KLA) and attached it to the apex of a TDN. They then loaded the anticancer medication doxorubicin (DOX). Corresponding experimental findings demonstrated that DOX may be efficiently delivered to mitochondria by the KLA-modified TDN, leading to cell death. In vitro anticancer effectiveness, mitochondrial targeting, cellular uptake, and 3KLA-TDN all indicated improvements. Vertex modifications at the vertex position of TDNs offer promising avenues for enhancing drug delivery specificity and efficacy in cancer treatment. These approaches leverage the unique properties of aptamers and other functional groups to target cancer cells more effectively, potentially leading to improved therapeutic outcomes.
3.2 Mosaic modification
Functionalized groups or molecules, such as SYBR Green I45 and other fluorescent labeling dyes46 or anticancer medicines,26 are inserted in the double helix structure of TDNs by conjugation in a mosaic modification. DOX, an anticancer drug, interferes with the macromolecular production of cancerous cells via the insertion of double strands of DNA, which shows promise for cancer therapy.44 DOX-loaded DNA nanostructures have many promising properties for the treatment of cancer and other illnesses, including targeted distribution, rapid release, reduced side effects, and the ability to overcome multidrug resistance. For drug delivery analysis, Kim et al.21 created DOX@Td, which is DOX loaded onto the side of the DNA tetrahedral double helix by the physical conjugation approach. By investigating the absorption mechanism of free DOX and the interaction of p-glycoprotein (P-gp) with cell membranes, it was discovered that DOX@Td entered cells by endocytosis and efficiently overcame multidrug resistance. A PTX/TDN drug delivery system was developed by Xie et al.26 The A549/T cells were able to effectively transport PTX/TDN via caveolin-dependent and exocytosis routes, sidestepping drug efflux pumps. Additionally, PTX/TDN has the potential to greatly reduce the number of wild-type and multidrug-resistant cells. TDNs may inhibit P-glycoprotein (P-gp) and mdr-1 gene expression. Mosaic modifications involving the insertion of functionalized groups or molecules within the double helix structure of TDNs offer promising avenues for targeted drug delivery and overcoming multidrug resistance in cancer therapy. The incorporation of molecules like DOX showcases the potential of TDNs to improve therapeutic outcomes and address challenges associated with drug resistance.
3.3 Capsule modification
Capsule modification involves the encapsulation of functionalized molecules inside a confined structure within a TDN. Erben et al.23 calculated that the inner space of the tetrahedron may hold a sphere with a radius of about 2.6 nm. The researchers attached cytochrome c to the 5′ end of an oligonucleotide and modified the sequence of the oligonucleotide to control the location (either internal or external) of cytochrome c to the TDN. This design has the potential to activate an apoptotic protease cascade. Zhang et al.47 synthesized nano-complexes with a core–shell structure by enclosing gold nanoparticles inside DNA cages. These complexes indicate great potential for use in treating tumors using photothermal, photodynamic, and immunotherapeutic approaches.48 Capsule modification involves encapsulating functionalized molecules within the confined space of TDNs. By encapsulating molecules such as cytochrome c or gold nanoparticles, researchers aim to trigger specific biological responses or enhance therapeutic effects. For example, Erben et al.23 explored the potential of attaching cytochrome c to TDNs to activate apoptotic protease cascades, highlighting the versatility of TDNs for targeted drug delivery and therapeutic applications.
3.4 Cantilever modification
Cantilever modification allows the suspension of functional molecules on the side arms of TDNs. To achieve this, the 5′ or 3′ ends that do not have complementary pairing stretch outwards to modify functional molecules, with the junction of these ends occurring on the edge (middle or another non-vertex) of the TDN. Using the DNA nanostructures' hydrophilicity and editability, Tian et al.19 created ANG-TDN, a modified version of TDN containing angiopep-2. This molecule exhibited high binding to the LRP-1 of glioma and BBB cells. At least 12 hours of serum stability was observed for ANG-TDN. Uppsala 87 malignant glioma (U87MG) cells and brain capillary endothelial cells might both benefit from a tweak to angiopep-2 that increases TDN absorption. The ANG-TDN was able to successfully target the U87MG human glioblastoma xenograft in naked mice and breach the blood–brain barrier, according to both in vitro and in vivo tests. Additional studies indicated that cantilever modification may be used to load siRNA or chemotherapy medicines onto TDNs,24,49 and that covalent bonds might be used to further improve TDN stability by replacing hydrogen bonds in branching DNA structures.
TDNs have been linked to many biomolecules for applications such as medication delivery, biological sensing, and imaging. However, it is still unclear if TDNs are capable of transporting molecules that have larger size and molecular weight than them. The complex balance of a TDN's shape-changing capabilities could be affected by changing a single biomolecule on its vertex or arm, which in turn can affect the stability, stiffness, and geometric structures of the assembled materials.50 Nanostructures of DNA may have different effects on their intracellular transport, final destination, and cellular absorption routes depending on their size, shape, and charge density.51 Despite the benefits of using smaller and less charged nucleic acids, more research into the situations in which their presence could hinder the absorption mechanism of TDNs is required.52 Cantilever modification allows the suspension of functional molecules on the side arms of TDNs. By attaching molecules such as angiopep-2 or siRNA to the side arms, researchers aim to enhance TDN targeting capabilities and therapeutic effects. For instance, Tian et al.19 created angiopep-2-modified TDNs that exhibited high binding to glioma cells and successfully breached the blood–brain barrier, demonstrating their potential for targeted drug delivery to brain tumors.
4. Applications of TDNs in tumor therapy
TDNs provide exceptional programmability, biocompatibility, and minimal biotoxicity, making them ideal drug carriers for surmounting challenges in cancer treatment. Recently, many nano-drug delivery systems for cancer treatment have been developed based on DNA tetrahedrons.
4.1 Targeted delivery
TDNs have been altered with different targeting molecules to develop a drug delivery system that enhances tissue selectivity and reduces non-specific interactions, hence minimizing undesired side effects. Aptamers have been extensively used for TDN modification because of their exceptional affinity and specificity towards their targets. “Systematic evolution of ligands by exponential enrichment” (SELEX) is a common method for producing aptamers, which are typically composed of short synthetic single-stranded DNA or RNA oligonucleotides.53 A variety of targets, from small molecules to whole cells, could be selectively bound to aptamers owing to their ability to assume a three-dimensional structure.54,55 The significance of the findings lies in their nuanced nature, necessitating further exploration to fully comprehend their implications for the field. Additional targeting agents, such as folic acid, cell-penetrating peptides, and Affibody molecules, have been used to increase the specificity of the TDN.56
4.2 Chemotherapy drugs
The loading of chemotherapy medications onto TDNs could be achieved by three primary techniques: chemical modification, intercalation, and electrostatic interaction.
4.2.1 Inhibition of proliferation.
Doxorubicin (DOX), an anthracycline medication, is frequently employed in cancer treatment because it effectively inhibits the proliferation of cancer cells by inserting itself between DNA strands and interacting with them, impeding the production of large macromolecules.57,58 However, the treatment's efficacy is diminished because of its substantial negative impact on healthy cells and organs.59 To tackle this issue, researchers have investigated drug delivery methods that use TDNs as carriers for DOX. Preliminary investigations revealed the increased buildup and effectiveness of DOX-loaded DNA tetrahedra, indicating the possibility of transdermal delivery.60 To enhance precision in delivering drugs, aptamers, such as MUC1 and sgc8c, were used, leading to the precise transportation of DOX to certain cancer cells. The use of aptamers such as MUC1 and AS1411 in dual or multiple targeting strategies significantly improves specificity and cellular absorption.61 The DNA tetrahedron, which has been engineered with a folate receptor (FR) and incorporates folic acid (FA), is effective in targeted treatment for cancer cells that overexpress FRs.62 The incorporation of tumor-penetrating peptides, monoclonal antibodies (cetuximab), platinum medicines, and metal complexes into DNA tetrahedron-based drug delivery systems has shown their capacity to provide precise and efficient cancer treatment.
Camptothecin (CPT), an effective quinoline alkaloid with cytotoxic properties that effectively inhibits DNA topoisomerase, is extensively used in the treatment of cancer.63 Zhang et al. used phosphorothioate DNA to attach CPT and form a DNA tetrahedron (CPT-TET) to create an accurate and manageable drug delivery system for the treatment of tumors.49 Modifying the quantity and locations of attached CPT molecules on the DNA structure makes it possible to enhance the drug loading capacity while maintaining the drug delivery system's ability to dissolve in water. The cellular absorption of CPT-TET was more efficient, resulting in increased cytotoxicity. Additionally, CPT-TET demonstrated a more effective inhibition of tumor development in a tumor mouse model.
4.2.2 Inhibition of cell division.
Paclitaxel (PTX), a naturally occurring antineoplastic agent, has been extensively used in the therapeutic management of diverse malignancies such as breast, ovarian, head and neck, and lung cancer.64 Lin et al. used electrostatic attraction to incorporate PTX onto tetrahedral DNA nanostructures (TDNs) as a method for combating drug-resistant lung cancer.26 The findings indicated that PTX/TDN might penetrate the cells via caveolae-dependent endocytosis and exit via exocytosis, circumventing the drug efflux pump and increasing the quantity of drug within the cells. The study showed that the combination of PTX/TDN effectively suppressed the expression of P-glycoprotein, offering great potential in overcoming medication resistance in lung cancer. Cai et al. used GMT8 and Gint4.T aptamers to modify a DNA tetrahedron to deliver PTX specifically for the treatment of glioblastoma multiforme (GBM).17 The delivery strategy significantly suppressed the growth, movement, and infiltration of GBM cells and triggered programmed cell death in U87MG cells.
Temozolomide (TMZ), a chemotherapeutic drug with the ability to cross the blood–brain barrier (BBB), is considered the most effective treatment for glioblastoma (GBM), anaplastic astrocytoma, and anaplastic oligoastrocytoma.65 Lin et al. used two aptamers (AS1411 and GS24) to modify a DNA tetrahedron and combined it with TMZ to create nanoparticles for the therapy of GBM.66 This nanoparticle can pass across the blood–brain barrier (BBB) and be absorbed by glioblastoma (GBM) cells. This leads to an increase in the effectiveness of temozolomide (TMZ) in killing GBM cells. Significantly, in contrast to free TMZ, the nanoparticle exhibited the ability to not only eliminate TMZ-sensitive cells but also overcome TMZ resistance by depleting O6-methylguanine-DNA-methyltransferase.
4.2.3 Inhibition of cytoskeletal components.
Maitansine (DM1) is a potent inhibitor of microtubules, specifically targeting β-tubulin. It efficiently prevents the clustering or separation of tubulin molecules.67 DM1 has a much stronger antitumor impact compared to other anti-microtubule medicines, with a magnitude ranging from 100 to 1000 times greater.68 Lin et al. chemically conjugated DM1 to three vertices of the DNA tetrahedron while modifying the HER2-targeting aptamer to the remaining vertex. This resulted in the creation of a new drug delivery vehicle called HTD, which is based on a DNA tetrahedron. The purpose of HTD is to treat HER2-positive breast cancer.69 They then used red blood cell membrane together with conventional liposomes to create a hybrid membrane that is both tumor-targeting and pH-sensitive. This membrane effectively enclosed HTD in a biomimetic camouflage and combined to make the ultimate hybrid delivery system. The delivery technique demonstrated a prolonged period of effectiveness inside a living organism and improved effectiveness in fighting against tumors.
5-Fluorouracil (5-FU) is a compound similar to pyrimidine that works by blocking the activity of thymidylate synthase and integrating its byproducts into RNA and DNA. It is extensively used in the treatment of different types of malignancies.70 Lin et al. published a study on the modification of a DNA tetrahedron-based nanomedicine using 5-FU and AS1411.42 This nanomedicine demonstrated increased therapeutic efficacy against breast cancer cells (MCF7 cell line), while not inducing any adverse effects on normal mammary cells (MCF10A cell line). Jorge et al. used a DNA tetrahedron as a novel vehicle to transport 5-fluoro-2′-deoxyuridine oligomers.71 Furthermore, the DNA tetrahedron was chemically altered by including cholesterol to augment its cellular absorption. The delivery technique demonstrated increased toxicity towards cells. Floxuridine, also known as 2′-deoxy-5-fluorouridine, is a fluoropyrimidine medication that is often used in therapeutic settings. When it is metabolized in the body, it becomes 5-FU.72 Zhu et al. used solid-phase synthesis to integrate floxuridine into DNA strands, which were then assembled into a DNA tetrahedron structure to create a drug delivery method known as DNA Trojan horses.28 Tetrahedron-structured DNA Trojan horses have shown enhanced anticancer efficacy, surpassing that of free floxuridine, in both in vitro and in vivo settings.
4.3 Combination therapy
Increasing research suggests that a majority of cancer treatments are no longer effective when using a single medication. Instead, combination therapy with numerous agents has emerged as a potential treatment method. This strategy could involve the use of a versatile medication delivery device. Chemotherapy medications could be incorporated into a DNA tetrahedron by intercalation inside the DNA double helix, chemical bonding, direct attachment to the DNA strand, or hybridization with the DNA strand. Furthermore, the DNA tetrahedron exhibits both biocompatibility and a notable absence of toxicity towards living species. Furthermore, it can efficiently load and dispense many medications concurrently.
4.3.1 Chemogene therapy.
The DNA tetrahedron has been used to encapsulate and disperse a synergistic blend of DOX and nucleic acid therapeutics to combat cancer. Ren et al.39 used an aptamer-modified DNA tetrahedron to concurrently administer DOX and DNAzyme, aiming to accomplish targeted and synergistic chemogene therapy. The aptamer and DNAzyme protruded from opposite corners of the DNA tetrahedron, while one side of the DNA tetrahedron had an i-motif sequence that exhibited remarkable sensitivity to variations in pH. This sensitivity facilitated the precise and regulated release of DOX. Employing this nanocarrier system has the potential to significantly enhance the efficacy of cancer cell treatment instead of utilizing a single chemical therapy.73
In a recent study, scientists used a DNA tetrahedron to co-load DOX and an ASO, together with gold nanoclusters (AuNCs), for self-assembly into a nanohybrid delivery system.57 This device was designed specifically to undergo controlled disassembly and was used for imaging tumors that were triggered, as well as for combined gene chemotherapy.74 The researchers used disulfide bonds to link three segments of the DNA tetrahedron, enabling the targeting of TK1 mRNA with the ASO. They then integrated DOX into the double helix of the DNA tetrahedron. Subsequently, the AuNCs were affixed to the DNA tetrahedron using electrostatic adsorption. The presence of legumain in cells enables the breakdown of the ligands of AuNCs, hence initiating cancer imaging. Similarly, elevated concentrations of glutathione may disrupt disulfide bridges, resulting in the release of the ASO and the consequent suppression of TK1 mRNA. The nanohybrid delivery system demonstrated antitumor effectiveness in both in vitro cell cultures and in vivo animal models.75
4.3.2 Chemoimmunotherapy.
Cancer chemoimmunotherapy is a treatment approach that combines chemotherapy with immunotherapy to enhance the effectiveness of tumor treatment.76 Wang et al.77 used a DNA tetrahedron as a vehicle to simultaneously transport the chemotherapeutic medication DOX and the immunological adjuvant CpG to achieve a synergistic effect in cancer treatment. They discovered that this approach amplified the immune-stimulating benefits of CpG while maintaining the tumor-destroying activity of DOX, suggesting a combined antitumor impact that is greater than using either treatment alone. Shen et al.78 used a DNA tetrahedron for the simultaneous delivery of DOX and CpG in the treatment of malignant melanoma. The DNA tetrahedron exhibited exceptional skin permeability in mice, enabling it to transport DOX and CpG more deeply into tumor tissue compared to free medicines by transdermal administration. This led to a significant suppression of tumor growth. Furthermore, the combination of the PD1 antibody with the treatment showed an enhanced therapeutic impact, significantly extending the life duration of the mice. Significantly, the study revealed enhanced immune responses against distant tumors in bilateral tumor models as a result of the generated systemic immune responses. Liu et al.79 used a similar method to assemble DNA tetrahedrons for the co-loading of DOX and CpG, which aided in the facilitation of chemoimmunotherapy associated with immunogenic cell death (ICD). Within this investigation, DOX functioned as both a chemotherapeutic drug and an inducer of ICD, whereas the immunostimulatory capabilities of CpG might enhance the effectiveness of DOX-induced immunotherapy. Thus, this delivery strategy demonstrated remarkable efficacy in inhibiting tumor growth and stimulating an immune response. Furthermore, the combined action of the two substances was far more potent when taken along with PD-L1.
4.3.3 Gene immunotherapy.
Tumor-associated macrophages (TAMs) are macrophages that are attracted from circulating monocytes to tumors. They have a significant involvement in tumor development, invasion, and metastasis.60,80 According to distinct activation modalities, TAMs are categorized into M1 and M2 phenotypic macrophages.81 M1 macrophages release proinflammatory cytokines, which can stimulate immunological responses and possess the capacity to destroy tumors.82 On the other hand, M2 macrophages release anti-inflammatory cytokines that inhibit the body's ability to respond to threats and encourage the development and advancement of tumors.82 Regrettably, macrophages present in the tumor microenvironment (TME) frequently have M2 macrophage characteristics. Hence, the conversion of M2 macrophages to M1 macrophages holds great potential as a therapeutic approach in the treatment of human malignancies.
Cheng et al.83 employed a DNA tetrahedron to concurrently administer siRNA and CpG (DNA tetrahedron-siRNA/CpG) to convert M2 into M1 macrophages and hence release proinflammatory cytokines to target tumors. The findings demonstrated that DNA tetrahedron-siRNA/CpG exhibited a high level of macrophage absorption efficiency under laboratory conditions. The presence of CpG and siRNA enabled the reprogramming of macrophages, specifically causing M2 macrophages to convert into M1 macrophages. In addition, this delivery mechanism had the potential to distribute in parallel and caused significant apoptosis and necrosis of tumor cells. Significantly, in the 4T1 xenograft tumor-bearing mouse model, the administration of DNA tetrahedron-siRNA/CpG into the 4T1 xenograft tumor-bearing animals reliably and consistently suppressed tumor development while causing minimal harm to vital organs. Qian et al. used a DNA tetrahedron to simultaneously transport siRNA and CpG.84 This approach effectively transformed TAMs into the M1 phenotype and triggered substantial anticancer immune responses. Moreover, the breast cancer xenograft mice model exhibited remarkable antitumor activity without any notable systemic adverse effects.
4.4 Photodynamic therapy
Photodynamic therapy (PDT) is a unique approach to cancer treatment that has been developed in recent years. When exposed to light, the photosensitizer (PS) may undergo either a type I or type II reaction, leading to the production of free radicals and reactive oxygen species.85 These substances induce oxidative stress and cause damage to cells. Several photosensitizers, including phthalocyanine, hypericin, methylene blue (MB), and pheophorbide, could be used in photodynamic therapy (PDT). Nevertheless, the majority of photosensitizers exhibit limited solubility, inadequate cell/tissue permeability, and a deficiency in selectivity, resulting in diminished effectiveness of photodynamic therapy. To address these limitations, several nanocarriers have been used to resolve these issues.86,87 In 2016, Tang et al.88 reported that a TDN can improve the absorption of MB by cells and boost the stability of MB in a reducing intracellular environment. The TDN with a high concentration of MB could elicit cytotoxicity when exposed to light, both in laboratory settings (in vitro) and in living organisms (in vivo). Furthermore, PDT could be used to augment the synergistic effects of other therapies. Obstoy et al.86 created a DNA nanodevice-based technique to identify and eliminate circulating tumor cells using a combination of chemotherapy and photodynamic treatment (PDT) as a demonstration of its feasibility.
Quazi et al.89 reported a peptide/DNA nanocomplex for targeted co-delivery of chemotherapy drugs and photosensitizers to cancer cells. The AS1411 aptamer extends from the three vertices of the DNA tetrahedron, and the G-quadruplex structure formed by AS1411 binds to the photosensitizer (TMPyP4). After DOX was loaded on the DNA tetrahedron, the synthesized polypeptide cationic polymer mPEG-PAsp (TECH) wrapped the DNA tetrahedron to form the final nanostructure composite.90 They found that the nanocomposite was efficiently taken up by HeLa cells and escaped from the lysosome efficiently. Under 660 nm laser irradiation, TMPyP4 induced the upregulation of intracellular ROS, which, in combination with DOX, effectively inhibited HeLa cell growth and showed excellent chemophotodynamic synergistic anticancer activity.61
This functional DNA nanocarrier could effectively penetrate the cell membrane and subsequently transport the drug into the subcellular organelles (mitochondria and nucleus), where the drug efficiently silenced the gene expression and significantly inhibited cancer cell growth. The chemical composition and pharmacodynamics of small-molecule drugs and macromolecular biologics are fundamentally different, so it remains challenging to load these two drugs into suitable nanocarriers for synergistic cancer therapy.91 Guo et al.92 chemically grafted a pheophytin A (PPA) photosensitizer to ssDNA pre-designed with phosphorothioate modification sites, and the four DNA strands grafted with PPA were assembled into a DNA tetrahedron. The DNA tetrahedron was further hybridized with siRNA (PD-L1) containing cohesive ends to form siRNA and PPA co-loaded DNA nanogels. With dual therapeutic agents inside, DNA nanogels could photodynamically kill tumor cells and induce significant ICD. By silencing PD-L1 mRNA in tumor cells, this strategy also significantly promoted antitumor immune responses, which synergistically enhanced antitumor efficacy. In addition, the results of hematoxylin–eosin staining showed that the DNA nanogels were nontoxic to other tissues and organs and exhibited excellent biosafety and biocompatibility (Fig. 2).
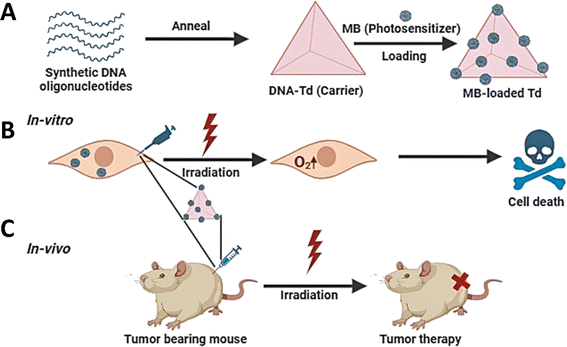 |
| Fig. 2 Schematic representation of the intracellular delivery of methylene blue (MB) using a DNA tetrahedron as a carrier. (A) Synthesis of a DNA tetrahedron and loading of MB on the DNA-Td. (B) Evaluation of the cellular uptake efficiency and photodynamic therapy (PDT) effect by MB delivered with the DNA tetrahedron at the cellular level, leading to successful translocation of MB into cells and enhanced potency in vitro. (C) Subsequent demonstration of the effectiveness of MB delivery by the DNA nanocarrier at the in vivo level, resulting in enhanced potency compared to free MB. | |
4.5 Immunotherapy
Tumor immunotherapy has emerged as a very promising approach to the treatment of cancer in recent years. Immunotherapy differs from conventional radiation and chemotherapy in that it uses the body's immune system to eradicate cancer cells, hence preventing the spread of cancer and its reappearance. CpG ODNs induce immune cells to generate proinflammatory cytokines, including TNF-α and IL-6, which activate the innate immune system via TLR9, hence boosting antitumor response.93,94
The effectiveness of immunotherapy is contingent upon the dosage of immunomodulatory sequences, which poses difficulty in administering a substantial dosage inside a single carrier. Materials that have been modified to have certain motifs with immunomodulatory activity could trigger targeted changes in the immune system. CpG ODN, a vaccination adjuvant that is both safe and efficient, has been extensively used in the field of tumor immunotherapy research and clinical studies.95
In recent times, scientists have investigated the use of DNA nanostructures as carriers for delivering CpG, therefore improving stability and targeting. Liu et al.96 used a TDN to simultaneously transport CpG and streptavidin (as a representative antigen), resulting in a more robust immune response compared to using a TDN alone. Li et al.97 used a TDN functionalized with CpG patterns to activate the immunological response in macrophage-like RAW264.7 cells. The findings demonstrated that cells internalized the functionalized TDN, which remained intact for 8 hours. This internalization process led to the production of cytokines, namely TNF-α, IL-6, and IL-12. The immunostimulatory activity of the TDN was further boosted by the presence of multivalent CpG motifs, hence increasing its importance in the field of tumor immunotherapy.
These investigations emphasize the potential of the TDN as a flexible carrier for therapeutic drugs, functioning as a stable vehicle for precise delivery to immune or tumor cells (Fig. 3).
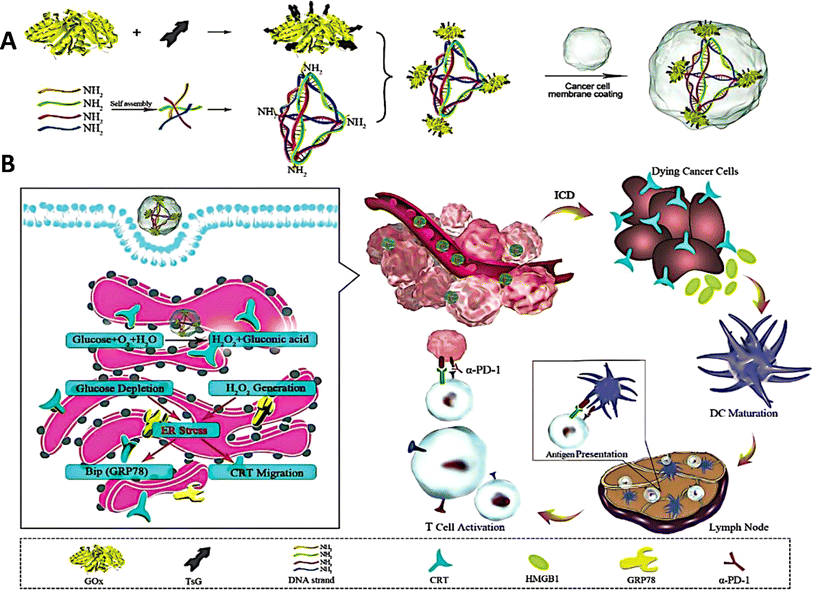 |
| Fig. 3 Schematic representation of the functionalized DNA tetrahedron nanoregulator designed to induce endoplasmic reticulum (ER) stress for tumor immunogen exposure and enhanced immunotherapy. (A) The tetrahedron structure, modified with Gox as the ER-targeting ligand (TsG → Gox), facilitates anchoring to ER organelles via binding to cell sulfonamide receptors. Subsequently, Gox-conjugated DNA tetrahedrons are camouflaged by cancer cell membranes to form the nanoregulator. (B) Upon accumulation in ER organelles, the catalytic reaction initiated by Gox depletes glucose and generates hydrogen peroxide (H2O2). This induces ER stress, evidenced by upregulated glucose-regulated protein 78 kD (GRP78), triggering immunogenic cell death (ICD) of cancer cells to expose damage-associated molecular patterns (DAMPs), including calreticulin (CRT) proteins and high mobility group box 1 (HMGB1). These DAMPs promote dendritic cell (DC) maturation, characterized by high expression of CD80 and CD86, which in turn present antigens to stimulate T cell proliferation and infiltration, leading to cancer cell destruction. Combined with an immune checkpoint inhibitor (α-PD-1), the nanoregulator demonstrates significant suppression of breast cancer and melanoma growth in tumor-bearing mouse models. | |
4.6 Protein and peptide drugs
Most malignancies are associated with aberrant protein expression or functional alterations, which makes protein-based cancer treatments particularly attractive owing to their targeted effectiveness and low toxicity.98,99 Proteins encounter obstacles such as enzymatic breakdown and the issue of entering cell membranes, which restricts their potential use.100 Researchers have created DNA origami nanostructures to serve as carriers for delivering proteins to specific cellular regions.101 Protein distribution using DNA tetrahedron nanocarriers has been recently investigated. Frokjaer et al. developed a hybrid platform comprising a streptavidin-mirror DNA tetrahedron (STV-Td) to transport enzymes into cells.102 The biotin-modified enzymes were bound to STV-Td by the strong interaction with B-SA, efficiently inhibiting tumor growth in a mouse model by using STV-Td as a carrier for delivering caspase-3 (CASP3).
Tang et al. proposed a genetically engineered DNA tetrahedron nano-regulator that stimulates “endoplasmic reticulum (ER)” stress, hence enhancing cancer immunotherapy.103 The nano-regulator was created by immobilizing “glucose oxidase” (GOx) onto the corners of the DNA tetrahedron and then encapsulating it with the cancer cell membrane. Glucose oxidase (GOx) accumulated in the endoplasmic reticulum (ER) and initiated a catalytic reaction that utilized glucose and generated hydrogen peroxide (H2O2). This mechanism led to the activation of immunogenic cell death (ICD). When combined with the immune checkpoint inhibitor α-PD-1, this nano-modulator demonstrated substantial inhibitory effects on both breast cancer and melanoma.
Li et al.104 used an aptamer-modified DNA tetrahedron as the container to encapsulate the protein therapeutic RNase A, aiming to achieve targeted delivery to cancer cells. The DNA tetrahedron was used to protect and encapsulate protein medicines, protecting them from degradation. Moreover, modifying aptamers improved the selectivity of drug transport and elevated the efficiency of cellular absorption. Glutathione in cancer cells selectively cleaves disulfide bonds to release RNase A, leading to death in certain cancer cells.
Kong et al.105 described a method using a DNA tetrahedron to create a cascade of enzymatic reactions to enhance cancer treatment in living organisms. It involved the attachment of glucose oxidase (GOx) and horseradish peroxidase (HRP) onto a DNA tetrahedron. This complex was successfully taken up by cells to trigger cancer starvation therapy and increase the levels of hydrogen peroxide in the immediate vicinity. HRP catalyzed the synthesis of indol-3-acetic acid (IAA) by reacting with H2O2. The occurrence of this reaction led to the creation of unpaired electrons, known as free radicals, which later caused the demise of cancerous cells. The spatial encapsulation of the DNA tetrahedron enhanced the efficiency of delivering intermediate metabolites across enzyme cascades, therefore demonstrating potent and mutually reinforcing cancer therapy in both laboratory studies and living organisms.
Tian et al.106 conducted research in which they used a DNA tetrahedron (TDN) containing melittin to specifically target and cure melanoma. The mechanism was denoted as a “nano-bee”. Upon encountering the target protein on the cell membrane, the DNA tetrahedron framework underwent a conformational shift, resulting in the release of melittin. As a result, the binding of melittin to the framework caused an escalation in its toxicity towards target cells while simultaneously enhancing its selectivity in comparison to unbound melittin. The molecular dynamics study verified that the DNA tetrahedron effectively enclosed melittin, hence reducing the adverse impacts of the nanobee.
4.7 Nucleic acid drugs
4.7.1 Cytosine–phosphate–guanine (CpG).
Mammalian immune systems are activated by unmethylated CpG sequences, which are often found in bacteria but seldom present in vertebrate DNA.107 The recognition of toll-like receptor 9 (TLR9) triggers both innate and adaptive immune responses.108 TLR9 is expressed in several immune cells, including macrophages, dendritic cells, and B cells. The presence of these cells triggers the production of proinflammatory cytokines, such as TNF-α and interleukin-6 (IL-6).109 Both fundamental and applied research have shown that CpG is a potent and reliable immune adjuvant, particularly in the environment of cancer immunotherapy.110 However, the task of introducing and retaining unbound CpG oligonucleotides inside cells is challenging, necessitating the use of a suitable carrier.
Fan et al.97 used a DNA tetrahedron as a carrier to encapsulate and transport several CpG motifs.97 The study revealed that the DNA tetrahedron nanocomposite exhibited resilience against nuclease degradation, maintaining its structural integrity in fetal bovine serum (FBS) for 24 hours and in cells for 8 hours.
The CpG sequences have a DNA composition that allows them to easily integrate into DNA tetrahedron (DTN) structures, which increases stability and improves targeted cellular absorption. The CpG-functionalized DNA tetrahedron effectively penetrated macrophage-like RAW264.7 cells without the need for a transfection agent. TLR9, situated intracellularly, recognizes CpG and initiates the significant release of proinflammatory cytokines such as TNF-α, IL-6, and IL-12. An unequivocal correlation was established between the amounts of secretion and the amount of CpG motifs present on the DNA tetrahedron.
Another modification was done by Fan and colleagues to transport an unmethylated CpG motif using a multivalent DNA nanostructure.97 The immunostimulatory effects are caused by toll-like receptor 9, which recognizes these patterns in bacterial and viral DNA. Results showed that CpG-TDN became more effective than CpG alone in inducing the release of inflammatory cytokines such as tumor necrosis factor-α and interleukins (IL-6 and IL-12). The results also showed that TDN acts as a nanocarrier for the transfer of functional nucleic acids and can protect CpG against nuclease degradation. The utilization of gene expression suppression holds particular relevance in the realm of cancer treatment, complementing the advancements in immunotherapy discussed in preceding sections. While CpG motifs and DNA nanostructures serve as potent immunostimulatory agents, the precise targeting offered by gene suppression techniques enhances the therapeutic efficacy while minimizing adverse effects. This synergy underscores the multifaceted approach adopted in contemporary cancer therapy, wherein the integration of diverse modalities aims to maximize treatment outcomes.
4.7.2 Small-interfering RNA (siRNA).
siRNA, a 21–23 base pair double-stranded non-coding RNA,111 is essential for gene silencing via the RNA interference pathway.112 Due to the promise of siRNA in efficiently treating malignancies caused by abnormal gene expression, it is crucial to transport synthesized siRNA into cancer cells.113 Nevertheless, siRNAs encounter obstacles such as enzymatic breakdown, limited cellular penetration caused by their negatively charged characteristics, and fast elimination by the kidneys.114 To tackle these problems, it is necessary to have appropriate transportation methods, and DNA tetrahedrons (DTNs) have been identified as efficient carriers for siRNA in the field of cancer therapy.
Anderson et al. became the initial group to employ DNA tetrahedra to deliver siRNA into cells. This development represents a significant advancement in the field of gene silencing inside tumors, as seen in ref. 24. To achieve the best delivery of siRNA, at least three folate (FA) molecules per DNA tetrahedron were required. The effectiveness of gene silencing relied on the accurate arrangement of folate molecules in terms of their location and timing. The DNA tetrahedron, which was carrying siRNA, demonstrated an extended duration of blood circulation in comparison to siRNA that was not bound. Cheng et al. successfully delivered siRNA to glioma cells by modifying a DNA tetrahedron with the AS1411 aptamer, resulting in the induction of apoptosis.115 Similarly, Xiao et al.90 used an AS1411 aptamer-modified DNA tetrahedron to transport anti-Braf siRNA, leading to a more effective cleavage of Braf mRNA in comparison to siRNA that is not bound to the aptamer.
Han et al.116 synthesized DNA tetrahedron nanocages by integrating an i-motif with therapeutic siRNA. Their work demonstrated the efficient disruption of EGFR expression in cancer cells by these nanocages. Song et al.117 used a DNA tetrahedron as an instrument to deliver CTR1 mRNA-targeting siRNA into pancreatic cancer cells, leading to a significant inhibition of cancer progression.
The flexibility of the DNA tetrahedron enables the simultaneous loading and distribution of two distinct siRNAs, resulting in a synergistic approach to cancer therapy. As an example, Han et al. used an FA-modified DNA tetrahedron to consecutively provide siRNAs that specifically target PD-L1 and PCSK9, leading to a synergistic treatment for colorectal cancer.118 The co-delivery technology demonstrated exceptional tumor accumulation and therapeutic effectiveness in mice without any adverse consequences.
By altering the dimensions of the DNA tetrahedron and combining it with diverse sugar backbones, precise organ targeting was accomplished. Ahn et al. developed a DNA tetrahedron to selectively target the liver and successfully reduce the expression of the ApoB1 protein.119 In addition, researchers devised a delivery system specifically designed to target the kidneys and distribute siRNA. This system used an L-DNA tetrahedron and successfully delivered siRNA into kidney cells, as shown in a study.25
Cai et al. developed a closed tetrahedron nanobox to overcome problems related to nuclease degradation and uncontrolled release. This nanobox effectively protects siRNA from degradation and successfully achieves gene silencing in both laboratory studies and living organisms.120
In addition to serving as carriers, DTNs may be organized into complex nanostructures to deliver siRNA. Zhang et al. used DNA tetrahedrons as fundamental units to construct a DNA nanogel to deliver siRNA, showcasing the potential of noncationic carriers for RNA delivery in the field of cancer treatment.121
Lee et al.24 established the notion of using folate and siRNA to construct TDNs that could silence the target luciferase gene in tumors. Following injection into the nude mice's tail vein, in vivo studies revealed that tumor and kidney tissues collected the highest amounts of folate-modified TDN with anti-luciferase siRNA, whereas the heart, lungs, spleen, and liver tissues gathered the lowest amounts. By monitoring luciferase activity in tumor xenograft mice with folate receptor overexpression in KB cells, they found that these modified TDNs can decrease the bioluminescence intensity by up to 60% at 48 hours post-injection. However, no such effect was observed with folic acid-conjugated antiluciferase siRNAs.
4.7.3 DNAzyme.
DNAzymes are short strands of nucleotides that possess enzymatic capabilities, allowing them to catalyze a range of processes such as cleaving, ligating, and phosphorylating DNA and RNA molecules.122 DNAzymes exhibit activity that is contingent upon distinct cofactors, such as metal ions and proteins, as well as particular sequences. Consequently, DNAzymes are found to be extensively useful in biosensing and gene silencing treatment.123 Nevertheless, the negative charge of DNAzymes hinders their ability to pass through the cell membrane, resulting in difficulties in effectively delivering DNAzymes into cells.
Using a DNA tetrahedron is regarded as an extremely promising approach for delivering DNAzymes since it may effectively penetrate cells without the need for transfection chemicals. Meng et al.124 presented Dz13, a DNAzyme, that specifically separates the mRNA of c-Jun and inhibits the development of squamous cell carcinomas by directly attaching its sequence to the 5′ terminus of a single-stranded DNA. This modified DNA was then combined to create tetrahedron nanostructures known as TDN-Dz13. TDN-Dz13 demonstrated superior cellular absorption and effectively cleaved intracellular target c-Jun mRNA, resulting in the suppression of cell growth, as compared to free Dz13. Additionally, several illnesses have potential therapeutic agents in the form of deoxyribozymes or DNAzymes, which are nucleic acids with catalytic activity. These agents may identify and cleave their substrates. Meng and colleagues used a TDN to transport the DNAzyme Dz13 for use in gene regulation.125,126 The DNAzyme can break the mRNA of the cell-proliferating protein c-Jun. The researchers discovered that TDN-Dz13 had a high level of cellular penetration and reduced cell proliferation via c-Jun mRNA-silencing.
4.7.4 Antisense oligonucleotides (ASOs).
Antisense oligonucleotides (ASOs) are artificially created short chains of nucleotides, usually consisting of 12–30 units. They are designed to match and bind to certain mRNA sequences, leading to the suppression of gene expression.127 ASOs may be used to regulate gene expression and show significant promise as a gene therapy tool for malignant tumors. However, ASOs are also subject to the intrinsic limitations of nucleic acid medicines. Specifically, unmodified ASOs undergo fast degradation in biological matrices and/or are eliminated by the kidneys due to their low cell membrane permeability.128 Therefore, it is essential to develop a highly effective ASO delivery system to overcome these constraints.
In their first investigation, Zhao et al. used a double-bundle DNA tetrahedron as a carrier for transmitting antisense oligonucleotides (ASOs) to suppress the protooncogene c-raf inside viable cells.135 The double-bundle DNA tetrahedron, in contrast to the standard one, can transmit a larger quantity of ASO, leading to an augmentation in the effective concentration. Furthermore, the ASO, which includes disulfide, was located on the inside surface of the double-stranded “DNA tetrahedron”, resulting in efficient protection against enzyme degradation. Furthermore, the double-stranded DNA tetrahedron was altered by including a “nuclear localization signal” (NLS) peptide to improve the specificity of the ASO's targeting. The results indicated that the ASO delivery strategy effectively reduced the levels of c-raf mRNA and protein expression. By using a double-bundle TDN, Zhao and colleagues can decrease the growth of cancer cells by delivering an antisense oligonucleotide (ASO) against the protooncogene c-raf.135 The disulfide link in the ASO will be broken and liberated from the TDN in a reducing environment. This will then target c-raf mRNA, which inhibits cell growth.
4.8 Hybrid delivery systems utilizing DNA tetrahedra
Hybrid drug delivery systems, consisting of TDNs and other nanomaterials, are frequently employed for the delivery of “anticancer medications”. Future applications that have the potential to combine the benefits of several nanomaterials could involve hybrid medication delivery systems.
4.8.1 High-molecular-weight polymers.
Yan et al.129 used redox-responsive “polyethyleneimine” (PEI) that has been cross-linked with disulfide bonds to coat DNA tetrahedrons loaded with DOX. This resulted in the formation of a new kind of nano-complex called PSP/TDNs@DOX NCs. The enzymatic degradation of PSP/TDNs@DOX NCs was reduced, resulting in enhanced stability of the serum. This enables DOX to penetrate cancer cells and tissues more effectively, hence conquering multidrug-resistant malignancy.
Wang et al.130 described the use of a combination of “cationic conjugated polymers” (CCPs) and “DNA tetrahedron” as a hybrid carrier for the simultaneous delivery of DOX and ASO (G3139) to specific targets. It was discovered that the combination of CCPs and DNA tetrahedron resulted in the formation of a distinct drug-carrying pocket, which facilitated the loading of DOX. The hybrid delivery method effectively administered the combined treatment of DOX and G3139, resulting in exceptional anticancer efficacy both in laboratory experiments and in vivo subjects.
4.8.2 Liposomes.
Shi et al.17 used pH-sensitive liposomes to encapsulate a DOX-loaded DNA tetrahedron to create a “liposome-based nanostructured drug” (LNSD) with programmable properties for the treatment of drug-resistant tumors. The LNSD demonstrated a notable ability to minimize DOX leakage during administration, resulting in a substantial enhancement in the effectiveness of anticancer treatment in mice with drug-resistant breast tumors, exceeding the results achieved with DOX and liposome-based DOX.
Ren et al. developed an intelligent nano-prodrug called Dox-MPK@MDL by sequentially constructing a dendrimer using a DNA tetrahedron, loading pH-sensitive Dox-MPK prodrugs, and coating liposomes and macrophage membranes. This was achieved by hierarchical self-assembly.39 The findings demonstrated that Dox-MPK@MDL selectively homed in on lung metastases of breast cancer by exploiting the biomimetic metastasis flocking effect (Fig. 4).
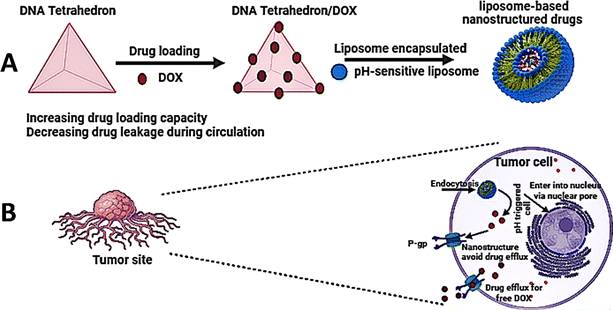 |
| Fig. 4 Schematic representation of the formation of pH-sensitive liposomes encapsulating nanostructured DOX (LNSD), highlighting its benefits in overcoming drug resistance in tumor treatment. (A) An LNSD is formed by loading DOX into transdermal patches (TD) to create small nanostructured DOX (TD/DOX), which is then encapsulated into pH-sensitive liposomes. (B) The resulting LNSD demonstrates enhanced cellular uptake of DOX, sustained drug concentration within resistant tumor cells, improved penetration into cell nuclei, and accumulation at tumor sites via the enhanced permeability and retention (EPR) effect of liposomes. Antitumor efficacy and reversal of DOX resistance were assessed using MCF-7/ADR cells and DOX-resistant breast tumor models. | |
4.8.3 Metal nanomaterials.
Willner et al. proposed hybrid nanostructures that combine DNA tetrahedra with metal–organic frameworks (MOFs), which can detect changes in pH and miRNA levels. The nanostructures were purposefully designed to facilitate the transportation of fluorescent dyes and chemotherapeutic drugs.131 The DNA tetrahedron gates played a crucial role in ensuring the security of the system, while the pH and miRNA-triggered reactions served as the mechanism for activating the hybrid system and releasing the therapeutic payload. This novel method merged the considerable drug-carrying capability of porous MOFs with the effective cell-penetrating characteristics of DNA tetrahedra. Further investigation conducted by Willner et al. examined hybrid nanostructures that are sensitive to ATP and VEGF.132
Meng et al. devised a nanoparticle system using “tetrahedral DNA nanostructures” (TDNs) and “gold nanoparticles (AuNPs)” to tailor the size for the treatment of deeply located cancers inside the body.133 This mechanism effectively disassembled and discharged DOX when the pH transitioned from normal tissues to the tumor microenvironment (TME) and cancerous intracellular regions. He et al.134 introduced a nanocomposite composed of a DNA tetrahedron containing DOX and “gold nanorods” (GNRs) for chemophotothermal therapy. The AS1411 aptamer-conjugated DNA tetrahedra, which contained DOX, were then condensed by “PEI-coated gold nanorods” (GNRs). This led to the acquisition of photothermal characteristics and enhanced ability to avoid the lysosomal compartment. The nanocomposite efficiently induced apoptosis and tissue necrosis in cancer cells upon exposure to “near-infrared (NIR) radiation”, demonstrating the significant synergistic effect of photothermal therapy and chemotherapy in eradicating cancer cells.
4.9 Gene silencing
Gene silencing is a technique used to control gene expression, which involves the use of several tools such as antisense oligonucleotides, ribozymes, and small interfering RNAs (siRNAs).135 SiRNAs, which are often used in genetic therapy, impede gene expression and protein synthesis by attaching to mRNA molecules that are complementary to them.136 Nevertheless, the fragility and vulnerability to deterioration of these substances impose restrictions on their use in living organisms, often necessitating the use of nanoparticles for transportation. Conventional nanomaterials have drawbacks such as toxicity and immunological reactions. However, the DNA tetrahedron has emerged as a biocompatible vector for gene silencing.137
Long et al.61 used a DNA tetrahedron to fabricate a siRNA delivery device aimed at suppressing the expression of genes in tumor cells. The technology enabled accurate manipulation of siRNA spatial orientation and cancer-targeting ligands.138 Nanostructures coupled with folic acid (FA) exhibited effective gene silencing, surpassing the limitations of siRNA, improving the effectiveness of RNA interference, and remaining stable in the bloodstream. This underscores the capacity of DNA tetrahedron structures to surmount obstacles related to siRNA transportation for biological purposes.139
5. Future directions and challenges in TDN-based cancer treatment
The future of TDN-based cancer treatment presents both challenges and promising avenues for advancement. Foremost among these challenges is the limited payload capacity of TDNs, particularly in delivering larger therapeutic molecules or combinations of drugs. Additionally, concerns persist regarding the biocompatibility and potential toxicity of TDNs, necessitating further investigation to ensure their safety for clinical use. Achieving efficient intracellular transport and precise targeting of cancer cells remain ongoing challenges crucial for optimizing therapeutic outcomes. Moreover, ensuring the stability and scalability of TDN synthesis and assembly processes is paramount for their practical application in cancer therapy on a larger scale. One major concern includes the fate of TDNs after entering the cells, the answer to which will revolutionize the field.
However, exciting opportunities exist for advancement in the field. Future research efforts could focus on enhancing the payload capacity of TDNs through innovative structural designs or the development of novel assembly techniques, thereby enabling the delivery of larger therapeutic cargoes or multiple drugs simultaneously. Advances in materials science and biotechnology may lead to the development of biocompatible TDNs with enhanced stability in biological environments, facilitating their translation into clinical practice. The enhanced stability will also help in reducing the clearance of TDNs from the kidneys in vivo. Incorporating advanced targeting ligands or functionalizing TDNs with stimuli-responsive elements could enable precise targeting of cancer cells, minimizing off-target effects and enhancing therapeutic efficacy. Integration of TDNs with emerging technologies such as precision medicine and gene editing techniques holds promise for personalized cancer treatments tailored to individual patient profiles, potentially leading to more effective and personalized therapeutic interventions. Moreover, addressing the regulatory challenges and streamlining the clinical translation process for TDN-based cancer therapies are essential steps towards realizing their full potential in clinical settings. Collaboration between researchers, clinicians, and regulatory agencies will be crucial in navigating these challenges and expediting the translation of TDN-based therapies from bench to bedside. By addressing these challenges and capitalizing on these potential advancements, TDN-based cancer treatment stands poised to revolutionize cancer therapy and improve patient outcomes.
6. Conclusions
Exploration of DNA nanostructures as drug carriers shows immense potential across a range of medical and pharmaceutical advancements. The major advantage of these structures is their availability for functional modifications. They can deliver a wide spectrum of compounds ranging from small molecules to nucleic acids into cells. These structures have promising applications in not only cancer immunotherapy but also autoimmune diseases. The ease of synthesis of these structures will help scale up the structures for translation into clinics. Despite challenges like improving production efficiency and overcoming biological barriers, progress is underway through molecular design and innovative applications. Ongoing efforts are expected to yield intelligent drug delivery systems with broader applications, making DNA nanostructures a promising player in advancing precise and efficient drug administration. This field represents a compelling avenue for future research, holding the promise of transformative developments in drug delivery systems.
Conflicts of interest
The authors have no conflict of interest to declare.
Acknowledgements
We sincerely thank all the members of the DB lab for their critical reading of the manuscript and their feedback. The work in the group is supported by SERB-DST, Gujcost, GSBTM, and MoES-STARS. PW thanks UGC for the research fellowship.
References
-
Cancer, S. N, https://www.cancer.gov/about-cancer/understanding/statistics, accessed 2024-02-13.
- H. Daraee, A. Etemadi, M. Kouhi, S. Alimirzalu and A. Akbarzadeh, Application of Liposomes in Medicine and Drug Delivery, Artif. Cells, Nanomed., Biotechnol., 2016, 44(1), 381–391, DOI:10.3109/21691401.2014.953633.
- Z. A. B. A. Aziz, A. Ahmad, S. H. Mohd-Setapar, H. Hassan, D. Lokhat, M. A. Kamal and G. Md. Ashraf, Recent Advances in Drug Delivery of Polymeric Nano-Micelles, Curr. Drug Metab., 2017, 18(1), 16–29, DOI:10.2174/1389200217666160921143616.
- D. Ma, Q.-M. Lin, L.-M. Zhang, Y.-Y. Liang and W. Xue, A Star-Shaped Porphyrin-Arginine Functionalized Poly(L-Lysine) Copolymer for Photo-Enhanced Drug and Gene Co-Delivery, Biomaterials, 2014, 35(14), 4357–4367, DOI:10.1016/j.biomaterials.2014.01.070.
- X. Xia, M. Yang, L. K. Oetjen, Y. Zhang, Q. Li, J. Chen and Y. Xia, An Enzyme-Sensitive Probe for Photoacoustic Imaging and Fluorescence Detection of Protease Activity, Nanoscale, 2011, 3(3), 950–953, 10.1039/c0nr00874e.
- S. N. Baker and G. A. Baker, Luminescent Carbon Nanodots: Emergent Nanolights, Angew Chem. Int. Ed. Engl., 2010, 49(38), 6726–6744, DOI:10.1002/anie.200906623.
- I. I. Slowing, J. L. Vivero-Escoto, C.-W. Wu and V. S.-Y. Lin, Mesoporous Silica Nanoparticles as Controlled Release Drug Delivery and Gene Transfection Carriers, Adv. Drug Delivery Rev., 2008, 60(11), 1278–1288, DOI:10.1016/j.addr.2008.03.012.
- D. Yang, Y. Tu, X. Wang, C. Cao, Y. Hu, J. Shao, L. Weng, X. Mou and X. Dong, A Photo-Triggered Antifungal Nanoplatform with Efflux Pump and Heat Shock Protein Reversal Activity for Enhanced Chemo-Photothermal Synergistic Therapy, Biomater. Sci., 2021, 9(9), 3293–3299, 10.1039/D1BM00457C.
- Y. Li, Z. Cai, W. Ma, L. Bai, E. Luo and Y. Lin, A DNA Tetrahedron-Based Ferroptosis-Suppressing Nanoparticle: Superior Delivery of Curcumin and Alleviation of Diabetic Osteoporosis, Bone Res., 2024, 12(1), 1–13, DOI:10.1038/s41413-024-00319-7.
- D. Bhatia, C. Wunder and L. Johannes, Self-Assembled, Programmable DNA Nanodevices for Biological and Biomedical Applications, ChemBioChem, 2021, 22(5), 763–778, DOI:10.1002/cbic.202000372.
- C. Guan, X. Zhu and C. Feng, DNA Nanodevice-Based Drug Delivery Systems, Biomolecules, 2021, 11(12), 1855, DOI:10.3390/biom11121855.
- T. Chen, L. Ren, X. Liu, M. Zhou, L. Li, J. Xu and X. Zhu, DNA Nanotechnology for Cancer Diagnosis and Therapy, Int. J. Mol. Sci., 2018, 19(6), 1671, DOI:10.3390/ijms19061671.
- R. P. Goodman, R. M. Berry and A. J. Turberfield, The Single-Step Synthesis of a DNA Tetrahedron, Chem. Commun., 2004,(12), 1372–1373, 10.1039/B402293A.
- Z. Chen, C. Liu, F. Cao, J. Ren and X. Qu, DNA Metallization: Principles, Methods, Structures, and Applications, Chem. Soc. Rev., 2018, 47(11), 4017–4072, 10.1039/C8CS00011E.
- A. Rajwar, S. R. Shetty, P. Vaswani, V. Morya, A. Barai, S. Sen, M. Sonawane and D. Bhatia, Geometry of a DNA Nanostructure Influences Its Endocytosis: Cellular Study on 2D, 3D, and in Vivo Systems, ACS Nano, 2022, 16(7), 10496–10508, DOI:10.1021/acsnano.2c01382.
- X. Peng, S. Fang, B. Ji, M. Li, J. Song, L. Qiu and W. Tan, DNA Nanostructure-Programmed Cell Entry via Corner Angle-Mediated Molecular Interaction with Membrane Receptors, Nano Lett., 2021, 21(16), 6946–6951, DOI:10.1021/acs.nanolett.1c02191.
- S. Shi, W. Fu, S. Lin, T. Tian, S. Li, X. Shao, Y. Zhang, T. Zhang, Z. Tang, Y. Zhou, Y. Lin and X. Cai, Targeted and Effective Glioblastoma Therapy via Aptamer-Modified Tetrahedral Framework Nucleic Acid-Paclitaxel Nanoconjugates That Can Pass the Blood–Brain Barrier, Nanomedicine, 2019, 21, 102061, DOI:10.1016/j.nano.2019.102061.
- R. P. Goodman, I. A. T. Schaap, C. F. Tardin, C. M. Erben, R. M. Berry, C. F. Schmidt and A. J. Turberfield, Rapid Chiral Assembly of Rigid DNA Building Blocks for Molecular Nanofabrication, Science, 2005, 310(5754), 1661–1665, DOI:10.1126/science.1120367.
- T. Tian, J. Li, C. Xie, Y. Sun, H. Lei, X. Liu, J. Xia, J. Shi, L. Wang, W. Lu and C. Fan, Targeted Imaging of Brain Tumors with a Framework Nucleic Acid Probe, ACS Appl. Mater. Interfaces, 2018, 10(4), 3414–3420, DOI:10.1021/acsami.7b17927.
- N. Oh and J.-H. Park, Endocytosis and Exocytosis of Nanoparticles in Mammalian Cells, Int. J. Nanomed., 2014, 9(Suppl 1), 51–63, DOI:10.2147/IJN.S26592.
- K.-R. Kim, D.-R. Kim, T. Lee, J. Y. Yhee, B.-S. Kim, I. C. Kwon and D.-R. Ahn, Drug Delivery by a Self-Assembled DNA Tetrahedron for Overcoming Drug Resistance in Breast Cancer Cells, Chem. Commun., 2013, 49(20), 2010–2012, 10.1039/C3CC38693G.
- P. Charoenphol and H. Bermudez, Aptamer-Targeted DNA Nanostructures for Therapeutic Delivery, Mol. Pharm., 2014, 11(5), 1721–1725, DOI:10.1021/mp500047b.
- C. M. Erben, R. P. Goodman and A. J. Turberfield, Single-Molecule Protein Encapsulation in a Rigid DNA Cage, Angew Chem. Int. Ed. Engl., 2006, 45(44), 7414–7417, DOI:10.1002/anie.200603392.
- H. Lee, A. K. R. Lytton-Jean, Y. Chen, K. T. Love, A. I. Park, E. D. Karagiannis, A. Sehgal, W. Querbes, C. S. Zurenko, M. Jayaraman, C. G. Peng, K. Charisse, A. Borodovsky, M. Manoharan, J. S. Donahoe, J. Truelove, M. Nahrendorf, R. Langer and D. G. Anderson, Molecularly Self-Assembled Nucleic Acid Nanoparticles for Targeted in Vivo siRNA Delivery, Nat. Nanotechnol., 2012, 7(6), 389–393, DOI:10.1038/nnano.2012.73.
- H. B. D. Thai, K.-R. Kim, K. T. Hong, T. Voitsitskyi, J.-S. Lee, C. Mao and D.-R. Ahn, Kidney-Targeted Cytosolic Delivery of siRNA Using a Small-Sized Mirror DNA Tetrahedron for Enhanced Potency, ACS Cent. Sci., 2020, 6(12), 2250–2258, DOI:10.1021/acscentsci.0c00763.
- X. Xie, X. Shao, W. Ma, D. Zhao, S. Shi, Q. Li and Y. Lin, Overcoming Drug-Resistant Lung Cancer by Paclitaxel Loaded Tetrahedral DNA Nanostructures, Nanoscale, 2018, 10(12), 5457–5465, 10.1039/C7NR09692E.
- T. Wu, J. Liu, M. Liu, S. Liu, S. Zhao, R. Tian, D. Wei, Y. Liu, Y. Zhao, H. Xiao and B. Ding, A Nanobody-Conjugated DNA Nanoplatform for Targeted Platinum-Drug Delivery, Angew Chem. Int. Ed. Engl., 2019, 58(40), 14224–14228, DOI:10.1002/anie.201909345.
- Q. Mou, Y. Ma, G. Pan, B. Xue, D. Yan, C. Zhang and X. Zhu, DNA Trojan Horses: Self-Assembled Floxuridine-Containing DNA Polyhedra for Cancer Therapy, Angew Chem. Int. Ed. Engl., 2017, 56(41), 12528–12532, DOI:10.1002/anie.201706301.
- K.-R. Kim, D. Bang and D.-R. Ahn, Nano-Formulation of a Photosensitizer Using a DNA Tetrahedron and Its Potential for in Vivo Photodynamic Therapy, Biomater. Sci., 2016, 4(4), 605–609, 10.1039/C5BM00467E.
- Z. Liu, H. Pei, L. Zhang and Y. Tian, Mitochondria-Targeted DNA Nanoprobe for Real-Time Imaging and Simultaneous Quantification of Ca2+ and pH in Neurons, ACS Nano, 2018, 12(12), 12357–12368, DOI:10.1021/acsnano.8b06322.
- M. I. Setyawati, R. V. Kutty and D. T. Leong, DNA Nanostructures Carrying Stoichiometrically Definable Antibodies, Small, 2016, 12(40), 5601–5611, DOI:10.1002/smll.201601669.
- X. Liu, Y. Xu, T. Yu, C. Clifford, Y. Liu, H. Yan and Y. Chang, A DNA Nanostructure Platform for Directed Assembly of Synthetic Vaccines, Nano Lett., 2012, 12(8), 4254–4259, DOI:10.1021/nl301877k.
- N. Y. Wong, C. Zhang, L. H. Tan and Y. Lu, Site-Specific Attachment of Proteins onto a 3D DNA Tetrahedron through Backbone-Modified Phosphorothioate DNA, Small, 2011, 7(10), 1427–1430, DOI:10.1002/smll.201100140.
- J. Yan, J. Chen, N. Zhang, Y. Yang, W. Zhu, L. Li and B. He, Mitochondria-Targeted Tetrahedral DNA Nanostructures for Doxorubicin Delivery and Enhancement of Apoptosis, J. Mater. Chem. B, 2020, 8(3), 492–503, 10.1039/C9TB02266J.
- T. Wu, Q. Liu, Y. Cao, R. Tian, J. Liu and B. Ding, Multifunctional Double-Bundle DNA Tetrahedron for Efficient Regulation of Gene Expression, ACS Appl. Mater. Interfaces, 2020, 12(29), 32461–32467, DOI:10.1021/acsami.0c08886.
- Z. Xia, P. Wang, X. Liu, T. Liu, Y. Yan, J. Yan, J. Zhong, G. Sun and D. He, Tumor-Penetrating Peptide-Modified DNA Tetrahedron for Targeting Drug Delivery, Biochemistry, 2016, 55(9), 1326–1331, DOI:10.1021/acs.biochem.5b01181.
- K.-R. Kim, T. Lee, B.-S. Kim and D.-R. Ahn, Utilizing the Bioorthogonal Base-Pairing System of L-DNA to Design Ideal DNA Nanocarriers for Enhanced Delivery of Nucleic Acid Cargos, Chem. Sci., 2014, 5(4), 1533–1537, 10.1039/C3SC52601A.
- M. Liu, W. Ma, Q. Li, D. Zhao, X. Shao, Q. Huang, L. Hao and Y. Lin, Aptamer-Targeted DNA Nanostructures with Doxorubicin to Treat Protein Tyrosine Kinase 7-Positive Tumours, Cell Proliferation, 2019, 52(1), e12511, DOI:10.1111/cpr.12511.
- T. Ren, Z. Deng, H. Liu, X. Li, J. Li, J. Yuan, Y. He, Q. Liu, Y. Yang and S. Zhong, Co-Delivery of DNAzyme and a Chemotherapy Drug Using a DNA Tetrahedron for Enhanced Anticancer Therapy through Synergistic Effects, New J. Chem., 2019, 43(35), 14020–14027, 10.1039/C9NJ02818H.
- R. Schlapak, J. Danzberger, D. Armitage, D. Morgan, A. Ebner, P. Hinterdorfer, P. Pollheimer, H. J. Gruber, F. Schäffler and S. Howorka, Nanoscale DNA Tetrahedra Improve Biomolecular Recognition on Patterned Surfaces, Small, 2012, 8(1), 89–97, DOI:10.1002/smll.201101576.
- Z. Wang, Q. Xue, W. Tian, L. Wang and W. Jiang, Quantitative Detection of Single DNA Molecules on DNA Tetrahedron Decorated Substrates, Chem. Commun., 2012, 48(77), 9661–9663, 10.1039/C2CC35208G.
- Y. Zhan, W. Ma, Y. Zhang, C. Mao, X. Shao, X. Xie, F. Wang, X. Liu, Q. Li and Y. Lin, DNA-Based Nanomedicine with Targeting and Enhancement of Therapeutic Efficacy of Breast Cancer Cells, ACS Appl. Mater. Interfaces, 2019, 11(17), 15354–15365, DOI:10.1021/acsami.9b03449.
- S. Soundararajan, W. Chen, E. K. Spicer, N. Courtenay-Luck and D. J. Fernandes, The Nucleolin Targeting Aptamer AS1411 Destabilizes Bcl-2 Messenger RNA in Human Breast Cancer Cells, Cancer Res., 2008, 68(7), 2358–2365, DOI:10.1158/0008-5472.CAN-07-5723.
- E. M. Reyes-Reyes, Y. Teng and P. J. Bates, A New Paradigm for Aptamer Therapeutic AS1411 Action: Uptake by Macropinocytosis and Its Stimulation by a Nucleolin-Dependent Mechanism, Cancer Res., 2010, 70(21), 8617–8629, DOI:10.1158/0008-5472.CAN-10-0920.
- Y. Ding, X. Liu, J. Zhu, L. Wang and W. Jiang, Quantitative Single-Molecule Detection of Protein Based on DNA Tetrahedron Fluorescent Nanolabels, Talanta, 2014, 125, 393–399, DOI:10.1016/j.talanta.2014.03.032.
- H. Ozhalici-Unal and B. A. Armitage, Fluorescent DNA Nanotags Based on a Self-Assembled DNA Tetrahedron, ACS Nano, 2009, 3(2), 425–433, DOI:10.1021/nn800727x.
- C. Zhang, X. Li, C. Tian, G. Yu, Y. Li, W. Jiang and C. Mao, DNA Nanocages Swallow Gold Nanoparticles (AuNPs) to Form AuNP@DNA Cage Core-Shell Structures, ACS Nano, 2014, 8(2), 1130–1135, DOI:10.1021/nn406039p.
- Z. Xiao, C. Ji, J. Shi, E. M. Pridgen, J. Frieder, J. Wu and O. C. Farokhzad, DNA Self-Assembly of Targeted near-Infrared-Responsive Gold Nanoparticles for Cancer Thermo-Chemotherapy, Angew Chem. Int. Ed. Engl., 2012, 51(47), 11853–11857, DOI:10.1002/anie.201204018.
- J. Zhang, Y. Guo, F. Ding, G. Pan, X. Zhu and C. Zhang, A Camptothecin-Grafted DNA Tetrahedron as a Precise Nanomedicine to Inhibit Tumor Growth, Angew Chem. Int. Ed. Engl., 2019, 58(39), 13794–13798, DOI:10.1002/anie.201907380.
- J. Yan, X. Zhan, Z. Zhang, K. Chen, M. Wang, Y. Sun, B. He and Y. Liang, Tetrahedral DNA Nanostructures for Effective Treatment of Cancer: Advances and Prospects, J. Nanobiotechnol., 2021, 19(1), 412, DOI:10.1186/s12951-021-01164-0.
- S. E. A. Gratton, P. A. Ropp, P. D. Pohlhaus, J. C. Luft, V. J. Madden, M. E. Napier and J. M. DeSimone, The Effect of Particle Design on Cellular Internalization Pathways, Proc. Natl. Acad. Sci. U. S. A., 2008, 105(33), 11613–11618, DOI:10.1073/pnas.0801763105.
- M. L. J. Carter, D. A. Rusling, S. Gurr, T. Brown and K. R. Fox, Stability of the Different Arms of a DNA Tetrahedron and Its Interaction with a Minor Groove Ligand, Biophys. Chem., 2020, 256, 106270, DOI:10.1016/j.bpc.2019.106270.
- C. Tuerk and L. Gold, Systematic Evolution of Ligands by Exponential Enrichment: RNA Ligands to Bacteriophage T4 DNA Polymerase, Science, 1990, 249(4968), 505–510, DOI:10.1126/science.2200121.
- H. Kaur, J. G. Bruno, A. Kumar and T. K. Sharma, Aptamers in the Therapeutics and Diagnostics Pipelines, Theranostics, 2018, 8(15), 4016–4032, DOI:10.7150/thno.25958.
- G. Zhu, H. Zhang, O. Jacobson, Z. Wang, H. Chen, X. Yang, G. Niu and X. Chen, Combinatorial Screening of DNA Aptamers for Molecular Imaging of HER2 in Cancer, Bioconjugate Chem., 2017, 28(4), 1068–1075, DOI:10.1021/acs.bioconjchem.6b00746.
- R. Duangrat, A. Udomprasert and T. Kangsamaksin, Tetrahedral DNA Nanostructures as Drug Delivery and Bioimaging Platforms in Cancer Therapy, Cancer Sci., 2020, 111(9), 3164–3173, DOI:10.1111/cas.14548.
- J. L. Nitiss, Targeting DNA Topoisomerase II in Cancer Chemotherapy, Nat. Rev. Cancer, 2009, 9(5), 338–350, DOI:10.1038/nrc2607.
- R. L. Momparler, M. Karon, S. E. Siegel and F. Avila, Effect of Adriamycin on DNA, RNA, and Protein Synthesis in Cell-Free Systems and Intact Cells, Cancer Res., 1976, 36(8), 2891–2895 CAS.
- C. Carvalho, R. X. Santos, S. Cardoso, S. Correia, P. J. Oliveira, M. S. Santos and P. I. Moreira, Doxorubicin: The Good, the Bad and the Ugly Effect, Curr. Med. Chem., 2009, 16(25), 3267–3285, DOI:10.2174/092986709788803312.
- Y. Chen, Y. Song, W. Du, L. Gong, H. Chang and Z. Zou, Tumor-Associated Macrophages: An Accomplice in Solid Tumor Progression, J. Biomed. Sci., 2019, 26(1), 78, DOI:10.1186/s12929-019-0568-z.
- Q. Long, X. Tian, H. Wang, N. Zhang, T. Han, Z. Li and S. Jiang, Applications of DNA Tetrahedron Nanostructure in Cancer Diagnosis and Anticancer Drugs Delivery, Nanotechnol. Rev., 2023, 12(1), 20220553, DOI:10.1515/ntrev-2022-0553.
- G. L. Zwicke, G. A. Mansoori and C. J. Jeffery, Utilizing the Folate Receptor for Active Targeting of Cancer Nanotherapeutics, Nano Rev., 2012, 3, 18496, DOI:10.3402/nano.v3i0.18496.
- N. Khaiwa, N. R. Maarouf, M. H. Darwish, D. W. M. Alhamad, A. Sebastian, M. Hamad, H. A. Omar, G. Orive and T. H. Al-Tel, Camptothecin's Journey from Discovery to WHO Essential Medicine: Fifty Years of Promise, Eur. J. Med. Chem., 2021, 223, 113639, DOI:10.1016/j.ejmech.2021.113639.
- E. K. Rowinsky and R. C. Donehower, Paclitaxel (Taxol), N. Engl. J. Med., 1995, 332(15), 1004–1014, DOI:10.1056/NEJM199504133321507.
- A. Karachi, F. Dastmalchi, D. A. Mitchell and M. Rahman, Temozolomide for Immunomodulation in the Treatment of Glioblastoma, Neuro-Oncology, 2018, 20(12), 1566–1572, DOI:10.1093/neuonc/noy072.
- W. Fu, C. You, L. Ma, H. Li, Y. Ju, X. Guo, S. Shi, T. Zhang, R. Zhou and Y. Lin, Enhanced Efficacy of Temozolomide Loaded by a Tetrahedral Framework DNA Nanoparticle in the Therapy for Glioblastoma, ACS Appl. Mater. Interfaces, 2019, 11(43), 39525–39533, DOI:10.1021/acsami.9b13829.
- A. E. Prota, K. Bargsten, J. F. Diaz, M. Marsh, C. Cuevas, M. Liniger, C. Neuhaus, J. M. Andreu, K.-H. Altmann and M. O. Steinmetz, A New Tubulin-Binding Site and Pharmacophore for Microtubule-Destabilizing Anticancer Drugs, Proc. Natl. Acad. Sci. U. S. A., 2014, 111(38), 13817–13821, DOI:10.1073/pnas.1408124111.
- M. Lopus, Antibody-DM1 Conjugates as Cancer Therapeutics, Cancer Lett., 2011, 307(2), 113–118, DOI:10.1016/j.canlet.2011.03.017.
- W. Ma, Y. Yang, J. Zhu, W. Jia, T. Zhang, Z. Liu, X. Chen and Y. Lin, Biomimetic Nanoerythrosome-Coated Aptamer–DNA Tetrahedron/Maytansine Conjugates: pH-Responsive and Targeted Cytotoxicity for HER2-Positive Breast Cancer, Adv. Mater., 2022, 34(46), 2109609, DOI:10.1002/adma.202109609.
- D. B. Longley, D. P. Harkin and P. G. Johnston, 5-Fluorouracil: Mechanisms of Action and Clinical Strategies, Nat. Rev. Cancer, 2003, 3(5), 330–338, DOI:10.1038/nrc1074.
- A. F. Jorge, A. Aviñó, A. A. C. C. Pais, R. Eritja and C. Fàbrega, DNA-Based Nanoscaffolds as Vehicles for 5-Fluoro-2′-Deoxyuridine Oligomers in Colorectal Cancer Therapy, Nanoscale, 2018, 10(15), 7238–7249, 10.1039/C7NR08442K.
- J. A. van Laar, Y. M. Rustum, S. P. Ackland, C. J. van Groeningen and G. J. Peters, Comparison of 5-Fluoro-2’-Deoxyuridine with 5-Fluorouracil and Their Role in the Treatment of Colorectal Cancer, Eur. J. Cancer, 1998, 34(3), 296–306, DOI:10.1016/s0959-8049(97)00366-3.
- W. Tang, L. Han, S. Duan, X. Lu, Y. Wang, X. Wu, J. Liu and B. Ding, An Aptamer-Modified DNA Tetrahedron-Based Nanogel for Combined Chemo/Gene Therapy of Multidrug-Resistant Tumors, ACS Appl. Bio Mater., 2021, 4(10), 7701–7707, DOI:10.1021/acsabm.1c00933.
- H. Sun, T. Wang, W. Ma, J. Huang, B. Chen, H. Cheng, S. Duan, X. He, L. Jian and K. Wang, A Stable DNA Tetrahedra-AuNCs Nanohybrid: On-Site Programmed Disassembly for Tumor Imaging and Combination Therapy, Biomaterials, 2022, 288, 121738, DOI:10.1016/j.biomaterials.2022.121738.
- Q. Wang, J. Cheng, F. Liu, J. Zhu, Y. Li, Y. Zhao, X. Li, H. Zhang, Y. Ju, L. Ma, X. Hui and Y. Lin, Modulation of Cerebrospinal Fluid Dysregulation via a SPAK and OSR1 Targeted Framework Nucleic Acid in Hydrocephalus, Adv. Sci., 2024, 1, 2306622, DOI:10.1002/advs.202306622.
- D. Salas-Benito, J. L. Pérez-Gracia, M. Ponz-Sarvisé, M. E. Rodriguez- Ruiz, I. Martínez-Forero and E. Castañón, Paradigms on Immunotherapy Combinations with Chemotherapy, Cancer Discovery, 2021, 11(6), 1353–1367 CrossRef CAS.
- Q. Wang, Y. Ma, Z. Lu, H. Yu and Z. Li, Co-Delivery of Chemotherapeutic Drugs and Immune Adjuvants by Nanoscale DNA Tetrahedrons for Synergistic Cancer Therapy, ACS Appl. Nano Mater., 2022, 5(1), 101–116 CrossRef CAS.
- F. Shen, L. Sun, L. Wang, R. Peng, C. Fan and Z. Liu, Framework nucleic acid immune adjuvant for transdermal delivery based chemoimmunotherapy for malignant melanoma treatment, Nano Lett., 2022, 22(11), 4509–4518 CrossRef CAS.
- M. Liu, L. Hao, D. Zhao, J. Li and Y. Lin, Self-Assembled Immunostimulatory Tetrahedral Framework Nucleic Acid Vehicles for Tumor Chemo-Immunotherapy, ACS Appl. Mater. Interfaces, 2022, 14(34), 38506–38514 CrossRef CAS.
- L. Cassetta and J. W. Pollard, Tumor-associated macrophages, Curr. Biol., 2020, 30(6), 246–248 CrossRef.
- M. Locati, G. Curtale and A. Mantovani, Diversity, Mechanisms, and Significance of Macrophage Plasticity, Annu. Rev. Phytopathol., 2020, 15(1), 123–147 CrossRef CAS.
- A. Shapouri-Moghaddam, S. Mohammadian, H. Vazini, M. Taghadosi, S.-A. Esmaeili, F. Mardani, B. Seifi, A. Mohammadi, J. T. Afshari and A. Sahebkar, Macrophage Plasticity, Polarization, and Function in Health and Disease, J. Cell. Physiol., 2018, 233(9), 6425–6440 CrossRef CAS.
- J. Cheng, S. Wang, Q. Min, J. Song and Y. Tian, Reconstructed Adoptivemacrophages with DNA-Tetrahedron-CpG/siRNA for Synergistic Solid Tumor Immunotherapy, Colloids Surf., A, 2022, 637, 128184 CrossRef CAS.
- H. Qian, T. Zhou, Y. Fu, M. Guo, W. Yang and D. Zhang, Selfassembled Tetrahedral Framework Nucleic Acid Mediates Tumorassociated Macrophage Reprogramming and Restores Antitumor Immunity, Mol. Ther.–Nucleic Acids, 2022, 27, 763–773 CrossRef CAS.
- T. A. Debele, S. Peng and H. C. Tsai, Drug Carrier for Photodynamic Cancer Therapy, Int. J. Mol. Sci., 2015, 16, 22094–22136 CrossRef CAS.
- B. Obstoy, M. Salaun, P. Bohn, L. Veresezan, R. Sesboue and L. Thiberville, Photodynamic Therapy Using Methylene Blue in Lung Adenocarcinoma Xenograft and Hamster Cheek Pouch Induced Squamous Cell Carcinoma, Photodiagn. Photodyn. Ther., 2016, 15, 109–114 CrossRef CAS.
- M. Barathan, V. Mariappan, E. M. Shankar, B. J. Abdullah, K. L. Goh and J. Vadivelu, Hypericin-Photodynamic Therapy Leads to Interleukin-6 Secretion by HepG2 Cells and Their Apoptosis via Recruitment of BH3 Interacting-Domain Death Agonist and Caspases, Cell Death Dis., 2013, 4, e697 CrossRef CAS.
- P. M. Tang, X. Z. Liu, D. M. Zhang, W. P. Fong and K. P. Fung, Pheophorbide a Based Photodynamic Therapy Induces Apoptosis via Mitochondrial-Mediated Pathway in Human Uterine Carcinosarcoma, Cancer Biol. Ther., 2009, 8, 533–539 CrossRef CAS.
- M. Z. Quazi and N. Park, DNA Hydrogel-Based Nanocomplexes with Cancer-Targeted Delivery and Light-Triggered Peptide Drug Release for Cancer-Specific Therapeutics, Biomacromolecules, 2023, 24(5), 2127–2137, DOI:10.1021/acs.biomac.3c00021.
- D. Xiao, Y. Li, T. Tian, T. Zhang, S. Shi, B. Lu, Y. Gao, X. Qin, M. Zhang, W. Wei and Y. Lin, Tetrahedral Framework Nucleic Acids Loaded with Aptamer AS1411 for siRNA Delivery and Gene Silencing in Malignant Melanoma, ACS Appl. Mater. Interfaces, 2021, 13(5), 6109–6118, DOI:10.1021/acsami.0c23005.
- L. Chen, J. Zhang, Z. Lin, Z. Zhang, M. Mao, J. Wu, Q. Li, Y. Zhang and C. Fan, Pharmaceutical Applications of Framework Nucleic Acids, Acta Pharm. Sin. B, 2022, 12(1), 76–91, DOI:10.1016/j.apsb.2021.05.022.
- Y. Guo, Q. Zhang, Q. Zhu, J. Gao, X. Zhu, H. Yu, Y. Li and C. Zhang, Copackaging Photosensitizer and PD-L1 siRNA in a Nucleic Acid Nanogel for Synergistic Cancer Photoimmunotherapy, Sci. Adv., 2022, 8(16), 2941 CrossRef.
- E. Latz, A. Verma, A. Visintin, M. Gong, C. M. Sirois, D. C. G. Klein, B. G. Monks, C. J. McKnight, M. S. Lamphier, W. P. Duprex, T. Espevik and D. T. Golenbock, Ligand-Induced Conformational Changes Allosterically Activate Toll-Like, Nat. Immunol., 2007, 8(1), 772–779 CrossRef CAS.
- A. M. Krieg, CpG Motifs in Bacterial DNA and Their Immune Effects, Annu. Rev. Immunol., 2002, 20, 709–760 CrossRef CAS.
- D. M. Klinman, Immunotherapeutic Uses of CpG Oligodeoxynucleotides, Nat. Rev. Immunol., 2004, 4(4), 249–258, DOI:10.1038/nri1329.
- X. W. Liu, Y. Xu, T. Yu, C. Clifford, Y. Liu, H. Yan and Y. Chang, A DNA Nanostructure Platform for Directed Assembly of Synthetic Vaccines, Nano Lett., 2012, 12, 4254–4259 CrossRef CAS.
- J. Li, H. Pei, B. Zhu, L. Liang, M. Wei, Y. He, N. Chen, D. Li, Q. Huang and C. Fan, Self-Assembled Multivalent DNA Nanostructures for Noninvasive Intracellular Delivery of Immunostimulatory CpG Oligonucleotides, ACS Nano, 2011, 5, 8783–8789 CrossRef CAS.
- T. Jiang, S. Shen, T. Wang, M. Li, B. He and R. Mo, A Substrate-Selective Enzyme-Catalysis Assembly Strategy for Oligopeptide Hydrogelassisted Combinatorial Protein Delivery, Nano Lett., 2017, 17(12), 7447–7454 CrossRef CAS.
- Y. Jiang, J. Zhang, F. Meng and Z. Zhong, Apolipoprotein E Peptidedirected Chimeric Polymersomes Mediate an Ultrahigh-Efficiency Targeted Protein Therapy for Glioblastoma, ACS Nano, 2018, 12(11), 11070–11079 CrossRef CAS.
- B. Leader, Q. J. Baca and D. E. Golan, Protein Therapeutics:
A Summary and Pharmacological Classification, Nat. Rev. Drug Discovery, 2008, 7(1), 21–39 CrossRef CAS.
- S. M. Douglas, I. Bachelet and G. M. Church, A Logic-Gated Nanorobot for Targeted Transport of Molecular Payloads, Science, 2012, 335(6070), 831–834 CrossRef CAS.
- S. Frokjaer and D. E. Otzen, Protein drug stability: a formulation challenge, Nat. Rev. Drug Discovery, 2005, 4(4), 298–306 CrossRef CAS.
- Y. Li, X. Zhang, X. Wan, X. Liu, W. Pan, N. Li and B. Tang, Inducing Endoplasmic Reticulum Stress to Expose Immunogens: A DNA Tetrahedron Nanoregulator for Enhanced Immunotherapy, Adv. Funct. Mater., 2020, 30(48), 2000532, DOI:10.1002/adfm.202000532.
- D. Li, X. Li, F. Yang, R. Yuan and Y. Xiang, Targeted Delivery of DNA Framework-Encapsulated Native Therapeutic Protein into Cancer Cells, ACS Appl. Mater. Interfaces, 2020, 12(49), 54489–54496 CrossRef CAS.
- G. Kong, M. Zhang, M. Xiong, M. Chen, C. Yang and Y. Zhao, Framework Nucleic Acid-Based Confined Enzyme Cascade for Efficient Synergistic Cancer Therapy in Vivo, Sci. China: Chem., 2021, 64(4), 660–665 CrossRef CAS.
- T. Tian, D. Xiao, T. Zhang, Y. Li, S. Shi and W. A. Zhong, Framework Nucleic Acid Based Robotic Nanobee for Active Targeting Therapy, Adv. Funct. Mater., 2021, 31(5), 2007342 CrossRef CAS.
- Z. Dongye, J. Li and Y. Wu, Toll-like Receptor 9 Agonists and Combination Therapies: Strategies to Modulate the Tumour Immune Microenvironment for Systemic Anti-Tumour Immunity, Br. J. Cancer, 2022, 127(9), 1584–1594, DOI:10.1038/s41416-022-01876-6.
- J. Zhou and G.-M. Deng, The Role of Bacterial DNA Containing CpG Motifs in Diseases, J. Leukocyte Biol., 2021, 109(5), 991–998 CrossRef CAS.
- S. Federico, L. Pozzetti, A. Papa, G. Carullo, S. Gemma, S. Butini, G. Campiani and N. Relitti, Modulation of the Innate Immune Response by Targeting Toll-like Receptors: A Perspective on Their Agonists and Antagonists, J. Med. Chem., 2020, 63(22), 13466–13513, DOI:10.1021/acs.jmedchem.0c01049.
- W. Chen, M. Jiang, W. Yu, Z. Xu, X. Liu and Q. Jia, CpG-Based Nanovaccines for Cancer Immunotherapy, Int. J. Nanomed., 2021, 16, 5281–5299 CrossRef.
- E. Bernstein, A. A. Caudy, S. M. Hammond and G. J. Hannon, Role for a Bidentate Ribonuclease in the Initiation Step of RNA Interference, Nature, 2001, 409(6818), 363–366 CrossRef CAS.
- G. J. Hannon, RNA interference, Nature, 2002, 418(6894), 244–251 CrossRef CAS.
- M. Jinek and J. A. Doudna, A Three-Dimensional View of the Molecular Machinery of RNA Interference, Nature, 2009, 457(7228), 405–412 CrossRef CAS.
- D. M. Dykxhoorn, C. D. Novina and P. A. Sharp, Killing the messenger: short RNAs that silence gene expression, Nat. Rev. Mol. Cell Biol., 2003, 4(6), 457–467 CrossRef CAS.
- Y. Zhou, Q. Yang, F. Wang, Z. Zhou, J. Xu and S. Cheng, Selfassembled DNA Nanostructure as a Carrier for Targeted siRNA Delivery in Glioma Cells, Int. J. Nanomed., 2021, 16, 1805–1817 CrossRef.
- X. Han, X. Xu, Z. Wu, Z. Wu and X. Qi, Synchronous Conjugation of I-Motif DNA and Therapeutic siRNA on the Vertexes of Tetrahedral DNA Nanocages for Efficient Gene Silence, Acta Pharm. Sin. B, 2021, 11(10), 3286–3296 CrossRef CAS.
- G. Song, H. Dong, D. Ma, H. Wang, X. Ren, Y. Qu, H. Wu, J. Zhu, W. Song, Y. Meng, L. Wang, T. Liu, X. Shen, Y. Zhao and C. Zhu, Tetrahedral Framework Nucleic Acid Delivered RNA Therapeutics Significantly Attenuate Pancreatic Cancer Progression via Inhibition of CTR1-Dependent Copper Absorption, ACS Appl. Mater. Interfaces, 2021, 13(39), 46334–46342, DOI:10.1021/acsami.1c13091.
- W. Guo, H. Gao, H. Li, S. Ge, F. Zhang, L. Wang, H. Shi and A. Han, Self-Assembly of a Multifunction DNA Tetrahedron for Effective Delivery of Aptamer PL1 and Pcsk9 siRNA Potentiate Immune Checkpoint Therapy for Colorectal Cancer, ACS Appl. Mater. Interfaces, 2022, 14(28), 31634–31644, DOI:10.1021/acsami.2c06001.
- K.-R. Kim, H. Jegal, J. Kim and D.-R. Ahn, A Self-Assembled DNA Tetrahedron as a Carrier for in Vivo Liver-Specific Delivery of siRNA, Biomater. Sci., 2020, 8(2), 586–590 RSC.
- Y. Gao, X. Chen, T. Tian, T. Zhang, S. Gao, X. Zhang, Y. Yao, Y. Lin and X. Cai, A Lysosome-Activated Tetrahedral Nanobox for Encapsulated siRNA Delivery, Adv. Mater., 2022, 34(46), e2201731, DOI:10.1002/adma.202201731.
- H. Xue, F. Ding, J. Zhang, Y. Guo, X. Gao, J. Feng, X. Zhu and C. Zhang, DNA Tetrahedron-Based Nanogels for siRNA Delivery and Gene Silencing, Chem. Commun., 2019, 55(29), 4222–4225, 10.1039/c9cc00175a.
- D. Morrison, M. Rothenbroker and Y. Li, DNAzymes: Selected for Applications, Small Methods, 2018, 2(3), 1700319 CrossRef.
- S. Khan, B. Burciu, C. D. M. Filipe, Y. Li, K. Dellinger and T. F. Didar, DNAzymebased Biosensors: Immobilization Strategies, Applications, and Future Prospective, ACS Nano, 2021, 15(9), 13943–13969 CrossRef CAS.
- L. Meng, W. Ma, S. Lin, S. Shi, Y. Li and Y. Lin, Tetrahedral DNA Nanostructure- Delivered DNAzyme for Gene Silencing to Suppress Cell Growth, ACS Appl. Mater. Interfaces, 2019, 11, 6850–6857 CrossRef CAS.
- L. Gong, Z. Zhao and Y.-F. Lv, DNAzyme-Based Biosensors and Nanodevices, Chem. Commun., 2015, 51, 979–995 RSC.
- C. W. S. Chan and L. M. Khachigian, DNAzymes and Their Therapeutic Possibilities, Intern. Med. J., 2009, 39(4), 249–251, DOI:10.1111/j.1445-5994.2009.01799.x.
- C. F. Bennett, Therapeutic Antisense Oligonucleotides Are Coming of Age, Annu. Rev. Med., 2019, 70(1), 307–321 CrossRef CAS.
- E. C. Kuijper, A. J. Bergsma, W. W. M. P. Pijnappel and A. Aartsma-Rus, Opportunities and Challenges for Antisense Oligonucleotide Therapies, J. Inherited Metab. Dis., 2021, 44(1), 72–87 CrossRef CAS.
- J. Yan, N. Zhang, Z. Zhang, W. Zhu, B. Li, L. Li, Y. Pu and B. He, Redox-Responsive Polyethyleneimine/Tetrahedron DNA/Doxorubicin Nanocomplexes for Deep Cell/Tissue Penetration to Overcome Multidrug Resistance, J. Controlled Release, 2021, 329, 36–49, DOI:10.1016/j.jconrel.2020.11.050.
- C. Wang, Y. Yu, M. Irfan, B. Xu, J. Li, L. Zhang, Z. Qin, C. Yu, H. Liu and X. Su, Rational Design of DNA Framework-Based Hybrid Nanomaterials for Anticancer Drug Delivery, Small, 2020, 16(44), e2002578, DOI:10.1002/smll.202002578.
- P. Zhang, Y. Ouyang, Y. S. Sohn, R. Nechushtai, E. Pikarsky, C. Fan and I. Willner, pH- and miRNA-Responsive DNA-Tetrahedra/Metal-Organic Framework Conjugates: Functional Sense-and-Treat Carriers, ACS Nano, 2021, 15(4), 6645–6657, DOI:10.1021/acsnano.0c09996.
- P. Zhang, A. Fischer, Y. Ouyang, J. Wang, Y. S. Sohn, R. Nechushtai, E. Pikarsky, C. Fan and I. Willner, Aptamer-Modified DNA Tetrahedra-Gated Metal–Organic Framework Nanoparticle Carriers for Enhanced Chemotherapy or Photodynamic Therapy, Chem. Sci., 2021, 12(43), 14473–14483, 10.1039/D1SC04229G.
- D. Gu, Y. Qiao, H. Fu, H. Zhao, X. Yue, S. Wang, Y. Yin, R. Xi, X. Fu, X. Zhao and M. Meng, Size-Controllable DNA Origami-Stacked Gold Nanoparticles for Deep Tumor-Penetrating Therapy, ACS Appl. Mater. Interfaces, 2022, 14(33), 38048–38055, DOI:10.1021/acsami.2c05750.
- Z. He, Q. Wang, N. Zhang, J. Yan, L. Li, J. Cao and B. He, Gold Nanorods/Tetrahedral DNA Composites for Chemo-Photothermal Therapy, Regener. Biomater., 2022, 9, rbac032, DOI:10.1093/rb/rbac032.
- M. Zhao, R. Wang, K. Yang, Y. Jiang, Y. Peng, Y. Li, Z. Zhang, J. Ding and S. Shi, Nucleic Acid Nanoassembly-Enhanced RNA Therapeutics and Diagnosis, Acta Pharm. Sin. B, 2023, 13(3), 916–941, DOI:10.1016/j.apsb.2022.10.019.
- N. Ebrahimi, M. S. Manavi, A. Nazari, A. Momayezi, F. Faghihkhorasani, A.-H. Rasool Riyadh Abdulwahid, F. Rezaei-Tazangi, M. Kavei, R. Rezaei, H. Mobarak, A. R. Aref and W. Fang, Nano-Scale Delivery Systems for siRNA Delivery in Cancer Therapy: New Era of Gene Therapy Empowered by Nanotechnology, Environ. Res., 2023, 239(Pt 2), 117263, DOI:10.1016/j.envres.2023.117263.
- H. Huang, S. Gao and X. Cai, DNA-Based Nanomaterials in the Immunotherapy, Curr. Drug Metab., 2023, 24(5), 367–384, DOI:10.2174/1389200224666230413082047.
- N. Uddin, D. W. Binzel, D. Shu, T.-M. Fu and P. Guo, Targeted Delivery of RNAi to Cancer Cells Using RNA-Ligand Displaying Exosome, Acta Pharm. Sin. B, 2023, 13(4), 1383–1399, DOI:10.1016/j.apsb.2022.11.019.
- W. Szymanowski, A. Szymanowska, A. Bielawska, G. Lopez-Berestein, C. Rodriguez-Aguayo and P. Amero, Aptamers as Potential Therapeutic Tools for Ovarian Cancer: Advancements and Challenges, Cancers, 2023, 15(21), 5300, DOI:10.3390/cancers15215300.
|
This journal is © The Royal Society of Chemistry 2024 |