Biomass@MOF nanohybrid materials for competitive drug adsorption: analysis by conventional macroscopic models and statistical physical models†
Received
18th November 2023
, Accepted 5th February 2024
First published on 16th February 2024
Abstract
This study discloses the design of nanohybrid Biomass@MOF resulting from the functionalization of a hydrochar (HC) through hydrothermal treatment (HT) of corn cob residues and MIL-53(Al). The nanohybrid, composed of 71 wt% Biomass and 29 wt% MOF, demonstrates stability and effectiveness after leaching and stability tests. Physicochemical characterization (SEM, BET, TGA, FTIR, XRD, XRF, and XPS) of the resulting hybrid confirms the development of a new nanostructure formed via in situ growth of MOF crystals by hybridizing reticular oxygen species present on the HC surface. HC@MIL-53(Al) served as an adsorbent for pharmaceutical compounds ketorolac (KTC) and naproxen (NPX) in a two-component and competing system. Under extreme conditions, HC@MIL-53(Al) proves to be a high-performing adsorbent, removing 100% of KTC and NPX in a mixture when their concentration is up to 150 ppm. Conventional isotherm models for bicomponent systems along with physical-statistical microscopic models revealed that the complex structure of the new composite utilizes functional groups of HC (–OH) and MIL-53(Al) (C
C) for physisorption of both contaminants, leading to the formation of adsorbate–adsorbate multilayers. These characteristics are further validated by a desorption study, demonstrating the ability of recovered HC@MIL-53(Al) to adsorb both contaminants with approximately 90% of their initial capacity after 5 cycles.
Environmental significance
Adsorption is a readily scalable technology in engineering for water decontamination. It addresses issues related to dyes, heavy metals, personal care products, agrochemicals, and, notably, pharmaceuticals – a challenging group of pollutants that often exhibit resistance to degradation, leading to increased concentrations in water sources. Additionally, the possibility to remove different pharmaceuticals in wastewater via competitive adsorption is a significant challenge in environmental remediation. Our recent research has focused on the design of novel nanohybrid materials combining optimum properties of biomass with metal organic frameworks for a number of environmental remediation applications (i.e. adsorption, photodegradation, etc.). This innovative approach offers several advantages, addressing challenges presented by both materials individually – improved adsorption capacity in biomass and simplified recovery of MOFs after use and high cost. Based on these premises, this contribution has been aimed to the preparation and utilization of a biomass@MOF nanohybrid in the competitive removal of two pharmaceuticals in wastewater, namely ketorolac (KTC) and naproxen (NPX).
|
1. Introduction
The design of novel nanomaterials holds significant promise, offering solutions to challenges that traditional materials alone cannot address. Over the past decade, various complex materials have emerged, contributing to advancements in physics, chemistry, and medicine. For instance, in physics, these materials enhance thermal processes and aid in designing space materials.1–3 In chemistry, they play a crucial role in environmental decontamination and the conversion of raw materials into value-added products.4,5 Moreover, in medicine, these materials facilitate drug transport within the human body.6 Among the emerging classes of porous materials, metal–organic frameworks (MOFs) stand out, with over 12
000 different structures recognized by the Cambridge Database. Since their introduction in 1995,7 MOFs have shown great promise in various applications in chemistry.
Unlike other physical–chemical processes, adsorption is a readily scalable technology in engineering for water decontamination. It addresses issues related to dyes, heavy metals, personal care products, agrochemicals, and, notably, pharmaceuticals a challenging group of pollutants that often exhibit resistance to degradation, leading to increased concentrations in water sources.8 The versatility of MOFs, particularly their ability to be modified post-synthetically (PSM),9,10 has expanded their applications in adsorption.11–13 Our recent research focuses on enhancing the structure and applications of adsorption through the functionalization of residual biomasses of lignocellulosic origin with MOFs.14–16 This innovative approach offers several advantages, addressing challenges presented by both materials individually improved adsorption capacity in biomass and simplified recovery of MOFs after use. The resulting hybrid material exhibits significant technical and economic potential compared to pure MOF powders, making it an attractive option for industrial applications. While MIL-53(Al) has been extensively studied as an adsorbent in aqueous matrices due to its hydrophobic properties and stability in water,17 impregnating it onto support surfaces, especially heat-treated biomass with no available functionalities, remains a challenge.
In this context, novel biomass/MOF hybrids pose a significant challenge with high expectations, particularly in pharmaceutical removal. Omer et al.18 synthesized a hybrid from the functionalization of carbon nanotubes with MIL-125(Ti) and MIL-53(Fe) encapsulated in microspheres obtained from algae waste with a high reuse capacity in the removal of tetracycline in water bodies. In the same way, ZIF-67 has also been tested as a functionalization agent for bio-structures, specifically with aerogels obtained from wood residues to capture tetracycline in water.19 On the other hand Ouyang et al.20 developed a composite of biochar from agro-industrial waste and UiO-66 for the effective removal of sulfamethoxazole in water. Studies have also demonstrated the deposition of UiO-66 on cellulose foams for the removal of aspirin and tetracycline in water.21 All these examples share a common result adsorption capacity of the new hybrids exceeds the capacities of their separate precursors. However, as in all these cases as well as in several other different application studies on biomass/MOF functionalization, they often omit to report on the final composition of the hybrid (%wt biomass and %wt MOF) and its stability in aqueous media before and after use. These aspects are critical due to the potential leaching of metal species and pollution that these new composites could generate. Another common issue in the published articles is the negligible mass composition of these new composites for the supporting bioadsorbent. These limitations hinder the consideration of new functionalization techniques using MOFs as a promising alternative to improve the use of waste biomasses in water treatment processes, such as chemical surface activation in the case of biochars.
MOF synthesis, despite its high yields, is known to be costly,22 making the application of these pristine materials economically unattractive. Given these challenges and with the aim of presenting a technically and economically viable alternative for water treatment processes using adsorption as a model technology, this study aimed to design Biomass@MOF, combining hydrochar (HC) obtained from the hydrothermal decomposition of corn cobs as bioadsorbent with MIL-53(Al). The resulting nanohybrid, denoted as HC@MIL-53(Al), was tested in the bicomponent adsorption of KTC and NPX drugs. The study evaluates the competition effects of both adsorbates at both macro and microscopic levels.
2. Experimental
2.1. Materials and chemical supplies
For the synthesis of pristine MOF, terephthalic acid (1,4-BDC), hexahydrated aluminum chloride (AlCl3·6H2O), N,N-dimethylformamide (DMF), and anhydrous methanol were obtained from SIGMA ALDRICH. Analytical standards of naproxen and ketorolac tromethamine, sodium hydroxide, hydrochloric acid, acetonitrile, and glacial acetic acid were acquired from the MERCK group. Additionally, were also obtained from. Corn cob of the local variety was collected from an experimental field located in Rocafuerte, Manabí province, Ecuador. All chemical reagents were used without any further purification, and the experimental tests were conducted at the Laboratory of Chemical and Biotechnological Analysis of the Technical University of Manabí.
KTC and NPX concentrations were measured by a high-performance liquid chromatograph (HPLC, Accela ThermoFisher USA) outfitted with a quadrupole pump and a diode array detector. A Column C18 (Thermo Fisher model 25.005-104.630) separated the analyte, according to the technique suggested by Zakeri-Milani, Barzegar-Jalali, Tajerzadeh, Azarmi and Valizadeh23 and Kalariya, Namdev, Srinivas and Gananadhamu24 for NPX and KTC respectively.
2.2. Corn cob valorization in hydrochar (HC)
Hydrocarbons (HC) from corn cob (Zea mays) were obtained through hydrothermal carbonization or hydrothermal treatment (HTC). Initially, the dried corn cob was reduced in size by cutting it into approximately 2 cm thick discs, which were then subjected to multiple washes to remove impurities from their surface. Biomass was then dried in a Memmert (Germany) oven at 80 °C for 24 hours to reduce the moisture content (<5%) in the sample. Subsequently, 20 g of biomass was weighed and placed inside a 250 ml capacity Teflon reactor, followed by the addition of 190 ml of water. The reactor was sealed and placed in a muffle furnace at 190 °C for 24 hours. After the HTC process, a solid phase fraction corresponding to the hydrocarbon and a liquid phase fraction containing a significant portion of degradable organic components were obtained. Solid phase fraction was subjected to multiple cycles of washing with water and dried in an 80 °C oven to reduce the moisture content (<3%). Finally, deep brown porous HC was sieved to obtain particles with a size of 250 microns.
2.3. Synthesis of MIL-53(Al) MOF
MIL-53(Al) was synthesized following the procedure reported by Isaeva, Chernyshev, Tarasov, Lobova, Kapustin and Davshan,17 with certain modifications to the solvothermal method. The reagents used in the synthesis were AlCl3·6H2O and 1,4-BDC in a molar ratio of 1
:
2. 242 mg of AlCl3·6H2O and 332 mg of 1,4-BDC were weighed separately and dissolved in 5 mL of deionized water (solution A) and 55 mL of DMF (solution B) respectively, using a magnetic stirrer. Solution B was slowly poured into solution A while maintaining its transparent condition. The resulting mixture of solutions was placed in a 250 ml volume Teflon-coated synthesis reactor and placed in a muffle furnace at 130 °C for 24 hours. The obtained white liquid was washed for 10 cycles with deionized water using centrifugation at 4000 rpm for 5 min/cycle to remove the presence of the solvent (DMF) and excess 1,4-BDC. Finally, the obtained material was mixed with methanol for activation and to remove residual DMF through evaporation. It was then placed in an oven and dried at 90 °C for 12 hours.
2.4. Synthesis of nanohybrid composite HC@MIL-53(Al)
Nanohybrid material was synthesized via in situ growth of MOF crystals on the surface of HC. The same methodology explained in the previous section (precursor MOF synthesis) was used, with the addition of 0.5 g of HC to the AlCl3·6H2O solution (solution A) during the synthesis steps (Fig. 1). Subsequently, the 1,4-BDC/DMF solution (solution B) was mixed in the same manner as in the precursor MOF synthesis. The phase separation, washing, and drying process were identical to those used for MIL-53(Al). The resulting material, named HC@MIL-53(Al), exhibited a black color under high humidity conditions and a gray color under low humidity conditions, resembling ashes and carbonization residues.
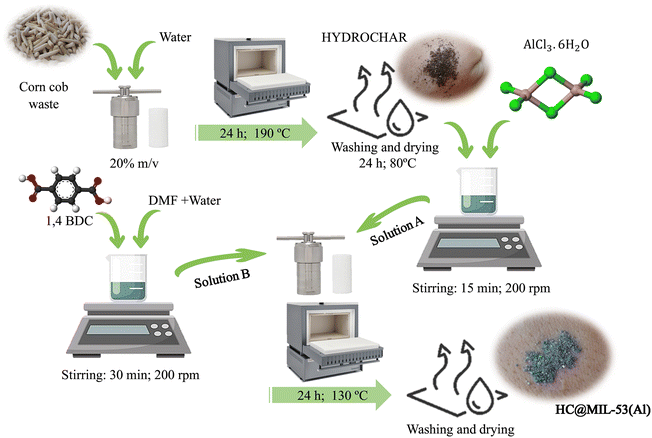 |
| Fig. 1 Schematization of the synthesis of the new HC@MIL-53(Al) nanohybrid. | |
2.4.1. Impregnation and leaching tests of MIL-53(Al) on HC.
Inductively coupled plasma atomic emission spectroscopy (ICP-OES) was the technique used to measure the concentration of leached Al3+ after each washing cycle. EPA Method 3015A: “Microwave Assisted Acid Digestion of Aqueous Samples and Extracts was used for this purpose”. Ten washes were performed on 5 mg of the material (in 50 mL of water solution) to quantify the amount of pristine MIL-53(Al) that was not supported within the HC structure. Mass loss after each wash was recorded from the initial mass, and aluminum content in wash water was determined only to confirm the presence of detached MOF in solution that could cause secondary contamination problems after use.
2.5. Material characterization
Materials characterization was already disclosed in a previous study proposed by our research group on a similar material.25 For this purpose, the following characterization techniques were used:
N2 adsorption and desorption measurement (BET isotherms) was conducted with a Micromeritics ASAP 2460 instrument (accelerated surface area and porosimetry system). Before measurements, samples were outgassed under flowing nitrogen for 8 h at 100 °C.
Thermogravimetric analysis (TGA) of each of the samples was carried out in the TGA-1-STAR System equipment of METTLER TOLEDO brand. The samples were heated at a rate of 10 °C min−1 in N2 atmosphere (50 mL min−1) in a temperature range of 30–800 °C.
X-ray diffraction (XRD) was carried out on a D8 advanced diffractometer (Bruker AXS) with Lynxeye detector at room temperature. XRD patterns were scanned at 2θ with a range of 10° to 70°, a step size of 0.02°, and a counting time of 0.2 s. Phase and peak identifications were supported by the Cambridge database for MOF identification using VESTA software.
Fourier Transform Infrared Spectra (FTIR) were obtained on a Thermo Scientific Nicolet Summit Pro with Thermo Scientific Everest Diamond ATR (Attenuated total reflection) Accessory. The spectra were averaged over 32 scans in the range of 4000 to 600 cm−1.
X-ray photoelectron spectroscopy (XPS) was performed using a SpecsTM ultra-high vacuum (UHV) multipurpose surface analysis system equipped with a Phoibos 150-MCD energy detector. The analysis was performed using a conventional X-ray source (XR-50, Specs, Al/Mg-Kα, hv = 1253.6 eV, 1 eV = 1.603 × 10−19 J) at a pressure below 10−10 mbar. XPS CASA program was used to deconvolute the obtained curves and quantify more important elements of the synthesized material.
2.6. Batch adsorption in a bicomponent system
Initially, the adsorption capacity (Qe) of the two pristine as well as the hybrid material were compared. All tests proposed in this manuscript were developed in a discontinuous and bicomponent system with equimolar solutions of both adsorbates to ensure the same number of molecules of each of them without creating a competition effect. The experimentally obtained data were processed for a kinetic study under the Pseudo First Order (PFO), Pseudo Second Order (PSO), Bangham, Avrami Fractionary and Intraparticle Diffusion or Weber-Morris (WM) models as described in Table 1.
Table 1 Kinetic models used in the fitting of experimental dataa
Models |
Non-linear shape |
Q
t and Qe are the adsorption capacities (mg g−1), t is the time (min), k represents the kinetic rate constant in each model with its respective unit (depending on the model) and n, m, and C are constants specific to each model.
|
PFO |
q
t = qe(1 − ek1·t) |
PSO |
|
Avrami fractionary |
q
t = 1 − e(−k)(t)n |
Bangham |
q
t = kγ·t1/m |
Intraparticle diffusion o WM |
q
t = kid·t1/2 + C |
Conventional macroscopic models of Extended Langmuir, Extended Freundlich, and Extended Sips were employed exclusively for bicomponent ADS systems (Table 2) and the thermodynamic parameters ΔG, ΔH and ΔS, ΔG by linearization and use of the Van't Hoff equation. On the other hand, the advanced statistical physics microscopic models employed in this study were the models of 1 adsorption site with the formation of 1 layer (SPM1), 2 adsorption sites with the formation of 1 layer (SPM2), and 2 adsorption sites with the formation of 2 layers (SPM3) with their respective binding energies as shown in Table 2.
Table 2 Equilibrium isotherms based on conventional macroscopic models and advanced microscopic models based on statistics-physics with their respective thermodynamic parameters
Models |
Non-linear shape |
Energy parameters |
Conventional bicomponent macroscopic models where i represents the first species and j represents the second species. The fit parameters of each model are qmax, qm, KF, and b.
Microscopic models where n and Nm are stearic parameters representing the type of deposition of adsorbate molecules and the density of the adsorption layer, respectively.
|
Langmuir extendeda |
|
ΔG° = −RT ln KD |
Freundlich extendeda |
|
Sips extendeda |
|
SPM 1b |
|
|
SPM 2b |
|
|
SPM 3b |
|
For the mathematical modeling of the adsorption kinetics StatSoft, Inc. (2007). STATISTICA (data analysis software system), version 8.0. was used and for the equilibrium and thermodynamic modeling both for the conventional macroscopic isotherms based on bicomponent systems and for the microscopic models based on the statistical physics models the MICROSOFT EXCEL SOLVER add-in was used. The best-fit models were statistically chosen based on the minimization of the Marquardt standard deviation error (MPSD) in congruence with the R2 value.
2.7. Competitive adsorption between KTC and NPX
To better analyze the competitive effect of both adsorbates, all possible combinations of the chosen initial concentrations from 0.1 mM to 0.9 mM were used as shown in Table S1.† These results were analyzed using a contour plot, which illustrates the influence of increasing the concentration of each adsorbate on the removal of the other. In addition to revealing the existence of synergy and/or antagonism between the molecules, this analysis provides the opportunity to understand and depict with greater precision the adsorption mechanism of KTC and NPX on the surface of HC@MIL-53(Al) in a binary system, along with the analysis of results from conventional macroscopic and microscopic statistical physics models.
2.8. Strategies for the proposed ADS mechanism
In addition to the analysis of the results of conventional macroscopic models and physico-statistical microscopic models, HC@MIL-53(Al) has been strategically characterized by FTIR before and after the ADS process. For this purpose, the material has been saturated at different equimolar concentrations of KTC and NPX and the changes in the main bands and vibrations in infrared spectroscopy have been analyzed. In transmittance, it is understood that the reduction of the vibration of the bands is the consequence of a greater absorption of the IR light by the functional groups. For this, the presence of the same functional group must increase to be able to absorb more IR light and transmit less signal. The opposite effect occurs when the presence of the functional group decreases, in this case, the signal as a consequence of band vibration increases since a part of the IR light is absorbed by the functional group present while the rest is transmitted.
2.9. Material recycling
After each adsorption cycle, the ability of the material to interact again with a solution of contaminants at the same starting concentration was evaluated. For this purpose, the solid material was separated after each use by centrifugation at T = 25 °C and 3000 rpm for 3 min. The solid material was then washed three times with a methanol solution for the same time, temperature, and recovery rate. Removal performance was quantified considering the starting parameters.
3. Results and discussion
3.1. Physicochemical characteristics of the material
Our previous study,25 described all the characteristics of the nanohybrid material, briefly summarized herein. In addition, all raw data from the experiments are in Mendeley Data (https://doi.org/10.17632/4ypxsm5gy3.1).
3.1.1. Textural properties of the material.
HC@MIL-53(Al) experiences a reduction in weight up to the fifth wash, directly correlated to leached MOF in the water after each cycle (Fig. 2a). The gravimetric results reveal a decrease in MOF release with each wash, stabilizing from the fifth to the tenth cycle and becoming nearly unquantifiable. The Al3+ concentration, determined through digestion and quantification, further supports the diminishing MOF release trend. X-ray fluorescence (XRF) analysis of HC@MIL-53(Al) after all washes (depicted in Fig. 2a) confirms the substantial MOF content in the hybrid, evidenced by the presence of Al3+. The findings support the technical feasibility of the MOF impregnation process in the surface structure of HT-treated biomass. Ultimately, a Biomass@MOF nanohybrid was successfully synthesized, comprising 71 wt% biomass (HC) and 29 wt% MOF.
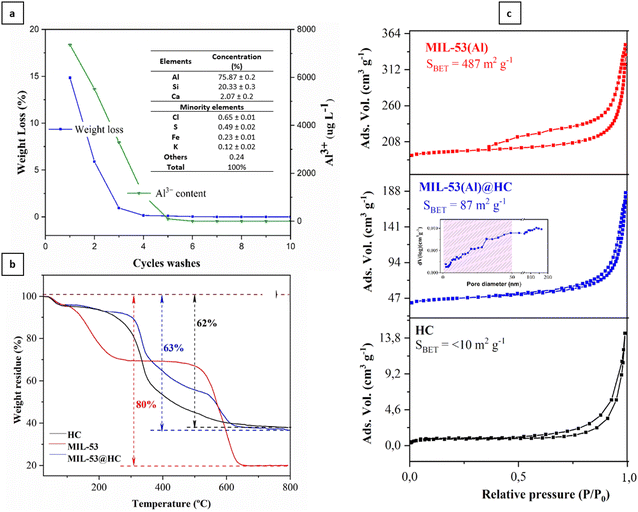 |
| Fig. 2 MIL-53(Al) leaching is directly represented by Al3+ quantification after each wash including XRD analysis (a). Characterization of precursors and hybrid separately by: thermogravimetric analysis (b), BET surface area (c), and pore size (including in c). | |
The thermal decomposition curve (Fig. 2b) validates the synthesis of the nanohybrid via HC functionalization with MIL-53(Al). The single, extended loss curve of HC, a thermally treated biomass, spans from 220 °C to 600 °C, with the most significant interval occurring from 220 °C to 350 °C. This interval corresponds to the decomposition and carbonization of all functionalities within the HC structure post-HT treatment. HC experiences losses of around 40% in the initial fast zone (220–350 °C), reaching 62% at the highest decomposition temperature (600 °C). Additionally, MIL-53(Al) undergoes a 30% mass loss in the initial stage (130 °C to 230 °C) due to the removal of residual DMF and uncross-linked 1,4-BDC in the structure. From 230 °C to 800 °C, the decomposition peak is attributed to cross-linked 1,4-BDC in the MOF structure, eventually carbonizing and forming metal oxides. Consequently, HC@MIL-53(Al) exhibits enhanced stability to thermal decomposition, owing to its biomass structural skeleton which can enhance mechanical properties and promote uniform surface heat distribution.
N2 adsorption–desorption shows a type IV isotherm for three materials (Fig. 3c). According to the classification proposed by IUPAC (International Union of Pure and Applied Chemistry) this isotherm is associated with mesoporous materials with high surface areas and low toxicity. This isotherm also presents a hysteresis loop typical of a mesoporous structure, allowing N2 entry and retention, as well as resistance to gas desorption. Initially, HC and MIL-53(Al) separately have surface areas of 8 and 487 m2 g−1 respectively. As is well known, hydrochar from lignocellulosic biomasses has a poor surface area nourished mainly by acid radicals.26,27 On the other hand, MIL-53(Al) reduces its surface area after impregnation as a consequence of its composition (71 wt% HC and 29 wt% MOF). This phenomenon is associated with the anchoring of the metal–organic scaffolds on HC through their available sites and the agglutination of the dust particles among themselves occupying available sites and forming granules.15 MIL-53(Al)@HC pore size distribution of BJH included in Fig. 3c shows that 72% of the pores are distributed in the 2–50 nm range (mesoporous range), properties that favor the adsorption of larger molecules.
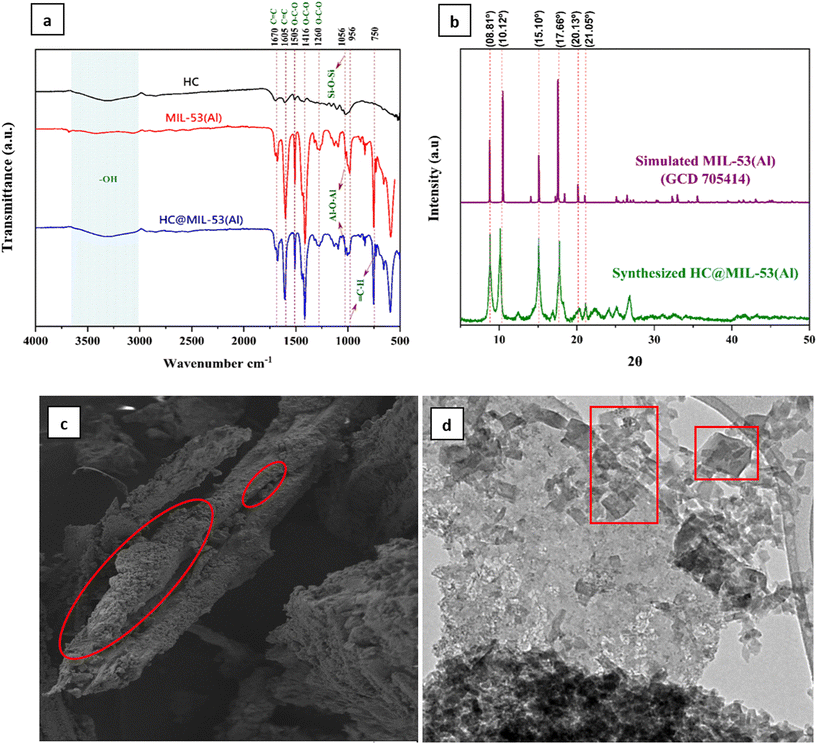 |
| Fig. 3 Comparison of FTIR spectra of HC, MIL-53(Al), and HC@MIL-53(HC) separately (a) and crystallographic comparison of simulated MIL-53(Al) (GCD 705414) and synthesized HC@MIL-53(Al) (b). SEM (c) and TEM (d) images of hybrid. | |
3.1.2. Functionalities (FTIR) and crystallinity (XRD).
HC showed characteristic peaks including carboxyl groups illustrated by an intense stretching around 1700 cm−1 and the hydroxyl group (–OH) represented in the broadband of 3000 and 3600 cm−1. In addition, HC shows a stretching in the 1056 cm−1 band characteristic of the siloxane group (Si–O–Si) due to biomass decomposition resulting from hydrothermal treatment (HT) (Fig. 3a, black line).
MIL-53(Al) exhibits clear vibrations at 1670 and 1605 cm−1, which correspond to the vibrations of the C
C double bond of the aromatic ring of the MOF. The elongations of the Al3+-coordinated 1,4-BDC ligands of the porous network in carboxylate (–OCO) are about 1510 cm−1 and 1416 cm−1, and the Al–O–Al bonds of the same network are at about 1000 cm−1 showing the correct synthesis of raw MOFs. Finally, out-of-plane
C–H bond vibrations were observed at 750 cm−1 and 990 cm−1, which correspond to the aromatic 1,4-BDC ring of the MOF (Fig. 3a, red line). The analysis of these results is fully consistent with previous literature results7,14,28 in the synthesis of rice husk biomass composites loaded with the same organometallic framework used in this study.
HC@MIL-53(Al) presented a combination of the functional groups of its precursors separately mainly in the broad –O–H band corresponding to the Al–OH (AlO4(OH)2) groups of the crystalline corners. The bands of the Si–O–Si groups also appear in the hybrid probably in the same form or the form of Al–O–Al and Al–O–Si. This hypothesis considers that the main functional groups of HC serve as active and free sites for hybridization forming a support structure for MIL-53(Al) growth. The fingerprint area of the IR spectra of MIL-53(Al) and HC compared with the IR spectrum of HC@MIL-53(Al) in OMNIC Paradigm software demonstrate a percentage similarity of 97% and 62%, respectively. These interesting percentages of similarity are due to the fact that HC, like any carbon, is an inert material whose functionality is represented only by the C, H, and O groups, and these are the ones that serve as anchors for the growth of the MIL-53(Al) crystals, which has a greater number of functional groups occupying the few active sites of the HC support.
XRD patterns in HC@MIL-53(Al) demonstrate the presence of the main diffraction peaks for MIL-53(Al) reported in the Cambridge database (GCD 705414).29 The characteristic peaks for this MOF are indexed in Fig. 3b by a comparison that demonstrates the similarity of simulated MIL-53(Al) and synthesized HC@MIL-53(Al), with the diffraction peaks located at 2theta: 8.81°, 10.12° 15.1°, 17.66°, 20.13° and 21.05° standing out which demonstrates the successful synthesis of the new hybrid. Although crystal growth is evident in the new structure, it is necessary to mention that the diffraction pattern of HC@MIL-53(Al) possesses perturbations and decrease in the mentioned crystallinity peaks, this is mainly due to the presence of HC which composes most of the material.28 The hydrothermal (HT) synthesis of HC@MIL-53(Al) leads to the formation of 1D orthorhombic planes structured by the bonding of 3D octahedral Al3+ crystals because of the spatial distribution of AlO6/AlO4(OH)29,30 represented by the crystalline peaks of the structure and showing coherence with the mentioned FTIR results and SEM (Fig. 3c) and TEM (Fig. 3d) images of hybrid. Judging from the images, SEM shows that the carbonaceous HC fibers resulting from HT have been the support for the growth of the MOF, while TEM clearly shows its crystalline form contrasting the arguments already raised in the course of the characterization.
3.1.3. Chemical state on the hybrid surface (XPS).
Fig. 4 presents a comprehensive XPS scan, illustrating the functionalization of HC with pure MOF following washing tests. This scan provides an in-depth analysis of the chemical state of all elements that constitute the HC@MIL-53(Al) structure. It also explores potential interactions to understand the binding capability. A full XPS scan (Fig. 4a) reveals the typical composition of MIL-53(Al): Al (13.68%), C (58.79%), and O (25.34%). It also includes the Si, Ca, and N structures of HC. The qualitative data for all elements align perfectly with the XRF analysis results of HC@MIL-53(Al) (Fig. 4b). The study places significant emphasis on the deconvolution of the XPS spectrum of O 1s at 531 eV. This is because it signifies the presence of lattice oxygen, denoted as H. Oxygen forms covalent bonds with other atoms. The intensity of O 1s is primarily attributed to the presence of HC, a product of HTC, known for its richness in O2− groups.31
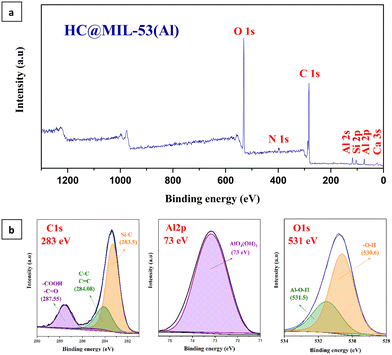 |
| Fig. 4 XPS spectra of HC@MIL-53(Al) (a) and of the most important elements that make up its structure (b) with their interactions. | |
This work confirms the hypothesis proposed earlier in FTIR characterization and demonstrates that H+ bonding with reactive O2− groups of HC is a possible mechanism of functionalization between HC and MIL-53(Al). Unlike most articles dedicated to studying and testing composites from biomass and MOF published to date, this work does not report peaks above 532 eV. This evidences that the crosslinking is mostly given by the energy of –OH bonds of the water present in the medium (due to exposure and ex situ analysis of the material) and of the –OH radicals of HC in the range of 530.6 and 531.5 eV respectively, as reported by Idriss32 in his analysis on the incorrect assignment of the XPS O 1s signal at 531–532 eV attributed to oxygen vacancies in catalysts.
C 1s spectrum at 283 eV reveals key carbon interactions, with a peak at 283.5 eV indicating the silane binding energy (C–Si) of the lignocellulosic biomass-derived material. The energy at 284.08 eV is linked to the C–C and C
C bonds of HC's carbonaceous skeleton33,34 and possibly the benzene rings of BDC of MIL-53(Al). This interaction is often observed at 287.55 eV in MOF materials due to the carboxylic acid (–COOH) from terephthalic acid (BDC). The Al 2p presence is signified by a 73 eV peak, attributed to Al3+ in the AlO4(OH)2 groups of the MIL-53 (Al) octahedral framework, as previously noted in the FTIR spectra and XRD patterns.
3.2. Batch adsorption in the bicomponent system
Adsorption results in Fig. S1† show that MIL-53(Al) and HC@MIL-53(Al) do not possess significant statistical differences between them and outperform HC in terms of Qe. Although the nanohybrid material has a Qe similar to that of pure MOF, issues with particle size, and cost-competitiveness (less expensive material than the precursor MOF given its large biomass content) are circumvented, adding value to lignocellulosic waste in the form of a benign-by-design nanomaterial.15
3.2.1. ADS parameters: pH, dose, and stirring speed.
The parameters of the adsorption process are shown in Fig. S2.† HC@MIL-53(Al) can adsorb more KTC and NPX at pH between 2 to 6 (Fig. S2a†). In this sense, none of the adsorption mechanisms for both pollutants corresponds with electrostatic interactions. This approach is supported by analyzing the pHpzc and pKα results of KTC and NPX shown in Fig. S3.† At pH 6 and 8, HC@MIL-53(Al) has negative charges on its surface, so it should repel NPX and KTC, however these are well-adsorbed. The experiments were selected and continued at pH 5 since it is the natural pH of the solution (system) and Qe did not have any significant differences with pH 4. Regarding the dose of the new material, Fig. S2c† shows that 4 mg mL−1 concentration (200 mg in 50 ml of solution) was enough to stabilize the removal of the adsorbates present in the bicomponent solution composed of 0.5 mM KTC (127 ppm) and 0.5 mM NPX (126 ppm). Increasing the stirring speed from 200 rpm to 300 rpm differed, although to a lesser extent, in the KTC ADS process (Fig. S2b†) which may be possible due to the increase in the kinetic energy of the system and consequent increase in adsorbate-adsorbent contact enhancing mass transfer zones. The adsorption capacity of NPX and KTC in our work reaches values around 30 mg g−1 for each of them in a mixed system. A comparison with similar studies is crucial. While literature offers limited work on bicomponent systems, especially with these specific adsorbates, monocomponent systems provide insights into certain composites' contaminant removal capacity. Zhang et al.35 synthesized an adsorbent by hybridizing MCNTs and UiO-66(NH2), similar to our work's composition: 20 wt% MCNTs and 80 wt% MOF. This hybrid material was tested on NPX removal, achieving a maximum adsorption capacity of 40 mg g−1. The difference in adsorption capacities likely stems from MOF's nature and properties including surface area and pore volume, not the support type. Xing et al.36 developed a composite between ZnO (33 wt%), SA sodium alginate (16 wt%), and HKUST-1 (51 wt%), reaching close to 80 mg g−1 of NPX adsorption. The high MOF composition in this work directly impacts pollutant adsorption capacity, as it's the main capture and removal agent.
For KTC adsorption, similar molecules such as Ketoprofen have been adsorbed by MOF and LDH-based composites. Wang and Zhang37 synthesized ZIF-8@NiAl-LDHs, but the maximum adsorption capacity did not exceed 10 mg g−1, indicating the complexity of these adsorbates requires specific attention and generic materials. Lastly, Sompornpailin et al.38 developed a similar material comprising functionalized alginate (extracted from algae) with MIL-53(Al) containing a mass composition of 75% and 25% respectively. The separate adsorption of Naproxen and Ketoprofen yielded removal values of 18 and 17 mg g−1, respectively, lower than our results. The difference may be due to a key factor: the support structure's synergy with the MOF. Based on these results, kinetic and thermodynamic equilibrium studies were subsequently performed at pH 5, using 200 mg in a solution volume of 50 mL and at a stirring speed of 200 rpm.
3.2.2. Equilibrium time and adsorption kinetics.
The fitting curves of KTC and NPX experimental data for Pseudo First Order (PFO), Pseudo Second Order (PSO), Fractional Avrami, Bangham, and Intraparticle Diffusion for certain initial concentrations of the bicomponent system (for representation only) are shown in Fig. 5. Equilibration times in ADS of KTC and NPX were 60 min and 20 min respectively, showing differences related to selectivity criteria, chemical affinity, and structure to the benefit of NPX. Tables S2 and S3† show the reference values (MPSD and R2) as well as the fit parameters for each model. As mentioned above, all adsorption experiments were performed in equimolar bicomponent solutions of KTC and NPX.
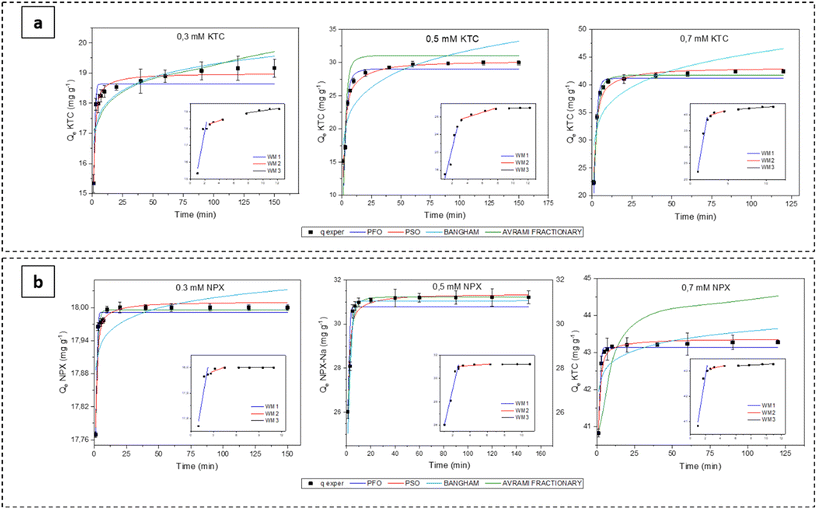 |
| Fig. 5 Pseudo-first order (PFO), pseudo-second-order (PSO), Bangham, Avrami Fractionary and intra-particle diffusion (WM) kinetic models (within each graph) for KTC (a) and NPX (b). | |
MPSD and R2 values show that the kinetic data of KTC and NPX were mainly fitted to the PSO model. This model assumes that the amount of adsorbed KTC and NPX depends only on the number of active sites of the adsorbent (HC@MIL-53(Al)) and not on their concentration in the medium. However, there is an increase in Qe (both experimental and calculated) with increasing concentration of KTC and NPX in bicomponent solution. This phenomenon leads to the assumption that Qe is being influenced by C0 from the beginning of the ADS process, as a consequence of a hypothetical formation of multilayers in equilibrium. The kinetic constant k2 increases with the first concentrations during the adsorbate-adsorbent interaction and decreases with the final concentrations when HC@MIL-53(Al) surface becomes saturated (this response could be influenced by the formation of adsorbate-adsorbate multilayers, a slower and low energy adsorption phenomenon). Both KTC and NPX experience a decrease in k2 with increasing adsorbate concentration. NPX shows a rapid decrease in its rate constant compared to KTC, which would position it as a candidate molecule for the formation of the adsorbate–adsorbate layer. A layer of NPX–NPX or NPX–KTC would be formed for each NPX molecule adsorbed on the surface of HC@MIL-53(Al) by a possible interaction via hydrogen bonding. Another hypothesis that could be attributed to these results is that it is not necessary to occupy all the active sites of the adsorbent material to initiate the multilayer formation process. It is important to mention that the kinetic model fit does not define the nature or type of adsorption of the system and only provides a clear idea of the time it takes for the adsorbates to saturate the adsorbent surface.
3.2.3. Macroscopic studies: equilibrium classic isotherms and process thermodynamics.
Equilibrium data (Qevs. Ce) were fitted to traditional bicomponent isotherms extended Langmuir (monolayers and homogeneous surfaces), extended Freundlich (multilayers and heterogeneous surfaces) and extended Sips (combined adsorption of extended Langmuir and extended Freundlich) at temperatures of 298 K, 313 K and 333 K (Fig. 6). The values of the fitting parameters of each model are presented in Table S4† as well as the MPSD and R2 error values that represent the statistical criteria for model selection. The extended Freundlich model presents a better fit for equimolar bicomponent adsorption of KTC. This model applies to adsorption systems where the adsorbent presents a heterogeneous surface arranged for multilayer formation. This model exhibits a convex curve (1 < n < 10) representing a favorable adsorption process for KTC as temperature increases. The increase in temperature favors the energetic heterogeneity of the adsorbent surface (and with it the equilibrium reach of the process) mainly because the mass transfer zone is shorter.39KF value, which represents the measure of the strength with which KTC adsorbs on the adsorbent surface, is also favored at increasing temperatures.
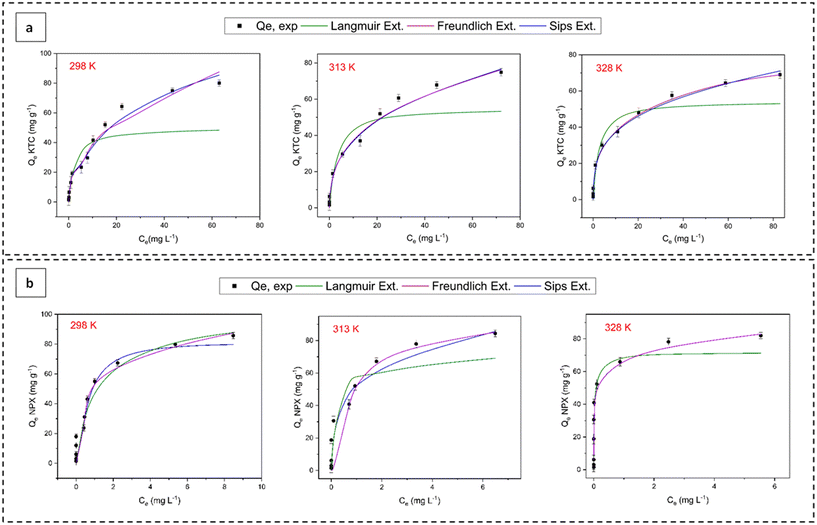 |
| Fig. 6 Equilibrium isotherms for two-component systems of the macroscopic models of extended Langmuir, extended Freundlich, and extended sips for KTC (a) and NPX (b). | |
For NPX, the experimental data are also described under the extended Freudlich model (Table S5†) and in addition to experiencing fast adsorption kinetics NPX forms a plateau at lower equilibrium concentrations than KTC. The increase in KF as a function of temperature between NPX and HC@MIL-53(Al) is greater with respect to that obtained for KTC. These results show a potential positive affinity effect between NPX and the active sites of HC@MIL-53(Al), achieving the formation of a prolonged multilayer thus confirming the hypothesis previously stated in the kinetic study.
The values of the thermodynamic variables shown in Table 3 confirmed the adsorption process of KTC is spontaneous (ΔG < 0). The adsorption enthalpy of KTC is less than 40 kJ mol−1, indicating a physisorption phenomenon mainly controlled by low molecular energy interactions. The adsorption of KTC on HC@MIL-53(Al) is primarily endothermic and favored at increasing temperatures, as mentioned in the previous sections.
Table 3 Thermodynamic parameters in bicomponent adsorption of KTC and NPX
KTC |
T (K) |
K
F (L mg−1) |
ΔG (kJ mol−1) |
ΔH (kJ mol−1) |
ΔS (kJ mol−1 K−1) |
298 |
14.50 |
−6626.13 |
7.72 |
0.04 |
313 |
10.03 |
−6001.70 |
|
|
328 |
19.61 |
−8116.86 |
|
|
NPX |
T (K) |
K
F (L mg−1) |
ΔG (kJ mol−1) |
ΔH (kJ mol−1) |
ΔS (kJ mol−1 K−1) |
298 |
55.73 |
−9961.42 |
27.11 |
0.12 |
313 |
54.38 |
−10 398.80 |
|
|
328 |
154.40 |
−13 742.81 |
|
|
The adsorption of NPX is also spontaneous (ΔG < 0) with an adsorption enthalpy below 40 kJ mol−1, indicating a similar physical adsorption process as in the case of KTC. The entropy values for NPX are higher than that of KTC suggesting an increase in the disorder of the molecules in the system and further confirming its position as the candidate molecule for multilayer formation. Importantly, these results also pointed to the versatility and relevance of the nanohybrid for adsorption processes due to the lack of chemical interactions between surface-adsorbate.
3.2.4. Microscopic study: statistical physical modeling and energetic parameters interpretations.
Tables S6 and S7† summarize the experimental data fitting of the 3 statistical physics models selected by means of R2 statistical parameters and MSPD minimization. Two active sites model with a monolayer formation appropriately describe the data obtained, with the exception of NPX data in which bilayer formation is preferred. Based on Table S8,† bond energies in the ADS of KTC and NPX do not exceed 40 kJ mol−1, in agreement with thermodynamics of conventional macroscopic models on mechanisms governed by physical type interactions (electrostatic, Van der Waals or probably hydrogen bonding).40,41
According to Fig. 7, the variation of bond energy for KTC decreases as a function of temperature. Site 1 despite having a higher energy level, decreases more than that of site 2 as temperature increases. The increase in temperature increases the kinetic energy of the molecules in the system and can break the intermolecular forces provided by both active sites. This is a very common feature of intermolecular bonds such as hydrogen bridges and non-covalent π–π interactions.42 On the other hand, if it is assumed that the energy of site 1 is the same for both contaminants as well as the energy of site 2 (Fig. 8) where the binding energies (at least that of site 1) for KTC are reflected would be contradicting the aforementioned statements. However, it can be suggested that being an ADS process in a bicomponent system a competition effect of both adsorbates for the active sites could change the behavior of the energy with which they bind to the HC@MIL-53(Al) surface. Based on this suggestion it can be proposed that the affinity demonstrated by NPX so far could be due to its ability to form a second adsorbate–adsorbate layer possibly through the same binding sites since the KTC energy for both sites is higher. At T = 318 K the binding energies of sites 1 and 2 for KTC decrease while for NPX increases (Fig. 7a and b). The kinetic energy would cause the bond breaking of both interactions in KTC as it is the one more strongly bound to HC@MIL-53(Al) (and therefore closer to its core) while NPX is favored by the increased kinetic energy of the system as it is farther away from the adsorbent surface. Finally, at T = 323 K the binding energy of binding sites 1 and 2 for KTC continue to decrease while sites 1 and 2 for NPX increase and decrease their energy respectively. If binding site 1 corresponds to the energy of π–π bond interactions and binding site 2 to the energy of –OH bonds, this behavior would be in full agreement with all that has been substantiated so far. The increase in kinetic energy would enhance the formation of the second layer for NPX through the π–π interaction but not through the hydrogen bonds which at T = 323 K break down decreasing their binding energy.
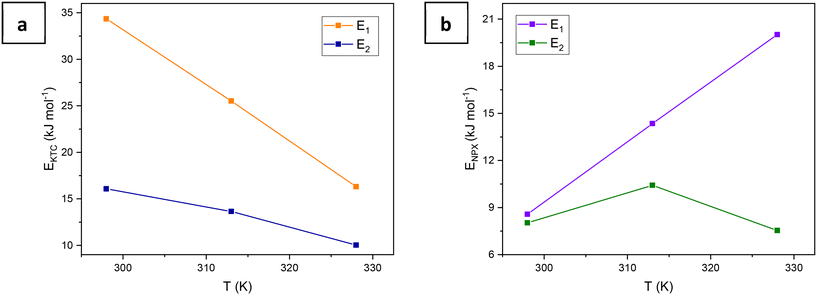 |
| Fig. 7 Effect of temperature on the adsorption energy between adsorbate and: KTC (a) and NPX (b). | |
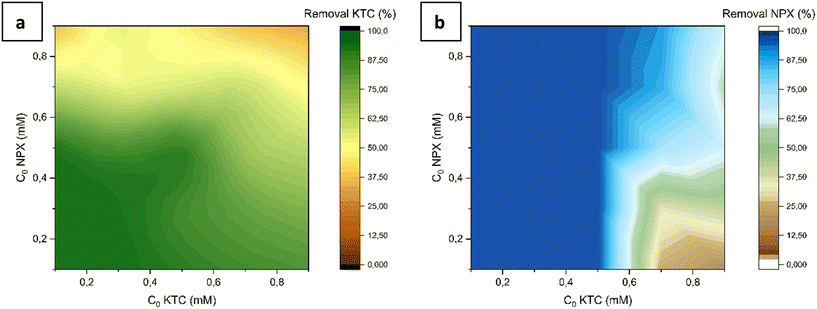 |
| Fig. 8 KTC (a) and NPX (b) removal behavior in a mixing system with different concentration combinations. | |
3.3. Competitive adsorption of KTC and NPX drugs on HC@MIL-53(Al)
The competitive nature of the two drugs was examined with the results of all possible combinations in Table S9† by evaluating the parameter Rq of a contaminant species (RqKTC or RqNPX) per incidence of Rq determines the incidence of ADS in the mixture (RqMIXTURE/RqSINGLE). For this purpose three possible behaviors are identified: the first assumes a synergistic effect if Rq > 1, the second assumes an antagonistic effect when Rq < 1, and the third indicates that there is no interaction when Rq ≈ 1. For a better understanding, Fig. 8 summarizes all the above-mentioned interactions in terms of removal (%R) when this has been influenced by the effect of the combination of the initial concentrations (C0) of both adsorbates. The contour plot for KTC removal (Fig. 8a) demonstrates the ADS potential that HC@MIL-53(Al) has for this contaminant when the initial concentration of KTC and NPX is 0.3 mM (76 and 75 ppm respectively). From the increase of this concentration in both species the removal of KTC decreases. These results are in full agreement with the values in Table S9† where it is observed that concentrations above 0.5 mM NPX affect the decrease of RqKTC (<1) due to the effect of the presence of NPX. If C0KTC is kept fixed and C0NPX is increased, Rq also decreases as an indication of the antagonistic effect of NPX. In short, it is understood that %RKTC decreases with increasing NPX concentration considering that NPX has a faster ADS kinetics with a comparatively better affinity to HC@MIL-53(Al).
Fig. 8b also shows a completely different behavior during NPX removal. The contour plot suggests that for all C0NPX an increase in C0KTC up to 0.5 mM keeps the %RNPX at the maximum and that increasing its concentration above this value decreases its removal at least for the lowest C0NPX. This behavior is completely reasonable if one thinks that the available HC@MIL-53(Al) sites are depleted, yet at higher initial NPX concentrations the increase in KTC concentration has significantly less effect than at lower initial NPX concentrations. This phenomenon is due to the fact that when C0NPX is the minimum and C0KTC is the maximum, the dominance in the number of KTC molecules can remove NPX from the active sites for its binding. The values of RqNPX are in Table S9.† show that if C0NPX is kept fixed and C0KTC is varied in increase, the value of RqNPX (>1) also increases proportionally which determines that KTC possesses a synergistic effect on NPX ADS. The results of this study contribute to a better understanding of the ADS mechanism of a mix of both pollutants on HC@MIL-53(Al) since, according to NPX kinetics, NPX adsorbs faster showing higher chemical affinity, with such effect probably resulting from the formation of a second adsorbate–adsorbate layer (NPX–NPX).
3.4. Adsorption mechanism: FTIR-based strategies
Fig. S4† confirms the reduction in the band vibration at 1670 cm−1 as a consequence of the increase of C
C groups due to the coupling between KTC and NPX molecules on HC@MIL-53(Al). KTC-HC@MIL-53(Al) and NPX-HC@MIL-53(Al) stackings respond to π-π type interactions affected by the aromatic rings of both structures. Likewise, the broadband belonging to the -OH groups between 3000–3600 cm−1 is also reduced by the presence of these functional groups, specifically by the interaction between the hydrogen bridges and KTC or NPX. On the other hand, the adsorption of these structures on the surface of the adsorbent has as a response to the increase of other vibrations characteristic of its functional composition. This happens because their functional vibration changes in plane by the displaced interaction of those functional groups as in the case of the stacking of the displaced benzene.43 Under this analysis, KTC and NPX feature substituted aromatic structures capable of interacting via π–π type bonds with the adsorbent material by stacking (not only in a sandwich but in a displaced manner). This would lead to the stackings causing a change in the original vibrational shape of the unsaturated HC@MIL-53(Al) functional groups. The increase of the vibration in the
C–H bending out-of-plane around 990 cm−1 further confirms the presence of the aromatic rings of KTC and NPX which vibrate linked to the aromatic rings of the material and could have changed their bending to only in-plane thereby reducing the absorption of light (increasing the transmittance in their absence).
3.5. Adsorption mechanism: proposal based on stearic parameters of statistical physics models
Fig. 9 depicts a plausible ADS process of KTC and NPX in a bicomponent system from the stearic parameters of Tables S6 and S7.† The parameter “n1” for KTC represents the way this molecule adsorbs on active site 1 (C
C) and suggests a non-parallel orientation at T = 298 K, multimolecular occupying one adsorption site and allowing the attachment of other molecules on the same active site. At T = 313 K and T = 328 K KTC is deposited parallel on the adsorbent surface interacting with at least two adsorption sites. Likewise “n2” for KTC represents the way in which this molecule adsorbs on adsorption site 2 (–OH) and suggests a mixed orientation at T = 298 K and T = 313 K. The increase in T influences KTC to adsorb on HC@MIL-53(Al) binding site 2 in parallel occupying two active sites at the same time. This phenomenon may be based on the increase of the kinetic energy and the breaking of the hydrogen bonds of the system. Given this effect and to continue interacting with the –OH groups, KTC is forced to occupy more than one adsorption site of this type. On the other hand, “n1 and n2” for NPX at all temperatures confer a multimolecular deposition on both active sites of the composite. This feature allows NPX to interact better with the active sites of HC@MIL-53(Al) compared to KTC, reducing the equilibrium time (kinetics) and making the adsorbate–adsorbate interaction through π–π stacking (NPX–NPX) and hydrogen bonding (NPX–NPX and NPX–KTC). The temperature increase favors the interaction of the NPX molecule with the active sites always adsorbing by non-parallel orientation with the adsorbent surface. This phenomenon differs in the way KTC is deposited on the surface of the material by interaction with hydrogen bridges. As mentioned above with increasing temperature the hydrogen bridges are broken and the adsorbates are forced to interact with more than one –OH group (parallel deposition) to continue to stay on the adsorbent surface. NPX does not experience such phenomenology since the main group responsible for its adsorption are interactions with C
C groups. Whenever NPX is deposited on HC@MIL-53(Al) by interaction with the –OH groups (in dependence on temperature) it does so in a non-parallel manner. As the temperature of the system increases NPX stops interacting with –OH groups, free to interact with KTC in parallel orientation.
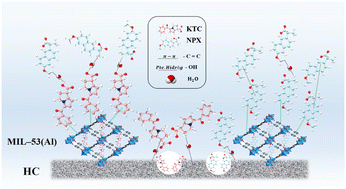 |
| Fig. 9 Mechanism of adsorption of KTC and NPX in mixture at T = 25 °C based on the results of macroscopic, microscopic models and FTIR analysis of saturated HC@MIL-53(Al) at different concentrations of both contaminants. | |
The proposed mechanism seamlessly aligns with other reported adsorption (ADS) studies on MIL 53(Al).41–43 The chemical nature of the adsorbate dictates the type of ADS system. For instance, Zambrano-Intriago et al., in their investigation of glyphosate ADS using a MIL-53(Al)-based composite, identified chelation interactions in their mechanism. This was substantiated by the high enthalpy value (129 kJ mol−1) of the process, attributed to the interaction between the Al3+ of the octahedral corner of the MOF and the phosphonate group in the glyphosate molecule.16 Conversely, Yan et al.44 synthesized a MIL-53(Fe)-based material in As5+ ADS and the mechanism suggested chemisorption through chelation between MOF nodes (metal) and the adsorbate (it appears that the metal cluster within the crystalline MOF is responsible for the chemical ADS). Hence, this study underscores the importance of enhancing the efficiency of MOF-biomass functionalization. This approach shifts the focus towards a physical adsorbate–adsorbate interaction, thereby bolstering the material's applicability in mass transfer and separation processes e.g. ADS.
3.5.1. Nanohybrid reuse.
The number of cycles demonstrating the reusability of HC@MIL-53(Al) supports the physisorption mechanism inferred from thermodynamic parameters and adsorption (ADS) binding energies for both KTC and NPX. In each cycle, Qe of NPX slightly surpasses that of KTC (Fig. S5†), indicating facile adsorption and desorption of NPX molecules on the material's surface. Regarding removal, in the fifth cycle, KTC and NPX exhibit an approximately 8% and 7% reduction, respectively, in their initial capacity. These findings were in good agreement with the binding energies of KTC (E1: 34.35 and E2: 16.07 kJ mol−1) and NPX (E1: 8.57 and E2: 8.03 kJ mol−1) at T = 298 K. The carbonaceous HC skeleton supporting the MOF predominantly contributes to this improvement.45 This modification prevents MIL-53(Al) leaching while maintaining high efficiency in the composite after each wash. The stability and robustness of the nanohybrid were demonstrated, highlighting the advantages of Biomass@MOF materials in pollutant adsorption (ADS) processes.
4. Conclusions
Biomass@MOF nanohybrids exhibit significant potential for drug adsorption, being particularly useful in the competitive adsorption (ADS) of KTC and NPX. The hybrid adsorbent presents a structure formed mostly by biomass (79 wt%), providing advantages from an economic and industrial viewpoint. Quantitative removal of both pollutants was achieved within 60 minutes. The hybrid could completely remove each pollutant in a mixture of around 200 ppm (100 ppm concentration each), concentrations that are well below those reported in literature for water bodies for this class of emerging pollutants. Microscopic modeling revealed that, in addition to addressing the drawbacks of the pristine materials individually, the functionalization of both precursors contributes to a synergistic adsorption mechanism. Each pristine component provides specific functional groups (–OH and C
C) during KTC and NPX removal. The weight composition, structural characteristics, pharmaceutical adsorption capacity, and high recyclability rate, as well as the low cost (compared to MOFs alone) position HC@MIL-53(Al) as a highly functional and economically attractive material for water treatment processes. This is especially relevant in scenarios where conventional technologies and materials may have difficulty removing emerging contaminants, including pharmaceuticals and other recalcitrant compounds.
Conflicts of interest
Authors declare that they have no conflicting interests.
Acknowledgements
This work received financial support from the Agencia Andaluza de Cooperación Internacional para el Desarrollo AACID (projects INVODES 2021UC001). This publication has been supported by RUDN University Strategic Academic Leadership Program (R. Luque).
References
- J. Mohapatra, S. Nigam, J. George, A. C. Arellano, P. Wang and J. P. Liu, Principles and applications of magnetic nanomaterials in magnetically guided bioimaging, Mater. Today Phys., 2023, 32, 101003, DOI:10.1016/j.mtphys.2023.101003.
- J. Kang, C. Zheng, K. Khan, A. K. Tareen, M. Aslam and B. Wang, Two dimensional nanomaterials-enabled smart light regulation technologies: Recent advances and developments, Optik, 2020, 220, 165191, DOI:10.1016/j.ijleo.2020.165191.
- M. Hujjatul Islam, M. T. Y. Paul, O. S. Burheim and B. G. Pollet, Recent developments in the sonoelectrochemical synthesis of nanomaterials, Ultrason. Sonochem., 2019, 59, 104711, DOI:10.1016/j.ultsonch.2019.104711.
- B. A. Yusuf, W. Yaseen, J. Xie, A. A. Babangida, A. I. Muhammad, M. Xie and Y. Xu, Rational design of noble metal-based multimetallic nanomaterials: A review, Nano Energy, 2022, 104, 107959, DOI:10.1016/j.nanoen.2022.107959.
- E. O. Oseghe, S. O. Akpotu, E. T. Mombeshora, A. O. Oladipo, L. M. Ombaka, B. B. Maria, A. O. Idris, G. Mamba, L. Ndlwana, O. S. Ayanda, A. E. Ofomaja, V. O. Nyamori, U. Feleni, T. T. I. Nkambule, T. A. M. Msagati, B. B. Mamba and D. W. Bahnemann, Multi-dimensional applications of graphitic carbon nitride nanomaterials – A review, J. Mol. Liq., 2021, 344, 117820, DOI:10.1016/j.molliq.2021.117820.
- L. Chen, Z. Mao, Y. Wang, Y. Kang, Y. Wang, L. Mei and X. Ji, Edge modification facilitated heterogenization and exfoliation of two-dimensional nanomaterials for cancer catalytic therapy, Sci. Adv., 2022, 8, eabo7372, DOI:10.1126/sciadv.abo7372.
- O. M. Yaghi and H. Li, Hydrothermal Synthesis of a Metal-Organic Framework Containing Large Rectangular Channels, J. Am. Chem. Soc., 1995, 117, 10401–10402, DOI:10.1021/ja00146a033.
- C. A. Morales-Paredes, J. M. Rodríguez-Díaz and N. Boluda-Botella, Pharmaceutical compounds used in the COVID-19 pandemic: A review of their presence in water and treatment techniques for their elimination, Sci. Total Environ., 2022, 814, 152691, DOI:10.1016/j.scitotenv.2021.152691.
- Z. Xu, W. Deng and X. Wang, 3D Hierarchical Carbon-Rich Micro-/Nanomaterials for Energy Storage and Catalysis, Electrochem. Energy Rev., 2021, 4, 269–335, DOI:10.1007/s41918-021-00094-7.
- G.-R. Xu, Z.-H. An, K. Xu, Q. Liu, R. Das and H.-L. Zhao, Metal organic framework (MOF)-based micro/nanoscaled materials for heavy metal ions removal: The cutting-edge study on designs, synthesis, and applications, Coord. Chem. Rev., 2021, 427, 213554, DOI:10.1016/j.ccr.2020.213554.
- M. Shabani Nashtaei, A. Mollahosseini, M. Rabbani, P. Shabannashtaei and S. Parvaz, Preparing PAN/MOF nanofiber composite by electrospinning method for carbon dioxide adsorption, Inorg. Chem. Commun., 2023, 153, 110731, DOI:10.1016/j.inoche.2023.110731.
- N. Faaizatunnisa, R. Ediati, H. Fansuri, H. Juwono, S. Suprapto, A. R. P. Hidayat and L. L. Zulfa, Facile green synthesis of core–shell magnetic MOF composites (Fe3O4@SiO2@HKUST-1) for enhanced adsorption capacity of methylene blue, Nano-Structures Nano-Objects, 2023, 34, 100968, DOI:10.1016/j.nanoso.2023.100968.
- S. Miao, J. Guo, Z. Deng, J. Yu and Y. Dai, Adsorption and reduction of Cr(VI) in water by iron-based metal-organic frameworks (Fe-MOFs) composite electrospun nanofibrous membranes, J. Cleaner Prod., 2022, 370, 133566, DOI:10.1016/j.jclepro.2022.133566.
- L. A. Zambrano-Intriago, E. V. Daza-López, A. Fernández-Andrade, R. Luque, C. G. Amorim, A. N. Araújo, J. M. Rodríguez-Díaz and M. C. B. S. M. Montenegro, Application of a novel hybrid MIL-53(Al)@rice husk for the adsorption of glyphosate in water: Characteristics and mechanism of the process, Chemosphere, 2023, 327, 138457, DOI:10.1016/j.chemosphere.2023.138457.
- B. F. Rivadeneira-Mendoza, O. A. Estrela Filho, K. J. Fernández-Andrade, F. Curbelo, F. Fred da Silva, R. Luque and J. M. Rodríguez-Díaz, MOF@biomass hybrids: Trends on advanced functional materials for adsorption, Environ. Res., 2023, 216, 114424, DOI:10.1016/j.envres.2022.114424.
- K. J. Fernández-Andrade, A. A. Fernández-Andrade, L. Á. Zambrano-Intriago, L. E. Arteaga-Perez, S. Alejandro-Martin, R. J. Baquerizo-Crespo, R. Luque and J. M. Rodríguez-Díaz, Microwave-assisted MOF@biomass layered nanomaterials: Characterization and applications in wastewater treatment, Chemosphere, 2023, 314, 137664, DOI:10.1016/j.chemosphere.2022.137664.
- V. I. Isaeva, V. V. Chernyshev, A. L. Tarasov, A. A. Lobova, G. I. Kapustin and N. A. Davshan, Conditions for the Formation of Microporous Metal–Organic Framework Mil-53(Al), Russ. J. Phys. Chem. A, 2018, 92, 2386–2390, DOI:10.1134/S0036024418120166.
- A. M. Omer, E. M. Abd El-Monaem, G. M. El-Subruiti, M. M. Abd El-Latif and A. S. Eltaweil, Fabrication of easy separable and reusable MIL-125(Ti)/MIL-53(Fe) binary MOF/CNT/Alginate composite microbeads for tetracycline removal from water bodies, Sci. Rep., 2021, 11, 23818, DOI:10.1038/s41598-021-03428-z.
- G. Chen, S. He, G. Shi, Y. Ma, C. Ruan, X. Jin, Q. Chen, X. Liu, H. Dai, X. Chen and D. Huang, In-situ immobilization of ZIF-67 on wood aerogel for effective removal of tetracycline from water, Chem. Eng. J., 2021, 423, 130184, DOI:10.1016/j.cej.2021.130184.
- J. Ouyang, J. Chen, S. Ma, X. Xing, L. Zhou, Z. Liu and C. Zhang, Adsorption removal of sulfamethoxazole from water using UiO-66 and UiO-66-BC composites, Particuology, 2022, 62, 71–78, DOI:10.1016/j.partic.2021.04.012.
- J. Cui, X. Xu, L. Yang, C. Chen, J. Qian, X. Chen and D. Sun, Soft foam-like UiO-66/Polydopamine/Bacterial cellulose composite for the removal of aspirin and tetracycline hydrochloride, Chem. Eng. J., 2020, 395, 125174, DOI:10.1016/j.cej.2020.125174.
- C. Y. Chuah, H. Lee and T.-H. Bae, Recent advances of nanoporous adsorbents for light hydrocarbon (C1 – C3) separation, Chem. Eng. J., 2022, 430, 132654, DOI:10.1016/j.cej.2021.132654.
- P. Zakeri-Milani, M. Barzegar-Jalali, H. Tajerzadeh, Y. Azarmi and H. Valizadeh, Simultaneous determination of naproxen, ketoprofen and phenol red in samples from rat intestinal permeability studies: HPLC method development and validation, J. Pharm. Biomed. Anal., 2005, 39, 624–630, DOI:10.1016/j.jpba.2005.04.008.
- P. D. Kalariya, D. Namdev, R. Srinivas and S. Gananadhamu, Application of experimental design and response surface technique for selecting the optimum RP-HPLC conditions for the determination of moxifloxacin HCl and ketorolac tromethamine in eye drops, J. Saudi Chem. Soc., 2017, 21, S373–S382, DOI:10.1016/j.jscs.2014.04.004.
- J. M. N. Mendoza, B. F. R. Mendoza, J. C. Mendoza, A. M. Balu, R. Luque, L. A. Z. Intriago and J. M. Rodríguez-Díaz, MIL-53(Al)@HC nanohybrid for bicomponent adsorption of ibuprofen and metsulfuron-methyl: Application of macro- and microscopic models and competition between contaminants, Environ. Res., 2023, 117492, DOI:10.1016/j.envres.2023.117492.
- Y. Liu, L. Wang, X. Wang, F. Jing, R. Chang and J. Chen, Oxidative ageing of biochar and hydrochar alleviating competitive sorption of Cd(II) and Cu(II), Sci. Total Environ., 2020, 725, 138419, DOI:10.1016/j.scitotenv.2020.138419.
- T. Zhang, X. Wu, X. Fan, D. C. W. Tsang, G. Li and Y. Shen, Corn waste valorization to generate activated hydrochar
to recover ammonium nitrogen from compost leachate by hydrothermal assisted pretreatment, J. Environ. Manage., 2019, 236, 108–117, DOI:10.1016/j.jenvman.2019.01.018.
- D. Guo, R. Wen, M. Liu, H. Guo, J. Chen and W. Weng, Facile fabrication of g-C3N4/MIL-53(Al) composite with enhanced photocatalytic activities under visible-light irradiation, Appl. Organomet. Chem., 2015, 29, 690–697, DOI:10.1002/aoc.3352.
- M. Meilikhov, K. Yusenko and R. A. Fischer, The adsorbate structure of ferrocene inside [Al(OH)(bdc)]x (MIL-53): a powder X-ray diffraction study, Dalton Trans., 2009, 600–602, 10.1039/B820882B.
- T. Loiseau, C. Serre, C. Huguenard, G. Fink, F. Taulelle, M. Henry, T. Bataille and G. Férey, A Rationale for the Large Breathing of the Porous Aluminum Terephthalate (MIL-53) Upon Hydration, Chem. – Eur. J., 2004, 10, 1373–1382, DOI:10.1002/chem.200305413.
- H. S. Kambo and A. Dutta, A comparative review of biochar and hydrochar in terms of production, physico-chemical properties and applications, Renewable Sustainable Energy Rev., 2015, 45, 359–378, DOI:10.1016/j.rser.2015.01.050.
- H. Idriss, On the wrong assignment of the XPS O1s signal at 531–532 eV attributed to oxygen vacancies in photo- and electro-catalysts for water splitting and other materials applications, Surf. Sci., 2021, 712, 121894, DOI:10.1016/j.susc.2021.121894.
- L. Zhang, Q. Wang, F. Xu, Z. Wang and G. Zhang, Insights into the evolution of chemical structures in hydrochars from hydrothermal carbonization of PVC, J. Energy Inst., 2022, 105, 323–333, DOI:10.1016/j.joei.2022.09.004.
- A. T. Kozakov, A. G. Kochur, N. Kumar, K. Panda, A. V. Nikolskii and A. V. Sidashov, Determination of sp2 and sp3 phase fractions on the surface of diamond films from C1s, valence band X-ray photoelectron spectra and CKVV X-ray-excited Auger spectra, Appl. Surf. Sci., 2021, 536, 147807, DOI:10.1016/j.apsusc.2020.147807.
- X. Zhang, C. Gao, R. Wang and R. Han, Remediation of ibuprofen and naproxen in water by a green composite material of magnetic carbon nanotube–metal–organic framework, J. Environ. Chem. Eng., 2023, 11, 111090, DOI:10.1016/j.jece.2023.111090.
- L. Xing, K. M. Haddao, N. Emami, F. Nalchifard, W. Hussain, H. Jasem, A. H. Dawood, D. Toghraie and M. Hekmatifar, Fabrication of HKUST-1/ZnO/SA nanocomposite for Doxycycline and Naproxen adsorption from contaminated water, Sustainable Chem. Pharm., 2022, 29, 100757, DOI:10.1016/j.scp.2022.100757.
- Y. Wang and L. Zhang, Improved performance of 3D hierarchical NiAl-LDHs micro-flowers via a surface anchored ZIF-8 for rapid multiple-pollutants simultaneous removal and residues monitoring, J. Hazard. Mater., 2020, 395, 122635, DOI:10.1016/j.jhazmat.2020.122635.
- D. Sompornpailin, P. Mongconpattarasuk, C. Ratanatawanate, R. Apiratikul, K. H. Chu and P. Punyapalakul, Adsorption of nonsteroidal anti-inflammatory drugs onto composite beads of a 1D flexible framework MIL-53(Al): Adsorption mechanisms and fixed-bed study, J. Environ. Chem. Eng., 2022, 10, 108144, DOI:10.1016/j.jece.2022.108144.
- Q. Hu, R. Lan, L. He, H. Liu and X. Pei, A critical review of adsorption isotherm models for aqueous contaminants: Curve characteristics, site energy distribution and common controversies, J. Environ. Manage., 2023, 329, 117104, DOI:10.1016/j.jenvman.2022.117104.
- H. Hanafy, L. Sellaoui, P. S. Thue, E. C. Lima, G. L. Dotto, T. Alharbi, H. Belmabrouk, A. Bonilla-Petriciolet and A. B. Lamine, Statistical physics modeling and interpretation of the adsorption of dye remazol black B on natural and carbonized biomasses, J. Mol. Liq., 2020, 299, 112099, DOI:10.1016/j.molliq.2019.112099.
- L. Sellaoui, É. C. Lima, G. L. Dotto, S. L. Dias and A. B. Lamine, Physicochemical modeling of reactive violet 5 dye adsorption on home-made cocoa shell and commercial activated carbons using the statistical physics theory, Results Phys., 2017, 7, 233–237 CrossRef.
- R. Munir, S. Zaib, I. Khan, Z. t. Islam, R. M. Gomila, C. J. McAdam, C. H. S. Yeow, J. M. White, T. Hökelek, A. A. Al-Askar, E. B. Elkaeed and A. Frontera, Complex interplay of hydrogen bonding, halogen bonding and π-interactions in methyl 2-(7-chloro-1H-pyrazolo[3,4-b]quinolin-1-yl)acetate: Synthesis, X-ray crystallography, energetic features and anti-urease efficacy, J. Mol. Struct., 2023, 1287, 135625, DOI:10.1016/j.molstruc.2023.135625.
- S. Yu, X. Wang, Y. Ai, X. Tan, T. Hayat, W. Hu and X. Wang, Experimental and theoretical studies on competitive adsorption of aromatic compounds on reduced graphene oxides, J. Mater. Chem. A, 2016, 4, 5654–5662, 10.1039/C6TA00890A.
- G. Yan, X. Qi, H. Wang and J. Shi, Magnetic MnFe2O4-MIL-53 (Fe) composite as an effective adsorbent for As(V) adsorption in wastewater, Microporous Mesoporous Mater., 2022, 346, 112290, DOI:10.1016/j.micromeso.2022.112290.
- L. Qi, H. Jiang, T. Lin, X. Chang and B. Jiang, Fabrication of MIL-53(Al) based composites from biomass activated carbon (AC) for efficient p-nitrophenol adsorption from aqueous solution, J. Taiwan Inst. Chem. Eng., 2021, 127, 220–227, DOI:10.1016/j.jtice.2021.08.020.
|
This journal is © The Royal Society of Chemistry 2024 |