DOI:
10.1039/D3AN02047A
(Paper)
Analyst, 2024,
149, 1132-1140
Covalently attached ionophores extend the working range of potentiometric pH sensors with poly(decyl methacrylate) sensing membranes†
Received
25th November 2023
, Accepted 13th December 2023
First published on 19th December 2023
Abstract
The pH working range of solid-contact ion-selective electrodes (ISEs) with plasticizer-free poly(decyl methacrylate) sensing membranes is shown to be expanded by covalent attachment of H+ ionophores to the polymeric membrane matrix. In situ photopolymerization not only incorporates the ionophores into the polymer backbone, but at the same time also attaches the sensing membranes covalently to the underlying inert polymer and nanographite solid contact, minimizing sensor drift and preventing failure by membrane delamination. A new pyridine-based H+ ionophore, 3-(pyridine-3-yl)propyl methacrylate, has lower basicity than trialkylamine ionophores and expands the upper detection limit. This reduces in particular the interference from hydrogen phthalate, which is a common component of commercial pH buffers. Moreover, the lower detection limit is improved by replacing the CH2CH2 spacer of previously reported dialkylaminoethyl methacrylates with a (CH2)10 spacer, which increases its basicity. Notably, for the more basic and highly cation-selective ionophore 10-(diisopropylamino)decyl methacrylate, the extent of counterion interference from hydrogen phthalate shifted the upper detection limit to lower pH by nearly one pH unit when the crosslinker concentration was decreased.
1 Introduction
Glass electrodes exhibit high H+ selectivity and are used for a wide range of applications1,2 but they are inherently fragile, exhibit high resistance, are difficult to miniaturize, and have a high affinity for proteins, requiring frequent cleaning and maintenance.3,4 Moreover, their working range is limited by the so-called acid and alkaline errors at low and high pH values, respectively.5 Many of these limitations can be addressed by the use of ion-selective electrodes (ISEs) with an ionophore-doped polymeric membrane.6–11 In particular, ionophores with different H+ affinities can be chosen to tune the working range for specific applications.12–20 Polymeric membrane ISEs are not without their flaws, though, including the loss of selectivity and sensitivity due to leaching of ionophores and, in the case of plasticized sensing membranes, plasticizers into samples.21–28 To overcome these limitations, several types of plasticizer-free polymeric ISE membranes with either covalently attached ionophores or covalently attached ionic sites have been introduced.29–38 Notably, attachment of both the ionic sites and ionophores to the matrix polymer results in sensing membranes with excessively high resistances and slow response times,35 an outcome that could probably only be avoided if the ionophore groups are close enough to one another so that ion hopping is feasible. Whether it is preferable to covalently attach the ionophore or the ionic sites to the polymer depends on the stability and stoichiometry of the ionophore complexes.35
The upper and lower detection limits of an ionophore-based H+ ISE depend on the basicity of the ionophore. Less basic ionophores typically shift both the lower and upper detection limits to a similar extent to lower pH,20 although exceptions to this rule have been observed when failure of counter-ion exclusion (also referred to as Donnan failure) is preceded by a super-Nernstian response region.39,40 At the upper detection limit, target ions and counter-ions are co-extracted into the sensing membrane, which is facilitated by the strong binding of the target ion to the ionophore as well as a high activity and lipophilicity of the counter ions.19,41 For interfering ions that have the same charge sign as the target ions, the lower detection limit is limited by the activities of these ions and the selectivity of the ISE for H+ with respect to these ions.42–44 The latter again depends on the stability of the complexes of the ionophore with the target ion, H+, and interfering ions. Therefore, the affinity of the ionophore for H+ controls the upper and lower ends of the sensing range. For example, conventional plasticized poly(vinyl chloride) doped with tridodecylamine17 exhibited a pH sensing range from >12 to 5.5 while the less basic 4-nonadecylpyridine (ETH 1907)14 responds linearly in the pH range of 9 to 2.
Importantly, the use of an ionophore with more than one group that can be protonated or a combination of two different ionophores does not extend the pH working range, as the upper and lower detection limits depend inherently on the ionic site concentration.45,46 On one hand, for sensor membranes with a low ionic site concentration, a more basic ionophoric group limits the upper detection limit to a pH higher than it would be the case for a less basic ionophore.47 On the other hand, for membranes with an ionic site concentration sufficiently high so that the less basic ionophore controls (and thereby improves) the upper detection limit, the lower detection limit suffers from the lower selectivity over interfering ions as provided by that less basic ionophore. Therefore, to permit analysis in a wide pH range, two electrodes with different ionophores need to be used in parallel.
Herein, we report on ISEs with plasticizer-free poly(decyl methacrylate) (PDMA) membranes using covalently attachable ionophores with a pyridine or tertiary amino group to cover a wider pH range than with previously reported 2-dialkylaminoethyl methacrylates. We report on the potentiometric response, interference from ions with a charge sign opposite to that of the measured ion, and how the weight percent of sensing components influences the upper detection limits.
2 Experimental section
2.1 Materials
Nanographite powder (GS-4827, particle size distribution from 0.10 µm to 10 µm; BET surface area 165 m2 g−1)48 was purchased from Alfa Aesar (Tewksbury, MA, USA). The ionophore 3-(pyridin-3-yl)propyl methacrylate was prepared by following a modified literature procedure49,50 by reaction of 3-(pyridin-3-yl)propanol with methacryloyl chloride in the presence of triethylamine. Upon extraction of the product from the reaction solution with dichloromethane, 100 ppm 4-methoxyphenol was added before further processing to prevent untimely polymerization. The ionophore 10-(diisopropylamino)decyl methacrylate was obtained in a three-step synthesis by (i) reaction of diisopropylamine with methyl sebacoyl chloride to give an amide ester, (ii) simultaneous reduction of the two carbonyl groups in the resulting compound with LiAlH4 to give a primary alcohol with a diisopropylamino substituent, and (iii) ester formation of this alcohol with methacryloyl chloride in the presence of triethylamine. Here too, upon extraction of the product from the reaction solution with dichloromethane, 100 ppm 4-methoxyphenol was added. For details of the syntheses and 1H NMR spectra of these ionophores as well as specifics on other reagents, see the ESI.†
2.2 Preparation of precursor solutions
Precursor solutions for PDMA membranes with the covalently attachable ionophore comprising a pyridine group were prepared by mixing 1.4 wt% 2,2-dimethoxy-2-phenylacetophenone (DMPA; photoinitiator), 90.0 wt% decyl methacrylate (DMA), 1.4 wt% 1,6-hexanediol dimethacrylate (crosslinker), 3.8 wt% potassium tetrakis(pentafluorophenyl)borate (ionic sites), and 3.3 wt% 3-(pyridin-3-yl)propyl methacrylate (ionophore, 300 mol% with respect to the ionic sites).
For PDMA membranes with the covalently attachable ionophore with a tertiary amino group, a precursor solution was prepared containing (unless noted otherwise) 1.4 wt% DMPA, 88.7 wt% DMA, 1.4 wt% 1,6-hexanediol dimethacrylate, 3.8 wt% potassium tetrakis(pentafluorophenyl)borate, and 4.7 wt% 10-(diisopropylamino)decyl methacrylate.
For PDMA membranes with mobile ionic sites but no ionophore, a precursor solution was prepared containing 1.5 wt% DMPA, 93 wt% DMA, 1.5 wt% 1,6-hexanediol dimethacrylate, and 4 wt% potassium tetrakis(pentafluorophenyl)borate.
2.3 Polymerization and grafting of PDMA membranes onto functionalized inert polymer bodies and solid contact
Solid-contact ISEs with PDMA membranes were prepared in an analogous manner as previously reported51 for the direct attachment of the sensing membrane to both underlying inert polymeric substrates and solid contact carbon. To improve potential stability, nanographite with a high surface area (165 m2 g−1)48 was applied as a high-capacity solid-contact material onto a gold-coated stainless steel pin serving as the underlying electron conductor.35,52,53 The cylindrical electrode bodies used for this purpose were custom-made from polypropylene, with a gold-coated stainless steel pin (diameter 2.5 mm) protruding at the end.51 Unlike in the case of commonly used gold electrodes for cyclic voltammetry, the gold pin was recessed by 0.5 mm with respect to the end of the polypropylene cylinder, and an additional groove of 0.5 mm depth surrounded the end of the pin, forming a small cavity, into which a 21 μL aliquot of a nanographite suspension in tetrahydrofuran (THF) was deposited to cover the exposed gold surface.51 The suspension used for this purpose was prepared by sonicating 50 mg of nanographite in 1 mL of THF for 30 min.
To attach the surface initiator to polypropylene and nanographite, a THF solution of 2,2-dimethoxy-2-phenylacetophenone (DMPA) was dropcast onto the nanographite and the surrounding polypropylene body. The photoinitiator was grafted onto the polypropylene and nanographite solid contact by UV irradiation (peak output: 365 nm) over 20 min. To graft the sensing membrane onto the functionalized polymer and solid contact surface, the precursor solution was dropcast onto the nanographite and the area of the polypropylene body previously activated with the photoinitiator. The electrodes were then placed into a well-sealed box covered by a UV-transparent quartz glass plate, and the box was flushed with argon for 10 min. The membranes were polymerized under UV irradiation (peak output: 365 nm) for 20 min. An 8-watt 3UV ultraviolet lamp (254/302/365 nm) from Analytik Jena (Jena, Germany) was used for all photopolymerization reactions.
2.4 Potentiometry
Potentiometric measurements were carried out in stirred solutions with an EMF-16 potentiometer (Lawson Labs, Malvern, PA, USA) against a double-junction reference electrode with AgCl-saturated 3.00 M KCl reference electrolyte and 1.0 M KCl bridge electrolyte (DX200, Mettler Toledo, Switzerland). A pH glass electrode (InLab 201, Mettler Toledo, Columbus, OH, USA) was used to separately determine the pH of all solutions. To measure the pH responses of sensing membranes, the sample pH was adjusted by adding aliquots of 1 M NaOH or 1 M HCl while monitoring the pH with the glass electrode. Selectivity coefficients against Na+, K+, and Li+ were measured using the fixed interference method (FIM) or, when indicated, the fixed primary ion method (FPIM).54 Long-term stabilities were measured in a temperature-controlled chamber at 25 °C with an AgCl-coated Ag wire directly inserted into the sample solution as the reference electrode.
2.5 Chronopotentiometry
Chronopotentiometry was used for resistance measurements. A gold electrode coated with nanographite and an ion-selective membrane was used as the working electrode, 10 mM sodium phosphate buffer solution (pH = 7.1) was used as the aqueous electrolyte, an AgCl/Ag electrode with AgCl-saturated 3.00 M KCl reference electrolyte and a 1.0 M KCl bridge electrolyte served as the double-junction reference electrode, and a Pt wire was used as the counter electrode. A constant current of +0.1 nA was applied to the ISE for 100 s, followed by a reverse current of the same magnitude for the same length of time.55 The specific capacitance of this nanographite solid contact was thus determined to be 1500 mF g−1.
3 Results and discussion
3.1 PDMA membranes with covalently attached pyridine and trialkylamine ionophores
With a view to improve the robustness and longevity of ionophore-doped pH-sensitive ISEs, in our previous work, we grafted ISE membranes to underlying inert electrode body materials and electron conductors, and we covalently attached an ionophore to the crosslinked PDMA sensing membrane.51 For this purpose, we used the commercially available compounds 2-(dimethylamino)ethyl methacrylate, 1, and 2-(diisopropylamino)ethyl methacrylate, 2, as ionophores (see Fig. 1). While these electrodes performed well in many aspects, there was room for the improvement of their lower detection limit (i.e., at high pH). Moreover, their upper detection limit was affected by Donnan failure when samples contained hydrophobic ions such as the very hydrophobic hydrogen phthalate, which is commonly used in commercial pH buffers. To this end, we report here on the new ionophores 3-(pyridin-3-yl)propyl methacrylate, 3, and 10-(diisopropylamino)decyl methacrylate, 4 (see Fig. 1) to improve the upper and lower detection limits, respectively. A brief description of the synthesis is given in the Experimental section; for further details, see the ESI.† The photoinitiated graft polymerization of the sensing membranes (see Fig. 2 and S6†) followed the same procedure as in our earlier work.50
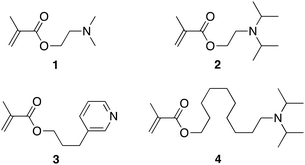 |
| Fig. 1 Structures of methacrylate-based H+ ionophores. | |
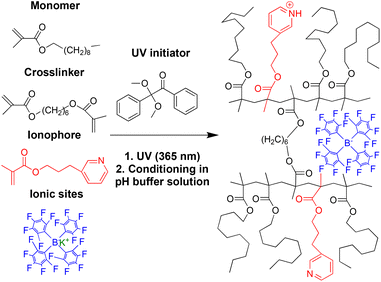 |
| Fig. 2 Photopolymerization of PDMA membranes with covalent attachment of an ionophore. Matrix monomer: decyl methacrylate; crosslinker: hexanediol dimethacrylate; ionic sites: potassium tetrakis(pentafluorophenyl)borate (blue); UV-initiator: 2,2-dimethoxy-2-phenylacetophenone; illustrated here for ionophore 3 (red). | |
3.2 Cation selectivities of PDMA membranes
Table 1 lists the cation selectivities of the ISEs prepared with one of the ionophores 1, 2, 3, or 4. Because ionophore 3 was chosen to be less basic than the trialkylamine ionophores to improve the counter ion interference at low pH, it is not surprising that the ISE membranes doped with 3 exhibit smaller discrimination of Li+, Na+, and K+ than the ISMs doped with 1, 2, or 4. Notably, the cation selectivities of ISMs doped with 3 are close to those of ISEs based on the previously reported ionophores ETH 1778 and ETH 1907, which are also pyridine derivatives but were covalently attached to a polymer backbone. Indeed, even though the selectivities for ETH 1778 and ETH 1907 from the literature as listed in Table 1 are for PVC membranes plasticized with o-nitrophenyl octyl ether (oNPOE), the cation selectivities of ETH 1778, 3, and ETH 1907 follow the same sequence as their estimated pKa values56–58 of 3.2, 5.4, and 6.1, respectively, in water.
Table 1 Working ranges and selectivities (FIM, unless noted otherwise) of pH ISEs comprising PDMA membranes with a covalently attached ionophore comprising a pyridine or tertiary amino group, along with data for comparable ISEs with plasticized PVC membranes and free ionophores
|
PDMA, no ionophore, mobile ionic sites (this work) |
PDMA, covalently attached ionophore 3 (this work) |
PDMA, covalently attached ionophore 1 35 |
PDMA, covalently attached ionophore 2 35 |
PDMA, covalently attached ionophore 4 (this work) |
PDMA, free ionophore, covalently attached ionic sites35 |
PVC/oNPOE, free ionophore14 |
PVC/oNPOE, free ionophore18 |
R: CH2CH2O(C O)C(CH3)CH2.
4-Nonadecylpyridine.
Octadecyl isonicotinate.
pKa of the neutral ionophore in water, as estimated using the Advanced Chemistry Development (ACD) acid dissociation calculator.56–58
Determined using 10 mM sodium phosphate buffer by adjusting the pH with 1.0 M HCl or 1.0 M NaOH.
Determined using 100 mM potassium phosphate buffer by pH adjustment with 1.0 M HCl or 1.0 M NaOH.
Determined using 60 mM LiOH, 6.6 mM citric acid, 11.0 mM boric acid, and 4 N HCl.
Microelectrodes.
FPIM (pH 2.3).
This work.
|
Ionophore |
n.a. |
3-Pyridyl-CH2Ra |
(CH3)2NRa |
[(CH3)2CH]2NRa |
[(CH3)2CH]2N–(CH2)8Ra |
N(C12H25)3 |
ETH 1907b |
ETH 1778c |
pKa in waterd |
n.a. |
5.4 |
8.2 |
9.3 |
11.0 |
9.8 |
6.1 |
3.2 |
Response slope (mV per decade) |
−50.3 ± 1.2 |
−56.7 ± 0.4 |
−55.7 ± 1.0 |
−58.7 ± 0.5 |
−59.2 ± 0.2 |
−55.0 ± 0.4 |
−56.7 |
−57.2 |
Working range (pH) |
n.a. |
<0.8–7.8e |
1.9–9.2e |
1.8–10.0e |
3.8–12.7e |
4.8–13.4e |
2–9f |
0–8g |
Electrical resistance |
n.a. |
54.0 ± 3.2 MΩ |
495 ± 174 MΩ |
125 ± 80 MΩ |
54.0 ± 3.2 MΩ |
4 to 31 GΩ |
35 ± 9 GΩh |
Not reported |
log KpotH,Li |
−0.5 ± 0.1i |
−7.2 ± 0.1 |
−9.3 ± 0.1j |
−10.3 ± 0.1 |
−12.3 ± 0.2 |
−11.4 ± 0.2 |
Not reported |
−6.9 |
log KpotH,Na |
−0.3 ± 0.1i |
−7.5 ± 0.1 |
−8.8 ± 0.1j |
−11.0 ± 0.3 |
−12.6 ± 0.1 |
−12.4 ± 0.7 |
−9.7 |
−5.6 |
log KpotH,K |
+0.4 ± 0.1i |
−7.7 ± 0.2 |
−8.0 ± 0.1j |
−10.7 ± 0.3 |
−12.4 ± 0.1 |
−12.7 ± 0.2 |
−8.7 |
−4.4 |
The cation selectivities for ISMs doped with 1, 2, or 4 also follow the predicted pKa values of these ionophores. As we described earlier, the increase in ionophore basicity from 1 to 2 by approximately one pKa unit results from the replacement of the methyl groups attached to the amino nitrogen with isopropyl groups. It is consistent with the extension of the lower detection limit by one pH unit. The further improvement of the detection limit by about two pH units when going from ionophore 3 to ionophore 4 is consistent with an estimated further increase in pKa of nearly two units. The critical change in the ionophore structure that explains this improvement is the replacement of the alkanoyloxy group at the β position of the amino group with an alkyl substituent. Notably, the selectivities of ISMs doped with 4 for H+ over Na+, K+, and Li+ were very high and similar to PDMA ISMs doped with tridodecylamine, resulting in very similar lower detection limits for the two ISEs, but offering the added advantage of resistance to leaching due to the covalent attachment.
Interestingly, the three selectivity coefficients for Li+, Na+, and K+ for ionophore-free PDMA membranes doped with mobile ionic sites fall in a rather narrow range. The same is true for each of the ISMs doped with ionophores 1, 2, 3, or 4, while selectivities for different electrodes vary substantially. In comparison, the selectivity coefficients of the oNPOE-plasticized ISMs with ETH 1778 cover a wider selectivity range, as it is often observed for H+ ionophores that do not interact with these metal cations. This suggests that the three metal cations interact with the carbonyl groups of PDMA, with the free energies of interaction falling in the order of Li+ > Na+ > K+, flattening the Li+ < Na+ < K+ selectivity pattern caused by the different hydration energies of these three cations (i.e., the Hofmeister series).
3.3 Upper detection limit of PDMA membranes
Donnan failure, that is, the interference of ions of the opposite charge sign than the target ion at high target ion concentrations, is a well-known phenomenon.11,19,20,41 In the context of H+ ISEs, an anion of particular concern is hydrogen phthalate. With its low hydrophilicity, as evident from the high selectivity of ionophore-free ion exchanger electrodes,59 and its high concentrations in many commercial pH buffer solutions, hydrogen phthalate is a challenging interferent for polymer membrane ISEs. While phthalic acid and its mono- and deprotonated forms are present in few real life samples, it became popular for the calibration of pH glass electrodes many decades before polymer membrane ISEs were even invented. A trivial approach would be to recommend that hydrogen phthalate-containing buffers should not be used for the calibration of polymer membrane ISE, but many users will find this inconvenient, given currently available commercial pH buffers. Moreover, reducing the interference of hydrogen phthalate for H+ ISEs will at the same time also reduce interferences of other anions of low hydrophilicity, such as salicylate, iodide, and bromide, to name a few.
Hydrogen phthalate counter ion interference is illustrated in Fig. 3, which shows the pH response of an ISE based on the more basic ionophore 2, either by addition of aliquots of HCl or phthalic acid (Fig. 3c and d, respectively). Note that the upper detection limit is not marked by a gradual decrease in response slope, as this is often observed for Donnan failure. Instead, the eventual decrease in response slope is preceded by a pH range with a width of about 2 pH units in which the response slope is super-Nernstian. This phenomenon has been observed previously14,39,60 but is still poorly understood. Notably, when hydrogen phthalate is present, the upper end of the linear response occurs at pH 5.5, a much higher pH than pH 3.8 that is observed when only chloride and phosphate are present in the sample. (Note that for quantitative purposes we define here the upper end of the linear response as the pH at which the measured potential is 5 mV higher than what is predicted by a linear regression in the linear response range. In the presence of a super-Nernstian response region the extrapolation of the linear response curve to the detection limit, as similarly recommended by IUPAC for the lower detection limit,61 would clearly not be adequate.)
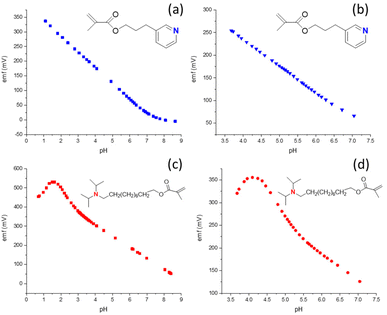 |
| Fig. 3 pH response of DMA-based membranes (n = 3) with covalently attached pyridine and tertiary amine ionophores. (a and c) pH adjusted by addition of 1.0 M HCl or 1.0 M NaOH to 10 mM sodium phosphate buffer background (pH 7.1). (b and d) pH adjusted by addition of 1.0 M potassium hydrogen phthalate to 1.0 mM sodium borate buffer and 10 mM potassium hydrogen phthalate (pH = 8.5). The pH shown on the x-axis was measured using a pH glass electrode, and emf values were measured relative to a free-flowing double junction reference electrode. | |
A further complication from counter ion interference is the emf drift that results not only in the pH region in which Donnan failure is observed, as for the exposure to 50 mM phthalate buffer of pH 4, but also the drift that is observed over a significant amount of time after the return of the ISE to a solution in which no Donnan failure is observed at equilibrium. This is illustrated in Fig. 4a. The emf only returns to a constant value when the phthalic acid has left the ISM.
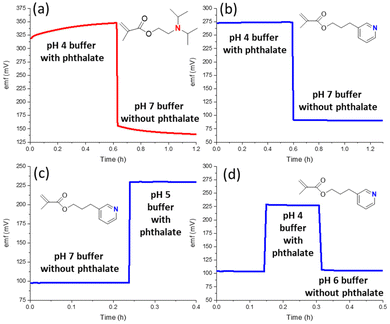 |
| Fig. 4 EMF of pH ISEs comprising PDMA membranes doped with (a) 2-(diisopropylamino)ethyl methacrylate and (b–d) 3-(pyridine-3-yl)propyl methacrylate. Buffers: pH 4, 50 mM potassium hydrogen phthalate, pH adjusted with HCl; pH 5, 50 mM potassium hydrogen phthalate/NaOH; pH 6, 50 mM phosphate buffer (K2HPO4, KH2PO4, HCl); pH 7, 50 mM phosphate buffer (KH2PO4/NaOH). | |
The smaller extent of interference from hydrophobic counter ions that results from the use of the less basic ionophore 3 is shown in Fig. 3. When the pH is adjusted by HCl addition to a 10 mM sodium phosphate buffer (Fig. 3a), the response is linear up to pH 0.8. This excellent resistance to error at low pH is particularly notable as acid error for some glass electrodes has been shown to occur already at pH < 2 and was found to worsen with increased anion concentrations.62 ISEs with 3 as the ionophore also exhibit in the presence of hydrogen phthalate a linear pH response up to pH 3.5. Importantly, experiments with hydrogen phthalate at a lower pH than 3.5 are neither needed nor possible because phthalic acid precipitates from the sample solutions at this low pH. Consistent with these observations, the emf of ISMs with ionophore 3 was also very stable when switching between different buffers with or without hydrogen phthalate (Fig. 4b–d). In addition, the calibration curves of these electrodes were linear and independent of nitrate, chloride, and hydrogen phthalate (see Fig. S5†).
For ISEs with 4 as the ionophore, experiments were also performed to explore whether the crosslinker concentration affects the pH at which Donnan failure occurs. Interestingly, the upper detection limits shifted by nearly a pH unit from pH 5.8 to 5.0 with decreasing crosslinker concentration. This result may be explained kinetically and is being investigated further.
3.4 Long-term EMF stability and effect of heat and nitric acid
The main advantages of the covalent attachment of the ISM to the underlying substrate and the covalent attachment of the ionophore to the ISM matrix polymer come from the prevention of ISM delamination and ionophore leaching, resulting in improved sensor lifetimes. Indeed, good long-term stabilities of the emf of ISEs based on ionophores 3 and 4 were observed in this work, as illustrated in Fig. 5.
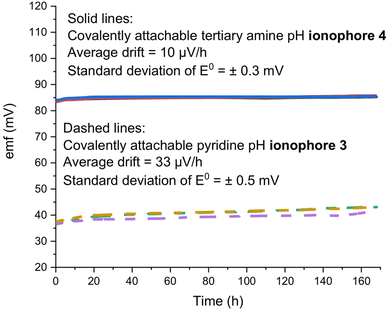 |
| Fig. 5 Long-term stability of PDMA membranes doped with covalently attached ionophore 4 (solid lines) or 3 (dash lines), as measured relative to an AgCl-coated Ag wire in 10.0 mM pH 7.1 phosphate buffer that contained 1.0 mM NaCl in a temperature-controlled Faraday cage at 25 °C. | |
To demonstrate the robustness of ISEs with PDMA membranes doped with ionophore 2, in our earlier work, electrodes were exposed to 90 °C heat for 2 h, 10 wt% ethanol solution for 1 day, or sterilization in an autoclave at 121 °C and 2.0 atm (for 30 min), and the electrodes maintained excellent sensor responses.35,51 In this work, ISEs with 3 or 4 as ionophores were exposed to 2% w/w nitric acid for 30 min. After 2% nitric acid exposure for 30 min, the electrodes exhibited unchanged working ranges and selectivities (Table 2). Moreover, no diminishment in response slopes and working ranges was observed upon two consecutive immersions of the ISEs for 30 min into water at 90 °C (Table 2). We note that for applications in which leaching of the ionic sites along with H+ into samples is of concern,11 the tetrakis(pentafluorophenyl)borate used in this work could be replaced by a more hydrophobic tetraphenylborate derivative.
Table 2 Potentiometric responses of ISEs with PDMA ISMs doped with covalently attached ionophores before and after two exposures to water at 90 °C or nitric acid (2 wt%) for 30 min each. Standard deviation of
: ±0.2
Ionophore |
Exposure |
Slope (mV per decade) |

|

|

|
3
|
Before |
−56.7 ± 0.4 |
−7.5 |
−7.7 |
−7.2 |
90 °C |
−56.4 ± 0.8 |
−7.6 |
−7.5 |
−7.7 |
HNO3 |
−55.7 ± 0.3 |
−7.3 |
−7.6 |
−7.5 |
4
|
Before |
−59.2 ± 0.2 |
−12.6 |
−12.4 |
−12.3 |
90 °C |
−58.9 ± 0.1 |
−12.5 |
−12.5 |
−12.3 |
HNO3 |
−58.2 ± 0.3 |
−12.4 |
−12.5 |
−12.1 |
4 Conclusions
To extend the working range of ISEs with plasticizer-free PDMA membranes, new covalently attachable pyridine and tertiary amine-based ionophores with different H+ affinities were synthesized. The ISE membranes doped with the pyridine-based ionophore showed a working range and selectivity very similar to membranes with a commercially available pyridine-based ionophore that cannot be covalently attached to the membrane polymer. Importantly, the less basic character of the pyridine ionophore not only shifts the upper detection limits to lower pH, but also reduces or even eliminates co-ion interference from very hydrophobic ions, such as hydrogen phthalate. In the case of ISEs doped with the tertiary amine-based ionophore, a very high selectivity, comparable to that of the conventional H+ ionophore tridodecylamine, was found. In addition, compared to previously investigated covalently attachable methacrylate-based tertiary amines, the replacement of the alkanoyloxy substituent at the β position of the amino group with an alkyl group makes the new ionophore more basic, resulting in a shift of the lower detection limit to higher pH. Moreover, we confirmed that these sensors show an excellent resistance to repeated heating to 90 °C or to 2 wt% of nitric acid solution for 30 min.
Author contributions
The manuscript was written through the contributions of all authors. All authors have given approval to the final version of the manuscript. We thank Ye Lin and Kelly Mitzel for thoughtful discussions of results from this project.
Conflicts of interest
The authors declare the following competing financial interests: K. R. C. and P. B. are inventors of the patent application 63/672,289 filed with the United States Patent and Trademark Office. P. B. is the inventor of U.S. Patent 11,567,030 B2; January 31, 2023.
Acknowledgements
The authors acknowledge funding for this project from Emerson Automation Solutions, Shakopee, MN, USA.
References
- W. Vonau and U. Guth, pH Monitoring: A Review, J. Solid State Electrochem., 2006, 10, 746–752, DOI:10.1007/s10008-006-0120-4.
- R. W. Burnett, A. K. Covington, N. Fogh-Andersen, W. R. Külpmann, A. H. J. Maas, O. Müller-Plathe, A. L. Van Kessel, P. D. Wimberley, W. G. Zijlstra, O. Siggaard-Andersen and H. F. Weisberg, Approved IFCC Recommendation on Definitions of Quantities and Conventions Related to Blood Gases and pH, Eur. J. Clin. Chem. Clin. Biochem., 1995, 33, 399–404, DOI:10.1515/cclm.1995.33.6.399.
- E. Lindner and R. E. Gyurcsányi, Quality Control Criteria for Solid-Contact, Solvent Polymeric Membrane Ion-Selective Electrodes, J. Solid State Electrochem., 2009, 13, 51–68, DOI:10.1007/s10008-008-0608-1.
-
W. Simon, D. Ammann, P. Anker, U. Oesch and D. M. Band, Ion Selective Electrodes and Their Clinical Application in Continuous Ion-Monitoring, in New Technologies in Clinical Laboratory Science: Proceedings of the fifth ECCLS Seminar held at Siena, Italy, 23–25 May 1984, ed. N. K. Shinton, Springer Netherlands, Dordrecht, 1985, pp. 115–121. DOI:10.1007/978-94-009-4928-7_14.
- F. G. K. Baucke, The Modern Understanding of the Glass Electrode Response, Fresenius’ J. Anal. Chem., 1994, 349, 582–596, DOI:10.1007/BF00323462.
- J. Bobacka, A. Ivaska and A. Lewenstam, Potentiometric Ion Sensors, Chem. Rev., 2008, 108, 329–351, DOI:10.1021/cr068100w.
- A. Michalska, All-Solid-State Ion Selective and All-Solid-State Reference Electrodes, Electroanalysis, 2012, 24, 1253–1265, DOI:10.1002/elan.201200059.
- G. A. Crespo and E. Bakker, Dynamic Electrochemistry with Ionophore Based Ion-Selective Membranes, RSC Adv., 2013, 3, 25461–25474, 10.1039/c3ra43751e.
- T. Yin and W. Qin, Applications of Nanomaterials in Potentiometric Sensors, TrAC, Trends Anal. Chem., 2013, 51, 79–86, DOI:10.1016/j.trac.2013.06.009.
-
P. Bühlmann and L. D. Chen, Ion-Selective Electrodes With Ionophore-Doped Sensing Membranes, in Supramolecular Chemistry: From Molecules to Nanomaterials; Volume 5: Self-Assembly and Supramolecular Devices, ed. J. W. Steed and P. A. Gale, John Wiley & Sons, 2012, pp. 2539–2580. DOI:10.1002/9780470661345.smc097.
- E. Bakker, P. Bühlmann and E. Pretsch, Carrier-Based Ion-Selective Electrodes and Bulk Optodes. 1. General Characteristics, Chem. Rev., 1997, 97, 3083–3132, DOI:10.1021/cr940394a.
- P. Anker, D. Ammann and W. Simon, Blood pH Measurement with a Solvent Polymeric Membrane Electrode in Comparison with a Glass Electrode, Mikrochim. Acta, 1983, 79, 237–242, DOI:10.1007/BF01303909.
- C. Espadas-Torre, E. Bakker, S. Barker and M. E. Meyerhoff, Influence of Nonionic Surfactants on the Potentiometric Response of Hydrogen Ion-Selective Polymeric Membrane Electrodes, Anal. Chem., 1996, 68, 1623–1631, DOI:10.1021/ac951017g.
- P. Chao, D. Ammann, U. Oesch, W. Simon and F. Lang, Extra- and Intracellular Hydrogen Ion-Selective Microelectrode Based on Neutral Carriers with Extended pH Response Range in Acid Media, Pfluegers Arch., 1988, 411, 216–219, DOI:10.1007/BF00582318.
- E. Bakker, P. Bühlmann and E. Pretsch, Polymer Membrane Ion-Selective Electrodes-What Are the Limits?, Electroanalysis, 1999, 11, 915–933, DOI:10.1002/(SICI)1521-4109(199909)11:13<915::AID-ELAN915>3.0.CO;2-J.
- A. Michalska, A. Hulanicki and A. Lewenstam, All Solid-State Hydrogen Ion-Selective Electrode Based on a Conducting Poly(pyrrole) Solid Contact, Analyst, 1994, 119, 2417–2420, 10.1039/AN9941902417.
- P. Schulthess, Y. Shijo, H. V. Pham, E. Pretsch, D. Ammann and W. Simon, A Hydrogen Ion-Selective Liquid-Membrane Electrode Based on Tri-n-dodecylamine as Neutral Carrier, Anal. Chim. Acta, 1981, 131, 111–116, DOI:10.1016/S0003-2670(01)93540-8.
- U. Oesch, Z. Brzózka, A. Xu, B. Rusterholz, G. Suter, H. V. Pham, D. H. Welti, D. Ammann, E. Pretsch and W. Simon, Design of Neutral Hydrogen Ion Carriers for Solvent Polymeric Membrane Electrodes of Selected pH Range, Anal. Chem., 1986, 58, 2285–2289, DOI:10.1021/ac00124a037.
- P. Bühlmann, S. Amemiya, S. Yajima and Y. Umezawa, Co-Ion Interference for Ion-Selective Electrodes Based on Charged and Neutral Ionophores: A Comparison, Anal. Chem., 1998, 70, 4291–4303, DOI:10.1021/ac9805145.
- E. Bakker, A. Xu and E. Pretsch, Optimum Composition of Neutral Carrier Based pH Electrodes, Anal. Chim. Acta, 1994, 295, 253–262, DOI:10.1016/0003-2670(94)80230-0.
- I. Bedlechowicz, M. Maj-Zurawska, T. Sokalski and A. Hulanicki, Effect of a Plasticizer on the Detection Limit of Calcium-Selective Electrodes, J. Electroanal. Chem., 2002, 537, 111–118, DOI:10.1016/S0022-0728(02)01256-1.
- B. Paczosa-Bator, R. Piech and A. Lewenstam, Determination of the Leaching of Polymeric Ion-Selective Membrane Components by Stripping Voltammetry, Talanta, 2010, 81, 1003–1009, DOI:10.1016/j.talanta.2010.01.048.
- A. Radu, S. Anastasova-Ivanova, B. Paczosa-Bator, M. Danielewski, J. Bobacka, A. Lewenstam and D. Diamond, Diagnostic of Functionality of Polymer Membrane-Based Ion Selective Electrodes by Impedance Spectroscopy, Anal. Methods, 2010, 2, 1490–1498, 10.1039/c0ay00249f.
- E. M. Zahran, A. New, V. Gavalas and L. G. Bachas, Polymeric Plasticizer Extends the Lifetime of PVC-Membrane Ion-Selective Electrodes, Analyst, 2014, 139, 757–763, 10.1039/c3an01963b.
- U. Oesch and W. Simon, Lifetime of Neutral Carrier Based Ion-Selective Liquid-Membrane Electrodes, Anal. Chem., 1980, 52, 692–700, DOI:10.1021/ac50054a024.
- P. Bühlmann, Y. Umezawa, S. Rondinini, A. Vertova, A. Pigliucci and L. Bertesago, Lifetime of Ion-Selective Electrodes Based on Charged Ionophores, Anal. Chem., 2000, 72, 1843–1852, DOI:10.1021/ac991130x.
- E. Bakker and E. E. Pretsch, Lipophilicity of Tetraphenylborate Derivatives as Anionic Sites in Neutral Carrier-Based Solvent Polymeric Membranes and Lifetime of Corresponding Ion-Selective Electrochemical and Optical Sensors, Anal. Chim. Acta, 1995, 309, 7–17, DOI:10.1016/0003-2670(95)00077-D.
- O. Dinten, U. E. Spichiger, N. Chaniotakis, P. Gehrig, B. Rusterholz, W. E. Morf and W. Simon, Lifetime of Neutral-Carrier-Based Liquid Membranes in Aqueous Samples and Blood and the Lipophilicity of Membrane Components, Anal. Chem., 1991, 63, 596–603, DOI:10.1021/ac00006a009.
- D. N. Reinhoudt, J. F. J. Engbersen, Z. Brzózka, H. H. van den Viekkert, G. W. N. Honig, H. A. J. Hoiterman and U. H. Verkerk, Development of Durable K+-Selective Chemically Modified Field Effect Transistors with Functionalized Polysiloxane Membranes, Anal. Chem., 1994, 66, 3618–3623, DOI:10.1021/ac00093a014.
- P. D. van der Wal, E. J. R. Sudhölter, B. A. Boukamp, H. J. M. Bouwmeester and D. N. Reinhoudt, Impedance Spectroscopy and Surface Study of Potassium-Selective Silicone Rubber Membranes, J. Electroanal. Chem., 1991, 317, 153–168, DOI:10.1016/0022-0728(91)85010-M.
- L. Y. Heng and E. A. H. Hall, Producing “Self-plasticizing” Ion-Selective Membranes, Anal. Chem., 2000, 72, 42–51, DOI:10.1021/ac9904765.
- Y. Qin, S. Peper, A. Radu, A. Ceresa and E. Bakker, Plasticizer-Free Polymer Containing a Covalently Immobilized Ca2+-Selective Ionophore for Potentiometric and Optical Sensors, Anal. Chem., 2003, 75, 3038–3045, DOI:10.1021/ac0263059.
- Y. H. Lee and E. A. H. Hall, One-Step Synthesis of K+-Selective Methacrylic-Acrylic Copolymers Containing Grafted Ionophore and Requiring No Plasticizer, Electroanalysis, 2000, 12, 178–186, DOI:10.1002/(SICI)1521-4109(200002)12:3<178::AID-ELAN178>3.0.CO;2-Z.
- L. Y. Heng and E. A. H. Hall, Assessing a Photocured Self-Plasticised Acrylic Membrane Recipe for Na+ and K+ Ion Selective Electrodes, Anal. Chim. Acta, 2001, 443, 25–40, DOI:10.1016/S0003-2670(01)01195-3.
- K. R. Choi, X. V. Chen, J. Hu and P. Bühlmann, Solid-Contact pH Sensor with Covalent Attachment of Ionophores and Ionic Sites to a Poly(decyl methacrylate) Matrix, Anal. Chem., 2021, 93, 16899–16905, DOI:10.1021/acs.analchem.1c03985.
- S. Daunert and L. G. Bachas, Ion-Selective Electrodes Using an Ionophore Covalently Attached to Carboxylated Poly(vinyl chloride), Anal. Chem., 1990, 62, 1428–1431, DOI:10.1021/ac00213a016.
- K. Kimura, T. Sunagawa, S. Yajima, S. Miyake and M. Yokoyama, Neutral Carrier-Type Ion Sensors Based on Sol-Gel-Derived Membranes Incorporating a Bis(crown ether) Derivative by Covalent Bonding, Anal. Chem., 1998, 70, 4309–4313, DOI:10.1021/ac9805706.
- P. C. Hobby, G. J. Moody and J. D. R. Thomas, Calcium Ion-Selective Electrode Studies: Covalent Bonding of Organic Phosphates and Phosphonates to Polymer Matrices, Analyst, 1983, 108, 581–590 RSC.
- X. V. Chen, M. P. S. Mousavi and P. Bühlmann, Fluorous-Phase Ion-Selective pH Electrodes: Electrode Body and Ionophore Optimization for Measurements in the Physiological pH Range, ACS Omega, 2020, 5, 13621–13629, DOI:10.1021/acsomega.0c00582.
- E. L. Anderson, N. M. Gingery, P. G. Boswell, X. V. Chen, J. Rábai and P. Bühlmann, Ion Aggregation and R3N+-C(R)-H⋯NR3 Hydrogen Bonding in a Fluorous Phase, J. Phys. Chem. B, 2016, 120, 11239–11246, DOI:10.1021/acs.jpcb.6b07299.
- S. Ogawara, J. L. Carey, X. U. Zou and P. Bühlmann, Donnan Failure of Ion-Selective Electrodes with Hydrophilic High-Capacity Ion-Exchanger Membranes, ACS Sens., 2016, 1, 95–101, DOI:10.1021/acssensors.5b00128.
- E. Bakker and E. Pretsch, Potentiometric Determination of Effective Complex Formation Constants of Lipophilic Ion Carriers within Ion-Selective Electrode Membranes, J. Electrochem. Soc., 1997, 144, L125–L127, DOI:10.1149/1.1837633.
- E. Bakker and E. Pretsch, Ion-Selective Electrodes Based on Two Competitive Ionophores for Determining Effective Stability Constants of Ion-Carrier Complexes in Solvent Polymeric Membranes, Anal. Chem., 1998, 70, 295–302, DOI:10.1021/ac970878h.
- A. Ceresa and E. Pretsch, Determination of Formal Complex Formation Constants of Various Pb2+ Ionophores in the Sensor Membrane Phase, Anal. Chim. Acta, 1999, 395, 41–52, DOI:10.1016/S0003-2670(99)00311-6.
- S. C. Ma and M. E. Meyerhoff, Potentiometric pH Response of Membranes Prepared with Various Aminated-Poly(vinyl chloride) Products, Mikrochim. Acta, 1990, 100, 197–208, DOI:10.1007/BF01244842.
- R. Yuan, Y. Q. Chai and R. Q. Yu, Poly(vinyl chloride) Matrix Membrane PH Electrode Based on 4,4′-Bis[(N,N-dialkylamino)methyl]azobenzene with a Wide Linear pH Response Range, Analyst, 1992, 117, 1891–1893, 10.1039/AN9921701891.
- T. J. Cardwell, R. W. Cattrall, L. W. Deady and K. A. Murphy, Investigation of the Range of a Plastic pH Sensor Based on a Dibasic Ionophore, Aust. J. Chem., 1992, 45, 435–438, DOI:10.1071/CH9920435.
- C. R. Rousseau, Y. E. Chipangura, A. Stein and P. Bühlmann, Effect of Ion Identity on Capacitance and Ion-to-Electron Transduction in Ion-Selective Electrodes with Nanographite and Carbon Nanotube Solid Contacts, Langmuir, 2024, 40 DOI:10.1021/acs.langmuir3c03027.
- M. Słowikowska, A. J. Wójcik, K. Wolski, A. Hatalak and S. Zapotoczny, Light-Promoted Synthesis of Surface-Grafted Polymers Bearing Pyridine Groups by Metal-Free ATRP in Microliter Volumes, Polymer, 2021, 234, 124244, DOI:10.1016/j.polymer.2021.124244.
- L. Cui and G. Lattermann, Synthesis and Characterization of New Acrylate and Methacrylate Monomers with Pendant Pyridine Groups, Macromol. Chem. Phys., 2002, 203, 2432–2437, DOI:10.1002/macp.200290020.
- K. R. Choi, B. K. Troudt and P. Bühlmann, Ion-Selective Electrodes With Sensing Membranes Covalently Attached to Both the Inert Polymer Substrate and Conductive Carbon Contact, Angew. Chem., Int. Ed., 2023, 202304674, DOI:10.1002/anie.202304674.
- J. Hu, A. Stein and P. Bühlmann, Rational Design of All-Solid-State Ion-Selective Electrodes and Reference Electrodes, TrAC, Trends Anal. Chem., 2016, 76, 102–114, DOI:10.1016/j.trac.2015.11.004.
- C. R. Rousseau and P. Bühlmann, Calibration-Free Potentiometric Sensing with Solid-Contact Ion-Selective Electrodes, TrAC, Trends Anal. Chem., 2021, 140, 116277, DOI:10.1016/j.trac.2021.116277.
- E. Bakker, E. Pretsch and P. Bühlmann, Selectivity of Potentiometric Ion Sensors, Anal. Chem., 2000, 72, 1127–1133, DOI:10.1021/ac991146n.
- J. Bobacka, Potential Stability of All-Solid-State Ion-Selective Electrodes Using Conducting Polymers as Ion-to-Electron Transducers, Anal. Chem., 1999, 71, 4932–4937, DOI:10.1021/ac990497z.
- M. Meloun and S. Bordovská, Benchmarking and Validating Algorithms That Estimate pKa Values of Drugs Based on Their Molecular Structures, Anal. Bioanal. Chem., 2007, 389, 1267–1281, DOI:10.1007/s00216-007-1502-x.
- Acid Dissociation Calculator, Advanced Chemistry Development, https://www.acdlabs.com/products/percepta/predictors/pka/#features, accessed June 30, 2019.
- SciFinder, Chemical Abstracts Service, https://www.cas.org/SCIFINDER, accessed August 16, 2023.
- N. Negash, G. Moges and B. S. Chandravanshi, Liquid Membrane Electrode Based on Brilliant Green-Hydrogen Phthalate Ion Pair, Chem. Anal., 1997, 42, 579–588 CAS.
- P. G. Boswell, C. Szíjjártó, M. Jurisch, J. A. Gladysz, J. Rábai and P. Bühlmann, Fluorophilic Ionophores for Potentiometric pH Determinations with Fluorous Membranes of Exceptional Selectivity, Anal. Chem., 2008, 80, 2084–2090, DOI:10.1021/ac702161c.
- G. G. Guilbault, R. A. Durst, M. S. Frant, H. Freiser, E. H. Hansen, T. S. Light, E. Pungor, G. Rechnitz, N. M. Rice, T. J. Rohm, W. Simon and J. D. R. Thomas, Recommendations for Nomenclature of Ion-Selective Electrodes, Pure Appl. Chem., 1976, 48, 127, DOI:10.1351/pac197648010127.
- R. Mauger, J. Chopindumas and J. C. Pariaud, Acid Error of the Glass Electrode in Aqueous Solution. 3. Mixed Acid and Alkaline Salt Solutions, J. Electroanal. Chem., 1978, 86, 369–382 CrossRef CAS.
Footnote |
† Electronic supplementary information (ESI) available: Experimental information on reagents as well as the synthesis and characterization of the new ionophores; upper detection limits of pH ISEs based on a pyridine derivative as the ionophore in backgrounds comprising different counterions; a schematic for the photopolymerization of DMA membranes with covalent attachment of a tertiary amine-based ionophore; working ranges of DMA-based pH ISEs based on a covalently attachable tertiary amine-based ionophore. See DOI: https://doi.org/10.1039/d3an02047a |
|
This journal is © The Royal Society of Chemistry 2024 |