DOI:
10.1039/D1CP04831G
(Perspective)
Phys. Chem. Chem. Phys., 2022,
24, 10684-10711
Recent trends in covalent functionalization of 2D materials
Received
22nd October 2021
, Accepted 19th April 2022
First published on 20th April 2022
Abstract
Covalent functionalization of the surface is more crucial in 2D materials than in conventional bulk materials because of their atomic thinness, large surface-to-volume ratio, and uniform surface chemical potential. Because 2D materials are composed of two surfaces with no dangling bond, covalent functionalization enables us to improve or precisely modify the electrical, mechanical, and chemical properties. In this review, we summarize the covalent functionalization methods and related changes in properties. First, we discuss possible sites for functionalization. Consequently, functionalization techniques are introduced, followed by the direct synthesis of functionalized 2D materials and characterization methods of functionalized 2D materials. Finally, we suggest how the issues may be solved to enlarge the research area and understanding of the chemistry of 2D materials. This review will help in understanding the functionalization of 2D materials.
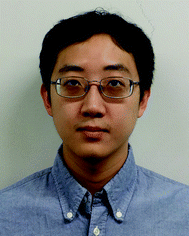
Jae Hwan Jeong
| Jae Hwan Jeong is a graduate student at Yonsei University under the supervision of Prof. Gwan-Hyoung Lee. |
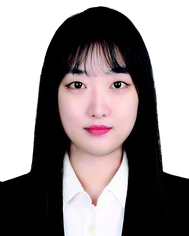
Sojung Kang
| Dr Sojung Kang is a postdoctoral researcher at Seoul National University under the supervision of Prof. Gwan-Hyoung Lee. She received a PhD in Materials Science and Engineering from Yonsei University. |
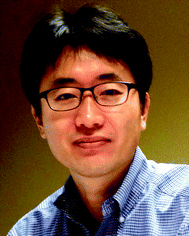
Namwon Kim
| Namwon Kim is an associate professor of Ingram School of Engineering at Texas State University. He received his PhD from the Department of Mechanical Engineering at Louisiana State University in 2009 and worked as a postdoctoral researcher at Columbia University and the Center for BioModular Multi-Scale Systems. His research interests focus upon advanced multi-scale manufacturing and surface engineering, experimental microfluidics and optical measurements, and transport phenomena as related to micro/nano-systems for bio-medical/analytical applications. |
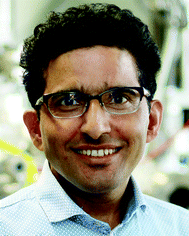
Rakesh Joshi
| Rakesh Joshi FRSC is an Associate Professor at the School of Materials Science and Engineering, UNSW Sydney, leading the graphene research group. Before joining UNSW, he was a Marie Curie International Fellow at the University of Manchester. A/Prof. Joshi is among a select group of researchers who have been awarded each of the world's most prestigious relevant International Research Fellowships; the JSPS Invitation Fellowship, the Humboldt Fellowship and the Marie Curie International Fellowship. Rakesh Joshi has over 95 journal articles (and 4 patents), with over 75 articles as the first/corresponding author. |
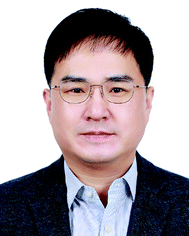
Gwan-Hyoung Lee
| Gwan-Hyoung Lee is an associate professor at Seoul National University. He received a PhD in Materials Science and Engineering from SNU. Then, he worked for Samsung Electronics. In 2010, he joined Columbia University as a postdoctoral researcher. After five years at Yonsei University, he is now with SNU since 2019. His research activities include the investigations of electrical, mechanical, and optical properties of 2D materials as well as 2D-material-based heterostructure devices for electrical and optoelectronic applications. |
1. Introduction
Research on two-dimensional (2D) materials has exploded since the mechanical exfoliation of graphene using a scotch tape method was invented.1 After graphene, other 2D materials, such as transition metal dichalcogenides (TMDCs),2,3 hexagonal boron nitride (hBN),4,5 black phosphorous (BP),6–8 MXene,9–11 and 2D oxides,12,13 have been found and studied. The unique properties of 2D materials, such as their atomic thinness, van der Waals (vdW) gap, dangling-bond-free surface, and high crystallinity, allow precise control of properties by electrical modulation and surface absorption, leading to extensive research into the properties and applications of 2D materials.14–22
However, wide application of 2D materials has been hindered by their limitations. Atomic-level thinness and large surface area result in high defect sensitivity in 2D materials. Exfoliation or dispersion of 2D materials, which are mandatory for most chemical modification processes, can introduce many defects.23–25 Several 2D materials, such as BP, are susceptible in the ambient environment and degrade over time.26,27 Therefore, making 2D materials more versatile has been an attractive issue to researchers for some time. With proper modification or treatment on 2D materials, more intriguing properties can be achieved for wide-ranging application. Compared with conventional bulk materials, 2D materials are more likely to be affected by traditional surface modification techniques, such as substitutional element doping,28,29 defects generation,30–32 composite formation with polymers,33–35 decoration with metal nanoparticles,36,37 and functionalization by physisorption.38–40 Conversely, 2D materials allow new modification methods that are inapplicable to traditional 3D materials, including assembly of stacked heterostructures,41–50 the intercalation of other ions or molecules between layers,51–54 and the modification of structures by ripple55–57 or rolling.57–59 Among them, covalent functionalization, which introduces covalent bonds between functional groups and 2D materials, has been widely studied for its versatility. Covalent functionalization of many 2D materials, such as graphene,60–67 graphene oxide (GO),60,68,69 TMDCs,70–74 BP,75–77 hBN,78,79 MXene,80 2D oxides,72–74,81 and others,40,82 have been extensively studied for a variety of applications, including biology,73,75,81,83–95 polymer composites,33–35,96 environmental technology,35,97–99 energy,60,100–103 electrical,100,104–108 optics,60 and catalytic engineering.106,109–112
There are plenty of review papers that cover the covalent functionalization of 2D materials. However, most of them are focused on specific 2D materials, applications, functionalization techniques, or functional groups, and there was no review paper dedicated to covalent functionalization techniques for general 2D materials. In this paper, we review various covalent functionalization methods and their related applications. As shown in Fig. 1, the discussion begins with the functional group at different locations, followed by individual functionalization techniques and the direct synthesis of functionalized 2D materials. Finally, common characterization techniques for functionalized 2D materials will be briefly introduced. From here on, the term covalent functionalization indicates functionalization by forming a covalent bond between 2D materials and functional groups. Other surface modification techniques, such as non-covalent and substitutional doping, are not discussed in this paper.
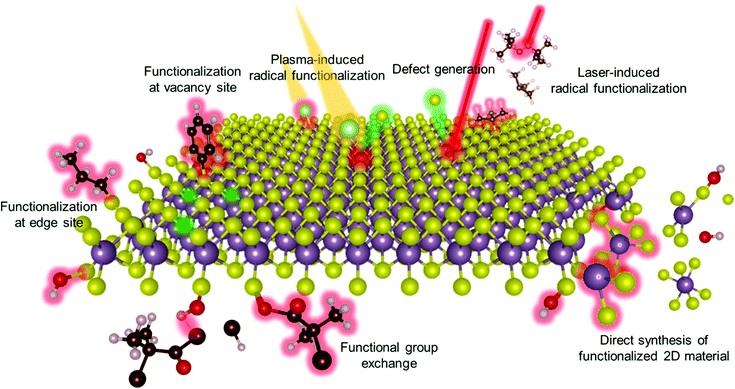 |
| Fig. 1 Schematic illustration of functionalization sites and various techniques for covalent functionalization of 2D Mater. | |
2. Sites for covalent functionalization
Location is a critical factor for the functionalization of 2D materials because different reaction sites require different reaction mechanisms between functional groups and 2D materials. In this section, we focus on the reaction mechanisms at each site, with the functionalization examples order of edge, vacancy, grain boundary, basal plane, local strain, and existing functional group.
2.1 Edge
Despite the fact that the surfaces of 2D materials are dangling-bond-free, their inevitable edges have dangling bonds. The high chemical potential of the edges can be utilized as catalysts for hydrogen evolution reactions (HER)113,114 and active sites to attach to the functional group.115,116
The introduction of more edge sites to 2D materials has been widely studied117,118 for relatively stable 2D materials, such as graphene and hBN,115,116 where the edges spontaneously bond with other molecules or atoms. For example, sonication with water119,120 or ball milling with NaOH solution121 can functionalize hBN with a hydroxyl group (–OH). Other groups or elements, such as halogen (F, Cl, Br, I),122,123 hydrogen,124 nitrogen,125 and NH2126 are similarly functionalize the edge using the ball-mill method under selected environmental conditions. These naturally or intentionally generated functional groups become highly concentrated at the edge site rather than at other locations, allowing edge-specific functionalization with complex molecules through the functional group exchange mechanism, which will be discussed in more detail at Section 2.6.
The functionalization reaction at the edge or other specific sites can be limited depending on the molecule's shape and size.127–129 For example, 1,2-dithiolanes only bond to the edge of MoS2 with two adjacent S vacant spaces,127 and dibenzothiophene selectively bonding to the point corner site of the MoS2 triangle.128 Furthermore, edge functionalization facilitates the exfoliation of 2D materials as the edge functional groups repulse each other, inducing delamination and allowing the surrounding solvent to penetrate between layers.122,130–132Fig. 2(a) shows edge-functionalization-induced delamination of graphene with maleimide as a functional group.131
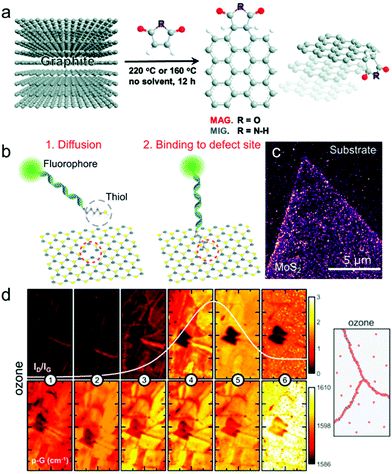 |
| Fig. 2 (a) Schematic drawing of edge functionalization and delamination of graphite edge by Diels–Alder reaction with maleimide (reprinted with permission from ref. 131 Copyright 2014 Chemical Communications). (b) Schematic drawing of selective chemisorption of a thiol group to S vacancy site of MoS2. (c) Fluorescent microscope image of fluorophore attached to MoS2 vacancy site (reprinted with permission from ref. 136 Copyright 2021 ACS Nano). (d) (left) G peak position Raman map of ozone functionalized graphene near grain boundary, for six different oxidation steps. (right) Schematic of defect (red) accumulated at grain boundary (reprinted with permission from ref. 156 Copyright 2015 2D Mater.). | |
2.2 Vacancy defect
Even in 2D materials with high crystallinity, vacancy defects are inevitable. In addition to intrinsic vacancies, extrinsic vacancies can be introduced by annealing, plasma treatment, ion bombardment, chemical/mechanical exfoliation, or electron beam (e-beam) irradiation.30–32,111
The vacancy on the 2D material surface has high chemical potential, attracting other molecules, and forming a covalent bond spontaneously.133–135 Molecules with thiol groups (–SH) are a notable example of vacancy functionalization, which are favorable for bonding with S vacancies of MoS2 unless the chemical bonding is disturbed by the molecule's geometry.136–142 (Fig. 2(b)) Zhang et al.136 fabricated fluorophore-attached thiol molecules to illuminate S vacancies using selective bonding between thiol groups and vacancies to make vacancies clearly visualized using an optical fluorescent microscope (Fig. 2(c)). Healing or passivation of defects also can be achieved via vacancy functionalization. For example, the S vacancy of the MoS2 surface can be repaired by thiol functionalization followed by breakage between the S and C bond.137,138
2.3 Grain boundary
Chemical vapor deposition (CVD) is one of the most widely used methods to synthesize 2D materials,143,144 for large-area single-layer 2D material films with sizable grain sizes and high crystallinity. However, the formation of grain boundaries (GBs) is inevitable for CVD-synthesized 2D material films unless a particular technique is used to align the crystal orientation, such as a single crystal substrate or super slow precursor supply.145–147 GBs are reported to affect the electronic and mechanical properties of 2D materials,148–150 and the additional properties are expected to be observed from GB functionalization.151
Consequently, many studies have attempted to improve or modify the properties of GBs, including the addition of metal nanoparticles152,153 or nanowires154 to them.
GBs are susceptible to chemical reactions, including functionalization and chemical etching, because of their high chemical potential.155Fig. 2(d) clearly shows that ozone exposure produces highly localized oxygen functionalization at the GB.156 This mechanism can be applied to the functionalization of GB for visual observation.157,158 Several theoretical studies predict the possibility of emerging electronic,151,156,159 magnetic,160 and mechanical161,162 properties by functionalizing GB. To our knowledge, however, experimental results regarding the additional properties are not yet reported.
2.4 Basal plane
This section covers the functionalization of the intrinsic basal plane of 2D materials without any defects. However, most molecules used for functionalization may include other active groups on the other side of the molecule, which increases the variety of structures and properties, including the physical properties and applications of functionalized 2D materials. Therefore, this section will focus on reactions between the 2D basal plane and the bonding part of the functional molecules to avoid unnecessary complexity.
Chemically stable 2D material surfaces and molecules can react with additional energy inputs, such as plasma, electric fields, and photon irradiation. On the other hand, when 2D materials or reacting molecules are chemically unstable and reactive enough, defects or other sites with high chemical potentials are not mandatory.
Functionalization of carbon nanotube (CNT)163–165 and fullerene (C60)166–169 by attacking the C–C bond at the carbon ring has a long history of research, and most of them are currently utilized to functionalize graphene with a few exceptions, such as reactions with some metal complexes.170–172 Most of the defect-free covalent functionalization of graphene can be categorized into two types—that is, cycloaddition and free-radical addition.
2.4.1 Cycloaddition.
Cycloaddition is a chemical reaction that adds a new ring to the graphene surface. The number of atoms in the newly formed ring is between 3 and 6, the ring being composed of carbon, nitrogen, and/or oxygen. Fig. 3 shows a simple schematic of the possible cycloaddition reactions for graphene. There is a total of 12 cycloadditions, three 2 + 1 reactions (Bingel reaction, carbene addition, and nitrene addition), one 2 + 2 reaction, six 2 + 3 reactions (pyrazoline adduct, Prato reaction, pyrrole adduct, isoxazoline adduct, zwitterion-mediated, and oxolane adduct), and two 2 + 4 reactions (Diels–Alder reaction by both dienophile and diene).
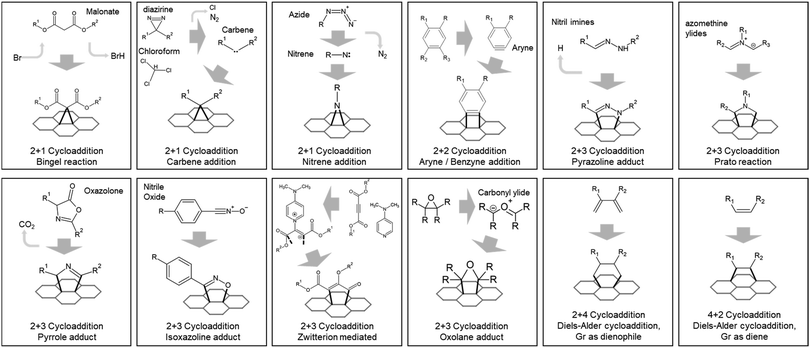 |
| Fig. 3 Reaction diagrams of cycloaddition reactions to defect-free, intrinsic graphene surface. | |
The Bingel reaction produces three carbon atom rings, using bromo-substituted malonate.173,174 Bingel reactions occur with either carbonate catalyst (Na2CO3, K2CO3, or NaHCO3)174 or external energy by heating or microwave.173
The carbene intermediate reaction produces a ring of three carbon atoms. Catalyst,175 heat, or ultraviolet light (UV)176 activates carbene intermediate from diazirine or chloroform.175
The reaction with nitrene and graphene produces a ring of two carbon atoms and one nitrogen atom. Molecules with azide groups are popular for generating nitrene intermediates, with thermal (approximately 90 °C) or photochemical activation (UV exposure).177–180
The 2 + 2 cycloaddition reaction between graphene and aryne (benzyne)181,182 produces four carbon atoms ring. Intermediate aryne is formed chemically,181 by UV,183 or by microwave-assistance.182
Reaction with nitrilimines, a 1,3-dipolar cycloaddition, produces a pyrazoline structure with two nitrogen atoms and three carbon atoms. Owing to its high threshold energy, the reaction demands either days of heating at high temperatures or hours of microwave irradiation.184
The Prato reaction is also the 1,3-dipolar cycloaddition,185,186 creating a pyrrolidine structure with one nitrogen atom and four carbon atoms. Azomethine ylide is commonly used as a precursor,185 but other derivatives, such as sarcosine, can also be used.186
The reaction with oxazolone produces a pyrrole structure, a ring of one nitrogen atom, and four carbon atoms, with one N–C double bond. The reaction requires a sealed container in solvent-free condition.187,188
The 1,3-dipolar cycloaddition reaction with nitrile oxide produces an isoxazoline structure, a five-atom ring with one oxygen atom, one nitrogen atom, and three carbon atoms. Similar to cycloaddition by nitrilimine, it requires a high temperature (170 °C) or external assistance such as microwave irradiation to overcome its high activation energy.189
The zwitterion-mediated cycloaddition reaction produces a carbon monocyclic five-atom ring. 4-dimethylaminopyridine and acetylene dicarboxylate react with each other to produce reactive zwitterion intermediates and functionalized graphene.190
The cycloaddition reaction with carbonyl ylide—formed by the epoxide ring-opening process—produces an oxolane structure, a five-atom ring with one oxygen atom, and four carbon atoms. This reaction occurs between graphene and tetracyanoethylene oxide under moderate heating conditions (140 °C–160 °C).191,192
Diels–Alder cycloaddition is a commonly used reaction in graphene chemistry because of its wide reaction temperature range, broad solvent selection, and relatively short reaction time. This method produces a ring with six carbon atoms via the reaction between dienophile and diene. In graphene functionalization, graphene can act as both dienophile193–197 and diene,131,194,196,198 depending on the molecule it reacts to.
2.4.2 Free radical addition.
Free-radical addition is a functionalization method that involves the reaction between 2D materials and molecules with unpaired electrons. Fig. 4 shows a simple list of possible functionalization reactions by free radicals.
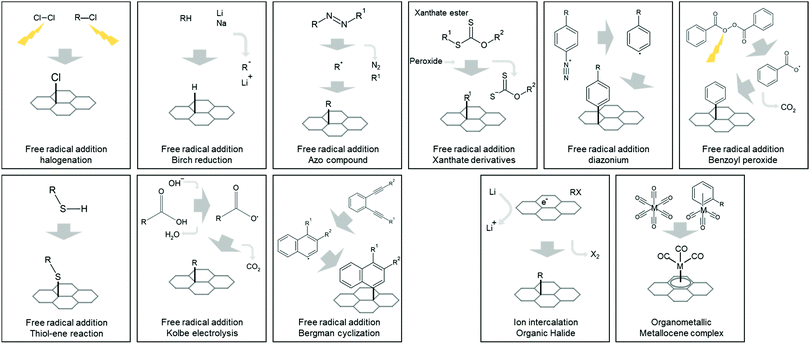 |
| Fig. 4 Reaction diagrams of 9 radical functionalization reactions and two uncategorized functionalization reactions to defect-free, intrinsic graphene surface. | |
Free-radical halogenation is one of the simplest functionalization methods for 2D materials. Halogen radicals Dissociating diatomic halogen gas or organic halides produces free halogen radicals. External energy is required to generate radicals such as plasma,199–203 UV,204–206 electrochemical,207,208 e-beam,209 gamma-ray,210 microwave,211 laser,212 and thermal heating.213–216 Some molecules (e.g., XeF2) are unstable enough to produce halogen radicals and react with graphene without external energy.199,201,217–220
Birch reduction is a hydrogenation method for graphene, using alkali metals (Li, Na, or K) as an electron source. Alcohol or water is usually used as a hydrogen source, with ammonia as the solvent to dissolve metal.221–225
Azo compounds are well-known initiators for free-radical polymerization. Under low-temperature heating in an organic solvent, they decompose to radicals, releasing nitrogen gas.226
Xanthate is another common molecule involved in radical reactions. With a peroxide initiator, xanthate breaks the C–S bond and releases a radical group. Reaction with xanthate derivatives is beneficial for controlling the reaction because of (1) controlled reaction activation timing by radical initiator triggering, and (2) self-regulating consistent functionalization reaction by reversible radical generation from the degenerative exchange behavior of the xanthate group.227 It also has several other attractive features, such as the broad availability of functional groups, solvents, and reacting concentration.228
The radical reaction by diazonium salt is one of the most popular functionalization methods—not only for graphene but also for other 2D materials—because of its generous reaction requirement.229 Diazonium salt can spontaneously become radical when exposed to low heat or accelerated electrochemically230–233 under both dry and solution conditions. Aryl diazonium,230,231,234–236 azide molecules,237,238 and others232,233,239,240 can be applied to this method.
Peroxide is a well-known for radical initiator and photoinitiator. Due to its low stability, peroxide can decompose in many ways, including low heat, light, and UV exposure. Benzoyl peroxide is often used for patterned functionalization by light-initiated decomposition.228,241,242
The thiol–ene reaction is a C–S bond-forming reaction between the thiol group and C–C bond, with the help of a radical initiator or catalyst. Although several methods can remove hydrogen from the thiol group and produce thiyl radical–catalysts, heat, light, and the radical initiator – method using azo compound azobisisobutyronitrile (AIBN) as a thermal radical initiator has been reported as major method for functionalizing graphene. Since AIBN decomposes to radicals at a low temperature of approximately 40 °C, thiol–ene functionalization methods with AIBN require mild reaction conditions, usually around 70 °C in the organic solvent.243–247
The Kolbe electrolysis reaction (not the Kolbe–Schmitt reaction) is an electrochemical decarboxylation reaction. Radical formation from carboxyl group dissociation under hydroxyl ions and electric potential (RCOOH + OH− → R* + H2O + CO2 + e−).248
Functionalization by Bergman cyclization—also called the Masamune–Bergman reaction—uses a radical intermediate generated from the cyclization of enediyne. Few Bergman cyclization reactions use low temperatures, such as 150 °C,249 but the most cases require the high cyclization temperature (up to 200 °C), limiting the selection of a solvent.
2.4.3 Other addition reactions.
Some other addition functionalization processes do not belong to cycloaddition or free-radical addition.
Similar to the Birch reduction methods, negatively charged 2D material—a common byproduct of metal ion intercalated liquid exfoliation—can act as a radical and take halogen atoms from organic halides.250–254 Moreover, modulating the metal ion ratio during the intercalation process allows the control of functionalization density.254
Several metal atoms—such as Fe, Cr, Mo, and W—can bond with five or six carbon atom rings by a haptic covalent bond (hexahapto(η6)-metal bond) to form metallocene complexes (sandwich or half-sandwich compounds). In the same way, these organometallic complexes—such as Cr(CO)6 and (η6-benzene)Cr(CO)3—can bond to carbon rings at the graphene surface.255–257 Metal complexes other than Cr, like Mo and W, have also been also tried.258,259 Functionalization by the η6-metal bond is beneficial for mild modification because it does not fully convert C–C hybridized bond.
2.4.4 Reactions for other 2D materials.
The many of abovementioned functionalization can also be applied to other 2D materials. Several free-radical addition reactions—diazonium,260–262 azo compounds,263 and nitrene intermediate264—have been used for BP functionalization. Fig. 5(a) shows one example of diazonium functionalization of BP surface.260 Besides free radical reactions, the chemical instability of BP makes several other reactions affordable, such as reaction with polymers,265–269 alkyl halide,270 and metal ligands.271–273 Furthermore, BP is reactive even at ambient conditions, and starts to decompose just by being exposed to ambient air274 and moisture.275 Consequently, increasing its environmental stability is one of the primary goals for functionalizing BP.260,263,264,273,274,276,277
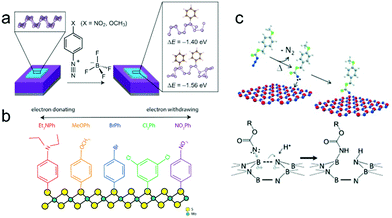 |
| Fig. 5 (a) Reaction between BP surface and diazonium derivative and DFT calculated structure of the following distortion (reprinted with permission from ref. 260 Copyright 2016 nature chemistry). (b) Schematic drawing of various functional groups attached to MoS2 arranged in order of doping (reprinted with permission from ref. 280 Copyright 2017 Journal of American Chemical Society). (c) Schematic illustration of reaction mechanism between nitrene and hBN (reprinted with permission from ref. 287 Copyright 2012 Chem. – Eur. J.). | |
Diazonium functionalization was also applied to TMDC.278–280Fig. 5(b) shows diazonium-functionalized MoS2 with different attached groups to control the doping levels of MoS2 from p-type to n-type.280 Other functionalization methods using organic halide with ion intercalation281–284 and Michael addition reactions using maleimide285 have also been reported for functionalization of TMDC.
Although hBN is less likely to be functionalized owing to its high chemical stability, some radical reactions like carbene intermediate286 and nitrene intermediate287 can react with hBN (Fig. 5(c)).
Halogenation of 2D materials other than graphene requires much more consideration because they readily disintegrate when exposed to halogenation gases such as XeF2.217 However, halogenating BP or hBN without disintegrating is possible by several different approaches, such as using a stable fluorine source (polymer288,289 or ammonium fluoride290–293) or controlling the reaction speed (temperature,294 electric potential,295 or ion beam irradiation296).
2.5 Local strain
2D materials with ripples, wrinkles, Moiré patterns, or 2D materials that are transferred onto the substrate with nanoparticles or patterned structures, has disturbed strain field in it, and local strain field is known to change the local chemical potential.201,234,297 As a result, functional groups are more likely to attach to the strained area201,234,298,299 (Fig. 6(a)).
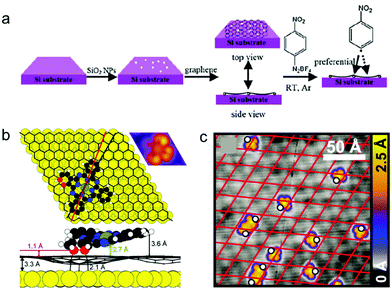 |
| Fig. 6 (a) Schematic drawing of diazonium radical functionalization on locally strained graphene (reprinted with permission from ref. 234 Copyright 2012 Chemical Communications). (b) DFT calculated structure of Iron phthalocyanine (FePc) on graphene/Ir(111) surface, bonded onto Moiré position. (c) STM topograph image of FePc on Iron phthalocyanine (FePc) on graphene/Ir(111) surface, arranged with Moiré pattern (reprinted with permission from ref. 198 Copyright 2015 J. Am. Chem. Soc.). | |
The Moiré structure is a repeated pattern observed in stacked 2D materials with misalignment or 2D material on a crystalline substrate, which gives periodic strain and potential wave.300–302 The size and form of the Moiré pattern are determined by the lattice constant difference or twisted angle of the stacked materials.303 Despite that Moiré pattern gives relatively small strain, several studies have revealed that the chemical bonding onto 2D material surfaces is favorable along with the Moiré pattern.198,304–306Fig. 6(b and c) show a clear example of selective bonding between monolayer graphene on the Ir(111) surface and iron phthalocyanine (FePc) molecules.198
2.6 Functional group
Intrinsically or intentionally formed functional groups on 2D material surfaces are great locations for chemical reactions and further functionalization due to their high chemical potential.
However, as pointed at Section 2.4, practically limitless numbers of molecules can become functional group of 2D materials, thus it is almost impossible to cover every related reaction that substitute or add other molecules to existing group. Therefore, for clarity and simplicity, we will discuss simple and common groups for 2D materials, such as epoxide, ketone, hydroxyl, carboxylic,307 hydrogen, and halogen. Fig. 7 and 8 show simplified schematic diagrams of the substitution and addition reactions.
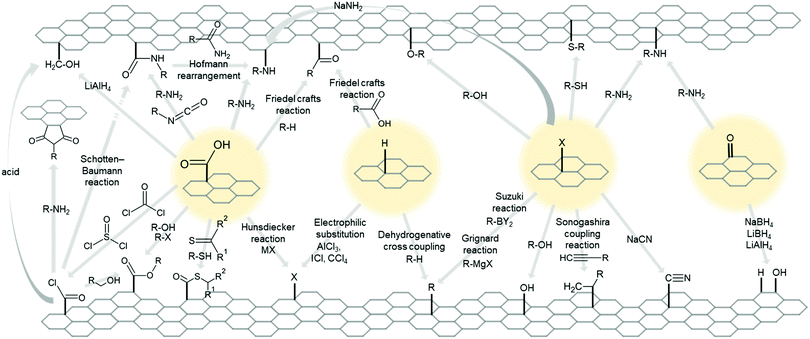 |
| Fig. 7 Schematic diagram of possible reactions for functional groups, carboxylic (–COOH), hydrogen (–H), halogen (–X), and ketone ( O). X is for halogen, and M is for metal. | |
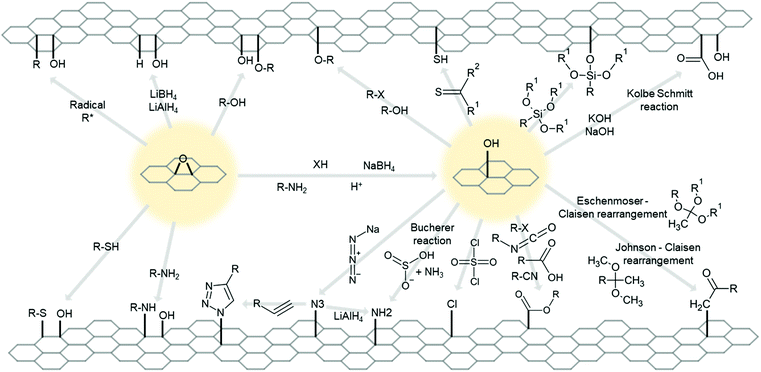 |
| Fig. 8 Schematic diagram of possible reactions for functional groups, hydroxyl (–OH) and epoxide (–O–). X stands for halogen. | |
2.6.1 Carboxylic group.
The carboxylic group (–COOH) is commonly found at the edges and surfaces of GO.307
The acyl chloride group (–COCl) is well-known as an intermediate step to introduce several other groups. It can be synthesized from the carboxylic group through reaction with SOCl2,308–314 COCl2,315 or PCl3.316 Several conversion processes are applied to acyl chloride, such as alcohol to an ester (–COOR),312 amine to amide (Schotten–Baumann reaction),308,317–320 phosphorous acid to phosphonate,316 and the ring-closing reaction of amine (R–(COCl)2 + R′–NH2 → pyrrole-R).315,317,321,322
There are two known carboxylic to ester conversion processes—that is, the Fischer esterification reaction with alcohol/hydroxyl initiated by carbodiimide/acid condition,323–325 and Williamson ether synthesis by alkyl halide.326,327 Carbodiimide is also used as a coupling agent for conversion reactions, from carboxyl to thioester (–COSR)328,329 and the amide (–CONR) group.330–337
Carboxyl reacts with thiol/thiourea to produce a thioester structure.
The reaction with an amino group and a carboxyl group produces an amide. Amino groups require a coupling agent such as hydroxybenzotriazole330 to react with carboxyl and make amides, while some reactive molecules such as hydrazine327 and isocyanate338 can react without a coupling agent. The fabricated primary amide group can be exchanged to the amine by the Hofmann rearrangement reaction (–CONH2 → –NH2).320
Friedel–Crafts acylation is a reaction that produces a ketone bond between the carboxyl group and the aromatic ring, with AlCl3 as a catalyst. This reaction is used when attach ferrocene to GO.339
Several other conversion reactions occur in the carboxyl group. For example, the hydroxymethyl group (–CH2OH) can be produced by LiAlH4,340 and the Hunsdiecker reaction (–COOH + MX → –X) can exchange carboxyl to a halogen atom.341,342
2.6.2 Hydrogen.
Hydrogen atoms on hydrogenated graphene—usually synthesized by annealing or plasma processes—can be substituted with halogen, alkyl groups, and ketone bonds.
Electrophilic substitution reactions can halogenate hydrogenated graphene. For example, AlCl3, ICl, or CCl4343 were used to synthesize chlorinated graphene. Likewise, bromosuccinimide344 was used to synthesize brominated graphene.
Alkyl groups can be grafted with ketone bonds by Friedel–Crafts acylation, where graphene acts as an aromatic ring.345 Alkyl group also can directly bond to the hydrogenated graphene surface in the presence of a proper oxidant through a dehydrogenative cross-coupling reaction.346
2.6.3 Halogen.
The halogen exchange reaction has attracted much attention because the reaction process is simple and does not cause severe damage to the graphene surface. Among them, fluorinated graphene is the most widely studied for functionalization by halogen exchange processes. Currently reported halogen exchange reactions on graphene surface are reaction with thiol (–S–R/thioethers),347,348 NaNH2 (–NH2, –NR/primary and secondary amine),348–353 cyanide (–CN/cyano group),354 and alcohol (–OH/hydroxyl).355,356
The Sonogashira coupling reaction,357 Grignard reaction, and Suzuki coupling reaction displace halogen atom to an alkyl group. The Grignard reaction uses an alkyl metal halide to exchange halogens with other groups. It has been applied to fluorinated358,359 and chlorinated graphene.360,361 The Suzuki coupling reaction (or Suzuki Miyamura reaction) is a halogen exchange reaction (–X → –R) in the presence of a palladium complex catalyst. It has been used to brominated graphene.362,363
Atom transfer radical polymerization is another promising application for halogen-functionalized 2D materials. Halogen atoms at the surface of 2D materials or the end of a functional group can be used as an initiator for atom transfer radical polymerization, such as polymer functionalization of 2D materials and continuous polymerization by the halogen exchange process.313,364–366
2.6.4 Epoxide group.
The epoxide group (–O–) is another frequent group on GO surfaces. Several epoxide ring-opening reactions can introduce a variety of functional groups with additional hydroxyl groups. Radical (–O– + R*→ –OH + –R),367 amine (–O– + R–NH2 → –NH–R + –OH),368–370 thiol (–O– + R–SH → –S–R + –OH),371,372 and lithium hydride (–OH + –H)340 have been employed in the epoxide ring-opening reaction. If the epoxide group reacts with molecules that supply an additional electron—such as HBr,373 sodium borohydride,340,374 hydrazine,375 and acid376—a direct conversion reaction to a single hydroxyl group rather than a ring-opening reaction is possible.
2.6.5 Hydroxyl group.
The hydroxyl group might be the most abundant group on both surfaces of GO and at the edge of other 2D materials, especially when 2D materials are exposed to ambient air or water.
When sodium azide reacts with a hydroxyl group, it exchanges the hydroxyl group with the azido group (–N3).377–379 Due to the high reactivity of the azido group, it can readily react with other molecules. For example, LiAlH4379 converts the azido group into an amine group, and the azide-alkyne Huisgen cycloaddition reaction produces a triazole group (a ring with three nitrogen atoms and two carbon atoms).379,380
Organic halides,342,366,375 alcohol,381 and carboxylic373 can form an epoxide bond with the hydroxyl group through a dehydration reaction.
Hydrogen exchange reaction replaces hydrogen atom at the hydroxyl group to bond with other molecules such as cyanide (Pinner reaction)338,382,383 and siloxane (silanization reaction).384–389 Many silanization cases allow each siloxane group to interconnect to create a dense network of siloxane molecules or composites.
Similar with epoxide bond formation reactions, ester bond formation is possible through the Claisen rearrangement reaction. Two slightly different Claisen rearrangement reactions—that is, the Eschenmoser–Claisen rearrangement390 and Jonson–Claisen rearrangement391—convert hydroxyl groups to ester bonds with functional molecules.
The Kolbe–Schmitt reaction, reaction by alkaline metal hydroxide and CO2 (–OH → –OH + –COOH), attaches another carboxyl group adjacent to the hydroxyl group on the graphene surface.392
There are some substitution reactions for the hydroxyl groups. Specifically, SO2Cl2 produces chlorine (–Cl),311 thiourea produces sulfhydryl (–SH),393 and hydrazine327 produces amide. Conversion onto amine (–NH2) is performed by both the Bucherer-like reaction394 and amine.395,396
2.6.6 Other groups.
The ketone groups (
O) are rarer than the other groups on the graphene surface. Similar to the epoxide group, the ketone group can be exchanged with a hydroxyl group (–OH + –H) by reacting with NaBH4, LiBH4, or LiAlH4.340 Moreover, hydrazine converts ketone to amide (
N–NH2).397 NaBH4, LiBH4, and LiAlH4 can also react to another uncommon group, converting aldehyde group (–COH) to hydroxymethyl group (–CH2OH).340
Oxygen-containing groups on graphene surfaces can bond with metal complexes, such as Pd,398 Co,399 and Pt,400,401 but specific reacting groups have not been identified.
2D material edges usually contain –H or hydroxyl groups. Therefore, if the surface is sufficiently free of defects and no group is attached, these intrinsic groups can be used as targets for edge-selective functionalization.315,343,402
2.7 Intrinsic dangling bonds
Not all 2D materials are vdW layered materials. Many ‘bulk’ materials, which form atomic bonds to all three dimension directions and no vdW gap, can form a 2D structure by well-controlled synthesis or exfoliation methods.403–405 Several metal oxides (In, Sn, Ti, Zn, W, Co, Ce, Gd, Eu), metal chalcogenides (MX, M = Cd, Zn, Pb, In, Cr, Cu, X = S, Se, Te), metals (Au, Rh, Pd, Ru, Te, Se, Ge), are known to form non-vdW 2D structure. Unlike layered vdW materials, those non vdW 2D materials have intrinsic active sites and dangling bonds, making those materials great candidates for catalyst and energy storage applications.406–409
The surface of non-vdW 2D materials is usually –H or –OH group terminated from the ambient environment, or metal ion terminated from the exfoliation or synthesis process. However, research about non-vdW 2D materials is still in early stage, and surface passivation/encapsulation is currently in main interest, further functionalization is not widely reported yet.
Metal–organic framework nanosheet (MON) is another 2D structured material group, which is the porous compound that is composed of periodically interconnected organic ligand and metal ion/cluster.410–414 Similar to other non-vdW 2D materials, MON has intrinsic active sites at both metal and organic ligand, which make MON suitable material for various applications such as sensor and catalyst.415–418 Plus, owing to its unique porous structure, filter, absorbent, or molecular separation also attract much interest as a promising application.412,414,415,419
Various groups can attach to active sites of MON during or post-synthesis. Currently, functionalizing MON is mainly aiming for several applications such as increasing/modifying catalytic activity, pore functionalization to modulate molecular selectivity for filtration, or increasing exfoliation yield after synthesis using functionalized ligand as synthesis precursor.420
2.8 Outlook
Functionalizing molecule reacts with every possible active site in 2D materials. That is, most functionalization reactions introduced in basal plane sections will react with defect sites also, even though the reaction works on clean crystal surfaces and does not require defect sites. Therefore, in many cases, the functionalization location depends on many parameters such as kind/status of 2D materials and functional group, rather than choice specific location exclusively. For example, for the mass-produced liquid dispersed 2D materials, the edge should be considered as a prominent functionalization location, and the local strain-dependent reaction would be neglected.
Functionalizing reaction to an existing group is beneficial in this manner, because functionalization density, uniformity, and location can be controlled. Beginning the functionalization process by attaching a simple group makes many problems easier, including avoiding unwanted groups or locations. For the molecules that cannot bond directly with 2D materials, application of another functionalization step is applicable.
Among all functionalizing reactions, the free radical reaction would be the most versatile choice. They are less selective than other reactions, but work with almost every 2D materials, with or without defect, and accept many other functionalization techniques beyond solution chemistry, including various initiation methods.
3. Functionalization techniques
Among the various functionalization techniques for 2D materials, the solution process is mandatory for most techniques, regardless of which chemical reaction is performed in the solution. For example, most chemical reactions described in Section 2 are solution-phase chemical reactions.
Therefore, in this section, we will discuss specific techniques for functionalizing 2D materials besides that solution-phase chemical processes.
3.1 Plasma
Plasma is a simple method for activating molecules into free radicals, and modifying 2D materials by plasma treatment is widely studied.421,422 Plasma modifies 2D materials via two major routes. First, highly reactive ionized molecules react more easily with the 2D material surface. Second, ions accelerate to the target surface during the plasma process, providing additional energy to activate the reaction, or create defects by knock-off surface atoms.30,423 Collision energy and movement of ions in the plasma chamber can be controlled by modulating the chamber design and working conditions.424 Various methods have been studied to avoid damage to the material by ion bombardment, including a remote plasma technique that generates plasma separately from the sample425,426 or by covering samples with a metal mesh.427
Light-weight diatomic molecules, hydrogen,199,202 oxygen,426,428,429 or halogen200,430,431 are commonly used in plasma functionalization technique because large molecules tend to dissociate, making it difficult to control the reaction result (Fig. 9(a)).
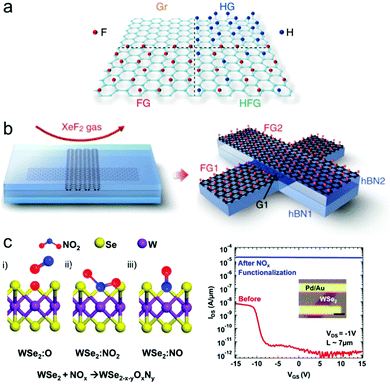 |
| Fig. 9 (a) Patterned graphene surface functionalization by plasma hydrogenation and fluorination by XeF2 (reprinted with permission from ref. 199 Copyright 2019 Advanced Materials). (b) Etching and functionalization process of graphene/hBN heterostructure by XeF2. hBN layers are etched away while graphene is fluorinated (reprinted with permission from ref. 217 Copyright 2018 nature communications). (c) Three possible reaction configurations between Se vacancy of WSe2 and NO2 gas (left) and transfer curve before/after NO2 treatment (right) (reprinted with permission from ref. 135 Copyright 2014 ACS Nano). | |
3.2 Gas exposure
If the gas or 2D material surface is chemically reactive, a simple gas exposure can functionalize the 2D material without further treatment.
Graphene fluorination by XeF2 is a well-known example of functionalization by reactive gas.199,201,217–220 In addition, using reactive XeF2 gas can do more than functionalize graphene. Son et al.217 fabricated an electronic device while etching off the hBN layer adjacent to the graphene layer, as shown in Fig. 9(b), to use graphene as an atomic-level etching block while successfully functionalizing graphene into fluorinated graphene using XeF2 exposure.
As discussed in Section 2, 2D material surfaces become reactive with vacancies or other defects, and reactive surfaces can bond with relatively chemically stable gas molecules. Fig. 9(c) shows an example of gas functionalization by surface vacancy defects, the chemical bond between NO2 gas molecules, and WSe2 at selenium vacancy.135
For less stable materials such as BP, oxygen or moisture acts as ‘reactive gas’ for functionalization, even without surface defects.274,275
3.3 Heating
Many functionalization processes, especially solution reaction processes, require a specific temperature range for the reaction. The reaction temperatures are determined by several aspects—that is, the threshold energy for the reaction, the boiling temperature of the solvent, the material thermal stability, and reaction speed.
Some functionalization methods require a much higher temperature than the boiling point of most solvents (>300 °C)—that is, sufficiently high that the thermal stability of 2D materials becomes an important issue. Fig. 10(b) shows a schematic diagram for one of the high-temperature functionalization processes, steam-mediated hydroxylation of hBN.213,432 Likewise, high temperature treatment can generate physical defects. For example, thermal treatment with iron nanoparticles cracks and expose defects to hBN433 (Fig. 10(c)).
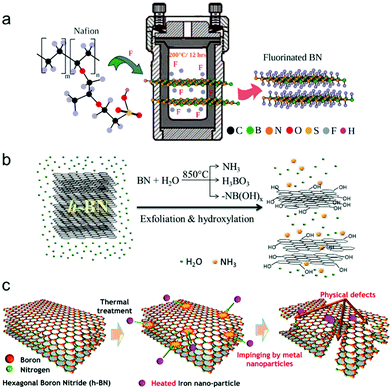 |
| Fig. 10 (a) Schematic image of hydrothermal fluorination of hBN method (reprinted with permission from ref. 289 Copyright 2017 Science Advances). (b) Schematic drawing of high-temperature steam-assisted hydroxylation and exfoliation process of hBN (reprinted with permission from ref. 432 Copyright 2015 Advanced Materials). (c) schematic drawing of defect engineering of hBN by high-temperature annealing with iron nanoparticles (reprinted with permission from ref. 433 Copyright 2016 RSC Adv.). | |
Some functional groups—especially halogens on the graphene surface—can be attached or detached simply by heating.206 For example, fluorinated graphene can be converted into hydroxylated graphene by heating with mixed alkali powder in air at 180 °C.355
3.4 Hydrothermal synthesis
Hydrothermal (water-based solution) or solvothermal (non-water solution) synthesis is a reaction method that uses high temperatures and pressures in a confined container with a non-reacting wall surface. High temperatures and pressures allow many reactions possible that are barely occur under other conditions. Another advantage of using the hydrothermal reaction method is the possibility of continuous reactor design434 with continuous precursor supply and the removal of by-products. Hydrothermal functionalization has been reported for various 2D materials and functional groups—that is, graphene (amine,370,394,435 fluorine,436,437 and bromine438), MoS2 (carboxyl439 and thiol ligands440), hBN (hydroxyl441–444 and fluorine289,290,292), and BP (hydroxyl445). Fig. 10(a) shows one example of hydrothermal synthesis process for fluorinated hBN, using polymer Nafion as a fluorine source.
3.5 Laser
Laser irradiation is a simple method for applying high energy into confined spaces using high-intensity collimated light through photothermal212 or photochemical effects. For instance, the laser can increase the local temperature to generate defects or cracks, or even disintegrate 2D materials. Fig. 11(a) shows a schematic of the functionalization mechanism—the reaction between the defected TMDC surfaces and the dissociated solvent molecule—by laser irradiation.446
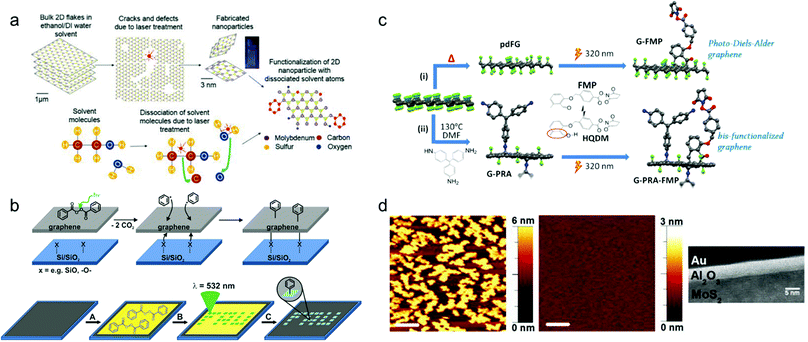 |
| Fig. 11 (a) Schematic illustration of laser-induced functionalization of 2D materials for defect generation (top) and dissociation of the solvent molecule (bottom) (reprinted with permission from ref. 446 Copyright 2018 Advanced Optical Materials). (b) Schematic drawing for the mechanism of laser-induced patterned functionalization of graphene using peroxide molecule (reprinted with permission from ref. 241 Copyright 2020 Angewandte Chemie International Edition). (c) schematic drawing of a process for UV-initiated functionalization of fluorinated graphene (reprinted with permission from ref. 197 Copyright 2019 Carbon). (d) AFM images of ALD grown Al2O3 on MoS2 (left), UV-ozone-functionalized MoS2 (center) and cross section HRTEM image of Al2O3 deposited on functionalized MoS2 (reprinted with permission from ref. 448 Copyright 2014 Appl. Phys. Lett.). | |
Laser is also an excellent tool for initiating photochemical reactions, mainly when used for patterned functionalization. Radical reactions using photoinitiators, commonly azo compounds and peroxides, can be activated by light illumination.241,242Fig. 11(b) shows a schematic of the functionalization process using peroxide by patterned laser irradiation on graphene.241
3.6 UV light
Depending on the wavelength of the light, UV light can break the bonds of several molecules to create reactive radicals. Owing to the high photon energy of UV light, UV irradiation is widely used in chemical reactions to activate them or generate radicals.
For example, several cycloaddition reactions can be activated by UV irradiation.176,177,183,197 One photo-induced cycloaddition example is shown in Fig. 11(c). In this research, Diels–Alder cycloaddition onto fluorinated graphene is activated by 320 nm UV, which isomerizes molecules into diene.197
Furthermore, depending on the wavelength of the light, UV light can break the bonds of several molecules to create reactive radicals. Several UV-activated radical functionalization cases involving azide,178 ozone,157,447–449 and halogen204–206 have been reported.
UV ozone is a popular method for light oxidation. Owing to its relatively low reactivity, UV-ozone oxidation has been used in several studies to functionalize or etch GBs selectively, to visualize GBs optically.157,158 Another popular application of soft functionalization by UV-ozone is to improve the layer uniformity of the deposited film.448,449 Uniform chemical potential at 2D material surfaces restricts thin film growth by atomic layer deposition (ALD). Consequently, surface functionalization of 2D materials is a promising method to improve the adhesion between 2D material surfaces and deposited material without severely damaging the electrical properties. Fig. 11(d) shows a clear example of improved film quality on MoS2 by UV-ozone treatment before the ALD.448 In Fig. 11(d), the deposited Al2O3 film shows a significant difference in roughness between with and without UV-ozone treatment, even with the same amount of deposition.
3.7 Liquid exfoliation
Liquid dispersion of 2D materials is a widely studied topic23–25,450,451 as it is critical because many functionalization methods are solution-based processes or include solution steps. Among the liquid exfoliation methods, mechanical methods such as sonication and jet milling are known to breaking apart 2D materials into tiny pieces along with delamination.23–25,450 2D materials are functionalized as soon as a new defect or edge sites are exposed during the liquid process unless an inert organic solvent is selected for the purpose. For example, 2D materials are hydroxyl-functionalized when exfoliated in water.119,120,395,452
Liquid exfoliation through chemical intercalation methods is preferable to mechanical methods for the larger flake size and defect generation. However, it still introduces defects and other modifications, including phase transition.453,454 Conversely, liquid chemical exfoliation is mandatory for some functionalization techniques. As discussed in Section 2.4, metal ion intercalation-induced liquid exfoliation induces electric charging of 2D materials, which is critical for several functionalization reactions.221–225,250–254,455 In these functionalization processes, modulating the metal intercalation process controls functionalization activation and density.254,455
3.8 Ball mill
The conventional ball-mill method has been widely applied to 2D materials for its simplicity and effectiveness.24,98,450,456 However, ball milling also introduces many defects and exposed edges with delamination, similar to liquid-phase mechanical exfoliation methods.121,456
Functionalization by ball-mill exfoliation is similar to that by liquid exfoliation for functionalization by defect generation. However, the versatility of environmental control during the process and additional energy supply for the chemical reaction from the ball's kinetic energy makes the ball-mill method a more versatile tool for functionalization.456,457 As shown in Fig. 12(a), performing the ball-mill process under the desired gas or liquid environment functionalizes 2D materials with selected groups. Various groups and elements, such as S,458,459 N,125 O,124,458 OH,460–462 carboxyl,463 halogen,122,123,130,293 NH2,126 polymer,269 and C60,276 have been used to functionalize 2D materials. Moreover, a ball mill with some additives such as acid, base, or salt, can accelerate exfoliation, breakage, and hydroxyl functionalization of 2D materials, even for chemically stable materials such as hBN464–470 and graphene.471
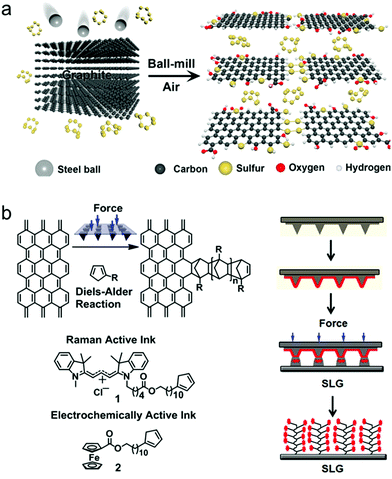 |
| Fig. 12 (a) Schematic illustration of edge functionalization process of graphene by ball milling (reprinted with permission from ref. 458 Copyright 2014 ACS Nano). (b) Molecular structure of Diels–Alder cycloaddition reaction for mechanical force accelerated graphene functionalization (left) and schematic drawing for patterned functionalization process (right) (reprinted with permission from ref. 193 Copyright 2013 J. Am. Chem. Soc.). | |
3.9 Other techniques
There are few reports for functionalization using other techniques.
Bian et al.193 reported a pressure-induced Diels–Alder cycloaddition reaction to functionalize graphene and patterned polymer chain growth on the surface (Fig. 12(b)).
Many functionalization processes can be activated or assisted by microwave irradiation. Microwaves activate reactions in two ways—that is, by heating or supplying energy directly to reacting molecules.173,182,184,189,206,211,472,473 Microwave heating works more efficiently than other conventional heating methods because it supplies thermal energy directly to the required location. Moreover, it is more effective for non-water solvents or no-solvent conditions, in which microwave energy is not absorbed by the solvent. For example, just a few seconds of microwave irradiation can increase the temperature of graphite with liquid halogen under cryogenic conditions by more than 800 °C, producing exfoliated and halogenated graphene.211
High energy ion irradiation can also be used for functionalization. Entani et al.296 reported that 2.4 MeV Cu ion irradiation could successfully fluorinate hBN covered by LiF layer.
3.10 Outlook
Some functionalization techniques (e.g., hydrothermal reaction, liquid exfoliation, ball mill) are practical when focusing on the productivity. Functionalization by liquid exfoliation or ball mill produces 2D material liquid dispersion simultaneously with functionalization, removing the demand for a separate functionalization step.
Other techniques are fitted for flake or film structured 2D materials on the chip. Most of them functionalize 2D materials by supplying energy for the initiation of the reaction, which also can damage 2D materials not only activate functionalizing reaction. Therefore, minimizing damage on 2D materials becomes one of the most critical factors for choosing a technique.
Specifically, two points are mainly considered; the benefit from functionalization would be large enough for taking a risk of damage, or damage from functionalization can be minimized. An example of a high benefit would be patterned functionalization, or when the technique for a specific functional group is limited (e.g., halogenation or hydrogenation). Otherwise, the remote plasma technique, or using low energy photon laser, just above to excite photoinitiator, is attempting to lower damage to 2D materials.
4. Direct synthesis of functionalized 2D materials
Although CVD is the most popular method for synthesizing high-quality 2D materials,143,144 there are several other synthesis methods,144,474,475 many of which support the possibility of the direct synthesis of functionalized 2D materials without a separate functionalization process.
4.1 Laser
Graphene476–480 and TMDCs481–483 can be synthesized by laser irradiation by local high-temperature heating from the photothermal effect. When the laser power is sufficiently strong to carbonize the precursor before it reacts with oxygen in the air and burns, the carbon-containing material thermally carbonizes and becomes laser-induced graphene (LIG).484–486 LIG has abundant oxygen, hydroxyl, and carboxyl functional groups on its surface and edge sites. The density of the functional group in LIG decreases as the laser power increases. Polymers are often selected as precursors for synthesis of LIG because of their uniform and controllable chemical composition, but other carbon-containing materials such as paper, wood, and fabric, can also be synthesized to LIG.487,488
4.2 Nanotube unzipping
Nanoribbons can be synthesized by unzipping nanotubes, such as CNTs489–491 or hBN nanotubes.492 Furthermore, several unzipping methods leave the functionalized group at the edge of the synthesized nanoribbon. A prominent example is a carboxyl and hydroxyl edge-functionalized graphene nanoribbon (GNR), synthesized by intercalation-assisted unzipping with KNO3 and KMnO4 (Fig. 13(a)).493–495
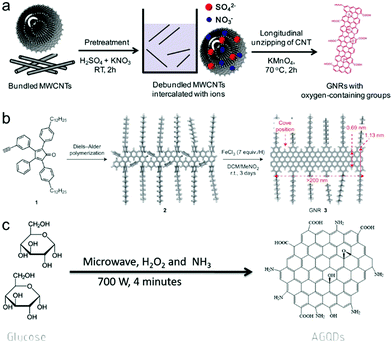 |
| Fig. 13 (a) Schematic drawing of carboxyl functionalized GNR procedure by ion intercalation assisted CNT unzipping (reprinted with permission from ref. 493 Copyright 2015 ACS Applied Materials & Interfaces) (b) Schematic drawing of a synthesis route for alkyl functionalized GNR via polymerization (reprinted with permission from ref. 526 Copyright 2014 Nature Chemistry). (c) Schematic drawing for reaction route to microwave-assisted solution synthesis method for amine-functionalized graphene quantum dot (reprinted with permission from ref. 527 Copyright 2019 C. R. Chim.). | |
As explained in the liquid exfoliation section, the unzipping process exposes non-passivated new edge sites, which bond with the surrounding molecules to be functionalized. The hBN nanoribbons fabricated by sonication unzipping are alkyl-chain functionalized if sonicated with alcohol,496 and NH2 functionalized when sonicated with ammonia.497
4.3 Hydrothermal synthesis
Hydrothermal (solvothermal) synthesis has been widely studied for the synthesis of 2D materials.434,498,499 Like liquid exfoliated 2D materials, the hydrothermal method synthesizes O or OH group-functionalized 2D materials from water as a solvent in the synthesis process.500 Groups other than OH are also possible, with wisely chosen additives for the reaction. For example, synthesis of amino-functionalized graphene501 and polymer-functionalized MoS2 with polyethylene glycol (PEG)502–504 and polyvinylpyrrolidone (PVP),505 are reported.
Hydrothermal synthesis is more suitable for synthesizing small particles than large and thin sheets. Functionalized 2D material quantum dots (QDs) are commonly synthesized using the hydrothermal process.506 The primary purpose for in situ functionalization for QD synthesis is to improve dispersion in solution and stability.440,507–511
4.4 Solution synthesis
Synthesis of 2D materials by solution chemistry to synthesize functionalized GNR,512–517 graphene,518–522 and TMDC523–525 using various molecules as precursors has been studied for a long time. During the synthesis of functionalized 2D materials, functional groups at the edge can be designed to modify the material properties and limit the size of the product.525
Carefully designed precursors and reactions can produce high-quality functionalized 2D materials with well-defined geometries (Fig. 13(b)). Complex geometry restricts the reaction and bonding mechanism between precursors, making the functional group and geometry of the product controllable.526 By contrast, simple primary precursors synthesize 2D materials with more defects and smaller sizes, such as QD527 (Fig. 13(c)).
4.5 Outlook
Nanotube unzipping and solution synthesis with large, complex precursors can produce highly crystalline 2D materials with low defect density.
However, other synthesis techniques in this section produce low crystallinity 2D materials. Therefore, in many cases, the synthesis of functionalized 2D materials is one of two cases; functionalized unavoidably during the synthesis process (LIG, unzip) or designed to assist the synthesis process (hydrothermal, solution).
5. Characterization
Many of the techniques that are used for characterizing 2D materials also gave information about the functionalization states of 2D materials. This section will cover general techniques to characterize groups, density, and location of functionalization on 2D materials. Techniques designed for specific applications (gas absorption, liquid dispersion stability, HER, and others) will not be discussed.
5.1 Optical spectroscopy
Optical spectroscopy is one of the most widely used technique for characterizing 2D materials for its simplicity. Among the known optical spectroscopy methods, Raman spectroscopy, photoluminescence (PL), Fourier-transform infrared spectroscopy (FT-IR), and Ultraviolet-visible spectroscopy (UV-vis) are favored for characterizing the functionalization state of 2D materials.
Raman spectroscopy is simple, non-destructive measurement technique. It is considered as one of the most fundamental characterization techniques for the research of 2D materials. Raman scattering signal is sensitive to various modifications, such as strain,15 doping,528 and defect.31 For this reason, almost every 2D material functionalization studies use Raman spectroscopy in various forms, including spatial mapping, tip-enhanced Raman spectroscopy,529,530 polarized Raman spectroscopy,531 and so forth.
PL also is a well-known technique for showing the band-related properties of 2D materials, which is easily influenced by the functionalization state. Although it can only be applied to materials with a bandgap less than 3 eV like TMDCs, and does not give a direct form of information for the band structure as Ultraviolet photoelectron spectroscopy (UPS) does,184 this simple method is widely used for characterizing the band structure of materials, including modified charge density and new band state.20,28,532,533
Other optical spectroscopy techniques including UV-vis312,362,511,534 and FT-IR286,323,379,455,511,535 are absorption spectroscopy methods that allow the chemical analysis of functionalized 2D materials. Those are usually utilized to track the functionalization and chemical reactions with organic molecules during and after the functionalization process. Both FT-IR and UV-vis share similar limitations. That is, the bonding status between functional groups cannot be measured, and a large quantity of 2D materials are required to be dispersed in solid powder or liquid medium for the analysis.
5.2 High energy spectroscopy
High energy spectroscopy,536 such as X-ray photoelectron spectroscopy (XPS),189,232,393,537 electron energy loss spectroscopy (EELS),286,538 energy-dispersive X-ray spectroscopy (EDS or EDX),286 and Auger electron spectroscopy (AES),192 are direct methods to analyze element composition of 2D materials using high energy beam radiation (e.g., photon, electron, or ion).
Because they give the most straightforward results about chemical compositions of target sample, high energy spectroscopy methods are widely used for characterizing the functionalization of 2D materials, even with several limitations – expensive equipment, difficult sample preparation step, difficult/impossible to measure light element (H or Li), and risk of damaging the sample.
Different spectroscopy techniques are favored for different 2D materials and functional groups. XPS is more suitable when the sample has various functional groups (e.g., GO) or a large organic functional group because it can detect the bonding status of the element. EDX or EELS can be a better option when high spatial resolution is required. Since EDX and EELS are attachable measurement options of electron microscopy, scanning electron microscope (SEM) and transmission electron microscope (TEM), they share similar requirements and limitations of SEM/TEM. Likewise, a sample with a single element functional group (e.g., halogenated graphene) would give the best result from EDX and EELS since a high-energy electron beam easily damages large organic molecules.
5.3 Scanning probe microscopy
Scanning probe microscopy (SPM) techniques are a substantial option for characterizing the surface property of functionalized 2D materials.
Observing the topography of an individual functional group might give the most straightforward image data about functionalization. However, it is applied to limited cases only, because of its tricky measurement and sample preparation.128,304 Roughness,230 which can be derived from relatively simple atomic force microscopy (AFM), may give indirect information about functionalization density.
Besides topography, from various measuring modes of AFM, one can distinguish changed 2D materials surfaces properties after functionalization. Friction measurement199,389 would be the easiest method that clearly shows the functionalization state. Although AFM friction cannot give quantitative results about the exact type of functional group itself, it can clearly distinguish the area with different functional groups or bare surfaces. Kelvin probe force microscopy (KPFM)61 also shows a clear contrast between different functionalization groups, in addition to Fermi level shift, without the risk of damaging the sample surface.
5.4 Others
Thermogravimetric analysis (TGA)185,187,189,228,232,247,252,323,362,379,472,524 is a convenient tool for getting brief information about the chemical bonding states of functional groups, especially for large organic functional groups. It also can be coupled with mass spectroscopy to get more information about functional groups by measuring the exact mass of dissociated byproducts.
Mass spectroscopy, both coupled with TGA and time-of-flight secondary ion mass spectrometry (TOF-SIMS),199,286,336,364 is another direct element analysis technique. Unlike XPS or EDX, they can detect hydrogen or other light elements, becoming almost the sole technique for characterizing hydrogenated 2D materials.
In addition, unlike TGA and TGA-coupled mass spectroscopy, TOF-SIMS does not require a large amount of sample and ion beam irradiation is controllable, allowing element analysis with space distribution, which is greatly beneficial, especially for atomic thickness 2D materials.
5.5 Outlook
Characterization methods for functionalized 2D materials can be selected considering several factors.
For a sample of flake or film structured 2D materials, which can be supported by chip or TEM grid, Raman, PL, EDX, AFM, and EELS are possible options. When the film is large enough, XPS can also be chosen. FT-IR, UV-vis, and TGA generally require a large quantity of sample and TGA can be selected when the sample damage is not a critical issue.
The functional group is also an important factor. XPS, UV-vis, FT-IR, and TGA are widely selected when 2D materials are functionalized with large organic molecules, especially the chemistry of the functional group itself is important, and 2D materials act as supporting substrates. By comparison, when the specific element of the functional group has to be detected, such as from single or few atom groups (e.g., halogenated graphene), EDX, EELS, XPS, and mass spectroscopy can be the proper option.
When the precise chemistry of the functional group is already known by previous studies or from other measurements, fast, approximate measurement may be enough for characterization. In this case, Raman or AFM can be the simplest and fastest option.
Raman, PL, AFM, EDX, and EELS provide characterization with the spatial distribution, which is highly demanding for many 2D material studies. Other methods, such as FT-IR or XPS may also support spatial analysis, but it is limited in resolution (FT-IR) or requires a difficult setup (XPS).
6. Conclusions
In this review, we discussed the currently reported covalent functionalization processes for 2D materials. Applying additional functionality to 2D materials by chemical modification has been a popular topic from the early stages of research. Furthermore, because of the continuous discovery of new 2D materials and increased demand for them, the importance of proper functionalization techniques for 2D materials continues to grow.
In conventional 3D materials, surface functionalization only changes its surface properties, and bulk properties are considered to remain intact. Consequently, surface functionalization adaptation for bulk materials is limited to particular applications, such as control surface reactions and biocompatibility. However, for 2D materials with a large surface-to-volume ratio, importance of surface modification by functionalization greatly increases, because it directly affects their properties.
Proper functionalization is critical for eliciting the potential of 2D materials, even including finding new 2D materials or synthesizing high-quality 2D materials. Apparently, an appropriate method should be carefully selected to expedite proper functionalization and improve product quality with high yield and low cost.
An enormous variety of molecules can be used to functionalize 2D materials using direct or group exchange methods. This versatility widens applications of 2D materials by controlling the doping level, forming a composite with polymer/nanoparticle/other 2D layers, and bonding with other biomolecules. However, 2D materials and their functionalization methods for the industry are still a long way off, with several limitations that need to be solved.
(1) It is difficult to control the exact bonding sites of the functional group. The molecules react simultaneously at different sites. For example, vacancy-targeted functionalization reactions also occur at the edge or at other defect locations, and many group exchange reactions occur with several different groups or locations. Even for the reactions designed for specific types of locations, only the density or distribution of functional groups can be controlled for most functionalization methods.
(2) Several functionalization methods result in unwanted defects, thereby lowering the quality of the end-product. For this reason, the energy supply during functionalization processes and defect generation must be well controlled so as not to damage the 2D materials to minimize material quality deterioration. Even non-defect-mediated pure chemical reactions under mild conditions cause reactive chemicals and byproducts during the process, which can attack and damage 2D materials. Moreover, even when the functionalization process does not cause unwanted defects, it inevitably disturbs other physical properties, such as electron mobility or mechanical strength.
(3) Many functionalization methods have been studied only for specific reaction conditions, materials, or material configurations (e.g., liquid dispersion, exfoliated flakes, or bulk). Although every 2D material shares some physical properties, such as clean surfaces and layered structures, but they differ in chemistry and reactivity, making it almost impossible to develop a universal 2D material functionalization process.
Currently, more research is required to solve or at least mitigate all the aforementioned problems. Every new 2D material functionalization research will add a step to fulfill the list of possible functionalization processes for every 2D material. Even though the process of completing this list is tedious, step-by-step efforts without a direct outcome, each step will bring the impractical dream of ‘perfect 2D functionalization methods’ a little closer to reality.
Moreover, research for completing the list will be more than just developing convenient experimental procedures or industrial applications. We believe that researching the functionalization of 2D materials will benefit the entire field of 2D materials, lead to a better understanding of the chemistry of 2D materials and eventually open new research areas full of unexplored territory.
Author contributions
Jae Hwan Jeong did every investigation and writing of this paper. Sojung Kang and Gwan-Hyoung Lee did review and editing. Namwon Kim and Rakesh Joshi review this work. Whole writing is done under Gwan-Hyoung Lee's supervise.
Conflicts of interest
There are no conflicts to declare.
Acknowledgements
This work was supported by Creative-Pioneering Researchers Program through Seoul National University (SNU), Brain Pool program funded by the Ministry of Science and ICT through the National Research Foundation of Korea (NRF-2021H1D3A2A01045033), and Basic Science Research Program through the National Research Foundation of Korea (NRF) funded by the Ministry of Science, ICT & Future Planning (NRF-2021R1A2C3014316).
Notes and references
- K. S. Novoselov, A. K. Geim, S. V. Morozov, D. Jiang, Y. Zhang, S. V. Dubonos, I. V. Grigorieva and A. A. Firsov, Science, 2004, 306, 666–669 CrossRef CAS PubMed.
- S. Manzeli, D. Ovchinnikov, D. Pasquier, O. V. Yazyev and A. Kis, Nat. Rev. Mater., 2017, 2, 17033 CrossRef CAS.
- W. Choi, N. Choudhary, G. H. Han, J. Park, D. Akinwande and Y. H. Lee, Mater. Today, 2017, 20, 116–130 CrossRef CAS.
- K. L. Zhang, Y. L. Feng, F. Wang, Z. C. Yang and J. Wang, J. Mater. Chem. C, 2017, 5, 11992–12022 RSC.
- A. Pakdel, Y. Bando and D. Golberg, Chem. Soc. Rev., 2014, 43, 934–959 RSC.
- Y. Xu, Z. Shi, X. Shi, K. Zhang and H. Zhang, Nanoscale, 2019, 11, 14491–14527 RSC.
- B. Li, C. Lai, G. Zeng, D. Huang, L. Qin, M. Zhang, M. Cheng, X. Liu, H. Yi, C. Zhou, F. Huang, S. Liu and Y. Fu, Small, 2019, 15, e1804565 CrossRef PubMed.
- X. Ling, H. Wang, S. Huang, F. Xia and M. S. Dresselhaus, Proc. Natl. Acad. Sci. U. S. A., 2015, 112, 4523–4530 CrossRef CAS PubMed.
- B. Anasori, M. R. Lukatskaya and Y. Gogotsi, Nat. Rev. Mater., 2017, 2, 16098 CrossRef CAS.
- Y. Gogotsi and B. Anasori, ACS Nano, 2019, 13, 8491–8494 CrossRef CAS PubMed.
- N. K. Chaudhari, H. Jin, B. Kim, D. S. Baek, S. H. Joo and K. Lee, J. Mater. Chem. A, 2017, 5, 24564–24579 RSC.
- K. Kalantar-zadeh, J. Z. Ou, T. Daeneke, A. Mitchell, T. Sasaki and M. S. Fuhrer, Appl. Mater. Today, 2016, 5, 73–89 CrossRef.
- T. Yang, T. T. Song, M. Callsen, J. Zhou, J. W. Chai, Y. P. Feng, S. J. Wang and M. Yang, Adv. Mater. Interfaces, 2018, 6, 1801160 CrossRef.
- A. Klein and W. Jaegermann, ECS J. Solid State Sci. Technol., 2020, 9, 093012 CrossRef CAS.
- G. G. Naumis, S. Barraza-Lopez, M. Oliva-Leyva and H. Terrones, Rep. Prog. Phys., 2017, 80, 096501 CrossRef PubMed.
- Q. H. Wang, K. Kalantar-Zadeh, A. Kis, J. N. Coleman and M. S. Strano, Nat. Nanotechnol., 2012, 7, 699–712 CrossRef CAS PubMed.
- B. Liu, A. Abbas and C. Zhou, Adv. Electron. Mater, 2017, 3, 1700045 CrossRef.
- B. Peng, P. K. Ang and K. P. Loh, Nano Today, 2015, 10, 128–137 CrossRef CAS.
- K. Zollner, P. E. Faria and J. Fabian, Phys. Rev. B, 2019, 100, 195126 CrossRef CAS.
- H. N. Li, M. E. Pam, Y. M. Shi and H. Y. Yang, Flatchem, 2017, 4, 48–53 CrossRef CAS.
- J. H. Kim, J. H. Jeong, N. Kim, R. Joshi and G. H. Lee, J. Phys. D: Appl. Phys, 2019, 52, 083001 CrossRef.
- D. Akinwande, C. J. Brennan, J. S. Bunch, P. Egberts, J. R. Felts, H. Gao, R. Huang, J.-S. Kim, T. Li, Y. Li, K. M. Liechti, N. Lu, H. S. Park, E. J. Reed, P. Wang, B. I. Yakobson, T. Zhang, Y.-W. Zhang, Y. Zhou and Y. Zhu, Extreme Mech. Lett, 2017, 13, 42–77 CrossRef.
- C. X. Huo, Z. Yan, X. F. Song and H. B. Zeng, Sci. Bull., 2015, 60, 1994–2008 CrossRef CAS.
- X. Cai, Y. Luo, B. Liu and H. M. Cheng, Chem. Soc. Rev., 2018, 47, 6224–6266 RSC.
- H. Tao, Y. Zhang, Y. Gao, Z. Sun, C. Yan and J. Texter, Phys. Chem. Chem. Phys., 2017, 19, 921–960 RSC.
- Q. Li, Q. H. Zhou, L. Shi, Q. Chen and J. L. Wang, J. Mater. Chem. A, 2019, 7, 4291–4312 RSC.
- G. Abellan, S. Wild, V. Lloret, N. Scheuschner, R. Gillen, U. Mundloch, J. Maultzsch, M. Varela, F. Hauke and A. Hirsch, J. Am. Chem. Soc., 2017, 139, 10432–10440 CrossRef CAS PubMed.
- K. H. Zhang and J. Robinson, MRS Adv., 2019, 4, 2743–2757 CrossRef CAS.
- L. Y. Loh, Z. P. Zhang, M. Bosman and G. Eda, Nano Res., 2021, 14, 1668–1681 CrossRef CAS.
- J. Jiang, T. Xu, J. Lu, L. Sun and Z. Ni, Research, 2019, 2019, 4641739 CAS.
- Z. Lin, B. R. Carvalho, E. Kahn, R. T. Lv, R. Rao, H. Terrones, M. A. Pimenta and M. Terrones, 2D Mater., 2016, 3, 022002 CrossRef.
- J. Xiong, J. Di, J. X. Xia, W. S. Zhu and H. M. Li, Adv. Funct. Mater., 2018, 28, 1801983 CrossRef.
- X. Q. Ji, Y. H. Xu, W. L. Zhang, L. Cui and J. Q. Liu, Composites, Part A, 2016, 87, 29–45 CrossRef CAS.
- W. Liu, B. Ullah, C. C. Kuo and X. K. Cai, Adv. Polym. Technol., 2019, 2019, 1–15 Search PubMed.
- X. X. Zhan, C. Si, J. Zhou and Z. M. Sun, Nanoscale Horiz., 2020, 5, 235–258 RSC.
- J. Deng, D. Deng and X. Bao, Adv. Mater., 2017, 29, 1606967 CrossRef PubMed.
- Z. C. Wang, Y. J. Ping, Q. Fu and C. X. Pan, MRS Adv., 2018, 3, 849–854 CrossRef CAS.
- B. Cai, S. L. Zhang, Z. Yan and H. B. Zeng, ChemNanoMat, 2015, 1, 542–557 CrossRef CAS.
- A. Kumar, K. Banerjee and P. Liljeroth, Nanotechnology, 2017, 28, 082001 CrossRef PubMed.
- L. Daukiya, J. Seibel and S. De Feyter, Adv. Phys.: X, 2019, 4, 1625723 CAS.
- A. L. Zhao, H. Li, X. J. Hu, C. Wang, H. Zhang, J. G. Lu, S. C. Ruan and Y. J. Zeng, J. Phys. D: Appl. Phys., 2020, 53, 293002 CrossRef CAS.
- X. Liu and M. C. Hersam, Adv. Mater., 2018, 30, e1801586 CrossRef PubMed.
- Z. Hu, Z. B. Liu and J. G. Tian, Chin. J. Chem., 2020, 38, 981–995 CrossRef CAS.
- K. S. Novoselov, A. Mishchenko, A. Carvalho and A. H. Castro Neto, Science, 2016, 353, aac9439 CrossRef CAS PubMed.
- B. V. Lotsch, Annu. Rev. Mater. Res., 2015, 45, 85–109 CrossRef CAS.
- D. S. Schulman, A. J. Arnold, A. Razavieh, J. Nasr and S. Das, IEEE Nanatechnol. Mag., 2017, 11, 6–17 Search PubMed.
- P. Solis-Fernandez, M. Bissett and H. Ago, Chem. Soc. Rev., 2017, 46, 4572–4613 RSC.
- S. Carr, D. Massatt, S. Fang, P. Cazeaux, M. Luskin and E. Kaxiras, Phys. Rev. B, 2017, 95, 075420 CrossRef.
- S. Carr, S. Fang and E. Kaxiras, Nat. Rev. Mater., 2020, 5, 748–763 CrossRef CAS.
- A. Nimbalkar and H. Kim, Nano-Micro Lett., 2020, 12, 126 CrossRef CAS PubMed.
- M. S. Stark, K. L. Kuntz, S. J. Martens and S. C. Warren, Adv. Mater., 2019, 31, e1808213 CrossRef PubMed.
- M. Rajapakse, B. Karki, U. O. Abu, S. Pishgar, M. R. K. Musa, S. M. S. Riyadh, M. Yu, G. Sumanasekera and J. B. Jasinski, npj 2D Mater. Appl., 2021, 5, 30 CrossRef CAS.
- L. Daukiya, M. N. Nair, M. Cranney, F. Vonau, S. Hajjar-Garreau, D. Aubel and L. Simon, Prog. Surf. Sci., 2019, 94, 1–20 CrossRef CAS.
- J. Wan, S. D. Lacey, J. Dai, W. Bao, M. S. Fuhrer and L. Hu, Chem. Soc. Rev., 2016, 45, 6742–6765 RSC.
- W. Chen, X. Gui, L. Yang, H. Zhu and Z. Tang, Nanoscale Horiz., 2019, 4, 291–320 RSC.
- S. K. Deng and V. Berry, Mater. Today, 2016, 19, 197–212 CrossRef CAS.
- W. F. Zhang, Y. Zhang, J. K. Qiu, Z. H. Zhao and N. Liu, InfoMat, 2021, 3, 133–154 CrossRef CAS.
- E. Ji, J. Son, J. H. Kim and G. H. Lee, Flatchem, 2018, 7, 26–33 CrossRef CAS.
- W. Deng, C. You, X. Chen, Y. Wang, Y. Li, B. Feng, K. Shi, Y. Chen, L. Sun and Y. Zhang, Small, 2019, 15, e1901544 CrossRef PubMed.
- D. W. Chang and J. B. Baek, Mater. Res. Express, 2016, 3, 044001 CrossRef.
- A. J. Clancy, H. Au, N. Rubio, G. O. Coulter and M. S. P. Shaffer, Dalton Trans., 2020, 49, 10308–10318 RSC.
- Y. Wang, S. S. Li, H. Y. Yang and J. Luo, RSC Adv., 2020, 10, 15328–15345 RSC.
- G. Bottari, M. A. Herranz, L. Wibmer, M. Volland, L. Rodriguez-Perez, D. M. Guldi, A. Hirsch, N. Martin, F. D'Souza and T. Torres, Chem. Soc. Rev., 2017, 46, 4464–4500 RSC.
- T. Kuila, S. Bose, A. K. Mishra, P. Khanra, N. H. Kim and J. H. Lee, Prog. Mater Sci., 2012, 57, 1061–1105 CrossRef CAS.
- I. A. Vacchi, C. Menard-Moyon and A. Bianco, Phys. Sci. Rev., 2017, 2, 20160103 Search PubMed.
- G. H. Yang, D. D. Bao, H. Liu, D. Q. Zhang, N. Wang and H. T. Li, J. Inorg. Organomet. Polym. Mater., 2017, 27, 1129–1141 CrossRef CAS.
- A. Criado, M. Melchionna, S. Marchesan and M. Prato, Angew. Chem., Int. Ed., 2015, 54, 10734–10750 CrossRef CAS PubMed.
- M. Park, N. Kim, J. Lee, M. Gu and B. S. Kim, Mater. Chem. Front., 2021, 5, 4424–4444 RSC.
- D. R. Dreyer, S. Park, C. W. Bielawski and R. S. Ruoff, Chem. Soc. Rev., 2010, 39, 228–240 RSC.
- S. Presolski and M. Pumera, Mater. Today, 2016, 19, 140–145 CrossRef CAS.
- X. Chen and A. R. McDonald, Adv. Mater., 2016, 28, 5738–5746 CrossRef CAS PubMed.
- Z. Y. Hai and S. Zhuiykov, Adv. Mater. Interfaces, 2018, 5, 1701385 CrossRef.
- J. Azadmanjiri, P. Kumar, V. K. Srivastava and Z. Sofer, ACS Appl. Nano Mater., 2020, 3, 3116–3143 CrossRef CAS.
- Z. Wei and S. Zhuiykov, Nanoscale, 2019, 11, 15709–15738 RSC.
- M. Pica and R. D’Amato, Inorganics, 2020, 8, 29 CrossRef CAS.
- W. Lei, G. Liu, J. Zhang and M. Liu, Chem. Soc. Rev., 2017, 46, 3492–3509 RSC.
- S. Thurakkal and X. Zhang, Adv. Sci., 2020, 7, 1902359 CrossRef CAS PubMed.
- Q. Weng, X. Wang, X. Wang, Y. Bando and D. Golberg, Chem. Soc. Rev., 2016, 45, 3989–4012 RSC.
- Z. Zheng, M. Cox and B. Li, J. Mater. Sci., 2017, 53, 66–99 CrossRef.
- R. Fang, C. Lu, A. Chen, K. Wang, H. Huang, Y. Gan, C. Liang, J. Zhang, X. Tao, Y. Xia and W. Zhang, ChemSusChem, 2020, 13, 1409–1419 CrossRef CAS PubMed.
- B. Ren, Y. Wang and J. Z. Ou, J. Mater. Chem. B, 2020, 8, 1108–1127 RSC.
- W. L. B. Huey and J. E. Goldberger, Chem. Soc. Rev., 2018, 47, 6201–6223 RSC.
- J. R. Choi, K. W. Yong, J. Y. Choi, A. Nilghaz, Y. Lin, J. Xu and X. Lu, Theranostics, 2018, 8, 1005–1026 CrossRef CAS PubMed.
- M. Emanet, O. Sen, I. C. Taskin and M. Culha, Front. Bioeng. Biotechnol., 2019, 7, 363 CrossRef PubMed.
- S. A. Zhu, L. J. Gong, J. N. Xie, Z. J. Gu and Y. L. Zhao, Small Methods, 2017, 1, 1700220 CrossRef.
- X. Li, J. Shan, W. Zhang, S. Su, L. Yuwen and L. Wang, Small, 2017, 13, 1602660 CrossRef PubMed.
- X. Zhou, H. Sun and X. Bai, Front. Bioeng. Biotechnol, 2020, 8, 236 CrossRef PubMed.
- H. Chen, T. Liu, Z. Su, L. Shang and G. Wei, Nanoscale Horiz., 2018, 3, 74–89 RSC.
- Z. Li and S. L. Wong, Mater. Sci. Eng., C, 2017, 70, 1095–1106 CrossRef CAS PubMed.
- J. Chen, H. Meng, Y. Tian, R. Yang, D. Du, Z. Li, L. Qu and Y. Lin, Nanoscale Horiz., 2019, 4, 321–338 RSC.
- Y. Chen, C. Tan, H. Zhang and L. Wang, Chem. Soc. Rev., 2015, 44, 2681–2701 RSC.
- D. Chimene, D. L. Alge and A. K. Gaharwar, Adv. Mater., 2015, 27, 7261–7284 CrossRef CAS PubMed.
- T. T. Hu, X. Mei, Y. J. Wang, X. S. Weng, R. Z. Liang and M. Wei, Sci. Bull., 2019, 64, 1707–1727 CrossRef CAS.
- Y. H. Wang, K. J. Huang and X. Wu, Biosens. Bioelectron., 2017, 97, 305–316 CrossRef CAS PubMed.
- C. Zhu, D. Du and Y. Lin, Biosens. Bioelectron., 2017, 89, 43–55 CrossRef CAS PubMed.
- A. Olean-Oliveira, G. O. A. Brito, C. X. Cardoso and M. F. S. Teixeira, Chemosensors, 2021, 9, 149 CrossRef CAS.
- T. H. Le, Y. Oh, H. Kim and H. Yoon, Chemistry, 2020, 26, 6360–6401 CrossRef CAS PubMed.
- H. H. Lyu, B. Gao, F. He, C. Ding, J. C. Tang and J. C. Crittenden, ACS Sustainable Chem. Eng., 2017, 5, 9568–9585 CrossRef CAS.
- Y. J. Zhang, L. Wang, N. N. Zhang and Z. J. Zhou, RSC Adv., 2018, 8, 19895–19905 RSC.
- Y. F. Wang, Y. H. Xu, M. L. Hu, H. Ling and X. Zhu, Nanophotonics, 2020, 9, 1601–1620 CrossRef CAS.
- K. Kannan, K. K. Sadasivuni, A. M. Abdullah and B. Kumar, Catalysts, 2020, 10, 495 CrossRef CAS.
- E. Singh, K. S. Kim, G. Y. Yeom and H. S. Nalwa, RSC Adv., 2017, 7, 28234–28290 RSC.
- G. Speranza, C, 2019, 5, 84 Search PubMed.
- Z. Hu, T. Niu, R. Guo, J. Zhang, M. Lai, J. He, L. Wang and W. Chen, Nanoscale, 2018, 10, 21575–21603 RSC.
- J. Liu, Z. Liu, C. J. Barrow and W. Yang, Anal. Chim. Acta, 2015, 859, 1–19 CrossRef CAS PubMed.
- X. T. Jiang, A. V. Kuklin, A. Baev, Y. Q. Ge, H. Agren, H. Zhang and P. N. Prasad, Phys. Rep., 2020, 848, 1–58 CrossRef CAS.
- J. Meyer, S. Hamwi, M. Kroger, W. Kowalsky, T. Riedl and A. Kahn, Adv. Mater., 2012, 24, 5408–5427 CrossRef CAS PubMed.
- K. S. Kumar, N. Choudhary, Y. Jung and J. Thomas, ACS Energy Lett., 2018, 3, 482–495 CrossRef CAS.
- L. L. Yu, J. Z. Qin, W. J. Zhao, Z. G. Zhang, J. Ke and B. J. Liu, Int. J. Photoenergy, 2020, 2020, 1–11 CrossRef CAS.
- D. W. Chen, Y. Q. Zou and S. Y. Wang, Mater. Today Energy, 2019, 12, 250–268 CrossRef.
- G. He, T. Dong, Z. Yang and P. Ohlckers, Chem. Mater., 2019, 31, 9917–9938 CrossRef CAS.
- J. Liu, W. Peng, Y. Li, F. Zhang and X. Fan, Trans. Tianjin Univ., 2020, 26, 149–171 CrossRef CAS.
- H. I. Karunadasa, E. Montalvo, Y. Sun, M. Majda, J. R. Long and C. J. Chang, Science, 2012, 335, 698–702 CrossRef CAS PubMed.
- T. F. Jaramillo, K. P. Jorgensen, J. Bonde, J. H. Nielsen, S. Horch and I. Chorkendorff, Science, 2007, 317, 100–102 CrossRef CAS PubMed.
- A. Bellunato, H. Arjmandi Tash, Y. Cesa and G. F. Schneider, ChemPhysChem, 2016, 17, 785–801 CrossRef CAS PubMed.
- X. Jia, J. Campos-Delgado, M. Terrones, V. Meunier and M. S. Dresselhaus, Nanoscale, 2011, 3, 86–95 RSC.
- W. Xu, S. Li, S. Zhou, J. K. Lee, S. Wang, S. G. Sarwat, X. Wang, H. Bhaskaran, M. Pasta and J. H. Warner, ACS Appl. Mater. Interfaces, 2018, 10, 4630–4639 CrossRef CAS PubMed.
- Y. Zhang, Q. Ji, G. F. Han, J. Ju, J. Shi, D. Ma, J. Sun, Y. Zhang, M. Li, X. Y. Lang, Y. Zhang and Z. Liu, ACS Nano, 2014, 8, 8617–8624 CrossRef CAS PubMed.
- Y. Lin, T. V. Williams, T. B. Xu, W. Cao, H. E. Elsayed-Ali and J. W. Connell, J. Phys. Chem. C, 2011, 115, 2679–2685 CrossRef CAS.
- M. Nurunnabi, M. Nafiujjaman, S. J. Lee, I. K. Park, K. M. Huh and Y. K. Lee, Chem. Commun., 2016, 52, 6146–6149 RSC.
- D. Lee, B. Lee, K. H. Park, H. J. Ryu, S. Jeon and S. H. Hong, Nano Lett., 2015, 15, 1238–1244 CrossRef CAS PubMed.
- J. Xu, I. Y. Jeon, J. M. Seo, S. Dou, L. Dai and J. B. Baek, Adv. Mater., 2014, 26, 7317–7323 CrossRef CAS PubMed.
- I. Y. Jeon, M. J. Ju, J. T. Xu, H. J. Choi, J. M. Seo, M. J. Kim, I. T. Choi, H. M. Kim, J. C. Kim, J. J. Lee, H. K. Liu, H. K. Kim, S. X. Dou, L. M. Dai and J. B. Baek, Adv. Funct. Mater., 2015, 25, 1170–1179 CrossRef CAS.
- I. Y. Jeon, H. J. Choi, S. M. Jung, J. M. Seo, M. J. Kim, L. Dai and J. B. Baek, J. Am. Chem. Soc., 2013, 135, 1386–1393 CrossRef CAS PubMed.
- I. Y. Jeon, H. J. Choi, M. J. Ju, I. T. Choi, K. Lim, J. Ko, H. K. Kim, J. C. Kim, J. J. Lee, D. Shin, S. M. Jung, J. M. Seo, M. J. Kim, N. Park, L. Dai and J. B. Baek, Sci. Rep., 2013, 3, 2260 CrossRef PubMed.
- W. Lei, V. N. Mochalin, D. Liu, S. Qin, Y. Gogotsi and Y. Chen, Nat. Commun., 2015, 6, 8849 CrossRef CAS PubMed.
- R. Canton-Vitoria, T. Scharl, A. Stergiou, A. Cadranel, R. Arenal, D. M. Guldi and N. Tagmatarchis, Angew. Chem., Int. Ed., 2020, 59, 3976–3981 CrossRef CAS PubMed.
- A. Tuxen, J. Kibsgaard, H. Gobel, E. Laegsgaard, H. Topsoe, J. V. Lauritsen and F. Besenbacher, ACS Nano, 2010, 4, 4677–4682 CrossRef CAS PubMed.
- T. Wang, R. Zhu, J. Zhuo, Z. Zhu, Y. Shao and M. Li, Anal. Chem., 2014, 86, 12064–12069 CrossRef CAS PubMed.
- M. Park, I. Y. Jeon, J. Ryu, H. Jang, J. B. Back and J. Cho, Nano Energy, 2016, 26, 233–240 CrossRef CAS.
- J. M. Seo and J. B. Baek, Chem. Commun., 2014, 50, 14651–14653 RSC.
- Z. Ji, J. Chen, L. Huang and G. Shi, Chem. Commun., 2015, 51, 2806–2809 RSC.
- R. A. Bueno, J. I. Martinez, R. F. Luccas, N. R. Del Arbol, C. Munuera, I. Palacio, F. J. Palomares, K. Lauwaet, S. Thakur, J. M. Baranowski, W. Strupinski, M. F. Lopez, F. Mompean, M. Garcia-Hernandez and J. A. Martin-Gago, Nat. Commun., 2017, 8, 15306 CrossRef CAS PubMed.
- V. Kumar, N. Joshi, B. Dhara, P. K. Jha, S. Rana, P. Ghosh and N. Ballav, J. Phys. Chem. C, 2018, 122, 21076–21082 CrossRef CAS.
- P. Zhao, D. Kiriya, A. Azcatl, C. Zhang, M. Tosun, Y. S. Liu, M. Hettick, J. S. Kang, S. McDonnell, K. C. Santosh, J. Guo, K. Cho, R. M. Wallace and A. Javey, ACS Nano, 2014, 8, 10808–10814 CrossRef CAS PubMed.
- M. Zhang, M. Lihter, T. H. Chen, M. Macha, A. Rayabharam, K. Banjac, Y. Zhao, Z. Wang, J. Zhang, J. Comtet, N. R. Aluru, M. Lingenfelder, A. Kis and A. Radenovic, ACS Nano, 2021, 15, 7168–7178 CrossRef CAS PubMed.
- M. Makarova, Y. Okawa and M. Aono, J. Phys. Chem. C, 2012, 116, 22411–22416 CrossRef CAS.
- Z. Yu, Y. Pan, Y. Shen, Z. Wang, Z. Y. Ong, T. Xu, R. Xin, L. Pan, B. Wang, L. Sun, J. Wang, G. Zhang, Y. W. Zhang, Y. Shi and X. Wang, Nat. Commun., 2014, 5, 5290 CrossRef CAS PubMed.
- S. Ippolito, A. G. Kelly, R. Furlan de Oliveira, M. A. Stoeckel, D. Iglesias, A. Roy, C. Downing, Z. Bian, L. Lombardi, Y. A. Samad, V. Nicolosi, A. C. Ferrari, J. N. Coleman and P. Samori, Nat. Nanotechnol., 2021, 16, 592–598 CrossRef CAS PubMed.
- X. Chen, N. C. Berner, C. Backes, G. S. Duesberg and A. R. McDonald, Angew. Chem., Int. Ed., 2016, 55, 5803–5808 CrossRef CAS PubMed.
- Q. Ding, K. J. Czech, Y. Zhao, J. Zhai, R. J. Hamers, J. C. Wright and S. Jin, ACS Appl. Mater. Interfaces, 2017, 9, 12734–12742 CrossRef CAS PubMed.
- D. M. Sim, M. Kim, S. Yim, M. J. Choi, J. Choi, S. Yoo and Y. S. Jung, ACS Nano, 2015, 9, 12115–12123 CrossRef CAS PubMed.
- A. T. Hoang, K. Qu, X. Chen and J. H. Ahn, Nanoscale, 2021, 13, 615–633 RSC.
- R. Kumar, S. Sahoo, E. Joanni, R. K. Singh, R. M. Yadav, R. K. Verma, D. P. Singh, W. K. Tan, A. P. del Pino, S. A. Moshkalev and A. Matsuda, Nano Res., 2019, 12, 2655–2694 CrossRef CAS.
- W. Yao, B. Wu and Y. Liu, ACS Nano, 2020, 14, 9320–9346 CrossRef CAS PubMed.
- A. Zavabeti, A. Jannat, L. Zhong, A. A. Haidry, Z. Yao and J. Z. Ou, Nano-Micro Lett, 2020, 12, 66 CrossRef CAS PubMed.
- S. Najmaei, Z. Liu, W. Zhou, X. Zou, G. Shi, S. Lei, B. I. Yakobson, J. C. Idrobo, P. M. Ajayan and J. Lou, Nat. Mater., 2013, 12, 754–759 CrossRef CAS PubMed.
- A. Isacsson, A. W. Cummings, L. Colombo, L. Colombo, J. M. Kinaret and S. Roche, 2D Mater., 2016, 4, 012002 CrossRef.
- O. V. Yazyev and Y. P. Chen, Nat. Nanotechnol., 2014, 9, 755–767 CrossRef CAS PubMed.
- A. W. Cummings, D. L. Duong, V. L. Nguyen, D. Van Tuan, J. Kotakoski, J. E. Barrios Vargas, Y. H. Lee and S. Roche, Adv. Mater., 2014, 26, 5079–5094 CrossRef CAS PubMed.
- G. Cuniberti, L. M. Sandonas, R. Gutierrez, A. Pecchia and A. Dianat, J. Self-Assembly Mol. Electron., 2015, 2015, 1–20 Search PubMed.
- K. Kim, H. B. Lee, R. W. Johnson, J. T. Tanskanen, N. Liu, M. G. Kim, C. Pang, C. Ahn, S. F. Bent and Z. Bao, Nat. Commun., 2014, 5, 4781 CrossRef CAS PubMed.
- J. Hong, J. B. Lee, S. Lee, J. Seo, H. Lee, J. Y. Park, J. H. Ahn, T. I. Seo, T. Lee and H. B. R. Lee, NPG Asia Mater., 2016, 8, e262 CrossRef CAS.
- Y. Wei, S. Chen, X. Dong, Y. Lin and L. Liu, Carbon, 2017, 113, 395–403 CrossRef CAS.
- J. Y. Lee, J. H. Lee, M. J. Kim, J. K. Dash, C. H. Lee, R. Joshi, S. Lee, J. Hone, A. Soon and G. H. Lee, Carbon, 2017, 115, 147–153 CrossRef CAS.
- M. Seifert, J. E. B. Vargas, M. Bobinger, M. Sachsenhauser, A. W. Cummings, S. Roche and J. A. Garrido, 2D Mater., 2015, 2, 024008 CrossRef.
- T. H. Ly, M. H. Chiu, M. Y. Li, J. Zhao, D. J. Perello, M. O. Cichocka, H. M. Oh, S. H. Chae, H. Y. Jeong, F. Yao, L. J. Li and Y. H. Lee, ACS Nano, 2014, 8, 11401–11408 CrossRef CAS PubMed.
- Y. Rong, K. He, M. Pacios, A. W. Robertson, H. Bhaskaran and J. H. Warner, ACS Nano, 2015, 9, 3695–3703 CrossRef CAS PubMed.
- J. E. Barrios Vargas, J. T. Falkenberg, D. Soriano, A. W. Cummings, M. Brandbyge and S. Roche, 2D Mater., 2017, 4, 025009 CrossRef.
- W. J. Yin, S. H. Wei and Y. Yan, Phys. Chem. Chem. Phys., 2013, 15, 8271–8275 RSC.
- N. N. Li, Z. D. Sha, Q. X. Pei and Y. W. Zhang, J. Phys. Chem. C, 2014, 118, 13769–13774 CrossRef CAS.
- Y. F. Li, D. Datta and Z. H. Li, Carbon, 2015, 90, 234–241 CrossRef CAS.
- D. Tasis, N. Tagmatarchis, A. Bianco and M. Prato, Chem. Rev., 2006, 106, 1105–1136 CrossRef CAS PubMed.
- H. C. Wu, X. L. Chang, L. Liu, F. Zhao and Y. L. Zhao, J. Mater. Chem., 2010, 20, 1036–1052 RSC.
- G. Clavé and S. Campidelli, Chem. Sci, 2011, 2, 1887 RSC.
- F. Diederich and C. Thilgen, Science, 1996, 271, 317–323 CrossRef CAS.
- A. Djordjevic, B. Srdjenovic, M. Seke, D. Petrovic, R. Injac and J. Mrdjanovic, J. Nanomater., 2015, 2015, 1–15 CrossRef.
- K. Itami, Chem. Rec., 2011, 11, 226–235 CrossRef CAS PubMed.
- D. K. Bohme, Philos. Trans. R. Soc. London, Ser. A, 2016, 374, 20150321 CrossRef PubMed.
- J. B. Cui, M. Burghard and K. Kern, Nano Lett., 2003, 3, 613–615 CrossRef CAS.
- J. M. Hawkins, Acc. Chem. Res., 2002, 25, 150–156 CrossRef.
- P. J. Fagan, J. C. Calabrese and B. Malone, Acc. Chem. Res., 2002, 25, 134–142 CrossRef.
- S. P. Economopoulos, G. Rotas, Y. Miyata, H. Shinohara and N. Tagmatarchis, ACS Nano, 2010, 4, 7499–7507 CrossRef CAS PubMed.
- B. Jin, J. Shen, R. Peng, C. Chen, Q. Zhang, X. Wang and S. Chu, Ind. Eng. Chem. Res., 2015, 54, 2879–2885 CrossRef CAS.
- C. K. Chua, A. Ambrosi and M. Pumera, Chem. Commun., 2012, 48, 5376–5378 RSC.
- Z. Hu, C. H. Song, Q. Shao, J. Li and Y. D. Huang, Compos. Sci. Technol., 2016, 135, 21–27 CrossRef CAS.
- L. H. Liu, M. M. Lerner and M. Yan, Nano Lett., 2010, 10, 3754–3756 CrossRef CAS PubMed.
- L. H. Liu and M. D. Yan, J. Mater. Chem., 2011, 21, 3273–3276 RSC.
- J. Choi, K. J. Kim, B. Kim, H. Lee and S. Kim, J. Phys. Chem. C, 2009, 113, 9433–9435 CrossRef CAS.
- T. A. Strom, E. P. Dillon, C. E. Hamilton and A. R. Barron, Chem. Commun., 2010, 46, 4097–4099 RSC.
- X. Zhong, J. Jin, S. Li, Z. Niu, W. Hu, R. Li and J. Ma, Chem. Commun., 2010, 46, 7340–7342 RSC.
- M. V. Sulleiro, S. Quiroga, D. Pena, D. Perez, E. Guitian, A. Criado and M. Prato, Chem. Commun., 2018, 54, 2086–2089 RSC.
- M. Yu, C. Chen, Q. Liu, C. Mattioli, H. Sang, G. Shi, W. Huang, K. Shen, Z. Li, P. Ding, P. Guan, S. Wang, Y. Sun, J. Hu, A. Gourdon, L. Kantorovich, F. Besenbacher, M. Chen, F. Song and F. Rosei, Nat. Chem., 2020, 12, 1035–1041 CrossRef CAS PubMed.
- M. Barrejon, M. J. Gomez-Escalonilla, J. L. Fierro, P. Prieto, J. R. Carrillo, A. M. Rodriguez, G. Abellan, M. C. Lopez-Escalante, M. Gabas, J. T. Lopez-Navarrete and F. Langa, Phys. Chem. Chem. Phys., 2016, 18, 29582–29590 RSC.
- M. Quintana, K. Spyrou, M. Grzelczak, W. R. Browne, P. Rudolf and M. Prato, ACS Nano, 2010, 4, 3527–3533 CrossRef CAS PubMed.
- A. Wang, W. Yu, Z. Xiao, Y. Song, L. Long, M. P. Cifuentes, M. G. Humphrey and C. Zhang, Nano Res., 2014, 8, 870–886 CrossRef.
- G. Neri, A. Scala, E. Fazio, P. G. Mineo, A. Rescifina, A. Piperno and G. Grassi, Chem. Sci., 2015, 6, 6961–6970 RSC.
- G. Neri, E. Fazio, P. G. Mineo, A. Scala and A. Piperno, Nanomaterials, 2019, 9, 1236 CrossRef CAS PubMed.
- H. Uceta, M. Vizuete, J. R. Carrillo, M. Barrejon, J. L. G. Fierro, M. P. Prieto and F. Langa, Chemistry, 2019, 25, 14644–14650 CrossRef CAS PubMed.
- X. Y. Zhang, W. R. Browne and B. L. Feringa, RSC Adv., 2012, 2, 12173–12176 RSC.
- X. J. Peng, Y. Li, G. L. Zhang, F. B. Zhang and X. B. Fan, J. Nanomater., 2013, 2013, 1–5 Search PubMed.
- L. V. Frolova, I. V. Magedov, A. Harper, S. K. Jha, M. Ovezmyradov, G. Chandler, J. Garcia, D. Bethke, E. A. Shaner, I. Vasiliev and N. G. Kalugin, Carbon, 2015, 81, 216–222 CrossRef CAS PubMed.
- S. Bian, A. M. Scott, Y. Cao, Y. Liang, S. Osuna, K. N. Houk and A. B. Braunschweig, J. Am. Chem. Soc., 2013, 135, 9240–9243 CrossRef CAS PubMed.
- S. Sarkar, E. Bekyarova, S. Niyogi and R. C. Haddon, J. Am. Chem. Soc., 2011, 133, 3324–3327 CrossRef CAS PubMed.
- J. Li, M. Li, L. L. Zhou, S. Y. Lang, H. Y. Lu, D. Wang, C. F. Chen and L. J. Wan, J. Am. Chem. Soc., 2016, 138, 7448–7451 CrossRef CAS PubMed.
- S. Sarkar, E. Bekyarova and R. C. Haddon, Acc. Chem. Res., 2012, 45, 673–682 CrossRef CAS PubMed.
- H. Bares, A. Bakandritsos, M. Medved, J. Ugolotti, P. Jakubec, O. Tomanec, S. Kalytchuk, R. Zboril and M. Otyepka, Carbon, 2019, 145, 251–258 CrossRef CAS.
- S. J. Altenburg, M. Lattelais, B. Wang, M. L. Bocquet and R. Berndt, J. Am. Chem. Soc., 2015, 137, 9452–9458 CrossRef CAS PubMed.
- J. Son, N. Buzov, S. Chen, D. Sung, H. Ryu, J. Kwon, S. Kim, S. Namiki, J. Xu, S. Hong, K. Watanabe, T. Taniguchi, W. P. King, G. H. Lee and A. M. van der Zande, Adv. Mater., 2019, 31, e1903424 CrossRef PubMed.
- S. Deng, D. Rhee, W. K. Lee, S. Che, B. Keisham, V. Berry and T. W. Odom, Nano Lett., 2019, 19, 5640–5646 CrossRef CAS PubMed.
- K. Drogowska-Horna, V. Vales, J. Plsek, M. Michlova, J. Vejpravova and M. Kalbac, Carbon, 2020, 164, 207–214 CrossRef CAS.
- R. H. Vervuurt, B. Karasulu, M. A. Verheijen, W. E. Kessels and A. A. Bol, Chem. Mater., 2017, 29, 2090–2100 CrossRef CAS PubMed.
- J. Wu, L. Xie, Y. Li, H. Wang, Y. Ouyang, J. Guo and H. Dai, J. Am. Chem. Soc., 2011, 133, 19668–19671 CrossRef CAS PubMed.
- K. Gopalakrishnan, K. S. Subrahmanyam, P. Kumar, A. Govindaraj and C. N. R. Rao, RSC Adv., 2012, 2, 1605–1608 RSC.
- B. Li, L. Zhou, D. Wu, H. Peng, K. Yan, Y. Zhou and Z. Liu, ACS Nano, 2011, 5, 5957–5961 CrossRef CAS PubMed.
- D. Bousa, J. Luxa, V. Mazanek, O. Jankovsky, D. Sedmidubsky, K. Klimova, M. Pumera and Z. Sofer, RSC Adv., 2016, 6, 66884–66892 RSC.
- W. Li, Y. Li and K. Xu, Nano Lett., 2021, 21, 1150–1155 CrossRef CAS PubMed.
- M. Zhao, X. Y. Guo, O. Ambacher, C. E. Nebel and R. Hoffmann, Carbon, 2015, 83, 128–135 CrossRef CAS.
- S. Ryu, M. Y. Han, J. Maultzsch, T. F. Heinz, P. Kim, M. L. Steigerwald and L. E. Brus, Nano Lett., 2008, 8, 4597–4602 CrossRef CAS PubMed.
- W. Chen, Z. Zhu, S. Li, C. Chen and L. Yan, Nanoscale, 2012, 4, 2124–2129 RSC.
- J. Zheng, H. T. Liu, B. Wu, C. A. Di, Y. L. Guo, T. Wu, G. Yu, Y. Q. Liu and D. B. Zhu, Sci. Rep., 2012, 2, 662 CrossRef PubMed.
- W. H. Lee, J. W. Suk, H. Chou, J. Lee, Y. Hao, Y. Wu, R. Piner, D. Akinwande, K. S. Kim and R. S. Ruoff, Nano Lett., 2012, 12, 2374–2378 CrossRef CAS PubMed.
- H. L. Poh, P. Simek, Z. Sofer and M. Pumera, Chemistry, 2013, 19, 2655–2662 CrossRef CAS PubMed.
- F. Withers, M. Dubois and A. K. Savchenko, Phys. Rev. B: Condens. Matter Mater. Phys., 2010, 82, 073403 CrossRef.
- K. Guérin, J. P. Pinheiro, M. Dubois, Z. Fawal, F. Masin, R. Yazami and A. Hamwi, Chem. Mater., 2004, 16, 1786–1792 CrossRef.
- S. H. Cheng, K. Zou, F. Okino, H. R. Gutierrez, A. Gupta, N. Shen, P. C. Eklund, J. O. Sofo and J. Zhu, Phys. Rev. B: Condens. Matter Mater. Phys., 2010, 81, 205435 CrossRef.
- J. Son, J. Kwon, S. Kim, Y. Lv, J. Yu, J. Y. Lee, H. Ryu, K. Watanabe, T. Taniguchi, R. Garrido-Menacho, N. Mason, E. Ertekin, P. Y. Huang, G. H. Lee and A. M. Van der Zande, Nat. Commun., 2018, 9, 3988 CrossRef PubMed.
- K. J. Jeon, Z. Lee, E. Pollak, L. Moreschini, A. Bostwick, C. M. Park, R. Mendelsberg, V. Radmilovic, R. Kostecki, T. J. Richardson and E. Rotenberg, ACS Nano, 2011, 5, 1042–1046 CrossRef CAS PubMed.
- J. T. Robinson, J. S. Burgess, C. E. Junkermeier, S. C. Badescu, T. L. Reinecke, F. K. Perkins, M. K. Zalalutdniov, J. W. Baldwin, J. C. Culbertson, P. E. Sheehan and E. S. Snow, Nano Lett., 2010, 10, 3001–3005 CrossRef CAS PubMed.
- R. R. Nair, W. Ren, R. Jalil, I. Riaz, V. G. Kravets, L. Britnell, P. Blake, F. Schedin, A. S. Mayorov, S. Yuan, M. I. Katsnelson, H. M. Cheng, W. Strupinski, L. G. Bulusheva, A. V. Okotrub, I. V. Grigorieva, A. N. Grigorenko, K. S. Novoselov and A. K. Geim, Small, 2010, 6, 2877–2884 CrossRef CAS PubMed.
- R. A. Schafer, J. M. Englert, P. Wehrfritz, W. Bauer, F. Hauke, T. Seyller and A. Hirsch, Angew. Chem., Int. Ed., 2013, 52, 754–757 CrossRef PubMed.
- A. Y. Eng, H. L. Poh, F. Sanek, M. Marysko, S. Matejkova, Z. Sofer and M. Pumera, ACS Nano, 2013, 7, 5930–5939 CrossRef CAS PubMed.
- Z. Yang, Y. Sun, L. B. Alemany, T. N. Narayanan and W. E. Billups, J. Am. Chem. Soc., 2012, 134, 18689–18694 CrossRef CAS PubMed.
- A. Y. Eng, Z. Sofer, S. Huber, D. Bousa, M. Marysko and M. Pumera, Chemistry, 2015, 21, 16828–16838 CrossRef CAS PubMed.
- K. E. Whitener, W. K. Lee, P. M. Campbell, J. T. Robinson and P. E. Sheehan, Carbon, 2014, 72, 348–353 CrossRef CAS.
- X. Y. Zhang, M. S. Han, S. Chen, L. Bao, L. Li and W. J. Xu, RSC Adv., 2013, 3, 17689–17692 RSC.
- B. Quiclet-Sire and S. Z. Zard, Chemistry, 2006, 12, 6002–6016 CrossRef CAS PubMed.
- F. Pennetreau, O. Riant and S. Hermans, Chemistry, 2014, 20, 15009–15012 CrossRef CAS PubMed.
- M. Sandomierski and A. Voelkel, J. Inorg. Organomet. Polym. Mater., 2021, 31, 1–21 CrossRef CAS.
- J. Greenwood, T. H. Phan, Y. Fujita, Z. Li, O. Ivasenko, W. Vanderlinden, H. Van Gorp, W. Frederickx, G. Lu, K. Tahara, Y. Tobe, I. H. Uji, S. F. Mertens and S. De Feyter, ACS Nano, 2015, 9, 5520–5535 CrossRef CAS PubMed.
- Z. Xia, F. Leonardi, M. Gobbi, Y. Liu, V. Bellani, A. Liscio, A. Kovtun, R. Li, X. Feng, E. Orgiu, P. Samori, E. Treossi and V. Palermo, ACS Nano, 2016, 10, 7125–7134 CrossRef CAS PubMed.
- B. D. Ossonon and D. Belanger, Carbon, 2017, 111, 83–93 CrossRef CAS.
- G. Ambrosio, A. Brown, L. Daukiya, G. Drera, G. Di Santo, L. Petaccia, S. De Feyter, L. Sangaletti and S. Pagliara, Nanoscale, 2020, 12, 9032–9037 RSC.
- Q. Wu, Y. Wu, Y. Hao, J. Geng, M. Charlton, S. Chen, Y. Ren, H. Ji, H. Li, D. W. Boukhvalov, R. D. Piner, C. W. Bielawski and R. S. Ruoff, Chem. Commun., 2013, 49, 677–679 RSC.
- E. Bekyarova, M. E. Itkis, P. Ramesh, C. Berger, M. Sprinkle, W. A. de Heer and R. C. Haddon, J. Am. Chem. Soc., 2009, 131, 1336–1337 CrossRef CAS PubMed.
- M. Z. Hossain, M. A. Walsh and M. C. Hersam, J. Am. Chem. Soc., 2010, 132, 15399–15403 CrossRef CAS PubMed.
- N. Kong, J. Park, X. Yang, O. Ramström and M. Yan, ACS Appl. Bio Mater., 2018, 2, 284–291 CrossRef PubMed.
- S. Bose and L. T. Drzal, Carbon Lett., 2015, 16, 101–106 CrossRef.
- C. Gómez-Anquela, M. Revenga-Parra, J. M. Abad, A. G. Marín, J. L. Pau, F. Pariente, J. Piqueras and E. Lorenzo, Electrochim. Acta, 2014, 116, 59–68 CrossRef.
- Y. Z. Lu, Y. Y. Jiang, W. T. Wei, H. B. Wu, M. M. Liu, L. Niu and W. Chen, J. Mater. Chem., 2012, 22, 2929–2934 RSC.
- K. F. Edelthalhammer, D. Dasler, L. Jurkiewicz, T. Nagel, S. Al-Fogra, F. Hauke and A. Hirsch, Angew. Chem., Int. Ed., 2020, 59, 23329–23334 CrossRef CAS PubMed.
- H. Liu, S. Ryu, Z. Chen, M. L. Steigerwald, C. Nuckolls and L. E. Brus, J. Am. Chem. Soc., 2009, 131, 17099–17101 CrossRef CAS PubMed.
- M. Castelain, G. Martinez, C. Marco, G. Ellis and H. J. Salavagione, Macromolecules, 2013, 46, 8980–8987 CrossRef CAS.
- Y. L. Li, L. Bao, Q. L. Zhou, E. C. Ou and W. J. Xu, ChemistrySelect, 2017, 2, 9284–9290 CrossRef CAS.
- N. D. Luong, H. Sinh le, L. S. Johansson, J. Campell and J. Seppala, Chemistry, 2015, 21, 3183–3186 CrossRef PubMed.
- M. Castelain, G. Martinez, G. Ellis and H. J. Salavagione, Chem. Commun., 2013, 49, 8967–8969 RSC.
- M. Liras, O. Garcia, I. Quijada-Garrido, G. Ellis and H. J. Salavagione, J. Mater. Chem. C, 2014, 2, 1723–1729 RSC.
- S. Sarkar, E. Bekyarova and R. C. Haddon, Angew. Chem., Int. Ed., 2012, 124, 4985–4988 CrossRef.
- X. Ma, F. Li, Y. Wang and A. Hu, Chem. – Asian J., 2012, 7, 2547–2550 CrossRef CAS PubMed.
- M. Z. Hossain, H. Yoko and M. A. Jusoh, Chem. Commun., 2016, 52, 14380–14383 RSC.
- M. Biswal, X. Zhang, D. Schilter, T. K. Lee, D. Y. Hwang, M. Saxena, S. H. Lee, S. Chen, S. K. Kwak, C. W. Bielawski, W. S. Bacsa and R. S. Ruoff, J. Am. Chem. Soc., 2017, 139, 4202–4210 CrossRef CAS PubMed.
- J. M. Englert, C. Dotzer, G. Yang, M. Schmid, C. Papp, J. M. Gottfried, H. P. Steinruck, E. Spiecker, F. Hauke and A. Hirsch, Nat. Chem., 2011, 3, 279–286 CrossRef CAS PubMed.
- K. C. Knirsch, J. M. Englert, C. Dotzer, F. Hauke and A. Hirsch, Chem. Commun., 2013, 49, 10811–10813 RSC.
- T. Morishita, A. J. Clancy and M. S. P. Shaffer, J. Mater. Chem. A, 2014, 2, 15022–15028 RSC.
- S. Sarkar, S. Niyogi, E. Bekyarova and R. C. Haddon, Chem. Sci., 2011, 2, 1326 RSC.
- S. Sarkar, H. Zhang, J. W. Huang, F. Wang, E. Bekyarova, C. N. Lau and R. C. Haddon, Adv. Mater., 2013, 25, 1131–1136 CrossRef CAS PubMed.
- S. M. Avdoshenko, I. N. Ioffe, G. Cuniberti, L. Dunsch and A. A. Popov, ACS Nano, 2011, 5, 9939–9949 CrossRef CAS PubMed.
- X. J. Tian, S. Sarkar, M. L. Moser, F. H. Wang, A. Pekker, E. Bekyarova, M. E. Itkis and R. C. Haddon, Mater. Lett., 2012, 80, 171–174 CrossRef CAS.
- M. G. Chen, A. Pekker, W. X. Li, M. E. Itkis, R. C. Haddon and E. Bekyarova, Carbon, 2018, 129, 450–455 CrossRef CAS.
- C. R. Ryder, J. D. Wood, S. A. Wells, Y. Yang, D. Jariwala, T. J. Marks, G. C. Schatz and M. C. Hersam, Nat. Chem., 2016, 8, 597–602 CrossRef CAS PubMed.
- M. van Druenen, F. Davitt, T. Collins, C. Glynn, C. O’Dwyer, J. D. Holmes and G. Collins, Chem. Mater., 2018, 30, 4667–4674 CrossRef CAS.
- S. Thurakkal and X. Zhang, Mater. Chem. Front., 2021, 5, 2824–2831 RSC.
- H. Hu, H. Gao, L. Gao, F. Li, N. Xu, X. Long, Y. Hu, J. Jin and J. Ma, Nanoscale, 2018, 10, 5834–5839 RSC.
- Y. Liu, P. Gao, T. Zhang, X. Zhu, M. Zhang, M. Chen, P. Du, G. W. Wang, H. Ji, J. Yang and S. Yang, Angew. Chem., Int. Ed., 2019, 58, 1479–1483 CrossRef CAS PubMed.
- V. Kumar, J. R. Brent, M. Shorie, H. Kaur, G. Chadha, A. G. Thomas, E. A. Lewis, A. P. Rooney, L. Nguyen, X. L. Zhong, M. G. Burke, S. J. Haigh, A. Walton, P. D. McNaughter, A. A. Tedstone, N. Savjani, C. A. Muryn, P. O'Brien, A. K. Ganguli, D. J. Lewis and P. Sabherwal, ACS Appl. Mater. Interfaces, 2016, 8, 22860–22868 CrossRef CAS PubMed.
- L. Zhou, C. Liu, Z. Sun, H. Mao, L. Zhang, X. Yu, J. Zhao and X. Chen, Biosens. Bioelectron., 2019, 137, 140–147 CrossRef CAS PubMed.
- M. Lee, Y. H. Park, E. B. Kang, A. Chae, Y. Choi, S. Jo, Y. J. Kim, S. J. Park, B. Min, T. K. An, J. Lee, S. I. In, S. Y. Kim, S. Y. Park and I. In, ACS Omega, 2017, 2, 7096–7105 CrossRef CAS PubMed.
- Y. Li, Z. Liu, Y. Hou, G. Yang, X. Fei, H. Zhao, Y. Guo, C. Su, Z. Wang, H. Zhong, Z. Zhuang and Z. Guo, ACS Appl. Mater. Interfaces, 2017, 9, 25098–25106 CrossRef CAS PubMed.
- C. Sun, L. Wen, J. Zeng, Y. Wang, Q. Sun, L. Deng, C. Zhao and Z. Li, Biomaterials, 2016, 91, 81–89 CrossRef CAS PubMed.
- Z. Sofer, J. Luxa, D. Bousa, D. Sedmidubsky, P. Lazar, T. Hartman, H. Hardtdegen and M. Pumera, Angew. Chem., Int. Ed., 2017, 56, 9891–9896 CrossRef CAS PubMed.
- L. Wu, J. Wang, J. Lu, D. Liu, N. Yang, H. Huang, P. K. Chu and X. F. Yu, Small, 2018, e1801405, DOI:10.1002/smll.201801405.
- Z. Sun, Y. Zhao, Z. Li, H. Cui, Y. Zhou, W. Li, W. Tao, H. Zhang, H. Wang, P. K. Chu and X. F. Yu, Small, 2017, 13, 28060458 Search PubMed.
- Y. Zhao, H. Wang, H. Huang, Q. Xiao, Y. Xu, Z. Guo, H. Xie, J. Shao, Z. Sun, W. Han, X. F. Yu, P. Li and P. K. Chu, Angew. Chem., Int. Ed., 2016, 55, 5003–5007 CrossRef CAS PubMed.
- M. T. Edmonds, A. Tadich, A. Carvalho, A. Ziletti, K. M. O'Donnell, S. P. Koenig, D. F. Coker, B. Ozyilmaz, A. H. Neto and M. S. Fuhrer, ACS Appl. Mater. Interfaces, 2015, 7, 14557–14562 CrossRef CAS PubMed.
- Z. Hu, Q. Li, B. Lei, Q. Zhou, D. Xiang, Z. Lyu, F. Hu, J. Wang, Y. Ren, R. Guo, E. Goki, L. Wang, C. Han, J. Wang and W. Chen, Angew. Chem., Int. Ed., 2017, 56, 9131–9135 CrossRef CAS PubMed.
- X. Zhu, T. Zhang, D. Jiang, H. Duan, Z. Sun, M. Zhang, H. Jin, R. Guan, Y. Liu, M. Chen, H. Ji, P. Du, W. Yan, S. Wei, Y. Lu and S. Yang, Nat. Commun., 2018, 9, 4177 CrossRef PubMed.
- S. Fan, W. Shen, J. Liu, H. Hei, R. Hu, C. Hu, D. Zhang, X. Hu, D. Sun, J. H. Chen, W. Ji and J. Liu, ACS Appl. Mater. Interfaces, 2018, 10, 39890–39897 CrossRef CAS PubMed.
- X. M. S. Chu, A. Yousaf, D. O. Li, A. L. A. Tang, A. Debnath, D. Ma, A. A. Green, E. J. G. Santos and Q. H. Wang, Chem. Mater., 2018, 30, 2112–2128 CrossRef CAS.
- K. C. Knirsch, N. C. Berner, H. C. Nerl, C. S. Cucinotta, Z. Gholamvand, N. McEvoy, Z. Wang, I. Abramovic, P. Vecera, M. Halik, S. Sanvito, G. S. Duesberg, V. Nicolosi, F. Hauke, A. Hirsch, J. N. Coleman and C. Backes, ACS Nano, 2015, 9, 6018–6030 CrossRef CAS PubMed.
- E. E. Benson, H. Zhang, S. A. Schuman, S. U. Nanayakkara, N. D. Bronstein, S. Ferrere, J. L. Blackburn and E. M. Miller, J. Am. Chem. Soc., 2018, 140, 441–450 CrossRef CAS PubMed.
- J. I. Paredes, J. M. Munuera, S. Villar-Rodil, L. Guardia, M. Ayan-Varela, A. Pagan, S. D. Aznar-Cervantes, J. L. Cenis, A. Martinez-Alonso and J. M. D. Tascon, ACS Appl. Mater. Interfaces, 2016, 8, 27974–27986 CrossRef CAS PubMed.
- S. Manjunatha, S. Rajesh, P. Vishnoi and C. N. R. Rao, J. Mater. Res., 2017, 32, 2984–2992 CrossRef CAS.
- P. Vishnoi, A. Sampath, U. V. Waghmare and C. N. Rao, Chemistry, 2017, 23, 886–895 CrossRef CAS PubMed.
- D. Voiry, A. Goswami, R. Kappera, C. e Silva Cde, D. Kaplan, T. Fujita, M. Chen, T. Asefa and M. Chhowalla, Nat. Chem., 2015, 7, 45–49 CrossRef CAS PubMed.
- M. Vera-Hidalgo, E. Giovanelli, C. Navio and E. M. Perez, J. Am. Chem. Soc., 2019, 141, 3767–3771 CrossRef CAS PubMed.
- T. Sainsbury, A. O'Neill, M. K. Passarelli, M. Seraffon, D. Gohil, S. Gnaniah, S. J. Spencer, A. Rae and J. N. Coleman, Chem. Mater., 2014, 26, 7039–7050 CrossRef CAS.
- T. Sainsbury, A. Satti, P. May, A. O'Neill, V. Nicolosi, Y. K. Gun'ko and J. N. Coleman, Chemistry, 2012, 18, 10808–10812 CrossRef CAS PubMed.
- Y. L. Hsieh, W. H. Su, C. C. Huang and C. Y. Su, ACS Appl. Mater. Interfaces, 2020, 12, 37375–37383 CrossRef CAS PubMed.
- S. Radhakrishnan, D. Das, A. Samanta, C. A. de Los Reyes, L. Deng, L. B. Alemany, T. K. Weldeghiorghis, V. N. Khabashesku, V. Kochat, Z. Jin, P. M. Sudeep, A. A. Marti, C. W. Chu, A. Roy, C. S. Tiwary, A. K. Singh and P. M. Ajayan, Sci. Adv., 2017, 3, e1700842 CrossRef PubMed.
- A. ul Ahmad, H. Liang, Q. Abbas, S. Ali, M. Iqbal, A. Farid, A. Abbas and Z. Farooq, Ceram. Int., 2019, 45, 19173–19181 CrossRef CAS.
- Y. Xue, Q. Liu, G. He, K. Xu, L. Jiang, X. Hu and J. Hu, Nanoscale Res. Lett., 2013, 8, 49 CrossRef PubMed.
- M. Du, X. Li, A. Wang, Y. Wu, X. Hao and M. Zhao, Angew. Chem., Int. Ed., 2014, 53, 3645–3649 CrossRef CAS PubMed.
- Y. Q. Bai, J. Zhang, Y. F. Wang, Z. Y. Cao, L. L. An, B. Zhang, Y. L. Yu, J. Y. Zhang and C. M. Wang, ACS Appl. Nano Mater., 2019, 2, 3187–3195 CrossRef CAS.
- J. Plutnar, J. Šturala, V. Mazánek, Z. Sofer and M. Pumera, Adv. Funct. Mater., 2018, 28, 1801438 CrossRef.
- X. Tang, H. Chen, J. S. Ponraj, S. C. Dhanabalan, Q. Xiao, D. Fan and H. Zhang, Adv. Sci., 2018, 5, 1800420 CrossRef PubMed.
- S. Entani, K. V. Larionov, Z. I. Popov, M. Takizawa, M. Mizuguchi, H. Watanabe, S. Li, H. Naramoto, P. B. Sorokin and S. Sakai, Nanotechnology, 2020, 31, 125705 CrossRef CAS PubMed.
- H. Li, A. W. Contryman, X. Qian, S. M. Ardakani, Y. Gong, X. Wang, J. M. Weisse, C. H. Lee, J. Zhao, P. M. Ajayan, J. Li, H. C. Manoharan and X. Zheng, Nat. Commun., 2015, 6, 7381 CrossRef CAS PubMed.
- D. W. Boukhvalov and M. I. Katsnelson, J. Phys. Chem. C, 2009, 113, 14176–14178 CrossRef CAS.
- X. Gao, Y. Wang, X. Liu, T. L. Chan, S. Irle, Y. Zhao and S. B. Zhang, Phys. Chem. Chem. Phys., 2011, 13, 19449–19453 RSC.
- H. Yoo, R. Engelke, S. Carr, S. Fang, K. Zhang, P. Cazeaux, S. H. Sung, R. Hovden, A. W. Tsen, T. Taniguchi, K. Watanabe, G. C. Yi, M. Kim, M. Luskin, E. B. Tadmor, E. Kaxiras and P. Kim, Nat. Mater., 2019, 18, 448–453 CrossRef CAS PubMed.
- D. Halbertal, N. R. Finney, S. S. Sunku, A. Kerelsky, C. Rubio-Verdu, S. Shabani, L. Xian, S. Carr, S. Chen, C. Zhang, L. Wang, D. Gonzalez-Acevedo, A. S. McLeod, D. Rhodes, K. Watanabe, T. Taniguchi, E. Kaxiras, C. R. Dean, J. C. Hone, A. N. Pasupathy, D. M. Kennes, A. Rubio and D. N. Basov, Nat. Commun., 2021, 12, 242 CrossRef CAS PubMed.
- J. S. Alden, A. W. Tsen, P. Y. Huang, R. Hovden, L. Brown, J. Park, D. A. Muller and P. L. McEuen, Proc. Natl. Acad. Sci. U. S. A., 2013, 110, 11256–11260 CrossRef CAS PubMed.
- F. He, Y. Zhou, Z. Ye, S. H. Cho, J. Jeong, X. Meng and Y. Wang, ACS Nano, 2021, 15, 5944–5958 CrossRef CAS PubMed.
- A. Kumar, K. Banerjee, M. Dvorak, F. Schulz, A. Harju, P. Rinke and P. Liljeroth, ACS Nano, 2017, 11, 4960–4968 CrossRef CAS PubMed.
- J. J. Navarro, S. Leret, F. Calleja, D. Stradi, A. Black, R. Bernardo-Gavito, M. Garnica, D. Granados, A. L. Vazquez de Parga, E. M. Perez and R. Miranda, Nano Lett., 2016, 16, 355–361 CrossRef CAS PubMed.
- J. J. Navarro, F. Calleja, R. Miranda, E. M. Perez and A. L. Vazquez de Parga, Chem. Commun., 2017, 53, 10418–10421 RSC.
- R. Yuge, M. Zhang, M. Tomonari, T. Yoshitake, S. Iijima and M. Yudasaka, ACS Nano, 2008, 2, 1865–1870 CrossRef CAS PubMed.
- X. Z. Tang, W. J. Li, Z. Z. Yu, M. A. Rafiee, J. Rafiee, F. Yavari and N. Koratkar, Carbon, 2011, 49, 1258–1265 CrossRef CAS.
- R. Rajesh, S. S. Kumar and R. Venkatesan, New J. Chem., 2014, 38, 1551–1558 RSC.
- P. Kumar, G. Singh, D. Tripathi and S. L. Jain, RSC Adv., 2014, 4, 50331–50337 RSC.
- C. B. Wang, J. W. Zhou and L. L. Chu, RSC Adv., 2015, 5, 52466–52472 RSC.
- D. Yu, Y. Yang, M. Durstock, J. B. Baek and L. Dai, ACS Nano, 2010, 4, 5633–5640 CrossRef CAS PubMed.
- S. T. Sun, Y. W. Cao, J. C. Feng and P. Y. Wu, J. Mater. Chem., 2010, 20, 5605–5607 RSC.
- Y. Cao, Z. Lai, J. Feng and P. Wu, J. Mater. Chem., 2011, 21, 9271 RSC.
- R. Sekiya, Y. Uemura, H. Murakami and T. Haino, Angew. Chem., Int. Ed., 2014, 53, 5619–5623 CrossRef CAS PubMed.
- K. Zelechowska, M. Przesniak-Welenc, M. Lapinski, I. Kondratowicz and T. Miruszewski, Beilstein J. Nanotechnol., 2017, 8, 1094–1103 CrossRef CAS PubMed.
- Y. F. Xu, Z. B. Liu, X. L. Zhang, Y. Wang, J. G. Tian, Y. Huang, Y. F. Ma, X. Y. Zhang and Y. S. Chen, Adv. Mater, 2009, 21, 1275–1279 CrossRef CAS.
- F. D. Liu, L. Wu, Y. J. Song, W. G. Xia and K. K. Guo, RSC Adv., 2015, 5, 45987–45995 RSC.
- A. Wolk, M. Rosenthal, S. Neuhaus, K. Huber, K. Brassat, J. K. N. Lindner, R. Grothe, G. Grundmeier, W. Bremser and R. Wilhelm, Sci. Rep., 2018, 8, 5843 CrossRef PubMed.
- W. B. Zhang, J. Z. Ma, D. G. Gao, Y. X. Zhou, C. M. Li, J. Zha and J. Zhang, Prog. Org. Coat., 2016, 94, 9–17 CrossRef CAS.
- R. Sekiya, Y. Uemura, H. Naito, K. Naka and T. Haino, Chemistry, 2016, 22, 8198–8206 CrossRef CAS PubMed.
- X. Zhang, Y. Huang, Y. Wang, Y. Ma, Z. Liu and Y. Chen, Carbon, 2009, 47, 334–337 CrossRef CAS.
- G. Q. Xu, P. W. Xu, D. J. Shi and M. Q. Chen, RSC Adv., 2014, 4, 28807–28813 RSC.
- L. M. Veca, F. Lu, M. J. Meziani, L. Cao, P. Zhang, G. Qi, L. Qu, M. Shrestha and Y. P. Sun, Chem. Commun., 2009, 2565–2567, 10.1039/b900590k.
- M. Cano, U. Khan, T. Sainsbury, A. O'Neill, Z. M. Wang, I. T. McGovern, W. K. Maser, A. M. Benito and J. N. Coleman, Carbon, 2013, 52, 363–371 CrossRef CAS.
- G. Zhao, F. G. Zhao, J. Q. Sun, W. Wang, Y. Lu, W. S. Li and Q. Y. Chen, Carbon, 2015, 94, 114–119 CrossRef CAS.
- Z. Seibers, M. Orr, G. S. Collier, A. Henriquez, M. Gabel, M. L. Shofner, V. La Saponara and J. Reynolds, Polym. Eng. Sci., 2020, 60, 86–94 CrossRef CAS.
- Y. Cao, P. Wang, J. Fan and H. Yu, Ceram. Int., 2021, 47, 654–661 CrossRef CAS.
- Y. L. Zu, C. Y. He, D. M. Liu and L. Chen, Dyes Pigm., 2020, 173, 107841 CrossRef CAS.
- M. Quintana, A. Montellano, A. E. del Rio Castillo, G. Van Tendeloo, C. Bittencourt and M. Prato, Chem. Commun., 2011, 47, 9330–9332 RSC.
- L. Kou, H. K. He and C. Gao, Nano-Micro Lett., 2010, 2, 177–183 CrossRef CAS.
- H. Bao, Y. Pan, Y. Ping, N. G. Sahoo, T. Wu, L. Li, J. Li and L. H. Gan, Small, 2011, 7, 1569–1578 CrossRef CAS PubMed.
- J. T. Robinson, S. M. Tabakman, Y. Liang, H. Wang, H. S. Casalongue, D. Vinh and H. Dai, J. Am. Chem. Soc., 2011, 133, 6825–6831 CrossRef CAS PubMed.
- M. Park, K. Song, T. Lee, J. Cha, I. Lyo and B. S. Kim, ACS Appl. Mater. Interfaces, 2016, 8, 21595–21602 CrossRef CAS PubMed.
- M. Park, T. Lee and B. S. Kim, Nanoscale, 2013, 5, 12255–12260 RSC.
- H. Hwang, P. Joo, M. S. Kang, G. Ahn, J. T. Han, B. S. Kim and J. H. Cho, ACS Nano, 2012, 6, 2432–2440 CrossRef CAS PubMed.
- B. Park, W. Lee, E. Lee, S. H. Min and B. S. Kim, ACS Appl. Mater. Interfaces, 2015, 7, 3329–3334 CrossRef CAS PubMed.
- S. Stankovich, R. D. Piner, S. T. Nguyen and R. S. Ruoff, Carbon, 2006, 44, 3342–3347 CrossRef CAS.
- M. B. Avinash, K. S. Subrahmanyam, Y. Sundarayya and T. Govindaraju, Nanoscale, 2010, 2, 1762–1766 RSC.
- O. Jankovsky, P. Simek, J. Luxa, D. Sedmidubsky, I. Tomandl, A. Mackova, R. Miksova, P. Malinsky, M. Pumera and Z. Sofer, ChemPlusChem, 2015, 80, 1399–1407 CrossRef CAS PubMed.
- O. Jankovsky, M. Lojka, J. Luxa, D. Sedmidubsky, O. Tomanec, R. Zboril, M. Pumera and Z. Sofer, Chemistry, 2017, 23, 10473–10479 CrossRef CAS PubMed.
- R. Xing, Y. Li and H. Yu, Chem. Commun., 2016, 52, 390–393 RSC.
- Y. Z. Tan, B. Yang, K. Parvez, A. Narita, S. Osella, D. Beljonne, X. Feng and K. Mullen, Nat. Commun., 2013, 4, 2646 CrossRef PubMed.
- S. Q. Lai, Y. Jin, X. P. Sun, J. Z. Pan, W. N. Du and L. J. Shi, Res. Chem. Intermed., 2018, 44, 3523–3536 CrossRef CAS.
- C. K. Chua and M. Pumera, Chem. – Asian J., 2012, 7, 1009–1012 CrossRef CAS PubMed.
- C. K. Chua, Z. Sofer and M. Pumera, Angew. Chem., Int. Ed., 2016, 55, 10751–10754 CrossRef CAS PubMed.
- V. Urbanova, K. Hola, A. B. Bourlinos, K. Cepe, A. Ambrosi, A. H. Loo, M. Pumera, F. Karlicky, M. Otyepka and R. Zboril, Adv. Mater., 2015, 27, 2305–2310 CrossRef CAS PubMed.
- K. E. Whitener, R. Stine, J. T. Robinson and P. E. Sheehan, J. Phys. Chem. C, 2015, 119, 10507–10512 CrossRef CAS.
- C. Bosch-Navarro, M. Walker, N. R. Wilson and J. P. Rourke, J. Mater. Chem. C, 2015, 3, 7627–7631 RSC.
- X. Ye, L. Ma, Z. Yang, J. Wang, H. Wang and S. Yang, ACS Appl. Mater. Interfaces, 2016, 8, 7483–7488 CrossRef CAS PubMed.
- R. Stine, J. W. Ciszek, D. E. Barlow, W. K. Lee, J. T. Robinson and P. E. Sheehan, Langmuir, 2012, 28, 7957–7961 CrossRef CAS PubMed.
- B. Li, T. He, Z. Wang, Z. Cheng, Y. Liu, T. Chen, W. Lai, X. Wang and X. Liu, Phys. Chem. Chem. Phys., 2016, 18, 17495–17505 RSC.
- J. Sturala, J. Luxa, J. Plutnar, Z. Janousek, Z. Sofer and M. Pumera, 2D Mater., 2019, 6, 034006 CrossRef CAS.
- A. Bakandritsos, M. Pykal, P. Blonski, P. Jakubec, D. D. Chronopoulos, K. Polakova, V. Georgakilas, K. Cepe, O. Tomanec, V. Ranc, A. B. Bourlinos, R. Zboril and M. Otyepka, ACS Nano, 2017, 11, 2982–2991 CrossRef CAS PubMed.
- P. Gong, J. Wang, W. Sun, D. Wu, Z. Wang, Z. Fan, H. Wang, X. Han and S. Yang, Nanoscale, 2014, 6, 3316–3324 RSC.
- J. Tucek, K. Hola, A. B. Bourlinos, P. Blonski, A. Bakandritsos, J. Ugolotti, M. Dubecky, F. Karlicky, V. Ranc, K. Cepe, M. Otyepka and R. Zboril, Nat. Commun., 2017, 8, 14525 CrossRef CAS PubMed.
- D. D. Chronopoulos, M. Medved, P. Blonski, Z. Novacek, P. Jakubec, O. Tomanec, A. Bakandritsos, V. Novotna, R. Zboril and M. Otyepka, Chem. Commun., 2019, 55, 1088–1091 RSC.
- V. Mazanek, A. Libanska, J. Sturala, D. Bousa, D. Sedmidubsky, M. Pumera, Z. Janousek, J. Plutnar and Z. Sofer, Chemistry, 2017, 23, 1956–1964 CrossRef CAS PubMed.
- D. D. Chronopoulos, A. Bakandritsos, P. Lazar, M. Pykal, K. Cepe, R. Zboril and M. Otyepka, Chem. Mater., 2017, 29, 926–930 CrossRef CAS PubMed.
- X. Z. Sun, B. Li and M. X. Lu, J. Solid State Chem., 2017, 251, 194–197 CrossRef CAS.
- M. Z. Hossain, M. B. A. Razak, H. Noritake, Y. Shiozawa, S. Yoshimoto, K. Mukai, T. Koitaya, J. Yoshinobu and S. Hosaka, J. Phys. Chem. C, 2014, 118, 22096–22101 CrossRef CAS.
- Y. J. Yao, J. Gao, F. Bao, S. F. Jiang, X. Zhang and R. Ma, RSC Adv., 2015, 5, 42754–42761 RSC.
- J. Gao, F. Bao, Q. D. Zhu, Z. F. Tan, T. Chen, H. H. Cai, C. Zhao, Q. X. Cheng, Y. D. Yang and R. Ma, Polym. Chem., 2013, 4, 1672–1679 RSC.
- H. Au, N. Rubio and M. S. P. Shaffer, Chem. Sci., 2018, 9, 209–217 RSC.
- S. H. Lee, D. R. Dreyer, J. An, A. Velamakanni, R. D. Piner, S. Park, Y. Zhu, S. O. Kim, C. W. Bielawski and R. S. Ruoff, Macromol. Rapid Commun., 2010, 31, 281–288 CrossRef CAS PubMed.
- X. Yang, L. Ma, S. Wang, Y. Li, Y. Tu and X. Zhu, Polymer, 2011, 52, 3046–3052 CrossRef CAS.
- N. N. Chai, J. Zeng, K. G. Zhou, Y. L. Xie, H. X. Wang, H. L. Zhang, C. Xu, J. X. Zhu and Q. Y. Yan, Chemistry, 2013, 19, 5948–5954 CrossRef CAS PubMed.
- C. S. Shan, L. N. Wang, D. X. Han, F. H. Li, Q. X. Zhang, X. D. Zhang and L. Niu, Thin Solid Films, 2013, 534, 572–576 CrossRef CAS.
- I. A. Vacchi, C. Spinato, J. Raya, A. Bianco and C. Menard-Moyon, Nanoscale, 2016, 8, 13714–13721 RSC.
- Y. Y. Wang, X. R. Cui, Y. J. Wang, W. J. Shan, Z. N. Lou and Y. Xiong, New J. Chem., 2020, 44, 16285–16293 RSC.
- G. Du, L. Nie, G. Gao, Y. Sun, R. Hou, H. Zhang, T. Chen and J. Fu, ACS Appl. Mater. Interfaces, 2015, 7, 3003–3008 CrossRef CAS PubMed.
- H. R. Thomas, A. J. Marsden, M. Walker, N. R. Wilson and J. P. Rourke, Angew. Chem., Int. Ed., 2014, 53, 7613–7618 CrossRef CAS PubMed.
- Y. Liu, R. Deng, Z. Wang and H. Liu, J. Mater. Chem., 2012, 22, 13619 RSC.
- J. Chen, W. Zhang, Y. Sun, Y. Zheng, N. Tang and Y. Du, Sci. Rep., 2016, 6, 26862 CrossRef CAS PubMed.
- Z. Liu, S. Zhu, Y. Li, Y. Li, P. Shi, Z. Huang and X. Huang, Polym. Chem., 2015, 6, 311–321 RSC.
- T. Taniguchi, S. Kurihara, H. Tateishi, K. Hatakeyama, M. Koinuma, H. Yokoi, M. Hara, H. Ishikawa and Y. Matsumoto, Carbon, 2015, 84, 560–566 CrossRef CAS.
- C. E. Halbig, P. Rietsch and S. Eigler, Molecules, 2015, 20, 21050–21057 CrossRef CAS PubMed.
- S. Eigler, Y. Hu, Y. Ishii and A. Hirsch, Nanoscale, 2013, 5, 12136–12139 RSC.
- R. Salvio, S. Krabbenborg, W. J. Naber, A. H. Velders, D. N. Reinhoudt and W. G. van der Wiel, Chemistry, 2009, 15, 8235–8240 CrossRef CAS PubMed.
- W. Li, Y. Li and K. Xu, Nano Lett., 2020, 20, 534–539 CrossRef CAS PubMed.
- E. Husamelden and H. Fan, J. Polym. Res., 2019, 26, 42 CrossRef.
- F. B. Meng, H. Ishida and X. B. Liu, RSC Adv., 2014, 4, 9471–9475 RSC.
- B. T. McGrail, B. J. Rodier and E. Pentzer, Chem. Mater., 2014, 26, 5806–5811 CrossRef CAS.
- S. F. Hou, M. L. Kasner, S. J. Su, K. Patel and R. Cuellari, J. Phys. Chem. C, 2010, 114, 14915–14921 CrossRef CAS.
- H. L. Su, Z. F. Li, Q. S. Huo, J. Q. Guan and Q. B. Kan, RSC Adv., 2014, 4, 9990–9996 RSC.
- S. Verma, M. Aila, S. Kaul and S. L. Jain, RSC Adv., 2014, 4, 30598–30604 RSC.
- X. W. Ou, L. Jiang, P. L. Chen, M. S. Zhu, W. P. Hu, M. H. Liu, J. F. Zhu and H. X. Ju, Adv. Funct. Mater., 2013, 23, 2422–2435 CrossRef CAS.
- H. Q. Yao, L. Jin, H. J. Sue, Y. Sumi and R. Nishimura, J. Mater. Chem. A, 2013, 1, 10783–10789 RSC.
- J. F. Ou, Y. Wang, J. Q. Wang, S. Liu, Z. P. Li and S. R. Yang, J. Phys. Chem. C, 2011, 115, 10080–10086 CrossRef CAS.
- W. R. Collins, W. Lewandowski, E. Schmois, J. Walish and T. M. Swager, Angew. Chem., Int. Ed., 2011, 50, 8848–8852 CrossRef CAS PubMed.
- S. A. Sydlik and T. M. Swager, Adv. Funct. Mater., 2013, 23, 1873–1882 CrossRef CAS.
- A. Y. Eng, Z. Sofer, D. Sedmidubsky and M. Pumera, ACS Nano, 2017, 11, 1789–1797 CrossRef CAS PubMed.
- C. K. Chua and M. Pumera, ACS Nano, 2015, 9, 4193–4199 CrossRef CAS PubMed.
- C. K. Chua, Z. Sofer, J. Luxa and M. Pumera, Chemistry, 2015, 21, 8090–8095 CrossRef CAS PubMed.
- K. Wu, C. X. Lei, W. X. Yang, S. G. Chai, F. Chen and Q. Fu, Compos. Sci. Technol., 2016, 134, 191–200 CrossRef CAS.
- C. V. S. Rosely, P. Shaiju and E. B. Gowd, J. Phys. Chem. B, 2019, 123, 8599–8609 CrossRef CAS PubMed.
- C. K. Chua and M. Pumera, Chem. Commun., 2016, 52, 72–75 RSC.
- H. Yan, H. Cheng, H. Yi, Y. Lin, T. Yao, C. Wang, J. Li, S. Wei and J. Lu, J. Am. Chem. Soc., 2015, 137, 10484–10487 CrossRef CAS PubMed.
- H. Yan, X. Zhao, N. Guo, Z. Lyu, Y. Du, S. Xi, R. Guo, C. Chen, Z. Chen, W. Liu, C. Yao, J. Li, S. J. Pennycook, W. Chen, C. Su, C. Zhang and J. Lu, Nat. Commun., 2018, 9, 3197 CrossRef PubMed.
- S. H. Sun, G. X. Zhang, N. Gauquelin, N. Chen, J. G. Zhou, S. L. Yang, W. F. Chen, X. B. Meng, D. S. Geng, M. N. Banis, R. Y. Li, S. Y. Ye, S. Knights, G. A. Botton, T. K. Sham and X. L. Sun, Sci. Rep, 2013, 3, 1775 CrossRef.
- H. Yan, Y. Lin, H. Wu, W. Zhang, Z. Sun, H. Cheng, W. Liu, C. Wang, J. Li, X. Huang, T. Yao, J. Yang, S. Wei and J. Lu, Nat. Commun., 2017, 8, 1070 CrossRef PubMed.
- I. Y. Jeon, D. S. Yu, S. Y. Bae, H. J. Choi, D. W. Chang, L. M. Dai and J. B. Baek, Chem. Mater., 2011, 23, 3987–3992 CrossRef CAS.
- C. Tan and H. Zhang, Nat. Commun., 2015, 6, 7873 CrossRef CAS PubMed.
- F. Wang, Z. Wang, T. A. Shifa, Y. Wen, F. Wang, X. Zhan, Q. Wang, K. Xu, Y. Huang, L. Yin, C. Jiang and J. He, Adv. Funct. Mater., 2017, 27, 1603254 CrossRef.
- N. Zhou, R. Yang and T. Zhai, Mater. Today Nano, 2019, 8, 100051 CrossRef.
- Y. Dou, L. Zhang, X. Xu, Z. Sun, T. Liao and S. X. Dou, Chem. Soc. Rev., 2017, 46, 7338–7373 RSC.
- Y. Wang, Z. Zhang, Y. Mao and X. Wang, Energy Environ. Sci., 2020, 13, 3993–4016 RSC.
- H. Wang, J. Chen, Y. Lin, X. Wang, J. Li, Y. Li, L. Gao, L. Zhang, D. Chao, X. Xiao and J. M. Lee, Adv. Mater., 2021, 33, e2008422 CrossRef PubMed.
- C. Jin and L. Kou, J. Phys. D: Appl. Phys., 2021, 54, 413001 CrossRef CAS.
- R. Sakamoto, K. Takada, T. Pal, H. Maeda, T. Kambe and H. Nishihara, Chem. Commun., 2017, 53, 5781–5801 RSC.
- D. J. Ashworth and J. A. Foster, J. Mater. Chem. A, 2018, 6, 16292–16307 RSC.
- M. Zhao, Y. Huang, Y. Peng, Z. Huang, Q. Ma and H. Zhang, Chem. Soc. Rev., 2018, 47, 6267–6295 RSC.
- J. Duan, Y. Li, Y. Pan, N. Behera and W. Jin, Coord. Chem., 2019, 395, 25–45 CrossRef CAS.
- G. Chakraborty, I. H. Park, R. Medishetty and J. J. Vittal, Chem. Rev., 2021, 121, 3751–3891 CrossRef CAS PubMed.
- J. Nicks, K. Sasitharan, R. R. R. Prasad, D. J. Ashworth and J. A. Foster, Adv. Funct. Mater., 2021, 31, 2103723 CrossRef CAS.
- Y. Xue, G. Zhao, R. Yang, F. Chu, J. Chen, L. Wang and X. Huang, Nanoscale, 2021, 13, 3911–3936 RSC.
- M. V. Varsha and G. Nageswaran, J. Electrochem. Soc, 2020, 167, 136502 CrossRef CAS.
- D. Zhu, M. Qiao, J. Liu, T. Tao and C. Guo, J. Mater. Chem. A, 2020, 8, 8143–8170 RSC.
- M. A. Solomos, F. J. Claire and T. J. Kempa, J. Mater. Chem. A, 2019, 7, 23537–23562 RSC.
- J. A. Foster, S. Henke, A. Schneemann, R. A. Fischer and A. K. Cheetham, Chem. Commun., 2016, 52, 10474–10477 RSC.
- Z. J. Han, A. T. Murdock, D. H. Seo and A. Bendavid, 2D Mater., 2018, 5, 032002 CrossRef.
- H. Nan, R. Zhou, X. Gu, S. Xiao and K. Ken Ostrikov, Nanoscale, 2019, 11, 19202–19213 RSC.
- Z. Hu, Z. Wu, C. Han, J. He, Z. Ni and W. Chen, Chem. Soc. Rev., 2018, 47, 3100–3128 RSC.
- S. Kang, Y. S. Kim, J. H. Jeong, J. Kwon, J. H. Kim, Y. Jung, J. C. Kim, B. Kim, S. H. Bae, P. Y. Huang, J. C. Hone, H. Y. Jeong, J. W. Park, C. H. Lee and G. H. Lee, ACS Appl. Mater. Interfaces, 2021, 13, 1245–1252 CrossRef CAS PubMed.
- N. McEvoy, H. Nolan, N. A. Kumar, T. Hallam and G. S. Duesberg, Carbon, 2013, 54, 283–290 CrossRef CAS.
- D. C. Kim, D. Y. Jeon, H. J. Chung, Y. Woo, J. K. Shin and S. Seo, Nanotechnology, 2009, 20, 375703 CrossRef PubMed.
- C. H. Huang, C. Y. Su, C. S. Lai, Y. C. Li and S. Samukawa, Carbon, 2014, 73, 244–251 CrossRef CAS.
- A. Felten, A. Eckmann, J. J. Pireaux, R. Krupke and C. Casiraghi, Nanotechnology, 2013, 24, 355705 CrossRef CAS PubMed.
- T. Gokus, R. R. Nair, A. Bonetti, M. Bohmler, A. Lombardo, K. S. Novoselov, A. K. Geim, A. C. Ferrari and A. Hartschuh, ACS Nano, 2009, 3, 3963–3968 CrossRef CAS PubMed.
- T. Lim and S. Ju, Surf. Coat. Technol., 2017, 328, 89–93 CrossRef CAS.
- C. Shen, G. Huang, Y. Cheng, R. Cao, F. Ding, U. Schwingenschlogl and Y. Mei, Nanoscale Res. Lett., 2012, 7, 268 CrossRef PubMed.
- F. Xiao, S. Naficy, G. Casillas, M. H. Khan, T. Katkus, L. Jiang, H. Liu, H. Li and Z. Huang, Adv. Mater., 2015, 27, 7196–7203 CrossRef CAS PubMed.
- J. H. Jung, M. Kotal, M. H. Jang, J. Lee, Y. H. Cho, W. J. Kim and I. K. Oh, RSC Adv., 2016, 6, 73939–73946 RSC.
- U. Alli, S. J. Hettiarachchi and S. Kellici, Chemistry, 2020, 26, 6447–6460 CrossRef CAS PubMed.
- J. Xu, Y. Li, M. Q. Qian, J. Pan, J. Ding and B. H. Guan, Appl. Catal., B, 2019, 256, 117797 CrossRef CAS.
- S. Z. Huang, Y. Li, Y. Y. Feng, H. R. An, P. Long, C. Q. Qin and W. Feng, J. Mater. Chem. A, 2015, 3, 23095–23105 RSC.
- O. Jankovsky, P. Simek, D. Sedmidubsky, S. Matejkova, Z. Janousek, F. Sembera, M. Pumera and Z. Sofer, RSC Adv., 2014, 4, 1378–1387 RSC.
- O. Jankovsky, P. Simek, K. Klimova, D. Sedmidubsky, S. Matejkova, M. Pumera and Z. Sofer, Nanoscale, 2014, 6, 6065–6074 RSC.
- Z. H. Cheng, B. Z. He and L. Zhou, J. Mater. Chem. A, 2015, 3, 1042–1048 RSC.
- A. Vadivelmurugan, R. Anbazhagan and H. C. Tsai, Mater. Lett., 2018, 218, 285–289 CrossRef CAS.
- B. H. Xie, X. Huang and G. J. Zhang, Compos. Sci. Technol., 2013, 85, 98–103 CrossRef CAS.
- M. L. Liu, Y. H. Xu, Y. Wang, X. Chen, X. Q. Ji, F. S. Niu, Z. Q. Song and J. Q. Liu, Adv. Opt. Mater., 2017, 5, 1600661 CrossRef.
- L. Y. Wu, K. Wu, D. Y. Liu, R. Huang, J. L. Huo, F. Chen and Q. Fu, J. Mater. Chem. A, 2018, 6, 7573–7584 RSC.
- L. Fu, T. Wang, J. H. Yu, W. Dai, H. Y. Sun, Z. D. Liu, R. Sun, N. Jiang, A. M. Yu and C. T. Lin, 2D Mater., 2017, 4, 025047 CrossRef.
- Y. Zhang, W. P. Sun, Z. Z. Luo, Y. Zheng, Z. W. Yu, D. Zhang, J. Yang, H. T. Tan, J. X. Zhu, X. L. Wang, Q. Y. Yan and S. X. Dou, Nano Energy, 2017, 40, 576–586 CrossRef CAS.
- K. H. Ibrahim, M. Irannejad, B. Wales, J. Sanderson, M. Yavuz and K. P. Musselman, Adv. Opt. Mater., 2018, 6, 1701365 CrossRef.
- S. Huh, J. Park, Y. S. Kim, K. S. Kim, B. H. Hong and J. M. Nam, ACS Nano, 2011, 5, 9799–9806 CrossRef CAS PubMed.
- A. Azcatl, S. McDonnell, K. C. Santosh, X. Peng, H. Dong, X. Y. Qin, R. Addou, G. I. Mordi, N. Lu, J. Kim, M. J. Kim, K. Cho and R. M. Wallace, Appl. Phys. Lett., 2014, 104, 111601 CrossRef.
- A. Azcatl, S. Kc, X. Peng, N. Lu, S. McDonnell, X. Qin, F. de Dios, R. Addou, J. Kim, M. J. Kim, K. Cho and R. M. Wallace, 2D Mater., 2015, 2, 014004 CrossRef.
- M. Yi and Z. G. Shen, J. Mater. Chem. A, 2015, 3, 11700–11715 RSC.
- J. H. Han, M. Kwak, Y. Kim and J. Cheon, Chem. Rev., 2018, 118, 6151–6188 CrossRef CAS PubMed.
- J. S. Y. Chia, M. T. T. Tan, P. S. Khiew, J. K. Chin, H. Lee, D. C. S. Bien and C. W. Siong, Chem. Eng. J., 2014, 249, 270–278 CrossRef CAS.
- J. Xia, J. Wang, D. Chao, Z. Chen, Z. Liu, J. L. Kuo, J. Yan and Z. X. Shen, Nanoscale, 2017, 9, 7533–7540 RSC.
- G. Eda, H. Yamaguchi, D. Voiry, T. Fujita, M. Chen and M. Chhowalla, Nano Lett., 2011, 11, 5111–5116 CrossRef CAS PubMed.
- L. Zhang, L. F. Gao, L. X. Li, C. X. Hu, Q. Q. Yang, Z. Y. Zhu, R. Peng, Q. Wang, Y. Peng, J. Jin and H. L. Zhang, Mater. Chem. Front., 2018, 2, 1700–1706 RSC.
- X. L. Fan, D. W. Chang, X. L. Chen, J. B. Baek and L. M. Dai, Curr. Opin. Chem. Eng., 2016, 11, 52–58 CrossRef.
- L. Burk, M. Gliem and R. Mülhaupt, Macromol. Mater. Eng., 2018, 304, 1800496 CrossRef.
- J. Xu, J. Shui, J. Wang, M. Wang, H. K. Liu, S. X. Dou, I. Y. Jeon, J. M. Seo, J. B. Baek and L. Dai, ACS Nano, 2014, 8, 10920–10930 CrossRef CAS PubMed.
- I. Y. Jeon, S. Zhang, L. Zhang, H. J. Choi, J. M. Seo, Z. Xia, L. Dai and J. B. Baek, Adv. Mater., 2013, 25, 6138–6145 CrossRef CAS PubMed.
- I. Y. Jeon, S. Y. Bae, J. M. Seo and J. B. Baek, Adv. Funct. Mater., 2015, 25, 6961–6975 CrossRef CAS.
- X. Zhu, T. Zhang, Z. Sun, H. Chen, J. Guan, X. Chen, H. Ji, P. Du and S. Yang, Adv. Mater., 2017, 29, 1605776 CrossRef PubMed.
- L. Yan, M. M. Lin, C. Zeng, Z. Chen, S. Zhang, X. M. Zhao, A. G. Wu, Y. P. Wang, L. M. Dai, J. Qu, M. M. Guo and Y. Liu, J. Mater. Chem., 2012, 22, 8367–8371 RSC.
- I. Y. Jeon, Y. R. Shin, G. J. Sohn, H. J. Choi, S. Y. Bae, J. Mahmood, S. M. Jung, J. M. Seo, M. J. Kim, D. Wook Chang, L. Dai and J. B. Baek, Proc. Natl. Acad. Sci. U. S. A., 2012, 109, 5588–5593 CrossRef CAS PubMed.
- O. S. Kwon, D. Lee, S. P. Lee, Y. G. Kang, N. C. Kim and S. H. Song, RSC Adv., 2016, 6, 59970–59975 RSC.
- D. Lee, S. Lee, S. Byun, K. W. Paik and S. H. Song, Composites, Part A, 2018, 107, 217–223 CrossRef CAS.
- B. P. Liu, S. H. Yan, A. T. Zhang, Z. Q. Song, Q. X. Sun, B. B. Huo, W. R. Yang, C. J. Barrow and J. Q. Liu, ChemNanoMat, 2019, 5, 784–791 CrossRef CAS.
- D. L. Fan, J. Feng, J. Liu, T. Y. Gao, Z. X. Ye, M. Chen and X. M. Lv, Ceram. Int., 2016, 42, 7155–7163 CrossRef CAS.
- C. C. Cao, Y. M. Xue, Z. Y. Liu, Z. Zhou, J. W. Ji, Q. Q. Song, Q. Hu, Y. Fang and C. C. Tang, 2D Mater., 2019, 6, 035014 CrossRef CAS.
- D. Ji, Z. Wang, Y. Zhu and H. Yu, Ceram. Int., 2020, 46, 21084–21089 CrossRef CAS.
- H. R. Zhao, J. H. Ding and H. B. Yu, Ind. Eng. Chem. Res., 2018, 57, 14096–14105 CrossRef CAS.
- Y. Arao, R. Kuwahara, K. Ohno, J. Tanks, K. Aida, M. Kubouchi and S. Takeda, Nanoscale Adv., 2019, 1, 4955–4964 RSC.
- C. C. Caliman, A. F. Mesquita, D. F. Cipriano, J. C. C. Freitas, A. A. C. Cotta, W. A. A. Macedo and A. O. Porto, RSC Adv., 2018, 8, 6136–6145 RSC.
- L. L. Li, J. Ji, R. Fei, C. Z. Wang, Q. Lu, J. R. Zhang, L. P. Jiang and J. J. Zhu, Adv. Funct. Mater., 2012, 22, 2971–2979 CrossRef CAS.
- M. Bosi, RSC Adv., 2015, 5, 75500–75518 RSC.
- S. Das, M. Kim, J. W. Lee and W. Choi, Crit. Rev. Solid State Mater. Sci., 2014, 39, 231–252 CrossRef CAS.
- S. K. Hyeong, K. H. Choi, S. W. Park, S. Bae, M. Park, S. Ryu, J. H. Lee and S. K. Lee, Appl. Sci. Converg. Technol., 2019, 28, 148–154 CrossRef.
- S. Uddin and Y. W. Song, Appl. Sci., 2021, 11, 2768 CrossRef CAS.
- G. J. Li, J. Appl. Phys., 2020, 127, 010901 CrossRef CAS.
- F. C. Wang, K. D. Wang, B. X. Zheng, X. Dong, X. S. Mei, J. Lv, W. Q. Duan and W. J. Wang, Mater. Technol., 2018, 33, 340–356 CrossRef CAS.
- Y. Zhao, Q. Han, Z. H. Cheng, L. Jiang and L. T. Qu, Nano Today, 2017, 12, 14–30 CrossRef CAS.
- O. A. Abbas, A. H. Lewis, N. Aspiotis, C. C. Huang, I. Zeimpekis, D. W. Hewak, P. Sazio and S. Mailis, Sci. Rep., 2021, 11, 5211 CrossRef CAS PubMed.
- Y. Z. Chen, H. Medina, T. Y. Su, J. G. Li, K. Y. Cheng, P. W. Chiu and Y. L. Chueh, ACS Nano, 2015, 9, 4346–4353 CrossRef CAS PubMed.
- C. C. Huang, H. Medina, Y. Z. Chen, T. Y. Su, J. G. Li, C. W. Chen, Y. T. Yen, Z. M. Wang and Y. L. Chueh, Nano Lett., 2016, 16, 2463–2470 CrossRef CAS PubMed.
- J. Lin, Z. Peng, Y. Liu, F. Ruiz-Zepeda, R. Ye, E. L. Samuel, M. J. Yacaman, B. I. Yakobson and J. M. Tour, Nat. Commun., 2014, 5, 5714 CrossRef CAS PubMed.
- Z. C. Zhang, M. M. Song, J. X. Hao, K. B. Wu, C. Y. Li and C. G. Hu, Carbon, 2018, 127, 287–296 CrossRef CAS.
- S. Lee, M. F. Toney, W. Ko, J. C. Randel, H. J. Jung, K. Munakata, J. Lu, T. H. Geballe, M. R. Beasley, R. Sinclair, H. C. Manoharan and A. Salleo, ACS Nano, 2010, 4, 7524–7530 CrossRef CAS PubMed.
- R. Ye, Y. Chyan, J. Zhang, Y. Li, X. Han, C. Kittrell and J. M. Tour, Adv. Mater., 2017, 29, 1702211 CrossRef PubMed.
- Y. Chyan, R. Ye, Y. Li, S. P. Singh, C. J. Arnusch and J. M. Tour, ACS Nano, 2018, 12, 2176–2183 CrossRef CAS PubMed.
- D. V. Kosynkin, A. L. Higginbotham, A. Sinitskii, J. R. Lomeda, A. Dimiev, B. K. Price and J. M. Tour, Nature, 2009, 458, 872–876 CrossRef CAS PubMed.
- L. Jiao, L. Zhang, X. Wang, G. Diankov and H. Dai, Nature, 2009, 458, 877–880 CrossRef CAS PubMed.
- H. Santos, L. Chico and L. Brey, Phys. Rev. Lett., 2009, 103, 086801 CrossRef CAS PubMed.
- K. J. Erickson, A. L. Gibb, A. Sinitskii, M. Rousseas, N. Alem, J. M. Tour and A. K. Zettl, Nano Lett., 2011, 11, 3221–3226 CrossRef CAS PubMed.
- C. Wang, Y. S. Li, J. Jiang and W. H. Chiang, ACS Appl. Mater. Interfaces, 2015, 7, 17441–17449 CrossRef CAS PubMed.
- Y. S. Li, J. L. Liao, S. Y. Wang and W. H. Chiang, Sci. Rep., 2016, 6, 22755 CrossRef CAS PubMed.
- Y. S. Li, X. Ao, J. L. Liao, J. J. Jiang, C. D. Wang and W. H. Chiang, Electrochim. Acta, 2017, 249, 404–412 CrossRef CAS.
- D. Kim, S. Nakajima, T. Sawada, M. Iwasaki, S. Kawauchi, C. Zhi, Y. Bando, D. Golberg and T. Serizawa, Chem. Commun., 2015, 51, 7104–7107 RSC.
- Y. L. Liao, Z. F. Chen, J. W. Connell, C. C. Fay, C. Park, J. W. Kim and Y. Lin, Adv. Funct. Mater., 2014, 24, 4497–4506 CrossRef CAS.
- X. Zhang, Z. Lai, C. Tan and H. Zhang, Angew. Chem., Int. Ed., 2016, 55, 8816–8838 CrossRef CAS PubMed.
- H. S. Matte, A. Gomathi, A. K. Manna, D. J. Late, R. Datta, S. K. Pati and C. N. Rao, Angew. Chem., Int. Ed., 2010, 49, 4059–4062 CrossRef CAS PubMed.
- B. Singh, G. Kaur, P. Singh, K. Singh, B. Kumar, A. Vij, M. Kumar, R. Bala, R. Meena, A. Singh, A. Thakur and A. Kumar, Sci. Rep., 2016, 6, 35535 CrossRef CAS PubMed.
- T. V. Tam and W. M. Choi, Curr. Appl. Phys., 2018, 18, 1255–1260 CrossRef.
- S. Wang, Y. Chen, X. Li, W. Gao, L. Zhang, J. Liu, Y. Zheng, H. Chen and J. Shi, Adv. Mater., 2015, 27, 7117–7122 CrossRef CAS PubMed.
- W. Yin, J. Yu, F. Lv, L. Yan, L. R. Zheng, Z. Gu and Y. Zhao, ACS Nano, 2016, 10, 11000–11011 CrossRef CAS PubMed.
- S. Wang, K. Li, Y. Chen, H. Chen, M. Ma, J. Feng, Q. Zhao and J. Shi, Biomaterials, 2015, 39, 206–217 CrossRef CAS PubMed.
- H. L. Yang, J. L. Zhao, C. Y. Wu, C. Q. Ye, D. W. Zou and S. G. Wang, Chem. Eng. J., 2018, 351, 548–558 CrossRef CAS.
- K. P. Musselman, K. H. Ibrahim and M. Yavuz, APL Mater., 2018, 6, 120701 CrossRef.
- H. Huang, C. C. Du, H. Y. Shi, X. Feng, J. Li, Y. L. Tan and W. B. Song, Part. Part. Syst. Char., 2015, 32, 72–79 CrossRef CAS.
- B. Liu, S. Yan, Z. Song, M. Liu, X. Ji, W. Yang and J. Liu, Chemistry, 2016, 22, 18899–18907 CrossRef CAS PubMed.
- B. Huo, B. Liu, T. Chen, L. Cui, G. Xu, M. Liu and J. Liu, Langmuir, 2017, 33, 10673–10678 CrossRef CAS PubMed.
- S. Angizi, A. Hatamie, H. Ghanbari and A. Simchi, ACS Appl. Mater. Interfaces, 2018, 10, 28819–28827 CrossRef CAS PubMed.
- M. P. More, P. H. Lohar, A. G. Patil, P. O. Patil and P. K. Deshmukh, Mater. Chem. Phys., 2018, 220, 11–22 CrossRef CAS.
- A. Narita, Z. Chen, Q. Chen and K. Mullen, Chem. Sci., 2019, 10, 964–975 RSC.
- M. Kolmer, A. K. Steiner, I. Izydorczyk, W. Ko, M. Engelund, M. Szymonski, A. P. Li and K. Amsharov, Science, 2020, 369, 571–575 CrossRef CAS PubMed.
- J. Gao, F. J. Uribe-Romo, J. D. Saathoff, H. Arslan, C. R. Crick, S. J. Hein, B. Itin, P. Clancy, W. R. Dichtel and Y. L. Loo, ACS Nano, 2016, 10, 4847–4856 CrossRef CAS PubMed.
- T. H. Vo, M. Shekhirev, D. A. Kunkel, M. D. Morton, E. Berglund, L. Kong, P. M. Wilson, P. A. Dowben, A. Enders and A. Sinitskii, Nat. Commun., 2014, 5, 3189 CrossRef PubMed.
- A. Jolly, D. Miao, M. Daigle and J. F. Morin, Angew. Chem., Int. Ed., 2020, 59, 4624–4633 CrossRef CAS PubMed.
- G. Li, K. Y. Yoon, X. Zhong, X. Zhu and G. Dong, Chemistry, 2016, 22, 9116–9120 CrossRef CAS PubMed.
- L. Chen, Y. Hernandez, X. Feng and K. Mullen, Angew. Chem., Int. Ed., 2012, 51, 7640–7654 CrossRef CAS PubMed.
- V. S. Iyer, M. Wehmeier, J. D. Brand, M. A. Keegstra and K. Müllen, Angew. Chem., Int. Ed. Engl., 1997, 36, 1604–1607 CrossRef CAS.
- U. Beser, M. Kastler, A. Maghsoumi, M. Wagner, C. Castiglioni, M. Tommasini, A. Narita, X. Feng and K. Mullen, J. Am. Chem. Soc., 2016, 138, 4322–4325 CrossRef CAS PubMed.
- Z. Qiu, A. Narita and K. Mullen, Faraday Discuss., 2021, 227, 8–45 RSC.
- X. Yan, B. Li, X. Cui, Q. Wei, K. Tajima and L. S. Li, J. Phys. Chem. Lett., 2011, 2, 1119–1124 CrossRef CAS PubMed.
- A. Kozhakhmetov, R. Torsi, C. Y. Chen and J. A. Robinson, J. Phys.: Mater, 2020, 4, 012001 Search PubMed.
- C. Altavilla, M. Sarno and P. Ciambelli, Chem. Mater., 2011, 23, 3879–3885 CrossRef CAS.
- W. Jung, S. Lee, D. Yoo, S. Jeong, P. Miro, A. Kuc, T. Heine and J. Cheon, J. Am. Chem. Soc., 2015, 137, 7266–7269 CrossRef CAS PubMed.
- A. Narita, X. Feng, Y. Hernandez, S. A. Jensen, M. Bonn, H. Yang, I. A. Verzhbitskiy, C. Casiraghi, M. R. Hansen, A. H. Koch, G. Fytas, O. Ivasenko, B. Li, K. S. Mali, T. Balandina, S. Mahesh, S. De Feyter and K. Mullen, Nat. Chem., 2014, 6, 126–132 CrossRef CAS PubMed.
- T. Van Tam, T. M. Altahtamouni, V. Le Minh, H. K. P. Ha, N. T. K. Chung and D. Van Thuan, C.R. Chim., 2019, 22, 822–828 CrossRef CAS.
- R. Beams, L. Gustavo Cancado and L. Novotny, J. Phys.: Condens. Matter, 2015, 27, 083002 CrossRef CAS PubMed.
-
M. Rahaman and D. R. T. Zahn, Recent Developments in Atomic Force Microscopy and Raman Spectroscopy for Materials Characterization, 2022, ch. 12 DOI:10.5772/intechopen.99817.
- L. M. Malard, L. Lafeta, R. S. Cunha, R. Nadas, A. Gadelha, L. G. Cancado and A. Jorio, Phys. Chem. Chem. Phys., 2021, 23, 23428–23444 RSC.
- J. Kim, J. U. Lee and H. Cheong, J. Phys.: Condens. Matter, 2020, 32, 343001 CrossRef CAS PubMed.
- M. R. Molas, Ł. Macewicz, A. Wieloszyńska, P. Jakóbczyk, A. Wysmołek, R. Bogdanowicz and J. B. Jasinski, npj 2D Mater. Appl., 2021, 5, 83 CrossRef CAS.
- T. Mueller and E. Malic, npj 2D Mater. Appl., 2018, 2, 29 CrossRef.
- M. Abdolkarimi-Mahabadi, A. Bayat and A. Mohammadi, Theor. Exp. Chem., 2021, 57, 191–198 CrossRef CAS.
- E. Fuente, J. A. Menéndez, M. A. Díez, D. Suárez and M. A. Montes-Morán, J. Phys. Chem. B, 2003, 107, 6350–6359 CrossRef CAS.
- A. Kroner and T. Hirsch, Front Chem., 2019, 7, 927 CrossRef PubMed.
- R. Addou and R. M. Wallace, J. Electron Spectrosc. Relat. Phenom., 2019, 231, 94–103 CrossRef CAS.
- M. J. Zachman, J. A. Hachtel, J. C. Idrobo and M. Chi, Angew. Chem., Int. Ed., 2020, 59, 1384–1396 CrossRef CAS PubMed.
|
This journal is © the Owner Societies 2022 |
Click here to see how this site uses Cookies. View our privacy policy here.