DOI:
10.1039/D1SC04687J
(Edge Article)
Chem. Sci., 2021,
12, 14182-14188
Enantioselective palladaelectro-catalyzed C–H olefinations and allylations for N–C axial chirality†
Received
24th August 2021
, Accepted 4th October 2021
First published on 13th October 2021
Abstract
Enantioselective palladaelectro-catalyzed C–H alkenylations and allylations were achieved with easily-accessible amino acids as transient directing groups. This strategy provided access to highly enantiomerically-enriched N–C axially chiral scaffolds under exceedingly mild conditions. The synthetic utility of our strategy was demonstrated by a variety of alkenes, while the versatility of our approach was reflected by atroposelective C–H allylations. Computational studies provided insights into a facile C–H activation by a seven-membered palladacycle.
Introduction
Organic electrocatalysis has surfaced as an increasingly powerful platform in the molecular sciences.1 Despite indisputable advances, asymmetric electro-organic synthesis continues to be underdeveloped.2 Particularly, the development of highly enantioselective electrocatalysis remains a challenge,3 while gaining full selectivity control is paramount for synthetically useful C–H transformations.4 In this context, we have recently developed the asymmetric metallaelectro-catalyzed C–H activation,5 largely leading to C–C axially chiral binaphthyls.6
N–C axially chiral heterobiaryls are key structural motifs in chiral catalysts, ligands, and natural products (Fig. 1a).7,8 Despite significant advances in the synthesis of chiral biaryls,9 the enantioselective assembly of N–C axially chiral molecules continues to be scarce.10 As a consequence, recent focus has shifted towards methods for the preparation of N–C axially chiral scaffolds, with key contributions by Wencel-Delord/Colobert,8 Xie11 and Shi,12 among others. However, the kinetic resolution with sterically-crowded N-arylindoles was restricted to the use of superstoichiometric amounts of toxic and cost-intensive silver salts in expensive fluorinated solvents, significantly jeopardizing the sustainability of the overall approach (Fig. 1b).11 Within our program on sustainable C–H activations,13 we have now unraveled a electrochemical kinetic resolution14 of N-arylindoles in the presence of a transient directing group (TDG)15 (Fig. 1c).
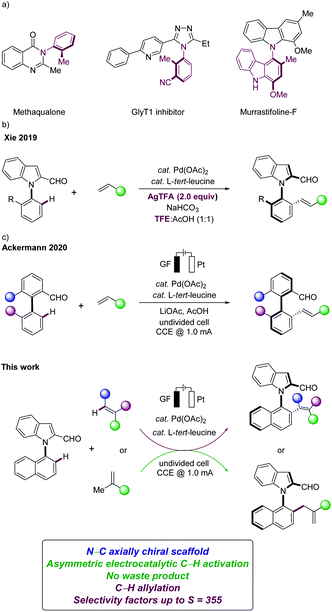 |
| Fig. 1 Evolution of electrocatalytic C–H activation towards N–C axial chirality. (a) Examples of N–C axially chiral compounds; (b) atroposelective palladacatalysis; (c) enantioselective metallaelectrocatalysis. | |
Notable features of our findings include (a) enantioselective organometallic C–H activation16 for the electrocatalytic assembly of N–C axially chiral scaffolds in the absence of toxic chemical oxidants, (b) easily-accessible transient directing groups in electrochemical C–H activations, (c) a wide substrate scope of maleimides and fluorinated alkenes, and (d) key mechanistic insights by computation, (e) leading to atroposelective electrochemical C–H allylations.
Results and discussion
We initiated our studies with L-tert-leucine as additive for the envisioned atroposelective electrochemical kinetic resolution of racemic N-arylindoles 1 for the synthesis of N–C axially chiral motifs (Tables 1 and S1 in the ESI†).17 Hence, racemic indole 1a was reacted with n-butyl acrylate (2a) in the presence of catalytic amounts of Pd(OAc)2 and L-tert-leucine as well as LiOAc in AcOH at 60 °C to provide the desired alkene 3 with 97% ee, while the recovered starting material 1a was obtained in 70% ee (entry 1). We were delighted to find that L-tert-leucine diethyl amide provided an excellent selectivity factor of S = 264, albeit at a lower conversion (entry 2). In contrast, L-proline provided the C3 alkenylated product, highlighting the challenges to overcome the innate C3 reactivity of indole.17 We did not observe reactivity, when TFE was used as the solvent (entry 3). Low conversions were obtained in a solvent mixture of TFE and AcOH (entry 4). Control experiments revealed the necessity of the TDG, and the palladium catalyst for the atroposelective electrochemical resolution of N-arylindole 1a (entries 5–7), while electricity proved to be important for improving the catalytic efficacy (Table S1†). Notably, the reaction worked efficiently under N2 atmosphere, albeit with lower conversion (entry 8). However, the addition of benzoquione as redox mediator failed to improve the conversion (entry 9). Thus, the metallaelectrocatalysis proceeded efficiently under mild conditions in redox-mediator-free condition.
Table 1 Optimization of the N–C atroposelective C–H olefinationa
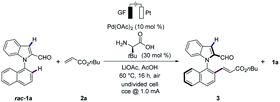
|
Entry |
Deviation from standard conditions |
Conv.b (%) |
ee (3) |
S
|
Reaction conditions: undivided cell, rac-1a (0.20 mmol), 2a (0.60 mmol), [Pd] (10 mol%), L-tert-leucine (30 mol%), LiOAc (2.0 equiv.), AcOH (4.5 mL), 60 °C, constant current at 1.0 mA, 16 h, graphite felt (GF) anode, Pt-plate cathode.
Calculated conversion, C = ee1a/(ee1a + ee3), ee1a = ee of 1a and ee3 = ee of 3.
Selectivity (S) = ln[(1 − C)(1 − ee1a)]/ln[(1 − C)(1 + ee1a)].
C3 alkenylated product was isolated.
1,4-Benzoquinone (10 mol%) as additive.
|
1 |
None |
42 |
97 |
138 |
2 |
L-tert-Leucine diethyl amide as TDG |
23 |
99 |
264 |
3 |
TFE as solvent, no LiOAc |
— |
— |
— |
4 |
TFE/AcOH as solvent, no LiOAc |
38 |
94 |
56 |
5 |
L-tert-Leucine (20 mol%) |
37 |
97 |
118 |
6 |
No palladium |
— |
— |
— |
7 |
No L-tert-leucine |
12d |
— |
— |
8 |
Under N2 |
26 |
99 |
280 |
9 |
1,4-Benzoquinone |
36e |
99 |
347 |
On/off experiments clearly showed the essential role of the electricity to improve the efficacy of the electrocatalytic C–H activation (Fig. 2).
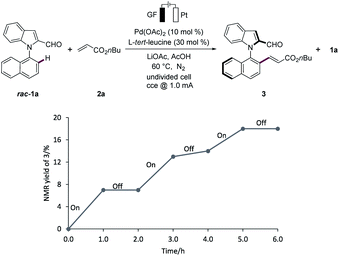 |
| Fig. 2 On/off electricity experiment. | |
With the optimized reaction conditions in hand, we next explored the scope of the palladaelectro-catalyzed N–C atroposelective olefination (Scheme 1). Thus, a wide variety of alkenes 2 provided the desired products 3–11 with excellent selectivity factors (S). Specifically, n-butyl acrylate (2a) and t-butyl acrylate (2b) provided the desired olefinated products 3 and 4 with 97% ee and 92% ee, respectively. Vinyl phosphonate (2c) and vinyl sulfone (2d) were also identified as suitable substrates to deliver the desired products 5 and 6, respectively, with high S-factors. An arene with an electron-donating methoxy group 2e likewise performed well, providing the olefinated product 7 with 92% ee. The bromo-substituted arene 2f was well tolerated in the N–C atroposelective alkenylation with an excellent S-factor of 355, which should prove invaluable for further late-stage modification. The palladaelectrocatalysis proceeded likewise with electron-rich 5-methyl substituted N-arylindole 1b with a wide range of alkenes 2. In contrast, unactivated alkenes gave thus far less satisfactory results, under otherwise identical conditions.
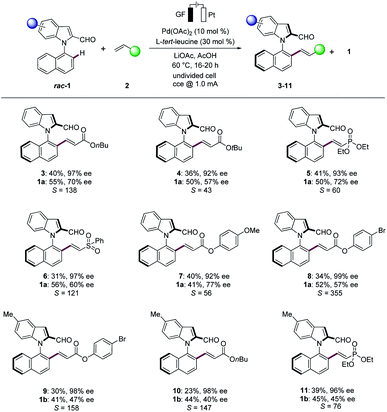 |
| Scheme 1 N–C atroposelective C–H olefination of N-arylindoles rac-1. | |
Maleimides represent key structural motifs in several natural products and drug candidates.18 Hence, we next examined the versatility of the N–C atroposelective transformations with maleimides 12 (Scheme 2). We were pleased to find that the robust electrocatalysis was viable for maleimides 12. A variation of the substitution pattern on the nitrogen atom was explored, to give the methyl (13), cyclohexyl (14) and phenyl (15) substituted products with excellent S-factors. To our delight, L-tert-leucine diethyl amide provided the desired product 13 with an excellent S-factor of 272. The absolute configuration of product 15 was unambiguously assigned by X-ray diffraction analysis.
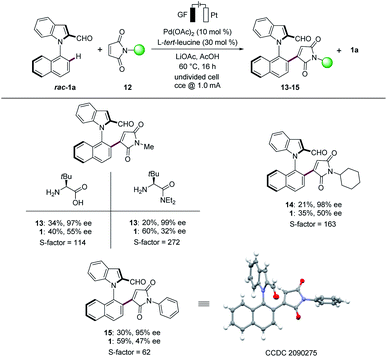 |
| Scheme 2 N–C atroposelective palladaelectro-catalyzed C–H olefination with maleimides 12. | |
To gain insight into the N–C atroposelective C–H alkenylation, the reaction mechanism was probed by means of DFT calculations at the PW6B95-D4/def2-TZVP+SMD(AcOH)//PBE0-D3BJ/def2-SVP level of theory for the alkylation with maleimides 12 (Fig. 3).19 In the C–H activation step, both the pathways giving origin to the five- and seven-membered ring cyclometalated species were considered. Not only the intermediate I-1 but also TS(1–2), leading to the seven-membered cyclometalated complex revealed to be more favorable over the five-membered ring pathway by 6.7 and 2.3 kcal mol−1 in I-1 and TS(1–2), respectively. Additionally, for the seven-membered ring pathway both the N- and O-coordination of the TDG to the palladium were considered. The O-coordinated pathway is strongly stabilized over the N-coordinated path by 13.1 and 11.4 kcal mol−1 in I-1 and TS(1–2) respectively. Thus, the C–H activation proceeds through an energetically preferred seven-membered ring pathway with a favored O-coordination of the TDG to the palladium in a facile fashion with a barrier of 12.6 kcal mol−1. The C–H activation step is followed by the coordination of the maleimide giving origin to the formation of intermediate I-4, which undergoes migratory insertion through TS(4–5) with an energy barrier of 13.6 kcal mol−1. These results indicate that C–H activation is not the rate determining-step.
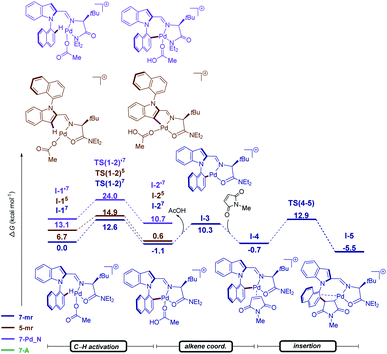 |
| Fig. 3 Computed relative Gibbs free energies (ΔG333.15) in kcal mol−1 between the C–H activation and insertion elementary steps at the PW6B95-D4/def2-TZVP+SMD(AcOH)//PBE0-D3BJ/def2-SVP level of theory. Superscripts 5 and 7 relate to structures, which lead to the formation of the 7-membered and the 5-membered cyclometalated intermediates. | |
Since the directed C3 position selectivity was observed with L-proline, the C–H activation elementary step was explored by computation (Fig. S5, see the ESI†).17 DFT calculations demonstrate that the C–H elementary step proceeded in a facile fashion at C3 of the indole with a low barrier of 18.2 kcal mol−1. This barrier is higher than the one calculated for the cationic pathway (Fig. 3). This may be due to the presence of a hydrogen bond stabilization between the L-proline NH and the acetate in intermediate I-1P, which needs to be disrupted upon acetate rotation, to give rise to transition state TS(1-2)P.
Fluorinated organic compounds have gained considerable recent attention in pharmaceutical and agrochemical industries, among others, due to their improved lipophilicities.20 Hence, we were pleased to find that the palladaelectro-catalyzed kinetic resolution proved to be amenable to fluorinated alkenes 16 for the synthesis of synthetically useful N–C axially chiral fluorinated motifs (Scheme 3). Thereby, various perfluorinated alkenes 16 provided the desired fluorinated compounds 17–22. Likewise, terminal perfluroalkylalkenes with different chain lengths 16a–16c reflected the versatility with excellent selectivity factors. Interestingly, the presence of bromine in perfluoroalkylalkene 16d did not alter the course of the electrocatalysis. Thus, the corresponding product 20 was obtained in 27% yield and 98% ee. Additionally, similar reactivities were observed when alkenes 16e and 16f were employed, providing the desired products 21 and 22 with S-factors of 99 and 156, respectively.
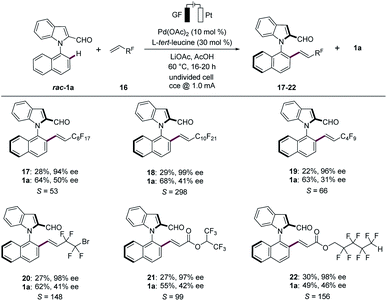 |
| Scheme 3 N–C atroposelective palladaelectro-catalyzed C–H olefination with fluorinated alkenes 16. | |
Finally, the N–C atroposelective electrocatalysis was not limited to monosubstituted alkenes. Indeed, unprecedented allylic selectivity21 was observed with 1,1-disubstituted alkenes22 for the synthesis of atroposelective N–C axially chiral heterobiaryls 24–29 (Scheme 4). The electrocatalysis proceeded efficiently with ethyl methacrylate (23a) to provide the corresponding product 24 with complete allylic selectivity in 35% yield and 92% ee. Similarly, fluorinated methacrylates 23b and 23c were found to be suitable substrates, providing the desired allylated products 25 and 26, respectively, with high enantioselectivities. Importantly, thiophenyl methacrylate (23d) was also successfully explored. We were pleased to find that methyl butenone (23e) was efficiently converted, providing the allylation product 28 with 91% ee. The reactivity was not limited to 1,1-disubstituted alkenes conjugated with electron withdrawing groups, since methylallyl ester 23f likewise delivered the allylated product 29 with high enantioselectivity.
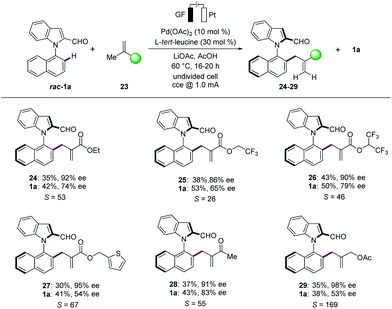 |
| Scheme 4 N–C atroposelective palladaelectro-catalyzed C–H allylation. | |
Furthermore, we probed the electrochemical C–H olefination by means of cyclic voltammetric analysis (Fig. 4 and S2–S4†).17 The oxidation peak at 1.1 V vs. SCE appeared after the first negative sweep of Pd(OAc)2, which corresponds to the oxidation of palladium(0) to palladium(II) (Fig. S2†). This redox event is significantly lower than the oxidation potential of the other components, being suggestive of the current regenerating the catalytically active palladium species (Fig. 4).
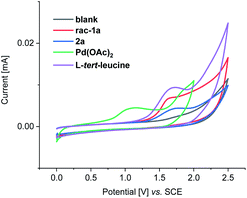 |
| Fig. 4 Cyclic voltammogram of several reactants in AcOH with nBu4NPF6 (0.1 M) at 100 mV s−1. | |
Based on our experimental and computational findings, we proposed the N–C atroposelective palladaelectro-catalyzed C–H olefination to commence with the coordination of the in situ generated imine 1a′′ with the palladium catalyst A leading to the formation of intermediate B, which subsequently undergoes facile C–H metalation (Scheme 5). Coordination of the alkene gives palladium allyl species D by migratory insertion, which is followed by β-H elimination to form complex E. The latter leads to the formation of a palladium(0) complex F which subsequently undergoes anodic oxidation to regenerate the palladium(II) catalytically active species A.23
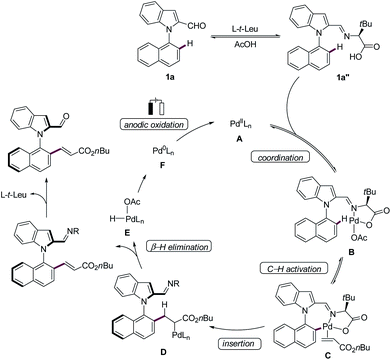 |
| Scheme 5 Proposed catalytic cycle. | |
Conclusions
In summary, we have reported on the unprecedented atroposelective palladaelectro-catalyzed C–H activation for the synthesis of N–C axially chiral indole biaryls. The atroposelective organometallic C–H activation was realized by means of a chiral amino acid under mild conditions. Thus, N–C axially chiral scaffolds were obtained with good to excellent selectivity factors in the absence of chemical oxidants. A wealth of alkenes was found to be compatible with this strategy, including nonactivated and perfluorinated alkenes. The first N–C atroposelective palladium(II)-catalyzed C–H allylation was achieved with challenging 1,1-disubstituted alkene, while computational studies on the metallaelectrocatalysis unraveled a facile C–H activation.
Data availability
All experimental data, procedures for data analysis and pertinent data sets are provided in the ESI.†
Author contributions
U. D., T. W. and L. A. conceived the project. U. D., T. W. and X. H. performed the experiments. X. H. performed CV studies. B. Y. and J. C. A. O. performed DFT calculations. U. D. and L. A. wrote the manuscript. All of the authors discussed the results and contributed to the preparation of the final manuscript.
Conflicts of interest
There are no conflicts to declare.
Acknowledgements
Generous support by the DFG (Gottfried-Wilhelm-Leibniz award and SPP 1807), the DAAD (fellowship to U. D.), and the CSC (scholarships to X. H. and B. Y.) is gratefully acknowledged. The authors thank Dr Christopher Golz (University of Göttingen) for assistance with the X-ray diffraction analysis and Ms Agnese Zangarelli (University of Göttingen) for early experimentation and starting material synthesis.
Notes and references
-
(a) L. F. T. Novaes, J. Liu, Y. Shen, L. Lu, J. M. Meinhardt and S. Lin, Chem. Soc. Rev., 2021, 50, 7941–8002 RSC;
(b) C. Zhu, N. W. J. Ang, T. H. Meyer, Y. Qiu and L. Ackermann, ACS Cent. Sci., 2021, 7, 415–431 CrossRef CAS PubMed;
(c) D. Pollok and S. R. Waldvogel, Chem. Sci., 2020, 11, 12386–12400 RSC;
(d) J. C. Siu, N. Fu and S. Lin, Acc. Chem. Res., 2020, 53, 547–560 CrossRef CAS PubMed;
(e) P. Xiong and H.-C. Xu, Acc. Chem. Res., 2019, 52, 3339–3350 CrossRef CAS PubMed;
(f) J.-i. Yoshida, A. Shimizu and R. Hayashi, Chem. Rev., 2018, 118, 4702–4730 CrossRef CAS PubMed;
(g) G. S. Sauer and S. Lin, ACS Catal., 2018, 8, 5175–5187 CrossRef CAS;
(h) K. D. Moeller, Chem. Rev., 2018, 118, 4817–4833 CrossRef CAS PubMed;
(i) M. Yan, Y. Kawamata and P. S. Baran, Chem. Rev., 2017, 117, 13230–13319 CrossRef CAS PubMed;
(j) R. Francke and R. D. Little, Chem. Soc. Rev., 2014, 43, 2492–2521 RSC;
(k) T. R. Cook, D. K. Dogutan, S. Y. Reece, Y. Surendranath, T. S. Teets and D. G. Nocera, Chem. Rev., 2010, 110, 6474–6502 CrossRef CAS PubMed;
(l) J.-i. Yoshida, K. Kataoka, R. Horcajada and A. Nagaki, Chem. Rev., 2008, 108, 2265–2299 CrossRef CAS PubMed;
(m) A. Jutand, Chem. Rev., 2008, 108, 2300–2347 CrossRef CAS PubMed.
-
(a) T. H. Meyer, I. Choi, C. Tian and L. Ackermann, Chem, 2020, 6, 2484–2496 CrossRef CAS;
(b) X. Chang, Q. Zhang and C. Guo, Angew. Chem., Int. Ed., 2020, 59, 12612–12622 CrossRef CAS PubMed;
(c) Q. Lin, L. Li and S. Luo, Chem.–Eur. J., 2019, 25, 10033–10044 CrossRef CAS PubMed;
(d) M. Ghosh, V. S. Shinde and M. Rueping, Beilstein J. Org. Chem., 2019, 15, 2710–2746 CrossRef CAS PubMed.
-
(a) S. G. Robinson, X. Wu, B. Jiang, M. S. Sigman and S. Lin, J. Am. Chem. Soc., 2020, 142, 18471–18482 CrossRef CAS PubMed;
(b) L. Song, N. Fu, B. G. Ernst, W. H. Lee, M. O. Frederick, R. A. DiStasio and S. Lin, Nat. Chem., 2020, 12, 747–754 CrossRef CAS PubMed;
(c) H. Qiu, B. Shuai, Y.-Z. Wang, D. Liu, Y.-G. Chen, P.-S. Gao, H.-X. Ma, S. Chen and T.-S. Mei, J. Am. Chem. Soc., 2020, 142, 9872–9878 CrossRef CAS PubMed;
(d) P.-S. Gao, X.-J. Weng, Z.-H. Wang, C. Zheng, B. Sun, Z.-H. Chen, S.-L. You and T.-S. Mei, Angew. Chem., Int. Ed., 2020, 59, 15254–15259 CrossRef CAS PubMed;
(e) X. Huang, Q. Zhang, J. Lin, K. Harms and E. Meggers, Nat. Catal., 2019, 2, 34–40 CrossRef CAS;
(f) T. J. DeLano and S. E. Reisman, ACS Catal., 2019, 9, 6751–6754 CrossRef CAS PubMed;
(g) N. Kise, Y. Hamada and T. Sakurai, Org. Lett., 2014, 16, 3348–3351 CrossRef CAS PubMed;
(h) N. Kise, H. Ozaki, N. Moriyama, Y. Kitagishi and N. Ueda, J. Am. Chem. Soc., 2003, 125, 11591–11596 CrossRef CAS PubMed.
-
(a) O. Vyhivskyi, A. Kudashev, T. Miyakoshi and O. Baudoin, Chem.–Eur. J., 2021, 27, 1231–1257 CrossRef CAS PubMed;
(b) T. K. Achar, S. Maiti, S. Jana and D. Maiti, ACS Catal., 2020, 10, 13748–13793 CrossRef CAS;
(c) G. Meng, N. Y. S. Lam, E. L. Lucas, T. G. Saint-Denis, P. Verma, N. Chekshin and J.-Q. Yu, J. Am. Chem. Soc., 2020, 142, 10571–10591 CrossRef CAS PubMed;
(d) G. Liao, T. Zhou, Q.-J. Yao and B.-F. Shi, Chem. Commun., 2019, 55, 8514–8523 RSC;
(e) Ł. Woźniak and N. Cramer, Trends Chem., 2019, 1, 471–484 CrossRef;
(f) J. Loup, U. Dhawa, F. Pesciaioli, J. Wencel-Delord and L. Ackermann, Angew. Chem., Int. Ed., 2019, 58, 12803–12818 CrossRef CAS PubMed;
(g) J. Diesel and N. Cramer, ACS Catal., 2019, 9, 9164–9177 CrossRef CAS;
(h) C. G. Newton, S.-G. Wang, C. C. Oliveira and N. Cramer, Chem. Rev., 2017, 117, 8908–8976 CrossRef CAS PubMed;
(i) D.-W. Gao, Q. Gu, C. Zheng and S.-L. You, Acc. Chem. Res., 2017, 50, 351–365 CrossRef CAS PubMed;
(j) J. Wencel-Delord, A. Panossian, F. R. Leroux and F. Colobert, Chem. Soc. Rev., 2015, 44, 3418–3430 RSC.
-
(a) R. C. Samanta, T. H. Meyer, I. Siewert and L. Ackermann, Chem. Sci., 2020, 11, 8657–8670 RSC;
(b) K.-J. Jiao, Y.-K. Xing, Q.-L. Yang, H. Qiu and T.-S. Mei, Acc. Chem. Res., 2020, 53, 300–310 CrossRef CAS PubMed;
(c) P. Gandeepan, L. H. Finger, T. H. Meyer and L. Ackermann, Chem. Soc. Rev., 2020, 49, 4254–4272 RSC;
(d) Y. Qiu, J. Struwe and L. Ackermann, Synlett, 2019, 30, 1164–1173 CrossRef CAS;
(e) N. Sauermann, T. H. Meyer, Y. Qiu and L. Ackermann, ACS Catal., 2018, 8, 7086–7103 CrossRef CAS;
(f) N. Sauermann, T. H. Meyer and L. Ackermann, Chem.–Eur. J., 2018, 24, 16209–16217 CrossRef CAS PubMed;
(g) C. Ma, P. Fang and T.-S. Mei, ACS Catal., 2018, 8, 7179–7189 CrossRef CAS;
(h) M. D. Kärkäs, Chem. Soc. Rev., 2018, 47, 5786–5865 RSC.
- U. Dhawa, C. Tian, T. Wdowik, J. C. A. Oliveira, J. Hao and L. Ackermann, Angew. Chem., Int. Ed., 2020, 59, 13451–13457 CrossRef CAS PubMed.
- T. Sugane, T. Tobe, W. Hamaguchi, I. Shimada, K. Maeno, J. Miyata, T. Suzuki, T. Kimizuka, S. Sakamoto and S.-i. Tsukamoto, J. Med. Chem., 2013, 56, 5744–5756 CrossRef CAS PubMed.
- J. Frey, A. Malekafzali, I. Delso, S. Choppin, F. Colobert and J. Wencel-Delord, Angew. Chem., Int. Ed., 2020, 59, 8844–8848 CrossRef CAS PubMed.
-
(a) X. Wu, R. M. Witzig, R. Beaud, C. Fischer, D. Häussinger and C. Sparr, Nat. Catal., 2021, 4, 457–462 CrossRef CAS;
(b) B.-B. Zhan, L. Wang, J. Luo, X.-F. Lin and B.-F. Shi, Angew. Chem., Int. Ed., 2020, 59, 3568–3572 CrossRef CAS PubMed;
(c) H. Song, Y. Li, Q.-J. Yao, L. Jin, L. Liu, Y.-H. Liu and B.-F. Shi, Angew. Chem., Int. Ed., 2020, 59, 6576–6580 CrossRef CAS PubMed;
(d) L. Jin, Q.-J. Yao, P.-P. Xie, Y. Li, B.-B. Zhan, Y.-Q. Han, X. Hong and B.-F. Shi, Chem, 2020, 6, 497–511 CrossRef CAS;
(e) Q. Wang, Z.-J. Cai, C.-X. Liu, Q. Gu and S.-L. You, J. Am. Chem. Soc., 2019, 141, 9504–9510 CrossRef CAS PubMed;
(f) J. Luo, T. Zhang, L. Wang, G. Liao, Q.-J. Yao, Y.-J. Wu, B.-B. Zhan, Y. Lan, X.-F. Lin and B.-F. Shi, Angew. Chem., Int. Ed., 2019, 58, 6708–6712 CrossRef CAS PubMed;
(g) G. Liao, H.-M. Chen, Y.-N. Xia, B. Li, Q.-J. Yao and B.-F. Shi, Angew. Chem., Int. Ed., 2019, 58, 11464–11468 CrossRef CAS PubMed;
(h) G. Liao, Q.-J. Yao, Z.-Z. Zhang, Y.-J. Wu, D.-Y. Huang and B.-F. Shi, Angew. Chem., Int. Ed., 2018, 57, 3661–3665 CrossRef CAS PubMed;
(i) G. Liao, B. Li, H.-M. Chen, Q.-J. Yao, Y.-N. Xia, J. Luo and B.-F. Shi, Angew. Chem., Int. Ed., 2018, 57, 17151–17155 CrossRef CAS PubMed;
(j) Q. Dherbassy, J.-P. Djukic, J. Wencel-Delord and F. Colobert, Angew. Chem., Int. Ed., 2018, 57, 4668–4672 CrossRef CAS PubMed;
(k) Q.-J. Yao, S. Zhang, B.-B. Zhan and B.-F. Shi, Angew. Chem., Int. Ed., 2017, 56, 6617–6621 CrossRef CAS PubMed;
(l) J. Zheng, W.-J. Cui, C. Zheng and S.-L. You, J. Am. Chem. Soc., 2016, 138, 5242–5245 CrossRef CAS PubMed;
(m) Q. Dherbassy, G. Schwertz, M. Chessé, C. K. Hazra, J. Wencel-Delord and F. Colobert, Chem.–Eur. J., 2016, 22, 1735–1743 CrossRef CAS PubMed;
(n) J. Zheng and S.-L. You, Angew. Chem., Int. Ed., 2014, 53, 13244–13247 CrossRef CAS PubMed;
(o) C. K. Hazra, Q. Dherbassy, J. Wencel-Delord and F. Colobert, Angew. Chem., Int. Ed., 2014, 53, 13871–13875 CrossRef CAS PubMed;
(p) T. Wesch, F. R. Leroux and F. Colobert, Adv. Synth. Catal., 2013, 355, 2139–2144 CrossRef CAS.
-
(a) J. K. Cheng, S.-H. Xiang, S. Li, L. Ye and B. Tan, Chem. Rev., 2021, 121, 4805–4902 CrossRef CAS PubMed;
(b) A. Link and C. Sparr, Chem. Soc. Rev., 2018, 47, 3804–3815 RSC;
(c) B. Zilate, A. Castrogiovanni and C. Sparr, ACS Catal., 2018, 8, 2981–2988 CrossRef CAS;
(d) J. Wencel-Delord and F. Colobert, Synthesis, 2016, 48, 2981–2996 CrossRef CAS.
- J. Zhang, Q. Xu, J. Wu, J. Fan and M. Xie, Org. Lett., 2019, 21, 6361–6365 CrossRef CAS PubMed.
-
(a) Y.-J. Wu, P.-P. Xie, G. Zhou, Q.-J. Yao, X. Hong and B.-F. Shi, Chem. Sci., 2021, 12, 9391–9397 RSC;
(b) Q.-J. Yao, P.-P. Xie, Y.-J. Wu, Y.-L. Feng, M.-Y. Teng, X. Hong and B.-F. Shi, J. Am. Chem. Soc., 2020, 142, 18266–18276 CrossRef CAS PubMed;
(c) S. Zhang, Q.-J. Yao, G. Liao, X. Li, H. Li, H.-M. Chen, X. Hong and B.-F. Shi, ACS Catal., 2019, 9, 1956–1961 CrossRef CAS.
-
(a) L. Ackermann, Acc. Chem. Res., 2020, 53, 84–104 CrossRef CAS PubMed;
(b) L. Ackermann, Acc. Chem. Res., 2014, 47, 281–295 CrossRef CAS PubMed.
-
M. R. Maddani, J.-C. Fiaud and H. B. Kagan, in Separation of Enantiomers: Synthetic Methods ed. M. Todd, Wiley-VCH, Weinheim, 2014, pp. 13–74 Search PubMed.
-
(a) G. Liao, T. Zhang, Z.-K. Lin and B.-F. Shi, Angew. Chem., Int. Ed., 2020, 59, 19773–19786 CrossRef CAS PubMed;
(b) P. Gandeepan and L. Ackermann, Chem, 2018, 4, 199–222 CrossRef CAS;
(c) Q. Zhao, T. Poisson, X. Pannecoucke and T. Besset, Synthesis, 2017, 49, 4808–4826 CrossRef CAS.
-
(a) T. Rogge, N. Kaplaneris, N. Chatani, J. Kim, S. Chang, B. Punji, L. L. Schafer, D. G. Musaev, J. Wencel-Delord, C. A. Roberts, R. Sarpong, Z. E. Wilson, M. A. Brimble, M. J. Johansson and L. Ackermann, Nat. Rev. Dis. Primers, 2021, 1, 43 CrossRef;
(b) U. Dhawa, N. Kaplaneris and L. Ackermann, Org. Chem. Front., 2021, 8, 4886–4913 RSC;
(c) S. Rej, Y. Ano and N. Chatani, Chem. Rev., 2020, 120, 1788–1887 CrossRef CAS PubMed;
(d) A. Dey, S. K. Sinha, T. K. Achar and D. Maiti, Angew. Chem., Int. Ed., 2019, 58, 10820–10843 CrossRef CAS PubMed;
(e) Y. Park, Y. Kim and S. Chang, Chem. Rev., 2017, 117, 9247–9301 CrossRef CAS PubMed;
(f) J. He, M. Wasa, K. S. L. Chan, Q. Shao and J.-Q. Yu, Chem. Rev., 2017, 117, 8754–8786 CrossRef CAS PubMed;
(g) S. Agasti, A. Dey and D. Maiti, Chem. Commun., 2017, 53, 6544–6556 RSC;
(h) O. Daugulis, J. Roane and L. D. Tran, Acc. Chem. Res., 2015, 48, 1053–1064 CrossRef CAS PubMed;
(i) G. Rouquet and N. Chatani, Angew. Chem., Int. Ed., 2013, 52, 11726–11743 CrossRef CAS PubMed;
(j) D. A. Colby, A. S. Tsai, R. G. Bergman and J. A. Ellman, Acc. Chem. Res., 2012, 45, 814–825 CrossRef CAS PubMed;
(k) L. Ackermann, R. Vicente and A. R. Kapdi, Angew. Chem., Int. Ed., 2009, 48, 9792–9826 CrossRef CAS PubMed.
- For detailed information, see supporting information†.
-
(a) E. M. Sletten and C. R. Bertozzi, Angew. Chem., Int. Ed., 2009, 48, 6974–6998 CrossRef CAS PubMed;
(b) C. Peifer, T. Stoiber, E. Unger, F. Totzke, C. Schächtele, D. Marmé, R. Brenk, G. Klebe, D. Schollmeyer and G. Dannhardt, J. Med. Chem., 2006, 49, 1271–1281 CrossRef CAS PubMed;
(c) P. Sellès, Org. Lett., 2005, 7, 605–608 CrossRef PubMed.
-
(a) E. Caldeweyher, S. Ehlert, A. Hansen, H. Neugebauer, S. Spicher, C. Bannwarth and S. Grimme, J. Chem. Phys., 2019, 150, 154122 CrossRef PubMed;
(b) S. Grimme, S. Ehrlich and L. Goerigk, J. Comput. Chem., 2011, 32, 1456–1465 CrossRef CAS PubMed;
(c) S. Grimme, J. Antony, S. Ehrlich and H. Krieg, J. Chem. Phys., 2010, 132, 154104 CrossRef PubMed;
(d) F. Weigend, Phys. Chem. Chem. Phys., 2006, 8, 1057–1065 RSC;
(e) Y. Zhao and D. G. Truhlar, J. Phys. Chem. A, 2005, 109, 5656–5667 CrossRef CAS PubMed;
(f) F. Weigend and R. Ahlrichs, Phys. Chem. Chem. Phys., 2005, 7, 3297–3305 RSC;
(g) J. M. L. Martin and A. Sundermann, J. Chem. Phys., 2001, 114, 3408–3420 CrossRef CAS;
(h) M. Ernzerhof and G. E. Scuseria, J. Chem. Phys., 1999, 110, 5029–5036 CrossRef CAS;
(i) C. Adamo and V. Barone, J. Chem. Phys., 1999, 110, 6158–6170 CrossRef CAS;
(j) A. Schäfer, C. Huber and R. Ahlrichs, J. Chem. Phys., 1994, 100, 5829–5835 CrossRef;
(k) A. Schäfer, H. Horn and R. Ahlrichs, J. Chem. Phys., 1992, 97, 2571–2577 CrossRef;
(l) M. Dolg, U. Wedig, H. Stoll and H. Preuss, J. Chem. Phys., 1987, 86, 866–872 CrossRef CAS.
-
(a) T. Furuya, A. S. Kamlet and T. Ritter, Nature, 2011, 473, 470–477 CrossRef CAS PubMed;
(b) K. Müller, C. Faeh and F. Diederich, Science, 2007, 317, 1881–1886 CrossRef PubMed.
-
(a) S. Dutta, T. Bhattacharya, D. B. Werz and D. Maiti, Chem, 2021, 7, 555–605 CrossRef CAS;
(b) U. Dhawa, C. Tian, W. Li and L. Ackermann, ACS Catal., 2020, 10, 6457–6462 CrossRef CAS;
(c) A. Baccalini, S. Vergura, P. Dolui, S. Maiti, S. Dutta, S. Maity, F. F. Khan, G. K. Lahiri, G. Zanoni and D. Maiti, Org. Lett., 2019, 21, 8842–8846 CrossRef CAS PubMed;
(d) T. K. Achar, X. Zhang, R. Mondal, M. S. Shanavas, S. Maiti, S. Maity, N. Pal, R. S. Paton and D. Maiti, Angew. Chem., Int. Ed., 2019, 58, 10353–10360 CrossRef CAS PubMed;
(e) T. Yamaguchi, Y. Kommagalla, Y. Aihara and N. Chatani, Chem. Commun., 2016, 52, 10129–10132 RSC;
(f) S. Maity, P. Dolui, R. Kancherla and D. Maiti, Chem. Sci., 2017, 8, 5181–5185 RSC;
(g) S. Maity, R. Kancherla, U. Dhawa, E. Hoque, S. Pimparkar and D. Maiti, ACS Catal., 2016, 6, 5493–5499 CrossRef CAS;
(h) G. Cera, T. Haven and L. Ackermann, Angew. Chem., Int. Ed., 2016, 55, 1484–1488 CrossRef CAS PubMed.
- B.-B. Zhan, Z.-S. Jia, J. Luo, L. Jin, X.-F. Lin and B.-F. Shi, Org. Lett., 2020, 22, 9693–9698 CrossRef CAS PubMed.
- C. Amatore, C. Cammoun and A. Jutand, Adv. Synth. Catal., 2007, 349, 292–296 CrossRef CAS.
Footnotes |
† Electronic supplementary information (ESI) available. CCDC 2090275. For ESI and crystallographic data in CIF or other electronic format see DOI: 10.1039/d1sc04687j |
‡ These authors contributed equally to this work. |
|
This journal is © The Royal Society of Chemistry 2021 |