A cerium-based MOFzyme with multi-enzyme-like activity for the disruption and inhibition of fungal recolonization†
Received
4th April 2020
, Accepted 16th June 2020
First published on 27th July 2020
Abstract
A cerium-based metal–organic framework (Ce-MOF, denoted as AU-1) was synthesized using a solvothermal method by employing 4,4′,4′′-nitrilotribenzoic acid (H3NTB) as the linker and cerium clusters as the metal center. The material was considered as a MOFzyme based on the peroxidase-like activity of Ce-MOF that could eliminate and kill fungi, such as Aspergillus flavus, Aspergillus niger, Aspergillus terreus, Candida albicans, and Rhodotorula glutinis. Ce-MOF showed high antifungal activity against airborne opportunistic human pathogens isolated from the outside of a hospital. The antifungal activity of CeMOF was evaluated using the colony-forming units, dry mass method, soluble proteins, and microscopic imaging. It exhibited an inhibition efficiency of 93.3–99.3% based on the colony-forming unit method. The Ce-MOF caused extensive deformation of the conidiophores, vesicles, and phialides with growth inhibition between 7.55–77.41% (based on the dry mass method). Ce-MOF showed different efficiencies in inhibiting the different fungal species. The biological activity of Ce-MOF was due to its enzymatic activity, such as those of antioxidants, catalase, superoxide dismutase, and peroxidase. Ce-MOF exhibited excellent enzymatic activity towards the fungal cells. Our results may facilitate the design of a MOFzyme system and pave the way for more profound applications of nanozymes.
Introduction
Metal–organic frameworks (MOFs)1,2 are fascinating porous materials with unique properties compared with other families of porous materials.3–12 They have several advanced applications,13–21 including MOF-based nanozymes (MOFzymes). MOFzymes are promising for applications, such as sensing and cancer therapy,22 and biomimetic catalysts.3 MOFzymes demonstrate significant advantages, such as facile synthesis procedure, low price, long-term storage stability, and high environmental stability.23 Several MOFs, including cerium-based MOFs, have been reported with enzymatic properties. It has been reported that ligand metal charge transfer (LMCT) is favorable only in Ce-based MOFs.4 A Ce-based MOF has been reported as an enzyme with deoxyribonuclease (DNase)- and peroxidase-mimetic activities that could combat biofilms.24 Cerium (Ce)-doped zeolitic imidazolate framework-8 (ZIF-8) showed simultaneous antibacterial and anti-inflammatory capabilities.25 A mixed-valence Ce-MOF (Ce-BPyDC) was applied for ascorbic acid (AA) detection using a sensitive and selective colorimetric method.26
Fungi are a heterogeneous group of microorganisms, which inhabit various environments, such as plants, debris, soils, rivers, lakes, seas and even extreme environments.27 They release their spores into the air and thus can be called as airborne fungi.28 Inhalation of hyphal fragments, spores, and by-products of fungi by human leads to serious respiratory problems, and may cause asthma.29 The airborne fungal spores pose a huge risk to human health, especially immunocompromised individuals and allergy-prone people,29 especially hospitalised patients, who are very susceptible to the infections caused by the spores.30Aspergillus is a well-known opportunistic human pathogen associated with aspergillosis, allergy, asthma, rhinitis, and conjunctivitis.31Aspergillus strains, such as Aspergillus flavus, have been reported as causal agents of aspergillosis, keratitis, otitis, invasive sinusitis, and other systemic infections.32 Yeasts, such as Candida albicans, colonize the skin and gastrointestinal tract of human as opportunistic pathogens.33 Nanotechnology has been employed in several advanced applications, including the development of antimicrobial agents.34–41 Nanoparticles exhibit high potential in the treatment of gastrointestinal tract42 and as antimicrobial materials against resistant microbial infections.
Herein, a new cerium-based MOF, AU-1 (referring to Assiut University-1), was synthesized using a solvothermal method. The crystallinity and phase purity of the synthesized material was confirmed using X-ray diffraction (XRD). The chemical bonds and coordination within the frame were verified via Fourier transform infrared spectroscopy (FT-IR) and X-ray photoelectron spectroscopy (XPS). The morphology and particle size were estimated using transmission electron microscopy (TEM), high-resolution TEM (HR-TEM), and scanning electron microscopy (SEM). According to the thermal analysis performed using thermogravimetric analysis (TGA) and differential thermal analysis (DTA), AU-1 exhibited high thermal stability. The Ce-MOF showed enzyme-mimicking activities and offered promising biological activity against airborne opportunistic fungi. It caused deformation and oxidative stress in the investigated fungal cells.
Materials and methods
Aluminum chloride anhydrous (AlCl3), triphenylamine, CH2Cl2, Na2SO4, 1,4-dioxane, bromine, cerium nitrate, and sodium hydroxide were purchased from Sigma-Aldrich (Germany). All chemical reagents were used without purification.
Synthesis of organic linker
The synthesis of the organic linker is systematically presented in Fig. 1a. The procedure involved two steps.
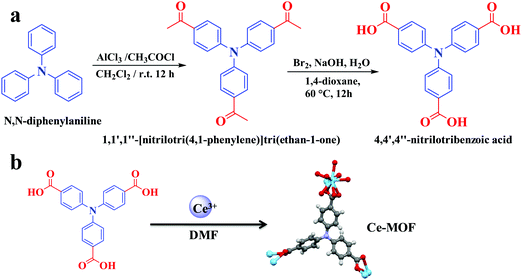 |
| Fig. 1 Schematic representation of the synthesis of the (a) organic linker and (b) Ce-MOF AU-1. | |
Synthesis of 4,4′,4′′-Triacetyltriphenylamine (1,1′,1′′-[nitrilotri(4,1-phenylene)]tri(ethan-1-one)).
A solution of aluminum chloride anhydrous (AlCl3, 3.6 g, 26.9 mmol) was prepared in dry CH2Cl2 (150 mL). The solution was cooled down to 273 K and stirred for 20 min. A solution of triphenylamine (2.0 g (8.1 mmol) was dissolved in 30 mL of dry CH2Cl2) was added dropwise to the solution of AlCl3. The color of the solution turned deep blue. After that, a solution of acetyl chloride (prepared via mixing 3.9 mL (56.2 mmol) in 30 mL of dry CH2Cl2) was added dropwise at 273 K. Then, the reaction mixture was stirred for 12 h at room temperature. The aqueous layer was extracted with CH2Cl2 (3 × 150 mL) and brine. The organic layers were dried over anhydrous Na2SO4. The solution was concentrated under reduced pressure to give the crude product, which was purified using flash column chromatography on silica gel with hexane/CH2Cl2 (1
:
10) as the eluent. The purified material was obtained a yellow solid with a yield of 92% (2.8 g). 1H NMR (400 MHz, CDCl3) chemical shift (δ) 7.90 ppm (d, 6H, J = 8 Hz), 7.14 ppm (d, 6H, J = 8 Hz), and 2.58 ppm (s, 9H) (Fig. S1, ESI†). 13C NMR (100 MHz, CDCl3) δ: 196.5 ppm, 150.3 ppm, 132.8 ppm, 130.1 ppm, 123.9 ppm, and 26.4 ppm (Fig. S2, ESI†).
4,4′,4′′-nitrilotribenzoic acid (H3NTB).
A solution of 4,4′,4′′-triacetyltriphenylamine (1.7 g, 4.7 mmol) was prepared in 1,4-dioxane (50 mL). The solution was cooled down on an ice bath. Bromine (2.4 mL, 47.2 mmol) was added dropwise to a solution of NaOH (6.2 g, 156.0 mmol) in H2O (30 mL) cooled on an ice bath with stirring for 20 min and then added dropwise to the above solution. The reaction mixture was stirred at room temperature for 12 h at 60 °C. Then, the mixture was cooled on an ice bath. A saturated solution of hydroxylamine hydrochloride was added. The solution was acidified with HCl. A solid precipitate was filtered and dried under vacuum to afford the pure product as a yellowish-white solid. Proton nuclear magnetic resonance (1H NMR, 400 MHz, DMSO-d6) chemical shifts (δ) of 12.8 ppm (bs, 3H), 7.92 ppm (d, 6H, J = 8 Hz), and 7.16 ppm (d, 6H, J = 8 Hz, Fig. S3, ESI†). 13C NMR (100 MHz, DMSO-d6) δ: 167.2 ppm, 150.3 ppm, 131.6 ppm, 126.4 ppm, 124.2 ppm (Fig. S4, ESI†).
Synthesis of the cerium-MOF
The cerium MOF, AU-1, was synthesized using a solvothermal method (Fig. 1b). Ce(NO3)3·6H2O (0.14 g) and 4,4′,4′′-nitrilotribenzoic acid (H3NTB, 0.12 g) were dissolved in a mixture of DMF (10 mL), H2O (1 mL), and HCl (0.1 M, 2 mL) in a sealed glass vial (Fig. 1b). The solution was sonicated for 20 min. Then, it was heated at 85 °C for 16 h. A yellow crystalline powder was obtained using filtration. The product was characterized using XRD before and after washing with DMF and ethanol (3 × 25 mL).
Characterization instruments
X-ray diffraction (XRD) was recorded using Phillips 1700 X’Pert (Cu Kα radiation). The structure solution and simulation of the XRD pattern were carried out using EXPO2014 and the diffraction-crystal module of the mercury (Hg) program version 3.1, which is available free of charge (http://www.ccdc.cam.ac.uk). The X-ray photoelectron spectra (XPS) were recorded using a Thermo Fisher (K-alpha) instrument with monochromated micro-focused Al Kα radiation (1486.6 eV). The C1s peak at 284.4 eV was used as the reference. The transmission electron microscopy (TEM) and high-resolution TEM (HR-TEM) images were recorded using TEM-2100 (JEOL, Japan, operated at accelerating voltage 200 kV). Scanning electron microscopy (SEM) was performed using JSM-6010LV (JEOL, Japan). The Ce-MOF sample was coated with Pt-Pd using a JEC-3000FC auto fine coater (JEOL, Japan). Fourier transform infrared spectroscopy (FT-IR) was carried out using a Nicolet spectrophotometer (model 6700). Thermogravimetric analysis (TGA) and differential thermal analysis (DTA) were performed using a TA 60 thermal analyzer (Shimadzu, Japan) at a heating rate of 10 °C min−1 and an airflow rate of 30 mL min−1. The proton (1H) and carbon (13C) analyses of the organic linker were conducted on Bruker 400 MHz instruments using the residual signals for CDCl3 at chemical shifts (δ) of 7.26 ppm and 77.0 ppm, and for DMSO-d6 at δ = 2.50 ppm and 39.4 ppm.
Microorganisms and inoculum preparation
Aspergillus flavus (Link, Af-10), Aspergillus niger (van Tieghem, An-14), Aspergillus terreus (Thom, At-40), Candida albicans (Robin, Berkhout, Ca-6) and Rhodotorula glutinis (Fresenius, Harrison, Ro-39) were isolated from the air spores present outside of a public hospital in the Assiut Governorate using Czapek's dextrose agar medium supplemented with chloramphenicol (250 mg mL−1) as the bacteriostatic. The climatic conditions were 17.2 km h−1 wind speed and 36% relative humidity with the minimum and maximum temperatures of 22 °C and 26.7 °C, respectively. The plates containing the medium were maintained open for 10 min outside the hospital in different places, then transferred directly to the laboratory and incubated at 28 ± 1 °C in aerobic conditions for 7 days. The developed isolates were identified and stored at 4 ± 1 °C on potato dextrose agar (PDA) slants.43 Before the experiment, the microbial inoculums were prepared via scraping of 5 and 3 days for Aspergillus sp. and C. Albicans or R. glutinis, respectively. The cells were cultured on PDA and suspended in sterilized distilled water fortified with 0.1% Triton X100 to at a concentration of 2 × 105 spore mL−1.
The effect of AU-1 on the growth of opportunistic pathogenic fungi
The Czapek's broth medium (g L−1) was prepared using glucose (30.0 g), KH2PO4 (1.0 g), yeast extract (5.0 g), MgSO4·7H2O (0.5 g), NaNO3 (3.0 g), KCl (0.5 g), FeSO4·7H2O (0.01 g) and distilled water (1 L) and autoclaved for 20 min at a pressure of 1.5 atm and a temperature of 121 °C. Chloramphenicol sterilized using a 0.22 mm membrane filter was added as a bacteriostatic. AU-1 (0, 10, 20, 30 and 40 μg mL−1) was tested in Czapek's broth (50 mL). The inoculums (1%, v/v) were added and incubated at 28 ± 1 °C in a rotary shaker (150 rpm) for 7 days. After fungal growth, the Aspergillus biomass was filtered using Whatman filter paper and washed twice with distilled water for drying at 70 °C in a hot air oven for dry mass estimation.44 For yeast, the biomass was collected using centrifugation (4000 rpm) for 10 min. The harvested biomass was then washed with distilled water (2 × 50 mL) to remove residues. The experiments were repeated at least three times to ensure repeatability and reproducibility.
The effect of AU-1 on the morphology of pathogenic fungi
All the cultures at different concentrations were examined using a microscope (Olympus CX41, Japan) for changes in cell morphology. Typically, samples from the growing cultures were trapped using a needle, stained with lactophenol cotton blue, and directly subjected to the microscopic imaging. The effect of the amount of Ce-MOF on the viability of the spores at the highest Ce-MOF concentration (40 μg mL−1) was recorded by inoculating the spore suspension from the Czapek's broth culture in sterilized Czapek's agar medium and determining the viability and ability of these spores to grow again. Meanwhile, the total counts of the yeast cells and fungal spores were determined via microbial counting on a hemocytometer.
Oxidative stress generated by AU-1 on the opportunistic fungi
The culture flasks containing AU-1 and inoculums were prepared in the broth medium, as described previously. Afterward, the biomass was collected using filtration (for Aspergillus) or centrifugation (for C. Albicans, and R. glutinis). The separated biomass was washed with double distilled water (3 × 50 mL) and disrupted mechanically via grinding in a cold mortar containing liquid nitrogen to ensure complete cellular breakage. The extracted cells were treated using potassium phosphate buffer supplemented with ethylenediaminetetraacetic acid (EDTA) and polyvinyl pyrrolidone (PVP, pH = 7.8). The microbial extracts were centrifuged at 4 °C (18
000 rpm, 10 min). The supernatants were collected for the following biological tests: total antioxidant activity (TA), soluble proteins (SP), superoxide dismutase (SOD), catalase (CAT), and peroxidase (POD).45
Analysis of enzymatic activity
The total antioxidants were estimated using the phosphomolybdenum method at 695 nm. The results were calculated as mg g−1 soluble protein using a standard curve of ascorbic acid.46 The soluble proteins were measured using the Folin reagent method (colorimetric method using absorbance at 750 nm). The amount of soluble protein (mg g−1) was calculated using a standard curve of bovine serum albumin. Superoxide dismutase (EC: 1.15.1.1) was assayed using the epinephrine autoxidation method at 480 nm for 1 min. Catalase (EC: 1.11.1.6) was evaluated via the measurement of hydrogen peroxide consumption for 1 min at 240 nm. Peroxidase (EC: 1.11.1.7) was determined using guaiacol (1.5 mM) at 470 for 1 min. All colorimetric assays were performed using a T60 UV spectrophotometer split beam.
The effect of H2O2 on the UV-Vis absorption of Ce-MOF was recorded using FLAME-S-UV-VIS (200-850 nm, Rochester, NY 14607 USA). Ce-MOF (8 mg) was dispersed in deionized water (8 mL) using ultrasonication. Hydrogen peroxide (0.05 mL) was added. A UV-cuvette was filled with 4 mL of the solution and the UV-Vis spectra of the solution were recorded over time (0–80 min).
Results and discussion
Material characterization
The solvothermal reaction between cerium nitrate and the tritopic carboxylate linker H3NTB (Fig. 1a) in DMF formed a Ce-MOF, namely AU-1 (Fig. 1b). The XRD of the as-synthesized Ce-MOF contained a strong diffraction Bragg's peak at 2θ around 25.3°, which was attributable to the linker H3NTB molecules residing inside the pores (Fig. S5, ESI†). The XRD pattern of the purified and activated frameworks showed no peak for the occluded linker molecules (Fig. 2). The synthesized crystals were small-sized, making it very difficult to directly determine their crystal structure using single-crystal diffraction measurements. Therefore, we relied on powder X-ray diffraction (PXRD) analysis to elucidate the Ce-MOF structure (Fig. 2). Based on the data, the AU-1 structure was first indexed to the trigonal R32 space group.47AU-1 crystallized in the hexagonal space group P63/mcm with the following cell parameters: lengths – a = 24.803 Å and c = 13.33 Å and angles – α = β = 90° and γ = 120°.47
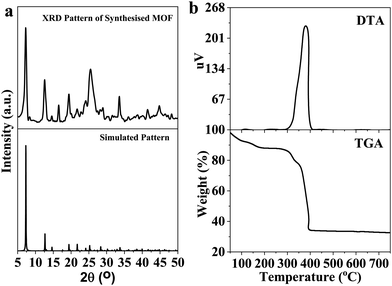 |
| Fig. 2 (a) Simulated XRD and experimental XRD patterns of AU-1, and (b) thermal analysis using TGA and DTA. Note that the low resolution of the lab X-ray data precluded the use of Rietveld refinement to determine the atomic coordinates further accurately. | |
The elemental composition and chemical oxidation state of the Ce-MOF was investigated using XPS spectra (Fig. 3). The XPS survey spectrum showed peaks related to the elements Ce, N, C, and O (Fig. 3a). The analysis of the C1s region showed peaks at the binding energies of 284.2 eV, 285.7 eV, and 287.9 eV corresponding to C–C, C–O–C, and C–C
O, respectively (Fig. 3b). The spectrum of N1s showed a peak at the binding energy of 399.5 eV for C–N (Fig. 3c). The analysis of the O1s spectrum showed peaks at the binding energies of 531.1 eV and 533.1 eV representing C
O and C–O, respectively (Fig. 3d). The XPS spectra of Ce3d indicated the presence of a mixed-valence state (Ce3+/Ce4+) in the Ce-MOF, and the ratio of Ce4+/Ce3+ was found to be 6% (Fig. 3e).48 The peaks at the binding energy values of 881.5 eV, 886.9 eV, 900.0 eV, and 912.3 eV were associated with Ce4+, while the peaks at 885.0 eV and 903.7 eV were associated with Ce3+.48 The XPS analysis confirmed the successful formation of Ce-MOF (Fig. 3f).
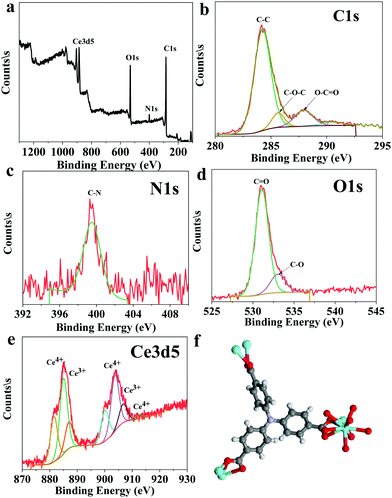 |
| Fig. 3 XPS analysis of the Ce-MOF: (a) element survey, (b) C1s, (c) N1s, (d) O1s, (e) Ce3d5, and (f) the chemical structure of the Ce-MOF. | |
The FT-IR spectra of the organic linker H3NTB and AU-1 confirmed the formation of coordination bonds within the framework (Fig. S6, ESI†). The FT-IR spectrum of H3NTB showed a strong vibrational peak at the wavenumber of 1693 cm−1 referring to the carbonyl group C
O (Fig. S7, ESI†). After coordination with Ce3+, the organic linker exhibited strong absorption peaks at wavenumbers 1595 cm−1 and 1410 cm−1 corresponding to the asymmetric and symmetric stretching vibrations of COO− (Fig. S7, ESI†). The results from the FT-IR analysis confirmed the complete activation of the compound (Fig. S7, ESI†). AU-1 had a hexagonal nanorod morphology, as confirmed by the TEM image (Fig. S8, ESI†). The material weakly diffracted in electron diffraction (Fig. S9, ESI†). The morphology of the Ce-MOF was confirmed using SEM images (Fig. S10, ESI†). The SEM images showed rods with a hexagonal morphology at the end (Fig. S10, ESI†).
To investigate the thermal stability of AU-1, TGA was performed in the range of 25–700 °C under an air atmosphere (Fig. 2b). It could be concluded that the material (empirical formula, C21H12CeNO7, and molecular weight 530.44 g mol−1) was stable up to 390 °C. The first weight loss (40–183 °C, 12 wt%) occurred because of the removal of the guest molecules. In the temperature range of 300–330 °C, the second weight loss took place due to the removal of the occluded DMF molecules. Above the temperature of 370 °C, the material started to decompose due to the loss of the framework linker. The residual value 32.2% corresponded to CeO2 (calculated value = 32.5%). The TGA curve confirmed the chemical formula of AU-1 (C21H12CeNO7). The differential thermal analysis (DTA) showed a major exothermic band at 380 °C corresponding to linker volatilization (Fig. 2b).
Biological activity of AU-1
Antifungal activity of AU-1: disruption and inhibiting recolonization of fungi.
The antifungal properties of the Ce-MOF were investigated against five fungal pathogens, namely Candida albicans, Aspergillus niger, Aspergillus flavus, Aspergillus terreus, Candida albicans, and Rhodotorula glutinis. C. Albicans is a dimorphic fungus i.e. forms both yeast and filamentous cells. A. niger is a filamentous Ascomycete fungus that can infect humans and plants and cause food contamination or fermentation. It can be found in bakery products, cheeses, and preserved fruits. A. flavus is a pathogenic fungus that can colonize cereal grains, legumes, and tree nuts. A. terreus is a mold that can be found in soil. Both A. flavus and A. terreus are saprotrophic fungi. R. glutinis colonizes foods, animals, and environment-based materials. All these fungi are spread worldwide. Thus, they were used as models to investigate the antifungal activity of the Ce-MOF, AU-1.
The antifungal activity of AU-1 against A. niger, A. flavus, A. terreus, C. Albicans and R. glutinis is presented in Fig. 4, 5 and Fig. S11–S13 (ESI†). The bioactivity of AU-1 was evaluated based on dry mass (Fig. 4a), soluble proteins (SP, Fig. 4b), microscopic images (Fig. 5), and colony-forming units (CFU, Fig. S11–S13, ESI†). The activities were measured using different concentrations of AU-1 (0–40 μg mL−1).
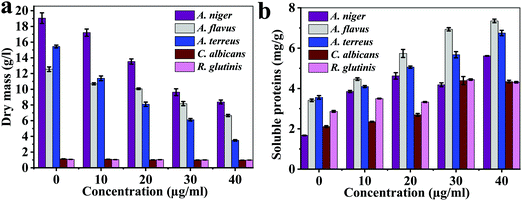 |
| Fig. 4 (a) Dry mass and (b) soluble proteins of the fungi upon treatment with different concentrations of AU-1 (0–40 μg mL−1). | |
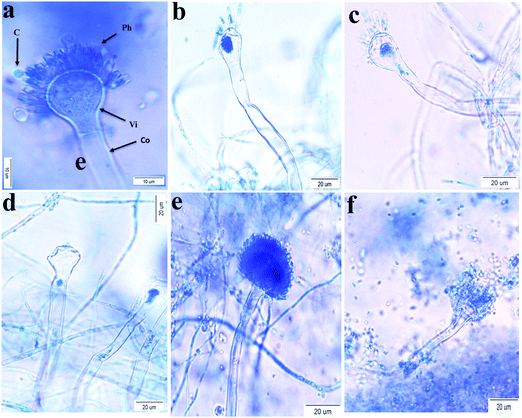 |
| Fig. 5 Microscopic images of A. flavus in (a) the medium and (b–d) after incubation with AU-1 (10, 20, and 40 μg mL−1, respectively); images of A. terreus in (e) the medium and (f) after incubation with AU-1 (40 μg mL−1). Co – conidiophore; Vi – vesicle; Ph – phialid; C – conidia. | |
The dry mass of fungi upon incubation with AU-1 is reported (Fig. 4a). The dry mass decreased with an increase in the AU-1 concentration (Fig. 4a). The dry mass of A. flavus (12.55 ± 0.39 g L−1), A. niger (19.05 ± 0.68 g L−1), A. terreus (15.45 ± 0.17 g L−1), C. Albicans (1.13 ± 0.02 g L−1), and R. glutinis (1.06 ± 0.03 g L−1) decreased to 6.65 ± 0.14 g L−1, 8.38 ± 0.24 g L−1, 3.49 ± 0.09 g L−1, 0.97 ± 0.05 g L−1, and 0.98 ± 0.02 g L−1, respectively (Fig. 4a). The change in dry mass was higher for A. flavus, A. niger, and A. terreus, compared to C. Albicans and R. glutinis (Fig. 4a). The decrease in dry mass was due to the antifungal activity of Ce-MOF, which inhibited fungal growth and disrupted their components. AU-1 showed high antifungal activity and inhibited the growth of the pathogenic fungi with inhibition efficiencies between 7.55–77.41% (Fig. 4a).
The soluble proteins of the fungi after treatment with different concentrations of AU-1 were estimated (Fig. 4b). The amount of soluble proteins (mg g−1 fresh weight) increased with an increase in the concentration of AU-1 (Fig. 4b). The maximum amount of soluble proteins was observed at the highest concentration of AU-1, indicating the disruption of fungal cells due to the oxidative stress generated by the Ce-MOF (Fig. 4b). Thus, the amount of soluble proteins is increased upon treatment with Ce-MOF (Fig. 4b).
The effect of the concentration (0–40 μg mL−1) of AU-1 on the morphology of pathogenic fungi was tested (Fig. 5). There was no dramatic change in the morphology of A. niger, C. Albicans and R. glutinis at all concentrations. On the other hand, changes in the morphology of A. flavus and A. terreus were observed at a high concentration of AU-1 (40 μg mL−1, Fig. 5) in the conidiophores, vesicles, and phialides. These morphological changes confirmed the disruption of cell wall and release of content due to the toxic effect of high concentrations of the Ce-MOF. These changes also verified the alterations in the physiology and metabolic activities of the cells. These fungal deformations were attributed to the toxic effects at high concentrations due to the accumulation of reactive oxygen species (ROS) in the cells, which may lead to cell death.
The colony-forming units (CFU) of the fungi were also used to evaluate the cytotoxicity of the Ce-MOF (Fig. S11–S13, ESI†). The spores generated after the treatment with Ce-MOF were still viable and could grow again in the medium (Fig. S11, ESI†). However, the total counts of the spores and yeast cells decreased dramatically compared with those from the non-treated samples (Fig. S13, ESI†). Data analysis for A. flavus, A. niger, A. terreus, C. Albicans, and R. glutinis showed the efficiencies of 93.3%, 96.3%, 99.3%, 93.3%, and 96%, respectively (Fig. S13, ESI†). The CFU method revealed that the Ce-MOF exhibited high antifungal activity (Fig. S13, ESI†), which varied between the different fungi due to their properties. However, the 6% difference in efficiency among them was insignificant, indicating that the Ce-MOF is a broad-spectrum antifungal agent (Fig. S13, ESI†).
Enzymatic activity of AU-1 against fungi: mechanism of antifungal activity.
The antifungal activity of AU-1 could be due to the well-known enzymatic activity of cerium-based nanomaterials. Thus, the enzymatic activity of AU-1 was recorded via measuring the total antioxidant (TA), catalase (CAT), superoxide dismutase (SOD), and peroxidase (POD) activities (Fig. 6a–d).
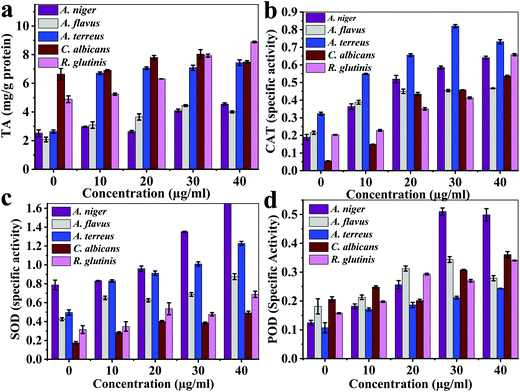 |
| Fig. 6 The multi-enzymatic activity of the Ce-MOF: (a) total antioxidant activity, (b) catalase enzyme, (c) superoxide dismutase enzyme, and (d) peroxidase enzyme. | |
The total antioxidant values (μg g−1 protein) reached the maximum of 4.44 ± 0.06 (30 μg mL−1) for A. flavus, 4.55 ± 0.09 (40 μg mL−1) for A. niger, 7.34 ± 0.19 (40 μg mL−1) for A. terreus, 8.03 ± 0.31 (30 μg mL−1) for C. Albicans, 8.88 ± 0.05 (40 μg mL−1) for R. glutinis, which were much lower compared with those of the control samples: 2.08 ± 0.16 mg g−1, 2.53 ± 0.22 mg g−1, 2.63 ± 0.11 mg g−1, 6.64 ± 0.35 mg g−1, and 4.88 ± 0.24 mg g−1 protein, respectively (Fig. 6a). The maximum catalase enzyme (CAT) values were 0.46 ± 0.002, 0.64 ± 0.008, 0.82 ± 0.009, 0.54 ± 0.004, and 0.66 ± 0.006 specific units for A. flavus, A. niger, A. terreus, C. Albicans, and R. glutinis, respectively (Fig. 6b). The maximum superoxide dismutase enzyme (SOD) specific activity values were 0.88 ± 0.03, 1.68 ± 0.01, 1.23 ± 0.21, 0.49 ± 0.01, and 0.69 ± 0.03 for A. flavus, A. niger, A. terreus, C. Albicans, and R. glutinis, respectively (Fig. 6c). The maximum peroxidase enzyme (POD) activity of AU-1 values were 0.34 ± 0.01, 0.51 ± 0.01, 0.24 ± 0.001, 0.36 ± 0.01, and 0.34 ± 0.001 U g−1 protein for A. flavus, A. niger, A. terreus, C. Albicans, and R. glutinis, respectively (Fig. 6d). Thus, AU-1 generated oxidative stress in the fungal cells and further caused generation of reactive oxygen species (ROS), which damaged the cell membrane.
There is no single mechanism that can support the redox regeneration mechanism of a Ce-MOF, such as AU-1.49 However, X-ray photoelectron spectroscopy (XPS) revealed the presence of both Ce3+ and Ce4+ ions in the framework of AU-1.50 It has been reported that cerium-based materials with a high ratio of Ce3+/Ce4+ exhibited better SOD activity.51,52 We also observed the formation of H2O2, which is one of the products of SOD-catalyzed reactions. The cerium center with two oxidation states (Ce4+ and Ce3+) was intrinsically formed during the synthesis of AU-1. A mechanism explaining the efficiency of AU-1 is proposed in Fig. 7 without involving the auto-regeneration of Ce3+ on AU-1.
 |
| Fig. 7 Superoxide dismutation in the AU-1 framework. | |
To support the antioxidant activity, the UV-Vis absorption spectra of Ce-MOF without and with a mild oxidant e.g. hydrogen peroxide (H2O2) were recorded (Fig. 8). The Ce-MOF solution displayed absorption at wavelengths 246 nm and 356 nm corresponding to π–π* and n–π*, respectively (Fig. 8a).53,54 The absorption intensities of both peaks increased with time (Fig. 8b). The increment in the absorbance was due to the oxidation of the Ce nodes upon interaction with H2O2. The absorbance intensity reached a steady-state within a very short time (10 min). This observation indicated the fast response of the Ce-MOF toward the oxidant, namely H2O2 (Fig. 8b).
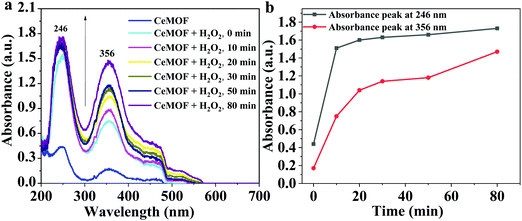 |
| Fig. 8 (a) UV-Vis absorption spectra of Ce-MOF without and with H2O2 over time, and (b) the absorbance intensities at wavelengths 246 nm and 356 nm. | |
MOFs are effective antifungal agents (Table 1). Copper-based MOFs, such as Cu-BTC (BTC = 1,3,5-benzenetricarboxylate)55 and HKUST-1,56 have been reported as antifungal agents against C. Albicans and Saccharomyces cerevisiae, respectively (Table 1). Cu-BTC could inhibit the spores of C. Albicans, Fusarium oxysporum, and Aspergillus oryzae up to 100% and 30% (for F. oxysporum, A. oryzae), without significant activity against A. niger even after 7 days of treatment (Table 1).55 The antifungal activity of Co(II)-coordination polymers (Co-CPs) containing glutarates and bipyridyl ligands was shown to be the highest at an optimum concentration of 2.0 mg mL−1 (Table 1).57 In this study, the reported inhibition rates were 44–62% and 90–99.98% for A. niger and C. Albicans (Table 1).57 The antifungal activity of Co-MOF was due to the generation of reactive nitrogen oxide species (NO) and H2O2. The Ce-MOF exhibited high activity at a comparatively low concentration of 40 μg mL−1.57 Voriconazole-incorporated zinc 2-methylimidazolates frameworks (V-ZIF) exhibited high penetration of C. Albicans biofilms with an antifungal efficiency of 100% (Table 1).58 MOF-based antifungal agents are promising and offer several advantages, such as high efficiency, low agent loading, and the possibility to be modified with other agents, such as nanoparticles (Table 1). AU-1 showed enzyme-like activities, including peroxidase-like activity, and could kill fungi with 93.3–99.3% efficiency (Table 1). Thus, it could prevent the recolonization of the fungi. The cerium-based MOF exhibited excellent ROS-scavenging activity and mimicked superoxide dismutase and catalase.59 Thus, it can be used further for biomedical applications, such as biocatalysis, biosensing, and drug delivery.
Table 1 A summary of MOF-based nanomaterials as antifungal agents
Materials |
Synthesis |
Microorganism |
Efficiency |
Optimal conc. |
Ref. |
Notes: Glu, glutarate; bpy, 4,4′-bipyridine; bpe, 1,2-bis(4-pyridyl)ethylene; bpymh, N,N′-bis((pyridine-4-ylmethylene)hydrazine). |
[Co2(Glu)2(μ-bpa)2]·(H2O)4, [Co4(Glu)4(μ-bpp)2], and [Co2(Glu)2(μ-bpe)2]·(H2O)0.5 |
Solvothermal synthesis, 100 °C for 72 h |
Candida albicans and Aspergillus niger |
44–62% (C. albicans) |
2.0 mg mL−1 |
57
|
90–99.98% (A. niger) |
Cu-BTC |
Solvothermal synthesis, 110 °C for 24 h |
C. Albicans, F. oxysporum, A. oryzae |
100% (C. albicans) |
500 ppm |
55
|
30% (F. oxysporum and A. oryzae) |
V-ZIF |
Stirred at ambient temperature for 30 min |
C. albicans
|
100% |
0.78 μg mL−1 |
58
|
CeMOF, AU-1 |
Solvothermal synthesis, 85 °C for 16 h |
Aspergillus flavus, Aspergillus niger, Aspergillus terreus, Candida albicans, and Rhodotorula glutinis |
93.3–99.3% |
40 μg mL−1 |
This study |
Conclusions
The solvothermal synthesis of a Ce-MOF, AU-1, was achieved using a tri-topic carboxylic linker and cerium as the metal center. The material had a crystalline phase with good thermal stability up to 380 °C. It contained Ce in mixed-valence states (Ce4+ and Ce3+). The antifungal activity of AU-1 was evaluated using different bioanalytical methods, including dry mass, soluble proteins, microscopic images, and colony-forming units. The data revealed high species-dependent antifungal activity of the Ce-MOF. The antifungal activity of the Ce-MOF increased with an increase in material concentration. AU-1 possessed good enzymatic activities, such as superoxide dismutation, catalase, and peroxidase, and may be useful as a new anticancer, antioxidant, and antimicrobial platform for other biomedical applications. This work promotes the application of MOFs in nanomedicine and biomaterials.
Conflicts of interest
There are no conflicts to declare.
Acknowledgements
The authors thank the Ministry of Higher Education and Scientific Research (Egypt), Assiut University, and Institutional Review Board (IRB) of the Faculty of Science at Assiut University, Egypt for support.
References
- H.-C. Zhou, J. R. Long and O. M. Yaghi, Chem. Rev., 2012, 112, 673–674 CrossRef CAS.
- J. Lee, O. K. Farha, J. Roberts, K. A. Scheidt, S. T. Nguyen and J. T. Hupp, Chem. Soc. Rev., 2009, 38, 1450 RSC.
- Z.-Y. Gu, J. Park, A. Raiff, Z. Wei and H.-C. Zhou, ChemCatChem, 2014, 6, 67–75 CrossRef CAS.
- X.-P. Wu, L. Gagliardi and D. G. Truhlar, J. Am. Chem. Soc., 2018, 140, 7904–7912 CrossRef CAS.
- H. N. Abdelhamid, Mater. Today Chem., 2020, 15, 100222 CrossRef CAS.
- A. A. Kassem, H. N. Abdelhamid, D. M. Fouad and S. A. Ibrahim, Int. J. Hydrogen Energy, 2019, 44, 31230–31238 CrossRef CAS.
- K.-J. Kim, H.-J. Kim, H.-G. Park, C.-H. Hwang, C. Sung, K.-S. Jang, S.-H. Park, B.-G. Kim, Y.-K. Lee, Y.-H. Yang, J. H. Jeong and Y.-G. Kim, Sci. Rep., 2016, 6, 24489 CrossRef CAS.
- H. N. Abdelhamid, Z. Huang, A. M. El-Zohry, H. Zheng and X. Zou, Inorg. Chem., 2017, 56, 9139–9146 CrossRef CAS.
- H. N. Abdelhamid, Microchim. Acta, 2018, 185, 200 CrossRef.
- H. E. Emam, H. N. Abdelhamid and R. M. Abdelhameed, Dyes Pigm., 2018, 159, 491–498 CrossRef CAS.
- M. N. Goda, H. N. Abdelhamid and A. E.-A. A. Said, ACS Appl. Mater. Interfaces, 2020, 12, 646–653 CrossRef CAS PubMed.
- A. A. Kassem, H. N. Abdelhamid, D. M. Fouad and S. A. Ibrahim, Microporous Mesoporous Mater., 2020, 305, 110340 CrossRef CAS.
- H. N. Abdelhamid, A. M. El-Zohry, J. Cong, T. Thersleff, M. Karlsson, L. Kloo and X. Zou, R. Soc. Open Sci., 2019, 6, 190723 CrossRef.
- L. Valencia and H. N. Abdelhamid, Carbohydr. Polym., 2019, 213, 338–345 CrossRef CAS.
- H. N. Abdelhamid, M. Wilk-Kozubek, A. M. El-Zohry, A. Bermejo Gómez, A. Valiente, B. Martín-Matute, A.-V. Mudring and X. Zou, Microporous Mesoporous Mater., 2019, 279, 400–406 CrossRef CAS.
- A. F. Abdel-Magied, H. N. Abdelhamid, R. M. Ashour, X. Zou and K. Forsberg, Microporous Mesoporous Mater., 2019, 278, 175–184 CrossRef CAS.
- S. Sultan, H. N. Abdelhamid, X. Zou and A. P. Mathew, Adv. Funct. Mater., 2018, 1805372 Search PubMed.
- H. N. Abdelhamid, M. Dowaidar and Ü. Langel, Microporous Mesoporous Mater., 2020, 110200 CrossRef CAS.
- H. N. Abdelhamid, Macromol. Chem. Phys., 2020, 221, 2000031 CrossRef CAS.
- H. N. Abdelhamid, M. Dowaidar, M. Hällbrink and Ü. Langel, Microporous Mesoporous Mater., 2020, 300, 110173 CrossRef CAS.
- H. N. Abdelhamid, Dalton Trans., 2020, 49, 4416–4424 RSC.
- X. Zhang, G. Li, D. Wu, X. Li, N. Hu, J. Chen, G. Chen and Y. Wu, Biosens. Bioelectron., 2019, 137, 178–198 CrossRef CAS.
- W. Song, B. Zhao, C. Wang, Y. Ozaki and X. Lu, J. Mater. Chem. B, 2019, 7, 850–875 RSC.
- Z. Liu, F. Wang, J. Ren and X. Qu, Biomaterials, 2019, 208, 21–31 CrossRef CAS PubMed.
- X. Li, M. Qi, C. Li, B. Dong, J. Wang, M. D. Weir, S. Imazato, L. Du, C. D. Lynch, L. Xu, Y. Zhou, L. Wang and H. H. K. Xu, J. Mater. Chem. B, 2019, 7, 6955–6971 RSC.
- L. Luo, L. Huang, X. Liu, W. Zhang, X. Yao, L. Dou, X. Zhang, Y. Nian, J. Sun and J. Wang, Inorg. Chem., 2019, 58, 11382–11388 CrossRef CAS.
-
C. J. Alexopoulos, C. W. Mims and M. Blackwell, Introductory Mycology, Wiley, New York, 4th edn, 1996, p. 868 Search PubMed.
- The Fifth Kingdom, http://www.mycolog.com/fifthtoc.html, (accessed 8 December 2019).
- H. Ogawa, M. Fujimura, Y. Takeuchi and K. Makimura, Clin. Exp. Allergy, 2012, 42, 1540–1541 CrossRef CAS.
- S. N. Baxi, J. M. Portnoy, D. Larenas-Linnemann, W. Phipatanakul, C. Barnes, S. Baxi, C. Grimes, W. E. Horner, K. Kennedy, D. Larenas-Linnemann, E. Levetin, J. D. Miller, W. Phipatanakul, J. M. Portnoy, J. Scott and P. B. Williams, J. Allergy Clin. Immunol. Pract., 2016, 4, 396–404 CrossRef.
- C. J. Schwab and D. C. Straus, Adv. Appl. Microbiol., 2004, 55, 215–238 CAS.
- M. T. Hedayati, A. C. Pasqualotto, P. A. Warn, P. Bowyer and D. W. Denning, Microbiology, 2007, 153, 1677–1692 CrossRef CAS PubMed.
- J. M. Achkar and B. C. Fries, Clin. Microbiol. Rev., 2010, 23, 253–273 CrossRef.
- M. Ramasamy and J. Lee, BioMed Res. Int., 2016, 2016, 1–17 CrossRef PubMed.
- P. Zhu, L. Zhou, Y. Song, L. Cai, M. Ji, J. Wang, G. Ruan and J. Chen, J. Mater. Chem. B, 2020, 8, 4899–4907, 10.1039/D0TB00106F.
- D. M. O’Brien, C. Vallieres, C. Alexander, S. M. Howdle, R. A. Stockman and S. V. Avery, J. Mater. Chem. B, 2019, 7, 5222–5229 RSC.
- I. Franco Castillo, E. García Guillén, J. M. de la Fuente, F. Silva and S. G. Mitchell, J. Mater. Chem. B, 2019, 7, 6412–6419 RSC.
- Y. N. Albayaty, N. Thomas, P. D. Ramírez-García, T. P. Davis, J. F. Quinn, M. R. Whittaker and C. A. Prestidge, J. Mater. Chem. B, 2020, 8, 1672–1681 RSC.
- H. N. Abdelhamid, Future Microbiol., 2020, fmb-2019-0260 Search PubMed.
-
H. N. Abdelhamid, Nanotoxicity, Elsevier, 2020, pp. 195–214 Search PubMed.
- S. Kumaran, H. N. Abdelhamid, N. Hasan and H.-F. Wu, Nanosci. Nanotechnol., 2020, 10, 80–85 CAS.
- Z. Liu, J. Liu, R. Wang, Y. Du, J. Ren and X. Qu, Biomaterials, 2015, 56, 206–218 CrossRef CAS.
- G. Abd-Elmonsef Mahmoud, A. S. A. Zidan, A. A. M. Aly, H. K. Mosbah and A. B. M. Ibrahim, Appl. Organomet. Chem., 2019, 33, e4740 CrossRef.
- N. A. Nafady, M. M. K. Bagy, M. H. Abd-Alla, F. M. Morsy and G. A.-E. Mahmoud, Rend. Lincei, 2015, 26, 335–344 CrossRef.
- S. Espín, E. Martínez-López, P. Jiménez, P. María-Mojica and A. J. García-Fernández, Environ. Res., 2014, 129, 59–68 CrossRef PubMed.
- P. Prieto, M. Pineda and M. Aguilar, Anal. Biochem., 1999, 269, 337–341 CrossRef CAS PubMed.
- Q. Yao, A. Bermejo Gómez, J. Su, V. Pascanu, Y. Yun, H. Zheng, H. Chen, L. Liu, H. N. Abdelhamid, B. Martín-Matute and X. Zou, Chem. Mater., 2015, 27, 5332–5339 CrossRef CAS.
- M. Das, S. Patil, N. Bhargava, J.-F. Kang, L. M. Riedel, S. Seal and J. J. Hickman, Biomaterials, 2007, 28, 1918–1925 CrossRef CAS PubMed.
- C. Xu and X. Qu, NPG Asia Mater., 2014, 6, e90 CrossRef CAS.
- R. Dalapati, B. Sakthivel, M. K. Ghosalya, A. Dhakshinamoorthy and S. Biswas, CrystEngComm, 2017, 19, 5915–5925 RSC.
- C. Korsvik, S. Patil, S. Seal and W. T. Self, Chem. Commun., 2007, 1056 RSC.
- E. G. Heckert, A. S. Karakoti, S. Seal and W. T. Self, Biomaterials, 2008, 29, 2705–2709 CrossRef CAS PubMed.
- H. N. Abdelhamid, A. Bermejo-Gómez, B. Martín-Matute and X. Zou, Microchim. Acta, 2017, 184, 3363–3371 CrossRef CAS.
- Y. Yang, K. Shen, J. Lin, Y. Zhou, Q. Liu, C. Hang, H. N. Abdelhamid, Z. Zhang and H. Chen, RSC Adv., 2016, 6, 45475–45481 RSC.
- S. Bouson, A. Krittayavathananon, N. Phattharasupakun, P. Siwayaprahm and M. Sawangphruk, R. Soc. Open Sci., 2017, 4, 170654 CrossRef.
- C. Chiericatti, J. C. Basilico, M. L. Zapata Basilico and J. M. Zamaro, Microporous Mesoporous Mater., 2012, 162, 60–63 CrossRef CAS.
- H.-C. Kim, S. Mitra, M. Veerana, J.-S. Lim, H.-R. Jeong, G. Park, S. Huh, S.-J. Kim and Y. Kim, Sci. Rep., 2019, 9, 14983 CrossRef.
- L. Su, Y. Li, Y. Liu, R. Ma, Y. Liu, F. Huang, Y. An, Y. Ren, H. C. Mei, H. J. Busscher and L. Shi, Adv. Funct. Mater., 2020, 2000537 CrossRef CAS.
- S. W. Choi and J. Kim, ACS Appl. Nano Mater., 2020, 3, 1043–1062 CrossRef CAS.
Footnote |
† Electronic supplementary information (ESI) available. See DOI: 10.1039/d0tb00894j |
|
This journal is © The Royal Society of Chemistry 2020 |