Survey of green building water systems reveals elevated water age and water quality concerns†
Received
22nd September 2015
, Accepted 5th November 2015
First published on 5th November 2015
Abstract
Widespread adoption of innovative water conservation strategies has potential unintended consequences for aesthetics and public health. A cross-section of green buildings were surveyed and compared to typical conventional buildings in terms of water retention time (i.e., water age), water chemistry, and levels of opportunistic pathogen genetic markers. Water age was estimated to be 2–6.7 months in an off-grid office, an average of 8 days in a Leadership in Environmental Engineering Design certified healthcare suite, and was increased to 2.7 days from 1 day due to installation of a solar “pre-heat” water tank in a net-zero energy house. Chlorine and chloramine residuals were often completely absent in the green building systems, decaying up to 144 times faster in premise plumbing with high water age when compared to distribution system water. Concentration of 16S rRNA and opportunistic pathogen genus level genetic markers were 1–4 orders of magnitude higher in green versus conventional buildings. This study raises concerns with respect to current green water system practices and the importance of considering potential public health impacts in the design of sustainable water systems.
Water impact
Here, we demonstrate that high water age is inherent to some green plumbing designs, and it has the potential to negatively impact the chemical and microbiological quality of drinking water in building plumbing systems. More work is needed to help achieve water conservation goals without compromising water quality or public health.
|
1. Introduction
The number of buildings marketed as “green” is increasing exponentially, with >75
000 certified Leadership in Energy and Environmental Design (LEED) projects in 2014 versus <7500 in 2010.1 Within 10 years the annual global market for energy efficient building products is predicted to exceed $620 billion, with 40–48% of new non-residential construction classified as green by 2015.2,3 Green potable water systems are operationally defined as those achieving at least a 20% overall decrease in potable water use compared to designs that implement no water conservation/efficiency strategies while also meeting applicable plumbing codes. For instance, LEED offers “points” toward certification for reducing potable water demand by 20–50% through use of water efficient landscapes and low flow fixtures.4 However, consequences to public health that may result from increased stagnation in premise plumbing (i.e., increased premise plumbing water age) due to reduced water demand is a generally overlooked aspect of water conservation.
It is well established that high water age is problematic in main distribution systems, contributing to problems with corrosion, taste and odors, and microbial regrowth.5 High water age in premise plumbing can further amplify such problems and also instigate proliferation of human pathogens.6–9 Synergistic effects can be incurred when both distribution and premise plumbing system water ages are high, as demonstrated in a few copper pitting corrosion case studies.10
Water age is likely to increase in both distribution and premise plumbing systems as water conservation practices are adopted at the community level. One study reported total utility system water demand decreased between 1997 and 2010 in 70% of municipalities surveyed (n = 436), with 15% of those systems decreasing by at least 25% between 2007 and 2010.11 These trends have given rise to concerns that widespread adoption of green building and water conservation practices will create another “conservation conundrum” and further detract from potable water quality in buildings.11–14
Higher water age also has possible implications for opportunistic pathogens in premise plumbing (OPPPs), including Legionella spp. (especially L. pneumophila), Mycobacterium avium complex (non-tuberculosis mycobacteria), Pseudomonas aeruginosa, Acanthamoeba spp., and Naegleria fowleri, which are now the waterborne pathogens of highest concern in the U.S.8,15,16 OPPPs can thrive under conditions created by premise plumbing, including the presence of materials that rapidly react with disinfectants or leach nutrients to water, inherently high plumbing surface area to volume ratios, widely variable flow conditions, numerous dead ends, impaired ability to achieve and maintain thermal targets that discourage microbial growth, and high water age.6–8,17–20 OPPPs are integral members of potable water microbial communities and have complex life cycles, including amplification and enhanced virulence when hosted by free-living amoebae such as Vermamoeba vermiformis.21L. pneumophila causes about 90% of reported premise plumbing related disease outbreaks, with other ecologically-related Legionella spp. also a cause for health concern.7,8,15,22
Only a few prior studies have touched upon the potential for OPPPs to proliferate in green water systems. In two conventional plumbing systems fitted with electronic-eye or hands-free metered faucets, higher colonization rates of Legionella spp. and P. aeruginosa were observed relative to conventional faucets.23,24 Another study showed higher colonization rates in faucets with larger hot and cold mixing volumes.25 In off-grid rainwater buildings, the occurrence of OPPPs, in particular L. pneumophila, has been documented and roof-collected rainwater system contamination has been serologically linked to Legionnaires' disease.26–30 However, prior studies did not investigate or report basic system features expected to influence OPPPs such as storage methods, plumbing materials, water chemistry, water demand, or water treatment in such facilities. Further, pathogen occurrence data is needed to inform quantitative microbial risk assessment models and raise awareness if a health concern is justified.27,31
The purpose of this study was to conduct the first survey of a cross-section of cutting-edge green buildings in order to establish a baseline understanding of water age, water quality characteristics, and design differences relative to conventional buildings. Water systems were characterized in terms of disinfectant residual, disinfectant decay rates, and levels of genetic markers corresponding to total bacteria, Legionella spp., L. pneumophila, M. avium, and V. vermiformis using quantitative polymerase chain reaction (qPCR).
2. Experimental
2.1 Description of field sites
Four buildings with a cross-section of energy and water conservation strategies were surveyed and sampled to explore effects of green plumbing systems on water quality (Table 1). The buildings included a conventional house with no conservation features (Fig. 1a), a healthcare suite (Fig. 1b), a net-zero energy house (Fig. 1c), and a net-zero energy and net-zero water office building (Fig. 1d).
Table 1 Summary of key factors regarding water age in three field sites and a baseline comparison
Parameter |
Healthcare suite |
Net-zero energy house |
Net-zero office |
Conventional home |
Estimated based on discussions with employees and observations made in the field.
Average annual use for American families.
|
Building type |
10 000 sqft LEED-Gold outpatient healthcare facility |
Net-zero energy single family household |
Small net-zero water and net-zero energy office |
Conventional household, no green features |
Total storage volume |
80 gallon water heater |
160 gallons hot water due to solar water heater |
3000 gallon rainwater cistern |
80 gallons hot water |
Approximate demand |
2627 gallons in 2013 (7.2 gallons per day on average) |
26 000 gallons per year (65–80 gallons per day) |
5000–8000 gallons per yeara |
110 000 gallons per yearb (>300 gallons per day) |
Water age estimate |
8 days on average |
Hydraulic retention time = 2.7 days in hot water storage tanks; higher in infrequently used lines |
1–6 months, dependent on rainfall and use |
Hydraulic retention time ~1 day in water heater |
Water system |
Cold line: plug flow hot lines: continuous recirculation |
Plug flow |
Continuously mixed, air–water interface storage |
Plug flow |
Disinfectant Residual |
Chloramine |
Chlorine |
N/A |
Chlorine |
Primary Plumbing Material |
Copper |
Copper to manifold; PEX after manifold |
Copper |
Copper |
In-building treatment |
N/A |
N/A |
20 μm + 5 μm + GAC filter + UV inactivation |
N/A |
Residual (as Cl2) 1st draw |
Cold |
0.01–0.04 mg L−1 |
0.04 mg L−1 |
N/A |
0.71 mg L−1 |
Hot |
0.0–0.03 mg L−1 |
0.02 mg L−1 |
N/A |
0.06 mg L−1 |
Residual (as Cl2) flushed |
Cold |
<0.25 mg L−1 3 min; 1.12 mg L−1 80 min |
0.17 mg L−1 3 min; 1.15 mg L−1 30 min |
N/A |
1.04 mg L−1 3 min |
Hot |
0.02–0.03 mg L−1 3 min |
0.41 mg L−1 5 min; 0.96 mg L−1 50 min |
N/A |
0.06 mg L−1 3 min |
Cause of high water age |
Long service pipe within building, high number of fixtures, infrequent use |
Solar water heater with storage to pre-heat water before heat pump |
Storage in cistern necessary, water age dependent on rainfall, use, and maintenance |
Normal water age |
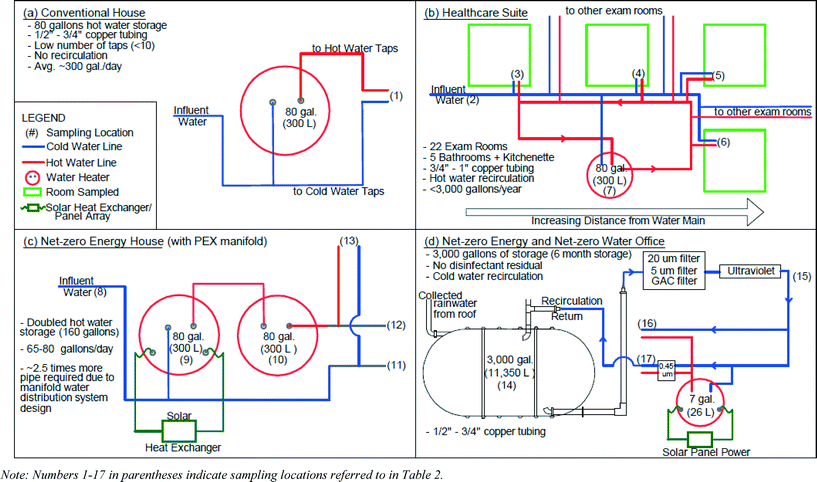 |
| Fig. 1 Plumbing schematics and sampling locations for each field site. | |
The net-zero energy home was an unoccupied single-family dwelling laboratory, simulating typical U.S. residential hot water demand of 245–300 L per day−1 (65–80 gallons per day) to demonstrate the feasibility of achieving net-zero energy in a residential setting. Water was supplied by a public utility with free chlorine residual. A solar-powered water heater (80 gallons) was installed upstream of an electric water heater (80 gallons) to “pre-heat” water and reduce the amount of electricity required by the electric tank. Both hot and cold water plumbing was 3/8′′ diameter flexible cross-linked polyethylene (PEX) tubing, with a maximum pipe volume of 1.1 L (0.3 gallons) to distal taps (equivalent to 12 seconds of flow with a 5.7 L min−1 (1.5 gpm) showerhead). The conventional single family home sampled as a control had 3/4′′ copper plumbing, four residents, an 80 gallon water heater, was served by the same public utility at the net-zero house, and no water conservation features.
The healthcare suite occupied the first floor of a LEED-Gold building. Water was supplied by a public utility with monochloramine residual. The suite had 20 exam rooms, each with a manually operated faucet for hand-washing (≤5.7 L min−1; ≤1.5 gpm flow rate), five bathrooms with electronic faucets (1.9 L min−1; 0.5 gpm flow rate), and one kitchenette sink. The minimum pipe diameters (1/2–1′′) specified in the plumbing code ensured adequate supply capacity for the large number of fixtures, but resulted in a high volume of water storage relative to the demand in this facility, which was essentially limited to bathroom use and hand-washing. All plumbing in this facility was copper. Hot water was supplied to two individual wings of this facility by 80 gallon water heaters.
The small net-zero energy and net-zero water office building collected, treated, and stored rainwater for all potable and non-potable uses. This building minimized water demand by using composting toilets, installing 1.0 gpm fixtures on all taps, and having no outdoor irrigation. Supply lines were 1/2 or 3/4′′ copper pipe and hot water was supplied to individual taps by 7 gallon water heaters.
2.2 Sampling routine
A sampling plan was developed for each field site based on building performance data, investigation of building plumbing blueprints, and field observations. The sampling plan was designed to capture profiles of water quality as it was flushed from taps. In particular, samples collected at each building were representative of the lowest and highest water age (i.e., influent and the portions of the plumbing system that were most distal to the influent water). The net-zero energy house was visited twice: once before the start of the net-zero energy experiment when the water had been relatively stagnant for two months and again after it was used regularly for two months. During the first visit to the net-zero energy house, water was flushed extensively until it was representative of water from the main distribution system, as indicated by steady cold water temperatures and chlorine residuals, but this was not possible during the second visit because of an energy experiment that was then in progress. Therefore, water from three showering events, which were part of the energy study, were sampled instead.
At the healthcare suite, hot and cold water was sampled in four exam rooms at the end of the plumbing system. Starting with the first flush, grab samples were collected until a disinfectant residual consistent with the main distribution system was obtained. Afterwards, the taps were shut off and water was allowed to sit stagnant and low volume samples (~35 mL) were collected at regular intervals during stagnation for physical and chemical parameters.
At the net-zero rainwater office, only stagnant and moderately flushed (3 minutes) water samples were collected from the taps because the facility was not connected to a water main. Therefore, additional flushing would not have a beneficial impact on water quality typically expected in systems connected to water utilities.
2.3 Water quality analysis
Temperature, pH, ammonia, nitrite, and total chlorine were measured in the field at the time of collection using a pH 110-Series meter (Oakton Research, Vernon Hills, Il) or a DR2700 spectrophotometer (Hach, Loveland, CO) according to standard methods. Aliquots were transported to the lab on ice and analyzed using USEPA Method 300.1 for anions. Cations were measured by inductively coupled plasma mass spectrometry after acidification with 2% nitric acid (v/v) and >24 hours holding time. Samples of unacidified water were passed through a 0.45 μm pore size filter in the field to differentiate “soluble” metals and later acidified with 2% nitric acid (% v/v). Biological activity reaction tests (BARTs; Hach, Loveland, CO) indicating the presence/absence of viable nitrifying bacteria were used for select samples.
2.4 Biological sample processing
Water samples were transported on ice and filtered through a 0.22 μm pore-size mixed cellulose ester filters (Millipore, Billerica, MA) within 36 hours of collection. Bottles were pre-dosed with filter-sterilized sodium thiosulfate to remove disinfectant residuals where applicable. Stagnant water samples (first draw) were 250–500 mL while flushed samples (following a three minute flush) were 1 L. FastSpin DNA extraction kits (MP Biomedicals, LLC) were used, and protocols are described elsewhere.31
2.5 Quantitative Polymerase Chain Reaction (qPCR) and statistical analysis
Legionella spp. (Leg 23S rRNA gene), L. pneumophila (macrophage infectivity potentiator (mip) gene), V. vermiformis (18S rRNA gene), M. avium (16S rRNA gene), and total bacteria (16S rRNA gene) were quantified using previously developed and reported methods using primers targeting the gene markers noted parenthetically for each target.31 The optimal dilution for minimizing the effect of PCR inhibitors was identified by performing a dilution series with positive control spikes on a subset of samples. The lowest dilution that yielded consistent quantification was chosen (in this case, 1
:
10). All values are reported as log (gene copies per mL + 1). Samples with detectable but non-quantifiable numbers were recorded as 0.5
log gene copies per mL. Non-parametric Mann–Whitney U-tests were performed on non-transformed qPCR data to identify statistically significant differences in the concentrations of bacterial genes where applicable (p-value < 0.05).
3. Results
This study provided detailed documentation of the potable water systems in three innovative green buildings (Fig. 1). After reviewing the characteristics of each water system and closely examining trends in disinfectant residuals within the buildings, we provide an overview of microbial sampling from each building.
3.1 Survey of building water systems
3.1.1 Water age.
A survey of the three green buildings revealed extremely high water age associated with innovative features intended to conserve water and energy. The net-zero rainwater office building offered on-site storage of up to 11
350 L (3000 gallons) to provide sufficient water through regular periods without rain. Demand was estimated to be 1700 L month−1 (450 gallons per month) during fall/spring and up to 5500 L month−1 (1450 gallons per month) during the summer, resulting in water age ranging from 2–6.7 months. The healthcare suite used only 10.6 L m−2 year−1 (0.26 gallons per ft 2 year−1), which is 60 times lower than typical commercial buildings.32 Very low use at each tap in patient exam rooms, coupled with large diameter pipes stipulated by plumbing code, resulted in an average overall premise plumbing water age of 8 days. At the net-zero energy house, the solar water heater increased the hot water storage and hot water age from <1 day to ~2.7 days. The conventional home used more than 4 times more water than the net-zero energy house studied.
3.1.2 Temperature profiles.
Temperature profiling of the green buildings generally revealed the need for very extensive flushing to draw fresh distribution system water to the point of use. In general, temperatures were in the ideal growth range for OPPPs (35–42 °C).22 In the healthcare facility, with 17 °C water in the distribution main, 70 minutes of continuous cold water flushing was required for water temperatures to drop below maximum cold water recommendations for OPPPs control (20 °C).33 The cold water lines at the net-zero energy house reached lower temperatures much faster (<5 minutes) than the healthcare facility, likely owing to the fact that it is a much smaller building. However, after thoroughly flushing the hot water system, the temperature in the solar “pre-heat” tank did not increase (on a partly cloudy day), whereas the electric water heater downstream recovered to its set point of 49 °C within an hour. Clearly, in the solar water heater there were long periods during which the water temperature was not sufficient to control regrowth (i.e., >55 °C).34 The net-zero rainwater office building was characterized by warm temperatures, even in the cold water, and flushing did not lower the temperature for this building. During the summer sampling, water temperatures were consistently >23 °C, whereas groundwater systems are typically <20 °C.35
3.1.3 In-building treatment at the net-zero rainwater office.
The net-zero rainwater office provided an opportunity to examine the effects of in-building treatment, which is a common strategy for managing water quality in green buildings. During normal operation, rainwater at the net-zero rainwater office was collected and passed through a 20 μm filter, a 5 μm filter, a granular activated carbon (GAC) filter, and then irradiated with ultra violet light at 253 nm at a dose of 30 mJ cm−2. An additional 0.45 μm filter was installed at each potable tap (bathroom and kitchenette sinks). Water was stored in an 11
350 L (3000 gallon) cistern that was recirculated at 19 L min−1 (5 gpm) through the treatment sequence twice a day for two hours (2270 L (600 gallons) recirculated each time). This configuration created a completely mixed water system as opposed to the approximate plug flow that exists in conventional buildings. Twice a year, the water was treated with a high concentration of bleach (>50 ppm) for 24 hours, after which time the water was completely drained and primed with 5680 L (1500 gallons) of groundwater from an on-grid building nearby. Thus, the office building was not truly “net-zero” water, as advertised, due to extensive water demands for maintenance procedures.
3.1.4 Water sources in the net zero office.
Water quality analysis revealed that alkalinity, calcium, and magnesium (~110 ppm as CaCO3, ~12 ppm Mg2+, and ~30 ppm Ca2+) were much higher than expected for rainwater and were actually more representative of the groundwater used for maintenance. This validated the estimation that groundwater, rather than rainwater, made up 38–58% of total water use in the facility. This estimate was based on interviews with occupants and observations made during sampling (Table S1†).
3.2 Disinfectant residual decay
A disinfectant residual was generally not maintained in the plumbing of the green buildings. At the healthcare suite, >80 minutes of flushing was necessary to establish a chloramine residual similar to that present in the main distribution system (Fig. 2a). Taps at these locations are typically used for 15–30 seconds at a time; therefore, it is unlikely that a chloramine residual is ever present in portions of this plumbing system. Approximately 30 minutes of flushing was required at the net-zero energy house to establish a residual in the cold water system during the first site visit. In contrast, the conventional home had a residual of 0.71 mg L−1 chlorine as Cl2 in stagnant cold water. The water heaters at the net-zero house had <0.15 mg L−1 chlorine as Cl2 during a 20 minute flush due to the added storage volume and water age associated with the solar heater (Fig. 1c).
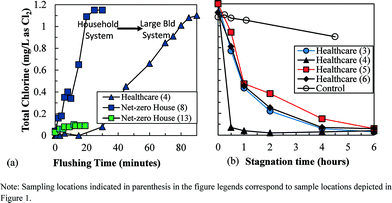 |
| Fig. 2 Total chlorine residual concentrations (a) as a function of stagnation in one room at the healthcare suite (large building system above), a tap near the head of the building at the net-zero house (household system above), and during three showering events at net-zero house, and (b) as a function of stagnation in several rooms at FS#1 (control represents identical water placed in a glass container with no head space). | |
During the second site visit at the net-zero house, influent cold water had a residual of 0.95 mg L−1 as Cl2, indicating enough water was being used to maintain a residual during regular periods of low use such as during the night; however, this did not translate to chlorine residuals in the hot water system or at the point of use. Disinfectant residual never increased during the three showering events monitored (net-zero house – location 13 in Fig. 2a). Assuming the hot water had no residual, the 30 gallons of 40 °C water used in the shower (of approximately 10 gallons of cold water at 20 °C + 20 gallons of hot water at 49 °C) is predicted to have a disinfectant residual of 0.31 mg L−1 as Cl2. Instead, the highest concentration observed was 0.10 mg L−1 as Cl2, which is 68% lower than expected. Therefore, the chlorine residual was either rapidly decaying as it flowed through the short pipes to the shower (via reactions with the pipe material or biofilms) or an instantaneous chlorine demand was present in water from the hot water system.
The healthcare suite also showed evidence of very rapid disinfectant residual decay. In all four exam rooms monitored, the first-order decay rate of chloramine in the plumbing was 20–144 times greater than in well-flushed (>80 minutes) water collected from the same tap and placed into a glass container with no headspace (i.e., “control,” Fig. 2b). The highest rate of decay (sample location 4) was observed in a sink that had been recently replaced, where the newer plumbing material may have been more reactive than older materials.6
3.3 Microbial constituents
16S rRNA genes, V. vermiformis, Legionella spp., and M. avium were detectable at all green building field sites (Table 2). Total bacterial levels, as represented by 16S rRNA genes, ranged from 400 gene copies per mL in well-flushed water at the conventional house to 6.46 × 107 gene copies per mL (5-log higher) in a stagnant cold water sample at the net-zero energy house. V. vermiformis, Legionella spp., and M. avium ranged from below detection in well-flushed samples to 2.57 × 105, 9.33 × 105, and 4.90 × 105 gene copies per mL, respectively, in stagnant or hot water samples. L. pneumophila was detected in nine samples but was never above the quantification limit.
Table 2 Log of qPCR results (log of gene copies per mL) for samples at all field locations
Location |
Sample description |
Sample locationa |
16S rRNA |
V. vermiformis
|
Legionella spp. |
M. avium
|
Sampling location numbers refer to locations depicted in Fig. 1.
Average (and median) of the four exam rooms is reported.
BD = below detection.
BQL = below quantification limit.
|
Healthcare |
Cold water |
Influent |
2 |
4.00 |
BDc |
BD |
BD |
Cold water |
Stagnant |
3–6 |
7.33 (7.08)b |
4.87 (4.30) |
5.20 (5.29) |
BQL |
Flushed |
3–6 |
6.84 (4.25) |
3.59 (1.82) |
4.68 (4.46) |
BQL |
Hot water |
Top of heater |
7 |
4.10 |
BD |
BQL |
BQL |
Bottom of heater |
7 |
3.07 |
0.78 |
3.16 |
3.43 |
Hot water |
Stagnant |
3–6 |
6.74 (6.66) |
4.61 (4.44) |
5.13 (5.00) |
3.22 (3.31) |
Net-zero house |
Influent |
Flushed |
8 |
3.30 |
BD |
BD |
BD |
Solar heater |
Visit #1 |
9 |
5.08 |
BQLd |
3.81 |
2.98 |
Visit #2 |
9 |
4.18 |
BD |
BQL |
BQL |
Water heater |
Visit #1 |
10 |
5.40 |
BQL |
3.54 |
3.11 |
Visit #2 |
10 |
5.03 |
BD |
BQL |
BQL |
Cold manifold |
Stagnant, visit #1 |
11 |
7.81 |
BQL |
4.67 |
BQL |
Stagnant, visit #2 |
11 |
6.68 |
3.44 |
3.73 |
BQL |
Hot manifold |
Stagnant, visit #1 |
12 |
7.59 |
2.88 |
4.30 |
3.16 |
Stagnant, visit #2 |
12 |
7.69 |
BD |
5.97 |
BQL |
Simulated shower |
Stagnant |
13 |
5.44 |
2.86 |
3.11 |
BQL |
Flushed (10 gallons) |
13 |
4.16 |
2.40 |
2.57 |
BQL |
Flushed (30 gallons) |
13 |
3.60 |
BQL |
BQL |
BQL |
Net-zero office |
Cistern (storage) |
N/A |
14 |
6.96 |
2.60 |
4.36 |
2.00 |
Post-treatment |
Flushed 3 min |
15 |
6.36 |
3.43 |
4.26 |
2.61 |
Cold potable |
Stagnant |
17 |
6.77 |
4.49 |
4.58 |
2.73 |
Flushed 3 min |
17 |
6.51 |
3.00 |
3.48 |
BQL |
Hot potable |
Stagnant |
17 |
6.90 |
4.20 |
4.28 |
2.65 |
Flushed 3 min |
17 |
6.52 |
2.26 |
3.34 |
3.32 |
Cold non-potable |
Stagnant |
16 |
6.95 |
2.93 |
3.32 |
3.69 |
Conventional house |
Cold water |
Stagnant |
1 |
5.32 |
BQL |
BQL |
BQL |
Flushed 3 min |
1 |
2.61 |
BD |
BD |
BD |
Hot water |
Stagnant |
1 |
2.91 |
BQL |
BQL |
BQL |
Flushed 3 min |
1 |
2.83 |
BD |
BD |
BD |
3.3.1 Increased OPPP gene marker detection during stagnation.
At all field sites, there were higher total bacterial genetic markers in stagnant samples than flushed samples. The median increase in 16S rRNA gene copies per mL was 2.8
log (p-value 0.007; nstagnant = 18, nflushed = 10). At the healthcare suite, there was also a median increase of 1.64
log in 16S rRNA gene copies per mL in distal hot water sample taps (sample sites 3–6) compared to water collected directly from the water heater (sampling site 7; Table 2), but the number of samples was insufficient to conduct statistical confidence testing.
The effects of prolonged stagnation in newly commissioned plumbing systems are effectively illustrated by comparing the first (prolonged stagnation before regular use) and second (regular use) site visits at the net-zero energy house. Overall levels of bacterial genetic markers decreased by up to 1.13
logs with regular water use at sample locations 9–11, but not location 12 (the hot water manifold). The conventional house receiving the same municipal water with a 2-fold reduction in water age yielded >20× less 16S rRNA gene copies per mL in stagnant cold water samples relative to the net-zero energy house during regular use. In addition, levels of Legionella spp., M. avium and V. vermiformis gene copies in the conventional house were below the quantification limit in stagnant samples and below the detection limit in flushed samples, whereas detectable levels of these genetic markers were frequently present in hot and cold stagnant and flushed shower samples in the net-zero energy house (Table 2). Concentrations of target markers decreased multiple orders of magnitude (1.84–3.11
log gene copies per mL) during the shower event flushing at the net-zero energy house (Table 2). In contrast, changes in median bacterial 16S rRNA genes between stagnant and flushed samples at the net-zero rainwater office were small in magnitude (0.41
log gene copies per mL), indicating that this universal marker for bacteria was more evenly spread throughout the system (sample sites 14–17; Table 2).
3.3.2 Targeted sampling of water heater at the healthcare facility.
The top and bottom of the water heater at the healthcare suite was sampled, but such samples were not accessible at the other facilities. Interestingly, samples from the bottom of the water heater had high concentrations of genetic markers of both M. avium (>2600 gene copies per mL) and Legionella spp. (>1500 gene copies per mL), while concentrations were below the quantification limit in water at the top of the water heater. Although this was not confirmed by sampling in this building, one hypothesis for elevated levels of Legionella spp. and M. avium in the bottom of the water heater is that this environment is conducive to regrowth due to warm (not hot) temperatures and high levels of nutrients.37,38
3.3.3 High prevalence of OPPPs at the net-zero rainwater office.
Quantifiable levels of V. vermiformis and Legionella spp. gene copies were detectable in all samples and M. avium was above the quantification limit in 86% (n = 7) of samples at the net-zero rainwater office. Collectively across the other sites, only 55%, 68%, and 26% (n = 31) of samples were above the quantification limit for gene copies corresponding to V. vermiformis, Legionella spp., and M. avium, respectively.
4. Discussion
This study reveals cause for concern about the public health implications of green building water systems, particularly with respect to potential creation of conditions ideal for the proliferation of OPPPs. Given the rapid expansion of green building construction at a time when OPPPs are now the primary source of waterborne disease outbreaks, fundamental research is needed to guide green building science down a path that protects public health while also conserving water and energy.
4.1 Rapid residual decay
Absence of disinfectant residuals and its rapid decay in green buildings was a major finding of this study. Several factors might have contributed to the high rate of chloramine decay in the healthcare suite in particular, including increased abiotic decay due to elevated temperatures, reactions with cupric ions, plumbing materials, or biofilm and biotic decay due to microbial growth.6,9,39–46 In the healthcare suite, temperatures at cold taps were sometimes elevated beyond room temperature (>5 °C) and there was evidence of low levels of nitrification (Table S2†). Although no direct quantification of biofilms was made, there were sometimes over 10
000 times more 16S rRNA genes in stagnant versus flushed samples, confirming significant levels of microbial activity in the stagnant pipes serving the taps compared to the water main.47
It was previously thought that rapid residual decay is only rarely observed in buildings;40,48 however, these results, in concert with Nguyen et al. (2012), give rise to the concern it may be commonplace in premise plumbing with high water age. Overall, disinfectant residual decay rates in these buildings were 2–16 times greater than reported in previous work in a green plumbing system with a chloramine residual, for which serious problems with lead and consumer complaints of the taste and odor of the water had been reported.6 It is clear that the reputation chloramine has earned in providing a stable residual for main distribution systems does not always extend to building plumbing systems.40
4.2 Total bacterial levels
Levels of 16S rRNA genes in stagnant samples from green buildings were orders of magnitude higher than what is typically observed in premise plumbing. A companion survey of distal taps in conventional residences across two chloramine distribution systems using the same qPCR assay yielded levels that were 2–4 orders of magnitude lower than those measured in the green buildings.31 A study comparing total bacteria via flow cytometry in stagnant to flushed water samples showed up to a 2
log reduction from 8 × 105 cell counts per mL in total bacteria over a 40 L flushing volume in cold water taps.49 The cold water in the conventional home, sampled herein as a control, also harbored about three orders of magnitude less overall 16S rRNA genes than the green buildings. It is apparent that as water age approaches that of conventional buildings overall bacterial regrowth approaches the same low levels. Building designers should be aware of designs that increase water age and water storage, and minimize their effect where possible.
4.3 Occurrence of Legionella markers
The levels of Legionella spp. gene markers detected at all three green building sites were high. The average Legionella spp. level in this work was 8.91 × 104 gene copies per mL compared to an average of 100 (n = 54) and 2.3 × 103 (n = 90) gene copies per mL in samples with quantifiable levels in conventional homes in two distribution systems reported in the companion survey mentioned in section 4.2.31 This trend is expected, given the higher water age and lack of disinfectant residuals in the green buildings compared to the conventional residences (<0.15 mg L−1 as Cl2 in this work compared to >2 mg L−1 as Cl2 in the survey of conventional homes).31
The higher prevalence of Legionella detected in the rainwater system is of particular concern, as only a small number of studies on the presence of OPPPs in rainwater have been conducted.27,50,51 The focus of these studies has been L. pneumophila, and concentrations are typically very low (<10 cells per mL) in comparison to those of Legionella spp. observed in this study. Considering there are up to 25 infectious species of Legionella, and given serologic links of disease outbreak to rainwater cisterns, the potential for rainwater storage to contribute to Legionella infections merits further examination.22,29,30 Extensive colonization of the rainwater system with V. vermiformis is also of concern, as it is an established host for Legionella amplification.21 Even if pathogenic microbes are inactivated by the UV treatment in this facility, the amoeba downstream could serve as a means for re-amplification. It should be noted that UV treatment systems can damage DNA such that it is no-longer detectable by qPCR, but the DNA can sometimes be repaired by surviving bacteria.36
4.4 Temperature and aesthetics
Temperature is also a critical overarching factor that influences the growth rates of microorganisms; for example, Legionella growth is reportedly inhibited below 20 °C and above 52 °C.33 Temperature profiles in buildings can fail to achieve their targeted settings; therefore, characterizing a system's temperature profile is suggested as an informative first step in identifying areas of high risk.20 Risks associated with lower temperature settings and storing warm/hot water must be assessed and balanced with conservation goals, in recognition that there are some necessary compromises at the nexus of public health, sustainable water, and sustainable energy. International plumbing codes have recently put limits on the amount of hot water volume in pipes to distal taps, which might help to reduce water age and associated problems in the future, especially if similar considerations are made for cold water lines as well.52,53 However, smaller diameter pipes have higher surface area to volume ratios, which might create another set of problems relative to biofilm formation and disinfectant residual demand.
Aesthetics can also be a critical factor in the ultimate success of green building water systems. For example, waterless urinals have received poor consumer reviews and, in many cases, are replaced well before reaching their design life.54 Other aesthetic factors could also hinder the success of green building water systems if not considered carefully in advance. In this study, aesthetics of the warm (23–29 °C) ambient temperature of the cold water in the rainwater office could be undesirable to some users who are accustomed to cooler water for drinking, especially compared to the lower temperatures provided from conventional on-demand well systems.55 In addition, there is increased likelihood to develop taste- and odor-causing compounds due to increased water age and microbial growth at elevated temperatures.47,56
4.5 A temporary practical solution and limitations
Regular flushing of water is one practical approach to addressing water quality and public health concerns in green buildings connected to water mains. Prior work in a LEED-Gold certified building with very high water age solved problems with elevated lead and microbial growth by regularly flushing a small volume of water (3 minutes of flushing every 6 hours, <1% of the total daily flow in the green building) to regularly introduce some fresh water with disinfectant into the system.6,57 While flushing and wasting water may be viewed to conflict with the conservation goals of green buildings, it may serve as a temporary solution to maintaining a disinfectant residual within buildings and assist microbial regrowth control. In the prior case study, temporary flushing provided semi-permanent benefits. Consistently maintaining a disinfectant residual is a known master variable governing microbial quality of drinking water and microbes recover quickly after the residual decays.58
Microbial regrowth control in off-grid net-zero water facilities, on the other hand, may prove to be an even greater challenge because simple flushing will not introduce a disinfectant residual from the water main. Intermittent/continuous recirculation in off-grid systems, which would decrease water stagnation but not water age, is not expected to improve water quality since microbes can accumulate and are not as readily washed from the system compared to conventional plug–flow configurations. Increased Legionella spp. growth has been observed in continuously recirculating versus completely stagnant conditions in a controlled pipe loop at room temperature with no disinfectant residual, conditions that are similar to the system layout observed at the net-zero rainwater office.59 Other work also suggests that recirculation can be a determining factor associated with increased detection of Legionella.60 In such circumstances, maintaining higher hot water temperatures and dosing a disinfectant within the building may be necessary to control OPPP regrowth, though there are concerns related to efficacy and practicality of in-building disinfection systems.61–64
5. Conclusions
This work raises concerns with respect to the chemical and microbiological stability of water quality in systems with high water age. The green buildings sampled had exceptionally high water age, and it appears that elevated water age is inherent to achieving sustainability goals of many green building plumbing systems.
The rapid rate of disinfectant loss in buildings with high water age needs to be better understood and addressed. A temporary solution to water quality problems in green buildings connected to drinking water mains involves routine flushing to maintain disinfectant residuals, improve corrosion control, and achieve temperature targets. Long-term solutions include determining if specific water chemistries employed by the utility can retain chemical and microbial stability of water in the distribution and premise plumbing system. Further, research is needed to determine if there are specific premise plumbing devices or plumbing materials that should be avoided.
Conservation strategies employed at each green building created both hot and cold water temperature profiles in plumbing that are conducive to OPPP growth during stagnation. Therefore, strategies for effectively maintaining target temperature profiles in buildings need to be explored. Design of green buildings with water conservation features should minimize overall water age and eliminate unnecessary water storage and should give special attention to avoiding conditions conducive to OPPPs. Determining how severe these anticipated problems are, and how widespread they are likely to become, is a high research priority given the massive investment society is making in green buildings.
Acknowledgements
This research was partially funded by the Water Research Foundation on project 4383 Green Building Design: Water Quality Considerations with project advisory committee members John Consolvo (Philadelphia Water Department), Mike Schock (USEPA), France Lemieux (Health Canada), and Pete Greiner (NSF), as well as the Alfred P. Sloan Foundation Microbiology of the Built Environment program and NSF CBET Award #1336650. The final Water Research Foundation final report can be found at waterrf.org. Any opinions, findings, conclusions, or recommendations expressed here are those of the authors and do not necessarily reflect the views of the sponsors.
References
-
United States Green Build Council (USGBC), Directory–Projects, http://www.usgbc.org/projects (accessed December 2014).
-
Navigant Research, Energy Efficient Buildings: Global Outlook Advanced HVAC, Lighting, Water Efficiency, Building Controls, Energy Management Systems, and Energy Service Companies: Global Market Analysis and Forecasts, 201, http://www.prnewswire.com/news-releases/energy-efficient-buildings-global-outlook-advanced-hvac-lighting-water-efficiency-building-controls-energy-management-systems-and-energy-service-companies-global-market-analysis-and-forecasts-300013046.html (accessed May 19, 2015).
-
McGraw Hill Construction, Green Outlook: Green Trends Driving Growth. McGraw Hill Construction Outlook 2011: Industry Forecasts and Trends, 2011, http://aiacc.org/wp-content/uploads/2011/06/greenoutlook2011.pdf (accessed December 2014).
-
United States Green Build Council (USGBC), Guide to LEED Certification, http://www.usgbc.org/cert-guide (accessed January 2015).
-
United States Environmental Protection Agency (USEPA), Effects of Water Age on Distribution System Water Quality. Office of Ground Water and Drinking Water: Cincinnati, OH, 2002, http://www.epa.gov/ogwdw/disinfection/tcr/pdfs/whitepaper_tcr_waterdistribution.pdf (accessed May 2015).
- C. Nguyen, C. Elfland and M. A. Edwards, Impact of advanced water conservation features and new copper pipe on rapid chloramine decay and microbial regrowth, Water Res., 2012, 46(3), 611–621, DOI:10.1016/j.watres.2011.11.006.
-
A. Pruden, M. A. Edwards and J. O. Falkinham III, Research Needs for Opportunistic Pathogens in Premise Plumbing: Experimental Methodology, Microbial Ecology and Epidemiology. Water Research Foundation Project 4379, Denver, CO, 2012, http://www.waterrf.org/PublicReportLibrary/4379.pdf (accessed September, 2015).
-
National Research Council (NRC), Drinking water distribution systems: assessing and reducing risk, Washington, DC, 2006 Search PubMed.
- K. M. Kelley, A. Stenson, R. Dey and A. J. Whelton, Water Res., 2014, 67, 19–32 CrossRef PubMed.
-
P. Scardina, M. A. Edwards, D. Bosch, G. Loganathan and S. Dwyer, S. Assessment of Non-Uniform Corrosion in Copper Piping, AWWA Research Foundation, Denver, CO, 2008 Search PubMed.
-
J. Hughes, M. Tiger, S. Eskaf, S. I. Berahzer, S. Royster, C. Boyle, D. Batten, P. Brandt and C. Noyes. Defining a Resilient Business Model For Water Utilities. Water Research Foundation Project 4366, Denver, CO, 2014.
-
K. Gordon and W. Olson, Retail Cost Recovery and Rate Design in a Restructure Environment, Technical Report for Edison Electric Institute, Washington, D.C., 2004, http://www.eei.org (accessed May 19, 2015) Search PubMed.
- W. J. Rhoads, A. Pruden, A. Pearce and M. A. Edwards, J. - Am. Water Works Assoc., 2015, 107(4), 50–56, 10.5942/jawwa.2015.107.0058 CrossRef.
-
M. A. Edwards, W. J. Rhoads, A. Pruden, A. Pearce and J. O. Falkinham, III, Green water systems and opportunistic premise plumbing pathogens, Plumbing Engineer, 2014, Available: http://plumbingengineer.com/nov_14/edwards_feature.php (accessed May 2015) Search PubMed.
-
Centers for Disease Control (CDC), Legionellosis -United States, 2000–2009, Morbidity and Mortality Weekly Report, 2011, vol. 60, issue 32, pp. 1083–1086 Search PubMed.
- T. A. Bartrand, J. J. Causey and J. L. Clancy, J. - Am. Water Works Assoc., 2014, 106(10), 418–432 CrossRef.
- C. K. Nguyen, K. A. Powers, M. A. Raetz, J. L. Parks and M. A. Edwards, Water Res., 2011, 45(16), 5302–5312 CrossRef CAS PubMed.
- R. H. Brazeau and M. A. Edwards, Journal of Green Building, 2011, 6(4), 77–95 CrossRef.
-
M. A. Edwards, D. Bosch, G. V. Loganathan and A. M. Dietrich, The future challenge of controlling distribution system water quality and protecting plumbing infrastructure: focusing on consumers, In Proceedings of the International Water Association Leading Edge Conference, Noordwijk, Netherlands, 2003 Search PubMed.
- E. Bédard, S. Fey, D. Charron, C. Lalancette, P. Cantin, P. Dolcé, C. Laferrière, E. Déziel and M. Prévost, Water Res., 2015, 71, 244–256, DOI:10.1016/j.watres.2015.01.006.
- J. M. Thomas and N. J. Ashbolt, Environ. Sci. Technol., 2011, 45, 860–869 CrossRef CAS PubMed.
-
World Health Organization (WHO), Legionella and the Prevention of Legionellosis. ISBN 92 4 156297 8. Available: http://www.who.int/water_sanitation_health/emerging/legionella.pdf (accessed December 19, 2014).
- H. Yapicioglu, T. G. Gokmen, D. Yildizdas, F. Koksal, F. Ozlu, E. Kale-Cekinmez, K. Mert, B. Mutlu, M. Satar, N. Narli and A. Candevir, J. Paediatr. Child Health, 2012, 48(5), 430–434 CrossRef PubMed.
- E. R. Sydnor, G. Bova, A. Gimburg, S. E. Cosgrove, T. M. Perl and L. L. Maragakis, Infect. Control Hosp. Epidemiol., 2012, 33(3), 235–240 CrossRef PubMed.
- D. Charron, E. Bédard, C. Lalancette, C. Laferriere and M. Prevost, Infect. Control Hosp. Epidemiol., 2014, 36(3), 311–319 CrossRef PubMed.
- W. Ahmed, F. Huygens, A. Goonetilleke and T. Gardner, Appl. Environ. Microbiol., 2008, 74(17), 5490–5496 CrossRef CAS PubMed.
- W. Ahmed, A. Viertiz, A. Goonetilleke and T. Gardner, Appl. Environ. Microbiol., 2010, 76(22), 7382–7391, DOI:10.1128/AEM.00944-10.
- H. Albrechtsen, Water Sci. Technol., 2002, 46(6–7), 311–316 CAS.
- G. Simmons, S. Jury, C. Thornley, D. Harte, J. Mohiuddin and M. A. Taylor, Water Res., 2008, 42(6), 1449–1458 CrossRef CAS PubMed.
- W. F. Schlech, G. W. Gorman, M. C. Payne and C. V. Broome, Arch. Intern. Med., 1985, 145, 2076–2079 CrossRef PubMed.
- H. Wang, M. A. Edwards, J. O. Falkinham, III and A. Pruden, Appl. Environ. Microbiol., 2012, 78(17), 6285–6294 CrossRef CAS PubMed.
-
Building Owners and Managers Association Building Environment Standards (BOMA BEst), BOMA BEst Energy and Environment Report – The National Green Building Report, Toronto, ON, 2014. http://www.bomabest.com/wp-content/uploads/BBEER-2014-Full-Report.pdf (accessed December 2014).
- Legionella Control Guideline. American Society for Heating, Refrigeration, and Air-Conditioning Engineers (ASHRAE), Atlanta, GA, 2000 Available: http://legionella.org/publications/non-visible/ashrae-guideline-12-2000/ (accessed December 2014).
- J. Darelid, S. Löfgren and B. E. Malmvall, J. Hosp. Infect., 2002, 50(3), 213–219 CrossRef CAS PubMed.
-
United States Environmental Protection Agency (USEPA), Average Temperature of Shallow Ground Water. U.S. Environmental Protection Agency Ecosystems Research Webpage, 2013. Available: http://www.epa.gov/athens/learn2model/part-two/onsite/ex/jne_henrys_map.html (accessed December 2014).
- C. W. McKinney and A. Pruden, Environ. Sci. Technol., 2012, 46(24), 13393–13400, DOI:10.1021/es303652q.
- M. Alary and J. R. Joly, Appl. Environ. Microbiol., 1991, 57(8), 2360–2367 CAS.
- R. H. Brazeau and M. A. Edwards, Environ. Eng. Sci., 2011, 30(10), 617–627 CrossRef PubMed.
- A. Sathasivan, J. Chiang and P. Nolan, Water Sci. Technol., 2009, 9(5), 493–499 CAS.
- Y. Zhang and M. A. Edwards, J. - Am. Water Works Assoc., 2009, 101(11), 51–62 CAS.
- Y. Zhang, N. Love and M. A. Edwards, Crit. Rev. Environ. Sci. Technol., 2009, 39(3), 153–208 CrossRef CAS.
- P. J. Vikesland, K. Ozekin and R. L. Valentine, Water Res., 2001, 35(7), 1766–1776 CrossRef CAS PubMed.
- D. W. Margerum, L. M. Schurter, J. Hobson and E. E. Moore, Environ. Sci. Technol., 1994, 28(2), 331–337 CrossRef CAS PubMed.
- M. W. LeChevallier, C. D. Lowry and R. G. Lee, J. - Am. Water Works Assoc., 1990, 82(7), 87–99 CAS.
- N. B. Hallam, J. R. West, C. F. Forster and J. Simms, Water Res., 2001, 35(17), 4063–4071 CrossRef CAS PubMed.
-
K. A. Powers, Aging and copper corrosion by-product release: role of common anions, impact of silica and chlorine and Mitigating release in New pipe, Master's Thesis, Virginia Tech, Blacksburg, VA, 2001 Search PubMed.
- K. Lautenschlager, N. Boon, Y. Wang, T. Egli and F. Hammes, Water Res., 2010, 44(17), 4868–4877 CrossRef CAS PubMed.
-
M. A. Edwards, B. Marshall, Y. Zhang and Y. J. Lee, Unintended consequences of chloramine hit home, In proceedings of the Water Environment Federation, 2005, vol. 1, pp. 240–256 Search PubMed.
- P. Lipphaus, F. Hammes, S. Kötzsch, J. Green, S. Gillespie and A. Nocker, Environ. Technol., 2014, 35(5), 620–628 CrossRef CAS PubMed.
- M. Kobayashi, K. Oana and Y. Kawakami, Microbiol. Immunol., 2014, 58, 15–21 CrossRef CAS PubMed.
- W. Ahmed, A. Goonetilleke and T. Gardner, Can. J. Microbiol., 2010, 56, 471–479 CrossRef CAS PubMed.
-
International Code Council, Inc., 2012 International Green Construction Code (IgCC), Club Hills, IL, ISBN: 978-1-60983-059-5 Search PubMed.
-
International Association of Plumbing and Mechanical Officials (IAPMO), 2012 Green Plumbing & Mechanical Code Supplement, 2nd Edition, Ontario, CA, Available: http://www.iapmo.org (accessed May 2015).
-
B. Chambers, Understanding the Selection and Use of Water Related Innovations in Green Buildings, Master's Thesis, Virginia Tech, Blacksburg, VA, December 2013. Available: https://vtechworks.lib.vt.edu/handle/10919/25298 (accessed May 2015) Search PubMed.
- A. J. Whelton and A. M. Dietrich, Water Res., 2004, 38(6), 1604–1614 CrossRef CAS PubMed.
-
J. Malleville and I. Suffet, Identification and Treatment of Tastes and Odours in Drinking Water, American Water Works Association Cooperative Report, AWWA, Denver, CO, 1987 Search PubMed.
- C. Elfland, P. Scardina and M. A. Edwards, J. - Am. Water Works Assoc., 2010, 102(11), 66–76 CAS.
- E. Bédard, D. Charron, C. Lalancette, E. Déziel and M. Prévost, FEMS Microbiol. Lett., 2014, 356, 226–234, DOI:10.1111/1574-6968.12494.
- Z. Liu, Y. E. Lin, J. E. Stout, C. C. Hwang, R. D. Vidic and V. L. Yu, J. Appl. Microbiol., 2006, 101(2), 437–442 CrossRef CAS PubMed.
- M. Pryor, S. Springthorpe, S. Riffard, T. Brooks, Y. Huo, G. Davis and S. A. Sattar, Water Sci. Technol., 2004, 50(1), 83–90 CAS.
- P. W. Muraca, V. L. Yu and A. Goetz, Infect. Control Hosp. Epidemiol., 1990, 11(2), 79–88 CrossRef CAS PubMed.
- Y. Lin, J. Stout, V. L. Yu and R. Vidic, Semin. Respir. Infect., 1998, 13(2), 147–159 CAS.
- B. R. Kim, J. E. Anderson, S. A. Mueller, W. A. Gaines and A. M. Kendall, Water Res., 2002, 36(18), 4433–4444 CrossRef CAS PubMed.
- W. J. Rhoads, A. Pruden and M. A. Edwards, Water Environ. Res., 2014, 86(6), 540–549, DOI:10.2175/106143014X13975035524989.
Footnote |
† Electronic supplementary information (ESI) available. See DOI: 10.1039/c5ew00221d |
|
This journal is © The Royal Society of Chemistry 2016 |