DOI:
10.1039/D4MA00219A
(Review Article)
Mater. Adv., 2024, Advance Article
Electrospun nanofibers based on plant extract bioactive materials as functional additives: possible sources and prospective applications
Received
5th March 2024
, Accepted 23rd August 2024
First published on 10th September 2024
Abstract
Electrospun nanofibers based on plant extracts have garnered increasing interest as valuable bioactive materials for medicinal and packaging applications. This concise review examines recent studies on functional plant extract nanofibers, emphasizing their fabrication techniques, antimicrobial characteristics, and potential applications. Plant extracts having bioactive compounds are generally obtained from diverse natural sources that can be incorporated into electrospinning solutions to develop functional nanofibers with enhanced germicidal activities. Key findings suggest that nanofibers integrated with natural bioactive materials possess adequate antibacterial, antioxidant, anti-inflammatory, and anticancer properties and are considered expedient biocompatible materials for use in biomedical and food packaging. The potential biomedical applications of these nanofibers include wound healing, drug delivery, and tissue engineering owing to their germicidal activity, biodegradability and biocompatibility, while packaging applications leverage antibacterial and food preservation capabilities. However, some constraints, including insolubility of some extracts, insufficient mechanical robustness for electrospinning, and lack of green solvents to mitigate bio-toxicity, have hindered their diversified applications. The current review, therefore, summarizes future research avenues concerning the scope of overcoming the limitations in this burgeoning field. Overall, plant extract functional nanofibers demonstrate their potential for utilization in biomedical and food packaging applications, but more research is needed to scale up production and make these eco-friendly biocomposite materials commercially available.
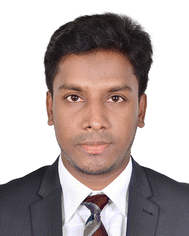 Md Nur Uddin | Md Nur Uddin is currently a PhD student in Fiber and Polymer Science at Wilson College of Textiles at NC State University. In 2023, he earned his MSc in Fiber and Biopolymer from Texas Tech University, where he worked on hemp processing innovations. He obtained his BSc in textile engineering from Dhaka University of Engineering and Technology in 2019. His research interests include nonwovens, electrospinning, biomaterials, nanotechnology, and nanofibers/nanocomposites. Uddin has consistently been writing scholarly works, participating in conference presentations, and fulfilling the role of a peer reviewer for several academic journals. |
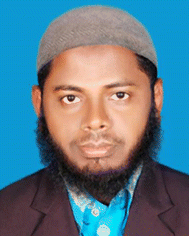 Ayub Ali | Ayub Ali is currently a PhD candidate in the Department of Materials Science and Engineering at UNSW, Sydney, Australia. He is also serving as an Assistant Professor in the Department of Textile Engineering, DUET. In 2014 and 2019, Mr Ali completed his bachelor's and master's, respectively, in the field of textile engineering. At the undergraduate level, Mr Ali achieved several prestigious awards due to his outstanding academic achievements. His key research and publications include the development and applications of electrospun nanofibers in solving various problems. His current research interests include fibrous materials, technical textiles, and waste valorization. |
 Md Jobaer | Md Jobaer is currently serving as a Senior Sub-Assistant Engineer at Bangladesh Railway. He earned his BSc in Electrical and Electronic Engineering from Northern University Bangladesh. His research interests encompass electrospinning, biomedical, and signal management. |
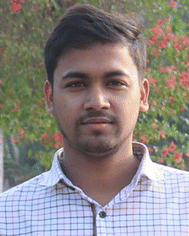 Sajjatul Islam Mahedi | Sajjatul Islam Mahdi is currently enrolled as a third-year student at Eastern Medical College in Comilla, where he is pursuing a Bachelor of Medicine and a Bachelor of Surgery. His research interests encompass electrospun nanofibers, medicine, and medical textiles. |
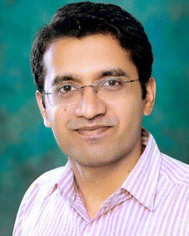 Anand Krishnamoorthy | Dr Anand Krishnamoorthy conducted his research at the Rubber Research Institute of India (RRII) and received his PhD from Cochin University of Science and Technology (CUSAT). He was a Senior Research Fellow in a Rubber Board-funded project on “Nanodispersions in Latex Technology.” Before completing his PhD, he became an Assistant Professor of Chemistry at Amal Jyothi College of Engineering. After six years in academia, he worked as an Executive at Apcotex Industries Limited and Deputy Manager at Primus Gloves Pvt. Ltd. He is now an Associate Professor at Adi Shankara Institute of Engineering and Technology. His research interests include polymer science, rubber technology, and nanotechnology. |
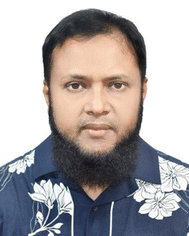 M. A. Rahman Bhuiyan | Dr M A Rahman Bhuiyan is currently working as a Professor in the Department of Textile Engineering at Dhaka University of Engineering & Technology, Gazipur, Bangladesh. Dr Bhuiyan received his PhD from the School of Fashion and Textiles, RMIT University, Australia, specializing in protective clothing systems. His research focuses on performance textiles, fiber-reinforced composites, functional nanofibrous materials, textile coloration, and wastewater management. He also works on sustainable textile processing and analysis of the environmental impact of an apparel life cycle, focusing on the reduction opportunities of the environmental impact associated with apparel manufacturing in developing countries like Bangladesh. |
Introduction
The emergence of electrospinning technology to produce fibrous structures with micro and nano dimensions has drawn significant attention in recent years due to its predominant advantages over other techniques.1 This electrofluidodynamic technique involves the interaction of the polymer solution moving at a constant flow rate with a high-voltage electric field at the tip of the needle that is directly connected to a power supply.2 A significant number of natural and synthetic polymeric materials, including polyamide, polyester, PCL, polyacrylonitrile, chitosan, collagen, starch, PVC, PEO, and PVA, have been used individually and collectively in electrospinning, maintaining the required processing parameters for particular application.3,4
The formation of nano-inscribed biomaterials utilizing this method is a contemporary issue because of their potential to provide professional protection in health care, military personnel, and other day-to-day emergency response applications.5–13 Electrospun nanofibers can also act as effective barriers against microorganisms, particles, and liquids, as well as enhance the mechanical, thermal, and chemical properties of the protective products.14,15 For example, electrospun nanofibers can be used to fabricate masks, respirators, and personal protective equipment (PPE) for medical and healthcare applications. Conventionally, the development of nano biomaterials with various functionalities, including germicidal activity, involves the usage of metal nanoparticles and synthetic antibiotics. However, their detrimental effects on the environment and human health, and/or bacterial resistance issues have triggered a surge in the use of natural antimicrobial compounds which are expected to be non-toxic, sustainable, and less prone to creating resistant bacteria.16,17
Plant extracts, with the advantage of their inherent medicinal properties, have been used for numerous purposes, especially in treating various diseases from time immemorial, and are considered to be the most prominent sources of biomolecules, which can be screened from different parts of a plant, for example, seeds, leaves, stems, flowers, etc.18 With the advent of various solvents and advanced extraction methods, the extraction of such biomolecules from medicinal plants has become more effective in terms of quantity and purity for their use in specific purposes. The commonly used techniques for plant extraction include maceration,18 digestion,19 decoction,20 infusion,21 percolation,22 Soxhlet extraction,23 superficial extraction,24 ultrasound-assisted extraction,25 and microwave-assisted extraction.26 Successful extraction also involves employing various solvents of different polarity, including polar to nonpolar, with a reasonable quantity for appropriate and excellent yields of extracts and biomolecules.27
Several studies have proposed that solvents such as ethyl acetate,28 hexane,29 dichloromethane,30 chloroform,31 acetone,32 ethanol,33 methanol,34 and butanol,35 and/or a combination of solvents in suitable ratios are the best solvent systems for extracting plant extracts. Because of improved biocompatibility, biodegradability, low toxicity, and intrinsically large surface area, nanofibrous mats are currently fabricated by incorporating such medicinal extracts as functional additives for enhanced biological activities and have emerged as novel materials for various biomedical applications, such as wound dressing,36 tissue engineering,37 and drug delivery.38–40 A significant number of research studies are therefore conducted on developing plant extract-based nanofibers to explore their formation, characterization, and potential applications in various fields. For instance, preparation of electrospun nanofibrous mats incorporating Azadirachta indica,41 Curcumin longa,42 chitosan,43 henna,44 Aloe vera,45 moringa,46 sericin,47 lignin,48 honey,49 ginger,50 keratin,51 propolis,52 etc. in combination with various carrier polymers for wound dressing and other biomedical purposes has been rigorously investigated in several contemporary research studies. Increasing efforts towards the process optimization and functionalization of the nanomats are also being made to diversify their use. To date, fewer than 200 experiments have focused on developing plant extract-based nanofibers, a small number compared to the extensive research on electrospinning post-commercialization (Fig. 1a). This figure is expected to grow as interest in sustainable and bioactive materials increases. Fig. 1b illustrates the diverse research interests in electrospinning technology, with a significant emphasis on polymer science (41%), highlighting its foundational role in nanofiber production. The substantial focus on agricultural and food chemistry (18%) and biomedical materials (16%) underscores the potential of electrospun nanofibers in biodegradable packaging and advanced medical applications, respectively. The notable portions dedicated to biochemistry and pharmaceutics (15%) and nanoscience (10%) indicate ongoing efforts to enhance pharmaceutical formulations and understand nanofiber properties at the nanoscale, demonstrating the technology's multidisciplinary impact. The novelty of this paper lies in its comprehensive examination of the integration of plant extracts into electrospinning solutions, highlighting the innovative techniques used for fabricating advanced functional nanofibers and their potential applications in biomedical and packaging fields. Additionally, it provides a detailed discussion of the benefits of green electrospinning as a sustainable alternative to metallic nanoparticles.
 |
| Fig. 1 Published research articles relevant to plant extract-based nanofibers: (a) quantity of papers published based on year and (b) data analyses according to subject area (data obtained from SciFinder, searching for “plant extract nanofibrous”). | |
Considering the current research gap, the purpose of the present review is to compile the existing research on plant extract bioactive materials and their integration into electrospinning solutions for creating functional nanofibers. This review aims to highlight the fabrication techniques, potential applications, and challenges in developing plant extract-based nanofibers, offering insights into overcoming these limitations for future advancements. Besides, the adverse effects of metallic nanoparticles and the importance of green electrospinning, followed by the formation and characterization of functional nanofibers, have been explained meticulously. Various challenges in fabricating plant extract-based nanofibers and potential scopes to overcome the limitations have also been discussed in detail.
Electrospinning fundamentals
Electrospinning is defined as a process of fabricating ultrafine nanofibers (nanometer size) by ejecting a charged polymer solution or melting through a spinneret under a high-voltage electric field, followed by its solidification in the form of filament.53,54 Key steps include preparing the polymer solution, loading it into a syringe, applying high voltage to create a Taylor cone, forming nanofibers as the solution is ejected towards a grounded collector, and collecting the fibers on a non-woven mat.55 Challenges such as controlling fiber diameter and morphology, scalability, and ensuring uniform nanoparticle distribution are crucial considerations. The electrospinning technique was initially invented by J. F. Cooley in 1900, and W. J. Morton improved the design of the electrospinning setup immediately thereafter, in 1902.56,57 Later on, J. Zeleny described the behavior of fluid droplets at the extremity of metal capillaries, and since then, the utilization of needle-equipped spinnerets has become a common practice.58 The work of A. Formhals, who patented 22 improvements of the electrospinning technique between 1931 and 1944, significantly enhanced the field.59–61 This work not only dealt with the process itself but also resulted in an improved apparatus for preparing nanothreads. In 1938, N. Alber, N. D. Rosenblum, and I. Kurchatov promoted the first commercial application of nanofibers.62 They developed filter materials known as “Petryanov filters” from electrospun fibers and were awarded the Stalin Prize for their efforts.58,60,63
The theory behind electrospinning was developed by Sir G. I. Taylor in 1969 and was the first mathematical modeling of electrified fluids.64 He modeled the behavior of fluid droplets under the effect of an electric field and mathematically explained the formation of the cone, later named the Taylor cone.64 In the last 20 years, a number of patents and patent applications have been developed on the topic of electrospinning by large industry players such as Elmarco, Dienes, eSpin Technologies, Nano Technics, KATO, Donaldson Company, Freudenberg Tech, etc. As of now, around fifty thousand (50
000) articles, patents, and books have been published on electrospinning.65
The incorporation of plant extracts into nanofibers presents significant challenges and limitations, primarily due to the potential for variability in extract composition and concentration, which can affect the consistency and reproducibility of the nanofibers' properties. Additionally, the stability of plant extracts during the electrospinning process and their long-term stability within the nanofibers are critical issues. Various electrospinning techniques also pose challenges, including controlling fiber diameter and morphology, scalability for industrial production, and ensuring uniform distribution of nanoparticles within the fibers.66 These limitations necessitate ongoing research and optimization to fully leverage the benefits of plant extract nanofibers.
Classification of electrospinning
The electrospinning process can be classified into four broad types: solvent-free electrospinning, electrospinning from solution, electrospinning from ionic liquids (ILs), and colloid electrospinning, also known as suspension electrospinning. Several techniques of electrospinning are illustrated in Fig. 2 (with needle) and Fig. 3 (needleless). Solvent-free electrospinning is particularly well suited for tissue engineering and wound dressing materials since the produced fibrous materials are suitable for further treatment. However, only a few researchers have paid attention to this solvent-free electrospinning process.67
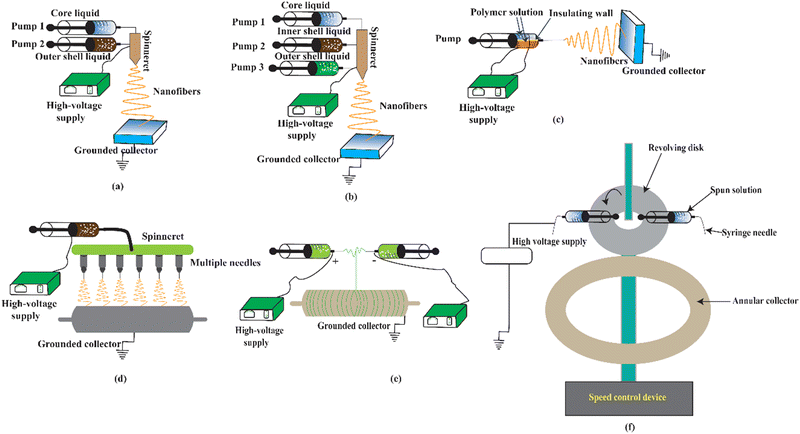 |
| Fig. 2 Schematic diagram of forming nanofibers using different electrospinning techniques with needle: (a) co-axial, (b) tri-axial, (c) side-by-side bi-component, (d) multi-needle based, (e) conjugate, and (f) centrifugal electrospinning. | |
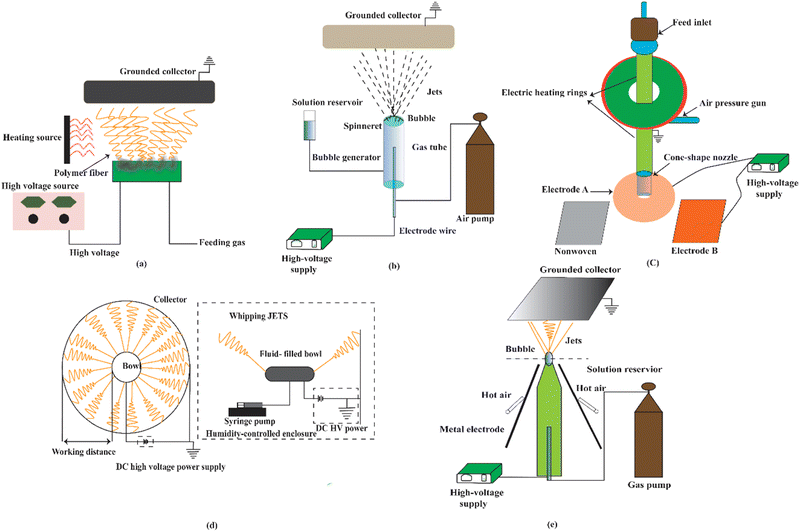 |
| Fig. 3 Schematic diagram of forming nanofibers using different needleless electrospinning techniques: (a) multi-bubble, (b) single-bubble, (c) gas-assisted melt differential, (d) edge, and (e) blown bubble electrospinning. | |
Melt electrospinning, supercritical CO2-assisted electrospinning, anion-curing electrospinning, UV-curing electrospinning, and thermo-curing electrospinning are all examples of solvent-free electrospinning. The most significant benefits of these systems are efficient, controlled, and environmentally friendly processes that produce harmful residue-free ultrafine fiber. As a result, it is very engaged in biomedicine, tissue engineering, and textile engineering. However, because of the high setup requirements due to the precursor's comparatively greater viscosity, large diameter fibers due to no solvent evaporation, and electrical bending instability, this technique is not widely employed.67
Solution electrospinning, the most extensively used method, requires the use of suitable solvents to solubilize the polymers and form homogeneous polymer solutions. It has a number of distinct advantages, including the availability of solvents, an elevated level of interest in biomaterial manufacturing, and the ability to commercialize the technique for bulk nanofiber manufacture.36,68 Because most biopolymers do not dissolve in water, this method of electrospinning requires the use of appropriate solvents. Even though some polymers are dissolvable in water, the surface tension of water might make it difficult to obtain smooth nanofibers. However, this method has notable drawbacks, including challenges related to the dielectric constant, conductivity, and volatility of the solvents used.68
Green solvents, such as ionic liquids (ILs), are an emerging research area in biopolymer dissolution. Environmentally friendly nature, effective dissolving of carbohydrate materials, good thermal stability, low vapor pressure, and variable viscosity are only a few of the benefits of ILs.69–72 The different types of ionic liquids result from how various forces work together, such as coulombic, van der Waals, and hydrogen bonding forces. These bonds and forces allow properties including viscosity, density, solubility, melting temperature, and hydrophobicity to be tailored to fulfill a variety of processing needs.73 These solvents also have the benefit of being able to be separated and recovered fast, unlike traditional solvents.
In addition to the abovementioned electrospinning methods, colloid or suspension electrospinning is a unique method of producing nanofibers. Like the solution electrospinning, this system requires three essential components: application of high voltage, needle, and collector. The fundamental benefit of this technique is that it keeps colloids in fibers immobilized. Colloids in the electrospinning feed, on the other hand, complicate the prediction of a system's theoretical features of physicochemical parameters.74
Physiological and ecological aspects of metal nanoparticles
In healthcare applications, such as cosmetics, pharmaceuticals, and food industries, synthetic antibiotics and metallic compounds utilized in biomedical nanofibers have been linked to elevated health and environmental risk.17 To date, several investigations on metal particle-based nanofibers for biomedical applications have been conducted. Silver, gold, and zinc particles are the most used metal particles, as shown in Table 1. These particles have no negative impact on the human body when used in moderate amounts.75,76 Nonetheless, these nanoparticles have a number of distinct advantages when used in biomedical electrospun nanomats. Silver nanoparticles (AgNPs) are the most extensively employed substance in nanomats. The efficacy and antibacterial and bactericidal effects of AgNPs have been studied in a number of investigations. The efficiency of these nanoparticles against multi-resistant and biofilm-forming bacteria was their main advantage.77–79 Bergin and Adhaya et al. showed that AgNPs and silver sulfadiazine were beneficial in treating chronic wound and burn infections.80,81
Table 1 Health hazards associated with metallic nanoparticles
Metallic particles |
Potential adverse effects |
Ref. |
Silver nanoparticles and silver nitrate |
Tissue toxicity, skin diseases such as argyria, cell death, and dose-dependent DNA damage, highly toxic to mammalian cells, sperm cell damage |
81–89 |
Zinc oxide nanoparticles |
Hazardous to mammalian cells |
83, 84, 90 and 91 |
Gold nanoparticles |
They can easily enter the cell membrane and assemble into vacuoles |
84, 92 and 93 |
Iron oxide nanoparticles |
DNA damage, oxidative stress, mitochondrial membrane dysfunction, and changes in gene expression |
88 and 94 |
Piroxicam |
Ulcers, bleeding, or holes in the stomach or intestine |
95 |
Titanium dioxide nanoparticles |
Vesicle formation after internalization, apoptosis, cytotoxic |
96 |
Silicon dioxide nanoparticles |
Reactive oxygen species (ROS) generation, lipid peroxidation – oxidative stress |
97 |
Copper oxide nanoparticles |
Dose-dependent oxidative stress, genotoxic effects, kidney, liver, and spleen are the target organs |
98 and 99 |
However, despite these benefits, metallic compounds have several detrimental impacts on health and the environment. According to Adhaya et al., these chemicals could cause tissue toxicity.81 Furthermore, some researchers claim that silver-based biomedical nanomaterials cause blue-gray coloration on the skin after a prolonged time due to increased melanin production caused by silver, resulting in skin diseases such as argyria100 and reoccurrence of silver-resistant bacteria.101–103 Szmyd et al. reported that high AgNP concentrations impair keratinocyte viability, metabolism, and migration by activating caspase 3 and 7, which are implicated in cell death and dose-dependent DNA damage.82
A series of studies have demonstrated that AgNPs are highly toxic to mammalian cells104–106 including brain cells,107 liver cells,107 and stem cells.104 McAuliffe et al. revealed in another significant investigation that AgNPs harmed male reproduction due to their high toxicity. According to this study, AgNPs pass the blood–testes barrier and are deposited in the testes, causing sperm cell damage.108 Takenaka et al. found in their studies that AgNPs can cause damage to the cardiovascular system. They looked at the pulmonary and systemic distribution of inhaled ultrafine elemental AgNPs in rats and discovered silver in the lungs after the exposure had finished. They also discovered that Ag was present in significant amounts in the blood, kidney, spleen, nose, brain, and heart.109 Because of their large surface area and small particle size, AgNPs can interact with membrane surfaces and propagate throughout the human body. This contact with membranes may produce extremely reactive and harmful radicals, such as reactive oxygen species, which can induce inflammation and damage mitochondrial cells. It has also been connected to neurological issues, gastrointestinal distress, headache, and fatigue.110
Similarly, ZnONPs are widely used in various hydrogel-based wound dressing materials.90,111 ZnONPs, on the other hand, have been reported to be less hazardous to mammalian cells than AgNPs.112,113 Coatings, cosmetics, packaging, and medicinal applications are only a few of the applications of these nanoparticles.114,115 Most investigations looked into the cytotoxicity of ZnONPs concerning their extracellular dissolution.116 This occurs because of an increased level of [Zn2+]. A study revealed that 300 mg kg−1 ZnONPs cause cellular damage in the liver of mice after 14 days of subacute oral therapy. In addition to high alanine aminotransferase and alkaline phosphatase levels, there were pathological lesions in the liver. ZnONPs increase lipid peroxidation to demonstrate oxidative stress. The liver was subjected to oxidative stress, which generated Fpg-specific DNA damage.117
AuNPs are also employed in nanofiber fabrication. These particles are used in wound therapy because of their chemical stability. They have antibacterial and healing properties both in vitro and in vivo, as investigated by Arafat et al.118,119 AuNPs are usually considered to be bioinert, but questions have been raised regarding their safety. However, Lu et al. found that these particles’ cytotoxicity is linked to their high concentration. AuNPs trapped in the liver can affect the function of this organ.120 The deleterious consequences of AuNPs were investigated by Nadine et al., who showed that particles as small as 14 nm could easily enter the cell membrane and assemble into vacuoles. In dermal fibroblasts, this penetration resulted in aberrant actin filaments and extracellular matrix constructs.121 Research indicates that cells can endocytose nanoparticles of gold and form cytotoxic aggregates. HL7702 cells were used to study the interaction between gold nanoparticles and glutathione, and the impact of this interaction on apoptotic signaling (human liver cell line).122
Titanium dioxide nanoparticles (TiO2NPs) exhibit unique physical and chemical properties in cosmetics and pharmaceuticals because of their high surface-to-mass ratio. However, researchers used TiO2NPs on rat and human glial cells to study the activities of the nervous system (C6 and U373). They found that the immunolocalization of F-actin is inhibited by TiO2NPs.123 Long-term inhalation of TiO2NPs may harm the brain.96 Animals exposed to TiO2NPs develop lung inflammation. The effects of intratracheal TiO2NPs on chronic pulmonary injury were evaluated for ninety days. The results suggested that the usage of TiO2NPs irritated the lungs and caused hemorrhage. TiO2NPs increased lung antioxidant capacity and lipid peroxidation in rats.124
Copper oxide nanoparticles (CuONPs) are widely employed in material development, including nanofabrication. CuONPs are lethal to mammalian cells due to the induction of oxidative stress. It is still unknown whether CuONPs constitute a genotoxicity risk to people. There is a correlation between p53 and the lung epithelial cell toxicity of CuONPs (A549). CuONPs reduced, in a dose-dependent manner, the viability of cells exposed to them. CuONPs result in glutathione depletion, lipid oxidation, an increase in catalase activity, and the activation of superoxide dismutase. They promoted the activation of the inflammation detecting Hsp70 protein. CuONPs had a similar positive effect on Rad51 and MSH2 DNA repair proteins. CuONPs have been shown to induce A549 cells to perish as a result of oxidative stress.98 The Hodge and Sterner scale classifies CuONPs as moderately hazardous. They target the kidneys, liver, and spleen, among other organs. These nanoparticles damaged the kidneys, liver, and spleen of mice; however, micro copper particles had no effect.99
Despite the health issues, these nanoparticles are linked to threats to the environment and biological organisms.125 The utilization of nanoparticles has enhanced artificial synthesis. Increased exposure of modified nanoparticles to biotic and abiotic ecosystem components will result from their rapid production and utilization. While nanomaterials are widely used, their long-term presence and prolonged exposure can be harmful to the environment. To examine the environmental implications of nanoparticles, it is crucial to comprehend their negative effects, how their dissolution in water influences their toxicity, their propensity to aggregate or settle, and their fate during wastewater treatment and incineration. However, it is necessary to study the acute and long-term effects of customized nanoparticles on the skin, gastrointestinal tract, and respiratory system.126 The use of metal nanoparticles in electrospun nanofibers for biomedical and food packaging applications poses significant risks, including oxidative stress, DNA damage, and ecological disruption.127 Rigorous safety assessments are crucial, encompassing comprehensive evaluations of biodistribution, metabolism, long-term effects, and both acute and chronic toxicity. In pharmaceuticals and cosmetics, detailed characterization and toxicological evaluations are essential to ensure that the benefits outweigh the risks. Continuous research, strict regulatory adherence, and proactive risk management are vital for safely harnessing nanotechnology's advantages.
In essence, nanotechnology has been utilized in both commercial and medical applications. Following this technology, metallic nanoparticles are widely used in healthcare applications despite having an elevated risk to health and the environment. The physicochemical features of nanoparticles facilitate medical and industrial applications. They are utilized by the electro/photocatalytic-environmental-space-cosmetic-medical-pharmaceutical industries.
Therefore, it is necessary to understand how the released nanoparticles interact with their surroundings, which requires a thorough understanding of the long-term effects of nanotechnology-designed particles on human health, as well as the detection and evaluation of nanotechnology's dangers and risks.125 Alternative ways, such as incorporating green materials and processes, could be a viable means to address their health and environmental issues. The following section illustrates the green electrospinning technique to avoid the utilization of these metallic nanoparticles.
Green electrospinning is a novel technique for increasing the production of electrospun fibers with little or no toxicity, particularly for medical, filtration, tissue, and food engineering (Fig. 4). It is an environmentally friendly and safe method and is particularly useful in medical and food applications. According to a number of studies, natural and synthetic polymers used in green electrospinning include PVA,128 PEO,129 PVP,128 collagen,130 silk,131 fibroin,132 polyimide,133 and PAA.134
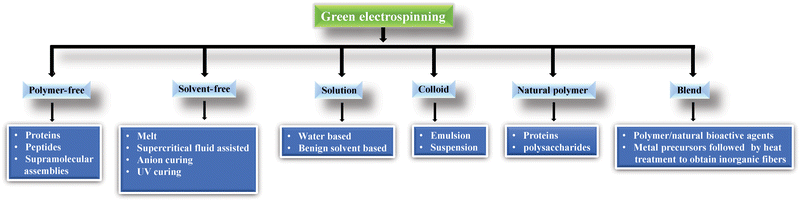 |
| Fig. 4 Classification of green electrospinning.135 | |
Water or mild solvents such as acetic acid might be used to make a solution to avoid toxicity; only the Class 3 solvent tested was capable of sufficient polymer solubilization and subsequent fiber formation. However, many biopolymers, such as chitin, chitosan, and cellulose, are not dissolved in water. As we discussed previously, metallic compounds have tremendous detrimental effects on health and the environment, and organic solvents are also responsible for health and environmental issues.136 Most often, electrospun biomaterial productions require toxic, hazardous fluorinated solvents, including HFIP (hexafluoro isopropanol), FA (ferulic acid), chloroform, and TFA (trifluoroacetic acid). DMF (N,N-dimethylformamide) and HFP (hexafluoropropylene) are very harmful to both humans and the environment. On the other hand, toxic or flammable organic solvents are still used in emulsion electrospinning, limiting its utility in in vivo medicinal and agricultural applications.135 These volatile organic compounds may affect the product user and producer and evaporate into the air, affecting water, air, and soil quality.137–139
However, research conducted by Hsieh et al. demonstrated the water solubility of chitosan after carboxymethylation and subsequent electrospinning with water-soluble PVA.140,141 Velankar et al. showed the use of oil–water emulsion electrospinning using polystyrene and PEO liquid phase. However, emulsion electrospinning still employs toxic or flammable organic solvents, limiting its utility in in vivo medicinal and agricultural applications.142
As a result, scholars are motivated to develop nanomats using harmless substances to reduce the aforementioned problems associated with health, safety, and the environment. Over the last decade, there have been significant advances in plant extract-based nanofibers. Due to the negative side effects of synthetic compounds reported by Srindhar et al.,143 plant extract encapsulated nanofibers have been developed for cosmetics, medicine, and the food industry.
Plants, indeed, contain a wide variety of bioactive chemical compounds possessing antimicrobial properties144,145 and with the potential to kill bacteria that cause infections. A range of phytochemicals are derived from plants and classified by Cowan et al. as phenolics, terpenoids, and alkaloids.146–148 These compounds can target pathogens by disrupting cell membranes, binding to cellular components, depriving the cells of essential nutrients, and inhibiting enzyme activity.131 The plant's crude extract can be derived from the plant's leaf, bark, stem, seeds, and flowers using a variety of organic solvents such as ethanol,149 methanol,150 and ethyl acetate.151
Because plant extracts are too brittle and have inadequate mechanical qualities to be electrospun alone, numerous biopolymers are utilized as carrier polymers to provide good mechanical properties, such as PVA,128 PEO,129 PVP,128 collagen,130 silk fibroin,132 polyimide,133 PAA,134 alginate,152 and gelatin.153 Cellulose,154 chitin,155 and chitosan156 are some of the natural and synthetic biopolymers. The fibrous structure, due to its nano- to micro-scale thickness, lacks the mechanical strength needed for use in clothing, so the fibrous web is usually laminated to enhance its durability and provide adequate reinforcement.5 However, all synthetic biopolymers cannot be dissolved in non-toxic solvents, limiting their use in medical applications. Embedding nanofibers in traditional clothing greatly improves the functional properties of self-cleaning, water repellency, and flame retardancy.157
Sources of bio-based materials forming nanofibers
Plant sources
Exploring a wide range of plant sources for bioactive agent-infused nanofibers offers a highly promising opportunity to develop advanced materials.158 The substances derived from different botanical sources such as leaves, seeds, stems, flowers, oils, and fruits have a crucial part in the complex creation of nanofibers, enhancing their capabilities. Several extracts found in the plant include those derived from Azadirachta indica (neem), which is well known for its strong antimicrobial properties; Achillea lycaonica, recognized for its noticeable anti-inflammatory effects; and Lawsonia inermis (henna), which possesses distinct medicinal qualities.159 Seeds, such as Trigonella foenum-graecum (fenugreek) and Allium sativum (garlic), coupled with mucilage, are important sources of bioactive compounds. The stems of plants such as Curcuma longa (turmeric), Zingiber officinale (ginger), and Glycyrrhiza glabra (licorice) contain unique bioactive compounds that have potential applications.
The use of bioactive agents from flowers such as Matricaria recutita (chamomile), Isatis tinctoria, and Helianthus annuus (sunflower) broadens the spectrum of bioactive compounds that can be incorporated into nanofibers. The inclusion of Eugenia caryophyllata (clove) and Olea europaea (olive) oils in nanofibers can enhance their intrinsic capabilities due to the presence of unique bioactive components. Fruits such as Garcinia mangostana, Citrus sinensis (orange), Ananas comosus (pineapple), and Momordica charantia add to the variety of bioactive substances that can be used to create nanofibers.
Animal sources
The importance of animal sources in the production of bioactive nanofibers should not be underestimated, especially when combined with plant-derived materials. Proteins like silk fibroin, gelatin, and keratin possess exceptional characteristics that can be strategically utilized for specific purposes. Silk fibroin, obtained from silkworms, demonstrates exceptional tensile strength and compatibility with living organisms.160 Gelatin, derived from collagen, offers flexibility and is known for its inherent biodegradability. Keratin, obtained from several animal sources, enhances the mechanical robustness and stability of nanofibers, hence broadening their range of functionalities.
Mineral sources
The use of mineral sources expands the range of materials accessible for the fabrication of bioactive nanofibers. Polysaccharides such as cellulose and chitin, obtained from plants and animals respectively, play a crucial role in enhancing the structural stability of nanofibers. Zein, a protein component, adds complexity to mineral sources for bio-based nanofibers.161,162
The incorporation of these many natural sources into electrospun nanofibers not only guarantees biocompatibility but also harmonizes effortlessly with environmentally sustainable methods. The resulting materials exhibit great potential for a wide range of applications in fields such as medicine, agriculture, and environmental remediation, highlighting the extensive possibilities and adaptability of nanofibers that contain bioactive agents.
Humans have used plant-based medications since prehistoric times. Since bioagents make it possible to cross-link scaffolds, the use of bioactive materials in tissue engineering has grown over the past few decades. Materials with bioactive compounds integrated offer special properties for tissue engineering matrices. Plants provide therapeutic substances and are the source of these compounds: leaves, stems, seeds, bark, flowers, and oils.163
The extraction process has employed biomaterials from these natural plant sources to prevent the development of acute or chronic toxicity associated with biocompatible and biodegradable materials derived from them. Natural polysaccharides, including alginate, cellulose, and starch, have been extensively used in tissue engineering. Protein extracts from plants and animals are also important sources of biomaterials.164 Plant extract cannot be electrospun alone due to numerous challenges; consequently, a variety of synthetic and natural biopolymers are loaded to create nanofibrous fibers (Fig. 5).
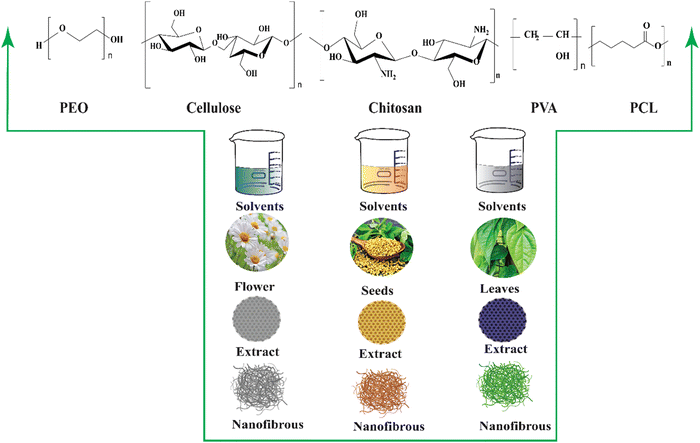 |
| Fig. 5 Carrier biopolymers (PEO, cellulose, chitosan, PVA, PCL) and different bioactive compounds extracted from plant sources (flowers, seeds, leaves) using appropriate solvents for converting them into nanofibrous materials for various applications. | |
The overview of plant-extract bioactive nanofibrous production conditions, opportunities, and difficulties of applying the nanomembrane for biomedical applications are the main points of the review. The development of the nanofibrous process, including its bioactive compounds and several plant-derived medicinal extracts from diverse plant parts, including leaves, stems, flowers, seeds, and oil, is discussed in the following section.
Bioactive agents or extract
Leaves
The Aloe vera plant belongs to the Liliaceae family, and it possesses several properties such as anti-inflammatory, antiarthritis, antibacterial, and antifungal properties. It contains several compounds such as amino acids, vitamins, sugars, minerals, and enzymes. In the Indian subcontinent, Azadirachta indica, often known as neem, contains more than 140 bioactive ingredients and has antimalarial, antiulcer, antibacterial, antifungal, antiviral, antioxidant, anti-inflammatory, and anticarcinogenic properties.165–167 Achillea lycaonica, a common species found in Turkey and a member of the Asteraceae family, has been claimed to have antioxidant, wound healing, antibacterial, and cytotoxic characteristics by many researchers.168,169 Reneta Gevrenova et al. used ethyl acetate, methanol, and water extracts of the aerial parts and roots of two Achillea species (A. alecppica and A. santolinoides) in another investigation. They discovered that hydroxybenzoic and hydroxycinnamic acids, phenolic acid glycosides and sugar esters, acylquinic acids, O-glycosyl flavones and flavonols, and flavonoid aglycons are present as bioactive constituents in these species. The extracts (Fig. 6) of these species may have the phytotherapeutic potential for Alzheimer's disease and industrial applications.170
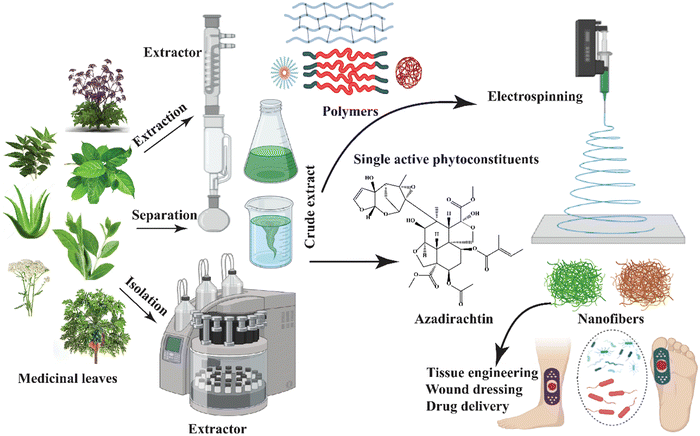 |
| Fig. 6 Schematic diagram of producing bioactive nanofibers utilizing extracts from medicinal plant leaves. | |
Korean angelica is another name for these small plants, mostly used as a medicinal herb in Asia. The dried roots of this plant are commonly used to treat anemia, pain, infection, and articular rheumatism. The medicinal dose is prepared by boiling the dried roots in water. Decursinol angelate is the most common medicinal compound of this plant, and various studies have shown that it can treat cancers, such as bladder cancer, colon cancer, leukemia, lung cancer, melanoma, myeloma, prostate cancer, and sarcoma.171–176 Carica papaya is a type of papaya fruit that belongs to the Caricia genus included in the Caricaceae family.177 It contains phenolics, flavonoids, and alkaloids (tryptanthrin and isatin), among other phytochemical substances (Fig. 7). It has antioxidant, antibacterial, anticancer, anti-inflammatory, antiulcer, antidiabetic, and many other properties due to these chemical substances.178 The medicinal plant Gymnema sylvestre is a perennial woody vine native to Asia, Africa, and Australia. It contains phytochemical compounds such as gymnemasaponins, anthraquinones, flavones, phytin, lupeol, and stigmasterol. Therefore, this plant is utilized for many purposes like microbial infection, wound healing, inflammation, obesity, arthritis, constipation, and cancer.179–182
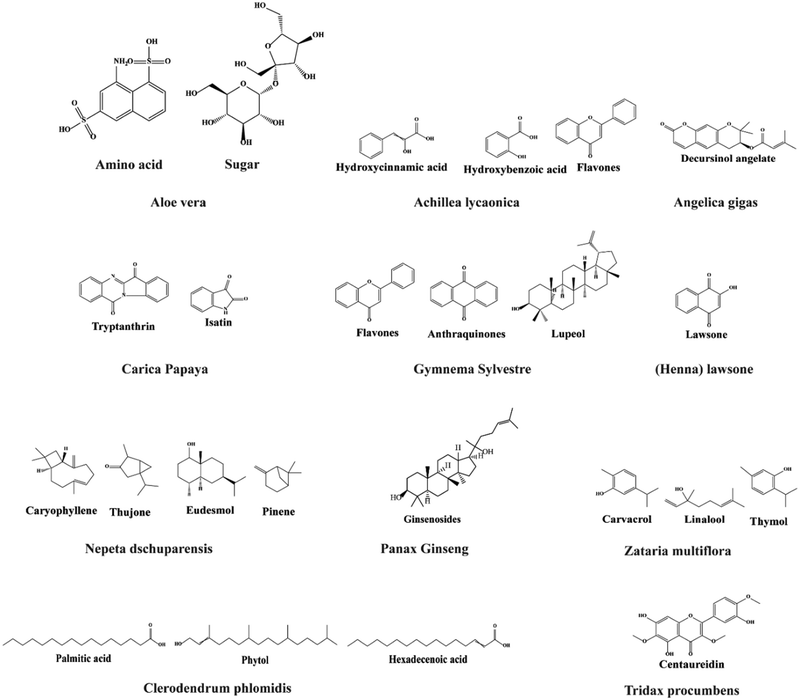 |
| Fig. 7 Bioactive compounds extracted from various plant leaves for developing functional nanofibers. | |
A natural red-orange dye, also known as hennotannic acid, is derived from the henna plant.136 This plant has been used for natural hair and skin dyes for over 5000 years. It contains lawsone (2-hydroxy-1,4-naphthoquinone) and exhibits various biological activities such as antioxidant, analgesic, anti-inflammatory, antibacterial, antifungal, and anticancer.183–185 Nepeta dschuparensis is a herbaceous aromatic plant that belongs to the Lamiaceae family. It is a flavonoid-rich plant that is used mostly in traditional herbal therapy. These plants contain flavonoids such as caryophyllene, 1,8-cineole, thujone, eudesmol, and pinene, which demonstrate antibacterial, antioxidant, and anti-inflammatory properties.186–188
Ginseng is found in Korea, China, and Siberia and it belongs to the Panax genus. Ginseng root contains polysaccharides, amino acids, polyacetylene alcohols and fatty acids that are all found in most ginseng species. Ginsenoside ingredients are the main active chemical compounds, and they have the structure of triterpenoid dammarane saponins. Approximately 30 ginsenosides have been obtained until now, although additional substances have also been found. In addition to its antioxidant properties, ginseng has been shown to have impacts on the cardiovascular system, immune system, and central nervous system, and it has anticancer properties.189 It has been used in medical applications for a long time. Its pharmacological activity in the human body, including the skin, cardiovascular, immunological, endocrine, and neurological systems, has been described in a number of investigations. It also promotes collagen synthesis in dermal fibroblast cells.190–193
The Lamiaceae family includes Zataria multiflora, widely grown in Iran, Pakistan, and Afghanistan. Carvacrol, thymol-carvacrol, and linalool are found in the essential oil of Zataria multiflora. However, the earlier studies found only carvacrol and thymol.194 These therapeutic constituents can help in the improvement of infectious wounded areas in the human body. They possess a wide range of biological activities, including spasmolytic, antinociceptive, antioxidant, anti-inflammatory, antifungal, and antibacterial effects.194–196 Terpenoids, flavonoids, phytol, hexadecenoic acid, and palmitic acid are among the more than 40 phytochemical components found in the flowering plant Clerodendrum phlomidis, which belongs to the Lamiaceae family. The Tridax procumbens flowering plant, also referred to as coatbuttons or coat buttons, is a member of the Asteraceae family; it is a pest and widespread weed plant. Although it is native to the subtropical America, it has been found everywhere. The aerial part of Tridax procumbens contains procumbenetin. It also contains flavonoids (centaureidin and centaurein), alkyl esters, sterols, pentacyclic triterpenes, fatty acids, and polysaccharides.197–200
The leaves of Tridax procumbens are used to treat wound healing and blood clotting.201–204 Its drink is effective against bronchitis, diarrhea, and dysentery. The active ingredients of this plant extract possess antihyperuricemia, antioxidant, antibacterial, antifungal, and antileishmanial activities.197–200 Therefore, Sharbidre et al. utilized this against SARS-CoV-2 by implementing computational methodologies.205 The Moringaceae family of flowering plants contains only one genus of Moringa, which has been used for generations to cure wounds and various ailments, such as diabetes and cold. A few phytoconstituents in Moringa species include alkaloids, saponins, tannins, steroids, phenolic acids, glucosinolates, flavonoids, and terpenes. The broad variety of phytochemicals in this species are useful in various medicinal applications.206
Seeds
Fenugreek, also known as methi, is a Leguminosae family member commonly used in cuisine preparations, especially in south Asian countries. The main constituents of fenugreek seeds include nonvolatile and volatile oil (in small amount) (Fig. 9). These seeds are rich in sugars, proteins, lipids, alkaloids, flavonoids, steroid saponins, sterols, vitamins, and minerals.207 The major bioactive compounds present in fenugreek seeds are diosgenin, rhaponticin, and isovitexin, as shown in Fig. 8.208 These seeds demonstrate hypoglycemic209, hypocholesterolemic,210 antioxidant, and anti-ulcerogenic effects.211 Flavonoids and polyphenols are bioactive constituents in plant-derived mucilage, which is made up of various phenolic compounds (Fig. 8). These bioactive substances prevent diseases related to oxidative damage.212,213 Moreover, a glue-type polysaccharide found in most plants exhibited several biological activities such as antibacterial, antioxidant, and anti-inflammatory. It is also found in Trigonella foenum-graecum and exhibits antioxidative, antimicrobial, and anti-inflammatory properties.213–215 Mucilage from cress seeds possesses a high mannose and galactose ratio. However, due to the different chain conformations and repulsive forces among the poly anions in solution, its application in electrospinning is limited.216 However, Bonino et al. reported that PVA and PEO were employed to minimize repulsive forces.217
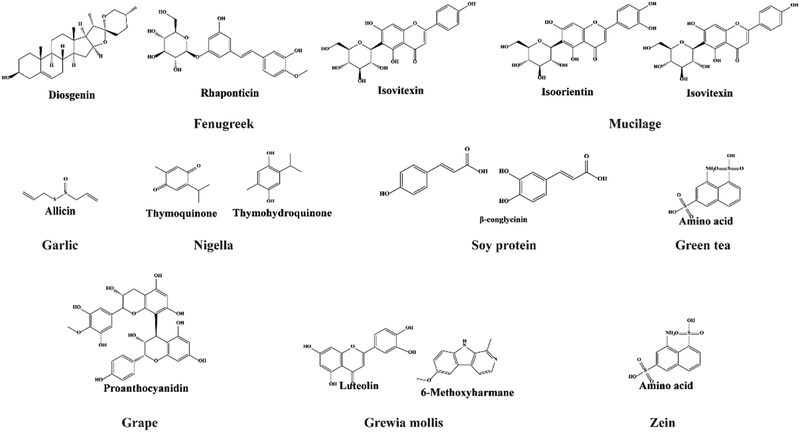 |
| Fig. 8 Molecular structures of bioactive compounds extracted from various seeds and incorporated into electrospun nanofibers. | |
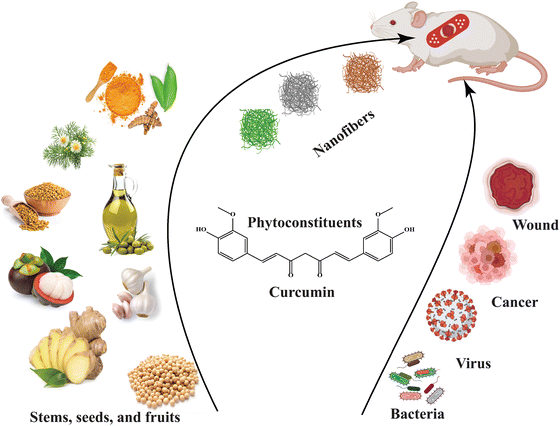 |
| Fig. 9 Functional electrospun nanofibers incorporated with stems, seeds, and fruit extract and their biomedical applications. The functionality and performance of the nanofibrous mat increased considerably after adding bioactive compounds into nanofibers during the fabrication. | |
Garlic is a bulbous flowering plant that belongs to the genus Allium. Allicin, the main phytochemical component of garlic, has a variety of biological actions, including antibacterial, antioxidant, antibiotic, anticancer, and antiviral properties.218–220 Garlic contains organosulfur compounds that are water- and oil-soluble.221 The latest work conducted by Yongxu et al. using CS/PVA/GO with allicin showed its excellent long-lasting antibacterial property against S. aureus bacteria and was suggested as a potential wound dressing material. Furthermore, the nanofiber was found to be highly hydrophilic, with a high capacity for natural retention and pH-responsiveness.222
The ancient medicinal seed Nigella sativa, which belongs to the Ranunculaceae family, is widely available. Thymoquinone (TQ) and thymohydroquinone (THQ) are the major important therapeutic constituents in nigella. Due to the presence of these groups in Nigella sativa, it has anti-inflammatory, antioxidant, antibacterial, immunological, antidiabetic, hepatoprotective, anticestodal, and antiaflatoxin properties.223–225 Soybean protein isolates are another name for soy protein containing β-conglycinin and glycinin as the major important chemical constituents. Usually, the glycinin peptide is superior to β-conglycinin in terms of antibacterial activity. The compound has the potential to be used in biomedical applications.226,227 Antimicrobial peptides kill microbes by penetrating and damaging the cell membrane of microbes. Soy protein is an example of an antimicrobial peptide derived from soybeans, and it contains protein levels of 53%, 74%, and 93% in different forms.228 It also contains significant reactive functional groups, such as NH2, OH, and SH, and is applied extensively in biomedical applications.229
Green tea is made from Camellia sinensis, which originated in China and later spread out throughout East Asia. It contains flavanols, flavonoids, amino acids (theanine or 5-N-ethylglutamine, glutamic acid, tryptophan, glycine, serine, aspartic acid, tyrosine, valine, leucine, threonine, arginine, and lysine), and phenolic acids. Due to these functional groups in green tea, it provides a wide range of health benefits such as prevention of cancer and cardiovascular diseases and anti-inflammatory, antiarthritic, antibacterial, antiangiogenic, antioxidative, antiviral, neuroprotective, and cholesterol-lowering effects.230
A series of papers reported the fabrication of nanofibers from green tea extracts for healthcare applications. Sadri et al. developed three types of nanofiber composites using CS and PEO for wound healing applications that showed antibacterial activity against Gram-positive and Gram-negative bacteria with good moisture performance (Table 2).231 PCL was also electrospun with green tea extract for cancer therapy, which showed an outstanding inhibition effect on tumor cells.232 Pusporini et al. incorporated PVP to fabricate nanofibers for antioxidant activities a few years ago. All these studies showed the fine fiber production of green tea extract-loaded nanofibers. Grape seeds are utilized to create grape seed extract. Numerous health advantages are related to blueberries' antioxidants and oligomeric proanthocyanidin complexes, in addition to treating high cholesterol, atherosclerosis, macular degeneration, and nerve damage.
Table 2 Plant sources, extraction, fabrication, and findings of nanofibers
Plant name |
Extractor |
Carrier polymers |
Solvents |
Electrospinning condition |
Antibacterial activity |
Properties |
Application |
Ref. |
Voltage (kV) |
(TCD) (cm) |
Feed rate (mL h−1) |
Fiber diameter (nm) |
Section 1: leaf extract nanofiber formation conditions, properties, and applications |
Aloe vera |
— |
Zein/collagen/PCL |
Ethanol/chloroform |
15 |
10 |
1.00 |
50–150 |
S. aureus and E. coli |
Cytocompatibility/suitable thermal stability and mechanical properties |
Wound healing |
233 |
Aloe vera |
— |
PLGA/PVA |
HFIP/DIW |
12 |
12 |
1.00 |
112–562 |
S. aureus and S. epidermidis |
Hydrophilic/antibacterial |
Wound healing/wound dressing |
234 |
Aloe vera |
— |
PLGA |
HFIP |
10 |
8 |
2.7 |
420–340 |
— |
Good handling properties |
Wound healing |
235 |
Azadirachta indica (neem) |
Methanol |
PVA/CS |
AA/DIW |
20 |
16 |
3.00 |
152–298 |
S. aureus |
Bi-layered/antibacterial/good tensile properties/good thermal and absorbance properties |
Wound dressing |
167 |
Azadirachta indica (neem) |
Ethanol |
PVA |
DIW |
20 |
15 |
3.50–4.00 |
185 |
S. aureus |
Biocompatibility/antibacterial |
Wound dressing |
149 |
Achillea lycaonica |
Ethanol/water |
PLA |
DMSO/chloroform |
24–32 |
17 |
9–12 |
124–1094 |
— |
Antibacterial/controlled drug release/good tensile strength/no risk of melting in the human body |
Tissue engineering |
150 |
Angelica gigas |
Ethanol |
PVA |
DIW |
25 |
15 |
1.00 |
75–170 |
— |
Fast dissolving mat |
Oral cancer |
236 |
Cariica papaya |
Ethanol/water |
PVA/Gel |
AA/chloroform |
10–15 |
10–15 |
0.45–0.60 |
140–160 |
S. aureus and E. coli |
Hydrophilic, antibacterial, and anti-inflammatory |
Scaffold/wound healing |
237 |
Carica papaya/honey |
— |
PU |
DMF/chloroform |
16 |
15 |
0.75 |
19–190 |
— |
Hydrophilic/porous morphology |
Burn injury |
238 |
Clerodendrum phlomidis |
n-hexane, ethyl acetate, ethanol, and methanol |
PCL |
Chloroform |
12 |
12 |
1.00 |
300–400 |
S. aureus, E. coli, P. aeruginosa, and S. typhi |
Antibacterial and antioxidant activity/superior mechanical, thermal and moisture properties |
Wound dressing |
239 |
Gymnema sylvestre |
Ultrasonic extraction |
PCL |
TFE |
12 |
12 |
1.00 |
79–377 |
S. aureus, E. coli, P. aeruginosa, and S. typhi |
Biocompatibility/antibacterial |
Wound dressing |
41 |
Gymnema sylvestre |
Methanol |
PCL |
Chloroform/methanol |
13 |
12 |
1.00 |
50–206 |
S. aureus and P. aeruginosa |
Antibacterial/infection control/superior mechanical properties and good wettability |
Wound dressing |
240 |
(Henna) lawsone |
DMF |
PCL/gel |
TFE |
10–22 |
12–18 |
0.30 |
10–297 |
S. aureus and P. aeruginosa |
Antibacterial/cell proliferation |
Wound healing, tissue engineering |
241 |
(Henna) lawsone |
— |
PLLA/Gel |
Chloroform/DMF/AA |
16–18 |
15 |
0.40 |
196–520 |
E. coli and S. aureus |
Biocompatibility/antibacterial |
Wound dressing |
242 |
(Henna) lawsone |
Ethanol |
CS/PEO |
AA |
25 |
10–20 |
0.10–1.50 |
64–87 |
E. coli and S. aureus |
Antibacterial/cell viability |
Tissue engineering |
243 |
Nepeta dschuparensis/honey |
Ethanol |
PVA/CS |
DIW/HCL |
17 |
— |
0.50 |
95–150 |
— |
Antibacterial/antifungal/anti-inflammatory |
Wound healing |
244 |
Panax ginseng |
Ethanol |
PCL |
DCM |
16 |
12 |
1.50 |
250–660 |
— |
Higher proliferation/hydrophilic |
Bone tissue engineering |
245 |
Zataria multiflora |
Water |
PVA/CS/Gel |
DIW/AA/ |
21 |
— |
0.20 |
58–218 |
S. aureus, P. aeruginosa, and C. albicans |
Antibacterial/antifungal/anti-inflammatory |
Wound healing |
195 |
Tridax procumbens |
DIW |
PCL |
DCM/DMF |
— |
— |
— |
100–124 |
E. coli and S. aureus |
Antibacterial |
Wound healing |
202 |
Moringa |
— |
PAN |
— |
20 |
10 |
0.15 |
|
E. coli and S. aureus |
Cost-effective and biodegradable nanofibers, antibacterial |
Wound healing |
246 |
|
Section 2: seed extract nanofiber formation conditions, properties, and applications |
Fenugreek |
HFIP |
Fibroin |
HFIP |
25 |
10 |
0.50 |
38–339 |
— |
Good mechanical and thermal properties/antibacterial/biocompatibility |
Wound dressing/scaffold |
247 |
Garlic |
Grinding |
PU |
DMF |
10.5 |
15 |
0.20 |
150–578 |
— |
Biocompatible |
Wound dressing |
248 |
Mucilage/chan and linaza |
DIW |
PVA |
Acetone |
30 |
20 |
1.00 |
350 |
— |
Antibacterial, antioxidant, anti-inflammatory and promoter of cell growth |
Tissue engineering |
249 |
Nigella |
Methanol |
PVA |
AA |
20 |
16 |
2.50 |
178–188 |
S. aureus and E. coli |
Biocompatibility/hydrophilic/antibacterial |
Wound dressing |
223 |
Nigella |
— |
PCL/PLA |
DMF/DCM |
10–20 |
15 |
0.80 |
638–707 |
S. aureus and E. coli |
Good handling properties/antibacterial |
Wound dressing |
250 |
Nigella |
Methanol |
PVA |
AA |
20 |
15 |
1.5 |
223 |
S. aureus |
Antibacterial/good moisture management and thermal properties |
Wound dressing |
251 |
Soy protein |
Ethanol |
Fibroin |
FA |
— |
— |
— |
71–160 |
— |
Anticancerous/anti-inflammatory/good moisture management |
Tissue engineering |
252 |
Green tea |
— |
CS/PEO |
AA |
17–20 |
10 |
0.3–0.5 |
100 |
S. aureus and E. coli |
Keeps wound surface moist, reduces inflammation and increases the speed of recovery and healing |
Wound dressing |
231 |
Grape |
— |
Silk/PEO |
tert-Butyl 29 hydroperoxide (t-BHP) |
14 |
15 |
0.3 |
420 |
|
Grape extract can be released from the nanofibers in a sustained manner |
Skin care, tissue regeneration and wound healing |
253 |
Grewia mollis |
Methanol |
PLGA |
DCM/DMF |
15 |
10 |
— |
— |
S. aureus and E. coli |
Potential effective antimicrobial membrane |
Wound dressing |
254 |
Zein |
Citric acid |
HA |
— |
17.5 |
15 |
1 |
100–300 |
— |
Cell proliferation |
Bone tissue engineering |
255 |
|
Section 3: stem extract nanofiber formation conditions, properties, and applications |
Curcumin |
— |
PCL/GT |
AA |
15 |
15 |
1.00 |
23–288 |
S. aureus |
Better healing performance/antibacterial |
Wound healing |
256 and 257 |
Curcumin |
— |
CA |
DMAc/acetone |
17.5 |
15 |
1.00 |
314–340 |
S. aureus and E. coli |
Anti-tumor/antioxidant/anti-inflammatory |
Wound healing |
258 |
Curcumin |
Methanol/ethanol |
PCL/PVA |
DMF/DCM |
12–24 |
16 |
1–3 |
— |
S. aureus and E. coli |
Water absorbability, antibacterial, and biocompatibility |
Wound dressing |
259 |
Curcumin |
— |
PVA |
AA |
15 |
10 |
1.00 |
47–138 |
S. aureus and E. coli |
Good mechanical properties/hydrophilic |
Wound dressing |
260 |
Curcumin |
DIW |
PVA |
DIW |
15 |
20 |
0.50 |
250–350 |
S. aureus and E. coli |
More even fibers/drug release/antioxidant/anti-inflammatory |
Biomedical |
261 |
Curcumin |
AA |
Gel |
DIW/EDC |
26 |
12 |
1.70 |
500 |
E. coli |
Antibacterial and anti-inflammatory |
Wound healing |
262 |
Curcumin |
— |
CA/PU/GO |
DMF/THF/acetone |
17 |
15 |
0.40 |
44–678 |
S. aureus |
Antibacterial and anti-inflammatory |
Wound healing |
263 |
Curcumin/honey |
Ethyl acetate |
PVA |
DIW |
20 |
15 |
— |
340 |
S. aureus |
Antibacterial, anti-inflammatory/good moisture management |
Wound healing |
151 |
Ginger |
NaOH |
CS/PVA/cellulose |
AA |
— |
— |
— |
100–200 |
B. cereus, E. coli, S. aureus and S. typhimurium |
Antibacterial, high mechanical strength |
Medical and packaging areas |
264 |
Licorice |
|
PVA |
|
25 |
15 |
|
245 |
S. aureus |
Better moisture absorbing material |
Wound healing |
265 |
|
Section 4: flower extract nanofiber formation conditions, properties, and applications |
Chamomile |
— |
CECS/PCL/PVA |
AcA/DIW/AA |
15–18 |
18 |
0.20 |
19–248 |
S. aureus and C. albicans |
Superior mechanical properties and great phosphate buffer uptake/controllable release behavior/antibacterial and antioxidant |
Wound dressing |
266 |
Chamomile |
— |
PCL/PS |
DMF/chloroform |
18 |
15.50 |
0.46 |
175 |
S. aureus and C. albicans |
Antibacterial and antifungal/drug release |
Wound healing |
267 |
Isatis tinctoria |
Alcohol |
PVP |
— |
10 |
5–8 |
— |
— |
S. aureus and E. coli |
Antibacterial, excellent wetting surface |
Wound healing |
268 |
Sunflower |
DIW |
PVA |
NaOH |
20 |
15 |
0.5–0.75 |
304–400 |
|
Hydrophobic and hydrophilic nanofibers |
Aqueous food systems |
269 |
|
Section 5: oil-based nanofiber formation conditions, properties, and applications |
Eugenol (clove oil) |
— |
PCL/PVA/CS |
DMF/AA/chloroform |
75 |
13 |
— |
162–387 |
S. aureus and P. aeruginosa |
Biocompatibility/hydrophilic/antibacterial |
Wound dressing |
270 |
Olive oil |
— |
PEO/CS/PCL |
AA/methanol/DCM |
15–25 |
7–20 |
0.20–1.00 |
95–131 |
S. aureus and E. coli |
Antibacterial/antifungal/anti-inflammatory |
Wound dressing |
271 |
Cinnamomum zeylanicum |
DIW |
PVA |
— |
17 |
15 |
0.5 |
80 |
— |
— |
Highly effective for use in stored product pest control |
272 |
Lavender oil |
— |
PU |
THF/DMF |
15 |
15 |
0.5 |
371–979 |
S. aureus and E. coli |
Excellent bactericidal properties, multifunctional wound dressings |
Wound dressing, promoting the regeneration of new tissue |
273 |
Cinnamon oil |
— |
PEO/cyclodextrin |
|
25 |
12 |
0.6 |
350–450 |
B. cereus |
Extends the shelf life of beef |
Active food packaging |
273 |
Grewia mollis, a floral species indigenous to Yemen and Oman, is widespread within the Malvaceae family and is indigenous to tropical Africa. It was shown that ethanol stem bark extracts, including tannic acid, phenolic components, and tannic acid and saponins, have antibacterial and anti-inflammatory activities.274 Typically, zein protein has been utilized as a film or nanofibrous scaffold for wound healing and tissue engineering purposes. Higher quantities of amino acids, including glutamic acid, proline, leucine, and alanine, and lower levels of basic and acidic amino acids can be found in zein proteins.
Stems
Curcumin is a yellow pigment found in turmeric, a flowering plant of the ginger family which is commonly used as a curry spice, especially in south Asian countries. There are three major types of curcuminoids found in turmeric: curcumin or diferuloylmethane (responsible for yellow color), demethoxycurcumin, and bisdemethoxycurcumin275 (Fig. 10). Many investigations have been conducted to determine the phytochemical constituents of turmeric that result in antioxidant, anti-tumorigenic, anti-inflammatory, anticancer, and many other properties.238 Due to these therapeutic properties turmeric has been used for a long time to heal many diseases like cough, rheumatism, diabetes, biliary disorders, anorexia, sinusitis, hepatic disorders, cancer, and alzheimers.276,277 Traditional civilizations have used ginger for a range of purposes. Chemicals like phenolic (gingerols, shogaols, and paradols) and terpene (β-bisabolene, α-curcumene, zingiberene, α-farnesene, and β-sesquiphellandrene) compounds are abundant in ginger. For thousands of years, ginger has been used in Chinese and Indian medicine to treat illnesses, including stomach pains and nausea. Ginger has antiviral, antibacterial, and anti-inflammatory properties. It is commonly known that ginger has medicinal qualities. Licorice is a flowering Fabaceae plant called Glycyrrhiza glabra and is well known for its fragrant, sweet root. Sugars, carbohydrates, bitters, resins, essential oils, tannins, inorganic salts, and tiny amounts of nitrogenous substances like proteins, amino acids, and nucleic acids can all be found in licorice extract.
 |
| Fig. 10 Bioactive compounds isolated from various plant stems and incorporated into electrospun nanofibers. | |
Flower
Chamomile is a daisy-like plant that belongs to the Asteraceae family and is commonly used to make herbal infusions for beverages. Apigenin, quercetin, patuletin, luteolin, and glucosides are phenolic and flavonoid compounds found in this plant.278 Its flower yields an essential oil that possesses antibacterial, anti-inflammatory, and antioxidant activities due to the presence of these functional compounds. A series of research studies have indicated that it could be used in cancer therapy,279–281 diabetic wound healing,282 and periodontal injury repair.283 Isatis tinctoria, a flowering plant from the Brassicaceae family, has remarkable therapeutic properties due to the presence of a diverse spectrum of compounds and is primarily found in Asian countries. Among the bioactive substances are alkaloids (tryptanthrin and isatin), flavonoids (isoorientin and isovitexin), polysaccharides, glucosinolates, carotenoids, fatty acids, and volatile components. A great deal of investigation reported that it has good antibacterial and anti-inflammatory activities.284–289
Sunflower (Helianthus annuus L.) contains active ingredients. The roots, stems, leaves, and seeds of the sunflower plant, as well as the flower, contain flavonoids and phenols. These chemical constituents have antimicrobial and antioxidant activity, and hydrophobic and hydrophilic nanofibers were developed by Shaneazadeh et al. (Table 2).
Oil
Cloves are members of the Myrtaceae family and are mostly found in Indonesia. Clove oil, also known as eugenol, is a phenolic substance with antioxidant, antibacterial, antifungal, and anti-inflammatory properties290 (Fig. 11). The predominant component, eugenol, has an inhibitory effect on oxidative stress due to radical scavenging property. The ancient olive fruits belong to the Oleaceae family and contain the major medicinal components, including phenolic and polyphenolic compounds such as oleuropein and hydroxytyrosol,291 resulting in antioxidant, anti-inflammatory,291 and antibacterial activity against S. aureus and S. typhimurium bacteria.292
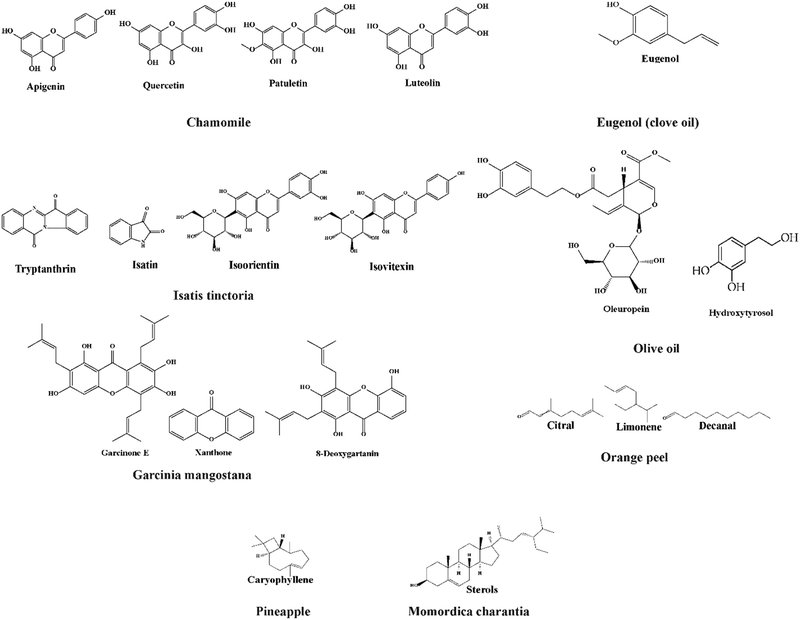 |
| Fig. 11 Chemical structures of medicinal compounds derived from fruits, oils, and flowers for nanofiber production. | |
Fruits
Garcinia mangostana is a member of the Guttiferae family and is known as the “Queen of Fruits” in Southeast Asia. Xanthone, α, β, and γ-mangostins, garcinone E, 8-deoxygartanin, and gartanin are all found in Garcinia mangostana.278 Much research reported its antioxidant, antitumoral, antiallergic, anti-inflammatory, antibacterial, and antiviral activities. Due to these properties, it has been used in a wide variety of illness treatments such as dysentery,293 diarrhea,294 hemorrhoids,295 food allergies,295 arthritis,295 wounds,296 tuberculosis,297 inflammation,298 ulcers,299 micosis,298 cholera,300 leucorrhea,301 fever,302 and suppuration.301 Oranges are the fruit of several citrus species in the Rutaceae family. Orange peels, the main byproduct of the citrus processing industry, are low in protein and high in pectin, cellulose, and hemicellulose. Additionally, the weight of fresh fruit is virtually entirely made up of orange peels. Ananas comosus, the pineapple, is economically the most significant Bromeliaceae plant. Pineapples have been cultivated for centuries and are native to South America. Flavonoids, phenolic acids, and other polyphenolic chemicals are present in pineapple which contribute to antioxidant activity. Momordica charantia from Cucurbitaceae is a tropical and subtropical vine used for its delicious fruit in Asia, Africa, and the Caribbean. This fruit has many bioactive substances categorized as carbs, proteins, fats, and more in the literature. Triterpenoids, saponins, polypeptides, flavonoids, alkaloids, and sterols are found in Momordica charantia.
Prospective applications of plant-based electrospun nanofibers
Biomedical applications
Recent interest has been drawn to quantitative research of electrospinning based on PLA, PCL, PEO, PVA, gelatin, chitosan, starch, etc., which is not surprising given the significance of material development for biomedical applications.303–305 The outcomes of this study will help improve the production of bio-based nanofibers. Polycaprolactone (PCL) fibers incorporated with Inula graveolens (L.) plant extract were fabricated by an electrospinning process.306 The resultant nanofibers had a ribbon-like twisted form and showed no toxic effect on cell cultures (Fig. 12). After evaluating the tensile properties, hydrophilicity, cell toxicity, and biocompatibility, the authors claimed that the Inula graveolens (L.) plant extract/PCL scaffold could be used as a potential material for wound healing and tissue regeneration applications. Ferulic acid is a naturally occurring polyphenolic plant constituent. It was isolated from the Parthenium hysterophorus plant and encapsulated in the PLGA/PEO electrospun nanofibrous matrix.307 Microscopic analysis showed that ferulic acid was found in the core of PLGA/PEO fibers.
 |
| Fig. 12 Mode of the bacterial killing mechanism. This figure shows how nanoparticles inhibit the growth of Gram-positive and Gram-negative bacteria. The nanoparticle adheres to bacteria's surfaces, disrupting membrane structure and function. DNA damage and protein inactivation occur when nanoparticles enter bacterial cells. It illustrates how nanoparticles interact with lipoprotein, peptidoglycan, lipoteichoic acid, lipopolysaccharide, and flagellin in the bacterial cell wall and how nanoparticles damage bacteria's electron transport chains, reducing energy production. | |
The ferulic acid encapsulated PLGA/PEO nanofibers exhibited better cytotoxic potential against HepG2 cells. Seyed and co-workers studied the effect of electric potential on the morphology of polyvinyl alcohol (PVA)/sodium alginate (SAlg) electrospun nanofibers containing herbal extracts of Calendula officinalis.308 The produced nanofiber mats have high porosity and a high surface-to-volume ratio. The diameters of Calendula-induced nanofibers were more compared to Calendula-free nanofibers for all applied potentials (5, 10, 15, and 20 kV).
Nanofibrous scaffolds of polylactic-co-glycolic acid (PLGA)/chitosan were synthesized through the emulsion electrospinning technique.309 This technique doesn’t require common solvents for either polymers or grafting. Furthermore, this allows the blending of polymers from natural and synthetic origins at different ratios. PLGA/chitosan scaffolds are hydrophilic due to the presence of chitosan and are mechanically strong because of having PLGA. Polyblends are highly useful in biomedical fields, like skin tissue engineering applications, as they have improved cell–scaffold interactions.
The development of nanofibrous hydrogel membranes by electrospinning has received tremendous attention in the research community in recent years. The hydrogel nanofibrous membranes are scaffolds that are extensively studied for their application in drug delivery systems, regenerative biomaterials for skin and tissue engineering, bone repair, etc.310,311 The advances in the synthesis and development of PVA-polysaccharide-based electrospun membranes are extensively reviewed recently.312
The inclusion of bioactive additives, nanoparticle additives, medicaments, and medicinal plants in hydrogel nanofibrous membranes was also discussed. The advances in the usage of natural therapeutic compound loaded electrospun nanofiber membranes for wound dressing applications are highlighted in a mini-review by Younes et al.313 Trends in tissue repair and regeneration using herbal scaffolds have been reviewed recently.314 The fabrication techniques, such as electrospinning, solvent casting, freeze-drying, additive manufacturing, and hydrogel formation, are discussed.
Packaging applications
The potential usage of functional plant-based nanofibers for food packaging applications is getting wider acceptance among the research community in recent days. Electrospun nanofibers encapsulated with natural antioxidants/ingredients are considered as a prospective packaging material for preserving the food materials and releasing nutrients to the food during preservation (Fig. 13).
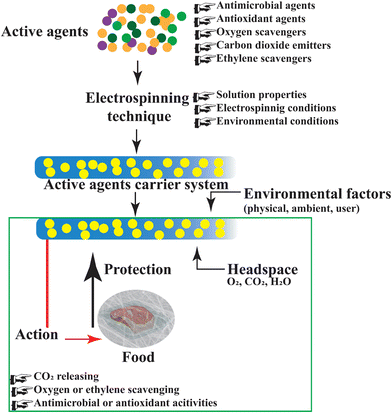 |
| Fig. 13 Antibacterial activities of bioactive plant extract nanofibers in food packaging.315 Bioactive nanofibers are used to fabricate smart food packaging that extends shelf life and improves food safety. Plant-based electrospun functional nanofibers, as packaging materials, regulate ambient gases (O2, CO2, H2O), diminish oxygen or ethylene, release carbon dioxide, and protect food by utilizing their antibacterial or antioxidant properties. These mechanisms reduce spoilage germs, slow down lipid and vitamin oxidation, and preserve food color, flavor, and texture efficiently. | |
Cinnamaldehyde (CNMA) is a bioactive chemical and packaging systems based on electrospun nanofibers with the inner area encapsulated with CNMA and the outer layer with compression-molded polyhydroxybutyrate (PHB) are reported.316 The virucidal activity of CNMA was tested against norovirus surrogates, murine norovirus (MNV), feline calicivirus (FCV), and hepatitis A virus (HAV). The study reported that the CNMA-incorporated multilayer films inactivated FCV and offered better protection against MNV and HAV. An interesting study on strawberry preservation with conventional low-density polyethylene (LDPE) vs. electrospun PVA/cinnamon essential oil (CEO)/β-cyclodextrin (β-CD) was reported by Wen et al.317 Unlike other essential oils such as clove and eucalyptus, CEO was chosen in their study as it was anticipated to give better food preservation and inhibit bacterial colony formation. The efficacy of fibrous PVA/CEO/β-CD mats against E. coli and S. aureus showed that the inhibition zones were 29 and 30 mm, respectively, and the fruits preserved with PVA/CEO/β-CD were stable without losing their flavor even after six days.
Cyclodextrin inclusion complexes (CD-ICs) are plant extracts used in the food industry. They are generally used as drug carriers and can form complexes with hydrophobic compounds. To improve the shelf-life and minimize the volatility of natural organic compounds, CD-ICs were used in nanofibers as carriers of plant extracts in food packaging applications.17 A detailed review of electrospun nanofibers having antioxidant, antibacterial, and antifungal properties for food packaging applications is available.318
Corn zein prolamine, in addition to chitosan ultrathin electrospun water resistant nanofibers, has intriguing utilization in food technology due to the bioactive and antimicrobial film-forming properties, such as a reinforcing fiber in plastic food packaging and as an edible carrier for encapsulating food additives or changing food qualities.319 Neo et al. also incorporated zein prolamine co-loaded with garlic acid; the resulting nanofibers demonstrated potential applications for food packaging materials owing to their antibacterial and antioxidant properties.320
Red raspberry extract with bioactive anthocyanins loaded with soy protein isolate was studied by Wang et al. for nanofiber development.321 The resultant fibers demonstrated excellent potential for active packaging due to their greater concentration of bioactive anthocyanins and improved antibacterial activity against Staphylococcus epidermidis in thermoplastic wheat gluten films electrospun from whey and soy protein isolate and zein loaded multilayer nanofibers developed by Farba et al.322 Encapsulated alpha-tocopherol release was prolonged and the water vapor barrier performance of the wheat gluten film was improved, highlighting the potential of hybrid hydrocolloid structures with active chemicals in the creation of innovative multifunctional food packaging materials (Fig. 14). Ethyl cellulose and soy protein were loaded with orange peel extract to develop nanofibers for antimicrobial, antioxidant, and safe food packaging materials as reported by Rashidi et al.323
 |
| Fig. 14 Bioactive nanofibrous membrane creating barrier layers to prevent harmful microbes from getting into food packaging. The nanolayer, which is composed of microscopic structures with bactericidal characteristics, shields encapsulated food from harmful microorganisms by either blocking their entry or neutralizing them. The figure shows a piece of meat with fresh veggies inside the nanolayer barrier, illustrating how versatile this technology is. The nanolayer is ideal for a wide variety of food packaging applications due to its transparency and flexibility. This infographic highlights how nanotechnology has the ability to completely change the food industry and make food security much more reliable. | |
By combining glutenin and PVA to form composite nanofibers, Han et al. were able to increase the average diameter and homogeneity while lowering the diameter and quantity of beads and showed that PVA/glutenin nanofibers were more elongated and could absorb more water than pure PVA nanofibers.324 Gelatin and zein were encapsulated with curcumin extract with the addition of green tea extract for bioactive food packaging application as reported by Ali et al.325 While the gelatin coatings demonstrated promising release behavior when exposed to fatty meal simulants, the results indicated that zein-based coatings would be better suited for packaging foods with high water content.
A recent study reported the fabrication of nanofibers employing lavender oil loaded with PVP for active food packaging applications.326 Nanofiber mats infused with lavender have antioxidant properties in vitro. Testing on minced lamb in situ confirmed this influence. Meat's shelf life was extended by lavender oil-loaded PVP nanofibers, lowering yeast molds, psychotropic bacteria, and aerobic mesophilic bacteria that cause damage to meat.
Similarly, another study showed the development of nanofibers loaded with PLA/Ag for wound dressing applications that exhibited enhanced tensile strength, absorbency, and antibacterial properties with no cytotoxicity effects.327 Mi Li et al. produced biodegradable active packaging materials that demonstrated a hydrophobic nature with a eugenol layer inside and exhibited remarkable antioxidant and antibacterial activity against Gram-positive and Gram-negative bacteria.328
Yadong et al. developed edible packaging materials in addition to gelatin with chamomile essential oil.329 The results showed that increasing the oil concentration led to an increase in fiber diameter while still maintaining uniform fibers. The developed samples exhibited excellent antioxidant and antibacterial activities. The latest study by Ceylan et al. developed nanofibers loaded with grape oil to produce high-quality products in the food industry. Aslaner et al. used grape extract with PEO and flour for electrospinning. The fibers were suggested to be utilized in protective multilayer packaging.330 The insights derived from recent research indicate a notable trend toward embracing nanofibers for advanced applications in food packaging. The encapsulation of natural antioxidants through electrospinning emerges as a favored strategy for preserving food materials and facilitating controlled nutrient release.
However, in biomedical contexts, the U.S. Food and Drug Administration (FDA) and the European Medicines Agency (EMA) require comprehensive preclinical and clinical evaluations for nanoparticles in medical devices and pharmaceuticals. This includes extensive toxicity testing, biodistribution studies, and detailed risk assessments to ensure that therapeutic benefits outweigh potential adverse effects.331,332 For food packaging, agencies like the FDA and the European Food Safety Authority (EFSA) focus on migration testing, toxicological evaluations, and comprehensive safety assessments to ensure that nanoparticles do not pose health risks to consumers. These guidelines mandate thorough risk assessments, including data on the potential migration of nanoparticles into food, their toxicokinetic properties, and any health impacts. Comparing the toxic effects and biocompatibility of different types of nanoparticles, such as silver, zinc oxide, gold, and titanium dioxide, allows for selecting the safest materials for specific applications.333 By adhering to these regulatory guidelines and safety standards, the risks associated with the use of metal nanoparticles in biomedical and food packaging applications can be effectively managed, ensuring that nanoparticles are thoroughly tested and evaluated for safety before reaching consumers. Continuous research and strict adherence to these guidelines are essential to harnessing the benefits of nanotechnology while safeguarding public health and the environment.
Challenges, prospects, and conclusions
While plant-based polymeric nanofibers offer a wide range of characteristics suitable for biomedical applications, they also present certain challenges and limitations. Plant-based bioactive compounds can be sensitive to geographical locations and seasons, potentially causing allergic reactions and adverse effects.334 One significant drawback is the insolubility of many plant materials, which limits their ability to be electrospun on their own. This issue is often mitigated by using copolymers.259 Additionally, surface tension can hinder smooth fiber production, as demonstrated by Ali et al., who utilized a cationizer to reduce the surface tension of the solution.167 Poor mechanical properties are another concern; thus, several biopolymers are combined with plant extracts to enhance cellular uptake.335 For example, soy-based scaffolds have low molecular weight, which complicates thorough characterization and optimization for biomedical applications.336
Although plant extracts are integrated into various biopolymers such as PVA, PLA, PCL, PGA, cellulose, and chitin, not all these biopolymers dissolve in water, necessitating the use of harmful chemical solvents like DMAc, HFEP, and HFP. These solvents, which evaporate during fiber manufacture, pose health risks and may leave residues that cause problems during applications.336 Intensive research can overcome these obstacles. While plant extracts are brittle and cannot be electrospun alone, synthetic and natural biopolymers can mitigate this limitation. Additionally, green solvents, such as ionic liquids or deep eutectic solvents, can be employed to avoid using harmful organic solvents.337,338
This review highlights the significant potential of bioactive electrospun nanofibers derived from plant extracts for biomedical and food packaging applications. Various plant materials, including leaves, seeds, stems, flowers, and fruits, have been used to extract bioactive chemicals with antibacterial, antioxidant, anti-inflammatory, and anticancer properties. These bioactive chemicals are effectively integrated into nanofibers using biopolymers as carriers.
The electrospinning process offers a flexible method to customize the structure and size of nanofibers, enabling specific properties. The resulting nanofibers find use in various applications such as enhanced wound healing, regulated drug administration, scaffolds for tissue engineering, food packaging protection, and antibacterial filtering. However, challenges such as the natural fragility of plant extracts, insufficient mechanical strength, and the necessity for carrier polymers and organic solvents during manufacturing must be addressed.
Future developments should focus on improving the structural durability of nanofibers through novel composite architectures and ensuring the continuous release of bioactive substances. Comprehensive in vivo testing is crucial to assess the long-term safety and effectiveness of these nanofibers in living organisms. Additionally, employing environmentally friendly electrospinning processes to scale up manufacturing will facilitate the commercialization of these eco-friendly nanomaterials. In summary, bioactive electrospun nanofibers from plants represent a highly promising field that bridges nanotechnology and natural phytotherapy.
Future research on bioactive electrospun nanofibers must prioritize several key efforts. These include developing advanced multi-polymer composites and hybrid nanofibers to enhance mechanical strength, exploring synergistic combinations of plant extracts to boost therapeutic benefits, and creating carrier matrices for prolonged release of bioactive compounds. To ensure safety and efficacy, extensive testing in animal models should precede human trials. This focused trajectory involves scaling up electrospinning rigs for large-scale production, utilizing environmentally friendly solvents, and commercializing plant extract-based electrospun products.
Abbreviations
AA | Acetic acid |
AcA | Acrylic acid |
BC | Bacterial cellulose |
CS | Chitosan |
CECS | Carboxyethyl chitosan |
CA | Cellulose acetate |
DIW | Deionized water |
DMAc/DMA | Dimethylacetamide |
DMF | Dimethylformamide |
DCM | Dichloromethane |
DMSO | Dimethyl sulfoxide |
EDC | N-Ethylenecarbodiimide |
EDTA | Ethylenediaminetetraacetic acid |
ECM | Extracellular matrix |
FA | Formic acid |
Gel | Gelatin |
AuNPs | Gold nanoparticles |
GT | Gum tragacanth |
GO | Graphene oxide |
HFIP | Hexafluoroisopropanol |
ILs | Ionic liquids |
PCL | Poly(caprolactone) |
PVP | Poly(vinyl pyrrolidone) |
PLGA | Polylactic-co-glycolic acid |
PU | Poly(urethane) |
PEO | Poly(ethylene oxide) |
PS | Poly(styrene) |
PLA | Poly(lactic acid) |
PVA | Poly(vinyl alcohol) |
PS | Poly(styrene) |
PCL | Poly(caprolactone) |
PAN | Poly(acrylonitrile) |
AgNPs | Silver nanoparticles |
THF | Tetrahydrofuran |
TFE | Trifluoroethanol |
ZnONPs | Zinc oxide nanoparticles |
Ethical approval
This article does not contain any studies with human participants performed by any of the authors.
Data availability
The data supporting the findings of this study are available within the article and its supplementary materials. Data can also be requested from the corresponding authors [Md Nur Uddin, Ayub Ali, and Abdur Rahman Bhuiyan at nur@duet.ac.bd, ayubali@duet.ac.bd, and arahman@duet.ac.bd, respectively], subject to reasonable request and any applicable privacy or ethical restrictions.
Conflicts of interest
The authors declare no potential conflict of interest.
Acknowledgements
We would like to thank all the individuals who contributed to this research, especially the authors and advisors, for their invaluable feedback and support throughout the study. The research team did not receive grants or funds from public, private, or commercial organizations.
References
- A. Rodrigo-Navarro, S. Sankaran, M. J. Dalby, A. del Campo and M. Salmeron-Sanchez, Nat. Rev. Mater., 2021, 6, 1175–1190 CrossRef.
- J.-h Wu, T.-g Hu, H. Wang, M.-h Zong, H. Wu and P. Wen, J. Agric. Food Chem., 2022, 8207–8221 CrossRef CAS PubMed.
- I. Pastoriza-Santos, C. Kinnear, J. Pérez-Juste, P. Mulvaney and L. M. Liz-Marzán, Nat. Rev. Mater., 2018, 3, 375–391 CrossRef.
- M. R. Bhuiyan, L. Wang, R. A. Shanks, Z. A. Ara and T. Saha, Cellulose, 2020, 27, 10501–10517 CrossRef CAS.
- M. Faccini, C. Vaquero and D. Amantia, J. Nanomater., 2012, 892894 CrossRef.
- S. Shi, Y. Si, Y. Han, T. Wu, M. I. Iqbal, B. Fei, R. K. Li, J. Hu and J. Qu, Adv. Mater., 2021, 2107938 Search PubMed.
- S. Lee and S. Kay Obendorf, J. Appl. Polym. Sci., 2006, 102, 3430–3437 CrossRef CAS.
- C. Li, S. Shu, R. Chen, B. Chen and W. Dong, J. Appl. Polym. Sci., 2013, 130, 1524–1529 CrossRef CAS.
- A. Raza, Y. Li, J. Sheng, J. Yu and B. Ding, Electrospun nanofibers for energy and environmental applications, Springer, 2014, pp. 355–369 Search PubMed.
- S. Shahidi, Cellulose, 2014, 21, 757–768 CrossRef CAS.
- M. D. Teli and B. N. Annaldewar, J. Text. Inst., 2017, 108, 460–466 CrossRef CAS.
- L. Chen, L. Bromberg, H. Schreuder-Gibson, J. Walker, T. A. Hatton and G. C. Rutledge, J. Mater. Chem., 2009, 19, 2432–2438 RSC.
- P. P. Kalelkar, M. Riddick and A. J. García, Nat. Rev. Mater., 2022, 7, 39–54 CrossRef CAS PubMed.
- B. Abadi, N. Goshtasbi, S. Bolourian, J. Tahsili, M. Adeli-Sardou and H. Forootanfar, Front. Bioeng. Biotechnol., 2022, 10, 986975 CrossRef PubMed.
- Y. Shi, C. Zhang, F. Jiang, L. Zhou, L. Cai, H. Ruan and J. Chen, Electrospun Polymeric Nanofibers: Insight into Fabrication Techniques and Biomedical Applications, Springer International Publishing, Cham, 2022, pp. 313–334 Search PubMed.
- G. W. Peterson, D. T. Lee, H. F. Barton, T. H. Epps and G. N. Parsons, Nat. Rev. Mater., 2021, 6, 605–621 CrossRef CAS.
- W. Zhang, S. Ronca and E. Mele, Nanomaterials, 2017, 7, 42 CrossRef PubMed.
- E. El Maaiden, S. Bouzroud, B. Nasser, K. Moustaid, A. El Mouttaqi, M. Ibourki, H. Boukcim, A. Hirich, L. Kouisni and Y. El Kharrassi, Molecules, 2022, 27, 2074 CrossRef CAS PubMed.
- K. Kowalik, M. Polak-Berecka, M. Prendecka-Wróbel, D. Pigoń-Zając, I. Niedźwiedź, D. Szwajgier, E. Baranowska-Wójcik and A. Waśko, Molecules, 2022, 27, 1006 CrossRef CAS PubMed.
- N. Bibi Sadeer, K. I. Sinan, Z. Cziáky, J. Jekő, G. Zengin, R. Jeewon, H. H. Abdallah, Y. AlDhaheri, A. H. Eid and M. F. Mahomoodally, Molecules, 2022, 27, 2000 CAS.
- C. Soto-Maldonado, B. Fernández-Araya, V. Saavedra-Sánchez, J. Santis-Bernal, L. Alcaíno-Fuentes, A. Arancibia-Díaz and M. E. Zúñiga-Hansen, Electron. J. Biotechnol., 2022, 56, 47–53 CrossRef CAS.
- S. V. Shilov, G. O. Ustenova, L. N. Kiyekbayeva, I. S. Korotetskiy, N. V. Kudashkina, N. V. Zubenko, R. A. Parenova, A. B. Jumagaziyeva, Z. A. Iskakbayeva and S. T. Kenesheva, Int. J. Biomater., 2022, 4427804 CAS.
- H. Boussak, S. Demim, S. Hammadou and L. L. Seiad, Mater. Today Proc., 2022, 49, 1041–1045 CrossRef CAS.
- A. Augusto, A. Miranda, L. Costa, J. Pinheiro, M. J. Campos, D. Raimundo, R. Pedrosa, G. Mitchell, K. Niranjan and S. F. Silva, J. Food Process. Preserv., 2022, e16630 CAS.
- I. M. Yusoff, Z. M. Taher, Z. Rahmat and L. S. Chua, Food Res. Int., 2022, 111268 CrossRef CAS PubMed.
- V. Mandal, Y. Mohan and S. Hemalatha, Phcog. Rev., 2007, 1, 7–18 CAS.
- R. M. Cywar, N. A. Rorrer, C. B. Hoyt, G. T. Beckham and E. Y.-X. Chen, Nat. Rev. Mater., 2022, 7, 83–103 CrossRef CAS.
- M. Binsalah, S. Devanesan, M. S. AlSalhi, A. Nooh, O. Alghamdi and N. Nooh, Microorganisms, 2022, 10, 789 CrossRef CAS PubMed.
- M. F. Ferdosi, A. Javaid and I. H. Khan, Bangladesh J. Bot., 2022, 51, 393–399 CrossRef.
- Y.-X. Liu, X.-L. An, Y.-N. Xu, Y.-J. Hao, X.-C. Piao, M.-Y. Jin and M.-L. Lian, LWT, 2022, 162, 113438 CrossRef CAS.
- L. Fitria, I. C. P. Gunawan, W. B. T. Sanjaya and M. I. Meidianing, Trop. Biodivers. Biotechnol., 2022, 7, 69389 CrossRef.
- K. A. Asiry, J. Vector Ecol., 2022, 47, 1–8 Search PubMed.
- M. Narayanan, A. Kiran, D. Natarajan, S. Kandasamy, S. Shanmugam, M. Alshiekheid, H. S. Almoallim and A. Pugazhendhi, Process Biochem., 2022, 112, 234–240 CrossRef CAS.
- M. Nabi, M. I. Zargar, N. Tabassum, B. A. Ganai, S. U. D. Wani, S. Alshehri, P. Alam and F. Shakeel, Plants, 2022, 11, 1667 CrossRef CAS PubMed.
- M. Hanfer, Z. Benramdane, T. Cheriet, D. Sarri, A. Menad, I. Mancini, R. Seghiri and S. Ameddah, Nat. Prod. Res., 2022, 36, 3124–3128 CrossRef CAS PubMed.
- Y. Lu, A. A. Aimetti, R. Langer and Z. Gu, Nat. Rev. Mater., 2016, 2, 1–17 Search PubMed.
- G. L. Koons, M. Diba and A. G. Mikos, Nat. Rev. Mater., 2020, 5, 584–603 CrossRef CAS.
- N. Mitrousis, A. Fokina and M. S. Shoichet, Nat. Rev. Mater., 2018, 3, 441–456 CrossRef CAS.
- A. Misra, S. Ganesh, A. Shahiwala and S. P. Shah, J. Pharm. Pharm. Sci., 2003, 6, 252–273 CAS.
- J. Wang, Y. Li and G. Nie, Nat. Rev. Mater., 2021, 6, 766–783 CrossRef CAS PubMed.
- N. A. Patil, P. M. Gore, N. J. Prakash, P. Govindaraj, R. Yadav, V. Verma, D. Shanmugarajan, S. Patil, A. Kore and B. Kandasubramanian, Chem. Eng. J., 2021, 416, 129152 CrossRef CAS PubMed.
- S. Mitra, T. Mateti, S. Ramakrishna and A. Laha, JOM, 2022, 1–16 Search PubMed.
- M. Bagheri, M. Validi, A. Gholipour, P. Makvandi and E. Sharifi, Bioeng. Transl. Med., 2022, 7, e10254 CrossRef CAS PubMed.
- S. Ravichandran, R. Jegathaprathaban, J. Radhakrishnan, R. Usha, V. Vijayan and A. Teklemariam, Bioinorg. Chem. Appl., 2022, 2335443 CrossRef CAS PubMed.
- S. Baghersad, A. Hivechi, S. H. Bahrami, P. B. Milan, R. A. Siegel and M. Amoupour, J. Drug Delivery Sci. Technol., 2022, 74, 103536 CrossRef CAS.
- C. Huang, X. Xu, J. Fu, D.-G. Yu and Y. Liu, Polymers, 2022, 14, 3266 CrossRef CAS PubMed.
- A. Kongprayoon, G. Ross, N. Limpeanchob, S. Mahasaranon, W. Punyodom, P. D. Topham and S. Ross, Polym. Chem., 2022, 13(22), 3343–3357 RSC.
- D. Kim, A. Bahi, L.-Y. Liu, T. Bement, S. Rogak, S. Renneckar, F. Ko and P. Mehrkhodavandi, ACS Sustainable Chem. Eng., 2022, 10, 2772–2783 CrossRef CAS.
- N. Bahari, N. Hashim, A. Md Akim and B. Maringgal, Nanomater., 2022, 12, 2560 CrossRef CAS PubMed.
- G. El Fawal, M. M. Abu-Serie and A. M. Omar, Polym. Bull., 2022, 1–22 Search PubMed.
- M. Khumalo, B. Sithole, T. Tesfaye and P. Lekha, J. Nanomater., 2022, 7080278 CrossRef CAS.
- Z. Zelca, S. Kukle, S. Janceva and L. Vilcena, Molecules, 2022, 27, 2311 CrossRef CAS PubMed.
- P. Gibson, H. Schreuder-Gibson and D. Rivin, Colloids Surf., A, 2001, 187, 469–481 CrossRef.
- W. Zhong, Advances in Smart Medical Textiles, Elsevier, 2016, pp. 57–70 Search PubMed.
- C. J. Angammana and S. H. Jayaram, Particul. Sci. Technol., 2016, 34, 72–82 CrossRef CAS.
- W. Morton and U. Patent, Method of dispersing fluids, 1902 Search PubMed.
- M. L. F. Nascimento, E. S. Araujo, E. R. Cordeiro, A. H. P. de Oliveira and H. P. de Oliveira, Recent Pat. Nanotechnol., 2015, 9, 76–85 CrossRef CAS PubMed.
- J. Zeleny, Phys. Rev., 1914, 3, 69 CrossRef.
- A. L. Szentivanyi, H. Zernetsch, H. Menzel and B. Glasmacher, Int. J. Artif. Organs, 2011, 34, 986–997 CrossRef CAS PubMed.
- N. Tucker, J. J. Stanger, M. P. Staiger, H. Razzaq and K. Hofman, J. Eng. Fibers Fabr., 2012, 7 DOI:10.1177/155892501200702S10.
- I. S. Chronakis, Micromanuf. Eng. Technol., 2010, 264–286 Search PubMed.
- A. Formhals, US Pat., 2116942, 1938.
- K. R. Spurny and J. C. Marijnissen, Nicolai Albertowich Fuchs: the pioneer of aerosol science biography, Delft Univ. Press, Delft, 1998 Search PubMed.
- W. Teo and S. Ramakrishna, Nanotechnology, 2005, 16, 1878 CrossRef CAS.
- Scifinder, Electrospinning, 2022 Search PubMed.
- C. Luo, S. D. Stoyanov, E. Stride, E. Pelan and M. Edirisinghe, Chem. Soc. Rev., 2012, 41, 4708–4735 RSC.
- B. Zhang, X. Yan, H.-W. He, M. Yu, X. Ning and Y.-Z. Long, Polym. Chem., 2017, 8, 333–352 RSC.
- C. Salas, Electrospun nanofibers, Elsevier, 2017, pp. 73–108 Search PubMed.
- P. Bonhote, A.-P. Dias, N. Papageorgiou, K. Kalyanasundaram and M. Grätzel, Inorg. Chem., 1996, 35, 1168–1178 CrossRef CAS PubMed.
- S. Zhu, Y. Wu, Q. Chen, Z. Yu, C. Wang, S. Jin, Y. Ding and G. Wu, Green Chem., 2006, 8, 325–327 RSC.
- M. Amde, J.-F. Liu and L. Pang, Environ. Sci. Technol., 2015, 49, 12611–12627 CrossRef CAS PubMed.
- A. Jordan and N. Gathergood, Chem. Soc. Rev., 2015, 44, 8200–8237 RSC.
- B. A. da Silva, R. de Sousa Cunha, A. Valerio, A. D. N. Junior, D. Hotza and S. Y. G. González, Eur. Polym. J., 2021, 147, 110283 CrossRef.
- D. Crespy, K. Friedemann and A. M. Popa, Macromol. Rapid Commun., 2012, 33, 1978–1995 CrossRef CAS PubMed.
- M. Ip, S. L. Lui, V. K. Poon, I. Lung and A. Burd, J. Med. Microbiol., 2006, 55, 59–63 CrossRef CAS PubMed.
- S. Sarkar, A. D. Jana, S. K. Samanta and G. Mostafa, Polyhedron, 2007, 26, 4419–4426 CrossRef CAS.
- T. H. Nguyen, Y. H. Kim, H. Y. Song and B. T. Lee, J. Biomed. Mater. Res., B: Appl. Biomater., 2011, 96, 225–233 CrossRef PubMed.
- N. Silvestry-Rodriguez, E. E. Sicairos-Ruelas, C. P. Gerba and K. R. Bright, Rev. Environ. Contam. Toxicol., 2007, 23–45 CAS.
- B. S. Atiyeh, M. Costagliola, S. N. Hayek and S. A. Dibo, Burns, 2007, 33, 139–148 CrossRef PubMed.
- S. Bergin and P. Wraight, Cochrane Database Syst. Rev., 2006,(1), CD005082 CAS.
- A. Adhya, J. Bain, O. Ray, A. Hazra, S. Adhikari, G. Dutta, S. Ray and B. K. Majumdar, J. Basic Clin. Pharm., 2014, 6, 29 CrossRef PubMed.
- R. Szmyd, A. G. Goralczyk, L. Skalniak, A. Cierniak, B. Lipert, F. L. Filon, M. Crosera, J. Borowczyk, E. Laczna and J. Drukala, Biol. Chem., 2013, 394, 113–123 CrossRef CAS PubMed.
- S. Hamdan, I. Pastar, S. Drakulich, E. Dikici, M. Tomic-Canic, S. Deo and S. Daunert, ACS Cent. Sci., 2017, 3, 163–175 CrossRef CAS PubMed.
- V. Vijayakumar, S. K. Samal, S. Mohanty and S. K. Nayak, Int. J. Biol. Macromol., 2019, 122, 137–148 CrossRef CAS PubMed.
- S. Pal, R. Nisi, M. Stoppa and A. Licciulli, ACS Omega, 2017, 2, 3632–3639 CrossRef CAS PubMed.
- M. Radulescu, E. Andronescu, G. Dolete, R. C. Popescu, O. Fufă, M. C. Chifiriuc, L. Mogoantă, T.-A. Bălşeanu, G. D. Mogoşanu and A. M. Grumezescu, Materials, 2016, 9, 345 CrossRef PubMed.
- P.-o Rujitanaroj, N. Pimpha and P. Supaphol, Polymer, 2008, 49, 4723–4732 CrossRef CAS.
- R.-H. Dong, Y.-X. Jia, C.-C. Qin, L. Zhan, X. Yan, L. Cui, Y. Zhou, X. Jiang and Y.-Z. Long, Nanoscale, 2016, 8, 3482–3488 RSC.
- J. Radwan-Pragłowska, L. Janus, M. Piątkowski, D. Bogdał and D. Matýsek, Polymers, 2020, 12, 159 CrossRef PubMed.
- P. Sudheesh Kumar, V.-K. Lakshmanan, T. Anilkumar, C. Ramya, P. Reshmi, A. Unnikrishnan, S. V. Nair and R. Jayakumar, ACS Appl. Mater. Interfaces, 2012, 4, 2618–2629 CrossRef CAS PubMed.
- M. I. Khan, S. K. Behera, P. Paul, B. Das, M. Suar, R. Jayabalan, D. Fawcett, G. E. J. Poinern, S. K. Tripathy and A. Mishra, Med. Microbiol. Immunol., 2019, 208, 609–629 CrossRef CAS PubMed.
- S. Mârza, K. Magyari, S. Bogdan, M. Moldovan, C. Peştean, A. Nagy, F. Tăbăran, E. Licarete, S. Suarasan and A. Dreanca, Biomed. Mater., 2019, 14, 025011 CrossRef PubMed.
- M. G. Arafa, R. F. El-Kased and M. M. Elmazar, Sci. Rep., 2018, 8, 1–16 CAS.
- S. P. Singh, M. Rahman, U. Murty, M. Mahboob and P. Grover, Toxicol. Appl. Pharmacol., 2013, 266, 56–66 CrossRef CAS PubMed.
- U. Paaver, I. Tamm, I. Laidmäe, A. Lust, K. Kirsimäe, P. Veski, K. Kogermann and J. Heinämäki, BioMed. Res. Int., 2014, 789765 Search PubMed.
- S. G. Márquez-Ramírez, N. L. Delgado-Buenrostro, Y. I. Chirino, G. G. Iglesias and R. López-Marure, Toxicology, 2012, 302, 146–156 CrossRef PubMed.
- I. Passagne, M. Morille, M. Rousset, I. Pujalté and B. L’azou, Toxicol., 2012, 299, 112–124 CrossRef CAS PubMed.
- M. Ahamed, M. A. Siddiqui, M. J. Akhtar, I. Ahmad, A. B. Pant and H. A. Alhadlaq, Biochem. Biophys. Res. Commun., 2010, 396, 578–583 CrossRef CAS PubMed.
- Z. Chen, H. Meng, G. Xing, C. Chen, Y. Zhao, G. Jia, T. Wang, H. Yuan, C. Ye and F. Zhao, Toxicol. Lett., 2006, 163, 109–120 CrossRef CAS PubMed.
- X. Chen and H. J. Schluesener, Toxicol. Lett., 2008, 176, 1–12 CrossRef CAS PubMed.
- E. G. d Nascimento, T. B. M. Sampaio, A. C. Medeiros and E. P. d Azevedo, Acta Cirurg. Bras., 2009, 24, 460–465 CrossRef PubMed.
- L. B. Rice, Curr. Opin. Microbiol., 2009, 12, 476–481 CrossRef CAS PubMed.
- L. R. Peterson, Clin. Infect. Dis., 2009, 49, 992–993 CrossRef PubMed.
- L. Braydich-Stolle, S. Hussain, J. J. Schlager and M.-C. Hofmann, Toxicol. Sci., 2005, 88, 412–419 CrossRef CAS PubMed.
- H.-C. Wen, Y.-N. Lin, S.-R. Jian, S.-C. Tseng, M.-X. Weng, Y.-P. Liu, P.-T. Lee, P.-Y. Chen, R.-Q. Hsu and W.-F. Wu, J. Phys., 2007, 89 Search PubMed.
- P. Gopinath, S. K. Gogoi, A. Chattopadhyay and S. S. Ghosh, Nanotechnology, 2008, 19, 075104 CrossRef CAS PubMed.
- S. Hussain, K. Hess, J. Gearhart, K. Geiss and J. Schlager, Toxicol. In Vitro, 2005, 19, 975–983 CrossRef CAS PubMed.
- M. E. McAuliffe and M. J. Perry, Nanotoxicol., 2007, 1, 204–210 CrossRef CAS.
- S. Takenaka, E. Karg, C. Roth, H. Schulz, A. Ziesenis, U. Heinzmann, P. Schramel and J. Heyder, Environ. Health Perspect., 2001, 109, 547–551 CAS.
- R. Senjen, Friends Earth Aus., 2007 Search PubMed.
- S. Kumar, V.-K. Lakshmanan, M. Raj, R. Biswas, T. Hiroshi, S. V. Nair and R. Jayakumar, Pharm. Res., 2013, 30, 523 CrossRef PubMed.
- T. Jamnongkan, S. K. Sukumaran, M. Sugimoto, T. Hara, Y. Takatsuka and K. Koyama, J. Polym. Eng., 2015, 35, 575–586 CrossRef CAS.
- O. Bondarenko, K. Juganson, A. Ivask, K. Kasemets, M. Mortimer and A. Kahru, Arch. Toxicol., 2013, 87, 1181–1200 CrossRef CAS PubMed.
- M. J. Osmond and M. J. Mccall, Nanotoxicology, 2010, 4, 15–41 CrossRef CAS PubMed.
- B. N. Snyder-Talkington, Y. Qian, V. Castranova and N. L. Guo, J. Toxicol. Environ. Health Part B, 2012, 15, 468–492 CAS.
- M. Pandurangan and D. H. Kim, J. Nanopart. Res., 2015, 17, 1–8 CrossRef CAS.
- V. Sharma, P. Singh, A. K. Pandey and A. Dhawan, Mutat. Res. Gen. Toxicol. Env. Mutagen., 2012, 745, 84–91 CrossRef CAS PubMed.
- M. M. Mihai, M. B. Dima, B. Dima and A. M. Holban, Materials, 2019, 12, 2176 CrossRef CAS PubMed.
- K. Niska, E. Zielinska, M. W. Radomski and I. Inkielewicz-Stepniak, Chem. – Biol. Interact., 2018, 295, 38–51 CrossRef CAS PubMed.
- S. Lu, D. Xia, G. Huang, H. Jing, Y. Wang and H. Gu, Colloids Surf., B, 2010, 81, 406–411 CrossRef CAS PubMed.
- N. Pernodet, X. Fang, Y. Sun, A. Bakhtina, A. Ramakrishnan, J. Sokolov, A. Ulman and M. Rafailovich, Small, 2006, 2, 766–773 CrossRef CAS PubMed.
- W. Cui, J. Li, Y. Zhang, H. Rong, W. Lu and L. Jiang, Nanomed. Nanotechnol. Biol. Med., 2012, 8, 46–53 CrossRef CAS PubMed.
- S. Hackenberg, G. Friehs, K. Froelich, C. Ginzkey, C. Koehler, A. Scherzed, M. Burghartz, R. Hagen and N. Kleinsasser, Toxicol. Lett., 2010, 195, 9–14 CrossRef CAS PubMed.
- G. Gao, Y. Ze, B. Li, X. Zhao, T. Zhang, L. Sheng, R. Hu, S. Gui, X. Sang and Q. Sun, J. Hazard. Mater., 2012, 243, 19–27 CrossRef CAS PubMed.
- A. Gajewicz, B. Rasulev, T. C. Dinadayalane, P. Urbaszek, T. Puzyn, D. Leszczynska and J. Leszczynski, Adv. Drug Delivery Rev., 2012, 64, 1663–1693 CrossRef CAS PubMed.
- C. Som, P. Wick, H. Krug and B. Nowack, Environ. Int., 2011, 37, 1131–1142 CrossRef CAS PubMed.
- W. Huang, F. Tao, F. Li, M. Mortimer and L.-H. Guo, NanoImpact, 2020, 20, 100268 CrossRef.
- U. Bunyatova, Z. Rzayev, İ. Koçum, M. Simsek and M. Yuruksoy, Acta Phys. Pol., A, 2016, 129(4), 431–435 CrossRef.
- K. Bubel, Y. Zhang, Y. Assem, S. Agarwal and A. Greiner, Macromolecules, 2013, 46, 7034–7042 CrossRef CAS.
- D. A. Castilla-Casadiego, M. Maldonado, P. Sundaram and J. Almodovar, MRS Commun., 2016, 6, 402–407 CrossRef CAS.
- X. Yang, L. Fan, L. Ma, Y. Wang, S. Lin, F. Yu, X. Pan, G. Luo, D. Zhang and H. Wang, Mater. Des., 2017, 119, 76–84 CrossRef CAS.
- S. Jiang, H. Hou, S. Agarwal and A. Greiner, ACS Sustainable Chem. Eng., 2016, 4, 4797–4804 CrossRef CAS.
- R. Sridhar, S. Sundarrajan, A. Vanangamudi, G. Singh, T. Matsuura and S. Ramakrishna, Macromol. Mater. Eng., 2014, 299, 283–289 CrossRef CAS.
- X. Wang, Y. Yuan, X. Huang and T. Yue, J. Appl. Polym. Sci., 2015, 132(16) DOI:10.1002/app.41811.
- N. Horzum, M. M. Demir, R. Muñoz-Espí and D. Crespy, Green Electrospinning, De Gruyter Berlin, Germany, 2019 Search PubMed.
- M. R. Bhuiyan, A. Islam, A. Ali and M. N. Islam, J. Clean. Prod., 2017, 167, 14–22 CrossRef CAS.
- W.-E. Teo, R. Inai and S. Ramakrishna, Sci. Technol. Adv. Mater., 2011, 12(1), 013002 CrossRef PubMed.
- M. Bläsing and W. Amelung, Sci. Total Environ., 2018, 612, 422–435 CrossRef PubMed.
- A. A. Horton, A. Walton, D. J. Spurgeon, E. Lahive and C. Svendsen, Sci. Total Environ., 2017, 586, 127–141 CrossRef CAS PubMed.
- S. Agarwal and A. Greiner, Polym. Adv. Technol., 2011, 22, 372–378 CrossRef CAS.
- J. Du and Y.-L. Hsieh, Nanotechnology, 2008, 19, 125707 CrossRef PubMed.
- M. Angeles, H. L. Cheng and S. S. Velankar, Polym. Adv. Technol., 2008, 19, 728–733 CrossRef CAS.
- R. Sridhar, R. Lakshminarayanan, K. Madhaiyan, V. A. Barathi, K. H. C. Lim and S. Ramakrishna, Chem. Soc. Rev., 2015, 44, 790–814 RSC.
- K. A. Hammer, C. F. Carson and T. V. Riley, J. Appl. Microbiol., 1999, 86, 985–990 CrossRef CAS PubMed.
- M. Kahkonen, A. Hopia, H. Vuorela, J. Rouha, K. Pihlaja and T. Kujala, J. Agric. Food Chem., 1999, 47, 3954–3962 CrossRef CAS PubMed.
- N. Sagarzazu, G. Cebrián, R. Pagán, S. Condón and P. Mañas, Innov. Food Sci. Emerg. Technol., 2010, 11, 283–289 CrossRef CAS.
- H. D. Dorman and S. G. Deans, J. Appl. Microbiol., 2000, 88, 308–316 CrossRef CAS PubMed.
- M. M. Cowan, Clin. Microbiol. Rev., 1999, 12, 564–582 CrossRef CAS PubMed.
- A. Ali and M. A. Shahid, J. Polym. Environ., 2019, 27, 2933–2942 CrossRef CAS.
- M. E. Cam, S. Cesur, T. Taskin, G. Erdemir, D. S. Kuruca, Y. M. Sahin, L. Kabasakal and O. Gunduz, Eur. Polym. J., 2019, 120, 109239 CrossRef CAS.
- M. A. Shahid, A. Ali, M. N. Uddin, S. Miah, S. M. Islam, M. Mohebbullah and M. S. I. Jamal, J. Ind. Text., 2020, 1528083720904379 Search PubMed.
- S. I. Jeong, M. D. Krebs, C. A. Bonino, S. A. Khan and E. Alsberg, Macromol. Biosci., 2010, 10, 934–943 CrossRef CAS PubMed.
- Z.-M. Huang, Y. Zhang, S. Ramakrishna and C. Lim, Polymer, 2004, 45, 5361–5368 CrossRef CAS.
- A. Isogai, T. Saito and H. Fukuzumi, Nanoscale, 2011, 3, 71–85 RSC.
- S. Ifuku and H. Saimoto, Nanoscale, 2012, 4, 3308–3318 RSC.
- K. Ohkawa, K.-I. Minato, G. Kumagai, S. Hayashi and H. Yamamoto, Biomacromolecules, 2006, 7, 3291–3294 CrossRef CAS PubMed.
- A. Baji, K. Agarwal and S. V. Oopath, Polymers, 2020, 12, 492 CrossRef CAS PubMed.
- D. Sundhari, N. Dhineshbabu, S. Sutha and M. R. Saravanan, Mater. Today: Proc., 2021, 46, 2682–2685 CAS.
- M. Bhuiyan, A. Bakkar, A. Ali, M. N. Uddin, A. R. Talha and R. M. Mohammad, Fibers Polym., 2024, 1–11 Search PubMed.
- B. Kundu, R. Rajkhowa, S. C. Kundu and X. Wang, Adv. Drug Delivery Rev., 2013, 65, 457–470 CrossRef CAS PubMed.
- A. C. Jaski, F. Schmitz, R. P. Horta, L. Cadorin, B. J. G. da Silva, J. Andreaus, M. C. D. Paes, I. C. Riegel-Vidotti and L. M. Zimmermann, Ind. Crops Prod., 2022, 186, 115250 CrossRef CAS.
- M. N. Uddin, M. S. I. Jamal, M. Y. Ali, M. A. Darda and S. I. Mahedi, Emerg. Mater, 2023, 1–13 Search PubMed.
- S. Kumar, G. J. Dobos and T. Rampp, J. Evid. Based Complem. Altern. Med., 2017, 22, 494–501 CrossRef PubMed.
- A. Indurkar, A. Pandit, R. Jain and P. Dandekar, Bioprinting, 2021, 21, e00127 CrossRef.
- R. Salam, J. Khokon and S. Mussa, Int. J. Nat. Soc., 2014, 1, 52–57 Search PubMed.
- R. Subapriya and S. Nagini, Curr. Med. Chem. Anticancer Agents, 2005, 5, 149–156 CrossRef CAS PubMed.
- A. Ali, M. A. Shahid, M. D. Hossain and M. N. Islam, Int. J. Biol. Macromol., 2019, 138, 13–20 CrossRef CAS PubMed.
- O. T. Agar, M. Dikmen, N. Ozturk, M. A. Yilmaz, H. Temel and F. P. Turkmenoglu, Molecules, 2015, 20, 17976–18000 CrossRef CAS PubMed.
- A. D. Azaz, T. Arabaci, M. K. Sangun and B. Yildiz, Asian J. Chem., 2008, 20, 1238 CAS.
- R. Gevrenova, G. Zengin, K. I. Sinan, E. Yıldıztugay, D. Zheleva-Dimitrova, C. Picot-Allain, M. F. Mahomoodally, M. Imran and S. Dall’Acqua, Antioxidants, 2021, 10, 1180 CrossRef CAS PubMed.
- J. Jang, S.-J. Jeong, H.-Y. Kwon, J. H. Jung, E. J. Sohn, H.-J. Lee, J.-H. Kim, S.-H. Kim, J. H. Kim and S.-H. Kim, J. Evid. Based Complem. Altern. Med., 2013, 1, 506324 Search PubMed.
- C. Jiang, J. Guo, Z. Wang, B. Xiao, H.-J. Lee, E.-O. Lee, S.-H. Kim and J. Lu, Breast Cancer Res. Treat., 2007, 9, 1–12 Search PubMed.
- H. H. Kim, S. S. Bang, J. S. Choi, H. Han and I.-H. Kim, Cancer Lett., 2005, 223, 191–201 CrossRef CAS PubMed.
- B. S. Kim, H. Seo, H.-J. Kim, S. M. Bae, H.-N. Son, Y. J. Lee, S. Ryu, R.-W. Park and J.-O. Nam, J. Med. Food, 2015, 18, 1121–1127 CrossRef CAS PubMed.
- J. Lü, S.-H. Kim, C. Jiang, H. Lee and J. Guo, Acta Pharmacol. Sin., 2007, 28, 1365–1372 CrossRef PubMed.
- J. Zhang, L. Li, C. Jiang, C. Xing, S.-H. Kim and J. Lu, Anticancer Agents Med. Chem., 2012, 12, 1239–1254 CrossRef CAS PubMed.
- M. Chávez-Pesqueira and J. Núñez-Farfán, Front. Ecol. Environ., 2017, 5, 155 CrossRef.
- A. Sharma, A. Bachheti, P. Sharma, R. K. Bachheti and A. Husen, Curr. Res. Biotechnol., 2020, 2, 145–160 CrossRef.
- A. B. A. Ahmed, A. Rao and M. Rao, Phytomedicine, 2010, 17, 1033–1039 CrossRef PubMed.
- G. Di Fabio, V. Romanucci, M. Zarrelli, M. Giordano and A. Zarrelli, Molecules, 2013, 18, 14892–14919 CrossRef CAS PubMed.
- B. Chodisetti, K. Rao and A. Giri, Nat. Prod. Res., 2013, 27, 583–587 CrossRef CAS PubMed.
- P. Tiwari, B. Mishra and N. S. Sangwan, Biomed. Res. Int., 2014, 830285 Search PubMed.
- R. Pradhan, P. Dandawate, A. Vyas, S. Padhye, B. Biersack, R. Schobert, A. Ahmad and F. H. Sarkar, Curr. Drug Targets, 2012, 13, 1777–1798 CrossRef CAS PubMed.
- B. Alia, A. Bashir and M. Tanira, Pharmacology, 1995, 51, 356–363 CrossRef PubMed.
- D. K. Singh, S. Luqman and A. K. Mathur, Ind. Crops Prod., 2015, 65, 269–286 CrossRef CAS.
- S. A. Bandh, A. N. Kamili, B. A. Ganai, B. A. Lone and S. Saleem, J. Pharm. Res, 2011, 4, 3141–3142 Search PubMed.
- F. B. Bejestani, Novelty Biomed., 2018, 6, 61–67 Search PubMed.
- B. Salehi, M. Valussi, A. K. Jugran, M. Martorell, K. Ramírez-Alarcón, Z. Z. Stojanović-Radić, H. Antolak, D. Kręgiel, K. S. Mileski and M. Sharifi-Rad, Trends Food Sci. Technol., 2018, 80, 104–122 CrossRef CAS.
- J. D. Park, D. K. Rhee and Y. H. Lee, Phytochem. Rev., 2005, 4, 159–175 CrossRef CAS.
- J. Lee, E. Jung, J. Lee, S. Huh, J. Kim, M. Park, J. So, Y. Ham, K. Jung and C.-G. Hyun, J. Ethnopharmacol., 2007, 109, 29–34 CrossRef CAS PubMed.
- D.-Y. Kim, M.-S. Jung, Y.-G. Park, H. D. Yuan, H. Y. Quan and S.-H. Chung, BMB Eep, 2011, 44, 659–664 CAS.
- Y. G. Park, H.-Y. Quan, S. J. Kim, M. S. Jung and S. H. Chung, Fitoterapia, 2012, 83, 215–222 CrossRef PubMed.
- P. Wang, X. Wei, F. Zhang, K. Yang, C. Qu, H. Luo and L. He, Phytomedicine, 2014, 21, 177–183 CrossRef CAS PubMed.
- K. Zomorodian, M. Saharkhiz, M. Rahimi, A. Bandegi, G. Shekarkhar, A. Bandegani, K. Pakshir and A. Bazargani, Pharmacogn. Mag., 2011, 7, 53 CrossRef CAS PubMed.
- N. T. Ardekani, M. Khorram, K. Zomorodian, S. Yazdanpanah, H. Veisi and H. Veisi, Int. J. Biol. Macromol., 2019, 125, 743–750 CrossRef CAS PubMed.
- H. Sajed, A. Sahebkar and M. Iranshahi, J. Ethnopharmacol., 2013, 145, 686–698 CrossRef PubMed.
- S. M. Jachak, R. Gautam, C. Selvam, H. Madhan, A. Srivastava and T. Khan, Fitoterapia, 2011, 82, 173–177 CrossRef PubMed.
- A. Taddei and A. Rosas-Romero, Phytomedicine, 2000, 7, 235–238 CrossRef CAS PubMed.
- C. C. Ikewuchi, J. C. Ikewuchi and M. O. Ifeanacho, Food Nutr. Sci., 2015, 6, 992 CAS.
- A. B. Thalkari, P. N. Karwa, P. S. Shinde, C. S. Gawli and P. S. Chopane, J. Pharmacogn. Phytochem, 2020, 12, 27–30 Search PubMed.
- J. Ravindran, V. Arumugasamy and A. Baskaran, Wound Med., 2019, 27, 100170 CrossRef.
- M. Suryamathi, C. Ruba, P. Viswanathamurthi, V. Balasubramanian and P. Perumal, Macromol. Res., 2019, 27, 55–60 CrossRef CAS.
- S. Ambulkar, P. Ambulkar, M. P. Deshmukh and A. B. Budhrani, Indian J. Forensic Med. Toxicol., 2020, 14(4), 6579–6584 Search PubMed.
- A. Shrivastav, A. K. Mishra, M. Abid, A. Ahmad, M. Fabuzinadah and N. A. Khan, Wound Med., 2020, 29, 100185 CrossRef.
- A. Sharbidre, P. Dhage, H. Duggal and R. Meshram, Biointerface Res. Appl. Chem, 2021, 11, 12120–12148 CAS.
- N. Z. Abd Rani, K. Husain and E. Kumolosasi, Front. Pharmacol., 2018, 9, 108 CrossRef PubMed.
- H. S. Snehlata and D. R. Payal, Int. J. Curr. Pharm. Rev. Res., 2012, 2, 169–187 Search PubMed.
- P. Sowmya and P. Rajyalakshmi, Plant Foods Hum. Nutr., 1999, 53, 359–365 CrossRef CAS PubMed.
- M. A. Ajabnoor and A. K. Tilmisany, J. Ethnopharmacol., 1988, 22, 45–49 CrossRef CAS PubMed.
- M. Sigalas, R. Biswas and K. M. Ho, Microw. Opt. Technol. Lett., 1996, 13, 205–209 CrossRef.
- R. S. Pandian, C. Anuradha and P. Viswanathan, J. Ethnopharmacol., 2002, 81, 393–397 CrossRef PubMed.
- J. Safaei-Ghomi, A. H. Ebrahimabadi, Z. Djafari-Bidgoli and H. Batooli, Food Chem., 2009, 115, 1524–1528 CrossRef CAS.
- J. Wang, S. Hu, S. Nie, Q. Yu, M. Xie and J. Wang, Crit. Rev. Food Sci. Nutr., 2016, S60–S84 Search PubMed.
- A. Ubeyitogullari and O. N. Ciftci, Food Hydrocolloids, 2020, 102, 105597 CrossRef CAS.
- A. Roche, E. Ross, N. Walsh, K. O'Donnell, A. Williams, M. Klapp, N. Fullard and S. Edelstein, Crit. Rev. Food Sci. Nutr., 2017, 57, 1089–1096 CrossRef CAS PubMed.
- C. Santos, C. J. Silva, Z. Büttel, R. Guimarães, S. B. Pereira, P. Tamagnini and A. Zille, Carbohydr. Polym., 2014, 99, 584–592 CrossRef CAS PubMed.
- C. A. Bonino, M. D. Krebs, C. D. Saquing, S. I. Jeong, K. L. Shearer, E. Alsberg and S. A. Khan, Carbohydr. Polym., 2011, 85, 111–119 CrossRef CAS.
- D. Edikresnha, T. Suciati, M. M. Munir and K. Khairurrijal, RSC Adv., 2019, 9, 26351–26363 RSC.
- K. Prasad, V. A. Laxdal, M. Yu and B. L. Raney, Mol. Cell. Biochem., 1995, 148, 183–189 CrossRef CAS PubMed.
- M. Focke, A. Feld and H. K. Lichtenthaler, FEBS Lett., 1990, 261, 106–108 CrossRef CAS PubMed.
- W. A. Sarhan, H. M. Azzazy and I. M. El-Sherbiny, ACS Appl. Mater. Interfaces, 2016, 8, 6379–6390 CrossRef CAS PubMed.
- Y. Liu, R. Song, X. Zhang and D. Zhang, Int. J. Biol. Macromol., 2020, 161, 1405–1413 CrossRef CAS PubMed.
- A. Ali, M. Mohebbullah, M. A. Shahid, S. Alam, M. N. Uddin, M. S. Miah, M. S. I. Jamal and M. S. Khan, J. Text. Inst., 2021, 112, 1611–1621 CrossRef CAS.
- M. N. Uddin, M. Mohebbullah, S. M. Islam, M. A. Uddin and M. Jobaer, Prog. Biomater., 2022, 11, 431–446 CrossRef CAS PubMed.
- A. Ali, M. R. Bhuiyan, M. Mohebbullah, M. F. Hossain, M. R. Alam, M. N. Uddin, M. A. Islam, M. A. Hossain, A. Rahman and M. G. M. Limon, AATCC J. Res., 2024, 24723444241237316 Search PubMed.
- M. Shahruzzaman, S. Hossain, T. Ahmed, S. F. Kabir, M. M. Islam, A. Rahman, M. S. Islam, S. Sultana and M. M. Rahman, Biological Macromolecules, Elsevier, 2022, pp. 165–202 Search PubMed.
- M. N. Uddin, M. Jobaer, S. I. Mahedi and A. Ali, J. Tex. Inst., 2023, 114, 1592–1617 CrossRef CAS.
- D. Cho, O. Nnadi, A. Netravali and Y. L. Joo, Macromol. Mater. Eng., 2010, 295, 763–773 CrossRef CAS.
- K. Ramji and R. N. Shah, J. Biomater. Appl., 2014, 29, 411–422 CrossRef PubMed.
- S. M. Chacko, P. T. Thambi, R. Kuttan and I. Nishigaki, Chin. Med., 2010, 5, 1–9 CrossRef PubMed.
- M. Sadri, S. Arab-Sorkhi, H. Vatani and A. Bagheri-Pebdeni, Fiber Polym., 2015, 16, 1742–1750 CrossRef CAS.
- S. Shao, L. Li, G. Yang, J. Li, C. Luo, T. Gong and S. Zhou, Int. J. Pharm., 2011, 421, 310–320 CrossRef CAS PubMed.
- M. Ghorbani, P. Nezhad-Mokhtari and S. Ramazani, Int. J. Biol. Macromol., 2020, 153, 921–930 CrossRef CAS PubMed.
- I. Garcia-Orue, G. Gainza, F. B. Gutierrez, J. J. Aguirre, C. Evora, J. L. Pedraz, R. M. Hernandez, A. Delgado and M. Igartua, Int. J. Pharm., 2017, 523, 556–566 CrossRef CAS PubMed.
- I. Garcia-Orue, G. Gainza, P. Garcia-Garcia, F. B. Gutierrez, J. J. Aguirre, R. M. Hernandez, A. Delgado and M. Igartua, Int. J. Pharm., 2019, 556, 320–329 CrossRef CAS PubMed.
- S. Nam, J.-J. Lee, S. Y. Lee, J. Y. Jeong, W.-S. Kang and H.-J. Cho, Int. J. Pharm., 2017, 526, 225–234 CrossRef CAS PubMed.
- J. Ahlawat, V. Kumar and P. Gopinath, Mater. Sci. Eng., C, 2019, 103, 109834 CrossRef CAS PubMed.
- A. Balaji, S. K. Jaganathan, A. F. Ismail and R. Rajasekar, Int. J. Nanomed., 2016, 11, 4339 CrossRef CAS PubMed.
- S. Ravichandran, J. Radhakrishnan, P. Jayabal and G. D. Venkatasubbu, Appl. Surf. Sci., 2019, 484, 676–687 CrossRef CAS.
- R. Ramalingam, C. Dhand, C. M. Leung, S. T. Ong, S. K. Annamalai, M. Kamruddin, N. K. Verma, S. Ramakrishna, R. Lakshminarayanan and K. D. Arunachalam, Mater. Sci. Eng., C, 2019, 98, 503–514 CrossRef CAS PubMed.
- M. Adeli-Sardou, M. M. Yaghoobi, M. Torkzadeh-Mahani and M. Dodel, Int. J. Biol. Macromol., 2019, 124, 478–491 CrossRef CAS PubMed.
- S. Vakilian, M. Norouzi, M. Soufi-Zomorrod, I. Shabani, S. Hosseinzadeh and M. Soleimani, Tissue Cell, 2018, 51, 32–38 CrossRef CAS PubMed.
- I. Yousefi, M. Pakravan, H. Rahimi, A. Bahador, Z. Farshadzadeh and I. Haririan, Mater. Sci. Eng., A, 2017, 75, 433–444 CrossRef CAS PubMed.
- A. Naeimi, M. Payandeh, A. R. Ghara and F. E. Ghadi, Carbohydr. Polym., 2020, 240, 116315 CrossRef CAS PubMed.
- S. Pajoumshariati, S. K. Yavari and M. A. Shokrgozar, Ann. Biomed. Eng., 2016, 44, 1808–1820 CrossRef PubMed.
- O. E. Fayemi, A. C. Ekennia, L. Katata-Seru, A. P. Ebokaiwe, O. M. Ijomone, D. C. Onwudiwe and E. E. Ebenso, ACS Omega, 2018, 3, 4791–4797 CrossRef CAS PubMed.
- S. Selvaraj and N. N. Fathima, ACS Appl. Mater. Interfaces, 2017, 9, 5916–5926 CrossRef CAS PubMed.
- M. P. Mani and S. K. Jaganathan, J. Text. Inst., 2019, 110, 1615–1623 CrossRef CAS.
- H. Urena-Saborio, E. Alfaro-Viquez, D. Esquivel-Alvarado, S. Madrigal-Carballo and S. Gunasekaran, Int. J. Biol. Macromol., 2018, 115, 1218–1224 CrossRef CAS PubMed.
- M. Sharifi, S. H. Bahrami, N. H. Nejad and P. B. Milan, J. Appl. Polym. Sci., 2020, 137, 49528 CrossRef CAS.
- A. Ali, S. M. Islam, M. Mohebbullah, M. N. Uddin, M. T. Hossain, S. K. Saha and M. S. I. Jamal, J. Text. Inst., 2021, 112, 561–567 CrossRef CAS.
- N. Varshney, A. K. Sahi, S. Poddar and S. K. Mahto, Int. J. Biol. Macromol., 2020, 160, 112–127 CrossRef CAS PubMed.
- S. Lin, M. Chen, H. Jiang, L. Fan, B. Sun, F. Yu, X. Yang, X. Lou, C. He and H. Wang, Colloids Surf., B, 2016, 139, 156–163 CrossRef CAS PubMed.
- H. M. Al-Youssef, M. Amina, S. Hassan, T. Amna, J. W. Jeong, K.-T. Nam and H. Y. Kim, Macromol. Res., 2013, 21, 589–598 CrossRef CAS.
- M. Zhang, Y. Liu, Y. Jia, H. Han and D. Sun, J. Bionic Eng., 2014, 11, 115–124 CrossRef.
- M. Ranjbar-Mohammadi and S. H. Bahrami, Int. J. Biol. Macromol., 2016, 84, 448–456 CrossRef CAS PubMed.
- M. Ranjbar-Mohammadi, S. Rabbani, S. H. Bahrami, M. Joghataei and F. Moayer, Mater. Sci. Eng., C, 2016, 69, 1183–1191 CrossRef PubMed.
- O. Suwantong, P. Opanasopit, U. Ruktanonchai and P. Supaphol, Polymer, 2007, 48, 7546–7557 CrossRef CAS.
- S. M. Saeed, H. Mirzadeh, M. Zandi and J. Barzin, Prog. Biomater., 2017, 6, 39–48 CrossRef CAS PubMed.
- M. M. Mahmud, S. Zaman, A. Perveen, R. A. Jahan, M. F. Islam and M. T. Arafat, J. Drug Delivery Sci. Technol., 2020, 55, 101386 CrossRef CAS.
- X.-Z. Sun, G. R. Williams, X.-X. Hou and L.-M. Zhu, Carbohydr. Polym., 2013, 94, 147–153 CrossRef CAS PubMed.
- A. S. Kulkarni, D. D. Gurav, A. A. Khan and V. S. Shinde, Colloids Surf., B, 2020, 189, 110885 CrossRef CAS PubMed.
- E. Esmaeili, T. Eslami-Arshaghi, S. Hosseinzadeh, E. Elahirad, Z. Jamalpoor, S. Hatamie and M. Soleimani, Int. J. Biol. Macromol., 2020, 152, 418–427 CrossRef CAS PubMed.
- J. Jacob, G. Peter, S. Thomas, J. T. Haponiuk and S. Gopi, Int. J. Biol. Macromol., 2019, 129, 370–376 CrossRef CAS PubMed.
- M. A. Shahid and M. S. Khan, Polym. Compos., 2022, 30, 09673911221109422 CAS.
- M. Shokrollahi, S. H. Bahrami, M. H. Nazarpak and A. Solouk, Int. J. Biol. Macromol., 2020, 147, 547–559 CrossRef CAS PubMed.
- B. Motealleh, P. Zahedi, I. Rezaeian, M. Moghimi, A. H. Abdolghaffari and M. A. Zarandi, J. Biomed. Mater. Res. B: Appl. Biomater, 2014, 102, 977–987 CrossRef PubMed.
- W.-H. Dong, J.-X. Liu, X.-J. Mou, G.-S. Liu, X.-W. Huang, X. Yan, X. Ning, S. J. Russell and Y.-Z. Long, Colloids Surf., B, 2020, 188, 110766 CrossRef CAS PubMed.
- E. Shanesazzadeh, M. Kadivar and M. Fathi, Int. J. Biol. Macromol., 2018, 119, 1–7 CrossRef CAS PubMed.
- C. Mouro, M. Simões and I. C. Gouveia, Adv. Polym. Technol., 2019, 9859506 CAS.
- A. Zarghami, M. Irani, A. Mostafazadeh, M. Golpour, A. Heidarinasab and I. Haririan, Fiber. Polym., 2015, 16, 1201–1212 CrossRef CAS.
- V. Mahdavi, H. Rafiee-Dastjerdi, A. Asadi, J. Razmjou, B. F. Achachlouei and S. G. Kamita, Am. J. Potato Res., 2017, 94, 647–657 CrossRef CAS.
- H. S. Sofi, T. Akram, A. H. Tamboli, A. Majeed, N. Shabir and F. A. Sheikh, Int. J. Pharm., 2019, 569, 118590 CrossRef CAS PubMed.
- E. I. Nep, P. O. Odumosu, N. C. Ngwuluka, P. O. Olorunfemi and N. A. Ochekpe, J. Polym., 2013, 938726 Search PubMed.
- A. Dasgupta, Translational Inflammation, Elsevier, 2019, pp. 69–91 Search PubMed.
- B. B. Aggarwal, A. Kumar and A. C. Bharti, Anticancer Res., 2003, 23, 363–398 CAS.
- D. Peschel, R. Koerting and N. Nass, J. Nutr. Biochem., 2007, 18, 113–119 CrossRef CAS PubMed.
- B. F. Adamu, J. Gao, A. K. Jhatial and D. M. Kumelachew, Mater. Des., 2021, 209, 109942 CrossRef CAS.
- J. K. Srivastava and S. Gupta, J. Agric. Food Chem., 2007, 55, 9470–9478 CrossRef CAS PubMed.
- I. Z. Matić, Z. Juranić, K. Šavikin, G. Zdunić, N. Nađvinski and D. Gođevac, Phytother. Res., 2013, 27, 852–858 CrossRef PubMed.
- A. M. Kamali, M. Nikseresht, H. Delaviz, M. J. Barmak, M. Servatkhah, M. T. Ardakani and R. Mahmoudi, Life Sci., 2014, 11, 403–406 Search PubMed.
- C. Weidner, S. J. Wowro, M. Rousseau, A. Freiwald, V. Kodelja, H. Abdel-Aziz, O. Kelber and S. Sauer, PLoS One, 2013, 8, e80335 CrossRef CAS PubMed.
- M. Moro, M. Silveira Souto, G. Franco, M. Holzhausen and C. Pannuti, J. Periodontal Res., 2018, 53, 288–297 CrossRef CAS PubMed.
- W. J. Kong, Y. L. Zhao, L. M. Shan, X. H. Xiao and W. Y. Guo, Chin. J. Chem., 2008, 26, 113–115 CrossRef CAS.
- L.-x Zhang, Y.-p Bai, P.-h Song, L.-p You and D.-q Yang, Chin. J. Integr. Med., 2009, 15, 141–144 CrossRef PubMed.
- H.-M. Cheng, Y.-C. Wu, Q. Wang, M. Song, J. Wu, D. Chen, K. Li, E. Wadman, S.-T. Kao and T.-C. Li, BMC Complement. Altern. Med., 2017, 17, 1–11 CrossRef PubMed.
- R. A. Muluye, Y. Bian and P. N. Alemu, J. Tradit. Complem. Med., 2014, 4, 93–98 CrossRef PubMed.
- W. Zhou and X.-Y. Zhang, Am. J. Chin. Med., 2013, 41, 743–764 CrossRef CAS PubMed.
- L.-W. He, X. Li, J.-W. Chen, D.-D. Sun, W.-Z. Jü and K.-C. Wang, Acta Pharm. Sin., 2006, 41, 1193–1196 CAS.
- R. Amorati, M. C. Foti and L. Valgimigli, J. Agric. Food Chem., 2013, 61, 10835–10847 CrossRef CAS PubMed.
- R. Heidari-Soureshjani, Z. Obeidavi, V. Reisi-Vanani, S. Ebrahimi Dehkordi, N. Fattahian and A. Gholipour, Adv. Herb. Med., 2016, 3(3), 13–19 Search PubMed.
- L. Guo, S. Gong, Y. Wang, Q. Sun, K. Duo and P. Fei, Foodborne Pathog. Dis., 2020, 17, 396–403 CrossRef CAS PubMed.
- M. Garnett and S. Sturton, Chin. Med. J., 1932, 46, 969–973 Search PubMed.
- J. F. Morton, Fruits Warm Climates, JF Morton, 1987 Search PubMed.
- C. Pierce Salguero, Thai Herbal: Traditional Recipes for Health and Harmony, Findhorn Press Ltd., Scotland, 2003, vol. 118 Search PubMed.
- W. Mahabusarakam, P. Wiriyachitra and W. C. Taylor, J. Nat. Prod., 1987, 50, 474–478 CrossRef CAS.
- B. Puri and A. Hall, Phytochemical dictionary: a handbook of bioactive compounds from plants, CRC press, 1998 Search PubMed.
- W. Chuakul, P. Saralamp, W. Paonil, R. Temsirirkkul and T. Clayton, Medicinal plants in Thailand, 1997, vol. II Search PubMed.
- J. B. Harborne, H. Baxter and G. P. Moss, Pythochemical Dictionary: A Handbook of Bioactive Compounds from Plants, Taylor and Francis Ltd, 1999 Search PubMed.
- A. Sen, K. Sarkar, P. Majumder and N. Banerji, Delhi, 1980, 19, 1008 Search PubMed.
- P. Moongkarndi, N. Kosem, S. Kaslungka, O. Luanratana, N. Pongpan and N. Neungton, J. Ethnopharmacology, 2004, 90, 161–166 CrossRef PubMed.
- P. Yates and G. H. Stout, J. Am. Chem. Soc., 1958, 80, 1691–1700 CrossRef CAS.
- S. Y. Gu and J. Ren, Macromol. Mater. Eng., 2005, 290, 1097–1105 CrossRef CAS.
- T. Padmanabhan, V. Kamaraj, L. Magwood Jr and B. Starly, J. Manuf. Process., 2011, 13, 104–112 CrossRef.
- M. N. Uddin, M. Mohebbullah, S. M. Islam, M. Jobaer, S. I. Mahedi and A. Ali, J. Sol–gel Sci. Technol., 2023, 108, 84–97 CrossRef CAS.
- W. J. Al-Kaabi, S. Albukhaty, A. J. Al-Fartosy, H. K. Al-Karagoly, S. Al-Musawi, G. M. Sulaiman, Y. H. Dewir, M. S. Alwahibi and D. A. Soliman, Appl. Sci., 2021, 11, 828 CrossRef CAS.
- P. Vashisth, N. Kumar, M. Sharma and V. Pruthi, Biotechnol. Rep., 2015, 8, 36–44 CrossRef PubMed.
- S. R. Tahami, N. Hasanzadeh Nemati, H. Keshvari and M. T. Khorasani, J. Modern Processes Manuf. Prod., 2020, 9, 43–56 Search PubMed.
- F. Ajalloueian, H. Tavanai, J. Hilborn, O. Donzel-Gargand, K. Leifer, A. Wickham and A. Arpanaei, Biomed. Res. Int., 2014, 475280 Search PubMed.
- A. C. Daly, L. Riley, T. Segura and J. A. Burdick, Nat. Rev. Mater., 2020, 5, 20–43 CrossRef CAS PubMed.
- L. Moroni, J. A. Burdick, C. Highley, S. J. Lee, Y. Morimoto, S. Takeuchi and J. J. Yoo, Nat. Rev. Mater., 2018, 3, 21–37 CrossRef CAS PubMed.
- E. A. Kamoun, S. A. Loutfy, Y. Hussein and E.-R. S. Kenawy, Int. J. Biol. Macromol., 2021, 187, 755–768 CrossRef CAS PubMed.
- Y. Pilehvar-Soltanahmadi, M. Dadashpour, A. Mohajeri, A. Fattahi, R. Sheervalilou and N. Zarghami, Mini Rev. Med. Chem., 2018, 18, 414–427 CrossRef CAS PubMed.
- T. Agarwal, S.-A. Tan, V. Onesto, J. X. Law, G. Agrawal, S. Pal, W. L. Lim, E. Sharifi, F. D. Moghaddam and T. K. Maiti, Adv. Biomed. Eng., 2021, 2, 100015 CrossRef.
- C. Zhang, Y. Li, P. Wang and H. Zhang, Compr. Rev. Food Sci. Food Saf., 2020, 19, 479–502 CrossRef CAS PubMed.
- M. J. Fabra, J. L. Castro-Mayorga, W. Randazzo, J. Lagarón, A. López-Rubio, R. Aznar and G. Sánchez, Food Environ. Virol., 2016, 8, 125–132 CrossRef CAS PubMed.
- P. Wen, D.-H. Zhu, H. Wu, M.-H. Zong, Y.-R. Jing and S.-Y. Han, Food Control, 2016, 59, 366–376 CrossRef CAS.
- F. Topuz and T. Uyar, Food Res. Int., 2020, 130, 108927 CrossRef CAS PubMed.
- S. Torres-Giner, A. Martinez-Abad, M. J. Ocio and J. M. Lagaron, J. Food Sci., 2010, 75, N69–N79 CrossRef CAS PubMed.
- Y. P. Neo, S. Swift, S. Ray, M. Gizdavic-Nikolaidis, J. Jin and C. O. Perera, Food Chem., 2013, 141, 3192–3200 CrossRef CAS PubMed.
- S. Wang, M. F. Marcone, S. Barbut and L.-T. Lim, Food Res. Int., 2013, 52, 467–472 CrossRef CAS.
- M. J. Fabra, A. López-Rubio, E. Sentandreu and J. M. Lagaron, Starch-Stärke, 2016, 68, 603–610 CrossRef CAS.
- M. Rashidi, S. S. Mansour, P. Mostashari, S. Ramezani, M. Mohammadi and M. Ghorbani, Int. J. Biol. Macromol., 2021, 193, 1313–1323 CrossRef CAS PubMed.
- Y. Han and H. Chen, Polym. Sci. Ser., 2013, 55, 320–326 CrossRef CAS.
- M. S. Ali, M. Haq, V. C. Roy, T. C. Ho, J.-S. Park, J.-M. Han and B.-S. Chun, Colloids Surf., B, 2023, 226, 113320 CrossRef CAS PubMed.
- C. Doğan, N. Doğan, M. Gungor, A. K. Eticha and Y. Akgul, Food Packag. Shelf Life, 2022, 34, 100942 CrossRef.
- S. Alippilakkotte, S. Kumar and L. Sreejith, Colloids Surf. Physicochem. Eng. Aspects, 2017, 529, 771–782 CrossRef CAS.
- M. Li, H. Yu, Y. Xie, Y. Guo, Y. Cheng, H. Qian and W. Yao, LWT, 2021, 139, 110800 CrossRef CAS.
- Z. Ceylan, N. Kutlu, R. Meral, M. M. Ekin and Y. E. Kose, Food Biosci., 2021, 42, 101076 CrossRef CAS.
- G. Aslaner, G. Sumnu and S. Sahin, Food Bioproc. Tech., 2021, 14, 1118–1131 CrossRef CAS.
- S. Bhatia and S. Bhatia, Nat. Polym. Drug Delivery Syst., 2016, 33–93 Search PubMed.
- M. R. Marques, Q. Choo, M. Ashtikar, T. C. Rocha, S. Bremer-Hoffmann and M. G. Wacker, Adv. Drug Delivery Rev., 2019, 151, 23–43 CrossRef PubMed.
- A. B. Sengul and E. Asmatulu, Environ. Chem. Lett., 2020, 18, 1659–1683 CrossRef CAS.
- R. F. Pereira and P. J. Bartolo, Adv. Wound Care, 2016, 5, 208–229 CrossRef PubMed.
- E. Ramazan, Advanced Textiles for Wound Care, Elsevier, 2019, pp. 509–540 Search PubMed.
- S. Ahn, Biomimetic and Estrogenic Plant-Based Nanofibrous Wound Dressings, Doctoral dissertation, Harvard University, 2019 Search PubMed.
- R. A. Mantz, D. M. Fox, J. M. Green, P. A. Fylstra, C. Hugh and P. C. Trulove, Z. Naturforsc. A, 2007, 62, 275–280 CrossRef CAS.
- M. Sharma, C. Mukesh, D. Mondal and K. Prasad, RSC Adv., 2013, 3, 18149–18155 RSC.
Footnote |
† Current address: Wilson College of Textiles, NC State University, Raleigh, NC 27695, USA. |
|
This journal is © The Royal Society of Chemistry 2024 |