DOI:
10.1039/C8SC01016A
(Edge Article)
Chem. Sci., 2018,
9, 3860-3865
Transition-metal-free decarboxylative bromination of aromatic carboxylic acids†
Received
2nd March 2018
, Accepted 24th March 2018
First published on 26th March 2018
Abstract
Methods for the conversion of aliphatic acids to alkyl halides have progressed significantly over the past century, however, the analogous decarboxylative bromination of aromatic acids has remained a longstanding challenge. The development of efficient methods for the synthesis of aryl bromides is of great importance as they are versatile reagents in synthesis and are present in many functional molecules. Herein we report a transition metal-free decarboxylative bromination of aromatic acids. The reaction is applicable to many electron-rich aromatic and heteroaromatic acids which have previously proved poor substrates for Hunsdiecker-type reactions. In addition, our preliminary mechanistic study suggests that radical intermediates are not involved in this reaction, which is in contrast to classical Hunsdiecker-type reactivity. Overall, the process demonstrates a useful method for producing valuable reagents from inexpensive and abundant starting materials.
Introduction
Aryl bromides are the substrate of choice when performing transition metal-catalysed cross-coupling reactions1 or preparing Grignard and organolithium reagents.2 They are also used in a variety of other transformations, such as nucleophilic substitution and HalEx reactions,3 and are the core structures in many natural products and dyes.4 Consequently, developing efficient methods for the synthesis of aryl bromides remains an important objective.5 The ability to directly substitute a carboxyl group with a bromo group has interested the synthetic community for many years. This transformation was first demonstrated by Borodine over a century ago, but came to bear the name “The Hunsdiecker Reaction” during the 1940's and is now a fundamental reaction in organic synthesis.6,7 The reaction involves the mixing of an aliphatic carboxylic acid with bromine in the presence of a silver salt to produce the desired alkyl halide. Various developments in this area were made during the latter half of the 20th century,8 however, the applicability of all these methods was limited due to the requirement of stoichiometric transition metal salts and/or poor generality. It is only recently that significant progress has been made in the decarboxylative bromination of aliphatic acids (Scheme 1A). Namely, the groups of Glorius9 and Li10 have demonstrated that primary, secondary and tertiary carboxylic acids can be converted into the corresponding alkyl bromides in the presence of either an iridium or silver catalyst.
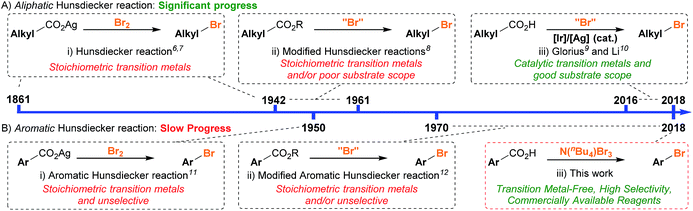 |
| Scheme 1 Timeline of the contrasting progress in (A) aliphatic vs. (B) aromatic decarboxylative bromination. | |
Conversely, the decarboxylative bromination of aromatic acids has suffered from a slower pace of development (Scheme 1B). Early findings demonstrated that the bromination of aromatic acids could indeed proceed, however, the reaction with electron-rich substrates was unselective and electron-deficient aromatics gave varying yields (Scheme 2).11 Many have looked to solve these issues, but current procedures still suffer as they (a) require stoichiometric transition metals, (b) are of poor generality, and/or (c) are unselective.12 This is disappointing as decarboxylative bromination holds potential as an economic route for aryl halide formation;13 benzoic acids are inexpensive and abundant, and the bromide group is a handle for selective transformations. We were eager to reinvestigate the aromatic Hunsdiecker reaction in order to establish a more efficient and selective route for aryl bromide formation. Herein we reveal the development of a transition metal-free decarboxylative bromination that is applicable to a variety of electron-rich aromatic acids. In addition, preliminary investigations begin to shed light on the mechanism of this reaction.
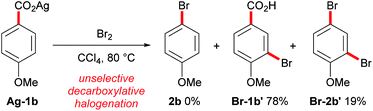 |
| Scheme 2 Current status of the aromatic Hunsdiecker reaction. | |
Results and discussion
We have recently reported a transition-metal-free decarboxylative iodination of aromatic acids.14 The success of this procedure lies in the ability to prepare a variety of aryl iodides, simply by heating a benzoic acid in the presence of I2. We therefore questioned whether a similarly efficient and low-cost decarboxylative bromination could be developed. We began our investigation by exposing the benzoic acid 1a to our previous conditions, but switching the halogen source from I2 to Br2 (Table 1). Unfortunately, this resulted in the undesired formation of the brominated acid 1a′ and dibrominated product 2a′ and none of the desired product 2a (Table 1, entry 1). This reactivity is comparable with the aromatic Hunsdiecker reaction shown in Scheme 2 and demonstrates the challenges for achieving a selective bromination. By lowering the equivalents of Br2 the selectivity could be improved, however, a large amount of the brominated acid 2a′ was still produced (entry 2). We then investigated less electrophilic bromine sources, such as NBS (N-bromosuccinimide) and DBH (1,3-dibromo-5,5-dimethylhydantoin), however a mixture of products was still obtained and the desired product was formed in low yield (entries 3 and 4). We then turned to the use of tribromide reagents as bromine sources for this transformation. Although pyridinium tribromide performed poorly in this reaction (entry 5), we found that tetraalkyl ammonium tribromide salts, N(Me4)Br3 and N(nBu4)Br3, displayed good reactivity and high selectivity for the desired decarboxylative bromination (entries 6 and 7). N(nBu4)Br3 was chosen as the brominating reagent of choice, allowing the product to be isolated in 90% yield. Further control experiments revealed that the reaction does not proceed in the absence of a base (entry 8), but that performing the reaction in the dark or adding one equivalent of water had little effect on the reaction (entries 9 and 10).15
Table 1 Optimisation of the transition metal free decarboxylative brominationa
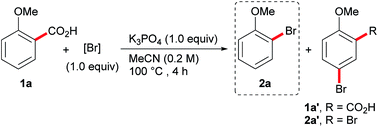
|
Entry |
[Br] |
1a
|
2a
|
1a′
|
2a′
|
Reaction conditions: 1a (0.2 mmol), [Br] (0.2 mmol, 1.0 equiv.), K3PO4 (0.2 mmol, 1.0 equiv.), MeCN (1.0 mL), 100 °C, 4 h.
Br2 (0.6 mmol, 3.0 equiv.).
N-bromosuccinimide.
1,3-Dibromo-5,5-dimethylhydantoin.
Pyridinium tribromide.
Yield in parenthesis is of isolated material. Isolated as a mixture with 2a′ (2a : 2a′, >150 : 1).
No K3PO4 added.
Performed in the dark.
1.0 equiv. H2O added.
|
1b |
Br2 |
Trace |
0 |
23 |
72 |
2 |
Br2 |
8 |
26 |
56 |
3 |
3 |
NBSc |
58 |
21 |
9 |
Trace |
4 |
DBHd |
6 |
40 |
32 |
7 |
5 |
PyHBr3e |
39 |
6 |
54 |
0 |
6 |
N(Me4)Br3 |
6 |
85 |
5 |
2 |
7 |
N(nBu4)Br3 |
5 |
91 (90)f |
1 |
Trace |
8g |
N(nBu4)Br3 |
100 |
0 |
0 |
0 |
9h |
N(nBu4)Br3 |
11 |
84 |
2 |
Trace |
10i |
N(nBu4)Br3 |
10 |
85 |
Trace |
3 |
Having demonstrated an efficient transition metal-free decarboxylative bromination, we then turned to exploring the scope of this reaction (Scheme 3). Previously, the decarboxylative bromination of 4-methoxybenzoic acid under Hunsdiecker-type conditions resulted in a mixture of products (Scheme 2), therefore we were impressed to observe the formation of the aryl bromide 2b in high yield and high selectivity. This clearly demonstrates the advantages of our procedure over previous techniques. The desired product, 2c, was not observed when using 3-methoxybenzoic acid, suggesting the position of decarboxylation must be sufficiently nucleophilic for the decarboxylative bromination to occur. Other highly electron-rich substrates (1d–h) could also undergo the desired transformation, including non-ortho-substituted benzoic acids (1b, 1h), which are generally unreactive in transition metal-mediated decarboxylative functionalisations. The procedure can also be applied on a large scale, thus, 5.5 g of the brominated product 2e was prepared using the standard conditions on the bench top at room temperature, without requiring column chromatography. Polymethylated benzoic acids are poorly reactive substrates in transition metal-mediated decarboxylations, but they, and even simple toluic acid, showed good reactivity under our conditions (2i–2l). The procedure could also be applied to napthoic acids, despite a slight loss in selectivity (2m, 2n). The position of dibromination is indicated in both Schemes 3 and 4 by a red asterisk. A range of halogenated and trifluoromethylated benzoic acids were successfully decarboxylated, however, the presence of a methoxy group was necessary to maintain efficient reactivity (2o–2y). Benzoic acids that do not bear electron-donating substituents were unreactive under these conditions (2z–2ac). In light of this, we were surprised to observe good reactivity with electron-poor polyfluorinated benzoic acids (2ad–2af). This goes against the general trend of reactivity in this reaction and we are currently investigating the cause of this unique behaviour.
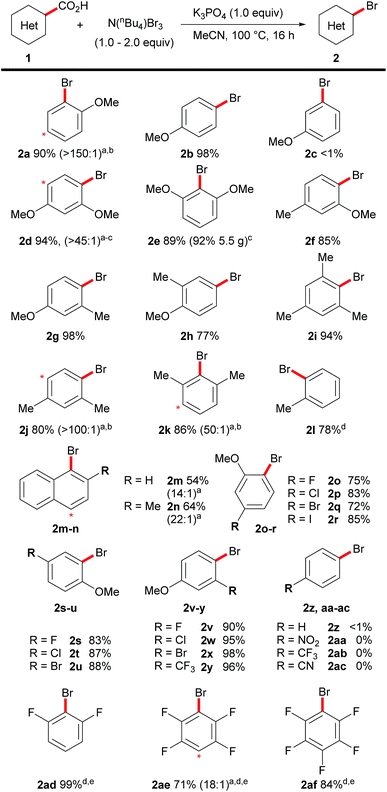 |
| Scheme 3 Scope of the decarboxylative bromination of aromatic acids. Reactions carried out on a 0.5 mmol scale. aRatios in brackets indicate mono : dibrominated material by NMR analysis before separation. Asterisk indicates position of dibromination. bIsolated as mixture. cRoom temperature. dYields determined by NMR analysis. eN(nBu4)Br3 (4.0 equiv.). | |
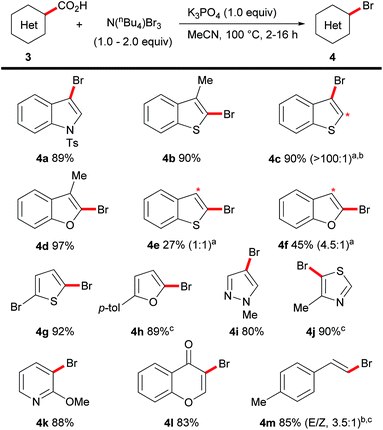 |
| Scheme 4 Scope of the decarboxylative bromination of heteroaromatic acids. Reactions carried out on a 0.5 mmol scale aRatios in brackets indicate mono : dibrominated material by NMR analysis before separation. Asterisk indicates position of dibromination. bIsolated as mixture. c50 °C. | |
The procedure could also be applied to a range of heteroaromatic acids (Scheme 4). The bromination of heteroaromatic acids is highly limited as elevated temperatures (>160 °C) and stoichiometric transition metals are generally necessary.16 Our conditions were applicable to indoles (4a), benzothiophenes (4b, 4c) and benzofurans (4d). Unfortunately, under standard reaction conditions benzothiophene-(4e) and, to an extent, benzofuran-2-carboxylic acid (4f) afforded undesirable levels of dihalogenation. This side-reaction, namely the β-bromination of benzo-fused five-membered heterocycles, is well-known to proceed readily at room-temperature with a variety of brominating agents; thus representing a limitation of our methodology. A range of 5-membered heterocycles (4g–4j) as well as pyridine (4k), chromone (4l) and cinnamic acid (4m) derivatives all underwent the desired decarboxylative bromination selectively.
Having established an efficient protocol for the decarboxylative bromination of aromatic acids, we began a preliminary mechanistic investigation. Our initial experiment involved the use of the oxyallyl-substituted benzoic acid 1A as a radical clock (Fig. 1a). The formation of cyclised products, via attack of an aryl radical on the pendent allyl chain of this compound, is an extremely fast process (k = 8 × 109 s−1),17 therefore, if cyclised products were to be observed in this reaction a radical mechanism could be suggested. Upon exposing oxyallyl-substituted benzoic acid 1A to our standard reaction conditions we only observed the formation of the aryl bromide 2A and none of the cyclised product. In light of this, we can suggest that either the reaction does not proceed through a radical mechanism or, if radicals are involved, then the rate at which the product is formed from the radical intermediate is an exceptionally fast process. This is an interesting observation as similar experiments that have previously been conducted on Hunsdiecker-type decarboxylations of aliphatic carboxylic acids have strongly supported a radical mechanism.18 Likewise, previous Hunsdiecker-type decarboxylations of aromatic acids have also been proposed to proceed through radical intermediates.12k-l,15b,18 Overall, although the above result does not definitively rule out a radical mechanism, it calls for a more thorough evaluation of Hunsdiecker-type reactivity.
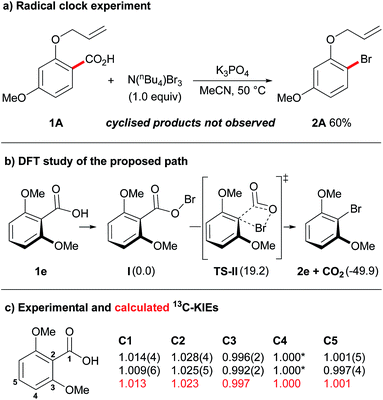 |
| Fig. 1 Mechanistic investigations. (a) Standard reaction conditions with benzoic acid 1A at 50 °C for 30 min. (b) Energies calculated in acetonitrile (B3LYP-D3BJ/6-31+G(d)).24 Gibbs free energies (G) in kcal mol−1. (c) Experimental KIEs (in black), the uncertainty on the last figure is reported in brackets. For C4 a KIE of 1.000 was assumed.25 Calculated KIEs (in red) for the proposed path.26 | |
Our previous work on decarboxylative iodinations established a concerted decarboxylation-iodination process, via a 4-membered transition state, as a possible non-radical pathway for decarboxylative halogenations.14 Following a similar protocol (Fig. 1b), our DFT study found a pathway for decarboxylative bromination proceeding through an analogous concerted decarboxylation-bromination transition state, TS-II. Thus, our current mechanistic hypothesis is as follows: the benzoic acid is initially transformed into the hypobromite species I upon exposure to K3PO4 and N(nBu4)Br3.19 The hypobromite then undergoes decarboxylation via a 4-membered transition state (TS-II), to provide the product 2e with concomitant loss of CO2. The barrier for this transformation was calculated to be 19.2 kcal mol−1, which is consistent with a process that proceeds at room temperature.20
We further probed the mechanism of this reaction by conducting 13C/12C KIE experiments. Heavy atom isotope effects have been widely used as a means to study decarboxylation events in chemical and enzymatic processes.21 The KIE at the C1 position for decarboxylation processes can be accurately determined at natural abundance by measuring the isotopic composition of the evolved CO2 through mass-spectroscopy technique.22 Unfortunately, with this method no information is gained on the other carbon atoms. Exploiting quantitative 13C-NMR, Singleton and co-workers have devised a useful procedure for determining intermolecular competitive 13C/12C KIEs at all positions at natural abundance.23 Over the course of the reaction, the starting material is progressively enriched in the slowest reacting isotopologues. By evaluating the isotopic composition in the starting material before and after the reaction the KIEs can be determined. This has proved a powerful tool in elucidating reaction mechanisms and we were eager to test its value on our system. Two independent experiments were performed on 2,6-dimethoxybenzoic acid (1e) under standard reaction conditions at 30 °C for 70 minutes (Fig. 1c). Remarkably, a primary KIE was observed at both C1 and C2 positions: KIEs of 1.014 ± 0.004 and 1.009 ± 0.006 were obtained for C1, while larger KIEs of 1.028 ± 0.004 and 1.025 ± 0.005 were measured for C2. These values are consistent with the proposed pathway in which a concerted decarboxylation-bromination transition state is involved in the product determining step (Fig. 1b). The lower KIE for C1 in comparison to C2 suggests either an early transition state,27 or that another kinetically relevant step is occurring prior to the product determining step.22e Examination of the reaction path by DFT (TS-II to 2e) revealed an early formation of the C1–Br bond, resulting in an “hidden” Wheland intermediate.28 Extrusion of CO2 from this transient species took place late along the reaction coordinates, thus in agreement with the experimental observations.29 To further probe our mechanistic hypothesis, the KIE values for the proposed path were calculated (Fig. 1c). Computed and experimental values were found to be in excellent agreement, lending strong support to the proposed concerted decarboxylation-bromination pathway.
We have conducted a preliminary mechanistic study of the developed decarboxylative bromination of aromatic acids. At present, we have strong evidence that excludes the intermediacy of aryl radicals (Fig. 1a). By measuring the 13C/12C KIEs and performing DFT calculations we identified a concerted decarboxylation-bromination as a possible pathway for this transformation (Fig. 1b and c). This represents an alternative mechanism for Hunsdiecker-type reactivity, as radicals are usually considered key intermediates in similar processes. While further investigations are necessary to better establish the mechanism of this reaction, we believe that these initial studies highlight previously unrealised features of our system. We hope that these results inspire future studies that may greatly impact this and related procedures, and lead to the development of more efficient decarboxylative technologies.
Conclusions
Due to slow progress and issues with selectivity, the utility of the aromatic Hunsdiecker reaction has previously failed to be fully realised. In this report we have detailed the successful development of a high yielding aromatic Hunsdiecker-type reaction. This has led to the development of a decarboxylative bromination of electron-rich aromatic acids using low-cost and abundant starting materials. The avoidance of transition metals and the ability to scale-up the reaction make the process attractive for its simplicity and low cost. The Hunsdiecker reaction is commonly proposed to proceed via a radical pathway, however, our combined experimental and theoretical mechanistic study has suggested an alternative mechanism that does not involve radical intermediates. These results directly challenge a long-held view of Hunsdiecker-type reactivity. Further studies are necessary, but we hope that future investigations will better elucidate this mechanism. Overall, we believe that this report demonstrates the potential of decarboxylative halogenation as an efficient route to value-added chemical commodities.
Conflicts of interest
There are no conflicts to declare.
Acknowledgements
We gratefully acknowledge the Engineering and Physical Sciences Research Council for funding (EP/L014017/2 and EP/K039547/1). We thank Dr Ralph Adams (UoM) for the quantitative NMR experiments.
References
-
(a) J. Hassan, M. Sévignon, C. Gozzi, E. Schulz and M. Lemaire, Chem. Rev., 2002, 102, 1359–1470 CrossRef CAS PubMed;
(b) C. C. C. Johansson Seechurn, M. O. Kitching, T. J. Colacot and V. Snieckus, Angew. Chem., Int. Ed., 2012, 51, 5062–5085 CrossRef CAS PubMed;
(c) C. Bolm, J. P. Hildebrand, K. Muniz and N. Hermanns, Angew. Chem., Int. Ed., 2001, 40, 3284–3308 CrossRef CAS.
-
(a) P. Knochel, W. Dohle, N. Gommermann, F. F. Kneisel, F. Kopp, T. Korn, I. Sapountzis and V. A. Vu, Angew. Chem., Int. Ed., 2003, 42, 4302–4320 CrossRef CAS PubMed;
(b) D. Tilly, F. Chevallier, F. Mongin and P. C. Gros, Chem. Rev., 2014, 114, 1207–1257 CrossRef CAS PubMed;
(c)
Handbook of Grignard Reagents, ed. G. S. Silverman and P. E. Rakita, Dekker, New York, NY, 1996 Search PubMed;
(d) N. Sotomayor and E. Lete, Curr. Org. Chem., 2003, 7, 275–300 CrossRef CAS.
- For nucleophilic substitution see
(a) J. F. Burnett and R. E. Zahler, Chem. Rev., 1951, 49, 273–412 CrossRef;
(b) J. F. Hartwig, Synlett, 2006, 1283–1294 CrossRef CAS. Halogen Exchange see:
(c) A. Klapars and S. L. Buchwald, J. Am. Chem. Soc., 2002, 124, 14844–14845 CrossRef CAS PubMed;
(d) M. Chen, S. Ichikawa and S. L. Buchwald, Angew. Chem., Int. Ed., 2015, 54, 263–266 CrossRef CAS PubMed;
(e) T. D. Sheppard, Org. Biomol. Chem., 2009, 7, 1043–1052 RSC. Aryl radical formation see
(f) W. P. Neumann, Synthesis, 1987, 665–683 CrossRef CAS;
(g) H. Kim and C. Lee, Angew. Chem., Int. Ed., 2012, 51, 12303–12306 CrossRef CAS PubMed;
(h) I. Ghosh, T. Ghosh, J. I. Bardagi and B. Konig, Science, 2014, 346, 725–728 CrossRef CAS PubMed. Aryne generation see
(i) H. Pellissier and M. Santelli, Tetrahedron, 2003, 59, 701–730 CrossRef CAS;
(j)
R. A. Moss, M. S. Platz and M. Jones Jr, in Reactive Intermediate Chemistry, ed. M. Winkler, H. H. Wenk and W. Sander, John Wiley & Sons, Inc., Hoboken, NJ, USA, 2005, pp. 741–794 Search PubMed.
-
(a) A. Butler and J. V. Walker, Chem. Rev., 1993, 93, 1937–1944 CrossRef CAS;
(b) J. L. Wolk and A. A. Frimer, Molecules, 2010, 15, 5473–5580 CrossRef CAS PubMed;
(c) J. L. Wolk and A. A. Frimer, Molecules, 2010, 15, 5561–5580 CrossRef CAS PubMed;
(d) G. W. Gribble, J. Chem. Educ., 2004, 81, 1441–1449 CrossRef CAS.
- For selected approaches for the preparation of aryl bromides see ref. 3e and
(a)
R. Taylor, Electrophilic Aromatic Substitution, John Wiley, New York, 1990 Search PubMed;
(b) V. Snieckus, Chem. Rev., 1990, 90, 879–933 CrossRef CAS;
(c) A. Roglans, A. Pla-Quintana and M. Moreno-Mañas, Chem. Rev., 2006, 106, 4622–4643 CrossRef CAS PubMed;
(d) D. Kalyani, A. R. Dick, W. Q. Anani and M. A. Sanford, Org. Lett., 2006, 8, 2523–2526 CrossRef CAS PubMed;
(e) C. J. Teskey, A. Y. W. Lui and M. F. Greaney, Angew. Chem., Int. Ed., 2015, 54, 11677–11680 CrossRef CAS PubMed.
-
(a) A. Borodine, Justus Liebigs Ann. Chem., 1861, 119, 121–123 CrossRef;
(b) H. Hunsdiecker and C. Hunsdiecker, Ber. Dtsch. Chem. Ges. B, 1942, 75, 291–297 CrossRef;
(c)
C. Wilson, in Org. React., John Wiley & Sons, Inc., Hoboken, NJ, USA, 2011, pp. 332–387 Search PubMed.
-
(a)
D. Crich, in Comprehensive Organic Synthesis, ed. B. M. Trost and I. Fleming, Pergamon Press, 1991, vol. 7, pp. 717–734 Search PubMed;
(b)
D. Crich and K. Sasaki, in Comprehensive Organic Synthesis II, ed. P. Knochel and G. A. Molander, Elsevier, 2014, pp. 818–836 Search PubMed;
(c)
J. J. Li, in Name Reactions. A Collection of Detailed Reaction Mechanisms, Springer-Verlag Berlin Heidelberg, 3rd edn, 2006, pp. 310–311 Search PubMed;
(d)
L. Kürti and B. Czakó, in Strategic Applications of Named Reactions in Organic Synthesis, Elsevier, California, SD, USA, 2005, pp. 218–219 Search PubMed.
-
(a) S. Cristol and W. J. Firth Jr, J. Org. Chem., 1961, 26, 280 CrossRef CAS;
(b) J. K. Kochi, J. Am. Chem. Soc., 1965, 87, 2500–2502 CrossRef CAS;
(c) D. H. R. Barton, H. P. Faro, E. P. Serebryakov and N. F. Woolsey, J. Chem. Soc., 1965, 2438–2444 RSC;
(d) D. H. R. Barton, D. Crich and W. B. Motherwell, Tetrahedron Lett., 1983, 24, 4979–4982 CrossRef CAS;
(e) D. H. R. Barton, D. Crich and W. B. Motherwell, J. Chem. Soc., Chem. Commun., 1983, 939–941 RSC;
(f) J. I. Concepcion, C. G. Francisco, R. Freire, R. Hernandez, J. A. Salazar and E. Suarez, J. Org. Chem., 1986, 51, 402–404 CrossRef CAS;
(g) P. Camps, A. E. Lukach, X. Pujol and S. Vázquez, Tetrahedron, 2000, 56, 2703–2707 CrossRef CAS.
- L. Candish, E. A. Standley, A. Gómez-Suárez, S. Mukherjee and F. Glorius, Chem.–Eur. J., 2016, 22, 9971–9974 CrossRef CAS PubMed.
- X. Tan, T. Song, Z. Wang, H. Chen, L. Cui and C. Li, Org. Lett., 2017, 19, 1634–1637 CrossRef CAS PubMed.
-
(a) W. G. Dauben and H. Tilles, J. Am. Chem. Soc., 1950, 72, 3185–3187 CrossRef CAS;
(b) R. A. Barnes and R. J. Prochaska, J. Am. Chem. Soc., 1950, 72, 3188–3191 CrossRef CAS;
(c) J. W. H. Oldham, J. Chem. Soc., 1950, 100–108 RSC.
- For reports that require stoichiometric transition metals see ref. 11 and:
(a) J. S. Kiely, L. L. Nelson and P. J. Boudjouk, Org. Chem., 1977, 42, 1480 CrossRef CAS;
(b) S. Uemura, S. Tanaka, M. Okano and M. Hamana, J. Org. Chem., 1983, 48, 3297–3301 CrossRef CAS;
(c) Y. Luo, X. Pan and J. Wu, Tetrahedron Lett., 2010, 51, 6646–6648 CrossRef CAS;
(d) J. Cornella, M. Rosillo-Lopez and I. Larrosa, Adv. Synth. Catal., 2011, 353, 1359–1366 CrossRef CAS;
(e) X. Peng, X.-F. Shao and Z.-Q. Liu, Tetrahedron Lett., 2013, 54, 3079–3081 CrossRef CAS;
(f) Z. Fu, Z. Li, Y. Song, R. Yang, Y. Liu and H. Cai, J. Org. Chem., 2016, 81, 2794–2803 CrossRef CAS PubMed. For reports that show limited scope and/or poor selectivity see:
(g) D. H. R. Barton, B. Lacher and S. Z. Zard, Tetrahedron Lett., 1985, 26, 5939–5942 CrossRef CAS;
(h) D. H. R. Barton, B. Lacher and S. Z. Zard, Tetrahedron, 1987, 43, 4321–4328 CrossRef CAS;
(i) B.-S. Koo, E.-H. Kim and K.-J. Lee, Synth. Commun., 2002, 32, 2275–2286 CrossRef CAS;
(j) H. Hamamoto, S. Hattori, K. Takemaru and Y. Miki, Synlett, 2011, 2011, 1563–1566 CrossRef;
(k) Z. Li, K. Wang and Z.-Q. Liu, Synlett, 2014, 25, 2508–2512 CrossRef CAS;
(l) J. Fang, D. Wang, G.-J. Deng and H. Gong, Tetrahedron Lett., 2017, 58, 4503–4506 CrossRef CAS.
- For selected reviews on decarboxylative activation see:
(a) L. J. Gooßen, K. Gooßen, N. Rodríguez, M. Blanchot, C. Linder and B. Zimmermann, Pure Appl. Chem., 2008, 80, 1725–1733 CrossRef;
(b) L. J. Gooßen, N. Rodríguez and K. Gooßen, Angew. Chem., Int. Ed., 2008, 47, 3100–3120 CrossRef PubMed;
(c) L. J. Gooßen, F. Collet and K. Gooßen, Isr. J. Chem., 2010, 50, 617–629 CrossRef;
(d) R. Shang and L. Liu, Sci. China: Chem., 2011, 54, 1670–1687 CrossRef CAS;
(e) N. Rodríguez and L. J. Gooßen, Chem. Soc. Rev., 2011, 40, 5030–5048 RSC;
(f) W. I. Dzik, P. P. Lange and L. J. Gooßen, Chem. Sci., 2012, 3, 2671–2678 RSC;
(g) J. Cornella and I. Larrosa, Synthesis, 2012, 44, 653–676 CrossRef CAS;
(h)
L. J. Gooßen and K. Gooßen, Decarboxylative Coupling Reactions, in Topics in Organometallic Chemistry, ed. L. J. Gooßen, Springer-Verlag Berlin Heidelberg, 2013, vol. 44, pp. 121–142 Search PubMed;
(i) G. J. P. Perry and I. Larrosa, Eur. J. Org. Chem., 2017, 2017, 3517–3527 CrossRef CAS PubMed;
(j) Y. Wei, P. Hu, M. Zhang and W. Su, Chem. Rev., 2017, 117, 8864–8907 CrossRef CAS PubMed.
- G. J. P. Perry, J. M. Quibell, A. Panigrahi and I. Larrosa, J. Am. Chem. Soc., 2017, 139, 11527–11536 CrossRef CAS PubMed.
- Previous decarboxylative halogenation procedures have required irradiation see ref. 8f and g and:
(a) A. I. Meyers and M. P. Fleming, J. Org. Chem., 1979, 44, 3405–3406 CrossRef CAS;
(b) K. Kulbitski, G. Nisnevich and M. Gandelman, Adv. Synth. Catal., 2011, 353, 1438–1442 CrossRef CAS.
- See ref. 12b, d–f.
-
(a) L. J. Johnston, J. Lusztyk, D. D. M. Wayner, A. N. Abeywickreyma, A. L. J. Beckwith, J. C. Scaiano and K. U. Ingold, J. Am. Chem. Soc., 1985, 107, 4594–4596 CrossRef CAS;
(b)
M. Newcomb, Encyclopedia of Radicals in Chemistry, Biology and Materials, John Wiley & Sons, Ltd., Chichester, UK, 2012; pp. 1–19 Search PubMed.
- See ref. 6c and R. G. Johnson and R. K. Ingham, Chem. Rev., 1956, 56, 219–269 CrossRef CAS.
- Attempts to establish benzoyl hypobromite I as a definite intermediate were unsuccessful. However, the formation of such acyl hypohalites are reported in the literature, and it is therefore reasonable to suggest its presence in this system. See:
(a) J. J. Kleinberg, Chem. Educ., 1946, 23, 559–562 CrossRef CAS;
(b) R. A. Zingaro, J. E. Goodrich, J. Kleinberg and A. J. Vanderwerf, J. Am. Chem. Soc., 1949, 71, 575–576 CrossRef CAS;
(c)
D. D. Tanner, N. J. Bunce, in Acyl Halides, ed. S. Patai, John Wiley & Sons, Ltd., Chichester, UK, 1972, pp. 455–500 Search PubMed;
(d) D. H. R. Barton, H. P. Faro, E. P. Serebryakov and N. F. J. Woolsey, J. Chem. Soc., 1965, 2438–2444 RSC;
(e) P. C. Srivastava, P. Singh, M. Tangiri, A. Sinha and S. J. Bajpai, J. Indian Chem. Soc., 1997, 74, 443–445 CAS.
- C. Colletto, S. Islam, F. Julia-Hernandez and I. Larrosa, J. Am. Chem. Soc., 2016, 138, 1677–1683 CrossRef CAS PubMed.
-
(a)
L. Melander and W. H. Saunders, Reaction Rates of Isotopic Molecules, John Wiley & Sons, Ltd., New York, NY, 1980 Search PubMed;
(b)
M. Wolsfberg, W. A. Van Hook, P. Paneth and L. P. N. Rebelo, Isotope effects in the Chemical, Geological, and Bio Sciences;Springer, Dodrecht, 2010 Search PubMed.
-
(a) B. W. Bromley, G. D. Hegeman and W. Meinschein, Anal. Biochem., 1982, 126, 436–446 CrossRef CAS PubMed;
(b) J. F. Marlier and M. H. O'Leary, J. Am. Chem. Soc., 1984, 106, 5054–5057 CrossRef CAS;
(c) J. F. Marlier and M. H. O'Leary, J. Am. Chem. Soc., 1986, 108, 4896–4899 CrossRef CAS;
(d) H. Gu and S. Zhang, Molecules, 2013, 18, 9278–9292 CrossRef CAS PubMed;
(e) A. A. Vandersteen, G. W. Howe, B. S. Lollar and R. Kluger, J. Am. Chem. Soc., 2017, 139, 15049–15053 CrossRef CAS PubMed.
-
(a) D. A. Singleton and A. A. Thomas, J. Am. Chem. Soc., 1995, 117, 9357–9358 CrossRef CAS . For applications see ref. 20 and:;
(b) A. E. Keating, S. R. Merrigan, D. A. Singleton and K. N. Houk, J. Am. Chem. Soc., 1999, 121, 3933–3938 CrossRef CAS;
(c) D. A. Singleton, S. R. Merrigan, B. J. Kim, P. Beak, L. M. Phillips and J. K. Lee, J. Am. Chem. Soc., 2000, 122, 3296–3300 CrossRef CAS;
(d) D. A. Singleton, Y. Wang, H. W. Yang and D. Romo, Angew. Chem., Int. Ed., 2002, 41, 1572–1575 CrossRef CAS.
- For information on the solvent correction model (SMD) see:
(a) V. Marenich, C. J. Cramer and D. G. Truhlar, J. Phys. Chem. B, 2009, 113, 6378–6396 CrossRef PubMed. For the dispersion correction (DFT-D3(BJ)) see:
(b) S. Grimme, J. Antony, S. Ehrlich and H. Krieg, J. Chem. Phys., 2010, 132, 154104–154119 CrossRef PubMed;
(c) S. Grimme, S. Ehrlich and L. Goerigk, J. Comput. Chem., 2011, 32, 1456–1465 CrossRef CAS PubMed . See ESI† for further details.
- The signal of C4 and C6 was used as “internal standard” in the determination of the isotopic composition of the starting material before and after the reaction. For further information see ESI† and ref. 23a.
- Kinetic isotope effects were calculated using ISOEFF98 according to the Bigeleisen equation.
(a) V. Anisinov and P. Paneth, J. Math. Chem., 1999, 26, 75–86 CrossRef;
(b)
V. Anisinov, P. Paneth, ISOEFF98, Lodz, Poland, 1998 Search PubMedTunnelling correction was applied using Pyquiver according to Bell's infinite-parabola model. The effect was found to be negligible
(c)
T. L. Anderson and E. E. Kwan, PyQuiver, 2016 Search PubMed;
(d) R. P. Bell, Chem. Soc. Rev., 1974, 3, 513–544 RSC . For further information see ESI.†.
- In decarboxylation reactions the KIE on C1 is found to increase as the transition state becomes more product-like. See ref. 22b–e and
(a) J. Bigeleisen, J. Chem. Phys., 1949, 17, 675–678 CrossRef CAS;
(b) C. Lewis, P. Paneth, M. H. O'Leary and D. Hilvert, J. Am. Chem. Soc., 1993, 115, 1410–1413 CrossRef CAS;
(c) G. G. B. Tcherkez, G. D. Farquhar and T. J. Andrews, Proc. Natl. Acad. Sci. U. S. A., 2006, 103, 7246–7251 CrossRef CAS PubMed.
- After the C1–Br bond is formed the gradient drops approaching 0, giving rise to a so-called “hidden intermediate”. For information see:
(a) E. Kraka and D. Cremer, Acc. Chem. Res., 2010, 43, 591–601 CrossRef CAS PubMed;
(b) H. S. Rzepa and C. Wentrup, J. Org. Chem., 2013, 78, 7565–7574 CrossRef CAS PubMed;
(c) D. Roca-Lopez, V. Polo, T. Tejero and P. Merino, J. Org. Chem., 2015, 80, 4076–4083 CrossRef CAS PubMed.
- See ESI† for further details.
Footnotes |
† Electronic supplementary information (ESI) available. See DOI: 10.1039/c8sc01016a |
‡ G. J. P. P. and J. M. Q. contributed equally to this work. |
|
This journal is © The Royal Society of Chemistry 2018 |
Click here to see how this site uses Cookies. View our privacy policy here.