DOI:
10.1039/D3SU00487B
(Critical Review)
RSC Sustain., 2024,
2, 1246-1268
Biogenic derived egg shell and its derivatives as solid base heterogeneous catalysts for organic transformations: a comprehensive review
Received
24th December 2023
, Accepted 4th April 2024
First published on 22nd April 2024
Abstract
The implication of employing green chemistry in synthetic chemistry has opened new doors of sustainability and innovation. The field has attracted tremendous attention in the area of the production of biodiesel, transesterification, carbon–carbon bond formation, condensation and isomerization. Increase in environmental pollution has encouraged researchers to shed light on environmentally benign and sustainable processes to combat the contemporary issues that arise in the current era. Overall, heterogeneous catalysis is proven to be advantageous over homogeneous catalysis as a green process because of the ease of catalyst separation from the reaction mixture fewer stages and less product contamination. The present review emphasizes the pivotal application of egg shell powder as an efficient biocatalyst for organic transformation reactions. Egg shell catalyzed reactions with high chemical selectivity, reduction of the catalyst usage, and high throughput have gained attention in the current scenario for all the above-mentioned reasons. The exact significance of these reactions has been explained thoroughly in the article keeping in mind a greener and economic future.
Sustainability spotlight
Biogenic derived egg shell and its derivatives as solid base heterogeneous catalysts for organic transformations underscore a pioneering commitment to sustainability and eco-friendly practices in the realm of catalysis. This comprehensive review delves into the transformative potential of egg-shell derived waste as a solid base heterogeneous catalyst, marking a significant stride towards green chemistry. The eggshell catalyst exhibits remarkable versatility, finding applications in crucial domains such as biodiesel production, carbon–carbon bond formation, and various other named reactions. What sets this catalyst apart is its origin in circular chemistry, aligning with a sustainable ethos by repurposing egg shell waste as a valuable resource. In doing so, it not only addresses waste management concerns but also mitigates potential environmental hazards that conventional catalysts might pose.
|
1. Introduction
According to modern perspectives, environmental, social and economic dimensions in chemistry, both processes and products, are conceptualized by universally acknowledged terms, “green and sustainable chemistry”. Green chemistry is traditionally achieved by applying environmentally benign protocols; meanwhile, their sustainability is distinguished by processes and products ensuring long-term efficiency so that the future generations can also use them as alternatives for existing systems. Research programs based on environmentally benign chemical techniques are gaining importance in academic and industrial research laboratories and recent developments in green synthesis of organic compounds allow introducing new “thinking” in laboratories and the chemical industry.1 In green chemistry, e-factors2 measure the environmental impact of chemical processes, focusing on resource efficiency and waste generation. Green chemistry aims to create products and processes that minimize pollution and enhance sustainability.3 E-factors quantify environmental performance, showing the ratio of waste to desired product mass. Lower e-factors signify better sustainability. They guide the development of eco-friendly alternatives by assessing waste generation per product unit, aiding in waste minimization and resource optimization. E-factor assessment considers non-recoverable materials, solvents, catalysts, and unwanted by-products. A smaller e-factor indicates progress towards zero waste, promoting greener practices in chemical processes. The e-factor can be mathematically represented as follows:
All these sets of laws governed by the principles of green chemistry can be followed by emphasizing research on nanoscience and nanotechnology, without compromising in potential for any particular application. Especially, nanoparticles that are made of biowaste materials are of immense interest due to cost efficiency and easy availability. Worldwide, livestock production is the largest user of agricultural land and it is leaving a noteworthy imprint on the environment.4,5 Chicken livestock has become a major hot spot for synthetic chemists since, it is the most common food globally and the egg-shell waste has many catalytic applications. Eggs are part of chicken livestock and according to the Food and Agricultural Organization (FAO) 2019 statistics, China stands on top for its production (∼63 million tons) and India got the 3rd place with a production of ∼12 million tons of eggs, which led to production of egg shell (ES) as a biowaste. The effective treatment and utilization of biowaste has to be emphasized due to environmental and economic concerns. Thus, bioconversion of waste into useable energy will significantly boost the sustainable economic developments. ES waste consists of 95% calcium carbonate, 0.3% phosphorus, 0.3% magnesium and traces of zinc, manganese, iron and copper along with amino acids, the protein membrane and collagen. Traditional disposal of it involves throwing away in landfills without any pretreatment, which ultimately creates serious environmental issues. ES that can be converted to nanoparticles possesses promising advantages like chemical stability, biocompatibility and bioactivity, high porosity, antibacterial and anti-inflammatory characteristics and it is considered to be a value-added product and used as an adsorbent, and also has therapeutic, nutraceutical, metallurgical and bioremediation applications.6,7
Several reviews are already available on ES applications and some of them are included here. Carvalho et al. gave an overview on the development of ES by-products, which can be used as low-cost adsorbents.8 The review had concentrated on comparison of ES by-products with other low-cost adsorbents, namely, bagasse pith, maize cob, hen feathers, egg shell waste, rice husk, coconut shell, waste carbon slurries, metal hydroxide sludge, clay materials, starch and other biosorbents along with activated carbons for heavy metal ion and organic dye removal. It also emphasized the entire characterization of ES (both physical and chemical) as well as sorption kinetics models. A. M. King'ori covered most of the reports on uses of egg shells and egg shell membranes.9 ES for nutrition and medicines, as a natural source of calcium, and as a plant fertilizer, liming source and soil stabilizer is covered. The use of ES membranes consisting of a type of fibrous protein called collagen, which connects and supports tissues of skin, bone, muscles, tendons and cartilage is included. A complete ultrastructure, biomineralization and other related applications were reviewed by Partha Sarathi Guru and Sukalyan Dash.10
There have been reviews on use of egg shell waste focusing on synthesis of syngas, dimethyl carbonate, and waste water11 and also on biodiesel production from green waste and nonedible cooking oil waste in particular.12 The present review has concentrated on the applications of ES in several organic transformation reactions. ES powder is proved to be an interesting solid base catalyst in heterogeneous catalysis. Very little attention is devoted to solid base catalysts compared to solid acid catalysts,13 especially, on ES as a catalyst. A liquid base catalyst requires a stoichiometric amount to carry out the reaction, whereas a solid base catalyst offers easy separation and purification of the product as well as reusability leading to a cleaner green and sustainable environment.
2. Egg shell powder as a catalyst for organic transformations
2.1 Suzuki and Heck coupling reactions
Use of environmentally benign supports such as cellulose, chitosan or any other biopolymers for catalytic organic conversions due to their non-toxicity is an innovative step towards sustainability.
An ongoing challenge in Suzuki and Heck coupling is to devise methods that are not only highly efficient but also economically viable and environmentally sustainable. Many established approaches rely on heat, excess reagents, and dilute conditions, which not only raise environmental concerns but also present opportunities for low e-factor alternatives.14 To address these issues, the development of eco-friendly catalysts for coupling reactions has emerged as a promising solution. These catalysts have not only reduced waste generation but also improved other crucial parameters such as reaction speed and scalability in many cases. In this regard, Yang et al.15 fabricated a catalyst by immersing the egg shell membrane into palladium acetate Pd(OAc)2 and palladium chloride PdCl2 with 0.6 mmol of Pd. During this process, the egg shell decolorized to yellow and brown when dipped in Pd(OAc)2 for PdCl2 solutions, respectively. The membranes were subsequently washed with deionized water (3 × 20 mL) and acetone (3 × 20 mL) and dried for 24 h at room temperature, after which the obtained catalyst was labelled ESM–Pd(OAc)2 and ESM–PdCl2. The group focused on the Suzuki reaction in water, as it is environmentally benign and readily accessible and also the model substrate used for Suzuki coupling was bromobenzene 1 with phenyl boronic acid 2 with a highest yield of 91%. It is worth noting that bases play a crucial role in the Suzuki reaction, and different bases such as cesium oxide, potassium phosphate, triethyl amine and tributyl ammonium bromide resulted in poor yields. Meanwhile potassium dichromate and sodium acetate improved the yield to 88 and 91%, respectively. Other reaction parameters such as reaction temperature and time were also evaluated; a temperature of 40 °C and a time of 60 min were found to be compatible. Only pristine palladium salts such as Pd(OAc)2, PdCl2, palladium diacetate, and tris(dibenzylideneacetone)dipalladium were analyzed as control catalysts. It was proven that the yield procured by these palladium salts was less than those of ESM–Pd(OAc)2 and ESM–PdCl2. But on comparing both ESM–Pd(OAc)2 and ESM–PdCl2, the former was more active within a shorter reaction time (Scheme 1a). The atom economy of reaction giving product 3 is 58% with an e-factor of 17.9. The meta and para substituted aromatic derivatives were more reactive than ortho substituted groups, which confirm that the steric hindrance of the coupling partners in the reactions is the influencing factor for the Suzuki coupling reaction (Scheme 1b).
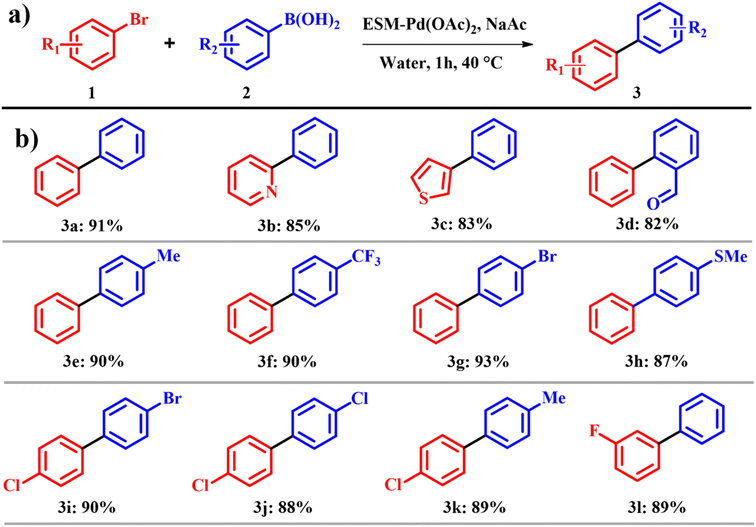 |
| Scheme 1 (a) Suzuki C–C cross coupling reactions with the ESM–Pd complex catalyst and (b) scope of the highly yielded derivatives (adapted from ref. 15). | |
The Heck reaction in water was also carried out and the best yield was obtained in the case of ESM–PdCl2 with a reaction temperature of 70 °C for 24 h with a yield of 93% (Scheme 2a). Different alkynes were studied for the Heck coupling reaction, out of which styrene derivatives and acrylate esters promised the desired cross-coupling products in high yields as shown in Scheme 2b.15 The atom economy of reaction giving product 5 is 69.34% with an e-factor of 27.4. Employing ESM–Pd in Suzuki and Heck coupling reactions instead of traditional coupling catalysts has led to a notable enhancement in atom efficiency under mild conditions. This advancement maintains high yields while significantly reducing overall waste generation in the reaction, resulting in lower e-factors.
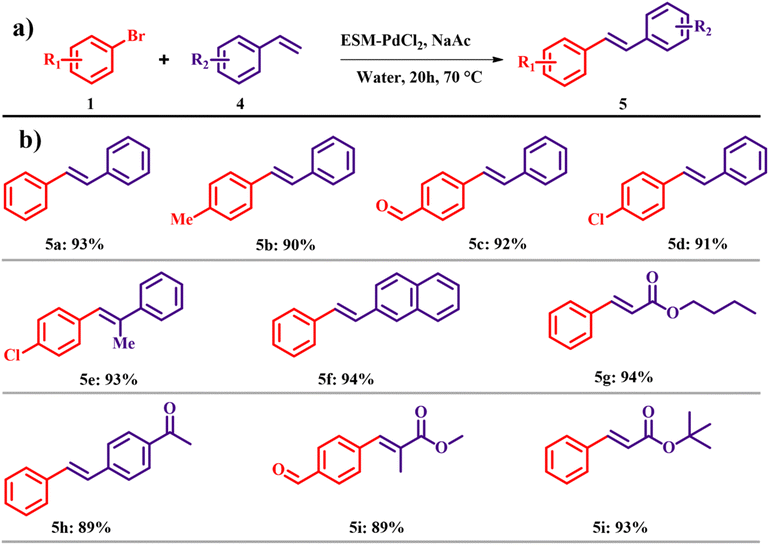 |
| Scheme 2 (a) Heck reactions with the ESM–Pd complex catalyst and (b) scope of the highly yielded derivatives (adapted from ref. 15). | |
2.2 Schiff base synthesis
Out of many chief attributes in synthetic chemistry, designing an organic reaction under the norms of green chemistry and abiding to the facets of sustainability is of prime importance. Among numerous facets of green chemistry, the solvent-free synthesis approach stands with utmost significance. It offers selectivity, reduced reaction time and a simplified purification process because it is a greener approach.16,17 ES can be used as a catalyst where the use of solvents can be greatly reduced. ES powder was used as a catalyst for the synthesis of Schiff bases under solvent free conditions.18 These will bond coordinately with metals to form metal complexes and are used as models for biological systems.19 Numerous research studies have explored the synthesis of Schiff bases,20,21 but they faced constraints such as long reaction time, low yield, use of toxic solvents and tedious workup procedures. A traditional synthesis of imines invented by Schiff involved condensation of carbonyl compounds with an amine followed by removal of water.22 An in situ dehydration strategy is used nowadays to remove water in the second step.23,24 Depending on the same protocol, Patil et al.18 used calcined ES as a dehydrating agent as shown in Scheme 3a. To set the scope and limitations of calcined ES catalyzed synthesis of Schiff bases, several reactions were carried out with structurally diverse carbonyl compounds 6 and substituted amines 7 and were observed to result in moderate to good yields 8 in most of the cases (Scheme 3b). The atom economy of reaction giving product 8 is 93.02% with an e-factor of 1.1. The solvent-free approach showcased remarkable progress in waste management, with an e-factor as low as 1.1, indicating substantial waste reduction. Analysis of the e-factor underscores the potential of this method to provide a greener alternative for synthesizing Schiff-based derivatives. On comparison with traditional methods, use of calcined ES emerged as a green method for the synthesis of Schiff bases.
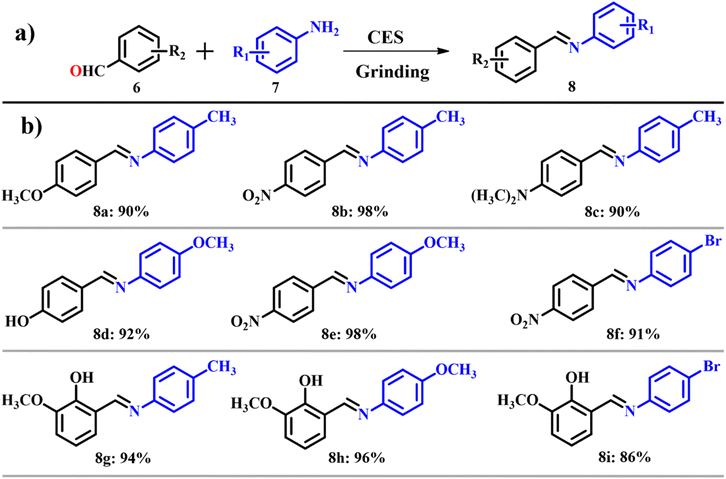 |
| Scheme 3 (a) Synthesis of a Schiff base catalyzed by calcined eggshell and (b) scope of the highly yielded derivatives (adapted from ref. 18). | |
2.3 Knoevenagel condensation reaction
Knoevenagel condensation is one of the significant and imperative synthetic organic chemistry reactions involving a nucleophilic addition reaction between methylene and α,β-unsaturated carbonyl groups followed by dehydration. The as procured products were subsequently used for biologically active chemicals, therapeutic drugs, flavors, perfume, etc.25 Appaturi et al. extended the work to explore Knoevenagel condensation reactions catalyzed by calcined ES under aqueous conditions.25,26 Quite a lot of attempts have been made for both acid catalyzed27,28 and base catalyzed29,30 Knoevenagel condensation reactions under different reaction conditions. Despite several trials, few of those methods are eco-friendly and have solvent free conditions, and completion of reactions with high yield is achieved at high temperature and long reaction time. Patil et al. continued to consider calcined ES as a catalyst for the Knoevenagel condensation reaction between aromatic and heteroaromatic aldehydes and active methylene compounds with a focus on C–C bond formation and achieved attractive yields for most of the reactions, and hence, calcined ES has been proved to be a potential substitute for soluble bases. Recently, El-Shahat and Abdelhameed fabricated a calcium-benzene tricarboxylate (Ca-BTC) MOF using ES as a calcium source (Scheme 4a). Ca-BTC was explored for Knoevenagel condensation with different catalyst loadings, solvent screening and influence of temperature on the conversion. For a test reaction, benzaldehyde 6 was employed as the carbonyl source and malononitrile 7 served as the methylene derivative. It was observed that in the absence of the catalyst no condensation product was procured. Among many solvents used, ethanol was found to produce 10 in a yield of 99% in 1 h at 60 °C, confirming that the reaction occurs smoothly in polar protic solvents over polar aprotic solvents. However, pristine calcium acetate was added, and 35% product conversion was observed. This is because of the electron transfer observed between BTC and Ca in the Ca-BTC MOF that enables 100% conversion. From the proposed mechanism in Scheme 4c, the lone pair of electrons from the carbonyl group of benzaldehyde links with the calcium ions, to form a charged surface, and malononitrile was added to the complex of Ca-BTC and benzaldehyde. Eventually benzaldehyde was condensed with the help of the catalyst to yield the desired product.31 The atom economy of reaction giving product 10 is 80.66% with an e-factor of 4.2. In Knoevenagel condensation reactions, employing malononitrile and reducing solvent usage result in lower e-factors.
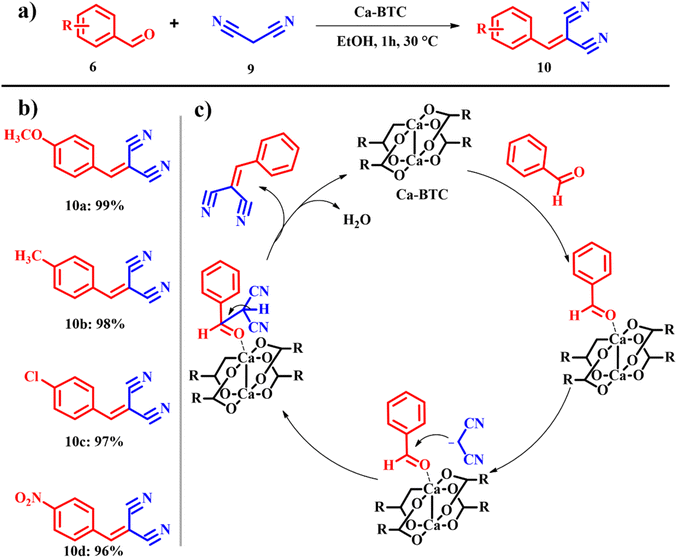 |
| Scheme 4 (a) Knoevenagel condensation catalyzed by the (Ca-BTC) MOF, (b) scope of the highly yielded derivatives and (c) catalytic cycle (adapted from ref. 31). | |
The integration of metal cations and functional moieties could endow MOFs with synergies and for enhancing the catalytic functionalities, metal oxides are incorporated to procure a composite catalyst. One such study was reported by Hassan et al. where a one-pot scalable non-precious CaO composite NH2-MIL-101(Cr) MOF hierarchical material was fabricated. Using the composite catalyst, the catalytic Knoevenagel reaction was carried out as a probe reaction using a CaO/NH2-MIL-101(Cr) MOF catalyst. The incorporation of CaO enhanced the strength and volume of the Lewis base sites from 0.31 to 1.34 mmol g−1 with a maximum product yield of 99% as a function of increased dosage of the MOF and 0.04 mmol g−1 of CaO. On adding only pure MOF and CaO, a product yield of 11 and 20% was obtained, respectively, which proved that CaO/MOF having acid–base features boosted the reaction. The CaO in the composite catalyst aided in the formation of carbanion intermediates and also the unsaturated Cr(VI) sites enabled the development of carbocations from the carbonyl moiety of benzaldehyde.32 CaO derived from egg shell delivers basic sites that extract protons from methylene of malononitrile resulting in the formation of carbanions followed by a dehydration reaction. Also, the CaO composite catalyst falls under the category of heterogeneous catalysts, having the advantage of being easily recyclable with long durability. Therefore, ES-derived calcium sources are found to be environmentally benign and also efficient in organic conversions proving the potential candidature of CaO-based catalysts for commercial purposes.
2.4 Henry reaction
The Henry reaction, also known as the nitro-aldol reaction or nitroalkene addition, is an important transformation reaction in organic synthesis. Named after the British chemist Edward Henry, this reaction involves the addition of a nitroalkane to an aldehyde or ketone, leading to the formation of β-nitro alcohols.33 This reaction is notable for its versatility and its applicability in the synthesis of various organic compounds, including pharmaceuticals, agrochemicals, and natural products.34 However, its limitations, such as substrate specificity, reactivity issues, and environmental concerns, must be considered when planning and conducting reactions.35 Addressing these limitations and exploring innovative strategies are essential for advancing the utility and efficiency of the Henry reaction in modern organic chemistry. Overcoming this, Swati and co-workers reported a sustainable, green and efficient process for the synthesis of 2-nitroalcohol derivatives 12 using 2 mmol aldehydes 6 and 6 mmol of nitroalkane 11 by stirring at ambient temperature using calcined ES as the catalyst (Scheme 5a). When aromatic aldehydes substituted with electron withdrawing groups such as nitro benzaldehyde, bromobenzaldehyde and chlorobenzaldehyde are taken, the reaction proceeded smoothly in excellent yields of the respective products. However, when using substrates with electron donating groups such as 4-methylbenzaldehyde and 4-methoxy benzaldehyde the progress of the reaction was relatively sluggish (Scheme 5b). Catalyst calcined ES behaves as a base generating carbanions (i) from the nitroalkane; the nucleophilic attack of the carbanion on the carbonyl carbon of aldehyde forms the adduct (ii) regenerating calcined ES (Scheme 5c).36 The reported calcined ES catalyst is found to be highly efficient for Henry's reaction under ambient conditions using a renewable catalyst source with zero-emission of pollutants. Since the starting compound was used in a 1
:
1 molar ratio and no dehydrated side-product was observed in this case, the atom economy reached 100%. Additionally, the e-factor was determined to be 4.1. These measured outcomes confirm the sustainability and environmental friendliness of the protocol.
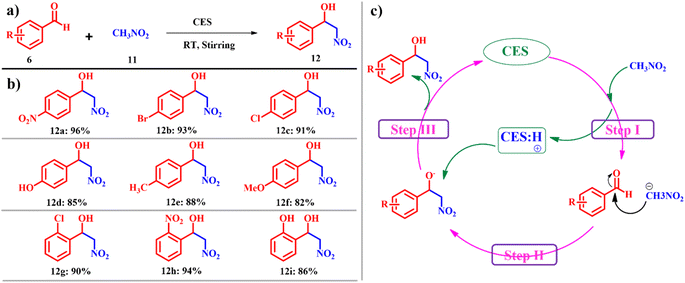 |
| Scheme 5 (a) Synthesis of 2-nitroethanols and (b) derivative of 2-nitro alcohol and (c) plausible mechanism for the synthesis of 2-nitro alcohol (adapted from ref. 36). | |
2.5 Aldol condensation reaction
Sodium hydroxide, potassium hydroxide, pyridine and other bases having nitrogen are used as catalysts in traditional aldol condensation reactions, but are highly toxic and corrosive.37 T. Clarina and co-workers demonstrated ES mediated aldol condensation to prepare a variety of chalcones.38 4-Hydroxy-4-phenylbutan-2-one 15 was synthesized (Scheme 6) under optimized catalytic reaction conditions; however, the best yield was obtained for NaOH catalyzed reactions. But, ES and CaCO3 resulted in the best yields within less reaction time with an e-factor of 12.8. Since, ES is eco-friendly and considered as a green catalyst, the ES catalyzed synthesis was concluded to be the best one.
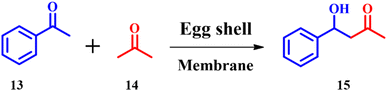 |
| Scheme 6 Synthesis of 4-hydroxy-4-phenylbutan-2-one (adapted from ref. 38). | |
2.6 Synthesis of heterocyclic derivatives
2-Aminochromenes are a class of heterocyclic organic compounds used in pigments, cosmetics, biodegradable agrochemicals and natural products.39 These are the products of three component coupling of an aromatic aldehyde, activated phenol and malononitrile in the presence of a base catalyst.40 Even though many reaction procedures are reported with varying parameters (reaction conditions and modified reagents/reactants/solvents), they suffer from usual problems associated with reaction time and difficult workup procedures. 7,8-Dihydro-4H-chromen-5(6H)-ones 17 were prepared (yields of 90–98%) by Mosaddegh and Hassankhani (Scheme 7a) by the reaction of a suitable mixture of aldehyde 6, malononitrile 9 and dimedone 16 in the presence of ES in ethanol under ambient temperature. On increasing the reaction temperature, the number of hydrogen bonds formed decreases, thereby increasing the entropy of the system. This causes interaction between the ES and the solvent blocking the active sites on the ES, which causes diminished catalytic activity. Products were prepared by using the ES solid base catalyst and on substituting the electron withdrawing group such as nitro and halides yielded products slowly because of electronegative induction of the substituted groups. These substituents pull away the electrons from the aromatic ring via a carbon–halogen sigma bond, leading to deactivation of the aromatic ring yielding low product yields (Scheme 7b).41 The atom economy of reaction giving product 17 is 93.87% with an e-factor of 7.6.
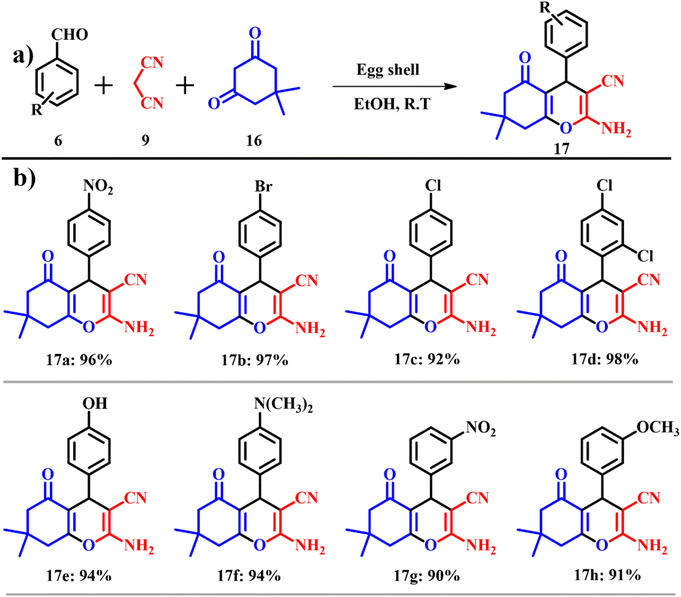 |
| Scheme 7 (a) Synthesis of 7,8-dihydro-4H-chromen-5(6H)-ones by using the ES catalyst and (b) 7,8-dihydro-4H-chromen-5(6H)-one derivatives (adapted from ref. 41). | |
Similarly, Paul and co-workers prepared a highly magnetic solid base, CaO based magnetic support modified with 20 wt% potassium fluoride (KF) [KF(20)CaOHC–Fe3O4/TiO2(400)] and silver immobilized KF modified CaO based magnetic catalyst [Ag@KF(20)CaOHC–Fe3O4/TiO2(400)] using ES. In order to obtain highly active CaO, calcination-, hydration-, and dehydration treatment was used. To standardize fitting reaction conditions for the synthesis of tetrahydro-4H-chromenes 17, similar to the previously cited literature41 in this work a one-pot three component reaction of benzaldehyde 6, malononitrile 9 and dimedone 16 was opted for as the model system but in place of ethanol, water was used as the solvent (Scheme 8a). A product yield of 93% was procured within a time scale of 15 min. KF(20)CaOHC–Fe3O4/TiO2(400) afforded a yield of 94% with a catalyst loading of 0.15 g using 5 mL of water and 1 mmol of the reactants. The atom economy of reaction giving product 17 is 93.87% with an e-factor of 15.1.
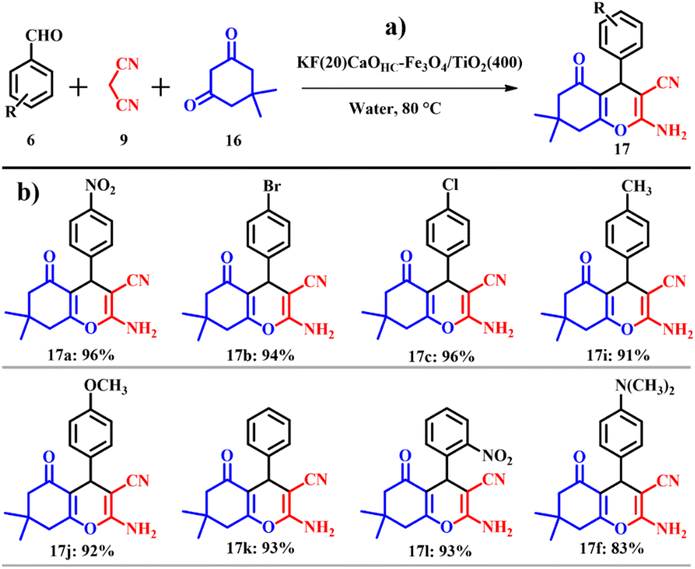 |
| Scheme 8 (a) KF(20)CaOHC–Fe3O4/TiO2(400) catalyzed one-pot synthesis of tetrahydro-4H-chromenes and (b) tetrahydro-4H-chromene derivatives (adapted from ref. 42). | |
The substrate scope of the reaction was studied using both electron withdrawing and electron donating substituents along with heterocyclic compounds giving admirable product yields as depicted in Scheme 8b. The entire catalytic activity is accredited to the KCaF3 being formed, which is proven by XRD analysis, the fluoride ion present in the catalyst acts as a base activating malononitrile to initiate nucleophilic attack on the aldehyde to generate β-hydroxy intermediate-A, followed by liberation of water to give the Knoevenagel intermediate (arylidenemalononitrile-B). The next steps set in with the activation of dimedone by the fluoride ion to undergo Michael addition with B giving intermediate C. Later, intermediate C undergoes tautomerization followed by intermolecular cyclisation resulting in the formation of the intended product tetrahydro-4H-chromenes as shown in Scheme 9.42
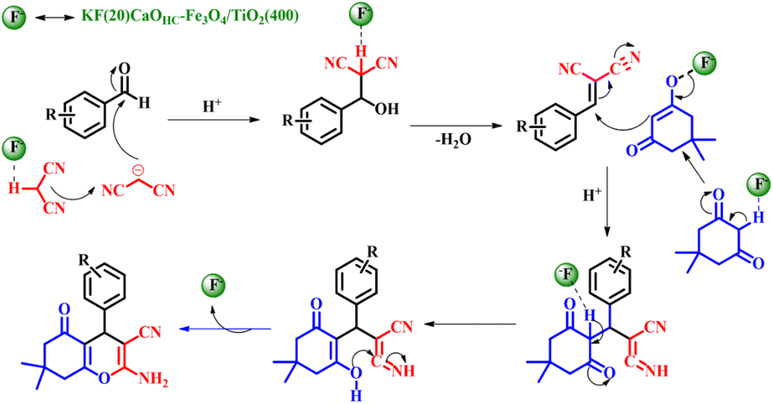 |
| Scheme 9 Plausible mechanism for the KF(20)CaOHC–Fe3O4/TiO2(400) catalyzed one-pot synthesis of tetrahydro-4H-chromenes (adapted from ref. 42). | |
Mosaddegh reported the application of nano-ES powder prepared via ultrasound irradiation in the catalytic green synthesis of 2-aminochromenes 19a and 19b (Scheme 10) through one pot three-component condensation of α- or β-naphthol 18a and 18b, malononitrile 9 and different aromatic aldehydes 6 at 120 °C under solvent free conditions. In the absence of the catalyst, only alkenes were formed even after a prolonged reaction time of 10 h.41,43
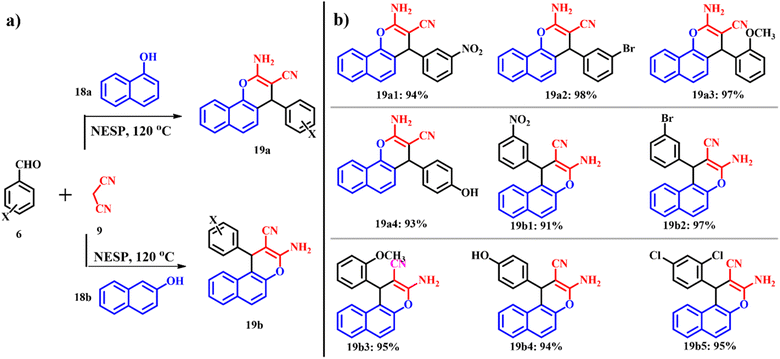 |
| Scheme 10 (a) Synthesis of 2-aminochromene-2-carbonitriles by using an ES catalyst and (b) 2-aminochromene-2-carbonitrile derivatives (adapted from ref. 43). | |
The same research group extended their work to synthesize pyran derivatives,44 which are known for pharmacological and biological activities.45 Usual synthesis of pyran derivatives involves one pot multicomponent condensation of aryl aldehydes 6, malononitrile 9 and 4-hydroxy-6-methyl-2H-pyran-2-one 20 in the presence of various auxiliary reagents.46,47 Again, general drawbacks like prolonged reaction time and relatively lower product yields are associated with this type of reaction. A calcined ES catalyst was used to synthesize 2-amino-7-methyl-5-oxo-4-phenyl-4,5-dihydropyrano[4,3-b]pyran-3-carbonitrile derivatives 21 as shown in Scheme 11a and the possible mechanism is shown in Scheme 11c. The experimental conditions were optimized by varying temperature and catalyst dosage. A catalyst amount of 0.1 g at 120 °C was considered as optimum and above or below the optimum conditions, the product yield decreased with decrease in reaction rates. The group was successful in solvent free synthesis of pyran derivatives with an exceptional e-factor of 1.3.
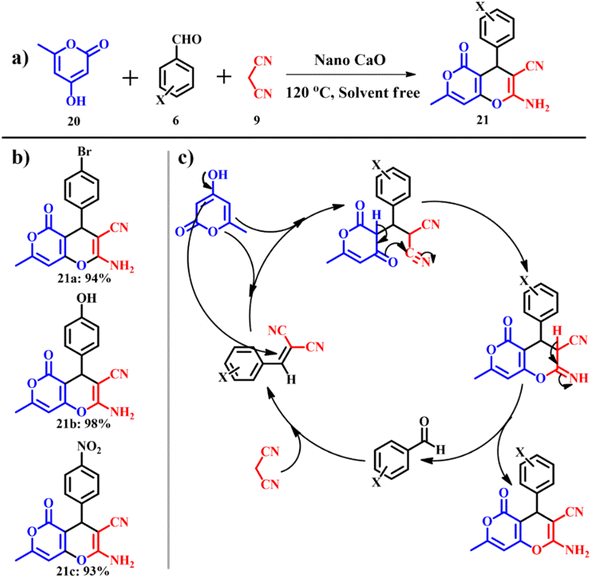 |
| Scheme 11 (a) Synthesis of 2-amino-7-methyl-5-oxo-4-phenyl-4,5-dihydropyrano[4,3-b]pyran-3-carbonitrile, (b) derivatives and (c) plausible mechanistic pathway for the synthesis of pyrano[4,3-b]pyran derivatives (adapted from ref. 44). | |
This work was inspired by Miri and co-workers. They continued to synthesize pyranoquinoline derivatives 26 (Scheme 12a), which are found to be potential anti-cancer agents. The product was procured via an efficient and environmentally benign method for the synthesis of pyranoquinoline. Aromatic aldehyde 6, Meldrum's acid 22 and 4-hydroxyquinolin-2(1H)-one 23 were catalyzed by ES in ethanol to attain the motif 26, on carrying out other solvents' screening, ethanol gave the highest yield. The model pathway and the possible mechanism are shown in Scheme 12c. The protocol occurs by a mechanism of Knoevenagel condensation, Michael addition, intramolecular cyclization, and isomerization.48 The presented work has scope in combinatorial chemistry, diversity-oriented synthesis and drug discovery.
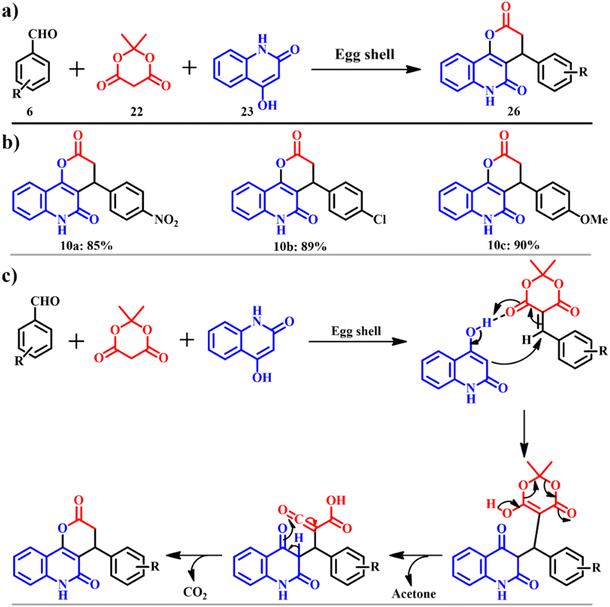 |
| Scheme 12 (a) The model reaction to synthesise pyranoquinoline and (b) derivatives and (c) plausible mechanism for synthesis of pyranoquinoline ring systems in the presence of eggshell as a catalyst (adapted from ref. 48). | |
As far as the catalytic efficiency of Ag@KF(20)CaOHC–Fe3O4/TiO2(400) is concerned, it is used in the synthesis of benzopyran pyrimidines 28 using salicylaldehyde 27, malononitrile 9 and piperidine as the model substrates (Scheme 13). The previous catalyst ([KF(20)CaOHC–Fe3O4/TiO2(400)]) did not show efficient completion of the reaction, whilst the silver-based catalyst showed a good yield within a short reaction time signifying the role of silver in the organic conversion. With cyclic secondary amines such as pyridines and morpholine, good product yields were obtained; however, in the case of aliphatic amines such as diethyl amine, diphenyl amine and dibutyl amine poor product yields were recorded, whereas with primary amines such as 4-methoxyaniline no product was formed even after a long reaction time. Also, it is observed that electron deficient groups gave higher product yields than electron donating groups (Scheme 13b). This could increase the electrophilicity of the carbonyl carbon, which in turn enhances the reaction rate. Ag@KF(20)CaOHC–Fe3O4/TiO2(400) showed uncompromised catalytic efficiency for 5 consequent cycles without compromise in the product yield with an e-factor of 12.5.42
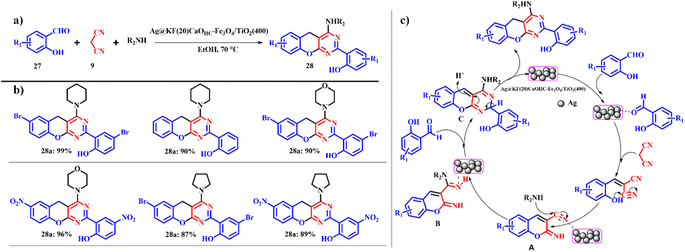 |
| Scheme 13 (a) Ag@KF(20)CaOHC–Fe3O4/TiO2(400) catalyzed one-pot synthesis of benzopyranopyrimidines and (b) derivatives and (c) plausible mechanism for the Ag@KF(20)CaOHC–Fe3O4/TiO2(400) synthesis of benzopyranopyrimidines (adapted from ref. 42). | |
Analogously, 1,4-dihydropyridines 31 and polyhydroquinolines 32 were synthesized via multicomponent reactions, which offer the respective products without isolation of the intermediates and thus possess high atom-economy. Customary syntheses of these derivatives include cyclocondensation of aldehydes 6, β-ketones 29 and ammonia in the presence of acetic acid or alcohols. Unfortunately, this process was not successful to synthesize different substituted 1,4-dihydropyridines. Several modifications and diverse catalysts were used to attempt the synthesis of substituted 1,4-dihydropyridines and polyhydroquinolines.50–52 Although potential reagents are used and most of the reactions provide high yields, use of expensive and strong oxidative and toxic transition metal reagents leads to environmentally unfriendly methods. Such drawbacks were overcome by making use of ES as a catalyst.49 The common model reaction mode is shown in Scheme 14a, which provides a new synthetic protocol for symmetrical and unsymmetrical Hantzsch condensation. Morbale et al. were successful in synthesizing almost 20 derivatives of 1,4-dihydropyridines and polyhydroquinolines that are shown in Scheme 14b.
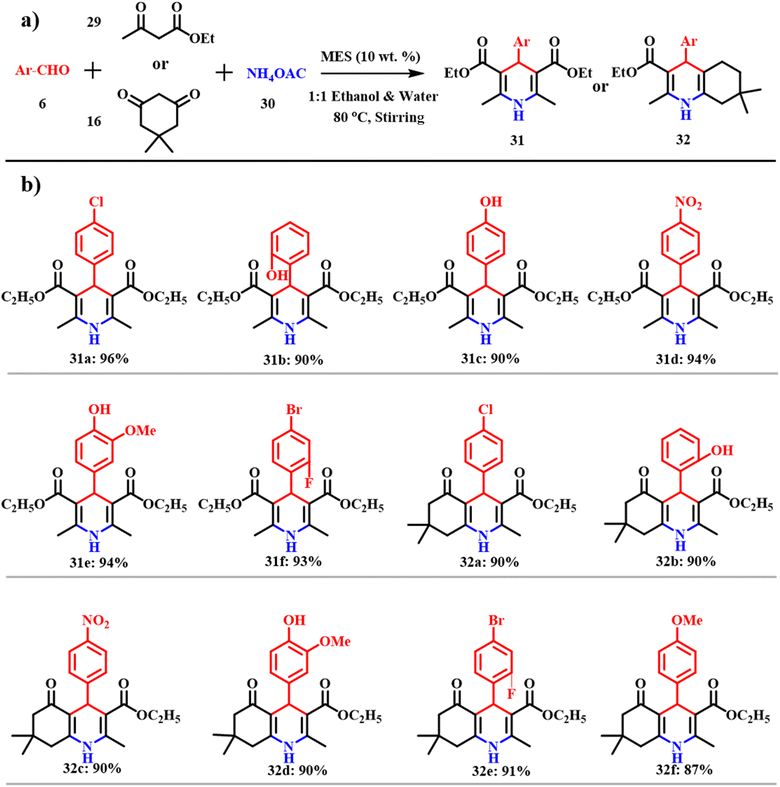 |
| Scheme 14 (a) MES catalyzed synthesis of 1,4-dihydropyridines and polyhydroquinolines and (b) their derivatives (adapted from ref. 49). | |
Nano-eggshell–titanium IV (NEST) was developed as a natural catalyst for the synthesis of dihydropyrano[2,3-c]pyrazole (DHPP) derivatives 35 (Scheme 15). To optimize the procedure involved in the synthesis of DHPPs, 4-chlorobenzaldehyde, malononitrile, ethyl acetoacetate, and hydrazine hydrate were taken in a molar ratio of 1
:
1
:
1
:
2. To initiate the reaction 0.06 g of NEST was added at room temperature under solvent free conditions and the best yield of 6-amino-4-(4-chlorophenyl)-3-methyl-1,4-dihydropyrano[2,3-c]pyrazole-5-carbonitrile was produced with an e-factor of 1.2. According to the proposed mechanism, hydrazine hydrate 34 and ethyl acetoacetate 33 react forming the intermediate 36 in the presence of NEST serving as a Lewis acid. The Knoevenagel condensation between malononitrile 9 and aromatic aldehyde 6 gives rise to intermediate 38, followed by Michael addition between intermediates 37 and 38 leading to the formation of intermediate 39 with subsequent cyclisation and tautomerization to yield DHPPs 35 in good yields. The durability of the catalyst was assessed by adding acetone to the reaction mixture followed by filtration, and the recovered NEST was then washed with dichloromethane and dried at ambient temperature. In the recycling tests, NEST showed no significant loss with constant catalytic activity for at least 4 cycles.53
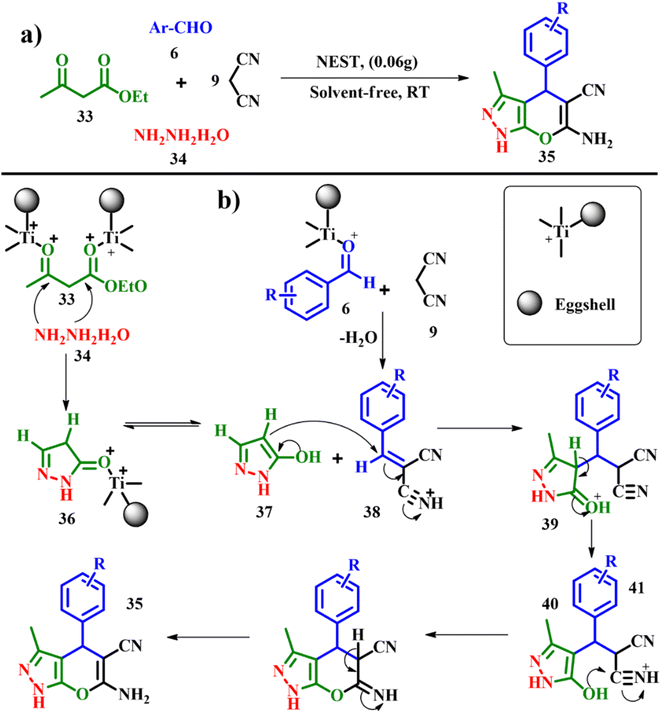 |
| Scheme 15 (a) Synthesis of dihydropyrano[2,3-c]pyrazoles catalyzed by nano-eggshell/Ti(IV) and (b) proposed mechanism for the synthesis of DHPPs (adapted from ref. 53). | |
Mosaddegh and co-workers fabricated a novel magnetic magnetite (Fe3O4) nanoparticle coated on porous eggshell waste as a competent catalyst in the alkali-free synthesis of 1,8-dioxo-octahydroxanthene 41 as depicted in Scheme 16a. The catalytic activity of Fe3O4/ES was tested using one-pot three-component Knoevenagel condensation of dimedone 16 with different aromatic aldehydes 6 under solvent free and ambient thermal conditions to obtain the consistent 1,8-dioxo-octahydroxanthene 41 product. In the absence of Fe3O4, the reaction resulted in no product formation with no cyclisation product even after 4 h. Later, optimum catalytic dosage was evaluated over a range of 0.02–0.2 g of Fe3O4/ES nanoparticle, and it was found that 0.1 g of Fe3O4/ES gave the maximum yield of the product, beyond which there was no considerable effect on the yield and reaction time. Also the influence of Fe3O4/ES, pristine Fe3O4 and plain ES on the reaction was investigated along with Fe3O4/ES, where plain ES was the best catalyst and the physically mixed ES and Fe3O4 could not improve the catalytic activity much while the chemically obtained Fe3O4 and ES enhanced the catalytic activity of ES. Since ES mainly constitutes of CaCO3 with a high surface area, porosity and active sites of the catalyst affirming high productivity in comparison to other catalysts. The scope of the reaction was evaluated using different aldehydes and in all the cases excellent product yields > 90% were obtained with an exceptional e-factor of 1.3 (Scheme 16b). Also, the catalyst was easily recovered magnetically after each run, washed with ethanol 3 times and dried at 120 °C in an oven and tested for subsequent activity. The catalyst showed no loss of activity for five cycles proving the recyclability of Fe3O4/ES.54
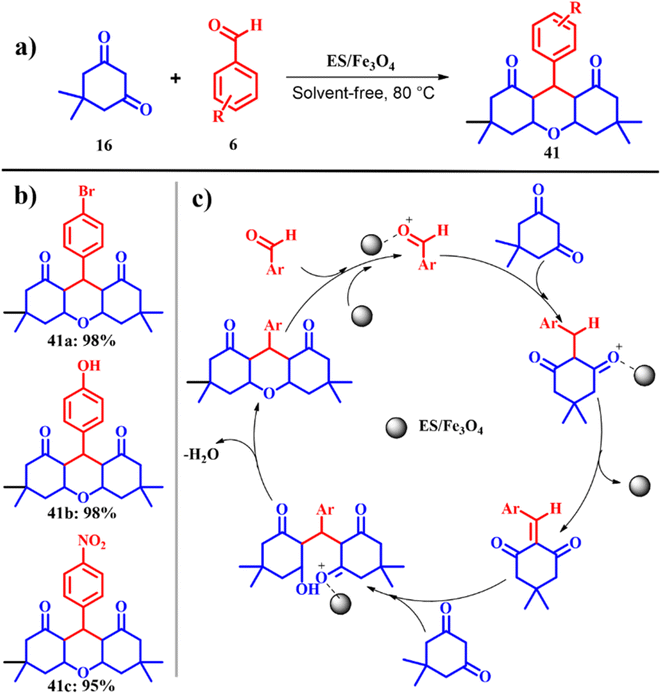 |
| Scheme 16 (a) and (b) Nano-ES/Fe3O4-catalyzed synthesis of 3,3,6,6-tetramethyl-9-phenyl-1,8-dioxo-1,2,3,4,5,6,7,8-octahydroxanthene and (c) proposed mechanism (adapted from ref. 54). | |
The cost effective, cleaner and green procedure for the one-pot synthesis of 1,8-dioxo-octahydroxanthene 44 using Fe3O4/ES shows the novel catalyst designed with low-cost materials reducing the environmental problems. Jonnalagadda and co-workers developed an eco-friendly and green protocol for the synthesis of sixteen pyrazolo-phthalazine derivatives using pristine eggshell powder (ESP) as the heterogeneous catalyst (Scheme 17a). A one-pot multi-component condensation reaction involving phthalic anhydride 42, hydrazine hydrate 34, malononitrile 43 and trimethoxy benzaldehyde 6 was explored using ESP as the catalyst. A catalyst ESP of 0.02 g was found to give the best yield 44 of the product in 95% using water as the solvent for a reaction time of 30 min at 60 °C. Other polar protic solvents such as ethanol and methanol provided a noteworthy yield of 63 and 51% for a time scale of 60 and 90 min, respectively. This solvent effect could be attributed to the swelling behavior of ESP in the presence of water, creating highly active sites. Also, other calcium salts such as calcium oxide, calcium carbonate and calcium hydroxide were also individually verified for their catalytic properties, but a low amount of product was obtained. Out of the sixteen derivatives, fourteen are new molecules with an excellent yield of up to >90%. The active sites on ESP initiate and accelerate the rate of the reaction. ESP tends to activate the carbonyl carbon of phthalic anhydride and aryl aldehydes. The hydrazine hydride triggers the nucleophilic attack on the activated carbon of carbonyl leading to the formation of intermediates shown in Scheme 17c. Subsequently, a cascade of Knoevenagel reactions fastened by the active methylene group with the active aryl aldehydes generate unsaturated intermediates. Further Michael addition of phthalhydrazide key intermediate occurs at the conjugated C
C bond site. Finally the intermolecular cyclisation followed by tautomerization of the adduct generates the desired product 44 with an e-factor of 15.3.55 The specialty of this work lies in the use of water as the solvent, being an economic alternative to organic solvents, and column free easy work-up, shorter reaction time, and biodegradable ESP have attracted interest in this work.
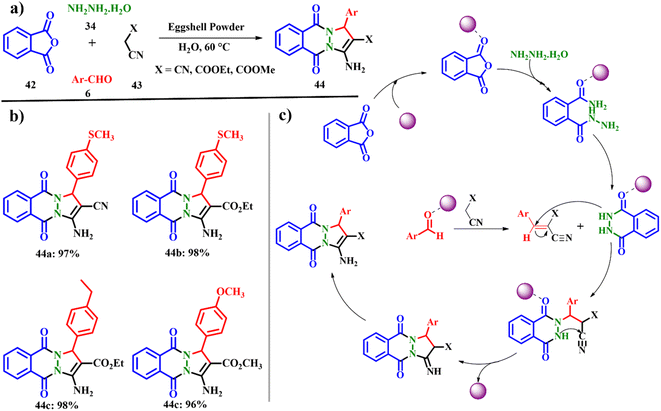 |
| Scheme 17 (a) Synthesis of pyrazolo-phthalazine derivatives, (b) eggshell powder-catalyzed phthalazine derivatives, and (c) a plausible mechanism for the formation of pyrazolo-phthalazines (adapted from ref. 55). | |
Benzimidazoles, benzoxazoles and benzothiazoles are other interesting classes of organic molecules, which gained interest in pharmacological and biological applications.56,57 Abdeljabar Haboub and colleagues showcased the electrochemical synthesis of benzimidazoles 46 (Scheme 18a), achieving an impressive atom economy of 91.12% and an e-factor of 44.6. Similarly, the synthesis of benzoxazoles 47 (Scheme 18b) yielded a notable atom economy of 91.66% with an e-factor of 55.1. Moreover, the production of benzothiazoles 48 (Scheme 18c) demonstrated a high atom economy of 92.18% alongside an e-factor of 41.1. ES representing a biogenic source of calcium carbonate was used in the synthesis of benzimidazoles, benzoxazoles and benzothiazoles. The product synthesis was modified using different solvents such as chloroform, methanol, tetrahydrofuran etc. But good product yields were achieved on using tetrahydro furan and solvent-free reactions. The calcined ES was impregnated with zinc chloride and copper(II) bromide. With the employment of calcined ES doped with MX2 (CuBr2 and ZnCl2) type Lewis acids, the products were isolated in the range of moderate to excellent yields (52–98%). After completion of the reaction protocol, the isolated catalyst was washed with dichloromethane and reactivated at 400 °C for 2 h, and was reused four times; after four recycles the catalytic activity of CEM was almost the same as that of the freshly prepared catalyst.58
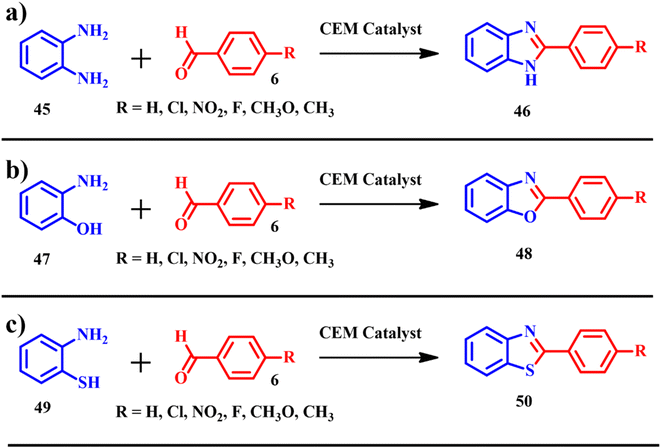 |
| Scheme 18 Synthesis of (a) benzimidazoles 46, (b) benzoxazoles 48 and (c) benzothiazoles 50 catalyzed by CEM, ZnCl2/CEM and CuBr2/CEM (adapted from ref. 58). | |
When compared to all the heterocyclic derivatives synthesized above, the solvent-free synthesis of heterocyclic derivatives using eggshell and its derivative demonstrates superior performance, yielding high product yields with e-factors consistently equal to or greater than 1. This approach has brought about significant improvements, not only in waste reduction but also in several other key parameters such as catalytic loading, reaction speed, and scalability. By utilizing eggshell and its derivative as catalysts in a solvent-free environment, this method minimizes the environmental footprint of the synthesis process. The absence of solvent not only reduces waste generation but also eliminates the need for solvent disposal, contributing to a more sustainable approach. Moreover, the high catalytic efficiency of eggshell-derived catalysts allows for lower catalytic loading, further enhancing the environmental and economic viability of the process.
2.7 Hydrogenation reaction
Mallampati and Valiyaveettil reported silver and gold nanoparticles immobilized on an ES membrane catalyst offering a larger surface area, stability, catalytic activity and recyclability. The efficacy of the ES membrane was evaluated using two organic conversions: (i) reduction of nitrophenols and (ii) one-pot synthesis of functionalized propargylamines 55. The ES membrane catalyst was synthesized by immersing the previously washed and cleaned ES membrane and immersing the same into an aqueous solution of chloroauric acid (HAuCl4) and silver nitrate (AgNO3) to initiate reduction on the surface of the ESM giving Au–ESM and Ag–ESM, respectively. The membrane changed color from white to red for gold solution and gray to silver solution with no additional reducing agents, that are further used for catalytic studies. Aromatic nitro-compounds are conventionally reduced by stoichiometric reduction and catalytic hydrogenation with the latter giving high yields of amines. In the absence of Au–ESM and Ag–ESM for a mixture of p-nitrophenol 51 and sodium borohydride, there was no reduction product observed. However, on the addition of Au/Ag–ESM, there was a slight change in the yellow color of p-nitrophenol 52 confirming the catalytic conversion to p-aminophenol. It is also observed that the rate of p-nitrophenol reduction catalyzed by Au/Ag–ESM is of the order p-nitrophenol > o-nitrophenol > m-nitrophenol. The p- and o-nitrophenolate ions formed during resonance are stabilized, which is not observed for m-phenolate ions as the –NO2 group cannot take part in direct resonance stabilization of oxygen thereby exerting a weak negative inductive effect. Thereby Ag–ESM shows higher activity than Au–ESM. The nanoparticle ESM also showed strong potential towards coupling reactions of aldehyde 6, amines 54 and alkynes 53 (A3), which are beneficial and are active intermediate compounds for biologically and pharmaceutically active agents. The catalyst successfully produced the desired product 55 with a yield of 99%, also confirming that the reaction proceeds through terminal alkyne C–H bond activation initiated by the nanoparticles on the ESM as depicted in Scheme 19. The calculated e-factors based on organic solvent usage are very high, typically only 1–2. The catalyst membrane was recovered by washing the membrane with toluene via filtration assistance. Ag–ESM and Au–ESM being heterogeneous systems are active up to 5 cycles of nitrophenol reduction. Leaching studies were carried out by analyzing the post reaction mixture by ICP-OES, indicating no significant Au or Ag residues from the ESM.59 Moreover, the calculated e-factors exhibit a notably high average, typically at 53.5. Taking into account the catalyst's attributes such as reusability, abundant availability, minimal toxicity, biodegradable membrane, and ease of handling, ES-based catalysts emerge as sustainable materials for catalytic applications. However, when considering the e-factor, their sustainability is somewhat diminished in this case.
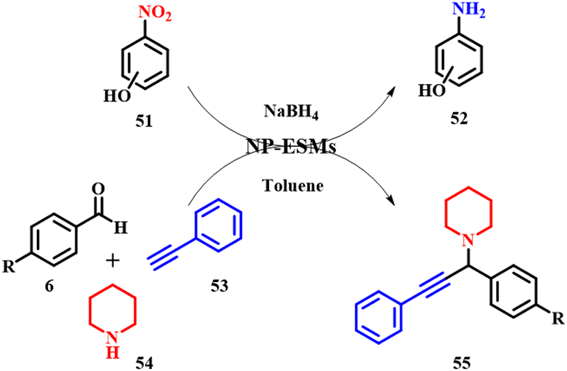 |
| Scheme 19 Au–ESM catalyzed reduction of p-nitrophenol and coupling reactions to form propargylamine derivatives (adapted from ref. 59). | |
At the same time, Claus and co-workers prepared varying molar ratios of Pd/Ag on eggshell as a catalyst for selective hydrogenation of acetylene under tail-end conditions performed using an advanced TEMKIN-reactor. The acetylene conversions decrease with an elevated dosage of silver content and compromised Pd/Ag ratio as expected. Pd100/Al2O3 shows the highest conversion of about 82%, which decreases to 35% for Pd5–Ag95/Al2O3.60
2.8 Synthesis of organic carbonates
The development of cleaner methods for organic conversions is a major challenge in green chemistry and can be handled by using ES as the catalyst. Dimethyl sulfate and methyl halides in methylation reactions and phosgene in polycarbonate and isocyanate synthesis can be replaced by dimethyl carbonate (DMC) 59, which has negligible ecotoxicity and low bioaccumulation.61 Usually, DMC is prepared in two steps as shown in Scheme 20; the first step involves carbonylation of methanol that uses naturally available CO256 and is considered to be a green synthesis. Meanwhile the second step, transesterification between methanol and propylene or ethylene carbonate, uses either acid or base catalysts such as alkali alcoholates or hydroxides and trialkyl amines. These catalysts induce a problem of separation, purification and reusability and hence, much attention has been given to solve this issue.62 Yuan Gao and Chunli Xu used calcined ES powder as a catalyst for the same.63 On varying the catalyst concentration, it is concluded that at a higher concentration of catalyst the reaction mixture becomes highly viscous and decreases efficiency. The experiments showed a 10
:
1 molar ratio of methanol to propylene carbonate 58 with addition of 0.8 wt% ES catalyst as the optimum concentration at room temperature and 1 atm pressure. Further, 80% conversion and 75% yield demonstrate the reusability of the catalyst four times proving the efficiency of the ES catalyst.
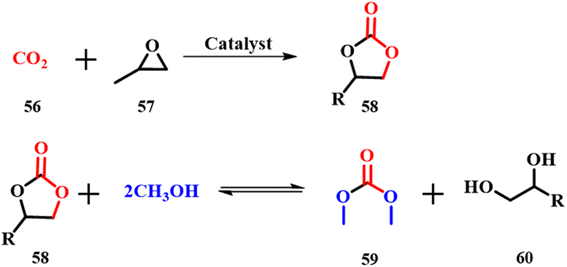 |
| Scheme 20 Calcined ES catalyzed synthesis of dimethyl carbonate (adapted from ref. 63). | |
2.9 Transformation of sugars
Analogously, an interesting valued organic derivative in both food and health care industries is lactulose (4-O-b-D-galactopyranosyl-D-fructose), which is produced from lactose 61.64 Either homogeneous or heterogeneous catalytic pathways are employed for the treatment of lactose with alkaline solution via Lobry de Bruyn–Alberda van Ekenstein transformation to get lactulose 62 (Scheme 21).65,66 Numerous catalysts such as sodium hydroxide, calcium hydroxide, potassium hydroxide, magnesium oxides, tertiary amines, borates and many other catalysts were considered for the isomerisation of lactose to lactulose. However, these conditions yield undesirable side products, which restrict the applicability of the method for industrial applications. ES and milk ultrafiltrate were used as raw materials for the production of lactulose. A minimal quantity of catalyst (ES) provided higher levels of isomerisation with lower amounts of side products.
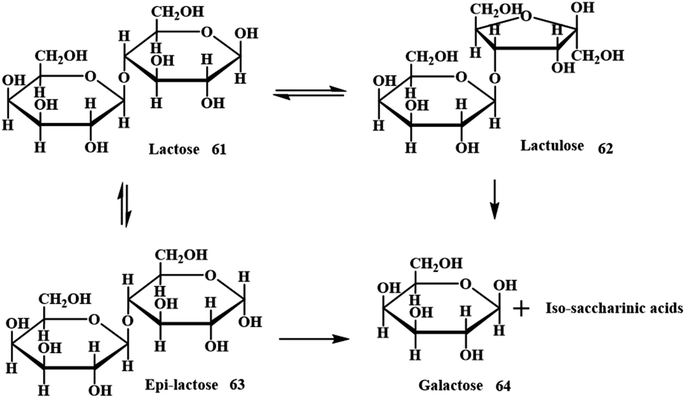 |
| Scheme 21 Simplified model of the alkaline isomerization of lactose (adapted from ref. 66). | |
2.10 Biodiesel synthesis
The current section mainly focuses on the parameters and its influence on the production of biodiesel using long chain fatty-acids for a transesterification reaction using cost effective eggshell waste as a precursor for CaO. Though there is high yield produced in homogeneous catalysis, many other parameters such as saponification, use of excess chemicals, and high expenditure for separation of the catalyst to avoid saponification need priority in the current scenario. Because biodiesel is appealing due to its environmental benefits and shows promise as a viable alternative fuel in the near future, many scientists have taken the initiative to build a variety of acceptable materials that could aid in the manufacture of biodiesel. In general, biodiesel is produced by catalytic reactions involving transesterification of animal fats or vegetable oils with short chain alcohols especially methanol 61.67,68 Traditional catalysts for such a kind of reaction are homogeneous strong acids and bases such as H2SO4, alkali metal hydroxides and alkoxides. The homogeneous acid catalyzed reactions cause corrosion and environment related issues along with difficulty in operating conditions and recycling of the catalyst. Also, the base catalyzed homogeneous reactions with free fatty acids produce unwanted fatty acids, which require an additional step of expensive separation procedures.69,70 Hence, use of a solid base heterogeneous catalyst for these types of reactions may solve the separation and toxicity associated issues. However, synthesis of an active solid base catalyst is relatively complex compared to the aforementioned homogeneous catalysts and requires expertise to handle the reactions.71 Transesterification of triglyceride 60 with methanol 61 using calcined eggshell (CES) waste as a solid base catalyst was investigated.72 The activity of ES is assessed by calcinating it at different temperatures. The size and shape of natural ES powder were irregular and they almost remained the same up to 700 °C, above which, the particle size of ES reduced with an ordered shape. These changes are attributed to change in composition upon calcination. ES loses CO2 when calcined between 700 and 800 °C, which was confirmed by decomposition temperature observed in thermogravimetric analysis (TGA). The X-ray diffraction (XRD) patterns of ES calcined at > 700 °C showed the major peak for CaO, whereas the one calcined at < 700 °C showed peaks for both CaCO3 and CaO as major and minor phases, respectively. The calcination temperature also affected the transesterification process, 97–99% yield was observed for reactions where CaO is the major phase and it reduced to 30% for reactions with CaCO3 as the major phase. The higher compositions of reactants lead to the formation of a viscous slurry consisting of mixtures, which reduced the efficiency of the catalyst. The catalyst was reused 17 times and after that, the catalytic efficiency decreased due to change in structural morphologies.
In a similar manner, researchers such as Wei et al.72 and Viriya-Empikul et al. have employed eggshells as catalysts in the transesterification process of vegetable oil. In a specific study, Viriya-Empikul et al. conducted transesterification on palmolein oil at 60 °C for 2 hours, utilizing a methanol to oil ratio of 15
:
1 and a 10% eggshell catalyst. This resulted in a substantial 90% biodiesel yield.73 Y. C. Sharma and co-workers reported ES employed biodiesel synthesis from Pongamia pinnata oil.74 The weight loss in the temperature range 400 to 480 °C was attributed to the decomposition of Ca(OH)2 and it indicates that CaO reacted with atmospheric moisture. The next weight loss at 700 °C was attributed to the decomposition of CaCO3. The dissolution of CaO (soluble up to 0.035 wt%) leads to the formation of OH− and O2− as shown in eqn (1) and (2), respectively. These ions further produce methoxide anions (eqn (3) and (4)), which act as a catalyst for the transesterification reaction.
| O2− + CH3OH ↔ OH− + CH3O− | (3) |
| OH− + CH3OH ↔ H2O + CH3O− | (4) |
The ES powder was modified in three steps, calcination–hydration–dehydration, to get CaO, and the catalytic activity is compared with that of commercial CaO via transesterification of waste frying oil.75 Commercial CaO showed a methyl ester 62 conversion of 67.57%, whereas ES–CaO showed a conversion of 94.52%. The key parameters of biodiesel like the density, flash point, cloud point and kinematic viscosity showed the feasibility of the synthesized product as biodiesel. Another group of researchers employed the same method of transesterification for palm oil, coconut oil and sunflower oil (Scheme 22) for biodiesel production.76 The catalyst was reused four times and the yield was not appropriate for palm and coconut oils, because of leaching problems. The obtained product was subjected to ASTM D6751 standards to examine the fuel properties and it was concluded that they can be used in compression–ignition engines.
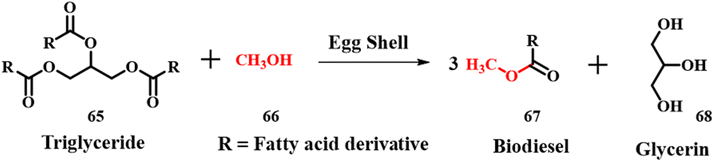 |
| Scheme 22 Synthesis of biodiesel (adapted from ref. 73). | |
Buasri et al. prepared an ES based heterogeneous catalyst from duck and chicken ES. The catalytic performance in transesterification of palm oil in the presence of methanol was studied. Both duck shell and ES showed a catalytic activity of 80 and 81 up to 4 cycles with negligible compromise in efficiency, respectively. Other governing factors such as the reaction time, temperature of the reaction, methanol
:
oil ratio, catalyst dosage and recyclability aspect were evaluated.77 Mayvan and co-workers studied waste chicken eggshell-derived CaO particles synthesized via thermal decomposition at 900 °C for 4 h. The as-synthesized compound was further used as an ultrasonic assisted-CaO catalyst for biodiesel production from waste cooking oil (WCO). It was found that the biodiesel product increases with decrease in CaO loading from 8.5 to 6 wt% and increasing the ultrasonic power from 150 to 300 W. However, increasing beyond 8.5 to 12 wt%, the biodiesel production surged. An effective quantification of the catalyst used not only enhances the biodiesel production, but also avoids side reactions (e.g.: saponification and hydrolysis), which also increases on account of increased viscosity of the medium with CaO concentration. The decrease in biodiesel production at higher dosages of CaO is attributed to lessened contact on the catalyst due to the necessity of higher ultrasonic influence for the same cavitation production and increased shielding effect of the catalyst on propagation of ultrasonic waves. In simple terms, a higher dosage of catalyst causes a larger number of transverse waves, which are weaker than longitudinal waves. The optimal reaction conditions reported for the current reported literature are a catalyst loading of 6.04 w/w% with an ultrasonic power of 299.66 W, and a reaction time of 39.84 min, generating a biodiesel 62 with a yield of 98.62% possessing a specific energy consumption of 5.01 kJ g−1.78 Ashine and co-workers worked on production of biodiesel from Argemone mexicana oil using an ES derived CaO catalyst. A catalyst loading of 3.05 wt% was found to be optimum for biodiesel generation, while further increase in catalyst loading causes hydrolysis as observed in the above report and also causes problems with improper mass transfer of methanol
:
oil and the catalyst. Further, methanol being a low-cost alcohol reacts voluntarily with oil during transesterification, and methanol is found to trigger the active sites on the catalyst. Beyond a certain limit, methanol could inactivate the catalyst and shift the reaction equilibrium to the left, decreasing the yield of biodiesel. This study showed a maximum yield of biodiesel at a 12
:
1 molar ratio of methanol and a reaction time of 3.01 h and the group obtained a biodiesel production yield of 99.07%. The catalyst showed significant recyclability > 4 times, which is a key parameter for economic and ecological concerns, and biodiesel yields of 84.52, 78.98, and 65.75%. The slight compromise in yield could be attributed to the catalytic poison by the oil, blocking the active sites, thereby reducing the biodiesel yield. Also, during catalyst recovery, traces of CaO could be leached out during methanol washing.79 Kirubakkaran and Selvan studied the effect of micro- and nano-eggshell catalysts on waste chicken fat for biodiesel production. The ES that was just dried, powdered and sieved to the micrometer scale was termed micro-eggshell, while the ES that is powdered followed by calcination to convert calcium carbonate to calcium oxide (catalyst) was labelled nano-ES. Since the nano-catalyst has a smaller crystalline size, high surface area and larger pore size compared to micro-ES, the catalyst disperses completely in the reaction medium facilitating biodiesel production. At a catalyst loading of 7.5 wt% of the oil 60, a maximum biodiesel 62 yield of 96.7% was obtained. With a slightly higher catalyst loading than 7.5 wt%, the non-homogeneity of the reaction medium, high viscosity (in turn reducing the efficiency of reaction mixing), lack of mass transfer and saponification result in compromised quantity of biodiesel production to 65%. However, lower catalyst loading resulted in abatement of biodiesel yield on account of incomplete catalytic reaction.80 In conclusion, the chicken ES derived CaO nano-catalyst is found to be eco-friendly, environmentally benign and cost-effective for the production of biodiesel. Upon extensive literature survey, we hereby reveal that eggshell derived CaO is a sustainable catalyst towards the production of biodiesel using various oil sources.
2.11 Oxidative reactions
Another class of reactions where eggshell is found to be a suitable candidate is oxidative reactions. This class of reactions has attracted a lot of attention due to their ability to degrade unsafe organic compounds and enhance the biodegradability of these hazardous compounds from industrial effluents. The use of a catalyst in wet oxidation (CWO) is an apt alternative to eliminate these organic molecules. Various heterogeneous catalysts were synthesized using solid supports. One of the most commonly used materials is metal particles as nano-catalysts. Keeping this demand in mind, Oulego et al. synthesized eggshell-supported 5% iron and 15% copper for catalytic oxidation of humic acid (HA) via CWO. The catalytic efficiency was evaluated in wet oxidation tests to degrade HA and was analyzed by chemical oxygen demand (COD) with respect to time along with the biodegradable index. A high performance up to 82.3% for 15% copper and 75.1% for 5% iron in COD reduction and 58% for pristine eggshell was observed.81 Another group of researchers, Nath et al., fabricated ESP and explored its oxidative degradation of crystal violet (CV) dye in aqueous medium under hydrogen peroxide (H2O2) medium at RT. As previously discussed, eggshell is a rich source of carbonate (CO3−2). This CO32− eventually forms carbonate anion radicals (CO3˙−) in the presence of H2O2. CO3˙− radicals, though weaker oxidants than ˙OH, competently serve as selective oxidants. The synergistic effect of both CO3˙− and ˙OH facilitates CV dye degradation, at an ESP dose of 10 g L−1 with an 91% removal of CV (10 mg L−1) with H2O2 (441 mM) until seven cycles. The effect of pH on the catalyst was also studied in the range of 3–12. The experimental results showed that at pH 3 the removal efficiency was 78% and at pH 5 and 8 it was close to 88%. Furthermore, at pH 12 the color of CV was completely changed without the addition of H2O2. At temperatures ranging from 310 K, 320 K and 330 K, the degradation efficiency was found to increase with increase in temperature up to 91%.82 On building a bridge between nanomaterials and eggshell waste, Wang et al. designed eggshell-supported copper oxide and used a peroxymonosulfate (PMS) activator for the removal of reactive blue 19 (RB19). The CuO/eggshell catalyst showed a high degradation efficiency of approximately 100% with 20 mg L−1 of RB19, 0.2 g L−1 of CuO/eggshell, 0.36 mM of PMS and a pH of 7.12 within 20 min. The influence of ions such as nitrates, chlorides, HA and carbonates on the degradation of RB19 over CuO/eggshell and PMS was inspected. Chlorides and nitrates were found to scavenge ˙OH and SO4˙− radicals having a detrimental effect on the degradation of RB19. Also, with the addition of HA the dye degradation was greatly affected because (i) HA behaves as a radical (˙OH and SO4˙−) scavenger and (ii) HA also forms strong π–π interaction with the catalyst blocking its active sites for RB19 degradation.83 Smith et al. designed waste eggshell-derived calcium ferrite (CaFe2O4) for the removal of the most persistent herbicide chlorophenol. CaFe2O4 with an iron loading of 7.1 wt% showed a 2-chlorophenol removal efficacy of 86.1% after an exposure time of 180 min under UV-vis light radiation. After 3 cycles the efficacy of CaFe2O4 was compromised to 70.5% without the demand for regeneration (either washing or re-calcination). Electron spin resonance (ESR) was employed to study the reactive oxygen species (OH˙, O2˙−) and holes generated on account of photogeneration using aqueous 5,5-dimethylpyrroline N-oxide. On running the scavenger test it was found that, with addition of radical scavengers the removal efficiency of 2-chlorophenol was lowered reflecting the scavenger reactions (Scheme 23).84 It is important to note that amongst various other biowaste being generated around the globe, eggshell has attracted considerable attention of scientists in all fields, along with proving its expertise too. The global availability and non-perilous nature are a boon of all times as far as green chemistry and sustainable chemical reactions are concerned (Scheme 23).
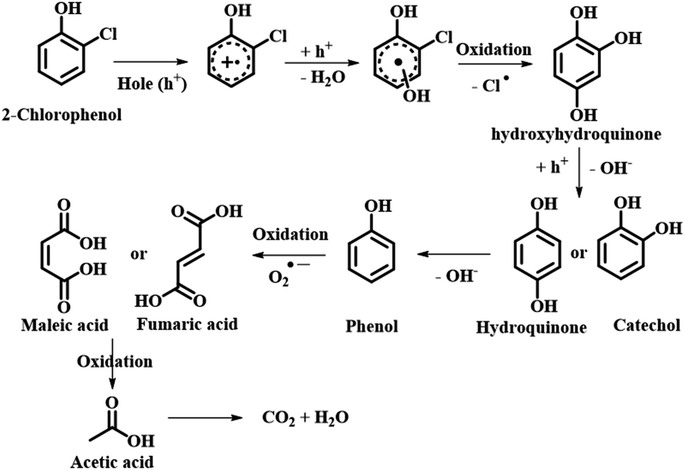 |
| Scheme 23 Photocatalytic degradation pathway of 2-CP over 30Fe/QES (adapted from ref. 84). | |
3. Summary and outlook
Eggshell, an efficient bio-based renewable catalyst, has paved its way through heterogeneous catalysis proving its benevolent service to the majority of organic transformation reactions. The conventional metal oxide-based catalysts are prone to obvious drawbacks; therefore there is a need to seek affordable, robust and sustainable catalysts. The naturally occurring eggshell is not only economically feasible and easily available but also sound in terms of reduction, dispersion and stabilization of nanoparticles due to the presence of groups such as carboxylic acid, amine and hydroxyl groups. The current review gives insights into the use of eggshell as an efficient support, template and catalyst involved in organic conversions. The structural morphology along with the hierarchical arrangement of atoms in the catalyst aids in mass and energy transfer. The employment of an eggshell mediated base catalyzed reaction is a win–win strategy for both organic chemists and environmentalists as far as environmental pollution remediation is concerned. The below Table 1 discusses the advantages and disadvantages of catalytic processes, which have been overcome by using eggshell as the catalyst. The search doesn't end here, there is still room for scope and invention not only for eggshell as a base-heterogeneous catalyst, but also for other bio-waste derived catalysts needing more consideration and implementation.
Table 1 Advantages and disadvantages of catalytic processes
Sl. no. |
Processes |
Advantages |
Disadvantages |
Ref. |
1 |
Suzuki and Heck coupling reaction |
Easy synthesis, production and isolation of the Pd–eggshell catalyst |
Slight leeching of the Pd catalyst into the product during crystallization |
85
|
Operation simplicity |
High cost of Pd and use of harsh solvents |
Reaction time |
|
2 |
Schiff base synthesis |
Solvent-free synthesis of Schiff's base using eggshell |
High solvent consumption in conventional methods |
16 and 17 |
3 |
Knoevenagel condensation reaction |
Catalyst is easy to separate and reuse |
Long reaction time and large amounts of organic solvent |
31
|
4 |
Henry reaction |
Reaction can be carried out without protecting the hydroxyl group |
Substrate specificity, reactivity issues, and environmental concerns |
35
|
Mild reaction conditions |
5 |
Aldol condensation reaction |
Replacement of the conventional catalysts with eggshell an environmentally friendly and efficient base catalyst |
The use of sodium hydroxide, potassium hydroxide, pyridine and other bases having nitrogen as a catalyst in traditional aldol condensation reactions |
37
|
6 |
Synthesis of heterocyclic derivatives (one-pot process) |
Efficient and ecofriendly |
Reaction time and difficult workup procedures |
41
|
Natural and heterogeneous |
7 |
Hydrogenation reactions |
ES-based catalysts offer a larger surface area, stability, catalytic activity and recyclability |
Undesired products |
59
|
8 |
Synthesis of organic carbonates |
Replacing hazardous chemicals with less toxic chemicals such as dimethyl carbonate (DMC) |
Dimethyl sulfate and methyl halides in methylation reaction of phosgene in polycarbonate are hazardous |
61
|
9 |
Transformation of sugars |
Eggshell based catalysts provided higher levels of isomerization with lower levels of side products |
Sodium hydroxide, calcium hydroxide, potassium hydroxide, magnesium oxides, tertiary amines, and borates are a few bases used in transformation of lactose to lactulose, yielding undesirable side products |
66
|
10 |
Synthesis of biodiesel |
Solid heterogeneous base catalyst for biodiesel synthesis may solve the separation and toxicity associated issues |
Conventional homogeneous acid catalyzed reactions, expensive separation procedure, corrosion and environment related issues |
86
|
11 |
Oxidation reactions |
Oxidative reaction using eggshell facilitates degradation of a wide spectrum of noxious and non-degradable pollutants in water, no sludge waste, hence being theoretically cost effective |
Reported photocatalysts have some drawbacks, requiring expensive and high-purity precursors in multi-step preparation processes |
84
|
The latest advancements in utilizing biogenic derived eggshell as a solid base heterogeneous catalyst for organic transformations show significant promise. This approach, employed in processes such as Schiff base formation, Knoevenagel condensation, Henry reactions, and synthesizing heterocyclic derivatives, exhibits the lowest e-factors and highest yields. Biogenic derived eggshell catalysts are preferred due to their minimal or zero waste production and high reusability. Their ability to be reused multiple times contributes to efficient organic transformations, reducing the need for new catalysts and lowering waste generation per unit of desired product mass. This efficient recyclability decreases overall waste output during the process, thus lowering e-factors. Lower e-factors indicate enhanced conversion rates, meaning less waste relative to the yield achieved, aligning with sustainability objectives and optimizing resource utilization.
4. Conclusions
This review of literature suggests the potential candidature of ES as a notable biocatalyst for a range of organic transformations, as it avoids the formation of side products in many of the reactions, provides solvent free conditions, has shorter reaction time by increasing the reaction rate and provides easy product separation and purification stages. It also concludes that, even though ES is used in many of the applications such as water treatment and nutrition, its usage as a solid base catalyst for heterogeneous reactions can be explored further as only a small number of papers have been reported to date. Owing to its bio-derived source of calcium, eggshell is expected to have tremendous usage as a heterogeneous catalyst and as a support material for various catalytic systems. The basic nature, porous structure, easy availability and high shelf life makes eggshell membranes and their derivatives an appropriate material in organic conversions.
Conflicts of interest
There are no conflicts to declare.
Acknowledgements
The authors thank the Center for Nano and Material Sciences, JAIN (Deemed-to-be University), Bangalore, India and postdoctoral fellowship grant (JU/APP/PDF/2022/611) for financial support.
References
- I. B. Solangi, F. Özcan, G. Arslan and M. Ersöz, Sep. Purif. Technol., 2013, 118, 470–478 CrossRef CAS.
- R. A. Sheldon, Chem. Commun., 2008, 3352–3365, 10.1039/b803584a.
- P. Anastas and N. Eghbali, Chem. Soc. Rev., 2010, 39, 301–312 RSC.
- M. Ye, M. Sun, X. Chen, Y. Feng, J. Wan, K. Liu, D. Tian, M. Liu, J. Wu, A. P. Schwab and X. Jiang, Waste Manage., 2017, 63, 275–283 CrossRef CAS PubMed.
- M. B. Kannan and K. Ronan, Waste Manage., 2017, 67, 67–72 CrossRef CAS PubMed.
- R. Bencheikh-Latmani, A. Obraztsova, M. R. Mackey, M. H. Ellisman and B. M. Tebo, Environ. Sci. Technol., 2007, 41, 214–220 CrossRef CAS PubMed.
- A. Senol, Sep. Sci. Technol., 2015, 50, 1010–1022 CrossRef CAS.
- J. Carvalho, J. Araujo and F. Castro, Waste Biomass Valorization, 2011, 2, 157–167 CrossRef.
- A. M. King'ori, Int. J. Poult. Sci., 2011, 10, 908–912 CrossRef.
- P. S. Guru and S. Dash, Adv. Colloid Interface Sci., 2014, 209, 49–67 CrossRef CAS PubMed.
- A. Laca, A. Laca and M. Diaz, J. Environ. Manage., 2017, 197, 351–359 CrossRef CAS PubMed.
- N. Mansir, S. H. Teo, U. Rashid, M. I. Saiman, Y. P. Tan, G. A. Alsultan and Y. H. Taufiq-Yap, Renewable Sustainable Energy Rev., 2018, 82, 3645–3655 CrossRef CAS.
- Y. Ono and T. Baba, Catal. Today, 1997, 38, 321–337 CrossRef CAS.
- R. A. Sheldon, Green Chem., 2007, 9, 1273–1283 RSC.
- S. Wu, H. Jiang, H. Zhang, L. Zhao, P. Yuan, Y. Zhang, Q. Su, Y. Wang, L. Wu and Q. Yang, J. Organomet. Chem., 2020, 925, 121496 CrossRef CAS.
- J. O. Metzger, Angew. Chem., Int. Ed., 1998, 37, 2975–2978 CrossRef CAS PubMed.
- T.-P. Loh, J.-M. Huang, S.-H. Goh and J. J. Vittal, Org. Lett., 2000, 2, 1291–1294 CrossRef CAS PubMed.
- S. Patil, S. D. Jadhav and S. K. Shinde, Org. Chem. Int., 2012, 2012, 1–5 CrossRef.
-
G. Wilkinson, Comprehensive Coordination Chemistry: The Synthesis, Reactions, Properties & Applications of Coordination Compounds, Pergamon Press, New York, NY, USA, 1987, vol. 1 Search PubMed.
- R. Ando, T. Yagyu and M. Maeda, Inorg. Chim. Acta, 2004, 357, 2237–2244 CrossRef CAS.
- D. Sanz, A. Perona, R. M. Claramunt and J. Elguero, Tetrahedron, 2005, 61, 145–154 CrossRef CAS.
- H. Schiff, Justus Liebigs Ann. Chem., 1864, 131, 118–119 CrossRef.
- B. E. Love and J. Ren, J. Org. Chem., 1993, 58, 5556–5557 CrossRef CAS.
- A. Vass, J. Dudás and R. S. Varma, Tetrahedron Lett., 1999, 40, 4951–4954 CrossRef CAS.
- J. N. Appaturi, R. Ratti, B. L. Phoon, S. M. Batagarawa, I. U. Din, M. Selvaraj and R. J. Ramalingam, Dalton Trans., 2021, 50, 4445–4469 RSC.
- S. Patil, S. D. Jadhav and M. B. Deshmukh, J. Chem. Sci., 2013, 125, 851–857 CrossRef CAS.
- A. V. Narsaiah and K. Nagaiah, Synth. Commun., 2003, 33, 3825–3832 CrossRef CAS.
- Y. M. Ren and C. Cai, Synth. Commun., 2007, 37, 2209–2213 CrossRef CAS.
- G. Cardillo, S. Fabbroni, L. Gentilucci, M. Gianotti and A. Tolomelli, Synth. Commun., 2003, 33, 1587–1594 CrossRef CAS.
- A. V. Narsaiah, A. K. Basak, B. Visali and K. Nagaiah, Synth. Commun., 2004, 34, 2893–2901 CrossRef CAS.
- M. El-Shahat and R. M. Abdelhameed, Appl. Catal., A, 2022, 635, 118558 CrossRef CAS.
- H. I. Aljaddua, M. S. Alhumaimess and H. M. A. Hassan, Arabian J. Chem., 2022, 15, 103588 CrossRef CAS.
- X. Bao, X. Li, C. Jiang, W. Xiao, G. Chen and A. Polyzos, Aust. J. Chem., 2022, 75, 806–819 CrossRef CAS.
- L. Dong and F. E. Chen, RSC Adv., 2020, 10, 2313–2326 RSC.
- S. Zhang, Y. Li, Y. Xu and Z. Wang, Chin. Chem. Lett., 2018, 29, 873–883 CrossRef CAS.
- S. D. Jadhav, R. C. Patil, A. A. Jagdale and S. S. Patil, Res. Chem. Intermed., 2021, 48, 593–606 CrossRef.
-
A. T. Nielsen and W. J. Houlihan, Organic Reaction, New Jersey, 1968, DOI:10.1002/0471264180.or016.01.
- T. Clarina, B. M. Valentina and V. Rama, Int. J. Sci. Res., 2014, 3, 2399–2403 Search PubMed.
- M. Kidwai, S. Saxena, M. K. Khan and S. S. Thukral, Bioorg. Med. Chem. Lett., 2005, 15, 4295–4298 CrossRef CAS PubMed.
- B. C. Ranu, A. Hajra and U. Jana, Tetrahedron Lett., 2000, 41, 531–533 CrossRef CAS.
- E. Mosaddegh and A. Hassankhani, Catal. Commun., 2013, 33, 70–75 CrossRef CAS.
- S. Sharma, S. Sharma, N. Sharma, S. Sharma and S. Paul, Catal. Lett., 2023, 154, 532–552 CrossRef.
- E. Mosaddegh, Ultrason. Sonochem., 2013, 20, 1436–1441 CrossRef CAS PubMed.
- E. Mosaddegh and A. Hassankhani, Chin. J. Catal., 2014, 35, 351–356 CrossRef CAS.
- H. Takao, K. Murai, N. Yasudomi, T. Goto, N. Umetsu and T. Horie, J. Pestic. Sci., 1994, 19, 151–156 CrossRef CAS.
- X.-S. Z. Wang, Z. Sen, Y.-L. Li, D.-Q. Shi and S.-J. Tu, Arkivoc, 2006, 2006, 107–113 Search PubMed.
- D. Rajguru, B. S. Keshwal and S. Jain, Med. Chem. Res., 2013, 22, 5934–5939 Search PubMed.
- L. A. Youseftabar-Miri, F. Akbari and F. Ghraghsahar, Iran. J. Catal., 2014, 4, 86–89 Search PubMed.
- S. T. S. Morbale, S. S. Sachin, S. D. Jadhav, M. B. Deshmukh and S. S. Patil, Der Pharmacia Lettre, 2015, 7, 169–182 Search PubMed.
- D. B. Biswanath, B. Ravikant, R. Ramu and B. Vittalrao, Chem. Pharm. Bull., 2006, 54, 1044–1045 CrossRef PubMed.
- M. M. Majid, M. Heravi, K. Bakhtiari, V. Zadsirjan, M. Saeedi and F. F. Bamoharram, Iran J. Org. Chem., 2010, 2, 298–302 Search PubMed.
- C. G. Evans and J. E. Gestwicki, Org. Lett., 2009, 11, 2957–2959 CrossRef CAS PubMed.
- A. Dehghani Tafti, B. B. F. Mirjalili, A. Bamoniri and N. Salehi, BMC Chem., 2021, 15, 6 CrossRef CAS PubMed.
- E. Mosaddegh, F. A. Hosseininasab and A. Hassankhani, RSC Adv., 2015, 5, 106561–106567 RSC.
- N. Kerru, L. Gummidi, S. V. H. S. Bhaskaruni, S. N. Maddila and S. B. Jonnalagadda, Res. Chem. Intermed., 2020, 46, 3067–3083 CrossRef CAS.
- I. Yildiz-Oren, I. Yalcin, E. Aki-Sener and N. Ucarturk, Eur. J. Med. Chem., 2004, 39, 291–298 CrossRef CAS PubMed.
- P. A. Thakurdesai, S. G. Wadodkar and C. T. Chopade, Pharmacologyonline, 2007, 1, 314–329 Search PubMed.
- A. Haboub, Am. J. Environ. Prot., 2015, 4, 28–32 Search PubMed.
- R. Mallampati and S. Valiyaveettil, ACS Sustain. Chem. Eng., 2014, 2, 855–859 CrossRef CAS.
- M. Kuhn, M. Lucas and P. Claus, Ind. Eng. Chem. Res., 2015, 54, 6683–6691 CrossRef CAS.
- M. Sankar, S. Satav and M. Palanichamy, ChemSusChem, 2010, 3, 575–578 CrossRef CAS PubMed.
- G. Stoica, S. Abello and J. Perez-Ramirez, ChemSusChem, 2009, 2, 301–304 CrossRef CAS PubMed.
- Y. Gao and C. Xu, Catal. Today, 2012, 190, 107–111 CrossRef CAS.
- A. Farhadi, A. L. I. Banan, J. Fields and A. L. I. Keshavarzian, J. Gastroenterol. Hepatol., 2003, 18, 479–497 CrossRef PubMed.
- F. Zokaee, T. Kaghazchi, M. Soleimani and A. Zare, J. Chin. Inst. Chem. Eng., 2002, 33, 307–314 CAS.
- F. Zokaee, T. Kaghazchi, A. Zare and M. Soleimani, Process Biochem., 2002, 37, 629–635 CrossRef CAS.
- M. Di Serio, R. Tesser, L. Pengmei and E. Santacesaria, Energy Fuels, 2008, 22, 207–217 CrossRef CAS.
- G. W. Huber, S. Iborra and A. Corma, Chem. Rev., 2006, 106, 4044–4098 CrossRef CAS PubMed.
- B. Dasilveiraneto, M. Alves, A. Lapis, F. Nachtigall, M. Eberlin, J. Dupont and P. Suarez, J. Catal., 2007, 249, 154–161 CrossRef CAS.
- D. Lopez, K. Suwannakarn, D. Bruce and J. Goodwinjr, J. Catal., 2007, 247, 43–50 CrossRef CAS.
- M.-H. Zong, Z.-Q. Duan, W.-Y. Lou, T. J. Smith and H. Wu, Green Chem., 2007, 9, 434–437 RSC.
- Z. Wei, C. Xu and B. Li, Bioresour. Technol., 2009, 100, 2883–2885 CrossRef CAS PubMed.
- N. Viriya-Empikul, P. Krasae, B. Puttasawat, B. Yoosuk, N. Chollacoop and K. Faungnawakij, Bioresour. Technol., 2010, 101, 3765–3767 CrossRef CAS PubMed.
- Y. C. Sharma, B. Singh and J. Korstad, Energy Fuels, 2010, 24, 3223–3231 CrossRef CAS.
- S. Niju, K. M. Meera, S. Begum and N. Anantharaman, J. Saudi Chem. Soc., 2014, 18, 702–706 CrossRef.
- A. Renita, P. P. Chowdhury, P. Sulthana, P. Phukan and A. Hannan, Int. J. Pharm. Pharm. Sci., 2016, 8, 143–146 CAS.
- N. C. A. Buasri, L. Vorrada, W. Chaiwat and S. Khamsrisuk, Sustainable Energy, 2013, 1, 7–13 Search PubMed.
- A. Attari, A. Abbaszadeh-Mayvan and A. Taghizadeh-Alisaraei, Biomass Bioenergy, 2022, 158, 106357 CrossRef CAS.
- F. Ashine, Z. Kiflie, S. V. Prabhu, B. Z. Tizazu, V. Varadharajan, M. Rajasimman, S.-W. Joo, Y. Vasseghian and M. Jayakumar, Fuel, 2023, 332, 126166 CrossRef CAS.
- M. Kirubakaran and V. Arul Mozhi Selvan, Bioresour. Technol. Rep., 2021, 14, 100658 CrossRef CAS.
- P. Oulego, A. Laca, S. Calvo and M. Díaz, Water, 2019, 12, 100 CrossRef.
- A. Nath, S. Biswas and A. Pal, Mater. Chem. Phys., 2023, 303, 127785 CrossRef CAS.
- J. Xu, S. Hu, L. Min and S. Wang, Water Sci. Technol., 2022, 85, 3271–3284 CrossRef CAS PubMed.
- S. Chaveanghong, T. Kobkeatthawin, J. Trakulmututa, T. Amornsakchai, P. Kajitvichyanukul and S. M. Smith, RSC Adv., 2023, 13, 17565–17574 RSC.
- X. Liu and D. Astruc, Adv. Synth. Catal., 2018, 360, 3426–3459 CrossRef CAS.
- M. Çelik Tolu and H. Oğuz, International Journal of Energy Applications and Technologies, 2018, 5, 147–152 CrossRef.
|
This journal is © The Royal Society of Chemistry 2024 |
Click here to see how this site uses Cookies. View our privacy policy here.