DOI:
10.1039/D3QM01048A
(Review Article)
Mater. Chem. Front., 2024,
8, 9-40
Nano-based theranostic approaches for infection control: current status and perspectives
Received
27th September 2023
, Accepted 16th November 2023
First published on 22nd November 2023
Abstract
Infections caused by bacteria and fungi are a substantial healthcare burden worldwide, leading to great socioeconomic impacts. Current treatments using antibiotics are facing challenges arising from antimicrobial resistance and new drug deficiency. Recent advances in photo/sonotherapy provide novel and efficient approaches to controlling infections, while the utilization of sensitive and rapid detection methods enables early prevention of microbial infections, maximizing the chances of successful treatment outcomes. Meanwhile, the development of nanotechnology provides an innovative strategy to integrate the therapeutic and diagnostic modalities into one system, and overcome their inherent physical or chemical drawbacks. In this review, the most updated strategies for controlling infection and detecting bacteria and fungi are elaborated. As different nanomaterials have varied natures and properties, the designing principles and action mechanism of representative nanotheranostic platforms published within the last seven years are summarized and discussed. This review also highlights the perspectives and relevant rational designs of nano-based theranostic platforms for infection control, and addresses the potential bottlenecks in clinical translation.
1. Introduction
Since birth, even as early as the embryo, the human body is constantly exposed to a wide variety of microbial cells that interact with the host. While most microbes are considered harmless, some of them can potentially result in life-threatening events in people.1,2 From easily neglected chronic infections to the most frequently diagnosed flu, septicemia, pneumonia or peritonitis, diseases caused by pathogenic microorganisms pose a serious threat to public health and may lead to great socioeconomic impacts. There are mainly three types of microbial infections: (1) bacterial, (2) fungal and (3) virus infections. Out of the three, diseases induced by bacteria and fungi are perhaps the most frequently occurring. According to a recent study, the estimated number of deaths caused by microbial infections in 2019 was approximately 13.7 million, of which 7.7 million were associated with bacteria, making them the second leading cause of death after ischemic heart disease.3 Following that, fungal infections have often been overlooked. Every year, at least 150 million cases are accounted for severe fungal infections and 1.5 million deaths are reported.4 They have a high incident rate and mortality in aged and immunocompromised individuals, in which the Candida and Aspergillus species are the most common fungal pathogens responsible for the majority of fungi-infected cases.5 Additionally, patients with severe COVID-19 infection also have a high risk of fungal co-infections due to modern clinical interventions (e.g., mechanical ventilation and application of dexamethasone).6 Thus, bacterial and fungal infections with high prevalence and economic setbacks have caused significant healthcare burdens worldwide, and immediate actions need to be taken to tackle these issues effectively.
Currently, use of antibiotics is the primary strategy for controlling microbial infections. Yet, with the increasing consumption and abuse of antibiotics, the antimicrobial resistance (AMR) crisis has become an extremely urgent issue on a global scale. In fact, the excessive use of antibiotics has contributed to the development of antibiotic-resistant strains and accelerated the emergence of superbugs.7 As such, with the rapid evolution of multi-drug-resistant, extensively drug-resistant and pandrug-resistant microorganisms, the discovery of new antimicrobial agents and strategies is critical to providing effective clinical infection management.
In response to these growing concerns, researchers are exploring new bioactive natural or synthetic small molecules, including antimicrobial peptides (AMPs), natural products and LpxC inhibitors, to expand the scope of potential drug candidates.8 Alternatively, the development and employment of antimicrobial adjuvants offer a new solution to the problem. In present clinical practice, three classes of adjuvants have been utilized: β-lactamase inhibitors, efflux pump inhibitors and membrane permeabilizers. These adjuvants, alongside antibiotics, work synergetically to reduce or reverse the resistance by restoring the microbial sensitivity to antibiotics.9 Despite demonstrating some promising results,10 the advancement of new drugs cannot keep pace with the rise of superbugs, and relying on the adjuvants alone may not be sufficient to address the challenges posed by AMR and its resulting crisis. Recent advances in photo/sonotherapy like photodynamic therapy (PDT), sonodynamic therapy (SDT) and photothermal therapy (PTT) provide novel approaches to controlling infections by producing reactive oxygen species (ROS) or heat to eradicate pathogens and their drug-resistant strains. Yet, their efficiency for clinical applications is still limited due to some physical and chemical barriers. In addition to suppressing microbial growth, monitoring pathogen activities may facilitate infection management as well. Specifically, sensitive and rapid detection of microbes enable early infection prevention, maximizing the chances of successful treatment outcomes. Taken together, the incorporation of both therapeutic and diagnostic (theranostic) strategies may significantly improve treatment efficacy by enhancing microbial targeting and monitoring abilities during the treatment process while exhibiting potent antimicrobial effects.11 Therefore, innovative methods that possess the above features may be crucial to achieving better treatment performance.
Nanomaterials have been widely celebrated for their potential in the biomedical field over the last few decades. Depending on their unique physicochemical properties, various nanomaterials with remarkable features can conveniently serve as versatile theranostic agents or stimuli-responsive nanosystems after surface modification and drug encapsulation.12 Notably, some nanomaterials exhibiting inhibitory activities per se against pathogenic microbes can facilely inherit antimicrobial effects derived from small molecules or metal ions and function as novel adjuvant carriers for delivering antibiotics, imaging moieties or microbe-targeting systems. In addition, as multifunctional vehicles, they can assist in the delivery of PDT/SDT/PTT sensitizers to designated lesions without hindering their therapeutic performance. Ultimately, they overcome the limitations of photo/sonotherapy by improving the bioavailability and targeting specificity, significantly boosting the efficacy of these strategies in infection control. Moreover, when administered in conjunction with antibiotics or other antimicrobial agents, they can act as potent nanomedicines to eliminate pathogens and their drug-resistant strains. With rational design and appropriate modifications, nanomedicines can even interact with the host cells upon trigger exposure and modulate the immuno-responses to manage infection.13 Collectively, nanomaterials as specific, traceable and highly efficient multipurpose platforms for microbial eradication may shed new light on constructing innovative theranostic nanomedicines to combat hard-to-treat infections and the current AMR crisis.14
This review aims to share an updated overview of the current strategies for controlling infection and detecting microbes, especially bacteria and fungi. We present a comprehensive summary of theranostic nanoplatforms developed from different nanomaterials published within the last seven years, and elaborate on the underlying principles behind the design/synthesis rationales of each nanomedicine (Scheme 1). Notably, we explore how they can be utilized for eradicating microbes, discriminating/labeling pathogens and imaging cells. The future prospects of nano-based theranostic platforms in clinical translation are also included to promote more explorations, solutions and actions in reducing the global health burdens.
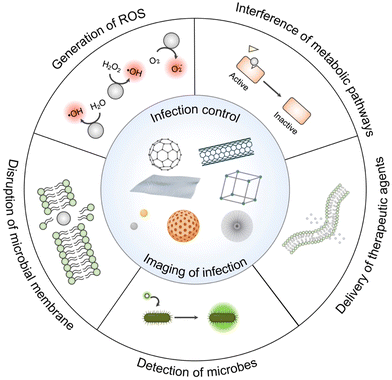 |
| Scheme 1 Illustrative scheme of various nanomaterials utilized as theranostic agents for infection control or management with representative action mechanisms of pathogen eradication and detection. | |
2. Current strategies for controlling infections and detecting microbes
2.1. Effects and mechanisms of antimicrobial therapies
2.1.1. Chemotherapy.
Antimicrobial chemotherapy refers to the utilization of natural or synthetic small molecules, such as antibiotics, to manage the growth of various pathogenic microbes. Generally, its mechanisms of action involve (i) inhibiting the synthesis of cell walls/nucleic acids, (ii) disrupting cell membrane integrity, (iii) generating oxidative stress and (iv) perturbing metabolism through interrupting enzyme/protein activity.15
Antibiotics, including both natural and synthesized types, have been considered one of the most important antimicrobial chemotherapeutic agents in the global market since their first discovery in the 1920s. Despite the increasing demands for antibiotics, the emergence of AMR has outpaced the development of new drugs. Meanwhile, natural antimicrobials, especially phytochemicals, are usually derived from the defense systems of plants to tackle the invasion of different microorganisms. The compounds, like phenolic acids, flavonoids, saponins, anthraquinones, alkaloids, and essential oils, have demonstrated antibacterial, antifungal and antiviral effects to some extent.16 For example, berberine, an isoquinoline alkaloid extracted from multiple medicinal plants (e.g., Coptis chinensis and Phellodendron chinense), possesses a potent and broad spectrum of antimicrobial activities by damaging the cell membranes and inhibiting protein synthesis.17 However, the application of these compounds is limited due to low water solubility and bioavailability, as well as high cytotoxicity, which ultimately affects their resultant antimicrobial efficiency.
Apart from the aforementioned pharmaceutical antimicrobials, many metal ions (e.g., Ag+, Au+, Ca2+, Mg2+, Zn2+, Ni2+, Cu2+ and Bi3+) also exhibit potent inhibitory effects on different microbes. Specifically, these ions exhibit a natural affinity for certain cellular components/proteins, which can affect the activity of enzymes and disturb the metabolic process in microbes.18 As such, some of the metal salts or organo-metallic compounds have been utilized as antimicrobial agents or antimicrobial adjuvants for enhanced therapeutic effects. For instance, bismuth salts have demonstrated high efficacy in treating gastrointestinal diseases associated with the Gram-negative (G−) pathogen Helicobacter pylori for over three centuries.19 The ions present in treatment can inhibit protein/ATP synthesis while disrupting the membrane function. Lately, some studies have shown that bismuth exhibits promising antibacterial and antiviral effects on the keystone periodontopathogen Porphyromonas gingivalis20,21 and SARS-CoV-2.22 Besides being a promising candidate for antimicrobial agents, metal ions can also reduce the development of AMR and restore the sensitivity of microbes to the antibiotics when co-administered,18 suggesting that different chemotherapeutic agents applied in combination could greatly enhance the treatment outcomes.
Currently, many challenges still need to be addressed in order to balance the efficacy of antimicrobial chemotherapeutic agents and their toxicity/side effects on patients. Thereby, it is highly warranted to develop new administration forms of the antimicrobial agents (i.e., nanomedicines) or therapeutic approaches, like photo/sonodynamic- and immuno-therapies, to tackle the emerging AMR crisis and overcome the issues of improving pharmaceutical performance and reducing cytotoxicity related to the conventional treatments.
2.1.2. Photo/sonodynamic therapy.
Light and sound, the two essential forms of energy, are being utilized in PDT and SDT for non-invasive antibiotic-independent therapeutic modalities in tackling microbial infections. Adopting a similar action mode, PDT and SDT have garnered much interest due to their ability to rapidly eliminate a wide range of microbes and their antibiotic-resistant strains (Fig. 1A).23–25 Generally, three key components are required to ensure appropriate functionality of PDT/SDT: (i) a non-toxic dye known as a photosensitizer (PS)/sonosensitizer (SS), (ii) exposure to light/ultrasound at a specific wavelength and (iii) the presence of oxygen molecules. Specifically, the electrons absorbed by the sensitizers upon irradiation undergo a transition to a higher energy triplet state and react with biomolecules in Type I and II pathways, leading to the generation of radicals/reactive oxygen species (ROS) and singlet oxygen (1O2) through energy transfer, respectively (Fig. 1B). Meanwhile, the type of radical generated can also be modulated since the ratio between the Type I and Type II pathways mainly depends on the microenvironment and the type of sensitizer used.26
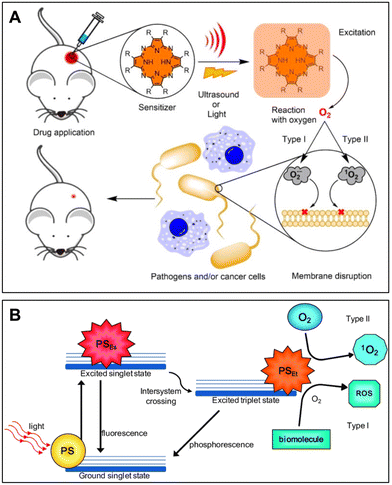 |
| Fig. 1 (A) Simplified mechanism of PDT and SDT. Reprinted with permission from ref. 25. Copyright 2022, Wiley-VCH. (B) Schematic Jablonski's diagram showing the energy transfer and mechanism of action of Type I and Type II pathways in PDT. Reproduced with permission from ref. 26. Copyright 2016, MDPI. | |
When designing PDT for the management of a localized infection, it is essential to take the following factors of photosensitizers into account, including (i) the specificity toward the microbes and host tissues, (ii) potential toxicity in the absence of light, (iii) delivery efficiency of light to the infected region and (iv) photochemical reactivity. According to the structures, the commonly used PS can be classified into phenothiazinium (e.g., methylene blue, toluidine blue, and Rose Bengal), natural compounds (e.g., curcumin and hypericin), tetra-pyrrole (e.g., porphyrin and phthalocyanine) and nanomaterials (e.g., fullerenes and titanium dioxide).24,27 Currently, all these four types of PS are reported and applied for PDT-related infection control.28–30 It is noted that cationic PSs are preferred over anionic PSs to tackle bacteria because the negatively charged surface of bacterial cells can more readily take up the PS with positively charged functional groups.24 Nevertheless, the selectivity of PSs can simultaneously be affected by both the cellular environment and the complex biological matrix present in vivo, and the permeability of the external light sources is still limited. Thus, introducing an alternative energy source without being perturbed by the local environment and developing long-wavelength light-responsive PSs are essential to address the limitations.
Referring to SDT, although its exact mechanism has not yet been fully revealed, it is commonly recognized that SS and ultrasound play an essential role in the successful generation of ROS. Similar to PDT, SDT can employ the same photosensitizing organic molecules and inorganic nanoparticles upon ultrasound exposure. In addition, ultrasound-responsive inorganic nanomaterials, especially metallic oxide nanoparticles composed of zinc oxide or titanium dioxide, exhibit excellent efficacy for eradicating microbes.31 In comparison to light, ultrasound offers significant advantages in terms of tissue penetration and phototoxicity reduction. Particularly, SDT can treat deep-seated lesions beyond the reach of light via targeted delivery and accumulation of SS. Moreover, ultrasound per se can cause cavitation, sonoporation and thermal effects, enabling further enhancement of the antimicrobial efficiency alongside the ROS produced by SS. In fact, the mechanical force from cavitations can physically damage the outer layer of microbes, while the resulting microstreams and shockwaves can disrupt the biofilm structures.32 Regarding the effects of sonoporation, drugs or SS can be easily transferred and accumulated in the microbial cells through the formation of small pores in cell membranes, consequently improving the treatment efficiency.33
In spite of demonstrating promising results in treating cancer and controlling infection, PDT and SDT as novel therapeutic strategies still require further adjustments before they can be implemented for clinical practice. Importantly, the generation of ROS in specific areas depends on the oxygen levels in the microenvironment for both therapies. As the functions of these therapies are restrained in the hypoxic microenvironment, they may consequently demonstrate little effect on anaerobic pathogens. Additionally, the treatment efficiency of PDT and SDT depends on the amount and distribution of sensitizers accumulated in lesioned regions. Thus, maintaining oxygen levels and increasing the targeting of sensitizers in microbe-infected areas still remain the major objectives in the current PDT/SDT research field.
Recently, PDT and SDT incorporating nano-based approaches have shown great potential in practical usage. As the therapeutic performance is governed by the local distribution and accumulation of sensitizers, photosensitizing nanocarriers, like up-conversion nanoparticles conjugated with a PS, have the capability to absorb infrared light and emit visible light, allowing deep tissue penetration of external light and effectively triggering the production of ROS. In the meantime, targeting ligand-conjugated carriers can readily improve the safety and efficacy of PSs by lowering their toxicity and enhancing their specificity.34 Regarding the deficiency under hypoxic conditions, nanocomplexes combined with PSs can tackle the hurdles in deep tissues by encapsulating oxygen donors induced by a near-infrared (NIR) two-photon laser.35 Likewise, nanoenzymic systems adjoining SS can coordinately relieve local hypoxia and promote angiogenesis to enhance SDT efficiency.36 Notably, these nanosystems can be optimized through various modifications, including surface charge,34 antibodies37 and molecules capable of binding to microbial membrane structures,38 thereby increasing the accumulation of sensitizers in microbial cells or biofilms. Compared to free sensitizers, nano-based approaches have been adopted to assist PDT/SDT for better therapeutic outcomes. Based on the positive outcomes in pre-clinical studies, it is highly feasible to use PDT for treating infections in easily accessible body cavities (e.g., the ear, mouth and nasal cavity), as well as wound infections near surfaces like cornea and skin, whereas SDT is more appropriate for curing the deep-seated infected areas.
2.1.3. Photothermal therapy.
Similar to PDT, PTT is a light-activated, photosensitizer-based therapy that constitutes an important part of antibiotic-independent strategies for controlling infection and managing AMR, while the lethal factor involved in PTT is hyperthermia. In comparison to photo/sonodynamic treatments, PTT is capable of functioning regardless of the oxygen level, making it a suitable therapeutic candidate in hypoxic environments. Precisely, light acts on photothermal agents (PTA) to convert light energy into heat for deactivating microbial nucleic acids and proteins, simultaneously stimulating acute inflammation in the environment and activating the host immune defense against infections.39,40
As a core component of PTT, PTA, either in the form of organic or inorganic materials, has made significant contributions to the advancement of PTT in preclinical studies. For organic PTA, a variety of organic dyes (e.g., cyanines, diketopyrrolopyrroles, croconaine and porphyrins) and some polymeric nanoparticles, as well as carbon-based materials (e.g., carbon nanotubes, graphene oxide and carbon dots), can generate heat in response to the long-wavelength light irradiation and work as theranostic agents after modifications.41 Regarding inorganic PTA, several types of nanomaterials have been developed to convert light to heat in a highly efficient manner, including noble metal nanomaterials (e.g., Au, Ag and Pt) and metal oxide/sulfide nanoparticles (e.g., Fe3O4, CuS, MoS2, MoOx and WS2).42 As a result, by incorporating microbial targeting molecules/modifications with these nanomaterials, PTT as an innovative approach can be incorporated into thermal-responsive smart delivery systems and avoid non-specific thermal damage to host tissues.43 Importantly, an improved therapeutic with exceptional antimicrobial effects can be attained by integrating PTT with PDT, SDT or antimicrobial chemotherapy approaches.40
2.1.4. Immunotherapy.
Immunotherapy is a type of therapeutic approach that employs the host defense system to combat various types of diseases.44 In brief, the treatment takes advantage of pattern recognition receptors (PRRs), such as toll-like receptors (TLRs), found in host cells to activate the related signaling pathways and initiate the inflammatory responses by expressing the pro-inflammatory cytokines. Furthermore, this approach is also responsible for activating the microbicidal mechanism of phagocytic cells and coordinately producing AMPs and toxic oxidants (e.g., reactive nitrogen species and ROS) to eliminate pathogenic intruders.45
To date, numerous immunomodulating TLR agonists have been reported for their efficiency in clearing bacterial infections by activating various TLRs.46,47 For example, studies have shown that the employment of MPLA, a TLR4 agonist, can substantially improve the survival rates of mice infected with Staphylococcus epidermidis, Escherichia coli, Moraxella catarrhalis or Haemophilus influenzae.48,49 Meanwhile, co-administrations of TLR agonists with antibiotics may generate synergetic effects.13 Although innate immunomodulators are effective in infection control, their therapeutic performances can be further improved by maintaining their bioactivity via prolonging circulation, escaping phagocytosis by immune cells and increasing biocompatibility in body fluids.
Apart from immunomodulators, AMPs, a class of peptides with diverse sequences and structures, offer several key advantages over antibiotics due to high potency, ability to tackle a broad spectrum of microbes, multiple modes of action and low risk of AMR development.50–53 These peptides, particularly the ones with cationic or anionic charges, can disturb the membrane of bacteria, interrupt the enzyme activity, or interact with their intracellular components, causing apoptosis or necrosis through various models, depending on the bacterial strains.54 Nonetheless, AMPs as therapeutic agents may be restricted due to their instability in physiological salt concentrations, susceptibility to protease degradation, hemolytic activity, inconsistency in their impacts on bacterial membranes and toxicity to mammalian cells.55 Meanwhile, developing an ideal peptide can be expensive and time-consuming, and often has a low success rate.
Taken together, additional assistance of novel techniques, such as incorporating multifunctional nano-based systems,56 may be crucial to enhancing the efficiency of immunotherapy and improving therapeutic performances, which will be further discussed in the following sections.
2.1.5. Combined therapy.
As different antimicrobial drugs or approaches have their own drawbacks, combined therapy has been frequently employed in order to overcome these shortcomings and maximize treatment outcomes by obtaining synergetic effects. In a typical manner, combined therapy involves the incorporation of two or more antimicrobial agents or therapeutic approaches, which in turn helps increase the sensitivity of microbes to the treatments, reduce or reverse the antibiotic resistance and enhance treatment efficiency. Based on the design concepts, nanoparticle-derived delivery systems integrated with multiple antimicrobial agents or sensitizers are considered ideal candidates to work jointly for effectively combating a variety of microbe-induced infections.
It has been observed that there is a growing trend of combining chemotherapy with photo/sonotheray to achieve synergetic effects in tackling the current AMR crisis. When applied collectively, these approaches can generate different yet complementary antimicrobial mechanisms of action, working as multiple lines of the defense system against noxious microbes. Notably, PTT is frequently utilized with chemotherapy or other ROS-involved methods in combined therapy, as the heat generated could improve the permeability of the microbial membranes and accelerate the intracellular accumulation of the chemotherapeutic agents or ROS. Simultaneously, the interdependent combination allows reduced dosage of antimicrobial agents and laser density for PDT/SDT, thereby diminishing potential tissue injury and adverse side effects.39 As a result, some inorganic PTA nanomaterials, such as gold nanoparticles57 and platinum nanoparticles,58 are synthesized and further surface-modified with antibiotics for controlling drug-resistant bacteria or fungi. Moreover, some metal ions, acting as antimicrobial agents or antimicrobial adjuvants, can be constructed into porous materials like metal–organic frameworks (MOFs) to combat microbes in synergy with encapsulated antibiotics.59
Since nanomaterials fabricated from various reagents are able to result in diverse properties, their utilization in the combined therapy can vary according to the designs and incorporated compounds. Further details of synergetic effects produced by all-in-one nanosystems in infection control are discussed in Section 3.
2.2. Detection and diagnosis of microbes
Until now, the conventional method of inoculating microbial cells on culture plates is still the gold standard for microbe detection in the human body. However, the process of culturing microbes on agar plates tends to be labor-intensive and time-consuming, and the method may be susceptible to false negatives due to its inability to accurately identify viable but non-culturable pathogens. Particularly for in vivo studies, effective detection of microbes is of great importance to improve the specificity and the efficacy of the theranostic methods. This section provides a brief overview of the most common approaches for targeting microbes, specifically focusing on strategies that are potentially compatible with the in vivo nano-system for infection control.
2.2.1. Non-specific interactions for detecting microbial cells.
Bacteria can be classified into Gram-positive (G+) and Gram-negative (G−) according to the composition of their cell wall. Generally, G− bacteria possess a thin layer of peptidoglycans located beneath the outer membrane containing lipopolysaccharides (LPS), phospholipids and lipoproteins, whereas G+ bacteria without the outer membrane have a relatively thicker layer of peptidoglycan.60 Since the cell walls in these two types of bacteria are composed of peptidoglycan, which has a net negative charge on the surface of the bacterial membrane, various cationic probes have been designed and synthesized to enhance probe–microbe interactions.61 Previously, Li et al. introduced the development of a cationic PS named 4TPA-BQ, which exhibits aggregation-induced emission (AIE) features for targeting both G+ and G− bacteria such as S. epidermidis and E. coli, respectively. In particular, the PS was found to be highly effective against ampicillin-resistant E. coli during in vivo experiments, killing the bacteria within 15 min while demonstrating good biocompatibility.62 In another study conducted by Shi et al., they presented a series of AIE-based cationic imidazolium compounds that are capable of binding to bacteria via electrostatic and hydrophobic interactions.63 As such, the construction of a positively charged probing nanosystem can be a practical approach to non-specifically interacting with bacterial membranes via electrostatic force.
Nevertheless, the sole reliance on the charge of the cationic probes for bacterial detection via non-specific electrostatic interaction makes it almost impossible to discriminate different bacterial species. Additionally, when used for antimicrobial photodynamic therapy, the non-specificity of the electrostatic-based method may also impose toxicity to mammalian cells and cannot be degraded by the cells even over extended periods of incubation. For example, the time-dependent fluorescence study reported by Li and colleagues showed that a small amount of cationic AIEgen 4TPA-BQ could still be found in normal cells after 8 h incubation by giving a weak orange fluorescence.62 Furthermore, the non-specific electrostatic forces may also be disrupted by the presence of other biomolecules and a complicated in vivo environment at the physiological salt concentration. Therefore, the utilization of a more specific and selective method, together with fast clearance in the host cells, may also need to be taken into consideration when designing onsite fluorescence agents for bacterial discrimination.
2.2.2. Specific detection of microbes.
Various methods for improving detecting and targeting specificities have been employed to capture bacterial cells in a complicated biological environment.64 Besides attaching to bacteria through electrostatic interactions, some probes have been strategically built to form covalent bonds for more potent and selective bindings with specific types of bacterial cells. Precisely, probes functionalized with boronic acid and its derivatives generally have higher specificity to bacteria by binding to the diol chain of 3-deoxy-D-manno-oct-2-ulosonic acid, which is a component of LPS in a reversible manner. Meanwhile, when being applied in conjunction with other therapeutic agents, targeted antimicrobial treatment with fluorescence labeling can be achieved. For example, when the acid is combined with PS, it has been found that the PS also has the capability to effectively treat E. coli in both planktonic and biofilm modes.65,66 In another study, Wulff-type boronic acid-functionalized quantum dots developed by Ye and co-workers can selectively target E. coli over a wide range of pH.67 Similarly, poly(amidoamine) (PAMAM), a chemically modified dendrimer with five phenylboronic acid groups at the terminus, demonstrates a promising ability to recognize G− bacteria.68 While most boronic acid-based probes have shown great potential in targeting the LPS on the membrane surface of G− bacteria, they can also be used for detecting G+ bacterial cells. Recently, a dendrimer modified with phenylboronic acid alongside dipicolylamine groups showed that the dendrimer possesses selectivity toward S. aureus at different pH values.69
Antibodies, on the other hand, are among the most popular recognition elements for microbe detection due to their high specificity and affinity. To date, antibodies with potent activities against selected bacterial strains have been jointly utilized with nanomaterials and developed into active immuno-biosensors.70–72 For example, the anti-S. aureus polyclonal antibody-modified poly(lactic-co-glycolic acid) (PLGA) nanoparticles with rifampicin loading could precisely eliminate the G+ bacterium at its planktonic, sessile and intracellular forms.72 Importantly, it has been observed that the bioactivity and bio-recognizing specificity of the antibodies can still be maintained even after conjugation. Despite showing promising selectivity for microbes, antibodies generally suffer from low stability and fast degradation in body fluids, and the complexity of their manufacturing processes may pose significant difficulties in finding a suitable nanomaterial for their joint use. As such, another type of biomacromolecule with binding capacity, named binding peptides, is emerging for interacting with the components or receptors located at microbial cell membranes/walls, and has been further applied as nanomaterial decoration for microbial detection.73,74 Binding peptides with smaller sizes than antibodies exhibit higher stability at diverse pH levels,75 while the peptide cyclization into the nanobody forms can further improve their proteolytic stability.76
Similar to antibodies, aptamers not only have excellent specificity and affinity to cellular targets but also possess key advantages, including high stability, low cost and flexibility to incorporate chemical modification for modulating the binding affinity. The selection of deoxyribonucleic acid (DNA) aptamers against bacterial cells can be achieved by an in vitro process known as the systematic evolution of ligands by exponential enrichment (SELEX).77 Previous studies have successfully identified aptamers that explicitly target Enterococcus faecalis,78 and other selected aptamers could bind with the LPS of E. coli and inhibit LPS-induced macrophage polarization.79 The use of aptamers for the detection of pathogenic bacteria has been reviewed by Li et al. and is further discussed in the cited article.80
In recent years, bacteriophages (also known as phages), which are viruses that only infect bacterial cells, have also been employed as recognition elements for targeting bacteria in vivo for super-antibacterial performance against specific strains of bacteria, such as the multi-drug resistant Pseudomonas aeruginosa.81 This phage-guided method is highly specific and can be utilized with other novel theranostic strategy, such as aggregation-induced emission-based PDT treatment, to enable point-of-care diagnosis and significantly reduce the toxicity issues that arise from the non-specific interaction of the PS with the surrounding normal cells and biomolecules.82
3. Design principles and representative nanomaterials
3.1. Carbonaceous nanomaterials
Carbonaceous nanomaterials, such as graphene/graphene oxide (GO) derivatives, carbon nanotubes (CNTs), carbon dots (CDs) and fluorenes, have been widely utilized owing to their antibacterial effectiveness in combating bacterial growth. Apart from being efficient antimicrobial agents, many carbon-based nanomaterials, besides CDs having inherent fluorescence, are often utilized in conjunction with the aforementioned agents like antibodies or fluorescent moieties for microbial detection applications due to their high surface area and electroconductivity. As such, for theranostic purposes, they are mainly used for their antimicrobial effects, whereas early detection of the disease often plays a supportive role.
3.1.1. Graphene-based materials.
Graphene-based materials, including graphene, graphene oxide and their derivatives, are thin sheets composed of sp2 carbon atoms. Their physiochemical properties (e.g., size, shape, quality, layer counts, defect density and hydrophilicity/hydrophobicity) grant them unique discriminating and antimicrobial activities when interacting with a broad spectrum of microorganisms.83 While the efficiency of inhibiting and eliminating microbial cells varies depending on the in-built characteristics of the as-synthesized nanomaterials, the graphitic layers, in general, can impair bacterial cells by mechanically seesawing the lipid membrane through oscillation or generating ROS to induce oxidative stress in living systems. Nevertheless, given that the antimicrobial and labeling abilities of the material itself may be minimal, further modifications for more versatile functions are necessary to achieve better theranostic performance. Favorably, these sheets exhibiting excellent electrical/mechanical properties often flourish with oxygen-containing functional groups (e.g., carbonyl, carboxyl and epoxy), which may allow additional surface functionalization by forming either covalent or non-covalent bonds with compounds demonstrating antimicrobial or fluorescent activities. Moreover, when graphene and its derivatives are combined with metal ions as well as their nanoforms, they may confer the ability to completely inhibit/eliminate the growth of bacteria in both planktonic and biofilm modes.84 Previously, graphene oxide nanocomposites with lanthanum hydroxide (La@GO) designed by Zheng and colleagues have offered an alternative solution to prevent the emergence of secondary resistance bacteria (Fig. 2A and B).85 The amalgamated nanoplatform could synergetically attack all resistant strains of the bacteria selected by disrupting the components at the bacterial cell membrane, and its long-time exposure to E. coli could effectively stop the evolution of secondary resistance compared with conventional treatments. Another study done by Parandhaman et al. synthesized graphene and silver nanocomposites with AMP functionalization (GAAP) to combat S. aureus-induced skin infections in mice (Fig. 2C).86 Indeed, the graphene displaying good biocompatibility and aqueous dispersibility gives an ideal platform for the conjugation of the metal nanoparticles and AMP, overcoming the limitations of these supporting materials and enhancing the overall outcomes in joint treatments. These all-in-one graphene-based nanoplatforms enable effective bactericidal effects upon contact with the noxious pathogen and acquire multiple mechanisms to eradicate bacteria and their resulting biofilms without compromising the original biocompatibility of the nanomaterials.
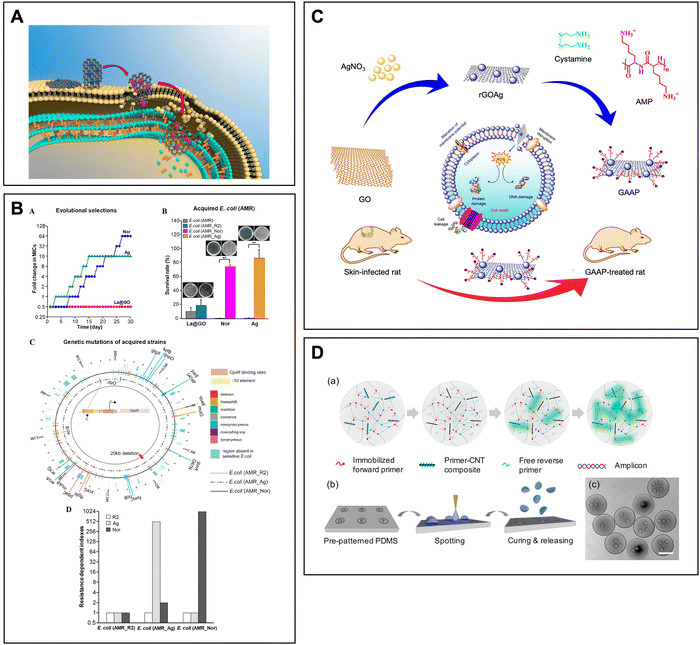 |
| Fig. 2 (A) Illustrative scheme showing that La@GO is capable of disrupting the components on the cell membrane by utilizing the extracellular multitarget invasion mechanism. (B) The La@GO can effectively prevent the evolution of secondary resistance. Reprinted with permission from ref. 85. Copyright 2019, American Chemical Society. (C) Rational design and application of the synthesized GAAP as a biofilm-disturbing agent in bacterial skin infection. Reproduced with permission from ref. 86. Copyright 2021, American Chemical Society. (D) PIN qPCR with primer-CNT composites (cPIN qPCR). Reprinted with permission from ref. 108. Copyright 2017, Elsevier B.V. | |
Concerning their antifungal properties, graphene and graphene oxides have demonstrated promising results as fungal inhibitors. They work by interfering with the metabolic processes of fungi, leading to lower production of several important enzymes essential for the survival of fungal cells. Nevertheless, their efficacy and mode of action can vary depending on the fungi species and may be influenced by physiological and genetic variations within the same fungal population. For instance, a study conducted by Nguyen et al. discovered that both graphene and graphene oxides could inhibit the growth of Aspergillus species by inducing oxidative stress and increasing the sensitivity of both species. Interestingly, their effects varied on the subspecies level, with graphene oxides proving more effective at increasing the sensitivity of A. niger, while graphene showed greater efficacy against A. flavus.87 Taken together, the graphene-based nanosystem with high specificity may also be an invaluable tool for effectively controlling fungal-induced infections.
Additional efforts have been made to adopt graphene-based nanomaterials for detecting the presence of microbes in diagnostic applications. As graphene-based nanomaterials do not exhibit fluorescence themselves, they usually require the assistance of fluorescent dyes or antibodies to indicate their location upon contact with microbes.88,89 A previous work carried out by Shen and colleagues added AIE-active luminogens (AIEgens) to GO to develop a highly sensitive complex that could identify and classify six different microbes, namely Saccharomyces cerevisiae, C. albicans, E. coli, P. aeruginosa, S. aureus and Bacillus subtilis via competitive interactions.90 The luminogens offer a wide range of probe selections, while GO could serve as a fluorescence quencher and a platform to assist the adhesion of biomolecules. Consequentially, the AIEgens and GO in combination could conveniently minimize the background noise from the luminescent agents and improve the microbial discrimination accuracy by producing different fluorescence intensities distinguishable from the naked eye upon the introduction of various microbial lysates, making them pioneering monitoring agents for differentiating complex bioanalysts. Thereby, graphene and its derivatives have often served as the initial building blocks before being further modified with other functional agents for multipurpose biomedical applications.91
3.1.2. Fullerenes.
Fullerenes are a different type of molecular structure consisting of hollow sp2 carbon clusters. These structures are identified by the notation Cn, in which n indicates the number of carbon atoms present (e.g., buckminsterfullerene, C60). Additional functionalization can modulate the solubility of fullerenes according to the type of aqueous medium and enhance their performance in controlling microbial growth through multiple mechanisms. Particularly, owing to their distinct structural orientation, these molecules can easily bind to bacteria via electrostatic interactions, and their high surface hydrophobicity enables them to effortlessly interact with the lipophilic domain of the microbial membrane. Formerly, a non-cytotoxicity amine-modified C70, synthesized using ethylenediamine, has been effective in killing super-bacteria like multidrug-resistant (MDR) E. coli and S. aureus.92 The C70 fullerene itself displayed hydrophobic properties, enabling it to form strong interactions with the bacteria and trigger cytoplasmic leakages, ultimately leading to bacterial cell death. Additionally, the subsequent in vivo experiments demonstrated that the amine-modified fullerenes could treat the area affected by bacterial infection and sequentially facilitate the wound healing process by modulating the immune response by promoting the secretion of growth factors. While fullerenes can physically eradicate bacteria by direct contact, they can also function as photosensitizers to generate ROS upon light exposure.93,94 At high concentrations, fullerenes can increase the uptake of O2 alongside H2O2 conversion, thereby impeding the respiratory chain and leading to lipid peroxidation and degradation of the bacterial cell membranes. Zhang and colleagues conducted a study on a hydrogel system that utilized self-assembled amphiphilic small peptides non-covalently linked to fullerene with PDT to eliminate MRSA.95 The fullerene-derived nanoparticles showed improved mechanical properties of the peptides on hydrogels and had synergetic effects on the survival of MRSA both in vitro and in vivo.
When it comes to microbial detection, they are often conjugated with other materials, such as modified fluorescent polymers and carbon nanotubes, to accelerate the electron transfer rate and enhance the detection limits.96 For instance, Chen and coworkers synthesized a universal amperometric DNA biosensor with C60 that could detect Mycobacterium tuberculosis with high sensitivity.96 Mainly, as the successful employment of electrochemical DNA biosensors using an enzyme-assisted amplification strategy for detecting a specific DNA sequence needs both capture and assistant probes, gold nanoparticle-decorated fullerene together with polyaniline-conjugated carbon nanotubes displaying large surface area and high conductivity can aid the transfer of electrons and amplify multiple electrochemical signals. The same group further synthesized a C60 nanoparticle (C60NP)-based diagnostic sandwich-like aptasensor and explored the effects of its binding ability towards M. tuberculosis antigen MPT64.97 Specifically, the C60NP nanosystem, consisting of nitrogen-doped carbon nanotubes, GO, aptamers and gold nanoparticles, exhibits exceptional redox activity, conductivity and stability and can accurately label the MPT64 antigen with the assistance of metal–organic frameworks, further boosting the analytical capabilities of the aptasensor. Together as an early diagnostic tool, they can provide an innovative method to effectively monitor the pathogenesis of tuberculosis induced by M. tuberculosis. Consequentially, as biocatalysts and nanocarriers, they hold great potential in various biomedical applications, particularly in the delivery of amino acids and drugs for effective antimicrobial therapeutic agents.98
3.1.3. Carbon nanotubes.
Similar to fullerenes, carbon nanotubes (CNTs) are also hollowed cylindrical skeletons comprised of the same carbon sheets. Depending on the thickness of the layer, they can be categorized as single-walled (SWCNTs)99,100 or multi-walled (MWCNTs).101 Despite belonging to the same CNT family, they undergo different modification processes during their synthesis. Indeed, the functionalization of SWCNTs leads to the sacrifice of multiple C
C bonds to form vacancies in the nanotube structure, subsequently altering the physiochemical properties of the material, whereas the same setting can only affect the outer wall of MWCNTs. In this regard, SWCNTs usually have a greater capacity for loading than MWCTNs.102 While SWCNTs and MWCNTs may behave differently after being functionalized, both CNTs with/without additional surface tailoring can suppress the growth of microbes in both planktonic and biofilm modes in a concentration-dependent manner, and share several common key factors to determine their antimicrobial performance, including length, dopants, functional groups, suspension states and the stage of biofilm formation.103,104 For example, SWCNTs coated with mesoporous silica (mSiO2) have been developed as an antibacterial nanoplatform to deliver silver ions (SWCNTs@mSiO2-TSD@Ag).104 As discussed, the SWCNTs with shorter lengths and diameters, acting like “needles”, can enhance the CNT–microbe interactions, leading to additional damage to the bacterial cell membrane. Jointly with the metal dopants, the SWCNTs@mSiO2-TSD@Ag nanosystem exhibits potent bactericidal effects on multi-drug-resistant bacteria in vitro by disrupting the structural skeleton of the bacterial cell membrane and releasing silver ions. Meanwhile, MWCNTs ultrasonically anchored on thermoplastic polyurethane (TPU) electrospun nanofibers (TPU/MWCNT) together with vancomycin (Van) as a TPU/Van-MWCNT have shown improved antimicrobial performance against S. aureus.105 The MWCNTs alone could eliminate both G+ and G− bacteria, and when used in combination, the conjunction with the antibiotic (TPU/Van-MWCNT) was found to hinder the biosynthesis of the bacterial cell wall, peptidoglycan and RNA. Specifically, the nanoplatform demonstrates a 2-fold reduction in its effective concentration to inhibit the growth of the bacterial cells while preventing their adhesion within 24 h, making it a promising material for wound dressing applications.
Regarding the use of CNTs as a supplementary fungicide, they have been explored for enhancing the overall antimycotic activities equivalent to the materials designed for antibacterial applications. In one study by Mohamed and co-workers, the MWCNTs with amino groups deposited on chitosan derivatives have been more effective in limiting the growth of fungal species, such as A. niger, Cryptococcus neoformans and C. tropicalis, compared to chitosan and AmB alone.106 The presence of MWCNTs could reduce the self-aggregation tendency of chitosan through multiple electrostatic interactions, thus creating a larger contact area between the nanocomposite and the microorganism cell wall. These studies highlight the potential of CNTs as a valuable tool in customizing nanomaterials with enhanced antimicrobial properties and offer new solutions to applications in various fields.
In addition, when CNTs are used for diagnostic applications, they may also assist materials like graphene to achieve better electrochemical targeting performance. Singh et al. synthesized a microfluidic chip comprised of sandwiched MWCNTs and GO alongside the immobilized antibodies that could selectively detect Salmonella typhimurium synergetically.107 Particularly, the diminished conductivity of GO after reduction can be addressed by employing CNTs. This integration could facilitate the separation of GO sheets and decrease their overall resistance, consequently causing an increase in the conductivity of the resulting composite. Meanwhile, due to its large surface area and embedded functional groups, the GO in the microfluidic chip could contribute to the system by serving as a carrier for transporting antibodies and other biomolecules. Correspondingly, the CNTs wrapping around GO nanosheets could improve the sensing ability and detection limits compared to GO alone. On the other hand, CNTs are capable of expanding the applications of a primer-incorporated network (PIN), allowing the amplification of low detection signals while reducing the unwanted non-specific byproducts through enhanced immobilization of the primers with separate reaction chambers. Previously, Jung's group proposed a composite utilizing SWCNTs to differentiate various pathogens for the real-time polymerase chain reaction (qPCR) (Fig. 2D).108 The SWCNT-based nanoplatform conjugated with specific primers could efficiently amplify the signals for detecting pathogenic DNAs, and the released companion primer could grant wide-ranging bacterial DNA analysis. Collectively, these systems can amplify the target gene effectively and precisely label for bacteria by providing an easily adjustable system for quantifying bacterial cells and identifying infectious diseases at an early stage.
3.1.4. Carbon dots.
Leaving aside the graphitic layers, carbon dots (CDs), sometimes described as carbon nanoparticles or carbon/graphene quantum dots, are a class of fluorescent spherical carbon-based nanomaterials with an average diameter of less than 10 nm.109–111 Owing to their affordability, easy preparation, environmental friendliness, excellent optical properties, high functionality, good biocompatibility and low cytotoxicity, they have been widely utilized in numerous biomedical fields, including detection,112 diagnostic,113 antibacterial,114 antifungal115 and antiviral116 theranostic applications. As a bioimaging agent, CDs with or without additional dopants (e.g., sulfur, nitrogen and other metal ions) are capable of emitting fluorescence across a wide range of wavelengths.117,118 Previously, Lu's team synthesized nitrogen and phosphorus co-doped carbon dots for bioimaging to assay the viability of bacterial cells.119 By changing the chemical reagents used for synthesis, the surface of the particles could be adjusted to weak or strong negative charges depending on the amount of nitrogen embedded. In particular, the system can accurately discriminate dead E. coli within 2 h, significantly reducing the time for staining bacteria when compared with the traditional plate counting method. Meanwhile, with the assistance of a microbial targeting agent, CDs can be transformed into a water-soluble and biocompatible yet highly specific fluorescent tracking system. As demonstrated in Fig. 3A, fluorescent CDs encapsulated in organosilica nanocapsules could effectively label S. aureus even at low concentrations. In order to achieve targeted labeling for the bacteria, the S. aureus-specific antigen was modified on the breakable nanocapsules, creating a responsive nanosystem that utilized antibody–antigen interactions for immunofluorescence.113 Upon contact with the bacteria, the CDs with blue emission could be released, subsequently serving as promising fluorescent biolabeling agents for both in vitro and in vivo models.
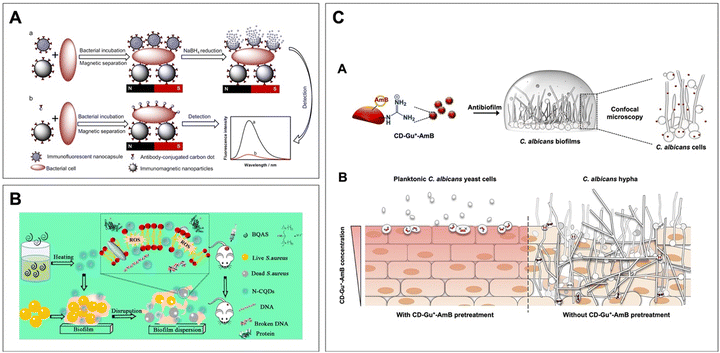 |
| Fig. 3 (A) Detection of bacteria by NaBH4 reduction. Reprinted with permission from ref. 113. Copyright 2018, American Chemical Society. (B) The N-doped CDs can generate ROS to eliminate bacteria and biofilm through multiple mechanisms. Reprinted with permission from ref. 114. Copyright 2019, American Chemical Society. (C) The red-emissive guanylated carbon dots functionalized with amphotericin B (CD-Gu+-AmB) can inhibit the two-day-old C. albicans biofilms and accumulate in the fungal cells. Applying CD-Gu+-AmB on reconstituted human oral epithelia could form a shielding layer to prevent the invasion of C. albicans. Reprinted with permission from ref. 115. Copyright 2019, American Chemical Society. | |
In addition, CDs are also highly regarded for their antimicrobial effects against a broad spectrum of microbes via direct or indirect incorporation of antimicrobial agents/functional groups as well as the implementation of various therapies.120,121 Specifically, the conjugation of pharmaceutical compounds with known bactericidal/antifungal effects on CDs can significantly improve the hydrophilicity of the drugs while maintaining their original activities. In fact, it has been suggested that cationic CDs synthesized with bis-quaternary ammonium salts exhibited potent antimicrobial activities against both G+ and G− antibiotic-resistant pathogens, as well as their biofilms and persister cells (Fig. 3B).114 Referring to their effects on fungal cells, the positively charged guanylated (Gu+) CDs linked to amphotericin B (AmB) could enhance the antifungal performance in an aqueous solution. These CDs displaying red fluorescence on the membrane-disrupted fungal cells and pretreated human oral epithelia could be conveniently monitored by simply using a fluorescent microscope and effectively preventing the invasion of C. albicans (Fig. 3C).115 Moreover, CDs with intrinsic optical properties have also been considered effective aPDT agents. A recent research work by Cheng's team showed that CDs conjugated with hemin and amino groups in abundance exhibited specific chemiluminescence imaging for identifying the pathogenic cells and enhanced PDT activities for synergetically combating bacterial infections.122 The as-designed hemin-modified CDs (H-CDs) featuring imaging-guided characteristics without causing aggregation-induced quenching could self-supply O2 and enhance the inherent PDT activities. Comprehensively, since hemin exhibits peroxidase-like functions, it plays a pivotal role in certain physiological signaling pathways and can be applied to the theranostic nanosystem in an H2O2-responsive manner. Taken together, the conjugation not only enables tracking of the location infected by the bacterial cells but can also rapidly react to the overly expressed H2O2 in the environment to jointly eliminate the pathogenic cells. Ultimately, CDs, as a novel agent with adjustable fluorescence and highly modifiable surfaces, enable additional conjugation of antimicrobial compounds. Together with the therapies mentioned earlier, they are capable of demonstrating enhanced theranostic ability for further theranostic applications and can offer an effective platform for translating the particles to clinical studies.
3.2. Metal/metal oxide nanoparticles
As mentioned, metal ions as a type of chemotherapy may contribute to the disruption of biofilms and quorum sensing, as well as the production of ROS that can damage bacterial components. Thereby, benefiting from their inherent antimicrobial activities, various metals have been constructed into different forms of nanoparticles to serve as metal reservoirs, enabling the gradual release of metal ions.123 In addition, to assist their role in diagnostic microbiology, the utilization of detecting agents is often necessary. In this following section, we briefly summarize a few commonly seen metal oxides that have been employed to tackle and monitor various types of microbes in the current biomedical fields in hopes of providing a general understanding for designing versatile therapeutic platforms.
Silver and silver oxide nanoparticles (AgNPs) with potent antimicrobial properties are one of the most commonly seen metallic nanomaterials in combating a broad spectrum of microbes, especially against MDR strains.124,125 Like many metal-based nanomaterials, the suppression activities of AgNPs may involve multiple mechanisms. At the membrane level, AgNPs can cause leakage of cellular content by penetrating the microbial membrane and interacting with sulfur-containing proteins in the microbial cell walls. In an intracellular manner, the binding to thiol groups may induce the production of ROS and free radicals, subsequently altering the respiratory chain and activating apoptosis. Despite exhibiting inhibitory effects on pathogenic microbial cells, AgNPs usually face challenges related to their tendency to self-aggregate, considerably limiting their effective potency. As such, supplementary modifications with other materials like chitosan, gelatin and GO are often required to restore and promote the inherent antimicrobial activities of AgNPs while improving their dispersion in aqueous solution. For instance, research work conducted by Ye and colleagues demonstrated that AgNPs with the support of other polymeric compounds (i.e., chitosan and gelatin) could stabilize the metal nanoparticles in the environment for an extended period.126 The polymer-based nanocomposites loaded with AgNPs not only increase the dispersibility of the metal nanoparticles at the infected site but also accelerate the wound healing process. Similarly, the AgNPs synthesized by Peng's team incorporated GO could also reduce their tendency to aggregate.127 The introduction of GO enables AgNPs to maintain their broad-spectrum antimicrobial potency, and the findings indicate that the incorporation could enhance the bactericidal effects against common oral pathogens such as S. mutans, Lactobacillus acidophilus, Aggregatibacter actinomycetemcomitans and C. albicans in comparison to using the AgNPs or GO alone. Furthermore, as theranostic agents, silver nanomaterials alongside other nanocarriers with high biocompatibility may maintain their antimicrobial effects while showing fluorescence due to the quantum confinement effects. In particular, the ultrasmall silver nanoclusters (AgNCs) with both antimicrobial activity and strong luminescence are favorable theranostic candidates for infection control. Previously, a self-assembled nanogel system constructed from chitosan and thiolated AgNCs displays potent luminescence and bactericidal effects for theranostic purposes.128 In fact, when a high concentration of AgNCs is combined with the thiolate ligands presented in the chitosan matrix, the resulting complex can introduce AIE, sequentially enhancing the luminescence and enabling tracking of the bacteria, while the Ag ions deposited on the chitosan can be released to suppress bacterial growth.
Gold nanoparticles (AuNPs), on the other hand, have been widely celebrated for their easy preparation, bioconjugation potential and biological inertness.129–131 Therefore, they have become the ideal candidates for various biomedical applications, including diagnostics, drug delivery and biocatalysis. Nevertheless, in order to utilize the materials for antimicrobial applications, they are usually accompanied by compounds demonstrating potent antimicrobial activities. In a previous study, Chowdhury et al. utilized AuNPs incorporated with AMPs to tackle Salmonella infection (Fig. 4A).132 By serving as a water-based nanoplatform, AuNPs facilitate the delivery of VG16KRKP AMPs without causing any substantial cytotoxicity. Other than increasing the dispersibility of the peptides, this combination can cause membrane damage upon interaction with LPS and lead to ion/metabolic release by forming pores in the bacterial cell membrane, resulting in bacterial cell lysis and death. It is worth noting that while AuNPs have minimal inhibition effects, they are capable of providing great contrast for photoacoustic (PA) imaging. It has been reported that the positively charged Au(I)–disulfide nanoparticles could bind to S. aureus through surface charge interaction and quantify the bacterial cells in a manner that is inversely related to their concentrations.133 In addition, when combined with photo- or chemo-therapeutic agents, they can take advantage of the approaches to generating antimicrobial effects, ultimately establishing a highly versatile platform for killing bacteria through multiple mechanisms.134 For example, the silver-coated gold nanorods have the ability to facilitate the recovery of PA signals by exposing the gold nanorods after the degradation of the Ag layer, simultaneously releasing Ag+ and leading to the killing of MRSA and E. coli.135 A similar rational design can also be found in synthesizing Ag shell-coated Pd-tipped gold nanorods for determining the H2O2 concentration at the inflammatory area and working as antibacterial agents. The etching of the Ag shell and release of Ag+ ions due to H2O2 at the inflammatory tissues could lead to the eradication of bacteria and PA imaging at 1260 and 700 nm.136 Recently, Hajfayjalian et al. reported dextran-coated gold-in-gold cage nanoparticles with the PA imaging effect in vivo, which could be activated by a NIR laser and enable spatial-controlled oral biofilm eradication via PTT.137 Taken together, by incorporating other antimicrobial agents and targeting molecules, AuNPs could serve as promising theranostic platforms for accurately labeling and tackling hard-to-kill pathogenic microbial cells.
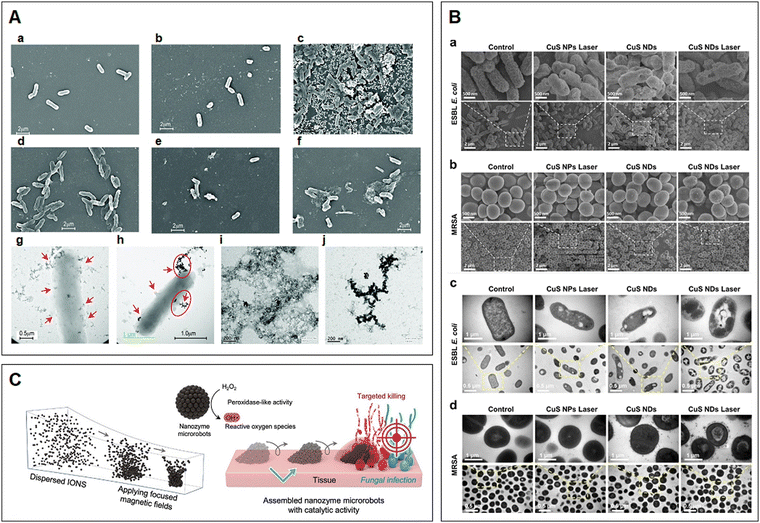 |
| Fig. 4 (A) SEM and TEM images showing the interaction of Au-VG16KRKP with S. Typhi Ty2 cells and LPS: (a) untreated cells, (b) free VG16KRP and (c) AuNPs only. SEM images of S. Typhi Ty2 treated with 1×, 2× and 5× MICs of Au-VG16KRKP for 2 h (d–f, respectively). (g)–(h) TEM images of 2× MIC of Au-VG16KRKP showing clear localization of the particles in the cell membrane, along with membrane disruption and blebbing (red arrows). TEM images of LPS micelles (i) alone and (j) in the presence of Au-VG16KRKP demonstrated clear disruption of the mesh-like arrangement and formation of dense nanoparticle-coated aggregates. Reproduced with permission from ref. 132. Copyright 2017, The Royal Society of Chemistry. (B) Bacteria's inner structure disruption and potential mechanism of the CuS ND PTT antibacterial effect. SEM images of (a) ESBL E. coli and (b) MRSA treated with different materials (45 μg mL−1) upon laser irradiation (2.5 W cm−2, 10 min). TEM images of (c) ESBL E. coli and (d) MRSA treated with different materials (45 μg mL−1) upon laser irradiation (2.5 W cm−2, 10 min). Reprinted with permission from ref. 142. Copyright 2019, American Chemical Society. (C) On-site assembly of individual nanozymes into catalytically active superstructures. The motion dynamics, morphology, and the location of catalysis of the structured assemblies can be controlled creating nanozyme microrobots for targeting fungal infection. Reprinted with permission from ref. 155. Copyright 2023, Wiley-VCH. | |
Copper nanoparticles (CuNPs), thanks to their facile synthesis, stability, thermal resistance and low cost, have been recognized as promising candidates for various biomedical applications, particularly those used to inhibit microbial growth (e.g., E. coli, P. aeruginosa, S. aureus, E. faecalis and Trichophyton rubrum).138–140 They are capable of establishing direct contact with microbes while releasing Cu2+ ions and generating ROS, which consequently resulting in damage to microbial membranes, cell leakage and eventually microbial cell death. Some studies have shown that the employment of copper sulfide (CuS) nanosystems can suppress the growth and eradicate MDR microbes in the infected area.141,142 Meanwhile, CuNPs also exhibit photocatalytic activity that can lead to the inactivation of bacteria. According to Qiao and co-workers, the as-synthesized CuS nanoplatforms as a novel nanomedicine could completely limit bacterial survival through PPT/PDT strategies (Fig. 4B), simultaneously accelerating the wound healing process at the bacteria-infected site when exposed to laser irradiation, proposing a potent antibacterial therapeutic approach to precisely and effectively tackling chronic infectious wounds. 142 When used for detection purposes, CuNPs as fluorescent DNA-templated copper nanoclusters (CuNCs) play a supporting role as they exhibit photocatalytic activities and AIE.143,144 A previous work reported by Qing et al. demonstrated that the resulting CuNCs could be applied to detect the presence of micrococcal nuclease, an enzyme secreted by S. aureus to degrade nucleic acid, therefore successfully identifying the G+ pathogen at the site of infection.145 In a similar manner, the magnetic CuNCs with fluorescence prepared by Zhou's group could also effectively detect the G− pathogen E. coli in a complex environment.146 Collectively, these fluorescent CuNCs as biosensors display promising potential for rapidly detecting a wide range of pathogens with high specificity and sensitivity, and may ultimately serve as a screening tool for in vitro applications.
Among all types of metallic nanoparticles, iron oxides (e.g., hematite, α-Fe2O3) possess magnetic properties, non-toxicity, biocompatibility and biodegradability.147–150 Their functionalities are closely linked to the size, morphology, stability and coating applied, which can be manipulated by applying various synthetic methods. Due to the diverse antimicrobial mechanisms exhibited by nanomaterials and the increasing demand for multipurpose smart platforms in biomedical therapeutic applications, the aforementioned nanoparticles are usually used in conjunction or as composites with one another. In fact, other materials can be granted magnetic and magnetic resonance imaging properties by integrating iron oxide nanoparticles.151,152 Importantly, the deposition of iron oxides onto other nanomaterials can facilitate the construction of microbe modulation agents with dual bactericidal and fungicidal properties. For instance, Ji and co-workers developed a type of glucose oxidase-modified iron oxide to combat persistent endodontic infections. The combined use of the nanomaterials, especially after the employment of a magnetic field, could effectively suppress the activities of E. faecalis and C. albicans, the key root canal-associated pathogens, in both planktonic and biofilm forms.153 Meanwhile, because of their catalytic activities, these magnetic nanoparticles have also been considered as nanoenzymes for various biochemical reactions, and are further utilized for theranostic applications, including immunoassays, biosensing and medical diagnoses.154 The self-assembled enzyme-like structures as micro-robotics with magnetic properties could precisely target and rapidly accumulate in C. albicans to generate ROS and eliminate the fungal cells (Fig. 4C).155 Furthermore, as a detection instrument, the surface of the particles has been modified with microbe-specific molecules to selectively track the desired microbes. Previously, a dual-recognition nanosystem was developed from vancomycin-modified Fe3O4@Au magnetic nanoparticles with surface modification of aptamer-functionalized surface-enhanced Raman scattering tags. This nanosystem is able to detect and quantify S. aureus and E. coli by capturing the microbes and isolating them using a magnetic force.156 By adding other novel phototherapeutic approaches, similar systems could rapidly target, collect and differentiate microbes followed by inactivation via PTT.157
Compared with other metal oxides, titanium dioxide (TiO2), a low-cost, high-abundance material with high chemical stability and biocompatibility, has been extensively studied and commercialized for its photocatalytic activity.158,159 In particular, TiO2 NPs have the ability to absorb ultraviolet (UV) light, making them a highly efficient PS for generating ROS and a PTA for converting laser energy to heat and inducing hyperthermia.160,161 As such, they have been constructed to tackle a wide range of microbes, including E. coli, S. aureus and Ustilago tritici (a common fungus responsible for wheat fungal disease).161,162 Nevertheless, since TiO2 alone may only be capable of absorbing UV photons, some studies have been exploring methods of immobilizing TiO2 NPs onto a suitable matrix and introducing additional transition metal ions to shift their photosensitivity from the UV to the visible region, allowing the design of multifunctional nanoplatforms that can be used for therapeutic purposes. For example, Zhang and colleagues have demonstrated that TiO2 and NIR light irradiation jointly can synergetically eradicate the single-species biofilms of E. coli and S. aureus by involving PTT/PDT and physically killing bacteria both in vitro and in vivo.161 This TiO2 nanoplatform could be highly efficient in orthopedic and dental-related applications for treating bone biofilm infection and promoting bone formation around implants, demonstrating great potential clinical use. Additionally, through the incorporation of other metal ions, the hybrid TiO2 composites can be further modified to expand the range of light exposure, thereby increasing the penetration into the targeted sites.163 Indeed, they have been utilized as a solar light-sensitive layer, providing extra adaptability for various applications. Previously, Marin-Caba et al. developed different forms of sunlight-sensitive TiO2 composites decorated with AuNPs to combat E. coli in both planktonic and biofilm modes under NIR light exposure.160 Importantly, besides exhibiting antibacterial effects through multiple mechanisms, the nanocomposites could trigger photodegradation of Rhodamine B and ROS production, efficiently eliminating the bacterial cells as well as their biofilm.
Zinc nanoparticles (ZnNPs) possess remarkable optical and semiconductive properties, making them highly useful in a variety of products, including plastics, paints and ceramics. They can also serve as effective antimicrobial agents against a broad spectrum of drug-resistant strains, including E. coli, S. aureus, P. aeruginosa, S. epidermidis, A. fumigatus and Candida spp., and have been explored for their potential in antimicrobial, drug delivery and theranostic applications.164–166 Their ion forms (Zn2+), especially in acidic environments, can easily infiltrate the bacterial cell wall and interact with different molecules (e.g., lipids, proteins and nucleic acids), subsequently interfering with critical microbial metabolic pathways and disrupting cellular functions. When ZnNPs are used in combination with other nanoparticles or drugs, they are capable of producing synergetic effects. For example, Punjabi et al. have found that ZnNPs alongside gentamicin have a fractional inhibitory concentration (FIC) value of 0.5 when treated against MRSA, indicating that the co-administration could decrease the toxic concentration and give synergism.166 Likewise, in another study conducted by Aditya and co-workers, ZnNPs dispersed in 1-butyl-3-methylimidazolium chloride (IL2) showed enhanced antibacterial activities against G+ S. epidermidis overall.167 In particular, the IL2 ionic liquids, besides being a dispersion medium, could also impart antimicrobial effects. Together with ZnNPs, they can increase the solubility of the particles in aqueous media and promote stronger interactions between the particles and bacterial cells. Regarding their capability for detection, ZnNPs have been prepared with other nanomaterials to form superparticles. Previously, a type of cobalt-doped ZnNP biomarker has been synthesized using MOFs as a template for selectively identifying the 3-hydroxy-2-butanone (3H-2B) biomarker, a highly toxic microbial volatile organic compound secreted by Listeria monocytogenes.168 The ZnNPs with various cobalt ratios could be used as a platform due to their large surface area that gives extra oxygen vacancies for enhancing gas sensing performance. As a result, these engineered particles could quickly respond to the 3H-2B biomarker of L. monocytogenes with high sensitivity and selectivity while maintaining stability, providing insight into constructing a noninvasive and accurate technique for labeling microbial toxins in the environment.
3.3. Metal–organic frameworks
Metal–organic frameworks (MOFs), highly coordinated porous crystals comprising of metal nodes and organic ligands, have garnered much attention due to their customizable features and diverse biomedical applications, including imaging, sensing and drug delivery.169–171 Currently, more than 90
000 MOFs are being synthesized.172 While their synthetic methods may vary, their antimicrobial activities, as well as monitoring abilities, typically originated from the embedded metals or ligands (e.g., antimicrobials and porphyrins), which may detect microbial cells while facilitating the generation of ROS to inhibit the growth of microbes, including E. coli, S. aureus, MRSA and C. albicans.173–176 As such, they have often been designed into a multifunctional platform by simply adjusting the starting reagents with known properties prior to further surface modifications. For instance, Huang et al. reported a rapid synthesis of Bi-MOFs with aligned crystallinity of CAU-17, demonstrated their potent antimicrobial effect on P. gingivalis and elaborated the action mechanisms including the clearance of H2S and binding with S-, N- and O-containing functional groups in biomacromolecules.176 The reaction between the Bi-MOF and H2S can be utilized to detect H2S-enriched antibiotic-tolerant S. aureus, and the endogenously generated reaction product, Bi2S3, enables PA imaging for in situ and precise theranostic infection control (Fig. 5).177 The MOFs constructed from Zn ion and porphyrin derivatives (Zn–TCPP) have a nanorod shape and display fluorescence in response to the acidic environment at the wound area. Notably, these Zn–TCPP nanorods could initiate fluorescence-imaging-guided PDT inactivation of microbes in a highly efficient way.178
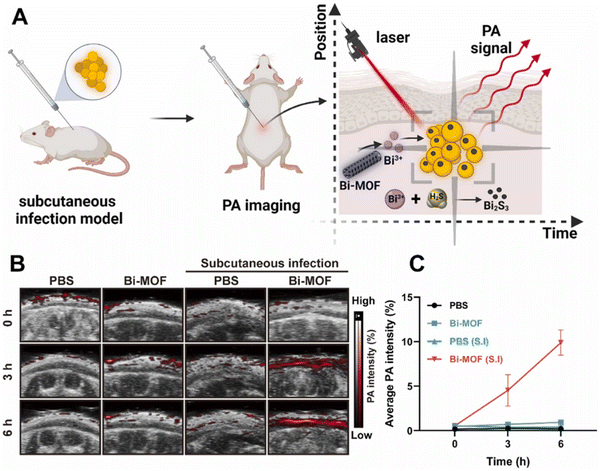 |
| Fig. 5 Bi-MOF realizes real-time in situ diagnoses of infection by laser-triggered photoacoustic (PA) imaging. (A). Schematic illustration of the diagnostic application of the Bi-MOF in a murine subcutaneous infection model. (B) Representative in vivo photoacoustic images. Normal mice or mice with subcutaneous infection were injected with PBS and 200 μg mL−1 Bi-MOF in the subcutaneous cavity. Photoacoustic imaging was monitored at 0, 3, and 6 h postinjection. (C) Quantitative analysis of the average PA intensity in the subcutaneous cavity of the mice in (B). Values are means ± SDs (n = 3). Reprinted with permission from ref. 177. Copyright 2022, Elsevier B.V. | |
Additionally, as MOFs contain cavities, they are commonly utilized in conjunction with other fluorescent dyes, antimicrobial/anti-inflammatory agents, or metal dopants in order to synergistically enhance their theranostic activities. Studies have shown that supplementary dopants or antimicrobials may create multiple pathways to eliminate bacteria and prevent the emergence of MDR pathogens. For example, Prussian blue (PB), with a chemical formula of Fe4[Fe(CN)6]3 clinically approved by the US Food and Drug Administration, has been considered for the synthesis of Fe-based MOFs owing to its remarkable biocompatibility, minimal cytotoxicity and PPT activities.179,180 When the structures were doped with other metal ions (e.g., zinc), the as-synthesized Fe-PB MOFs with cubical morphologies have the ability to slowly release Zn2+, Fe2+ and Fe3+. These cubes can penetrate bacteria and disturb intracellular metabolic pathways, simultaneously enhancing antimicrobial efficiency. As a result, MOFs are usually preferred in wound repair applications caused by bacterial infections. Similarly, PCN-224, a type of Zr-based porphyrin MOF, demonstrates that Zr and porphyrin, together with PB, can produce combined effects.181 According to Luo et al., the metal ions and organic ligands can retain their oxygen conversion abilities even after the formation of the frameworks. Other studies have incorporated peptides labeled with fluorescent dyes to identify and discriminate multiple microbial cells.182 As suggested in the work conducted by Sun and coworkers, the ultrathin two-dimensional (2D) Zn-based MOFs were capable of electrostatically binding to peptides with different properties (e.g., hydrophobicity and charges). Since 2D MOFs can quench fluorescence upon the release of the peptides and produce various fluorescence responses, the collective nanosheets can serve as a suitable nanoplatform for determining microbial pathogens in a reversible manner according to the as-generated fluorescence intensity patterns.
On account of their porous structures, MOFs accommodating imaging or therapeutic agents have been synthesized as coating layers on other nanomaterials, such as NIR-triggered nitric oxide (NO) nanogenerators. Recently, the nanogenerator, designed for treating infection and restoring angiogenesis in diabetic ulcers, contains an upconversion nanoparticle (UCNP) as the core and surface coating of ZIF-8 MOFs loaded with the sodium nitroprusside NO donor to jointly create a core–shell structure. Then, a layer of ROS-responsive polymer is coated on the shell to prevent the premature release of the NO donor, followed by another loading of two fluorescent dyes through π–π stacking. The results indicate that the as-synthesized nanogenerator can increase the HIF-1α level by suppressing its ubiquitin process, detect the infection using fluorescence or PA imaging and kill the bacteria in the wound area via releasing NO and ROS.183 A similar ‘shielding’ design strategy is also used to synthesize ZIF-67-armored ZnO2 nanotheranostic agents, which include ZnO2 as a core coated with the porous ZIF-67 shell and loaded with an organic NIR probe. This multifunctional MOF-armored nanotheranostic can generate both O2 and H2O2 from the core ZnO2, while the Co2+in the MOF layer can catalyze the ROS generation from H2O2via a Fenton-like reaction. The loaded NIR dye as a methylene blue derivative could react with peroxynitrite, which is enriched at the bacterial infection region as a biomarker, and produce methylene blue as a PS for detection and enhanced bactericidal treatment (Fig. 6A).184
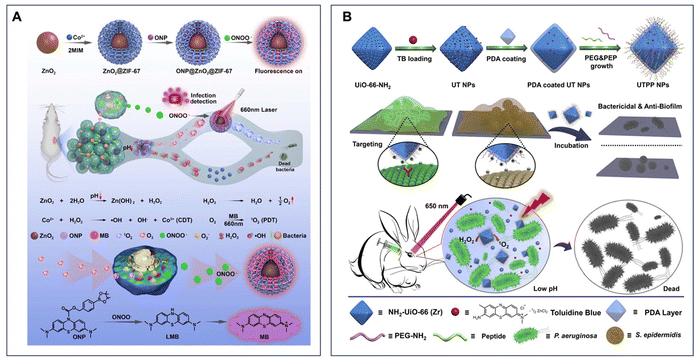 |
| Fig. 6 (A) Preparation of ONP@ZnO2@ZIF-67 and the mechanism of the nanosystem for infection detection and treatment. Reprinted with permission from ref. 184. Copyright 2022, Elsevier B.V. (B) Schematic of the fabrication process of TB@UiO-66-NH2@PEP&PEG NPs for application in integrated theranostics. Reprinted with permission from ref. 186. Copyright 2020, Elsevier B.V. | |
In regard to their employment in theranostic applications, MOFs with the aforementioned features can offer highly sensitive and real-time targeting of the pathogens yet exhibit bactericidal activities. For instance, Fe-based MOFs, such as MIL-100, could serve as nanocarriers to deliver 3-azido-D-alanine (D-AzAla) and nanoenzymes to catalyze the degradation of H2O2. This MOF-based nanosystem could specifically accumulate at the bacteria-infected inflammatory region followed by the H2O2-induced decomposition and release of D-Azala. The alanine was then integrated with the bacteria and added an unnatural azide group to their cell walls. Ultimately, the injected dibenzocyclooctyne-modified PS nanoparticles selectively labeled and eradicated the bacteria via bioorthogonal reaction and PDT.185 Another type of MOF material, UiO-66-NH2, is applied in combination with the LPS-targeting polypeptide and toluidine blue as PS for in situ self-imaging and managing infectious endophthalmitis. The synthesized UiO-66-NH2 nanoparticles featured in a pH-responsive manner can degrade at the acidic bacterial infection region and release Zr ions from the MOF structure and cargo PS for the rapid and synergistic elimination of bacterial infections, while the LPS-targeting polypeptides enhance the interactions of nanoparticles with P. aeruginosa and S. epidermidis and avoid the potential side effects to the retinal pigment epithelial cells (Fig. 6B).186 Collectively, MOFs are a class of highly versatile materials that can be easily tailored by modifying different preparation parameters, resulting in a wide range of theranostic applications.
3.4. Silica/silicon nanoparticles
Silica nanoparticles, as one of the most frequently adopted inorganic nanomaterials in the biomedical field, have displayed many remarkable characteristics, including an easily modifiable surface, tunable pore size and variable morphology. In particular, their porous forms (mesoporous silica nanoparticles, MSNs) with pore sizes ranging from 2–50 nm exhibit considerably large surface areas for accommodating guest molecules. Accordingly, they have been constructed into stimuli-responsive or targeted delivery platforms, making them potential candidates for multipurpose vehicles to deliver various therapeutic agents, such as antibiotics,187 antiseptics188 and photo/thermal-responsive compounds.189 Remarkably, the employment of these nanoparticles allows effective infection control to inhibit the growth of pathogenic microbial cells and their biofilm formation by encapsulating or covalently modifying different antimicrobial agents with limited water solubility and bioavailability. Meanwhile, silica nanoparticles with good biocompatibility can also be applied in diagnosis by incorporating diverse imaging moieties or masking other toxic or non-toxic nanomaterials. For example, a type of MSN doped with phosphorescence imaging ions (Eu3+ and Gd3+) was loaded with curcumin and further demonstrated inhibitory effects on Zika virus in vitro.190 Following that, other luminescent nanodots like α-NaYF4:Yb:Er, ZnGa2O4:Cr3+ (Fig. 7A) and SrAl2O4:Eu2+ (Fig. 7B) can be coated by MSNs via the core–shell structure or in situ deposited on MSNs for imaging purposes.191–193
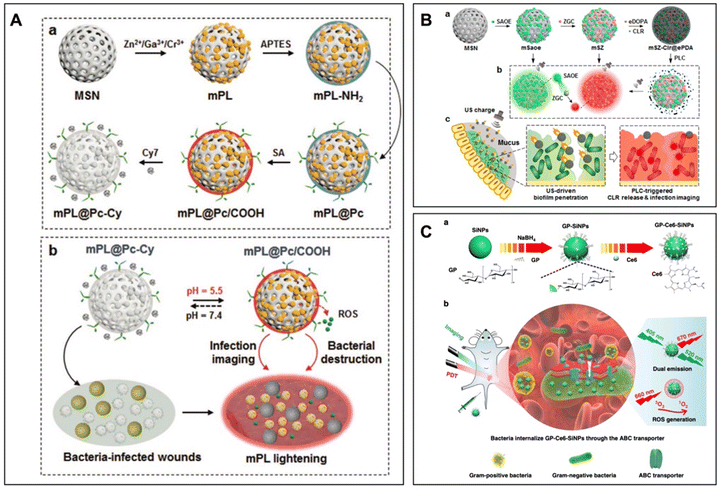 |
| Fig. 7 (A) Schematic illustration of the fabrication process and working principle of mPL@Pc-Cy NPs. (a) ZGC NDs are in situ grown on MSNs and amino groups are introduced on mPL NPs, followed by surface grafting of Si-Pc. Amino groups are reacted with succinic anhydride to obtain mPL@Pc/COOH, and Cy7 is electrostatically assembled to prepare mPL@Pc-Cy NPs. (b) In response to the acidic microenvironment, fluorescence emissions of NPs are restored for monitoring bacterial infection. In the meantime, the afterglow emission excites Si-Pc to persistently generate ROS for bacterial destruction. Reprinted with permission from ref. 192. Copyright 2022, Wiley-VCH. (B) Preparation process and theranostic mechanism of mSZ-Clr@ePDA NPs. (a) SAOE and ZGC NDs are in situ deposited on MSNs to prepare mSZ NPs, followed by CLR adsorption and surface coating with ePDA layers to obtain mSZ-Clr@ePDA NPs. (b) US activation of mSaoe NPs generates green luminescence, which excites ZGC NDs to produce red emissions from mSZ NPs. ePDA coatings of mSZ-Clr@ePDA NPs quench the emissions, and PLC-triggered breakdown of ePDA layers quickly restores the emission from mSZ NPs. (c) After oral administration of mSZ-Clr@ePDA NPs, US drives NP penetration across mucus and biofilm barriers. PLC triggers the local release of CLR to destruct bacteria and restore mSZ emission for precise imaging of H. pylori infection. Reprinted with permission from ref. 193. Copyright 2022, American Chemical Society. (C) Design of multifunctional nanoagents for detection and photodynamic treatment of bacterial infections. (a) Synthetic route of nanoagents of GP-Ce6-SiNPs. GP-Ce6-SiNPs are composed of SiNPs conjugated to GP and loaded with Ce6 molecules. (b) Imaging and treatment of Gram-negative and Gram-positive bacterial infections by GP-Ce6-SiNPs. GP-Ce6-SiNPs are robustly internalized by ABC transporters, which are only present in bacterial cells but not in mammalian cells. Under 405 nm excitation, GP-Ce6-SiNPs exhibit two typical emission maximum peaks at 520 (SiNPs) and 670 nm (Ce6). Under 660 nm irradiation, ROS of 1O2 is produced from Ce6, triggering PDT against bacteria. Reprinted with permission from ref. 198. Copyright 2019, Springer Nature. | |
Because of their easily modifiable surface, silica nanoparticles are commonly utilized as stimuli-responsive nanosystems. For instance, a smart bandage constructed by Chen et al. showed that the luminescent porous silica particles could respond to ROS and pH changes in the wound area. Specifically, the emission of the luminescent particles was consequently altered via the Förster resonance energy transfer (FRET) effect, while the antibiotics loaded as the cargo could be released to eradicate the local bacteria.194 Furthermore, silica nanoparticle-based systems may be capable of directly responding to the bacteria per se. Zhao and co-workers developed bacteria-activated theranostic nanoprobes by directly coating the vancomycin-modified polyelectrolyte–cypate complexes on the silica nanoparticles. This coating could be dissociated and then drawn to the bacteria, subsequently turning the cypate from an ‘off’ to an ‘on’ state.195 Ultimately, the resultant bacteria-induced ‘turn-on’ NIR fluorescence, together with the photothermal elimination effect, suggests the versatile roles of silica nanoparticles applied as theranostic nanomedicines for infection control.
Apart from the silica nanoparticles, silicon-based nanomaterials have also received much focus because of their intriguing physicochemical properties, including high luminescence and photostability. Given the different cell wall structures of the G− and G+ bacteria, the modification of silicon nanoparticles (SiNPs) with particular antibiotics is able to facilitate the accumulation of nanoparticles in the specific pathogens and achieve selective targeting and inhibition. For instance, SiNPs obtained by reducing (3-aminopropyl)trimethoxysilane using 1,8-naphthalimide could be applied as fluorescent probes followed by conjugation with vancomycin for selective accumulation in G+ S. aureus and inhibit its growth.196,197 Alternatively, the modification of the glucose polymer and the loading of red-emissive chlorin e6 on the green fluorescent silicon nanoparticles can facilitate the rapid internalization of nanomaterials by both G− and G+ bacteria using a mechanism dependent on an ATP-binding cassette transporter pathway. Eventually, the resultant nanoagents with dual emission enable the in vivo detection of bacteria at a low concentration (i.e., 105 colony-forming units) and PDT-based antibacterial treatment with efficiencies around 98% against S. aureus and P. aeruginosa (Fig. 7C).198
In summary, these luminescent nanoparticles loaded/grafted with photosensitizers or antibiotics followed by the stimuli-responsive polymer coating can further function as turn-on probes to efficiently eradicate bacteria for theranostic treatment.
3.5. AIE-based nanosystems
Recently, aggregation-induced emission (AIE) has received much attention for its employment in theranostic applications. In fact, AIE-active luminogens (AIEgens) exhibit many attractive characteristics compared with the traditional fluorophores/luminogens, such as strong fluorescence, a large Stokes shift, good photostability and enhanced ROS generation in the aggregate state. Although these properties make AIEgens an ideal candidate for infection control through PDT, the hydrophobicity of AIEgens may restrict their utilization in biomedical areas. Therefore, the AIEgens can be constructed into the nanosystems with improved bioavailability for effective imaging-guided treatments.199
In general, AIEgens can be encapsulated and delivered by inorganic or organic particles, as well as MOFs.185,200–203 Meanwhile, structure modifications of AIEgens, like the conjugation with hydrophilic groups or polymers, enable the formation of the polymeric AIE-active nanodots/nanocomposites via self-assembly.204–206 Notably, some of these nanosystems demonstrating specific selectivity to fungal cells can also target the infections caused by particular microbe types. As G− and G+ bacteria have different cell wall structures, the AIEgens functionalized with either positively charged quaternary ammonium structures or hydrophobic interactive morpholine moieties can easily bind to the thick peptidoglycan carrying a negative charge at the outer cell wall. Collectively, they can achieve fluorescence ‘turn-on’ detection and the resultant selective antimicrobial effects on the G+ bacteria, such as B. subtilis, S. aureus and its antibiotic-resistant strain, MRSA.206–208 Hu and colleagues reported an AIEgen named DPNAP with a hydroxyl-modified salicylaldehyde Schiff-base structure, which could selectively bind with G+ bacteria and fungi and only enlighten fungi cells, resulting in the efficient PDT-based inhibitory effects on fungi, such as C. albicans and S. cerevisiae.209
Meanwhile, AIE-based treatments are usually applied in the combination of PDT with other approaches, including PTT,210 chemo-dynamic therapy,211,212 and nitric oxide213 for the synergetic therapeutic outcomes in the infection control treatments. Additionally, AIEgen-modified polymers can deliver antibiotics to eliminate the intracellular bacteria, and track the drug release via the FRET effect.214
3.6. Polymeric, biomacromolecule and lipid-based nanoparticles
Polymers, including both natural and synthetic ones, possess several exceptional features in chemical, physical, mechanical and structural aspects. Besides being able to be self-assembled into nanomaterials, they can actively contribute as an additive component after being further modified on other nanomaterials, making them great carriers for biomedical applications. Notably, the polymeric nanoparticles as nanotheranostics have been fabricated into biodegradable and stimuli-responsive platforms to deliver small molecules or even biomacromolecules (e.g., proteins, peptides and nucleic acids) for favorably targeting pathogenic microbes as well as their biofilms.215
In a typical manner, polymeric nanoparticles could be endowed with antimicrobial effects through three approaches: (1) synthesizing from antimicrobial cationic/anionic polymers, (2) encapsulating antimicrobial agents (e.g., antibiotics, AMPs and metal/metal oxide nanoparticles) or (3) incorporating sensitizers for generating ROS or heat. Subsequently, by combining different approaches together, they, as one nanosystem, may have synergistic effects on eradicating microbes and their biofilms. For instance, a pH-sensitive PPEG-MA-b-P(DPA-co-HEMA)-Ce6 nanotheranostic system could shift its overall surface potential from negative to highly positive (+11.6 mV) at pH 6.0, enabling effective binding and labeling of the bacteria with red fluorescence. Importantly, the nanoparticles could inhibit the growth of E. coli and S. aureus with the help of light irradiation at 660 nm through combined cationic chemo- and photodynamic therapies (Fig. 8A).216
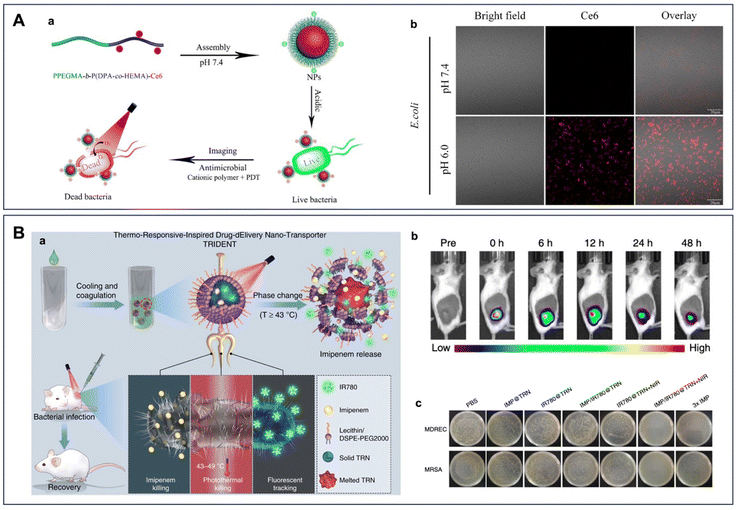 |
| Fig. 8 (A) (a) Schematic illustration of the PPEGMA-b-P(DPA-co-HEMA)-Ce6 NPs as theranostic antimicrobial agents. (b) Fluorescence confocal microscopy of E. coli after incubation with the PPEGMA-b-P(DPA-co-HEMA)-Ce6 NPs (0.1 mg mL−1) under different pH conditions for 3 h. For each panel, the images from left to right show the bright field of bacteria, Ce6 fluorescence (red), and the overlay of two images. Scale bar is 20 μm. Reprinted with permission from ref. 216. Copyright 2018, American Chemical Society. (B) (a) Near infrared (NIR)-activated TRIDENT for antibiotic-resistant bacteria killing. (b) Fluorescence images showing the retention of TRIDENTs in the infected skin after the local injection. The high fluorescence intensity lasts for a long time at the infected site. (c) Photographs of plated bacterial colonies obtained from infected skin tissues of mice in the seven treatment groups. Reprinted with permission from ref. 235. Copyright 2019, Springer Nature. | |
As microbes are negatively charged, polymers carrying a positive charge may electrostatically attract microbial cells and easily accumulate in the microbe-rich area. Based on this concept, polymeric nanoparticles can be either synthesized from positively charged polymers, such as ε-poly-L-lysine217 and polycaprolactone,218 or constructed with switchable surface coatings upon the stimulation of certain conditions, including acidic, ROS or specific enzyme-rich microenvironments.216,219–221 Precisely, the conversion of polymer surface charge at different pH values allows the nanoparticles to bind with the microbes in a designated region or gather in the biofilms for more efficient therapeutic effects. For instance, the polyaniline and glycol chitosan grafted nanoparticles exhibiting persistent luminescence could quickly respond to the bacterial-infected acid area by switching their surface charge to positive, followed by binding the bacteria for eradication.219 In regard to designing polymeric nanoparticles with stimuli-responsive features, the surface charge can be altered in a pH-dependent manner for cleaving caging groups220 or by introducing enzymes to induce degradation of a negatively charged coating layer to expose the positively charged function groups lying underneath the polymer.221 In addition, like other nanomaterials, the polymeric nanoparticles modified with vancomycin could also increase their pathogen-targeting effects and generate synergistic effects with phototherapy both in vitro and in vivo.222
Referring to biomacromolecules, they can either be incorporated with other nanomaterials as hybrid nanotheranostics or form nanostructures per se. In previous sections, aptamers as detection probes in nanotheranostic applications have been elaborated on, from the working mechanism to the practical utilization of modification on various nanomaterials. In addition to aptamers, DNA nanostructures have also obtained increasing attention because of their utilization in the biomedical field. However, it is worth noting that DNA nanostructures are more frequently employed as theranostic agents in anti-cancer studies. In fact, when DNA nanostructures are modified with aptamers, they can also deliver PS or anticancer drugs such as doxorubicin for the targeted tumor growth inhibition.223 For their applications in controlling infections, there are fewer relevant studies than the anti-cancer ones. Nevertheless, some proof-of-concept works have demonstrated the potential of DNA nanostructures/nanoparticles in this area. For instance, DNA nanostructures could incorporate with antisense peptide nucleic acids against specific genes to interfere with the replication of MRSA224 or absorb AMPs via electrostatic interactions and enhance the antimicrobial effects of AMP by preventing the degradation of the peptides in a protease-rich environment.225 Furthermore, DNA could be constructed into the groove-rich nanoparticles with increased surface area to load the antibiotic named netropsin for sustained and controlled release, providing a new form of DNA nanoparticles for infection control.226 Other research work combines the DNA tetrahedron containing a hairpin structure with a PET pair for a fluorescence switchable biosensor to detect a protein and siRNA complex (Ago2/miR-21 complex).227 Although there has been no report of a DNA nanostructure-based theranostic platform for controlling infections so far, current findings have suggested the possibility of their future use in this area.
The lipid-based nanomaterials, including liposomes and solid lipid nanoparticles, have excellent biocompatibility, which could directly fuse with the microbes for a highly efficient pathogen eradication or integrate with the host cell membrane to deliver therapeutic agents for combating the intracellular microbes while reducing the inflammatory responses.228,229 Previously, liposomes containing calcofluor white and voriconazole have demonstrated superior efficiency for drug-delivering applications of the nanomaterial in vitro. This nanomaterial, which specifically targets fungi, could be utilized for treating animal models infected with C. albicans and prolonging the drug release time, making them promising therapeutic options for the management of fungal infections.230 When being utilized as radiolabeled nanotheranostics to deliver various antimicrobial agents, they are available for positron emission tomography imaging by introducing the radioactive isotope ions, such as 64Cu2+, 99mTc and 89Zr.231–233 Given the convenient incorporation of various agents into the structures, the lipid nanoparticles/nanoliposomes can be functionalize maltohexaose234 or folic acid229 for better targeting of bacteria or bacteria-infected macrophages. Notably, the construction of lauric acid and stearic acid-based thermo-responsive nanostructures with IR780 and imipenem loading and lecithin/DSPE-PEG2000 coatings could bring novel theranostic platforms with irradiation-induced phase change, leading to imipenem killing, photothermal eradication and fluorescence tracking of the MDR bacteria (Fig. 8B).235
4. From lab to clinic
As discussed in previous sections, compared to conventional methods, multifunctional nano-based systems with detectable and antimicrobial features offer a promising approach to combating hard-to-kill microbes and preventing disease progression at an early stage, providing new insights into the development of various theranostic applications. Additionally, these nanoplatforms can overcome the disabilities of the original drug (e.g., poor water solubility) and synergetically enhance the efficiency of the overall treatments with reduced cytotoxicity in animal studies. At present, only a limited class of nanomaterials have advanced to clinical trials, including liposomes, polymeric nanoparticles, iron oxides, AgNPs and TiO2 NPs, and even fewer of them have successfully become available in the market for commercial use.236–241 A list of current NPs for pathogen-related clinical studies conducted in the last 10 years is summarized in Table 1. Among all documented nanomaterials, lipid-based nanoparticles are the most promising candidates for safe clinical use. Recently, Badawi and colleagues conducted a clinical study by loading metronidazole (MTZ) with solid lipid nanoparticles (SLNs) as MTZ-SLNs to manage bacterial vaginosis (BV) and its recurrence.242 The MTZ-SLNs were applied to sixty female patients who have been diagnosed with BV compared to the marketed vaginal gel Metron®, suggesting their feasibility as an alternative to traditional treatments. Meanwhile, SLNs also facilitate the delivery of fluconazole to treat Malassezia species-induced pityriasis versicolor, and the fluconazole-loaded SLN topical gel displayed enhanced therapeutic outcomes with the reference of market-available Canistan® cream in a randomized controlled clinical trial.243 Similarly, an inhaled liposomal ciprofloxacin (ARD-3150) was administrated to individuals with bronchiectasis and chronic P. aeruginosa infection over a period of 48 weeks.244,245 The phase 3 trials demonstrated that a daily dose of ARD-3150 exhibiting similar safety and tolerability profiles to the placebo has significant antibacterial effects against the G− pathogen in the airway, thereby reducing the bacterial load and alleviating the respiratory symptoms in patients with lung infection.
Table 1 Nanoparticle-based therapeutics conducted clinically for managing microbes in the last 7 years
Types of nanoparticles |
Target pathogens |
Study type |
Clinical trial number |
AgNPs |
Oral pathogens |
Interventional |
NCT02761525 |
AgNPs |
Multidrug-resistant bacteria (MRSA and vancomycin-resistant S. aureus) |
Observational |
NCT04431440 |
PLGA NPs with ciprofloxacin |
E. faecalis
|
Interventional |
NCT05475444 |
As mentioned, nanoparticles have also emerged as a promising tool in the field of bacterial detection, offering high sensitivity, specificity, and rapidity. Specifically, the use of nanoparticles in detecting clinically isolated bacteria has gained significant attention due to their potential applications in translational studies. By utilizing the unique properties of nanoparticles, such as their large surface area-to-volume ratio and surface plasmon resonance, researchers have developed various nanoparticle-based assays that can precisely identify and quantify specific bacterial strains. The NIR fluorescent nanosensors, constructed from tailored SWCNTs, were able to remotely fingerprint bacteria by detecting the metabolites and specific virulence factors. After integrating nine different nanosensors into the hydrogel array, this array could identify and distinguish six clinically isolated bacteria by generating different NIR images.246 At the same time, a colorimetric nanodiagnostic platform has been developed using a multicomponent nucleic acid enzyme (MNAzyme)–gold nanoparticle that provides a rapid, sensitive and efficient approach to identifying clinical pathogens and their antimicrobial-resistant strains. In particular, different clinical samples were collected for DNA extraction and isothermal amplification followed by the MNAzyme–gold nanoparticle readout. The amplified targeted DNA led to the cleavage of linker DNA by MNAzyme, which in turn released the monodispersed gold nanoparticles for colorimetric detection.247 For fungal detection, the modified carboxylated iron oxide superparamagnetic particles with the conjugation of aminated DNA oligonucleotides can function as a T2 contrast agent in T2 magnetic resonance for detecting fungal cells in whole blood. The developed nanodiagnostic approach significantly simplified the detection of clinically isolated Candida species by shortening the detecting time and increasing the precision to 1 CFU mL−1.248,249 These advancements in nanoparticle-based detection systems have the potential to revolutionize bacterial diagnosis and treatment, leading to improved patient outcomes and reduced healthcare costs.
Despite showing great promise in nano-based theranostic approaches for infection control, there is still significant room for improvement in translating current research findings into clinical applications. In fact, while significant advancements in generating new nanomaterials with antibacterial activity have been made to revolutionize the drug delivery systems and contribute to optimizing the original drug properties, only a few nanomaterials have proceeded to clinical trials and entered the market due to the challenges associated with (1) biocompatibility and toxicity of NPs, (2) reduced antimicrobial performance after administration and (3) the lack of standardized clinical protocols. Furthermore, the majority of nanoparticles/nanosystems tested in clinical trials have been mainly designed for bacterial detection or eradication, while fungi-targeted nanotherapeutics or nanodiagnostics are relatively rare. Additionally, it is worth noting that current nanoparticle-related clinical trials are focusing on targeting either therapy or diagnosis of microbial infections. Yet, none of the known nanotheranostic systems has been translated into clinical trials, despite some of them showing superior effects on managing microbial infections both in vitro and in vivo. As such, more actions should be taken to promote the clinical translation of promising nanotheranostic systems for future clinical applications.
5. Summary and perspectives
To date, infections caused by bacteria and fungi have become huge healthcare and socioeconomic burdens on a global scale. Fortunately, thanks to the rapid development of pharmaceutical engineering, nanotechnology and biotechnology, novel drugs and therapeutic approaches are emerging to tackle the problems in the infection control process. Novel nanomaterials have served as potential tools for giving alternative options to overcome the shortcomings of the conventional therapies. Importantly, these newly developed nanosystems can provide additional tailoring of the material with some of them carrying inherited antimicrobial effects. Although some promising therapeutic effects have been obtained in the pre-clinical studies, there is still a gap between basic research and clinical translation.
As research in microbial etiology advances, various factors and aspects involved in the pathogenesis of different diseases reveal sophisticated interaction profiles of microflora and host. Apart from the most frequently mentioned challenges posed by AMR, recurrent or persistent infections also require great attention and innovative theranostic treatments. As the most recalcitrant cases may be predominantly associated with the resistance, tolerance and persistence of microbes along with compromised host immune responses, multimodality therapy should be considered for efficient pathogen elimination and host immuno-modulation simultaneously. In addition, the microbial symbiosis at specific body niches (e.g., skin, oral cavity, gastrointestinal tract, and genitourinary tract) plays an essential role in maintaining the well-being and health status of the host, while the dysbiosis commonly accompanied by inflammation contributes to the onsets of various diseases. Notably, the shifting of microbial symbiosis to dysbiosis is usually attributed to the proliferation of one or several opportunistic pathogens. However, current treatments (i.e., antibiotics and ROS generation) lacking specificity towards microbial species may result in the disruption of microbiota and overgrowth of resistant/tolerant pathogens. Meanwhile, the detection and imaging of pathogens at genus and species levels can greatly enhance the precise management and early diagnosis of infections. Therefore, we suggest focusing on the following aspects for refinement and optimization in future studies:
(1) Nanotheranostic systems with dual microbe eradication and inflammation modulation effects.
As infections usually happen together with either acute or chronic inflammation of the host, reducing the concentration of pro-inflammatory cytokines and the number of microbes loaded locally or systemically can remarkably alleviate infection symptoms and ensure speedy recovery of patients.
(2) Pathogen genus/species-specific nanotheranostic platforms for precisely eradicating noxious microbes and restoring microbial symbiosis.
In view of the pivotal role of microbial symbiosis in maintaining human health, it is crucial to accurately detect and track the relative abundance changes for certain opportunistic pathogens to enable the precise regulation of microflora imbalance at various body niches.
(3) Stimuli-responsive nanotheranostic systems as ‘smart’ agents for enhancing/maintaining the overall antimicrobial treatment upon contacting microbes and reducing the adverse side effects of the host.
Since nanomaterials have been widely celebrated as promising options for constructing stimuli-responsive delivery platforms, with appropriate synthesis and modification, they can work as microbial-triggered systems in response to pathogen-specific biological molecules.
(4) Most importantly, ensuring biocompatibility and safety should be the first priority when designing and synthesizing nanotheranostic systems.
In order to achieve this, the constructing material components should be carefully selected from biocompatible elements, polymers, natural antimicrobial reagents or compounds with minimal side effects. As a result, the nanosystems can work in a synergetic manner to eradicate pathogens for a more promising outcome.
(5) As for diagnostic applications, further research is needed to make significant contributes to the field.
By functionalizing a more specific targeting nanosystem using aptamers or antibodies, the accuracy of diagnosis can be increased. Additionally, extensive development of ‘turn-on’ fluorescence/luminescence imaging by the nanosystems can greatly simplify the detection process for nanotheranostics.
Taken together, nanotechnology in combination with newly developed biotechnology exhibits great potential in the management and diagnosis of bacterial and fungal infections. Importantly, it could significantly benefit the translation to clinical applications by designing nanotheranostic agents with high biocompatibility, therapeutic efficacy and selectivity to pathogens while exhibiting a low detection limit and reduced side effects concurrently. In conclusion, current studies in basic sciences involving microbiology, immunology, pharmaceutical engineering and bio-nanomaterials can strongly support the development of novel nanotheranostic agents and vigorously promote their clinical translations.
Abbreviations
2D | Two-dimensional |
3H-2B | 3-Hydroxy-2-butanone |
AgNCs | Silver nanoclusters |
AgNPs | Silver and silver oxide nanoparticles |
AIE | Aggregation-induced emission |
AIEgens | AIE-active luminogens |
AmB | Amphotericin B |
AMPs | Antimicrobial peptides |
AMR | Antimicrobial resistance |
ARD-3150 | Inhaled liposomal ciprofloxacin |
AuNPs | Gold nanoparticles |
BV | Bacterial vaginosis |
C60NPs | C60 nanoparticles |
CD-Gu+-AmB | Guanylated carbon dots functionalized with amphotericin B |
CDs | Carbon dots |
CNTs | Carbon nanotubes |
CuNCs | Copper nanoclusters |
CuNPs | Copper nanoparticles |
D-AzAla | 3-Azido-D-alanine |
DNA | Deoxyribonucleic acid |
FIC | Fractional inhibitory concentration |
FRET | Förster resonance energy transfer |
G− | Gram-negative |
G+ | Gram-positive |
GAAP | Graphene and silver nanocomposites with antimicrobial peptide functionalization |
GO | Graphene oxide |
Gu+ | Positively charged guanylated groups |
H-CDs | Hemin-modified CDs |
IL2 | 1-Butyl-3-methylimidazolium chloride |
La@GO | Graphene oxide nanocomposites with lanthanum hydroxide |
LPS | Lipopolysaccharides |
MDR | Multidrug-resistant |
MOFs | Metal–organic frameworks |
mSiO2 | Mesoporous silica |
MSNs | Mesoporous silica nanoparticles |
MTZ | Metronidazole |
MWCNTs | Multi-walled carbon nanotubes |
NIR | Near-infrared |
PA | Photoacoustic |
PAMAM | Poly(amidoamine) |
PB | Prussian blue |
PDT | Photodynamic therapy |
PIN | Primer-incorporated network |
PLGA | Poly(lactic-co-glycolic acid) |
PRRs | Pattern recognition receptors |
PS | Photosensitizers |
PTA | Photothermal agents |
PTT | Photothermal therapy |
qPCR | Real-time polymerase chain reaction |
ROS | Reactive oxygen species |
SDT | Sonodynamic therapy |
SELEX | Systematic evolution of ligands by exponential enrichment |
SiNPs | Silicon nanoparticles |
SLNs | Solid lipid nanoparticles |
SS | Sonosensitizers |
SWCNTs | Single-walled carbon nanotubes |
TLRs | Toll-like receptors |
TPU | Thermoplastic polyurethane |
UCNP | Upconversion nanoparticle |
UV | Ultraviolet |
Van | Vancomycin |
ZnNPs | Zinc nanoparticles |
Conflicts of interest
There are no conflicts to declare.
Acknowledgements
This work was supported by the Health and Medical Research Fund from Hong Kong Food and Health Bureau [20190682] to X. Li, as well as Hong Kong Baptist University [RC-KRPS-20-21/02, SKLP_2223_P02, and RMGS-2022-13-07], the Guangdong Province Zhu Jiang Talents Plan [2016ZT06C090] and the Guangzhou City Talents Plan [CYLJTD-201609] to K. C. F. Leung.
References
- S. Fraune, R. Augustin, F. Anton-Erxleben, J. Wittlieb, C. Gelhaus, V. B. Klimovich, M. P. Samoilovich and T. C. Bosch, In an early branching metazoan, bacterial colonization of the embryo is controlled by maternal antimicrobial peptides, Proc. Natl. Acad. Sci. U. S. A., 2010, 107, 18067–18072 CrossRef CAS.
- J. Libertucci and V. B. Young, The role of the microbiota in infectious diseases, Nat. Microbiol., 2019, 4, 35–45 CrossRef CAS.
- G. B. D. A. R. Collaborators, Global mortality associated with 33 bacterial pathogens in 2019: a systematic analysis for the Global Burden of Disease Study 2019, Lancet, 2022, 400, 2221–2248 CrossRef PubMed.
- J. T. Loh and K.-P. Lam, Fungal infections: immune defense, immunotherapies and vaccines, Adv. Drug Delivery Rev., 2023, 196, 114775 CrossRef CAS PubMed.
- F. Bongomin, S. Gago, R. O. Oladele and D. W. Denning, Global and multi-national prevalence of fungal diseases—estimate precision, J. Fungi, 2017, 3, 57 CrossRef.
- J.-P. Gangneux, Fungal infections in mechanically ventilated patients with COVID-19 during the first wave: the French multicentre MYCOVID study, Lancet Respir. Med., 2022, 10, 180–190 CrossRef CAS.
- V. J. Morley, R. J. Woods and A. F. Read, Bystander selection for antimicrobial resistance: implications for patient health, Trends Microbiol., 2019, 27, 864–877 CrossRef CAS PubMed.
- U. Theuretzbacher, K. Outterson, A. Engel and A. Karlen, The global preclinical antibacterial pipeline, Nat. Rev. Microbiol., 2020, 18, 275–285 CrossRef PubMed.
- G. D. Wright, Antibiotic adjuvants: rescuing antibiotics from resistance, Trends Microbiol., 2016, 24, 862–871 CrossRef CAS.
- H. Douafer, V. Andrieu, O. Phanstiel IV and J. M. Brunel, Antibiotic adjuvants: make antibiotics great again!, J. Med. Chem., 2019, 62, 8665–8681 CrossRef CAS.
- S. Jeelani, R. J. Reddy, T. Maheswaran, G. Asokan, A. Dany and B. Anand, Theranostics: a treasured tailor for tomorrow, J. Pharm. BioAllied Sci., 2014, 6, S6 CrossRef CAS.
- H. Liu, W. Zhong, X. Zhang, D. Lin and J. Wu, Nanomedicine as a promising strategy for the theranostics of infectious diseases, J. Mater. Chem. B, 2021, 9, 7878–7908 RSC.
- C. T. Perciani, L. Y. Liu, L. Wood and S. A. MacParland, Enhancing immunity with nanomedicine: employing nanoparticles to harness the immune system, ACS Nano, 2020, 15, 7–20 CrossRef PubMed.
- L. Jiang, L. Ding and G. Liu, Nanoparticle formulations for therapeutic delivery, pathogen imaging and theranostic applications in bacterial infections, Theranostics, 2023, 13, 1545 CrossRef CAS PubMed.
-
P. Davey, M. H. Wilcox, W. Irving and G. Thwaites, Antimicrobial Chemotherapy, Oxford University Press, USA, 2015 Search PubMed.
- R. Gyawali and S. A. Ibrahim, Natural products as antimicrobial agents, Food Control, 2014, 46, 412–429 CrossRef CAS.
- F. Jamshaid, J. Dai and L. X. Yang, New development of novel berberine derivatives against bacteria, Mini-Rev. Med. Chem., 2020, 20, 716–724 CrossRef CAS.
- A. Frei, A. D. Verderosa, A. G. Elliott, J. Zuegg and M. A. Blaskovich, Metals to combat antimicrobial resistance, Nat. Rev. Chem., 2023, 7, 202–224 CrossRef CAS PubMed.
- M. P. Dore, H. Lu and D. Y. Graham, Role of bismuth in improving Helicobacter pylori eradication with triple therapy, Gut, 2016, 65, 870–878 CrossRef CAS.
- T. F. Cheng, Y. T. Lai, C. Wang, Y. Wang, N. Jiang, H. Li, H. Z. Sun and L. J. Jin, Bismuth drugs tackle Porphyromonas gingivalis and attune cytokine response in human cells, Metallomics, 2019, 11, 1207–1218 CrossRef CAS.
- C. Wang, X. Li, T. Cheng, H. Sun and L. Jin, Eradication of Porphyromonas gingivalis persisters through colloidal bismuth subcitrate synergetically combined with metronidazole, Front. Microbiol., 2021, 12, 748121 CrossRef.
- S. Yuan, R. Wang, J. F.-W. Chan, A. J. Zhang, T. Cheng, K. K.-H. Chik, Z.-W. Ye, S. Wang, A. C.-Y. Lee and L. Jin, Metallodrug ranitidine bismuth citrate suppresses SARS-CoV-2 replication and relieves virus-associated pneumonia in Syrian hamsters, Nat. Microbiol., 2020, 5, 1439–1448 CrossRef CAS PubMed.
- S. P. Songca and Y. Adjei, Applications of antimicrobial photodynamic therapy against bacterial biofilms, Int. J. Mol. Sci., 2022, 23, 3209 CrossRef CAS PubMed.
- G. Jaber, R. Dariush, A. Shahin, T. Alireza and B. Abbas, Photosensitizers in antibacterial photodynamic therapy: an overview, Laser Ther., 2018, 27, 293–302 CrossRef.
- M. Wysocki, B. Czarczynska-Goslinska, D. Ziental, M. Michalak, E. Güzel and L. Sobotta, Excited state and reactive oxygen species against cancer and pathogens: a review on sonodynamic and sono-photodynamic therapy, ChemMedChem, 2022, 17, e202200185 CrossRef CAS.
- G. M. Calixto, J. Bernegossi, L. M. De Freitas, C. R. Fontana and M. Chorilli, Nanotechnology-based drug delivery systems for photodynamic therapy of cancer: a review, Molecules, 2016, 21, 342 CrossRef PubMed.
- E. Polat and K. Kang, Natural photosensitizers in antimicrobial photodynamic therapy, Biomedicines, 2021, 9, 584 CrossRef CAS PubMed.
- N. Kashef, G. Ravaei Sharif Abadi and G. E. Djavid, Photodynamic inactivation of primary human fibroblasts by methylene blue and toluidine blue O, Photodiagn. Photodyn. Ther., 2012, 9, 355–358 CrossRef CAS PubMed.
- T. Maisch, C. Bosl, R. M. Szeimies, N. Lehn and C. Abels, Photodynamic effects of novel xf porphyrin derivatives on prokaryotic and eukaryotic cells, Antimicrob. Agents Chemother., 2005, 49, 1542–1552 CrossRef CAS PubMed.
- M. Grinholc, B. Szramka, J. Kurlenda, A. Graczyk and K. P. Bielawski, Bactericidal effect of photodynamic inactivation against methicillin-resistant and methicillin-susceptible Staphylococcus aureus is strain-dependent, J. Photochem. Photobiol., B, 2008, 90, 57–63 CrossRef CAS.
- Q. Xu, W. Xiu, Q. Li, Y. Zhang, X. Li, M. Ding, D. Yang, Y. Mou and H. Dong, Emerging nanosonosensitizers augment sonodynamic-mediated antimicrobial therapies, Mater. Today Bio, 2023, 19, 100559 CrossRef CAS.
- M. Zupanc, Ž. Pandur, T. S. Perdih, D. Stopar, M. Petkovšek and M. Dular, Effects of cavitation on different microorganisms: the current understanding of the mechanisms taking place behind the phenomenon. A review and proposals for further research, Ultrason. Sonochem., 2019, 57, 147–165 CrossRef CAS PubMed.
- H. Horsley, J. Owen, R. Browning, D. Carugo, J. Malone-Lee, E. Stride and J. L. Rohn, Ultrasound-activated microbubbles as a novel intracellular drug delivery system for urinary tract infection, J. Controlled Release, 2019, 301, 166–175 CrossRef CAS PubMed.
- M. Klausen, M. Ucuncu and M. Bradley, Design of photosensitizing agents for targeted antimicrobial photodynamic therapy, Molecules, 2020, 25, 5239 CrossRef CAS PubMed.
- H. Cao, L. Wang, Y. Yang, J. Li, Y. Qi, Y. Li, Y. Li, H. Wang and J. Li, An assembled nanocomplex for improving both therapeutic efficiency and treatment depth in photodynamic therapy, Angew. Chem., Int. Ed., 2018, 130, 7885–7889 CrossRef.
- W. Li, Y. Song, X. Liang, Y. Zhou, M. Xu, Q. Lu, X. Wang and N. Li, Mutual-reinforcing
sonodynamic therapy against Rheumatoid Arthritis based on sparfloxacin sonosensitizer doped concave-cubic rhodium nanozyme, Biomaterials, 2021, 276, 121063 CrossRef CAS PubMed.
- R. Wang, C. Song, A. Gao, Q. Liu, W. Guan, J. Mei, L. Ma and D. Cui, Antibody-conjugated liposomes loaded with indocyanine green for oral targeted photoacoustic imaging-guided sonodynamic therapy of Helicobacter pylori infection, Acta Biomater., 2022, 143, 418–427 CrossRef CAS.
- Y. Wang, Y. Jin, W. Chen, J. Wang, H. Chen, L. Sun, X. Li, J. Ji, Q. Yu and L. Shen, Construction of nanomaterials with targeting phototherapy properties to inhibit resistant bacteria and biofilm infections, Chem. Eng. J., 2019, 358, 74–90 CrossRef CAS.
- J. Huo, Q. Jia, H. Huang, J. Zhang, P. Li, X. Dong and W. Huang, Emerging photothermal-derived multimodal synergetic therapy in combating bacterial infections, Chem. Soc. Rev., 2021, 50, 8762–8789 RSC.
- Y. Chen, Y. Gao, Y. Chen, L. Liu, A. Mo and Q. Peng, Nanomaterials-based photothermal therapy and its potentials in antibacterial treatment, J. Controlled Release, 2020, 328, 251–262 CrossRef CAS.
- H. S. Jung, P. Verwilst, A. Sharma, J. Shin, J. L. Sessler and J. S. Kim, Organic molecule-based photothermal agents: an expanding photothermal therapy universe, Chem. Soc. Rev., 2018, 47, 2280–2297 RSC.
- N. Fernandes, C. F. Rodrigues, A. F. Moreira and I. J. Correia, Overview of the application of inorganic nanomaterials in cancer photothermal therapy, Biomater. Sci., 2020, 8, 2990–3020 RSC.
- Y. Wang, Y. Wen, Y. Qu, Z. Pei and Y. Pei, Pillar [5] arene based glyco-targeting nitric oxide nanogenerator for hyperthermia-induced triple-mode cancer therapy, J. Colloid Interface Sci., 2022, 615, 386–394 CrossRef CAS PubMed.
- P. Dobosz and T. Dzieciątkowski, The intriguing history of cancer immunotherapy, Front. Immunol., 2019, 10, 2965 CrossRef CAS PubMed.
- K. Bedard and K. H. Krause, The NOX family of ROS-generating NADPH oxidases: physiology and pathophysiology, Physiol. Rev., 2007, 87, 245–313 CrossRef CAS PubMed.
- A. S. Debrie, N. Mielcarek, S. Lecher, X. Roux, J. C. Sirard and C. Locht, Early protection against pertussis induced by live attenuated Bordetella pertussis BPZE1 depends on TLR4, J. Immunol., 2019, 203, 3293–3300 CrossRef CAS.
- L. Matarazzo, F. Casilag, R. Porte, F. Wallet, D. Cayet, C. Faveeuw, C. Carnoy and J. C. Sirard, Therapeutic synergy between antibiotics and pulmonary toll-like receptor 5 stimulation in antibiotic-sensitive or -resistant pneumonia, Front. Immunol., 2019, 10, 723 CrossRef CAS.
- J. J. Chase, W. Kubey, M. H. Dulek, C. J. Holmes, M. G. Salit, F. C. Pearson, 3rd and E. Ribi, Effect of monophosphoryl lipid A on host resistance to bacterial infection, Infect. Immun., 1986, 53, 711–712 CrossRef CAS PubMed.
- T. Hirano, S. Kodama, T. Kawano, K. Maeda and M. Suzuki, Monophosphoryl lipid A induced innate immune responses via TLR4 to enhance clearance of nontypeable Haemophilus influenzae and Moraxella catarrhalis from the nasopharynx in mice, FEMS Microbiol. Immunol., 2011, 63, 407–417 CrossRef CAS.
- É. Pardoux, D. Boturyn and Y. Roupioz, Antimicrobial peptides as probes in biosensors detecting whole bacteria: a review, Molecules, 2020, 25, 1998 CrossRef.
- C. Zhong, N. Zhu, Y. Zhu, T. Liu, S. Gou, J. Xie, J. Yao and J. Ni, Antimicrobial peptides conjugated with fatty acids on the side chain of D-amino acid promises antimicrobial potency against multidrug-resistant bacteria, Eur. J. Pharm.
Sci., 2020, 141, 105123 CrossRef CAS PubMed.
- S.-U. Gorr, H. V. Brigman, J. C. Anderson and E. B. Hirsch, The antimicrobial peptide DGL13K is active against drug-resistant Gram-negative bacteria and sub-inhibitory concentrations stimulate bacterial growth without causing resistance, PLoS One, 2022, 17, e0273504 CrossRef CAS PubMed.
- C. H. Chen and T. K. Lu, Development and challenges of antimicrobial peptides for therapeutic applications, Antibiotics, 2020, 9, 24 CrossRef CAS.
- W. C. Wimley, Describing the mechanism of antimicrobial peptide action with the interfacial activity model, ACS Chem. Biol., 2010, 5, 905–917 CrossRef CAS PubMed.
- M. Erdem Büyükkiraz and Z. Kesmen, Antimicrobial peptides (AMPs): a promising class of antimicrobial compounds, J. Appl. Microbiol., 2022, 132, 1573–1596 CrossRef.
- M. Chamundeeswari, J. Jeslin and M. L. Verma, Nanocarriers for drug delivery applications, Environ. Chem. Lett., 2019, 17, 849–865 CrossRef CAS.
- H. Wang, Z. Song, S. Li, Y. Wu and H. Han, One stone with two birds: functional gold nanostar for targeted combination therapy of drug-resistant Staphylococcus aureus infection, ACS Appl. Mater. Interfaces, 2019, 11, 32659–32669 CrossRef CAS PubMed.
- X. Ji, H. Yang, W. Liu, Y. Ma, J. Wu, X. Zong, P. Yuan, X. Chen, C. Yang, X. Li, H. Lin, W. Xue and J. Dai, Multifunctional parachute-like nanomotors for enhanced skin penetration and synergetic antifungal therapy, ACS Nano, 2021, 15, 14218–14228 CrossRef CAS.
- Z. Song, Y. Wu, Q. Cao, H. Wang, X. Wang and H. Han, pH-responsive, light-triggered on-demand antibiotic release from functional metal-organic framework for bacterial infection combination therapy, Adv. Funct. Mater., 2018, 28, 1800011 CrossRef.
- T. J. Silhavy, D. Kahne and S. Walker, The bacterial cell envelope, Cold Spring Harbor Perspect. Biol., 2010, 2, a000414 Search PubMed.
- W. W. Wilson, M. M. Wade, S. C. Holman and F. R. Champlin, Status of methods for assessing bacterial cell surface charge properties based on zeta potential measurements, J. Microbiol. Methods, 2001, 43, 153–164 CrossRef CAS.
- Q. Li, Y. Li, T. Min, J. Gong, L. Du, D. L. Phillips, J. Liu, J. W. Y. Lam, H. H. Y. Sung, I. D. Williams, R. T. K. Kwok, C. L. Ho, K. Li, J. Wang and B. Z. Tang, Time-dependent photodynamic therapy for multiple targets: a highly efficient AIE-active photosensitizer for selective bacterial elimination and cancer cell ablation, Angew. Chem., Int. Ed., 2020, 59, 9470–9477 CrossRef CAS.
- J. Shi, M. Wang, Z. Sun, Y. Liu, J. Guo, H. Mao and F. Yan, Aggregation-induced emission-based ionic liquids for bacterial killing, imaging, cell labeling, and bacterial detection in blood cells, Acta Biomater., 2019, 97, 247–259 CrossRef CAS PubMed.
- X. Zhou, Z. Hu, D. Yang, S. Xie, Z. Jiang, R. Niessner, C. Haisch, H. Zhou and P. Sun, Bacteria detection: from powerful SERS to its advanced compatible techniques, Adv. Sci., 2020, 7, 2001739 CrossRef CAS PubMed.
- H. Xu, F. Tang, J. Dai, C. Wang and X. Zhou, Ultrasensitive and rapid count of Escherichia coli using magnetic nanoparticle probe under dark-field microscope, BMC Microbiol., 2018, 18, 100559 Search PubMed.
- Y.-C. Kuo, Q. Wang, C. Ruengruglikit, H. Yu and Q. Huang, Antibody-conjugated CdTe quantum dots for Escherichia coli detection, J. Phys. Chem. C, 2008, 112, 4818–4824 CrossRef CAS.
- S. Ye, T. Han, M. Cheng and L. Dong, Wulff-type boronic acid-functionalized quantum
dots for rapid and sensitive detection of Gram-negative bacteria, Sens. Actuators, B, 2022, 356, 131332 CrossRef CAS.
- Y. Tsuchido, R. Horiuchi, T. Hashimoto, K. Ishihara, N. Kanzawa and T. Hayashita, Rapid and selective discrimination of Gram-positive and Gram-negative bacteria by boronic acid-modified poly(amidoamine) dendrimer, Anal. Chem., 2019, 91, 3929–3935 CrossRef CAS PubMed.
- A. Mikagi, K. Manita, A. Yoyasu, Y. Tsuchido, N. Kanzawa, T. Hashimoto and T. Hayashita, Rapid bacterial recognition over a wide pH range by boronic acid-based ditopic dendrimer probes for Gram-positive bacteria, Molecules, 2021, 27, 256 CrossRef PubMed.
- Y. Zhong, X. T. Zheng, Q. L. Li, X. J. Loh, X. Su and S. Zhao, Antibody conjugated Au/Ir@Cu/Zn-MOF probe for bacterial lateral flow immunoassay and precise synergetic antibacterial treatment, Biosens. Bioelectron., 2023, 224, 115033 CrossRef CAS.
- Y. Zhang, M. Gong, X. Li, H. Liu, P. Liang, S. Cui, L. Zhang, C. Zhou, T. Sun, M. Zhang, C. Y. Wen and J. Zeng, Au-Fe3O4 heterodimer multifunctional nanoparticles-based platform for ultrasensitive naked-eye detection of Salmonella typhimurium, J. Hazard. Mater., 2022, 436, 129140 CrossRef CAS.
- L. G. Miranda Calderon, T. Alejo, S. Santos, G. Mendoza, S. Irusta and M. Arruebo, Antibody-functionalized polymer nanoparticles for targeted antibiotic delivery in models of pathogenic bacteria infecting human macrophages, ACS Appl. Mater. Interfaces, 2023, 15, 40213–40227 CrossRef CAS.
- J. I. Lee, S. C. Jang, J. Chung, W.-K. Choi, C. Hong, G. R. Ahn, S. H. Kim, B. Y. Lee and W.-J. Chung, Colorimetric allergenic fungal spore detection using peptide-modified gold nanoparticles, Sens. Actuators, B, 2021, 327, 128894 CrossRef CAS.
- M. Tanaka, I. H. Harlisa, Y. Takahashi, N. A. Ikhsan and M. Okochi, Screening of bacteria-binding peptides and one-pot ZnO surface modification for bacterial cell entrapment, RSC Adv., 2018, 8, 8795–8799 RSC.
- B. L. Bray, Large-scale manufacture of peptide therapeutics by chemical synthesis, Nat. Rev. Drug Discovery, 2003, 2, 587–593 CrossRef CAS PubMed.
- A. Breine, K. Van Holsbeeck, C. Martin, S. Gonzalez, M. Mannes, E. Pardon, J. Steyaert, H. Remaut, S. Ballet and C. Van Der Henst, Bypassing the need for cell permeabilization: nanobody CDR3 peptide improves binding on living bacteria, Bioconjugate Chem., 2023, 34, 1234–1243 CrossRef CAS PubMed.
- K. Sefah, D. Shangguan, X. Xiong, M. B. O'Donoghue and W. Tan, Development of DNA aptamers using Cell-SELEX, Nat. Protoc., 2010, 5, 1169–1185 CrossRef CAS PubMed.
- C. Kolm, I. Cervenka, U. J. Aschl, N. Baumann, S. Jakwerth, R. Krska, R. L. Mach, R. Sommer, M. C. DeRosa, A. K. T. Kirschner, A. H. Farnleitner and G. H. Reischer, DNA aptamers against bacterial cells can be efficiently selected by a SELEX process using state-of-the art qPCR and ultra-deep sequencing, Sci. Rep., 2020, 10, 20917 CrossRef CAS PubMed.
- D. Yilmaz, T. Muslu, A. Parlar, H. Kurt and M. Yuce, SELEX against whole-cell bacteria resulted in lipopolysaccharide binding aptamers, J. Biotechnol., 2022, 354, 10–20 CrossRef CAS.
- D. Li, L. Liu, Q. Huang, T. Tong, Y. Zhou, Z. Li, Q. Bai, H. Liang and L. Chen, Recent advances on aptamer-based biosensors for detection of pathogenic bacteria, World J. Microbiol. Biotechnol., 2021, 37, 45 CrossRef CAS.
- X. He, Y. Yang, Y. Guo, S. Lu, Y. Du, J.-J. Li, X. Zhang, N. L. C. Leung, Z. Zhao, G. Niu, S. Yang, Z. Weng, R. T. K. Kwok, J. W. Y. Lam, G. Xie and B. Z. Tang, Phage-guided targeting, discriminative imaging, and synergetic killing of bacteria by AIE bioconjugates, J. Am. Chem. Soc., 2020, 142, 3959–3969 CrossRef CAS.
- M. Y. Wu, L. Chen, Q. Chen, R. Hu, X. Xu, Y. Wang, J. Li, S. Feng, C. Dong, X. L. Zhang, Z. Li, L. Wang, S. Chen and M. Gu, Engineered phage with aggregation-induced emission photosensitizer in cocktail therapy against sepsis, Adv. Mater., 2023, 35, e2208578 CrossRef.
- W. Han, Z. Wu, Y. Li and Y. Wang, Graphene family nanomaterials (GFNs)—promising materials for antimicrobial coating and film: a review, Chem. Eng. J., 2019, 358, 1022–1037 CrossRef CAS.
- W. Yang, G. Chata, Y. Zhang, Y. Peng, J. E. Lu, N. Wang, R. Mercado, J. Li and S. Chen, Graphene oxide-supported zinc cobalt oxides as effective cathode catalysts for microbial fuel cell: high catalytic activity and inhibition of biofilm formation, Nano Energy, 2019, 57, 811–819 CrossRef CAS.
- H. Zheng, Z. Ji, K. R. Roy, M. Gao, Y. Pan, X. Cai, L. Wang, W. Li, C. H. Chang, C. Kaweeteerawat, C. Chen, T. Xia, Y. Zhao and R. Li, Engineered graphene oxide nanocomposite capable of preventing the evolution of antimicrobial resistance, ACS Nano, 2019, 13, 11488–11499 CrossRef CAS.
- T. Parandhaman, P. Choudhary, B. Ramalingam, M. Schmidt, S. Janardhanam and S. K. Das, Antibacterial and antibiofouling activities of antimicrobial peptide-functionalized graphene–silver nanocomposites for the inhibition and disruption of Staphylococcus aureus biofilms, ACS Biomater. Sci. Eng., 2021, 7, 5899–5917 CrossRef CAS PubMed.
- H. N. Nguyen, C. Chaves-Lopez, R. C. Oliveira, A. Paparella and D. F. Rodrigues, Cellular and metabolic approaches to investigate the effects of graphene and graphene oxide in the fungi Aspergillus flavus and Aspergillus niger, Carbon, 2019, 143, 419–429 CrossRef CAS.
- X. Cheng, S. Zheng, W. Wang, H. Han, X. Yang, W. Shen, C. Wang and S. Wang, Synthesis of two-dimensional graphene oxide-fluorescent nanoprobe for ultrasensitive and multiplex immunochromatographic detection of respiratory bacteria, Chem. Eng. J., 2021, 426, 131836 CrossRef CAS.
- W. Zhao, Y. Xing, Y. Lin, Y. Gao, M. Wu and J. Xu, Monolayer graphene chemiresistive biosensor for rapid bacteria detection in a microchannel, Sens. Actuators Rep., 2020, 2, 100004 CrossRef.
- J. Shen, R. Hu, T. Zhou, Z. Wang, Y. Zhang, S. Li, C. Gui, M. Jiang, A. Qin and B. Z. Tang, Fluorescent sensor array for highly efficient microbial lysate identification through competitive interactions, ACS Sens., 2018, 3, 2218–2222 CrossRef CAS.
- Y. Liu, Q. Zhang, N. Zhou, J. Tan, J. Ashley, W. Wang, F. Wu, J. Shen and M. Zhang, Study on a novel poly (vinyl alcohol)/graphene oxide-citicoline sodium-lanthanum wound dressing: biocompatibility, bioactivity, antimicrobial activity, and wound healing effect, Chem. Eng. J., 2020, 395, 125059 CrossRef CAS.
- J. Zhang, J. Xu, H. Ma, H. Bai, L. Liu, C. Shu, H. Li, S. Wang and C. Wang, Designing an amino-fullerene derivative C70–(EDA)8 to fight superbacteria, ACS Appl. Mater. Interfaces, 2019, 11, 14597–14607 CrossRef CAS.
- D. Wang, W. Wang, H. Lu, C. You, L. Liang, C. Liu, H. Xiang and Y. Chen, Charge transfer of ZnTPP/C60 cocrystal-hybridized bioimplants satisfies osteosarcoma eradication with antitumoral, antibacterial and osteogenic performances, Nano Today, 2022, 46, 101562 CrossRef CAS.
- L. Xiao, R. Huang, Y. Zhang, T. Li, J. Dai, N. Nannapuneni, T. R. Chastanet, M. Chen, F. H. Shen, L. Jin, H. C. Dorn and X. Li, A new formyl peptide receptor-1 antagonist conjugated fullerene nanoparticle for targeted treatment of degenerative disc diseases, ACS Appl. Mater. Interfaces, 2019, 11, 38405–38416 CrossRef CAS.
- Y. Zhang, H. Zhang, Q. Zou, R. Xing, T. Jiao and X. Yan, An injectable dipeptide–fullerene supramolecular hydrogel for photodynamic antibacterial therapy, J. Mater. Chem. B, 2018, 6, 7335–7342 RSC.
- Y. Chen, S. Guo, M. Zhao, P. Zhang, Z. Xin, J. Tao and L. Bai, Amperometric DNA biosensor for Mycobacterium tuberculosis detection using flower-like carbon nanotubes-polyaniline nanohybrid and enzyme-assisted signal amplification strategy, Biosens. Bioelectron., 2018, 119, 215–220 CrossRef CAS.
- Y. Chen, X. Liu, S. Guo, J. Cao, J. Zhou, J. Zuo and L. Bai, A sandwich-type electrochemical aptasensor for Mycobacterium tuberculosis MPT64 antigen detection using C60NPs decorated N-CNTs/GO nanocomposite coupled with conductive PEI-functionalized metal-organic framework, Biomaterials, 2019, 216, 119253 CrossRef CAS PubMed.
- G. Gülseren, A. Saylam, A. Marion and S. Özçubukçu, Fullerene-based mimics of biocatalysts show remarkable activity and modularity, ACS Appl. Mater. Interfaces, 2021, 13, 45854–45863 CrossRef.
- A. Payan, A. Akbar Isari and N. Gholizade, Catalytic decomposition of sulfamethazine antibiotic and pharmaceutical wastewater using Cu-TiO2@functionalized SWCNT ternary porous nanocomposite: influential factors, mechanism, and pathway studies, Chem. Eng. J., 2019, 361, 1121–1141 CrossRef CAS.
- A. A. Isari, F. Hayati, B. Kakavandi, M. Rostami, M. Motevassel and E. Dehghanifard, N, Cu co-doped TiO2@functionalized SWCNT photocatalyst coupled with ultrasound and visible-light: an effective sono-photocatalysis process for pharmaceutical wastewaters treatment, Chem. Eng. J., 2020, 392, 123685 CrossRef CAS.
- J. Akhtari, R. Faridnia, H. Kalani, R. Bastani, M. Fakhar, H. Rezvan and A. K. Beydokhti, Potent in vitro antileishmanial activity of a nanoformulation of cisplatin with carbon nanotubes against Leishmania major, J. Global Antimicrob. Resist., 2019, 16, 11–16 CrossRef.
- D. H. Carrales-Alvarado, R. Leyva-Ramos, I. Rodríguez-Ramos, E. Mendoza-Mendoza and A. E. Moral-Rodríguez, Adsorption capacity of different types of carbon nanotubes towards metronidazole and dimetridazole antibiotics from aqueous solutions: effect of morphology and surface chemistry, Environ. Sci. Pollut. Res., 2020, 27, 17123–17137 CrossRef CAS.
- X. Yan, S. Li, J. Bao, N. Zhang, B. Fan, R. Li, X. Liu and Y.-X. Pan, Immobilization of highly dispersed ag nanoparticles on carbon nanotubes using electron-assisted reduction for antibacterial performance, ACS Appl. Mater. Interfaces, 2016, 8, 17060–17067 CrossRef CAS.
- Y. Zhu, J. Xu, Y. Wang, C. Chen, H. Gu, Y. Chai and Y. Wang, Silver nanoparticles-decorated and mesoporous silica coated single-walled carbon nanotubes with an enhanced antibacterial activity for killing drug-resistant bacteria, Nano Res., 2020, 13, 389–400 CrossRef CAS.
- C. Liu, H. Shi, H. Yang, S. Yan, S. Luan, Y. Li, M. Teng, A. F. Khan and J. Yin, Fabrication of antibacterial electrospun nanofibers with vancomycin-carbon nanotube via ultrasonication assistance, Mater. Des., 2017, 120, 128–134 CrossRef CAS.
- N. A. Mohamed and N. A. Abd El-Ghany, Novel aminohydrazide cross-linked chitosan filled with multi-walled carbon nanotubes as antimicrobial agents, Int. J. Biol. Macromol., 2018, 115, 651–662 CrossRef CAS.
- C. Singh, M. A. Ali, V. Reddy, D. Singh, C. G. Kim, G. Sumana and B. D. Malhotra, Biofunctionalized graphene oxide wrapped carbon nanotubes enabled microfluidic immunochip for bacterial cells detection, Sens. Actuators, B, 2018, 255, 2495–2503 CrossRef CAS.
- S. Jung, J. Kim, J. Kim, S. H. Yang and S. K. Kim, Extensible multiplex real-time PCR for rapid bacterial identification with carbon nanotube composite microparticles, Biosens. Bioelectron., 2017, 94, 256–262 CrossRef CAS PubMed.
- C. Xia, S. Zhu, T. Feng, M. Yang and B. Yang, Evolution and synthesis of carbon dots: from carbon dots to carbonized polymer dots, Adv. Sci., 2019, 6, 1901316 CrossRef CAS.
- S. Y. Lim, W. Shen and Z. Gao, Carbon quantum dots and their applications, Chem. Soc. Rev., 2015, 44, 362–381 RSC.
- F. Wu, J. Chen, Z. Li, H. Su, K. C.-F. Leung, H. Wang and X. Zhu, Red/near-infrared emissive metalloporphyrin-based nanodots for magnetic resonance imaging-guided photodynamic therapy in vivo, Part. Part. Syst. Charact., 2018, 35, 1800208 CrossRef.
- V. D. Dang, A. B. Ganganboina and R.-A. Doong, Bipyridine- and copper-functionalized N-doped carbon dots for fluorescence turn off–on detection of ciprofloxacin, ACS Appl. Mater. Interfaces, 2020, 12, 32247–32258 CrossRef CAS PubMed.
- L. Yang, W. Deng, C. Cheng, Y. Tan, Q. Xie and S. Yao, Fluorescent immunoassay for the detection of pathogenic bacteria at the single-cell level using carbon dots-encapsulated breakable organosilica nanocapsule as labels, ACS Appl. Mater. Interfaces, 2018, 10, 3441–3448 CrossRef CAS.
- H. Wang, Z. Song, J. Gu, S. Li, Y. Wu and H. Han, Nitrogen-doped carbon quantum dots for preventing biofilm formation and eradicating drug-resistant bacteria infection, ACS Biomater. Sci. Eng., 2019, 5, 4739–4749 CrossRef CAS.
- X. Li, R. Huang, F. K. Tang, W. C. Li, S. S. W. Wong, K. C.-F. Leung and L. Jin, Red-emissive guanylated polyene-functionalized carbon dots arm oral epithelia against invasive fungal infections, ACS Appl. Mater. Interfaces, 2019, 11, 46591–46603 CrossRef CAS PubMed.
- H. Lei, A. Alu, J. Yang, X. He, C. He, W. Ren, Z. Chen, W. Hong, L. Chen, X. He, L. Yang, J. Li, Z. Wang, W. Wang, Y. Wei, S. Lu, G. Lu, X. Song and X. Wei, Cationic crosslinked carbon dots-adjuvanted intranasal vaccine induces protective immunity against Omicron-included SARS-CoV-2 variants, Nat. Commun., 2023, 14, 2678 CrossRef CAS.
- N. Tejwan, A. K. Saini, A. Sharma, T. A. Singh, N. Kumar and J. Das, Metal-doped and hybrid carbon dots: a comprehensive review on their synthesis and biomedical applications, J. Controlled Release, 2021, 330, 132–150 CrossRef CAS.
- Q. Zhang, R. Wang, B. Feng, X. Zhong and K. Ostrikov, Photoluminescence mechanism of carbon dots: triggering high-color-purity red fluorescence emission through edge amino protonation, Nat. Commun., 2021, 12, 6856 CrossRef CAS.
- F. Lu, Y. Song, H. Huang, Y. Liu, Y. Fu, J. Huang, H. Li, H. Qu and Z. Kang, Fluorescent carbon dots with tunable negative charges for bio-imaging in bacterial viability assessment, Carbon, 2017, 120, 95–102 CrossRef CAS.
- X. Dong, W. Liang, M. J. Meziani, Y.-P. Sun and L. Yang, Carbon dots as potent antimicrobial agents, Theranostics, 2020, 10, 671–686 CrossRef CAS PubMed.
- P. Li, L. Sun, S. Xue, D. Qu, L. An, X. Wang and Z. Sun, Recent advances of carbon dots as new antimicrobial agents, SmartMat, 2022, 3, 226–248 CrossRef CAS.
- K. Cheng, H. Wang, S. Sun, M. Wu, H. Shen, K. Chen, Z. Zhang, S. Li and H. Lin, Specific chemiluminescence imaging and enhanced photodynamic therapy of bacterial infections by hemin-modified carbon dots, Small, 2023, 19, 2207868 CrossRef CAS.
- S. M. Dizaj, F. Lotfipour, M. Barzegar-Jalali, M. H. Zarrintan and K. Adibkia, Antimicrobial activity of the metals and metal oxide nanoparticles, Mater. Sci. Eng., C, 2014, 44, 278–284 CrossRef CAS.
- J. Chen, L. Yang, J. Chen, W. Liu, D. Zhang, P. Xu, T. Dai, L. Shang, Y. Yang, S. Tang, Y. Zhang, H. Lin, Z. Chen and M. Huang, Composite of silver nanoparticles and photosensitizer leads to mutual enhancement of antimicrobial efficacy and promotes wound healing, Chem. Eng. J., 2019, 374, 1373–1381 CrossRef CAS.
- X. Q. Kang, Y. Qiao, X. Y. Lu, S. P. Jiang, W. S. Li, X. J. Wang, X. L. Xu, J. Qi, Y. H. Xiao and Y. Z. Du, Tocopherol polyethylene glycol succinate-modified hollow silver nanoparticles for combating bacteria-resistance, Biomater. Sci., 2019, 7, 2520–2532 RSC.
- H. Ye, J. Cheng and K. Yu, In situ reduction of silver nanoparticles by gelatin to obtain porous silver nanoparticle/chitosan composites with enhanced antimicrobial and wound-healing activity, Int. J. Biol. Macromol., 2019, 121, 633–642 CrossRef CAS.
- J. M. Peng, J. C. Lin, Z. Y. Chen, M. C. Wei, Y. X. Fu, S. S. Lu, D. S. Yu and W. Zhao, Enhanced antimicrobial activities of silver-nanoparticle-decorated reduced graphene nanocomposites against oral pathogens, Mater. Sci. Eng., C, 2017, 71, 10–16 CrossRef CAS.
- J. Liu, L. Liu, S. Li, Q. Kang, R. Zhang and Z. Zhu, Self-assembled nanogels of luminescent thiolated silver nanoclusters and chitosan as bactericidal agent and bacterial sensor, Mater. Sci. Eng., C, 2021, 118, 111520 CrossRef CAS PubMed.
- K. C.-F. Leung, X.-B. Li, X. Li, S.-F. Lee, J. C. Yu, P. M. Mendes, K. E. Hermann and M. A. Van Hove, Soft nanohand grabs a growing nanoparticle, Mater. Chem. Front., 2019, 3, 1555–1564 RSC.
- L. Wang, M. Natan, W. Zheng, W. Zheng, S. Liu, G. Jacobi, I. Perelshtein, A. Gedanken, E. Banin and X. Jiang, Small molecule-decorated gold nanoparticles for preparing antibiofilm fabrics, Nanoscale Adv., 2020, 2, 2293–2302 RSC.
- L. Wang, S. Li, J. Yin, J. Yang, Q. Li, W. Zheng, S. Liu and X. Jiang, The density of surface coating can contribute to different antibacterial activities of gold nanoparticles, Nano Lett., 2020, 20, 5036–5042 CrossRef CAS.
- R. Chowdhury, H. Ilyas, A. Ghosh, H. Ali, A. Ghorai, A. Midya, N. R. Jana, S. Das and A. Bhunia, Multivalent gold nanoparticle–peptide conjugates for targeting intracellular bacterial infections, Nanoscale, 2017, 9, 14074–14093 RSC.
- Y. Guo, Y. Zheng, Y. Liu, X. Feng, Q. Dong, J. Li, J. Wang and C. Zhao, A concise detection strategy of Staphylococcus aureus using N-succinyl-chitosan-dopped bacteria-imprinted composite film and AIE fluorescence sensor, J. Hazard. Mater., 2022, 423, 126934 CrossRef CAS.
- C. Zhang, D.-T. Shi, K.-C. Yan, A. C. Sedgwick, G.-R. Chen, X.-P. He, T. D. James, B. Ye, X.-L. Hu and D. Chen, A glycoconjugate-based gold nanoparticle approach for the targeted treatment of Pseudomonas aeruginosa biofilms, Nanoscale, 2020, 12, 23234–23240 RSC.
- T. Kim, Q. Zhang, J. Li, L. Zhang and J. V. Jokerst, A gold/silver hybrid nanoparticle for treatment and photoacoustic imaging of bacterial infection, ACS Nano, 2018, 12, 5615–5625 CrossRef CAS.
- J. Ye, Z. Li, Q. Fu, Q. Li, X. Zhang, L. Su, H. Yang and J. Song, Quantitative photoacoustic diagnosis and precise treatment of inflammation in vivo using activatable theranostic nanoprobe, Adv. Funct. Mater., 2020, 30, 2001771 CrossRef CAS.
- M. Hajfathalian, C. R. De Vries, J. C. Hsu, A. Amirshaghaghi, Y. C. Dong, Z. Ren, Y. Liu, Y. Huang, Y. Li, S. A. B. Knight, P. Jonnalagadda, A. Zlitni, E. A. Grice, P. L. Bollyky, H. Koo and D. P. Cormode, Theranostic gold-in-gold cage nanoparticles enable photothermal ablation and photoacoustic imaging in biofilm-associated infection models, J. Clin. Invest., 2023, 133, e168485 CrossRef PubMed.
- C. Ma, J. Borgatta, R. De La Torre-Roche, N. Zuverza-Mena, J. C. White, R. J. Hamers and W. H. Elmer, Time-dependent transcriptional response of tomato (Solanum lycopersicum L.) to Cu nanoparticle exposure upon infection with Fusarium oxysporum f. sp. lycopersici, ACS Sustainable Chem. Eng., 2019, 7, 10064–10074 CrossRef CAS.
- A. B. Alayande, M. Obaid and I. S. Kim, Antimicrobial mechanism of reduced graphene oxide-copper oxide (rGO-CuO) nanocomposite films: the case of Pseudomonas aeruginosa PAO1, Mater. Sci. Eng., C, 2020, 109, 110596 CrossRef CAS PubMed.
- H. Qamar, S. Rehman, D. K. Chauhan, A. K. Tiwari and V. Upmanyu, Green synthesis, characterization and antimicrobial activity of copper oxide nanomaterial derived from Momordica charantia, Int. J. Nanomed., 2020, 15, 2541–2553 CrossRef CAS.
- X. Huang, N. Hu, X. Wang, Y. S. Zhang and R. Sun, Copper sulfide nanoparticle/cellulose composite paper: room-temperature green fabrication for nir laser-inducible ablation of pathogenic microorganisms, ACS Sustainable Chem. Eng., 2017, 5, 2648–2655 CrossRef CAS.
- Y. Qiao, Y. Ping, H. Zhang, B. Zhou, F. Liu, Y. Yu, T. Xie, W. Li, D. Zhong, Y. Zhang, K. Yao, H. A. Santos and M. Zhou, Laser-activatable CuS nanodots to treat multidrug-resistant bacteria and release copper ion to accelerate healing of infected chronic nonhealing wounds, ACS Appl. Mater. Interfaces, 2019, 11, 3809–3822 CrossRef CAS.
- H.-B. Wang, B.-B. Tao, A.-L. Mao, Z.-L. Xiao and Y.-M. Liu, Self-assembled copper nanoclusters structure-dependent fluorescent enhancement for sensitive determination of tetracyclines by the restriction intramolecular motion, Sens. Actuators, B, 2021, 348, 130729 CrossRef CAS.
- K. T. Prakash, N. Singh and V. Venkatesh, Synthesis of novel luminescent copper nanoclusters with substituent driven self-assembly and aggregation induced emission (AIE), Chem. Commun., 2019, 55, 322–325 RSC.
- T. Qing, C. Long, X. Wang, K. Zhang, P. Zhang and B. Feng, Detection of micrococcal nuclease for identifying Staphylococcus aureus based on DNA templated
fluorescent copper nanoclusters, Microchim. Acta, 2019, 186, 248 CrossRef.
- Z. Zhou, Y. Zhang, M. Guo, K. Huang and W. Xu, Ultrasensitive magnetic DNAzyme-copper nanoclusters fluorescent biosensor with triple amplification for the visual detection of E. coli O157:H7, Biosens. Bioelectron., 2020, 167, 112475 CrossRef CAS PubMed.
- A. C. K. Chung, X. Li, W.-C. Li, T. Wang, H.-K. Lee, L. Jin, Z. Cai and K. C.-F. Leung, Mass spectrometry imaging and monitoring of in vivo glutathione-triggered cisplatin release from nanoparticles in the kidneys, Nanoscale Adv., 2020, 2, 5857–5865 RSC.
- L. Zhang, X. Y. Tian, C. K. W. Chan, Q. Bai, C. K. Cheng, F. M. Chen, M. S. H. Cheung, B. Yin, H. Yang, W.-Y. Yung, Z. Chen, F. Ding, K. C.-F. Leung, C. Zhang, Y. Huang, J. Y. W. Lau and C. H. J. Choi, Promoting the delivery of nanoparticles to atherosclerotic plaques by DNA coating, ACS Appl. Mater. Interfaces, 2019, 11, 13888–13904 CrossRef CAS.
- Z. Yu, X. Li and J. Guo, Combat antimicrobial resistance emergence and biofilm formation through nanoscale zero-valent iron particles, Chem. Eng. J., 2022, 444, 136569 CrossRef CAS.
- Z. Liu, X. Zhao, B. Yu, N. Zhao, C. Zhang and F.-J. Xu, Rough carbon–iron oxide nanohybrids for near-infrared-II light-responsive synergetic antibacterial therapy, ACS Nano, 2021, 15, 7482–7490 CrossRef CAS PubMed.
- G. Liu, Q. Xiong, Y. Xu, Q. Fang, K. C.-F. Leung, M. Sang, S. Xuan and L. Hao, Magnetically separable MXene@Fe3O4/Au/PDA nanosheets with photothermal-magnetolytic coupling antibacterial performance, Appl. Surf. Sci., 2022, 590, 153125 CrossRef CAS.
- C. Xu, O. U. Akakuru, J. Zheng and A. Wu, Applications of iron oxide-based magnetic nanoparticles in the diagnosis and treatment of bacterial infections, Front. Bioeng. Biotechnol., 2019, 7, 141 CrossRef.
- Y. Ji, Z. Han, H. Ding, X. Xu, D. Wang, Y. Zhu, F. An, S. Tang, H. Zhang, J. Deng and Q. Zhou, Enhanced eradication of bacterial/fungi biofilms by glucose oxidase-modified magnetic nanoparticles as a potential treatment for persistent endodontic infections, ACS Appl. Mater. Interfaces, 2021, 13, 17289–17299 CrossRef CAS.
- G. H. Yu, Z. L. Chi, A. Kappler, F. S. Sun, C. Q. Liu, H. H. Teng and G. M. Gadd, Fungal nanophase particles catalyze iron transformation for oxidative stress removal and iron acquisition, Curr. Biol., 2020, 30, 2943–2950 CrossRef CAS.
- M. J. Oh, S. Yoon, A. Babeer, Y. Liu, Z. Ren, Z. Xiang, Y. Miao, D. P. Cormode, C. Chen, E. Steager and H. Koo, Nanozyme-based robotics approach for targeting fungal infection, Adv. Mater., 2023, 11, 2300320 CrossRef PubMed.
- C. Zhang, C. Wang, R. Xiao, L. Tang, J. Huang, D. Wu, S. Liu, Y. Wang, D. Zhang, S. Wang and X. Chen, Sensitive and specific detection of clinical bacteria via vancomycin-modified Fe3O4@Au nanoparticles and aptamer-functionalized SERS tags, J. Mater. Chem. B, 2018, 6, 3751–3761 RSC.
- W. Zhao, D. Zhang, T. Zhou, J. Huang, Y. Wang, B. Li, L. Chen, J. Yang and Y. Liu, Aptamer-conjugated magnetic Fe3O4@Au core-shell multifunctional nanoprobe: a three-in-one aptasensor for selective capture, sensitive SERS detection and efficient near-infrared light triggered photothermal therapy of Staphylococcus aureus, Sens. Actuators, B, 2022, 350, 130879 CrossRef CAS.
- J. Shi, J. Li, Y. Wang and C. Y. Zhang, TiO2-based nanosystem for cancer therapy and antimicrobial treatment: a review, Chem. Eng. J., 2022, 431, 133714 CrossRef CAS.
- V. Kumaravel, K. M. Nair, S. Mathew, J. Bartlett, J. E. Kennedy, H. G. Manning, B. J. Whelan, N. S. Leyland and S. C. Pillai, Antimicrobial TiO2 nanocomposite coatings for surfaces, dental and orthopaedic implants, Chem. Eng. J., 2021, 416, 129071 CrossRef CAS.
- L. Marín-Caba, G. Bodelón, Y. Negrín-Montecelo and M. A. Correa-Duarte, Sunlight-sensitive plasmonic nanostructured composites as photocatalytic coating with antibacterial properties, Adv. Funct. Mater., 2021, 31, 2105807 CrossRef.
- X. Zhang, G. Zhang, M. Chai, X. Yao, W. Chen and P. K. Chu, Synergetic antibacterial activity of physical-chemical multi-mechanism by TiO2 nanorod arrays for safe biofilm eradication on implant, Bioact. Mater., 2021, 6, 12–25 CAS.
- N. M. Alabdallah, M. A. Irshad, M. Rizwan, R. Nawaz, A. Inam, M. Mohsin, I. Khurshid, H. F. Alharby, A. A. Bamagoos and S. Ali, Synthesis, characterization and antifungal potential of titanium dioxide nanoparticles against fungal disease (Ustilago tritici) of wheat (Triticum aestivum L.), Environ. Res., 2023, 228, 115852 CrossRef CAS PubMed.
- J. Chen, S. Dai, L. Liu, M. F. Maitz, Y. Liao, J. Cui, A. Zhao, P. Yang, N. Huang and Y. Wang, Photo-functionalized TiO2 nanotubes decorated with multifunctional Ag nanoparticles for enhanced vascular biocompatibility, Bioact. Mater., 2021, 6, 45–54 CAS.
- S. Sonia, K. Ruckmani and M. Sivakumar, Antimicrobial and antioxidant potentials of biosynthesized colloidal zinc oxide nanoparticles for a fortified cold cream formulation: a potent nanocosmeceutical application, Mater. Sci. Eng., C, 2017, 79, 581–589 CrossRef CAS.
- H. Chandra, D. Patel, P. Kumari, J. S. Jangwan and S. Yadav, Phyto-mediated synthesis of zinc oxide nanoparticles of Berberis aristata: characterization, antioxidant activity and antibacterial activity with special reference to urinary tract pathogens, Mater. Sci. Eng., C, 2019, 102, 212–220 CrossRef CAS PubMed.
- K. Punjabi, S. Mehta, R. Chavan, V. Chitalia, D. Deogharkar and S. Deshpande, Efficiency of biosynthesized silver and zinc nanoparticles against multi-drug resistant pathogens, Front. Microbiol., 2018, 9, 2207 CrossRef.
- A. Aditya, S. Chattopadhyay, D. Jha, H. K. Gautam, S. Maiti and M. Ganguli, Zinc oxide nanoparticles dispersed in ionic liquids show high antimicrobial efficacy to skin-specific bacteria, ACS Appl. Mater. Interfaces, 2018, 10, 15401–15411 CrossRef CAS.
- M.-M. Pan, D. Feng, Y. Ouyang, D. Yang, X. Yu, L. Xu and I. Willner, MOF-templated synthesis of cobalt-doped zinc oxide superparticles for detection of the 3-hydroxy-2-butanone microbial biomarker, Sens. Actuators, B, 2022, 358, 131482 CrossRef CAS.
- Z. Lin, J. J. Richardson, J. Zhou and F. Caruso, Direct synthesis of amorphous coordination polymers and metal–organic frameworks, Nat. Rev. Chem., 2023, 7, 273–286 CrossRef CAS.
- S. Abednatanzi, P. Gohari Derakhshandeh, H. Depauw, F. X. Coudert, H. Vrielinck, P. Van Der Voort and K. Leus, Mixed-metal metal–organic frameworks, Chem. Soc. Rev., 2019, 48, 2535–2565 RSC.
- Q. Fang, Y. Sun, J. Duan, L. Bai, K. Xu, Q. Xiong, H. Xu, K. C.-F. Leung, A. Hui and S. Xuan, ZIF-8 self-etching method for Au/polydopamine hybrid cubic microcapsules with modulated nanostructures, CrystEngComm, 2019, 21, 6935–6944 RSC.
- S. M. Moosavi, A. Nandy, K. M. Jablonka, D. Ongari, J. P. Janet, P. G. Boyd, Y. Lee, B. Smit and H. J. Kulik, Understanding the diversity of the metal-organic framework ecosystem, Nat. Commun., 2020, 11, 4068 CrossRef CAS PubMed.
- J. Li, X. Liu, L. Tan, Z. Cui, X. Yang, Y. Liang, Z. Li, S. Zhu, Y. Zheng, K. W. K. Yeung, X. Wang and S. Wu, Zinc-doped Prussian blue enhances photothermal clearance of Staphylococcus aureus and promotes tissue repair in infected wounds, Nat. Commun., 2019, 10, 4490 CrossRef.
- H. N. Rubin, B. H. Neufeld and M. M. Reynolds, Surface-anchored metal–organic framework–cotton material for tunable antibacterial copper delivery, ACS Appl. Mater. Interfaces, 2018, 10, 15189–15199 CrossRef CAS.
- L. Su, Y. Li, Y. Liu, R. Ma, Y. Liu, F. Huang, Y. An, Y. Ren, H. C. Mei, H. J. Busscher and L. Shi, Antifungal-inbuilt metal–organic-frameworks eradicate Candida albicans biofilms, Adv. Funct. Mater., 2020, 30, 2000537 CrossRef CAS.
- R. Huang, Z. Zhou, X. Lan, F. K. Tang, T. Cheng, H. Sun, K. C.-F. Leung, X. Li and L. Jin, Rapid synthesis of bismuth-organic frameworks as selective antimicrobial materials against microbial biofilms, Mater. Today Bio, 2023, 18, 100507 CrossRef CAS PubMed.
- K. Yuan, K. Huang, Y. Yang, Y. Lin, Y. Liu, F. Li, Y. Liang, H. Chang, Y. Chen, T. Tang and S. Yang, Multi-roles of nanoscale bismuth metal-organic frameworks: infectious photoacoustic probe and inhibitor of antibiotics tolerant bacteria via targeting endogenous H2S, Nano Today, 2022, 47, 101683 CrossRef CAS.
- L. Zhang, M. Ouyang, Y. Zhang, L. Zhang, Z. Huang, L. He, Y. Lei, Z. Zou, F. Feng and R. Yang, The fluorescence imaging and precise suppression of bacterial infections in chronic wounds by porphyrin-based metal–organic framework nanorods, J. Mater. Chem. B, 2021, 9, 8048–8055 RSC.
- Q. Hu, X. Huang, Z. Wang, G. Li, Z. Han, H. Yang, P. Liao, X. Ren, Q. Zhang, J. Liu and C. He, Slower removing ligands of metal organic frameworks enables higher electrocatalytic performance of derived nanomaterials, Small, 2020, 16, e2002210 CrossRef.
- S. Wei, Y. Qiao, Z. Wu, X. Liu, Y. Li, Z. Cui, C. Li, Y. Zheng, Y. Liang, Z. Li, S. Zhu, H. Wang, X. Wang, R. Che and S. Wu, Na+ inserted metal-organic framework for rapid therapy of bacteria-infected osteomyelitis through microwave strengthened Fenton reaction and thermal effects, Nano Today, 2021, 37, 101090 CrossRef CAS.
- Y. Luo, X. Liu, L. Tan, Z. Li, K. W. K. Yeung, Y. Zheng, Z. Cui, Y. Liang, S. Zhu, C. Li, X. Wang and S. Wu, Enhanced photocatalytic and photothermal properties of ecofriendly metal-organic framework heterojunction for rapid sterilization, Chem. Eng. J., 2021, 405, 126730 CrossRef CAS.
- Z. Sun, S. Wu, Y. Peng, M. Wang, M. Jalalah, M. S. Al-Assiri, F. A. Harraz, J. Yang and G. Li, Sensor array for rapid pathogens identification
fabricated with peptide-conjugated 2D metal-organic framework nanosheets, Chem. Eng. J., 2021, 405, 126707 CrossRef CAS.
- Y. Yang, K. Huang, M. Wang, Q. Wang, H. Chang, Y. Liang, Q. Wang, J. Zhao, T. Tang and S. Yang, Ubiquitination flow repressors: enhancing wound healing of infectious diabetic ulcers through stabilization of polyubiquitinated hypoxia-inducible factor-1α by theranostic nitric oxide nanogenerators, Adv. Mater., 2021, 33, e2103593 CrossRef PubMed.
- K. Huang, F. Li, K. Yuan, Y. Yang, H. Chang, Y. Liang, X. Yan, J. Zhao, T. Tang and S. Yang, A MOF-armored zinc-peroxide nanotheranostic platform for eradicating drug resistant bacteria via image-guided and in situ activated photodynamic therapy, Appl, Mater. Today, 2022, 28, 101513 CrossRef.
- D. Mao, F. Hu, Kenry, S. Ji, W. Wu, D. Ding, D. Kong and B. Liu, Metal-organic-framework-assisted in vivo bacterial metabolic labeling and precise antibacterial therapy, Adv. Mater., 2018, 30, e1706831 CrossRef.
- Y. Jin, Y. Wang, J. Yang, H. Zhang, Y.-W. Yang, W. Chen, W. Jiang, J. Qu, Y. Guo and B. Wang, An Integrated theranostic nanomaterial for targeted photodynamic therapy of infectious endophthalmitis, Cell Rep. Phys. Sci., 2020, 1, 100173 CrossRef CAS.
- B. Ma, Y. Chen, G. Hu, Q. Zeng, X. Lv, D. H. Oh, X. Fu and Y. Jin, Ovotransferrin antibacterial peptide coupling mesoporous silica nanoparticle as an effective antibiotic delivery system for treating bacterial infection in vivo, ACS Biomater. Sci. Eng., 2021, 8, 109–118 CrossRef PubMed.
- X. Li, C.-H. Wong, T.-W. Ng, C.-F. Zhang, K. C.-F. Leung and L. Jin, The spherical nanoparticle-encapsulated chlorhexidine enhances anti-biofilm efficiency through an effective releasing mode and close microbial interactions, Int. J. Nanomed., 2016, 11, 2471 CAS.
- X. Li, C. Wang, L. Wang, R. Huang, W. C. Li, X. Wang, S. S. W. Wong, Z. Cai, K. C.-F. Leung and L. Jin, A glutathione-responsive silica-based nanosystem capped with in-situ polymerized cell-penetrating poly(disulfide)s for precisely modulating immuno-inflammatory responses, J. Colloid Interface Sci., 2022, 614, 322–336 CrossRef CAS PubMed.
- T.-H. Lo, Z.-Y. Wu, S.-Y. Chen, F.-Y. Meng, P.-T. Chou, C.-M. Wang and H.-M. Lin, Curcumin-loaded mesoporous silica nanoparticles with dual-imaging and temperature control inhibits the infection of Zika virus, Microporous Mesoporous Mater., 2021, 314, 110886 CrossRef CAS.
- Z. Zhang, H. Yan, B. Qiu, P. Ran, W. Cao, X. Jia, K. Huang and X. Li, Persistent luminescence–based theranostics for real-time monitoring and simultaneously launching photodynamic therapy of bacterial infections, Small, 2022, 18, 2200813 CrossRef CAS.
- D. Zhou, Z. Zhang, B. Qiu, D. Zhang, S. Xie, K. Huang and X. Li, Ultrasound-activated persistent luminescence imaging and bacteria-triggered drug release for Helicobacter pylori infection theranostics, ACS Appl. Mater. Interfaces, 2022, 14, 26418–26430 CrossRef CAS.
- M. C. Grüner, M. S. Arai, M. Carreira, N. Inada and A. S. de Camargo, Functionalizing the mesoporous silica shell of upconversion nanoparticles to enhance bacterial targeting and killing via photosensitizer-induced antimicrobial photodynamic therapy, ACS Appl. Bio Mater., 2018, 1, 1028–1036 CrossRef PubMed.
- X. Chen, F. Wo, Y. Jin, J. Tan, Y. Lai and J. Wu, Drug-porous silicon dual luminescent system for monitoring and inhibition of wound infection, ACS Nano, 2017, 11, 7938–7949 CrossRef CAS PubMed.
- Z. Zhao, R. Yan, X. Yi, J. Li, J. Rao, Z. Guo, Y. Yang, W. Li, Y.-Q. Li and C. Chen, Bacteria-activated theranostic nanoprobes against methicillin-resistant Staphylococcus aureus infection, ACS Nano, 2017, 11, 4428–4438 CrossRef CAS PubMed.
- X. Zhai, B. Song, B. Chu, Y. Su, H. Wang and Y. He, Highly fluorescent, photostable, and biocompatible silicon theranostic nanoprobes against Staphylococcus aureus infections, Nano Res., 2018, 11, 6417–6427 CrossRef CAS.
- L. Zhang, X. Ji, Y. Su, X. Zhai, H. Xu, B. Song, A. Jiang, D. Guo and Y. He, Fluorescent silicon nanoparticles-based nanotheranostic agents for rapid diagnosis and treatment of bacteria-induced keratitis, Nano Res., 2021, 14, 52–58 CrossRef.
- J. Tang, B. Chu, J. Wang, B. Song, Y. Su, H. Wang and Y. He, Multifunctional nanoagents for ultrasensitive imaging and photoactive killing of Gram-negative and Gram-positive bacteria, Nat. Commun., 2019, 10, 4057 CrossRef PubMed.
- X. Lin, W. Li, Y. Wen, L. Su and X. Zhang, Aggregation-induced emission (AIE)-Based nanocomposites for intracellular biological process monitoring and photodynamic therapy, Biomaterials, 2022, 287, 121603 CrossRef CAS.
- H. Yuan, Z. Li, H. Bai, Z. Chen, C. Yao, S. Jia, L. Li, R. Qi and C.-H. Zhao, Aggregation-induced emission nanoparticles with NIR and photosensitizing characteristics for resistant bacteria elimination and real-time tracking, Mater. Chem. Front., 2021, 5, 6611–6617 RSC.
- H. Wu, Y. Fang, L. Tian, X. Liu, X. Zhou, X. Chen, H. Gao, H. Qin and Y Liu, AIE nanozyme-based long persistent chemiluminescence and fluorescence for POCT of pathogenic bacteria, ACS Sens., 2023, 8, 3205–3214 CrossRef CAS PubMed.
- Q. Li, Y. Wu, H. Lu, X. Wu, S. Chen, N. Song, Y.-W. Yang and H. Gao, Construction of supramolecular nanoassembly for responsive bacterial elimination and effective bacterial detection, ACS Appl. Mater. Interfaces, 2017, 9, 10180–10189 CrossRef CAS.
- R. Chen, C. Ren, M. Liu, X. Ge, M. Qu, X. Zhou, M. Liang, Y. Liu and F. Li, Early detection of SARS-CoV-2 seroconversion in humans with aggregation-induced near-infrared emission nanoparticle-labeled lateral flow immunoassay, ACS Nano, 2021, 15, 8996–9004 CrossRef CAS.
- B. Ren, K. Li, Z. Liu, G. Liu and H. Wang, White light-triggered zwitterionic polymer nanoparticles based on an AIE-active photosensitizer for photodynamic antimicrobial therapy, J. Mater. Chem. B, 2020, 8, 10754–10763 RSC.
- A. Panigrahi, V. N. Are, S. Jain, D. Nayak, S. Giri and T. K. Sarma, Cationic organic nanoaggregates as AIE luminogens for wash-free imaging of bacteria and broad-spectrum antimicrobial application, ACS Appl. Mater. Interfaces, 2020, 12, 5389–5402 CrossRef CAS.
- T. Gao, H. Zeng, H. Xu, F. Gao, W. Li, S. Zhang, Y. Liu, G. Luo, M. Li and D. Jiang, Novel self-assembled organic nanoprobe for molecular imaging and treatment of Gram-positive bacterial infection, Theranostics, 2018, 8, 1911 CrossRef CAS PubMed.
- W. Wang, Y. Gao, M. Zhang, Y. Li and B. Z. Tang, Neutrophil-like biomimic AIE nanoparticles with high-efficiency inflammatory cytokine targeting enable precise photothermal therapy and alleviation of inflammation, ACS Nano, 2023, 17, 7394–7405 CrossRef CAS.
- R. Hu, F. Zhou, T. Zhou, J. Shen, Z. Wang, Z. Zhao, A. Qin and B. Z. Tang, Specific discrimination of Gram-positive bacteria and direct visualization of its infection towards mammalian cells by a DPAN-based AIEgen, Biomaterials, 2018, 187, 47–54 CrossRef CAS.
- R. Hu, Q. Deng, Q. Tang, R. Zhang, L. Wang, B. Situ, C. Gui, Z. Wang and B. Z. Tang, More is less: creation of pathogenic microbe-related theranostic oriented AIEgens, Biomaterials, 2021, 271, 120725 CrossRef CAS.
- W. Wang, F. Wu, Q. Zhang, N. Zhou, M. Zhang, T. Zheng, Y. Li and B. Z. Tang, Aggregation-induced emission nanoparticles for single near-infrared light-triggered photodynamic and photothermal antibacterial therapy, ACS Nano, 2022, 16, 7961–7970 CrossRef CAS PubMed.
- J. Zhang, F. Zhou, Z. He, Y. Pan, S. Zhou, C. Yan, L. Luo and Y. Gao, AIEgen intercalated nanoclay-based photodynamic/chemodynamic theranostic platform for ultra-efficient bacterial eradication and fast wound healing, ACS Appl. Mater. Interfaces, 2022, 14, 30533–30545 CrossRef CAS.
- K. Xue, C. Yang, C. Wang, Y. Liu, J. Liu, L. Shi and C. Zhu, An exceptional broad-spectrum nanobiocide for multimodal and synergetic inactivation of drug-resistant bacteria, CCS Chem., 2022, 4, 272–285 CrossRef CAS.
- Y. Zhou, W. Wu, P. Yang, D. Mao and B. Liu, Near-infrared chemiluminescent nanoprobes for deep imaging and synergetic photothermal-nitric-oxide therapy of bacterial infection, Biomaterials, 2022, 288, 121693 CrossRef CAS PubMed.
- M. Chen, J. He, S. Xie, T. Wang, P. Ran, Z. Zhang and X. Li, Intracellular bacteria destruction via traceable enzymes-responsive release and deferoxamine-mediated ingestion of antibiotics, J. Controlled Release, 2020, 322, 326–336 CrossRef CAS.
- V. A. Spirescu, C. Chircov, A. M. Grumezescu and E. Andronescu, Polymeric nanoparticles for antimicrobial therapies: an up-to-date overview, Polymers, 2021, 13, 724 CrossRef CAS.
- P. Liu, L. Q. Xu, G. Xu, D. Pranantyo, K.-G. Neoh and E.-T. Kang, pH-sensitive theranostic nanoparticles for targeting bacteria with fluorescence imaging and dual-modal antimicrobial therapy, ACS Appl. Nano Mater., 2018, 1, 6187–6196 CrossRef CAS.
- W. Fan, H. Han, Z. Lu, Y. Huang, Y. Zhang, Y. Chen, X. Zhang, J. Ji and K. Yao, ε-poly-L-lysine-modified polydopamine nanoparticles for targeted photothermal therapy of drug-resistant bacterial keratitis, Bioeng. Transl. Med., 2023, 8, e10380 CrossRef CAS.
- R. Ghosh, M. Malhotra, R. R. M. Sathe and M. Jayakannan, Biodegradable polymer theranostic fluorescent nanoprobe for direct visualization and quantitative determination of antimicrobial activity, Biomacromolecules, 2020, 21, 2896–2912 CrossRef CAS.
- L. X. Yan, L. J. Chen, X. Zhao and X. P. Yan, pH switchable nanoplatform for in vivo persistent luminescence imaging and precise photothermal therapy of bacterial infection, Adv. Funct. Mater., 2020, 30, 1909042 CrossRef CAS.
- C. Wang, W. Zhao, B. Cao, Z. Wang, Q. Zhou, S. Lu, L. Lu, M. Zhan and X. Hu, Biofilm-responsive polymeric nanoparticles with self-adaptive deep penetration for in vivo photothermal treatment of implant infection, Chem. Mater., 2020, 32, 7725–7738 CrossRef CAS.
- H. Zhou, D. Tang, X. Kang, H. Yuan, Y. Yu, X. Xiong, N. Wu, F. Chen, X. Wang and H. Xiao, Degradable pseudo conjugated polymer nanoparticles with NIR-II photothermal
effect and cationic quaternary phosphonium structural bacteriostasis for anti-infection therapy, Adv. Sci., 2022, 9, 2200732 CrossRef CAS.
- X. Guo, B. Cao, C. Wang, S. Lu and X. Hu,
In vivo photothermal inhibition of methicillin-resistant Staphylococcus aureus infection by in situ templated formulation of pathogen-targeting phototheranostics, Nanoscale, 2020, 12, 7651–7659 RSC.
- F. Nicolson, A. Ali, M. F. Kircher and S. Pal, DNA nanostructures and DNA-functionalized nanoparticles for cancer theranostics, Adv. Sci., 2020, 7, 2001669 CrossRef CAS.
- Y. Zhang, W. Ma, Y. Zhu, S. Shi, Q. Li, C. Mao, D. Zhao, Y. Zhan, J. Shi, W. Li, L. Wang, C. Fan and Y. Lin, Inhibiting methicillin-resistant Staphylococcus aureus by tetrahedral DNA nanostructure-enabled antisense peptide nucleic acid delivery, Nano Lett., 2018, 18, 5652–5659 CrossRef CAS PubMed.
- Y. Liu, Y. Sun, S. Li, M. Liu, X. Qin, X. Chen and Y. Lin, Tetrahedral framework nucleic acids deliver antimicrobial peptides with improved effects and less susceptibility to bacterial degradation, Nano Lett., 2020, 20, 3602–3610 CrossRef CAS.
- H. Jeon, H. Nam and J. B. Lee, Sustained release of minor-groove-binding antibiotic netropsin from calcium-coated groove-rich DNA particles, Pharmaceutics, 2019, 11, 387 CrossRef CAS PubMed.
- K. Zhang, W. Huang, Y. Huang, H. Li, K. Wang, X. Zhu and M. Xie, DNA tetrahedron based biosensor for argonaute2 assay in single cells and human immunodeficiency virus type-1 related ribonuclease H detection in vitro, Anal. Chem., 2019, 91, 7086–7096 CrossRef CAS.
- M. Ferreira, M. Ogren, J. N. Dias, M. Silva, S. Gil, L. Tavares, F. Aires-da-Silva, M. M. Gaspar and S. I. Aguiar, Liposomes as antibiotic delivery systems: a promising nanotechnological strategy against antimicrobial resistance, Molecules, 2021, 26, 2047 CrossRef CAS.
- J. Zheng, R. Hu, Y. Yang, Y. Wang, Q. Wang, S. Xu, P. Yao, Z. Liu, J. Zhou and J. Yang, Antibiotic-loaded reactive oxygen species-responsive nanomedicine for effective management of chronic bacterial prostatitis, Acta Biomater., 2022, 143, 471–486 CrossRef CAS PubMed.
- W. Liu, M. Li, B. Tian, X. Yang, W. Du, X. Wang, H. Zhou, C. Ding and S. Sai, Calcofluor white-cholesteryl hydrogen succinate conjugate mediated liposomes for enhanced targeted delivery of voriconazole into Candida albicans, Biomater. Sci., 2023, 11, 307–321 RSC.
- A. S. Clausen, D. E. Østergaard, P. Holmberg, J. R. Henriksen, J. Tham, P. P. Damborg, A. I. Jensen, A. Kjaer, A. E. Hansen and T. L. Andresen, Quantitative determination of 64Cu-liposome accumulation at inflammatory and infectious sites: potential for future theranostic system, J. Controlled Release, 2020, 327, 737–746 CrossRef CAS.
- M. Karpuz, A. Temel, E. Ozgenc, Y. Tekintas, G. Erel-Akbaba, Z. Senyigit and E. Atlihan-Gundogdu, 99mTc-labeled, colistin encapsulated, theranostic liposomes for Pseudomonas aeruginosa infection, AAPS PharmSciTech, 2023, 24, 77 CrossRef CAS PubMed.
- S.-H. Cheng, M. R. Groseclose, C. Mininger, M. Bergstrom, L. Zhang, S. C. Lenhard, T. Skedzielewski, Z. D. Kelley, D. Comroe and H. Hong, Multimodal imaging distribution assessment of a liposomal antibiotic in an infectious disease model, J. Controlled Release, 2022, 352, 199–210 CrossRef CAS.
- X. Pang, Q. Xiao, Y. Cheng, E. Ren, L. Lian, Y. Zhang, H. Gao, X. Wang, W. Leung and X. Chen, Bacteria-responsive nanoliposomes as smart sonotheranostics for multidrug resistant bacterial infections, ACS Nano, 2019, 13, 2427–2438 CAS.
- G. Qing, X. Zhao, N. Gong, J. Chen, X. Li, Y. Gan, Y. Wang, Z. Zhang, Y. Zhang and W. Guo, Thermo-responsive triple-function nanotransporter for efficient chemo-photothermal therapy of multidrug-resistant bacterial infection, Nat. Commun., 2019, 10, 4336 CrossRef.
- J. P. Clancy, L. Dupont, M. W. Konstan, J. Billings, S. Fustik, C. H. Goss, J. Lymp, P. Minic, A. L. Quittner, R. C. Rubenstein, K. R. Young, L. Saiman, J. L. Burns, J. R. W. Govan, B. Ramsey and R. Gupta, Phase II studies of nebulised Arikace in CF patients with Pseudomonas aeruginosa infection, Thorax, 2013, 68, 818–825 CrossRef CAS PubMed.
- O. O. Okusanya, S. M. Bhavnani, J. P. Hammel, A. Forrest, C. C. Bulik, P. G. Ambrose and R. Gupta, Evaluation of the pharmacokinetics and pharmacodynamics of liposomal amikacin for inhalation in cystic fibrosis patients with chronic pseudomonal infections using data from two phase 2 clinical studies, Antimicrob. Agents Chemother., 2014, 58, 5005–5015 CrossRef.
- K. V. Houser, G. L. Chen, C. Carter, M. C. Crank, T. A. Nguyen, M. C. Burgos Florez, N. M. Berkowitz, F. Mendoza, C. S. Hendel, I. J. Gordon, E. E. Coates, S. Vazquez, J. Stein, C. L. Case, H. Lawlor, K. Carlton, M. R. Gaudinski, L. Strom, A. R. Hofstetter, C. J. Liang, S. Narpala, C. Hatcher, R. A. Gillespie, A. Creanga, M. Kanekiyo, J. E. Raab, S. F. Andrews, Y. Zhang, E. S. Yang, L. Wang, K. Leung, W.-P. Kong, A. W. Freyn, R. Nachbagauer, P. Palese, R. T. Bailer, A. B. McDermott, R. A. Koup, J. G. Gall, F. Arnold, J. R. Mascola, B. S. Graham, J. E. Ledgerwood, J. Casazza, L. Holman, A. O. Ola, P. Costner, J. Cunningham, B. Larkin, L. Novik, J. Saunders, W. Whalen, X. Wang, A. M. Eshun, A. Taylor, K. C. Cheng, C. Laurencot, J. H. Cox, S. Sitar, L. Le, I. Pittman, O. Vasilenko, R. S. Rothwell, G. Yamshchikov, E. Burch, S. Hickman, O. Trofymenko, C. Boyd, P. Apte, C. T. Cartagena, R. Hicks, P. Williams, Z. Blaku and C. Tran, Safety and immunogenicity of a ferritin nanoparticle H2 influenza vaccine in healthy adults: a phase 1 trial, Nat. Med., 2022, 28, 383–391 CrossRef CAS.
- S. J. Thomas, E. D. Moreira, N. Kitchin, J. Absalon, A. Gurtman, S. Lockhart, J. L. Perez, G. Pérez Marc, F. P. Polack, C. Zerbini, R. Bailey, K. A. Swanson, X. Xu, S. Roychoudhury, K. Koury, S. Bouguermouh, W. V. Kalina, D. Cooper, R. W. Frenck, L. L. Hammitt, Ö. Türeci, H. Nell, A. Schaefer, S. Ünal, Q. Yang, P. Liberator, D. B. Tresnan, S. Mather, P. R. Dormitzer, U. Şahin, W. C. Gruber and K. U. Jansen, Safety and efficacy of the BNT162b2 mRNA Covid-19 vaccine through 6 months, N. Engl. J. Med., 2021, 385, 1761–1773 CrossRef CAS PubMed.
- P. T. Heath, E. P. Galiza, D. N. Baxter, M. Boffito, D. Browne, F. Burns, D. R. Chadwick, R. Clark, C. Cosgrove, J. Galloway, A. L. Goodman, A. Heer, A. Higham, S. Iyengar, A. Jamal, C. Jeanes, P. A. Kalra, C. Kyriakidou, D. F. McAuley, A. Meyrick, A. M. Minassian, J. Minton, P. Moore, I. Munsoor, H. Nicholls, O. Osanlou, J. Packham, C. H. Pretswell, A. San Francisco Ramos, D. Saralaya, R. P. Sheridan, R. Smith, R. L. Soiza, P. A. Swift, E. C. Thomson, J. Turner, M. E. Viljoen, G. Albert, I. Cho, F. Dubovsky, G. Glenn, J. Rivers, A. Robertson, K. Smith and S. Toback, Safety and efficacy of NVX-CoV2373 Covid-19 vaccine, N. Engl. J. Med., 2021, 385, 1172–1183 CrossRef CAS.
- H. Valizadeh, S. Abdolmohammadi-Vahid, S. Danshina, M. Ziya Gencer, A. Ammari, A. Sadeghi, L. Roshangar, S. Aslani, A. Esmaeilzadeh, M. Ghaebi, S. Valizadeh and M. Ahmadi, Nano-curcumin therapy, a promising method in modulating inflammatory cytokines in COVID-19 patients, Int. Immunopharmacol., 2020, 89, 107088 CrossRef CAS.
- N. M. Badawi, M. A. Elkafrawy, R. M. Yehia and D. A. Attia, Clinical comparative study of optimized metronidazole loaded lipid nanocarrier vaginal emulgel for management of bacterial vaginosis and its recurrence, Drug Delivery, 2021, 28, 814–825 CrossRef CAS PubMed.
- S. El-Housiny, M. A. Shams Eldeen, Y. A. El-Attar, H. A. Salem, D. Attia, E. R. Bendas and M. A. El-Nabarawi, Fluconazole-loaded solid lipid nanoparticles topical gel for treatment of pityriasis versicolor: formulation and clinical study, Drug Delivery, 2018, 25, 78–90 CrossRef CAS.
- J. D. Chalmers, D. Cipolla, B. Thompson, A. M. Davis, A. O'Donnell, G. Tino, I. Gonda, C. Haworth and J. Froehlich, Changes in respiratory symptoms during 48-week treatment with ARD-3150 (inhaled liposomal ciprofloxacin) in bronchiectasis: results from the ORBIT-3 and -4 studies, Eur. Respir. J., 2020, 56, 2000110 CrossRef CAS.
- C. S. Haworth, D. Bilton, J. D. Chalmers, A. M. Davis, J. Froehlich, I. Gonda, B. Thompson, A. Wanner and A. E. O'Donnell, Inhaled liposomal ciprofloxacin in patients with non-cystic fibrosis bronchiectasis and chronic lung infection with Pseudomonas aeruginosa (ORBIT-3 and ORBIT-4): two phase 3, randomised controlled trials, Lancet Respir. Med., 2019, 7, 213–226 CrossRef CAS PubMed.
- R. Nißler, O. Bader, M. Dohmen, S. G. Walter, C. Noll, G. Selvaggio, U. Groß and S. Kruss, Remote near infrared identification of pathogens with multiplexed nanosensors, Nat. Commun., 2020, 11, 5995 CrossRef.
- M. A. Abdou Mohamed, H. N. Kozlowski, J. Kim, K. Zagorovsky, M. Kantor, J. J. Feld, S. Mubareka, T. Mazzulli and W. C. W. Chan, Diagnosing antibiotic resistance using nucleic acid enzymes and gold nanoparticles, ACS Nano, 2021, 15, 9379–9390 CrossRef CAS.
- E. Mylonakis, C. J. Clancy, L. Ostrosky-Zeichner, K. W. Garey, G. J. Alangaden, J. A. Vazquez, J. S. Groeger, M. A. Judson, Y. M. Vinagre, S. O. Heard, F. N. Zervou, I. M. Zacharioudakis, D. P. Kontoyiannis and P. G. Pappas, T2 magnetic resonance assay for the rapid diagnosis of candidemia in whole blood: a clinical trial, Clin. Infect. Dis., 2015, 60, 892–899 CrossRef CAS.
- L. A. Neely, M. Audeh, N. A. Phung, M. Min, A. Suchocki, D. Plourde, M. Blanco, V. Demas, L. R. Skewis, T. Anagnostou, J. J. Coleman, P. Wellman, E. Mylonakis and T. J. Lowery, T2 magnetic resonance enables nanoparticle-mediated rapid detection of candidemia in whole blood, Sci. Transl. Med., 2013, 5, 182ra154 Search PubMed.
|
This journal is © the Partner Organisations 2024 |
Click here to see how this site uses Cookies. View our privacy policy here.