DOI:
10.1039/D3QI01576A
(Review Article)
Inorg. Chem. Front., 2024,
11, 29-49
Local structural environment of single-atom catalysts
Received
14th August 2023
, Accepted 7th November 2023
First published on 9th November 2023
Abstract
Single-atom catalysts (SACs) provide opportunities for bridging the gap between homogeneous and heterogeneous catalysis, facilitating the precise structural identification of the catalytically active sites, and offering new opportunities for interpreting the structure–performance relationship from the atomistic perspective. In view of this, the background of catalysis, catalysts and the history of the development of SACs are introduced in sequence. Subsequently, the correlation between the structure and performance of SACs is reviewed with respect to aspects of their local structural environment including metal single-atoms (MSA), amount of MSA loaded, electronegativity, oxidation state, coordination atom, and coordination number. By combining theoretical and experimental analyses, a deep understanding of the correlation between the structure and catalytic mechanism will shed light on the optimization and design of SACs toward high-efficiency chemical energy conversion. Finally, the whole review of SACs is summarized and future prospects are outlined.
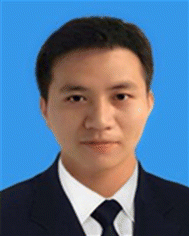 Zheng Chen | Zheng Chen obtained his PhD degree from RWTH Aachen University (Germany) in 2021, and currently works as a post-doctoral researcher at Fujian Institute of Research on the Structure of Matter, Chinese Academy of Sciences (CAS). His research focuses on the design and synthesis of novel inorganic materials and their applications in (photo)electrochemical energy conversion. |
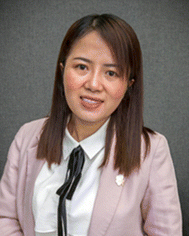 Lili Han | Lili Han obtained her PhD degree from Tianjin University. After that, she worked at Tianjin University of Technology, Brookhaven National Laboratory and the University of California, Irvine, before transferring to Research on the Structure of Matter, CAS, as a group leader in 2021. Her research interests are focused on transmission electron microscopy, three-dimensional reconstruction of electron tomography, and single-atom/nano-sized materials for energy conversion applications. |
Introduction
Catalysis, as the soul of chemistry, is one of the essential cornerstones of modern materials science and industrial production.1,2 It works as the engine of the chemical industry, being employed in more than 80% of the energy, chemical, medicine, and food production processes required for life in modern society.3,4 The realization of these processes is inseparable from recognizing catalytic reactions and understanding the essential properties of catalysts to develop new catalysts.
Nanotechnology has flourished in the past decades, driving the development of nanocatalysis science and technology and resulting in nanocatalysts emerging as the times require.5–8 Compared with traditional catalysts, the size of the active species of nanocatalysts is nanoscale, therefore nanocatalysts have higher specific surface areas with more exposed reaction sites.9–11 Due to the unique physicochemical properties of catalysts at the nanoscale, such as crystal face effects, size effects and synergistic effects, nanocatalysts exhibit excellent catalytic performances in many chemical reactions.12–14 Sub-nanoscale clusters outperform nanoscale particles in terms of catalytic activity and/or selectivity as demonstrated by theoretical and experimental findings.15 Therefore, downsizing the catalyst particles or clusters to single-atoms is extremely desirable for catalytic reactions because atomically isolated sites often function as active sites.14–21
Single metal atoms are ubiquitous in natural biological enzymes as active centers;22e.g. the active centers of chlorophyll, heme and nitrogenase are Mg, Fe and Mo atoms, respectively.23–25 These active single atoms combine and cooperate with surrounding biomolecules, and play a crucial catalytic role in life activities.26,27 The design and fabrication of isolated metal atoms as active species can be traced back to the pioneering work of Thomas et al. in 1995.28,29 They reported an oxygen-coordinated Ti-catalyst with a very high dispersion of isolated Ti atoms and they quantitatively determined the atomic environment of the active sites.29 In 2000, Heiz et al. prepared Pd clusters supported on MgO substrate as a catalyst and determined that one Pd atom was enough for the catalytic cyclotrimerization of acetylene.30 In 2003, Flytzani-Stephanopoulos et al. developed catalysts with nonmetallic gold or platinum species as the active species loaded on CeO2.31 A significant milestone was established by John Meurig Thomas, who introduced the concept of the single-site heterogeneous catalyst (SSHC) by immobilizing well-defined organometallic compounds on uniform supports like zeolites in 2005.32 In 2007, Lee et al. fabricated a single-site mesoporous Pd1/Al2O3 catalyst that was confirmed by atomic-level-resolution electron microcopy and extended X-ray absorption fine structure (EXAFS) analysis.33 Based on pioneering works, which include those of Heiz and Flytzani-Stephanopoulos, Thomas further proposed the hypothesis of single metal atoms as active sites for heterogeneous catalysis in 2011.31,34,35 In the same year, a SAC that consisted of isolated single Pt atoms anchored to FeOx was synthesized by Zhang et al.36 This catalyst has extremely high atom-efficiency, exhibiting excellent stability and high activity for CO oxidation and attracting extensive research interests.36 After that, numerous SACs,37–46 including non-noble metal and multiple-metal SACs,47–56 have been developed as excellent catalysts with special catalytic properties for application in photocatalysis,57–60 electrocatalysis,61–66 and thermocatalysis67–76 (Fig. 1).
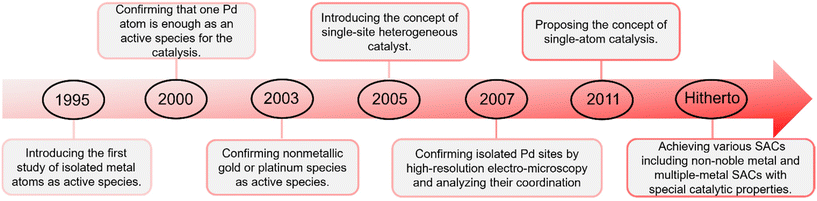 |
| Fig. 1 Schematic illustration of the timeline for the history of the monatomic catalyst. | |
SACs with atomically dispersed metal atoms anchored on supports offer a fundamental but powerful platform for probing and determining their structure–performance relationships at the atomic scale.37,77–85 However, there is still a lack of a universal design principle that can provide a universal interpretation of the relationship between the intrinsic properties of the active sites and the catalytic activity of supported SACs, as well as a set of unified guiding principles that govern the formation of SACs.28,86–94 Thus, analyses of individual elements of SACs not only provide unified principles to understand the nature of active sites in different kinds of SAC, but also inspire deeper insights into the SAC formation mechanism and shed light on carefully rationally designing SACs with effective targeting performance.
Regulation of the metal center of SACs
Metal single-atoms
Although metals are less abundant elements on Earth and expensive, their presence in catalysts is inherent to modulate chemical reactions through electrocatalysis, thermocatalysis and photocatalysis for energy conversion and storage, environmental treatment, and organic electrosynthesis (Table 1).95–99 Thus, there is longstanding interest in fabricating metal-containing catalysts, especially SACs featuring atomically dispersed metal atoms as powerful catalytically active centers with 100% atom-utilization efficiency (Fig. 2).100
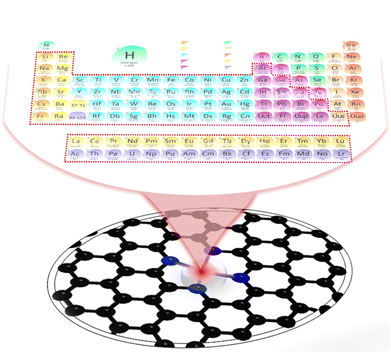 |
| Fig. 2 Schematic illustration of SACs across the periodic table. | |
Table 1 Various representative metal-based SACs with unique advantages towards high-efficiency electrochemical, photochemical and thermochemical applications
Metal |
Support |
Application |
Performance |
Ref. |
Mg |
Graphitic carbon nitride |
Electrochemical CO2 reduction reaction (CO2RR) to CO |
CO faradaic efficiency (FE): >90%, turnover frequency (TOF): 18 000 h−1, |
124
|
Al |
Nitrogen-doped carbon |
Photochemical CO2 cycloaddition |
Conversion efficiency: ≈95%, reaction rate: 3.52 mmol g−1 h−1 |
125
|
Ca |
N- and O- coordinated carbon matrix |
Electrochemical oxygen reduction reactions (ORRs) |
Half-wave potential (E1/2): 0.77 V, power density: 218 mW cm−2 |
126
|
Sc |
Nitrogen-doped carbon |
Electrochemical nitrogen reduction reaction (NRR) to ammonia |
Yield rate (YR): 20.4 μg cm−2 h−1, FE: 81.3% at −0.68 V |
127
|
Ti |
Reduced graphene oxide |
Cathodic reduction in hybrid photovoltaics |
Power conversion efficiencies: 20.6% |
128
|
V |
3D nitrogen-doped hierarchical carbon |
Na–S batteries |
Reversible capacity: 445 mA h g−1 over 800 cycles; rate capability: 224 mA h g−1 |
129
|
Cr |
Nitrogen-doped carbon |
Electrochemical ORR |
E
1/2: 0.773 V |
130
|
Mn |
Graphitic carbon nitride |
Electrochemical CO2RR to CO |
Current density: 14.0 mA cm−2, FE: 98.8%, |
131
|
Fe |
Nitrogen-doped carbon |
Electrochemical ORR |
E
1/2: 0.78 V |
129
|
Co |
nitrogen-doped carbon |
Electrochemical ORR |
Current density: 0.022 A cm−2 at 0.9 ViR-free, power density: 0.64 W cm−2 in 1.0 bar H2/O2 |
132
|
Ni |
MoS2 |
Electrochemical oxygen evolution reaction (OER) |
Overpotential at 20 mA cm−2 decreases from 465 to 174 mV |
133
|
Cu |
N- and O- coordinated carbon |
Selective oxidation of benzene |
Conversion efficiencies: 83.7%, selectivity of phenol: 98.1% |
134
|
Zn |
Nitrogen-doped carbon |
Electrochemical ORR |
E
1/2: 0.746 V in an acidic medium |
135
|
Ga |
N-, P- and S-coordinated carbon |
Electrochemical CO2RR to CO |
FE: 92% |
136
|
Y |
Nitrogen-doped carbon |
Electrochemical CO2RR to CO |
YR: 23.2 μg cm−2 h−1, FE: 88.3% at −0.58 V |
127
|
Zr |
N- and O- coordinated carbon matrix |
Zinc–air battery |
power density: 324 mW cm−2 |
137
|
Nb |
Graphitic carbon nitride |
Photocatalytic degrading amoxicillin |
Enhanced from 15.3% to 69.1% compared to pure g-C3N4 |
138
|
Mo |
Nitrogen-doped porous carbon |
Electrochemical NRR to NH3 |
YR: 34.0 ± 3.6 μg h−1 mgcat.−1, FE: 14.6 ± 1.6% in 0.1 M KOH |
139
|
Ru |
Nitrogen-doped carbon |
Thermochemical propane dehydrogenation |
Selectivity of propylene: around 92% |
140
|
Rh |
Nitrogen-doped carbon |
Electrochemical formic acid oxidation |
28- and 67- fold Enhancements compared with state-of-the-art Pd/C and Pt/C |
141
|
Pd |
TiO2 |
Hydrogenation of C C bonds |
100% styrene conversion |
142
|
Ag |
C3N4 nanotubes |
Photochemical CO2RR to CO |
YR: 0.32 μmol h−1, selectivity: >94% |
143
|
Cd |
N- and S- coordinated carbon matrix |
Electrochemical CO2RR to CO |
Current density: 182.2 mA cm−2, FE: 99.7%, TOF: 73 000 h−1 |
144
|
In |
Nitrogen-doped carbon |
Electrochemical CO2RR to formate |
Current density: 8.87 mA cm−2 FE: 96%, TOF: 12 500 h−1 |
145
|
Sn |
N- and O- coordinated carbon fibre |
Electrochemical CO2RR to CO |
Current density: over 240 mA cm−2, FE: 92.1% |
146
|
Sb |
Nitrogen-doped carbon |
Electrochemical CO2RR to CO |
TOF: 16 500 h−1 at −0.9 V |
147
|
Ba |
Pd1/Al2O3 |
Hydrogenation reaction |
Least increased 4-fold than Pd1/Al2O3 in activity |
148
|
La |
MoO3−x |
Photocatalytic NRR |
YR: 209.0 μmol h−1 g−1 |
149
|
Hf |
Two-dimensional extended porphyrin |
Electrochemical NRR to ammonia |
Onset potential: −0.53 V |
150
|
Ta |
Nitrogen-doped carbon |
Electrochemical NRR to ammonia |
YR: 19.97 mg h−1 mgcat.−1, FE: 8.52% |
151
|
W |
Nitrogen-doped carbon |
Photochemical water vapor oxidation |
YR of C1 oxygenates: 4956 μmol gcat.−1 |
152
|
Re |
Carbon black |
Electrochemical ORR |
E
1/2: 0.72 V |
153
|
Os |
sulfur-functionalized MXene Ti2C |
Low-temperature CO oxidation |
CO oxidation with a reaction barrier energy of only 0.74 eV, the highest stability |
154
|
Ir |
Nickel–iron sulfide nanosheet arrays |
Electrochemical OER |
Overpotential: ∼170 mV in 1.0 M KOH, TOF: 9.85 s−1 |
155
|
Pt |
Nitrogen-doped carbon |
Electrochemical HER |
Overpotential: 19 mV in 0.5 M H2SO4 |
156
|
Au |
CdS |
Photochemical CO2RR |
160- and 113-fold enhancement compared with pristine CdS for CO and CH4 generation |
157
|
Hg |
Three-dimensional Ag aerogel |
Oxidase-like reaction |
A specific enhancement of the surface-enhanced Raman spectroscopy effect |
158
|
Pb |
Polydopamine |
Thermal decomposition of cyclotrimethylenetrinitramine |
4.8 times faster than that without polydopamine-supported Pb-SACs |
159
|
Bi |
Nitrogen-doped carbon networks |
Electrochemical CO2RR to CO |
FE: 97%, current density: 3.9 mA cm−2, TOF: 5535 h−1 at 0.39 V |
160
|
By preparing the samples through different precursor reactions, SACs can be formed with various channels and anchoring groups as well as distribution of metals. One type of metal precursor is anchored on the support and forms atomic dispersions of monometallic active species, i.e. monometallic SACs.101–109 If an insufficient amount of a metal precursor is injected, some uncoordinated organic ligands will be retained and enable the coordination of another species of metal atoms, thus resulting in the formation of SAC with multi-metallic active species, i.e. multi-metallic SAC.110 Catalysts with an atomically bimetallic structure can deliver superior catalytic performance to that of monometallic SAC due to cooperation between the two metal atoms.111–114 The reported atomically bimetallic catalysts mostly feature supported diatomic-pair structures with M–M bonds or diatomic-ensemble structures with M–X–M (M′) linkages.115–123 For example, Hu et al. reported the synergistic effect of two sets of single-atom sites, specifically for Ni1 and Ru1 anchored on CeO2 for the dry reforming of CH4.55 Complementary theoretical analysis revealed that Ni1 activated CH4 while Ru1 dissociated CO2 in the catalytic reaction. Han et al. developed SACs based on 37 monometallic elements, including 1-, 2-, 8- and 12-metal SACs.54 Each metallic atom in the 12-metal SAC with Sc, Mn, Fe, Co, Ni, Cu, Zn, Y, Zr, Pd, Hf and W overcame aggregation with not only itself but also any of the other metallic atoms. This provides a demonstration of complex multimetallic phase for SACs and demonstrates that employing single atom anchor sites as structural units to construct concentration-complex SAC materials can be achievable and has no fundamental limit when the complex concentrations of down to 12 different elements.
These results demonstrate that metals especially the presence of MSAs in catalysts drive superior catalytic activities, as confirmed by complementary analyses of density functional theory (DFT) calculations and experiments. Meanwhile, regulating the metal centers of SACs, spanning from monometallic to concentration-complex multi-metallic materials, offers the opportunity to diversify the design of SACs.
Amount of MSA loaded
The amount of MSA loaded is a key factor as it dominates the density of active sites for targeted catalytic applications. Therefore, increasing the loading of MSA is one of the aspects to increase the number of active sites to boost their activity. It is well-accepted that the amount of MSA loaded is positively correlated to the ratios of metal precursor in all precursor and end up to the largest ratios of that, indicating that SACs in preparation have the available metal precursor ratios and the amount of metal precursors employed as the active centers can be adjusted to upper limit in a certain extent.99,120
In general, the amount of MSA in each SACs is usually near 0.1 wt%, because the obtainable SACs have a low loading of MSA to avoid aggregation due to the high surface energy of atomically dispersed atoms. To a large degree, this occurrence results from the lack of anchoring groups on the support to atomically stabilize the metal atoms or the relatively weak interactions between the anchoring groups of the support and the metal atoms. Karim et al. successfully synthesized an Ir-SAC with various amounts of Ir. The Ir single-atoms supported on MgAl2O4 exhibited outstanding catalytic behavior for CO oxidation when the amount of Ir loaded was 0.0025 wt%.161 Whilst Ir nanoparticles were clearly observed when the amount of Ir loaded was increased up to 1 wt%. In 2016, Li and co-workers made a significant breakthrough to accomplish an order-of-magnitude improvement in metal loading up to 4 wt% via rationally designing and preparing suitable supports to form structures with numerous anchoring groups and strong coordination environments; this holds great promise for achieving the practical application of SACs.162 Zeng et al. demonstrated that the amount of Pt supported on MoS2 obviously influenced the catalytic activity and selectivity for CO2 hydrogenation.45 Neighboring Pt monomers can be achieved by increasing the amount of Pt loaded up to 7.5 wt% while maintaining atomically dispersed Pt. Notably, for the basis of kinetic experiments, the activation energy for 7.5 wt% loading of Pt supported on MoS2 is 72.3 kJ mol−1, much lower than that of 124.7 kJ mol−1 for 0.2 wt% loading of Pt supported on MoS2. These results supposed that the higher loading of atomically isolated Pt monomers was favorable for CO2 hydrogenation compared to those with low loading.
A high amount of MSA is essential to maximize their potential in the catalytic industry.26,110,162–166 It is noteworthy that Lu et al. reported a versatile approach combining impregnation and two-step annealing to synthesize ultra-high-density SACs with up to 23 wt% loading of MSA for 15 SACs utilizing a standardized, automated protocol (Fig. 3). Drastically enhanced reactivity was observed upon increasing the amount of MSA loaded. Surprisingly, Li et al. successfully developed a Fe-SAC that could be easily synthesized at the gram scale with Fe loaded up to a record value of 30 wt%, which overcame the aggregation of single-atom sites, showing unprecedented catalytic performance and meeting the requirement for practical applications.165 Additionally, Han et al. developed 12-metal SACs with high metal loadings.54 The total metal loading for the 12-metal SAC reached a high value of 7.53 wt%, exhibiting higher activity than those of the mixed and individual monometallic SACs for the OER as well as demonstrating that there was no fundamental limit to assembling concentration-complex multi-metallic SAC with a high metal loading.
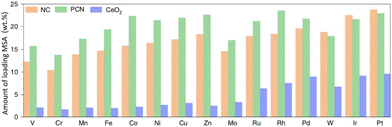 |
| Fig. 3 Loading of MSA achieved in this study on nitrogen-doped carbon (NC), polymeric carbon nitride (PCN) and CeO2 supports for 15 SACs. Reproduced with permission from ref. 163. Copyright 2022 Nature Publishing Group. | |
Regulating the amount of MSA loaded will affect the site density of SACs thus modulating the potential properties of SACs, e.g., the ORR and the cathodic process of fuel cell membrane electrodes. Recent breakthroughs in synthesizing SACs have led to MSA loadings above 10 wt%; these catalysts surpass the performance of the benchmark Pt/C (20 wt%) catalyst for the ORR. Noteworthily, Yu et al. synthesized a series of isolated Fe–N4 type SACs with different amounts of MSA loaded and demonstrated that strong interactions between adjacent Fe–N4 moieties altered the electronic structure when the inter-site distance was less than about 1.2 nm with the increased MSA loading, resulting in improved intrinsic ORR activity (Fig. 4).167 The noteworthy improvement in site performance proceeds until adjacent Fe atoms become as close as about 0.7 nm. Their results highlight the significance of identifying the fundamental mechanism of the inter-site distance effect with the amounts of MSA loaded in Fe–N4 type catalysts for the ORR, which promotes the development of targeted SACs with a high amount of MSA loaded.
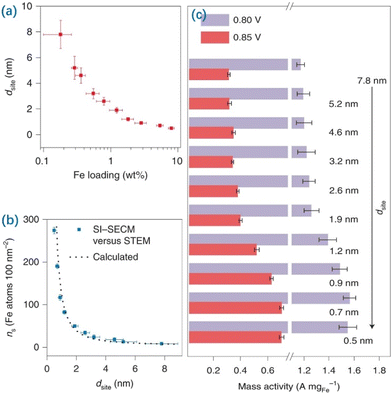 |
| Fig. 4 Correlation of Fe contents and site densities with different dsite values. (a) Calibration of dsite with statistical distributions against the corresponding Fe loading. The error bars correspond to standard deviations of Fe contents (x-axis) and dsite (y-axis) for each sample prepared in five independent trials. (b) The plot of Fe atom density (ns, Fe atoms per 100 nm−2) versus dsite. Error bars indicate standard deviations for ns in five experiments. The dotted curve shows the values for ns calculated according to assumed rectangular models. (c) Apparent ORR activity: the mass current density at 0.85 and 0.80 V normalized by the total weight of Fe in different samples. The error bars correspond to standard deviations of mass activity calculations from five groups of data for each sample. Reproduced with permission from ref. 167. Copyright 2021 Nature Publishing Group. | |
These results indicate that utilizing supports with a low metal loading could realize the atomic dispersion of mononuclear metal and precursors and reducing the metal loading could be a direct and effective approach to resist the agglomeration of metal species into nanoparticles. Furthermore, rationally designing and preparing suitable supports to form structures with numerous anchoring groups and strong coordination environments are beneficial for stabilizing metal atoms to achieve a much higher MSA loading, ensuring abundant active sites to target subsequent catalytic reactions.
Electronegativity
Electronegativity is a chemical property that describes the ability of an atom to attract an electron to itself. It is used to predict whether there is a bond between atoms and quantify the electron affinity of the element when the corresponding bond is formed.168 The electronegativity difference generated by different metal atoms in the catalyst induces significant charge redistribution around the metal atom, thus generating different interactions with multiple intermediates on various sites of the SAC system.44,168,169 The construction of controllable multifunctional active sites on the surface of the SAC by tailoring the electronegativity of the metal composition benefits the adsorption of relevant intermediates, stabilizing the intermediates and greatly enhancing the efficiency of the catalytic reaction. Simultaneously, creating highly active sites on the surface of the SAC by tailoring the electronegativity of the metal composition could optimize energy barriers and overcome kinetics issues for multistep reactions, accelerating the rates of reaction pathways during the catalytic process.
For example, regarding the correlation of the adsorption free energies of intermediates (ΔGOH* and ΔGH*) for the ORR, OER and HER with the electronegativity of atoms, Xu et al. plotted ΔGOH* and ΔGH*versus the descriptors θd * EM/EO and θd * EM/EH (Fig. 5), respectively (where θd, ΔGOH* and ΔGH* represent valence electrons in the d-orbitals of the metal, the free energy for OH* adsorption and the free energy for H* adsorption, respectively; EM, EO and EH represent the electronegativity of the metal, oxygen and hydrogen, respectively).169 The plots show that the descriptors have a strong correlation with ΔGOH* and ΔGH* for SACs under certain conditions (Fig. 5), suggesting that electronegativity and valence electrons in the d-orbitals of the metal can be used as response factors to evaluate the adsorption strength between the adsorbate and active metal center and affect the catalytic activity of the SACs for the HER, OER and ORR.
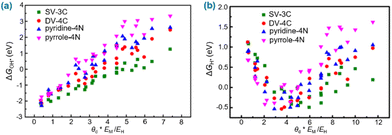 |
| Fig. 5 (a) ΔGOH* described by θd * EM/EO. (b) ΔGH* described by θd * EM/EH. Reproduced with permission from ref. 169. Copyright 2018 Nature Publishing Group. | |
Similarly, Guan et al. demonstrated that the catalytic activity and selectivity toward the CO2RR on transition-metals (TMs) among SACs were strongly correlated with the intrinsic properties and could be well described with a single descriptor, ψ, which was calculated based on a formula containing the valence-electron number and the electronegativity of metals:
| ψ = (Πi=1NSvi)2/N/(Πi=1Nχi)1/N | (1) |
where
N is the number of metals and neighboring nitrogen atoms;
Svi and
χi are the sum of the valence-electron number and the electronegativity of the metal and anchored nitrogen dopants, respectively.
170 In the research, TM-doped pyridine-like graphene was chosen as the test system, of which TMs surrounded with four pyridine N atoms, named M–N
4–G SACs. Complementary analyses of DFT calculations and experiments demonstrated that the reactivity and selectivity of M–N
4–G SACs were in good agreement with the trend Co–N
4–G > Fe–N
4–G > Mn–N
4–G > Cu–N
4–G > Ni–N
4–G, suggesting that the catalytic activity of the SACs for the CO
2RR could be predicted with the aid of the corresponding metal electronegativity.
Simultaneously, Han et al. found that the largest SAC metal precursor ratio was related to its metal electronegativity through a parabolic relationship; the ratio initially increases then flattens and finally decreases as the electronegativity increases (Fig. 6a).54 A parabolic relationship between electronegativity and MSA loading is not as obvious as the relationship between electronegativity and metal precursor ratio (Fig. 6b), since the amount of MSA loaded is not always proportional to the metal precursor ratio.110 This suggests that tailoring the metal electronegativity can achieve the corresponding SAC regulation.
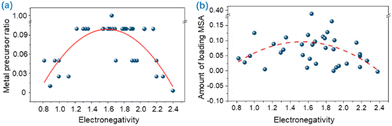 |
| Fig. 6 (a) Dependence of the largest SAC metal precursor ratios on electronegativity. (b) Dependence of the monometallic SAC loading of MSA on electronegativity. Reproduced with permission from ref. 54. Copyright 2022 Nature Publishing Group. | |
These results indicate that the local environment of the active metal center can impact on the catalytic activities of SACs, owing to the difference in adsorption free energies among SACs in different coordination environments with different charge distributions. Tailoring the electronegativity of the metal composition can achieve charge redistribution, leading to adjustments of the catalytic activities of SACs; this is also a powerful option for addressing the scaling relationship challenge.
Oxidation state
Although there is still confusion over how the oxidation state affects the catalytic activity for SACs clearly,162 it is undeniable that the oxidation state of the metal element of SACs is key for the local environment of the active sites and dominates the catalytic performance toward targeted reactions.171 In view of the electronic structure of SACs, the oxidation state of their metal sites can reflect electron transfer between metals and intermediates, which potentially has a relationship with the redox reaction activity.172
Gu et al. reported a Fe-SAC with dispersed Fe(III) ions coordinated with pyrrolic N atoms of the N-doped carbon support.173 Electrochemical results suggested that the superior activity of Fe3+ sites was derived from faster CO2 adsorption and weaker CO absorption compared to that of conventional Fe2+ sites while the iron ions maintained a +3 oxidation state during electrocatalysis. Jin et al. constructed a Ni-SAC with partially oxidized Ni single-atom sites in polymeric carbon nitride. XANES revealed that the oxidation state of Ni single-atom sites increased with the deepening of oxidation, getting closer to the oxidation state of Ni2+.174 X-ray photoelectron spectroscopy (XPS) further revealed that the oxidation state of the Ni single-atom active site was actually an intermediate oxidation state with a ratio of 2
:
1 for Ni2+
:
Ni0. Electron paramagnetic resonance spectroscopy (EPR) further found that the oxidized Ni single-atom active site had more d-orbitals with unpaired electrons occupying the valence band, which was positively correlated with the catalytic performance of Ni single-atom active sites, indicating that the unpaired electrons had a significant promoting effect on the photolysis of water for hydrogen production. X-ray absorption near-edge structure (XANES) and XPS revealed the oxidation state changes to the Ni single-atom active sites during the reaction. The catalyst was gradually oxidized during the reaction, affecting the catalytic activity. After the oxidation annealing process of the catalyst, the Ni single-atom active sites returned to the oxidation state before the reaction, and the photocatalytic performance was also restored. These results proved that the Ni single-atom active sites in the partially oxidized state were effective for carbon nitride photolysis with the best catalytic effect of hydrogen production from water.
Cao et al. developed a series of Os-SACs with oxidation states ranging from +0.9 to +2.9 produced by modulation of the coordination environments (Fig. 7).94 The Os-SACs showed a volcano relationship between oxidation state and HER activity, with an upsurge of activity achieved at a moderate oxidation state of +1.3 (Os-N3S1). Mechanistic analysis illustrated that increasing the oxidation states strengthened H atom adsorption on Os owing to increased energy levels and decreased occupancy of Os–H antibonding states, whereas further increasing the oxidation states weakened H atom adsorption due to the decreased occupancy of the Os–H bonding state (Fig. 7). This demonstrated the essential roles of oxidation states in manipulating catalytic activity, which was conducive to the rational design of SACs.
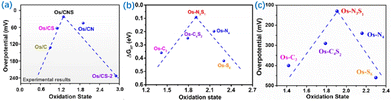 |
| Fig. 7 (a) The relationship between oxidation state and overpotential obtained from experiments for different Os-SAC. (b) DFT calculations. ΔGH* as a function of the oxidation state of Os-SACs. (c) Theoretical over-potential at 10 mA cm−2 as a function of oxidation state for different Os-SACs. Reproduced with permission from ref. 94. Copyright of 2022 Nature Publishing Group. | |
These results indicate that regulating the oxidation state offers an approach for tailoring SACs, which further provides a significant chance to rationally form SACs with their targeted activities. Furthermore, modulating the oxidation state is the key to understanding the structure–performance relationship and revealing the redox reaction mechanisms.
Regulation of the coordination environment for SACs
Coordination atoms
Except the active central metal is critical to driving a specific catalytic reaction, the coordination components of supports on the surface have essential roles in modulating the catalytic performances of SACs.175–183 This has prompted intense efforts toward the precise design of tailored structures by engineering the coordination environment with coordination atoms, from the first coordination shell to the second shell or higher for SACs.184–193 The first coordination shell refers to those atoms directly bonded to the central MSAs, while the second coordination shell refers to atoms bonded to the first-shell atoms but not to the central MSAs. The engineering of the first or higher coordination shells by precisely tailoring the heterogeneity of coordination atoms offers a great opportunity to tune the properties of SACs.194–202 Compared to engineering the first coordination shell, engineering the second and/or higher coordination shells would alter the distribution of the electron density over the central single metal sites indirectly and more moderately through long-range electron delocalization, thus tuning the catalytic performance of SACs.181 The effects of engineering the coordination shell is not just to redistribute uneven charge and thus optimize the adsorption energies of intermediate species, but also to modify the conductivity of the supported framework and thus improve charge conduction.203 The numerous types and variety of coordination elements (e.g. N, O, P, and S), as well as dual or multiple heteroatoms being simultaneously employed to coordinate with the MSA, offer a broad range of coordination configurations to rationally build SACs with targeting activities.44,204
Nitrogen possesses a lone pair of electrons, so its specific electric structure offers the potential to bond and stabilize the MSAs, forming M–Nx configurations and effectively regulating the electronic structure, light absorption, charge transfer, reaction barrier, etc.110 The stabilization effect mainly results from the strong coordination interactions between the lone pair of electrons of N and the d-orbitals of metals.169 Numerous SACs coordinated to N have been fulfilled. The incorporated N aids in activating the supporting materials for trapping electrons or protons.204 For example, Zhou et al. reported that Pt single-atom-coordinated ultrathin MOF nanosheets showed notable promotion of electron transfer and a decrease in the Gibbs free energy for the adsorbed H atoms, by constructing surface active sites for support materials through M–Nx configurations.164,204 In addition, Zhang et al. reported that the controllable synthesis of single cobalt atoms could achieve superior electrochemical activity by tuning C/N hybrid coordination precisely, because C/N hybrid coordination could enhance electron transfer to improve the adsorption and reaction kinetics of the intermediates.205,206 Zhang et al. established high-purity pyrrole-type and pyridine-type Fe–N4-based SACs, of which the coordinated N atoms were pyrrolic-N and pyridinic-N, respectively (Fig. 8). The pyrrole-type showed obviously better ORR activity than the pyridine-type in an acidic medium.207
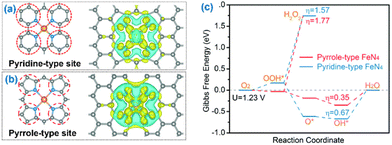 |
| Fig. 8 Illustrations of the (a) pyridine-type Fe–N4 and (b) pyrrole-type Fe–N4 geometries and their corresponding electron accumulation (yellow) and electron depletion (blue). (c) Free energy diagram of the oxygen reduction reaction on pyrrole-type Fe–N4 and pyridine-type Fe–N4. Reproduced with permission from ref. 207. Copyright 2020 Royal Society of Chemistry. | |
For O-coordinated SACs, their MSAs are anchored by coordinating with O atoms. Metal oxides as typical oxygenated compound supports are employed to stabilize the MSAs, where the coordinating O atoms are generally the lattice oxygen.208–214 The first report on the concept of SACs was the successful preparation of O-coordinated SACs with a structure in which an isolated single Pt atom was anchored to the FeOx support by forming a bond with two FeOx lattice O atoms.36 The strong bonding interaction with negatively charged O in the unsaturated state empowered the formation and stability of a positively charged Pt single-atom possessing partly empty d-orbitals. Distinguishing metal-oxides as support for the formation of SACs, some compounds do not contain oxygen intrinsically, but they can also anchor MSAs through metal–oxygen bonds because they possess incorporated O atoms or oxidized-functional groups after oxide-treated. For example, Wu et al. found that graphene oxide (GO) had dangling oxygen groups that possessed sufficient power to pluck the MSAs (Fe, Co, Ni, and Cu) from their corresponding bulk metal configurations with the assistance of sonication to form the target GO-supported SACs (Fig. 9).215
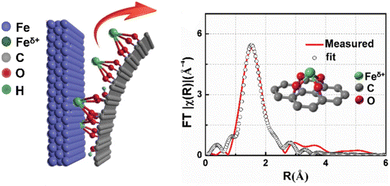 |
| Fig. 9 Schematic illustration of the construction and EXAFS fitting curve of Fe-single-atoms/GO, inset is the proposed Fe–O4 coordination environment. Reproduced with permission from ref. 215. Copyright 2019 John Wiley and Sons. | |
Phosphorus, as a group VA element resembling N, possesses a lone pair of electrons, making it a promising alternative to coordinate the metal atom. Different from N, P has a larger atomic radius and weaker electronegativity, which leads to the metal sites of the M–Px configuration having a greater electron density than those of the M–Nx configurations.204 Based on the properties of P, the P-ligand might work as a channel to transfer electrons from the supports to metal sites, thus promoting electron accumulation at the single metal sites. This electron-enriching behavior of M–Px benefits reduction processes at the active metal sites.216–218 For example, Zhou et al. developed a SAC with P-coordinated Pd single-atoms supported on g-C3N4 nanosheets.219 Compared to the corresponding N-coordinated SAC, its Pd single-atoms were less oxidized, and its Pd–P bond was longer than the Pd–N bond. Moreover, coordination with P atoms led to a decreased electron density within the supports, thus showing superior photocatalytic HER activity. Similarly, Zhou et al. developed a SAC with P-coordinated Co single-atoms supported on CdS (Fig. 10).220 The incorporated P can induce the decreased electron density of the CdS support and increase the electron density of CoP3 sites, tuning the electronic states of the active sites and thus exhibiting an amazing photocatalytic activity toward the dehydrogenation of formic acid.
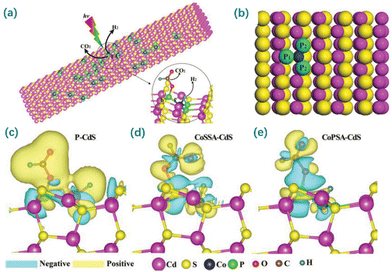 |
| Fig. 10 (a) Mechanism of formic acid dehydrogenation and hydrogen production for atomically dispersed Co–P3 species on CdS nanorods (CoPSA-CdS). (b) Geometric structure of CoPSA-CdS at the atomic level from the first-principles simulation. (c–e) The charge density difference maps between the adsorbed formic acid and CdS for P-CdS, sulfur-coordinated Co single atom-loaded CdS nanorods (CoSSA-CdS) and CoPSA-CdS. Reproduced with permission from ref. 220. Copyright 2019 John Wiley and Sons. | |
Sulfur, as another p-block element with moderate electronegativity, shows promising coordination and the ability to stabilize MSAs by forming the M–Sx configurations,204 especially when metal sulfides are used as supports to anchor the MSAs.45,221–225 This type of substitution would tune the electron density and modulate the activity of the catalytic site. For example, Bao et al. developed an S-coordinated SAC, where the Pt atoms substituted Mo sites and were atomically dispersed within MoS (Fig. 11).223 A suitable hydrogen adsorption energy was tuned by forming Pt–S–Mo, effectively boosting the HER activity. Additionally, the doped S atoms employed to stabilize MSAs can also be observed to form MSxHx (H = heteroatom).182,226,227 Tang et al. developed a Mo-SAC with isolated Mo atoms dispersed in the O and S codoped graphene matrix to form a mixture configuration (MoOx, MoSx, and MoOxSx), where the isolated Mo atoms were anchored by O and S co-coordination.182 After tailoring the coordination environment surrounding the single Mo atoms by incorporating S, excellent catalytic activity toward the OER was exhibited.
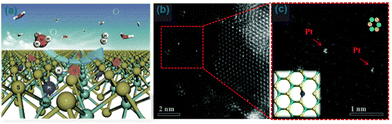 |
| Fig. 11 (a) Schematic illustration of the construction. (b) HAADF-STEM images of Pt-MoS2 showing that the single Pt atoms uniformly disperse in the 2D MoS2 plane. (c) Magnified domain of the red dashed rectangle in (b) showing a honeycomb arrangement of MoS2, and the single Pt atoms occupying the exact positions of the Mo atoms (marked by red arrows). Reproduced with permission from ref. 223. Copyright 2015 Royal Society of Chemistry. | |
In addition to the above-mentioned N, O, P and S doping, some studies have attempted doping with other nonmetallic atoms, like B, F and Cl, and identified a new synergistic mechanism.228–230 Typically, B, with an atomic radius close to that of carbon as well as the unique tri-electron structure, can be doped into the carbon skeleton without affecting its overall structure and introduce electron-deficient sites on the carbon substrate, achieving B-doped carbon-based SACs with higher conductance and ion adsorption capacity.231
These above results indicate that the catalytic activity of SACs is susceptible to their local coordination environment because the MSAs as the catalytically active centers are stabilized on the supports through coordination interactions from the viewpoint of coordination chemistry. Engineering the coordination environment with various coordination atoms, such as N, O, P and S, makes sense for developing effective SACs with optimal electronic structures for their targeted catalytic applications.
Coordination number
The coordination number of SACs, as a crucial parameter in the modulation of the coordination microenvironment, would significantly affect the electronic and geometric structure of active centers in SACs, playing important roles in regulating catalytic activity and selectivity.204,232–236
Despite tremendous advances in developing SACs, an effective strategy for precisely regulating coordination numbers without interfering with the single dispersed metal centers is lacking.237–240 MOFs have been well-recognized as one of the most promising candidates for the precise regulation of the coordination environment of SACs.241–244 The normally adopted strategy used to modulate the coordination number is exclusively restricted to the one-step pyrolysis of metal-doped MOF precursors, for which a higher temperature results in lower N coordination numbers for MOF-derived M–N type SACs. Given that MOF-derived SAC formation is synchronous with pyrolysis, complex synthesis parameters, such as pre-reduction and pyrolysis temperature, have a severe influence on the flexibility and universality of regulating SACs. Thus, it is possible that a promising and manageable solution to manipulate the coordination microenvironment of SACs could come from the decoupling of MOF carbonization and single-atom decoration, which would avoid complicated variables for pyrolysis.178
Wang et al. developed Mo–Nx–C (x = 2, 3, 4) type Mo-SACs by controlling the Mo–Nx coordination numbers (Fig. 12a).245 These three Mo–Nx–C catalysts containing similar Mo contents but different N contents were obtained by tailoring host–guest templates of Mo-doped ZIF-8 precursor at 800 °C, 900 °C and 1000 °C, and these catalysts were named Mo–N4–C, Mo–N3–C and Mo–N2–C, respectively. The Mo–N3–C catalyst was identified as the most promising nanozyme candidate with exclusive peroxidase-like behavior, geometrical structure differences and different orientation relationships of the frontier molecular orbitals (Fig. 12b and c). Similarly, Wang et al. developed a series of Co-SACs with different nitrogen coordination numbers of the Co center (Fig. 12d), by regulating the pyrolysis temperature.246 Among the three different types of Co–Nx moieties (x = 2, 3, 4), the CO2RR activity for Co–N2 was significantly higher than those for the others (Fig. 12e and f). Jiang et al. developed a facile post-synthetic metal substitution strategy to achieve the tailored synthesis of Ni-SAC with a low coordination number on N-doped carbon support.247 The Ni-SAC was synthesized by pyrolysis of the Zn-MOFs at high temperature to vaporize their low boiling point Zn atoms and then treating them in acidic media to remove Zn atoms from the Zn–N3–C sites, followed by treatment to refill abundant Zn vacancies with Ni atoms (Fig. 12g). The formed Ni–N3–C type SAC exhibited superior performance under certain conditions compared with Ni–N4–C (Fig. 12h and i). Xiao et al. successfully synthesized a Cu-SAC supported on g-C3N4 planes with two types of Cu–Nx configurations.248 The Cu–N3 configuration was constructed between a single Cu atom and three N atoms of the monolayer of carbon nitride, while the Cu–N4 configuration was constructed between a single Cu atom and four N atoms of two layers of carbon nitride. The simultaneous presence of Cu–N3 and Cu–N4 dramatically exhibited superior charge transfer and thus improved the performance of the solar-driven HER.181,248
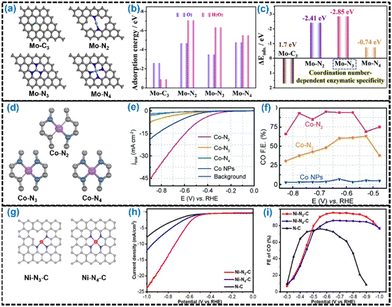 |
| Fig. 12 (a) Different potential peroxidase-like models with optimized top Mo–C3, Mo–N2, Mo–N3, and Mo–N4 structures by DFT calculations. (b) Adsorption energies for O2 and H2O2 adsorption on Mo–C3, Mo–N2, Mo–N3 and Mo–N4 structures. (c) Different values of H2O2 and O2 adsorption energies on Mo–C3, Mo–N2, Mo–N3 and Mo–N4 structures. Reproduced with permission from ref. 245. Copyright 2021 Elsevier Inc. (d) Schematic illustration of the geometric configurations of Co–N2, Co–N3 and Co–N4. (e) LSV curves of Co–N2, Co–N3, Co–N4 and Co nanoparticles (NPs) and pure carbon paper as a background. (f) CO faradaic efficiencies at different applied potentials for different catalysts. Reproduced with permission from ref. 246. Copyright 2018 John Wiley and Sons. (g) Schematic illustration of the geometric configurations of Ni–N3–C and Ni–N4–C. (h) LSV curves and (i) faradaic efficiencies of Ni–N3−C, Ni–N4−C and N–C. Reproduced with permission from ref. 247. Copyright 2021 John Wiley and Sons. | |
These results support the importance of the coordination number. The variation in the number of coordinated atoms in SACs can induce different distributions of local electron densities of the central MSA leading to catalytic activation sites being jointly constructed from coordinated atoms and MSAs, which significantly impacts on the adsorption and desorption of reaction intermediates, thus modulating the catalytic activity and selectivity of SACs.
Conclusions and perspectives
The SAC has become one of the most active research frontiers in the field of catalysis. Herein, the nature of catalysis has been reviewed, for which the catalysts, from bulk materials, nanoparticles, and clusters to single-atoms, play a fundamental role and sequentially possess higher atomic utilization and special activity. Benefiting from the unique physicochemical properties of SACs, important developments for SACs have been reviewed chronologically and their corresponding opportunities have been introduced.
Given that catalytic reactions require catalysts with appropriate active sites, the unique advantages of SACs are obvious in terms of their catalytic activity. The library of SACs includes various SACs that offer a database showing the relationship between their structure and special catalytic activity. Here we systematically elaborated on the catalytic properties of SACs in terms of their structural elements including MSA, amount of MSA, metal electronegativity, oxidation state, coordination atoms, and coordination number. Thus, analyzing individual formation elements of SACs aids in the identification of the real active sites and unveils the catalytic mechanisms for single-atom catalysis, while summarizing the unified principles sheds light on rationally designing SACs with special performances.
With the development of research, the knowledge and understanding of SACs have achieved remarkable progress. Considering the enormous potential of SACs, plenty of opportunities have sprung up and pushed the rapid development further. Specifically, future improvement in this field can be tied to the following aspects:
The success of synthesizing various SACs with unique interactions between the supports and the active sites enables them to exhibit unique catalytic activities, which surpass those of traditional catalysts. However, to further enhance catalytic processes, precisely regulating the electric structures is highly desirable; this holds enormous promise for modifying and optimizing the specific performance of SACs. Apart from precisely regulating SAC structures with dual or multiple metals as active centers and regulating the coordination environment with different types of coordinated atoms and numbers from the first shell to those beyond, the supports should also be considered to achieve the rational regulation of their electric structures and thus can create possible synergistic effects that potentially further enhance atom utilization and reduce energy barriers as well as improve catalytic efficiency for target catalytic applications.
In situ characterization technologies help to understand the catalytic process of SACs, the mechanism of interaction with reactants and the dynamic evolution of the active site. However, understanding their dynamics in practical working states is still inadequate. Therefore, continued efforts should be devoted to investigating the structure–performance relationship and catalytic mechanisms at the atomic level by employing the SAC library as model systems with the assistance of theoretical simulations and analyses combined with more accurate in situ studies including in situ electron microscopy and in situ spectra analyses. These are urgently required to elucidate and provide deep insights into the catalytic mechanism and dynamics of SACs in the reaction system.
To meet the demand for fundamental research and practical applications of catalysis, improving the MSA loading with uniform dispersion and stability while achieving large-scale and green synthesis processes are major vital avenues. Exploring SACs with a high loading of MSA with uniform dispersion is an important pathway to further enhance the catalytic performance. On the other hand, the stability of currently available single-atom-based catalysts is normally unsatisfactory because the MSAs are extremely active, causing them to rapidly react with the surrounding reactants to form other undesired species. In view of this, it is significant to explore and develop novel strategies to controllably synthesize SACs with a high loading of MSA and robustness. Additionally, the synthesis of SACs for large-scale production usually involves preparing precursors and multiple subsequent processes, which may entail harsh reaction conditions, the generation of toxic byproducts and high production costs. Thus, optimizing the chemical production process, alleviating environmental pollution and reducing production costs are highly desired to achieve SACs via “green” technology for large-scale manufacturing.
Overall, SACs hold great potential for advancing the development of various catalytic applications and pushing them toward commercialization. Much effort should be continuously devoted to the SAC area, achieving the design of specific catalysts for the targeted reactions and promoting the atomic-economic green catalytic process leading to an improvement in our daily lives in the future.
Conflicts of interest
There are no conflicts to declare.
Acknowledgements
The authors gratefully acknowledge the National Key Research and Development Program of China for Young Scientists (2022YFA1505700) and the National Natural Science Foundation of China (22205232) for financial support.
References
- K. Jiang, S. Back, A. J. Akey, C. Xia, Y. Hu, W. Liang, D. Schaak, E. Stavitski, J. K. Nørskov, S. Siahrostami and H. Wang, Highly selective oxygen reduction to hydrogen peroxide on transition metal single atom coordination, Nat. Commun., 2019, 10, 5–6 CrossRef.
- I. Fechete, Y. Wang and J. C. Védrine, The past, present and future of heterogeneous catalysis, Catal. Today, 2012, 189, 2–27 CrossRef CAS.
-
K. Ding and Y. Uozumi, Handbook of Heterogeneous Asymmetric Phase-Transfer New Frontiers in Asymmetric, 2008 Search PubMed.
- Z. Li, S. Ji, Y. Liu, X. Cao, S. Tian, Y. Chen, Z. Niu and Y. Li, Well-Defined Materials for Heterogeneous Catalysis: From Nanoparticles to Isolated Single-Atom Sites, Chem. Rev., 2020, 120, 623–682 CrossRef CAS.
- Y. Zhang, Z. Shen, C. Liu, X. Mao, Y. Zhuang, S. Chen and Y. Tang, A Quadrupole Receiving Coil With Series-Connected Diode Rectifiers for Interoperability of Nonpolarized and Polarized Transmitting Coils, IEEE Trans. Power Electron., 2023, 38, 8000–8004 Search PubMed.
- Y. Zhang, Y. Wu, C. Liu, Z. Shen, H. Wang, W. Pan, X. Mao and Z. Li, Passive Paralleling of Multi-Phase Diode Rectifier for Wireless Power Transfer Systems, IEEE Trans. Circuits Syst. II Express Briefs, 2023, 70, 646–649 Search PubMed.
- X. Yuan and M. Zhu, Recent advances in atomically precise metal nanoclusters for electrocatalytic applications, Inorg. Chem. Front., 2023, 10, 3995–4007 RSC.
- S. Electrocatalysts, X. Yang, W. Song, T. Zhang, Z. Huang, J. Zhang and J. Ding, Review of Emerging Atomically Precise Composite Site-Based Electrocatalysts, Adv. Energy Mater., 2023, 13, 2301737 CrossRef.
- J. Guo, Y. Zheng, Z. Hu, C. Zheng, J. Mao, K. Du, M. Jaroniec, S. Z. Qiao and T. Ling, Direct seawater electrolysis by adjusting the local reaction environment of a catalyst, Nat. Energy, 2023, 8, 264–272 CAS.
- K. Du, L. Zhang, J. Shan, J. Guo, J. Mao, C. C. Yang, C. H. Wang, Z. Hu and T. Ling, Interface engineering breaks both stability and activity limits of RuO2 for sustainable water oxidation, Nat. Commun., 2022, 13, 1–9 Search PubMed.
- Y. Yang, C. Hu, J. Shan, C. Cheng, L. Han, X. Li, R. Wang, W. Xie, Y. Zheng and T. Ling, Electrocatalytically Activating and Reducing N2 Molecule by Tuning Activity of Local Hydrogen Radical, Angew. Chem., Int. Ed., 2023, 62, e202300989 CrossRef CAS.
- M. Luo and S. Guo, Strain-controlled electrocatalysis on multimetallic nanomaterials, Nat. Rev. Mater., 2017, 21, 17059 CrossRef.
- J. N. Kuhn, W. Huang, C. K. Tsung, Y. Zhang and G. A. Somorjai, Structure sensitivity of carbon-nitrogen ring opening: Impact of platinum particle size from below 1 to 5 nm upon pyrrole hydrogenation product selectivity over monodisperse platinum nanoparticles loaded onto mesoporous silica, J. Am. Chem. Soc., 2008, 130, 14026–14027 CrossRef CAS.
- S. Cao, F. F. Tao, Y. Tang, Y. Li and J. Yu, Size- and shape-dependent catalytic performances of oxidation and reduction reactions on nanocatalysts, Chem. Soc. Rev., 2016, 45, 4747–4765 RSC.
- L. Liu and A. Corma, Metal Catalysts for Heterogeneous Catalysis: From Single Atoms to Nanoclusters and Nanoparticles, Chem. Rev., 2018, 118, 4981–5079 CrossRef CAS.
- G. F. S. R. Rocha, M. A. R. da Silva, A. Rogolino, G. A. A. Diab, L. F. G. Noleto, M. Antonietti and I. F. Teixeira, Carbon nitride based materials: more than just a support for single-atom catalysis, Chem. Soc. Rev., 2023, 52, 4878–4932 RSC.
- T. Bai, D. Li, S. Xiao, F. Ji, S. Zhang, C. Wang, J. Lu, Q. Gao and L. Ci, Recent progress on single-atom catalysts for lithium-air battery applications, Energy Environ. Sci., 2023, 16, 1431–1465 RSC.
- S. Wang, L. Wang, D. Wang and Y. Li, Recent advances of single-atom catalysts in CO2 conversion, Energy Environ. Sci., 2023, 16, 2759–2803 RSC.
- P. Zhang, K. Chen, J. Li, M. Wang, M. Li, Y. Liu and Y. Pan, Bifunctional Single Atom Catalysts for Rechargeable Zinc–Air Batteries: From Dynamic Mechanism to Rational Design, Adv. Mater., 2023, 2303243 CrossRef CAS.
- Y. Gao, B. Liu and D. Wang, Microenvironment Engineering of Single/Dual–Atom Catalysts for Electrocatalytic Application, Adv. Mater., 2023, 35, 2209654 CrossRef CAS.
- H. Zhong, Y. Su, Y. Wu, J. Gu, R. Ma, Y. Luo, H. Lin, M. Tao, J. Chen, Z. Liang, K. Wang, X. Zheng, Z. Chen, J. Peng, Z. Lv, Z. Gong, J. Huang and Y. Yang, Long-Life and High-Loading All-Solid-State Li–S Batteries Enabled by Acetylene Black with Dispersed Co-N4 as Single Atom Catalyst, Adv. Energy Mater., 2023, 13, 2300767 CrossRef CAS.
- Y. Wang, J. Mao, X. Meng, L. Yu, D. Deng and X. Bao, Catalysis with Two-Dimensional Materials Confining Single Atoms: Concept, Design, and Applications, Chem. Rev., 2019, 119, 1806–1854 CrossRef CAS.
- B. K. Burgess and D. J. Lowe, Mechanism of molybdenum nitrogenase, Chem. Rev., 1996, 96, 2983–3011 CrossRef CAS.
- J. R. Black, Q. Z. Yin, J. R. Rustad and W. H. Casey, Magnesium isotopic equilibrium in chlorophylls, J. Am. Chem. Soc., 2007, 129, 8690–8691 CrossRef CAS.
- W. Nam, High-valent iron (IV)-oxo complexes of heme and non-heme ligands in oxygenation reactions, Acc. Chem. Res., 2007, 40, 522–531 CrossRef CAS.
- A. Wang, J. Li and T. Zhang, Heterogeneous single-atom catalysis, Nat. Rev. Chem., 2018, 2, 65–81 CrossRef CAS.
- B. J. A. Osborn, G. Wilkinson, J. F. Young, R. D. Gillard, J. A. Osborn, P. B. Stockwell and P. Chem, Mild Hydroformylation of Olefins using Rhodium Catalysts, Chem. Commun., 1964, 17 Search PubMed.
- J. Xi, H. S. Jung, Y. Xu, F. Xiao, J. W. Bae and S. Wang, Synthesis Strategies, Catalytic Applications, and Performance Regulation of Single-Atom Catalysts, Adv. Funct. Mater., 2021, 31, 2008318 CrossRef CAS.
- M. Thomas, R. Fernando, S. Gopinathan and T. John Meurig, Heterogeneous catalysts obtained by grafting metallocene complexes onto mesoporous silica, Nature, 1995, 378, 159–162 CrossRef.
- S. Abbet, A. Sanchez, U. Heiz, W. D. Schneider, A. M. Ferrari, G. Pacchioni and N. Rösch, Acetylene cyclotrimerization on supported size-selected Pdn clusters (1 ≤ n ≤ 30): One atom is enough!, J. Am. Chem. Soc., 2000, 122, 3453–3457 CrossRef CAS.
- Q. Fu, H. Saltsburg and M. Flytzani-Stephanopoulos, Active nonmetallic Au and Pt species on ceria-based water-gas shift catalysts, Science, 2003, 301, 935–938 CrossRef CAS.
- J. M. Thomas, R. Raja and D. W. Lewis, Single-site heterogeneous catalysts, Angew. Chem.,
Int. Ed., 2005, 44, 6456–6482 CrossRef CAS.
- S. F. J. Hackett, R. M. Brydson, M. H. Gass, I. Harvey, A. D. Newman, K. Wilson and A. F. Lee, High-activity, single-site mesoporous Pd/Al2O3 catalysts for selective aerobic oxidation of allylic alcohols, Angew. Chem., Int. Ed., 2007, 46, 8593–8596 CrossRef CAS.
- J. M. Thomas, Z. Saghi and P. L. Gai, Can a single atom serve as the active site in some heterogeneous catalysts?, Top. Catal., 2011, 54, 588–594 CrossRef CAS.
- S. Abbet, A. M. Ferrari, L. Giordano, G. Pacchioni, H. Häkkinen, U. Landman and U. Heiz, Pd1/MgO(100): a model system in nanocatalysis, Surf. Sci., 2002, 514, 249–255 CrossRef CAS.
- B. Qiao, A. Wang, X. Yang, L. F. Allard, Z. Jiang, Y. Cui, J. Liu, J. Li and T. Zhang, Single-atom catalysis of CO oxidation using Pt1/FeOx, Nat. Chem., 2011, 3, 634–641 CrossRef CAS.
- S. F. Hung, A. Xu, X. Wang, F. Li, S. H. Hsu, Y. Li, J. Wicks, E. G. Cervantes, A. S. Rasouli, Y. C. Li, M. Luo, D. H. Nam, N. Wang, T. Peng, Y. Yan, G. Lee and E. H. Sargent, A metal-supported single-atom catalytic site enables carbon dioxide hydrogenation, Nat. Commun., 2022, 13, 819 CrossRef CAS.
- M. Flytzani-Stephanopoulos, Gold atoms stabilized on various supports catalyze the water-gas shift reaction, Acc. Chem. Res., 2014, 47, 783–792 CrossRef CAS.
- T. Cui, L. Li, C. Ye, X. Li, C. Liu, S. Zhu, W. Chen and D. Wang, Heterogeneous Single Atom Environmental Catalysis: Fundamentals, Applications, and Opportunities, Adv. Funct. Mater., 2022, 32, 2108381 CrossRef CAS.
- Z. Li, Y. Chen, S. Ji, Y. Tang, W. Chen, A. Li, J. Zhao, Y. Xiong, Y. Wu, Y. Gong, T. Yao, W. Liu, L. Zheng, J. Dong, Y. Wang, Z. Zhuang, W. Xing, C. T. He, C. Peng, W. C. Cheong, Q. Li, M. Zhang, Z. Chen, N. Fu, X. Gao, W. Zhu, J. Wan, J. Zhang, L. Gu, S. Wei, P. Hu, J. Luo, J. Li, C. Chen, Q. Peng, X. Duan, Y. Huang, X. M. Chen, D. Wang and Y. Li, Iridium single-atom catalyst on nitrogen-doped carbon for formic acid oxidation synthesized using a general host–guest strategy, Nat. Chem., 2020, 12, 764–772 CrossRef CAS.
- X. Wu, H. Zhang, S. Zuo, J. Dong, Y. Li, J. Zhang and Y. Han, Engineering the Coordination Sphere of Isolated Active Sites to Explore the Intrinsic Activity in Single-Atom Catalysts, Nano-Micro Lett., 2021, 13, 1–28 Search PubMed.
- A. Beniya and S. Higashi, Towards dense single-atom catalysts for future automotive applications, Nat. Catal., 2019, 2, 590–602 CrossRef.
- X. Sun, C. Chen, S. Liu, S. Hong, Q. Zhu, Q. Qian, B. Han, J. Zhang and L. Zheng, Aqueous CO2 Reduction with High Efficiency Using α-Co(OH)2-Supported Atomic Ir Electrocatalysts, Angew. Chem., Int. Ed., 2019, 58, 4669–4673 CrossRef CAS.
- Y. Wang, J. Mao, X. Meng, L. Yu, D. Deng and X. Bao, Catalysis with Two-Dimensional Materials Confining Single Atoms: Concept, Design, and Applications, Chem. Rev., 2019, 119, 1806–1854 CrossRef CAS.
- H. Li, L. Wang, Y. Dai, Z. Pu, Z. Lao, Y. Chen, M. Wang, X. Zheng, J. Zhu, W. Zhang, R. Si, C. Ma and J. Zeng, Synergetic interaction between neighbouring platinum monomers in CO2 hydrogenation, Nat. Nanotechnol., 2018, 13, 411–417 CrossRef CAS.
- Y. Shang, X. Xu, B. Gao, S. Wang and X. Duan, Single-atom catalysis in advanced oxidation processes for environmental remediation, Chem. Soc. Rev., 2021, 50, 5281–5322 RSC.
- B. Chen, T. Wang, S. Zhao, J. Tan, N. Zhao, S. P. Jiang, Q. Zhang, G. Zhou and H. M. Cheng, Efficient Reversible Conversion between MoS2 and Mo/Na2S Enabled by Graphene-Supported Single Atom Catalysts, Adv. Mater., 2021, 33, 2007090 CrossRef CAS.
- E. Luo, H. Zhang, X. Wang, L. Gao, L. Gong, T. Zhao, Z. Jin, J. Ge, Z. Jiang, C. Liu and W. Xing, Single-Atom Cr−N4 Sites Designed for Durable Oxygen Reduction Catalysis in Acid Media, Angew. Chem., Int. Ed., 2019, 131, 12599–12605 CrossRef.
- Q. Li, W. Chen, H. Xiao, Y. Gong, Z. Li, L. Zheng, X. Zheng, W. Yan, W. C. Cheong, R. Shen, N. Fu, L. Gu, Z. Zhuang, C. Chen, D. Wang, Q. Peng, J. Li and Y. Li, Fe Isolated Single Atoms on S, N Codoped Carbon by Copolymer Pyrolysis Strategy for Highly Efficient Oxygen Reduction Reaction, Adv. Mater., 2018, 30, 1800588 CrossRef.
- H. Yang, S. F. Hung, S. Liu, K. Yuan, S. Miao, L. Zhang, X. Huang, H. Y. Wang, W. Cai, R. Chen, J. Gao, X. Yang, W. Chen, Y. Huang, H. M. Chen, C. M. Li, T. Zhang and B. Liu, Atomically dispersed Ni(i) as the active site for electrochemical CO2 reduction, Nat. Energy, 2018, 3, 140–147 CrossRef CAS.
- L. Zhang, Y. Jia, G. Gao, X. Yan, N. Chen, J. Chen, M. T. Soo, B. Wood, D. Yang, A. Du and X. Yao, Graphene Defects Trap Atomic Ni Species for Hydrogen and Oxygen Evolution Reactions, Chem, 2018, 4, 285–297 CAS.
- K. Qi, X. Cui, L. Gu, S. Yu, X. Fan, M. Luo, S. Xu, N. Li, L. Zheng, Q. Zhang, J. Ma, Y. Gong, F. Lv, K. Wang, H. Huang, W. Zhang, S. Guo, W. Zheng and P. Liu, Single-atom cobalt array bound to distorted 1T MoS2 with ensemble effect for hydrogen evolution catalysis, Nat. Commun., 2019, 10, 5231 CrossRef.
- G. Liu, A. W. Robertson, M. M. J. Li, W. C. H. Kuo, M. T. Darby, M. H. Muhieddine, Y. C. Lin, K. Suenaga, M. Stamatakis, J. H. Warner and S. C. E. Tsang, MoS2 monolayer catalyst doped with isolated Co atoms for the hydrodeoxygenation reaction, Nat. Chem., 2017, 9, 810–816 CrossRef CAS.
- L. Han, H. Cheng, W. Liu, H. Li, P. Ou, R. Lin, H. T. Wang, C. W. Pao, A. R. Head, C. H. Wang, X. Tong, C. J. Sun, W. F. Pong, J. Luo, J. C. Zheng and H. L. Xin, A single-atom library for guided monometallic and concentration-complex multimetallic designs, Nat. Mater., 2022, 21, 681–688 CrossRef CAS.
- Y. Tang, Y. Wei, Z. Wang, S. Zhang, Y. Li, L. Nguyen, Y. Li, Y. Zhou, W. Shen, F. F. Tao and P. Hu, Synergy of single-atom Ni1 and Ru1 sites on CeO2 for dry reforming of CH4, J. Am. Chem. Soc., 2019, 141, 7283–7293 CrossRef CAS.
- R. Jiang, L. Li, T. Sheng, G. Hu, Y. Chen and L. Wang, Edge-Site Engineering of Atomically Dispersed Fe-N4 by Selective C-N Bond Cleavage for Enhanced Oxygen Reduction Reaction Activities, Journal, 2018, 140, 11594–11598 CrossRef CAS.
- W. Zhong, R. Sa, L. Li, Y. He, L. Li, J. Bi, Z. Zhuang, Y. Yu and Z. Zou, A Covalent Organic Framework Bearing Single Ni Sites as a Synergistic Photocatalyst for Selective Photoreduction of CO2 to CO, J. Am. Chem. Soc., 2019, 141, 7615–7621 CrossRef CAS.
- J. Xia, B. Wang, J. Di, Y. Li, S. Z. Yang, H. Li and S. Guo, Construction of single-atom catalysts for electro-, photo- and photoelectro-catalytic applications: State-of-the-art, opportunities, and challenges, Mater. Today, 2022, 53, 217–237 CrossRef CAS.
- Z. Jiang, W. Sun, W. Miao, Z. Yuan, G. Yang, F. Kong, T. Yan, J. Chen, B. Huang, C. An and G. A. Ozin, Living Atomically Dispersed Cu Ultrathin TiO2 Nanosheet CO2 Reduction Photocatalyst, Adv. Sci., 2019, 6, 1900289 CrossRef.
- H. Liu, M. Cheng, Y. Liu, J. Wang, G. Zhang, L. Li, L. Du, G. Wang, S. Yang and X. Wang, Single atoms meet metal-organic frameworks: collaborative efforts for efficient photocatalysis, Energy Environ. Sci., 2022, 15, 3722–3749 RSC.
- C. Kim, H. Min, J. Kim and J. H. Moon, Boosting electrochemical methane conversion by oxygen evolution reactions on Fe-N-C single atom catalysts, Energy Environ. Sci., 2023, 16, 3158–3165 RSC.
- X. Q. Xiao, X. Hu, Q. Liu, Y. Zhang, G. J. Zhang and S. Chen, Single-atom nanozymes as promising catalysts for biosensing and biomedical applications, Inorg. Chem. Front., 2023, 10, 4289–4312 RSC.
- Y. Wu, X. Wang, B. Tian, W. Shuang, Z. Bai and L. Yang, Optimizing reaction intermediate adsorption by engineering the coordination structure of single-atom Fe–N5–C electrocatalysts for efficient oxygen reduction, Inorg. Chem. Front., 2023, 10, 4209–4220 RSC.
- L. Shi, Y. Yan, Y. Wang, T. Bo, W. Zhou, X. Ren and Y. Li, Efficient and selective photocatalytic CO2 reduction over Ga single atom decorated quantum dots under visible light, Inorg. Chem. Front., 2023, 10, 2731–2741 RSC.
- L. Xiong, H. Qi, S. Zhang, L. Zhang, X. Liu, A. Wang and J. Tang, Highly Selective Transformation of Biomass Derivatives to Valuable Chemicals by Single-Atom Photocatalyst Ni/TiO2, Adv. Mater., 2023, 35, 1–9 Search PubMed.
- C. Peng, R. Pang, J. Li and E. Wang, Current Advances on the Single–Atom Nanozyme and its Bio–Applications, Adv. Mater., 2023, 2211724 CrossRef.
- L. Lin, S. Yao, R. Gao, X. Liang, Q. Yu, Y. Deng, J. Liu, M. Peng, Z. Jiang, S. Li, Y. W. Li, X. D. Wen, W. Zhou and D. Ma, A highly CO-tolerant atomically dispersed Pt catalyst for chemoselective hydrogenation, Nat. Nanotechnol., 2019, 14, 354–361 CrossRef CAS.
- Y. Zheng and S. Z. Qiao, Metal-organic framework assisted synthesis of single-atom catalysts for energy applications, Natl. Sci. Rev., 2018, 5, 626–627 CrossRef CAS.
- C. Riley, S. Zhou, D. Kunwar, A. De La Riva, E. Peterson, R. Payne, L. Gao, S. Lin, H. Guo and A. Datye, Design of Effective Catalysts for Selective Alkyne Hydrogenation by Doping of Ceria with a Single-Atom Promotor, J. Am. Chem. Soc., 2018, 140, 12964–12973 CrossRef CAS.
- Y. Kwon, T. Y. Kim, G. Kwon, J. Yi and H. Lee, Selective Activation of Methane on Single-Atom Catalyst of Rhodium Dispersed on Zirconia for Direct Conversion, J. Am. Chem. Soc., 2017, 139, 17694–17699 CrossRef CAS.
- J. Zhang, X. Wu, W. C. Cheong, W. Chen, R. Lin, J. Li, L. Zheng, W. Yan, L. Gu, C. Chen, Q. Peng, D. Wang and Y. Li, Cation vacancy stabilization of single-atomic-site Pt1/Ni(OH)x catalyst for diboration of alkynes and alkenes, Nat. Commun., 2018, 9, 1002 CrossRef.
- F. Huang, Y. Deng, Y. Chen, X. Cai, M. Peng, Z. Jia, P. Ren, D. Xiao, X. Wen, N. Wang, H. Liu and D. Ma, Atomically Dispersed Pd on Nanodiamond/Graphene Hybrid for Selective Hydrogenation of Acetylene, J. Am. Chem. Soc., 2018, 140, 13142–13146 CrossRef CAS.
- F. Huang, Y. Deng, Y. Chen, X. Cai, M. Peng, Z. Jia, J. Xie, D. Xiao, X. Wen, N. Wang, Z. Jiang, H. Liu and D. Ma, Anchoring Cu1 species over nanodiamond-graphene for semi-hydrogenation of acetylene, Nat. Commun., 2019, 10, 4431 CrossRef.
- Z. Li, M. Zhang, X. Dong, S. Ji, L. Zhang, L. Leng, H. Li, J. H. Horton, Q. Xu and J. Zhu, Strong electronic interaction of indium oxide with palladium single atoms induced by quenching toward enhanced hydrogenation of nitrobenzene, Appl. Catal., B, 2022, 313, 121462 CrossRef CAS.
- G. Lei, H. Pan, H. Mei, X. Liu, G. Lu, C. Lou, Z. Li and J. Zhang, Emerging single atom catalysts in gas sensors, Chem. Soc. Rev., 2022, 51, 7260–7280 RSC.
- P. Kumar, T. A. Al-Attas, J. Hu and M. G. Kibria, Single Atom Catalysts for Selective Methane Oxidation to Oxygenates, ACS Nano, 2022, 16, 8557–8618 CrossRef CAS.
- V. Giulimondi, A. Ruiz–Ferrando, A. H. Clark, S. K. Kaiser, F. Krumeich, A. J. Martín, N. López and J. Pérez–Ramírez, Catalytic Synergies in Bimetallic Ru-Pt Single–Atom Catalysts via Speciation Control, Adv. Funct. Mater., 2022, 32, 2206513 CrossRef CAS.
- K. Liu, B. Badamdorj, F. Yang, M. J. Janik and M. Antonietti, Water acting as a catalytic promoter for electron-proton transfer in the Pt single atom catalyzed environmental reduction reactions, Appl. Catal., B, 2022, 316, 121641 CrossRef CAS.
- Y. Li, Q. Guan, G. Huang, D. Yuan, F. Xie, K. Li, Z. Zhang, X. San and J. Ye, Low Temperature Thermal and Solar Heating Carbon-Free Hydrogen Production from Ammonia Using Nickel Single Atom Catalysts, Adv. Energy Mater., 2022, 12, 2202459 CrossRef CAS.
- C. Liu, G. Pan, N. Liang, S. Hong, J. Ma and Y. Liu, Ir Single Atom Catalyst Loaded on Amorphous Carbon Materials with High HER Activity, Adv. Sci., 2022, 9, 2105392 CrossRef CAS.
- X. Liu, L. Zheng, C. Han, H. Zong, G. Yang, S. Lin, A. Kumar, A. R. Jadhav, N. Q. Tran, Y. Hwang, J. Lee, S. Vasimalla, Z. Chen, S. G. Kim and H. Lee, Identifying the Activity Origin of a Cobalt Single-Atom Catalyst for Hydrogen Evolution Using Supervised Learning, Adv. Funct. Mater., 2021, 31, 2100547 CrossRef CAS.
- W. Da Zhang, H. Dong, L. Zhou, H. Xu, H. R. Wang, X. Yan, Y. Jiang, J. Zhang and Z. G. Gu, Fe single-atom catalysts with pre-organized coordination structure for efficient electrochemical nitrate reduction to ammonia, Appl. Catal., B, 2022, 317, 121750 CrossRef.
- M. Jiao, Z. Chen, N. Wang and L. Liu, DFT calculation screened CoCu and CoFe dual-atom catalysts with remarkable hydrogen evolution reaction activity, Appl. Catal., B, 2023, 324, 122244 CrossRef CAS.
- N. Karmodak, S. Vijay, G. Kastlunger and K. Chan, Computational Screening of Single and Di-Atom Catalysts for Electrochemical CO2 Reduction, ACS Catal., 2022, 12, 4818–4824 CrossRef CAS.
- C. Wang, K. Wang, Y. Feng, C. Li, X. Zhou, L. Gan, Y. Feng, H. Zhou, B. Zhang, X. Qu, H. Li, J. Li, A. Li, Y. Sun, S. Zhang, G. Yang, Y. Guo, S. Yang, T. Zhou, F. Dong, K. Zheng, L. Wang, J. Huang, Z. Zhang and X. Han, Co and Pt Dual-Single-Atoms with Oxygen-Coordinated Co–O–Pt Dimer Sites for Ultrahigh Photocatalytic Hydrogen Evolution Efficiency, Adv. Mater., 2021, 33, 2003327 CrossRef CAS.
- X. He, H. Zhang, X. Zhang, Y. Zhang, Q. He, H. Chen, Y. Cheng, M. Peng, X. Qin, H. Ji and D. Ma, Building up libraries and production line for single atom catalysts with precursor-atomization strategy, Nat. Commun., 2022, 13, 5721 CrossRef CAS.
- Y. Ling, H. Ge, J. Chen, Y. Zhang, Y. Duan, M. Liang, Y. Guo, T. S. Wu, Y. L. Soo, X. Yin, L. Ding and L. Wang, General Strategy toward Hydrophilic Single Atom Catalysts for Efficient Selective Hydrogenation, Adv. Sci., 2022, 9, 2202144 CrossRef CAS.
- Y. Zhang, L. Jiao, W. Yang, C. Xie and H. L. Jiang, Rational Fabrication of Low-Coordinate Single-Atom Ni Electrocatalysts by MOFs for Highly Selective CO2 Reduction, Angew. Chem., Int. Ed., 2021, 60, 7607–7611 CrossRef CAS.
- L. Su, P. Wang, X. Ma, J. Wang and S. Zhan, Regulating Local Electron Density of Iron Single Sites by Introducing Nitrogen Vacancies for Efficient Photo-Fenton Process, Angew. Chem., Int. Ed., 2021, 60, 21261–21266 CrossRef CAS.
- H. Zhang, X. Jin, J.-M. Lee and X. Wang, Tailoring of Active Sites from Single to Dual Atom Sites for Highly Efficient Electrocatalysis, ACS Nano, 2022, 16, 17572–17592 CrossRef CAS.
- G. Di Liberto, L. A. Cipriano and G. Pacchioni, Universal Principles for the Rational Design of Single Atom Electrocatalysts? Handle with Care, ACS Catal., 2022, 12, 5846–5856 CrossRef CAS.
- W. Yang, F. Polo-garzon, H. Zhou, Z. Huang and M. Chi, Boosting the Activity of Pd Single Atoms by Tuning Their Local Environment on Ceria for Methane Combustion, Angew. Chem., Int. Ed., 2023, 62, e202217323 CrossRef CAS.
- K. Maiti, S. Maiti, M. T. Curnan, H. J. Kim and J. W. Han, Engineering Single Atom Catalysts to Tune Properties for Electrochemical Reduction and Evolution Reactions, Adv. Energy Mater., 2021, 11, 2101670 CrossRef CAS.
- D. Cao, H. Xu, H. Li, C. Feng, J. Zeng and D. Cheng, Volcano-type relationship between oxidation states and catalytic activity of single-atom catalysts towards hydrogen evolution, Nat. Commun., 2022, 13, 5843 CrossRef CAS.
- C. Xie, Z. Niu, D. Kim, M. Li and P. Yang, Surface and Interface Control in Nanoparticle Catalysis, Chem. Rev., 2020, 120, 1184–1249 CrossRef CAS.
- A. Kulkarni, S. Siahrostami, A. Patel and J. K. Nørskov, Understanding Catalytic Activity Trends in the Oxygen Reduction Reaction, Chem. Rev., 2018, 118, 2302–2312 CrossRef CAS.
- X. Zhang, H. Shi and B. Q. Xu, Catalysis by gold: Isolated surface Au3+ ions are active sites for selective hydrogenation of 1,3-butadiene over Au/ZrO2 catalysts, Angew. Chem., Int. Ed., 2005, 44, 7132–7135 CrossRef CAS.
- S. T. Hunt and Y. Román-Leshkov, Principles and Methods for the Rational Design of Core-Shell Nanoparticle Catalysts with Ultralow Noble Metal Loadings, Acc. Chem. Res., 2018, 51, 1054–1062 CrossRef CAS.
- J. Liu, Catalysis by Supported Single Metal Atoms, ACS Catal., 2017, 7, 34–59 CrossRef CAS.
- S. K. Kaiser, Z. Chen, D. Faust Akl, S. Mitchell and J. Pérez-Ramírez, Single-Atom Catalysts across the Periodic Table, Chem. Rev., 2020, 120, 11703–11809 CrossRef CAS.
- N. Wang, X. Zhao, R. Zhang, S. Yu, Z. H. Levell, C. Wang, S. Ma, P. Zou, L. Han, J. Qin, L. Ma, Y. Liu and H. L. Xin, Highly Selective Oxygen Reduction to Hydrogen Peroxide on a Carbon-Supported Single-Atom Pd Electrocatalyst, ACS Catal., 2022, 12, 4156–4164 CrossRef CAS.
- L. Han, M. Hou, P. Ou, H. Cheng, Z. Ren, Z. Liang, J. A. Boscoboinik, A. Hunt, I. Waluyo, S. Zhang, L. Zhuo, J. Song, X. Liu, J. Luo and H. L. Xin, Local Modulation of Single-Atomic Mn Sites for Enhanced Ambient Ammonia Electrosynthesis, ACS Catal., 2021, 11, 509–516 CrossRef CAS.
- L. Han, Z. Ren, P. Ou, H. Cheng, N. Rui, L. Lin, X. Liu, L. Zhuo, J. Song, J. Sun, J. Luo and H. L. Xin, Modulating Single-Atom Palladium Sites with Copper for Enhanced Ambient Ammonia Electrosynthesis, Angew. Chem., Int. Ed., 2021, 60, 345–350 CrossRef CAS.
- L. Zhang, L. Han, H. Liu, X. Liu and J. Luo, Potential-Cycling Synthesis of Single Platinum Atoms for Efficient Hydrogen Evolution in Neutral Media, Angew. Chem., Int. Ed., 2017, 56, 13694–13698 CrossRef CAS.
- F. Lü, S. Zhao, R. Guo, J. He, X. Peng, H. Bao, J. Fu, L. Han, G. Qi, J. Luo, X. Tang and X. Liu, Nitrogen-coordinated single Fe sites for efficient electrocatalytic N2 fixation in neutral media, Nano Energy, 2019, 61, 420–427 CrossRef.
- L. Han, S. Song, M. Liu, S. Yao, Z. Liang, H. Cheng, Z. Ren, W. Liu, R. Lin, G. Qi, X. Liu, Q. Wu, J. Luo, H. L. Xin, X. Liu, Q. Wu, J. Luo and H. L. Xin, Stable and Efficient Single-Atom Zn Catalyst for CO2 Reduction to CH4, J. Am. Chem. Soc., 2020, 142, 12563–12567 CrossRef CAS.
- X. Peng, S. Zhao, Y. Mi, L. Han, X. Liu, D. Qi, J. Sun, Y. Liu, H. Bao, L. Zhuo, H. L. Xin, J. Luo and X. Sun, Trifunctional Single-Atomic Ru Sites Enable Efficient Overall Water Splitting and Oxygen Reduction in Acidic Media, Small, 2020, 16, 1–10 Search PubMed.
- L. Han, X. Liu, J. Chen, R. Lin, H. Liu, L. U. Fang, S. Bak, Z. Liang, S. Zhao, E. Stavitski, J. Luo, R. R. Adzic and H. L. Xin, Atomically Dispersed Molybdenum Catalysts for Efficient Ambient Nitrogen Fixation, Angew. Chem., Int. Ed., 2019, 58, 2321–2325 CrossRef CAS.
- Y. Zhang, G. Li, Z. Zhao, L. Han, Y. Feng, S. Liu, B. Xu, H. Liao, G. Lu, H. L. Xin and X. Huang, Atomically Isolated Rh Sites within Highly Branched Rh2Sb Nanostructures Enhance Bifunctional Hydrogen Electrocatalysis, Adv. Mater., 2021, 33, 1–9 Search PubMed.
- S. Ji, Y. Chen, X. Wang, Z. Zhang, D. Wang and Y. Li, Chemical Synthesis of Single Atomic Site Catalysts, Chem. Rev., 2020, 120, 11900–11955 CrossRef CAS.
- J. Zhang, Q. an Huang, J. Wang, J. Wang, J. Zhang and Y. Zhao, Supported dual-atom catalysts: Preparation, characterization, and potential applications, Chin. J. Catal., 2020, 41, 783–798 CrossRef CAS.
- E. Guan, J. Ciston, S. R. Bare, R. C. Runnebaum, A. Katz, A. Kulkarni, C. X. Kronawitter and B. C. Gates, Supported Metal Pair-Site Catalysts, ACS Catal., 2020, 10, 9065–9085 CrossRef CAS.
- J. Fu, J. Dong, R. Si, K. Sun, J. Zhang, M. Li, N. Yu, B. Zhang, M. G. Humphrey, Q. Fu and J. Huang, Synergistic effects for enhanced catalysis in a dual single-atom catalyst, ACS Catal., 2021, 11, 1952–1961 CrossRef CAS.
- H. Bai, D. Chen, Q. Ma, R. Qin, H. Xu, Y. Zhao, J. Chen and S. Mu, Atom Doping Engineering of Transition Metal Phosphides for Hydrogen Evolution Reactions, Electrochem. Energy Rev., 2022, 5, 24 CrossRef CAS.
- Z. Lu, B. Wang, Y. Hu, W. Liu, Y. Zhao, R. Yang, Z. Li, J. Luo, B. Chi, Z. Jiang, M. Li, S. Mu, S. Liao, J. Zhang and X. Sun, An Isolated Zinc–Cobalt Atomic Pair for Highly Active and Durable Oxygen Reduction, Angew. Chem., Int. Ed., 2019, 58, 2622–2626 CrossRef CAS.
- M. Zhang, Z. Hu, L. Gu, Q. Zhang, L. Zhang, Q. Song, W. Zhou and S. Hu, Electrochemical conversion of CO2 to
syngas with a wide range of CO/H2 ratio over Ni/Fe binary single-atom catalysts, Nano Res., 2020, 13, 3206–3211 CrossRef CAS.
- Y. Zhao, K. R. Yang, Z. Wang, X. Yan, S. Cao, Y. Ye, Q. Dong, X. Zhang, J. E. Thorne, L. Jin, K. L. Materna, A. Trimpalis, H. Bai, S. C. Fakra, X. Zhong, P. Wang, X. Pan, J. Guo, M. Flytzani-Stephanopoulos, G. W. Brudvig, V. S. Batista and D. Wang, Stable iridium dinuclear heterogeneous catalysts supported on metal-oxide substrate for solar water oxidation, Proc. Natl. Acad. Sci. U. S. A., 2018, 115, 2902–2907 CrossRef CAS.
- Y. Yang, Y. Yang, Y. Qian, H. Li, Z. Zhang, Y. Mu, D. Do, B. Zhou, J. Dong, W. Yan, Y. Qin, L. Fang, R. Feng, J. Zhou, P. Zhang, J. Dong, G. Yu, Y. Liu, X. Zhang, X. Zhang, X. Fan and X. Fan, O-coordinated W-Mo dual-atom catalyst for pH-universal electrocatalytic hydrogen evolution, Sci. Adv., 2020, 6, 1–14 Search PubMed.
- H. Wang, J. X. Liu, L. F. Allard, S. Lee, J. Liu, H. Li, J. Wang, J. Wang, S. H. Oh, W. Li, M. Flytzani-Stephanopoulos, M. Shen, B. R. Goldsmith and M. Yang, Surpassing the single-atom catalytic activity limit through paired Pt-O-Pt ensemble built from isolated Pt1 atoms, Nat. Commun., 2019, 10, 3808 CrossRef.
- Y. Pan, C. Zhang, Z. Liu, C. Chen and Y. Li, Structural Regulation with Atomic-Level Precision: From Single-Atomic Site to Diatomic and Atomic Interface Catalysis, Matter, 2020, 2, 78–110 CrossRef.
- X. Zhu, D. Zhang, C. J. Chen, Q. Zhang, R. S. Liu, Z. Xia, L. Dai, R. Amal and X. Lu, Harnessing the interplay of Fe–Ni atom pairs embedded in nitrogen-doped carbon for bifunctional oxygen electrocatalysis, Nano Energy, 2020, 71, 104597 CrossRef CAS.
- J. Jiao, R. Lin, S. Liu, W. C. Cheong, C. Zhang, Z. Chen, Y. Pan, J. Tang, K. Wu, S. F. Hung, H. M. Chen, L. Zheng, Q. Lu, X. Yang, B. Xu, H. Xiao, J. Li, D. Wang, Q. Peng, C. Chen and Y. Li, Copper atom-pair catalyst anchored on alloy nanowires for selective and efficient electrochemical reduction of CO2, Nat. Chem., 2019, 11, 222–228 CrossRef CAS.
- J. Wang, Z. Huang, W. Liu, C. Chang, H. Tang, Z. Li, W. Chen, C. Jia, T. Yao, S. Wei, Y. Wu and Y. Li, Design of N-Coordinated Dual-Metal Sites: A Stable and Active Pt-Free Catalyst for Acidic Oxygen Reduction Reaction, J. Am. Chem. Soc., 2017, 139, 17281–17284 CrossRef CAS.
- Q. Wang, K. Liu, J. Fu, C. Cai, H. Li, Y. Long, S. Chen, B. Liu, H. Li, W. Li, X. Qiu, N. Zhang, J. Hu, H. Pan and M. Liu, Atomically Dispersed s-Block Magnesium Sites for Electroreduction of CO2 to CO, Angew. Chem., Int. Ed., 2021, 60, 25241–25245 CrossRef CAS.
- Q. Yang, H. Peng, Q. Zhang, X. Qian, X. Chen, X. Tang, S. Dai, J. Zhao, K. Jiang, Q. Yang, J. Sun, L. Zhang, N. Zhang, H. Gao, Z. Lu and C. Liang, Atomically Dispersed High-Density Al–N4 Sites in Porous Carbon for Efficient Photodriven CO2 Cycloaddition, Adv. Mater., 2021, 33, 2103186 CrossRef CAS.
- Z. Lin, H. Huang, L. Cheng, W. Hu, P. Xu, Y. Yang, J. Li, F. Gao, K. Yang, S. Liu, P. Jiang, W. Yan, S. Chen, C. Wang, H. Tong, M. Huang, W. Zheng, H. Wang and Q. Chen, Tuning the p-Orbital Electron Structure of s-Block Metal Ca Enables a High-Performance Electrocatalyst for Oxygen Reduction, Adv. Mater., 2021, 33, 1–10 Search PubMed.
- J. Liu, X. Kong, L. Zheng, X. Guo, X. Liu and J. Shui, Rare Earth Single-Atom Catalysts for Nitrogen and Carbon Dioxide Reduction, ACS Nano, 2020, 14, 1093–1101 CrossRef CAS.
- S. Liang, C. Zhu, N. Zhang, S. Zhang, B. Qiao, H. Liu, X. Liu, Z. Liu, X. Song, H. Zhang, C. Hao and Y. Shi, A Novel Single-Atom Electrocatalyst Ti1/rGO for Efficient Cathodic Reduction in Hybrid Photovoltaics, Adv. Mater., 2020, 32, 1–8 Search PubMed.
- Y. Jiang, Z. Yu, X. F. Zhou, X. Cheng, H. Huang, F. Liu, Y. Yang, S. He, H. Pan, H. Yang, Y. Yao, X. Rui and Y. Yu, Single-Atom Vanadium Catalyst Boosting Reaction Kinetics of Polysulfides in Na–S Batteries, Adv. Mater., 2023, 35, 1–10 Search PubMed.
- E. Luo, H. Zhang, X. Wang, L. Gao, L. Gong, T. Zhao, Z. Jin, J. Ge, Z. Jiang, C. Liu and W. Xing, Single-Atom Cr−N4 Sites Designed for Durable Oxygen Reduction Catalysis in Acid Media, Angew. Chem., Int. Ed., 2019, 58, 12469–12475 CrossRef CAS.
- J. Feng, H. Gao, L. Zheng, Z. Chen, S. Zeng, C. Jiang, H. Dong, L. Liu, S. Zhang and X. Zhang, A Mn-N3 single-atom catalyst embedded in graphitic carbon nitride for efficient CO2 electroreduction, Nat. Commun., 2020, 11, 1–8 CrossRef.
- X. Xie, C. He, B. Li, Y. He, D. A. Cullen, E. C. Wegener, A. J. Kropf, U. Martinez, Y. Cheng, M. H. Engelhard, M. E. Bowden, M. Song, T. Lemmon, X. S. Li, Z. Nie, J. Liu, D. J. Myers, P. Zelenay, G. Wang, G. Wu, V. Ramani and Y. Shao, Performance enhancement and degradation mechanism identification of a single-atom Co–N–C catalyst for proton exchange membrane fuel cells, Nat. Catal., 2020, 3, 1044–1054 CrossRef CAS.
- T. Sun, Z. Tang, W. Zang, Z. Li, J. Li, Z. Li, L. Cao, J. S. Dominic Rodriguez, C. O. M. Mariano, H. Xu, P. Lyu, X. Hai, H. Lin, X. Sheng, J. Shi, Y. Zheng, Y. R. Lu, Q. He, J. Chen, K. S. Novoselov, C. H. Chuang, S. Xi, X. Luo and J. Lu, Ferromagnetic single-atom spin catalyst for boosting water splitting, Nat. Nanotechnol., 2023, 18, 763–771 CrossRef CAS.
- T. Zhang, Z. Sun, S. Li, B. Wang, Y. Liu, R. Zhang and Z. Zhao, Regulating electron configuration of single Cu sites via unsaturated N, O-coordination for selective oxidation of benzene, Nat. Commun., 2022, 13, 1–8 Search PubMed.
- J. Li, S. Chen, N. Yang, M. Deng, S. Ibraheem, J. Deng, J. Li, L. Li and Z. Wei, Ultrahigh–Loading Zinc Single–Atom Catalyst for Highly Efficient Oxygen Reduction in Both Acidic and Alkaline Media, Angew. Chem., Int. Ed., 2019, 131, 7109–7113 CrossRef.
- Z. Zhang, J. Zhu, S. Chen, W. Sun and D. Wang, Liquid Fluxional Ga Single Atom Catalysts for Efficient Electrochemical CO2 Reduction, Angew. Chem., Int. Ed., 2022, 62, e202215136 CrossRef.
- X. Wang, Y. An, L. Liu, L. Fang, Y. Liu, J. Zhang, H. Qi, T. Heine, T. Li, A. Kuc, M. Yu and X. Feng, Atomically Dispersed Pentacoordinated-Zirconium Catalyst with Axial Oxygen Ligand for Oxygen Reduction Reaction, Angew. Chem., Int. Ed., 2022, 61, e202209746 CrossRef CAS.
- J. Luo, H. Han, X. Wang, X. Qiu, B. Liu, Y. Lai, X. Chen, R. Zhong, L. Wang and C. Wang, Single-atom Nb anchored on graphitic carbon nitride for boosting electron transfer towards improved photocatalytic performance, Appl. Catal., B, 2023, 328, 122495 CrossRef CAS.
- L. Han, X. Liu, J. Chen, R. Lin, H. Liu, F. Lü, S. Bak, Z. Liang, S. Zhao, E. Stavitski, J. Luo, R. R. Adzic and H. L. Xin, Atomically Dispersed Molybdenum Catalysts for Efficient Ambient Nitrogen Fixation, Angew. Chem., Int. Ed., 2019, 131, 2343–2347 CrossRef.
- Y. Zhou, F. Wei, H. Qi, Y. Chai, L. Cao, J. Lin, Q. Wan, X. Liu, Y. Xing, S. Lin, A. Wang, X. Wang and T. Zhang, Peripheral-nitrogen effects on the Ru1 centre for highly efficient propane dehydrogenation, Nat. Catal., 2022, 5, 1145–1156 CrossRef CAS.
- Y. Xiong, J. Dong, Z. Q. Huang, P. Xin, W. Chen, Y. Wang, Z. Li, Z. Jin, W. Xing, Z. Zhuang, J. Ye, X. Wei, R. Cao, L. Gu, S. Sun, L. Zhuang, X. Chen, H. Yang, C. Chen, Q. Peng, C. R. Chang, D. Wang and Y. Li, Single-atom Rh/N-doped carbon electrocatalyst for formic acid oxidation, Nat. Nanotechnol., 2020, 15, 390–397 CrossRef CAS.
- P. Liu, Y. Zhao, R. Qin, S. Mo, G. Chen, L. Gu, D. M. Chevrier, P. Zhang, Q. Guo, D. Zang, B. Wu, G. Fu and N. Zheng, Photochemical route for synthesizing atomically dispersed palladium catalysts, Science, 2016, 352, 797–801 CrossRef CAS.
- X. Zhang, L. Shang, Z. Yang and T. Zhang, A Rhenium Single-Atom Catalyst for the Electrocatalytic Oxygen Reduction Reaction, ChemPlusChem, 2021, 86, 1635–1639 CrossRef CAS.
- Y. Wu, C. Chen, X. Yan, X. Sun, Q. Zhu, P. Li, Y. Li, S. Liu, J. Ma, Y. Huang and B. Han, Boosting CO2 Electroreduction over a Cadmium Single-Atom Catalyst by Tuning of the Axial Coordination Structure, Angew. Chem., Int. Ed., 2021, 60, 20803–20810 CrossRef CAS.
- H. Shang, T. Wang, J. Pei, Z. Jiang, D. Zhou, Y. Wang, H. Li, J. Dong, Z. Zhuang, W. Chen, D. Wang, J. Zhang and Y. Li, Design of a Single–Atom Indiumδ+ –N4 Interface for Efficient Electroreduction of CO2 to Formate, Angew. Chem., Int. Ed., 2020, 132, 22651–22655 CrossRef.
- B. Wulan, X. Cao, D. Tan, X. Shu, J. Ma, S. Hou and J. Zhang, Atomic Bridging of Sn Single Atom with Nitrogen and Oxygen Atoms for the Selective Electrocatalytic Reduction of CO2, CCS Chem., 2023, 5, 2415–2425 CrossRef CAS.
- M. Jia, S. Hong, T. S. Wu, X. Li, Y. L. Soo and Z. Sun, Single Sb sites for efficient electrochemical CO2 reduction, Chem. Commun., 2019, 55, 12024–12027 RSC.
- Y. Zhang, Y. Cheng, X. Wang, Q. Sun, X. He and H. Ji, Enhanced Hydrogenation Properties of Pd Single Atom Catalysts with Atomically Dispersed Ba Sites as Electronic Promoters, ACS Catal., 2022, 12, 15091–15096 CrossRef CAS.
- X. Liu, Y. Luo, C. Ling, Y. Shi, G. Zhan, H. Li, H. Gu, K. Wei, F. Guo, Z. Ai and L. Zhang, Rare earth La single atoms supported MoO3-x for efficient photocatalytic nitrogen fixation, Appl. Catal., B, 2022, 301, 120766 CrossRef CAS.
- C. Huang, S. Lv, C. Li, B. Peng and G. Li, Single-atom catalysts besed on two-dimensional metalloporphyrin monolayers for ammonia synthesis under amient aonditions, Nano Res., 2022, 15, 4039–4047 CrossRef CAS.
- S. Wang, M. Sun, L. Zheng and S. Zhou, On the promising performance of single Ta atom in efficient nitrogen fixation, Chem Catal., 2021, 1, 1322–1330 CrossRef CAS.
- Y. Wang, J. Zhang, W. X. Shi, G. L. Zhuang, Q. P. Zhao, J. Ren, P. Zhang, H. Q. Yin, T. B. Lu and Z. M. Zhang, W Single-Atom Catalyst for CH4 Photooxidation in Water Vapor, Adv. Mater., 2022, 34, 1–10 Search PubMed.
- X. Zhang, L. Shang, Z. Yang and T. Zhang, A Rhenium Single-Atom Catalyst for the Electrocatalytic Oxygen Reduction Reaction, ChemPlusChem, 2021, 86, 1635–1639 CrossRef CAS.
- Y. Meng, J. X. Liang, C. Zhu, C. Q. Xu and J. Li, Theoretical studies of MXene-supported single-atom catalysts: Os1/Ti2CS2 for low-temperature CO oxidation, Sci. China Mater., 2022, 65, 1303–1312 CrossRef CAS.
- Z. Lei, W. Cai, Y. Rao, K. Wang, Y. Jiang, Y. Liu, X. Jin, J. Li, Z. Lv, S. Jiao, W. Zhang, P. Yan, S. Zhang and R. Cao, Coordination modulation of iridium single-atom catalyst maximizing water oxidation activity, Nat. Commun., 2022, 13, 1–10 Search PubMed.
- S. Fang, X. Zhu, X. Liu, J. Gu, W. Liu, D. Wang, W. Zhang, Y. Lin, J. Lu, S. Wei, Y. Li and T. Yao, Uncovering near-free platinum single-atom dynamics during electrochemical hydrogen evolution
reaction, Nat. Commun., 2020, 11, 1–8 CrossRef.
- Y. Cao, L. Guo, M. Dan, D. E. Doronkin, C. Han, Z. Rao, Y. Liu, J. Meng, Z. Huang, K. Zheng, P. Chen, F. Dong and Y. Zhou, Modulating electron density of vacancy site by single Au atom for effective CO2 photoreduction, Nat. Commun., 2021, 12, 1–10 CrossRef.
- D. Liu, H. Gao, W. Jiang, S. Yan, H. Liu, J. Chen, S. Wen, W. Zhang, X. Wang, B. Zhao and W. Song, Ag Aerogel-Supported Single-Atom Hg Nanozyme Enables Efficient SERS Monitoring of Enhanced Oxidase-Like Catalysis, Anal. Chem., 2023, 95, 4335–4343 CrossRef CAS.
- W. Qu, S. Niu, D. Sun, H. Gao, Y. Wu, Z. Yuan, X. Chen, Y. Wang, T. An, G. Wang and F. Zhao, Pb Single Atoms Enable Unprecedented Catalytic Behavior for the Combustion of Energetic Materials, Adv. Sci., 2021, 8, 1–8 Search PubMed.
- E. Zhang, T. Wang, K. Yu, J. Liu, W. Chen, A. Li, H. Rong, R. Lin, S. Ji, X. Zheng, Y. Wang, L. Zheng, C. Chen, D. Wang, J. Zhang and Y. Li, Bismuth Single Atoms Resulting from Transformation of Metal-Organic Frameworks and Their Use as Electrocatalysts for CO2 Reduction, J. Am. Chem. Soc., 2019, 141, 16569–16573 CrossRef CAS PubMed.
- Y. Lu, J. Wang, L. Yu, L. Kovarik, X. Zhang, A. S. Hoffman, A. Gallo, S. R. Bare, D. Sokaras, T. Kroll, V. Dagle, H. Xin and A. M. Karim, Identification of the active complex for CO oxidation over single-atom Ir-on-MgAl2O4 catalysts, Nat. Catal., 2019, 2, 149–156 CrossRef CAS.
- Y. Chen, S. Ji, C. Chen, Q. Peng, D. Wang and Y. Li, Single-Atom Catalysts: Synthetic Strategies and Electrochemical Applications, Joule, 2018, 2, 1242–1264 CrossRef CAS.
- X. Hai, S. Xi, S. Mitchell, K. Harrath, H. Xu, D. F. Akl, D. Kong, J. Li, Z. Li, T. Sun, H. Yang, Y. Cui, C. Su, X. Zhao, J. Li, J. Pérez-Ramírez and J. Lu, Scalable two-step annealing method for preparing ultra-high-density single-atom catalyst libraries, Nat. Nanotechnol., 2022, 17, 174–181 CrossRef CAS.
- Q. Zuo, T. Liu, C. Chen, Y. Ji, X. Gong, Y. Mai and Y. Zhou, Ultrathin Metal–Organic Framework Nanosheets with Ultrahigh Loading of Single Pt Atoms for Efficient Visible-Light-Driven Photocatalytic H2 Evolution, Angew. Chem., Int. Ed., 2019, 58, 10198–10203 CrossRef CAS.
- Y. Xiong, W. Sun, P. Xin, W. Chen, X. Zheng, W. Yan, L. Zheng, J. Dong, J. Zhang, D. Wang and Y. Li, Gram-Scale Synthesis of High-Loading Single-Atomic-Site Fe Catalysts for Effective Epoxidation of Styrene, Adv. Mater., 2020, 32, 2000896 CrossRef CAS.
- Z. Pu, I. S. Amiinu, R. Cheng, P. Wang, C. Zhang, S. Mu, W. Zhao, F. Su, G. Zhang, S. Liao and S. Sun, Single-Atom Catalysts for Electrochemical Hydrogen Evolution Reaction: Recent Advances and Future Perspectives, Nano-Micro Lett., 2020, 12, 1–29 CrossRef.
- Z. Jin, P. Li, Y. Meng, Z. Fang, D. Xiao and G. Yu, Understanding the inter-site distance effect in single-atom catalysts for oxygen electroreduction, Nat. Catal., 2021, 4, 615–622 CrossRef CAS.
- J. Hao, Z. Zhuang, K. Cao, G. Gao, C. Wang, F. Lai, S. Lu, P. Ma, W. Dong, T. Liu, M. Du and H. Zhu, Unraveling the electronegativity-dominated intermediate adsorption on high-entropy alloy electrocatalysts, Nat. Commun., 2022, 13, 2662 CrossRef CAS.
- H. Xu, D. Cheng, D. Cao and X. C. Zeng, A universal principle for a rational design of single-atom electrocatalysts, Nat. Catal., 2018, 1, 339–348 CrossRef CAS.
- X. Guan, C. Zhao, X. Liu, S. Liu, W. Gao and Q. Jiang, Universal Principle to Describe Reactivity and Selectivity of CO2 Electroreduction on Transition Metals and Single-Atom Catalysts, J. Phys. Chem. C, 2020, 124, 25898–25906 CrossRef CAS.
- A. Walsh, A. A. Sokol, J. Buckeridge, D. O. Scanlon and C. R. A. Catlow, Oxidation states and ionicity, Nat. Mater., 2018, 17, 958–964 CrossRef CAS.
- Y. Chen, S. Ji, S. Zhao, W. Chen, J. Dong, W. C. Cheong, R. Shen, X. Wen, L. Zheng, A. I. Rykov, S. Cai, H. Tang, Z. Zhuang, C. Chen, Q. Peng, D. Wang and Y. Li, Enhanced oxygen reduction with single-atomic-site iron catalysts for a zinc-air battery and hydrogen-air fuel cell, Nat. Commun., 2018, 9, 5422 CrossRef CAS.
- J. Gu, C. S. Hsu, L. Bai, H. M. Chen and X. Hu, Atomically dispersed Fe3+ sites catalyze efficient CO2 electroreduction to CO, Science, 2019, 364, 1091–1094 CrossRef CAS.
- X. Jin, R. Wang, L. Zhang, R. Si, M. Shen, M. Wang, J. Tian and J. Shi, Electron Configuration Modulation of Nickel Single Atoms for Elevated Photocatalytic Hydrogen Evolution, Angew. Chem., Int. Ed., 2020, 132, 6894–6898 CrossRef.
- X. Rong, H. J. Wang, X. L. Lu, R. Si and T. B. Lu, Controlled Synthesis of a Vacancy-Defect Single-Atom Catalyst for Boosting CO2 Electroreduction, Angew. Chem., Int. Ed., 2020, 59, 1961–1965 CrossRef CAS.
- L. Jiao, J. Wang and H. L. Jiang, Microenvironment Modulation in Metal-Organic Framework-Based Catalysis, Acc. Mater. Res., 2021, 2, 327–339 CrossRef CAS.
- F. Li, G. F. Han, Y. Bu, H. J. Noh, J. P. Jeon, T. J. Shin, S. J. Kim, Y. Wu, H. Y. Jeong, Z. Fu, Y. Lu and J. B. Baek, Revealing Isolated M-N3C1 Active Sites for Efficient Collaborative Oxygen Reduction Catalysis, Adv. Mater., 2020, 132, 23886–23891 Search PubMed.
- H. Fei, J. Dong, D. Chen, T. Hu, X. Duan, I. Shakir, Y. Huang and X. Duan, Single atom electrocatalysts supported on graphene or graphene-like carbons, Chem. Soc. Rev., 2019, 48, 5207–5241 RSC.
- X. Wang, Y. Jia, X. Mao, D. Liu, W. He, J. Li, J. Liu, X. Yan, J. Chen, L. Song, A. Du and X. Yao, Edge-Rich Fe−N4 Active Sites in Defective Carbon for Oxygen Reduction Catalysis, Adv. Mater., 2020, 32, 2000966 CrossRef CAS.
- K. Jiang, S. Back, A. J. Akey, C. Xia, Y. Hu, W. Liang, D. Schaak, E. Stavitski, J. K. Nørskov, S. Siahrostami and H. Wang, Highly selective oxygen reduction to hydrogen peroxide on transition metal single atom coordination, Nat. Commun., 2019, 10, 3997 CrossRef.
- Y. Zhu, J. Sokolowski, X. Song, Y. He, Y. Mei and G. Wu, Engineering Local Coordination Environments of Atomically Dispersed and Heteroatom-Coordinated Single Metal Site Electrocatalysts for Clean Energy-Conversion, Adv. Energy Mater., 2020, 10, 1902844 CrossRef CAS.
- C. Tang, Y. Jiao, B. Shi, J. N. Liu, Z. Xie, X. Chen, Q. Zhang and S. Z. Qiao, Coordination Tunes Selectivity: Two-Electron Oxygen Reduction on High-Loading Molybdenum Single-Atom Catalysts, Angew. Chem., Int. Ed., 2020, 59, 9171–9176 CrossRef CAS.
- X. Li, R. Hongpan, J. Zhang, D. Wang and Y. Li, Modulating the local coordination environment of single-atom catalysts for enhanced catalytic performance, Nano Res., 2020, 13, 1842–1855 CrossRef CAS.
- Z. Chen, X. Liao, C. Sun, K. Zhao, D. Ye, J. Li, G. Wu, J. Fang, H. Zhao and J. Zhang, Enhanced performance of atomically dispersed dual-site Fe-Mn electrocatalysts through cascade reaction mechanism, Appl. Catal., B, 2021, 288, 120021 CrossRef CAS.
- W. Tan, S. Xie, D. Le, W. Diao, M. Wang, K. Bin Low, D. Austin, S. Hong, F. Gao, L. Dong, L. Ma, S. N. Ehrlich, T. S. Rahman and F. Liu, Fine-tuned local coordination environment of Pt single atoms on ceria controls catalytic reactivity, Nat. Commun., 2022, 13, 7070 CrossRef CAS.
- F. Chen, X. L. Wu, C. Shi, H. Lin, J. Chen, Y. Shi, S. Wang and X. Duan, Molecular Engineering toward Pyrrolic N-Rich M-N4 (M = Cr, Mn, Fe, Co, Cu) Single-Atom Sites for Enhanced Heterogeneous Fenton-Like Reaction, Adv. Funct. Mater., 2021, 31, 2007877 CrossRef CAS.
- J. Wei, S. N. Qin, J. Yang, H. L. Ya, W. H. Huang, H. Zhang, B. J. Hwang, Z. Q. Tian and J. F. Li, Probing Single-Atom Catalysts and Catalytic Reaction Processes by Shell-Isolated Nanoparticle-Enhanced Raman Spectroscopy, Angew. Chem., Int. Ed., 2021, 60, 9306–9310 CrossRef CAS.
- L. Zong, K. Fan, P. Li, F. Lu, B. Li and L. Wang, Promoting Oxygen Reduction Reaction on Atomically Dispersed Fe Sites via Establishing Hydrogen Bonding with the Neighboring P Atoms, Adv. Energy Mater., 2022, 2203611 Search PubMed.
- B. Schulze Lammers, N. López-Salas, J. Stein Siena, H. Mirhosseini, D. Yesilpinar, J. Heske, T. D. Kühne, H. Fuchs, M. Antonietti and H. Mönig, Real-Space Identification of Non-Noble Single Atomic Catalytic Sites within Metal-Coordinated Supramolecular Networks, ACS Nano, 2022, 16, 14284–14296 CrossRef CAS.
- L. Li, W. Yu, W. Gong, H. Wang, C. L. Chiang, Y. Lin, J. Zhao, L. Zhang, J. M. Lee and G. Zou, Sulfur-induced electron redistribution of single molybdenum atoms promotes nitrogen electroreduction to ammonia, Appl. Catal., B, 2023, 321, 122038 CrossRef CAS.
- H. Jin, P. Li, P. Cui, J. Shi, W. Zhou, X. Yu, W. Song and C. Cao, Unprecedentedly high activity and selectivity for hydrogenation of nitroarenes with single atomic Co1-N3P1 sites, Nat. Commun., 2022, 13, 723 CrossRef CAS.
- X. Sui, L. Zhang, J. Li, K. Doyle-Davis, R. Li, Z. Wang and X. Sun, Advanced Support Materials and Interactions for Atomically Dispersed Noble-Metal Catalysts: From Support Effects to Design Strategies, Adv. Energy Mater., 2022, 12, 2102556 CrossRef CAS.
- J. Leverett, R. Daiyan, L. Gong, K. Iputera, Z. Tong, J. Qu, Z. Ma, Q. Zhang, S. Cheong, J. Cairney, R. S. Liu, X. Lu, Z. Xia, L. Dai and R. Amal, Designing Undercoordinated Ni-Nx and Fe-Nx on Holey Graphene for Electrochemical CO2 Conversion to Syngas, ACS Nano, 2021, 15, 12006–12018 CrossRef CAS.
- F. Zhang, Y. Zhu, C. Tang, Y. Chen, B. Qian, Z. Hu, Y. C. Chang, C. W. Pao, Q. Lin, S. A. Kazemi, Y. Wang, L. Zhang, X. Zhang and H. Wang, High-Efficiency Electrosynthesis of Hydrogen Peroxide from Oxygen Reduction Enabled by a Tungsten Single Atom Catalyst with Unique Terdentate N1O2 Coordination, Adv. Funct. Mater., 2022, 32, 2110224 CrossRef CAS.
- J. Wei, K. Xiao, Y. Chen, X. P. Guo, B. Huang and Z. Q. Liu, In situ precise anchoring of Pt single atoms in spinel Mn3O4 for a highly efficient hydrogen evolution reaction, Energy Environ. Sci., 2022, 15, 4592–4600 RSC.
- C. E. Creissen and M. Fontecave, Keeping sight of copper in single-atom catalysts for electrochemical carbon dioxide reduction, Nat. Commun., 2022, 13, 2280 CrossRef CAS.
- X. Cheng, Y. Wang, Y. Lu, L. Zheng, S. Sun, H. Li, G. Chen and J. Zhang, Single-atom alloy with Pt-Co dual sites as an efficient electrocatalyst for oxygen reduction reaction, Appl. Catal., B, 2022, 306, 121112 CrossRef CAS.
- Y. Kim, G. Collinge, M. Lee, K. Khivantsev, S. J. Cho, V. Glezakou, R. Rousseau, J. Szanyi and J. H. Kwak, Surface Density Dependent Catalytic Activity of Single Palladium Atoms Supported on Ceria, Angew. Chem., Int. Ed., 2021, 60, 22769–22775 CrossRef CAS.
- X. Zhou, J. Gao, Y. Hu, Z. Jin, K. Hu, K. M. Reddy, Q. Yuan, X. Lin and H. J. Qiu, Theoretically Revealed and Experimentally Demonstrated Synergistic Electronic Interaction of CoFe Dual-Metal Sites on N-doped Carbon for Boosting Both Oxygen Reduction and Evolution Reactions, Nano Lett., 2022, 22, 3392–3399 CrossRef CAS.
- S. Yang, X. Li, T. Tan, J. Mao, Q. Xu, M. Liu, Q. Miao, B. B. Mei, P. Qiao, S. Gu, F. Sun, J. Ma, G. Zeng and Z. Jiang, A fully-conjugated covalent organic framework-derived carbon supporting ultra-close single atom sites for ORR, Appl. Catal., B, 2022, 307, 121147 CrossRef CAS.
- Y. Fang, Q. Zhang, H. Zhang, X. Li, W. Chen, J. Xu, H. Shen, J. Yang, C. Pan, Y. Zhu, J. Wang, Z. Luo, L. Wang, X. Bai, F. Song, L. Zhang and Y. Guo, Dual Activation of Molecular Oxygen and Surface Lattice Oxygen in Single Atom Cu1/TiO2 Catalyst for CO Oxidation, Angew. Chem., Int. Ed., 2022, 61, 1433–7851 Search PubMed.
- W. Tang, H. Zhang, X. Yang, Z. Dai, Y. Sun, H. Liu, Z. Hu and X. Zheng, Ru single atom catalyst with dual reaction sites for efficient fenton-like degradation of organic contaminants, Appl. Catal., B, 2023, 320, 121952 CrossRef CAS.
- H. Yang, Z. Chen, S. Kou, G. Lu, D. Chen and Z. Liu, Carbon-supported catalysts with atomically dispersed metal sites for oxygen electroreduction: present and future perspectives, J. Mater. Chem. A, 2021, 9, 15919–15936 RSC.
- H. Sun, Y. Ma, Q. Zhang and C. Su, Engineering the Local Coordination Environment of Single-Atom Catalysts and Their Applications in Photocatalytic Water Splitting: A Review, Trans. Tianjin Univ., 2021, 27, 313–330 CrossRef CAS.
- Z. L. Wang, X. F. Hao, Z. Jiang, X. P. Sun, D. Xu, J. Wang, H. X. Zhong, F. L. Meng and X. B. Zhang, C and N Hybrid Coordination Derived Co-C-N Complex as a Highly Efficient Electrocatalyst for Hydrogen Evolution Reaction, J. Am. Chem. Soc., 2015, 137, 15070–15073 CrossRef CAS.
- P. Yin, T. Yao, Y. Wu, L. Zheng, Y. Lin, W. Liu, H. Ju, J. Zhu, X. Hong, Z. Deng, G. Zhou, S. Wei and Y. Li, Single Cobalt Atoms with Precise N-Coordination as Superior Oxygen Reduction Reaction Catalysts, Angew. Chem., Int. Ed., 2016, 55, 10800–10805 CrossRef CAS.
- N. Zhang, T. Zhou, M. Chen, H. Feng, R. Yuan, C. Zhong, W. Yan, Y. Tian, X. Wu, W. Chu, C. Wu and Y. Xie, High-purity pyrrole-type FeN4 sites as a superior oxygen reduction electrocatalyst, Energy Environ. Sci., 2020, 13, 111–118 RSC.
- L. Zeng, C. Dai, B. Liu and C. Xue, Oxygen-assisted stabilization of single-atom Au during photocatalytic hydrogen evolution, J. Mater. Chem. A, 2019, 7, 24217–24221 RSC.
- L. Wang, S. Zhang, Y. Zhu, A. Patlolla, J. Shan, H. Yoshida, S. Takeda, A. I. Frenkel and F. Tao, Catalysis and in situ studies of Rh1/Co3O4 Nanorods in Reduction of NO with H2, ACS Catal., 2013, 3, 1011–1019 CrossRef CAS.
- R. Lang, T. Li, D. Matsumura, S. Miao, Y. Ren, Y. Cui, Y. Tan, B. Qiao, L. Li, A. Wang, X. Wang and T. Zhang, Hydroformylation of Olefins by a Rhodium Single-Atom Catalyst with Activity Comparable to RhCl(PPh3)3, Angew. Chem., Int. Ed., 2016, 128, 16288–16292 CrossRef.
- J. Lin, A. Wang, B. Qiao, X. Liu, X. Yang, X. Wang, J. Liang, J. Li, J. Liu and T. Zhang, Remarkable performance of Ir1/FeOx single-atom catalyst in water gas shift reaction, J. Am. Chem. Soc., 2013, 135, 15314–15317 CrossRef CAS.
- L. Wang, W. Zhang, S. Wang, Z. Gao, Z. Luo, X. Wang, R. Zeng, A. Li, H. Li, M. Wang, X. Zheng, J. Zhu, W. Zhang, C. Ma, R. Si and J. Zeng, Atomic-level insights in optimizing reaction paths for hydroformylation reaction over Rh/CoO single-atom catalyst, Nat. Commun., 2016, 71, 14036 CrossRef.
- J. Jones, H. Xiong, A. T. DeLaRiva, E. J. Peterson, H. Pham, S. R. Challa, G. Qi, S. Oh, M. H. Wiebenga, X. I. P. Hernández, Y. Wang and A. K. Datye, Thermally stable single-atom platinum-on-ceria catalysts via atom trapping, Science, 2016, 353, 150–154 CrossRef CAS.
- Y. Li, Z. S. Wu, P. Lu, X. Wang, W. Liu, Z. Liu, J. Ma, W. Ren, Z. Jiang and X. Bao, High-Valence Nickel Single-Atom Catalysts Coordinated to Oxygen Sites for Extraordinarily Activating Oxygen Evolution Reaction, Adv. Sci., 2020, 7, 1903089 CrossRef CAS.
- Y. Qu, L. Wang, Z. Li, P. Li, Q. Zhang, Y. Lin, F. Zhou, H. Wang, Z. Yang, Y. Hu, M. Zhu, X. Zhao, X. Han, C. Wang, Q. Xu, L. Gu, J. Luo, L. Zheng and Y. Wu, Ambient Synthesis of Single-Atom Catalysts from Bulk Metal via Trapping of Atoms by Surface Dangling Bonds, Adv. Mater., 2019, 31, 1904496 CrossRef CAS.
- K. Yuan, D. Lützenkirchen-Hecht, L. Li, L. Shuai, Y. Li, R. Cao, M. Qiu, X. Zhuang, M. K. H. Leung, Y. Chen and U. Scherf, Boosting Oxygen Reduction of Single Iron Active Sites via Geometric and Electronic Engineering: Nitrogen and Phosphorus Dual Coordination, J. Am. Chem. Soc., 2020, 142, 2404–2412 CrossRef CAS.
- S. Guo, P. Yuan, J. Zhang, P. Jin, H. Sun, K. Lei, X. Pang, Q. Xu and F. Cheng, Atomic-scaled cobalt encapsulated in P,N-doped carbon sheaths over carbon nanotubes for enhanced oxygen reduction electrocatalysis under acidic and alkaline media, Chem. Commun., 2017, 53, 9862–9865 RSC.
- Y. Li, M. Kong, J. Hu and J. Zhou, Carbon-Microcuboid-Supported Phosphorus-Coordinated Single Atomic Copper with Ultrahigh Content and Its Abnormal Modification to Na Storage Behaviors, Adv. Energy Mater., 2020, 10, 2000400 CrossRef CAS.
- P. Zhou, N. Li, Y. Chao, W. Zhang, F. Lv, K. Wang, W. Yang, P. Gao and S. Guo, Thermolysis of Noble Metal Nanoparticles into Electron-Rich Phosphorus-Coordinated Noble Metal Single Atoms at Low Temperature, Angew. Chem., Int. Ed., 2019, 58, 14184–14188 CrossRef CAS.
- P. Zhou, Q. Zhang, Z. Xu, Q. Shang, L. Wang, Y. Chao, Y. Li, H. Chen, F. Lv, Q. Zhang, L. Gu and S. Guo, Atomically Dispersed Co–P3 on CdS Nanorods with Electron-Rich Feature Boosts Photocatalysis, Adv. Mater., 2020, 32, 1904249 CrossRef CAS.
- A. W. Robertson, Y. C. Lin, S. Wang, H. Sawada, C. S. Allen, Q. Chen, S. Lee, G. D. Lee, J. Lee, S. Han, E. Yoon, A. I. Kirkland, H. Kim, K. Suenaga and J. H. Warner, Atomic Structure and Spectroscopy of Single Metal (Cr, V) Substitutional Dopants in Monolayer MoS2, ACS Nano, 2016, 10, 10227–10236 CrossRef CAS.
- X. Wu, H. Zhang, J. Dong, M. Qiu, J. Kong, Y. Zhang, Y. Li, G. Xu, J. Zhang and J. Ye, Surface step decoration of isolated atom as electron pumping: Atomic-level insights into visible-light hydrogen evolution, Nano Energy, 2018, 45, 109–117 CrossRef CAS.
- J. Deng, H. Li, J. Xiao, Y. Tu, D. Deng, H. Yang, H. Tian, J. Li, P. Ren and X. Bao, Triggering the electrocatalytic hydrogen evolution activity of the inert two-dimensional MoS2 surface via single-atom metal doping, Energy Environ. Sci., 2015, 8, 1594–1601 RSC.
- Z. Luo, Y. Ouyang, H. Zhang, M. Xiao, J. Ge, Z. Jiang, J. Wang, D. Tang, X. Cao, C. Liu and W. Xing, Chemically activating MoS2 via spontaneous atomic palladium interfacial doping towards efficient hydrogen evolution, Nat. Commun., 2018, 9, 2120 CrossRef.
- Y. C. Lin, D. O. Dumcenco, H. P. Komsa, Y. Niimi, A. V. Krasheninnikov, Y. S. Huang and K. Suenaga, Properties of individual dopant atoms in single-layer MoS2: Atomic structure, migration, and enhanced reactivity, Adv. Mater., 2014, 26, 2857–2861 CrossRef CAS.
- J. Zhang, M. Zhang, Y. Zeng, J. Chen, L. Qiu, H. Zhou, C. Sun, Y. Yu, C. Zhu and Z. Zhu, Single Fe Atom on Hierarchically Porous S, N-Codoped Nanocarbon Derived from Porphyra Enable Boosted Oxygen Catalysis for Rechargeable Zn-Air Batteries, Small, 2019, 15, 1900307 CrossRef.
- J. Zhang, Y. Zhao, C. Chen, Y. C. Huang, C. L. Dong, C. J. Chen, R. S. Liu, C. Wang, K. Yan, Y. Li and G. Wang, Tuning the Coordination Environment in Single-Atom Catalysts to Achieve Highly Efficient Oxygen Reduction Reactions, J. Am. Chem. Soc., 2019, 141, 20118–20126 CrossRef CAS PubMed.
- Y. Sun, X. Liu, M. Zhu, Z. Zhang, Z. Chen, S. Wang, Z. Ji, H. Yang and X. Wang, Non-noble metal single atom-based catalysts for electrochemical reduction of CO2: Synthesis approaches and performance evaluation, DeCarbon, 2023, 2, 100018 CrossRef.
- B. Zhang, J. Zhang, J. Shi, D. Tan, L. Liu, F. Zhang, C. Lu, Z. Su, X. Tan, X. Cheng, B. Han, L. Zheng and J. Zhang, Manganese acting as a high-performance heterogeneous electrocatalyst in carbon dioxide reduction, Nat. Commun., 2019, 10, 1–8 CrossRef.
- M. Yi, N. Li, B. Lu, L. Li, Z. Zhu and J. Zhang, Single-atom Pt decorated in heteroatom (N, B, and F)-doped ReS2 Grown on Mo2CTx for efficient pH-universal hydrogen evolution reaction and flexible Zn–air batteries, Energy Storage Mater., 2021, 42, 418–429 CrossRef.
- Y. Wu, Z. Zhuang, C. Chen, J. Li, F. Xiao and C. Chen, Atomic-level regulation strategies of single-atom catalysts: Nonmetal heteroatom doping and polymetallic active site construction, Chem. Catal., 2023, 3, 100586 CrossRef CAS.
- L. Wang, C. Zhu, M. Xu, C. Zhao, J. Gu, L. Cao, X. Zhang, Z. Sun, S. Wei, W. Zhou, W. X. Li and J. Lu, Boosting activity and stability of metal single-atom catalysts via regulation of coordination number and local composition, J. Am. Chem. Soc., 2021, 143, 18854–18858 CrossRef CAS.
- D. Huang, N. He, Q. Zhu, C. Chu, S. Weon, K. Rigby, X. Zhou, L. Xu, J. Niu, E. Stavitski and J. H. Kim, Conflicting roles of coordination number on catalytic performance of single-atom pt catalysts, ACS Catal., 2021, 11, 5586–5592 CrossRef CAS.
- P. Liu and N. Zheng, Coordination chemistry of atomically dispersed catalysts, Natl. Sci. Rev., 2018, 5, 636–638 CrossRef CAS.
- X. Liang, D. Wang, Z. Zhao, T. Li, Y. Gao and C. Hu, Coordination Number Dependent Catalytic Activity of Single-Atom Cobalt Catalysts for Fenton-Like Reaction, Adv. Funct. Mater., 2022, 32, 2203001 CrossRef CAS.
- W. Liu, L. Zhang, X. Liu, X. Liu, X. Yang, S. Miao, W. Wang, A. Wang and T. Zhang, Discriminating Catalytically Active FeNx Species of Atomically Dispersed Fe-N-C Catalyst for Selective Oxidation of the C-H Bond, J. Am. Chem. Soc., 2017, 139, 10790–10798 CrossRef CAS.
- X. Wang, Z. Chen, X. Zhao, T. Yao, W. Chen, R. You, C. Zhao, G. Wu, J. Wang, W. Huang, J. Yang, X. Hong, S. Wei, Y. Wu and Y. Li, Regulation of Coordination Number over Single Co Sites: Triggering the Efficient Electroreduction of CO2, Angew. Chem., Int. Ed., 2018, 130, 1962–1966 CrossRef.
- Y. Pan, K. Sun, S. Liu, X. Cao, K. Wu, W. C. Cheong, Z. Chen, Y. Wang, Y. Li, Y. Liu, D. Wang, Q. Peng, C. Chen and Y. Li, Core-Shell ZIF-8@ZIF-67-Derived CoP Nanoparticle-Embedded N-Doped Carbon Nanotube Hollow Polyhedron for Efficient Overall Water Splitting, J. Am. Chem. Soc., 2018, 140, 2610–2618 CrossRef CAS.
- C. Yan, H. Li, Y. Ye, H. Wu, F. Cai, R. Si, J. Xiao, S. Miao, S. Xie, F. Yang, Y. Li, G. Wang and X. Bao, Coordinatively unsaturated nickel-nitrogen sites towards selective and high-rate CO2 electroreduction, Energy Environ. Sci., 2018, 11, 1204–1210 RSC.
- A. S. Varela, W. Ju, A. Bagger, P. Franco, J. Rossmeisl and P. Strasser, Electrochemical Reduction of CO2 on Metal-Nitrogen-Doped Carbon Catalysts, ACS Catal., 2019, 9, 7270–7284 CrossRef CAS.
- Y. Z. Chen, R. Zhang, L. Jiao and H. L. Jiang, Metal–organic framework-derived porous materials for catalysis, Coord. Chem. Rev., 2018, 362, 1–23 CrossRef CAS.
- C. Reviews, Introduction to Metal−Organic Frameworks, Chem. Rev., 2012, 112, 673–674 CrossRef.
- T. Islamoglu, S. Goswami, Z. Li, A. J. Howarth, O. K. Farha and J. T. Hupp, Postsynthetic Tuning of Metal-Organic Frameworks for Targeted Applications, Acc. Chem. Res., 2017, 50, 805–813 CrossRef CAS.
- H. Li, L. Li, R.-B. Lin, W. Zhou, Z. Zhang, S. Xiang and B. Chen, Porous metal-organic frameworks for gas storage and separation: Status and challenges, EnergyChem, 2019, 1, 100006 CrossRef.
- Y. Wang, G. Jia, X. Cui, X. Zhao, Q. Zhang, L. Gu, L. Zheng, L. H. Li, Q. Wu, D. J. Singh, D. Matsumura, T. Tsuji, Y. T. Cui, J. Zhao and W. Zheng, Coordination Number Regulation of Molybdenum Single-Atom Nanozyme Peroxidase-like Specificity, Chem, 2021, 7, 436–449 CAS.
- X. Wang, Z. Chen, X. Zhao, T. Yao, W. Chen, R. You, C. Zhao, G. Wu, J. Wang, W. Huang, J. Yang, X. Hong, S. Wei, Y. Wu and Y. Li, Regulation of Coordination Number over Single Co Sites: Triggering the Efficient Electroreduction of CO2, Angew. Chem., Int. Ed., 2018, 57, 1944–1948 CrossRef CAS.
- Y. Zhang, L. Jiao, W. Yang, C. Xie and H.-L. Jiang, Rational Fabrication of Low-Coordinate Single-Atom Ni Electrocatalysts by MOFs for Highly Selective CO2 Reduction, Angew. Chem., Int. Ed., 2021, 60, 7607–7611 CrossRef CAS.
- X. Xiao, Y. Gao, L. Zhang, J. Zhang, Q. Zhang, Q. Li, H. Bao, J. Zhou, S. Miao, N. Chen, J. Wang, B. Jiang, C. Tian and H. Fu, A Promoted Charge Separation/Transfer System from Cu Single Atoms and C3N4 Layers for Efficient Photocatalysis, Adv. Mater., 2020, 32, 2003082 CrossRef CAS.
|
This journal is © the Partner Organisations 2024 |
Click here to see how this site uses Cookies. View our privacy policy here.