DOI:
10.1039/D3NR05524H
(Review Article)
Nanoscale, 2024,
16, 8216-8235
Microorganism-derived extracellular vesicles: emerging contributors to female reproductive health
Received
31st October 2023
, Accepted 27th March 2024
First published on 28th March 2024
Abstract
Extracellular vesicles (EVs) are cell-derived nanoparticles that carry small molecules, nucleic acids, and proteins long distances in the body facilitating cell–cell communication. Microorganism-derived EVs mediate communication between parent cells and host cells, with recent evidence supporting their role in biofilm formation, horizontal gene transfer, and suppression of the host immune system. As lipid-bound bacterial byproducts, EVs demonstrate improved cellular uptake and distribution in vivo compared to cell-free nucleic acids, proteins, or small molecules, allowing these biological nanoparticles to recapitulate the effects of parent cells and contribute to a range of human health outcomes. Here, we focus on how EVs derived from vaginal microorganisms contribute to gynecologic and obstetric outcomes. As the composition of the vaginal microbiome significantly impacts women's health, we discuss bacterial EVs from both healthy and dysbiotic vaginal microbiota. We also examine recent work done to evaluate the role of EVs from common vaginal bacterial, fungal, and parasitic pathogens in pathogenesis of female reproductive tract disease. We highlight evidence for the role of EVs in women's health, gaps in current knowledge, and opportunities for future work. Finally, we discuss how leveraging the innate interactions between microorganisms and mammalian cells may establish EVs as a novel therapeutic modality for gynecologic and obstetric indications.
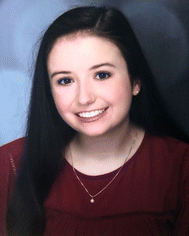 Kaitlyn A. Moore | Kaitlyn A. Moore is a PhD student in the Fischell Department of Bioengineering at the University of Maryland, College Park. |
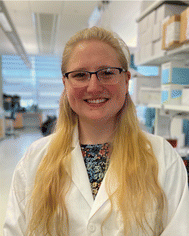 Alyssa P. Petersen | Alyssa P. Petersen is a PhD student in the Department of Chemical & Biomolecular Engineering at the University of Maryland, College Park. |
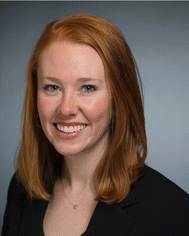 Hannah C. Zierden | Hannah C. Zierden, PhD is an Assistant Professor in the Department of Chemical & Biomolecular Engineering at the University of Maryland, College Park. |
Significance
Extracellular vesicles are an emerging contributor to obstetric and gynecologic health. This review details recent work done to understand the role of microorganism-derived EVs in the female reproductive tract, with important implications for engineering next generation therapies for women's health.
Introduction
The human body contains more bacterial cells than mammalian cells.1 This “human microbiome” plays a role in a range of health outcomes across several organ systems, from the gastrointestinal tract to the central nervous system. The female reproductive tract, too, is home to a unique microenvironment which significantly impacts gynecologic and obstetric outcomes. Unlike the diversity seen in the gastrointestinal environment, an optimal vaginal microbiome is one dominated by a single Lactobacillus spp.2 High-throughput screening studies have determined five primary community state types (CSTs), four of which are dominated by a single species of Lactobacillus (CST I by L. crispatus, CST II L. gasseri, CST III L. iners, CST V L. jensenii).3 Conversely, CST IV describes a polymicrobial environment marked by an overgrowth of facultative anaerobes. Clinically, CST IV is referred to as bacterial vaginosis (BV), a condition which affects ∼30% of women in the United States and is associated with an increased risk for pelvic inflammatory disease, urinary tract infections, infertility, acquisition of sexually transmitted infections, and preterm birth.4–9 The mechanisms that facilitate these disease manifestations have been explored through both clinical and pre-clinical studies, but are still not well understood.
Communication between microbes and mammalian cells is facilitated through the production of small molecules and proteins which elicit host immune responses and trigger functional changes in host tissues.10–13Lactobacillus spp. are considered protective in the vaginal environment, as they produce lactic acid and hydrogen peroxide, both thought to prevent the growth of pathogenic microbes.14–16Gardnerella vaginalis, Mobiluncus mulieris, and Prevotella bivia, on the other hand, produce mucus degrading enzymes, shed lipopolysaccharide, and secrete cytolysins, contributing to the onset of adverse health events.17–19 In recent studies, the microbiology field has turned its attention to extracellular vesicles (EVs) as a mode of microbe-host communication.20–22 Here, we use the term “EVs” to describe the biological particles produced by both Gram negative and Gram positive bacteria, as well as fungal species and parasites which inhabit the vaginal microenvironment.23 This term is used to encompass all naturally released particles from a cell, including membrane vesicles, outer membrane vesicles, outer–inner membrane vesicles, cytoplasmic membrane vesicles, and explosive outer membrane vesicles that, according to the International Society for Extracellular Vesicles (ISEV), “are delimited by a lipid bilayer and cannot replicate.”24 EVs are nano-sized (50–1000 nm, see Table 1) particles with different classifications used to describe their biogenesis (Fig. 1).25 With a thick cell wall, EVs produced by Gram positive bacteria are called membrane vesicles or cytoplasmic membrane vesicles and are thought to be produced via bubbling cell death.26,27 Gram negative bacteria have an inner and outer membrane, resulting in the production of several classes of EVs. Outer membrane vesicles are the result of stress causing blebbing of the outer membrane. Explosive outer membrane vesicles and outer–inner membrane vesicles are produced after weakening of the peptidoglycan layer.26,27 These varied mechanisms of EV production result in differences in EV cargoes, including small molecules, nucleic acids, and proteins.28 These cargoes are protected from degradation and dilution in vivo by a lipid membrane, often decorated with surface proteins which facilitate tissue targeting and cellular uptake.29,30 EVs have innate barrier crossing abilities, which further enhance their ability to communicate with host cells.31 For example, previous work from our group demonstrates that the cervicovaginal mucus barrier has a mesh size of ∼300 nm, which is too small for whole microbes to efficiently penetrate.32,33 In contrast, EVs from vaginal microbes are 100–300 nm and may be able to evade steric and adhesive interactions with mucins, permitting them to reach female reproductive tract tissues to deliver differential signals to host cells.31 The growing interest in microbial EVs has highlighted the many unanswered questions regarding the role and use of microbial EVs in the context of female reproductive health.
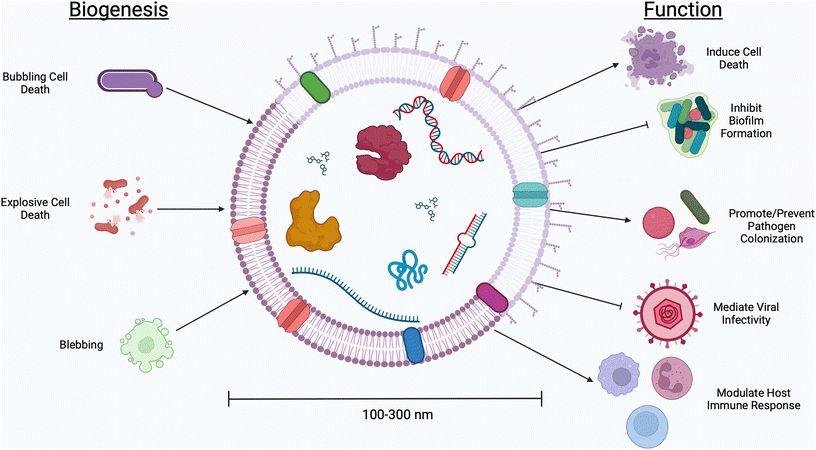 |
| Fig. 1 Bacteria and microorganism-derived EVs have demonstrated effects on host cells. Vaginal microbes generate different classifications of EVs, with Gram positive bacteria producing EVs via bubbling cell death and Gram negative bacteria producing outer membrane vesicles, explosive outer membrane vesicles, and outer–inner membrane vesicles via cell blebbing. Different classes of EVs have different biological functions ranging from inducing host cell death, inhibiting biofilm formation, decreasing viral infectivity, and eliciting host immune responses. | |
Table 1 EVs are produced by microorganisms in the female reproductive tract. Differences in physical characteristics and function may be artifacts of differences in isolation and handling of EVs. As work in the EV field continues to grow, careful consideration should be taken to follow the minimal reporting guidelines as defined by the International Society of Extracellular Vesicles45,50
Parent cell |
Parent cell source |
Parent cell strain |
Isolation method |
Storage method |
Size |
Concentration |
Cargo analysis |
Function |
Ref. |
L. crispatus
|
Patient-derived isolates |
BC5 |
Ultracentrifugation |
PBS at −80 °C |
89.3 ± 49.2 nm |
34.70 ± 2.31 × 109 particles per mL |
Total protein analysis, nucleic acid analysis |
Affected bacterial adhesion to cervical cells |
69
|
L. gasseri
|
Patient-derived isolates |
BC12 |
129.1 ± 51.9 nm |
30.60 ± 1.05 × 109 particles per mL |
L. crispatus
|
Patient-derived isolates |
BC3 |
Ultracentrifugation |
PBS at 4 °C |
133.14 ± 2.90 nm |
3.26 ± 0.11 × 1010 particles per mL |
Total protein analysis, metabolic analysis, proteomics |
Suppressed HIV-1 replication |
56
|
BC5 |
141.26 ± 9.78 nm |
1.18 ± 0.32 × 1010 particles per mL |
No protection against HIV-1 infection |
L. gasseri
|
Patient-derived isolates |
BC12 |
∼140 nm |
5.87 ± 0.20 × 1010 particles per mL |
Suppressed HIV-1 replication |
BC13 |
∼140 nm |
1.32 ± 0.44 × 1011 particles per mL |
No protection against HIV-1 infection |
S. aureus
|
ATCC |
12600 |
Ultracentrifugation |
PBS at 4 °C |
231.97 ± 11.24 nm |
3.52 ± 1.79 × 1011 particles per mL |
Proteomics |
Suppressed HIV-1 replication |
77
|
E. faecium
|
ATCC |
19434 |
∼200 nm |
3.78 ± 1.61 × 1011 particles per mL |
E. faecalis
|
ATCC |
19433 |
∼190 nm |
2.27 ± 0.88 × 1012 particles per mL |
G. vaginalis
|
ATCC |
14018 |
179.07 ± 17.22 nm |
5.42 ± 1.65 × 1011 particles per mL |
L. gasseri
|
ATCC |
9857 |
Ultracentrifugation, filtration |
PBS at −80 °C |
85 nm |
1.6 × 1010 to 2.2 × 1011 particles per mL |
Total protein analysis, nucleic acid analysis, proteomics |
Inhibited T. vaginalis cytoadhesion |
55
|
G. vaginalis
|
ATCC |
14018 |
115 nm |
3.21 × 1010 to 1.5 × 1011 particles per mL |
Enhanced T. vaginalis cytoadhesion |
L. gasseri
|
ATCC |
19992 |
Ultracentrifugation |
PBS at −20 °C |
295–458 nm |
— |
Total protein analysis |
Disrupted G. vaginalis and S. aureaus biofilm formation |
72
|
L. crispatus
|
BCRC |
14618 |
Ultracentrifugation, filtration |
PBS |
110.8 ± 3.2 nm |
— |
Total protein analysis |
Decreased oxidative stress-induced trophoblast death |
67
|
A. muciniphila
|
ATCC |
BAA-835 |
Ultracentrifugation |
PBS at −80 °C |
119.2 ± 3.4 nm |
— |
Total protein analysis, nucleic acid analysis, RT-qPCR, 16S rDNA gene sequencing, RNA sequencing |
Decreased pre-eclamptic symptoms in mice and cultured trophoblasts |
68
|
G. vaginalis
|
ATCC |
14019 |
Ultracentrifugation |
PBS at −20 °C |
120–260 nm |
— |
Total protein analysis, proteomics |
Increased cytotoxicity and inflammation in vaginal epithelial cells |
49
|
G. vaginalis
|
ATCC |
14019 |
Ultracentrifugation |
PBS (pH 7.4) at −20 °C |
190–250 nm at pH 6.5, 190–615 nm at pH 3.5 |
— |
Total protein analysis, proteomics |
Decreased viability of and increased IL-8 production in vaginal epithelial cells |
66
|
C. albicans
|
ATCC |
90028 |
Centrifugation |
PBS (pH 7.4) |
135 nm; 100–250 nm, 300–350 nm, 420–480 nm |
61.63 × 106 particles per mL |
Total protein analysis, total lipid analysis |
Decreased biofilm formation |
71
|
ATCC |
10231 |
195 nm; 100 to 400 nm |
36.2 × 106 particles per mL |
ATCC |
SC5314 |
175 nm; 100 to 400 nm |
120.5 × 106 particles per mL |
C. albicans
|
— |
SN152 |
Size exclusion chromatography |
PBS (pH 7.2) |
EVs: 30–200 nm, 200–1000 nm; biofilm EVs: 30–200 nm |
∼1 × 106 particles per mL in biofilms |
Proteomics, carbohydrate analysis, lipidomics |
Enhanced biofillm formation and antifungal drug resistance |
75
|
S. agalactiae (GBS) |
Patient-derived isolates |
GB00020, sequence type 1, serotype cpsV |
Ultracentrifugation |
PBS at −80 °C |
— |
∼0.05–0.10 particles per CFU |
Proteomics |
— |
88
|
GB00037, sequence type 1, serotype cpsV |
— |
∼0.05–0.10 particles per FU |
GB00112, sequence type 17, serotype cpsIII |
— |
∼0.05–0.15 particles per FU |
GB00411, sequence type 17, serotype cpsIII |
— |
∼0.05–0.15 particles per FU |
GB00653, sequence type 12, serotype cpsII |
— |
∼0.05–0.15 particles per FU |
GB01455, sequence type 12, serotypeII |
— |
∼0.15–0.20 particles per FU |
S. agalactiae (GBS) |
Patient-derived isolates |
A909-derived ΔcovR |
Ultracentrifugation |
PBS |
— |
— |
Total protein analysis |
Caused hemolysis in human neutrophils, decreased neonate surivival in mice |
89
|
A909-derived ΔcovRΔcylE |
— |
— |
Non-hemolytic |
S. agalactiae (GBS) |
Patient-derived isolates |
A909 (serotype IA) |
Ultracentrifugation |
PBS (pH 7.2) |
<50 nm; 150–300 nm |
1.7 × 104 particles per mL |
Total protein analysis, proteomics, nucleic acid analysis, lipidomics |
Degraded collagen and reduced fetal membrane stiffness preterm birth and intrauterine demise |
90
|
C. trachomatis
|
|
serovar F/Cal-I-13 |
Nitrogen cavitation, density gradient ultracentrifugation |
PBS at 4 °C or −80 °C |
700–2000 nm |
— |
western blot |
Immune modulating |
95
|
N. gonorrhoeae
|
Patient-derived isolates |
FA1090, F62, MS11, and 1291 |
Ultracentrifugation |
PBS |
— |
— |
Proteomics |
— |
100
|
N. gonorrhoeae
|
|
WS1, F62 (serum-susceptible) FA638, JS1 (serum-resistant) |
Ultracentrifugation, filtration |
— |
— |
— |
Electrophoresis |
Serum-resistant EVs protected serum-susceptible strains |
101
|
N. gonorrhoeae
|
Meningococcal Reference Unit |
K454 |
Differential ultracentrifugation |
PBS at −80 °C |
100 ± 20 nm |
— |
— |
Immunosuppressive; Reduced T-cell proliferation |
104
|
T. vaginalis
|
— |
B7RC2 |
Density gradient utracentrifugation |
PBS at −20 °C |
95 nm; 50–150 nm |
— |
Total protein analysis, proteomics, total RNA analysis |
Immune modulating, increased parasite adherence to cells |
106
|
T. vaginalis
|
— |
B7RC2 |
Ultracentrifugation |
PBS |
105 nm |
2.74 × 1012 particles per mL |
Total protein analysis, nucleic acid analysis, RNA-seqencing |
— |
107
|
T. vaginalis
|
— |
B7RC2 ((PA) and Jt wild type) |
Centrifugation |
PBS + 1X HALT protease inhibitors |
MVs: 380 nm; Exosomes: 63 nm |
— |
Proteomics |
Taken up by parasites and cervical cells |
54
|
T. vaginalis
|
Patient-derived isolate |
GT-21 |
Centrifugation |
PBS (pH 7) + protease inhibitors |
50–100 nm |
— |
Total protein analysis, nucleic acid analysis |
Immune modulating |
108
|
T. vaginalis
|
ATCC 50167 |
B7RC2 |
Density gradient utracentrifugation |
PBS (pH 7.4) at −20 °C |
— |
— |
Total protein analysis |
Internalized via clathrin-independent, lipid raft-mediated endocytosis |
109
|
C. albicans
|
ATCC MYA-2876 |
SC5314 |
Centrifugation |
PBS |
≤50 nm; 50–100 nm |
— |
Lipidomics |
— |
112
|
C. albicans
|
ATCC |
90028 |
Centrifugation |
PBS (pH 7.4) |
50–100 nm; 450–850 nm |
— |
Proteomics, total protein analysis, lipidomics |
Internalized by macrophages, immune modulating |
114
|
Patient-derived isolate |
11 |
50–100 nm; 350–450 nm |
— |
Proteomics, total protien analysis, lipidomics |
Internalized by macrophages, immune modulating |
C. albicans
|
Patient-derived isolate |
SC5314 (71) |
Ultracentrifugation |
PBS + TEAB buffer |
50–450 nm, 100–200 nm |
— |
Total protein analysis, proteomics |
Induced cytotoxicity in human macrophages |
116
|
In this review, we examine recent studies which provide evidence for the role of bacterial EVs in mediating female reproductive health. We discuss bacterial EVs from both healthy and dysbiotic vaginal microbiota, and EVs from common vaginal bacterial, fungal, and parasitic pathogens, highlighting evidence for their role in gynecologic health, as well as gaps in current knowledge and opportunities for future work. Finally, we discuss how leveraging the innate interactions between microorganisms and mammalian cells may establish EVs as a novel therapeutic modality for gynecologic and obstetric indications. It is worth noting that a growing body of work details how mammalian cell-derived EVs participate in female reproductive tract physiology, ranging from infertility and miscarriages, to gynecologic cancers and homeostasis during pregnancy.34–38 There are several thorough and recent reviews which detail how EVs from cervical, placental, uterine, and endometrial cells contribute to important gynecologic and obstetric function.39–41 While EVs produced by mammalian cells likely enable host–microbe communication, and likely serve as a response to signaling from microorganisms in the female reproductive tract, the focus of this review is on EVs produced by microorganisms. We hope that by understanding EVs produced by microorganisms and their effect on the female reproductive tract, future studies may begin to ask more complicated questions regarding host–microbe crosstalk facilitated by EVs.
Considerations for isolation and characterization of extracellular vesicles
Throughout EV research, variability in isolation, storage, and characterization protocols makes it difficult to compare across studies (Table 1).42,43 Even the tubes used to store EVs throughout experiments may alter observed results.44 ISEV has up-to-date recommendations for isolation procedures and reporting guidelines that will help in comparing results across future studies.45 Nonetheless, here we make an effort to highlight experimental procedures as we assess differences in EVs from vaginal microbes that may contribute to differential clinical outcomes.
Several emerging techniques are used to efficiently isolate pure populations of EVs.46 First, it is necessary to remove parent cells from cultured media or biological matrix, often done via low-speed centrifugation (2000 to 15
000 rcf) or filtration (0.2 or 0.45 μm). Sequential ultracentrifugation (100
000 to 200
000 rcf) or density ultracentrifugation is used to isolate particles in the size range of EVs. Ultracentrifugation typically generates high-purity, but low-recovery EV samples. Size exclusion chromatography is used to separate the components of conditioned media based on size, with large particulates eluting from the column in earlier fractions than small particulates. Chromatography methods suffer from impurity issues, where lipoproteins and other small non-EV cell byproducts may elute in the same fractions as EVs. Given their lipid membrane and net negative surface charge, EVs are amenable to isolation using anion exchange chromatography. Ultrafiltration, as well as precipitation with a number of commercially available reagents, allows for concentration of isolated EVs. Differences in isolation protocols may account for variability in experimental observations regarding EV function, so it is typically necessary to optimize isolation protocols for EVs from different parent cells (Table 1).
After isolation, the physical characteristics of EVs can be measured using nanoparticle tracking analysis (NTA) and dynamic light scattering (DLS).47 NTA and DLS measure the diffusivity of particles in solution and calculate a hydrodynamic radius of individual particles based on principles of Brownian motion. NTA presents an improvement over DLS, which may overestimate the size of large particles. Transmission electron microscopy (TEM) is commonly used to visualize and measure EVs, specifically assessing structure and morphology. Protein concentration is also part of the ISEV recommended reporting guidelines, with techniques such as western blot and flow cytometry being used to detect specific surface markers. Escherichia coli-derived EVs have been detected using the surface marker OmpA.48 While less work has been done to determine surface markers of EVs from vaginal microbes, future work could use surface layer proteins or something more specific, such as the cytolytic protein vaginolysin which may be found on the surface of G. vaginalis EVs.45,49,50 Generally, studies report size, concentration, morphology, and protein content of EVs, with some also reporting the surface charge (ζ-potential).
Where the size, ζ-potential, and morphology of EVs may affect stability in the vaginal environment, membrane composition (lipids) and targeting proteins may impact EV uptake into female reproductive tract cells (Fig. 1). Proteomics and lipidomic studies can give insight into membrane compositions that affect uptake. EVs are taken up via several mechanisms: they may bind to specific cell receptors; they may enter host cells by direct fusion with the cell membrane; or they may enter via an endocytic uptake (clathrin-mediated or caveolin-mediated endocytosis, phagocytosis, micropinocytosis).51–53 Studies demonstrate that route of uptake may vary based on size, as well as macromolecules within the EV membrane. Specific to female reproductive tract cells, G. vaginalis-derived EVs adhere to the cell surface and are internalized by vaginal epithelial cells.49T. vaginalis EVs are taken up by mammalian cells via caveolae-dependent endocytosis.54 By further exploring mechanisms of cellular uptake that facilitate microbe-host communication by EVs, future work may advance our understanding of the biological effects of EVs, as well as their use as therapeutic agents.
Once EVs reach a target host cell, internal cargoes are released into the cytoplasm, allowing for unique biological cargoes to be delivered to host cells.49,55,56 These cargoes have immunomodulatory properties, both in and outside the context of gynecologic and obstetric health. RNA cargoes (mRNA, siRNA, miRNA) can activate host immune response, regulate bacterial growth, and weaken host biological barriers (Table 1). DNA cargoes may enable horizontal gene transfer with the potential to increase antibacterial resistance (Fig. 2).57 Protein cargoes may mediate bacterial virulence and immune system evasion.49,58 In addition to nucleic acids and proteins, Lactobacillus-derived EVs were found to carry metabolites with antiviral activity, including lactic acid.56 Understanding differences in EV cargoes will not only reveal differences in the biological function of EVs, but may help to identify therapeutic opportunities using EVs or mimicking EV interactions with host tissues using synthetic lipid nanoparticles.
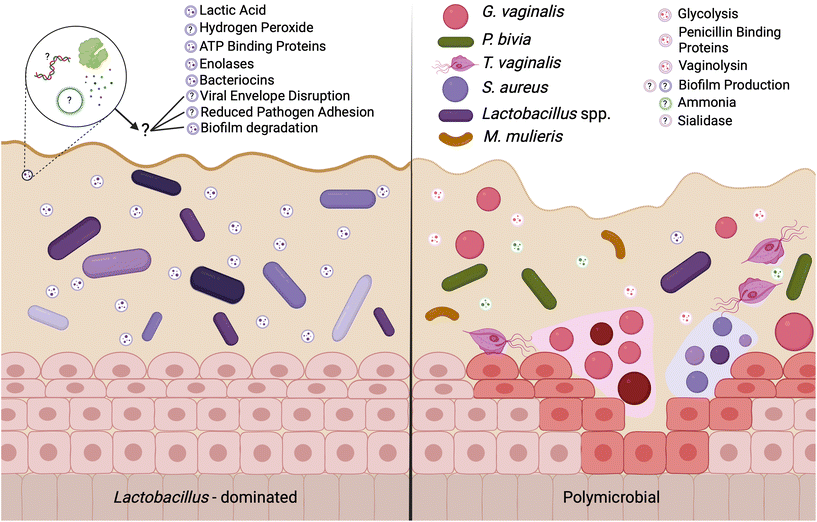 |
| Fig. 2 EVs may mediate differences in female reproductive outcomes are associated with the composition of the vaginal microbiome. The composition of the vaginal microbiome is associated with differential gynecologic and obstetric outcomes, where a lactobacilli-dominated microenvironment is clinically associated with healthy outcomes and a polymicrobial environment is associated with adverse outcomes including preterm birth, infertility, urinary tract infections, and increased risk for sexually transmitted infections. Recent work suggests that some of these outcomes are facilitated by microorganism-derived EVs. EVs from probiotic bacteria carry antimicrobial factors, including lactic acid, hydrogen peroxide, and antimicrobial peptides to protect against pathogens and promote the growth of healthy-associated microbes. In contrast, pathogen-derived EVs carry cytolytic proteins and mucin degrading enzymes, allowing for evasion and suppression of the host immune system. The current evidence supports further efforts in understanding EV cargoes and EV-mediated outcomes in the female reproductive tract. | |
Vaginal bacterial extracellular vesicles mediate female reproductive outcomes
A Lactobacillus spp. dominated vaginal microbiome is considered protective, playing an important role in maintaining a healthy environment (Fig. 2). Lactobacillus spp. produce lactic acid, which lowers the pH of the vagina, preventing the growth of pathogenic bacteria and reducing inflammation in the reproductive tract. These microbes also produce antimicrobial compounds, including hydrogen peroxide, bacteriocins, and enzymes. In contrast, dysbiosis in the vaginal microenvironment is associated with an increased risk for a broad spectrum of gynecologic and obstetric disease.2–4,59 The pathogenesis of BV begins with the introduction of G. vaginalis, P. bivia, and A. vaginae into the vaginal environment, synergistically displacing lactobacilli (Fig. 2). As G. vaginalis forms a biofilm, the secretion of proteolytic enzymes and production of amino acids promotes the growth of P. bivia which produces ammonia, thereby continuing the cycle of biofilm formation.17,18 Sialidase produced by these microbes degrades the mucus barrier, allowing bacterial invasion into the vaginal epithelium.60–62 Despite improved fundamental understanding of the vaginal microbiome, promoting a healthy vaginal microbiome and healthy reproductive outcomes remains clinically challenging. It has been proposed that bacteria may ascend from the vaginal environment into upper levels of the female reproductive tract, causing inflammation and adverse health outcomes. However, our recent work demonstrates that the pore size of the cervicovaginal mucus barrier is too small for whole bacteria to penetrate (Fig. 3A–C).32,33 Given their small size, it may be more likely that EVs penetrate cervicovaginal mucus to deliver proteins, nucleic acids, or small molecules that initiate reproductive outcomes (Fig. 3D). Supporting this hypothesis, separate work suggests that first-pass meconium may contain bacterial EVs from the maternal microbiome, indicating that bacterial EVs may be able to ascend from the vaginal environment, reach the uterine cavity, and traverse the placental barrier to impart differential signals that may influence pregnancy and neonatal outcomes.63,64 Another study suggests that EVs may be a source of microbial DNA in the placenta, with evidence of in vitro uptake of bacterial EVs by trophoblasts.65 In this section, we present recent evidence to support the role of vaginal bacterial EVs in female reproductive outcomes (Tables 1 and 2).
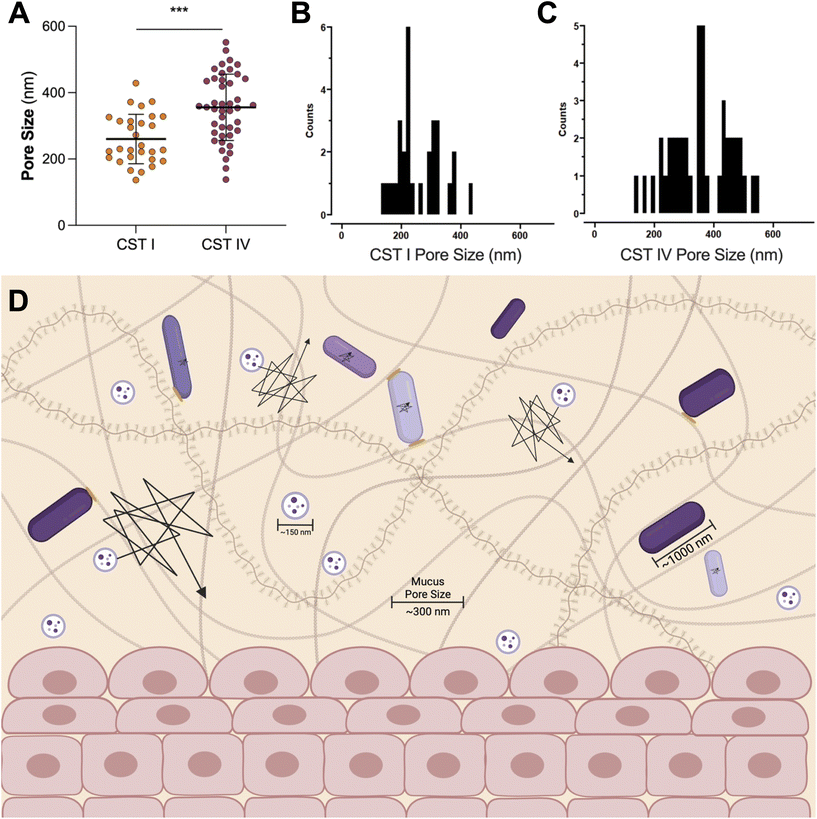 |
| Fig. 3 The pore size of cervicovaginal mucus may be permissive of EVs. (A) The pore size of cervicovaginal mucus differs based on the composition of the vaginal microbiome, where a polymicrobial environment has an increased pore size. (B) The distribution of pore sizes in CST I samples, and (C) CST IV samples shows a range of pore sizes for pathogens or EVs to move through mucus. Data republished with permission from ref. 33. (D) Even the increased pore size observed in CST IV samples is not large enough for whole bacteria to penetrate. In contrast, even the less permissive mucus barrier observed in the case of CST I samples (pore size ∼300 nm) is large enough for EVs ∼100–200 nm in size to move through mucus without significant steric hindrance. In vitro and ex vivo evidence suggests that EVs may mediate female reproductive tract disease, potentially by penetrating the vaginal mucus barrier and ascending into upper levels of the female reproductive tract. This proposed mobility makes EVs useful as carriers for vaginally delivered therapeutics. Black lines represent the trajectories of particles or bacteria through mucus, where a larger trajectory is indicative of a more mobile particle. Bacteria are hindered through steric interactions with mucus pores, represented by yellow ovals. | |
Table 2 Bacteria-free supernatants demonstrate effects on host cells. Bacteria-free supernatants have been used to examine the effects of bacterial byproducts on host cells. While these studies do not explicitly investigate the contribution of EVs to host responses, the supernatants used throughout the experiments undoubtedly contain EVs. The results of these studies support further investigation into the effects of EVs on female reproductive tract cells
Parent cell |
Parent cell source |
Parent cell strain |
Function |
Ref. |
L. crispatus
|
Patient-derived isolates |
BC3 |
Decreased HIV-1 replication and infectivity in ex vivo |
76
|
BC5 |
L. gasseri
|
Patient-derived isolates |
BC12 |
BC13 |
G. vaginalis
|
Patient-derived isolates |
BC16 |
BC17 |
— |
L. crispatus
|
ATCC |
33197 |
Decreased C. trachomatis infectivity |
78
|
ATCC |
33820 |
ATCC |
55844 |
PTA |
10138 |
L. jensenii
|
ATCC |
25258 |
L. iners
|
ATCC |
55195 |
Did not alter C. trachomatis infectivity |
L. crispatus
|
Patient-derived isolates |
— |
Decreased C. albicans growth and propagation in a vulvovaginal candidiasis murine model |
79
|
L. gasseri
|
Patient-derived isolates |
— |
Decreased growth of C. albicans in vitro |
L. jensenii
|
Patient-derived isolates |
— |
|
L. crispatus
|
ATCC |
33197 |
Significantly increased tight junctions in cervicovaginal epithelial cells |
80
|
L. jensenii
|
ATCC |
25258 |
|
L. iners
|
ATCC |
55195 |
Mildly increased tight junctions in cervicovaginal epithelial cells |
G. vaginalis
|
ATCC |
14018 |
Did not alter tight junctions in cervicovaginal epithelial cells |
L. crispatus
|
Patient-derived isolates |
— |
Immune modulating, decreased ectocervical cell permeability |
81
|
L. iners
|
ATCC |
55195 |
Increased cervical cell permeability, pro-inflammatory |
G. vaginalis
|
ATCC |
14018 |
|
L. crispatus
|
ATCC |
33197 |
Decreased matrix metalloproteinases in female reproductive tract cells |
82
|
G. vaginalis
|
ATCC |
14019 |
Increased matrix metalloproteinases in female reproductive tract cells |
L. crispatus
|
ATCC |
33197 |
Decreased cervical cell permeability |
84
|
G. vaginalis
|
ATCC |
14018 |
Increased cell death permeability |
M. mulieris
|
ATCC |
35243 |
Increased cell permeability and cytokine secretion |
83
|
BV is associated with increased reproductive tract inflammation. One recent study demonstrated the pro-inflammatory and cytotoxic activity of G. vaginalis EVs in vaginal epithelial cells in vitro.49 Cellular uptake of EVs induced cell blebbing and a dose-responsive decrease in cell viability. EV surface proteins associated with pathogenesis, virulence, antibiotic resistance, and protection against the host immune system caused functional changes in vaginal epithelial cells in vitro. Vaginolysin, a pore forming toxin associated with the virulence of G. vaginalis, was also detected in the isolated EVs.49 As a follow-up, the same group investigated the effects of a decreased pH on G. vaginalis growth and EV production as a proxy for understanding how a lactobacilli-dominated vaginal environment may change the effects of G. vaginalis EVs on epithelial cells.66 In addition to decreased viability of G. vaginalis, the size, morphology, and protein content of G. vaginalis EVs were significantly altered at pH 3.5. Of note, vaginolysin was no longer found in the EVs from G. vaginalis cultured at pH 3.5. Authors attributed this change to a degradation of vaginolysin prior to EV release. Interestingly, EVs from G. vaginalis cultured at pH 3.5 still increased IL-8 production from vaginal epithelial cells, indicating a potential role for G. vaginalis-derived EVs in modulating the host immune system in an acidic vaginal environment.66 Artuyants, et al. similarly reported vaginolysin as the most significantly enriched protein found in EVs from G. vaginalis cultures.55 However, in this study, treatment with G. vaginalis EVs did not alter human ectocervical cell viability. Future work could evaluate mechanisms of cellular uptake that differ based on culture conditions and strain-level differences in G. vaginalis cultures.
A Lactobacillus-dominated vaginal environment is associated with healthy pregnancy outcomes. Recent work investigated the effects of L. crispatus EVs on placental function in response to oxidative stress.67 While oxidative stress in the first trimester initiates important adaptations to pregnancy, chronic or severe oxidative stress may result in pregnancy loss, preterm birth, preeclampsia, fetal growth restriction, or hypertension. Given the association of L. crispatus with healthy pregnancies, Wang and coworkers investigated the role of L. crispatus EVs on mitigating the effects of hydrogen peroxide-induced oxidative stress on placental cells in vitro. L. crispatus EVs rescued cells from hydrogen peroxide-induced death by recovering Akt phosphorylation and inhibiting mitochondrial fission.67 Another preclinical study investigated Akkermansia muciniphila-derived EVs as prophylactic treatment of preeclampsia.68A. muciniphila is a gut microbe found in lower levels in patients who develop preeclampsia. In mice, daily oral administration of A. muciniphila EVs prevented intrauterine growth restriction, lowered blood pressure, and alleviated placental dysfunction.68 The mechanisms underlying this attenuation of disease remain unknown, so future work may investigate the protective properties of EVs by assessing protein and nucleic acid cargoes.
In addition to microbe-host communication, bacterial EVs mediate microbe–microbe communication in the female reproductive tract. Lactobacillus spp. EVs have been found to promote the colonization of Lactobacillus spp., while reducing the adhesion of pathogenic species.69 By pretreating cervical cells with L. crispatus- and L. gasseri-derived EVs, Croatti, et al. demonstrated increased adhesion of lactobacilli in vitro, accompanied by reduced adhesion of Escherichia coli, Staphylococcus aureus, Streptococcus agalactiae, and Enterococcus faecalis.69 Beyond bacterial adhesion, some evidence suggests that EVs may contribute to high recurrence rates of BV by facilitating the formation of biofilms in the vagina (Fig. 4).70–75 Khan, et al. investigated the role of L. gasseri derived EVs in disrupting biofilms formed by G. vaginalis and S. aureus.72 Following 48 h of biofilm formation, G. vaginalis and S. aureus cultures were treated with L. gasseri-derived EVs. The authors observed a dose responsive decrease in biofilm thickness and integrity.72 Further work could be done to determine which specific cargoes may play a role in these observations.69
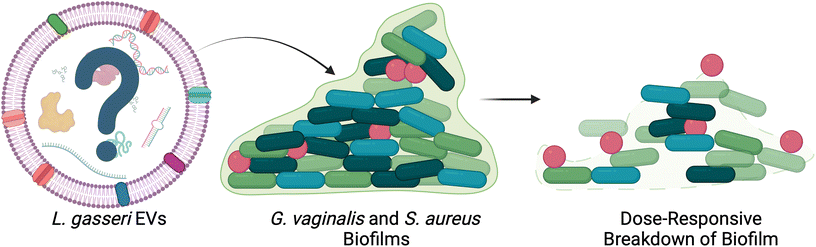 |
| Fig. 4
L. gasseri EVs disrupt biofilm formation in vitro. G. vaginalis and S. aureus biofilms were disrupted after treatment with L. gasseri EVs in a dose-responsive manner. This work presents a potential therapeutic avenue for bacterial EVs in the female reproductive tract. Adapted with permission from.72 | |
A Lactobacillus spp.-dominated vaginal microbiome is associated with a decreased risk of sexually transmitted infections, including HIV-1. These protective properties are facilitated through several biological mechanisms: maintenance of the mucosal barrier, inactivation of HIV-1 via acidification of the vagina, and induction of host immune response against HIV-1. Building upon prior work using bacteria-free supernatants from cultures of lactobacilli,76 a recent study investigated the ability of EVs from two strains of each L. crispatus and L. gasseri to inhibit HIV-1 infection both in vitro and ex vivo. Authors observed strain-specific reduction of HIV-1 replication in T cells after incubation with EVs.56 The authors found similar results in human tonsillar and cervicovaginal tissues ex vivo. As there were no physical differences in EVs (similar size and production rates), the authors hypothesized their findings may be attributed to differences in cargo. Indeed, amino acids, lactic acid, and several proteins were hallmarks of the EVs which inhibited HIV-1 replication.56 Interestingly, the authors found similar results using EVs from G. vaginalis, S. aureus, Enterococcus faecium, and E. faecalis.77 As pretreatment of cells with EVs did not prevent HIV-1 replication in vitro, these findings suggest that bacterial EVs inhibit infection through direct interactions with HIV-1 virions rather than indirect interactions with viral target cells.56 In a separate study, Artuyants, et al. evaluated microbe-pathogen interactions, specifically studying the effects of L. gasseri and G. vaginalis EVs on T. vaginalis cytoadhesion. Consistent with clinical observations, EVs from L. gasseri inhibited T. vaginalis cytoadhesion, whereas G. vaginalis EVs enhanced cytoadhesion.55 While the exact mechanisms behind these observations remain unclear, together these studies are suggestive of the role of lactobacilli-derived EVs in supporting a healthy vaginal microenvironment.
As the EV-field continues to grow, we can look to studies which investigate the effects of bacteria-free supernatants on female reproductive tract cells (Table 2). Bacteria-free supernatants are typically generated by centrifugation at a relatively low speed to remove whole cells without removing other byproducts, similar to the first step of EV isolation. While other bacterial byproducts are certainly present in bacteria-free supernatants, these samples undoubtedly also contain EVs. Despite these studies not explicitly mentioning EVs, we believe they support the idea that EVs play a role in the microbe-host communication that underlies microbiome-associated diseases. Edwards, et al. investigated Lactobacillus spp. supernatants on Chlamydia trachomatis infectivity and observed a decrease in human cervical epithelial cell infection after pre-treatment with lactic acid, or L. crispatus or L. jensenii, but not L. iners, bacteria-free supernatants.78 The authors observed more persistent protection with bacteria-free supernatants than with lactic acid alone, suggesting that there may be important bacterial byproducts which contribute to protection against C. trachomatis.78 Jang, et al. observed that L. crispatus bacteria-free supernatants reduced the growth of Candida albicans.79 Certainly more work should be done to investigate the mechanisms underlying these observations, but the current evidence supports that a bacterial byproduct, perhaps EVs, may contribute to increased protection against vaginal infections.
Investigating the effects of bacterial byproducts on cervical remodeling, Delgado-Diaz, et al. tested the effects of lactic acid on tight junctions in ectocervical cells, demonstrating increased barrier integrity and altered gene expression related to epithelial barrier properties.80 Interestingly, when treated with bacteria-free supernatants tight junction genes were further increased (Table 2). While the commercially available lactic acid and bacteria-free supernatants were not directly compared, the apparent increase in expression of barrier function genes associated with supernatant treatment may be attributed to other secreted factors in the conditioned media. In a similar study, Anton and coauthors investigated the effects of microbial supernatants on cervical cell permeability, as a representation of how bacteria may contribute to premature cervical remodeling and preterm birth (Table 2).81 The authors demonstrated that L. crispatus bacteria-free supernatants protected in vitro cervical barriers from degradation by lipopolysaccharide (LPS). The supernatants reduced IL-6 and IL-8 secretions, and were protective against G. vaginalis-induced changes relevant to cervical remodeling.81 Interestingly, L. crispatus supernatants elicited a stronger anti-inflammatory response than live bacteria across all three cell types, indicating that a byproduct of the parent cells may be responsible for the protective nature of L. crispatus in the female reproductive tract.82 In a follow-up study, authors observed differing effects of L. crispatus and G. vaginalis supernatants on vaginal epithelial, ectocervical, and endocervical cells.82–84 Where L. crispatus supernatants were protective of barrier function, G. vaginalis supernatants caused degradation of the cervical barrier in vitro, accompanied by an increase in cytokine production and release of matrix metalloproteinases (Table 2).81,84 In a later study, the group investigated bacteria-free supernatants from M. mulieris, another bacterium highly associated with preterm birth.83M. mulieris supernatants significantly increased cervical cell degradation and production of inflammatory cytokines IL-6 and IL-8, as compared to non-conditioned media.83 While these studies did not specifically investigate the role of EVs on cervical remodeling and preterm birth, and the mechanisms linking bacterial EVs and preterm birth need to be further explored, there is convincing evidence for investigating how bacterial byproducts (EVs, in particular) may mediate microbe-host communications in the context of gynecologic and obstetric health. As lipid-bound bacteria-produced nanoparticles, EVs may aid in the transport and uptake of cargoes that contribute to adverse gestational outcomes. We propose that, in addition to small molecules and secreted proteins, the bacteria-free supernatants evaluated in the aforementioned studies contained bacterial EVs and suggest that future studies utilize isolated particles to directly probe the effects of EVs on epithelial integrity, as well as cervical remodeling and related mechanisms of parturition.
Many questions remain regarding the role of bacterial EVs in the vaginal microenvironment and reproductive outcomes, including infertility, recurrent pregnancy loss, miscarriage, pelvic inflammatory disease, and more. With clinical evidence linking Lactobacillus spp. with improved fertility, gestational, and gynecologic outcomes, and pathogenic species with adverse outcomes, there are likely many pathways in which EVs potentiate clinical outcomes. We can look to work done using EVs from other species to inform hypotheses regarding EV function, but it is clear that species- and strain-level differences must be evaluated to fully understand microbe-host communication facilitated by bacterial EVs in the vaginal environment.
Extracellular vesicles enable pathogen:host communication
Beyond the adverse health outcomes associated with BV, the female reproductive tract is susceptible to infection by other pathogenic bacteria, parasites, and fungi. Recent studies examined the role of EVs in promoting pathogenesis of these microorganisms in the vaginal environment and female reproductive tract.
Group B streptococcus
Streptococcus agalactiae, or group B streptococcus (GBS), resides in the rectovaginal area of up to 30% of adults.85–87 While generally considered harmless, GBS can cause severe infection and disease in neonates. For this reason, pregnant people are screened for GBS at around 36 weeks of pregnancy. If positive, patients are given antibiotics at time of delivery. As all the serotypes of GBS are known to produce EVs (Table 1), the effects of GBS may persist even after antibiotic treatment.88 These biological nanoparticles may also be able to ascend into the uterine compartment, leading to adverse obstetric outcomes prior to antibiotic treatment. Several studies have investigated the production of EVs across different, clinically relevant strains of GBS.88–90 One study systematically evaluated EVs from six strains of GBS, demonstrating differences in production, physical properties, and protein cargoes, where nearly 30% of protein cargoes were detected across all six strains of GBS.88 Variable abundance of peptidase, hyaluronidase, and sialidase were observed across the three sequence types analyzed in this study, highlighting the potential for a strain-specific EV contribution to GBS virulence.88
A separate study evaluated the hemolytic activity of EVs from hemolytic and nonhemolytic GBS.89 Authors demonstrated that the EVs protected GBS against host defenses, specifically reactive oxygen species. In vivo work demonstrated that intraperitoneal injection of hemolytic and nonhemolytic EVs into neonatal mice caused morbidity and mortality due to neonatal lung injury, consistent with clinical manifestations of GBS disease in human neonates.89 A third study evaluated EVs from GBS in their ability to cause preterm birth and intrauterine fetal demise.90 The authors found that EVs caused a dose-dependent decrease in cervical cell viability in vitro, led to a breakdown of murine chorio-decidual membranes ex vivo, and were able to ascend into the murine uterus within 6 h of vaginal administration. Intraamniotic injections of GBS EVs caused breakdown of the chorio-decidual epithelium, an influx of neutrophils and lymphocytes into the amniotic sacs (reflective of chorioamnionitis), and a significant and dose-dependent induction of preterm birth.90 These in vivo studies are certainly interesting and suggestive of the ability of GBS-derived EVs to lead to adverse gynecologic outcomes. Future work could better mimic the human case by investigating fetal response to EVs after maternal vaginal administration.
Sexually transmitted bacterial infections
Chlamydia trachomatis and Neisseria gonorrhoeae, two microbes which cause sexually transmitted infections, produce EVs (Table 1).91C. trachomatis is an intracellular bacterium that causes chlamydia, the most common sexually transmitted infection. Chlamydia can lead to cervicitis, pelvic inflammatory disease, and infertility in females. Neonates exposed to C. trachomatis may develop pneumonia or conjunctivitis.92 Infection occurs when elementary bodies are phagocytosed and use cellular nutrients to develop reticulate bodies, eventually producing more elementary bodies.93 As cell nutrients are taken for C. trachomatis growth, the host cell will rupture, releasing new elementary bodies to infect other cells. As the pathogen develops into reticulate bodies, it communicates with the host cell, in part, through the production of EVs.94–96 EVs from C. trachomatis were first described based on observed changes to the bacterial membrane in response to stressors, such as antibiotics, nutrient deprivation, and co-infections.97 Physical perturbations in the outer membrane of the bacteria led to the production of EVs blebbing from the membrane of the inclusions. TEM images of C. trachomatis-infected human endocervical cells revealed EVs present within inclusion bodies within infected cells, and Frohlich and coworkers were able to isolate C. trachomatis EVs from infected mouse fibroblast cells.94 EV cargo analysis revealed cytotoxic proteins present in the isolated vesicles, supporting the hypothesis that EVs play a role in modulating the host immune response. Albeit mild, EVs from C. trachomatis caused an increase in IL-6 from mammalian cells in vitro, suggesting that EVs may aid in evasion of the host immune system.95 Understanding EVs from intracellular bacteria is challenging, as it is difficult to differentiate between EVs produced by host cells and those produced by infectious bacteria. However, as technology in the EV field develops there will be more opportunities to understand how EVs play a role in infection and how they may be harnessed to treat and prevent infections.
N. gonorrhoeae causes gonorrhea, another potentially asymptomatic sexually transmitted infection that can lead to significant complications in neonates, usually corneal infection that can progress to blindness.98 Outer membrane blebs from N. gonorrhoeae were first described by Dorward and Judd in 1989.99 EVs were observed to be released during the log-phase of growth and to have similar immunogenic properties as parent microbes. More recently, Zielke and coworkers assessed the protein composition of N. gonorrhoeae cell envelopes and EVs.100 Authors found differences across strains, but identified a large fraction of proteins responsible for biogenesis and cellular metabolism. While the authors did not investigate the function of specific proteins, follow-up studies have built upon this work to develop vaccines and therapies for preventing gonorrhea (see more in section “Extracellular vesicles as a therapeutic platform”).
A 1992 study investigated the role of N. gonorrhoeae EVs in protecting bacteria against human serum immunoglobulins.101,102 Pettit and Judd found differences in host recognition of EVs produced by serum-resistant and serum-susceptible strains of N. gonorrhoeae.102 Hypothesizing that the EVs may be able to remove antibodies against the parent microbe from serum, authors demonstrated that incubating serum-susceptible bacteria with EVs from resistant strains increased the viability of the bacteria.101 Similarly, a 2007 study by Lee and coworkers demonstrated that opacity-associated (Opa) proteins on the surface of N. gonorrhoeae EVs mediated binding to human carcinoembryonic antigen-related cellular adhesion molecules (CEACAMs).103,104 CEACAM-specific EVs caused a reduction in T-cell proliferation and inhibited expression of CD69 in T-cells. These studies highlight the immunosuppressive nature of EVs and help to identify design criteria for effective vaccines using bacterial EVs.
Trichomonas vaginalis
Trichomonas vaginalis is a protozoan parasite which causes trichomoniasis.105 A sexually transmitted disease, trichomoniasis is usually asymptomatic in males, but causes itching, discharge, and painful urination and vaginal intercourse in females. The first study to report on T. vaginalis EVs identified cup-shaped particles between 50–100 nm in size that transferred both RNA and protein cargoes to ectocervical cells.106 Nievas, et al. found different cargo signatures in different sizes of EVs, demonstrating the heterogeneity of EV biogenesis, loading, and function.54 Artuyants, et al. isolated T. vaginalis EVs and identified small RNA cargoes with high abundance of tRNAs that were delivered to host cells.107 Twu, et al. observed a proinflammatory response in ectocervical cells caused by T. vaginalis EVs comparable to the response induced by ectocervical cell exposure to T. vaginalis parasite.106 Furthermore, treatment with EVs led to a dose-responsive increase in adherence of parasite to ectocervical cells.106 In a separate study, Olmos-Ortiz and coworkers observed that mice vaginally pre-treated with T. vaginalis EVs had significantly more viable trophozoites than mice that did not receive pre-treatment with EVs.108 Authors observed a significant increase in IL-10 secretion in vaginal lavage, and a significant decrease in IL-13 and IL-17 secretion, after exposure to T. vaginalis EVs, suggesting that EVs may reduce the host immune response to allow for persistence of the parasite.108
Specifically investigating host cell uptake of T. vaginalis EVs into mammalian cells, Rai and Johnson identified heparan sulfate proteoglycans on the surface of host cells as critical mediators of EV internalization.109 Inhibitors for clathrin-mediated endocytosis, phagocytosis, and micropinocytosis showed no effect on EV uptake, whereas inhibition of caveolae-dependent endocytosis significantly reduced EV uptake.109 Understanding mechanisms of uptake, as well as immunomodulatory properties of EVs may guide the development of therapeutics for female reproductive tract disease.
Candida albicans
Candida albicans is a commensal fungus found in the skin, mouth, gastrointestinal tract, and vagina.110 In the female reproductive tract, C. albicans causes vulvovaginal candidiasis, which causes vaginal itching, soreness, pain during sexual intercourse and urination, and abnormal discharge. Recurrent vulvovaginal candidiasis, in particular, severely affects the quality of life of women.111 Albuquerque, et al. first isolated EVs from C. albicans, and used TEM analysis to measure particles in the 100–200 nm range.112 Vargas and coworkers later identified differences in EV populations from different strains of C. albicans, where cargo proteins were involved in pathways including entry and adhesion into host cells, gluconeogenesis, glycolysis, and biofilm formation.113,114 As biofilms are a primary mechanism of fungal pathogenesis, several studies have evaluated the role of EVs in biofilm adhesion and matrix biogenesis. Building upon work identifying phospholipids as a primary component of C. albicans biofilms, Zarnowski and coworkers hypothesized that EVs may contribute to biofilm formation.74,75 As the endosomal sorting complexes required for transport (ESCRT) pathway is responsible for the production of EVs in other eukaryotes, authors developed mutants with deletion of ESCRT subunits. In addition to observing a decrease in the number of EVs produced by this library of mutants, authors reported increased biofilm susceptibility to antifungal treatments.74 By reintroducing EVs, the susceptibility was reversed to near-control levels, indicating that the observed effect was due to a lack of EVs produced by the fungus. In a follow-up study, the same group evaluated EV cargoes for specific contributions to drug susceptibility, biofilm adhesion, and biofilm dispersion, and highlighted specific protein cargoes as potentially useful therapeutic targets.115
In addition to EVs secreted from C. albicans biofilms, Martínez-López, et al. identified differences in EVs secreted from yeast compared to EVs secreted from hyphal cells.116 The yeast to hypha transition is critical for C. albicans virulence, where hyphal cells are better equipped for invasion of host tissues and yeast typically allow for dispersion and adhesion. EVs secreted by yeast were larger than those secreted by hyphal cells and carried different protein cargoes which contributed to differences in host cytotoxicity, where hyphal EVs increased cell death and TNFα secretion. The virulence factors that affect cellular immune response have been observed in other studies, where in an invertebrate model of C. albicans infection, pre-treatment with EVs reduced fungal colony forming units, suggesting that EVs may prime the host system to prevent fungal growth.116
Extracellular vesicles show potential as biomarkers and therapeutics
Given their role as communicators in vivo, EVs have gained interest as a modality for reporting and treating disease (Fig. 5).20 Reflective of parent cell function, EVs derived from human samples may give insight into cellular function and, in the case of bacteria, important strain level differences. Advances must be made in isolation protocols for this to be a clinical reality, but at least one other study isolated EVs from respiratory mucus.117 Similarly, characterization of EVs from human samples is challenging based on the heterogeneity of EVs represented in a given biofluid, as well as changes to bacteria based on host pressures (i.e., hormones, stress, environmental exposures). Engineering technologies to enhance the detection of surface proteins and single-particle technologies will advance our use of EVs as biomarkers of female reproductive tract disease.
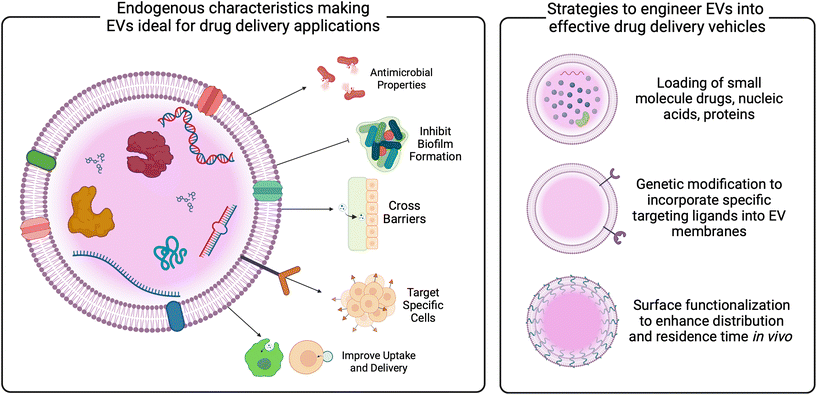 |
| Fig. 5 Improving the understanding of EVs will enable the translation of next generation EV therapeutics for gynecologic and obstetric indications. EVs present an interesting modality for therapeutic delivery. With the ability to cross biological barriers, target specific cells, and improve cellular uptake, EVs may display benefits over synthetic nanoparticles. Established methods demonstrate the ability for EVs to be loaded with small molecule drugs, nucleic acids, and proteins either pre- or post-isolation. Technological developments may help to improve targeting and residence time in vivo. | |
As a therapeutic platform, EVs from bacteria present benefits over mammalian cell-derived EVs. Bacteria are easily scalable and rapidly proliferate. Further, there are established and easy gene editing protocols for cargo loading. Clinical studies have used bacterial EVs as vaccines, and pre-clinical work highlights EV functionality as carriers for small molecules, nucleic acids, and proteins.118–122 These cargoes may help to prime a host immune system to ward off infections by parent pathogens, making them especially interesting as vaccine agents. Other reviews describe bacterial EVs as vaccines in more detail, but briefly, a meningococcal B EV-based vaccine was used with varying efficacy in Cuba, Norway, and New Zealand to vaccinate against meningitis.121–123 Using cultures of single strains of N. meningitidis may have limited the efficacy, as multiple strains are typically behind epidemic outbreaks. A retrospective study suggested that this vaccine may also protect against gonorrhea, given the overlap in genetic homology between N. meningitidis and N. gonorrhoeae; however, it is difficult to make assessments based on the longitudinal data.118,123 Future studies could evaluate vaccine effectiveness in a priori designed trials, and expand EV-based vaccines to prime the host immune system against BV-associated bacteria, for example.
In addition to vaccines, bacterial EVs have been proposed as novel drug delivery vehicles. Compared to synthetic carriers, EVs demonstrate advantages, including enhanced biocompatibility, reduced immunogenicity, innate barrier-crossing abilities, and specific targeting.22,29,31,124–127 Studies have demonstrated the loading of bacterial EVs with both hydrophobic and hydrophilic small molecule drugs, as well as nucleic acid and protein cargoes.128–130 These data reveal the role of EV membrane composition on loading efficiencies, indicating the need for tailored protocols for EVs from different parent cells. Loading techniques vary, and are described in more detail elsewhere,131–133 but have not been widely studied in EVs relevant to the female reproductive tract. Given the significant role of the vaginal microbiome in gynecologic outcomes, as well as the growing evidence of EVs mediating these outcomes discussed above, vaginal bacterial EVs may be useful as carriers of therapeutics for gynecologic and obstetric health (Fig. 4 and 5).
Innovation in genetic modification of bacteria, as well as methods for enhancement of EVs post-production may improve targeting and residence time in vivo (Fig. 5). Described in more detail elsewhere,20,134,135 current work has investigated surface modification via PEGylation of EVs as a method for improving circulation and penetration through biological barriers,136,137 as well as the addition of targeting ligands to enhance specific cell uptake.138,139 Based on studies of synthetic particles for gynecologic indications, PEGylation may enhance EV mobility through cervicovaginal mucus.140–142 Similarly, EV membranes could be fused with anti-oxytocin receptor antibodies for targeting myometrial contractions,143 or with chondroitin sulfate A-binding peptides to target trophoblast cells for placental drug delivery.144 Additional concerns for the translation of bacterial EVs lie with the production and manufacturing of EVs. Advances in scalable manufacturing must be made to enable the clinical translation of EV therapeutics. From other parent cells, media components and stressors have been investigated for increasing the production of EVs.145–148 Recent work demonstrated that genetic manipulation of both Gram positive and Gram negative microbes could increase EV secretion.149 Similarly, improvements in isolation procedures that increase both recovery and purity are needed and currently being studied. While ultracentrifugation is an industry standard,150,151 advances in tangential flow filtration, anion exchange chromatography, and immunocapture assays may increase yield, purity, and reproducibility of EV isolations.152,153 Specific work should focus on the use of vaginal bacterial EVs as therapeutics and how to improve EV isolation from relevant parent cells.
Based on their endogenous interactions with host cells in the female reproductive tract, bacterial EVs may prove to be an optimal platform for vaginally delivered therapies. Vaginal drug delivery is optimal for targeting female reproductive tract tissues.141,142 By directly exposing target tissues to therapeutic cargoes, treatment efficacy is improved. Vaginal drug delivery bypasses the harsh gastrointestinal environment and the hepatic first pass, which are challenges to traditional oral and systemic formulations.140,141,154 However, vaginally administered formulations must evade adhesive and steric interactions with the protective cervicovaginal mucus barrier. Recent work demonstrated that the pore size of cervicovaginal mucus is ∼300–400 nm, with a significant increase in the average pore size of polymicrobial (CST IV) samples.32,33 While EVs are small enough to penetrate the mucus mesh, it is unclear how their surface properties may affect adhesive interactions with carboxyl and sulfate groups and hydrophobic regions of the mucus mesh. Future studies should investigate the adhesive interactions between bacterial EVs and mucus. However, given preclinical in vivo evidence of particle ascension and the proposed functionality of EVs in mediating gynecologic and obstetric outcomes, it seems possible, if not likely, that EVs are able to penetrate the cervicovaginal mucus barrier to reach the underlying epithelium. Taken together, there is strong support for utilizing EVs as a vaginal drug delivery platform, however, as bacterial byproducts, regulatory studies must be done to understand the use of EVs in the clinic. For example, it is important to understand how culture-derived EVs differ from endogenously produced EVs, and how these differences may impact EV uptake and function in vivo.
Concluding remarks
EVs enable communication between cells across all forms of life. Given that the human microbiome so significantly impacts human health outcomes, recent interests have been directed towards understanding the functionality of bacterial EVs, with specific attention to EVs from the gut microbiome. A smaller, but no less important, body of work examines bacterial and pathogen-derived EVs relevant to the female reproductive tract. Whether these biological nanoparticles are protective against the invasion of pathogenic bacteria and viruses, as in the case of L. crispatus-derived EVs, or are immunosuppressive and aid in the pathogenicity of BV or other sexually transmitted infections, there is clear evidence for the role of bacteria- and pathogen-derived EVs in women's health. Further study of microorganism-derived EVs as mediators of disease and as a novel therapeutic platform for treating gynecologic and obstetric indications are needed and warranted. With the many unanswered questions surrounding mechanisms that contribute to female reproductive tract disease, and the significant need for new therapies to treat these indications, EVs may prove to be an important modality in understanding and improving women's health outcomes.
Author contributions
All authors contributed to manuscript drafting, editing, and revision, and approved the submitted version.
Conflicts of interest
There are no conflicts to declare.
Acknowledgements
Figures were created using BioRender.com. K.A.M. and A.P.P. were supported by Clark Fellowships through the University of Maryland.
References
- L. K. Ursell, J. L. Metcalf, L. W. Parfrey and R. Knight, Defining the human microbiome, Nutr. Rev., 2012, 70(Suppl 1), S38–S44 CrossRef PubMed.
- B. Ma, L. J. Forney and J. Ravel, Vaginal microbiome: rethinking health and disease, Annu. Rev. Microbiol., 2012, 66, 371–389 CrossRef CAS PubMed.
- J. Ravel,
et al., Vaginal microbiome of reproductive-age women, Proc. Natl. Acad. Sci. U. S. A., 2011, 108(Suppl 1), 4680–4687 CrossRef CAS PubMed.
- E. H. Koumans,
et al., The prevalence of bacterial vaginosis in the United States, 2001–2004; associations with symptoms, sexual behaviors, and reproductive health, Sex. Transm. Dis., 2007, 34, 864–869 CrossRef PubMed.
- D. A. Eschenbach, Bacterial vaginosis and anaerobes in obstetric-gynecologic infection, Clin. Infect. Dis, 1993, 16(Suppl 4), S282–S287 CrossRef PubMed.
- J. Atashili, C. Poole, P. M. Ndumbe, A. A. Adimora and J. S. Smith, Bacterial vaginosis and HIV acquisition: a meta-analysis of published studies, AIDS, 2008, 22, 1493–1501 CrossRef PubMed.
- A. S. Dingens, T. S. Fairfortune, S. Reed and C. Mitchell, Bacterial vaginosis and adverse outcomes among full-term infants: a cohort study, BMC Pregnancy Childbirth, 2016, 16, 278 CrossRef PubMed.
- D. B. Nelson, A. L. Hanlon, G. Wu, C. Liu and D. N. Fredricks, First Trimester Levels of BV-Associated Bacteria and Risk of Miscarriage Among Women Early in Pregnancy, Matern. Child Health J., 2015, 19, 2682–2687 CrossRef PubMed.
- D. N. Fredricks, T. L. Fiedler and J. M. Marrazzo, Molecular identification of bacteria associated with bacterial vaginosis, N. Engl. J. Med., 2005, 353, 1899–1911 CrossRef CAS PubMed.
- J. L. McCarville, G. Y. Chen, V. D. Cuevas, K. Troha and J. S. Ayres, Microbiota Metabolites in Health and Disease, Annu. Rev. Immunol., 2020, 38, 147–170 CrossRef CAS PubMed.
- D. Paik,
et al., Human gut bacteria produce Tau(Eta)17-modulating bile acid metabolites, Nature, 2022, 603, 907–912 CrossRef CAS PubMed.
- C. Ceccarani,
et al., Diversity of vaginal microbiome and metabolome during genital infections, Sci. Rep., 2019, 9, 14095 CrossRef PubMed.
- B. Vitali,
et al., Vaginal microbiome and metabolome highlight specific signatures of bacterial vaginosis, Eur. J. Clin. Microbiol. Infect. Dis., 2015, 34, 2367–2376 CrossRef CAS PubMed.
- S. E. Hawes,
et al., Hydrogen peroxide-producing lactobacilli and acquisition of vaginal infections, J. Infect. Dis., 1996, 174, 1058–1063 CrossRef CAS PubMed.
- S. J. Klebanoff, S. L. Hillier, D. A. Eschenbach and A. M. Waltersdorph, Control of the microbial flora of the vagina by H2O2-generating lactobacilli, J. Infect. Dis., 1991, 164, 94–100 CrossRef CAS PubMed.
- S. S. Witkin,
et al., Influence of vaginal bacteria and D- and L-lactic acid isomers on vaginal extracellular matrix metalloproteinase inducer: implications for protection against upper genital tract infections, mBio, 2013, 4, e00460–13 CrossRef PubMed.
- J. R. Schwebke, C. A. Muzny and W. E. Josey, Role of Gardnerella vaginalis in the pathogenesis of bacterial vaginosis: a conceptual model, J. Infect. Dis., 2014, 210, 338–343 CrossRef PubMed.
- C. A. Muzny,
et al., An Updated Conceptual Model on the Pathogenesis of Bacterial Vaginosis, J. Infect. Dis., 2019, 220, 1399–1405 CrossRef CAS PubMed.
- J. L. Patterson, P. H. Girerd, N. W. Karjane and K. K. Jefferson, Effect of biofilm phenotype on resistance of Gardnerella vaginalis to hydrogen peroxide and lactic acid, Am. J. Obstet. Gynecol., 2007, 197(170), e171–e177 Search PubMed.
- J. Xie, Q. Li, F. Haesebrouck, L. Van Hoecke and R. E. Vandenbroucke, The tremendous biomedical potential of bacterial extracellular vesicles, Trends Biotechnol., 2022, 40, 1173–1194 CrossRef CAS PubMed.
- N. Diaz-Garrido, J. Badia and L. Baldoma, Microbiota-derived extracellular vesicles in interkingdom communication in the gut, J. Extracell. Vesicles, 2021, 10, e12161 CrossRef PubMed.
- M. Bittel,
et al., Visualizing transfer of microbial biomolecules by outer membrane vesicles in microbe-host-communication
in vivo, J. Extracell. Vesicles, 2021, 10, e12159 CrossRef CAS PubMed.
- K. W. Witwer and C. Thery, Extracellular vesicles or exosomes? On primacy, precision, and popularity influencing a choice of nomenclature, J. Extracell. Vesicles, 2019, 8, 1648167 CrossRef PubMed.
- M. Toyofuku, N. Nomura and L. Eberl, Types and origins of bacterial membrane vesicles, Nat. Rev. Microbiol., 2019, 17, 13–24 CrossRef CAS PubMed.
- H. Liu,
et al., Bacterial extracellular vesicles as bioactive nanocarriers for drug delivery: Advances and perspectives, Bioact. Mater., 2022, 14, 169–181 CAS.
- S. N. Dean, D. H. Leary, C. J. Sullivan, E. Oh and S. A. Walper, Isolation and characterization of Lactobacillus-derived membrane vesicles, Sci. Rep., 2019, 9, 877 CrossRef PubMed.
- B. György,
et al., Membrane vesicles, current state-of-the-art: emerging role of extracellular vesicles, Cell. Mol. Life Sci., 2011, 68, 2667–2688 CrossRef PubMed.
- M. Yanez-Mo,
et al., Biological properties of extracellular vesicles and their physiological functions, J. Extracell. Vesicles, 2015, 4, 27066 CrossRef PubMed.
- E. J. Jones,
et al., The Uptake, Trafficking, and Biodistribution of Bacteroides thetaiotaomicron Generated Outer Membrane Vesicles, Front. Microbiol., 2020, 11, 57 CrossRef PubMed.
- H. Parker, K. Chitcholtan, M. B. Hampton and J. I. Keenan, Uptake of Helicobacter pylori outer membrane vesicles by gastric epithelial cells, Infect. Immun., 2010, 78, 5054–5061 CrossRef PubMed.
- G. Kwak,
et al., Extracellular vesicles enhance pulmonary transduction of stably associated adeno-associated virus following intratracheal administration, J. Extracell. Vesicles, 2023, 12, e12324 CrossRef PubMed.
- T. Hoang,
et al., The cervicovaginal mucus barrier to HIV-1 is diminished in bacterial vaginosis, PLoS Pathog., 2020, 16, e1008236 CrossRef PubMed.
- H. C. Zierden,
et al., Cervicovaginal mucus barrier properties during pregnancy are impacted by the vaginal microbiome, Front. Cell. Infect. Microbiol., 2023, 13, 1015625 CrossRef CAS PubMed.
- L. B. James-Allan,
et al., A novel technique using chronic infusion of small extracellular vesicles from gestational diabetes mellitus causes glucose intolerance in pregnant mice, Clin. Sci., 2022, 136, 1535–1549 CrossRef CAS PubMed.
- H. C. Zierden,
et al., Extracellular vesicles are dynamic regulators of maternal glucose homeostasis during pregnancy, Sci. Rep., 2023, 13, 4568 CrossRef CAS PubMed.
- S. Sheller-Miller, J. Trivedi, S. M. Yellon and R. Menon, Exosomes Cause Preterm Birth in Mice: Evidence for Paracrine Signaling in Pregnancy, Sci. Rep., 2019, 9, 608 CrossRef PubMed.
- L. J. Liang,
et al., Tumor-secreted exosomal Wnt2B activates fibroblasts to promote cervical cancer progression, Oncogenesis, 2021, 10, 30 CrossRef CAS PubMed.
- Y. Xiao,
et al., Macrophage-derived extracellular vesicles regulate follicular activation and improve ovarian function in old mice by modulating local environment, Clin. Transl. Med., 2022, 12, e1071 CrossRef CAS PubMed.
- S. Nair and C. Salomon, Extracellular vesicles and their immunomodulatory functions in pregnancy, Semin. Immunopathol., 2018, 40, 425–437 CrossRef CAS PubMed.
- A. Nakahara,
et al., Circulating Placental Extracellular Vesicles and Their Potential Roles During Pregnancy, Ochsner J., 2020, 20, 439–445 CrossRef PubMed.
- A. E. Morelli and Y. Sadovsky, Extracellular vesicles and immune response during pregnancy: A balancing act, Immunol. Rev., 2022, 308, 105–122 CrossRef CAS PubMed.
- A. Gorgens,
et al., Identification of storage conditions stabilizing extracellular vesicles preparations, J. Extracell. Vesicles, 2022, 11, e12238 CrossRef CAS PubMed.
- S. Gelibter,
et al., The impact of storage on extracellular vesicles: A systematic study, J. Extracell. Vesicles, 2022, 11, e12162 CrossRef CAS PubMed.
- E. G. Evtushenko, D. V. Bagrov, V. N. Lazarev, M. A. Livshits and E. Khomyakova, Adsorption of extracellular vesicles onto the tube walls during storage in solution, PLoS One, 2020, 15, e0243738 CrossRef CAS PubMed.
- K. W. Witwer,
et al., Updating MISEV: Evolving the minimal requirements for studies of extracellular vesicles, J. Extracell. Vesicles, 2021, 10, e12182 CrossRef CAS PubMed.
- Y. T. Tang,
et al., Comparison of isolation methods of exosomes and exosomal RNA from cell culture medium and serum, Int. J. Mol. Med., 2017, 40, 834–844 CrossRef CAS PubMed.
- T. Arab,
et al., Characterization of extracellular vesicles and synthetic nanoparticles with four orthogonal single-particle analysis platforms, J. Extracell. Vesicles, 2021, 10, e12079 CrossRef CAS PubMed.
- R. Imamiya,
et al., Escherichia coli-Derived Outer Membrane Vesicles Relay Inflammatory Responses to Macrophage-Derived Exosomes, mBio, 2023, 14, e0305122 CrossRef PubMed.
- P. Shishpal, N. Kasarpalkar, D. Singh and V. M. Bhor, Characterization of Gardnerella vaginalis membrane vesicles reveals a role in inducing cytotoxicity in vaginal epithelial cells, Anaerobe, 2020, 61, 102090 CrossRef CAS PubMed.
- C. Thery,
et al., Minimal information for studies of extracellular vesicles 2018 (MISEV2018): a position statement of the International Society for Extracellular Vesicles and update of the MISEV2014 guidelines, J. Extracell. Vesicles, 2018, 7, 1535750 CrossRef PubMed.
- L. A. Mulcahy, R. C. Pink and D. R. Carter, Routes and mechanisms of extracellular vesicle uptake, J. Extracell. Vesicles, 2014, 3, 24641 CrossRef PubMed.
- B. S. Joshi, M. A. de Beer, B. N. G. Giepmans and I. S. Zuhorn, Endocytosis of Extracellular Vesicles and Release of Their Cargo from Endosomes, ACS Nano, 2020, 14, 4444–4455 CrossRef CAS PubMed.
- C. Verdera, H. Gitz-Francois, J. J. Schiffelers and R. M. Vader, Cellular uptake of extracellular vesicles is mediated by clathrin-independent endocytosis and macropinocytosis, J. Controlled Release, 2017, 266, 100–108 CrossRef PubMed.
- Y. R. Nievas,
et al., Membrane-shed vesicles from the parasite Trichomonas vaginalis: characterization and their association with cell interaction, Cell. Mol. Life Sci., 2018, 75, 2211–2226 CrossRef CAS PubMed.
- A. Artuyants, J. Hong, P. Dauros-Singorenko, A. Phillips and A. Simoes-Barbosa, Lactobacillus gasseri and Gardnerella vaginalis produce extracellular vesicles that contribute to the function of the vaginal microbiome and modulate host–Trichomonas vaginalis interactions, Mol. Microbiol., 2023, 15130 CrossRef PubMed.
- R. A. Ñahui Palomino,
et al., Extracellular vesicles from symbiotic vaginal lactobacilli inhibit HIV-1 infection of human tissues, Nat. Commun., 2019, 10, 5656 CrossRef PubMed.
- L. T. Zhu,
et al., Diverse functional genes harboured in extracellular vesicles from environmental and human microbiota, J. Extracell. Vesicles, 2022, 11, e12292 CrossRef PubMed.
- M. Gurung,
et al., Staphylococcus aureus produces membrane-derived vesicles that induce host cell death, PLoS One, 2011, 6, e27958 CrossRef CAS PubMed.
- S. L. Hillier,
et al., Association between bacterial vaginosis and preterm delivery of a low-birth-weight infant, N. Engl. J. Med., 1995, 333, 1737–1742 CrossRef CAS PubMed.
- A. M. Briselden, B. J. Moncla, C. E. Stevens and S. L. Hillier, Sialidases (neuraminidases) in bacterial vaginosis and bacterial vaginosis-associated microflora, J. Clin. Microbiol., 1992, 30, 663–666 CrossRef CAS PubMed.
- K. Agarwal and A. L. Lewis, Vaginal sialoglycan foraging by Gardnerella vaginalis: mucus barriers as a meal for unwelcome guests?, Glycobiology, 2021, 31, 667–680 CrossRef CAS PubMed.
- A. L. Lewis and W. G. Lewis, Host sialoglycans and bacterial sialidases: a mucosal perspective, Cell. Microbiol., 2012, 14, 1174–1182 CrossRef CAS PubMed.
- J. Turunen,
et al., Presence of distinctive microbiome in the first-pass meconium of newborn infants, Sci. Rep., 2021, 11, 19449 CrossRef CAS PubMed.
- J. Turunen,
et al., Bacterial extracellular vesicles in the microbiome of first-pass meconium in newborn infants, Pediatr. Res., 2023, 93, 887–896 CrossRef CAS PubMed.
- R. Menon,
et al., Amplification of microbial DNA from bacterial extracellular vesicles from human placenta, Front. Microbiol., 2023, 14, 1213234 CrossRef PubMed.
- P. Shishpal, V. Patel, D. Singh and V. M. Bhor, pH Stress mediated alteration in protein composition and reduction in cytotoxic potential of Gardnerella vaginalis membrane vesicles, Front. Microbiol., 2021, 12, 723909 CrossRef PubMed.
- L.-M. Wang, B.-H. Lee, C.-Y. Hou, W.-H. Hsu and C.-J. Tai, Probiotics-Derived Extracellular Vesicles Protect Oxidative Stress against H2O2 Induction in Placental Cells, Fermentation, 2022, 8, 74 CrossRef CAS.
- Y. Chen,
et al., Extracellular vesicles derived from Akkermansia muciniphila promote placentation and mitigate preeclampsia in a mouse model, J. Extracell. Vesicles, 2023, 12, e12328 CrossRef PubMed.
- V. Croatti,
et al., Lactobacilli extracellular vesicles: potential postbiotics to support the vaginal
microbiota homeostasis, Microb. Cell Fact., 2022, 21, 1–11 CrossRef PubMed.
- Y.-R. Ho,
et al., The enhancement of biofilm formation in Group B streptococcal isolates at vaginal pH, Med. Microbiol. Immunol., 2013, 202, 105–115 CrossRef CAS PubMed.
- L. Honorato,
et al., Extracellular vesicles regulate biofilm formation and yeast-to-hypha differentiation in Candida albicans, mBio, 2022, 13, e00301–e00322 CrossRef PubMed.
- S. Khan, P. Shishpal and V. M. Bhor, Membrane vesicles of Lactobacillus gasseri ATCC 19992 disrupt biofilms of vaginal pathogens, Anaerobe, 2023, 82, 102761 CrossRef CAS PubMed.
- D. Lahiri,
et al., Exosome-associated host–pathogen interaction: a potential effect of biofilm formation, J. Anal. Sci. Technol., 2021, 12, 1–8 CrossRef.
- R. Zarnowski,
et al., Coordination of fungal biofilm development by extracellular vesicle cargo, Nat. Commun., 2021, 12, 6235 CrossRef CAS PubMed.
- R. Zarnowski,
et al., Candida albicans biofilm–induced vesicles confer drug resistance through matrix biogenesis, PLoS Biol., 2018, 16, e2006872 CrossRef PubMed.
- R. A. Ñahui Palomino, S. Zicari, C. Vanpouille, B. Vitali and L. Margolis, Vaginal Lactobacillus Inhibits HIV-1 Replication in Human Tissues Ex Vivo, Front. Microbiol., 2017, 8, 906 CrossRef PubMed.
- P. E. Costantini,
et al., Extracellular vesicles generated by Gram-positive bacteria protect human tissues ex vivo from HIV-1 infection, Front. Cell. Infect. Microbiol., 2022, 11, 1470 Search PubMed.
- V. L. Edwards,
et al., The Cervicovaginal Microbiota-Host Interaction Modulates Chlamydia trachomatis Infection, mBio, 2019, 10, e01548–19 CAS.
- S. J. Jang, K. Lee, B. Kwon, H. J. You and G. Ko, Vaginal lactobacilli inhibit growth and hyphae formation of Candida albicans, Sci. Rep., 2019, 9, 8121 CrossRef PubMed.
- D. J. Delgado-Diaz,
et al., Lactic acid from vaginal microbiota enhances cervicovaginal epithelial barrier integrity by promoting tight junction protein expression, Microbiome, 2022, 10, 1–16 CrossRef PubMed.
- L. Anton,
et al., Common cervicovaginal microbial supernatants alter cervical epithelial function: mechanisms by which Lactobacillus crispatus contributes to cervical health, Front. Microbiol., 2018, 9, 2181 CrossRef PubMed.
- K. D. Gerson,
et al., Gardnerella vaginalis induces matrix metalloproteinases in the cervicovaginal epithelium through TLR-2 activation, J. Reprod. Immunol., 2022, 152, 103648 CrossRef CAS PubMed.
- C. M. Dude, A. Saylany, A. Brown, M. Elovitz and L. Anton, Microbial supernatants from Mobiluncus mulieris, a bacteria strongly associated with spontaneous preterm birth, disrupts the cervical epithelial barrier through inflammatory and miRNA mediated mechanisms, Anaerobe, 2020, 61, 102127 CrossRef CAS PubMed.
- L. Anton,
et al., Gardnerella vaginalis alters cervicovaginal epithelial cell function through microbe-specific immune responses, Microbiome, 2022, 10, 119 CrossRef CAS PubMed.
- R. Creti,
et al., Neonatal group B Streptococcus infections, J. Pediatr. Infect. Dis., 2017, 36, 256–262 CrossRef PubMed.
- J. M. Edwards,
et al., Group B Streptococcus (GBS) Colonization and Disease among Pregnant Women: A Historical Cohort Study, Infect. Dis. Obstet. Gynecol., 2019, 2019, 5430493 CrossRef PubMed.
- B. P. Gonçalves,
et al., Group B streptococcus infection during pregnancy and infancy: estimates of regional and global burden, Lancet Global Health, 2022, 10, e807–e819 CrossRef PubMed.
- C. R. McCutcheon,
et al., Production and composition of group B streptococcal membrane vesicles vary across diverse lineages, Front. Microbiol., 2021, 12, 770499 CrossRef PubMed.
- B. Armistead,
et al., Hemolytic membrane vesicles of group B Streptococcus promote infection, J. Infect. Dis., 2021, 223, 1488–1496 CrossRef CAS PubMed.
- M. V. Surve,
et al., Membrane vesicles of group B Streptococcus disrupt feto-maternal barrier leading to preterm birth, PLoS Pathog., 2016, 12, e1005816 CrossRef PubMed.
- O. T. Van Gerwen, C. A. Muzny and J. M. Marrazzo, Sexually transmitted infections and female reproductive health, Nat. Microbiol., 2022, 7, 1116–1126 CrossRef CAS PubMed.
- G. S. L. Manasseh, S. Amarakoon, V. Photiou, N. Arruti and A. D. Borman, Approach to conjunctivitis in newborns, Br. Med. J., 2022, 376, e068023 CrossRef PubMed.
- C. Elwell, K. Mirrashidi and J. Engel, Chlamydia cell biology and pathogenesis, Nat. Rev. Microbiol., 2016, 14, 385–400 CrossRef CAS PubMed.
- K. Frohlich, Z. Hua, J. Wang and L. Shen, Isolation of Chlamydia trachomatis and membrane vesicles derived from host and bacteria, J. Microbiol. Methods, 2012, 91, 222–230 CrossRef CAS PubMed.
- K. M. Frohlich,
et al., Membrane vesicle production by Chlamydia trachomatis as an adaptive response, Front. Cell. Infect. Microbiol., 2014, 4, 73 Search PubMed.
- M. A. Scidmore, E. R. Fischer and T. Hackstadt, Restricted fusion of Chlamydia trachomatis vesicles with endocytic compartments during the initial stages of infection, Infect. Immun., 2003, 71, 973–984 CrossRef CAS PubMed.
- T. R. Paul, S. T. Knight, J. E. Raulston and P. B. Wyrick, Delivery of azithromycin to Chlamydia trachomatis-infected polarized human endometrial epithelial cells by polymorphonuclear leucocytes, J. Antimicrob. Chemother., 1997, 39, 623–630 CrossRef CAS PubMed.
- L. E. Edwards, M. I. Barrada, A. A. Hamann and E. Y. Hakanson, Gonorrhea in pregnancy, Am. J. Obstet. Gynecol., 1978, 132, 637–641 CrossRef CAS PubMed.
- D. W. Dorward, C. F. Garon and R. C. Judd, Export and intercellular transfer of DNA via membrane blebs of Neisseria gonorrhoeae, J. Bacteriol., 1989, 171, 2499–2505 CrossRef CAS PubMed.
- R. A. Zielke, I. H. Wierzbicki, J. V. Weber, P. R. Gafken and A. E. Sikora, Quantitative proteomics of the Neisseria gonorrhoeae cell envelope and membrane vesicles for the discovery of potential therapeutic targets, Mol. Cell. Proteomics, 2014, 13, 1299–1317 CrossRef CAS PubMed.
- R. K. Pettit and R. C. Judd, The interaction of naturally elaborated blebs from serum-susceptible and serum-resistant strains of Neisseria gonorrhoeae with normal human serum, Mol. Microbiol., 1992, 6, 729–734 CrossRef CAS PubMed.
- R. K. Pettit and R. C. Judd, Characterization of naturally elaborated blebs from serum-susceptible and serum-resistant strains of Neisseria gonorrhoeae, Mol. Microbiol., 1992, 6, 723–728 CrossRef CAS PubMed.
- H. S. Lee, M. A. Ostrowski and S. D. Gray-Owen, CEACAM1 dynamics during neisseria gonorrhoeae suppression of CD4+ T lymphocyte activation, J. Immunol., 2008, 180, 6827–6835 CrossRef CAS PubMed.
- H. S. Lee,
et al., Neisserial outer membrane vesicles bind the coinhibitory receptor carcinoembryonic antigen-related cellular adhesion molecule 1 and suppress CD4+ T lymphocyte function, Infect. Immun., 2007, 75, 4449–4455 CrossRef CAS PubMed.
- N. Kalia, J. Singh and M. Kaur, Microbiota in vaginal health and pathogenesis of recurrent vulvovaginal infections: a critical review, Ann. Clin. Microbiol. Antimicrob., 2020, 19, 5 CrossRef PubMed.
- O. Twu,
et al., Trichomonas vaginalis exosomes deliver cargo to host cells and mediate hostratioparasite interactions, PLoS Pathog., 2013, 9, e1003482 CrossRef CAS PubMed.
- A. Artuyants,
et al., Extracellular vesicles produced by the protozoan parasite Trichomonas vaginalis contain a preferential cargo of tRNA-derived small RNAs, Int. J. Parasitol., 2020, 50, 1145–1155 CrossRef CAS PubMed.
- L. Olmos-Ortiz,
et al., Trichomonas vaginalis exosome–like vesicles modify the cytokine profile and reduce inflammation in parasite–infected mice, Parasite Immunol., 2017, 39, e12426 CrossRef PubMed.
- A. K. Rai and P. J. Johnson, Trichomonas vaginalis extracellular vesicles are internalized by host cells using proteoglycans and caveolin-dependent endocytosis, Proc. Natl. Acad. Sci. U. S. A., 2019, 116, 21354–21360 CrossRef CAS PubMed.
- Z. Sun,
et al., Vulvovaginal candidiasis and vaginal microflora interaction: Microflora changes and probiotic therapy, Front. Cell. Infect. Microbiol., 2023, 13, 1123026 CrossRef CAS PubMed.
- J. D. Sobel, Recurrent vulvovaginal candidiasis, Am. J. Obstet. Gynecol., 2016, 214, 15–21 CrossRef PubMed.
- P. C. Albuquerque,
et al., Vesicular transport in Histoplasma capsulatum: an effective mechanism for trans-cell wall transfer of proteins and lipids in ascomycetes, Cell. Microbiol., 2008, 10, 1695–1710 CrossRef CAS PubMed.
- M. L. Rodrigues,
et al., Analysis of Yeast Extracellular Vesicles, Methods Mol. Biol., 2016, 1459, 175–190 CrossRef CAS PubMed.
- G. Vargas,
et al., Compositional and immunobiological analyses of extracellular vesicles released by C andida albicans, Cell. Microbiol., 2015, 17, 389–407 CrossRef CAS PubMed.
- R. Zarnowski,
et al., A common vesicle proteome drives fungal biofilm development, Proc. Natl. Acad. Sci. U. S. A., 2022, 119, e2211424119 CrossRef CAS PubMed.
- R. Martínez-López,
et al., Candida albicans hyphal extracellular vesicles are different from yeast ones, carrying an active proteasome complex and showing a different role in host immune response, Microbiol. Spectrum, 2022, 10, e00698–e00622 Search PubMed.
- F. Wu,
et al., Smoking Induced Extracellular Vesicles Release and Their Distinct Properties in Non-Small Cell Lung Cancer, J. Cancer, 2019, 10, 3435–3443 CrossRef CAS PubMed.
- W. E. Abara,
et al., Effectiveness of a serogroup B outer membrane vesicle meningococcal vaccine against gonorrhoea: a retrospective observational study, Lancet Infect. Dis., 2022, 22, 1021–1029 CrossRef CAS PubMed.
- C. Chbib, S. M. Shah, R. P. Gala and M. N. Uddin, Potential Applications of Microparticulate-Based Bacterial Outer Membrane Vesicles (OMVs) Vaccine Platform for Sexually Transmitted Diseases (STDs): Gonorrhea, Chlamydia, and Syphilis, Vaccines, 2021, 9, 1245 CrossRef CAS PubMed.
- M. J. H. Gerritzen, D. E. Martens, R. H. Wijffels, L. van der Pol and M. Stork, Bioengineering bacterial outer membrane vesicles as vaccine platform, Biotechnol. Adv., 2017, 35, 565–574 CrossRef CAS PubMed.
- I. Leduc,
et al., The serogroup B meningococcal outer membrane vesicle-based vaccine 4CMenB induces cross-species protection against Neisseria gonorrhoeae, PLoS Pathog., 2020, 16, e1008602 CrossRef CAS PubMed.
- E. A. Semchenko and K. L. Seib, Outer membrane vesicle vaccines for Neisseria gonorrhoeae, Nat. Rev. Urol., 2022, 19, 5–6 CrossRef CAS.
- J. Paynter,
et al., Effectiveness of a group B outer membrane vesicle meningococcal vaccine in preventing hospitalization from gonorrhea in New Zealand: a retrospective cohort study, Vaccines, 2019, 7, 5 CrossRef.
- L. Alvarez-Erviti,
et al., Delivery of siRNA to the mouse brain by systemic injection of targeted exosomes, Nat. Biotechnol., 2011, 29, 341–345 CrossRef CAS.
- K. Ridder,
et al., Extracellular vesicle-mediated transfer of genetic information between the hematopoietic system and the brain in response to inflammation, PLos Biol., 2014, 12, e1001874 CrossRef PubMed.
- P. Dalvi, B. Sun, N. Tang and L. Pulliam, Immune activated monocyte exosomes alter microRNAs in brain endothelial cells and initiate an inflammatory response through the TLR4/MyD88 pathway, Sci. Rep., 2017, 7, 9954 CrossRef.
- R. Stentz,
et al., A bacterial homolog of a eukaryotic inositol phosphate signaling enzyme mediates cross-kingdom dialog in the mammalian gut, Cell Rep., 2014, 6, 646–656 CrossRef CAS PubMed.
- G. Fuhrmann, A. Serio, M. Mazo, R. Nair and M. M. Stevens, Active loading into extracellular vesicles significantly improves the cellular uptake and photodynamic effect of porphyrins, J. Controlled Release, 2015, 205, 35–44 CrossRef CAS PubMed.
- L. Pascucci,
et al., Paclitaxel is incorporated by mesenchymal stromal cells and released in exosomes that inhibit in vitro tumor growth: a new approach for drug delivery, J. Controlled Release, 2014, 192, 262–270 CrossRef CAS PubMed.
- D. Sun,
et al., A novel nanoparticle drug delivery system: the anti-inflammatory activity of curcumin is enhanced when encapsulated in exosomes, Mol. Ther., 2010, 18, 1606–1614 CrossRef CAS PubMed.
- X. Zhang,
et al., Engineered Extracellular Vesicles for Cancer Therapy, Adv. Mater., 2021, 33, e2005709 CrossRef PubMed.
- P. Vader, E. A. Mol, G. Pasterkamp and R. M. Schiffelers, Extracellular vesicles for drug delivery, Adv. Drug Delivery Rev., 2016, 106, 148–156 CrossRef CAS PubMed.
- P. Fu,
et al., Extracellular vesicles as delivery systems at nano-/micro-scale, Adv. Drug Delivery Rev., 2021, 179, 113910 CrossRef CAS PubMed.
- M. Richter, P. Vader and G. Fuhrmann, Approaches to surface engineering of extracellular vesicles, Adv. Drug Delivery Rev., 2021, 173, 416–426 CrossRef CAS PubMed.
- X. Jia, J. Tang, C. Yao and D. Yang, Recent Progress of Extracellular Vesicle Engineering, ACS Biomater. Sci. Eng., 2021, 7, 4430–4438 CrossRef CAS PubMed.
- S. A. A. Kooijmans,
et al., PEGylated and targeted extracellular vesicles display enhanced cell specificity and circulation time, J. Controlled Release, 2016, 224, 77–85 CrossRef CAS PubMed.
- L. Patras,
et al., Trojan horse treatment based on PEG-coated extracellular vesicles to deliver doxorubicin to melanoma in vitro and in vivo, Cancer Biol. Ther., 2022, 23, 1–16 CrossRef CAS PubMed.
- W. Huang,
et al., Modified bacterial outer membrane vesicles induce autoantibodies for tumor therapy, Acta Biomater., 2020, 108, 300–312 CrossRef CAS PubMed.
- L. Chen,
et al., Outer membrane vesicles displaying engineered glycotopes elicit protective antibodies, Proc. Natl. Acad. Sci. U. S. A., 2016, 113, E3609–E3618 CrossRef CAS PubMed.
- T. Hoang,
et al., Development of a mucoinert progesterone nanosuspension for safer and more effective prevention of preterm birth, J. Controlled Release, 2019, 295, 74–86 CrossRef CAS PubMed.
- H. C. Zierden,
et al., Avoiding a Sticky Situation: Bypassing the Mucus Barrier for Improved Local Drug Delivery, Trends Mol. Med., 2021, 27, 436–450 CrossRef CAS PubMed.
- H. C. Zierden,
et al., Enhanced drug delivery to the reproductive tract using nanomedicine reveals therapeutic options for prevention of preterm birth, Sci. Transl. Med., 2021, 13, eabc6245 CrossRef CAS PubMed.
- J. W. Paul,
et al., Drug delivery to the human and mouse uterus using immunoliposomes targeted to the oxytocin receptor, Am. J. Obstet. Gynecol., 2017, 216, 283.e1–283.e14 CrossRef CAS PubMed.
- B. Zhang,
et al., Placenta-specific drug delivery by trophoblast-targeted nanoparticles in mice, Theranostics, 2018, 8, 2765–2781 CrossRef CAS PubMed.
- A. J. McBroom and M. J. Kuehn, Release of outer membrane vesicles by Gram-negative bacteria is a novel envelope stress response, Mol. Microbiol., 2007, 63, 545–558 CrossRef CAS PubMed.
- M. R. Loeb, Bacteriophage T4-mediated release of envelope components from Escherichia coli, J. Virol., 1974, 13, 631–641 CrossRef CAS PubMed.
- D. Grenier and M. Belanger, Protective effect of Porphyromonas gingivalis outer membrane vesicles against bactericidal activity of human serum, Infect. Immun., 1991, 59, 3004–3008 CrossRef CAS PubMed.
- N. D. Allan and T. J. Beveridge, Gentamicin delivery to Burkholderia cepacia group IIIa strains via membrane vesicles from Pseudomonas aeruginosa PAO1, Antimicrob. Agents Chemother., 2003, 47, 2962–2965 CrossRef CAS PubMed.
- Y. Liu,
et al., A Universal Strategy to Promote Secretion of G+/G- Bacterial Extracellular Vesicles and Its Application in Host Innate Immune Responses, ACS Synth. Biol., 2023, 12, 319–328 CrossRef CAS PubMed.
- S. Ayala-Mar, J. Donoso-Quezada, R. C. Gallo-Villanueva, V. H. Perez-Gonzalez and J. González-Valdez, Recent advances and challenges in the recovery and purification of cellular exosomes, Electrophoresis, 2019, 40, 3036–3049 CrossRef CAS PubMed.
- J. Chen,
et al., Review on Strategies and Technologies for Exosome Isolation and Purification, Front. Bioeng. Biotechnol., 2021, 9, 811971 CrossRef PubMed.
- N. Zarovni,
et al., Integrated isolation and quantitative analysis of exosome shuttled proteins and nucleic acids using immunocapture approaches, Methods, 2015, 87, 46–58 CrossRef CAS PubMed.
- M. Logozzi, R. Di Raimo, D. Mizzoni and S. Fais, Immunocapture-based ELISA to characterize and quantify exosomes in both cell culture supernatants and body fluids, Methods Enzymol., 2020, 645, 155–180 CAS.
- H. C. Zierden, R. L. Shapiro, K. DeLong, D. M. Carter and L. M. Ensign, Next generation strategies for preventing preterm birth, Adv. Drug Delivery Rev., 2021, 174, 190–209 CrossRef CAS PubMed.
|
This journal is © The Royal Society of Chemistry 2024 |
Click here to see how this site uses Cookies. View our privacy policy here.