DOI:
10.1039/D3NR05488H
(Minireview)
Nanoscale, 2024,
16, 6402-6428
Flexible and stretchable implantable devices for peripheral neuromuscular electrophysiology
Received
30th October 2023
, Accepted 24th February 2024
First published on 29th February 2024
Abstract
The peripheral nervous and muscular system, a cornerstone of human physiology, plays a pivotal role in ensuring the seamless functioning of the human body. This intricate network, comprising nerves and muscles extending throughout the body, is essential for motor control, sensory feedback, and the regulation of autonomic bodily functions. The qualified implantable peripheral interface can accurately monitor the biopotential of the target tissue and conduct treatment with stimulation, enhancing the human–machine interaction and new achievements in disease cure. Implantable electrodes have revolutionized the field of neuromuscular interfaces, offering precise bidirectional communication between the neuromuscular system and external devices. They enable natural control for individuals with limb loss, bridging the gap between mind and machine and aiding neuromuscular rehabilitation. In research and medical diagnostics, implantable electrodes provide invaluable tools for studying neuromuscular function and the development of therapies. However, traditional rigid electrodes face challenges due to the dynamic nature of the peripheral neuromuscular system. Flexible and stretchable devices show immense promise in accommodating dynamic alterations, offering adaptability, and accurate monitoring of electrophysiological signals. This review delves into the challenges associated with the peripheral interface, primarily focusing on monitoring and stimulation. It then provides a summary of common materials and structural design optimizations, discusses technologies for enhancing interface adhesion and surface functionalization, and explores encapsulation methods for implanted devices. Recent advancements in energy supply and the applications of implantable, flexible, and stretchable devices are also comprehensively reviewed, with due consideration given to ethical concerns and signal analysis. The promising directions are finally presented to provide enlightenment for high-performance sensor–tissue interfaces in the future, which will promote profound progress in clinical and human–machine interaction research. Flexible and stretchable devices are at the forefront of healthcare, with the potential to transform the treatment of neuromuscular disorders and enhance human augmentation, blurring the lines between natural and artificial limbs. They represent a promising avenue for the future, with exciting applications in healthcare, science, and technology, promising to bring us closer to the seamless integration of human and machine in the realm of neuromuscular interfaces.
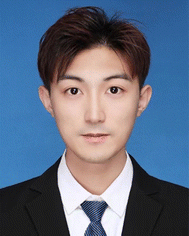 Hanfei Li | Hanfei Li is a doctoral student at Shandong University. At present, he is jointly trained in the Neural Engineering Centre of the Chinese Academy of Sciences, Shenzhen Advanced Technology Research Institute. His main research direction is the development and preparation of multi-functional flexible stretchable electrodes for biological interfaces. |
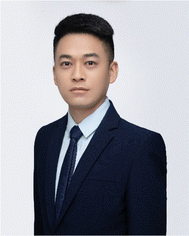 Fei Han | Fei Han received his M.S. degree from the University of Science and Technology of China in 2018 and his Ph.D. degree from Fudan University in 2021. Dr Han joined the Shenzhen Institute of Advanced Technology, Chinese Academy of Sciences, as a postdoctoral fellow in 2021 and transitioned to an assistant researcher after completing the postdoctoral program in 2023. He focuses on research related to developing multifunctional flexible and stretchable electrodes and sensors for biomedical engineering application. |
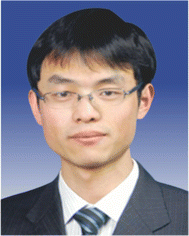 Zhiyuan Liu | Zhiyuan Liu is currently a professor at Shenzhen Institute of Advanced Technology, Chinese Academy of Sciences, China. He received his B.S. from Harbin Institute of Technology and was awarded his Ph.D. in Materials Science and Engineering (MSE) in 2017 from Nanyang Technological University (NTU), Singapore. During that time, he worked with Prof. Xiaodong Chen in Singapore and Prof. Zhenan Bao at Stanford University. His research interests are related to soft and stretchable bio-interface sensors. |
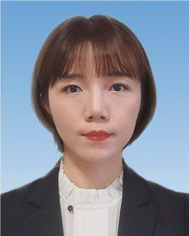 Qiong Tian | Qiong Tian obtained her doctoral degree from Xi'an Jiaotong University in 2017. After being a Research Associate at the City University of Hong Kong from 2018 to 2020, she joined the Shenzhen Institute of Advanced Technology, Chinese Academy of Sciences (SIAT, CAS), as an Assistant Professor in 2020. She currently serves as an Associate Professor at the Neural Engineering Centre, SIAT, CAS. Her primary research interests revolve around the development of flexible and stretchable sensors on bio-interfaces. |
1. Introduction
The peripheral nervous and muscular system stands as a cornerstone of human physiology, playing a pivotal role in ensuring the seamless functioning of the human body. This intricate network, comprising nerves and muscles that extend throughout the body, is essential for various aspects of daily life. It facilitates motor control, allowing us to perform movements ranging from the simplest tasks like walking to the most complex actions such as playing musical instruments or engaging in athletic endeavors. Furthermore, it serves as a conduit for sensory feedback, relaying information on touch, temperature, and pain, enabling us to interact with and respond to our surrounding environment, thereby maintaining our safety and comfort. Moreover, it exerts influence over the autonomic nervous system, regulating automatic bodily functions like heart rate, respiration, digestion, and circulation, thus maintaining internal equilibrium and stability. Additionally, in cases of injury or neuromuscular disorders, the system's functionality can be enhanced through rehabilitation and physical therapy, leading to improvements in recovery and overall quality of life. Finally, as a subject of scientific inquiry, research into the peripheral nervous and muscular system is vital for advancing our understanding of neuromuscular diseases, kinesiology, biomechanics, and medicine.
Implantable electrodes offer a wealth of advantages and hold immense significance when it comes to interfacing with peripheral nerves and muscles.1,2 Their role in medical and technological advancements cannot be overstated. One of the primary benefits of implantable electrodes lies in their ability to establish a direct and stable connection with peripheral nerves and muscles.3,4 This connection is pivotal in the field of neuromuscular interfaces, enabling a precise bidirectional communication between the nervous system and external devices. Excitable cells undergo continuous electric potential changes during physiological activities. The placement of electrodes on the designated interface facilitates the transfer of a bipotential, which is subsequently detected through a rear amplifier circuit.5 Additionally, electrical stimulation triggers a functional response by depolarizing cell membranes with charge injection, facilitated by the flow of ionic current between the electrodes, especially when one is in close proximity to the target tissue. When the charge injection is high enough to activate voltage-gated ion channels by a single square pulse, allowing the influx of sodium into the cell,6 it depolarizes the cell membrane, thereby eliciting an action potential. The commonly followed criterion is to maintain the electrode polarization within the water electrolysis window.7 For individuals with conditions such as limb loss, implantable electrodes can be integrated into prosthetic limbs, offering the potential for natural and intuitive control.4,8 These electrodes can pick up neural signals, allowing users to regain mobility and dexterity, effectively bridging the gap between mind and machine. Moreover, implantable electrodes have also revolutionized the field of neuromuscular rehabilitation. They play a crucial role in devices like functional electrical stimulation systems, which help individuals with muscle weakness or paralysis regain movement.9,10 By stimulating the appropriate muscles in response to neural signals, these electrodes can restore functions and improve the quality of life for those with neuromuscular disorders.11,12 In research and medical diagnostics, implantable electrodes provide invaluable tools for studying neuromuscular function.13 They allow for the precise measurement and modulation of neural activity, aiding in the development of therapies for conditions ranging from chronic pain to movement disorders. Furthermore, the integration of implantable electrodes into prosthetics and orthotics has opened up exciting possibilities for enhanced human augmentation.8 These devices enable not only control but also sensory feedback, allowing users to perceive touch and pressure, further blurring the lines between natural and artificial limbs.
While traditional rigid electrodes have their place in certain applications, they often face challenges in real-time monitoring and stimulation within the peripheral neuromuscular system due to deformation and compatibility issues.14 The significance of stretchable electrodes lies in their potential to revolutionize the landscape of neurostimulation and recording technologies. By accommodating the dynamic mechanical properties of biological tissues, these electrodes promise improved longevity, reduced tissue damage, and enhanced signal quality in comparison with their rigid counterparts. Understanding the current state-of-the-art in stretchable electrode technology is crucial for researchers and practitioners working on next-generation medical devices and neuro-prosthetics. Unlike the brain, the peripheral neuromuscular system is subject to constant motion and changes, underscoring the need for devices that offer the required flexibility to accommodate such dynamic alterations. Alongside this, there are growing demands for these devices to be not only accurate in monitoring electrophysiological signals but also highly adaptable to the dynamic nature of the peripheral neuromuscular system. The efficacy of implantable devices has been substantiated by numerous studies, underscoring the advantages of stretchability and flexibility. Specifically, flexible devices, in contrast to their rigid counterparts, have demonstrated a reduction in neuroinflammatory responses.15 The incorporation of stretchability provides electrodes with greater adaptability to the surrounding tissues.16 This brings to the forefront the critical importance of flexibility and stretchability. Whether for a better understanding of the workings of the nervous system or the development of the next generation of treatment modalities, these flexible and stretchable devices show immense promise. By delving into their materials synthesis, morphological design, and applications in monitoring and stimulating, we can gain a deeper understanding of their role in the peripheral neuromuscular system and their impact on the future of healthcare.
The current stretchable neural electrodes face challenges in achieving true synchronization with the irregular motion of the tissues, thereby impacting the quality of monitored electrophysiological signals. The mechanical mismatch between neural electrodes and tissues, leading to continuous pressure on the tissues and secondary damage, is explicitly acknowledged. The critical importance of the morphological optimization of electrodes in neural interfaces is underscored in this review. Moreover, the transition from invasive implantation methods, marked by tissue damage and application difficulties, to more minimally invasive approaches is discussed. Moving away from conventional large-window implantation towards methods involving injections and sutures is highlighted as a promising direction. Furthermore, the adverse effects of poor interface compatibility, including fibrous encapsulation due to immune responses and the resulting failure of long-term implanted devices, are discussed. This review will focus on the significance of flexibility and stretchability in implantable electrophysiological monitoring and stimulation devices, especially in the context of the peripheral neuromuscular system as shown in Fig. 1. We will explore how these devices cope with the dynamic nature of the peripheral nervous system and their potential applications in addressing neuromuscular-related disorders and conditions. We first present an overview of emerging materials synthesis and morphological design which significantly accelerate the progress in flexible and stretchable implanted electrodes. Subsequently, solutions targeting the interface adhesion and surface functionalization for bio-interface fusion between the implanted electrode and the organism are summarized. Recent advancements in energy supply and the applications of implantable, flexible, and stretchable devices are also comprehensively reviewed, with due consideration given to ethical concerns and signal analysis. The promising directions are finally presented to provide enlightenment for high-performance sensor–tissue interfaces in the future, which will promote profound progress in clinical and human–machine interaction research.
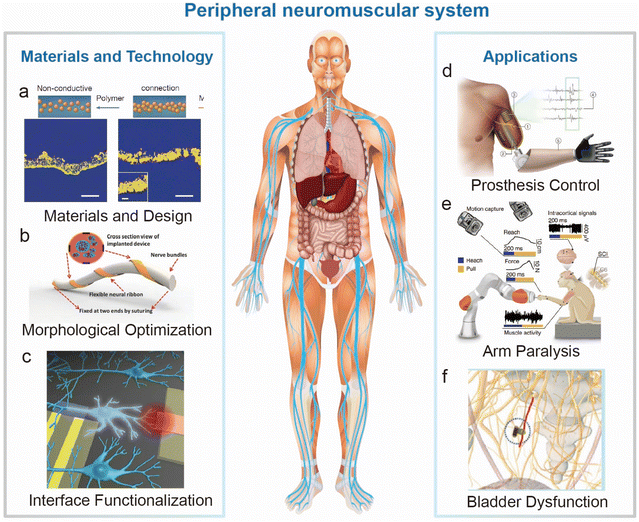 |
| Fig. 1 Overall diagram of the materials, technology and applications of electrodes for peripheral nerves and muscles. (a–c) Emerging materials and technology in electrode preparation, shape design and interface functionalization. (a) Microcracked and self-adhesive SEBS–Au interface illustration for stretchable electronics.17 This figure has been reproduced from ref. 17 with permission from Springer Nature; copyright: 2023. (b) Flexible and biocompatible neural ribbon electrode with a unique spiral wrapping design.18 This figure has been reproduced from ref. 18 with permission from WILEY-VCH Verlag GmbH & Co. KGaA; copyright: 2015. (c) Functionalization of the interface between electrode devices and biological individuals.19 This figure has been reproduced from ref. 19 with permission from AMER CHEMICAL SOC; copyright: 2016. (d) Advanced bionic limb technologies for muscle reinnervation with the mechanical and neural interfacing of bionic limbs with the body.8 This figure has been reproduced from ref. 8 with permission from Springer Nature; copyright: 2023. (e) Paralyzed monkey performing a robotic reach, grasp and pull task with the help of implanted electrodes.9 This figure has been reproduced from ref. 9 with permission from Springer Nature; copyright: 2022. (f) Bladder dysfunction curing by bladder nerve stimulation.10 This figure has been reproduced from ref. 10 with permission from WILEY-VCH Verlag GmbH & Co. KGaA; copyright: 2017. | |
2. Design and technology
2.1. Electronic materials
2.1.1. Stretchable wires.
Fig. 2 shows the materials of flexible and stretchable electrodes for implantation: the establishment of stretchable wires, the exposed part in contact with the tissues, and the functional materials introduced. With the advancement of stretchable electronics, various methods have been introduced to enhance the design of implantable electrodes. These include typical designs for stretchable substrates, such as serpentines,20 wavy structures,21,22 conductive microcracks,17,23 and liquid metals24,25 as shown in Fig. 2a–e. Due to the irregular motion exhibited by the tissues, uniaxial or biaxial stretchable electrodes face limitations in achieving complete synchronization with the dynamic tissue movements, thereby impacting the fidelity of the monitored electrophysiological signals. In response to these challenges, design strategies advocating for omnidirectional stretchability have been posited. Minev et al.23 prepared microcracked chromium/gold (Cr/Au) electrodes that sustain millions of mechanical stretch cycles, electrical stimulation pulses, and chemical injections in Fig. 2a. Li et al.22 subsequently enhanced the stretchability by a biaxial pre-stretching strategy, and a monitoring resolution of 10 μm at cell-level is unprecedented, realized on the stretchable electrode in Fig. 2b. In Fig. 2c, Ji et al.20 designed stretchable parylene-C electrodes with delicate serpentine structures. After 5000 repetitive stretching cycles, the electrochemical impedance of the microelectrodes remains in a steady state. In Fig. 2d, Qi et al.26 provided a new perspective on the highly stretchable and mechanically stable conductive polymer electrode. The prepared wavy polypyrrole (PPy) nanowire electrodes perform both high stretchability and good electrode–substrate adhesion. In addition, a low Young's modulus of 450 kPa, excellent recycling stability of 10
000 cycles, and high conductivity were also achieved in this electrode. In Fig. 2e, Dong et al.24 fabricated a highly stretchable electrode array (SEA) based on the liquid metal–polymer conductor (MPC), achieving high mechanical flexibility and good cytocompatibility for neural interfaces.
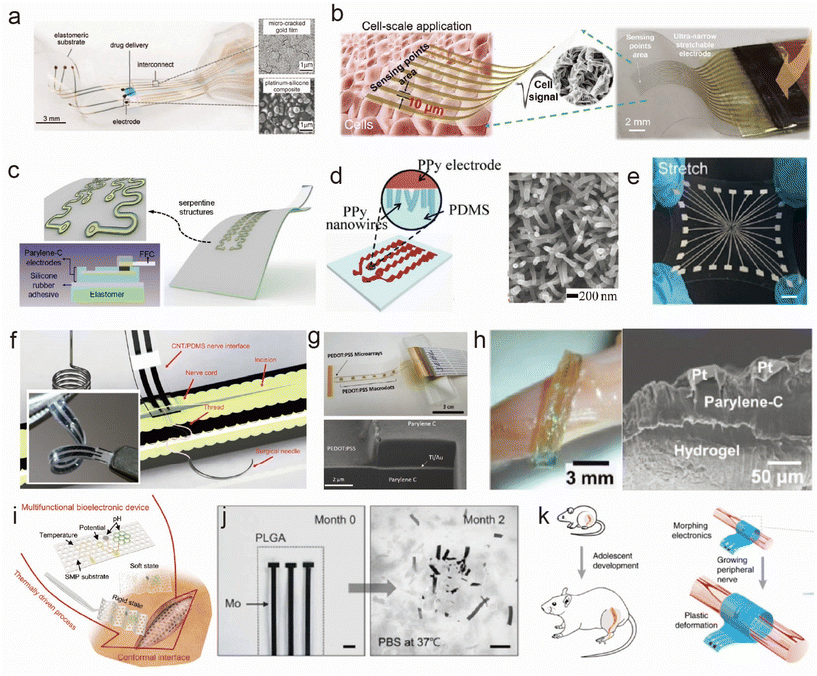 |
| Fig. 2 Materials of flexible and stretchable electrodes for implantation. (a–e) Typical design for a stretchable substrate: (a) stretchable film electrodes with microcrack Au.23 This figure has been reproduced from ref. 23 with permission from the American Association for the Advancement of Science; copyright: 2015. (b) Stretchable electrodes by a biaxial pre-stretching strategy.22 This figure has been reproduced from ref. 22 with permission from WILEY-VCH Verlag GmbH & Co. KGaA; copyright: 2023. (c) Serpentine-patterned stretchable electrodes.20 This figure has been reproduced from ref. 20 with permission from Elsevier; copyright: 2020. (d) Wavy PPy electrode.26 This figure has been reproduced from ref. 26 with permission from WILEY-VCH Verlag GmbH & Co. KGaA; copyright: 2017. (e) Stretchable electrode composed of liquid metal.24 This figure has been reproduced from ref. 24 with permission from WILEY-VCH Verlag GmbH & Co. KGaA; copyright: 2021. (f–h) Surface-exposed part of the electrode: (f) CNT/PDMS electrodes with 10 000 stretching cycles up to 20% strain.35 This figure has been reproduced from ref. 35 with permission from AMER INST PHYSICS; copyright: 2020. (g) Conductive polymer.38 This figure has been reproduced from ref. 38 with permission from WILEY-VCH Verlag GmbH & Co. KGaA; copyright: 2017. (h) Interfacing with a hydrogel.47 This figure has been reproduced from ref. 47 with permission from WILEY-VCH Verlag GmbH & Co. KGaA; copyright: 2018. (i–k) Functional materials for special conditions: (i) flexible electrodes on shape-memory substrates.53 This figure has been reproduced from ref. 53 with permission from WILEY-VCH Verlag GmbH & Co. KGaA; copyright: 2023. (j) Bioresorbable electrode.56,57 This figure has been reproduced from ref. 56 with permission from the American Association for the Advancement of Science; copyright: 2022. This figure has been reproduced from ref. 57 with permission from WILEY-VCH Verlag GmbH & Co. KGaA; copyright: 2021. (k) Self-adaptive electrode which can grow with the tissues.58 This figure has been reproduced from ref. 58 with permission from Springer Nature; copyright: 2020. | |
2.1.2. Conductive interfacing materials.
Even though the encapsulated stretchable wires are discussed, the parts facing the tissues are requirements for biocompatibility and stability. Biocompatibility and stability are crucial for success of transmission between the neuromuscular surface and electrode during monitoring and stimulating. Moreover, the stimulating stability can maintain a high current and voltage. A major challenge when designing electrodes for peripheral tissues is the mechanical mismatch between the tissues and the device, which can lead to non-conformal contact,27,28 tissue damage,15,16 and inefficient stimulation due to current leakage.29 With the advancement of flexible electronics, noble metals, carbon materials, conductive polymers, and hydrogels can all achieve performance matching with tissue interfaces through chemical modification and structural control (Fig. 2f–h).
Noble metals and their oxides are used as electrode surface materials for interfacing with tissues due to their excellent chemical inertness. Many studies also develop flexible electrodes using these materials, such as polydimethylsiloxane (PDMS)/Au,30 parylene-C/IrOx31 and polyimide (PI)/tungsten
:
titanium (W
:
Ti),32 to understand how different material combinations and electrode configurations influence neural stimulation. Based on this, some studies have sought to enhance the charge injection capacity by increasing the specific surface area of the electrode material. Ashok et al.33 designed flexible and low-impedance mesoporous Au electrodes highly suitable for biological sensing applications because the large surface areas formed within the mesoporous network allow for a high current density. Lienemann et al.34 developed stretchable cuff electrodes based on silicone rubber, Au nanowire conductors and Pt-coated nanowire electrodes. Excellent stability for 50% strain cycling and one million stimulation pulses were achieved. These materials can exhibit excellent biocompatibility and demonstrate tremendous potential in the development of flexible electrodes. Their characteristics include good mechanical stability, flexibility, and stretchability, as well as excellent electrode performance, such as low impedance and high current density. These advantages make inert metals valuable and widely applicable in neural stimulation and biosensing applications.
Carbon nanotubes (CNTs) have been strategically incorporated into the design of neural interfaces to enhance their mechanical and electrochemical properties. For instance, Terkan et al.35 developed a nerve interface by embedding CNTs in PDMS electrodes, as shown in Fig. 2f. They rigorously assessed the mechanical and electrochemical stability of this interface through extensive testing, which included subjecting it to 10
000 stretching cycles at up to 20% strain and more than 4 million biphasic stimulation pulses. In another study, Zhou et al.36 revealed the remarkable properties of CNTs in promoting neural cell growth and axon organization. They ingeniously integrated CNTs with biodegradable polycaprolactone fumarate (PCLF). Their findings showed that electrical stimulation not only enhanced cell proliferation and neurite extension but also facilitated essential cellular processes, such as migration and intracellular connections, crucial for nerve regeneration. Furthermore, the incorporation of CNTs into stretchable electrodes not only improved the film homogeneity and packing density but also conferred a higher degree of surface roughness. This adjustment not only enhanced the biocompatibility by mitigating the immune responses but also increased the effective surface area, thus improving charge transfer at the electrode–tissue interface.18
Conjugated polymers have been demonstrated to be an ideal bioelectronic interface for the treatment of a wide range of chronic diseases due to their outstanding mechanical flexibility, mixed-conducting electrical properties, and remarkable chemical tunability.37 Lee and colleagues introduced a stretchable neuromorphic implant based on poly(3,4-ethylenedioxythiophene):poly(styrenesulfonate) (PEDOT:PSS), which successfully restores coordinated and fluid motions in the limbs of mice afflicted with neurological motor disorders, enabling them to engage in activities such as playing with a ball, walking, or running. Additionally, as depicted in Fig. 2g, Ganji and collaborators38 applied a coating of PEDOT:PSS to the surface of microelectrode arrays, showcasing the safe and high-fidelity intraoperative monitoring of electrophysiology. PEDOT:PSS microelectrodes exhibited the capability to detect significant differential neural modulation under a variety of clinically relevant conditions. This highlights their potential for bioelectronic applications in treating chronic diseases.
Hydrogels emerge as an excellent choice for interface materials, given their soft mechanical properties that closely match those of body tissues. This characteristic allows hydrogels to achieve conformal contact with tissues, thereby enhancing signal transduction while minimizing the risk of an autoimmune inflammatory response.39 However, there is a common tradeoff between the modulus and fatigue resistance/stretchability of these hydrogels.40 Supramolecular41 and double-network42 hydrogels are developed be stretched to 10–100 times longer than their original length. Minimal signal attenuation over an extended time was realized by utilizing microgels as large crosslinking centers in hydrogel networks.41 This is successfully demonstrated for use as long-term implantable sensory devices. Mechanically tough hydrogels with a tissue-like modulus are also developed as outstanding bioadhesive hydrogel neural electrodes due to the assembly of a highly conductive PEDOT:PSS network.43,44
When the electrode's charge injection capacity is exceeded, irreversible electrochemical processes occur, releasing toxic byproducts directly into the nerves and muscles, leading to biological tissue damage.45 Recent studies have found that the hydrogel coating on the electrodes enhances the overall charge injection limit and provides a mechanically flexible interface.46 In Fig. 2h, Huang et al.'s47in situ gelation of four-arm-polyethylene glycol (PEG) grafted catechol hydrogels induced by oxidation using Fe3+ produces a conformal adhesive contact with the underlying hydrogel-based multielectrode arrays (MEAs), robust adhesion to electronic sub-structures, and the rapid dissolution of the water-soluble sacrificial release layers. MEAs are integrated on hydrogel-based substrates to produce free-standing ultra-compliant neural probes, which are then laminated to the surface of the dorsal root ganglia in feline subjects to record single-unit neural activity. Zhao et al.48 use salt/PEG aqueous two-phase systems to create customizable hydrogel ionic circuits. These electronics offer properties such as transparency, stretchability, a completely aqueous-based interface, a distribution of ionic electrical signals between engineered and biological systems, and the avoidance of tissue damage from electrical stimulation. Moreover, many efforts have involved composite preparations by combining hydrogels with other functional materials to achieve superior biocompatibility and improved tissue integration. For example, conductive materials, such as microgel41 and graphene oxide,49 were incorporated with hydrogels to enhance the fatigue performance and provide a good chemomechanical match to the tissues. Conductive materials, like CNT50 and silver nanowires,51 were blended with biocompatible hydrogel to enhance the stimulating performance, minimizing signal dissipation without causing an obvious inflammatory response. Induced pluripotent stem cell (iPSC)–derived myocytes were grafted onto a flexible fibrin hydrogel electrode array.52 Enhanced resolution and electrical recording were also demonstrated.
2.2. Functional polymer substrates
With the continuous advancement of medical science, the requirements for implanted electrodes have also evolved. On the basis of the regular research on stretchability and chemical stability during monitoring and stimulating, additional functions are used to minimize the destruction during operation and optimize the interface signal transmission. The integration of shape-memory,53 self-healing and adaptive electrodes54,55 paves the way for responsive and customizable neural interfaces. In parallel, the development of bioresorbable devices56,57 and growing electronics that harmonize with growing tissues58 signify a transition towards more biocompatible and sustainable neural interface technologies. As shown in Fig. 2i, Jiao et al.53 employed a fast-responding shape-memory polymer substrate to prepare a flexible bioelectronic device with switchable rigidity and a reconfigurable shape, achieving self-adaptive contact in the soft state at body temperature. Shape reconfiguration allows the device to be implanted through small incisions and recover in limited spaces to envelop biological surfaces. In Fig. 2j, Lee et al.56 reported a bioresorbable nerve stimulator that enabled the local electrical stimulation of peripheral nerves without the need for secondary removal after pain relief. Furthermore, through material composition, design choices, and manufacturing methods, the device possesses thin, flexible form factors and fully bioresorbable characteristics, thereby bypassing many of the inherent disadvantages of previous technologies in specific use cases, ensuring excellent biocompatibility of the device. In Fig. 2k, Liu et al.58 design and fabricate multilayered morphing self-adaptive electronics, consisting of viscoplastic electrodes and a strain sensor that eliminate the stress at the interface between the electronics and growing tissue, potentially avoiding additional surgeries for infants, children and adolescents after they grow up. These diverse approaches encompass an exciting landscape of innovation in peripheral neuromuscular electrode design and hold the potential to transform the field of neuro-prosthetics and disease treatment.
2.3. Shape and morphology
Fig. 3 shows the flexible and stretchable implantable electrodes with different shapes for peripheral neuromuscular electrophysiological applications. In the realm of neural interfaces and implantable electrodes, a diverse array of shape and morphology configurations has been devised to enhance their functionality and biocompatibility. The field of neural interfacing has witnessed a remarkable evolution in electrode design and implantation techniques. A diverse array of electrode types, from stretchable film electrodes to self-folding cuff electrodes, and even injectable conductive hydrogels, has emerged to address various challenges associated with interfacing with neural tissues. These innovations aim to improve signal quality, reduce invasiveness, enhance long-term stability, and ultimately broaden the scope of neural interface applications. In this context, the development of novel electrode designs and materials is paving the way for safer, more effective, and less disruptive neural interventions.
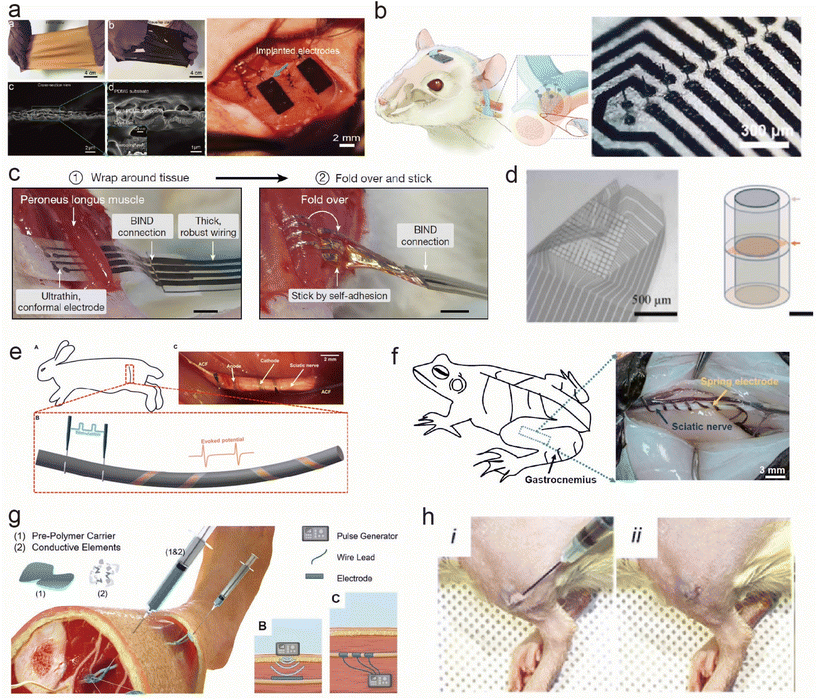 |
| Fig. 3 Flexible and stretchable implantable electrodes with different shapes for peripheral neuromuscular electrophysiological applications. (a) PDMS/Au membrane electrode sewn to the tissues.59 This figure has been reproduced from ref. 59 with permission from WILEY-VCH Verlag GmbH & Co. KGaA; copyright: 2019. (b) Implantation of a microneedle nerve array film in a rodent autonomic nerve.62 This figure has been reproduced from ref. 62 with permission from WILEY-VCH Verlag GmbH & Co. KGaA; copyright: 2022. (c) Cuff-like stretchable electrode wrapping the tissues.17 This figure has been reproduced from ref. 17 with permission from Springer Nature; copyright: 2023. (d) Sieve-like stretchable electrode placed on the cross-section of the nerves.68 This figure has been reproduced from ref. 68 with permission from Elsevier SCI Ltd; copyright: 2021. (e) Plant-inspired micro-ribbon.69 This figure has been reproduced from ref. 69 with permission from the American Association for the Advancement of Science; copyright: 2019. (f) Spring-like flexible electrodes.70 This figure has been reproduced from ref. 70 with permission from the National Academy of Sciences; copyright: 2020. (g) Injectable conductive elastomer electrode.72 This figure has been reproduced from ref. 72 with permission from WILEY-VCH Verlag GmbH & Co. KGaA; copyright: 2019. (h) Injectable hydrogel electrode.73 This figure has been reproduced from ref. 73 with permission from WILEY-VCH Verlag GmbH & Co. KGaA; copyright: 2023. | |
Membranes have been commonly used to interface with the muscle to monitor the electromyographic signals. Liu et al.59,60 prepared a stretchable film electrode with an interlocking layer between PDMS and Au. The electrodes were sewn to the muscles for long-term implantation to monitor intramuscular electric signals as shown in Fig. 3a. Moreover, Nguyen et al.61 developed an ultra-thin, flexible electrode based on SiC/SiO2 biointegrated nanomembranes with semiconductor properties, introducing thin-film electrode technology with the capability to interact with the neural tissues while maintaining outstanding stability in biofluid environments for several decades. Steins et al.60 decorated a flexible thin-film electrode with a three-dimensional (3D) protruding microelectrode array to enhance the signal quality by transversely penetrating the nerves and reducing the distance to the axons. Furthermore, Yan et al.62 developed a flexible microneedle nerve array with PDMS as the substrate, which was implanted into the rat peroneal nerve using a long-term, cuff-free, and suture-free fixation method (Fig. 3b). In summary, thin-film electrodes possess properties of flexibility, stretchability, and extreme thinness, enabling excellent integration with muscle or neural tissues for the long-term monitoring of electrical signals or interaction with neural tissues. Some thin-film electrodes also exhibit semiconductor characteristics, allowing for stability in biological fluid environments. Through improved design, signal quality can be enhanced and interaction efficiency with the neural tissues can be increased, offering wide-ranging prospects for application.
Cuff electrodes offer a conventional yet effective design for interfacing with peripheral nerves.63,64 Recently, functional materials and novel structures were explored to develop smart cuff electrodes. A self-folding cuff electrode with soft and stretchable printing resins as substrates and a microcracked gold film as a conductive layer targeting small peripheral nerves was developed.65 This folding is achieved by a highly swelling sodium acrylate hydrogel. These electrodes can be bent for ease of implantation when stretched (>20%). Additionally, innovative approaches like the nanoclip design66 and the microfluidic electrolyte gel cuff46 showcase the evolution of this concept. Chen et al.17 applied a self-adhesive and stretchable electrode to wrap around the tissue and nerves without damage to the targeting tissues, as shown in Fig. 3c. Sieve structures and their combination with cuffs, along with needle-based approaches,62,67,68 expand the toolkit for precise neural access for further analyzing the cross-section signal transmitting (Fig. 3d). Zhang et al.69 integrated stretchable mesh serpentine PI wires onto a flexible shape-memory substrate to fabricate a proposed 3D twining electrode. It can naturally self-climb onto nerves, driven by 37 °C normal saline, and form 3D flexible neural interfaces with minimal constraint on the deforming nerves (Fig. 3e). The spring shape also shows promise in muscle interfaces. Zhang et al.70 prepared a stretchable spring electrode with tremendous tensile strain (>1000%) using a 3D direct-ink printing (Fig. 3f). These printed materials formed asymmetric microribbons composed of directionally self-assembled two-dimensional nanoflakes in a polymeric matrix, and these microribbons could spontaneously transform into ultrastretchable springs with a controllable helical architecture upon stimulation. These springs are compatible with soft and dynamic biological tissues, making them suitable for use as neural electrodes.
Furthermore, in order to minimize the trauma during the implantation, fiber-based implants71 and injectable forms of flexible and stretchable electrodes are explored for the accurate and safe targeting of deep-seated body organs. Trevathan et al.72 developed a novel electrode called the Injectrode, based on a silicone–metal–particle composite material. In this process, they injected an uncured, flowable prepolymer around neuroanatomical targets and cured it in vivo. It can conform to the target structures, forming an electrically conductive interface that is much less stiff than traditional neuromodulation electrodes, reducing the need for surgical manipulation (Fig. 3g). J. Park et al.73 developed an injectable conductive hydrogel that further imparts tunable degradability to the injectable electrode. The hydrolyzable and stable conductive hydrogels maintained their shape for 3 days and 7 days, respectively, after in vivo administration. Compared with injected nonconductive hydrogel and epidermal electrodes, this injectable conductive hydrogel electrode significantly improved the quality of electromyography signals in rats (Fig. 3h).
3. Interface adhesion
3.1. Structure enhanced adhesion
Structural design-based interfacial adhesion holds significant value in the realm of flexible physiological electrodes. Common structural configurations encompass mechanical interlocking structures, curling and enveloping structures, as well as stent-mounted structures (Stentrode).
Mechanical interlocking structures rely on the shape and structural characteristics of a surface to facilitate an adhesion between objects. This mechanism hinges on the interlocking and fitting of microstructures, yielding robust adhesion forces.74 For example, certain insects boast microstructures on their foot appendages, enabling them to traverse both vertical and inclined surfaces. This concept has further been embraced for the development of biomimetic devices such as suction cups, serpent-like robots, and insect-inspired robotic systems. Inspired by the endoparasitic worm Pomphorhynchus laevis, Professor Jeffrey M. Karp's research team has developed a biphasic microneedle array.75 By utilizing expandable microneedle tips that mechanically interlock with the tissue, this novel approach enhances the adhesion strength by 3.5 times compared with the conventional staples used in skin graft fixation, resulting in a removal force of approximately 4.5 N cm−2 from intestinal mucosal tissue. H. Lee and colleagues have introduced a biologically inspired microneedle with enhanced tissue adhesion,76 fabricated using digital light processing 3D printing technology (Fig. 4a). The microneedles are designed with barbs generated by the gradient in cross-linking density within the photopolymer. Experimental evidence demonstrates that microneedles with these barbs exhibit an 18-fold increase in tissue adhesion capability compared with those without barbs. Additionally, these barbed microneedles possess the capability for sustained drug release. The ongoing development of mechanical interlocking structures hints at their immense potential within the domain of flexible bioelectronic materials and devices.
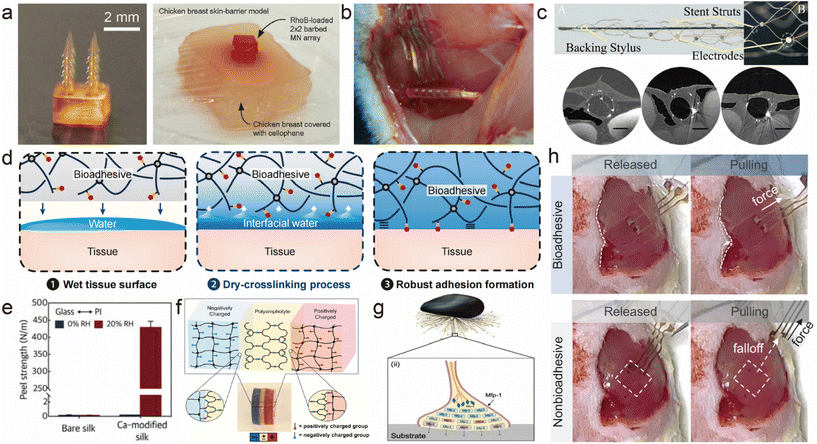 |
| Fig. 4 Various adhesion methods for flexible implantable bioelectronics. (a) 3D printed microneedle with backward-facing curved barbs, and the photograph of an ex vivo drug release test with the chicken breast skin-barrier model.76 This figure has been reproduced from ref. 76 with permission from WILEY-VCH Verlag GmbH & Co. KGaA; copyright: 2020. (b) Self-closing stretchable cuff electrode wrapped around a rat sciatic nerve for electrophysiological signal monitoring.63 This figure has been reproduced from ref. 63 with permission from the American Chemical Society; copyright: 2023. (c) Image of a generation 1 preclinical animal Stentrode™,78 and high-resolution ex vivo synchrotron X-ray images of the time-dependent vessel wall incorporation of the Stentrode struts at day 0, and three weeks and four months after implantation in the superior sagittal sinus.80 Scale bars, 2 mm. This figure has been reproduced from ref. 78 with permission from TAYLOR & FRANCIS Ltd; copyright: 2019. This figure has been reproduced from ref. 80 with permission from Springer Nature; copyright: 2016. (d) Mechanisms of instant and tough wet adhesion formation.89 This figure has been reproduced from ref. 89 with permission from the National Academy of Sciences; copyright: 2020. (e) Average peel strengths of bare silk and the Ca-modified silk from the PI film at 0 and 20% RH (12 h attaching time) with standard deviation (n = 3).109 This figure has been reproduced from ref. 109 with permission from WILEY-VCH Verlag GmbH & Co. KGaA; copyright: 2018. (f) Demonstration of the self-adjustable adhesion of a PA hydrogel to charged hydrogels.94 This figure has been reproduced from ref. 94 with permission from WILEY-VCH Verlag GmbH & Co. KGaA; copyright: 2015. (g) Mussel adhesion in seawater.105 This figure has been reproduced from ref. 105 with permission from WILEY-VCH Verlag GmbH & Co. KGaA; copyright: 2020. (h) Photographs that show a fully bioadhesive OECT attached to the GM muscle maintaining stable contact during mechanical agitation, and the comparison with a non-bioadhesive OECT.108 This figure has been reproduced from ref. 108 with permission from the American Association for the Advancement of Science; copyright: 2023. | |
In contrast to conventional thin-film or needle electrodes, the typical structure of flexible electrodes employing curling and wrapping techniques achieves precise positioning through its coiled configuration, ensuring a close fit to the target nerve (Fig. 4b).63,69,77 This enables accurate localization, minimizing mechanical distortions and wire failures, while significantly reducing the impact on adjacent nerves and tissues during stimulation of the target point.
The Stentrode, a compact stent-mounted electrode array pioneered by Dr Opie and his team, allows the collection of intracranial electroencephalographic activity without the need for invasive open-brain surgery (Fig. 4c).78 This groundbreaking electrode design effectively mitigates the inflammation and tissue responses typically associated with craniotomy and has been validated across a spectrum of medical applications.79 Multiple electrodes are distributed along an elongated stent, which expands within the target region upon insertion into the patient's brain via the vascular system. The electrodes establish a robust attachment to the monitoring sites through stent expansion, thus enhancing the electrode's adherence to the monitoring points while simultaneously diminishing the interface impedance and elevating the quality of the collected electrophysiological signals. Building upon the advancements of Stentrode applications in the field of neuroscience (Fig. 4c),80 we envision its potential for extensive development in the electrophysiological monitoring and stimulation of peripheral systems in the future.
3.2. Hydrogel material enhanced adhesion
Over the past few decades, hydrogel materials, composed of substances with dynamic water-absorbing networks, have attracted considerable attention due to their outstanding biocompatibility, high elasticity, and dynamic self-healing properties. Building upon the intrinsic wetting characteristics of hydrogel materials, their adhesion to tissues can be significantly augmented through modifications or surface treatments.81–83 This heightened adhesion not only effectively mitigates the performance instability during the usage of hydrogel materials, such as changes in interfacial impedance and interface separation in the context of hydrogel electrodes, but also helps prevent adverse consequences resulting from detachment (Fig. 4d). This section summarizes the four main methods currently used to enhance the adhesion of hydrogels.
3.2.1. Hydrogen bond adhesion.
Hydrogen bonds, with a binding energy of approximately 0.5 to 1.8 kcal mol−1, are weak non-covalent bonds. Through the interaction of hydrogen bonds, adhesion, attachment, or bonding between interfaces can be achieved (Fig. 4e). However, due to the low individual bonding energy of a single hydrogen bond, the mechanical performance of hydrogels cross-linked through hydrogen bonds tends to be weak. For instance, Hou et al. designed an environmentally friendly hydrogen-bond-crosslinked hydrogel network,84 where the hydrogen bond interaction between branched starch (Amy) molecular chains and water forms the backbone of the hydrogel network. Simultaneously, Na+ dissolved in the Amy/water network serve as carriers for transmitting electrical signals, resulting in an injectable conductive hydrogel. Due to the presence of dynamically repairing hydrogen bonds in the network, this hydrogel can rapidly heal within 2 to 3 seconds at room temperature with a healing efficiency of 98%. However, the weak bonding energy in the Amy/water network leads to a low mechanical strength (800 Pa) of the physically crosslinked hydrogel, limiting its practical application. Dynamic and reversible hydrogen bonds provide the hydrogen bond-crosslinked hydrogel with fast self-healing properties.
When hydrogen bonds in a hydrogel network aggregate into hydrogen bond clusters, strong physical interaction points, namely multi-hydrogen bond crosslinking points, further enhance the mechanical properties of the hydrogel. Chaoxia Jin's team proposed a novel strategy for preparing high-strength double-crosslinked gels by introducing multiple hydrogen bonds.85 This strategy involved utilizing the multiple hydrogen bond interactions between polymers and the natural polyphenolic compound tannic acid (TA). They introduced a polymer/tannic acid crosslinked network into the existing polymer gel network, resulting in a polymer/tannic acid double-crosslinked hydrogel. Compared with the original single-network gel, the polymer/tannic acid double-network gel exhibited an order of magnitude increase in breaking strength and a several-fold increase in breaking elongation. Moreover, the dynamic nature of the hydrogen bonds endowed the gel with a rapid self-repair capability. The polyphenolic structure of TA, similar to the catechol groups in polydopamine (PDA), contributed to the high adhesion (100 kPa) of the double-crosslinked hydrogel to various substrates.86–88 However, the susceptibility of the phenolic hydroxyl groups in catechol to oxidation into quinones resulted in a significant decrease in adhesion strength.
It should be noted that hydrogels containing carboxyl groups are affected by reliability under physiological saline conditions because the dissociation of carboxyl hydrogen bonds occurs at higher pH levels.89 Under moist conditions, dry tapes quickly form physical crosslinks with damp tissues due to their rapid absorption of interfacial moisture, leading to a significant reduction in their adhesion energy.90 To enhance hydrogen bond adhesion in a moist environment, Cui et al. developed a Janus hydrogel,91 where the distribution of the polyelectrolyte complex gradually decreased from a completely neutralized carboxylic acid layer to a less neutralized layer. Consequently, the highly neutralized layer lacks adhesion, while the less neutralized carboxylic acid layer retains adhesive properties. When attached to a moist surface, this layer can maintain intimate contact with the tissue without relying on interfacial moisture, owing to its inherent hydrophobicity inherited from the polyelectrolyte complex. Similarly, employing a hydrophobic strategy with self-adjusting interfacial molecules imparts excellent underwater adhesion to the hydrogel. After immersion in an Fe3+ solution, the negatively charged headgroups of dodecyl sulfate (SDS) rearrange through electrostatic interactions, while the alkyl chains aggregate through hydrophobic interactions. Subsequently, after removing free SDS, the hydrophobic hydrogel surface repels water molecules to ensure the direct formation of hydrogen bonds between the adhesive ends.92
3.2.2. Electrostatic interactions and topological adhesion.
Moreover, electrostatic interactions and topological adhesion are common forms of physical adhesion (Fig. 4f). Since many hydrogels and biological surfaces carry net negative charges, they repel each other upon contact. To overcome this issue, introducing both positive and negative charges into the hydrogel simultaneously can achieve non-specific adhesion.93 Under the influence of an external electric field, the formation of ion bonds and adhesion induced by polarization can increase the adhesion forces.94,95 However, it is important to note that merely increasing the ion monomer concentration of polyelectrolyte hydrogels does not always directly correlate with improved interfacial adhesion.
Topological adhesion relies on the shape and structural features of an object's surface to achieve adhesion through the special arrangement of the surface microstructures. Typically, this adhesion involves the interlocking or mutual fitting of microstructures, enabling objects to adhere firmly. For instance, this form of adhesion can be used in the design of biomimetic devices to achieve the adhesion and manipulation of tiny devices or to study the surface modification of materials to enhance their topological adhesion properties. In hydrogels, the trigger causes the polymer chains to entwine with the nearby adherent network, forming a closely bonded interface.96–98 Temperature, pH, iron ions, and organic solvents are typically effective triggers. In topological adhesion, this physically crosslinked wet adhesion also exhibits reversibility as the trigger conditions change.96,97,99
3.2.3. Covalent bond adhesion.
The majority of hydrogels contain a multitude of functional groups, such as hydroxyl, ether, amino, carboxyl, or catechol groups, which enable the hydrogel to ensure interfacial adhesion by forming covalent bonds with the tissue surface. Two typical interfacial reactions are the Schiff base reaction and the Michael addition reaction. The Schiff base reaction is a condensation reaction that occurs between amino groups and carbonyl compounds, such as ketones or aldehydes.82,100,101 The Michael addition is a versatile chemical reaction that involves a nucleophilic reagent (usually an enamine or enamine) adding to α,β-unsaturated ketone compounds.102,103 Based on the covalent bonding formed by aldehyde groups and amino groups, the adhesion strength at the interface can be significantly enhanced. This is achieved by preparing hydrogels containing aldehyde groups and allowing them to react with amino groups present in the tissue. Additionally, the characteristics of the interfacial surface morphology, mechanical strength, and adhesion strength can be controlled by adjusting the aldehyde/amine ratio. Photosensitive ortho-nitrobenzene can be converted to ortho-nitrobenzaldehyde under UV irradiation, and by reacting with the nitro groups on the tissue surface, covalent bonds are formed between the hydrogel and the tissue interface, resulting in a significant improvement in adhesion.104
3.2.4. Non-covalent bond adhesion.
Non-covalent interactions encompass a wide range of interactions, including the mechanically interlocking structure, hydrogen bonding, and electrostatic adsorption, as described earlier. These interactions are generally considered forms of non-covalent interaction. Additionally, there are several other typical non-covalent interactions, such as van der Waals interactions, dipole–dipole attraction, and cation–π interactions, among others. These non-covalent interactions are typically weaker and reversible. For instance, marine mussels adhere to various substrates through multiple mechanisms, including electrostatic interactions, hydrogen bonds, and cation–π interactions (Fig. 4g).105 Furthermore, in many scenarios, both covalent and non-covalent interactions act simultaneously. For example, tannic acid, used for modifying hydrogel adhesion, can simultaneously form hydrogen bonds and covalent interactions with the interfacing tissue.106,107 Moreover, in addition to modifying the adhesion of conductive electrodes on flexible biological interfaces, researchers have designed bio-adhesive polymer semiconductor films (Fig. 4h).108 These films feature a dual-network structure formed by bio-adhesive brush-like polymers and redox-active semiconductor polymers. This fully bio-adhesive transistor sensor enables high-quality and stable electrophysiological recording on isolated rat hearts and in vivo rat muscles.
In general, structurally enhanced adhesion typically relies on the physical interaction between flexible materials and the target. This method places higher demands on the structural design of materials, requiring more precise manufacturing techniques. In addition, hydrogels themselves possess excellent elasticity and stretchability, and their adhesion to tissues can be fine-tuned by altering functional groups within the gel. Furthermore, hydrogels allow for adhesion and de-adhesion transitions through external conditions such as light exposure or heating. Similarly, hydrogels may have certain drawbacks in specific application scenarios. For instance, their tendency to swell upon contact with liquids can result in volume changes, potentially leading to instability or inappropriate adhesive forces. Besides, the relative softness of hydrogels may impact the stability in high mechanical stress or friction environments. Thus, considering the distinct advantages and limitations of both structurally enhanced adhesion and hydrogel-based adhesive materials, appropriate choices can be made based on specific needs.
4. Surface functionalization
Surface modifications of flexible bioelectrodes are of great significance because they can significantly enhance the performance of bioelectrodes, especially at the interface with biological organisms. These surface modifications include impedance reduction, multifunctionality, amphiphilic modification, and protein modification, all of which have a significant impact on the application and effectiveness of bioelectrodes. This section will primarily focus on recent advancements in these four types of functional surface modification.
4.1. Surface modifications for enhanced electrical properties and multi-functionalization abilities
Bioelectrodes are used for monitoring bioelectric signals or establishing interfaces between biological organisms and electronic devices. However, at biological interfaces, high impedance is often a challenge. This high impedance results in decreased signal quality, reduced energy efficiency, and decreased affinity between the electrode and the tissue. Common methods to reduce interface impedance include using highly conductive materials, increasing the surface area, and surface modification of the electrode.110 Surface modification is a simple and effective method for this purpose. Typical materials used for modifying bioelectrode surfaces include carbon materials, gel materials, and emerging two-dimensional materials. For example, platinum black (Fig. 5a) and iridium oxide-modified flexible electrodes exhibit significantly reduced impedance.111 When used as stimulation electrodes, they show enhanced charge injection capacity and charge storage capacity by orders of magnitude. Carbonization titanium, zinc oxide, and others are also commonly used for neural interface electrodes. In recent years, two-dimensional materials like MXenes have gained significant development in the field of neural interfaces. They combine the advantages of a hydrophilic surface and metallic conductivity, offering excellent mechanical, electrochemical (high conductivity and capacitance), photothermal, and physical properties. Researchers have developed high-throughput microfabrication processes to create MXene-based neural electronic devices (Fig. 5b).112 Compared with standard metal microelectrodes, MXene-based neural electrodes show significantly lower impedance. Moreover, they exhibit lower baseline noise, a higher signal-to-noise ratio, and lower sensitivity to 60 Hz interference during in vivo neural recordings. Through various surface modifications, neural interface electronic materials not only achieve a significant reduction in impedance but also improve their interference resistance and biocompatibility. This enhances the quality of detected biological signals and expands the applications of bioelectrodes.
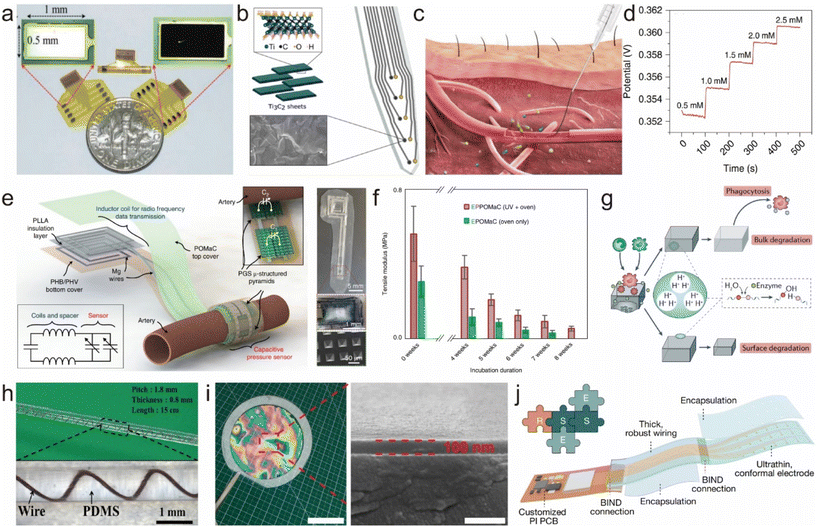 |
| Fig. 5 Strategies for surface modification and device encapsulation. (a) Photograph of fabricated nerve cuff electrodes with sputtered Pt or electroplated Pt black.111 This figure has been reproduced from ref. 111 with permission from WILEY-VCH Verlag GmbH & Co. KGaA; copyright: 2017. (b) Ti3C2 MXene decorated electrode for high-resolution neural interfaces.112 This figure has been reproduced from ref. 112 with permission from the American Chemical Society; copyright: 2018. (c and d) Schematics showing the injection of the fiber into a blood vessel, and the open-circuit potential responses at different concentrations of the analyte.113 This figure has been reproduced from ref. 113 with permission from Springer Nature; copyright: 2019. (e) Illustration of the sensor with an exposed view of the bilayer coil structure for wireless data transmission and the cuff-type pulse sensor wrapped around the artery.118 This figure has been reproduced from ref. 118 with permission from Springer Nature; copyright: 2019. (f) POMaC elastomer in vitro degradation study.118 This figure has been reproduced from ref. 118 with permission from Springer Nature; copyright: 2019. (g) The degradation of synthetic polymers occurs in vivo through hydrolysis, enzymatic degradation and oxidation. Two modes of degradation are involved: surface and bulk degradation.119 This figure has been reproduced from ref. 119 with permission from Springer Nature; copyright: 2019. (h) The cable encapsulated by PDMS.120 This figure has been reproduced from ref. 120 with permission from Springer; copyright: 2011. (i) Photograph of a freestanding SEBS film with a thickness of 100 nm.121 This figure has been reproduced from ref. 121 with permission from Springer; copyright: 2022. (j) Stretchable encapsulation strategy for device assembly using ultra-thin SESB films.17 This figure has been reproduced from ref. 17 with permission from Springer; copyright: 2023. | |
In the realm of implantable electrophysiological signal recording and stimulation, the pursuit of multifunctionality stands out as a crucial trend in future advancements. Beyond the fundamental role of capturing physiological electrical signals, researchers have successfully implemented electrode functionalization, facilitating the detection of electrolyte ion concentrations, pH levels, glucose levels, and various chemical components within bodily fluids. The transformative potential of these multifunctional bioelectrodes extends to revolutionizing disease diagnosis and prevention, and advancing personalized medical research. For example, researchers have designed fiber-like electrochemical sensors with a multi-level spiral structure, mimicking the structure of biological muscles (Fig. 5c and d).113 In this device, polyaniline, as a controllable acid–base-sensitive polymer, undergoes a balance shift between its oxidized and reduced states under different pH conditions. Its application on the surface of flexible electrodes serves the function of monitoring changes in pH values. These sensors have a lower bending internal stress compared with traditional implant materials like Au wires or PDMS. By using injection methods adapted to the one-dimensional structure of the fibers, these sensors can be accurately implanted into the target areas. These multi-level fiber sensors with axial or radial structures enable the detection of the substance distribution at different positions or the detection of different chemical substances at the same site. They have been successfully used to monitor blood glucose and calcium levels in real-time, showing results comparable to commercial methods. In conclusion, research on electrode multi-functionalization is of great significance for monitoring electrolyte balance, disease diagnosis and prevention, and advancing medical research towards comprehensive information and personalized medicine.
4.2. Amphiphilic modification and protein modification
Amphiphilic modification involves introducing functional groups on the electrode surface that possess both hydrophilic and hydrophobic properties, thereby enhancing the electrode's interface adaptability.114 For instance, San-Yuan Chen and colleagues developed a novel conductive nanogel neural interface composed of amphiphilic chitosan-modified poly(3,4-ethylenedioxythiophene) (PMSDT).115 This neural interface exhibits a biomimetic structure/mechanical performance, as well as ionic/electronic conductivity comparable to gold (Au). Wenbing Wan's team reported using nanoscale microtubules (MTs) as a scaffold template and self-assembling amphiphilic cell membrane fragments with hydrophobic multi-walled carbon nanotubes (MWNTs).116 This mixture was cross-linked to form a conductive scaffold. Finally, polyaniline (PANI) was added to the nanocomposite material to enhance its conductivity. As an electrode, the resulting cell-based conductive gel increased the interface surface area and enhanced the material's conductivity. Amphiphilic modification can improve the performance of electrodes within complex biological systems. Since electrodes typically need to interact with aqueous bodily fluids and biological tissues, amphiphilic-modified electrodes can better adapt to these interfaces. This helps reduce the instability between the electrode and the biological entity, ultimately improving the stability and affinity of signal transmission.
Protein modification involves introducing specific proteins or biomolecules onto the electrode surface to achieve specific interactions with biological entities. For example, a brain-derived neuronal specific cell adhesion molecule, L1, was covalently bound to the neural electrode array surface by Prof. Xinyan Cui's group.117 Reduced glial activation was induced and higher neuronal density and axonal regeneration were promoted around the implants. The electrode–tissue interface was maintained with high quality. Compared with uncoated arrays, laminar electrode arrays coated with brain-derived neuron-specific cell adhesion molecules enhanced the overall visual-evoked single-unit (SU) yield and SU amplitude within a 0–1500 μm depth in the mouse brain, as well as the signal-to-noise ratio (SNR). The improvement in recordings was most significant in the hippocampal region, where the control group exhibited a severe reduction in recording yield one week after implantation, while the coated implants maintained higher SU yields throughout the entire 16 weeks. These results collectively confirm the effectiveness of biomimetic coatings based on brain-derived neuron-specific cell adhesion molecules in reducing inflammatory tissue reactions and enhancing the quality and longevity of neural recordings. Furthermore, protein modification imparts electrodes with specific biological recognition functions. By immobilizing enzymes or antibodies, electrodes can be used for biosensing applications, such as detecting glucose levels in blood glucose monitoring. These protein-modified electrodes can achieve specific biomolecule recognition, enabling highly targeted detection and analysis.
5. Encapsulation strategies
Flexible and stretchable physiological electrodes represent a revolutionary biomedical device that integrates the latest advances in microelectronics, biology, and materials science. They aim to achieve high-precision monitoring and intervention in human physiological activities. These electrodes provide innovative tools for scientific research and bring new hopes and opportunities to the healthcare field, contributing to the forefront of personalized medicine and medical technology. By combining flexibility, biocompatibility, and high-precision monitoring, flexible physiological electrodes have become a remarkable technology in the biomedical field, profoundly impacting the future of medical and biological research.
Given the rapid development of flexible and stretchable physiological electrodes in recent decades, their encapsulation methods have garnered increasing attention.122,123 The primary reason is that encapsulation failure can lead to various issues, such as reduced device stability, leakage, short circuits, device failures, and even damage to biological tissues. Therefore, electrode encapsulation is crucial to ensure its performance, stability, and biocompatibility. Carefully designing and selecting appropriate encapsulation materials is a key factor for the successful application and long-term reliability of electrodes. Additionally, encapsulation needs to consider the specific requirements of particular applications, such as implantable medical devices, biosensors, or brain–machine interfaces, to ensure that the electrodes can effectively operate in various environments. Compared with the encapsulation of wearable flexible electronics, implantable flexible electronics impose higher demands on encapsulation materials in terms of biocompatibility, flexibility, long-term stability, and waterproofing. Therefore, this section primarily introduces the advances in encapsulation for implantable and degradable biodevices, along with methods for their stretchable encapsulation.
5.1. Long-term encapsulation strategies
In the context of implantable devices, long-term implantable biomedical devices are employed to monitor parameters within the human body, providing real-time data to assist doctors in adjusting treatment plans and enhancing patients’ quality of life. In long-term implantable biomedicine, encapsulation is of paramount importance because it needs to ensure that electronic devices remain stable in long-term contact with biological fluids and can closely interface with soft, curved biological tissues. The development of this technology holds potential for achieving semi-permanent biomedical applications within the human body, including sensing biological signals, and stimulating tissues or organs for disease diagnosis and treatment.123
These encapsulation layers are typically made from organic or inorganic materials and involve different deposition techniques and material types. Achieving ultralow water permeability (water vapor transmission rate (WVTR) < 10−6 g m−2 day−1 at 25 °C) is essential to ensure multi-decade lifetimes under physiological conditions of temperature and pH.124 Polymer layers are usually cost-effective but require thicker layers due to their high WVTR. Inorganic encapsulation layers typically have higher quality but come at a higher cost. However, their WVTR is very low, providing a better encapsulation performance. Nonetheless, even with an acceptable encapsulation quality from ALD or CVD deposition, achieving perfect encapsulation throughout the entire biomedicine surface is nearly impossible due to limitations in the laboratory environment, contamination, or defects. Hence, researchers are exploring new defect-free materials and multilayer encapsulation to achieve more perfect encapsulation. Research results indicate that thermally grown silicon dioxide (t-SiO2) exhibits excellent encapsulation quality,125 leading to long-term stability in biological fluids. For example, Roger's research group used t-SiO2 for device encapsulation, achieving 25 days of intracranial pressure monitoring with implantable devices. Other researchers using similar techniques have realized long-term implantable electrophysiological monitoring lasting for several hundred days. It is worth noting that the encapsulation layers need further improvement to delay ion diffusion and extend the lifespan of the biological encapsulation layer. By employing multilayer encapsulation techniques, such as the additional deposition of aluminum oxide or hafnium oxide, researchers have successfully reduced the ion diffusion and extended the lifespan of the encapsulation layers. Compared with t-SiO2, single-crystal silicon carbide is an excellent long-term implantable encapsulation material due to its extremely low water and ion permeability.
5.2. Bioresorbable encapsulation strategies
Conventional implantable electronic devices often require secondary surgeries for removal, imposing additional physical burdens and health risks on patients. In contrast, biodegradable flexible bioelectrodes offer numerous advantages. For example, they gradually degrade after fulfilling their intended function, eliminating the need for secondary surgery and reducing the patient's burden. Additionally, biodegradable electrodes typically exhibit good biocompatibility, reducing the risk of infection or immune rejection. Their degradation rate can also be adjusted through material selection and design to meet the needs of different applications.126
In this context, the biodegradability of the encapsulation layer is also crucial for these devices. Common biodegradable materials include inorganic materials, polymer materials, and natural materials. Inorganic biodegradable materials used for encapsulation layers include silicon dioxide, metal oxides, silicon nitride, magnesium, and magnesium oxide. Silicon dioxide layers are typically prepared using methods like chemical vapor deposition and provide excellent barrier properties, forming an effective insulating layer between the device and the complex external environment. Common biodegradable polymer materials include polylactic acid, poly(lactic-co-glycolic acid), and polycaprolactone.127–130 Polylactic acid has a longer degradation period, polycaprolactone has a slower degradation rate, and the degradation rate of poly(lactic-co-glycolic acid) can be adjusted by changing the composition ratio. For example, Fig. 5e presents the design of a pressure sensor, constructed entirely from biodegradable materials and based on fringe-field capacitor technology, for measuring arterial blood flow in both contact and non-contact modes. The sensor is operated wirelessly through inductive coupling, demonstrating minimal hysteresis, rapid response times, excellent cycling stability, and high robustness. It enables easy mounting and eliminates the need for removal, thus reducing the risk of vessel trauma. Compared with inorganic and polymer materials, natural materials demonstrate superior performance. For instance, silk and spider silk-derived silk fibroin exhibit good biocompatibility and tunable degradation rates. However, further research is needed to control their mechanical properties. Materials such as cellulose, starch, and chitosan are also frequently employed for biodegradable natural material encapsulation layers (Fig. 5f and g). Additionally, the degradation of the encapsulation layer can also play a role in releasing drugs for therapeutic purposes.131
Considering the degradation period, rate, and mechanical properties of different biodegradable materials, in conjunction with the application scenarios of flexible and stretchable electronic devices, selecting the appropriate biodegradable encapsulation layer not only reduces the patient's burden but also unlocks more possibilities for medical research and disease treatment.
5.3. Stretchable encapsulation strategies
Numerous tissues and organs in the human body undergo periodic deformations, including the rhythmic pulsation of the heart, the cyclic expansion and contraction of the lungs during respiration, and the stretching of the muscles. These physiological movements present a significant challenge for implanted electronic devices, as conventional rigid encapsulation layers are ill-suited to accommodate such dynamic deformations. This limitation can lead to damage and reduced performance of the implanted devices. In response to this challenge, the development of stretchable encapsulation materials has become paramount in the realm of flexible and stretchable implantable bioelectronics. These materials offer the potential to allow electronic devices to seamlessly integrate with the body's natural movements, thereby mitigating damage and ensuring long-term device functionality.
Typically, flexible materials used for encapsulation employ an elastic substrate, such as PDMS (Fig. 5h), Ecoflex, polyurethane, or SEBS (styrene ethylene butylene styrene). These materials enable the fabrication of ultra-thin films through various techniques, which are employed to encapsulate the electronic components. This approach not only maintains the structural integrity and electrical performance of the devices but also permits their adaptation to dynamic physiological changes.120,132 In recent years, our research group has conducted extensive investigations into base materials and encapsulation materials using SEBS, PDMS, and Ecoflex. Leveraging methods such as air–liquid interfacial drop-casting, we have successfully developed ultra-thin SEBS films, known for their remarkable self-adhesiveness, enabling the encapsulation of flexible, stretchable bioelectronic devices based on SEBS substrates (Fig. 5i and j).17,121 This unique encapsulation method not only shields the device from external liquids, preserving its performance, but also ensures the seamless adhesion of the encapsulation layer to the device, preserving its overall structural integrity. Moreover, we have explored the use of spin-coating to apply ultra-thin PDMS and Ecoflex films, providing an effective encapsulation method. A diluted PDMS solution, mixed with organic solvents, is applied to the substrate after the electrodes have been prepared. Following curing, the resulting ultra-thin encapsulation layer integrates seamlessly with the device, encapsulating the conductive pathways while preserving the electrical properties and robust performance of the internal electrodes. This encapsulation strategy has been found to maintain stable electrical characteristics while protecting the device from environmental factors such as temperature and humidity fluctuations. In addition to these approaches, gel materials, as a class of wet-stretchable materials, have found valuable applications in implantable flexible bioelectronics. Their unique properties enable them to accommodate dynamic physiological changes and environmental influences effectively.
6. Energy supply method for implantable flexible stretchable equipment
The way the flexible stretchable device is powered is critical because it directly affects the service life, performance and feasibility of the device.133–141 In current methods, many implantable devices use built-in lithium batteries or other rechargeable batteries to provide power.142–148 These batteries usually have a long life and can provide a long-term energy supply for the device.149–156 The patient or medical professional can maintain the power to the device through external wireless charging or periodic battery replacement. In addition, some devices use wireless charging technology to transfer power from an external charging device to a battery inside the device. This method can extend the service life of the device and reduce the need to replace the battery.157 Wireless charging can also improve the tightness of the device and reduce the risk of infection.158 Finally, some implantable devices use energy-harvesting techniques, such as heat, mechanical vibration or light energy, to capture energy from the surrounding environment to supply the device.159 These technologies, while promising, are currently limited by their limited energy-harvesting efficiency and often fail to provide enough power to meet the needs of energy-intensive devices.
In the future, one trend may be the use of biofuel cells, which convert biochemical reactions in living organisms into electricity.160,161 This approach is expected to provide a longer service life and reduce the frequency of battery replacement. Second, advances in nanotechnology could be widely used in implantable flexible devices, tiny devices that can generate electrical energy through mechanical vibration or movement in the body.162 This technology is expected to provide an automatic way of supplying power without external intervention.163–166 Bojing Shi et al. propose a body-integrated self-powered system (BISS) that is a simple, efficient, and cost-effective way to harvest energy from human movement (Fig. 6a). Biomechanical energy for moving the human body can be obtained through a piece of electrode attached to the skin. The basic principle of BISS is inspired by the combined effect of friction electrification between the sole and the floor and electrification of the human body.167 A. J. Bandodkar et al. reported a biocompatible sweat-activated battery technology that can be embedded in a soft microfluidic platform (Fig. 6b). The battery can be used in a detachable electronic module that contains a wireless communication and power management system and is capable of continuously recording physiological signals on the skin.168 Qiang Zheng et al. report on a biodegradable triboelectric nanogenerator (BD-TENG) for in vivo biomechanical energy harvesting that can be degraded and reabsorbed in animals after completing its working cycle without any adverse long-term effects (Fig. 6c).169 The BD-TENG, designed with a multilayer structure, demonstrates remarkable electrical output power and converts in vivo biomechanical energy into electricity for use in implantable medical devices. Philipp Gutruf et al. describe a highly miniaturized wireless energy harvesting and digital communication electronic device for a thin, small pacemaker platform weighing 110 mg with a subcutaneous implantable capability and tolerance to more than 200
000 multi-axial strain cycles without compromising the electrical or optical performance (Fig. 6d).170 Yang et al. reported a stretchable TEG (S-TEG) (more than 50% stretchability of the entire device) that is geometrically suitable for a variety of complex and dynamic heat source surfaces (Fig. 6e). The S-TEG consists of thermocouple arrays of the thermocouple types p-(Sb2Te3) and n-(Bi2Te3) in hot-pressed nanolayers, and utilizes a wavy serpentine interconnect to integrate all the units. The S-TEG collects energy from the dynamic surface of human skin, offering a potential energy solution for health monitoring in wearable devices.171 In addition, future implantable flexible devices may be powered in smarter ways, such as drawing energy from a remote power source. This may involve using an external power source or wireless energy transfer with an outside device to ensure that the device always has enough power.172
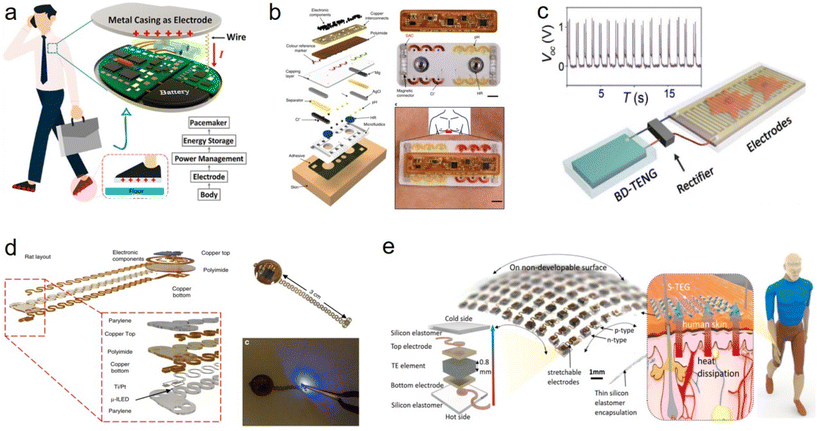 |
| Fig. 6 Power supply scheme for flexible and stretchable implant devices. (a) Diagram of the self-powered pacemaker based on the implantable BISS.167 This figure has been reproduced from ref. 167 with permission from the American Chemical Society; copyright: 2019. (b) Flexible and biocompatible sweat-activated cell (SAC)-powered, skin-interfaced hybrid microfluidic–microelectronic system.168 This figure has been reproduced from ref. 168 with permission from Springer Nature; copyright: 2020. (c) Electrical stimulation of nerve cells powered by BD-TENG, rectified electrical output of BD-TENG and schematic diagram of the self-powered nerve cell stimulation system.169 This figure has been reproduced from ref. 169 with permission from the American Association for the Advancement of Science; copyright: 2016. (d) Wireless, battery-free, fully implantable pacemakers with electrical and optical stimulation capabilities. These images are rendered images of the layered design of devices configured for rats and mice, images of devices, and photoimages of devices activated by photogenetic stimulation.170 This figure has been reproduced from ref. 170 with permission from Springer Nature; copyright: 2019. (e) Design of the S-TEG. The schematic diagram shows the energy harvesting of the S-TEG from the waste heat of human skin; in the diagram, an optical image and an exploded schematic of the device show the 10 × 10 array p–n couples of TE materials and the components of one unit, respectively.171 This figure has been reproduced from ref. 171 with permission from the American Chemical Society; copyright: 2020. | |
In short, the energy supply method of implantable flexible stretchable devices is constantly evolving, and it is expected to achieve more durable and intelligent energy supply methods in the future to meet the needs of different application fields. These new technologies will help improve the feasibility, performance and portability of devices.
7. Application of flexible and stretchable implantable devices in monitoring and electrical stimulation
7.1. Application in monitoring
Implantable flexible stretchable devices have potential applications in the electrophysiological monitoring of peripheral muscles and nerves, especially in rehabilitation therapy monitoring.18,22,25,26,173–178 In terms of device types, implantable flexible stretchable devices typically include flexible electrode arrays, biocompatible packages, micro-electronics, and data transmission systems. Rongyu Tang et al. developed a fluidic cuff electrode using a gallium-based liquid metal (LM) conductor as a prototype artificial peripheral nerve (Fig. 7a). After being implanted and connected to the sciatic nerve, the LM electrodes within the freely moving rats’ bodies survive repeated body stretching and retain their long-term effectiveness in transmitting sciatic nerve signals with a high signal-to-noise ratio during two-week experiments. They demonstrated that the LM electrodes meet the requirements of peripheral nerve signal recording and stimulation for long-term implantation, and have the potential to become a new generation of artificial peripheral nerve devices to interface with, supplement, or even enhance and replace the real peripheral nerve.25 Sanghoon Lee et al. argue that a reliable neural interface between peripheral nerves and implantable devices is central to advanced neural repair and bioelectronic medicine (Fig. 7b). They studied the effect of a flexible split-ring electrode on the selective recording and stimulation of the sciatic nerve. This design makes implanting active electrodes on the sciatic nerve simple and reliable, with minimal pressure on the nerve, but still providing good electrical contact with the nerve. In addition, partial induced neural signals from the nerves were also recorded using the transverse differential bipolar configuration, demonstrating differential recording capabilities. In addition, they found that in terms of signal-to-noise ratio (SNR), the quality of neural signals recorded by the split-ring electrode was higher than that recorded by the commercial cuff electrode.179 Sahar Elyahoodayan et al. have suggested that the outer nerve sleeves are one of the least invasive peripheral nerve interfaces because they are located outside the nerve. They developed an electrode that can improve selectivity and sensitivity while maintaining the sleeve shape (Fig. 7c). They focus on demonstrating the in vivo function of microfluidics and microelectrodes in acute preparations, where they assess the ability to locally remove connective tissue and record and stimulate neural activity in rat sciatic nerves with microchannel-embedded microelectrodes. In vivo electrical evaluation showed that microelectrodes placed in microfluidic channels could successfully stimulate and record the compound action potentials of rat sciatic nerve. They proved that it is feasible to use an integrated microelectrode and microfluidic cuff to stimulate, record and administer the localized dissolution of the epineural layer.180 Mei Yu et al. proposed a self-closing stretchable cuff electrode, which is able to self-close onto the bundles of tissues after dropping water onto it. For in vivo testing, both sciatic nerve stimulation to drive the muscles and electromyographic signal monitoring around a rat's extensor digitorum longus for 1 month prove that their proposed electrode conforms well to the curved surface of biological tissue (Fig. 7d).63 Amy E. Rochford et al. reported the long-term survival and functional integration of a biohybrid device carrying human iPSC-derived cells with the forearm nerve bundle of freely moving rats, following 4 weeks of implantation. By improving the tissue–electronics interface with an intermediate cell layer, they demonstrated enhanced resolution and electrical recording in vivo as a first step toward restorative therapies using regenerative bioelectronics (Fig. 7e).181 These devices are typically designed to fit tightly into muscle tissue for effective electrophysiological monitoring. In terms of implant location, these devices can be implanted into muscle tissue, usually located near the muscular area of interest, such as the thigh, abdomen, back, arms, etc. In terms of electrophysiological monitoring functions, these devices are able to record the electrical activity of muscles, including electromyography (EMG), which provides information on muscle activity patterns, strength, fatigue and coordination.182 This is important for rehabilitation, sports science, evaluation of athlete performance, and diagnosis and treatment of neuromuscular diseases.183,184 In terms of flexibility and stretchability, muscle tissue can stretch and deform during exercise, so these devices must be flexible and stretchy to adapt to the biomechanical properties of the muscle while avoiding discomfort or damage to the muscle tissue.185 In terms of data transmission, these devices are usually equipped with built-in data transmission systems that can transmit electrophysiological data to external devices, such as computers or monitoring devices, for real-time analysis.186 In summary, implantable flexible stretchable devices have many potential applications in the electrophysiological monitoring of muscles and nerves throughout the body, providing critical information about muscle function and performance.
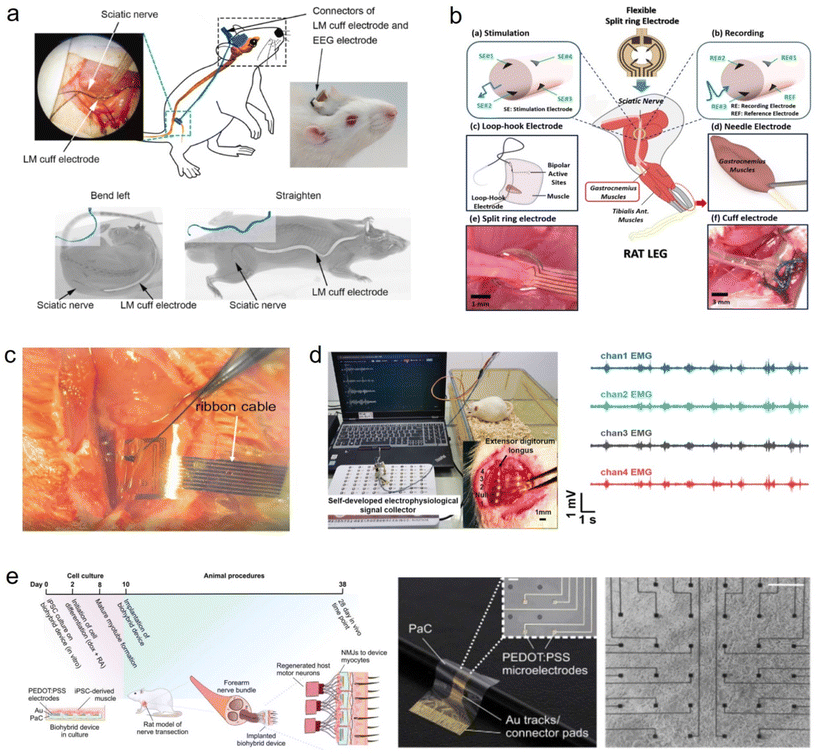 |
| Fig. 7 Application of flexible and stretchable implantable devices in monitoring. (a) Characterization of the implanted LM cuff electrode and EEG electrode array: a photographic image of the LM cuff electrode attached to the sciatic nerve during the surgical procedure, a sketch showing the positions of the LM cuff electrode and EEG electrode array inside the rat body, a photographic image of the assembled electrode connector on a rat's head after surgery, and the CT images showing the positions of the implanted LM cuff electrode and EEG electrode array when the rat bends its body or straightens up, with insets showing the LM cuff electrode outlined in blue.25 This figure has been reproduced from ref. 25 with permission from Elsevier Advanced Technology; copyright: 2022. (b) Schematic diagram of the experimental setup. Schematic diagram of the implanted flexible split ring electrode on the sciatic nerve for stimulation and recording. Schematic diagram of an intra-muscular loop–hook bipolar electrode implanted in either the tibialis anterior or gastrocnemius muscle. Schematic diagram of subdermal electrical stimulation of the hind limb using a needle electrode. Picture of the implanted flexible split ring electrode and commercial cuff electrode (Microprobe Inc., Gaithersburg, MD, USA) on the sciatic nerve in rats.179 This figure has been reproduced from ref. 179 with permission from SAGE Publications INC; copyright: 2017. (c) A lyse-and-attract cuff electrode (LACE) locked around the sciatic nerve.180 This figure has been reproduced from ref. 180 with permission from Elsevier; copyright: 2020. (d) In vivo recording animal experiments with the self-closing stretchable cuff electrode. On the left is the experimental setup for the self-closing electrode wrapped around a rat extensor digitorum longus for long-term EMG monitoring. On the right are the EMG signals detected by the self-closing cuff electrode at the 24th day after implantation.63 This figure has been reproduced from ref. 63 with permission from the American Chemical Society; copyright: 2023. (e) This is an in vivo biohybrid device design customized for the peripheral nervous system. Experimental timeline showing the fabrication and implantation of the biohybrid device from an in vitro cell culture step into an animal model. Cells are seeded onto the flexible biohybrid devices at day 0. After 48 hours (day 2), the differentiation process is initiated. At day 8, the myotubes are mature; therefore, between days 8 and 10 is the optimal timing for the implantation of the biohybrid devices into a peripheral nerve rat model. The devices are then implanted for a period of 4 weeks.181 This figure has been reproduced from ref. 181 with permission from the American Association for the Advancement of Science; copyright: 2023. | |
7.2. Application in electrical stimulation
Implantable flexible stretchable devices play a key role in neuroregulatory electrical stimulation therapy. These devices are used to mimic or tune the electrical activity of neurons to treat a variety of neurological diseases or symptoms.138,179,187 In terms of implantation location, these devices can be implanted near peripheral nerve tissue to provide electrical stimulation when needed.188 The location of the implant depends on the specific purpose of the treatment, and may include the brain, spinal cord, nerve roots or deep nerves in addition to the peripheral nerves.34,189–191 In terms of the therapeutic function of electrical stimulation, implantable devices can be used to control the excitation or inhibition of neurons by transmitting electrical stimulation signals, so as to reduce pain, improve motor control, manage epilepsy, treat Parkinson's disease, reduce depression symptoms, etc. In terms of the electrical stimulation parameters, the effectiveness of treatment depends on the parameters of electrical stimulation, such as frequency, amplitude, pulse width, etc. Doctors can adjust these according to the needs and reactions of patients. In terms of data transmission, these devices often have built-in data transmission systems that can monitor the patient's status and record the effects of treatment. The data help doctors make personalized adjustments. In terms of data analysis, the success of treatment often requires monitoring and analyzing large amounts of data to ensure the effectiveness and safety of electrical stimulation. Jiahui Wang et al. reported a muscle electrical stimulation device, which is powered directly by stacked layer triboelectric nanogenerators (TENG) via flexible multi-channel intramuscular electrodes. This multi-channel intramuscular electrode can map sparsely distributed motor neurons in muscle tissue, enabling efficient muscle stimulation by the TENG (Fig. 8a).192 Their work validates the possibility of direct TENG muscle stimulation. In addition, the involvement of the multiple-channel intramuscular electrode provides guidance for practical applications of using this self-powered system, as it allows stimulation efficiency optimization. With the recent development of TENGs, we believe this self-powered system can be used for electrical muscle stimulation to treat muscle function loss. Yeongjun Lee et al. reported a stretchable neuromorphic implant that restores coordinated and smooth motions in the legs of mice with neurological motor disorders, enabling the animals to kick a ball, walk or run (Fig. 8b). The neuromorphic implant acts as an artificial efferent nerve by generating electrophysiological signals from excitatory post-synaptic signals and by providing proprioceptive feedback. The device operates at low power (∼1/150 that of a typical microprocessor system), and consists of hydrogel electrodes connected to a stretchable transistor incorporating an organic semiconducting nanowire (acting as an artificial synapse), connected via an ion gel to an artificial proprioceptor incorporating a carbon nanotube strain sensor (acting as an artificial muscle spindle).3 This work shows that advanced functions of coordinated and complex leg motions can be elicited in living mammals via soft neural interfaces and stretchable electronic systems. This is a step towards a future artificial nerve system that could serve as a low-power neuromorphic prosthetic device that enables limb movement via motor-cortex-driven signals. In the future, simple systems such as the SNEN that use the principle of neuroplasticity may represent a promising bioengineering technology for the generation of voluntary motion in animals with motor disorders, obviating the need for heavy and complicated electronic devices. Sanghoon Lee et al. proposed a novel flexible neural clip (FNC) that can be used to interface with a variety of different peripheral nerves (Fig. 8c). To illustrate the flexibility of the design, this study stimulates the pelvic nerve, the vagus nerve, and branches of the sciatic nerve and evaluates the feasibility of the design in modulating the function of each of these nerves. It is found that this FNC allows a fine-tuning of physiological processes such as micturition, heart rate, and muscle contractions. Furthermore, this study also tests the ability of the wirelessly powered FNC to enable the remote modulation of visceral pelvic nerves located deep in the body. These results show that the FNC can be used with a range of different nerves, providing one of the critical pieces in the field of bioelectronics medicines.10 This interface does not only provide sciatic nerves interfacing in a paradigm-shift manner, but also paves the way for doing neural modulation for bioelectronic medicine that requires a reliable modulation of small peripheral somatic and visceral nerves, thus advancing implantable bioelectronics toward an untapped potential of neuromodulation. Rui Guo et al. presented and fabricated a flexible neural microelectrode array system based on a liquid metal Ga–In alloy (75.5% Ga and 24.5% In weight). The alloy has a unique low melting point (10.35 °C), excellent electrical conductivity and high compliance, which is conducive to being used as an implantable flexible neural electrode. In conceptual experiments with electrical stimulation of the sciatic nerve in animals, dead bullfrogs implanted with a flexible neural microelectrode array were even able to rhythmically contract and move their lower limbs when electrically stimulated by the implant (Fig. 8d). This demonstrates a highly efficient method for rapidly restoring biological neural function. In addition, a series of biological experiments have proved that liquid metal materials have good biocompatibility. A liquid metal electrode has lower resistance properties compared with a platinum electrode, its resistance value range is far less than the range of the platinum electrode and the electrodes are conducive to the protection of the electrodes and tissues, and thus increased the cathode electric charge storage capacity. The experiments of biocompatibility and bullfrog sciatic nerve electrical stimulation showed that liquid metal electrode has no significant destructive effect on cells and has similar effects to platinum electrodes. In the future, this liquid metal mode for nerve stimulation is expected to play an important role as a bioelectrode to overcome the fundamental mismatch between the mechanics of biological tissues and electronic devices.193 Ai-Ping Yu et al. investigated the effect of implantation of electrodes at different contact points on the regeneration of the sciatic nerve after resection (Fig. 8e). The models of sciatic nerve resection and microsurgical repair were randomly divided into four groups (point contact, 1/4 circle contact; the whole circle touches; no electrodes as a control). Electrical stimulation, electrophysiology, morphology, and histology were performed to examine the sciatic nerve and muscle at 4 and 10 weeks after implantation, respectively. The ischiatic functional index, compound muscle action potential amplitude and motor nerve conduction velocity in the point and 1/4 circle contact groups were significantly higher than those in the contact group at 4 weeks and 10 weeks after implantation. The point and 1/4 circle contact pattern promoted sciatic nerve regeneration, reduced muscle contraction, and lessened mechanical injury. Changes in the nerve trunk were observed at weeks 4 and 10 when compared with the full circle contact group. Electrodes with point contacts and 1/4 round contacts represent an effective alternative to portable electrical stimulation for the regeneration of the injured sciatic nerve and the reduction of subsequent muscle atrophy, which may provide a promising approach for the treatment of peripheral nerve injuries.194 The use of implantable flexible stretchable devices has made significant progress in neuroregulatory therapy, but further research and clinical validation are still needed to ensure safety and efficacy.
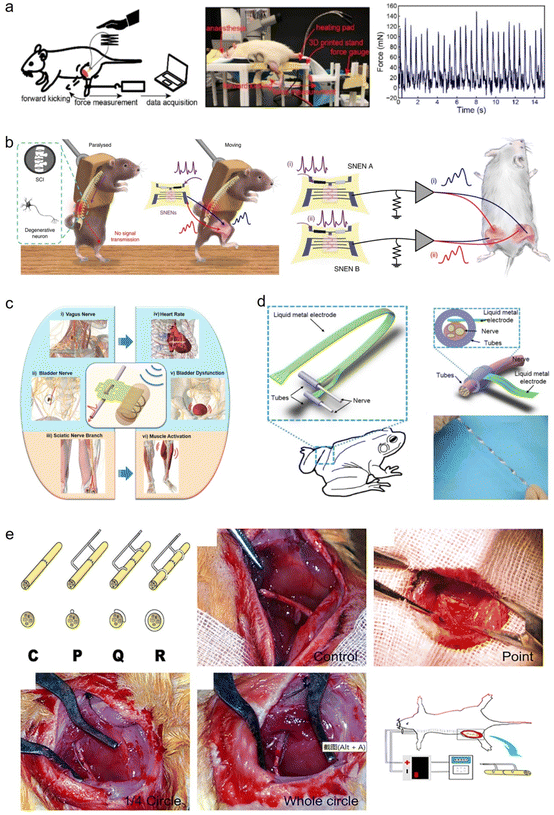 |
| Fig. 8 Application of flexible and stretchable implantable devices in electrical stimulation. (a) In vivo mapping of a stacked-layer TENG stimulation efficiency. Schematic of the testing setup with force measurement. In vivo testing setup. Force profile measured when the TENG directly powers muscle stimulation.192 This figure has been reproduced from ref. 192 with permission from the American Chemical Society; copyright: 2019. (b) Bipedal walking locomotion. Schematic of a paralysed mouse afflicted by SCI or MND (left) and a mouse that had recovered voluntary motor function by using SNEN (right). Practical locomotion is demonstrated with coordinated stimulation of the muscles by post-synaptic signals of the SNEN and patterned pre-synaptic Ap inputs. Configuration of the mouse for bipedal walking locomotion.3 This figure has been reproduced from ref. 3 with permission from Springer Nature; copyright: 2022. (c) Schematic diagram of peripheral nerves and modulated functions using a wireless FNC. Schematic diagram of different applications of a wireless FNC interface for the wireless modulation of nerves to achieve different organ or tissue outputs. (i) Vagus nerve stimulation (VNS), (ii) bladder nerve stimulation, and (iii) stimulation of sciatic nerve branches for the modulation of (iv) heart rate (HR), (v) bladder dysfunction, and (vi) leg muscles, respectively.10 This figure has been reproduced from ref. 10 with permission from WILEY-VCH Verlag GmbH & Co. KGaA; copyright: 2017. (d) The schematic diagram of the liquid metal nerve electrodes in vivo. A silicone tube was used to fix the electrodes on the nerve. The pictures of the liquid metal nerve electrodes.193 This figure has been reproduced from ref. 193 with permission from IOP PUBLISHING Ltd; copyright: 2017. (e) The design and contact patterns of the point electrode, 1/4 circle electrode and whole circle electrode used as an implanted flexible electrode. The dissected sciatic nerve was repaired as a control. Point contact electrode implantation. 1/4 circle contact electrode implantation. Whole-circle contact electrode implantation. Schematic illustration of the animal procedures: a nickel–titanium alloy wire was placed into the proximal and distal nerve segments with three contact patterns of the electrodes and buried behind the neck through the subcutaneous tunnel, respectively.194 This figure has been reproduced from ref. 194 with permission from Elsevier IRELAND Ltd; copyright: 2019. | |
8. Ethics, signal analysis and processing
In the field of implantable flexible stretchable devices for electrophysiological signal monitoring and electrical stimulation, issues related to biosafety, ethics, laws and regulations, and signal analysis and processing are crucial. These factors directly relate to the safety, privacy, and legality of future patients, and are worthy of attention and attention.
At the ethical and moral level, as implantable devices can monitor patients’ physiological signals and other sensitive data in real-time, maintaining patients’ privacy is crucial. Device manufacturers and medical professionals must take measures to ensure data security, such as encryption, authentication, and access control. Patients should be informed about how their data are used and whether they are shared with other institutions. There are autonomous decision-making and informed consent issues: before implanting a device, patients should fully understand the risks and benefits of implanting the device so that they can make informed consent decisions. Medical professionals need to provide detailed information to help patients understand the potential benefits of implantable devices, but they also need to honestly introduce the potential risks and complications. Patients have the right to decide whether to accept implanted devices to ensure that their autonomy and dignity are respected.
For signal analysis and processing, current practices include the use of various algorithms and techniques to analyze physiological signal data collected from devices. These methods aim to detect abnormalities, monitor physiological parameters, and provide real-time feedback to support medical decision-making. Future trends include more advanced signal analysis and processing methods, such as artificial intelligence and machine learning. These technologies are expected to improve the interpretability, accuracy, and predictability of the data. At the same time, the data cloud connection of implantable devices will also accelerate the development of remote monitoring and remote medical services.
Overall, the development of implantable electrophysiological signal monitoring and electrical stimulation flexible stretchable devices is continuously driving the forefront of biomedical engineering. Future development will benefit from innovative technologies and interdisciplinary cooperation to achieve safer and more efficient implantable device applications.
9. Conclusions and outlook
Implantable electrodes are at the forefront of advancing our ability to interface with peripheral nerves and muscles. Their precision, stability, and therapeutic potential have far-reaching implications, promising improved mobility, function, and quality of life for individuals with neuromuscular challenges. Additionally, they are propelling innovation in the fields of rehabilitation, assistive technology, and neuromuscular research, offering a brighter future for those in need of these technologies.
Looking ahead to the future, implantable electrodes show enormous potential. The advancement of technology is expected to redefine the field of prosthetics and rehabilitation, making it more natural and intuitive. These electrodes not only provide better control over biomimetic limbs, but also provide sensory feedback, thereby promoting closer interaction with prosthetics. This development direction is expected to promote the realization of fully functional and realistic prosthetic devices, bringing new possibilities to the field of neurological rehabilitation.
Although significant progress has been made in the development of implantable and stretchable electrophysiological electrodes, there are still some challenges. One of the main issues is to extend the biocompatibility and stability of these electrodes in physiological environments to ensure their long-term effective operation. It is crucial to achieve a seamless interface between electrodes and biological tissues, as mechanical mismatches can lead to non-conformal contact, tissue damage, and inefficient stimulation. In this regard, material selection and the development of packaging technology play a crucial role. Addressing potential issues such as packaging failures, leakage, and short circuits remains a major challenge. In addition, improving the stretchability of electrodes to adapt to the dynamic deformation of body tissues is also a continuous challenge that requires innovative materials and packaging strategies. Realizing precise and selective stimulation or recording specific physiological signals remains a key obstacle, and further improvements in electrode design and signal processing algorithms are needed.
Addressing these challenges is crucial for the sustained progress and successful clinical translation of implantable and stretchable electrophysiological electrodes. To overcome these obstacles, interdisciplinary collaboration is particularly important, covering materials science, engineering, biology, and clinical expertise. The ongoing research aims to improve the performance and safety of implantable electrophysiological electrodes for applications in neurorecording, neuroregulation, and other bioelectronic therapies. A promising direction for future research is to develop intelligent and adaptive materials that can dynamically respond to changes in physiological environments. The integration of reactive polymers is a potential research direction that can alter mechanical properties based on specific biological cues, achieving alignment with natural tissue movement. Combining advanced sensors for the continuous monitoring of biochemical markers can provide valuable insights into complex physiological processes. This multimodal sensing combined with adaptive stimulation capability is expected to completely change the way treatment interventions are carried out, achieving personalized and precise control. Meanwhile, striving to improve the energy efficiency of equipment and exploring innovative power sources or energy-harvesting mechanisms can help reduce the need for frequent replacement or charging. Overall, these directions have paved the way for the development of the next generation of implantable electrophysiological electrodes, making it possible to seamlessly integrate their functions with the human body.
It is worth noting that currently, active electrodes, in conjunction with synthetic biology, are rapidly evolving. The primary distinction between active electrodes and traditional ones is their controllable electrode activity. Traditional electronic devices are designed and assembled based on non-living materials (inorganic/organic), with well-defined working physical mechanisms. Their inherent drawbacks include static behavior and limited adaptability to the environment. In contrast, devices based on active materials, such as DNA, RNA, proteins, lipids, and cells, inspired by the activity of living organisms, possess potential characteristics such as self-repair, self-replication, self-renewal, dynamic adjustability, self-sustainability, and environmental adaptability after implantation. Therefore, the development of flexible implantable devices based on active materials will facilitate seamless integration with dynamic living organisms. The in-depth advancement of these active electronic devices will lead to a profound fusion of human–machine interfaces, advance research in artificial intelligence neural networks, and enable the replication of neural networks in living cell networks.
This represents the cutting edge of neural engineering, poised to drive progress in the field of neuroscience, deepening our comprehension of the nervous system and its disorders. Through ongoing research and development, we can envision a future where these technologies offer enhanced precision, extended lifespans, and broader applications. They hold the potential to improve the lives of countless individuals, offering newfound hope and opportunities for those facing neuromuscular challenges, while also providing more personalized treatment options.
Conflicts of interest
The authors declare no conflict of interest.
Acknowledgements
This work was supported by National Key R&D Program of China (2021YFF0501600, 2021YFF0501602), National Natural Science Foundation of China (62101544, 62201558, 62101545, and 62201559), Shenzhen Science and Technology Program (KQTD20210811090217009), and Guangdong Basic and Applied Basic Research Foundation (2022A1515010879).
References
- C. Kathe, M. A. Skinnider, T. H. Hutson, N. Regazzi, M. Gautier, R. Demesmaeker, S. Komi, S. Ceto, N. D. James, N. Cho, L. Baud, K. Galan, K. J. E. Matson, A. Rowald, K. Kim, R. Wang, K. Minassian, J. O. Prior, L. Asboth, Q. Barraud, S. P. Lacour, A. J. Levine, F. Wagner, J. Bloch, J. W. Squair and G. Courtine, Nature, 2022, 611, 540–547 CrossRef CAS PubMed.
- M. Ortiz-Catalan, E. Mastinu, P. Sassu, O. Aszmann and R. Brånemark, N. Engl. J. Med., 2020, 382, 1732–1738 CrossRef PubMed.
- Y. Lee, Y. Liu, D.-G. Seo, J. Y. Oh, Y. Kim, J. Li, J. Kang, J. Kim, J. Mun, A. M. Foudeh, Z. Bao and T.-W. Lee, Nat. Biomed. Eng., 2022, 7, 511–519 CrossRef PubMed.
- S. Raspopovic, G. Valle and F. M. Petrini, Nat. Mater., 2021, 20, 925–939 CrossRef CAS PubMed.
- Y. Cui, F. Zhang, G. Chen, L. Yao, N. Zhang, Z. Liu, Q. Li, F. Zhang, Z. Cui, K. Zhang, P. Li, Y. Cheng, S. Zhang and X. Chen, Adv. Mater., 2021, 33, 2100221 CrossRef CAS PubMed.
- C. Neudorfer, C. T. Chow, A. Boutet, A. Loh, J. Germann, G. J. B. Elias, W. D. Hutchison and A. M. Lozano, Brain Stimul., 2021, 14, 513–530 CrossRef PubMed.
- G. Schiavone, X. Kang, F. Fallegger, J. Gandar, G. Courtine and S. P. Lacour, Neuron, 2020, 108, 238–258 CrossRef CAS PubMed.
- D. Farina, I. Vujaklija, R. Brånemark, A. M. J. Bull, H. Dietl, B. Graimann, L. J. Hargrove, K.-P. Hoffmann, H. Huang, T. Ingvarsson, H. B. Janusson, K. Kristjánsson, T. Kuiken, S. Micera, T. Stieglitz, A. Sturma, D. Tyler, R. F. F. Weir and O. C. Aszmann, Nat. Biomed. Eng., 2023, 7, 473–485 CrossRef PubMed.
- B. Barra, S. Conti, M. G. Perich, K. Zhuang, G. Schiavone, F. Fallegger, K. Galan, N. D. James, Q. Barraud, M. Delacombaz, M. Kaeser, E. M. Rouiller, T. Milekovic, S. Lacour, J. Bloch, G. Courtine and M. Capogrosso, Nat. Neurosci., 2022, 25, 924–934 CrossRef CAS PubMed.
- S. Lee, W. Y. X. Peh, J. Wang, F. Yang, J. S. Ho, N. V. Thakor, S. C. Yen and C. Lee, Adv. Sci., 2017, 4, 1700149 CrossRef PubMed.
- D. Afanasenkau, D. Kalinina, V. Lyakhovetskii, C. Tondera, O. Gorsky, S. Moosavi, N. Pavlova, N. Merkulyeva, A. V. Kalueff, I. R. Minev and P. Musienko, Nat. Biomed. Eng., 2020, 4, 1010–1022 CrossRef PubMed.
- Y. S. Choi, Y.-Y. Hsueh, J. Koo, Q. Yang, R. Avila, B. Hu, Z. Xie, G. Lee, Z. Ning, C. Liu, Y. Xu, Y. J. Lee, W. Zhao, J. Fang, Y. Deng, S. M. Lee, A. Vázquez-Guardado, I. Stepien, Y. Yan, J. W. Song, C. Haney, Y. S. Oh, W. Liu, H.-J. Yoon, A. Banks, M. R. MacEwan, G. A. Ameer, W. Z. Ray, Y. Huang, T. Xie, C. K. Franz, S. Li and J. A. Rogers, Nat. Commun., 2020, 11, 5990 CrossRef CAS PubMed.
- S. Mondal, N. Zehra, A. Choudhury and P. K. Iyer, ACS Appl. Bio Mater., 2020, 4, 47–70 CrossRef PubMed.
- B. Llerena Zambrano, A. F. Renz, T. Ruff, S. Lienemann, K. Tybrandt, J. Vörös and J. Lee, Adv. Healthcare Mater., 2020, 10, 2001397 CrossRef PubMed.
- J. K. Nguyen, D. J. Park, J. L. Skousen, A. E. Hess-Dunning, D. J. Tyler, S. J. Rowan, C. Weder and J. R. Capadona, J. Neural Eng., 2014, 11, 056014 CrossRef PubMed.
- Y. Li, N. Li, W. Liu, A. Prominski, S. Kang, Y. Dai, Y. Liu, H. Hu, S. Wai, S. Dai, Z. Cheng, Q. Su, P. Cheng, C. Wei, L. Jin, J. A. Hubbell, B. Tian and S. Wang, Nat. Commun., 2023, 14, 4488 CrossRef CAS PubMed.
- Y. Jiang, S. Ji, J. Sun, J. Huang, Y. Li, G. Zou, T. Salim, C. Wang, W. Li, H. Jin, J. Xu, S. Wang, T. Lei, X. Yan, W. Y. X. Peh, S.-C. Yen, Z. Liu, M. Yu, H. Zhao, Z. Lu, G. Li, H. Gao, Z. Liu, Z. Bao and X. Chen, Nature, 2023, 614, 456–462 CrossRef CAS PubMed.
- Z. Xiang, S. C. Yen, S. Sheshadri, J. Wang, S. Lee, Y. H. Liu, L. D. Liao, N. V. Thakor and C. Lee, Adv. Mater., 2015, 28, 4472–4479 CrossRef PubMed.
- D. T. Simon, E. O. Gabrielsson, K. Tybrandt and M. Berggren, Chem. Rev., 2016, 116, 13009–13041 CrossRef CAS PubMed.
- B. Ji, Z. Xie, W. Hong, C. Jiang, Z. Guo, L. Wang, X. Wang, B. Yang and J. Liu, J. Mater., 2020, 6, 330–338 Search PubMed.
- D.-Y. Khang, H. Jiang, Y. Huang and J. A. Rogers, Science, 2006, 311, 208–212 CrossRef CAS PubMed.
- H. Li, F. Han, L. Wang, L. Huang, O. W. Samuel, H. Zhao, R. Xie, P. Wang, Q. Tian, Q. Li, Y. Zhao, M. Yu, J. Sun, R. Yang, X. Zhou, F. Li, G. Li, Y. Lu, P. Guo and Z. Liu, Adv. Funct. Mater., 2023, 33, 2300859 CrossRef CAS.
- I. R. Minev, P. Musienko, A. Hirsch, Q. Barraud, N. Wenger, E. M. Moraud, J. Gandar, M. Capogrosso, T. Milekovic, L. Asboth, R. F. Torres, N. Vachicouras, Q. Liu, N. Pavlova, S. Duis, A. Larmagnac, J. Vörös, S. Micera, Z. Suo, G. Courtine and S. P. Lacour, Science, 2015, 347, 159–163 CrossRef CAS PubMed.
- R. Dong, X. Liu, S. Cheng, L. Tang, M. Chen, L. Zhong, Z. Chen, S. Liu and X. Jiang, Adv. Healthcare Mater., 2021, 10, 2000641 CrossRef CAS PubMed.
- R. Tang, C. Zhang, B. Liu, C. Jiang, L. Wang, X. Zhang, Q. Huang, J. Liu and L. Li, Biosens. Bioelectron., 2022, 216, 114600 CrossRef CAS PubMed.
- D. Qi, Z. Liu, Y. Liu, Y. Jiang, W. R. Leow, M. Pal, S. Pan, H. Yang, Y. Wang, X. Zhang, J. Yu, B. Li, Z. Yu, W. Wang and X. Chen, Adv. Mater., 2017, 29, 1702800 CrossRef PubMed.
- F. Fallegger, G. Schiavone and S. P. Lacour, Adv. Mater., 2019, 32, 1903904 CrossRef PubMed.
- S. P. Lacour, G. Courtine and J. Guck, Nat. Rev. Mater., 2016, 1, 16063 CrossRef CAS.
- Y. H. Cho, Y. G. Park, S. Kim and J. U. Park, Adv. Mater., 2021, 33, 2005805 CrossRef CAS PubMed.
- P. Chen, P. Wu, X. Wan, Q. Wang, C. Xu, M. Yang, J. Feng, B. Hu and Z. Luo, Nano Energy, 2021, 86, 106123 CrossRef CAS.
- R. Caldwell, M. G. Street, R. Sharma, P. Takmakov, B. Baker and L. Rieth, Biomaterials, 2020, 232, 119731 CrossRef CAS PubMed.
- C. Silveira, E. Brunton, E. Escobedo-Cousin, G. Gupta, R. Whittaker, A. O'Neill and K. Nazarpour, IEEE Trans. Neural Syst. Rehabil. Eng., 2020, 28(10), 2136–2143 Search PubMed.
- A. Ashok, T. K. Nguyen, M. Barton, M. Leitch, M. K. Masud, H. Park, T. A. Truong, Y. V. Kaneti, H. T. Ta, X. Li, K. Liang, T. N. Do, C. H. Wang, N. T. Nguyen, Y. Yamauchi and H. P. Phan, Small, 2023, 19, 2204946 CrossRef CAS PubMed.
- S. Lienemann, J. Zötterman, S. Farnebo and K. Tybrandt, J. Neural Eng., 2021, 18, 045007 CrossRef PubMed.
- K. Terkan, F. Zurita, T. Jamal Khalaf, P. Rinklin, T. Teshima, T. Kohl and B. Wolfrum, APL Mater., 2020, 8, 101111 CrossRef CAS.
- Z. Zhou, X. Liu, W. Wu, S. Park, A. L. Miller Ii, A. Terzic and L. Lu, Biomater. Sci., 2018, 6, 2375–2385 RSC.
- Y. Liu, V. R. Feig and Z. Bao, Adv. Healthcare Mater., 2021, 10, 2001916 CrossRef CAS PubMed.
- M. Ganji, E. Kaestner, J. Hermiz, N. Rogers, A. Tanaka, D. Cleary, S. H. Lee, J. Snider, M. Halgren, G. R. Cosgrove, B. S. Carter, D. Barba, I. Uguz, G. G. Malliaras, S. S. Cash, V. Gilja, E. Halgren and S. A. Dayeh, Adv. Funct. Mater., 2017, 28, 1700232 CrossRef.
- M. Zhu, H. Wang, S. Li, X. Liang, M. Zhang, X. Dai and Y. Zhang, Adv. Healthcare Mater., 2021, 10, 2100646 CrossRef CAS PubMed.
- H. Yuk, B. Lu and X. Zhao, Chem. Soc. Rev., 2019, 48, 1642–1667 RSC.
- Q. Liang, X. Xia, X. Sun, D. Yu, X. Huang, G. Han, S. M. Mugo, W. Chen and Q. Zhang, Adv. Sci., 2022, 9, 2201059 CrossRef CAS PubMed.
- G. Liu, C. Zhou, W. L. Teo, C. Qian and Y. Zhao, Angew. Chem., Int. Ed., 2019, 58, 9366–9372 CrossRef CAS PubMed.
- M. Yang, P. Chen, X. Qu, F. Zhang, S. Ning, L. Ma, K. Yang, Y. Su, J. Zang, W. Jiang, T. Yu, X. Dong and Z. Luo, ACS Nano, 2023, 17, 885–895 CrossRef CAS PubMed.
- J. Chong, C. Sung, K. S. Nam, T. Kang, H. Kim, H. Lee, H. Park, S. Park and J. Kang, Nat. Commun., 2023, 14, 2206 CrossRef CAS PubMed.
- X. S. Zheng, C. Tan, E. Castagnola and X. T. Cui, Adv. Healthcare Mater., 2021, 10, 2100119 CrossRef CAS PubMed.
- R. Thakur, F. P. Aplin and G. Y. Fridman, Micromachines, 2021, 12, 1522 CrossRef PubMed.
- W. C. Huang, X. C. Ong, I. S. Kwon, C. Gopinath, L. E. Fisher, H. Wu, G. K. Fedder, R. A. Gaunt and C. J. Bettinger, Adv. Funct. Mater., 2018, 28, 1801059 CrossRef.
- S. Zhao, P. Tseng, J. Grasman, Y. Wang, W. Li, B. Napier, B. Yavuz, Y. Chen, L. Howell, J. Rincon, F. G. Omenetto and D. L. Kaplan, Adv. Mater., 2018, 30, 1800598 CrossRef PubMed.
- K. Yang, J. Lee, J. S. Lee, D. Kim, G.-E. Chang, J. Seo, E. Cheong, T. Lee and S.-W. Cho, ACS Appl. Mater. Interfaces, 2016, 8, 17763–17774 CrossRef CAS PubMed.
- J. Ding, Z. Chen, X. Liu, Y. Tian, J. Jiang, Z. Qiao, Y. Zhang, Z. Xiao, D. Wei, J. Sun, F. Luo, L. Zhou and H. Fan, Mater. Horiz., 2022, 9, 2215–2225 RSC.
- Z. Chen, X. Liu, J. Ding, Y. Tian, Y. Zhang, D. Wei, J. Sun, F. Luo, L. Zhou and H. Fan, Carbohydr. Polym., 2022, 296, 119923 CrossRef CAS PubMed.
- A. E. Rochford, A. Carnicer-Lombarte, M. Kawan, A. Jin, S. Hilton, V. F. Curto, A. L. Rutz, T. Moreau, M. R. N. Kotter, G. G. Malliaras and D. G. Barone, Sci. Adv., 2023, 9, 8162 CrossRef PubMed.
- Y. Jiao, Y. Zhang, H. Feng, H. Li, Z. Wang, P. Wang, Y. Wang, N. Zheng, T. Xie, Y. Ma and X. Feng, Adv. Electron. Mater., 2023, 9, 2201343 CrossRef CAS.
- H. Seo, S. I. Han, K. I. Song, D. Seong, K. Lee, S. H. Kim, T. Park, J. H. Koo, M. Shin, H. W. Baac, O. K. Park, S. J. Oh, H. S. Han, H. Jeon, Y. C. Kim, D. H. Kim, T. Hyeon and D. Son, Adv. Mater., 2021, 33, 2007346 CrossRef CAS PubMed.
- K.-I. Song, H. Seo, D. Seong, S. Kim, K. J. Yu, Y.-C. Kim, J. Kim, S. J. Kwon, H.-S. Han, I. Youn, H. Lee and D. Son, Nat. Commun., 2020, 11, 4195 CrossRef PubMed.
- G. Lee, E. Ray, H.-J. Yoon, S. Genovese, Y. S. Choi, M.-K. Lee, S. Şahin, Y. Yan, H.-Y. Ahn, A. J. Bandodkar, J. Kim, M. Park, H. Ryu, S. S. Kwak, Y. H. Jung, A. Odabas, U. Khandpur, W. Z. Ray, M. R. MacEwan and J. A. Rogers, Sci. Adv., 2022, 8, 9169 CrossRef PubMed.
- H. Guo, D. D'Andrea, J. Zhao, Y. Xu, Z. Qiao, L. E. Janes, N. K. Murthy, R. Li, Z. Xie, Z. Song, R. Meda, J. Koo, W. Bai, Y. S. Choi, S. W. Jordan, Y. Huang, C. K. Franz and J. A. Rogers, Adv. Funct. Mater., 2021, 31, 2102724 CrossRef CAS PubMed.
- Y. Liu, J. Li, S. Song, J. Kang, Y. Tsao, S. Chen, V. Mottini, K. McConnell, W. Xu, Y.-Q. Zheng, J. B. H. Tok, P. M. George and Z. Bao, Nat. Biotechnol., 2020, 38, 1031–1036 CrossRef CAS PubMed.
- Z. Liu, H. Wang, P. Huang, J. Huang, Y. Zhang, Y. Wang, M. Yu, S. Chen, D. Qi, T. Wang, Y. Jiang, G. Chen, G. Hu, W. Li, J. Yu, Y. Luo, X. J. Loh, B. Liedberg, G. Li and X. Chen, Adv. Mater., 2019, 31, 1901360 CrossRef PubMed.
- H. Steins, M. Mierzejewski, L. Brauns, A. Stumpf, A. Kohler, G. Heusel, A. Corna, T. Herrmann, P. D. Jones, G. Zeck, R. von Metzen and T. Stieglitz, Microsyst. Nanoeng., 2022, 8, 131 CrossRef CAS PubMed.
- T.-K. Nguyen, M. Barton, A. Ashok, T.-A. Truong, S. Yadav, M. Leitch, T.-V. Nguyen, N. Kashaninejad, T. Dinh, L. Hold, Y. Yamauchi, N.-T. Nguyen and H.-P. Phan, Proc. Natl. Acad. Sci. U. S. A., 2022, 119, 2203287119 CrossRef PubMed.
- D. Yan, A. A. Jiman, E. C. Bottorff, P. R. Patel, D. Meli, E. J. Welle, D. C. Ratze, L. A. Havton, C. A. Chestek, S. W. P. Kemp, T. M. Bruns, E. Yoon and J. P. Seymour, Small, 2022, 18, 2200311 CrossRef CAS PubMed.
- M. Yu, C. Wang, H. Cui, J. Huang, Q. Yu, P. Wang, C. Huang, G. Li, Y. Zhao, X. Du and Z. Liu, ACS Appl. Mater. Interfaces, 2023, 15, 7663–7672 CrossRef CAS PubMed.
- S. Lienemann, M. J. Donahue, J. Zötterman, S. Farnebo and K. Tybrandt, Adv. Mater. Technol., 2023, 8, 2201322 CrossRef CAS.
- L. Hiendlmeier, F. Zurita, J. Vogel, F. Del Duca, G. Al Boustani, H. Peng, I. Kopic, M. Nikić, T. F. Teshima and B. Wolfrum, Adv. Mater., 2023, 35, 2210206 CrossRef CAS PubMed.
- T. M. Otchy, C. Michas, B. Lee, K. Gopalan, V. Nerurkar, J. Gleick, D. Semu, L. Darkwa, B. J. Holinski, D. J. Chew, A. E. White and T. J. Gardner, Nat. Commun., 2020, 11, 4191 CrossRef PubMed.
- B. Park, J.-W. Jang and S. Kim, Micro Nano Syst. Lett., 2022, 10, 1–7 CrossRef.
- A. Veith, X. Li, H. Modi, A. Abbaspour, L. Luan, C. Xie and A. B. Baker, Biomaterials, 2021, 275, 120924 CrossRef CAS PubMed.
- Y. Zhang, N. Zheng, Y. Cao, F. Wang, P. Wang, Y. Ma, B. Lu, G. Hou, Z. Fang, Z. Liang, M. Yue, Y. Li, Y. Chen, J. Fu, J. Wu, T. Xie and X. Feng, Sci. Adv., 2019, 5, 1066 CrossRef PubMed.
- M. Zhang, R. Guo, K. Chen, Y. Wang, J. Niu, Y. Guo, Y. Zhang, Z. Yin, K. Xia, B. Zhou, H. Wang, W. He, J. Liu, M. Sitti and Y. Zhang, Proc. Natl. Acad. Sci. U. S. A., 2020, 117, 14667–14675 CrossRef PubMed.
- X. S. Zheng, A. Y. Griffith, E. Chang, M. J. Looker, L. E. Fisher, B. Clapsaddle and X. T. Cui, Acta Biomater., 2020, 103, 81–91 CrossRef CAS PubMed.
- J. K. Trevathan, I. W. Baumgart, E. N. Nicolai, B. A. Gosink, A. J. Asp, M. L. Settell, S. R. Polaconda, K. D. Malerick, S. K. Brodnick, W. Zeng, B. E. Knudsen, A. L. McConico, Z. Sanger, J. H. Lee, J. M. Aho, A. J. Suminski, E. K. Ross, J. L. Lujan, D. J. Weber, J. C. Williams, M. Franke, K. A. Ludwig and A. J. Shoffstall, Adv. Healthcare Mater., 2019, 8, 1900892 CrossRef CAS PubMed.
- J. Park, S. Lee, M. Lee, H. S. Kim and J. Y. Lee, Small, 2023, 19, 2300250 CrossRef CAS PubMed.
- M. Zhu, F. Zhang and X. Chen, Small Struct., 2020, 1, 2000045 CrossRef.
- S. Y. Yang, E. D. O'Cearbhaill, G. C. Sisk, K. M. Park, W. K. Cho, M. Villiger, B. E. Bouma, B. Pomahac and J. M. Karp, Nat. Commun., 2013, 4, 1702 CrossRef PubMed.
- D. Han, R. S. Morde, S. Mariani, A. A. La Mattina, E. Vignali, C. Yang, G. Barillaro and H. Lee, Adv. Funct. Mater., 2020, 30, 1909197 CrossRef CAS.
- C. Russell, A. D. Roche and S. Chakrabarty, Int. J. Intell. Rob. Appl., 2019, 3, 11–18 CrossRef.
- S. E. John, D. B. Grayden and T. Yanagisawa, Expert Rev. Med. Devices, 2019, 16, 841–843 CrossRef CAS PubMed.
- A. Deshmukh, L. Brown, M. F. Barbe, A. S. Braverman, E. Tiwari, L. Hobson, S. Shunmugam, O. Armitage, E. Hewage, M. R. Ruggieri and J. Morizio, J. Neurosci. Methods, 2020, 333, 108562 CrossRef PubMed.
- T. J. Oxley, N. L. Opie, S. E. John, G. S. Rind, S. M. Ronayne, T. L. Wheeler, J. W. Judy, A. J. McDonald, A. Dornom, T. J. H. Lovell, C. Steward, D. J. Garrett, B. A. Moffat, E. H. Lui, N. Yassi, B. C. V. Campbell, Y. T. Wong, K. E. Fox, E. S. Nurse, I. E. Bennett, S. H. Bauquier, K. A. Liyanage, N. R. van der Nagel, P. Perucca, A. Ahnood, K. P. Gill, B. Yan, L. Churilov, C. R. French, P. M. Desmond, M. K. Horne, L. Kiers, S. Prawer, S. M. Davis, A. N. Burkitt, P. J. Mitchell, D. B. Grayden, C. N. May and T. J. O'Brien, Nat. Biotechnol., 2016, 34, 320–327 CrossRef CAS PubMed.
- X. Zhang, Y. Jiang, L. Han and X. Lu, Biosurf. Biotribol., 2021, 7, 163–179 CrossRef.
- J. Xu, Y. Liu and S.-H. Hsu, Molecules, 2019, 24, 3005 CrossRef CAS PubMed.
- X. Yao, S. Zhang, L. Qian, N. Wei, V. Nica, S. Coseri and F. Han, Adv. Funct. Mater., 2022, 32, 2204565 CrossRef CAS.
- J. Hou, M. Liu, H. Zhang, Y. Song, X. Jiang, A. Yu, L. Jiang and B. Su, J. Mater. Chem. A, 2017, 5, 13138–13144 RSC.
- H. Fan, J. Wang and Z. Jin, Macromolecules, 2018, 51, 1696–1705 CrossRef CAS.
- C. Chen, X. Yang, S.-J. Li, C. Zhang, Y.-N. Ma, Y.-X. Ma, P. Gao, S.-Z. Gao and X.-J. Huang, Green Chem., 2021, 23, 1794–1804 RSC.
- Z. Han, S. Chen, L. Deng, Q. Liang, X. Qu, J. Li, B. Wang and H. Wang, ACS Appl. Mater. Interfaces, 2022, 14, 45954–45965 CrossRef CAS PubMed.
- A. Aguzin, G. C. Luque, L. I. Ronco, I. del Agua, G. Guzmán-González, B. Marchiori, A. Gugliotta, L. C. Tomé, L. M. Gugliotta, D. Mecerreyes and R. J. Minari, ACS Biomater. Sci. Eng., 2022, 8, 2598–2609 CrossRef CAS PubMed.
- X. Chen, H. Yuk, J. Wu, C. S. Nabzdyk and X. Zhao, Proc. Natl. Acad. Sci. U. S. A., 2020, 117, 15497–15503 CrossRef CAS PubMed.
- S. J. Wu, H. Yuk, J. Wu, C. S. Nabzdyk and X. Zhao, Adv. Mater., 2021, 33, 2007667 CrossRef CAS PubMed.
- C. Cui, T. Wu, X. Chen, Y. Liu, Y. Li, Z. Xu, C. Fan and W. Liu, Adv. Funct. Mater., 2020, 30, 2005689 CrossRef CAS.
- L. Han, M. Wang, L. O. Prieto-López, X. Deng and J. Cui, Adv. Funct. Mater., 2019, 30, 1907064 CrossRef.
- A. Xin, R. Zhang, K. Yu and Q. Wang, J. Mech. Phys. Solids, 2019, 125, 1–21 CrossRef CAS.
- C. K. Roy, H. L. Guo, T. L. Sun, A. B. Ihsan, T. Kurokawa, M. Takahata, T. Nonoyama, T. Nakajima and J. P. Gong, Adv. Mater., 2015, 27, 7344–7348 CrossRef CAS PubMed.
- A. V. Dobrynin, R. H. Colby and M. Rubinstein, J. Polym. Sci., Part B: Polym. Phys., 2004, 42, 3513–3538 CrossRef CAS.
- J. Steck, J. Kim, J. Yang, S. Hassan and Z. Suo, Extreme Mech. Lett., 2020, 39, 100803 CrossRef.
- J. Yang, R. Bai and Z. Suo, Adv. Mater., 2018, 30, 1800671 CrossRef PubMed.
- J.-N. Zhang, H. Zhu, T. Liu, Y. Chen, C. Jiao, C. He and H. Wang, Polymer, 2020, 206, 122845 CrossRef CAS.
- J. Yang, R. Bai, J. Li, C. Yang, X. Yao, Q. Liu, J. J. Vlassak, D. J. Mooney and Z. Suo, ACS Appl. Mater. Interfaces, 2019, 11, 24802–24811 CrossRef CAS PubMed.
- J. Liu, J. Li, F. Yu, Y.-X. Zhao, X.-M. Mo and J.-F. Pan, Int. J. Biol. Macromol., 2020, 147, 653–666 CrossRef CAS PubMed.
- J. Ren, H. Yang, Y. Wu, S. Liu, K. Ni, X. Ran, X. Zhou, W. Gao, G. Du and L. Yang, RSC Adv., 2022, 12, 15241–15250 RSC.
- T. Feng, W. Ji, Y. Zhang, F. Wu, Q. Tang, H. Wei, L. Mao and M. Zhang, Angew. Chem., Int. Ed., 2020, 59, 23445–23449 CrossRef CAS PubMed.
- C. Cui, C. Fan, Y. Wu, M. Xiao, T. Wu, D. Zhang, X. Chen, B. Liu, Z. Xu, B. Qu and W. Liu, Adv. Mater., 2019, 31, 1905761 CrossRef CAS PubMed.
- H. Yuk, J. Wu and X. Zhao, Nat. Rev. Mater., 2022, 7, 935–952 CrossRef CAS.
- C. Xie, X. Wang, H. He, Y. Ding and X. Lu, Adv. Funct. Mater., 2020, 30, 1909954 CrossRef CAS.
- I. Perkucin, K. S. K. Lau, C. M. Morshead and H. E. Naguib, Biomed. Mater., 2022, 18, 015020 CrossRef PubMed.
- J. Zhang, M. Hu, X. Zheng, H. Wang, X. Li, X. Zhang and H. Yang, Adv. Mater. Technol., 2023, 8, 2300243 CrossRef CAS.
- N. Li, Y. Li, Z. Cheng, Y. Liu, Y. Dai, S. Kang, S. Li, N. Shan, S. Wai, A. Ziaja, Y. Wang, J. Strzalka, W. Liu, C. Zhang, X. Gu, J. A. Hubbell, B. Tian and S. Wang, Science, 2023, 381, 686–693 CrossRef CAS PubMed.
- J. W. Seo, H. Kim, K. Kim, S. Q. Choi and H. J. Lee, Adv. Funct. Mater., 2018, 28, 1800802 CrossRef.
- Q. Zeng and Z. Huang, Adv. Funct. Mater., 2023, 33, 2301223 CrossRef CAS.
- Y. J. Lee, H. J. Kim, J. Y. Kang, S. H. Do and S. H. Lee, Adv. Healthcare Mater., 2017, 6, 1601022 CrossRef PubMed.
- N. Driscoll, A. G. Richardson, K. Maleski, B. Anasori, O. Adewole, P. Lelyukh, L. Escobedo, D. K. Cullen, T. H. Lucas, Y. Gogotsi and F. Vitale, ACS Nano, 2018, 12, 10419–10429 CrossRef CAS PubMed.
- L. Wang, S. Xie, Z. Wang, F. Liu, Y. Yang, C. Tang, X. Wu, P. Liu, Y. Li, H. Saiyin, S. Zheng, X. Sun, F. Xu, H. Yu and H. Peng, Nat. Biomed. Eng., 2019, 4, 159–171 CrossRef PubMed.
- J. Goding, C. Vallejo-Giraldo, O. Syed and R. Green, J. Mater. Chem. B, 2019, 7, 1625–1636 RSC.
- W.-C. Huang, Y.-C. Lo, C.-Y. Chu, H.-Y. Lai, Y.-Y. Chen and S.-Y. Chen, Biomaterials, 2017, 122, 141–153 CrossRef CAS PubMed.
- K. Wu, J. Tao, Q. Liao, S. Chen and W. Wan, Mater. Sci. Eng., C, 2020, 113, 110971 CrossRef CAS PubMed.
- A. Golabchi, K. M. Woeppel, X. Li, C. F. Lagenaur and X. T. Cui, Biosens. Bioelectron., 2020, 155, 112096 CrossRef CAS PubMed.
- C. M. Boutry, L. Beker, Y. Kaizawa, C. Vassos, H. Tran, A. C. Hinckley, R. Pfattner, S. Niu, J. Li, J. Claverie, Z. Wang, J. Chang, P. M. Fox and Z. Bao, Nat. Biomed. Eng., 2019, 3, 47–57 CrossRef CAS PubMed.
- C. Li, C. Guo, V. Fitzpatrick, A. Ibrahim, M. J. Zwierstra, P. Hanna, A. Lechtig, A. Nazarian, S. J. Lin and D. L. Kaplan, Nat. Rev. Mater., 2019, 5, 61–81 CrossRef.
- S. H. Kim, J.-H. Moon, J. H. Kim, S. M. Jeong and S.-H. Lee, Biomed. Eng. Lett., 2011, 1, 199–203 CrossRef.
- R. Xie, Q. Li, L. Teng, Z. Cao, F. Han, Q. Tian, J. Sun, Y. Zhao, M. Yu, D. Qi, P. Guo, G. Li, F. Huo and Z. Liu, npj Flexible Electron., 2022, 6, 75 CrossRef CAS.
- E. Song, J. Li and J. A. Rogers, APL Mater., 2019, 7, 050902 CrossRef.
- M. Sang, K. Kim, J. Shin and K. J. Yu, Adv. Sci., 2022, 9, 2202980 CrossRef CAS PubMed.
- M. Mariello, K. Kim, K. Wu, S. P. Lacour and Y. Leterrier, Adv. Mater., 2022, 34, 2201129 CrossRef CAS PubMed.
- J. Shin, Y. Yan, W. Bai, Y. Xue, P. Gamble, L. Tian, I. Kandela, C. R. Haney, W. Spees, Y. Lee, M. Choi, J. Ko, H. Ryu, J.-K. Chang, M. Pezhouh, S.-K. Kang, S. M. Won, K. J. Yu, J. Zhao, Y. K. Lee, M. R. MacEwan, S.-K. Song, Y. Huang, W. Z. Ray and J. A. Rogers, Nat. Biomed. Eng., 2018, 3, 37–46 CrossRef PubMed.
- C. Y. Kim, M. J. Ku, R. Qazi, H. J. Nam, J. W. Park, K. S. Nam, S. Oh, I. Kang, J.-H. Jang, W. Y. Kim, J.-H. Kim and J.-W. Jeong, Nat. Commun., 2021, 12, 535 CrossRef CAS PubMed.
- T. A. Pham, T. K. Nguyen, R. K. Vadivelu, T. Dinh, A. Qamar, S. Yadav, Y. Yamauchi, J. A. Rogers, N. T. Nguyen and H. P. Phan, Adv. Funct. Mater., 2020, 30, 2004655 CrossRef CAS.
- A. A. La Mattina, S. Mariani and G. Barillaro, Adv. Sci., 2020, 7, 1902872 CrossRef CAS PubMed.
- E. J. Dierings de Souza, D. H. Kringel, A. R. Guerra Dias and E. da Rosa Zavareze, Carbohydr. Polym., 2021, 265, 118068 CrossRef CAS PubMed.
- G. Lee, S. K. Kang, S. M. Won, P. Gutruf, Y. R. Jeong, J. Koo, S. S. Lee, J. A. Rogers and J. S. Ha, Adv. Energy Mater., 2017, 7, 1700157 CrossRef.
- S. K. Prajapati, A. Jain, A. Jain and S. Jain, Eur. Polym. J., 2019, 120, 109191 CrossRef CAS.
- C. Gao, Y. Liu, F. Gu, Z. Chen, Z. Su, H. Du, D. Xu, K. Liu and W. Xu, Chem. Eng. J., 2023, 460, 141769 CrossRef CAS.
- W. Babatain, U. Buttner, N. El-Atab and M. M. Hussain, ACS Nano, 2022, 16, 20305–20317 CrossRef CAS PubMed.
- H. U. Chung, B. H. Kim, J. Y. Lee, J. Lee, Z. Xie, E. M. Ibler, K. Lee, A. Banks, J. Y. Jeong, J. Kim, C. Ogle, D. Grande, Y. Yu, H. Jang, P. Assem, D. Ryu, J. W. Kwak, M. Namkoong, J. B. Park, Y. Lee, D. H. Kim, A. Ryu, J. Jeong, K. You, B. Ji, Z. Liu, Q. Huo, X. Feng, Y. Deng, Y. Xu, K.-I. Jang, J. Kim, Y. Zhang, R. Ghaffari, C. M. Rand, M. Schau, A. Hamvas, D. E. Weese-Mayer, Y. Huang, S. M. Lee, C. H. Lee, N. R. Shanbhag, A. S. Paller, S. Xu and J. A. Rogers, Science, 2019, 363, 947 CrossRef PubMed.
- W. Gao, H. Ota, D. Kiriya, K. Takei and A. Javey, Acc. Chem. Res., 2019, 52, 523–533 CrossRef CAS PubMed.
- R. Ghaffari, J. Choi, M. S. Raj, S. Chen, S. P. Lee, J. T. Reeder, A. J. Aranyosi, A. Leech, W. Li, S. Schon, J. B. Model and J. A. Rogers, Adv. Funct. Mater., 2019, 30, 1907269 CrossRef.
- S. A. Hashemi, S. Ramakrishna and A. G. Aberle, Energy Environ. Sci., 2020, 13, 685–743 RSC.
- D. Jiang, B. Shi, H. Ouyang, Y. Fan, Z. L. Wang and Z. Li, ACS Nano, 2020, 14, 6436–6448 CrossRef CAS PubMed.
- Y. Liu, L. Wang, L. Zhao, K. Yao, Z. Xie, Y. Zi and X. Yu, Adv. Electron. Mater., 2019, 6, 1901174 CrossRef.
- O. Macherey and R. P. Carlyon, Curr. Biol., 2014, 24, R878–R884 CrossRef CAS PubMed.
- Y. Yang and W. Gao, Chem. Soc. Rev., 2019, 48, 1465–1491 RSC.
- Y. Liu, L. Wang, L. Zhao, X. Yu and Y. Zi, InfoMat, 2020, 2, 318–340 CrossRef CAS.
- W. J. Song, S. Yoo, G. Song, S. Lee, M. Kong, J. Rim, U. Jeong and S. Park, Batteries Supercaps, 2019, 2, 181–199 CrossRef.
- N. Xu, J. A. Wilson, Y.-D. Wang, T. Su, Y. Wei, J. Qiao, X.-D. Zhou, Y. Zhang and S. Sun, Appl. Catal., B, 2020, 272, 118953 CrossRef CAS.
- J. Yan, Z. Tang, B. Li, D. Bi, Q. Lai and Y. Liang, ACS Sustainable Chem. Eng., 2019, 7, 17817–17824 CrossRef CAS.
- Y. Zhang, Y.-P. Deng, J. Wang, Y. Jiang, G. Cui, L. Shui, A. Yu, X. Wang and Z. Chen, Energy Storage Mater., 2021, 35, 538–549 CrossRef.
- D. Zhou, L. Xue, L. Wang, N. Wang, W.-M. Lau and X. Cao, Nano Energy, 2019, 61, 435–441 CrossRef CAS.
- Y. H. Zhu, X. Y. Yang, T. Liu and X. B. Zhang, Adv. Mater., 2019, 32, 1901961 CrossRef PubMed.
- C. Chen, S. Xu, Y. Kuang, W. Gan, J. Song, G. Chen, G. Pastel, B. Liu, Y. Li, H. Huang and L. Hu, Adv. Energy Mater., 2019, 9, 1802964 CrossRef.
- H.-J. Peng, J.-Q. Huang and Q. Zhang, Chem. Soc. Rev., 2017, 46, 5237–5288 RSC.
- D.-Y. Wang, C.-Y. Wei, M.-C. Lin, C.-J. Pan, H.-L. Chou, H.-A. Chen, M. Gong, Y. Wu, C. Yuan, M. Angell, Y.-J. Hsieh, Y.-H. Chen, C.-Y. Wen, C.-W. Chen, B.-J. Hwang, C.-C. Chen and H. Dai, Nat. Commun., 2017, 8, 14283 CrossRef CAS PubMed.
- W. P. Wang, J. Zhang, Y. X. Yin, H. Duan, J. Chou, S. Y. Li, M. Yan, S. Xin and Y. G. Guo, Adv. Mater., 2020, 32, 2000302 CrossRef CAS PubMed.
- Y. Wang, C. Chen, H. Xie, T. Gao, Y. Yao, G. Pastel, X. Han, Y. Li, J. Zhao, K. Fu and L. Hu, Adv. Funct. Mater., 2017, 27, 1703140 CrossRef.
- Z. Yu, H. Wang, X. Kong, W. Huang, Y. Tsao, D. G. Mackanic, K. Wang, X. Wang, W. Huang, S. Choudhury, Y. Zheng, C. V. Amanchukwu, S. T. Hung, Y. Ma, E. G. Lomeli, J. Qin, Y. Cui and Z. Bao, Nat. Energy, 2020, 5, 526–533 CrossRef CAS.
- G. Zhou, D.-W. Wang, F. Li, P.-X. Hou, L. Yin, C. Liu, G. Q. Lu, I. R. Gentle and H.-M. Cheng, Energy Environ. Sci., 2012, 5, 8901 RSC.
- G. Zhou, Q. Wang, S. Wang, S. Ling, J. Zheng, X. Yu and H. Li, J. Power Sources, 2018, 384, 172–177 CrossRef CAS.
- E. Song, Z. Xie, W. Bai, H. Luan, B. Ji, X. Ning, Y. Xia, J. M. Baek, Y. Lee, R. Avila, H.-Y. Chen, J.-H. Kim, S. Madhvapathy, K. Yao, D. Li, J. Zhou, M. Han, S. M. Won, X. Zhang, D. J. Myers, Y. Mei, X. Guo, S. Xu, J.-K. Chang, X. Yu, Y. Huang and J. A. Rogers, Nat. Biomed. Eng., 2021, 5, 759–771 CrossRef CAS PubMed.
- J. Zhang, J. Liu, H. Su, F. Sun, Z. Lu and A. Su, Sens. Actuators, B, 2021, 341, 130046 CrossRef CAS.
- Y. Liu, X. Huang, J. Zhou, C. K. Yiu, Z. Song, W. Huang, S. K. Nejad, H. Li, T. H. Wong, K. Yao, L. Zhao, W. Yoo, W. Park, J. Li, Y. Huang, H. R. Lam, E. Song, X. Guo, Y. Wang, Z. Dai, L. Chang, W. J. Li, Z. Xie and X. Yu, Adv. Sci., 2022, 9, 2104635 CrossRef CAS PubMed.
- X. Huang, L. Zhang, Z. Zhang, S. Guo, H. Shang, Y. Li and J. Liu, Biosens. Bioelectron., 2019, 124–125, 40–52 CrossRef CAS PubMed.
- J. Zhang, X. Huang, L. Zhang, Y. Si, S. Guo, H. Su and J. Liu, Sustainable Energy Fuels, 2020, 4, 68–79 RSC.
- F. Xu, S. Dong, G. Liu, C. Pan, Z. H. Guo, W. Guo, L. Li, Y. Liu, C. Zhang, X. Pu and Z. L. Wang, Nano Energy, 2021, 88, 106247 CrossRef CAS.
- Z. Li, G. Zhu, R. Yang, A. C. Wang and Z. L. Wang, Adv. Mater., 2010, 22, 2534–2537 CrossRef CAS PubMed.
- T. Mudgal, M. Tiwari and D. Bharti, ACS Appl. Energy Mater., 2022, 5, 15881–15889 CrossRef CAS.
- M. Wu, K. Yao, D. Li, X. Huang, Y. Liu, L. Wang, E. Song, J. Yu and X. Yu, Mater. Today Energy, 2021, 21, 100786 CrossRef.
- X. Wu, W. Ren, Y. Wen, S. Ono, J. Zhu, J. W. Evans and A. C. Arias, ACS Sens., 2023, 8, 2740–2749 CrossRef CAS PubMed.
- B. Shi, Z. Liu, Q. Zheng, J. Meng, H. Ouyang, Y. Zou, D. Jiang, X. Qu, M. Yu, L. Zhao, Y. Fan, Z. L. Wang and Z. Li, ACS Nano, 2019, 13, 6017–6024 CrossRef CAS PubMed.
- A. J. Bandodkar, S. P. Lee, I. Huang, W. Li, S. Wang, C. J. Su, W. J. Jeang, T. Hang, S. Mehta, N. Nyberg, P. Gutruf, J. Choi, J. Koo, J. T. Reeder, R. Tseng, R. Ghaffari and J. A. Rogers, Nat. Electron., 2020, 3, 554–562 CrossRef CAS.
- Q. Zheng, Y. Zou, Y. Zhang, Z. Liu, B. Shi, X. Wang, Y. Jin, H. Ouyang, Z. Li and Z. L. Wang, Sci. Adv., 2016, 2, 1501478 CrossRef PubMed.
- P. Gutruf, R. T. Yin, K. B. Lee, J. Ausra, J. A. Brennan, Y. Qiao, Z. Xie, R. Peralta, O. Talarico, A. Murillo, S. W. Chen, J. P. Leshock, C. R. Haney, E. A. Waters, C. Zhang, H. Luan, Y. Huang, G. Trachiotis, I. R. Efimov and J. A. Rogers, Nat. Commun., 2019, 10, 5742 CrossRef CAS PubMed.
- Y. Yang, H. Hu, Z. Chen, Z. Wang, L. Jiang, G. Lu, X. Li, R. Chen, J. Jin, H. Kang, H. Chen, S. Lin, S. Xiao, H. Zhao, R. Xiong, J. Shi, Q. Zhou, S. Xu and Y. Chen, Nano Lett., 2020, 20, 4445–4453 CrossRef CAS PubMed.
- L. Ortega, A. Llorella, J. P. Esquivel and N. Sabaté, Microsyst. Nanoeng., 2019, 5, 3 CrossRef PubMed.
- J. Shi and Y. Fang, Adv. Mater., 2018, 31, 1804895 CrossRef PubMed.
- C. Wang, K. Xia, H. Wang, X. Liang, Z. Yin and Y. Zhang, Adv. Mater., 2018, 31, 1801072 CrossRef PubMed.
- L. Xiang, X. Zeng, F. Xia, W. Jin, Y. Liu and Y. Hu, ACS Nano, 2020, 14, 6449–6469 CrossRef CAS PubMed.
- R. Yin, D. Wang, S. Zhao, Z. Lou and G. Shen, Adv. Funct. Mater., 2020, 31, 2008936 CrossRef.
- X. Yu, Z. Xie, Y. Yu, J. Lee, A. Vazquez-Guardado, H. Luan, J. Ruban, X. Ning, A. Akhtar, D. Li, B. Ji, Y. Liu, R. Sun, J. Cao, Q. Huo, Y. Zhong, C. Lee, S. Kim, P. Gutruf, C. Zhang, Y. Xue, Q. Guo, A. Chempakasseril, P. Tian, W. Lu, J. Jeong, Y. Yu, J. Cornman, C. Tan, B. Kim, K. Lee, X. Feng, Y. Huang and J. A. Rogers, Nature, 2019, 575, 473–479 CrossRef CAS PubMed.
- K. Wang, C. L. Frewin, D. Esrafilzadeh, C. Yu, C. Wang, J. J. Pancrazio, M. Romero-Ortega, R. Jalili and G. Wallace, Adv. Mater., 2019, 31, 1805867 CrossRef PubMed.
- S. Lee, S. Sheshadri, Z. Xiang, I. Delgado-Martinez, N. Xue, T. Sun, N. V. Thakor, S.-C. Yen and C. Lee, Sens. Actuators, B, 2017, 242, 1165–1170 CrossRef CAS.
- S. Elyahoodayan, C. Larson, A. M. Cobo, E. Meng and D. Song, J. Neurosci. Methods, 2020, 336, 108634 CrossRef CAS PubMed.
- A. E. Rochford, A. Carnicer-Lombarte, M. Kawan, A. Jin, S. Hilton, V. F. Curto, A. L. Rutz, T. Moreau, M. R. N. Kotter, G. G. Malliaras and D. G. Barone, Sci. Adv., 2023, 9, 8162 CrossRef PubMed.
- M. Cai, W. Hong, S. Nie, C. Wang and J. Song, Flexible Printed Electron., 2022, 7, 035002 CrossRef.
- K. Tybrandt, D. Khodagholy, B. Dielacher, F. Stauffer, A. F. Renz, G. Buzsáki and J. Vörös, Adv. Mater., 2018, 30, 1706520 CrossRef PubMed.
-
X. Zhao, Z. Wang, R. Xu and D. Ming, presented in part at the 2021 IEEE International Conference on Robotics and Biomimetics (ROBIO), 2021, 785–789.
- P. R. Patel, H. Zhang, M. T. Robbins, J. B. Nofar, S. P. Marshall, M. J. Kobylarek, T. D. Y. Kozai, N. A. Kotov and C. A. Chestek, J. Neural Eng., 2016, 13, 066002 CrossRef PubMed.
- J.-F. Wang, H.-H. Tian and Y. Fang, Chin. J. Anal. Chem., 2019, 47, 1549–1558 CAS.
- Y. Song, J. Min and W. Gao, ACS Nano, 2019, 13, 12280–12286 CrossRef CAS PubMed.
- M. Eickenscheidt, T. Herrmann, M. Weisshap, A. Mittnacht, L. Rudmann, G. Zeck and T. Stieglitz, Biosens. Bioelectron., 2022, 205, 114090 CrossRef CAS PubMed.
- S. Lee, H. Wang, W. Y. Xian Peh, T. He, S.-C. Yen, N. V. Thakor and C. Lee, Nano Energy, 2019, 60, 449–456 CrossRef CAS.
- E. A. Cuttaz, C. A. R. Chapman, O. Syed, J. A. Goding and R. A. Green, Adv. Sci., 2021, 8, 2004033 CrossRef CAS PubMed.
- Z. Zhao, X. Li, F. He, X. Wei, S. Lin and C. Xie, J. Neural Eng., 2019, 16, 035001 CrossRef PubMed.
- J. Wang, H. Wang, N. V. Thakor and C. Lee, ACS Nano, 2019, 13, 3589–3599 CrossRef CAS PubMed.
- R. Guo and J. Liu, J. Micromech. Microeng., 2017, 27, 104002 CrossRef.
- A.-P. Yu, Y.-J. Shen, Y.-Q. Qiu, J. Li, Y.-D. Shen, X.-M. Wang, M. Cong, Q.-R. He, Q.-Z. Chen and S. Jiang, Neurosci. Res., 2019, 145, 22–29 CrossRef PubMed.
|
This journal is © The Royal Society of Chemistry 2024 |
Click here to see how this site uses Cookies. View our privacy policy here.