DOI:
10.1039/D3QI00596H
(Research Article)
Inorg. Chem. Front., 2023,
10, 5098-5110
Oxygen vacancies confined in hierarchically porous CsPbBr3@Pb-MOF through in situ structural transformation for promoting photocatalytic CO2 reduction†
Received
30th March 2023
, Accepted 11th July 2023
First published on 25th July 2023
Abstract
All-inorganic perovskite (CsPbBr3) nanocrystals (NCs) are exceptional candidates for photocatalysis due to their optimal band structure, high molar extinction coefficient, and long charge-carrier diffusion lengths. However, their inevitable instability and low charge transfer efficiency have prevented their widespread applications. A strategy that can improve both stability and charge separation efficiency is desperately required. Herein, by introducing an in situ structural transformation strategy, hydrophobic hierarchically porous CsPbBr3@Pb-MOF is obtained which exhibits high durability in water for more than 7 weeks and appealing thermal stability and resistance to anion intrusion. Meanwhile, abundant oxygen vacancies are detected which efficiently suppress the photogenerated charge recombination. As a result, impressive CO2 photoreduction activity is achieved with CsPbBr3@Pb-MOF as a catalyst. Through a gas–solid reaction (without sacrificial agents and photosensitizers), this CsPbBr3@Pb-MOF-2 composite exhibits a CO yield of 107 μmol g−1 h−1 with 99.2% selectivity under visible-light (λ > 420 nm) irradiation, surpassing most reported CsPbX3-based photocatalysts under similar conditions.
1. Introduction
Excessive carbon dioxide (CO2) emissions have greatly affected the balance of the natural carbon cycle, causing a greenhouse effect.1 The direct conversion of CO2 has been considered as a potential strategy for alleviating both global warming and energy shortages.2 Lead halide perovskite (CsPbBr3) nanocrystals (NCs) have emerged as an encouraging photocatalyst for various reactions including CO2 reduction,3–5 pollutant degradation,6–8 hydrogen production,9 and organic synthesis10,11 due to their optimal band structure, high molar extinction coefficient, and long charge-carrier diffusion lengths.12–14 However, pure CsPbBr3 still faces two major obstacles including the inherent low stability and serious photogenerated electron–hole recombination, which limit its applications in photocatalysis.15–17 To overcome instability problems, encapsulating pure CsPbBr3 into a protective matrix is considered to be a promising strategy. For example, SiO2,18,19 zeolites,20 glass,21–23 and polymers24,25 have been used as matrixes for the protection of all-inorganic perovskites. Concerning photogenerated charge recombination, the construction of CsPbBr3-based heterojunctions has been proven to be an efficacious route. Metal oxides,26–28 g-C3N4,29 and graphene30 have been coupled with CsPbBr3 to reduce charge recombination. However, a strategy that simultaneously improves the stability and charge separation of pure CsPbBr3 is still underexplored.
Recently, metal–organic frameworks (MOFs) have emerged as photocatalysts, photocatalytic hosts, and cocatalysts for CO2 photoreduction, garnering significant attention. This interest stems from their remarkable attributes such as distinctive electronic band structures, exceptional CO2 adsorption capacities, and customizable light-absorption capabilities.31–33 MOFs have recently been established as promising host matrixes to protect and enhance the physicochemical properties of pure CsPbBr3 NCs because of their tunable porous structure and chemical functionality.34–36 As we know, the preparation technique largely determines the structures and performance of perovskite@MOF composites. Normally, the preparation methods of perovskite@MOFs can be broadly classified into four categories: (1) “ship-in-bottle” (ship refers to perovskites, bottle refers to MOFs), (2) “bottle-around-ship”, (3) Pb-MOF conversion, and (4) one-pot strategy.35 The most prevalent “ship-in-bottle” strategy involves introducing precursors of a perovskite (e.g., Pb, Cs, Br, ions) into a prepared beforehand MOF inside which the perovskite can assemble subsequently.37 To implement this methodology, Lu et al.38 employed pre-synthesized Fe-doped PCN-221 and subjected it to treatment involving a PbI2 solution, resulting in the formation of PbI2@PCN-221(Fex). In the subsequent step, the PbI2@PCN-221(Fex) composite underwent further treatment, this time utilizing an ethanolic solution of CH3NH3I, leading to the creation of the exquisite hybrid MAPbI3@PCN-221(Fex). However, the diffusion of perovskite precursors into MOFs is largely restricted by the microporous structure of MOFs, which often results in the generation of substantial quantities of perovskites on the surface of MOFs rather than within their pores. Concerning the “bottle-around-ship” strategy, the pre-prepared perovskite is distributed in the precursor solution of the MOF. Subsequently, the assembly of the MOF is initiated to embed the perovskite. For example, Su et al.39 achieved the growth of a zinc/cobalt zeolite imidazole coating on the surface of CsPbBr3 quantum dots (QDs). High temperatures and polar solvents are precisely indispensable in the nucleation and building of MOFs but are harmful to the perovskite structure. In contrast, the “one-pot strategy” of premixing both MOFs and perovskite precursors to produce MOFs and perovskites simultaneously or sequentially is a highly desirable strategy due to the time, energy, and cost savings. To prepare MAPbBr3@ZIF-8, all components required for the synthesis of ZIF-8 (Zn(NO3)2 and 2-methylimidazole) and MAPbBr3 (PbBr2 and MABr) were mixed and stirred in methanol/DMF solvent at room temperature by Mollick et al.40 However, with the “one-pot strategy”, the location and size of the perovskite generated in the MOF cannot be easily manipulated. Recently, through “Pb-MOF conversion”, the lead metal nodes of Pb-MOF are picked as the source of Pb for perovskite construction, which facilitates the preparation of composites, minimizes perovskite aggregation, and shortens the electron transportation path.41–43 Using this approach, Li et al.41 reported the growth of perovskite nanocrystals (NCs) within the confines of the Pb-MOF structure through a direct conversion reaction between halide salt and the Pb-MOF. Nevertheless, the in situ growth of a perovskite in Pb-MOF may occupy and destroy the porous structure and affect the applications requiring porosity. Therefore, the development of a strategy that facilitates the introduction of perovskites into MOFs while preserving the porous structure and rendering the composite functionalities remains the central task.
Herein, via an in situ structural transformation strategy, hydrophobic hierarchically porous CsPbBr3@Pb-MOF with oxygen vacancies is successfully prepared based on a lead bromide-based MOF (PbBr-MOF) and the corresponding cesium bromide salt (CsBr). As expected, the hydrophobic Pb-MOF host significantly increased the stability of pristine CsPbBr3 NCs. More importantly, the oxygen vacancies confined in CsPbBr3@Pb-MOF effectively inhibit the photogenerated electron–hole recombination of CsPbBr3. The produced CsPbBr3@Pb-MOF is used for CO2 photocatalytic reduction through the gas–solid mode without sacrificial agents and photosensitizers. This composite exhibits an exceptional CO yield of 107 μmol g−1 h−1 with 99.2% selectivity under visible light irradiation (λ > 420 nm), representing an improvement of 8.9 times over the yield observed with pure CsPbBr3 (12 μmol g−1 h−1, 88% selectivity). The possible mechanisms responsible for the photoreduction of CO2 are well studied.
2. Experimental
2.1 Materials
Lead bromide (PbBr2, 99.9%), lead nitrate (Pb(NO)3, 99%), cesium bromide (CsBr, 99%), terephthalic acid (TPA, >99%), trimesic acid (BTC, C6H3(CO2H)3, 98%), oleic acid (OA, 80%–90%), oleylamine (OM, 90%), triethylamine (C6H15N, 99.0%), toluene (C7H8, 99%), N,N-dimethylformamide (DMF, 99.5%), methanol (CH3OH, 99.5%), fuming nitric acid (HNO3, ≥95%), ethanol (CH3CH2OH, 99.5%), and n-hexane (C6H14, >99%) were purchased from Aladdin Co. Ltd.
2.2 Synthesis
2.2.1 Preparation of PbBr-MOF.
The solvothermal approach was used to create PbBr-MOF with a little change to the prior procedure.44 For the typical synthesis of PbBr-MOF 0.1835 g PbBr2 (0.5 mmol), 0.2640 g terephthalic acid (1.60 mmol), 120 μL nitric acid, ethanol (1.5 mL), and DMF (2.5 mL) were mixed introduced into a 20 mL Teflon reactor, kept at 100 °C for 72 h, and then cool down naturally. Finally, the obtained rod-like crystals were rinsed with DMF and ethanol and dried in a natural environment.
2.2.2 Solvothermal preparation of CsPbBr3@Pb-MOF.
To prepare CsPbBr3@Pb-MOF, PbBr-MOF (20 mg), CsBr (15, 30, 45 mg), methyl alcohol (4 mL), and deionized water (0.1 mL) were loaded into a 25 mL Teflon reactor, reacted at 190 °C for 2 h, then cooled. The precipitates generated were repeatedly rinsed with H2O. We have labeled the samples with different concentrations of CsPbBr3 as CsPbBr3@Pb-MOF-1, CsPbBr3@Pb-MOF-2, and CsPbBr3@Pb-MOF-3, respectively.
2.2.3 Preparation of CsPbBr3@Pb-MOF without oxygen vacancy.
CsPbBr3@Pb-MOF without oxygen vacancies was synthesized using a one-pot ultrasonic method. In detail, Pb(NO)3 (0.1 mmol), BTC (0.1 mmol), CsBr (0.1 mmol), and OM (4 mL) were sequentially added to deionized water (8 mL) and subjected to 30 minutes of sonication. The resulting oil phase solution was separated and washed multiple times with n-hexane. Afterward, the obtained precipitate was dried at 80 °C for 4 hours. The resulting sample was named CsPbBr3@Pb-MOF-WOV.
2.2.4 Preparation of Pb-MOF.
To prepare Pb-MOF, PbBr-MOF (20 mg), methyl alcohol (4 mL), and deionized water (0.1 mL) were loaded into a 25 mL Teflon reactor, reacted at 190 °C for 2 h, and then cooled. The precipitates generated were repeatedly rinsed with H2O.
2.2.5 Preparation of pure CsPbBr3.
CsPbBr3 NCs were synthesized using a process modified from a reported work.45 At room temperature, PbBr2 (0.2 mmol) and CsBr (0.2 mmol) were dispersed in DMF (8 mL). After complete dissolution, OM (2 mL) and OA (2 mL) were added to the aforementioned solution. To synthesize CsPbBr3 NCs, the precursor was promptly added to toluene (5 mL). The synthesis of CsPb(BrCl)3 and CsPb(BrI)3 was comparable to that of CsPbBr3. The difference in the preparation was that CsBr2 was replaced by CsCl2 or CsI2.
2.2.6 Synthesis of general Pb-MOF.
General Pb-MOF was synthesized using the procedure of a reported work.46 The mixture of Pb(NO)3 (0.331 g, 1.0 mmol), terephthalic acid (0.166 g, 1.0 mmol), H2O (10 mL), and ethanol (2 mL) was placed in a 20 mL Teflon reactor. Then, to achieve a pH of about 4.0 in the solution, triethylamine (0.4 mL) was added. The Teflon reactor was placed in an oven at 160 °C for 4 days and then cooled down to the surrounding ambient temperature. We could obtain the pale-yellow crystals after washing them with H2O several times.
2.3 Photocatalytic CO2 reduction experiments
A solid–gas mode was used to test the CO2 photoreduction performances of the as-prepared samples. Firstly, 10 mg catalysts were dispersed in 1 mL hexane and dropped on a 1 cm × 2 cm glass substrate. Next, to avoid interference with the experimental results, excess organic solvents were removed by vacuum drying. The prepared films and 100 μL water as a reducing agent were put in a 50 mL sealed Pyrex bottle. Before illumination, the reactor atmosphere was replaced with CO2 (99.99%) for 30 min to remove other gases. As the light source, a 300 W Xe lamp (cut-off 420 nm) was employed and the light intensity was maintained at 120 mW cm−2. In order to detect CO during the reaction, 0.5 mL of the photocatalyzed mixed gas was fed into a flame ionization detector (FID) for gas chromatography. 1 mL of the gas was injected into a gas chromatography-thermal conductivity detector (GC-TCD) to detect H2. The isotope examination was performed under identical photocatalytic reaction conditions and 13CO was determined by GC-MS. Cycling experiments were performed under the same conditions and the samples were directly transferred and reused in the next cycle.
2.4 Electrochemical measurements
A CHI760E electrochemical analyzer with a three-electrode setup was used to conduct each photoelectrochemical measurement. As the working electrode, fluorine-doped tin oxide (FTO) glass (1 × 1 cm2) was covered with a photocatalyst. Ag/AgCl (in saturated KCl) and a Pt slice (1 × 1 cm2) were adopted as reference electrodes and counter electrodes, respectively. The 1-butyl-3-methylimidazolium hexafluorophosphate (TBAPF6) CH2Cl2 solution (0.2 mol) worked as the electrolyte.
2.5 Material characterization
Powder X-ray diffraction (PXRD) patterns were performed on a Rigaku Ultima IV using Cu Kα radiation (λ = 1.54056 Å). A JEM-2010 was used to capture transmission electron microscopy (TEM) and high-resolution transmission electron microscopy (HRTEM) images. Scanning electron microscopy (SEM) and energy-dispersive X-ray spectroscopy (EDS) were conducted using SU8010. N2 adsorption–desorption isotherms were collected using a MIC-ASAP 2460 instrument at 77 K. Surface wettability was measured using a Phoenix 300. CO2 adsorption isotherms were collected using a MIC-ASAP 2460 instrument at 298 K. X-ray photoelectron spectroscopy (XPS) was conducted on an Escalab 250 instrument. Using a TU-1900 UV-Vis spectrophotometer, UV-Vis diffuse reflectance spectra (UV-vis DRS) were recorded. The emission lifetime was analyzed using a FLS1000 fluorescence spectrometer. The gas product was collected using a GC-9800 gas chromatograph. 13CO2 and H218O isotope experiments were conducted using an HP 6890GC-5973MSD gas chromatography-mass spectrometer. Using a Bruker EMXplus instrument, electron paramagnetic resonance (EPR) signals were acquired. Inductively coupled plasma mass spectrometry (ICP-MS) was tested using an Agilent 7900 instrument.
3. Results and discussion
3.1 Design and fabrication of CsPbBr3@Pb-MOF
A lead bromide-based MOF (PbBr-MOF) was deliberately selected as the matrix MOF and prepared according to a reported method with adjustments (Fig. S1†).44 In the structures, zigzag [Pb2Br3]+ chains as the secondary building units (SBUs) are coordinated by terephthalic acid (Fig. S2†). CsPbBr3 encapsulated composites were synthesized using PbBr-MOF and CsBr as raw materials under solvothermal conditions (Fig. 1). In comparison with the method used by Lu et al.38 for preparing the composites using the “ship-in-bottle” approach, our method offers a simpler preparation process, while the “ship-in-bottle” approach has advantages in controlling the proportions of each component. Strong emissions were observed under a UV lamp (365 nm) excitation, implying that CsPbBr3 may be formed (Fig. S3†). While keeping the amount of PbBr-MOF constant, we varied the dosage of CsBr (15, 30, and 45 mg) to obtain samples with different concentrations of CsPbBr3 within Pb-MOF, that were respectively denoted as CsPbBr3@Pb-MOF-1, CsPbBr3@Pb-MOF-2, and CsPbBr3@Pb-MOF-3. Furthermore, the content of Cs in the CsPbBr3@Pb-MOF composite materials was determined using inductively coupled plasma mass spectrometry (ICP-MS), yielding values of 0.8% (CsPbBr3@Pb-MOF-1), 2.7% (CsPbBr3@Pb-MOF-2), and 3.3% (CsPbBr3@Pb-MOF-3), respectively.
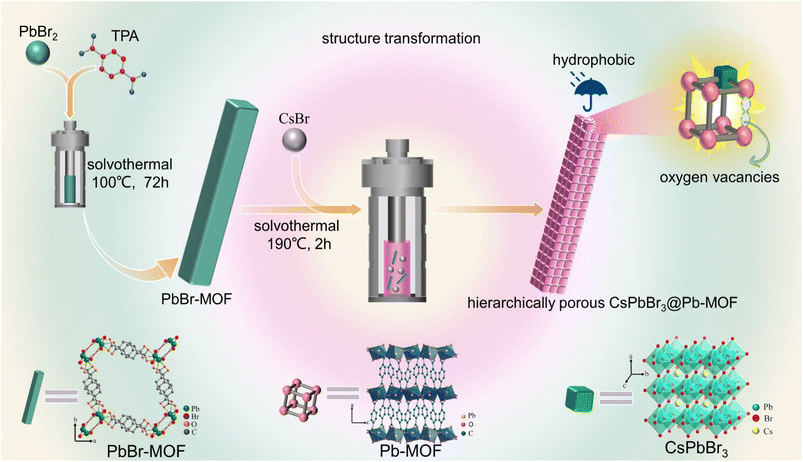 |
| Fig. 1 A schematic diagram for the synthesis of CsPbBr3@Pb-MOF. | |
3.2 Characterization of CsPbBr3@Pb-MOF
Initially, the crystal structures of CsPbBr3, Pb-MOF, and CsPbBr3@Pb-MOF composites were investigated using powder X-ray diffraction (PXRD) analysis (Fig. 2a and Fig. S4†). For sample CsPbBr3@Pb-MOF-1, no characteristic peaks corresponding to CsPbBr3 were observed, due to its low concentration. For both CsPbBr3@Pb-MOF-2 and CsPbBr3@Pb-MOF-3, the successful combination of CsPbBr3 NCs was indicated by powder X-ray diffraction (PXRD), in which three distinctive peaks at 21.4, 26.3, and 43.5° corresponded to the (110), (111), and (220) planes of cubic CsPbBr3 (PDF#75-0412), respectively.47 A notable observation was the significantly stronger intensity of the characteristic peaks of CsPbBr3 in CsPbBr3@Pb-MOF-3 compared to CsPbBr3@Pb-MOF-2, attributed to the higher content of CsPbBr3 in CsPbBr3@Pb-MOF-3 compared to CsPbBr3@Pb-MOF-2. However, to our surprise, the characteristic diffraction peaks belonging to the PbBr-MOF matrix almost disappeared and are replaced by a new set of diffraction peaks. In order to clarify this issue, the parent MOF was subjected to solvothermal conditions. The obtained powder samples were tested by PXRD and it was found that their diffraction peaks basically corresponded to the diffraction peaks in the perovskite composites, suggesting that the structure of PbBr-MOF was transformed under solvothermal synthesis (Fig. S5 and S6†).44,46 The elements and their contents in the transformed materials were examined by energy-dispersive X-ray (EDX). In EDX, Pb, C, and O were detected in equal distribution, suggesting that Br ions of PbBr-MOF are shed during the structural transformation (Fig. S7†). Combined with the PXRD results, we boldly speculate that PbBr-MOF is likely to be transformed into Pb-MOF. To confirm the structural stability, we study the PXRD pattern of PbBr-MOF and Pb-MOF after water immersion (Fig. S8†). The major peaks of PbBr-MOF disappeared after being immersed for 10 min in water, while the peaks of Pb-MOF remained intact, suggesting that Pb-MOF is extremely stable in water. During the test, we found that powdery Pb-MOF can float on the surface of the water. We speculate that the excellent water stability may result from its hydrophobicity. The water contact angle of 140° verifies this prediction (Fig. S9†).48 Pb-MOF with a hydrophobic interface makes it a promising candidate for the protection of CsPbBr3. For comparison, we tried to synthesize this MOF using Pb(NO)3 and terephthalic acid as the precursor. Fortunately, crystals of this MOF (denoted as general Pb-MOF, see more details in Experimental sections) were obtained and its structure was resolved in which Pb ions are octahedrally coordinated by terephthalic acid. By comparing its PXRD patterns, we could find that they matched each other very well, indicating that the transformed structure is Pb-MOF (Fig. S10†). The generated composite was therefore named CsPbBr3@Pb-MOF. We speculate that the solvothermally induced in situ structural transformation provides a prerequisite for the growth of the perovskite in CsPbBr3@Pb-MOF. The broken Pb–Br bond releases occupied Pb sites, which are crucial for the formation of the CsPbBr3 perovskite, meanwhile, the detached halogen is also considered an important component of the perovskite.
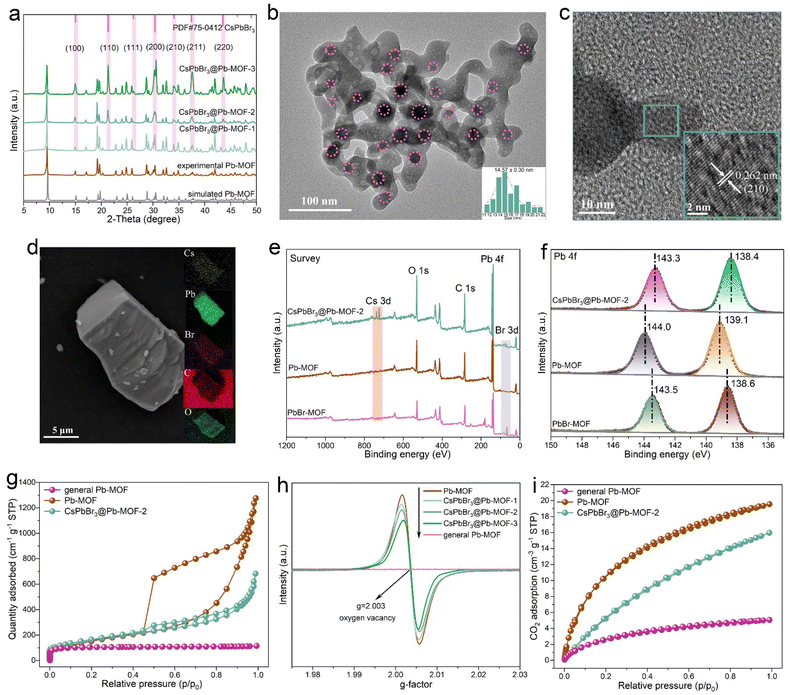 |
| Fig. 2 (a) PXRD patterns of CsPbBr3@Pb-MOF. (b) TEM image of CsPbBr3@Pb-MOF-2, dark dots in the figure are CsPbBr3 NCs. Inset of (b) shows particle size distributions of CsPbBr3 NCs. (c) The HRTEM image of CsPbBr3@Pb-MOF. Inset of (c) shows the lattice fringe of CsPbBr3 NCs. (d) SEM image and EDX mapping of CsPbBr3@Pb-MOF-2. XPS analysis of the samples: (e) survey spectra and (f) Pb 4f spectra. (g) N2 adsorption–desorption isotherms of general Pb-MOF, Pb-MOF, and CsPbBr3@Pb-MOF-2 at 77 K. (h) Electron paramagnetic resonance spectra of general Pb-MOF, Pb-MOF, and CsPbBr3@Pb-MOF. (i) CO2 adsorption–desorption isotherms of general Pb-MOF, Pb-MOF, and CsPbBr3@Pb-MOF-2 at 298 K. | |
The structure of the composite was further understood by transmission electron microscopy (TEM), high-resolution transmission electron microscopy (HRTEM), energy dispersive X-ray (EDX) spectroscopy, X-ray photoelectron spectroscopy (XPS), electron paramagnetic resonance (EPR) and gas adsorption. TEM and HRTEM measurements were performed and depicted in Fig. 2b and c. In Fig. 2b, we can see that the CsPbBr3 NCs marked with circles, indicating a size of 14.75 ± 0.30 nm, are uniformly embedded in the Pb-MOF host without significant aggregations. As shown in Fig. S11,† pure CsPbBr3 NCs exhibit uniform cubic shapes with an average particle size of 10.24 ± 0.07 nm. As illustrated in Fig. 2c, HRTEM images show lattice spaces of 0.262 nm, which are a good match with the (210) plane of cubic CsPbBr3.49 In Fig. 2d, the EDX mapping of CsPbBr3@Pb-MOF-2 indicates that Cs, Pb, Br, C, and O are uniformly distributed in the matrix. XPS measurements are shown in Fig. 2e and f. In the comparison between PbBr-MOF and Pb-MOF, the Br 3d signal belonging to PbBr-MOF is not seen for Pb-MOF. In addition, when compared with Pb-MOF, signals for new Br and Cs species are observed in CsPbBr3@Pb-MOF-2 composites. For a more detailed analysis, high-resolution XPS spectra of Pb 4f are presented in Fig. 2f. For PbBr-MOF, Pb 4f spectra show two prominent peaks at 143.5 (4f5/2) and 138.6 eV (4f7/2).50 In addition, the Pb 4f5/2 and Pb 4f7/2 peaks of Pb-MOF were shifted to 144.0 and 139.1 eV, respectively, due to the change in the coordination environment of the Pb atom during the structural transformation (the breaking of Pb–Br bonds and reorganization of Pb with carboxylic acid ligands). Of note, in comparison with Pb-MOF, the Pb 4f bands of CsPbBr3@Pb-MOF-2 demonstrates a 0.7 eV shift to the lower binding energy after the growth of CsPbBr3 in Pb-MOF. This phenomenon indicates that the Pb node in Pb-MOF is coordinated with Br to produce a new Pb–Br bond belonging to CsPbBr3.41,51
N2 adsorption–desorption isotherms of general Pb-MOF, Pb-MOF, and CsPbBr3@Pb-MOF-2 were collected at 77 K to evaluate porosity and the specific surface area, as illustrated in Fig. 2g. The curves for general Pb-MOF are a type I isotherm, signifying the presence of microporosity.52 Moreover, the N2 adsorption–desorption isotherms of Pb-MOF reveal a combination mode of type I and type IV, implying the simultaneous existence of micropores and mesopores.52,53 On the other hand, intrinsic micropores (<2 nm) are maintained, while mesoporous features (concentrated at 4 nm) appear in Pb-MOF in the pore size distribution curve (Fig. S12†). The Brunauer–Emmett–Teller (BET) surface area of Pb-MOF is determined to be 904.58 m2 g−1, which is bigger than the general Pb-MOF (398.43 m2 g−1). The rich mesoporous and larger surface area of Pb-MOF are expected to favor mass transport and enhance the utilization of active sites.47,54 In Fig. 2g, a combination mode of type I and type IV of the N2 adsorption–desorption isotherm is observed for CsPbBr3@Pb-MOF-2.52 The result confirms the presence of micropores and mesopores in CsPbBr3@Pb-MOF-2.52,53 Likewise, the pore size distributions of CsPbBr3@Pb-MOF-2 agree with this conclusion (Fig. S13†). Meanwhile, compared with Pb-MOF (904.58 m2 g−1), CsPbBr3@Pb-MOF (587.68 m2 g−1) shows lower BET surface areas. Furthermore, apparent mesopores were observed in Pb-MOF on comparing the TEM of general Pb-MOF (Fig. S14†). The generation of mesopores may be ascribed to the in situ structural transformation of MOFs which leads to the missing linker defects (Fig. S15†).53,55,56
Subsequently, electron paramagnetic resonance (EPR) was used to detect oxygen vacancy, as shown in Fig. 2h. Compared with general Pb-MOF, a symmetrical signal of the oxygen vacancy (g = 2.003) is exhibited in the EPR spectra of Pb-MOF.57 These results unambiguously suggest the existence of oxygen vacancies in Pb-MOF together with coordinatively unsaturated Pb atoms. Obviously, the symmetric signal belonging to the oxygen vacancy is also revealed in the CsPbBr3@Pb-MOF composite.57 However, as the content of CsPbBr3 increased in Pb-MOF, a downward trend was observed in the intensity of oxygen vacancies. Such oxygen vacancies can act as electron capture traps that can facilitate photogenerated charge separation.58 CO2 adsorption is a prerequisite for photocatalytic CO2 reduction. CO2 capture capacities of general Pb-MOF, Pb-MOF, and CsPbBr3@Pb-MOF-2 were evaluated from CO2 adsorption isotherms at 297 K, shown in Fig. 2i. The general Pb-MOF and Pb-MOF display CO2 uptakes of 5 and 19.5 cm3 g−1, respectively. We ascribed the enhanced CO2 adsorption to a higher specific surface area and mesoporous channel in Pb-MOF.59,60 Correspondingly, the CO2 adsorption amount of CsPbBr3@Pb-MOF-2 (15.9 cm2 g−1) is between general Pb-MOF and Pb-MOF. The good water stability, abundant oxygen vacancy, mesoporous structure, and higher specific surface area make Pb-MOF a promising host to assist CsPbBr3 for photocatalytic CO2 reduction.48,54,61
3.3 Optical properties
Along with the structural characteristics mentioned above, the optical characteristics of CsPbBr3@Pb-MOF-2 composites, pure CsPbBr3 NCs and Pb-MOF were also studied, as illustrated in Fig. 3. As shown in Fig. 3a, with ultraviolet-visible (UV-Vis) diffuse reflectance spectroscopy (DRS) the light absorption properties of pure CsPbBr3, Pb-MOF and CsPbBr3@Pb-MOF-2 composites were evaluated. The absorption band edges of Pb-MOF (425 nm), pure CsPbBr3 (520 nm) and CsPbBr3@Pb-MOF-2 (543 nm) samples are in the visible range. However, on coupling CsPbBr3 with Pb-MOF, the absorption edge of CsPbBr3@Pb-MOF-2 was markedly red-shifted, resulting in an apparent enhancement of the sample's light-absorbing capacity. This is of significant importance for the utilization of photocatalysts in a practical setting. The band gap of the samples was calculated using the Kubelka–Munk function,28 as depicted in Fig. 3b. These calculated values are consistent with previous literature results.14,20 Based on the reaction thermodynamics, the energy band structure is also critical in photocatalytic performance. Herein, Mott–Schottky (MS) analyses of CsPbBr3 and Pb-MOF were performed and depicted in Fig. 3c and d. Firstly, as shown in Fig. 3b, the bandgaps (Egs) of CsPbBr3 and Pb-MOF were evaluated to be 2.42 eV and 3.08 eV, respectively. In the MS curve, the slope of CsPbBr3 and Pb-MOF demonstrates a positive value, suggesting the samples are n-type characteristic photocatalysts. As known, the MS curve is assessed as a flat band potential and the flat band potential of an n-type semiconductor is 0.1 V lower than its valence band (ECB).62 According to the MS curve, the flat band potential of CsPbBr3 is about −1.10 V vs. Ag/AgCl (≈−0.90 V vs. NHE) (flat band potential of Pb-MOF is about −0.17 V vs. NHE). Therefore, the ECB of CsPbBr3 is −1.00 V vs. NHE (ECB of Pb-MOF is −0.27 V vs. NHE) and the valence band (EVB) of CsPbBr3 was calculated as 1.42 V vs. NHE (EVB of Pb-MOF is 2.81 V vs. NHE), based on the formula of ECB = EVB − Eg. Analyzing the energy level, as illustrated in Fig. 3e, the ECB of CsPbBr3 (−1.00 V vs. NHE) is more negative than CO2/CO (−0.52 V vs. NHE) and O2/˙O2− (−0.33 V vs. NHE) and the EVB of CsPbBr3 (0.85 V vs. NHE) is more positive than H2O/O2 (0.81 V).12 As for Pb-MOF, the ECB of Pb-MOF (−0.27 V vs. NHE) is not more negative than CO2/CO (−0.52 V vs. NHE) and O2/˙O2− (−0.33 V vs. NHE). But the EVB of Pb-MOF (2.81 V vs. NHE) is more positive than H2O/O2 (0.81 V), H2O/˙OH (1.99 V) and OH−/˙OH (2.34 V). To further verify the energy band structure of CsPbBr3@Pb-MOF-2, CsPbBr3 and Pb-MOF, electron paramagnetic resonance (EPR) analysis was executed. For EPR studies, the sample was dissolved in 5,5-dimethyl-1-pyrroline N-oxide (DMPO, capture agent of the spin electron) to identify ˙O2− and ˙OH, respectively.26,63 In Fig. 3f, it can be seen that under visible-light irradiation conditions, Pb-MOF did not exhibit the characteristic peaks of DMPO-˙O2−. In comparison, the characteristic signals of DMPO-˙O2− are successfully detected in CsPbBr3@Pb-MOF-2 and CsPbBr3 NCs. In addition, the peak intensity of CsPbBr3@Pb-MOF-2 is much stronger than CsPbBr3 NCs, suggesting CsPbBr3@Pb-MOF-2 possesses a higher electron transfer efficiency. As shown in Fig. 3g, on irradiating Pb-MOF, CsPbBr3 NCs and CsPbBr3@Pb-MOF-2, no typical peak of DMPO-˙OH is detected, indicating the possible formation of a Z-scheme heterojunction between CsPbBr3 and Pb-MOF.64 This observation highlights the essential role of oxygen vacancies in Pb-MOF in facilitating the transfer of photogenerated electrons between CsPbBr3 and Pb-MOF. Meanwhile, the band positions of CsPbBr3@Pb-MOF and CsPbBr3 satisfy the thermodynamic requirements for reduction and oxidation reactions, indicating it might be an appropriate catalyst for CO2 reduction.
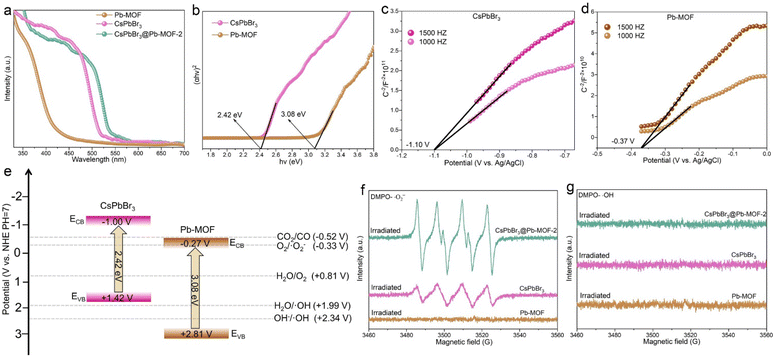 |
| Fig. 3 (a) UV-vis diffuse reflectance spectra of Pb-MOF, CsPbBr3 and CsPbBr3@Pb-MOF-2. (b) The band gap spectra of CsPbBr3 and Pb-MOF. Mott–Schottky plots of (c) CsPbBr3 and (d) Pb-MOF. (e) Schematic illustration of the potential energy diagram for CsPbBr3 and Pb-MOF. Electron paramagnetic resonance spectra of (f) DMPO-˙O2− and (g) DMPO-˙OH in the presence of CsPbBr3@Pb-MOF-2, CsPbBr3 and Pb-MOF. | |
3.4 Water stability, thermal stability, and preventing anion intrusion studies
To check the potential of CsPbBr3@Pb-MOF for photocatalytic applications, we systematically investigated the stability of CsPbBr3@Pb-MOF-2 including water stability, thermal stability, and preventing anion intrusion (Fig. 4). Being ionic crystals, CsPbBr3 NCs readily dissolve in the presence of water, which quenches their luminescence. The PL intensities of CsPbBr3@Pb-MOF-2 remained consistent, displaying approximately 82.6% of their initial strength even after 49 days of immersion in water, as depicted in Fig. 4a (left side) and Fig. S16.† In sharp contrast, when 1 ml of water was added to pure CsPbBr3 NCs dispersed in hexane for 20 min, the fluorescence value of the CsPbBr3 sample was only 1% of the initial value (Fig. 4a, right side). This is attributed to the excellent hydrophobicity exhibited by CsPbBr3@Pb-MOF-2 (water contact angle 140.2°), (Fig. S17†). Next, the thermal stability of CsPbBr3@Pb-MOF-2 was investigated during heating–cooling in the range of 20–120 °C (Fig. 3b). After heating at 120 °C for 30 min, CsPbBr3@Pb-MOF-2 could retain about 52% of its original PL intensity. Impressively, the PL emission value progressively recovered after cooling (green line), eventually reaching the original value. PXRD of CsPbBr3@Pb-MOF-2 after immersion in water and after heating remains intact further proving their high stability (Fig. S18†). The anion intrusion issue of CsPbBr3 is another shortcoming in the application.65 To illustrate the behavior of CsPbBr3@Pb-MOF-2, samples containing single and two halogens including CsPbBr3@Pb-MOF-2 and CsPb(BrCl)3, and CsPbBr3@Pb-MOF-2 and CsPb(BrI)3, were mixed and subjected to the environment. As shown in Fig. 4c and d, the position and intensity of the fluorescence peaks of the samples did not change after 24 hours. The excellent water stability, thermal stability, and prevention of anion exchange of this CsPbBr3@Pb-MOF-2 composite can be attributed to the hydrophobic Pb-MOF walls that protect CsPbBr3 from various chemical conditions.66
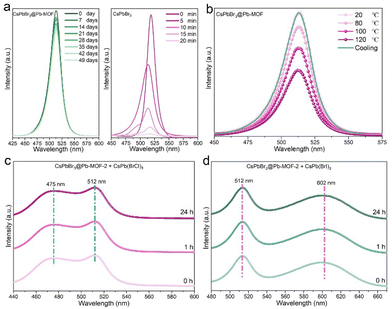 |
| Fig. 4 (a) PL intensity changes of CsPbBr3@Pb-MOF-2 and CsPbBr3 with time. (b) The PL spectra of CsPbBr3@Pb-MOF-2 as a function of temperature. PL spectra of (c) CsPbBr3@Pb-MOF-2 and CsPb(BrCl)3 and (d) CsPbBr3@Pb-MOF-2 and CsPb(BrI)3 mixed powders at different time intervals. | |
3.5 Photoinduced charge separation and transfer dynamics
As known, the photogenerated charge transfer and separation efficiency of the sample have a crucial effect on the photocatalytic efficiency.12–17 On account of this point, photoelectrochemical, electrochemical, and photoluminescence experiments were performed, as presented in Fig. 5. Firstly, the photocurrent response of CsPbBr3@Pb-MOF-2 and pure CsPbBr3 NCs were measured under turn-on–off cycles of visible-light (λ > 420 nm) irradiation. As demonstrated in Fig. 5a, the CsPbBr3@Pb-MOF-2 sample exhibits a significantly higher photocurrent density compared to CsPbBr3 NCs. This result indicates that CsPbBr3@Pb-MOF-2 has a more efficient transfer of photogenerated charge. In other words, oxygen vacancies in Pb-MOF act as a reservoir to receive the photogenerated charge generated by CsPbBr3. Furthermore, the charge transport behaviors of CsPbBr3@Pb-MOF-2 and CsPbBr3 were probed using electrochemical impedance spectroscopy (EIS), as depicted in Fig. 5b. In the Nyquist plot, CsPbBr3@Pb-MOF-2 exhibits smaller semicircle radii owing to small charge transfer resistance.27 In comparison, pure CsPbBr3 NCs show a larger charge transfer resistance, demonstrating the superb carrier mobility between CsPbBr3 NCs and Pb-MOF. Furthermore, the charge transfer dynamics of CsPbBr3@Pb-MOF and CsPbBr3 NCs are evaluated by steady-state photoluminescence (PL). The fluorescence intensity of CsPbBr3@Pb-MOF-2 was dramatically quenched compared to CsPbBr3 NCs (Fig. 5c), verifying that the recombination of photogenerated charges of CsPbBr3@Pb-MOF-2 was effectively inhibited. Furthermore, the time-resolved PL spectra of CsPbBr3@Pb-MOF-2 and CsPbBr3 NCs were recorded (Fig. 5d). The average lifetime of the samples was observed to decline significantly, from 66.8 ns for pure CsPbBr3 NCs to 12.3 ns for CsPbBr3@Pb-MOF (Table S1†).30,39 From all the above experiments, we believe that the enhanced photogenerated charge separation in CsPbBr3@Pb-MOF-2 may be due to the introduced oxygen vacancies acting as charge capture centers.
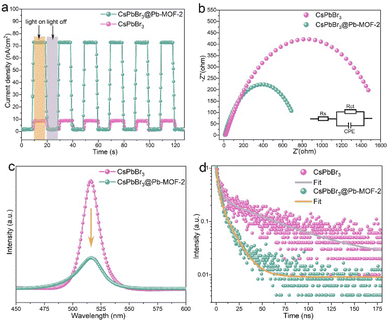 |
| Fig. 5 (a) Photocurrent responses of the samples at −0.4 V vs. Ag/AgCl under visible light irradiation (λ > 420 nm). (b) Electrochemical impendence spectra of the samples. The inset of (b) shows the equivalent circuit diagram, Rs: solution resistance, Rct: interface charge transfer resistance, CPE: constant phase element. (c) Steady-state PL spectra with a 365 nm excitation wavelength. (d) Time-resolved PL decay plots with a 365 nm excitation wavelength and a 516 nm emission wavelength. | |
3.6 Photocatalyst activity tests
This as-synthesized CsPbBr3@Pb-MOF, CsPbBr3@Pb-MOF-WOV (Fig. S19†), pure CsPbBr3 NCs, and pure Pb-MOF were further explored by CO2 photoreduction in the gas–solid mode with H2O as the reductant, without using any sacrificial reagent or photosensitizers (Fig. S20†). Under visible-light (λ > 420 nm) irradiation, for CsPbBr3@Pb-MOF, CsPbBr3@Pb-MOF-WOV and CsPbBr3 NCs, CO is the main reduction product along with a trace of H2. CsPbBr3@Pb-MOF exhibits ≥99.2% selectivity towards CO production, while pure CsPbBr3 NCs and CsPbBr3@Pb-MOF-WOV only show 88% and 90.0 selectivities (Table S2†). The high selectivity of CsPbBr3@Pb-MOF may be attributed to its hydrophobic surface depressing H2 production (Fig. S21†).67 In Fig. 6a, CsPbBr3@Pb-MOF-2 displays the highest CO production rate of 107 μmol g−1 h−1, which is 8.9 times higher than that of pure CsPbBr3 NCs (12 μmol g−1 h−1). Simultaneously, the CO production of CsPbBr3@Pb-MOF-2 was about 3.7 and 1.2 times higher than that of CsPbBr3@Pb-MOF-1 (29 μmol g−1 h−1) and CsPbBr3@Pb-MOF-3 (89 μmol g−1 h−1), indicating that an appropriate concentration of oxygen vacancies and perovskite content can optimize CO yield. In contrast, the CO production of CsPbBr3@Pb-MOF-WOV was only 18 μmol g−1 h−1, highlighting the crucial role of oxygen vacancies in enhancing the CO yield. As for Pb-MOF, no CO or H2 could be observed. The CO yield of CsPbBr3@Pb-MOF-2 is also comparable to the reported highest perovskite-based photocatalysts in gas–solid phase and liquid–solid phase photocatalysis (Table S3†). To confirm the actual roles of each component during the photocatalytic reduction of CO2, control experiments were conducted, as shown in Fig. 6b. When H2O was removed from the system, the CO yield decreased to 30 μmol g−1 h−1, indicating a significant contribution of H2O as the electron/proton source. The absence of light and photocatalyst resulted in no detection of CO, affirming the crucial role of both light and photocatalyst in the photoreduction of CO2. When replacing CO2 with Ar, no CO was observed, indicating CO did not originate from the organic matter in the MOF. To demonstrate this perspective, isotope labeling tests were carried out (Fig. 6c). On replacing CO2 with 13CO2 (m/z = 45) as the feedstock, an obvious peak of 13CO (m/z = 29) was detected. Therefore, CO was confirmed to be obtained from the photoreduction of CO2. Moreover, the photocatalytic reaction was also investigated using H218O as a replacement for H2O, and the MS results are shown in Fig. S22.† The corresponding signals at m/z = 28 and m/z = 30 were observed, indicating the formation of C16O and C18O, respectively. These findings suggest that the O atom in the produced CO is not solely derived from CO2, but can also originate from H2O. This observation is consistent with the findings reported by Zhang et al.68 Based on the experimental results and the findings of Zhang et al., two possible pathways (Fig. S23 and S24†) for photocatalytic CO2 reduction to produce CO can be presented as follows.
CO2 + 2e− + 2H+ → CO + H2O |
H2O + CO2 + 2e− + 2H+ → CO + 2H2O |
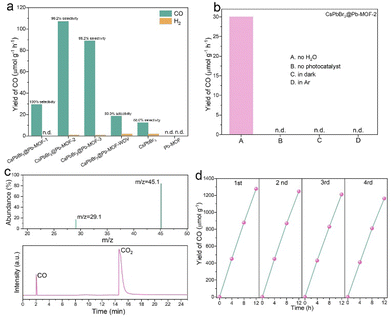 |
| Fig. 6 (a) Photoreduction CO2 performances of the samples. (b) The photocatalytic performance of CsPbBr3@Pb-MOF-2 under different conditions. (c) The GC chromatogram and GC-mass result of an isotopic experiment using 13CO2. (d) 48 h recycle photocatalytic CO2 reduction using CsPbBr3@Pb-MOF-2. | |
Given the significance of the stability in the practical application of photocatalysts, a cycling test was performed on CsPbBr3@Pb-MOF-2. As shown in Fig. 6d, only a 9% loss of photocatalytic activity is incurred after 48 h of recycling of the reaction. Moreover, after a 48 h stability test, CsPbBr3@Pb-MOF-2 was subjected to characterization through PXRD, XPS, TEM, and UV-Vis (Fig. S25 and S28†). The results demonstrate the exceptional stability of CsPbBr3@Pb-MOF-2.
3.7 The possible photocatalytic mechanism
A possible mechanism for CO2 photoreduction over CsPbBr3@Pb-MOF is proposed based on the above results and analyses, as described in Fig. 7. Firstly, the opportune specific surface area and mesoporous structure of Pb-MOF can benefit from the adsorption and concentration of CO2 and supply abundant active sites, thereby promoting the subsequent photoreduction reaction. Secondly, under light irradiation, CsPbBr3 was excited and released photogenerated electrons (e−) and photogenerated holes (h+). Later, the oxygen vacancies in the Pb-MOF function act as a photogenerated electron acceptor from CsPbBr3. The improved charge transfer between CsPbBr3 and Pb-MOF inhibits the radiative recombination of the photogenerated electrons and holes. Thirdly, the enriched electrons on the surface of Pb-MOF undergo a 2-electron reaction to reduce CO2 to CO. Meanwhile, a Pb-MOF hydrophobic surface environment with a high concentration of CO2 boosts the selectivity for CO2 photoreduction, suppressing the generation of the H2 byproduct. Finally, the performance of CsPbBr3 NCs is improved, owing to the synergistic effect of Pb-MOF.
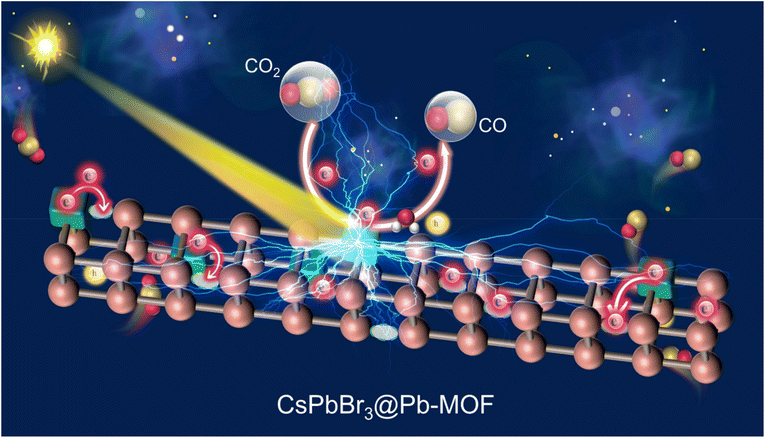 |
| Fig. 7 The possible mechanism of CO2 photoreduction for CsPbBr3@Pb-MOF. | |
4. Conclusions
In summary, we developed a facile and low-cost in situ structural transformation strategy to introduce both CsPbBr3 and oxygen vacancies into hydrophobic hierarchically porous Pb-MOF by reacting PbBr-MOF with CsBr. During the structural transformation from PbBr-MOF to Pb-MOF, the exposure of Pb metal nodes in Pb-MOF is involved in the construction of CsPbBr3, which facilitates CsPbBr3@Pb-MOF preparation, minimizes CsPbBr3 aggregation, and shortens the photogenerated electron transportation path. Meanwhile, the organic linker omission in structural transformation results in a hierarchically porous structure and abundant oxygen vacancies which enables fast mass transport and accelerates the photogenerated charge separation. The enhanced stability and outstanding electron transfer efficiency permit CsPbBr3@Pb-MOF-2 to exhibit a significantly impressive photocatalytic performance for CO2 reduction to CO (107 μmol g−1 h−1, 99.2% selectivity) which is an 8.9-fold improvement over that of pure CsPbBr3 (12 μmol g−1 h−1, 88% selectivity) under visible-light (λ > 420 nm). Our research provides new insights and offers novel perspectives for the development of efficient lead-based perovskite MOF composite photocatalysts.
Author contributions
The manuscript was written through the contributions of all authors.
Conflicts of interest
There are no conflicts of interest to declare.
Acknowledgements
This work was financially supported by the NSFC of China (no. 22271023 and 21971032), the Education Department of Jilin Province (no. JJKH20221153KJ) and Jilin Provincial Department of Science and Technology (no. 20230508108RC).
References
- O. S. Bushuyev, P. De Luna, C. T. Dinh, L. Tao, G. Saur, J. van de Lagemaat, S. O. Kelley and E. H. Sargent, What should we make with CO2 and how can we make it?, Joule, 2018, 2, 825–832 CrossRef CAS
.
- Z. Zhang, Y. Zheng, L. Qian, D. Luo, H. Dou, G. Wen, A. Yu and Z. Chen, Emerging trends in sustainable CO2-management materials, Adv. Mater., 2022, 34, 2201547 CrossRef CAS PubMed
.
- Y.-F. Xu, M.-Z. Yang, B.-X. Chen, X.-D. Wang, H.-Y. Chen, D.-B. Kuang and C.-Y. Su, A CsPbBr3 perovskite quantum dot/graphene oxide composite for photocatalytic CO2 reduction, J. Am. Chem. Soc., 2017, 139, 5660–5663 CrossRef CAS PubMed
.
- J. Hou, S. Cao, Y. Wu, Z. Gao, F. Liang, Y. Sun, Z. Lin and L. Sun, Inorganic colloidal perovskite quantum dots for robust solar CO2 reduction, Chem. – Eur. J., 2017, 23, 9481–9485 CrossRef CAS PubMed
.
- S.-H. Guo, J. Zhou, X. Zhao, C.-Y. Sun, S.-Q. You, X.-L. Wang and Z.-M. Su, Enhanced CO2 photoreduction via tuning halides in perovskites, J. Catal., 2019, 369, 201–208 CrossRef CAS
.
- G. Gao, Q. Xi, H. Zhou, Y. Zhao, C. Wu, L. Wang, P. Guo and J. Xu, Novel inorganic perovskite quantum dots for photocatalysis, Nanoscale, 2017, 9, 12032–12038 RSC
.
- D. Cardenas-Morcoso, A. F. Gualdrón-Reyes, A. B. Ferreira Vitoreti, M. García-Tecedor, S. J. Yoon, M. Solis de la Fuente, I. Mora-Seró and S. Gimenez, Photocatalytic and photoelectrochemical degradation of organic compounds with all-Inorganic metal halide perovskite quantum dots, J. Phys. Chem. Lett., 2019, 10, 630–636 CrossRef CAS PubMed
.
- H. Hu, M. Chen, N. Yao, L. Wu, Q. Zhong, B. Song, M. Cao and Q. Zhang, Highly stable CsPbBr3 colloidal nanocrystal clusters as photocatalysts in polar solvents, ACS Appl. Mater. Interfaces, 2021, 13, 4017–4025 CrossRef CAS PubMed
.
- Z. Guan, Y. Wu, P. Wang, Q. Zhang, Z. Wang, Z. Zheng, Y. Liu, Y. Dai, M.-H. Whangbo and B. Huang, Perovskite photocatalyst CsPbBr3−xIx with a bandgap funnel structure for H2 evolution under visible light, Appl. Catal., B, 2019, 245, 522–527 CrossRef CAS
.
- X. Zhu, Y. Lin, J. San Martin, Y. Sun, D. Zhu and Y. Yan, Lead halide perovskites for photocatalytic organic synthesis, Nat. Commun., 2019, 10, 1–10 CrossRef PubMed
.
- X. Zhu, Y. Lin, Y. Sun, M. C. Beard and Y. Yan, Lead-halide perovskites for photocatalytic α-Alkylation of aldehydes, J. Am. Chem. Soc., 2019, 141, 733–738 CrossRef CAS PubMed
.
- J. Wang, Y. Shi, Y. Wang and Z. Li, Rational design of metal halide perovskite nanocrystals for photocatalytic CO2 reduction: recent advances, challenges, and prospects, ACS Energy Lett., 2022, 7, 2043–2059 CrossRef CAS
.
- P. Chen, W.-J. Ong, Z. Shi, X. Zhao and N. Li, Pb-Based halide perovskites: recent advances in photo(electro)catalytic applications and looking beyond, Adv. Funct. Mater., 2020, 30, 1909667 CrossRef CAS
.
- S. H. Teo, C. H. Ng, Y. H. Ng, A. Islam, S. Hayase and Y. H. Taufiq-Yap, Resolve deep-rooted challenges of halide perovskite for sustainable energy development and environmental remediation, Nano Energy, 2022, 99, 107401 CrossRef CAS
.
- H. Huang, B. Pradhan, J. Hofkens, M. B. J. Roeffaers and J. A. Steele, Solar-driven metal halide perovskite photocatalysis: design, stability, and performance, ACS Energy Lett., 2020, 5, 1107–1123 CrossRef CAS
.
- J. T. DuBose and P. V. Kamat, Efficacy of perovskite photocatalysis: challenges to overcome, ACS Energy Lett., 2022, 7, 1994–2011 CrossRef CAS
.
- J. Fu, K. Jiang, X. Qiu, J. Yu and M. Liu, Product selectivity of photocatalytic CO2 reduction reactions, Mater. Today, 2020, 32, 222–243 CrossRef CAS
.
- D. Yan, T. Shi, Z. Zang, S. Zhao, J. Du and Y. Leng, Stable and low-threshold whispering-gallery-mode lasing from modified CsPbBr3 perovskite quantum dots@SiO2 sphere, Chem. Eng. J., 2020, 401, 126066 CrossRef CAS
.
- Z. Hu, Z. Liu, Y. Bian, S. Li, X. Tang, J. Du, Z. Zang, M. Zhou, W. Hu, Y. Tian and Y. Leng, Enhanced two-photon-pumped emission from In situ synthesized nonblinking CsPbBr3/SiO2 nanocrystals with excellent stability, Adv. Opt. Mater., 2018, 6, 1700997 CrossRef
.
- Y. Tong, Q. Wang, E. Mei, X. Liang, W. Gao and W. Xiang, One-pot synthesis of CsPbX3 (X = Cl, Br, I)@Zeolite: a potential material for wide–color–gamut backlit displays and upconversion emission, Adv. Opt. Mater., 2021, 9, 2100012 CrossRef CAS
.
- B. Ai, C. Liu, J. Wang, J. Xie, J. Han and X. Zhao, Precipitation and optical properties of CsPbBr3 quantum dots in phosphate glasses, J. Am. Ceram. Soc., 2016, 99, 2875–2877 CrossRef CAS
.
- X. Huang, Q. Guo, D. Yang, X. Xiao, X. Liu, Z. Xia, F. Fan, J. Qiu and G. Dong, Reversible 3D laser printing of perovskite quantum dots inside a transparent medium, Nat. Photonics, 2020, 14, 82–88 CrossRef CAS
.
- S. Liu, G. Shao, L. Ding, J. Liu, W. Xiang and X. Liang, Sn-doped CsPbBr3 QDs glasses with excellent stability and optical properties for WLED, Chem. Eng. J., 2019, 361, 937–944 CrossRef CAS
.
- H. Zhang, X. Wang, Q. Liao, Z. Xu, H. Li, L. Zheng and H. Fu, Embedding perovskite nanocrystals into a polymer matrix for tunable luminescence probes in cell imaging, Adv. Funct. Mater., 2017, 27, 1604382 CrossRef
.
- Q. Zhou, Z. Bai, W. Lu, Y. Wang, B. Zou and H. Zhong, In situ fabrication of halide perovskite nanocrystal-embedded polymer composite films with enhanced photoluminescence for display backlights, Adv. Mater., 2016, 28, 9163–9168 CrossRef CAS PubMed
.
- Y. Jiang, J.-F. Liao, H.-Y. Chen, H.-H. Zhang, J.-Y. Li, X.-D. Wang and D.-B. Kuang, All-solid-state Z-scheme α-Fe2O3/Amine-RGO/CsPbBr3 hybrids for visible-light-driven photocatalytic CO2 reduction, Chem, 2020, 6, 766–780 CAS
.
- Y. Jiang, J.-F. Liao, Y.-F. Xu, H.-Y. Chen, X.-D. Wang and D.-B. Kuang, Hierarchical CsPbBr3 nanocrystal-decorated ZnO nanowire/macroporous graphene hybrids for enhancing charge separation and photocatalytic CO2 reduction, J. Mater. Chem. A, 2019, 7, 13762–13769 RSC
.
- X. Yue, L. Cheng, J. Fan and Q. Xiang, 2D/2D BiVO4/CsPbBr3 S-scheme heterojunction for photocatalytic CO2 reduction: Insights into structure regulation and Fermi level modulation, Appl. Catal., B, 2022, 304, 120979 CrossRef CAS
.
- Y. Zhao, H. Shi, X. Hu, E. Liu and J. Fan, Fabricating CsPbX3/CN heterostructures with enhanced photocatalytic activity for penicillins 6-APA degradation, Chem. Eng. J., 2020, 381, 122692 CrossRef CAS
.
- Y.-H. Chen, J.-K. Ye, Y.-J. Chang, T.-W. Liu, Y.-H. Chuang, W.-R. Liu, S.-H. Liu and Y.-C. Pu, Mechanisms behind photocatalytic CO2 reduction by CsPbBr3 perovskite-graphene-based nanoheterostructures, Appl. Catal., B, 2021, 284, 119751 CrossRef CAS
.
- L. Jiao and H.-L. Jiang, Metal-organic frameworks for catalysis: Fundamentals and future prospects, Chin. J. Catal., 2023, 45, 1–5 CrossRef CAS
.
- K. Sun, Y. Qian and H.-L. Jiang, Metal-Organic Frameworks for Photocatalytic Water Splitting and CO2 Reduction, Angew. Chem., 2023, 135, e202217565 CrossRef
.
- J. Li, H. Huang, W. Xue, K. Sun, X. Song, C. Wu, L. Nie, Y. Li, C. Liu, Y. Pan, H.-L. Jiang, D. Mei and C. Zhong, Self-adaptive dual-metal-site pairs in metal-organic frameworks for selective CO2 photoreduction to CH4, Nat. Catal., 2021, 4, 719–729 CrossRef CAS
.
- S. K. Yadav, G. K. Grandhi, D. P. Dubal, J. C. Mello, M. Otyepka, R. Zbořil, R. A. Fischer and K. Jayaramulu, Metal halide perovskite@metal–organic framework hybrids: synthesis, design, properties, and applications, Small, 2020, 16, 2004891 CrossRef CAS PubMed
.
- J. Hou, Z. Wang, P. Chen, V. Chen, A. K. Cheetham and L. Wang, Intermarriage of halide perovskites and metal–organic framework crystals, Angew. Chem., 2020, 132, 19602–19617 CrossRef
.
- C. Zhang, W. Li and L. Li, Metal halide perovskite nanocrystals in metal–organic framework host: not merely enhanced stability, Angew. Chem., 2021, 133, 7564–7577 CrossRef
.
- G.-Y. Qiao, D. Guan, S. Yuan, H. Rao, X. Chen, J.-A. Wang, J.-S. Qin, J.-J. Xu and J. Yu, Perovskite quantum dots encapsulated in a mesoporous metal–organic framework as synergistic photocathode materials, J. Am. Chem. Soc., 2021, 143, 14253–14260 CrossRef CAS PubMed
.
- L.-Y. Wu, Y.-F. Mu, X.-X. Guo, W. Zhang, Z.-M. Zhang, M. Zhang and T.-B. Lu, Encapsulating Perovskite Quantum Dots in Iron-Based Metal–Organic Frameworks (MOFs) for Efficient Photocatalytic CO2 Reduction, Angew. Chem., Int. Ed., 2019, 58, 9491–9495 CrossRef CAS PubMed
.
- Z.-C. Kong, J.-F. Liao, Y.-J. Dong, Y.-F. Xu, H.-Y. Chen, D.-B. Kuang and C.-Y. Su, Core@shell CsPbBr3@Zeolitic imidazolate framework nanocomposite for efficient photocatalytic CO2 reduction, ACS Energy Lett., 2018, 3, 2656–2662 CrossRef CAS
.
- S. Mollick, T. N. Mandal, A. Jana, S. Fajal, A. V. Desai and S. K. Ghosh, Ultrastable luminescent hybrid bromide perovskite@MOF nanocomposites for the degradation of organic pollutants in water, ACS Appl. Nano Mater., 2019, 2, 1333–1340 CrossRef CAS
.
- C. Zhang, B. Wang, W. Li, S. Huang, L. Kong, Z. Li and L. Li, Conversion of invisible metal-organic frameworks to luminescent perovskite nanocrystals for confidential information encryption and decryption, Nat. Commun., 2017, 8, 1138 CrossRef PubMed
.
- H. Tsai, H. Huang, J. Watt, C. Hou, J. Strzalka, J. Shyue, L. Wang and W. Nie, Cesium lead halide perovskite nanocrystals assembled in metal–organic frameworks for stable blue light emitting diodes, Adv. Sci., 2022, 9, 2105850 CrossRef CAS PubMed
.
- H. Tsai, S. Shrestha, R. A. Vilá, W. Huang, C. Liu, C.-H. Hou, H.-H. Huang, X. Wen, M. Li, G. Wiederrecht, Y. Cui, M. Cotlet, X. Zhang, X. Ma and W. Nie, Bright and stable light-emitting diodes made with perovskite nanocrystals stabilized in metal–organic frameworks, Nat. Photonics, 2021, 15, 843–849 CrossRef
.
- C. Peng, X. Song, J. Yin, G. Zhang and H. Fei, Intrinsic white-light-emitting metal–organic frameworks with structurally deformable secondary building units, Angew. Chem., Int. Ed., 2019, 58, 7818–7822 CrossRef CAS PubMed
.
- X. Li, Y. Wu, S. Zhang, B. Cai, Y. Gu, J. Song and H. Zeng, CsPbX3 Quantum Dots for Lighting and Displays: Room-Temperature Synthesis, Photoluminescence Superiorities, Underlying Origins and White Light-Emitting Diodes, Adv. Funct. Mater., 2016, 26, 2435–2445 CrossRef CAS
.
- Y.-X. Tan, F.-Y. Meng, M.-C. Wu and M.-H. Zeng, Two Pb(II) dicarboxylates constructed by rigid terephthalate or flexible d(+)-camphorate with different 3D motif based on cooperative effect of steric hindrance of ligand and lone pair electrons, J. Mol. Struct., 2009, 928, 176–181 CrossRef CAS
.
- B. N. Choi, J. Y. Seo, Z. An, P. J. Yoo and C.-H. Chung, An in situ spectroscopic study on the photochemical CO2 reduction on CsPbBr3 perovskite catalysts embedded in a porous copper scaffold, Chem. Eng. J., 2022, 430, 132807 CrossRef CAS
.
- K. Jayaramulu, F. Geyer, A. Schneemann, Š. Kment, M. Otyepka, R. Zboril, D. Vollmer and R. A. Fischer, Hydrophobic metal–organic frameworks, Adv. Mater., 2019, 31, 1900820 CrossRef PubMed
.
- J. Cao, C. Yan, C. Luo, W. Li, X. Zeng, Z. Xu, X. Fu, Q. Wang, X. Chu, H. Huang, X. Zhao, J. Lu and W. Yang, Cryogenic–temperature thermodynamically suppressed and strongly confined CsPbBr3 quantum dots for deeply blue light–emitting diodes, Adv. Opt. Mater., 2021, 9, 2100300 CrossRef CAS
.
- X. Chen, Y. Yu, C. Yang, J. Yin, X. Song, J. Li and H. Fei, Fabrication of robust and porous lead chloride-based metal–organic frameworks toward a selective and sensitive smart NH3 sensor, ACS Appl. Mater. Interfaces, 2021, 13, 52765–52774 CrossRef CAS PubMed
.
- B. Xu, Z. Gao, S. Yang, H. Sun, L. Song, Y. Li, W. Zhang, X. Sun, Z. Wang, X. Wang and X. Meng, Multicolor random lasers based on perovskite quantum dots embedded in intrinsic Pb–MOFs, J. Phys. Chem. C, 2021, 125, 25757–25764 CrossRef CAS
.
- M. Thommes, K. Kaneko, A. V. Neimark, J. P. Olivier, F. Rodriguez-Reinoso, J. Rouquerol and K. S. W. Sing, Physisorption of gases, with special reference to the evaluation of surface area and pore size distribution (IUPAC Technical Report), Pure Appl. Chem., 2015, 87, 1051–1069 CrossRef CAS
.
- L. Feng, S. Yuan, L.-L. Zhang, K. Tan, J.-L. Li, A. Kirchon, L.-M. Liu, P. Zhang, Y. Han, Y. J. Chabal and H.-C. Zhou, Creating hierarchical pores by controlled linker thermolysis in multivariate metal–organic frameworks, J. Am. Chem. Soc., 2018, 140, 2363–2372 CrossRef CAS PubMed
.
- K. Cheng, F. Svec, Y. Lv and T. Tan, Hierarchical micro–and mesoporous Zn–based metal–organic frameworks templated by hydrogels: their use for enzyme immobilization and catalysis of knoevenagel reaction, Small, 2019, 15, 1902927 CrossRef CAS PubMed
.
- G. Cai, X. Ma, M. Kassymova, K. Sun, M. Ding and H.-L. Jiang, Large-scale production of hierarchically porous metal–organic frameworks by a reflux-Assisted post-synthetic ligand substitution strategy, ACS Cent. Sci., 2021, 7, 1434–1440 CrossRef CAS PubMed
.
- L. Feng, K.-Y. Wang, X.-L. Lv, T.-H. Yan and H.-C. Zhou, Hierarchically porous metal–organic frameworks: synthetic strategies and applications, Natl. Sci. Rev., 2020, 7, 1743–1758 CrossRef CAS PubMed
.
- R. Xu, Q. Ji, P. Zhao, M. Jian, C. Xiang, C. Hu, G. Zhang, C. Tang, R. Liu, X. Zhang and J. Qu, Hierarchically porous UiO-66 with tunable mesopores and oxygen vacancies for enhanced arsenic removal, J. Mater. Chem. A, 2020, 8, 7870–7879 RSC
.
- F. Lei, Y. Sun, K. Liu, S. Gao, L. Liang, B. Pan and Y. Xie, Oxygen vacancies confined in ultrathin indium oxide porous sheets for promoted visible-light water splitting, J. Am. Chem. Soc., 2014, 136, 6826–6829 CrossRef CAS PubMed
.
- S.-Y. Kim, A.-R. Kim, J. W. Yoon, H.-J. Kim and Y.-S. Bae, Creation of mesoporous defects in a microporous metal-organic framework by an acetic acid-fragmented linker co-assembly and its remarkable effects on methane uptake, Chem. Eng. J., 2018, 335, 94–100 CrossRef CAS
.
- Y. Mao, D. Chen, P. Hu, Y. Guo, Y. Ying, W. Ying and X. Peng, Hierarchical mesoporous metal–organic frameworks for enhanced CO2 capture, Chem. – Eur. J., 2015, 21, 15127–15132 CrossRef CAS PubMed
.
- S. Zhang, M. Du, Z. Xing, Z. Li, K. Pan and W. Zhou, Defect-rich and electron-rich mesoporous Ti-MOFs based NH2-MIL-125 (Ti)@ZnIn2S4/CdS hierarchical tandem heterojunctions with improved charge separation and enhanced solar-driven photocatalytic performance, Appl. Catal., B, 2020, 262, 118202 CrossRef CAS
.
- M. Dong, J. Zhou, J. Zhong, H. Li, C. Sun, Y. Han, J. Kou, Z. Kang, X. Wang and Z. Su, CO2 dominated bifunctional catalytic sites for efficient industrial exhaust conversion, Adv. Funct. Mater., 2022, 32, 2110136 CrossRef CAS
.
- H. Guo, S. Wan, Y. Wang, W. Ma, Q. Zhong and J. Ding, Enhanced photocatalytic CO2 reduction over direct Z-scheme NiTiO3/g-C3N4 nanocomposite promoted by efficient interfacial charge transfer, Chem. Eng. J., 2021, 412, 128646 CrossRef CAS
.
- M. Zhang, M. Lu, Z. Lang, J. Liu, M. Liu, J. Chang, L. Li, L. Shang, M. Wang, S. Li and Y. Lan, Semiconductor/Covalent–Organic–Framework Z–Scheme Heterojunctions for Artificial Photosynthesis, Angew. Chem., Int. Ed., 2020, 59, 6500–6506 CrossRef CAS PubMed
.
- V. K. Ravi, R. A. Scheidt, A. Nag, M. Kuno and P. V. Kamat, To exchange or not to exchange. suppressing anion exchange in cesium lead halide perovskites with PbSO4−oleate capping, ACS Energy Lett., 2018, 3, 1049–1055 CrossRef CAS
.
- L. Xie, M. Xu, X. Liu, M. Zhao and J. Li, Hydrophobic metal–organic frameworks: assessment, construction, and diverse applications, Adv. Sci., 2020, 7, 1901758 CrossRef CAS PubMed
.
- A. Li, Q. Cao, G. Zhou, B. V. K. J. Schmidt, W. Zhu, X. Yuan, H. Huo, J. Gong and M. Antonietti, Three-Phase Photocatalysis for the Enhanced Selectivity and Activity of CO2 Reduction on a Hydrophobic Surface, Angew. Chem., Int. Ed., 2019, 58, 14549–14555 CrossRef CAS PubMed
.
- Y. Zheng, H. Yao, R. Di, Z. Xiang, Q. Wang, F. Lu, Y. Li, G. Yang, Q. Ma and Z. Zhang, Water coordinated on Cu(I)-based catalysts is the oxygen source in CO2 reduction to CO, Nat. Commun., 2022, 13, 2577 CrossRef CAS PubMed
.
|
This journal is © the Partner Organisations 2023 |
Click here to see how this site uses Cookies. View our privacy policy here.