DOI:
10.1039/D3EE01008B
(Perspective)
Energy Environ. Sci., 2023,
16, 4280-4304
A roadmap for achieving scalable, safe, and low-cost direct air carbon capture and storage†
Received
30th March 2023
, Accepted 14th July 2023
First published on 19th July 2023
Abstract
Direct air carbon capture and storage (DACCS) involves a set of approaches for capturing CO2 directly from the air and its subsequent long-term storage. DACCS is at an early stage of technical development and currently faces a variety of challenges, including high cost and energy requirements. Building on publicly available data, this paper provides: (i) an overview and classification of DACCS systems, (ii) a harmonization of technical and economic performance of direct air capture technologies, (iii) a comprehensive list of technical- and infrastructure-based obstacles to scaling DACCS systems, and (iv) a roadmap and list of priority initiatives for research, development, demonstration, and deployment of DACCS. Our intent is to drive progress against high-impact priority actions, with a focus on accelerating research, development, and deployment of safe, scalable, and low cost DACCS as a component of the broader carbon dioxide removal portfolio.
Broader context
Direct air carbon capture and storage (DACCS) is one of the carbon dioxide removal solutions that are currently the focus of many efforts to help deliver the negative greenhouse gas emissions required for climate stability. Even though DACCS offers large potential scalability, relative ease of monitoring, reporting and verification, and high durability, it still faces many uncertainties, limitations and obstacles that prevent the required gigaton-scale deployment.
In this work, we introduce a new hierarchy for classifying DACCS technologies that supports understanding of the key DAC performance indicators and the quality of the underlying data. We then harmonized available energy and cost data, to allow for a like-for-like comparison. Based on our own obtained first-of-a-kind plant cost values, we derived five obstacles categories that naturally drive high-priority initiatives targeting scalable, safe, low-cost, and low-energy DACCS by 2050. These are compiled in a technology roadmap, where lead actors, critical paths and milestones, and investment cost ranges are provided for all identified priority initiatives. The development of the roadmap was conducted in close consultation with a series of stakeholders, including academia, industry, investment, government and policy making, which ensured that our roadmap resonates with the key players in the field.
|
1 Introduction
The Intergovernmental Panel on Climate Change suggests that, in addition to rapidly reducing CO2 emissions, we will need to remove between 1.3–29 GtCO2 from the atmosphere annually by 2050 to limit global warming to 1.5 °C above pre-industrial temperatures.1 Carbon dioxide removal (CDR) encompasses a set of solutions that accomplishes this task by drawing down atmospheric CO2 and durably storing it. Many CDR solutions, however, are not yet ready for large-scale deployment as there is significant uncertainty regarding their mechanics, cost, and broader impact. Further, deployment of these solutions may require long lead times that will need to be compressed in order to mitigate climate change on relevant time scales. Thus, this decade is a decisive period to test and develop CDR options that will eventually deliver the negative greenhouse gas (GHG) emissions required for climate stability.
Broadly, CDR solutions can be grouped into three categories. There are varying naming conventions for these three groups within the CDR community and a consensus has not yet emerged on how to best label them. For the purposes of this paper, we refer to biogenic, geochemical and novel CDR. Biogenic CDR entails harnessing natural carbon fixation processes through living organisms, such as photosynthesis. Geochemical CDR harnesses carbon fixation processes that occur and are geochemical in nature, such as weathering, mineralization, and the transfer of carbon between the air and ocean. Novel CDR (nCDR) includes novel applications and combinations of chemistry, geochemistry, physics, and biology that do not occur widely in nature, such as most approaches commonly referred to as direct air capture and direct ocean capture.
Direct air carbon capture and (permanent) storage (DACCS), is a valuable component of this portfolio. DACCS' distinctive positive attributes include large potential scalability (in terms of physical requirements), relative ease of monitoring, reporting and verification (MRV), and high durability of carbon storage. In direct air capture (DAC), CO2 is chemically or physically separated from other gas components in air in facilities that can be switched on and off, making DAC technologies highly controllable. Moreover, geological storage and in situ mineralization, the two most prominently discussed options to store CO2 captured at scale from DAC facilities, are both durable storage solutions for which MRV is straightforward and reliable, compared to other forms of CDR that take place in open environments.
However, DACCS faces certain uncertainties, limitations and obstacles. To date, only one DAC technology has reached commercial deployment and few technologies have reached pilot plant scale (technology readiness level (TRL) of 6 or higher2). Moreover, all known technologies face the challenge of high current energy requirements and cost (measured plant performance data: heat, 1500 kWh per tCO2;3,4 electricity, 500 kWh per tCO2;3,4 and cost, USD600–1000 per tCO25–7). For DACCS to play a major role in the climate response, both energy and cost must be reduced. Previous studies indicate that cost must be reduced by a factor of approximately 3–10 in order to achieve values of USD100–200 per ton of net CO2 removed in a long term.1,8 This means that for a targeted 10 gigaton (Gt) net CO2 removal annually, the world will need to invest $1 trillion per year, if DAC cost is to be reduced to USD100 per tCO2.
Accordingly, it is critical that developments and improvements for a range of DAC technologies are rapidly expedited this decade. This advancement requires a greater understanding of the current state of DACCS and identification of existing obstacles to scaling at the pace required to meaningfully contribute to climate targets.
This paper aims to surface opportunities to accelerate DACCS research, testing, development, and deployment, and to prioritize activities designed to remedy current key gaps and obstacles. We build on previous literature6,8–14 and on discussions with experts to provide: (i) an overview and classification of DACCS systems, (ii) an overview and harmonization of technical and economic performance of DAC technologies, based on publicly available data, (iii) a comprehensive list of technical and infrastructure-based obstacles to scaling DACCS systems, and (iv) a list of priority initiatives, including a roadmap to 2050, for unlocking research, development, demonstration and deployment (RDD&D) of DACCS. In (iv), we define priority initiatives as RDD&D activities necessary to overcome identified obstacles. This paper focuses on RDD&D obstacles only, as obstacles related to other supporting elements of a high-functioning CDR ecosystem such as demand, human capital and social acceptance15 are expected to apply to CDR solutions more generally and not to DACCS systems specifically.
2 Methodology
For DAC technology classification, we first screened available literature and collected information on the current state of DAC deployment. We assessed reported DAC process designs, input (e.g., materials, energy supply systems) and output (e.g., CO2 product stream, waste) of DAC units, required equipment to enable DAC operation, required land and additional resources to enable DAC plant development, and current DAC companies and TRLs. The latest reported DAC process designs helped us classify the technologies by their capture mechanism and release driver (see Section 3).
We then developed a harmonized techno-economic analysis (TEA) framework supporting a techno-economic comparison of DAC technologies as: (i) the most well-known obstacle to de-risk and enable large-scale implementation of DAC is the current high cost, (ii) current cost drivers can pinpoint technology obstacles and areas for technology improvement, thus priority initiatives, and (iii) cost comparison of technologies on a like-for-like basis was challenging when based on the available data. The harmonization allows for the comparison of different DAC processes from different data sources. The harmonized TEA framework uses identical assumptions, system boundary definitions, and assessment procedure to deal with different TRLs. As a result, we derived our own first-of-a-kind (FOAK) plant cost values using inputs from the publicly available data (see Section 4), which differ from those in the original sources, but are comparable among each others.
Next, we categorized obstacles across five main areas: (i) materials for carbon capture, (ii) DAC process designs, (iii) essential equipment for the operation of a DAC unit, (iv) DACCS system integration, and (v) infrastructure for enabling a large-scale DACCS deployment. By system integration, we refer to the integration of DACCS into the wider industrial and energy networks and into society.
Priority initiatives were then derived, resulting in our proposed DACCS' Roadmap to Gigaton Scale. We involved an advisory board constituted of representatives from academia, industry, investment, government and policy making in defining the approach to roadmapping and the identification and framing of obstacles and priority initiatives. Throughout the roadmapping process, we convened the advisory board to: (i) brainstorm the best approach for the roadmapping exercise, and (ii) review obstacles and priority initiatives for DACCS. For the roadmap, lead actors (i.e., academia, industry, government), critical paths and milestones to short (by 2030), medium (by 2040), and long (by 2050) term, and investment cost ranges are provided for all identified priority initiatives.
3 Overview and classification of DACCS systems
We classified DAC processes into different technology categories for comparison across energy, cost, and specific RDD&D requirements.
3.1 Defining the system boundary of a DAC plant
We define a DAC process as the process of capturing CO2 from open atmospheres, i.e., separating the ultra-dilute CO2 (today around 420 ppm) over other gases directly from atmospheric air. This definition excludes carbon capture from closed atmospheres, like carbon capture from heating, ventilation and air conditioning (HVAC) systems, and the steps in which captured CO2 is transported and stored or further conditioned to meet specific requirements imposed by the storage option. We refer to the latter as DACCS. A DAC plant mainly consists of a DAC unit, which interacts with the existing energy supply system, the material supply chain, and the CO2 transport and storage infrastructure (if CO2 is permanently stored). The considered system boundary of a DAC plant is illustrated in Fig. 1. In general, the capture of CO2 from the air requires input of both materials and energy, which can be heat and/or electricity. As output, DAC processes deliver a more concentrated CO2 stream, while they may also create waste (e.g., degraded solvent or sorbent material) and/or produce direct CO2 emissions itself (‘emitted CO2 from flue gas’ in Fig. 1). The purity of CO2 output streams may vary from single percentages by volume16,17 to almost pure CO2,3 depending on the technology used.
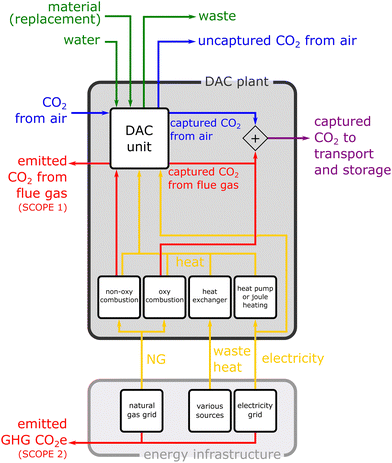 |
| Fig. 1 Schematic representation of the considered system boundary: DAC plant. The different elements of the DAC plant are: (i) the DAC unit, which captures CO2 from the air (blue arrows); (ii) the upstream energy supply infrastructure (yellow arrows); (iii) the downstream CO2 transport and storage infrastructure (purple arrows); (iv) the material supply chain (green arrows); and (v) the Scope 1 (direct emissions from the operation of the DAC unit) and Scope 2 (indirect emissions from the operation of the DAC unit) emissions (red arrows). Different energy sources and energy supply systems that provide heat and/or electricity to the DAC unit are considered. | |
DAC processes piloted to date generally use either natural gas, waste heat, and/or electricity to supply the required energy. Different energy sources will impact the efficiency and economics of a DAC plant.
3.2 Classification of DAC technologies
Here, DAC technologies are structured according to four main CO2 separation mechanisms: physical and/or chemical binding to either (i) a liquid or (ii) a solid material; (iii) separation based on differences in gas diffusivities; and (iv) separation by differences in freezing point (Fig. 2). DAC technologies that bind CO2 to a liquid or to a solid material can be further categorized by their respective CO2 regeneration driver (i.e., the driver that releases the CO2 from the material it is bound to). Similarly, the separation by differences in diffusivity can be distinguished between pressure-driven processes (membrane as a sieve) and voltage-driven processes (electrochemical separation). For separation by freezing point (cryogenic separation), we solely focus on vapor-solid separation of CO2. This classification leads to 11 (non-exhaustive) distinct DAC technologies, listed in Table 1. Detailed descriptions of all technologies are provided in Section S1 in ESI.† This particular classification approach was chosen to span the technological landscape of DAC processes and highlight specific obstacles associated with each technology. This classification also allows for future incorporation of yet unknown research, development and demonstration (RD&D) actions.
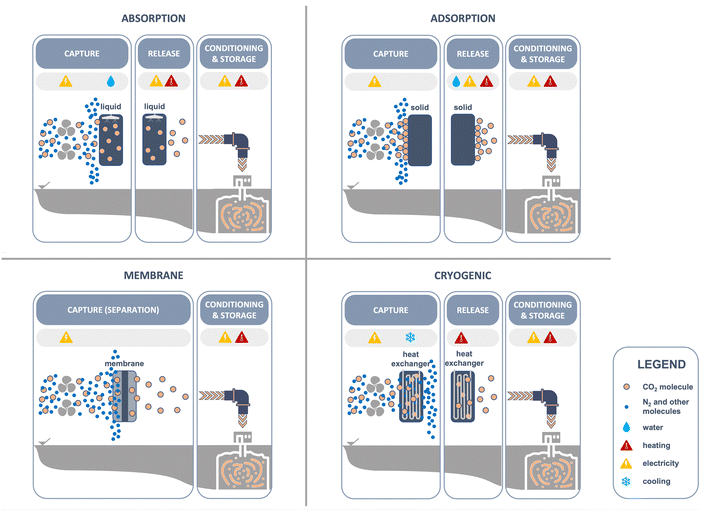 |
| Fig. 2 The four primary separation mechanisms in DAC processes. (i) ‘Absorption’, which works by binding the CO2 within a liquid; (ii) ‘Adsorption’, which works by binding the CO2 to the surface of a solid; (iii) ‘Membrane’ that separates via different diffusion abilities; and (iv) ‘Cryogenic’ process that desublimates (phase change from gas directly to solid without an intermediate liquid phase) CO2 based on the higher freezing point compared to other atmospheric gasses. The CO2 produced from the main separation processes is directed for further conditioning and storage. In absorption and adsorption technologies different drivers for the regeneration of the material can be applied, including water (steam or humidity), electricity, and heat, which results in 11 DAC technology categories (see Table 1). | |
Table 1 Classification of DAC processes based on the CO2 capture mechanism and release drivers. For each DAC technology (i.e., from 1A to 4A), the known capture material, existing companies pursuing the technology, and a data quality assessment are provided. The quality of the publicly available data is assessed based on studies that provide mass, energy, and cost values for each DAC technology. In the ‘process data’ (technical performance data, i.e., mass and energy balance) and ‘plant data’ (economic performance data) columns, colors are represented as: (i) green, high confidence (multiple peer-reviewed studies, theoretical understanding with experimental validation); (ii) yellow, medium confidence (multiple modeling or simulation studies, lack of testing and validation); (iii) red, low confidence (single study, multiple news articles, mostly incomplete data derived from simulations or concept studies); and (iv) grey: no data is found
Capture mechanism |
Release driver |
Capture material |
DAC Companies |
Process data |
Plant data |
1: absorption (with liquid solvent) |
1A: high-grade heat |
Hydroxide (KOH) |
Carbon engineering20 (1PointFive)21 |
Green22 |
Green23–25 |
1B: crystallization & low-grade heat |
Amino acid |
— |
Red26–28 |
Grey |
1C: low-grade heat stripping |
Monoethanolamine (MEA) |
— |
Yellow29–31 |
Yellow30–32 |
Amino acid |
— |
1D: voltage |
Hydroxide (KOH/NaOH) |
Carbon Blade33 |
Green31,37 |
Grey |
CO2CirculAir (SMART-DAC)34 |
Unknown |
E-Quester35 |
Mission Zero Technologies36 |
|
2: adsorption (with solid sorbent) |
2A: high-grade heat |
Hydroxide (Ca(OH)2) |
Heirloom,38 Origen39/8 Rivers40 |
Green41,42 |
Green41 |
2B: humidity |
Ammonium resins |
Avnos,43 Infinitree,44 Carbon Collect (MechanicalTreesTM)45 |
Red47,48 |
Grey |
Alkali carbonate |
Clairity46 |
2C: low-grade heat and/or vacuum and/or steam |
Amine14 |
Carbyon,49 Climeworks,18 Emissol,50 Global Thermostat,19 Hydrocell51 |
Green3,4,68,69 |
Green3 |
MOF |
AirthenaTM,52 Carbon Infinity,53 AspiraDAC54 |
Zeolites |
TerraFixing,55 GreenCap solutions56 (Removr57) |
Akali carbonate |
Sustaera58 (Susteon spinout59) |
Unknown |
Air View Engineering,60 BlancAir,61 Carbon Capture,62 DAC City,63 InnoSepra,64 Noya,65 ReCarbn,66 Skytree67 |
|
2D: voltage |
Redox-active molecule (e.g., Quinone) |
Verdox,16 Holy Grail70 |
Red67,74 |
Grey |
|
|
Ion-exchange fiber |
Carbominer71 |
|
|
Hydroxide (Ca(OH)2)72 |
ParallelCarbon73 |
|
3: membrane separation |
3A: pressure gradient |
Polymer membrane |
— |
Red17 |
Grey |
|
3B: voltage |
Ion exchange membrane75 |
RepAir76 |
Yellow77,78 |
Grey |
|
4: cryogenic separation |
4A: phase change (vapor–solid) |
N/A |
HighHopesTM79 |
Red80–83 |
Grey |
Within each of the 11 DAC technologies (see Table 1), multiple configurations can exist, which can differ by material, equipment, process layout, and energy vector. Configurations reflect the different process layouts, realized either by different DAC companies (or research organizations) pursuing a similar technology, e.g., Climeworks18 and Global Thermostat19 both use adsorption with a temperature vacuum swing process, or by different energy vector, e.g., Carbon Engineering20 looking at both natural gas-fired and electric calciners. A list of these configurations is provided in Table 1 in ESI.†
Table 1 further lists existing DAC companies (not exhaustive), grouped by the 11 technologies. This assignment was done based on our best knowledge using publicly available information and patents. We have not listed DAC companies in the table for which central aspects for classification are proprietary or publicly unknown (e.g., AirCapture,84 Airmyne,85 Hago Energetics86). We also excluded companies mainly focused on integrating DAC into existing infrastructure (e.g., Cedar Carbon,87 CO2Rail,88,89 NeoCarbon,90 Soletair Power91) and DAC companies which are primarily focused on the production of e-fuels instead of generating negative emissions (e.g., Aircela,92 Prometheus,93 and RedoxNRG,94 Terraform Industries95).
3.3 Technology readiness and data quality
For technology analysts, policy and decision makers, and potential investors interested in DAC, a good understanding of the current state of technology development is highly valuable, yet access to good public data on technology performance is currently scarce or non-existent.
As presented in Table 1, most current companies are pursuing a solid sorbent-based approach using temperature vacuum swing adsorption (TVSA), denoted here as Technology 2C. Climeworks18 has deployed this technology at scale.96 Liquid solvent paired with an electrically-driven DAC process (Technology 1D) is second, whereas only one or two companies pursue each of the other technologies. Technologies 1B, 1C, and 3A are not pursued by any company at present. For 1B this is due to the very early stage of the technology, limiting it to laboratory research. On the other hand, 1C is mature but mainly applied to point-source capture from a stack, and similarly, 3A is not yet pursued for DAC applications at high TRL.
Eight out of the 11 technologies have yet to reach the pilot plant stage (TRL 6, following the U.S. Department of Energy definition2). No operational plant data exist, and the only information available comes from lab-scale experiments (TRL 3–4), small lab pilots (TRL 5) and/or from modeling. This implies that most DAC technologies still have significant lead time to reach commercialisation. Due to low TRLs for the majority of DAC technologies, most public data sources limit their scope to the capture process itself, and do not include any auxiliary material and energy requirements, or equipment costs for the deployment of the process within a DAC plant. Accordingly, in Table 1 the available data for each of the 11 technologies is separated into two different categories: (i) mass and energy balance (MEB) data of the capture process (e.g., system boundary is placed around the DAC unit, and not around the DAC plant as of Fig. 1) and, (ii) additional data required for the deployment of a DAC plant (system boundary as defined in Fig. 1). The former allows for an approximation of performance for the different technologies (see Section 4.1), whereas the latter provides sufficient information for a (rudimentary) techno-economic assessment (see Section 4.2).
The quality of the publicly available data is indicated through colors in Table 1, grouped into low, medium, and high confidence.
Table 1 highlights that good quality, independent, and complete data for DAC plants is scarce, if not lacking from the public domain. The publicly available Stripe/Frontier applications68 are one of the rare resources for data on the rapidly increasing number of startup DAC companies. There is a general lack of technical performance data for 6 technologies (highlighted in red in the ‘process data’ column of Table 1), and only complete and sound technical and economic data is available for 3 technologies (highlighted in green in the ‘plant data’ column of Table 1).
Publicly available data for each of the different technologies is compiled and presented in Table 2. This data represents the baseline for the identification of techno-economic drivers (see Section 4), obstacles (see Section 5), and the formulation of priority initiatives (see Section 6).
Table 2 Publicly available data for the considered DAC technologies. Data on maximum TRLs, energy requirements (i.e., natural gas, waste heat and electricity), water dependency, effect of humidity, raw material requirements, produced wastes, and estimated land use are provided per identified DAC configuration. The 11 DAC technologies (listed in Table 1) have been further classified into configurations based on their difference in material (m), equipment (q), process layout (p), or energy vector (e)
DAC configuration |
Max TRL |
CO2 purity [%] |
Natural gas [GJ per tCO2] |
Waste heat [GJ per tCO2] |
Electricity [GJ per tCO2] |
Water dependency |
Humidity |
Raw materials |
Waste |
Estimated land use |
Electricity demand for low-grade heat pumps. Heat demand follows from multiplication with COP, here assumed to be 2.
Electricity demand for resistive heaters to provide low-grade heat (COP of 1).
|
1A |
(e)NG |
6 |
97 |
9.8 |
— |
— |
High (around 4.38 t per tCO2, as 500 t per hour for 1 MtCO2 per year plant) |
Drier areas have larger water requirements (increased evaporation) |
KOH and CaCO3 solutions |
Waste CaCO3 fines (0.03 t per tCO2, as 3.4 t per hour for 1 Mt per year CO2 captured), degraded KOH |
>0.016 km2 per Mt per year97 |
(e)Electric |
? |
97 |
5.3 |
— |
1.3 |
(e)Syngas |
? |
97 |
5.3 |
— |
0.3 |
1B |
(m)PyBIG |
3 |
100 |
— |
— |
3.25a |
Water intake expected but magnitude unknown |
Same as 1A |
Glycine and sarcosine amino acids |
Degraded amino acids, waste M-BBIG (an organic molecule) |
No data |
1C |
(m)MEA |
1–2 |
100 |
— |
— |
15.9 (10.7b) |
High |
Same as 1A |
MEA solution |
Degraded MEA, volatile MEA – emissions to the atmosphere |
Large plant footprint |
(m)Amino acid |
4 |
100 |
— |
— |
16.6 (12.6b) |
High |
Same as 1A |
Amino-acid salt solution |
Degraded amino-acid salt solution |
Large plant footprint |
1D |
(q)BPMED |
4 |
90 |
— |
— |
22.4 |
Same as 1A |
Same as 1A |
KOH solution |
Degraded membrane and KOH |
No data |
2A |
(m)MgO looping |
6 |
? |
5.9–8.0 |
— |
0.3 |
Depending on mineral and hydration, N/A for MgO, 2.5–5 t per tCaO98 |
High humidity increases reaction rate |
Fines makeup (MgCO3) |
Loss of fines to environment |
6 km2 per Mt per year (large area needed for MgO spreading) |
2B |
(p)Passive air flow |
4 |
5 |
— |
— |
1.2 |
High water demand for CO2 release and material regeneration |
Very low humidity required |
Ion exchange resin powder (I200) from polypropylene with ammonium |
Degraded ion exchange resin |
0.08 km2 per Mt per year97 (unknown spacing criteria of mechanical trees) |
2C |
(p)Waste heat |
9 |
99 |
— |
9.8 |
1 |
Cooling to condense water |
Humidity will impact performance. For one specific sorbent, higher humidities negatively impact cost. |
Amine-based solid sorbent |
Degraded amine-based solid sorbent |
0.1–2 km2 per Mt per year97 |
(q)Heat pump |
|
99 |
|
— |
5.9 (4.9a) |
2D |
(q)Bed100 |
3–4 |
100 |
— |
— |
1 |
N/A |
No effect |
Redox-active materials (e.g., quinones) |
No data |
No data |
3A |
(p)DeltaP50 |
1–2 |
40–60 |
— |
— |
44–62 |
N/A |
No data |
Organic polymer membranes |
No data |
No data |
3B |
(m)Shorted membrane |
3–4 |
? |
— |
— |
41–62 |
N/A |
No data |
PiperIon PAP-TP-85 ionomeric membrane and conductive additives (e.g. CNTs, Vulcan XC-72R) |
No data |
No data |
4A |
(p)Heat exchanger |
2 |
? |
— |
— |
5 |
N/A |
Air is usually dry below freezing |
Aluminum for heat exchanger |
N/A |
Limited to arctic regions |
4 Techno-economic drivers
We compared all technologies, apart from cryogenic,§ based on their techno-economic data. For each, we extracted insights from the regional variation in price and carbon intensity (Section 4.1) and, when possible, from full TEA of FOAK DAC plants (Section 4.2). The results of this work drive our analysis on the obstacles, and more specifically obstacles primarily linked to the high energy and cost requirements of DAC technologies and their current development state. When obstacles are identified from this analysis, they are marked following the complete list of obstacles presented in Section 5. Obstacles are denoted as ‘#A’, where ‘A’ refers to the number assigned to each obstacle.
4.1 Insights from energy prices and carbon emissions
The capture cost of a DAC plant depends on its location due to wider system integration and local ambient conditions. DAC is often proposed to be renewable electricity or waste heat powered. This may be a justifiable assumption for a future where the electricity grid is fully decarbonized, or for small plants that can tap into limited available waste heat. Here, however, we analyze DAC technologies assuming existing energy supply systems, and translated the energy demand values from Table 2 to operational costs for different regions in the world,¶ using costs and emission intensities for four case study countries and one ‘best case’ scenario (see Table 3). The ‘best case’ scenario combines the lowest observed energy prices (reported in the USA) with the lowest observed natural gas leakage rates (reported in UK and Germany) and renewable electricity production. The methodology of the calculation is detailed in Section S2 in ESI.†
Table 3 Energy-related foreground data for 5 case studies. Energy prices,100,101 carbon intensity,102,103 natural gas (NG) leakage,104 and associated global warming potential (GWP) on a 100 year time horizon for four case study countries and one hypothetical ‘best case’, which combines the lowest energy prices with the minimal leakage rates and renewable electricity production
Case study |
NG pricea (USD per MW per h) |
Electricity pricea (USD per MW per h) |
Carbon intensity of electricity (gCO2e per kW per h) |
Natural gas leakage (%) |
GWP100 for leaked methaneb (gCO2e per kW per h) |
Costing year of 2019, exchange rate USD to GBP of 1.28.99
Global warming potential (GWP) assessed on a 100 year time horizon for methane, assuming 100% share in natural gas.
|
USA |
12.9 |
68.2 |
388 |
1.3 |
25.3 |
UK |
27.6 |
147.5 |
225 |
0.3 |
6.7 |
Germany |
29.0 |
149.6 |
311 |
0.3 |
5.3 |
France |
40.8 |
117.9 |
51 |
1.7 |
34.2 |
Best case |
12.9 |
68.2 |
12 |
0.3 |
5.3 |
The different case studies allow us to assess the impact of energy price and carbon intensity on capture costs. Despite its large fugitive methane leakage, USA generally shows the lowest costs (Fig. 3), which indicates that energy price is a stronger driver for net captured costs than CO2 intensity and/or natural gas leakage. In comparison to Germany, USA values are always lower despite having higher upstream carbon emissions. In France, low-carbon electricity strongly favors electrically-powered configurations but still leads to higher costs than the USA case. This underpins the relevance of minimizing energy requirement, further discussed in Section 5 (#2, #5, #6, #7, #12).
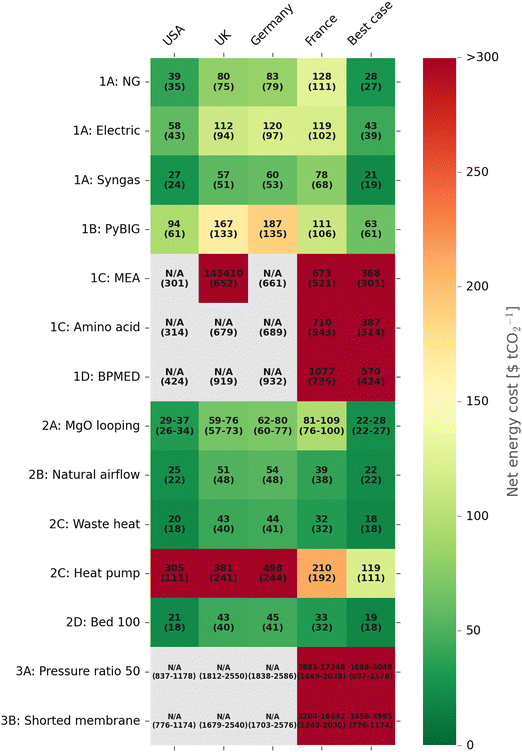 |
| Fig. 3 Net energy and gross energy (in brackets) costs in USD per tCO2. The costs are provided for the 14 DAC configurations and for 5 electricity mix cases: USA, UK, Germany, France, and ‘best case’. ‘N/A’ denotes net positive emissions due to an emissions factor (i.e., amount of CO2 emitted over amount of CO2 captured) of >1 (see Section S2 in ESI†). | |
Looking at the differences in net and gross costs across selected countries, the carbon intensity of electricity has a stronger impact on costs than methane leakage rates of natural gas. This is not to say that methane leakage is generally less important; the selected countries lie on the low end for natural gas leakage rates, where values of 2.2% (Brazil, China), 7.2% (Turkey), 15.6% (Greece), and 21.1% (Slovakia) exist.104 The variability in carbon intensity for electricity among our five case studies is larger than the variability in methane leakage rates, which translates to a stronger impact of the electricity supply on the net costs. Or in other words, reducing the energy requirements of electrically-powered configurations has a higher impact on net-removal costs than energy reduction for processes powered by natural gas. This dependency on low carbon electricity and minimal methane leakage rate presents a clear obstacle to scaling (#20). Furthermore, across considered configurations, five (i.e., ‘1C: MEA’, ‘1C: Amino acid’, 1D, 3A, and 3B) would not lead to net-negative emissions when tying into current energy supply systems, with the exception of that of France. This further underlines the need for large scale low-carbon electricity (#20), and reductions in energy consumption, where the energy consumption can improve through selection of optimal material (#2), process design (#5, #6, #7), equipment (#10, #11), system integration (#16, #17), and process learning from deployment (doing, experience) (#8, #9, #17).
The results for configurations 1A and 2A also show that renewable energy is not a prerequisite for DAC. Oxy-fired natural gas-driven processes show competitive energy costs. Neither 1A nor 2A lead to net-positive emissions. Moreover, the natural gas configurations are at the lower end of the cost ranges compared to the other technologies. This shows that fossil-powered DACCS may have a place in the transition to a zero carbon grid.
Based on the best case scenario in Fig. 3 and only accounting for the price of electricity and natural gas, the energy cost could range between USD20–1000 per t of net CO2 removed. These numbers are solely indicative due to inhomogeneous data quality, missing independent validation of reported performance (#4, #8, #9, #12) or unknown effects of geographical temperature and humidity variation (#3, #16), but provide baseline threshold values.
We believe that inhomogeneous data quality has the strongest effect on prohibiting a fair comparison on energy-related costs illustrated in Fig. 3. The most extreme example is Technology 2B. Humidity Swing produces a significantly less concentrated stream of CO2 as a product (5%) than other technologies. It is expected that significant energy will be required to condense large amounts of water and to concentrate CO2 so it can be plugged into current permanent storage options, or that alternative storage options for low-purity DAC have to be developed. Technology 2B also assumes that passive air flow is sufficient for allowing the atmospheric air to pass through the air contactor unit. If this is not the case, additional energy will be required.
4.2 Insights from first-of-a-kind DAC plant economics
As indicated in Table 1, sufficient data is available for technologies 1A, 1C, 2A, and 2C to conduct a harmonized TEA, for which the results are presented in Fig. 4 and 5. Fig. 4 presents the levelized cost of gross and net CO2 captured of the FOAK commercial plants for these 4 technologies (total of 6 configurations) and using US energy prices. The costs of CO2 transport and storage (T&S) are excluded, as to focus on the capture technologies. T&S costs are important, although they can be assumed roughly identical for the 4 technologies as each of them produces highly concentrated CO2 streams (above 95% purity). For interested readers, Young et al.105 present values including T&S costs.
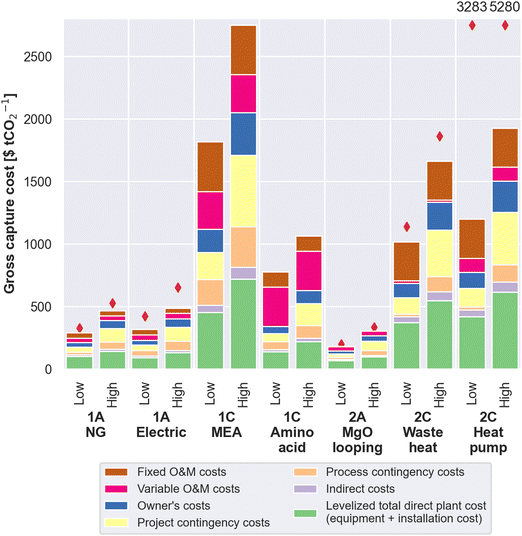 |
| Fig. 4 Estimated levelized FOAK gross and net capture cost for 7 configurations using our harmonized TEA framework. The 7 configurations are presented in Table 2 and described in Section S1 in ESI.† Note: the low and high estimates result from using upper and lower values for contingencies (see Tables S3 and S4 in ESI†), and the upper and lower data values provided by the original sources. The bars are presented here are based on energy prices for the USA and represent gross capture cost. The costs for net CO2 removal are larger and available as a diamond when they fall within the scale of the graph, based on the respective DAC process emissions: ‘1A: NG’, +13%; ‘1A: Electric’, +34%; ‘1C: MEA’, net positive, ‘1C: Amino acid’, net positive (see Fig. 3); ‘2A: MgO looping’, +10%; ‘2C: TVSA waste heat’, +12%; ‘2C: TVSA heat pump’, +174%. | |
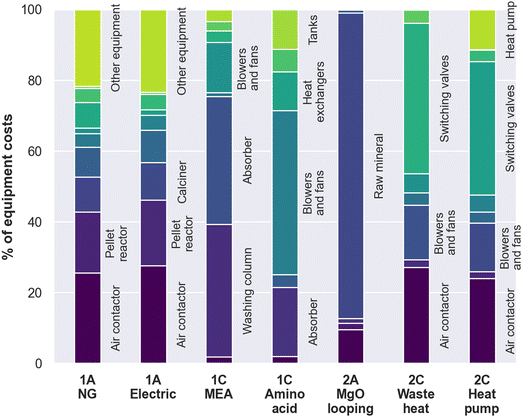 |
| Fig. 5 Equipment cost breakdown for 7 configurations resulting from the harmonized TEA. The 7 configurations are presented in Table 2 and described in ESI,† Section S1. Any pieces of equipment which make up more than 10% of the total equipment cost are labeled. | |
The estimated gross capture costs in Fig. 4 range between USD60-2750 per tCO2 where two cost values are presented for each configuration due to different assumptions for process and project contingencies, and the range in available cost data. Technology 2A currently presents the lowest cost (i.e., around USD60-300 per tCO2) and a high spread between the estimated cost values, which mainly originates from the large difference of the two plant sizes presented in McQueen et al.41 Technology 2A is also the only technology that currently has the potential to cost below USD100 per tCO2, but values can reach up to USD300 per tCO2. In addition to the gross cost values, Fig. 4 presents net cost values (as diamonds), incorporating the carbon intensity of the US energy supply. The respective values, are found to be 19% to 48% higher. For Technology 1C, regardless of the configuration, the emissions from the DAC process in the US context would be larger than the captured CO2, resulting in a net increase of CO2 emissions instead of a reduction (no diamonds for Technology 1C in Fig. 4).
Fig. 4 also shows that the largest share of the cost for a FOAK plant is attributed to capital cost items: project and process contingencies (see Fig. 4) contribute to 17–39%, depending on the configuration. The process contingencies represent the likely cost increase a technology will undergo when moving from lower TRL to a FOAK commercial plant (as a result of issues that require further engineering, the addition of auxiliary or balance of plant equipment, inefficiencies during scale up, among others).106 Other large cost contributors are direct equipment and installation costs (green bars), ranging from 16 to 40%, and owners costs (blue bars), ranging from 7 to 14%. High equipment costs highlight the urgency and importance in research, development and construction of fit-for-purpose equipment, which is currently lacking (#10), for significant reduction in capital expenditure (CAPEX). Operational costs represent a smaller share of the total capture costs for some technologies (i.e., 1C and 2C) and a higher share for others (i.e., 1A and 2A). As we move from FOAK to the nth-of-a-kind (NOAK) plant, it is expected that capital costs will fall more rapidly than operational costs as a result of learning by doing and scaling.105 Reducing operational costs requires reducing energy use, which is limited by physical boundaries. A second law efficiency of >30% is seldom seen in CO2 separation technologies107 while recent modeling has shown it is unlikely that solid sorbent DAC second law efficiencies larger than 20% will be reached, even with the most optimal material-process combinations.108 To achieve further operational cost reduction, insights and breakthroughs in material science are also crucial (#1). Technologies 1C and 2C could highly benefit from this area of research, innovation, and development (see operational costs in Fig. 4).
For capital costs of the TVSA configuration, we used quotes from interviews with Climeworks founders. They state that USD10–15 M was required to build the Orca plant (with removal capacity of 4000 tCO2 per year)18 and USD3–4 M for building the Hinwil plant (with removal capacity of 900 tCO2 per year).18 In addition, independent academic assessments and breakdown of the capital costs are beginning to be published from IEAGHG,10 Young et al.,105 Sendi et al.,109 Valentine et al.110 There are still significant uncertainties, especially with respect to the air contactor cost, as completely novel designs are required. However, capital costs are estimated at 40–60% of the total investment cost for a FOAK plant and about 20% for a NOAK plant.10,105
Additionally, it is important to highlight that technologies 1A and 2A exhibit the lowest FOAK costs. Both of these technologies utilize significant economies of scale compared to 2C, for example, which is more modular. This leads to lower capital costs, particularly for the FOAK plant. Conversely, this also means that fewer opportunities are available to bring down the capital cost via technological learning.105 As a result, these technologies will not necessarily demonstrate the lowest costs for a NOAK plant. Beyond the scale of the technology, technology 2A also benefits from an uncomplicated process design and low-cost sorbent. However, there are limits to the scalability of this technology based on sorbent availability. But, this can be overcome by using other minerals such as CaO.
In summary, Fig. 4 presents four points: (i) current DAC technologies have net removal costs well above USD100 per tCO2 (this is in line with reported cost for DAC technologies of >USD300 per tCO26), (ii) the spread between higher and lower cost estimates is large, denoting the current level of DAC deployment and the uncertainty around reported technology performance, (iii) some DAC technologies would render net positive emissions when deployed in todays US energy system (or in similar electricity grids), and (iv) large cost shares of CAPEX indicate expected large future cost reduction for NOAK plants.
Fig. 5 breaks down equipment cost presented in Fig. 4. It shows that air contactors and absorbers for absorption-based technologies (i.e., 1A and 1C) have a high contribution to equipment cost. Lack of optimal, fit-for-purpose air contactors or other separation units make this cost share more significant (#10). According to Keith et al.,23 the high cost for air contactors (for Technology 1A) is associated with their size and slow transfer of gaseous CO2 into the solution. These are also expected barriers for the case of absorbers and water wash columns (Technology 1C), where limited lab-scale and absence of pilot-scale testing make it more difficult to accelerate the design of optimal DAC separation units both at process design (#8, #10, #11) and system integration levels (#17). Additionally, pellet reactors and calciners, for Technology 1A, and washing columns and blowers/fans, for Technology 1C, also represent a significant share of CAPEX. The cost for pellet reactors and calciners maybe overcome with specialized supply chains (#14).
The equipment cost estimates for the ‘1C: MEA’ and ‘1C: Amino acid’ configurations are based on the work of Kiani et al.30 For the ‘1C: MEA’ configuration, Kiani et al.30 estimated that the water washing column and the absorber account for 73% of the capital cost. The ‘1C: Amino acid’ configuration does not require a washing section, which reduces its capital costs (see Fig. 4). The main cost driver for the ‘1C: Amino acid’ configuration is the blower and fans, at 46% of the total equipment cost. The second largest contributor is the absorber, which accounts for 20% of the equipment cost. However, Kiani et al.30 assumed cost reductions for the absorber for the application in DAC. If no cost reductions are applied (and a conventional absorber is used), then the absorber would have been the largest equipment cost, at 59% share.
For configuration ‘2A: MgO looping’, the cost of purchasing the mineral and the operational expenditure (OPEX) associated with moving them are the biggest cost contributors. Switching from MgCO3 to a cheaper, more abundant mineral (e.g., CaCO3) could significantly decrease the cost of this technological solution. The calciner is the second most important cost item in Technology 2A. Heirloom is pursuing an electrically-powered calciner, which is different from the here-used study by McQueen et al.41 It is not clear if an electric calciner will reduce the equipment cost but it is expected to have an impact on the net CO2 removal cost and OPEX.
The four main cost drivers of adsorption-based technologies using a temperature vacuum swing process (Technology 2C) are: (i) high capital costs for the air contactor, the blowers and fans, and the switching valves; (ii) high fixed operational cost; and (iii) high variable operational cost, for the ‘heat pump’ configuration. The primary energy driver for adsorption-based systems is the energy required for sorbent regeneration. The crux here is that in current adsorption systems like Technology 2C, most energy is not needed to break the bond between the sorbent surface and CO2, but for heating packing material and desorbing co-adsorbed water. The development of materials with higher CO2 mass transfer rates (to increase adsorbent loading), reduced CO2 heats of adsorption, a higher heat transfer rate, lower water adsorbed amount, and a lower heat capacity have all been shown to reduce the energy penalty of solid adsorbent TVSA processes, and thus provide a pathway for sorbent development.108 The second biggest energy driver for adsorption-based systems is the energy required for passing large amounts of air through the air contactor unit (due to the resistance to flow that the air contactor and the material impose, known as backpressure) (#10). The higher the backpressure the greater the energy requirement is.
5 Obstacles
Based on the insights obtained from publicly available data on DAC technologies, our TEA harmonization, and our consultation sessions with the advisory board, we developed a list of obstacles categorized into: (i) materials, (ii) process design, (iii) equipment, (iv) system integration, and (v) infrastructure obstacles (see Table 4). A comprehensive list of these obstacles is provided in Table S5 in ESI,† and they are further analyzed in this section.
Table 4 List of technology- and infrastructure-related obstacles. Technology related obstacles are further classified into: (i) materials, (ii) process, (iii) equipment, and (iv) system integration obstacles
No. |
Category |
Obstacle |
#1 |
Material |
Unexplored materials |
#2 |
Material |
Unexplored material benefits |
#3 |
Material |
Unknown/unreported stability and recyclability |
#4 |
Material |
Missing independent validation of reported performance |
#5 |
Process design |
Lack of detailed process designs and evaluation |
#6 |
Process design |
Unexplored synergies between material and process development |
#7 |
Process design |
Unexplored process potential |
#8 |
Process design |
Missing validation of process operation at scale |
#9 |
Process design |
Lack of public data and independent performance assessment |
#10 |
Equipment |
Lack of fit-for-purpose equipment |
#11 |
Equipment |
Unexplored process intensification |
#12 |
Equipment |
Lack of comparative studies in DAC settings |
#13 |
Equipment |
Designs for crucial DAC equipment are proprietary |
#14 |
Material & equipment |
Lack of specialized supply chains |
#15 |
System integration |
Lack of TEA and LCA studies |
#16 |
System integration |
Lack of studies on DAC integration into local/regional climates |
#17 |
System integration |
Lack of upscaling studies |
#18 |
System integration |
Lack of studies on regional environmental and socio-economic impacts |
#19 |
System integration |
Lack of DAC integration into industrial clusters |
#20 |
Infrastructure |
Competition for access to low-carbon energy |
#21 |
Infrastructure |
High water footprint |
#22 |
Infrastructure |
Land transformation and high footprint |
#23 |
Infrastructure |
Lack of existing CO2 transport infrastructure |
#24 |
Infrastructure |
Lack of existing CO2 storage sites |
5.1 Materials
The most advanced DAC technologies primarily rely on commercially available materials (e.g., potassium and calcium hydroxide or polymer resins) around which a working concept has been formulated. This approach has helped to reduce technical risk and support accelerated pilot, and commercial scaling.18–20 There are numerous existing materials and millions of hypothetical ones that can be generated in silico to be explored for DAC applications.111–115 Their properties (e.g., density, solubility, permeability, volatility, viscosity, porosity, heat of absorption, heat of adsorption, thermal conductivity) cover a broad design space, making their exploration challenging (#1). Further materials research could help reduce the energy consumption or increase CO2 removal efficiency (#2). For example, material properties such as specific heat capacity and thermal conductivity have a direct impact on the process energy requirements and, consequently, the OPEX of the DAC process.112 Additionally, material's ability to select CO2 over other components in air, such as nitrogen, oxygen, and water, is expected to affect its CO2 removal efficiency and can impact not only the OPEX but also the CAPEX of the process. However, even for existing materials, tradeoffs between material properties are largely unexplored. Lack of such studies and established frameworks for guiding the selection of materials are currently an obstacle in enabling an advanced and rapid deployment of DAC technologies (#2).
Material stability (thermal, chemical or mechanical) and recyclability (#3) are crucial in reducing the economic and environmental impact of the DAC unit. Using materials which are not stable or reusable for many capture and release cycles may increase operational costs for material replacement, and a higher environmental impact, due to a greater demand for material synthesis, disposal and, in some cases, degradation due to oxidation or thermal decomposition.116–121 For most materials there are questions on stability when operating in a real-world environment, especially as a result of exposure to high oxygen concentrations, humidity or other impurities in air.120,122 The degradation of materials may also lead to atmospheric release of unwanted compounds which could require additional remediation cost.118,119 However, there is currently a lack of studies on the mechanisms that cause material aging, degradation, and loss to the atmosphere. Also, it has been shown that different environments have a varying effect on materials performance, especially productivity (#3). For example, some adsorption materials may perform well in terms of CO2 removal efficiency in dry environments, but are expected to perform poorly under high humidity (e.g., zeolites, K2CO3).69,120,122–124 The lack of repeated cyclic exposure to capture and release conditions is a crucial obstacle to scale and is related to the current state of DAC: early stage of R&D, advanced RD&D comes from companies rather than academic groups and research organizations, and limited availability of test trials.
Lack of reported/measured properties for existing materials or theoretical optimal properties make it difficult to build a framework for what makes an “ideal” material for DAC applications. There is also a lack of independent validation of reported performance (#4), given that many inventions are further developed within company walls, limiting public dissemination of performance data.
5.2 Process design
For 5 out of the 11 considered DAC technologies in this study, detailed process designs are not yet formulated (#5). Process designs that do exist often cover the heart of the separation process (i.e., CO2 capture and release) with constrained boundaries neglecting other elements/units/systems crucial for scaling up DACCS (e.g., integrated energy systems, heat and electricity storage layouts, CO2 conditioning processes and equipment needed for CO2 utilization/conversion/storage). Depending on the selected CO2 destination option, different specifications (e.g., CO2 purity, maximum level of impurities, temperature and pressure) of the CO2 product stream will be required and those are often not taken into account in process designs. Consequently, for Technologies 1B, 2B, 2D, 3A, and 4A, ‘process data’ is very limited (see Table 1). For the remaining technologies, independent assessment of process design and performance is also missing, but available for technologies (i) 1A on KOH with calcium looping,23 (ii) 1C on amine-based carbon capture,30 (iii) 1D on KOH with electrochemical regeneration,31 and (iv) 2C on solid sorbent TVSA.3,105 Where independent assessment of process design and performance is present, it is probable that there is room to further optimize process design and operation.105
However, detailed process models that use traditional performance criteria, such as CO2 removal efficiency – in the case of a CO2 separation process – and energy consumption can lead to incorrect conclusions in assessing the suitability of a material and a process (#5). For example, thermodynamic models that exclude mass and heat transfer effects cannot be used to predict productivity and simple metrics such as working capacity and may fail to indicate the right material and process. Other examples include mechanisms where models largely differ from observed data and are therefore unsuitable to guide the process design, e.g.; the kinetic interaction of CO2 and water on the adsorbents. Other studies have shown that metrics beyond material and process design, such as capital and OPEX (their evaluation requires a TEA of the process) and climate change (its evaluation requires a life cycle assessment (LCA) over the entire supply chain), may lead to different conclusions on the suitability of a material and/or a process.111 There are currently limited studies that go beyond material metrics and explore the synergies between thousands of materials and different process designs with the help of a computer (#6).
Exploring the synergy between material and process is crucial. Studies have shown that a lack of such a harmonization can lead to expensive delays in identifying optimally tailored materials.111–115,117 Also, no framework exists for advising on optimal material and process characteristics needed to catalyze large-scale implementation of DAC. This obstacle is observed among all DAC techniques, even those currently at higher TRL.
Understanding of key cycle steps and sequences, regeneration methods (e.g., indirect heating, Joule heating, microwave, electric calciner) and bed configurations (e.g., fixed beds, moving beds, fluidized beds) and packing for DAC processes (#7) is currently limited.117 For example, in adsorption systems the regeneration method for a DACCS system cannot be easily selected as constraints on CO2 purity might be imposed by the CO2 destination option.111 If a TVSA process is considered (Technology 2C), there is no straightforward process configuration to ensure the desired specifications in the product stream can be met at the lowest possible cost. Alternative heating methods such as indirect heating, steam injection, or an electrified method could significantly improve the process performance and reduce the energy consumption of DAC.125,126 Similarly, process heat integration can offer great potential to minimise sensible heat requirement for a TVSA process and is explored by Climeworks.127
Successful demonstrations at TRL 6 or higher are also critical to increasing process fidelity and de-risking technologies. There are currently few technologies that operate beyond lab-scale (e.g., 1A, 2A, 2B, and 2C) and few others that have secured funding for their first pilot (e.g., 1C) (#8). Moving to a pilot/demonstration phase will enable the verification of DAC systems and the investigation of gaps that can only be addressed at scale (e.g., the spacing of air contactors to avoid taking in CO2 depleted air from a neighboring contactor). For all technologies except 1A and 2C, verification of successful operation beyond lab-scale is absent. To date, there is a caveat in reported pilot or commercial scale operations, either because technologies have yet to reach TRL 6, or because plant data is not shared publicly. Small but important exceptions include Deutz and Bardow3 and Schellevis and Brilman.128
Equally, the lack of public data on DAC technology operational performance and experimental data on verification of scaled operation limits independent performance assessment (#9). This is a barrier to DAC innovation and deployment as it limits researchers from pursuing concept innovation, companies from refining and optimizing solutions, and governments, investors, and policy makers from making the most informed policy and funding decisions.
5.3 Equipment
Components and materials used in the few existing pilot or commercial DAC plants are either general purpose products or expensive purpose-built solutions. For nearly all DAC technologies, there is an absence of DAC-specific mass produced equipment or large scale availability of advanced materials. Examples include MOFs, air contactors, fans/blowers, CO2 compressors, and energy units/sources, e.g., heat pumps, heat exchangers, photovoltaics, and energy storage layouts (#10).23,129–131 Apart from the air contactors, the other equipment are already used at commercial scale and, therefore, their conversion to DAC-specific design is expected to be more straightforward, even though there is still room for improvement. For example, for technologies requiring low-temperature heat, there is room to further improve low-temperature heat pumps, opening up the use of electricity for provision of regeneration heat.129
Air contactors are key equipment components for DAC due to the significant airflow required for scaled CO2 capture. To the best of our knowledge, there are currently no published comparative studies on contactor designs (e.g., conventional contactors, cross-flow columns, membrane contactors) and their performance in a DAC-specific setting (#11) preventing fair comparison between different air contactor designs or passive air contacting methods, which limits the pace of DAC deployment and equipment optimization.
Carbon Engineering has made significant headway in increasing the productivity of air contactors for liquid solvents.23,132 If DAC is to scale, productivity must improve to reduce the number of units needed, reducing plant footprint and overall liquid solvent DAC plants costs (see Section 4). Additional work is needed to optimize other parameters for improved air contactor designs/configurations, such as low pressure drop, high CO2 uptake, fast mass and heat transfer, low footprint, and low capital cost. For instance, Carbyon, developed a rotating contactor for optimizing these characteristics,49 Global Thermostat designed a rotating multi-monolith moving bed,133 and Climeworks developed a packed bed system with appropriate flow distributor plates.134 For membranes the design of optimal modules remains an open question, for electrochemical regeneration of alkaline solvents an alternative cell design (e.g., high pressure cells) will potentially overcome cell resistance (as a result of CO2 bubble formation) and thereby lower energy consumption. An additional challenge is that existing air contactor designs from Carbon Engineering, Climeworks and Global Thermostat are proprietary (#13). Ideally, air contactors would be developed by general equipment providers to serve other DAC companies, lowering capital costs across the DAC portfolio.
For technologies where solid minerals are regenerated, there is a lack of rigorous comparison and development of different calcination methods (e.g., oxy-fired, post-calcination CO2 capture, solar calcination, electric calcination, or calcination using alternative fuels like biogas). Equally, air contacting strategies for solid minerals (heaps, trays, reactors, spreading on land) require further investigation and comparison.
Process, equipment, and material design are highly interdependent and, therefore, an integrated optimization is important. This can be done through a process intensification that explores the synergy between different unit operations and processes for efficient performance and low energy consumption. However, this work is currently unexplored for DAC technologies or lacks publicly available information (#12).
For DAC to scale at a rate adequate to address the pace of climate change, plants must be brought online rapidly. In large industrial settings this will likely require this generally requires the development of specialized equipment and material supply chains (#14). These do not yet exist, in part because most technologies have yet to scale to TRL 6 or higher.
5.4 System integration
In Section 4, the lack of real plant data as inputs for TEAs, the use of different assumptions and system boundaries across studies, the exclusion of auxiliary and balance of plant equipment, et cetera, have highlighted the challenges associated with current DAC TEA studies (#15). Comprehensive LCA studies are further challenged, as the system boundaries are broader (e.g., material synthesis, material reuse or disposal, heat and electricity storage systems, and integration of CO2 transportation and storage infrastructure) and, therefore, more data is required. Few studies exist assessing the overall environmental impact of low-carbon energy sources or the material depletion using a wide set of environmental impact categories.3,135 Further, there are currently limited studies on the evaluation of different electricity (e.g., grid, photovoltaic power and nuclear) and heat sources (e.g., electricity, waste and solar heat) for DAC technologies in TEA and LCA studies. For these systems, the integration of high-temperature heat pumps, a Fresnel solar heat plant, thermal energy and electricity storage systems may be needed and are rarely present (except from Terlouw et al.130) to allow for a holistic analysis of the DACCS system. DAC(CS) LCAs have shown that energy consumption of DAC is a crucial element in terms of environmental impact. Therefore, the consideration of different energy sources is important for identifying the optimal set of DACCS solutions per location. For instance, the availability and use of waste heat is considered an optimal heat source as it is available at low cost and it comes almost burden-free in terms of environmental impacts.
Due to the current lack of DAC demonstration plants, LCAs based on real-world conditions are limited. Existing work has focused on Technologies 1A and 2C3,130,136,137 and comprehensive LCAs of the other technologies are critically missing, limiting their ability to guide their development to minimal environmental impact. There is also lack of integration of DAC with the processes needed for CO2 transportation and storage based on the geographical storage location: energy needs for compression, infrastructure requirements, drilling of boreholes, electricity for the injection of CO2, CO2 leakage from CO2 transportation in pipelines. LCAs that exclude advanced environmental impact categories, such as material resources and depletion, or important infrastructure aspects such as land transformation and water availability, are lacking key information. The absence of such system-level assessments will delay understanding the future role and limitations of DACCS in the climate response and may delay the design of novel systems (e.g., heat and electricity storage) that allow for (near-)autonomous DAC operation in proximity of, for instance, geological CO2 storage sites.
Understanding how local/regional climates affect the performance, cost and environmental footprint of the different DAC technologies is a key knowledge gap. Relevant sensitivity studies are missing for all technologies, with the exceptions of KOH with calcium looping138 and solid sorbents TVSA109,139 (#16). Across these studies, the net removed cost varies by a factor of over 2. Climate sensitivity studies are crucial to making informed siting decisions for DAC, to understanding their performance and resource needs across regions, and also to adjusting and developing materials and processes to suit specific climates. Terlouw et al.130 have shown that the deployment of DACCS at geographic locations with CO2-intensive grid electricity mixes leads to net GHG emissions instead of GHG removal.
It is expected that upscaling will be crucial for near-future DAC units instead of large-scale roll-outs. However, there is currently limited work and no publicly available data on the performance of demonstration plants (#17). Technology scaling will partially depend on minimum viable size. Naturally, economies of scale lead to lower costs if the plant is larger. Young et al.105 showed that for both KOH with calcium looping and MgO looping, the costs increase rapidly if the starting scale is below 0.5 MtCO2 per year. Meanwhile, the starting scale does not seem to be as critical for the modular technologies, such as temperature vacuum swing adsorption or KOH with BPMED regeneration, as it is expected that they can easily be scaled up (or scaled out). However, there are many important insights that are currently missing and can be extracted from the operation of these plants, e.g., material replacement, material degradation, optimal spacing between air contactors,140 process response and operational control, that can be beneficial for optimizing the DAC unit and whole systems setting. Also, the starting scale is expected to impact how many doublings can be achieved in a technologys development, which is critical to reducing cost via technological learning.
Likewise, there are currently limited studies on regional environmental and socio-economic impacts (#18). For example, the integration of aim scenarios into local energy system models are crucial for determining the future carbon removal potential of DACCS systems. Effectively pursuing this requires a good understanding of DAC technologies technical, economic, and environmental performance. This performance data can then be fed into local/regional energy system models138,141 and IAMs for assessing the contribution of DACCS in the low-carbon energy transition.
Lastly, studies on integrating DAC into existing industry clusters are largely absent (#19). DACCS could benefit if integrated into already existing industrial clusters by either using waste heat, if available from other industrial processes, or delivering conditioned CO2 to already existing CCUS infrastructure. There are several transport and storage projects currently in development, which are associated with industrial clusters (e.g., four transport and storage projects linked to five industrial clusters in the UK, the Porthos project in the Netherlands for transporting CO2 to offshore storage sites, and the Houston CCS storage hub in the US) that DACCS could benefit from by plugging in. Although integration into existing industrial clusters appears beneficial, it may also present tradeoffs given opportunities to optimize waste heat integration within the cluster before it is dedicated to DAC operations. However, the lack of studies on how this integration (including land availability and requirements, energy system integration, CO2 conditioning, etc.) could work puts DACCS early scaled deployment at risk. Promisingly, there is ongoing research on the integration of DAC into existing airflow systems and equipment such as cooling towers, wind turbines, moving vehicles or heating, ventilation, and air conditioning systems,142–145 but detailed work that goes beyond equipment-level analysis has not yet been conducted.
5.5 Infrastructure
There are multiple common infrastructure-related obstacles across DACCS systems: competition for access to low-carbon energy (#20), high water footprint (#21), land transformation and high footprint (#22), lack of existing CO2 transport infrastructure (#23), and lack of existing CO2 storage sites (#24).
Competition for access to low-carbon energy.
Availability of low-carbon energy sources is crucial for DAC deployment. DACCS at scale will likely compete with other industries and with households for access to low-carbon energy, creating challenges in prioritizing access. Additionally, as shown from our harmonized TEA results, powering a DAC system with a carbon intensive grid electricity mix would alter the cost of net-removed tons of CO2 significantly, even if the net removal efficiency of the DAC unit is high and negative emissions are achieved.
Building out renewable electricity supply is one option to provide low-carbon energy for DAC(CS). Another is nuclear energy, given its low carbon footprint and its continuous operational capacity. Reliance on natural gas and existing natural gas infrastructure to power high-grade heat DAC systems (i.e., Technology 1A could use an oxy-fired calciner for solvent regeneration) is also possible, however it may be met with resistance as the call to end fossil fuel use grows.
High water footprint.
High water use is an obstacle for certain DAC approaches (e.g., absorption-based DAC technologies, humidity swing) or DACCS systems (depending on material synthesis procedure or CO2 storage option). For example, technologies 1A and 1C require a great amount of make-up water (4.38 tons per ton of CO223) due to solvent volatility and/or water losses during process operation. Consequently, a 1Mt DAC facility could require nearly 13
000 tonnes of make-up water a day in a dry region. Lack of geographical inventories and water risk assessments, including information on scarcity, is an obstacle for understanding and evaluating the scale and relevance of the potential environmental impacts related to water use. However, DACCS may rate favorably in terms of water usage compared to other CDR solutions, such as BECCS, where it may present an even higher burden for massive scale-up.146,147
Land transformation and high footprint.
It is unclear how much land area DACCS installations require given that available data generally focuses on the DAC unit and not on the wider DACCS system (see Table 2). The physical footprint depends not only on the removal efficiency of the DAC unit and its design characteristics, but also on DACCS system-level configuration and integration. For instance, if land requirements of energy production are taken into consideration, overall land use may be quite significant (e.g., use of photovoltaics to power DAC units). Also, due to an expected increase in population and the electrification of energy systems, it is likely that DACCS will compete with agriculture for land availability and with other industries on land transformation. If DACCS is integrated into existing industrial clusters, waste heat, if available from other industrial processes, can be used to support the DAC unit operation. It may also be possible to supply the CO2 product stream into existing CCUS chains by integrating DACCS and CCUS. However, studies from such integrations are not currently unavailable.
Lack of existing CO2 transport infrastructure.
To enable permanent storage, CO2 can be either transported via pipelines or shipped to storage sites (either geological/in situ mineralization storage sites or to plants for integration into durable products), which creates the need for reliable and low cost transport. In the first case, pipelines and compression stations along the pipelines are needed.130 Availability of multi-user CO2 pipeline networks is key for reducing the cost of CO2 transport and for the rapid deployment of DACCS. Existing examples include the Midwest Carbon Express in the United States, an offshore CO2 pipeline connecting Belgium with Norway, and the Delta Corridor connecting parts of Germany and the Netherlands.148
The key obstacles to pipeline buildout are permitting timelines, capital costs, and public/political support. CO2 shipping offers safe, reliable, and flexible CO2 transportation well-suited to short distances and low to medium volume (around 2000 t or less149), especially in cases where pipeline transport is not an option. Shipping may be more suitable near-term for DACCS where low volumes of CO2 are produced, excluding the integration of DACCS into industrial clusters and DAC Hub projects.
However, there are still obstacles to CO2 shipping related to the CO2 pressurization levels as different conditioning (i.e., phase, temperature and pressure) is required for different transportation options and parameters (e.g., amount of cargo set to be transported, the distance to the final storage site, the arrangement for offloading, and the level of impurities and condition of the captured CO2). For instance, the design of specialized tanks for piping and refrigeration systems for the transportation of liquified CO2 is needed.
Lack of existing CO2 storage sites (geological storage and in situ mineralization).
Plans to build CCS and DACCS facilities worldwide have created a gap between anticipated demand for dedicated CO2 storage sites and the pace of development of storage facilities. As of today, only a few large-scale geological storage reservoirs have been accessed for CO2 storage in the US.150 Furthermore, apart from a few examples worldwide,151 these reservoirs are not optimally organized to support integration.
Permitting timelines, capital costs, and public/political support are also obstacles to storage buildout. Streamlined permitting regimes for storage reservoirs do not yet exist and site-specific geologic data and assessments are very limited. This creates a significant market entry barrier for storage project developers. Furthermore, a lack of continuous monitoring, measurement, and verification technologies for the stored CO2 are currently an obstacle for DACCS as well as CCS.152
Given the need for long-term monitoring of geological storage reservoirs to ensure permanent removal, enhanced in situ mineralization is increasingly attracting interest.153,154 It refers to injecting CO2 into subsurface basalts or peridotites, where it reacts with water to form solid carbonate minerals.155 These mafic and ultramafic rocks contain a high fraction of CO2-reactive minerals, allowing rapid carbonation and thus permanently storing the carbon on geological time scales.151In situ mineralization also offers the advantage of requiring less upfront capital investment and a smaller minimum viable scale compared to conventional geological storage due to shallower drilling.156,157 While the technology for this approach is advancing, further piloting and demonstration of CO2 mineralization is imperative for the scale-up of this storage method.158
6 Priority initiatives
In this section we present a roadmap (see Fig. 6) which includes a set of priority initiatives aimed at overcoming the 24 key obstacles presented in Section 5, and ultimately targeting the overall objective of scalable, safe, low-cost, and low-energy DACCS by 2050. Mirroring the structure of the identified obstacles, priority initiatives are grouped into the categories: (i) materials, (ii) process design, (iii) equipment, (iv) system integration, and (v) infrastructure priority initiatives.
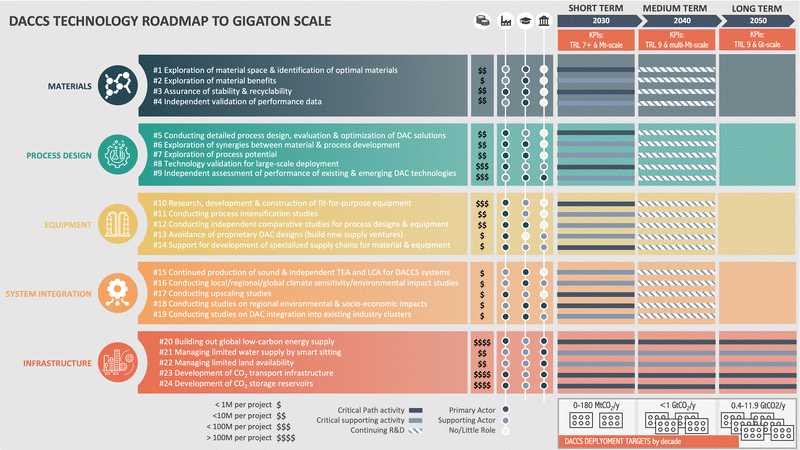 |
| Fig. 6 DACCS technology roadmap to gigaton scale. We list all priority initiatives across 5 main areas: (i) materials, (ii) process design, (iii) equipment, (iv) system integration, and (v) infrastructure. Expected investment costs, main actors across industry, academia and government, respectively, and critical activities in a short, medium and long term are assigned for every priority initiative. Key performance indicators (KPIs) – installed capacity by decade and DAC TRL levels – for ensuring success in DACCS technology deployment and scalability are presented per decade. Installed predicted capacities by 2030, 2040 and 2050 are obtained from Fuhrman et al.159 | |
Quantitative deployment targets for DACCS based on climate scenarios are crucial for coordinating and prioritizing actions within an industry roadmap. To determine critical paths, we relied on climate scenarios that estimate DACCS' contribution to total annual amount of CDR needed in 2030, 2040 and 2050 as provided by Fuhrman et al.159 Hitting a gigaton scale target by 2050 and assuming a scale-up factor of ten times growth every ten years (i.e., a growth rate similar to that of photovoltaics160) requires scaling at multi-megaton scale by 2040 and megaton scale by 2030. This deployment ramp up is depicted in Fig. 6 with the air contactor icons and is based on projections by Fuhrman et al.159
Given the time required to scale DACCS from a megaton to a gigaton industry, the first four categories (i.e., materials, process design, equipment, and system integration) need to be delivered largely by 2030, i.e., in less than seven years' time. Afterwards, DAC deployment (the construction of commercial plants) continues in tandem with R&D, using lessons learned from the constructed plants and, thereby, closing the feedback loop (the blue/white shaded continued R&D in Fig. 6 needed to deliver further technology improvement). The infrastructure initiatives require continuous delivery until 2050, to facilitate an expanding DACCS sector (e.g., transport & storage infrastructure needs to ramp-up in line with DAC ramping).
The DAC roadmap also includes key performance indicators (KPIs), the specifically required installed capacity by decade and DAC TRL levels, that can be used as metrics of success for DACCS technology deployment and scalability (see orange rectangles in Fig. 6). As previously mentioned, being in line with the gigaton targets by 2050 requires reaching megaton scale by 2030. Also, rapid deployment between 2030 and 2050 requires technologies that are expected to contribute to gigaton scale in 2050 to have been demonstrated or being in the process of demonstration by the end of the decade. This implies reaching a TRL of at least 7 by 2030.
In summary, DACCS gigaton-scale deployment targets by 2050 makes this decade the decisive decade for testing DAC and developing the technologies required for climate stability. A comprehensive list of all priority initiatives suggested, including a detailed breakout for all covered DAC technologies, is provided in Table S6 in ESI.†
6.1 Materials
We suggest four main initiatives aimed at overcoming obstacles related to CO2 capture materials: (i) exploration of the material space and identification of optimal materials (#1), (ii) exploration of material benefits (#2), (iii) assurance of stability and recyclability (#3), and (iv) independent validation of performance data (#4).
Exploration of material space and identification of optimal materials.
Involves accessing existing material databases or creating new databases and computer models to better understand material properties.111,115,161 Public access to these databases and models will accelerate knowledge transfer and provide the highest impact. Artificial intelligence and machine learning can be used to speed the exploration of materials.
Exploration of material benefits.
Highlights the need for identifying optimal trade offs between the properties of capture materials. In practice, this implies experimental (or computational, where experiments cannot be performed) measurements of all relevant material performance parameters. Each group of materials (e.g., solvents, sorbents, membranes) has a different set of material properties that need to be evaluated. These properties have been extensively covered in literature (e.g., ref. 120 and 162 for solvents, ref. 163 for membranes). An extensive list of material properties needed for characterizing solid sorbents can be found in Charalambous et al.135 Once the property data is collected, it can be used to model and evaluate the performance of these materials in the given separation process using metrics such as energy consumption or CO2 removal efficiency. To facilitate the application of the results in industry, frameworks for the selection of optimal materials that also cover technical, economic and environmental performance should be established.
Assurance of stability and recyclability.
Refers to the need to ensure long lifetimes for capture materials. To achieve this, long-term degradation studies for carbon capture materials, fundamental studies on the factors that cause material aging, degradation, and the loss of material to the atmosphere (e.g., presence of water, oxygen, or acid gasses) and studies on the effect of local/regional climates and operation conditions on the material lifetime have to be conducted.
Independent validation of performance data.
Flags the need to validate performance claims for proprietary technologies by independent research organizations and to develop a benchmark performance protocol for DAC material testing.
6.2 Process design
We suggest five main initiatives aimed at overcoming obstacles related to DAC processes: (i) conducting detailed process design, evaluation and optimization of DAC solutions (#5), (ii) exploration of synergies between material and process development (#6), (iii) exploration of process potential (#7), (iv) technology validation for large-scale deployment (#8), and (v) independent assessment of the performance of existing and emerging DAC technologies (#9).
Conducting detailed process design, evaluation and optimization of DAC solutions.
Refers to the need for conducting detailed process designs and evaluation taking into account auxiliary process elements (not only the capture and release of CO2), such as fans/blowers used for enabling the flow of atmospheric air through the air contactor, and transport phenomena and rate processes, such as momentum, mass and heat transfer. Such models will enable the extraction of more accurate insights from process optimization and the use of advanced process performance indicators (e.g., CO2 productivity, capital and operational expenditure, net CO2 removal efficiency) for the selection of both optimal materials and processes.
Exploration of synergies between material and process development.
Refers to the need for conducting synergistic material and process optimization to move beyond targeted materials selection and exploring the wider material space available. This opens up the potential to identify in silico novel structures that have not been previously considered for DAC applications.
Exploration of process potential.
Refers to the need for optimizing cycle steps and sequences or sequencing of unit operations in a DAC process and ensuring alternative process configurations are being explored. Examples include the study/development of alternative regeneration methods for sorbents and solvents (e.g., indirect heating, Joule heating, electric calciner, microwave), the usage of process waste heat through heat integration, or the testing of alternative bed configurations (e.g., fixed beds, moving beds, fluidized beds).
Technology validation for large-scale deployment.
Refers to the need for: (i) prototyping technologies that have reached proof of concept stage (i.e., 1B, 1C and 3A) in a lab environment (TRL 5), (ii) piloting more technologies beyond lab-scale (TRL 6+) and operating them for a sustained duration (minimum of one year), and (iii) continued plant build out for technologies that have been piloted (1A, 2A and 2C). So far, Climeworks TVSA technology (2C) is the only technology that has reached commercial scale (TRL 9), and it is imperative that rapid plant buildout continues. This buildout is expected to spark the deployment-led innovation needed to reduce costs.105,164,165 It is key to develop these plants across different geographical regions, which may come with their own technology implementation demands, requiring technology and or system customisation and therefore R&D.105,109,165,166 Further, establishing supply chains for TVSA-specific materials and equipment (see Section 6.3) requires a minimum equipment demand which can only be achieved through continuous deployment at scale.
Independent assessment of the performance of existing and emerging DAC technologies.
Refers to the need to have independent research organizations corroborate company disclosures of expected (or measured) performance. Also, it is critical for knowledge transfer that data from publicly-funded pilots and demonstrations is publicly disseminated and does not remain within companies walls.
6.3 Equipment
We suggest five main initiatives aimed at overcoming obstacles related to equipment: (i) research, development and construction of fit-for-purpose equipment (#10), (ii) conducting of process intensification studies (#11), (iii) conducting independent comparative studies for process designs and main equipment (#12), (iv) avoidance of proprietary DAC designs (#13), and (v) support for development of specialized supply chains for generic DAC equipment (#14).
Research, development and construction of fit-for-purpose equipment.
Refers to the need to design and create equipment specifically for DAC applications instead of using general purpose products, as this tends to vastly improve performance. Examples include oxy-fuelled or solar calciners for Technology 1A or robust and efficient heat pumps for Technology 2C.23,129–131 The key guiding principles to keep in mind when designing fit-for-purpose equipment is to aim for equipment whose components can be mass produced. Furthermore, it would be beneficial if equipment could be used across technologies to foster knowledge spillover and learning.
Conducting process intensification studies.
Highlights the need for studying synergistic effects between different unit operations/processes for an efficient performance and low energy consumption and the need to design process intensification strategies based on this.
Conducting independent comparative studies for process designs and main equipment.
Refers to the need for comparative assessment by independent research organizations of the most important DAC process design and system components. Examples could include different types of calciners or different contactor designs.
Avoidance of proprietary DAC designs.
Refers to the need to incentivize generic technology developers to develop DAC-specific equipment (e.g., packings, vacuum pumps, other contacting equipment).
Support for development of specialized supply chains for DAC material & equipment.
Refers to the need to define and disseminate the core elements of each DACCS technology so that industrial parties can start offering equipment and materials to DAC companies. This includes the need to establish large-scale supply of economic solvents and sorbents.
6.4 System integration
We suggest five main initiatives aimed at overcoming obstacles related the system integration: (i) continued independent production of sound and independent TEA and LCA for existing and new DACCS technologies (#15), (ii) conducting local/regional/global climate sensitivity/environmental impact studies (#16), (iii) conducting upscaling studies (#17), (iv) conducting studies on regional environmental and socio-economic impacts (#18), and (v) conducting studies on DAC integration into existing industry clusters (#19).
Continued production of sound and independent TEA and LCA for existing and new DACCS technologies.
Refers to the urgency of conducting independent TEA and LCA studies for emerging and already existing DAC technologies while ensuring that measured performance data (e.g., from pilot and demonstration plants) becomes publicly available to underpin TEA and LCA studies. Both types of studies rely on vast amounts of good quality data input and, therefore, it is critical that key system parameters and resource requirements are disseminated to the public domain. This becomes more important as commercial-scale plants come online so the field can quickly learn and evolve. Funders can accelerate this process by requiring extensive data disclosure for projects they support. As discussed in Sections 3–5, objective cost estimates, for instance, are lacking for most of the technologies currently in development.
Conducting local/regional/global climate sensitivity/environmental impact studies.
Refers to the need for conducting geospatial analysis of climate impacts on DACCS systems and modeling of DACCS cost sensitivity and environmental impact studies in different geographic environments. DAC siting must be optimized with respect to atmospheric conditions to enable a safe and responsible global DACCS deployment.
Conducting upscaling studies.
Refers to the near-future need of upscaling DAC units safely and responsibly. Modular units may be easier for scaling up, however, learnings from upscaling testing and reporting this, including, e.g., optimal spacing between the air contactors, are crucial for ensuring optimal DAC park layout designs.
Conducting studies on regional environmental and socio-economic impacts.
Refers to the need for producing models (e.g., integrated assessment models used for shared socio-economic pathway scenarios) for assessing regional environmental and socio-economic impacts (in contrast to global ones) on regional energy systems from large-scale DACCS deployment.
Conducting studies on DAC integration into existing industry clusters.
Refers to the necessity to conduct studies on how to integrate DACCS into local energy systems and existing industrial clusters to benefit from existing infrastructure. Because DAC generally requires the handling of large volumes of air, heat, and electricity, and a suitable storage site, it may benefit from co-siting with other industries or industrial clusters and these options must be explored. DACCS cannot only benefit from potentially available waste heat streams from industrial processes but also from storing large quantities of CO2 more securely by integrating into existing CCS infrastructure. Industries with which DAC can be integrated include thermal power plants, server farms, cement production or commercial HVAC handling systems.
6.5 Infrastructure
We suggest five main initiatives aimed at overcoming obstacles related to infrastructure: (i) building out global low-carbon energy supply (#20), (ii) managing limited water supply through smart siting and technology choices (#21), (iii) managing limited land availability (#22), (iv) development of CO2 transport infrastructure (#23), and (v) development of CO2 storage reservoirs (#24).
Building out global low-carbon energy supply.
Refers to the need for increasing global low-carbon energy supply (both on grid/off-grid electricity and alternative low-carbon energy resources). A rapid build out of renewable (green/clean) grid electricity will be needed to prevent DACCS from competing with other industries for low-carbon energy and thereby undermining the purpose of climate mitigation and slowing the overall energy transition. Moreover, large-scale and economically attractive DACCS deployment may require remote installations close to suitable sites for geologic storage. Therefore, autonomous off-grid system layouts, such as solar energy with energy storage, need to be planned and built specifically for DAC plants. On a national level, access to waste energy should be streamlined across different industries (e.g., nuclear, cement, steel, waste disposal, etc.).
Managing limited water supply through smart sitting and technology choices.
Refers to the fact that limited water supply is a common challenge across CDR approaches that can only be partially addressed through strategic siting decisions and appropriate DAC technology choices. Strategic siting implies siting DAC technologies with the potential to produce water (e.g., 2C) in more arid areas while refraining to do so for technologies with high water consumption (e.g., 1a, 1C, and 1D). Better siting decisions can be enabled by building out geographical inventories and water risk assessments.
Managing limited land availability.
Refers to the fact that limited land supply is a common challenge across CDR approaches that can only be partially addressed through strategic siting decisions and appropriate DAC technology choices. Integration of DAC into industrial clusters and infrastructure will most likely be key in preventing DACCS from displacing other industries, including food production and farming).
Development of CO2 transport infrastructure.
Refers to the need for transporting CO2 to storage sites for carbon sequestration. DACCS can benefit from available multi-user CO2 pipeline networks for reducing transport costs and to increase the rate of deployment. Also, efforts on optimizing costs and operations for CO2 conditioning (for both pipelines and shipping) and transportation (e.g., building compression stations along the pipelines) are necessary.
Development of CO2 storage reservoirs.
Is necessary to securely and permanently store CO2 to ensure both net negativity of the DACCS process and prevent short-term reversals to the atmosphere. Given long permitting timelines, sufficient storage reservoirs must be identified and developed today to provide availability both now, as DAC technologies are piloted, but particularly as these technologies begin to ramp up. Storage reservoirs will be needed across the globe to support integration with multiple climate-dependent DAC technologies, and to allow multiple geographies to benefit economically. These reservoirs could be set up as pay-to-use wells within hub networks, allowing several DAC companies to collectively store their CO2. Liability for maintaining and monitoring stored CO2 could be held by the storage partner, or alternatively, by a 3rd party, such as governments, to alleviate some of the risk involved. Furthermore, streamlined permitting regimes and publicly available site-specific geologic data and assessments would help de-risk storage projects for storage developers and reduce storage development timelines.
Both transport systems and storage reservoirs will need to include measurement and monitoring equipment to provide quantities of captured, compressed, transported and stored CO2, and account for any potential leaks in the process. Ultimately, determination of all carbon accounting for DACCS rests on the reliability of MRV tools, beginning with the initial measurements. Innovative forms of long-term monitoring of subsurface storage may be able to provide either more detailed surveillance or lower costs, but may need to be included as infrastructure is built, rather than after the fact.
In short.
This roadmap provides a menu of technical activities that need to be undertaken as a matter of urgency. It includes indicative costs for projects that address the corresponding obstacles as well as the most likely actor(s) to lead these. This way, investors, governments, and businesses can pick and choose the activities they feel they are best placed to support, and our hope is this roadmap will fast-track further DACCS advancement.
Also, it is important to remember that this roadmap focuses on the list of priority initiatives needed to achieve large-scale DAC deployment with permanent storage. DAC coupled with CO2 utilization (DACCU) is not expected to be, for the majority of the utilization pathways, a permanent CO2 storage solution, so it is not included in this roadmap. However, DACCU could be crucial for the deployment and testing of DAC technologies. For example, CO2 utilization in greenhouses or for the production of synthetic aviation fuels, chemicals or fertilizers could be critical enablers of DAC scale up and cost reductions.
7 Conclusions
In this perspective, we proposed a roadmap to 2050 for achieving scalable, safe and low-cost DACCS. The current body of knowledge on DAC technologies was synthesized and discussed with a focus on identifying and overcoming obstacles to large-scale deployment. We introduced a new hierarchy for classifying DAC technologies that supports understanding of the key DAC performance indicators and the quality of the underlying data, on which claims on energy requirements and future cost can be built. We then harmonized available energy and cost data, to allow for a like-for-like comparison between DAC technologies with available data. We conclude that there is a wide gap between companies future cost estimates of below USD100 per tCO2 removal and a path to achieve this objective.
For most DAC technologies, ‘plant data’ is unavailable, presenting a major obstacle to cost and energy use reduction efforts. For the technologies where ‘plant data’ does exist, future cost ranges of USD100–300 per tCO2 seem attainable, especially due to expected technological learning from continuous DAC deployment, penetration of low-carbon energy sources, and ongoing electrification of energy systems. Nevertheless, this cost range is a highly ambitious target requiring significant technology advancement and is necessary to keep required future DAC investment below $1 trillion.
We identified a set of critical priority initiatives that could help overcome the most important materials-, technical- and infrastructure-related obstacles. As deployment-led innovation alone is unlikely to make DACCS economically feasible, we propose simultaneous investments into research and development, deployment and the buildout of supporting infrastructure. Further research is needed to better quantify the cost of proposed priority initiatives and their attendant RD&D projects and to expand them to cover policy, investment, international collaboration, recommendations for the private sector, and stakeholder/community engagement overall.
Acronyms
BECCS | Bioenergy with carbon capture and storage |
CAPEX | Capital expenditure |
CCS | Carbon capture and storage |
CDR | Carbon dioxide removal |
DAC | Direct air capture |
DACCS | Direct air carbon capture and (permanent) storage |
DACCU | Direct air carbon capture and utilization |
FOAK | First-of-a-kind |
GBP | British pound sterling |
GHG | Greenhouse gas |
Gt | Gigaton |
IAM | Integrated assessment model |
LCA | Life cycle assessment |
MEB | Mass and energy balance |
MRV | Monitoring, reporting and verification |
nCDR | Novel carbon dioxide removal |
NOAK | Ith-of-a-kind |
OPEX | Operational expenditure |
RD&D | Research, development and demonstration |
RDD&D | Research, development, demonstration and deployment |
SI | Supplementary information |
TEA | Techno-economic analysis |
TRL | Technology readiness level |
TVSA | Temperature vacuum swing adsorption |
USD | U.S. dollar |
Data availability
Supplementary Information, containing details of the techno-economic evaluation, is available for this paper. Correspondence and requests for additional materials should be addressed to the corresponding author.
Author contributions
Lukas Küng: conceptualization, data curation, formal analysis, investigation, methodology, visualization, writing – original draft, writing – review & editing. Silvan Aeschlimann: conceptualization, formal analysis, investigation, methodology, visualization, writing – original draft, writing – review & editing. Charithea Charalambous: conceptualization, data curation, investigation, methodology, visualization, writing – review & editing. Fergus McIlwaine: data curation, formal analysis, investigation, visualization, methodology. John Young: data curation, formal analysis, investigation, visualization. Noah Shannon: conceptualization, visualization, writing – review & editing. Karen Strassel: data curation, investigation, writing – review & editing. Cara Nichole Maesano: data curation, investigation, writing – review & editing. Rudy Kashar: writing – review & editing. Daniel Pike: conceptualization, funding acquisition, project administration. Mijndert van der Spek: conceptualization, methodology, validation, writing – review & editing. Susana Garcia: conceptualization, methodology, project administration, validation, writing – review & editing.
Conflicts of interest
The authors declare no competing interests.
Acknowledgements
This roadmap is a product of collaborative effort between Heriot-Watt University and the non-governmental organization RMI. RMI acknowledges restricted grant funding from Meta (grant agreement no. INB2423455), Protocol Labs, and Total Energies. The co-authors thank the following advisory board members who played a critical role in shapping this roadmap: André Bardow, Niels Berghout, Alix Bouxin, Sara Budinis, Sabine Fuss, Howard Herzog, Dennis Jong, Aïcha El Khamlichi, Anu Khan, Frauke Kracke, Wilfried Maas, Tom Mikunda, Eli Mitchell-Larson, Berend Smit, and John Tuttle.
References
- IPCC, Climate Change 2022: Mitigation of Climate Change. Contribution of Working Group III to the Sixth Assessment Report of the Intergovernmental Panel on Climate Change, 2022.
- U.S. Department of Energy and National Energy Technology Laboratory (NETL), 2014 Technology Readiness Assessment – Overview, 2015.
- S. Deutz and A. Bardow, Life-cycle assessment of an industrial direct air capture process based on temperature-vacuum swing adsorption, Nat. Energy, 2021, 6, 203–213 CrossRef CAS.
- G. Leonzio, P. S. Fennell and N. Shah, Analysis of Technologies for Carbon Dioxide Capture from the Air, Appl. Sci., 2022, 12, 8321 CrossRef CAS.
- M. Bui,
et al., Carbon capture and storage (CCS): the way forward, Energy Environ. Sci., 2018, 11, 1062–1176 RSC.
- International Energy Agency, Direct Air Capture: A key technology for net zero, 2022.
-
S. Budinis Direct Air Capture – IEA, 2022; https://www.iea.org/reports/direct-air-capture.
- C. Bloch, R. Kahsar, J. Newcomb and G. Wohl, Direct Air Capture and the Energy Transition: Putting Potential Opportunity Costs in Perspective, RMI Insight Brief, 2022, 1–30 Search PubMed.
- Mission Innovation, Carbon Dioxide Removal Technology Roadmap: Innovation Gaps and Landscape Analysis, 2022.
- IEAGHG, Global Assessment of Direct Air Capture Costs, 2021.
-
D. Sandalow, J. Friedmann, C. McCormick and S. McCoy, Direct Air Capture of Carbon Dioxide: ICEF Roadmap 2018, 2018.
- National Academies of Sciences, Engineering, and Medicine, Negative Emissions Technologies and Reliable Sequestration: A Research Agenda, Washington, DC: The National Academies Press, Washington, DC, 2019 DOI:10.17226/25259.
-
J. Wilcox, B. Kolosz and J. Freeman, CDR Primer, 2021; https://cdrprimer.org/.
- M. Erans, E. S. S.-P. Rez, D. P. Hanak, Z. Clulow, D. M. Reiner and G. A. Mutch, Direct air capture: process technology, techno-economic and socio-political challenges, Energy Environ. Sci., 2022, 15, 1360 RSC.
- C. N. Maesano, J. S. Campbell, S. Foteinis, V. Furey, O. Hawrot, D. Pike, S. Aeschlimann, P. L. Reginato, D. R. Goodwin, L. L. Looger, E. S. Boyden and P. Renforth, Geochemical Negative Emissions Technologies: Part II. Roadmap, Front. Clim., 2022, 4, 162 Search PubMed.
- Verdox, https://verdox.com.
- S. Fujikawa, R. Selyanchyn and T. Kunitake, A new strategy for membrane-based direct air capture, Polym. J., 2021, 53, 111–119 CrossRef CAS.
- Climeworks, https://climeworks.com/.
- Global Thermostat, https://globalthermostat.com/.
- Carbon Engineering, https://carbonengineering.com/.
- 1pointfive, https://www.1pointfive.com.
- D. W. Keith, M. Ha-Duong and J. K. Stolaroff, Climate Strategy with CO2 Capture from the Air, Clim. Change, 2006, 74, 17–45 CrossRef CAS.
- D. W. Keith, G. Holmes, D. S. Angelo and K. Heidel, A Process for Capturing CO2 from the Atmosphere, Joule, 2018, 2, 1573–1594 CrossRef CAS.
- G. Holmes and D. W. Keith, An air-liquid contactor for large-scale capture of CO2 from air, Trans. R. Soc. A, 2012, 370, 4380–4403 CAS.
- APS, Direct Air Capture of CO2 with Chemicals: A Technology Assessment for the APS Panel on Public Affairs, 2011.
- F. M. Brethomé, N. J. Williams, C. A. Seipp, M. K. Kidder and R. Custelcean, Direct air capture of CO2 via aqueous-phase absorption and crystalline-phase release using concentrated solar power, Nat. Energy, 2018, 3, 553–559 CrossRef.
- R. Custelcean, Direct air capture of CO2 via crystal engineering, Chem. Sci., 2021, 12, 12518 RSC.
-
R. Custelcean, N. J. Williams and C. A. Seipp, Guanidine compounds for carbon dioxide capture, U.S. Pat., 10583387B2, 2020 Search PubMed.
- F. Barzagli, C. Giorgi, F. Mani and M. Peruzzini, Screening Study of Different Amine-Based Solutions as Sorbents for Direct CO2 Capture from Air. ACS Sustain, Chem. Eng., 2020, 8, 14013–14021 CAS.
- A. Kiani, K. Jiang and P. Feron, Techno-Economic Assessment for CO2 Capture From Air Using a Conventional Liquid-Based Absorption Process, Front. Energy Res., 2020, 1, 92 CrossRef.
- F. Sabatino, A. Grimm, F. Gallucci, M. van Sint Annaland, G. J. Kramer and M. Gazzani, A comparative energy and costs assessment and optimization for direct air capture technologies, Joule, 2021, 5, 2047–2076 CrossRef CAS.
- Rolls-Royce, CSIRO, Project ENCORE: Direct Air Capture, Final Report, 2021.
- Carbon Blade, https://www.carbon-blade.com.
- CO2CirculAir, https://www.co2circulair.com/how-smart-dac-works.html.
- E-quester, https://e-quester.com.
- Mission Zero Technologies, https://www.missionzero.tech.
- F. Sabatino, M. Mehta, A. Grimm, M. Gazzani, F. Gallucci, G. J. Kramer and M. van Sint Annaland, Evaluation of a Direct Air Capture Process Combining Wet Scrubbing and Bipolar Membrane Electrodialysis, Ind. Eng. Chem. Res., 2020, 59, 7007–7020 CrossRef CAS.
- Heirloom, https://www.heirloomcarbon.com.
- Origen, https://origencarbonsolutions.com.
- 8 Rivers Capital,; Origen Power, https://github.com/frontierclimate/carbon-removal-source-materials/blob/main/Project.
- N. McQueen, P. Kelemen, G. Dipple, P. Renforth and J. Wilcox, Ambient weathering of magnesium oxide for CO2 removal from air, Nat. Commun., 2020, 11, 3299 CrossRef CAS PubMed.
-
N. McQueen, M. Ghoussoub, J. Mills and M. Scholten, A scalable direct air capture process based on accelerated weathering of calcium hydroxide, 2022.
- Avnos, https://www.avnos.com.
- Infinitree, http://www.infinitreellc.com.
- Carbon Collect, https://mechanicaltrees.com.
- Clairity Technology, https://github.com/frontierclimate/carbon-removal-source-materials/blob/main/Project.
- T. Wang, K. S. Lackner and A. B. Wright, Moisture-swing sorption for carbon dioxide capture from ambient air: a thermodynamic analysis, Phys. Chem. Chem. Phys., 2013, 15, 504–514 RSC.
- M. Fasihi, O. Efimova and C. Breyer, Techno-economic assessment of CO2 direct air capture plants, J. Cleaner Prod., 2019, 224, 957–980 CrossRef CAS.
- Carbyon, https://carbyon.com.
- Emissol, https://github.com/frontierclimate/carbon-removal-source-materials/blob/main/Project.
- hydrocell, https://hydrocell.fi.
- Airthena, https://www.csiro.au/en/work-with-us/ip-commercialisation/marketplace/co2gen.
- Carbon Infinity, https://www.carboninfinity.com.
- AspiraDAC, https://www.aspiradac.com.
- TerraFixing, https://www.terrafixing.com/home.
-
G. Solutions
https://greencap-solutions.com/direct-air-capture/
.
- Removr, https://www.removr.no.
- Sustaera, https://www.sustaera.com.
- Susteon, https://susteon.com/projects/.
- Air View Engineering, https://www.airviewengineering.co.uk.
- BLANCAIR, https://www.blancair.com.
- Carbon Capture, https://www.carboncapture.com.
- DAC City, https://www.daccity.com/#intro.
- InnoSepra, https://www.innosepra.com/dac-main.
- Noya, https://www.noya.co.
- ReCarbn. https://recarbn.eu/.
- Skytree, https://skytree.eu/technology/.
- Frontier, GitHub Repository: carbon-removal-source-materials, 2022; https://github.com/frontierclimate/carbon-removal-source-materials.
- G. Santori, C. Charalambous, M.-C. Ferrari and S. Brandani, Adsorption artificial tree for atmospheric carbon dioxide capture, purification and compression, Energy, 2018, 162, 1158–1168, DOI:10.1016/j.energy.2018.08.090.
- Holy Grail, https://www.holygrail.ai.
- Carbominer, https://carbominer.com.
- Parallel Carbon, Stripe Application. https://github.com/frontierclimate/carbon-removal-source-materials/blob/main/Project.
- Parallel Carbon, https://www.parallelcarbon.com.
- S. Voskian and T. A. Hatton, faradaic electro-swing reactive adsorption for CO2 capture, Energy Environ. Sci., 2019, 12, 3530–3547 RSC.
- RepAir, Stripe Application. https://github.com/frontierclimate/carbon-removal-source-materials/blob/main/Project.
- RepAir, https://repair-carbon.com.
- L. Shi, Y. Zhao, S. Matz, S. Gottesfeld, B. P. Setzler and Y. Yan, A shorted membrane electrochemical cell powered by hydrogen to remove CO2 from the air feed of hydroxide exchange membrane fuel cells, Nat. Energy, 2022, 7, 238–247 CrossRef CAS.
-
Y. Yan, D. Yan, S. Matz, R. Razdan and B. Setzler, Electrochemically Driven Carbon Dioxide Separator, Patent WO/2021/236979, 2021 Search PubMed.
- High Hopes, https://www.highopeslabs.com.
- T. von Hippel, Thermal removal of carbon dioxide from the atmosphere: energy requirements and scaling issues, Clim. Change, 2018, 148, 491–501 CrossRef CAS.
-
L. Blain, High Hopes claims stratospheric breakthrough in direct air CO2 capture, 2021, https://newatlas.com/environment/high-hopes-carbon-capture-balloons/#:~:text=To%20reach%20just%20one%20million,and%20no%20punctures%20or%20tears.
-
D. Cooper High Hopes plans to extract atmospheric CO2 with hot air balloons. 2021; https://www.engadget.com/high-hopes-balloon-carbon-capture-120057079.html?guce_referrer=aHR0cHM6Ly93d3cuZ29vZ2xlLmNvbS8guce_referrer_sig=AQAAAJvymR3vkqAILvekPpsT6CJfsIOHP7myE65dBnMeAbLHjq6rQ3WwkXL01GaapuuyO2Xj38os479CRE5yLyy6pzjTCrvOnPyC3Cra_guc_consent_skip=1677795199.
-
E. Berlzon and J. Stonestreet, Sky's the limit: Israeli startup develops balloons to capture carbon, 2021; https://www.reuters.com/business/environment/skys-limit-israeli-startup-develops-balloons-capture-carbon-2021-11-04/.
- AirCapture, https://www.aircapture.com.
- Airmyne, https://www.airmyne.com.
- hago energetics, http://hagoenergetics.com/index.php/projects/direct-air-capture-project/.
- Cedar Carbon, https://www.cedarcarbon.co.
- CO2Rail, https://www.co2rail.com.
- CO2Rail, https://github.com/frontierclimate/carbon-removal-source-materials/blob/main/Project.
- NeoCarbon, https://www.neocarbon.tech.
- Soletair Power, https://www.soletairpower.fi.
- Aircela, https://www.aircela.com.
- Prometheus, https://www.prometheusfuels.com/technology.
- RedoxNRG, https://www.redoxnrg.com.
- Terraform Industries, https://terraformindustries.wordpress.com/2023/01/09/terraform-industries-whitepaper-2-0/.
- Climeworks, Orca: the first large-scale plant, 2021; https://climeworks.com/roadmap/orca.
- P. Viebahn, A. Scholz and O. Zelt, The Potential Role of Direct Air Capture in the German Energy Research Program—Results of a Multi-Dimensional Analysis, Energies, 2019, 12, 3443, DOI:10.3390/en12183443.
- M. Simoni, M. D. Wilkes, S. Brown, J. L. Provis, H. Kinoshita and T. Hanein, Decarbonising the lime industry: State-of-the-art, Renewable Sustainable Energy Rev., 2022, 168, 112765 CrossRef CAS.
- Exchange Rates UK, Compare Live Foreign Currency Exchange Rate & History, 2023; https://www.exchangerates.org.uk.
- BEIS, Statistical data set: International industrial energy prices, 2022; https://www.gov.uk/government/statistical-data-sets/international-industrial-energy-prices.
- NREL, Wind LCA Harmonization, 2013; https://www.nrel.gov/docs/fy13osti/57131.pdf.
- EIA, Emissions by plant and by region, 2023; https://www.eia.gov/electricity/data/emissions/.
- European Environment Agency, Greenhouse gas emission intensity of electricity generation by country. 2022; https://www.eea.europa.eu/data-and-maps/daviz/co2-emission-intensity-9/#tab-chart_2.
- J. Cooper, P. Balcombe and A. Hawkes, The quantification of methane emissions and assessment of emissions data for the largest natural gas supply chains, J. Cleaner Prod., 2021, 320, 128856 CrossRef CAS.
-
J. Young, N. McQueen, C. Charalambous, S. Foteinis, O. Hawrot, M. Ojeda, H. Pilorgé, J. Andresen, P. Psarras, P. Renforth, S. Garcia and M. van der Spek, The cost of direct air capture and storage can be reduced via strategic deployment but is unlikely to fall below stated cost targets, 2023; https://chemrxiv.org/engage/chemrxiv/article-details/637e3ebeebc1c76513d02d12.
- IEAGHG, Technical Reviews. 2021; http://documents.ieaghg.org/index.php/s/YKm6B7zikUpPgGA?path=.
- J. Wilcox, P. C. Psarras and S. Liguori, Assessment of reasonable opportunities for direct air capture, Environ. Res. Lett., 2017, 12, 065001 CrossRef.
- J. Young, F. Mcilwaine, B. Smit, S. Garcia and M. van der Spek, Process-informed adsorbent design guidelines for direct air capture, Chem. Eng. J., 2023, 456, 141035 CrossRef CAS.
- M. Sendi, M. Bui, N. M. Dowell and P. Fennell, Geospatial analysis of regional climate impacts to accelerate cost-efficient direct air capture deployment, One Earth, 2022, 5, 1153–1164 CrossRef.
-
J. Valentine, A. Zoelle, S. Homsy, H. Mantripragada, M. Woods, N. Roy, A. Kilstofte, M. Sturdivan, M. Steutermann and T. Fout, Direct Air Capture Case Studies: Sorbent System, 2022 DOI:10.2172/1879535.
- C. Charalambous, E. Moubarak, E. Sanchez-Fernandez, J. Schilling, A. Bardow, B. Smit and S. Garcia, Can we systematically rank the performance of any existing material for sorbent-based carbon capture?, Proceedings of the 16th Greenhouse Gas Control Technologies Conference (GHGT-16) 23-24 Oct, 2022 DOI:10.2139/ssrn.4286397.
- S. M. Moosavi, B. Á. Novotny, D. Ongari, E. Moubarak, M. Asgari, Ö. Kadioglu, C. Charalambous, A. Guerrero, A. H. Farmahini and L. Sarkisov,
et al., A data-science approach to predict the heat capacity of nanoporous materials, Nat. Mater., 2022, 21, 1419–1425 CrossRef CAS PubMed.
- E. Moubarak, S. M. Moosavi, C. Charalambous, S. Garcia and B. Smit, A Robust Framework for Generating Adsorption Isotherms to Screen Materials for Carbon Capture, Ind. Eng. Chem. Res., 2023, 62, 10252–10265, DOI:10.1021/acs.iecr.3c01358.
- C. Charalambous, F. Mcilwaine, E. Moubarak, B. Smit and S. Garcia, Can we systematically screen millions of chemical structures for cost-effective carbon capture?, Boletin Del Grupo Espanol Del Carbon, 2021, 61, 14–18 Search PubMed.
- P. G. Boyd,
et al., Data-driven design of metal-organic frameworks for wet flue gas CO2 capture, Nature, 2019, 576, 253–256 CrossRef CAS PubMed.
- K. Jablonka, C. Charalambous, E. Fernandez, G. Wiechers, J. Monteiro, P. Moser, B. Smit and S. Garcia, Machine learning for industrial processes: Forecasting amine emissions from a carbon capture plant, Sci. Adv., 2023, 9(1) DOI:10.1126/sciadv.adc9576.
- M.-Y. A. Low, L. V. Barton, R. Pini and C. Petit, Analytical review of the current state of knowledge of adsorption materials and processes for direct air capture, Chem. Eng. Res. Des., 2023, 189, 745–767 CrossRef CAS.
- P. Moser, G. Wiechers, S. Schmidt, J.-S. Monteiro, E. Goetheer, C. Charalambous, A. Saleh, M. van der Spek and S. Garcia, ALIGN-CCUS: Results of the 18 month test with aqueous AMP/PZ solvent at the pilot plant at Niederaussem - solvent management, emissions and dynamic behavior, Int. J. Greenh. Gas Control, 2021, 109, 103381, DOI:10.1016/j.ijggc.2021.103381.
- P. Moser, G. Wiechers, S. Schmidt, J. G. M.-S. Monteiro, C. Charalambous, S. Garcia and E. S. Fernandez, Results of the 18 month test with MEA at the post-combustion capture pilot plant at Niederaussem – new impetus to solvent management, emissions and dynamic behaviour, Int. J. Greenh. Gas Control, 2020, 95, 102945, DOI:10.1016/j.ijggc.2019.102945.
- E. S. Sanz-Pérezpérez, C. R. Murdock, S. A. Didas and C. W. Jones, Direct Capture of CO2 from Ambient Air, Chem. Rev., 2016, 116(19), 11840–11876, DOI:10.1021/acs.chemrev.6b00173.
- J. Wu, X. Zhu, F. Yang, R. Wang and T. Ge, Shaping techniques of adsorbents and their applications in gas separation: a review, J. Mater. Chem. A, 2022, 10, 22853–22895 RSC.
- A. Kumar, D. G. Madden, M. Lusi, K. J. Chen, E. A. Daniels, T. Curtin, J. J. Perry and M. J. Zaworotko, Direct Air Capture of CO2 by Physisorbent Materials, Angew. Chem., Int. Ed., 2015, 54, 14372–14377 CrossRef CAS PubMed.
- M. N. Naseer, A. A. Zaidi, K. Dutta, Y. A. Wahab, J. Jaafar, R. Nusrat, I. Ullah and B. Kim, Past, present and future of materials applications for CO2 capture: A bibliometric analysis, Energy Rep., 2022, 8, 4252–4264 CrossRef.
- C. Charalambous, G. Santori, E. Vilarrasa-Garcia, M. Bastos-Neto, C. Cavalcante and S. Brandani, Pure and Binary Adsorption of Carbon Dioxide and Nitrogen on AQSOA FAM Z02, J. Chem. Eng. Data, 2018, 63(3), 661–670, DOI:10.1021/acs.jced.7b00864.
- M. Gholami, T. R. V. Assche and J. F. Denayer, Temperature vacuum swing, a combined adsorption cycle for carbon capture, Curr. Opin. Chem. Eng., 2023, 39, 100891 CrossRef.
- Y. Gomez-Rueda, B. Verougstraete, C. Ranga, E. Perez-Botella, F. Reniers and J. F. Denayer, Rapid temperature swing adsorption using microwave regeneration for carbon capture, J. Chem. Eng., 2022, 446, 137345 CrossRef CAS.
-
C. Gebald, N. Repond and J. Wurzbacher, Steam assisted vacuum desorption process for carbon dioxide capture, U.S. Pat., 10279306B2, 2019 Search PubMed.
- M. Schellevis and W. Brilman, Experimental Campaign with a 1 kg Day−1 Fixed Bed DAC Unit Using Solid Amines, Proceedings of the 16th Greenhouse Gas Control Technologies Conference (GHGT-16) 23-24 Oct, 2022 DOI:10.2139/ssrn.4290007.
- G. Leonzio and N. Shah, Innovative Process Integrating Air Source Heat Pumps and Direct Air Capture Processes, Ind. Eng. Chem. Res., 2022, 61, 13221–13230 CrossRef CAS.
- T. Terlouw, K. Treyer, C. Bauer and M. Mazzotti, Life Cycle Assessment of Direct Air Carbon Capture and Storage with Low-Carbon Energy Sources, Environ. Sci. Technol., 2021, 55, 11397–11411 CrossRef CAS PubMed.
- N. McQueen, M. J. Desmond, R. H. Socolow, P. Psarras and J. Wilcox, Natural Gas vs. Electricity for Solvent-Based Direct Air Capture, Front. Clim, 2021, 2, 38 Search PubMed.
-
K. R. Heidel, J. A. Ritchie, A. P. S. Kainth and D. W. Keith, Recovering a caustic solution via calcium carbonate crystal aggregates, U.S. Pat., 8728428B1, 2014 Search PubMed.
-
P. Eisenberger, Rotating multi-monolith bed movement system for removing CO2 from the atmosphere, U.S. Pat., 9925488B2, 2018 Search PubMed.
-
C. Gebald, W. Meier; N. Repond; T. Ruesch and J. A. Wurzbacher, Direct air capture device, U.S. Pat., 10232305B2, 2019 Search PubMed.
- C. Charalambous, E. Moubarak, J. Schilling, E. S. Fernandez, J.-Y. Wang, L. Herraiz, F. Mcilwaine, K. M. Jablonka, S. M. Moosavi, J. V. Herck, G. Mouchaham, C. Serre, A. Bardow, B. Smit and S. Garcia, Shedding Light on the Stakeholders’ Perspectives for Carbon Capture, ChemRxiv, 2023 DOI:10.26434/chemrxiv-2023-sn90q.
- K. Madhu, S. Pauliuk, S. Dhathri and F. Creutzig, Understanding environmental trade-offs and resource demand of direct air capture technologies through comparative life-cycle assessment, Nat. Energy, 2021, 6, 1035–1044 CrossRef CAS.
- M. M. J. de Jonge, J. Daemen, J. M. Loriaux, Z. J. N. Steinmann and M. A. J. Huijbregts, Life cycle carbon efficiency of Direct Air Capture systems with strong hydroxide sorbents, Int. J. Greenh. Gas Control, 2019, 80, 25–31 CrossRef CAS.
- K. An, A. Farooqui and S. T. McCoy, The impact of climate on solvent-based direct air capture systems, Appl. Energy, 2022, 325, 119895 CrossRef CAS.
- J. F. Wiegner, A. Grimm, L. Weimann and M. Gazzani, Optimal Design and Operation of Solid Sorbent Direct Air Capture Processes at Varying Ambient Conditions, Ind. Eng. Chem. Res., 2022, 61, 12649–12667 CrossRef CAS.
-
S. Sadri-Irani and P. Luzzatto-Fegiz, Predicting Land Usage, Optimal Design and Performance of Large-Scale Deployments of Direct Air Capture Devices. 2022.
- C. Breyer, M. Fasihi and A. Aghahosseini, Carbon dioxide direct air capture for effective climate change mitigation based on renewable electricity: a new type of energy system sector coupling, Mitig. Adapt. Strateg. Glob. Chang, 2020, 25, 43–65 CrossRef.
- A. Sodiq, Y. Abdullatif, B. Aissa, A. Ostovar, N. Nassar, M. El-Naas and A. Amhamed, A review on progress made in direct air capture of CO2, Environ. Technol. Innov., 2023, 29, 102991 CrossRef CAS.
- L. Baus and S. Nehr, Potentials and limitations of direct air capturing in the built environment, Build. Environ., 2022, 208, 108629 CrossRef.
- P. Dong, X. Li, Y. Yu, Z. Zhang and J. Feng, Direct Air Capture via Natural Draft Dry Cooling Tower, Int. J. Greenh. Gas Control., 2021, 109, 103375 CrossRef CAS.
- C. Charalambous, E. Moubarak, J. Young, E. Fernandez, S. Moosavi, S. Majumdar, M. van der Spek, B. Smit and S. Garcia, CO2 recovery from ultra-dilute streams: Bridging a novel temperature vacuum swing adsorption model with materials screening, 2020 Virtual AIChE Annual Meeting, 2020 Search PubMed.
- Energy Futures Initiative, Surveying the BECCS Landscape, 2022; http://www.energyfuturesinitiative.org.
- M. Fajardy and N. M. Dowell, The energy return on investment of BECCS: is BECCS a threat to energy security?, Energy Environ. Sci., 2018, 11, 1581–1594 RSC.
- IEA, Energy Technology Perspective, 2023; https://iea.blob.core.windows.net/assets/a86b480e-2b03-4e25-bae1-da1395e0b620/EnergyTechnologyPerspectives2023.pdf.
- IEA, CO2 Transport and Storage. 2022; https://www.iea.org/reports/co2-transport-and-storage.
- STOREGGA, CO2 Storage Resource Catalogue - Cycle 3 Report. 2022.
- Carbfix, https://www.carbfix.com.
- IEA, CO2 Storage Resources and their Development, 2022.
- Carbfix, Permanent and Secure Geological Storage of CO2 by In-Situ Carbon Mineralization, 2022.
- CORDIS, Upscaling and optimizing subsurface, in situ carbon mineralization as an economically viable industrial option, 2017; https://cordis.europa.eu/project/id/764760.
- J. S. Campbell, S. Foteinis, V. Furey, O. Hawrot, D. Pike, S. Aeschlimann, C. N. Maesano, P. L. Reginato, D. R. Goodwin, L. L. Looger, E. S. Boyden and P. Renforth, Geochemical Negative Emissions Technologies: Part I. Review, Front. Clim, 2022, 4, 879133 CrossRef.
- P. Kelemen, S. M. Benson, H. Pilorgé, P. Psarras and J. Wilcox, An Overview of the Status and Challenges of CO2 Storage in Minerals and Geological Formations, Front. Clim, 2019, 1 DOI:10.3389/fclim.2019.00009.
- W. J. Schmelz, G. Hochman and K. G. Miller, Total cost of carbon capture and storage implemented at a regional scale: Northeastern and midwestern United States, Interface Focus, 2020, 10(5) DOI:10.1098/rsfs.2019.0065.
- U.S. Department of Energy, Project Selections for FOA 2610: CarbonSAFE Phase II - Storage Complex Feasibility, 2023; https://www.energy.gov/fecm/project-selections-foa-2610-carbonsafe-phase-ii-storage-complex-feasibility?utm_medium=emailutm_source=govdelivery.
- J. Fuhrman, A. Clarens, K. Calvin, S. C. Doney, J. A. Edmonds, P. O'Rourke, P. Patel, S. Pradhan, W. Shobe and H. McJeon, The role of direct air capture and negative emissions technologies in the shared socioeconomic pathways towards +1.5 and +2 futures, Environ. Res. Lett., 2021, 16, 114012 CrossRef CAS.
- Finance, B. B. N. E. Solar - 10 Predictions for 2022. https://about.bnef.com/blog/solar-10-predictions-for-2022/.
- D. Casaban, S. Ritchie and E. Tsalaporta, The impact of Direct Air Capture during the last two decades: A bibliometric analysis of the scientific research, part I, Sustainable Chem. Climate Action, 2022, 1, 100009 CrossRef.
-
F. Vega, M. Cano, S. Camino, L. M. G. Fernández; E. Portillo; B. Navarrete, F. Vega, M. Cano, S. Camino, L. M. G. Fernández, E. Portillo and B. Navarrete, Solvents for Carbon Dioxide Capture, 2018; https://www.intechopen.com/chapters/57510undefined/chapters/57510.
- D. Theodoros, A. Akrivi, K. Dimitrios, S. George, G. Chara, D. George, N. Lampros, B. Konstantinos, R. Hannes, L. Udo, E. Solon, P. Cristina, H. David and P. Grigorios, Solvents for Membrane-Based Post-Combustion CO2 Capture for Potential Application in the Marine Environment, Appl. Sci., 2022, 12, 6100 CrossRef.
- E. S. Rubin, C. Short, G. Booras, J. Davison, C. Ekstrom, M. Matuszewski and S. McCoy, A proposed methodology for CO2 capture and storage cost estimates, Int. J. Greenhouse Gas Control, 2013, 17, 488–503 CrossRef CAS.
- A. Malhotra, T. S. Schmidt and J. Huenteler, The role of inter-sectoral learning in knowledge development and diffusion: Case studies on three clean energy technologies, Technol. Forecase. Soc., 2019, 146, 464–487 CrossRef.
- F. Kong, G. Rim, M. G. Song, C. Rosu, P. Priyadarshini, R. P. Lively, M. J. Realff and C. W. Jones, Research needs targeting direct air capture of carbon dioxide: Material & process performance characteristics under realistic environmental conditions, Korean J. Chem. Eng., 2022, 39, 1–19 CrossRef CAS.
Footnotes |
† Electronic supplementary information (ESI) available. See DOI: https://doi.org/10.1039/d3ee01008b |
‡ The authors have contributed equally. |
§ It has been stated that the energy requirements of a cryogenic DAC unit are comparable to other DACs only when cryogenic refrigeration units reach efficiencies above 30% (currently around 15%) and heat exchangers operate with a temperature difference between the cold and warm air of less than 3 K at the cold end.80 These operating conditions are limited to arctic regions. Furthermore, these regions are exposed to seasonality, limiting the suitable operation time to 6 to 9 months per year. All these challenges make its scalability to Gt-scale extremely far more difficult than for other processes. Still, RD&D in cryogenic applications is vital, as cryogenic technologies become more competitive in post-combustion capture where CO2 concentrations are much higher. |
¶ We did not optimize any DAC configuration for different areas but rather used publicly available data. The impact of humidity and temperature on energy requirements or on general suitability of a configuration are neglected. Also, we likely underestimate the energy requirements by assuming the process values (in the case of limited data) are identical to those of a complete DAC plant. It is worth noting that this is a first order approximation to explore the costs of a DAC process for different infrastructure and energy supply designs. |
|
This journal is © The Royal Society of Chemistry 2023 |
Click here to see how this site uses Cookies. View our privacy policy here.