DOI:
10.1039/D2EN00547F
(Critical Review)
Environ. Sci.: Nano, 2022,
9, 3725-3741
Graphene-based nanomaterials and microbial communities: a review of their interactions, from ecotoxicology to bioprocess engineering perspectives
Received
10th June 2022
, Accepted 29th July 2022
First published on 8th September 2022
Abstract
Graphene-based materials (GBMs) are gaining more and more attention from the scientific community due to their unique properties. One of the major concerns is to deliver the safest material possible on the market. To address this challenge, assessing the GBM ecotoxicity is required. Microbial communities are present in every environmental compartment, are at the basis of every trophic chain and endorse many essential roles in the environment and in engineered systems such as element cycling. Studies conducted on GBMs' impact mainly focused on the effect of GBMs towards mono species cultures of bacteria and algae, which are not environmentally relevant. This review examines recent advances about GBM effects on complex microbial communities, bringing together ecotoxicology and bioprocess engineering research, two major and complementary fields in environmental sciences. This review reveals the potential of GBMs to disrupt the growth of the communities but also their structure and diversity in a broad spectrum of environmental conditions. Multiple studies reported the disruption of carbon and nitrogen cycling throughout the perturbation of related species. Electron transfer, on which relies many bioprocesses, was also found to be enhanced. The fate of antibiotic resistance genes was also influenced. Moreover, communities seemed to exhibit a form of resilience through composition modifications and also by reducing the oxidation state of the GBMs. Nevertheless, GBMs are pointed out as disruptors of the structure and activity of microbial communities, which could lead to larger environmental consequences as these communities are at the basis of every ecosystem's health.
Environmental significance
Microbial communities are ubiquitous in the environment and play major roles in the health and stability of our ecosystems via different bioprocess including element cycling. The functional potentialities of microbial communities have led to their domestication and intensification of their activities in controlled systems for scientific or industrial purposes. It is more and more recognized that the health of organisms and more globally ecosystems relies on the health of microbial communities. The disruption of these communities would lead to broad environmental and health problems. Therefore, it is essential to evaluate the impact of all types of new and emergent pollutants. GBMs have recently arrived on the market and this review aims to inform on their potential impact on microbial communities.
|
1. Introduction
Graphene, a two dimensional crystalline and allotropic form of carbon, was exfoliated from graphite for the first time by Novoselov and his co-workers in 2004.1 A variety of graphene-based materials (GBMs) have then been elaborated, such as graphene oxide (GO) and reduced graphene oxide (rGO), that can be classified according to their carbon/oxygen ratio, their number of graphene layers and their average width.2 GBMs have attracted the attention of the scientific community thanks to their unique properties. Their rigidity, elasticity, and electrical and thermal conduction have made them very coveted materials in many sectors such as electronics and photonics,3 energy production,4 drug delivery in nanomedicine5 and in waste treatment through the absorption of pollutants such as pharmaceutical drugs in waste waters.6
The unique properties of GBMs have led to an increase of their production over the last years and their market is predicted to reach more than 200 million USD in 2022.7 This increase will inevitably result in the release of GBMs in the environment including in the aquatic ecosystems. To date, the environmental concentrations of GBMs have not been measured due to the lack of operational analytic methods.8 According to De Marchi et al., the predicted environmental concentrations (PECs) would be similar to that of the carbon nanotubes (CNTs) since GBMs and CNTs share similar physical and chemical properties.9 The PECs of CNTs were estimated, using probabilistic modelling and means, to be 0.17 ng L−1 in surface waters, 3.6 ng L−1 in wastewater, 0.12 mg kg−1 in sewage sludge and 0.02 ng m−3 in the air. For environmental compartments which can retain GBMs such as soils and sediments, 5.1 ng kg−1 and 0.79 μg kg−1 are predicted to be accumulated each year, respectively.10
Microbial communities can be found in all environments and ecosystems. These highly diverse communities play a crucial role in the regulation of the biogeochemical cycles.11 They represent a source of carbon and nutrient for higher trophic levels, drive the formation and decomposition of organic matter,12 are responsible for the stabilisation of sediments in the aquatic ecosystems,13 endorse key activities in soil formation and promote plant growth.14 Disturbance of these communities could lead to large-scale environmental and ecological consequences. Effects of GBMs on microbes have been mainly investigated on mono species models of bacteria such as Staphylococcus epidermis, Escherichia coli, Staphylococcus aureus and Bacillus subtilis15–19 and eukaryotic micro algae such as Scenedesmus obliquus and Nitzschia palea.20,21 Results showed that GBMs had an antimicrobial activity due to oxidative stress. GBMs interact with the membranes and oxidate lipids, proteins and nucleic acids causing the disruption of biochemical reactions and leading to the production of different reactive oxygen species (ROS).17 Another mechanism suggested by Mejías Carpio et al.18 was the capacity of GBMs to disable bacteria to interact with their extracellular medium, preventing them from accessing to nutrients and therefore decreasing metabolic activities. However, some studies contested the antimicrobial activity of GBMs and evidenced beneficial effects of GO on the growth rates of microorganisms such as Escherichia coli.22 Some raised the hypothesis that Gram negative bacteria were more resistant thanks to their thicker layer of peptidoglycan,16 but others showed similar response between Gram negative and positive species.15 This led to the understanding that the effects of GBMs were species dependent but also material dependent. Indeed, behaviour of GBMs in the environment differs as a function of the properties of these materials, and is also influenced by environmental factors such as pH and ion concentrations.23 In studies conducted on bacteria, authors pointed out that the response of bacteria to GBMs exposure led to a feedback on GBMs themselves, through a modification of the biochemical properties of GBMs, concentration and fate. It was also revealed that certain bacterial and fungal species were able to biodegrade and reduce GBMs.24,25
Although knowledge was recently acquired on bacteria or microalgae and GBMs, microbial species never appear as a monoculture in the environment but are part of a microbial community. Microbial assemblies embedded in a self-produced matrix of extracellular polymeric substances result, for example in surface-attached biofilms, in a much more successful form of life.26 This form of life appears to offer many functions such as mechanic stability, adherence, water retention, the capacity to retain sources of energy, exchange of genetic information and a protection to environmental fluctuations and contaminations.27 The community also allows interactions and metabolic cooperation between a wide range of species.28 With all these functions and the fact that these communities have the capacity to adapt to a wide range of environmental conditions, the microbial community assembly can however be modulated by intrinsic and extrinsic drivers such as chemicals, metals and antibiotics.29 The potential harmful and beneficial effects on human and vertebrate gut microbiota by engineered nanomaterials such as GBMs were already put forward and was reviewed by Zhang et al. in 2020 (ref. 30) and more recently by Xie et al.31 and Utembe et al.32 in 2022. In this review, to add to the current understanding of the interactions between microbes and GBMs and contribute to a One Health approach, we focused on a broad range of systems that represent the diversity of microbial communities in environmental sciences.
Literature about the impact of GBMs on microbial environmental communities is limited. The topic has nonetheless emerged and the typology of recent studies will be described in the first section of this review. The second point is focused on the effects of GBMs on the structure of the different communities studied. The third point is focused on the effects of GBMs on the different activities of microbial communities and the different species, gene and enzymatic activities related: carbon and nitrogen cycling, host interactions and antibiotic resistance. Finally, the fourth point of this review will address interactions in the opposite direction, i.e. the effects of microbial communities on the fate of GBMs and the potential influence on their bioaccumulation.
2. Studies addressing interactions between GBMs and microbial communities
The following terms “ALL = (graphene) AND (ALL = (microbial communit*) OR ALL = (bacterial communit*) OR ALL = (prokaryotic communit*) OR ALL = (microbiome) OR ALL = (microbiota))” were chosen in order to cover the literature of this topic in Google Scholar and Web of Science™. The tool Web of Science™ displayed 328 publications for these keywords since 2013 (16/05/2022). The number of publications per year can be found in Fig. 1 and reveals the emergence of this topic. Among these 328 publications, literature was refined in order to keep publications dealing with microbial communities in environmental sciences, i.e. environmental compartments as well as bioprocesses. Publications working on mono species, human microbiota and which did not have environmental or bioprocess toxicity purposes were not kept to avoid overlapping with previous reviews. For instance, articles dealing with mice microbiota were discarded since they fit in human health framework.
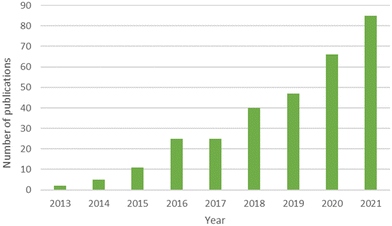 |
| Fig. 1 Number of publications per year since 2013 for the terms ALL = (graphene) AND (ALL = (microbial communit*) OR ALL = (bacterial communit*) OR ALL = (prokaryotic communit*) OR ALL = (microbiome) OR ALL = (microbiota)) indexed in Web of Science™. | |
2.1 Environmental compartments studied and experimental model
The literature documenting the interactions between GBMs and microbial communities (Table 1) is restricted to laboratory experiments in which environmental compartments or bioprocesses are mimicked in controlled conditions. The absence of publications derived from real systems (in natura or in full-scale bioreactors) is probably related to the absence of reliable methodology for GBMs quantification. The majority of the studies (47%) were conducted with microbial communities in bioprocesses (Fig. 2). Among studies focused on bioprocesses, activated sludge sampled from wastewater treatment plants was usually used as starting material, either as inoculum in aerobic batch reactors33–37 or as feeding in anaerobic batch reactors.38–40 The interaction between GBMs and microbial communities in anaerobic digesters was also investigated in reactors fed with swine manure,41,42 cattle manure43 or synthetic wastewater.44,45 Communities in aerobic granular sludges46–49 (strong compact structures embedding microbial cells) were also exposed to GBMs. In addition to the aforementioned bioprocesses that are designed to remove organic matter (and recover energy through methane production in the case of anaerobic digestion), anammox reactors carrying out anaerobic ammonium oxidation were also investigated.50–53 Among environmental compartments, soil was highly studied and represented 27% of the studies. The different types of soils studied were the following: paddy,54 urban,55,56 agriculture,57–62 forest,63 mountain64 and grassland65 soils. The aquatic environment was also studied using communities from surface waters such as rivers,66,67 lake,66 estuary68 and aquarium69,70 but also sediment communities.71,72 Those communities were exposed to GBMs in microcosm experiments of variable size, complexity and realism. Although most studies relied on small-scale (≤1 L) batch incubations, Evariste et al.69 used a more complex experimental setup combining a water and a sediment compartment as well as a reconstituted trophic chain. Such an experimental model integrates the interactions between pelagic and benthic microbial communities together and other aquatic organisms (decomposers, primary and secondary consumers). Reconstituted wetlands were also studied twice by the same authors in 2022.73,74 Less represented compartments in the literature were gut microbiota, here from zebrafish.75–77 The low representation of gut microbiota is in line with recent reviews highlighting that gut microbiota are understudied in spite of their key role in host and ecosystem response.78,79
Table 1 Summary of results obtained across the literature. α stands for the alpha diversity and β for the beta diversity. Four types of effects were reported regarding growth, diversity and activity: ↗ stands for an increase compared to control, ↘ for an inhibition compared to control. E stands for effect, NE for no effect. The “–” symbol means that this effect was not investigated during the study. EPS: exopolymeric substances. COD: carbon oxygen demand. OM: organic matter
Experimental conditions |
Observed effects |
Study |
Type of community |
Type of GBMs |
Concentrations |
Exposure time |
Growth |
Diversity |
Activity |
ARGs |
Impact on graphene |
33
|
Activated sludge |
GO |
10–20–50–100–200–300 mg L−1 |
5 h |
↘ |
— |
Nitrification: ↘ |
— |
— |
BOD5: ↘ |
34
|
Activated sludge |
GO–graphene |
5–100 mg L−1 |
45–60 d |
↘ |
α: Transitory ↘ |
Nitrification: ↘ |
— |
Reduction |
35
|
Activated sludge |
GO |
5–25 mg L−1 |
11 d |
— |
α: ↗/β: E |
Nitrification: ↗ |
— |
— |
36
|
Activated sludge |
GO–graphene |
1–5 mg L−1 |
10 d |
— |
β: E |
COD removal: ↘ |
— |
— |
Nitrification: ↘ |
37
|
Activated sludge |
rGO–rGO–nZVI |
10 mg L−1 |
115 d |
↗ |
β: E |
Nitrification: ↗ |
— |
— |
38
|
Anaerobic digester |
GO |
21.6–43.2 g L−1 |
55 d |
— |
— |
Methanogenesis: ↘ |
— |
— |
40
|
Anaerobic digester |
Graphene |
5–10–15 g L−1 g−1 L−1 |
8–80 d |
↗ |
α: ↗/β: E |
Methanogenesis: ↗ |
— |
— |
COD removal: ↗ |
39
|
Anaerobic digester |
GO–graphene |
3 mg L−1 |
13–18 d |
— |
α: Transitory ↘ |
Methanogenesis: ↗ |
↘ |
— |
β: E |
44
|
Anaerobic digester |
Graphene |
30–120 mg L−1 |
15–55 d |
— |
— |
Methanogenesis: E |
— |
— |
EPS: ↗ |
41
|
Anaerobic digester |
GO |
5–50–100–500 mg L−1 |
0–5–13–22 d |
— |
— |
Methanogenesis: ↘ |
↘ |
— |
42
|
Anaerobic digester |
GO with Cu |
100–800 mg L−1 |
5–15–30–50 d |
— |
— |
— |
↘ |
— |
43
|
Anaerobic digester |
rGO |
10–20–30 mg L−1 |
4–5 d |
— |
α: ↗/β: E |
Methanogenesis: ↗ |
|
— |
45
|
Anaerobic digester |
GO–rGO |
10–50–300 mg L−1 |
5–13–22 d |
— |
— |
Methanogenesis: ↘ |
— |
Reduction |
46
|
Aerobic granular sludge |
GO |
15–25–35–55–75–95 mg L−1 |
7 d |
↘ |
β: E |
Nitrification: ↘ |
— |
— |
COD removal: ↘ |
EPS: E |
47
|
Aerobic granular sludge |
GO |
15–35–55–75–95–115 mg L−1 (increase of dose every 8 d) |
112 d |
— |
— |
Nitrification: ↘ |
— |
— |
COD removal: ↘ |
EPS: ↗ |
48
|
Aerobic granular sludge |
GO |
50–100–150–200 mg L−1 |
5–30 d |
— |
β: E |
Nitrification: E |
— |
— |
EPS: E |
49
|
Aerobic granular sludge |
GO |
60 mg L−1 |
235 min |
— |
β: E |
Denitrification: NE |
— |
— |
EPS: ↗ |
50
|
Anammox reactor |
GO |
1–10 mg L−1 |
61 d |
— |
— |
Anammox: ↘ |
— |
— |
51
|
Anammox reactor |
GO |
0.05–0.1–0.15 g L−1 |
0–7–14–21–28–35–42 h |
— |
— |
Anammox: ↗ |
— |
— |
EPS: ↗ |
52
|
Anammox reactor |
Graphene |
10–25–50–100 mg L−1 |
48 h |
— |
β: E |
Anammox: ↗ |
— |
— |
EPS: E |
53
|
Anammox reactor |
rGO |
15 mg L−1 |
316 d |
↗ |
β: NE |
Anammox: ↗ |
— |
Degradation–oxidation |
55
|
Soil |
GO |
0.1–0.5–1 mg g−1 |
7–13–21–31–45–59 d |
↘ |
— |
Enzyme activity (OM degradation): ↗ |
— |
— |
59
|
Soil |
GO |
5 g kg−1 |
90 d |
— |
α: ↗/β: E |
— |
— |
Reduction |
60
|
Soil |
GO–rGO |
50 mg kg−1 |
90 d |
— |
α: ↘/β: E |
— |
— |
— |
54
|
Soil |
Graphene |
10–100–1000 mg kg−1 |
4–21–60 d |
↗ |
β: E |
— |
— |
— |
65
|
Soil |
Graphene |
1000 mg kg−1 |
1 y |
↘ |
— |
— |
— |
— |
63
|
Soil |
Graphene |
10–100–1000 mg kg−1 |
7–15–30–60–90 d |
↗ |
α: Transitory ↘/β: E |
— |
— |
— |
64
|
Soil |
GO with cd |
1–2 g kg−1 |
3–7–15–30–60 d |
— |
α: ↘ |
— |
— |
— |
58
|
Soil |
GO–rGO–aGO |
1 ng–1 μg–1 mg kg−1 |
7–14–30 d |
— |
α: NE/β: E |
— |
— |
— |
57
|
Soil |
GO |
1 ng–1 μg–1 mg kg−1 |
7–14–30 d |
— |
α: NE/β: E |
— |
— |
— |
56
|
Soil |
GO–Ag–GO |
100–500–1000 mg kg−1 |
7–14–21 d |
NE |
α: NE/β: E |
C cycling: ↘ |
|
— |
Nitrification: ↘ |
61
|
Soil |
Graphene |
300 mg kg−1 |
30–360 d |
— |
α: ↗/β: E |
— |
— |
— |
62
|
Soil |
Graphene |
0–0.1–100–1000 mg kg−1 |
20–39 d |
— |
β: E |
— |
— |
— |
71
|
Sediment |
GO–rGO |
0.1 g/20 mL |
1–2–3–5–7 d |
— |
α: ↘/β: E |
Denitrification: ↗ |
— |
— |
72
|
Sediment |
GO |
5–7.5–10–25–50 mg L−1 |
18 h |
— |
— |
Denitrification: E |
— |
— |
EPS: ↗ |
67
|
Surface water |
GO |
0.01–0.1–1 mg L−1 |
10 d |
— |
— |
— |
↘ |
— |
68
|
Surface water |
GO |
50–100–150–200–250–300 mg L−1 |
15 min |
— |
— |
— |
↘ |
— |
66
|
Surface water |
GO–Ag–GO |
10–50–100 mg L−1 |
1–24–48 h |
— |
— |
Nitrification: ↘ |
— |
— |
69
|
Surface water |
GO–rGO |
0.5–1 mg L−1 |
48–144 h |
— |
β: E |
— |
— |
— |
70
|
Surface water |
GO–rGO |
0.1–1–10 mg L−1 |
48–144 h |
↘ |
α: ↗/β: E |
— |
— |
— |
73
|
Constructed wetland |
GO |
0.5–5 mg L−1 |
30–60–90–120 d |
— |
β: E |
Nitrification: ↘ |
— |
— |
Denitrification: ↘ |
74
|
Constructed wetland |
GO |
0.5–5 mg L−1 |
30–60–90–120 d |
Transitory ↘ |
α: ↘ then ↗/β: E |
OM removal: NE |
— |
— |
75
|
Zebrafish microbiota |
GO |
0.05–0.5–5–50 mg L−1 |
25 d |
— |
α: E/β: E |
— |
— |
— |
76
|
Zebrafish microbiota |
Graphene–GO–rGO |
1 μg per day |
14 d |
— |
α: NE/β: E |
— |
— |
— |
77
|
Zebrafish microbiota |
FLG |
0.25 mg L−1 (approx. 48 mg kg−1 dry mass) |
48 h |
— |
α: NE/β: E |
— |
— |
— |
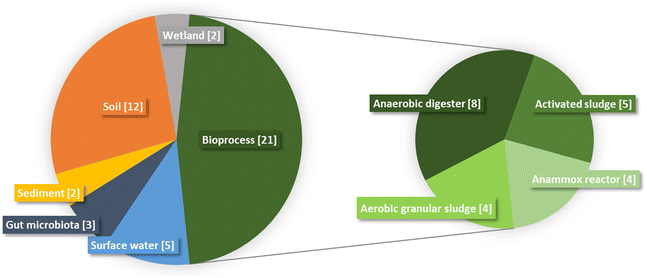 |
| Fig. 2 Distribution of studies documenting the interactions between GBMs and microbial communities as a function of the experimental model studied. For the bioprocess category, subcategories were distinguished and represented in the right panel. The number of articles contributing to each category or subcategory is indicated in brackets. | |
2.2 Graphene based materials used, concentrations and exposure time
Graphene oxide was the most represented material (32 studies) compared to graphene (12 studies), rGO (10 studies) and few layers graphene (FLG) (1 study; Fig. 3). This imbalance can be related to the higher toxicity of GO reported for mono species models of bacteria.17 Effects of graphene composite materials were also investigated in 4 studies, such as Ag–GO and rGO–nZVI (nano zerovalent iron). Co-contamination was just tested twice, bringing together GO and Cu in an anaerobic digester fed with swine manure42 and another study exposing Cd contaminated soils to GO.64
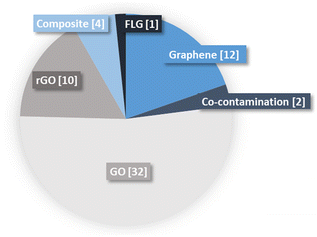 |
| Fig. 3 Types of GBMs and number of times they occurred in the literature. The number of articles contributing to each category is indicated in brackets. | |
The concentrations tested (Table 1, Fig. 4) in the same compartments are heterogeneous, especially in soils: the lowest concentration tested, 10−9 mg kg−1, is 13 orders of magnitude lower than the highest concentration tested, 5 g kg−1. Generally, the concentrations tested were higher than predictable concentrations mentioned previously in the introduction. In some cases, the concentrations tested in soil or surface water exceeded PECs by eight orders of magnitude.64,66 This can be explained by the fact that some studies aim to reproduce a spill in the environment, representing a “hotspot”, while PECs refer to a diffuse concentration in the environment. It is noteworthy that in some extreme cases in the field of bioprocess engineering, GBMs were not seen as contaminants but as a lever to improve process efficiency and were added at very high concentrations. For example, graphene was added up to 15 g L−1 to increase phenol removal during anaerobic digestion.40
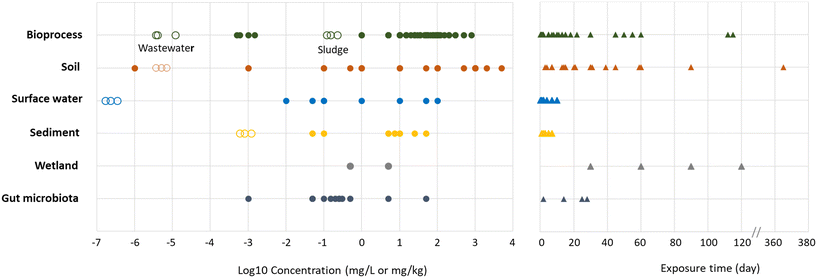 |
| Fig. 4 GBMs concentrations (left panel) and time of exposure (right panel) used in the studies. Full circles represent the concentrations experimentally tested in studies while empty circles represent CNTs PECs (GBMs PECs are still unknown but can be comparable to CNT PECs due to their similar properties9). PECs are only available for sediment, surface water, bioprocess and soil. Two series of PECs are represented in bioprocess, the lowest represents PECs in wastewaters effluent and the highest represents PECs in wastewater sludges. The three concentrations of PECs given by types of environmental compartments are the first, second and third quartile of the prediction model.10 For sediment and soils, concentrations of PECs represent a yearly accumulation in mg kg−1 per year. | |
The times of exposure were also highly heterogeneous (Table 1, Fig. 4). Times of exposure in soils were higher, starting at 3 days (ref. 64) and going up to one year of exposure.65 These longer time of exposures in soils are related with their characteristics to retain GBMs in the environment. In bioprocesses, exposure times were generally around a few hours, but some studies went up to 60 days of exposure, depending on the retention time in real processes (hours to few days for activated sludges when anaerobic digesters have higher retention time from 15 to 60 days). In aquatic environments such as surface waters and sediments, exposure was relatively short, never exceeding 10 days. These heterogeneous times of exposure are important because, combined with appropriate concentrations, they represent different types of pollutions in the environment.
2.3 Study goals: from ecotoxicology to process engineering
The recent results included in this review span both ecotoxicological studies in environmental compartments and studies on the effects on microbial communities in engineered systems such as nitrification, denitrification, anaerobic ammonium oxidation (anammox) and anaerobic digestion reactors. In both types of studies, microbial communities were exposed to GBMs in experimental setups that mimic actual systems, by using microcosms for natural environment such as surface waters,67,69 or using laboratory scale reactors to mimic full scale reactors: sequential batch reactors,34 anaerobic digestors38,41,42,44,45 and anammox up flow column reactor.51 The specificity of the studies focused on engineered systems is that they systematically targeted the functions intensified in such reactors by measuring bioprocess efficiency together with, generally, documenting the related functional guilds of microbes. On the contrary, studies carried out in microcosms mimicking environmental compartments do not necessarily investigate the effects of GBMs on functional counterparts to bioprocess efficiency, which are the activities contributing to natural biogeochemical cycles. The lack of functional data in such studies is probably due to the coexistence of multiple interacting activities in the natural environment, whereas bioprocesses are highly constrained to intensify one activity. Therefore, even if ecotoxicology and bioprocess engineering refer to distinct scientific communities, combining both perspectives in this review was found to be relevant since bioprocesses can be seen as a tool to a better understanding of the relationships between GBMs and the structure and function of microbial communities.
3. Effect of GBMs on the microbial community structure
3.1 Effect on growth, viability and biomass
One of the main endpoints studied on microbial communities is the growth rate. It can be expressed as the evolution of the number of cells, the biomass or even the quantity of DNA. GBMs tend to affect negatively the growth of microbial communities. For instance, in activated sludges, it was shown that GO had an impact on the growth as soon as five hours of exposure at 50 mg L−1, and decreased viability at 100, 200 and 300 mg L−1.33 The quantity of DNA in aerobic granules was also found to be decreased after 7 days of exposure to 35 and 95 mg L−1 of GO.46 The decrease of cell viability of communities exposed to 100 mg L−1 in a sequential batch reactor during 45 and 60 days demonstrated an acute toxicity of long term exposure to high concentrations of GO.34 Decrease of biomass (determined by DNA quantity) was also reported on soil microbial communities exposed for a year to graphene at 1 g kg−1.65 A decrease of microbial biomass was additionally reported in soil communities after 7 days of exposure to GO at 0.5 and 1 g kg−1, but was interestingly followed by a recovery after 13 to 59 days.55 In a biofilm composed of diatoms and bacteria, the bacterial growth rate was found to be highly diminished compared to the control (up to 75%) by the presence of 1 and 10 mg L−1 of GO after 48 and 144 h of exposure, whereas rGO impacted only slightly the growth rate at 10 mg L−1.70 This study highlighted different effects of different GBMs on the same community. Like reported on monocultures of bacteria,16 the overall oxidative stress was enhanced in microbial communities exposed to GBMs33,50,66 which could explain the observed negative effects on the growth rates in these different studies.
Although negative effects were generally measured, it is noteworthy that positive effects were also reported with GBMs. An increase of biomass in soil was observed after 4 days of exposure to 10 and 100 mg kg−1 of graphene.54 These results were supported in soil by Song et al. in 2018 (ref. 63) who observed, after a slight decrease at day 7, an increase of biomass after exposure to 10 and 100 mg kg−1 of graphene for the following days and until the end of exposure, day 90. On the other hand, in the same study, 1000 mg kg−1 of graphene affected negatively the biomass after 60 days of exposure. A beneficial effect of GO at 10 mg L−1 on the growth rates of diatoms was measured in a diatom–bacteria biofilm.70 In this same study, bacteria growth rate was decreased, showing contrasted effects of GO on different organisms in one same community. Taken as a whole, these results suggest an adaptivity or even a growth stimulation of some microorganisms at lower doses of graphene. Higher concentrations and longer exposures seem to generate a negative effect on the biomass. This form of adaptivity, and sometimes resilience, can be related with the expression of genes that are markers of the global health of cells. Mobility, wall and envelope biogenesis, repair, recombination and replication genes were investigated and were found to increase after an exposure to 1 and 10 mg L−1 of GO during 61 days.50 In the same study, the lactate dehydrogenase (LDH) activity, an indicator of stress and cell death when it increases, was found to decrease through time. At higher concentrations such as 1 and 2 g kg−1 of GO, the LDH activity was significantly promoted during the first 15 days of exposure but returned to normal between 30 and 60 days of exposure.64 It was also notified in constructed wetlands that exposition to GO led to the production of antioxidative enzymes such as superoxide reductase in order to counter oxidative damage from GO.74 Taken as a whole, these results support the idea of resilience, or even positive effects, that can be observed on growth.
3.2 Effect on the alpha diversity
The alpha diversity of microbial communities represents the richness and/or evenness of taxa within a community, commonly quantified through diversity indexes such as Chao1, Shannon and Simpson indexes.80 Alpha diversity indexes are commonly derived from metabarcoding datasets, i.e. high-throughput sequencing of 16S rRNA gene amplicons. The modification of the alpha diversity in communities exposed to GBMs did not show a common pattern. The absence of detectable effect on the alpha diversity was reported in shorter-term studies conducted with soils (below 30 days)56–58 and zebrafish microbiota (below 14 days).76,77 Increase of the alpha diversity, especially the Shannon index, was rare but occurred in anaerobic digesters exposed to 10, 20 and 30 mg L−1 of rGO43 and 5, 10 and 15 g L−1 of GO,40 in activated sludge exposed to 25 mg L−1 of GO35 and soils exposed to 5 g kg−1 of GO for 90 days59 and to 300 mg kg−1 of graphene for 360 days.61 Nevertheless, a decrease of the alpha diversity was observed in many studies. Interestingly, a co-contamination of Cd and 1 and 2 g kg−1 of GO only altered the alpha diversity (Shannon index) of soil after 60 days of exposure.64 This late effect on the Shannon index was also reported on communities in sequential batch reactors after 45 days of exposure to 100 mg L−1 of GO, but with recovery after 60 days.34 Meanwhile, a decrease of several indexes (Richness, Evenness, Shannon and Chao1) was also measured during a short exposure of 7 days to GO and rGO in sediments.71 Exposure to rGO 50 mg kg−1 for 90 days also decreased ACE (estimation of the number of species using sample coverage), Chao1 and Shannon indexes in a soil community, while GO at the same concentration had no effect on the alpha diversity.60 Transitional effects were also observed in soils: in 2018, Song et al.63 reported a decrease compared to control of the alpha diversity after an exposure of 7–15 days to 10 and 100 mg kg−1 of graphene, but an increase after a longer exposure. The recovery and increase were not observed for the highest concentration of 1000 mg L−1, displaying a form of resilience of the communities to lower concentrations of GBMs. After 18 days, a recovery of the alpha diversity (Chao, Shannon, Simpson and ACE) was also observed in anaerobic digesters exposed to 3 mg L−1 of graphene and GO.39 These results are consistent with observed effects on growth and biomass showing a transitory effect and the adaptivity of microorganisms exposed to lower concentrations. Different effects were also reported in the same study between the gut microbiota of female and male zebrafishes.75 Alpha diversity of microbiota exposed to 5 mg L−1 of GO was increased in the female and decreased in male. Microbiota composition was not the same between male and female individuals, showing a difference of response to GO as a function of the composition of the community.
3.3 Effect on the beta diversity
The beta diversity represents the dissimilarity between communities structure as usually determined by metabarcoding. When it was investigated and analysed between control communities and exposed communities, differences were always evidenced, but the temporal patterns of dissimilarity were not congruent. For instance, in soil communities exposed to graphene, a dose dependent effect was observed in the 7 first days with 10, 100 and 1000 mg kg−1, and a recovery was pointed out at day 21 and 60.54 This recovery was not observed in other soil experiments with the same concentrations.63 Instead, communities exposed to the highest dose of graphene (100 et 1000 mg kg−1) differed more from the control in the later stages. Soil56–58,60–62 and sediment71 communities exposed to GO, rGO and/or graphene showed similar results, demonstrating time, concentration and pollutant dependant shifts of microbial communities. In a complex biofilm composed of diatoms and bacteria, 10 mg L−1 of GO caused shifts in the structure of the communities exposed, whereas rGO did not impact the beta diversity.70 Finally, in a complex aquatic system containing several trophic levels with a microbial community at its basis, changes in the benthic and planktonic communities exposed to 0.05 and 0.1 mg L−1 of GO and rGO were revealed.69 This shows the capacity of GBMs to drive community structures by decreasing non-tolerant species, benefitting to more tolerant species. These changes in microbial communities can also have an impact on the interaction network of these communities, for example increasing negative interactions such as competition. The degradation of these interactions, highlighted by Lian et al.,34 can lead to disruptions of important functions of the communities, such as element cycling, and generate broader consequences for the entire ecosystem.
Although beta diversity is generally affected by GBMs, it is difficult to point out specific taxa which are particularly affected or tolerant in the presence of GBMs while all communities studied originated from highly diverse systems and experiments were conducted in highly diverse environmental conditions. Nevertheless, a common pattern was the identification of changes in taxa with recognized roles in major biochemical cycles such as nitrogen and carbon cycles.
4. Microbial activities affected by GBMs and related taxa affected
4.1 Nitrogen cycle
Microbial communities regulate nitrogen cycling through multiple processes such as nitrification (oxidation of ammonia into nitrite and nitrate), denitrification (series of reductions leading to the transformation of nitrate into elemental nitrogen) and anammox reactions (nitrate and ammonia simultaneously converted into nitrogen and water).
4.1.1 Nitrification.
The nitrification process was found to be inhibited by GO at 50 to 300 mg L−1 in activated sludge after 5 h of exposure.33 This was also observed with a longer time of exposure to GO (48 h) in river and lake communities during fall, while surprisingly in the same study, no effect was observed during summer, underlining the difference of effect of GO influenced by the different environmental factors,66 and so different communities. Inhibition of NH4+ removal by the presence of 115 mg L−1 of GO was also underlined in aerobic granular sludge47 and by 3.64 and 5.26 g L−1 of GO in sequential batch reactors.36 Transient effects on the nitrification process were highlighted in aerobic granular sludge exposed to 150 and 200 mg L−1 of GO: after 5 days, the nitrification rate was increased, in parallel with a higher abundances of the Nitrospirae family and the Nitrosomonas genus, while after 30 days it was decreased.48 In other aerobic granules, the removal of NH4+ was found to be decreased after 7 days of exposure to 55 mg L−1 and up to 95 mg L−1 (decrease of 88.7%).46 Inhibition of nitrification was confirmed on communities exposed to 100 mg L−1 of GO and graphene in sequential batch reactor for 45 days, but after 60 days of exposure, the nitrification rate was recovered with GO.34 This recovery can be associated with changes and adaption of communities, promoting species responsible of the nitrification process. In soil communities, the nitrification rate was found to be decreased by 1 mg kg−1 of Ag–GO after 21 days of exposure.56 The decrease of nitrification was explained by the decrease of the urease enzyme activity observed64 in soil communities exposed to GO where a recovery of activity was observed after 30 days of exposure. This decrease can also be associated to the diminished relative abundance of Nitrospirae, which has a role in nitrification, and the Rhodospirillales order (N2 fixation). The relative abundance of Nitrospirae was also found to be diminished in soils exposed to graphene along with Planctomyces, capable of ammoniac oxidation.54 Although GBMs seem to usually impair nitrification, the ammonia removal by nitrification was found to be increased in activated sludge exposed to 5 and 25 mg L−1 of GO.35 It was also observed in wastewaters exposed to 10 mg L−1 of rGO37 explained by an increase of the amoA gene copies and an increase of the relative abundance of Nitrospirae.
4.1.2 Anammox process.
Upon exposure to GO at 1 and 10 mg L−1, the anammox rate exhibited a dose dependent short term decrease, but recovered after 15 days.50 The recovery could be associated to a higher relative abundance of anammox bacteria such as the Anammoxglobus specie. On the contrary, in a community fed with NH4+ and NO2− in batch, the anammox process was increased by 0.05; 0.1 and 0.15 g L−1 of GO after 42 h of exposure.51 Increases of the anammox rate was also observed in a community exposed to 15 mg L−1 of rGO for 316 days (ref. 53) and in a reactor exposed to 25, 50, 100 and 150 mg L−1 of graphene52 where higher relative abundances of anammox bacteria species were observed: Candidatus Brocadia and Candidatus Jettenia. Anammox relies on electron transfer and interestingly, it has been put forward the capacity of Candidatus Brocadia to use GO as an electron acceptor in the case of anaerobic oxidation of ammonium.81 This potential increase of electron transfer by GBMs could be an explanation to the increase of the anammox process.
4.1.3 Denitrification.
The denitrification process was also studied in soil communities exposed to GO and rGO. From day 1 to day 7, the nitrogen, particularly nitrate, removal efficiency was increased. These results were correlated with the increase of the relative abundance of Sulfuricella and Rhodobacter, both genera capable of denitrification. This was also correlated with the increase of the activity of different denitrification enzymes: nitrate reductase (NAR), nitrite reductase (NIR), nitric oxide synthase (NOS) and nitric oxide reductase (NOR).71 NAR and NIR activity was also enhanced in a sediment community exposed to 7.5 and 10 mg L−1 of GO for 18 h.72 As denitrification relies on extracellular electron transfer,82 this increase of denitrification is explained by the increase of the electron transport system thanks to GO. If those results show increases in denitrification and related taxa, it is noteworthy that taxa capable of converting nitrate in nitrite were found less abundant in soils exposed to pristine-GO,59 but unfortunately denitrification rates were note monitored. Other results showed that genera capable of removing nitrate such as Paracoccus and Klebsiella could be tolerant to GO in aerobic granules46 and others, such as Zoogloea, intolerant to GO in sequential batch reactors.36 Functional groups, predicted with FAPROTAX database, involved in nitrate reduction were found less abundant in the presence of 0.1 and 100 mg kg−1 of graphene.62
4.2 Carbon cycle
4.2.1 Methanogenesis.
One of the major roles of microbial communities in the carbon cycling is methane (CH4) formation through methanogenesis. On one hand, CH4 has a strong radiative effect and is thus related to climate change.83 Methanogenesis in the environment has therefore been extensively studied to better constrain CH4 emissions to the atmosphere. On the other hand, CH4 being an energy source, methanogenesis is also a crucial microbial process because it can be domesticated and intensified to produce energy in bioprocess engineering. The interactions between GBMs and methanogenesis have been mainly studied in the bioprocess engineering perspective. The effect of GO and rGO was investigated on the three different pathways of methanogenesis in batches:45 acetoclastic, methylotrophic and hydrogenotrophic. After 144 h of exposure, GO had beneficial impact on the acetoclastic and methylotrophic pathways at 10 and 50 mg L−1, while 300 mg L−1 impacted negatively acetoclastic and hydrogenotrophic ones. With rGO, methylotrophic methanogenesis was increased at all concentrations, acetoclastic at 50 and 300 mg L−1 and hydrogenotrophic at 500 mg L−1. This underlined the difference of effect between two GBMs and their concentrations in a same system. With longer times of exposure and in the presence of graphene, the acetoclastic methanogenesis was found to increase after 15 days of exposure at 30 and 120 mg L−1, but was decreased with 120 mg L−1 after 55 days.44 Surprisingly, the acetate kinase activity was decreased: the authors suggested that this loss of activity was compensated by the increase of the coenzyme F420. In the same study, relative abundances of genera implicated in methanogenesis were found to be affected: Methanosarcina (decrease) and Methanobacterium (increase), but also lactate producers such as Olsenalla (increase) and Lactococcus (decrease). Methane production was also enhanced in anaerobic digesters exposed to 3 mg L−1 of graphene and GO after 13 and 18 days (ref. 39) and to 10 mg L−1 of GO after 8 days.40 10, 20 and 30 mg L−1 of rGO for 45 days also increased methane production in an anaerobic digester and was related with the increase of the relative abundance of the Methanoculleus genus, capable of hydrogenotrophic methanogenesis.43 The increase of methanogenic activity was not found in all studies. GO at 2.16 and 43.2 mg L−1 was found to decrease the total methane production, affecting the methylotrophic pathway. It was highlighted that GO had a high affinity with the organic substrates, leading to a decrease of their bioavailability.38 GO at 5, 50, 100 and 500 mg L−1 decreased the total methane production from swine manure. In the same experiment, the key gene mcrA was quantified and interestingly found to be increased and the abundance of the genera Methanobrevibacter and Methanosphaera were found to be decreased. The authors suggested that the membrane wrapping, oxidative stress and cell death due to GO addition caused the methanogenesis inhibition.41 There seem to be no general trend in the effects of GBMs on methanogenesis. Possible increases or decreases seem to be related to the different degree of resistance to GBMs of taxas capable of this bioprocess. An explanation of the increase could be the enhancement of electron transfer by the materials. Interestingly, Igarashi et al.84 exposed a co-culture of Geobacter (electron producer) and Methanoasarcina (electron consumer) to GO. They underlined the fact that in the absence of GO, methane production did not occur, while in the presence of GO, acetate was produced from ethanol and then consumed to produce methane. This suggests the capacity of GO to promote the “direct interspecies electron transfer” by playing the hypothetic role of electron carrier. However, it has not been proven that this electron transfer between species with possible enhancement of methanogenesis occurs in more complex communities.
4.2.2 Degradation of organic matter.
Evariste et al.70 measured potential activities related to organic matter degradation in aquatic microbial community exposed to 0.1, 1 and 10 mg L−1 of rGO and GO. The authors revealed an increase of the specific activity of the communities exposed to 10 mg L−1 of GO for 48 hours. This increase was not noticeable after 144 h of exposure. In the same study, the genus Silanimonas (degradation of benzene) was found to be tolerant to GO along with the genus Acidovorax (degradation of organic matter and aromatic compounds). Acidovorax genus was also identified as tolerant in aerobic granules exposed to GO.46 The presence of 10 to 300 mg L−1 of GO in activated sludge decreased of over 50% of the BOD5, revealing a high loss of organic compound removal.33 In the same way, in aerobic granular sludges, after 7 days of exposure to GO ranging from 35 to 95 mg L−1, the COD (Chemical Oxygen Demand) removal was highly decreased in a dose dependent manner (up to 73.7% decrease).46 A decrease of the COD removal was also found in aerobic granular sludge exposed to 115 mg L−1 of GO47 and in sequential batch reactors exposed to 3,64 and 5.26 g L−1 of GO for 8 days.36 On the contrary the COD removal increased in the presence of 10 mg L−1 of GO in anaerobic digesters.40 Functional groups involved in cellulolysis, as predicted with FAPROTAX database, were found more abundant in the presence of 0.1 and 100 mg kg−1 of graphene.62 Chung et al.55 exposed soil communities to 0.1, 0.5 and 1 mg g−1 of GO and investigated enzyme activities related to organic matter degradation. Xylosidase and β-1,4-N-acetylglucosamidase activities decreased in the first 21 days and then a recovery and even an increase of activity was reported after 45 days of exposure. In another soil community, Ag–GO at 1 mg kg−1 significantly impacted the activity of cellobiohydrolase, β-glucosidase and xylosidase.56 At the level of gene expression, it was shown that genes encoding for transport, metabolism of carbohydrates and amino acids and signal transduction mechanisms were impacted by acute and chronic exposure of GO.50
Other studies during which activities related to the carbon cycle were not directly investigated also found changes in taxa with carbon-related activities. The relative abundance of genera involved in the biodegradation of organic pollutants were found to be enhanced by the presence of graphene in soil communities: Bacillus, Arthrobacter and Geobacter.54Sphingomonas relative abundance, also capable of organic pollutant biodegradation, was found to increase in other soil communities co-exposed to GO and Cu along with Actinobacteria, involved in decomposition of organic matter such as chitin and cellulose.64Actinobacteria was also found to be more abundant in soils exposed to graphene for 30 days.61 In sequential batch reactors exposed to graphene and GO, an increase of the relative abundance of Saccharibacteria, capable of metabolizing organic macromolecules, was observed compared to control.34 In an anaerobic system, changes in the relative abundances of genera with a role in the carbon cycle such as Alkaliflexus (utilisation of carbohydrates), Syntrophomanas (degradation of butyrate) or Lactobacillus (fermentation) were observed.40 In an anaerobic digestor exposed to rGO, the relative abundance of Defluvitoga, capable of converting complex carbohydrates in acetate, ethanol, H2 and CO2, was found to be increased.43 Soil communities during two studies, were exposed to graphite, rGO and/or GO57,58 with no focus on the carbon or nitrogen cycle. Nevertheless, the results also highlighted modifications of relative abundances of genera with roles in the carbon cycle such as Acidobacterium (use of glucose and lactose) and Chlorobiales (use of CO2) and the specie Clonostachys candelabrum (fermentation). In soils, changes in the relative abundances of functional groups predicted using FAPROTAX database (REFFF) after exposition to 50 mg kg−1 of GO and rGO were observed. These functional groups were related to organic matter degradation (chemoheterotrophy and fermentation). There is no shared trend in these overall results, but changes in the taxa composition of microbial communities and in their activities show the potential of GBMs to modulate the degradation of organic matter.
4.2.3 Exopolymeric substance production.
Microbial communities have also a role in the carbon cycle through the production of EPS in biofilms. Few studies were focused on EPS production but all congruently demonstrated an increase of production. GO at 0.05 and 0.1 g L−1 induced an increase of total EPS, but no increase was revealed at 0.15 g L−1 after 42 h of exposure in an anammox reactor.51 In a sediment community exposed to GO, the total EPS content was also increased after 18 h of exposition.72 In an anaerobic digester fed with wastewater and exposed to graphene for 55 days, the total EPS production also increased at 30 and 120 mg L−1.44 The relative content of carbohydrates increased while the relative protein content decreased. The EPS content also increased in aerobic granular sludge exposed to 60 mgGO L−1.49 In other aerobic granules exposed for 7 days, the production of EPS was found to be increased at the lowest concentration tested (15, 25 and 35 mgGO L−1) while higher concentrations (55, 75 and 95 mg L−1) decreased the production of EPS.46 In the same study, the content of the EPS was also changed. The protein content was increased at the lowest concentrations and decreased at the highest while the carbohydrates content was decreased at each concentration. The protein content of EPS was also increased in an annamox reactor exposed to 10 mg L−1 of graphene52 and in aerobic granular sludge exposed to 35 and 50 mg L−1.47 On the contrary, it was observed in aerobic granular sludge, after an increase of the protein content at day 5, a decrease of the protein content and total EPS production after 30 days of exposure to 150 and 200 mg L−1 of GO.48 This showed the capacity of graphene-based materials to modify not only the quantity but also the quality of EPS.
4.3 Microbiota and host interactions
Gut microbiota endorse vital roles in health and metabolism of their hosts.85 In the past two decades, tremendous advances were made in understanding the influence of microbiota in human health, mostly using mouse and rat as experimental models.86 In line with these advances, the interactions between nanoparticles, human gut microbiota models and human health have been explored and have been reviewed extensively.30–32 However, in environmental sciences and more specifically in ecotoxicology, gut microbiota is just emerging.78,79 Therefore, literature remains scarce and almost inexistent about GBMs ecotoxicity towards gut microbiota of hosts that are representative of aquatic or terrestrial ecosystems, except for three studies conducted on zebrafishes. Studies that focused on gut microbiota of zebrafishes revealed decreases in Fusobacteria and Bacteroidetes phyla which have a major role in the production of vitamins and the degradation of complex molecules.87 The same study also revealed an increase in the Proteobacteria phylum, which was in this case associated with dysbiosis and toxin liberation88 and which was correlated with weight gain of zebrafishes exposed to GO.75 The relative abundance of Proteobacteria was also decreased in zebrafish microbiota exposed to FLG.77 Inflammatory responses caused by GBMs were also reported in zebrafishes and was associated with dysbiosis: the increase of Fusobacteria, Cetobacterium and Lactobacillus and the decrease of Firmicutes and Pseudomonas.76 GBMs have the potential to modulate gut microbiota and thus be a threat for hosts of these unbalanced communities. Nevertheless, the use of one only host model is questionable. More research is needed to better constrain the effects of GBMs on gut microbiota of environmental hosts that would better represent the biodiversity in terrestrial and aquatic ecosystems.
4.4 Antibiotics resistance
The effect of GBMs, especially GO, was also tested on antibiotic resistance and revealed a decrease of abundance and dissemination of antibiotic resistance genes (ARGs) in communities. In a freshwater microcosm exposed to 0.01, 0.1 and 1 mg L−1, the abundance of intI, sul1 and sul2 genes were found to decrease after 4 days of exposure up to the end of experiment: 10 days. The authors explained that this decrease was due to the formation of DNA–GO complexes as revealed by Gold View 1, a nucleic acid marker. This complex disabled the access to DNA and thus its horizontal transfer.67 The dynamics of four antibiotic resistance genes (tetA, sul2, ermB and ampC) were also studied on an estuary community cultivated in a Luria-Bertani broth medium. The decrease of gene abundances was effective at the lowest concentration tested, 50 μg L−1, and in a dose dependent manner up to the highest concentration tested, 300 μg L−1.68 This confirms previous results and extends their relevance at lower concentrations. In an anaerobic digestor fed with swine manure containing 50, 100 and 500 mg L−1 of GO, total abundance of ARGs decreased compared to that in control. It is noteworthy that a majority of ARGs decreased (ermB, tetM, tetG, suI1 and blaTEM). This decrease could be related to the changes in the microbial community, showing a decrease of potential host populations.41 During another experiment with anaerobic digestion of swine manure containing 100 and 800 mg L−1 of GO, similar results were obtained as the overall abundance of ARGs was lower than in control conditions, and the same was observed for mobile genetic elements.42 This generally observed decrease could be explained by the formation of DNA–GO complexes as revealed by Gold View 1, a nucleic acid marker,67 and disabling the horizontal gene transfer (HGT) of this complexed DNA. The partial disabling of HGT within microbial communities was supported by a study on tetracycline resistance in a coculture of a donor strain (E. coli HB101 with a RP4 plasmid) and a recipient strain (E. coli NK5449). It was reported that the survival rate of bacteria exposed to tetracycline diminished in the presence of 10, 80 and 100 mg L−1 of GO, explained by a decrease of gene copies (tetA and aphA).89 A higher removal of antibiotic resistance genes such as blaOXA-1, ermB, ermF, tetQ and tetX was also reported after 18 days in an anaerobic digestor exposed to 3 mg L−1 of GO.39 These decreases of antibiotic resistance genes abundances in presence of GO can be explained by the capacity of GO to damage DNA structure, cleavage and also intercalate into plasmidic DNA and form GO–DNA complexes which inhibits horizontal transfer.90 On the contrary, conjugative transfer of tetA and aphA, that does not involve free DNA in the medium, was found to be increased in a community exposed to 0.1, 1, 10, 50 and 100 mg L−1 of GO.89
5. Effect of microbial communities on graphene-based materials
5.1 Degradation, reduction and oxidation of GBMs by microbial communities
The capacity of microbial communities to reduce the size and the oxidation state of GBMs was reported in several recent studies (Table 1). After exposing soil communities to GO, Du et al.59 showed different changes in the GO properties. Scanning electron microscopy images showed rougher texture and transmission electron microscopy images revealed a lower transparency due to aggregation of minerals such as N, Mg, Al, Si, K, Ca and Fe, leading to thicker nanomaterials. Investigations also showed a decrease in C–O (from 29% to 18%) and C
O (from 26% to 8%) functional groups. This reduction was also notified in anaerobic digestors45 and sequential batch reactors.34 This reduction can be explained by GO acting as an acceptor of electron during reduction process such as respiration91 and glycolysis.16 The capacity of GO to be reduced while enhancing direct interspecies electron transfer during anaerobic processes was also reported. This phenomenon lead to the formation of conductive complexes composed of GO and species.84 These two processes have only been investigated in monospecific or coculture tests, but similar processes can be expected in complex communities where the reduction of GBMs was reported. At the opposite of reducing, the capacity to oxidize FLG into a GO-like material was also reported in axenic monocultures of wood degrading and saprotrophic fungi.92
The capacity of naphthalene degrading bacteria to degrade graphite, GO and rGO was previously reviewed25 and was also observed in anammox reactors, where the oxidation of rGO was also evidenced.53 The degradation of graphene by different microbial peroxidase enzymes was also highlighted: lignin peroxidase produced by white rot fungus93 but also horseradish peroxidase and myeloperoxidase.24 Furthermore, in the presence of graphene, the relative abundance of bacterial strains capable of the biodegradation of complex carbon pollutants were found to be enhanced,34,54,64,70 suggesting that this capacity of biodegradation could also be effective on GBMs. To our knowledge, this oxidative activity has not been demonstrated in complex communities.
5.2 Bioaccumulation and fate of GBMs after interactions with microbial communities
The fate of GBMs in the environment is driven by their physical and chemical properties. The changes in the GBMs oxidation state reviewed in paragraph 5.1 by microbial communities will therefore modulate their fate and bioavailability. Furthermore, microbial communities were found to accumulate different GBMs such as GO in wastewater sludge,33 but also FLG in diatom biofilms.21 This phenomenon could lead to a higher bioavailability of GBMs for higher trophic level, for example biofilm-feeding grazers. The trophic transfer of graphene was highlighted in simplified aquatic food chains, in particular between E. coli bacteria and T. thermophila protozoa.94 Once GBMs are up taken by higher trophic species such as zebrafish, they were found to accumulate in the gut and induce dysbiosis.75–77 The accumulation of GBMs in microbial assemblies could represent a threat to trophic chains and therefore to the stability of ecosystems. Literature about bioaccumulation of GBMs is still poorly represented and needs to be explored further.
6. Conclusion and perspectives
GBMs are well known to impact monocultures of microbial organisms. Studies now try to focus on microbial communities from different compartments of the environment, but also in engineered systems, in order to assess broader environmental impacts. This review gathers recent knowledge and understandings of interactions between microbial communities and GBMs and highlights the diversity of mechanisms behind these interactions. Results mainly exhibit negative impacts of GBM on the growth, generally due to oxidative stress, but some studies did underline forms of resilience after exposure to low concentrations and even some beneficial effects. Microbial communities endorse many roles throughout different activities that were found to be influenced by the presence of GBMs. Indeed, activities related to biochemical cycles, such as carbon and nitrogen cycles, were affected in different manners. Bioprocess yields were increased or decreased, but no shared patterns could be observed. It seems that the effect comes from the disruption of the community structure leading to the promotion or decrease of species related to particular activities, subsequently influencing the gene and enzyme activity and the overall bioprocess. Even if the carbon (methanogenesis, EPS production and composition and carbon uptake) and nitrogen (nitrification, denitrification and anammox) cycles have been put forward in recent literature, there is no reason why other cycles such as the phosphorus one would not be affected. Literature remains scarce but impacts of GBMs on phosphorus removal46 and related enzymes55 were reported. If the increase of species with roles in these different bioprocesses is an explanation of the increases of the different yields, the potential electron transfer by GBMs could also be one. Bioprocesses such as methanogenesis, denitrification or anammox rely on electron transfer and graphene nanomaterials seem to increase the direct interspecies electron transfer. Effects of GBMs on antibiotic resistance was also highlighted, generally decreasing horizontal gene transfer and related gene copies number due to the interaction of GBMs with genetic material and potentially mitigating their transfer and causing damage and cleavage. The structure and oxidation state of GBMs was also impacted by the interaction with microbial communities: reduction, oxidation and potential biodegradation of the materials by specific bacteria. The potential retention of GBMs by communities is also a none negligible mediator of the environmental fate of GBMs and increases their potential bioaccumulation across higher trophic chains.
Some results show similar trends but some are contradictory. Apart from the difference of concentrations tested, the heterogeneity of results probably originates from the fact that all studies started their experiments with different community compositions from different environmental compartments, but also exposed those communities in different conditions. Environmental factors have been shown to mediate the fate and toxicity of GBMs in the environment,23 which could also contribute to the difference of results. Moreover, it is obvious that the variability of the results can be influenced by the variety of the characteristics of the GBM itself. The multiple processes for synthesis, oxidation and reduction of these nanomaterials are likely to lead to large variations in the composition, the structure or the level of surface functionalization of these 2D materials, introducing an even higher level of variability after exposure in a biological matrix. This great variability of materials contributes to make the comparison between various studies particularly difficult or even uncertain.
Various results were put forward in the literature but gaps to fill are still remaining. First of all, research efforts have been mainly focused on bioprocesses and soil communities while the water environment is slightly understudied although this compartment is a major receptacle of GBMs such as GO, due to their high oxidation state and thus great stability in water.95,96 Microbial communities are not only composed by bacteria. Studies mainly focused on the bacterial and archaeal compartment, while fungi or even eukaryotic microalgae such as diatoms were poorly studied. Besides, there is a lack of realism of the environmental conditions. Only one study relied on complex experimental conditions with reconstituted trophic chains.69 When it comes to the types of GBMs tested, GO was mainly studied which creates a gap of knowledge about the effects of rGO or graphene. Moreover, GBMs have the characteristic to interact and adsorb different pollutants such as heavy metals.97 Only few studies are oriented towards co-contamination, whereas this case is the most representative of the environmental conditions nowadays. The concentrations tested during studies were in ranges exceeding predicted values. Even if the actual concentrations of GBMs in the natural environment cannot be measured, probabilistic models of CNTs concentrations in different environmental compartments were elaborated but substantially higher concentrations of nanomaterials were generally tested. The discrepancy between environmental concentrations of nanomaterials and concentrations used in studies was already put forward, underlining a lack of environmental relevance.98 There is a real need to develop an operational analytical method in order to assess environmental concentrations and obtain realistic results. The need of coordination between studies in nanomaterials analysis, fate, transport modelling and hazard assessment was already discussed by Holden et al.99 along with the importance of the environmental relevance. Even if the literature on GBMs exhibits different types of exposure such as chronic and acute, very few have taken these points into account and therefore share few similarities in order for them to be comparable and environmentally relevant.
Nevertheless, GBMs were pointed out as disruptors of microbial communities, in terms of both composition and functions (indirectly and directly). As a matter of fact, all lowest concentrations tested in every compartment induced effects on the composition or activity of the microbial communities exposed. Recent studies have shown that plant and human pathogens abundance could be mediated by GBMs57,58 which could lead to ecological disruptions and increases in plant and human diseases. Gut microbiota can also be disrupted by GBMs and lead to dysbiosis in hosts, leading to a broader environmental problem by causing diseases to species bearing unstable microbiota. In a world where the global health depends on the health of all three components (humans, environment and animals), known under the concept of “One Health”,100 and where microbial communities play a crucial role within each of the three components,101 the possible disturbance of these communities could lead to broad consequences.
Author contributions
Paul Braylé: conceptualization; visualization; investigation; writing – original draft. Eric Pinelli: conceptualization; supervision; writing – review & editing. Laury Gauthier: funding acquisition; project administration; writing – review & editing. Florence Mouchet: funding acquisition; writing – review & editing. Maialen Barret: conceptualization, visualization; supervision; writing – review & editing.
Conflicts of interest
All authors have declared that this review was carried out without any commercial and financial relationships that could be interpreted as a potential conflict of interest.
Acknowledgements
Authors would like to thank the European Union's Horizon 2020 research and innovation program under grant agreement n°881603 and the French Ministry of Higher Education and Research for the PhD grant n°2020-11.
References
- K. S. Novoselov, A. K. Geim, S. V. Morozov, D. Jiang, Y. Zhang and S. V. Dubonos, et al., Electric Field Effect in Atomically Thin Carbon Films, Science, 2004, 306(5696), 666–669 CrossRef CAS PubMed.
- P. Wick, A. E. Louw-Gaume, M. Kucki, H. F. Krug, K. Kostarelos and B. Fadeel,
et al., Classification Framework for Graphene-Based Materials, Angew. Chem., Int. Ed., 2014, 53(30), 7714–7718 CrossRef CAS PubMed.
- P. Avouris and C. Dimitrakopoulos, Graphene: synthesis and applications, Mater. Today, 2012, 15(3), 86–97 CrossRef CAS.
- M. Liang, B. Luo and L. Zhi, Application of graphene and graphene-based materials in clean energy-related devices, Int. J. Energy Res., 2009, 33(13), 1161–1170 CrossRef CAS.
- Y. Pan, N. G. Sahoo and L. Li, The application of graphene oxide in drug delivery, Expert Opin. Drug Delivery, 2012, 9(11), 1365–1376 CrossRef CAS PubMed.
- A. C. Sophia, E. C. Lima, N. Allaudeen and S. Rajan, Application of graphene based materials for adsorption of pharmaceutical traces from water and wastewater – a review, Desalin. Water Treat., 2016, 57(57), 27573–27586 Search PubMed.
- L. Lin, H. Peng and Z. Liu, Synthesis challenges for graphene industry, Nat. Mater., 2019, 18(6), 520–524 CrossRef CAS.
- D. G. Goodwin, A. S. Adeleye, L. Sung, K. T. Ho, R. M. Burgess and E. J. Petersen, Detection and Quantification of Graphene-Family Nanomaterials in the Environment, Environ. Sci. Technol., 2018, 52(8), 4491–4513 CrossRef CAS PubMed.
- L. De Marchi, C. Pretti, B. Gabriel, P. A. A. P. Marques, R. Freitas and V. Neto, An overview of graphene materials: Properties, applications and toxicity on aquatic environments, Sci. Total Environ., 2018, 631–632, 1440–1456 CrossRef CAS PubMed.
- T. Y. Sun, F. Gottschalk, K. Hungerbühler and B. Nowack, Comprehensive probabilistic modelling of environmental emissions of engineered nanomaterials, Environ. Pollut., 2014, 185, 69–76 CrossRef CAS.
- P. G. Falkowski, T. Fenchel and E. F. Delong, The Microbial Engines That Drive Earth's Biogeochemical Cycles, Science, 2008, 320(5879), 1034–1039 CrossRef CAS PubMed.
-
L. Condron, C. Stark, M. O'Callaghan, P. Clinton and Z. Huang, The Role of Microbial Communities in the Formation and Decomposition of Soil Organic Matter, in Soil Microbiology and Sustainable Crop Production [Internet], ed. G. R. Dixon and E. L. Tilston, Springer Netherlands, Dordrecht, 2010, pp. 81–118, DOI:10.1007/978-90-481-9479-7_4.
-
L. V. Evans, Biofilms: Receent Advances in their Study and Control, CRC Press, 2003, p. 507 Search PubMed.
-
R. Koshila Ravi, S. Anusuya, M. Balachandar and T. Muthukumar, Microbial Interactions in Soil Formation and Nutrient Cycling, in, Mycorrhizosphere and Pedogenesis [Internet], ed. A. Varma and D. K. Choudhary, Springer, Singapore, 2019, pp. 363–382, DOI:10.1007/978-981-13-6480-8_21.
- S. Kang, M. S. Mauter and M. Elimelech, Microbial Cytotoxicity of Carbon-Based Nanomaterials: Implications for River Water and Wastewater Effluent, Environ. Sci. Technol., 2009, 43(7), 2648–2653 CrossRef CAS PubMed.
- O. Akhavan and E. Ghaderi, Toxicity of Graphene and Graphene Oxide Nanowalls Against Bacteria, ACS Nano, 2010, 4(10), 5731–5736 CrossRef CAS PubMed.
- S. Liu, T. H. Zeng, M. Hofmann, E. Burcombe, J. Wei and R. Jiang,
et al., Antibacterial Activity of Graphite, Graphite Oxide, Graphene Oxide, and Reduced Graphene Oxide: Membrane and Oxidative Stress, ACS Nano, 2011, 5(9), 6971–6980 CrossRef CAS PubMed.
- I. E. Mejías Carpio, C. M. Santos, X. Wei and D. F. Rodrigues, Toxicity of a polymer–graphene oxide composite against bacterial planktonic cells, biofilms, and mammalian cells, Nanoscale, 2012, 4(15), 4746 RSC.
- F. Perreault, A. F. de Faria, S. Nejati and M. Elimelech, Antimicrobial Properties of Graphene Oxide Nanosheets: Why Size Matters, ACS Nano, 2015, 9(7), 7226–7236 CrossRef CAS PubMed.
- S. Du, P. Zhang, R. Zhang, Q. Lu, L. Liu and X. Bao,
et al., Reduced graphene oxide induces cytotoxicity and inhibits photosynthetic performance of the green alga Scenedesmus obliquus, Chemosphere, 2016, 164, 499–507 CrossRef CAS PubMed.
- M. Garacci, M. Barret, F. Mouchet, C. Sarrieu, P. Lonchambon and E. Flahaut,
et al., Few Layer Graphene sticking by biofilm of freshwater diatom Nitzschia palea as a mitigation to its ecotoxicity, Carbon, 2017, 113, 139–150 CrossRef CAS.
- O. N. Ruiz, K. A. S. Fernando, B. Wang, N. A. Brown, P. G. Luo and N. D. McNamara,
et al., Graphene Oxide: A Nonspecific Enhancer of Cellular Growth, ACS Nano, 2011, 5(10), 8100–8107 CrossRef CAS PubMed.
- J. Zhao, Z. Wang, J. C. White and B. Xing, Graphene in the Aquatic Environment: Adsorption, Dispersion, Toxicity and Transformation, Environ. Sci. Technol., 2014, 48(17), 9995–10009 CrossRef CAS PubMed.
- M. Chen, X. Qin and G. Zeng, Biodegradation of Carbon Nanotubes, Graphene, and Their Derivatives, Trends Biotechnol., 2017, 35(9), 836–846 CrossRef CAS.
- L. Liu, C. Zhu, M. Fan, C. Chen, Y. Huang and Q. Hao,
et al., Oxidation and degradation of graphitic materials by naphthalene-degrading bacteria, Nanoscale, 2015, 7(32), 13619–13628 RSC.
- H. C. Flemming and J. Wingender, The biofilm matrix, Nat. Rev. Microbiol., 2010, 8(9), 623–633 CrossRef CAS PubMed.
- H. C. Flemming, T. R. Neu and D. J. Wozniak, The EPS Matrix: The ‘House of Biofilm Cells’, J. Bacteriol., 2007, 189(22), 7945–7947 CrossRef CAS.
- S. J. Pamp, C. Sternberg and T. Tolker-Nielsen, Insight into the microbial multicellular lifestyle via flow-cell technology and confocal microscopy, Cytometry, Part A, 2009, 75(2), 90–103 CrossRef.
-
C. Callieri, E. M. Eckert, A. Di Cesare and F. Bertoni, Microbial Communities, in Encyclopedia of Ecology [Internet], Elsevier, 2019, pp. 126–134, Available from: https://linkinghub.elsevier.com/retrieve/pii/B9780124095489112229 Search PubMed.
- Y. Zhang, M. Mortimer and L. H. Guo, Interplay between engineered nanomaterials and microbiota, Environ. Sci.: Nano, 2020, 7(9), 2454–2485 RSC.
- J. Xie, M. Zhao, C. Wang, Y. Yong and Z. Gu, Recent advances in understanding the effects of nanomaterials on gut microbiota, Chem. Eng. J., 2022, 435, 134976 CrossRef CAS.
- W. Utembe, N. Tlotleng and A. Kamng'ona, A systematic review on the effects of nanomaterials on gut microbiota, Curr. Res. Microb. Sci., 2022, 3, 100118 CAS.
- F. Ahmed and D. F. Rodrigues, Investigation of acute effects of graphene oxide on wastewater microbial community: A case study, J. Hazard. Mater., 2013, 256–257, 33–39 CrossRef CAS PubMed.
- S. Lian, Y. Qu, S. Li, Z. Zhang, H. Zhang and C. Dai,
et al., Interaction of graphene-family nanomaterials with microbial communities in sequential batch reactors revealed by high-throughput sequencing, Environ. Res., 2020, 184, 109392 CrossRef CAS PubMed.
- Y. Sha, J. Liu, J. Yu, S. Xu, W. Yan and Z. Li,
et al., Effect of Graphene Oxide on the Ammonia Removal and Bacterial Community in a Simulated Wastewater Treatment Process, J. Environ. Eng., 2020, 146(9), 04020097 CrossRef CAS.
- H. N. Nguyen and D. F. Rodrigues, Chronic toxicity of graphene and graphene oxide in sequencing batch bioreactors: A comparative investigation, J. Hazard. Mater., 2018, 343, 200–2077 CrossRef CAS PubMed.
- M. M. Alam, A. Masud, B. Scharf, I. Bradley and N. Aich, Long-Term Exposure and Effects of rGO–nZVI Nanohybrids and Their Parent Nanomaterials on Wastewater-Nitrifying Microbial Communities, Environ. Sci. Technol., 2022, 56(1), 512–524 CrossRef CAS PubMed.
- B. Dong, Z. Xia, J. Sun, X. Dai, X. Chen and B. J. Ni, The inhibitory impacts of nano-graphene oxide on methane production from waste activated sludge in anaerobic digestion, Sci. Total Environ., 2019, 646, 1376–1384 CrossRef CAS PubMed.
- P. Wang, Y. Zheng, P. Lin, J. Li, H. Dong and H. Yu,
et al., Effects of graphite, graphene, and graphene oxide on the anaerobic co-digestion of sewage sludge and food waste: Attention to methane production and the fate of antibiotic resistance genes, Bioresour. Technol., 2021, 339, 125585 CrossRef CAS PubMed.
- Y. Li, Q. Wang, L. Liu, S. Tabassum, J. Sun and Y. Hong, Enhanced phenols removal and methane production with the assistance of graphene under anaerobic co-digestion conditions, Sci. Total Environ., 2021, 759, 143523 CrossRef CAS PubMed.
- J. Zhang, Z. Wang, Y. Wang, H. Zhong, Q. Sui and C. Zhang,
et al., Effects of graphene oxide on the performance, microbial community dynamics and antibiotic resistance genes reduction during anaerobic digestion of swine manure, Bioresour. Technol., 2017, 245, 850–859 CrossRef CAS PubMed.
- R. Zhang, J. Gu, X. Wang, Y. Li, J. Liu and C. Lu,
et al., Response of antibiotic resistance genes abundance by graphene oxide during the anaerobic digestion of swine manure with copper pollution, Sci. Total Environ., 2019, 654, 292–299 CrossRef CAS PubMed.
- H. Muratçobanoğlu, Ö. B. Gökçek, R. A. Mert, R. Zan and S. Demirel, The impact of reduced graphene oxide (rGO) supplementation on cattle manure anaerobic digestion: Focusing on process performance and microbial syntrophy, Biochem. Eng. J., 2021, 173, 108080 CrossRef.
- T. Tian, S. Qiao, X. Li, M. Zhang and J. Zhou, Nano-graphene induced positive effects on methanogenesis in anaerobic digestion, Bioresour. Technol., 2017, 224, 41–47 CrossRef CAS PubMed.
- J. I. Bueno-López, C. H. Nguyen, J. R. Rangel-Mendez, R. Sierra-Alvarez, J. A. Field and F. J. Cervantes, Effects of graphene oxide and reduced graphene oxide on acetoclastic, hydrogenotrophic and methylotrophic methanogenesis, Biodegradation, 2020, 31(1), 35–45 CrossRef.
- A. Kedves, L. Sánta, M. Balázs, P. Kesserű, I. Kiss and A. Rónavári,
et al., Chronic responses of aerobic granules to the presence of graphene oxide in sequencing batch reactors, J. Hazard. Mater., 2020, 389, 121905 CrossRef CAS PubMed.
- A. Kedves, A. Rónavári and Z. Kónya, Long-term effect of graphene oxide on the aerobic granular sludge wastewater treatment process, J. Environ. Chem. Eng., 2021, 9(1), 104853 CrossRef CAS.
- Y. Tian, D. Yu, Y. Wang and G. Chen, Performance and responses of aerobic granular sludge at different concentrations of graphene oxide after a single administered dose, Water Environ. Res., 2021, 93(10), 2210–2222 CrossRef CAS PubMed.
- C. Guo, Y. Wang, Y. Luo, X. Chen, Y. Lin and X. Liu, Effect of graphene oxide on the bioactivities of nitrifying and denitrifying bacteria in aerobic granular sludge, Ecotoxicol. Environ. Saf., 2018, 156, 287–293 CrossRef CAS PubMed.
- H. Li, Z. Chi and B. Yan, Long-term impacts of graphene oxide and Ag nanoparticles on anammox process: Performance, microbial community and toxic mechanism, J. Environ. Sci., 2019, 79, 239–247 CrossRef CAS PubMed.
- D. Wang, G. Wang, G. Zhang, X. Xu and F. Yang, Using graphene oxide to enhance the activity of anammox bacteria for nitrogen removal, Bioresour. Technol., 2013, 131, 527–530 CrossRef CAS PubMed.
- A. Elreedy, S. Ismail, M. Ali, S. Q. Ni, M. Fujii and M. Elsamadony, Unraveling the capability of graphene nanosheets and γ-Fe2O3 nanoparticles to stimulate anammox granular sludge, J. Environ. Manage., 2021, 277, 111495 CrossRef CAS PubMed.
- M. Tomaszewski, G. Cema, S. Ciesielski, D. Łukowiec and A. Ziembińska-Buczyńska, Cold anammox process and reduced graphene oxide – Varieties of effects during long-term interaction, Water Res., 2019, 156, 71–81 CrossRef CAS.
- W. Ren, G. Ren, Y. Teng, Z. Li and L. Li, Time-dependent effect of graphene on the structure, abundance, and function of the soil bacterial community, J. Hazard. Mater., 2015, 297, 286–294 CrossRef CAS PubMed.
- H. Chung, M. J. Kim, K. Ko, J. H. Kim, H. Kwon and I. Hong,
et al., Effects of graphene oxides on soil enzyme activity and microbial biomass, Sci. Total Environ., 2015, 514, 307–313 CrossRef CAS PubMed.
- M. J. Kim, D. Ko, K. Ko, D. Kim, J. Y. Lee and S. M. Woo,
et al., Effects of silver-graphene oxide nanocomposites on soil microbial communities, J. Hazard. Mater., 2018, 346, 93–102 CrossRef CAS PubMed.
- C. Forstner, T. G. Orton, A. Skarshewski, P. Wang, P. M. Kopittke and P. G. Dennis, Effects of graphene oxide and graphite on soil bacterial and fungal diversity, Sci. Total Environ., 2019, 671, 140–148 CrossRef CAS PubMed.
- C. Forstner, T. G. Orton, P. Wang, P. M. Kopittke and P. G. Dennis, Effects of carbon nanotubes and derivatives of graphene oxide on soil bacterial diversity, Sci. Total Environ., 2019, 682, 356–363 CrossRef CAS PubMed.
- J. Du, X. Hu and Q. Zhou, Graphene oxide regulates the bacterial community and exhibits property changes in soil, RSC Adv., 2015, 5(34), 27009–27017 RSC.
- J. Du, Q. Zhou, J. Wu, G. Li, G. Li and Y. Wu, Soil bacterial communities respond differently to graphene oxide and reduced graphene oxide after 90 days of exposure, Soil Ecol. Lett., 2020, 2(3), 176–179 CrossRef CAS.
- F. Wu, S. Jiao, J. Hu, X. Wu, B. Wang and G. Shen,
et al., Stronger impacts of long-term relative to short-term exposure to carbon nanomaterials on soil bacterial communities, J. Hazard. Mater., 2021, 410, 124550 CrossRef CAS PubMed.
- Y. Ge, C. Shen, Y. Wang, Y. Q. Sun, J. P. Schimel and J. L. Gardea-Torresdey,
et al., Carbonaceous Nanomaterials Have Higher Effects on Soybean Rhizosphere Prokaryotic Communities During the Reproductive Growth Phase than During Vegetative Growth, Environ. Sci. Technol., 2018, 52(11), 6636–6646 CrossRef CAS PubMed.
- J. Song, C. Duan, Y. Sang, S. Wu, J. Ru and X. Cui, Effects of Graphene on Bacterial Community Diversity and Soil Environments of Haplic Cambisols in Northeast China, Forests., 2018, 9(11), 677 CrossRef.
- T. Xiong, X. Yuan, H. Wang, L. Leng, H. Li and Z. Wu,
et al., Implication of graphene oxide in Cd-contaminated soil: A case study of bacterial communities, J. Environ. Manage., 2018, 205, 99–106 CrossRef CAS PubMed.
- Y. Ge, J. H. Priester, M. Mortimer, C. H. Chang, Z. Ji and J. P. Schimel,
et al., Long-Term Effects of Multiwalled Carbon Nanotubes and Graphene on Microbial Communities in Dry Soil, Environ. Sci. Technol., 2016, 50(7), 3965–3974 CrossRef CAS.
- K. Ko, M. J. Kim, J. Y. Lee, W. Kim and H. Chung, Effects of graphene oxides and silver-graphene oxides on aquatic microbial activity, Sci. Total Environ., 2019, 651, 1087–1095 CrossRef CAS PubMed.
- X. Zou, L. Zhang, Z. Wang and Y. Luo, Mechanisms of the Antimicrobial Activities of Graphene Materials, J. Am. Chem. Soc., 2016, 138(7), 2064–2077 CrossRef CAS.
- W. Yu, S. Zhan, Z. Shen, Q. Zhou and D. Yang, Efficient removal mechanism for antibiotic resistance genes from aquatic environments by graphene oxide nanosheet, Chem. Eng. J., 2017, 313, 836–846 CrossRef CAS.
- L. Evariste, A. Mottier, L. Lagier, S. Cadarsi, M. Barret and C. Sarrieu,
et al., Assessment of graphene oxide ecotoxicity at several trophic levels using aquatic microcosms, Carbon, 2020, 156, 261–271 CrossRef CAS.
- L. Evariste, P. Braylé, F. Mouchet, J. Silvestre, L. Gauthier and E. Flahaut,
et al., Graphene-based nanomaterials modulate internal biofilm interactions and microbial diversity, Front. Microbiol., 2021, 12 Search PubMed , Available from: https://www.frontiersin.org/articles/10.3389/fmicb.2021.623853/abstract.
- Y. Li, Y. Lu, W. Zhang, H. Wu, C. Zhang and L. Wang,
et al., Enhanced biological nitrogen removal from sediment by graphene derivative-mediated community assembly, Bioresour. Technol., 2020, 306, 123187 CrossRef CAS PubMed.
- Y. Liao, S. Li, X. Zhu, Z. Dang, S. Tang and G. Ji, The promotion and inhibition effect of graphene oxide on the process of microbial denitrification at low temperature, Bioresour. Technol., 2021, 340, 125636 CrossRef CAS PubMed.
- C. Yan, J. Huang, Y. Wang, X. Lin, C. Cao and X. Qian, Assessment on the treatment of nitrogen contaminant by constructed wetland exposed to different concentrations of graphene oxide, J. Cleaner Prod., 2022, 338, 130567 CrossRef CAS.
- C. Yan, J. Huang, C. Cao, Y. Wang, X. Lin and X. Qian, Response of constructed wetland for wastewater treatment to graphene oxide: Perspectives on plant and microbe, J. Hazard. Mater., 2022, 422, 126911 CrossRef CAS PubMed.
- P. P. Jia, T. Sun, M. Junaid, Y. H. Xiong, Y. Q. Wang and L. Liu,
et al., Chronic exposure to graphene oxide (GO) induced inflammation and differentially disturbed the intestinal microbiota in zebrafish, Environ. Sci.: Nano, 2019, 6(8), 2452–2469 RSC.
- M. Zheng, J. Lu, G. Lin, H. Su, J. Sun and T. Luan, Dysbiosis of gut microbiota by dietary exposure of three graphene-family materials in zebrafish (Danio rerio), Environ. Pollut., 2019, 254, 112969 CrossRef CAS.
- K. Lu, S. Dong, E. J. Petersen, J. Niu, X. Chang and P. Wang,
et al., Biological Uptake, Distribution, and Depuration of Radio-Labeled Graphene in Adult Zebrafish: Effects of Graphene Size and Natural Organic Matter, ACS Nano, 2017, 11(3), 2872–2885 CrossRef CAS PubMed.
- S. Duperron, S. Halary, A. Gallet and B. Marie, Microbiome-Aware Ecotoxicology of Organisms: Relevance, Pitfalls, and Challenges, Front. Public Health., 2020, 8 Search PubMed , Available from: https://www.frontiersin.org/articles/10.3389/fpubh.2020.00407/full.
- L. Evariste, M. Barret, A. Mottier, F. Mouchet, L. Gauthier and E. Pinelli, Gut microbiota of aquatic organisms: A key endpoint for ecotoxicological studies, Environ. Pollut., 2019, 248, 989–999 CrossRef CAS PubMed.
- T. C. J. Hill, K. A. Walsh, J. A. Harris and B. F. Moffett, Using ecological diversity measures with bacterial communities, FEMS Microbiol. Ecol., 2003, 43(1), 1–11 CrossRef CAS.
- D. R. Shaw, M. Ali, K. P. Katuri, J. A. Gralnick, J. Reimann and R. Mesman,
et al., Extracellular electron transfer-dependent anaerobic oxidation of ammonium by anammox bacteria, Nat. Commun., 2020, 11(1), 2058 CrossRef CAS PubMed.
- M. Hao, H. Chen, Y. He, X. Wang, Y. Zhang and H. Lao,
et al., Recycling sludge-derived hydrochar to facilitate advanced denitrification
of secondary effluent: Role of extracellular electron transfer, Chemosphere, 2022, 291, 132683 CrossRef CAS PubMed.
-
G. Myhre, D. Shindell and J. Pongratz, Anthropogenic and Natural Radiative Forcing, in Climate change 2013: the physical science basis; Working Group I contribution to the fifth assessment report of the Intergovernmental Panel on Climate Change [Internet], ed. T. Stocker, Ludwig-Maximilians-Universität München, Cambridge, 2014 [cited 2021 Jun 9], pp. 659–740, Available from: https://epub.ub.uni-muenchen.de/68564/ Search PubMed.
- K. Igarashi, E. Miyako and S. Kato, Direct Interspecies Electron Transfer Mediated by Graphene Oxide-Based Materials, Front. Microbiol., 2020, 10 Search PubMed , Available from: https://www.frontiersin.org/articles/10.3389/fmicb.2019.03068/full?report=reader.
- V. Tremaroli and F. Bäckhed, Functional interactions between the gut microbiota and host metabolism, Nature, 2012, 489(7415), 242–249 CrossRef CAS PubMed.
- Y. Fan and O. Pedersen, Gut microbiota in human metabolic health and disease, Nat. Rev. Microbiol., 2021, 19(1), 55–71 CrossRef CAS PubMed.
- G. Gibiino, L. R. Lopetuso, F. Scaldaferri, G. Rizzatti, C. Binda and A. Gasbarrini, Exploring Bacteroidetes: Metabolic key points and immunological tricks of our gut commensals, Dig. Liver Dis., 2018, 50(7), 635–639 CrossRef.
- N. R. Shin, T. W. Whon and J. W. Bae, Proteobacteria: microbial signature of dysbiosis in gut microbiota, Trends Biotechnol., 2015, 33(9), 496–503 CrossRef CAS PubMed.
- M. T. Guo and G. S. Zhang, Graphene oxide in the water environment could affect tetracycline-antibiotic resistance, Chemosphere, 2017, 183, 197–203 CrossRef CAS PubMed.
- L. Xu, J. Zhao, Z. Liu, Z. Wang, K. Yu and B. Xing, Cleavage and transformation inhibition of extracellular antibiotic resistance genes by graphene oxides with different lateral sizes, Sci. Total Environ., 2019, 695, 133932 CrossRef CAS PubMed.
- Y. Jiao, F. Qian, Y. Li, G. Wang, C. W. Saltikov and J. A. Gralnick, Deciphering the Electron Transport Pathway for Graphene Oxide Reduction by Shewanella oneidensis MR-1, J. Bacteriol., 2011, 193(14), 3662–3665 CrossRef CAS.
- F. Candotto Carniel, L. Fortuna, D. Zanelli, M. Garrido, E. Vázquez and V. J. González,
et al., Grapheene environmental biodegradation: Wood degrading and saprotrophic fungi oxidize few-layer graphene, J. Hazard. Mater., 2021, 414, 125553 CrossRef CAS PubMed.
- G. Lalwani, W. Xing and B. Sitharaman, Enzymatic degradation of oxidized and reduced graphene nanoribbons by lignin peroxidase, J. Mater. Chem. B, 2014, 2(37), 6354–6362 RSC.
- S. Dong, T. Xia, Y. Yang, S. Lin and L. Mao, Bioaccumulation of 14C-Labeled Graphene in an Aquatic Food Chain through Direct Uptake or Trophic Transfer, Environ. Sci. Technol., 2018, 52(2), 541–549 CrossRef CAS PubMed.
- J. D. Lanphere, B. Rogers, C. Luth, C. H. Bolster and S. L. Walker, Stability and Transport of Graphene Oxide Nanoparticles in Groundwater and Surface Water, Environ. Eng. Sci., 2014, 31(7), 350–359 CrossRef CAS PubMed.
- K. He, G. Chen, G. Zeng, M. Peng, Z. Huang and J. Shi,
et al., Stability, transport and ecosystem effects of graphene in water and soil environments, Nanoscale, 2017, 9(17), 5370–5388 RSC.
- K. T. Chan, J. B. Neaton and M. L. Cohen, First-principles study of metal adatom adsorption on graphene, Phys. Rev. B: Condens. Matter Mater. Phys., 2008, 77(23), 235430 CrossRef.
- P. A. Holden, F. Klaessig, R. F. Turco, J. H. Priester, C. M. Rico and H. Avila-Arias,
et al., Evaluation of Exposure Concentrations Used in Assessing Manufactured Nanomaterial Environmental Hazards: Are They Relevant?, Environ. Sci. Technol., 2014, 48(18), 10541–10551 CrossRef CAS PubMed.
- P. A. Holden, J. Gardea-Torresdey, F. Klaessig, R.
F. Turco, M. Mortimer and K. Hund-Rinke,
et al., Considerations of Environmentally Relevant Test Conditions for Improved Evaluation of Ecological Hazards of Engineered Nanomaterials, Environ. Sci. Technol., 2016, 50(12), 6124–6145 CrossRef CAS.
- D. Destoumieux-Garzón, P. Mavingui, G. Boetsch, J. Boissier, F. Darriet and P. Duboz,
et al., The One Health Concept: 10 Years Old and a Long Road Ahead, Front. Vet. Sci., 2018, 5 Search PubMed , Available from: https://www.frontiersin.org/articles/10.3389/fvets.2018.00014/full?utm_source=ad&utm_medium=fb&utm_campaign=ba_sci_fvets.
- A. H. C. van Bruggen, E. M. Goss, A. Havelaar, A. D. van Diepeningen, M. R. Finckh and J. G. Morris, One Health – Cycling of diverse microbial communities as a connecting force for soil, plant, animal, human and ecosystem health, Sci. Total Environ., 2019, 664, 927–937 CrossRef CAS.
|
This journal is © The Royal Society of Chemistry 2022 |
Click here to see how this site uses Cookies. View our privacy policy here.