DOI:
10.1039/D1EE02118D
(Perspective)
Energy Environ. Sci., 2022,
15, 1034-1077
Perspective on the hydrogen economy as a pathway to reach net-zero CO2 emissions in Europe†
Received
9th July 2021
, Accepted 31st January 2022
First published on 2nd February 2022
Abstract
The envisioned role of hydrogen in the energy transition – or the concept of a hydrogen economy – has varied through the years. In the past hydrogen was mainly considered a clean fuel for cars and/or electricity production; but the current renewed interest stems from the versatility of hydrogen in aiding the transition to CO2 neutrality, where the capability to tackle emissions from distributed applications and complex industrial processes is of paramount importance. However, the hydrogen economy will not materialise without strong political support and robust infrastructure design. Hydrogen deployment needs to address multiple barriers at once, including technology development for hydrogen production and conversion, infrastructure co-creation, policy, market design and business model development. In light of these challenges, we have brought together a group of hydrogen researchers who study the multiple interconnected disciplines to offer a perspective on what is needed to deploy the hydrogen economy as part of the drive towards net-zero-CO2 societies. We do this by analysing (i) hydrogen end-use technologies and applications, (ii) hydrogen production methods, (iii) hydrogen transport and storage networks, (iv) legal and regulatory aspects, and (v) business models. For each of these, we provide key take home messages ranging from the current status to the outlook and needs for further research. Overall, we provide the reader with a thorough understanding of the elements in the hydrogen economy, state of play and gaps to be filled.
Broader context
The interest in hydrogen has soared in the last five years: many businesses, countries and organisations see clean hydrogen as indispensable for reaching the Paris Agreement target of below 2 °C and toward 1.5 °C global warming. However, today hydrogen production is a CO2 intensive process, accounting for about 2% of global CO2 emissions. Clearly, we cannot scale up towards a hydrogen economy by continuing with greenhouse gas-emitting processes. Hydrogen has to be produced with the lowest greenhouse gas footprint possible, but also affordably, likely requiring both the fossil route with CO2 capture and storage and the electrolysis route with renewable energy supply. How will these routes develop in the future, what is the competitive edge of one towards the other and when will price parity occur versus buying CO2 emissions certificates? How may hydrogen networks evolve and what regulatory and market designs are needed for successful implementation? These are questions we are grappling with at present and that we try to address in this perspective paper. No simple answers can be provided, but there are directions and signposts we can use to create a credible narrative on how this may develop. Here, we try to provide the reader with an experienced and cross-disciplinary view on what the hydrogen economy is and is not, and how it may develop over the next decades.
|
1. Introduction
Hydrogen is a versatile energy carrier and can in principle be used wherever fossil fuels are used today. This includes hard-to-abate sectors like industry and mobility. The interest in hydrogen has soared in the last five years: many businesses, countries and organisations see hydrogen as indispensable for reaching the Paris agreement target of below 2 °C and toward 1.5 °C global warming. Global production of hydrogen is about 90 mega tonnes per year (Mt/a), and applications of hydrogen are dominated by refining and industrial uses. Current hydrogen production is totally dominated by reforming of fossil fuels, most commonly natural gas, without integration with technologies to mitigate or reduce greenhouse gas (GHG) emissions. Today, the emissions caused by these operations account for approximately 900 Mt CO2–eq per year or about 2% of global CO2 emissions.1,2
Clearly, we cannot scale up towards the hydrogen economy by continuing with GHG-emitting processes. Hydrogen must be produced with the lowest GHG footprint possible but also affordably. To that end, hydrogen from natural gas with carbon dioxide capture, transport and storage (CCS) is often put forward, in addition to hydrogen production using renewable energy – both options often coined low-carbon hydrogen. How will these routes develop in the future, what is the competitive edge of one towards the other and when will price parity occur versus buying CO2 emissions certificates? These are questions we are grappling with at present. No simple answer can be provided, but there are directions and signposts we can use to create a credible narrative on how this will develop.
First, clearly we will need all available low-carbon hydrogen production pathways to fulfil the expected role of hydrogen in the energy transition; the scale and pace of decarbonisation simply requires this. We are much more likely to reach our targets if we can rely on multiple energy vectors rather than one, i.e., electricity, biomass, and natural gas (or other fossil fuels).
Second, we need to address the barriers to large-scale deployment of the hydrogen economy. For electrolysis, this is presently controlled by limitations of cheap and abundant supplies of renewable electricity. The question is not if electrolysis should be supplied by renewables, but how, especially as system analyses show that in a system where the electricity mix is dominated by non-renewable production, most new renewable production should be used for decarbonising the electricity mix, rather than producing hydrogen.3–6 This is usually referred to as the additionality principle. For the fossil fuel pathway, the limitation at present is access to CO2 transport and storage in the scale of hundreds of megatonnes per year, extending to gigatonnes per year.7
Third, there is the difference in scale of production methods. The need for a sizeable CCS infrastructure means that hydrogen production based on fossil fuels will only make sense at large scale, otherwise it will not be cost-efficient to connect the production unit to the CO2-transportation system. Electrolysis-based hydrogen, on the other hand, lends itself well to modular and smaller installations with simpler infrastructure needs, although also electrolysis is moving into the 100 megawatt (MW) scale now with several initiatives to make this happen (e.g., the Refhyne project8).
Fourth, we know that the price sensitivity of fuels varies by end-use market: price sensitivity is much lower in the transportation sector than in the industrial sector, meaning that certain markets may be more or less suitable as outlets for hydrogen suppliers and traders, e.g., based on the hydrogen's origin and the supplier's ability to supply flexibly. The above also means that we need to assess the hydrogen system as a whole, including sector coupling, instead of looking at specific end uses one by one.
Fifth, the often-forgotten issue of safety and acceptance of the hydrogen economy can constitute a barrier to large-scale deployment. It is often taken for granted that the introduction of a large-scale hydrogen economy will be uncontroversial. This assumption can be dangerous, the hydrogen economy must prove itself as safe and an economy that works for people, implying that the introduction and deployment of hydrogen as a significant energy vector in our society must be proven in the field. This in turn means that research and development of critical safety aspects, as well as living labs, will be important in the quest for climate neutrality by hydrogen.
The sixth question is how the market uptake and growth should be tailored. We must remember that the upscaling foreseen is tremendous: in the EU hydrogen strategy, the targets for H2 production capacity are 6 GW of electrolysis by 2024, equivalent to 1 million tonnes of H2 per year, and 40 GW by 2030, equivalent to 10 million tonnes of H2 per year.9 The 2030 target also presumes 40 GW of additional import to the EU. This is from a base of some 100 MW today, meaning scale-up by several hundreds. This ambition is a very hopeful and much-needed signal, but as in most value chains, supply must be matched by demand, meaning that also a vast demand must be created, and hydrogen use incentivised. Electrolysis-based hydrogen may help to create a market because of its positive image, giving confidence in hydrogen as a low-carbon solution. Fossil fuel-based hydrogen with CCS can then deliver at scale relatively fast and in a cost-effective fashion. While this is developing, Europe's electricity mix GHG footprint will significantly be reduced and the sustainable production of hydrogen from electrolysis will ramp up and may at some time surpass hydrogen from fossil fuels with CCS in volume. Within this century, hydrogen should ideally be dominated by electricity-based production (but only if sufficient renewable electricity can become available).
Finally, to combine production and markets, hydrogen transmission, distribution and storage need to be present at scale. Hydrogen infrastructure to support market creation and facilitate supply has a similar chicken-and-egg problem to, for example, CO2 transport and storage infrastructure: it needs to be installed before there is sufficient demand, thus economic rationale, for it. Whether re-purposing natural gas infrastructure or building new hydrogen infrastructure, it will have to be done in substantial steps that “leap-frog” demand. We do not have time for a more evolutionary transition as has been the case with electricity.
There are obviously many assumptions made in the above discussion. We do not currently know how and how fast the decarbonisation of the electricity mix will develop, the availability of space for renewables generation likely playing a significant role. How will net removal of CO2 play a role, and could biomass-produced hydrogen with CCS become an important step in making the production net negative in terms of greenhouse gas emissions? What will govern the ETS (European Trading System for CO2 emissions) price in the future? It is believed the EU ETS price thus far has been influenced by the shift from coal to natural gas in electricity production and it is assumed that new EU targets will facilitate low-carbon hydrogen production and removing CO2 from the atmosphere further down the road.10 Clearly, if the ETS price will continue to move towards the 100€ per tonne CO2 mark by 2030, this would be a huge incentive for companies to invest in hydrogen infrastructure and appliances: at this price, hydrogen will be close to parity with natural gas but also quite similar to “traditional” post-combustion CCS cost.7 These are uncertainties we need to work out as we go. However, there is no doubt that the hydrogen economy is needed to reach global warming mitigation targets.
We see this in the hydrogen strategies which have emerged the last years. Besides the EU hydrogen strategy, we have seen strategies emerge in European countries such as Germany, The Netherlands, Norway, Portugal, France and in the UK. The focus of all is to enable the hydrogen economy driven by the necessity to provide energy carriers not emitting greenhouse gases when utilised. The strategies differ in the focus on production methods, infrastructures whereas all want to develop a thriving industry and value creation based on hydrogen. It is clear that the countries that do not have own petroleum production have no particular interest in developing schemes for low carbon hydrogen from natural gas and CCS. But it could become a source of low carbon hydrogen imports as reflected in the EU hydrogen strategy and the German hydrogen strategy. Hydrogen also opens up for new better interaction with neighbouring regions to Europe and integrated energy systems. The possibilities for renewable sourced hydrogen in Africa and export to Europe is interesting besides catering for own electricity and domestic hydrogen demand in key African countries. This has been subject to studies working on a European hydrogen corridor with interconnectors to neighbouring economies.11
1.1. Aims, scope and paper organisation
The above discussion is a teaser for the rest of this perspective. We will thoroughly address these issues with the aim to provide the reader with our view on what the hydrogen economy is and is not, how it may develop over the next decades, which technologies, production methods and end-uses may come into play and why (not), what the legal and regulatory requirements are or should be and how the commercial end of the hydrogen economy should be designed. The rationale is to bring together many decades of cumulative experience in hydrogen research with the aim to provide a one-stop starting point for the reader who wants to catch up on this exciting and relevant topic. Outside the scope of this paper are the more fundamental science question on, e.g., materials design for electrolysers and hydrogen storage, reforming catalysts et cetera. It is hoped that this perspective will give the reader a thorough understanding of the elements in the hydrogen economy, state of play and gaps to be filled.
The paper is organized as follows. Section 2 describes hydrogen end-use technologies and applications (where hydrogen is needed, what technology can be used to convert it to the useful service, and what status and perspectives of applications and technologies are). Section 3 describes the different routes for producing low-carbon hydrogen (status and perspective of hydrogen from water electrolysis, hydrogen from fossil fuels with CCS and hydrogen from biomass). Thereby, Sections 2 and 3 set the boundaries of what the hydrogen economy can technically span. Section 4 addresses hydrogen transportation infrastructure and safety aspects, as well as what optimal hydrogen network development may look like. Section 5 discusses the legal and regulatory requirements to facilitate the development of a hydrogen economy, particularly hydrogen networks. Finally, Section 6 discusses hydrogen business models and how hydrogen markets should be organised given its societal value, including the need for a system perspective, market drivers, the public–private interface and synthesises this into an example business model for the United Kingdom. Every section starts with a synthesis of the key messages, that are then further elaborated, allowing the reader to obtain a quick view of our perspective on each of the discussed topics.
2. Hydrogen end-use technologies and applications
In this section, we provide our perspective on hydrogen end-use technologies and applications. First, we provide a historical context to understand how the (envisioned) role of hydrogen has evolved in time and how it has finally come to be viewed as key to cross-sector decarbonisation. We continue by looking at the different hydrogen conversion technologies, i.e., where hydrogen is converted to satisfy the demand for a certain service, and at the different end-use applications (e.g., providing industrial heat). Finally, we analyse the interplay between technologies, applications, and the system boundary conditions that control the transition to the hydrogen economy.
The envisioned role of hydrogen in the energy transition, and especially its final use, has varied through the years. The concept of the hydrogen economy was first introduced in 1970 by Bockris and Appleby,12 and since then, hydrogen has been at the centre of several research programs with focus on both production and use.13 The first works on hydrogen as fuel for heat and/or power generation dates back to the 1970s: following the successful deployment onboard spacecrafts, H2 was proposed as fuel for electricity generation in fuel cells (FC).14–16 A few years later, in the early 1980s, Japanese and Swiss engineers started to investigate the use of H2 in gas turbines (GT).17–20 Since then, the technological development of H2-fuelled power systems has proceeded with variable momentum, and has experienced multiple false starts. This stems from the several hurdles that must be tackled simultaneously when introducing hydrogen at scale, which include technological and infrastructure development, regulation, and standardisation, coordinated policy measures, and market creation. While in the past, hydrogen was mainly associated to fuel cells for transportation and/or electricity production, the current renewed interest stems from the versatility of hydrogen and the multiple roles that it can take in the transition to CO2 neutrality: low and high temperature heat provision, maritime, air and road transportation, energy storage for balancing the power grid and building block for chemicals synthesis and other industrial processes. Notably, the common denominator is the capability of hydrogen to tackle emissions from distributed applications and complex industrial processes. Within this framework, it is important to note that there is no absolute or preferred winner among the end-use applications; rather, each country – or region with similar socio-economic conditions – may follow different H2 implementation pathways and end-use distribution. Eventually, early applications will create hydrogen value chains which then support the emergence and deployment of other applications.
While technical challenges in the conversion of H2 certainly exist, they are often used in the public arena to argue against H2 deployment rather than to think about possible solutions. In fact, several commercial solutions do exist for the conversion of H2 in end-use applications and these are marketed by specific manufactures and can be normally acquired with performance warranties, depending on the application.21 To name a few: Ballard Power Systems, Bloom Energy, Solidpower, Fuel Cell Energy, and Panasonic (fuel cells), GE, Siemens, and Ansaldo (gas turbines), MAN and GE-Jenbacher (internal combustion engines), and Tenova and Danieli (Steel production). However, as these technologies were designed to run on natural gas as primary energy source, they do not perform equally well when running on H2, and have substantial room for improvement from a technical and cost perspective.21 This gap must be closed for end users to reap most benefits of the transition to hydrogen. Moreover, while hydrogen conversion technologies can be regarded as commercial, their integration within a certain application might not be. In other words, the technology readiness of the entire hydrogen chain is typically lower than that of the standalone technologies. This calls for demonstration at scale, considering chains relevant to a certain country or region.
Importantly, the specific technical features required by conversion technologies might be different, if not contrasting, when applied to different end use applications, e.g., fuel cells for power generation and for heat provision call for different performance characteristics, the same could be said for gas turbines for grid balancing versus for industrial cogeneration. This, however, poses a significant challenge for advancing technologies, as manufacturers can hardly afford to have multiple lines of development in a phase where market drivers are not yet consolidated. In Table 1, we have summarized specific needs of each technology to support a certain end use application. Moreover, for each combination we have indicated prospects of deployment during the transition to net-zero-CO2 systems. Both technologies and applications are further discussed in the following sections.
Table 1 Hydrogen technologies and end use applications: needed technical features and prospects for the transition to a CO2-neutral society. CHP: combined heat and power; Tcell: operating temperature of the fuel cell; Tout GT: outlet temperature of the gas turbine; T ICE: operating temperature of the internal combustion engine
Technology |
End use applications |
Heat provision needs |
Power generation and grid balancing needs |
Transport needs |
Low temperature fuel cells
|
Moderately fast dynamics |
Fast dynamics and start-up/shut down |
Light and compact design |
Operation at varying load and ambient conditions |
Multi-year stack lifetime |
Multi-year stack lifetime |
Multi-year stack lifetime |
Cost competitive with other balancing technologies |
Mass manufacturing |
System maintenance without highly specialized technicians |
Efficient coupling with electrolysers in power-to-gas configurations |
|
Be cost-competitive with heat pumps |
|
|
Low temperature heat (below Tcell)
|
High temperature heat
|
|
Road
|
Maritime
|
Guarantee demand at varying ambient conditions through the year |
Embedded efficient H2 post-combustion with no NOx |
|
Be broadly cost-competitive cost with batteries |
Moderately fast change in operating point |
|
|
|
Fast dynamics and start-up/shut down |
Design optimized for operation on board of ships (V–I curve) |
|
|
|
Easy maintainability |
|
|
|
|
|
|
|
Relevance for 2050 net-zero |
|
|
|
|
|
Residential/commercial CHP |
Small scale industrial processes with high electricity consumptions |
Grid balancing and seasonal energy |
Lorries and long-distance applications |
Small/medium ferries for passengers |
|
|
|
|
|
|
High temperature fuel cells
|
Balanced electricity and heat output |
Standard solutions for remote/island applications and backup systems (no grid balancing) |
Maritime
|
To operate at varying loads and ambient conditions |
Reliable and robust operation |
Light and compact design |
Multi-year stack lifetime |
|
Multi-year stack lifetime |
System maintenance without highly specialized technicians |
|
Competitive cost with respect to low temperature fuel cells and internal combustion engines |
|
|
|
Resistance to maritime environment |
Low temperature heat (below Tcell)
|
High temperature heat (around or above Tcell)
|
|
|
|
Guaranteed demand throughout seasons |
Matching of cell and process temperature |
|
|
|
Ability to provide heat flexibly |
or |
|
|
|
|
Embedded efficient H2 post-combustion with no NOx |
|
|
|
|
|
|
|
|
|
Relevance for 2050 net-zero |
|
|
|
|
Large commercial applications |
Industrial applications |
Island configurations and backup |
Mid-size and range ships (propulsion engine) or on-board power supply of large ships |
|
|
|
|
|
|
Gas Turbines
|
Efficient and cost-competitive micro and small-scale gas turbines |
Efficient and cost-competitive medium and large-scale gas turbines |
Light and compact design |
For CHP: ability to operate with positive primary energy saving index under varying conditions |
Fast dynamics and start-up/shut down |
Same or better fuel efficiency and performance than gas turbines fuelled with synthetic hydrocarbons |
Adaptability to seasonal variations |
No NOx generation |
|
|
|
|
Premixed combustors for (close to) 100% H2 |
|
|
Low temperature
|
High temperature (around or above Tout GT)
|
|
Aviation
|
Maritime
|
Efficient and compact heat exchange |
For high T: embedded efficient H2 post-combustion with no NOx |
|
Fast dynamic in power ramp up/down |
Moderately fast change in operating point and broad power range |
|
Efficient and compact heat exchange |
|
Noise control |
Resistance to maritime environment |
|
|
|
No flashback flame issues in the combustion at anytime |
|
Relevance for 2050 net-zero |
|
|
|
|
|
Large commercial applications (e.g., airports) |
Industrial applications |
Grid balancing |
Medium and large planes |
Large ships |
|
|
|
|
|
|
Internal combustion engines
|
Efficient and cost-competitive gas engines |
Efficient and cost-competitive engines at different scales |
Maritime
|
For CHP: capable of operating with positive primary energy saving under varying conditions |
Fast dynamics and start-up/shut down |
Light and compact design |
Simple maintenance |
No NOx generation |
Same or better fuel efficiency and performance than internal combustion engines fuelled with synthetic hydrocarbons |
Adaptability to seasonal variations |
|
|
Moderately fast change in operating point and broad power range |
Low temperature
|
High temperature (around or above T ICE)
|
|
|
|
Efficient and compact heat exchange |
For high T: embedded efficient H2 post-combustion with no NOx |
|
|
|
|
|
|
|
|
|
Relevance for 2050 net-zero |
|
|
|
|
|
|
Medium scale CHP in residential/commercial |
Small/medium industrial applications |
Grid balancing |
Medium-large ships |
|
|
|
|
|
|
|
Traffic light legend |
|
|
|
|
|
|
The technology-end use application combination is very relevant for 2050 net-zero scenario |
|
The technology-end use application combination is relevant for 2050 net-zero scenario |
|
The technology-end use application combination is somewhat relevant for 2050 net-zero scenario |
|
The technology-end use application combination is not very relevant for 2050 net-zero scenario |
2.1. Technologies for end use hydrogen conversion
As of today, we can recognize five main end-use technologies of H2: (i) fuel cells, which can be applied to electricity (and heat) generation for stationary as well as to mobile (e.g., road and maritime transport) applications; (ii) gas turbines and (iii) internal combustion engines (ICE), which can both be used for electricity (and heat) generation as well as maritime transport; (iv) industrial, commercial and residential boilers and furnaces for heat generation at different temperature levels, and (v) a plethora of chemical reactors where H2 is fed as chemical building block or reductant. It is important to note that the development trajectories – undertaken and required – for these conversion technologies are very different. While the vast majority of existing FC are inherently fit for H2 use – in fact they have been built for that purpose – GT, ICE, and burners have been originally designed to run on hydrocarbon fuels. Accordingly, while fuel cells mainly call for lowering capital costs and increasing lifetime, H2-fired GT, ICE and burners aim at reaching the same standards set by the machines running on carbonaceous fuels in terms of efficiency, cost and emissions. In the following, we discuss the different technologies in more detail.
Fuel cells.
From many perspectives, fuel cells are the optimal H2 conversion technology: they were specifically conceived to use H2 without suffering from the thermodynamic limitations of heat engines. Today, fuel cells can be regarded as commercial technologies: in 2019, 1.1 GW of fuel cell capacity, or about 70
000 units, were shipped globally.22 Thanks to the growing demand, the supply chain is consolidating throughout Europe, US and Asia. However, a few remarks are worth making when looking at fuel cell deployment within a hydrogen economy.
Several types of fuel cells exist (typically categorized according to the electrolyte) and can be adopted for different end-use H2 applications. We can recognize four main FC types. Polymeric fuel cells (PEMFC) dominate the market both by units and capacity (62% and 83%, respectively).22 The other three types include phosphoric acid fuel cells (PAFC), solid oxide fuel cells (SOFC), and molten carbonate fuel cells (MCFC). Looking at current applications, PEMFC are used in both stationary and ground transport applications, while PAFC, SOFC and MCFC are mainly designed for steady-state power and heat generation. This is a result of the operating temperature: thanks to the low operating temperature (80–100 °C), PEMFC can undergo fast cold starts while also offering high efficiency and power density, which makes PEMFC suitable for powertrain applications.23 As for stationary applications, all four types of cells are used, with preferred solutions depending on the end use. Here, PEMFC are mainly adopted for residential combined heat and power (CHP) applications, SOFC for commercial (and partially residential) CHP applications, and PAFC and MCFC for onsite (baseload) power applications.
When thinking of fuel cells as H2 conversion devices we must recognize that many existing cells are designed to operate in current applications with natural gas as fuel input, which must be reformed to H2 prior undergoing the electrochemical conversion. In fact, only PEMFC for powertrains have substantial operating hours with pure H2. It is therefore important to understand the implications of switching to pure hydrogen as input. Again, we can distinguish between two main cases depending on the operating temperature and on the capability of carrying out internal reforming of hydrocarbons.
In low-temperature FCs (PEMFC and PAFC, PAFC operates at around 150–210 °C), where no reforming is possible within the FC stack, the anode must be supplied with high or very high purity H2. Commercial PEM or PA cells running on natural gas are therefore equipped with all components required to make H2 available at the stack, and to keep contaminants, e.g., CO, largely below catalyst poisoning levels. When moving to H2 as fuel input, a substantial simplification of such systems is possible, which will lower capital expenditure (CAPEX) and will require minor tuning in the cell operation strategy (e.g., in the optimal voltage–current density working point and heat management).
In high-temperature FCs (MCFC and SOFC), where reforming can take place inside the stack, natural gas does not need to be fully converted to H2 outside the cell. In fact, the vast majority of high-temperature cells are designed with internal reforming, as it has proved superior compared to external reforming.24 For the reforming to effectively take place in the stack, the cell must be designed to enhance heat transfer between the anode membrane and the gas while limiting the temperature change along the stack. When running on pure H2, the cell heat balance is different, and the stack has to be redesigned for a new optimum operation. Moreover, there exist an intrinsic loss as the cell heat is not converted into highly valuable chemical energy, i.e., via reforming. Similar issues are already experienced when varying fuel composition, as different studies have pointed out.25–27 Challenges include, but are not limited to a change in the performance metrics and polarization curve, non-uniform stack internal profiles and a steep change in temperature gradients. While running on pure H2 will eventually simplify the overall system complexity and its CAPEX, the design optimization is a demanding process in term of costs and time which should not be underestimated, especially in light of the fuel cell manufacturers size. On the other hand, MCFC and SOFC may be particularly interesting in a H2 economy, as they are able to operate as net hydrogen generator units while producing electricity (and heat) when running on hydrocarbons at low fuel utilization factor. They could therefore be very useful in the transition from natural gas (NG) to a green H2-based society. Moreover, MCFC and SOFC offer a large variety of highly efficient processes integrated with carbon capture and storage (for more details see, e.g., ref. 28–30), allowing low carbon (albeit not net-zero over the life cycle) H2 production from natural gas, and potentially negative CO2 emissions when using a biogenic feedstock.
Two further issues may be relevant to the use of hydrogen FCs. First, the purity of the supplied hydrogen needs to adhere to strict constraints (e.g., 99.97% purity for PEMFC), as otherwise FC producers would waive their product guarantees. Given that large-scale hydrogen supply systems may not deliver at FC specifications, some form of on-site polishing may be required at for example vehicle filling stations.
Second, there is the issue of NOx formation in the FC stack after-burner: as FCs cannot fully convert their fuel input, some H2 is left in the anode outlet stream. While recovering it via a proper separation process is the preferred option in large plants,31 in small and medium applications the residual H2 is typically burned. Care must be taken in preventing NOx formation from this combustion, e.g., using catalytic converters (see also section 2.2.1), or proper NOx removal abatement.
Gas turbines.
H2 and H2-rich syngas can currently fuel commercial gas turbines. However, these machines are far from the very efficient state-of-the-art GTs that reach 60+ % electric efficiency in combined cycles, produce close to zero NOx emissions, and achieve fast ramp-up/down in operation. In fact, GT manufacturers offer syngas turbines for H2-rich blends that use diffusive combustors,32,33e.g., multi-nozzle quiet combustors, rather than the state-of-the-art premixed nozzle types (commercial machines using the latter combustor can be fed with only up to 5% H2).34 Diffusive combustors are used in combination with large amounts of diluents, such as nitrogen or steam (approximately 50 vol%), which prevent the formation of local hot spots in the combustion and the associated thermal NOx.35–37 Using diluents results however in lower efficiency, as it typically entails a reduction in the turbine inlet temperature, and in a reduced operability window.35 Moreover, the turbomachine design is mostly unchanged compared to natural gas-fueled machines (with exception of reducing the compressor stages for very low-LHV fuels) making their operation suboptimal.
The challenges that need addressing to use H2, or H2-rich syngas, in state-of-the-art gas turbines are diverse. First, there are challenges inherent to the combustion of H2; these include (i) thermal NOx formation because of the high H2 flame temperature, and (ii) high heat transfer coefficient in the expanding flue gas because of increased amount of water. Second, there are challenges linked to using H2 in gas turbines whose design is optimized for running on natural gas, e.g., handling the higher flue gas-to-air volumetric ratio, and the higher mass flow rate required for blade cooling. Practical solutions exist for these challenges, but they come at the expense of the machine efficiency and capacity of operating dynamically. If high performance is to be maintained, then different technical solutions are needed. Aerodynamic challenges can be solved by designing new blade shapes and resizing the turbomachines as best for H2 use. This can be implemented today but comes with high costs, and therefore needs sufficient market drivers.
On the other hand, suppression of NOx formation requires the development of ad hoc lean premixed combustors using (close to) 100% H2 as fuel (selective catalytic reduction would also be technically feasible,36 yet not economically viable given the large reduction required). Similarly, to those designed for natural gas, premixed combustors tailored for H2 would allow for high gas turbine efficiency and operability, while also limiting NOx formation to a few ppm (<10 ppm).34,35 The design for such components is very complex though, and exhibits safety issues because of the low ignition temperature of hydrogen and the risk of flashback (i.e., the flame moving back into the burner because the flame speed is higher than the gas flow velocity). Other challenges like low combustion efficiency and potentially higher CO or hydrocarbon emissions (when using a H2-rich syngas) need also to be kept under control.37 Improved designs are under way that exploit the flexibility of 3D printing and that allow for burning ultra-lean fuel/oxidant mixtures.38 Also, catalytic burners that lower the flame temperature have been tested for several gas-turbines types, including those of Siemens and GE.37
In fact, after years of R&D, the development of H2 premixed combustors has reached a turning point, and the leading gas turbine manufacturers39 have pledged to reach 100% hydrogen fuelled gas turbine within the next decade(s), e.g., Siemens and GE by 2030.40,41
Internal combustion engines.
H2-fuelled ICEs have long been investigated, with the first attempts already in the 19th century, and modern development starting in the late 20th century. Most of the R&D efforts have been focused on automotive applications. In 2009, Verhelst and Wallner provided a detailed review of H2-fueled ICEs for transportation purposes covering fundamentals of combustion, modeling, engine design, safety and applications.42 They concluded that (i) H2-fuelled ICEs are technically viable, and (ii) H2 is a desirable fuel for ICEs, but that it also poses challenges that have to be addressed with specific engine design, e.g., external gas recirculation (EGR). However, since this review paper was published, the automotive industry has been experiencing a disruptive transition towards electric engines, either battery- or fuel cell-powered. On the other hand, H2 is regarded as pivotal for large integration of intermittent renewables. From this perspective, stationary H2-fuelled ICEs represent an interesting alternative to FCs and GTs given their rather high electric efficiency (40–50%), prompt dynamics, robustness of the technology, and flexibility in running with natural gas–hydrogen mixtures. Similar to H2 GTs, several ICE manufacturers have been operating engines with high H2 content (up to 70 vol%), and have plans to quickly develop machines for 100% H2.43 As with H2 gas turbines, NOx formation remains a challenge, though it can be controlled either via EGR (but with an efficiency loss) or via high-pressure direct injection of H2 into the combustion chamber (yet to be fully developed).
Burners for heating provision.
The key challenge of using hydrogen for industrial heating, like its use in gas turbines, is the high temperature of combustion and inherent NOx formation beyond regulation limits.36 However, the boundary conditions of furnace and/or boiler burners make the combustion process easier compared to the complex fluid dynamic conditions found in gas turbines and ICEs. While the literature on H2 industrial burners is limited, a few examples and patent applications exist that provide robust evidence for using H2 in this context. Lowe and co-authors44 showed experimentally and numerically that switching industrial furnace burners from natural gas to hydrogen is possible from a technical viewpoint, while also keeping NOx emissions within regulation limits. More recently, Toyota has developed a general-purpose hydrogen burner for industrial use, where hydrogen–oxygen mixing is retarded, and the adiabatic flame temperature is lowered.45,46 The resulting NOx levels in the exhaust gas are claimed to be below that of natural gas burners of similar size.45
Chemical reactors for H2 conversion.
Generally speaking, H2-based chemical reactors are established and well-known technologies. Relevant examples include the Haber-Bosh ammonia synthesis and methanol synthesis, where several reactor configurations are available. These will remain important end conversion of H2 and will need to adapt to evolving materials, catalysts, design, et cetera. We see, however, an additional line of H2 conversion reactors becoming more and more important, namely H2–CO2 reactors, where CO2 is activated and converted into C-based fuels or chemicals (see further Section 2.2.4).
2.2 Hydrogen end use applications
2.2.1. Supply of industrial, commercial, and residential heat.
Hydrogen has the capability of supplying thermal energy to a process at a wide range of temperatures without producing direct CO2 emissions. Clearly, this makes it a pivotal element wherever heat is needed: from the low temperatures for residential and commercial buildings to (and foremost) the large variety of industrial processes (steam boilers, furnaces, process reactors). In the following we analyse the use of hydrogen as heat provider, starting from the industrial sector where typically heat is needed at high temperature (i.e., above 200 °C), and concluding with the built environment heat supply.
Provision of industrial high-temperature heat.
In 2014, direct and indirect emissions from industrial processes accounted for approximately 15 Gt CO2-eq per annum, roughly 28% of global GHG emissions.47 Around 40% of these emissions stem from fuel combustion for heating, with the remainder mainly in the form of process emissions and emissions for electricity generation.47 Although there are alternatives to hydrogen for the provision of low-temperature industrial heat (e.g., electrification through heat pumps, decarbonized heat networks),47 the majority of industrial heat is required at levels of several hundreds of degrees and higher, necessitating the combustion of a fuel.47,48 From this perspective, hydrogen has the potential to fully decarbonize medium- and high-temperature industrial heating. Steel making, a difficult-to-decarbonize industrial process, is probably the application that will benefit the most from using hydrogen. Here, hydrogen can be used in the direct reduction of iron ore to iron (the so-called DRI process) or as alternative to methane in the electric arc furnaces, and possibly in both (DRI and electric arc furnaces are complementary processes).49 DRI processes typically require new reactors for iron ore reduction, whose design need to be tailor-made for hydrogen use. Several industrial initiatives exist, with Hybrit50 and Energirion51 being the most advanced in terms of demonstration and commercialization. Not surprisingly, the challenge lies in the demonstration of the process at scale.52 Recently, a hydrogen-methane blend was successfully demonstrated as fuel in the forging process of industrial steelmaking without requiring plant or equipment (burners) modification and while also maintaining the quality of the end product.53
Potentially, hydrogen combustion – especially in small industrial plants – could be facilitated by including a catalytic combustion section. The catalyst, often platinum or another noble or transition metal,54 would then aid the lean-premixed fuel combustion by allowing oxidation at lower temperatures, thereby mitigating NOx formation. Advantages regarding fuel flexibility may also be present: it is expected that heavy industry may need to flexibly fire several fuels, where catalytic burners can also play a role.37
Provision of commercial and residential low temperature heat.
The role of hydrogen for heating in commercial and residential buildings is more controversial than in industrial applications. Because heat is needed at lower temperature, typically in the range of 60–90 °C, several technologies exist that can potentially fulfil this function without emitting CO2. These include heat pumps, district heating (e.g., coupled to waste plants, geothermal, or biomass), biogas, and hydrogen. The preferred pathway is once again dependent on the boundary conditions of the respective energy system and cannot be identified without accounting for the geographical, social and economic context. Looking at the use of hydrogen in commercial and residential buildings, fuel cells (co-producing electricity)55 and catalytic boilers are the technological options receiving most interest.54 Co-generation of heat and electricity is more likely applicable to the commercial building stock, since electricity and heat demands are higher, and economies of scale can be reached more easily. On the other hand, catalytic boilers may be more suitable for residential buildings, and to our knowledge, at least one model, by Giacomini, is commercially available and CE certified.54 Interestingly, catalytic boilers have limited operating temperature (around 400 °C) and, therefore, burns hydrogen virtually free of NOx. Other hydrogen boiler prototypes, e.g., by Baxi and Bosch, are ready for large-scale piloting.56,57 While residential hydrogen boilers are technically feasible and safe, questions remain to be answered around the implementation pathway and the integration with other CO2-free technologies for residential heating. In particular, high initial numbers are required and a gradual ramp up of production, like with normal new technologies, may not be acceptable. As a result, the production of the first large volume may need guaranteed off-take, e.g., through government backing, to decrease the risk for commercial boiler suppliers.54 Hydrogen for residential heating is not widely considered yet, but the UK§ for example, is betting strongly on it.58
2.2.2. Power generation and seasonal storage for balancing of intermittent renewable electricity production.
Hydrogen has historically been associated with clean power generation, particularly when photovoltaic panels (PV) and wind turbine (WT) were deemed too expensive to play a significant role in the energy transition. However, the perspective of hydrogen use in power generation has changed drastically during the last 5 years. On the one hand, there has been an extraordinary growth in wind and solar installations thanks to the fall of their levelized cost of electricity (LCOE) – solar PV and onshore wind are currently the cheapest source of new electricity generation capacity for two-thirds of the world population.59 On the other hand, the power generation sector can potentially benefit from multiple low, zero, or even negative CO2 emissions technologies, making hydrogen a contender but not an indispensable ingredient of the mix. As a result, H2 for electricity generation faces now the prospect of providing specific services rather than a solution for base-load production. These services include peak electricity generation, seasonal electricity storage, backup dispatchable plants, and combined heat and power systems (CHP) for centralised/decentralised applications.23 Accordingly, H2-based power technologies need to follow a development pathway targeted to acquire the key characteristics for these applications, going beyond the traditional high-efficiency and low-cost combination. In the following, we examine these services more in details.
Electricity grid balancing.
As mentioned above, hydrogen can provide grid balancing services to match power generation and demand.60 During periods of high energy demand, sudden demand peaks can be met by converting H2 in gas turbines, internal combustion engines, or low temperature fuel cells. Here the requirement is the capability of ramping-up operation within seconds (primary frequency control) and/or minutes (secondary frequency control). Hydrogen supply may or may not include hydrogen storage. In the latter case, the hydrogen used as a fuel needs to be readily available from a dedicated hydrogen grid, and its production, e.g., via steam methane reforming or water electrolysis, needs to be balanced with the overall use. This configuration would be viable only when other hydrogen end-uses are present, as grid balancing is likely to be needed for limited hours per year while still requiring significant infrastructure and conversion technologies. On the other hand, the use of hydrogen storage, which can span from hours- (short-term storage) to days- and months-equivalent (long-term, seasonal storage), would enable more steady state hydrogen production (further discussed in Section 4).61,62 Furthermore, when coupled with hydrogen production via water electrolysis, hydrogen storage allows full exploitation of the potential of renewable energy sources (RES) by absorbing the excess energy during periods of low energy demand (and reusing it during periods of high energy demand). Interestingly, the role of hydrogen as power-to H2-to power system is downplayed when a large exogenous hydrogen demand is present, e.g., for industrial feedstock or heating.63 Again, this shows that hydrogen deployment needs to be coordinated considering the different end-users and their evolving role during the energy transition, for example through “hubs” (see Section 4 for more details).
Optimal grid balancing services, and the highest energy efficiency, are obtained by coupling electricity storage in hydrogen with other forms of electricity storage, particularly batteries (see Fig. 1). This allows optimal exploitation of the technical features of the different storage options to deal with the variability of electricity generation and demand at different sizes and time scales.62,64 On the one hand, batteries are characterized by a round-trip efficiency that is high for short periods but decreases with time due to self-discharging losses, which makes them optimal for storing electricity during periods shorter than about three months.65 On the other hand, storage in hydrogen is characterized by negligible self-discharging losses, but lower values of overall round-trip efficiency (mostly due to the losses incurred during hydrogen production and re-electrification), making it more suited to seasonal energy storage.66,67 Furthermore, electricity storage in hydrogen is likely to use large-scale hydrogen production (e.g., electrolysers) and conversion (e.g., fuel cells) plants, making power-to-hydrogen cost-competitive with respect to batteries. The cost of the expensive components of a power-to-hydrogen system, electrolysers and fuel cells, scale with the rate of the charging/discharging power conversion processes (instead of the stored energy quantity). In other words, power-to-hydrogen allows for a better decoupling of energy and power than batteries.64
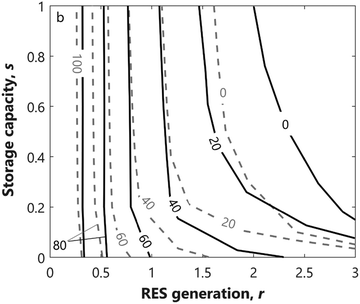 |
| Fig. 1 CO2 emissions isolines (in t per GWhel) at varying undispatchable renewable generation (x-axis) and storage capacity (y-axis) when considering a system with only H2 storage (solid lines) and H2 storage + batteries (dashed lines). Adapted from Gabrielli et al.64 | |
When CO2 emissions are to be minimized, RES need to be oversized with respect to the demand and coupled with large amounts of electricity storage. In such a case, the cost optimum solution features storage in hydrogen deployed and operated in a seasonal fashion, and batteries operated for short-term storage.64 The associated hydrogen storage needs to be large enough to enable seasonal operation but also flexible with respect to injection/withdrawal to exploit RES production peaks. Underground hydrogen storage in salt caverns, and possibly in depleted gas reservoirs and aquifers, is likely the most viable option (tank and in-grid storage might be used for short, local, buffer capacity).64
Combined heat and power.
Thanks to their modularity, hydrogen-based electrochemical technologies (i.e., fuel cells and electrolysers) can be deployed for centralised and decentralised applications covering both the macro (MW) and micro (kW) scale without significant change in the conversion performance.68,69 In domestic or urban energy systems, fuel cells contribute to increasing the efficiency of the energy supply system by cogenerating electricity and heat. At the same time, electrolysers can utilise electricity in moments of excess production, which allows for a wider deployment of renewable energy sources while avoiding local grid congestion. Overall, this helps to enhance the energy efficiency and self-sufficiency of decentralized energy systems and unlock the full potential of electricity distribution grids with an overall reduction of CO2 emissions. An important point in this application is the selection of the device that best matches the intended use. Within the framework of urban energy systems, where the cogeneration of electricity and heat is often driven by the heat demand,70 low-temperature conversion technologies (e.g., PEMFC) are the optimal choice due to their faster dynamics and their higher thermal efficiency. However, when energy end-users require a higher fraction of electrical energy, or when larger fractions of self-sufficiency are requested, high-temperature technologies such as SOFC and MCFC can be preferred.71 As a matter of fact, the optimal degree of decentralization of hydrogen-based power generation systems is still an open question, with possible solutions ranging from national and regional installations72 to neighbourhood and building units.73 The optimal solution depends on the specific cases of interest and on the entire hydrogen supply chain (see Section 4).
2.2.3. Low carbon (heavy) road transport, maritime shipping, and aviation.
Hydrogen can play a key role in tackling distributed CO2 emissions, which largely stem from the various forms of transportation. While hydrogen properties present both advantages and disadvantages depending on the specific application, hydrogen fulfils a key prerequisite: it fully eliminates direct CO2 emissions. This is evident when comparing hydrogen to fossil fuel powered systems, but it also leads to clear advantages with respect to renewable-based synthetic hydrocarbons, i.e., there is no need to re-capture carbon (or make carbon circular). In fact, the decarbonisation potential of H2-based transportation depends on the carbon-intensity of the hydrogen production pathway, which, in turn, depends on the energy system at large. At the same time, and especially when comparing to internal combustion engine vehicles (ICEV), hydrogen-fed fuel cell electric vehicles (FCEV) substantially eliminate pollutant emissions in the exhaust, thus improving local air quality in areas with high traffic volumes. While FCEV and battery electric vehicles (BEV) are often presented as competing solutions, their role in decarbonisation is rather complementary, especially when considering the 1.5 °C timeline (i.e., there is not enough time to develop the perfect battery). On the one hand, BEV seem to fulfil most user requirements at lower costs for motorised individual traffic, which is clearly reflected by current market trends and announcements of major car manufacturers.74,75 On the other hand, FCEV seem to be the most promising “clean” option in the heavy-duty road vehicles and (mid-range) maritime shipping sectors due to specific use profiles and associated requirements in terms of range, fuelling time and lifetime.76,77 An overview of ICEV/FCEV/BEV vehicles is shown in Table 2.
Table 2 Comparative overview of drivetrain technology characteristics and performance today (and expected mid-term trends in between brackets) from best (+) to worst (−)
|
ICEV (fossil fuel) |
FCEV |
BEV |
Depending on fuel supply – potentially large reductions compared to ICEV.
|
Investment costs |
+ (+) |
− (o) |
o (+) |
Operation costs |
o (o) |
− (+) |
+ (+) |
Additional infrastructure needs |
+ (+) |
− (o) |
o (o) |
GHG emissions (life cycle) |
− (−) |
o (+)a |
o (+)a |
Air pollutant emissions (tailpipe) |
− (o) |
+ (+) |
+ (+) |
Efficiency |
− (−) |
o (o) |
+ (+) |
Range |
+ (+) |
o (o) |
− (o) |
Fuelling time |
+ (+) |
o (o) |
− (−) |
Road transportation.
Today, fuel cell buses represent the most widely adopted hydrogen application in the transport sector with hundreds of vehicles in operation, mainly in Europe, China, Japan and the US.78,79 Most of them are publicly operated and exhibit predictable operation profiles (routes) requiring few fuelling stations, which facilitated their roll-out.78,80,81 Commercialisation of fuel cell (as well as battery electric) heavy-duty trucks is lagging behind. However, the first substantial numbers of FC trucks are going to hit the road in Switzerland and Norway82 with a few other European countries following suit. By 2025, 1600 Hyundai “Xcient Fuel Cell” trucks83 are foreseen to be operating on Swiss roads, and this will represent the first large-scale introduction of such heavy-duty vehicles in specific markets.84 Other truck manufacturers are developing similar vehicles. The rather slow development of the heavy trucking market segment can be attributed to high vehicle and hydrogen cost as well as the (currently) limited refuelling infrastructure.79
Maritime transportation.
The same obstacles present for road transportation hinder hydrogen deployment in maritime shipping today. On top of that, limited hydrogen storage density poses a problem for long-distance trips.85 Liquified hydrogen could improve this, but affecting both energy and infrastructure costs. When looking at present implementation, the first commercial fuel cell ferry is deployed in Scotland86 while the second is meant to start operating by the mid 2020's in San Francisco.87 Several pilot and demonstration projects are also ongoing.88–90 From a technology perspective, while hydrogen-fuelled road vehicles are generally equipped with low-temperature PEMFC, both high-temperature fuel cells and internal combustion engines represent interesting alternative solutions for ships, where also ammonia could be used as hydrogen storage medium.85,91
Aviation.
In aviation, a competition between hydrogen and liquid renewable-based synthetic hydrocarbons can be reasonably expected. While small aircraft for short-range applications could in principle use fuel cells (either alone or combined with, e.g., turbines), larger, commercial aircraft are likely to keep using gas turbines fuelled with hydrogen or synthetic hydrocarbons. Hydrogen propulsion could be more economic up to medium range aircraft segments compared to synfuels, which may be more cost competitive for long-range aircraft.92 Using hydrogen as aviation fuel would require an adaptation of the fuelling infrastructure within airports, though the challenge would be not as significant as transporting and distributing hydrogen throughout a nation or region. Finally, it is worth stressing that recently Airbus, the largest plane manufacturer along with Boeing, has released plans for transitioning to zero-emission aircrafts fed by hydrogen, which suggests that the aviation industry is actively looking, if not betting on hydrogen as alternative zero emission fuel.93
In addition to its different sectors of application, it is important to consider the economic and environmental aspects of hydrogen-fed vehicles. The economic competitiveness of FCEVs is still limited by high vehicle and fuel costs as well as substantial investments required for hydrogen transport and storage infrastructure. Current total costs of ownership (TCO) of FC vehicles – passenger vehicles, trucks, buses, ships – are higher than those of fossil fuelled counterparts.23,79,81,85,94,95 Perspectives in terms of reaching cost parity are uncertain: TCO projections vary over wide ranges and while some studies estimate cost competitiveness to be achieved within a few years (e.g. ref. 79 and 96), others are less optimistic and expect TCO parity not before 2030–2040.85,95,97,98 The key issue is the required reduction in purchase costs for FCEVs, which can only be achieved by substantially increasing production volumes. While this seems to be realistic for buses and freight transport vehicles, since the market for low-carbon alternatives is still underdeveloped, the recent development of BEV in the passenger vehicle segment represents a high entry barrier. Besides pure vehicle purchase costs, sufficient lifetime of FC stacks must be ensured to keep capital investments reasonable. Finally, hydrogen transport and distribution infrastructure can only become affordable if sufficient hydrogen can be sold (further discussed in Sections 4 and 6). Cost competitiveness also depends on the development of alternatives, both in terms of economic and technology performance. For example, substantially increasing oil prices or carbon taxes would make conventional vehicles less attractive, while new battery technologies with higher energy storage density and longer lifetimes would result in advantages for BEV.
The environmental performance exhibits less ambiguity. While local and regional air quality will profit from FCEV in any case – which is important for both urban areas and large ports – the environmental benefits (and potential trade-offs) of FCEV from a life-cycle perspective are mostly determined by the hydrogen production, transportation and storage pathways and not by the FCEV itself.76,80,91,97,99–101
Accelerating the deployment of FCEV requires targeted support measures. These can address environmental issues, e.g., CO2-emission performance standards for passenger vehicles102 and heavy-duty vehicles103 or larger low-emission zones,104 or directly set a plan for phasing out internal combustion engines.105 Measures can also aim at improving economic competitiveness of FCEV by enforcing the “polluter pays principle” via internalizing externalities resulting from impacts on human health due to release of air pollutants, GHG emissions, or noise, which would help electric vehicles reaching cost parity. Addressing local and global environmental damages in synergistic ways can help to effectively foster climate action in road transport while maintaining public acceptance and socially fair outcomes.106 Road toll exemptions for clean vehicles can also be granted, as currently in Switzerland. Finally, voluntary agreements in specific sectors, as recently set in place by the International Maritime Organization,107 can accelerate FCEV employment.
2.2.4. Carbon free and carbon-based synthetic products derived from hydrogen.
Currently, the largest end-use of hydrogen is in the synthesis of fuels and chemicals, above all ammonia production and oil refining. While the latter will gradually disappear, the former will likely expand – for fertilizers but also for energy applications – and various other opportunities will arise, where hydrogen may act as a feedstock, energy input or reducing agent to the synthesis reaction. Among the possible products, fuels represent the largest potential market. Most prominently, synthetic hydrocarbon fuels are being discussed as a replacement of current fossil fuels, especially for sectors in which direct electrification is difficult and that need high energy density, liquid fuels (like aviation).108 Typical synthesis routes involve the reduction of CO2 through hydrogen to form molecules containing a single carbon atom, such as formic acid, methanol or methane, or to synthesis gas (carbon monoxide and hydrogen) followed by a Fischer–Tropsch synthesis to form longer carbon chains. Also, base chemicals can be derived in this way, where existing as well as novel organic chemistry routes enable the synthesis of virtually all products of the modern chemical industry that are today derived from fossil feedstock.109 Besides renewables-based hydrocarbons, ammonia represents the most prominently discussed carbon-free hydrogen-based fuel,110,111 which could be used in a range of applications.112–114 In a similar way as synthetic hydrocarbon-based chemicals enable the sector coupling of the electricity and the chemical industry, ammonia acts as a link between the fuel and the fertilizer industry (and potentially transportation). As with hydrogen, there is strong advocacy for a “methanol economy”115 or an “ammonia economy”.116,117 However, the different synthetic fuel candidates have fundamentally different properties and fuels are used in such a wide variety of applications, that the ambition of finding the ideal synthetic fuel of the future seems unachievable. Important aspects include the chain efficiency of the fuel synthesis and use with “cradle-to-grave”-type system boundaries. In general, such indirect electrification via electricity-based synthetic fuels exhibits a two to 14 times lower energy efficiency than direct electrification, which is substantial, depending on end-use technologies (fuel cell, turbine, et cetera) and considering all conversion steps from electricity to end-use.108 For carbon-based fuels, such analysis must imply the closing of the carbon cycle, either through CO2 capture at the fuel use or through sourcing of the feedstock carbon from the atmosphere via biological means or direct air capture (DAC). Secondly, infrastructure requirements for the synthesis, transport, and storage of the synthetic fuels play an important role and existing fossil fuel infrastructure is a major argument for carbon-based synthetic fuels. Thirdly, health, safety and environmental aspects, such as toxicity of the fuels, explosion risks and the ease of handling have to be considered. These aspects should be assessed with a dynamic view on regulatory requirements and technological capabilities, as demonstrated by the emergence of a worldwide LNG transport and storage network over the course of the last two decades.
In the discussion of different fuels and their race for public and private investment, it is important to note that hydrogen is a central feedstock for all such fuels and products proposed. Making hydrogen economically and logistically available will enable the market to develop and test the different products and fuels. Large-scale applications that inevitably require specific products justify research and development of carbon-based and carbon-free alternatives. These applications include intercontinental air travel, which benefits most of the unrivalled volumetric energy density of hydrocarbon fuels, and fertilizers production, which requires ammonia as a means to supply nitrogen. These large-scale applications represent substantial markets and considering the lack of climate-neutral alternatives, they should be prioritized considering the (at least within the next few decades) limited availability of low-carbon hydrogen.108
2.3. Matching technologies, applications, and boundary conditions
While there often is a tendency to promote one specific end-use of hydrogen, independently of the boundary conditions, hydrogen will be needed across different sectors and applications. However, different economic, geographical, and societal macro areas will see different low-carbon implementation pathways, which translate into a different final portfolio of hydrogen end-uses. We therefore expect that one or two applications will catalyse the deployment of H2 for all relevant end users, and that regions with similar environmental conditions will experience similar pathways. To understand and quantify this better, a cross-sectorial analysis of the overall hydrogen value chain is required. For example, Fig. 2 illustrates the pan-European nature of the hydrogen infrastructure but also the different types of end-uses found in each country (from the ELEGANCY ACT project118). For the different countries, we see that:
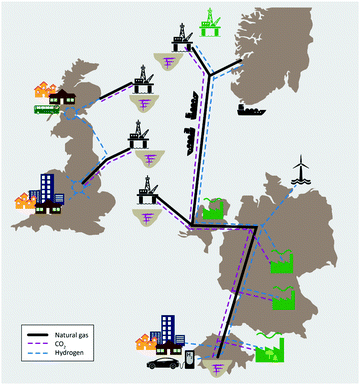 |
| Fig. 2 Possible European chains for H2, CO2 and natural gas production, transport, storage and usage. This map illustrates the large number of choices that must be made about the future energy system: where will H2 be produced, how will it be transported and used; how will CO2 be captured and transported, and where will it be stored? | |
In Germany, hydrogen is expected to play a key role in decarbonizing heavy industry and transportation. Critical open questions concern the optimal development and legal-social aspects of building a new infrastructure for hydrogen production (local/central/import), its integration with the natural gas grid, and the transportation of by-product CO2 to third countries for storage.119,120
In the Netherlands, hydrogen deployment will be triggered by industrial clusters such as the Rotterdam harbour, where it can be used as building block for chemicals production, as alternative feedstock in power plants, and for high or medium temperature heating. For this to commence, it is important to investigate industrial clusters from a coordinated, multi-actor perspective, so as to identify a joint masterplan for transition and exploit synergies among processes.121,122
In Switzerland, hydrogen demand is expected to be driven by road transportation, which has led to an interest in distributed H2 production. Distributed production implies relatively small volumes and typically high costs. It is therefore critical to identify optimal technologies and network configurations.4,5
In Norway, the hydrogen focus is on production and export based initially on natural gas reforming with CO2 capture and storage. It is important to evaluate the different options for the location of H2 production and CO2 separation as well as technology choices. Factors influencing the choices include the level of H2 demand, the existence of a CCS infrastructure and constraints on the H2-transportation infrastructure, and the expected cost reductions due to capacity development.123
Finally, in the UK, hydrogen deployment may be triggered by industrial and residential/commercial heating demand, with supporting applications in heavy goods transport and potentially power generation. Urban and suburban transition will have to be reconciled with the industrial clusters transition, such that most benefits are reaped.124,125
3. Hydrogen production methods
3.1. Introduction
As hydrogen is an energy carrier, it needs to be produced from other energy sources. Virtually all hydrogen currently stems from fossil fuels, roughly 70% from natural gas, with only a minority of approximately 2% (based on mass of total H2 produced, including the hydrogen as by-product from chloralkaline processes) produced via water or brine electrolysis.126 Other approaches include coal or oil gasification and/or biomass-based hydrogen production, mainly biomass gasification.
In this section, we provide our perspective on hydrogen production technologies by discussing typical characteristics, including their technology readiness level, feedstock availability, costs, life-cycle carbon footprint and net-zero potential and possible showstoppers. We discuss the current as well as the expected status at mid-century (except for costs), when many European economies aim to be net-zero CO2 (or GHG) emitters.127 Afterwards, we provide a more detailed overview of the characteristics of each technology, focussing more on the status of technology and potential technical hurdles that still need to be overcome for successful large-scale technology deployment. We include the technologies we think are most relevant in the energy system discourse, and which may play a role in the transition to net-zero GHG economies (i.e., up to 2050): water electrolysis (alkaline, polymer electrolyte membrane and solid oxide), natural gas reforming (steam reforming, autothermal reforming and partial oxidation), and biomass-based hydrogen production (reforming of biomethane and biomass gasification options). The main characteristics of the selected production technologies are summarised in Table 3, Fig. 3 and 4.
Table 3 Selected hydrogen production technologies and assessment characteristics, based on the analyses in the subsequent sections
Technology |
TRL current |
Expected TRL 2050 |
Feed/resource availability current |
Expected feed/resource availability 2050 |
Commensurate to net-zero current? |
Expected commensurate to net-zero 2050? |
Possible showstoppers? |
The 2017 global wind and solar electricity production was just 6% of the total global electricity production.128
Although uncertain and highly dependent on implementation of further decarbonisation policies, based on the current net-zero pledges, it is expected that sufficient renewable electricity for large-scale electrolysis may be available.129,130
Life cycle GHG footprint is non-negligible as production of assets (wind turbines, PV cells, electrolysers) is associated with GHG emissions, e.g., due to the combustion of coal during steel production.
If production of infrastructure (wind turbines, PV cells, electrolysers) can be made GHG neutral and the electricity grid at large reaches carbon neutrality.
Only if fugitive methane emissions of NG production and transport can be fully mitigated.
If scale up issues can be overcome, including financing of capital-intensive pilot and demonstration plants.
|
Alkaline electrolysis plus renewable electricity |
9 |
9 |
Limiteda |
Sufficientb |
Largelyc |
Likelyd |
Availability of low-carbon electricity |
Polymer electrolyte electrolysis plus renewable electricity |
8 |
9 |
Limiteda |
Sufficientb |
Largelyc |
Likelyd |
Availability of low-carbon electricity |
Solid oxide electrolysis plus renewable electricity |
6 |
9 |
Limiteda |
Sufficientb |
Largelyc |
Likelyd |
Availability of low-carbon electricity; insufficient technology progress |
Natural gas reforming with CO2 capture and storage |
8 |
9 |
Sufficient |
Sufficient |
No, but can be low carbon |
Possiblye |
Availability of CCS infrastructure, reduction of CH4 emissions from NG supply |
Biomethane reforming with CO2 capture and storage |
8 |
9 |
Very limited |
Limited |
Yes |
Yes |
Limited biomass resources, CCS availability |
Low temperature biomass gasification |
7 |
9 |
Very limited |
Limited |
Yes |
Yes |
Biomass/residue availability, CCS availability |
High temperature biomass gasification |
6 |
9f |
Very limited |
Limited |
Yes |
Yes |
Technology scale-up, dedicated bioenergy crop availability, CCS availability |
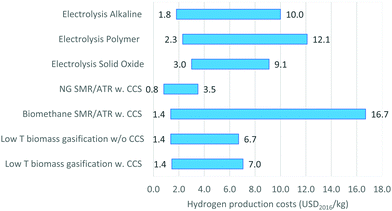 |
| Fig. 3 Current reported cost ranges for selected hydrogen production technologies. Ranges based on values reported in Speirs et al.,131 IEA,126 Binder et al.,132 Howes et al.,133 Bauer et al.,134 IEAGHG135 and Parkinson et al.136,137 and converted to 2016 USD per kg using an average 2016 USD/GPB exchange rate of 1.3552 and an average 2016 EUR/GBP exchange rate of 1.1068. | |
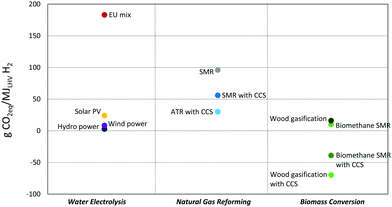 |
| Fig. 4 Lifecycle impacts on climate change (using the GWP100 indicator) of hydrogen produced via water electrolysis, natural gas reforming, and biomass conversion considering different types of electricity for electrolysis and several process configurations for natural gas reforming and biomass conversion. Data sources: electrolysis and NG reforming (Bauer et al., 2021),138 biomethane reforming (Antonini et al., 2020),5 wood gasification (Antonini et al., 2021).4 Methane emissions from natural gas supply represent current average European supply and amount to about 1.3% (Bauer et al., 2021). GHG emissions of electricity used for electrolysis represent typical values for run-of-river hydropower, wind power and solar photovoltaics in central Europe (ecoinvent, 2021).139 SMR with CCS includes ∼56% capture and storage of the plant-wide emissions produced, ATR with CCS includes ∼93% capture and storage of the plant-wide emissions produced. | |
The currently most mature technologies include alkaline (technology readiness level (TRL) 9) and polymer electrolyte (TRL 9) electrolysis and natural gas reforming (TRL 9). New deployment of the latter should critically include CO2 capture and storage, as moving towards the Paris climate agreement would prohibit constructing new CO2 emitting production facilities. It is therefore not surprising that most of the announced new hydrogen projects make use of natural gas reforming with CCS for large-scale facilities, and electrolysis for smaller scale projects.1 The issue with the latter is mainly the availability of low carbon electricity, as well as currently high costs, although the costs are expected to fall as a result of economies of scale and technological learning.136,140 The availability of renewable electricity production, however, continues to be an issue, as deployment of electrolysis capacity not running on renewables goes against the needed shift to lower GHG emissions: the 2020 global wind and solar electricity production was under 10% of the total global electricity production,141 and the share of renewables in 2040 is still projected to be smaller than 50%, with the make-up mainly fossil and some nuclear,128 raising serious doubts on the availability of renewable electricity for large-scale hydrogen production. Some announced projects, like the Dutch NortH2 project, do plan to use dedicated renewables, but this naturally leads to displacement of emissions from hydrogen production to other sectors of the economy, analogous to land use change for biomass production. We therefore expect the required, quick, cost-effective deployment of large hydrogen production capacity to come from fossil fuels, mainly natural gas, combined with CCS, where electrolysis will follow, rather than lead. Fig. 3 and 4 show that this option is not only currently the cheapest, but can have a life-cycle GHG footprint comparable with renewables-based electrolysis when two conditions are met: (i) advanced reforming technologies (e.g., autothermal reforming) are used with plant-wide CO2 removal rates approaching 100%, and (ii) methane emissions from natural gas supply need to be very low over the full production and supply chain, for example, in line with the current European standards.138 Questions have been raised to what extend this is realistic,142 but recent studies5,138 have suggested that natural-gas-based hydrogen production can capture and mitigate almost all CO2 emissions from reformers if the most advanced technologies are used.
The issue of fugitive methane emissions seems more of a threat, especially since low leakage European natural gas production in the North Sea region is gradually replaced with imports from non-European countries and Russia, which typically come with much higher leakage rates and are outside the control of European countries.143 For NG-based hydrogen to fit in a net-zero economy foreseen for 2050, the leakage from such production and supply chains needs to be dramatically reduced (actually brought to negligible values), and the during the 26th Conference of Parties (COP26) announced 30% leakage cut in 2030 is far from enough to achieve this.
The successful implementation of large-scale, low carbon NG-based hydrogen production also critically depends on the availability of CO2 transport and storage infrastructure,144 requiring strong policy measures to incentivise and/or facilitate this (see also Section 5). Further down the TRL scale we find solid oxide electrolysis and biomass gasification-based hydrogen production. The development and inherent cost reductions of the electrolysis technologies is well under way, as discussed below in Section 3.2. Biomass gasification has been demonstrated at pilot or demonstration scale, for instance in the Silvagas145 and GoBiGas plants,146 but still suffers from technical hurdles, especially in the case of high temperature, entrained flow gasification (Section 3.4), which on paper is the most suitable for direct hydrogen production. Reported cost projections for biomass-based hydrogen show a mixed picture, with values reported as low as NG-based hydrogen production (Fig. 3), but we believe these may be strongly underestimated. Biohydrogen based on residues or other sustainable biomass feedstock is essentially commensurate with net-zero targets and may deliver substantial amounts of negative emissions if coupled with CCS.4,5 However, we believe the constrained availability of biomass for bioenergy will be a serious limitation to large-scale deployment, as will be elaborated in Section 3.3.4.
In short, low-cost, low-carbon hydrogen production can commence today, as illustrated by the increased number of recently-announced large projects (see, e.g., ref. 147) and the technology pipeline has a full complement of technologies that can further increase capacity and flexibility at reduced costs and carbon footprint. However, there are still substantial challenges with the hydrogen production technologies themselves that need to be urgently addressed, including the availability of CO2 transport and storage infrastructure, the reduction of methane emissions along natural gas supply chains, and the availability of sufficient renewable electricity, and biomass for bioenergy.
3.2. Hydrogen production via electrolysis
The current production of hydrogen from electrochemical technologies amounts to around 2 Mt year,126 this is produced from plants with an installed capacity of 37.9 GW.148 Electrolysis is the process of splitting water into hydrogen and oxygen using an electric current. The overall concept can be understood by considering what happens when two electrodes (an anode and a cathode) between which a current flows, are placed in pure water. At the cathode, a reduction half-reaction occurs where protons are combined with electrons to form hydrogen gas. Similarly, at the anode, a balancing oxidation half-reaction occurs where water is split into oxygen, protons, and electrons. In practical technologies, electrolytes are used to improve the efficiency and membranes are used to separate the oxygen and hydrogen evolved.
There are three main practical technologies for water electrolysis: alkaline water electrolysis (AEC), polymer electrolyte membrane electrolysis (PEMEC), and solid oxide electrolysis (SOEC). Alkaline electrolysers typically use an electrolyte of potassium hydroxide, with electrodes separated by a thin membrane (“diaphragm”) through which hydroxide ions can travel. This technology has been used for many decades. Polymer electrolyte membranes are more recent and compact and use a solid polymer membrane which acts as an electrolyte while also being able to conduct protons. They use more expensive catalysts (platinum group metals) compared to AEC technology. Solid oxide electrolysers use a ceramic membrane which under the conditions of operation are oxide ion conducting.
While AEC and PEMEC can be considered as mature technologies, SOEC – which are operated under high temperatures around 800 °C and use steam as input – are not yet entirely commercialized. PEMEC are most flexible in their operation, i.e., can follow intermittent loads with high ramping rates while AEC are capable of modest ramping and SOEC best operated under steady conditions. Table 4 summarizes their key characteristics.
Table 4 Key characteristics of water electrolysis technologies
|
AEC |
PEMEC |
SOEC |
Electrolyte |
Liquid |
Solid (polymeric) |
Solid (ceramic) |
Operating temperature |
60–80 °C |
50–80 °C |
600–850 °C |
Typical output pressure |
1–30 bar |
20–30 (up to 80) bar |
1 (up to 25) bar |
Hydrogen purity |
>99.5% |
>99.5% |
>99.5% |
Catalyst used |
Ni/Co/Fe, Ni/C-Pt |
Iridium, Platinum |
Nonprecious metals, e.g., Ni |
Advantages |
Low capital costs |
Fast response – flexible operation |
High electrical efficiency, relies on abundant materials |
Disadvantages |
Slow dynamics, corrosive electrolyte |
Use of noble metals |
Mechanically unstable electrodes, less suited for intermittent loads |
Challenges |
Durability needs improvement |
Reduce noble metal content |
Structural changes in electrodes limiting lifetime |
Unlike traditional process technologies, water splitting by electrolysis is a process in which capacity is defined by surface area of electrodes. This places a limit on the capacity of individual modules, and capacity is built by “numbering up” and using multiple modules. This also facilitates flexible operation and robustness. The modularity of the technology means that cost reductions will arise through standardisation and mass production, rather than through economies of scale.
3.2.1. Efficiency and economics of electrolysis: current status and future trends.
The economic competitiveness of hydrogen production via electrolysis is mainly determined by investment costs and lifetimes of electrolysers, their utilisation rates as well as expenses for electricity supply, which are in turn driven by the efficiency of electricity-to-hydrogen conversion. Reported system efficiency depends on unit sizes and other parameters and can thus vary considerably. However, much of this variability in reported estimates is actually due to the different system boundaries considered. Total system efficiencies are often about 10% lower than the electrolysis efficiency alone, depending on the duty, accounting for rectification of current, purification, compression, and storage. The temperature of operation, capacity and age of the installation also impact efficiency.
Projections of improvements in efficiency are not expected to be as significant as those expected in capital cost. Schmidt et al.149 undertook a survey of experts to develop estimates of efficiency improvements for AEC and PEMEC. They found that improvements of approximately 10% higher heating value may be achievable with some of the drivers being thinner membranes, higher operating temperatures, and marginal improvements in balance of plant design (water pre-treatment, current rectification). In addition, efficiency, and thus electricity consumption per unit of hydrogen produced, is improved substantially with electrolyser capacity up to the MW or 10 s of MW range, after which marginal improvements are made, as shown in Fig. 5 for AEC and PEMEC. Table 5 provides efficiency ranges of the three electrolyser types today as well as expected ranges in 2050.
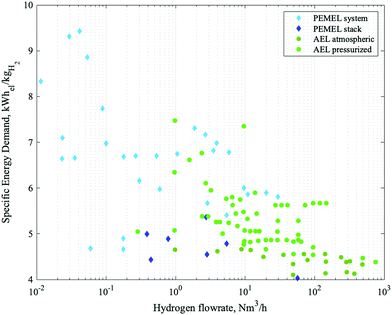 |
| Fig. 5 The dependency of specific energy demand on the production rate today. Based on Smolinka.150 | |
Table 5 Efficiency ranges (% LHV) of electrolyser systems today and in 2050 (expected). SOEC figures do not include energy for steam generation151,152
|
AEC |
PEMEC |
SOEC |
2020 |
58–70% |
58–65% |
81–83% |
2050 |
61–80% |
70–74% |
88–90% |
On the other hand, dramatic improvements are expected in CAPEX. Capital cost reductions were reviewed in Glenk et al.153 (Fig. 6), Schmidt et al.149 and here using data from the Potsdam Institute for Climate Impact Research (PIK)154 (Fig. 7). The Glenk review synthesised manufacturer data from interviews with grey and academic literature to give estimates of historical costs up to 2016 and projections up to 2030. There is understandably high variability in CAPEX historically as (particularly PEM) electrolysers mature. The Glenk study estimated average annual CAPEX declines of 4.8% for PEM and 3% for alkaline electrolysis; trend analyses for SOEC were not undertaken, thus we added them in Fig. 6 below.
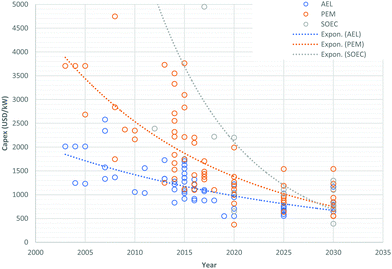 |
| Fig. 6 Examples of CAPEX estimates for different electrolyser types for the year of development. Source: Glenk & Reichelstein.155 | |
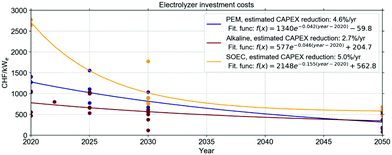 |
| Fig. 7 Expected cost reductions to 2050 of AEC, PEMEC, and SOEC, based on data from PIK.154 | |
In these analyses, there have been and are future expectations of significant cost reductions in PEMEC technology, based on its relative immaturity and headroom. Current costs of SOEC are high, but given the bill of materials, large cost reductions are expected in the future. The costs are expected to follow different trajectories, but Glenk expect them to end up being fairly similar by 2030 as shown in Table 6. Schmidt et al. estimate costs for 2020 at 992, 1440 and 3254 USD per kW for AEC, PEMEC and SOEC respectively, reducing to 697, 1115 and 2168 USD per kW by 2030,149 higher than the Glenk estimate. The most recent CAPEX review with projections up to 2050 has been performed by PIK (2021).154 We used their data to establish our own trend lines for AEC, PEMEC, and SOEC, as shown in Fig. 7.
Table 6 Average cost projections for electrolyser types. Source: Glenk et al.155
Year |
AEL (USD per kW) |
PEM (USD per kW) |
SOEC (USD per kW) |
2020 |
951 |
1138 |
1296 |
2025 |
816 |
997 |
1062 |
2030 |
768 |
899 |
835 |
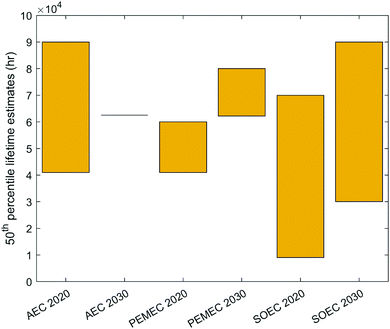 |
| Fig. 8 Projections of lifespans of each electrolyser technology for 2020 and 2030 across interviewed experts. Bars show the 50th percentile estimate ranges of the experts, based on Schmidt et al.149 Note that the AEC estimate for 2030 was from one expert only. | |
Capital costs are amortised over the equipment lifetime, which means increasing lifetime should lead to cost reductions. Schmidt et al.,149 used an expert elicitation approach to try to understand likely improvements in electrolyser lifetimes. A summary of their findings is illustrated in Fig. 8. SOEC are relatively new and hence their current expected lifetimes are relatively low. However, in the near future, the lifetimes of all technologies are expected to become relatively similar and attaining the range of life of 60
000–70
000 h by 2030.
3.2.2. Photocatalytic and photoelectrochemical hydrogen production.
In addition to the electrolysis technologies discussed above, hydrogen can be produced via sunlight driven water splitting devices, i.e., with photoelectrochemical (PEC) or photocatalytic (PC) devices.156–159 This technology family has the benefit of integrating in one device (with many possible configurations depending on the materials adopted) the photocurrent generation and the water electrolysis. While the technology is the natural evolution of a PV-electrolyser system (PV-E) and on the long run it could potentially offer lower costs and higher efficiency, the technology is far from becoming competitive with the other production routes, and will not play a significant role in the near- and mid-term hydrogen economy.160 We therefore do not discuss PEC or PC in this work, but redirect interested readers to specific publications.156–159,161
3.3. Low carbon hydrogen production from fossil fuels
As highlighted in Section 3.1, around 76% of the 70 Mt per year of today's H2 demand is produced from natural gas, with the remainder coming mainly from coal. The dependency on fossil fuels also means that significant CO2 emissions are associated with current hydrogen production, totalling about 830 MtCO2 per year.126 However, several solutions exist where CO2 from the process is not emitted but captured – from the syngas (typically prior to H2 purification) and/or flue gases – and safely stored in a geologic formation. Depending on the capture technology selected, CO2 capture (and subsequent storage) can make hydrogen production from fossil fuels close to CO2 neutral. We believe low carbon H2 production from natural gas will play an important role in the establishment of the hydrogen economy and in its consolidation, i.e., in the near (2030) and midterm future (2050).1 However, we expect the fossil-route to disappear in the long run, as fossil fuels will (have to) be fully phased out. It remains however difficult to predict when this will happen, as it will depend on a combination of several factors, among others the scale-up (and cost) of green H2 production.
An overview of the standard route for H2 production with CO2 capture is illustrated in option 1 in Fig. 9: the syngas generation section consists of a syngas production unit (e.g., reforming, partial oxidation, gasification) and of a syngas shift unit, where CO is further converted to H2; the resulting syngas undergoes different separations, where eventually high purity CO2 and H2 are produced. Several solutions exist where CO2 from the process is not emitted, but captured – from the syngas, typically prior H2 separation, and/or flue gases – and safely stored underground.162 A detailed discussion on the variety of specific processes and technologies that can be currently adopted is out-of-scope here, and can be found with great detail in different literature sources, e.g., the Ullmann Encyclopedia126,162 and Perry's Chemical Engineers Handbook163 and several review works on hydrogen production from fossil fuels.100,164,165 It is worth stressing that all technological components of the state-of-the-art route are mature and are offered commercially, at scale, and with guarantees by several engineering companies; this shall not be surprising because they are all present in UREA/methanol plants. Note that the cost of hydrogen increases when CCS is added; for example, IEA estimates an increase from 1.7 to 2.3 € per kg H2 in Europe, from 1.0 € per kg H2 to 1.5 € per kg H2 in the US, and from 1.8 € per kg H2 to 2.4 € per kg H2 in China (considering existing technologies).126 Equally important, the blue hydrogen production technology can already be deployed at scale to match a steep increase in hydrogen demand. Therefore, it is worth stressing that virtual CO2 neutral hydrogen from fossil fuels is already a viable possibility today, provided the CH4 emissions along the value chain are low.138
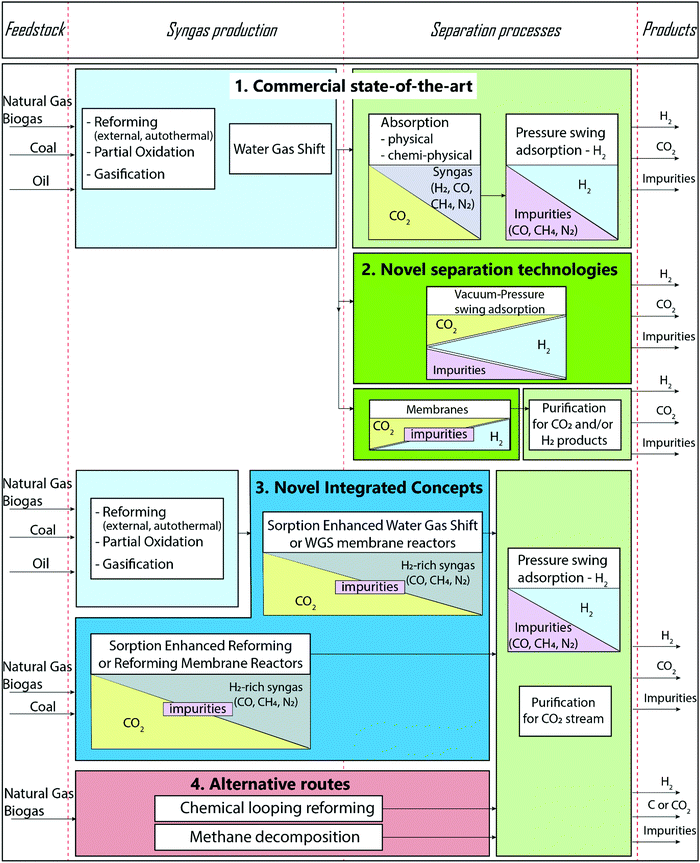 |
| Fig. 9 Hydrogen production from fossil fuels with carbon capture and storage, with highlight on current and future pathways relevant for CO2-neutrality in 2050. | |
Given that the blue hydrogen route will remain an important player for reasonably 30+ years, the technology will undergo further development and improvements. These should aim to reduce the (energy) cost of producing very low carbon merchant hydrogen, to maximise resource use and economic efficiency. Additionally, development should aim to move towards 100% capture of plant-wide CO2 emissions. However, this also means that many lines of current research will not find their way to full implementation as the transition to green hydrogen may happen before that. It is therefore important to understand how the technology can and needs to evolve in time to deliver cost- and environmentally optimal hydrogen while efficiently using R&D resources, especially time and budget. Fig. 9 provides an overview of four pathways to improve low carbon hydrogen production from fossil fuels, starting from improvement of today's state-of-the-art. These technologies are not representative of the whole landscape of research and development for clean hydrogen production from fossil fuels, but are those that we believe key for H2 deployment for a net-zero society by 2050. Whether or not all these four pathways will be deployed along the course of this century depends on a great number of variables, not in the least on how quickly electrolysis-based hydrogen production will develop and scale up.
Improvements in the state-of-the-art route.
Existing hydrogen production processes will further advance by employing new materials, novel reactor configurations, or better process integration. For the syngas production units, efforts need to be focused on the development of better integrated reformers, for example reusing high temperature gas from the autothermal reformer (ATR) to heat up a gas heated reformer (GHR).166,167 Moreover, they should allow for increasing the hydrogen production scale, thus overcoming current limits of SMR, which are too complex to scale-up further in a cost-effective manner. This means that for the scale of hydrogen production foreseen, we expect to see a move from classical steam methane reforming to autothermal and gas heated reforming, and/or partial oxidation. When looking at the syngas separation section, novel solvents and advanced process configurations for CO2 separation have the potential to decrease the energy consumption and the capital cost of CO2 capture. Moreover, one further critical development is that the CO2 removal from the hydrogen production plant should approach 100%. This is possible already with today's CO2 capture technology168–170 but requires the process to be designed such that all sources of CO2 emissions in the hydrogen plant are addressed, e.g., including furnaces and heaters.
Development of novel separation processes.
Two concepts alternative to liquid scrubbing are particularly promising: (i) the use of adsorption cycles at ambient temperature to carry out the CO2 and H2 separations in one single process using a vacuum pressure swing adsorption (VPSA) cycle,171–173 and (ii) the use of membranes to separate hydrogen from a pressurized syngas with low energy consumption. The former is of particular interest for small to medium size applications and for retrofitting existing PSA; future R&D efforts should focus on demonstrating the technology at relevant scale and in real industrial conditions. For the latter, efforts must be focused on developing better material frameworks that possess the right combination of selectivity and permeability under practical conditions, but also be easy to manufacture defect-free on a porous support with enough mechanical strength.174,175
Development of novel integrated concepts.
These routes exploit Le Chatelier's principle: by applying a change in the product concentrations, a more favourable chemical equilibrium is established. This can be achieved via either immobilizing one of the products in a solid phase, or via continuous removal of one of the products (see Fig. 1 in ESI†). At present, the sorption-enhanced water-gas shift (SEWGS) concept is the closest to commercialization (TRL 7) among the different integrated concepts.176–179 Another concept that is attracting significant interest thanks to the process intensification potential is the sorption enhanced reforming (SER), where CO2 is removed as the methane reforming takes place.180–182 Finally, intensified hydrogen production routes include membrane reactors, where membranes can be integrated in the reforming or in the water-gas-shift step.25,183–186 These technologies are further discussed in the ESI.†
Development of alternative H2 production routes.
In addition to the previous developments, interesting concepts are being investigated for compact and efficient production of hydrogen. These include chemical-looping reforming (and all associated configurations) and thermal methane decomposition. Hydrogen production with chemical looping offers potential for high energy efficiency and for a compact system, possibly integrating the CO2 separation.187,188 Different challenges remain to be addressed for industrial deployment though, which are specific to the reactor configuration and reaction pathway, and include erosion of the reactor by the oxygen carrier, solid–solid heat integration, carbon deposition, and mechanical stability of the oxygen carrier. Accordingly, we see these technologies as important improvements if large-scale production of low carbon hydrogen from fossil fuels is still needed in the second half of this century, but do not expect them to contribute significantly before.
3.4. Hydrogen production from biogenic sources
Hydrogen production from biogenic sources is of particular interest for future hydrogen production, as biomass is a renewable feedstock and when the biomass to hydrogen value chain is managed well, it can approach net-zero GHG emissions or, if the CO2 produced during the hydrogen manufacturing is captured and permanently stored, biohydrogen may be a means to reach net-negative CO2 emissions.4,5 Biogenic hydrogen production mostly involves the gasification of biomass, and possibly the reforming of biogas or biomethane.189 Although biomass gasification has advanced substantially, and some (fluidized bed) gasifiers are operating at higher TRL (TRL 7–8), the development of large-scale equipment is also characterised by technical setbacks (e.g., the German Choren entrained flow gasifier, that failed in 2009 because commissioning took longer than its investors could bear¶). Furthermore, sufficient biomass availability for bioenergy production is far from guaranteed, briefly discussed in Section 3.4.3, and the amount that is available might well be directed to energy outlets other than hydrogen.
3.4.1. Hydrogen production routes via biomass gasification.
Biomass gasification is the key step for production of hydrogen from biomass. It is a thermochemical process commencing at elevated temperature and sometimes elevated pressure, breaking down the cellulose, hemicellulose and lignin compounds that are the building blocks of biomass, into a product gas, mainly consisting of carbon monoxide, hydrogen and water, but also methane and other hydrocarbons can be produced, including tars and char.189 Biomass gasification involves several overlapping processes, including drying, pyrolysis (the conversion of solid biomass into chars, liquids, and gases), partial oxidation, and reforming (the conversion of hydrocarbons to syngas).146
Biomass gasification is a widely studied process including many different biomass sources as feedstock, as well as reactor types and reactor conditions.145,146,189,190 A typical division of biomass gasification processes can be in terms of temperature, distinguishing between high-temperature processes operating over 1300 °C and low-temperature processes operating roughly between 700 and 1000 °C.145 The high temperature route typically uses oxygen blown entrained flow gasifiers that produce a very pure hydrogen stream free from tars but at the expense of comparatively lower biomass to hydrogen efficiencies and low fuel flexibility (requiring a very well defined biomass feed). In the 2000's and early 2010's there was much enthusiasm for high temperature wood gasification, but especially the very high costs of upscaling (not of the final commercial size technology) have proven a large hurdle and there are few activities currently. Low temperature biomass gasification in fluidized bed gasifiers has progressed more and larger plants have been built, including the 40 MWth Silvagas plant in the USA and the 32 MWth GoBiGas plant in Sweden with commercial units being sold by market parties,191 although these plants are still an order of magnitude smaller than what might be expected of commercial-scale hydrogen plants and the question is if the fluidised beds can be scaled enough to truly benefit from economies of scale. Another large drawback of the low temperature route is the production of tars which have to be removed or cracked, the latter also requiring high temperature catalytic conversion. There are also other concepts emerging that either try to leverage the benefits of low-temperature and high-temperature, such as the transport integrated gasification (TRIG)192,193 and biomass chemical and/or calcium looping.194 For more in-depth discussions of novel biomass gasification technologies, the reader is referred to the supplementary information and to dedicated reviews.146,189,190,195
3.4.2. Hydrogen production routes via biogas reforming.
Besides natural gas, biogas and biomethane can also be fed into steam or autothermal reformers,196 without significantly changing performance – a slightly lower efficiency is observed due to absence of higher hydrocarbons, but this also removes the need for a pre-reforming step. Raw biogas can be cleaned of CO2 and fed as biomethane, but could also be fed to a reformer directly, i.e., without upgrading,197,198 allowing for additional CO2 capture and storage, increasing the potential for net CO2 removal from the atmosphere. The issue with direct feeding of biogas is therefore not so much of a technical, but rather of a logistic nature: as biogas production is very decentralised, the volumes are usually very small. The largest producers are typically wastewater treatment plants that produce biogas at the single MW scale. Moreover, raw biogas cannot be fed into the natural gas pipe network, because of the higher density of CO2 and the risk of the carbon dioxide creating ‘heavy pockets’ in the pipes. Biohydrogen production from raw biogas will necessarily be limited to small scale. If a larger scale is foreseen, then the suggested option is to locally remove the CO2 from the biogas, and feed biomethane to the natural gas network, while the reforming plants are ensured to buy biomethane, e.g., through a certificate of origin (like current renewable-electricity contracts for electricity users).
3.4.3. Cost of hydrogen production from biomass.
There is large uncertainty on the cost of hydrogen production from biomass with CCS, as few studies have been published, while more studies can be found for hydrogen from biomass without CCS. The work from the Sustainable Gas Institute (SGI) summarises some of these studies and complements this with their own estimates.131,136 Ecofys,133 the IEAGHG135 and Bauer et al.,134 recently also presented their estimates, where the former two do in fact include CCS. The SGI presents values for biomass gasification without CCS in the range of 1.4 to 3 US$2016 per kg H2 and with CCS in between 1.4 and 3.6 US$2016 per kg H2, on par with NG-based H2 production with CCS, but do report that these costs are probably underestimated, as biomass gasification is currently too far from commercialisation to get to grips with real potential costs. This statement is underpinned by the Ecofys, IEAGHG and Bauer studies, which place biomass-based hydrogen without CCS (respectively 3.4–5.1, 5.6 and 2.5–6.7 US$2016 per kg H2) and with CCS (respectively 3.9–7, and 6.1–6.3 US$2016 per kg H2) at substantially higher values than NG-based hydrogen. The IEAGHG study stresses that only very recently, some high-level costs have become available of operating small-scale biofuels plants, highlighting indeed the uncertainty of the above numbers. Costs for biohydrogen may fall once deployed at large-scale due to learning by doing and economies of scale, but for large costs reductions, the high-temperature, high-pressure technology (or the TRIG technology) should become available, which is currently still far away, as discussed in Section 3.4.1. Furthermore, reported costs of hydrogen from biomethane range from 1.4 to 16.7,131,134 a very large spread indeed, mostly driven by different assumptions on the cost of anaerobic digestion; costs will most likely be higher than the solid-biomass gasification route. In short, this brief discussion implies that bio-based hydrogen will likely have a cost disadvantage towards NG-based hydrogen, at least in the foreseeable future, with large uncertainty on how it may develop towards the middle of the century.
3.4.4. Availability of biomass as limitation for biohydrogen production.
Possibly the key limiting factor for biomass-based hydrogen may be the availability of biomass for bioenergy. Bioenergy competes with food, feed, and nature conservation and/or other ecosystem services, and the remaining biomass potential for hydrogen may be limited. There seems to be a consensus that these other biomass uses should be prioritised over bioenergy, although some argue199 that feed for cattle should be considered less relevant, as the world should move towards a more plant-based diet and would need biomass for energy. Furthermore, the future availability depends on the evolution of a multitude of social, political and economic factors including land tenure and regulation, trade and technology.200,201
Fig. 10 provides an overview of global bioenergy potentials quoted in four studies.202–205 The estimates for 2050 differ, ranging from about 45 EJ per year to around 300 EJ per year, where Fuss et al.205 quote very optimistic estimates that go as high as 1548 EJ per year, but the latter also includes large-scale algae production for bioenergy and uses very technology-optimistic assumptions. Jones and Albanito201 argue that the different estimates of biomass availability derive from differing use of the same FAO (the UN Food and Agriculture Organisation) data and the use of different spatial and temporal factors, affecting biomass production potential. The more conservative estimates assume bioenergy crops only (or mostly) on so-called marginal or degraded land, while completely safeguarding currently existing natural parks (forests, wetlands, etcetera) and thereby have stronger emphasis on ecological and biophysical concerns and natural limits. When productive land is also included, minimum potentials increase to 130–160 EJ per year and maxima of 216 and 267 EJ per year. The optimistic estimates quoted in Fuss205 start at 350 EJ per year and range to 1548 EJ per year, but these come from economic optimisation models that assume significant technological change to improve yields, and the aforementioned inclusion of algae. Note that the 2010 production of biomass for bioenergy was approximately 50 EJ per year,203 so although there is some increase to be expected, we believe it more realistic to count on a doubling or trebling over the next decades, rather than a major increase that might support the very large volumes of biohydrogen needed in a net-zero-emissions world.
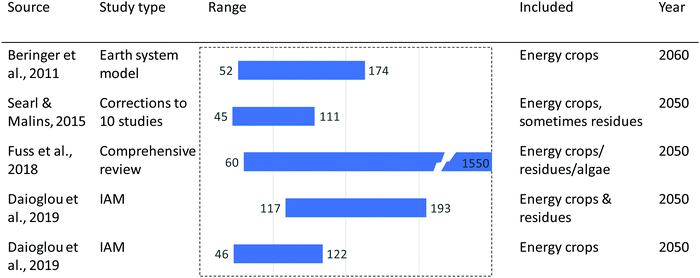 |
| Fig. 10 Concise overview of biomass potentials as reported in the academic literature.202–204 All sources report the so-called sustainable potential, minding the conservation of forests, wetlands and so on, as well as sufficient space for food and feed production. Estimates include energy crops and, where indicated, biomass waste/residues; the studies quoted by Fuss et al. with the highest estimates include large-scale algae production and assume aggressive technology and agricultural production improvements. | |
3.5. Effects of hydrogen impurities on CO2 storage
Finally, as noted in Sections 3.3 and 3.4, clean hydrogen production from fossil fuels or biomass produces a CO2 stream which, if not utilised in a chemical process, must be transported and stored in a suitable geological sink. The technology required to achieve this is well developed and already implemented in a small but growing number of CCS projects. The technical issues that arise when CCS is applied to hydrogen production are mainly associated with the presence of H2 impurity in the CO2 stream directed to transport and geological storage. A certain level of H2 impurity in the CO2 stream is the inevitable consequence of imperfect separation processes and high net marginal cost associated with improving CO2 purity. The actual level of H2 impurity in the CO2 stream will depend upon the exact processes used to produce and separate the H2, as constrained by technical, economic and environmental factors, and upon commercial agreements between the parties operating H2 production and CO2 transport and storage. A working assumption is that the CO2 stream may contain up to 2 mol% H2, and the question to be addressed what influence this has on the design and operation of transport and storage processes.
To obtain adequate pipeline capacity, long-distance CO2 transportation will operate at pressures above the CO2 critical pressure of 7.4 MPa, such that the stream is in a liquid or dense super-critical state (‘dense phase’), depending upon temperature in relation to the CO2 critical temperature of 304 K. In a long pipeline, pressure drops are such that compressor stations are required periodically to boost the pressure and maintain the desired dense single-phase state. In this context, the influence of impurities is seen mainly in the phase behaviour of the CO2 stream and, to a lesser extent, in phase properties, such as density and viscosity, that affect pipeline capacity and pressure drops. Fig. 11 shows the phase envelope for the mixture (0.98 CO2 + 0.02 H2) in comparison with both the vapour–pressure curve of pure CO2 and the phase envelope of (0.98 CO2 + 0.02 N2).206 It can be seen that H2 as an impurity has a much greater influence than N2 at the same level. This is an important factor to consider when determining pipeline entry pressure and the location and specification of compressor stations. It has also been found that H2 impurity can substantially reduce the density at temperatures in the vicinity of the CO2 critical point207 and this must be accounted for in determining pipeline capacity and compressor duty.208 Seevam et al.,209 have simulated the effects of impurities on the pressure drop and temperature profiles along pipelines. For one scenario involving a 3 mol% H2 impurity, the maximum distance between compressor stations was found to decrease from 300 km to 105 km. In the techno-economic study of Skaugen et al.,210 it was found that 5 mol% air impurity increased the overall cost of CO2 transportation over 500 km by about €2.3 per tonne of CO2 when the pipeline was optimally designed. Larger cost increases were found when repurposing existing pipelines that were optimised for transportation of pure CO2. The influence of impurities of re-liquefaction during cryogenic ship transportation has also been studied.211 H2 and other impurities in the CO2 also affect pipeline safety. The increased bubble-point pressure is a main parameter in the assessment of running-ductile fracture and leads to stricter requirements on the pipe wall thickness or material toughness.212
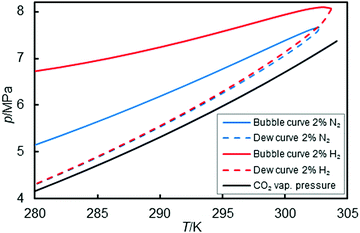 |
| Fig. 11 Dew and bubble curves of impure CO2 in comparison with the vapour–pressure curve of pure CO2. Equation-of-state model fitted to experimental data, adapted from ref. 206. | |
Geological storage of CO2 can be regarded as a well-established technology supported by strong scientific understanding of the fundamental processes of structural, residual and solubility trapping.213 The greatest available storage capacity is found in saline aquifers, followed by depleted oil and gas fields, and these sinks provide more than sufficient capacity for large-scale deployment of geological carbon storage.214 The presence of low-level H2 impurity will most likely have little immediate effect but there is evidence that, over the long term, some negative artefacts can arise. It is known that H2 is much less soluble than CO2 in connate waters215 meaning that, over time, the non-aqueous phase may become enriched in H2. This will lead to changes in physical properties (for example, an increase in the interfacial tension against brine) but such changes are not expected to influence storage capacity significantly. However, there is some evidence that subsurface microbial communities may be able to utilise H2,216 possibly leading to biofilm growth and reductions in the reservoir permeability and injectivity. To date there is a lack of definitive evidence concerning this risk.
4. Hydrogen transport and storage networks
4.1. Introduction
80% of H2 in Europe is produced in sites that are categorised as either “captive” or “merchant” H2 production, and where H2 is generated as a by-product and consumed in the same location.217 This has meant that H2 networks have been limited in size and scope, with technologies such as pipelines only spanning across 10 s of km in a region, serving a single user or multiple users in industrial clusters. Altogether, H2 pipelines cover approximately 5000 km in length across the world;218 this is expected to increase drastically with the development of infrastructure over the coming decades. For example, the European Hydrogen Backbone intends to extend the H2 infrastructure to create a pan-European network, with a length of approximately 40
000 km by 2040. Moreover, H2 transport and storage networks facilitate the integration between suppliers and consumers in both domestic and international markets, thereby expanding the hydrogen economy.11
At the system-level, the most appropriate type of network infrastructure to deploy in each country depends on the extent to which H2 is expected to penetrate the energy system both locally and internationally. Nations may have a competitive advantage in large-scale production of low-carbon H2 if they have access to low-cost CO2 storage, natural gas, biomass, or renewable electricity (see Section 3). Such geographical advantages may enable these nations to ramp up their production capacity cost-effectively to serve the growing market whilst creating additional jobs and stimulating the growth of their economy.219 However, these advantages can only be realised as economic benefits and strategic opportunities through coordinated policy development and regulatory alignment on network infrastructure (Sections 5 and 6).
This section critically discusses the technical feasibility of H2 networks, given their importance in connecting H2 suppliers with storage providers and end-users during system expansion. In this instance, the term “H2 networks” is assumed to include both pipeline and non-pipeline transport along with H2 storage infrastructure.
The current lack of a large-scale H2 network infrastructure may represent a barrier to widespread H2 deployment.220 Blending H2 into existing natural gas grids with up to 20% by volume can help to overcome this barrier initially to enable the scale-up of domestic H2 production, albeit with an emissions reduction of only 7%.221 This is made possible as existing gas-based transport and end-use technologies can operate with such blends of H2.222,223 Blending removes the need for ancillary investments in dedicated H2 transport and storage infrastructure and provides an offtaker for H2 use, until the scale is reached where dedicated infrastructure is necessary. However, additional costs may be incurred due to modifications to the existing pipeline integrity management systems, but this is expected to be lower than the cost of new transport infrastructure.224 Blending provides an important benefit to the system in the near-term: scale-up of low-carbon H2 production using existing infrastructure whilst increasing public acceptance.225 This can support the rollout of production technologies and induce cost reductions from technology learning, and a falling cost of H2 supply enables nascent markets to develop, thereby reducing the level of financial support required for commercialisation of subsequent investments.226
The overall cost of H2 supply can be reduced through economies of scale effects, especially through increases in production capacity from modular construction.227 Concurrent with blending, industrial fuel switching using pure H2 may present a unique opportunity to scale-up production through clusters of end-users.228 Regional clusters of high demand enable the deployment of larger production modules and reduce the initial need for investment in large-scale transport infrastructure.125 Additionally, the use of H2 in industry can serve as an anchor for investments in CCS infrastructure, especially as industrial demands are largely time-invariant, necessitating a steady supply of fuel.229
In particular, low-carbon H2 production through methane reforming coupled with CCS is cleaner (approximately 3 kg CO2-eq per kg H2)230 and more economical compared to water electrolysis when the carbon intensity of the electricity supply is high.231 Reforming-based H2 production can operate without supply-side interruptions, thereby eliminating the need for grid-scale H2 storage, whilst delivering scalable emissions reduction. In contrast, renewable H2 production will require access to large-scale storage infrastructure, which could complicate the design of the corresponding H2 supply chains, as it requires defining the optimal operation of additional components. For example, the H21 North of England study found that a cost-effective offshore wind-based electrolysis system requires 19.5 TW h of H2 storage to supply an annual average heating demand of 74.5 TW h per year in contrast to 8.05 TW h for methane reforming with CCS.57
Co-Locating natural gas-based H2 production in industrial clusters is also beneficial as the total system cost of CO2 transport and storage can be reduced through broader integration with CCS networks. This is being explored in more detail in projects such as Porthos|| in the Port of Rotterdam, Netherlands. The optimal design of such CCS networks, as well as the location of natural gas-based H2 production, depend on the availability of CO2 storage and on the nature of the adopted capture and transport technologies.231 Oversizing initial investments in both H2 and CO2 transport and storage are likely to reduce the overall cost of CO2 avoidance for the industrial sector, as CCS networks can abate industrial process emissions unrelated to fuel switching (e.g., emissions from the iron & steel sector).232
A greater degree of H2 deployment across power, transport, and buildings sectors would, however, require substantial investments in large-scale transport and storage infrastructure, especially for domestic consumers.125 Key factors, such as the scale of H2 demand and the transport distance, influence the selection of the most suitable transport technologies (Table 7).126 New, dedicated H2 infrastructure may be integrated with repurposed gas networks to support the market development for H2 and in such cases, regulatory alignment will be especially important.233 Transportation technologies such as pipelines, road tankers, and ships can transport H2 and offer different services in a mature H2 economy.
The North of Europe is currently responsible for 60% of the region's demand for hydrogen, whilst also hosting the largest industrial ports in Europe.234 There is a vast potential for renewable energy in the North Sea to support Europe's ambitions for 40 GW of low-carbon hydrogen production capacity by 2030.235 The global electrolyser capacity is dubbed to increase to 54 GW by 2030 through projects under construction and planning, with the potential to increase further to 91 GW by 2030 if projects in early planning phase were also accounted.1 The 54 GW figure is regionally disaggregated as follows: Europe (22 GW), Australia (21 GW), Latin America (5 GW), Middle East (3 GW), amongst others. Given these developments, Europe is likely to benefit from international trade with suppliers and regions that can produce low-cost hydrogen. This will ensure the development of a resilient hydrogen economy, which is less exposed to supply and demand shocks. Moreover, there is a well-developed natural gas pipeline infrastructure which connect ports in Europe with its industrial hubs; this infrastructure could be repurposed to support hydrogen delivery from outside of Europe.
Table 7 Transport and storage network considerations for different H2 supply technologies and end-use sectors in a net-zero compliant energy system
H2 supply technologies |
End-use sector |
Power |
Heat |
Transportation |
Industry |
Export market |
Considerations applying to renewable-based water electrolysis |
Storage infrastructure is important to balance variable power demands with a variable supply of H2. |
Storage infrastructure is needed to balance variable heat demands with a variable supply of H2. Linepack storage is needed for 100–1000 s of homes in addition to aboveground tank storage. Conversions at a larger scale will require access to cheaper, large-scale H2 storage such as geological caverns and more storage resources will be required relative to methane reforming with CCS or nuclear-based electrolysis. |
The transport network may involve pipelines or road tankers. If the purity level of H2 in the pipeline network is lower than the electrolyser output, then road tanker transport may be more suitable depending on the economic viability of that project. |
Large-scale storage infrastructure is necessary as the industrial demands are mostly time invariant, but the supply of renewables is intermittent. |
Ships are better suited for exports over longer distances, and the degree of purity needed may be decided based on standards, regulations, or negotiated contracts. |
|
|
|
Storage infrastructure is needed to meet the transportation demand and it may involve tank storage or geological caverns. |
|
|
Considerations applying to methane/biomethane reforming with CCS |
Storage infrastructure is needed to balance variable power demands and a steady supply of H2. |
Storage infrastructure is needed to balance variable heat demands and a steady supply of H2. Linepack storage may suffice for 100–1000 s of homes, along with aboveground tank storage. Conversions at a larger scale will require cheaper storage such as geological caverns. |
Storage infrastructure may be needed but it is dependent on the scale of H2 adoption in the transportation sector and it may be served by pipe storage. |
Large-scale storage infrastructure is unnecessary as the industrial demands are mostly time invariant, and they can be supplied steadily. |
Same as above |
|
Purification is unlikely to be necessary as it is directly combusted. |
Purification is unlikely to be necessary as it is directly combusted. |
Further purification is likely to be needed at the consumer location to make it suitable for application in FCEVs. |
Further purification is unlikely to be necessary for industrial applications. |
|
Considerations applying to all supply technologies |
Few transport network connections (likely pipelines) are needed as gas turbines are large, centralized, users of H2 (compared to, e.g., residential heating where many connections/pipes are required) |
The extent of the pipeline network depends on the number of households and buildings converted to H2. Smaller-scale distribution networks are suitable for 10–100 s of homes, and larger pipeline networks are necessary for conversions at a greater scale. |
The extent of the pipeline network depends on the number of H2 refueling stations operating across a given region. |
Few transport network connections (likely pipelines) are needed as furnaces, boilers and industrial processes are clustered users of H2. |
The type of transport infrastructure needed will be a function of the desired export distance as pipelines are suitable for distances in the 1000 km range, but ships are economical for longer distances. |
4.2. Key transport technologies
Road tankers and ships can transport liquefied H2 at ambient conditions, with a higher volumetric energy density compared to gaseous H2. But, liquefaction of H2 is energy-intensive and requires almost one-third of its embodied energy as fuel in comparison to 10% for natural gas.236 A liquid boil-off rate of 0.3–0.6% per day is not considered as significant for road tankers due to the short delivery time frames, spanning several hours or days.237 But it may be relevant for ships although the boil-offs can be used as a power source. The energy density of liquefied H2 (2.6 kW h l−1) is lower than fuels such as gasoline (9.1 kW h l−1) and LNG (6.9 kW h l−1), necessitating the usage of larger transport vessels.238–240 At very short distances, the transport of liquefied H2 through road tankers is more affordable compared to pipelines. This form of transport is especially relevant for areas with low demands and a distributed mode of production. In contrast, ships may be cheaper than pipelines for transport over very long distances (>5000 km), with an approximate cost of USD 3 per kg H2, rendering them suitable for international exports,126 but the export market may only emerge following the initial expansion of the domestic hydrogen market. Owing to its ability to transport large volumes of H2 over medium to long distances economically (0.11–0.21 EUR per kg for a transport distance of 1000 km),11 pipeline transportation may be appropriate for most applications.241
Pipelines are capital intensive with long operational lifetimes (30–50 years) and as such, revenue certainty is particularly important for securing investor confidence (see also Section 6). The potential to use repurposed natural gas networks to transport H2 is an attractive prospect as it eliminates the need for further capital investments to reach the consumers. In principle, both natural gas transmission and distribution infrastructure can be repurposed to transport H2 with adjustments to the pipe materials. High-strength steels, which are commonly used for natural gas transmission at high pressures,242 undergo embrittlement upon exposure to H2. Therefore, transport of H2 in high-pressure environments could increase the likelihood of pipe failure243,244 and should not be recommended until safe operating pressure ranges are established. Carbon steels of a low-strength grade have been used for H2 transport with few practical issues due to their increased resistance to H2 migration.245 However, the impact of cyclic loading on the fatigue strength of low-strength steels is uncertain and needs further investigation. Due to these uncertainties, the transmission of H2 in existing high-pressure gas pipelines is unlikely to be viable without modifications. Consequently, new H2 transmission infrastructure may be needed to support deployment and to meet the projected hydrogen demand across a range of end-use sectors as suggested by the Council of European Energy Regulators.233 This will be accompanied by the need to establish a commercial and regulatory framework which guides system expansion, whilst accounting for the evolution of networks from their initial use in industrial clusters to multiple end-use applications over time.
Unlike high-pressure gas transmission, the majority of the low-pressure distribution networks can transport H2 with only minor upgrades.246 Typically, the size of the low-pressure distribution network (218
000 km in the UK) is much larger than the high-pressure transmission network (19
600 km in the UK).57 Consequently, its conversion offers more value for the transportation of H2, greatly reducing costs for domestic end-use applications. Gas distribution pipes are often constructed from materials such as polyethylene or reinforced polymers, which eliminate concerns related to metallic corrosion and dust formation.247 However, the permeation rate for H2 was estimated to be 4–5 times greater than for methane in the polymer pipes used in the United States natural gas distribution system.224 Even though polyethylene pipes are more porous to H2 than natural gas, the total leakages amount to 0.001% of the transported volume.248 In contrast, leakages in steel and iron pipelines mainly occurs through the seals, threads and mechanical joints. Nonetheless, there is a three-fold greater leakage volume for H2 relative to natural gas.224 Overall, there are no significant technical barriers limiting the deployment of H2 transport infrastructure.
4.3. Storage infrastructure
H2 production using renewable electricity will reduce GHG emissions in the transition towards net-zero emissions. However, for renewable-based H2 supply, there may be periods of time where there is a mismatch between H2 production and consumption due to the spatio-temporal availability of renewable energy sources. Such supply gaps can occur on different time scales, from hourly to seasonal, and hence require access to storage infrastructure including both short- and long-term energy storage technologies.64 The former can be supplied using electricity storage, e.g., via batteries, which is a suitable option to store energy over periods lasting up to a month. The latter can be provided by different forms of hydrogen storage, namely medium-size, near-surface bulk storage249 and large-scale underground hydrogen storage.250 Furthermore, the storage infrastructure can reinforce the flexibility of the power and hydrogen transmission system, especially in instances where the supply of renewable energy may be distant from the consumption sites.251 The synergies between electricity and hydrogen storage can be exploited, namely high round-trip efficiency for the former, and low self-discharge losses and specific costs for the latter,** to reduce the overall costs of renewable-based H2 supply.64 Additionally, storage infrastructure can be combined with inflexible production processes such as conventional steam reformers to dispatch H2 at times of peak demand, thereby reducing an oversizing of production capacity.125
Medium-size, near-surface hydrogen storage can be provided by pipe storage (i.e., linepack capacity), which provides high operational flexibility†† but is less cost-competitive than underground hydrogen storage. The latter offers the required capacity and conditions to store hydrogen on seasonal time scales.250 Among the various stationary forms of hydrogen storage, salt caverns are considered most relevant, with operational units in the UK and the United States. The salt deposits are near-impermeable to high pressure gases and the saline environment prevents the onset of biochemical reactions which could otherwise consume the stored hydrogen. Furthermore, salt caverns can be operated with comparatively high injection and withdrawal rates depending on the geology, and are typically characterized by limited construction costs (estimated as approximately 350 EUR per MW h of H2 storage capacity),57 especially if they already exist in a location.252 Overall, salt caverns are well-suited for large-scale storage installations, where the required capacities are such that hydrogen storage becomes cost-competitive with electricity storage.64
The integration of hydrogen storage across different sectors is widely investigated in the scientific literature, though most studies focus on the role of hydrogen storage within the design of national energy systems. Some studies focus on 100% renewable energy systems while not considering the spatial resolution of the energy system, see, e.g., ref. 253, 254 and 255, whereas others consider the spatial and temporal variation of renewable energy generation, e.g., in Germany256–258 Italy,259 or the UK.260 Findings for Germany show that a H2 supply chain based on renewable electricity results in a hydrogen cost below 9.5 EUR per kg H2 (which is the current H2 price at German fuelling stations). However, this cost would increase by 25% if large-scale hydrogen storage in salt caverns is not available,258 highlighting the relevance of large-scale storage for the economic viability of hydrogen networks.
4.4. Cost-effective pipeline network design
Mathematical modelling tools can aid the development of new network configurations for large-scale decarbonisation, whilst explicitly accounting for the economic and environmental performance of the designed systems. The delivery requirement for H2, imposed by end-use applications across the various sectors of the economy, is a key determinant of the size of the network, its tortuosity, and its operating characteristics. On a higher heating value basis, H2 has a volumetric energy density of 12.7 MJ m−3 compared to 40.0 MJ m−3 for natural gas at similar temperature and pressure.261 Thus, H2 pipelines need to operate at higher flow rates to compensate for its lower volumetric energy density relative to natural gas. This is achievable through pipelines with larger diameters and/or pressure drops (approximately 20 bar over a distance of 80–100 km), albeit with greater material and compression costs. Alternatively, the installation of multiple parallel pipelines has the potential to reduce the need for higher operating pressures by reducing the material flow per individual pipe, thereby lowering compression costs at the expense of an increase in overall project CAPEX.262
Existing natural gas transmission networks use a range of pipeline diameters such as 600 mm, 900 mm, and 1200 mm with the larger pipe sizes used in regions with comparatively higher demands. The H21 project in the UK has proposed for the installation of a new H2 transmission infrastructure with 1200 mm pipelines covering over 75% of the network routes in the North of England region.57 An extension of the analysis by Sunny et al.,125 shows that the peak demand for the heating sector can be met economically using an H2 supply at comparable pressures to natural gas transmission as depicted in Fig. 12. In this instance, larger pipelines with diameters of 1050–1200 mm are deployed to a greater extent across the network for H2 (87%) compared to existing natural gas networks (29%). Thereby, indicating the economic value from increasing pipe diameters instead of building multiple pipelines in parallel. A lower-pressure H2 transmission network of greater length can integrate distributed modes of production whereas higher-pressure networks are more cost effective for large centralised production of H2. Importantly, systems analysis can identify robust network designs through an explicit assessment of biomass, H2, electricity and CO2 infrastructure in a region.
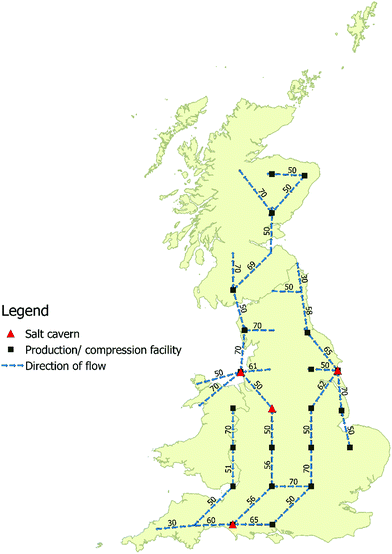 |
| Fig. 12 Transmission of H2 to supply peak heat demands for Great Britain based on Sunny et al.125 The direction of transmission is illustrated with the numeric labels representing the average operating pressure of the transmission line measured in bar. Salt caverns and production facilities dispense H2, and booster compressors increase the transmission pressures to satisfy periods of high demand. | |
4.5. Network safety considerations
The actual routing of pipeline infrastructure for any application not only depends on optimal economics, but also on the terrain, rights of way, location of the H2 production facilities, H2 or CO2 storage, etc. New H2 pipelines may follow similar routes to the natural gas transmission network because of the prevalence of existing connection points.54 The safety distance (minimum distance between pipelines and sensitive areas to reflect a minimal risk of impact) for H2 transmission will increase with an increase in the operating pressures and pipe sizes.263 Similar to the case of natural gas, pipelines can be buried underground to reduce the safety distance. The “NaturalHy” project has found that the failure frequency of existing distribution pipelines transporting up to 50% H2 by volume is comparable to that of natural gas.224 However, blend compositions above 20% of H2 did increase the severity of vented explosions, whilst marginally reducing the fire hazard. In particular, adding H2 to an existing pipeline increases the risk to an individual nearby to the pipeline, but decreases the extent of the hazardous region.224 Overall, the risk from leakage of H2 in transmission and distribution networks is comparable to that of natural gas, mainly as H2 is less dense and able to disperse rapidly upon release.57 However, as with any pipeline project, risk analysis should be performed on a case-by-case basis using prevailing risk assessment practices where this finding is subject to further investigation.57 Most importantly, practical concerns related to on-site fault repair and leakage prevention must be resolved before investment due to the greater risk for H2 relative to natural gas in the near vicinity of the pipeline. Notably, recent projects such as “H2HoWi” in Germany and H100 in the UK are developing the evidence base surrounding the use of pipelines for the supply of pure H2 for domestic applications.264
The overall safety of the transportation network can be ensured through robust mechanical design, material selection, use-specific hazard considerations, etcetera. H2 is characterised as a flammable gas within Category “E” of the European Standard pipeline design code BS-EN-14161:2011. The Published Document 8010-3:2009 provides guidance on the design and routing of steel pipelines on land to ensure minimal risks to the external environment from the transport of flammables. Nonetheless, new functional specifications must be developed for other pipelines such as polyethylene, incorporating design standards on operating characteristics and monitoring to ensure its safe and sustainable operation in the long-term. Overall, there are no major technical barriers to the deployment of H2 transport and storage infrastructure and their overall feasibility depends on societal acceptance and the rate of market expansion.
5. Legal and regulatory requirements for enabling the development of hydrogen infrastructures
To play their role in the transition to a low carbon energy system, low carbon energy carriers like hydrogen will depend among other elements on sound infrastructure regulations. While many industrial actors have expanded their views on hydrogen, legislators and regulators now need to decide how to develop an enabling and consistent legal framework for the gas infrastructure and for the gas market, balancing climate ambitions, flexibility, security of supply, cost effectiveness, safety, and fairness for final customers.
The gas market design (physical and financial) builds on some key regulatory principles. The question investigated here is to know whether such principles will need to be adapted and complemented by others in the context of energy system integration and decarbonised gas networks, and if so, how. The Gas Directive 2009/73/EC already envisaged the possibility of injecting other types of gas into the natural-gas networks system. Therefore, the same regulatory principles could be sufficient to integrate small volumes of hydrogen. However, in the context of higher shares of hydrogen, sector coupling and more decentralised production, the gas market design needs to be adapted. In 2021 the European Commission has started a two-step process for revising the relevant EU legislative framework as part of the European Green Deal implementation. First, as part of the so-called “fit for 55” package, the Commission has proposed a revision of the Renewable Energy Directive (EU) 2018/2001. Second, as part of the “Hydrogen and Gas markets Decarbonisation” package, the Commission has put forward a proposal for a more comprehensive revision (recast) of the Gas Market Directive265 and the Gas Regulation (EC) No 715/2009.266 The second package on gas markets contains the most relevant proposals for the purpose of gas market regulation.
The recommendation made by the present author is that the redesign of the gas market should be based on the following four key guiding principles, in order to enable a higher share of hydrogen within the energy system:267
• Integrated energy system planning as well as integrated governance tools (Section 5.1).
• Efficient and coordinated permitting procedures for infrastructure projects (Section 5.2).
• Transparent and enabling regime for access to the grid and for gas grid conversion (Section 5.2).
• Safe, effective and smart operation of transport networks and related infrastructures (Section 5.3).
Before developing each of these guiding principles, the question of the regulatory approach to be applied to a sector still at its early stage must be raised. Legal frameworks have an important enabling role, but they should also consider the state of development of the sector. For this reason, regulatory models existing in mature markets cannot be copied and duplicated to still emerging markets. This calls for a progressive regulatory approach, based on dynamic regulation.233 Good regulatory principles, including the ones described hereafter, should serve as guidance, and applied gradually, with possible temporary exemption as practiced under previous EU gas and electricity directives.
5.1. Integrated energy system planning, infrastructure development and governance tools
To enable the integration of hydrogen (and other gases with a low GHG footprint) into the energy market and the coupling of different sectors, it is necessary to think in terms of an “energy system” approach from the inception of energy policy, which means applying integrated energy system planning.
Integrated energy system planning.
Energy planning is the process of designing and implementing policies regarding the development of the energy system. Energy planning can be undertaken at a local, national, regional or even global level. A major and growing challenge for energy planning is to ensure consistency between different policy objectives and different planning levels. While energy modelling is often the basis of energy planning policies, the need to clarify processes, objectives and responsibilities through legal requirements arises rapidly. Historically, countries have developed their national energy planning framework differently, both in terms of legal bindingness (from a simple guidance document to a binding legal framework) and content (from few general objectives and targets to full programming law). Examples of comprehensive legal frameworks on energy planning are, however, rare. Recently, long-term energy planning has become a more common tool supported by dedicated legal requirements, which may contribute to spreading good practices and to harmonisation, at least within the EU.
At the EU level, Regulation (EU) 2018/1999 on the Governance of the Energy Union (the Governance Regulation) promotes integrated energy system planning as a new common tool for achieving the objectives of the Energy Union and the Union's 2030 Climate and Energy Framework. The Governance Regulation defines different instruments as part of the ‘governance mechanism’,268 two of which being the integrated national energy and climate plans (NECPs) and the long-term strategies (LTSs).268 The NECPs and the LTSs offer an opportunity to advance in the effort of integrated energy system planning. However, the current focus of the Governance Regulation is primarily on the formal integration of the processes for the elaboration of the energy and climate plans, and on the identification of interlinkages between the five dimensions of the Energy Union269 with a target compliance perspective rather than on the energy system integration. The requirements in terms of integrated energy system planning remain quite general and indirect, whilst they are not part of the assessment criteria for the elaboration and assessment of the NECPs and LTSs. An option for improvement would be to incorporate a requirement to systematically assess the potential for energy system integration, including for the different energy carriers/vectors such as H2 (and for the decarbonisation strategies, like CCUS).
Integrated grid planning.
To reach the envisaged volumes of hydrogen within the European energy system, it will be necessary to ensure enough capacity in transport infrastructures and to develop an appropriately designed infrastructure network connecting the relevant supply and demand points, as discussed in Section 4.4 above. As existing gas grids can be used to transport hydrogen blended with or as a substitute for natural gas, this raises the question of gas grid planning with an increased share of hydrogen.
The Gas Directive 2009/73/EC already allows for the possibility of transporting other types of gases than natural gas in the natural gas system, subject to compatibility and safety requirements and in accordance with the principle of non-discrimination. This entails that hydrogen will fall under the scope of application of the Gas Directive, under the conditions set by the latter. The Gas Directive establishes common rules for the transmission, distribution, supply, and storage of natural gas.270 Because hydrogen can be produced and used in diverse ways and is at the interface between the electricity and the gas systems, the legal treatment of hydrogen storage is still to be clarified. Already existing infrastructure planning procedures could be used to better integrate hydrogen. Hydrogen uses, sector coupling and repurposing opportunities for gas assets could be part of the National Development Plans (NDPs), the Ten-Year Development Plans (TYDPs) and the Gas Target Model.
Regulation of pure hydrogen networks.
Hydrogen transport through the natural gas network is clearly covered by the Gas Directive. However, transporting it through a pipeline dedicated to hydrogen, i.e., without any link to the natural gas network, seems to fall outside the scope of the Gas Directive, since the Directive is applicable to other types of gases ‘as far as such gases can technically and safely be injected into, and transported through, the natural gas system’.270 In the current state of the legislation, there are no EU law provisions specifically addressing a transportation system purely dedicated to hydrogen. This does not prevent developing hydrogen transportation pipeline infrastructures, but it certainly brings legal uncertainty and may lead to different national approaches. In the context of an integrated internal market for energy, including for hydrogen, a minimum harmonisation of hydrogen transport networks regulation at EU level is both necessary and proportionate, justifying EU intervention. Current shortcomings could be addressed either by making the current Gas Directive a ‘gases directive’ applicable to a broad range of gases, or by adopting a new legal act dedicated to hydrogen. The first approach seems to be the most appropriate to respect the current architecture of the EU energy legislation, and the upcoming revision of the Gas Directive provides an opportunity to do so. This also seems to be the approach taken by the European Commission in its proposal for revising the Gas Directive and Regulation.
5.2. Efficient and coordinated infrastructure permitting procedures (project level)
The complexity of existing permitting procedures for H2 (alone or in combination with CCS to deliver low-carbon H2) is a risk factor in the development of H2 projects. As of now, a number of separate permits must be obtained through the whole supply chain: permits for H2 production, grid connection and transmission, H2 storage licenses (if this is part of the infrastructure), emission permits, building permit under urban and land planning legislation, and safety and civil protection permits. Most of these permits require a preliminary environmental impact assessment and public consultation rounds. Parallel permitting procedures is a well-known risk of delays for such projects. It increases administrative and financial burdens for project developers. This is particularly true in cases of interrelated, overlapping activities which require permissions from several agencies responsible for separate elements of the supply chain. Some countries have developed good practices, but the permitting procedures are still cumbersome in most EU countries.
Simplification of the permitting process for H2 – and related activities such as CCS – could be achieved through conditional or unconditional permit exemptions for certain elements of the supply chain. Simplification could also be achieved through the creation of a single regulatory body (‘one-stop shop’ or single permit) to oversee permitting processes for the entire supply chain, or through mandated coordination between existing regulatory agencies responsible for permitting processes. There is a need to streamline permitting processes at both the national and the EU level to ensure that the various levels of consent are considered in a timely and cost-effective manner. As a positive example, EU cross-border energy infrastructure projects selected as Projects of Common Interest deal with a single national authority for obtaining permits and benefit from an accelerated permit granting process.271
A common requirement for permitting procedures, such as under the Renewable Energy Directive (EU) 2018/2001 (REDII), is that they are necessary, transparent and proportionate.272 This includes ensuring coordination between agencies, implementing concrete time limits for decisions (with sanctions for overruns), transparency with regard to process and cost, as well as the obligation to designate one-stop shops for authorisation, certification and licensing.272 It may be valuable for similar legislative requirements to be developed for the H2 supply chain, particularly in relation to the permits required for various sectoral applications of H2.
A final important consideration is the role of permitting processes in increasing public support and acceptance of H2 activities. Requirements for the inclusion of impact assessments, consultation processes and compensation mechanisms or ownership for local populations could ensure the feasibility of the project, particularly regarding supply chains involving H2 storage, recharging stations or large-scale H2 production trials, by offering a platform to communicate benefits and cement public support before construction. The respect and improvement of public consultation procedures is therefore fundamental for avoiding local opposition and related litigation risks. It may also result in additional benefits for both the project developer and local populations.
5.3. Access to gas grid and grid conversion
Access to the gas grid.
Although grid connections requirements exist, uncertainties around grid access conditions may remain, particularly for new types of energy generation capacity like hydrogen. Grid access is a significant factor for integrating hydrogen into the energy market and in determining the viability – including commercial – of new energy projects. To reduce this uncertainty, specific legislative measures have been introduced, specifically priority or guaranteed access and dispatch. Priority access to the grid provides assurance to connected energy generators that they will be able to sell and transmit their energy when the source is available. Guaranteed access ensures that all energy that is already sold (because integrated into the sport market) obtains access to the grid. Those two measures must therefore be seen as counter parts. They have already been used in the context of the Renewable Energy Directive 2009/28/EC (now repealed) for enabling the integration of electricity generation from renewable energy sources into the internal energy market.
Under existing rules, the EU allows States to grant mandatory priority or guaranteed access to the grid for renewable energy, indigenous fuels, and CHP-applications (combined with guaranteed transmission of electricity). This access can be granted provided operations are in accordance with the national electricity permitting scheme and the Electricity Directive. There is therefore precedent of allowing priority or guaranteed access to the grid for low-carbon energy sources. Importantly, the Energy Efficiency Directive (also under revision) states that priority access for CHP to the grid must not endanger renewable energy expansion. This tends to indicate that EU rules favour the deployment of renewable energy (including therefore renewable H2) over alternative low-carbon energy sources (such as fossil H2 with CCS), for the purpose of supporting primarily renewable energy sources. This call for a review of the grid access regime in the view of integrating larger volumes of low carbon hydrogen – including green hydrogen – into the energy market, as envisaged in the EU Hydrogen Strategy and most of the newly released national hydrogen strategies.
Third party access (TPA) regime and tariffs conditions.
TPA is commonly defined as the legally enforceable right of economically independent undertakings to access and use, under specific terms (regulated or negotiated), various energy network facilities owned by other companies.273 TPA is a fundamental regulatory instrument to address natural monopolies in network industries. In the current state of development of the hydrogen markets, a strict TPA regime seems disproportionate and may even refrain the development of hydrogen infrastructures. When hydrogen is blended with natural gas in natural gas networks, the TPA regime under the gas legislation will normally apply, but the legislative does not foresee yet a clear TPA regime for pure hydrogen networks. To that respect, the Hydrogen and decarbonised gas market package contains several concrete proposals for improvement, such as the application of a negotiated access until 2030, followed up by a regulated access after 2030. Tariffs conditions, including at injection points and for cross-border interconnections, are also made more precise.
Grid conversion.
The conversion of gas grids to hydrogen should be a priority measure in the integration of hydrogen into the energy market. It has a series of benefits, notably in terms of cost saving, local acceptance, and environmental protection, by avoiding the duplicating of transport infrastructures. This has been duly taken into account in the Hydrogen and decarbonised gas market package, but the extent to which gas grid operators investing in grid conversion will be allowed to recover their costs through e.g., tariffs, still need to be further clarified. Notably, the cross-subsidisation of H2 networks by natural gas network revenues could be allowed subject to conditions. Grid conversion operations should also be subject to safety requirements.
5.4. Operation of networks and related infrastructures
The deployment of hydrogen is at the same time benefiting from the existing regime for gas, as it is facing the constraints of that same regime. Operation of hydrogen networks and related installations or services – such as storage – are good examples of this. Certain transmission or distribution system operators may be interested and well placed for taking operator responsibility for hydrogen transport and storage infrastructure and commercial operations. However, they may be prevented to do so by unbundling rules. At the EU level, both the Electricity and Gas Directives define requirements for the unbundling of upstream/downstream ownership structures and functions. The objective of the unbundling regime is to foster competition on the energy market by separating the activities of energy generation and supply from the operation of transmission and distribution. There is currently a lack of legal clarity with regards to the grid operators’ unbundling requirements between commercial and grid activities regarding new activities such as hydrogen storage. Key to the deployment of hydrogen projects and the integration of hydrogen into the internal energy market will be to precise the legal qualification of hydrogen storage activities, including the applicability of the unbundling regime.
6. Business models for initial infrastructure development
6.1. Introduction
Although there are several technical challenges to the deployment and use of hydrogen that are widely acknowledged, investment barriers to delivery of first-of-a-kind large-scale hydrogen infrastructure in Europe lie in a combination of commercial, legal, financial and policy constraints. These barriers can be overcome, however, by government intervention if there is a real coherent strategic rationale for investment propositions along the value chain supported by society and implemented in collaborative business models with the private sector.
Previous chapters have highlighted that use of hydrogen from electrolysis and reformed methane as an energy carrier will require substantial effort and time to transform the existing gas system at scale. The urgency of the physical implementation of emissions reductions pathway to net zero in a period of less than 30 years means facilitating new and modified regulations (Chapter 5), new markets and market functioning, infrastructure (Chapter 4), and long-term planning in both the public and private sectors.274 While there is a clear societal objective to transition away from fossil fuels, business models and business cases for project and infrastructure investment during the transitioning of the energy system will need to build on a likely continuing interim role of natural gas.275
There are numerous definitions of business models in the literature but in simple terms, business models describe how a business or organisational entity creates, delivers, and captures value.276 In the context of deploying new or re-purposed large-scale infrastructure serving multiple sectors of the economy, a business model can be considered as the means to organise and structure all the relevant and material elements of investment, market development and asset operation that can deliver the combined objectives of public and private sector sponsoring parties.
This section explores how to create the conditions for successful investment in first-of-a-kind hydrogen infrastructure as well as CCS infrastructure where it is combined with hydrogen production and/or synergistic emissions reduction in industrial clusters. Selecting and implementing business models to achieve this goal is a complex exercise. Research conducted in two pan-European projects277,278 has led to the following conclusions which are discussed in more detail in the sub-sections below:
1. To remove investment barriers for both public and private stakeholders, the techniques of systems thinking need to be applied to business models so they can deliver optimal integrated outcomes for multiple economic/market sectors.
2. Metrics for assessing and deciding on projects and investments need to be broadened away from standard cost-benefit-analysis or technology cost reduction to include the greater value for society created by undertaking these investments.
3. Business models for first-of-a-kind investments should ensure three key outcomes can be met: initial revenue from medium to long term stable anchor users; both government and private stakeholders committed to invest with no or low regret; and flexibility and optionality provided for future evolution of markets, technology, and utilisation.
4. Initial market development for hydrogen end-use must be led primarily by government and/or public bodies with assistance from the private sector where it has specific expertise.
5. The selection of first-of-a-kind projects for government support should be made based on an integrated system view that uses an appropriate mix of collaboration and competition between stakeholders, projects, regions, or industrial clusters to ensure cost effectiveness and new barriers are not created for expansion and utilisation over time (cf. point 3).
6. Risk allocation and sharing between the public and private sectors based on an accurate assessment of which stakeholder is best placed to mitigate and manage the risk is essential to structuring collaborative business models. The risk sharing is dealt with through four principal business model components: assets and rights ownership, capital sourcing, market development, and physical delivery.
6.2. Shaping a business and investment rationale for hydrogen infrastructure
6.2.1. Systems thinking applied to business models.
Recent research on business models for large-scale hydrogen (and CCS) value chains has concluded that there is a need to differentiate between system or macroeconomic business models and business segment or micro-economic business models.277 System business models are the principal means for the mitigation of exogenous risks (including political, policy, social and outcome risks) across multiple integrated business activities and markets. These are risks which cannot in general be managed by the private sector alone. They require a macroeconomic solution that can overcome barriers to investment by both the public and private sectors into the various operational segments of a full hydrogen chain with or without CCS infrastructure. Operational business models assist in the mitigation of endogenous risks and therefore focus on the risks and delivery of the outputs and services for a particular business segment within the hydrogen/CCS chain.
Although operational business models are ‘components’ of the system business model, the system business model is greater than the sum of the parts. It is designed holistically ‘top-down’ so that barriers to investment are removed, end-use market development is facilitated, and segment businesses perform in a unified fashion to deliver the system objective.279 Infrastructure investment is quite distinctive and requires business models that can address its specific characteristics: requirement for major upfront capital investment, long term revenue streams, public involvement, natural monopolies, and complex value delivery (economic, social, environmental benefit in addition to financial returns).
Integrated energy system planning (Section 5.1.1) can provide a generic structure in which to develop policy and legal frameworks for hydrogen but enabling infrastructure investability also requires practical removal of barriers to the financing and physical delivery of objectives and outcomes. For example, unlike renewable electricity, which can simply enter mature electricity networks and markets, low/zero-carbon hydrogen requires substantial change in the end-use consumer markets and new or re-purposed infrastructure (possibly including CO2 transport and storage). Hence, the development and selection of sector- or project-specific business models becomes dependent on an over-arching system (integrated) business model that, at a minimum, should address the following:
a. System-level (economy-wide) strategic rationale and objectives.
b. Cross-sectoral synergies and sector coupling.
c. Development of ‘low-carbon’ end-use product and service markets.
d. Enduring system governance and oversight until markets are self-sustaining.
e. Public-private risk sharing reflecting system characteristics/properties.
f. Public-private collaboration and capacity/capability building.
g. Societal and social acceptance with removal of moral hazard.
h. Flexibility to select different low-regrets decarbonisation options over time.
6.2.2. Valuing societal benefits not only evaluating costs.
To date there has been a strong focus in European policies on cost reduction for hydrogen technologies (including production with CCS), and project and infrastructure investment. Also, energy system transformation pathways tend to be assessed from a traditional least cost perspective using sectoral levelized cost of energy metrics. However, in the context of achieving net zero emissions with hydrogen as one of the energy carriers, the key metrics need to be broadened to include the greater value for society realised by undertaking these investments. The system outcomes are not only about economic growth or value for money, but rather sustainable growth and a circular economy with minimal dependence on fossil fuels. Evaluation of policy, investment, and financing decisions which influence, and are influenced by, business models therefore require including:
a. The cost of pollution on health, environmental degradation, and long-term societal impact.
b. The economic multiplier effects of different activities and sectors.
c. The value created by flexibility and optionality for future technology deployments.
d. The facilitation of synergies between different energy consuming activities and economic sectors (this also includes the utility of CCS infrastructure within industrial regions).
e. The management of the decline in the fossil fuel industry at the same time as creating new opportunities for technology development and an alternative energy system.
These principles and metrics are among the practical aspects of implementing the European Green Deal ‘just transition’ mechanism280 through public-private collaborative business models.
6.2.3. First-of-a-kind investment – three key principles: ‘anchor’ users, low-regrets, and optionality.
Governments are still learning how to create a flexible decarbonisation pathway with emergent options that can develop over time and adapt to technology performance and cost improvements, societal preferences, and consumer demand. For example, hydrogen transport infrastructure will be needed for hydrogen markets whether production comes from electrolysis, methane reforming, or other means. Business models that facilitate large-scale implementation of hydrogen infrastructure irrespective of production technology, and which can deliver economies of scale along with supporting new and evolving markets, will help create low-regrets options for the future. ‘Low-regrets’ actions and investments are those that have zero to small future penalty (such as cost or performance) for changing between the different outcomes and options that they can possibly deliver.
The concept of sustained ‘anchor’ demand (e.g., low carbon industrial clusters or transport hubs) is an essential requirement to justify first investments with minimum regrets and maximum optionality. The choice of the ‘right’ strategic early infrastructure users will allow selection of business models and creation of business cases which can be supported by local communities, society and private investors. Key factors are:
a. Minimising complexity of implementation (e.g., number of users, regulatory changes, technical risks, skills development, and training).
b. Selecting market(s) where investment costs can be socialised (e.g., domestic heating).
c. Selecting government support mechanisms or levies that do not penalise intermediate or final use and do not disadvantage low-carbon products.
d. Minimising risk of stranded assets from volume and demand uncertainty.
6.2.4. Market development.
The overriding problem to solve with business models is how to develop (and to what extent) the various hydrogen markets, while ensuring low-regrets investments and optionality at decision points along a country's decarbonisation pathway. Market maturity, and who is responsible for market development where the market is immature or does not exist, dictates the capacity of the economic system to remunerate or create value for the public and private participants. Remuneration ranges from direct and/or indirect support from fully government-based revenue to fully market-based revenue (no support).
Many industrial companies operate in competitive international markets. Currently, there is insufficient premium (if any) and demand for low carbon ‘green’ products across Europe for companies to justify the additional investment costs and risks for fuel switching to hydrogen and/or carbon capture without government support and guarantees – even if the gas or CO2 infrastructure were available. Pro-active and managed end-use market development is therefore critical to create demand for new low carbon products and services based on hydrogen, and to transition from an early phase of government-supported infrastructure development to a sustainable market-driven expansion at a pace commensurate with a 2050 net zero target.
6.2.5. Collaboration versus competition.
An essential requirement of business models for investing in hydrogen infrastructure (also where it includes CCS) as well as initial end-use consumer markets, is the choice of collaboration versus competition between stakeholders, projects, regions, or industrial clusters. A healthy amount of collaboration is occurring between private sector companies, public utilities, research institutions and governments across Europe for innovation, feasibility, and concept definition projects. Nevertheless, questions remain as to how European governments, including the European Commission, will utilise a mix of collaboration and competition to support viability of the first infrastructure projects, including where they will be located and how they will be expanded and utilised over time.
Feedback from stakeholder meetings and workshops in two European research projects277,278 held in collaboration with the European Zero Emissions Platform (ZEP) highlighted that:
a. Each private sector party is generally only interested in their core business and expects other parties to deliver the other segments of the infrastructure.
b. Large-scale hydrogen-CCS infrastructure development will require multiple regional organisations to be involved to create low-regrets solutions for both industrial decarbonisation and hydrogen use such as domestic and commercial heating.
c. There is a need for inter-regional leadership, governance, cooperation, and cross-sectoral integration to develop a complete energy system decarbonisation framework.
6.3. Public-private collaboration: removing investment barriers and sharing risk
6.3.1. Risk allocation.
Risk allocation determines the attractiveness for equity, debt and government investors of a given project (acceptable rate of return, financeability, value-for-money), as well as the ability to remain viable through to the end of a long-term contract. In infrastructure projects risks are often mitigated by a combination of measures from both public and private sectors which may also change over time.
In contrast to the mature natural gas markets in Europe, deployment of the first hydrogen (and CCS) infrastructure and operations may require some bundling of business activities to remove investment barriers, provide market-making certainty for operators, handle policy and regulatory gaps, and generally reduce commercial risks, including counterparty risk. This would only be for a period sufficient to create a self-sustaining market demand. For example, the business of hydrogen retailing could be combined with the business of hydrogen distribution in a regional hydrogen conversion. This would remove commercial and engineering risks while enabling efficient management of the physical delivery such as matching customer appliance conversion with supply network operation. Already, the preferred business model for CO2 infrastructure in the UK is to combine transport and storage in one regulated business.281
This business optionality can also assist with managing interfaces between a hydrogen-CCS chain and other parts of the energy system that will influence delivery and scale of the two networks. For example, various possible business combinations within the hydrogen network will interact with the transport sector; and various business combinations within a CCS network will interact with industrial utilisation of CO2 and H2 as feedstock (see Chapter 4). Furthermore, there may be business and risk mitigation benefits from cross-ownership of different segments of the value chain to facilitate investment and operability. Such ownership structures have been used effectively in the international LNG industry.
Whilst the first large-scale infrastructure required to deploy hydrogen may benefit substantially from business segment bundling or cross-ownership, this would require business models that are in contravention of the EU Gas Market Directive 2009/73/EC and its amendments (see Chapter 5) with respect to unbundling energy suppliers from network operators. Hence, if regulations are not fit-for-purpose to deliver the first hydrogen infrastructure in a cost-effective and optimal manner, then carve-outs may be needed until such time as sufficient hydrogen and/or CO2 disposal market maturity materialises.
6.3.2. Business model structures.
The main driver for selecting a business model structure is the degree of transfer of responsibility and risks from the public sector to the private sector. There are many possible variations in the detail of these structures and they are also the subject of continuous innovation to adapt to the external investment environment, jurisdiction, and macro-economic conditions. In all cases, however, they can be categorised according to four main components of the transfer of responsibility: ownership, financing, market development (including revenue structure), and physical delivery including operational activities.
At one end of the spectrum, the public sector retains all responsibility for the ownership, financing, and all the physical activities as well as assuming all associated risks. Historically this was a very common structure for delivering public services such as gas and electricity, water, railways, bus networks and waste disposal. At the other end of the spectrum, the private sector carries all the risks and responsibility for the infrastructure assets and service, and there is no government involvement and support. This can work in the case of fully liberalised mature markets such as cable and mobile phone networks (Europe), wholesale gas supply (UK) and petrol retail (Europe). The private sector model is designed to ensure high levels of competition with high efficiencies and innovation with no financial burden on the government. In between, there are many types of arrangements where those responsibilities are split between the public and private sectors.
The nature of infrastructure investment with long-term capital requirements, value beyond traditional financial returns, and monopolistic nature therefore requires synergies and/or collaboration between the public and private sectors to overcome those challenges. These ‘collaborative’ structures are generally known as ‘public-private-partnerships’ or PPPs. The PPP approach282 is designed to overcome both the market failures in critical infrastructure investment from the private sector (e.g., market power) and public sector failures (e.g., inefficiencies and financing constraints). These business models allow the transfer of appropriate risks to private investors who can access significant pools of capital funds and achieve better operating efficiencies whilst retaining the necessary government control over pricing and infrastructure development resulting in added value for all stakeholders.
The preceding sections have emphasised the need for public and private sector collaboration to overcome investment barriers and business risks for the hydrogen value chain, both with and without CCS infrastructure. Of paramount importance is managing the risk associated with the immaturity of the markets for hydrogen use, ‘low-carbon’ consumer products, and CO2 disposal – all with significant uncertainty over the future demand prospects.
6.4. An example business model
In this section an example system business model (Fig. 13) is presented for the first phase of the UK H21 North of England regional hydrogen conversion roadmap.57,283 The first phase of this roadmap proposes delivering a hydrogen-CCS infrastructure network for domestic and commercial heating plus some possible industrial fuel switching or power generation across the northern regions of England in the period to 2034. The business model is based on collaboration between the government, and public and private sector institutions taking account of realistic risk sharing and strategic drivers consistent with the UK government's legal 2050 net zero emissions target. It offers one perspective for addressing the requirements presented in Section 6.2.
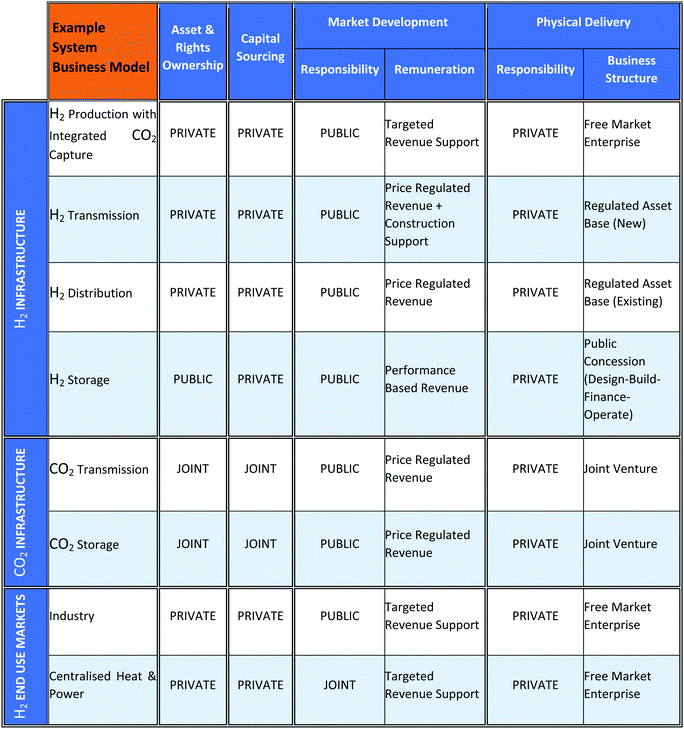 |
| Fig. 13 Example of H2-CCS system business model for the first phase of the UK H21 Roadmap with component segment business models. | |
This business model was developed with input from extensive stakeholder engagement that focussed on an assessment of the system-level investment barriers and risks, and the mitigation measures for the early phases of market creation and hydrogen-CCS infrastructure deployment in England. Hydrogen production is planned from centralised autothermal reforming of natural gas and integrated carbon capture.283
The H21 North of England roadmap was a private sector initiative and has been followed by a detailed UK Hydrogen Strategy published by the government's Department of Business, Energy, and Industrial Strategy in August 2021.284 The strategy includes an in-depth look at the types of issues presented in this Section 6, and what actions and policies the government can undertake to support the development of hydrogen infrastructure and markets. The strategy's objectives could be achieved with the H21 business model example provided here, although other variations are possible. The main observation to make is that this example H21 business model is not a theoretical one, but rather one feasible solution applicable to the UK government Hydrogen Strategy.
The following sub-sections summarise the four components of the H21 example system business model structure that were described in Section 6.3.2. They contain a brief description of the reasoning for allocating risks and responsibility in the sectoral business models in Fig. 13, and provide some high-level insights into how the public and private sectors could collaborate.
6.4.1. Asset and rights ownership.
Private ownership is envisaged for most sectors – this solution builds on the established ownership models in England for gas transmission, distribution and supply, gas processing and power generation but recognising that in the first phase of the H21 roadmap there will be little to no free-market competition other than for appliance conversions, and project supply chains such as engineering and construction.
A joint public-private ownership is suggested for the CO2 transport and storage (T&S) infrastructure to facilitate the management and delivery of this critical chain component for the overall outcome of system decarbonisation. At a minimum, government underwriting of uninsurable CO2 storage risks and assistance with the structuring of a storage financial security is essential to remove the investment barriers for the private sector. A joint venture can also allow government to steer infrastructure expansion, have full transparency over performance and pricing, ensure fair access to all users at the appropriate cost and collaboration with all the regions of the north of England. The government can also demonstrate its commitment to long term infrastructure use and net zero policy delivery to facilitate investor participation and capital sourcing from the private markets.
Until such time as end-use markets for hydrogen have reached a critical level of demand and become self-sustaining the investment in, and provision of, hydrogen storage services will be highly uncertain and risky. In this business model, government ownership of initial geological hydrogen storage facilities is proposed as a ‘public-good’ infrastructure with a public concession awarded to the private sector through a competitive bid process.
6.4.2. Capital sourcing.
Private capital sourcing is envisaged, though government intervention may be required for the CO2 transport and storage infrastructure and for the H2 production facility, as necessary to support private capital sourcing and reduce investors’ and banks’ financial exposure.
6.4.3. Market development.
In all markets, the government would be responsible for the market development of both hydrogen and CO2 through the implementation of several government interventions via policy, regulatory, fiscal, and financial means.
1. Hydrogen infrastructure remuneration.
i. Hydrogen production in this business model follows a similar free enterprise model as with power generation supported by targeted revenue support in a form similar to a contract for difference with an entity such as the Low Carbon Contracts Company (LCCC),‡‡ with all the capital and operating and maintenance (O&M) costs of the facility covered initially. Because the period to 2034 is focussed on heating and fuel switching, the gate price of hydrogen would be the same or lower than that of natural gas. The business model could include some contractual flexibility for initial underperformance of the facility (especially the carbon capture component) and performance and availability-based incentives/penalties.
ii. Hydrogen transmission and distribution would use an analogous price regulated revenue model as currently in place for natural gas – possibly with some additional construction support for the new parts of the hydrogen transmission system. This approach can be taken because the pipeline network will be part of a well-defined and planned system conversion of domestic and commercial customers. Investment in low and medium pressure networks will include re-purposing of existing infrastructure assets.
2. CO2 infrastructure remuneration.
i. CO2Transport & Storage infrastructure would be structured through a public/private sector joint venture with price regulated revenue to cover the capital investment cost and O&M costs for the initial oversized capacity at an agreed rate of return. Government partial ownership would offer flexibility in the negotiation of risk/return with the co-investors and regulatory backed revenue would provide guarantees for the investors regarding the market demand uncertainty, i.e., infrastructure usage. Regulation would define the apportionment of the service charges between the initial hydrogen production facility and additional and future users.
3. Hydrogen end use markets.
i. Commercial and domestic customers would be serviced by free enterprise retail businesses for hydrogen supply that will be the same or similar to those currently existing for natural gas and electricity. In this business model example initial bundling of distribution and retail services is not required because of the prescribed regional conversion of the consumer market and the largely completed distribution network replacement programme (using polyethylene pipes – see Section 4.3). Retailers would be able to negotiate their own pricing arrangements with the hydrogen producer(s).
ii. Industrial decarbonisation through hydrogen fuel switching would be the responsibility of privately-owned industrial companies. The combination of hydrogen being offered at the same cost as natural gas, EU ETS (or similar UK ETS) pressures, branding and reputational value may be sufficient to justify the fuel switching capital expenditure. Additional support may be necessary in the form of capital grants for specific industries. This approach would allow ‘green’ low-carbon products to be competitively priced in consumer markets.
iii. Hydrogen (or blended) power generation would be structured on the traditional free enterprise business model already established in the power-generation sector. The investors in a new or refurbished facility would be remunerated using a targeted revenue support mechanism based on the existing Contract-for-Difference mechanism with LCCC and the capacity market to cover the additional CAPEX and O&M costs. Commissioning delays and technical issues related to the technology could be handled contractually, and contractual mechanisms could be defined to cover the risks of plant unavailability (or higher emission costs if natural gas is burnt) due to hydrogen unavailability.
6.4.4. Physical delivery.
Delivery of the infrastructure, facilities and services is allocated to private investors, and is consistent with the established models in the UK. The technologies proposed are relatively proven and there has been interest expressed in the ongoing sponsored projects supported by experience and expertise available in the private sector, including supply chains.
6.4.5. Summary.
The above example is a very cursory presentation of the full system business model for the H21 roadmap. Nevertheless, it demonstrates that, although there may be choices for selecting different mitigation measures when sharing the risks and responsibilities between public and private stakeholders, no individual sector or part of a new hydrogen value chain can be facilitated without taking account of other activities within the chain. Furthermore, the extent and type of government financial and revenue support for new activities or infrastructure is highly dependent on the sustainability of market development, which in turn depends on government policies and mechanisms for establishing end use consumer and industrial demand. It is only through taking a system perspective that these outcomes can be achieved.
7. Summary, key messages and outlook
In this paper, we have tried to provide the reader with our, hopefully balanced, perspective on the status of the hydrogen economy, and on what is needed to deploy the hydrogen economy as part of Europe's (but equally other regions’) drive towards net-zero-CO2 societies. From the above, we have tried to convey the message that the emergence of a full-fledged hydrogen economy will most certainly not happen by itself, but rather needs strong support and clever design, first and foremost from governments at multiple levels (regional, national, local) but also from many industry players, including the producers of base commodities like steel, electricity, and chemicals. Without this, we may see some bilateral production-consumption combinations, e.g., within an industrial cluster or at fuelling stations for bus fleets, but no large-scale rollout. Second, hydrogen will unlikely be the, or the only, energy vector of the future, but if it receives the integrated support we envision, it may become a pivotal component of future energy systems, especially with respect to sector coupling. We will here briefly summarize the key messages and give an outlook for further work.
Hydrogen end-use technologies and applications
The first key message on end-use technologies is that commercial technologies exist in most of the envisioned end-use applications: hydrogen fuel cells, gas turbines, internal combustion engines and steel production technologies are all sold today by large and credible parties. This implies that large sectors of the economy can transition away from fossil fuels once sufficient hydrogen production becomes available. But, the hydrogen-fuelled equipment is not yet on par with incumbent fossil-fired equipment so using hydrogen would come at a penalty on, e.g., efficiency. Furthermore, when applied to different end-use applications, the same H2 conversion technology may need different technology features, which may conflict and hinder further development in the absence of established markets.
The outlook for hydrogen use is, however, positive. It can be expected that gas turbines designed to run on (almost) pure hydrogen will reach the market in the next decade, with performance that is on par with their natural gas-fired counterparts. At the same time, tailor-made, efficient hydrogen burners will be demonstrated for specific high temperature processes in industry (e.g., steelmaking, glassmaking). We also expect hydrogen fuel cells to develop quickly further, not only due to their uptake in transport applications, but also in industrial cogeneration and low temperature heating. This will trigger a positive reinforcing cycle supported by inherent learning by doing. For this to happen, it is important to proceed fast with large-scale demonstration of integrated hydrogen end-use systems (the technology implemented into its technical/commercial context) to identify and tackle end-user specific issues that H2 conversion technologies may experience.
Another key message is that hydrogen will take multiple roles in the transition to CO2 neutrality. While today H2 is mainly used in the chemical industry, we expect that high temperature heat provision (possibly also low), road transportation (heavy goods transport), and energy storage for balancing the power grid will most likely catalyse the transition to a full hydrogen economy. Further down the line there may be applications in air and ship transportation, as well as chemical building blocks alongside recycled CO2 for CO2-neutral carbon-based fuels and materials. Importantly, we do not foresee a preferred global winner among hydrogen uses, but rather each country – or region with similar socio-economic conditions – following different H2 implementation pathways and end-use distribution. Eventually, early applications will create hydrogen value chains which then support the emergence and deployment of other applications.
Hydrogen production methods
The key message on low-carbon hydrogen production is that scalable production technology is available today, i.e., reforming with CO2 capture as well as water electrolysis. However, large-scale production of low-carbon hydrogen is hindered by system limitations, notably the unavailability of CO2 transport and storage networks for the former and sufficient renewable electricity for the latter. A second key message is that, in the interest of a timely transition to a CO2 net-zero society, H2 production routes need to be evaluated from a life cycle perspective avoiding any dogmatic prejudice: it is found that the GHG footprint of hydrogen from fossil fuels can be as low as, or lower than, that from electrolysis with current technologies, depending on the GHG intensity of the used electricity and natural gas. H2 from natural gas with CCS is therefore a key enabler for a green hydrogen-based future.
If the limitations of renewable electricity and CCS networks at scale can be addressed, the outlook for hydrogen production is positive too: where the price of low-carbon hydrogen produced today is still around 50% (or more) higher than traditional (GHG intensive) hydrogen, it is projected to come down significantly through new technology maturing, economies of scale and learning by doing. For example, several routes for improving fossil-based hydrogen exist at TRL7+, focussing on either reforming or on H2/CO2 separation or on integration of the two steps. This means that by 2050, when GHG neutrality should be reached, these solutions should be ready. On a less positive note, we believe technology for hydrogen production from biomass will have a hard time scaling up, especially due to the many complexities of biomass gasification, but also given the low expected availability of biomass for bioenergy, which may likely be used to produce other energy vectors or products. In this context, biohydrogen is not likely to play a large role in the global transition to net-zero in 2050 but may play a role in specific small regional contexts.
Hydrogen transport and storage networks
The key message on hydrogen networks is that their implementation needs careful system design and planning, considering expected local end-uses. Early investment in dedicated hydrogen infrastructure may be unrealistic due to high costs compared to the relatively modest offtake but blending hydrogen into the NG infrastructure can kick-start the hydrogen economy by allowing production to ramp up without the need of dedicated hydrogen transport networks. Also, bilateral production and use within an industrial site or cluster can likely form important seeds for substantial development of hydrogen assets. In the design of hydrogen networks, one needs to carefully plan for emergence of production and consumption locations and types: a network with more distributed production would benefit from lower transmission pressures, while a network with large centralised production benefits from higher pressures. Finally, although the safety considerations around hydrogen pipelines are very comparable to natural gas pipes, the risks vary slightly, which may at points lead to a different safety distance.
Looking ahead, once hydrogen starts to also penetrate the transport sector and the built environment, dedicated hydrogen networks are indispensable, and their construction will likely go region by region to maximise network utilisation. Notably, some local NG distribution networks can transport 100% hydrogen with relatively minor modifications. Hydrogen storage will become critical to overcome periods of low production once more intermittent renewables-based hydrogen will be produced. H2 storage is particularly attractive when salt caverns or underground salt layers are available.
Legal and regulatory requirement for enabling the development of hydrogen infrastructures
The key message on legal and regulatory requirements for Europe (among others) is that carbon-free energy carriers like hydrogen will depend among other elements on sound infrastructure regulations. Especially, the gas market design needs to be adapted if we are to allow for higher shares of sustainable gases in transport networks, sector coupling and more decentralised production of hydrogen, whereas the existing EU regulatory principles could be sufficient to integrate small volumes of hydrogen into the existing natural gas infrastructure. The gas market redesign should be based on four key principles:
1. Integrated energy system planning as well as integrated governance tools.
2. Efficient and coordinated permitting procedures for infrastructure projects.
3. Transparent and enabling regime for access to the grid and for gas grid conversion.
4. Safe, effective and smart operation of transport networks and related infrastructures.
The current outlook for fit-for-purpose regulation in the European Economic Area that will facilitate investment in, and growth of, hydrogen infrastructure and markets in a manner that can maximise its contribution to a net zero economy is judged to be neutral to slightly negative over the next five to ten years. The complexity of adapting the European gas market design needs to be reduced by taking pragmatic steps in phases, such as starting with hydrogen blending and selected end-use safety regulations. The UK on the other hand has already advanced the adaptation and development of its gas regulatory environment through collaboration between the private sector, the gas market regulator and the health and safety regulator. Thus, the outlook for timely and effective regulation of hydrogen (and CCS) value chains in the UK is judged as very positive.
Business models for initial infrastructure development
The key message on business models is that there are still major investment barriers to delivery of first-of-a-kind hydrogen infrastructure in Europe, stemming from a combination of commercial, legal, financial and policy constraints. These barriers need to be removed by developing business models that create room for durable revenues (and profits) while distributing risk over the actors that can bear it best. To create the conditions for successful investment in initial large scale hydrogen infrastructure, six items are imperative:
1. Systems thinking applied to business models to deliver optimal integrated outcomes for multiple economic/market sectors.
2. Societal value should lead investment decisions, instead of standard cost-benefit analysis.
3. Three key outcomes should be met: initial revenue from medium to long term stable anchor users; both government and private stakeholders can commit to invest with no or low regret; and flexibility and optionality is provided for future evolution of markets, technology, and utilisation.
4. Initial market development for hydrogen end-use must be led primarily by government and/or public bodies with assistance from the private sector where it has specific expertise.
5. The selection of first-of-a-kind projects for government support is based on an integrated system view using an appropriate mix of collaboration and competition between stakeholders, projects, regions, or industrial clusters to ensure cost effectiveness.
6. Risk allocation and sharing between the public and private sectors based on an accurate assessment of which stakeholder is best placed to mitigate and manage the risk.
The outlook for implementation of effective business models to deliver hydrogen and CCS infrastructure and markets in the European Economic Area in the period to 2030 is judged as neutral to positive. Although national-level business models in countries such as The Netherlands and Norway will help deliver initial projects, system business models that support a wider sectoral and regional growth of hydrogen production, transport and use will be challenging. The outlook is more positive in the UK where the government has increased its effort to facilitate hydrogen (including CCS) in the energy mix using public-private business models and support mechanisms across the full value chain. Nevertheless, it too will face the difficulty of integrating production, infrastructure, and markets into a functioning system across regions and market sectors.
Conflicts of interest
There are no conflicts to declare.
Acknowledgements
ACT ELEGANCY, Project No 271498, has received funding from DETEC (CH), BMWi (DE), RVO (NL), Gassnova (NO), BEIS (UK), Gassco, Equinor and Total, and is cofounded by the European Commission under the Horizon 2020 programme, ACT Grant Agreement No 691712.
References
-
IEA, Global Hydrogen Review 2021, 2021 DOI:10.1787/39351842-en.
- J. Tollefson, COVID curbed carbon emissions in 2020—but not by much, Nature, 2021, 589, 343 CrossRef CAS PubMed.
- E. F. Bødal, D. Mallapragada, A. Botterud and M. Korpås, Decarbonization synergies from joint planning of electricity and hydrogen production: a Texas case study, Int. J. Hydrogen Energy, 2020, 45, 32899–32915 CrossRef.
- C. Antonini, K. Treyer, E. Moioli, C. Bauer, T. J. Schildhauer and M. Mazzotti, Hydrogen from wood gasification with CCS – a techno-environmental analysis of production and use as transport fuel, Sustain. Energy Fuels, 2021, 5, 2602–2621 RSC.
- C. Antonini, K. Treyer, A. Streb, M. van der Spek, C. Bauer and M. Mazzotti, Hydrogen production from natural gas and biomethane with carbon capture and storage – a techno-environmental analysis, Sustain. Energy Fuels, 2020, 4, 2967–2986 RSC.
-
L. van Cappellen, H. Croezen and F. Rooijers, Feasibility study into blue hydrogen; Technical, economic & sustainability analysis, 2018 Search PubMed.
- IFP Energies Nouvelles & AS, S. E. Hydrogen4EU. Charting pathways to enable net zero, 2021.
- Shell. Shell starts up Europe's largest PEM green hydrogen electrolyser, Shell, 2021, https://www.shell.com/media/news-and-media-releases/2021/shell-starts-up-europes-largest-pem-green-hydrogen-electrolyser.html (Accessed: 5th July 2021).
- European Commission, A hydrogen strategy for a climate-neutral Europe. COM(2020) 301 final, 2020.
-
M. Lewis, Deep Decarbonization. Green hydrogen, net zero, and the future of the EU-ETS, 2020 Search PubMed.
-
J. Jens, A. Wang, K. Van der Leun, D. Peters and M. Buseman, Extending the European Hydrogen Backbone. Gas for Climate, 2021 Search PubMed.
- J. O. M. Bockris and A. J. Appleby, The hydrogen economy: an ultimate economy?, Environ. This Mon., 1972, 1, 29 Search PubMed.
- J. O. M. Bockris, The hydrogen economy: its history, Int. J. Hydrogen Energy, 2013, 38, 2579–2588 CrossRef CAS.
-
L. J. Nuttall, Solid polymer electrolysis fuel cell status report, in Energy 10; Annual Intersociety Energy Conversion and Engineering Conference 210–217, 1975.
-
L. J. Nuttall, Status of solid polymer electrolyte electrochemical cell technology for electrolytic hydrogen generation and fuel cell power generation, Ocean thermal energy for the 80's, 1979, vol. 2, p. 3B-3/1 Search PubMed.
- R. L. A. Fernandes and L. J. Nuttall, Advanced Electrolysis Development for Hydrogen-Cyde Peak Shaving for Electric Utilities, Proc. IEEE, 1983, 71, 1086–1088 CAS.
- B. Basler and A. Korosi, Combustion Of Fuels With Low Hydrogen Content In Bbc Gas Turbines, Brown Boveri Rev., 1980, 67(12), 705–714 CAS.
- H. Hiroyasu, M. Arai, T. Kadota and J. Yoso, An Experimental Study on Kerosene-Hydrogen Hybrid Combustion in a Gas Turbine Combustor, Bull. JSME, 1980, 23, 1655–1662 CrossRef CAS.
- Y. Tsujikawa and T. Sawada, On the utilization of hydrogen as a fuel for gas turbine: 1st report, on the utilization of low temperature exergy of liquid hydroden, Trans. Jap. Soc. Mech. Eng., 1980, 46(401), 163–170 CrossRef CAS.
- Y. Tsujikawa and T. Sawada, On the Utilization of Hydrogen as a Fuel for Gas Turbine: 2nd Report, An Analysis of a Precooled Gas Turbine with an Auxiliary Cycle, Trans. Jpn. Soc. Mech. Eng., B, 1982, 48(432), 1567–1576 CrossRef.
- B. Emerson, T. Lieuwen, B. Noble and N. Espinoza, Hydrogen substitution for natural gas in turbines: Opportunities, issues, and challenges, Power Eng., 2021 Search PubMed , https://www.power-eng.com/gas/hydrogen-substitution-for-natural-gas-in-turbines-opportunities-issues-and-challenges/?utm_medium=email&utm_source=pe_weekly_newsletter&utm_campaign=2021-06-22.
-
D. Hart, F. Lehner, S. Jones and J. Lewis, The Fuel Cell Industry Review 2019, 2019 Search PubMed.
- I. Staffell, D. Scamman, A. Velazquez Abad, P. Balcombe, P. E. Dodds, P. Ekins, N. Shah and K. R. Ward, The role of hydrogen and fuel cells in the global energy system, Energy Environ. Sci., 2019, 12, 463–491 RSC.
- S. Campanari and M. Gazzani, High efficiency SOFC power cycles with indirect natural gas reforming and CO2 capture, Proc. ASME Turbo Expo, 2014, 3A Search PubMed.
- A. Amiri, S. Tang, R. Steinberger-Wilckens and M. O. Tadé, Evaluation of fuel diversity in Solid Oxide Fuel Cell system, Int. J. Hydrogen Energy, 2018, 43, 23475–23487 CrossRef CAS.
- N. F. Harun, D. Tucker and T. A. Adams, Impact of fuel composition transients on SOFC performance in gas turbine hybrid systems, Appl. Energy, 2016, 164, 446–461 CrossRef CAS.
- Y. Yi, A. D. Rao, J. Brouwer and G. S. Samuelsen, Fuel flexibility study of an integrated 25 kW SOFC reformer system, J. Power Sources, 2005, 144, 67–76 CrossRef CAS.
- S. Campanari, L. Mastropasqua, M. Gazzani, P. Chiesa and M. C. Romano, Predicting the ultimate potential of natural gas SOFC power cycles with CO2 capture – Part A: methodology and reference cases, J. Power Sources, 2016, 324, 598–614 CrossRef CAS.
- S. Campanari, L. Mastropasqua, M. Gazzani, P. Chiesa and M. C. Romano, Predicting the ultimate potential of natural gas SOFC power cycles with CO2 capture - Part B: applications, J. Power Sources, 2016, 325, 194–208 CrossRef CAS.
- L. Barelli, G. Bidini, S. Campanari, G. Discepoli and M. Spinelli, Performance assessment of natural gas and biogas fueled molten carbonate fuel cells in carbon capture con fi guration, J. Power Sources, 2016, 320, 332–342 CrossRef CAS.
- P. Chiesa, S. Campanari and G. Manzolini, CO2 cryogenic separation from combined cycles integrated with molten carbonate fuel cells, Int. J. Hydrogen Energy, 2010, 36, 10355–10365 CrossRef.
-
G. E. Power. Powering the Future with Gas Power Systems: Offerings 2018, 2018, p. 153.
-
J. Goldmeer, Power to Gas: Hydrogen for Power Generation – Fuel Flexible Gas Turbines as Enablers for a Low or Reduced Carbon Energy Ecosystem, 2019, pp. 1–19 Search PubMed.
- S. Taamallah, K. Vogiatzaki, F. M. Alzahrani, E. M. A. Mokheimer, M. A. Habib and A. F. Ghoniem, Fuel flexibility, stability and emissions in premixed hydrogen-rich gas turbine combustion: technology, fundamentals, and numerical simulations, Appl. Energy, 2015, 154, 1020–1047 CrossRef CAS.
- M. Gazzani, P. Chiesa, E. Martelli, S. Sigali and I. Brunetti, Using hydrogen as gas turbine fuel: Premixed versus diffusive flame combustors, J. Eng. Gas Turbines Power, 2014, 136(GTP-13-1381) DOI:10.1115/1.4026085.
- P. Chiesa, G. Lozza and L. Mazzocchi, Using hydrogen as gas turbine fuel, J. Eng. Gas Turbines Power, 2005, 127, 73–80 CrossRef CAS.
- M. H. Du Toit, A. V. Avdeenkov and D. Bessarabov, Reviewing H2 Combustion: A Case Study for Non-Fuel-Cell Power Systems and Safety in Passive Autocatalytic Recombiners, Energy Fuels, 2018, 32, 6401–6422 CrossRef CAS.
- D. Flin, Working toward 100% hydrogen, Gas Turbine World, 2019, 10–13 Search PubMed.
-
S. Patel, High-Volume Hydrogen Gas Turbines Take Shape. Power Mag, 2019. Available at: https://www.powermag.com/high-volume-hydrogen-gas-turbines-take-shape/, (Accessed: 29th May 2020).
-
N. Lindstrand, This Swedish scientist works towards fulfilling Siemens’ 2030 hydrogen pledge. Siemens, 2019. Available at: https://new.siemens.com/global/en/company/stories/energy/hydrogen-capable-gas-turbine.html, (Accessed: 29th May 2020).
-
F. Simon, GE eyes 100% hydrogen-fuelled power plants by 2030. Euractiv (2021), Available at: https://www.euractiv.com/section/energy/news/ge-eyes-100-hydrogen-fuelled-power-plants-by-2030/, (Accessed: 4th June 2021).
- S. Verhelst and T. Wallner, Hydrogen-fueled internal combustion engines, Prog. Energy Combust. Sci., 2009, 35, 490–527 CrossRef CAS.
- S. Laiminger, M. Url, K. Payrhuber and M. Schneider, Hydrogen as Future Fuel for Gas Engines, MTZ Worldw, 2020, 81, 64–69 CrossRef.
- C. Lowe, N. Brancaccio, D. Batten, C. Leung and D. Waibel, Technology assessment of hydrogen firing of process heaters, Energy Procedia, 2011, 4, 1058–1065 CrossRef.
-
K. Hirata, D. Sakuma and K. Mimura, HYDROGEN GAS BURNER STRUCTURE, AND HYDROGEN GAS BURNER DEVICE INCLUDING THE SAME. European Patent Office, 2017 Search PubMed.
- Toyota Develops World's First General-purpose Hydrogen Burner for Industrial Use, 2018, Available at: https://global.toyota/en/newsroom/corporate/25260001.html.
-
A. de Pee
et al.
, Decarbonization of industrial sectors: the next frontier, McKinsey & Company, 2018 Search PubMed.
- H-Vision. Blue hydrogen as accelerator and pioneer for energy transition in the industry, 2019.
-
P. Duarte, Hydrogen-based steelmaking, 2019.
- M. Pei, M. Petäjäniemi, A. Regnell and O. Wijk, Toward a fossil free future with hybrit: Development of iron and steelmaking technology in Sweden and Finland, Metals, 2020, 10, 1–11 Search PubMed.
- Energiron. Hydrogen. Energiron Available at: https://www.energiron.com/hydrogen/, (Accessed: 24th June 2021).
-
O. Olsson and B. Nykvist, Bigger is sometimes better: Demonstrating hydrogen steelmaking at scale, 2020 Search PubMed.
-
M. P. Bailey, BREAKTHROUGH TESTING FOR H2/NATURAL-GAS BLEND IN INDUSTRIAL STEELMAKING. Chemical Engineering, 2021. Available at: https://www.chemengonline.com/breakthrough-testing-for-h2-natural-gas-blend-in-industrial-steelmaking/?oly_enc_id=7021H0637790B9R. (Accessed: 4th June 2021).
- Northern Gas Networks, H21 Leeds city gate, 2016.
-
IEA. Technology Roadmap. Hydrogen and Fuel Cells, 2015 DOI:10.1007/SpringerReference_7300.
-
BEIS. Hy4Heat Engagement Event, 2020 DOI:10.1017/CBO9781107415324.004.
- Northern Gas Networks & Equinor & Cadent. H21 North of England Report., 2018.
- J. W. Fawcett, H21 North of England, Notes Queries, 1919, s12-V, 317–318 Search PubMed.
- Bloomberg New Energy Finance. Scale-up of Solar and Wind Puts Existing Coal, Gas at Risk, 2020. Available at: https://about.bnef.com/blog/scale-up-of-solar-and-wind-puts-existing-coal-gas-at-risk/. (Accessed: 19th May 2021).
- B. Zakeri and S. Syri, Electrical energy storage systems: A comparative life cycle cost analysis, Renewable Sustainable Energy Rev., 2015, 42, 569–596 CrossRef.
- S. Samsatli and N. J. Samsatli, The role of renewable hydrogen and inter-seasonal storage in decarbonising heat – Comprehensive optimisation of future renewable energy value chains, Appl. Energy, 2019, 233–234, 854–893 CrossRef.
- P. Gabrielli, M. Gazzani, E. Martelli and M. Mazzotti, Optimal design of multi-energy systems with seasonal storage, Appl. Energy, 2018, 219, 408–424 CrossRef.
- L. Weimann, P. Gabrielli, A. Boldrini, G. J. Kramer and M. Gazzani, Optimal hydrogen production in a wind-dominated zero-emission energy system, Adv. Appl. Energy, 2021, 100032 CrossRef.
- P. Gabrielli, A. Poluzzi, G. J. Kramer, C. Spiers, M. Mazzotti and M. Gazzani, Seasonal energy storage for zero-emissions multi-energy systems via underground hydrogen storage, Renewable Sustainable Energy Rev., 2020, 121, 109629 CrossRef CAS.
- I. Petkov and P. Gabrielli, Power-to-hydrogen as seasonal energy storage: an uncertainty analysis for optimal design of low-carbon multi-energy systems, Appl. Energy, 2020, 274, 115197 CrossRef CAS.
- R. Amirante, E. Cassone, E. Distaso and P. Tamburrano, Overview on recent developments in energy storage: Mechanical, electrochemical and hydrogen technologies, Energy Convers. Manage., 2017, 132, 372–387 CrossRef CAS.
- H. Blanco and A. Faaij, A review at the role of storage in energy systems with a focus on Power to Gas and long-term storage, Renewable Sustainable Energy Rev., 2018, 81, 1049–1086 CrossRef.
- B. Nastasi and G. Lo Basso, Power-to-Gas integration in the Transition towards Future Urban Energy Systems, Int. J. Hydrogen Energy, 2017, 42, 23933–23951 CrossRef CAS.
- Y. Matsuo, S. Endo, Y. Nagatomi, Y. Shibata, R. Komiyama and Y. Fujii, A quantitative analysis of Japan’s optimal power generation mix in 2050 and the role of CO2-free hydrogen, Energy, 2018, 165, 1200–1219 CrossRef CAS.
- S. Ferrari, F. Zagarella, P. Caputo and A. D’Amico, Results of a literature review on methods for estimating buildings energy demand at district level, Energy, 2019, 175, 1130–1137 CrossRef.
- P. Gabrielli, M. Gazzani and M. Mazzotti, Electrochemical conversion technologies for optimal design of decentralized multi-energy systems: Modeling framework and technology assessment, Appl. Energy, 2018, 221, 557–575 CrossRef CAS.
- O. Tlili, C. Mansilla, M. Robinius, K. Syranidis, M. Reuss, J. Linssen, J. André, Y. Perez and D. Stolten, Role of electricity interconnections and impact of the geographical scale on the French potential of producing hydrogen via electricity surplus by 2035, Energy, 2019, 172, 977–990 CrossRef.
-
P. Murray, K. Orehounig and J. Carmeliet, Optimal design of multi-energy systems at different degrees of decentralization. Energy Procedia, Elsevier Ltd, 158, pp. 4204–4209, 2019 Search PubMed.
-
S. Amelang, Mercedes-Benz gives up on fuel cell cars – report. Clean Energy Wire, Available at: https://www.cleanenergywire.org/news/mercedes-benz-gives-fuel-cell-cars-report.
- Volkswagen. Battery or fuel cell, that is the question. Volkswagen Newsroom. Available at: https://www.volkswagen-newsroom.com/en/stories/battery-or-fuel-cell-that-is-the-question-5868.
- E. A. Bouman, E. Lindstad, A. I. Rialland and A. H. Strømman, State-of-the-art technologies, measures, and potential for reducing GHG emissions from shipping – A review, Transp. Res. Part D Transp. Environ., 2017, 52, 408–421 CrossRef.
- R. Sacchi, C. Bauer and B. L. Cox, Does Size Matter? The Influence of Size, Load Factor, Range Autonomy, and Application Type on the Life Cycle Assessment of Current and Future Medium? The Heavy-Duty Vehicles, Environ. Sci. Technol., 2021, 55, 5224–5235 CrossRef CAS PubMed.
- R. Rath, P. Kumar, S. Mohanty and S. K. Nayak, Recent advances, unsolved deficiencies, and future perspectives of hydrogen fuel cells in transportation and portable sectors, Int. J. Energy Res., 2019, 43, 8931–8955 CrossRef.
-
B. Deloitte, Fueling the Future of Mobility Hydrogen and fuel cell solutions for transportation, vol. 1, 2020 Search PubMed.
- A. Lozanovski, N. Whitehouse, N. Ko and S. Whitehouse, Sustainability assessment of fuel cell buses in public transport, Sustainability, 2018, 10, 1480–1495 CrossRef.
- M. Mahmoud, R. Garnett, M. Ferguson and P. Kanaroglou, Electric buses: A review of alternative powertrains, Renewable Sustainable Energy Rev., 2016, 62, 673–684 CrossRef.
- Nel ASA: Establishes joint venture to supply green hydrogen to Hyundai trucks in Norway. NEL. Available at: https://nelhydrogen.com/press-release/nel-asa-establishes-joint-venture-to-supply-green-hydrogen-to-hyundai-trucks-in-norway/.
- Hyundai, For a better tomorrow, Available at: https://hyundai-hm.com/en/.
- Unique hydrogen ecosystem for emission-free mobility, Available at: https://hydrospider.ch/en/unique-hydrogen-ecosystem-for-emission-free-mobility/.
- L. van Biert, M. Godjevac, K. Visser and P. V. Aravind, A review of fuel cell systems for maritime applications, J. Power Sources, 2016, 327, 345–364 CrossRef CAS.
-
M. Lewis, EGEB: Scotland trials world's first hydrogen ferry. Electrek, 2020. Available at: https://electrek.co/2020/10/14/egeb-scotland-hydrogen-ferry-microwave/. (Accessed: 29th June 2021).
- Golden Gate Zero Emission Marine. Available at: https://watergoround.com/.
-
M. Steen, H. Bach, O. Bjorgum, T. Hansen and A. Kenzhegaliyeva, Report Greening the fleet: A technological innovation system (TIS) analysis of hydrogen, battery electric, liquefied biogas, and biodiesel in the maritime sector, 2020.
-
Toshiba. Toshiba Delivers Mobile Hydrogen Fuel Cell System to Fuel Cell Ship. Available at: https://www.toshiba-energy.com/en/info/info2019_1227.htm.
- HySeas III. H2020 project website, Available at: https://www.hyseas3.eu/.
-
P. Eckle, A. Langguth and C. Nakhle, Towards net-zero. INNOVATING FOR A CARBON FREE FUTURE OF SHIPPING IN THE NORTH AND BALTIC SEA, 2019 Search PubMed.
- Clean Sky 2 Joint Undertaking & Fuel Cells and Hydrogen 2 Joint Undertaking. Hydrogen-powered aviation - A fact-based study of hydrogen technology, economics, and climate impact by 2050. Publications Office of the European Union, 2020. DOI:10.2843/766989.
- Airbus reveals new zero-emission concept aircraft. Airbus, 2020. Available at: https://www.airbus.com/newsroom/press-releases/en/2020/09/airbus-reveals-new-zeroemission-concept-aircraft.html. (Accessed: 13th March 2021).
- P. Balcombe, J. Brierley, C. Lewis, L. Skatvedt, J. Speirs, A. Hawkes and I. Staffell, How to decarbonise international shipping: Options for fuels, technologies and policies, Energy Convers. Manage., 2019, 182, 72–88 CrossRef CAS.
- J. Ally and T. Pryor, Life cycle costing of diesel, natural gas, hybrid and hydrogen fuel cell bus systems: an Australian case study, Energy Policy, 2016, 94, 285–294 CrossRef CAS.
- J. Jones, A. Genovese and A. Tob-Ogu, Hydrogen vehicles in urban logistics: a total cost of ownership analysis and some policy implications, Renewable Sustainable Energy Rev., 2020, 119, 109595 CrossRef.
- B. Cox, C. Bauer, A. Mendoza Beltran, D. P. van Vuuren and C. L. Mutel, Life cycle environmental and cost comparison of current and future passenger cars under different energy scenarios, Appl. Energy, 2020, 269, 115021 CrossRef.
- M. Miotti, J. Hofer and C. Bauer, Integrated environmental and economic assessment of current and future fuel cell vehicles, Int. J. Life Cycle Assess., 2017, 22, 94–110 CrossRef CAS.
- B. L. Cox and C. L. Mutel, The environmental and cost performance of current and future motorcycles, Appl. Energy, 2018, 212, 1013–1024 CrossRef.
- S. E. Hosseini and M. A. Wahid, Hydrogen production from renewable and sustainable energy resources: promising green energy carrier for clean development, Renewable Sustainable Energy Rev., 2016, 57, 850–866 CrossRef CAS.
- IPCC, Summary for Policymakers, in Global Warming of 1.5°C. An IPCC Special Report on the impacts of global warming of 1.5°C above pre-industrial levels and related global greenhouse gas emission pathways, in the context of strengthening the global response to the threat of climate change, sustainable development, and efforts to eradicate poverty, ed. V. Masson-Delmotte, P. Zhai, H.-O. Pörtner, D. Roberts, J. Skea, P. R. Shukla, A. Pirani, W. Moufouma-Okia, C. Péan, R. Pidcock, S. Connors, J. B. R. Matthews, Y. Chen, X. Zhou, M. I. Gomis, E. Lonnoy, T. Maycock, M. Tignor and T. Waterfield, 2018.
- European Union, REGULATION (EU) 2019/631 OF THE EUROPEAN PARLIAMENT AND OF THE COUNCIL, 2019, 2016, 48–119 Search PubMed.
- European Union, REGULATION (EU) 2019/1242 OF THE EUROPEAN PARLIAMENT AND OF THE COUNCIL, 2019, 2019, 202–240 Search PubMed.
- Low Emission Zones, Urban Access Regulations in Europe Available at: https://urbanaccessregulations.eu/low-emission-zones-main.
-
M. Kemp, M. Ballesteros and S. O’Brien, Phasing-out sales of internal combustion engine vehicles, 2020 Search PubMed.
- F. Creutzig, A. Javaid, N. Koch, B. Knopf, G. Mattioli and O. Edenhofer, Adjust urban and rural road pricing for fair mobility, Nat. Clim. Chang., 2020, 591–594 CrossRef.
- International Maritime Organzation, ADOPTION OF THE INITIAL IMO STRATEGY ON REDUCTION OF GHG EMISSIONS.
- F. Ueckerdt, C. Bauer, A. Dirnaichner, J. Everall, R. Sacchi and G. Luderer, Potential and risks of hydrogen-based e-fuels in climate change mitigation, Nat. Clim. Chang., 2021, 11, 384–393 CrossRef CAS.
- A. Kätelhön, R. Meys, S. Deutz, S. Suh and A. Bardow, Climate change mitigation potential of carbon capture and utilization in the chemical industry, Proc. Natl. Acad. Sci. U. S. A., 2019, 166, 11187–11194 CrossRef PubMed.
- A. Valera-Medina, H. Xiao, M. Owen-Jones, W. I. F. David and P. J. Bowen, Ammonia for power, Prog. Energy Combust. Sci., 2018, 69, 63–102 CrossRef.
- A. Klerke, C. H. Christensen, J. K. Nørskov and T. Vegge, Ammonia for hydrogen storage: Challenges and opportunities, J. Mater. Chem., 2008, 18, 2304–2310 RSC.
- S. Giddey, S. P. S. Badwal, C. Munnings and M. Dolan, Ammonia as a Renewable Energy Transportation Media, ACS Sustainable Chem. Eng., 2017, 5, 10231–10239 CrossRef CAS.
- M. C. Chiong,
et al., Advancements of combustion technologies in the ammonia-fuelled engines, Energy Convers. Manage., 2021, 244, 114460 CrossRef CAS.
- J. S. Cardoso,
et al., Ammonia as an energy vector: Current and future prospects for low-carbon fuel applications in internal combustion engines, J. Clean. Prod., 2021, 296, 126562 CrossRef CAS.
- G. A. Olah, Beyond Oil and Gas: The Methanol Economy, Angew. Chem., Int. Ed., 2005, 44, 2636–2639 CrossRef CAS PubMed.
- N. Morlanés,
et al., A technological roadmap to the ammonia energy economy: Current state and missing technologies, Chem. Eng. J., 2021, 408, 127310 CrossRef.
- D. R. MacFarlane,
et al., A Roadmap to the Ammonia Economy, Joule, 2020, 4, 1186–1205 CrossRef CAS.
-
S. T. Munkejord
et al.
, ELEGANCY - Enabling a low-carbon economy via hydrogen and CCS in 11th Trondheim CCS Conference, June 2021 (Sintef, 2021).
-
D. Benrath, S. Flamme, S. Glanz and F. M. Hoffart, D5.5.2 German Case Study: Final Design and First Results Date, 2018 Search PubMed.
-
S. Glanz and A.-L. Schonauer, H2/CCS chains in Germany – Social Perception and Acceptance. Sintef, 2019. Available at: https://blog.sintef.com/sintefenergy/h2-ccs-chains-germany-social-perception-acceptance/. (Accessed: 4th June 2021).
-
R. de Kler and R. Bhardwaj, D5.2.1. Report describing the current industrial cluster in Rotterdam with its socio-economic contribution, CO2 emissions and target setting for emission reduction, 2018.
-
R. Bhardwaj, R. de Kler and O. Partenie, D5.2.2 Needed H2 production facilities, integration in port infrastructure, possible ownership structure and CAPEX/OPEX estimates, 2020.
- S. Roussanaly and R. Anantharaman, D5.6.2 Scenarios for Norwegian H2 value chain, Publishable summary, 2019 Search PubMed.
-
W. Goldthorpe and L. Avignon, D5.4.3 Report H21 Leeds and North of England risk matrix, business case template and risk reduction strategies, 2020.
- N. Sunny, N. MacDowell and N. Shah, What is needed to deliver carbon-neutral heat using hydrogen and CCS?, Energy Environ. Sci., 2020, 13, 4204–4224 RSC.
- International Energy Agengy, The future of hydrogen. Seizing today's opportunities., 2019 DOI:10.1016/S1464-2859(12)70027-5.
- J. B. Wevers, L. Shen and M. van der Spek, What does it take to go net-zero-CO2? A life cycle assessment on long-term storage of intermittent renewables with chemical energy carriers, Front. Energy Res., 2020, 8, 104 CrossRef.
-
IEA. World Energy Outlook, 2018 DOI:10.1049/ep.1977.0180.
- International Renewable Energy Agency. IRENA (2019), Global Energy Transformation: A Roadmap to 2050. Global Energy Transformation. A Roadmap to 2050, 2019.
-
IEA. Net Zero by 2050: A Roadmap for the Global Energy Sector. International Energy Agency, 2021.
-
J. Speirs
et al.
, A greener gas grid: what are the options?, 2017 Search PubMed.
- M. Binder, M. Kraussler, M. Kuba and M. Luisse, Hydrogen from biomass gasification, IEA Bioenergy, 2018 Search PubMed.
-
J. Howes
et al.
, Innovation Needs Assessment for Biomass Heat. Final Report, 2018.
-
C. Bauer
et al.
, Electricity storage and hydrogen – Technologies, costs and environmental burdens, 2021 Search PubMed.
- IEAGHG, Biorefineries with CCS, 2021-01, 2021.
- B. Parkinson, P. Balcombe, J. F. Speirs, A. D. Hawkes and K. Hellgardt, Levelized cost of CO2 mitigation from hydrogen production routes, Energy Environ. Sci., 2019, 12, 19–40 RSC.
- B. Parkinson,
et al., Hydrogen production using methane: Techno-economics of decarbonizing fuels and chemicals, Int. J. Hydrogen Energy, 2018, 43, 2540–2555 CrossRef CAS.
- C. Bauer,
et al., On the climate impacts of blue hydrogen production, Sustain. Energy Fuels, 2022, 6, 66–75 RSC.
- G. Wernet, C. Bauer, B. Steubing, J. Reinhard, E. Moreno-Ruiz and B. Weidema, The ecoinvent database version 3 (part I): overview and methodology, Int. J. Life Cycle Assess., 2016, 21, 1218–1230 CrossRef.
-
A. Louwen, S. Krishnan, M. Derks and M. Junginger, Deliverable D3.2 Comprehensive Report on Experience Curves, 2018 Search PubMed.
-
IEA. World Energy Outlook 2021, 2021 Search PubMed.
- R. W. Howarth and M. Z. Jacobson, How green is blue hydrogen?, Energy Sci. Eng., 2021, 00, 1–12 Search PubMed.
- International
Energy Agency (IEA). Methane Tracker 2020, 2020.
- J. Lane, C. Greig and A. Garnett, Uncertain storage prospects create a conundrum for carbon capture and storage ambitions, Nat. Clim. Chang, 2021, 11, 925–936 CrossRef.
- B. Batidzirai, G. Schotman, M. Van der Spek, M. Junginger and A. Faaij, Techno-economic performance of sustainable international bio-SNG production and supply chains on short and longer term, Biofuels, Bioprod. Biorefin., 2018, 13, 325–357 CrossRef.
- V. S. Sikarwar,
et al., An overview of advances in biomass gasification, Energy Environ. Sci., 2016, 9, 2939–2977 RSC.
- Global CCS Institute. Global Status of CCS 2020, 2020.
- A. Buttler and H. Spliethoff, Current status of water electrolysis for energy storage, grid balancing and sector coupling via power-to-gas and power-to-liquids: a review, Renewable Sustainable Energy Rev., 2018, 82, 2440–2454 CrossRef CAS.
- O. Schmidt,
et al., Future cost and performance of water electrolysis: An expert elicitation study, Int. J. Hydrogen Energy, 2017, 42, 30470–30492 CrossRef CAS.
-
T. Smolinka, Water electrolysis: status and potential for development in Joint NOW GmbH – FCH JU Water Electrolysis Day Brussels (BE), April 03, 2014, 2014.
-
ICCT. Assessment of Hydrogen Production Costs from Electrolysis: United States and Europe, 2020.
- PIK. Price of Hydrogen: Data 28.01.2021, 2021. Available at: https://h2foroveralls.shinyapps.io/H2Dash/. Accessed: 28th January 2021.
- G. Glenk and S. Reichelstein, Economics of converting renewable power to hydrogen, Nat. Energy, 2019, 4, 216–222 CrossRef CAS.
- PIK, Price of Hydrogen: Data 28.01.2021, 2021.
- G. Glenk and S. Reichelstein, Economics of converting renewable power to hydrogen, Nat. Energy, 2019, 4, 216–222 CrossRef CAS.
- S. D. Tilley, Recent Advances and Emerging Trends in Photo-Electrochemical Solar Energy Conversion, Adv. Energy Mater., 2019, 9, 1–13 Search PubMed.
- R. Abe, Recent progress on photocatalytic and photoelectrochemical water splitting under visible light irradiation, J. Photochem. Photobiol., C, 2010, 11, 179–209 CrossRef CAS.
- J. H. Kim, D. Hansora, P. Sharma, J. W. Jang and J. S. Lee, Toward practical solar hydrogen production-an artificial photosynthetic leaf-to-farm challenge, Chem. Soc. Rev., 2019, 48, 1908–1971 RSC.
- C. Jiang, S. J. A. Moniz, A. Wang, T. Zhang and J. Tang, Photoelectrochemical devices for solar water splitting-materials and challenges, Chem. Soc. Rev., 2017, 46, 4645–4660 RSC.
- A. Grimm, W. A. de Jong and G. J. Kramer, Renewable hydrogen production: A techno-economic comparison of photoelectrochemical cells and photovoltaic-electrolysis, Int. J. Hydrogen Energy, 2020, 45, 22545–22555 CrossRef CAS.
- M. R. Shaner, H. A. Atwater, N. S. Lewis and E. W. McFarland, A comparative technoeconomic analysis of renewable hydrogen production using solar energy, Energy Environ. Sci., 2016, 9, 2354–2371 RSC.
-
Ullmann's Encyclopedia of Industrial Chemistry. Wiley, 2000, Online ISBN: 9783527306732 DOI:10.1002/14356007.
-
R. H. Perry and D. W. Green, Perry's chemical engineers’ handbook, McGraw-Hill, 2019 Search PubMed.
- R. M. Navarro, M. A. Peña and J. L. G. Fierro, Hydrogen production reactions from carbon feedstocks: Fossil fuels and biomass, Chem. Rev., 2007, 107, 3952–3991 CrossRef CAS PubMed.
- M. Voldsund, K. Jordal and R. Anantharaman, Hydrogen production with CO2 capture, Int. J. Hydrogen Energy, 2016, 41, 4969–4992 CrossRef CAS.
-
P. E. J. Abbot and M. J. Fernie, Process for the production of hydrocarbons, 2006 Search PubMed.
-
N. B. Jakobsson, A. H. Hansen and P. A. Han, PROCESS FOR REFORMING HYDROCARBONS, 2014 Search PubMed.
- P. Moser, G. Wiechers, S. Schmidt, J. Garcia Moretz-Sohn Monteiro, E. Goetheer, C. Charalambous, A. Saleh, M. van der Spek and S. Garcia, ALIGN-CCUS: Results of the 18-Month Test with Aqueous AMP/PZ Solvent at the Pilot Plant at Niederaussem – Solvent Management, Emissions and Dynamic Behavior, Int.
J. Greenh. Gas Control, 2021, 109, 103381 CrossRef CAS.
- C. Antonini, J.-F. Pérez-Calvo, M. van der Spek and M. Mazzotti, Optimal design of an MDEA CO2 Capture Plant for Clean Hydrogen Production - A rigorous process optimization approach, Sep. Purif. Technol., 2022, 279, 119715 CrossRef.
- P. Brandl, M. Bui, J. P. Hallett and N. Mac Dowell, Beyond 90% capture: Possible, but at what cost?, Int. J. Greenh. Gas Control, 2021, 105, 103239 CrossRef CAS.
- A. Streb, M. Hefti, M. Gazzani and M. Mazzotti, Novel Adsorption Process for Co-Production of Hydrogen and CO2 from a Multicomponent Stream, Ind. Eng. Chem. Res., 2019, 58, 17489–17506 CrossRef CAS.
- M. Asgari, A. Streb, M. van der Spek, W. Queen and M. Mazzotti, Synergistic material and process development: application of a metal-organic framework, Cu-TDPAT, in single-cycle hydrogen purification and CO2 capture from synthesis gas, Chem. Eng. J., 2021, 414, 128778 CrossRef CAS.
- A. Streb and M. Mazzotti, Adsorption for efficient low carbon hydrogen production: part 2—Cyclic experiments and model predictions, Adsorption, 2021, 59, 10093–10109 Search PubMed.
- P. Li,
et al., Recent developments in membranes for efficient hydrogen purification, J. Membr. Sci., 2015, 495, 130–168 CrossRef CAS.
- N. A. Al-Mufachi, N. V. Rees and R. Steinberger-Wilkens, Hydrogen selective membranes: a review of palladium-based dense metal membranes, Renewable Sustainable Energy Rev., 2015, 47, 540–551 CrossRef CAS.
- M. Gazzani, M. C. Romano and G. Manzolini, CO2 capture in integrated steelworks by commercial-ready technologies and SEWGS process, Int. J. Greenh. Gas Control, 2015, 41, 249–267 CrossRef CAS.
- M. Gazzani, E. Macchi and G. Manzolini, CO2 capture in integrated gasification combined cycle with SEWGS - Part A: Thermodynamic performances, Fuel, 2013, 105, 206–219 CrossRef CAS.
- G. Manzolini,
et al., Techno-economic assessment of SEWGS technology when applied to integrated steel-plant for CO2 emission mitigation, Int. J. Greenh. Gas Control, 2020, 94, 102935 CrossRef CAS.
- Stepwise H2020 project.
- L. Barelli, G. Bidini, F. Gallorini and S. Servili, Hydrogen production through sorption-enhanced steam methane reforming and membrane technology: a review, Energy, 2008, 33, 554–570 CrossRef CAS.
- I. Martínez, M. C. Romano, P. Chiesa, G. Grasa and R. Murillo, Hydrogen production through sorption enhanced steam reforming of natural gas: Thermodynamic plant assessment, Int. J. Hydrogen Energy, 2013, 38, 15180–15199 CrossRef.
- L. Riva,
et al., Techno-economic analysis of the Ca–Cu process integrated in hydrogen plants with CO2 capture, Int. J. Hydrogen Energy, 2018, 43, 15720–15738 CrossRef CAS.
- G. Bang,
et al., High-purity hydrogen production via a water-gas-shift reaction in a palladium-copper catalytic membrane reactor integrated with pressure swing adsorption, Chem. Eng. J., 2021, 411, 128473 CrossRef CAS.
- G. Bruni,
et al., On the energy efficiency of hydrogen production processes via steam reforming using membrane reactors, Int. J. Hydrogen Energy, 2019, 44, 988–999 CrossRef CAS.
- E. Fernandez,
et al., Palladium based membranes and membrane reactors for hydrogen production and purification: An overview of research activities at Tecnalia and TU/e, Int. J. Hydrogen Energy, 2017, 42, 13763–13776 CrossRef CAS.
- F. Gallucci, E. Fernandez, P. Corengia and M. van Sint Annaland, Recent advances on membranes and membrane reactors for hydrogen production, Chem. Eng. Sci., 2013, 92, 40–66 CrossRef CAS.
- I. Martínez, D. Armaroli, M. Gazzani and M. C. Romano, Integration of the Ca–Cu Process in Ammonia Production Plants, Ind. Eng. Chem. Res., 2017, 56, 2526–2539 CrossRef.
- M. Luo,
et al., Review of hydrogen production using chemical-looping technology, Renewable Sustainable Energy Rev., 2018, 81, 3186–3214 CrossRef CAS.
- V. S. Sikarwar, M. Zhao, P. S. Fennell, N. Shah and E. J. Anthony, Progress in biofuel production from gasification, Prog. Energy Combust. Sci., 2017, 61, 189–248 CrossRef.
- A. Arregi, M. Amutio, G. Lopez, J. Bilbao and M. Olazar, Evaluation of thermochemical routes for hydrogen production from biomass: a review, Energy Convers. Manage., 2018, 165, 696–719 CrossRef CAS.
- E. Van Der Roest, M. Van Der Spek, A. Ramirez, B. Van Der Zwaan and G. Rothenberg, Converting Waste Toilet Paper into Electricity: A First- Stage Technoeconomic Feasibility Study, Energy Technol., 2017, 5, 2189–2197 CrossRef.
- E. D. Larson,
et al., Design and analysis of a low-carbon lignite/biomass-to-jet fuel demonstration project, Appl. Energy, 2020, 260, 114209 CrossRef CAS.
-
S. Ariyapadi, P. Shires, M. Bhargava and D. Ebbern, KBR's transport gasifier (TRIG) - An advanced gasification technology for sng production from low-rank coals in 25th Annual International Pittsburgh Coal Conference, PCC – Proceedings, 2008.
- J. Adánez,
et al., Chemical looping combustion of solid fuels, Prog. Energy Combust. Sci., 2018, 65, 6–66 CrossRef.
- A. Molino, S. Chianese and D. Musmarra, Biomass gasification technology: the state of the art overview, J. Energy Chem., 2016, 25, 10–25 CrossRef.
-
L. Rosa and M. Mazzotti, Potential for hydrogen production from sustainable biomass with carbon capture and storage in Europe (in review), 2021.
-
C. Antonini
et al.
Elegancy D1.3.1. Report on optimal plants for production of low-carbon H2 with state of the art technologies, 2018.
- G. Di Marcoberardino, D. Vitali, F. Spinelli and M. Binotti, Green Hydrogen Production from Raw Biogas: A Techno-Economic Investigation of Conventional Processes Using Pressure Swing Adsorption Unit, Processes, 2018, 6, 19 CrossRef.
- A. Muscat, E. M. de Olde, I. J. M. de Boer and R. Ripoll-Bosch, The battle for biomass: a systematic review of food-feed-fuel competition, Glob. Food Sec., 2020, 25, 100330 CrossRef.
- N. E. Vaughan and C. Gough, Expert assessment concludes negative emissions scenarios may not deliver, Environ. Res. Lett., 2016, 11, 095003 CrossRef.
- M. B. Jones and F. Albanito, Can biomass supply meet the demands of bioenergy with carbon capture and storage (BECCS)?, Glob. Chang. Biol., 2020, 26, 5358–5364 CrossRef PubMed.
- V. Daioglou, J. C. Doelman, B. Wicke, A. Faaij and D. P. van Vuuren, Integrated assessment of biomass supply and demand in climate change mitigation scenarios, Glob. Environ. Chang, 2019, 54, 88–101 CrossRef.
- T. Beringer, W. Lucht and S. Schaphoff, Bioenergy production potential of global biomass plantations under environmental and agricultural constraints, GCB Bioenergy, 2011, 3, 299–312 CrossRef CAS.
- S. Searle and C. Malins, A reassessment of global bioenergy potential in 2050, GCB Bioenergy, 2015, 7, 328–336 CrossRef.
- S. Fuss, W. F. Lamb, M. W. Gallaghan, J. Hilair, F. Creutzig, T. Amann, T. Beringer, W. De Oliveira Garcia, J. Hartmann, T. Khanna, G. Luderer, G. F. Nemet, J. Rogelj, P. Smith, J. L. Vicente, J. Wilcox, M. Del Mar Zamora Dominguez and J. C. Minx, Negative emissions – Part 2: Costs, potentials and side effects, Environ. Res. Lett., 2018, 13, 063002 CrossRef.
- O. Fandiño, J. P. M. Trusler and D. Vega-Maza, Phase behavior of (CO2 + H2) and (CO2 + N2) at temperatures between (218.15 and 303.15) K at pressures up to 15 MPa, Int. J. Greenh. Gas Control, 2015, 36, 78–92 CrossRef.
- Y. Sanchez-Vicente, T. C. Drage, M. Poliakoff, J. Ke and M. W. George, Densities of the carbon dioxide + hydrogen, a system of relevance to carbon capture and storage, Int. J. Greenh. Gas Control, 2013, 13, 78–86 CrossRef CAS.
- C. Okezue and D. Kuvshinov, A comprehensive study of the effect of chemical impurities on selection and sizing of centrifugal machines for supercritical carbon dioxide transport pipelines, Appl. Energy, 2018, 230, 816–835 CrossRef CAS.
-
P. N. Seevam, J. M. Race, M. J. Downie and P. Hopkins, Transporting the Next Generation of CO2 for Carbon, Capture and Storage: The Impact of Impurities on Supercritical CO2 Pipelines. in 2008 7th International Pipeline Conference, ASME, 2009, vol 1.
- G. Skaugen, S. Roussanaly, J. Jakobsen and A. Brunsvold, Techno-economic evaluation of the effects of impurities on conditioning and transport of CO2 by pipeline, Int. J. Greenh. Gas Control, 2016, 54, 627–639 CrossRef CAS.
- S. H. Jeon and M. S. Kim, Effects of impurities on re-liquefaction system of liquefied CO2 transport ship for CCS, Int. J. Greenh. Gas Control, 2015, 43, 225–232 CrossRef CAS.
- S. T. Munkejord, M. Hammer and S. W. Løvseth, CO2 transport: Data and models – A review, Appl. Energy, 2016, 169, 499–523 CrossRef CAS.
- M. Bui, C. Adjiman, A. Bardow, E. J. Anthony, A. Boston, S. Brown, P. S. Fennell, S. Fuss, G. Amparo, L. A. Hacket, J. P. Hallet, H. J. Herzog, G. Jackson, J. Kemper, S. Krevor, G. C. Maitland, M. Matuszewski, I. S. Metcalfe, C. Petit, G. Puxty, J. Reimer, D. M. Reiner, E. S. Rubin, S. A. Scott, N. Shah, B. Smit, J. P. M. Trusler, P. Webley, J. Wilcox and N. Mac Dowell, Carbon capture and storage (CCS): the way forward, Energy Environ. Sci., 2018, 11, 1062–1176 RSC.
- C. Zahasky and S. Krevor, Global geologic carbon storage requirements of climate change mitigation scenarios, Energy Environ. Sci., 2020, 13, 1561–1567 RSC.
- G. A. Torín-Ollarves and J. P. M. Trusler, Solubility of hydrogen in sodium chloride brine at high pressures, Fluid Phase Equilib., 2020, 539, 113025 CrossRef.
- S. P. Gregory, M. J. Barnett, L. P. Field and A. E. Milodowski, Subsurface microbial hydrogen cycling: Natural occurrence and implications for industry, Microorganisms, 2019, 7(2), 53 CrossRef CAS PubMed.
- Hydrogen Europe, Clean Hydrogen Monitor 2020, Hydrogen Europe, 2020.
- H2tools, Hydrogen pipelines, Available at: https://h2tools.org/hyarc/hydrogen-data/hydrogen-pipelines. (Accessed: 29th June 2021).
- M. C. Slattery, E. Lantz and B. L. Johnson, State and local economic impacts from wind energy projects: texas case study, Energy Policy, 2011, 39, 7930–7940 CrossRef.
-
International Energy Agency, The Future of Hydrogen, 2019 Search PubMed.
- C. J. Quarton and S. Samsatli, Should we inject hydrogen into gas grids? Practicalities and whole-system value chain optimisation, Appl. Energy, 2020, 275, 115172 CrossRef CAS.
- J. Wahl and J. Kallo, Quantitative valuation of hydrogen blending in European gas grids and its impact on the combustion process of large-bore gas engines, Int. J. Hydrogen Energy, 2020, 45, 32534–32546 CrossRef CAS.
- Ademe, GRHYD, 2021. Available at: https://grhyd.fr/mise-oeuvre-taux-variable-dhydrogene/. (Accessed: 17th May 2021).
-
M. Melaina, O. Antonia and M. Penev, Blending Hydrogen into Natural Gas Pipeline Networks: A Review of Key Issues, 2013. DOI:10.2172/1068610.
- T. Isaac, HyDeploy: The UK's First Hydrogen Blending Deployment Project, Clean Energy, 2019, 3, 114–125 CrossRef.
- M. Jansen,
et al., Offshore wind competitiveness in mature markets without subsidy, Nat. Energy, 2020, 5, 614–622 CrossRef.
-
A. Spyroudi
et al.
, Offshore Wind and Hydrogen: Solving the Integration Challenge, 2020 Search PubMed.
- Climate Change Committee, The Sixth Carbon Budget: The UK's path to Net Zero. Committee on Climate Change, 2020.
- E. Energy, Industrial Fuel Switching Market Engagement Study - Final report for Business, Energy Industrial Strategy, 2018 Search PubMed.
- A. Al-Qahtani, B. Parkinson, K. Hellgardt, N. Shah and G. Guillen-Gosalbez, Uncovering the true cost of hydrogen production routes using life cycle monetisation, Appl. Energy, 2021, 281, 115958 CrossRef CAS.
- P. Gabrielli, F. Charbonnier, A. Guidolin and M. Mazzotti, Enabling low-carbon hydrogen supply chains through use of biomass and carbon capture and storage: a Swiss case study, Appl. Energy, 2020, 275, 115245 CrossRef CAS.
-
IEAGHG. Understanding the Cost of Retrofitting CO2capture in an Integrated Oil Refinery, 2017.
- ACER & CEER, When and How to Regulate Hydrogen Networks?, 2021.
- IEA, Hydrogen in North-Western Europe - a vision towards 2030, 2021.
- European Commission, A hydrogen strategy for a climate-neutral Europe. Paper Knowledge. Toward a Media History of Documents, 2020.
-
K. Ohlig and L. Decker, The latest developments and outlook for hydrogen liquefaction technology. in AIP Conference Proceedings, American Institute of Physics Inc., 1573, pp. 1311–1317, 2014 Search PubMed.
-
S. Hawkins, Technological Characterisation of Hydrogen Storage and Distribution Technologies UKSHEC Social Science Working Paper No. 21, 2006.
- A. Demirbaş, Fuel properties of hydrogen, liquefied petroleum gas (LPG), and compressed natural gas (CNG) for transportation, Energy Sources, 2002, 24, 601–610 CrossRef.
-
P. E. Dodds and W. Mcdowall, A review of hydrogen delivery technologies for energy system models, 2012.
- Energy Information Administration (EIA), Few transportation fuels surpass the energy densities of gasoline and diesel, Today in Energy, 2013.
- M. Pudukudy, Z. Yaakob, M. Mohammad, B. Narayanan and K. Sopian, Renewable hydrogen economy in Asia – Opportunities and challenges: an overview, Renewable Sustainable Energy Rev., 2014, 30, 743–757 CrossRef.
- P. E. Dodds and S. Demoullin, Conversion of the UK gas system to transport hydrogen, Int. J. Hydrogen Energy, 2013, 38, 7189–7200 CrossRef CAS.
-
O. Florrison, I. Alliat, B. Lowesmith and G. Hankinson, The value of the existing natural gas system for hydrogen, the sustainable future energy carrier (progress obtained in the NATURALHY-project). in 23rd World Gas Conference, Amsterdam, 2006.
-
P. Castello, E. Tzimas, P. Moretto and S. D. Peteves, Techno-economic assessment of hydrogen transmission and distribution systems in Europe in the medium and long term (Technical Report), 2005.
- B. C. C. van der Zwaan, K. Schoots, R. Rivera-Tinoco and G. P. J. Verbong, The cost of pipelining climate change mitigation: An overview of the economics of CH4, CO2 and H2 transportation, Appl. Energy, 2011, 88, 3821–3831 CrossRef.
-
M. Haines, E. Polman and J. Delaat, Reduction of CO2 emissions by addition of hydrogen to natural gas. Greenhouse Gas Control Technologies, Elsevier, 2005, 7, pp. 337–345. DOI:10.1016/B978-008044704-9/50035-5.
-
H. Iskov, Field test of hydrogen in the natural gas grid, 2010 Search PubMed.
- D. Haeseldonckx and W. D’haeseleer, The use of the natural-gas pipeline infrastructure for hydrogen transport in a changing market structure, Int. J. Hydrogen Energy, 2007, 32, 1381–1386 CrossRef CAS.
-
V. Tietze and S. Luhr, Near-Surface Bulk Storage of Hydrogen. Transition to Renewable Energy Systems, Wiley-VCH Verlag GmbH & Co. KGaA, 2013, pp. 659–690. DOI:10.1002/9783527673872.ch32.
-
O. Kruck, R. Prelicz and T. Rudolph, Assessment of the potential, the actors and relevant business cases for large scale and seasonal storage of renewable electricity by hydrogen under- ground storage in Europe, 2014.
- A. Antenucci and G. Sansavini, Extensive CO2 recycling in power systems via Power-to-Gas and network storage, Renewable Sustainable Energy Rev., 2019, 100, 33–43 CrossRef CAS.
- A. S. Lord, P. H. Kobos and D. J. Borns, Geologic storage of hydrogen: Scaling up to meet city transportation demands, Int. J. Hydrogen Energy, 2014, 39, 15570–15582 CrossRef CAS.
- B. V. Mathiesen,
et al., Smart Energy Systems for coherent 100% renewable energy and transport solutions, Appl. Energy, 2015, 145, 139–154 CrossRef.
- G. Krajačić,
et al., Planning for a 100% independent energy system based on smart energy storage for integration of renewables and CO2 emissions reduction, Appl. Therm. Eng., 2011, 31, 2073–2083 CrossRef.
- B. Sørensen, A renewable energy and hydrogen scenario for northern Europe, Int. J. Energy Res., 2008, 32, 471–500 CrossRef.
- M. Robinius,
et al., Linking the Power and Transport Sectors—Part 1: The Principle of Sector Coupling, Energies, 2017, 10, 956 CrossRef.
- M. Robinius,
et al., Linking the Power and Transport Sectors—Part 2: Modelling a Sector Coupling Scenario for Germany, Energies, 2017, 10, 957 CrossRef.
- L. Welder,
et al., Spatio-temporal optimization of a future energy system for power-to-hydrogen applications in Germany, Energy, 2018, 158, 1130–1149 CrossRef.
- G. Guandalini, M. Robinius, T. Grube, S. Campanari and D. Stolten, Long-term power-to-gas potential from wind and solar power: A country analysis for Italy, Int. J. Hydrogen Energy, 2017, 42, 13389–13406 CrossRef CAS.
- S. Samsatli, I. Staffell and N. J. Samsatli, Optimal design and operation of integrated wind-hydrogen-electricity networks for decarbonising the domestic transport sector in Great Britain, Int. J. Hydrogen Energy, 2016, 41, 447–475 CrossRef CAS.
-
E. W. Lemmon, M. O. McLinden and D. G. Friend, ‘Thermophysical Properties of Fluid Systems’ in NIST Chemistry WebBook, Reference Database Number 69. NIST Chemistry WebBook, Reference Database Number 69, 2013. Available at: https://webbook.nist.gov/chemistry/fluid/.
-
I. Walker, B. Madden and T. Foaad, Hydrogen supply chain evidence base, 2018.
- S. Sklavounos and F. Rigas, Estimation of safety distances in the vicinity of fuel gas pipelines, J. Loss Prev. Process Ind, 2006, 19, 24–31 CrossRef.
- E.ON. Unique project in Germany: Natural gas pipeline is converted to pure hydrogen, 2020.
- European Commission. Proposal for a directive of the European Parliament and of the Council on common rules for the internal markets in renewable and natural gases and in hydrogen. (European Commission, 2021).
- European Commission. Proposal for a Regulation of the European Parliament and of the Council on the internal markets for renewable and natural gases and for hydrogen (recast), 2021.
- C. Banet, Hydrogen and CCUS Compatible Gas Networks: Identifying Legal Principles for Gas Market Re-Design, OGEL, 2021, 19(2) Search PubMed.
- European Parliament. REGULATION (EU) 2018/1999 OF THE EUROPEAN PARLIAMENT AND OF THE COUNCIL. art 1.1 (European Parliament, 2018).
- European Parliament. REGULATION (EU) 2018/1999 OF THE EUROPEAN PARLIAMENT AND OF THE COUNCIL. art. 3.3(b) (European Parliament, 2018).
- The European Parliament and the Council of the European Union. DIRECTIVE 2009/73/EC OF THE EUROPEAN PARLIAMENT AND OF THE COUNCIL of 13 July 2009 concerning common rules for the internal market in natural gas and repealing Directive 2003/55/EC. (European Parliament, 2009).
- The European Parliament and the Council of the European Union. REGULATION (EU) No 347/2013 OF THE EUROPEAN PARLIAMENT AND OF THE COUNCIL of 17 April 2013 on guidelines for trans-European energy infrastructure. (European Parliament, 2013).
- The European Parliament and the Council of the European Union. DIRECTIVE (EU) 2018/2001 OF THE EUROPEAN PARLIAMENT AND OF THE COUNCIL of 11 December 2018 on the promotion of the use of energy from renewable sources (recast). (European Parliament, 2018).
- A. Kotlowski, Third-Party Access Rights in the Energy Sector: A Competition Law Perspective, Util. law Rev, 2007, 16, 101 Search PubMed.
-
J. P. Stern, Narratives for Natural Gas in Decarbonising European Energy Markets. 2019 Search PubMed.
-
J. Stern, The future of gas in decarbonising European energy markets – the need for a new approach. Oxfort Institute of Energy Studies, 2017.
-
J. Bryson, A. Pike, C. Walsh and T. Foxon, Infrastructure Business Models (IBM) Working Paper. Infrastructure Business Models (IBM), 2014.
-
W. Goldthorpe and L. Avignon, D3.3.3 Interim report detailing the development of business models and commercial structures Actual, 2020.
-
J. Andreas, W. Goldthorpe and L. Avignon, Deliverable Nr. D5.6.1 Commercial methodologies for early CO2 cluster development and expansion, 2020.
-
W. Goldthorpe and L. Avignon, A Systems Approach to Business Models and Public-private Risk Sharing for Large Scale CCS Deployment. in 15th International Conference on Greenhouse Gas Control Technologies, GHGT-15 15th 18th March 2021 Abu Dhabi, UAE 9 (SSRN Electronic Journal, 2021). DOI:10.2139/ssrn.3816435.
- Commission of the European communities. The Just Transition Mechanism: making sure no one is left behind. Available at: https://ec.europa.eu/info/strategy/priorities-2019-2024/european-green-deal/actions-being-taken-eu/just-transition-mechanism_en. (Accessed: 12th January 2021).
- BEIS. Carbon Capture, Usage and Storage An update on business models for Carbon Capture, Usage and Storage, 2020.
- World Bank Group. Public Private Partnership Legal Resource Centre (PPPLRC), 2019. Available at: https://ppp.worldbank.org/public-private-partnership/standardized-agreements-bidding-documents-and-guidance-manuals, (Accessed: 12th January 2021).
-
W. Goldthorpe and L. Avignon, D5.4.3 Report H21 Leeds and North of England risk matrix, business case template and risk reduction strategies, 2020.
- HM Government. UK Hydrogen Strategy, 2021.
Footnotes |
† Electronic supplementary information (ESI) available. See DOI: 10.1039/d1ee02118d |
‡ Current affiliation: Climeworks AG, 8050, Zürich, Switzerland. |
§ The UK department for Business, Energy and Industrial Strategy is running Hy4Heat programme, which focuses on Government support and comprehensive stimulation policies, safety, certification and appliance design.58 |
¶ See, e.g., https://energycentral.com/c/ec/what-happened-choren. |
|| https://www.porthosCOu003csubu003e2u003c/subu003e.nl/en/. |
** Electric batteries typically operate with around 0.9 round trip efficiency and a self-discharge factor in the range of 0.05–0.01% energy content per hour. Hydrogen storage in underground salt caverns has negligible, if any, loss in time but hydrogen can be lost during injection/extraction through the well casing (typical charge and discharge material efficiency is around 95%). Underground hydrogen storage in existing cavern is expected to cost around 1 € kW−1 h−1 while batteries are currently around 100–150 € kW−1 h−1. The salt cavern cost will increase, but remaining in the low range, when caverns need to be mined.63,64 |
†† The linepack flexibility is the amount of gas in the grid that can be managed flexibly by varying the grid pressure within a minimum and maximum value; as such, it is particularly suited to accommodate short-lived imbalances between demand and supply. While the linepack flexibility is an inherent property of the grid, changing its magnitude implies changing the grid design, and therefore comes at high cost. On the other hand, salt caverns need to be operated so that the pressure gradient between the wall and the cavern never puts the wall integrity at risks. As such, the cavern fits better medium and long-term storage where changes in the cavern-wall pressure gradient are limited. |
‡‡ The Low Carbon Contracts Company is a limited company owned by the UK Government with the function of being the public sector manager of, and counterparty on, contracts for difference entered into with electricity power generators. |
|
This journal is © The Royal Society of Chemistry 2022 |
Click here to see how this site uses Cookies. View our privacy policy here.