DOI:
10.1039/D1RA01947C
(Review Article)
RSC Adv., 2021,
11, 35392-35407
Nanotechnology-based strategies for gastric cancer imaging and treatment
Received
11th March 2021
, Accepted 4th October 2021
First published on 2nd November 2021
Abstract
Gastric cancer is the second biggest cause of cancer-related deaths worldwide. Despite the improvement in deciphering molecular mechanisms, advances of detection and imaging, implementation of prevention programs, and personalized treatment, the overall curative rate remains low. In particular, with the emergence of nanomaterials, different imaging modalities can be integrated into one single platform, and combined therapies with synergetic effects against gastric cancer were established. Moreover, the development of theranostic strategies with simultaneous diagnostic and therapeutic ability was boosted by multifunctional nanoparticles. Herein, we present a comprehensive review of major nanotechnology-based breakthroughs for gastric cancer imaging and treatment. We will describe the superiority of nanomaterials used in gastric cancer and summarize nanotechnology applications for the improvement of cancer imaging and therapeutic efficacy.
1 Introduction
Gastric cancer is one of the most common malignancies worldwide,1 with approximately 400
000 new cases in 2019.2 Despite the predicted decline in incidence, gastric cancer is still a leading cause of cancer-related deaths at present, with the fifth highest morbidity and the third highest mortality among all diagnosed malignancies.3 The high incidence and unfavorable prognosis of gastric cancer remain a public health issue, especially in East Asia.4,5 Most patients are diagnosed at the advanced stage and succumb to a worse prognosis and quality of life. The World Health Organization divided gastric cancer into papillary, tubular, mucinous, and poorly cohesive carcinomas. However, a clear biological classification scheme has not been achieved, and clinical utility is limited.6 It is urgent to translate our understanding into relevant clinical advances.
Nano platform has emerged as a promising strategy for tumor theranostics and surgery guidance due to its versatile imaging and therapeutic abilities.7,8 Based on their unique nanoscale, nanoparticles are employed as contrast agent and drug delivery carrier. For instance, ascribe to the leaky vasculature and poor lymphatic drainage of tumor tissue, nanoparticles are expected to enter the interstitium and prolong tumor retention.9 It is of great significance to deal with the obstacles such as in vivo stability and rapid clearance of contrast agent along,10 as well as low efficiency and side effects of the naked drug.11 Additionally, some nanoparticles were functionalized and modified with targeted ligands. They provide better sensitivity and high resolution and protect image payloads from self-quenching. All these properties indicate that nanoparticles can be modified in many different ways for gastric cancer diagnosis and treatment.
Nevertheless, image-guided surgery has made great strides in recent years.12,13 In parallel with the sophisticated infrastructure and prohibitive cost of traditional imaging modalities, nanoparticles with sufficient sensitivity and specificity can identify surgical margins and local metastasis in real time.14 A large number of nanoparticles sought to conquer the unfavorable prognosis of cancer were developed. Some of them were involved in clinical trials.15–19
Therefore, we review the recent progress of nanoparticles implemented in gastric cancer diagnosis and treatment to depict the nanotechnology from design to application targeted at gastric cancer. Firstly, we briefly discussed the superiority of nanomaterials involved in gastric cancer management. Secondly, the available nanomaterials for gastric cancer imaging was introduced. Moreover, functional nanomaterials constructed for different therapies and theranostics were discussed. Finally, we paid attention to the challenges and limitations and had envisioned a promising improvement in the near future.
2 Nanotechnology approaching gastric cancer
2.1 Enhanced gastric cancer targeted imaging/drug delivery
In clinical practice, traditional imaging examinations including magnetic resonance imaging (MRI), computed tomography (CT), positron emission computed tomography (PET), single-photon emission computed tomography (SPECT), and PET-CT is extensively used in gastric cancer diagnosis. However, the contrast agents are restricted to poorly targeted biodistribution, single imaging modality, rapid clearance, and other unwilling side effects. Diverse types of nanoparticles with inherent characteristics or functional modification shed light on the development of novel enhanced imaging pathways for gastric cancer diagnosis. They generally hold the same advantages consisting of real-time imaging, specific accumulation in tumor and local metastasis, superior tumor-background ratio, high sensitivity as well as high resolution.
2.1.1 Integrin receptor. In terms of gastric cancer tissue, its biomarkers have been taken into consideration in constructing new nanoparticles. Integrins are a family of transmembrane receptors taking part in cellular interaction with an extracellular matrix. αvβ3 integrin is overexpressed on the surface of several types of cancer (including gastric cancer) cells and activated endothelial cells of tumor neovasculature, while its expression level in normal cells is extremely low.20 Hence, arginine–glycine–aspartic acid (RGD) peptide, targeting αvβ3 integrin with numerous advantages of peptide implemented for biomedicals such as easy chemical synthesis, tiny size, high stability, and low immunogenicity, has been widely utilized as the positive targeting strategy in the rational design of nanoparticles for gastric cancer.21 RGD peptide-decorated nanoparticles achieved sufficiently satisfied performance beneficial from targeting tumor site.22An integrin-targeting RGD-indocyanine green (RGD-ICG) molecular probe with a surgical navigation system was developed by Cheng et al.23 With this RGD decorated nanoprobe, the sensitivity and specificity of their diagnosis method raised to 93.93% and 100% respectively, with a diagnostic index (DI) of 193.93% and a diagnostic accuracy rate of 93.93%. Furthermore, compared with the conventionally-treated control group, the minimum tumor diameter measured was 1.8 mm during the surgery and the operative time was shortened by 3.26-fold. These results showed this RGD-ICG probe not only improved the diagnostic accuracy rate for gastric cancer, shorten the operative time, but also improved the quality of the surgery for gastric cancer.
Besides, researchers still have been making efforts to figure out a high affinity and capability sequence to use in biomedical areas among various motifs and RGD, such as cRGDyk, a cyclic RGD peptide. Mao et al. fabricated chlorin e6 conjugated silk fibroin-based nanoparticles with cRGDyk as targeting ligand for drug delivery (5-fluorouracil, 5-FU) and photodynamic therapy for gastric cancer. This active tumor cell targeting nanoparticle manifested sustained release, perfect PDT potentials. In MGC-803 cells loaded mice, tumor burden was greatly reduced with excellent biocompatibility and safety.
2.1.2 HER2. Overexpressed human epithelial growth factor receptor 2 (HER2) associated with tumor invasion, metastasis, chemoresistance, and poor prognosis has been proved in numerous cancers including breast cancer, gastric cancer, and ovarian cancer.24 Its significant overexpression in gastric cancer takes a proportion of about 23%, which endows it another target spot for nanotechnology.25 Peptides with high affinity combining extracellular HER2 such as MARSGL has been reported.26 On this basis, Kanazaki et al. designed iron oxide nanoparticles conjugated with anti-HER2 moieties, which was consist of whole IgG, single fragment variable, and the above-mentioned peptide.27 Results demonstrated high affinity and specific binding to HER2 expressing cells of the nanoparticles, indicating the potential promising application as an in vivo tumor imaging probe. Other HER2 targeting strategies were also proposed in the nanotechnology realm.28–32 Christopher et al. reported dual HER2 targeting nanoparticles with trastuzumab and liposomal-encapsulated doxorubicin (MM-302) demonstrated synergistic targeting and anti-tumor activity in breast cancer and gastric cancer.33 By targeting different domains of the HER2 receptor, MM-302 and trastuzumab could simultaneously bind to HER2-overexpressing tumor cells. Moreover, this novel combination therapy that efficiently targets HER2-overexpressing cells through multiple mechanisms theoretically supported a randomized phase II clinical trial.
2.1.3 Other biomarkers. The phenomenon of increased glucose uptake in gastric carcinoma was believed to be facilitated by glucose transporters (Gluts).34 Increased expression of gluts accelerate cell proliferation and indicate higher UICC stage and inferior prognosis.35 Accordingly, Ding et al. reported a “Sweet Tooth”-oriented SN38 prodrug delivery nanoplatform for targeted gastric cancer therapy.36 Various biomarkers have been utilized for targeted delivery. Increased expression level of carcinoembryonic antigen (CEA) in gastric cancer inspired its antibody conjugated liposomes.37,38 Iinuma et al. developed CEA-targeted adenovirus-mediated gene delivery.39 A cisplatin-encapsulated transferrin–polyethylene glycol liposome were utilized for peritoneal dissemination of gastric cancer.40 As a human monoclonal antibody, GAH positively reacts to >90% cancerous stomach tissues, but negatively to all normal tissues. In a phase I and pharmacokinetic study, Matsumura et al. reported that an immunoliposome-encapsulated doxorubicin showed superior cytotoxic activity against several GC cell lines and the toxicities were mild.41 These strategies optimized drug distribution and targeted delivery.
2.1.4 Tumor microenvironment. Apart from overexpressed biomarkers, tumor microenvironment was another vital aspect taken into consideration, in which the cellular metabolism, acidic environment, and concentration of some biological substances are significantly different from normal tissue. Therefore, environmental response nanoparticles which can respond to specific tumor microenvironment was extensively developed and utilized in cancer diagnosis and treatment. Glutathione (GSH) is a natural agent with the ability to reduce disulfide bonds. More importantly, it is indispensable in regulating cell growth as well as maintaining redox homeostasis with a 4-fold higher concentration in tumors than that in normal cells.42,43 Additionally, GSH concentration is at sub-millimole levels intracellularly but at micromole levels extracellular.44 In this scenario, stimuli-responsive nanoplatforms towards GSH for tailorable drug-releasing or therapeutic payloads delivery became a possible pathway to improve the efficacy for gastric tumors with low toxicity to normal tissue. Shi et al. synthesized RGD-decorated polyethylene glycol (PEG)–paclitaxel (PTX) conjugates with disulfide linkage.45 These amphiphilic PEG–PTX conjugates assembled into micelles and would be decomposed under the reduction of GSH and ultimately release PTX in weakly acidic conditions of the intracellular environment. It was proved that the release of PTX from the micelles in response to GSH, and the micelles could be delivered to the tumor site and inhibit the tumor growth efficiently with low side effects. Zhang et al. reported a sequential catalytic platform based on Au/Pt star targeting tumor microenvironment containing a GSH-sensitive disulfide bond.46 In xenografted murine mode, the probe specifically targeted the tumor sites, then the disulfide linker was cleaved by GSH and the IR780 molecules could be released for both photo-thermal therapy and photodynamic therapy. Later, glucose oxidase loaded in the probe effectively catalyzed intracellular glucose in the manner of consuming oxygen to generate H2O2 and enhance the local acidity, then the Pt layer exhibited peroxidase-like property and catalyzed H2O2 producing toxic ·OH for tumor oxidative damage. GSH-sensitive release and real-time imaging ability were simultaneously possessed in this nano-platform. Novel molecular target pots and relevant strategies are emerging and paving the way for personal therapy (Fig. 1).47,48
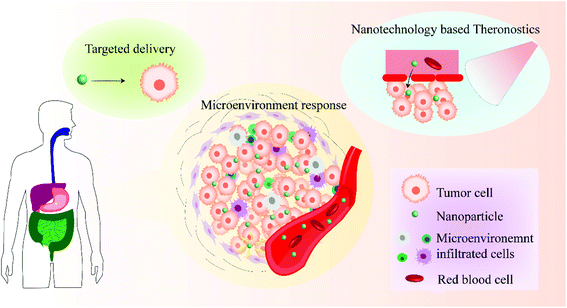 |
| Fig. 1 Nanoparticles designed for gastric cancer theronostics. | |
2.2 Enhanced multi-level gastric cancer management
When it comes to gastric cancer treatment, nanotechnology likewise revealed more and more its versatility even at different stages. The majority of patients with gastric cancer who diagnosed at an advanced stage resulted in a dismal prognosis, while nanotechnology serves as the potential solution to address this issue. Nanoparticles with high spatial resolution can realize an early diagnosis and precise therapy at the millimeter level.49 By taking advantage of nanoparticles, the evaluation manifested that visible or microtumor peritoneal metastasis lesions could also be detected, which enhanced the survival rate.50
2.2.1 Early diagnosis. Furthermore, because early gastric cancer is not manually palpable owing to its shallow invasion depth. Deciding an adequate gastric resection range by localizing techniques as well as surgeon's experience and visual/tactile cues is inevitable. Wang et al. evaluated the imaging efficacy of charcoal nanoparticles during laparoscopic surgery for gastrointestinal cancer.51 The nanoparticles served as a tattoo agent and located tumors within a short time. Subsequent pathologic examination revealed that tumors in all removed tissues, which indicated nanotechnology contributed to a precise removal of gastric cancer.
2.2.2 Lymph node metastasis detection. Lymph node metastasis is another important reason for disappointing prognosis and low survival rate.52 Lymph-bored metastasis could occupy over half gastric cancer metastasis, to which radiotherapy and regional lymph nodes dissection serve as the main strategy. Consequently, thoroughness of regional lymph node dissection is of significant importance for survival improvement. However, due to the limited time available during surgery, detailed pathological examinations including multisection and immunohistochemical were not always performed.53 Nanoparticles detecting lymph node metastasis were reported by Shi et al.54 The resulting strong fluorescence and CT contrast in metastatic lymph nodes compared to normal lymph nodes can precisely differentiate lymph node metastasis of gastric cancer.There are several other reasons for taking advantage of nanoparticles in countering gastric cancer. Nanoparticles could be used in detecting surgical margins while a negative surgical margin was associated with decreasing rates of local recurrence and improving survival. And nanotechnology does not interfere with the surgical workflow. These characteristics indicate the brilliant prospect for nanoparticles to fulfill immense potential in gastric cancer diagnosis and treatment.
2.3 Administration of nanomedicines
Most nanomedicines were administrated systemically. Because they can specifically located at tumor site for diagnosis and therapy with high bioavailability. There are also novel modes of administration, such as percutaneous microneedle and in situ hydrogel injection. In consideration of hydrochloric acid in gastric juice, oral administration is difficult. Nevertheless, an in vivo activatable pH-responsive graphitic nanozyme for selective treatment of H. pylori was reported. In clinical practice, surgeons inject tracer (e.g., ICG) by endoscopy preoperatively or intraoperatively for precise tumor resection and lymph node dissection. However, in preclinical study, experimental animals are not suitable for endoscopy.
3 Nanoparticles designed for the imaging of gastric cancer
3.1 Fluorescence imaging
As a remarkable imaging modality, fluorescence imaging holds prominent merits such as excellent sensitivity, real-time mode, fast imaging, flexible instrument, high safety, and low cost.55 The near-infrared (NIR) window from 700 to 1300 nm is superior to the visible wavelength. Imaging with light below 700 nm is strongly absorbed by hemoglobin as well as other endogenous molecules, and scattering in human tissues. Imaging with light above 1300 nm is retarded by the lipid and water absorbance.56 Among the NIR fluorophores, indocyanine green (ICG), which has been approved by the US Food and Drug Administration (FDA), thanks to its relatively lower tissue absorption and higher quantum yield.57 It is the first adoption for in vivo imaging in the human body.58 Ding et al. reported the PEGylated liposome-encapsulated ICG with RGD peptide conjugated covalently not only present higher UV absorbance spectrum and stability than free-ICG in vitro but also accumulated more in the tumor tissues of SGC7901 tumor-bearing mice.59 Hironori et al. established ICG-loaded lactosome (ICGm) nanoparticle and investigated its theranostic value using a murine draining lymph node metastasis model of gastric cancer.60 In vivo imaging revealed the metastatic lymph nodes in the ICGm-treated mice while in the ICG-treated group the metastatic lymph nodes didn't appear. Wang et al. synthesized a novel ICG conjugated gold nanoshells which effectively accumulated in both subcutaneously transplanted and peritoneal metastasis models.50 Near-infrared imaging provided sufficient optical contrast and accurate detection of both visible and microtumor lesions (<3 mm) for preoperative guidance and intraoperative resection.
Although there are other types of nanoparticles-based fluorophores developed for gastric cancer diagnosis, such as FDA approved 5-aminolevulinic acid (5-ALA) and other cyanine-based fluorophores,61 the inherent limitations cannot be ignored. Due to the fluorophores visible emission profile, imaging light scattering, and tissue penetration remain expected to be ameliorated. Indeed, the NIR window can be further divided into NIR I and NIR II. Because the magnitude of scattering is inversely proportional to the wavelength of light, NIR II fluorophores have piqued researchers' interest.62 Moreover, when imaging at gradually longer wavelengths, the quality of photon attenuation, tissue autofluorescence, and scattering are all significantly reduced.63 Several NIR II nanoparticles aimed at tumor imaging have been investigated,64 but the current application for gastric tumors is rigorously limited.
3.2 Photoacoustic imaging
Photoacoustic (PA) imaging is one of the promising imaging modalities with the ability to provide high sensitivity and resolution 2D and 3D imaging.65 In PA imaging, non-ionizing pulse lasers directly irradiate toward biological tissue, and the energy is absorbed by exogenous nanoparticles or endogenous molecules such as hemoglobin. The energy converts into heat and confers on the resultant thermal expansion, which is related to the physiological properties of the tissue and the generated ultrasonic waves are measured by an ultrasound transducer. These results are ultimately analyzed and reconstructed as PA images.
Wang et al. coated RGD-conjugated silica on the surface of carbon nanotubes to gastric cancer-bearing nude mice models.66 Observed by an optoacoustic imaging system, results showed the nanotubes targeted gastric cancer cells in vivo and obtained strong photoacoustic imaging in the nude model. Kengo et al. designed iron oxide nanoparticles conjugated with anti-HER2 moieties for PA tumor imaging.27 The nanoparticles selectively visualized HER2-positive tumors in PA imaging studies and are a potential PA contrast agent for gastric cancer imaging. Liang et al. prepared gold nanostars-based PEGylated nanoprobes with conjugated CD44v6 monoclonal antibodies as the targeting ligands.67 PA imaging revealed that the nanoprobes could target gastric cancer vascular system actively at 4 h post-injection. Yang et al. packaged the novel photosensitizers (IR780) and metformin in PEG-PCL liposomes, whose corresponding 3D imaging video demonstrated that nanoparticles could reach capillary vessels around tumor tissues over time, depict the tumor vasculature through PA imaging, and further monitor the treatment effect more clearly.68
3.3 Computed tomography
CT is a widely used non-radioactive examination for tumor diagnosis. Though safe and mature imaging technologies were achieved in the clinic, poor targeting and dissatisfied sensitivity remain the main limitation. Nanotechnology is regarded as a glorious improvement strategy. Zhang et al. conducted a clinical study and used contrast-enhanced CT with targeting nanoparticles contrast agent to diagnose early-stage gastric cancer.69 Compared with single CT detection, contrast-enhanced CT with targeting nanoparticles improves not only the accuracy of CT but also the diagnostic confidence in patients with suspected gastric cancer. Similar results were reported in esophageal cancer.70
3.4 Magnetic resonance imaging
Magnetic resonance imaging (MRI) is another common detection means. Among which, inorganic nanoparticles take the main place and superparamagnetic iron oxide nanoparticles (SPIONs) are dominated.71–74 Yan et al. reported MRI/optical dual-modality molecular probe.75 The nanoprobe was conjugated coupling polyethylene glycol (PEG)-modified nano-Fe3O4 with specific targeted cyclopeptide GX1 and near-infrared fluorescent dyes Cy5.5. The nanoparticles could selectively gather in gastric cancer loaded mice and the distribution model was consistent with the distribution of new vessels. Guo et al. present the development of a biological nanoprobe with high biocompatibility by bonding β-CD onto the surface of iron oxide-gold nanoclusters (Fe3O4@Au@β-CD).76 This nanoprobe could be selectively uptaken by gastric cancer cells (MGC-803) and exhibited red fluorescence in the cells. Nevertheless, it showed a low r2/r1 ratio and could be a potential T2 contrast agent for MRI. However, the limitations of SPIONs including genotoxicity should not be neglected,77 and other types of nanoparticles for MRI have been exploited. A Gd-doping CuS micellar nanoparticles conjugated with cRGD and a metalloprotease-2 (MMP-2) – cleavable fluorescent substrate was reported by Shi et al.78 In response to MMP-2, this nanoprobe displays a high r1 relaxivity (∼60.0 mM−1 s−1 per Gd3+ at 1 T) and a large NIR fluorescence turn-on ratio (∼185-fold). This study highlights the versatility of designing tumor-targeting, and activatable nanoprobes with improved selectivity for gastric cancer MRI in vivo (Fig. 2).
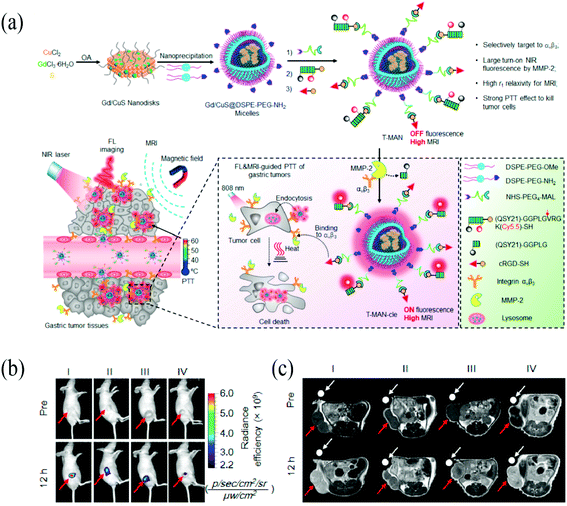 |
| Fig. 2 (a) Scheme illustration of tumor-targeting and MMP-2 activatable fluorescent nanoprobe (T-MAN), which can selectively enter and accumulate in the gastric tumors via αvβ3 integrin mediated active delivery. (b) Fluorescence image of T-MAN. (c) MR imaging of T-MAN. Reprinted with permission from ref. 78. Copyright 2019, American Chemical Society. | |
3.5 Multimodal imaging
Above mentioned imaging strategies certainly provided improvements in gastric cancer detection, while every single imaging strategy was hindered by its inbuilt limitations. However, merely single imaging technology imperative cannot reveal all desired information. By combing several imaging strategies, clinicians have access to multiple, complementary, and integrated diagnosis cues and trumpet the strengths and counteract potentially negative effects of different tools simultaneously. For instance, clinically established including MRI, CT, and PET are generally utilized preoperatively and postoperatively for high sensitivity and deep depth imaging, while real-time modalities including fluorescence imaging and PA imaging can export high contrast imaging intraoperatively though limited from a low depth of detection.
With the ability to deliver multiple payloads, nanoparticles are propitious to play the carrier role in multimodal imaging. Several nanoparticles armed with multimodal imaging potential have been explored.79,83 Compared to a single imaging device, SPECT/CT with dual validation can minimize false signals. A novel targeted nuclear imaging agent, diethylenetriaminepentaacetic acid (DTPA)/glucose-regulated protein 78 (GRP78BP)-conjugated micelles with radioisotope indium-111 (111In) showed higher radioactive signals than non-targeted 111In-labeled micelles.80 The efficiency of DTPA/GRP78BP-conjugated micelles with 111In reached 93%. Another phospholipid-shelled nanodroplets encapsulating perfluoropentane (PFP) with copper-64 (64Cu) labeled were evaluated for PET/CT and ultrasound imaging.81 These nanomaterials contribute to the diagnosis by fusing the science and technology of different imaging modalities. Shi et al. designed the mesoporous silica gadolinium-loaded gap-enhanced Raman tags for both preoperative and intraoperative imaging.82 They exhibited strong attenuation property for CT imaging, high T1 relaxivity for MRI capability and surface-enhanced Raman spectroscopy (SERS) signal with outstanding dispersity and stability, presented multi-mode imaging performance of gastric tumor. Nanoparticles are expected to make more progress in gastric tumor detection in a multimodal way (Fig. 3).
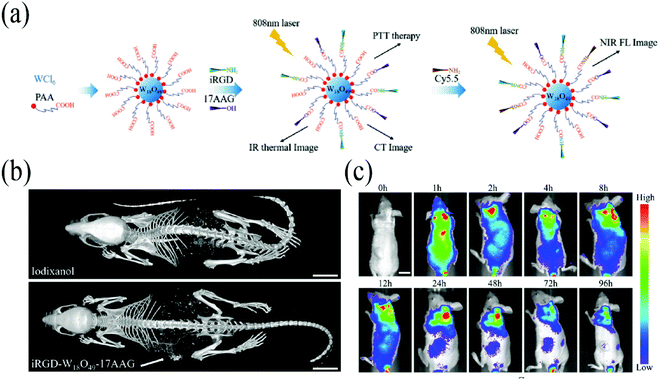 |
| Fig. 3 (a) Schematic diagram of the synthetic process of iRGD-W18O49-17AAG. (b) In vivo CT image of iodixanol and iRGD-W18O49-17AAG nanoparticles. (c) In vivo fluorescence image of iRGD-W18O49-17AAG. Reprinted with permission from ref. 83. Copyright 2019, John Wiley and Sons. | |
4 Strategies of nanoparticles in the treatment of gastric cancer
4.1 Chemotherapy
With the advantages such as reliable biocompatibility and biodegradability, nanomedicines are feasible for chemotherapeutics delivery. As a second-line therapy for locally advanced or metastatic gastric cancer, PTX is highly lipophilic and not suitable for injection. Abraxane consists of albumin nanoparticles and PTX with a particle size of approximately 130 mm. The nanoformulation maintains treatment benefits and decreases the toxicities simultaneously. Moreover, aside from the EPR effect, by transendothelial transport via albumin-binding protein, nanoparticles accumulate more in tumor tissue.84 Abraxane has been approved by FDA for several cancers including metastasis breast cancer, lung cancer, and pancreatic cancer, and clinical trials for the efficacy and safety in gastric cancer therapy were conducted, showing promising activity and manageable toxicities.85 Shi et al. reported a novel type of PTX nanoparticles with RGD-decorated and disulfide linkage, endowing the polymer–PTX with active target and environment response ability.45 In vivo results indicated that the nanoparticles were delivered to the tumor site, inhibited the tumor growth efficiently by releasing PTX, and exhibited low side effects. Tetrandrine (Tet) is a bisbenzylisoquinoline alkaloid with potential antitumor effects in several cancers and proved to enhance the antitumor activity of PTX in gastric cancer synergistically. Li et al. encapsulated Tet into self-assemble PTX nanofibers and acquired novel PTX and Tet co-loaded nanofibers.22 The self-assembled nanofibers depicted an improvement of mitochondrial apoptosis level and a strong antitumor effect both in vitro and in vivo, enhancing the clinical efficacy and reducing the side effect of Ptx in gastric cancer.
As one of the first-line gastric cancer antineoplastics, 5-fluorouracil (5-FU) is a fluorinated pyrimidine uracil analog with the cytotoxicity related to thymidylate synthase binding and inhibition. In the studies of Elisabete et al.86 the nanoparticles co-loaded with 5-FU and PTX were surface-functionalized with a monoclonal antibody targeting a glycan mediating hematogenous metastasis named sialyl-Lewis A. As a result, this nano-vehicle was successfully developed to deliver 5-FU and PTX therapeutic agents to metastatic gastric cancer cells. Collectively, nanoparticles are anticipated to establish improved therapeutics against gastric cancer.
Besides, irinotecan and doxorubicin were widely applicated in clinical practice and show single-agent activity. An irinotecan hydrochloride curcumin nanosystem showed more toxic than single components, and GC cells had more absorption and higher toxicity to nanoparticles under slightly acidic conditions.87 Zhou et al. encapsulated doxorubicin into a pH-sensitive and long circulation nanoparticle for chemo-photothermal synergistic treatment.88 The suppression of this nanosystem was significantly better than single chemotherapy or photothermal treatment. Dual-targeting hybrid nanoparticles for SN38 delivery to Her2 and CD44 overexpressed gastric cancer was reported by Yang and his colleagues.89 Upon encapsulating SN38 agent in the NPs, the anti-Her2 peptide and hyaluronic acid on the surface allow preferential delivery of the nanoparticle to gastric cancer cells. Nanoformulations facilitate chemotherapy against gastric cancer.
Resistance to chemotherapy remains a major hurdle for gastric cancer administration which attributes to tumor relapse and failure of chemotherapy.90 Based on their unique physicochemical properties originated from nanoscale size, nanoparticles are allowed to penetrate the cell membrane and accumulate more into tumor sites than routine drugs, suggesting the potential application against drug resistance.91 Azimee et al. used TiO2 nanoparticles for improving the chemotherapeutic effects of 5-FU in human AGS gastric cells.92 TiO2 nanoparticles increase reactive oxygen species (ROS) production, block autophagy flux, and subsequently induced cytotoxic and apoptotic effects of 5-FU improvement in AGS cells. By inhibiting the expression of P-glycoprotein (P-gp), Yang et al. found another way to combat drug resistance.93 The SPION functionalized with chemosensitizing agent XMD8-92 could carry anticancer drug doxorubicin (DOX) to multiple drug resistance gastric cancer cells (SCG 7901/VCR). In comparison with DOX treatment, the nanoparticles both in vitro and in vivo exhibited higher efficient tumor inhibition. Nanoparticles may pave the way to offer a possible solution for circumventing the resistance of chemotherapy in gastric cancer.
4.2 Radiotherapy
Radiotherapy producing high energy ionizing radiation may cause lethal damage in tumor tissue, inhibit microscopic tumor and regional lymph nodes extensions.94 Along with the advances in tumor imaging, nanoparticles played an immense role in radiotherapy. Compared with radiation alone, nanoparticles served as radiosensitizers could bring better therapeutic benefits.95 Zhang et al. explored the chitosan-modified gold nanoparticles (CS-GNPs) in the applications of gastric cancer cell's response to X-ray irradiation.96 MTT results revealed the biocompatibility of CS-GNPs while the survival rates under irradiation comparison with irradiation alone confirmed the enhancement of cell radiation therapeutic sensitivity, indicating the potential application in gastric cancer irradiation therapy. Likewise, Huang et al. synthesized biocompatible Ag microspheres with bovine serum albumin (BSA).97 The individual Ag microsphere composed of nanoscale Ag assemblies showed enhanced radiation effects in gastric cancer cells. Collectively, due to their high atomic number and electron densities, gold and silver nanoparticles were widely applied as radiation sensitizers to increase energy deposition into tumor site and enhance the efficacy of radiotherapy as a result.98
For advanced gastric cancer, with the feature of a high tendency of invasion and metastasis, the adjuvant chemo-radiotherapy treatment seemed to be a viable option. However, severe general toxicity retarded standard chemo-radiotherapy application in clinical practice. Therefore, the demand for better therapeutic efficacy and fewer side effects drove the development of novel therapeutic strategies. Based on the above-mentioned Abraxane and human neutrophils (NEs) with radiotherapy is present by Ju et al.99 Human NEs were developed to carry Abraxane and confirmed to maintain their intrinsic functions. In this system, modest radiation was applied to exert tumor disruption and increase the inflammatory factors released, which in turn guided the NEs homing to tumoral sites. Then, Abraxane/NEs were activated by inflammatory factors and formed neutrophil extracellular traps, leading to a burst release of Abraxane and finally induced superior tumor suppression. Nanoparticles for adjuvant chemo-radiotherapy may provide more opportunities for patients with advanced gastric cancer.
4.3 Phototherapy
Phototherapy, namely photo-triggered therapeutic modalities, holds the superiorities of selective, non-invasive, and repeatable operation and is mainly consist of photodynamic therapy (PDT) and photothermal therapy (PTT).100 Besides, photo-induced chemotherapy101,102 and photoimmunotherapy (PIT)103 also attracted significant attention in recent years. Given the hydrophobic nature of most photosensitizers which impedes their systemic administration, nanoparticles could serve as perfect carriers to deliver photosensitizers located in tumor sites and improve their biodistribution. Moreover, light could be easily tuned and focused to achieve precise treatment, which decreases damage to healthy tissue. IR780 is a widely used photosensitizer response to irradiation and generates ROS and heat. However, because of its high hydrophobicity and photosensitivity, IR780 is almost insoluble in water and needs encapsulation. Deng et al. utilized an amphiphilic macromolecular conjugate (sericin–cholesterol) with folic acid (FA) as a target ligand to encapsulate IR780. Such an amphiphilic macromolecule, which was able to form stable micelles by self-assembly, greatly raised the solubility and photo-stability of IR780. After absorbed by FA-positive gastric cancer cells (BGC-823), The FA-Ser-Chol/IR780 micelles depicted remarkable PDT and PTT cytotoxicity under laser irradiation. We previously reported IR780 and metformin packaged in PEG-PCL liposomes and exploited a step-by-step therapy.68 Upon such nanoparticles accumulated in tumor tissues and were irradiated by 808 nm laser, ROS were generated while metformin and IR780 were further released. Then metformin can directly inhibit complex I located in the mitochondrial electron transport chain. Resultant cell respiration inhibited overcame tumor hypoxia and ultimately enhanced PDT and PTT for gastric cancer. These works announced the application of nanoparticles in photosensitizers delivering and gastric cancer phototherapy assistance.
Apart from organic nanoparticles, other types of nanomaterials such as CuS nanoparticles,61,78 graphene nanoparticles,104 and gold nanoparticles,105–107 were developed for photothermal therapy. Yang et al. developed the tumor-targeting nanoparticles comprising carboxyl-group-functionalized W18O49 nanoparticles, iRGD, and HSP90-inhibitor 17AAG.83 The W18O49 nanoparticles showed excellent PTT and CT imaging contrast, in which 17AAG can inhibit the heat-shock response and overcome the thermoresistance, thus increasing the curative effect of PTT and reducing the chance of tumor recurrence. Both in vivo and in vitro, the potential of W18O49 nanoparticles in gastric cancer targeting, dual-modality imaging, and remarkable enhanced PTT is highlighted. Gold nanoparticles are highly efficient in the conversion of optical energy into heat. Zhang et al. reported the mesoporous carbon–gold hybrid nanoprobes for real-time imaging, PTT/PDT, and nanozyme oxidative therapy.106 Because of the large surface area and numerous –COOH groups, the nanoparticles were available for surface chemically modification with numerous targeting molecules and loading abundant NIR dye IR780, the carbon–gold hybrid nanoprobes revealed excellent tumor targeting efficacy, long tumor retention, and favorably therapeutic effect for gastric tumor.
4.4 Combination therapies and theranostics
Novel therapies, such as targeted therapy, gene therapy, and immunotherapy, emerged in recent years and promote anticancer treatments. Though trastuzumab and ramucirumab were approved by the FDA and European Union for targeted therapy against advanced gastric cancer, most patients do not get a benefit.108 Combination therapies may be a preferred choice for better outcomes. In a clinical trial, trastuzumab plus chemotherapy exhibited a significantly longer overall survival. The development of gene therapy in combination with anticancer drugs also provided an unprecedented opportunity to improve treatment outcomes.109 For instance, an aptamer-siRNA chimera/5-FU combined collagen membrane can specifically bind to gastric cancer cells, targeted delivered 5-FU, and silenced drug-resistant gene.110 Combining immunotherapy with chemotherapy is another attractive putative therapeutic strategy.111 TfR1 binding with H-ferritin nanocarrier may be a new option, which achieves prognostic diagnosis and enhances the therapeutic efficacy in clinical gastric cancer.112 Moreover, a combination of PTT/PDT with chemotherapy and adjuvant immunotherapy was also established and promoted strong antitumor immune responses.113
As well as multimodal imaging and combination therapy, by taking advantage of the versatility of nanoparticles, there are more and more nano-based designs of the co-delivering imaging unit and the therapeutic unit at once, which means theranostic nanoparticles. Previously, clinicians mainly detected tumor before or after therapeutic measures, while theranostic nanoparticles endow us with the power of synchronous diagnosis and treatment. Moreover, some frameworks of nanoparticles themselves armed with the abilities of imaging, such as gold nanoparticles for CT and SPIONs for MRI, were also excellent candidates for theranostic system construction. Nevertheless, most photosensitizers, such as IR780 and chlorin e6 were both possessed of imaging abilities and tumor toxicities. That is because photosensitizers can serve as the vector not only to absorb NIR and excite fluorescence but also to generate ROS and/or produce heat.114 The other type of theranostic nanoparticles is to envelop diagnosis and treatment payloads into the same nanoparticles. However, more complex structure and assembling may be credited for increasing the difficulty of quality control and additional cost. To date, there are batch to batch nano-based theranostic systems have been reported and showed their potentials in gastric cancer management (Table 1).
Table 1 Representative nanotechnology-based theranostic agents for gastric cancera
Nanoparticles |
Imaging strategy |
Therapeutic strategy |
References |
Abbreviations: PA, photoacoustic; PTT, photothermal therapy; PDT, photodynamic therapy. |
ICG-loaded lactosome |
Fluorescence |
PTT |
60 |
Carbon–gold hybrid nanoparticles |
Fluorescence |
PTT |
106 |
Ternary copper-based chalcogenide nanoplatform CuS–NiS2 nanomaterials |
MRI |
PTT/PDT |
125 |
Hyaluronidase-sensitive mesoporous silica nanoparticles |
Fluorescence |
PDT |
68 and 126 |
Chlorin e6 functionalized silk fibroin nanoparticles |
Fluorescence |
Chemotherapy |
126 and 127 |
Folic acid–sericin–cholesterol/IR780 micelles |
Fluorescence |
PTT/PDT |
114 and 127 |
IR820/paclitaxel/imiquimod/encapsulated thermosensitive liposome |
Fluorescence |
PTT/PDT/chemotherapy |
113 and 114 |
Cisplatin/ICG loaded PLGA-(DSPE-PEG2000) nanoparticles |
Fluorescence |
Chemotherapy |
113 and 128 |
Oxaliplatin–Au–Fe3O4–herceptin nanoparticles |
MRI |
Chemotherapy |
29 and 128 |
RGD-CuS-Cy5.5 nanoparticles |
CT/MRI |
PTT |
29 and 61 |
W18O49 nanoparticles |
CT/fluorescence |
PTT |
61 and 83 |
PEGPCL-IR780-MET nanoparticles |
PA/fluorescence |
PTT |
68 and 83 |
PTX-R837-IR820@TSL |
Fluorescence |
PTT/PDT/chemotherapy/Immunotherapy |
68 and 113 |
DOX-IR820 nanoparticles |
Fluorescence |
PTT/chemotherapy |
88 |
5 Challenges and prospectives
Among in excess of 50 nanomedicines approved by the FDA, few are available for gastric cancer treatment.115 A fundamental obstacle remains in the complicacy of aberrant molecule characteristics of gastric cancer patients. The world health organization divided gastric cancer into papillary, tubular, mucinous, and poorly cohesive carcinomas. However, a clear biologic classification scheme has not been achieved, and clinical utility is limited.6 Moreover, the molecular pathway of gastric cancer is still unclear. Deciphering molecular mechanisms and identifying potent biomarkers of gastric cancer are believed to promote signal background ratio and facilitate theranostic performance (Fig. 4).
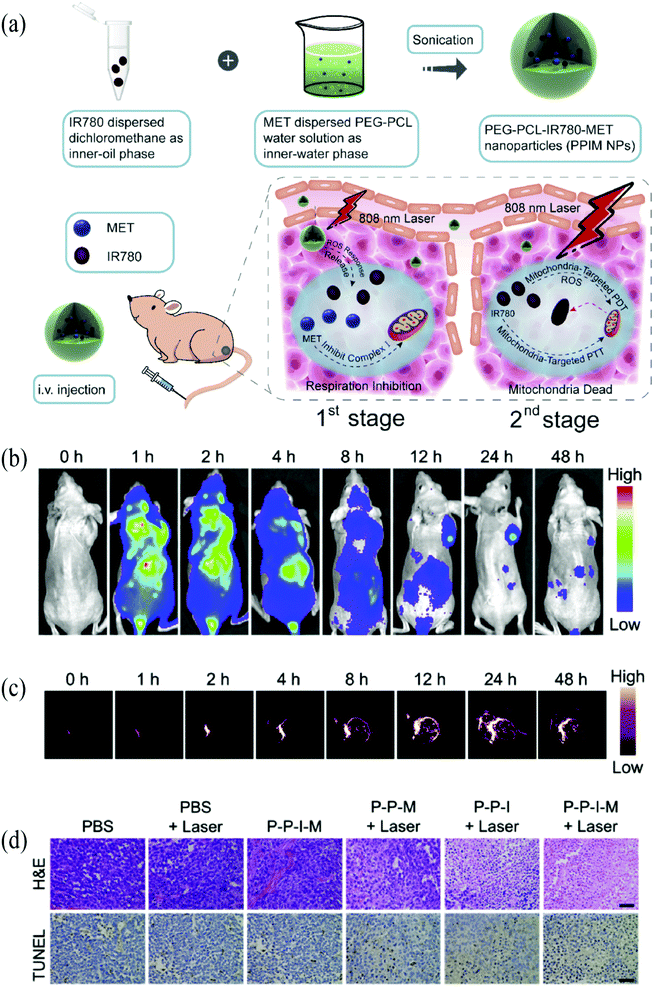 |
| Fig. 4 (a) Schematic illustration of PEG-PCL-IR780-MET NPs. (b) In vivo fluorescence image of PEG-PCL-IR780-MET NPs. (c) Photoacoustic image of PEG-PCL-IR780-MET NPs (d) H&E staining and TUNEL staining tumor sections. Copyright with permission from ref. 68. Copyright 2020 Elsevier. | |
The applications and limitations of available nanoparticles are as above-mentioned. Limited tissue penetration depth retarded the utility of NIR dyes. Signal of quantum dots (QDs) can detect in deeper depth though, the biocompatibility and safety are worried. Some strategies have been proposed to pave the way for better performance. Multiplexed NIR-II probes, consists of donor–acceptor–donor (DAD) dyes and NIR-II imaging probes, were reported by Rui et al. and demonstrated an excellent imaging approach to guide sentinel lymph node surgery in various cancer models.116 On the one hand, NIR-II fluorescence imaging with longer wavelengths reduces scattering and tissue auto-fluorescence; on the other hand, the identical molar amount of QDs displayed superior brightness and photo-stability. This combination of dual-NIR II imaging-guided surgery can be performed under bright light and adding to its potential and convenience in clinical use. Another naked eye and fluorescence dual-mode imaging agent was synthesized for lymph node tumor metastasis diagnosis.117 However, the relevant researches approaching gastric cancer are limited.
Despite the mounting studies in this field, most of them are in vivo ones. Because of the metabolisms in the body, the peculiarities of the nanoparticles, and the high degree of tumor heterogeneity, the biodistribution and targeting ability of nanoparticles may be different in preclinical studies and clinical practice. For example, proteins including antibodies may be absorbed on the surface of nanoparticles and form the protein corona.118 Denatured proteins in the corona of nanoparticles impact the targeting and antitumor activities in vivo.119,120 Biomimetic nanoparticles are supposed to address this issue.121 Extracellular vesicles are natural nanoscale membrane vesicles. Engineered extracellular vesicles with unique physiological and biochemical properties, including prolonged retention circulation time, improved tissue and organ targeting specificity, and enhanced cytoplasmic delivery efficiency, are contributing to tumor image tracing and treatment.122 Likewise, membrane coated nanoparticles were shown to be promising nanocarriers. Combined with RGD peptide or HER-2 antibody, biomimetic nano-platforms are leading the way to conquer the off-target effect and prolong in vivo circulation.21,123
Multifunctional nanoparticles were endowed with additional capabilities such as targeting and image contrast enhancement. However, additional functionality leads to additional synthetic steps and costs. Furthermore, more complex behavior and effects in vivo and greater regulatory hurdles are concerned.124 Taken the great heterogeneity of gastric cancer, it is of significance to understand the molecular underpinnings of gastric cancer progression, and design good manufacturing practice (GMP)-grade nanoparticles.
In summary, nanotechnology is a promising strategy for early diagnosis and imaging-directed therapy against gastric cancer, one of the most common malignant tumors worldwide. Series of materials with different imaging and therapeutic capabilities were enrolled in nanoparticles design and synthesis and achieved inspiring outcomes. Though some obstacles have not been conquered and there is a long way from preclinical studies to clinical translation, comprehensive understanding and systematic view may promote rational designing and translational medical research. We believe that nano-based technology facilitates imaging and therapeutic advances and shows a bright future.
Abbreviations
MRI | Magnetic resonance imaging |
CT | Computed tomography |
PET | Positron emission computed tomography |
SPECT | Single-photon emission computed tomography |
RGD | Arginine–glycine–aspartic |
ICG | Indocyanine green |
PDT | Photodynamic therapy |
HER2 | Human epithelial growth factor receptor 2 |
GSH | Glutathione |
5-Fu | 5-Fluorouracil |
PEG | Polyethylene glycol |
PTX | Paclitaxel |
NIR | Near-infrared |
5-ALA | 5-Aminolevulinic acid |
PA | Photoacoustic |
SPION | Superparamagnetic iron oxide nanoparticle |
DTPA | Diethylenetriaminepentaacetic acid |
ROS | Reactive oxygen species |
PTT | Photothermal therapy |
PIT | Photoimmunotherapy |
Consent for publication
All authors agree to publish.
Availability of data and material
The datasets used and analyzed during the current study are available from the corresponding author on reasonable request.
Funding
This work was supported by the National Natural Science Foundation of China (81602103) and the Natural Science Foundation of Jiangsu Province (BK20200052).
Conflicts of interest
All authors have no conflict of interest.
References
- F. Bray, J. Ferlay, I. Soerjomataram, R. L. Siegel, L. A. Torre and A. Jemal, Global cancer statistics 2018: GLOBOCAN estimates of incidence and mortality worldwide for 36 cancers in 185 countries, Ca-Cancer J. Clin., 2018, 68(6), 394–424 CrossRef PubMed.
- R. L. Siegel, K. D. Miller and A. Jemal, Cancer statistics, 2019, Ca-Cancer J. Clin., 2019, 69, 7–34 CrossRef PubMed.
- M. Arnold, J. Y. Park, M. C. Camargo, N. Lunet, D. Forman and I. Soerjomataram, Is gastric cancer becoming a rare disease? A global assessment of predicted incidence trends to 2035, Gut, 2020, 69(5), 823–829 CrossRef PubMed.
- P.-A. Leake, R. Cardoso, R. Seevaratnam, L. Lourenco, L. Helyer, A. Mahar, C. Law and N. G. Coburn, A systematic review of the accuracy and indications for diagnostic laparoscopy prior to curative-intent resection of gastric cancer, Gastric Cancer, 2012, 15, S38–S47 CrossRef PubMed.
- L. Yang, Incidence and mortality of gastric cancer in China, World J. Gastroenterol., 2006, 12(1), 17–20 CrossRef PubMed.
- I. D. Nagtegaal, R. D. Odze, D. Klimstra, V. Paradis, M. Rugge, P. Schirmacher, K. M. Washington, F. Carneiro and I. A. Cree, Edito WHOCT: The 2019 WHO classification of tumours of the digestive system, Histopathology, 2020, 76(2), 182–188 CrossRef PubMed.
- C. Y. Kuo, Y. Chao and C. P. Li, Update on treatment of gastric cancer, J. Chin. Med. Assoc., 2014, 77(7), 345–353 CrossRef PubMed.
- R. Duncan and R. Gaspar, Nanomedicine(s) under the Microscope, Mol. Pharm., 2011, 8(6), 2101–2141 CrossRef CAS PubMed.
- B. Haley and E. Frenkel, Nanoparticles for drug delivery in cancer treatment, Urol. Oncol.: Semin. Orig. Invest., 2008, 26(1), 57–64 CrossRef CAS PubMed.
- S. K. Golombek, J.-N. May, B. Theek, L. Appold, N. Drude, F. Kiessling and T. Lammers, Tumor targeting via EPR: Strategies to enhance patient responses, Adv. Drug Delivery Rev., 2018, 130, 17–38 CrossRef CAS PubMed.
- W. Volmar, K. P. Tracy and M. Nicole, Nanopharmaceuticals (part 1): products on the market, Int. J. Nanomed., 2014, 9, 4357–4373 Search PubMed.
- I. S. Alam, I. Steinberg, O. Vermesh, N. S. van den Berg, E. L. Rosenthal, G. M. van Dam, V. Ntziachristos, S. S. Gambhir, S. Hernot and S. Rogalla, Emerging Intraoperative Imaging Modalities to Improve Surgical Precision, Mol. Imaging Biol., 2018, 20(5), 705–715 CrossRef PubMed.
- N. E. Wojtynek and A. M. Mohs, Image-guided tumor surgery: the emerging role of nanotechnology, Wiley Interdisciplinary Reviews Nanomedicine and Nanobiotechnology, 2020, p. e1624 Search PubMed.
- K. R. Tringale, J. Pang and Q. T. Nguyen, Image-guided surgery in cancer: a strategy to reduce incidence of positive surgical margins, Wiley Interdiscip. Rev. Nanomed., 2018, 10(3), 18 Search PubMed.
- C. Daxiang, M. Lijun, Z. Xiao and Z. Chunlei, Advances and Prospects of Nanotheranostic Technology for Gastric Cancer, Nano Biomed. Eng., 2016, 219–239 Search PubMed.
- R. T. Li, B. R. Liu and J. H. Gao, The application of nanoparticles in diagnosis and theranostics of gastric cancer, Cancer Lett., 2017, 386, 123–130 CrossRef CAS PubMed.
- Z. M. Avval, L. Malekpour, F. Raeisi, A. Babapoor, S. M. Mousavi, S. A. Hashemi and M. Salari, Introduction of magnetic and supermagnetic nanoparticles in new approach of targeting drug delivery and cancer therapy application, Drug Metab. Rev., 2020, 52(1), 157–184 CrossRef PubMed.
- Q. Y. Jia, Z. Y. Zhao, K. Liang, F. C. Nan, Y. Li, J. Wang, J. C. Ge and P. F. Wang, Recent advances and prospects of carbon dots in cancer nanotheranostics, Mater. Chem. Front., 2020, 4(2), 449–471 RSC.
- S. Tong, H. Zhu and G. Bao, Magnetic iron oxide nanoparticles for disease detection and therapy, Mater. Today, 2019, 31, 86–99 CrossRef CAS PubMed.
- C. Jin, B. N. Zhang, Z. Wei, B. Ma, Q. Pan and P. Hu, Effects of WD-3 on tumor growth and the expression of integrin αvβ3 and ERK1/2 in mice bearing human gastric cancer using the 18F-RGD PET/CT imaging system, Mol. Med. Rep., 2017, 16(6), 9295–9300 CrossRef CAS PubMed.
- M. Alipour, M. Baneshi, S. Hosseinkhani, R. Mahmoudi, A. J. Arabzadeh, M. Akrami, J. Mehrzad and H. Bardania, Recent progress in biomedical applications of RGD-based ligand: from precise cancer theranostics to biomaterial engineering: a systematic review, J. Biomed. Mater. Res., Part A, 2020, 108(4), 839–850 CrossRef CAS PubMed.
- X. L. Li, N. Yu, J. Li, J. N. Bai, D. Ding, Q. Y. Tang and H. E. Xu, Novel “Carrier-Free” Nanofiber Codelivery Systems with the Synergistic Antitumor Effect of Paclitaxel and Tetrandrine through the Enhancement of Mitochondrial Apoptosis, ACS Appl. Mater. Interfaces, 2020, 12(9), 10096–10106 CrossRef CAS PubMed.
- H. D. Cheng, C. W. Chi, W. T. Shang, R. G. W. Sha, J. X. Cui, J. Z. Ye, S. X. Jiang, Y. M. Mao, C. T. Zeng and H. P. Huo, et al., Precise integrin-targeting near-infrared imaging-guided surgical method increases surgical qualification of peritoneal carcinomatosis from gastric cancer in mice, Oncotarget, 2017, 8(4), 6258–6272 CrossRef PubMed.
- S. Lorenzen and F. Lordick, How will human epidermal growth factor receptor 2-neu data impact clinical management of gastric cancer?, Curr. Opin. Oncol., 2011, 23(4), 396–402 CrossRef CAS PubMed.
- W. Tai, R. Mahato and K. Cheng, The role of HER2 in cancer therapy and targeted drug delivery, J. Controlled Release, 2010, 146(3), 264–275 CrossRef CAS PubMed.
- M. Houimel, P. Schneider, A. Terskikh and J. P. Mach, Selection of peptides and synthesis of pentameric peptabody molecules reacting specifically with ErbB-2 receptor, Int. J. Cancer, 2001, 92(5), 748–755 CrossRef CAS PubMed.
- K. Kanazaki, K. Sano, A. Makino, Y. Shimizu, F. Yamauchi, S. Ogawa, N. Ding, T. Yano, T. Temma and M. Ono, Development of anti-HER2 fragment antibody conjugated to iron oxide nanoparticles for in vivo HER2-targeted photoacoustic tumor imaging, Nanomedicine, 2015, 11(8), 2051–2060 CrossRef CAS PubMed.
- H. Zolata, H. Afarideh and F. A. Davani, Triple Therapy of HER2(+) Cancer Using Radiolabeled Multifunctional Iron Oxide Nanoparticles and Alternating Magnetic Field, Cancer Biother.Radiopharm., 2016, 31(9), 324–329 CrossRef CAS PubMed.
- D. R. Liu, X. W. Li, C. L. Chen, C. Li, C. B. Zhou, W. D. Zhang, J. G. Zhao, J. Fan, K. Cheng and L. Chen, Target-specific delivery of oxaliplatin to HER2-positive gastric cancer cells in vivo using oxaliplatin-Au-Fe3O4-herceptin nanoparticles, Oncol. Lett., 2018, 15(5), 8079–8087 Search PubMed.
- M. Truffi, M. Colombo, L. Sorrentino, L. Pandolfi, S. Mazzucchelli, F. Pappalardo, C. Pacini, R. Allevi, A. Bonizzi and F. Corsi, et al., Multivalent exposure of trastuzumab on iron oxide nanoparticles improves antitumor potential and reduces resistance in HER2-positive breast cancer cells, Sci. Rep., 2018, 8(1), 6563 CrossRef PubMed.
- J. Peng, J. Chen, F. Xie, W. Bao, H. Xu, H. Wang, Y. Xu and Z. Du, Herceptin-conjugated paclitaxel loaded PCL-PEG
worm-like nanocrystal micelles for the combinatorial treatment of HER2-positive breast cancer, Biomaterials, 2019, 222, 119420 CrossRef CAS PubMed.
- M. Satpathy, L. Wang, R. J. Zielinski, W. Qian, Y. A. Wang, A. M. Mohs, B. A. Kairdolf, X. Ji, J. Capala and M. Lipowska, Targeted Drug Delivery and Image-Guided Therapy of Heterogeneous Ovarian Cancer Using HER2-Targeted Theranostic Nanoparticles, Theranostics, 2019, 9(3), 778–795 CrossRef CAS PubMed.
- C. W. Espelin, S. C. Leonard, E. Geretti, T. J. Wickham and B. S. Hendriks, Dual HER2 Targeting with Trastuzumab and Liposomal-Encapsulated Doxorubicin (MM-302) Demonstrates Synergistic Antitumor Activity in Breast and Gastric Cancer, Cancer Res., 2016, 76(6), 1517–1527 CrossRef CAS PubMed.
- T. Kawamura, T. Kusakabe, T. Sugino, K. Watanabe, T. Fukuda, A. Nashimoto, K. Honma and T. Suzuki, Expression of glucose transporter-1 in human gastric carcinoma: association with tumor aggressiveness, metastasis, and patient survival, Cancer, 2001, 92(3), 634–641 CrossRef CAS PubMed.
- H. Schlößer, U. Drebber, A. Urbanski, S. Haase, C. Baltin, F. Berlth, S. Neiß, M. von Bergwelt-Baildon, U. Fetzner and U. Warnecke-Eberz, et al., Glucose transporters 1, 3, 6, and 10 are expressed in gastric cancer and glucose transporter 3 is associated with UICC stage and survival, Gastric Cancer, 2017, 20(1), 83–91 CrossRef PubMed.
- N. Ding, S. Xu, S. Zheng, Q. Ye, L. Xu, S. Ling, S. Xie, W. Chen, Z. Zhang and M. Xue, et al., “Sweet tooth”-oriented SN38 prodrug delivery nanoplatform for targeted gastric cancer therapy, J. Mater. Chem. B, 2021, 9(12), 2816–2830 RSC.
- I. Uyama, K. Kumai, T. Yasuda, T. Tagawa, K. Ishibiki, M. Kitajima and T. Tadakuma, Improvement of therapeutic effect by using Fab' fragment in the treatment of carcinoembryonic antigen-positive human solid tumors with adriamycin-entrapped immunoliposomes, Jpn. J. Cancer Res., 1994, 85(4), 434–440 CrossRef CAS PubMed.
- X. Li, L. Shao, X. Lu, Z. Yang, S. Ai, F. Sun, M. Wang, W. Guan and S. Liu, Risk factors for lymph node metastasis in gastric neuroendocrine tumor: a retrospective study, BMC Surg., 2021, 21(1), 174 CrossRef CAS PubMed.
- T. Tanaka, J. Huang, S. Hirai, M. Kuroki, M. Kuroki, N. Watanabe, K. Tomihara, K. Kato and H. Hamada, Carcinoembryonic antigen-targeted selective gene therapy for gastric cancer through FZ33 fiber-modified adenovirus vectors, Clin. Cancer Res., 2006, 12(12), 3803–3813 CrossRef CAS PubMed.
- H. Iinuma, K. Maruyama, K. Okinaga, K. Sasaki, T. Sekine, O. Ishida, N. Ogiwara, K. Johkura and Y. Yonemura, Intracellular targeting therapy of cisplatin-encapsulated transferrin-polyethylene glycol liposome on peritoneal dissemination of gastric cancer, Int. J. Cancer, 2002, 99(1), 130–137 CrossRef CAS PubMed.
- Y. Matsumura, M. Gotoh, K. Muro, Y. Yamada, K. Shirao, Y. Shimada, M. Okuwa, S. Matsumoto, Y. Miyata and H. Ohkura, et al., Phase I and pharmacokinetic study of MCC-465, a doxorubicin (DXR) encapsulated in PEG immunoliposome, in patients with metastatic stomach cancer, Ann. Oncol., 2004, 15(3), 517–525 CrossRef CAS PubMed.
- C. Schlüsener, M. Xhinovci, S. J. Ernst, A. Schmitz, N. Tannert and C. Janiak, Solid-solution mixed-linker synthesis of isoreticular Al-based MOFs for an easy hydrophilicity tuning in water-sorption heat transformations, Chem. Mater., 2019, 31, 4051 CrossRef.
- P. Chao, X. Huanhuan, F. Xiushuang, X. Yuan, L. Jing and W. Erkang, Glutathione Regulated Inner Filter Effect of MnO Nanosheets on Boron Nitride Quantum Dots for Sensitive Assay, Anal. Chem., 2019, 91(9), 5762–5767 CrossRef PubMed.
- R. Cheng, F. Feng, F. Meng, C. Deng, J. Feijen and Z. Zhong, Glutathione-responsive nano-vehicles as a promising platform for targeted intracellular drug and gene delivery, J. Controlled Release, 2011, 152(1), 2–12 CrossRef CAS PubMed.
- J. W. Shi, S. P. Liu, Y. Yu, C. Y. He, L. J. Tan and Y. M. Shen, RGD peptide-decorated micelles assembled from polymer-paclitaxel conjugates towards gastric cancer therapy, Colloids Surf., B, 2019, 180, 58–67 CrossRef CAS PubMed.
- A. Zhang, Q. Zhang, G. Alfranca, S. Pan, Z. Huang, J. Cheng, Q. Ma, J. Song, Y. Pan and J. Ni, GSH-triggered sequential catalysis for tumor imaging and eradication based on star-like Au/Pt enzyme carrier system, Nano Res., 2020, 13(1), 160–172 CrossRef CAS.
- A. J. Bass, V. Thorsson, I. Shmulevich, S. M. Reynolds, M. Miller, B. Bernard, T. Hinoue, P. W. Laird, C. Curtis and H. Shen, et al., Comprehensive molecular characterization of gastric adenocarcinoma, Nature, 2014, 513(7517), 202–209 CrossRef PubMed.
- H. Liu, S. H. Shin, H. Chen, T. Liu, Z. Li, Y. Hu, F. Liu, C. Zhang, D. J. Kim and K. Liu, CDK12 and PAK2 as novel therapeutic targets for human gastric cancer, Theranostics, 2020, 10(14), 6201–6215 CrossRef CAS PubMed.
- M. Weiwei, J. Dandan, M. Shengjun, L. Shuang, L. Yongjun and Z. Na, Promoting Early Diagnosis and Precise Therapy of Hepatocellular Carcinoma by Glypican-3-Targeted Synergistic Chemo-Photothermal Theranostics, ACS Appl. Mater. Interfaces, 2019, 11(26), 23591–23604 CrossRef PubMed.
- S. H. Wang, C. W. Chi, H. D. Cheng, X. T. Pan, S. S. Li, F. R. Zhang, S. R. Gaowa, J. Z. Ye, Y. M. Mao and K. S. He, et al., Photothermal Adjunctive Cytoreductive Surgery for Treating Peritoneal Metastasis of Gastric Cancer, Small Methods, 2018, 2(4), 7 CrossRef.
- Y. Wang, J. Ma, S. Huang, R. Wang, C. S. Jiang, L. Yu, N. Lin, W. J. Yang and W. Wang, Safety and Efficacy of Preoperative Tattooing with Charcoal Nanoparticles for Laparoscopic Resection of Gastric Tumors, J. Nanosci. Nanotechnol., 2016, 16(7), 7290–7294 CrossRef CAS.
- F. Jacques, S. Isabelle, D. Rajesh, E. Sultan, M. Colin, R. Marise, D. M. Parkin, F. David and B. Freddie, Cancer incidence and mortality worldwide: sources, methods and major patterns in GLOBOCAN 2012, Int. J. Cancer, 2015, 136(5), E359–E386 CrossRef PubMed.
- S. Kamiya, H. Takeuchi, T. Nakahara, M. Niihara, R. Nakamura, T. Takahashi, N. Wada, H. Kawakubo, Y. Saikawa, T. Omori, K. Murakami and Y. Kitagawa, et al., Auxiliary diagnosis of lymph node metastasis in early gastric cancer using quantitative evaluation of sentinel node radioactivity, Gastric Cancer, 2016, 19(4), 1080–1087 CrossRef CAS PubMed.
- H. Shi, R. Yan, L. Wu, Y. Sun, S. Liu, Z. Zhou, J. He and D. Ye, Tumor-targeting CuS nanoparticles for multimodal imaging and guided photothermal therapy of lymph node metastasis, Acta Biomater., 2018, 72, 256–265 CrossRef CAS PubMed.
- H. Gao, P. Bao, S. Dai, R. Liu, S. Ji, S. Zeng, J. Shen, Q. Liu and D. Ding, Far-Red/Near-Infrared Emissive (1,3-Dimethyl)barbituric Acid-Based AIEgens for High-Contrast Detection of Metastatic Tumors in the Lung, Chem.–Asian J., 2019, 14(6), 871–876 CrossRef CAS PubMed.
- T. K. Hill and A. M. Mohs, Image-guided tumor surgery: will there be a role for fluorescent nanoparticles?, Wiley Interdiscip. Rev.: Nanomed. Nanobiotechnol., 2016, 8(4), 498–511 CAS.
- M. Aya, T. Eiichi, C. Hak Soo, H. W. Joshua, K. Vida, G. Sylvain, G. L. Rita and V. F. John, Real-time intra-operative near-infrared fluorescence identification of the extrahepatic bile ducts using clinically available contrast agents, Surgery, 2010, 148(1), 87–95 CrossRef PubMed.
- T. Ishizawa, N. Fukushima, J. Shibahara, K. Masuda, S. Tamura, T. Aoki, K. Hasegawa, Y. Beck, M. Fukayama and N. Kokudo, Real-Time Identification of Liver Cancers by Using Indocyanine Green Fluorescent Imaging, Cancer, 2009, 115(11), 2491–2504 CrossRef PubMed.
- J. Ding, M. Feng, F. Wang, H. Wang and W. X. Guan, Targeting effect of PEGylated liposomes modified with the Arg-Gly-Asp sequence on gastric cancer, Oncol. Rep., 2015, 34(4), 1825–1834 CrossRef CAS PubMed.
- H. Tsujimoto, Y. Morimoto, R. Takahata, S. Nomura, K. Yoshida, S. Hiraki, H. Horiguchi, H. Miyazaki, S. Ono and D. Saito, et al., Theranostic Photosensitive Nanoparticles for Lymph Node Metastasis of Gastric Cancer, Ann. Surg. Oncol., 2015, 22(Suppl. 3), S923–S928 CrossRef PubMed.
- H. Shi, R. Q. Yan, L. Y. Wu, Y. D. Sun, S. Liu, Z. Y. Zhou, J. He and D. J. Ye, Tumor-targeting CuS nanoparticles for multimodal imaging and guided photothermal therapy of lymph node metastasis, Acta Biomater., 2018, 72, 256–265 CrossRef CAS PubMed.
- X. J. Li, J. J. Zhou, C. R. Liu, Q. R. Xiong, H. W. Duan and P. C. K. Cheung, Stable and Biocompatible Mushroom beta-Glucan Modified Gold Nanorods for Cancer Photothermal Therapy, J. Agric. Food Chem., 2017, 65(43), 9529–9536 CrossRef CAS PubMed.
- S. J. Zhu, R. Tian, A. L. Antaris, X. Y. Chen and H. J. Dai, Near-Infrared-II Molecular Dyes for Cancer Imaging and Surgery, Adv. Mater., 2019, 31(24), 25 Search PubMed.
- R. Tian, H. L. Ma, Q. L. Yang, H. Wan, S. J. Zhu, S. Chandra, H. T. Sun, D. O. Kiesewetter, G. Niu and Y. Y. Liang, Rational design of a super-contrast NIR-II fluorophore affords high-performance NIR-II molecular imaging guided microsurgery, Chem. Sci., 2019, 10(1), 326–332 RSC.
- G. Ku and L. H. V. Wang, Deeply penetrating photoacoustic tomography in biological tissues enhanced with an optical contrast agent, Opt. Lett., 2005, 30(5), 507–509 CrossRef PubMed.
- C. Wang, C. C. Bao, S. J. Liang, H. L. Fu, K. Wang, M. Deng, Q. D. Liao and D. X. Cui, RGD-conjugated silica-coated gold nanorods on the surface of carbon nanotubes for targeted photoacoustic imaging of gastric cancer, Nanoscale Res. Lett., 2014, 9, 10 CrossRef PubMed.
- S. J. Liang, C. Li, C. L. Zhang, Y. S. Chen, L. Xu, C. C. Bao, X. Y. Wang, G. Liu, F. C. Zhang and D. X. Cui, CD44v6 Monoclonal Antibody-Conjugated Gold Nanostars for Targeted Photoacoustic Imaging and Plasmonic Photothermal Therapy of Gastric Cancer Stem-like Cells, Theranostics, 2015, 5(9), 970–984 CrossRef CAS PubMed.
- Z. Yang, J. Wang, S. Liu, X. Li, L. Miao, B. Yang, C. Zhang, J. He, S. Ai and W. Guan, Defeating relapsed and refractory malignancies through a nano-enabled mitochondria-mediated respiratory inhibition and damage pathway, Biomaterials, 2020, 229, 119580 CrossRef CAS PubMed.
- K. M. Zhang, X. J. Du, K. H. Yu, K. Y. Zhang and Y. C. Zhou, Application of novel targeting nanoparticles contrast agent combined with contrast-enhanced computed tomography during screening for early-phase gastric carcinoma, Exp. Ther. Med., 2018, 15(1), 47–54 CrossRef CAS PubMed.
- J. J. Gai, Z. L. Gao, L. Q. Song, Y. Y. Xu, W. X. Liu and C. X. Zhao, Contrast-enhanced computed tomography combined with Chitosan-Fe3O4 nanoparticles targeting fibroblast growth factor receptor and vascular endothelial growth factor receptor in the screening of early esophageal cancer, Exp. Ther. Med., 2018, 15(6), 5344–5352 Search PubMed.
- Z. Bakhtiary, A. A. Saei, M. J. Hajipour, M. Raoufi, O. Vermesh and M. Mahmoudi, Targeted superparamagnetic iron oxide nanoparticles for early detection of cancer: Possibilities and challenges, Nanomedicine, 2016, 12(2), 287–307 CrossRef CAS PubMed.
- P. Wang, Y. Z. Qu, C. Li, L. Yin, C. F. Shen, W. Chen, S. M. Yang, X. W. Bian and D. C. Fang, Bio-functionalized dense-silica nanoparticles for MR/NIRF imaging of CD146 in gastric cancer, Int. J. Nanomed., 2015, 10, 15 Search PubMed.
- Y. T. Chen, G. D. Lian, C. D. Liao, W. W. Wang, L. J. Zeng, C. C. Qian, K. H. Huang and X. T. Shuai, Characterization of polyethylene glycol-grafted polyethylenimine and superparamagnetic iron oxide nanoparticles (PEG-g-PEI-SPION) as an MRI-visible vector for siRNA delivery in gastric cancer in vitro and in vivo, J. Gastroenterol., 2013, 48(7), 809–821 CrossRef CAS PubMed.
- R. Hachani, M. A. Birchall, M. W. Lowdell, G. Kasparis, L. D. Tung, B. B Manshian, S. J. Soenen, W. Gsell, U. Himmelreich, C. A. Gharagouzloo, S. Sridhar and N. T. K. Thanh, et al., Assessing cell-nanoparticle interactions by high content imaging of biocompatible iron oxide nanoparticles as potential contrast agents for magnetic resonance imaging, Sci. Rep., 2017, 7(1), 7850 CrossRef PubMed.
- X. J. Yan, X. Y. Song and Z. B. Wang, Construction of specific magnetic resonance imaging/optical dual-modality molecular probe used for imaging angiogenesis of gastric cancer, Artif. Cells, Nanomed., Biotechnol., 2017, 45(3), 399–403 CrossRef CAS PubMed.
- H. E. Guo, Y. X. Zhang, W. T. Liang, F. F. Tai, Q. C. Dong, R. P. Zhang, B. F. Yu and W. Y. Wong, An inorganic magnetic fluorescent nanoprobe with favorable biocompatibility for dual-modality bioimaging and drug delivery, J. Inorg. Biochem., 2019, 192, 72–81 CrossRef CAS PubMed.
- M. Mahmoudi, H. Hofmann, B. Rothen-Rutishauser and A. Petri-Fink, Assessing the in vitro and in vivo toxicity of superparamagnetic iron oxide nanoparticles, Chem. Rev., 2012, 112(4), 2323–2338 CrossRef CAS PubMed.
- H. Shi, Y. Sun, R. Yan, S. Liu, L. Zhu, S. Liu, Y. Feng, P. Wang, J. He and Z. Zhou, et al., Magnetic Semiconductor Gd-Doping CuS Nanoparticles as Activatable Nanoprobes for Bimodal Imaging and Targeted Photothermal Therapy of Gastric Tumors, Nano Lett., 2019, 19(2), 937–947 CrossRef CAS PubMed.
- Z. Li, S. Yin, L. Cheng, K. Yang, Y. Li and Z. Liu, Magnetic Targeting Enhanced Theranostic Strategy Based on Multimodal Imaging for Selective Ablation of Cancer, Adv. Funct. Mater., 2014, 24(16), 2312–2321 CrossRef CAS.
- C.-C. Cheng, C.-F. Huang, A.-S. Ho, C.-L. Peng, C.-C. Chang, F.-D. Mai, L.-Y. Chen, T.-Y. Luo and J. Chang, Novel targeted nuclear imaging agent for gastric cancer diagnosis: glucose-regulated protein
78 binding peptide-guided 111In-labeled polymeric, micelles, Int. J. Nanomed., 2013, 8(1), 1385–1391 CrossRef PubMed.
- U. Shin, J. Kim, J. Lee, D. Park, C. Lee, H. C. Jung, J. Park, K. Lee, M. W. Lee and S. W. Kim, et al., Development Of (Cu)-C-64-Loaded Perfluoropentane Nanodroplet: A Potential Tumor Theragnostic Nano-Carrier And Dual-Modality Pet-Ultrasound Imaging Agents, Ultrasound Med. Biol., 2020, 46(10), 2775–2784 CrossRef PubMed.
- B. W. Shi, B. Y. Zhang, Y. Q. Zhang, Y. Q. Gu, C. Zheng, J. Yan, W. B. Chen, F. H. Yan, J. Ye and H. Zhang, Multifunctional gap-enhanced Raman tags for preoperative and intraoperative cancer imaging, Acta Biomater., 2020, 104, 210–220 CrossRef CAS PubMed.
- Z. Y. Yang, J. F. Wang, S. Liu, F. Sun, J. Miao, E. Xu, L. Tao, Y. X. Wang, S. C. Ai and W. X. Guan, Tumor-Targeting W18O49 Nanoparticles for Dual-Modality Imaging and Guided Heat-Shock-Response-Inhibited Photothermal Therapy in Gastric Cancer, Part. Part. Syst. Charact., 2019, 36(7), 12 CrossRef.
- K. Jang, S. Yoon, S. E. Kim, J. Y. Cho, S. H. Yoon, K. S. Lim, K. S. Yu, I. J. Jang and H. Lee, Novel nanocrystal formulation of megestrol acetate has improved bioavailability compared with the conventional micronized formulation in the fasting state, Drug Des., Dev. Ther., 2014, 8, 851–858 CrossRef CAS PubMed.
- H. Bando, H. Shimodaira, K. Fujitani, A. Takashima, K. Yamaguchi, N. Nakayama, T. Takahashi, E. Oki, M. Azuma, T. Nishina, S. Hironaka, Y. Komatsu and K. Shitara, et al., A phase II study of nab-paclitaxel in combination with ramucirumab in patients with previously treated advanced gastric cancer, Eur. J. Cancer, 2018, 91, 86–91 CrossRef CAS PubMed.
- E. Fernandes, D. Ferreira, A. Peixoto, R. Freitas, M. Relvas-Santos, C. Palmeira, G. Martins, A. Barros, L. L. Santos and B. Sarmento, et al., Glycoengineered nanoparticles enhance the delivery of 5-fluorouracil and paclitaxel to gastric cancer cells of high metastatic potential, Int. J. Pharm., 2019, 570, 12 CrossRef PubMed.
- H. M. Liu, M. H. Yuan, Y. S. Liu, Y. P. Guo, H. J. Xiao, L. Guo and F. Liu, Self-Monitoring and Self-Delivery of Self-Assembled Fluorescent Nanoparticles in Cancer Therapy, Int. J. Nanomed., 2021, 16, 2487–2499 CrossRef PubMed.
- Y. Zhou, X. Z. Sun, L. S. Zhou and X. Z. Zhang, pH-Sensitive and Long-Circulation Nanoparticles for Near-Infrared Fluorescence Imaging-Monitored and Chemo-Photothermal Synergistic Treatment Against Gastric Cancer, Front. Pharmacol., 2020, 11, 14 CrossRef PubMed.
- Z. Yang, H. Y. Luo, Z. Cao, Y. Chen, J. B. Gao, Y. Q. Li, Q. Jiang, R. H. Xu and J. Liu, Dual-targeting hybrid nanoparticles for the delivery of SN38 to Her2 and CD44 overexpressed human gastric cancer, Nanoscale, 2016, 8(22), 11543–11558 RSC.
- H. J. Chang, M. Y. Choi, M. H. Cho, K. E. Lee and S. N. Lee, Molecular mechanism of chemoresistance and restoration in human gastric cancer cells, J. Clin. Oncol., 2019, 37(15), 1 Search PubMed.
- M. P. Vinardell and M. Mitjans, Antitumor Activities of Metal Oxide Nanoparticles, Nanomaterials, 2015, 5(2), 1004–1021 CrossRef CAS PubMed.
- S. Azimee, M. Rahmati, H. Fahimi and M. A. Moosavi, TiO2 nanoparticles enhance the chemotherapeutic effects of 5-fluorouracil in human AGS gastric cancer cells via autophagy blockade, Life Sci., 2020, 248, 9 CrossRef PubMed.
- C. X. Yang, X. Pang, W. H. Chen, X. Y. Wang, G. Lin, C. C. Chu, X. Z. Zhang, X. M. Deng, X. Y. Chen and G. Liu, Environmentally responsive dual-targeting nanotheranostics for overcoming cancer multidrug resistance, Sci. Bull., 2019, 64(10), 705–714 CrossRef CAS.
- Y. Liu, P. Zhang, F. Li, X. Jin, J. Li, W. Chen and Q. Li, NanoEnhancersMetal-based for Future Radiotherapy: Radiosensitizing and Synergistic Effects on Tumor Cells, Theranostics, 2018, 8(7), 1824–1849 CrossRef CAS PubMed.
- S. Azizi, A. Ghasemi, H. Asgarian-Omran, Z. Zal, A. Montazeri, H. Yazdannejat and S. J. Hosseinimehr, Cerium oxide nanoparticles sensitize non-small lung cancer cell to ionizing radiation, Marmara Pharm. J., 2018, 22(2), 307–313 CrossRef CAS.
- C. Zhang, P. Huang, L. Bao, M. He, T. Luo, G. Gao and D. Cui, Enhancement of gastric cell radiation sensitivity by chitosan-modified gold nanoparticles, J. Nanosci. Nanotechnol., 2011, 11(11), 9528–9535 CrossRef CAS PubMed.
- P. Huang, D. P. Yang, C. L. Zhang, J. Lin, M. He, L. Bao and D. X. Cui, Protein-directed one-pot synthesis of Ag microspheres with good biocompatibility and enhancement of radiation effects on gastric cancer cells, Nanoscale, 2011, 3(9), 3623–3626 RSC.
- S. Batooei, A. Khajeali, R. Khodadadi and J. P. Islamian, Metal-based nanoparticles as radio-sensitizer in gastric cancer therapy, J. Drug Delivery Sci. Technol., 2020, 56, 6 Search PubMed.
- C. Y. Ju, Y. J. Wen, L. P. Zhang, Q. Q. Wang, L. J. Xue, J. Shen and C. Zhang, Neoadjuvant Chemotherapy Based on Abraxane/Human Neutrophils Cytopharmaceuticals with Radiotherapy for Gastric Cancer, Small, 2019, 15(5), 10 Search PubMed.
- S. Gai, G. Yang, P. Yang, F. He, J. Lin, D. Jin and B. Xing, Recent advances in functional nanomaterials for light–triggered cancer therapy, Nano Today, 2018, 19, 146–187 CrossRef CAS.
- Y. B. Wang, W. B. Wu, D. Mao, C. Teh, B. Wang and B. Liu, Metal-Organic Framework Assisted and Tumor Microenvironment Modulated Synergistic Image-Guided Photo-Chemo Therapy, Adv. Funct. Mater., 2020, 30(28), 2002431 CrossRef CAS.
- W. Zuo, D. Chen, Z. Fan, L. Chen, Z. Zhu, Q. Zhu and X. Zhu, Design of Light/ROS Cascade-Responsive Tumor-Recognizing Nanotheranostics for Spatiotemporally Controlled Drug Release in Locoregional Photo-Chemotherapy, Acta Biomater., 2020, 327–340 CrossRef CAS PubMed.
- C. H. Wu, X. T. Guan, J. M. Xu, Y. X. Zhang, Q. Y. Liu, Y. Tian, S. Li, X. Qin, H. Yang and Y. Y. Liu, Highly efficient cascading synergy of cancer photo-immunotherapy enabled by engineered graphene quantum dots/photosensitizer/CpG oligonucleotides hybrid nanotheranostics, Biomaterials, 2019, 205, 106–119 CrossRef CAS PubMed.
- J. Chen, G. M. He, G. Y. Xian, X. Q. Su, L. L. Yu and F. Yao, Mechanistic biosynthesis of SN-38 coated reduced graphene oxide sheets for photothermal treatment and care of patients with gastric cancer, J. Photochem. Photobiol., B, 2020, 204, 7 CrossRef PubMed.
- M. Singh, D. C. C. Harris-Birtill, Y. Zhou, M. E. Gallina, A. E. G. Cass, G. B. Hanna and D. S. Elson, Application of Gold Nanorods for Photothermal Therapy in Ex Vivo Human Oesophagogastric Adenocarcinoma, J. Biomed. Nanotechnol., 2016, 12(3), 481–490 CrossRef CAS PubMed.
- A. M. Zhang, S. J. Pan, Y. H. Zhang, J. Chang, J. Cheng, Z. C. Huang, T. L. Li, C. L. Zhang, J. M. de la Fuentea and Q. Zhang, Carbon-gold hybrid nanoprobes for real-time imaging, photothermal/photodynamic and nanozyme oxidative therapy, Theranostics, 2019, 9(12), 3443–3458 CrossRef CAS PubMed.
- W. W. Zhang, X. Y. Ding, H. Cheng, C. Y. Yin, J. Yan, Z. P. Mou, W. Y. Wang, D. X. Cui, C. D. Fan and D. D. Sun, Dual-Targeted Gold Nanoprism for Recognition of Early Apoptosis, Dual-Model Imaging and Precise Cancer Photothermal Therapy, Theranostics, 2019, 9(19), 5610–5625 CrossRef CAS PubMed.
- M. Apicella, S. Corso and S. Giordano, Targeted therapies for gastric cancer: failures and hopes from clinical trials, Oncotarget, 2017, 8(34), 57654–57669 CrossRef PubMed.
- X. Dai and C. Tan, Combination of microRNA therapeutics with small-molecule anticancer drugs: mechanism of action and co-delivery nanocarriers, Adv. Drug Deliv. Rev., 2015, 81, 184–197 CrossRef CAS PubMed.
- W. Chen, S. Yang, X. Wei, Z. Yang, D. Liu, X. Pu, S. He and Y. J. Zhang, Ahm: Construction of Aptamer-siRNA Chimera/PEI/5-FU/Carbon Nanotube/Collagen Membranes for the Treatment of Peritoneal Dissemination of Drug-Resistant Gastric Cancer, Adv. Healthcare Mater., 2020, 9(21), e2001153 CrossRef PubMed.
- M. Davern and J. Lysaght, Cooperation between chemotherapy and immunotherapy in gastroesophageal cancers, Cancer Lett., 2020, 495, 89–99 CrossRef CAS PubMed.
- X. J. Cheng, K. L. Fan, L. Wang, X. J. Ying, A. J. Sanders, T. Guo, X. F. Xing, M. Zhou, H. Du and Y. Hu, et al., TfR1 binding with H-ferritin nanocarrier achieves prognostic diagnosis and enhances the therapeutic efficacy in clinical gastric cancer, Cell Death Dis., 2020, 11(2), 13 Search PubMed.
- X. B. Meng, K. Wang, L. Lv, Y. Zhao, C. Sun, L. J. Ma and B. Zhang, Photothermal/Photodynamic Therapy with Immune-Adjuvant Liposomal Complexes for Effective Gastric Cancer Therap, Part. Part. Syst. Charact., 2019, 36(6), 9 CrossRef.
- L. Z. Deng, W. H. Guo, G. X. Li, Y. F. Hu and L. M. Zhang, Hydrophobic IR780 loaded sericin nanomicelles for phototherapy with enhanced antitumor efficiency, Int. J. Pharm., 2019, 566, 549–556 CrossRef CAS PubMed.
- F. Farjadian, A. Ghasemi, O. Gohari, A. Roointan, M. Karimi and M. R. Hamblin, Nanopharmaceuticals and nanomedicines currently on the market: challenges and opportunities, Nanomedicine, 2019, 14(1), 93–126 CrossRef CAS PubMed.
- R. Tian, H. L. Ma, S. J. Zhu, J. Lau, R. Ma, Y. J. Liu, L. S. Lin, S. Chandra, S. Wang and X. F. Zhu, Multiplexed NIR-II Probes for Lymph Node-Invaded Cancer Detection and Imaging-Guided Surgery, Adv. Mater., 2020, 32(11), 10 Search PubMed.
- W. J. Zhang, S. C. Song, H. X. Wang, Q. Wang, D. Li, S. Z. Zheng, Z. Y. Xu, H. T. Zhang, J. Wang and J. Sun, In vivo irreversible albumin-binding near-infrared dye conjugate as a naked-eye and fluorescence dual-mode imaging agent for lymph node tumor metastasis diagnosis, Biomaterials, 2019, 217, 11 Search PubMed.
- S. Liu, X. Jiang, X. Tian, Z. Wang, Z. Xing, J. Chen, J. Zhang, C. Wang and L. Dong, A method to measure the denatured proteins in the corona of nanoparticles based on the specific adsorption of Hsp90ab1, Nanoscale, 2020, 12(29), 15857–15868 RSC.
- H. Wang, T. Ding, J. Guan, X. Liu, J. Wang, P. Jin, S. Hou, W. Lu, J. Qian and W. Wang, Interrogation of Folic Acid-Functionalized Nanomedicines: The Regulatory Roles of Plasma Proteins
Reexamined, ACS Nano, 2020, 14(11), 14779–14789 CrossRef PubMed.
- S. Liu, Z. Wang, X. Jiang, J. Gan, X. Tian, Z. Xing, Y. Yan, J. Chen, J. Zhang and C. Wang, et al., Denatured corona proteins mediate the intracellular bioactivities of nanoparticles via the unfolded protein response, Biomaterials, 2021, 265, 120452 CrossRef CAS PubMed.
- J. W. Yoo, D. J. Irvine, D. E. Discher and S. Mitragotri, Bio-inspired, bioengineered and biomimetic drug delivery carriers, Nat. Rev. Drug Discovery, 2011, 10(7), 521–535 CrossRef CAS PubMed.
- P. Wu, B. Zhang, D. K. W. Ocansey, W. Xu and H. Qian, Extracellular vesicles: A bright star of nanomedicine, Biomaterials, 2020, 120467 Search PubMed.
- R. Molinaro, C. Corbo, J. O. Martinez, F. Taraballi, M. Evangelopoulos, S. Minardi, I. K. Yazdi, P. Zhao, E. De Rosa and M. B. Sherman, Biomimetic proteolipid vesicles for targeting inflamed tissues, Nat. Mater., 2016, 15(9), 1037–1046 CrossRef CAS PubMed.
- Z. Cheng, A. Al Zaki, J. Z. Hui, V. R. Muzykantov and A. Tsourkas, Multifunctional Nanoparticles: Cost Versus Benefit of Adding Targeting and Imaging Capabilities, Science, 2012, 338(6109), 903–910 CrossRef CAS PubMed.
- J. Chen, R. J. Zhang, C. Tao, X. F. Huang, Z. D. Chen, X. P. Li, J. L. Zhou, Q. Zeng, B. X. Zhao and M. M. Yuan, CuS-NiS2 nanomaterials for MRI guided phototherapy of gastric carcinoma via triggering mitochondria-mediated apoptosis and MLKL/CAPG-mediated necroptosis, Nanotoxicology, 2020, 14(6), 774–787 CrossRef CAS PubMed.
- H. Yao, K. K. Xu, J. H. Zhou, L. Zhou and S. H. Wei, A Tumor Microenvironment Destroyer for Efficient Cancer Suppression, ACS Biomater. Sci. Eng., 2020, 450–462 CrossRef CAS PubMed.
- S. M. Towsif Abtab, D. Alezi, P. M. Bhatt, A. Shkurenko, Y. Belmabkhout, H. Aggarwal, Weseliński ŁJ, N. Alsadun, U. Samin and M. N. Hedhili, et al., Reticular chemistry in action: A hydrolytically stable MOF capturing twice its weight in adsorbed water, Chem, 2018, 4, 94 CAS.
- T. Y. Shi, L. S. Gu, Y. Sun, S. L. Wang, C. Q. You, X. Y. Zhang, J. Zhu and B. W. Sun, Enhanced legumain-recognition and NIR controlled released of cisplatin-docyanine nanosphere against gastric carcinoma, Eur. J. Pharmacol., 2017, 794, 184–192 CrossRef CAS PubMed.
|
This journal is © The Royal Society of Chemistry 2021 |
Click here to see how this site uses Cookies. View our privacy policy here.