DOI:
10.1039/D0SC03690K
(Edge Article)
Chem. Sci., 2020,
11, 10367-10377
Surface hydration for antifouling and bio-adhesion†
Received
5th July 2020
, Accepted 8th August 2020
First published on 10th August 2020
Abstract
Antifouling properties of materials play crucial roles in many important applications such as biomedical implants, marine antifouling coatings, biosensing, and membranes for separation. Poly(ethylene glycol) (or PEG) containing polymers and zwitterionic polymers have been shown to be excellent antifouling materials. It is believed that their outstanding antifouling activity comes from their strong surface hydration. On the other hand, it is difficult to develop underwater glues, although adhesives with strong adhesion in a dry environment are widely available. This is related to dehydration, which is important for adhesion for many cases while water is the enemy of adhesion. In this research, we applied sum frequency generation (SFG) vibrational spectroscopy to investigate buried interfaces between mussel adhesive plaques and a variety of materials including antifouling polymers and control samples, supplemented by studies on marine animal (mussel) behavior and adhesion measurements. It was found that PEG containing polymers and zwitterionic polymers have very strong surface hydration in an aqueous environment, which is the key for their excellent antifouling performance. Because of the strong surface hydration, mussels do not settle on these surfaces even after binding to the surfaces with rubber bands. For control samples, SFG results indicate that their surface hydration is much weaker, and therefore mussels can generate adhesives to displace water to cause dehydration at the interface. Because of the dehydration, mussels can foul on the surfaces of these control materials. Our experiments also showed that if mussels were forced to deposit adhesives onto the PEG containing polymers and zwitterionic polymers, interfacial dehydration did not occur. However, even with the strong interfacial hydration, strong adhesion between mussel adhesives and antifouling polymer surfaces was detected, showing that under certain circumstances, interfacial water could enhance the interfacial bio-adhesion.
Introduction
Antifouling properties are important for many extensively used materials in various applications and devices such as biomedical implants, drug delivery vehicles, marine antifouling coatings, sensors, and membranes.1–4 Extensive research demonstrated that zwitterionic polymers and polymers containing poly(ethylene glycol) components (referred to as “PEG materials” in this article) exhibit excellent antifouling activities.5–9 It is believed that the strong surface hydration of such polymers mediates their excellent antifouling performance.10–13 With strong surface hydration, it is difficult for biological media (e.g., protein molecules, bacteria, marine organisms, etc.) to displace strongly bonded surface water molecules to stick to (or to foul on) the surfaces of zwitterionic polymers and PEG materials. In contrast, for non-antifouling materials, surface hydration is not as strong.12,13 Then biological media can displace or repel surface water molecules to dehydrate the surface to stick to the surface. It is reasonable to believe that strong interfacial hydration leads to antifouling, while interfacial dehydration results in fouling.
Similarly, almost all the man-made adhesives and glues work on a dry surface but fail to stick to a wet hydrated surface, because such adhesives cannot dehydrate the surface. The hard, dry adhesives of insects and geckos also stick well when dry and less well or not at all when substrates are underwater.14,15 Different from insects and geckos, a trip to the beach will find mussels, barnacles, tube worms, sea stars, oysters, and many more animals bonding to rocks that are quite wet. Presumably, marine biology has devised clever ways clearing water from surfaces to dehydrate the surface in order to create adhesive–substrate bonds.16–18 In addition to the understanding on the molecular mechanisms of antifouling, understanding on bio-adhesion in wet environments is equally important, which will greatly aid in the design and development of underwater glues for many important medical and other applications. We believe that bio-adhesion is also mediated by interfacial hydration/dehydration.
However, it is difficult to test the above “beliefs” or “hypothesis” on the relationships between “interfacial hydration/dehydration” and “antifouling/fouling” experimentally because it is challenging to study buried solid/liquid interfaces in situ nondestructively to probe interfacial hydration or examine buried solid/solid interfaces to test interfacial dehydration. Traditional surface sensitive techniques require high vacuum to operate. It is therefore impossible to use them to test surface structures in a liquid environment. Recently, sum frequency generation (SFG) vibrational spectroscopy has been developed into a powerful tool to probe buried solid/liquid and solid/solid interfaces in situ in real time at the molecular level.19–22 We have applied SFG to study the interfacial molecular behavior of proteins including adhesive proteins.23–26 Here in order to elucidate the effects of interfacial hydration/dehydration on antifouling and bio-adhesion, we probed buried interfaces between mussel adhesive plaques and a variety of antifouling and fouling materials using SFG, in combination with mussel behavior study and adhesion measurements. Our results demonstrated that strong hydration is the key to achieve excellent antifouling performance of a polymer material, and dehydration leads to fouling. Surprisingly, we also found that hydration can enhance bio-adhesion under certain circumstances.
Experimental
Surface preparation for mussel attachment and adhesion measurements
Fused silica and glass slides, 3 inch × 1 inch × 1 mm, were purchased from VWR. Aluminum T6061 sheets of 1/8 inch thickness were ordered from Farmers Copper (Galveston, TX). Poly(methyl methacrylate) (PMMA) and polystyrene (PS) sheets of 1/8 inch thickness were bought from United States Plastic Corporation (Lima, Ohio). Aluminum, PMMA and PS were cut into 3 inch × 1 inch pieces. All substrates were cleaned with soap and rinsed with deionized water and ethanol. Aluminum, fused silica, and glass received an additional rinse of acetone. These substrates were suitable for mussel deposition and adhesion testing.
For each coating, two substrates (3 inch × 1 inch) were held together with binder clips with one aluminum piece underneath for support (see ESI, Fig. S1†). Two rubber bands were used to hold each animal in place. Combining two substrates together allowed for more stability and a larger area for animals to deposit adhesives. When substrates are listed in the order of surface energy, water contact angles from our own measurements and the literature were used for aluminum,27 fused silica,28 polysulfobetaine methacrylate (SBMA), oligo(ethylene glycol) methacrylate (OEGMA),10 PMMA,29 and PS.30
Surface preparation for SFG spectroscopy
When making thin films suitable for SFG spectroscopy, PMMA (Mw = 75
000 g per mole), PS (Mw = 280
000 g per mole), and toluene were purchased from Sigma-Aldrich. Thin films of PMMA and PS were prepared by spin coating a 1% (w/w) toluene solution on clean fused silica prisms at 3000 rpm for 40 s using a P-6000 spin coater (Speedline Technologies) followed by annealing at 95 °C for 24 h. The brushes of OEGMA and SBMA were prepared on fused silica prisms via atomic transfer radical polymerization (ATRP) according to a previous report.10 The thicknesses of the identical polymer brushes prepared on silicon wafers were measured to be 25–30 nm using an alpha-SE ellipsometer (J. A. Woollam).
Animal handling
One blue mussel (Mytilus edulis) was placed on each plate and held in place with two rubber bands. Mussels used were adults of ∼6–8 cm length. Five mussels, one on each plate, were contained in a 10 gallon aquarium in 4 °C, 3.5 g/100 mL salinity water with constant aeration. Additional details of the laboratory aquarium system can be found in a previous report.31
Mussel plaque collection for SFG spectroscopy
Animals deposited adhesive plaques onto uncoated surfaces of fused silica and polymer coatings of PS, PMMA, SBMA, and OEGMA. For each type of surface, the substrates were adhered to a larger piece of glass with double sided tape or epoxy to provide a larger area for animals to deposit glue. One mussel was held in place on each surface with a rubber band. Animals were contained separately in a 10 gallon aquarium and exposed to surfaces for a minimum of 5 days. The adhesive was collected until several plaques were deposited onto each surface. For the surfaces of OEGMA and SBMA, animals and surfaces were placed on PVC stands to encourage plaque deposition on coatings rather than on the glass aquarium tank bottom.
Surface spectroscopy
Sum frequency generation (SFG) spectroscopy is a nonlinear optical spectroscopic method well suited to examine chemistry at interfaces.19–22 Selection rules allow observation of only species immediately at a surface or within an interface for which there is no inversion symmetry. For example, the available inversion symmetry of free water does not bring about an SFG signal whereas water bound at the interface with order does appear in spectra.19–22
SFG spectroscopy was implemented according to the protocol reported previously.13 Visible and infrared (IR) input beams were overlapped spatially and temporally at the interfaces between mussel adhesive plaques and the substrates immersed in water, or the interfaces between the substrates and water without the plaques. All SFG spectra were collected using the ssp (s-polarized sum frequency output, s-polarized visible input, and p-polarized IR input) polarization combination.32 More introduction to SFG spectroscopy can be found in the ESI.†
Adhesion data collection
Adhesion testing was carried out three days after placement of mussels and plates into aquaria. Animals on plates were removed from aquaria and their threads were cut leaving behind attached plaques and threads attached to surfaces. Photographs were taken of plaques to determine the area by digital image analysis. Removal force was collected on an Instron 5544 materials testing system. Each thread was pulled perpendicular to the surface until failure. Adhesion of plaques was calculated by dividing the removal force by plaque area. Additionally, adhesive plaque production (Table S1†) and material failure modes (Table S3†) were recorded. At least 10 animals were examined for each surface (fused silica, PMMA, PS, OEGMA, and SBMA) and 75 animals for aluminum controls. Adhesion was gathered in at least 2 rounds where each round contained five mussels on a separate plate per coating type.
Adhesion for the first round of coatings was collected over 6 consecutive days, testing each coating type independently. Aluminum controls were tested on the same day of each surface for consistency. A second round of adhesion testing was gathered over 6 consecutive days with controls. Animals deposited less plaques on the surfaces of PMMA, OEGMA and SBMA and therefore a third round of adhesion testing was performed. Here, data were gathered over 4 consecutive days. During the second and third rounds of testing, animals on plates were placed on top of PVC stands to encourage plaque deposition onto test surfaces rather than the glass tanks.
Combining all rounds of adhesion testing, 10 animals were examined on each surface of fused silica and PS. Due to the additional round of testing, 15 animals were examined on each of PMMA, OEGMA, and SBMA surfaces. Aluminum controls were tested alongside all coatings thus resulting in the collection of more animals and plaques than on other surfaces.
Results and discussion
Mussel behavior
Mussels were placed atop a series of substrates ranging from high to low surface energies. The animals attached by depositing a flowing adhesive precursor that cures into a final plaque formation.33 Control materials investigated in this study on which biofouling occurs included fused silica (water contact angle of ∼20°), PMMA (∼71°), aluminum (∼75°), and PS (∼87°). Also included in this research were the antifouling zwitterionic polymer (grafted SBMA, ∼20°) and PEG material (grafted OEGMA, 42°).10 As discussed above, zwitterionic34 and OEGMA35 surfaces have gained a measure of fame for antifouling properties (i.e., preventing adhesion) within biomedical as well as marine shipping contexts.
When placed onto surfaces, marine mussels attach by depositing their adhesive plaques (Fig. 1a and b).36 Prior to settlement, the animals may move around in search of the most appealing location. Consequently, we used rubber bands to keep the animals in one place and force adhesion to a given substrate. For the aluminum (Fig. 1c), fused silica, PS, and PMMA substrates, mussels remained in place and attached with their plaques. The zwitterionic SBMA (Fig. 1d) and OEGMA surfaces, by contrast, resulted in different animal behavior. Most of the mussels were able to work their way off from these antifouling substrates. In order to understand the origin of this unusual action, we monitored the animals over several days. ESI Video 1† shows that the mollusks opened and closed their shells repeatedly. In doing so, they were able to wiggle free from their constraints, but only when on the zwitterionic SBMA and OEGMA surfaces.
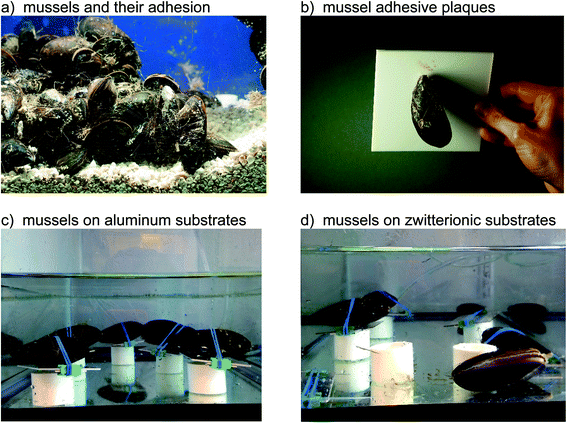 |
| Fig. 1 Mussel adhesive and animal behavior on different surfaces. (a) Mussels using an adhesive for attaching to each other and the side of an aquarium tank. (b) A mussel bonding to a sheet of polytetrafluoroethylene (Teflon), showing the adhesive plaques and threads. (c) Photograph showing mussels two days after binding to aluminum substrates. Note how all animals remain attached. Top down views of mussels on the substrates are provided in Fig. S1 (ESI†). Each mussel and substrate is resting on a piece of plastic pipe to prevent the animal from placing its adhesive on the more stable aquarium bottom. (d). Mussels after two days on a zwitterionic SBMA surface. Most animals have moved off the substrates. | |
The above observation of the mussel behavior demonstrated clearly that animals could easily settle on the four “control” or “fouling” surfaces including silica, PMMA, PS, and aluminum, but do not want to settle down on the two antifouling polymer SBMA and OEGMA surfaces. We believe that the drastically different animal behaviors on fouling and antifouling surfaces are caused by different surface hydration on these materials. Likely the control materials have weak surface hydration, while the antifouling SBMA and OEGMA have strong surface hydration, which will be investigated in more detail below.
Surface hydration in water
SFG spectra could be collected from the material/water interfaces in situ in real time to probe the molecular structure of a solid/liquid interface. Extensive research has been performed to investigate the interfacial water structure using SFG.37–45 Typically two broad peaks centered around 3200 and 3400 cm−1 could be detected, sometimes along with a relatively narrower peak at around 3700 cm−1.37–45 It is agreed that the ∼3700 peak is due to the free OH stretching, while debates still exist for the assignment for the other two peaks. It is generally believed that the 3200 cm−1 and 3400 cm−1 peaks come from the strongly and weakly (or loosely) hydrogen bonded water molecules at the interface.
We have collected SFG spectra from a variety of surfaces in water.11,46–51 It was found in our previous research that stronger surface hydration leads to better antifouling behavior.10–13,52,53 The strong hydration is characterized by a strong 3200 cm−1 peak, a weak (or absence of) 3400 cm−1 signal, and a strong overall water signal intensity.10–13 For example, SFG spectra collected from the antifouling SBMA and OEGMA surfaces in water are both dominated by the ∼3200 cm−1 peak, showing that the surfaces are covered by strongly bonded water molecules, leading to excellent antifouling properties.10 For a control PMMA surface, both 3200 cm−1 and 3400 cm−1 peaks were detected, indicating that the PMMA surface has a substantial amount of loosely bonded water molecules and thus PMMA is not a good antifouling material.12
Fig. 2a shows a picture of a mussel adhesive plaque on a surface, while Fig. 2b shows the schematic of the SFG sample geometry. SFG spectra can be collected from the sample/water interface (position A in Fig. 2b) and the sample/mussel adhesive plaque interface (position B in Fig. 2b).
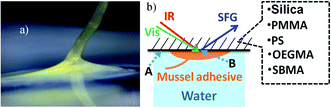 |
| Fig. 2 Mussel adhesive plaque and SFG sample geometry. (a) Picture of a mussel adhesive plaque on a surface. (b) Schematic showing the SFG sample geometry used to collect SFG spectra from the sample/water interface (position A) and the sample/mussel adhesive plaque interface (position B). The input laser beams penetrate the substrates (silica or silica with polymer thin films) to reach the sample/water and the sample/mussel adhesive interfaces. Aluminum was not studied because it is not transparent for the input laser beams. | |
Fig. 3 shows SFG spectra collected from the surfaces and interfaces of five samples, while aluminum was not included owing to a lack of optical transparency. Note that the y-axes are on different scales to aid visualization of spectral features. We first discuss the SFG spectra collected from the material/water interface in Fig. 3, shown in blue. It is worth mentioning that these SFG spectra were not collected from the “clean” material/water interface, instead, they were detected from the samples after the mussel deposition experiments, from the surface regions where there were no adhesives deposited (e.g., position A in Fig. 2b).
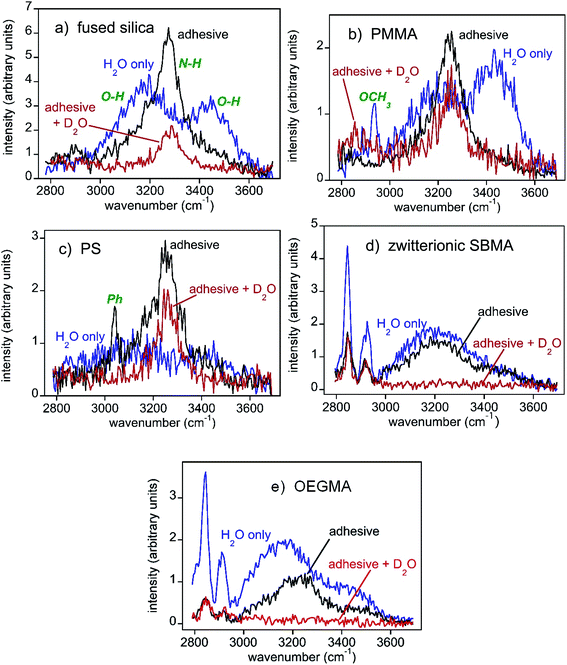 |
| Fig. 3 SFG spectra of the substrate/water interface (region A in Fig. 2(b), blue curves), substrate/mussel adhesive interface (region B in Fig. 2(b), black curves), and the substrate/mussel adhesive interface after placing the sample in D2O for a while (red curves). Note that the y-axes differ between panels in order to better visualize differences in spectra. The SFG intensities in different panels are different because of the different water orientation and ordering at various interfaces due to different interfacial interactions. SFG spectra collected from mussel adhesive plaque/substrate interfaces for the different plaques on the same type of substrate are similar and the results are reproducible. (a) Fused silica. General water and amine peak assignments are shown in green. These same assignments apply to all other spectra shown. (b) PMMA. In the water spectrum, prior to adhesive deposition, a substrate methyl peak was observed. (c) PS. The adhesive spectrum also shows a phenyl peak from the substrate. (d) Zwitterionic SBMA. (e) OEGMA. | |
The O–H stretching SFG spectra collected from three control samples, fused silica, PMMA, and PS in water show some common features (blue spectra in Fig. 3a–c). In addition to the signals at around ∼3200 cm−1, all three blue spectra have strong ∼3400 cm−1 signals, showing substantial coverage of loosely bonded water molecules on the surfaces, or weak hydration. This observation matches our previous observation on the “clean” PMMA/water interface well.12 In contrast, SFG spectra collected from the SBMA and OEGMA surfaces are dominated by the ∼3200 cm−1 signal (blue spectra in Fig. 3d and e), showing strong surface hydration, similar to those observed from the “clean” SBMA/water and OEGMA/water interfaces.10 The surface hydration detected by SFG can be well correlated with the above animal behavior on various sample surfaces: On the weakly hydrated silica, PMMA, and PS surfaces, mussels easily settled and deposited adhesives. On the strongly hydrated SBMA and OEGMA surfaces, mussel tried to move away from the surfaces, even after tying with rubber bands.
Our previous research shows that for SBMA, protein molecules cannot disrupt the surface hydration of SBMA at the SBMA/protein solution interface,10 due to the strong SBMA surface hydration. On the OEGMA/protein solution interfaces, protein molecules could disrupt the interfacial water structure and loosely deposit onto the surface, which can be easily washed off by water. Both SBMA and OEGMA are antifouling materials. For fouling surfaces, protein molecules can disrupt the surface hydration and deposit to the surfaces permanently. Here instead of protein molecules, we used SFG to study interfacial hydration between various surfaces and mussel adhesives deposited by real live animals, the results of which will be presented below.
Dehydration at interfaces between mussel adhesives and control surfaces
To understand bio-adhesion and biofouling in detail, SFG spectra were collected from the buried interfaces between the three control samples (silica, PMMA, and PS) and mussel adhesives respectively (black spectra in Fig. 3a–c). After mussel attachment and partial plaque coverage, the interfacial SFG spectra of all three buried solid/solid interfaces between the control samples and adhesives exhibit some common features: They all shifted from showing strong water signals (O–H stretching at ∼3200 cm−1 and ∼3450 cm−1) to those of amines from the protein-based adhesive (N–H stretching at ∼3280 cm−1). Spectral fitting results in Fig. S2 (ESI†) showed that a single amine peak can describe the spectra well, indicating that no ordered water exists at the three control material/mussel adhesive interfaces, and likely dehydration occurred at these interfaces.
SFG detects signals from a medium with no inversion symmetry. The absence of the SFG water signal at the control sample/mussel adhesive interfaces indicates that there is no ordered water at the interfaces. To confirm the interfacial dehydration and to exclude the existence of disordered water (e.g., water molecules adopting a random orientation) at the interfaces, the above three samples were placed in D2O and SFG spectra were then collected. The SFG spectral regions shown in Fig. 3 will not detect O-D and N-D stretching signals. If an interface is hydrated by disordered water molecules, then changing a sample from residing in H2O (black spectra) to D2O (red spectra) will diminish the interfacial N–H signals because of the H-D exchange between the N–H groups and D2O molecules accessing the interfaces. Placement of adhesive-fused silica into D2O (Fig. 3a) shrank the amine N–H signal with some intensity persisting. This change indicated that water (D2O here) could access some of the interface to exchange protons with proteins (e.g., N–H to N–D). The remaining N–H signal indicated that water could not access the entire interface with the rest of the system being dehydrated, without ordered or disordered water. For PMMA, the amine N–H peak for the adhesive/sample interface only decreased a little (Fig. 3b), showing that the buried PMMA/mussel adhesive interface is mostly dehydrated and cannot be accessed by D2O, preventing the H-D exchange for interfacial N–H groups. Further support for such a conclusion was obtained by having the animals deposit glue atop deuterated PMMA to yield similar results (Fig. S2, ESI†). PS (Fig. 3c) was generally similar to PMMA – a small N–H decrease when in D2O, and analogous results when using a deuterated plastic surface (Fig. S2, ESI†). The H–D exchange experiments using D2O clearly demonstrated the dehydration of the buried interfaces between the three control samples and mussel adhesives. Therefore we concluded that for biofouling to occur, mussels displaced the water on the sample surface and dehydrated the surface while depositing adhesives on the surface. These happened when a material surface does not have strong hydration.
Hydration at interfaces between mussel adhesives and antifouling SBMA as well as OEGMA
As presented above, most mussels left the SBMA or OEGMA surfaces without depositing adhesive plaques even when tied with rubber bands. Only a small amount of mussels stayed on the SBMA or OEGMA surfaces and deposited adhesives. SFG spectra were collected from the buried interfaces between these mussel adhesives and antifouling polymers, SBMA or OEGMA. For zwitterionic SBMA (Fig. 3d), plaque deposition decreased the SFG water signal, but only to a small extent. Furthermore, no prominent amine N–H stretching peak was observed. Quantitative spectral fitting results in Fig. S2 (ESI†) attested to the absence of the amide N–H signal. A water signal at ∼3200 cm−1 indicated water that was hydrogen bonded strongly. These data showed hydrogen bonded interfacial water residing between the mussel adhesive and the surface. From the OEGMA spectra (Fig. 3e), it can be seen that plaque binding decreased the water signal to yield a continued presence of water with a weak N–H contribution (Fig. S2, ESI†). Here, too, interfacial water appeared to persist between the adhesive and surface.
Soaking the adhesive–SBMA sample in D2O eliminated almost all of the SFG signal (red spectrum in Fig. 3d), indicating that the entire interface was accessible by water (or D2O). Furthermore, there appeared to be no dehydrated, ordered N–H functionalities at this adhesive–SBMA interface. A similar result was found for OEGMA (Fig. 3e). A weak N–H may have been present for adhesive–OEGMA (Fig. S2, Table S6, ESI†). Nonetheless, the entire SBMA and OEGMA systems appeared to be water accessible, with no regions of dehydration. The interfacial hydration between mussel adhesives and control samples vs. antifouling materials is drastically different. At the mussel adhesive/control sample interfaces, dehydration occurred. At the antifouling polymer/mussel adhesive interfaces, dehydration was not observed.
Adhesion testing
To further understand antifouling and bio-adhesion, we measured the adhesion strengths of mussel adhesives on control surfaces and antifouling surfaces. The adhesive performance of mussel glue on each substrate was quantified using an established method (Fig. 4).36,54 Briefly, adhesion values (in kPa) were obtained by pulling each plaque perpendicular to the surface until failure and the maximum force was divided by the plaque contact area on the surface. Data in Fig. 4 and Table S1† show that this adhesive functioned in a generally classic manner, with stronger binding to substrates with higher surface energies. Such behavior is in agreement with how adhesives stick better to, for example, metals than hydrophobic plastics.55 However, mussel adhesion does not track perfectly with surface energy. Aluminum brings about particularly high strengths, possibly due to observations of changes in the surface (e.g., shiny to dull) after hours of residence in sea water.36 Condition index tests ensured that animal health was not influenced by any substrate (Table S2†).56,57
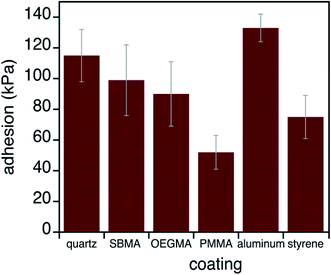 |
| Fig. 4 Performance of mussel adhesives on different substrates. Average adhesion of mussel plaques on each surface. The substrates are ordered from the highest to lowest surface energies. Error bars shown are 99% confidence intervals. | |
A particularly interesting finding here is that, in the rare instances when mussels attached to the OEGMA and zwitterionic SBMA substrates, adhesion strengths were quite substantial. This was different from what we expected. Since most adhesives and glues fail in wet environments, we thought that the interfacial hydration would lead to weak adhesion between mussel adhesives and SBMA or OEGMA. This was not observed and will be discussed in more detail below.
Discussion on antifouling
Our previous studies demonstrated that the surface hydration is well correlated with the antifouling activity of a material.10–13 For control samples or materials which do not possess antifouling properties, surface hydration is weak, as evidenced by the observation of both 3200 cm−1 and 3400 cm−1 peaks. Mussels can easily settle on such surfaces, dehydrate the surfaces, and deposit adhesives. Interfacial dehydration was observed in situ using SFG between mussel adhesives and the three control samples. The dehydration process and the adhesive deposition process could occur at the same time: The adhesive proteins are deposited onto the surface to replace interfacial water molecules. Adhesive proteins could also bind water molecules initially, but when they came into contact with the control sample, they more favorably interacted with the control sample surface directly, squeezing the initially bound water from the protein and the adhesive/control material interface, leading to interfacial dehydration. For the two antifouling materials SBMA and OEGMA, strong surface hydration was observed, as evidenced by the dominating SFG signals at 3200 cm−1. With strong surface hydration, mussels do not like to settle on these surfaces and try to escape even when bound by rubber bands. The established antifouling properties of these surfaces may derive primarily from mussels avoiding the hydrating surfaces. Once attached, however, bonding tracked well with the high surface energies, which is not related to the antifouling behavior of the material.
Discussion on bio-adhesion
How might we explain the attachment strength of mussels to substrates without consistently strong protein peaks and without completely dehydrated surfaces? The first column of Table 1 lists these five substrates in the order of decreasing surface energy or increasing water contact angles (column 2). After mussel attachment, the SFG spectra collected from the buried mussel adhesive/sample interfaces were fit to approximate relative intensities of water O–H versus protein amine N–H signals (columns 3 and 4). Measured adhesion values are in column 5. Quite striking is that, contrary to what one might expect, adhesion did not correlate with the detected presence of protein (N–H signal) on all surfaces. We can explain this underwater bonding with either water O–H or protein N–H signals. Such results lead to the conclusion that adhesion may be brought about either by the protein alone, which can dehydrate some substrates,16–18 or a combination of disordered protein and ordered interfacial water. Such ordered interfacial water can be the initial hydration layer on the SBMA or OEGMA surface and/or the protein bound water at the interface (protein bound water in the adhesive bulk should not contribute the SFG signal due to inversion symmetry). Water appears to help adhesion on some surfaces.
Table 1 Examination of substrates as functions of water contact angles, SFG signal intensities, and mussel adhesion. Arbitrary units are denoted by “a.u.”
Surface |
Water contact angle (°) |
Water intensity with plaque (a.u.) |
N–H intensity with plaque (a.u.) |
Adhesion (kPa) |
Fused silica |
20 |
0 |
2.2 ± 0.2 |
115 ± 17 |
SBMA |
20 |
1.2 ± 0.2 |
0 |
99 ± 23 |
OEGMA |
42 |
1.1 ± 0.1 |
0 |
90 ± 21 |
PMMA |
71 |
0 |
1.4 ± 0.1 |
52 ± 11 |
PS |
87 |
0 |
1.5 ± 0.2 |
75 ± 14 |
Speaking generally, water inhibits most adhesion phenomena. Water bound to surfaces prevents proteins or polymers from generating strong contacts. Cohesive forces can also be eliminated by hydration, thus “hiding” proteins or polymers from the counterparts and minimizing interactions. Reducing the amount of water present at surfaces appears to be a logical means of generating adhesive contacts. However, the practical aspects of such drying are not easy to achieve. Placement of hydrophobic species such as oils atop submerged high energy substrates may be able to remove bulk hydration, but water remains attached at the surface.58–60 Our current understanding of the importance of removing water to gain adhesive function may be in flux. Having a hydrophobic polymer appears to be helpful, if not essential, for displacing water and achieving strong adhesion between submerged substrates.61 One of the highest performing underwater adhesives is made from an all hydrophobic polymer host with pendant catechol groups.62 Yet some data indicate that increasing the polymer dielectric constant aids underwater bonding.63 These results may imply that hydrophilicity is actually beneficial for underwater bonding. Results found here indicate that viewing surfaces to be only wet and bad at adhesion versus dry and good for bonding is likely too simplistic of a view.
The hard, dry adhesives of insects and geckos provide a potential analogy to explain how interfacial water might contribute to the bonding of a wet, flowing adhesive. These terrestrial organisms attach temporarily to surfaces, via van der Waals forces, using hard and structured materials often shaped into nanoscale hair64 or pad65 structures. In general, they stick well when dry and less well or not at all when substrates are underwater.14,15 However, when in between dry and submerged, high humidity environments can actually increase the bonding of hard bioadhesives.66,67 Just the right amount of water fills gaps between the hard adhesive and substrate, contributing capillary forces and strengthening bonding.15 At an even smaller scale, nanoconfined water between the surface and adsorbate can be structured, no longer behaving in the way that we usually think of bulk solvent and possibly illustrating how surface hydration can be beneficial.68 With data shown here, we may now be seeing the first known instance of interfacial hydration contributing to the underwater bonding of a curing bio-adhesive.
Conclusion
In this research, SFG has been applied to investigate the molecular structures of buried solid/liquid and solid/solid interfaces in situ in real time to understand antifouling and bio-adhesion, supplemented by the marine animal (mussel) activity study and adhesion measurements. It was found that both antifouling zwitterionic polymer SBMA and PEG containing polymer OEGMA have strong surface hydration in water. Because of the strong surface hydration, mussels do not want to settle on the SBMA or OEGMA surface to deposit adhesive plaque (Fig. 5a). Oppositely, on control sample surfaces, only weak surface hydration was detected. It is easy for mussels to settle on such surfaces, dehydrate the surfaces, and deposit adhesive plaques on these surfaces. Therefore surface hydration is important for antifouling activity of a material.
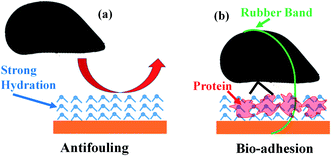 |
| Fig. 5 Schematics (not drawn to scale) showing proposed mechanisms of antifouling (a) and strong bio-adhesion (b) of mussels on SBMA or OEGMA surfaces. Because of the strong surface hydration, mussels do not want to stay on the SBMA or OEGMA surface, leading to the excellent antifouling performance of SBMA and OEGMA (a). If mussels are forced to stay on SBMA or OEGMA (e.g., tied with a rubber band), the interfacial water can strongly interact with both surface and mussel adhesive proteins, resulting in strong bio-adhesion (b). | |
It is widely observed that bio-adhesion is weak in water. Our SFG studies show that dehydration occurs when mussels deposit adhesives on control sample surfaces, leading to strong adhesion, as expected. It has been shown in the literature that mussel adhesive proteins can replace interfacial water.16,69 When interfacial dehydration occurred, the interfacial SFG water O–H stretching signal was replaced by the interfacial protein N–H stretching signal. Interestingly, it was found that for a small amount of mussels which deposited adhesives on SBMA or OEGMA, interfacial dehydration did not occur. Under such circumstances, strong adhesion was also measured between the mussel adhesive and SBMA or OEGMA surface, with the presence of interfacial water. We therefore concluded that under certain conditions, interfacial water can enhance the interfacial adhesion, with the help of interfacial proteins (Fig. 5b).
The detailed mechanism on how water and mussel adhesive protein interact at the interface to enhance adhesion needs further investigation in the future. It is well known that cross-linking of 3,4-dihydroxylphenylalanine (DOPA) containing mussel adhesive proteins plays an important role in bio-adhesion of mussels. We believe that on SBMA and OEGMA, interfacial water molecules can be strongly hydrogen bonded with both cross-linked mussel adhesive proteins and polymer surfaces, leading to strong bio-adhesion. Certainly other related or different effects such as chelating or capillary forces may also play roles.15,66,67,70
This study demonstrates the power of using SFG to probe interfacial hydration/dehydration, and the importance of surface hydration/dehydration for antifouling and bio-adhesion. The knowledge obtained from this study will help the design and development of antifouling materials with improved performance and polymer materials with desired bio-adhesion properties.
Conflicts of interest
The authors declare no competing interests.
Acknowledgements
This work was supported by the Office of Naval Research grants N00014-15-1-2277, N00014-16-1-3084, N00014-19-1-2063 (to SJ), N00014-16-1-3115, N00014-19-1-2171, N00014-20-1-2234 (to ZC), N00014-13-1-0245, N00014-16-1-2709 (to JJW), and the National Science Foundation grant CHE-0952928 (to JJW).
References
- A. Dworak, A. Utrata-Wesolek, L. Otulakowski and B. Trzebicka, Polymers in Medicine – Direction of Development, Polymer, 2019, 64, 645–655 CAS
.
- S. R. Puniredd, D. Janczewski, D. P. Go, X. Y. Zhu, S. F. Guo, S. L. M. Teo, S. S. C. Lee and G. J. Vancso, Imprinting of Metal Receptors into Multilayer Polyelectrolyte Films: Fabrication and Applications in Marine Antifouling, Chem. Sci., 2015, 6, 372–383 RSC
.
- C. Jiang, G. Wang, R. Hein, N. Liu, X. Luo and J. J. Davis, Antifouling Strategies for Selective In Vitro and In Vivo Sensing, Chem. Rev., 2020, 120, 3852–3889 CrossRef CAS
.
- D. Rana and T. Matsuura, Surface Modifications for Antifouling Membranes, Chem. Rev., 2010, 110, 2448–2471 CrossRef CAS
.
- H. Y. Wu, C. J. Lee, H. F. Wang, Y. Hu, M. Young, Y. Han, F. J. Xu, H. B. Cong and G. Cheng, Highly Sensitive and Stable Zwitterionic Poly(sulfobetaine-3,4-ethylenedioxythiophene) (PSBEDOT) Glucose Biosensor, Chem. Sci., 2018, 9, 2540–2546 RSC
.
- Y. J. Han, Z. F. Yuan, P. Zhang and S. Y. Jiang, Zwitterlation Mitigates Protein Bioactivity Loss in Vitro over PEGylation, Chem. Sci., 2018, 9, 8561–8566 RSC
.
- Q. Q. Guo, Y. Y. Wang, L. M. Zhang, P. Zhang, Y. J. Yu, Y. Q. Zhang, C. X. Li, S. Y. Jiang and X. G. Zhang, In Situ Real-time Tracing of Hierarchical Targeting Nanostructures in Drug Resistant Tumors Using Diffuse Fluorescence Tomography, Chem. Sci., 2019, 10, 7878–7886 RSC
.
- B. W. Li, P. Jain, J. R. Ma, J. K. Smith, Z. F. Yuan, H. C. Hung, Y. W. He, X. J. Lin, K. Wu, J. Pfaendtner and S. Y. Jiang, Trimethylamine N-oxide-derived Zwitterionic Polymers: A New Class of Ultralow Fouling Bioinspired Materials, Sci. Adv., 2019, 5, eaaw9562 CrossRef CAS
.
- S. Lowe, N. M. O'Brien-Simpson and L. A. Connal, Antibiofouling Polymer Interfaces: Poly(ethylene glycol) and Other Promising Candidates, Polym. Chem., 2015, 6, 198–212 RSC
.
- C. Leng, H.-C. Hung, S. Sun, D. Wang, Y. Li, S. Jiang and Z. Chen, Probing the Surface Hydration of Nonfouling Zwitterionic and PEG Materials in Contact with Proteins, ACS Appl. Mater. Interfaces, 2015, 7, 16881–16888 CrossRef CAS
.
- C. Leng, S. Sun, K. Zhang, S. Jiang and Z. Chen, Molecular Level Studies on Interfacial Hydration of Zwitterionic and Other Antifouling Polymers in Situ, Acta Biomater., 2016, 40, 6–15 CrossRef CAS
.
- C. Leng, H. Hung, O. A. Sieggreen, Y. Li, S. Jiang and Z. Chen, Probing the Surface Hydration of Nonfouling Zwitterionic and Poly(ethylene glycol) Materials with Isotopic Dilution Spectroscopy, J. Phys. Chem. C, 2015, 119, 8775–8780 CrossRef CAS
.
- C. Leng, X. Han, Q. Shao, Y. Zhu, Y. Li, S. Jiang and Z. Chen,
In situ Probing the Surface Hydration of Zwitterionic Polymer Brushes: Structural and Environmental Effects, J. Phys. Chem. C, 2014, 118, 15840–15845 CrossRef CAS
.
- A. Y. Stark, T. W. Sullivan and P. H. Niewiarowski, The Effect of Surface Water and Wetting on Gecko Adhesion, J. Exp. Biol., 2012, 215, 3080–3086 CrossRef
.
- P. Dittsche and A. P. Summers, Aquatic versus Terrestrial Attachment: Water Makes a Difference, Beilstein J. Nanotechnol., 2014, 5, 2424–2439 CrossRef
.
- W. Wei, L. Petrone, Y. Tan, H. Cai, J. N. Israelachvili, A. Miserez and J. H. Waite, An Underwater Surface-Drying Peptide Inspired by a Mussel Adhesive Protein, Adv. Funct. Mater., 2016, 26, 3496–3507 CrossRef CAS
.
- G. P. Maier, M. V. Rapp, J. H. Waite, J. N. Israelachvili and A. Butler, Adaptive Synergy Between Catechol and Lysine Promotes Wet Adhesion by Surface Salt Displacement, Science, 2015, 348, 628–632 CrossRef
.
- S. A. Mian, L. C. Saha, J. Jang, L. Wang, X. Gao and S. Nagase, Density Functional Theory Study of Catechol Adhesion on Silica Surfaces, J. Phys. Chem. C, 2010, 114, 20793–20800 CrossRef CAS
.
- Z. Chen, Investigating Buried Polymer Interfaces Using Sum Frequency Generation Vibrational Spectroscopy, Prog. Polym. Sci., 2010, 35, 1376–1402 CrossRef CAS
.
- G. L. Richmond, Molecular Bonding and Interactions at Aqueous Surfaces as Probed by Vibrational Sum Frequency Spectroscopy, Chem. Rev., 2002, 102, 2693–2724 CrossRef CAS
.
- Y. R. Shen and V. Ostroverkhov, Sum-Frequency Vibrational Spectroscopy on Water Interfaces: Polar Orientation of Water Molecules at Interfaces, Chem. Rev., 2006, 106, 1140–1154 CrossRef CAS
.
- D. Lis, E. H. G. Backus, J. Hunger, S. H. Parekh and M. Bonn, Liquid Flow Along a Solid Surface Reversibly Alters Interfacial Chemistry, Science, 2014, 344, 1138–1142 CrossRef CAS
.
- J. Wang, M. A. Even, Z. Chen, A. H. Schmaier, J. H. Waite and Z. Chen, Detection of Amide I Signals of Interfacial Proteins in situ Using SFG, J. Am. Chem. Soc., 2003, 125, 9914–9915 CrossRef CAS
.
- M. A. Even, J. Wang and Z. Chen, Structural Information of Mussel Adhesive Protein Mefp-3 Acquired at Various Polymer/Mefp-3 Solution Interfaces, Langmuir, 2008, 24, 5795–5801 CrossRef CAS
.
- C. Zhang, J. Jasensky, C. Leng, C. D. Grosso, G. D. Smith, J. J. Wilker and Z. Chen, Sum Frequency Generation Vibrational Spectroscopic Studies on Buried Heterogeneous Biointerfaces, Opt. Lett., 2014, 39, 2715–2718 CrossRef CAS
.
- B. Ding, J. Jasensky, Y. Li and Z. Chen, Engineering and Characterization of Peptides and Proteins at Surfaces and Interfaces: A Case Study in Surface-Sensitive Vibrational Spectroscopy, Acc. Chem. Res., 2016, 49, 1149–1157 CrossRef CAS
.
- S. M. Smith, B. S. Taft and J. Moulton, Contact Angle Measurements for Advanced Thermal Management Technologies, Front. Heat Mass Transfer, 2014, 5, 1–9 Search PubMed
.
- B. Jańczuk, E. Chibowski and T. Bialopiotrowicz, Time Dependence Wettability of Quartz with Water, Chem. Pap., 1986, 40, 349–356 Search PubMed
.
- C. J. van Oss, R. J. Good and H. J. Busscher, Estimation of the Polar Surface-Tension Parameters of Glycerol and Formamide, for Use in Contact-Angle Measurements on Polar Solids, J. Dispersion Sci. Technol., 1990, 11, 75–81 CrossRef CAS
.
- A. Carre, Polar Interactions at Liquid/Polymer Interfaces, J. Adhes. Sci. Technol., 2007, 21, 961–981 CrossRef CAS
.
- J. R. Burkett, J. L. Wojtas, J. L. Cloud and J. J. Wilker, A Method for Measuring the Adhesion Strength of Marine Mussels, J. Adhesion, 2009, 85, 601–615 CrossRef CAS
.
- J. Wang, C. Y. Chen, S. M. Buck and Z. Chen, Molecular Chemical Structure on Poly(methyl methacrylate) (PMMA) Surface Studied by Sum Frequency Generation (SFG) Vibrational Spectroscopy, J. Phys. Chem. B, 2001, 105, 12118–12125 CrossRef CAS
.
- A. Hagenau, M. H. Suhre and T. R. Scheibel, Nature as a Blueprint for Polymer Material Concepts: Protein Fiber-Reinforced Composites as Holdfasts of Mussels, Prog. Polym. Sci., 2014, 39, 1564–1583 CrossRef CAS
.
- J. B. Schlenoff, Zwitteration: Coating Surfaces with Zwitterionic Functionality to Reduce Nonspecific Adsorption, Langmuir, 2014, 30, 9625–9636 CrossRef CAS
.
- C. S. Gudipati, J. A. Finlay, J. A. Callow, M. E. Callow and K. L. Wooley, The Anti-Fouling and Fouling-Release Performance of Unique Hyperbranched Fluoropolymer (HBFP)-Poly(ethylene glycol) (PEG) Composite Coatings Evaluated by Protein Adsorption and the
Settlement of Zoospores of the Green Fouling Alga Ulva (syn. Enteromorpha), Langmuir, 2005, 21, 3044–3053 CrossRef CAS
.
- J. R. Burkett, J. L. Wojtas, J. L. Cloud and J. J. Wilker, A Method for Measuring the Adhesion Strength of Marine Mussels, J. Adhesion, 2009, 85, 601–615 CrossRef CAS
.
- C. S. Tian and Y. R. Shen, Structure and Charging of Hydrophobic Material/Water Interfaces Studied by Phase-Sensitive Sum-Frequency Vibrational Spectroscopy, Proc. Natl. Acad. Sci. U. S. A., 2009, 106, 15148–15153 CrossRef CAS
.
- F. G. Moore and G. L. Richmond, Integration or Segregation: How Do Molecules Behave at Oil/Water Interfaces?, Acc. Chem. Res., 2008, 41, 739–748 CrossRef CAS
.
- A. Perry, C. Neipert, B. Space and P. B. Moore, Theoretical modeling of interface specific vibrational spectroscopy: Methods and applications to aqueous interfaces, Chem. Rev., 2006, 106, 1234–1258 CrossRef CAS
.
- A. Mafi, D. Hu and K. C. Chou, Interactions of Sulfobetaine Zwitterionic Surfactants with Water on Water Surface, Langmuir, 2016, 32, 10905–10911 CrossRef CAS
.
- D. Lis, E. H. G. Backus, J. Hunger, S. H. Parekh and M. Bonn, Liquid Flow Along a Solid Surface Reversibly Alters Interfacial Chemistry, Science, 2014, 344, 1138–1142 CrossRef CAS
.
- S. Pullanchery, T. Yang and P. S. Cremer, Introduction of Positive Charges into Zwitterionic Phospholipid Monolayers Disrupts Water Structure Whereas Negative Charges Enhances It, J. Phys. Chem. B, 2018, 122, 12260–12270 CrossRef CAS
.
- J. D. Cyran, M. A. Donovan, D. Vollmer, F. S. Brigiano, S. Pezzotti, D. R. Galimberti, M. Gaigeot, M. Bonn and E. H. G. Backus, Molecular Hydrophobicity at a Macroscopically Hydrophilic Surface, Proc. Natl. Acad. Sci. U. S. A., 2019, 116, 1520–1525 CrossRef CAS
.
- S. Urashima, A. Myalitsin, S. Nihonyanagi and T. Tahara, The Topmost Water Structure at a Charged Silica/Aqueous Interface Revealed by Heterodyne-Detected Vibrational Sum Frequency Generation Spectroscopy, J. Phys. Chem. Lett., 2018, 9, 4109–4114 CrossRef CAS
.
- N. Ji, V. Ostroverkhov, C. S. Tian and Y. R. Shen, Characterization of Vibrational Resonances of Water-Vapor Interfaces by Phase-Sensitive Sum-Frequency Spectroscopy, Phys. Rev. Lett., 2008, 100, 096102 CrossRef CAS
.
- J. Wang, S. E. Woodcock, S. M. Buck, C. Y. Chen and Z. Chen, Different Surface Restructuring Behaviors of Polymethacrylates Detected by SFG in Water, J. Am. Chem. Soc., 2001, 123, 9470–9471 CrossRef CAS
.
- J. Wang, Z. Paszti, M. A. Even and Z. Chen, Measuring Polymer Surface Ordering Differences in Air and in Water by Sum Frequency Generation (SFG) Vibrational Spectroscopy, J. Am. Chem. Soc., 2002, 124, 7016–7023 CrossRef CAS
.
- M. L. Clarke, C. Chen, J. Wang and Z. Chen, Molecular Level Structures of Poly(n-Alkyl Methacrylate)s with Different Side Chain Lengths at the Polymer/Air and Polymer/Water Interfaces, Langmuir, 2006, 22, 8800–8806 CrossRef CAS
.
- J. M. Hankett, X. Lu, Y. Liu, E. Seeley and Z. Chen, Interfacial Molecular Restructuring of Plasticized Polymers in Water, Phys. Chem. Chem. Phys., 2014, 16, 20097–20106 RSC
.
- J. M. Hankett, Y. Liu, X. Zhang, C. Zhang and Z. Chen, Molecular Level Studies of Polymer Behaviors at the Water Interface Using Sum Frequency Generation Vibrational Spectroscopy, J. Polym. Sci., Part B: Polym. Phys., 2013, 51, 311–328 CrossRef CAS
.
- X. Lu, C. Zhang, N. Ulrich, M. Xiao, Y.-H. Ma and Z. Chen, Studying Polymer Surfaces and Interfaces with Sum Frequency Generation Vibrational Spectroscopy, Anal. Chem., 2017, 89, 466–489 CrossRef CAS
.
- C. Leng, H. G. Buss, R. A. Segalman and Z. Chen, Surface Structure and Hydration of Sequence-specific Amphiphilic Polypeptoids for Antifouling/Fouling Release Applications, Langmuir, 2015, 31, 9306–9311 CrossRef CAS
.
- M. E. Barry, E. C. Davidson, C. Zhang, A. L. Patterson, B. Yu, A. K. Leonardi, N. Duzen, K. Malaviya, J. L. Clarke, J. A. Finlay, A. S. Clare, Z. Chen, C. K. Ober and R. A. Segalman, The Role of Hydrogen Bonding in Peptoid-Based Marine Antifouling Coatings, Macromolecules, 2019, 52, 1287–1295 CrossRef
.
- C. A. Del Grosso, T. W. McCarthy, C. L. Clark, J. L. Cloud and J. J. Wilker, Managing Redox Chemistry To Deter Marine Biological Adhesion, Chem. Mater., 2016, 28, 6791–6796 CrossRef CAS
.
-
A. J. Kinloch, Adhesion and Adhesives Science and Technology, Springer, New York, 1987 Search PubMed
.
- J. Davenport and X. Chen, A Comparison of Methods for the Assessment of Condition in the Mussel (Mytilus edulis L.), J. Mollus. Stud., 1987, 53, 293–297 CrossRef
.
- R. H. Baird, Measurement of Condition in Mussels and Oysters, J. Cons., Cons. Int. Explor. Mer, 1957, 23, 249–257 CrossRef
.
- H. R. Baker, P. B. Leach, C. R. Singleterry and W. A. Zisman, Cleaning by Surface Displacement of Water and Oils, Ind. Eng. Chem., 1967, 59, 29–40 CrossRef CAS
.
- A. Hodgson and S. Haq, Water Adsorption and the Wetting of Metal Surfaces, Surface Sci. Rep., 2009, 64, 381–451 CrossRef CAS
.
- P. A. Thiel and T. E. Madey, The Interaction of Water with Solid Surfaces: Fundamental Aspects, Surface Sci. Rep., 1987, 7, 211–385 CrossRef CAS
.
- S. Kaur, A. Narayan, S. Dalvi, Q. Liu, A. Joy and A. Dhinojwala, Direct Observation of the Interplay of Catechol Binding and Polymer Hydrophobicity in a Mussel-Inspired Elastomeric Adhesive, ACS Cent. Sci., 2018, 4, 1420–1429 CrossRef CAS
.
- M. A. North, C. A. D. Grosso and J. J. Wilker, High Strength Underwater Bonding with Polymer Mimics of Mussel Adhesive Proteins, ACS Appl. Mater. Interfaces, 2017, 9, 7866–7872 CrossRef CAS
.
- Y. Mu, X. Wu, D. Pei, Z. Wu, C. Zhang, D. Zhou and X. Wan, Contribution of the Polarity of Mussel-Inspired Adhesives in the Realization of Strong Underwater Bonding, ACS Biomater. Sci. Eng., 2017, 3, 3133–3340 CrossRef CAS
.
-
K. Autumn, in Biological Adhesives, ed. Smith A. M. and Callow J. A., Springer, Berlin, 2006, pp. 225–256 Search PubMed
.
-
S. N. Gorb, in Advances in Insect Physiology: Insect Mechanics and Control, ed. Casas, J., Elsevier, San Diego, 2007, pp. 81–115 Search PubMed
.
- A. Y. Stark, M. R. Klittich, M. Setti, P. H. Niewiarowski and A. Dhinojwala, The Effect of Temperature and Humidity on Adhesion of a Gecko-Inspired Adhesive: Implications for the Natural System, Sci. Rep., 2016, 6, 30936 CrossRef
.
- J. O. Wolff and S. N. Gorb, The Influence of Humidity on the Attachment Ability of the Spider Philodromus dispar (Araneae, Philodromidae), Proc. R. Soc. B, 2012, 279, 139–143 CrossRef
.
- R. M. Ziolek, F. Fraternali, A. Dhinojwala, M. Tsige and C. D. Lorenz, Structure and Dynanics of Nanoconfined Water Between Surfactant Monolayers, Langmuir, 2020, 36, 447–455 CrossRef CAS
.
- Y. Akdogan, W. Wei, K. –Y. Huang, Y. Kageyama, E. W. Danner, D. R. Miller, N. R. M. Rodriguez, J. H. Waite and S. Han, Intrinsic Surface-Drying Properties of Bioadhesive Proteins, Angew. Chem., Int. Ed., 2014, 53, 11253–11256 CrossRef CAS
.
- S. A. Mian, L.-M. Yang, L. C. Saha, E. Ahmed, M. Ajmal and E. Ganz, A Fundamental Understanding of Catechol and Water Adsorption on a Hydrophilic Silica Surface: Exploring the Underwater Adhesion Mechanism of Mussels on An Atomic Scale, Langmuir, 2014, 30, 6906–6914 CrossRef CAS
.
Footnotes |
† Electronic supplementary information (ESI) available: More detailed experimental procedures and data analysis methods, supplementary tables, figures, and one video. See DOI: 10.1039/d0sc03690k |
‡ These authors contributed equally to this work. |
§ Currently at Meinig School of Biomedical Engineering, Cornell University, USA. |
|
This journal is © The Royal Society of Chemistry 2020 |
Click here to see how this site uses Cookies. View our privacy policy here.