DOI:
10.1039/D0QM00303D
(Review Article)
Mater. Chem. Front., 2020,
4, 2517-2547
Recent advances of hollow-structured sulfur cathodes for lithium–sulfur batteries
Received
7th May 2020
, Accepted 21st June 2020
First published on 22nd June 2020
Abstract
Lithium–sulfur batteries (LSBs) have attracted increasing interest due to their high theoretical energy density and low-cost sulfur. Challenges are still faced in the development of sulfur cathodes, due to the insulating properties of sulfur and lithium sulfide, diffusion of soluble polysulfides and slow redox kinetics of electrochemical conversion reactions. Hollow-structured materials with features such as high specific surface area, tunable pore structure, and controllable morphology and composition have shown great potential to be applied in high performance sulfur cathodes for LSBs. To promote further breakthroughs in this amazing field, this review highlights on the recent advances of hollow-structured sulfur cathodes, with an emphasis on polar inorganic/organic materials that exhibit strong interactions with polysulfides, thus suppressing the unfavourable shuttle effect, and/or efficient catalytic activity towards sulfur conversion reactions, thus improving the redox kinetics. Material design principles, experimental methods and the subsequent effects on electrochemical performance are discussed. The remaining challenges and perspectives associated with sulfur cathode design and battery evaluation are also presented.
1 Introduction
Lithium-ion batteries (LIBs) have powered the revolution of our modern society, becoming more and more indispensable, from portable to large-scale electronic devices such as mobile phones and vehicles.1 After three decades of intensive research, the energy density of LIBs is approaching their physical limit (500–600 W h kg−1) based on intercalation/deintercalation reactions of Li ions.2,3 Besides, the high cost of LIBs hampers the popularity of electric vehicles.4 The ever-growing demands for batteries with higher energy density and lower cost have triggered the (re)exploration of alternative electrochemistry, such as lithium–air,5 metal–sulfur,6 and metal-ion (Na, K, Mg, Ca, Al) batteries.7 Among these electrochemical energy storage systems, lithium–sulfur batteries (LSBs) composed of a lithium anode and a sulfur cathode, which deliver a high theoretical energy density of 2600 W h kg−1 and are of low cost owing to the earth abundant and eco-friendly sulfur, have been regarded as one of the most promising candidates.8 Hence, it is not surprising that the study of LSBs is dated back to the 1960s.9 Nevertheless, in early studies, challenges including low sulfur utilization caused by the intrinsic insulating nature of sulfur and its reduction products (lithium sulfides), and short cycling life owing to the highly soluble lithium polysulfide intermediates (LiPSs), hindered the in-depth research on LSBs. The interest in LSBs was soon shadowed under the effulgence of more reliable LIBs. There remained sporadic research efforts focusing on identifying the redox couples in LSBs operated in different electrolyte systems (solvents and salts).10,11
In recent years, with the rapid development of materials science and nanotechnology, the research on LSBs has made much progress.12,13 In 2009, Nazar's group applied a highly ordered mesoporous carbon nanostructure (CMC-3) as the sulfur host for the first time, which dramatically improved the capacity from less than 500 mA h g−1 to 1320 mA h g−1 with an impressive sulfur utilization of 80%.14 Since then, the electrical conductivity and the LiPS trapping ability have become criteria for the design of sulfur host materials. The LSB community has focused on carbonaceous materials featuring high conductivity and high specific area (SSA), providing an efficient conductive framework and physical confinement to LiPSs.15 Later, it was realized that to achieve long-term cycling performance of >200 cycles, the weak interaction between the non-polar carbon and polar LiPSs was not enough.16 Then, it was found that various metal oxides/sulfides/nitrides and metal–organic-frameworks (MOFs) could form strong chemical bond with LiPSs, which greatly enhanced the long-term cycling stability (up to 1000 cycles).17,18 Thereupon, the strong chemical interaction with LiPSs became an essential requirement for sulfur hosting materials.19–21 More recently, electrocatalysis in LSBs, which has been proved to be able to facilitate the conversion kinetics of sulfur species and reduce the redox overpotential and thus increase the sulfur utilization and prolong the battery cycling life, is becoming a new focus for sulfur host design.22–24
Along with an understanding of the chemistry of sulfur hosting materials, studying the morphology and microstructure of these materials also plays a significant role in LSB research.25 A wide spectrum of sulfur hosts with a variety of morphologies, including porous/hollow nano-spheres, nanowires/nanotubes, nanosheets/nanoplates and hierarchical structures, have been developed.26,27 Hollow-structured nanomaterials stand out as there are plenty of void spaces for accommodating sulfur or lithium sulfide, buffering the huge volume expansion of sulfur during the charge process and physically confining LiPSs.28–30 In its first phase, the development of various carbon hollow structures using different hard- and soft-templating methods has provided tremendous opportunities for sulfur cathode design, due to their high specific surface area, tuneable porosity, and controllable morphology.15 Many kinds of hollow carbon-based sulfur cathodes have been demonstrated with improved sulfur utilization and cycling stability.31–36 Although hollow carbon structures can provide physical confinement to sulfur in the pore channels, the weak interaction between the nonpolar carbon and polar LiPSs cannot effectively immobilize LiPSs in a long run. Subsequently, other strategies, such as doping with heteroatoms (e.g., N or S) or decorating with inorganic polar species, have thus been developed as efficient methods to enhance the chemisorption between LiPSs and the carbon host.19,36–38 Recent studies on hollow carbon structures in LSBs have been summarised in another review paper.39
Hollow-structured materials with high conductivity, strong LiPS adsorption capability and efficient electrocatalytic activity are supposed to boost the electrochemical performance of LSBs. In this review, we summarize the recent progress and the foremost findings on hollow structured sulfur cathodes. In particular, we focus on hollow structures containing polar inorganic/organic materials, emphasizing the synthesis methods, and the relationship between material microstructures and electrochemical performance of LSBs. In an attempt to provide guidance towards the rational design of practical sulfur cathodes, challenges and perspectives for future development of LSBs are provided.
2 An overview of LSBs and hollow-structured sulfur cathodes
2.1 The redox chemistries and challenges of LSBs
A typical LSB consists of a lithium anode, sulfur cathode, polypropylene separator, and ether-based electrolyte. The typical galvanostatic discharge/charge profile and cyclic voltammogram are shown in Fig. 1a and b, respectively. During the discharge process, the solid S8 undergoes stepwise reduction reactions towards Li2S with a series of soluble polysulfide intermediates.40 In detail, S8 is initially reduced to Li2S8, and the solid–liquid process generates the first plateau at a potential of 2.2–2.3 V vs. Li+/Li (Fig. 1a). Li2S8 is then reduced to shorter-chain LiPSs, such as Li2S6 and Li2S4, and the liquid–liquid transformation generates a slope in the discharge curve (Fig. 1a). The theoretical capacity of these two processes is 419 mA h g−1,41 corresponding to the first cathodic peak at around 2.2–2.4 V vs. Li+/Li in the CV curves (Fig. 1b). The short-chain LiPSs can be either directly reduced to solid Li2S or follow stepwise reduction from Li2S2 to Li2S, generating the long-plateau at a potential of 1.9–2.1 V vs. Li+/Li. These processes correspond to the second cathodic peak at around 2.0 V vs. Li+/Li in the CV curves (Fig. 1b). The low-potential steps contribute three quarters of the overall theoretical capacity (1256 mA h g−1).41 During the charge process, lithium sulfides are reversibly oxidised back to sulfur.
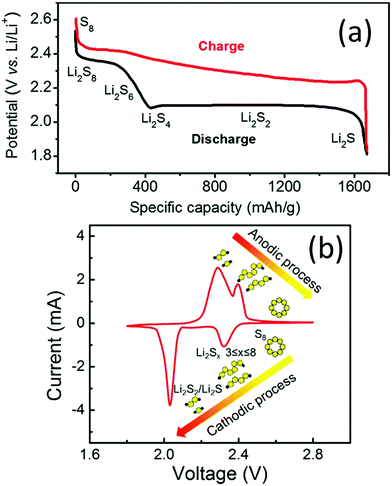 |
| Fig. 1 (a) Typical two-plateau discharge/charge curves of LSBs in ether-based electrolytes. Reproduced with permission.12 Copyright 2016, the Royal Society of Chemistry. (b) CV profile of LSBs in ether-based electrolytes. Reproduced with permission.42 Copyright 2017 Elsevier. | |
Despite the high theoretical energy density, there are several critical issues hindering the functioning of LSBs. For the sulfur cathodes, firstly, the insulating nature (both ionically and electronically) of the solid sulfur species (S8, Li2S2 and Li2S) blocks the charge/ion transfer in the active species. Adding conductive additives is one possible way to make batteries work. However, the quantity of additives has to be strictly controlled, as any excess amount of conductive additives is detrimental to the overall energy density. Moreover, the accumulation of sulfur on the electrode surface during repeated charge and discharge cycles increases the cell polarization and leads to higher possibility of device failure caused by the blocking of Li+ or e− pathways.43 Secondly, the soluble LiPSs shuttle between two electrodes and chemically react with lithium, causing active material loss, lithium corrosion, and electrolyte consumption. For Li anodes, inhomogeneous lithium deposition and lithium dendrite growth lead to repeated breaking and formation of the solid–electrolyte interface (SEI), which consumes lithium and the electrolyte.44 Moreover, lithium dendrites may cause short-circuit, leading to safety issues.45 For the electrolyte, the widely used ether-based electrolytes consist of flammable solvents and oxidative LiNO3, raising safety concerns for practical application.46
2.2 Hollow-structured sulfur cathodes
The design of hollow structured sulfur cathodes is a primary way for improving the performance of LSBs. Generally, there are two approaches to prepare hollow structured sulfur composites. One is infiltrating sulfur into the prefabricated hollow nanomaterials via a melt-diffusion or a dissolving–evaporation process;14,47 the other is encapsulating the prefabricated sulfur nanoparticles by in situ growing materials on the surface of sulfur. Benefiting from the significant progress of the design and synthesis of hollow structures, the first method is convenient, low cost and widely applicable to a wide spectrum of hollow-structured materials. The melt-diffusion approach usually involves a heat treatment process of the mixture of hosts and sulfur in a sealed vial at around 155 °C, when sulfur exhibits low viscosity and can easily infiltrate into or disperse onto the host matrix.48 Sometimes, an additional heat treatment step at around 300 °C is applied to remove the sulfur remaining on the outer surface of the host. The dissolving approach is realised by dissolving sulfur in carbon disulfide (CS2) or other solvents with high sulfur solubility, followed by either immersing the host into the sulfur-containing solution or directly dropping a specific amount of solution onto the hosts.47 CS2 can be easily removed via evaporation at room temperature under stirring. Although sulfur can infiltrate into the hollow structure by the dissolving approach, it is difficult to avoid sulfur aggregation. Moreover, sulfur species can spread out from the host.49 To better control the sulfur dispersion, sulfur nanoparticles offer an option. There are two methods widely used to prepare nano-sized sulfur particles. One is the acid catalysed precipitation of sodium thiosulphate (eqn (1)). Dilute HCl or H2SO4 is predominantly used as the acid catalyst, and the surfactants are added to control the size of sulfur particles.50 Another one is a microemulsion technique, using sodium polysulfide and HCl as the reactants (eqn (2)).51 | Na2S2O3 + 2HCl → 2NaCl + SO2↑ + S↓ + H2O | (1) |
| Na2Sx + 2HCl → 2NaCl + (x − 2)S↓ + 2H2S↑ | (2) |
Hollow-structured sulfur cathodes summarized in this review are divided into two categories: inorganic hollow-structured sulfur cathodes, which apply hollow metal compounds or metal compound decorated hollow carbons as sulfur host materials, and organic hollow-structured sulfur cathodes, which utilize conductive polymers and organic frameworks.
3 Inorganic hollow-structured sulfur cathodes
Compared to nonpolar carbon, inorganic materials with polar surfaces have a better ability to anchor polysulfides. It's worth mentioning that many materials that chemically interact with LiPSs simultaneously possess electrocatalytic activity.24,52 A wide range of inorganic hollow structures, such as metal oxides, metal sulfides, metal nitrides, metal hydroxides, metal phosphides, and their hybrids with hollow carbons, have been investigated as sulfur-hosting materials.
3.1 Metal oxides
Metal oxides with different morphologies have been widely studied and used as sulfur hosts, or cathode mediators in LSBs.53 Metal oxides contain a strong polar surface owing to the oxygen anion (O2−), and thus they typically exhibit strong chemical interactions with LiPSs.21 The low-cost and easy fabrication of metal oxides greatly promoted their application in LSBs.54 The major drawback of metal oxides is their poor conductivity, which seriously affects the rate performance of LSBs. To improve their conductivity, metal oxides have been incorporated into different hollow carbon structures, such as carbon hollow nanofibers, nanoboxes, and nanospheres. Furthermore, developing other phases (such as Magnéli phase), or creating oxygen vacancies is another approach to increase the intrinsic conductivity of metal oxides. In this part, MnO2 and TiO2, which are among the most widely studied metal oxides in LSBs are used as examples to demonstrate the rational design of highly efficient hollow-structured metal oxide sulfur hosts.
3.1.1 MnO2.
MnO2 is widely known as the electrode material for Zn–MnO2 batteries. Nazar's group initially demonstrated that MnO2 acted as an efficient polysulfide mediator to immobilize LiPSs via reacting with LiPSs, forming surface-bound intermediates of thiosulfate (Fig. 2f).53 In their work, MnO2 nanosheets were fabricated through the reaction of graphene oxide and KMnO4. The melt-diffusion process was applied to incorporate sulfur into the MnO2 host, generating 75S/MnO2 nanosheet composites (Fig. 2a, sulfur content 75 wt%). The superior polysulfide entrapment ability of MnO2 nanosheets was confirmed by an in situ visual electrochemical measurement of 75S/MnO2 and 75S/KB (using Ketjenblack carbon as the host). The electrolyte of 75S/MnO2 remained light yellow at the end of the discharge–charge process (after 12 h), which was in sharp contrast to that of 75S/KB (Fig. 2d). Taking advantage of the hollow structure, the authors further constructed a sulfur–core MnO2 shell architecture by in situ growing of MnO2 nanosheets onto the sulfur particles (Fig. 2b) by a simple redox reaction between sulfur and potassium permanganate (Fig. 2e).55 To buffer the volume expansion of sulfur electrode, the yolk-shelled S–MnO2 structure (Fig. 2c) was created by partially dissolving the sulfur core using toluene. The yolk–shell S–MnO2 composite (sulfur content 82 wt%) achieved a high capacity of 950 mA h g−1 after 300 cycles at 0.5C, which was 88% of its initial capacity. The capacity of the yolk–shell S–MnO2 cathode remained at 315 mA h g−1 after 1700 cycles at 2C, corresponding to a capacity decay rate of 0.039% per cycle. Since then, MnO2 with different structures have been developed as sulfur host materials.56–58 A core–shell composite with λ-MnO2 nanorod encapsulated sulfur was prepared, which exhibited a capacity retention of 82% for 300 cycles at 0.5C.59 The enhanced high-rate performance was attributed to the unique one-dimensional λ-MnO2 nanorods, which acted as large tunnels to incorporate Li ions, preventing the deposition of solid lithium sulfide. It was further revealed that the capacity degradation could be attributed to the phase transformation from λ-MnO2 to Mn3O4.
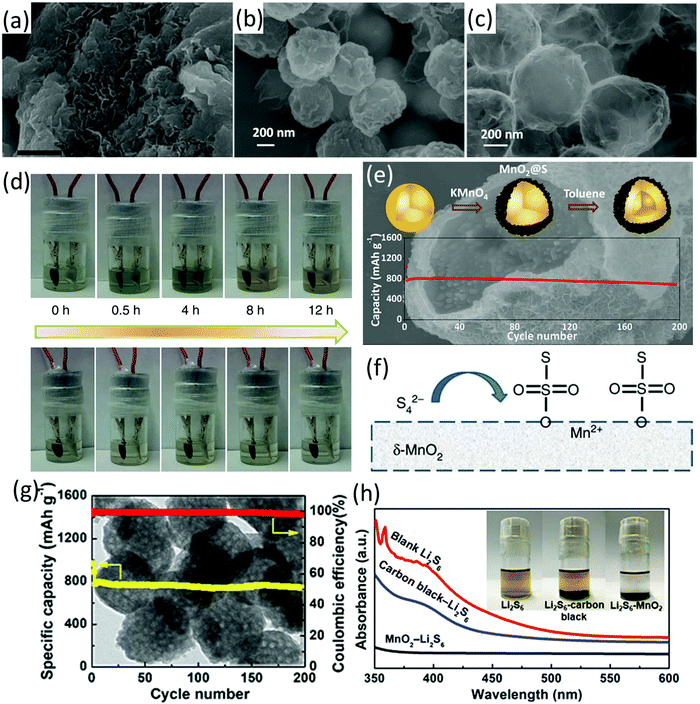 |
| Fig. 2 SEM images of (a) 75S/MnO2 nanosheet composite (scale bars, 50 nm), (b) spherical sulfur nanoparticles, and (c) rinsed (by toluene) NS-core/MnO2 with a sulfur content of 80 wt%, (d) visual in situ electrochemical measurement at C/20, 75S/KB (up) and 75S/MnO2 (down), (e) schematic illustration of the preparation process, SEM image and cycling performance of the core–shell S–MnO2 composite, (f) schematic for the interaction-reaction mechanism of MnO2 and LiPSs with the formation of surface-bound intermediates of thiosulfate. (a, d and f) Reproduced with permission.53 Copyright 2015 Springer Nature. (b, c and e) Reproduced with permission.55 Copyright 2016 American Chemical Society. (g) SEM image and cycling performance of the S–mesoporous MnO2 nanosphere composite, (h) visual polysulfide adsorption test using mesoporous MnO2 nanosphere and carbon black as the adsorbents (after 0.5 h) and the corresponding UV-vis spectra of the supernatant. Reproduced with permission.60 Copyright 2018 American Chemical Society. | |
Besides encapsulating the prefabricated sulfur nanoparticles in MnO2, mesoporous MnO2 nanospheres were fabricated and used as a sulfur host (Fig. 2g).60 To prepare MnO2 nanospheres, polyaniline coated SiO2 spheres were firstly prepared (PANI@SiO2). MnO2 was formed on the surface of PANI@SiO2via the reaction between KMnO4 and PANI. The mesoporous MnO2 nanospheres were finally obtained after purification by annealing and the removal of SiO2 using NaOH solution. MnO2 nanospheres did not have obvious morphology change after the melt-diffusion process, suggesting their robust structure. The strong interaction of the mesoporous MnO2 nanospheres and LiPSs was confirmed by the visual LiPS adsorption test using MnO2 as the adsorbent and UV-vis measurements (Fig. 2h). Benefiting from the strong LiPSs entrapment from both chemical interaction and physical confinement, the MnO2-based sulfur electrode delivered an initial capacity of 1349.3 mA h g−1 and exhibited a capacity decay rate of 0.073% per cycle for 500 cycles at 1C.
Despite the impressive polysulfide entrapment of MnO2, its poor conductivity remains a concern. In this regard, MnO2 has been integrated with different hollow carbon structures. For example, carbon hollow nanofibers filled with MnO2 nanosheets (MnO2@HCF, Fig. 3b) were prepared using MnO2 nanowires as hard templates.61 As shown in Fig. 3a, MnO2 nanowires were firstly coated with SiO2 and resorcinol formaldehyde (RF) resin (MnO2@SiO2@RF), followed by annealing and removal of the SiO2 interlayer to achieve MnO2@HCF. At a sulfur content of 71 wt% and a sulfur loading of 3.5 mg cm−2, the MnO2@HCF-based sulfur electrode delivered a capacity of 662 mA h g−1 after 300 cycles at 0.5C, corresponding to an areal capacity of 2.3 mA h cm−2. The hollow carbon nanofibers enabled a fast electron and ion transfer, while the polysulfide diffusion was suppressed by the strong chemical bonding of MnO2 and physical confinement of the hollow structure. Based on a similar methodology, MnO2@carbon hollow nanoboxes were prepared using MnCO3 nanocubes as the template and precursor (Fig. 3c and d).62 In these situations, Mn-based species were used as both template and precursor, leading to the formation of hybrids with MnO2 filled inside the hollow structure. In another study, MnO2 nanosheets were in situ grown on carbon fibres via the reaction of KMnO4 solution and carbon (eqn (3)), forming coaxial C/MnO2 hollow nanofibers.63 The coaxial C/MnO2 hollow nanofiber-based sulfur cathode exhibited a low capacity decay rate of 0.044% and 0.051% per cycle over 1000 cycles at 1C and 2C, respectively. A dual sulfur@carbon@MnO2 core–shell structure (Fig. 3e–g) has been designed and synthesized by the same group, delivering an impressive rate-performance of 465 mA h g−1 at 4C, and a low capacity decay rate of 0.052% per cycle for 1000 cycles at 3C.64
| 3C + 4MnO4− + H2O → 4MnO2 + 2HCO3− + CO32− | (3) |
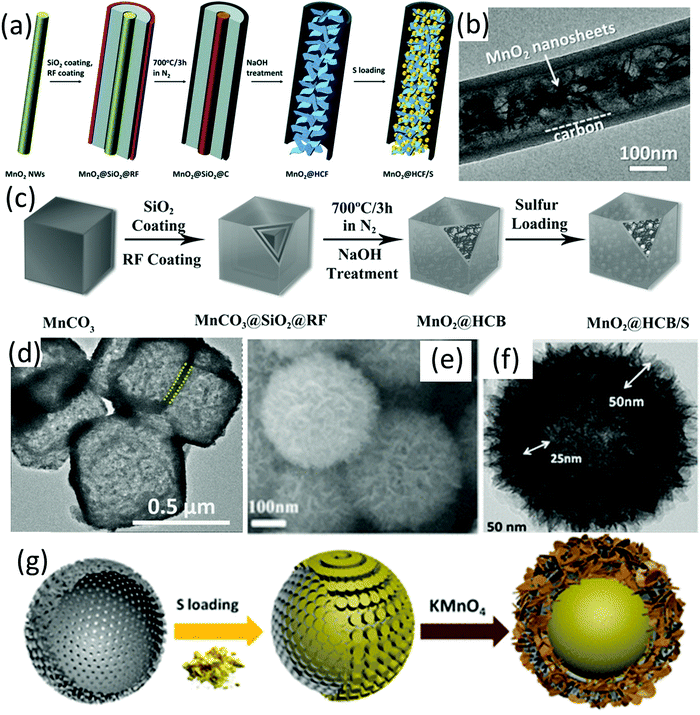 |
| Fig. 3 (a) Schematic for MnO2@HCF/S preparation, (b) TEM image of MnO2@HCF, reproduced with permission.61 Copyright 2015 Wiley-VCH. (c) Schematic for MnO2@HCB/S nanobox preparation, (d) TEM image of MnO2@HCB nanoboxes, Reproduced with permission.62 Copyright 2017 Wiley-VCH. (e) SEM and (f) TEM images and, (g) schematic for the preparation of a S@C@MnO2 dual core–shell hybrid, Reproduced with permission.64 Copyright 2017 American Chemical Society. | |
Besides the versatile carbon matrixes, conductive polymers are another choice to improve the conductivity of sulfur–MnO2 composites. For example, a hybrid hollow structure composed of MnO2 nanosheet coating layer and sulfur@poly(3,4-ethylenedioxythiophene) nanospheres (MnO2@sulfur@PEDOT) was fabricated.65 The hybrid structure with a superior electrolyte wettability delivered a capacity of 827 mA h g−1 at 0.2C after 200 cycles, which was superior to that of sulfur@PEDOT (551 mA h g−1). Last but not the least, it was reported that the chemical interaction of MnO2 with LiPSs can be enhanced via creating oxygen vacancies.66 The oxygen vacancies generated by annealing MnO2 hollow spheres in a reducing atmosphere (H2/N2: 8/92 at volume) not only provided more surface-active sites for LiPS adsorption and solid lithium sulfide precipitation, but also facilitated the transformation of LiPSs to thiosulfate and polythionate intermediates. LSBs using MnO2 with oxygen vacancies as the sulfur host delivered a capacity of 551 mA h g−1 after 400 cycles at 2C, which was better than that of 410 mA h g−1 using pristine MnO2 as the sulfur host.
3.1.2 TiO2.
TiO2 is another metal oxide which has long been used as a cathode additive or sulfur host material for LSBs owing to its strong interaction with LiPS and its nature abundance and nontoxicity. TiO2 with different phases has been investigated both experimentally and theoretically.67,68 With an emphasis to create enough space to buffer the huge volume expansion of sulfur during lithiation, a sulfur–TiO2 yolk–shell has been initially proposed by Cui and co-workers (Fig. 4a and b).69 Monodispersed sulfur nanoparticles with a diameter of around 800 nm were firstly prepared via the reaction of sodium thiosulfate and hydrochloric acid. A TiO2 layer with a thickness of around 15 nm was coated on the as-obtained sulfur spheres through a controllable hydrolysis of titanium diisopropoxide bis(acetylacetonate) in a solution of alkaline/aqueous isopropanol. The yolk–shell nanoarchitecture was created after partial dissolution of the inner sulfur using toluene. The as-obtained sulfur–TiO2 yolk–shell composite (sulfur content 71 wt%) exhibited an initial capacity of 1030 mA h g−1 at 0.5C with a capacity retention of 81% and 67% after 500 and 1000 cycles, respectively. The yolk–shell structure allowed the volume expansion of sulfur electrode, and the intact TiO2 shell with a small pore size of around 0.3 nm effectively minimized the diffusion of polysulfide, leading to a superior electrochemical performance to those of bare sulfur and sulfur–TiO2 core–shell counterparts.
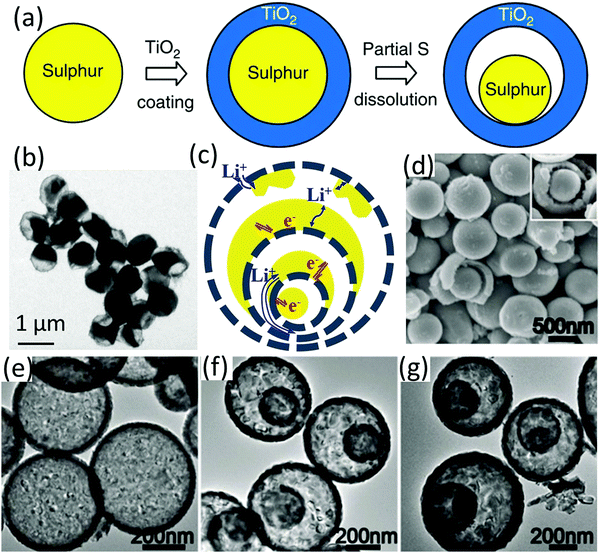 |
| Fig. 4 (a) Schematic of the synthesis route, and (b) TEM image of the sulfur–TiO2 yolk–shell nanostructure. Reproduced with permission.69 Copyright 2013 Springer Nature. (c) Schematic for the lithiation/delithiation process of sulfur@HoMS, (d) SEM image of 3S-TiO2−x HoMSs, and TEM images of (e) 1S-TiO2−x HoMSs, (f) 2S-TiO2−x HoMSs, and (g) 3S-TiO2−x HoMSs. Reproduced with permission.70 Copyright 2019 Wiley-VCH. | |
More recently, the application of TiO2 microboxes as sulfur host has been reported.71 CaTiO3 microcubes, the self-sacrificial templates for the synthesis of TiO2 microboxes, were firstly prepared via a solvothermal process, followed by the generation of H2TiO3 layer on the surface of CaTiO3 and then TiO2 coated CaTiO3via Na2EDTA-assisted ion exchange of CaTiO3 (eqn (4)).72 The formation of the hollow structure was driven by the ion exchange between CaTiO3 and H+ at a different outward and inward diffusion rate, respectively.73 TiO2 microboxes exhibited efficient polysulfide adsorption ability, as revealed by visualized LiPS adsorption and the following UV-vis measurement.71 Infiltrating sulfur into the TiO2 microboxes via melt-diffusion generated the TiO2/S composite (sulfur content 70 wt%), whose capacity was maintained at 600 mA h g−1 after 500 cycles at 1C. However, obvious voltage humps were observed at the initial charge curves due to the low conductivity of TiO2.71
| CaTiO3 + H2O + Na2EDTA → TiO2 + CaEDTA + 2NaOH | (4) |
Developing other phases (such as the Magnéli phase, Ti
nO
2n−1) and creating oxygen vacancies have been widely used to increase the conductivity of metal oxides.
74,75 In addition, multi-shelled hollow structures have demonstrated attracting properties for a wide range of applications (such as energy storage and conversion), which have gained increasing research interest.
76–78 For example, multi-shelled hollow TiO
2−x (TiO
2−x HoMSs) prepared
via a sequential templating approach (STA) has been demonstrated as an efficient sulfur carrier (
Fig. 4c–g).
70 To prepare TiO
2 HoMSs, carbonaceous microspheres (CMS) were firstly synthesized
via the emulsion polymerization of sucrose through a hydrothermal process, followed by a controlled hydrolysis of TiCl
4 in the solution containing the as-obtained CMS solution, and a subsequent calcination step in air. The number of shells can be controlled
via tuning the aging conditions and annealing process. The surface hydrogenated TiO
2−x HoMSs were prepared
via annealing the as-obtained TiO
2 HoMSs in an Ar/H
2 (1
![[thin space (1/6-em)]](https://www.rsc.org/images/entities/char_2009.gif)
:
![[thin space (1/6-em)]](https://www.rsc.org/images/entities/char_2009.gif)
9) atmosphere, generating oxygen vacancies and Ti
3+ ions. Owing to oxygen vacancies and Ti
3+ ions, TiO
2−x HoMSs not only provided multiple spatial confinement and chemical interactions with LiPSs, but also enhanced the electron mobility and electrolyte penetration. The electrochemical properties of TiO
2−x HoMSs with one, two and three shells (1S-TiO
2−x HoMSs, 2S-TiO
2−x HoMSs, and 3S-TiO
2−x HoMSs, respectively) were investigated, among which the triple-shelled one presented the best performance with an initial capacity of 903 mA h g
−1 at 0.5C and a superior capacity retention of 79% for 1000.
Lou and co-workers designed a hybrid hollow structure composed of highly conductive TiO and carbon shells (TiO@C-HSs).79 TiO presented almost an order of magnitude higher electrical conductivity than the Magnéli phase (TinO2n−1). The preparation process is shown in Fig. 5a–d. TiO2 and polydopamine were sequentially coated on the polystyrene surface, generating PS@TiO2 and PS@TiO2@PDA, respectively. TiO@C-HSs were obtained after annealing PS@TiO2@PDA in N2/H2 (95/5) flow, during which TiO2 was reduced to conductive TiO, PS was decomposed and a carbon layer derived from PDA was formed on the surface. It was worth noting that without PDA, the PS@TiO2 core–shell structure would totally collapse after the annealing process, suggesting that PDA played an essential role in the formation of the robust shells. Benefiting from the superior conductivity of the TiO@C shells, the TiO@C-HS based sulfur electrode delivered a capacity of 750 mA h g−1 and 630 mA h g−1 after 500 cycles at 0.2C and 0.5C, respectively. The performance was superior to that of using TiO2−x@C-nanoparticles, TiO2-nanoparticles and C-HS as the sulfur hosts. However, a higher content carbon additive of 20 wt% (vs. 10 wt%) was essential to build a conductive network when the sulfur loading was increased to 4.0 mg cm−2. Learning from LIBs, to construct an efficient electrode, micrometre-scale particles need a smaller amount of binder and conductive additives than nanoparticles. In this regard, the authors further designed a hierarchical sulfur electrode composed of highly packed TiO hollow nanospheres and a carbon sheath, which simultaneously mitigated the issues of aggregated polysulfide dissolution and an inefficient conductive network in a thick sulfur electrode (GC-TiO@CHF, Fig. 5e–h).80 The grape-like electrode was prepared via electrospinning SiO2@TiO2 into polyacrylonitrile (PAN), followed by annealing and etching steps. Using GC-TiO@CHF as the sulfur host, the microscale electrode exhibited a superior cycling stability for more than 400 cycles at a high sulfur loading of 5.0 mg cm−2.
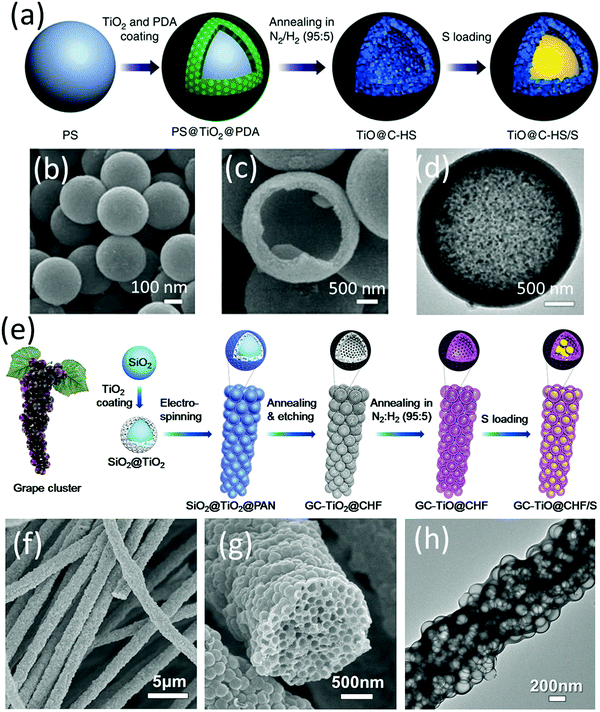 |
| Fig. 5 (a) Schematic for TiO@C-HS/S fabrication, (b and c) SEM, and (d) TEM images of TiO@C-HS. Reproduced with permission.79 Copyright 2016 Springer Nature. (e) Schematic for GC-TiO@CHF/S fabrication, (f and g) SEM, and (h) TEM images of GC-TiO@CHF. Reproduced with permission.80 Copyright 2017 Elsevier. | |
3.1.3 Other metal oxides.
Besides TiO2 and MnO2 based hollow structures, a wide range of hollow-structured metal oxides or their compounds have been designed and used as sulfur hosts, from single-shelled hollow structures, such as hollow MoO2 spheres,81 hollow NiCo2O4 nanofibers,82 jujube pit like Fe3O4–carbon composites,83 CeO2–carbon nanospheres,82 and V2O3 spheres,84 to various double-shelled hollow structures, such as SnO2@C nanospheres,85 defect-rich Co3O4−x microspheres,86 and NiO–NiCo2O4–carbon heterostructures,87 and finally to multi-shelled hybrids, such as Fe-doped Co3O4 hollow microspheres88 and V-decorated V2O5 microspheres (V/V2O5).89 Integrating metal oxides with highly conductive hollow carbons and polymers and creating defects or oxygen vacancies in these metal oxides are the general and applicable approaches to make better hollow-structured metal oxide sulfur hosts for high-performance LSBs.
3.2 Metal sulfides
Different from most of the metal oxides, which are semiconductors, metal sulfides typically consist of a wide range of semimetal or metallic phases.90 Furthermore, many metal sulfides exhibit strong LiPS interactions and efficient electrocatalysis for the sulfur conversion reactions. Despite these desirable merits for being a sulfur host for LSBs, the list of metal sulfides used as sulfur hosts is obviously shorter than that of metal oxides.54,90 Among these hollow-structured metal sulfides, although not much, cobalt sulfides of different phases, such as CoS2, Co3S4, and Co8S9, have gained particular attention. This is not only because of their good conductivity and superior electrocatalysis, but also benefited from the well-developed fabrication process using Co-based MOF/ZIF as the templates for the rational design of hollow structures. In this part, representative cobalt sulfide-based hollow sulfur hosts are summarized, with a focus on material design, preparation procedure and the electrochemical properties of the corresponding LSBs.
3.2.1 CoS2.
CoS2, a stable and metallic mineral, exhibits efficient electrocatalysis for hydrogen evolution reaction and oxygen reduction.91,92 Zhang and co-workers integrated micro-sized CoS2 with graphene as a sulfur host for LSBs.93 The CoS2–electrolyte interface served as polysulfide adsorption and activation sites, which promoted the redox reactions of polysulfide and the energy efficiency of LSBs. However, the nonporous bulk CoS2 particles unavoidably limited the exposed active sites to fully fulfil the properties of LiPS adsorption and redox acceleration. To make a better use of CoS2, a brain-coral-like mesoporous hollow nitrogen-doped graphitic carbon structure decorated with the in situ grown CoS2 nanoparticles (CoS2@NGCNs) was rationally designed and prepared through a four-step process (Fig. 6a).94 The hollow ZIF-67 spheres were firstly prepared using a room-temperature solution process (with CH3OH and SO42− ions). Secondly, the spherical Co@NGCNs was generated by a pyrolysis process of ZIF-67 under Ar/H2 (95
:
5 by volume) at 650 °C. Thirdly, the Co/CoS2@NGCNs was obtained by thermal sulfidation of the Co@NGCNs by sulfur at 300 °C. CoS2@NGCNs was finally obtained after chemically etching out the Co of Co/CoS2@NGCNs using HCl solution, when mesopores with an average size of around 9 nm were created on the carbon shell. The stepwise morphology evolution and the formation mechanisms of the unique structure are demonstrated in Fig. 6a and b, respectively. The unique nanostructure exhibited multiple advantages when used as a sulfur host, including (a) the mesoporous surface acted as the conductive pathways and storeroom for sulfur species, allowing enhanced sulfur utilization and high sulfur loading, (b) the void space in the centre could accommodate sulfur, buffer the volume expansion and provide physical confinement to the polysulfide, and (c) the polar CoS2 nanoparticles and nitrogen-doped carbon matrix could chemically immobilize polysulfide. CoS2@NGCNs presented the strongest LiPS adsorption ability among various control samples (Fig. 6c). Besides, CoS2@NGCNs-based LSBs achieved a higher sulfur utilization and lower polarization (Fig. 6d), and a positive shift in the reduction peaks and a negative shift of the oxidation peaks (Fig. 6e), suggesting the promoted redox kinetics of sulfur conversion. With a sulfur content of 75 wt%, the CoS2@NGCNs based sulfur electrode delivered a capacity of 900.3 mA h g−1 after 100 cycles at 0.1C and 519 mA h g−1 after 300 cycles at 1C.
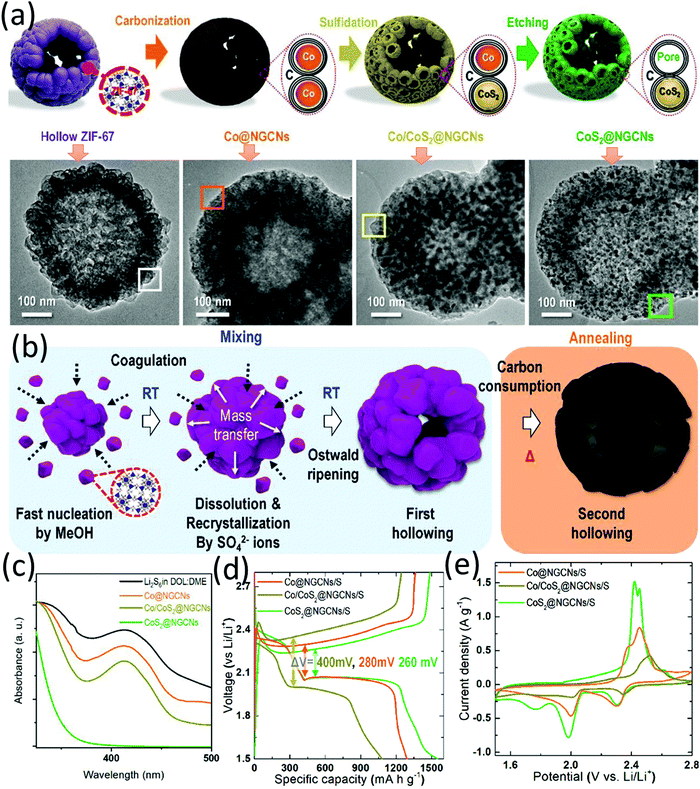 |
| Fig. 6 (a) Schematic for the four-step fabrication of CoS2@NGCNs, and TEM images of the corresponding intermediates, (b) proposed formation mechanisms of CoS2@NGCNs, (c) UV-vis spectrum of the original Li2S6, and the supernatant solutions after adsorption test using different adsorbents for 2 h, (d) galvanostatic discharge/charge profiles at 0.1C, and (e) CV profiles at 0.1 mV s−1 of hollow Co@NGCNs, Co/CoS2@NGSNs, and CoS2@NGCNs based LSBs. Reproduced with permission.94 Copyright 2019 Wiley-VCH. | |
3.2.2 Co3S4.
Co3S4 presents a high conductivity of 330 S cm−1 at room temperature95 and has been applied as sulfur hosts with various material integration and structure designs. Co3S4 polyhedra prepared using ZIF-67 as a self-sacrificing template96 were integrated with activated carbon nanofibers (ACNF) and sulfur via vacuum filtration, forming a compact film electrode.97 The compact sulfur electrode delivered high areal capacities of 5.25 mA h cm−2 (701 mA h g−1) and 7.47 mA h cm−2 (550 mA h g−1) after 100 cycles at 0.5C when the sulfur loading was 7.5 mg cm−2 and 13.5 mg cm−2, respectively. The superior performance was attributed to the highly-conductive hollow Co3S4 polyhedra which not only chemically trapped polysulfide and allowed quick electron transport, but also provided sufficient void space for LiPS storage.
The catalytic effect of Co3S4 for the sulfur conversion reactions in LSBs was initially investigated by Zhang and co-workers,98 inspired by the earlier reports that Co3S4 demonstrated 2–3 times higher electrocatalytic activity for oxygen reduction reactions than CoS2.99 In Zhang's work, Co3S4 nanotubes were firstly synthesized by a two-step hydrothermal process. Specifically, the first hydrothermal process (CoCl2·6H2O + CO(NH2)2) generated the nano-needle Co(CO3)0.35Cl0.20(OH)1.10 with a diameter of around 80–90 nm, and the nano-needle Co(CO3)0.35Cl0.20(OH)1.10 transformed into Co3S4 nanotubes due to the Kirkendall effect during the hydrothermal sulfuration process. Co3S4@S was obtained by a melt-diffusion process. The Co3S4@S cathode delivered an initial capacity of 517 mA h g−1 at 5C with 305 mA h g−1 left after 1000 cycles, corresponding to a capacity retention of 59% and a capacity decay rate of 0.041% per cycle. The Li2S4 adsorption test revealed the strong interaction between Co3S4 and LiPSs, and the CV results using symmetrical cells demonstrated the superior catalysis of Co3S4 on the conversion of Li2S6.
A hybrid nanocomposite of carbon nanotube intercalated hollow Co3S4 nanoboxes (CNT/Co3S4-NBs Fig. 7c) was prepared via a self-template method, which presented as an efficient sulfur host.100 The composite of sulfur and CNT/Co3S4-NBs (S@CNT/Co3S4-NBs, Fig. 7b and d) was prepared as shown in Fig. 7a. Specifically, ZIF-67 nanocubes were firstly in situ nucleated onto a –COOH-functionalized CNT network (CNTs/ZIF-67), followed by solvothermal sulfuration and a subsequent carbonization process. The difference in diffusion rates of sulfur species and cobalt (Kirkendall effect) was the driving force for the formation of hollow Co3S4-NBs. Combining the three-dimensional CNT conductive network with the conductive, polar hollow Co3S4 nanoboxes, the hybrid exhibited superior charge transfer ability, and synergistic chemical immobilization and physical confinement of LiPSs. Owing to these properties, the S@CNT/Co3S4-NBs cathode with a sulfur loading of 3.5 mg cm−2 delivered an initial areal capacity of 3.32 mA h cm−2 (1012 mA h g−1) at 0.2C, and 2.87 mA h cm−2 (820 mA h g−1) was maintained after 150 cycles. To uncover the mechanism of the superior performance, coin cells with different sulfur cathodes (S@CNT/Co3S4-NBs, S@CNT, S@Co3S4-NBs) were disassembled after 30 cycles (0.2C) at the discharged state, and the cathodes were soaked in the electrolyte solvent for 4 h (Fig. 7e). The transparent colour of the S@CNT/Co3S4-NBs containing solution and the corresponding UV-vis absorption spectra confirmed that the shuttle effect was efficiently suppressed by CNT/Co3S4-NBs.
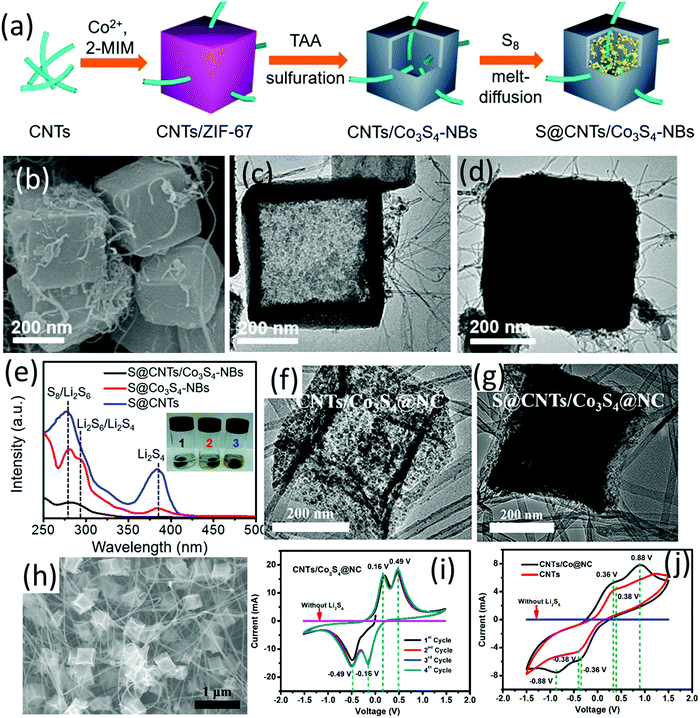 |
| Fig. 7 (a) Schematic for S@CNTs/Co3S4-NBs preparation, (b) SEM image of S@CNTs/Co3S4-NBs, TEM images of (c) CNTs/Co3S4-NBs, and (d) S@CNTs/Co3S4-NBs, (e) UV-vis absorption spectra of the electrolyte solvent (DOL/DME) with the soaked cycled cathode: (1) S@CNTs/Co3S4-NBs, (2) S@Co3S4-NBs and (3) S@CNTs. The inset photograph compares the colour of the solutions containing the corresponding cathodes. Reproduced with permission.100 Copyright 2017 American Chemical Society. TEM images of (f) CNTs/Co3S4@NC and (g) S@CNTs/Co3S4@NC, (h) SEM image of CNTs/Co3S4@NC, and CV curves of the symmetric cells with and without Li2S6 at 10 mV s−1 using (i) CNTs/Co3S4@NC, (j) CNTs/Co@NC and CNTs as the electrodes. Reproduced with permission.101 Copyright 2019 American Chemical Society. | |
More recently, a hierarchical carbon nanostructure composed of highly-dispersed Co3S4 nanoparticles, ZIF-67 and CNT sponge (CNTs/Co3S4@NC, Fig. 7f and h) was developed as a sulfur host.101 The S@CNTs/Co3S4@NC (Fig. 7g) cathode exhibited impressive high-rate performance with 720 mA h g−1 retained after 1000 cycles at 5C (85% capacity retention) and a low voltage polarization (refer to the distance of the charge and discharge plateaus at half capacity). The impressive high-rate performance was attributed to the excellent catalytic capability of CNTs/Co3S4@NC. As revealed by the CV curves of the symmetric cells, sharp peaks with narrow separation was obtained using CNTs/Co3S4@NC as the electrodes (Fig. 7i), compared to the much broader peaks with widened separation observed in the case of using CNTs/Co@NC and CNTs (Fig. 7j).
3.2.3 Co8S9.
Metallic Co8S9 with a high conductivity of 290 S cm−1 at room temperature102 is among the most widely studied metal sulfides in LSBs. Nazar et al. have demonstrated the dual-interaction of polysulfide with graphene-like Co8S9 nanosheets (S22−–Coδ+ and Li+–Sδ−) through both theoretical calculation and spectroscopic studies.103 It is known that the polar–polar interaction between the host and polysulfide is generally based on monolayered chemical adsorption.104 Despite the strong chemical interaction and large exposed adsorption sites of the polar host materials, it might be difficult to trap all LiPSs in a long run relying on two-dimensional nanosheets.28 In this regard, a highly-conductive honeycomb-like spherical structure assembled from hollow Co9S8 tubes was designed as a sulfur host. The well-aligned hollow tubes allow a fast ion transfer and provide a buffer room for the volume expansion of the sulfur electrode during the lithiation process, and the polar Co9S8 effectively bonds the polysulfide for long-term cycling.105 The two-step fabrication process is shown in Fig. 8a. The first step is the preparation of the urchin-like precursor with a diameter of around 10 μm (Fig. 8b), which was constructed from Co(CO3)0.5OH·0.11H2O nanotubes with a diameter of about 100 nm (Fig. 8c and d). The solid nanorods transformed into hollow nanotubes due to the anion exchange. The honeycomb-like Co9S8 was obtained after the reaction between Na2S and the as-prepared precursor under a hydrothermal process. The S@Co9S8 composite with sulfur infiltrated into the hollow Co9S8 tubes (Fig. 8e) was obtained through the melt-diffusion process. Compared to the pure sulfur electrode, the S@Co9S8 cathode exhibited a higher capacity, a lower voltage polarization and sharper redox peaks of the CV curves. In a long-term cycling process, the S@Co9S8 cathode delivered a capacity of 756.6 mA h g−1 after 600 cycles at 1C, corresponding to a capacity degradation rate of 0.026% per cycle. Based on a similar sulfur host design methodology, Co9S8/C nanopolyhedra were prepared via sulfurization of ZIF-67 nanopolyhedra using thioacetamide (TAA), followed by an annealing process at 350 °C in a N2 atmosphere.106 Large internal cavities were created due to the different ion diffusion rates of sulfur ions in TAA and cobalt ions in ZIF-67. Benefitting from the large void space and the polar Co9S8/C shell that provided both spatial and chemical confinement of polysulfide, the Co9S8/C based sulfur electrode presented a capacity of 560 mA h g−1 after 1000 cycles at 2C, corresponding to a low capacity rate of 0.041% per cycle.
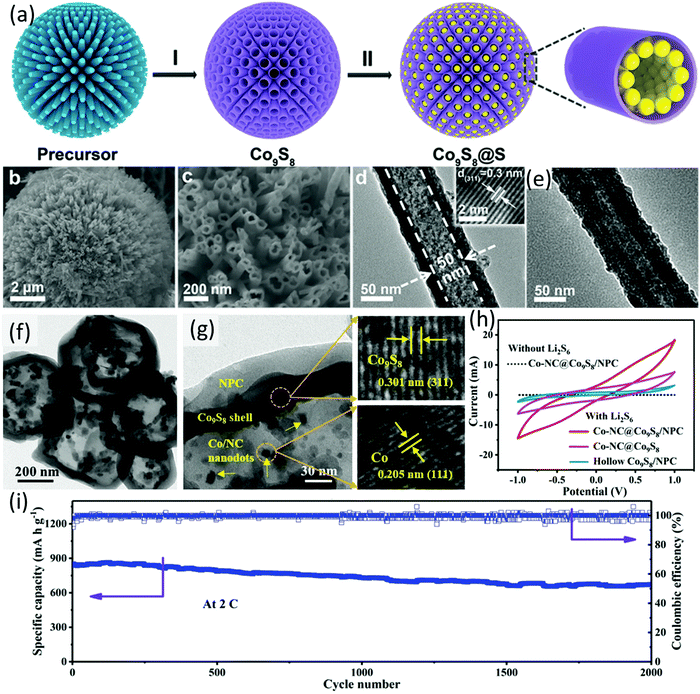 |
| Fig. 8 (a) Schematic for S@Co9S8 preparation, (b and c) SEM images, (d) TEM image of honeycomb-like Co9S8, and (e) TEM image of S@Co9S8. Reproduced with permission.105 Copyright 2018 Wiley-VCH. (f) TEM and (g) HRTEM images of Co-NC@Co9S8/NPC, and (h) CV profiles of the symmetric cells using different hosts as electrodes, (i) long-term cycling performance of Co-NC@Co9S8/NPC-S at a sulfur loading of 1.8 mg cm−2. Reproduced with permission.107 Copyright 2020 Wiley-VCH. | |
More recently, a double-shelled hollow polyhedron composed of a core of cobalt nanoparticles encapsulated in nitrogen-doped carbon, an inner Co9S8 shell, and an outer nitrogen-doped porous carbon layer (Co/NC@Co9S8/NPC, Fig. 8f and g) was developed as a sulfur host.107 Compared to Co/NC@ Co9S8 and the hollow Co9S8/NPC, the Co/NC@Co9S8/NPC presented an enhanced chemisorption ability to LiPSs and catalytic activity to sulfur conversion reactions. The author systematically investigated the formation process of the Co/NC@Co9S8/NPC. The imidazolium-based ionic polymer (ImIP)-encapsulated ZIF-67 (ZIF-67@ImIP), used as the core–shell precursor, was firstly prepared, followed by refluxing the precursor in thioacetamide (TAA) solution, during which the inner CoS shell was generated based on the reaction of TAA and ZIF-67, generating the yolk-double-shelled ZIF-67@CoS/ImIP. The final product was obtained after annealing the intermediate in a N2 atmosphere at 600 °C, during which the amorphous CoS converted to Co9S8, the ZIF-67 converted to Co/NC nanodots, and ImIP converted to porous nitrogen-doped carbon simultaneously. To verify the catalytic effect of the hosts, symmetrical cells composed of identical electrode (the hosts) and Li2S6 catholyte were assembled. CV measurements using the symmetrical cell presented the redox current trend of Co-NC@Co9S8/NPC > Co-NC@Co9S8 > Co9S8@NPC, suggesting the superior catalytic activity of NC@Co9S8/NPC (Fig. 8h). Benefiting from the elaborate double-shelled structure and the intrinsic material chemistry, the sulfur electrode using Co/NC@Co9S8/NPC as the sulfur host (Co/NC@Co9S8/NPC-S) maintained a superior capacity of 670 mA h g−2 after 2000 cycles at 2C, corresponding to a low capacity decay rate of 0.011 each cycle (Fig. 8i). Moreover, both of the sulfur cathode and lithium anode remained intact without yellow-coloured LiPSs on their surface, suggesting that the shuttle effect was effectively suppressed. Even without LiNO3 in the electrolyte, the Co/NC@Co9S8/NPC-S electrode exhibited a low capacity decay rate of 0.032% for 500 cycles at 2C. At a high sulfur loading of 4.5 mg cm−2, Co/NC@Co9S8/NPC-S delivered an initial capacity of 946 mA h g−1, which was maintained at 806 mA h g−1 after 500 cycles at 0.5C.
3.2.4 Other metal sulfides.
Besides the above-mentioned cobalt sulfides which were prepared using ZIF-67 as the template, there are other hollow-structured metal sulfides applied as sulfur hosts using SiO2 spheres as the hard templates. For example, NiS-nanoparticle decorated spherical hollow carbon was prepared with SiO2 spheres as the template.108 SiO2 spheres (450 nm) were firstly prepared, then SiO2@Ni–silicate@RF spheres (530 nm) were generated by adding nickel nitrate solution and resorcinol formaldehyde resin (RF). After annealing the as-obtained product in a N2 atmosphere, SiO2@Ni@C spheres were obtained. NiS@C-HSs were generated via the reaction between SiO2@Ni@C and Na2S, when Ni was converted to NiS nanoparticles and SiO2 was etched away by OH− generated from the hydrolysis of S2− ions. LSBs with NiS@C-HSs as the sulfur host delivered a capacity of 695 mA h g−1 after 300 cycles at 0.5C, corresponding to a low capacity degradation rate of 0.013% per cycle. A hollow carbon structure with sulfur-deficient MoS2 nanosheets anchored on the inner side (MoS2−x/HMC) was achieved, where SiO2 spheres were used as the templates for the preparation of HMC.109 MoS2 nanosheets were grown on the HMC (MoS2/HMC) via a hydrothermal process. The sulfur-deficient MoS2−x was generated by further annealing the as-obtained MoS2/HMC in H2/Ar (5/95) flow. LSBs using MoS2−x/HMC as a sulfur host delivered a capacity of 754 mA h g−1 after 100 cycles at 0.2C, corresponding to a capacity retention of 70%, which was higher than those of MoS2/HMC (58.5%) and HMC (51.1%). The enhanced performance was attributed to sulfur vacancies which promoted the chemical interaction of MoS2 and polysulfide. Besides creating sulfur vacancies, taking advantage of metallic 1T-MoS2 is another approach to enhance the performance of MoS2 as a sulfur host. A porous graphene/MoS2 hybrid with N-doped graphene wrapped 1T-MoS2 nanotube was synthesized as a sulfur host.110 The nanotube was constructed from vertically oriented few-layered MoS2, which self-assembled through a spray-drying approach. Benefiting from the strong LiPS adsorption and efficient catalytic activity of 1T-MoS2 and the graphene conductive network, the S-graphene/MoS2 cathode exhibited a high capacity of 1214 mA h g−1 after 200 cycles at 0.2C.
It is noticed that most of the metal sulfides used in LSBs are mono-metal sulfides. Inspired by the outstanding electrochemical properties of NiCo2S4 for oxygen reduction and hydrogen evolution reactions111,112 and other energy storage systems,113,114 Xia and co-workers initially designed NiCo2S4 yolk–shell hollow spheres as the sulfur host.115 The NiCo2S4 yolk–shell hollow spheres were synthesized via sulfurization of NiCo-glycerate spheres using thioacetamide (TAA) under a hydrothermal process. The S/NiCo2S4 electrode presented a low capacity rate of 0.074% per cycle for 500 cycles at 0.5C. Compared to Ni3S4 and Co3S4 with a similar morphology, NiCo2S4 yolk–shell hollow spheres exhibited a high current density in the CV curves measured by symmetrical cells, demonstrating the most efficient catalytic activity to sulfur conversion reactions.
Compared to the abundant hollow metal oxides family, fewer hollow metal sulfides have been developed for sulfur hosts, while significant progress has been made in using metal sulfides for other energy storage systems and energy conversion.116,117 The impressive electrochemical performance of the hollow metal sulfide-based LSBs encourages more research efforts on the design and investigation of hollow metal sulfides for LSBs.
3.3 Metal nitrides
Metal nitrides typically present a better conductivity than their metal oxide counterparts, which is highly desirable when used as sulfur hosts.118 Many metal oxides can be readily transformed into relevant metal nitrides through an ammonization process, with the morphology of the metal oxide well inherited by metal nitrides, and vice versa.119 Most of the hollow metal nitride sulfur hosts are obtained via annealing the corresponding metal oxides in a NH3 atmosphere at high temperature.
3.3.1 TiN.
The conductivity of TiN (from 4 × 103 to 5.5 × 105 S cm−1) is comparable to those of metals, which is highly desirable for a sulfur host.119,120 Goodenough et al. have initially investigated the electrochemical properties of LSBs using mesoporous TiN as the sulfur host, revealing strong chemical interactions between TiN and LiPSs.121 Later, the chemical interaction and catalytic mechanisms of TiN have been confirmed by several other groups.122,123 TiN of different morphologies can be readily synthesized by annealing TiO2 with desirable morphologies.124,125 For example, a hollow TiN nanosphere was synthesized by annealing a hollow TiO2 nanosphere in ammonia gas, and the S–TiN cathode with a high sulfur loading of 3.6 mg cm−2 exhibited a capacity of 710 mA h g−1 after 100 cycles at 0.2C.126 The enhanced electrochemical performance was attributed to the highly conductive TiN shells, which provided strong chemical interactions with polysulfide, and the void space in the hollow spheres, which buffered the volume expansion of the sulfur electrode during the discharge process. Due to enhanced conductivity and efficient electrocatalysis of TiN, these TiO2-derived TiN hollow structures when used as sulfur hosts typically allowed superior electrochemical performance to that using the original TiO2.127,128 Hollow porous TiN tubes were prepared via ammonizing the pre-synthesized hollow TiO2 tubes, and the TiN–sulfur composite (TiN/S) was obtained through the melt-diffusion process.129 Owing to the high metallic conductivity of TiN and its high affinity for LiPSs, the TiN/S cathode delivered a capacity of 890 mA h g−1 after 100 cycles at 0.5C and 740 mA h g−1 after 450 cycles at 1C.
3.3.2 VN.
Li and co-workers have demonstrated the strong LiPS anchoring effect and fast LiPS conversion enabled by using VN/Graphene composites as the current collector for LSBs.130 To prepare a sulfur host that meets the requirements of high conductivity, sufficient void space for sulfur accommodation, volume buffering and LiPS confinement, strong chemical interactions with LiPSs, and efficient electrocatalytic activity for sulfur conversion, porous-shell VN nanobubbles were designed and synthesized through an organometallic conversion and a subsequent thermal nitridation process (Fig. 9a).131 Specifically, V2O5 nanobubbles (V2O5-NBs) were firstly prepared with carbon nanospheres as the sacrificial templates. VN-NBs (Fig. 9b) were then obtained after a heat treatment of the V2O5-NBs in NH3/Ar flow. The infiltration of sulfur into the VN-NBs (S@VN-NBs, Fig. 9c) was achieved via a melt-diffusion process. The S@VN-NBs cathode with a sulfur loading of 1.2 mg cm−2 maintained a capacity of 837 and 704 mA h g−1 after 1000 cycles at 1 and 2C, corresponding to a capacity decay rate of 0.024% and 0.027%, respectively. When the sulfur loading was increased to 3.3, 5.4 and 6.8 mg cm−2, the S@VN-NBs cathode delivered a capacity of 951, 799, and 563 mA h g−1 after 200 cycles at 0.5C, respectively. The areal capacity of S@VN-NBs with 5.4 mg cm−2 was among the best reported at that time, suggesting that the rational design of ordered nanostructures was an efficient approach to achieve high-performance LSBs. With a similar material design methodology for a superior sulfur host, a hybrid structure of cobalt-doped VN yolk–shell nanosphere encapsulated in carbon (Co-VN@C) was designed through a template-free solvothermal process with a subsequent nitridation step (Fig. 9e inset).132 Benefiting from the synergistic effect of cobalt, vanadium nitride, and carbon matrix, Co-VN@C/S exhibited a superior rate performance and long-term cycling stability compared to Co-VN@/S and VN/S (Fig. 9e). Even with a high sulfur loading of 4.07 mg cm−2, Co-VN@C/S delivered a high capacity of 830 mA h g−1 after 100 cycles at 0.2C.
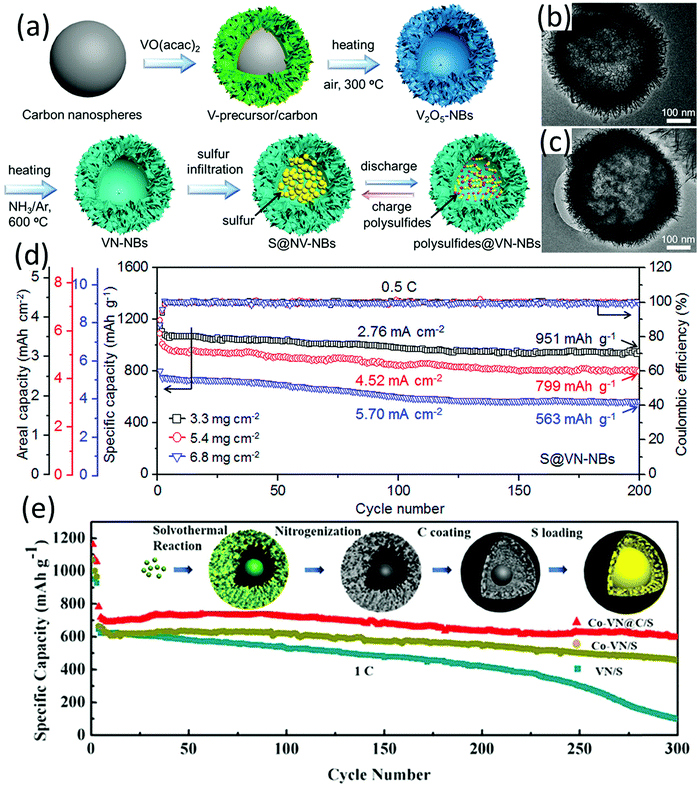 |
| Fig. 9 (a) Schematic for preparation of VN-NBs and S@VN-NBs and the delithiation/lithiation process, TEM images of (b) VN-NBs and (c) S@VN-NBs, and (d) cycling capabilities of S@VN-NBs cathodes with different sulfur loadings at 0.5C. Reproduced with permission.131 Copyright 2017 American Chemical Society. (e) Cycling performance of VN/S, Co-VN/S, and Co-VN@C/S at 1C; inset: schematic illustration for Co-VN@C/S fabrication. Reproduced with permission.132 Copyright 2018 American Chemical Society. | |
Despite these attractive properties of metal nitrides and the well-known merits of hollow structures, the hollow-structured metal nitrides developed for sulfur hosts are fewer than the hollow-structured metal sulfides. This may be due to the difficulty and high-cost for the synthesis of metal nitride hollow structures. More efforts are desirable for the development of facile, efficient and cost-effective approaches to fabricate hollow-structured metal nitrides.
3.4 Metal carbides
Nazar and co-workers are the pioneers who used the delaminated MXene phase (the early-transition-metal carbides or carbonitrides) as sulfur hosts.133 Two-dimensional Ti2C and Ti3C2 nanosheets were demonstrated as efficient sulfur hosts, which enabled stable cycling performance of LSBs.134 Coupling the polar metallic metal carbide with the hollow carbon spheres, a hybrid hollow structure with double-shelled carbon with encapsulated Mo2C nanoparticles (Mo2C/C@C) was developed as the sulfur host.135 The inner carbon shell was mainly used for encapsulation, while the outer carbon layer was for surface modification, thus leading to a better battery performance than that with a single carbon shell (Mo2C/C) host.
A hybrid hollow structure with MoSe2 and Mo2C encapsulated into hollow F-doped carbon fibers (MoSe2@FC@Mo2C, Fig. 10a) was synthesized through electrospinning (ZIF-67 was incorporated into the fibre precursor), followed by carbonization and a subsequent hydrothermal process.136 MoSe2@FC@Mo2C allowed stepwise electrocatalysis of different sulfur species with F-doped carbon fibers (FC) accelerating the transformation of S8 and Li2S4, while the MoSe2 and Mo2C promoted the conversion between Li2S4 and Li2S. Benefitting from these advantages, the MoSe2@FC@Mo2C/S cathode exhibited a high capacity of 802 mA h g−1 at 5C, which was superior to those of FC/S and MoSe2@FC/S (Fig. 10b). Under a challenging electrolyte/sulfur ratio of 5 mL g−1 and a high sulfur loading of 5.5 mg cm−2, MoSe2@FC@Mo2C/S presented a low capacity decay rate of 0.029% each cycle for 1000 cycles at 2C (Fig. 10c).
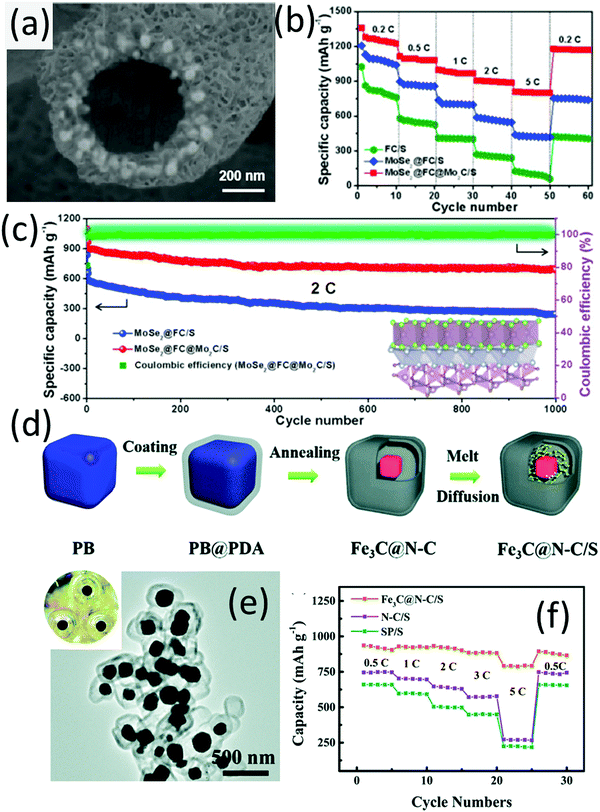 |
| Fig. 10 (a) SEM image of MoSe2@FC@Mo2C, (b) rate-performances of LSBs using different sulfur hosts, and (c) long-term cycling of the MoSe2@FC@Mo2C/S cathode. Reproduced with permission.136 Copyright 2020 Elsevier. (d) Schematic for frogspawn-like hollow Fe3C@N–C preparation, (e) TEM image of Fe3C@N–C; the inset is the photo of frogspawn, (f) rate-performance of Fe3C@N–C/S, super P/S, and N–C/S (nitrogen-doped carbon). Reproduced with permission.137 Copyright 2019 the Royal Society of Chemistry. | |
Different from most of the hybrids where metal compounds are generally anchored on either the outer surface or the inner surface of the carbon shells, a frogspawn-like structure composed of a nitrogen-doped carbon shell and a Fe3C particle core (Fe3C@N–C, Fig. 10e) was designed and presented as an efficient sulfur host.137 The Fe3C@N–C was obtained by annealing polydopamine (PDA) coated cubic Prussian blue (PB), when the metal-like Fe3C generated from the PB precursor was encapsulated in nitrogen-doped carbon shells derived from PDA (Fig. 10d). Fe3C cores acted as a centre for polysulfide trapping, while the void space provided sufficient room to buffer the volume expansion of sulfur during discharge. The Fe3C@N–C/S electrode delivered an impressive high-rate capacity of 792 mA h g−1 at 5C (Fig. 10f). The electrode maintained a capacity of 586 mA h g−1 after 400 cycles at 0.5C, corresponding to a low capacity decay rate of 0.08% per cycle.
The number of hollow-structured metal carbides applied as sulfur hosts are relatively limited, possibly due to the high-temperature annealing process, which is essential for the synthesis of metal carbides but detrimental for the hollow structure. To prepare highly-efficient sulfur hosts, integrating the metallic and polar metal carbides with hollow carbons should be a solution.
3.5 Metal phosphide
Wang and co-workers are among the pioneering researchers who applied metal phosphides as sulfur host materials and investigated their chemical interaction with LiPSs and electrocatalysis for sulfur conversion in LSBs.138 They initially proposed the surface oxidation-induced polysulfide-binding mechanism which is generally applicable to a wide range of transition metal phosphides.139 A sulfur cathode containing CNT supported CoP nanoparticles (CoP-CNT) with a high sulfur loading of 7.0 mg cm−2 maintained a high areal capacity of 5.6 mA h cm−2 for 200 cycles.139 When MoP-CNT was used as the host material, the sulfur cathode realized a stable areal capacity of 5.0 mA h cm−2 under a lean electrolyte condition of 4 mLE gS−1 (electrolyte/sulfur).140 To integrate the advantages of transition metal phosphides (for LiPSs immobilization and conversion) with the hollow structure (to carry sulfur, buffer the volume expansion and confine LiPSs), a Ni2P yolk–shell structure (Ni2P-YS) was prepared via a multistep process (Fig. 11a). Ni-yolk–shell (Ni-YS) was first prepared with a one-pot solvothermal process. The morphology evolution of the Ni-YS along with the solvothermal process (3, 6, 9, 12 h) is shown in Fig. 11b–e, revealing the stepwise hollowing out of the yolk–shell structure (Fig. 11f). Ni2P-YS composed of a core with a dimeter of 276 nm and a spherical shell with a dimeter of 855 nm (Fig. 11g and h) was obtained by annealing Ni-SY (glycerol) in PH3/Ar flow. The Ni2P-YS based sulfur cathode delivered an impressive high-rate performance with a capacity of 439 mA h g−1 at 10C, which was maintained at 394 mA h g−1 after 1000 cycles at 5C (Fig. 11i).141 It was revealed that the conductive polar Ni2P not only facilitated the ion transportation but also improved the polysulfide nucleation. Besides, the yolk–shell structure could accommodate sulfur species and provided buffer room for the correlated volume expansion.
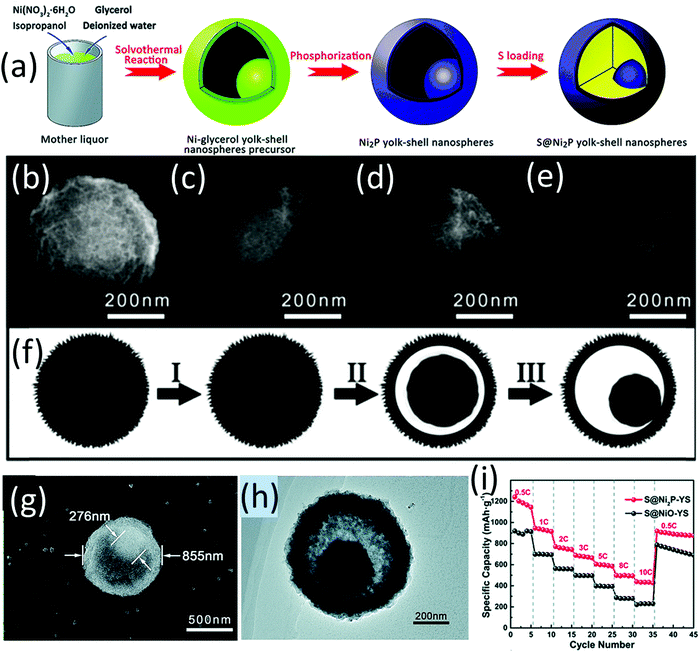 |
| Fig. 11 (a) Schematic for the preparation of hollow yolk–shell Ni2P-YS and S@Ni2P-YS composites, SEM images of Ni-YS precursor/intermediates generated at different stages of the solvothermal process: (b) 3 h, (c) 6 h, (d) 9 h, and (e) 12 h, (f) schematic for the stepwise formation of the Ni-glycerate yolk–shell structure, (g) SEM, and (h) TEM of NiP-YS, (i) rate performance of S@Ni2P-YS and S@NiO-YS. Reproduced with permission.141 Copyright 2017 the Royal Society of Chemistry. | |
A hybrid structure composed of CoP nanoparticles, hollow carbon polyhedra and carbon nanotube (CoP@HPCN) was prepared using ZIF-8 and ZIF-67 as the templates and metal ion sources. After a successive carbonization, oxidization and phosphorization process, Co@HPCN, Co3O4@HPCN, and the final CoP@HPCN were generated, respectively.142 The CoP@HPCN/S cathode with a high sulfur loading of 3.7 mg cm−2 presented a low self-discharge constant of 0.03% per day for 60 days. This was mainly attributed to the high porosity and cavity of the hollow architecture, which can accommodate sulfur and buffer its volume expansion, and the uniformly dispersed CoP nanoparticles, which not only chemically immobilized LiPSs but also acted as an electrocatalyst to promote the redox kinetics of sulfur species. More recently, Ru–Mo4P3 nanoparticle-decorated hollow carbon spheres (HCS-Ru–Mo4P3) were demonstrated as good sulfur hosts, and the corresponding S/HCS-Ru–Mo4P3 cathode achieved a high capacity of 660 mA h g−1 at 4C and a high areal capacity of 5.6 mA h cm−2 after 50 cycles at a sulfur loading of 6.6 mg cm−2.143 The performance was among the best of LSBs using transition metal phosphides as the sulfur host materials. It was revealed that Ru induced the phase transformation from MoP to Mo4P3, which possessed higher electrocatalytic activity towards sulfur conversion than RuP2 and MoP. The synergistic effect of Mo4P3 and Ru made Ru–Mo4P3 highly active sites for LiPS adsorption and conversion. Combining the advantages of the hollow carbon structure as a sulfur carrier, the S/HCS-Ru–Mo4P3 cathode achieved superior electrochemical properties.
Transition metal phosphides have long been used as efficient catalysts for photoelectrochemical, hydro-processing.144,145 The application of transition metal phosphides for LSBs is still in its infancy. More efforts on the preparation and application of novel metal phosphides for LSBs are desirable.
3.6 Metal hydroxides
Metal hydroxides, such as Co(OH)2, have been demonstrated as efficient stabilizers for high-performance LSBs, presenting good capability to suppress the shuttle effect of LiPSs.146 Huang and co-workers demonstrated that a drastically enhanced performance (with negligible capacity decay for 500 cycles) was achieved by encapsulating a conventional S8-carbon black composite (S8@CB) in nickel nitrate hydroxide, Ni3(NO3)2(OH)4.147 The Ni3(NO3)2(OH)4 layer not only served as a physical barrier to trap LiPSs, but also reacted with lithium to generate an ion-conductive, polar/hydrophilic-rich layer for ion transportation and LiPS trapping. These results suggest metal hydroxides are potential sulfur hosts to achieve high-performance LSBs. Layered double hydroxides (LDHs) have long been applied as catalysts for a wide range of areas, such as oxygen evolution reactions148 and photodegradation of organic waste.149,150 Lou et al. designed various hollow-structure LDHs as sulfur host materials, and investigated the electrochemical properties of S-LDHs composites. For example, double-shelled nanocages composed of a layered double hydroxide outer shell and a Co(OH)2 inner shell were prepared (CH@LDH), which could not only accommodate a high sulfur content of 75 wt%, but also suppress the diffusion of polysulfide via a self-functionalized surface as chemical adsorption sites, maximizing the advantages of the hollow structure as a sulfur host.151 When used as a sulfur host, the CH@LDH-based sulfur electrode with a high sulfur loading of 3.0 mg cm−2 delivered an initial capacity of 747 mA h g−1 at 0.5C, which was maintained at 491 mA h g−1 after 100 cycles. The preparation process and TEM images of the corresponding intermediates are shown in Fig. 12a. Specifically, ZIF-67 was firstly synthesized as a sacrificial template, followed by reaction with Ni(NO3)2, forming ZIF-67@LDH. After the reaction of ZIF-67@LDH with Na2MoO4 solution, double-shelled CH@LDH was obtained. More recently, authors from the same group further demonstrated that hollow nickel-ion layered double hydroxide polyhedra (Ni/Fe LDH, Fig. 12b and c) were suitable for a sulfur host.152 MIL-88A was used as a template for Ni/Fe LDH. S@Ni/Fe LDH was prepared via the melt-diffusion process, which well inherited the morphology of Ni/Fe LDH except that the void in the core was filled with sulfur (Fig. 12b–e). S@Ni/Fe LDH with a sulfur loading of 2–3 mg cm−2 presented an initial capacity of 844 mA h g−1 at 1C, which was maintained at 501 mA h g−1 after 1000 cycles, corresponding to a decay rate of 0.04% each cycle.
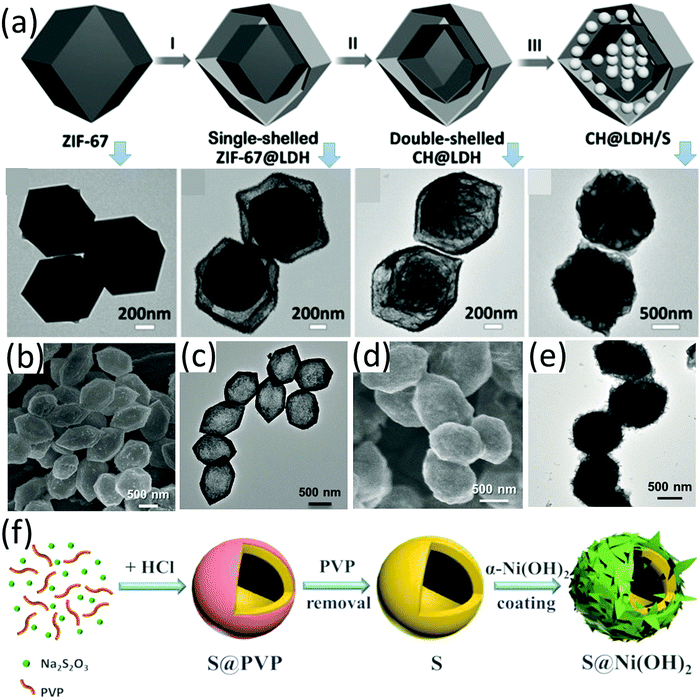 |
| Fig. 12 (a) Schematic for synthesis of CH@LDH and TEM images of the corresponding intermediates. Reproduced with permission.151 Copyright 2016 Wiley-VCH. (b) SEM and (c) TEM images of Ni/Fe LDH, and (d) SEM and (e) TEM images of S@Ni/Fe LDH. Reproduced with permission.152 Copyright 2018, Wiley-VCH. (f) Schematic for the synthesis of double-shelled hollow S@Ni(OH)2 spheres. Reproduced with permission.153 Copyright 2017 Elsevier. | |
Besides infiltrating sulfur into a pre-synthesized hollow structure, sulfur can be encapsulated in metal hydroxides, forming core–shell or hollow yolk–shell sulfur composites. A α-Ni(OH)2 nanosheet encapsulated nanosized sulfur (S@Ni(OH)2) composite was prepared via a two-step method (Fig. 12f).153 Hollow sulfur nanospheres were firstly synthesized based on the reaction of Na2S2O3, polyvinylpyrrolidone (PVP) and HCl, followed by the growth of α-Ni(OH)2 nanosheets (3.5 nm thick) on the surface of the as-obtained sulfur nanospheres based on the reaction of C6H12N4 and Ni(NO3)2·6H2O. The as-prepared S@Ni(OH)2 (sulfur content of 81 wt%) exhibited a maximum capacity of 708 mA h g−1 at 1C after a few activating cycles, which was maintained at 422 mA h g−1 after 1000 cycles, corresponding to a capacity decay rate of 0.04% per cycle and a capacity retention of 58.8%.
3.7 Metals
Metal catalysts with high electrical conductivity, such as Pt and Co, were demonstrated with excellent capability to enhance polysulfide anchoring and increase the kinetics of the polysulfide conversion reaction.23 To promote the dispersion of metal catalysts and achieve a better sulfur distribution, hybrid hollow structures with metallic nanoparticles decorated on the surface of hollow carbon matrix are desirable. For example, a cobalt nanoparticle-decorated porous nitrogen-doped graphitic carbon polyhedron (Co–N-GC) was prepared by thermal annealing ZIF-67 in a N2 atmosphere.154 Co–N-GC well inherited the polyhedron morphology of ZIF-67 with a similar size of 350 nm. Cobalt particles with a calculated size of 22.09 nm were uniformly dispersed on the carbon surface. The hollow-structured sulfur electrode (sulfur content 70 wt%) was obtained via melt-diffusion, which delivered an initial capacity of 1440 mA h g−1 at 0.2C which was maintained at 850 mA h g−1 after 200 cycles, corresponding to a decay rate of 0.023% per cycle. A nitrogen-doped hollow carbon microflower with embedded cobalt nanoparticles (H-Co-NCM) was prepared through carbonizing metanilic acid intercalated cobalt–aluminum layered double hydroxides (CoAl LDHs), followed by an acid etching step when only strongly bonded Co functionalities by nitrogen atoms remained.47 Co/N heteroatom-doping not only promoted conductivity of the carbon matrix, but also synergistically served as active catalysts and adsorption sites. S@H-Co-NCM was prepared by a sulfur dissolving and evaporation process in CS2, followed by a heat treatment at 200 °C under N2 flow. The S@H-Co-NCM cathode delivered a capacity of 611 mA h g−1 at 2C, and achieved a capacity decay rate of 0.069% each cycle over 500 cycles at 0.5C.
Palladium has long been used as a catalyst in different areas, such as alkynylation,155 coupling reactions of aryl chlorides,156 oxygen reduction and hydrogen oxidation in full cells.157 Zuo and co-workers initially investigated the catalysis of palladium on the sulfur conversion reaction in LSBs.158 Pd-Nanocrystal-embedded hollow carbon spheres (Pd@HCS, Fig. 13c and d) were designed as the sulfur host, which were prepared through the process as shown in Fig. 13a. Combining the hollow nanostructure with Pd nanocrystals, LiPSs were synergistically trapped by physical confinement and chemical adsorption, achieving rapid redox reactions for sulfur conversion reactions (Fig. 13b). Both Li2S6 adsorption test and theoretical calculations confirmed the strong interaction between LiPSs and Pd@HCS. The catalytic ability of Pd NPs was further testified by CV tests using symmetrical coin cells, with much higher redox currents when Pd@HCS was used as the electrode compared to that of using HCS. To further investigate the electrocatalysis of Pd NPs, a CV test based on semi-liquid cells was conducted. Compared to Pd@HCS, HCS exhibited much broader peaks and larger voltage hysteresis with lower cathodic onset potential and higher anodic onset potential (Fig. 13e). Owing to the advantages of Pd@HCS, the Pd@HCS/S cathode with a high sulfur loading of 5.88 mg cm−2 delivered an initial capacity of 873 mA h g−1 at 0.2C, with a capacity retention of 85% after 100 cycles.
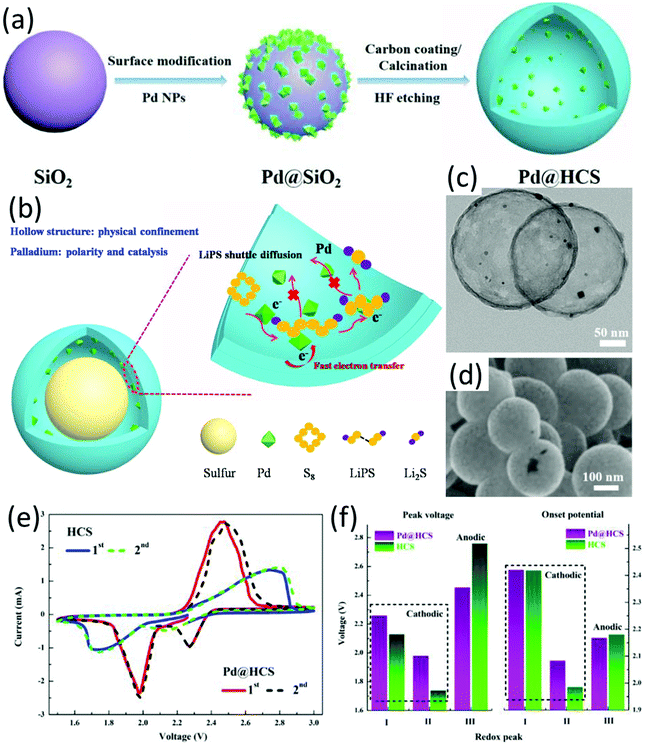 |
| Fig. 13 (a) Schematic for the synthesis of hollow Pd@HCS microspheres, (b) schematic of the hollow Pd@HCS/S structure and the discharge process, (c) TEM, and (d) SEM images of Pd@HCS, (e) CV curves of the semi-liquid LSBs using Li2S6 as the active material, and (f) the corresponding peak voltages and onset potentials of the asymmetrical LSBs. Reproduced with permission.158 Copyright 2019 Elsevier. | |
Single-atom catalysts have attracted ever growing interest due to their potential to achieve 100% atom utilization when used as catalysts.159 By annealing Fe-ZIF-8 in an argon atmosphere, Wang et al. prepared an iron single-atom-decorated nitrogen-rich carbon nanocage (FeSA-CN).160 Using FeSA-CN as the sulfur host, the FeSA-CN/S cathode with a sulfur content of 68.7 wt% delivered a high capacity of 605 mA h g−1 at 4C and exhibited a capacity retention of 70% for 500 cycles, corresponding to a capacity decay rate of 0.06% per cycle. The cycled battery using FeSA-CN as the sulfur host presented a much smoother lithium anode. The enhanced performance was attributed to the synergetic effect of the single-atom iron catalyst and nitrogen-doped carbon polyhedron. FeSA greatly facilitated the redox kinetics and thus the shuttle effect of polysulfide was mitigated. Moreover, the nitrogen-doped carbon polyhedron provided good conductivity and physical confinement to the polysulfide.
3.8 Other inorganic metal compounds
The conventional transition metal compounds, such as metal oxides/sulfides/nitrides, have attracted huge interest as sulfur host materials. The abundant material chemistries provide sufficient choices for sulfur hosts. Efforts to explore a wider range of materials as the sulfur host materials are desirable to further promote the performance of LSBs. There are some other inorganic hollow-structured materials, such as LiFePO4 microspheres,161 Prussian blue nanocubes,162 and metal fluoride-based hybrid hollow structures,163 reported as sulfur host materials and demonstrated some desirable properties. For example, hollow LiFePO4 microspheres (LFP) were investigated as the sulfur carrier, which suppressed the shuttle effect via chemical adsorption and catalytically promoted the redox kinetics of conversion between long-chain and short-chain polysulfides.161 Combining with holey graphene (HG), LFP enabled the corresponding sulfur electrode to exhibit a high capacity of 748 mA h g−1 at 5C, and it maintained a capacity of 831 mA h g−1 at 1C after 500 cycles at a high sulfur loading of 4.3 mg cm−2.
Prussian blue nanocubes (Na2Fe[Fe(CN)6]) could trap polysulfide through both spatial confinement and Lewis acid–base interactions. When a conductive poly(3,4-ethylenedioxythiophene) (PEDOT) layer was coated onto Na2Fe[Fe(CN)6], a high-performance sulfur host ((Na2Fe[Fe(CN)6])@PEDOT) was obtained.162 The corresponding sulfur electrode delivered a high rate performance of 683 mA h g−1 at 5C, which was maintained at 544 mA h g−1 after 200 cycles. In another study, a thin AlF3 layer was deposited on a core–shell sulfur-hollow carbon composite (S@HCS), generating S@HCS@AlF3. The as-obtained S@HCS@AlF3 delivered a capacity of 702 mA h g−1 after 500 cycles at 1C, corresponding to a capacity decay rate of 0.052% per cycle.163 The inner carbon shell not only enhanced the electrode conductivity but also provided a physical barrier to the soluble polysulfide, while the outer AlF3 layer further improved the overall electrode conductivity and immobilized the polysulfide via chemical bonding.
4 Organic hollow-structured sulfur cathodes
4.1 Conductive polymers
Conductive polymers have been widely used for sulfur cathode design in LSBs, which can not only increase the electrode conductivity but also provide strong chemical adsorption to LiPSs. Hollow polymer-sulfur composites can be constructed by either encapsulating the prefabricated sulfur particle templates with various conductive polymers on the sulfur surface, or infiltrating sulfur into the prefabricated hollow-structured polymers via a melt-diffusion process.
A core–shell sulfur-polypyrrole (S-PPy) composite was prepared by coating a conductive polypyrrole layer on sulfur nanospheres.164 The spherical sulfur nanoparticles were prepared by a reaction of sodium thiosulfate and p-toluenesulfonic acid (pTSA). With the help of a cationic surfactant, decyltrimethylammonium bromide (DeTAB), polypyrrole could be readily nucleated and then formed a polypyrrole layer with a thickness of around 100 nm onto sulfur spheres. The capacity of the as-obtained S-PPy composite (sulfur content 65.8 wt%) was maintained at around 600 mA h g−1 at 0.5C and 400 mA h g−1 at 2C after 50 cycles. However, SEM images revealed that sulfur spheres were not uniformly covered by the polymer layer. Another core–shell PPy encapsulated nanosized sulfur sphere (around 150 nm) was prepared via a one-pot process (S@PPy, Fig. 14a).165 The nanosized sulfur spheres were firstly fabricated by the reaction of Na2S, sulfur powder and Triton X-100 solution. Then, HCl and FeCl3 were added sequentially into the as-formed sulfur solution, followed by washing and immersing the mixture into H3PO4 solution for PO43− doping. The as-prepared S@PPy with a sulfur content of 80 wt% (Fig. 14c) exhibited an initial discharge capacity of 1142 mA h g−1 at 0.1C, which was maintained at 805 mA h g−1 after 50 cycles. Besides encapsulating sulfur with polymers, melt-diffusion has also been applied to prepare polymer-sulfur hollow composites. Hollow polypyrrole nanospheres (PHNS) with wrinkled, ultra-thin shell were firstly prepared through in situ polymerization of pyrrole on the surface of Fe3O4 nanospheres (PPy@Fe3O4), followed by removal of the Fe3O4 template using HCl solution (Fig. 14b).166 S@PHNS composites were obtained via a melt-diffusion process. The conductive PHNS shells served as channels which enabled fast ion and electron transport, and promoted the redox reactions. Benefiting from these advantages, S@PHNS delivered a high capacity of 536.5 mA h g−1 at 5C.
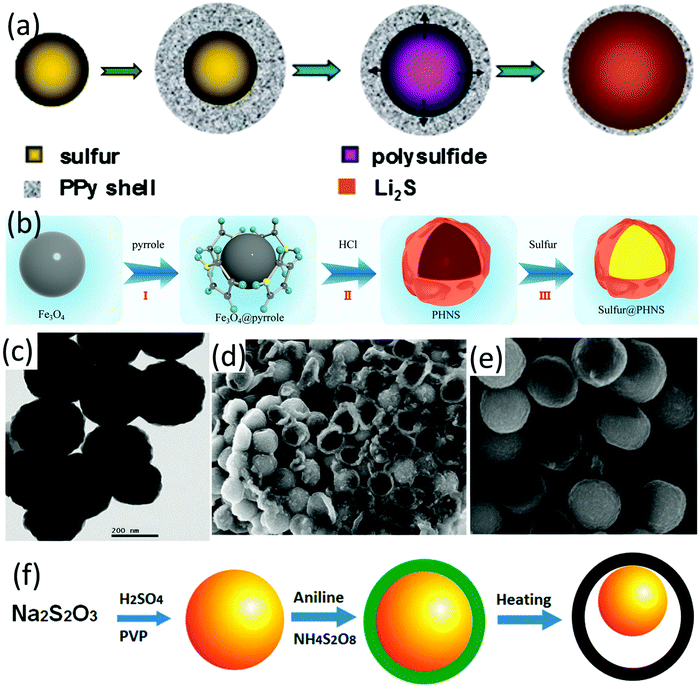 |
| Fig. 14 (a) Schematic for the synthetic process of core–shell structured S@PPy. Reproduced with permission.165 Copyright 2016 Elsevier. (b) Schematic for the synthesis of the S@PHNS composite. Reproduced with permission.166 Copyright 2018, Elsevier. (c) TEM image of core–shell structured S@PPy. Reproduced with permission.165 Copyright 2016 Elsevier. SEM images of (d) core–shell- and (e) yolk–shell-based S-Pani composites after five cycles, (f) schematic for the two-step synthesis process of the core–shell and yolk–shell S-Pani composites. Reproduced with permission.49 Copyright 2013 American Chemical Society. | |
Despite the enhanced electrochemical properties, such as sulfur utilization and rate performance, it is difficult for the core–shell structure to accommodate the huge volume expansion of suflur during the lithiation process, which might lead to unsatisfactory cycling stability. Creating a yolk–shell structure with reserved interior void space is an effective way to buffer the volume change of the conversion-type electrode materials.69 Yolk–shell structured sulfur cathodes can be achieved by partially removing the inner sulfur of the core–shell structures via dissolving or thermal evaporation. A yolk–shell polyaniline-sulfur composite (S-Pani) was prepared via the process shown in Fig. 14f.49 Instead of partially dissolving sulfur by toluene as in Cui's work,69 the yolk–shell structure was created via a heat treatment. On one hand, it was found that the S-Pani core–shell was destroyed by toluene. On the other hand, the author proposed something that was worth pondering – if sulfur could leach out from the TiO2 shell via dissolving in toluene, polysulfide could leach out from the shell as it dissolved in the electrolyte. The core–shell S-Pani (sulfur content of 82 wt%) based LSBs suffered from fast capacity decay with only 280 mA h g−1 left after 125 cycles. In comparison, the yolk–shell S-Pani (sulfur content of 58 wt%) based electrodes exhibited a high capacity of 765 mA h g−1 after 200 cycles at 0.2C, corresponding to a capacity retention of 69.5%. The SEM images of the cycled sulfur electrode revealed that about half of the core–shell S-Pani particles were either broken or shrunk (Fig. 14d), while the yolk–shell S-Pani particles were well preserved (Fig. 14e). These results suggested that void space in the yolk–shell structure was essential for long-term cycling stability. Despite the overwhelming cycling stability of the yolk–shell S-Pani based LSB over that of core–shell, it is unreasonable to attribute all these achievements to the yolk–shell structure, considering different sulfur contents in these two systems (82 wt% vs. 69.5 wt%). As is known, sulfur contents can greatly affect the battery performance.
4.2 Organic-frameworks
Owing to the high specific area and tuneable pore sizes, porous aromatic frameworks (PAFs) and metal organic frameworks (MOFs) have gained intensive research interest and have been applied in a wide spectrum of areas.167,168 However, due to their low conductivity, both PAFs and MOFs are typically considered not suitable for sulfur host materials. Despite the shortfall of low conductivity, the confining effect of the porous structure and the strong Lewis acid–base interaction between LiPSs and MOFs endowed them with the potential as efficient sulfur host.169–171 Instead of being used as the sacrifice template for the preparation of hollow structures, hollow ZIF-67 was applied as the sulfur carrier, considering the strong Lewis acid–base bonding between Co2+ and S2−. To increase the conductivity, the ZIF-67-S composite was coated with PPy through a water-phase chemical oxidative polymerization process (ZIF-67-S-PPy).172 The fabrication is as shown in Fig. 15a. The ZIF-67-S-PPy composite with a sulfur content of 60 wt% (Fig. 15b and c) achieved the best performance among various control samples, with an initial capacity of 1092.5 mA h g−1 and 353.6 mA h g−1 remained after 200 cycles. Although the performance is not as impressive as many other reports, this work provides some guidance for constructing conductive hollow MOF-based composites for sulfur hosts. Considering the rich chemistries of the MOF family, more efforts on applying MOFs as sulfur host materials may bring some new findings. As an emerging family of advanced materials, organic frameworks have gained increasing interest. Using polystyrene (PS) microspheres as sacrificial templates, porphyrin organic framework hollow spheres (POF-HSs) with regulated shell thickness and void size were prepared and used as a sulfur host (Fig. 15f and g).173 Owing to polar POF shells and a hollow architecture, POF-HSs exhibited strong LiPS adsorption ability (Fig. 15d). Benefiting from dual chemical adsorption and physical confinement of POF-HSs, the shuttle effect of LSBs was efficiently suppressed, leading to a superior rate performance with a high capacity of 800 mA h g−1 at 4C (Fig. 15d), and stable cycling performance with 773 mA h g−1 remained after 200 cycles at 0.5C. The enhanced performance of the POF-HS based LSB suggests that organic framework engineering is an applicable approach for the preparation of efficient sulfur host materials.
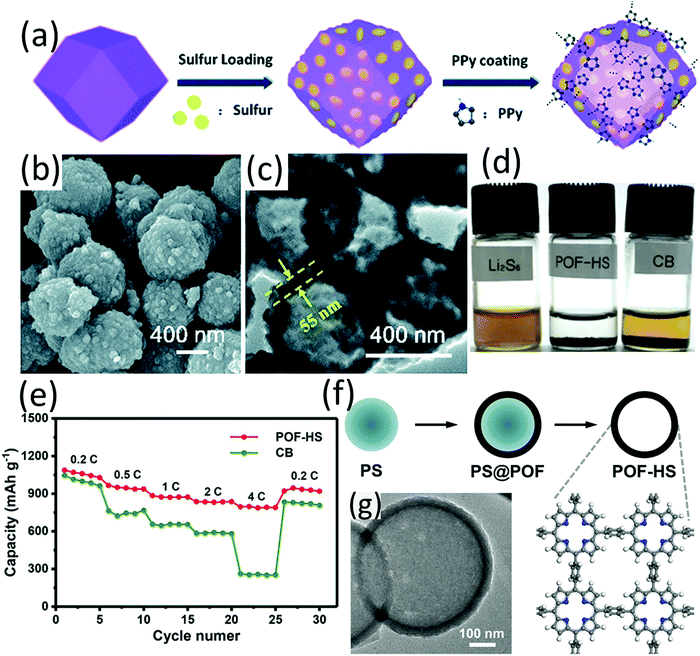 |
| Fig. 15 (a) Schematic for the synthesis of ZIF-67-S-PPy, (b) SEM, and (c) TEM images of ZIF-67-S-PPy. Reproduced with permission.172 Copyright 2019 the Royal Society of Chemistry. (d) Visualized adsorption test of polysulfides (after 24 h) using POF-HS or CB as the adsorbents, (e) rate performance of POF-HS/S and CB/S cathodes, (f) schematic for the preparation of POF-HSs, (g) TEM image of the hollow spherical POF-HS. Reproduced with permission.173 Copyright 2018 Wiley-VCH. | |
5 Summary and perspectives
The design of sulfur hosts relies on the knowledge of materials chemistry and nanostructure engineering. In brief, from the materials chemistry perspective, good electric conductivity, strong polysulfide interactions and efficient electrocatalytic activity are the key requirements; and from the nanostructure engineering angle, creating suitable porosity and void space to accommodate S8/Li2S, to buffer volume expansion and confine polysulfide, are critical. Because the hollow-structured materials possess a high specific surface area and tunable pore structure, and their morphology and composition are highly controllable, they have shown great potential to undertake the role of sulfur hosts in the cathode of LSBs.
In this review, we have summarised the recent advances in the design of hollow-structured sulfur cathodes for LSBs. In particular, hollow structures containing polar materials including inorganic or organic compounds and their hybrids with hollow carbons are highlighted by analysing their synthesis methods, structures and electrochemical performance in LSBs. Carbon hollow structured materials show high sulfur utilization and cycling performance due to the physical confinement of the pore channels; however, the nonpolar carbon surface is not efficient to immobilize LiPSs for long-term cycling. In contrast, inorganic or organic hollow structures including metal oxides, sulfides, nitrides, carbides, phosphides, hydroxides, conductive polymers and organic frameworks show a better ability to anchor polysulfides due to the strong interaction between polar surfaces and LiPSs, and a higher electrocatalytic activity for the sulfur conversion reactions. Nevertheless, some of these inorganic/organic compounds face the issue of low conductivity or/and the difficulty in fabricating the hollow structure. Hybrid hollow structures integrating carbonaceous conductive frameworks with inorganic/organic polar species have provided a variety of solutions to tackle the above-mentioned issue. A comparison of some representative hollow-structured sulfur cathodes is summarised in Table 1. At the early stage when carbon materials were predominantly used as sulfur hosts, to improve the performance of LSBs, research effort was mainly focused on optimizing the geometrical structures of the host, such as the porosity, pore size distribution, pore volume, specific surface area, and the ratio between sulfur and the host.28 For example, when the pores of carbon materials are small enough to confine small sulfur molecules, the cycling stability of LSBs can be significantly improved owing to the direct solid–solid reaction without generating soluble LiPSs.174–176 Once the sulfur content exceeded the pore volume of the host materials, the performance of LSBs degenerated quickly. Xiao et al. reported that hollow carbon spheres with a pore size of 2.8 nm exhibited better sulfur impregnation than those with a pore size of 4.1 nm and 3.2 nm.177 Nazar et al. demonstrated that an improved cycling stability of LSBs could be achieved via deliberately controlling the shell porosity of the hollow carbon spheres.67 Differently, the rational design of polar hollow-structured composites has mainly focused on materials’ chemistry, such as chemical interactions between the host and LiPSs and the catalytic effect on the redox reactions, when the physical confinement of the hollow structure is usually taken for granted with a few efforts on further optimization of the geometrical properties. The performance of LSBs is supposed to be further improved via optimizing both the geometrical and chemical properties of the hollow-structured sulfur cathode.70,151
Table 1 Comparison of representative hollow-structured sulfur electrodes
A |
B |
C |
D |
E |
F |
G |
H |
Note: the capital letters in the header refer to the following: A stands for the host materials, B the sulfur infiltration method, C the sulfur content of the sulfur-host composites (wt%), D the sulfur content of the sulfur electrode (wt%), E the areal sulfur loading (mg cm−2), F the electrolyte/sulfur ratio (μL mg−1), G the capacity (mA h g−1)/cycles/C-rate, and H the reference. A carbon nanofiber membrane was added as an up-current collector. Refers to a double-shelled hollow polyhedron with inlaid cobalt nanoparticles encapsulated in nitrogen-doped carbon (Co/NC) nanodots. Refers to MoSe2 and Mo2C encapsulated into hollow F-doped carbon fibres. |
Yolk–shell S–MnO2 |
Nano-S template |
80 |
64 |
1.5 |
n/a |
315/1700/2.0 |
55
|
Core–shell S/γMnO2 |
Nano-S template |
61 |
43 |
2 |
n/a |
374/400/2.0 |
59
|
MnO2 nanospheresa |
Melt-diffusion |
71 |
0.50 |
1.2 |
15 |
721/500/1.0 |
60
|
Hollow C nanofibers-MnO2 |
Melt-diffusion |
71 |
0.50 |
3.5 |
n/a |
662/300/0.5 |
61
|
C/MnO2 hollow nanofibers |
Melt-diffusion |
70 |
49 |
2.5 |
24 |
629/300/1.0 |
63
|
Core–shell C@MnO2 |
Melt-diffusion |
58 |
41 |
3.0 |
19 |
550/500/1.0 |
64
|
Yolk–shell S–TiO2 |
Nano-S template |
71 |
53 |
0.4–0.6 |
n/a |
690/1000/0.5 |
69
|
TiO2 microboxes |
Melt-diffusion |
70 |
48 |
1.0 |
n/a |
626/500/1.0 |
71
|
Multi-shelled hollow TiO2−x |
Melt-diffusion |
56 |
39 |
0.5 |
n/a |
713/1000/0.5 |
70
|
TiO2@hollow C sphere |
Melt-diffusion |
70 |
56 |
1.5 |
n/a |
630/500/0.5 |
79
|
TiO–C hollow fiber |
Vapor phase infusion |
73 |
58 |
5.0 |
n/a |
680/400/0.2 |
80
|
Hollow CoS2@N-doped Carbon |
Melt-diffusion |
75 |
n/a |
∼1.3 |
n/a |
519/300/1.0 |
94
|
Co3S4 nanotubes |
Melt-diffusion |
74 |
59 |
∼2 |
40 μL per cell |
305/1000/5.0 |
98
|
CNTs threaded hollow Co3S4 nanoboxes |
CS2 dissolution, melt-diffusion + evaporation |
70 |
49 |
3.5 |
15 |
820/150/0.2 |
100
|
70 |
56 |
CNT/Co3S4 N-doped carbon nanocubes |
Melt-diffusion + evaporation |
74 |
74 |
7.4 |
10 |
810/1000/5.0 |
101
|
Co9S8 tubules |
Melt-diffusion CS2 dissolution |
70 |
52 |
n/a |
n/a |
894/600/1.0 |
105
|
Co9S8-hollow C-polyhedra |
CS2 dissolution + melt diffusion + evaporation |
77 |
32 |
3.0 |
15 μL per cell |
680/300/0.5 |
178
|
Co-NC@Co9S8/NPCb |
CS2 dissolution + melt-diffusion + evaporation |
75 |
60 |
4.5 |
10 |
607/500/1.0 |
107
|
NiS-hollow carbon spheres |
Melt-diffusion |
72 |
50 |
1.0 |
20 |
695/300/0.5 |
108
|
1T-MoS2 nanotube-N-doped graphene |
Melt-diffusion |
80 |
64 |
5.2 |
n/a |
629/100/0.2 |
110
|
Hollow porous TiN tubes |
Melt-diffusion |
74 |
52 |
1.0 |
n/a |
840/450/0.5 |
129
|
Porous-shell VN nanobubbles |
Melt-diffusion + evaporation |
78 |
62 |
5.4 |
n/a |
799/200/0.5 |
131
|
Co-doped VN yolk–shell |
Melt-diffusion |
70 |
49 |
4.1 |
n/a |
830/100/0.2 |
132
|
MoSe2@FC@Mo2Cc |
Melt-diffusion + evaporation |
72 |
50 |
5.5 |
5 |
688/1000/2.0 |
136
|
Hollow Fe3C–N-doped carbon |
Melt-diffusion |
70 |
56 |
1.5 |
n/a |
586/400/0.5 |
137
|
Ni2P yolk–shell |
Melt-diffusion + evaporation |
65 |
52 |
n/a |
n/a |
394/1000/5.0 |
141
|
Ni/Fe LDH hollow polyhedron |
Melt-diffusion |
70 |
49 |
2–3 |
30 |
501/1000/1.0 |
152
|
α-Ni(OH)2 hollow spheres |
Nano-S template |
81 |
57 |
2.2 |
n/a |
422/1000/1.0 |
153
|
Co–N-doped hollow carbon |
Melt-diffusion |
70 |
49 |
2.0 |
35 μL per cell |
625/500/1.0 |
154
|
Pd-hollow carbon |
CS2 dissolution and melt-diffusion |
76 |
57 |
5.88 |
15 |
742/100/0.2 |
158
|
Despite the impressive electrochemical performance enabled by using a wide range of advanced hollow structures as sulfur hosts, there is still a long way to go to commercialize LSBs. In terms of hollow-structured material design and preparation, cost-effective, eco-friendly and scalable fabrication methodologies are highly desirable and need more research efforts in the future. As is known, the well-controlled fabrication process in laboratory, which leads to delicate nanoarchitectures, might not work in an industry-scale production. Thus, the reproducibility of the promoted battery performance achieved at the laboratory scale remains a concern, when the materials are scaled up to the industry level.179 Besides, the cost of raw materials and the delicate synthesis procedures are generally not a problem in academic research, while these remain another concern for practical applications. Similar to catalysts used in industry, long-term stability of the sulfur host is an important criterion to evaluate their performance, and thus the characterization of the sulfur hosts after long-term electrochemical cycling is essential. Battery is an application-driven technology, and thus, it is essential to keep the criteria for practical application in mind for the design of sulfur hosts and evaluation of battery performance.180
From the aspect of performance evaluation, to achieve high performance LSBs with both energy density and cost superior to those of the state-of-the-art LIBs, the sulfur content and mass loading, the electrolyte to sulfur ratio, and the amount of lithium anode have to be strictly controlled.181,182 Although there is a growing number of research papers presenting these parameters, the lack of standard LSB systems makes it difficult to achieve a reasonable, comprehensive, and convenient comparison of various home-made systems. Without a standard system, the merits of some specific components may be overstated at the cost of other properties. For example, LSBs with low sulfur content, using a flooded electrolyte and an overdose of lithium at the anode could still achieve high specific capacity. A standard LSB can not only make the real good ones stand out and the shortfalls visible, but also act as a lighthouse to provide a practical direction for the research community. Moreover, most of the studies only demonstrated the champion performance. It is widely known that LSBs are very sensitive to the fabrication and testing conditions, and hence it is hard to make a conclusion that some specific optimization works according to a single impressive battery. In this regard, to make a more reasonable conclusion, the statistic distribution of the performance of a batch cell is required.
Lastly, as mentioned above, the sulfur cathode, lithium anode, electrolyte and the separator all play critical roles in the battery operation. The research efforts have predominantly focused on the cathode parts. Despite the growing research work on the other components, such as solid-state electrolytes and lithium metal anodes, research on the entire LSB systems is still lacking.183–186 Furthermore, the interplay of different components has not yet been well understood. LSBs are a complicated system, and a step closer to practical application of LSBs may lie in the incorporation of the advances in different components, and a better understanding of the interplay among them.
Conflicts of interest
There are no conflicts to declare.
References
- M. Li, J. Lu, Z. Chen and K. Amine, 30 years of lithium-ion batteries, Adv. Mater., 2018, 30, 1800561 CrossRef PubMed.
- V. Kolosnitsyn and E. Karaseva, Lithium-sulfur batteries: Problems and solutions, Russ. J. Electrochem., 2008, 44, 506–509 CrossRef CAS.
- J. Janek and W. G. Zeier, A solid future for battery development, Nat. Energy, 2016, 1, 1–4 Search PubMed.
- Z. P. Cano, D. Banham, S. Ye, A. Hintennach, J. Lu, M. Fowler and Z. Chen, Batteries and fuel cells for emerging electric vehicle markets, Nat. Energy, 2018, 3, 279–289 CrossRef.
- L. Grande, E. Paillard, J. Hassoun, J. B. Park, Y. J. Lee, Y. K. Sun, S. Passerini and B. Scrosati, The lithium/air battery: still an emerging system or a practical reality?, Adv. Mater., 2015, 27, 784–800 CrossRef CAS PubMed.
- S. H. Chung and A. Manthiram, Current status and future prospects of metal–sulfur batteries, Adv. Mater., 2019, 31, 1901125 CrossRef PubMed.
- J. Muldoon, C. B. Bucur and T. Gregory, Quest for nonaqueous multivalent secondary batteries: magnesium and beyond, Chem. Rev., 2014, 114, 11683–11720 CrossRef CAS PubMed.
- P. G. Bruce, S. A. Freunberger, L. J. Hardwick and J.-M. Tarascon, Li–O 2 and Li–S batteries with high energy storage, Nat. Mater., 2012, 11, 19–29 CrossRef CAS PubMed.
-
H. Danuta, U. Juliusz, D. Herbert and J. Ulam, Electric dry cells and storage batteries, US Pat., 3043896, 1962 Search PubMed.
- H. Yamin, J. Penciner, A. Gorenshtain, M. Elam and E. Peled, The electrochemical behavior of polysulfides in tetrahydrofuran, J. Power Sources, 1985, 14, 129–134 CrossRef CAS.
- H. Yamin and E. Peled, Electrochemistry of a nonaqueous lithium/sulfur cell, J. Power Sources, 1983, 9, 281–287 CrossRef CAS.
- Z. W. Seh, Y. Sun, Q. Zhang and Y. Cui, Designing high-energy lithium–sulfur batteries, Chem. Soc. Rev., 2016, 45, 5605–5634 RSC.
- A. Manthiram, Y. Fu, S.-H. Chung, C. Zu and Y.-S. Su, Rechargeable lithium–sulfur batteries, Chem. Rev., 2014, 114, 11751–11787 CrossRef CAS PubMed.
- X. Ji, K. T. Lee and L. F. Nazar, A highly ordered nanostructured carbon–sulphur cathode for lithium–sulphur batteries, Nat. Mater., 2009, 8, 500–506 CrossRef CAS PubMed.
- Z. Li, Y. Huang, L. Yuan, Z. Hao and Y. Huang, Status and prospects in sulfur–carbon composites as cathode materials for rechargeable lithium–sulfur batteries, Carbon, 2015, 92, 41–63 CrossRef CAS.
- Q. Pang, X. Liang, C. Y. Kwok and L. F. Nazar, Advances in lithium–sulfur batteries based on multifunctional cathodes and electrolytes, Nat. Energy, 2016, 1, 1–11 Search PubMed.
- Z. Zeng and X. Liu, Sulfur immobilization by “chemical anchor” to suppress the diffusion of polysulfides in lithium–sulfur batteries, Adv. Mater. Interfaces, 2018, 5, 1701274 CrossRef.
- J. Xu, T. Lawson, H. Fan, D. Su and G. Wang, Updated Metal Compounds (MOFs, S, OH, N, C) Used as Cathode Materials for Lithium–Sulfur Batteries, Adv. Energy Mater., 2018, 8, 1702607 CrossRef.
- Y. Wang, X. Huang, S. Zhang and Y. Hou, Sulfur hosts against the shuttle effect, Small Methods, 2018, 2, 1700345 CrossRef.
- W. Ren, W. Ma, S. Zhang and B. Tang, Recent advances in shuttle effect inhibition for lithium sulfur batteries, Energy Storage Mater., 2019, 23, 707–732 CrossRef.
- T. Tang and Y. Hou, Chemical Confinement and Utility of Lithium Polysulfides in Lithium Sulfur Batteries, Small Methods, 2019, 1900001 Search PubMed.
- D. Liu, C. Zhang, G. Zhou, W. Lv, G. Ling, L. Zhi and Q. H. Yang, Catalytic effects in lithium–sulfur batteries: promoted sulfur transformation and reduced shuttle effect, Adv. Sci., 2018, 5, 1700270 CrossRef PubMed.
- H. Al Salem, G. Babu, C. V. Rao and L. M. R. Arava, Electrocatalytic polysulfide traps for controlling redox shuttle process of Li–S batteries, J. Am. Chem. Soc., 2015, 137, 11542–11545 CrossRef CAS PubMed.
- W. G. Lim, S. Kim, C. Jo and J. Lee, A comprehensive review of materials with catalytic effects in Li–S batteries: enhanced redox kinetics, Angew. Chem., Int. Ed., 2019, 58, 18746–18757 CrossRef CAS PubMed.
- J. Park, S.-H. Yu and Y.-E. Sung, Design of structural and functional nanomaterials for lithium-sulfur batteries, Nano Today, 2018, 18, 35–64 CrossRef CAS.
- J. Zhang, H. Huang, J. Bae, S. H. Chung, W. Zhang, A. Manthiram and G. Yu, Nanostructured host materials for trapping sulfur in rechargeable Li–S batteries: structure design and interfacial chemistry, Small Methods, 2018, 2, 1700279 CrossRef.
- M. Jana, R. Xu, X.-B. Cheng, J. S. Yeon, J. M. Park, J.-Q. Huang, Q. Zhang and H. S. Park, Rational design of two-dimensional nanomaterials for lithium–sulfur batteries, Energy Environ. Sci., 2020, 13, 1049–1075 RSC.
- Z. Li, H. B. Wu and X. W. D. Lou, Rational designs and engineering of hollow micro-/nanostructures as sulfur hosts for advanced lithium–sulfur batteries, Energy Environ. Sci., 2016, 9, 3061–3070 RSC.
- J. Wang, Y. Cui and D. Wang, Design of hollow nanostructures for energy storage, conversion and production, Adv. Mater., 2019, 31, 1801993 CrossRef PubMed.
- J. Zhao, M. Yang, N. Yang, J. Wang and D. Wang, Hollow Micro-/Nanostructure Reviving Lithium-sulfur Batteries, Chem. Res. Chin. Univ., 2020, 36, 313–319 CrossRef CAS.
- Q. Sun, B. He, X.-Q. Zhang and A.-H. Lu, Engineering of Hollow Core–Shell Interlinked Carbon Spheres for Highly Stable Lithium–Sulfur Batteries, ACS Nano, 2015, 9, 8504–8513 CrossRef CAS PubMed.
- Z. Yu, M. Liu, D. Guo, J. Wang, X. Chen, J. Li, H. Jin and Z. Yang, X. a. Chen and S. Wang, Radially Inwardly Aligned Hierarchical Porous Carbon for Ultra-Long-Life Lithium–Sulfur Batteries, Angew. Chem., Int. Ed., 2020, 59, 6406–6411 CrossRef CAS PubMed.
- J. Zang, T. An, Y. Dong, X. Fang, M. Zheng, Q. Dong and N. Zheng, Hollow-in-hollow carbon spheres with hollow foam-like cores for lithium–sulfur batteries, Nano Res., 2015, 8, 2663–2675 CrossRef CAS.
- Y. Zhong, Q. Lu, Y. Zhu, Y. Zhu, W. Zhou, S. Wang and Z. Shao, Fructose-Derived Hollow Carbon Nanospheres with Ultrathin and Ordered Mesoporous Shells as Cathodes in Lithium–Sulfur Batteries for Fast Energy Storage, Adv. Sustainable Syst., 2017, 1, 1700081 CrossRef.
- C. Zhang, H. B. Wu, C. Yuan, Z. Guo and X. W. Lou, Confining Sulfur in Double-Shelled Hollow Carbon Spheres for Lithium–Sulfur Batteries, Angew. Chem., Int. Ed., 2012, 51, 9592–9595 CrossRef CAS PubMed.
- N. Jayaprakash, J. Shen and S. S. Moganty, A. Corona and L. A. Archer, Porous Hollow Carbon@Sulfur Composites for High-Power Lithium–Sulfur Batteries, Angew. Chem., Int. Ed., 2011, 50, 5904–5908 CrossRef CAS PubMed.
- W. Zhou, C. Wang, Q. Zhang, H. D. Abruña, Y. He, J. Wang, S. X. Mao and X. Xiao, Tailoring pore size of nitrogen-doped hollow carbon nanospheres for confining sulfur in lithium–sulfur batteries, Adv. Energy Mater., 2015, 5, 1401752 CrossRef.
- M. Zhang, C. Yu, C. Zhao, X. Song, X. Han, S. Liu, C. Hao and J. Qiu, Cobalt-embedded nitrogen-doped hollow carbon nanorods for synergistically immobilizing the discharge products in lithium–sulfur battery, Energy Storage Mater., 2016, 5, 223–229 CrossRef.
- A. Fu, C. Wang, F. Pei, J. Cui, X. Fang and N. Zheng, Recent advances in hollow porous carbon materials for lithium–sulfur batteries, Small, 2019, 15, 1804786 CrossRef PubMed.
- M. Barghamadi, A. S. Best, A. I. Bhatt, A. F. Hollenkamp, M. Musameh, R. J. Rees and T. Rüther, Lithium–sulfur batteries-the solution is in the electrolyte, but is the electrolyte a solution?, Energy Environ. Sci., 2014, 7, 3902–3920 RSC.
- Y.-S. Su, Y. Fu, T. Cochell and A. Manthiram, A strategic approach to recharging lithium-sulphur batteries for long cycle life, Nat. Commun., 2013, 4, 1–8 Search PubMed.
- L. Kong, H.-J. Peng, J.-Q. Huang, W. Zhu, G. Zhang, Z.-W. Zhang, P.-Y. Zhai, P. Sun, J. Xie and Q. Zhang, Beaver-dam-like membrane: a robust and sulphifilic MgBO2 (OH)/CNT/PP nest separator in Li-S batteries, Energy Storage Mater., 2017, 8, 153–160 CrossRef.
- H. Pan, J. Chen, R. Cao, V. Murugesan, N. N. Rajput, K. S. Han, K. Persson, L. Estevez, M. H. Engelhard and J.-G. Zhang, Non-encapsulation approach for high-performance Li–S batteries through controlled nucleation and growth, Nat. Energy, 2017, 2, 813–820 CrossRef CAS.
- D. Lin, Y. Liu and Y. Cui, Reviving the lithium metal anode for high-energy batteries, Nat. Nanotechnol., 2017, 12, 194–206 CrossRef CAS PubMed.
- X. Zhang, A. Wang, X. Liu and J. Luo, Dendrites in lithium metal anodes: suppression, regulation, and elimination, Acc. Chem. Res., 2019, 52, 3223–3232 CrossRef CAS PubMed.
- S. Zhang, K. Ueno, K. Dokko and M. Watanabe, Recent advances in electrolytes for lithium–sulfur batteries, Adv. Energy Mater., 2015, 5, 1500117 CrossRef.
- S. Chen, X. Han, J. Luo, J. Liao, J. Wang, Q. Deng, Z. Zeng and S. Deng, In situ transformation of LDH into hollow cobalt-embedded and N-doped carbonaceous microflowers as polysulfide mediator for lithium-sulfur batteries, Chem. Eng. J., 2020, 385, 123457 CrossRef.
- B. Meyer, Elemental sulfur, Chem. Rev., 1976, 76, 367–388 CrossRef CAS.
- W. Zhou, Y. Yu, H. Chen, F. J. DiSalvo and H. C. D. Abruña, Yolk–shell structure of polyaniline-coated sulfur for lithium–sulfur batteries, J. Am. Chem. Soc., 2013, 135, 16736–16743 CrossRef CAS PubMed.
- R. G. Chaudhuri and S. Paria, Synthesis of sulfur nanoparticles in aqueous surfactant solutions, J. Colloid Interface Sci., 2010, 343, 439–446 CrossRef CAS PubMed.
- Y. Guo, J. Zhao, S. Yang, K. Yu, Z. Wang and H. Zhang, Preparation and characterization of monoclinic sulfur nanoparticles by water-in-oil microemulsions technique, Powder Technol., 2006, 162, 83–86 CrossRef CAS.
- G. Zhou, H. Tian, Y. Jin, X. Tao, B. Liu, R. Zhang, Z. W. Seh, D. Zhuo, Y. Liu and J. Sun, Catalytic oxidation of Li2S on the surface of metal sulfides for Li–S batteries, Proc. Natl. Acad. Sci. U. S. A., 2017, 114, 840–845 CrossRef CAS PubMed.
- X. Liang, C. Hart, Q. Pang, A. Garsuch, T. Weiss and L. F. Nazar, A highly efficient polysulfide mediator for lithium–sulfur batteries, Nat. Commun., 2015, 6, 1–8 Search PubMed.
- A. N. Arias, A. Y. Tesio and V. Flexer, Non-Carbonaceous Materials as Cathodes for Lithium-Sulfur Batteries, J. Electrochem. Soc., 2018, 165, A6119 CrossRef CAS.
- X. Liang and L. F. Nazar, In Situ Reactive Assembly of Scalable Core–Shell Sulfur–MnO2 Composite Cathodes, ACS Nano, 2016, 10, 4192–4198 CrossRef CAS PubMed.
- K. Kim, P. J. Kim, J. P. Youngblood and V. G. Pol, Surface Functionalization of Carbon Architecture with Nano-MnO2 for Effective Polysulfide Confinement in Lithium–Sulfur Batteries, ChemSusChem, 2018, 11, 2375–2381 CrossRef CAS PubMed.
- X. Zhao, H. Wang, G. Zhai and G. Wang, Facile Assembly of 3D Porous Reduced Graphene Oxide/Ultrathin MnO2 Nanosheets-S Aerogels as Efficient Polysulfide Adsorption Sites for High-Performance Lithium–Sulfur Batteries, Chem. – Eur. J., 2017, 23, 7037–7045 CrossRef CAS PubMed.
- J. Liu, C. Wang, B. Liu, X. Ke, L. Liu, Z. Shi, H. Zhang and Z. Guo, Rational synthesis of MnO2@ CMK/S composite as cathode materials for lithium–sulfur batteries, Mater. Lett., 2017, 195, 236–239 CrossRef CAS.
- L. Ni, Z. Wu, G. Zhao, C. Sun, C. Zhou, X. Gong and G. Diao, Core–Shell Structure and Interaction Mechanism of γ-MnO2 Coated Sulfur for Improved Lithium-Sulfur Batteries, Small, 2017, 13, 1603466 CrossRef PubMed.
- S. Tu, X. Zhao, M. Cheng, P. Sun, Y. He and Y. Xu, Uniform Mesoporous MnO2 Nanospheres as a Surface Chemical Adsorption and Physical Confinement Polysulfide Mediator for Lithium–Sulfur Batteries, ACS Appl. Mater. Interfaces, 2019, 11, 10624–10630 CrossRef CAS PubMed.
- Z. Li, J. Zhang and X. W. Lou, Hollow carbon nanofibers filled with MnO2 nanosheets as efficient sulfur hosts for lithium–sulfur batteries, Angew. Chem., Int. Ed., 2015, 54, 12886–12890 CrossRef CAS PubMed.
- S. Rehman, T. Tang, Z. Ali, X. Huang and Y. Hou, Integrated design of MnO2@ carbon hollow nanoboxes to synergistically encapsulate polysulfides for empowering lithium sulfur batteries, Small, 2017, 13, 1700087 CrossRef PubMed.
- L. Ni, G. Zhao, Y. Wang, Z. Wu, W. Wang, Y. Liao, G. Yang and G. Diao, Coaxial Carbon/MnO2 Hollow Nanofibers as Sulfur Hosts for High-Performance Lithium-Sulfur Batteries, Chem. – Asian J., 2017, 12, 3128–3134 CrossRef CAS PubMed.
- L. Ni, G. Zhao, G. Yang, G. Niu, M. Chen and G. Diao, Dual core–shell-structured S@ C@ MnO2 nanocomposite for highly stable lithium–sulfur batteries, ACS Appl. Mater. Interfaces, 2017, 9, 34793–34803 CrossRef CAS PubMed.
- M. Yan, Y. Zhang, Y. Li, Y. Huo, Y. Yu, C. Wang, J. Jin, L. Chen, T. Hasan, B. Wang and B.-L. Su, Manganese dioxide nanosheet functionalized sulfur@PEDOT core–shell nanospheres for advanced lithium–sulfur batteries, J. Mater. Chem. A, 2016, 4, 9403–9412 RSC.
- J. Wu, Q. Ma, C. Lian, Y. Yuan and D. Long, Promoting polythionate intermediates formation by oxygen-deficient manganese oxide hollow nanospheres for high performance lithium-sulfur batteries, Chem. Eng. J., 2019, 370, 556–564 CrossRef CAS.
- S. Evers, T. Yim and L. F. Nazar, Understanding the nature of absorption/adsorption in nanoporous polysulfide sorbents for the Li–S battery, J. Phys. Chem. C, 2012, 116, 19653–19658 CrossRef CAS.
- C. Yuan, S. Zhu, H. Cao, L. Hou and J. Lin, Hierarchical sulfur-impregnated hydrogenated TiO2 mesoporous spheres comprising anatase nanosheets with highly exposed (001) facets for advanced Li-S batteries, Nanotechnology, 2015, 27, 045403 CrossRef PubMed.
- Z. Wei Seh, W. Li, J. J. Cha, G. Zheng, Y. Yang, M. T. McDowell, P.-C. Hsu and Y. Cui, Sulphur–TiO2 yolk–shell nanoarchitecture with internal void space for long-cycle lithium–sulphur batteries, Nat. Commun., 2013, 4, 1331 CrossRef PubMed.
- E. H. M. Salhabi, J. Zhao, J. Wang, M. Yang, B. Wang and D. Wang, Hollow Multi-Shelled Structural TiO2- x with Multiple Spatial Confinement for Long-Life Lithium–Sulfur Batteries, Angew. Chem., Int. Ed., 2019, 131, 9176–9180 CrossRef.
- J. Ni, L. Jin, M. Xue, J. Zheng, J. P. Zheng and C. Zhang, TiO2 microboxes as effective polysufide reservoirs for lithium sulfur batteries, Electrochim. Acta, 2019, 296, 39–48 CrossRef CAS.
- X. Gao, G. Li, Y. Xu, Z. Hong, C. Liang and Z. Lin, TiO2 Microboxes with Controlled Internal Porosity for High-Performance Lithium Storage, Angew. Chem., Int. Ed., 2015, 54, 14331–14335 CrossRef CAS PubMed.
- W. Yang, L. Zhang, Y. Hu, Y. Zhong, H. B. Wu and X. W. Lou, Microwave-Assisted Synthesis of Porous Ag2S–Ag Hybrid Nanotubes with High Visible-Light Photocatalytic Activity, Angew. Chem., Int. Ed., 2012, 51, 11501–11504 CrossRef CAS PubMed.
- X. Tao, J. Wang, Z. Ying, Q. Cai, G. Zheng, Y. Gan, H. Huang, Y. Xia, C. Liang, W. Zhang and Y. Cui, Strong Sulfur Binding with Conducting Magnéli-Phase TinO2n–1 Nanomaterials for Improving Lithium–Sulfur Batteries, Nano Lett., 2014, 14, 5288–5294 CrossRef CAS PubMed.
- Q. Pang, D. Kundu, M. Cuisinier and L. F. Nazar, Surface-enhanced redox chemistry of polysulphides on a metallic and polar host for lithium-sulphur batteries, Nat. Commun., 2014, 5, 4759 CrossRef CAS PubMed.
- J. Wang, J. Wan, N. Yang, Q. Li and D. Wang, Hollow multishell structures exercise temporal–spatial ordering and dynamic smart behaviour, Nat. Rev. Chem., 2020, 4, 159–168 CrossRef.
- J. Wang, H. Tang, H. Wang, R. Yu and D. Wang, Multi-shelled hollow micro-/nanostructures: promising platforms for lithium-ion batteries, Mater. Chem. Front., 2017, 1, 414–430 RSC.
- D.-S. Bin, Z.-X. Chi, Y. Li, K. Zhang, X. Yang, Y.-G. Sun, J.-Y. Piao, A.-M. Cao and L.-J. Wan, Controlling the Compositional Chemistry in Single Nanoparticles for Functional Hollow Carbon Nanospheres, J. Am. Chem. Soc., 2017, 139, 13492–13498 CrossRef CAS PubMed.
- Z. Li, J. Zhang, B. Guan, D. Wang, L.-M. Liu and X. W. D. Lou, A sulfur host based on titanium monoxide@ carbon hollow spheres for advanced lithium–sulfur batteries, Nat. Commun., 2016, 7, 1–11 Search PubMed.
- Z. Li, B. Y. Guan, J. Zhang and X. W. D. Lou, A compact nanoconfined sulfur cathode for high-performance lithium-sulfur batteries, Joule, 2017, 1, 576–587 CrossRef CAS.
- X. Wu, Y. Du, P. Wang, L. Fan, J. Cheng, M. Wang, Y. Qiu, B. Guan, H. Wu and N. Zhang, Kinetics enhancement of lithium–sulfur batteries by interlinked hollow MoO2 sphere/nitrogen-doped graphene composite, J. Mater. Chem. A, 2017, 5, 25187–25192 RSC.
- W. Qi, W. Jiang, F. Xu, J. Jia, C. Yang and B. Cao, Improving confinement and redox kinetics of polysufides through hollow NC@ CeO2 nanospheres for high-performance lithium-sulfur batteries, Chem. Eng. J., 2020, 382, 122852 CrossRef CAS.
- L. Fan, H. Wu, X. Wu, M. Wang, J. Cheng, N. Zhang, Y. Feng and K. Sun, Fe-MOF derived jujube pit like Fe3O4/C composite as sulfur host for lithium-sulfur battery, Electrochim. Acta, 2019, 295, 444–451 CrossRef CAS.
- M. Zhu, S. Li, J. Liu and B. Li, Promoting polysulfide conversion by V2O3 hollow sphere for enhanced lithium-sulfur battery, Appl. Surf. Sci., 2019, 473, 1002–1008 CrossRef CAS.
- B. Cao, D. Li, B. Hou, Y. Mo, L. Yin and Y. Chen, Synthesis of double-shell SnO2@ C hollow nanospheres as sulfur/sulfide cages for lithium–sulfur batteries, ACS Appl. Mater. Interfaces, 2016, 8, 27795–27802 CrossRef CAS PubMed.
- J. Wang, W. Wang, Y. Zhang, Z. Bakenov, Y. Zhao and X. Wang, Synthesis of highly defective hollow double-shelled Co3O4- x microspheres as sulfur host for high-performance lithium-sulfur batteries, Mater. Lett., 2019, 255, 126581 CrossRef CAS.
- L. Hu, C. Dai, H. Liu, Y. Li, B. Shen, Y. Chen, S. J. Bao and M. Xu, Double-Shelled NiO-NiCo2O4 Heterostructure@ Carbon Hollow Nanocages as an Efficient Sulfur Host for Advanced Lithium–Sulfur Batteries, Adv. Energy Mater., 2018, 8, 1800709 CrossRef.
- W. Wang, Y. Zhao, Y. Zhang, J. Wang, G. Cui, M. Li, Z. Bakenov and X. Wang, Defect-Rich Multishelled Fe-Doped Co3O4 Hollow Microspheres with Multiple Spatial Confinements to Facilitate Catalytic Conversion of Polysulfides for High-Performance Li–S Batteries, ACS Appl. Mater. Interfaces, 2020, 12, 12763–12773 CrossRef PubMed.
- J.-X. Lin, Y.-X. Mo, P.-F. Zhang, Y.-Y. Li, Y.-J. Wu, S.-J. Zhang, Z.-G. Gao, J.-D. Chen, W.-F. Ren, J.-T. Li, Y. Zhou, L. Huang and S.-G. Sun, Ultrahigh sulfur content up to 93 wt% encapsulated in multilayer nanoshell of V/V2O5 composite to suppress shuttle effect of lithium–sulfur battery with high-performance, Mater. Today Energy, 2019, 13, 267–276 CrossRef.
- X. Liu, J. Q. Huang, Q. Zhang and L. Mai, Nanostructured metal oxides and sulfides for lithium–sulfur batteries, Adv. Mater., 2017, 29, 1601759 CrossRef PubMed.
- J. S. Jirkovsky, A. Björling and E. Ahlberg, Reduction of oxygen on dispersed nanocrystalline CoS2, J. Phys. Chem. C, 2012, 116, 24436–24444 CrossRef CAS.
- M. S. Faber, R. Dziedzic, M. A. Lukowski, N. S. Kaiser, Q. Ding and S. Jin, High-performance electrocatalysis using metallic cobalt pyrite (CoS2) micro-and nanostructures, J. Am. Chem. Soc., 2014, 136, 10053–10061 CrossRef CAS PubMed.
- Z. Yuan, H.-J. Peng, T.-Z. Hou, J.-Q. Huang, C.-M. Chen, D.-W. Wang, X.-B. Cheng, F. Wei and Q. Zhang, Powering Lithium–Sulfur Battery Performance by Propelling Polysulfide Redox at Sulfiphilic Hosts, Nano Lett., 2016, 16, 519–527 CrossRef CAS PubMed.
- S. D. Seo, D. Park, S. Park and D. W. Kim, Brain-Coral-Like Mesoporous Hollow CoS2@ N-Doped Graphitic Carbon Nanoshells as Efficient Sulfur Reservoirs for Lithium–Sulfur Batteries, Adv. Funct. Mater., 2019, 29, 1903712 CrossRef.
- R. Bouchard, P. Russo and A. Wold, Preparation and electrical properties of some thiospinels, Inorg. Chem., 1965, 4, 685–688 CrossRef CAS.
- Z.-F. Huang, J. Song, K. Li, M. Tahir, Y.-T. Wang, L. Pan, L. Wang, X. Zhang and J.-J. Zou, Hollow Cobalt-Based Bimetallic Sulfide Polyhedra for Efficient All-pH-Value Electrochemical and Photocatalytic Hydrogen Evolution, J. Am. Chem. Soc., 2016, 138, 1359–1365 CrossRef CAS PubMed.
- H. Xu and A. Manthiram, Hollow
cobalt sulfide polyhedra-enabled long-life, high areal-capacity lithium-sulfur batteries, Nano Energy, 2017, 33, 124–129 CrossRef CAS.
- J. Pu, Z. Shen, J. Zheng, W. Wu, C. Zhu, Q. Zhou, H. Zhang and F. Pan, Multifunctional Co3S4@sulfur nanotubes for enhanced lithium-sulfur battery performance, Nano Energy, 2017, 37, 7–14 CrossRef CAS.
- H. Behret, H. Binder and G. Sandstede, Electrocatalytic oxygen reduction with thiospinels and other sulphides of transition metals, Electrochim. Acta, 1975, 20, 111–117 CrossRef CAS.
- T. Chen, Z. Zhang, B. Cheng, R. Chen, Y. Hu, L. Ma, G. Zhu, J. Liu and Z. Jin, Self-Templated Formation of Interlaced Carbon Nanotubes Threaded Hollow Co3S4 Nanoboxes for High-Rate and Heat-Resistant Lithium–Sulfur Batteries, J. Am. Chem. Soc., 2017, 139, 12710–12715 CrossRef CAS PubMed.
- H. Zhang, M. Zou, W. Zhao, Y. Wang, Y. Chen, Y. Wu, L. Dai and A. Cao, Highly Dispersed Catalytic Co3S4 among a Hierarchical Carbon Nanostructure for High-Rate and Long-Life Lithium–Sulfur Batteries, ACS Nano, 2019, 13, 3982–3991 CrossRef CAS PubMed.
- N. Kumar, N. Raman and A. Sundaresan, Synthesis and properties of cobalt sulfide phases: CoS2 and Co9S8, Z. anorg. allg. Chem., 2014, 640, 1069–1074 CrossRef CAS.
- Q. Pang, D. Kundu and L. F. Nazar, A graphene-like metallic cathode host for long-life and high-loading lithium–sulfur batteries, Mater. Horiz., 2016, 3, 130–136 RSC.
- X. Tao, J. Wang, C. Liu, H. Wang, H. Yao, G. Zheng, Z. W. Seh, Q. Cai, W. Li and G. Zhou, Balancing surface adsorption and diffusion of lithium-polysulfides on nonconductive oxides for lithium–sulfur battery design, Nat. Commun., 2016, 7, 11203 CrossRef CAS PubMed.
- C. Dai, J. M. Lim, M. Wang, L. Hu, Y. Chen, Z. Chen, H. Chen, S. J. Bao, B. Shen and Y. Li, Honeycomb-Like Spherical Cathode Host Constructed from Hollow Metallic and Polar Co9S8 Tubules for Advanced Lithium–Sulfur Batteries, Adv. Funct. Mater., 2018, 28, 1704443 CrossRef.
- T. Chen, L. Ma, B. Cheng, R. Chen, Y. Hu, G. Zhu, Y. Wang, J. Liang, Z. Tie and J. Liu, Metallic and polar Co9S8 inlaid carbon hollow nanopolyhedra as efficient polysulfide mediator for lithium–ulfur batteries, Nano Energy, 2017, 38, 239–248 CrossRef CAS.
- Z. Li, Z. Xiao, P. Li, X. Meng and R. Wang, Enhanced Chemisorption and Catalytic Effects toward Polysulfides by Modulating Hollow Nanoarchitectures for Long-Life Lithium–Sulfur Batteries, Small, 2020, 16, 1906114 CrossRef CAS PubMed.
- C. Ye, L. Zhang, C. Guo, D. Li, A. Vasileff, H. Wang and S. Z. Qiao, A 3D hybrid of chemically coupled nickel sulfide and hollow carbon spheres for high performance lithium–sulfur batteries, Adv. Funct. Mater., 2017, 27, 1702524 CrossRef.
- H.-E. Wang, X. Li, N. Qin, X. Zhao, H. Cheng, G. Cao and W. Zhang, Sulfur-deficient MoS 2 grown inside hollow mesoporous carbon as a functional polysulfide mediator, J. Mater. Chem. A, 2019, 7, 12068–12074 RSC.
- B. Yu, Y. Chen, Z. Wang, D. Chen, X. Wang, W. Zhang, J. He and W. He, 1T-MoS2 nanotubes wrapped with N-doped graphene as highly-efficient absorbent and electrocatalyst for Li–S batteries, J. Power Sources, 2020, 447, 227364 CrossRef CAS.
- Q. Liu, J. Jin and J. Zhang, NiCo2S4@ graphene as a bifunctional electrocatalyst for oxygen reduction and evolution reactions, ACS Appl. Mater. Interfaces, 2013, 5, 5002–5008 CrossRef CAS PubMed.
- A. Sivanantham, P. Ganesan and S. Shanmugam, Hierarchical NiCo2S4 nanowire arrays supported on Ni foam: an efficient and durable bifunctional electrocatalyst for oxygen and hydrogen evolution reactions, Adv. Funct. Mater., 2016, 26, 4661–4672 CrossRef CAS.
- L. Shen, J. Wang, G. Xu, H. Li, H. Dou and X. Zhang, NiCo2S4 nanosheets grown on nitrogen-doped carbon foams as an advanced electrode for supercapacitors, Adv. Energy Mater., 2015, 5, 1400977 CrossRef.
- J. Xiao, L. Wan, S. Yang, F. Xiao and S. Wang, Design hierarchical electrodes with highly conductive NiCo2S4 nanotube arrays grown on carbon fiber paper for high-performance pseudocapacitors, Nano Lett., 2014, 14, 831–838 CrossRef CAS PubMed.
- X. Tan, X. Wang, X. Wang, Y. Wang, C. Li and D. Xia, NiCo2S4 yolk–shell hollow spheres with physical and chemical interaction toward polysulfides for advanced lithium-sulfur batteries, Ionics, 2019, 25, 4047–4056 CrossRef CAS.
- X. Y. Yu, L. Yu and X. W. Lou, Metal sulfide hollow nanostructures for electrochemical energy storage, Adv. Energy Mater., 2016, 6, 1501333 CrossRef.
- L. Zhou, Z. Zhuang, H. Zhao, M. Lin, D. Zhao and L. Mai, Intricate hollow structures: controlled synthesis and applications in energy storage and conversion, Adv. Mater., 2017, 29, 1602914 CrossRef PubMed.
- N. Mosavati, S. O. Salley and K. S. Ng, Characterization and electrochemical activities of nanostructured transition metal nitrides as cathode materials for lithium sulfur batteries, J. Power Sources, 2017, 340, 210–216 CrossRef CAS.
- X. Lu, G. Wang, T. Zhai, M. Yu, S. Xie, Y. Ling, C. Liang, Y. Tong and Y. Li, Stabilized TiN nanowire arrays for high-performance and flexible supercapacitors, Nano Lett., 2012, 12, 5376–5381 CrossRef CAS PubMed.
- B. Avasarala and P. Haldar, Electrochemical oxidation behavior of titanium nitride based electrocatalysts under PEM fuel cell conditions, Electrochim. Acta, 2010, 55, 9024–9034 CrossRef CAS.
- Z. Cui, C. Zu, W. Zhou, A. Manthiram and J. B. Goodenough, Mesoporous Titanium Nitride-Enabled Highly Stable Lithium–Sulfur Batteries, Adv. Mater., 2016, 28, 6926–6931 CrossRef CAS PubMed.
- T.-G. Jeong, D. S. Choi, H. Song, J. Choi, S.-A. Park, S. H. Oh, H. Kim, Y. Jung and Y.-T. Kim, Heterogeneous catalysis for lithium–sulfur batteries: enhanced rate performance by promoting polysulfide fragmentations, ACS Energy Lett., 2017, 2, 327–333 CrossRef CAS.
- X. Xiao, H. Wang, W. Bao, P. Urbankowski, L. Yang, Y. Yang, K. Maleski, L. Cui, S. J. Billinge and G. Wang, Two-Dimensional Arrays of Transition Metal Nitride Nanocrystals, Adv. Mater., 2019, 31, 1902393 CrossRef PubMed.
- W. G. Lim, C. Jo, A. Cho, J. Hwang, S. Kim, J. W. Han and J. Lee, Approaching ultrastable high-rate Li–S batteries through hierarchically porous titanium nitride synthesized by multiscale phase separation, Adv. Mater., 2019, 31, 1806547 CrossRef PubMed.
- X. Liu, Y. Zhang, T. Wu and J. Huang, Hierarchical nanotubular titanium nitride derived from natural cellulose substance and its electrochemical properties, Chem. Commun., 2012, 48, 9992–9994 RSC.
- C. Li, J. Shi, L. Zhu, Y. Zhao, J. Lu and L. Xu, Titanium nitride hollow nanospheres with strong lithium polysulfide chemisorption as sulfur hosts for advanced lithium-sulfur batteries, Nano Res., 2018, 11, 4302–4312 CrossRef CAS.
- J. Luo, J. Zheng and B. Dang, Hollow TiN Nanospheres as Advanced Host Materials for High Performance Lithium-Sulfur Batteries, ChemistrySelect, 2019, 4, 14027–14030 CrossRef CAS.
- L. Chen, W. Yang, H. Zhang, J. Liu and Y. Zhou, Self-templated preparation of hollow mesoporous TiN microspheres as sulfur host materials for advanced lithium–sulfur batteries, J. Mater. Sci., 2018, 53, 10363–10371 CrossRef CAS.
- D.-R. Deng, T.-H. An, Y.-J. Li, Q.-H. Wu, M.-S. Zheng and Q.-F. Dong, Hollow porous titanium nitride tubes as a cathode electrode for extremely stable Li–S batteries, J. Mater. Chem. A, 2016, 4, 16184–16190 RSC.
- Z. Sun, J. Zhang, L. Yin, G. Hu, R. Fang, H.-M. Cheng and F. Li, Conductive porous vanadium nitride/graphene composite as chemical anchor of polysulfides for lithium-sulfur batteries, Nat. Commun., 2017, 8, 1–8 CrossRef PubMed.
- L. Ma, H. Yuan, W. Zhang, G. Zhu, Y. Wang, Y. Hu, P. Zhao, R. Chen, T. Chen and J. Liu, Porous-shell vanadium nitride nanobubbles with ultrahigh areal sulfur loading for high-capacity and long-life lithium–sulfur batteries, Nano Lett., 2017, 17, 7839–7846 CrossRef CAS PubMed.
- W. Ren, L. Xu, L. Zhu, X. Wang, X. Ma and D. Wang, Cobalt-doped vanadium nitride yolk–shell nanospheres@ carbon with physical and chemical synergistic effects for advanced Li–S batteries, ACS Appl. Mater. Interfaces, 2018, 10, 11642–11651 CrossRef CAS PubMed.
- X. Liang, A. Garsuch and L. F. Nazar, Sulfur Cathodes Based on Conductive MXene Nanosheets for High-Performance Lithium–Sulfur Batteries, Angew. Chem., Int. Ed., 2015, 54, 3907–3911 CrossRef CAS PubMed.
- X. Liang, Y. Rangom, C. Y. Kwok, Q. Pang and L. F. Nazar, Interwoven MXene Nanosheet/Carbon-Nanotube Composites as Li–S Cathode Hosts, Adv. Mater., 2017, 29, 1603040 CrossRef PubMed.
- Z. Wang, X. Xu, Z. Liu, S. Ji, S. O. A. Idris and J. Liu, Hollow spheres of Mo2C@ C as synergistically confining sulfur host for superior Li–S battery cathode, Electrochim. Acta, 2020, 332, 135482 CrossRef CAS.
- Y. Xiao, Y. Liu, G. Qin, P. Han, X. Guo, S. Cao and F. Liu, Building MoSe2-Mo2C incorporated hollow fluorinated carbon fibers for Li-S batteries, Composites, Part B, 2020, 108004 CrossRef CAS.
- H. Zhang, H. Cui, J. Li, Y. Liu, Y. Yang and M. Wang, Frogspawn inspired hollow Fe3C@ N–C as an efficient sulfur host for high-rate lithium–sulfur batteries, Nanoscale, 2019, 11, 21532–21541 RSC.
- Y. Mi, W. Liu, X. Li, J. Zhuang, H. Zhou and H. Wang, High-performance Li–S battery cathode with catalyst-like carbon nanotube-MoP promoting polysulfide redox, Nano Res., 2017, 10, 3698–3705 CrossRef CAS.
- Y. Zhong, L. Yin, P. He, W. Liu, Z. Wu and H. Wang, Surface Chemistry in Cobalt Phosphide-Stabilized Lithium–Sulfur Batteries, J. Am. Chem. Soc., 2018, 140, 1455–1459 CrossRef CAS PubMed.
- Y. Yang, Y. Zhong, Q. Shi, Z. Wang, K. Sun and H. Wang, Electrocatalysis in lithium sulfur batteries under lean electrolyte conditions, Angew. Chem., Int. Ed., 2018, 130, 15775–15778 CrossRef.
- J. Cheng, D. Zhao, L. Fan, X. Wu, M. Wang, N. Zhang and K. Sun, Ultra-high rate Li–S batteries based on a novel conductive Ni2P yolk–shell material as the host for the S cathode, J. Mater. Chem. A, 2017, 5, 14519–14524 RSC.
- Z. Ye, Y. Jiang, J. Qian, W. Li, T. Feng, L. Li, F. Wu and R. Chen, Exceptional adsorption and catalysis effects of hollow polyhedra/carbon nanotube confined CoP nanoparticles superstructures for enhanced lithium–sulfur batteries, Nano Energy, 2019, 64, 103965 CrossRef CAS.
- F. Ma, X. Wang, J. Wang, Y. Tian, J. Liang, Y. Fan, L. Wang, T. Wang, R. Cao and S. Jiao, Phase-transformed Mo4P3 nanoparticles as efficient catalysts towards lithium polysulfide conversion for lithium–sulfur battery, Electrochim. Acta, 2020, 330, 135310 CrossRef CAS.
- M. Sharon and G. Tamizhmani, Transition metal phosphide semiconductors for their possible use in photoelectrochemical cells and solar chargeable battery (Saur Viddyut Kosh V), J. Mater. Sci., 1986, 21, 2193–2201 CrossRef CAS.
- S. T. Oyama, Novel catalysts for advanced hydroprocessing: transition metal phosphides, J. Catal., 2003, 216, 343–352 CrossRef CAS.
- X.-Q. Niu, X.-L. Wang, D.-H. Wang, Y. Li, Y.-J. Zhang, T. Yang, T. Yu and J.-P. Tu, Metal hydroxide–a new stabilizer for the construction of sulfur/carbon composites as high-performance cathode materials for lithium–sulfur batteries, J. Mater. Chem. A, 2015, 3, 17106–17112 RSC.
- J. Jiang, J. Zhu, W. Ai, X. Wang, Y. Wang, C. Zou, W. Huang and T. Yu, Encapsulation of sulfur with thin-layered nickel-based hydroxides for long-cyclic lithium–sulfur cells, Nat. Commun., 2015, 6, 8622 CrossRef CAS PubMed.
- F. Song and X. Hu, Exfoliation of layered double hydroxides for enhanced oxygen evolution catalysis, Nat. Commun., 2014, 5, 1–9 Search PubMed.
- G. Fan, F. Li, D. G. Evans and X. Duan, Catalytic applications of layered double hydroxides: recent advances and perspectives, Chem. Soc. Rev., 2014, 43, 7040–7066 RSC.
- K.-H. Goh, T.-T. Lim and Z. Dong, Application of layered double hydroxides for removal of oxyanions: a review, Water Res., 2008, 42, 1343–1368 CrossRef CAS PubMed.
- J. Zhang, H. Hu, Z. Li and X. W. Lou, Double-shelled nanocages with cobalt hydroxide inner shell and layered double hydroxides outer shell as high-efficiency polysulfide mediator for lithium–sulfur batteries, Angew. Chem., Int. Ed., 2016, 55, 3982–3986 CrossRef CAS PubMed.
- J. Zhang, Z. Li, Y. Chen, S. Gao and X. W. Lou, Nickel–iron layered double hydroxide hollow polyhedrons as a superior sulfur host for lithium–sulfur batteries, Angew. Chem., Int. Ed., 2018, 57, 10944–10948 CrossRef CAS PubMed.
- C. Dai, L. Hu, M.-Q. Wang, Y. Chen, J. Han, J. Jiang, Y. Zhang, B. Shen, Y. Niu and S.-J. Bao, Uniform α-Ni (OH) 2 hollow spheres constructed from ultrathin nanosheets as efficient polysulfide mediator for long-term lithium-sulfur batteries, Energy Storage Mater., 2017, 8, 202–208 CrossRef.
- Y.-J. Li, J.-M. Fan, M.-S. Zheng and Q.-F. Dong, A novel synergistic composite with multi-functional effects for high-performance Li–S batteries, Energy Environ. Sci., 2016, 9, 1998–2004 RSC.
- E.-i. Negishi and L. Anastasia, Palladium-Catalyzed Alkynylation, Chem. Rev., 2003, 103, 1979–2018 CrossRef CAS PubMed.
- A. F. Littke and G. C. Fu, Palladium-Catalyzed Coupling Reactions of Aryl Chlorides, Angew. Chem., Int. Ed., 2002, 41, 4176–4211 CrossRef CAS PubMed.
- E. Antolini, Palladium in fuel cell catalysis, Energy Environ. Sci., 2009, 2, 915–931 RSC.
- S. Ma, L. Wang, Y. Wang, P. Zuo, M. He, H. Zhang, L. Ma, T. Wu and G. Yin, Palladium nanocrystals-imbedded mesoporous hollow carbon spheres with enhanced electrochemical kinetics for high performance lithium sulfur batteries, Carbon, 2019, 143, 878–889 CrossRef CAS.
- X.-F. Yang, A. Wang, B. Qiao, J. Li, J. Liu and T. Zhang, Single-atom catalysts: a new frontier in heterogeneous catalysis, Acc. Chem. Res., 2013, 46, 1740–1748 CrossRef CAS PubMed.
- C. Wang, H. Song, C. Yu, Z. Ullah, Z. Guan, R. Chu, Y. Zhang, L. Zhao, Q. Li and L. Liu, Iron single-atom catalyst anchored on nitrogen-rich MOF-derived carbon nanocage to accelerate polysulfide redox conversion for lithium sulfur batteries, J. Mater. Chem. A, 2020, 8, 3421–3430 RSC.
- B. Wang, F. Jin, Y. Xie, H. Luo, F. Wang, T. Ruan, D. Wang, Y. Zhou and S. Dou, Holey graphene modified LiFePO4 hollow microsphere as an efficient binary sulfur host for high-performance lithium-sulfur batteries, Energy Storage Mater., 2020, 26, 433–442 CrossRef.
- D. Su, M. Cortie, H. Fan and G. Wang, Prussian Blue Nanocubes with an Open Framework Structure Coated with PEDOT as High-Capacity Cathodes for Lithium–Sulfur Batteries, Adv. Mater., 2017, 29, 1700587 CrossRef PubMed.
- M. Ashuri, H. Dunya, Z. Yue, D. Alramahi, X. Mei, K. Kucuk, S. Aryal, C. U. Segre and B. K. Mandal, Enhancement in Electrochemical Performance of Lithium-Sulfur Cells through Sulfur Encapsulation in Hollow Carbon Nanospheres Coated with Ultra-Thin Aluminum Fluoride Layer, ChemistrySelect, 2019, 4, 12622–12629 CrossRef CAS.
- Y. Fu and A. Manthiram, Core-shell structured sulfur-polypyrrole composite cathodes for lithium-sulfur batteries, RSC Adv., 2012, 2, 5927–5929 RSC.
- Y. Xie, H. Zhao, H. Cheng, C. Hu, W. Fang, J. Fang, J. Xu and Z. Chen, Facile large-scale synthesis of core–shell structured sulfur@ polypyrrole composite and its application in lithium–sulfur batteries with high energy density, Appl. Energy, 2016, 175, 522–528 CrossRef CAS.
- Y. Liu, W. Yan, X. An, X. Du, Z. Wang, H. Fan, S. Liu, X. Hao and G. Guan, A polypyrrole hollow nanosphere with ultra-thin wrinkled shell: Synergistic trapping of sulfur in Lithium-Sulfur batteries with excellent elasticity and buffer capability, Electrochim. Acta, 2018, 271, 67–76 CrossRef CAS.
- T. Ben, C. Pei, D. Zhang, J. Xu, F. Deng, X. Jing and S. Qiu, Gas storage in porous aromatic frameworks (PAFs), Energy Environ. Sci., 2011, 4, 3991–3999 RSC.
- L. J. Murray, M. Dincă and J. R. Long, Hydrogen storage in metal–organic frameworks, Chem. Soc. Rev., 2009, 38, 1294–1314 RSC.
- B. Guo, T. Ben, Z. Bi, G. M. Veith, X.-G. Sun, S. Qiu and S. Dai, Highly dispersed sulfur in a porous aromatic framework as a cathode for lithium–sulfur batteries, Chem. Commun., 2013, 49, 4905–4907 RSC.
- J. Zheng, J. Tian, D. Wu, M. Gu, W. Xu, C. Wang, F. Gao, M. H. Engelhard, J.-G. Zhang, J. Liu and J. Xiao, Lewis Acid–Base Interactions between Polysulfides and Metal Organic Framework in Lithium Sulfur Batteries, Nano Lett., 2014, 14, 2345–2352 CrossRef CAS PubMed.
- M. Du, Q. Li, Y. Zhao, C.-S. Liu and H. Pang, A review of electrochemical energy storage behaviors based on pristine metal–organic frameworks and their composites, Coord. Chem. Rev., 2020, 416, 213341 CrossRef CAS.
- P. Geng, S. Cao, X. Guo, J. Ding, S. Zhang, M. Zheng and H. Pang, Polypyrrole coated hollow metal–organic framework composites for lithium–sulfur batteries, J. Mater. Chem. A, 2019, 7, 19465–19470 RSC.
- B. Q. Li, S. Y. Zhang, L. Kong, H. J. Peng and Q. Zhang, Porphyrin organic framework hollow spheres and their applications in lithium–sulfur batteries, Adv. Mater., 2018, 30, 1707483 CrossRef PubMed.
- B. Zhang, X. Qin, G. Li and X. Gao, Enhancement of long stability of sulfur cathode by encapsulating sulfur into micropores of carbon spheres, Energy Environ. Sci., 2010, 3, 1531–1537 RSC.
- Z. Li, L. Yuan, Z. Yi, Y. Sun, Y. Liu, Y. Jiang, Y. Shen, Y. Xin, Z. Zhang and Y. Huang, Insight into the electrode mechanism in lithium-sulfur batteries with ordered microporous carbon confined sulfur as the cathode, Adv. Energy Mater., 2014, 4, 1301473 CrossRef.
- Y. Dong and T. Ben, Impregnated Sulfur in Carbonized Nitrogen-containing Porous Organic Frameworks as Cathode with High Rate Performance and Long Cycle Life for Lithium-sulfur Batteries, Chem. Res. Chin. Univ., 2019, 35, 654–661 CrossRef CAS.
- G. He, S. Evers, X. Liang, M. Cuisinier, A. Garsuch and L. F. Nazar, Tailoring Porosity in Carbon Nanospheres for Lithium–Sulfur Battery Cathodes, ACS Nano, 2013, 7, 10920–10930 CrossRef CAS PubMed.
- T. Chen, L. Ma, B. Cheng, R. Chen, Y. Hu, G. Zhu, Y. Wang, J. Liang, Z. Tie, J. Liu and Z. Jin, Metallic and polar Co9S8 inlaid carbon hollow nanopolyhedra as efficient polysulfide mediator for lithium–sulfur batteries, Nano Energy, 2017, 38, 239–248 CrossRef CAS.
- Y. Ye, F. Wu, S. Xu, W. Qu, L. Li and R. Chen, Designing realizable and scalable techniques for practical lithium sulfur batteries: a perspective, J. Phys. Chem. Lett., 2018, 9, 1398–1414 CrossRef CAS PubMed.
- A. Eftekhari and D.-W. Kim, Cathode materials for lithium–sulfur batteries: a practical perspective, J. Mater. Chem. A, 2017, 5, 17734–17776 RSC.
- J. Lochala, D. Liu, B. Wu, C. Robinson and J. Xiao, Research progress toward the practical applications of lithium–sulfur batteries, ACS Appl. Mater. Interfaces, 2017, 9, 24407–24421 CrossRef CAS PubMed.
- A. Bhargav, J. He, A. Gupta and A. Manthiram, Lithium-sulfur batteries: attaining the critical metrics, Joule, 2020, 4, 285–291 CrossRef.
- H. Yuan, J. Liu, Y. Lu, C. Zhao, X. Cheng, X. Nan, Q. Liu, J. Huang and Q. Zhang, Toward Practical All-solid-state Batteries with Sulfide Electrolyte: A Review, Chem. Res. Chin. Univ., 2020, 36, 377–385 CrossRef CAS.
- M. Rana, B. Luo, M. Kaiser, I. Gentle and R. Knibbe, The role of functional materials to produce high areal capacity lithium sulfur battery, J. Energy Chem., 2020, 46, 195–209 CrossRef.
- M. Rana, S. Ahad, M. Li, B. Luo, L. Wang, I. Gentle and R. Knibbe, Review on areal capacities and long-term cycling performances of lithium sulfur battery at high sulfur loading, Energy Stor. Mater., 2019, 18, 289–310 CrossRef.
- H. Wang and Y. Tang, Artificial Solid Electrolyte Interphase Acting as “Armor” to Protect the Anode Materials for High-performance Lithium-ion Battery, Chem. Res. Chin. Univ., 2020, 36, 402–409 CrossRef CAS.
|
This journal is © the Partner Organisations 2020 |
Click here to see how this site uses Cookies. View our privacy policy here.