DOI:
10.1039/D0QI00529K
(Review Article)
Inorg. Chem. Front., 2020,
7, 3217-3246
Supramolecular chemistry of substituted cucurbit[n]urils
Received
7th May 2020
, Accepted 25th July 2020
First published on 27th July 2020
Abstract
Since the first example of substituted cucurbit[n]urils (Q[n]), decamethylcucurbit[5]uril (Me10Q[5]), was reported in 1992, numerous substituted Q[n]s have been synthesized and studied. In this review article, we focus on the recognition properties of the substituted Q[n]s toward a variety of organic species as well as the coordination chemistry of substituted Q[n]s with different metal ions, including alkali/alkaline-earth metals, transition metals, and lanthanides. Applications of substituted Q[n]s, and the self-assembly processes affording mechanically interlocked molecules (MIMs) are also described. The main purpose of this review is to highlight important advances in the blossoming field of substituted Q[n]s, which will likely be of interest to researchers in supramolecular chemistry.
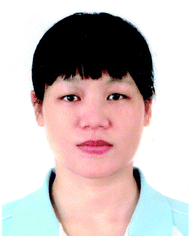 Rui-Lian Lin | Rui-Lian Lin graduated from Gannan Normal University in 1995, and received her MS degree from Anhui University of Technology in 2012. She became an associate professor at Anhui University of Technology in 2016. Her research focuses on supramolecular chemistry and coordination chemistry based on cucurbit[n]urils. |
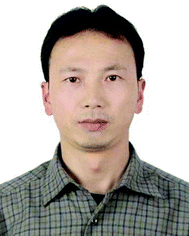 Jing-Xin Liu | Jing-Xin Liu graduated from Gannan Normal University in 1995, received his MS degree from Guizhou University in 2004, and received his PhD degrees from Xiamen University in 2007. He became a professor of chemistry at Anhui University of Technology in 2011. His current research focuses on properties and applications of cucurbit[n]urils. |
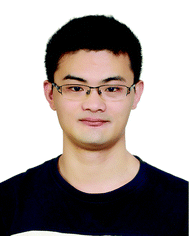 Kai Chen | Kai Chen studied Chemistry at the Guizhou University, where he completed his Master degree with honors in 2011 under the supervision of Prof. Zhu Tao. And then he received his Ph.D. from Nanjing University in 2015 under the supervision of Prof. Wei-Yin Sun. In May 2015 he joined Nanjing University of Information Science & Technology as a lecture, where he is working on the development of new chemical environmental materials based on cucurbit[n]urils. |
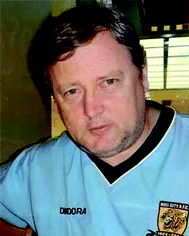 Carl Redshaw | Carl Redshaw studied chemistry (BSc, PhD) at Newcastle University, was a Welch Fellow at the University of Texas (Austin), a PDRA with Sir G. Wilkinson at Imperial College, a PDRA at Durham and later a Levehulme Fellow at Imperial College. He was Lecturer, Senior lecturer and Reader at UEA before becoming Chair of Inorganic Materials at the University of Hull. He holds a Visiting Professorship at Northwest University, Xi'an (100 Talents Plan), and has >350 research publications, mostly in coordination chemistry and catalysis. His group also has interests in calixarenes, cucurbiturils and anti-cancer agents. |
1. Introduction
Since the first macrocyclic polyether, dibenzo[18]crown-6, was reported by Pedersen back in 1967,1,2 host–guest/supramolecular chemistry has experienced rapid development. Host–guest/supramolecular chemistry usually involves the molecular recognition and inclusion of substrates by macrocyclic compounds, such as cyclodextrins (α, β and γ), calix[n]arenes, pillar[n]arenes, and various cyclophanes.3–9 Cucurbit[n]uril (n = 5–8, 10, 13–15, commonly abbreviated as Q[n]s or CB[n]s, Fig. 1),5,10–20 a family of artificial macrocyclic hosts, have in recent years played a central role in supramolecular chemistry, which reflects their excellent properties in molecular recognition and molecular assembly. The journey for Q[n]s started in 1905, when a white solid (Q[6]), was prepared by Behrend and co-workers, although its composition and structure was not known at that time.21 Seventy-five years later, Freeman et al. restudied the synthesis of the white solid and ascertained the structure of Q[6] via the use of X-ray diffraction.22 Since then, Q[6] has been used for both metal ion coordination and small molecule inclusion. After the discovery of a series of Q[n] homologues in 2000 and 2001 by Kim and Day,23,24 research activity in Q[n] chemistry progressed greatly, and has impacted on a wide range of fields, including sensing, catalysis, drug delivery, nano-materials and stimuli-responsive systems.
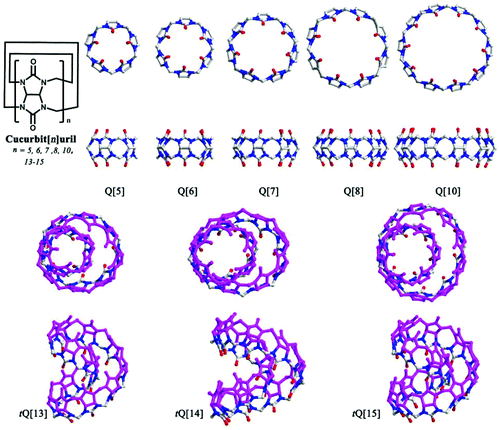 |
| Fig. 1 X-ray crystal structures of cucurbit[n = 5–8, 10, 13–15]urils. | |
The common Q[n] homologues comprise n glycoluril units linked by 2n methylene (Fig. 1). They feature a hydrophobic cavity with different sizes, two polar carbonyl portals with negative electrostatic potential, and a circular outer surface with positive electrostatic potential. Based on these structural features, Q[n]s can accommodate hydrophobic molecules or groups of suitable size in their cavities, coordinate to various metal ions at their portals, and connect with electronegative species through their outer surfaces. As a result, many research groups have reported a large number of Q[n] complexes with different metal ions, anions and charged organic molecules.5,10–20 Although Q[n] homologues are outstanding macrocyclic hosts for fundamental and applied molecular recognition, three main shortcomings still hinder their research and applications. Firstly, Q[n] homologues have poor solubility in pure water and in most common organic solvents, especially the Q[6], Q[8], and Q[10] systems. Most host–guest chemistry research on Q[n] homologues has to be performed in acid solution or in the presence of alkali metal ion, which strongly affects the binding affinity between the host and the guest, as illustrated by Buschmann,25 Kaifer,26 Nau,27 and Kim28et al. Secondly, although the sizes and shapes of the guests are diverse, Q[n] homologues (except for Q[13–15]) possess high symmetrical cavities. Consequently, Q[n] homologues fail to selectively recognize some guest molecules with special shape.75–77,142,152 Thirdly, in comparison with other macrocyclic hosts, for example the calix[n]arenes, Q[n] homologues are difficult to functionalize. To overcome these shortcomings, significant efforts have been invested on the design and synthesis of substituted Q[n]s.
Over the past two decades or so, various substituted Q[n]s have been prepared.29–60 As will be discussed later, the host–guest/supramolecular properties exhibited by substituted Q[n]s reveal advantages versus Q[n]s. (1) Substitutions greatly increase solubility of substituted Q[n]s by decreasing solid-state packing. (2) Unsymmetrical substitutions deform the cavity symmetry and open up better selectivity to guest sequestration.39,40,50,56 It should be noted that Q[n]s are amongst some of the most selective hosts because of their different cavity sizes. (3) Substitutions greatly increase the functionalizability of Q[n] homologues. (4) By creating substituted Q[n]s and increasing the diversification of the structures, more complex chemical systems such as complicated molecular machines and smart sensors can be built.
Although great progress has been achieved in Q[n]s chemistry and numerous reviews have appeared,10–20 few focus on the field of substituted Q[n]s. This review article will highlight advances in field of substituted Q[n]s. We will begin by discussing the synthesis of classical substituted Q[n]s, such as fully and partially methyl-substituted Q[n]s, cyclohexano-substituted Q[n]s, cyclopentano-substituted Q[n]s, hydroxy-substituted Q[n]s, and substituted Q[n]s with mixed substitutions. We will then focus on the host–guest complexation properties of the substituted Q[n]s, their inclusion and coordination compounds, plus mechanically interlocked molecules (MIMs) and molecular devices, including many examples from our own group's efforts.
2. Synthesis of substituted Q[n]s
Back in 1992, Stoddart and co-workers reported the first example of a substituted Q[n], namely decamethylcucurbit[5]uril (Me10Q[5]).29 The reaction of 2,3-butanedione with urea under acidic conditions leads to dimethylglycoluril. Condensation of dimethylglycoluril with formaldehyde in acidic medium generates a white precipitate of Me10Q[5] (Table 1). Since then, a variety of Q[n] derivatives, including fully and partially alkyl-substituted Q[n], hemicucurbit[n]uril,30,31 biotin[6]uril,32 bambus[6]uril,33 and acyclic Q[n] congeners,34,35 have been synthesized by Day, Isaacs, Kim, Sindelar, Tao and other groups. Note that the present review focuses on substituted Q[n]s, those Q[n] derivatives where the basic skeleton is preserved, as shown in Table 1.
Table 1 Synthesis of substituted Q[n]s
No. |
Synthetic strategy |
Yields |
Ref. |
1 |
|
Me10Q[5]: 16% |
29
|
2 |
|
CyH5Q[5]: 16% |
36
|
CyH6Q[6]: 2% |
3 |
|
CyP5Q[5]: 3.2% |
37
|
CyP6Q[6]: 5.2% |
CyP7Q[7]: 1.4% |
4 |
|
CyB5Q[5]: 10% |
38
|
CyB6Q[6]: 13% |
CyB7Q[7]: 4% |
CyB8Q[8]: 1% |
5 |
|
TMeQ[6]: 30% |
39
|
6 |
|
OMeQ[6]: 3.4% |
40
|
7 |
|
o-TMeQ[6]: 3.4% |
41
|
8 |
|
m-Me4Q[8]: 4% |
42
|
9 |
|
HMe5Q[5]: 6.4% |
43
|
HMe6Q[6]: 5.8% |
10 |
|
CyH2Q[6]: 30% |
46
|
11 |
|
CyH3Q[6]: 39.6% |
47
|
12 |
|
o-Me4Q[8]: 11% |
50
|
o-CyH2Q[8]: 4% |
13 |
|
(HO)10Q[5]: 42% |
51
|
(HO)12Q[6]: 45% |
(HO)14Q[7]: 5% |
(HO)16Q[8]: 4% |
14 |
|
Q[7]-NHCO-CH2CH3: 96% |
52
|
Q[7]-NHCO-CHCH2: 87% |
Q[7]-NHCO-CH2CN: 94% |
Q[7]-NHCO-CH2CO2H: 84% |
Q[7]-NHCO-C6H5: 63% |
Q[7]-NHCO-(CH2)2Br: 73% |
Q[7]-O-(CH2)3OH: 76% |
Q[7]-O-(CH2)3Br: 63% |
15 |
|
(HO)1Q[6]: 12% |
54
|
16 |
|
Me2Q[7]: 31% |
55
|
CyHQ[7]: 18% |
MePhQ[7]: 3% |
17 |
|
(HO)1Q[5]: 95% |
57
|
(HO)1Q[6,7]: 95–100% |
(HO)1Q[8]: 90% |
18 |
|
PheQ[6]: 0.2% |
60
|
In 2001, Kim et al. synthesized fully substituted cyclohexanoQ[n]s (abbreviated as CyHnQ[n]s, n = 5, 6), which are soluble not only in water but also in some organic solvents.36 Day and coworkers synthesized two other kinds of fully substituted Q[n], the cyclopentanoQ[n]37 (abbreviated as CyPnQ[n], n = 5, 6, 7) in 2012 and the cyclobutanoQ[n]38 (abbreviated as CyBnQ[n], n = 5–8) in 2017. The syntheses of these fully substituted Q[n]s have similar procedures: the synthesis of the substituted glycoluril (precursor), and condensation of substituted glycoluril with formaldehyde in acidic medium.
The Tao group prepared the first example of a partially methyl-substituted Q[n], the symmetrical tetramethylcucurbit[6]uril (TMeQ[6]) in 2004.39 The synthetic procedure involved the diether of dimethylglycoluril and the glycoluril dimer. Using similar strategies and appropriate building blocks, the Tao group later prepared a series of partially methyl-substituted Q[n]s, such as the symmetrical octamethyl-substituted cucurbit[6]uril40 (OMeQ[6]), ortho-tetramethyl-substituted cucurbit[6]uril41 (o-TMeQ[6]), meta-tetramethyl-substituted cucurbit[8]uril42 (m-Me4Q[8]). Moreover, the same group has recently synthesized a series of hemimethyl-substituted cucurbit[n]urils (HMenQ[n]s, n = 5, 6, 7) using the precursor 3α-methylglycoluril.43–45 A series of partially cyclohexano-substituted cucurbit[6]uril such as the para-dicyclohexanocucurbit[6]uril46 (CyH2Q[6]) and meta-tricyclohexanocucurbit[6]uril47 (CyH3Q[6]) and some substituted Q[n]s with mixed substituents48 were isolated. Concomitantly, the toxicity of HMe7Q[7] was studied by Wang and co-workers, revealing that HMe7Q[7] exhibited a good biocompatibility profile.49 Other groups also reported the synthesis of methyl- and cyclohexano-substituted Q[n]. The Isaacs group in 2015 presented a building block strategy for the synthesis of ortho-tetramethyl-substituted cucurbit[8]uril (o-Me4Q[8]) and ortho-dicyclohexano-substituted cucurbit[8]uril (o-CyH2Q[8]) by condensation of glycoluril hexamer with bis(cyclic ethers) under well-defined conditions.50
One of the shortcomings of the Q[n] homologues is that they are difficult to functionalize. To overcome this long-standing problem, Kim et al. created an effective procedure in 2003 via the oxidation the Q[n] homologues with K2S2O8 in water to produce their corresponding hydroxy-substituted (HO)mQ[n]s (n = 5–8, m = 10, 12, 14 and 16), which can then be further modified to afford tailored Q[n] derivatives.51 The Kim group recently demonstrated a superacid-mediated conversion of the hydroxyl group on Q[n]s (n = 6 and 7) to yield other important functional groups in high yields.52 Additionally, they found that the resulting substituted Q[n] can be readily conjugated to an enzyme to afford a Q[n]-conjugated enzyme which is useful in protein blotting assays.53
Other groups also have made significant progress towards functionalizing Q[n] homologues. In 2012, Scherman and co-workers prepared monohydroxylated Q[6] through the controlled oxidation of Q[6] in the presence of a tailor-made bisimidazolium guest.54 In the same year, the Isaacs group presented a building-block approach for mono-functionalization of some substituted Q[7]s.55 Interestingly, mono-functionalized Q[7] exhibits self-association into tetrameric aggregates in aqueous solution, as shown in Fig. 2. In 2015, the Isaacs group synthesized hydrophobic monofunctionalized Q[7], which can undergo self-inclusion complexation and form vesicle-type assemblies.56 Ouari and Bardelang et al. reported a photochemical method for producing hydroxy-substituted (HO)mQ[n]s using hydrogen peroxide and UV light.57,58 Very recently, Kaifer, Dong and coworkers obtained monohydroxylated cucurbit[7]uril ((HO)1Q[7]) through the direct oxidation of Q[7].59
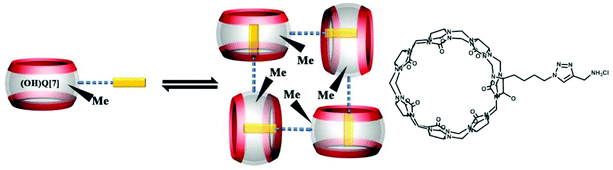 |
| Fig. 2 Mono-functionalized Q[7] self-associated into tetrameric aggregates. | |
By simply mixing glycoluril, paraformaldehyde, and another aldehyde, Sindelar and coworkers prepared a novel type of monosubstituted Q[6], mono(2-phenylethyl)cucurbit[6]uril (PheQ[6]).60 Different from the above mentioned substituted Q[n]s, the substituent is attached to one methylene bridge of the PheQ[6] (Fig. 3a and b). Impressively, the monosubstituted Q[6] macrocycles self-assembled into tetrameric aggregates (Fig. 3c) in the solid state. Compared to the PheQ[6], it is impossible for unsubstituted Q[n]s to self-associate into polymers without the assistance of other compounds.
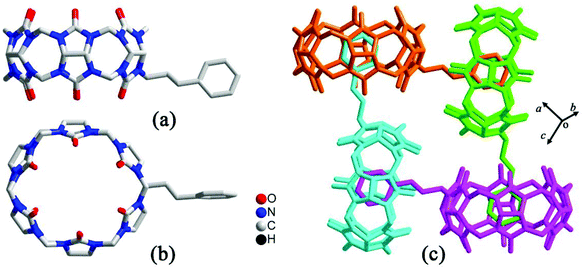 |
| Fig. 3 The crystal structures of mono(2-phenylethyl)cucurbit[6]uril (PheQ[6], top (a) and side (b) views) and the tetramer (c) based on PheQ[6]. | |
3. Molecular recognition of substituted Q[n]s
3.1. Binding behaviour of alkyldiammonium ions toward substituted Q[n]s
In their pioneering work, Collet, Cram, Rebek, et al. observed that molecular behaviour including conformations and reactivity in small spaces can be quite different from that in dilute solution.61–64 With this in mind, the molecular behaviour of different alkyl chains in the hydrophobic cavities of the Q[n] derivatives can also be regarded as another study of a kind of small space. Therefore, the binding interactions between a series of alkyldiammonium ions and a variety of substituted Q[6]s, including TMeQ[6],65,66 CyH2Q[6],67 CyH6Q[6],67 and CyP6Q[6]s68 has been investigated both in aqueous solution and in the solid state. NMR spectra and single-crystal X-ray diffraction analyses revealed that the binding behaviour varies depending upon the alkyl chain length. Here, the TMeQ[6] is taken as a representative example for discussion (Fig. 4). 1,2-Ethanediammonium resides outside the portals of the TMeQ[6] and binds exo to the host portals forming an exclusion complex. Other longer alkyldiammonium guests can be accommodated in the cavity of the TMeQ[6] forming 1
:
1 inclusion complexes. Interestingly, if the alkyl chain length is longer than the height of the cavity of the TMeQ[6], then the alkyl chains usually take a contorted conformation. Isothermal titration calorimetry (ITC) experiments indicated that the binding of the alkyldiammonium guests with the substituted Q[6] is mainly enthalpy driven, which benefits from hydrophobic effects and host–guest interactions. It is accepted that the main driving force for the assembly of the inclusion complex based on Q[n] is the hydrophobic effect.69 However, the binding of the charged alkyldiammonium guests with the substituted Q[6] should also take into account the host–guest interactions, including ion–dipole interactions and van der Waals interactions.
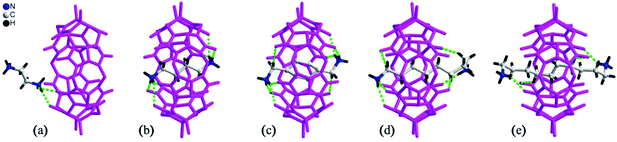 |
| Fig. 4 Extended and contorted conformations of alkanediammonium ions in the cavity of TMeQ[6]: (a) 1,2-ethanediammonium∩TMeQ[6]; (b) 1,4-butanediammonium@TMeQ[6]; (c) 1,6-hexanediammonium@TMeQ[6]; (d) 1,8-octanediammonium@TMeQ[6]; (e) 1,10-decanediammonium@TMeQ[6]. | |
HMe6Q[6] is soluble in water as well as in DMSO. The binding behaviour of HMe6Q[6] towards alkyldiammonium ions in aqueous solution is completely different from that observed in the organic solvent DMSO.70 In aqueous solution, the alkyl chains of the alkyldiammonium ions were encapsulated into the HMe6Q[6] cavity, forming an inclusion complex. In contrast, in DMSO, the HMe6Q[6] engulfs only the NH3+ groups of the alkyldiammonium ions, forming a head-inclusion complex, which can be switched to supramolecular polymers upon heating (Fig. 5). Recently, we also compared the binding behaviour of HMe7Q[7] with alkyldiammonium ions (protonated guests) and their corresponding uncharged alkyldiamines in aqueous solution.71 The results suggested that the driving force for the host–guest binding is related to the features of the guests.
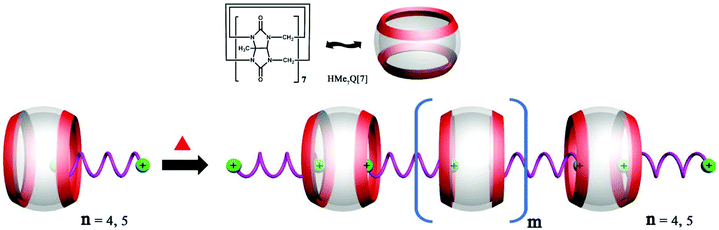 |
| Fig. 5 Supramolecular polymer aggregated from head-inclusion complex. | |
It is also noteworthy that ammonium ions can act as templates in Q[n] formation, influencing both the kinetics and the thermodynamics of the process. In particular, Anzenbacher Jr. and Isaacs and coworkers showed that the p-xylylenediammonium ion was capable of acting as a template in the reaction between glycoluril and formaldehyde (<2 equiv.) affording a methylene bridged glycoluril hexamer and bis-nor-seco-Q[10].72 Importantly, this methodology could be readily scaled up to deliver, without the need for chromatography, multigram quantities of the hexamer. Further reaction (macrocyclization) with phthalaldehydes allowed access to monofunctionalized Q[6] derivatives, for example derivatives containing NO2 or CO2H motifs, thereby opening up the possibility for further functionalization. The size of the template proved crucial in determining the product formed during the macrocyclization. For example, both the p-xylylenediammonium and hexanediammonium ions were found to slow down the formation of Q[6], instead preferring to favour formation of an intermediate containing an NCOCN-bridge which contains two stereogenic centers. Conducting the reaction between the hexamer and o-phthaldehyde in CF3CO2H allowed the intermediate to be characterized by mass spectrometry and 1H NMR spectroscopy.
Cao and Isaacs designed a monofunctionalized Q[6] derivative (symbol 1 in Fig. 6) that possessed a covalently bonded (added by Click chemistry via a propargyloxy substituent) isobutylammonium group.73 The structure comprised a mixture of cyclic dimers as evidenced by DOSY and ESI-MS experiments, and in water, they self-assemble into a cyclic daisy chain. This structure proved to be responsive to specific competing hosts, for example whilst Q[6] and Q[7] had little effect, the addition of Q[8] (1.5 equiv.) resulted in the formation of a new complex involving the monomeric isobutylammonium and Q[8]. 1H NMR studies (upfield shifts for the triazole and isobutylammonium protons) indicated that a back-folded conformation was present in the Q[8] cavity. Further addition of Q[6] reverses the process and gives back the daisy chain. Obviously, substituents in 1 also act as competitive guests.
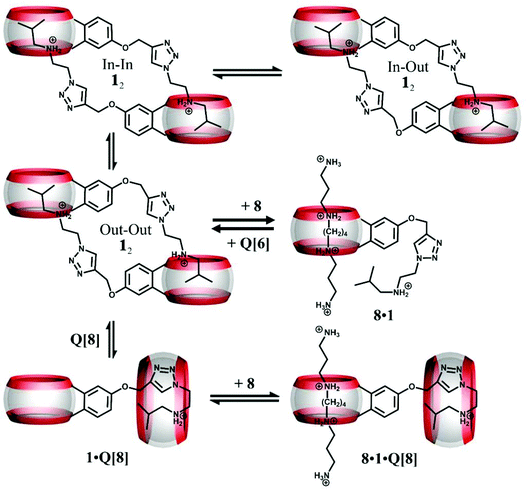 |
| Fig. 6 Depiction of the three isomers of 12 and their response to addition of spermine (symbol 8), Q[8], and Q[6]. | |
3.2 Binding behaviour of N-alkylated viologens with substituted Q[n]s
The series of N-alkylated viologens (methyl viologen MV2+, ethyl viologen EV2+, propyl viologen PV2+, butyl viologen BV2+, pentyl viologen FV2+ and heptyl viologen HV2+) are special redox-active organic guests with an alkyl chain and an aromatic group. A host–guest study involving N-alkylated viologens can help to understand the interdependence of redox processes and molecular selectivity. We and others have performed a series of experiments to study the binding interactions between a series of N-alkylated viologen guests and substituted Q[6]s, including (HO)1Q[7],55 CyH6Q[6],74 TMeQ[6],75 CyH2Q[6]76 and CyH3Q[6].76 As shown in Fig. 7, MV2+ and EV2+ form 1
:
1 complexes in which the bipyridinium aromatic nucleus is partially included inside the CyH6Q[6] cavity. PV2+, BV2+, FV2+, and HV2+ form 2
:
1 complexes with CyH6Q[6], in which each of the viologen aliphatic chains is included by a host molecule. The results suggested that the inclusion of the alkyl chains is favored compared to inclusion of the aromatic nucleus.74
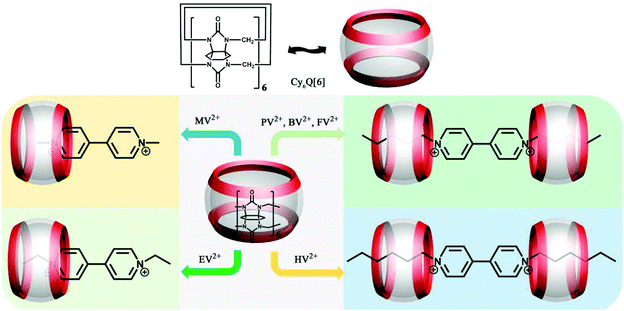 |
| Fig. 7 Binding behaviour of N-alkylated viologens with CyH6Q[6]. | |
3.3. Aniline-containing guests recognized by substituted Q[n]
In these Q[n] systems, it is usual to encounter shielding and deshielding effects of the host. For example, the hydrophobic cavity of a Q[n] is a proton-shielding region. When the guest is encapsulated into the Q[n] cavity, the proton signals of the guest undergo an upfield shift. In contrast, the outside of the portals of a Q[n] is a proton-deshielding region which would induce a downfield shift of the proton signals of guests located outside of the Q[n] portal. However, we recently encountered shielding and deshielding effects induced by a guest.75–77 When the aromatic motif of a guest, such as aniline-containing guests, is located inside of the TMeQ[6] cavity, the ellipsoidal cavity of the TMeQ[6] is complementary in both size and shape to the aromatic group of the guest. As a result, remarkable chemical shifts for the TMeQ[6] host protons were observed (Fig. 8). Similar situations were observed for other substituted Q[n]s with ellipsoidal cavity.76
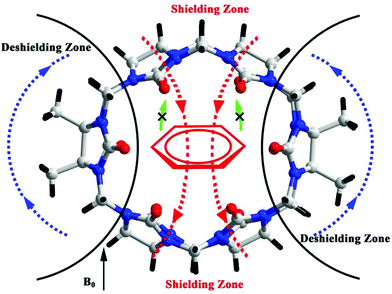 |
| Fig. 8 Aromatic ring-induced shielding and deshielding zones. | |
3.4. Recognition of enantiomeric amino acids by substituted Q[n]s
Amino acid recognition and determination is of great significance in fields as diverse as food testing, nutritional analysis, and medical diagnostics.78–80 In 2017, we reported a family of supramolecular complexes of TMeQ[6] which interacted with enantiomeric amino acids (D,L-Gln; D,L-Glu; D,L-Met; D,L-Ser; D-Val).81 The seven supramolecular complexes are classified into two structural types, inclusion and exclusion structures (Fig. 9), the adoption of which mainly depends on the length of the alkyl chain of the enantiomeric amino acid. Interestingly, the reaction of TMeQ[6] with L-Val doesn't produces a crystalline material, suggesting that TMeQ[6] can be used to separate D-Val from its enantiomer.
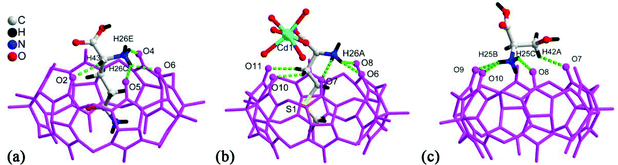 |
| Fig. 9 X-ray crystal structure of TMeQ[6] complexes with different amino acids showing inclusion and exclusion structural types: (a) D-Gln@TMeQ[6]; (b) D-Met@TMeQ[6]; (c) D-Ser∩TMeQ[6]. | |
3.5. Recognition of other organic guests by substituted Q[n]s
Haloalkanes are an important class of organic compound which possess various applications. In 2015, we reported the first example of haloalkane encapsulation inside the cavities of Q[n]s.82 X-ray crystallography and NMR spectroscopy were used to establish that the haloalkane 1-(3-chlorophenyl)-4-(3-chloropropyl)-piperazinium (PZ+) dihydrochloride forms a highly stable inclusion complex PZ+@TMeQ[6], with half of the chloropropyl group of the PZ+ residing within the TMeQ[6] cavity (Fig. 10).
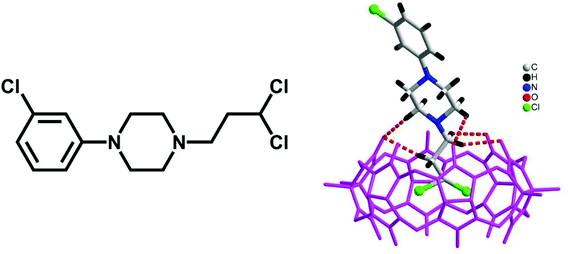 |
| Fig. 10 ORTEP diagram of the inclusion complex PZ+@TMeQ[6]. | |
The binding behaviour of HMe7Q[7] with 1,10-phenanthroline and its derivative 4,7-dimethyl-1,10-phenanthroline was investigated by NMR spectroscopy and ITC techniques, which suggested the formation of a half-inclusion complex and a double binding complex (Fig. 11).83 The latter complex exhibits two binding modes simultaneously: endo binding and exo binding, which is not observed in conventional host–guest complexes.
 |
| Fig. 11 Schematic representation of two binding modes. | |
As more substituted Q[n]s are synthesized, more organic guests are reported to be recognized and accommodated by these new substituted Q[n]s. For example, hymexazol,84 thiabendazole,85 6-benzyladenine86 were reported to form inclusion complexes with HMe6Q[6], and TMeQ[6]. Kaifer, Dong and coworkers recently studied the binding properties of (HO)1Q[7] with a series of selected guests.59 The results indicated that the binding affinities of the guests with the (HO)1Q[7] were slightly smaller than those of the parent Q[7].
4. Coordination chemistry of substituted Q[n]s with metal ions
The two identical carbonyl-laced portals that Q[n]s possess makes them fascinating coordination ligands. The coordination affinities of substituted Q[n]s are different from those of their parent Q[n]s because of the effects of the substituents. This is in part due to the new affinities displayed by the introduced substituents, which will become evident from the examples described below, but also because these substituents impart new properties such as increased solubility which can greatly enhance chemical reactivity. For fully and partially alkyl-substituted Q[n]s, the alkyl groups on the waist belong to electron-donating groups, which can enhance the polar properties of the carbonyl oxygen atoms at the portal, and enhance the electrostatic interactions between substituted Q[n]s and metal ions. In this section, we describe the outstanding coordination properties of the substituted Q[n]s, and their coordination compounds with a wide range of metal ions, including alkali metals, alkaline-earth metals, transition metals and lanthanides. These coordination compounds (Table 2) have led to many interesting structural features and applications, some of which are discussed herein.
Table 2 A list of the substituted Q[n]-based coordination compounds. For the sake of clarity, they are named according to the type of the metal ions contained in the compounds: A for alkali metal, AE for alkali-earth metal, TM for transition metal, and Ln for lanthanide metals
Compounds |
Formula |
Structural type |
Ref. |
A-1
|
{[K(H2O)](NO3@(HO)10Q[5])[K(μ-NO3)]}Cl(H3O)22+·7H2O |
2-D |
88
|
A-2
|
{[Rb3(μ2-H2O)(μ3-H2O)(H2O)5SO4]+(H2O@(HO)10Q[5])}Cl(H3O)+·2H2O |
1-D |
88
|
A-3
|
[Cs2(H2O@(HO)10Q[5])]SO42−(H3O)44+ |
2-D |
88
|
A-4
|
{Sr2(Cl@Me2Q[5]}3Cl·19H2O |
2-D |
89
|
A-5
|
{K2(H2O@1,2,4-CyH3Q[5])}2Cl·15.5H2O. |
3-D |
89
|
A-6
|
{[K2(1,2,4-Me6Q[5])]3(H2O)4}6+·6Cl−·47(H2O) |
3-D |
90
|
A-7
|
{[K2(CyH5Q[5])]34(H2O)}6+·6I−·23(H2O) |
3-D |
91
|
A-8
|
{K6(H2O)4[H2O@Me2Q[5]]3}·6I·39H2O |
2-D |
91
|
A-9
|
{K6(H2O)4[H2O@1,3-CyH2Q[5]]2[H2O@1,2,4-CyH3Q[5]]}·6I·46H2O |
2-D |
91
|
A-10
|
{K6(H2O)4[H2O@1,2,4-CyH3Q[5]]3}·6Cl·55H2O |
2-D |
91
|
A-11
|
{K6(H2O)4[H2O@1,2,3-CyH3Q[5]]3}·6I·26H2O |
2-D |
91
|
A-12
|
{K6(H2O)4[H2O@CyH5Q[5]]3}·6Cl·53H2O |
2-D |
91
|
A-13
|
{[Na2(H2O)6(H2O@TMeQ[6])]·2(C6H5NO3)·Cl2(H2O)10} |
1-D |
92
|
A-14
|
{[Na2(H2O)6(H2O@TMeQ[6])]·2(C6H5NO3)·Br2(H2O)10} |
1-D |
92
|
A-15
|
{[Na2(H2O)6(C6H5NO3)2(2H2O@TMeQ[6])]·6(C6H5NO3)}F2(H2O)4} |
1-D |
92
|
A-16
|
[K2(H2O)3((H2O)0.5@Me10Q[5])]·Cl2 |
1-D |
93
|
A-17
|
[K2(H2O)2(H2O@Me10Q[5])]·(ClO4)2·3H2O |
0-D |
93
|
A-18
|
[K2(H2O)2(SCN)(Me10Q[5])]·(SCN)·H2O |
0-D |
93
|
A-19
|
[Sr2Me10Q[5](H2O)4Cl]3+·3Cl−·2(HCl)·19H2O |
0-D |
94
|
A-20
|
[K(H2O)Me10Q[5]Cl]·[Zn(H2O)2Cl2]·[ZnCl4]2−·2(H3O)+·8H2O |
0-D |
94
|
A-21
|
[Na(H2O)(H2O)2@Me10Q[5]](C6H6O2)2Cl·8H2O |
0-D |
95
|
A-22
|
[K2(H2O)2(H2O)@Me10Q[5]](C6H6O2)2Cl2·7H2O |
1-D |
95
|
A-23
|
[Rb2(H2O)2(H2O)@Me10Q[5]](C6H6O2)2Cl2·7H2O |
1-D |
95
|
A-24
|
[Cs(H2O)2(H2O@Me10Q[5]](C6H6O2)2Cl·6H2O |
0-D |
95
|
A-25
|
{Na2(H2O)2Cl@CyP5Q[5]}·[ZnCl4]·H3O·9H2O |
0-D |
96
|
A-26
|
{K2(H2O)2Cl@CyP5Q[5]}·[ZnCl4]·H3O·10H2O |
0-D |
96
|
A-27
|
{Rb2CyP5Q[5]}·[ZnCl4]·3H2O |
0-D |
96
|
A-28
|
{Cs2CyP5Q[5]}·[ZnCl4]·3H2O |
1-D |
96
|
A-29
|
OMeQ[6]·[Cd2Cl7]·3H3O·10H2O |
0-D |
97
|
A-30
|
{K4(H2O)10OMeQ[6]}·[Cd2Cl8]·2H2O |
1-D |
97
|
A-31
|
OMeQ[6]·[CdCl4]·2H3O·11H2O |
0-D |
97
|
A-32
|
{CsOMeQ[6]}·[Cd2Cl7]·2H3O·5H2O |
0-D |
97
|
A-33
|
{Na2(H2O)6(p-Hyb)2OMeQ[6]}·2(p-Hyb)·2Cl·H2O |
0-D |
97
|
A-34
|
{Cs(H2O)OMeQ[6]}·4(p-Hyb)·3H3O·7H2O |
0-D |
97
|
A-35
|
{Na2(H2O)10(HO)1OMeQ[6]}·2[CdCl4]·2H3O·3H2O |
0-D |
98
|
A-36
|
{K2(H2O)10(HO)1OMeQ[6]}·2[CdCl4]·2H3O·2H2O |
1-D |
98
|
A-37
|
{Rb(H2O)8(HO)1OMeQ[6]}·2[CdCl4]·3H3O·H2O |
1-D |
98
|
A-38
|
{K2(H2O)6(1,3,5-Me6Q[6])}2+·Cr2O72−·15H2O |
0-D |
99
|
A-39
|
{Rb2(H2O)6(1,3,5-Me6Q[6])}2+·2Cl−·14H2O |
0-D |
99
|
A-40
|
{Cs2(H2O)6(1,3,5-Me6Q[6])}2+·2Cl−·11H2O |
1-D |
99
|
A-41
|
[Li2(H2O)4{H2O@Me10Q[5]}·PdCl4·4H2O |
0-D |
124
|
A-42
|
[Na2(H2O)5{H2O@Me10Q[5]}·PdCl4·5H2O |
0-D |
124
|
A-43
|
[K2(H2O)3{(H2O)0.5@Me10Q[5]}]·PdCl4 |
2-D |
124
|
A-44
|
[Rb2(H2O)3{(H2O)0.5@Me10Q[5]}]·PdCl4 |
2-D |
124
|
A-45
|
[Cs2(H2O)2{H2O@Me10Q[5]}]·PdCl4 |
0-D |
124
|
A-46
|
{[Ag(H2O)]2(H2O@Me10Q[5])}·2NO3·2H2O |
0-D |
125
|
AE-1
|
2{Sr2(H2O)8(HO)1OMeQ[6]}·3[CdCl4]·2Cl·31H2O |
1-D |
97
|
AE-2
|
{Ba2(H2O)8Cl[CdCl4](HO)1OMeQ[6]}·2Cl·H3O·22H2O |
1-D |
97
|
AE-3
|
2[Ca2(H2O)8(NO3@o-TMeQ[6])]·2(CdCl4)·4Cl·2H3O·21H2O |
1-D |
41
|
AE-4
|
2[Sr2(H2O)9(NO3@o-TMeQ[6])]·2(CdCl4)·2Cl·22H2O |
1-D |
41
|
AE-5
|
{(CaCl)(TMeQ[6])}·Cl·17.5H2O |
0-D |
111
|
AE-6
|
{(Sr2Cl2)[TMeQ[6]@H2O]}·Cl2·10H2O |
1-D |
111
|
AE-7
|
{Ca2(H2O)4[(NO3)@CyH5Q[5]]}2(NO3)6·26H2O |
0-D |
102
|
AE-8
|
{Sr2(H2O)3(NO3)[(NO3)@CyH5Q[5]]}(NO3)2·7H2O |
1-D |
102
|
AE-9
|
{Ba(H2O)[(H2O)2@CyH5Q[5]]}(NO3)2·11H2O |
1-D |
102
|
AE-10
|
{Mg(H2O)(Cl@CyP5Q[5])}·2[ZnCl4]·3H3O·15H2O |
0-D |
103
|
AE-11
|
{Ca2(Cl@CyP5Q[5])}·2[ZnCl4]·H3O·16H2O |
0-D |
103
|
AE-12
|
2{Sr2(H2O)5(Cl@CyP5Q[5])}·2[ZnCl4]·[Zn(H2O)Cl3]·Cl·H3O·8H2O |
0-D |
103
|
AE-13
|
2{Ba2(H2O)3(Cl@CyP5Q[5])}·3[ZnCl4]·23H2O |
1-D |
103
|
AE-14
|
{Mg(H2O)6@CyP6Q[6])}·2[Zn(H2O)Cl3]·9H2O |
0-D |
104
|
AE-15
|
{Ca2(H2O)8CyP6Q[6]}·2[ZnCl4]·13(H2O) |
1-D |
104
|
AE-16
|
{Sr2(H2O)10CyP6Q[6]}·2[ZnCl4]·6(H2O) |
1-D |
104
|
AE-17
|
{Ba2(H2O)10CyP6Q[6]}·2[ZnCl4]·6(H2O) |
1-D |
104
|
AE-18
|
{Ca(H2O)4@HMe6Q[6]}[CdCl4]·7H2O |
1-D |
105
|
AE-19
|
{Ba2(H2O)8@HMe6Q[6]}[CdCl4]·2Cl·12H2O |
1-D |
105
|
AE-20
|
{Ca1.5(H2O)5(HO)1Q[7]}·2[CdCl4]·H3O·35H2O |
1-D |
106
|
AE-21
|
{Sr1.5(H2O)5(HO)1Q[7]}·2[CdCl4]·H3O·35H2O |
1-D |
106
|
TM-1
|
{Cd6Cl14CyH6Q[6]}·(H3O)2·(H2O)2 |
0-D |
108
|
TM-2
|
(TMeQ[6])·(H3O)44+·(CuCl4)24−·8H2O |
0-D |
109
|
TM-3
|
[(TMeQ[6])(CuCl2)2(H2O)4]·10H2O |
1-D |
109
|
TM-4
|
TMeQ[6]·14H2O |
0-D |
109
|
TM-5
|
[Cu(H2O)4(C4H8O2)@(CyH6Q[6])]·2(NO3)·4(H2O) |
1-D |
110
|
TM-6
|
[Cu2(H2O)6Cl2(TMeQ[6])]·2Cl·15(H2O) |
1-D |
110
|
TM-7
|
[Cu2(H2O)9(TMeQ[6])]·2(SO4)·16(H2O) |
0-D |
110
|
TM-8
|
[(C6H10N2)@(TMeQ[6])]·CuCl4·7(H2O) |
0-D |
110
|
TM-9
|
[Cu(H2O)3·CyP5Q[5]]·(ClO4)2 |
1-D |
111
|
TM-10
|
[Zn2(H2O)4·(CyP5Q[5])2]·(ClO4)4 |
1-D |
111
|
TM-11
|
[Cu(H2O)2·CyP6Q[6]]·(ClO4)2 |
1-D |
111
|
TM-12
|
[Zn(H2O)2·CyP6Q[6]]·(ClO4)2·H2O |
0-D |
111
|
Ln-1–3
|
{LnCl2(H2O)3CyH5Q[5]}·NO3·18.5H2O Ln = La, Ce, Pr |
1-D |
115
|
Ln-4,5
|
{LnCl2(H2O)2CyH5Q[5]}·NO3·14H2O Ln = Nd, Sm |
1-D |
115
|
Ln-6
|
{Dy(H2O)2[Cl@CyH5Q[5]]Dy(H2O)6}·5Cl·20H2O |
0-D |
115
|
Ln-7,8
|
{LnCl(H2O)[Cl@CyH5Q[5]]Ln(H2O)6}·4Cl·13H2O Ln = Ho, Er |
0-D |
115
|
Ln-9–17
|
[Ln(H2O)4](NO3)3·CyH6Q[6]·nH2O, Ln = Y, Sm, Eu, Gd, Tb, Dy, Ho, Er, Yb |
1-D |
116
|
Ln-18
|
{Lu2(H2O)12CyH6Q[6]}·4NO3·2Cl·20H2O |
1-D |
116
|
Ln-19–21
|
{Ln4(H2O)16[NO3@CyH6Q[6]]2}·10NO3·nH2O, Ln = La, Ce, Nd |
0-D |
116
|
Ln-22
|
{Pr(H2O)3Cl2[NO3@CyH6Q[6]]}·25H2O |
0-D |
116
|
Ln-23
|
{Pr(H2O)3Cl2[NO3@CyH6Q[6]]}·23H2O |
0-D |
116
|
Ln-24–27
|
{Ln2(H2O)6(Cl@HMe5Q[5])}·2[CdCl4]·2H2O Ln = La, Ce, Pr, Nd |
0-D |
117
|
Ln-28–32
|
{HMe6Q[6]·[Ln(H2O)8]}·3Cl·nH2O n = 11–14, Ln = Tb, Dy, Ho, Er, Tm |
0-D |
117
|
Ln-33
|
{HMe6Q[6]·[Sm(H2O)8]}·3Cl·10H2O |
0-D |
117
|
Ln-34–40
|
{o-TMeQ[6]·[Ln(H2O)8]}·2(NO3)·Cl·9H2O Ln = Tb, Dy, Ho, Er, Tm, Yb, Lu |
0-D |
118
|
Ln-41
|
La(H2O)4OMeQ[6]}2·2(CdCl4)·[CdCl2(H2O)4]·16H2O |
1-D |
119
|
Ln-42
|
{(NO3)2@OMeQ[6]·Ce2(H2O)10}·2(CdCl4)·10H2O |
0-D |
119
|
Ln-43,44
|
{Cl@OMeQ[6]·Ln2(H2O)9}·2(CdCl4)·3Cl−·nH2O Ln = Pr, Eu |
0-D |
119
|
Ln-45
|
{OMeQ[6]·Nd2(H2O)12}·2(CdCl4)·2Cl−·4H2O |
0-D |
119
|
Ln-46
|
OMeQ[6]·Sm(H2O)8·(CdCl4)·NO3−·3H2O |
0-D |
119
|
Ln-47–49
|
OMeQ[6]·Ln(H2O)8·(CdCl4)·Cl−·nH2O Ln = Gd, Dy, Ho |
0-D |
119
|
Ln-50
|
{(C6H6O2)@OMeQ[6]·Ho2(H2O)10}·2(CdCl4)·(C6H6O2)·4Cl−·4(H3O)+·8H2O |
0-D |
119
|
Ln-51–53
|
{Ln(H2O)6·CyP6Q[6]}·2[ZnCl4]·Cl·2(H3O)·n(H2O) Ln = La, Ce, Sm |
0-D |
120
|
Ln-54–59
|
{Ln(H2O)5·CyP6Q[6]}·2[ZnCl4]·(H3O)·n(H2O) Ln = Pr, Nd, Eu, Gd, Tb, Dy |
0-D |
120
|
Ln-60
|
CyP6Q[6]·[Ho(H2O)8]·[ZnCl4]·[Zn(H2O)Cl3]·15(H2O) |
0-D |
120
|
Ln-61–69
|
{t(HO)2OMeQ[6]·[Ln(H2O)8]}·x(NO3)·yCl·zH2O Ln = Eu, Gd, Tb, Dy, Ho, Er, Tm, Yb, Lu |
0-D |
121
|
Ln-70–72
|
{Ln(H2O)5(NO3)(o-TMeQ[6])}·(NO3)2·6H2O Ln = Nd, Sm, Eu |
0-D |
122
|
Ln-73–80
|
{Ln(H2O)6(o-TMeQ[6])}·2(NO3)·Cl·nH2O Ln = Gd, Tb, Dy, Ho, Er, Tm, Yb, Lu |
0-D |
122
|
Ln-81–84
|
{Ln(H2O)6CyH6Q[6]}·2(CdCl4)·H3O·nH2O Ln = La, Ce, Pr, Nd |
1-D |
123
|
Ln-85
|
{Sm(H2O)5CyH6Q[6]}·2(CdCl4)·H3O·10H2O |
1-D |
123
|
Ln-86–88
|
{Ln(H2O)5(NO3)@CyH6Q[6]}·2(CdCl4)·2H3O·nH2O Ln = Gd, Tb, Dy |
1-D |
123
|
4.1. With alkali metal ions
Generally, the coordination of Q[n] homologues with alkali metal ions leads to discrete (closed or opened) molecular capsules or 1D chains because the Q[n] homologues contain only two opposite coordination orientations. For instance, we previously reported a series of discrete molecular capsules of unsubstituted Q[5] with various metal ions, such as alkali (K+), alkaline earth (Ba2+), transition (Cd2+), and lanthanide (La3+) metal ions.87 However, (HO)mQ[n] are a series of multi-dentate ligands with different coordination orientations. In 2011, we prepared and characterized three coordination polymers of alkali metal ions (K+, Rb+ and Cs+) with the (HO)10Q[5] ligand.88 Their crystal structures revealed that not only the carbonyl groups of the portals but also the hydroxy groups at the waist position of the (HO)10Q[5] ligand participated in the coordination, which led to 1D to 2D coordination polymers A-1–3 (Fig. 12).
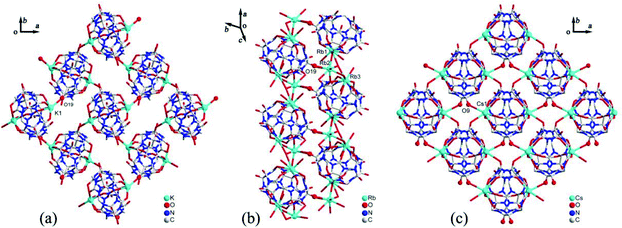 |
| Fig. 12 2D coordination polymers of (HO)10Q[5] with alkali metal ions K+ (a), Rb+ (b) and Cs+ (c). | |
In 2008 and 2010, Day, Lindoy, Tao, and Wei et al. reported three attractive catenane structures, which resulted from the coordination of three substituted Q[5]s (1,2,4-Me6Q[5], 1,2,4-CyH3Q[5] and CyH5Q[5]) with potassium ions.89,90 These catenated structures A-5–7 featured trigonal-planar units, each one involving three substituted Q[5] ligands and six bound K+ ions. Adjacent trigonal-planar basic units connected with each other through their bound K+ ions, generating a 10-ligand “bracelet” framework. Interpenetration of the “bracelet” framework led to a complicated 3D catenane structure (Fig. 13). Using a similar strategy, the Tao group also prepared a series of 2D networks A-8–12 by using the coordination of K+ ion with other substituted Q[5]s, including Me2Q[5], 1,3-CyH2Q[5], 1,2,3-CyH3Q[5] and 1,2,4-CyH3Q[5].91 Apparently, the electron donating effect of the substituents increased the electron density at the portals of these substituted Q[5] ligands, and hence enhanced their binding affinities.
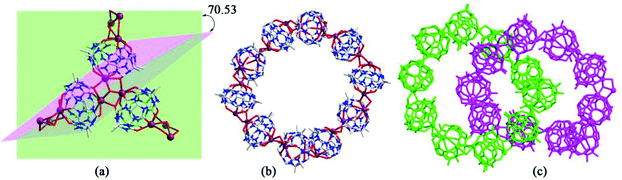 |
| Fig. 13 (a) Schematic illustrating that the dihedrals between any two K1 junction planes in the trigonal planar branch are identical at 70.53. (b) A single 10-membered 1,2,4-HMeQ[5] “bracelet”. (c) Catenation of two 10-membered 1,2,4-HMeQ[5] bracelets. | |
We and others have also reported coordination compounds of alkali metal ions (Na+, K+, Rb+ and Cs+) with both fully and partially alkyl-substituted Q[n]s, including TMeQ[6],92 Me10Q[5],93–95 CyP5Q[5],96 OMeQ[6],97 (HO)1OMeQ[6],98 1,3,5-Me6Q[6].99 For example, Chen, Tao and coworkers investigated the coordination of OMeQ[6] with alkali metal ions in the presence of polychloride cadmium anions, such as [Cd2Cl8]4−, [CdCl4]2−, and [Cd2Cl7]3− anions, or in the presence of p-hydroxybenzoic acid.97 The resulting structures A-29–34 indicated the presence of “outer-surface interactions”, which are a unique type of noncovalent interaction first proposed by Tao.100 For the OMeQ[6] molecules and the selected inorganic anions or aromatic molecules (Fig. 14), such interactions play an important role in the formation of different OMeQ[6]/A+-based supramolecular assemblies.
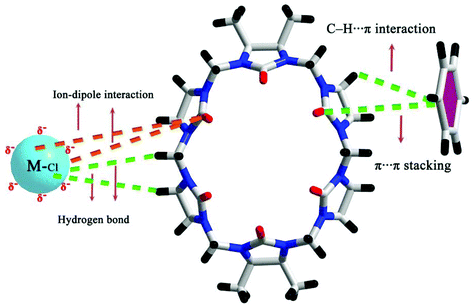 |
| Fig. 14 Outer-surface interactions of OMeQ[6] with inorganic anions (left) and aromatic moieties (right). | |
4.2. Interaction with alkaline earth metal ions
As early as 2008, the Tao group reported the coordination compounds AE-5 and AE-6 of TMeQ[6] which incorporated two alkaline earth metal ions, Ca2+ and Sr2+, which coordinated directly to the TMeQ[6] ligand.101 In 2012, we prepared and characterized three coordination compounds AE-7–9 of the CyH5Q[5] ligand with alkaline-earth metal ions (Ca2+, Sr2+, Ba2+).102 Analysis of the crystallographic data indicated that the radius (size) of the coordinated metal ions determines whether the molecular capsule is closed or opened (Fig. 15).
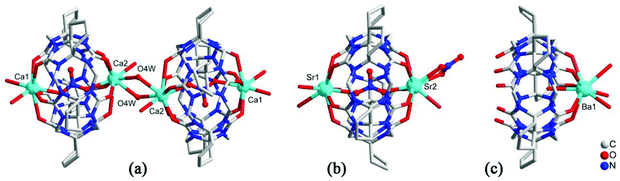 |
| Fig. 15 Crystal structures of three coordination compounds of CyH5Q[5] with alkaline-earth metal ions (Ca2+ (a), Sr2+ (b) and Ba2+ (c)) showing three kinds of molecular capsules. | |
Tao and Ma et al. investigated the coordination compounds AE-10–17 of CyP5Q[5] and CyP6Q[6] with a series alkaline-earth metal ions (AE2+ = Mg2+, Ca2+, Sr2+, Ba2+) in the presence of [ZnCl4]2− anions.103,104 X-ray diffraction analysis revealed that the [ZnCl4]2− anion acts as an inducer in the self-assembly of the CyP5Q[5]/CyP6Q[6]-based complexes. As shown in Fig. 16, for example, due to the ion–dipole interaction between the [ZnCl4]2− anions and the electropositive outer surface of the CyP5Q[5], the [ZnCl4]2− anions form a honeycomb-like framework, and the CyP5Q[5]-Mg2+ coordination complex occupy the cells of this framework. For coordination compounds of alkaline earth metal ions with other substituted Q[n]s, such as (HO)1OMeQ[6],99o-TMeQ[6],41 CyP6Q[6],104 HMe6Q[6]105 and (HO)1Q[7],106 the honeycomb effect is also observed, which originates from the inorganic anions [CdCl4]2− or [ZnCl4]2−.
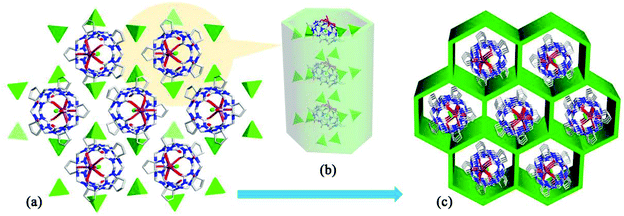 |
| Fig. 16 Binding interaction of Mg2+ with CyP5Q[5]. (a) Supramolecular assembly of Mg2+ cations, CyP5Q[5] molecules and [ZnCl4]2− anions. (b) Occupying the cells of the framework. (c) Honeycomb-like framework constructed of [ZnCl4]2− anions, with CyP5Q[5]-based chains. | |
Coordination compounds of substituted Q[n]s with alkaline-earth metal ions constructed in the presence of inorganic anions usually possess different porous structures, and display differing absorption of volatile compounds.103,105,106 For example, the HMe6Q[6]-Ca2+/Ba2+-[CdCl4]2− based coordination compounds AE-18 and AE-19 exhibit excellent thermal stability as well as permanent porosity. They also show high adsorption capacity and selectivity to Et2O and CH3OH.105 The porous materials constructed by the coordination compounds of (HO)1Q[7] with Ca2+ and Sr2+, AE-20 and AE-21, revealed a large sorption capacity for CH3OH.
4.3. Interaction with transition metal ions
Transition metal coordination complexes usually display special optical, electrical and magnetic properties. However, only a few examples of Q[n]-transition metal coordination complex have been reported.107–110 More often than not, transition metal ions prefer to form aqua complexes rather than to coordinate with Q[n] ligands in aqueous solution. This section presents some examples of substituted Q[n]s complexes with transition metal ions.
In 2010, the complexation behaviour of CyH6Q[6] with Cd2+ ions was investigated by electrochemical methods and X-ray crystallography.108 The host CyH6Q[6] displayed extraordinary binding affinity towards Cd2+ ions. It can form a 1
:
6 CyH6Q[6]/Cd2+ complex TM-1 in both aqueous solution and the solid state (Fig. 17). The strong binding affinity of CyH6Q[6] toward Cd2+ ions may be derived from to the electron donating property of its six substituted cyclohexano groups. The study suggests the potential utility of CyH6Q[6] as an effective chelator and extractant for toxic heavy metals.
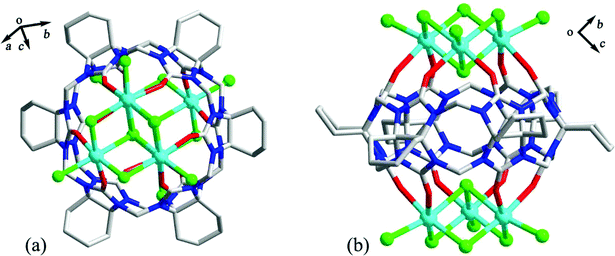 |
| Fig. 17 X-ray structure of CyH6Q[6]/Cd2+ complex: (a) top view; (b) side view. | |
Three years later, we studied the effect of chloride anion concentration on the self-assembly of supramolecular architectures based on TMeQ[6] and copper chloride.109 Under different chloride anion concentrations, three supramolecular architectures TM-2–4 were obtained through the reaction of TMeQ[6] and copper chloride. X-ray diffraction analysis revealed three tubular structures (Fig. 18), one of which is attributed to coordination bonds and the other two resulted from weak noncovalent interactions. In 2013, Zhang et al. described coordination compounds of CyP6Q[6] (TM-5) and TMeQ[6] (TM-6–8) with Cu2+ in the absence or presence of a third species.110 The resulting coordination compounds showed how the corresponding supramolecular assemblies can depend on the addition of the third species. Very recently, Ma and co-workers studied coordination compounds of CyP5Q[5] (TM-9 and TM-10) and CyP6Q[6] (TM-11 and TM-12) with two transition metal ions, namely Cu2+ and Zn2+.111
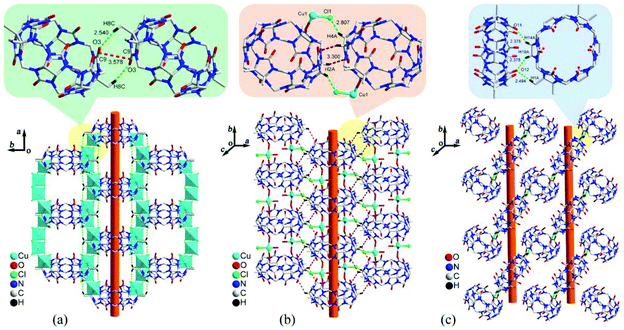 |
| Fig. 18 Three tubular structures self-assembled from TMeQ[6] and copper chloride under different chloride anion concentrations: (a) 1.0 M HCl; (b) 3.0 M HCl; (c) 6.0 M HCl. | |
4.4. Interaction with lanthanide metal ions
Due to their unique physicochemical properties, lanthanide ions are widely used in numerous technological devices.112–114 However, lanthanide ions are difficult to separate because of the poor shielding of the nuclear charge by filling of the 4f electron shell. The study of lanthanide coordination complexes here may lead to fascinating topological structures and promising applications. On comparison with other metal ions, lanthanide ions are the most studied in the coordination chemistry of substituted Q[n]s.115–123
Because of the effect of lanthanide contraction, the lanthanide complexes with substituted Q[n]s usually display interesting structural progressions. As early as 2012, we prepared eight coordination compounds Ln-1–8 of CyH5Q[5] with a number of lanthanide ions (La3+, Ce3+, Pr3+, Nd3+, Sm3+, Dy3+, Ho3+, and Er3+) by reactions of the corresponding lanthanide species with the CyH5Q[5] in aqueous solution.115 Crystal structure analysis revealed two coordination modes (Fig. 19). Close inspection of these crystal structures revealed some interesting structural variations, which were ascribed to the effect of lanthanide contraction.
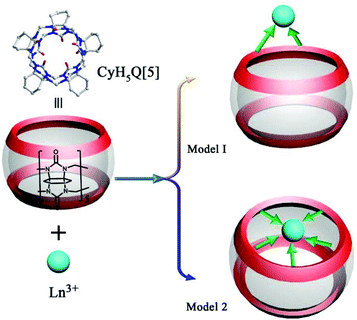 |
| Fig. 19 Two kinds of coordination mode observed for CyH5Q[5] with lanthanide ions. | |
In the following year, the effect of lanthanide contraction induced coordination architectures of CyH6Q[6] was also studied by Ni and Tao et al.116 The slightly different ionic radii of the lanthanide metal ions plays a key role in the formation of different coordination modes for CyH6Q[6]. As a result, fifteen coordination architectures Ln-9–23 of CyH6Q[6]-Ln were structurally classified into four groups, as shown in Fig. 20.
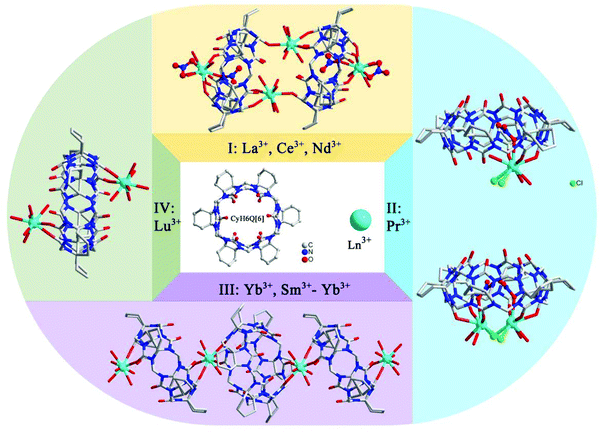 |
| Fig. 20 Coordination complexes of Cy6Q[6] with lanthanide ions, which were structurally classified into four groups. | |
On the other hand, each substituted Q[n] displays a different binding affinity to the same lanthanide ions. Some substituted Q[n]s only coordinate with light lanthanide ions, while others only coordinate with heavy lanthanide ions, and this provided a new method for separating lanthanide ions. For example, Zhu et al. investigated the interactions between a series of lanthanide ions and HMe5Q[5] and HMe6Q[6] in 2015.117 X-ray diffraction analysis revealed that HMe5Q[5] and HMe6Q[6] selectively interacted with certain lanthanide ions. In the presence of [CdCl4]2−, HMe5Q[5] crystallized with four light lanthanides, La3+, Ce3+, Pr3+, and Nd3+ and formed the coordination capsules Ln-24–27, whereas the other lanthanide ions remained in solution. Under the same conditions, HMe6Q[6] crystallized with aqua complexes of lanthanide cations ([Ln(H2O)8]3+, Ln = Gd–Lu) and formed the adducts Ln-28–32, whilst La3+, Ce3+, Pr3+, Nd3+, Sm3+, and Eu3+ remained in solution. It should be noted that no satisfactory data were collected for the ions Gd3+, Yb3+, and Lu3+. In neutral solution and in the absence of CdCl2, HMe6Q[6] crystallized with aqua complexes of lanthanide cations (Ln = Sm–Lu) to form adducts. Here, Ln-33 is a representative adduct. No solid crystals of HMe6Q[6] with La3+, Ce3+, Pr3+, and Nd3+ could be obtained. Energy-dispersive spectrometry (EDS) studies indicated that the lighter or heavier lanthanide ions could be isolated from their counterparts through the interaction with HMe5Q[5] and HMe6Q[6].
In the past five years, the coordination chemistry of lanthanide metal ions with other substituted Q[n]s, such as o-TMeQ[6],118,122 OMeQ[6],119 CyP6Q[6],120t(HO)2OMeQ[6],121 and CyH6Q[6],123 under different conditions were also studied. Table 3 lists the coordination conditions and results of all substituted Q[n]s on interaction with lanthanide metal ions.
Table 3 The coordination conditions and results of substituted Q[n]s with lanthanide ionsa
Ligand |
La |
Ce |
Pr |
Nd |
Sm |
Eu |
Gd |
Tb |
Dy |
Ho |
Er |
Tm |
Yb |
Lu |
Ref. |
Promethium is not discussed because it is a radioactive element. × No crystals were obtained, √ Obtained crystals contain lanthanide ions, — no information, ○ The obtained crystals without lanthanide ions. |
CyH5Q[5] in water |
√ |
√ |
√ |
√ |
√ |
— |
— |
— |
√ |
√ |
√ |
— |
— |
— |
115
|
CyH6Q[6] in water |
√ |
√ |
√ |
√ |
√ |
√ |
√ |
√ |
√ |
√ |
√ |
— |
√ |
√ |
116
|
HMe5Q[5] in [CdCl4]2− |
√ |
√ |
√ |
√ |
× |
× |
× |
× |
× |
× |
× |
× |
× |
× |
117
|
HMe6Q[6] in [CdCl4]2− |
× |
× |
× |
× |
× |
× |
√ |
√ |
√ |
√ |
√ |
√ |
√ |
√ |
117
|
HMe6Q[6] in water |
× |
× |
× |
× |
√ |
√ |
√ |
√ |
√ |
√ |
√ |
√ |
√ |
√ |
117
|
o-TMeQ[6] in neutral sol. |
× |
× |
× |
× |
× |
× |
× |
√ |
√ |
√ |
√ |
√ |
√ |
√ |
118
|
OMeQ[6] in [CdCl4]2− |
√ |
√ |
√ |
√ |
√ |
√ |
√ |
— |
√ |
√ |
× |
× |
× |
× |
119
|
CyP6Q[6] in [ZnCl4]2− |
√ |
√ |
√ |
√ |
√ |
√ |
√ |
√ |
√ |
√ |
√ |
○ |
○ |
○ |
120
|
(HO)2OMeQ[6] in water |
× |
× |
× |
× |
× |
√ |
√ |
√ |
√ |
√ |
√ |
√ |
√ |
√ |
121
|
o-TMeQ[6] in Cd(NO3)2 |
× |
× |
× |
√ |
√ |
√ |
√ |
√ |
√ |
√ |
√ |
√ |
√ |
√ |
122
|
CyH6Q[6] in [CdCl4]2− |
√ |
√ |
√ |
√ |
√ |
√ |
— |
√ |
√ |
○ |
× |
○ |
○ |
× |
123
|
5. Applications of substituted Q[n]s
It must be noted that applications of hydroxylated Q[n]s and their derivatives have been reviewed in our previous work,19 and will not be discussed in this review.
5.1. Catalysis
In 2013, the Cao group prepared a series of M-Pd-Me10Q[5] (M = Li, Na, K, Rb, and Cs) hybrid solid materials (Fig. 21), which displayed excellent catalytic performance and good recyclability as phosphine-free pre-catalysts for Heck cross-coupling reactions.124 Their studies revealed that the activated Pd(II) species were released from the crystalline hybrid pre-catalysts and were transformed into catalytically active Pd nanoparticles during the catalytic reactions. In the following year, the Cao group prepared a composite material Ag@Me10Q[5], in which spherical silver nanoparticles (Ag0 NPs) with an average size of ca. 4.4 nm were observed.125 As a heterogeneous catalyst for the reduction of various nitrophenols, the composite material Ag@Me10Q[5] exhibited excellent catalytic performance and remained active after several consecutive cycles.
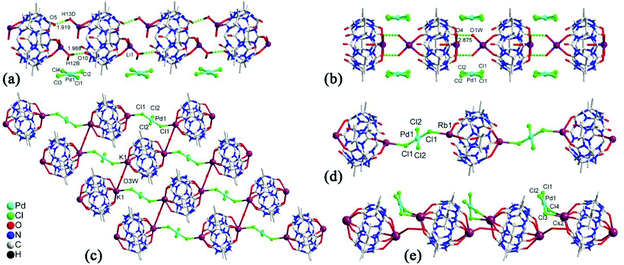 |
| Fig. 21 Crystal structures of a series of M-Pd-Me10Q[5] (M = Li (a), Na (b), K (c), Rb (d), and Cs (e)) hybrid solid materials. | |
5.2. Inorganic–organic hybrid materials
The redox catalysis and magnetism exhibited by inorganic polyoxometalates (POMs) has made them attractive for research.126 In 2009, Kögerler and coworkers reported an example of a hybrid supramolecular architecture based on POMs and Q[n].127 Since then, Cao, Tao, Zhang, Zhu and co-workers have demonstrated numerous hybrid compounds of POMs with substituted Q[n] derivatives.128–137 Very recently, Zhang and co-workers found that the self-assembly of (HO)12Q[6] and Keggin-type POMs (such as H3[PMo12O40] and H3[PW12O40]) under different concentrations of hydrochloric acid yields two types of supramolecular assemblies: one being hybrid compounds (HO)12Q[6]-POMs-HCl and the other (HO)12Q[6]-HCl (Fig. 22).137
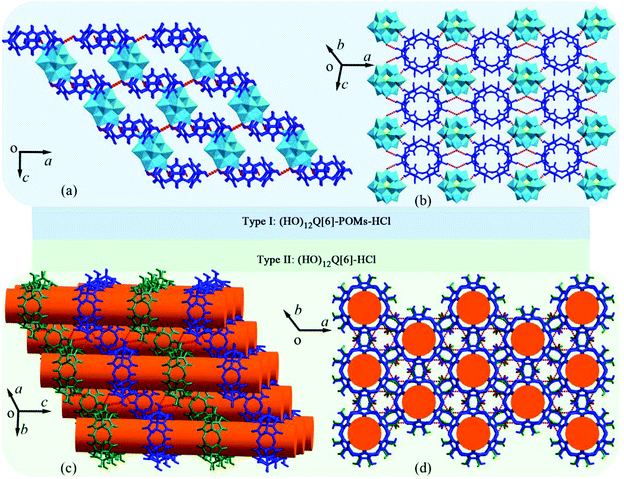 |
| Fig. 22 Two types of supramolecular assemblies in different directions: hybrid compounds (HO)12Q[6]-POMs-HCl ((a) and (b)) and accumulation body of (HO)12Q[6]-HCl ((c) and (d)). | |
They exhibited various interesting chemical and physical properties. It is worth mentioning that the complexation of POMs with Q[n]s or substituted Q[n]s is driven by “outer-surface interactions”, including ion–dipole interactions, hydrogen bonding, C–H⋯π interactions, as well as π⋯π stacking. Compared to Q[n]s, HO groups of the (HO)12Q[6] facilitate the formation of the “outer-surface interactions”, and enhance crystal stability of the supramolecular assemblies.
5.3. Fluorescent chemosensors
Using the host–guest interactions of TMeQ[6] and HMe6Q[6] with a fluorophore guest (Fig. 23) as a fluorescence indicator displacement (FID) system, Ni and co-workers constructed a new type of ratiometric fluorescent chemosensor for the sensing, detection, and recognition of two α-amino acids, namely lysine and methionine.138 As indicated in Fig. 23, the differing binding interactions of the two substituted Q[6]s towards the target analytes led to a useful ratiometric detection signal output for the discrimination of lysine and methionine versus the other tested α-amino acids.
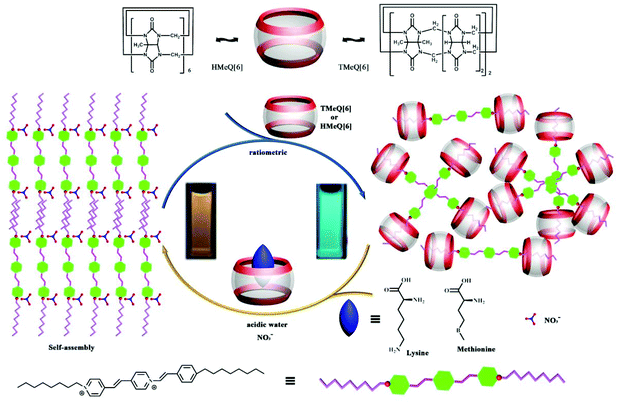 |
| Fig. 23 Illustration of the plausible fluorescence indicator displacement process based on host–guest interactions. | |
As part of their template studies (see section 3.1), Anzenbacher Jr. and Isaacs and coworkers isolated a Q[6] derivative with a covalently attached 2,3-dialkylnaphthalene fluorophore (symbol 19 in Fig. 24), which can emit fluorescence in response to UV irradiation.72 When combined with a number of different metals, only in the case of Eu3+ or Dy3+ was significant fluorescence quenching observed. The Eu3+ system was found to act as a sensor for the histamine shown below (Fig. 24, bottom, left). It was postulated that the histamine occupies the cavity and one ureidyl C
O portal and adopts a 1
:
1 binding model; the Eu3+ was thought to occupy the other ureidyl C
O portal. The addition of the histamine leads to displacement of the quenching Eu3+ or Dy3+, and recovers fluorescence.
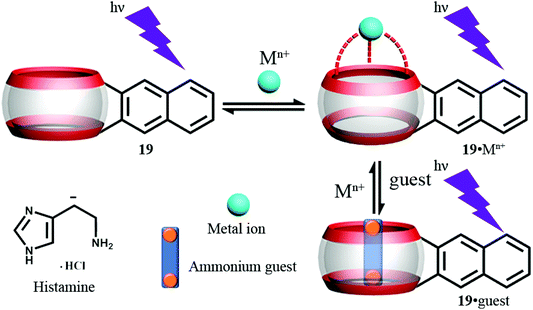 |
| Fig. 24 Structure and Schematic representation of fluorescence assay based on 19.72 | |
Urbach and coworkers reported an optical sensor based on the combination of Q[7] and tetramethylrhodamine.139 Equilibrium dissociation constants for this system matched those of the parent Q[7] despite the presence of the fluorophore. Cellular uptake was demonstrated using HT22 neurons, with localization at the cytoplasm and no disruption of cell growth over a 4 day period (concentration ≤2.2 μM).
5.4. Controlled “smart” SERS hot spot
One of the challenges that has faced single-molecule surface-enhanced Raman spectroscopy (SERS) is the ability to place the single molecule of interest reliably within a hot spot. In their pioneering work, Kim and co-workers demonstrated a novel strategy for locating and securing a single target analyte in a SERS hot spot at a plasmonic nanojunction.140 As shown in Fig. 25, a thiol-functionalized cucurbit[6]uril, mercaptopropyloxy-Q[6] (thiol-Q[6]), acts as dual-function building block: a molecular spacer to generate a nanogap between a Ag nanoparticle and a Ag substrate, and a binding pocket to accommodate the target molecule through host–guest interactions. It is noteworthy that the substituent of the thiol-Q[6] play an important role in linking a single silver nanoparticle (AgNP) to the Ag substrate. The position of the hot spot is controllable by adjusting the length of the polymethylene linker separating the target from the binding moiety, spermine.
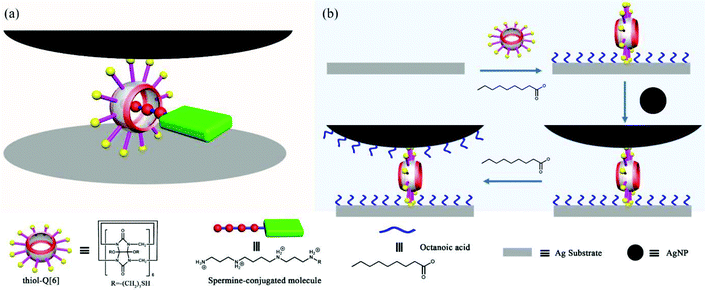 |
| Fig. 25 (a) Schematic representation of a SERS hot spot. (b) Fabrication process of a SERS hot spot. | |
5.5. Gas inclusion and adsorption
Huber et al. recently investigated the binding of three substituted Q[5]s with dioxygen O2.141 The study revealed that the (HO)10Q[5] is able to significantly bind dioxygen gas at physiological temperature, even in the presence of sodium chloride at the concentration of injectable solution in blood. The study suggests the potential utility of (HO)10Q[5] as a precursor host to transport O2 in a hemoglobin substitute solution.
We recently compared the binding abilities of the hosts CyH2Q[6] and CyH6Q[6] with the guests aniline, p-methylaniline, and p-phenyldiamine.142 The results suggested that both hosts can accommodate all guests to form stable inclusion complexes. Entrapment tests and thermogravimetric analyses showed that the CyH2Q[6] possessed higher removal efficiency for aniline than did the CyH6Q[6]. Presumably the size and shape of the CyH2Q[6] is more complementary to the aromatic rings of the guests than those of CyH6Q[6] (Fig. 26).
 |
| Fig. 26 X-ray crystal structures of CyH2Q[6] with guests aniline (a), p-methylaniline (b), and p-phenyldiamine (c). | |
In 2015, the Tao group synthesized HMe5Q[5]-Hydroquinone-based143 and HMe6Q[6]-based144 supramolecular assemblies which had large porous structures. These porous materials demonstrated selective sorption for methanol and ethanol. Zhang and Chen et al. recently prepared two supramolecular assemblies of (HO)12Q[6] under different concentrations of hydrochloric acid, which were found to possess different kinds of channels.145 These activated desolvated (HO)12Q[6]-based solid supramolecular assemblies demonstrated high absorption selectivities and capacities for polychloromethanes, including tetrachloromethane, trichloromethane, and dichloromethane.
6. Self-assembly processes using substituted Q[n]s
6.1. Self-assembly of (pseudo)rotaxane and poly(pseudo)rotaxane
(Pseudo)rotaxanes, poly(pseudo)rotaxanes, catenanes, dentrimers, and other MIMs have attracted much attention because they are precursors for molecular machines. From a structural point of view, Q[n]s and substituted Q[n]s are perfect candidates for MIMs because they possess different hydrophobic cavities and different binding affinities. About twenty years ago, Kim and co-workers demonstrated a useful approach for constructing polyrotaxanes.146 By using the same approach with minor modifications, some examples of MIMs based on substituted Q[n]s have been reported.
In 2011, we presented a novel strategy for synthesizing chiral helical polyrotaxanes. The achiral N,N′-bis(2-pyridylmethyl)-1,6-hexanediamine “string” contains two typical functional pyridyl groups and one 1,6-hexanediamine chain.147 In aqueous solution, the TMeQ[6] bead was held in the middle position of the “string”. The addition of AgNO3 resulted in a helical polyrotaxane, which was characterized by X-ray crystallography (Fig. 27).148 The chirality of the polyrotaxane originated from the twist of the long alkyl chain when bound within the TMeQ[6] cavity. Two opposite chiral helical polyrotaxanes were observed in the crystal structure, indicating that they crystallized as a racemic compound. When KI was added to the solution of helical polyrotaxane, a mass of white precipitate was formed immediately and all of the 1H NMR signals of the helical polyrotaxane disappeared, indicating that the polyrotaxane structure was thoroughly destroyed.
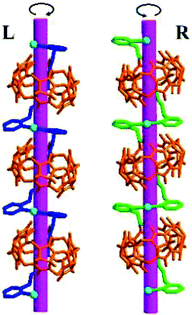 |
| Fig. 27 X-ray crystal structure of helical polyrotaxanes of TMeQ[6] with N,N′-bis(2-pyridylmethyl)-1,6-hexanediamine and Ag+. | |
Zhang et al. reported the synthesis of two polyrotaxanes by using TMeQ[6] (Fig. 28).149 The TMeQ[6] “bead” first reacted with the “string's” of N,N′-bis(3-pyridylmethyl)-1,4-butanediamine dichloride and N,N′-bis(3-pyridylmethyl)-1,6-hexane-diamine dichloride to form stable pseudorotaxanes. The reaction of the pseudorotaxanes with various transition metal ion Cd2+ then produced 1D polyrotaxanes. Another kind of polypseudorotaxanes, which involve the combination of multiple noncovalent interactions, including host–guest interactions, π–π stacking interactions, C–H⋯π interactions and metal–host coordination, were also reported.150
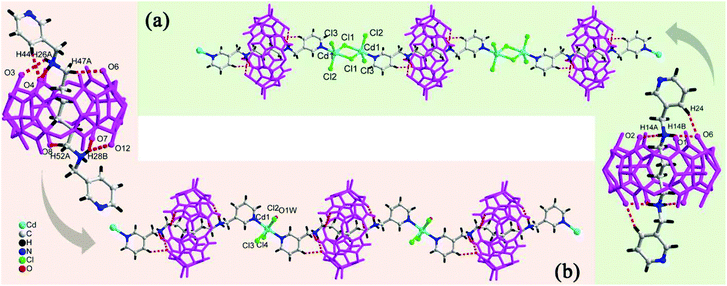 |
| Fig. 28 X-ray crystal structure of two polyrotaxanes of TMeQ[6] with N,N′-bis(3-pyridylmethyl)-1,4-butanediamine (a) and N,N′-bis(3-pyridylmethyl)-1,6-hexane-diamine (b). | |
6.2. Self-assembly of heterowheel pseudorotaxanes
The Day group designed and synthesized an intriguing tetraammonium chain, which performs the molecular machine function of contraction and expansion when bound with CyP6Q[6] and Q[7], respectively.151 They also demonstrated the construction of a hetero-[4]-pseudorotaxane (Fig. 29), which combined the binding function of the “wheels” CyP6Q[6] and Q[7] with the designed molecular “axle”.
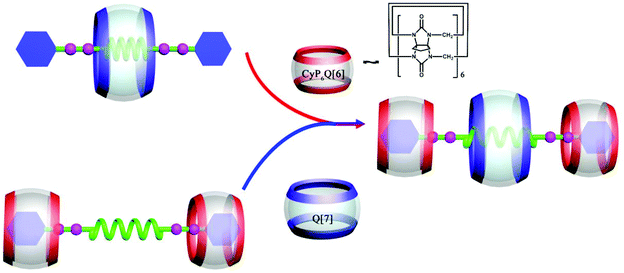 |
| Fig. 29 Schematic representation of the construction of a hetero[4]pseudorotaxane based on CyP6Q[6] and Q[7]. | |
Very recently, we constructed two novel heterowheel [4]pseudorotaxanes consisting of TMeQ[6] and Q[7] in which the Q[7] can rotate freely around the horizontal axis, while the TMeQ[6] cannot. In the construction process of the [4]pseudorotaxanes, the dethreading and movement of the wheels along the axle was observed (Fig. 30).152 Under alkaline conditions, the [4]pseudorotaxanes is destroyed. Thus, the construction and dissociation of the [4]pseudorotaxanes can be controlled through acid/base regulation.
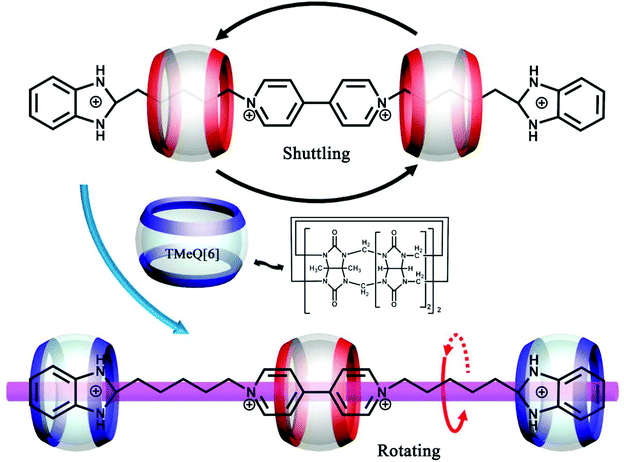 |
| Fig. 30 Schematic representation of the construction of a hetero[4]pseudorotaxane based on TMeQ[6] and Q[7]. | |
6.3. Molecular encapsulation and release by substituted Q[n]
Artificial macrocyclic hosts have potential applications in various fields such as fragrance sustained-release, nutrient preservation, and drug delivery, molecular encapsulation and controlled release. Very recently, we have synthesized 1-(4-carboxybenzyl)-4-[2-(4-pyridyl)-vinyl]-pyridinium chloride with trans- and cis-isomers.153 The trans- and cis-form of the guest can be controlled by encapsulation and release by the molecular container TMeQ[6] under light irradiation and heating (Fig. 31). The encapsulation of the trans-form into TMeQ[6] is attributed to strong host–guest interactions and hydrophobic effects while cis-form release from TMeQ[6] is attributed to a size effect.
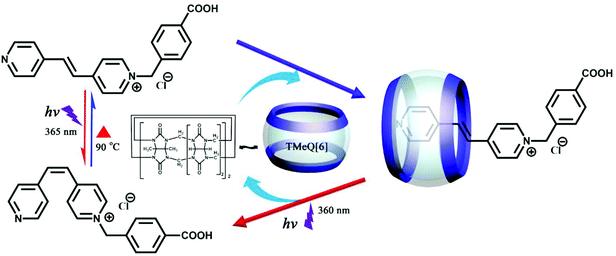 |
| Fig. 31 Guest encapsulation and release by TMeQ[6] under light irradiation and heating. | |
Very recently, we found another molecular encapsulation and release system based on CyH6Q[6] and 1,ω-bisbenzimidazolyl derivatives.154 Experimental results revealed that all guests can form 1
:
1 or 1
:
2 inclusion complexes with CyH6Q[6] residing over benzoimidazole groups of the guests. Interestingly, the guest was released from the CyH6Q[6] cavity at high pH value and encapsulated back into the CyH6Q[6] cavity at low pH value.
6.4. Molecular switches
In 2017, we designed and successfully constructed an electrochemically-driven molecular switch involving a special axle guest hexyldimethyl(ferrocenylmethyl)ammonium bromide.155 As shown in Fig. 32, both hosts CyH6Q[6] and Q[7] can form different stable [2]pseudorotaxanes with the axle guest in its different redox states. Most importantly, the combination and dissociation of the hosts with the guest as well as the binding location can be controlled by electrochemical means.
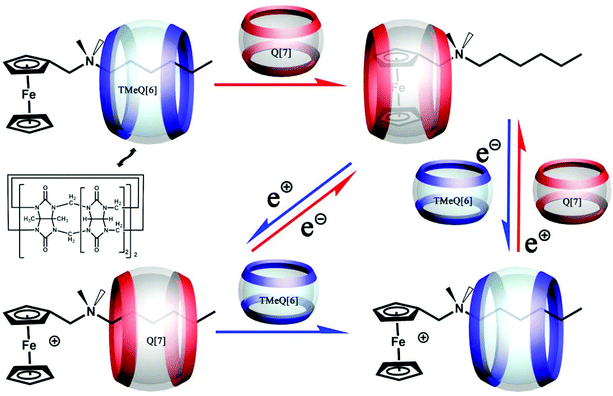 |
| Fig. 32 Electrochemically-driven molecular switch constructed by axle guest hexyldimethyl(ferrocenylmethyl)ammonium bromide with hosts CyH6Q[6] and Q[7]. | |
7. Summary and outlook
Almost two decades have passed since the first example of substituted Q[n] was reported by the Stoddart group. Numerous substituted Q[n]s have been designed and successfully synthesized, which addressed many long-standing concerns in cucurbituril chemistry. These substituted Q[n]s possess enhanced solubility in aqueous and organic media, good selectivity in molecular recognition, and high binding affinities to different metal ions. In particular, the synthesis of monohydroxylated Q[n]s provides an opportunity to further functionalize the substituted Q[n]s. In this review article, the recognition properties of the substituted Q[n]s toward many organic species have been demonstrated. The coordination structures and characteristics of substituted Q[n] with different metal ions, including alkali/alkaline-earth metals, transition metals, and lanthanides have also been identified. The supramolecular properties of the substituted Q[n]s have led to their applications in areas as diverse as catalysis, sensors, hybrid materials, and gas inclusion and adsorption. The substituted Q[n]s also have been used in the construction of MIMs and molecular devices.
With the synthesis of more substituted Q[n]s, cucurbituril chemistry has ushered in a new era of development. The intrinsic properties of the substituted Q[n]s-high binding affinities, high selectivity, fine solubility in aqueous and organic media, diversity of cavity size and shape-suggest that substituted Q[n]s will become important components in MIMs. New molecular machines involving substituted Q[n]s with unprecedented characteristics are expected. The potential applications of substituted Q[n]s can be envisioned in pharmaceutical sciences, functional material science and beyond.
Conflicts of interest
There are no conflicts of interest to declare.
Acknowledgements
We thank the National Natural Science Foundation of China (Grant No.: 21371004 and 21601090), Natural Science Foundation of Anhui Province of China (1808085MB43, 2008085MB36), the Natural Science Foundation of Jiangsu Province (BK20160943) and the Priority Academic Program Development of Jiangsu Higher Education Institutions (PAPD) for financial support. CR thanks the EPSRC for financial support (Grant no. EP/L012804/1 and EP/S025537/1).
References
- C. J. Pedersen, Cyclic polyethers and their complexes with metal salts, J. Am. Chem. Soc., 1967, 89, 2495–2496 CrossRef CAS.
- C. J. Pedersen, Cyclic polyethers and their complexes with metal salts, J. Am. Chem. Soc., 1967, 89, 7017–7036 CrossRef CAS.
-
F. Diederich, Cyclophanes, The Royal Society of Chemistry, Cambridge, 1991 Search PubMed.
- Z. Liu, S. K. M. Nalluri and J. F. Stoddart, Surveying macrocyclic chemistry: from flexible crown ethers to rigid cyclophanes, Chem. Soc. Rev., 2017, 46, 2459–2478 RSC.
- J. Murray, K. Kim, T. Ogoshi, W. Yao and B. C. Gibb, The aqueous supramolecular chemistry of cucurbit[n]urils, pillar[n]arenes and deep-cavity cavitands, Chem. Soc. Rev., 2017, 46, 2479–2496 RSC.
- M. J. Frampton and H. L. Anderson, Insulated molecular wires, Angew. Chem., Int. Ed., 2007, 46, 1028–1064 CrossRef CAS PubMed.
- G. Yu, K. Jie and F. Huang, Supramolecular Amphiphiles Based on Host–Guest Molecular Recognition Motifs, Chem. Rev., 2015, 115, 7240–7303 CrossRef CAS PubMed.
- L. Yang, X. Tan, Z. Wang and X. Zhang, Supramolecular Polymers: Historical Development, Preparation, Characterization, and Functions, Chem. Rev., 2015, 115, 7196–7239 CrossRef CAS PubMed.
- T. Ogoshi and T. Yamagishi, Pillar[5]- and pillar[6]arene-based supramolecular assemblies built by using their cavity-size-dependent host–guest interactions, Chem. Commun., 2014, 50, 4776–4787 RSC.
- O. A. Gerasko, D. G. Samsonenko and V. P. Fedin, Supramolecular chemistry of cucurbiturils, Russ. Chem. Rev., 2002, 71, 741–760 CrossRef CAS.
- J. W. Lee, S. Samal, N. Selvapalam, H. J. Kim and K. Kim, Cucurbituril homologues and derivatives: new opportunities in supramolecular chemistry, Acc. Chem. Res., 2003, 36, 621–630 CrossRef CAS PubMed.
- J. Lagona, P. Mukhopadhyay, S. Chakrabarti and L. Isaacs, The cucurbit[n]uril family, Angew. Chem., Int. Ed., 2005, 44, 4844–4870 CrossRef CAS PubMed.
- E. Masson, X. X. Ling, R. Joseph, L. Kyeremeh-Mensah and X. Y. Lu, Cucurbituril chemistry: a tale of supramolecular success, RSC Adv., 2012, 2, 1213–1247 RSC.
- X. L. Ni, X. Xiao, H. Cong, L. L. Liang, K. Chen, X. J. Cheng, N. N. Ji, Q. J. Zhu, S. F. Xue and Z. Tao, Cucurbit[n]uril-based coordination chemistry: from simple coordination complexes to novel poly-dimensional coordination polymers, Chem. Soc. Rev., 2013, 42, 9480–9508 RSC.
- J. Lü, J.-X. Lin, M.-N. Cao and R. Cao, Cucurbituril: a promising organic building block for the design of coordination compounds and beyond, Coord. Chem. Rev., 2013, 257, 1334–1356 CrossRef.
- A. E. Kaifer, Toward Reversible Control of Cucurbit[n]uril Complexes, Acc. Chem. Res., 2014, 47, 2160–2167 CrossRef CAS PubMed.
- K. I. Assaf and W. M. Nau, Cucurbiturils: from synthesis to high-affinity binding and catalysis, Chem. Soc. Rev., 2015, 44, 394–418 RSC.
- S. J. Barrow, S. Kasera, M. J. Rowland, J. D. Barrio and O. A. Scherman, Cucurbituril-based molecular recognition, Chem. Rev., 2015, 115, 12320–12406 CrossRef CAS PubMed.
- R. H. Gao, L. X. Chen, K. Chen, Z. Tao and X. Xiao, Coord. Chem. Rev., 2017, 348, 1–24 CrossRef CAS.
- X. J. Cheng, L. L. Liang, K. Chen, N. N. Ji, X. Xiao, J. X. Zhang, Y. Q. Zhang, S. F. Xue, Q. J. Zhu, X. L. Ni and Z. Tao, Twisted cucurbit[14]uril, Angew. Chem., Int. Ed., 2013, 52, 7252–7255 CrossRef CAS PubMed.
- R. Behrend, E. Meyer and F. Rusche, Condensation products of glycoluril and formaldehyde, Liebigs Ann. Chem., 1905, 339, 1–37 CrossRef.
- W. A. Freeman, W. L. Mock and N.-Y. Shih, Cucurbituril, J. Am. Chem. Soc., 1981, 103, 7367–7368 CrossRef CAS.
- J. Kim, I. S. Jung, S. Y. Kim, E. Lee, J. K. Kang, S. Sakamoto, K. Yamaguchi and K. Kim, New cucurbituril homologues: syntheses, isolation, characterization, and X-ray crystal structures of cucurbit[n]uril (n = 5, 7, and 8), J. Am. Chem. Soc., 2000, 122, 540–541 CrossRef CAS.
- A. I. Day, A. P. Arnold, R. J. Blanch and B. Snushall, Controlling factors in the synthesis of cucurbituril and its homologues, J. Org. Chem., 2001, 66, 8094–8100 CrossRef CAS PubMed.
- H. J. Buschmann, R. C. Meschke and E. Schollmeyer, Complex formation of crown ethers and cryptands with alkali metal and ammonium ions in chloroform, J. Solution Chem., 2009, 38, 209–217 CrossRef CAS.
- W. Ong and A. E. Kaifer, Salt Effects on the Apparent Stability of the Cucurbit[7]uril-Methyl Viologen Inclusion Complex, J. Org. Chem., 2004, 69, 1383–1385 CrossRef CAS PubMed.
- C. Márquez, R. R. Hudgins and W. M. Nau, Mechanism of Host–Guest Complexation by Cucurbituril, J. Am. Chem. Soc., 2004, 126, 5806–5816 CrossRef PubMed.
- Y. Kim, H. Kim, Y. H. Ko, N. Selvapalam, M. V. Rekharsky, Y. Inoue and K. Kim, Complexation of Aliphatic Ammonium Ions with a Water-Soluble Cucurbit[6]uril Derivative in Pure Water: Isothermal Calorimetric, NMR, and X-ray Crystallographic Study, Chem. – Eur. J., 2009, 15, 6143–6151 CrossRef CAS PubMed.
- A. Flinn, G. C. Hough, J. F. Stoddart and D. J. Williams, Decamethylcucurbit[5]uril, Angew. Chem., Int. Ed., 1992, 31, 1475–1477 CrossRef.
- Y. Miyahara, K. Goto, M. Oka and T. Inazu, Remarkably Facile Ring-Size Control in Macrocyclization: Synthesis of Hemicucurbit[6]uril and Hemicucurbit[12]uril, Angew. Chem., Int. Ed., 2004, 43, 5019–5022 CrossRef CAS PubMed.
- N. N. Andersen, M. Lisbjerg, K. Eriksen and M. Pittelkow, Hemicucurbit[n]urils and Their Derivatives-Synthesis and Applications, Isr. J. Chem., 2018, 58, 435–448 CrossRef CAS.
- M. Lisbjerg, B. M. Jessen, B. Rasmussen, B. E. Nielsen, A. Ø. Madsen and M. Pittelkow, Discovery of a cyclic 6+6 hexamer of D-biotin and formaldehyde, Chem. Sci., 2014, 5, 2647–2650 RSC.
- J. Svec, M. Necas and V. Sindelar, Bambus[6]uril, Angew. Chem., Int. Ed., 2010, 49, 2378–2381 CrossRef CAS PubMed.
- C. A. Burnett, D. Witt, J. C. Fettinger and L. Isaacs, Acyclic Congener of Cucurbituril: Synthesis and Recognition Properties, J. Org. Chem., 2003, 68, 6184–6191 CrossRef CAS PubMed.
- L. Gilberg, M. S. A. Khan, M. Enderesova and V. Sindelar, Cucurbiturils substituted on the methylene bridge, Org. Lett., 2014, 16, 2446–2449 CrossRef CAS PubMed.
- J. Z. Zhao, H. J. Kim, J. Oh, S. Y. Kim, J. W. Lee, S. Sakamoto, K. Yamaguchi and K. Kim, Cucurbit[n]uril derivatives soluble in water and organic solvents, Angew. Chem., Int. Ed., 2001, 40, 4233–4235 CrossRef CAS PubMed.
- F. Wu, L.-H. Wu, X. Xiao, Y.-Q. Zhang, S.-F. Xue, Z. Tao and A. I. Day, Locating the Cyclopentano Cousins of the Cucurbit[n]uril Family, J. Org. Chem., 2012, 77, 606–611 CrossRef CAS PubMed.
- Y. Zhao, V. Mandadapu, H. Iranmanesh, J. E. Beves and A. I. Day, The Inheritance Angle: A Determinant for the Number of Members in the Substituted Cucurbit[n]uril Family, Org. Lett., 2017, 19, 4034–4037 CrossRef CAS PubMed.
- Y. J. Zhao, S. F. Xue, Q. J. Zhu, Z. Tao, J. X. Zhang, Z. B. Wei, L. S. Long, M. L. Hu, H. P. Xiao and A. I. Day, Synthesis of a symmetrical tetrasubstituted cucurbit[6]uril and its host–guest inclusion complex with 2,2′-bipyridine, Chin. Sci. Bull., 2004, 49, 1111–1116 CrossRef CAS.
- J. J. Zhou, X. Yu, Y. C. Zhao, X. Xiao, Y. Q. Zhang, Q. J. Zhu, S. F. Xue, Q. J. Zhang, J. X. Liu and Z. Tao, Synthesis of a symmetrical octamethyl-substituted cucurbituril with a dimethyl-substituted glycoluril dimer, Tetrahedron, 2014, 70, 800–804 CrossRef CAS.
- J. J. Zhou, X. Yu, Y. C. Zhao, X. Xiao, Y. Q. Zhang, S. F. Xue, Z. Tao, J. X. Liu and Q. J. Zhu, Assemblies of alkaline-earth-metal ions with o-tetramethyl-substituted cucurbituril in the presence of the cadmium tetrachloride anion, Eur. J. Inorg. Chem., 2014, 23, 5771–5776 CrossRef.
- X. X. Wang, F. Y. Tian, M. Liu, K. Chen, Y. Q. Zhang, Q. J. Zhu and Z. Tao, A water soluble tetramethyl-substituted cucurbit[8]uril obtained from larger intermediates?, Tetrahedron, 2019, 75, 130488 CrossRef.
- J. X. Lin, Y. Q. Zhang, J. X. Zhang, S. F. Xue, Q. J. Zhu and Z. Tao, Synthesis of partially methyl substituted cucurbit[n]urils with 3a-methyl-glycoluril, J. Mol. Struct., 2008, 875, 442–446 CrossRef CAS.
- C. Z. Wang, W. X. Zhao, Y. Q. Zhang, S. F. Xue, Q. J. Zhu and Z. Tao, A supramolecular assembly of methyl-substituted cucurbit[5]uril and its potential applications in selective absorption, RSC Adv., 2015, 5, 17354–17357 RSC.
- W. X. Zhao, C. Z. Wang, Y. Q. Zhang, S. F. Xue, Q. J. Zhu and Z. Tao, Supramolecular assembly of a methyl-substituted cucurbit[6]uril and its potential applications in selective sorption, New J. Chem., 2015, 39, 2433–2436 RSC.
- L. M. Zheng, J. N. Zhu, Y. Q. Zhang, Q. J. Zhu, S. F. Xue, Z. Tao, J. X. Zhang, Z. Xin, Z. B. Wei, L. S. Long and A. I. Day, Opposing substitution in cucurbit[6]urils forms ellipsoid cavities: the symmetrical dicyclohexanocucurbit[6]uril is no exception highlighted by inclusion and exclusion complexes, Supramol. Chem., 2008, 20, 709–716 CrossRef CAS.
- X. L. Ni, Y. Q. Zhang, Q. J. Zhu, S. F. Xue and Z. Tao, Crystal structures of host–guest complexes of meta-tricyclohexyl cucurbit[6]uril with small organic molecules, J. Mol. Struct., 2008, 876, 322–327 CrossRef CAS.
- F. F. Shen, K. Chen, Y. Q. Zhang, Q. J. Zhu, Z. Tao and H. Cong, Mono- and dihydroxylated symmetrical octamethylcucurbiturils and allylated derivatives, Org. Lett., 2016, 18, 5544–5547 CrossRef CAS PubMed.
- X. Yang, W. Zhao, Z. Wang, Y. Huang, S. M. Y. Lee, Z. Tao and R. Wang, Toxicity of hemimethyl-substituted cucurbit[7]uril, Food Chem. Toxicol., 2017, 108, 510–518 CrossRef CAS PubMed.
- B. Vinciguerra, P. Y. Zavalij and L. Isaacs, Synthesis and Recognition Properties of Cucurbit[8]uril Derivatives, Org. Lett., 2015, 17, 5068–5071 CrossRef CAS PubMed.
- S. Y. Jon, N. Selvapalam, D. H. Oh, J. K. Kang, S. Y. Kim, Y. J. Jeon, J. W. Lee and K. Kim, Facile synthesis of cucurbit[n]uril derivatives via direct functionalization: expanding utilization of cucurbit[n]uril, J. Am. Chem. Soc., 2003, 125, 10186–10187 CrossRef CAS.
- S. K. Ghosh, A. Dhamija, Y. H. Ko, J. An, M. Y. Hur, D. R. Boraste, J. Seo, E. Lee, K. M. Park and K. Kim, Superacid-Mediated Functionalization of Hydroxylated Cucurbit[n]urils, J. Am. Chem. Soc., 2019, 141, 17503–17506 CrossRef CAS PubMed.
- G. Sung, S.-Y. Lee, M.-G. Kang, K. L. Kim, J. An, J. Sim, S. Kim, S. Kim, J. Ko, H.-W. Rhee, K. M. Park and K. Kim, Supra-blot: an accurate and reliable assay for detecting target proteins with a synthetic host molecule–enzyme hybrid, Chem. Commun., 2020, 56, 1549–1552 RSC.
- N. Zhao, G. O. Lloyd and O. A. Scherman, Monofunctionalized cucurbit[6]uril synthesis using imidazolium host–guest complexation, Chem. Commun., 2012, 48, 3070–3072 RSC.
- B. Vinciguerra, L. Cao, J. R. Cannon, P. Y. Zavalij, C. Fenselau and L. Isaacs, Synthesis and self-assembly processes of monofunctionalized cucurbit[7]uril, J. Am. Chem. Soc., 2012, 134, 13133–13140 CrossRef CAS.
- Y. Yu, J. Li, M. Zhang, L. Cao and L. Isaacs, Hydrophobic monofunctionalized cucurbit[7]uril undergoes self-inclusion complexation and forms vesicle-type assemblies, Chem. Commun., 2015, 51, 3762–3765 RSC.
- M. M. Ayhan, H. Karoui, M. Hardy, A. Rockenbauer, L. Charles, R. Rosas, K. Udachin, P. Tordo, D. Bardelang and O. Ouari, Comprehensive synthesis of monohydroxy-cucurbit[n]urils (n = 5, 6, 7, 8): high purity and high conversions, J. Am. Chem. Soc., 2015, 137, 10238–10245 CrossRef CAS PubMed.
- M. M. Ayhan, H. Karoui, M. Hardy, A. Rockenbauer, L. Charles, R. Rosas, K. Udachin, P. Tordo, D. Bardelang and O. Ouari, Correction to “Comprehensive synthesis of monohydroxy-cucurbit[n]urils (n = 5, 6, 7, 8): high purity and high conversions”, J. Am. Chem. Soc., 2016, 138, 2060–2061 CrossRef CAS PubMed.
- N. Dong, J. He, T. Li, A. Peralta, M. R. Avei, M. Ma and A. E. Kaifer, Synthesis and Binding Properties of Monohydroxycucurbit[7]uril: A Key Derivative for the Functionalization of Cucurbituril Hosts, J. Org. Chem., 2018, 83, 5467–5473 CrossRef CAS PubMed.
- L. Gilberg, M. S. A. Khan, M. Enderesova and V. Sindelar, Cucurbiturils Substituted on the Methylene Bridge, Org. Lett., 2014, 16, 2446–2449 CrossRef CAS.
- J. Canceill, M. Cesario, A. Collet, J. Guilhem and C. Pascard, A New Bis-Cyclotribenzyl Cavitand Capable of Selective Inclusion of Neutral Molecules in Solution - Crystal-Structure of Its CH2Cl2 Cavitate, J. Chem. Soc., Chem. Commun., 1985, 6, 361–363 RSC.
- D. J. Cram, S. Karbach, Y. H. Kim, L. Baczynskyj and G. W. Kalleymeyn, Shell Closure of 2 Cavitands Forms Carcerand Complexes with Components of the Medium as Permanent Guests, J. Am. Chem. Soc., 1985, 107, 2575–2576 CrossRef CAS.
- J. Rebek, Molecular behavior in small spaces, Acc. Chem. Res., 2009, 42, 1660–1668 CrossRef CAS PubMed.
- D. Ajami and J. Rebek, More chemistry in small spaces, Acc. Chem. Res., 2013, 46, 990–999 CrossRef CAS.
- B. Yang, L. M. Zheng, Z. Z. Gao, X. Xiao, Q. J. Zhu, S. F. Xue, Z. Tao, J. X. Liu and G. Wei, Extended and contorted conformations of alkanediammonium ions in symmetrical α,α′,δ,δ′-tetramethyl-cucurbit[6]uril cavity, J. Org. Chem., 2014, 79, 11194–11198 CrossRef CAS PubMed.
- C. L. Shan, B. Yang, W. Q. Sun, X. Xiao, Z. Tao and J. X. Liu, 1,3-propanediammonium and
1,12-dodecanediammonium encapsulated in the cavity of symmetrical α,α′,δ,δ′-tetramethyl-cucurbit[6]uril, Supramol. Chem., 2015, 27, 606–612 CrossRef CAS.
- G. S. Fang, W. Q. Sun, W. X. Zhao, R. L. Lin, Z. Tao and J. X. Liu, Host–guest complexation of di-cyclohexanocucurbit[6]uril and hexa-cyclohexano-cucurbit[6]uril with alkyldiammonium ions: A comparative study, Org. Biomol. Chem., 2016, 14, 674–679 RSC.
- Y. X. Qu, R. L. Lin, Y. Q. Zhang, K. Z. Zhou, Q. D. Zhou, Q. J. Zhu, Z. Tao, P. H. Ma, J. X. Liu and G. Wei,
Endo/exo binding of alkyl and aryl diammonium ions by cyclopentanocucurbit[6]uril, Org. Chem. Front., 2017, 4, 1799–1805 RSC.
- F. Biedermann, V. D. Uzunova, O. A. Scherman, W. M. Nau and A. D. Simone, Release of High-Energy Water as an Essential Driving Force for the High-Affinity Binding of Cucurbit[n]urils, J. Am. Chem. Soc., 2012, 134, 15318–15323 CrossRef CAS PubMed.
- L. J. Meng, X. Tian, S. Huang, R. L. Lin, X. H. Liu, Q. J. Zhu, Z. Tao and J. X. Liu, Solvent- and Heat-Dependent Binding Behaviors of HMeQ[6] with Alkyldiammonium Ions, ChemistrySelect, 2018, 3, 9211–9217 CrossRef CAS.
- W. X. Zhao, C. Z. Wang, L. X. Chen, X. W. Cui, R. L. Lin, Q. J. Zhu, Z. Tao and J. X. Liu, Host–guest complexation of HMeQ[7] with alkyldiammonium ions and alkyldiamines: A comparative study, RSC Adv., 2016, 6, 11937–11942 RSC.
- D. Lucas, T. Minami, G. Iannuzzi, L. Cao, J. B. Wittenberg, P. Anzenbacher Jr. and L. Isaacs, Templated Synthesis of Glycoluril Hexamer and Monofunctionalized Cucurbit[6]uril Derivatives, J. Am. Chem. Soc., 2011, 133, 17966–17976 CrossRef CAS PubMed.
- L. Cao and L. Isaacs, Daisy Chain Assembly Formed from a Cucurbit[6]uril Derivative, Org. Lett., 2012, 14, 3072–3075 CrossRef CAS PubMed.
- R. L. Lin, J. Q. Li, J. X. Liu and A. E. Kaifer, The Binding Interactions between Cyclohexanocucurbit[6]uril and Alkyl Viologens Give Rise to a Range of Diverse Structures in the Solid and the Solution Phases, J. Org. Chem., 2015, 80, 10505–10511 CrossRef CAS PubMed.
- R. L. Lin, Y. P. Dong, Y. F. Hu, J. X. Liu, L. S. Bai, J. Y. Gao, H. L. Zhu and J. Zhao, Inclusion of methylviologen in symmetrical α,α′,δ,δ′-tetramethyl-cucurbit[6]uril, RSC Adv., 2012, 2, 7754–7758 RSC.
- H. Shi, L. M. Zheng, R. L. Lin, G. S. Fang, W. Q. Sun and J. X. Liu, Binding behaviors of para-dicyclohexanocucurbit[6]uril and meta-tricyclohexanocucurbit[6]uril with dialkyl viologens, Supramol. Chem., 2018, 30, 713–721 CrossRef CAS.
- R. L. Lin, G. S. Fang, W. Q. Sun and J. X. Liu, Aniline-containing guests recognized by α,α′,δ,δ′-tetramethyl-cucurbit[6]uril host, Sci. Rep., 2016, 6, 39057 CrossRef PubMed.
- M. Qin, F. Y. Li, Y. Huang, W. Ran, D. Han and Y. L. Song, 20 Natural Amino Acids Identification by a Photochromic Sensor Chip, Anal. Chem., 2015, 87, 837–842 CrossRef CAS PubMed.
- Y. Zhou and J. Y. Yoon, Recent progress in fluorescent and colorimetric chemosensors for detection of amino acids, Chem. Soc. Rev., 2012, 41, 52–67 RSC.
- H. Heli, M. Hajjizadeh, A. Jabbari and A. A. Moosavi-Movahedi, Fine steps of electrocatalytic oxidation and sensitive detection of some amino acids on copper nanoparticles, Anal. Biochem., 2009, 388, 81–90 CrossRef CAS PubMed.
- P. H. Shan, S. C. Tu, R. L. Lin, Z. Tao, J. X. Liu and X. Xiao, Supramolecular complexes of α,α′,δ,δ′-tetramethyl-cucurbit[6]uril binding with enantiomeric
amino acids, CrystEngComm, 2017, 19, 2168–2171 RSC.
- X. Xiao, Z. Z. Gao, C. L. Shan, Z. Tao, Q. J. Zhu, S. F. Xue and J. X. Liu, Encapsulation of haloalkane 1-(3-Chlorophenyl)-4-(3-chloropropyl)-piperazinium in symmetrical α,α′,δ,δ′-tetramethyl-cucurbit[6]uril, Phys. Chem. Chem. Phys., 2015, 17, 8618–8621 RSC.
- L. J. Meng, W. X. Zhao, L. X. Chen, W. Q. Sun, R. L. Lin, Q. J. Zhu, Z. Tao and J. X. Liu, Single and Double Binding of 1,10-Phenanthroline and 4,7-Dimethyl-1,10-phenanthroline to HMeQ[7]: Contrasting pKa Shifts Induced by HMeQ[7], ChemistrySelect, 2018, 3, 1335–1341 CrossRef CAS.
- Y. Fan, R.-H. Gao, X. Xiao and Z. Tao, Inclusion Complexes of Hymexazol with Three Different Cucurbit[n]uril: Preparation, and Physicochemical and Antifungal Characterization, Isr. J. Chem., 2018, 58, 466–471 CrossRef CAS.
- Q. Liu, Q. Tang, Y. Y. Xi, Y. Huang, X. Xiao, Z. Tao, S. F. Xue, Q. J. Zhu, J. X. Zhang and G. Wei, Supramol. Chem., 2015, 27, 386–392 CrossRef CAS.
- H. Zhang, Y. Huang, S. F. Xue, Z. Tao and Q. J. Zhu, Host–guest interactions of 6-benzyladenine with normal and modified cucurbituril: 1H NMR, UV absorption spectroscopy and phase solubility methods, Supramol. Chem., 2011, 23, 527–532 CrossRef CAS.
- J. X. Liu, L. S. Long, R. B. Huang and L. S. Zheng, Molecular Capsules Based on Cucurbit[5]uril Encapsulating “Naked” Anion Chlorine, Cryst. Growth Des., 2006, 6, 2611–2614 CrossRef CAS.
- X. Xiao, Z. Tao, Q. J. Zhu, S. F. Xue, J. X. Liu and G. Wei, Coordination Polymers Constructed from Alkali Metal Ions and (HO)10cucurbit[5]uril, CrystEngComm, 2011, 13, 3794–3800 RSC.
- X. L. Ni, J. X. Lin, Y. Y. Zheng, W. S. Wu, Y. Q. Zhang, S. F. Xue, Q. J. Zhu, Z. Tao and A. I. Day, Supramolecular bracelets and interlocking rings elaborated through the interrelationship of neighboring chemical environments of alkyl-substitution on cucurbit[5]uril, Cryst. Growth Des., 2008, 8, 3446–3450 CrossRef CAS.
- Z. F. Li, F. Wu, F. G. Zhou, X. L. Ni, X. Feng, X. Xiao, Y. Q. Zhang, S. F. Xue, Q. J. Zhu, L. F. Lindoy, J. K. Clegg, Z. Tao and G. Wei, Approach to 10-Unit “Bracelet” Frameworks Based on Coordination of Alkyl-Substituted Cucurbit[5]urils and Potassium Ions, Cryst. Growth Des., 2010, 10, 5113–5116 CrossRef CAS.
- Z. F. Li, L. L. Liang, F. Wu, F. G. Zhou, X. L. Ni, X. Feng, X. Xiao, Y. Q. Zhang, S. F. Xue, Q. J. Zhu, J. K. Clegg, Z. Tao, L. F. Lindoy and G. Wei, An approach to networks based on coordination of alkyl-substituted cucurbit[5]urils and potassium ions, CrystEngComm, 2013, 15, 1994–2001 RSC.
- W.-J. Chen, L.-S. Long, R.-B. Huang and L.-S. Zheng, A Dihalide–Decahydrate Cluster of [X2(H2O)10]2− in a Supramolecular Architecture of {[Na2(H2O)6(H2O@TMEQ[6])]·2(C6H5NO3)}X2(H2O)10 (TMEQ[6]=α,α′,δ,δ′-Tetramethylcucurbit[6]uril; X=Cl, Br), Cryst. Growth Des., 2013, 13, 2507–2513 CrossRef CAS.
- J. Lin, J. Lü, M. Cao and R. Cao, Effects of Cocrystalline Subunits on the Supramolecular Chemistry of Me10Q[5]: From Simple Inorganic Anions to Cluster Anions, Cryst. Growth Des., 2011, 11, 778–783 CrossRef CAS.
- Y.-Q. Zhang, Q.-J. Zhu, S.-F. Xue and Z. Tao, Chlorine Anion Encapsulation by Molecular Capsules Based on Cucurbit[5]uril and Decamethylcucurbit[5]uril, Molecules, 2007, 12, 1325–1333 CrossRef CAS PubMed.
- Y. F. Hu, K. Chen, J. X. Liu, R. L. Lin, W. Q. Sun, Q. J. Zhu, S. F. Xue and Z. Tao, Complexation of Decamethylcucurbit[5]uril with Alkali Metal Ions, Polyhedron, 2012, 31, 632–637 CrossRef CAS.
- L. T. Wei, Y. Q. Zhang, K. Z. Zhou, L. L. Zhan, Y. X. Qu, Z. Tao and P. H. Ma, Coordination of fully substituted cyclopentano cucurbit[5]uril with alkali cation in the presence of tetrachloridezincate anion, Inorg. Chim. Acta, 2016, 445, 1–7 CrossRef CAS.
- F. F. Shen, J. L. Zhao, K. Chen, Z. Y. Hua, M. D. Chen, Y. Q. Zhang, Q. J. Zhu and Z. Tao, Supramolecular coordination assemblies of a symmetrical octamethyl-substituted cucurbituril with alkali metal ions based on the outer-surface interactions of cucurbit[n]urils, CrystEngComm, 2017, 19, 2464–2474 RSC.
- F. F. Shen, J. L. Zhao, K. Chen, J. Xu, Y. Wang, Z. Y. Hua, L. Wu, M. D. Chen, Y. Q. Zhang and Z. Tao, Coordination and supramolecular assemblies of mono-hydroxylated octamethylcucurbit[6]uril with alkali and alkaline earth metal ions in the presence of polychloride cadmium anions, CrystEngComm, 2017, 19, 4017–4024 RSC.
- H. T. Hou, W. J. Chen, Y. Q. Zhang, Q. J. Zhu, S. F. Xue and Z. Tao, Coordination and supramolecular assemblies of meta-hexamethyl-substituted cucurbit[6]uril with alkali metal ions, Polyhedron, 2015, 87, 117–121 CrossRef CAS.
- X. L. Ni, X. Xiao, H. Cong, Q. J. Zhu, S. F. Xue and Z. Tao, Self-assemblies based on the “outer-surface interactions” of cucurbit[n]urils: new opportunities for supramolecular architectures and materials, Acc. Chem. Res., 2014, 47, 1386–1395 CrossRef CAS PubMed.
- Y. Q. Zhang, L. M. Zhen, D.-H. Yu, Y.-J. Zhao, S.-F. Xue, Q.-J. Zhu and Z. Tao, Structures of supramolecular assemblies formed by substituted cucurbiturils and metal ions, J. Mol. Struct., 2008, 875, 435–441 CrossRef CAS.
- Y. F. Hu, K. Chen, R. L. Lin, W. Q. Sun, J. Zhu, J. X. Liu, Q. J. Zhu, S. F. Xue and Z. Tao, Metal-ion-induced self-assembly of closed/opened molecular capsule based on pentacyclopentanocucurbit[5]uril, RSC Adv., 2012, 2, 5663–5668 RSC.
- L. T. Wei, Y. Q. Zhang, K. Z. Zhou, L. L. Zhan, Y. X. Qu, Z. Tao and P. H. Ma, Coordination of fully substituted cyclopentano cucurbit[5]uril with alkaline earth cations in the presence of tetrachlorozincate anions, Inorg. Chim. Acta, 2016, 453, 277–283 CrossRef CAS.
- Y. X. Qu, Y. Q. Zhang, K. Z. Zhou, L. T. Wei, L. L. Zhan, S. Y. Cheng, Z. Tao and P. H. Ma, Interaction of Cyclopentano Cucurbit[6]uril with Alkaline Earth Cations and Supramolecular Assemblies with Aid of [ZnCl4]2−, ChemistrySelect, 2017, 2, 4360–4363 CrossRef CAS.
- C. Z. Wang, W. X. Zhao, F. F. Shen, Y. Q. Zhang, Q. J. Zhu, X. Xiao and Z. Tao, Methyl-substituted cucurbit[6]uril-based microporous supramolecular frameworks for highly selective Et2O/CH3OH adsorption, CrystEngComm, 2016, 18, 2112–2118 RSC.
- R. H. Gao, Z. Y. Hua, K. Chen, J. Xu, Q. J. Zhu, Z. Tao and J. L. Zhao, Coordination supramolecular assemblies of a monohydroxycucurbit[7]uril and their potential applications in gas sorption, Dalton Trans., 2018, 47, 1942–1947 RSC.
- J. X. Liu, C. H. Dong, L. S. Long, R. B. Huang and L. S. Zheng, From 1D zigzag chain to 1D tubular structure, weak field ligand-dependent assembly of cucurbit[6]uril-based
tubular coordination polymer, Dalton Trans., 2009, 36, 7344–7346 RSC.
- X. Feng, Z.-F. Li, Z. Tao, S.-F. Xue, Q.-J. Zhu, Y.-Q. Zhang and J. X. Liu, Complexation of Cyclohexanocucurbit[6]uril with Cadmium Ions: X-ray Crystallographic and Electrochemical Study, Inorg. Chem., 2010, 49, 7638–7640 CrossRef CAS PubMed.
- R. L. Lin, W. Q. Sun, W. R. Yao, J. Zhu and J. X. Liu, Anion Concentration Control in the Self-assembly of Symmetrical α,α′,δ,δ′-Tetramethyl-Cucurbit[6]uril-Based Tubular Architectures, RSC Adv., 2014, 4, 18323–18328 RSC.
- T. Zhang, Y. Q. Zhang, Q. J. Zhu and Z. Tao, Supramolecular assemblies based on the interaction of a copper dication with alky-substituted cucurbit[6]urils, Polyhedron, 2013, 53, 98–102 CrossRef CAS.
- W. W. Zhao, L. T. Wie, K. Z. Zhou, M. H. Chen, S. Y. Cheng, D. F. Jiang and P. H. Ma, A study on the coordination of fully substituted cyclopentano cucurbit[5, 6]uril with copper and zinc ions, ChemistrySelect, 2019, 4, 11674–11677 CrossRef CAS.
- A. Abdallah, S. Freslon, X. Fan, A. Rojo, C. Daiguebonne, Y. Suffren, K. Bernot, G. Calvez, T. Roisnel and O. Guillou, Lanthanide-Based Coordination Polymers With 1,4-Carboxyphenylboronic Ligand: Multiemissive Compounds for Multisensitive Luminescent Thermometric Probes, Inorg. Chem., 2019, 58, 462–475 CrossRef CAS PubMed.
- Y. Cui, J. Zhang, H. He and G. Qian, Photonic functional metal-organic frameworks, Chem. Soc. Rev., 2018, 47, 5740–5785 RSC.
- Y. Cui, B. Li, H. He, W. Zhou, B. Chen and G. Qian, Metal-Organic Frameworks as Platforms for Functional Materials, Acc. Chem. Res., 2016, 49, 483–493 CrossRef CAS PubMed.
- J. X. Liu, Y. F. Hu, R. L. Lin, W. Q. Sun, X. F. Chu, Q. J. Zhu, S. F. Xue and Z. Tao, Coordination Complexes Based on Pentacyclohexanocucurbit[5]uril and Lanthanide(III) Ions: Lanthanide Contraction Effect Induced Structural Variation, CrystEngComm, 2012, 14, 6983–6989 RSC.
- X. Qin, X. L. Ni, J. X. Hu, K. Chen, Y. Q. Zhang, C. Redshaw, Q. J. Zhu, S. F. Xue and Z. Tao, Macrocycle-based metal ion complexation: a study of the lanthanide contraction effect towards hexacyclohexanocucurbit[6]uil, CrystEngComm, 2013, 15, 738–744 RSC.
- C. Z. Wang, W. X. Zhao, Y. Q. Zhang, S. F. Xue, Z. Tao and Q. J. Zhu, Interaction of Ln3+ with Methyl-Substituted Cucurbit[n]urils (n = 5, 6) Derived from 3a-Methyl Glycoluril, ChemPlusChem, 2015, 80, 1052–1059 CrossRef CAS PubMed.
- X. Yu, J. K. Clegg, Y. Q. Zhang, S. F. Xue, Z. Tao, Q. J. Zhu, L. F. Lindoy and G. Wei, Adducts of aqua complexes of Ln3+ with ortho-tetramethyl substituted cucurbituril: Potential applications for isolation of heavier lanthanides, Polyhedron, 2015, 91, 150–154 CrossRef CAS.
- R. L. Lin, J. J. Zhou, F. H. Zhou, W. Q. Sun, J. X. Liu and K. Chen, Lanthanide contraction effect and organic additive impact the coordination structures of lanthanide ions with symmetrical octamethyl-substituted cucurbit[6]uril ligand, CrystEngComm, 2019, 21, 5641–5649 RSC.
- Y. X. Qu, K. Z. Zhou, K. Chen, Y. Q. Zhang, X. Xiao, Q. D. Zhou, Z. Tao, P. H. Ma and G. Wei, Coordination and Supramolecular Assemblies of Fully Substituted Cyclopentanocucurbit[6]uril with Lanthanide Cations in the Presence of Tetrachlorozincate Anions, and Their Potential Applications, Inorg. Chem., 2018, 57, 7412–7419 CrossRef CAS PubMed.
- F. F. Shen, K. Chen, Z. Y. Hua, Y. Wang, J. Xu, M. D. Chen, Y. Q. Zhang and Z. Tao, Adducts of aqua complexes of Ln3+ with a dihydroxylated symmetrical octamethyl-substituted cucurbituril: potential applications for isolation of heavier lanthanides, CrystEngComm, 2017, 19, 5635–5639 RSC.
- J. J. Zhou, X. Yu, Y. C. Zhao, X. Xiao, Y. Q. Zhang, Q. J. Zhu, S. F. Xue, Z. Tao, J. X. Liu and Q. J. Zhu, Coordination of Ln3+ in ortho-tetramethyl-substituted cucurbituril supramolecular assemblies formed in the presence of nitrate cadmium: potential applications for isolation of heavier lanthanides, CrystEngComm, 2014, 16, 10674–10680 RSC.
- L. M. Zheng and J. X. Liu, Lanthanide Contraction Effect on Crystal Structures of Lanthanide Coordination Polymers with Cyclohexanocucurbit[6]uril Ligand, J. Solid State Chem., 2017, 245, 45–49 CrossRef CAS.
- H. F. Li, J. Lü, J. X. Lin, Y. Huang, M. Cao and R. Cao, Crystalline Hybrid Solid Materials of Palladium and Decamethylcucurbit[5]uril as Recoverable Precatalysts for Heck Cross- Coupling Reactions, Chem. – Eur. J., 2013, 19, 15661–15668 CrossRef CAS PubMed.
- H. F. Li, J. Lü, J. X. Lin and R. Cao, Monodispersed Ag Nanoparticles as Catalyst: Preparation Based on Crystalline Supramolecular Hybrid of Decamethylcucurbit[5]uril and Silver Ions, Inorg. Chem., 2014, 53, 5692–5697 CrossRef CAS PubMed.
-
M. T. Pope and A. Müller, Polyoxometalates: From Platonic Solids to Antiviral Activity, Kluwer, Dordrecht, The Netherlands, 1994 Search PubMed.
- X. K. Fang, P. Kögerler, L. Isaacs, S. Uchida and N. Mizuno, Cucurbit[n]uril-polyoxoanion hybrids, J. Am. Chem. Soc., 2009, 131, 432–433 CrossRef CAS PubMed.
- J. X. Lin, J. Lü, R. Cao, J. T. Chen and C. Y. Su, Supramolecular assembly from decavanadate anion and decamethylcucurbit[5]uril, Dalton Trans., 2009, 38, 1101–1103 RSC.
- J. X. Lin, J. Lü, H. X. Yang and R. Cao, Construction of train-like supramolecular structures from decamethylcucurbit[5]uril and iso- or Hetero-Keggin-Type polyoxotungstates, Cryst. Growth Des., 2010, 10, 1966–1970 CrossRef CAS.
- J. X. Lin, J. Lü, M. N. Cao and R. Cao, Effects of cocrystalline subunits on the supramolecular chemistry of Me10Q[5]: from simple inorganic anions to cluster anions, Cryst. Growth Des., 2011, 11, 778–783 CrossRef CAS.
- L. W. Han, J. Lü, J. X. Lin and R. Cao, New types of hybrid solids of tetravanadate polyanions and cucurbituril, Dalton Trans., 2012, 41, 10080–10084 RSC.
- M. Cao, J. X. Lin, J. Lü, Y. You, T. Liu and R. Cao, Development of a polyoxometallate-based photocatalyst assembled with cucurbit[6]uril via hydrogen bonds for azo dyes degradation, J. Hazard. Mater., 2011, 186, 948–951 CrossRef CAS PubMed.
- J. Lü, J. X. Lin, X. L. Zhao and R. Cao, Photochromic hybrid materials of cucurbituril and polyoxometalates as photocatalysts under visible light, Chem. Commun., 2012, 48, 669–671 RSC.
- B. X. Han, C. Z. Wang, Y. Zhao, K. Chen, X. Xiao, Q. J. Zhu, S. F. Xue, Y. Q. Zhang and Z. Tao, [PMo12O40]3−-induced perhydroxycucurbit[5]uril-based porous supramolecular assemblies, Eur. J. Inorg. Chem., 2014, 23, 831–835 CrossRef.
- B. X. Han, C. Z. Wang, K. Chen, X. Xiao, Z. Tao, S. F. Xue, Y. Q. Zhang and Q. J. Zhu, Coordination and supramolecular assemblies of K+/Ln3+ to perhydroxycucurbit[5]uril in the presence of [PMo12O40]3−: potential application in isolation of light lanthanides, CrystEngComm, 2014, 16, 1615–1619 RSC.
- L. W. Han, J. X. Lin, Q. Yin, B. Karadeniz, H. F. Li, J. Lü and R. Cao, Sandwich-type Inorganic–Organic Hybrid Solids of Iso-polyvanadate Clusters and Decamethylcucurbit[5]uril, Cryst. Growth Des., 2016, 16, 1213–1217 CrossRef CAS.
- X. Xia, W. W. Ge, H. Chen, Z. Tao, Y. Zhang, G. Wei and K. Chen, Porous supramolecular assemblies and functional properties of perhydroxylated cucurbit[6]uril and polyoxometallates, New J. Chem., 2019, 43, 10297–10304 RSC.
- Q. Bai, S. Zhang, H. Chen, T. Sun, C. Redshaw, J. X. Zhang, X. L. Ni, G. Wei and Z. Tao, Alkyl Substituted Cucurbit[6]uril Assisted Competitive Fluorescence Recognition of Lysine and Methionine in Aqueous Solution, ChemistrySelect, 2017, 2, 2569–2573 CrossRef CAS.
- A. T. Bockus, L. C. Smith, A. G. Grice, O. A. Ali, C. C. Young, W. Mobley, A. Leek, J. L. Roberts, B. Vinciguerra, L. Isaacs and A. R. Urbach, Cucurbit[7]uril–Tetramethylrhodamine Conjugate for Direct Sensing and Cellular Imaging, J. Am. Chem. Soc., 2016, 138, 16549–16552 CrossRef CAS PubMed.
- N. H. Kim, W. Hwang, K. Baek, M. R. Rohman, J. Kim, H. W. Kim, J. Mun, S. Y. Lee, G. Yun, J. Murray, J. W. Ha, J. Rho, M. Moskovits and K. Kim, Smart SERS Hot Spots: Single Molecules Can Be Positioned in a Plasmonic Nanojunction Using Host–Guest Chemistry, J. Am. Chem. Soc., 2018, 140, 4705–4711 CrossRef CAS PubMed.
- G. Huber, P. Berthault, A. L. Nguyen, A. Pruvost, E. Barruet, J. Rivollier, M. P. Heck and B. Prieur, Cucurbit[5]uril derivatives as oxygen carriers, Supramol. Chem., 2019, 31, 668–675 CrossRef CAS.
- M. Zhang, H. Shi, D. Meng, K. Chen, R. L. Lin, W. Q. Sun and J. X. Liu, Encapsulation and removal of aniline by di-cyclohexanocucurbit[6]uril, New J. Chem., 2019, 43, 1487–1493 RSC.
- C. Z. Wang, W. X. Zhao, Y. Q. Zhang, S. F. Xue, Q. J. Zhu and Z. Tao, A supramolecular assembly of methyl-substituted cucurbit[5]uril and its potential applications in selective absorption, RSC Adv., 2015, 5, 17354–17357 RSC.
- W. X. Zhao, C. Z. Wang, Y. Q. Zhang, S. F. Xue, Q. J. Zhu and Z. Tao, Supramolecular assembly of a methyl-substituted cucurbit[6]uril and its potential applications in selective sorption, New J. Chem., 2015, 39, 2433–2436 RSC.
- X. Xia, K. Chen, Y. Q. Yao, C. Y. Shan, Z. Tao, Y. Q. Zhang, Q. D. Zhou and G. Wei, Highly selective absorption of polychloromethanes in perhydroxylated cucurbit[6]uril-based supramolecular assemblies, New J. Chem., 2018, 42, 802–806 RSC.
- K. M. Park, D. Whang, E. Lee, J. Heo and K. Kim, Transition Metal Ion Directed Supramolecular Assembly of One and Two-Dimensional Polyrotaxanes Incorporating Cucurbituril, Chem. – Eur. J., 2002, 8, 498–508 CrossRef CAS.
- L. He, J. P. Zeng, D. H. Yu, H. Cong, Y. Q. Zhang, Q. J. Zhu, S. F. Xue and Z. Tao, Kinetic and thermodynamic inclusion complexes of symmetric tetramethyl-substituted cucurbit[6]uril with HCl salts of N,N′-bis(pyridylmethyl)-1,6-hexanediamine, Supramol. Chem., 2010, 22, 619–628 CrossRef CAS.
- J. P. Zeng, H. Cong, K. Chen, Z. Tao, S. F. Xue, Q. J. Zhu, Y. Q. Zhang and J. X. Liu, Novel Strategy to Assemble Achiral Ligands to Chiral Helical Polyrotaxanes Structures, Inorg. Chem., 2011, 50, 6521–6525 CrossRef CAS PubMed.
- X. Wei, J. P. Zeng, H. Cong, Y. Q. Zhang, Z. Tao, Q. J. Zhu and S. F. Xue, Synthesis of supramolecular polyrotaxanes assemblies incorporating symmetrical α,α′,δ,δ′-tetramethyl-cucurbit[6]uril moieties using polychloride zinc(II) and cadmium(II) anions, Supramol. Chem., 2014, 26, 692–697 CrossRef CAS.
- Z. Xiao, R. L. Lin, Q. Y. Liu, Z. Tao, J. X. Liu and X. Xiao, Multiple noncovalent interactions constructed polymeric supramolecular crystals: recognition of butyl viologen by para-dicyclohexano cucurbit[6]uril and α,α′,δ,δ′-tetramethylcucurbit[6]uril, Org. Chem. Front., 2017, 4, 2422–2427 RSC.
- W. J. Wu, F. Wu and A. I. Day, Molecular Snuggle and Stretch of a Tetraammonium Chain in the Construction of a Hetero-[4]pseudorotaxane with CyclopentanoQ[6] and Classical Q[7], J. Org. Chem., 2017, 82, 5507–5515 CrossRef CAS.
- R. L. Lin, R. Li, H. Shi, K. Zhang, D. Meng, W. Q. Sun, K. Chen and J. X. Liu, Symmetrical-Tetramethyl-Cucurbit[6]uril-Driven Movement of Cucurbit[7]uril Gives Rise to Heterowheel [4]Pseudorotaxanes, J. Org. Chem., 2020, 85, 3568–3575 CrossRef CAS.
- K. Zhang, W. Q. Sun, R. L. Lin, X. Xiao, Z. Tao and J. X. Liu, Controlled encapsulation and release of an organic guest in the cavity of α,α′,δ,δ′-tetramethylcucurbit[6]uril, Eur. J. Org. Chem., 2019, 1503–1507 CrossRef CAS.
- L. M. Zheng, K. Zhang, R. L. Lin, X. F. Chu and J. X. Liu, Binding interactions of bisbenzimidazolyl derivatives with cyclohexanocucurbit[6]uril, J. Inclusion Phenom. Macrocyclic Chem., 2020, 96, 125–135 CAS.
- H. Shi, W. Q. Sun, R. L. Lin, C. H. Liu and J. X. Liu, Construction of a molecular switch and selector under electrochemical control, ACS Omega, 2017, 2, 4575–4580 CrossRef CAS.
|
This journal is © the Partner Organisations 2020 |
Click here to see how this site uses Cookies. View our privacy policy here.